- Department of Anorectal, Kunming Municipal Hospital of Traditional Chinese Medicine, The Third Affiliated Hospital of Yunnan University of Chinese Medicine, Kunming, Yunnan, China
Despite the increasing number of studies on nanomedicine-based cancer immunotherapy, the overall research trends in this field remain inadequately characterized. This study aims to evaluate the research trends and hotspots in nanomedicine-based cancer immunotherapy through a bibliometric analysis. As of March 31, 2024, relevant publications were retrieved from the Web of Science Core Collection. Analytical tools including VOSviewer, CiteSpace, and an online bibliometric analysis platform were employed. A total of 5,180 publications were analyzed. The study reveals geographical disparities in research output, with China and the United States being the leading contributors. Institutionally, the Chinese Academy of Sciences, University of Chinese Academy of Sciences, and Sichuan University are prominent contributors. Authorship analysis identifies key researchers, with Liu Zhuang being the most prolific author. “ACS Nano” and the “Journal of Controlled Release and Biomaterials” are identified as the leading journals in the field. Frequently occurring keywords include “cancer immunotherapy” and “drug delivery.” Emerging frontiers in the field, such as “mRNA vaccine,” “sonodynamic therapy,” “oral squamous cell carcinoma,” “STING pathway,”and “cGAS-STING pathway,” are experiencing rapid growth. This study aims to provide new insights to advance scientific research and clinical applications in nanomedicine-based cancer immunotherapy.
Introduction
According to statistics from the World Health Organization (WHO) in 2019, cancer was among the top two leading causes of death in 112 countries worldwide (1). In economically developed countries, mortality rates from stroke and coronary heart disease are declining, while cancer has emerged as the primary and sole disease impacting life expectancy (2). The majority of cancer cases were concentrated in East Asia, Western Europe, and the high-income North America region, collectively making up 63.7% of global cancer incidences (3).
Addressing cancer continues to be a significant challenge in global health, requiring ongoing exploration of innovative strategies for prevention, treatment, and enhancing patient outcomes (4). Recently, a range of treatment options have been employed in the fight against cancer, such as surgery, chemotherapy, radiation therapy, targeted therapy, immunotherapy, and hormonal therapy (5). Because cancer cells have developed various strategies to evade immune detection (6–8), leading to impaired immune cell function and the failure of anti-tumor immune responses, immunotherapy has emerged as a groundbreaking advancement in cancer treatment, transforming the landscape of oncology by enhancing the body’s natural defenses to eradicate cancerous cells (9). Cancer immunotherapy, tracing back a century (10), has recently experienced exponential growth, leading to the development of major types of treatment including oncolytic virus therapies (11) (12), cancer vaccines (13, 14), cytokine therapies (15, 16), adoptive cell transfer (17, 18), and immune checkpoint inhibitors (19, 20).
Despite notable breakthroughs, immunotherapy still struggles with issues like low solubility, poor stability, and short half-lives of agents (21), along with risks of severe allergy-related reactions (22). Moreover, delivering immune cells or agents into tumors within an immunosuppressive tumor microenvironment (iTME) remains a challenge (23). The term “nanomedicine” emerged in the late 1990s as nanoparticles gained attention in cancer therapy for altering drug pharmacokinetics and accumulating in tumors via the enhanced permeation and retention (EPR) effect (24). Nanomedicine contributes significantly to various stages of the cancer immune cycle (25). For instance, it facilitates tumor antigen releasing and presenting by addressing issues like rapid immune clearance and inefficient antigen delivery. One example is the development of mesoporous silica nanoparticles (MSNs) by Qian et al., which carry antigens out of necrotic tissue for selective entry into immune organs for immunotherapy (26). Additionally, in the stage of T cells priming and activation, nanomedicine plays a role in controlling cytokine delivery to enhance T-cell activation, as demonstrated by Tang et al.’s TCR-signaling-responsive nanoparticle (27). Moreover, nanoparticles designed to overcome both signaling and physical barriers are increasingly utilized as supplementary treatments to bolster checkpoint blockade immunotherapy (28, 29).
Extracellular vesicles (EVs) are nano-sized, membranous structures secreted into the extracellular space. They play a crucial role not only in various cancers (including head and neck, lung, gastric, breast, and hepatocellular carcinoma) but also in conditions such as diabetes, viral infections, autoimmune, and renal diseases (30). This underscores the potential advancements in molecular diagnostics and drug delivery, showcasing the broad applicability and promise of nanomedicine. Additionally, other inflammatory diseases, including cancer, such as cardiovascular diseases, allergies, autoimmune, and neuropsychiatric diseases, commonly feature dysregulation of the immune response. Immunotherapy has promising potential to treat these inflammatory disorders, such as rheumatoid arthritis (RA), intestinal inflammation, and pulmonary arterial hypertension (PAH) (31). For instance, Shen et al. developed spherical polyethyleneimine-coated mesoporous polydopamine nanoparticles loaded with the STING antagonist C-176 (PEI-PDA@C-176 NPs) for treating RA, illustrating Nanomaterials are also used in the treatment of various common diseases (32). By considering these comorbidities and predisposing factors, researchers can develop more comprehensive and effective nanomedicine-based treatments.
Expanding on extensive research in nanomedicine for cancer immunotherapy, there is an opportunity to conduct a detailed examination of its evolution and emerging trends using bibliometric analysis. Bibliometrics, widely adopted in the medical field, provides an objective and data-driven approach compared to traditional literature reviews (33). It enables the analysis of vast scientific literature, revealing nuanced research trends and academic networks (34). Recent bibliometric studies have covered diverse topics such as cancer (35), pain (36), and cardiovascular diseases (37). Considering the increasing complexity and growth of nanomedicine in cancer immunotherapy, a thorough bibliometric evaluation is justified.
Therefore, this study aims to utilize bibliometric visualization tools to analyze the research status, key areas of focus, and future directions of nanomedicine-based therapies in cancer immunotherapy from 2014 to 2024. The findings will be presented through a knowledge map, providing insights into the evolving landscape of nanomedicine in cancer immunotherapy research.
Materials and methods
Data retrieval
Bibliometric data were retrieved from Web of Science Core Collection (WoSCC) database on March 31, 2024, using the following keyword query: (TS= [(Immunotherapy) OR (Immunotherapies) OR (Immunotherapeutic)] AND TS= [(cancer) OR (tumor) OR (neoplasm) OR (malignanc) OR (oncology)] AND TS=(nano*)). The search phrase “nano*” was used to find all terms beginning with “nano,” including nanomedicine, nanoparticles, nanomaterials, nanocarriers, nanocomposites, nanotechnology, etc. The search was restricted to articles and reviews written in English between January 1, 2014, and March 31, 2024. Our search resulted in 6434 relevant papers (Figure 1).
Data processing
For the search outcomes described previously, the full records and cited references were exported in both plain text and tab-delimited formats. These plain text files underwent analysis using CiteSpace (version 6.1.R6), the tab-delimited files were subjected to analytical processing in VOSviewer (version 1.6.20.0) and the Online Analysis Platform for Bibliometrics (OALM) accessible at [http://bibliometric.com/].
Data analysis
This research utilized three bibliometric tools to thoroughly examine and support the findings. Specifically, CiteSpace was used for co-occurrence, cluster, and emergent analyses. VOSviewer was employed for co-occurrence and cluster analyses, while the OALM platform was utilized for relational network analysis. Additionally, the study involved documenting the journal name, impact factor (IF), and journal ranking (Q1 to Q4, indicating quartile within the category) based on the 2022 edition of the Journal Citation Reports (JCR). Microsoft Excel was used to illustrate the global production and evolutionary trends of relevant papers and to create charts representing the rankings of different factors.
Research ethics
The data sources utilized in our study were derived from publicly accessible databases. As such, obtaining approval from an ethics committee was deemed unnecessary.
Result
Analysis of annual publications
Over the past decade, nanomedicine-based cancer immunotherapy research has seen two phases. From 2014 to 2018, there was moderate growth, with fewer than 500 annual publications. However, from 2019 to 2023, there was a significant increase, totaling 5180 papers, representing 85.2% of the decade’s output. This rising interest is evident in Figure 2, with a polynomial curve fitting score of 0.9252, highlighting global academic attention towards nanomedicine-based cancer immunotherapy.
Analysis of countries/regions
Nanomedicine-based cancer immunotherapy research has received contributions from a total of 94 countries/regions. Among them, China emerged as the leading contributor, with 3839 papers published and the highest citation count of 117,364, followed by the United States of America (USA) with 1460 papers and 77,334 citations, and South Korea with 340 papers and 10,167 citations, as detailed in Table 1.
In the CiteSpace visual analysis, circles represent countries/regions with their sizes indicating publication volume, while lines between circles depict collaborative links. Nodes highlighted with purple rings denote high centrality, with the thickness of the ring reflecting the centrality’s magnitude. Among these countries/regions, China and Spain lead with the highest centrality scores of 0.23, followed by the USA, Germany, and Australia, each at 0.16, and India at 0.12. This is illustrated in Figure 3A. The map in Figure 3B further shows robust collaborations between China and the USA, while partnerships among other countries appear more dispersed.
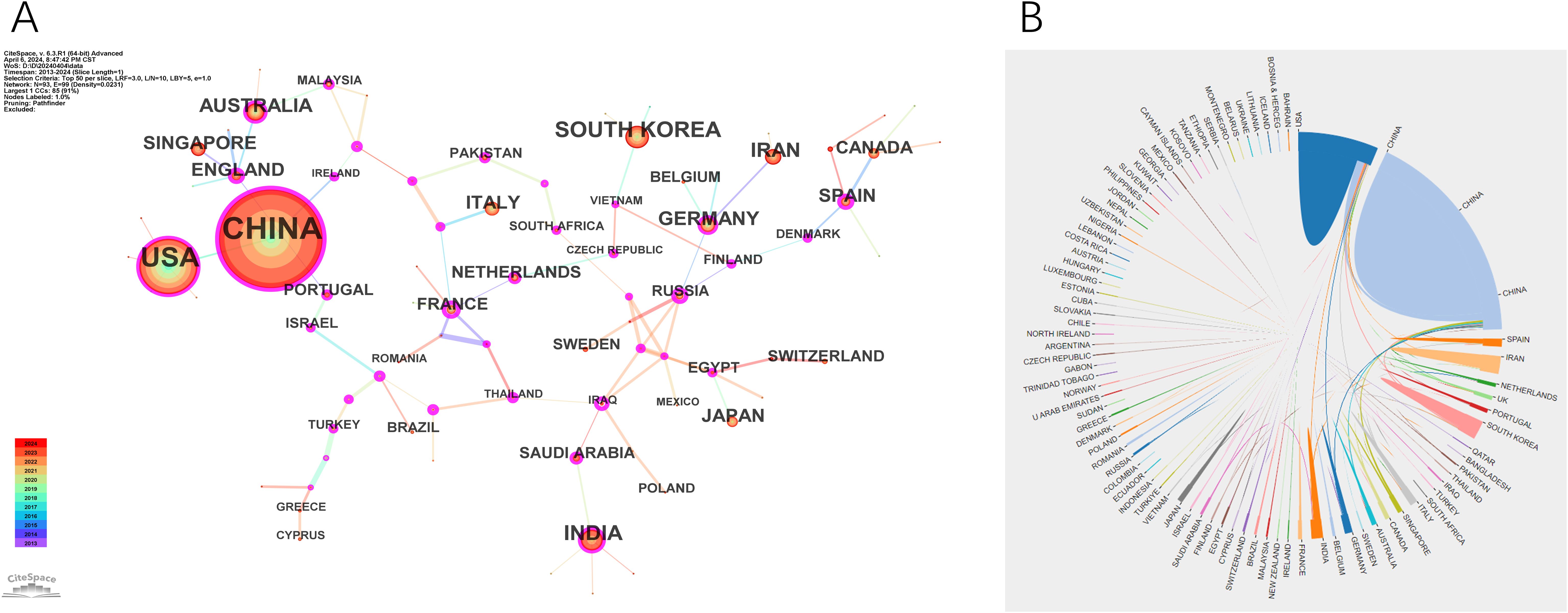
Figure 3. Visualization of countries. (A) The cooperation network map of countries. (B) The cooperation network diagram between countries.
Analysis of institution
A total of 4116 institutions have contributed to the publication landscape in this field, with the top ten most active institutions highlighted in Table 2. Leading the pack is the Chinese Academy of Sciences, with 519 publications and 22,716 citations, followed by the University of Chinese Academy of Sciences with 260 publications and 11,229 citations, and Sichuan University with 223 publications and 7,075 citations. The Chinese Academy of Sciences also boasts the highest TLS (Total Link Strength), establishing itself as a central node in fostering collaboration across the research community.
The institutions with the highest centrality, indicative of their pivotal role in the network of collaborations, are Shanghai Jiao Tong University (0.38), University College London (0.37), University of London (0.29), Institut National de la Santé et de la Recherche Médicale (Inserm) (0.28), and the National Institutes of Health (NIH) (0.27), as shown in Figure 4.
Analysis of journals
A total of 775 journals have contributed to research in this field, with 10 of them surpassing 120 papers each, as detailed in Table 3. Leading in output is ACS Nano, followed by Journal of Controlled Release and Biomaterials. Among the top 10 journals with the most publications, Advanced Materials boasts the highest Impact Factor (IF) at 29.40, securing the fourth position in publication quantity. All these prolific journals were classified as Q1 based on the 2022 Journal Citation Reports (JCR), indicating their high academic impact.
Co-citation relationships occur when journals are cited together in the same publication, indicating a connection in scholarly content quality. Table 3 shows that the top 3 co-cited journals remain ACS Nano, Biomaterials, and Journal of Controlled Release, with all top 10 co-cited journals also categorized as Q1 journals.
Analysis of authors and co-cited authors
Analysis of authors plays a crucial role in identifying key figures within a research field. Table 4 lists the top 10 authors in nanomedicine-based cancer immunotherapy research, highlighting their high productivity and citation rates. Notably, Liu Zhuang from Soochow University lead in paper publications (62), while Chen Qian, also from Soochow University, stands out with the most citations (1887).
Utilizing VOSviewer, we identified 27,766 researchers contributing to this field. Following Price’s law, which considers authors with over 6 papers as core authors, we found 1,075 core authors, as depicted in Figure 5. Despite this, these core authors collectively published 10,673 papers, accounting for only 18.23% of the total sample. This figure is notably below 50%, suggesting that scholars in this field are widely spread out and haven’t yet unified into a cohesive core author group. This lack of collaboration might have somewhat hindered the academic progress of nanomedicine-based cancer immunotherapy research.
Analysis of co-cited references and references bursts
Among the 528 co-cited references, we have identified the top ten in Table 5. Riley RS et al.’s (2019) work, “Delivery technologies for cancer immunotherapy,” stands out with the highest citation count of 427. This paper delves into a key challenge in cancer immunotherapy—the controlled modulation of the immune system. It discusses advancements in biomaterials and drug delivery systems, especially nanoparticles, and examines the opportunities and challenges of integrating delivery technologies into cancer immunotherapy.
Figure 6A presents the network visualization map of co-cited references, with a Q-value of 0.7024 and a mean S value of 0.8878. The top two references in terms of centrality, both with a centrality score of 0.67, were published by Kuai R et al. in 2017 and Xu J et al. in 2017.Kuai R et al.’s paper introduces a novel nano-vaccine system tailored for personalized neo-epitope vaccination, showcasing the first successful anti-tumor efficacy using personalized nanomedicine with tumor-specific neo-antigens. On the other hand, Xu J et al.’s work demonstrates nanoparticles capable of effectively eradicating primary tumors under light exposure, inhibiting distant light-inaccessible tumors, and preventing tumor recurrence through immune memory activation.
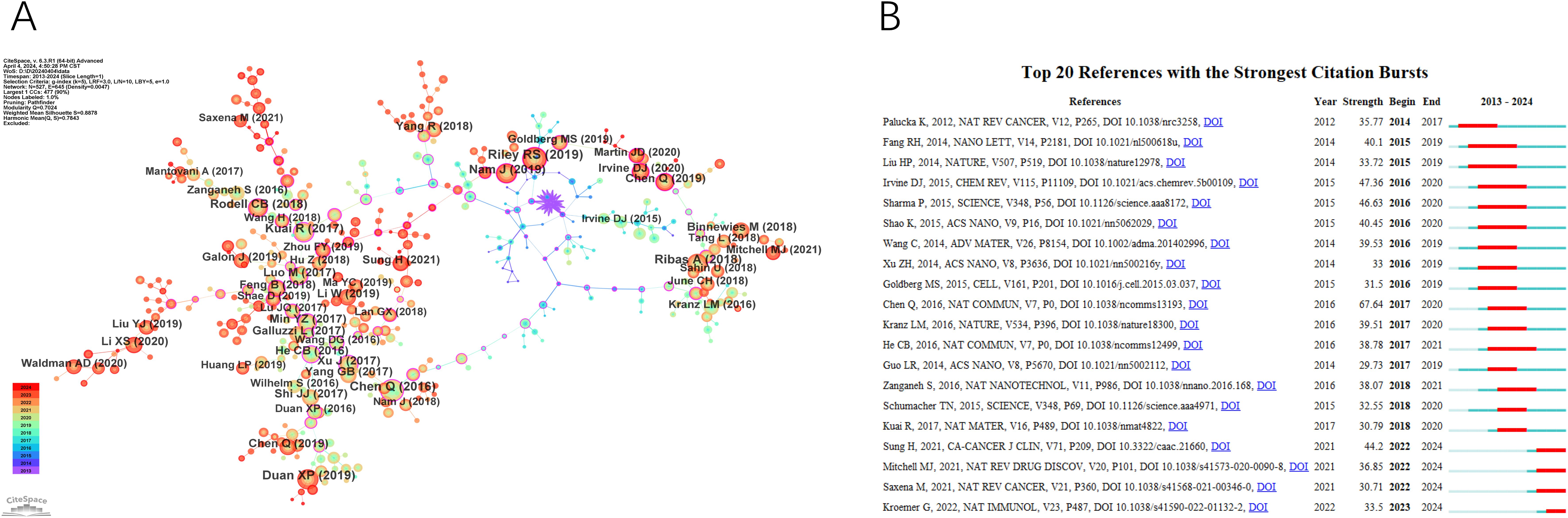
Figure 6. The main references. (A) The Co-cited References network. (B) Top 20 references with the strongest citation bursts.
A paper with a strong citation burst signifies a significant milestone in the field, indicating widespread recognition and impact. Figure 6B displays the top 20 references with the highest citation bursts, showing the time interval from 2013 to 2024 in blue and the burst duration in red. Among these, “Photothermal therapy with immune-adjuvant nanoparticles together with checkpoint blockade for effective cancer immunotherapy” by Chen Q et al. (2016) exhibited the most pronounced citation burst. Additionally, ongoing citation bursts are observed in specific articles, such as Sung H et al.’s paper in CA Cancer J Clin in 2021, Mitchell MJ et al.’s paper in Nat Rev Drug Discov in 2021, Saxena M et al.’s paper in Nat Rev Cancer in 2021, and Kroemer G et al.’s paper in Nat Immunol in 2022. This indicates that these research topics are likely to sustain their popularity in the future and could emerge as potential frontiers in the field of nanomedicine-based cancer immunotherapy research.
Analysis of keywords
Keywords act as condensed summaries, highlighting the main themes of a document and providing an indicative overview of its scientific content. Analyzing keywords can reveal the focal areas within a research field. Table 6 lists the top 20 most frequently occurring terms in this field. Notably, “drug delivery” emerged as the most popular keyword after filtering out initial high-frequency words lacking analytical significance.
The co-occurring keyword analysis in CiteSpace, spanning from 2013 to 2024 with yearly intervals, reveals a network visualized in Figure 7A, consisting of 481 nodes and 501 links. This network, indicating robust correlations among keywords, represents node size by frequency and line color by chronology, transitioning from blue (older) to orange (newer). Notably, the top 3 words in terms of centrality ranking, indicated by the thickness of the purple ring, are breast cancer (centrality: 0.64), cancer stem cell (centrality: 0.43), polymeric nanoparticles (centrality: 0.38), These high-centrality nodes underscore their significant impact, representing emerging trends in nanomedicine-based cancer immunotherapy research.
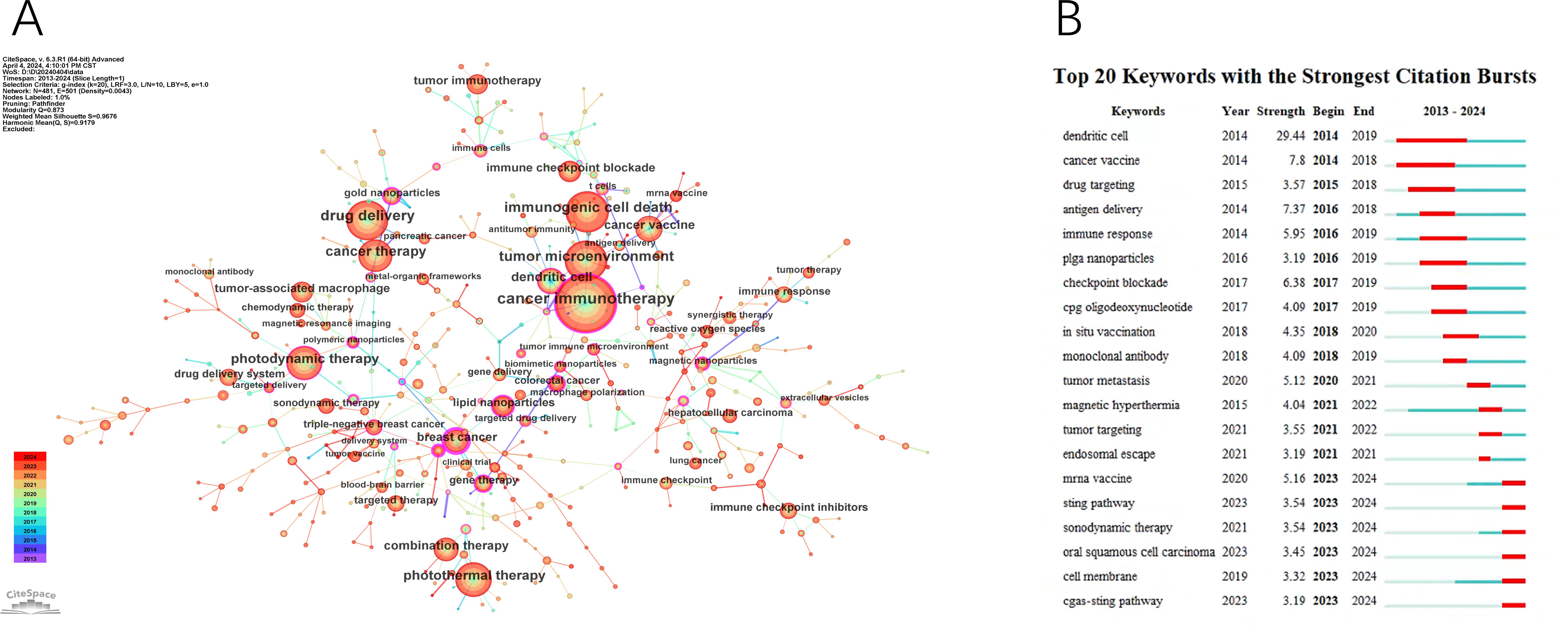
Figure 7. The main keywords. (A) The Keywords network. (B) Top 20 Keywords with the strongest citation bursts.
Using the keyword co-citation network, we conducted emergent word detection and present the top 20 keywords with the strongest citation bursts in this field in Figure 7B. The keyword “dendritic cell” had the most significant citation burst with a score of 29.44. Furthermore, keywords such as “mRNA vaccine,” “sonodynamic therapy,” “oral squamous cell carcinoma,” “STING pathway,” and “cGAS-STING pathway” continued to exhibit burstiness until 2024, suggesting that these research directions may continue to gain momentum in the future.
Clustering analysis was conducted on co-occurring keywords, resulting in 15 clusters with a quality index (Q) of 0.873 and a silhouette score (S) of 0.9676, indicating reliable and meaningful clustering outcomes (Figure 8A). The cluster labels revealed major themes in the research field. Figure 8B, generated by sorting Figure 8A based on the time zone, illustrates the historical progression of nanomedicine-based cancer immunotherapy research. The core terms of each cluster show varying levels of interest during different periods. Some topics persist and evolve, leading to more research directions over time (#0 cancer immunotherapy, #1 targeted delivery, #2 photoacoustic imaging, #3 immune response, #6 gene therapy, #7 triple-negative breast cancer, #8 cancer vaccine, #9 lipid nanoparticles, #10 targeted therapy), while others gradually lose prominence (#4 hepatocellular carcinoma, #5 photodynamic therapy, #11 drug delivery, #12 immune checkpoint blockade, #13 sonodynamic therapy, #14 tumor immunotherapy).
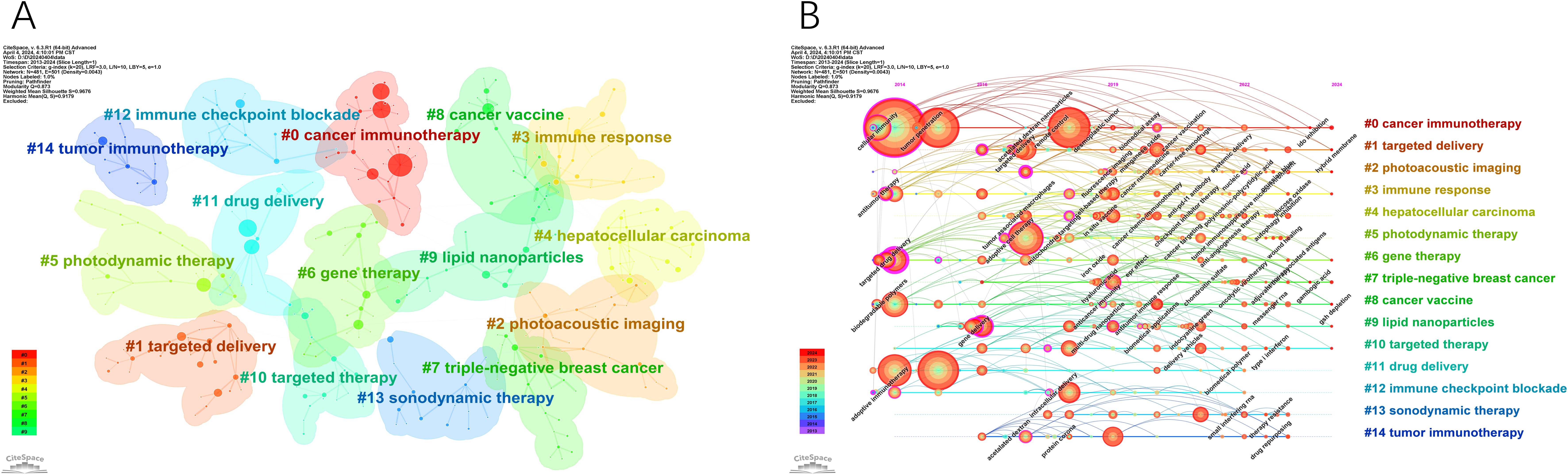
Figure 8. The main keywords clusters. (A) Keywords cluster analysis co-occurrence map. (B) Timeline of keywords cluster.
Discussion
General information
Research interest in nanomedicine-based cancer immunotherapy has significantly grown over the past decade, as indicated by rising annual publication figures and citation counts. Since 2018, the field has seen rapid clinical advancements, including the development of chimeric antigen receptor (CAR) T cells and the modulation of immune responses through the blocking of suppressive checkpoints (38). Moreover, various nanoparticles have been employed to deliver immunogenic cell death (ICD) promoters, enhancing anti-tumor immunity (39). Additionally, a considerable number of exogenous cancer vaccines are currently in clinical trials (40).
Results showed China leading with 3839 research publications from 2014 to 2024, followed by the United States with 1460 publications. Both countries dominated the top 10 most productive institutions. This leadership can be attributed to substantial investments. The US allocated $19.4 billion to nanotechnology from 2001 to 2014, with an additional $1.5 billion proposed in 2015. In contrast, China prioritized nanotechnology in its Medium and Long Term Development Plan 2006–2020, aiming for 2.5% of GDP invested in science and technology by 2020 (41). These investments propelled China and the US as global leaders in nanotechnology.
ACS Nano leads in both publication count and citations, highlighting its significance in nanoscience and nanotechnology. It’s an academic journal from the American Chemical Society (ACS). Notably, the top 10 journals by publication count and co-cited journals are all categorized as Q1, indicating their high quality and impact. This abundance of reputable journals underscores the substantial interest in Nanomedicine-based cancer immunotherapy research.
Liu Zhuang, from the Institute of Functional Nano & Soft Materials at Soochow University, is the most prolific author with 62 papers. Chen Qian, also from the same institute, leads in citations with 1887. Both focus on biomaterials and nanomedicine, addressing challenges in tumor diagnosis and treatment. Their work includes novel nanoprobes for biomolecular detection, molecular imaging (42, 43), and innovative strategies for tumor therapies like photodynamic therapy (44, 45), radiation therapy (46, 47), and immunotherapy (48, 49), leveraging nanotechnology and biomaterials.
Research hotspots
Centrality is a crucial metric for assessing the importance and impact of nodes within a research domain. Analyzing keyword centrality can also help predict research hotspots. The top 3 words with high centrality in this study are breast cancer, cancer stem cell, and polymeric nanoparticles.
According to the 2022 global cancer statistics by the International Agency for Research on Cancer (IARC), breast cancer, accounting for 11.6% of all cancers worldwide, ranks as the second most frequently diagnosed cancer that year (1). Nanoparticles play a crucial role in advancing three primary immunotherapeutic strategies for breast cancer treatment: immune checkpoint blockade, modulation of immunosuppressive pathways within the tumor microenvironment, and induction of immunogenic cancer cell death. For instance, ARAC (Antigen Release Agent and Checkpoint Inhibitor) has demonstrated its ability to selectively target and eliminate 4T1 breast cancer cells while prolonging the survival of mice with LLC-JSP tumors in the lungs (50). Additionally, cell-derived exosome nanoparticles (SMART-Exo) have shown promising results in engaging T cells to specifically target and kill HER2-positive breast cancer cells (51). Other examples include Hu et al.’s magnetic nanoparticle (MNP) based system designed to modulate macrophage polarization and inhibit tumor growth (52), as well as Zhou et al.’s 80 nm prodrug vesicle that significantly reduces recurrence and distant metastasis in 4T1 breast tumors (53). These nanoparticle-based approaches highlight the potential of nanotechnology in enhancing the efficacy of immunotherapies against breast cancer.
The presence of cancer stem cells (CSCs) in various solid tumors, including those in the breast (54), colon (55), brain (56), lung (57), and liver (58), significantly contributes to the challenges of cancer treatment, notably drug resistance and recurrence post-therapy. Traditional cancer treatments often lead to an increased proportion of CSCs, thereby enhancing their survival and spread (59). To address this issue, there is a growing interest in developing effective CSC-targeted therapies. Nanotechnology-based drug delivery systems, particularly nanocarrier-based therapeutic agents such as liposomal doxorubicin (60), albumin-bound paclitaxel (61), and PEGylated L-Asparaginase (62), have shown success in clinical applications. Additionally, innovative approaches involving multifunctional nanoparticles are being researched and developed. For example, Xie et al. developed a biodegradable nanoparticle with a human umbilical cord mesenchymal stem cell membrane, which targets tumors effectively, offering new possibilities for lung cancer treatment (63). Liu et al. created a magnetic nanoparticle that combines heat and chemotherapy to specifically destroy up to 98% of lung CSCs when exposed to an alternating magnetic field for 30 minutes (64). Additionally, Pan et al. engineered nanoparticles targeting breast cancer stem cells for co-delivery of doxorubicin and a specific enzyme, showing promise in overcoming drug resistance (65).
Polymeric nanoparticles consist of polymers and copolymers that protect a drug, whether it’s encapsulated within the particle, adsorbed on the surface, or chemically linked to it. In 2002, Discher and Eisenberg outlined the structure of these nanoparticles (66). They are biodegradable, breaking down into non-harmful alcohols and other small molecules as end products (67). This characteristic offers several advantages, including improved bloodstream circulation time, reduced premature leakage, higher encapsulation rates, and more controlled release kinetics (68–70). Moreover, their synthetic adjustability allows for the preparation of nanoparticles that can co-encapsulate various therapeutic agents, each with distinct release profiles. This capability enables the simultaneous delivery of different therapeutics or imaging agents (71, 72). Several examples of polymeric nanoparticles formulated to facilitate the delivery of essential therapeutic agents are presented here: GEM loaded lipid polymer hybrid nanoparticles (LPHNs), which have been shown to enhance antitumor efficacy in breast cancer treatment (73); LPHNPs, which represent a promising delivery system for the safe, stable, and controlled transport of methotrexate to cancer cells, leading to improved therapeutic outcomes (74); and plitidepsin-containing carriers, as investigated by Elisabetta Fedeli et al., demonstrating comparable anticancer activity to standard drug formulations across various cancer cell lines (75).
The references Co-citation analyses illuminate key research directions and developments in in this field. The most cited article, “Delivery technologies for cancer immunotherapy” (Nat Rev Drug Discov., 2019), underscores the efficacy of immunotherapy in cancer treatment. However, challenges persist in modulating the immune system effectively (23). Recent advancements focus on nanoparticle-based targeting of T cells, restoring T cell function (76), and developing a lipid-based nanoparticle mRNA vaccine for dendritic cell targeting without antibodies (77). These innovations aim to enhance immunotherapy potency while minimizing adverse effects.
The second most cited paper, authored by Qian Chen et al., presents a therapeutic approach that aims to eradicate primary tumors, suppress metastasis, and prevent tumor recurrence. This is achieved by integrating adjuvant nanoparticle-based photothermal therapy with checkpoint-blockade immunotherapy (78). Additionally, this paper has exhibited the most pronounced citation burst, underscoring its significance. The third most cited article is a review focused on nanoparticle-based treatment strategies aimed at inducing and augmenting immunogenic cell death to enhance cancer immunotherapy (39).
Future trends
Burst detection analysis identifies emerging research trends by highlighting keywords with significant citation spikes, indicating periods of intense scholarly interest. Five recent emerging trends in Nanomedicine-based cancer immunotherapy were determined according to the most recent keyword bursts; these keywords are listed as follows:
“mRNA vaccine”
The objective of mRNA-based vaccination is to elicit or enhance a robust anti-tumor immune response (79). During vaccination, naked or vehicle-loaded mRNA vaccines effectively express tumor antigens in antigen-presenting cells (APCs), promoting APC activation and stimulating both innate and adaptive immune responses (80). The ex vivo engineering of autologous dendritic cells with mRNA has been the preferred method for delivering tumor antigens; however, most mRNA vaccine strategies now emphasize direct mRNA administration using lipid nanoparticle formulations (81). Non-formulated mRNA is prone to rapid degradation by extracellular RNases (82). Consequently, various nanocarrier pharmaceutical systems, typically incorporating polymers such as peptides or lipids, have been developed to optimize mRNA stability and enhance its uptake by APCs (83). Examples of these include protamine-formulated mRNA-based cancer vaccines (84), mRNA-based lipoplex vaccines (85), mRNA-based lipid nanoparticle vaccines (86), and mRNA-based dendritic cell cancer vaccines (87).
“sonodynamic therapy”
Sonodynamic therapy (SDT), which combines low-intensity ultrasound with a chemotherapeutic agent (sonosensitizer), has emerged as a promising alternative for cancer treatment (88). The antitumor effects of SDT result from various physical and chemical processes during ultrasound-induced acoustic cavitationt (89). SDT effectively induces cancer immunogenic cell death (ICD), activating antitumor immune responses through the reactive oxygen species (ROS)-mediated apoptosis pathway (90). It shows particular efficacy in deep-seated malignant tumors. Additionally, low-intensity ultrasound exposure has minimal side effects, and cytotoxicity is limited to the transient overlap of sonosensitizers and ultrasound (91). The therapeutic efficacy of SDT can be optimized by targeting the tumor site and adjusting the ultrasound frequency, intensity, and irradiation duration (90). Recent advancements in nanotechnology and nanoscience have led to the development of enhanced sonosensitizers, which significantly improve the therapeutic outcomes of SDT-based immunotherapy. Well-designed sonosensitizers have increased the ROS yield by enhancing acoustic cavitation and alleviating tumor hypoxia (92, 93). Furthermore, modifiable nanocarriers have substantially boosted the bioavailability and tumor targeting of sonosensitizers by prolonging their circulation time in the blood and enhancing their accumulation in tumor tissues (94).
“oral squamous cell carcinoma”
Oral squamous cell carcinoma (OSCC) is a prevalent head and neck malignancy (95). As reported by the Global Cancer Observatory (GCO), there were 377,713 cases of OSCC worldwide in 2020, predominantly in Asia (96). The 5-year survival rate remains below 50% (97).
Nanodrug delivery systems (nano-DDS) show great promise for the prevention and treatment of OSCC (98). This technology enables precise drug targeting to specific organs, cells, or molecules. Wang et al. developed ligand-decorated, cancer-targeted CDDP-loaded PLGA-PEG/NR7 nanoparticles, demonstrating the potential of NR7 peptides for targeted delivery, rapid uptake by cells, and increased cytotoxicity in OSCC cells with receptor overexpression (99). Another study introduced MF-094@ZIF-8-PDA-PEGTK nanoparticles to create a molecular inhibitor delivery system, suggesting new strategies for clinical OSCC treatment (100). Additionally, bacterial therapy is gaining attention in tumor immunotherapy due to its ability to trigger antitumor immune responses through various mechanisms. Recent research has introduced a bacterial nanomedicine that shows promise for enhancing OSCC treatment, indicating its potential in clinical applications (101).
“STING pathway” and “cGAS-STING pathway”
The STING pathway is activated when the cyclic GMP-AMP synthase (cGAS) enzyme binds directly to DNA, producing the cyclic dinucleotide 2’−5’ cGAMP (102). Under basal conditions, the Stimulator of Interferon Genes (STING) protein resides in the endoplasmic reticulum (ER) membrane (103). Once cGAS is activated and synthesizes cGAMP, STING becomes active by directly binding to cGAMP (104). The activated cGAS-STING signaling cascade results in the upregulation of IRF3, non-canonical NF-κB, and canonical NF-κB pathways (105). Due to its strong immune-stimulatory properties, the cGAS–STING pathway is considered a promising target for cancer immunotherapy (106). In preclinical mouse models, injecting STING agonists has led to the rapid rejection of murine tumors (107). Researchers developed a dimeric ligand with enhanced STING binding affinity, which showed efficacy in the CT-26 colorectal cancer model (108). Before developing effective and safe drugs, it is crucial to address the challenge of locally increasing type I interferons (IFNs) in the tumor microenvironment (TME) to boost innate immunity (109). Castro et al. utilized nanoparticles (NPs) carrying IFN-γ, which upregulated costimulatory molecules on cell surfaces and promoted the secretion of pro-inflammatory cytokines (110). Additionally, inhaling these nanocarriers enhanced immunity against lung metastases. Liu et al. demonstrated that liposomes encapsulating cGAMP in mice models with lung metastases induced pro-inflammatory responses at metastatic sites (111).
By analyzing these four review articles with significant citation growth, a prominent research trend can be identified: the integration of advanced biotechnologies in cancer treatment and management. Among the notable burst references up to 2024 are four review articles: “Global Cancer Statistics 2020: GLOBOCAN Estimates of Incidence and Mortality Worldwide for 36 Cancers in 185 Countries (1),” which updates cancer incidence and mortality worldwide using GLOBOCAN 2020 data, thereby guiding the development of cancer prevention and control strategies; “Engineering Precision Nanoparticles for Drug Delivery (112),” which discusses advances in nanoparticle design to improve drug delivery and patient outcomes; “Therapeutic Cancer Vaccines (14),” which explores combining vaccine platforms with novel immunomodulatory approaches and standard treatments to enhance clinical efficacy; and “Immunogenic Cell Stress and Death (113),” which examines the evolution of immunogenic cell death, proposes strategies to enhance or restore it therapeutically, and reviews its role in autoimmune disorders. These studies focus on leveraging innovative technologies and methodologies to increase the precision and efficacy of cancer treatment, reflecting the deep exploration and interdisciplinary collaboration in the field of biomedical research on cancer.
These topics encompass advanced therapeutic approaches, key cellular mechanisms, and specific diseases, highlighting the interplay between novel treatments and fundamental biological processes.
Limitation
This study has several limitations, chiefly its reliance on English-language articles from the Web of Science Core Collection (WoSCC) database. Although WoSCC is comprehensive, it may omit high-quality research published in other languages or indexed in other databases. Furthermore, recent high-quality publications might be underrepresented due to citation delays, potentially affecting the accuracy of trend analyses. Despite these limitations, the study offers valuable insights into the evolution, hotspots, and emerging areas of nanomedicine-based cancer immunotherapy, thereby facilitating further research. The exclusive use of WoSCC, while potentially leading to incomplete data, is justified, as alternative databases like PubMed or Embase do not provide the full-text access and citation analysis necessary for a thorough bibliometric review.
Conclusion
The bibliometric analysis of nanomedicine-based cancer immunotherapy publications from 2014 to 2024 reveals significant contributions and emerging trends. Substantial economic investment has positioned China and the United States as the leading countries in research output. Journals such as ACS Nano are pivotal in disseminating key findings. Major research areas highlighted include cancer immunotherapy and targeted delivery. This study provides a comprehensive roadmap for future research, underscoring the importance of collaboration and emphasizing the potential of polymeric nanoparticles in nanomedicine-based cancer immunotherapy.
Author contributions
CC: Writing – original draft. PY: Writing – original draft. ZZ: Conceptualization, Writing – review & editing.
Funding
The author(s) declare that no financial support was received for the research, authorship, and/or publication of this article.
Conflict of interest
The authors declare that the research was conducted in the absence of any commercial or financial relationships that could be construed as a potential conflict of interest.
Publisher’s note
All claims expressed in this article are solely those of the authors and do not necessarily represent those of their affiliated organizations, or those of the publisher, the editors and the reviewers. Any product that may be evaluated in this article, or claim that may be made by its manufacturer, is not guaranteed or endorsed by the publisher.
References
1. Sung H, Ferlay J, Siegel RL, Laversanne M, Soerjomataram I, Jemal A, et al. Global cancer statistics 2020: GLOBOCAN estimates of incidence and mortality worldwide for 36 cancers in 185 countries. CA Cancer J Clin. (2021) 71:209–49. doi: 10.3322/caac.21660
2. Lin L, Yan L, Liu Y, Yuan F, Li H, Ni J. Incidence and death in 29 cancer groups in 2017 and trend analysis from 1990 to 2017 from the Global Burden of Disease Study. J Hematol Oncol. (2019) 12:96. doi: 10.1186/s13045-019-0783-9
3. Lin L, Li Z, Yan L, Liu Y, Yang H, Li H. Global, regional, and national cancer incidence and death for 29 cancer groups in 2019 and trends analysis of the global cancer burden, 1990-2019. J Hematol Oncol. (2021) 14:197. doi: 10.1186/s13045-021-01213-z
4. Kalager M, Adami HO, Lagergren P, Steindorf K, Dickman PW. Cancer outcomes research-a European challenge: measures of the cancer burden. Mol Oncol. (2021) 15:3225–41. doi: 10.1002/1878-0261.13012
5. Joshi SS, Badgwell BD. Current treatment and recent progress in gastric cancer. CA Cancer J Clin. (2021) 71:264–79. doi: 10.3322/caac.21657
6. Khong HT, Restifo NP. Natural selection of tumor variants in the generation of “tumor escape” phenotypes. Nat Immunol. (2002) 3:999–1005. doi: 10.1038/ni1102-999
7. Thomas DA, Massagué J. TGF-beta directly targets cytotoxic T cell functions during tumor evasion of immune surveillance. Cancer Cell. (2005) 8:369–80. doi: 10.1016/j.ccr.2005.10.012
8. Rabinovich GA, Gabrilovich D, Sotomayor EM. Immunosuppressive strategies that are mediated by tumor cells. Annu Rev Immunol. (2007) 25:267–96. doi: 10.1146/annurev.immunol.25.022106.141609
9. Zhang Y, Zhang Z. The history and advances in cancer immunotherapy: understanding the characteristics of tumor-infiltrating immune cells and their therapeutic implications. Cell Mol Immunol. (2020) 17:807–21. doi: 10.1038/s41423-020-0488-6
10. Hoos A, Britten C. The immuno-oncology framework: Enabling a new era of cancer therapy. Oncoimmunology. (2012) 1:334–9. doi: 10.4161/onci.19268
11. Andtbacka RH, Kaufman HL, Collichio F, Amatruda T, Senzer N, Chesney J, et al. Talimogene laherparepvec improves durable response rate in patients with advanced melanoma. J Clin Oncol. (2015) 33:2780–8. doi: 10.1200/JCO.2014.58.3377
12. Ma R, Li Z, Chiocca EA, Caligiuri MA, Yu J. The emerging field of oncolytic virus-based cancer immunotherapy. Trends Cancer. (2023) 9:122–39. doi: 10.1016/j.trecan.2022.10.003
13. Li WH, Li YM. Chemical strategies to boost cancer vaccines. Chem Rev. (2020) 120:11420–78. doi: 10.1021/acs.chemrev.9b00833
14. Saxena M, van der Burg SH, Melief CJM, Bhardwaj N. Therapeutic cancer vaccines. Nat Rev Cancer. (2021) 21:360–78. doi: 10.1038/s41568-021-00346-0
15. Propper DJ, Balkwill FR. Harnessing cytokines and chemokines for cancer therapy. Nat Rev Clin Oncol. (2022) 19:237–53. doi: 10.1038/s41571-021-00588-9
16. Liu Y, Adu-Berchie K, Brockman JM, Pezone M, Zhang DKY, Zhou J, et al. Cytokine conjugation to enhance T cell therapy. Proc Natl Acad Sci U S A. (2023) 120:e2213222120. doi: 10.1073/pnas.2213222120
17. Dudley ME, Rosenberg SA. Adoptive-cell-transfer therapy for the treatment of patients with cancer. Nat Rev Cancer. (2003) 3:666–75. doi: 10.1038/nrc1167
18. Rosenberg SA, Parkhurst MR, Robbins PF. Adoptive cell transfer immunotherapy for patients with solid epithelial cancers. Cancer Cell. (2023) 41:646–8. doi: 10.1016/j.ccell.2023.03.003
19. Carlino MS, Larkin J, Long GV. Immune checkpoint inhibitors in melanoma. Lancet. (2021) 398:1002–14. doi: 10.1016/S0140-6736(21)01206-X
20. Dall’Olio FG, Marabelle A, Caramella C, Garcia C, Aldea M, Chaput N, et al. Tumor burden and efficacy of immune-checkpoint inhibitors. Nat Rev Clin Oncol. (2022) 19:75–90. doi: 10.1038/s41571-021-00564-3
21. Waldmann TA. Cytokines in cancer immunotherapy. Cold Spring Harb Perspect Biol. (2018) 10:a028472. doi: 10.1101/cshperspect.a028472
22. Yong SB, Chung JY, Song Y, Kim J, Ra S, Kim YH. Non-viral nano-immunotherapeutics targeting tumor microenvironmental immune cells. Biomaterials. (2019) 219:119401. doi: 10.1016/j.biomaterials.2019.119401
23. Riley RS, June CH, Langer R, Mitchell MJ. Delivery technologies for cancer immunotherapy. Nat Rev Drug Discovery. (2019) 18:175–96. doi: 10.1038/s41573-018-0006-z
24. Chauhan VP, Jain RK. Strategies for advancing cancer nanomedicine. Nat Mater. (2013) 12:958–62. doi: 10.1038/nmat3792
25. Zuo S, Song J, Zhang J, He Z, Sun B, Sun J. Nano-immunotherapy for each stage of cancer cellular immunity: which, why, and what? Theranostics. (2021) 11:7471–87. doi: 10.7150/thno.59953
26. Qian M, Chen L, Du Y, Jiang H, Huo T, Yang Y, et al. Biodegradable mesoporous silica achieved via carbon nanodots-incorporated framework swelling for debris-mediated photothermal synergistic immunotherapy. Nano Lett. (2019) 19:8409–17. doi: 10.1021/acs.nanolett.9b02448
27. Tang L, Zheng Y, Melo MB, Mabardi L, Castaño AP, Xie YQ, et al. Enhancing T cell therapy through TCR-signaling-responsive nanoparticle drug delivery. Nat Biotechnol. (2018) 36:707–16. doi: 10.1038/nbt.4181
28. Goodwin TJ, Zhou Y, Musetti SN, Liu R, Huang L. Local and transient gene expression primes the liver to resist cancer metastasis. Sci Transl Med. (2016) 8:364ra153. doi: 10.1126/scitranslmed.aag2306
29. Sung YC, Jin PR, Chu LA, Hsu FF, Wang MR, Chang CC, et al. Delivery of nitric oxide with a nanocarrier promotes tumor vessel normalization and potentiates anti-cancer therapies. Nat Nanotechnol. (2019) 14:1160–9. doi: 10.1038/s41565-019-0570-3
30. Kumar MA, Baba SK, Sadida HQ, Marzooqi SA, Jerobin J, Altemani FH, et al. Extracellular vesicles as tools and targets in therapy for diseases. Signal Transduct Target Ther. (2024) 9:27. doi: 10.1038/s41392-024-01735-1
31. Xiao Q, Li X, Li Y, Wu Z, Xu C, Chen Z, et al. Biological drug and drug delivery-mediated immunotherapy. Acta Pharm Sin B. (2021) 11:941–60. doi: 10.1016/j.apsb.2020.12.018
32. Shen H, Jin L, Zheng Q, Ye Z, Cheng L, Wu Y, et al. Synergistically targeting synovium STING pathway for rheumatoid arthritis treatment. Bioact Mater. (2022) 24:37–53. doi: 10.1016/j.bioactmat.2022.12.001
33. Wei N, Xu Y, Li Y, Shi J, Zhang X, You Y, et al. A bibliometric analysis of T cell and atherosclerosis. Front Immunol. (2022) 13:948314. doi: 10.3389/fimmu.2022.948314
34. Wu F, Gao J, Kang J, Wang X, Niu Q, Liu J, et al. Knowledge mapping of exosomes in autoimmune diseases: A bibliometric analysis (2002-2021). Front Immunol. (2022) 13:939433. doi: 10.3389/fimmu.2022.939433
35. Jiang S, Liu Y, Zheng H, Zhang L, Zhao H, Sang X, et al. Evolutionary patterns and research frontiers in neoadjuvant immunotherapy: a bibliometric analysis. Int J Surg. (2023) 109:2774–83. doi: 10.1097/JS9.0000000000000492
36. Ye J, Ding H, Ren J, Xia Z. The publication trend of neuropathic pain in the world and China: a 20-years bibliometric analysis. J Headache Pain. (2018) 19:110. doi: 10.1186/s10194-018-0941-4
37. Zhang X, Yi K, Xu JG, Wang WX, Liu CF, He XL, et al. Application of three-dimensional printing in cardiovascular diseases: a bibliometric analysis. Int J Surg. (2024) 110:1068–78. doi: 10.1097/JS9.0000000000000868
38. Ribas A, Wolchok JD. Cancer immunotherapy using checkpoint blockade. Science. (2018) 359:1350–5. doi: 10.1126/science.aar4060
39. Duan X, Chan C, Lin W. Nanoparticle-mediated immunogenic cell death enables and potentiates cancer immunotherapy. Angew Chem Int Ed Engl. (2019) 58:670–80. doi: 10.1002/anie.201804882
40. Guo Y, Lei K, Tang L. Neoantigen vaccine delivery for personalized anticancer immunotherapy. Front Immunol. (2018) 9:1499. doi: 10.3389/fimmu.2018.01499
41. Gao Y, Jin B, Shen W, Sinko PJ, Xie X, Zhang H, et al. China and the United States–Global partners, competitors and collaborators in nanotechnology development. Nanomedicine. (2016) 12:13–9. doi: 10.1016/j.nano.2015.09.007
42. Chen Q, Liu X, Chen J, Zeng J, Cheng Z, Liu Z. A self-assembled albumin-based nanoprobe for in vivo ratiometric photoacoustic pH imaging. Adv Mater. (2015) 27:6820–7. doi: 10.1002/adma.201503194
43. Chen Q, Liang C, Sun X, Chen J, Yang Z, Zhao H, et al. H2O2-responsive liposomal nanoprobe for photoacoustic inflammation imaging and tumor theranostics via in vivo chromogenic assay. Proc Natl Acad Sci U S A. (2017) 114:5343–8. doi: 10.1073/pnas.1701976114
44. Chen Q, Wang C, Cheng L, He W, Cheng Z, Liu Z. Protein modified upconversion nanoparticles for imaging-guided combined photothermal and photodynamic therapy. Biomaterials. (2014) 35:2915–23. doi: 10.1016/j.biomaterials.2013.12.046
45. Gong H, Chao Y, Xiang J, Han X, Song G, Feng L, et al. Hyaluronidase to enhance nanoparticle-based photodynamic tumor therapy. Nano Lett. (2016) 16:2512–21. doi: 10.1021/acs.nanolett.6b00068
46. Chen Q, Chen J, Yang Z, Xu J, Xu L, Liang C, et al. Nanoparticle-enhanced radiotherapy to trigger robust cancer immunotherapy. Adv Mater. (2019) 31:e1802228. doi: 10.1002/adma.201802228
47. Gao M, Liang C, Song X, Chen Q, Jin Q, Wang C, et al. Erythrocyte-membrane-enveloped perfluorocarbon as nanoscale artificial red blood cells to relieve tumor hypoxia and enhance cancer radiotherapy. Adv Mater. (2017) 29(35):10.1002/adma.201701429. doi: 10.1002/adma.201701429
48. Chen Q, Chen M, Liu Z. Local biomaterials-assisted cancer immunotherapy to trigger systemic antitumor responses. Chem Soc Rev. (2019) 48:5506–26. doi: 10.1039/C9CS00271E
49. Fang H, Wu Y, Chen L, Cao Z, Deng Z, Zhao R, et al. Regulating the obesity-related tumor microenvironment to improve cancer immunotherapy. ACS Nano. (2023) 17:4748–63. doi: 10.1021/acsnano.2c11159
50. Wang R, Kumar P, Reda M, Wallstrum AG, Crumrine NA, Ngamcherdtrakul W, et al. Nanotechnology applications in breast cancer immunotherapy. Small. (2023) 21. doi: 10.1002/smll.202308639
51. Shi X, Cheng Q, Hou T, Han M, Smbatyan G, Lang JE, et al. Genetically engineered cell-derived nanoparticles for targeted breast cancer immunotherapy. Mol Ther. (2020) 28:536–47. doi: 10.1016/j.ymthe.2019.11.020
52. Hu A, Chen X, Bi Q, Xiang Y, Jin R, Ai H, et al. A parallel and cascade control system: magnetofection of miR125b for synergistic tumor-association macrophage polarization regulation and tumor cell suppression in breast cancer treatment. Nanoscale. (2020) 12:22615–27. doi: 10.1039/D0NR06060G
53. Zhou F, Feng B, Yu H, Wang D, Wang T, Ma Y, et al. Tumor microenvironment-activatable prodrug vesicles for nanoenabled cancer chemoimmunotherapy combining immunogenic cell death induction and CD47 blockade. Adv Mater. (2019) 31:e1805888. doi: 10.1002/adma.201805888
54. Pece S, Tosoni D, Confalonieri S, Mazzarol G, Vecchi M, Ronzoni S, et al. Biological and molecular heterogeneity of breast cancers correlates with their cancer stem cell content. Cell. (2010) 140:62–73. doi: 10.1016/j.cell.2009.12.007
55. Shimokawa M, Ohta Y, Nishikori S, Matano M, Takano A, Fujii M, et al. Visualization and targeting of LGR5+ human colon cancer stem cells. Nature. (2017) 545:187–92. doi: 10.1038/nature22081
56. Lathia JD, Mack SC, Mulkearns-Hubert EE, Valentim CL, Rich JN. Cancer stem cells in glioblastoma. Genes Dev. (2015) 29:1203–17. doi: 10.1101/gad.261982.115
57. Sourisseau T, Hassan KA, Wistuba I, Penault-Llorca F, Adam J, Deutsch E, et al. Lung cancer stem cell: fancy conceptual model of tumor biology or cornerstone of a forthcoming therapeutic breakthrough? J Thorac Oncol. (2014) 9:7–17. doi: 10.1097/JTO.0000000000000028
58. Sell S, Leffert HL. Liver cancer stem cells [published correction appears in J Clin Oncol. 2008 Aug 1;26(22): 3819]. J Clin Oncol. (2008) 26:2800–5. doi: 10.1200/JCO.2007.15.5945
59. Shen S, Xia JX, Wang J. Nanomedicine-mediated cancer stem cell therapy. Biomaterials. (2016) 74:1–18. doi: 10.1016/j.biomaterials.2015.09.037
60. Barenholz Y. Doxil®–the first FDA-approved nano-drug: lessons learned. J Control Release. (2012) 160:117–34. doi: 10.1016/j.jconrel.2012.03.020
61. Gradishar WJ, Tjulandin S, Davidson N, Shaw H, Desai N, Bhar P, et al. Phase III trial of nanoparticle albumin-bound paclitaxel compared with polyethylated castor oil-based paclitaxel in women with breast cancer. J Clin Oncol. (2005) 23:7794–803. doi: 10.1200/JCO.2005.04.937
62. Veronese FM, Pasut G. PEGylation, successful approach to drug delivery. Drug Discovery Today. (2005) 10:1451–8. doi: 10.1016/S1359-6446(05)03575-0
63. Xie L, Zhang C, Liu M, Huang J, Jin X, Zhu C, et al. Nucleus-targeting manganese dioxide nanoparticles coated with the human umbilical cord mesenchymal stem cell membrane for cancer cell therapy. ACS Appl Mater Interf. (2023) 15:10541–53. doi: 10.1021/acsami.3c01176
64. Liu D, Hong Y, Li Y, Hu C, Yip TC, Yu WK, et al. Targeted destruction of cancer stem cells using multifunctional magnetic nanoparticles that enable combined hyperthermia and chemotherapy. Theranostics. (2020) 10:1181–96. doi: 10.7150/thno.38989
65. Pan Y, Zhou S, Li Y, Parshad B, Li W, Haag R. Novel dendritic polyglycerol-conjugated, mesoporous silica-based targeting nanocarriers for co-delivery of doxorubicin and tariquidar to overcome multidrug resistance in breast cancer stem cells. J Control Release. (2021) 330:1106–17. doi: 10.1016/j.jconrel.2020.11.015
66. Discher DE, Eisenberg A. Polymer vesicles. Science. (2002) 297:967–73. doi: 10.1126/science.1074972
67. Bonifácio BV, Silva PB, Ramos MA, Negri KM, Bauab TM, Chorilli M. Nanotechnology-based drug delivery systems and herbal medicines: a review. Int J Nanomed. (2014) 9:1–15. doi: 10.2147/IJN.S52634
68. Krishnamurthy S, Vaiyapuri R, Zhang L, Chan JM. Lipid-coated polymeric nanoparticles for cancer drug delivery. Biomater Sci. (2015) 3:923–36. doi: 10.1039/C4BM00427B
69. Koseva NS, Rydz J, Stoyanova EV, Mitova VA. Hybrid protein-synthetic polymer nanoparticles for drug delivery. Adv Protein Chem Struct Biol. (2015) 98:93–119. doi: 10.1016/bs.apcsb.2014.12.003
70. Park J, Wrzesinski SH, Stern E, Look M, Criscione J, Ragheb R, et al. Combination delivery of TGF-β inhibitor and IL-2 by nanoscale liposomal polymeric gels enhances tumor immunotherapy. Nat Mater. (2012) 11:895–905. doi: 10.1038/nmat3355
71. Eckardt O, Pietsch C, Zumann O, von der Lühe M, Brauer DS, Schacher FH. Well-defined siO2@P(EtOx-stat-EI) core-shell hybrid nanoparticles via sol-gel processes. Macromol Rapid Commun. (2016) 37:337–42. doi: 10.1002/marc.201500467
72. He C, Lu J, Lin W. Hybrid nanoparticles for combination therapy of cancer. J Control Release. (2015) 219:224–36. doi: 10.1016/j.jconrel.2015.09.029
73. Yalcin TE, Ilbasmis-Tamer S, Takka S. Antitumor activity of gemcitabine hydrochloride loaded lipid polymer hybrid nanoparticles (LPHNs): In vitro and in vivo. Int J Pharm. (2020) 580:119246. doi: 10.1016/j.ijpharm.2020.119246
74. Tahir N, Madni A, Balasubramanian V, Rehman M, Correia A, Kashif PM, et al. Development and optimization of methotrexate-loaded lipid-polymer hybrid nanoparticles for controlled drug delivery applications. Int J Pharm. (2017) 533:156–68. doi: 10.1016/j.ijpharm.2017.09.061
75. Fedeli E, Lancelot A, Dominguez JM, Serrano JL, Calvo P, Sierra T. Self-assembling hybrid linear-dendritic block copolymers: the design of nano-carriers for lipophilic antitumoral drugs. Nanomater (Basel). (2019) 9:161. doi: 10.3390/nano9020161
76. Schmid D, Park CG, Hartl CA, Subedi N, Cartwright AN, Puerto RB, et al. T cell-targeting nanoparticles focus delivery of immunotherapy to improve antitumor immunity. Nat Commun. (2017) 8:1747. doi: 10.1038/s41467-017-01830-8
77. Kranz LM, Diken M, Haas H, Kreiter S, Loquai C, Reuter KC, et al. Systemic RNA delivery to dendritic cells exploits antiviral defense for cancer immunotherapy. Nature. (2016) 534:396–401. doi: 10.1038/nature18300
78. Chen Q, Xu L, Liang C, Wang C, Peng R, Liu Z. Photothermal therapy with immune-adjuvant nanoparticles together with checkpoint blockade for effective cancer immunotherapy. Nat Commun. (2016) 7:13193. doi: 10.1038/ncomms13193
79. Beck JD, Reidenbach D, Salomon N, Sahin U, Türeci Ö, Vormehr M, et al. mRNA therapeutics in cancer immunotherapy. Mol Cancer. (2021) 20:69. doi: 10.1186/s12943-021-01348-0
80. Miao L, Zhang Y, Huang L. mRNA vaccine for cancer immunotherapy. Mol Cancer. (2021) 20:41. doi: 10.1186/s12943-021-01335-5
81. Lorentzen CL, Haanen JB, Met Ö, Svane IM. Clinical advances and ongoing trials on mRNA vaccines for cancer treatment. Lancet Oncol. (2022) 23:e492. doi: 10.1016/S1470-2045(22)00372-2
82. Tsui NB, Ng EK, Lo YM. Stability of endogenous and added RNA in blood specimens, serum, and plasma. Clin Chem. (2002) 48:1647–53. doi: 10.1093/clinchem/48.10.1647
83. Midoux P, Pichon C. Lipid-based mRNA vaccine delivery systems. Expert Rev Vaccines. (2015) 14:221–34. doi: 10.1586/14760584.2015.986104
84. Sebastian M, Schröder A, Scheel B, Hong HS, Muth A, von Boehmer L, et al. A phase I/IIa study of the mRNA-based cancer immunotherapy CV9201 in patients with stage IIIB/IV non-small cell lung cancer. Cancer Immunol Immunother. (2019) 68:799–812. doi: 10.1007/s00262-019-02315-x
85. Sahin U, Oehm P, Derhovanessian E, Jabulowsky RA, Vormehr M, Gold M, et al. An RNA vaccine drives immunity in checkpoint-inhibitor-treated melanoma. Nature. (2020) 585:107–12. doi: 10.1038/s41586-020-2537-9
86. Cafri G, Gartner JJ, Zaks T, Hopson K, Levin N, Paria BC, et al. mRNA vaccine-induced neoantigen-specific T cell immunity in patients with gastrointestinal cancer. J Clin Invest. (2020) 130:5976–88. doi: 10.1172/JCI134915
87. Batich KA, Mitchell DA, Healy P, Herndon JE 2nd, Sampson JH. Once, twice, three times a finding: reproducibility of dendritic cell vaccine trials targeting cytomegalovirus in glioblastoma. Clin Cancer Res. (2020) 26:5297–303. doi: 10.1158/1078-0432.CCR-20-1082
88. Son S, Kim JH, Wang X, Zhang C, Yoon SA, Shin J, et al. Multifunctional sonosensitizers in sonodynamic cancer therapy. Chem Soc Rev. (2020) 49:3244–61. doi: 10.1039/C9CS00648F
89. Alphandéry E. Ultrasound and nanomaterial: an efficient pair to fight cancer. J Nanobiotechnol. (2022) 20:139. doi: 10.1186/s12951-022-01243-w
90. Yang Y, Huang J, Liu M, Qiu Y, Chen Q, Zhao T, et al. Emerging sonodynamic therapy-based nanomedicines for cancer immunotherapy. Adv Sci (Weinh). (2023) 10:e2204365. doi: 10.1002/advs.202204365
91. Shin Low S, Nong Lim C, Yew M, Siong Chai W, Low LE, Manickam S, et al. Recent ultrasound advancements for the manipulation of nanobiomaterials and nanoformulations for drug delivery. Ultrason Sonochem. (2021) 80:105805. doi: 10.1016/j.ultsonch.2021.105805
92. Chen J, Feng L, Jin P, Shen J, Lu J, Song Y, et al. Cavitation assisted endoplasmic reticulum targeted sonodynamic droplets to enhanced anti-PD-L1 immunotherapy in pancreatic cancer. J Nanobiotechnol. (2022) 20:283. doi: 10.1186/s12951-022-01459-w
93. Li C, Yang XQ, An J, Cheng K, Hou XL, Zhang XS, et al. Red blood cell membrane-enveloped O2 self-supplementing biomimetic nanoparticles for tumor imaging-guided enhanced sonodynamic therapy. Theranostics. (2020) 10:867–79. doi: 10.7150/thno.37930
94. Li M, Zhu Y, Yang C, Yang M, Ran H, Zhu Y, et al. Acoustic triggered nanobomb for US imaging guided sonodynamic therapy and activating antitumor immunity. Drug Deliv. (2022) 29:2177–89. doi: 10.1080/10717544.2022.2095058
95. Sachdeva A, Dhawan D, Jain GK, Yerer MB, Collignon TE, Tewari D, et al. Novel strategies for the bioavailability augmentation and efficacy improvement of natural products in oral cancer. Cancers (Basel). (2022) 15:268. doi: 10.3390/cancers15010268
96. Romano A, Di Stasio D, Petruzzi M, Fiori F, Lajolo C, Santarelli A, et al. Noninvasive imaging methods to improve the diagnosis of oral carcinoma and its precursors: state of the art and proposal of a three-step diagnostic process. Cancers (Basel). (2021) 13:2864. doi: 10.3390/cancers13122864
97. Woolgar JA, Triantafyllou A. A histopathological appraisal of surgical margins in oral and oropharyngeal cancer resection specimens. Oral Oncol. (2005) 41:1034–43. doi: 10.1016/j.oraloncology.2005.06.008
98. Tan Y, Wang Z, Xu M, Li B, Huang Z, Qin S, et al. Oral squamous cell carcinomas: state of the field and emerging directions. Int J Oral Sci. (2023) 15:44. doi: 10.1038/s41368-023-00249-w
99. Wang ZQ, Liu K, Huo ZJ, Li XC, Wang M, Liu P, et al. A cell-targeted chemotherapeutic nanomedicine strategy for oral squamous cell carcinoma therapy. J Nanobiotechnol. (2015) 13:63. doi: 10.1186/s12951-015-0116-2
100. Zhang X, Han Y, Liu S, Guo B, Xu S, He Y, et al. MF-094 nanodelivery inhibits oral squamous cell carcinoma by targeting USP30. Cell Mol Biol Lett. (2022) 27:107. doi: 10.1186/s11658-022-00407-8
101. Shi E, Shan T, Wang H, Mao L, Liang Y, Cao M, et al. A bacterial nanomedicine combines photodynamic-immunotherapy and chemotherapy for enhanced treatment of oral squamous cell carcinoma. Small. (2023) 19:e2304014. doi: 10.1002/smll.202304014
102. Sun L, Wu J, Du F, Chen X, Chen ZJ. Cyclic GMP-AMP synthase is a cytosolic DNA sensor that activates the type I interferon pathway. Science. (2013) 339:786–91. doi: 10.1126/science.1232458
103. Ishikawa H, Barber GN. STING is an endoplasmic reticulum adaptor that facilitates innate immune signaling [published correction appears in Nature. 2008 Nov 13;456(7219):274]. Nature. (2008) 455:674–8. doi: 10.1038/nature07317
104. Burdette DL, Monroe KM, Sotelo-Troha K, Iwig JS, Eckert B, Hyodo M, et al. STING is a direct innate immune sensor of cyclic di-GMP. Nature. (2011) 478:515–8. doi: 10.1038/nature10429
105. Todisco E, Gaipa G, Biagi E, Bonamino M, Gramigna R, Introna M, et al. CD40 ligand-stimulated B cell precursor leukemic cells elicit interferon-gamma production by autologous bone marrow T cells in childhood acute lymphoblastic leukemia. Leukemia. (2002) 16:2046–54. doi: 10.1038/sj.leu.2402672
106. Decout A, Katz JD, Venkatraman S, Ablasser A. The cGAS-STING pathway as a therapeutic target in inflammatory diseases. Nat Rev Immunol. (2021) 21:548–69. doi: 10.1038/s41577-021-00524-z
107. Corrales L, Glickman LH, McWhirter SM, Kanne DB, Sivick KE, Katibah GE, et al. Direct activation of STING in the tumor microenvironment leads to potent and systemic tumor regression and immunity. Cell Rep. (2015) 11:1018–30. doi: 10.1016/j.celrep.2015.04.031
108. Ramanjulu JM, Pesiridis GS, Yang J, Concha N, Singhaus R, Zhang SY, et al. Design of amidobenzimidazole STING receptor agonists with systemic activity. Nature. (2019) 570:E53. doi: 10.1038/s41586-019-1265-5
109. Wang Y, Luo J, Alu A, Han X, Wei Y, Wei X. cGAS-STING pathway in cancer biotherapy. Mol Cancer. (2020) 19:136. doi: 10.1186/s12943-020-01247-w
110. Castro F, Pinto ML, Almeida R, Pereira F, Silva AM, Pereira CL, et al. Chitosan/poly(γ-glutamic acid) nanoparticles incorporating IFN-γ for immune response modulation in the context of colorectal cancer. Biomater Sci. (2019) 7:3386–403. doi: 10.1039/C9BM00393B
111. Liu Y, Crowe WN, Wang L, Lu Y, Petty WJ, Habib AA, et al. An inhalable nanoparticulate STING agonist synergizes with radiotherapy to confer long-term control of lung metastases. Nat Commun. (2019) 10:5108. doi: 10.1038/s41467-019-13094-5
112. Mitchell MJ, Billingsley MM, Haley RM, Wechsler ME, Peppas NA, Langer R. Engineering precision nanoparticles for drug delivery. Nat Rev Drug Discovery. (2021) 20:101–24. doi: 10.1038/s41573-020-0090-8
Keywords: Citespace, VOSviewer, nano-oncology immunotherapy, emerging research trends, visualization analysis
Citation: Chen C, Yuan P and Zhang Z (2024) Nanomedicine-based cancer immunotherapy: a bibliometric analysis of research progress and prospects. Front. Immunol. 15:1446532. doi: 10.3389/fimmu.2024.1446532
Received: 24 June 2024; Accepted: 05 August 2024;
Published: 23 August 2024.
Edited by:
Abad Khan, University of Swabi, PakistanReviewed by:
Irfan Ullah, University of Minnesota Twin Cities, United StatesLateef Ahmad, University of Swabi, Pakistan
Copyright © 2024 Chen, Yuan and Zhang. This is an open-access article distributed under the terms of the Creative Commons Attribution License (CC BY). The use, distribution or reproduction in other forums is permitted, provided the original author(s) and the copyright owner(s) are credited and that the original publication in this journal is cited, in accordance with accepted academic practice. No use, distribution or reproduction is permitted which does not comply with these terms.
*Correspondence: Zhiyun Zhang, amlhbmNoaWRhb2RpXzIwMTBAMTYzLmNvbQ==