- 1Department of Biotechnology, School of Engineering and Applied Sciences, Bennett University, Greater Noida, Uttar Pradesh, India
- 2Gene Regulation Laboratory, National Institute of Immunology, New Delhi, India
- 3Azrieli Faculty of Medicine, Bar-Ilan University, Safed, Israel
- 4Biological Engineering and Sciences, Indian Institute of Technology Gandhinagar Palaj, Gandhinagar, Gujarat, India
The human microbiome is the complex ecosystem consisting of trillions of microorganisms that play a key role in developing the immune system and nutrient metabolism. Alterations in the gut microbiome have been linked to cancer initiation, progression, metastasis, and response to treatment. Accumulating evidence suggests that levels of vitamins and minerals influence the gut environment and may have implications for cancer risk and progression. Bifidobacterium has been reported to reduce the colorectal cancer risk by binding to free iron. Additionally, zinc ions have been shown to activate the immune cells and enhance the effectiveness of immunotherapy. Higher selenium levels have been associated with a reduced risk of several cancers, including colorectal cancer. In contrast, enhanced copper uptake has been implicated in promoting cancer progression, including colon cancer. The interaction between cancer and gut bacteria, as well as dysbiosis impact has been studied in animal models. The interplay between prebiotics, probiotics, synbiotics, postbiotics and gut bacteria in cancer offers the diverse physiological benefits. We also explored the particular probiotic formulations like VSL#3, Prohep, Lactobacillus rhamnosus GG (LGG), etc., for their ability to modulate immune responses and reduce tumor burden in preclinical models. Targeting the gut microbiome through antibiotics, bacteriophage, microbiome transplantation-based therapies will offer a new perspective in cancer research. Hence, to understand this interplay, we outline the importance of micronutrients with an emphasis on the immunomodulatory function of the microbiome and highlight the microbiome’s potential as a target for precision medicine in cancer treatment.
1 Introduction
The human microbiome comprises approximately 100 trillion microbial cells, with majority inhabiting the gut (1). The human gut microbiome refers to the total microbial population including archaea, fungi, viruses, and a complex hub of bacteria with diverse populations of Actinobacteria, Firmicutes, Proteobacteria, Bacteroides, Eubacteria, Bacteroidetes, Bifidobacteria, Cyanobacteria, Spirochaete, and many more (2–4). The makeup and diversity of the gut microbiome have been shown to influence numerous aspects of human health. Beyond the gastrointestinal (GI) tract, the gut microbiota has profound impact on immune surveillance, systemic inflammation, and metabolic pathways, all of which are linked to the initiation and progression of cancer (5). The gut microbiota influences the immune system and may interact with intratumoral microbiota, forming an immuno-oncology microbiome axis (6). Intestinal microbes interact with host cells to maintain intestinal barrier integrity and influence the bioavailability of therapeutic agents (7). The microbiome composition in cancer patients has been identified as a potential target for manipulation in both immunotherapy and cancer (8). Findings from the colitis-associated colorectal cancer (CAC) mice microbiota revealed the pivotal role in the predisposition of tumor development (9). The composition and diversity of the gut microbiome influence the cancer pathogenesis, progression, sustenance, and treatment outcomes. For example, a study found that greater diversity of gut flora in 55 cervical cancer patients undergoing chemotherapy and radiation therapy (CRT) was associated with better treatment outcomes. The outcome of the study suggested that benefited patients from CRT therapy have higher infiltration of activated CD4+ T cells and more diverse gut microbiome (10). High microbiome diversity in patients with rare long-term survival (LTS) for pancreatic ductal adenocarcinoma (PDAC) may reflect either the pancreas returns to healthy stasis or the host microbiome’s attempt to stifle unfavourable developments in the pancreas (11). In colorectal cancer (CRC) patients, the link between diet-microbiome interaction and composition supports the significance of the microbiome in cancer. A high-fat diet may alter the microbial composition, which in turn could influence cancer risk and prognosis. The negative correlations between the abundance of Bacteroides fragilis and the intake of dietary fats such as unprocessed pork (belly) and animal fats were studied. This suggests that higher consumption of these fats may be linked to lower levels of Bacteroides fragilis, potentially impacting cancer risk (12). Investigations in mouse models have revealed that bacterial strains such as Lactobacillus casei BL23 (13) and Lactobacillus acidophilus (14) can be used as probiotics to modify the microbiota composition for cancer treatment.
Micronutrients functions as anti-inflammatory and antioxidant agents through a variety of chemical mechanisms involving cell-signaling pathways. They exert their effects on cells by influencing epigenomic, transcriptomic, proteomic, and metabolomic alterations via gene expression modulation. Micronutrients also play a role in inhibiting cell proliferation, promoting apoptosis, and reduce angiogenesis by targeting the signaling pathways such as the PI3K/AKT/mTOR. Higher intake of micronutrients such as vitamin B12, vitamin D, folic acid, selenium, and antioxidants like carotenoids have been shown to significantly lower the risk of lung, CRC, prostate, and breast cancers (15, 16). Additionally, micronutrients are essential for the growth and function of gut microbes. Insufficient micronutrient intake can lead to dysbiosis by altering the composition and function of the gut microbiome (17, 18). These modifications may have an impact on immune response modulation, which is crucial in cancer development and progression (19). For example, patients with gastric cancer have been found to have lower blood levels of vitamin C, frequently in conjunction with Helicobacter pylori infection. Vitamin C is depleted as a result of H. pylori toxins interfering with its transportation inside the stomach lumen (20, 21). Supplementing with vitamin C has demonstrated promise in boosting immune responses and inhibiting tumor growth, underscoring its function in gut-host interactions that could impact the risk of cancer (22). Epidemiological studies present a positive correlation between micronutrient uptake and reduced cancer risk whereas the randomized controlled results concerning the effectiveness of dietary supplements in cancer prevention remain inconclusive. Moreover, the relationship between bacteria and tumors are intricate within the human body. Both direct and indirect degrees of interaction between gut microbiota and tumor have been investigated (23). H. pylori in GI malignancies and anaerobic bacterial species like Fusobacterium and Bacteroides fragilis in CRC are the two examples of direct connection (23–25). Indirect interactions between bacterial species have been observed in individuals with CRC and head and neck squamous cell carcinoma (HNSCC), respectively. Examples of these species are Candida albicans and Fusobacterium nucleatum (26, 27). Hence, outcomes to cancer disease such as survival and treatment response are affected by the gut microbiome with its diverse array of bacteria. By comprehensively understanding the mechanism through which different micronutrients interact with human gut flora and participate in cancer disease, researchers can develop new personalized therapies and treatment modalities for affected individuals. This review discusses the complex link between the gut microflora, micronutrients and cancer, showcasing the immense potential in harnessing microbiome-targeted strategies for enhancing cancer detection, prevention, and treatment to improve prognosis and patient longevity.
2 Gut microbiome and micronutrients in cancer
According to the statistical data from the International Agency for Research on Cancer (IARC), factors such as poor diet, lifestyle, smoking alongside overpopulation and aging, are expected to significantly escalate the worldwide burden of cancer by 2030 (28). The human gut microbiome not only affects the emergence of cancer (29) but also modifies the toxicity and efficacy of cancer therapies, including immunotherapy (30). The bacteria and fungus that make up the intratumor microbiome are involved in oncogenesis, tumor progression, and treatment response (31). Through a variety of processes, including DNA damage and alterations in gene expression and tumor microenvironment (TME), immune system, and host metabolism, microorganisms can either stimulate or inhibit cell growth and metastasis (32). Diet and nutrition play a key role in modulating the gut microbiome, impacting the bioavailability and metabolism of bioactive food constituents. Such interactions can eventually influence cancer development (33, 34). Vegetable-rich diets increase the abundance of fiber-degrading bacteria Firmicute and the synthesis of short-chain fatty acids (SCFAs) in the gut, impacting microbial functions (35). Differences in microbial functions were observed in 27 fecal samples from omnivorous, vegan, and vegetarian individuals, potentially impacting the development of colon cancer (36). With the improved cancer management in recent years, the uptake of micronutrient supplements like vitamins and minerals complements the standard therapy in patients (37). Research indicates that micronutrient deficiencies have an impact on nutritional status, immune function, and treatment tolerance in cancer patients. Malnutrition, particularly cachexia, is commonly observed among cancer patients and can lead to adverse treatment outcomes and reduced quality of life. Micronutrient supplementation, when appropriately selected and timed, can enhance patient compliance, reduce adverse effects, and improve treatment response, prognosis, and overall quality of life (38, 39). Studies have shown that micronutrients like selenium, vitamin D, and L-carnitine are crucial in supporting the immune functions and overall well-being of cancer patients.
2.1 Interactions between fat soluble vitamins, gut microbiome and cancer
2.1.1 Vitamin A
The human GI tract provides a hospitable environment for a diverse array of microbes. These microbes play a crucial role in altering the essential nutrients needed for their survival (40). The relationship between gut microbiome, vitamin A metabolism, and host immunity plays an important role in maintaining health and preventing disease. Animal models and meta-analysis studies have demonstrated the protective effects of vitamin A and its derivatives against lung (41) and prostate cancer (42). Vitamin A homeostasis disruptions significantly impact immune cell migration and gut environment activity, critical for maintaining immunological balance (43–45). Studies have established a direct correlation between the gut microbiome composition and vitamin A plasma concentrations, suggesting the potential role of vitamin A in shaping the gut microbial community (46–48). A study in six-week-old CT26 induced BALB/c mice supplemented with βeta-carotene, a precursor of vitamin A, demonstrated the inhibition of cancer cachexia-related changes as well as restored the diversity of gut microbiome. The anti-cancer property of βeta-carotene involves systemic inflammation and lipolysis inhibition (49). In another study, vitamin A supplementation has shown potential in improving health outcomes by fostering the growth of beneficial microbes like Lactobacillus and Bacteroidetes (50–52). Early childhood vitamin A supplementation significantly impacts the development of the gut microbiota, promoting the maturation of the intestinal microbial ecosystem (53). This modulation of gut flora suggests the potential of vitamin A as an adjuvant therapy for infectious disease (Figure 1).
2.1.2 Vitamin D
Emerging evidence suggests that vitamin D metabolism and functions are disrupted in numerous types of cancers, and it is crucial for treatment and prevention of cancer (54). Vitamin D directly controls tumor proliferation and differentiation while indirectly modulates the immune cells of TME, thereby demonstrating anti-cancer properties (55). In a study conducted on C57BL/6 mice, it was observed that increased availability of higher levels of vitamin D provides a greater display of immune-dependent resistance to cancer. In mice, vitamin D activity on intestinal cell lining is responsible for resistance as it modifies the microbiome composition in favor of Bacteroides fragilis and enhances the immune response against cancer (56). As studied in 74 CRC patients, vitamin D supplementation enhances the diversity of Bacteroides gallinarum, and Bacteroides clarus and shapes the gut microbiome (57). A high dosage of vitamin D3 treatment significantly decrease the prevalence of common opportunistic pathogen species, including Pseudomonas, Escherichia, and Shigella, leading to an increase in phylotype richness in human gut microbiome (58) (Figure 1).
2.1.3 Vitamin E
Lipid-soluble vitamin E contains eight naturally occurring isoforms: α, β, δ, and γ isoforms of tocopherol and α, β, δ, and γ isoforms of tocotrienol, contributing to the anti-cancerous effect of vitamin E (59). Reduced levels of vitamin E have been linked with a higher cancer risk. However, supplementation of α-tocopherol has a favorable effect on lowering cancer risk (60). In mice, tocotrienol has been demonstrated to modify the gut microbiome composition, possibly restoring gut health to a more favorable state. Mice infected with colon cancer when supplemented with δ-tocotrienol exhibit increased levels of Streptococcaceae, Bacteroides, and Lactococcus bacteria. This therapeutic effect of tocotrienol exerts anti-inflammatory action and provides benefit to the gut flora (61). In mice, the enhanced diversity of gut microbiota including Roseburia, Bacteroides and Lactococcus, was observed when δ-tocotrienol in conjunction with δTE-13 metabolite, was administered and demonstrated a beneficial impact in preventing colon cancer and reducing colitis in mice (62) (Figure 1).
2.1.4 Vitamin K
Vitamin K acts as a coenzyme and catalyzes the carboxylation reaction of vitamin K-dependent proteins (63). Vitamin K1 (phylloquinone) and vitamin K2 (menaquinone) are essential cofactors for γ-carboxylation and alterations in γ-carboxylation levels have significant implications in cancer development and progression (64). Anti-inflammatory and antioxidant effects of vitamin K prevents cellular senescence and has a potential anticancer effect in pancreatic and prostate cancer (65). A study in human colorectal cell line Caco-2 and mouse macrophage cell line RAW 264.7 tested the 86 different gut bacteria strains. The outcome of this study demonstrated the crucial role of vitamin K2 in inhibiting the secretion of pro-inflammatory cytokines like interleukin 8 (IL-8) in Caco-2 cells while interleukin 6 (IL-6) and tumor necrosis factor-α (TNF-α) in RAW 264.7 cells (66). In mice induced with azoxymethane (AOM), vitamin K2 administration lowered the susceptibility to colon carcinogenesis and decreased the presence of CRC-causing microbes such as H. apodemus and H. mesocricetorum, while an increase in certain species such as Curvibacter lanceolatus, Psychrobacter phenylpyruvicus, and Parasutterella excrementihominis were observed (67) (Figure 1).
2.2 Interactions between water soluble vitamins, gut microbiome and cancer
2.2.1 Vitamin B
Vitamin B complex plays a multifaceted role and helps in regulating immune cell activity, bacteria survival, and overall gut microbiome health. B Vitamins exhibit prebiotic potential, influencing the gut microbiome composition. Bifidobacterium and Lactobacillus species within the gut microbiome have been reported to synthesize several B vitamins (68, 69). Increased vitamin B2 concentrations in the blood and diet decrease the CRC risk as reported in a meta-analysis (70). Interestingly, thiamine supplementation has been shown to inhibit tumorigenesis in certain cancer types (71) and plays a role in developing immunotherapies for cancer eradication. A study in patient tissue samples infected with H. pylori showed lower levels of riboflavin plasma concentrations compared to the non-infected group. Thus, H. pylori was validated to be associated with riboflavin absorption and upregulation of stomach cancer in patients (72). Commensal bacteria lower the risk of CRC by producing the vitamin B2 precursor 5-OP-RU, which also enhances the intestinal mucosa’s defense against infections through immunological mechanisms (73, 74). Vitamin B5 originating from the gut microbiome stimulates the formation of Tc22 immune cells that produce interleukin 22 (IL-22) and have potent anticancer effects and strong immunotherapy responses (75). This diverse immunological and anti-cancer effects of B vitamins collectively highlight the potential therapeutic value of commensal bacterial strains capable of synthesizing these essential micronutrients (76).
2.2.2 Vitamin C
Vitamin C exhibits potent anti-tumor properties and directly induce apoptosis through the production of hydroxyl radicals via the Fenton reaction. The pro-oxidant properties of vitamin C contribute to tumor growth inhibition by regulating the gene expression (77). Vitamin C intake has been linked to reduce the risk of developing gastric cancer (78), bladder cancer (79), breast cancer (80), glioma (81), cervical tumors (82), prostate cancer (83), pancreatic cancer (84) and overall cancer incidence. It has been demonstrated that vitamin C can prevent hypoxia and oncogenic kinase signaling, reverse the epithelial-to-mesenchymal transition (EMT), and enhance the immune system (85). With its anti-microbial properties, vitamin C modulates the microbial diversity in the intestine (86). According to a study in human gastric biopsy specimens and six-week-old Mongolian gerbils inoculated with H. pylori, vitamin C inhibited the growth of 64 H. pylori strains isolated from human biopsy samples and similar inhibitory effect was also observed in an in vivo model after oral administration of vitamin C (87).
2.3 Role of essential minerals in gut microbiome and cancer
Microelements such as iron, zinc, and selenium play crucial roles in tissue functioning and cellular metabolism. Microbes constitute 200 grams of the total body mass of a healthy individual (88). Interactions between microelements and gut microbiome disturb the microbial niche, affecting gut health. The function and configuration of the gut flora are amended by the shortage or excess of mineral composition (89). The gut microbes have evolved the mechanism of transportation and binding microminerals with high affinity and it is crucial as the levels of bioavailable microminerals necessary for their growth are typically low (90). Studies in 30 male C57BL/6 mice fed with magnesium-deficient diet for six weeks reported a change in the microbial composition of the gut (91).
2.3.1 Iron
Iron (Fe) is required as a trace element for many different biological functions, such as energy consumption, cellular respiration, DNA replication, and iron-dependent signaling (92–94). Further, it plays a crucial role in cancer biology, especially in cancer stem cells (CSCs) and mesenchymal cancer cells (95). As reported by Andrews et. al, bacteria need 10-7 – 10-5 M of iron for optimal growth (96). Due to the poor solubility, iron remains unabsorbed in the colon and thereby promoting the proliferation of intestinal pathogens (97, 98). A study in 6–14-year-old African children stated that microbes such as Lactobacillus and Bifidobacterium require iron in very minute quantities for survival, whereas E. coli, Shigella, and Salmonella depend on iron for colonization and to enhance their virulence (99). Consumption of iron-enriched diets is associated with the higher levels of Proteobacteria which, in turn, reduces the microbial activity (100). However, due to the distinctive iron withholding mechanisms, the availability of iron to microorganisms is restricted and hence pathogen growth is inhibited (101). At the site of the large intestine, Bifidobacteriaceae family bacteria bind to the iron limiting the formulation of free radicals and reducing the risk of CRC (102). The growth of Bifidobacterium is promoted by the globular protein Lactoferrin that shows a high affinity towards iron and has bifidogenic properties (103) (Figure 2).
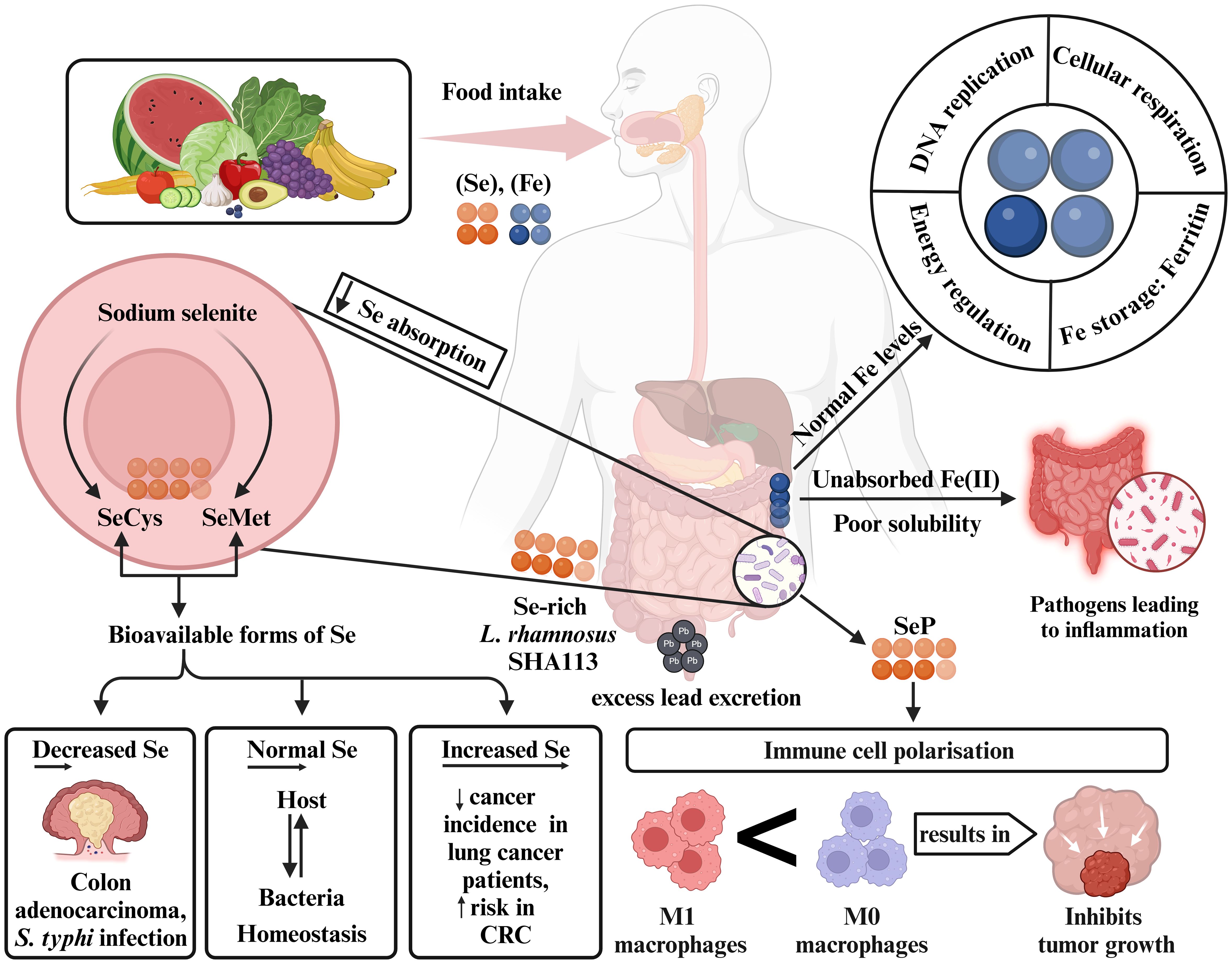
Figure 2. Modulation of gut microbiota based on Se and Fe levels. Adequate dietary intake supports Se and Fe biosynthesis. Low Se absorption by gut microbes leads to sodium selenite metabolizing into SeCys and SeMet. Deficient Se levels may increase colon adenocarcinoma and S. typhi infection risk, while elevated levels are linked to CRC. Normal Se levels promote homeostasis between host and microbiota, aided by Lactobacillus rhamnosus, which helps prevent colon issues by eliminating excess lead. SeP present in gut microbial genes influence immune cell polarization, reducing tumor progression. Unabsorbed Fe(II) can trigger gut inflammation, but normal Fe levels support essential functions like DNA replication, energy regulation, and cellular respiration. Se, selenium; Fe, iron; SeCys, selenocysteine; SeMet, selenomethionine; SeP, selenoproteins; Pb, lead; CRC, colorectal cancer.
2.3.2 Zinc
Zinc (Zn), an essential micronutrient required for several biological functions including DNA binding and synaptic transmission, is found in more than a thousand DNA-binding proteins (104). It is necessary for the action of Zn-containing DNA-repair enzymes, which are vital for mending damage to DNA. Lack of Zn can impair these enzymes capacity to fix damaged DNA, which can accelerate the onset of cancer (105). The metal transporter families, ZIP (SLC39) and ZnT (SLC30) are involved in maintaining Zn homeostasis, where ZIP increases the cytoplasmic Zn concentrations and the ZnT reduces the concentration (106, 107). Research conducted on Zn-deficient N-nitrosomethylbenzylamine (NMBA) rats revealed a higher frequency of esophageal cancer in humans. The development of endophytic tumor is permitted by the attenuated form of NMBA carcinogen in conjunction with a Zn shortage (108–110). Zn deficiency decreases the microbial population in the gut and results in the modification of SCFAs output (111). Bacterial species such as Haemophilus influenzae, E. coli, Campylobacter jejuni, and others require Zn for their virulence and colonization (112). A study in Indonesian preschool children reported that taking Zn supplements for 90 days along with the probiotic Lactobacillus plantarum greatly boosts the humoral immune response (113). Increased immunotherapy efficacy occurs through the activation of immune cells including dendritic and CD8 T cells, facilitated by the delivery of by Zn ions delivered into the TME. Mice with hepatocellular cancer (HCC) have been used to illustrate this (114). Zn supplementation causes a potent necrotic response in tumor cells and activates specific signaling pathways in prostate cancer, including ERK1/2 and protein kinase C (PKC), and prevents the division of tumor cells (115). The proliferation of bacteria, including Prevotella, Proteobacteria, and Actinobacteria, has been linked to zinc enrichment of the microbiome and benefit cancer therapy. The ability of Zn to regulate the gut epithelial wall helps in the regeneration of impaired intestinal epithelium (116, 117) (Figure 3).
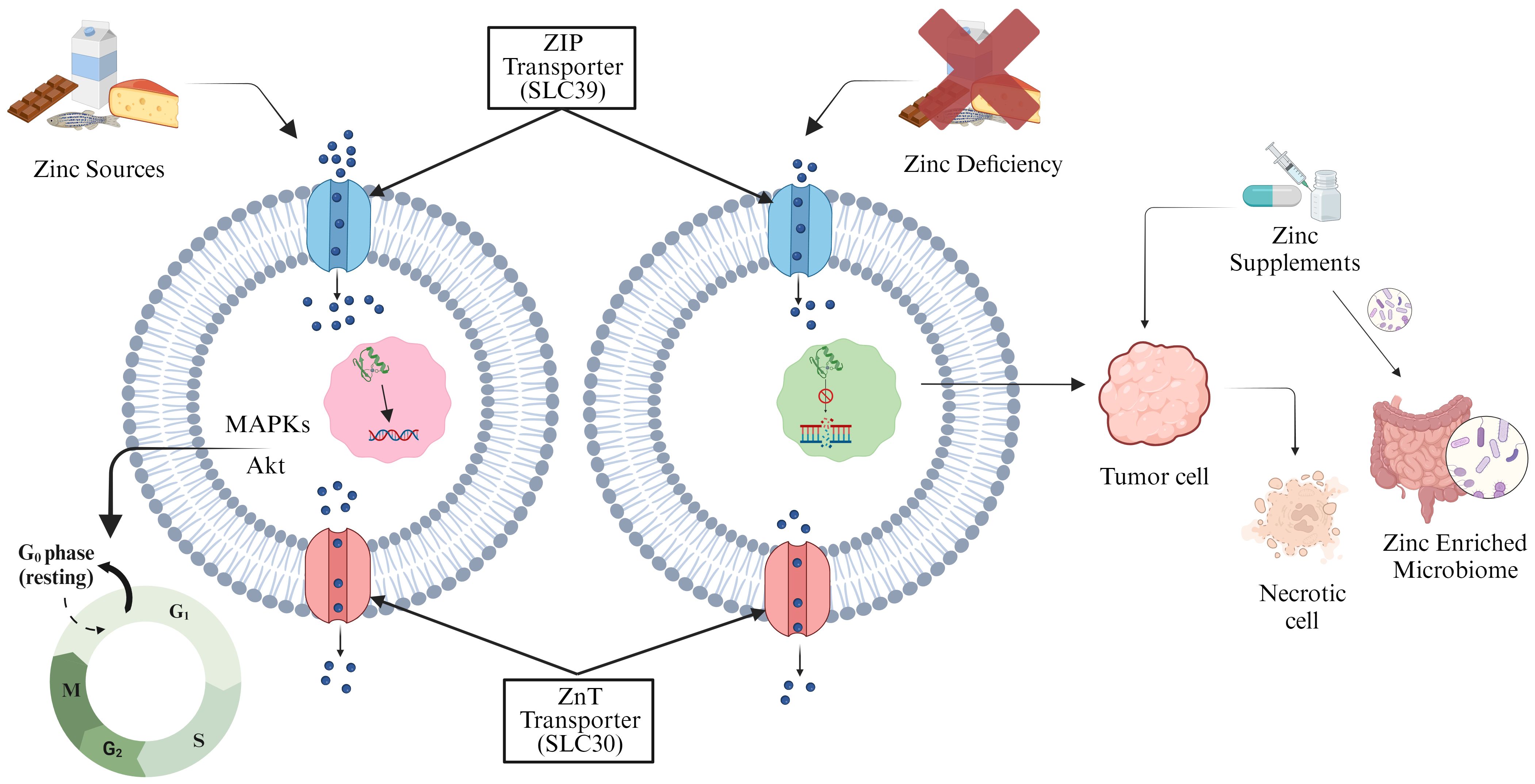
Figure 3. Zinc transporter proteins regulate cellular Zn storage and distribution. Adequate Zn levels maintain controlled phosphorylation-dependent MAPKs and Akt cell cycle signaling pathways (left). Zn deficiency induces DNA damage and cancer. Zn supplementation triggers necrotic response in tumor cells, inhibiting division, and enhances gut microflora, offering additional benefits (right).
2.3.3 Selenium
Selenium (Se), an important trace element of the human body plays a major role in cancer prevention through its essential chemical compounds, L-selenomethionine (SeMet), sodium selenite, and selenocysteine (SeCys) (118, 119). The low absorption of inorganic Se in human cells is typically caused by lactic acid-producing bacteria that convert sodium selenite into SeCys and SeMet, thus facilitating the production of bioavailable forms of Se (120). Lactobacillus increases the Se concentrations in human cells as reported in thyroid adenomas (121). Bacteria such as Enterobacteriaceae, C. difficile, and E. coli. are known to colonize the human gut because of the selenoproteins encoding genes (122). Findings from clinical trials and epidemiological studies on lung cancer patients suggest that increased Se levels reduce the risk of cancer recurrence and incidence (123). Elevated levels of selenoprotein P were adversely correlated with CRC risk, although lower expression of several selenoproteins was observed in colorectal adenoma and cancer tissues (124). Se-enriched yeast supplementation has shown encouraging outcomes in decreasing the occurrence and mortality of colorectal, prostate, and lung cancers (125). Se-rich Lactobacillus rhamnosus SHA113 aids in lead excretion through stools and prevents lead damage to the gut (126). Minimal Se consumption results to a gut microbiome pattern that is more susceptible to S. typhi infections (127). Se administration alters immune cell polarization within the TME, promoting the antitumor pro-inflammatory and anticancer M1 macrophage phenotype over the immunosuppressive M2 phenotype. Additionally, it increases the activity of neutrophils, natural killer (NK) cells, and CD8+ T lymphocytes, promoting antitumor immunity and potentially inhibiting tumor growth and progression (128, 129). A study in eight-week-old mice supplemented with Se showed an increase in the number of microbes belonging to the Lactobacillus genus along with Ruminococcaceae, Christensenellaceae, and Lachnospiraceae niche (130) (Figure 2).
2.3.4 Copper
The biochemistry of human beings depends heavily on the trace element copper (Cu) (131). In human body Cu is required as a micronutrient for several physiological processes, including oxygen transportation, enzyme functioning, and cell signaling (132). Cu plays a crucial role in cell proliferation and cancer progression, as evidenced by increased Cu levels in cancer cell lines, tissues, and patient serum (133–136). Cu-binding proteins, such as mitogen activated protein kinase 1 (MEK1) and UNC-51-like kinases 1 and 2 (ULK1/2), facilitate signal cascade activation, promoting tumorigenesis (137). Cu is an essential regulator of the autophagic kinases ULK1/2 to drive lung adenocarcinoma. Cu chelators such as tetrathiomolybdate (TTM) and Cu4 (2,4-di-tert-butyl-6-(1H-imidazo- [1, 10] phenanthrolin-2-yl)phenol)4]·10 CH3CN, a bioinorganic complex 2, have been proposed as potential cancer drugs due to their ability to inhibit Cu-dependent signaling pathways (138). Inflammatory cytokines, which mobilize Cu, are linked to Six-Transmembrane Epithelial Antigen of the Prostate 4 (STEAP4) activation, enhancing Cu uptake and X-Linked Inhibitory Apoptosis Protein (XIAP) activation, thereby suppressing apoptosis in colon cancer (139). Animal study on pigs shows that the excess of Zn and Cu leads to stability of the intestinal microbiome and provides resistance to the antimicrobial agents, and antibiotics (140). Shao et al. in his study observed that people living in metal-polluted regions have alterations in their gut microbiome due to long-term exposure to multiple metals including Cu (141). Toxic metals, including Cu, have a detrimental effect on the gut microbiota and an increase in its concentrations leads to the reduction of the diverse bacterial community (142).
3 The tripartite microbiome: bacterial, viral, and fungal interactions from gut to tumor
3.1 Bacterial influence in gut and tumor microenvironments
3.1.1 Fusobacterium nucleatum
In CRC, Fusobacterium species, particularly F. nucleatum, are observed to be more abundant as compared to healthy or precancerous colon tissues (29, 143, 144). Originating from the oral cavity, F. nucleatum is detected in over 40% of CRC patients’ oral samples, suggesting a link between oral and gut microbial shifts in CRC progression (145). In esophageal cancer tissues, the DNA levels of F. nucleatum was detected in 23% of cases, suggesting a potential association with cancer development. In patients positive for F. nucleatum, a higher cancer-specific mortality rate was observed with the bacterium linked to the activation of chemokines, particularly C-C motif chemokine ligand 20 (CCL20), which is involved in immune cell migration and tumor progression (146). A notable dominance of F. nucleatum subsp. animalis has been reported in CRC tissues. Microbiome analysis of human tumor tissue and metagenomic analysis of stool samples consistently indicates a clear enrichment of F. nucelatum animalis clade 2, especially in the proximal colon, with its presence varying across patients tissues from approximately 10% to 90% (147, 148). This observation has been validated across diverse geographic regions, sequencing cores, age groups, and computational approaches, all contributing to the same outcome that CRC creates a unique microenvironment that fosters Fusobacterium colonization. Using metagenomic sequencing, it was revealed that colorectal tumor may facilitate an environment that promotes Fusobacterium proliferation, potentially driving colorectal liver metastases (149). Transcript levels of F. nucleatum were found to be up to 400 times higher in CRC tumor tissues as compared to the healthy tissues, further underscoring its role in cancer development (144, 150, 151). Moreover, the association between F. nucleatum and liver metastasis expands the potential role of F. nucleatum in cancer (149). In mouse models, F. nucleatum reduces gut microbiota diversity and linked to the development of colorectal cancer liver metastasis (CRLM). This dysbiosis influences the immune response in the liver, further promoting cancer growth (152). Mechanistically, F. nucleatum connects with the host endothelial and epithelial cells through FadA adhesion protein, allowing internalization into host cells. This interaction triggers proinflammatory signaling pathways, including nuclear factor (NF)-kB and IL-6, promoting a microenvironment conducive to tumor growth (153, 154). In vitro studies have shown that outer membrane vesicle (OMV) secreted by F. nucleatum stimulate intestinal epidermal cells to produce IL-8 and TNF-α, initiating inflammatory response that further support tumorigenesis (155). Additionally, F. nucleatum localizes at tumor sites by binding through its Fap2 lectin to carbohydrate moiety present on the tumor surface in CRC (156) and breast cancer (157). This interaction potentially facilitates EMT, a process associated with cancer cell invasion, metastasizing, stemness, and therapy resistance (158).
3.1.2 Helicobacter pylori
H. pylori infection affects over 50% of the global population and is strongly linked to various GI diseases, including gastric cancers, peptic ulcers, and mucosa-associated lymphoid tissue (MALT) lymphomas (159–161). During H. pylori infection, MUC1, a transmembrane mucin glycoprotein, is upregulated and undergoes shedding (162). In this process, MUC1 exhibits altered glycosylation and impacts its protective barrier function (163). In gastric cancer, the change in function of MUC1 role from anti-inflammatory to pro-inflammatory contributes to chronic inflammation and cancer development (164). H. pylori recruit the polymorphonuclear neutrophils (PMNs) and indirectly alters the cancer progression, as outlined by the Correa pathway (165, 166). This pathway promotes a Th1-like immune response facilitated by pro-inflammatory cytokines such as interferon-γ (IFN-γ), interleukin-1β (IL-1β), and TNF-α, which further exacerbate inflammation and cancer development (167–169). The translocation of H. pylori into the host gastric epithelial cells is mediated by interactions between HopQ protein of the bacteria and host carcinoembryonic antigen-related cell adhesive molecules (CEACAM) (170, 171). Once inside the host epithelial cells, H. pylori deliver its cytotoxin-associated gene A (CagA) protein via a type 4 secretion system (T4SS), directly linking this mechanism to the development of gastric cancer (172). The injection of CagA leads to aberrant cellular signaling, including interactions with Src homology 2 (SH2) domain-containing protein tyrosine phosphatase 2 (SHP2) and phosphoinositide 3-kinase (PI3K), driving the transformation of normal epithelial cells into neoplastic ones (173).
3.1.3 Bacteroides fragilis
B. fragilis is Gram-negative anaerobic symbiont that constitutes about 25% of anaerobes in human colon. The enterotoxigenic B. fragilis (ETBF) strain is closely associated with the induction of colitis and colon tumorigenesis, as evidenced in both colorectal neoplasia patients and fecal samples of CRC patients (24, 174). Moreover, the ETBF strain is considered as an ‘alpha bug’ due to its direct involvement in cancer development, capability to manipulate microbial communities and its unique virulence factors that promote tumorigenesis (175). In murine model study, ductal colonization with ETBF significantly accelerates breast tumor development and metastatic progression (176). In CRC, the ETBF-encoded metalloprotease Bacteroides fragilis Toxin (BFT) results in tissue impairment and chronic intestinal inflammation by activating critical signaling pathways, including Wnt/β-catenin and NF-kB (177–179). This inflammation caused by ETBF is a driver of CRC, as the infection significantly alters the regulation of cellular ‘stemness.’ Additionally, BFT-positive B. fragilis contributes to localized bacterial dysbiosis by encouraging the proliferation of other cancer-promoting bacteria. This imbalance disrupts the host immune system and foster a pro-inflammatory environment conducive to CRC development (180). Through a toll-like receptor 4 (TLR4)-dependent pathway, ETBF increases levels of transcriptional and epigenetic regulators, which promote tumor growth both in vivo and in vitro (181). The resulting pro-inflammatory and tumorigenic environment in the gut highlights the dual role of B. fragilis in both normal gut symbiosis and cancer promotion within the TME.
3.1.4 Escherichia coli
The B2 and D phylogroups of E. coli are implicated in both intestinal and extra-intestinal diseases, contributing significantly to cancer initiation within the gut-tumor axis. These E. coli strains trigger cancer initiation and manipulation of host cell cycle by inducing inflammation, oxidative stress, and alteration in the cellular niche (182). Enteroaggregative E. coli (EAEC) is a significant intestinal pathogen that causes inflammation in the intestine (183). When EAEC binds to its intestinal receptor, MUC1, it enhances bacterial adhesion by interacting with adherence fimbriae (AAF) (184). The development of CRC has been largely attributed to the diversity of E. coli, particularly in individuals with mucosa-internalized and mucosa-associated CRC (185, 186). Additionally, eukaryotic epithelial cells are damaged by interstrand crosslinks and double-strand DNA breaks induced by colibactin, a protein secreted by pathogenic strains of E. coli and encoded by the pKs pathogenicity island (187–189). In CRC, E. coli enhances tumor growth by promoting cellular senescence and the secretion of growth factors that facilitate proliferation as demonstrated in both CRC mouse models (190, 191) and CRC human biopsies (191). E. coli-induced DNA damage responses are consistently observed in CRC, with a notable impact on Wnt signaling pathways, further driving cellular transformation and tumor growth (187, 191–193). Likewise, E. coli infection in the urinary bladder promotes chronic inflammation and activates the NF-κB pathway (194). This activation, driven by bacterial lipopolysaccharide (LPS), inhibits apoptosis and increases inflammation (195, 196).
3.1.5 Propionobacterium acnes
Propionobacterium acnes, is a Gram-positive bacterium that is commonly found in the sebaceous (oil) glands of the skin (197). The gut microbiome influences systemic inflammation and immune responses and affect skin conditions like acne. The gut-brain-axis suggests that stress and emotional factors can alter gut microbiota further impacting the skin health (198). P. acnes has been reported in prostate cancer in numerous investigations (199, 200). P. acnes strains isolated from prostate cancer tissues exhibited inflammatory response, characterized by elevated secretion of IL-6 and IL-8 when cocultured with RWPE1 prostate epithelial cells. This suggests that P. acnes play a role in promoting chronic inflammation within the TME (199). Similarly, Cohen et al. observed a substantial change in the surface characteristics of P. acnes strains present in prostatic tissues when compared with isolated skin samples, highlighting carcinogenic infection of P. acnes with a higher degree of prostatic inflammation (200). In an in vivo induced mouse model of prostate cancer, P. acnes infection further highlighted its role in exacerbating inflammation and promoting cell proliferation, contributing to tumor development (201).
3.2 Viral contributions to gut dysbiosis and tumor development
Apart from bacteria, a considerable number of viruses also coexist in the human gut and are collectively known as the gut virome. Single-stranded and double-stranded DNA and RNA viruses compose the healthy adult gut virome (202). The human virome is primarily composed of phages that can infect and lyse bacteria in the gut, and Myoviridae, Podoviridae, Siphoviridae, and Microviridae are present dominantly. Viruses that infect eukaryotic cells constitute less than 10% of the total virome volume (203). Additionally, Hannigan and colleagues studied individuals with healthy colons, adenomas, and CRC. They found that bacteriophages, belonging to the Siphoviridae and Myoviridae families, were more common in people with CRC. These findings suggest that the types of viruses present in the gut are linked to CRC and may contribute to the disease by affecting the bacterial populations (204). Researchers compared the viromes of fecal samples from CRC patients and non-CRC controls. They identified 22 viral genera that distinguished CRC patients from healthy control. Additionally, they found that the gut bacteriophage diversity was higher in CRC patients than in controls (205). In contrast, another research group observed a decreased virome diversity in a mouse model of colorectal neoplasia. However, this alteration occurred after the appearance of the first tumor. These findings suggest a correlation between tumor development and gut virome alteration. At the genus level, Brunovirus and Hpunavirus were positively correlated with tumor growth (206). Earlier studies of the virome faced several limitations, including low sensitivity of sequencing technologies, difficulty in establishing a causal role of the virome in disease pathogenesis, and lack of entire viral genome annotations (207). Genome or gene clustering approaches appear to be promising avenues for elucidating the phylogeny of newly discovered gut viruses (208).
3.3 Fungal microbiota: implications for gut health and tumor growth
Fungi have emerged as key players in the diagnosis and progression of cancer. A study by Liu et al. revealed a significant increase in the abundance of 93 out of 108 fungal species, such as Candida pseudohaemulonis, Aspergillus ochraceoroseus, A. rambellii, and Malassezia globose, in CRC patients compared to healthy individuals. These findings suggest that complex interactions between bacteria and fungi, including the upregulation of D-arginine and D-ornithine and the stimulation of the butanoate metabolism pathway, may contribute to the development and progression of CRC (209). Moreover, qPCR analysis targeting the fungal 5.8S ribosomal gene revealed elevated fungal loads in diverse tumor types, including breast, melanoma, lung, colon, ovary, pancreas, brain, and bone, compared to the respective negative controls. Notably, fungal load varied across the above-mentioned tumor types. Subsequent internal transcribed spacer 2 (ITS-2) amplicon sequencing confirmed increased fungal abundance in these cancers. Interestingly, statistical analysis revealed that five fungal taxa—Malasseziomycetes, Saccharomycetes, Diothideomycetes, Sordariomycetes, and Candida were significantly overrepresented across these cancer types (210). Furthermore, the PDAC mycobiome was found to be distinct from both the gut and normal pancreatic mycobiomes, with a significant enrichment of Malassezia species in both mice and humans. Experimental studies involving fungal adoptive transfer and ablation suggest that specific fungal species can promote PDAC progression. This mechanism involves oncogenic Kras-induced inflammation leading to fungal dysbiosis, which subsequently activates the mannose-binding lectin C3 (MBL-C3) cascade and accelerates tumor growth (211). Similarly, abundance of 15 fungal biomarkers was significantly elevated in gastric cancer patients compared to a control group. Specifically, Candida albicans and Alternaria species were enriched. Given its association with gastric cancer, C. albicans could potentially serve as a fungal marker for this disease. Zhong et al. hypothesized that C. albicans may contribute to gastric cancer pathogenesis by reducing fungal diversity (212). Therefore, the emerging field of mycobiome research holds significant promise for understanding the role of fungi in human health and disease. Consequently, future research may uncover novel fungal biomarkers and therapeutic targets to combat cancer.
4 Microbial dysbiosis in cancer pathogenesis
Dysbiosis is characterized by an altered composition of gut microbiome, which disrupts the physiological homeostasis within intestinal epithelial cells (213). Dietary changes, antibiotic use, and inflammatory bowel disease contribute to dysbiosis (214). Disruptions in gut microbial activity hinder the continuous synthesis of microbial primary metabolites such as amino acids, vitamins, and polysaccharides (215). Secondary metabolites such as alkaloids, phenols, antibiotics, and others are highly crucial for defining the function and specificity of the diverse gut microbiome. The microbiota alters the TME and immune responses, impacting the effectiveness of cancer therapies and contributing to treatment-induced dysbiosis (216). The imbalance in the gut microbiome reduces the number of beneficial bacteria while increasing the number of cancer-causing bacteria. Additionally, gut microbiota dysbiosis can lead to increased inflammation and cholestasis in the liver, further promoting the development of pancreatic and HCC cancer (217). As per study conducted in fecal samples of 61 lung cancer patients, the loss of microflora diversity was observed when compared with the healthy stool samples of 28 individuals. There was a significant decrease in anti-inflammatory and SCFAs-producing bacteria and an abundance of tumor-promoting bacteria in these patients (218). Gut microbial metabolites, differing significantly in lung cancer patients, may serve as biomarkers for non-invasive diagnosis and enhance immunotherapy efficacy by influencing lung immune status through the gut-lung axis (219). Similarly, another study unveiled the association between dysbiosis and cholangiocarcinoma (CCA) development where microbial taxa such as Bifidobacteriaceae, Enterobacteriaceae, and Enterococcaceae were linked. It was observed that abnormal microbiome diversity fuels the disease progression via creating a feedback loop and accumulation of genetic and epigenetic changes (220). In elderly patients with HCC, the levels of Firmicutes, Actinobacteria, and Synergites were low as compared to the normal group where the Proteobacteria, Fusobacteria, and Tenericutes were in abundance. According to Yang et al., the fecal microbiome diversity of Fusobacterium and Flavonifractor was linked to young-onset colorectal cancer (yCRC), whereas the abundance of Streptococcus was observed in old onset colorectal cancer (oCRC). The dominance of Flavonifractor plautii in yCRC and F. nucleatum as a crucial microbiome in both yCRC and oCRC was confirmed with the sequencing methods. The findings from the study concluded that CRC is more closely related to young people because of the connection between diet and lifestyle factors (221). The gut microbiota composition between the CRC patients and healthy patients revealed the dominance of Bacteroides and Prevotella group in cancer patients compared to normal individuals. In contrast, there were no alterations in other common gut bacteria, including C. leptum, C. coccoides, Lactobacillus/Leuconostoc/Pediococcus, Bifidobacterium, E. coli, and Faecalibacterium prausnitzii. Likewise, qPCR analysis of stool samples revealed a higher amount of genetic material from bacteroides in colon cancer patients (222). Research has demonstrated substantial differences in the microbiota of individuals with gastritis, intestinal metaplasia (IM), and gastric cancer, suggesting a potential involvement of dysbiosis in disease progression. The result from this study reveals the enrichment of pro-inflammatory oral bacterial species, lactic acid-producing bacteria, and SCFAs synthesis pathways as relevant mechanisms contributing to gastric cancer (223). To delve deeper into the potential role of microbiome led dysbiosis in distinguishing between tumor patients and healthy patients, further investigation is required with an emphasis on clinical validations and elucidation of underlying mechanisms.
5 Tweak to treat: biotics and microbiome crosstalk in cancer
5.1 Prebiotics
Prebiotics are characterized as selectively fermented non-digestable dietary fibers that support the growth of probiotic microorganisms and their role in maintaining the intestinal microbial homeostasis and alleviating gut dysbiosis contributes to overall host health. Prebiotics act in colon and modulate the levels of resident Lactobacilli and Bifidobacteria to produce SCFAs (224). These synthesized SCFAs regulate a range of gut and ex-gut functions, including gut epithelial and mucus barrier, immune function, glucose and lipid metabolism, energy expenditure, and satiety (224, 225). Majority of the prebiotics are derivatives of carbohydrate group and possess the ability to withstand stomach enzymes (226). Several prebiotics such as triterpenoid saponins from Gynostemma pentaphylum (GpS) lower the colon polyps. Likewise, jujube polysaccharide, galacto-oligosaccharides, inulin and Djulis (Chenopodium formosanum) exhibited prevention of CRC development (Figure 4) (227). Upon administration with a complex prebiotic composed of four different oligosaccharides (fructooligosaccharides, xylooligosaccharides, polydextrose and resistant dextrin), CRC patients showed increased serum levels of immunoglobulins (228). Also, higher level of beneficial bacteria like Bifidobacterium and Enterococcus were found in the perioperative period along with the increased abundance of Escherichia-Shigella in postoperative period (228). Pectin has exhibited anticancerous properties in various mice models and several cell lines, like Galactan rich pectin (RG-1) and modified citrus pectin inhibit cancer metastasis. Ohkami et. al, reported antitumor role of apple pectin in AOM induced colon carcinogenic rat (Table 1) (240).
5.2 Probiotics
According to the World Health Organization (WHO), probiotics are defined as ‘live microorganisms’ which when administered in adequate amount confer a healthy benefit on the host (241). Probiotics are widely used as standardized food supplements and recognized for their safety (242). Probiotics exert health benefits by the production of SCFAs, specifically butyrate, through the fermentation of polysaccharides by Clostridium butyricum and Akkermansia muciniphila (243). Butyrate exhibits wide-ranging effects, including the regulation of immune function, intestinal hormone production, and lipogenesis. Studies indicate that butyrate producing Firmicutes family plays a role in apoptosis by reducing cell proliferation. Additionally, Bifidobacterium bifidum increases butyrate production leading to apoptosis induction in malignant CRC cells (226, 244, 245). Beyond the well-established production of SCFAs, specific probiotic formulations, such as VSL#3 composed of eight different strains containing Bifidobacteria (three), Lactobacilli, (four) and Streptococcus (one), demonstrated the capability to synthesize conjugated linoleic acid (CLA). Interestingly, in an in vivo AOM-induced murine CRC model, VSL#3 administration resulted in the upregulation of colonic TNF-α compared to the untreated control group further suggesting a potential immunomodulatory role of VSL#3 linked to enhanced epithelial healing. Also, CLA has a role in inhibiting COX-2 expression and inducing apoptosis (229). A study by Li et al. investigated the therapeutic effects of a novel formulation of three strains designated as ‘Prohep’ further supports the potential of probiotics in HCC (230). ‘Prohep’ formulation includes Lactobacillus rhamnosus GG (LGG) as a keystone species present in the human gut, viable E. coli strain Nissle 1917 (EcN), and the immunomodulatory components of heat inactivated VSL#3. Notably, this study was performed in an in vivo model of HCC and demonstrated a significant tumor reduction within the treated mice by downregulating the interleukin 17 (IL-17) expression and further weakening the angiogenesis (Figure 4) (230). Studies also revealed a significant correlation between the abundance and diversity of intestinal flora and the effectiveness of PD-1 inhibitor treatment, ultimately impacting patient survival (246). LGG when administered orally or in combination with anti-PD-1 in melanoma and CRC-murine model, increases the anticancer function of dendritic cells (DC) via activated CD8+ T cells in the TME. This combination showed a significantly stronger effect to suppress tumor growth when given alongside immune checkpoint blockade (ICB) therapy. LGG triggers the production of Type 1 interferon- β (IFN- β) via cGAS/STING/TBK and interferon regulatory factor-7 (IRF-7) pathway (231).
Conversely, in colon cancer cells LGG treatment upregulated the expression of pro-apoptotic proteins Bax, caspase 3, and p53 (232). LGG cell-free supernatant (LGG-SN) demonstrated selective cytotoxicity towards HT-29, Caco-2 and HCT-116 colon cancer cell line and A375 metastatic melanoma cancer cells, inducing a G2/M cell cycle arrest and reducing the viability. Additionally, LGG-SN exhibits a synergistic effect when combined with chemotherapeutic agents like 5-Fluorouracil (5FU) and irinotecan, potentially enhancing their efficacy against cancer cells (247). Lactobacillus casei Shirota (LcS) has also been implicated in exhibiting anti-tumor properties. Studies suggest the potential efficacy of LcS against transplantable tumor models and chemically induced carcinogenesis. The underlying mechanism involves modulation of the host immune response, particularly via cellular arm enhancement. LcS administration has been shown to upregulate the production of cytokines such as IFN-γ, IL-1β, and TNF-α, which play a crucial role in activating immune effector cells to target and eliminate tumor cells (233). Furthermore, in vitro study using human gastric adenocarcinoma (AGS) and bladder (J253) cell lines demonstrated the ability of specific probiotic strains to induce apoptosis in cancer cells. Lactobacillus acidophilus La-14 (SD-5212) and Bifidobacterium longum subsp. Longum 35624 has been shown to induce morphological changes indicative of apoptosis suggesting a dose-dependent anti-proliferative effect (234). These same strains have also exhibited anti-angiogenic potential by downregulating COX-2 expression, an enzyme linked to tumorigenesis, blood vessel growth (angiogenesis), and cancer cell survival (234).
A study conducted by Sivan. et al. investigated the influence of gut microbiota on tumor growth employed genetically identical C57BL/6 mice harboring distinct gut microbial communities obtained from two sources: Jackson Laboratory (JAX) and Taconic Farms (TAC) (235). Notably, tumors grew significantly faster in TAC mice compared to JAX mice. This disparity correlated with a more robust tumor-specific T cell response and intratumoral accumulation of CD8+ T cells in JAX mice. To elucidate the potential immunomodulatory role of gut microbiota, researchers performed fecal microbiota transplantation (FMT) by orally administering fecal suspensions from JAX or TAC mice to TAC and JAX recipients before tumor implantation. FMT from JAX mice significantly delayed tumor growth in TAC recipients. Subsequent analysis revealed a strong association between Bifidobacterium abundance (400-fold higher in JAX-fed TAC mice) and the observed antitumor T cell response (235). This finding led to the investigation of a commercially available Bifidobacterium cocktail containing B. breve and B. longum. Oral administration of this cocktail, alone or combined with the immune checkpoint inhibitor (ICI) αPD-L1, to TAC mice with established tumors, resulted in significantly improved tumor control compared to untreated controls. This effect correlated with a robust induction of tumor-specific T cells in the periphery. Interestingly, the antitumor effect of Bifidobacterium extended beyond B16 melanoma, with similar observations in TAC mice bearing either B16 or MB49 bladder cancer cells. These findings suggest that Bifidobacterium may activate host dendritic cells, thereby enhancing the function of tumor specific CD8+ T cells, ultimately leading to improved tumor control (235).
Further, probiotics modulate the immune response by enhancing the intestinal barrier function through regulation of tight junction proteins, thereby promoting anti-inflammatory cytokine production, and potentially activating antigen-presenting cells (APCs) that prime tumor-specific T cells (248). This strengthened barrier prevents the translocation of harmful agents and dampens chronic inflammation, a known risk factor for cancer development (248). These studies highlight the complexity of probiotic-host interactions, emphasizing the need for further research to elucidate strain-specific effects and optimize probiotic interventions for cancer immunotherapy. Live biotherapeutic products (LBPs), the therapeutics based on probiotics and live bacteria, are developed to prevent, treat, or cure a human disease (242). Despite the numerous attempts by researchers, only Bacillus Calmette-Guérin (attenuated Mycobacterium bovis) has been approved for human use. Here, as an improved method, instead of live attenuated bacteria have been used to treat bladder cancer (249). In another ongoing human clinical study, the effects of a single strain LPB (Christensenella minuta) has been tested to treat obesity and other metabolic diseases (Table 1) (250).
5.3 Synbiotics
A mixture containing live microorganisms and substrate(s) that are selectively utilized by host microorganisms, confering health benefits on the host is known as synbiotics (251). A synbiotic containing probiotics VSL#3® (Streptococcus thermophilus BT01, B. breve BB02, B. animalis subsp. Lactis BL03, B. animalis susp lactis BI04, L. plantarum BP06, L. paracasei BP07, L. helveticus BD08) and prebiotic yacon based product (PBY) modulated the composition of mice intestinal microbiota (Figure 4). The symbiotic group experienced 38.1% reduction in the incidence of pre-neoplastic lesions in mice colon as compared to the control group. Also, biotic group of mice had high concentrations of SCFA as compared to control and probiotic mice. Upregulation of IL-2 and IL-4 was observed in synbiotic group, which helps in improvement of immune response and antitumor defense (236). Raftilose Synergy 1® composed of oligofructose enriched inulin and probiotics containing LGG and B. lactis Bb12, served as a synbiotic given to AOM induced colon cancer infected mice and significantly reduced tumors as compared to the control group. Also, significant reduction of Glutathione S-transferase placental enzyme pi type (GST-P) expression was observed (Table 1) (237).
5.4 Postbiotics
According to International Scientific Association of Probiotics and Prebiotics (ISAPP), postbiotics are described as preparation of inanimate microorganisms and/or their components that confers a health benefit on the host (252). The postbiotic metabolite (PM) produced by L. plantarum UL4 (Figure 4) demonstrated significant induction of apoptosis in human breast cancer cells MCF-7 (238). Also, after 48 hours cell cycle was arrested at G0/G1 phase. In AOM-dextran sulphate sodium (DSS)-induced carcinogenesis mouse model, ‘ferrichrome’ derived from L. casei ATCC 334, led to a reduction in tumor size. Ferrichrome increased the DNA damage inducible transcript 3 (DDIT3) signal and activated caspase-3 pathway, leading to DNA fragmentation. Also, ferichrome combined with 5FU significantly decreased the tumor size (Table 1) (239).
6 Targeting the gut microbiome for improved cancer therapy
The correlation between antibody treatment and reduced gut population has been observed in melanoma, and colon cancer. In melanoma (B16) and colon (MC38) mouse models, antibody administration has been reported to imperial the immunotherapy efficacy and reduce the inflammatory cytokine production using anti-IL-10/CpG oligodeoxynucleotides (253). Clinical data indicates that systemic use of antibiotics results in less effective ICI (254). In laboratory mice with MC-26 carcinoma cell implants, the efficacy of gemcitabine was found to be restored when it was directed against intratumoral bacteria (255). On the other hand, it has also been established that broad-spectrum antibiotic treatments have negative impacts on tumor therapy. In patients receiving antibiotics for non-small cell lung cancer (NSCLC) had lower survival rates both before and after the treatment, while patients receiving ICIs for advanced melanomas and allogenic hematopoietic cell transfer therapy reported evidence of antibiotic mediated loss of Bifidobacterium spp. or Akkermansia spp (256). Similarly, the negative effect of antibiotics in patients with hematologic malignancies was studied and the overall reduced survival rate was observed in patients receiving anti-Gram-positive antibiotics. Thus, methods are being developed to reduce the impact of antibiotics on the native microbiota. The use of ‘tailored microbiome therapy’ may help increase therapeutic response by selectively reducing the microbial communities. Current research focuses on developing new strategies that allow for the targeted removal of commensals that promote cancer while having little effect on the microbiome.
Bacteriophages have the ability to directly destroy bacteria and are known to target tumor microbiome and modulate immunity, making them the ideal treatment option for clinical applications (257). Systemic administration of three Myoviridae bacteriophages in 13 patients infected with S. aureus infection displayed preliminary effectiveness (258). It has been reported that use of phage preparations enables the targeted suppression of cancer promoting commensals, while having low impact on surrounding microbiome. For example, in the AGS human gastric adenocarcinoma cell line, H. pylori, a lytic bacteriophage demonstrated the synergistic activity of cancer-associated commensal. Further, H. pylori infected AGS cells showed reduced reactive oxygen species (ROS) accumulation when treated with Hp phage (259). According to Gogokhia et al., dsDNA from Caudovirales phages activates the innate immune response by interacting with TLR9. This shows that phages reduce the colitis and intestinal inflammation. Further, they also reduce colon cancer caused by bacteria and hence benefit the host immune system by stimulating TLR9 (260).
Dietary modifications and microbiome transplantation are also known to regulate the microbiome alteration that aids in cancer treatment. Dietary modification has a major influence on gut microbiome. For example, animal fat removal demonstrated a significant reduction in Bacteroidales (261) whereas a high-fiber diet consumption led to an increase in SCFAs-producing bacteria upon consuming a high-fiber diet. Such shifts to high-fiber diet, exhibited pro-apoptotic and anti-inflammatory responses in colorectal mouse cancer models (262). Similarly, it was observed that inulin administration in adenocarcinoma mouse models improved the effectiveness of anti-PD-1 therapy while simultaneously increasing the relative abundance of Roseburia, Lactobacillus, and Akkermansia (263). Microbiome transplantation has been widely accepted as a therapy where disease-associated microbiome is replaced with a healthy individual through FMT. Studies have supported the enhanced immnotherapy via transferring the patient fecal sample into antibiotic treated specific-pathogen free (SPF) mice (264). Microbes, in conjunction with ICIs or chemotherapy regimens can also be adopted in the treatment of cancer patients (265). Additionally, human clinical trials highlighted that in a fraction of FMT recipients, particularly melanoma patients who earlier responded to ICI treatment but later developed ICI-resistance, FMT restored the ICI non-responsiveness (266, 267). Thus, targeting the gut microbiome may represent a novel approach for cancer prevention and treatment (Figure 5).
7 Discussion
The interplay of the gut microbiome and dietary micronutrients holds a captivating avenue for exploring potential cancer prevention strategies and treatment. In this review, we delved into the intricate mechanisms and contributions of micronutrients and gut bacteria in cancer treatment. Micronutrients have specific characteristics like anti-inflammatory and antioxidant properties and modulate the gene expression with impact on cell proliferation and apoptosis. Adequate intake of vitamin B12, folic acid, vitamin D, selenium, and carotenoids have been reported to significantly reduce the risk of cancer. The human gut microbiome plays a pivotal role in nutrient metabolism, immune system development, and drug metabolism. Disruptions in dietary patterns and gut microbiome are linked to cancer progression. A fiber-rich diet promotes the growth of healthy bacteria like Firmicutes which leads to the production of SCFA with enhanced anticancer properties. Vitamin B complex exhibits prebiotic potential that influences beneficial bacterial growth. Similarly, minerals like zinc and selenium play a crucial role in gut health and influence cancer therapies. The tumor immune microenvironment plays a crucial role in cancer prognosis and treatment process, with recent studies indicating that the lung microbiome may influence immune cell behaviour and cancer progression (268). Studies reveal a strong correlation between the composition of gut microbiome and patient survival. For example, F. nucleatum, significantly abundant in CRC, promotes cell invasion and metastasis (148). Similarly, H. pylori infection, a major risk factor for gastric cancer, disrupts cellular pathways and actively involved in cell cycle regulation (159). Furthermore, particular strains of B. fragilis, highly enriched in CRC patients, contribute to impaired tissue integrity, chronic inflammation, and dysfunctional immune response, thereby promoting tumor growth and development (179). Additionally, pathogenic E. coli strains can induce inflammation, oxidative stress, and DNA damage in host cells. They can also manipulate the cell cycle, hence leading to tumor formation (182). Interestingly, even P. acnes, typically associated with acne, has been linked to prostate cancer, with certain strains triggering inflammation and promoting cell proliferation (201). However, the crosstalk between bacteria and cancer cells not always results in tumor development. For instance, bacteria such as Pseudomonas aeruginosa, secretes azurin in the presence of cancer cells, with a dose-dependent relationship and enhances patient survival in breast and melanoma cancer cases (269). Thus, understanding the distinct roles of cancer-associated bacteria in carcinogenesis can lead to the development of innovative treatments and strategies. The oral microbiome, alongside the gut microbiome, holds promise as a diagnostic and prognostic tool for various cancers and serve as a therapeutic target (270). Natural polyphenols have emerged as an effective cancer treatment therapy with their antioxidant-like properties (271). Prebiotics, probiotics, synbiotics and postbiotics exert beneficial effects through multiple mechanisms. One key mechanism involves the production of SCFAs, particularly butyrate, during dietary fiber fermentation by probiotic bacteria like C. butyricum and A. muciniphila. Butyrate-producing Firmicutes families are reported to induce apoptosis in cancer cells. Other probiotic formulations like VSL#3, synthesizes CLA and exhibits potential immunomodulatory effects. Furthermore, ‘Prohep’ demonstrates a significant reduction in tumor burden in HCC mice model. Recent findings highlight a critical link between the gut microbiome and the efficacy of PD-1/PD-L1 checkpoint inhibitor immunotherapy (246). The genetic modulation of gut microbiome using targeted dietary interventions or by probiotic supplementation and simultaneously optimized micronutrient intake may enhance the efficacy of the cancer treatment. By strategic modulation of the gut microbiome and targeted suppression of detrimental bacterial populations, while upregulation of beneficial bacteria, significant advancement can be achieved in cancer prevention.
8 Future perspective
Understanding, the gut bacteria influence on cancer metabolism and immune response is critical for developing targeted therapies. By deciphering these molecular pathways, novel therapeutic strategies can be unlocked. This requires isolation and characterization of specific probiotic strains with demonstrably positive effects on the gut microbiome composition and anti-tumor properties. Probiotic-based therapies can offer promising avenues for the treatment of cancer. Genetically engineered probiotics are capable of modulating immune responses against tumor cells by producing tumor-killing agents, which may offer enhanced efficacy and reduced collateral damage. The synergistic combination of prebiotics and probiotics can provide a nutrient-rich environment that supports beneficial bacteria, potentially optimizing treatment outcomes. Further, personalized probiotic therapy may facilitate the selection of the optimal and most effective probiotic strains for specific tumor types. Prebiotic interventions offer significant promise. By selectively promoting the growth of beneficial bacteria, prebiotics can contribute to a balanced microbiome required to foster a robust immune response and reduce inflammation. These factors are crucial in combating cancer, as a strong immune system and reduced inflammatory environment can help inhibit tumor growth and progression. Thus, the future of cancer therapy holds immense potential for leveraging the power of the gut microbiome. By carefully selecting and administering probiotics and prebiotics, we may be able to develop targeted therapies against tumors while safeguarding the health of vital organ systems. As our understanding of the microbiome-cancer axis continues to evolve, we can anticipate exciting advancements in personalized medicine and therapy. Further, meticulous characterization of these strains can pave the way for personalized cancer treatment strategies tailored to an individual’s unique gut microbiome. However, establishing the efficacy and safety of probiotic interventions is crucial. Well-designed clinical trials are essential for evaluating the potential of probiotic supplementation as an adjunct therapy in cancer prevention and treatment, as well as in managing side effects associated with chemotherapy. Thus, by pursuing this multifaceted approach, we can harness the power of the gut microbiome to revolutionize cancer care.
Author contributions
SC: Conceptualization, Formal analysis, Supervision, Validation, Writing – original draft, Writing – review & editing. KB: Conceptualization, Data curation, Formal analysis, Investigation, Writing – original draft, Writing – review & editing. KJ: Conceptualization, Data curation, Formal analysis, Investigation, Writing – original draft. ND: Data curation, Formal analysis, Writing – original draft. NP: Data curation, Formal analysis, Investigation, Writing – original draft, Writing – review & editing. GG: Data curation, Formal analysis, Writing – original draft. AmK: Data curation, Writing – original draft. AnK: Software, Writing – review & editing.
Funding
The author(s) declare that no financial support was received for the research, authorship, and/or publication of this article.
Acknowledgments
Figures 1-5 were created with BioRender.com.
Conflict of interest
The authors declare that the research was conducted in the absence of any commercial or financial relationships that could be construed as a potential conflict of interest.
Publisher’s note
All claims expressed in this article are solely those of the authors and do not necessarily represent those of their affiliated organizations, or those of the publisher, the editors and the reviewers. Any product that may be evaluated in this article, or claim that may be made by its manufacturer, is not guaranteed or endorsed by the publisher.
References
1. Qin J, Li R, Raes J, Arumugam M, Burgdorf KS, Manichanh C, et al. A human gut microbial gene catalogue established by metagenomic sequencing. Nature. (2010) 464:59–65. doi: 10.1038/nature08821
2. Brandi G, Frega G. Microbiota: overview and implication in immunotherapy-based cancer treatments. Int J Mol Sci. (2019) 20:2699. doi: 10.3390/ijms20112699
3. Hugon P, Dufour JC, Colson P, Fournier PE, Sallah K, Raoult D. A comprehensive repertoire of prokaryotic species identified in human beings. Lancet Infect Dis. (2015) 15:1211–9. doi: 10.1016/S1473-3099(15)00293-5
4. The Human Microbiome Project Consortium. Structure, function and diversity of the healthy human microbiome. Nature. (2012) 486:207–14. doi: 10.1038/nature11234
5. Wen X, Ye X, Yang X, Jiang R, Qian C, Wang X. The crosstalk between intestinal bacterial microbiota and immune cells in colorectal cancer progression. Clin Trans Oncol. (2022) 25:620–32. doi: 10.1007/s12094-022-02995-5
6. Yang L, Li A, Wang Y, Zhang Y. Intratumoral microbiota: roles in cancer initiation, development and therapeutic efficacy. Signal Transduct Target Ther. (2023) 8:35. doi: 10.1038/s41392-022-01304-4
7. Wang M, Zhang L, Chang W, Zhang Y. The crosstalk between the gut microbiota and tumor immunity: Implications for cancer progression and treatment outcomes. Front Immunol. (2023) 13. doi: 10.3389/fimmu.2022.1096551
8. Pham F, Moinard-Butot F, Coutzac C, Chaput N. Cancer and immunotherapy: a role for microbiota composition. Eur J Cancer. (2021) 155:145–54. doi: 10.1016/j.ejca.2021.06.051
9. Ashkenazi-Preiser H, Reuven O, Uzan-Yulzari A, Komisarov S, Cirkin R, Turjeman S, et al. The cross-talk between intestinal microbiota and MDSCs fuels colitis-associated cancer development. Cancer Res Commun. (2024) 4:1063–81. doi: 10.1158/2767-9764.CRC-23-0421
10. Sims TT, El Alam MB, Karpinets TV, Dorta-Estremera S, Hegde VL, Nookala S, et al. Gut microbiome diversity is an independent predictor of survival in cervical cancer patients receiving chemoradiation. Commun Biol. (2021) 4:237. doi: 10.1038/s42003-021-01741-x
11. Riquelme E, Zhang Y, Zhang L, Montiel M, Zoltan M, Dong W, et al. Tumor microbiome diversity and composition influence pancreatic cancer outcomes. Cell. (2019) 178:795–806. doi: 10.1016/j.cell.2019.07.008
12. Hoang T, Kim MJ, Park JW, Jeong SY, Lee J, Shin A. Nutrition-wide association study of microbiome diversity and composition in colorectal cancer patients. BMC Cancer. (2022) 22:656. doi: 10.1186/s12885-022-09735-6
13. Jacouton E, Michel ML, Torres-Maravilla E, Chain F, Langella P, Bermúdez-Humarán LG. Elucidating the immune-related mechanisms by which probiotic strain lactobacillus casei BL23 displays anti-tumoral properties. Front Microbiol. (2019) 9. doi: 10.3389/fmicb.2018.03281
14. Chen CC, Lin WC, Kong MS, Shi HN, Walker WA, Lin CY, et al. Oral inoculation of probiotics Lactobacillus acidophilus NCFM suppresses tumour growth both in segmental orthotopic colon cancer and extra-intestinal tissue. Br J Nutr. (2012) 107:1623–34. doi: 10.1017/S0007114511004934
15. Chakraborty A, Guha S, Chakraborty D. Micronutrients in preventing cancer: A critical review of research. Asian Pacific J Cancer Biol. (2020) 5:119–25. doi: 10.31557/apjcb.2020.5.3.119-125
16. Fagbohun OF, Gillies CR, Murphy KPJ, Rupasinghe HPV. Role of antioxidant vitamins and other micronutrients on regulations of specific genes and signaling pathways in the prevention and treatment of cancer. Int J Mol Sci. (2023) 24:6092. doi: 10.3390/ijms24076092
17. Barone M, D’Amico F, Brigidi P, Turroni S. Gut microbiome–micronutrient interaction: The key to controlling the bioavailability of minerals and vitamins? BioFactors. (2022) 48:307–14. doi: 10.1002/biof.v48.2
18. Beane KE, Redding MC, Wang X, Pan JH, Le B, Cicalo C, et al. Effects of dietary fibers, micronutrients, and phytonutrients on gut microbiome: a review. Appl Biol Chem. (2021) 64:36. doi: 10.1186/s13765-021-00605-6
19. Frąk M, Grenda A, Krawczyk P, Milanowski J, Kalinka E. Interactions between dietary micronutrients, composition of the microbiome and efficacy of immunotherapy in cancer patients. Cancers (Basel). (2022) 14:5577. doi: 10.3390/cancers14225577
20. Dabrowska-Ufniarz E, Dzieniszewski J, Jarosz M, Wartanowicz M. Vitamin C concentration in gastric juice in patients with precancerous lesions of the stomach and gastric cancer. Med Sci Monit. (2002) 8:CR96–103.
21. Correa P. Helicobacter pylori and gastric carcinogenesis. Am J Surg Pathol. (1995) 19 Suppl 1:S37–43.
22. Böttger F, Vallés-Martí A, Cahn L, Jimenez CR. High-dose intravenous vitamin C, a promising multi-targeting agent in the treatment of cancer. J Exp Clin Cancer Res. (2021) 40:343. doi: 10.1186/s13046-021-02134-y
23. Manzoor SS, Doedens A, Burns MB. The promise and challenge of cancer microbiome research. Genome Biol. (2020) 21:131. doi: 10.1186/s13059-020-02037-9
24. Boleij A, Hechenbleikner EM, Goodwin AC, Badani R, Stein EM, Lazarev MG, et al. The bacteroides fragilis toxin gene is prevalent in the colon mucosa of colorectal cancer patients. Clin Infect Dis. (2015) 60:208–15. doi: 10.1093/cid/ciu787
25. Kostic AD, Gevers D, Pedamallu CS, Michaud M, Duke F, Earl AM, et al. Genomic analysis identifies association of Fusobacterium with colorectal carcinoma. Genome Res. (2012) 22:292–8. doi: 10.1101/gr.126573.111
26. Vesty A, Gear K, Biswas K, Radcliff FJ, Taylor MW, Douglas RG. Microbial and inflammatory-based salivary biomarkers of head and neck squamous cell carcinoma. Clin Exp Dent Res. (2018) 4:255–62. doi: 10.1002/cre2.v4.6
27. Flemer B, Warren rD, Barrett MP, Cisek K, Das A, Jeffery IB, et al. The oral microbiota in colorectal cancer is distinctive and predictive. Gut Microbiota. (2017). doi: 10.1136/gutjnl-2017-314814
28. Thun MJ, DeLancey JO, Center MM, Jemal A, Ward EM. The global burden of cancer: priorities for prevention. Carcinogenesis. (2010) 31:100–10. doi: 10.1093/carcin/bgp263
29. Kostic AD, Chun E, Robertson L, Glickman JN, Gallini CA, Michaud M, et al. Fusobacterium nucleatum potentiates intestinal tumorigenesis and modulates the tumor-immune microenvironment. Cell Host Microbe. (2013) 14:207–15. doi: 10.1016/j.chom.2013.07.007
30. Roy S, Trinchieri G. Microbiota: a key orchestrator of cancer therapy. Nat Rev Cancer. (2017) 17:271–85. doi: 10.1038/nrc.2017.13
31. Fernandes MR, Aggarwal P, Costa RGF, Cole AM, Trinchieri G. Targeting the gut microbiota for cancer therapy. Nat Rev Cancer. (2022) 22:703–22. doi: 10.1038/s41568-022-00513-x
32. Garrett WS. Cancer and the microbiota. Sci (1979). (2015) 348:80–6. doi: 10.1126/science.aaa4972
33. Wu GD, Chen J, Hoffmann C, Bittinger K, Chen YY, Keilbaugh SA, et al. Linking long-term dietary patterns with gut microbial enterotypes. Sci (1979). (2011) 334:105–8. doi: 10.1126/science.1208344
35. De Filippis F, Pellegrini N, Vannini L, IB J, La SA, Laghi L, et al. High-level adherence to a Mediterranean diet beneficially impacts the gut microbiota and associated metabolome. Gut. (2016) 65:1812–21. doi: 10.1136/gutjnl-2015-309957
36. De Angelis M, Ferrocino I, Calabrese FM, De Filippis F, Cavallo N, Siragusa S, et al. Diet influences the functions of the human intestinal microbiome. Sci Rep. (2020) 10:4247. doi: 10.1038/s41598-020-61192-y
37. Micke O, Bruns F, Glatzel M, Schönekaes K, Micke P, Mücke R, et al. Predictive factors for the use of complementary and alternative medicine (CAM) in radiation oncology. Eur J Integr Med. (2009) 1:19–25. doi: 10.1016/j.eujim.2009.02.001
38. Fearon KC, Voss AC, Hustead DS. Definition of cancer cachexia: effect of weight loss, reduced food intake, and systemic inflammation on functional status and prognosis. Am J Clin Nutr. (2006) 83:1345–50. doi: 10.1093/ajcn/83.6.1345
39. Tisdale MJ. Mechanisms of cancer cachexia. Physiol Rev. (2009) 89:381–410. doi: 10.1152/physrev.00016.2008
40. Loh JS, Mak WQ, Tan LKS, Ng CX, Chan HH, Yeow SH, et al. Microbiota–gut–brain axis and its therapeutic applications in neurodegenerative diseases. Signal Transduct Target Ther. (2024) 9:37. doi: 10.1038/s41392-024-01743-1
41. Fritz H, Kennedy D, Fergusson D, Fernandes R, Doucette S, Cooley K, et al. Vitamin A and retinoid derivatives for lung cancer: A systematic review and meta analysis. PloS One. (2011) 6:e21107. doi: 10.1371/journal.pone.0021107
42. Loh W, Yin X, Kishida R, Chia S, Ong C, Seow W. Association between vitamin A and E forms and prostate cancer risk in the Singapore prostate cancer study. Nutrients. (2023) 15:2677. doi: 10.3390/nu15122677
43. Czarnewski P, Das S, Parigi S, Villablanca E. Retinoic acid and its role in modulating intestinal innate immunity. Nutrients. (2017) 9:68. doi: 10.3390/nu9010068
44. Coombes JL, Siddiqui KRR, Arancibia-Cárcamo CV, Hall J, Sun CM, Belkaid Y, et al. A functionally specialized population of mucosal CD103+ DCs induces Foxp3+ regulatory T cells via a TGF-β– and retinoic acid–dependent mechanism. J Exp Med. (2007) 204:1757–64. doi: 10.1084/jem.20070590
45. Hall JA, Cannons JL, Grainger JR, Dos Santos LM, Hand TW, Naik S, et al. Essential role for retinoic acid in the promotion of CD4+ T cell effector responses via retinoic acid receptor alpha. Immunity. (2011) 34:435–47. doi: 10.1016/j.immuni.2011.03.003
46. Lv Z, Wang Y, Yang T, Zhan X, Li Z, Hu H, et al. Vitamin A deficiency impacts the structural segregation of gut microbiota in children with persistent diarrhea. J Clin Biochem Nutr. (2016) 59:113–21. doi: 10.3164/jcbn.15-148
47. Li L, Krause L, Somerset S. Associations between micronutrient intakes and gut microbiota in a group of adults with cystic fibrosis. Clin Nutr. (2017) 36:1097–104. doi: 10.1016/j.clnu.2016.06.029
48. Mandal S, Godfrey KM, McDonald D, Treuren WV, Bjørnholt JV, Midtvedt T, et al. Fat and vitamin intakes during pregnancy have stronger relations with a pro-inflammatory maternal microbiota than does carbohydrate intake. Microbiome. (2016) 4:55. doi: 10.1186/s40168-016-0200-3
49. Kim Y, Jung S, Park G, Shin H, Heo SC, Kim Y. [amp]]beta;-Carotene suppresses cancer cachexia by regulating the adipose tissue metabolism and gut microbiota dysregulation. J Nutr Biochem. (2023) 114:109248. doi: 10.1016/j.jnutbio.2022.109248
50. Lee H, Ko G. Antiviral effect of vitamin A on norovirus infection via modulation of the gut microbiome. Sci Rep. (2016) 6:25835. doi: 10.1038/srep25835
51. Lee H, Ko G. New perspectives regarding the antiviral effect of vitamin A on norovirus using modulation of gut microbiota. Gut Microbes. (2017) 8:616–20. doi: 10.1080/19490976.2017.1353842
52. Liu J, Liu X, Xiong XQ, Yang T, Cui T, Hou NL, et al. Effect of vitamin A supplementation on gut microbiota in children with autism spectrum disorders - a pilot study. BMC Microbiol. (2017) 17:204. doi: 10.1186/s12866-017-1096-1
53. Huda MN, Ahmad SM, Kalanetra KM, Taft DH, Alam MJ, Khanam A, et al. Neonatal vitamin A supplementation and vitamin A status are associated with gut microbiome composition in Bangladeshi infants in early infancy and at 2 years of age. J Nutr. (2019) 149:1075–88. doi: 10.1093/jn/nxz034
54. Bikle DD. Extraskeletal actions of vitamin D. Ann N Y Acad Sci. (2016) 1376:29–52. doi: 10.1111/nyas.2016.1376.issue-1
55. Carlberg C, Velleuer E. Vitamin D and the risk for cancer: A molecular analysis. Biochem Pharmacol. (2022) 196:114735. doi: 10.1016/j.bcp.2021.114735
56. Giampazolias E, da Costa MP, Lam KC, Lim KHJ, Cardoso A, Piot C, et al. Vitamin D regulates microbiome-dependent cancer immunity. Sci (1979). (2024) 384:428–37. doi: 10.1126/science.adh7954
57. Bellerba F, Serrano D, Johansson H, Pozzi C, Segata N, NabiNejad A, et al. Colorectal cancer, Vitamin D and microbiota: A double-blind Phase II randomized trial (ColoViD) in colorectal cancer patients. Neoplasia. (2022) 34:100842. doi: 10.1016/j.neo.2022.100842
58. Bashir M, Prietl B, Tauschmann M, Mautner SI, Kump PK, Treiber G, et al. Effects of high doses of vitamin D3 on mucosa-associated gut microbiome vary between regions of the human gastrointestinal tract. Eur J Nutr. (2016) 55:1479–89. doi: 10.1007/s00394-015-0966-2
59. Abraham A, Kattoor AJ, Saldeen T, Mehta JL. Vitamin E and its anticancer effects. Crit Rev Food Sci Nutr. (2019) 59:2831–8. doi: 10.1080/10408398.2018.1474169
60. Yang CS, Luo P, Zeng Z, Wang H, Malafa M, Suh N. Vitamin E and cancer prevention: Studies with different forms of tocopherols and tocotrienols. Mol Carcinog. (2020) 59:365–89. doi: 10.1002/mc.23160
61. Kumareswaran A, Ekeuku SO, Mohamed N, Muhammad N, Hanafiah A, Pang KL, et al. The effects of tocotrienol on gut microbiota: A scoping review. Life. (2023) 13:1882. doi: 10.3390/life13091882
62. Yang C, Zhao Y, Im S, Nakatsu C, Jones-Hall Y, Jiang Q. Vitamin E delta-tocotrienol and metabolite 13’-carboxychromanol inhibit colitis-associated colon tumorigenesis and modulate gut microbiota in mice. J Nutr Biochem. (2021) 89:108567. doi: 10.1016/j.jnutbio.2020.108567
63. Hao Z, Jin DY, Stafford DW, Tie JK. Vitamin K-dependent carboxylation of coagulation factors: insights from a cell-based functional study. Haematologica. (2020) 105:2164–73. doi: 10.3324/haematol.2019.229047
64. Welsh J, Bak MJ, Narvaez CJ. New insights into vitamin K biology with relevance to cancer. Trends Mol Med. (2022) 28:864–81. doi: 10.1016/j.molmed.2022.07.002
65. Chen A, Li J, Shen N, Huang H, Hang Q. Vitamin K: New insights related to senescence and cancer metastasis. Biochim Biophys Acta (BBA) - Rev Cancer. (2024) 1879:189057. doi: 10.1016/j.bbcan.2023.189057
66. Smajdor J, Jedlińska K, Porada R, Górska-Ratusznik A, Policht A, Śróttek M, et al. The impact of gut bacteria producing long chain homologs of vitamin K2 on colorectal carcinogenesis. Cancer Cell Int. (2023) 23:268. doi: 10.1186/s12935-023-03114-2
67. Zhang Y, Ma C, Zhao J, Xu H, Hou Q, Zhang H. Lactobacillus casei Zhang and vitamin K2 prevent intestinal tumorigenesis in mice via adiponectin-elevated different signaling pathways. Oncotarget. (2017) 8:24719–27. doi: 10.18632/oncotarget.15791
68. Uebanso T, Shimohata T, Mawatari K, Takahashi A. Functional roles of B-vitamins in the gut and gut microbiome. Mol Nutr Food Res. (2020) 64. doi: 10.1002/mnfr.202000426
69. Bedani R, Cucick ACC, de Albuquerque MAC, LeBlanc JG, Saad SMI. B-group vitamins as potential prebiotic candidates: their effects on the human gut microbiome. J Nutr. (2024) 154:341–53. doi: 10.1016/j.tjnut.2023.12.038
70. Ben S, Du M, Ma G, Qu J, Zhu L, Chu H, et al. Vitamin B2 intake reduces the risk for colorectal cancer: a dose–response analysis. Eur J Nutr. (2019) 58:1591–602. doi: 10.1007/s00394-018-1702-5
71. Peterson CT, Rodionov DA, Osterman AL, Peterson SN. B vitamins and their role in immune regulation and cancer. Nutrients. (2020) 12:3380. doi: 10.3390/nu12113380
72. Matnuri M, Zheng C, Sidik D, Bai G, Abdukerim M, Abdukadier A, et al. Correlation analysis of riboflavin, RFT2 and Helicobater pylori in gastric carcinoma. Int J Clin Exp Pathol. (2015) 8:13339–45.
73. Legoux F, Bellet D, Daviaud C, El Morr Y, Darbois A, Niort K, et al. Microbial metabolites control the thymic development of mucosal-associated invariant T cells. Sci (1979). (2019) 366:494–9. doi: 10.1126/science.aaw2719
74. Yu LCH. Microbiota dysbiosis and barrier dysfunction in inflammatory bowel disease and colorectal cancers: exploring a common ground hypothesis. J BioMed Sci. (2018) 25:79. doi: 10.1186/s12929-018-0483-8
75. Bourgin M, Kepp O, Kroemer G. Immunostimulatory effects of vitamin B5 improve anticancer immunotherapy. Oncoimmunology. (2022) 11. doi: 10.1080/2162402X.2022.2031500
76. Villemin C, Six A, Neville BA, Lawley TD, Robinson MJ, Bakdash G. The heightened importance of the microbiome in cancer immunotherapy. Trends Immunol. (2023) 44:44–59. doi: 10.1016/j.it.2022.11.002
77. Vissers MCM, Das AB. Potential mechanisms of action for vitamin C in cancer: reviewing the evidence. Front Physiol. (2018). doi: 10.3389/fphys.2018.00809
78. Kong P, Cai Q, Geng Q, Wang J, Lan Y, Zhan Y, et al. Vitamin intake reduce the risk of gastric cancer: meta-analysis and systematic review of randomized and observational studies. PloS One. (2014) 9:e116060. doi: 10.1371/journal.pone.0116060
79. Chen F, Li Q, Yu Y, Yang W, Shi F, Qu Y. Association of vitamin C, vitamin D, vitamin E and risk of bladder cancer: a dose-response meta-analysis. Sci Rep. (2015) 5:9599. doi: 10.1038/srep09599
80. Zhang D, Xu P, Li Y, Wei B, Yang S, Zheng Y, et al. Association of vitamin C intake with breast cancer risk and mortality: a meta-analysis of observational studies. Aging. (2020) 12:18415–35. doi: 10.18632/aging.103769
81. Zhou S, Wang X, Tan Y, Qiu L, Fang H, Li W. Association between vitamin C intake and glioma risk: evidence from a meta-analysis. Neuroepidemiology. (2015) 44:39–44. doi: 10.1159/000369814
82. Cao D, Shen K, Li Z, Xu Y, Wu D. Association between vitamin C Intake and the risk of cervical neoplasia: A meta-analysis. Nutr Cancer. (2016) 68:48–57. doi: 10.1080/01635581.2016.1115101
83. Bai XY, Qu X, Jiang X, Xu Z, Yang Y, Su Q, et al. Association between dietary vitamin C intake and risk of prostate cancer: A meta-analysis involving 103,658 subjects. J Cancer. (2015) 6:913–21. doi: 10.7150/jca.12162
84. Fan H, Kou J, Han D, Li P, Zhang D, Wu Q, et al. Association between vitamin C intake and the risk of pancreatic cancer: a meta-analysis of observational studies. Sci Rep. (2015) 5:13973. doi: 10.1038/srep13973
85. Zhao L, Quan Y, Wang J, Wang F, Zheng Y, Zhou A. Vitamin C inhibit the proliferation, migration and epithelial-mesenchymal-transition of lens epithelial cells by destabilizing HIF-1α. Int J Clin Exp Med. (2015) 8:15155–63.
86. Mousavi S, Bereswill S, Heimesaat MM. Immunomodulatory and antimicrobial effects of vitamin C. Eur J Microbiol Immunol (Bp). (2019) 9:73–9. doi: 10.1556/1886.2019.00016
87. Zhang HM, Wakisaka N, Maeda O, Yamamoto T. Vitamin C inhibits the growth of a bacterial risk factor for gastric carcinoma:Helicobacter pylori. Cancer. (1997) 80:1897–903. doi: 10.1002/(SICI)1097-0142(19971115)80:10<1897::AID-CNCR4>3.0.CO;2-L
88. Sender R, Fuchs S, Milo R. Are we really vastly outnumbered? Revisiting the ratio of bacterial to host cells in humans. Cell. (2016) 164:337–40. doi: 10.1016/j.cell.2016.01.013
89. Knez M, Ranic M, Stangoulis JCR, Glibetic M. The mineral intake and microbiota. In: Comprehensive Gut Microbiota. Amsterdam, Netherlands: Elsevier (2022). p. 230–42.
90. Power SE, O’Toole PW, Stanton C, Ross RP, Fitzgerald GF. Intestinal microbiota, diet and health. Br J Nutr. (2014) 111:387–402. doi: 10.1017/S0007114513002560
91. Winther G, Jørgensen BMP, Elfving B, Nielsen DS, Kihl P, Lund S, et al. Dietary magnesium deficiency alters gut microbiota and leads to depressive-like behaviour. Acta Neuropsychiatr. (2015) 27:168–76. doi: 10.1017/neu.2015.7
92. Torti SV, Torti FM. Iron and cancer: more ore to be mined. Nat Rev Cancer. (2013) 13:342–55. doi: 10.1038/nrc3495
93. Torti SV, Manz DH, Paul BT, Blanchette-Farra N, Torti FM. Iron and cancer. Annu Rev Nutr. (2018) 38:97–125. doi: 10.1146/annurev-nutr-082117-051732
94. Jung M, Mertens C, Tomat E, Brüne B. Iron as a central player and promising target in cancer progression. Int J Mol Sci. (2019) 20:273. doi: 10.3390/ijms20020273
95. Rodriguez R, Schreiber SL, Conrad M. Persister cancer cells: Iron addiction and vulnerability to ferroptosis. Mol Cell. (2022) 82:728–40. doi: 10.1016/j.molcel.2021.12.001
96. Andrews SC, Robinson AK, Rodríguez-Quiñones F. Bacterial iron homeostasis. FEMS Microbiol Rev. (2003) 27:215–37. doi: 10.1016/S0168-6445(03)00055-X
97. Kortman GAM, Raffatellu M, Swinkels DW, Tjalsma H. Nutritional iron turned inside out: intestinal stress from a gut microbial perspective. FEMS Microbiol Rev. (2014) 38:1202–34. doi: 10.1111/1574-6976.12086
98. Kortman GAM, Dutilh BE, Maathuis AJH, Engelke UF, Boekhorst J, Keegan KP, et al. Microbial metabolism shifts towards an adverse profile with supplementary iron in the TIM-2 in vitro model of the human colon. Front Microbiol. (2016) 6. doi: 10.3389/fmicb.2015.01481
99. Zimmermann MB, Chassard C, Rohner F, N’Goran EK, Nindjin C, Dostal A, et al. The effects of iron fortification on the gut microbiota in African children: a randomized controlled trial in Côte d’Ivoire. Am J Clin Nutr. (2010) 92:1406–15. doi: 10.3945/ajcn.110.004564
100. Shearer J, Shah S, MacInnis MJ, Shen-Tu G, Mu C. Dose-responsive effects of iron supplementation on the gut microbiota in middle-aged women. Nutrients. (2024) 16:786. doi: 10.3390/nu16060786
101. Cassat JE, Skaar EP. Iron in infection and immunity. Cell Host Microbe. (2013) 13:509–19. doi: 10.1016/j.chom.2013.04.010
102. Kot E, Bezkorovainy A. Binding of ferric iron to the cell walls and membranes of bifidobacterium thermophilum: effect of free radicals. J Agric Food Chem. (1999) 47:4606–10. doi: 10.1021/jf990474l
103. Oda H, Wakabayashi H, Yamauchi K, Abe F. Lactoferrin and bifidobacteria. BioMetals. (2014) 27:915–22. doi: 10.1007/s10534-014-9741-8
104. Walsh CT, Sandstead HH, Prasad AS, Newberne PM, Fraker PJ. Zinc: health effects and research priorities for the 1990s. Environ Health Perspect. (1994) 102:5–46.
105. Ho E. Zinc deficiency, DNA damage and cancer risk. J Nutr Biochem. (2004) 15:572–8. doi: 10.1016/j.jnutbio.2004.07.005
106. Jeong J, Eide DJ. The SLC39 family of zinc transporters. Mol Aspects Med. (2013) 34:612–9. doi: 10.1016/j.mam.2012.05.011
107. Palmiter RD, Findley SD. Cloning and functional characterization of a mammalian zinc transporter that confers resistance to zinc. EMBO J. (1995) 14:639–49. doi: 10.1002/j.1460-2075.1995.tb07042.x
108. Newberne PM, Broitman S, Schrager TF. Esophageal carcinogenesis in the rat: zinc deficiency, DNA methylation and alkyltransf erase activity. Pathobiology. (1997) 65:253–63. doi: 10.1159/000164136
109. Fong L. Induction of esophageal tumors in zinc-deficient rats by single low doses of N-nitrosomethylbenzylamine (NMBA): analysis of cell proliferation, and mutations in H-ras and p53 genes. Carcinogenesis. (1997) 18:1477–84. doi: 10.1093/carcin/18.8.1477
110. Fong LYY, Li JX, Farber JL, Magee PN. Cell proliferation and esophageal carcinogenesis in the zinc-deficient rat. Carcinogenesis. (1996) 17:1841–8. doi: 10.1093/carcin/17.9.1841
111. Reed S, Neuman H, Moscovich S, Glahn R, Koren O, Tako E. Chronic zinc deficiency alters chick gut microbiota composition and function. Nutrients. (2015) 7:9768–84. doi: 10.3390/nu7125497
112. Gielda LM, DiRita VJ. Zinc competition among the intestinal microbiota. mBio. (2012) 3. doi: 10.1128/mBio.00171-12
113. Surono IS, Martono PD, Kameo S, Suradji EW, Koyama H. Effect of probiotic L. plantarum IS-10506 and zinc supplementation on humoral immune response and zinc status of Indonesian pre-school children. J Trace Elements Med Biol. (2014) 28:465–9. doi: 10.1016/j.jtemb.2014.07.009
114. Cen D, Ge Q, Xie C, Zheng Q, Guo J, Zhang Y, et al. ZnS@BSA nanoclusters potentiate efficacy of cancer immunotherapy. Adv Mater. (2021) 33. doi: 10.1002/adma.202104037
115. Li D, Stovall DB, Wang W, Sui G. Advances of zinc signaling studies in prostate cancer. Int J Mol Sci. (2020) 21:667. doi: 10.3390/ijms21020667
116. Usama U, Khan MJ, Fatima S, Fatima S. Role of zinc in shaping the gut microbiome; proposed mechanisms and evidence from the literature. J Gastrointest Dig Syst. (2018) 08. doi: 10.4172/2161-069X.1000548
117. Alam AN, Sarker SA, Wahed MA, Khatun M, Rahaman MM. Enteric protein loss and intestinal permeability changes in children during acute shigellosis and after recovery: effect of zinc supplementation. Gut. (1994) 35:1707–11. doi: 10.1136/gut.35.12.1707
118. Mahima, Verma AK, Kumar A, Rahal A, Kumar V, Roy D. Inorganic versus organic selenium supplementation: A review. Pakistan J Biol Sci. (2012) 15:418–25. doi: 10.3923/pjbs.2012.418.425
119. Barger JL, Kayo T, Pugh TD, Vann JA, Power R, Dawson K, et al. Gene expression profiling reveals differential effects of sodium selenite, selenomethionine, and yeast-derived selenium in the mouse. Genes Nutr. (2012) 7:155–65. doi: 10.1007/s12263-011-0243-9
120. Ferreira RLU, Sena-Evangelista KCM, de Azevedo EP, Pinheiro FI, Cobucci RN, Pedrosa LFC. Selenium in human health and gut microflora: bioavailability of selenocompounds and relationship with diseases. Front Nutr. (2021) 8. doi: 10.3389/fnut.2021.685317
121. Yang J, Mu X, Wang Y, Zhu D, Zhang J, Liang C, et al. Dysbiosis of the salivary microbiome is associated with non-smoking female lung cancer and correlated with immunocytochemistry markers. Front Oncol. (2018) 8. doi: 10.3389/fonc.2018.00520
122. Hrdina J, Banning A, Kipp A, Loh G, Blaut M, Brigelius-Flohé R. The gastrointestinal microbiota affects the selenium status and selenoprotein expression in mice. J Nutr Biochem. (2009) 20:638–48. doi: 10.1016/j.jnutbio.2008.06.009
123. Reid ME, Duffield-Lillico AJ, Garland L, Turnbull BW, Clark LC, Marshall JR. Selenium supplementation and lung cancer incidence: an update of the nutritional prevention of cancer trial. Cancer Epidemiol Biomarkers Prev. (2002) 11:1285–91.
124. Hughes DJ, Fedirko V, Jenab M, Schomburg L, Méplan C, Freisling H, et al. Selenium status is associated with colorectal cancer risk in the European prospective investigation of cancer and nutrition cohort. Int J Cancer. (2015) 136:1149–61. doi: 10.1002/ijc.v136.5
125. Hoffmann PR. Mechanisms by which selenium influences immune responses. Arch Immunol Ther Exp (Warsz). (2007) 55:289–97. doi: 10.1007/s00005-007-0036-4
126. Jin H, Riaz Rajoka MS, Xu X, Liao N, Pang B, Yan L, et al. Potentials of orally supplemented selenium-enriched Lacticaseibacillus rhamnosus to mitigate the lead induced liver and intestinal tract injury. Environ Pollution. (2022) 302:119062. doi: 10.1016/j.envpol.2022.119062
127. Zhai Q, Cen S, Li P, Tian F, Zhao J, Zhang H, et al. Effects of dietary selenium supplementation on intestinal barrier and immune responses associated with its modulation of gut microbiota. Environ Sci Technol Lett. (2018) 5:724–30. doi: 10.1021/acs.estlett.8b00563
128. Petrie HT, Klassen LW, Klassen PS, O’Dell JR, Kay HD. Selenium and the immune response: 2. Enhancement of murine cytotoxic T-lymphocyte and natural killer cell cytotoxicity in vivo. J Leukoc Biol. (1989) 45:215–20. doi: 10.1002/jlb.45.3.215
129. Masri DS. Microquantity for macroquality. J Pediatr Hematol Oncol. (2011) 33:e361–2. doi: 10.1097/MPH.0b013e31822d4d39
130. Ramírez-Acosta S, Huertas-Abril PV, Selma-Royo M, Prieto-Álamo MJ, Collado MC, Abril N, et al. The role of selenium in shaping mice brain metabolome and selenoproteome through the gut-brain axis by combining metabolomics, metallomics, gene expression, and amplicon sequencing. J Nutr Biochem. (2023) 117:109323. doi: 10.1016/j.jnutbio.2023.109323
131. Harris Z, Gitlin J. Genetic and molecular basis for copper toxicity. Am J Clin Nutr. (1996) 63:836S–41S. doi: 10.1093/ajcn/63.5.836
132. Denoyer D, Masaldan S, La Fontaine S, Cater MA. Targeting copper in cancer therapy: ‘Copper That Cancer. ’ Metallomics. (2015) 7:1459–76. doi: 10.1039/C5MT00149H
133. Shanbhag VC, Gudekar N, Jasmer K, Papageorgiou C, Singh K, Petris MJ. Copper metabolism as a unique vulnerability in cancer. Biochim Biophys Acta (BBA) - Mol Cell Res. (2021) 1868:118893. doi: 10.1016/j.bbamcr.2020.118893
134. Gupte A, Mumper RJ. Elevated copper and oxidative stress in cancer cells as a target for cancer treatment. Cancer Treat Rev. (2009) 35:32–46. doi: 10.1016/j.ctrv.2008.07.004
135. Khadem-Ansari MH, Asoudeh M, Gheshlaghi HFK, Nozari S, Zarringol M, Maroufi NF, et al. Copper and zinc in stage I multiple myeloma: relation with ceruloplasmin, lipid peroxidation, and superoxide dismutase activity. Horm Mol Biol Clin Investig. (2019) 37. doi: 10.1515/hmbci-2018-0055
136. Sun S, Cai J, Yang Q, Zhao S, Wang Z. The association between copper transporters and the prognosis of cancer patients undergoing chemotherapy: a meta-analysis of literatures and datasets. Oncotarget. (2017) 8:16036–51. doi: 10.18632/oncotarget.13917
137. Turski ML, Brady DC, Kim HJ, Kim BE, Nose Y, Counter CM, et al. A novel role for copper in ras/mitogen-activated protein kinase signaling. Mol Cell Biol. (2012) 32:1284–95. doi: 10.1128/MCB.05722-11
138. Brady DC, Crowe MS, Turski ML, Hobbs GA, Yao X, Chaikuad A, et al. Copper is required for oncogenic BRAF signalling and tumorigenesis. Nature. (2014) 509:492–6. doi: 10.1038/nature13180
139. Liao Yj, Chen Jm, Long J, Zhou Yj, Liang B, Zhou Y. Tanshinone IIA alleviates CCL2-induced leaning memory and cognition impairment in rats: A potential therapeutic approach for HIV-associated neurocognitive disorder. BioMed Res Int. (2020) 2020:1–15.
140. Yazdankhah S, Rudi K, Bernhoft A. Zinc and copper in animal feed – development of resistance and co-resistance to antimicrobial agents in bacteria of animal origin. Microb Ecol Health Dis. (2014) 25. doi: 10.3402/mehd.v25.25862
141. Shao M, Zhu Y. Long-term metal exposure changes gut microbiota of residents surrounding a mining and smelting area. Sci Rep. (2020) 10:4453. doi: 10.1038/s41598-020-61143-7
142. Dai J, Yang X, Yuan Y, Jia Y, Liu G, Lin N, et al. Toxicity, gut microbiota and metabolome effects after copper exposure during early life in SD rats. Toxicology. (2020) 433–434:152395. doi: 10.1016/j.tox.2020.152395
143. Marchesi JR, Dutilh BE, Hall N, Peters WHM, Roelofs R, Boleij A, et al. Towards the human colorectal cancer microbiome. PloS One. (2011) 6:e20447. doi: 10.1371/journal.pone.0020447
144. Castellarin M, Warren RL, Freeman JD, Dreolini L, Krzywinski M, Strauss J, et al. Fusobacterium nucleatum infection is prevalent in human colorectal carcinoma. Genome Res. (2012) 22:299–306. doi: 10.1101/gr.126516.111
145. Komiya Y, Shimomura Y, Higurashi T, Sugi Y, Arimoto J, Umezawa S, et al. Patients with colorectal cancer have identical strains of Fusobacterium nucleatum in their colorectal cancer and oral cavity. Gut. (2019) 68:1335–7. doi: 10.1136/gutjnl-2018-316661
146. Yamamura K, Baba Y, Nakagawa S, Mima K, Miyake K, Nakamura K, et al. Human microbiome fusobacterium nucleatum in esophageal cancer tissue is associated with prognosis. Clin Cancer Res. (2016) 22:5574–81. doi: 10.1158/1078-0432.CCR-16-1786
147. Zepeda-Rivera M, Minot SS, Bouzek H, Wu H, Blanco-Míguez A, Manghi P, et al. A distinct Fusobacterium nucleatum clade dominates the colorectal cancer niche. Nature. (2024) 628:424–32. doi: 10.1038/s41586-024-07182-w
148. Tahara T, Yamamoto E, Suzuki H, Maruyama R, Chung W, Garriga J, et al. Fusobacterium in colonic flora and molecular features of colorectal carcinoma. Cancer Res. (2014) 74:1311–8. doi: 10.1158/0008-5472.CAN-13-1865
149. Bullman S, Pedamallu CS, Sicinska E, Clancy TE, Zhang X, Cai D, et al. Analysis of Fusobacterium persistence and antibiotic response in colorectal cancer. Sci (1979). (2017) 358:1443–8. doi: 10.1126/science.aal5240
150. Kim GW, Kim YS, Lee SH, Park SG, Kim DH, Cho JY, et al. Periodontitis is associated with an increased risk for proximal colorectal neoplasms. Sci Rep. (2019) 9:7528. doi: 10.1038/s41598-019-44014-8
151. Yang SF, Huang HD, Fan WL, Jong YJ, Chen MK, Huang CN, et al. Compositional and functional variations of oral microbiota associated with the mutational changes in oral cancer. Oral Oncol. (2018) 77:1–8. doi: 10.1016/j.oraloncology.2017.12.005
152. Yin H, Miao Z, Wang L, Su B, Liu C, Jin Y, et al. Fusobacterium nucleatum promotes liver metastasis in colorectal cancer by regulating the hepatic immune niche and altering gut microbiota. Aging. (2022) 14:1941–58. doi: 10.18632/aging.203914
153. Han YW, Shi W, Huang GT, Kinder Haake S, Park NH, Kuramitsu H, et al. Interactions between periodontal bacteria and human oral epithelial cells: Fusobacterium nucleatum adheres to and invades epithelial cells. Infect Immun. (2000) 68:3140–6. doi: 10.1128/IAI.68.6.3140-3146.2000
154. Han YW, Redline RW, Li M, Yin L, Hill GB, McCormick TS. Fusobacterium nucleatum induces premature and term stillbirths in pregnant mice: implication of oral bacteria in preterm birth. Infect Immun. (2004) 72:2272–9. doi: 10.1128/IAI.72.4.2272-2279.2004
155. Engevik MA, Danhof HA, Ruan W, Engevik AC, Chang-Graham AL, Engevik KA, et al. Fusobacterium nucleatum secretes outer membrane vesicles and promotes intestinal inflammation. mBio. (2021) 12. doi: 10.1128/mBio.02706-20
156. Abed J, Emgård JEM, Zamir G, Faroja M, Almogy G, Grenov A, et al. Fap2 mediates fusobacterium nucleatum colorectal adenocarcinoma enrichment by binding to tumor-expressed gal-galNAc. Cell Host Microbe. (2016) 20:215–25. doi: 10.1016/j.chom.2016.07.006
157. Parhi L, Alon-Maimon T, Sol A, Nejman D, Shhadeh A, Fainsod-Levi T, et al. Breast cancer colonization by Fusobacterium nucleatum accelerates tumor growth and metastatic progression. Nat Commun. (2020) 11:3259. doi: 10.1038/s41467-020-16967-2
158. Zhang S, Li C, Liu J, Geng F, Shi X, Li Q, et al. Fusobacterium nucleatum promotes epithelial-mesenchymal transiton through regulation of the lncRNA MIR4435-2HG/miR-296-5p/Akt2/SNAI1 signaling pathway. FEBS J. (2020) 287:4032–47. doi: 10.1111/febs.v287.18
159. Cover TL, Blaser MJ. Helicobacter pylori in health and disease. Gastroenterology. (2009) 136:1863–73. doi: 10.1053/j.gastro.2009.01.073
160. Sugizaki K, Tari A, Kitadai Y, Oda I, Nakamura S, Yoshino T, et al. Anti- Helicobacter pylori therapy in localized gastric mucosa-associated lymphoid tissue lymphoma: A prospective, nationwide, multicenter study in Japan. Helicobacter. (2018) 23. doi: 10.1111/hel.2018.23.issue-2
161. Hooi JKY, Lai WY, Ng WK, Suen MMY, Underwood FE, Tanyingoh D, et al. Global prevalence of helicobacter pylori infection: systematic review and meta-analysis. Gastroenterology. (2017) 153:420–9. doi: 10.1053/j.gastro.2017.04.022
162. Guang W, Czinn SJ, Blanchard TG, Kim KC, Lillehoj EP. Genetic regulation of MUC1 expression by Helicobacter pylori in gastric cancer cells. Biochem Biophys Res Commun. (2014) 445:145–50. doi: 10.1016/j.bbrc.2014.01.142
163. Lindén SK, Sheng YH, Every AL, Miles KM, Skoog EC, Florin THJ, et al. MUC1 Limits Helicobacter pylori Infection both by Steric Hindrance and by Acting as a Releasable Decoy. PloS Pathog. (2009) 5:e1000617. doi: 10.1371/journal.ppat.1000617
164. Bose M, Mukherjee P. Microbe–MUC1 crosstalk in cancer-associated infections. Trends Mol Med. (2020) 26:324–36. doi: 10.1016/j.molmed.2019.10.003
165. Correa P. A model for gastric cancer epidemiology. Lancet. (1975) 306:58–60. doi: 10.1016/S0140-6736(75)90498-5
166. Correa P, Piazuelo MB. The gastric precancerous cascade. J Dig Dis. (2012) 13:2–9. doi: 10.1111/j.1751-2980.2011.00550.x
167. Bagheri N, Salimzadeh L, Shirzad H. The role of T helper 1-cell response in Helicobacter pylori-infection. Microb Pathog. (2018) 123:1–8. doi: 10.1016/j.micpath.2018.06.033
168. Guiney DG, Hasegawa P, Cole SP. Helicobacter pylori preferentially induces interleukin 12 (IL-12) rather than IL-6 or IL-10 in human dendritic cells. Infect Immun. (2003) 71:4163–6. doi: 10.1128/IAI.71.7.4163-4166.2003
169. Hafsi N, Voland P, Schwendy S, Rad R, Reindl W, Gerhard M, et al. Human dendritic cells respond to helicobacter pylori, promoting NK cell and th1-effector responses in vitro. J Immunol. (2004) 173:1249–57. doi: 10.4049/jimmunol.173.2.1249
170. Hamway Y, Taxauer K, Moonens K, Neumeyer V, Fischer W, Schmitt V, et al. Cysteine residues in helicobacter pylori adhesin hopQ are required for CEACAM–hopQ interaction and subsequent cagA translocation. Microorganisms. (2020) 8:465. doi: 10.3390/microorganisms8040465
171. Javaheri A, Kruse T, Moonens K, Mejías-Luque R, Debraekeleer A, Asche CI, et al. Helicobacter pylori adhesin HopQ engages in a virulence-enhancing interaction with human CEACAMs. Nat Microbiol. (2016) 2:16189. doi: 10.1038/nmicrobiol.2016.189
172. Odenbreit S, Püls J, Sedlmaier B, Gerland E, Fischer W, Haas R. Translocation of Helicobacter pylori CagA into Gastric Epithelial Cells by Type IV Secretion. Sci (1979). (2000) 287:1497–500. doi: 10.1126/science.287.5457.1497
173. Hatakeyama M. Structure and function of <i<Helicobacter pylori</i< CagA, the first-identified bacterial protein involved in human cancer. Proc Japan Academy Ser B. (2017) 93:196–219.
174. Haghi F, Goli E, Mirzaei B, Zeighami H. The association between fecal enterotoxigenic B. fragilis with colorectal cancer. BMC Cancer. (2019) 19:879. doi: 10.1186/s12885-019-6115-1
175. Sears CL, Pardoll DM. Perspective: alpha-bugs, their microbial partners, and the link to colon cancer. J Infect Dis. (2011) 203:306–11. doi: 10.1093/jinfdis/jiq061
176. Parida S, Wu S, Siddharth S, Wang G, Muniraj N, Nagalingam A, et al. A procarcinogenic colon microbe promotes breast tumorigenesis and metastatic progression and concomitantly activates notch and β-catenin axes. Cancer Discovery. (2021) 11:1138–57. doi: 10.1158/2159-8290.CD-20-0537
177. Wu S, Morin PJ, Maouyo D, Sears CL. Bacteroides fragilis enterotoxin induces c-Myc expression and cellular proliferation. Gastroenterology. (2003) 124:392–400. doi: 10.1053/gast.2003.50047
178. Wu S, Powell J, Mathioudakis N, Kane S, Fernandez E, Sears CL. Bacteroides fragilis Enterotoxin Induces Intestinal Epithelial Cell Secretion of Interleukin-8 through Mitogen-Activated Protein Kinases and a Tyrosine Kinase-Regulated Nuclear Factor-κB Pathway. Infect Immun. (2004) 72:5832–9. doi: 10.1128/IAI.72.10.5832-5839.2004
179. Cheng WT, Kantilal HK, Davamani F. The mechanism of bacteroides fragilis toxin contributes to colon cancer formation. Malaysian J Med Sci. (2020) 27:9–21. doi: 10.21315/mjms2020.27.4.2
180. Geis AL, Fan H, Wu X, Wu S, Huso DL, Wolfe JL, et al. Regulatory T-cell response to enterotoxigenic bacteroides fragilis colonization triggers IL17-dependent colon carcinogenesis. Cancer Discovery. (2015) 5:1098–109. doi: 10.1158/2159-8290.CD-15-0447
181. Liu QQ, Li CM, Fu LN, Wang HL, Tan J, Wang YQ, et al. Enterotoxigenic Bacteroides fragilis induces the stemness in colorectal cancer via upregulating histone demethylase JMJD2B. Gut Microbes. (2020) 12:1788900. doi: 10.1080/19490976.2020.1788900
182. Bonnet M, Buc E, Sauvanet P, Darcha C, Dubois D, Pereira B, et al. Colonization of the human gut by E. coli and colorectal cancer risk. Clin Cancer Res. (2014) 20:859–67. doi: 10.1158/1078-0432.CCR-13-1343
183. Steiner TS, Lima AAM, Nataro JP, Guerrant RL. Enteroaggregative escherichia coli produce intestinal inflammation and growth impairment and cause interleukin-8 release from intestinal epithelial cells. J Infect Dis. (1998) 177:88–96. doi: 10.1086/jid.1998.177.issue-1
184. Boll EJ, Ayala-Lujan J, Szabady RL, Louissaint C, Smith RZ, Krogfelt KA, et al. Enteroaggregative Escherichia coli Adherence Fimbriae Drive Inflammatory Cell Recruitment via Interactions with Epithelial MUC1. mBio. (2017) 8. doi: 10.1128/mBio.00717-17
185. Martin HM, Campbell BJ, Hart CA, Mpofu C, Nayar M, Singh R, et al. Enhanced Escherichia coli adherence and invasion in Crohn’s disease and colon cancer T. K. Korhonen (Division of General Microbiology, University of Helsinki, Finland), who kindly donated Escherichia coli IH11165; Professor J.-F. Colombel (Laboratoire de Recherche sur les Maladies Inflammatoire de l’Intestine, Centre Hospitalier Universitaire, Lille, France) and Professor A. Darfeuille-Michaud (Faculte de Pharmacie, Clermont-Ferrand, France). Gastroenterology. (2004) 127:80–93. doi: 10.1053/j.gastro.2004.03.054
186. Swidsinski A, Khilkin M, Kerjaschki D, Schreiber S, Ortner M, Weber J, et al. Association between intraepithelial Escherichia coli and colorectal cancer. Gastroenterology. (1998) 115:281–6. doi: 10.1016/S0016-5085(98)70194-5
187. Bossuet-Greif N, Vignard J, Taieb F, Mirey G, Dubois D, Petit C, et al. The colibactin genotoxin generates DNA interstrand cross-links in infected cells. mBio. (2018) 9. doi: 10.1128/mBio.02393-17
188. Buc E, Dubois D, Sauvanet P, Raisch J, Delmas J, Darfeuille-Michaud A, et al. High prevalence of mucosa-associated E. coli producing cyclomodulin and genotoxin in colon cancer. PloS One. (2013) 8:e56964.
189. Pleguezuelos-Manzano C, Puschhof J, Rosendahl Huber A, van Hoeck A, Wood HM, Nomburg J, et al. Mutational signature in colorectal cancer caused by genotoxic pks+ E. coli. Nature. (2020) 580:269–73. doi: 10.1038/s41586-020-2080-8
190. Arthur JC, Perez-Chanona E, Mühlbauer M, Tomkovich S, Uronis JM, Fan TJ, et al. Intestinal inflammation targets cancer-inducing activity of the microbiota. Sci (1979). (2012) 338:120–3. doi: 10.1126/science.1224820
191. Dalmasso G, Cougnoux A, Delmas J, Darfeuille-Michaud A, Bonnet R. The bacterial genotoxin colibactin promotes colon tumor growth by modifying the tumor microenvironment. Gut Microbes. (2014) 5:675–80. doi: 10.4161/19490976.2014.969989
192. Dziubańska-Kusibab PJ, Berger H, Battistini F, Bouwman BAM, Iftekhar A, Katainen R, et al. Colibactin DNA-damage signature indicates mutational impact in colorectal cancer. Nat Med. (2020) 26:1063–9. doi: 10.1038/s41591-020-0908-2
193. Iftekhar A, Berger H, Bouznad N, Heuberger J, Boccellato F, Dobrindt U, et al. Genomic aberrations after short-term exposure to colibactin-producing E. coli transform primary colon epithelial cells. Nat Commun. (2021) 12:1003. doi: 10.1038/s41467-021-21162-y
194. El-Mosalamy H, Salman TM, Ashmawey AM, Osama N. Role of chronic E. coli infection in the process of bladder cancer- an experimental study. Infect Agent Cancer. (2012) 7:19. doi: 10.1186/1750-9378-7-19
195. Pålsson-McDermott EM, O’Neill LAJ. Signal transduction by the lipopolysaccharide receptor, Toll-like receptor-4. Immunology. (2004) 113:153–62.
196. Faherty CS, Maurelli AT. Staying alive: bacterial inhibition of apoptosis during infection. Trends Microbiol. (2008) 16:173–80. doi: 10.1016/j.tim.2008.02.001
197. Lee YB, Byun EJ, Kim HS. Potential role of the microbiome in acne: A comprehensive review. J Clin Med. (2019) 8:987. doi: 10.3390/jcm8070987
198. Bowe WP, Logan AC. Acne vulgaris, probiotics and the gut-brain-skin axis - back to the future? Gut Pathog. (2011) 3:1. doi: 10.1186/1757-4749-3-1
199. Fassi Fehri L, Mak TN, Laube B, Brinkmann V, Ogilvie LA, Mollenkopf H, et al. Prevalence of Propionibacterium acnes in diseased prostates and its inflammatory and transforming activity on prostate epithelial cells. Int J Med Microbiol. (2011) 301:69–78. doi: 10.1016/j.ijmm.2010.08.014
200. Cohen RJ, Shannon BA, Mcneal JE, Shannon T, Garrett KL. Propionibacterium acnes associated with inflammation in radical prostatectomy specimens: A possible link to cancer evolution? J Urol. (2005) 173:1969–74. doi: 10.1097/01.ju.0000158161.15277.78
201. Shinohara DB, Vaghasia AM, Yu S, Mak TN, Brüggemann H, Nelson WG, et al. A mouse model of chronic prostatic inflammation using a human prostate cancer-derived isolate of Propionibacterium acnes. Prostate. (2013) 73:1007–15. doi: 10.1002/pros.22648
202. Cao Z, Sugimura N, Burgermeister E, Ebert MP, Zuo T, Lan P. The gut virome: A new microbiome component in health and disease. EBioMedicine. (2022) 81:104113. doi: 10.1016/j.ebiom.2022.104113
203. Tamayo-Trujillo R, Guevara-Ramírez P, Cadena-Ullauri S, Paz-Cruz E, Ruiz-Pozo VA, Zambrano AK. Human virome: Implications in cancer. Heliyon. (2023) 9:e14086. doi: 10.1016/j.heliyon.2023.e14086
204. Hannigan GD, Duhaime MB, Ruffin MT, Koumpouras CC, Schloss PD. Diagnostic potential and interactive dynamics of the colorectal cancer virome. mBio. (2018) 9. doi: 10.1128/mBio.02248-18
205. Nakatsu G, Zhou H, Wu WKK, Wong SH, Coker OO, Dai Z, et al. Alterations in enteric virome are associated with colorectal cancer and survival outcomes. Gastroenterology. (2018) 155:529–541.e5. doi: 10.1053/j.gastro.2018.04.018
206. Li Y, Zhang F, Zheng H, Kalasabail S, Hicks C, Fung K, et al. Fecal DNA virome is associated with the development of colorectal neoplasia in a murine model of colorectal cancer. Pathogens. (2022) 11:457. doi: 10.3390/pathogens11040457
207. Massimino L, Lovisa S, Lamparelli LA, Danese S, Ungaro F. Gut eukaryotic virome in colorectal carcinogenesis: Is that a trigger? Comput Struct Biotechnol J. (2021) 19:16–28.
208. Li J, Yang F, Xiao M, Li A. Advances and challenges in cataloging the human gut virome. Cell Host Microbe. (2022) 30:908–16. doi: 10.1016/j.chom.2022.06.003
209. Liu NN, Jiao N, Tan JC, Wang Z, Wu D, Wang AJ, et al. Multi-kingdom microbiota analyses identify bacterial–fungal interactions and biomarkers of colorectal cancer across cohorts. Nat Microbiol. (2022) 7:238–50. doi: 10.1038/s41564-021-01030-7
210. Narunsky-Haziza L, Sepich-Poore GD, Livyatan I, Asraf O, Martino C, Nejman D, et al. Pan-cancer analyses reveal cancer-type-specific fungal ecologies and bacteriome interactions. Cell. (2022) 185:3789–3806.e17. doi: 10.1016/j.cell.2022.09.005
211. Aykut B, Pushalkar S, Chen R, Li Q, Abengozar R, Kim JI, et al. The fungal mycobiome promotes pancreatic oncogenesis via activation of MBL. Nature. (2019) 574:264–7. doi: 10.1038/s41586-019-1608-2
212. Zhong M, Xiong Y, Zhao J, Gao Z, Ma J, Wu Z, et al. Candida albicans disorder is associated with gastric carcinogenesis. Theranostics. (2021) 11:4945–56. doi: 10.7150/thno.55209
213. DeGruttola AK, Low D, Mizoguchi A, Mizoguchi E. Current understanding of dysbiosis in disease in human and animal models. Inflammation Bowel Dis. (2016) 22:1137–50. doi: 10.1097/MIB.0000000000000750
214. Alshehri D, Saadah O, Mosli M, Edris S, Alhindi R, Bahieldin A. Dysbiosis of gut microbiota in inflammatory bowel disease: Current therapies and potential for microbiota-modulating therapeutic approaches. Bosn J Basic Med Sci. (2020). doi: 10.17305/bjbms.2020.5016
215. Rowland I, Gibson G, Heinken A, Scott K, Swann J, Thiele I, et al. Gut microbiota functions: metabolism of nutrients and other food components. Eur J Nutr. (2018) 57:1–24. doi: 10.1007/s00394-017-1445-8
216. Blake SJ, Wolf Y, Boursi B, Lynn DJ. Role of the microbiota in response to and recovery from cancer therapy. Nat Rev Immunol. (2024) 24:308–25. doi: 10.1038/s41577-023-00951-0
217. Marroncini G, Naldi L, Martinelli S, Amedei A. Gut–liver–pancreas axis crosstalk in health and disease: from the role of microbial metabolites to innovative microbiota manipulating strategies. Biomedicines. (2024) 12:1398. doi: 10.3390/biomedicines12071398
218. Qin X, Bi L, Yang W, He Y, Gu Y, Yang Y, et al. Dysbiosis of the gut microbiome is associated with histopathology of lung cancer. Front Microbiol. (2022) 13. doi: 10.3389/fmicb.2022.918823
219. Li X, Shang S, Wu M, Song Q, Chen D. Gut microbial metabolites in lung cancer development and immunotherapy: Novel insights into gut-lung axis. Cancer Lett. (2024) 598:217096. doi: 10.1016/j.canlet.2024.217096
220. Rao B, Ren T, Wang X, Wang H, Zou Y, Sun Y, et al. Dysbiosis in the human microbiome of cholangiocarcinoma. Front Physiol. (2021) 12. doi: 10.3389/fphys.2021.715536
221. Yang Y, Du L, Shi D, Kong C, Liu J, Liu G, et al. Dysbiosis of human gut microbiome in young-onset colorectal cancer. Nat Commun. (2021) 12:6757. doi: 10.1038/s41467-021-27112-y
222. Sobhani I, Tap J, Roudot-Thoraval F, Roperch JP, Letulle S, Langella P, et al. Microbial dysbiosis in colorectal cancer (CRC) patients. PloS One. (2011) 6:e16393. doi: 10.1371/journal.pone.0016393
223. Castaño-Rodríguez N, Goh KL, Fock KM, Mitchell HM, Kaakoush NO. Dysbiosis of the microbiome in gastric carcinogenesis. Sci Rep. (2017) 7:15957. doi: 10.1038/s41598-017-16289-2
224. Hardy H, Harris J, Lyon E, Beal J, Foey A. Probiotics, prebiotics and immunomodulation of gut mucosal defences: homeostasis and immunopathology. Nutrients. (2013) 5:1869–912. doi: 10.3390/nu5061869
225. Davani-Davari D, Negahdaripour M, Karimzadeh I, Seifan M, Mohkam M, Masoumi S, et al. Prebiotics: definition, types, sources, mechanisms, and clinical applications. Foods. (2019) 8:92. doi: 10.3390/foods8030092
226. Eslami M, Yousefi B, Kokhaei P, Hemati M, Nejad ZR, Arabkari V, et al. Importance of probiotics in the prevention and treatment of colorectal cancer. J Cell Physiol. (2019) 234:17127–43. doi: 10.1002/jcp.v234.10
227. Mahdavi M, Laforest-Lapointe I, Massé E. Preventing colorectal cancer through prebiotics. Microorganisms. (2021) 9:1325. doi: 10.3390/microorganisms9061325
228. Xie X, He Y, Li H, Yu D, Na L, Sun T, et al. Effects of prebiotics on immunologic indicators and intestinal microbiota structure in perioperative colorectal cancer patients. Nutrition. (2019) 61:132–42. doi: 10.1016/j.nut.2018.10.038
229. Bassaganya-Riera J, Viladomiu M, Pedragosa M, De Simone C, Hontecillas R. Immunoregulatory mechanisms underlying prevention of colitis-associated colorectal cancer by probiotic bacteria. PloS One. (2012) 7:e34676. doi: 10.1371/journal.pone.0034676
230. Li J, Sung CYJ, Lee N, Ni Y, Pihlajamäki J, Panagiotou G, et al. Probiotics modulated gut microbiota suppresses hepatocellular carcinoma growth in mice. Proc Natl Acad Sci. (2016) 113. doi: 10.1073/pnas.1518189113
231. Si W, Liang H, Bugno J, Xu Q, Ding X, Yang K, et al. Lactobacillus rhamnosus GG induces cGAS/STING- dependent type I interferon and improves response to immune checkpoint blockade. Gut. (2022) 71:521–33. doi: 10.1136/gutjnl-2020-323426
232. Gamallat Y, Meyiah A, Kuugbee ED, Hago AM, Chiwala G, Awadasseid A, et al. Lactobacillus rhamnosus induced epithelial cell apoptosis, ameliorates inflammation and prevents colon cancer development in an animal model. Biomed Pharmacother. (2016) 83:536–41. doi: 10.1016/j.biopha.2016.07.001
233. Matsuzaki T. Immunomodulation by treatment with Lactobacillus casei strain Shirota. Int J Food Microbiol. (1998) 41:133–40. doi: 10.1016/S0168-1605(98)00046-4
234. Nada HG, Sudha T, Darwish NHE, Mousa SA. Lactobacillus acidophilus and Bifidobacterium longum exhibit antiproliferation, anti-angiogenesis of gastric and bladder cancer: Impact of COX2 inhibition. PharmaNutrition. (2020) 14:100219. doi: 10.1016/j.phanu.2020.100219
235. Sivan A, Corrales L, Hubert N, Williams JB, Aquino-Michaels K, Earley ZM, et al. Commensal Bifidobacterium promotes antitumor immunity and facilitates anti–PD-L1 efficacy. Sci (1979). (2015) 350:1084–9. doi: 10.1126/science.aac4255
236. dos Santos Cruz BC, da Silva Duarte V, Giacomini A, Corich V, de Paula SO, da Silva Fialho L, et al. Synbiotic VSL3 and yacon-based product modulate the intestinal microbiota and prevent the development of pre-neoplastic lesions in a colorectal carcinogenesis model. Appl Microbiol Biotechnol. (2020) 104:8837–57. doi: 10.1007/s00253-020-10863-x
237. Femia AP. Antitumorigenic activity of the prebiotic inulin enriched with oligofructose in combination with the probiotics Lactobacillus rhamnosus and Bifidobacterium lactis on azoxymethane-induced colon carcinogenesis in rats. Carcinogenesis. (2002) 23:1953–60. doi: 10.1093/carcin/23.11.1953
238. Chuah LO, Foo HL, Loh TC, Mohammed Alitheen NB, Yeap SK, Abdul Mutalib NE, et al. Postbiotic metabolites produced by Lactobacillus plantarum strains exert selective cytotoxicity effects on cancer cells. BMC Complement Altern Med. (2019) 19:114. doi: 10.1186/s12906-019-2528-2
239. Iwama T, Fujiya M, Konishi H, Tanaka H, Murakami Y, Kunogi T, et al. Bacteria-derived ferrichrome inhibits tumor progression in sporadic colorectal neoplasms and colitis-associated cancer. Cancer Cell Int. (2021) 21:21. doi: 10.1186/s12935-020-01723-9
240. Ohkami H, Tazawa K, Yamashita I, Shimizu T, Murai K, Kobashi K, et al. Effects of apple pectin on fecal bacterial enzymes in azoxymethane-induced rat colon carcinogenesis. Japanese J Cancer Res. (1995) 86:523–9. doi: 10.1111/j.1349-7006.1995.tb02429.x
241. McFarland LV. From yaks to yogurt: the history, development, and current use of probiotics. Clin Infect Dis. (2015) 60:S85–90. doi: 10.1093/cid/civ054
242. O’Toole PW, Marchesi JR, Hill C. Next-generation probiotics: the spectrum from probiotics to live biotherapeutics. Nat Microbiol. (2017) 2:17057. doi: 10.1038/nmicrobiol.2017.57
243. Shen H, Zhao Z, Zhao Z, Chen Y, Zhang L. Native and engineered probiotics: promising agents against related systemic and intestinal diseases. Int J Mol Sci. (2022) 23:594. doi: 10.3390/ijms23020594
244. Requena T, Martínez-Cuesta MC, Peláez C. Diet and microbiota linked in health and disease. Food Funct. (2018) 9:688–704. doi: 10.1039/C7FO01820G
245. Fotiadis CI, Stoidis CN, Spyropoulos BG, Zografos ED. Role of probiotics, prebiotics and synbiotics in chemoprevention for colorectal cancer. World J Gastroenterol. (2008) 14:6453. doi: 10.3748/wjg.14.6453
246. Lu K, Dong S, Wu X, Jin R, Chen H. Probiotics in cancer. Front Oncol. (2021) 11. doi: 10.3389/fonc.2021.638148
247. Salemi R, Vivarelli S, Ricci D, Scillato M, Santagati M, Gattuso G, et al. Lactobacillus rhamnosus GG cell-free supernatant as a novel anti-cancer adjuvant. J Transl Med. (2023) 21:195. doi: 10.1186/s12967-023-04036-3
248. Śliżewska K, Markowiak-Kopeć P, Śliżewska W. The role of probiotics in cancer prevention. Cancers (Basel). (2020) 13:20.
249. Brevi A, Zarrinpar A. Live biotherapeutic products as cancer treatments. Cancer Res. (2023) 83:1929–32. doi: 10.1158/0008-5472.CAN-22-2626
250. Paquet JC, Claus SP, Cordaillat-Simmons M, Mazier W, Rawadi G, Rinaldi L, et al. Entering first-in-human clinical study with a single-strain live biotherapeutic product: input and feedback gained from the EMA and the FDA. Front Med (Lausanne). (2021) 8:716266. doi: 10.3389/fmed.2021.716266
251. Swanson KS, Gibson GR, Hutkins R, Reimer RA, Reid G, Verbeke K, et al. The International Scientific Association for Probiotics and Prebiotics (ISAPP) consensus statement on the definition and scope of synbiotics. Nat Rev Gastroenterol Hepatol. (2020) 17:687–701. doi: 10.1038/s41575-020-0344-2
252. Salminen S, Collado MC, Endo A, Hill C, Lebeer S, Quigley EMM, et al. The International Scientific Association of Probiotics and Prebiotics (ISAPP) consensus statement on the definition and scope of postbiotics. Nat Rev Gastroenterol Hepatol. (2021) 18:649–67. doi: 10.1038/s41575-021-00440-6
253. Iida N, Dzutsev A, Stewart CA, Smith L, Bouladoux N, Weingarten RA, et al. Commensal bacteria control cancer response to therapy by modulating the tumor microenvironment. Sci (1979). (2013) 342:967–70. doi: 10.1126/science.1240527
254. Pinato DJ, Howlett S, Ottaviani D, Urus H, Patel A, Mineo T, et al. Association of prior antibiotic treatment with survival and response to immune checkpoint inhibitor therapy in patients with cancer. JAMA Oncol. (2019) 5:1774. doi: 10.1001/jamaoncol.2019.2785
255. Geller LT, Barzily-Rokni M, Danino T, Jonas OH, Shental N, Nejman D, et al. Potential role of intratumor bacteria in mediating tumor resistance to the chemotherapeutic drug gemcitabine. Sci (1979). (2017) 357:1156–60. doi: 10.1126/science.aah5043
256. Derosa L, Hellmann MD, Spaziano M, Halpenny D, Fidelle M, Rizvi H, et al. Negative association of antibiotics on clinical activity of immune checkpoint inhibitors in patients with advanced renal cell and non-small-cell lung cancer. Ann Oncol. (2018) 29:1437–44. doi: 10.1093/annonc/mdy103
257. Kabwe M, Dashper S, Bachrach G, Tucci J. Bacteriophage manipulation of the microbiome associated with tumour microenvironments-can this improve cancer therapeutic response? FEMS Microbiol Rev. (2021) 45.
258. Petrovic Fabijan A, Lin RCY, Ho J, Maddocks S, Ben Zakour NL, Iredell JR, et al. Safety of bacteriophage therapy in severe Staphylococcus aureus infection. Nat Microbiol. (2020) 5:465–72. doi: 10.1038/s41564-019-0634-z
259. Cuomo P, Papaianni M, Fulgione A, Guerra F, Capparelli R, Medaglia C. An innovative approach to control H. pylori-induced persistent inflammation and colonization. Microorganisms. (2020) 8:1214. doi: 10.3390/microorganisms8081214
260. Gogokhia L, Buhrke K, Bell R, Hoffman B, Brown DG, Hanke-Gogokhia C, et al. Expansion of bacteriophages is linked to aggravated intestinal inflammation and colitis. Cell Host Microbe. (2019) 25:285–99. doi: 10.1016/j.chom.2019.01.008
261. Turnbaugh PJ, Ley RE, Mahowald MA, Magrini V, Mardis ER, Gordon JI. An obesity-associated gut microbiome with increased capacity for energy harvest. Nature. (2006) 444:1027–31. doi: 10.1038/nature05414
262. Donohoe DR, Holley D, Collins LB, Montgomery SA, Whitmore AC, Hillhouse A, et al. A gnotobiotic mouse model demonstrates that dietary fiber protects against colorectal tumorigenesis in a microbiota- and butyrate-dependent manner. Cancer Discovery. (2014) 4:1387–97. doi: 10.1158/2159-8290.CD-14-0501
263. Han K, Nam J, Xu J, Sun X, Huang X, Animasahun O, et al. Generation of systemic antitumour immunity via the in situ modulation of the gut microbiome by an orally administered inulin gel. Nat BioMed Eng. (2021) 5:1377–88. doi: 10.1038/s41551-021-00749-2
264. Zhang PF, Xie D. Targeting the gut microbiota to enhance the antitumor efficacy and attenuate the toxicity of CAR-T cell therapy: a new hope? Front Immunol. (2024) 15. doi: 10.3389/fimmu.2024.1362133
265. Huang J, Duan F, Xie C, Xu J, Zhang Y, Wang Y, et al. Microbes mediated immunogenic cell death in cancer immunotherapy. Immunol Rev. (2024) 321:128–42. doi: 10.1111/imr.v321.1
266. Baruch EN, Youngster I, Ben-Betzalel G, Ortenberg R, Lahat A, Katz L, et al. Fecal microbiota transplant promotes response in immunotherapy-refractory melanoma patients. Sci (1979). (2021) 371:602–9. doi: 10.1126/science.abb5920
267. Davar D, Dzutsev AK, McCulloch JA, Rodrigues RR, Chauvin JM, Morrison RM, et al. Fecal microbiota transplant overcomes resistance to anti–PD-1 therapy in melanoma patients. Sci (1979). (2021) 371:595–602. doi: 10.1126/science.abf3363
268. Shi S, Chu Y, Liu H, Yu L, Sun D, Yang J, et al. Predictable regulation of survival by intratumoral microbe-immune crosstalk in patients with lung adenocarcinoma. Microbial Cell. (2024) 11:29–40. doi: 10.15698/mic2024.02.813
269. Choi JK, Naffouje SA, Goto M, Wang J, Christov K, Rademacher DJ, et al. Cross-talk between cancer and Pseudomonas aeruginosa mediates tumor suppression. Commun Biol. (2023) 6:16. doi: 10.1038/s42003-022-04395-5
270. Inamura K. Oral–gut microbiome crosstalk in cancer. Cancers (Basel). (2023) 15:3396. doi: 10.3390/cancers15133396
Keywords: microbiome, cancer, micronutrients, probiotics, dysbiosis
Citation: Bhatnagar K, Jha K, Dalal N, Patki N, Gupta G, Kumar A, Kumar A and Chaudhary S (2024) Exploring micronutrients and microbiome synergy: pioneering new paths in cancer therapy. Front. Immunol. 15:1442788. doi: 10.3389/fimmu.2024.1442788
Received: 02 June 2024; Accepted: 18 November 2024;
Published: 29 November 2024.
Edited by:
Ofer Reizes, Cleveland Clinic, United StatesReviewed by:
Mukulika Bose, University of Texas MD Anderson Cancer Center, United StatesGeorge Grant, Independent Researcher, Aberdeen, United Kingdom
Copyright © 2024 Bhatnagar, Jha, Dalal, Patki, Gupta, Kumar, Kumar and Chaudhary. This is an open-access article distributed under the terms of the Creative Commons Attribution License (CC BY). The use, distribution or reproduction in other forums is permitted, provided the original author(s) and the copyright owner(s) are credited and that the original publication in this journal is cited, in accordance with accepted academic practice. No use, distribution or reproduction is permitted which does not comply with these terms.
*Correspondence: Sarika Chaudhary, c2FyaWthLmNoYXVkaGFyeUBiZW5uZXR0LmVkdS5pbg==
†These authors share first authorship