- The Bloomberg~Kimmel Institute for Cancer Immunotherapy, Johns Hopkins University School of Medicine, Baltimore, MD, United States
In recent years, immunotherapy has emerged as a crucial component of cancer treatment. However, its efficacy remains limited across various cancer types, highlighting unmet needs. Poliovirus receptor-related 2 (PVRL2) and Poliovirus receptor (PVR) are members of the Nectin and Nectin-like Molecules family, known for their role as cell-cell adhesion molecules. With the development of immunotherapy, their involvement in tumor immune mechanisms as immune checkpoint factors has garnered significant attention. PVRL2 and PVR are predominantly expressed on tumor cells and antigen-presenting cells, binding to PVRIG and TIGIT, respectively, which are primarily found on T and NK cells, thereby suppressing antitumor immunity. Notably, gynecological cancers such as ovarian and endometrial cancers exhibit high expression levels of PVRL2 and PVR, with similar trends observed in various other solid and hematologic tumors. Targeting these immune checkpoint pathways offers a promising therapeutic avenue, potentially in combination with existing treatments. However, the immunomodulatory mechanism involving these bindings, known as the DNAM-1 axis, is complex, underscoring the importance of understanding it for developing novel therapies. This article comprehensively reviews the immunomodulatory mechanisms centered on PVRL2 and PVR, elucidating their implications for various cancer types.
1 Introduction
In recent years, immunotherapy has become a new treatment modality for solid and non-solid cancers (1–3). It has become an essential pillar of cancer treatment, along with surgery, chemotherapy, and radiation therapy. Among immunotherapies, anti-CTLA4 and anti-PD-1/PD-L1 antibodies are the most common immune checkpoint inhibitors (ICIs) for solid tumors (3). Starting with the 2011 FDA approval of the anti-CTLA4 antibody, Ipilimumab, for metastatic melanoma (4), the anti-PD-1 antibodies (e.g., nivolumab, pembrolizumab, dostarlimab) and the anti-PD-L1 antibodies (e.g., atezolizumab, durvalumab, avelumab) have been FDA-approved for a variety of cancers (5). ICIs are a very promising treatment, sometimes affecting dramatically in cases that would have otherwise had no effective treatment (5). On the other hand, the therapeutic efficacy of existing ICIs is limited, and even in potentially effective carcinomas, response rates are limited to 20-40% (6). In addition, there are many cases in which acquired resistance develops during the treatment course (7, 8). Therefore, various attempts have been made to improve therapeutic efficacy, including developing new ICIs and combination therapy. Recently, combination therapy with a new ICI, anti-LAG3 antibody (relatlimab) and anti-PD-1 antibody (nivolumab) was shown to be effective in melanoma and was FDA-approved (9).
Recently, the Poliovirus receptor-related 2 (PVRL2)-Poliovirus receptor-related immunoglobulin (Ig) domain containing (PVRIG) pathway and the Poliovirus receptor (PVR)-T cell immunoreceptor with Ig and immunoreceptor tyrosine-based inhibitory motif (ITIM) domains (TIGIT) pathway have been focused on as potential new targets for cancer immunotherapy (10). These pathways are entirely different from the CTLA4 and PD-1/PD-L1 pathways, which have been previously targeted. The DNAX Accessory Molecule-1 (DNAM1) axis, including the PVRL2-PVRIG and PVR-TIGIT pathways, is a complex immunoregulatory mechanism, but recent studies have revealed much. This review article will focus on PVRL2 and PVR, which play vital roles in the DNAM1 axis, from the viewpoint of tumor immunity.
2 PVRL2 and PVR as the Nectin and Nectin-like molecule family
PVRL2, also known as Nectin-2, CD112, or PRR2, is a member of the Nectin family. PVR, also known as Necl-5, CD155, or TAGE4, has a domain structure like nectin and is one of the Nectin-like Molecules (Necls) (11–13). The Nectin and Necl Family have been identified to date as four types of Nectins (Nectin-1 to Nectin-4) and five types of Necls (Necl-1 to Necl-5), but they were discovered in different ways and given diverse names (11–13). In this article, the terminology is unified as PVRL2 and PVR.
Nectin and Necl family are immunoglobulin-like transmembrane cell adhesion molecules expressed in various cell types (14–16). Nectins are mainly involved in cell-cell adhesion, and Necls have a greater variety of cellular functions (14). They are involved in the organogenesis of sperm, eyes, inner ear, teeth, cerebral cortex, and nerves (12). Cell-cell adhesion can be involved in various diseases, among which the Nectin and Necl family is well known to be associated with Alzheimer's disease, mental disorders, viral infections, and cancer (12). In cancer, the expression of adhesion factors on the cell surface plays an essential role in dissemination, metastasis, and growth (17). The Nectin and Necl family also plays a vital role as a member of immunomodulatory mechanisms and is deeply involved in tumor progression (11).
Cell-cell adhesion is mediated by tight junctions, adherens junctions, and desmosomes, and the Nectin and Necl family localizes to adherens junctions (16). Nectin has three extracellular Ig-like domains (one variable region and two constant regions) followed by a transmembrane region and a cytoplasmic tail (Figure 1) (15). The cytoplasmic tail of nectin other than nectin-4 contains a conserved Afadin binding motif, Glu/Ala-X-Tyr-Val, to which the PDZ domain of Afadin binds, causing nectin to bind to actin filaments (18). Necls have the same domain structure as nectin but do not have an Afadin binding motif in their cytoplasmic tail (Figure 1) (19). PVR most closely resembles nectin compared to other Necls, with PVRL2 and PVR having 51.7% amino acid sequence identity in the extracellular region (12, 20).
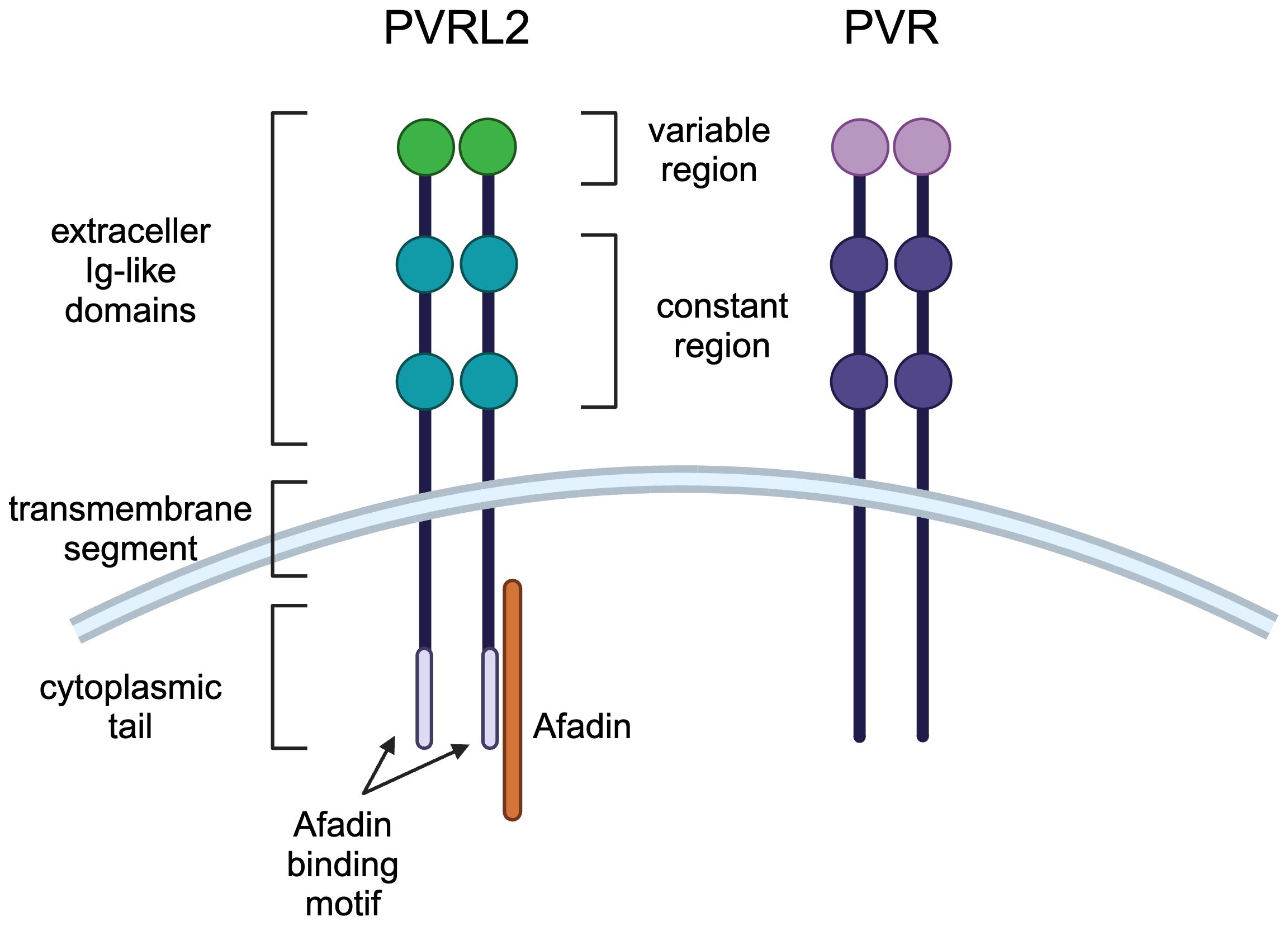
Figure 1 Molecular structures of PVRL2 and PVR. PVRL2 and PVR have the same structure of three extracellular Ig-like domains (one variable region and two constant regions) followed by a transmembrane region and a cytoplasmic tail. PVRL2 has an Afadin binding motif in the cytoplasmic tail, but PVR doesn't have it.
Cell adhesion molecules form trans dimers and mediate cell-cell adhesion by trans interaction (12). Cadherins form only trans homo dimers, whereas the Nectin and Necl families form not only trans homo dimers but also trans hetero dimers between family members (14, 19, 21). PVRL2 has homophilic and heterophilic and PVR has heterophilic cell-cell adhesion activity (12, 21). Unlike cadherins, the Nectin and Necl family is also expressed in immune cells such as T and NK cells and is characterized by its involvement in immune mechanisms (22).
3 The function of the PVRL2 and PVR in the immune system
While PVRL2 and PVR are known to be expressed on many tumor cells, they are also expressed on immune cells and widely regulate cell-cell interactions (22). However, most reports on PVRL2 and PVR in the immune system focus on the interaction of PVRL2 and PVR expressed in tumor cells with T and NK cells. There is a lack of reports on how T and NK cells interact with PVRL2 and PVR expressed in immune cells, especially myeloid cells. In this section, we mainly summarize the functions of PVRL2 and PVR expressed on tumor cells, focusing on their immune system regulation (Figure 2).
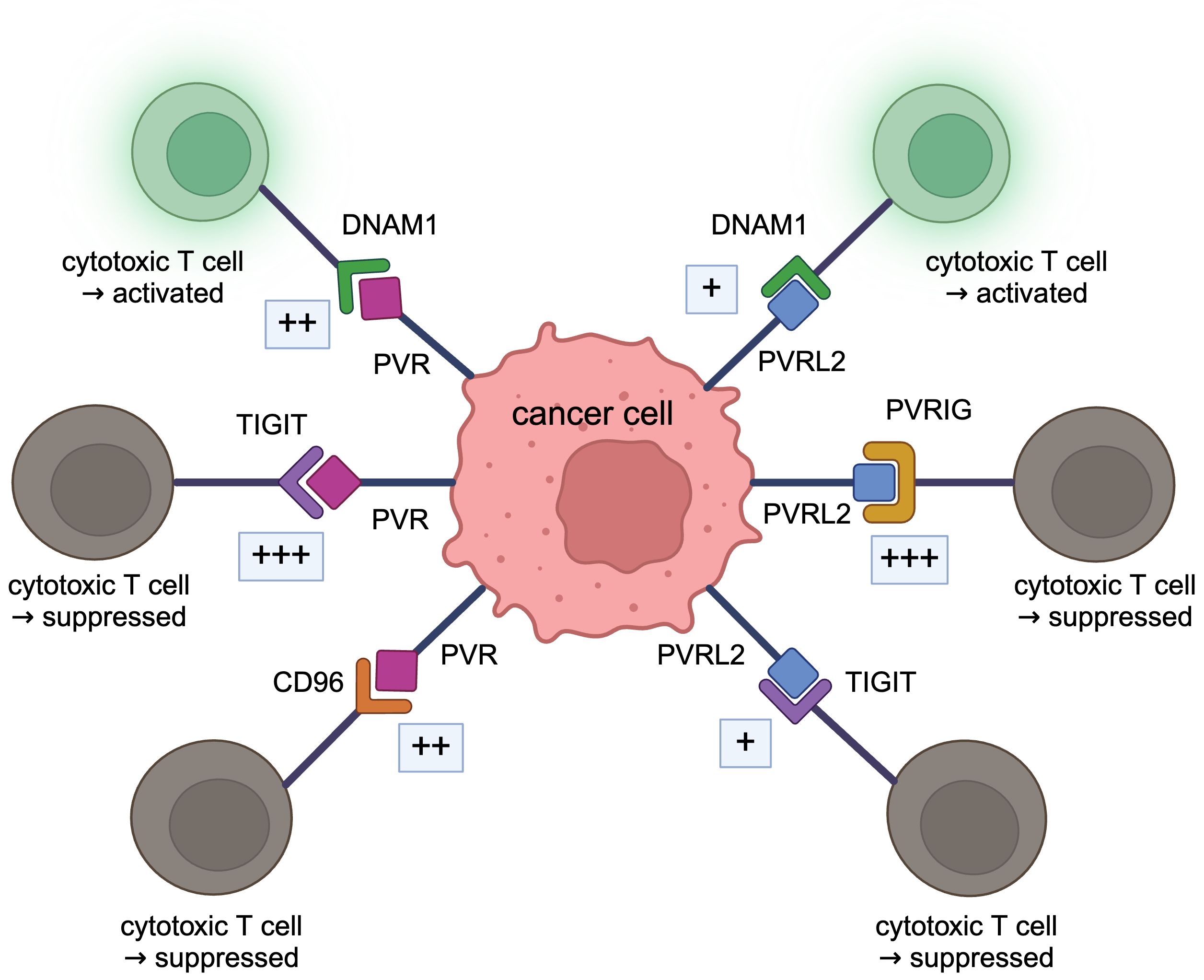
Figure 2 DNAM1 axis. PVRL2 binds to PVRIG and TIGIT, and PVR binds to TIGIT and CD96, acting in a tumor immunosuppressive manner. These bindings are stronger than the immunostimulatory bindings of DNAM1 to PVRL2 or PVR.
3.1 PVRL2 (Nectin-2, CD112)
PVRL2 was reported as Poliovirus Receptor Related 2 (PRR2), a cell surface molecule homologous to PVR (15). The gene encoding this molecule has two mRNA isoforms, a 3.0 kb short form and a 4.4 kb long form (15). PVRL2 is ubiquitously expressed in various cells, including epithelial cells, endothelial cells, smooth muscle cells, and fibroblasts (23, 24). However, PVRL2 expression in immune cells is specific to myeloid cells, with little expression in lymphocytes (15). PVRL2 is also characterized by high expression in Sertori and Leydig cells in the testis and is involved in spermatogenesis (25). Disruption of PVRL2 results in male infertility (25). PVRL2 is also expressed in vascular endothelial cells and regulates angiogenesis (23, 24).
In the tumor immune axis, PVRL2 is expressed on tumor cells and tumor-infiltrating immune cells, especially macrophages, and is involved in tumor growth and metastasis (12, 13). For example, PVRL2 is highly expressed in CD14+ cells (as tumor-associated macrophages) and CD45- cells (as tumor cells) in breast, endometrial, ovarian, lung, and liver cancers (26). Regarding regulation of expression on tumor cells, PVRL2 is primarily expressed in the cytoplasm in tumor cell lines, and inhibition of the ubiquitin pathway has been shown to increase surface expression of PVRL2 and increase tumor cell sensitivity to NK cell cytotoxicity (27).
3.2 PVR (Necl-5, CD155)
In 1989, it was reported that human PVR is a transmembrane protein with an amino acid and domain structure like nectins, which is characteristic of the Ig superfamily and is expressed in various human tissues (28). Like PVRL2, PVR is expressed on epithelial cells, endothelial cells, smooth muscle cells, and fibroblasts, as well as on immunocompetent cells such as Neutrophils, T cells, and plasma cells (29). Quantification of PVR mRNA in normal human tissues shows the highest expression in the liver (30). Soluble PVR isoforms are present in human serum and cerebrospinal fluid (30, 31).
PVR is involved in cell migration; PVR has been shown to interact with the platelet-derived growth factor (PDGF) receptor to regulate the interaction between the PDGF receptor and integrin, effectively inducing directional cell migration (32). PVR also attracts growing microtubules to the plasma membrane of the leading edge of moving cells (33). PVR is also involved in cell proliferation; PVR has been shown to promote activation of Ras-Raf-MEK-ERK signaling, increase cyclin D2 and E expression, decrease p27Kip1, and shorten the G0/G1 phase of the cell cycle (34). It has also been suggested that PVR may regulate angiogenesis by vascular endothelial growth factor (VEGF) (35). In the immune system, PVR is involved in negative selection in the thymus using a PVR-deficient mouse model (36). A pathway dependent on the DNA damage response is involved in the elevated expression of PVR (37). It has also been shown that DNA damage downregulates PVR expression (38, 39). Hedgehog signaling is often activated in cancer (40), and PVR is upregulated by the signaling (41). PVR expression is induced through the Raf-MEK-ERK-AP-1 pathway by upregulating fibroblast growth factor or KRAS (42).
3.3 Receptor expression and regulatory mechanisms
As receptors for PVRL2 in the immune system, Poliovirus receptor-related immunoglobulin domain containing (PVRIG, CD112R), T cell immunoreceptor with Ig and ITIM domains (TIGIT, VSTM3, VSIG9, WUCAM), DNAX Accessory Molecule-1 (DNAM1, CD226, TLiSA1) are known. The binding of PVRL2 to PVRIG or PVRL2 to TIGIT suppresses tumor immunity, whereas the binding of PVRL2 to DNAM1 promotes tumor immunity (10). TIGIT, DNAM1, and CD96 (TACTILE) are known receptors for PVR in the immune system; binding of PVR to TIGIT suppresses tumor immunity while binding to DNAM1 promotes tumor immunity; binding to CD96 acts both to suppress and promote tumor immunity (10).
PVRIG is a transmembrane protein consisting of an extracellular IgV domain, a transmembrane domain, and an intracellular ITIM-like motif with a high affinity for PVRL2 (43). PVRIG is expressed in human T and NK cells and functions as an immune checkpoint pathway (43). PVRIG is not expressed in dendritic cells, neutrophils, monocytes, or B cells (43). The expression of PVRIG in T cells can vary with the expression of other immune checkpoint factors. For example, PVRIG has been shown to correlate with the expression of T cell exhaustion markers such as PD-1 and TIGIT in CD8+ T cells and CD4+ T cells (26). It has also been shown that the blockade of PVRIG increases TIGIT expression, but the blockade of TIGIT or PD-1 does not alter PVRIG expression (26).
TIGIT is a member of the Ig superfamily and is a transmembrane protein that contains an extracellular IgV domain, a transmembrane domain, and a cytoplasmic tail containing an ITIM and an Ig tail tyrosine (ITT)-like motif (44). TIGIT is expressed on various T cell subsets, NK, and NKT cells in humans (44–50). TIGIT is a receptor for PVRL2 and PVR (44, 51). TIGIT is the most crucial receptor for PVR; compared to the binding of PVRL2 and TIGIT, the binding of PVR to TIGIT is very strong and strongly immunosuppressive (44). The TIGIT/PVR pathway suppresses IFN-γ production in NK cells (52). TIGIT is highly expressed predominantly in tumor-infiltrating T cells and plays a vital role in suppressing the activity of CD8+ T cells (53). TIGIT inhibits T cell cytotoxic function by competing with DNAM1, as discussed below (54). The binding of PVRL2 to TIGIT is much weaker than that of PVR to TIGIT, suggesting that it does not play a significant role in the tumor immune system.
CD96, another receptor for PVR, was reported in 1992 as a member of the Ig superfamily that activates T cells (55). CD96 is mainly expressed in T and NK cells (55, 56) and highly expressed in tumor-infiltrating CD8+ T cells (56, 57). It was also reported in 2014 that CD96 competes with and directly inhibits the binding of DNAM1 and PVR, thereby limiting NK cell function (58). CD96 is also expressed on tumor cells and is associated with chemotherapy resistance and poor prognosis (59).
DNAM1 is a member of the Ig superfamily of transmembrane proteins with two extracellular IgV domains and an intracellular ITIM-like motif (60). It is expressed in T, NK, B, and monocytes (60–64). It binds to both PVRL2 and PVR and promotes activation of T, NK, B, and monocytes (60–64). The expression of PVR enhances NK cell activity via the DNAM1 pathway (65). DNAM1, unlike PVRIG and TIGIT, exhibits antitumor activity by enhancing the cytotoxic activity of immune cells through ligand binding (66, 67). On the other hand, it has recently been reported that under inflammatory conditions, DNAM1 promotes IFN-γ secretion by conventional CD4+ T cells and contributes to tumorigenesis (68). The interaction between PVRL2 and DNAM1 requires the homodimerization or engagement of the homodimeric interface of PVRL2 IgV (22). DNAM1 has a soluble form and can bind to PVRL2 or PVR on tumor cells (69).
3.4 Relationships of PVRL2 and PVR in the DNAM1 axis
DNAM1 axis, including PVRL2 and PVR, is very complex (10), and the relationship needs to be well organized. PVRL2 and PVR are both ligands for DNAM1 (62, 63). Both PVRL2 and PVR, which are expressed on antigen-presenting cells, act in an immunostimulatory manner when bound to DNAM1 to activate T and NK cells (70), but the binding between PVR and DNAM1 is stronger than that between PVRL2 and DNAM1 (63). PVRL2 binds to PVRIG and is expressed on T and NK cells, acting immunosuppressively. Interestingly, the immunosuppressive binding of PVRL2 to PVRIG is stronger than the immunostimulatory binding of PVRL2 to DNAM1 (43). PVRIG binds specifically to PVRL2, and there are no reports of PVRIG acting with PVR. Both PVRL2 and PVR also bind to TIGIT and inhibit the cytotoxic activity of immune cells (46). The action of PVRL2 and TIGIT is the same as that of PVR and TIGIT (71). In the past, TIGIT expressed on human NK cells was shown to bind to PVR and PVRL2 and inhibit tumor killing by NK cells (46). Recently, however, it has been demonstrated that the binding of PVRL2 to TIGIT is very weak, with little interaction (26, 43, 51). In mice, binding to TIGIT is restricted to PVR (72). The binding of PVR to TIGIT is very strong and exhibits strong immunosuppressive activity (44). The binding of PVR to TIGIT is stronger than PVR to DNAM1 (44, 73). PVR also binds to CD96, expressed in T and NK cells (55). This binding has been shown to stimulate the cytotoxicity of activated NK cells and act in an immunostimulatory manner (74), while anti-CD96 antibodies stimulate the cytotoxic function of NK cells (75). Signaling through CD96 has also been shown to enhance the cytotoxic activity of CD8+ effector T cells (76). Therefore, whether this pathway acts in an immunostimulatory or inhibitory direction is not constant. The affinity between CD96 and PVR is higher than that between PVR and DNAM1 but lower than between PVR and TIGIT (43, 44).
Blockade of each pathway of the DNAM1 axis has been shown to affect the regulation of immune mechanisms. In PVRIG-deficient mouse models of melanoma and colorectal cancer, tumor growth is suppressed, and tumor-infiltrating CD8+ T cells are increased (77). PVRIG inhibition effectively suppresses tumor growth and prolongs the survival of tumor-bearing mice, which is associated with enhanced frequency and cytotoxicity of tumor-infiltrating NK cells (78). TIGIT inhibition also activates T cells (79). Although blockade of either PVRIG or TIGIT slightly increases mitosis and cytokine production in CD4+ T cells (43), double block of PVRIG and TIGIT has been shown to enhance significantly CD4+ T cell proliferation and cytokine secretion, including IFN-γ, IL-13, IL-10, IL-5, IL-13, IL-10, IL-5, IL-2, and enhances the cytotoxicity of CD8+ T cells (26, 43). It also reduces the cytotoxicity of T cells and NK cells by blocking DNAM1 (62, 63, 73). It has also been shown that increased CD8+ T cells with high DNAM1 expression improve response to anti-TIGIT therapy (80).
4 PVRL2 and PVR in cancer
So far, we have discussed the complex expression of PVRL2 and PVR and their immunomodulatory mechanisms. In this section, we summarize the expression of PVRL2 and PVR in cancer and their effects on tumor immunoregulatory mechanisms (Table 1).
In 2013, gene expression profiling and immunohistochemistry (IHC) showed that PVRL2 is overexpressed in breast and ovarian cancer clinical tissues (81). Subsequently, The Cancer Genome Atlas Program has published mRNA expression data for several cancers and found high expression of PVRL2 mRNA in breast, ovarian, prostate, endometrial, gastric, liver, pancreatic, and lung cancers, with no carcinoma specificity (26). Expression of PVRL2 is found in both PD-L1 negative and PD-L1 positive tumors (26).
PVR is overexpressed in many cancers compared to normal tissue and has been shown to correlate with poor prognosis (82, 83). It has also been demonstrated that PVR expression correlates with poor prognosis in many cancers (82). Even if there is no prognostic difference by PD-L1 expression, there may be a prognostic difference by PVR expression (84). It has also been shown that patients with lung, gastrointestinal, breast, and gynecological cancers have higher levels of soluble PVR in their serum than healthy donors (31).
Depending on the type of cancer, either PVRL2 or PVR may be expressed predominantly. Breast, ovarian, endometrial, and prostate cancers express PVRL2 predominantly, while melanoma, esophageal, and colorectal cancers express PVR predominantly (26). There are also many reports on ligands for PVRL2 and PVR in cancer. PVRIG, the ligand for PVRL2, is upregulated in various carcinomas, including kidney, ovary, lung, prostate, and endometrial cancer (26). As mentioned above, TIGIT functions particularly strongly as a ligand for PVR and is highly expressed in tumor-infiltrating lymphocytes in many cancers, including endometrial, breast, renal cell, non-small cell lung, and colon cancer (53). It has also been shown that TIGIT+ CD8+ T cells frequently co-express PD-1 in melanoma and ovarian high-grade serous carcinomas (82, 85).
Heterogeneity of expression of immune checkpoint pathways in tumors is important for considering tumor immunity, but there are still very few reports on the heterogeneity of DNAM1 axis expression so far. Only one report has shown that the expression of PVR, PVRL2, TIGIT, PD-1, and PD-L1 in lung adenocarcinoma is heterogeneous within and between tumors and varies according to tumor growth patterns (86), so future reports are expected. In addition, even though PVRL2 and PVR are known to be expressed on both tumor cells and antigen-presenting cells (especially macrophages), most studies have focused on the expression on tumor cells.
Another critical factor in cancer is the change in expression that occurs with treatment, such as chemotherapy. In vitro, Adriamycin treatment of breast cancer cell lines increases PVR expression (39). The combination of PVR knockdown and Adriamycin administration induces more cell death and inhibits tumor growth than either one (39). Myeloma cells treated with doxorubicin, melphalan, and bortezomib also upregulate PVRL2 and PVR expression and suppress the antitumor effects of NK cells (87). Thus, it is suggested that chemotherapy causes changes in the expression of PVR and PVRL2, but there are still only a few reports. However, these effects must be considered in clinical practice.
5 What is known in detail about each cancer type?
5.1 Gynecologic Müllerian cancer
As mentioned above, PVRL2 and PVR are highly expressed in ovarian and endometrial cancers. Therefore, the significance of PVRL2 and PVR in these gynecologic Müllerian cancers is very important.
5.1.1 Ovarian cancer
Ovarian cancer is one of the poorest prognoses among gynecologic cancers (88). Among epithelial ovarian cancer, about three-quarters are high-grade serous ovarian cancer (HGSOC) (89). HGSOC have a modest immunogenic repertoire (90). However, the tumor immune microenvironment is known to be highly suppressive (91). In the phase 3 clinical trials, ovarian cancer has a limited response to existing ICIs (92–96), and new therapeutic targets and combination therapies are expected to be developed.
In 2007, it was shown that PVR is expressed in ovarian cancer cells and enhances NK cell activity via the DNAM1 pathway (97). In clinical samples, PVR was also expressed well in tumor epithelial cells of HGSOC (82). In this report, PVR expression did not correlate with tumor-infiltrating lymphocytes, whereas PD-L1 was highly expressed on tumor-associated macrophages and positively correlated with TILs, indicating that PVR and PD-L1 have different expression patterns (82).
Recently, focal adhesion kinase (FAK) was reported to function in tumor immunosuppression of ovarian cancer via the PVR/TIGIT pathway (98). In this report, it was shown that FAK and PVR are coexpressed in tumor cells in HGSOC and that the combination of FAK inhibitors and anti-TIGIT antibodies maintains elevated TIL levels, decreases TIGIT-positive Tregs, prolongs survival, increases CXCL13, and leads to the formation of tertiary lymphoid structure in the ovary (98).
In 2013, gene expression profiling and immunohistochemical studies first demonstrated that PVRL2 is overexpressed in clinical ovarian cancer specimens and various human ovarian cancer cell lines (81). In another cohort, PVRL2 was identified as the highly expressed transcript, and immunohistochemistry confirmed that it is overexpressed in HGSOC samples compared to normal or benign samples (99). Similar results were also confirmed by analysis of gene expression data from TCGA (99). Bekes et al. reported that in a cohort of 60 patients with ovarian cancer, PVRL2 expression of patients with lymph node metastases or residual tumors after surgery had higher levels of PVRL2 gene expression than those with negative lymph nodes or complete tumor resection (100).
Regarding the tumor microenvironment, peritoneal biopsies showed clear co-localization of PVRL2 and CD31 in the vasculature, and PVRL2 expression was suppressed in the peritoneal endothelium of patients with high blood VEGF levels (100). In vitro, it has been reported that VEGF causes downregulation of PVRL2 and that PVRL2 knockdown in endothelial cells is associated with increased endothelial permeability (100). HGSOC often results in ascites accumulation, and PVRL2 may be a contributing factor.
However, the role of the DNAM1 axis in ovarian cancer through tumor immune mechanisms is still poorly understood. In addition, previous studies have focused on HGSOC. The frequency of endometriosis-associated ovarian cancers (EAOC), including clear cell carcinoma, is high in Japan and other Asian countries (101). The mechanism of occurrence of EAOC is entirely different from that of HGSOC (102). Clinical trials have suggested the efficacy of ICI for clear cell carcinoma (103, 104), and studies involving the DNAM1 axis are also expected.
5.1.2 Endometrial cancer
Endometrial cancer is the fourth most common cancer in women and the most common gynecologic cancer in the United States (105). Endometrial cancer is an important disease because, unlike many other cancers, the number of patients is increasing (106). Hypermutators due to POLE mutations are present in about 10% of cases of endometrial cancer (107). In endometrial cancer, the frequency of DNA mismatch repair deficiency or microsatellite instability-high is about 30%, the highest among all cancer types (108). These endometrial cancers have high neoantigen loads and many TILs and are highly immunogenic (109). The efficacy of anti-PD-1 antibodies against endometrial cancer has also been shown in a clinical trial (110), suggesting that endometrial cancer and immunotherapy are compatible.
Analysis using TCGA data shows that both PVRL2 and PVR are highly expressed in endometrial cancer, but PVRL2 expression is more predominant (26). PVR+PVRL2+ tumor cells are the most abundant compared to other cancers and have higher PVRIG expression (26). It has also been reported that cancer-associated fibroblasts derived from endometrial cancer have decreased PVR expression and more substantial suppression of the cytotoxic activity of NK cells (111).
However, even though the DNAM1 axis seems to be a promising therapeutic target for endometrial cancer, no detailed studies have been reported other than these, and future development is expected.
5.2 Other solid tumors
5.2.1 Liver cancer
Hepatocellular carcinoma (HCC) has a moderate tumor mutational burden (90). In the tumor immune microenvironment of HCC, PD-1 expression is upregulated in lymphocytes, and PD-L1 and PD-L2 expression is also upregulated in Kupffer cells and hepatic sinusoidal endothelial cells (112). Inflammatory response with PD-1 and PD-L1 overexpression is seen in 25% of HCC samples (112).
It has been shown by bulk and single-cell RNA sequencing and IHC that PVRL2 is overexpressed in HBV-associated hepatocellular carcinoma (113). A liver cancer model using PVRL2 knockout mice has been shown to restore T cell infiltration into tumors and reduce T cell exhaustion, suppressing tumor growth (113). On the other hand, a study of 159 human subjects diagnosed with hepatocellular carcinoma showed that PVRL2 expression in tumor specimens was lower than that in peritumoral liver tissue, and low PVRL2 expression was associated with poor prognosis (114), so the significance is controversial. Single-cell RNA seq analysis of hepatocellular carcinoma also suggests that blockade of the PVR/PVRL2 and TIGIT pathways may exert an antitumor effect (115).
5.2.2 Pancreas cancer
Pancreatic ductal carcinoma (PDA) is one of the tumors with extremely low immunogenicity (90). Despite promising Phase 1 clinical trials, subsequent trials have not shown the efficacy of existing anti-PD-1/PD-L1 or anti-CTLA4 antibodies, except in mismatch repair deficient cases (116). Thus, immunotherapy for PDA remains challenging.
PVRL2 expression is found in about half of cases of pancreatic cancer but not in benign lesions or normal pancreatic tissue (117). PVR expression is also higher in the tumor than adjacent normal tissue (118). PVRL2 and PVR expression have been reported to be poor prognostic factors (117, 119, 120). It has also been reported that PVRL2 expression correlates not with prognosis but with histologic grade (121).
Regarding tumor immunity, PVR expression was inversely correlated with tumor-infiltrating lymphocytes (119). The percentage of DNAM1+ and CD96+ NK cells is significantly lower in pancreatic cancer patients than in healthy controls, and reduced percentages of DNAM1+ and CD96+ NK cells are associated with tumor histologic grade and lymph node metastasis (118). In a pancreatic cancer pre-clinical model, combining a neoantigen vaccine and anti-TIGIT/anti-PD-1 antibody enhances anti-tumor effects (122). A detailed analysis of human samples showed that pancreatic cancer has many TRM cells with a PD-1+TIGIT+ phenotype, and the promising potential of double blockade of PD-1 and TIGIT has been suggested (123).
5.2.3 Gastric cancer
Esophagogastric adenocarcinoma (EGAC) is considered to have a moderate tumor mutational burden (90). Immunotherapy for EGAC is promising. Phase 3 clinical trials have shown the efficacy of anti-PD-1 antibodies, which are already FDA-approved (124).
TIGIT-positive and PD-1-positive tumor-infiltrating CD8-positive T cells are increased in gastric cancer (125, 126). In addition, gastric cancer tissues and cell lines overexpress PVRL2 and PVR (125, 126). Soluble PVR is significantly higher in gastric cancer patients and is reduced by surgical resection (30). High PVR, PVRL2, and TIGIT expression are associated with poor prognosis (126, 127). An immune checkpoint score system, including PVRL2 and PVR, can predict prognosis (128).
In vivo, PVR binds to TIGIT and inactivates CD8+ T cells, and targeting PVR-TIGIT promotes CD8+ T cell responses and improves survival in cancer-bearing mice (125). Furthermore, inhibition of both TIGIT and PD-1 further promotes CD8+ T cell activation and improves survival in tumor-bearing mice (125).
5.2.4 Colorectal cancer
Among colorectal cancers, mismatch repair deficient or microsatellite instability-high populations are highly immunogenic, and ICI is effective (129). However, for mismatch repair proficiency or microsatellite stability, existing ICIs are poorly effective, and new strategies are required (129).
PVR is highly expressed in patients with colorectal cancer (130). In 100 patients with stage III colorectal cancer, high expression of PVR and TIGIT were independent poor prognostic factors (131). Serum PVRL2 levels in patients with colorectal cancer were significantly higher than those in healthy controls (132). Expression of DNAM1 on CD8 T cells infiltrating liver metastases has also been shown to be a favorable prognostic factor (133).
In a colorectal cancer model, PD-L1 blockade inhibited tumor growth in PVRIG-deficient mice but not in wild-type mice (77). Furthermore, anti-PVRIG antibodies have been reported to inhibit tumor progression alone or in combination with PD-L1 inhibitors (77). These data are valuable because they demonstrate the efficacy of existing ICI and anti-PVRIG antibody combination therapy.
5.2.5 Breast cancer
Triple-negative breast cancer (TNBC), which accounts for 15-20% of all breast cancers, is one of the most promising cancers for immunotherapy (134). In the past, chemotherapy was the only option, but now, many anti-PD-1/PD-L1 and anti-CTLA4 antibodies are FDA-approved and used in clinical practice (134). However, their efficacy is still far from satisfactory, and there is a need for further development of immunotherapy.
In breast cancer patients, high PVR expression is associated with poor prognosis (135–137). In examining PVR expression and NK cell infiltration by IHC, PVR expressed on the plasma membrane strongly correlates with the tumor-infiltrating NK cells (138). PVR expression is also associated with a mesenchymal phenotype (135). PVR secreted by Brain metastasis cancer-associated fibroblasts has been shown to enhance the invasive potential of cancer cells (139). Serum-soluble PVR levels correlate with risk factors for breast cancer (140). In addition, TIGIT expression correlates with age and histologic grade in triple-negative breast cancer (141) and correlates with poor prognosis in invasive breast cancer (142). Single-cell RNA sequencing analysis suggests that the PVRL2-TIGIT pathway promotes immune escape and lymph node metastasis (143, 144).
In vitro analysis showed that blockade of PVRIG or TIGIT increased the number of IFN-γ-producing NK cells under co-culture of breast cancer cells, and the combination of anti-PVRIG and anti-TIGIT antibodies induced a further increase in IFN-γ-producing NK cells and improved cytotoxicity of NK cells (145). Although the direct relationship to immune mechanisms is unclear, the knockdown of PVR also induced mesenchymal-epithelial transition of TNBC cells, inhibited TNBC cell migration, invasion, and metastasis in vitro and in vivo, and inhibited TNBC cell growth and survival (135).
5.2.6 Melanoma
Melanoma is one of the most immunogenic tumors (90). Indeed, the development of immunotherapy has revolutionized the treatment of melanoma (146). Nevertheless, there is a need for different therapeutic strategies, as many cases do not respond to existing ICIs or become resistant to treatment at an early phase (146).
PVR is overexpressed in melanomas compared to melanocytes and benign nevi and correlates with known poor prognostic factors (147). Tumors with high PVR expression before treatment have a higher proportion of PD-1+ CD8+ T cells, which correlates with a lower response to anti-PD-1 and anti-PD-1/CTLA4 combination therapy (148).
PVR is a vital ligand recognized by DNAM1 in inhibiting NK cell-mediated melanoma metastasis (149). In vivo, soluble PVR inhibits DNAM1-mediated NK cell cytotoxic activity and promotes melanoma lung metastasis (150). TIGIT has also been well studied in melanoma and has been shown to regulate cytotoxic responses of T cells via the TIGIT-PVR pathway (85, 151). Analysis of tumor cell lines and paired TILs from ICI-treated melanoma patients showed high expression of PVR, TIGIT ligand, and TIGIT in tumor cell lines and tumor-infiltrating T cells, respectively, and functional assays showed that TIGIT blockade or PVR deletion activated T cells (10.1136/jitc-2021-003134). PVR expression was increased in surviving tumor cells after co-culture with TILs from the responder and inhibited TIGIT+ T cell activation (152). The combination of IL15 and TIGIT blockade has also been shown to restore the cytotoxicity of NK cells mediated by PVR (153).
5.2.7 Prostate cancer
Prostate cancer has low immunogenicity and a suppressive tumor immune microenvironment (154). Therefore, anti-PD-1/PD-L1 and anti-CTLA4 antibodies have minimal efficacy (154).
In castration-resistant prostate cancer, blocking the TIGIT/PVR pathway with an anti-TIGIT monoclonal antibody has been shown to enhance the antitumor effect of NK cells (155). In addition, there are reports on the expression of PVR and PVRL2 in many other cancers. The expression of PVR has been reported as a poor prognostic factor in head and neck cancer (156), esophageal cancer (157), lung cancer (84, 158–160), and bladder cancer (161, 162). In cervical cancer, PVR expression increases as cervical lesions progress (163). It has also been reported that miRNAs regulate PVR expression in lung adenocarcinomas (164). It has also been shown that PVRL2 expression is a poor prognostic factor in gallbladder cancer (165) or glial tumors of the brain (166). PVRL2 is highly expressed in esophageal squamous cell carcinoma and is associated with advanced-stage and histologic differentiation (167). It has also been shown that decreased PVR expression induces cell apoptosis and inhibits tumor cell growth by inhibiting PI3K/Akt and MAPK signaling pathways (168). However, none of these reports have directly demonstrated a role for PVRL2 or PVR in tumor immune mechanisms.
5.3 Hematologic tumor
5.3.1 Acute myeloid leukemia
TIGIT, PVR, and PVRL2 are highly expressed in AML patients, and high expression of PVR and PVRL2 correlates with poor prognosis (169–171). In vitro, inhibition of PVR2 or PVR with antibodies has a higher therapeutic effect on AML cell lines (170). Knockdown of TIGIT also restores CD8+ T cell dysfunction (169).
There are reports that PVRIG is expressed in NK cells of AML patients (172) and that DNAM1 is highly expressed in AML and is a prognostic factor (173).
5.3.2 Lymphoma
In cutaneous T-cell lymphoma (CTCL), PVR is highly expressed in tumor cells (174). It is interesting to note that the expression of DNAM1 on NK cells and CD8+ cells in the peripheral blood of CTCL patients was decreased, while DNAM1 levels in the serum were increased, strongly reflecting disease activity, suggesting that soluble DNAM1 in the serum was generated by the shedding of membrane-form DNAM1 (174).
6 Therapeutic applications and clinical trials
The front runner in therapies targeting the DNAM1 axis is an anti-TIGIT antibody. Several mechanisms have been proposed for the immunosuppressive mechanism of TIGIT: cell-extrinsic mechanisms include the interaction of TIGIT with PVR, which has been shown to regulate cytokine production by DCs and affect T cell activity (44). Cell-intrinsic mechanisms are also thought to be involved, and agonistic anti-TIGIT mAbs have been shown to inhibit human and mouse T cell proliferation and cytokine production via anti-CD3/anti-CD28 mAbs in the absence of antigen-presenting cells (47). Thus, TIGIT blockade may inhibit these mechanisms and activate T cell cytotoxicity. Since the introduction of anti-TIGIT antibodies in 2018 (175), many clinical trials are ongoing (10). TIGIT and PD-1 can be co-expressed in tumor-infiltrating lymphocytes, and inhibition of both checkpoint pathways leads to greater activation of CD8+ T cell effector functions (53). Therefore, the efficacy of combination therapy with anti-TIGIT and anti-PD-1/PD-L1 antibodies is also very promising. In non-small cell lung cancer, a phase II trial of anti-TIGIT antibody (tiragorumab) plus anti-PD-L1 antibody (atezolizumab) demonstrated an objective response rate of 31.3% vs. 16.2%, compared with placebo plus atezolizumab. Median progression-free survival was 5.4 months (95% CI: 4.2-not estimable) vs. 3.6 months (2.7-4.4) for placebo plus atezolizumab (176). Several phase III clinical trials are currently underway for anti-TIGIT antibodies. Although in the preclinical stage, the usefulness of TIGIT and PD-1 chimeric immune-checkpoint switch receptors has also been reported (177).
A highly reactive anti-PVRIG drug known as COM701 is in its first clinical trial phase (178). The combination of COM701 and COM902, an anti-TIGIT monoclonal antibody, has shown good antitumor effects in vivo and is expected to have clinical applications (179).
A clinical trial of DNAM1 agonist for solid tumors was also underway, but this study was terminated due to a strategic business decision made by the company (180).
The anti-PVRL2 monoclonal antibody has also been shown to exert anti-tumor effects by antibody-dependent cellular cytotoxicity in vitro or in vivo (81). Antibody-drug conjugates targeting PVRL2 therapeutically have also been shown to exert antitumor effects in a mouse xenograft model (181), and Fc2-modified anti-PVRL2 antibodies have been shown to exert antitumor effects with controlled adverse effects in monkeys (182). There is also a report that Bispecific anti-CD3 x anti-CD155 antibody is effective against hematologic cancers (183), which is an interesting new direction.
Clinical trials of PVSRIPO in patients with recurrent malignant glioblastomas (184) and unresectable melanoma treated with intratumoral PVSRIPO (185) have all shown promising results. Still, these are direct effects on the tumor cells.
7 Conclusions
Among the Nectin and Necl family members, PVRL2 and PVR are critical players in tumor immunity, and immune checkpoint pathways mediated by them are potential therapeutic targets. However, the antitumor effects mediated by PVRL2, PVR, and other DNAM1 axis members remain to be elucidated and require further study.
Author contributions
KM: Conceptualization, Writing – original draft. SG: Conceptualization, Writing – review & editing.
Funding
The author(s) declare that no financial support was received for the research, authorship, and/or publication of this article.
Acknowledgments
The figure was created with BioRender.com (https://www.biorender.com/).
Conflict of interest
The authors declare that the research was conducted in the absence of any commercial or financial relationships that could be construed as a potential conflict of interest.
Publisher’s note
All claims expressed in this article are solely those of the authors and do not necessarily represent those of their affiliated organizations, or those of the publisher, the editors and the reviewers. Any product that may be evaluated in this article, or claim that may be made by its manufacturer, is not guaranteed or endorsed by the publisher.
References
1. Salik B, Smyth MJ, Nakamura K. Targeting immune checkpoints in hematological Malignancies. J Hematol Oncol. (2020) 13:111. doi: 10.1186/s13045-020-00947-6
2. Kaushik I, Ramachandran S, Zabel C, Gaikwad S, Srivastava SK. The evolutionary legacy of immune checkpoint inhibitors. Semin Cancer Biol. (2022) 86:491–8. doi: 10.1016/j.semcancer.2022.03.020
3. Sun Q, Hong Z, Zhang C, Wang L, Han Z, Ma D. Immune checkpoint therapy for solid tumors: clinical dilemmas and future trends. Signal Transduct Target Ther. (2023) 8:320. doi: 10.1038/s41392-023-01522-4
4. Hodi FS, O'Day SJ, McDermott DF, Weber RW, Sosman JA, Haanen JB, et al. Improved survival with ipilimumab in patients with metastatic melanoma. N Engl J Med. (2010) 363:711–23. doi: 10.1056/NEJMoa1003466
5. Bagchi S, Yuan R, Engleman EG. Immune checkpoint inhibitors for the treatment of cancer: clinical impact and mechanisms of response and resistance. Annu Rev Pathol. (2021) 16:223–49. doi: 10.1146/annurev-pathol-042020-042741
6. Ribas A, Wolchok JD. Cancer immunotherapy using checkpoint blockade. Science. (2018) 359:1350–5. doi: 10.1126/science.aar4060
7. Syn NL, Teng MWL, Mok TSK, Soo RA. De-novo and acquired resistance to immune checkpoint targeting. Lancet Oncol. (2017) 18:e731–41. doi: 10.1016/S1470-2045(17)30607-1
8. Sharma P, Hu-Lieskovan S, Wargo JA, Ribas A. Primary, adaptive, and acquired resistance to cancer immunotherapy. Cell. (2017) 168:707–23. doi: 10.1016/j.cell.2017.01.017
9. Tawbi HA, SChadendorf D, Lipson EJ, Ascierto PA, Matamala L, Castillo Gutierrez E, et al. Relatlimab and nivolumab versus nivolumab in untreated advanced melanoma. N Engl J Med. (2022) 386:24–34. doi: 10.1056/NEJMoa2109970
10. Alteber Z, Kotturi MF, Whelan S, Ganguly S, Weyl E, Pardoll DM, et al. Therapeutic targeting of checkpoint receptors within the DNAM1 axis. Cancer Discovery. (2021) 11:1040–51. doi: 10.1158/2159-8290.CD-20-1248
11. Samanta D, Almo SC. Nectin family of cell-adhesion molecules: structural and molecular aspects of function and specificity. Cell Mol Life Sci. (2015) 72:645–58. doi: 10.1007/s00018-014-1763-4
12. Mandai K, Rikitake Y, Mori M, Takai Y. Nectins and nectin-like molecules in development and disease. Curr Top Dev Biol. (2015) 112:197–231. doi: 10.1016/bs.ctdb.2014.11.019
13. Huang K, Lui WY. Nectins and nectin-like molecules (Necls): Recent findings and their role and regulation in spermatogenesis. Semin Cell Dev Biol. (2016) 59:54–61. doi: 10.1016/j.semcdb.2016.01.034
14. Takai Y, Miyoshi J, Ikeda W, Ogita H. Nectins and nectin-like molecules: roles in contact inhibition of cell movement and proliferation. Nat Rev Mol Cell Biol. (2008) 9:603–15. doi: 10.1038/nrm2457
15. Lopez M, Aoubala M, Jordier F, Isnardon D, Gomez S, Dubreuil P. The human poliovirus receptor related 2 protein is a new hematopoietic/endothelial homophilic adhesion molecule. Blood. (1998) 92:4602–11. doi: 10.1182/blood.V92.12.4602
16. Takahashi K, Nakanishi H, Miyahara M, Mandai K, Satoh K, Satoh A, et al. Nectin/PRR: an immunoglobulin-like cell adhesion molecule recruited to cadherin-based adherens junctions through interaction with Afadin, a PDZ domain-containing protein. J Cell Biol. (1999) 145:539–49. doi: 10.1083/jcb.145.3.539
17. Chambers AF, Groom AC, MacDonald IC. Dissemination and growth of cancer cells in metastatic sites. Nat Rev Cancer. (2002) 2:563–72. doi: 10.1038/nrc865
18. Mandai K, Nakanishi H, Satoh A, Obaishi H, Wada M, Nishioka H, et al. Afadin: A novel actin filament-binding protein with one PDZ domain localized at cadherin-based cell-to-cell adherens junction. J Cell Biol. (1997) 139:517–28. doi: 10.1083/jcb.139.2.517
19. Takai Y, Ikeda W, Ogita H, Rikitake Y. The immunoglobulin-like cell adhesion molecule nectin and its associated protein afadin. Annu Rev Cell Dev Biol. (2008) 24:309–42. doi: 10.1146/annurev.cellbio.24.110707.175339
20. Lopez M, Eberle F, Mattei MG, Gabert J, Birg F, Bardin F, et al. Complementary DNA characterization and chromosomal localization of a human gene related to the poliovirus receptor-encoding gene. Gene. (1995) 155:261–5. doi: 10.1016/0378-1119(94)00842-G
21. Ikeda W, Kakunaga S, Itoh S, Shingai T, Takekuni K, Satoh K, et al. Tage4/Nectin-like molecule-5 heterophilically trans-interacts with cell adhesion molecule Nectin-3 and enhances cell migration. J Biol Chem. (2003) 278:28167–72. doi: 10.1074/jbc.M303586200
22. Liu J, Qian X, Chen Z, Xu X, Gao F, Zhang S, et al. Crystal structure of cell adhesion molecule nectin-2/CD112 and its binding to immune receptor DNAM-1/CD226. J Immunol. (2012) 188:5511–20. doi: 10.4049/jimmunol.1200324
23. Son Y, Lee B, Choi YJ, Jeon SA, Kim JH, Lee HK, et al. Nectin-2 (CD112) is expressed on outgrowth endothelial cells and regulates cell proliferation and angiogenic function. PloS One. (2016) 11:e0163301. doi: 10.1371/journal.pone.0163301
24. Russo E, Runge P, Jahromi NH, Naboth H, Landtwing A, Montecchi R, et al. CD112 regulates angiogenesis and T cell entry into the spleen. Cells. (2021) 10:169. doi: 10.3390/cells10010169
25. Bouchard MJ, Dong Y, McDermott BM Jr., Lam DH, Brown KR, Shelanski M, et al. Defects in nuclear and cytoskeletal morphology and mitochondrial localization in spermatozoa of mice lacking nectin-2, a component of cell-cell adherens junctions. Mol Cell Biol. (2000) 20:2865–73. doi: 10.1128/MCB.20.8.2865-2873.2000
26. Whelan S, Ophir E, Kotturi MF, Levy O, Ganguly S, Leung L, et al. PVRIG and PVRL2 are induced in cancer and inhibit CD8(+) T-cell function. Cancer Immunol Res. (2019) 7:257–68. doi: 10.1158/2326-6066.CIR-18-0442
27. Molfetta R, Milito ND, Zitti B, Lecce M, Fionda C, Cippitelli M, et al. The Ubiquitin-proteasome pathway regulates Nectin2/CD112 expression and impairs NK cell recognition and killing. Eur J Immunol. (2019) 49:873–83. doi: 10.1002/eji.201847848
28. Mendelsohn CL, Wimmer E, Racaniello VR. Cellular receptor for poliovirus: molecular cloning, nucleotide sequence, and expression of a new member of the immunoglobulin superfamily. Cell. (1989) 56:855–65. doi: 10.1016/0092-8674(89)90690-9
29. The human protein atlas. Available online at: https://www.proteinatlas.org/.
30. Baury B, Masson D, McDermott BM Jr., Jarry A, Blottiere HM, Blanchardie P, et al. Identification of secreted CD155 isoforms. Biochem Biophys Res Commun. (2003) 309:175–82. doi: 10.1016/S0006-291X(03)01560-2
31. Iguchi-Manaka A, Okumura G, Kojima H, Cho Y, Hirochika R, Bando H, et al. Increased soluble CD155 in the serum of cancer patients. PloS One. (2016) 11:e0152982. doi: 10.1371/journal.pone.0152982
32. Amano H, Ikeda W, Kawano S, Kajita M, Tamaru Y, Inoue N, et al. Interaction and localization of Necl-5 and PDGF receptor beta at the leading edges of moving NIH3T3 cells: Implications for directional cell movement. Genes Cells. (2008) 13:269–84. doi: 10.1111/j.1365-2443.2008.01167.x
33. Minami A, Mizutani K, Waseda M, Kajita M, Miyata M, Ikeda W, et al. Necl-5/PVR enhances PDGF-induced attraction of growing microtubules to the plasma membrane of the leading edge of moving NIH3T3 cells. Genes Cells. (2010) 15:1123–35. doi: 10.1111/j.1365-2443.2010.01450.x
34. Kakunaga S, Ikeda W, Shingai T, Fujito T, Yamada A, Minami Y, et al. Enhancement of serum- and platelet-derived growth factor-induced cell proliferation by Necl-5/Tage4/poliovirus receptor/CD155 through the Ras-Raf-MEK-ERK signaling. J Biol Chem. (2004) 279:36419–25. doi: 10.1074/jbc.M406340200
35. Kinugasa M, Amano H, Satomi-Kobayashi S, Nakayama K, Miyata M, Kubo Y, et al. Necl-5/poliovirus receptor interacts with VEGFR2 and regulates VEGF-induced angiogenesis. Circ Res. (2012) 110:716–26. doi: 10.1161/CIRCRESAHA.111.256834
36. Qiu Q, Ravens I, Seth S, Rathinasamy A, Maier MK, Davalos-Misslitz A, et al. CD155 is involved in negative selection and is required to retain terminally maturing CD8 T cells in thymus. J Immunol. (2010) 184:1681–9. doi: 10.4049/jimmunol.0900062
37. Ardolino M, Zingoni A, Cerboni C, Cecere F, Soriani A, Iannitto ML, et al. DNAM-1 ligand expression on Ag-stimulated T lymphocytes is mediated by ROS-dependent activation of DNA-damage response: relevance for NK-T cell interaction. Blood. (2011) 117:4778–86. doi: 10.1182/blood-2010-08-300954
38. Fionda C, Abruzzese MP, Zingoni A, Soriani A, Ricci B, Molfetta R, et al. Nitric oxide donors increase PVR/CD155 DNAM-1 ligand expression in multiple myeloma cells: role of DNA damage response activation. BMC Cancer. (2015) 15:17. doi: 10.1186/s12885-015-1023-5
39. Gao J, Zheng Q, Shao Y, Wang W, Zhao C. CD155 downregulation synergizes with adriamycin to induce breast cancer cell apoptosis. Apoptosis. (2018) 23:512–20. doi: 10.1007/s10495-018-1473-8
40. Hanna A, Shevde LA. Hedgehog signaling: modulation of cancer properties and tumor mircroenvironment. Mol Cancer. (2016) 15:24. doi: 10.1186/s12943-016-0509-3
41. Solecki DJ, Gromeier M, Mueller S, Bernhardt G, Wimmer E. Expression of the human poliovirus receptor/CD155 gene is activated by sonic hedgehog. J Biol Chem. (2002) 277:25697–702. doi: 10.1074/jbc.M201378200
42. Hirota T, Irie K, Okamoto R, Ikeda W, Takai Y. Transcriptional activation of the mouse Necl-5/Tage4/PVR/CD155 gene by fibroblast growth factor or oncogenic Ras through the Raf-MEK-ERK-AP-1 pathway. Oncogene. (2005) 24:2229–35. doi: 10.1038/sj.onc.1208409
43. Zhu Y, Paniccia A, Schulick AC, Chen W, Koenig MR, Byers JT, et al. Identification of CD112R as a novel checkpoint for human T cells. J Exp Med. (2016) 213:167–76. doi: 10.1084/jem.20150785
44. Yu X, Harden K, Gonzalez LC, Francesco M, Chiang E, Irving B, et al. The surface protein TIGIT suppresses T cell activation by promoting the generation of mature immunoregulatory dendritic cells. Nat Immunol. (2009) 10:48–57. doi: 10.1038/ni.1674
45. Boles KS, Vermi W, Facchetti F, Fuchs A, Wilson TJ, Diacovo TG, et al. A novel molecular interaction for the adhesion of follicular CD4 T cells to follicular DC. Eur J Immunol. (2009) 39:695–703. doi: 10.1002/eji.200839116
46. Stanietsky N, Simic H, Arapovic J, Toporik A, Levy O, Novik A, et al. The interaction of TIGIT with PVR and PVRL2 inhibits human NK cell cytotoxicity. Proc Natl Acad Sci U.S.A. (2009) 106:17858–63. doi: 10.1073/pnas.0903474106
47. Joller N, Hafler JP, Brynedal B, Kassam N, Spoerl S, Levin SD, et al. Cutting edge: TIGIT has T cell-intrinsic inhibitory functions. J Immunol. (2011) 186:1338–42. doi: 10.4049/jimmunol.1003081
48. Levin SD, Taft DW, Brandt CS, Bucher C, Howard ED, Chadwick EM, et al. Vstm3 is a member of the CD28 family and an important modulator of T-cell function. Eur J Immunol. (2011) 41:902–15. doi: 10.1002/eji.201041136
49. Joller N, Lozano E, Burkett PR, Patel B, Xiao S, Zhu C, et al. Treg cells expressing the coinhibitory molecule TIGIT selectively inhibit proinflammatory Th1 and Th17 cell responses. Immunity. (2014) 40:569–81. doi: 10.1016/j.immuni.2014.02.012
50. Zhang B, Zhao W, Li H, Chen Y, Tian H, Li L, et al. Immunoreceptor TIGIT inhibits the cytotoxicity of human cytokine-induced killer cells by interacting with CD155. Cancer Immunol Immunother. (2016) 65:305–14. doi: 10.1007/s00262-016-1799-4
51. Deuss FA, Gully BS, Rossjohn J, Berry R. Recognition of nectin-2 by the natural killer cell receptor T cell immunoglobulin and ITIM domain (TIGIT). J Biol Chem. (2017) 292:11413–22. doi: 10.1074/jbc.M117.786483
52. Li M, Xia P, Du Y, Liu S, Huang G, Chen J, et al. T-cell immunoglobulin and ITIM domain (TIGIT) receptor/poliovirus receptor (PVR) ligand engagement suppresses interferon-gamma production of natural killer cells via beta-arrestin 2-mediated negative signaling. J Biol Chem. (2014) 289:17647–57. doi: 10.1074/jbc.M114.572420
53. Johnston RJ, Comps-Agrar L, Hackney J, Yu X, Huseni M, Yang Y, et al. The immunoreceptor TIGIT regulates antitumor and antiviral CD8(+) T cell effector function. Cancer Cell. (2014) 26:923–37. doi: 10.1016/j.ccell.2014.10.018
54. Lozano E, Dominguez-Villar M, Kuchroo V, Hafler DA. The TIGIT/CD226 axis regulates human T cell function. J Immunol. (2012) 188:3869–75. doi: 10.4049/jimmunol.1103627
55. Wang PL, O'Farrell S, Clayberger C, Krensky AM. Identification and molecular cloning of tactile. A novel human T cell activation antigen that is a member of the Ig gene superfamily. J Immunol. (1992) 148:2600–8. doi: 10.4049/jimmunol.148.8.2600
56. Lepletier A, Lutzky VP, Mittal D, Stannard K, Watkins TS, Ratnatunga CN, et al. The immune checkpoint CD96 defines a distinct lymphocyte phenotype and is highly expressed on tumor-infiltrating T cells. Immunol Cell Biol. (2019) 97:152–64. doi: 10.1111/imcb.12205
57. Mittal D, Lepletier A, Madore J, Aguilera AR, Stannard K, Blake SJ, et al. CD96 is an immune checkpoint that regulates CD8(+) T-cell antitumor function. Cancer Immunol Res. (2019) 7:559–71. doi: 10.1158/2326-6066.CIR-18-0637
58. Chan CJ, Martinet L, Gilfillan S, Souza-Fonseca-Guimaraes F, Chow MT, Town L, et al. The receptors CD96 and CD226 oppose each other in the regulation of natural killer cell functions. Nat Immunol. (2014) 15:431–8. doi: 10.1038/ni.2850
59. Li J, Xia Q, Di C, Li C, Si H, Zhou B, et al. Tumor cell-intrinsic CD96 mediates chemoresistance and cancer stemness by regulating mitochondrial fatty acid beta-oxidation. Adv Sci (Weinh). (2023) 10:e2202956. doi: 10.1002/advs.202202956
60. Shibuya A, Campbell D, Hannum C, Yssel H, Franz-Bacon K, McClanahan T, et al. DNAM-1, a novel adhesion molecule involved in the cytolytic function of T lymphocytes. Immunity. (1996) 4:573–81. doi: 10.1016/S1074-7613(00)70060-4
61. Burns GF, Triglia T, Werkmeister JA, Begley CG, Boyd AW. TLiSA1, a human T lineage-specific activation antigen involved in the differentiation of cytotoxic T lymphocytes and anomalous killer cells from their precursors. J Exp Med. (1985) 161:1063–78. doi: 10.1084/jem.161.5.1063
62. Bottino C, Castriconi R, Pende D, Rivera P, Nanni M, Carnemolla B, et al. Identification of PVR (CD155) and Nectin-2 (CD112) as cell surface ligands for the human DNAM-1 (CD226) activating molecule. J Exp Med. (2003) 198:557–67. doi: 10.1084/jem.20030788
63. Pende D, Bottino C, Castriconi R, Cantoni C, Marcenaro S, Rivera P, et al. PVR (CD155) and Nectin-2 (CD112) as ligands of the human DNAM-1 (CD226) activating receptor: involvement in tumor cell lysis. Mol Immunol. (2005) 42:463–9. doi: 10.1016/j.molimm.2004.07.028
64. Wang H, Qi J, Zhang S, Li Y, Tan S, Gao GF. Binding mode of the side-by-side two-IgV molecule CD226/DNAM-1 to its ligand CD155/Necl-5. Proc Natl Acad Sci U.S.A. (2019) 116:988–96. doi: 10.1073/pnas.1815716116
65. Castriconi R, Dondero A, Corrias MV, Lanino E, Pende D, Moretta L, et al. Natural killer cell-mediated killing of freshly isolated neuroblastoma cells: critical role of DNAX accessory molecule-1-poliovirus receptor interaction. Cancer Res. (2004) 64:9180–4. doi: 10.1158/0008-5472.CAN-04-2682
66. Iguchi-Manaka A, Kai H, Yamashita Y, Shibata K, Tahara-Hanaoka S, Honda S, et al. Accelerated tumor growth in mice deficient in DNAM-1 receptor. J Exp Med. (2008) 205:2959–64. doi: 10.1084/jem.20081611
67. Gilfillan S, Chan CJ, Cella M, Haynes NM, Rapaport AS, Boles KS, et al. DNAM-1 promotes activation of cytotoxic lymphocytes by nonprofessional antigen-presenting cells and tumors. J Exp Med. (2008) 205:2965–73. doi: 10.1084/jem.20081752
68. Nakamura-Shinya Y, Iguchi-Manaka A, Murata R, Sato K, Vo AV, Kanemaru K, et al. DNAM-1 promotes inflammation-driven tumor development via enhancing IFN-gamma production. Int Immunol. (2022) 34:149–57. doi: 10.1093/intimm/dxab099
69. Hou S, Zheng X, Wei H, Tian Z, Sun R. Recombinant soluble CD226 protein directly inhibits cancer cell proliferation in vitro. Int Immunopharmacol. (2014) 19:119–26. doi: 10.1016/j.intimp.2014.01.012
70. Pende D, Castriconi R, Romagnani P, Spaggiari GM, Marcenaro S, Dondero A, et al. Expression of the DNAM-1 ligands, Nectin-2 (CD112) and poliovirus receptor (CD155), on dendritic cells: relevance for natural killer-dendritic cell interaction. Blood. (2006) 107:2030–6. doi: 10.1182/blood-2005-07-2696
71. Samanta D, Guo H, Rubinstein R, Ramagopal UA, Almo SC. Structural, mutational and biophysical studies reveal a canonical mode of molecular recognition between immune receptor TIGIT and nectin-2. Mol Immunol. (2017) 81:151–9. doi: 10.1016/j.molimm.2016.12.003
72. Stanietsky N, Rovis TL, Glasner A, Seidel E, Tsukerman P, Yamin R, et al. Mouse TIGIT inhibits NK-cell cytotoxicity upon interaction with PVR. Eur J Immunol. (2013) 43:2138–50. doi: 10.1002/eji.201243072
73. Tahara-Hanaoka S, Shibuya K, Kai H, Miyamoto A, Morikawa Y, Ohkochi N, et al. Tumor rejection by the poliovirus receptor family ligands of the DNAM-1 (CD226) receptor. Blood. (2006) 107:1491–6. doi: 10.1182/blood-2005-04-1684
74. Fuchs A, Cella M, Giurisato E, Shaw AS, Colonna M. Cutting edge: CD96 (tactile) promotes NK cell-target cell adhesion by interacting with the poliovirus receptor (CD155). J Immunol. (2004) 172:3994–8. doi: 10.4049/jimmunol.172.7.3994
75. Blake SJ, Stannard K, Liu J, Allen S, Yong MC, Mittal D, et al. Suppression of metastases using a new lymphocyte checkpoint target for cancer immunotherapy. Cancer Discovery. (2016) 6:446–59. doi: 10.1158/2159-8290.CD-15-0944
76. Chiang EY, de Almeida PE, de Almeida Nagata DE, Bowles KH, Du X, Chitre AS, et al. CD96 functions as a co-stimulatory receptor to enhance CD8(+) T cell activation and effector responses. Eur J Immunol. (2020) 50:891–902. doi: 10.1002/eji.201948405
77. Murter B, Pan X, Ophir E, Alteber Z, Azulay M, Sen R, et al. Mouse PVRIG has CD8(+) T cell-specific coinhibitory functions and dampens antitumor immunity. Cancer Immunol Res. (2019) 7:244–56. doi: 10.1158/2326-6066.CIR-18-0460
78. Li Y, Zhang Y, Cao G, Zheng X, Sun C, Wei H, et al. Blockade of checkpoint receptor PVRIG unleashes anti-tumor immunity of NK cells in murine and human solid tumors. J Hematol Oncol. (2021) 14:100. doi: 10.1186/s13045-021-01112-3
79. Lozano E, Mena MP, Diaz T, Martin-Antonio B, Leon S, Rodriguez-Lobato LG, et al. Nectin-2 expression on Malignant plasma cells is associated with better response to TIGIT blockade in multiple myeloma. Clin Cancer Res. (2020) 26:4688–98. doi: 10.1158/1078-0432.CCR-19-3673
80. Jin HS, Ko M, Choi DS, Kim JH, Lee DH, Kang SH, et al. CD226(hi)CD8(+) T cells are a prerequisite for anti-TIGIT immunotherapy. Cancer Immunol Res. (2020) 8:912–25. doi: 10.1158/2326-6066.CIR-19-0877
81. Oshima T, Sato S, Kato J, Ito Y, Watanabe T, Tsuji I, et al. Nectin-2 is a potential target for antibody therapy of breast and ovarian cancers. Mol Cancer. (2013) 12:60. doi: 10.1186/1476-4598-12-60
82. Smazynski J, Hamilton PT, Thornton S, Milne K, Wouters MCA, Webb JR, et al. The immune suppressive factors CD155 and PD-L1 show contrasting expression patterns and immune correlates in ovarian and other cancers. Gynecol Oncol. (2020) 158:167–77. doi: 10.1016/j.ygyno.2020.04.689
83. Zhang D, Liu J, Zheng M, Meng C, Liao J. Prognostic and clinicopathological significance of CD155 expression in cancer patients: a meta-analysis. World J Surg Oncol. (2022) 20:351. doi: 10.1186/s12957-022-02813-w
84. Lee JB, Hong MH, Park SY, Chae S, Hwang D, Ha SJ, et al. Overexpression of PVR and PD-L1 and its association with prognosis in surgically resected squamous cell lung carcinoma. Sci Rep. (2021) 11:8551. doi: 10.1038/s41598-021-87624-x
85. Chauvin JM, Pagliano O, Fourcade J, Sun Z, Wang H, Sander C, et al. TIGIT and PD-1 impair tumor antigen-specific CD8(+) T cells in melanoma patients. J Clin Invest. (2015) 125:2046–58. doi: 10.1172/JCI80445
86. Muller S, Mayer S, Moller P, Barth TFE, Marienfeld R. Spatial distribution of immune checkpoint proteins in histological subtypes of lung adenocarcinoma. Neoplasia. (2021) 23:584–93. doi: 10.1016/j.neo.2021.05.005
87. Soriani A, Zingoni A, Cerboni C, Iannitto ML, Ricciardi MR, Di Gialleonardo V, et al. ATM-ATR-dependent up-regulation of DNAM-1 and NKG2D ligands on multiple myeloma cells by therapeutic agents results in enhanced NK-cell susceptibility and is associated with a senescent phenotype. Blood. (2009) 113:3503–11. doi: 10.1182/blood-2008-08-173914
88. Siegel RL, Miller KD, Fuchs HE, Jemal A. Cancer statistics, 2021. CA Cancer J Clin. (2021) 71:7–33. doi: 10.3322/caac.21654
89. Labidi-Galy SI, Papp E, Hallberg D, Niknafs N, Adleff V, Noe M, et al. High grade serous ovarian carcinomas originate in the fallopian tube. Nat Commun. (2017) 8:1093. doi: 10.1038/s41467-017-00962-1
90. Yarchoan M, Hopkins A, Jaffee EM. Tumor mutational burden and response rate to PD-1 inhibition. N Engl J Med. (2017) 377:2500–1. doi: 10.1056/NEJMc1713444
91. Yang C, Xia BR, Zhang ZC, Zhang YJ, Lou G, Jin WL. Immunotherapy for ovarian cancer: adjuvant, combination, and neoadjuvant. Front Immunol. (2020) 11:577869. doi: 10.3389/fimmu.2020.577869
92. Moore KN, Bookman M, Sehouli J, Miller A, Anderson C, Scambia G, et al. Atezolizumab, bevacizumab, and chemotherapy for newly diagnosed stage III or IV ovarian cancer: placebo-controlled randomized phase III trial (IMagyn050/GOG 3015/ENGOT-OV39). J Clin Oncol. (2021) 39:1842–55. doi: 10.1200/JCO.21.00306
93. Pujade-Lauraine E, Fujiwara K, Ledermann JA, Oza AM, Kristeleit R, Ray-Coquard IL, et al. Avelumab alone or in combination with chemotherapy versus chemotherapy alone in platinum-resistant or platinum-refractory ovarian cancer (JAVELIN Ovarian 200): an open-label, three-arm, randomized, phase 3 study. Lancet Oncol. (2021) 22:1034–46. doi: 10.1016/S1470-2045(21)00216-3
94. Monk BJ, Colombo N, Oza AM, Fujiwara K, Birrer MJ, Randall L, et al. Chemotherapy with or without avelumab followed by avelumab maintenance versus chemotherapy alone in patients with previously untreated epithelial ovarian cancer (JAVELIN Ovarian 100): an open-label, randomized, phase 3 trial. Lancet Oncol. (2021) 22:1275–89. doi: 10.1016/S1470-2045(21)00342-9
95. Hamanishi J, Takeshima N, Katsumata N, Ushijima K, Kimura T, Takeuchi S, et al. Nivolumab versus gemcitabine or pegylated liposomal doxorubicin for patients with platinum-resistant ovarian cancer: open-label, randomized trial in Japan (NINJA). J Clin Oncol. (2021) 39:3671–81. doi: 10.1200/JCO.21.00334
96. Kurtz JE, Pujade-Lauraine E, Oaknin A, Belin L, Leitner K, Cibula D, et al. Atezolizumab combined with bevacizumab and platinum-based therapy for platinum-sensitive ovarian cancer: placebo-controlled randomized phase III ATALANTE/ENGOT-ov29 trial. J Clin Oncol. (2023) 41:4768–78. doi: 10.1200/JCO.23.00529
97. Carlsten M, Bjorkstrom NK, Norell H, Bryceson Y, van Hall T, Baumann BC, et al. DNAX accessory molecule-1 mediated recognition of freshly isolated ovarian carcinoma by resting natural killer cells. Cancer Res. (2007) 67:1317–25. doi: 10.1158/0008-5472.CAN-06-2264
98. Ozmadenci D, Shankara Narayanan JS, Andrew J, Ojalill M, Barrie AM, Jiang S, et al. Tumor FAK orchestrates immunosuppression in ovarian cancer via the CD155/TIGIT axis. Proc Natl Acad Sci U.S.A. (2022) 119:e2117065119. doi: 10.1073/pnas.2117065119
99. James NE, Miller K, LaFranzo N, Lips E, Woodman M, Ou J, et al. Immune modeling analysis reveals immunologic signatures associated with improved outcomes in high grade serous ovarian cancer. Front Oncol. (2021) 11:622182. doi: 10.3389/fonc.2021.622182
100. Bekes I, Lob S, Holzheu I, Janni W, Baumann L, Wockel A, et al. Nectin-2 in ovarian cancer: How is it expressed and what might be its functional role? Cancer Sci. (2019) 110:1872–82. doi: 10.1111/cas.13992
101. Murakami K, Kotani Y, Nakai H, Matsumura N. Endometriosis-associated ovarian cancer: the origin and targeted therapy. Cancers (Basel). (2020) 12:1676. doi: 10.3390/cancers12061676
102. Murakami K, Kanto A, Sakai K, Miyagawa C, Takaya H, Nakai H, et al. Frequent PIK3CA mutations in eutopic endometrium of patients with ovarian clear cell carcinoma. Mod Pathol. (2021) 34:2071–9. doi: 10.1038/s41379-021-00861-3
103. Hamanishi J, Mandai M, Ikeda T, Minami M, Kawaguchi A, Murayama T, et al. Safety and antitumor activity of anti-PD-1 antibody, nivolumab, in patients with platinum-resistant ovarian cancer. J Clin Oncol. (2015) 33:4015–22. doi: 10.1200/JCO.2015.62.3397
104. Sia TY, Manning-Geist B, Gordhandas S, Murali R, Marra A, Liu YL, et al. Treatment of ovarian clear cell carcinoma with immune checkpoint blockade: a case series. Int J Gynecol Cancer. (2022) 32:1017–24. doi: 10.1136/ijgc-2022-003430
105. Henley SJ, Ward EM, Scott S, Ma J, Anderson RN, Firth AU, et al. Annual report to the nation on the status of cancer, part I: National cancer statistics. Cancer. (2020) 126:2225–49. doi: 10.1002/cncr.32802
106. Lu KH, Broaddus RR. Endometrial cancer. N Engl J Med. (2020) 383:2053–64. doi: 10.1056/NEJMra1514010
107. Kandoth C, Schultz N, Cherniack AD, Akbani R, Liu Y, Shen H, et al. Integrated genomic characterization of endometrial carcinoma. Nature. (2013) 497:67–73. doi: 10.1038/nature12113
108. Bonneville R, Krook MA, Kautto EA, Miya J, Wing MR, Chen HZ, et al. Landscape of microsatellite instability across 39 cancer types. JCO Precis Oncol. (2017) 2017:PO.17.00073. doi: 10.1200/PO.17.00073
109. Howitt BE, Shukla SA, Sholl LM, Ritterhouse LL, Watkins JC, Rodig S, et al. Association of polymerase e-mutated and microsatellite-instable endometrial cancers with neoantigen load, number of tumor-infiltrating lymphocytes, and expression of PD-1 and PD-L1. JAMA Oncol. (2015) 1:1319–23. doi: 10.1001/jamaoncol.2015.2151
110. O'Malley DM, Bariani GM, Cassier PA, Marabelle A, Hansen AR, De Jesus Acosta A, et al. Pembrolizumab in patients with microsatellite instability-high advanced endometrial cancer: results from the KEYNOTE-158 study. J Clin Oncol. (2022) 40:752–61. doi: 10.1200/JCO.21.01874
111. Inoue T, Adachi K, Kawana K, Taguchi A, Nagamatsu T, Fujimoto A, et al. Cancer-associated fibroblast suppresses killing activity of natural killer cells through downregulation of poliovirus receptor (PVR/CD155), a ligand of activating NK receptor. Int J Oncol. (2016) 49:1297–304. doi: 10.3892/ijo.2016.3631
112. Sperandio RC, Pestana RC, Miyamura BV, Kaseb AO. Hepatocellular carcinoma immunotherapy. Annu Rev Med. (2022) 73:267–78. doi: 10.1146/annurev-med-042220-021121
113. Ho DW, Tsui YM, Chan LK, Sze KM, Zhang X, Cheu JW, et al. Single-cell RNA sequencing shows the immunosuppressive landscape and tumor heterogeneity of HBV-associated hepatocellular carcinoma. Nat Commun. (2021) 12:3684. doi: 10.1038/s41467-021-24010-1
114. Huang X, Qu P, Chen Y, Zhou X, Wu Y, Liu F, et al. Low expression of CD112 is associated with poor overall survival in patients with hepatocellular carcinoma. Hum Pathol. (2014) 45:1944–50. doi: 10.1016/j.humpath.2014.06.001
115. Li A, Ji B, Yang Y, Ye B, Zhu Q, Hu X, et al. Single-cell RNA sequencing highlights the role of PVR/PVRL2 in the immunosuppressive tumor microenvironment in hepatocellular carcinoma. Front Immunol. (2023) 14:1164448. doi: 10.3389/fimmu.2023.1164448
116. Morrison AH, Byrne KT, Vonderheide RH. Immunotherapy and prevention of pancreatic cancer. Trends Cancer. (2018) 4:418–28. doi: 10.1016/j.trecan.2018.04.001
117. Liang S, Yang Z, Li D, Miao X, Yang L, Zou Q, et al. The clinical and pathological significance of nectin-2 and DDX3 expression in pancreatic ductal adenocarcinomas. Dis Markers. (2015) 2015:379568. doi: 10.1155/2015/379568
118. Peng YP, Xi CH, Zhu Y, Yin LD, Wei JS, Zhang JJ, et al. Altered expression of CD226 and CD96 on natural killer cells in patients with pancreatic cancer. Oncotarget. (2016) 7:66586–94. doi: 10.18632/oncotarget.v7i41
119. Nishiwada S, Sho M, Yasuda S, Shimada K, Yamato I, Akahori T, et al. Clinical significance of CD155 expression in human pancreatic cancer. Anticancer Res. (2015) 35:2287–97.
120. Ma H, Chen X, Mo S, Mao X, Chen J, Liu Y, et al. The spatial coexistence of TIGIT/CD155 defines poorer survival and resistance to adjuvant chemotherapy in pancreatic ductal adenocarcinoma. Theranostics. (2023) 13:4601–14. doi: 10.7150/thno.86547
121. Izumi H, Hirabayashi K, Nakamura N, Nakagohri T. Nectin expression in pancreatic adenocarcinoma: nectin-3 is associated with a poor prognosis. Surg Today. (2015) 45:487–94. doi: 10.1007/s00595-015-1126-2
122. Peng H, Li L, Zuo C, Chen MY, Zhang X, Myers NB, et al. Combination TIGIT/PD-1 blockade enhances the efficacy of neoantigen vaccines in a model of pancreatic cancer. Front Immunol. (2022) 13:1039226. doi: 10.3389/fimmu.2022.1039226
123. Pearce H, Croft W, Nicol SM, Margielewska-Davies S, Powell R, Cornall R, et al. Tissue-resident memory T cells in pancreatic ductal adenocarcinoma coexpress PD-1 and TIGIT and functional inhibition is reversible by dual antibody blockade. Cancer Immunol Res. (2023) 11:435–49. doi: 10.1158/2326-6066.CIR-22-0121
124. Moehler M, Högner A, Wagner AD, Obermannova R, Alsina M, Thuss-Patience P, et al. Recent progress and current challenges of immunotherapy in advanced/metastatic esophagogastric adenocarcinoma. Eur J Cancer. (2022) 176:13–29. doi: 10.1016/j.ejca.2022.08.023
125. He W, Zhang H, Han F, Chen X, Lin R, Wang W, et al. CD155T/TIGIT signaling regulates CD8(+) T-cell metabolism and promotes tumor progression in human gastric cancer. Cancer Res. (2017) 77:6375–88. doi: 10.1158/0008-5472.CAN-17-0381
126. Xu D, Zhao E, Zhu C, Zhao W, Wang C, Zhang Z, et al. TIGIT and PD-1 may serve as potential prognostic biomarkers for gastric cancer. Immunobiology. (2020) 225:151915. doi: 10.1016/j.imbio.2020.151915
127. Liu X, Xu C, Guo T, Zhan S, Quan Q, Li M, et al. Clinical significance of CD155 expression and correlation with cellular components of tumor microenvironment in gastric adenocarcinoma. Front Immunol. (2023) 14:1173524. doi: 10.3389/fimmu.2023.1173524
128. Wang JB, Li P, Liu XL, Zheng QL, Ma YB, Zhao YJ, et al. An immune checkpoint score system for prognostic evaluation and adjuvant chemotherapy selection in gastric cancer. Nat Commun. (2020) 11:6352. doi: 10.1038/s41467-020-20260-7
129. Zhao W, Jin L, Chen P, Li D, Gao W, Dong G. Colorectal cancer immunotherapy-Recent progress and future directions. Cancer Lett. (2022) 545:215816. doi: 10.1016/j.canlet.2022.215816
130. Masson D, Jarry A, Baury B, Blanchardie P, Laboisse C, Lustenberger P, et al. Overexpression of the CD155 gene in human colorectal carcinoma. Gut. (2001) 49:236–40. doi: 10.1136/gut.49.2.236
131. Murakami D, Matsuda K, Iwamoto H, Mitani Y, Mizumoto Y, Nakamura Y, et al. Prognostic value of CD155/TIGIT expression in patients with colorectal cancer. PloS One. (2022) 17:e0265908. doi: 10.1371/journal.pone.0265908
132. Karabulut M, Gunaldi M, Alis H, Afsar CU, Karabulut S, Serilmez M, et al. Serum nectin-2 levels are diagnostic and prognostic in patients with colorectal carcinoma. Clin Transl Oncol. (2016) 18:160–71. doi: 10.1007/s12094-015-1348-1
133. Viot J, Abdeljaoued S, Vienot A, Seffar E, Spehner L, Bouard A, et al. CD8(+) CD226(high) T cells in liver metastases dictate the prognosis of colorectal cancer patients treated with chemotherapy and radical surgery. Cell Mol Immunol. (2023) 20:365–78. doi: 10.1038/s41423-023-00978-2
134. Keenan TE, Tolaney SM. Role of immunotherapy in triple-negative breast cancer. J Natl Compr Canc Netw. (2020) 18:479–89. doi: 10.6004/jnccn.2020.7554
135. Zheng Q, Gao J, Yin P, Wang W, Wang B, Li Y, et al. CD155 contributes to the mesenchymal phenotype of triple-negative breast cancer. Cancer Sci. (2020) 111:383–94. doi: 10.1111/cas.14276
136. Li YC, Zhou Q, Song QK, Wang RB, Lyu S, Guan X, et al. Overexpression of an immune checkpoint (CD155) in breast cancer associated with prognostic significance and exhausted tumor-infiltrating lymphocytes: A cohort study. J Immunol Res. (2020) 2020:3948928. doi: 10.1155/2020/3948928
137. Cabioglu N, Bayram A, Emiroglu S, Onder S, Karatay H, Oner G, et al. Diverging prognostic effects of CD155 and CD73 expressions in locally advanced triple-negative breast cancer. Front Oncol. (2023) 13:1165257. doi: 10.3389/fonc.2023.1165257
138. Triki H, Charfi S, Bouzidi L, Ben Kridis W, Daoud J, Chaabane K, et al. CD155 expression in human breast cancer: Clinical significance and relevance to natural killer cell infiltration. Life Sci. (2019) 231:116543. doi: 10.1016/j.lfs.2019.116543
139. Adhikari E, Liu Q, Johnson J, Stewart P, Marusyk V, Fang B, et al. Brain metastasis-associated fibroblasts secrete fucosylated PVR/CD155 that induces breast cancer invasion. Cell Rep. (2023) 42:113463. doi: 10.1016/j.celrep.2023.113463
140. Iguchi-Manaka A, Okumura G, Ichioka E, Kiyomatsu H, Ikeda T, Bando H, et al. High expression of soluble CD155 in estrogen receptor-negative breast cancer. Breast Cancer. (2020) 27:92–9. doi: 10.1007/s12282-019-00999-8
141. Boissière-Michot F, Chateau MC, Thézenas S, Guiu S, Bobrie A, Jacot W. Correlation of the TIGIT-PVR immune checkpoint axis with clinicopathological features in triple-negative breast cancer. Front Immunol. (2022) 13:1058424. doi: 10.3389/fimmu.2022.1058424
142. Guo C, Luo Z, Ismtula D, Bi X, Kong H, Wang Y, et al. TIGIT is a novel prognostic marker and correlate for immune infiltration in invasive breast cancer. Comb Chem High Throughput Screen. (2023) 26:639–51. doi: 10.2174/1386207325666220629162823
143. Xu K, Wang R, Xie H, Hu L, Wang C, Xu J, et al. Single-cell RNA sequencing reveals cell heterogeneity and transcriptome profile of breast cancer lymph node metastasis. Oncogenesis. (2021) 10:66. doi: 10.1038/s41389-021-00355-6
144. Zou Y, Ye F, Kong Y, Hu X, Deng X, Xie J, et al. The single-cell landscape of intratumoral heterogeneity and the immunosuppressive microenvironment in liver and brain metastases of breast cancer. Adv Sci (Weinh). (2023) 10:e2203699. doi: 10.1002/advs.202203699
145. Xu F, Sunderland A, Zhou Y, Schulick RD, Edil BH, Zhu Y. Blockade of CD112R and TIGIT signaling sensitizes human natural killer cell functions. Cancer Immunol Immunother. (2017) 66:1367–75. doi: 10.1007/s00262-017-2031-x
146. Ziogas DC, Theocharopoulos C, Koutouratsas T, Haanen J, Gogas H. Mechanisms of resistance to immune checkpoint inhibitors in melanoma: What we have to overcome? Cancer Treat Rev. (2023) 113:102499. doi: 10.1016/j.ctrv.2022.102499
147. Bevelacqua V, Bevelacqua Y, Candido S, Skarmoutsou E, Amoroso A, Guarneri C, et al. Nectin like-5 overexpression correlates with the Malignant phenotype in cutaneous melanoma. Oncotarget. (2012) 3:882–92. doi: 10.18632/oncotarget.v3i8
148. Lepletier A, Madore J, O'Donnell JS, Johnston RL, Li XY, McDonald E, et al. Tumor CD155 expression is associated with resistance to anti-PD1 immunotherapy in metastatic melanoma. Clin Cancer Res. (2020) 26:3671–81. doi: 10.1158/1078-0432.CCR-19-3925
149. Chan CJ, Andrews DM, McLaughlin NM, Yagita H, Gilfillan S, Colonna M, et al. DNAM-1/CD155 interactions promote cytokine and NK cell-mediated suppression of poorly immunogenic melanoma metastases. J Immunol. (2010) 184:902–11. doi: 10.4049/jimmunol.0903225
150. Okumura G, Iguchi-Manaka A, Murata R, Yamashita-Kanemaru Y, Shibuya A, Shibuya K. Tumor-derived soluble CD155 inhibits DNAM-1-mediated antitumor activity of natural killer cells. J Exp Med. (2020) 217:1. doi: 10.1084/jem.20191290
151. Inozume T, Yaguchi T, Furuta J, Harada K, Kawakami Y, Shimada S. Melanoma cells control antimelanoma CTL responses via interaction between TIGIT and CD155 in the effector phase. J Invest Dermatol. (2016) 136:255–63. doi: 10.1038/JID.2015.404
152. Kawashima S, Inozume T, Kawazu M, Ueno T, Nagasaki J, Tanji E, et al. TIGIT/CD155 axis mediates resistance to immunotherapy in patients with melanoma with the inflamed tumor microenvironment. J Immunother Cancer. (2021) 9:e003134. doi: 10.1136/jitc-2021-003134
153. Chauvin JM, Ka M, Pagliano O, Menna C, Ding Q, DeBlasio R, et al. IL15 stimulation with TIGIT blockade reverses CD155-mediated NK-cell dysfunction in melanoma. Clin Cancer Res. (2020) 26:5520–33. doi: 10.1158/1078-0432.CCR-20-0575
154. Noori M, Azizi S, Mahjoubfar A, Abbasi Varaki F, Fayyaz F, Mousavian AH, et al. Efficacy and safety of immune checkpoint inhibitors for patients with prostate cancer: a systematic review and meta-analysis. Front Immunol. (2023) 14:1181051. doi: 10.3389/fimmu.2023.1181051
155. Wang F, Liu S, Liu F, Xu T, Ma J, Liang J, et al. TIGIT immune checkpoint blockade enhances immunity of human peripheral blood NK cells against castration-resistant prostate cancer. Cancer Lett. (2023) 568:216300. doi: 10.1016/j.canlet.2023.216300
156. Lim SM, Hong MH, Ha SJ, Hwang D, Chae S, Koh YW, et al. Overexpression of poliovirus receptor is associated with poor prognosis in head and neck squamous cell carcinoma patients. J Cancer Res Clin Oncol. (2021) 147:2741–50. doi: 10.1007/s00432-021-03531-8
157. Zhao K, Ma L, Feng L, Huang Z, Meng X, Yu J. CD155 overexpression correlates with poor prognosis in primary small cell carcinoma of the esophagus. Front Mol Biosci. (2020) 7:608404. doi: 10.3389/fmolb.2020.608404
158. Nakai R, Maniwa Y, Tanaka Y, Nishio W, Yoshimura M, Okita Y, et al. Overexpression of Necl-5 correlates with unfavorable prognosis in patients with lung adenocarcinoma. Cancer Sci. (2010) 101:1326–30. doi: 10.1111/j.1349-7006.2010.01530.x
159. Oyama R, Kanayama M, Mori M, Matsumiya H, Taira A, Shinohara S, et al. CD155 expression and its clinical significance in non-small cell lung cancer. Oncol Lett. (2022) 23:166. doi: 10.3892/ol.2022.13286
160. Nishizawa N, Shimajiri S, Oyama R, Manabe T, Nemoto Y, Matsumiya H, et al. Prognostic factors of resected pathological stage I lung adenocarcinoma: evaluating subtypes and PD-L1/CD155 expression. Sci Rep. (2023) 13:21687. doi: 10.1038/s41598-023-47888-x
161. Zhang J, Zhu Y, Wang Q, Kong Y, Sheng H, Guo J, et al. Poliovirus receptor CD155 is up-regulated in muscle-invasive bladder cancer and predicts poor prognosis. Urol Oncol. (2020) 38:41 e11–8. doi: 10.1016/j.urolonc.2019.07.006
162. Mori K, Matsumoto K, Amano N, Koguchi D, Shimura S, Hagiwara M, et al. Expression of membranous CD155 is associated with aggressive phenotypes and a poor prognosis in patients with bladder cancer. Cancers (Basel). (2022) 14:1576. doi: 10.3390/cancers14061576
163. Liu L, Wang Y, Geng C, Wang A, Han S, You X, et al. CD155 promotes the progression of cervical cancer cells through AKT/mTOR and NF-kappaB pathways. Front Oncol. (2021) 11:655302. doi: 10.3389/fonc.2021.655302
164. Nakanishi T, Yoneshima Y, Okamura K, Yanagihara T, Hashisako M, Iwasaki T, et al. MicroRNA-326 negatively regulates CD155 expression in lung adenocarcinoma. Cancer Sci. (2023) 114:4101–13. doi: 10.1111/cas.15921
165. Miao X, Yang ZL, Xiong L, Zou Q, Yuan Y, Li J, et al. Nectin-2 and DDX3 are biomarkers for metastasis and poor prognosis of squamous cell/adenosquamous carcinomas and adenocarcinoma of gallbladder. Int J Clin Exp Pathol. (2013) 6:179–90.
166. Dekanić A, Babarović E, Kučan Brlić P, Knežić M, Savić Vuković A, Mazor M, et al. The prognostic significance of Nectin-2 and Nectin-4 expression in glial tumors. Pathol Res Pract. (2023) 244:154416. doi: 10.1016/j.prp.2023.154416
167. Li M, Qiao D, Pu J, Wang W, Zhu W, Liu H. Elevated Nectin-2 expression is involved in esophageal squamous cell carcinoma by promoting cell migration and invasion. Oncol Lett. (2018) 15:4731–6. doi: 10.3892/ol.2018.7953
168. Tang X, Yang J, Shi A, Xiong Y, Wen M, Luo Z, et al. CD155 cooperates with PD-1/PD-L1 to promote proliferation of esophageal squamous cancer cells via PI3K/Akt and MAPK signaling pathways. Cancers (Basel). (2022) 14:5610. doi: 10.3390/cancers14225610
169. Kong Y, Zhu L, Schell TD, Zhang J, Claxton DF, Ehmann WC, et al. T-cell immunoglobulin and ITIM domain (TIGIT) associates with CD8+ T-cell exhaustion and poor clinical outcome in AML patients. Clin Cancer Res. (2016) 22:3057–66. doi: 10.1158/1078-0432.CCR-15-2626
170. Stamm H, Klingler F, Grossjohann EM, Muschhammer J, Vettorazzi E, Heuser M, et al. Immune checkpoints PVR and PVRL2 are prognostic markers in AML and their blockade represents a new therapeutic option. Oncogene. (2018) 37:5269–80. doi: 10.1038/s41388-018-0288-y
171. Kaito Y, Hirano M, Futami M, Nojima M, Tamura H, Tojo A, et al. CD155 and CD112 as possible therapeutic targets of FLT3 inhibitors for acute myeloid leukemia. Oncol Lett. (2022) 23:51. doi: 10.3892/ol.2021.13169
172. Li J, Whelan S, Kotturi MF, Meyran D, D'Souza C, Hansen K, et al. PVRIG is a novel natural killer cell immune checkpoint receptor in acute myeloid leukemia. Haematologica. (2021) 106:3115–24. doi: 10.3324/haematol.2020.258574
173. ChashChina A, Marklin M, Hinterleitner C, Salih HR, Heitmann JS, Klimovich B. DNAM-1/CD226 is functionally expressed on acute myeloid leukemia (AML) cells and is associated with favorable prognosis. Sci Rep. (2021) 11:18012. doi: 10.1038/s41598-021-97400-6
174. Takahashi N, Sugaya M, Suga H, Oka T, Kawaguchi M, Miyagaki T, et al. Increased Soluble CD226 in Sera of Patients with Cutaneous T-Cell Lymphoma Mediates Cytotoxic Activity against Tumor Cells via CD155. J Invest Dermatol. (2017) 137:1766–73. doi: 10.1016/j.jid.2017.03.025
175. Dixon KO, Schorer M, Nevin J, Etminan Y, Amoozgar Z, Kondo T, et al. Functional anti-TIGIT antibodies regulate development of autoimmunity and antitumor immunity. J Immunol. (2018) 200:3000–7. doi: 10.4049/jimmunol.1700407
176. Cho BC, Abreu DR, Hussein M, Cobo M, Patel AJ, Secen N, et al. Tiragolumab plus atezolizumab versus placebo plus atezolizumab as a first-line treatment for PD-L1-selected non-small-cell lung cancer (CITYSCAPE): primary and follow-up analyses of a randomized, double-blind, phase 2 study. Lancet Oncol. (2022) 23:781–92. doi: 10.1016/S1470-2045(22)00226-1
177. Zhao J, Dong J, Deng C, Zhang Q, Sun S, Li H, et al. Enhancing T cell anti-tumor efficacy with a PD1-TIGIT chimeric immune-checkpoint switch receptor. Oncoimmunology. (2023) 12:2265703. doi: 10.1080/2162402X.2023.2265703
178. Vaena DA, Fleming GF, Chmielowski B, Sharma M, Hamilton EP, Sullivan RJ, et al. COM701 with or without nivolumab: Results of an ongoing phase 1 study of safety, tolerability and preliminary antitumor activity in patients with advanced solid Malignancies (NCT03667716). J Clin Oncol. (2021) 39:supple2504. doi: 10.1200/JCO.2021.39.15_suppl.2504
179. Hansen K, Kumar S, Logronio K, Whelan S, Qurashi S, Cheng HY, et al. COM902, a novel therapeutic antibody targeting TIGIT augments anti-tumor T cell function in combination with PVRIG or PD-1 pathway blockade. Cancer Immunol Immunother. (2021) 70:3525–40. doi: 10.1007/s00262-021-02921-8
180. ClinicalTrials.gov. Available online at: https://clinicaltrials.gov/study/NCT04099277.
181. Sim YH, Um YJ, Park JY, Seo MD, Park SG. A novel antibody-drug conjugate targeting nectin-2 suppresses ovarian cancer progression in mouse xenograft models. Int J Mol Sci. (2022) 23:12358. doi: 10.3390/ijms232012358
182. Oshima T, Miyashita H, Ishimura Y, Ito Y, Tanaka Y, Hori A, et al. Fc engineering of anti-Nectin-2 antibody improved thrombocytopenic adverse event in monkey. PloS One. (2018) 13:e0196422. doi: 10.1371/journal.pone.0196422
183. Ma L, Ma J, Sun X, Liu H. Bispecific anti-CD3xanti-CD155 antibody mediates T-cell immunotherapy in human hematologic Malignancies. Invest New Drugs. (2023) 41:522–31. doi: 10.1007/s10637-023-01367-2
184. Desjardins A, Gromeier M, Herndon JE 2nd, Beaubier N, Bolognesi DP, Friedman AH, et al. Recurrent glioblastoma treated with recombinant poliovirus. N Engl J Med. (2018) 379:150–61. doi: 10.1056/NEJMoa1716435
Keywords: cancer immunotherapy, DNAM1, immune checkpoint inhibitor, PVR, PVRIG, PVRL2, TIGIT
Citation: Murakami K and Ganguly S (2024) The Nectin family ligands, PVRL2 and PVR, in cancer immunology and immunotherapy. Front. Immunol. 15:1441730. doi: 10.3389/fimmu.2024.1441730
Received: 31 May 2024; Accepted: 22 July 2024;
Published: 02 August 2024.
Edited by:
Noha Mousaad Elemam, University of Sharjah, United Arab EmiratesReviewed by:
Peng Xu, Yangzhou University, ChinaWei Wang, University of Wisconsin-Madison, United States
Copyright © 2024 Murakami and Ganguly. This is an open-access article distributed under the terms of the Creative Commons Attribution License (CC BY). The use, distribution or reproduction in other forums is permitted, provided the original author(s) and the copyright owner(s) are credited and that the original publication in this journal is cited, in accordance with accepted academic practice. No use, distribution or reproduction is permitted which does not comply with these terms.
*Correspondence: Sudipto Ganguly, c2dhbmd1bDhAamhtaS5lZHU=