- 1School of Chemistry, Chemical Engineering and Life Science, Wuhan University of Technology, Wuhan, China
- 2Hubei Key Laboratory of Nanomedicine for Neurodegenerative Diseases, School of Chemistry, Chemical Engineering and Life Science, Wuhan University of Technology, Wuhan, China
- 3Hunan Key Laboratory of Typical Environmental Pollution and Health Hazards, School of Public Health, Hengyang Medical School, University of South China, Hengyang, China
- 4Institute of WUT-AMU, Wuhan University of Technology, Wuhan, China
- 5State Key Laboratory of Advanced Technology for Materials Synthesis and Processing, Wuhan University of Technology, Wuhan, China
AIM2, a cytosolic innate immune receptor, has the capability to recognize double-stranded DNA (dsDNA). This paper delineates the structural features of AIM2 and its mechanisms of activation, emphasizing its capacity to detect cytosolic DNA and initiate inflammasome assembly. Additionally, we explore the diverse functions of AIM2 in different cells. Insights into AIM2-mediated neuroinflammation provide a foundation for investigating novel therapeutic strategies targeting AIM2 signaling pathways. Furthermore, we present a comprehensive review of the roles of AIM2 in neurodegenerative diseases, including Alzheimer’s disease (AD) and Parkinson’s disease (PD). Finally, we discuss its therapeutic implications. In conclusion, a profound understanding of AIM2 in neurodegenerative diseases may facilitate the development of effective interventions to mitigate neuronal damage and slow disease progression.
1 Introduction
Neurodegenerative diseases are characterized by the progressive degeneration of neurons in the central nervous system (CNS), resulting in cognitive decline and/or motor dysfunction. These disorders present significant challenges to global healthcare systems and impose a substantial economic burden on society. Prominent examples of neurodegenerative diseases include Alzheimer’s disease (AD), Parkinson’s disease (PD) and amyotrophic lateral sclerosis (ALS). The etiology of neurodegenerative diseases is multifactorial, involving complex interactions between genetic and environmental factors. While genetic mutations predispose individuals to certain neurodegenerative diseases, environmental toxins and lifestyle factors also contribute to disease onset and progression (1).
Emerging evidence suggests neuroinflammation, including both innate and acquired immunity, plays crucial roles in the pathophysiology of these diseases, contributing to the initiation and progression of neurodegeneration (2–4). The innate immune system provides the first line of defense, primarily through the actions of microglia, the resident immune cells of the CNS (5). Meanwhile, the acquired immune system, involving adaptive immune responses, adds another layer of complexity to the immune dynamics in neurodegenerative conditions (6).
Innate immunity in the CNS is largely mediated by microglia, which are activated in response to neuronal injury, pathogens, and aggregated proteins commonly found in neurodegenerative diseases. In AD, microglia recognize amyloid-beta (Aβ) plaques through pattern recognition receptors (PRR) such as toll-like receptors (TLRs) and nucleotide-binding oligomerization domain (NOD)-like receptors (NLRs) (7). Upon activation, microglia initiate a cascade of pro-inflammatory responses, releasing cytokines, chemokines, and reactive oxygen species (ROS). While these responses aim to clear pathological proteins and debris, chronic activation can lead to sustained inflammation and neuronal damage (8). Similarly, in PD, microglia become activated in response to α-synuclein aggregates, contributing to the progressive loss of dopaminergic neurons in the substantia nigra (9).
Astrocytes, another key player in the CNS innate immune response, also become reactive in neurodegenerative diseases. Reactive astrocytes undergo morphological and functional changes, including the upregulation of glial fibrillary acidic protein (GFAP) and the release of pro-inflammatory mediators (10). In neurodegenerative diseases such as ALS, reactive astrocytes contribute to motor neuron degeneration by releasing toxic substances and exacerbating inflammatory responses. The interplay between microglia and astrocytes amplifies the neuroinflammatory environment, promoting further neuronal injury (11).
The acquired immune system, comprising T cells and B cells, also significantly impacts neurodegenerative disease progression. In the context of neurodegenerative diseases like AD and PD, there is increasing evidence of T cell infiltration into the CNS. These T cells can recognize and respond to neuronal antigens, contributing to the inflammatory milieu (6). For example, in AD, the presence of Aβ-reactive T cells has been detected, suggesting that adaptive immune responses may exacerbate neuroinflammation and neuronal damage (12). B cells and antibodies also play roles in neurodegenerative diseases. In AD, the role of B cells is less clear, but autoantibodies against neuronal components have been identified, indicating a potential contribution to disease progression (13).
2 Inflammasome
Neuroinflammation, characterized by the activation of immune cells and the release of pro-inflammatory mediators, is closely intertwined with inflammasome activation in the CNS. Inflammasomes are multiprotein complexes that include PRRs, adapter proteins where applicable, and inflammatory caspases. They are activated by pathogen-associated molecular patterns (PAMPs) or endogenous danger-associated molecular patterns (DAMPs). Upon activation, PRRs oligomerize, facilitating the binding and oligomerization of the adapter protein apoptosis-associated speck-like protein containing a caspase recruitment domain (ASC). ASC has two death domains (DDs), a pyrin domain (PYD) at the N-terminus and a caspase recruitment domain (CARD) at the C-terminus. ASC oligomerization subsequently activates caspase-1, leading to the maturation and release of the proinflammatory cytokines interleukin-1β (IL-1β) and interleukin-18 (IL-18) (14–16). These cytokines elicit robust inflammatory responses by activating the IL-1β receptor (IL-1βR)/IL-18 receptor (IL-18R)-myeloid differentiation primary response gene 88 (MyD88)-nuclear factor kappa-B (NFκB) pathway. Furthermore, active caspase-1 cleaves gasdermin D (GSDMD), resulting in the liberation of its N-terminus, which forms pores in the plasma membrane (PM) and triggers pyroptosis (14–16). Proper inflammasome activation is crucial for pathogen elimination and the clearance of damaged cell, whereas excessive inflammasomes activity contributes to various diseases, including inflammatory disorders and neurodegenerative diseases.
Based on their cellular localization, PRRs are classified into membrane-bound and cytoplasmic types. TLRs and the C-type lectin receptors (CLRs) are examples of membrane-bound PRRs, whereas NLRs, retinoic acid-inducible gene-I–like receptors (RLRs) and absent in melanoma 2 (AIM2)-like receptors (ALRs) are cytoplasmic PRRs. This review focuses on AIM2, a member of the ALR family, which belongs to the pyrin and hematopoietic, interferon inducible, and nuclear (HIN) domain (PYHIN) family, characterized by a pyrin domain (PYD) at the N-terminus and one or two HIN domains at the C-terminus.
AIM2 is a cytosolic receptor for double-stranded DNA (dsDNA). Previous studies suggested that in the absence of dsDNA, the HIN and PYD domains of AIM2 interact to maintain it in an autoinhibited state. Interaction of dsDNA with the HIN domain alleviates AIM2 from this autoinhibited state (17), promoting the formation of AIM2 PYD filament (18). Nevertheless, Sohn and his colleagues challenged this autoinhibitory model. They proposed that the AIM2 PYD domain is not involved in AIM2 autoinhibition but rather aids in both binding to dsDNA and self-association of AIM2. Additionally, the AIM2 PYD domain enhances the binding of AIM2 to dsDNA, whereas the AIM2 HIN domain’s interaction with dsDNA promotes AIM2 oligomerization (19). Clustering of AIM2 on dsDNA induces filamentous polymerization of AIM2 PYD.
3 The mechanisms of AIM2 activation
The HIN domain of AIM2 consists of two oligonucleotide/oligosaccharide binding (OB) folds, each binding to one DNA strand (17, 20, 21). This interaction is predominantly facilitated by electrostatic interactions between positively charged residues of the OB folds and the negatively charged phosphates on the ribose backbone of DNA molecules (17, 20, 21). The binding of AIM2 to dsDNA is sequence-independent due to the lack of interaction between the HIN domain of AIM2 and the DNA bases (22). However, the length of dsDNA is an important factor for efficient activation of the AIM2 inflammasome. A minimum length of 70 base pairs (bp) of dsDNA is required for AIM2 inflammasome activation. However, optimal AIM2 activation requires dsDNA of at least 200 bp in length (17). Moreover, the assembly of the AIM2 inflammasome is expedited by longer dsDNA molecules (23). A recent study suggested that the HIN domain of AIM2 does not exhibit a preference for dsDNA over other nucleic acids. Nonetheless, AIM2 demonstrates a greater affinity for binding to and oligomerizing on dsDNA. Additionally, although AIM2 can assemble filaments on various nucleic acids besides dsDNA, these filaments are poorly organized and fail to enhance ASC polymerization (24).
AIM2 activation has been observed in various pathological conditions, such as neurodegenerative diseases (25, 26), diabetes (27) and heart failure (28). Nevertheless, the mechanisms underlying AIM2 activation in these diseases remain elusive. Emerging evidence suggests that AIM2 can be activated by a variety of types of cytoplasmic DNA, including foreign DNA from virus/bacteria, or self-DNA herniated from the nuclear envelope or mitochondrial membrane in these pathological states.
3.1 Bacterial and virus infection
The activation of the AIM2 inflammasome during bacterial infections is primarily attributed to its ability to detect microbial dsDNA. Two essential prerequisites for AIM2 inflammasome activation in the context of bacterial infection are the access of bacteria to the cytoplasm and the subsequent release of bacterial DNA through bacteriolysis. The secretory effector protein SdhA of L. pneumophila is known to inhibit the release of bacterial DNA and thereby impede AIM2 inflammasome activation (29). Additionally, infection of macrophages with F. tularensis generates cytosolic DNA that specifically binds to AIM2, inducing AIM2 oligomerization, which subsequently leads to the activation of caspase-1 and cell death (30). Peng and colleagues demonstrated that mutations in certain bacterial strains result in increased intracellular lysis, leading to enhanced release of bacterial DNA into the host cell cytosol, thereby triggering AIM2 inflammasome activation (31). Furthermore, a mutation in the lmo2473 gene, which encodes a protein of unknown function, resulted in hyperactivation of the AIM2 inflammasome in L. monocytogenes infected macrophages. This hyperactivation was associated with impaired cell wall integrity in the lmo2473 mutant, leading to increased intracellular lysis and consequent DNA release into the cytosol, which in turn augmented AIM2 inflammasome activation (32).
Moreover, AIM2 can recognize cytosolic dsDNA viruses and form an inflammasome complex that enhances host defense by inducing pyroptosis. Classical dsDNA-containing viruses, such as mouse cytomegalovirus (MCMV) and vaccinia virus, are known to activate the AIM2 inflammasome in both mouse bone marrow-derived macrophages (BMDMs) and bone marrow-derived dendritic cells (BMDCs) (33). Human papillomaviruses, which are also dsDNA viruses, specifically infect human keratinocytes and promote the production of cytokines in an AIM2-dependent manner (34). Additionally, siRNA-mediated knockdown of AIM2 in human glomerular mesangial cells infected with hepatitis B virus results in decreased expression of IL-1β, IL-18, and caspase-1 (35).
3.2 The herniation of genomic DNA from the nuclear membrane
The herniation of genomic DNA from the nuclear membrane refers to the pathological protrusion or extrusion of nuclear DNA into the cytoplasm, typically resulting from nuclear envelope instability or cellular stress. Nelfinavir, an HIV aspartyl protease inhibitor, has been shown to induce inflammasome formation and trigger IL-1R-dependent inflammation in mice. This drug impairs the maturation of lamin A, a critical structural component of the nuclear envelope, thereby facilitating the release of DNA into the cytosol. Additionally, the absence of AIM2 diminishes nelfinavir-mediated inflammasome activation (36). Furthermore, AIM2 in myoepithelial cells (MECs) from the lacrimal gland can be directly activated by self-genomic DNA (self-gDNA), which might contribute to the pathogenesis of primary Sjögren’s syndrome (pSS) (37).
3.3 Mitochondrial dysfunction
Mitochondria dysfunction has emerged as a significant trigger for AIM2 activation. One of the key mechanisms leading to AIM2 activation is the release of mitochondrial DNA (mtDNA) into the cytoplasm. Once in the cytoplasm, mtDNA acts as a potent activator of AIM2 inflammasome. For example, oxidative stress occurring in hepatic steatosis leads to mitochondrial damage, release of mtDNA, and subsequent activation of the AIM2 inflammasome. Additionally, the nonsteroidal anti-inflammatory drug (NSAID)-activated gene-1 (NAG-1)/growth differentiation factor-15 (GDF15) prevents hepatic steatosis by inhibiting the activation of AIM2 (38). Furthermore, cholesterol induces the release of mtDNA and activates AIM2 in macrophages. Studies suggest that oxidized mtDNA activates the NLRP3 inflammasome, whereas non-oxidized mtDNA primarily stimulates the AIM2 inflammasome (39).
4 AIM2 protein interactions
Interactions between AIM2 and various protein partners play essential roles in modulating cellular functions beyond inflammasome assembly, ranging from immune regulation to cellular metabolism. These interactions provide insights into the multifaceted roles of AIM2 in neurodegenerative diseases.
One notable set of AIM2 interacting partners are the proteins related to the regulation of T-cell functions. In T follicular helper (TFH) cells, AIM2 regulates TFH differentiation by recruiting c-musculoaponeurotic fibrosarcoma (c-MAF). This interaction regulates c-MAF expression, enhances IL-21 production, and recruits hydroxymethyltransferase ten-eleven translocation 2 (TET2) to the AIM2 promoter, leading to DNA demethylation and increased AIM2 transcription (Figure 1A) (40). Additionally, AIM2 has been found to modulate the activity of regulatory T (Treg) cells through its interaction with activated protein C kinase 1 (RACK1). In Treg cells, AIM2 preserves Treg cell identity by recruiting RACK1, which induces AKT dephosphorylation by recruiting the phosphatase PP2A. This inhibition of AKT signaling shifts the metabolic program of Treg cells towards oxidative phosphorylation, thereby stabilizing the Treg cell lineage (Figure 1B) (41).
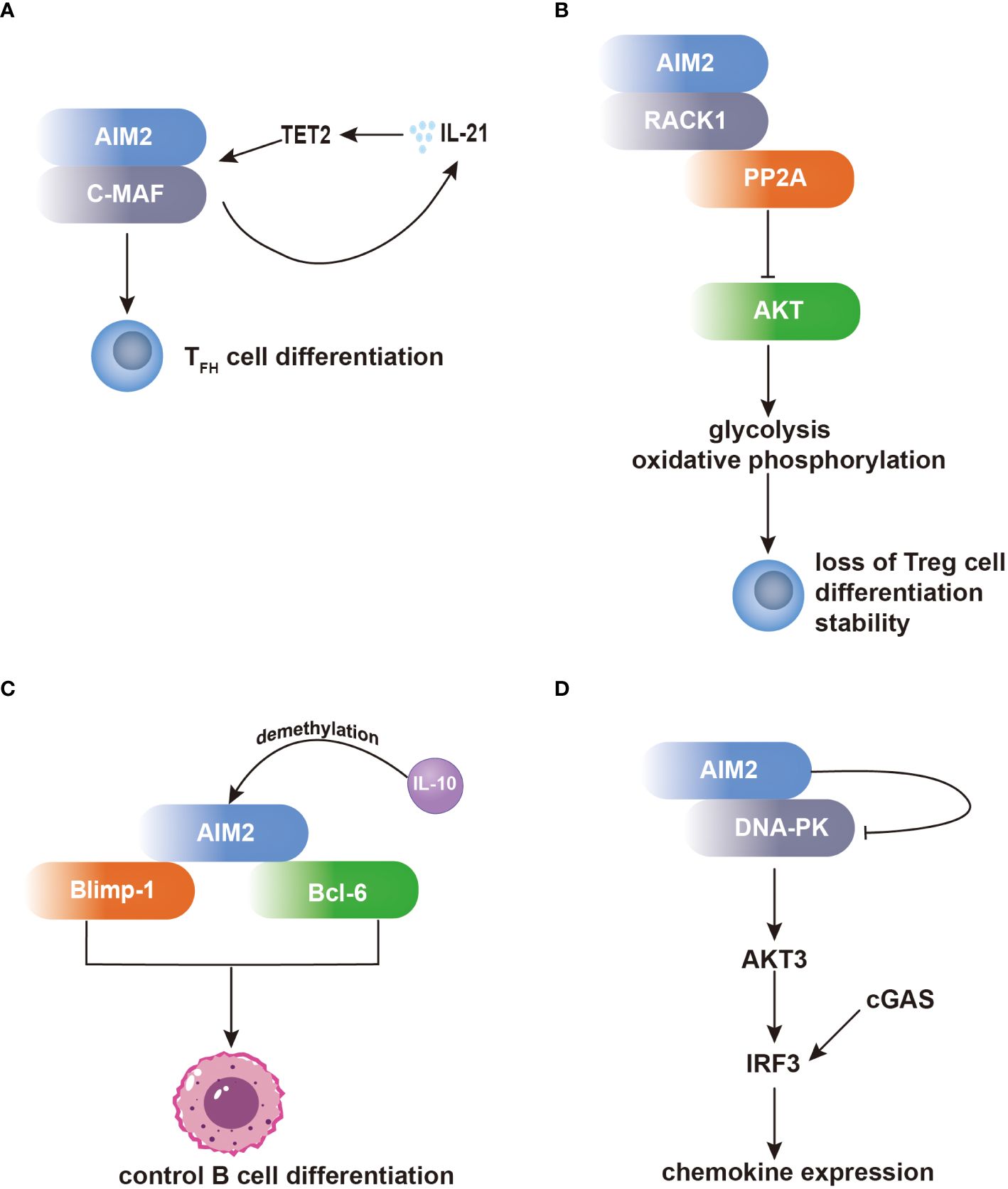
Figure 1 AIM2 modulates the differentiation of T cells and B cells independent of the inflammasome. (A) Within TFH cells, AIM2 orchestrates TFH differentiation through c-MAF recruitment, bolstering IL-21 production. Consequently, IL-21 facilitates TET2 recruitment to the AIM2 promoter, enhancing AIM2 transcription. (B) Within Treg cells, AIM2 preserves their identity via RACK1 interaction. RACK1 facilitates AKT dephosphorylation by recruiting PP2A, thereby inducing a metabolic shift towards oxidative phosphorylation, crucial for Treg cell stability. (C) AIM2 exerts influence over B-cell differentiation by interacting with Blimp-1 and Bcl-6. AIM2’s direct interaction with Bcl-6 upregulates Bcl-6 expression, whereas its binding to Blimp-1 downregulates Blimp-1 expression, impacting B-cell differentiation. Notably, both Blimp-1 and Bcl-6 are transcription factors pivotal in regulating B-cell differentiation. (D) Within microglia, AIM2 binds to DNA-PK, effectively suppressing its activity. Consequently, IRF3 phosphorylation is diminished, leading to inhibition of chemokine expression.
Furthermore, AIM2 participates in the regulation of B-cell differentiation through its interactions with transcription factors involved in B-cell fate determination. By directly binding to B lymphocyte-induced maturation protein 1 (Blimp-1) and B-cell lymphoma 6 protein (Bcl-6), AIM2 can modulate their expression levels, thereby influencing the balance between plasma cell differentiation and germinal center reactions. The dynamic interplay between AIM2 and these transcriptional regulators highlights the complexity of B-cell differentiation and the potential regulatory role of AIM2 in antibody production and immune responses (Figure 1C) (42).
Additionally, in microglia, AIM2 forms a complex with DNA-dependent protein kinase (DNA-PK), wherein DNA-PK acts as a negative regulator of AIM2-mediated inflammation. Through this interaction, DNA-PK suppresses the activity of AIM2, thereby inhibiting downstream signaling cascades involved in inflammatory gene expression. Specifically, DNA-PK inhibits the activation of AKT3 and subsequent phosphorylation of interferon regulatory factor 3 (IRF3), such effect inhibits cyclic GMP–AMP synthase (cGAS) and DNA-PK comediated-IRF3 activation, leading to the attenuation of microglial antiviral pathway-related inflammation (Figure 1D) (43).
Moreover, AIM2 interacts with proteins involved in the regulation of macrophage activation. In macrophages, the E3 ubiquitin ligase HECT, UBA and WWE domain-containing E3 ubiquitin protein ligase 1 (HUWE1) has been identified as an AIM2-interacting protein. Through its BH3 domain, HUWE1 binds to the HIN domain of AIM2, mediating AIM2 polyubiquitination and promoting its activation (Figure 2A) (44). This interaction underscores the importance of post-translational modifications in regulating AIM2 inflammasome activity in macrophages. Additionally, AIM2 interacts with tripartite motif containing 11 (TRIM11), leading to AIM2 auto-polyubiquitination and subsequent degradation via selective autophagy. This regulatory mechanism serves as a feedback loop to control AIM2 levels and prevent excessive inflammasome activation (Figure 2B) (45).
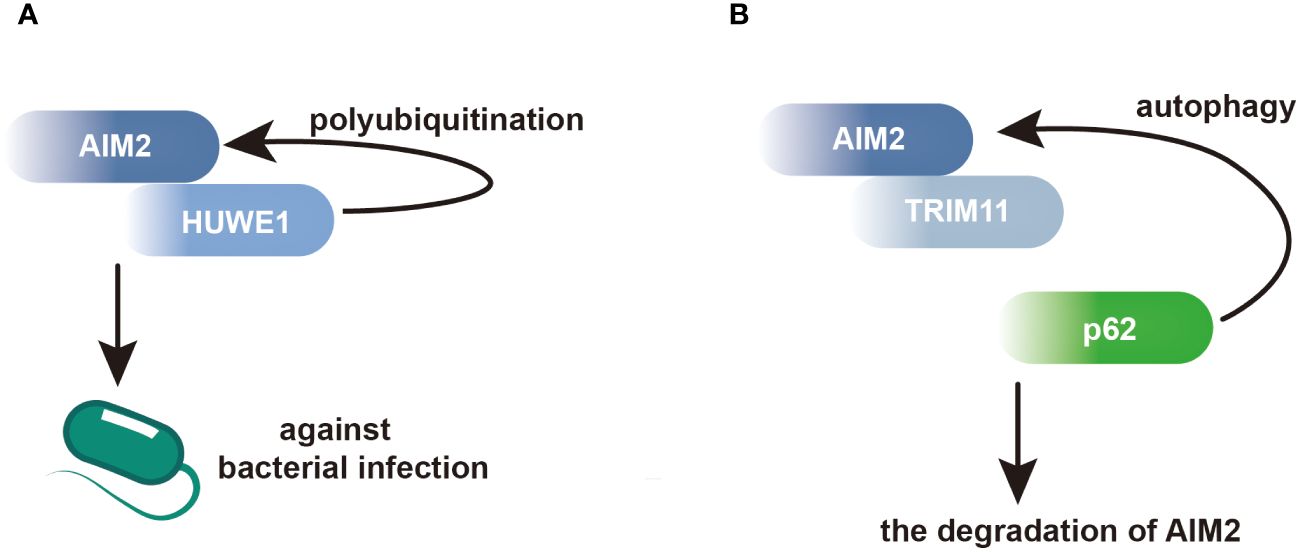
Figure 2 AIM2 modulates its activation and degradation by interacting with its partners in BMDMs. (A) Within BMDMs, HUWE1 has been identified as an AIM2-interacting protein, facilitating the polyubiquitination of AIM2 and triggering its activation. This activation enhances host defense against bacterial infections. (B) Through its PS domain, TRIM11 interacts with AIM2, facilitating the association between TRIM11 and the autophagic cargo receptor p62. This interaction leads to the degradation of AIM2.
5 Activation and roles of AIM2 inflammasome in different cells
5.1 Neurons
In recent years, researchers have consistently observed high expression of the AIM2 inflammasome in neurons across various CNS diseases (46, 47). Upon activation, the AIM2 inflammasome secretes caspase-1-cleaving inflammatory cytokines in neurons, ultimately inducing neuronal cell death via pannexin-1 channel opening (48). Additionally, the inhibition of pannexin-1 channels using probenecid in a rat model of subarachnoid hemorrhage reduced AIM2 inflammasome activation in neurons, resulting in the attenuation of brain edema and neurological deficits in rats (49). Furthermore, adding synthetic dsDNA to cultured neurons induces IL-1β secretion in an AIM2-dependent manner, which consequently enhances axon extension. Behavioral analyses further reveal that AIM2 deletion in mice induces anxious behaviors, and impairs locomotion and auditory fear memory (46).
5.2 Dendritic cells
In DCs, AIM2 plays a pivotal role in orchestrating immune responses against various pathogens and in shaping adaptive immunity. DCs are professional antigen-presenting cells required for initiating and modulating immune responses. Upon encountering pathogens, DCs recognize and capture antigens, process them, and present antigenic peptides to T cells, thereby activating adaptive immune responses. Studies have shown that the activation of AIM2 inflammasome in DCs is important for mounting effective immune responses against various pathogens, including bacteria, viruses, and parasites. For example, AIM2 activation in DCs is essential for controlling infections caused by pathogens such as Francisella novicida, Mycobacterial species and Adenovirus (50).
In addition, AIM2 activation in DCs has been implicated in cancer immunotherapy. The activation of AIM2 contributes to the formation of an immunosuppressive microenvironment within melanoma. Vaccination with AIM2-deficient DCs enhances the therapeutic efficacy of adoptive T-cell therapy and anti–programmed death-ligand 1 (PD-L1) immunotherapy in tumors unresponsive to current treatments. Similarly, mouse DCs treated with AIM2 siRNA in vivo and human DCs in vitro exhibit potent anti-tumor immune responses (51).
Moreover, differentiation of monocytes into DCs induced by CD137 ligand (CD137L) activates the AIM2 inflammasome, leading to the polarization of CD8+ T cells towards a cytotoxic T lymphocyte (Tc1) phenotype. This conversion enhances T-cell response against cancer‐associated viruses (52). Additionally, activation of AIM2 in plasmacytoid DCs induces Ca2+ release, triggering calpain activation and IL-1α release, thereby promoting tumor cell proliferation in the lung (53).
5.3 Monocyte
Monocytes can be categorized into two groups. One group consists of immature proinflammatory monocytes, which express CD115, lymphocyte antigen 6C (Ly6C), and C-C chemokine receptor type 2 (CCR2) on their cell membrane. Upon activation, these monocytes migrate to injury sites and differentiate into proinflammatory macrophages. The other group consists of regulatory/patrolling monocytes, which express low levels of Ly6C and high levels of CX3CR1, and do not express CCR2. Under healthy conditions, these monocytes differentiate into tissue-resident macrophages.
AIM2 activation in monocytes contributes to T cell death following tissue injuries, increasing susceptibility to life-threatening infections. Monocytes detect DNA released during injury via the AIM2 inflammasome, upregulating Fas ligand (FasL) expression and inducing apoptosis in extrinsic T cells (54). Furthermore, Infection of monocytes with severe acute respiratory syndrome-coronavirus-2 (SARS‐CoV‐2) triggers inflammatory responses via activation of the AIM2 inflammasome, thereby contributing to the pathogenesis of COVID-19 (55).
5.4 Macrophages/Microglia
Macrophages exhibit diverse functions including pathogen defense and wound healing. They are classified into “pro-inflammatory (M1)” and “anti-inflammatory (M2)” macrophages based on their activation conditions. Initially, this dichotomy was proposed to simplify the understanding of macrophage functions in various physiological and pathological contexts. However, recent research suggests that macrophages exhibit a much broader spectrum of activation states, influenced by the complex interplay of signals within their microenvironment. This continuum of activation challenges the binary classification, as macrophages can simultaneously express markers and functions characteristic of both M1 and M2 phenotypes or transition between states in response to dynamic changes in their environment. Consequently, the simplistic M1/M2 paradigm may not adequately capture the full functional diversity and plasticity of macrophages (56).
Considerable evidence supports the role of AIM2 activation in eliminating intracellular bacteria and DNA viruses within infected macrophages, such as Francisella tularensis, Listeria monocytogenes, and Murine cytomegalovirus (30, 33). AIM2 activation in macrophages also contributes to kidney injury, and renal carcinoma. In a mouse model of kidney injury induced by unilateral ureteric obstruction (UUO), the activation of AIM2 inflammasome in macrophages, triggered by DNA uptake, regulates renal inflammation and fibrosis (57). In renal carcinoma models, AIM2 enhances the polarization switch of tumor-associated macrophages (TAMs) from the anti-inflammatory M2 phenotype to the pro-inflammatory M1 phenotype (58). Additionally, AIM2 collaborates with adrenergic signaling, promoting macrophages to assume an immunosuppressive phenotype by increasing the expression of programmed death-ligand 1 (PD-L1) and indoleamine 2,3-dioxygenase (IDO). blocking either the AIM2 inflammasome or α1-adrenergic receptor (α1-AR) diminishes IL-1β release triggered by chimeric antigen receptor T-cell (CAR-T) therapy (59).
Microglia, often termed the “sentinels of the brain,” are resident immune cells of the CNS crucial for maintaining its homeostasis. Microglial AIM2 functions have also been investigated. In the 1-methyl-4-phenyl-1,2,3,6-tetrahydropyridine (MPTP) mouse model, microglial AIM2 activation diminishes cGAS - mediated antiviral inflammation. This inhibition occurs independently of the inflammasome and involves IRF3 phosphorylation suppression (25). Microglial AIM2 deficiency worsened behavioral and pathological symptoms in both MPTP-induced and transgenic PD mouse models (25). Additionally, microglial AIM2 alleviates symptoms of experimental autoimmune encephalomyelitis (EAE) independent of inflammasome activation. Absence of AIM2 enhances microglial activities and peripheral immune cell infiltration into the CNS, exacerbating neuroinflammation and demyelination during disease progression (43). Furthermore, microglial AIM2 contributes to age-related cognitive dysfunction by modulating complement-dependent microglial phagocytosis (60). Similarly, microglia exhibit heightened expression levels of AIM2 in AD mice. However, cognitive and synaptic dysfunction in AD mice were ameliorated upon conditional knockout of microglial AIM2. This knockout also led to a reduction in excessive microglial phagocytosis of synapses via the inhibition of complement activation (61).
5.5 B cells
B cells function in antibody production, antigen presentation and cytokine production. Antibody binding to targets can trigger antibody-dependent cellular cytotoxicity (ADCC) and complement-dependent cytotoxicity (CDC). Moreover, B cells perform antigen presentation by internalizing, processing and presenting antigens to CD4+ T cells. Additionally, B cells secrete both pro-inflammatory and anti-inflammatory cytokines in response to stimulation.
AIM2 expression is confined to mature B cells, specifically within the CD27 positive subset. In response to IFN-γ, these cells upregulate AIM2 mRNA expression, while activation of B-cell receptor by CD40 leads to downregulation of AIM2, enabling B-cell proliferation (62). AIM2 in B cells has been found to play a noncanonical role in regulating CXCL16 production. AIM2 knockout enhances CXCL16 production in B cells while reducing the expression of homing receptors [sphingosine-1-phosphate receptor 1 (S1PR1) and CD62 ligand (CD62L)] in CD8+ T cells, thereby increasing their infiltration and retention in chronic inflammatory tissues independently of the inflammasome (63). Additionally, AIM2 deficiency in B cells modulates B-cell differentiation through the Blimp-1-Bcl-6 axis, leading to the amelioration of systemic lupus erythematosus (SLE) (42).
5.6 T cells
T cells encompass helper, regulatory, cytotoxic and memory subsets. Helper T cells secrete cytokines to facilitate B cell differentiation upon stimulation. Regulatory T cells modulate immune responses. Cytotoxic T cells eliminate infected cells. Memory T cells, specific to antigens, swiftly transition into effector T cells upon re-exposed to the antigen.
AIM2 plays a cell-intrinsic role in CD4+ T-cell differentiation. AIM2 induces the differentiation of CD4+ T cells into FOXP3+ regulatory T cells (Treg). RNA sequencing reveals that Treg differentiation is not primarily driven by the expression of signature genes, but rather by the modulation of cell metabolism. Similarly, in a T-cell transfer model of colitis, T cells deficient in ATM2 preferentially differentiated into FOXP3+ cells in vivo (64). Moreover, AIM2 enhances Treg cell stability and protects mice from autoimmune encephalomyelitis and inflammatory colitis. Transcription factors associated with Treg cells, including RUNX1, ETS1, BCL11B and CREB can occupy AIM2 promoter. Moreover, AIM2 mitigates EAE symptoms by promoting the switch from glycolysis to oxidative phosphorylation in Treg cells (41). Additionally, AIM2 influences the development of SLE by regulating TFH cell differentiation through IL-21-induced TET2 recruitment and the interaction between AIM2 and c-MAF (40).
6 The roles of AIM2 in neurodegenerative diseases
6.1 AD
AD is the most prevalent neurodegenerative disease. While amyloid-β (Aβ) deposition in the brain has been proposed as the initiating factor in AD, accumulating evidence challenges this hypothesis. Conversely, elevated levels of inflammatory markers in AD patients and the identification of AD risk genes associated with innate immune functions imply that neuroinflammation may play a role in AD pathogenesis.
In AD, Aβ is generated through the sequential cleavage of two enzymes and cleared from the brain via transport into the cerebrospinal fluid (CSF). Upon surpassing a critical concentration threshold, Aβ aggregates into oligomers and fibrils, serving as DAMPs that trigger inflammasome activation. Research has demonstrated that Aβ can activate the AIM2 inflammasome in BV2 cells and primary cultured microglia, leading to increased mRNA and protein expression of AIM2 (65).
Numerous studies have demonstrated the implication of AIM2 in AD (Table 1). Deletion AIM2 reduces Aβ deposition and suppresses microglial activation in 5XFAD mice; However, it does not improve the memory and anxiety phenotypes of these mice. Interestingly, the knockout of AIM2 in 5XFAD mice leads to an increase in inflammatory cytokine expression (26). Conversely, deleting AIM2 in APP/PS1 mice improves spatial memory, promotes the induction of long-term potentiation (LTP) in hippocampal slices, and induces structural changes in dendrites. Transcriptional microarray analysis revealed that AIM2 deficiency in APP/PS1 mice alters the expression levels of various proteins, including Pten, Homer1, and Ppp2r3a (66). Moreover, ectopic expressing optineurin (OPTN) inhibits the activation of AIM2 inflammasomes induced by Aβ oligomers in APP/PS1 mice (65). Furthermore, AIM2 regulates microglial phagocytosis of synapses, triggering activation of the complement system through the classical pathway, ultimately contributing to cognitive impairment in Aβ1–42-induced AD mice (61).
6.2 PD
PD ranks as the second most common neurodegenerative disease, characterized by the gradual loss of dopaminergic neurons in the substantia nigra. Numerous studies have implicated neuroinflammation in the pathogenesis of PD (75, 76). Limited research has investigated the role of AIM2 in PD (Table 1). In MPTP-induced mouse models, the NLRP3 inflammasome exhibits significant activation in the nigrostriatal system, while the activities of AIM2 and other inflammasomes remain unaltered (77). Conversely, a separate study has shown that in the MPTP mouse model, microglial AIM2 exerts a negative regulatory effect on neuroinflammation independent of the inflammasome, and its deficiency exacerbates symptoms in both MPTP-induced and transgenic PD mouse models (25). Furthermore, crocin, one of herbal medicines, significantly reduced neuroinflammation and the loss of DA neurons in the SN of lipopolysaccharide (LPS)-induced PD models. Hematoxylin and eosin (H&E) staining revealed that crocin treatment reduced cell infiltration at the site of LPS injection, potentially through the inhibition of AIM2 expression (67).
6.3 TBI/SCI induced neurodegeneration
TBI and spinal cord injury (SCI) are significant causes of neurodegeneration, with neuroinflammation playing a central role in this process. Following TBI or SCI, there is an immediate activation of the innate immune response, characterized by the rapid infiltration of immune cells such as microglia and astrocytes to the site of injury. These cells release pro-inflammatory cytokines, chemokines, and ROS, which exacerbate neuronal damage. This sustained neuroinflammation not only contributes to secondary injury mechanisms, including excitotoxicity, oxidative stress, and blood-brain barrier (BBB) disruption, but also impedes the regenerative processes essential for neural recovery (78, 79). The interplay between neuroinflammation and neurodegeneration creates a detrimental feedback loop, where ongoing inflammation perpetuates neuronal loss and synaptic dysfunction, ultimately leading to progressive cognitive and motor deficits observed in TBI and SCI patients (78, 80).
Numerous studies indicate that AIM2 activation is crucial in TBI pathology (Table 1). Treatment with Poly(dA:dT) triggers ASC speck formation and IL-1β release in embryonic cortical neurons. Moreover, CSF from TBI patients can induce AIM2 activation and neuronal pyroptosis in vitro (48). In the controlled cortical impact (CCI) mouse model, brain microvascular endothelial cells (BMVECs) extracted from the injured cerebral cortex exhibit activation of both NLRs and AIM2 inflammasomes. Suppressing pyroptosis with Ac-YVAD-cmk mitigates TBI-induced brain edema and BBB leakage (68). Furthermore, TBI mice show elevated expression of NLRs and the AIM2 inflammasome in the cerebral cortex. VX765, a selective caspase-1 inhibitor, attenuates pyroptosis and inhibits the HMGB1/TLR4/NF-κB pathway (69). A recent study has discovered that AIM2 can serve as a serum marker reflecting the severity of human severe TBI and predicting poor outcomes (81).
Furthermore, in the healthy spinal cord, AIM2 expression is predominant in neurons, astrocytes, microglia, and oligodendrocytes, its expression increases following SCI (82). Additionally, in rat SCI models, both NLRs and AIM2 become activated 72 h post-injury. Hormonal therapy decreases AIM2 protein expression and signals associated with NLR synthesis and activation (70). Moreover, miR-672–5p, a crucial miRNA found in anti-inflammatory microglial exosomes, targets AIM2 gene expression. Applying miR-672–5p can repair SCI-induced damage (71).
In addition, pre-treatment with rapamycin has been demonstrated to ameliorate the outcome of cervical SCI in rats by modulating the AIM2 signaling pathway (72). Meanwhile, Schwann cell (SC) therapy has exhibited greater efficacy in dampening AIM2 inflammasome activation and the associated inflammatory pathway in SCI compared to Wharton’s jelly mesenchymal stem cells (WJ-MSCs) (73). Additionally, melanocortin receptor 4 (MC4R) has shown a protective effect on neurons by inhibiting AIM2 activation post-SCI, partly attributed to its regulation of mitochondrial homeostasis (74).
7 Targeting of AIM2 signaling pathways as a novel therapeutic approach in neurodegenerative diseases
Targeting of AIM2 signaling pathways presents a promising therapeutic avenue for neuroprotection in neurodegenerative diseases, with various strategies focusing on regulating AIM2 expression, activation, and downstream signaling pathways (Figure 3).
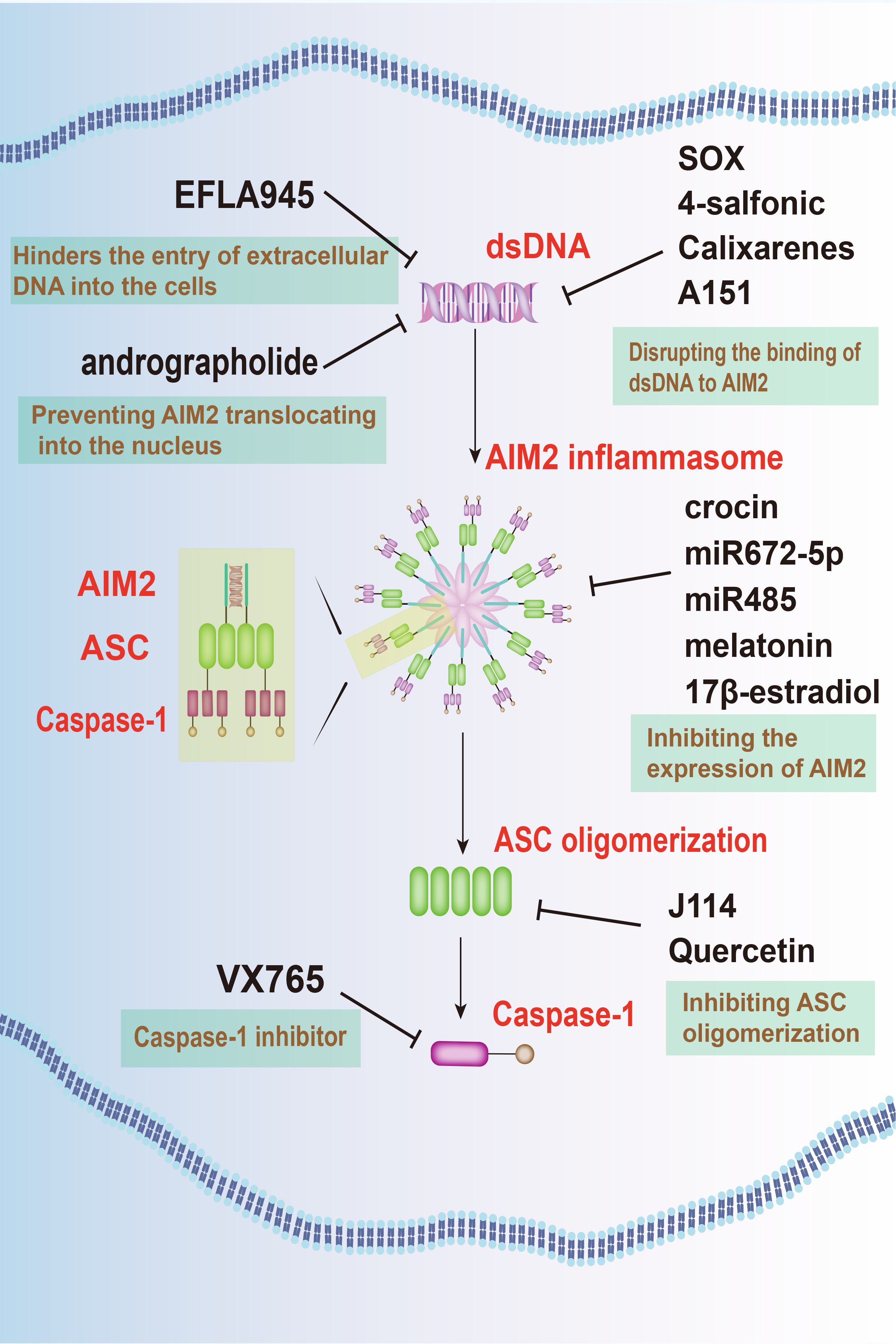
Figure 3 Targeting AIM2 signaling pathways offers a novel therapeutic approach for neurodegenerative diseases. Several agents, such as EFLA945, andrographolide, KSHV-encoded SOX protein, 4-sulfonic calixarenes, and A151, have been identified to inhibit AIM2 activation. Additionally, crocin, miRNAs, along with substances like melatonin and E2, are effective in inhibiting the expression of AIM2. Furthermore, J114 and quercetin are known to hinder ASC oligomerization, while VX-765 prevents the activation of caspase-1. These combined approaches offer a multi-faceted strategy to mitigate the detrimental effects of AIM2 in neurodegenerative conditions.
7.1 Inhibiting AIM2 activation
One such approach involves inhibiting AIM2 activation by targeting its upstream regulators. Agent like EFLA945 hinders the entry of extracellular DNA into the cells, thus preventing AIM2 activation and protecting against cell damage (83). In addition, andrographolide, an active component extracted from andrographis paniculate, effectively prevented AIM2 activation by preventing it from translocating into the nucleus to sense DNA damage. By this way, it ameliorates radiation-induced lung injury (RILI) (84). Furthermore, compounds like the kaposi’s sarcoma-associated herpesvirus (KSHV)-encoded SOX protein (85), 4-sulfonic calixarenes (86), and A151 (87) disrupt the binding of dsDNA to AIM2, inhibiting AIM2 inflammasome activation and mitigating inflammatory responses.
7.2 Inhibiting AIM2 expression
An alternative approach focuses on the inhibition of AIM2 expression within the CNS. Techniques such as microRNAs (miRNAs), along with substances like melatonin and 17β-estradiol (E2), offers targeted suppression of AIM2, potentially mitigating neuroinflammatory responses.
MiRNAs, found ubiquitously in organisms, possess the capability to selectively bind to target mRNA molecules, thereby regulating gene expression post-transcriptionally by inducing their degradation or inhibiting translation. MiR672–5p downregulates AIM2 expression, inhibiting neuronal pyroptosis via the AIM2/ASC/caspase-1 pathway (71). Similarly, miR-485 suppresses AIM2 expression by directly interacting with its 3’-UTR. Long non-coding RNA (lncRNA) maternally expressed gene 3 (MEG3) acts as a sponge for miR-485, ultimately leading to pyroptosis and inflammation during cerebral ischemia/reperfusion (I/R) mediated by AIM2 (88).
Moreover, hormonal therapy employing melatonin and E2 presents significant promise in addressing CNS injuries. Melatonin, primarily secreted by the pineal gland, confers protective effects against neuronal injury, while E2 demonstrates substantial anti-inflammatory properties. Research suggests that both melatonin and E2 reduce the upregulation of AIM2 expression, providing potential therapeutic pathways for neurodegenerative conditions (70, 89). In addition, crocin, an herbal medicine, also attenuates the LPS-induced neuroinflammation in PD by inhibiting AIM2 expression (67).
7.3 Inhibiting downstream signaling pathways of AIM2
Promising drug candidates targeting AIM2 signaling pathways have also emerged for potential clinical applications in neurodegenerative disease treatment. VX-765, for instance, acts as a selective caspase-1 inhibitor, effectively halting downstream inflammatory responses triggered by AIM2 inflammasome activation. Preclinical studies have confirmed VX-765’s efficacy in alleviating neuroinflammation and neuronal damage in animal models of TBI (69).
On the other hand, J114 disrupts the interaction between AIM2 and the adaptor protein ASC, hindering ASC oligomerization. This disruption leads to significant inhibitory effects on caspase-1 activation and IL-1β release in human THP-1 macrophages (90). Quercetin, a dietary flavonol abundant in various natural sources, stands out among polyphenols due to its potent antioxidant properties demonstrated in numerous studies. It interferes with ASC oligomerization, thereby impeding AIM2 inflammasome activation and preventing IL-1-mediated mouse vasculitis (91).
8 The limitations of current research on AIM2 and the challenges faced in therapeutic development
Despite significant advances in understanding the role of AIM2 in neuroinflammation and neurodegenerative diseases, several limitations and challenges persist in the current research landscape. One major limitation is the complexity of the AIM2 signaling pathways and their interactions with various cellular processes. AIM2 is known to modulate the differentiation and function of immune cells such as T cells, B cells, and microglia through both inflammasome-dependent and independent mechanisms. However, the precise molecular mechanisms underlying these interactions are not fully elucidated. This gap in knowledge hampers the development of targeted therapies, as the intricate network of signaling pathways must be thoroughly understood to design effective interventions.
Furthermore, the heterogeneity of neurodegenerative diseases adds another layer of complexity to AIM2 research. Diseases such as AD and PD exhibit diverse pathological features and progression patterns, influenced by genetic, environmental, and lifestyle factors. This heterogeneity complicates the identification of universal therapeutic targets within the AIM2 pathway. Additionally, patient-specific variations in AIM2 expression and activity further challenge the development of standardized treatments. Personalized medicine approaches, which tailor treatments based on individual patient profiles, may offer a solution but require extensive research to identify relevant biomarkers and develop corresponding therapeutic strategies.
In the context of therapeutic development, another critical issue is the delivery and specificity of AIM2-targeted drugs. Ensuring that these therapies can cross the BBB and reach affected regions in the brain at therapeutic concentrations is a significant challenge. Moreover, achieving specificity in targeting AIM2 without affecting other inflammasome pathways or immune functions is crucial to minimize potential side effects and off-target effects. Current therapeutic candidates, such as small molecule inhibitors and biologic agents, show promise in preclinical models, but their efficacy and safety in humans remain to be rigorously tested.
9 Conclusion
The investigation of AIM2 signaling in neurodegenerative diseases has unveiled its multifaceted roles in various pathological conditions. Its involvement in diverse cellular processes underscores its potential as a therapeutic target in these diseases. Several drug candidates targeting AIM2 signaling pathways offer promise for clinical applications in the treatment of neurodegenerative diseases. These candidates have demonstrated efficacy in preclinical models. However, further research is warranted to elucidate the precise mechanisms underlying AIM2-mediated neuroinflammation and its implications for therapeutic development.
Author contributions
KY: Conceptualization, Supervision, Writing – original draft. XW: Writing – original draft. HP: Writing – original draft. XQW: Writing – review & editing. YH: Writing – review & editing. YY: Writing – review & editing. XZ: Visualization, Writing – review & editing. TS: Conceptualization, Funding acquisition, Supervision, Writing – original draft.
Funding
The author(s) declare financial support was received for the research, authorship, and/or publication of this article. This work was supported by the National Natural Science Foundation of China to TS (51873168).
Conflict of interest
The authors declare that the research was conducted in the absence of any commercial or financial relationships that could be construed as a potential conflict of interest.
Publisher’s note
All claims expressed in this article are solely those of the authors and do not necessarily represent those of their affiliated organizations, or those of the publisher, the editors and the reviewers. Any product that may be evaluated in this article, or claim that may be made by its manufacturer, is not guaranteed or endorsed by the publisher.
References
1. Migliore L, Coppede F. Genetics, environmental factors and the emerging role of epigenetics in neurodegenerative diseases. Mutat Res. (2009) 667:82–97. doi: 10.1016/j.mrfmmm.2008.10.011
2. Andreasson KI, Bachstetter AD, Colonna M, Ginhoux F, Holmes C, Lamb B, et al. Targeting innate immunity for neurodegenerative disorders of the central nervous system. J Neurochem. (2016) 138:653–93. doi: 10.1111/jnc.13667
3. Chen X, Holtzman DM. Emerging roles of innate and adaptive immunity in alzheimer's disease. Immunity. (2022) 55:2236–54. doi: 10.1016/j.immuni.2022.10.016
4. Mason HD, McGavern DB. How the immune system shapes neurodegenerative diseases. Trends Neurosci. (2022) 45:733–48. doi: 10.1016/j.tins.2022.08.001
5. Gao C, Jiang J, Tan Y, Chen S. Microglia in neurodegenerative diseases: mechanism and potential therapeutic targets. Signal Transduct Target Ther. (2023) 8:359. doi: 10.1038/s41392-023-01588-0
6. DeMaio A, Mehrotra S, Sambamurti K, Husain S. The role of the adaptive immune system and T cell dysfunction in neurodegenerative diseases. J Neuroinflamm. (2022) 19:251. doi: 10.1186/s12974-022-02605-9
7. Tahara K, Kim HD, Jin JJ, Maxwell JA, Li L, Fukuchi K. Role of toll-like receptor signalling in abeta uptake and clearance. Brain. (2006) 129:3006–19. doi: 10.1093/brain/awl249
8. Subhramanyam CS, Wang C, Hu Q, Dheen ST. Microglia-mediated neuroinflammation in neurodegenerative diseases. Semin Cell Dev Biol. (2019) 94:112–20. doi: 10.1016/j.semcdb.2019.05.004
9. Bido S, Muggeo S, Massimino L, Marzi MJ, Giannelli SG, Melacini E, et al. Microglia-specific overexpression of alpha-synuclein leads to severe dopaminergic neurodegeneration by phagocytic exhaustion and oxidative toxicity. Nat Commun. (2021) 12:6237. doi: 10.1038/s41467-021-26519-x
10. Booth HDE, Hirst WD, Wade-Martins R. The role of astrocyte dysfunction in parkinson's disease pathogenesis. Trends Neurosci. (2017) 40:358–70. doi: 10.1016/j.tins.2017.04.001
11. Gleichman AJ, Carmichael ST. Glia in neurodegeneration: drivers of disease or along for the ride? Neurobiol Dis. (2020) 142:104957. doi: 10.1016/j.nbd.2020.104957
12. Monsonego A, Zota V, Karni A, Krieger JI, Bar-Or A, Bitan G, et al. Increased T cell reactivity to amyloid beta protein in older humans and patients with alzheimer disease. J Clin Invest. (2003) 112:415–22. doi: 10.1172/JCI18104
13. Lopez OL, Rabin BS, Huff FJ, Rezek D, Reinmuth OM. Serum autoantibodies in patients with alzheimer's disease and vascular dementia and in nondemented control subjects. Stroke. (1992) 23:1078–83. doi: 10.1161/01.STR.23.8.1078
14. Heneka MT, McManus RM, Latz E. Inflammasome signalling in brain function and neurodegenerative disease. Nat Rev Neurosci. (2018) 19:610–21. doi: 10.1038/s41583-018-0055-7
15. Mangan MSJ, Olhava EJ, Roush WR, Seidel HM, Glick GD, Latz E. Targeting the nlrp3 inflammasome in inflammatory diseases. Nat Rev Drug Discovery. (2018) 17:588–606. doi: 10.1038/nrd.2018.97
16. Swanson KV, Deng M, Ting JP. The nlrp3 inflammasome: molecular activation and regulation to therapeutics. Nat Rev Immunol. (2019) 19:477–89. doi: 10.1038/s41577-019-0165-0
17. Jin T, Perry A, Jiang J, Smith P, Curry JA, Unterholzner L, et al. Structures of the hin domain:DNA complexes reveal ligand binding and activation mechanisms of the aim2 inflammasome and ifi16 receptor. Immunity. (2012) 36:561–71. doi: 10.1016/j.immuni.2012.02.014
18. Lu A, Li Y, Yin Q, Ruan J, Yu X, Egelman E, et al. Plasticity in pyd assembly revealed by cryo-em structure of the pyd filament of aim2. Cell Discovery. (2015) 1:15013–. doi: 10.1038/celldisc.2015.13
19. Morrone SR, Matyszewski M, Yu X, Delannoy M, Egelman EH, Sohn J. Assembly-driven activation of the aim2 foreign-dsdna sensor provides a polymerization template for downstream asc. Nat Commun. (2015) 6:7827. doi: 10.1038/ncomms8827
20. Burckstummer T, Baumann C, Bluml S, Dixit E, Durnberger G, Jahn H, et al. An orthogonal proteomic-genomic screen identifies aim2 as a cytoplasmic DNA sensor for the inflammasome. Nat Immunol. (2009) 10:266–72. doi: 10.1038/ni.1702
21. Roberts TL, Idris A, Dunn JA, Kelly GM, Burnton CM, Hodgson S, et al. Hin-200 proteins regulate caspase activation in response to foreign cytoplasmic DNA. Science. (2009) 323:1057–60. doi: 10.1126/science.1169841
22. Wang B, Tian Y, Yin Q. Aim2 inflammasome assembly and signaling. Adv Exp Med Biol. (2019) 1172:143–55. doi: 10.1007/978-981-13-9367-9_7
23. Matyszewski M, Morrone SR, Sohn J. Digital signaling network drives the assembly of the aim2-asc inflammasome. Proc Natl Acad Sci USA. (2018) 115:E1963–E72. doi: 10.1073/pnas.1712860115
24. Garg A, Stallings CM, Sohn J. Filament assembly underpins the double-stranded DNA specificity of aim2-like receptors. Nucleic Acids Res. (2023) 51:2574–85. doi: 10.1093/nar/gkad090
25. Rui WJ, Li S, Yang L, Liu Y, Fan Y, Hu YC, et al. Microglial aim2 alleviates antiviral-related neuro-inflammation in mouse models of parkinson's disease. Glia. (2022) 70:2409–25. doi: 10.1002/glia.24260
26. Wu PJ, Hung YF, Liu HY, Hsueh YP. Deletion of the inflammasome sensor aim2 mitigates abeta deposition and microglial activation but increases inflammatory cytokine expression in an alzheimer disease mouse model. Neuroimmunomodulation. (2017) 24:29–39. doi: 10.1159/000477092
27. Nie L, Zhao P, Yue Z, Zhang P, Ji N, Chen Q, et al. Diabetes induces macrophage dysfunction through cytoplasmic dsdna/aim2 associated pyroptosis. J Leukoc Biol. (2021) 110:497–510. doi: 10.1002/JLB.3MA0321-745R
28. Onodi Z, Ruppert M, Kucsera D, Sayour AA, Toth VE, Koncsos G, et al. Aim2-driven inflammasome activation in heart failure. Cardiovasc Res. (2021) 117:2639–51. doi: 10.1093/cvr/cvab202
29. Ge J, Gong YN, Xu Y, Shao F. Preventing bacterial DNA release and absent in melanoma 2 inflammasome activation by a legionella effector functioning in membrane trafficking. Proc Natl Acad Sci USA. (2012) 109:6193–8. doi: 10.1073/pnas.1117490109
30. Fernandes-Alnemri T, Yu JW, Juliana C, Solorzano L, Kang S, Wu J, et al. The aim2 inflammasome is critical for innate immunity to francisella tularensis. Nat Immunol. (2010) 11:385–93. doi: 10.1038/ni.1859
31. Peng K, Broz P, Jones J, Joubert LM, Monack D. Elevated aim2-mediated pyroptosis triggered by hypercytotoxic francisella mutant strains is attributed to increased intracellular bacteriolysis. Cell Microbiol. (2011) 13:1586–600. doi: 10.1111/cmi.2011.13.issue-10
32. Sauer JD, Witte CE, Zemansky J, Hanson B, Lauer P, Portnoy DA. Listeria monocytogenes triggers aim2-mediated pyroptosis upon infrequent bacteriolysis in the macrophage cytosol. Cell Host Microbe. (2010) 7:412–9. doi: 10.1016/j.chom.2010.04.004
33. Rathinam VA, Jiang Z, Waggoner SN, Sharma S, Cole LE, Waggoner L, et al. The aim2 inflammasome is essential for host defense against cytosolic bacteria and DNA viruses. Nat Immunol. (2010) 11:395–402. doi: 10.1038/ni.1864
34. Reinholz M, Kawakami Y, Salzer S, Kreuter A, Dombrowski Y, Koglin S, et al. Hpv16 activates the aim2 inflammasome in keratinocytes. Arch Dermatol Res. (2013) 305:723–32. doi: 10.1007/s00403-013-1375-0
35. Zhen J, Zhang L, Pan J, Ma S, Yu X, Li X, et al. Aim2 mediates inflammation-associated renal damage in hepatitis B virus-associated glomerulonephritis by regulating caspase-1, il-1beta, and il-18. Mediators Inflammation. (2014) 2014:190860. doi: 10.1155/2014/190860
36. Di Micco A, Frera G, Lugrin J, Jamilloux Y, Hsu ET, Tardivel A, et al. Aim2 inflammasome is activated by pharmacological disruption of nuclear envelope integrity. Proc Natl Acad Sci USA. (2016) 113:E4671–80. doi: 10.1073/pnas.1602419113
37. Yang M, Delcroix V, Lennikov A, Wang N, Makarenkova HP, Dartt DA. Genomic DNA activates the aim2 inflammasome and sting pathways to induce inflammation in lacrimal gland myoepithelial cells. Ocul Surf. (2023) 30:263–75. doi: 10.1016/j.jtos.2023.09.012
38. Wang Y, Chen C, Chen J, Sang T, Peng H, Lin X, et al. Overexpression of nag-1/gdf15 prevents hepatic steatosis through inhibiting oxidative stress-mediated dsdna release and aim2 inflammasome activation. Redox Biol. (2022) 52:102322. doi: 10.1016/j.redox.2022.102322
39. Dang EV, McDonald JG, Russell DW, Cyster JG. Oxysterol restraint of cholesterol synthesis prevents aim2 inflammasome activation. Cell. (2017) 171:1057–71 e11. doi: 10.1016/j.cell.2017.09.029
40. Wu H, Deng Y, Long D, Yang M, Li Q, Feng Y, et al. The il-21-tet2-aim2-C-maf pathway drives the T follicular helper cell response in lupus-like disease. Clin Transl Med. (2022) 12:e781. doi: 10.1002/ctm2.781
41. Chou WC, Guo Z, Guo H, Chen L, Zhang G, Liang K, et al. Aim2 in regulatory T cells restrains autoimmune diseases. Nature. (2021) 591:300–5. doi: 10.1038/s41586-021-03231-w
42. Yang M, Long D, Hu L, Zhao Z, Li Q, Guo Y, et al. Aim2 deficiency in B cells ameliorates systemic lupus erythematosus by regulating blimp-1-bcl-6 axis-mediated B-cell differentiation. Signal Transduct Target Ther. (2021) 6:341. doi: 10.1038/s41392-021-00725-x
43. Ma C, Li S, Hu Y, Ma Y, Wu Y, Wu C, et al. Aim2 controls microglial inflammation to prevent experimental autoimmune encephalomyelitis. J Exp Med. (2021) 218. doi: 10.1084/jem.20201796
44. Guo Y, Li L, Xu T, Guo X, Wang C, Li Y, et al. Huwe1 mediates inflammasome activation and promotes host defense against bacterial infection. J Clin Invest. (2020) 130:6301–16. doi: 10.1172/JCI138234
45. Liu T, Tang Q, Liu K, Xie W, Liu X, Wang H, et al. Trim11 suppresses aim2 inflammasome by degrading aim2 via P62-dependent selective autophagy. Cell Rep. (2016) 16:1988–2002. doi: 10.1016/j.celrep.2016.07.019
46. Wu PJ, Liu HY, Huang TN, Hsueh YP. Aim 2 inflammasomes regulate neuronal morphology and influence anxiety and memory in mice. Sci Rep. (2016) 6:32405. doi: 10.1038/srep32405
47. Yuan B, Zhou XM, You ZQ, Xu WD, Fan JM, Chen SJ, et al. Inhibition of aim2 inflammasome activation alleviates gsdmd-induced pyroptosis in early brain injury after subarachnoid haemorrhage. Cell Death Dis. (2020) 11:76. doi: 10.1038/s41419-020-2248-z
48. Adamczak SE, de Rivero Vaccari JP, Dale G, Brand FJ 3rd, Nonner D, Bullock MR, et al. Pyroptotic neuronal cell death mediated by the aim2 inflammasome. J Cereb Blood Flow Metab. (2014) 34:621–9. doi: 10.1038/jcbfm.2013.236
49. Zheng Y, Tang W, Zeng H, Peng Y, Yu X, Yan F, et al. Probenecid-Blocked Pannexin-1 Channel Protects against Early Brain Injury Via Inhibiting Neuronal Aim2 Inflammasome Activation after Subarachnoid Hemorrhage. Front Neurol. (2022) 13:854671. doi: 10.3389/fneur.2022.854671
50. Eichholz K, Bru T, Tran TT, Fernandes P, Welles H, Mennechet FJ, et al. Immune-complexed adenovirus induce aim2-mediated pyroptosis in human dendritic cells. PloS Pathog. (2016) 12:e1005871. doi: 10.1371/journal.ppat.1005871
51. Fukuda K, Okamura K, Riding RL, Fan X, Afshari K, Haddadi NS, et al. Aim2 regulates anti-tumor immunity and is a viable therapeutic target for melanoma. J Exp Med. (2021) 218. doi: 10.1084/jem.20200962
52. Dharmadhikari B, Nickles E, Harfuddin Z, Ishak NDB, Zeng Q, Bertoletti A, et al. Cd137l dendritic cells induce potent response against cancer-associated viruses and polarize human cd8(+) T cells to tc1 phenotype. Cancer Immunol Immunother. (2018) 67:893–905. doi: 10.1007/s00262-018-2144-x
53. Sorrentino R, Terlizzi M, Di Crescenzo VG, Popolo A, Pecoraro M, Perillo G, et al. Human lung cancer-derived immunosuppressive plasmacytoid dendritic cells release il-1alpha in an aim2 inflammasome-dependent manner. Am J Pathol. (2015) 185:3115–24. doi: 10.1016/j.ajpath.2015.07.009
54. Roth S, Cao J, Singh V, Tiedt S, Hundeshagen G, Li T, et al. Post-injury immunosuppression and secondary infections are caused by an aim2 inflammasome-driven signaling cascade. Immunity. (2021) 54:648–59 e8. doi: 10.1016/j.immuni.2021.02.004
55. Junqueira C, Crespo A, Ranjbar S, de Lacerda LB, Lewandrowski M, Ingber J, et al. Fcgammar-mediated sars-cov-2 infection of monocytes activates inflammation. Nature. (2022) 606:576–84. doi: 10.1038/s41586-022-04702-4
56. Strizova Z, Benesova I, Bartolini R, Novysedlak R, Cecrdlova E, Foley LK, et al. M1/M2 macrophages and their overlaps - myth or reality? Clin Sci (Lond). (2023) 137:1067–93. doi: 10.1042/CS20220531
57. Komada T, Chung H, Lau A, Platnich JM, Beck PL, Benediktsson H, et al. Macrophage uptake of necrotic cell DNA activates the aim2 inflammasome to regulate a proinflammatory phenotype in ckd. J Am Soc Nephrol. (2018) 29:1165–81. doi: 10.1681/ASN.2017080863
58. Chai D, Zhang Z, Shi SY, Qiu D, Zhang C, Wang G, et al. Absent in melanoma 2-mediating M1 macrophages facilitate tumor rejection in renal carcinoma. Transl Oncol. (2021) 14:101018. doi: 10.1016/j.tranon.2021.101018
59. Liu D, Xu X, Dai Y, Zhao X, Bao S, Ma W, et al. Blockade of aim2 inflammasome or alpha1-ar ameliorates il-1beta release and macrophage-mediated immunosuppression induced by car-T treatment. J Immunother Cancer. (2021) 9. doi: 10.1136/jitc-2020-001466
60. Ye L, Shu S, Jia J, Sun M, Xu S, Bao X, et al. Absent in melanoma 2 mediates aging-related cognitive dysfunction by acting on complement-dependent microglial phagocytosis. Aging Cell. (2023) 22:e13860. doi: 10.1111/acel.13860
61. Ye L, Hu M, Mao R, Tan Y, Sun M, Jia J, et al. Conditional knockout of aim2 in microglia ameliorates synaptic plasticity and spatial memory deficits in a mouse model of alzheimer's disease. CNS Neurosci Ther. (2024) 30:e14555. doi: 10.1111/cns.14555
62. Svensson A, Patzi Churqui M, Schluter K, Lind L, Eriksson K. Maturation-dependent expression of aim2 in human B-cells. PloS One. (2017) 12:e0183268. doi: 10.1371/journal.pone.0183268
63. El-Zaatari M, Bishu S, Zhang M, Grasberger H, Hou G, Haley H, et al. Aim2-mediated/ifn-beta-independent regulation of gastric metaplastic lesions via cd8+ T cells. JCI Insight. (2020) 5. doi: 10.1172/jci.insight.94035
64. Lozano-Ruiz B, Tzoumpa A, Martinez-Cardona C, Moreno D, Aransay AM, Cortazar AR, et al. Absent in melanoma 2 (Aim2) regulates the stability of regulatory T cells. Int J Mol Sci. (2022) 23. doi: 10.3390/ijms23042230
65. Cao LL, Guan PP, Zhang SQ, Yang Y, Huang XS, Wang P. Downregulating expression of optn elevates neuroinflammation via aim2 inflammasome- and ripk1-activating mechanisms in app/ps1 transgenic mice. J Neuroinflamm. (2021) 18:281. doi: 10.1186/s12974-021-02327-4
66. Chen J, Shu S, Chen Y, Liu Z, Yu L, Yang L, et al. Aim2 deletion promotes neuroplasticity and spatial memory of mice. Brain Res Bull. (2019) 152:85–94. doi: 10.1016/j.brainresbull.2019.07.011
67. Alizadehmoghaddam S, Pourabdolhossein F, Najafzadehvarzi H, Sarbishegi M, Saleki K, Nouri HR. Crocin attenuates the lipopolysaccharide-induced neuroinflammation via expression of aim2 and nlrp1 inflammasome in an experimental model of parkinson's disease. Heliyon. (2024) 10:e25523. doi: 10.1016/j.heliyon.2024.e25523
68. Ge X, Li W, Huang S, Yin Z, Xu X, Chen F, et al. The pathological role of nlrs and aim2 inflammasome-mediated pyroptosis in damaged blood-brain barrier after traumatic brain injury. Brain Res. (2018) 1697:10–20. doi: 10.1016/j.brainres.2018.06.008
69. Sun Z, Nyanzu M, Yang S, Zhu X, Wang K, Ru J, et al. Vx765 attenuates pyroptosis and hmgb1/tlr4/nf-kappab pathways to improve functional outcomes in tbi mice. Oxid Med Cell Longev. (2020) 2020:7879629. doi: 10.1155/2020/7879629
70. Majidpoor J, Khezri Z, Rostamzadeh P, Mortezaee K, Rezaie MJ, Fathi F, et al. The expressions of nlrp1, nlrp3, and aim2 inflammasome complexes in the contusive spinal cord injury rat model and their responses to hormonal therapy. Cell Tissue Res. (2020) 381:397–410. doi: 10.1007/s00441-020-03250-5
71. Zhou Z, Li C, Bao T, Zhao X, Xiong W, Luo C, et al. Exosome-shuttled mir-672-5p from anti-inflammatory microglia repair traumatic spinal cord injury by inhibiting aim2/asc/caspase-1 signaling pathway mediated neuronal pyroptosis. J Neurotrauma. (2022) 39:1057–74. doi: 10.1089/neu.2021.0464
72. Xiao X, Chen XY, Dong YH, Dong HR, Zhou LN, Ding YQ, et al. Pre-treatment of rapamycin transformed M2 microglia alleviates traumatic cervical spinal cord injury via aim2 signaling pathway in vitro and in vivo. Int Immunopharmacol. (2023) 121:110394. doi: 10.1016/j.intimp.2023.110394
73. Kharazinejad E, Hassanzadeh G, Sahebkar A, Yousefi B, Reza Sameni H, Majidpoor J, et al. The comparative effects of schwann cells and wharton's jelly mesenchymal stem cells on the aim2 inflammasome activity in an experimental model of spinal cord injury. Neuroscience. (2023) 535:1–12. doi: 10.1016/j.neuroscience.2023.10.011
74. Wang Y, Fang N, Wang Y, Geng Y, Li Y. Activating mc4r promotes functional recovery by repressing oxidative stress-mediated aim2 activation post-spinal cord injury. Mol Neurobiol. (2024). doi: 10.1007/s12035-024-03936-9
75. Tan EK, Chao YX, West A, Chan LL, Poewe W, Jankovic J. Parkinson disease and the immune system - associations, mechanisms and therapeutics. Nat Rev Neurol. (2020) 16:303–18. doi: 10.1038/s41582-020-0344-4
76. Tansey MG, Wallings RL, Houser MC, Herrick MK, Keating CE, Joers V. Inflammation and immune dysfunction in parkinson disease. Nat Rev Immunol. (2022) 22:657–73. doi: 10.1038/s41577-022-00684-6
77. Huang S, Chen Z, Fan B, Chen Y, Zhou L, Jiang B, et al. A selective nlrp3 inflammasome inhibitor attenuates behavioral deficits and neuroinflammation in a mouse model of parkinson's disease. J Neuroimmunol. (2021) 354:577543. doi: 10.1016/j.jneuroim.2021.577543
78. Boulton M, Al-Rubaie A. Neuroinflammation and neurodegeneration following traumatic brain injuries. Anat Sci Int. (2024). doi: 10.1007/s12565-024-00778-2
79. Ziegler G, Grabher P, Thompson A, Altmann D, Hupp M, Ashburner J, et al. Progressive neurodegeneration following spinal cord injury: implications for clinical trials. Neurology. (2018) 90:e1257–e66. doi: 10.1212/WNL.0000000000005258
80. Faden AI, Wu J, Stoica BA, Loane DJ. Progressive inflammation-mediated neurodegeneration after traumatic brain or spinal cord injury. Br J Pharmacol. (2016) 173:681–91. doi: 10.1111/bph.13179
81. Chen SH, Hu FL, Wang G, Liang XS, He CJ. Importance of aim2 as a serum marker for reflecting severity and predicting a poor outcome of human severe traumatic brain injury: A prospective longitudinal cohort study. Clin Chim Acta. (2024) 559:119691. doi: 10.1016/j.cca.2024.119691
82. Wang SN, Guo XY, Tang J, Ding SQ, Shen L, Wang R, et al. Expression and localization of absent in melanoma 2 in the injured spinal cord. Neural Regener Res. (2019) 14:542–52. doi: 10.4103/1673-5374.245481
83. Chung IC, Yuan SN, OuYang CN, Hu SI, Lin HC, Huang KY, et al. Efla 945 restricts aim2 inflammasome activation by preventing DNA entry for psoriasis treatment. Cytokine. (2020) 127:154951. doi: 10.1016/j.cyto.2019.154951
84. Gao J, Peng S, Shan X, Deng G, Shen L, Sun J, et al. Inhibition of aim2 inflammasome-mediated pyroptosis by andrographolide contributes to amelioration of radiation-induced lung inflammation and fibrosis. Cell Death Dis. (2019) 10:957. doi: 10.1038/s41419-019-2195-8
85. Zhang X, Lan Q, Zhang M, Wang F, Shi K, Li X, et al. Inhibition of aim2 inflammasome activation by sox/orf37 promotes lytic replication of kaposi's sarcoma-associated herpesvirus. Proc Natl Acad Sci USA. (2023) 120:e2300204120. doi: 10.1073/pnas.2300204120
86. Green JP, El-Sharkawy LY, Roth S, Zhu J, Cao J, Leach AG, et al. Discovery of an inhibitor of DNA-driven inflammation that preferentially targets the aim2 inflammasome. iScience. (2023) 26:106758. doi: 10.1016/j.isci.2023.106758
87. Kaminski JJ, Schattgen SA, Tzeng TC, Bode C, Klinman DM, Fitzgerald KA. Synthetic oligodeoxynucleotides containing suppressive ttaggg motifs inhibit aim2 inflammasome activation. J Immunol. (2013) 191:3876–83. doi: 10.4049/jimmunol.1300530
88. Liang J, Wang Q, Li JQ, Guo T, Yu D. Long non-coding rna meg3 promotes cerebral ischemia-reperfusion injury through increasing pyroptosis by targeting mir-485/aim2 axis. Exp Neurol. (2020) 325:113139. doi: 10.1016/j.expneurol.2019.113139
89. Habib P, Harms J, Zendedel A, Beyer C, Slowik A. Gonadal hormones E2 and P mitigate cerebral ischemia-induced upregulation of the aim2 and nlrc4 inflammasomes in rats. Int J Mol Sci. (2020) 21. doi: 10.3390/ijms21134795
90. Jiao Y, Nan J, Mu B, Zhang Y, Zhou N, Yang S, et al. Discovery of a novel and potent inhibitor with differential species-specific effects against nlrp3 and aim2 inflammasome-dependent pyroptosis. Eur J Med Chem. (2022) 232:114194. doi: 10.1016/j.ejmech.2022.114194
Keywords: inflammasome, AIM2, neurodegenerative disease, Alzheimer’s disease, Parkinson’s disease
Citation: Yang K, Wang X, Pan H, Wang X, Hu Y, Yao Y, Zhao X and Sun T (2024) The roles of AIM2 in neurodegenerative diseases: insights and therapeutic implications. Front. Immunol. 15:1441385. doi: 10.3389/fimmu.2024.1441385
Received: 04 June 2024; Accepted: 01 July 2024;
Published: 15 July 2024.
Edited by:
Juan Pablo de Rivero Vaccari, University of Miami, United StatesReviewed by:
Rebecca Leigh Schmidt, Colorado Mountain College, United StatesMenglu Yang, Schepens Eye Research Institute and Harvard Medical School, United States
Hao Tang, University of Leicester, United Kingdom
Copyright © 2024 Yang, Wang, Pan, Wang, Hu, Yao, Zhao and Sun. This is an open-access article distributed under the terms of the Creative Commons Attribution License (CC BY). The use, distribution or reproduction in other forums is permitted, provided the original author(s) and the copyright owner(s) are credited and that the original publication in this journal is cited, in accordance with accepted academic practice. No use, distribution or reproduction is permitted which does not comply with these terms.
*Correspondence: Kai Yang, a3lhbmc0M0B3aHV0LmVkdS5jbg==; Taolei Sun, c3VudGxAd2h1dC5lZHUuY24=