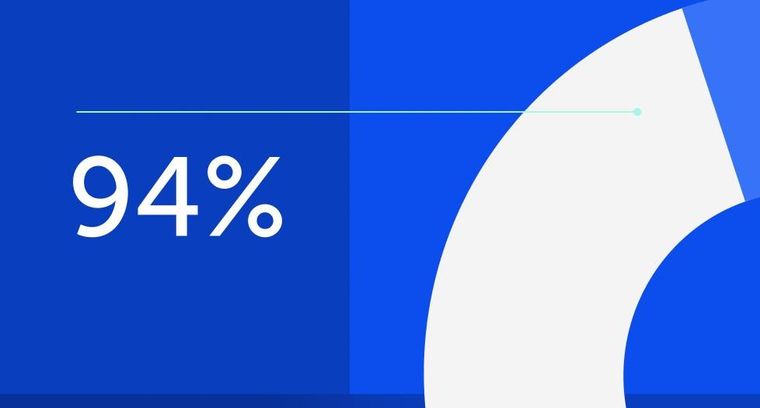
94% of researchers rate our articles as excellent or good
Learn more about the work of our research integrity team to safeguard the quality of each article we publish.
Find out more
ORIGINAL RESEARCH article
Front. Immunol., 18 July 2024
Sec. Parasite Immunology
Volume 15 - 2024 | https://doi.org/10.3389/fimmu.2024.1441131
Haptoglobin is a plasma protein of mammals that plays a crucial role in vascular homeostasis by binding free haemoglobin released from ruptured red blood cells. Trypanosoma brucei can exploit this by internalising haptoglobin-haemoglobin complex to acquire host haem. Here, we investigated the impact of haptoglobin deficiency (Hp-/-) on T. brucei brucei infection and the parasite´s capacity to internalise haemoglobin in a Hp-/- mouse model. The infected Hp-/- mice exhibited normal disease progression, with minimal weight loss and no apparent organ pathology, similarly to control mice. While the proteomic profile of mouse sera significantly changed in response to T. b. brucei, no differences in the infection response markers of blood plasma between Hp-/- and control Black mice were observed. Similarly, very few quantitative differences were observed between the proteomes of parasites harvested from Hp-/- and Black mice, including both endogenous proteins and internalised host proteins. While haptoglobin was indeed absent from parasites isolated from Hp-/-mice, haemoglobin peptides were unexpectedly detected in parasites from both Hp-/- and Black mice. Combined, the data support the dispensability of haptoglobin for haemoglobin internalisation by T. b. brucei during infection in mice. Since the trypanosomes knock-outs for their haptoglobin-haemoglobin receptor (HpHbR) internalised significantly less haemoglobin from Hp-/- mice compared to those isolated from Black mice, it suggests that T. b. brucei employs also an HpHbR-independent haptoglobin-mediated mode for haemoglobin internalisation. Our study reveals a so-far hidden flexibility of haemoglobin acquisition by T. b. brucei and offers novel insights into alternative haemoglobin uptake pathways.
African trypanosomes belonging to the complex of Trypanosoma brucei ecotypes are responsible for a number of serious diseases in humans and cattle (1). Being haem auxotrophs, trypanosomes cannot build this essential molecule from simpler building blocks via an endogenous haem biosynthetic pathway (2, 3). They need to secure the haem cofactors from external sources, i.e. from haemoglobin of their vertebrate hosts (4). Trypanosoma b. brucei is an extracellular parasite that predominantly localises in mammalian blood, where it multiplies as a bloodstream stage. To acquire haem, the bloodstream trypanosomes capture and internalise haemoglobin released from the lysed red blood cells, with haemolysis being an event concomitant to the T. b. brucei infection (5). The parasites internalise haemoglobin via recognition of the haptoglobin-haemoglobin (HpHb) complex, which is spontaneously formed in blood plasma. The interaction of the HpHb complex with T. b. brucei is mediated by a dedicated haptoglobin-haemoglobin receptor (HpHbR) expressed on the cell membrane of the bloodstream stage (6) and conserved across the Trypanosoma lineage (7–9). Concurrently, the HpHb complexes are sequestered from the blood by CD163-mediated clearance, i.e. the host self-defence mechanisms against an excessive build-up of extracellular haemoglobin (10). Deployment of HpHbR and CD163 by the parasite and the host, respectively, creates a micro-environment highly competitive for extracellular haemoglobin capture. This tug-of-war between the uptake of haemoglobin by trypanosomes and the host’s clearance mechanism represents a remarkable act of inter-organismal molecular interplay (Figure 1).
Figure 1 Overview of haemoglobin scavenging systems in human macrophages, mouse macrophages, and Trypanosoma b. brucei upon haemolysis. The source of extracellular haemoglobin in blood plasma is mostly ruptured red blood cells, setting a competition between the endogenous haemoglobin clearance mechanisms mediated by mice macrophages, and the haemoglobin salvage pathway of T. b. brucei in the infected mice. While we know that the mouse macrophages can bind free haemoglobin by the CD163 homologue, the impact of haptoglobin deficiency in host mice (Hp-/-) on T. b. brucei capability to internalise haemoglobin and retain infectivity is unknown. RBC, red blood cell; TbHpHbR, T. brucei haptoglobin-haemoglobin receptor; mCD163, mouse CD163 receptor; hCD163, human CD163 receptor.
Using an Hp-deficient mouse model, we elicited a new experimental framework, where the parasite-host interaction takes place in the background with no Hp-mediated processes. While the human homologue of CD163 binds only the HpHb complex and does not interact with free uncomplexed haemoglobin (10), the mouse homologue of CD163 binds both free haemoglobin as well as the HpHb complex (11). Since we wondered how the absence of plasma Hp affects haem biology and pathogenesis of T. b. brucei in mice, we aimed to address the following questions: i) what are the collateral effects of Hp deficiency on the blood plasma proteome (i.e. the proteomic landscape where the parasite-host interaction occurs)? ii) can T. b. brucei survive and proliferate in the blood of an Hp-deficient mouse? iii) can the parasite internalise from the mouse bloodstream and make efficient use of haemoglobin uncomplexed with Hp?
To address these questions, we have infected haptoglobin knock-out (Hp-/-) mice with T. b. brucei and performed a comprehensive analysis of this parasite-host system. We have characterised the blood plasma proteome of Hp-/- mice, followed the dynamics of their trypanosome infection, and examined the parasite’s strategies for haemoglobin acquisition in an Hp-lacking host. By showing that T. b. brucei not only survives but also replicates in the bloodstream of Hp-/- mice, our data challenge the conventional paradigm, in which Hp plays an essential role during infection. The nuanced interplay between this parasitic flagellate and its host, the latter lacking a molecule considered critical for the parasite’s survival, yet again demonstrates the enormous plasticity of T. b. brucei, which has evolved (an) alternative pathway(s) for haemoglobin uptake, revealing a built-in versatility in its survival strategies. This research not only improves our understanding of the molecular intricacies of the T. b. brucei infection, but also challenges established dogmas about the key role of Hp in host-parasite interactions.
Mice deficient for haptoglobin (Hp-\-) were generated by directed excision of the haptoglobin gene (Acc. no: Uniprot Q61646) by homologous recombination in the C57BL/6JRj genetic background (Lim et al., 1998). Following the cross of heterozygous parents, we screened the offspring for homozygosity and verified the target gene deletion (Supplementary Figure S1). Using label-free proteomics of plasma acquired from Hp-/- and control Black mice prior to infection, we confirmed a complete lack of the Hp protein in the former mice (Figure 2A), which was concomitant with an additional quantitative disbalance in the blood plasma proteome (Figure 2B). For example, α-1 anti-trypsin was found markedly (Fold Change > 74) elevated in the sera of Hp-/- mice, together with both heavy and light ferritin chains (Figures 2A, B). A full list of identified proteins is available as Supplementary Data S1. Vice versa, dozens of proteins were less abundant in the sera of Hp-/- mice, with certain immunoglobulin chains being particularly strongly down-regulated (Figure 2B).
Figure 2 Proteomics profiling of blood plasma from Hp-/- and Black mice. (A) A volcano plot of mice proteins identified in the plasma of Hp-/- and control Black mice (C57BL/6J), FDR = 0.01. Note that the level of haptoglobin (MS2 intensities) is detected only in Black mice, confirming the absence of Hp in the plasma of Hp-/- mice. Data are derived from four independent replicates; NaN = not identified. (B) A list of proteins that were either more or less abundant in the blood plasma from Hp-/- mice compared to Black mice; n = 4, t-test p < 0.05. A full list of identified proteins is available as Supplementary Data S1. (C) SDS-PAGE separation of mouse blood plasma proteins and western blot analysis using primary antibodies, fluorescently labelled with Alexa 488 (A488), against the heavy and light chains of mouse IgG, showing little, if any, impact of Hp deficiency on the amount of circulating IgGs. Please see individual heavy and light chains under reducing conditions. The bottom western blot using anti-Hp antibodies unequivocally confirms full Hp deficiency in Hp-/- mice.
To elaborate on these observations, we used western blot analysis to validate haptoglobin deficiency in Hp-/- mice and to determine the level of circulatory immunoglobulin IgGs in the sera of Hp-/- mice. Using fluorescently labelled anti-mouse IgGs, reacting with the heavy and light chains of mouse IgG, we revealed no net change in circulating IgGs in Hp-/- mice compared to Black mice (Figure 2C). Note that the level of haemoglobin chains also remained unaffected in the plasma of Hp-/- mice, indicating no increased haemolysis or elevated free haemoglobin levels. Collectively, these proteomics data validate the full Hp deficiency in Hp-/- mice and provide a perspective of the proteomic landscape of the blood plasma in the mouse models used in this study.
To evaluate the impact of a dysregulated blood plasma proteome on the course of T. b. brucei infection, we performed a comparative infection experiment in Hp-/- and Black mice and monitored the progression of parasitaemia. Trypanosomes were found not only to be viable in the Hp-/- mice bloodstream, but also progressed towards the parasitic wave, which peaked on day 5 post-infection, followed by swift clearance of the parasites from the bloodstream, similarly to the control mice (Figure 3A). In both mice strains, we documented no spleen or liver pathologies, as indicated by organ weight, during the parasitaemia peak (Figure 3B; Supplementary Figure S2). While the Black control mice (C57BL/6J) responded to trypanosomes with a partial weight loss, their Hp-/- counterparts displayed only a minor and insignificant weight loss in females, and a partial weight loss in males (Figure 3C). These data reveal that Hp-/- mice not only remain susceptible to T. b. brucei, but also retain mechanisms curbing excessive parasite load, despite inherent changes to the blood plasma proteome, including possible subtle immunoglobulin dysregulation.
Figure 3 Trypanosoma b. brucei infection in Black and Hp-/- mice. (A) The bloodstream trypanosomes were injected intraperitoneally into Black (blue line) and Hp-/- mice (red line) and the resulting parasitaemia was monitored daily; n = 8, mean and SD are shown. (B, C) Bar graphs of weights of spleen (B) and liver (C) from Black and Hp-/- mice obtained at day 4 post-infection (DPI); n = 4, mean and standard errors of the means (SEM) are shown. Representative images of the organs are shown as Supplementary Figure S2. (D) Weights of non-infected (black bars) and infected mice (grey bars) are shown at the indicated DPI; n ≥ 4, means and SEMs are shown; t test: * = p < 0.05, ** = p < 0.01.
To determine the responsive proteomic signatures of the Hp-/- and control mice sera to infection, as well as the behaviour of the T. b. brucei bloodstream stage in this specific host environment (Hp-/-), we concurrently harvested parasites and the mice blood plasma during the second parasitaemia wave, i.e. 12 days post-infection. The proteomic analysis of mice plasma samples revealed profound changes as a response to T. b. brucei infection in both Hp-/- and control mice (Figure 4A). In the latter, the parasites stimulated the expression of Hp, making it one of the most responsive plasma proteins to infection (Figure 4B). This enhances the validity of our model system, as it broadens the quantitative differences between Hp-/- mice lacking any Hp, and control mice with induced Hp in their sera. Furthermore, serum amyloid A-1 and A-2 proteins (SAA1 and SAA2), creatine kinase M type (MCK), and α(1)-acid glycoprotein (AGP) were plasma markers significantly upregulated in response to infection in Black mice (Figure 4B). In Black mice, T. b. brucei infection also induced expression of a large battery of plasma proteins, which were present exclusively in the plasma of infected mice, encompassing proteases (Napsin, Legumain, Cathepsin G, Cathepsin O), enzymes of the tyrosine degradation pathway (homogentisate 1,2-dioxygenase; 4-hydroxyphenylpyruvate dioxygenase), and a chitinase to name a few (Supplementary Data S2). In Hp-/- mice, in contrast, T. b. brucei infection caused very little plasma protein upregulation (Supplementary Data S2).
Figure 4 Dual proteomic analysis of plasma from non-infected and infected mice, and from T. b. brucei isolated from mice blood. (A) Principal component analysis of proteomics data of plasma obtained from Hp-/- and Black mice before and after infection. (B) A volcano plot of proteomics data of sera from control mice 12 days post-infection (p.i.), with significantly (Q value = 0.05) increased quantity of proteins responsive to the T. b. brucei infection. Haptoglobin (Hp) was identified in the plasma from Black mice as a T. b. brucei response marker with its level consistently elevated during infection (right inset) (n = 3). SAA1 and SSA2, serum amyloid A-1 and A-2 proteins, respectively; AGP, α(1)-acid glycoprotein; MCK, creatine kinase M type. (C) A list of proteins identified in T. b. brucei isolated from Hp-/- mice (n = 3), and not identified in parasites isolated from Black mice (n = 3). (D) A list of proteins identified in T. b. brucei isolated from Black mice (n = 3) and not identified in parasites isolated from Hp-/- mice (n = 3). (E) A proteomic quantification of mouse α-subunit haemoglobin (Acc. No. P01942) in T. b. brucei isolated from infected Black or Hp-/- mice. (F) Wild type parasites were purified from the blood of Hp-/- mice and imaged by transmission electron microscopy. Mouse haemoglobin was detected using α-haemoglobin antibodies and visualised with 10-nm gold nanoparticles coupled to protein A (arrows). Kinetoplast (K), flagellar pocket (FP), endosomes (E). Negative control, represented by an application of gold-labelled protein A only, produced no signal (data not shown), confirming the specificity of haemoglobin detection.
The comparison of proteomic spectra of the bloodstream stage trypanosomes, isolated concurrently from the Hp-/- and Black mice, revealed very few statistically significant differences (FC ≥ 5) (Supplementary Figure S3, Supplementary Data S3). Still, 7 and 10 T. b. brucei proteins were only present in parasites isolated from Hp-/- or Black mice, respectively (Figures 4C, D; Supplementary Data S3).When host proteins internalised by trypanosomes infecting Hp-/- mice were inspected, apart from the prominent absence of Hp, very few other proteins stood out, as if in these mice the parasites experienced no nutritional deprivation. Specifically, we observed no difference in the quantity of host haemoglobin in parasites isolated from Black and Hp-/- mice (Figure 4E), irrespective of whether host mice contained or lacked Hp in their blood. To investigate the origin of the haemoglobin peptides, we measured the MS2 intensities. While peptides derived from the mouse haemoglobin beta chain were exclusively of mouse origin, peptides from the alpha chain were a mixture of mouse and bovine haemoglobin. However, the vast majority originated from the mouse (MS2 intensities: mouse Hb 3.2 × 10^6 vs. bovine Hb 1.5 × 10^5). These data suggest that T. b. brucei can carry over a limited amount of host macromolecules from cultures. However, most haemoglobin molecules found in T. b. brucei 12 days post-infection are of immediate host origin. This validates our interpretation of similar haemoglobin uptake by T. b. brucei parasites in Black and Hp-/- mice. The full list of proteins, of both host and parasite origins, identified by proteomics in wild type T. b. brucei parasitising Hp-/- and Black mice is described in Supplementary Data S3. Note that the level of T. b. brucei HpHbR is identical in parasites isolated from Hp-/- and Black mice (Supplementary Data S3). To support our observation of identical quantities of haemoglobin in parasites isolated from Black and Hp-/- mice, immunodetection by western blotting (Supplementary Figure S4) and on parasite cryo-sections was carried out (Figure 4F). The latter confirms the internalisation of host haemoglobin at high resolution, the signal being localised to the cytoplasmic space near the flagellar pocket, i.e. a region characteristic for its endocytic/exocytic activity (Figure 4F). Altogether, these data reveal that T. b. brucei can induce a profound plasma proteome change in mice and that the parasite is endowed with the capacity to internalise haemoglobin without the prerequisite to form complexes with Hp in the blood.
In order to confirm that T. b. brucei can accumulate haem cofactors in both Black and Hp-/- mice, acetone extracts of purified parasites were analysed by HPLC. Intriguingly, we detected two haem peaks in T. b. brucei isolated from Black mice, but only a single (polar) haem in cells from Hp-/- mice (Figure 5A). As controls, extracts from the procyclic stage of T. b. brucei known to contain haem b and a (12)and from Escherichia coli known to contain haem b and o (13) were used to assign haem forms in the bloodstream stage of T. b. brucei. Both retention time and spectra (Figures 5B, C) unambiguously showed that the parasites from Black mice contained both haem b and o. Although the content of haem b varied, this measurement further supports the view that there was no deviation in haem b availability between the parasites isolated from Black and Hp-/- mice (Figure 5D). On the other hand, (farnesylated) haem o was present in parasites isolated from Black mice but was below the limit of detection in those purified from Hp-/- mice (Figure 5E).
Figure 5 Identification and quantification of haem species in T. b. brucei. (A) Parasites isolated from Black mice contain both haem b and o, while haem o is virtually absent in cells isolated from Hp -/- mice. (B) Haem species in the bloodstream stage of T. b. brucei were identified using control extracts from Escherichia coli and from the procyclic stage of T. b. brucei. The retention time and absorbance spectra (C) were used to determine the authenticity of the haem species, showing identical levels of haem b (D) between the bloodstream of T. b. brucei isolated from Hp-/- and Black mice, and the presence of haem o (E) only in the bloodstream of T. b. brucei isolated from Black mice; LOD, limit of detection.
Having established that the bloodstream stage acquires haem(oglobin) even from Hp-/- mice, we sought to reveal mechanisms behind this process. Therefore, we generated a haptoglobin-haemoglobin receptor (HpHbR) knock out cell line (HpHbR-KO) and subjected these parasites to the workflow described above. The HpHbR-KO T. b. brucei induced proteomic signatures in the blood plasma of Black mice 5 days post-infection, during the first parasitaemia peak (14), with clear segregation of mouse plasma proteomes prior to and post-infection (Figure 6A). Again, Hp was determined as an infection response marker of mouse plasma, even for the HpHbR-KO parasites (Supplementary Figure S5). The full list of plasma response markers to the HpHbR-KO infection in both Hp-/- and Black mice is described in Supplementary Data S4.
Figure 6 Assessment of haemoglobin internalisation by HpHbR-KO T. (b) brucei isolated from Hp-/- and Black mice. (A) Principal component analysis of proteomics data of plasma obtained from the Hp-/- and Black mice before and after infection (5 days post infection) by the HpHbR-KO parasites, n = 4. (B) The bar graph shows the Log2 quantity of haemoglobin internalised by HpHbR parasites isolated from Black and Hp-/- mice, as determined by proteomics. Means and SDs are shown; n = 5; t test p = 0.0004. (C) The HpHbR-KO parasites were isolated from the blood of Hp-/- mice for imaging by transmission electron microscopy coupled with immunodetection. Mouse haemoglobin was detected using α-haemoglobin antibodies and visualised through 10 nm gold nanoparticles-coupled with protein A (arrowheads). Kinetoplast (K), flagellar pocket (FP). (D) Reducing SDS-PAGE of haemoglobin pull-downs from mouse sera visualised in a stain-free mode; left gel indicates protein separation of starting material (diluted mice sera) and the supernatant after incubation with haemoglobin beads; right gel shows elution fraction from the beads: first two lanes show elution from haemoglobin beads after incubation with mice sera; blank indicates 8M elution from haemoglobin-beads after incubation with PBS only, instead of mice sera. (E) Membranes of electrophoretically separated serum proteins under native conditions; left, amido black staining for proteins; right, immunoblot against mouse haemoglobin; HMW, high molecular weight.
Furthermore, the proteomics of HpHbR-KO revealed that parasites isolated from Black and Hp-/- mice contained virtually identical (FC ≥ 5) spectra of endogenous and host proteins (Supplementary Figure S6). However, inspection of specific peptides of mouse haemoglobin revealed a statistically significant decrease in the amount of internalised haemoglobin in the HpHbR-KO parasites isolated from Hp-/- mice (Figure 6B). These data show that trypanosomes lacking HpHbR internalise lower amounts of mouse haemoglobin when in Hp-/- mice, but still contain a majority of total haemoglobin found in the parasites isolated from Black mice. This indicates that Hp also promotes internalisation of host haemoglobin via an HpHbR-independent route and that most of it is acquired without the need to exploit Hp-haemoglobin binding capacity. The full list of host and parasite proteins identified in wild type T. b. brucei isolated from Hp-/- and Black mice is available in Supplementary Data S5. To further confirm genuine internalisation of haemoglobin, and to rule out possible non-specific surface adherence, we subjected the HpHbR-KO parasites isolated from Hp-/- mice to immunogold labelling. Similarly to wild type trypanosomes, the HpHbR-KOs localised host haemoglobin in the vicinity of the flagellar pocket in these HpHbR parasites when in Hp-/- mice (Figure 6C).
To elaborate on the mode of internalisation, we explored the native binding partners of haemoglobin in the mouse sera. Using affinity precipitation with haemoglobin Sepharose beads, we were able to pull down several binding partners of haemoglobin from the mouse sera, such as apolipoprotein A-1 (apoA1) (Figure 6D), a major protein component of high-density lipoprotein (HDL) particles in blood plasma. To confirm whether haemoglobin was integrated into the high molecular weight lipoprotein particles, we electrophoretically separated the plasma proteins under native conditions, and subsequently confirmed the presence of haemoglobin by immunodetection. Indeed, apart from a faint haemoglobin signal co-migrating with albumin, most of the haemoglobin signal was associated with the high molecular weight complexes of blood plasma (Figure 6E). The proteomics data further documented the internalisation of the HDL components via the Hp-independent uptake pathway (when parasites isolated from Hp-/- or Black mice were compared), but indicates a role of HpHbR in the uptake of HDL particles, as wild type parasites contained 36 HDL components, while HpHbR-KO parasites contained 16HDL components (Supplementary Data S3, S5).
The presented findings reveal a complex and previously unexplored dimension to the interplay between haptoglobin (Hp), the blood plasma proteome, and the dynamics of T. b. brucei infection in a laboratory mouse model, disclosing a novel facet of the complex host-parasite interplay. Perhaps the most unexpected discovery is the capacity of trypanosomes to acquire and internalise haemoglobin in the absence of Hp, as it challenges the prevailing notion that Hp is a required binding partner for internalisation of haemoglobin by the parasites via their HpHbR (8). Previous work showed that the internalisation of fluorescently-labelled haemoglobin by the parasite was unequivocally conditioned by co-supplementation with Hp or an Hp-related protein (8). We argue that this experimental setup was based on a non-physiological assumption of the existence of free haemoglobin, which is non-existent in blood plasma, where extracellular haemoglobin readily associates with plasma components (15). Hence, our data are not in direct conflict with the earlier finding that Hp is essential for haemoglobin uptake (8), but rather extends the claim and contextualises it within the in vivo environment and dynamics. Since results from the in vitro binding experiments supported the notion that Hp promotes haemoglobin binding to HbHpR (7, 9, 16), which was further corroborated by the failure of HpHbR-KO bloodstream trypanosomes to acquire haem (8, 14), we interpret our data by assuming that haemoglobin is bound to other plasma proteins. Pull-downs with immobilised haemoglobin from the mouse blood plasma containing a negligible amount of Hp confirmed earlier observations that apolipoprotein-A1 is its predominant partner. Apolipoprotein-A1 is a dominant component of HDL nanoparticles, which contain more than 80 proteins in mice, including α and β haemoglobin chains (17).
The incorporation of haemoglobin into the HDL is likely to be apolipoprotein-A1-dependent, as their association can be prevented by apolipoprotein-A1 peptide mimetics (18). Indeed, haemoglobin binds apolipoprotein-A extremely efficiently (19). Apolipoprotein A1 can thus constitute a binding partner of haemoglobin with HDLs, which the parasites actively endocytose (20). In such a scenario, haemoglobin would be internalised, along with the HDL particles, by the parasite both through HpHbR-dependent and HpHbR-independent routes, as previously predicted (21). Combined, these observations reflect the complexity of haemoglobin fate when in blood, i.e. its complexing with Hp and clearance by CD163-mediated routes, as well as capture by the HDLs. Importantly, the HDL-associated haemoglobin may be internalised by T. b. brucei (22) and used for haem extraction to promote the parasite’s haem-dependent metabolic processes (14). This mode of internalisation of haemoglobin is likely complementary to its prevailing HpHbR-mediated acquisition. In humans, Hp is a natural antagonist of the HDL component, called trypanolytic factor 1 (TLF-1), which is toxic to T. brucei due to the presence of lytic apolipoprotein L1. The induction and upregulation of Hp during the acute phase reduces protection against trypanosomes. In contrast, Hp -/- mice showed higher TLF-1-mediated protection against T. b. brucei infection, suggesting that TLF-1 and Hp compete for the substrate in a dose-dependent manner (23).
The bioavailability of haem cofactors is critical for the regulation of trypanosomiasis in the vertebrate host. As shown recently, the absence of HpHbR in T. b. brucei resulted in a rapidly progressing parasitaemia caused by the absence of stumpy forms (14). Hence, due to the absence of Hp, we expected a similar parasitaemia surge to occur in Hp-/- mice. Surprisingly, the parasitaemia was comparable in both Hp-/- and Black mice with typical parasitic wave rises and falls. According to our measurements, regardless of the host’s genetic background, the parasites acquire haem b. As we have shown earlier (12) and as corroborated here, the haem chromatography profiles of the procyclic and bloodstream stages differ significantly, with haem o detected only in the latter, probably due to its immediate conversion to the final product. In contrast, haem a is not formed at all in the bloodstreams, while the haem o precursor accumulates (14). The in vivo system thus likely better reflects the complexity of the parasite - host interaction, including multiple routes of haem uptake. Accumulation of haem o in the bloodstream of T. b. brucei has not been reported so far and the role of this pigment remains enigmatic. Such plasticity and versatility in binding among the blood plasma molecules indicated in this work appears to be common and is not only specific for haemoglobin, upon Hp knock out, but also for haem, upon hemopexin (Hpx) knock out, whereby the haem buffering capacity of serum from adult Hp-/-Hpx-/- mice was found to be indistinguishable from that of control Hp+/+Hpx+/+ mice, suggesting that circulating proteins and/or macromolecules other than Hp and/or Hpx can scavenge labile haem (24).
The procyclic stage of T. brucei from the insect vector has a fully active mitochondrion and, therefore, contains more haemoproteins, such as subunits of the respiratory complexes (4), and takes up haem via the haem transporter HRG (25), which is well described in other haem auxotrophs such as ticks or Leishmania flagellates (26, 27). Since the bloodstream trypanosomes rely on glycolysis, with many mitochondrial haemoproteins downregulated or absent (25), the expression of HRG decreases in the mammalian host (25). Although in bacteria haem o can serve as a genuine cofactor of ubiquinol oxidases, eukaryotic alternative oxidases are di-iron proteins and mitochondrial terminal oxidases (COX) require haem a. In eukaryotes, haem o is thus known only as an intermediate of haem a biosynthesis (28). After uptake into the trypanosome cell, haem b is converted into haem a through the intermediate haem o, whose biosynthesis and role are poorly understood. However, the bloodstream T. b. brucei lacks a physiologically relevant level of COX (29) and, indeed, we did not detect haem a. Therefore, it is remarkable that a significant pool of acquired haem b is farnesylated. The mitochondrial biosynthesis of haem o and its likely relocation to the membranes indicates a signalling role. Why haem o does not accumulate in parasites in Hp -/- mice needs to be addressed in a future study.
The presented mouse model data cannot be fully extrapolated to humans because of inherent differences in Hp dynamics in their blood. Mice have, by default, very low levels of Hp in their bloodstream, in some strains even below the detection limit (30). In contrast, in human blood, Hp is a highly abundant plasma protein, present in about a gram per litre, which has profound consequences for the binding capacity of haemoglobin released into plasma during physiological and pathological haemolyses (10, 11). For these reasons, the role of mouse Hp in T. b. brucei infection might be conceptually questionable, but since elevated Hp levels are a concomitant feature of parasitaemia, we set out to explore its function in the Hp-/- mice. Human haemoglobin forms, with Hp, a high affinity HpHb complex (31), which is subsequently bound by a dedicated transmembrane receptor, CD163 (10). Recognition of the CD163-HpHb complex is necessary for its endocytosis and clearance from the bloodstream (10, 32). Similarly in mice, CD163 also recognises HpHb complexes but, unlike its human homologue, the mouse CD163 also binds extracellular haemoglobin, existing transiently to facilitate effective clearance of Hb (11). In fact, such removal of free haemoglobin from mouse blood is extremely efficient, with the capacity to clear 0.1 mg Hb per 10 g bodyweight (constituting 1 ‰ of the total Hb content in mice, i.e. a realistic estimate of the physiological situation) within minutes (11). Such an ability of mouse CD163 may have evolved due to the low default levels of its binding partner, Hp, to prevent toxic effects of extracellular haemoglobin by its swift clearance. Overall, the Hp-/- mouse, with a very short half-life of its free haemoglobin, provides a dynamic framework for the study of haemoglobin partitioning between T. b. brucei and its host.
The proteomic analysis determined broader changes in the plasma protein repertoire, extending beyond the expected absence of Hp in Hp-/- mice and known markers elicited by the T. b. brucei infection (33). The altered abundance of immunoglobulins, ferritin, and α-1 anti-trypsin, among others, suggests a compensatory response triggered by the ablation of Hp. It has been shown previously that the development of T- and B-cells is inhibited in mice subjected to this genetic manipulation, resulting in a significantly lower level of antigen-specific IgGs (34, 35). Thus, the haemoglobin-binding role of Hp is extended by its crucial contribution to the regulation of proliferation and functional differentiation of these immune system components. Indeed, our proteomics data of mouse sera also show substantially modulated levels of individual immunoglobulins, but the overall level of circulating IgGs appears unaffected. In contrast, Hp-/- mice show an increased concentration of ferritin heavy and light chains.
The highly significant inverse correlation between serum Hp and urinary (36) or epithelial ferritin (37) is likely a systemic response to the elevated levels of unbound haemoglobin, and thus of available haem. Additionally, we detected an increased level of gallectin-1, which is involved in pathogen recognition and the modulation of innate and adaptive host immune responses (38), including those against Trypanosoma cruzi (39). Moreover, it is worth noting that numerous host proteins, such as SAA1, SAA2, FABP, and AGP, are responsive (FC > 5) to T. b. brucei in both Hp-/- and Black mice. The SAA proteins are key components of the acute phase response following trauma, infection and other stimuli, during which, their serum levels rise dramatically (40). Due to their lipophilic nature, SAA proteins majorly partition into HDL particles (41).
In summary, our data challenge the conventional paradigm regarding the indispensability of Hp for successful T. b. brucei infection and, perhaps even more importantly, identify another element of the remarkable adaptability and flexibility of these blood parasites. We suggest that while the Hp-haemoglobin complexes appear transiently in the blood, they are internalised by T. b. brucei via HpHbR-mediated uptake. Displaying a longer half-life in blood, the lipoprotein complexes with Hb may thus represent a constant source of the haemoglobin-derived haem for trypanosomes.
Bloodstream T. b. brucei 90-13 were cultivated at 37 °C in HMI-9 medium (Thermo Fisher), supplemented with 10% heat-inactivated fetal bovine serum. Cell densities were measured using the Z2 Coulter counter (Beckman Coulter). Black and Hp-/- mice (four to six weeks old) were injected intraperitoneally with 1 × 105 90-13 strain T. b. brucei cells. Infection was followed daily by dilution of tail snip blood in TrypFix buffer (3.7% formaldehyde, 1×SSC buffer) and manual counting of trypanosomes in a Neubauer hemocytometer.
HpHbR-KO cell lines were generated by successive deletion of alleles with pPuro-KO or pHygro-KO, and pPhleo-KO constructs. Trypanosomes were transfected with linearized plasmid DNA (10 μg). Briefly, a total of 1 × 107 T.b. brucei cells was harvested by centrifugation at 1000 × g at 4°C for 10 min and washed 1 time with PBS. Cells were resuspended in 100 μL of AMAXA Human T-cell solution and electroporated with AMAXA Nucleofactor apparatus. Predefined program X-001 was used for electroporation. Selection markers were applied 6 h post-transfection, and clones were generated by limited dilution. The Hp-/- mouse strain was previously generated by allelic disruption through homologous recombination (Lim et al., 1998), and the heterozygotes were crossed by us to generate the full KO mouse strain.
Before the trypanosomes were recovered from the bloodstream, the mice were euthanised with Narketan/Xlazine solution and heparin (500 U per mouse) was administered intraperitoneally to prevent blood clotting. The parasites were separated from the erythrocytes on a diethylaminoethyl (DEAE) cellulose column according to a standard protocol. The purified trypanosomes were washed once with PBS and frozen at -80°C for MS/MS and HPLC analysis.
Before and during infection, blood was collected from the tail tip of the mice (9 uL) and mixed with 1uL of 109 mM sodium citrate (Sigma Aldrich, C8532), centrifuged at 1000 × g for 5 minutes and the upper layer (plasma) was frozen at -80°C for MS/MS and Western blot analysis.
Total DNA was extracted from the blood of Black and Hp-/- mice using a GeneAll kit (Exgene Clinic SV) and used as a template for PCR analysis of mouse haptoglobin (NM_017370) and β−actin (NM_007393) as a control with the following primers: b-actin_musFW1: GGCCGGGACCTGACAGACTACCTC, b-actin_musRV1:GTCACGCACGATTTCCCTCTCAGC, b-actin_musRV2: ACATCTGCTGGAAGGTGGACAGTG, haptoglobin_musFW1: TGTTGTCACTCTCCTGCTC, haptoglobin_musRV1: TCACCCATTGCTTCTCGTC, haptoglobin_musRV2: CACACACTGCCTCACATTC.
Lysates from 5 × 106 T. b. brucei cells were separated on a 12% SDS-polyacrylamide gel, transferred to a PVDF membrane, and probed with the polyclonal anti-Hp (ab256454, Abcam) and anti-Hb (MA5-32328, Invitrogen) antibodies at 1:2000 dilutions and with the monoclonal anti-alpha-tubulin (T9026, Sigma Aldrich) at 1:5000 dilution as a loading control. The anti-mouse and anti-rabbit secondary antibodies were horseradish peroxidase conjugated and detected by ECL substrate (#1705061, BioRad). The images were detected with BioRad Image Lab software.
CNBr-activated Sepharose™ 4B beads were incubated with haemoglobin in PBS and subsequently blocked with 1M Tris solution. These beads were incubated with sera C3N mice and subsequently washed with PBS before Urea (1M and 8M) elutions. Blank elution was performed on haemoglobin beads incubated with PBS only, without mouse sera.
Sera from mice were separated in pore-limit native PAGE in Tris-Borate-EDTA (TBE) buffer (0.09M Tris, 0.08M boric acid, 2mM EDTA). Samples were loaded in the running buffer supplemented with 10% (vol/vol) glycerol and 0.001% (w/vol) bromophenol blue. Samples were run in 4−16% Bis-Tris gel (Invitrogen) at 150 V in a cold room for 12 hr. Proteins were transferred onto nitrocellulose using a Trans-Blot Turbo system (BioRad) and used for Western blot analyses as described above and stained with Amido Black to visualise proteins.
Bloodstream cells were harvested by centrifugation at 1000 × g at 4°C for 10 min and washed 3 times with PBS on ice. Cells were resuspended in 30 μL H2O, extracted with 200 μL acetone/0.2% HCl, and the supernatant was collected after centrifugation at 1000 × g at 4°C for 5 min. The pellet was resuspended in 100 μL acetone/0.2% HCl and centrifuged as described above. Both supernatants were combined, and 150 μL of each sample was immediately injected into a high-performance liquid chromatography system (Infinity 1200, Agilent Technologies) and separated using a reverse-phase column (4 μm particle size, 3.9 × 75 mm) (Waters) with 0.1% trifluoroacetic acid and acetonitrile/0.1% trifluoroacetic acid as solvents A and B, respectively. Pigments were eluted with a linear gradient of solvent B (30–100% in 12 min) followed by 100% of B at a flow rate of 0.8 mL/min at 40°C. Haem species were detected by diode array detector (Infinity 1200, Agilent Technologies) and identified by retention time and absorbance spectra according to control extracts (see Figures 5B, C).
Blood from mouse tails (9 μL) was collected, mixed with 1 μL heparin and centrifuged at 4000x g for 5 minutes. The supernatant containing the blood plasma was collected and frozen at -80°C until analysis. Trypanosoma cell pellets were sonicated and treated with Benzonase Nuclease (Merck 70664-3) to isolate proteins. Cell proteins or mouse sera corresponding to approximately 100 microgram of protein were processed according to the SP4 glass bead-free protocol (42). Briefly, samples were solubilised with SDS [final concentration 1% (w/v)] in 100 mM TEAB (triethylammonium bicarbonate), reduced with TCEP [tris(2-carboxyethyl)phosphine], alkylated with CAA (chloroacetamide), and digested with trypsin (Trypsin Gold, Mass Spectrometry Grade, Promega V5280) over-night at 37°C at 1:50 ratio (trypsin:protein). Samples were desalted on Empore C18 columns, dried in a Speedvac and dissolved in 0.1% TFA + 2% acetonitrile. About 500 ng of peptide digests were separated on a 50 cm C18 column (EASY-Spray ES903, Thermo Fisher Scientific) using 120 min (sera 90 min) elution gradient (Dionex Ultimate 3000, flowrate 300 nL/min) and analysed in a DIA mode on an Orbitrap Exploris 480 mass spectrometer equipped with a FAIMS unit (Thermo Fisher Scientific). The resulting raw files were processed and visualised in Spectronaut 18.6 (Biognosys) software using the default setting with Precurson and Protein Q-value and PEP cutoff set at 0.01. Proteome fasta sequences used were: UP000000589_10090.fasta (mouse, UniProt release 2023_01) and TriTrypDB-64_TbruceiTREU927_AnnotatedProteins.fasta (tritrypdb.org, release 64).
Cells were fixed in 4% formaldehyde with 0.1% glutaraldehyde in 0.1 M HEPES pH 7.2 for 1 h at room temperature (RT). After washing in HEPES buffer, samples were embedded in 15% gelatin, cryoprotected in 2.3 M sucrose for 72 h at 4°C, and frozen by plunging into liquid nitrogen. Ultrathin cryosections were cut at -100°C, and pick-up with 1.15 M sucrose/1% methylcellulose solution (25 cp, Sigma). Sections were incubated for 1 h at RT in 1% fish skin gelatin (FSG) with 0.05% Tween20 and labelled with monoclonal rabbit IgG directed to haemoglobin (SN70-09, Invitrogen) diluted 1:30 in FSG for 1 h at RT. After washing in FSG, the sections were incubated with protein A coupled with 10 nm gold nanoparticles (British BioCell International) diluted 1:50 in FSG for 30 min at RT. Sections were washed in HEPES, postfixed for 5 min in 1% glutaraldehyde diluted in 0.1 M HEPES, washed in dH2O, and then contrasted/embedded in a mixture of 2% methylcellulose and 3% aq. uranyl acetate solution (9:1). Samples were observed using a JEM 1400 TEM (JEOL) equipped with a CMOS camera XAROSA (EMSIS GmbH). Control sections were incubated without the primary antibody.
Statistical significance of differences were analyzed for two datasets (knock-out and wild-type, or infected and non-infected) using GraphPad Prism 10 (GraphPad Software, CA) employing the t-test with p values < 0.05 considered as significant, with detailed descriptions in Figure legends.
The datasets presented in this study can be found in online repositories. The names of the repository/repositories and accession number(s) can be found below: PXD052778 (PRIDE).
The research on mice was approved by the Czech Ministry of Agriculture (project No. 28/2016 and 56-2022-P). All experimental procedures complied with the Czech law about Protection of Animals Against Cruelty (Act No. 246/1992, Decree No. 419/2012). The study was conducted in accordance with the local legislation and institutional requirements.
EH: Conceptualization, Data curation, Formal analysis, Investigation, Methodology, Writing – review & editing. MVr: Data curation, Formal analysis, Investigation, Methodology, Writing – review & editing. MT: Investigation, Methodology, Writing – review & editing. ES: Investigation, Methodology, Writing – review & editing. JPi: Investigation, Methodology, Writing – review & editing. ZV: Investigation, Methodology, Writing – review & editing. MVa: Formal analysis, Investigation, Methodology, Resources, Visualization, Writing – review & editing. RS: Data curation, Formal analysis, Investigation, Methodology, Visualization, Writing – review & editing. JL: Formal analysis, Funding acquisition, Project administration, Resources, Supervision, Writing – review & editing. JPe: Conceptualization, Formal analysis, Funding acquisition, Investigation, Project administration, Writing – original draft, Writing – review & editing.
The author(s) declare financial support was received for the research, authorship, and/or publication of this article. We acknowledge support from the Czech Science Foundation 22-01026S and 22-14356S (to JL), 22-18424M (to JPe), and Czech BioImaging grant LM2023050 (to MVa). Proteomics Service Laboratory at the Institute of Physiology and the Institute of Molecular Genetics of the Czech Academy of Sciences is acknowledged for the LC-MS/MS experiments.
We are grateful to Miguel Soares (Instituto Gulbenkian de Ciencia, Oeiras, Portugal) for providing us with the breeding pairs of the mice Hp-/- strain.
The authors declare that the research was conducted in the absence of any commercial or financial relationships that could be construed as a potential conflict of interest.
All claims expressed in this article are solely those of the authors and do not necessarily represent those of their affiliated organizations, or those of the publisher, the editors and the reviewers. Any product that may be evaluated in this article, or claim that may be made by its manufacturer, is not guaranteed or endorsed by the publisher.
The Supplementary Material for this article can be found online at: https://www.frontiersin.org/articles/10.3389/fimmu.2024.1441131/full#supplementary-material
1. Lukeš J, Kachale A, Votýpka J, Butenko A, Field MC. African trypanosome strategies for conquering new hosts and territories: the end of monophyly? Trends Parasitol. (2022) 38:724–36. doi: 10.1016/j.pt.2022.05.011
2. Kořený L, Oborník M, Lukeš J. Make it, take it, or leave it: heme metabolism of parasites. PloS Pathog. (2013) 9:e1003088. doi: 10.1371/journal.ppat.1003088
3. Cenci U, Moog D, Curtis BA, Tanifuji G, Eme L, Lukeš J, et al. Heme pathway evolution in kinetoplastid protists. BMC Evolutionary Biol. (2016) 16:109. doi: 10.1186/s12862-016-0664-6
4. Tripodi KEJ, Menendez Bravo SM, Cricco JA. Role of heme and heme-proteins in trypanosomatid essential metabolic pathways. Enzyme Research 2011. (2011) p:1–12. doi: 10.4061/2011/873230
5. Widener J, Nielsen MJ, Shiflett A, Moestrup S, Hajduk S. Haemoglobin is a co-factor of human trypanosome lytic factor. PloS Pathog. (2007) 3:e129. doi: 10.1371/journal.ppat.0030129
6. Higgins MK, Lane-Serff H, MacGregor P, Carrington M. A receptor’s tale: an eon in the life of a trypanosome receptor. PloS Pathog. (2017) 13:e1006055. doi: 10.1371/journal.ppat.1006055
7. Higgins MK, Tkachenko O, Brown A, Reed J, Raper J, Carrington M. Structure of the trypanosome haptoglobin–haemoglobin receptor and implications for nutrient uptake and innate immunity. Proc Natl Acad Sci. (2013) 110:1905–10. doi: 10.1073/pnas.1214943110
8. Vanhollebeke B, De Muylder G, Nielsen MJ, Pays A, Tebabi P, Dieu M. A haptoglobin-haemoglobin receptor conveys innate immunity to trypanosoma brucei in humans. Science. (2008) 320:677–81. doi: 10.1126/science.1156296
9. Lane-Serff H, MacGregor P, Peacock L, Macleod OJS, Kay C, Gibson W, et al. Evolutionary diversification of the trypanosome haptoglobin-haemoglobin receptor from an ancestral haemoglobin receptor. eLife. (2016) 5:e13044. doi: 10.7554/eLife.13044
10. Kristiansen M, Graversen JH, Jacobsen C, Sonne O, Hoffman H, Law SKA, et al. Identification of the haemoglobin scavenger receptor. Nature. (2001) 409:198–201. doi: 10.1038/35051594
11. Etzerodt A, Kjolby M, Nielsen MJ, Maniecki MB, Svendsen P, Moestrup SK, et al. Plasma clearance of haemoglobin and haptoglobin in mice and effect of CD163 gene targeting disruption. Antioxidants Redox Signaling. (2013) 18:2254–63. doi: 10.1089/ars.2012.4605
12. Changmai P, Horáková E, Long S, Černotíková-Stříbrná E, McDonald LM, Bontempi EJ, et al. Both human ferredoxins equally efficiently rescue ferredoxin deficiency in Trypanosoma brucei. Mol Microbiol. (2013) 89:135–51. doi: 10.1111/mmi.12264
13. Puustinen A, Wikström M. The heme groups of cytochrome o from Escherichia coli. Proc Natl Acad Sci. (1991) 88:6122–6. doi: 10.1073/pnas.88.14.6122
14. Horáková E, Lecordier L, Cunha P, Sobotka R, Changmai P, Langedijk CJM, et al. Heme-deficient metabolism and impaired cellular differentiation as an evolutionary trade-off for human infectivity in Trypanosoma brucei gambiense. Nat Commun. (2022) 13:7075. doi: 10.1038/s41467-022-34501-4
15. Watanabe J, Chou KJ, Liao JC, Miao Y, Meng H-H, Ge H, et al. Differential association of haemoglobin with proinflammatory high density lipoproteins in atherogenic/hyperlipidemic mice. J Biol Chem. (2007) 282:23698–707. doi: 10.1074/jbc.M702163200
16. Stødkilde K, Torvund-Jensen M, Moestrup SK, Andersen CBF. Structural basis for trypanosomal haem acquisition and susceptibility to the host innate immune system. Nat Commun. (2014) 5:5487. doi: 10.1038/ncomms6487
17. Pamir N, Pan C, Plubell DL, Hutchins PM, Tang C, Wimberger J, et al. Genetic control of the mouse HDL proteome defines HDL traits, function, and heterogeneity. J Lipid Res. (2019) 60:594–608. doi: 10.1194/jlr.M090555
18. Watanabe J, Grijalva V, Hama S, Barbour K, Berger FG, Navab M, et al. Haemoglobin and its scavenger protein haptoglobin associate with apoA-1-containing particles and influence the inflammatory properties and function of high density lipoprotein. J Biol Chem. (2009) 284:18292–301. doi: 10.1074/jbc.M109.017202
19. Du R, Winarsih I, Ho B, Ding JL. Lipid-free apolipoprotein A-I exerts an antioxidative role against cell-free haemoglobin. Am J Clin Exp Immunol. (2012) 1:33–48.
20. Vanhollebeke B, Uzureau P, Monteyne D, Pérez-Morga D, Pays E. Cellular and Molecular Remodeling of the Endocytic Pathway during Differentiation of Trypanosoma brucei Bloodstream Forms. Eukaryotic Cell. (2010) 9:1272–82. doi: 10.1128/EC.00076-10
21. Vanhollebeke B, Pays E. The trypanolytic factor of human serum: many ways to enter the parasite, a single way to kill. Mol Microbiol. (2010) 76:806–14. doi: 10.1111/j.1365-2958.2010.07156.x
22. Pays E, Vanhollebeke B, Uzureau P, Lecordier L, Pérez-Morga D. The molecular arms race between African trypanosomes and humans. Nat Rev Microbiol. (2014) 12:575–84. doi: 10.1038/nrmicro3298
23. Barker C, Barbour KW, Berger FG, Hajduk SL. Activity of human trypanosome lytic factor in mice. Mol Biochem Parasitol. (2001) 117:129–36. doi: 10.1016/S0166-6851(01)00339-5
24. Ramos S, Jeney V, Figueiredo A, Paixão T, Sambo MR, Quinhentos V, et al. Targeting circulating labile heme as a defense strategy against malaria. Life Sci Alliance. (2024) 7:e202302276. doi: 10.26508/lsa.202302276
25. Horáková E, Changmai P, Vancová M, Sobotka R, Abbeele JVD, Vanhollebeke B, et al. The Trypanosoma brucei TbHrg protein is a heme transporter involved in the regulation of stage-specific morphological transitions. J Biol Chem. (2017) 292:6998–7010. doi: 10.1074/jbc.M116.762997
26. Perner J, Hatalova T, Cabello-Donayre M, Urbanova V, Sojka D, Frantova H, et al. Haem-responsive gene transporter enables mobilization of host haem in ticks. Open Biol. (2021) 11:210048. doi: 10.1098/rsob.210048
27. Cabello-Donayre M, Orrego LM, Herráez E, García-Hernández R, Pérez-Victoria JM. New insights on heme uptake in leishmania spp. Int J Mol Sci. (2022) 23:10501. doi: 10.3390/ijms231810501
28. Swenson SA, Moore CM, Marcero JR, Medlock AE, Reddi AR, Khalimonchuk O, et al. From synthesis to utilization: the ins and outs of mitochondrial heme. Cells. (2020) 9:579. doi: 10.3390/cells9030579
29. Acestor N, Zíková A, Dalley RA, Anupama A, Panigrahi AK, Stuart KD, et al. Trypanosoma brucei mitochondrial respiratome: composition and organization in procyclic form. Mol Cell Proteomics. (2011) 10:M110.006908. doi: 10.1074/mcp.M110.006908
30. Peacock AC, Zíková A, Dalley RA, Anupama A, Panigrahi AK, Stuart KD, et al. Haptoglobin levels in serum of various strains of mice. Science. (1967) 158:1703–4. doi: 10.1126/science.158.3809.1703
31. Madsen M, Gelderman AH, Ragland RH, HA, HoffmaN. Molecular characterization of the haptoglobin·Haemoglobin receptor CD163. J Biol Chem. (2004) 279:51561–7. doi: 10.1074/jbc.M409629200
32. Moestrup S, Møller H. CD163: a regulated haemoglobin scavenger receptor with a role in the anti-inflammatory response. Ann Med. (2009) 36:347–54. doi: 10.1080/07853890410033171
33. Calomeno NA, Moreira RS, Fernandes LA, Batista F, Marques J, Wagner G, et al. Serum proteomic signature of Trypanosoma evansi –infected mice for identification of potential biomarkers. Veterinary Parasitol. (2021) 290:109342. doi: 10.1016/j.vetpar.2021.109342
34. Huntoon KM, Rybchyn MS, Easterbrook-Smith SB, Henriques C, Wilson MR. The acute phase protein haptoglobin regulates host immunity. J Leukocyte Biol. (2008) 84:170–81. doi: 10.1189/jlb.0208100
35. Huntoon KM, Russell L, Tracy E, Barbour KW, Li Q, Shrikant PA, et al. A unique form of haptoglobin produced by murine hematopoietic cells supports B-cell survival, differentiation and immune response. Mol Immunol. (2013) 55:345–54. doi: 10.1016/j.molimm.2013.03.008
36. Lipschitz DA, Allegre A, Cook JD. The clinical significance of ferritinuria. Blood. (1980) 55:260–4. doi: 10.1182/blood.V55.2.260.260
37. Balla J, Vercellotti GM, Jeney V, Yachie A, Varga Z, Jacob HS, et al. Heme, heme oxygenase, and ferritin: how the vascular endothelium survives (and dies) in an iron-rich environment. Antioxidants Redox Signaling. (2007) 9:2119–38. doi: 10.1089/ars.2007.1787
38. Liu F-T, Stowell SR. The role of galectins in immunity and infection. Nat Rev Immunol. (2023) 23:479–94. doi: 10.1038/s41577-022-00829-7
39. Rodriguez A, García GA, Bua J, Cerliani JP, Postan M, Tasso LM, et al. Galectin-1 prevents infection and damage induced by trypanosoma cruzi on cardiac cells. PloS Negl Trop Dis. (2015) 9:e0004148. doi: 10.1371/journal.pntd.0004148
40. Sack GH. Serum Amyloid A (SAA) Proteins. Springer: Subcellular Biochemistry, Vol. 94. (2020). pp. 421–36.
41. Noborn F, Ancsin JB, Ubhayasekera W, Kisilevsky R, Li J-P. Heparan sulfate dissociates serum amyloid A (SAA) from acute-phase high-density lipoprotein, promoting SAA aggregation. J Biol Chem. (2012) 287:25669–77. doi: 10.1074/jbc.M112.363895
Keywords: haptoglobin (Hp), infection, Trypanosoma, haemoglobin (Hb), blood markers, acute phase protein
Citation: Horáková E, Vrbacký M, Tesařová M, Stříbrná E, Pilný J, Vavrušková Z, Vancová M, Sobotka R, Lukeš J and Perner J (2024) Haptoglobin is dispensable for haemoglobin uptake by Trypanosoma brucei. Front. Immunol. 15:1441131. doi: 10.3389/fimmu.2024.1441131
Received: 31 May 2024; Accepted: 19 June 2024;
Published: 18 July 2024.
Edited by:
Romulo Dias Novaes, Federal University of Alfenas, BrazilReviewed by:
Eliziária Cardoso Santos, Universidade Federal dos Vales do Jequitinhonha e Mucuri, BrazilCopyright © 2024 Horáková, Vrbacký, Tesařová, Stříbrná, Pilný, Vavrušková, Vancová, Sobotka, Lukeš and Perner. This is an open-access article distributed under the terms of the Creative Commons Attribution License (CC BY). The use, distribution or reproduction in other forums is permitted, provided the original author(s) and the copyright owner(s) are credited and that the original publication in this journal is cited, in accordance with accepted academic practice. No use, distribution or reproduction is permitted which does not comply with these terms.
*Correspondence: Julius Lukeš, anVsYUBwYXJ1LmNhcy5jeg==; Jan Perner, cGVybmVyQHBhcnUuY2FzLmN6
Disclaimer: All claims expressed in this article are solely those of the authors and do not necessarily represent those of their affiliated organizations, or those of the publisher, the editors and the reviewers. Any product that may be evaluated in this article or claim that may be made by its manufacturer is not guaranteed or endorsed by the publisher.
Research integrity at Frontiers
Learn more about the work of our research integrity team to safeguard the quality of each article we publish.