- 1Department of Microbiology and Immunology, McGill University, Montréal, QC, Canada
- 2Infectious Diseases and Immunity in Global Health Program, Research Institute of the McGill University Health Centre, Montréal, QC, Canada
Introduction: Exosomes produced by the protozoan parasite Leishmania (LeishEXO) are well-established drivers of virulence, though mechanisms underlying their exacerbation of experimental leishmaniasis remain elusive. Expression of Annexin A1 (ANXA1), a protein implicated in exosome-mediated pathologies and viral internalization, has been shown to correlate with cutaneous leishmaniasis severity. Given ANXA1’s regulation of myeloid cells – the canonical hosts for Leishmania – we studied the potential role of ANXA1 and its receptors FPR1/2 in exerting LeishEXO’s effects.
Methods: Murine and in vitro ANXA1-/- models were used to study the generation of protective TH1 responses during experimental L. major infection with and without LeishEXO. Recruitment of inflammatory cells was assessed using a peritoneal cell recruitment assay and immunophenotyping, and production of inflammatory mediators was measured using a cytokine and chemokine array. Treatment of experimental models with FPR2 antagonist WRW4 and FPR1/2 agonist WKYMVm was used to delineate the role of the FPR/ANXA1 axis in LeishEXO-mediated hyperpathogenesis.
Results: We established that ANXA1 deficiency prohibits LeishEXO-mediated pathogenesis and myeloid cell infection, with minimal alterations to adaptive and innate immune phenotypes. FPR2 blockade with WRW4 similarly inhibited leishmanial hyperpathogenesis, while direct activation of FPRs with WKYMVm enhanced infection and recapitulated the LeishEXO-mediated phenotype. This research describes LeishEXO’s utilization of the ANXA1/FPR axis to facilitate parasitic internalization and pathogenesis, which may be leveraged in the development of therapeutics for leishmaniasis.
Introduction
Leishmaniases are an array of cutaneous and visceral pathologies caused by the protozoan parasite Leishmania, responsible for over 2 million new infections and 30,000 deaths annually (1). Infection typically occurs when a phlebotomine sandfly bearing the parasite takes a bloodmeal from a mammalian host, inoculating Leishmania into the host dermis (2). Parasites are then rapidly internalized by inflammatory myeloid cells recruited to the site of infection – most notably macrophages and neutrophils (2). While macrophages act as the canonical host cell for Leishmania, infected neutrophils become apoptotic before being, themselves, engulfed by macrophages, allowing the parasite to maximize its establishment through a “Trojan Horse” mechanism (2).
To ensure parasitic persistence, Leishmania promotes macrophage dysfunction by altering key signaling pathways and impairing the host cell’s ability to mount an effective parasitotoxic response (3, 4). Our previous research has established that critical leishmanial virulence factors, such as the surface metalloprotease GP63, are packaged within extracellular vesicles (EVs) which can, in turn, directly abrogate macrophage function and pre-emptively produce a favorable environment for parasitic uptake and persistence (3). Exosomes, which are ubiquitously-produced small EVs containing biologically active cargo (including proteins, nucleic acids, and lipids), have been the focus of numerous studies due to their implication in intercellular communication, and roles in the modulation of immunity, oncogenesis, and the immunopathogenesis of infectious disease (5–9).
We previously reported that Leishmania produces exosomes in the midgut of its sandfly vector, which are co-inoculated with parasites during the sandfly’s bloodmeal, in turn exerting an immunomodulatory effect (10). In fact, leishmanial exosomes isolated from in vitro cultures are as effective at modulating early macrophage and host inflammatory responses as whole Leishmania parasites (3, 11, 12). Furthermore, Leishmania EVs exacerbate cutaneous leishmaniasis lesions by inducing the overproduction of the inflammatory cytokine IL-23/IL-17, leading to the recruitment of neutrophils and pathogenic hyperinflammation (10, 11). However, while it is well-established that leishmanial exosomes are crucial modulators of macrophage function, potentiators of Leishmania infection, and drivers of immunopathogenesis, mechanisms underlying their effects remain elusive (3, 4, 10, 12).
The annexin-family protein Annexin A1 (ANXA1) has been implicated in EV-mediated exacerbation of non-communicable disease, including in the promotion of malignant cell proliferation and development of cardiovascular microcalcifications, notably through direct interaction with exosomal lipids and phospholipids, as well as through the stimulation of the downstream N-formyl peptide receptors (FPR) 1 and 2 (13–16). ANXA1 also has an established role in infectious disease, capable of facilitating viral entry into host cells (17). While ANXA1 has previously been reported to play a role in the regulation of myeloid cells at early time points following Leishmania infection, and expression levels of the protein have been shown to correlate with the severity of the histopathological features of cutaneous leishmaniasis, the effect of leishmanial exosome/ANXA1 interaction has yet to be studied (18, 19). Herein, we hypothesized that ANXA1 could be implicated in cellular events underlying leishmanial exosome-mediated exacerbation of cutaneous leishmaniasis. To study this, we utilized in vivo, ex vivo, and in vitro studies to provide evidence that leishmanial exosomes exploit the ANXA1/FPR axis to enhance myeloid cell invasion by Leishmania in the early stages of infection, driving immunopathogenesis. This research uncovers a novel role for this immunomodulatory mechanism, which may be leveraged in the development of therapeutics for leishmaniasis.
Materials and methods
Parasite culture
Leishmania major parasites used in the study were NIH S (MHOM/SN/74/Seidman) clone A2. Parasites were cultured at 25 ˚C, 5% CO2 in Schneider’s Drosophila Medium (SDM) supplemented with 10% heat-inactivated fetal bovine serum (FBS; Wisent, St-Bruno, QC, Canada), and 5 mg/mL Hemin. Cultures of promastigotes were passaged every 3–4 days to maintain logarithmic growth or were grown to stationary phase (day 6–8 post-passage) before being used in infections.
Extraction of L. major-derived vesicles
To extract L. major exosomes/extracellular vesicles (LeishEXO), 800 mL of late log phase parasite culture (1–4 × 108 parasites/mL) was centrifuged at 300 x g and pelleted. Parasites were then washed 3 times with PBS to remove dead cells and debris using centrifugation at 300 x g. Parasites were resuspended in FBS-free RPMI 1640 medium without phenol-red (Life Technologies), then incubated in a shaking incubator at ˚C for 4 hours, simulating egestion of parasites into mammalian hosts and stimulating EV production. Parasites were then separated from the vesicle-enriched supernatant using centrifugation at 300 x g for 5 minutes, then at 2000 x g for 10 minutes. The supernatant was filtered sequentially through a 0.45 μM syringe filter followed by a 0.20 μM syringe filter and centrifuged for 1 hour at 100,000 x g at 4°C using a Beckman Coulter Optima XPN-90™ ultracentrifuge and a SW32.Ti swinging rotor with open-top thin wall polypropylene tubes (16 x 102 mm; Beckman Coulter™, Brea, CA, USA). The supernatant was discarded, and pellets were collected, pooled together, and resuspended in exosome buffer (137 mM NaCl, 20 mM Hepes pH 7.5), before being centrifuged for 1 hour at 100,000 x g at 4 ˚C. The final exosome pellet was resuspended in exosome buffer in approximately 200-300 μL and stored at -80 ˚C.
Protein concentrations of the extracted samples were assessed using the microBCA Protein Assay kit according to manufacturer`s instructions (Thermo Scientific, catalogue number 23235). Particle size distribution and concentration of EV preparations were assessed by nanoparticle tracking analysis using an LM-10 Nanosight machine in the laboratory of Dr. Janus Rak at the Research Institute of the McGill University Health Centre, as previously described (3, 20). A dosage of 10 μg of Leishmania EVs/Exosomes was selected for in vivo and in vitro experiments following established protocols (10).
Chemicals
Chemical agonists/antagonists used in this study include the selective FPR2 antagonist WRW4 or WRWWWW (1μm; Tocris Biosciences, Ellisville, MO, USA), and the FPR agonist WKYMVm (1μm; Tocris Biosciences, Ellisville, MO, USA).
Animals and ethics
Male C57BL/6 mice were used in all experiments, and ANXA1-deficient mice were generously provided by Dr. Maziar Divangahi (McGill University) (16). Animal experiments were carried out in containment level 2 pathogen-free housing facilities in the Research Institute of the McGill University Health Center (RI-MUHC). Experiments were performed in accordance with the regulations of the Canadian Council of Animal Care Guidelines (CCAC), and McGill University Animal Care Committee (UACC) under ethics protocol numbers 7791 and 4859. Mice were housed socially in 3–5 mice per IVC cage, with food, water, and soft bedding, and were euthanized after 8–10 weeks using isoflurane and CO2 asphyxiation followed by cervical dislocation. Adult male C57BL/6 (6–8 weeks old) mice purchased from Charles River Laboratories (Wilmington, MA, USA) were used for all experiments.
Murine footpad infections
Groups of five male C57BL/6 mice were infected in the right hind footpad with 5 x 106 stationary-phase Leishmania major stationary phase promastigotes, either alone or with 10 μg of Leishmania EVs/Exosomes. Ten, 20, or 30 mice were used per experiment, separated between 2, 4, or 6 groups, as described in figure captions. Footpad swelling was measured weekly or bi-weekly with a metric caliper to monitor lesion development, with uninfected footpads used as a negative control. No randomization or blinding was used, and mice in each group were housed in the same cage for the duration of the experiment. Lesion progression was monitored for ten weeks, at the end of which footpads were processed using a limiting dilution assay to determine the parasite burden for select experiments. Mice were euthanized after ten weeks using isoflurane and CO2 asphyxiation followed by cervical dislocation.
Murine intraperitoneal inoculation
Mice (6–8 weeks old, male) were injected intraperitoneally with either endotoxin-free PBS (Wisent Inc, St-Bruno, QC), or 108 stationary phase L. major promastigotes, either alone or co-inoculated with Leishmania EVs/Exosomes (10 μg). All injections were prepared using endotoxin-free PBS (Wisent Inc, St-Bruno, QC) with a final volume of 250 μL per mouse. Three independent experiments were performed for each experimental design. Six hours post-intraperitoneal infection, mice were sacrificed and generously sprayed with 75% ethanol. The peritoneum was exposed, and 5 ml of cold endotoxin-free PBS (Wisent Inc, St- Bruno, QC, Canada) was injected into the peritoneal cavity, avoiding organ perforation. After injection, the peritoneum was gently massaged to loosen the attached cells into the PBS solution, and lavages were collected by moving the needle in the peritoneal cavity while aspirating. Samples were kept on ice, and the number of live cells in the lavages was counted using a hemocytometer and an optical microscope.
Harvested cells were prepared for microscopy using the Cytospin 4 cytocentrifuge (Thermo Scientific, Waltham, MA, USA). Cells were fixed and stained using the Differential Quik (Diff-Quik) stain kit (Ral Diagnostics, Martillac, France). The percentage and the total number of cell types found in the lavage were counted, along with the percentage of cells infected and the number of Leishmania amastigotes found within the cells. The peritoneal lavages were then used for in vitro culture and centrifugation to pellet the recruited cells and obtain cell-free supernatants for further analysis.
In vitro culture of myeloid cells
A volume of 200μl of peritoneal lavages was plated in 4-well chamber slides and supplemented with Dulbecco’s modified eagle medium (Wisent, St-Bruno, QC, Canada) with 10% FBS and 1% penicillin-streptomycin-glutamine. Cells were kept at 37 ˚C with 5% CO2 for 24–48 hours. Cells were then fixed and stained using Diff-Quik, and a minimum of 200 cells per slide were counted to obtain the percentage of infected cells and number of amastigotes per cell.
Multiplex cytokine/chemokine quantification assay
A volume of 200μl of the lavage supernatant was analyzed by a multiplex mouse cytokine array/chemokine array 44-plex assay (Eve Technologies, Calgary, AB, Canada), including eotaxin, Erythropoietin, 6Ckine, Fractalkine, G-CSF, GM-CSF, IFNB1, IFN-γ, IL-1α, IL-1β, IL-2, IL-3, IL-4, IL- 5, IL-6, IL-7, IL-9, IL-10, IL-11, IL-12 (p40), IL-12 (p70), IL-13, IL-15, IL-16, IL-17, IL-20, IP- 10, KC, LIF, LIX, MCP-1, MCP-5, M-CSF, MDC, MIG, MIP-1α, MIP-1β, MIP-2, MIP-3α, MIP-3B, RANTES, TARC, TIMP-1, TNF-α, and VEGF. Multiplex laser bead technology was employed, utilizing antibodies coupled to color-coded polystyrene beads, permitting quantification using lasers that excite the fluorescent conjugates. Cytokines and chemokines from the lavage fluid were quantified using data provided by Eve technologies.
Cell isolation and preparation for immunophenotyping
The right popliteal lymph node was isolated using mechanical disruption through a 70 μM strainer in a complete RPMI medium. Footpads were collected and digested in RPMI 1640 with 5% FBS (Wisent, Saint-Bruno, QC) containing collagenase D (0.5 mg/mL) in the presence of DNAse I (0.005 uM) (Sigma-Aldrich) for 60 minutes at 37 ˚C, then passed through a 70 μm cell strainer. Cells were counted using Trypan Blue (Gibco Thermo Fisher Scientific, Waltham, MA) and kept in complete RPMI medium. For cytokine analysis, 5 x 105 cells were plated in a 96 flat-well culture dish and exposed to Phorbol 12-myristate 13-acetate (PMA; Sigma-Aldrich), ionomycin (Sigma-Aldrich), and monensin (BD Golgi-Stop™, BD Biosciences) following manufacturer’s guidelines for 3 hours before staining.
Flow cytometry
Single-cell suspensions obtained from cell isolation were stained with the following fluorescence-conjugated monoclonal antibodies purchased from Thermo Fisher Scientific (Waltham, MA) unless otherwise stated: α-CD3 FITC (17A2), α-CD4–Alexa700 (GK1.5), α-CD8-V500 (53-6.7) (BD Biosciences). Intracellular stains included α-Foxp3-FITC or PECy7 (FJK-16s), α-Ki67-BUV395 (B56, BD Biosciences), α-IL17A-APC (eBio17B7), α-IFNγ-BUV737 (XMG1.2, BD Biosciences), and α-IL4-PE (11B11) using the Ebioscience™ Foxp3 staining kit (Thermo Fisher Scientific). Non-viable cells were excluded using fixable viability dye eFluor 780 reagents (Thermo Fisher Scientific). Data were acquired using a FACS Fortessa X-20 flow cytometer (BD Biosciences) and analyzed using FlowJo version 10 software (TreeStar, BD Biosciences).
Statistical analysis
Statistical significance between groups was determined using unpaired Student t-tests, corrected for multiple comparisons using the Holm-Sidak method. Brown-Forsythe and Welch ANOVA tests, with Games-Howell’s correction for multiple comparisons, or two-way ANOVA with multiple comparisons by uncorrected Fisher LSD’s Test or Holm-Sidak tests, were used when comparing multiple groups. P-values lower than 0.05 were considered significant. *P < 0.05, **P < 0.01, ***P < 0.001, and ****P < 0.0001.
Limiting dilution assay
Limiting dilution assays were performed on footpad lesions at ten weeks post-infection by processing infected tissue and diluting it in PBS. After counting parasites in the extraction solution, 20-fold serial dilutions were prepared in SDM supplemented with 10% FBS. A volume of 100 μL of each dilution was transferred into wells of a microtiter plate. A control plate contained serial dilutions of in vitro-cultured L. major promastigotes. After seven days of incubation at 26 ˚C, the number of positive wells (presence of motile parasites) and negative wells (absence of motile parasites) was identified by direct observation under an inverted light microscope, enabling the total number of parasites in footpad lesions to be estimated.
Results
ANXA1 is required for leishmanial exosome-mediated hyperpathogenesis during experimental L. major infection
Given the primary constituents of exosomes are lipids and phospholipids, which directly interact with negative-charged phospholipid-sensing annexin receptors, we hypothesized that ANXA1 was involved in the exacerbation of leishmaniasis by leishmanial exosomes (LeishEXO). To study this, we used C57BL/6 mice, which are genetically resistant to leishmaniasis, and mount a strong Th1 response against the parasite (21, 22). In a model of experimental leishmaniasis, footpad infections of wildtype and ANXA1-/- mice were performed using L. major either alone or in combination with LeishEXO. The purity of L. major exosome preparations was validated (Supplementary Figure S1), and the selected dose was 10 μg per mouse – an amount that we have previously reported to elicit an optimal response (10) and that corresponds to approximately 10 (12) particles (23). Strikingly, while wildtype mice responded to the co-inoculation of parasites and exosomes with the expected infective phenotype, mice lacking ANXA1 did not display increased footpad swelling compared to groups infected with parasites alone (Figure 1). These findings suggested that ANXA1 was required in the exosome-mediated exacerbation of experimental cutaneous leishmaniasis. This pronounced variance between co-inoculated wildtype and knockout mice was sustained over most of the course of infection, raising additional questions surrounding the effect of ANXA1 deficiency on the adaptive immune response.
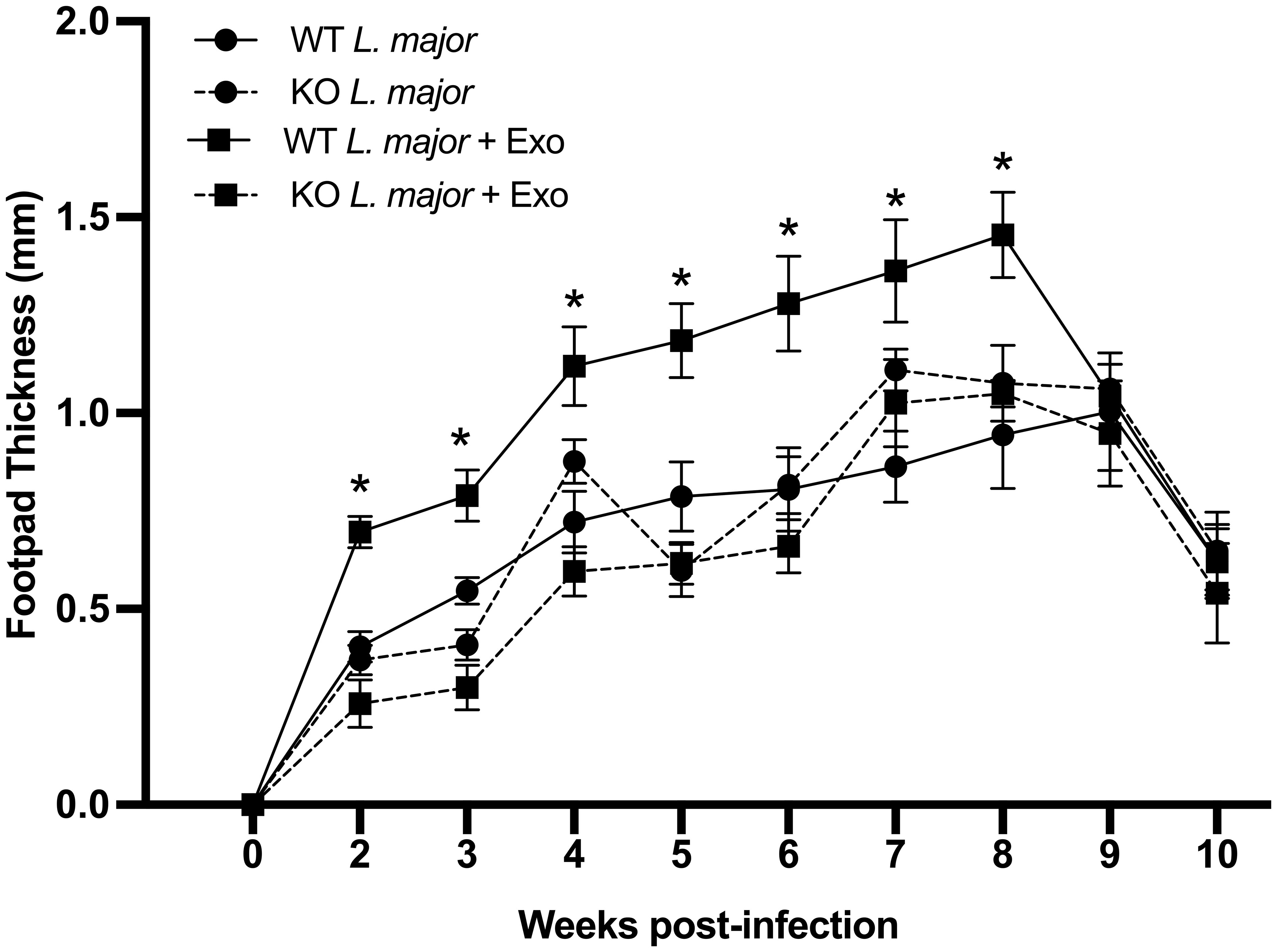
Figure 1 ANXA1 is required for leishmanial exosome-mediated immunopathogenesis during experimental L. major infection. L. major promastigotes were injected alone or in combination with LeishEXO into footpads of wildtype or ANXA1-/- mice. Lesion thickness was monitored weekly for ten weeks post-infection. Data are represented as mean ± SEM, n = 9. Differences were found to be significant using two-way ANOVA with Holm–Sidak’s correction. *P ≤ 0.05, **P ≤ 0.01, ***P ≤ 0.001, ****P ≤ 0.0001, ns, non-significant.
ANXA1 deficiency does not prohibit the generation of a cellular TH1 response during experimental L. major infection
An efficacious host response against L. major requires the polarization of CD4+ T helper 1 (TH1) cells, and the activation and migration of CD8+ T cells, which are critical for parasitic control and clearance (24). Inversely, a T helper 2 (TH2)-skewed response has been shown to enable persistent Leishmania infection (25, 26). To determine whether the absence of ANXA1 exerted an effect on the development of a productive leishmanicidal adaptive immune response, we sought to characterize immune cell populations and adaptive phenotypes over the course of experimental L. major infection.
T cells from the draining popliteal lymph node, a critical site for T cell differentiation, were assessed at various time points post-infection to measure immune polarization (27). Given data indicating that the frequency of IFN-γ+ CD4+ T cells peaked at week 5 post-infection, indicating that the TH1 response was established, we chose this final timepoint for subsequent experiments (Supplementary Figure S2).
When comparing T cell populations isolated from wildtype mice comparatively to their ANXA1-deficient counterparts following inoculation with L. major alone or in combination with leishmanial exosomes, no differences in the frequency of actively dividing Ki67+ T cells were observed between any groups (Figure 2A). Further, no statistical difference was observed in the total number of lymphocytes in the popliteal lymph nodes at weeks 1 or 5 between the groups (Figure 2B). As expected, IFN-γ- but not IL-4- or IL17A-producing T cells increased significantly in the popliteal lymph node of all groups over the course of infection as the adaptive response was established (Figures 2C–E). Extended data showed no difference in the mean fluorescence intensity (MFI) of IFN-γ-producing CD4+ T cells between the groups at weeks 1 or 5 (Supplementary Figure S3A). The abundance of Foxp3+ TREG cells did not vary over the course of infection, suggesting that the immune response was geared towards an effective clearance of the parasite in all groups (24) (Figure 2F). Interestingly, when measuring the frequency of IFN-γ-producing CD8+ T cells, which have been suggested to have a protective role during Leishmania infection (28), a clear increase was observed over time (Figure 2G). While this was independent of the presence of exosomes in the initial inoculum, a lower proportion of these cells was identified in knockout mice comparatively to wildtype controls, revealing a potential defect in the generation or retention of IFN-γ-producing CD8+ T cells associated with the absence of ANXA1. Notably, while there were no significant differences in the MFI of IFN-γ-producing CD8+ T cells at 1 week post-infection, wildtype mice infected with L. major alone or in combination with LeishEXO exhibited higher IFN-γ levels than ANXA1-deficient mice infected with the parasite alone at the 5 week time point (Supplementary Figure S3B). These findings agree with previous work indicating that ANXA1 is implicated in, but not critical to, the generation of a TH1 response (29). However, this difference likely exerts a limited effect considering our earlier footpad infection model demonstrated that both wildtype and knockout mice displayed similar footpad swelling response to parasites alone (Figure 1).
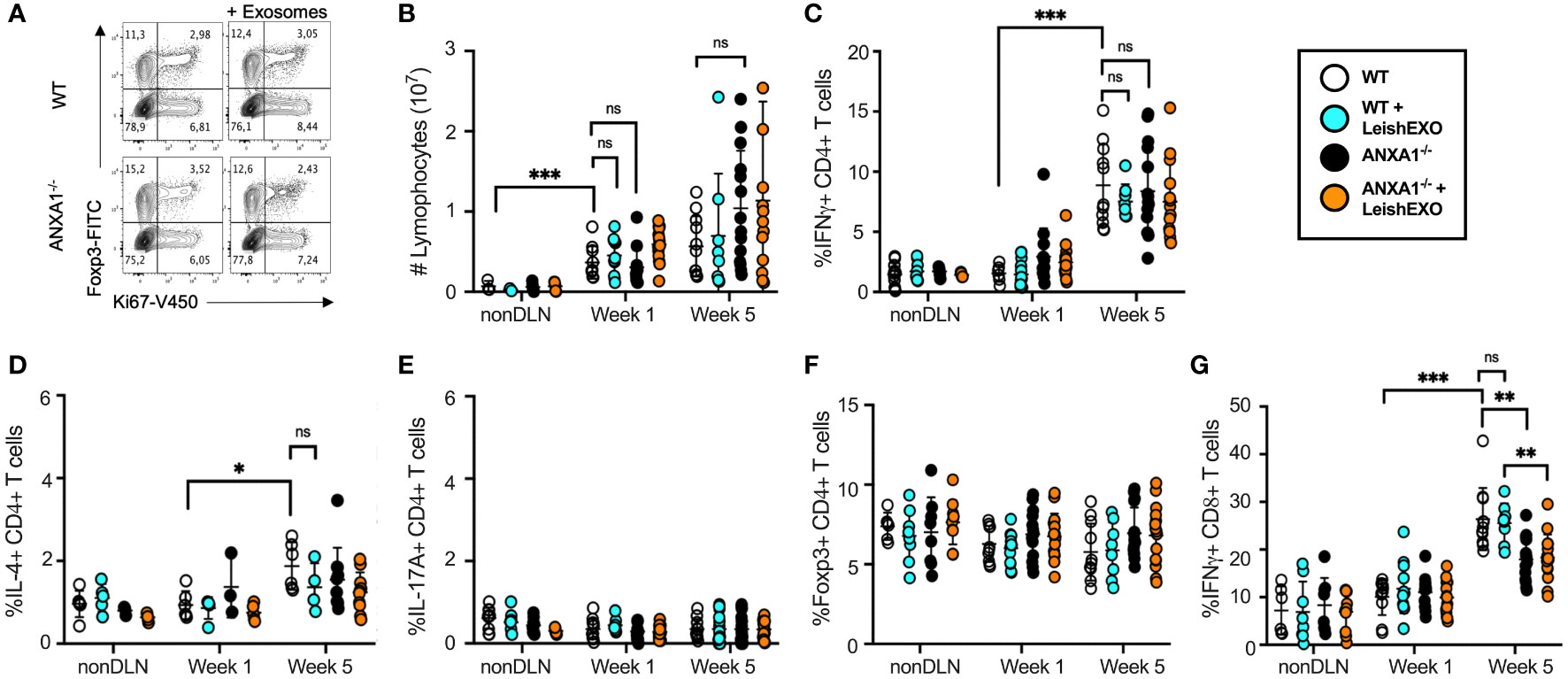
Figure 2 ANXA1 deficiency does not prohibit the generation of a cellular TH1 response during experimental L. major infection. L. major promastigotes were injected alone or in combination with LeishEXO into footpads of wildtype or ANXA1-/- mice. Mice were sacrificed at weeks 1 and 5 post-infection (See also Supplementary Figure S2, Supplementary Figure S3 and Supplementary Figure S4), and T cells were isolated from draining (DLN) and non-draining (nonDLN) popliteal lymph nodes. (A) Representative dot plot of Foxp3+ and Ki67+ CD3+CD4+ T cells from the DLN at week 5 post-infection. (B) Total lymphocytes collected from nonDLN and DLN at weeks 1 and 5 post-infection. Frequency of CD4+ T cells that are (C) IFN-γ-producing, (D) IL-4-producing, (E) IL-17A-producing, (F) Foxp3+, and of (G) IFN-γ-producing CD8+ T cells collected from nonDLN and DLN at weeks 1 and 5 post-infection. Data are represented as mean ± SEM, n = 3. Differences were found to be significant using two-way ANOVA with Holm–Sidak’s correction. *P ≤ 0.05, **P ≤ 0.01, ***P ≤ 0.001, ****P ≤ 0.0001, ns, non-significant.
Additional studies assessing the phenotypes of cells derived from the infected footpads showed no difference in the abundance of CD4+,CD8+, and γδ T cells in knockout mice by week 1 (Supplementary Figures 4A-C). Further, the co-inoculation of LeishEXO induced a significant increase in the frequency of CD11b+ cells in footpads of wildtype mice, suggesting that dendritic cells or macrophages were primarily affected by the presence of Leishmania-derived EVs (Supplementary Figure S2D). Though this trend was also observed in cell populations derived from ANXA1-deficient mice, it was not statistically significant.
Collectively, these observations indicate that the ANXA1-dependent effect of leishmanial exosomes does not significantly influence the generation of a protective adaptive immune response at later time points post-infection. This suggests that ANXA1 may alter the innate immune response during promastigote implantation and early inflammatory events.
ANXA1 deficiency does not affect Leishmania/LeishEXO-mediated cellular and humoral inflammation during acute experimental L. major infection
To further decipher ANXA1’s role in the immune response to leishmaniasis, we aimed to characterize its involvement in early inflammatory events following Leishmania infection. Peritoneal infections of wildtype and ANXA1-/- mice were performed using L. major alone or in combination with LeishEXO, following a well-established model of acute infection and inflammatory cell recruitment (30). Cells recruited to the peritoneal cavity were counted 6 hours post-infection, revealing an increase in total recruited cells in all infected groups, independent of the addition of LeishEXO (Figure 3A). Further, no significant differences were observed between total recruited cells in wildtype and ANXA1-/- mice.
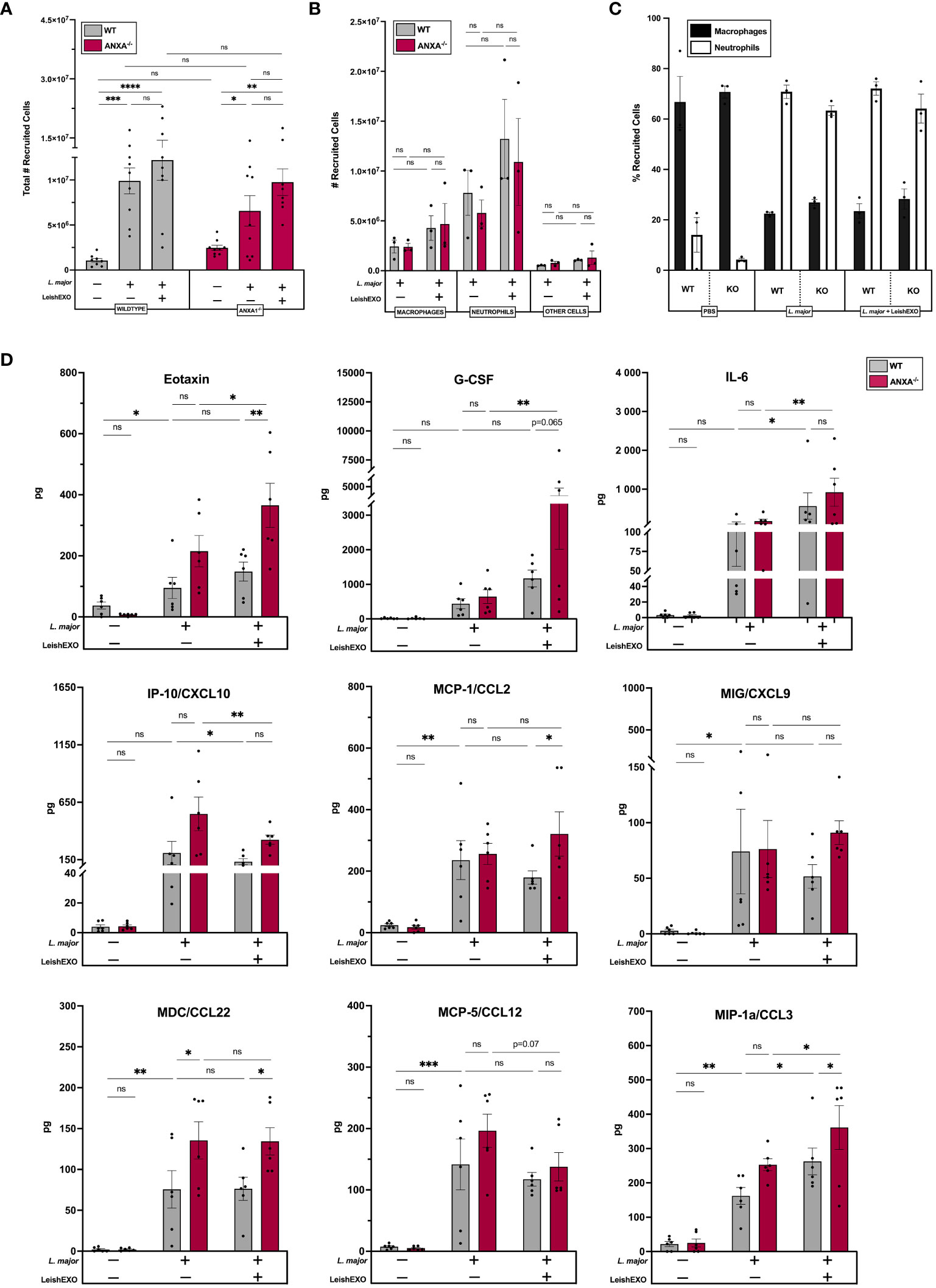
Figure 3 ANXA1 deficiency does not affect Leishmania/LeishEXO-mediated inflammatory cell recruitment or production of inflammatory mediators during acute experimental L. major infection. Wildtype or ANXA1-/- mice were injected intraperitoneally with L. major promastigotes, alone or in combination with LeishEXO for 6 hours. (A) Total recruited inflammatory cells, (B) total macrophages, neutrophils, or other cells recruited to the peritoneum, and (C) percentage of neutrophils and macrophages within recruited inflammatory cells. (D) Protein expression of inflammatory mediators in peritoneal lavage fluid (See also Supplementary Figure S5). Data are represented as mean ± SEM, (A–C) n = 3 and (D) n = 6. Differences were found to be significant using two-way ANOVA with Holm–Sidak’s correction. *P ≤ 0.05, **P ≤ 0.01, ***P ≤ 0.001, ****P ≤ 0.0001, ns, non-significant.
When further characterizing myeloid cell populations recruited to the peritoneal cavity, similar numbers of macrophages and neutrophils were identified in wildtype and ANXA1-/- mice (Figure 3B). While a trend indicated a slight increase in myeloid cell recruitment when Leishmania infection was accompanied with LeishEXO, this difference was not statistically significant. This observation was confirmed using the percentage of total recruited inflammatory cells that were macrophages and neutrophils, indicating that both L. major alone and in combination with leishmanial exosomes recruited more neutrophils than macrophages comparatively to a PBS control (Figure 3C). Similar percentages were observed in wildtype and ANXA1-/- mice. Together, these data suggest that ANXA1-deficiency plays a limited role in inflammatory cell recruitment during acute L. major infection.
We next sought to measure the effects of ANXA1 deficiency and of LeishEXO stimulation on the expression of inflammatory mediators to further characterize their role in early inflammatory events during Leishmania infection. The production of cytokines and chemokines was assessed using a 44-multiplex assay that measured levels of protein in the peritoneal lavage, revealing that eotaxin, G-CSF, IL-6, IP-10 (CXCL10), MCP-1/CCL2, MIG/CXCL9, MDC/CCL22, MCP-5/CCL12, and MIP-1a/CCL3 displayed notable differences between groups (Figure 3D). Levels of eotaxin, a potent chemoattractant for eosinophils (31), were elevated in both wildtype and ANXA1-/- mice infected with L. major alone. Further, co-inoculation with LeishEXO exhibited significantly increased eotaxin levels in ANXA1-deficient mice compared to their wildtype counterparts – a finding that was not observed in response to L. major infection alone. The peritoneal accumulation of G-CSF, a potent stimulator of granulocyte development in the bone marrow (31), was significantly increased by the addition of LeishEXO to the inoculum in ANXA1-deficient mice only. While a trend indicated that ANXA1-/- mice produced more G-CSF than their wildtype counterparts when stimulated with both L. major and LeishEXO, this finding was not statistically significant. Levels of IL-6, an essential player in the initiation of the inflammatory response (31), were shown to be significantly enhanced by the addition of LeishEXO to the L. major infection in both wildtype and knockout mice – though no difference in IL-6 accumulation was observed between the animals. Interestingly, levels of IP-10/CXCL10, a chemokine involved in Th1 polarization (31), decreased in both wildtype and ANXA1-deficient mice when inoculated with L. major and LeishEXO compared to mice infected with the parasite alone. Further, levels of the inflammatory chemokine MCP-1/CCL2 (31) were similarly increased in both models following L. major infection, although protein expression levels were much higher in ANXA1-/- mice than their wildtype counterparts when co-inoculated with LeishEXO. This same trend was observed in the peritoneal accumulation of the chemokine MIG/CXCL9, although not to statistically significant levels. When assessing levels of MDC/CCL22, involved in monocyte migration (31), protein accumulation was notably higher in ANXA1-deficient mice than in wildtype mice, in groups either having received L. major alone or in combination with LeishEXO (Figure 3D). The addition of LeishEXO to the L. major inoculum did not further enhance MDC/CCL22 protein expression. Levels of MCP-5/CCL12 detected in the peritoneal cavity followed a similar trend, although not to statistically significant levels. Finally, co-inoculation of LeishEXO with L. major significantly increased levels of the chemokine MIP-1a/CCL3 in both ANXA1-/- and wildtype mice comparatively to groups having received the parasite alone. Further, MIP-1a/CCL3 levels were higher in ANXA1-deficient mice than in their wildtype counterparts when inoculated with L. major/LeishEXO – a trend that was similarly observed in mice receiving L. major alone, though not statistically significant. Additional inflammatory mediators implicated in a robust leishmanicidal response, including IL-1β, IL-10, IL-12 (p70), IL-17, and TNF-α, did not display notable changes in response to the co-inoculation of LeishEXO or ANXA1 deficiency (Supplementary Figure S5). Altogether, these results suggest that ANXA1 deficiency does not affect inflammatory cell recruitment and only moderately affects inflammatory cytokine and chemokine production in acute L. major infection.
ANXA1 deficiency abrogates LeishEXO-mediated myeloid cell infection
We next aimed to address whether parasitemia and myeloid cell infection varied between wildtype and knockout mice. Infection rates of cells isolated from the peritoneal cavity were counted, revealing that the co-inoculation of LeishEXO in wildtype mice led to a significant increase in the number of infected neutrophils, which was not observed in their ANXA1-deficient counterparts (Figure 4A). Peritoneal macrophage infection, which initially showed no difference between wildtype and knockout animals 6 hours post-infection, was further dissected using an ex vivo culture model to account for potential longer required incubation times. Macrophages were harvested from infected ANXA1-/- and wildtype mice peritoneal lavages and cultured for 24–48 hours before counting (Figure 4B). The percentage of infected macrophages derived from wildtype mice increased over time and was significantly higher when animals received both L. major and LeishEXO. Interestingly, the proportion of infected macrophages derived from ANXA1-deficient mice was independent of LeishEXO co-inoculation and did not increase over time.
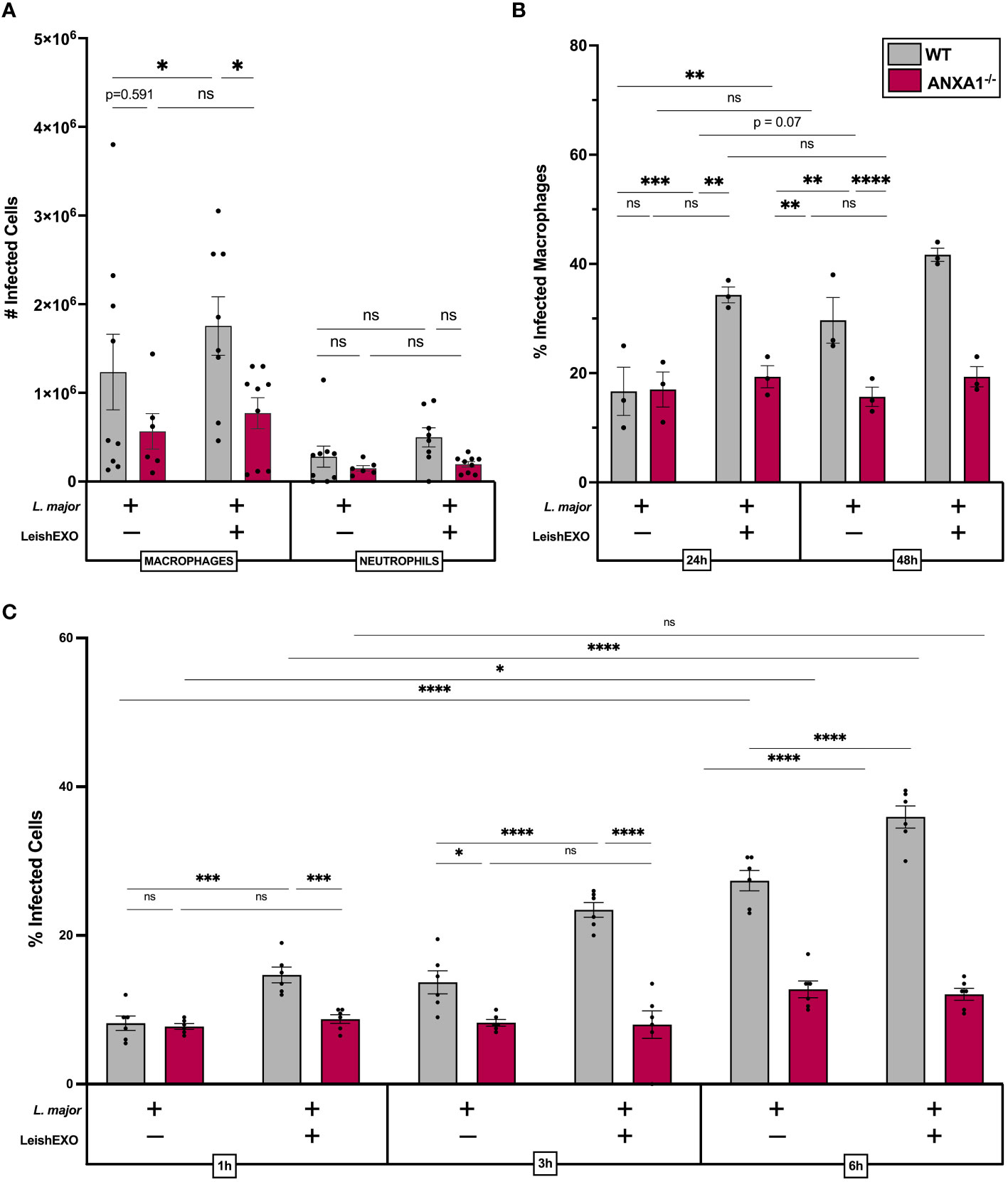
Figure 4 ANXA1 deficiency abrogates LeishEXO-mediated myeloid cell infection during acute experimental L. major infection. Wildtype or ANXA1-/- mice were injected intraperitoneally with L. major promastigotes, alone or in combination with LeishEXO for 6 hours. (A) Total infected myeloid cells in peritoneal lavage. (B) Percentage of infected macrophages derived from peritoneal lavage following 24–48 hours of in vitro culture. (C) Percentage of infected naïve peritoneal macrophages inoculated with L. major promastigotes alone or in combination with LeishEXO for 1, 3, or 6 hours. Data are represented as mean ± SEM, (A) n = 9, (B) n = 3, and (C) n = 6. Differences were found to be significant using two-way ANOVA with Holm–Sidak’s correction. *P ≤ 0.05, **P ≤ 0.01, ***P ≤ 0.001, ****P ≤ 0.0001, ns, non-significant.
To corroborate these findings, we aimed to decipher earlier time points post-stimulation in an in vitro model, using naïve cultured peritoneal macrophages isolated from both animal models. Cell cultures were inoculated with L. major alone or in combination with LeishEXO, and infection was counted over a short-term time course experiment (Figure 4C). At 1, 3, and 6 hours post-infection, co-inoculation of LeishEXO resulted in significantly higher percentages of infected wildtype macrophages. This effect was absent in macrophages derived from ANXA1-deficient mice, which displayed a constant percentage of infected cells across all time points and experimental groups. Overall, these data indicate that ANXA1 deficiency significantly abrogates myeloid cell infection by L. major, which is typically exacerbated by the presence of LeishEXO in wildtype animals.
FPR2 blockade inhibits LeishEXO-mediated hyperinfective L. major infection
We next sought to understand the mechanisms underlying ANXA1’s involvement in the LeishEXO-mediated infectivity of L. major to myeloid cells and associated cutaneous pathology. FPR2 is a G-protein coupled receptor (GPCR) expressed by myeloid cells (32), among many other cell types, which binds to the cleaved form of ANXA1. As FPR2 is an established downstream receptor of ANXA1, we aimed to assess the implication of the ANXA1/FPR2 receptor-ligand interaction in an in vivo model of experimental cutaneous leishmaniasis. Wildtype C57BL/6 mice were treated with the FPR2 antagonist WRW4 (1 µM) or PBS 30 minutes prior to infection with L. major alone or in combination with LeishEXO. While footpads of control mice developed the expected inflammatory phenotype when co-inoculated with L. major and LeishEXO, for which footpad thickness far exceeded that observed in mice infected with L. major alone, WRW4 pre-treated mice did not display enhanced LeishEXO-mediated pathology (Figure 5A). This unresponsiveness to LeishEXO when pre-treated with WRW4, displaying similar footpad inflammation in both L. major and L. major/LeishEXO infected groups, implicated FPR2 as a key player in LeishEXO-mediated pathogenesis. Measuring the parasite burden in footpads at 10 weeks post-infection with a limiting dilution assay indicated that, while PBS-treated control mice displayed higher parasitemia when inoculated with L. major in combination with LeishEXO than when infected with the parasite alone, this was not the case in WRW4 pre-treated mice (Figure 5B). The similar parasite burden measured in WRW4 pre-treated mice, independent of the presence of LeishEXO in the initial inoculum, further corroborated that FPR2 is involved in exosome-mediated pathogenesis of Leishmania.
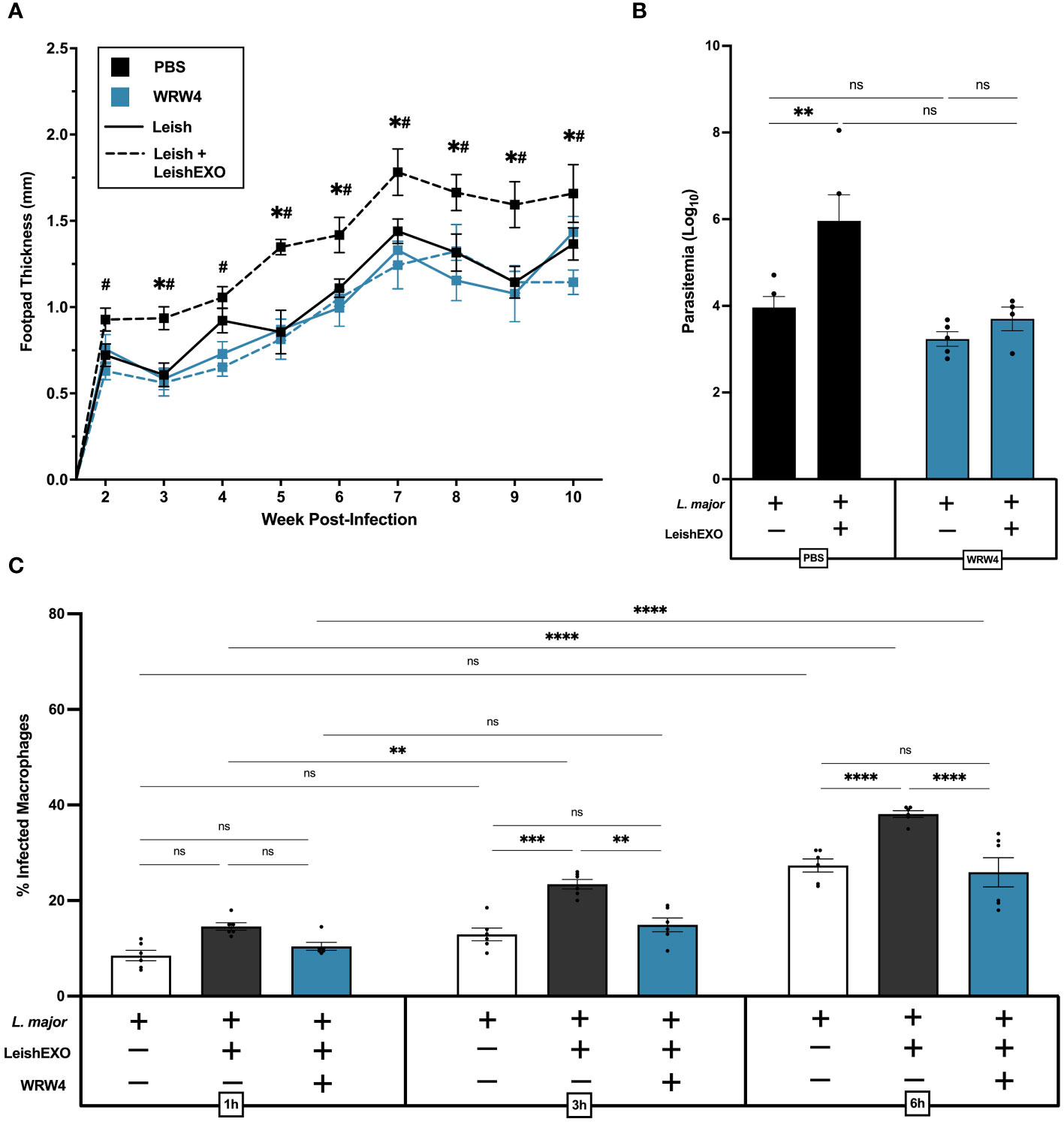
Figure 5 FPR2 blockade with antagonist WRW4 inhibits LeishEXO-mediated immunopathogenesis of L. major infection. L. major promastigotes were injected alone or in combination with LeishEXO into footpads of mice pre-treated with WRW4 or PBS. (A) Lesion thickness was monitored weekly for ten weeks post-infection. (B) Footpad parasitemia at 10 weeks post-infection. (C) Percentage of infected naïve peritoneal macrophages pre-treated with WRW4 or left untreated, inoculated with L. major promastigotes, alone or in combination with LeishEXO, for 1, 3, or 6 hours (See also Supplementary Figure S6). Data are represented as mean ± SEM, (A, B) n = 9 and (C) n = 6. Differences were found to be significant using two-way ANOVA with Holm–Sidak’s correction. *P ≤ 0.05, **P ≤ 0.01, ***P ≤ 0.001, ****P ≤ 0.0001, ns = non-significant. All significant (P ≤ 0.05) differences in footpad thickness are indicated for PBS-treated L. major vs. PBS-treated L. major/LeishEXO (#) and WRW4-treated L. major/LeishEXO vs. PBS-treated L. major/LeishEXO (+).
An in vitro peritoneal macrophage infection model was then used to validate the effect of WRW4 on the blockade of LeishEXO-mediated pathogenesis. Experimental groups included cells infected with L. major alone, co-inoculated with L. major and LeishEXO, or pre-treated with WRW4 followed by infection with L. major/LeishEXO (Figure 5C). In untreated cells, the addition of LeishEXO to the L. major inoculum induced a significantly higher proportion of infected macrophages at 3 and 6 hours post-infection – a trend that was visible as early as 1 hour post-infection, though not to statistically significant levels. Strikingly, cells pre-treated with WRW4 prior to the L. major/LeishEXO challenge displayed similar infection levels to untreated macrophages infected with L. major alone, indicating an unresponsiveness to LeishEXO. Interestingly, despite higher levels of infection, numbers of amastigotes per cell remained constant across all experimental groups (Supplementary Figure S6). Thus, these data show that the FPR2 antagonist WRW4 effectively inhibits a ligand-receptor interaction that is necessary for LeishEXO-mediated exacerbation of L. major pathogenesis. Altogether, this suggests that the ANXA1/FPR2 interaction in myeloid cells is a key contributor to skin pathology and parasitemia during cutaneous leishmaniasis.
FPR agonism enhances L. major infection independently of ANXA1 and LeishEXO
We next sought to determine whether direct activation of FPRs with the agonist WKYMVm could bypass the deficient LeishEXO/ANXA1 interaction in ANXA1-/- mice and result in a similar phenotype to that of L. major/LeishEXO co-inoculation in our wildtype in vivo model. To address this, wildtype and ANXA1-deficient C57BL/6 mice were infected with L. major alone, L. major in combination with LeishEXO, or L. major along with WKYMVm (Figure 6A). As expected, co-inoculation of L. major and LeishEXO resulted in significantly enhanced footpad swelling in wildtype mice comparatively to the parasite alone, though the severity of cutaneous pathology was surpassed by the group receiving L. major and WKYMVm. Strikingly, ANXA1-deficient mice, while unresponsive to the additive effects of LeishEXO inoculation along with L. major, displayed a hyperinfective phenotype and greater footpad swelling when L. major was co-inoculated with WKYMVm. This increase in footpad thickness indicates that the FPR agonist was capable of bypassing ANXA1 deficiency, directly stimulating the cellular receptor and downstream pathogenesis.
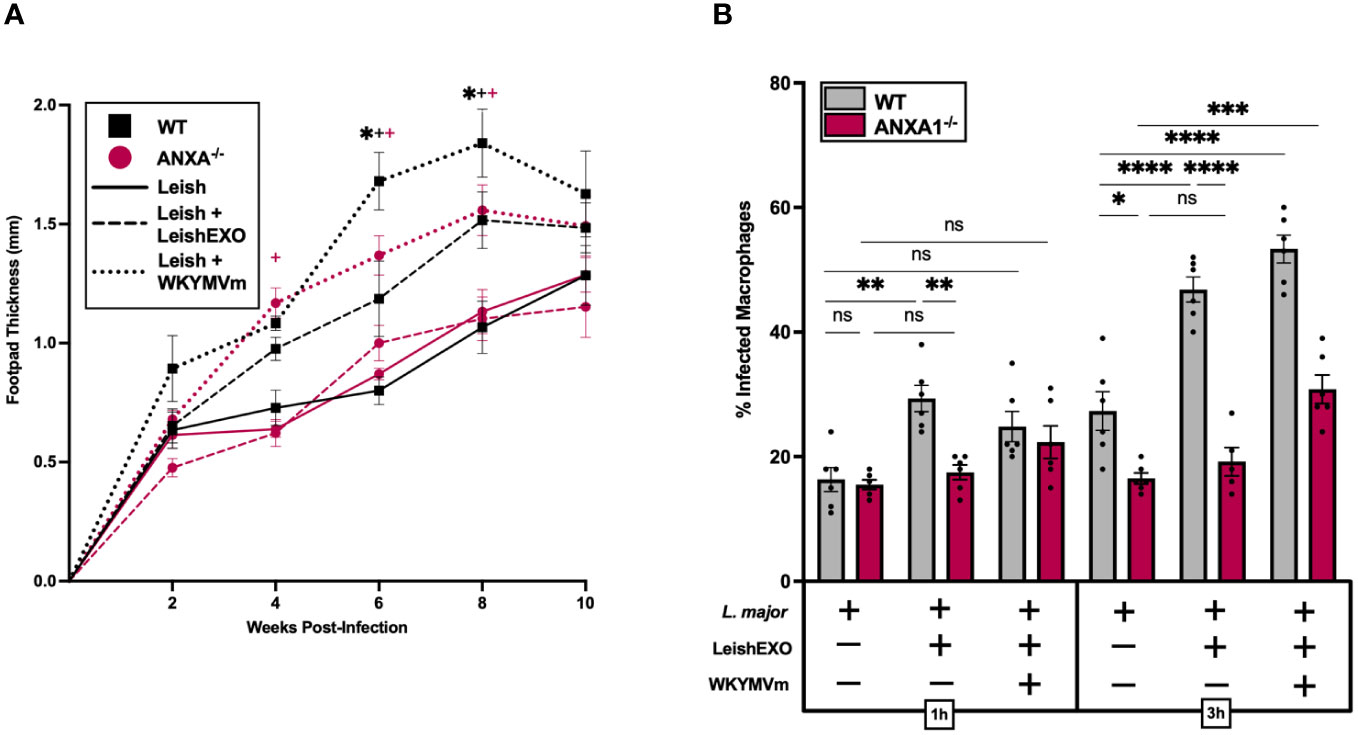
Figure 6 FPR agonism with WKYMVm enhances hyperinfective L. major infection independently of ANXA1 and LeishEXO. L. major promastigotes were injected alone, in combination with LeishEXO, or along with WKYMVm into footpads of wildtype or ANXA1-/- mice. (A) Lesion thickness was monitored bi-weekly for ten weeks post-infection. (B) Percentage of infected naïve peritoneal macrophages inoculated with L. major promastigotes alone, in combination with LeishEXO, or along with WKYMVm, for 1 or 3 hours. Data are represented as mean ± SEM, n = 6. Differences were found to be significant using two-way ANOVA with Holm–Sidak’s correction. *P ≤ 0.05, **P ≤ 0.01, ***P ≤ 0.001, ****P ≤ 0.0001, ns = non-significant. All significant (P ≤ 0.05) differences in footpad thickness are indicated for L. major vs. L. major/LeishEXO (#) and L. major/LeishEXO vs. L. major/WKYMVm (+).
An in vitro study of the effect of FPR agonism on myeloid cell infection by L. major was then performed using peritoneal macrophages isolated from both wildtype and ANXA1-/- mice. Cells received either L. major alone, in combination with LeishEXO, or along with WKYMVm, and infection rates were measured at 1 and 3 hours post-inoculation (Figure 6B). The addition of the FPR agonist led to an increase in the percentage of infected ANXA-deficient macrophages at 3 hours post-infection, albeit less pronounced than the effect observed in cells derived from wildtype mice. This significant increase in infected cells, particularly when compared to the unresponsiveness to LeishEXO, provides further evidence that activation of FPRs with WKYMVm bypasses the deficient ANXA1 signaling cascade that is implicated in LeishEXO recognition. Together, these data highlight the importance of FPRs in Leishmania-associated cutaneous pathology and indicate that stimulation of the ANXA1/FPR axis by LeishEXO is likely implicated in this process.
Discussion
Though exosomes produced by the protozoan parasite Leishmania are well-established drivers of virulence (3, 10, 12), mechanisms underlying their capacity to promote infection remain elusive. Our group has previously described that co-inoculation of L. major with leishmanial exosomes (LeishEXO) in a murine footpad model induces the development of cutaneous lesions far more severe than that caused by the parasite alone (33). In the present study, experiments using ANXA1-deficient C57BL/6 mice demonstrated that Annexin A1 (ANXA1) – a crucial player in various physiological and pathological processes (34–36) – is involved to this LeishEXO-mediated response.
ANXA1’s involvement in the adaptive immune response remains poorly described, with conflicting studies indicating that it can mediate both pro-inflammatory and anti-inflammatory responses (37–39). Also known as lipocortin, ANXA1 is constitutively produced by T cells (40, 41)and has been implicated in lymphocyte proliferation, differentiation, and activation (37–39). When ascertaining whether ANXA1 deficiency hindered the development of a Th1 response in a Th1-biased L. major murine infection model, we observed limited to no effect on the development of effector T cell subsets, including Th1 (IFNγ+ CD4+), Th2 (IL-4+ CD4+), Th17 (Il-17A+ CD4+), or regulatory (Foxp3+CD4+) T cells. Whereas some previous studies indicate that ANXA1 deficiency favors the development of Th2 T cells in a murine model of KLH-stimulation (42), others have observed an increase in Th1-associated inflammatory mediators of allergic inflammation (37, 43), suggesting that this process is antigen-specific. Further, most of these studies utilize autoimmune and allergy models (38) which cannot properly recapitulate the immunopathogenesis of infectious agents. While ANXA1-deficiency has been associated with higher T cell proliferation in vitro (42), we found similar expression levels of the proliferative marker Ki67 in populations derived from both wildtype and knockout mice. In addition, no variation in total lymphocyte counts was observed in the knockout model comparatively to its wildtype counterpart, indicating that ANXA1-/- does not inhibit T cell homing to the lymph node. However, functional studies of these subsets may be of interest, given evidence that ANXA1-deficient T cells exhibit impaired responses to TCR stimulation (42), along with transcriptomic studies, to conclusively determine that these knockout and wildtype lymphocyte populations are identical. Further, while B cells express particularly low levels of ANXA1/FPR2 (37) and their role in leishmaniasis is limited (44), immunophenotyping these populations may uncover differences in the adaptive immune response.
Variations were noted, however, when assessing the frequency and MFI of IFNγ-producing CD8+ T cells, which contribute to the generation of the Th1 response and are particularly important in the resistance against primary and secondary L. major infection (28). Higher frequencies of these cells in wildtype mice indicate a potential immune deficiency caused by the absence of ANXA1. Further, while infected footpads from both wildtype and ANXA1-/- mice displayed similar T cell populations, larger populations of Cd11b+ myeloid cells were identified in wildtype mice, suggesting innate immune involvement. Thus, given limited variations in adaptive immune profiles between wildtype and ANXA1-deficient mice, we determined that the innate immune response was likely responsible for the important differences observed in LeishEXO-mediated lesion pathology.
The role of ANXA1 is much better described in innate immune cascades, whereby its interaction with FPR2 leads to the recruitment of and differentiation of monocytes, and neutrophil apoptosis (39) – inflammatory events that recapitulate the “Trojan Horse” immunopathogenic mechanism of early Leishmania infection (2). To evaluate whether ANXA1-/- mice therefore exhibited variations in myeloid cell population recruitment compared to wildtype mice, we utilized an acute peritoneal L. major infection model. While infection and recruitment of entire myeloid cell populations in the footpad would be a particularly interesting way to study this, infiltrates at early time points post-infection are notoriously difficult to isolate due to limited accessibility of small cell populations during the retrieval process (45). In fact, very few studies assess innate inflammatory infiltrates in murine footpads and utilize histological sectioning and staining of tissues, which would be of limited use when studying small intracellular pathogens (46). Thus, the acute peritoneal infection model was chosen, given its established use to study the rapid onset of infection and the innate immune response (30) – which would not be as evident in a footpad model – allowing us to decipher early events in myeloid cell recruitment more effectively. Further, peritoneal macrophages display significantly less heterogeneity than tissue-specific populations throughout the footpad (45, 47), facilitating subsequent experimental steps. Interestingly, our data demonstrated that the total number of cells, including macrophages and neutrophils, as well macrophage to neutrophil recruitment ratios, were not significantly affected by the absence of ANXA1 regardless of LeishEXO stimulation – though further phenotypic studies of recruited cells could illuminate phenotypic differences between subpopulations. Given the ANXA1/FPR axis is one of many interactions involved in the recruitment of myeloid cells, many of which are directly upregulated by Leishmania, the injection of parasites into the peritoneal cavity likely stimulates multiple cascades at once, overshadowing any ANXA1-specific effect. Further characterization of the peritoneal microenvironment using a cytokine and chemokine multiplex assay of the lavage fluid indicated variations in few inflammatory mediators. Of these, levels of IL-6 and CXCL10 increased in both wildtype and knockout mice in response to LeishEXO co-inoculation, implicating Leishmania exosomes in inflammatory and lymphocyte recruitment processes, though these were not reflected in T cell populations. Similarly, despite consistent Treg populations, levels of the chemoattractant CCL22 were notably higher in ANXA1-/- mice regardless of the addition of LeishEXO. Protein expression of eotaxin, G-CSF, and CCL3 displayed an additive effect of ANXA1-/– and LeishEXO-dependent increase, though innate immune cell populations were similarly unaffected. Further, the expression of key cytokines involved in leishmaniasis-mediated inflammation, including IL-1β, IL-10, IL-12 and TNF-α (48), remained constant across all experimental groups. Together, these findings suggest that the addition of LeishEXO to L. major inoculum does not significantly alter myeloid cell recruitment or the production of inflammatory mediators during acute L. major infection, independently of ANXA1. Given the lack of explicit modulation of the adaptive or innate immune responses by LeishEXO in wildtype or ANXA1-/- mice, we next studied host cells.
Analysis of the infection rate of myeloid cells derived from wildtype or ANXA1-deficient mice by L. major indicated a significant LeishEXO-dependent increase in infectivity. The severity of cutaneous leishmaniasis has been shown to be a direct function of the number of internalized parasites during initial infection (49), with higher infection rates persisting over the course of disease. Previous work by our lab has shown that exosomes produced by Leishmania are co-egested along with the parasite into a mammalian host during the sandfly vector’s bloodmeal (10). Given that uptake of leishmanial exosomes is extremely rapid (50, 51), and that the half-life of EVs in circulation is in the order of 2–30 minutes (52), LeishEXO-mediated effects must occur immediately following inoculation and cause permanent changes, enabling persistence of the hyperinfective phenotype long after exosomes have degraded. Such would be the case if LeishEXO increased the number of myeloid cells infected at early timepoints post-infection, causing cutaneous pathology to be exacerbated accordingly and maintained over time, without necessarily altering the fate of the adaptive response in the later stages of the infection.
In addition, our data indicates that the LeishEXO-mediated increase in infectivity is dependent on the presence of ANXA1, therefore implicating the ANXA1/FPR axis in this process. ANXA1 is known to bind to and activate both FPR1 and FPR2, leading to various immune cascades which result in either pro-inflammatory or pro-resolving effects depending on ligands and context. Specifically, ANXA1/FPR1 interactions generally mediate wound closure, particularly in the context of bacterial infections (53, 54). Conversely, interaction with the FPR2 receptor is thought to regulate multiple inflammatory, anti-inflammatory, and pro-resolving effects, and exhibits greater sensitivity and responsiveness to ANXA1 (53, 55). Additionally, while less is known about FPR3, it is also a receptor for ANXA1, albeit with significantly less affinity, and may play a role in modulating immune responses (37). Of the FPRs, the interaction of ANXA1 with FPR2 is more extensively studied and documented in the context of inflammation and immune responses (56). Given that ANXA1 deficiency does not prohibit the generation of a cellular TH1 response to experimental L. major infection, the ANXA1/FPR1 interaction is likely not essential in our model, indicating that the ANXA1/FPR2 axis may be implicated in this immunopathogenic process.
Pre-treatment of wildtype C57BL/6 mice with the FPR2 antagonist WRW4 was capable of inhibiting LeishEXO-mediated immunopathogenesis, decreasing footpad swelling as well as parasitemia. In vitro work corroborated these findings, indicating that FPR2 blockade inhibits LeishEXO-mediated enhancement of macrophage infections, exhibiting similar infection rates to cells incubated with L. major alone. This is of notable interest, as while it has been put forward as a potential target for influenza infection due to its role in virion internalization and persistence (32, 57), this is the first report of a protozoan parasite exploiting the ANXA1/FPR2 axis to establish greater infection. Additional evidence indicates that the ANXA1/FPR2 interaction promotes endosomal transport (58) – a finding that is particularly interesting given Leishmania’s exploitation of endosomes to form parasitophorous vacuoles within host cells. Further, it is tempting to speculate that the therapeutic success FPR2 antagonists have displayed against influenza viruses in preclinical trials could be leveraged in the fight against leishmaniasis (32), although additional pre-clinical and clinical studies are required to assess their efficacy. Additional challenges arise, however, due to heterogenous immunopathogenesis mechanisms of different species of Leishmania, as while the antagonist may be beneficial to L. major infection, ANXA1 is associated with disease resolution in cutaneous L. (Viannia) braziliensis infection (18). Thus, species identification would be required prior to treatment, reducing its potential use in resource-limited settings. Further, the antagonist is not entirely specific to FPR2, exhibiting low affinity for other FPRs (59); thus, the potential influence of FPR1 and FPR3 cannot be entirely ruled out.
Different species of Leishmania employ diverse strategies to evade the immune response and establish infection. This can be directly observed through the action of their exosomes, whereby species-specific cargo of proteins, lipids, and nucleic acids may exert distinct effects on the immune system. For instance, while L. major exosomes have been shown to induce a pro-inflammatory response in macrophages, exosomes from L. (V.) braziliensis have been implicated in promoting an immunosuppressive environment, favoring parasite survival and persistence (11). These differences may explain divergent roles for ANXA1 in the immunopathogenesis of different Leishmania species. Thus, while the ANXA1/FPR axis, mediated by leishmanial exosomes, may be involved in greater parasite internalization in the context of L. major infection, future studies should assess whether these mechanisms are shared by other Leishmania species.
Experiments using the FPR agonist WKYMVm were used to assess whether direct activation of FPRs could bypass the effects of ANXA1 deficiency and replicate the phenotype generated by co-inoculation of LeishEXO in wildtype animals. Strikingly, the addition of WKYMVm to the L. major inoculum induced footpad inflammation in ANXA1-/-, very closely mirroring that of L. major/LeishEXO in their wildtype counterparts, indicating that direct stimulation of FPRs induces a LeishEXO-like phenotype. In vitro experiments further corroborated that the agonist was capable of partially rescuing the expected infectivity, although not to levels observed in wildtype animals. Although we were particularly interested in the effects of FPR2 stimulation, WKYMVm acts as a non-specific agonist for FPRs 1, 2, and 3 (59). While our study primarily attributes the observed effects to the interaction between ANXA1 and FPR2, we cannot exclude the possibility that WKYMV may also be acting through these receptors. Further studies using more selective agonists and antagonists for each FPR subtype are needed to delineate the specific contributions of FPR1, FPR2, and FPR3 in the context of LeishEXO’s effects on L. major infection. Of additional interest is the observation that, while the number of infected cells increases with LeishEXO/FPR stimulation, the number of parasites within infected myeloid cells is similar between experimental groups, indicating that the primary goal of this LeishEXO-mediated process is to enhance cell entry rather than increase parasite burden. The exposure of host cells to LeishEXO could trigger cellular responses that facilitate the entry of parasites into cells but limit subsequent parasite replication or survival within the cell, resulting in single-cell infection without increasing the overall parasite burden per cell. Additional studies surrounding the dynamics of parasite internalization will be essential to elucidating this process.
Altogether, these data implicate the ANXA1/FPR axis as a potential contributor to LeishEXO-mediated immunopathogenesis during L. major infection. Specifically, ANXA1 interacts with FPRs to enhance the recruitment and infection of myeloid cells, leading to increased early infection rates that persist over time and severe cutaneous lesions. Indeed, despite higher infectivity rates, variations in cytokine and chemokine levels suggest that the primary role of ANXA1/FPR in LeishEXO-mediated processes is to enhance cell entry rather than significantly alter the overall inflammatory profile. Given that FPR2 stimulation overlaps with downstream effectors of the TH2 response, the ANXA1/FPR2 interaction may promote persistence and chronicity following parasite uptake (56). Additional parasite-derived components are necessary, however, to the development of this phenotype, including the packaging of sufficient levels of the surface metalloprotease GP63 within the EVs, enabling downstream modulation of host cell signaling pathways, including the activation of SHP-1 and dephosphorylation of ERK1/2 (11, 33). Along with the modulation of cytoskeletal organization and of the phagocytic capacity of myeloid cells upon FPR2 activation, the interaction between host and parasite factors may explain, in part, the increase in the initial infection of host cells by Leishmania. Further, while the exact mechanisms by which ANXA1 interacts with FPRs are still under investigation, studies suggest that the binding of ANXA1 to lipids, particularly phospholipids, may influence its interaction with FPR2 indirectly (60). This may provide the missing link between Leishmania exosomes, which have an outer layer constituted primarily of lipids and phospholipids (33), and the ANXA1/FPR axis.
Moreover, we hypothesize that the parasitic virulence factor GP63, packaged within leishmanial exosomes, may directly contribute to LeishEXO-mediated stimulation of the ANXA1/FPR axis. As a non-specific metalloprotease, GP63 may cleave mammalian ANXA1, thereby enabling its interaction with the FPRs on monocytes, enhancing their recruitment and infection by the parasite (61). Given that it is primarily the cleaved form of ANXA1 that interacts with the receptor (37–39), this may modulate host signaling pathways, leading to greater parasite internalization and contributing to the hyperinfective phenotype and enhanced lesion severity, although additional studies are required to investigate this potential mechanism. This further implicates FRP2 specifically, as it is displays the greatest sensitivity to cleaved ANXA1, and is a promiscuous receptor capable of interacting with a variety of endogenous and exogenous ligands, such as exosome-derived lipids (62).
In summary, this study elucidates the role of the ANXA1/FPR axis in the exacerbation of cutaneous leishmaniasis mediated by leishmanial exosomes, whereby initial infection is enhanced and maintained over the course of infection, resulting in increased severity of disease. Through a comprehensive series of experiments utilizing ANXA1-deficient mice, as well as agonists and antagonists of FPRs, we delineated a potential mechanism underlying this phenomenon, and have provided novel insights into the involvement of ANXA1 in the immunopathogenesis of leishmaniasis, which may be leveraged in the development of therapeutics for leishmaniasis.
Data availability statement
The original contributions presented in the study are included in the article/Supplementary materials. Further inquiries can be directed to the corresponding author.
Ethics statement
The animal study was approved by McGill University Animal Care Committee (UACC) under ethics protocol numbers 7791 and 4859. The study was conducted in accordance with the local legislation and institutional requirements.
Author contributions
AdSLF: Conceptualization, Data curation, Formal analysis, Investigation, Methodology, Visualization, Writing – original draft, Writing – review & editing. AL: Investigation, Validation, Visualization, Writing – original draft, Writing – review & editing. FA: Formal analysis, Investigation, Validation, Visualization, Writing – review & editing. CP: Funding acquisition, Resources, Supervision, Writing – review & editing. MO: Conceptualization, Data curation, Funding acquisition, Project administration, Resources, Supervision, Writing – review & editing.
Funding
The author(s) declare financial support was received for the research, authorship, and/or publication of this article. This work was supported by grants from the Canadian Institutes of Health Research (CIHR) awarded to MO (Grants PJT-159765 and ER1-143489) and to CP (Grant PJT-148821, PJT-191999 and GA1-177693). AL is supported by a graduate fellowship from the Fonds de Recherches du Québec en Santé (FRQS) and AS is a recipient of a Brazilian CNPq Science without Borders studentship.
Acknowledgments
We thank the McGill Facility for Electron Microscopy Research (FEMR), the Immunophenotyping Platform and the Animal Facilities at the Research Institute of the McGill University Health Centre (RI-MUHC). Special thanks are due to Dr. Maziar Divangahi for providing ANXA1-deficient mice.
Conflict of interest
The authors declare that the research was conducted in the absence of any commercial or financial relationships that could be construed as a potential conflict of interest.
The author(s) declared that they were an editorial board member of Frontiers, at the time of submission. This had no impact on the peer review process and the final decision.
Publisher’s note
All claims expressed in this article are solely those of the authors and do not necessarily represent those of their affiliated organizations, or those of the publisher, the editors and the reviewers. Any product that may be evaluated in this article, or claim that may be made by its manufacturer, is not guaranteed or endorsed by the publisher.
Supplementary material
The Supplementary Material for this article can be found online at: https://www.frontiersin.org/articles/10.3389/fimmu.2024.1436151/full#supplementary-material
References
2. Laskay T, van Zandbergen G, Solbach W. Neutrophil granulocytes–Trojan horses for Leishmania major and other intracellular microbes? Trends Microbiol. (2003) 11:210–4. doi: 10.1016/s0966-842x(03)00075-1
3. Hassani K, Antoniak E, Jardim A, Olivier M. Temperature-induced protein secretion by leishmania mexicana modulates macrophage signalling and function. PloS One. (2011) 6:e18724. doi: 10.1371/journal.pone.0018724
4. Olivier M, Atayde VD, Isnard A, Hassani K, Shio MT. Leishmania virulence factors: focus on the metalloprotease GP63. Microbes Infect. (2012) 14:1377–89. doi: 10.1016/j.micinf.2012.05.014
5. Skotland T, Hessvik NP, Sandvig K, Llorente A. Exosomal lipid composition and the role of ether lipids and phosphoinositides in exosome biology. J Lipid Res. (2019) 60:9–18. doi: 10.1194/jlr.R084343
6. Puhka M, Takatalo M, Nordberg M-E, Valkonen S, Nandania J, Aatonen M, et al. Metabolomic profiling of extracellular vesicles and alternative normalization methods reveal enriched metabolites and strategies to study prostate cancer-related changes. Theranostics. 7. (2017) 7(16):3824–41. doi: 10.7150/thno.19890
7. Schey KL, Luther JM, Rose KL. Proteomics characterization of exosome cargo. Methods San Diego Calif. (2015) 87:75–82. doi: 10.1016/j.ymeth.2015.03.018
8. van den Boorn JG, Dassler J, Coch C, Schlee M, Hartmann G. Exosomes as nucleic acid nanocarriers. Adv Drug Deliv. Rev. (2013) 65:331–5. doi: 10.1016/j.addr.2012.06.011
9. Tai Y-L, Chen K-C, Hsieh J-T, Shen T-L. Exosomes in cancer development and clinical applications. Cancer Sci. (2018) 109:2364–74. doi: 10.1111/cas.13697
10. Atayde VD, Suau HA, Townsend S, Hassani K, Kamhawi S, Olivier M. Exosome secretion by the parasitic protozoan Leishmania within the sand fly midgut. Cell Rep. (2015) 13:957–67. doi: 10.1016/j.celrep.2015.09.058
11. Atayde VD, Hassani K, da Silva Lira Filho A, Borges AR, Adhikari A, Martel C, et al. Leishmania exosomes and other virulence factors: Impact on innate immune response and macrophage functions. Cell Immunol. (2016) 309:7–18. doi: 10.1016/j.cellimm.2016.07.013
12. Hassani K, Olivier M. Immunomodulatory impact of leishmania-induced macrophage exosomes: A comparative proteomic and functional analysis. PloS Negl Trop Dis. (2013) 7:e2185. doi: 10.1371/journal.pntd.0002185
13. Li Q, Liu W, Wang Z, Wang C, Ai Z. Exosomal ANXA1 derived from thyroid cancer cells is associated with Malignant transformation of human thyroid follicular epithelial cells by promoting cell proliferation. Int J Oncol. (2021) 59104. doi: 10.3892/ijo.2021.5284
14. Rogers MA, Buffolo F, Schlotter F, Atkins SK, Lee LH, Halu A, et al. Annexin A1–dependent tethering promotes extracellular vesicle aggregation revealed with single–extracellular vesicle analysis. Sci Adv. (2020) 6:eabb1244. doi: 10.1126/sciadv.abb1244
15. Pessolano E, Belvedere R, Bizzarro V, Franco P, Marco ID, Petrella F, et al. Annexin A1 contained in extracellular vesicles promotes the activation of keratinocytes by mesoglycan effects: an autocrine loop through FPRs. Cells. (2019) 8(7):753. doi: 10.3390/cells8070753
16. Tzelepis F, Verway M, Daoud J, Gillard J, Hassani-Ardakani K, Dunn J, et al. Annexin1 regulates DC efferocytosis and cross-presentation during Mycobacterium tuberculosis infection. J Clin Invest. (2015) 125:752–68. doi: 10.1172/JCI77014
17. Arora S, Lim W, Bist P, Perumalsamy R, Lukman HM, Li F, et al. Influenza A virus enhances its propagation through the modulation of Annexin-A1 dependent endosomal trafficking and apoptosis. Cell Death Differ. (2016) 23:1243–56. doi: 10.1038/cdd.2016.19
18. Oliveira LG, Souza-Testasicca MC, Vago JP, Figueiredo AB, Canavaci AMC, Perucci LO, et al. Annexin A1 Is Involved in the Resolution of Inflammatory Responses during Leishmania Braziliensis Infection. J Immunol Baltim. Md 1950. (2017) 198:3227–36. doi: 10.4049/jimmunol.1602028
19. Silva HALda, Lima GSde, Boité MC, Porrozzi R, Hueb M, Damazo AS. Expression of annexin A1 in Leishmania-infected skin and its correlation with histopathological features. Rev Soc Bras Med Trop. (2015) 48:560–7. doi: 10.1590/0037-8682-0183-2015
20. Vucetic A, Filho ADSL, Dong G, Olivier M. Isolation of extracellular vesicles from leishmania spp. In: Michels PAM, Ginger ML, Zilberstein D, editors. Trypanosomatids: methods and protocols methods in molecular biology. New York, USA: Springer US (2020). p. 555–74. doi: 10.1007/978-1-0716-0294-2_33
21. Reiner SL, Locksley RM. The regulation of immunity to leishmania major. Annu Rev Immunol. (1995) 13:151–77. doi: 10.1146/annurev.iy.13.040195.001055
22. Sacks D, Noben-Trauth N. The immunology of susceptibility and resistance to Leishmania major in mice. Nat Rev Immunol. (2002) 2:845–58. doi: 10.1038/nri933
23. Douanne N, Dong G, Amin A, Bernardo L, Blanchette M, Langlais D, et al. Leishmania parasites exchange drug-resistance genes through extracellular vesicles. Cell Rep. (2022) 40:111121. doi: 10.1016/j.celrep.2022.111121
24. Rogers KA, DeKrey GK, Mbow ML, Gillespie RD, Brodskyn CI, Titus RG. Type 1 and type 2 responses to Leishmania major. FEMS Microbiol Lett. (2002) 209:1–7. doi: 10.1111/fml.2002.209.issue-1
25. Alexander J, Brombacher F. T helper1/T helper2 cells and resistance/susceptibility to leishmania infection: is this paradigm still relevant? Front Immunol. (2012) 3. doi: 10.3389/fimmu.2012.00080
26. Poudel B, Yorek MS, Mazgaeen L, Brown SA, Kanneganti T-D, Gurung P. Acute IL-4 Governs Pathogenic T Cell Responses during Leishmania major Infection. ImmunoHorizons. (2020) 4:546–60. doi: 10.4049/immunohorizons.2000076
27. Poulter LW, Pandolph CR. Mechanisms of immunity to leishmaniasis. IV. Significance of lymphatic drainage from the site of infection. Clin Exp Immunol. (1982) 48:396.
28. Stäger S, Rafati S. CD8+ T cells in leishmania infections: friends or foes? Front Immunol. (2012) 3:5. doi: 10.3389/fimmu.2012.00005
29. D’Acquisto F, Merghani A, Lecona E, Rosignoli G, Raza K, Buckley CD, et al. Annexin-1 modulates T-cell activation and differentiation. Blood. (2007) 109:1095–102. doi: 10.1182/blood-2006-05-022798
30. Chan A, Ayala J-M, Alvarez F, Piccirillo C, Dong G, Langlais D, et al. The role of Leishmania GP63 in the modulation of innate inflammatory response to Leishmania major infection. PloS One. (2021) 16:e0262158. doi: 10.1371/journal.pone.0262158
31. Mesquita TGR, de Junior J, Silva do ES, da Silva GAV, de Araújo FJ, Pinheiro SK, et al. Distinct plasma chemokines and cytokines signatures in Leishmania guyanensis-infected patients with cutaneous leishmaniasis. Front Immunol. (2022) 13. doi: 10.3389/fimmu.2022.974051
32. Alessi M-C, Cenac N, Si-Tahar M, Riteau B. FPR2: A novel promising target for the treatment of influenza. Front Microbiol. (2017) 8. doi: 10.3389/fmicb.2017.01719
33. da Silva Lira Filho A, Fajardo EF, Chang KP, Clément P, Olivier M. Leishmania exosomes/extracellular vesicles containing GP63 are essential for enhance cutaneous leishmaniasis development upon co-inoculation of leishmania amazonensis and its exosomes. Front Cell Infect Microbiol. (2022) 11. doi: 10.3389/fcimb.2021.709258
34. Cirino G, Flower RJ, Browning JL, Sinclair LK, Pepinsky RB. Recombinant human lipocortin 1 inhibits thromboxane release from Guinea-pig isolated perfused lung. Nature. (1987) 328:270–2. doi: 10.1038/328270a0
35. Cloix JF, Colard O, Rothhut B, Russo-Marie F. Characterization and partial purification of “renocortins”: two polypeptides formed in renal cells causing the anti-phospholipase-like action of glucocorticoids. Br J Pharmacol. (1983) 79:313–21. doi: 10.1111/j.1476-5381.1983.tb10526.x
36. Alldridge LC, Harris HJ, Plevin R, Hannon R, Bryant CE. The annexin protein lipocortin 1 regulates the MAPK/ERK pathway *. J Biol Chem. (1999) 274:37620–8. doi: 10.1074/jbc.274.53.37620
37. Gavins FN, Hickey MJ. Annexin A1 and the regulation of innate and adaptive immunity. Front Immunol. (2012) 3. doi: 10.3389/fimmu.2012.00354
38. D’Acquisto F. On the adaptive nature of Annexin-A1. Curr Opin Pharmacol. (2009) 9:521–8. doi: 10.1016/j.coph.2009.04.007
39. Kelly L, McGrath S, Rodgers L, McCall K, Tulunay Virlan A, Dempsey F, et al. Annexin-A1: The culprit or the solution? Immunology. (2022) 166:2–16. doi: 10.1111/imm.13455
40. Spurr L, Nadkarni S, Pederzoli-Ribeil M, Goulding NJ, Perretti M, D’Acquisto F. Comparative analysis of Annexin A1-formyl peptide receptor 2/ALX expression in human leukocyte subsets. Int Immunopharmacol. (2011) 11:55–66. doi: 10.1016/j.intimp.2010.10.006
41. Morand EF, Hutchinson P, Hargreaves A, Goulding NJ, Boyce NW, Holdsworth SR. Detection of intracellular lipocortin 1 in human leukocyte subsets. Clin Immunol Immunopathol. (1995) 76:195–202. doi: 10.1006/clin.1995.1115
42. D’Acquisto F, Paschalidis N, Sampaio ALF, Merghani A, Flower RJ, Perretti M. Impaired T cell activation and increased Th2 lineage commitment in Annexin-1-deficient T cells. Eur J Immunol. (2007) 37:3131–42. doi: 10.1002/eji.200636792
43. Ng FSP, Wong KY, Guan SP, Mustafa FB, Kajiji TS, Bist P, et al. Annexin-1-deficient mice exhibit spontaneous airway hyperresponsiveness and exacerbated allergen-specific antibody responses in a mouse model of asthma. Clin Exp Allergy. (2011) 41:1793–803. doi: 10.1111/j.1365-2222.2011.03855.x
44. Scott P, Novais FO. Cutaneous leishmaniasis: immune responses in protection and pathogenesis. Nat Rev Immunol. (2016) 16:581–92. doi: 10.1038/nri.2016.72
45. Willenborg S, Roscito JG, Gerbaulet A, Roers A, Dahl A, Eming SA, et al. Isolation of macrophages from mouse skin wounds for single-cell RNA sequencing. STAR Protoc. (2022) 3:101337. doi: 10.1016/j.xpro.2022.101337
46. Schönlau F, Schlesiger C, Ehrehen J, Grabbe S, Sorg C, Sunderkötter C. Monocyte and macrophage functions in M-CSF-deficient op/op mice during experimental leishmaniasis. J Leukoc. Biol. (2003) 73:564–73. doi: 10.1189/jlb.12011003
47. Cassado A, dos A, D’Império Lima MR, Bortoluci KR. Revisiting mouse peritoneal macrophages: heterogeneity, development, and function. Front Immunol. (2015) 6. doi: 10.3389/fimmu.2015.00225
48. Scott P, Natovitz P, Coffman RL, Pearce E, Sher A. Immunoregulation of cutaneous leishmaniasis. T cell lines that transfer protective immunity or exacerbation belong to different T helper subsets and respond to distinct parasite antigens. J Exp Med. (1988) 168:1675–84. doi: 10.1084/jem.168.5.1675
49. Giraud E, Martin O, Yakob L, Rogers M. Quantifying leishmania metacyclic promastigotes from individual sandfly bites reveals the efficiency of vector transmission. Commun Biol. (2019) 2:1–12. doi: 10.1038/s42003-019-0323-8
50. Silverman JM, Reiner NE. Leishmania exosomes deliver preemptive strikes to create an environment permissive for early infection. Front Cell Infect Microbiol. (2012) 1:26. doi: 10.3389/fcimb.2011.00026
51. Silverman JM, Clos J, de’Oliveira CC, Shirvani O, Fang Y, Wang C, et al. An exosome-based secretion pathway is responsible for protein export from Leishmania and communication with macrophages. J Cell Sci. (2010) 123:842–52. doi: 10.1242/jcs.056465
52. Parada N, Romero-Trujillo A, Georges N, Alcayaga-Miranda F. Camouflage strategies for therapeutic exosomes evasion from phagocytosis. J Adv Res. (2021) 31:61–74. doi: 10.1016/j.jare.2021.01.001
53. Lee C, Han J, Jung Y. Formyl peptide receptor 2 is an emerging modulator of inflammation in the liver. Exp Mol Med. (2023) 55:325–32. doi: 10.1038/s12276-023-00941-1
54. de Paula-Silva M, da Rocha GHO, Broering MF, Queiroz ML, Sandri S, Loiola RA, et al. Formyl peptide receptors and annexin A1: complementary mechanisms to infliximab in murine experimental colitis and crohn’s disease. Front Immunol. (2021) 12:714138. doi: 10.3389/fimmu.2021.714138
55. Perretti M, Chiang N, La M, Fierro IM, Marullo S, Getting SJ, et al. Endogenous lipid- and peptide-derived anti-inflammatory pathways generated with glucocorticoid and aspirin treatment activate the lipoxin A4 receptor. Nat Med. (2002) 8:1296–302. doi: 10.1038/nm786
56. Bena S, Brancaleone V, Wang JM, Perretti M, Flower RJ. Annexin A1 interaction with the FPR2/ALX receptor. J Biol Chem. (2012) 287:24690–7. doi: 10.1074/jbc.M112.377101
57. Park EJ, Prajuabjinda O, Soe ZY, Darkwah S, Appiah MG, Kawamoto E, et al. Exosomal regulation of lymphocyte homing to the gut. Blood Adv. (2019) 3:1–11. doi: 10.1182/bloodadvances.2018024877
58. Rahman F, Chebbo M, Courtin N, Fotso Fotso A, Alessi M-C, Riteau B. The annexin A1 receptor FPR2 regulates the endosomal export of influenza virus. Int J Mol Sci. (2018) 19:1400. doi: 10.3390/ijms19051400
59. Lice I, Sanches JM, Correia-Silva RD, Corrêa MP, Icimoto MY, Silva AAR, et al. Effects of formyl peptide receptor agonists ac9-12 and WKYMV in in vivo and in vitro acute inflammatory experimental models. Cells. (2022) 11:228. doi: 10.3390/cells11020228
60. Shao G, Zhou H, Zhang Q, Jin Y, Fu C. Advancements of Annexin A1 in inflammation and tumorigenesis. OncoTargets. (2019) 12:3245–54. doi: 10.2147/OTT.S202271
61. Gomez MA, Contreras I, Hallé M, Tremblay ML, McMaster RW, Olivier M. Leishmania GP63 alters host signaling through cleavage-activated protein tyrosine phosphatases. Sci Signal. (2009) 2:ra58. doi: 10.1126/scisignal.2000213
Keywords: Leishmania, cutaneous leishmaniasis, extracellular vesicles, exosomes, Annexin A1, FPR2
Citation: da Silva Lira Filho A, Lafleur A, Alvarez F, Piccirillo CA and Olivier M (2024) Implication of the Annexin 1/FPR axis in leishmanial exosome-mediated Leishmania major skin hyperpathogenesis. Front. Immunol. 15:1436151. doi: 10.3389/fimmu.2024.1436151
Received: 21 May 2024; Accepted: 01 July 2024;
Published: 15 July 2024.
Edited by:
Diana Bahia, Federal University of Minas Gerais, BrazilReviewed by:
Claudio Vieira Da Silva, Federal University of Uberlandia, BrazilPeter Epeh Kima, University of Florida, United States
Lucas P. Carvalho, Gonçalo Moniz Institute (IGM), Brazil
Copyright © 2024 da Silva Lira Filho, Lafleur, Alvarez, Piccirillo and Olivier. This is an open-access article distributed under the terms of the Creative Commons Attribution License (CC BY). The use, distribution or reproduction in other forums is permitted, provided the original author(s) and the copyright owner(s) are credited and that the original publication in this journal is cited, in accordance with accepted academic practice. No use, distribution or reproduction is permitted which does not comply with these terms.
*Correspondence: Martin Olivier, bWFydGluLm9saXZpZXJAbWNnaWxsLmNh