- Department of Zoology, Faculty of Life Sciences, University of Okara, Okara, Pakistan
The Dengue virus (DENV), primarily spread by Aedes aegypti and also by Aedes albopictus in some regions, poses significant global health risks. Alternative techniques are urgently needed because the current control mechanisms are insufficient to reduce the transmission of DENV. Introducing Wolbachia pipientis into Ae. aegypti inhibits DENV transmission, however, the underlying mechanisms are still poorly understood. Innate immune effector upregulation, the regulation of autophagy, and intracellular competition between Wolbachia and DENV for lipids are among the theories for the mechanism of inhibition. Furthermore, mainly three immune pathways Toll, IMD, and JAK/STAT are involved in the host for the suppression of the virus. These pathways are activated by Wolbachia and DENV in the host and are responsible for the upregulation and downregulation of many genes in mosquitoes, which ultimately reduces the titer of the DENV in the host. The functioning of these immune pathways depends upon the Wolbachia, host, and virus interaction. Here, we summarize the current understanding of DENV recognition by the Ae. aegypti’s immune system, aiming to create a comprehensive picture of our knowledge. Additionally, we investigated how Wolbachia regulates the activation of multiple genes associated with immune priming for the reduction of DENV.
1 Introduction
Arboviruses are mainly transmitted by blood-feeding arthropods like Aedes mosquitoes. Predominantly transmitted by female Aedes aegypti, these viruses encode RNA genomes including dengue (DENV), Zika (ZIKV), chikungunya (CHIKV), yellow fever (YFV), and Ross River (RRV) viruses, etc. (1). Aedes-borne viruses are potentially deadly; they cause at least 40,000 deaths, each year (2). One of these viruses, DENV is endemic in over 141 countries, affects 390 million people, and claims 36,000 lives annually (3). As of right now, proper treatments for these viral diseases are unavailable. To combat this, different strategies focus on hosts, host-vector interaction, and the vectors themselves. From all these, vector control is a primary approach that involves chemical, environmental, and biological methods. Notably, one novel biological method is Wolbachia-based control, which may involve replacing wild-type mosquito populations with Wolbachia-infected variants. Additionally, Wolbachia can also inhibit viral proliferation in their host’s midguts, significantly reducing their ability to transmit viruses (4, 5). In the past two decades, establishing Wolbachia-infected Ae. aegypti population resistant to DENV, and investigating transgenic drivers for population replacement have substantially progressed (6, 7).
Naturally, Wolbachia inhabits around 65% of all insect species (8) and in arthropods, this bacterium exhibits both mutualistic and parasitic interactions with its hosts (9). It provides multiple approaches to disease suppression such as by reducing vector population through incompatible males, affecting the fitness of the host, inhibiting pathogen transmission (10–13), affecting reproduction through male killing (14), feminization (15), parthenogenesis (16), and primarily, by cytoplasmic incompatibility (CI) (17, 18). In insects, CI occurs in two forms: (a) Unidirectional CI, where infected males can mate with only infected females with the same strain and cross with wild females resulting in embryo lethality and (b) Bidirectional CI, where males and females infected with different strains of Wolbachia cannot produce viable off-springs (Figure 1) (19). Wolbachia-infected females gain an evolutionary edge by mating with uninfected males, yielding viable offspring (20, 21). Manipulation of reproduction seems promising as it suggests that once a Wolbachia strain invades a target vector population through host reproductive alteration, ongoing management by health authorities might be minimized.
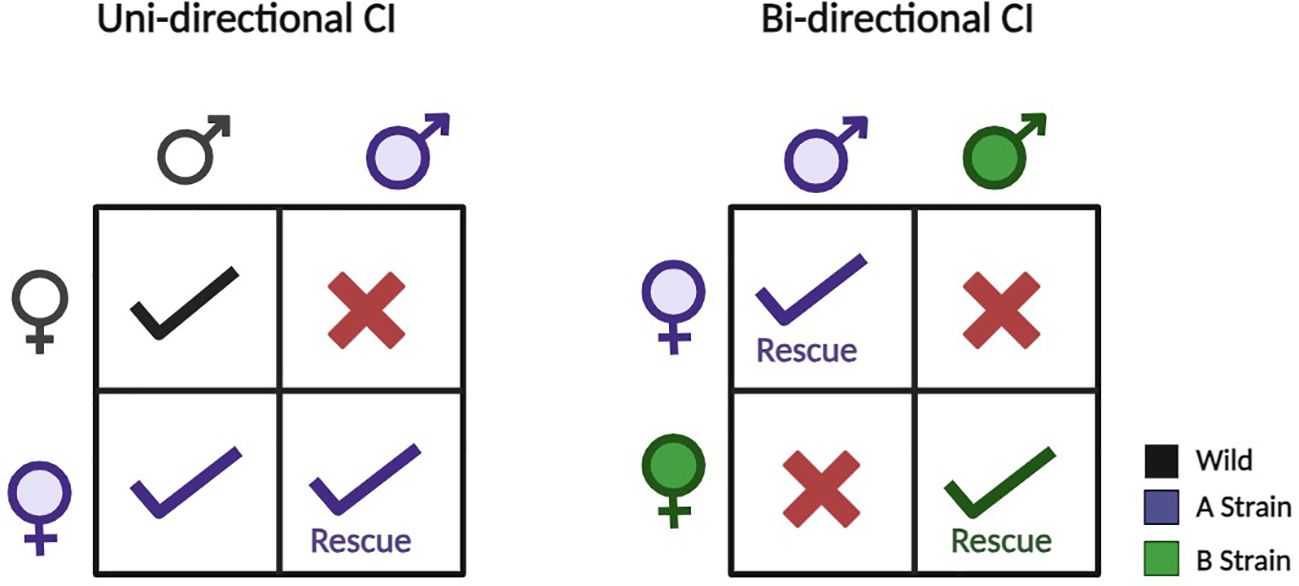
Figure 1 Uni- and bidirectional cytoplasmic incompatibility (CI) occur when crosses between infected and uninfected individuals lead to incompatible offspring. In unidirectional CI, only crosses with infected males and uninfected females are incompatible. In bidirectional CI, crosses between individuals infected with different CI-inducing strains are incompatible. Notably, unidirectional CI can sometimes occur between hosts infected with different bacterial strains. Figure created using BioRender.com.
Anti-pathogenic effects of Wolbachia have been observed by many authors when it transfected non-native hosts (10, 22, 23). Although Ae. aegypti lacks natural association with Wolbachia, however, an uninfected Ae. aegypti laboratory population can quickly become infected when Wolbachia-infected females are introduced to the population (24). Hoffmann et al. (4) artificially introduced the wMel strain of Wolbachia into Ae. aegypti mosquitoes. This strain induces CI, hampering breeding with Wolbachia-free mosquitoes. It was reported that it spreads quickly through mosquito populations, with little harm to the mosquitoes, and reaches high levels within just a few generations under semi-natural conditions. Most interestingly once a disease-blocking Wolbachia strain establishes itself in the target vector population, it can persist without further releases. It makes Wolbachia the best tool to inhibit arboviral transmission (25).
Investigating mosquito interactions with microorganisms, particularly with Wolbachia, reveals fascinating details about the defense mechanisms of the insects. Investigating mosquito interactions with microorganisms, particularly mosquitoes, like many insects, possess a vigorous innate immune system activated through pattern-recognition receptors (PRRs). In Ae. aegypti, both Toll and immune deficiency (IMD) signaling pathways induce antimicrobial peptides through transcription factors REL1 and REL2 (26). In transfected mosquito lines, the presence of Wolbachia activates the immune system, however, the precise function of these immune responses in establishing the symbiotic relationship between Wolbachia and the mosquitoes remains uncertain. Understanding how Wolbachia inhibits arboviruses is important for predicting factors that could affect both the viruses and mosquitoes. It will help to anticipate changes that might influence the effectiveness of Wolbachia-mediated inhibition. Gaining this understanding is essential to maximizing the Wolbachia-based control strategies’ durability and efficacy. This review article will go over how Wolbachia interferes with the way mosquito hosts, Ae. aegypti, interact with DENV, inhibits the entry and replication of viruses, reduces the amount of nutrients required for an arboviral infection, boosts immunity, produces reactive oxygen species (ROS), promotes cellular regeneration for a better midgut barrier, and controls genes involved in a range of cellular functions.
2 Defence systems of Ae. aegypti as a host
Pathogen-blocking mechanisms vary among host species, and a cellular process involved in pathogen blocking may not be generally applicable. It is commonly known that invertebrates, including Ae. aegypti, do not possess adaptive immunity. Mosquitoes employ defense mechanisms both within and outside their bodies to prevent pathogens and mainly rely on their innate immune system (27). It is now recognized that innate immunity in mosquitoes provides prompt defense against infections via humoral or cellular responses, which are typically brought on by the invasive microorganism. The cellular part involves special cells called hemocytes, while the humoral part includes various substances like PRR and anit-microbial peptides (AMPs). However, gene network analysis across insect species highlights strong connections between the pathways controlling the production of nutrients in the insect and the ability of viruses to replicate (28).
The genome of Ae. aegypti, known for its role as a disease vector, contains genes crucial for both viral infection and defense mechanisms. The most recent reference genome (AaegL5) reveals an expanded range of gene families, such as chemosensory receptors (related to the mosquito’s ability to sense chemicals), glutathione S-transferase (involved in detoxification processes), and C-type lectin (associated with immune responses), including specific genetic regions (chromosome 2) associated with viral susceptibility (29, 30). The presence of DENV, ZIKV, and CHIKV induces varying transcriptomic changes in Ae. aegypti (31). When these viruses infect Ae. aegypti, they trigger changes in the mosquito’s genetic activity in specific areas like cell structure, genetic processes, immune responses, stress reactions, and metabolic activities (32–34).
Wolbachia complicates the interaction between Ae. aegypti and arboviruses by disrupting the same molecular processes that are necessary for the viruses (33, 34–39). This interference causes cellular disturbances that harm the pathogen. Mosquitoes possess a natural defense mechanism against oxidative stress induced by blood meals. This defense involves activating antioxidants to protect their tissues. In DENV infection, mosquitoes produce ROS like mammalian cells but avoid the harmful effects associated with ROS accumulation (40). This unique ability is considered an evolutionary advantage, ensuring the successful transmission of the virus without compromising the mosquito’s health. DENV infection in mosquito cells (specifically C6/36 cells), causes endoplasmic reticulum (ER) stress by inducing the unfolded protein response, a cellular stress response mechanism. The chaperones GRP78/BiP and GRP94 are used as ER stress sensor genes, and their upregulation is observed against DENV in the cells of mosquitoes (41). Alterations in the mitochondrial membrane potential are linked to a noteworthy rise in GST (Glutathione S-Transferase) activity, suggesting the possibility of ER stress induction. Because mosquito cells have more GST activity, there may be less oxidative stress in the environment, which would facilitate viral propagation. Knocking down GST in DENV-infected cells elevates the concentration of superoxide dismutase, linking GST activity to oxidative stress regulation during DENV infection in mosquitoes (42, 43). GST also plays a significant role in minimizing cell death triggered by oxidative stress induced by DENV2 in mosquito cells (44). Additionally, eIF5A (an important protein involved in the complex process of protein synthesis) levels decrease during the aging of Ae. aegypti mosquitoes and its expression is upregulated in response to actively replicating DENV in the C6/36 cell line. It indicates a potential role for eIF5A in the cellular response to DENV infection (43, 45). Knowing all these cellular defense mechanisms in Ae. aegypti may help us to understand the mechanism behind Wolbachia-mediated control of DENV. Additionally, there is much evidence that the transinfection of various strains of Wolbachia into Ae. aegypti can prevent the spread of DENV (Table 1).
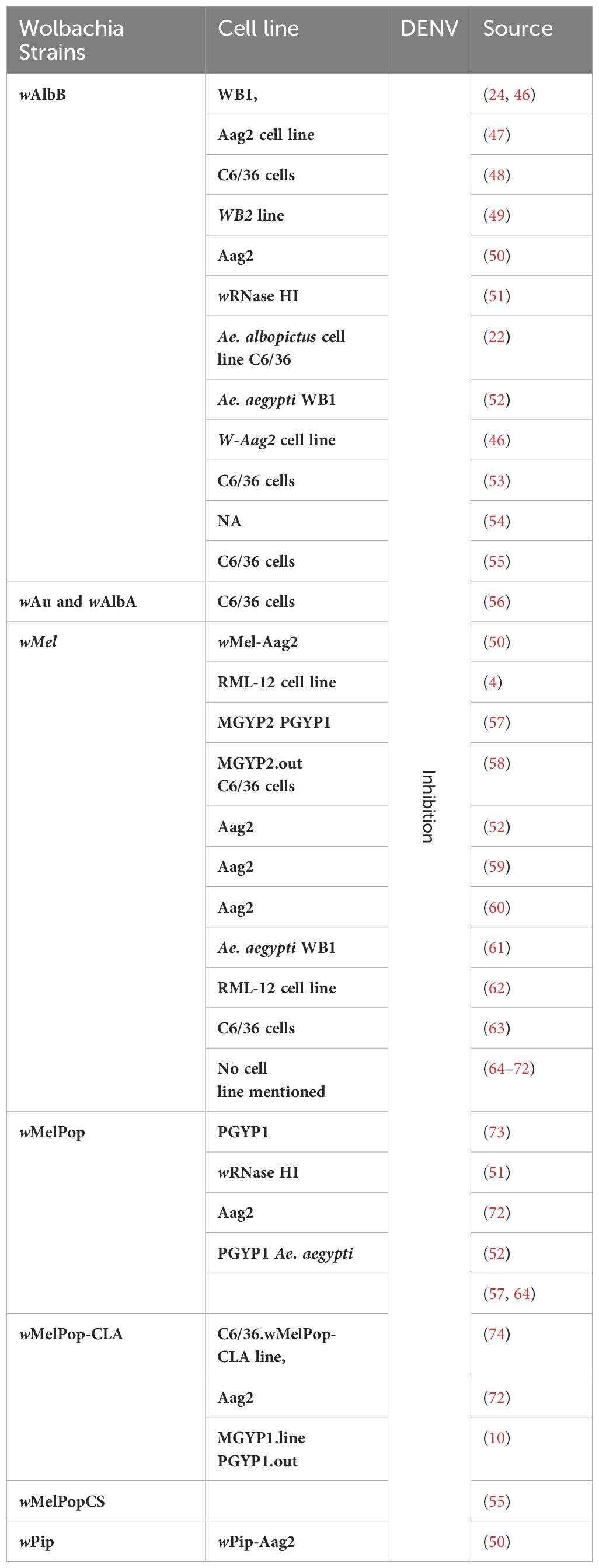
Table 1 Transaction of different Wolbachia strains into different cell lines results in DENV inhibition.
3 Wolbachia-Aedes-dengue association
The internal cellular structure of Ae. aegypti is required for arboviruses to successfully move through the stages of viral entrance, replication, assembly, and exit (75). This framework, referred to as the cytoskeleton, is made up of an actin filament and microtubule network. Arboviruses help build host cell structures for their survival, while Wolbachia does the opposite, weakening these structures to block the arboviral binding and entry (Figure 2) (9). In DENV-infected Ae. aegypti, genes for specific proteins such as dynein, vimentin, tubulin, actin, myosin, tropomyosin, and laminin are substantially expressed (76). Guo et al. (77) reported that actin and tubulin support DENV infection in vitro, while NS5 is associated with myosin in DENV infection (78). The introduction of the wAlbB strain appears to influence the cellular environment by reducing the levels of specific proteins associated with cell adhesion (dystroglycan) and cytoskeletal structure (beta-tubulin) in Ae. aegypti cells infected with DENV (79). This reveals a mechanism by which Wolbachia interferes with the virus’s development. This is a significant finding as it demonstrates how Wolbachia interferes with the early stages of arboviral infection (79).
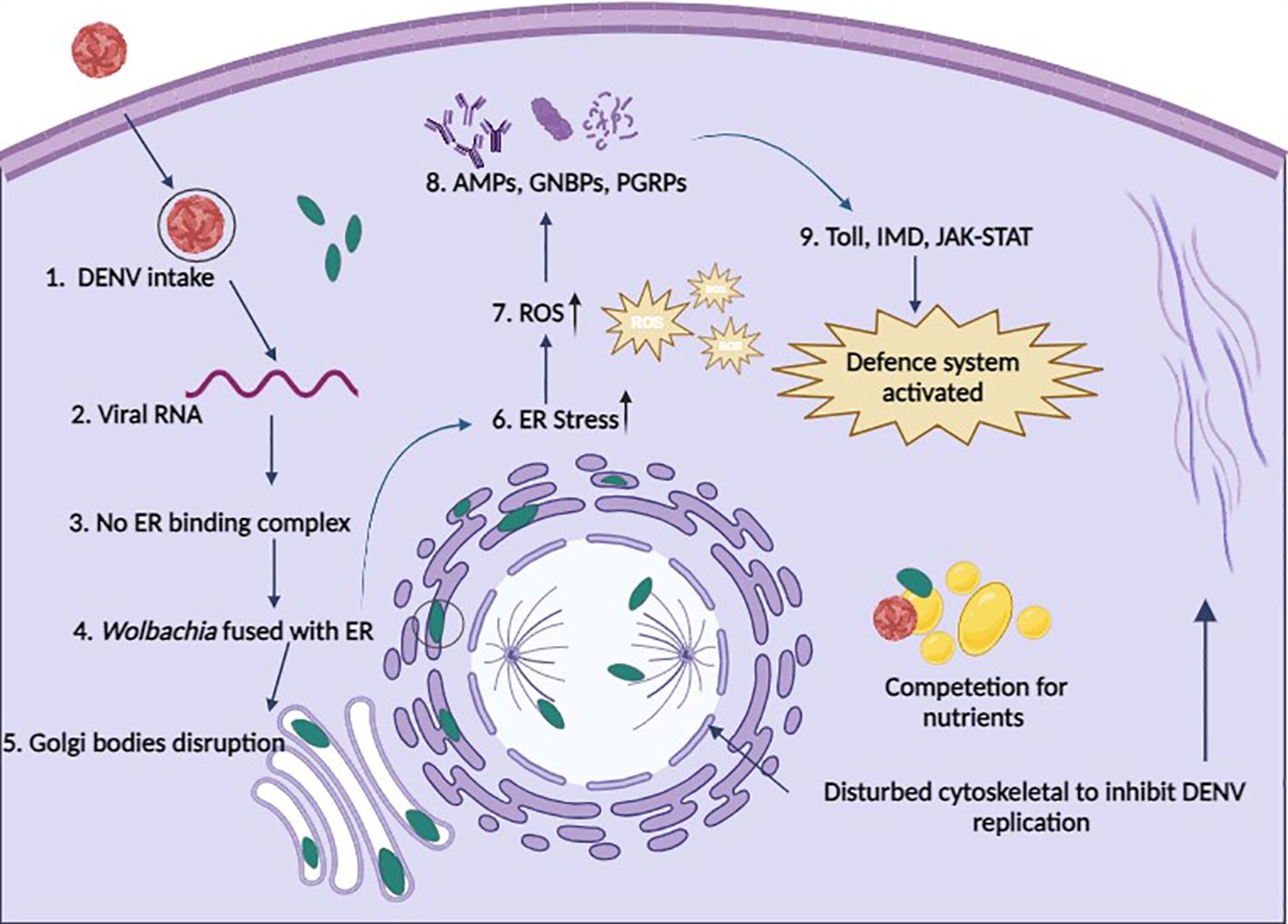
Figure 2 Possible defense systems of cells in the presence of Wolbachia. 1. DENV enters a Wolbachia-infected cell through endocytosis; 2. Viral RNA starts replication; 3. Replication of DENV is restricted because no binding complex forms on the ER membrane; 4. Wolbachia fused with the ER membrane and disturbs it; 5. No formation of Golgi vesicles due to disturbance of Golgi apparatus membrane by Wolbachia; 6. Wolbachia induces ER stress; 7. Wolbachia produces ROS to increase cellular stress; 8. Upregulation of AMPs, GNBPs, and PGRPs to boost immunity; 9. Immune pathways are activated to fight against pathogens (cells take Wolbachia as part of innate immunity); & Wolbachia also competes with DENV for nutrients and also disturbs the cytoskeleton to stop the movement of DENV and maturation.
4 How Wolbachia control DENV?
While Wolbachia is recognized for inhibiting certain viruses, its effectiveness is mainly observed against viruses with positive-sense or double-stranded RNA genomes (13). Its ability to inhibit negative-sense RNA viruses is less commonly reported. DENV, a positive-strand RNA virus, enters midgut cells following a blood meal (80). Many proteins, including replication factors, are produced when the viral RNA is translated into a polyprotein. Once DENV surpasses the midgut barrier, it can access other tissues like the fat body and the hemocytes. As soon as the virus enters the hemocoel, it can reproduce in the salivary gland cells and travel to the lumen of the glands. From there, the virus can be transmitted to a human host during subsequent mosquito blood-feeding. The exact mechanism behind Wolbachia-mediated blocking remains a mystery, primarily due to challenges in isolating the contributions of the three partners in the Wolbachia-Aedes-dengue association. The understanding of this process relies on observations of how these partners interact for a clear comprehension of the specific mechanisms involved (81). Some scientists suggest that Wolbachia may outcompete the virus for resources like lipids, enhance the mosquito’s immune system (82), and possibly this bacterium can lessen Ae. aegypti’s susceptibility to DENV (83). Additionally, there are many possible methods by which the transinfection of various strains of Wolbachia into Ae. aegypti can prevent the spread of disease. This section is all about how DENV affects the genes of Ae. aegypti and how the mosquito responds at the cellular level in the presence and absence of Wolbachia.
4.1 Competition for intracellular resources
Studies suggest that Wolbachia-induced metabolic changes in transinfected Ae. aegypti may elucidate the pathogen-blocking mechanism (84–86). New studies suggest that instead of simply struggling over lipids, there’s a more complicated relationship where changes in lipids might work against each other. In one study by Koh et al., when DENV infection alone leads to an abundance of lipids Wolbachia and DENV both want the same things inside the cells of mosquitoes (87). Wolbachia relies on various host factors for replication, transmission, and manipulation of the host. It depends on host-derived membranes (88), altering their morphology, and affecting cholesterol/lipid metabolism (85). Wolbachia strategically localizes itself within vesicles closely associated with the endoplasmic reticulum, to gain access to the host cell’s lipid-rich environment (89). On the other hand, the DENV also disturbs the internal membranes of the cell to produce specific locations where the virus can multiply (90). By manipulating the cell’s fatty acid synthesis pathway, DENV effectively increases the production of lipids to facilitate its replication, while Wolbachia often triggers a response against pathogens in arthropods by competing for cholesterol and iron, necessary for their growth (46). wMelPop or wMel infected cells of Ae. aegypti exhibit a significant reduction in total cholesterol (91) suggesting reliance on the host cell for lipid production due to lacking essential genes. This reduction in cholesterol impacts DENV replication, which also relies on cholesterol production. However, high Wolbachia abundance might consume excessive fatty acids, potentially disrupting normal cellular functions and virus replication. While the exact mechanism remains unclear, Wolbachia could be more resource-efficient than the virus, potentially enhancing mosquito immunity.
In Ae. aegypti, the Wolbachia strain or DENV disturbs cholesterol levels, resulting in increased cholesterol storage and localized lipid droplet accumulation (85). This dysregulation is marked by the upregulation of Niemann-Pick type C2, sterol carrier protein 2, and calnexin 99, associated with the downregulation of fatty acid synthase and LDL receptor proteins, indicative of compromised intracellular cholesterol transport. Specific lipids, like sphingomyelins and cardiolipins, are highly present in DENV3-infected mosquitoes but depleted when wMel is present, suggesting an indirect antagonistic effect (87). In another study, the interaction involves elevated acyl-carnitine lipids during DENV infection but a significant reduction in wMel-infected cells (92). Lowering acyl-carnitine increases wMel density while adding this lipid to wMel-infected cells boosts DENV. A recent study indicates that wMel-transinfected Ae. aegypti suppresses DENV and ZIKV through the downregulation of the insulin receptor, however exact mechanisms need to be defined (93). In simple words, the virus may seek a lipid-rich environment for replication, which Wolbachia disrupts. However, understanding how Wolbachia downregulates the DENV is a matter of interest that is unclear.
4.2 Immune priming
To prevent arboviral transmission, Wolbachia employs two strategies. For starters, it competes for limited host cellular resources with arboviruses. Second, when transmitted to non-native hosts, it uses immune priming, which is a preactivation of the host’s immune system. This strengthens the arthropod’s resistance to arboviral infections. Signaling pathways such as IMD, Toll, and JAK-STAT initiate immune priming (94). Rances et al. (95) found that Wolbachia activates immunological genes linked to Toll pathways, melanization, and AMPs. The JAK-STAT pathway, known for regulating antiviral immunity, has been proven effective in preventing DENV infection in Ae. aegypti (96). wAlbB-transinfected Ae. aegypti upregulates Toll (GNBP1, SPZ3B, MYD88) and IMD (PRGP-LE, REL2) pathway genes, triggering the release of AMPs (e.g., cecropins, defensins) during arboviral infection (33, 34, 46). This immune-priming effect can be observed in mosquito larvae exposed to dormant dengue virus, resulting in protection against the virus in maturity (97).
4.2.1 Wolbachia and Toll pathway
Vector-virus interactions have been studied since the initial Ae. aegypti genome sequence was made public (98). Ae. aegypti’s defense against DENV infection is mediated by this pathway, as demonstrated by early transcriptome analysis in conjunction with functional assessments (99). Immune genes of the Toll pathway are upregulated in response to DENV-2 infection, indicating Myeloid Differentiation factor 88 (MYD88) is responsible for the high level of DENV and its essential role in controlling mosquito defense against DENV (33). Wolbachia activation of the Toll pathway induces the host release of ROS, leading to the synthesis of AMPs and antioxidants as shown in Figure 3 (100). The silencing of the tissues of the midgut. In both the carcass and midgut tissue of Ae. aegypti infected with DENV, the AMP transcripts are highly marked (96). Furthermore, AMP gene expression is enhanced by the silencing of Cactus and Caspar (33). Viruses can modulate host arboviral susceptibility by downregulating AMP genes, as demonstrated in in-vitro and transcriptomic research on DENV-, ZIKV-, and CHIKV-infected mosquitoes (101). After infection, there’s a temporary increase in the expression of Spätzle (spz) and Rel1A, along with a transient rise in Cactus expression, which later decreases after 7 days (102). This upregulation indicates a robust immune response, with the pathway recognizing and combating the presence of the DENV in Ae. aegypti. When Ae. aegypti becomes infected with dengue, the Gram-negative binding proteins (GNBPs) may engage with virus particles or cellular debris, which could trigger immunological responses or directly neutralize virus particles, strengthening the mosquito’s defenses against DENV. Susceptibility has also been directly linked to several immune-related genes. Caicedo et al. (103) demonstrated that certain genes in Ae. aegypti significantly reduce the proliferation of DENV. These genes for specific proteins included Keratinocyte lectin (AAEL009842), GNBP (AAEL009176), Cathepsin-b (AAEL007585), and NPC2 (AAEL015136). This demonstrates the significance of these genes and their role in the functioning of DENV infection. In mosquitoes, the midgut serves as a primary site for the replication of the virus and now it’s clear that the Toll pathway activation by RNAi-mediated depletion of Cactus suppresses viral infection in the mosquito midgut.
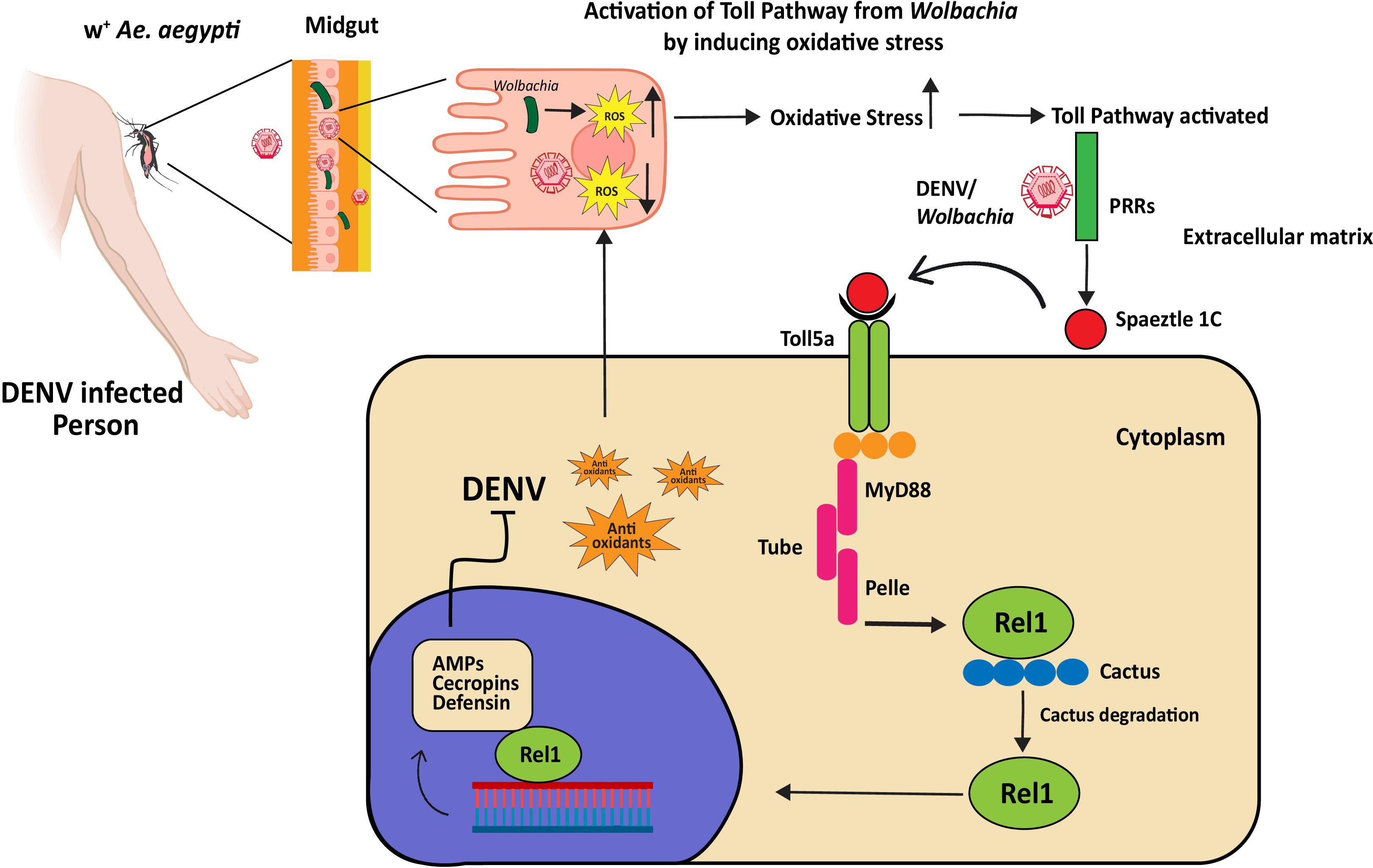
Figure 3 Dengue virus inhibition by Wolbachia-triggered Toll pathway activation in Ae. aegypti. Wolbachia produces ROS to favor its replication. To produce anti-oxidants to cope with oxidative stress, the Toll pathway is activated. The Toll pathway controls immune responses to Wolbachia and DENV through the systemic production of AMPs. PRRs recognize DENV or Wolbachia-associated molecular patterns and start maturation of spaetzle1C, it binds to Toll5a receptors and initiates the Toll pathway through adaptor proteins MyD88, Tube, and Pelle. The Cactus protein, a negative regulator of Rel1, is degraded by phosphorylation. Rel1 translocates into the nucleus and activates the transcription of genes encoding for AMPs, cecropin, and defending. These AMPs stop the replication of DENV (the exact mechanism is unknown).
Bonizzoni et al. (104–106) found that extracellular PRR attaches to pathogen-derived ligands to initiate the Toll pathway. It triggers a proteolytic cascade that causes the Spätzle processing enzyme (SPE) to convert pro-Spätzle to Spätzle (107). Effector gene transcription is started when Spz binds to the transmembrane receptor Toll, triggering a cytoplasmic cascade that results in the nuclear translocation of the NF-kB transcription factor Rel1a. This pathway is noticeably downregulated in response to DENV infection specifically, certain variants of DENV-2, found in the 3’ untranslated region 3’UTR, inhibit the Toll pathway within mosquito salivary glands by producing subgenomic flaviviral RNA (108). However, there’s evidence suggesting that Wolbachia induces oxidative stress within the mosquito, and this stress, in turn, triggers the Toll pathway (100).
In the mosquito’s antiviral defense, multiple immune pathways are engaged, with each pathway showing specificity toward particular viruses. DENV virus activates Toll pathway genes, and increased expression of AMPs has been observed in these mosquitoes but their specific function in antiviral defense has yet to be fully understood. Pan et al. (100) suggested that the Toll pathway is responsible for expressing antioxidants and AMPs such as defensins and cecropins. Defensins were originally assumed to target enveloped viruses by breaking the viral envelope. Their extracellular antiviral impact is indicated by the fact that they are generated in the fat body and released into the hemolymph. Wolbachia infection activates defensins, including DEFA and CECA, to limit DENV proliferation, as demonstrated in DEF/CEC transgenic Ae. aegypti (109). Hence to fully understand mosquito antiviral defenses and their significance in the fight against infectious illnesses, more research is necessary.
4.2.2 Wolbachia and IMD pathway
The IMD pathway is an important component of the insect defense system, particularly effective against gram-negative bacteria (110). Like the mammalian tumor necrosis factor signaling mechanism, the IMD pathway activates when membrane-bound PGRPs detect any pathogen. This triggers a signaling cascade involving the IMD protein, caspases, and kinases, ultimately leading to the activation of Rel2. It activates the transcription of AMPs and defense-related genes (111). When D. melanogaster gets infected by viruses, it activates the IMD pathway, which triggers the production of AMPs to fight off the invaders (112). Silencing key components of this pathway led to increased DENV titers in DENV-resistant mosquito strains, indicating its potential as an antiviral defense mechanism against this virus (113). Furthermore, Wolbachia activates this pathway, as a mechanism of defense in both natural host Drosophila and transinfected host Ae. aegypti mosquitoes (11, 22, 95).
Ye et al. (57) reported that boosting the IMD pathway leads to higher wAlbB titers while silencing it leads to a decrease. The mosquito’s innate immune system can detect wAlbB through PGRP-LE, acting as a PRR and this triggers the activation of the IMD pathway. It is similar to how PGRP-LE functions as an intracellular sensor of Gram-negative bacteria in Drosophila, inducing the IMD pathway (110). Enhanced immunity boosts the expression of molecules that stimulate rather than inhibit wAlbB proliferation in Ae. aegypti, possibly because these AMPs lack specific targets on the Wolbachia cell membrane (46). Immune boosting may lead to increased Wolbachia density via the production of molecules that support Wolbachia replication, such as antioxidants as in the case of the Toll pathway (100). Increased production of AMPs by these antioxidants may indirectly benefit Wolbachia by removing susceptible microbial flora, allowing Wolbachia to occupy new niches. This suggests a positive feedback loop between host immune system activation and Wolbachia growth, aiding the establishment of Wolbachia symbiosis in transinfected Ae. aegypti lines. However, by activating the IMD pathway Wolbachia inhibits the replication of DENV in mosquitoes (Figure 4).
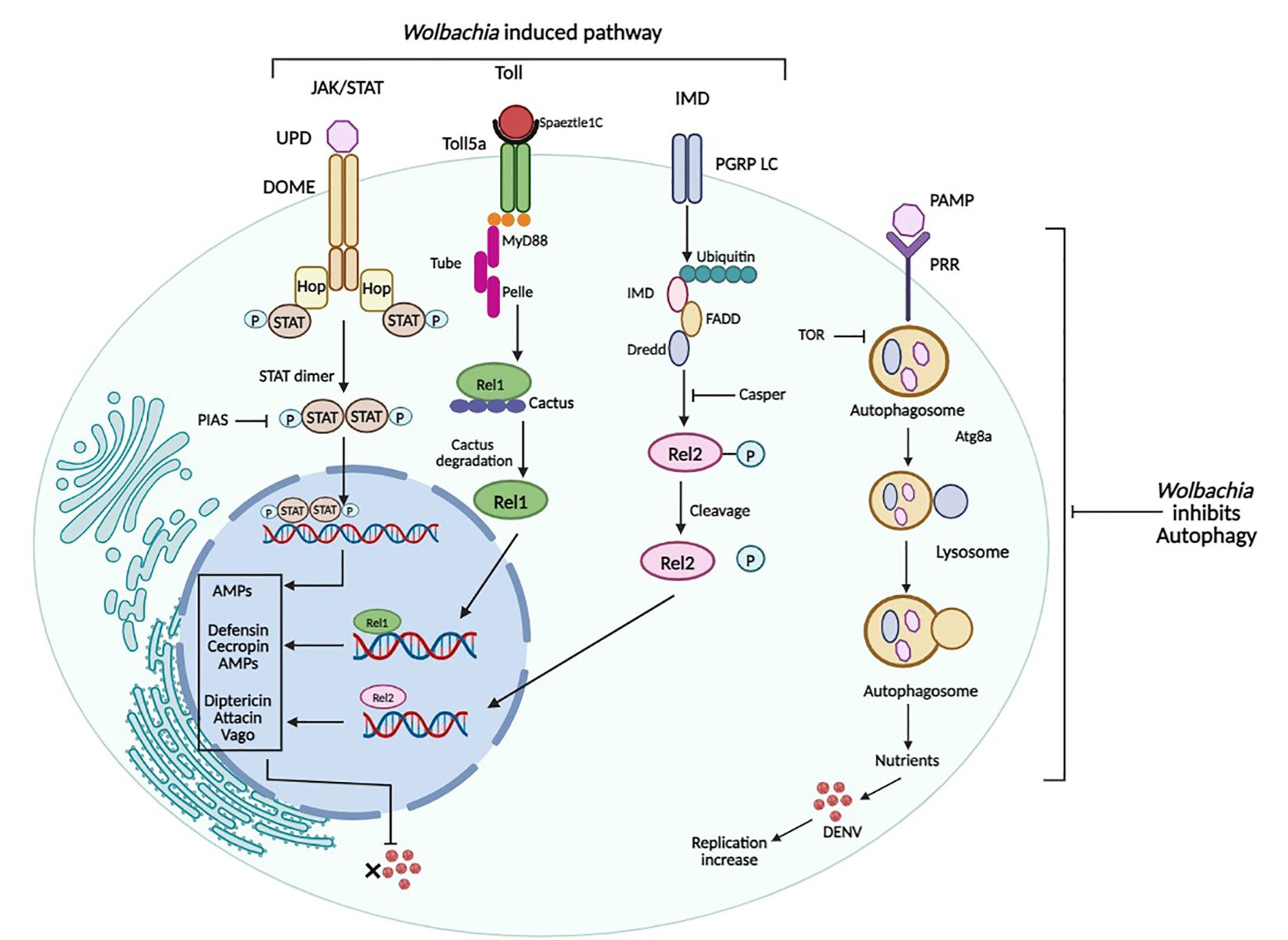
Figure 4 Immune pathways of Ae. aegypti and their regulation by Wolbachia. Wolbachia activates Toll, IMD, and JAK-STAT Pathway which in turn results in down-regulation of DENV. While Wolbachia inhibits autophagy, activated by DENV for its replication. Figure created in BioRender.com.
4.2.3 Wolbachia and JAK-STAT pathway
The JAK-STAT pathway is crucial in Ae. aegypti’s defense against DENV, as suppressing it leads to increased virus replication in the mosquito midgut (114) and its activation, on the other hand, reduces virus replication. In Ae. aegypti, high JAK/STAT activation limits DENV replication, but the pathway’s effectors and the mechanisms behind JAK-STAT pathway-mediated antiviral effects are poorly understood. In Ae. aegypti, this pathway is activated by ligands such as Unpaired (Upd), which binds to the Dome receptor, leading to downstream signaling activation. Suppressing the Dome receptor or its JAK homolog HOP through RNA interference increases mosquito susceptibility to DENV infection while blocking the negative regulator, a protein inhibitor of activated STAT (PIAS), enhances DENV resistance (114). Two putative effector genes, DVRF1 and DVRF2, have been identified in Ae. aegypti as dengue virus restriction factors, but their functions are uncharacterized. The activation of various immunity-related genes by the JAK-STAT pathway suggests its role as a non-classical innate immune defense against DENV. Souza-Neto, Sim, & Dimopoulos (114) reported that wMel induces the JAK/STAT pathway in Ae. aegypti, which controls DENV. However, the exact mechanism by which Wolbachia modulates the expression of the JAK/STAT pathways remains unclear.
4.2.4 Transfected Wolbachia and mosquito immune responses
Naturally occurring Wolbachia does not have a major effect on vector competence in mosquito species. For example, Ae. albopictus’ wAlbB is unable to produce resistance against DENV in its native host (22). Bourtzis et al. (115) demonstrated that the AMP transcripts in Ae. albopictus is not substantially affected by Wolbachia. Wolbachia-infected mosquitoes exhibit resistance to diseases, which is probably the result of an increased host immune response that balances any potential negative consequences resulting from the recently acquired parasite. The key point here is that Wolbachia-induced immune factors activate pre-invasion, contrasting with pathogen-induced factors that activate post-invasion. Compared to the Toll pathway’s later activation by DENV, Wolbachia increases its activity before DENV invasion, allowing it to play a more significant role in clearing invasive viruses (33).
Many researchers explained the mechanisms through which Wolbachia activates the immune system of its host. In the case of wAlbB infected Ae. aegypti, Pan et al. (100) reported increased hydrogen peroxide (H2O2) levels and a significant increase in the expression of genes that encode NADPH oxidase (NOXM) and Dual Oxidase 2 (DUOX2) enzymes. These enzymes play a role in the production of ROS (116, 117). However, an upregulation of antioxidant genes in Wolbachia-infected mosquitoes implies the activation of mechanisms to neutralize ROS. Brennan et al. (35) also show ROS generation and antioxidant protein expression in Wolbachia-infected Ae. albopictus cell. ROS serve as messengers, activating NF-κB, a central regulator, to control immunity, inflammation, and cell survival (118).
Pan et al. (46) demonstrated that Ae. aegypti’s IMD and Toll pathways respond to wAlbB introduction, influencing infection levels. Activation increases wAlbB titer, while silencing reduces it, and elevated infection persists through maternal transmission. Remarkably, immune system amplification strongly promotes the synthesis of chemicals that actively promote wAlbB development in Ae. aegypti rather than merely failing to inhibit it. This is likely because there are no specific targets for AMPs in the Wolbachia cell membrane. The mosquito immune pathways trigger the effector molecules, such as Wolbachia-AMPs DEF and CEC, but surprisingly don’t impede Wolbachia growth. This immune system boost serves as a survival signal for the successful establishment of a novel Wolbachia symbiosis.
4.3 Autophagy
When cells face stress or starvation, autophagy helps get rid of damaged organelles and large protein aggregates. It can be used to degrade invasive bacteria, viruses, and parasites in addition to its function in recycling cell components during development (119). Autophagy is important for iron scavenging and cellular homeostasis and DENV induces and relies on autophagy for efficient viral replication in mammalian cells, despite its antiviral functions (120). DENV-induced autophagy, specifically targeting lipid droplets, alters cell metabolism, leading to the release of free fatty acids. Inhibition of this autophagy pathway inhibits DENV replication, suggesting that this pathway creates a favourable environment for viral replication by providing energy (121). Additionally, When Wolbachia is present, it manipulates the cell’s autophagy, impacting the replication of arboviruses. This interference limits the nutrients available for the viruses, making it harder for them to grow.
Activating autophagy decreases bacteria whereas suppressing it boosts bacterial populations in many organisms. Wolbachia levels are regulated by autophagy in a range of hosts, indicating the bacteria’s adaptation to resist autophagy and stay inside host cells. Wolbachia secretes a protein that manipulates the autophagy initiation pathway (122). Recent demonstrations show that ATG8a, a protein indicating autophagy activation, is abundant in Brugia tissues with high Wolbachia levels (123). Activation of the autophagy pathway triggered by Wolbachia infection is controlled by TOR–Atg1 signaling pathway genetic modification (123). Modification of TOR-Atg1 results in increased lysosomal production within the cell. Wolbachia-containing vacuoles can be bound by these lysosomes and eliminated. However, using a substance called 3-MA, autophagy could be inhibited which causes an increase in the quantity of Wolbachia in both animals and cells. Wolbachia most likely evolved anti-autophagy mechanisms to live and proliferate inside host cells. Furthermore, the APG5 is the most important gene of the autophagy (123). A recent finding revealed that there was no significant effect of Wolbachia infection on APG5 expression. Even though the load of DENV is high with the suppression of APG5, the Wolbachia presence does not alter the level of APG5. This indicates that in the presence of a Wolbachia infection autophagy is acting independently, but is probably a crucial factor in the Ae. aegypti against DENV infection.
4.4 miRNA-dependent immune pathways
The miRNA-dependent immune route is the fourth mechanism and it regulates numerous cellular functions, including transposon silencing, antiviral defense, differentiation, timing, cell division, and death, and is greatly aided by miRNAs. This pathway controls arboviral infection in diverse mosquito vectors by regulating arthropod host genes. Hussain et al. (36) concentrated on comprehending the impact of the wMelPop on cellular miRNAs in female mosquitoes. aae-miR-2940-5p, a mosquito-specific miRNA, is substantially increased in wMelPop-CLA-infected mosquitoes as opposed to uninfected mosquitoes. Mature aae-miR-2940-5p and pelo transcripts were found to co-localize by (124), suggesting the potential, in Wolbachia-containing cells, for aae-miR-2940-5p to downregulate the pelo transcripts. The immune response to viral infections consists of RNA interference (RNAi), a protective mechanism (125) that protects mosquitoes against DENV. In Ae. aegypti RNAi is the most important antiviral pathway, shown to reduce the proliferation of multiple viruses (DENV, chikungunya, and Sindbis viruses) but seems less crucial for blocking pathogens in naturally Wolbachia-infected insects (126). Activated by viral dsRNA cleavage, this pathway employs siRNAs to degrade viral ssRNA via cellular machinery. R2D2 and Dicer-2 are essential components of this pathway and if silenced mosquitoes are more susceptible to DENV (126). The RNase III domain of Dicer-2 cleaves the dsRNA after binding of Dicer-2-R2D2 complex to the viral dsRNA, for the formation of siRNA of 21– 23 nucleotides long. Now the siRNA will initiate the RNAi machinery by binding with RNA-induced silencing complex (RISC) which breaks the double-stranded RNA and unwinds one of the siRNA strands keeping the other for targeted degradation of single-stranded viral RNA with sequence complementary to the siRNA by the host endonuclease, Argonaute-2 (Ago2). Although RNAi activates against DENV, it doesn’t always stop the virus completely, emphasizing its role but limited effectiveness. To ensure the long-term survival of infected mosquitoes, it might just modify the replication of the virus to maintain chronic viral infection.
4.5 Wolbachia and specific immunity of Ae. aegypti
Wolbachia infects various tissues in the host, leading to significant impacts on host physiology (127). These effects extend to the cellular, individual, and population levels, affecting gene expression (33, 39), macromolecule availability (128), and fecundity, (129). The diversity of Wolbachia’s effects on the host highlights the complexity of this symbiotic relationship. Wolbachia combines reproductive manipulation, like cytoplasmic incompatibility, with mutualistic benefits, such as pathogen protection. The relationship spans a range between parasitism and mutualism. This dual impact makes Wolbachia a promising tool for controlling vector-borne diseases, using its influence on host reproduction and immune enhancement to reduce disease transmission. The mechanism through which Wolbachia provides antiviral protection is still a subject of ongoing research and discussion.
The example of DENV replication being seriously disrupted in the presence of Wolbachia is arguably the most thoroughly researched. Authors examine whether the Chromodomain helicase DNA binding proteins (CHD) may play a role in the interactions among Wolbachia, Aedes, and DENV. CHD proteins are a type of proteins classified within the ATP-dependent chromatin modifiers, specifically belonging to the SNF2 superfamily. Experimental evidence by (130) supports AeCHD7, a host component in Ae. aegypti, supporting DENV replication, while Wolbachia’s downregulation of it may inhibit DENV replication. Reduction in the expression levels of AeCHD genes is observed in mosquitoes infected with Wolbachia. AeCHD7 promotes DENV replication, but Wolbachia reduces its expression in female Ae. aegypti, limiting the replication of DENV. This mechanism is only for female mosquitoes and not universally applicable across different hosts for Wolbachia to inhibit viral replication.
Asad et al. (131) discovered two vago proteins, AeVago1 and AeVago2, in Ae. aegypti. Vago is a special antiviral protein found in insects. They investigated AeVago1 production increased in Wolbachia-infected Ae. aegypti. Without changing the density of Wolbachia, AeVago1 knockdown in Wolbachia-infected cells boosted DENV replication. Based on the data, it appears that AeVago1 which Wolbachia induces in Aag2 cells, prevents DENV replication. Wu et al. (132) Lapidot et al. (133) have revealed the significance of the pelo protein for efficient viral replication, specifically for the Drosophila C virus and Tomato yellow leaf curl virus. Asad et al. (124) reported that wMelPop-CLA inhibits the pelo protein, and this inhibition might protect Ae. aegypti mosquitoes against DENV particles. Ae. aegypti’s tissues exhibit widespread expression of the pelo gene, with salivary gland expression being especially high but interestingly (134), but the presence of Wolbachia results in the suppression of pelo in various cell lines, salivary glands, muscles, and ovaries. In summary, the pelo protein promotes replication of DENV and on the other hand, Wolbachia inhibits the pelo protein in female Ae. aegypti mosquitoes, which may reduce DENV in these mosquitoes.
4.6 Unique miRNA expression in Wolbachia infection
Despite extensive research on Wolbachia biology, numerous unexplored mechanisms exist in its interactions with other organisms, suggesting manipulation of the host’s environment to ensure its survival. One such mechanism is the differential expression of mosquito cellular miRNAs due to Wolbachia infection (36). miRNAs function as post-transcriptional regulators, controlling multiple genes. In the presence of microbes, some miRNAs are dysregulated, while others are exclusively expressed, altering mosquito host responses as microbes persist within cells (135). Different Wolbachia strains have substantiated effects on Ae. aegypti’s miRNA profile such as in Wolbachia-infected mosquitoes, wMelpop-CLA induces exclusive miRNA expression, notably elevating miR-2940, which targets genes regulating Wolbachia density (36, 136). miR-2940 enhances wMelpop-CLA replication by upregulating metalloprotease m41 ftsh and arginine methyl transferase 3 (AaArgM3) genes while inhibiting it reduces target gene expression and Wolbachia levels. Metalloprotease genes like m41 ftsh are upregulated by miR-2940 in DENV-infected Ae. aegypti (106), and this miRNA is downregulated in WNV-infected cells (137). It suggests that Wolbachia may exploit host miRNAs to control essential host genes. On the other hand, miR-2940 inhibits DNA methyltransferase (AaDnmt2) in wMelpop-CLA-infected mosquitoes (136). This gene is responsible for host defense and genome stability and is present abundantly in DENV-infected mosquitoes that are negative for Wolbachia. It indicates that Wolbachia creates a cellular environment incompatible with the virus by downregulating AaDnmt2 in Ae. aegypti (136).
Other miRNAs, can influence host autophagy and viral replication. For instance, aae-miR-12 miRNAs, induced by wMelPop-CLA in Aag2 cells, can influence host autophagy and viral replication. For instance, aae-miR-12 suppresses monocarboxylate transporter (MCT1) and DNA replication licensing factor (MCM6), potentially impacting autophagy pathways (138). MCT1’s involvement in autophagy is a matter of interest, which is exploited by DENV and ZIKV to evade host immune defenses (139). Further investigation is needed to determine if Wolbachia-produced miRNAs can modulate MCT1 activity and autophagy. Wolbachia infection also triggers the expression of aae-miR-981, resulting in the downregulation of importin b-4 in wMelPop-CLA-infected Aag2 cells (37). This reduction in importin b-4 activity inhibits the translocation of AGO1 to the nucleus. While the exact advantage of hindering AGO1 translocation for Wolbachia’s viral blocking remains unclear, importin b is known to assist in the nuclear migration of DENV and ZIKV non-structural proteins for optimal replication (140, 141). It suggests that the downregulation of importin b during Wolbachia infection may hinder viral transcription. Additionally WsRNA-46, a Wolbachia-derived miRNA in infected Ae. aegypti, promotes dynein expression, required for cellular transport and maintaining density in both Wolbachia and arboviruses, indicating an overlapping requirement for host cellular factors (142).
4.7 Fight for cytoskeletal components
Studies link Wolbachia’s pathogen-blocking effect to decreased viral load, but the mechanism and timing of interference in the virus life cycle are unclear. It was reported that Wolbachia interacts with the host cytoskeleton in two ways: by secreting effector molecules that bind to cytoskeletal structures to maintain optimal density and ensure transmission, and by regulating the expression of cytoskeletal proteins like dystroglycan and tubulin, crucial for arboviral infection (79). Arboviruses upregulate cytoskeletal structures for viral processes while the transinfected wAlbB strain in Ae. aegypti (Aag2) cells infected with DENV show downregulation of cytoskeletal membrane proteins, dystroglycan, and beta-tubulin. Silencing these cytoskeletal proteins inhibits DENV binding to Aag2 cells. This indicates Wolbachia’s direct involvement in hindering DENV binding and entry by targeting host cytoskeletal proteins utilized by the virus (106, 143).
4.8 Phenoloxidase cascade
The third mechanism disrupts arboviral transmission by triggering the phenoloxidase (PO) cascade. Melanin is produced by this cascade, which involves the enzyme phenoloxidase. Melanin exhibits antipathogenic properties when it accumulates around invasive pathogens and at wound sites (144, 145). The mosquito’s innate immune response to arboviruses depends on this process. Studies reveal that Wolbachia increases melanization in native and non-native arthropod vectors using phenoloxidase activities. Therefore, the phenoloxidase cascade that Wolbachia induces is probably a defense mechanism against different arboviral infections (146).
5 Discussion
This study analyzed different studies on the effect of Wolbachia on the immune system of hosts and offers an appealing mechanistic explanation for pathogen blocking. Recent field studies have demonstrated the effectiveness of Wolbachia in suppressing vector-borne disease transmission (4, 10, 13, 56, 72, 146, 147). These studies utilize three main strategies: (a) introducing Wolbachia-infected males to induce CI with uninfected females (4), (b) deploying Wolbachia strains that reduce mosquito fitness, such as by shortening lifespan, especially in regions with seasonal variation, (73) and (c) introducing Wolbachia strains that inhibit viral transmission by reducing vector competence (10, 13, 23, 72). These strategies, implemented in various countries including Australia, China, Indonesia, Brazil, and Vietnam, have shown promising results in controlling Aedes-borne viral infections.
Wolbachia-infected mosquitoes have been successfully used in over 14 countries, initially proven effective in Cairns, Australia in 2011 (4). In Brazil, after a resurgence of dengue in 1981, large-scale releases of Wolbachia-infected mosquitoes resulted in a notable 38% reduction in dengue and a 10% reduction in chikungunya (69). Yogyakarta, Indonesia also saw a significant 77% decrease in dengue transmission with Wolbachia-infected mosquito releases, accompanied by an 83% reduction in severe dengue cases (61, 148). In the USA, Myanmar, Malaysia, and China, the introduction of wAlbB-infected Ae. aegypti led to reduced human dengue incidence (54, 148, 149). Singapore’s release of the wAlbB strain in 2018 resulted in a 71-88% decrease in dengue cases (150). Cost-effectiveness analyses proposed implementing Wolbachia in high-risk urban areas of Vietnam, estimating significant reductions in dengue cases and associated economic benefits over 20 years (151). While field studies have demonstrated the effectiveness of Wolbachia in controlling mosquito-borne diseases, understanding the underlying mechanisms is crucial for optimizing its use.
Several mechanisms have been proposed to explain how Wolbachia inhibits virus replication in mosquitoes. Early in DENV infection, mosquitoes enhance innate immune genes, but as the infection progresses, it can suppress mosquito defenses, through the inhibition of immune-related genes (33, 76). However, the mosquitoes’ ability to compete with viruses can be modified by their microbiota. Wolbachia, a microbe, inhibits disease transmission by vectors, either by directly blocking virus transmission or reducing mosquito lifespan but the exact mechanism remains unclear due to Wolbachia’s inability to be cultured in a lab. Experimental evidence has repeatedly shown that Wolbachia is effective at preventing the replication of different flaviviruses, such as CHIKV, ZIKV, WNV, and DENV (10, 152, 153) with numerous studies demonstrating its significant inhibition of DENV replication (22, 136, 154). Transinfecting Wolbachia into Ae. aegypti, a vector not naturally hosting it, effectively inhibited DENV and CHIKV replication (155). Though much research has been done, the true mechanism or mechanisms through which Wolbachia inhibits viral reproduction in its host environment are still predominantly unknown.
Wolbachia, in general, boosts immune responses and increases resistance to viruses in mosquitoes (156). Additionally, mosquitoes infected with the wMelPop strain feed less as they age due to a bent proboscis, leading to reduced bite rates (157). Furthermore, the wMel strain has been successfully transinfected into Ae. aegypti, inducing CI, ensuring high maternal transmission, and blocking the transmission of DENV (158). Wolbachia is believed to induce pathogen interference by activating the host’s innate immune system, particularly immune genes in the IMD and Toll pathways, such as REL1 and REL2 (10, 22, 23). Upregulation of immune effector genes is shown by wMelPop-CLA (10) and wAlbB (46) infected Ae. aegypti, by activation of IMD and Toll pathway. This activation increases the density of Wolbachia while turning off these pathways reduces it. The density increase may result from effector molecules that support Wolbachia replication and enhance the immune system. Such as the production of ROS that in turn initiates the Toll pathway (100) that is responsible for the inhibition of DENV. It demonstrates a positive feedback loop between the host immune system and Wolbachia density.
Another possibility for the observed effects of Wolbachia on DENV could be related to competition for essential nutrients. Cholesterol is recognized as a key fatty acid essential for the successful replication of DENV and Wolbachia. Substantive evidence suggests that wMel competes with the DENV for limited sub-cellular fatty acid resources crucial for viral replication (4). Besides this when mosquitoes get infected with DENV there’s a natural defense system called autophagy that usually helps the virus grow. Chen and Smartt (159) discovered a surprising twist that this defense system might fight against the virus. DENV uses autophagy to help it grow. This special kind of autophagy focuses on lipid droplets and changes how the cell works. Interestingly, the Wolbachia hijack the cell’s internal process, to acquire nutrients it needs from the host. ATG8a, which indicates activation of autophagy, is found in large amounts in tissues of the host, where Wolbachia is also abundant (123). The reason is that Wolbachia relies on host cells for unsaturated fatty acids and may deplete these fatty acids, upsetting DENV replication. The hypothesis suggests that Wolbachia’s presence at high densities could inhibit viruses by competing for cholesterol, but experimental testing is needed for confirmation. Several aspects of immunity have been changed by Wolbachia in Ae. aegypti have been included in this review. Several other mechanisms are still not clear like, the connection between the lncRNA and the Toll pathway as Wolbachia uses lncRNA to activate the Toll pathway.
A radical effort is underway to combat dengue by using Wolbachia for long-term biological control. Recent studies are looking at immunity more completely. The lack of anti-dengue drugs highlights the importance of understanding how Wolbachia inhibits viral growth, which could inform new drug development. Wolbachia interferes with viruses by altering host factors necessary for viral replication. Future research should focus on identifying and characterizing these host factors, which could lead to novel strategies for controlling mosquito-borne diseases. Mosquito strains, carrying Wolbachia are currently bred and experimentally released in areas with a high public health burden of DENV transmission (59, 160). The ongoing Wolbachia releases offer a unique opportunity to predict the evolutionary impacts on the bacterium, virus, and mosquito host. This includes potential scenarios like the DENV partly evading transmission blockage and Wolbachia reducing its harmful effects on mosquitoes. These predictions aim to improve future forecasting and strategies. In the future, studies will try to understand how Wolbachia deals with the immune system, hormones, metabolism, and behavior of the host. To project the long-term stability of Wolbachia–Ae. aegypti mosquito system that controls mosquitoes and prevents dengue, we need to understand how Wolbachia and the host’s immunity work together.
6 Conclusions
Manipulation of mosquitoes’ innate immunity by Wolbachia to control diseases like malaria, dengue, chikungunya, and Zika is a rising strategy these days. However, its successful implementation relies on a thorough understanding of mosquito immunity and interactions with Wolbachia and viruses. This review concludes that Ae. aegypti’s innate immune response is essential to its ability to spread DENV, and using Wolbachia to boost immunity helps prevent DENV transmission. Even while the understanding of the host-Wolbachia-virus relationship has advanced significantly, there are still gaps in our understanding. Although the precise mechanism of antiviral defense is unknown. Determining the mechanism of Wolbachia-induced viral inhibition requires an understanding of mosquito innate immune responses in the presence of Wolbachia. This information is crucial for a major plan against arboviruses.
Author contributions
IMus: Conceptualization, Data curation, Investigation, Writing – original draft. MS: Conceptualization, Data curation, Supervision, Validation, Writing – review & editing. IMun: Investigation, Writing – review & editing.
Funding
The author(s) declare that no financial support was received for the research, authorship, and/or publication of this article.
Conflict of interest
The authors declare that the research was conducted in the absence of any commercial or financial relationships that could be construed as a potential conflict of interest.
Publisher’s note
All claims expressed in this article are solely those of the authors and do not necessarily represent those of their affiliated organizations, or those of the publisher, the editors and the reviewers. Any product that may be evaluated in this article, or claim that may be made by its manufacturer, is not guaranteed or endorsed by the publisher.
References
2. Bhatt S, Gething PW, Brady OJ, Messina JP, Farlow AW, Moyes CL, et al. The global distribution and burden of dengue. Nature. (2013) 496:504–7. doi: 10.1038/nature12060
3. World Mosquito Program. (2022). Available online at: www.worldmosquitoprogram.org.
4. Hoffmann AA, Montgomery BL, Popovici J, Iturbe-Ormaetxe I, Johnson PH, Muzzi F, et al. Successful establishment of Wolbachia in Aedes populations to suppress dengue transmission. Nature. (2011) 476:454–7. doi: 10.1038/nature10356
5. Joubert DA, Walker T, Carrington LB, De Bruyne JT, Kien DHT, Hoang NLT, et al. Establishment of a Wolbachia superinfection in Aedes aEgypti mosquitoes as a potential approach for future resistance management. PloS Pathog. (2016) 12:e1005434. doi: 10.1371/journal.ppat.1005434
6. Chen C-H, Huang H, Ward CM, Su JT, Schaeffer LV, Guo M, et al. A synthetic maternal-effect selfish genetic element drives population replacement in Drosophila. science. (2007) 316:597–600. doi: 10.1126/science.1138595
7. Sinkins SP, Gould F. Gene drive systems for insect disease vectors. Nat Rev Genet. (2006) 7:427–35. doi: 10.1038/nrg1870
8. Werren JH, Baldo L, Clark ME. Wolbachia: master manipulators of invertebrate biology. Nat Rev Microbiol. (2008) 6:741–51. doi: 10.1038/nrmicro1969
9. Reyes JIL, Suzuki Y, Carvajal T, Muñoz MNM, Watanabe K. Intracellular interactions between arboviruses and Wolbachia in Aedes aEgypti. Front Cell Infect Microbiol. (2021) 11:690087. doi: 10.3389/fcimb.2021.690087
10. Moreira LA, Iturbe-Ormaetxe I, Jeffery JA, Lu G, Pyke AT, Hedges LM, et al. A Wolbachia symbiont in Aedes aEgypti limits infection with dengue, Chikungunya, and Plasmodium. Cell. (2009) 139:1268–78. doi: 10.1016/j.cell.2009.11.042
11. O’Connor L, Plichart C, Sang AC, Brelsfoard CL, Bossin HC, Dobson SL. Open release of male mosquitoes infected with a Wolbachia biopesticide: field performance and infection containment. PloS Negl Trop Dis. (2012) 6:e1797. doi: 10.1371/journal.pntd.0001797
12. Rasic G, Endersby NM, Williams C, Hoffmann AA. Using Wolbachia-based release for suppression of Aedes mosquitoes: insights from genetic data and population simulations. Ecol Appl. (2014) 24:1226–34. doi: 10.1890/13-1305.1
13. Teixeira L, Ferreira A, Ashburner M. The bacterial symbiont Wolbachia induces resistance to RNA viral infections in Drosophila melanogaster. PloS Biol. (2008) 6:e2. doi: 10.1371/journal.pbio.1000002
14. Riparbelli MG, Giordano R, Ueyama M, Callaini G. Wolbachia-mediated male killing is associated with defective chromatin remodeling. PloS One. (2012) 7:e30045. doi: 10.1371/journal.pone.0030045
15. Rousset F, Bouchon D, Pintureau B, Juchault P, Solignac M. Wolbachia endosymbionts responsible for various alterations of sexuality in arthropods. Proc R Soc London Ser B: Biol Sci. (1992) 250:91–8. doi: 10.1098/rspb.1992.0135
16. Weeks AR, Breeuwer JA. Wolbachia-induced parthenogenesis in a genus of phytophagous mites. Proc: Biol Sci. (2001) 268:2245–51. doi: 10.1098/rspb.2001.1797
17. Blagrove MS, Arias-Goeta C, Di Genua C, Failloux A-B, Sinkins SP. A Wolbachia wMel transinfection in Aedes albopictus is not detrimental to host fitness and inhibits Chikungunya virus. PloS Negl Trop Dis. (2013) 7:e2152. doi: 10.1371/journal.pntd.0002152
18. Blagrove MS, Arias-Goeta C, Failloux AB, Sinkins SP. Wolbachia strain wMel induces cytoplasmic incompatibility and blocks dengue transmission in Aedes albopictus. Proc Natl Acad Sci United States America. (2012) 109:255–60. doi: 10.1073/pnas.1112021108
19. Stouthamer R, Breeuwer JA, Hurst GD. Wolbachia pipientis: microbial manipulator of arthropod reproduction. Annu Rev Microbiol. (1999) 53:71–102. doi: 10.1146/annurev.micro.53.1.71
20. Turelli M, Hoffmann AA. Rapid spread of an inherited incompatibility factor in California Drosophila. Nature. (1991) 353:440–2. doi: 10.1038/353440a0
21. Yen JH, Barr AR. The etiological agent of cytoplasmic incompatibility in Culex pipiens. J Invertebrate Pathol. (1973) 22:242–50. doi: 10.1016/0022-2011(73)90141-9
22. Bian G, Xu Y, Lu P, Xie Y, Xi Z. The endosymbiotic bacterium Wolbachia induces resistance to dengue virus in Aedes aEgypti. PloS Pathog. (2010) 6:e1000833. doi: 10.1371/journal.ppat.1000833
23. Kambris Z, Cook PE, Phuc HK, Sinkins SP. Immune activation by life-shortening Wolbachia and reduced filarial competence in mosquitoes. science. (2009) 326:134–6. doi: 10.1126/science.1177531
24. Xi Z, Khoo CC, Dobson SL. Wolbachia establishment and invasion in an Aedes aEgypti laboratory population. science. (2005) 310:326–8. doi: 10.1126/science.1117607
25. Ross PA, Turelli M, Hoffmann AA. Evolutionary ecology of wolbachia releases for disease control. Annu Rev Genet. (2019) 53:93–116. doi: 10.1146/annurev-genet-112618-043609
26. Waterhouse RM, Kriventseva EV, Meister S, Xi Z, Alvarez KS, Bartholomay LC, et al. Evolutionary dynamics of immune-related genes and pathways in disease-vector mosquitoes. science. (2007) 316:1738–43. doi: 10.1126/science.1139862
27. Kumar A, Srivastava P, Sirisena P, Dubey SK, Kumar R, Shrinet J, et al. Mosquito innate immunity. Insects. (2018) 9:95. doi: 10.3390/insects9030095
28. Lindsey AR, Bhattacharya T, Hardy RW, Newton IL. Wolbachia and virus alter the host transcriptome at the interface of nucleotide metabolism pathways. mBio. (2021) 12:e03472–03420. doi: 10.1128/mBio.03472-20
29. Adelman ZN, Myles KM. The C-type lectin domain gene family in Aedes aEgypti and their role in arbovirus infection. Viruses. (2018) 10:367. doi: 10.3390/v10070367
30. Matthews BJ, Dudchenko O, Kingan S, Koren S, Antoshechkin I, Crawford JE, et al. Improved Aedes aEgypti mosquito reference genome assembly enables biological discovery and vector control. BioRxiv. (2017) 563:501–7. doi: 10.1038/s41586-018-0692-z
31. Mukherjee D, Das S, Begum F, Mal S, Ray U. The mosquito immune system and the life of dengue virus: what we know and do not know. Pathogens. (2019) 8:77. doi: 10.3390/pathogens8020077
32. Ramirez JL, Dimopoulos G. The Toll immune signaling pathway control conserved anti-dengue defenses across diverse Ae. aEgypti strains and against multiple dengue virus serotypes. Dev Comp Immunol. (2010) 34:625–9. doi: 10.1016/j.dci.2010.01.006
33. Xi Z, Ramirez JL, Dimopoulos G. The Aedes aEgypti toll pathway controls dengue virus infection. PloS Pathog. (2008) 4:e1000098. doi: 10.1371/journal.ppat.1000098
34. Zhao L, Alto BW, Jiang Y, Yu F, Zhang Y. Transcriptomic analysis of aedes aEgypti innate immune system in response to ingestion of chikungunya virus. Int J Mol Sci. (2019) 20:3133. doi: 10.3390/ijms20133133
35. Brennan LJ, Keddie BA, Braig HR, Harris HL. The endosymbiont Wolbachia pipientis induces the expression of host antioxidant proteins in an Aedes albopictus cell line. PloS One. (2008) 3:e2083. doi: 10.1371/journal.pone.0002083
36. Hussain M, Frentiu FD, Moreira LA, O’Neill SL, Asgari S. Wolbachia uses host microRNAs to manipulate host gene expression and facilitate colonization of the dengue vector Aedes aEgypti. Proc Natl Acad Sci United States America. (2011) 108:9250–5. doi: 10.1073/pnas.1105469108
37. Hussain M, O’Neill SL, Asgari S. Wolbachia interferes with the intracellular distribution of Argonaute 1 in the dengue vector Aedes aEgypti by manipulating the host microRNAs. RNA Biol. (2013) 10:1868–75. doi: 10.4161/rna.27392
38. Lindsey AR, Bhattacharya T, Newton IL, Hardy RW. Conflict in the intracellular lives of endosymbionts and viruses: a mechanistic look at Wolbachia-mediated pathogen-blocking. Viruses. (2018) 10:141. doi: 10.3390/v10040141
39. Zheng Y, Wang JL, Liu C, Wang CP, Walker T, Wang YF. Differentially expressed profiles in the larval testes of Wolbachia infected and uninfected Drosophila. BMC Genomics. (2011) 12:595. doi: 10.1186/1471-2164-12-595
40. Birben E, Sahiner UM, Sackesen C, Erzurum S, Kalayci O. Oxidative stress and antioxidant defense. World Allergy Organ J. (2012) 5:9–19. doi: 10.1097/WOX.0b013e3182439613
41. Chen TH, Chiang YH, Hou JN, Cheng CC, Sofiyatun E, Chiu CH, et al. XBP1-mediated biP/GRP78 upregulation copes with oxidative stress in mosquito cells during dengue 2 virus infection. BioMed Res Int. (2017) 2017:3519158. doi: 10.1155/2017/3519158
42. Chen T-H, Tang P, Yang C-F, Kao L-H, Lo Y-P, Chuang C-K, et al. Antioxidant defense is one of the mechanisms by which mosquito cells survive dengue 2 viral infection. Virology. (2011) 410:410–7. doi: 10.1016/j.virol.2010.12.013
43. Lin CC, Yang CF, Tu CH, Huang CG, Shih YT, Chuang CK, et al. A novel tetraspanin C189 upregulated in C6/36 mosquito cells following dengue 2 virus infection. Virus Res. (2007) 124:176–83. doi: 10.1016/j.virusres.2006.11.002
44. Balakrishnan B, Su S, Wang K, Tian R, Chen M. Identification, expression, and regulation of an omega class glutathione S-transferase in rhopalosiphum padi (L.) (Hemiptera: aphididae) under insecticide stress. Front Physiol. (2018) 9:427. doi: 10.3389/fphys.2018.00427
45. Shih YT, Yang CF, Chen WJ. Upregulation of a novel eukaryotic translation initiation factor 5A (eIF5A) in dengue 2 virus-infected mosquito cells. Virol J. (2010) 7:214. doi: 10.1186/1743-422X-7-214
46. Pan X, Pike A, Joshi D, Bian G, McFadden MJ, Lu P, et al. The bacterium Wolbachia exploits host innate immunity to establish a symbiotic relationship with the dengue vector mosquito Aedes aEgypti. ISME J. (2018) 12:277–88. doi: 10.1038/ismej.2017.174
47. Lu P, Bian G, Pan X, Xi Z. Wolbachia induces density-dependent inhibition to dengue virus in mosquito cells. PloS Negl Trop Dis. (2012) 6:e1754. doi: 10.1371/journal.pntd.0001754
48. Hugo LE, Rašić G, Maynard AJ, Ambrose L, Liddington C, Thomas CJ, et al. Wolbachia wAlbB inhibit dengue and Zika infection in the mosquito Aedes aEgypti with an Australian background. PloS Negl Trop Dis. (2022) 16:e0010786. doi: 10.1371/journal.pntd.0010786
49. Liu W-L, Yu H-Y, Chen Y-X, Chen B-Y, Leaw SN, Lin C-H, et al. Lab-scale characterization and semi-field trials of Wolbachia strain wAlbB in a Taiwan Wolbachia introgressed Ae. aEgypti strain. PloS Negl Trop Dis. (2022) 16:e0010084. doi: 10.1371/journal.pntd.0010084
50. Loterio RK, Monson EA, Templin R, de Bruyne JT, Flores HA, Mackenzie JM, et al. Antiviral Wolbachia strains associate with Aedes aEgypti endoplasmic reticulum membranes and induce lipid droplet formation to restrict dengue virus replication. mBio. (2023) 15:e02495–02423. doi: 10.1128/mbio.02495-23
51. Hussain M, Zhang G, Leitner M, Hedges LM, Asgari S. Wolbachia RNase HI contributes to virus blocking in the mosquito Aedes aEgypti. Iscience. (2023) 26:105836. doi: 10.1016/j.isci.2022.105836
52. Axford JK, Ross PA, Yeap HL, Callahan AG, Hoffmann AA. Fitness of wAlbB Wolbachia infection in Aedes aEgypti: parameter estimates in an outcrossed background and potential for population invasion. Am J Trop Med Hygiene. (2016) 94:507–16. doi: 10.4269/ajtmh.15-0608
53. Ahmad NA, Mancini M-V, Ant TH, Martinez J, Kamarul GM, Nazni WA, et al. Wolbachia strain wAlbB maintains high density and dengue inhibition following introduction into a field population of Aedes aEgypti. Philos Trans R Soc B. (2021) 376:20190809. doi: 10.1098/rstb.2019.0809
54. Nazni WA, Hoffmann AA, NoorAfizah A, Cheong YL, Mancini MV, Golding N, et al. Establishment of Wolbachia Strain wAlbB in Malaysian Populations of Aedes aEgypti for Dengue Control. Curr Biol. (2019) 29:4241–4248.e4245. doi: 10.1016/j.cub.2019.11.007
55. Flores HA, Taneja de Bruyne J, O’Donnell TB, Tuyet Nhu V, Thi Giang N, Thi Xuan Trang H, et al. Multiple Wolbachia strains provide comparative levels of protection against dengue virus infection in Aedes aEgypti. PloS Pathog. (2020) 16:e1008433. doi: 10.1371/journal.ppat.1008433
56. Ant TH, Herd CS, Geoghegan V, Hoffmann AA, Sinkins SP. The Wolbachia strain wAu provides highly efficient virus transmission blocking in Aedes aEgypti. PloS Pathog. (2018) 14:e1006815. doi: 10.1371/journal.ppat.1006815
57. Ye YH, Woolfit M, Rances E, O’Neill SL, McGraw EA. Wolbachia-associated bacterial protection in the mosquito Aedes aEgypti. PloS Negl Trop Dis. (2013) 7:e2362. doi: 10.1371/journal.pntd.0002362
58. Frentiu FD, Zakir T, Walker T, Popovici J, Pyke AT, van den Hurk A, et al. Limited dengue virus replication in field-collected Aedes aEgypti mosquitoes infected with Wolbachia. PloS Negl Trop Dis. (2014) 8:e2688. doi: 10.1371/journal.pntd.0002688
59. O’Neill SL, Ryan PA, Turley AP, Wilson G, Retzki K, Iturbe-Ormaetxe I, et al. Scaled deployment of Wolbachia to protect the community from dengue and other Aedes transmitted arboviruses. Gates Open Res. (2018) 2:36. doi: 10.12688/gatesopenres.12844.3
60. Indriani C, Tantowijoyo W, Rancès E, Andari B, Prabowo E, Yusdi D, et al. Reduced dengue incidence following deployments of Wolbachia-infected Aedes aEgypti in Yogyakarta, Indonesia: a quasi-experimental trial using controlled interrupted time series analysis. Gates Open Res. (2020) 4:50. doi: 10.12688/gatesopenres.13122.1
61. Utarini A, Indriani C, Ahmad RA, Tantowijoyo W, Arguni E, Ansari MR, et al. Efficacy of Wolbachia-infected mosquito deployments for the control of dengue. New Engl J Med. (2021) 384:2177–86. doi: 10.1056/NEJMoa2030243
62. Gu X, Ross PA, Rodriguez-Andres J, Robinson KL, Yang Q, Lau MJ, et al. A wMel Wolbachia variant in Aedes aEgypti from field-collected Drosophila melanogaster with increased phenotypic stability under heat stress. Environ Microbiol. (2022) 24:2119–35. doi: 10.1111/1462-2920.15966
63. Pacidônio EC, Caragata EP, Alves DM, Marques JT, Moreira LA. The impact of Wolbachia infection on the rate of vertical transmission of dengue virus in Brazilian Aedes aEgypti. Parasites Vectors. (2017) 10:1–6. doi: 10.1186/s13071-017-2236-z
64. Ferguson NM, Hue Kien DT, Clapham H, Aguas R, Trung VT, Bich Chau TN, et al. Modeling the impact on virus transmission of Wolbachia-mediated blocking of dengue virus infection of Aedes aEgypti. Sci Trans Med. (2015) 7:279ra237–279ra237. doi: 10.1126/scitranslmed.3010370
65. Carrington LB, Tran BCN, Le NTH, Luong TTH, Nguyen TT, Nguyen PT, et al. Field-and clinically derived estimates of Wolbachia-mediated blocking of dengue virus transmission potential in Aedes aEgypti mosquitoes. Proc Natl Acad Sci. (2018) 115:361–6. doi: 10.1073/pnas.1715788115
66. Ryan PA, Turley AP, Wilson G, Hurst TP, Retzki K, Brown-Kenyon J, et al. Establishment of wMel Wolbachia in Aedes aEgypti mosquitoes and reduction of local dengue transmission in Cairns and surrounding locations in northern Queensland, Australia. Gates Open Res. (2019) 3:1547. doi: 10.12688/gatesopenres.13061.2
67. Ford SA, Allen SL, Ohm JR, Sigle LT, Sebastian A, Albert I, et al. Selection on Aedes aEgypti alters Wolbachia-mediated dengue virus blocking and fitness. Nat Microbiol. (2019) 4:1832–9. doi: 10.1038/s41564-019-0533-3
68. Pinto SB, Riback TI, Sylvestre G, Costa G, Peixoto J, Dias FB, et al. Effectiveness of Wolbachia-infected mosquito deployments in reducing the incidence of dengue and other Aedes-borne diseases in Niterói, Brazil: A quasi-experimental study. PloS Negl Trop Dis. (2021) 15:e0009556. doi: 10.1371/journal.pntd.0009556
69. Ribeiro dos Santos G, Durovni B, Saraceni V, Souza Riback TI, Pinto SB, Anders KL, et al. Estimating the impact of the wMel release program on dengue and chikungunya incidence in Rio de Janeiro, Brazil. Medrxiv. (2022) 22:P1587–1595. doi: 10.1016/S1473-3099(22)00436-4
70. Velez ID, Tanamas SK, Arbelaez MP, Kutcher SC, Duque SL, Uribe A, et al. Reduced dengue incidence following city-wide wMel Wolbachia mosquito releases throughout three Colombian cities: Interrupted time series analysis and a prospective case-control study. PloS Negl Trop Dis. (2023) 17:e0011713. doi: 10.1371/journal.pntd.0011713
71. Hue KDT, Goncalves DS, Vi TT, Long VT, Nhu VTT, Giang NT, et al. Wolbachia wMel-mediated effects on dengue virus vertical transmission from Aedes aEgypti to their offspring. Parasites Vectors. (2023) 308:308. doi: 10.1186/s13071-023-05921-y
72. Walker T, Johnson P, Moreira L, Iturbe-Ormaetxe I, Frentiu F, McMeniman C, et al. The wMel Wolbachia strain blocks dengue and invades caged Aedes aEgypti populations. Nature. (2011) 476:450–3. doi: 10.1038/nature10355
73. McMeniman CJ, Lane RV, Cass BN, Fong AW, Sidhu M, Wang Y-F, et al. Stable introduction of a life-shortening Wolbachia infection into the mosquito Aedes aEgypti. science. (2009) 323:141–4. doi: 10.1126/science.1165326
74. Frentiu FD, Robinson J, Young PR, McGraw EA, O’Neill SL. Wolbachia-mediated resistance to dengue virus infection and death at the cellular level. PloS One. (2010) 5:e13398. doi: 10.1371/journal.pone.0013398
75. Foo KY, Chee H-Y. Interaction between flavivirus and cytoskeleton during virus replication. BioMed Res Int. (2015) 2015:427814. doi: 10.1155/2015/427814
76. Sim S, Dimopoulos G. Dengue virus inhibits immune responses in Aedes aEgypti cells. PloS One. (2010) 5:e10678. doi: 10.1371/journal.pone.0010678
77. Guo X, Xu Y, Bian G, Pike AD, Xie Y, Xi Z. Response of the mosquito protein interaction network to dengue infection. BMC Genomics. (2010) 11:380. doi: 10.1186/1471-2164-11-380
78. Paingankar MS, Gokhale MD, Deobagkar DN. Dengue-2-virus-interacting polypeptides involved in mosquito cell infection. Arch Virol. (2010) 155:1453–61. doi: 10.1007/s00705-010-0728-7
79. Lu P, Sun Q, Fu P, Li K, Liang X, Xi Z. Wolbachia inhibits binding of dengue and Zika viruses to mosquito cells. Fronters Microbiol. (2020) 11:1750. doi: 10.3389/fmicb.2020.01750
80. Salazar MI, Richardson JH, Sanchez-Vargas I, Olson KE, Beaty BJ. Dengue virus type 2: replication and tropisms in orally infected Aedes aEgypti mosquitoes. BMC Microbiol. (2007) 7:9. doi: 10.1186/1471-2180-7-9
81. Johnson KN. The impact of wolbachia on virus infection in mosquitoes. Viruses. (2015) 7:5705–17. doi: 10.3390/v7112903
83. Edenborough KM, Flores HA, Simmons CP, Fraser JE. Using Wolbachia to eliminate dengue: Will the virus fight back? J Virol. (2021) 95:e02203–02220. doi: 10.1128/JVI.02203-20
84. Cui Y, Liu P, Mooney BP, Franz AW. Quantitative proteomic analysis of chikungunya virus-infected Aedes aEgypti reveals proteome modulations indicative of persistent infection. J Proteome Res. (2020) 19:2443–56. doi: 10.1021/acs.jproteome.0c00173
85. Geoghegan V, Stainton K, Rainey SM, Ant TH, Dowle AA, Larson T, et al. Perturbed cholesterol and vesicular trafficking associated with dengue blocking in Wolbachia-infected Aedes aEgypti cells. Nat Commun. (2017) 8:526. doi: 10.1038/s41467-017-00610-8
86. Leier HC, Weinstein JB, Kyle JE, Lee J-Y, Bramer LM, Stratton KG, et al. A global lipid map defines a network essential for Zika virus replication. Nat Commun. (2020) 11:3652. doi: 10.1038/s41467-020-17433-9
87. Koh C, Islam MN, Ye YH, Chotiwan N, Graham B, Belisle JT, et al. Dengue virus dominates lipid metabolism modulations in Wolbachia-coinfected Aedes aEgypti. Commun Biol. (2020) 3:518. doi: 10.1038/s42003-020-01254-z
88. White PM, Serbus LR, Debec A, Codina A, Bray W, Guichet A, et al. Reliance of wolbachia on high rates of host proteolysis revealed by a genome-wide RNAi screen of drosophila cells. Genetics. (2017) 205:1473–88. doi: 10.1534/genetics.116.198903
89. Cho K-O, Kim G-W, Lee O-K. Wolbachia bacteria reside in host Golgi-related vesicles whose position is regulated by polarity proteins. PloS One. (2011) 6:e22703. doi: 10.1371/journal.pone.0022703
90. Perera R, Riley C, Isaac G, Hopf-Jannasch AS, Moore RJ, Weitz KW, et al. Dengue virus infection perturbs lipid homeostasis in infected mosquito cells. PloS Pathog. (2012) 8:e1002584. doi: 10.1371/journal.ppat.1002584
91. Caragata EP, Rancès E, O’Neill SL, McGraw EA. Competition for amino acids between Wolbachia and the mosquito host, Aedes aEgypti. Microb Ecol. (2014) 67:205–18. doi: 10.1007/s00248-013-0339-4
92. Manokaran G, Flores HA, Dickson CT, Narayana VK, Kanojia K, Dayalan S, et al. Modulation of acyl-carnitines, the broad mechanism behind Wolbachia-mediated inhibition of medically important flaviviruses in Aedes aEgypti. Proc Natl Acad Sci United States America. (2020) 117:24475–83. doi: 10.1073/pnas.1914814117
93. Haqshenas G, Terradas G, Paradkar PN, Duchemin JB, McGraw EA, Doerig C. A role for the insulin receptor in the suppression of dengue virus and zika virus in wolbachia-infected mosquito cells. Cell Rep. (2019) 26:529–535 e523. doi: 10.1016/j.celrep.2018.12.068
94. Kamtchum-Tatuene J, Makepeace BL, Benjamin L, Baylis M, Solomon T. The potential role of Wolbachia in controlling the transmission of emerging human arboviral infections. Curr Opin Infect Dis. (2017) 30:108–16. doi: 10.1097/QCO.0000000000000342
95. Rances E, Ye YH, Woolfit M, McGraw EA, O’Neill SL. The relative importance of innate immune priming in Wolbachia-mediated dengue interference. PloS Pathog. (2012) 8:e1002548. doi: 10.1371/journal.ppat.1002548
96. Jupatanakul N, Sim S, Anglero-Rodriguez YI, Souza-Neto J, Das S, Poti KE, et al. Engineered aedes aEgypti JAK/STAT pathway-mediated immunity to dengue virus. PloS Negl Trop Dis. (2017) 11:e0005187. doi: 10.1371/journal.pntd.0005187
97. Vargas V, Cime-Castillo J, Lanz-Mendoza H. Immune priming with inactive dengue virus during the larval stage of Aedes aEgypti protects against the infection in adult mosquitoes. Sci Rep. (2020) 10:6723. doi: 10.1038/s41598-020-63402-z
98. Nene V, Wortman JR, Lawson D, Haas B, Kodira C, Tu ZJ, et al. Genome sequence of Aedes aEgypti, a major arbovirus vector. science. (2007) 316:1718–23. doi: 10.1126/science.1138878
99. Weaver SC, Vasilakis N. Molecular evolution of dengue viruses: contributions of phylogenetics to understanding the history and epidemiology of the preeminent arboviral disease. Infect Genet Evol. (2009) 9:523–40. doi: 10.1016/j.meegid.2009.02.003
100. Pan X, Zhou G, Wu J, Bian G, Lu P, Raikhel AS, et al. Wolbachia induces reactive oxygen species (ROS)-dependent activation of the Toll pathway to control dengue virus in the mosquito Aedes aEgypti. Proc Natl Acad Sci United States America. (2012) 109:E23–31. doi: 10.1073/pnas.1116932108
101. Carvalho-Leandro D, Ayres C, Guedes D, Suesdek L, Melo-Santos M, Oliveira CF, et al. Immune transcript variations among Aedes aEgypti populations with distinct susceptibility to dengue virus serotype 2. Acta Tropica. (2012) 124:113–9. doi: 10.1016/j.actatropica.2012.07.006
102. Souza-Neto JA, Powell JR, Bonizzoni M. Aedes aEgypti vector competence studies: A review. Infect Genet Evol. (2019) 67:191–209. doi: 10.1016/j.meegid.2018.11.009
103. Caicedo PA, Serrato IM, Sim S, Dimopoulos G, Coatsworth H, Lowenberger C, et al. Immune response-related genes associated to blocking midgut dengue virus infection in Aedes aEgypti strains that differ in susceptibility. Insect Sci. (2019) 26:635–48. doi: 10.1111/1744-7917.12573
104. Bonizzoni M, Dunn WA, Campbell CL, Olson KE, Marinotti O, James AA. Complex modulation of the Aedes aEgypti transcriptome in response to dengue virus infection. PloS One. (2012) 7:e50512. doi: 10.1371/journal.pone.0050512
105. Luplertlop N, Surasombatpattana P, Patramool S, Dumas E, Wasinpiyamongkol L, Saune L, et al. Induction of a peptide with activity against a broad spectrum of pathogens in the Aedes aEgypti salivary gland, following Infection with Dengue Virus. PloS Pathog. (2011) 7:e1001252. doi: 10.1371/journal.ppat.1001252
106. Sim S, Ramirez JL, Dimopoulos G. Dengue virus infection of the Aedes aEgypti salivary gland and chemosensory apparatus induces genes that modulate infection and blood-feeding behavior. PloS Pathog. (2012) 8:e1002631. doi: 10.1371/journal.ppat.1002631
107. DeLotto Y, DeLotto R. Proteolytic processing of the Drosophila Spatzle protein by easter generates a dimeric NGF-like molecule with ventralising activity. Mech Dev. (1998) 72:141–8. doi: 10.1016/s0925-4773(98)00024-0
108. Pompon J, Manuel M, Ng GK, Wong B, Shan C, Manokaran G, et al. Dengue subgenomic flaviviral RNA disrupts immunity in mosquito salivary glands to increase virus transmission. PloS Pathog. (2017) 13:e1006535. doi: 10.1371/journal.ppat.1006535
109. Kokoza V, Ahmed A, Woon Shin S, Okafor N, Zou Z, Raikhel AS. Blocking of Plasmodium transmission by cooperative action of Cecropin A and Defensin A in transgenic Aedes aEgypti mosquitoes. Proc Natl Acad Sci United States America. (2010) 107:8111–6. doi: 10.1073/pnas.1003056107
110. Kaneko T, Silverman N. Bacterial recognition and signalling by the Drosophila IMD pathway. Cell Microbiol. (2005) 7:461–9. doi: 10.1111/j.1462-5822.2005.00504.x
111. Stöven S, Silverman N, Junell A, Hedengren-Olcott M, Erturk D, Engström Y, et al. Caspase-mediated processing of the Drosophila NF-κB factor Relish. Proc Natl Acad Sci. (2003) 100:5991–6. doi: 10.1073/pnas.1035902100
112. Avadhanula V, Weasner BP, Hardy GG, Kumar JP, Hardy RW. A novel system for the launch of alphavirus RNA synthesis reveals a role for the Imd pathway in arthropod antiviral response. PloS Pathog. (2009) 5:e1000582. doi: 10.1371/journal.ppat.1000582
113. Xi Z, Gavotte L, Xie Y, Dobson SL. Genome-wide analysis of the interaction between the endosymbiotic bacterium Wolbachia and its Drosophila host. BMC Genomics. (2008) 9:1–12. doi: 10.1186/1471-2164-9-1
114. Souza-Neto JA, Sim S, Dimopoulos G. An evolutionary conserved function of the JAK-STAT pathway in anti-dengue defense. Proc Natl Acad Sci United States America. (2009) 106:17841–6. doi: 10.1073/pnas.0905006106
115. Bourtzis K, Pettigrew M, O’Neill SL. Wolbachia neither induces nor suppresses transcripts encoding antimicrobial peptides. Insect Mol Biol. (2000) 9:635–9. doi: 10.1046/j.1365-2583.2000.00224.x
116. Kawahara T, Quinn MT, Lambeth JD. Molecular evolution of the reactive oxygen-generating NADPH oxidase (Nox/Duox) family of enzymes. BMC Ecol Evol. (2007) 7:109. doi: 10.1186/1471-2148-7-109
117. Kumar S, Molina-Cruz A, Gupta L, Rodrigues J, Barillas-Mury C. A peroxidase/dual oxidase system modulates midgut epithelial immunity in Anopheles Gambiae. science. (2010) 327:1644–8. doi: 10.1126/science.1184008
118. Morgan MJ, Liu ZG. Crosstalk of reactive oxygen species and NF-kappaB signaling. Cell Res. (2011) 21:103–15. doi: 10.1038/cr.2010.178
119. Merkling SH, van Rij RP. Beyond RNAi: antiviral defense strategies in Drosophila and mosquito. J Insect Physiol. (2013) 59:159–70. doi: 10.1016/j.jinsphys.2012.07.004
120. Lee YR, Lei HY, Liu MT, Wang JR, Chen SH, Jiang-Shieh YF, et al. Autophagic machinery activated by dengue virus enhances virus replication. Virology. (2008) 374:240–8. doi: 10.1016/j.virol.2008.02.016
121. Heaton NS, Randall G. Dengue virus-induced autophagy regulates lipid metabolism. Cell Host Microbe. (2010) 8:422–32. doi: 10.1016/j.chom.2010.10.006
122. Niu H, Xiong Q, Yamamoto A, Hayashi-Nishino M, Rikihisa Y. Autophagosomes induced by a bacterial Beclin 1 binding protein facilitate obligatory intracellular infection. Proc Natl Acad Sci. (2012) 109:20800–7. doi: 10.1073/pnas.1218674109
123. Voronin D, Cook DA, Steven A, Taylor MJ. Autophagy regulates Wolbachia populations across diverse symbiotic associations. Proc Natl Acad Sci United States America. (2012) 109:E1638–1646. doi: 10.1073/pnas.1203519109
124. Asad S, Hussain M, Hugo L, Osei-Amo S, Zhang G, Watterson D, et al. Suppression of the pelo protein by Wolbachia and its effect on dengue virus in Aedes aEgypti. PloS Negl Trop Dis. (2018) 12:e0006405. doi: 10.1371/journal.pntd.0006405
125. Blair CD. Mosquito RNAi is the major innate immune pathway controlling arbovirus infection and transmission. Future Microbiol. (2011) 6:265–77. doi: 10.2217/fmb.11.11
126. Sanchez-Vargas I, Scott JC, Poole-Smith BK, Franz AW, Barbosa-Solomieu V, Wilusz J, et al. Dengue virus type 2 infections of Aedes aEgypti are modulated by the mosquito’s RNA interference pathway. PloS Pathog. (2009) 5:e1000299. doi: 10.1371/journal.ppat.1000299
127. Dobson SL, Bourtzis K, Braig HR, Jones BF, Zhou W, Rousset F, et al. Wolbachia infections are distributed throughout insect somatic and germ line tissues. Insect Biochem Mol Biol. (1999) 29:153–60. doi: 10.1016/s0965-1748(98)00119-2
128. Molloy JC, Sommer U, Viant MR, Sinkins SP. Wolbachia modulates lipid metabolism in aedes albopictus mosquito cells. Appl Environ Microbiol. (2016) 82:3109–20. doi: 10.1128/AEM.00275-16
129. Stouthamer R, Luck R. Influence of microbe-associated parthenogenesis on the fecundity of Trichogramma deion and T. pretiosum. Entomol Experimentalis Applicata. (1993) 67:183–92. doi: 10.1111/j.1570-7458.1993.tb01667.x
130. Asad S, Hall-Mendelin S, Asgari S. Downregulation of Aedes aEgypti chromodomain helicase DNA binding protein 7/Kismet by Wolbachia and its effect on dengue virus replication. Scencei Rep. (2016) 6:36850. doi: 10.1038/srep36850
131. Asad S, Parry R, Asgari S. Upregulation of Aedes aEgypti Vago1 by Wolbachia and its effect on dengue virus replication. Insect Biochem Mol Biol. (2018) 92:45–52. doi: 10.1016/j.ibmb.2017.11.008
132. Lapidot M, Karniel U, Gelbart D, Fogel D, Evenor D, Kutsher Y, et al. A novel route controlling begomovirus resistance by the messenger RNA surveillance factor pelota. PloS Genet. (2015) 11:e1005538. doi: 10.1371/journal.pgen.1005538
133. Wu X, He W-T, Tian S, Meng D, Li Y, Chen W, et al. Pelo is required for high efficiency viral replication. PloS Pathog. (2014) 10:e1004034. doi: 10.1371/journal.ppat.1004034
134. Shamsadin R, Adham IM, von Beust G, Engel W. Molecular cloning, expression and chromosome location of the human pelota gene PELO. Cytogenet Cell Genet. (2000) 90:75–8. doi: 10.1159/000015667
135. Feng X, Zhou S, Wang J, Hu W. microRNA profiles and functions in mosquitoes. PloS Negl Trop Dis. (2018) 12:e0006463. doi: 10.1371/journal.pntd.0006463
136. Zhang G, Hussain M, O’Neill SL, Asgari S. Wolbachia uses a host microRNA to regulate transcripts of a methyltransferase, contributing to dengue virus inhibition in Aedes aEgypti. Proc Natl Acad Sci United States America. (2013) 110:10276–81. doi: 10.1073/pnas.1303603110
137. Slonchak A, Hussain M, Torres S, Asgari S, Khromykh AA. Expression of mosquito microRNA Aae-miR-2940-5p is downregulated in response to West Nile virus infection to restrict viral replication. J Virol. (2014) 88:8457–67. doi: 10.1128/JVI.00317-14
138. Osei-Amo S, Hussain M, O’Neill SL, Asgari S. Wolbachia-induced aae-miR-12 miRNA negatively regulates the expression of MCT1 and MCM6 genes in Wolbachia-infected mosquito cell line. PloS One. (2012) 7:e50049. doi: 10.1371/journal.pone.0050049
139. Velentzas PD, Zhang L, Das G, Chang T-K, Nelson C, Kobertz WR, et al. The proton-coupled monocarboxylate transporter hermes is necessary for autophagy during cell death. Dev Cell. (2018) 47:281–293. e284. doi: 10.1016/j.devcel.2018.09.015
140. Ji W, Luo G. Zika virus NS5 nuclear accumulation is protective of protein degradation and is required for viral RNA replication. Virology. (2020) 541:124–35. doi: 10.1016/j.virol.2019.10.010
141. Pryor MJ, Rawlinson SM, Butcher RE, Barton CL, Waterhouse TA, Vasudevan SG, et al. Nuclear localization of dengue virus nonstructural protein 5 Through Its Importin α/β–recognized nuclear localization sequences is integral to viral infection. Traffic (Copenhagen Denmark). (2007) 8:795–807. doi: 10.1111/j.1600-0854.2007.00579.x
142. Baldridge GD, Baldridge AS, Witthuhn BA, Higgins L, Markowski TW, Fallon AM. Proteomic profiling of a robust Wolbachia infection in an Aedes albopictus mosquito cell line. Mol Microbiol. (2014) 94:537–56. doi: 10.1111/mmi.12768
143. Sim S, Jupatanakul N, Ramirez JL, Kang S, Romero-Vivas CM, Mohammed H, et al. Transcriptomic profiling of diverse Aedes aEgypti strains reveals increased basal-level immune activation in dengue virus-refractory populations and identifies novel virus-vector molecular interactions. PloS Negl Trop Dis. (2013) 7:e2295. doi: 10.1371/journal.pntd.0002295
144. Rodriguez-Andres J, Rani S, Varjak M, Chase-Topping ME, Beck MH, Ferguson MC, et al. Phenoloxidase activity acts as a mosquito innate immune response against infection with Semliki Forest virus. PloS Pathog. (2012) 8:e1002977. doi: 10.1371/journal.ppat.1002977
145. Thomas P, Kenny N, Eyles D, Moreira LA, O’Neill SL, Asgari S. Infection with the wMel and wMelPop strains of Wolbachia leads to higher levels of melanization in the hemolymph of Drosophila melanogaster, Drosophila simulans and Aedes aEgypti. Dev Comp Immunol. (2011) 35:360–5. doi: 10.1016/j.dci.2010.11.007
146. Rainey SM, Shah P, Kohl A, Dietrich I. Understanding the Wolbachia-mediated inhibition of arboviruses in mosquitoes: progress and challenges. J Gen Virol. (2014) 95:517–30. doi: 10.1099/vir.0.057422-0
147. Hoffmann AA, Ross PA, Rasic G. Wolbachia strains for disease control: ecological and evolutionary considerations. Evolutionary Applications. (2015) 8(8):751–68.
148. Indriani C, Tanamas SK, Khasanah U, Ansari MR, Rubangi Tantowijoyo W. Impact of randomised wmel Wolbachia deployments on notified dengue cases and insecticide fogging for dengue control in Yogyakarta City. Glob Health Action. (2023) 16(1):2166650.
149. Crawford JE, Clarke DW, Criswell V, Desnoyer M, Cornel D, Deegan B, et al. Efficient production of male Wolbachia-infected Aedes aegypti mosquitoes enables large-scale suppression of wild populations. Nature Biotechnology. (2020) 38(4):482–92.
150. Zheng X, Zhang D, Li Y, Yang C, Wu Y, Liang X, et al. Incompatible and sterile insect techniques combined eliminate mosquitoes. Nature. (2019) 572(7767):56–61.
151. Tantowijoyo W, Tanamas SK, Nurhayati I, Setyawan S, Budiwati N, Fitriana I, et al. Aedes aegypti abundance and insecticide resistance profiles in the applying Wolbachia to eliminate dengue trial. PLoS Neglected Tropical Diseases. (2022) 16(4):e0010284.
152. Dutra HL, Rocha MN, Dias FB, Mansur SB, Caragata EP, Moreira LA. Wolbachia blocks currently circulating zika virus isolates in Brazilian aedes aEgypti mosquitoes. Cell Host Microbe. (2016) 19:771–4. doi: 10.1016/j.chom.2016.04.021
153. Hussain M, Lu G, Torres S, Edmonds JH, Kay BH, Khromykh AA, et al. Effect of Wolbachia on replication of West Nile virus in a mosquito cell line and adult mosquitoes. J Virol. (2013) 87:851–8. doi: 10.1128/JVI.01837-12
154. Hughes H, Britton NF. Modelling the use of Wolbachia to control dengue fever transmission. Bull Math Biol. (2013) 75:796–818. doi: 10.1007/s11538-013-9835-4
155. McMeniman CJ, Lane AM, Fong AW, Voronin DA, Iturbe-Ormaetxe I, Yamada R, et al. Host adaptation of a Wolbachia strain after long-term serial passage in mosquito cell lines. Appl Environ Microbiol. (2008) 74:6963–9. doi: 10.1128/AEM.01038-08
156. Zhang D, Wang Y, He K, Yang Q, Gong M, Ji M, et al. Wolbachia limits pathogen infections through induction of host innate immune responses. PloS One. (2020) 15:e0226736. doi: 10.1371/journal.pone.0226736
157. Turley AP, Moreira LA, O’Neill SL, McGraw EA. Wolbachia infection reduces blood-feeding success in the dengue fever mosquito, Aedes aEgypti. PloS Negl Trop Dis. (2009) 3:e516. doi: 10.1371/journal.pntd.0000516
158. Walker T, Johnson PH, Moreira LA, Iturbe-Ormaetxe I, Frentiu FD, McMeniman CJ, et al. The wMel Wolbachia strain blocks dengue and invades caged Aedes aegypti populations. Nature. (2011) 476(7361):450–3.
159. Chen TY, Smartt CT. Activation of the autophagy pathway decreases dengue virus infection in Aedes aEgypti cells. Parasites Vectors. (2021) 14:551. doi: 10.1186/s13071-021-05066-w
160. Carrington LB, Tran BCN, Le NTH, Luong TTH, Nguyen TT, Nguyen PT, et al. Field- and clinically derived estimates of Wolbachia-mediated blocking of dengue virus transmission potential in Aedes aegypti mosquitoes. Proceedings of the National Academy of Sciences of the United States of America. (2018) 115(2):361–6.
Keywords: dengue virus, Aedes aegypti, Wolbachia, innate immune pathways, toll pathway, IMD pathway, JAK/STAT pathway
Citation: Mushtaq I, Sarwar MS and Munzoor I (2024) A comprehensive review of Wolbachia-mediated mechanisms to control dengue virus transmission in Aedes aegypti through innate immune pathways. Front. Immunol. 15:1434003. doi: 10.3389/fimmu.2024.1434003
Received: 17 May 2024; Accepted: 16 July 2024;
Published: 08 August 2024.
Edited by:
Luwen Zhang, University of Nebraska-Lincoln, United StatesReviewed by:
André Alves Dias, Oswaldo Cruz Foundation (Fiocruz), BrazilKíssila Rabelo, Rio de Janeiro State University, Brazil
Copyright © 2024 Mushtaq, Sarwar and Munzoor. This is an open-access article distributed under the terms of the Creative Commons Attribution License (CC BY). The use, distribution or reproduction in other forums is permitted, provided the original author(s) and the copyright owner(s) are credited and that the original publication in this journal is cited, in accordance with accepted academic practice. No use, distribution or reproduction is permitted which does not comply with these terms.
*Correspondence: Muhammad Sajjad Sarwar, bXNzcmF2aWFuQGdtYWlsLmNvbQ==
†These authors have contributed equally to this work and share first authorship