- 1Stem Cell Transplant and Cellular Immunotherapy Program, Georgetown Lombardi Comprehensive Cancer Center, Washington, DC, United States
- 2Center for Gene and Cellular Immunotherapy, Washington University in Saint Louis, Saint Louis, MO, United States
Chimeric antigen receptor (CAR) T-cell therapy has revolutionized the treatment of hematologic malignancies, offering remarkable remission rates in otherwise refractory conditions. However, its expansion into broader oncological applications faces significant hurdles, including limited efficacy in solid tumors, safety concerns related to toxicity, and logistical challenges in manufacturing and scalability. This review critically examines the latest advancements aimed at overcoming these obstacles, highlighting innovations in CAR T-cell engineering, novel antigen targeting strategies, and improvements in delivery and persistence within the tumor microenvironment. We also discuss the development of allogeneic CAR T cells as off-the-shelf therapies, strategies to mitigate adverse effects, and the integration of CAR T cells with other therapeutic modalities. This comprehensive analysis underscores the synergistic potential of these strategies to enhance the safety, efficacy, and accessibility of CAR T-cell therapies, providing a forward-looking perspective on their evolutionary trajectory in cancer treatment.
1 Introduction
The concept of chimeric antigen receptor (CAR) T-cell therapy represents a transformative advance in cancer immunotherapy, bridging the innate power of cellular immunity with the precision of molecular targeting. The genesis of this revolutionary therapy dates back to the late eighties when the first CAR was engineered (1, 2), signifying the inception of a new era in targeted cancer therapy. These early constructs laid the foundational framework for what would become a series of iterative and transformative advancements in the field (3).
Over the ensuing decades, CAR T-cell therapy has evolved dramatically, propelled by significant technological innovations and a deeper understanding of cancer immunology. Initial clinical successes were most notable in hematologic malignancies, such as acute lymphoblastic leukemia (4, 5) and diffuse large B-cell lymphoma (6), where remission rates previously unattainable with traditional therapies were achieved. This was evidenced by landmark clinical trials that demonstrated profound responses in patients resistant to conventional treatments, establishing CAR T-cells as a pillar of modern oncological therapy (7–12).
Despite these impressive outcomes, expanding CAR T-cell therapy to broader oncological applications, particularly solid tumors, has encountered significant challenges (13). Key obstacles include the immunosuppressive tumor microenvironment (TME), antigen escape variants, and the physical barriers that impede CAR T-cell infiltration and function (13). Moreover, systemic toxicities such as cytokine release syndrome (CRS) and neurotoxicity pose severe risks, limiting the therapy’s widespread application (14, 15). Furthermore, the individualized manufacturing process for CAR T-cell therapy introduces logistical and economic hurdles (16), including high costs and variability in the quality of patients’ T cells.
Recognizing these hurdles, the scientific community has embarked on a quest to refine and evolve CAR T-cell strategies to overcome these barriers. This review examines emerging strategies shaping the future of CAR T-cell therapy, including next-generation CAR constructs, improved manufacturing processes, and novel combination therapies. It highlights the potential of these strategies to broaden the applicability of CAR T-cell therapies across a wider range of cancers providing a forward-looking perspective on their evolution in cancer treatment. Additionally, this review critically analyzes the field’s current gaps, controversies, and future directions, emphasizing the multifaceted challenges and opportunities in advancing CAR T-cell therapies Table 1 and Figure 1.
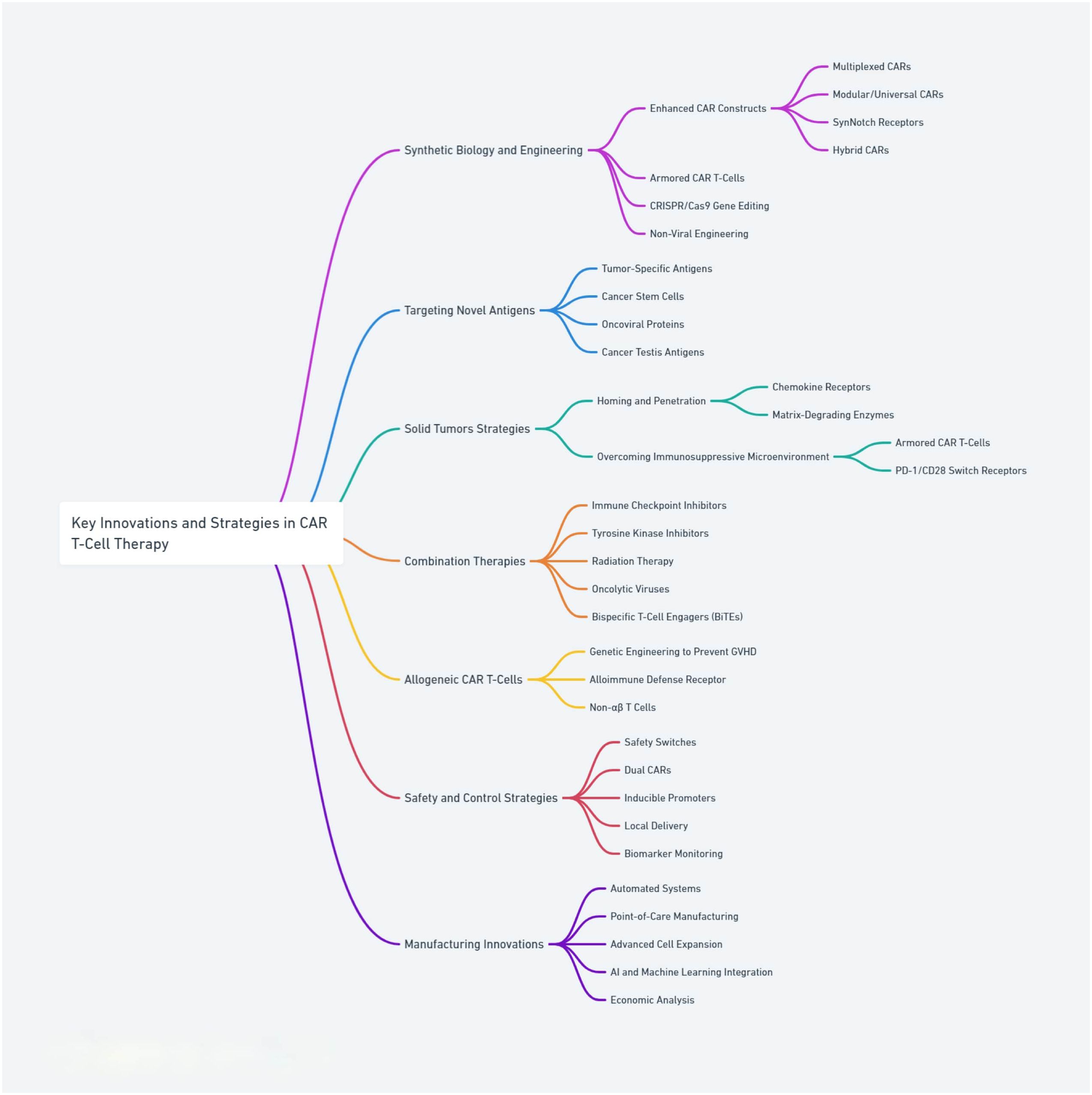
Figure 1. Core strategies and innovations in CAR T-cell therapies, highlighting advancements in design, engineering, and safety features.
2 Core strategies and innovations in CAR T-cell therapy
2.1 Synthetic biology and engineering: redefining CAR T-cell design and manufacturing
Despite its promise, the efficacy of CAR T-cell therapy remains limited, with durable remissions being achieved in only 40% of diffuse large B-cell lymphoma patients (17). Enhancing the design of chimeric antigen receptor (CAR) constructs is a critical area of research aimed at augmenting the efficacy of CAR T-cell therapies. This effort involves the engineering of CARs with advanced signaling capabilities and the integration of safety features to address the limitations observed with first-and second-generation CAR T cells (18).
A range of innovative strategies focusing on the refinement of CAR constructs and optimization of manufacturing protocols have emerged. For example, multiplexed CARs targeting multiple tumor-associated antigens aim to forestall tumor escape mechanisms by addressing antigen loss or heterogeneity (19, 20). Similarly, the development of “modular” or “universal” CAR systems offers adaptable targeting capabilities through switchable, bispecific adaptors, enhancing the precision of CAR T-cell engagement with diverse tumor antigens (21–26). Furthermore, synthetic Notch (SynNotch) receptors represent a cutting-edge advancement in CAR T cell engineering, employing a dual antigen recognition strategy for activation. This novel approach uses SynNotch receptors to initiate transcription of a CAR after interacting with a predefined primary antigen, ensuring activation is strictly tumor specific (27–29). This two-step activation process allows for more precise tumor targeting and minimizes off-tumor activity, enhancing safety and reducing potential toxicities associated with conventional CAR T cell therapies. In addition to these innovations, Hybrid CARs technologies combine features from both T-cell receptors (TCRs) and CARs to enhance the targeting of cancer-specific antigens and reduce tonic signaling, enabling the engineered cells to recognize and engage with intracellular antigens presented at ultra-low densities on cancer cells by MHC molecules (30–36). This advancement will potentially broaden the therapeutic applicability of CAR technology, allowing it to target the entire proteome of a cancer cell.
Advancements in CAR T-cell design have led to the development of “third generation” CAR T cells, which represent a next-generation approach aiming to enhance the efficacy and longevity of these therapies. By integrating multiple co-stimulatory molecules into the CAR structure, these sophisticated constructs are designed to provide enhanced activation and sustained support to the CAR T cells (37, 38). Additionally, “armored” CAR T cells are genetically modified to enhance their efficacy and persistence. These modifications allow armored CAR T-cells to secrete active cytokines or express other pro-inflammatory ligands to enhance their anti-tumor activity, helping them better survive, disrupt, and modulate the tumor microenvironment, improving their function in hostile conditions (39–41).
In the pursuit of enhancing the efficacy and persistence of CAR T-cell therapies, researchers have innovated and refined manufacturing protocols. The integration of CRISPR/Cas9 gene editing represents a revolutionary stride in CAR T cell manufacturing. This precise gene-editing technology allows for the specific deletion of CAR T genes in an effort to bolster CAR T cell persistence and functionality, such as the knockout of genes encoding inhibitory receptors (42), epigenetic modifiers (43), and those mediating CAR T exhaustion (44). In parallel, advancements in vector technology have substantially improved the delivery of genetic material into T cells (45). Viral vectors, known for their high efficiency in gene delivery, have been optimized to enhance transduction rates while minimizing the risk of insertional oncogenesis—a concern with earlier-generation vectors especially retroviral vectors (46). Modern lentiviral and retroviral vectors offer stable gene transfer, which is crucial for the long-term expression of CAR genes (47). Non-viral engineering strategies have also progressed, offering more scalable and potentially safer alternatives to viral methods (48). These include transposon-based systems like Sleeping Beauty (49), which enable stable gene integration, and mRNA electroporation (50), which provides a transient expression that can be advantageous for safety. Additionally, non-viral methods such as the CRISPR complex delivery system for manufacturing CAR T cells offers enhanced safety by avoiding viral vector risks and ensuring precise gene editing without affecting other genome areas, thus minimizing off-target effects. The technology is also versatile, allowing for both gene knock-ins and knock-outs, enhancing the functional capabilities of CAR T cells and their therapeutic efficacy and safety (51, 52) Table 2.
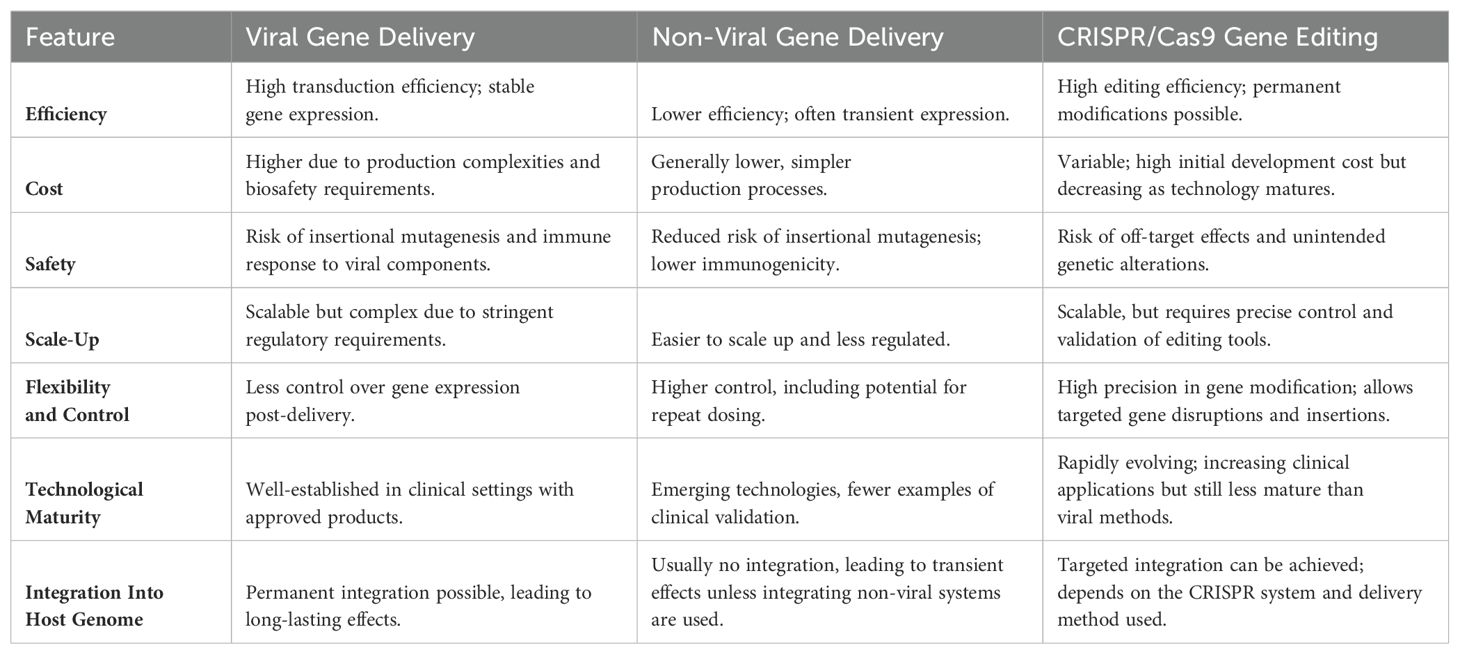
Table 2. Comparison of viral, non-viral gene delivery, and CRISPR/Cas9 Gene Editing in CAR T-Cell Therapy.
The strategic selection of specific T cell subsets is pivotal in honing the efficacy of CAR T cell therapies. Central memory T cells (Tcm) and stem cell-like memory T cells (Tscm) are being employed due to their inherent longevity, robust proliferative abilities, and potent antitumor responses (53). These cells are known for their self-renewal capacity and long-term memory, providing a persistent immunological presence against tumors. Leveraging these subsets is instrumental in creating a pool of CAR T cells with superior proliferative capacity, long-term persistence, and potent antitumor activity (54, 55). Additionally, the inclusion of both CD4+ and CD8+ T cells could further enhance the therapeutic potential of CAR T-cell constructs. CD4+ T cells, often termed helper T cells, are crucial for their supportive role in immune modulation and enhancing the function of CD8+ T cells, which are primarily responsible for executing cytotoxic actions against tumor cells. Incorporating both subtypes not only facilitates a robust and sustained antitumor immune response but also capitalizes on the synergistic interactions between them to maximize therapeutic outcomes (56–58) Table 3.
Interdisciplinary approaches are crucial in advancing CAR T-cell therapy by integrating insights from bioinformatics, materials science, immunology, single-cell studies, and various omics technologies. Bioinformatics plays a pivotal role in analyzing large-scale genomic and proteomic data to identify new targets (59) and understand complex cellular behaviors (60, 61). Furthermore, single-cell technologies and omics analyses enable the detailed study of individual cell behaviors and responses, providing a more nuanced understanding of the variability and efficacy of CAR T-cell therapies (62). These interdisciplinary efforts are vital for tailoring therapies to individual patients’ unique biological contexts, ultimately enhancing the precision and effectiveness of CAR T-cell treatments (Figure 2).
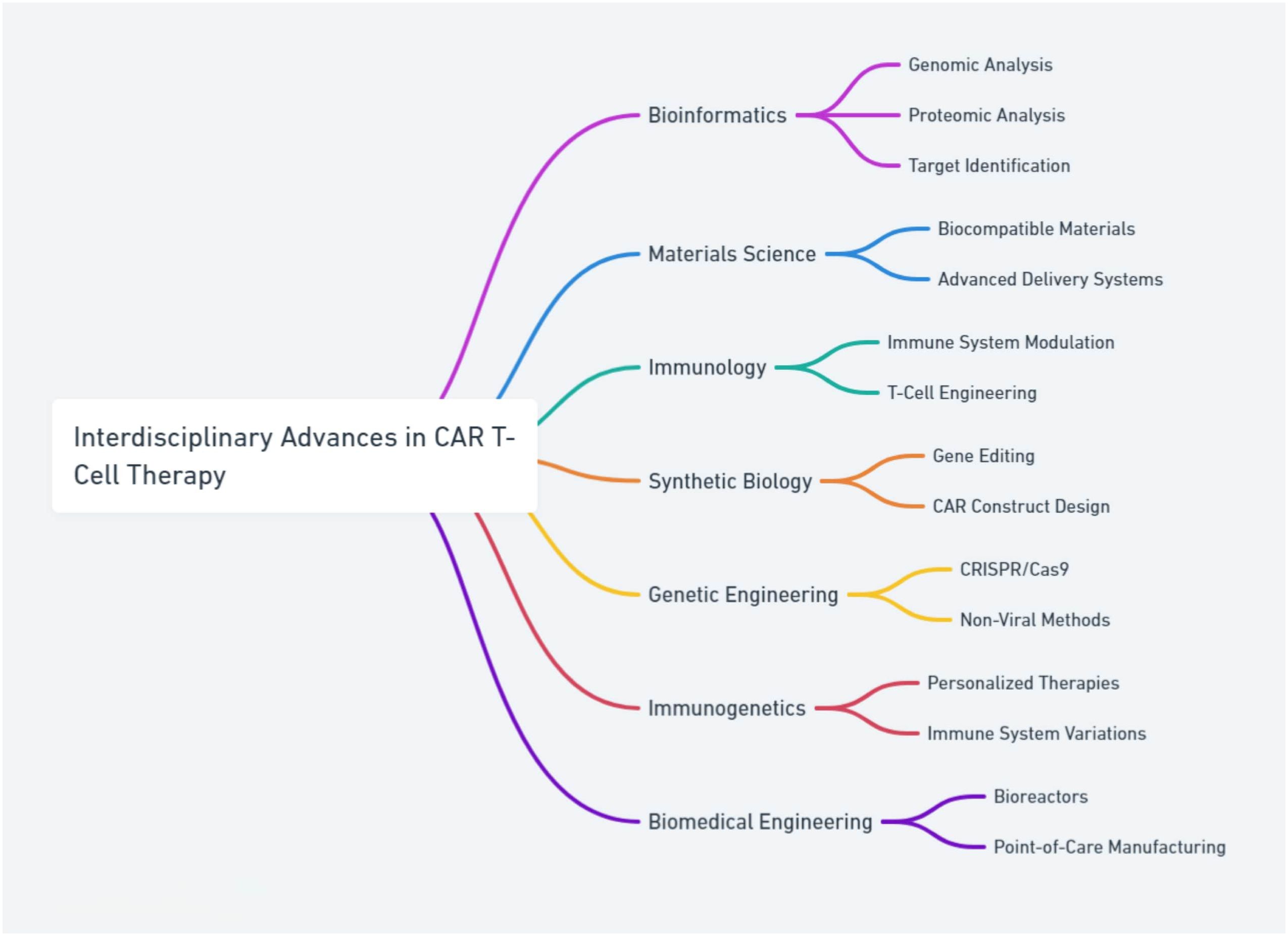
Figure 2. The integration of interdisciplinary approaches in advancing CAR T-cell therapy, combining insights from bioinformatics, materials science, immunology, single-cell studies, and omics technologies to enhance the precision and effectiveness of treatments.
The integration of synthetic biology and advanced engineering in CAR T-cell therapy brings forth transformative prospects for improving therapeutic efficacy and safety. However, this progress is accompanied by substantial challenges and controversies. Innovations such as multiplexed, modular, and SynNotch CAR systems provide unprecedented precision in tumor targeting, potentially reducing off-target effects and enhancing treatment specificity. Despite these advancements, the complexity and novel nature of these designs prompt concerns about their predictability and consistent performance in diverse clinical scenarios. The scientific community continues to debate the ideal balance between the sophistication of these designs and the practicalities of manufacturing, scalability, and regulatory approvals. Ethical considerations and the dynamic nature of regulatory standards further complicate the rapid adoption and implementation of these advanced therapies. Importantly, there is a critical need for comprehensive clinical trials to rigorously evaluate the long-term safety and efficacy of these next-generation CAR T cells. Such trials are essential to ensure that these innovative treatments can be safely integrated into clinical practice and can deliver sustained benefits to patients. As research progresses, streamlining manufacturing processes and establishing robust and more cost-effective regulatory frameworks will be pivotal in overcoming current limitations. Together, these steps will help to unlock the full potential of CAR T-cell therapies, extending their benefits beyond hematologic malignancies to include the effective treatment of solid tumors.
2.2 Expanding the horizon: targeting novel antigens for enhanced specificity and efficacy in CAR T-cell therapy
In the advancement of CAR T-cell therapy, a key focus has been on identifying and targeting new antigens to expand the therapy’s efficacy across diverse cancer types. Researchers are exploring tumor-specific antigens (TSAs) and neoantigens that are unique to cancer cells, minimizing off-target effects and personalizing treatment (63–67). Oncoviral proteins (68, 69) and cancer testis antigens (CTAs) and other embryonic antigens (70, 71) provide new targets, especially in cancers associated with viral infections and limited normal tissue expression, respectively.
Targeting cancer stem cells (CSCs) with CAR T cell therapy is an emerging strategy aimed at eradicating the root of tumor regrowth and metastasis. CSCs are elusive targets due to their low abundance and expression of conventional antigens, but their role in driving tumor progression and recurrence makes them logical targets (72, 73). CAR T cells are being engineered to recognize CSC-specific antigens to selectively target and eliminate these progenitor cells, potentially leading to more durable responses and reducing the likelihood of cancer relapse (74–77).
The pursuit of targeting novel antigens in CAR T-cell therapy represents a cutting-edge approach that holds promise for enhancing treatment specificity and expanding efficacy across diverse cancer types. While the use of tumor-specific antigens and neoantigens offers a pathway toward more personalized and less toxic treatments, significant challenges remain. Identifying and validating effective targets that are unique to cancer cells without affecting normal tissue is complex and requires rigorous testing. Furthermore, the targeting of cancer stem cells, though promising for preventing recurrence, presents issues of selectivity and safety due to their low abundance and heterogeneity. Continued advancements in antigen discovery, coupled with an integrative approach that combines CAR T-cell therapy with other treatment modalities, are critical for overcoming current limitations and fully realizing the potential of this powerful therapeutic tool.
2.3 Strategies to enhance CAR T-cell therapy in solid tumors: homing, penetration, and overcoming immunosuppressive microenvironment
Addressing the formidable challenges posed by solid tumors necessitates innovative strategies in CAR T-cell therapy, focusing on two critical areas: enhancing homing and penetration, and overcoming the immunosuppressive tumor microenvironment (TME). Solid tumors present a unique set of barriers, including a dense extracellular matrix and a hostile TME characterized by immune-suppressive cells and factors. To improve CAR T-cell homing, research has pivoted towards engineering CAR T cells to express specific chemokine receptors that match the chemokines secreted by tumors, thereby enhancing their trafficking and infiltration capabilities (78, 79). Furthermore, modifications are being explored to facilitate penetration through the tumor’s dense matrix by incorporating matrix-degrading enzymes into CAR T-cell designs (80, 81). Another emerging strategy is targeting tumor vasculature with CAR T cells engineered to attack specific endothelial markers which offers a dual benefit: direct tumor starvation and enhanced immune cell infiltration, boosting antitumor efficacy (82).
Simultaneously, strategies to counteract the immunosuppressive effects of the TME are critical. This includes the development of “armored” CAR T cells capable of secreting cytokines to modulate the TME, making it more conducive to T-cell activity, as discussed in the previous section (39–41). Additionally, various genetic modifications are being considered, including the design of PD-1–CD28 switch receptors (83), which are engineered to convert inhibitory signals into stimulatory ones, and the knockdown of intracellular inhibitors (84) to maintain CAR T-cell activation and effector functions. Metabolic adaptation is also key, where CAR T cells are engineered to withstand the nutrient deprived and hypoxic TME (85). Enhancements to the CAR T metabolic pathways enable them to maintain functionality and survive in the harsh tumor conditions (86, 87). In addition, there’s a growing interest in inhibiting tumor-derived exosomes, which often carry immunosuppressive molecules (88). By preventing these exosomes from reaching and impairing CAR T cells, it’s possible to preserve the T cells’ vigor and anti-tumor activity, further bolstering their effectiveness against solid tumors.
Local delivery of CAR T cells is an innovative method where CAR T cells are administered directly into the tumor site, offering a concentrated attack while potentially reducing systemic toxicity (89, 90). This targeted approach may improve the efficiency of CAR T-cell penetration and functionality within the solid tumor microenvironment. Advancing this concept, researchers are leveraging nanotechnology to develop novel delivery systems. These nano-carriers can protect CAR T cells during transit, enhance their migration and infiltration into tumors, and provide controlled release mechanisms, which could lead to improved persistence and efficacy of the CAR T cells (91, 92).
The innovative strategies being developed to enhance CAR T-cell therapy in solid tumors—focusing on improved homing, penetration, and overcoming the immunosuppressive microenvironment—represent significant advances in the field. While engineering CAR T cells to express specific chemokine receptors and matrix-degrading enzymes shows promise in enhancing infiltration into solid tumors, the complexity and heterogeneity of these tumors pose substantial challenges. The development of ‘armored’ CAR T cells and other genetically modified cells intended to modulate the hostile TME is progressing, yet concerns about safety, specificity, and long-term effects remain. Debates within the scientific community regarding the practicality of these complex interventions versus simpler, more robust approaches underscore the need for a balanced exploration of these technologies. Extensive clinical trials and continuous technological improvements are crucial to validate these strategies and ensure they can be safely and effectively integrated into patient care.
2.4 Synergistic combination therapies: enhancing CAR T-cell efficacy through multimodal treatment approaches
The integration of CAR T-cell therapy with other cancer treatment modalities is at the forefront of innovative strategies aimed at overcoming existing barriers in immunotherapy and CAR T cell therapy. Research is actively pursuing the synergistic potential of CAR T therapy alongside immune checkpoint inhibitors (ICIs), which are known to rejuvenate exhausted T cells and enhance the immunogenicity of the tumor microenvironment (93, 94). This combination is particularly promising in solid tumors, where ICIs alone have shown limited efficacy. Numerous clinical trials are evaluating the efficacy of combining CAR T cells with ICIs like PD-1 and CTLA-4 inhibitors to potentiate the anti-tumor response while aiming to mitigate immune-related adverse events (95, 96).
Moreover, the strategic use of targeted therapies such as tyrosine kinase inhibitors (TKIs) alongside CAR T cells is another area of exploration (97). TKIs can modulate key signaling pathways within both tumor cells and T cells, potentially enhancing the CAR T cells’ ability to persist and remain active in hostile tumor environments (97, 98). This approach is being tested in various hematologic and solid tumors, with early trials showing promising enhancements in CAR T cell functionality and overall survival rates (98) (NCT04257578, NCT04484012).
Additionally, other targeted therapies like DNA damage repair inhibitors, which sensitize cancer cells to immunotherapy and promote infiltration (99), and angiogenesis inhibitors, which can alter the tumor microenvironment to improve T cell infiltration and function (100), are being investigated. The combination of CAR T cells with BRAF and MEK inhibitors (101), EZH2 inhibitors (102), lenalidomide (103) and others could potentially lead to synergistic anti-tumor effects, further enhancing the efficacy of CAR T cell therapies in complex oncological landscapes.
Low-dose chemotherapy is also employed in combination with CAR T therapy as a bridging therapy, conditioning regimen, or neoadjuvant and adjuvant treatment (104). Lymphodepletion prior to CAR T cell therapy not only creates space for CAR T cells to expand but also reduces the immunosuppressive regulatory cells, thereby boosting the efficacy of CAR T cells post-infusion (105, 106). The timing, dosage, and type of chemotherapeutic agents are critical aspects currently under clinical investigation to optimize this synergy.
Radiation therapy, used concurrently with CAR T-cell therapy, is believed to promote effector T cell recruitment, remodel the tumor vasculature, enhance T cell infiltration, and alter the suppressive nature of the tumor environment (107). This combination is particularly examined in solid tumors to increase local control and potentially generate systemic immune responses (108, 109). However, fractionation, dosing, and timing of radiation should be optimized to maximize the potential therapeutic benefits while minimizing potential risks like radiation-induced T cell apoptosis.
Oncolytic viruses and bispecific T-cell engagers (BiTEs) represent innovative combination partners for CAR T cells as well. Oncolytic viruses can lyse tumor cells, alter the TME to make it more susceptible to immune cell mediated attack, and provoke an innate immune response that may prime the tumor for CAR T cell therapy (110). Multiple studies have demonstrated various degrees of success and the potential of this combinatory approach (111–113). Meanwhile, BiTEs can bridge CAR T cells to tumor cells by targeting two different antigens simultaneously, which could reduce antigen escape and enhance the specificity of the CAR T-cell response (114, 115).
In summary, combining CAR T-cell therapy with other cancer treatments such as ICIs, TKIs, and other targeted therapies is a promising frontier in oncology. While these combinations show potential in enhancing efficacy and overcoming resistance, substantial challenges remain. These include the complexity of treatment regimens, potential for increased toxicity, and the need for meticulously designed clinical trials to determine optimal dosing and scheduling. Further research and interdisciplinary collaboration are crucial to balance innovation with careful evaluation, ensuring these therapies can be safely and effectively integrated into clinical practice, ultimately improving patient outcomes.
2.5 Allogeneic CAR T-cell therapy: innovations for on-demand use and strategies to prevent graft-versus-host disease and rejection
Autologous CAR T-cell therapies, while highly personalized, face significant limitations, including treatment delays, complexity, and variability in T-cell quality and quantity, which can affect efficacy. These therapies involve a time-consuming process where a patient’s own T cells are harvested and engineered to express CARs, introducing accessibility issues for patients with rapidly progressing diseases or insufficient T-cell counts. In contrast, allogeneic CAR T cells, derived from healthy donors and manufactured in bulk, provide a ready-to-use solution that can be standardized, potentially leading to more consistent therapeutic outcomes across different patients. This “off-the-shelf” approach offers immediate availability, reduces manufacturing time and costs, and enables rapid deployment in acute clinical settings, making it a more accessible and cost-effective treatment option for a wider patient population Table 4.
However, the application of allogeneic CAR T cells introduces the risk of graft-versus-host disease (GVHD), a serious complication stemming from the donor immune cells attacking the recipient’s body. To mitigate this risk, sophisticated genetic engineering strategies are being employed. These include the knockout of the T-cell receptor (TCR) alpha chain gene or beta chain gene (116–118) to prevent the recognition of host cells by the infused CAR T cells, the use of virus-specific T cells with more restricted TCR repertoire as a source of generating allogeneic CAR T cells (119), and the use of non-αβ T cells, such as NK cells, invariant NK (iNKT), γδ T cells, or CD4/CD8 double negative T cells to engineer CAR cells (120–124).
Allogeneic CAR T-cell therapies face significant challenges in ensuring their evasion of the host immune surveillance and effective expansion and persistence in patients–pivotal for achieving sustained antitumor responses. To specifically target this issue, researchers have innovated by integrating an alloimmune defense receptor (ADR) which targets activated T and NK cells expressing the 4-1BB activation marker, thereby evading immune-mediated rejection while maintaining antitumor efficacy (125). This approach not only enhances CAR T-cell persistence but also minimizes the risk of graft-versus-host disease. Additionally, optimizing lymphodepletion and refining gene editing for T-cell robustness are crucial for overcoming these expansion and persistence challenges in allogeneic CAR T-cell therapy (117, 126).
The transformative potential of allogeneic CAR T-cell therapy lies in its ability to provide standardized, ready-to-use treatments that can be rapidly deployed. Nonetheless, this approach necessitates advanced genetic engineering to prevent immune rejection and GVHD. Addressing these challenges involves navigating complex ethical and regulatory concerns while ensuring long-term safety and efficacy through rigorous clinical trials. Balancing innovation with patient safety will be essential to realize the full potential of allogeneic CAR T cells, making them a viable option for a broader spectrum of patients globally.
2.6 Advanced strategies to mitigate toxicities in CAR T-cell therapy: engineering safety switches and enhancing control
In the quest to overcome the toxicities associated with CAR T-cell immunotherapy, several innovative strategies are being employed to enhance safety and control. The integration of “safety switches”, such as inducible caspase-9 (127–130) and antibody-dependent cell-mediated cytotoxicity (ADCC) switches (131), enables the rapid elimination of CAR T cells in the event of severe side effects. Additionally, small molecule-based safety switches have been developed, allowing clinicians to rapidly deactivate the cells if adverse effects occur (132–134). Another promising approach is the use of Split, Universal, and Programmable (SUPRA) CARs, which divide the CAR system into two distinct components that must interact for activation. This design enhances control, enables more precise targeting, and reduces unintended T-cell activation (135, 136).
Similarly, Dual CARs employ a strategy where CAR T cells are engineered to express two distinct receptors, requiring recognition of two specific antigens for activation. This dual recognition system enhances the specificity of CAR T cells, significantly reducing the risk of off-target effects and increasing the safety profile of these therapies (137). Control of CAR expression is achieved using inducible promoters (138, 139) or drug-responsive elements (140, 141), and innovative methods like sound (142) or light (143) activation, allowing for precise temporal and spatial control over CAR expression. This controlled approach helps to minimize the risk of overactivation and associated toxicities.
Local delivery of CAR T cells, which involves administering these cells directly to the tumor site, minimizes systemic exposure and reduces the risk of widespread toxicities often associated with broader systemic administration (89, 144). Prophylactic use of medications such as tocilizumab and anakinra is explored to preemptively reduce the severity of cytokine release syndrome (CRS) and neurotoxicity (145–147). Techniques like biomarker monitoring (148) and fractionated dosing (149) are under investigation to better predict and manage toxic responses by moderating CAR T-cell activity. Furthermore, advancements in cellular engineering, such as CRISPR/Cas9 gene editing, are aimed at enabling CAR T cells to resist activation by certain cytokines that contribute to toxicities (150, 151). Mouse models are also being developed to study the pathogenesis of CRS and neurotoxicity in CAR T-cell therapy, aiding in the identification of key inflammatory pathways and the testing of new safety interventions (14, 152, 153).
The implementation of advanced safety strategies such as safety switches, controlled activation systems, and localized delivery methods represents a significant advancement in mitigating the inherent toxicities of CAR T-cell therapy. These innovations offer enhanced control over CAR T-cell function, potentially reducing severe side effects and improving patient safety. However, these sophisticated mechanisms also introduce greater complexity into therapy design and application, which could impact both the reliability and cost of treatments. The integration of such advanced features necessitates rigorous clinical trials to confirm their efficacy and safety, alongside ethical considerations regarding access and cost. As research progresses, the challenge will be to refine these technologies to ensure they enhance therapeutic outcomes without compromising efficacy, paving the way for safer, more effective CAR T-cell therapies accessible to a wider range of patients.
2.7 Optimizing production, enhancing accessibility and reducing cost: innovative strategies in CAR T-cell therapy manufacturing
To tackle the challenges of manufacturing and accessibility in CAR T-cell therapy, researchers are deploying multiple innovative strategies aimed at streamlining production, enhancing efficiency, and reducing costs. Automated manufacturing systems are a pivotal advancement, utilizing closed-system bioreactors that standardize the production process, diminish the risk of contamination, and minimize labor costs. These systems can significantly cut down on the time required to produce therapeutic doses of CAR T cells (154–156), Table 5. Point-of-care manufacturing involves the development of compact, on-site production units within hospital settings, which reduces logistical complexities associated with the transport of cellular materials and shortens the turnaround time from collection to infusion. This approach not only speeds up the treatment process but also aims to lower overall therapy costs (157, 158).
Advanced cell expansion techniques are being developed to improve the yield and functionality of CAR T cells, including optimizing the growth media (159, 160) and conditions in bioreactors (161). Artificial Intelligence (AI) and Machine Learning (ML) have transformative potential in optimizing the production, enhancing accessibility, and reducing the costs of CAR T cell therapy. AI can streamline manufacturing processes through automation and precise control, ensuring consistency and quality while reducing labor costs (162–164). Machine learning models can predict patient outcomes from pre-infusion transcriptomes, outperforming traditional methods (165). Additionally, neural networks help design CAR constructs with optimal signaling motifs, streamlining the development and enhancing the accessibility of these therapies (166).
Economic analysis is integral to the strategies for optimizing CAR T-cell therapy manufacturing, enhancing accessibility, and reducing costs (16, 167). The high cost of CAR T-cell therapies primarily stems from the complexity of the production processes and the personalized nature of the treatments. Strategies to reduce these costs include streamlining manufacturing protocols, employing automated systems, and developing scalable batch processes which can reduce labor costs and minimize errors (16). Economic benefits also arise from shortening production times and reducing the footprint of manufacturing facilities through point-of-care production technologies (157, 158). Furthermore, adopting non-viral gene transfer methods and utilizing less costly reagents can significantly cut production expenses (48). By lowering the cost of goods and improving manufacturing efficiency, these therapies can become more accessible, particularly in low- and middle-income countries, where the burden of treatment costs is most pronounced. Engaging in cooperative strategies with global partners and governments to establish local manufacturing facilities can also reduce transportation costs and tariffs, further driving down prices and expanding access (168, 169). These economic considerations are essential for the widespread adoption of CAR T-cell therapies and require ongoing innovation and investment to ensure that these life-saving treatments are affordable and available to all patients in need, regardless of geographic location.
Efforts to streamline regulatory approvals for new manufacturing facilities and methods are crucial. Engaging with regulatory bodies to simplify and expedite the review and approval processes can significantly decrease the time and financial burden associated with bringing CAR T-cell therapies to market (170–172). Additionally, addressing regulatory and ethical considerations is essential, especially as CAR T-cell therapies involve complex genetic manipulations and personalized treatment protocols. Regulatory frameworks must ensure patient safety, manage ethical concerns related to genetic editing, and handle the implications of using donor cells in allogeneic therapies. Ethical considerations also extend to ensuring equitable access to these potentially life-saving therapies, preventing disparities in healthcare outcomes. Collaborative dialogues with ethicists, patient advocacy groups, and regulators are necessary to navigate these aspects effectively, ensuring that CAR T-cell therapies are not only scientifically sound but also socially responsible and accessible to all segments of the population.
In summary, the innovative strategies aimed at optimizing the production, enhancing accessibility, and reducing the cost of CAR T-cell therapies present significant advancements in the field. Automated manufacturing systems, point-of-care production units, and global manufacturing networks have the potential to standardize treatments, reduce production time, and make therapies more accessible, especially in underserved regions. However, these advances bring complexities, including high initial costs, operational challenges, and significant regulatory hurdles. Moreover, the integration of AI and machine learning promises further optimization but requires careful implementation to ensure quality and efficacy are maintained. As the field progresses, a balanced approach that addresses these technological, regulatory, and ethical challenges will be crucial for realizing the full potential of CAR T-cell therapies, making them a viable option for a broader range of patients globally. This comprehensive strategy will need to continue evolving, guided by ongoing research and adaptation to new insights and technological advancements.
3 Discussion
This review underscores the transformative strides being made in CAR T-cell therapy, with a particular emphasis on overcoming limitations that have restricted its application beyond hematologic malignancies. As we venture into novel territories, such as solid tumors and non-cancerous diseases, the synthesis of interdisciplinary advances has paved the way for potential breakthroughs, yet it also presents a complex landscape of challenges and opportunities.
3.1 Interdisciplinary innovation and its implications
The integration of bioinformatics, materials science, immunology, synthetic biology, genetic engineering, immunogenetics, and biomedical engineering has ushered in a new era of precision in CAR T-cell therapy. The utilization of computational tools to design CAR constructs and predict therapeutic outcomes is revolutionizing how treatments are personalized. Synthetic biology and genetic engineering are enhancing the specificity and efficacy of these constructs, while immunogenetics helps tailor therapies to individual immune system variations. Biomedical engineering contributes to the development of biocompatible materials and advanced delivery systems that improve the in vivo functionality of therapeutic cells. However, the translation of these complex designs from the bench to bedside necessitates innovations in manufacturing processes that can accommodate such personalized approaches at scale. Future research should focus on developing modular platforms that can be easily adapted to incorporate new discoveries and patient-specific data.
3.2 Economic and ethical considerations in global access
While technological advancements promise to enhance efficacy and safety, their real-world application raises significant economic and ethical questions. The high cost of these therapies remains a formidable barrier to access in low- and middle-income countries. Future initiatives should explore the development of cost-effective production methods such as the use of automated and decentralized manufacturing units. Ethically, there is a need to establish frameworks that ensure equitable access to these therapies globally, perhaps through international collaborations and policy reforms.
3.3 Regulatory evolution
As CAR T-cell therapies evolve, so too must the regulatory frameworks that govern their development and deployment. The rapid pace of innovation challenges current regulatory paradigms, which are often ill-equipped to handle the nuances of advanced gene and cell therapies. An ongoing dialogue between regulators, researchers, and industry stakeholders is essential to develop more adaptive regulatory approaches that can keep pace with technological advancements while ensuring patient safety.
3.4 Emerging areas of research
Looking forward, CAR T-cell therapies are expanding into exciting new territories. For autoimmune diseases, these therapies show promise in conditions like systemic lupus erythematous (173), multiple sclerosis (174) and type 1 diabetes (175), where they may modulate immune responses similarly to their actions against malignant cells. In the realm of aging and degenerative diseases, CAR T-cells are being investigated for their potential to modify the aging process and treat age-related ailments (176, 177). Additionally, the adaptation of CAR T-cell therapies for infectious diseases suggests a new frontier in managing chronic infections that resist conventional treatments (178). This expansion not only broadens the therapeutic potential of CAR T-cell therapies but also highlights the innovative cross-disciplinary approaches being undertaken to overcome current limitations and explore new applications.
4 Conclusion
The path forward for CAR T-cell therapy involves not only scientific and technological innovation but also a concerted effort to address the logistical, economic, and ethical challenges that come with such profound medical advancements. As we continue to push the boundaries of what is possible in medical science, a balanced approach that incorporates clinical needs, patient safety, and equitable access will be crucial for realizing the full potential of CAR T-cell therapies.
Author contributions
AA: Conceptualization, Formal analysis, Funding acquisition, Investigation, Resources, Writing – original draft, Writing – review & editing. JFD: Conceptualization, Funding acquisition, Supervision, Writing – review & editing.
Funding
The author(s) declare financial support was received for the research, authorship, and/or publication of this article. Leukemia Society of America SCOR (JFD), NCI Leukemia SPORE, P50 CA171963 (JFD); NIH/NCI: R35 CA210084 NCI Outstanding Investigator Award (JFD). The preparation of this review was generously supported by a contribution from M. G. Gibby (AA).
Acknowledgments
Figures 1 and 2 were created using Whimsical.
Conflict of interest
JFD: Consulting/Advisory Committees: Rivervest, Bioline, Incyte, NeoImmuneTech, Macrogenics, Vertex, Bluebird Bio, hC Bioscience; Employment/Salary: Washington University; Ownership Investment: Magenta, WUGEN.
The remaining author declares that the research was conducted in the absence of any commercial or financial relationships that could be construed as a potential conflict of interest.
Publisher’s note
All claims expressed in this article are solely those of the authors and do not necessarily represent those of their affiliated organizations, or those of the publisher, the editors and the reviewers. Any product that may be evaluated in this article, or claim that may be made by its manufacturer, is not guaranteed or endorsed by the publisher.
Glossary
CAR T-cell Therapy: A type of cancer treatment where T cells are modified to express chimeric antigen receptors (CARs) that target specific cancer cells
Chimeric Antigen Receptor (CAR): Engineered receptors grafted onto T cells to give them the ability to target specific proteins on cancer cells
Autologous CAR T-cells: CAR T-cells derived from a patient’s own T cells
Allogeneic CAR T-cells: CAR T-cells derived from healthy donor T cells, designed for off-the-shelf use
Tumor Microenvironment (TME): The environment surrounding a tumor, including blood vessels, immune cells, fibroblasts, signaling molecules, and the extracellular matrix
Cytokine Release Syndrome (CRS): A potentially severe side effect of CAR T-cell therapy involving a large, rapid release of cytokines into the blood
Neurotoxicity: Toxicity that affects the nervous system, potentially a side effect of CAR T-cell therapy
Multiplexed CARs: CARs that target multiple antigens to reduce the likelihood of tumor escape
Modular/Universal CARs: CAR systems designed for adaptability through switchable, bispecific adaptors to target diverse antigens
SynNotch Receptors: A dual antigen recognition system where an initial antigen interaction triggers the expression of a CAR targeting a second antigen
Hybrid CARs: CARs combining elements of T-cell receptors (TCRs) and CARs to enhance targeting of cancer-specific antigens
Third Generation CARs: CARs with multiple co-stimulatory molecules, designed to enhance activation, proliferation, and antitumor efficacy
Armored CARs: Genetically modified CARs that secrete cytokines or express ligands to enhance survival and anti-tumor activity in the tumor microenvironment
CRISPR/Cas9: A gene-editing technology used to modify CAR T-cells for improved functionality and persistence
Transposon-Based Systems (e.g., Sleeping Beauty): Non-viral gene transfer methods used to integrate CAR genes into T cells
Central Memory T cells (Tcm): T cell subsets known for their longevity and robust proliferative ability, used in CAR T-cell therapies for enhanced efficacy
Stem Cell-like Memory T cells (Tscm): T cell subsets with long-term self-renewal capacity, providing durable antitumor responses
Inducible Caspase-9 (iCasp9): A safety switch that triggers CAR T-cell apoptosis upon activation by a specific drug
Antibody-Dependent Cell-Mediated Cytotoxicity (ADCC): A mechanism by which antibodies promote the destruction of target cells by immune cells
Split, Universal, and Programmable (SUPRA) CARs: CARs divided into distinct components for enhanced control and precision in targeting
Dual CARs: CAR T-cells engineered to require recognition of two distinct antigens for activation, enhancing specificity and safety
Lymphodepletion: Pre-treatment with chemotherapy to prepare the patient’s body for CAR T-cell infusion by reducing immunosuppressive regulatory cells
Oncolytic Viruses: Viruses engineered to selectively infect and kill cancer cells, used in combination with CAR T-cell therapy
Bispecific T-cell Engagers (BiTEs): Molecules that link CAR T-cells to tumor cells by targeting two different antigens, enhancing CAR T-cell specificity and activity
Graft-versus-Host Disease (GVHD): A condition where donor immune cells attack the recipient’s body, a risk in allogeneic CAR T-cell therapy
Alloimmune Defense Receptor (ADR): A receptor integrated into CAR T-cells to target and eliminate activated host immune cells, preventing rejection
Checkpoint Inhibitors (ICIs): Drugs that block proteins which inhibit T cell activity, used in combination with CAR T-cell therapy to enhance antitumor response
Tyrosine Kinase Inhibitors (TKIs): Drugs that inhibit enzymes involved in signaling pathways, enhancing CAR T-cell persistence and activity
Bioinformatics: The application of computational tools to analyze biological data, crucial for designing CAR constructs and predicting outcomes
Artificial Intelligence (AI): Technology used to optimize manufacturing processes and predict patient outcomes in CAR T-cell therapy
Economic Analysis: Assessing the cost-effectiveness of CAR T-cell therapies to enhance accessibility and affordability
Regulatory Frameworks: Guidelines and standards set by regulatory bodies to ensure the safety and efficacy of CAR T-cell therapies
Point-of-Care Manufacturing: On-site production of CAR T-cells in hospital settings, reducing logistical complexities and treatment delays
Nano-Carriers: Nanotechnology-based delivery systems to enhance CAR T-cell migration, infiltration, and functionality in tumors
Senolytic CAR T-cells: CAR T-cells designed to target and eliminate senescent cells, potentially used in treating age-related diseases
References
1. Kuwana Y, Asakura Y, Utsunomiya N, Nakanishi M, Arata Y, Itoh S, et al. Expression of chimeric receptor composed of immunoglobulin-derived V regions and T-cell receptor-derived C regions. Biochem Biophys Res Commun. (1987) 149:960–8. doi: 10.1016/0006-291X(87)90502-X
2. Gross G, Waks T, Eshhar Z. Expression of immunoglobulin-T-cell receptor chimeric molecules as functional receptors with antibody-type specificity. Proc Natl Acad Sci U S A. (1989) 86:10024–8. doi: 10.1073/pnas.86.24.10024
3. Mitra A, Barua A, Huang L, Ganguly S, Feng Q, He B. From bench to bedside: the history and progress of CAR T cell therapy. Front Immunol. (2023) 14:1188049. doi: 10.3389/fimmu.2023.1188049
4. Brentjens RJ, Davila ML, Riviere I, Park J, Wang X, Cowell LG, et al. CD19-targeted T cells rapidly induce molecular remissions in adults with chemotherapy-refractory acute lymphoblastic leukemia. Sci Transl Med. (2013) 5:177ra38. doi: 10.1126/scitranslmed.3005930
5. Grupp SA, Kalos M, Barrett D, Aplenc R, Porter DL, Rheingold SR, et al. Chimeric antigen receptor-modified T cells for acute lymphoid leukemia. N Engl J Med. (2013) 368:1509–18. doi: 10.1056/NEJMoa1215134
6. Kochenderfer JN, Wilson WH, Janik JE, Dudley ME, Stetler-Stevenson M, Feldman SA, et al. Eradication of B-lineage cells and regression of lymphoma in a patient treated with autologous T cells genetically engineered to recognize CD19. Blood. (2010) 116:4099–102. doi: 10.1182/blood-2010-04-281931
7. Maude SL, Laetsch TW, Buechner J, Rives S, Boyer M, Bittencourt H, et al. Tisagenlecleucel in children and young adults with B-cell lymphoblastic leukemia. N Engl J Med. (2018) 378:439–48. doi: 10.1056/NEJMoa1709866
8. Locke FL, Ghobadi A, Jacobson CA, Miklos DB, Lekakis LJ, Oluwole OO, et al. Long-term safety and activity of axicabtagene ciloleucel in refractory large B-cell lymphoma (ZUMA-1): a single-arm, multicentre, phase 1-2 trial. Lancet Oncol. (2019) 20:31–42. doi: 10.1016/S1470-2045(18)30864-7
9. Wang M, Munoz J, Goy A, Locke FL, Jacobson CA, Hill BT, et al. KTE-X19 CAR T-cell therapy in relapsed or refractory mantle-cell lymphoma. N Engl J Med. (2020) 382:1331–42. doi: 10.1056/NEJMoa1914347
10. Abramson JS, Palomba ML, Gordon LI, Lunning MA, Wang M, Arnason J, et al. Lisocabtagene maraleucel for patients with relapsed or refractory large B-cell lymphomas (TRANSCEND NHL 001): a multicentre seamless design study. Lancet. (2020) 396:839–52. doi: 10.1016/S0140-6736(20)31366-0
11. Munshi NC, Anderson LD Jr., Shah N, Madduri D, Berdeja J, Lonial S, et al. Idecabtagene vicleucel in relapsed and refractory multiple myeloma. N Engl J Med. (2021) 384:705–16. doi: 10.1056/NEJMoa2024850
12. Martin T, Usmani SZ, Berdeja JG, Agha M, Cohen AD, Hari P, et al. Ciltacabtagene autoleucel, an anti-B-cell maturation antigen chimeric antigen receptor T-cell therapy, for relapsed/refractory multiple myeloma: CARTITUDE-1 2-year follow-up. J Clin Oncol. (2023) 41:1265–74. doi: 10.1200/JCO.22.00842
13. Albelda SM. CAR T cell therapy for patients with solid tumours: key lessons to learn and unlearn. Nat Rev Clin Oncol. (2024) 21:47–66. doi: 10.1038/s41571-023-00832-4
14. Morris EC, Neelapu SS, Giavridis T, Sadelain M. Cytokine release syndrome and associated neurotoxicity in cancer immunotherapy. Nat Rev Immunol. (2022) 22:85–96. doi: 10.1038/s41577-021-00547-6
15. Flugel CL, Majzner RG, Krenciute G, Dotti G, Riddell SR, Wagner DL, et al. Overcoming on-target, off-tumour toxicity of CAR T cell therapy for solid tumours. Nat Rev Clin Oncol. (2023) 20:49–62. doi: 10.1038/s41571-022-00704-3
16. Cliff ERS, Kelkar AH, Russler-Germain DA, Tessema FA, Raymakers AJN, Feldman WB, et al. High cost of chimeric antigen receptor T-cells: challenges and solutions. Am Soc Clin Oncol Educ Book. (2023) 43:e397912. doi: 10.1200/EDBK_397912
17. Cappell KM, Kochenderfer JN. Long-term outcomes following CAR T cell therapy: what we know so far. Nat Rev Clin Oncol. (2023) 20:359–71. doi: 10.1038/s41571-023-00754-1
18. Giordano Attianese GMP, Ash S, Irving M. Coengineering specificity, safety, and function into T cells for cancer immunotherapy. Immunol Rev. (2023) 320:166–98. doi: 10.1111/imr.13252
19. Spiegel JY, Patel S, Muffly L, Hossain NM, Oak J, Baird JH, et al. CAR T cells with dual targeting of CD19 and CD22 in adult patients with recurrent or refractory B cell Malignancies: a phase 1 trial. Nat Med. (2021) 27:1419–31. doi: 10.1038/s41591-021-01436-0
20. Bagley SJ, Logun M, Fraietta JA, Wang X, Desai AS, Bagley LJ, et al. Intrathecal bivalent CAR T cells targeting EGFR and IL13Rα2 in recurrent glioblastoma: phase 1 trial interim results. Nat Med. (2024). doi: 10.1038/s41591-024-02893-z
21. Loff S, Dietrich J, Meyer JE, Riewaldt J, Spehr J, von Bonin M, et al. Rapidly switchable universal CAR-T cells for treatment of CD123-positive leukemia. Mol Ther Oncolytics. (2020) 17:408–20. doi: 10.1016/j.omto.2020.04.009
22. Borrok MJ, Li Y, Harvilla PB, Vellalore Maruthachalam B, Tamot N, Prokopowitz C, et al. Conduit CAR: redirecting CAR T-cell specificity with A universal and adaptable bispecific antibody platform. Cancer Res Commun. (2022) 2:146–57. doi: 10.1158/2767-9764.CRC-21-0150
23. Wermke M, Kraus S, Ehninger A, Bargou RC, Goebeler ME, Middeke JM, et al. Proof of concept for a rapidly switchable universal CAR-T platform with UniCAR-T-CD123 in relapsed/refractory AML. Blood. (2021) 137:3145–8. doi: 10.1182/blood.2020009759
24. Urbanska K, Lanitis E, Poussin M, Lynn RC, Gavin BP, Kelderman S, et al. A universal strategy for adoptive immunotherapy of cancer through use of a novel T-cell antigen receptor. Cancer Res. (2012) 72:1844–52. doi: 10.1158/0008-5472.CAN-11-3890
25. Ma JS, Kim JY, Kazane SA, Choi SH, Yun HY, Kim MS, et al. Versatile strategy for controlling the specificity and activity of engineered T cells. Proc Natl Acad Sci U.S.A. (2016) 113:E450–8. doi: 10.1073/pnas.1524193113
26. Rodgers DT, Mazagova M, Hampton EN, Cao Y, Ramadoss NS, Hardy IR, et al. Switch-mediated activation and retargeting of CAR-T cells for B-cell Malignancies. Proc Natl Acad Sci U.S.A. (2016) 113:E459–68. doi: 10.1073/pnas.1524155113
27. Ruffo E, Butchy AA, Tivon Y, So V, Kvorjak M, Parikh A, et al. Post-translational covalent assembly of CAR and synNotch receptors for programmable antigen targeting. Nat Commun. (2023) 14:2463. doi: 10.1038/s41467-023-37863-5
28. Choe JH, Watchmaker PB, Simic MS, Gilbert RD, Li AW, Krasnow NA, et al. SynNotch-CAR T cells overcome challenges of specificity, heterogeneity, and persistence in treating glioblastoma. Sci Transl Med. (2021) 13. doi: 10.1126/scitranslmed.abe7378
29. Roybal KT, Rupp LJ, Morsut L, Walker WJ, McNally KA, Park JS, et al. Precision tumor recognition by T cells with combinatorial antigen-sensing circuits. Cell. (2016) 164:770–9. doi: 10.1016/j.cell.2016.01.011
30. Walseng E, Köksal H, Sektioglu IM, Fåne A, Skorstad G, Kvalheim G, et al. A TCR-based chimeric antigen receptor. Sci Rep. (2017) 7:10713. doi: 10.1038/s41598-017-11126-y
31. Liu M, Akahori Y, Fujiwara H, Miyahara Y eds. 284 A novel hybrid T-cell receptor form of CAR-T cells showed enhanced activity against solid tumors. BMJ Publishing Group Ltd (2023).
32. Mog BJ, Dinapoli SR, Hwang MS, Nichakawade TD, Douglass J, Hsiue EH-C, et al. Abstract LB095: Hybrid TCR-CAR design surpasses conventional CARs and patient-derived TCRs in targeting an ultra-low-density neoantigen. Cancer Res. (2023) 83:LB095–LB. doi: 10.1158/1538-7445.AM2023-LB095
33. Helsen CW, Hammill JA, Lau VWC, Mwawasi KA, Afsahi A, Bezverbnaya K, et al. The chimeric TAC receptor co-opts the T cell receptor yielding robust anti-tumor activity without toxicity. Nat Commun. (2018) 9:3049. doi: 10.1038/s41467-018-05395-y
34. Mansilla-Soto J, Eyquem J, Haubner S, Hamieh M, Feucht J, Paillon N, et al. HLA-independent T cell receptors for targeting tumors with low antigen density. Nat Med. (2022) 28:345–52. doi: 10.1038/s41591-021-01621-1
35. Xu Y, Yang Z, Horan LH, Zhang P, Liu L, Zimdahl B, et al. A novel antibody-TCR (AbTCR) platform combines Fab-based antigen recognition with gamma/delta-TCR signaling to facilitate T-cell cytotoxicity with low cytokine release. Cell Discovery. (2018) 4:62. doi: 10.1038/s41421-018-0066-6
36. Liu Y, Liu G, Wang J, Zheng ZY, Jia L, Rui W, et al. Chimeric STAR receptors using TCR machinery mediate robust responses against solid tumors. Sci Transl Med. (2021) 13. doi: 10.1126/scitranslmed.abb5191
37. Ramos CA, Rouce R, Robertson CS, Reyna A, Narala N, Vyas G, et al. In vivo fate and activity of second- versus third-generation CD19-specific CAR-T cells in B cell non-Hodgkin's lymphomas. Mol Ther. (2018) 26:2727–37. doi: 10.1016/j.ymthe.2018.09.009
38. Enblad G, Karlsson H, Gammelgård G, Wenthe J, Lövgren T, Amini RM, et al. A phase I/IIa trial using CD19-targeted third-generation CAR T cells for lymphoma and leukemia. Clin Cancer Res. (2018) 24:6185–94. doi: 10.1158/1078-0432.CCR-18-0426
39. Ma X, Shou P, Smith C, Chen Y, Du H, Sun C, et al. Interleukin-23 engineering improves CAR T cell function in solid tumors. Nat Biotechnol. (2020) 38:448–59. doi: 10.1038/s41587-019-0398-2
40. Adachi K, Kano Y, Nagai T, Okuyama N, Sakoda Y, Tamada K. IL-7 and CCL19 expression in CAR-T cells improves immune cell infiltration and CAR-T cell survival in the tumor. Nat Biotechnol. (2018) 36:346–51. doi: 10.1038/nbt.4086
41. Lange S, Sand LGL, Bell M, Patil SL, Langfitt D, Gottschalk S. A chimeric GM-CSF/IL18 receptor to sustain CAR T-cell function. Cancer Discovery. (2021) 11:1661–71. doi: 10.1158/2159-8290.CD-20-0896
42. Nakazawa T, Natsume A, Nishimura F, Morimoto T, Matsuda R, Nakamura M, et al. Effect of CRISPR/cas9-mediated PD-1-disrupted primary human third-generation CAR-T cells targeting EGFRvIII on in vitro human glioblastoma cell growth. Cells. (2020) 9. doi: 10.3390/cells9040998
43. Yoshikawa T, Wu Z, Inoue S, Kasuya H, Matsushita H, Takahashi Y, et al. Genetic ablation of PRDM1 in antitumor T cells enhances therapeutic efficacy of adoptive immunotherapy. Blood. (2022) 139:2156–72. doi: 10.1182/blood.2021012714
44. Zhang X, Zhang C, Qiao M, Cheng C, Tang N, Lu S, et al. Depletion of BATF in CAR-T cells enhances antitumor activity by inducing resistance against exhaustion and formation of central memory cells. Cancer Cell. (2022) 40:1407–22.e7. doi: 10.1016/j.ccell.2022.09.013
45. Bulcha JT, Wang Y, Ma H, Tai PWL, Gao G. Viral vector platforms within the gene therapy landscape. Signal Transduct Target Ther. (2021) 6:53. doi: 10.1038/s41392-021-00487-6
46. Chen F, Qi X, Zhang R, Wu ZY, Yan CE, Li J, et al. Episomal lentiviral vectors confer erythropoietin expression in dividing cells. Plasmid. (2017) 90:15–9. doi: 10.1016/j.plasmid.2017.02.001
47. Jadlowsky JK, Leskowitz R, McKenna S, Karar J, Ma Y, Dai A, et al. Long-term stability of clinical-grade lentiviral vectors for cell therapy. Mol Ther Methods Clin Dev. (2024) 32:101186. doi: 10.1016/j.omtm.2024.101186
48. Moretti A, Ponzo M, Nicolette CA, Tcherepanova IY, Biondi A, Magnani CF. The past, present, and future of non-viral CAR T cells. Front Immunol. (2022) 13:867013. doi: 10.3389/fimmu.2022.867013
49. Monjezi R, Miskey C, Gogishvili T, Schleef M, Schmeer M, Einsele H, et al. Enhanced CAR T-cell engineering using non-viral Sleeping Beauty transposition from minicircle vectors. Leukemia. (2017) 31:186–94. doi: 10.1038/leu.2016.180
50. VanderBurgh JA, Corso TN, Levy SL, Craighead HG. Scalable continuous-flow electroporation platform enabling T cell transfection for cellular therapy manufacturing. Sci Rep. (2023) 13:6857. doi: 10.1038/s41598-023-33941-2
51. Zhang J, Hu Y, Yang J, Li W, Zhang M, Wang Q, et al. Non-viral, specifically targeted CAR-T cells achieve high safety and efficacy in B-NHL. Nature. (2022) 609:369–74. doi: 10.1038/s41586-022-05140-y
52. Hamilton JR, Chen E, Perez BS, Sandoval Espinoza CR, Kang MH, Trinidad M, et al. In vivo human T cell engineering with enveloped delivery vehicles. Nat Biotechnol. (2024). doi: 10.1038/s41587-023-02085-z
53. Gattinoni L, Speiser DE, Lichterfeld M, Bonini C. T memory stem cells in health and disease. Nat Med. (2017) 23:18–27. doi: 10.1038/nm.4241
54. Arcangeli S, Bove C, Mezzanotte C, Camisa B, Falcone L, Manfredi F, et al. CAR T cell manufacturing from naive/stem memory T lymphocytes enhances antitumor responses while curtailing cytokine release syndrome. J Clin Invest. (2022) 132. doi: 10.1172/JCI150807
55. Arcangeli S, Falcone L, Camisa B, De Girardi F, Biondi M, Giglio F, et al. Next-generation manufacturing protocols enriching T(SCM) CAR T cells can overcome disease-specific T cell defects in cancer patients. Front Immunol. (2020) 11:1217. doi: 10.3389/fimmu.2020.01217
56. Turtle CJ, Hanafi LA, Berger C, Hudecek M, Pender B, Robinson E, et al. Immunotherapy of non-Hodgkin's lymphoma with a defined ratio of CD8+ and CD4+ CD19-specific chimeric antigen receptor-modified T cells. Sci Transl Med. (2016) 8:355ra116. doi: 10.1126/scitranslmed.aaf8621
57. Galli E, Bellesi S, Pansini I, Di Cesare G, Iacovelli C, Malafronte R, et al. The CD4/CD8 ratio of infused CD19-CAR-T is a prognostic factor for efficacy and toxicity. Br J Haematol. (2023) 203:564–70. doi: 10.1111/bjh.19117
58. Sommermeyer D, Hudecek M, Kosasih PL, Gogishvili T, Maloney DG, Turtle CJ, et al. Chimeric antigen receptor-modified T cells derived from defined CD8+ and CD4+ subsets confer superior antitumor reactivity in vivo. Leukemia. (2016) 30:492–500. doi: 10.1038/leu.2015.247
59. Gottschlich A, Thomas M, Grünmeier R, Lesch S, Rohrbacher L, Igl V, et al. Single-cell transcriptomic atlas-guided development of CAR-T cells for the treatment of acute myeloid leukemia. Nat Biotechnol. (2023) 41:1618–32. doi: 10.1038/s41587-023-01684-0
60. Melenhorst JJ, Chen GM, Wang M, Porter DL, Chen C, Collins MA, et al. Decade-long leukaemia remissions with persistence of CD4(+) CAR T cells. Nature. (2022) 602:503–9. doi: 10.1038/s41586-021-04390-6
61. Fraietta JA, Lacey SF, Orlando EJ, Pruteanu-Malinici I, Gohil M, Lundh S, et al. Determinants of response and resistance to CD19 chimeric antigen receptor (CAR) T cell therapy of chronic lymphocytic leukemia. Nat Med. (2018) 24:563–71. doi: 10.1038/s41591-018-0010-1
62. Yang J, Chen Y, Jing Y, Green MR, Han L. Advancing CAR T cell therapy through the use of multidimensional omics data. Nat Rev Clin Oncol. (2023) 20:211–28. doi: 10.1038/s41571-023-00729-2
63. Xie N, Shen G, Gao W, Huang Z, Huang C, Fu L. Neoantigens: promising targets for cancer therapy. Signal Transduct Target Ther. (2023) 8:9. doi: 10.1038/s41392-022-01270-x
64. Wang Z, Cao YJ. Adoptive cell therapy targeting neoantigens: A frontier for cancer research. Front Immunol. (2020) 11:176. doi: 10.3389/fimmu.2020.00176
65. O’Rourke DM, Nasrallah MP, Desai A, Melenhorst JJ, Mansfield K, Morrissette JJD, et al. A single dose of peripherally infused EGFRvIII-directed CAR T cells mediates antigen loss and induces adaptive resistance in patients with recurrent glioblastoma. Sci Trans Med. (2017) 9:eaaa0984. doi: 10.1126/scitranslmed.aaa0984
66. Posey AD Jr., Schwab RD, Boesteanu AC, Steentoft C, Mandel U, Engels B, et al. Engineered CAR T cells targeting the cancer-associated Tn-glycoform of the membrane mucin MUC1 control adenocarcinoma. Immunity> . (2016) 44:1444–54. doi: 10.1016/j.immuni.2016.05.014
67. Wilkie S, van Schalkwyk MC, Hobbs S, Davies DM, van der Stegen SJ, Pereira AC, et al. Dual targeting of ErbB2 and MUC1 in breast cancer using chimeric antigen receptors engineered to provide complementary signaling. J Clin Immunol. (2012) 32:1059–70. doi: 10.1007/s10875-012-9689-9
68. Tashiro H, Brenner MK. Immunotherapy against cancer-related viruses. Cell Res. (2017) 27:59–73. doi: 10.1038/cr.2016.153
69. Maus MV, Fraietta JA, Levine BL, Kalos M, Zhao Y, June CH. Adoptive immunotherapy for cancer or viruses. Annu Rev Immunol. (2014) 32:189–225. doi: 10.1146/annurev-immunol-032713-120136
70. Jakobsen MK, Gjerstorff MF. CAR T-cell cancer therapy targeting surface cancer/testis antigens. Front Immunol. (2020) 11:1568. doi: 10.3389/fimmu.2020.01568
71. Ai H, Yang H, Li L, Ma J, Liu K, Li Z. Cancer/testis antigens: promising immunotherapy targets for digestive tract cancers. Front Immunol. (2023) 14:1190883. doi: 10.3389/fimmu.2023.1190883
72. Saygin C, Matei D, Majeti R, Reizes O, Lathia JD. Targeting cancer stemness in the clinic: from hype to hope. Cell Stem Cell. (2019) 24:25–40. doi: 10.1016/j.stem.2018.11.017
73. Codd AS, Kanaseki T, Torigo T, Tabi Z. Cancer stem cells as targets for immunotherapy. Immunology. (2018) 153:304–14. doi: 10.1111/imm.12866
74. Alhabbab RY. Targeting cancer stem cells by genetically engineered chimeric antigen receptor T cells. Front Genet. (2020) 11:312. doi: 10.3389/fgene.2020.00312
75. Watanabe Y, Tsukahara T, Murata K, Hamada S, Kubo T, Kanaseki T, et al. Development of CAR-T cells specifically targeting cancer stem cell antigen DNAJB8 against solid tumours. Br J Cancer. (2023) 128:886–95. doi: 10.1038/s41416-022-02100-1
76. Casucci M, Nicolis Di Robilant B, Falcone L, Camisa B, Norelli M, Genovese P, et al. CD44v6-targeted T cells mediate potent antitumor effects against acute myeloid leukemia and multiple myeloma. Blood. (2013) 122:3461–72. doi: 10.1182/blood-2013-04-493361
77. Porcellini S, Asperti C, Corna S, Cicoria E, Valtolina V, Stornaiuolo A, et al. CAR T cells redirected to CD44v6 control tumor growth in lung and ovary adenocarcinoma bearing mice. Front Immunol. (2020) 11:99. doi: 10.3389/fimmu.2020.00099
78. Lesch S, Blumenberg V, Stoiber S, Gottschlich A, Ogonek J, Cadilha BL, et al. T cells armed with C-X-C chemokine receptor type 6 enhance adoptive cell therapy for pancreatic tumours. Nat BioMed Eng. (2021) 5:1246–60. doi: 10.1038/s41551-021-00737-6
79. Cadilha BL, Benmebarek MR, Dorman K, Oner A, Lorenzini T, Obeck H, et al. Combined tumor-directed recruitment and protection from immune suppression enable CAR T cell efficacy in solid tumors. Sci Adv. (2021) 7. doi: 10.1126/sciadv.abi5781
80. Caruana I, Savoldo B, Hoyos V, Weber G, Liu H, Kim ES, et al. Heparanase promotes tumor infiltration and antitumor activity of CAR-redirected T lymphocytes. Nat Med. (2015) 21:524–9. doi: 10.1038/nm.3833
81. Zhao R, Cui Y, Zheng Y, Li S, Lv J, Wu Q, et al. Human hyaluronidase PH20 potentiates the antitumor activities of mesothelin-specific CAR-T cells against gastric cancer. Front Immunol. (2021) 12:660488. doi: 10.3389/fimmu.2021.660488
82. Akbari P, Katsarou A, Daghighian R, van Mil L, Huijbers EJM, Griffioen AW, et al. Directing CAR T cells towards the tumor vasculature for the treatment of solid tumors. Biochim Biophys Acta Rev Cancer. (2022) 1877:188701. doi: 10.1016/j.bbcan.2022.188701
83. Liu H, Lei W, Zhang C, Yang C, Wei J, Guo Q, et al. CD19-specific CAR T cells that express a PD-1/CD28 chimeric switch-receptor are effective in patients with PD-L1-positive B-cell lymphoma. Clin Cancer Res. (2021) 27:473–84. doi: 10.1158/1078-0432.CCR-20-1457
84. Prinzing B, Zebley CC, Petersen CT, Fan Y, Anido AA, Yi Z, et al. Deleting DNMT3A in CAR T cells prevents exhaustion and enhances antitumor activity. Sci Transl Med. (2021) 13:eabh0272. doi: 10.1126/scitranslmed.abh0272
85. Li W, Pan X, Chen L, Cui H, Mo S, Pan Y, et al. Cell metabolism-based optimization strategy of CAR-T cell function in cancer therapy. Front Immunol. (2023) 14:1186383. doi: 10.3389/fimmu.2023.1186383
86. Chan JD, Scheffler CM, Munoz I, Sek K, Lee JN, Huang YK, et al. FOXO1 enhances CAR T cell stemness, metabolic fitness and efficacy. Nature. (2024) 629:201–10. doi: 10.1038/s41586-024-07242-1
87. Simula L, Fumagalli M, Vimeux L, Rajnpreht I, Icard P, Birsen G, et al. Mitochondrial metabolism sustains CD8(+) T cell migration for an efficient infiltration into solid tumors. Nat Commun. (2024) 15:2203. doi: 10.1038/s41467-024-46377-7
88. Zhong W, Xiao Z, Qin Z, Yang J, Wen Y, Yu Z, et al. Tumor-derived small extracellular vesicles inhibit the efficacy of CAR T cells against solid tumors. Cancer Res. (2023) 83:2790–806. doi: 10.1158/0008-5472.CAN-22-2220
89. Brown CE, Hibbard JC, Alizadeh D, Blanchard MS, Natri HM, Wang D, et al. Locoregional delivery of IL-13Rα2-targeting CAR-T cells in recurrent high-grade glioma: a phase 1 trial. Nat Med. (2024) 30:1001–12. doi: 10.1038/s41591-024-02875-1
90. Agliardi G, Liuzzi AR, Hotblack A, De Feo D, Núñez N, Stowe CL, et al. Intratumoral IL-12 delivery empowers CAR-T cell immunotherapy in a pre-clinical model of glioblastoma. Nat Commun. (2021) 12:444. doi: 10.1038/s41467-020-20599-x
91. Grosskopf AK, Labanieh L, Klysz DD, Roth GA, Xu P, Adebowale O, et al. Delivery of CAR-T cells in a transient injectable stimulatory hydrogel niche improves treatment of solid tumors. Sci Adv. (2022) 8:eabn8264. doi: 10.1126/sciadv.abn8264
92. Nguyen NT, Huang K, Zeng H, Jing J, Wang R, Fang S, et al. Nano-optogenetic engineering of CAR T cells for precision immunotherapy with enhanced safety. Nat Nanotechnol. (2021) 16:1424–34. doi: 10.1038/s41565-021-00982-5
93. Grosser R, Cherkassky L, Chintala N, Adusumilli PS. Combination immunotherapy with CAR T cells and checkpoint blockade for the treatment of solid tumors. Cancer Cell. (2019) 36:471–82. doi: 10.1016/j.ccell.2019.09.006
94. Lv Y, Luo X, Xie Z, Qiu J, Yang J, Deng Y, et al. Prospects and challenges of CAR-T cell therapy combined with ICIs. Front Oncol. (2024) 14:1368732. doi: 10.3389/fonc.2024.1368732
95. Adusumilli PS, Zauderer MG, Rivière I, Solomon SB, Rusch VW, O'Cearbhaill RE, et al. A phase I trial of regional mesothelin-targeted CAR T-cell therapy in patients with Malignant pleural disease, in combination with the anti-PD-1 agent pembrolizumab. Cancer Discovery. (2021) 11:2748–63. doi: 10.1158/2159-8290.CD-21-0407
96. Kverneland AH, Pedersen M, Westergaard MCW, Nielsen M, Borch TH, Olsen LR, et al. Adoptive cell therapy in combination with checkpoint inhibitors in ovarian cancer. Oncotarget. (2020) 11:2092–105. doi: 10.18632/oncotarget.v11i22
97. Liu J, Jiao X, Ma D, Fang Y, Gao Q. CAR-T therapy and targeted treatments: Emerging combination strategies in solid tumors. Med. (2024). doi: 10.1016/j.medj.2024.03.001
98. Heczey A. Alliance of the titans: an effective combination of a TKI with CAR T cells. Mol Ther. (2019) 27:1348–9. doi: 10.1016/j.ymthe.2019.07.008
99. Ji F, Zhang F, Zhang M, Long K, Xia M, Lu F, et al. Targeting the DNA damage response enhances CD70 CAR-T cell therapy for renal carcinoma by activating the cGAS-STING pathway. J Hematol Oncol. (2021) 14:152. doi: 10.1186/s13045-021-01168-1
100. Bocca P, Di Carlo E, Caruana I, Emionite L, Cilli M, De Angelis B, et al. Bevacizumab-mediated tumor vasculature remodelling improves tumor infiltration and antitumor efficacy of GD2-CAR T cells in a human neuroblastoma preclinical model. Oncoimmunology. (2017) 7:e1378843. doi: 10.1080/2162402X.2017.1378843
101. Dörrie J, Babalija L, Hoyer S, Gerer KF, Schuler G, Heinzerling L, et al. BRAF and MEK inhibitors influence the function of reprogrammed T cells: consequences for adoptive T-cell therapy. Int J Mol Sci. (2018) 19. doi: 10.3390/ijms19010289
102. Kailayangiri S, Altvater B, Lesch S, Balbach S, Göttlich C, Kühnemundt J, et al. EZH2 inhibition in ewing sarcoma upregulates G(D2) expression for targeting with gene-modified T cells. Mol Ther. (2019) 27:933–46. doi: 10.1016/j.ymthe.2019.02.014
103. Wang Z, Zhou G, Risu N, Fu J, Zou Y, Tang J, et al. Lenalidomide enhances CAR-T cell activity against solid tumor cells. Cell Transplant. (2020) 29:963689720920825. doi: 10.1177/0963689720920825
104. Wang AX, Ong XJ, D'Souza C, Neeson PJ, Zhu JJ. Combining chemotherapy with CAR-T cell therapy in treating solid tumors. Front Immunol. (2023) 14:1140541. doi: 10.3389/fimmu.2023.1140541
105. Lickefett B, Chu L, Ortiz-Maldonado V, Warmuth L, Barba P, Doglio M, et al. Lymphodepletion - an essential but undervalued part of the chimeric antigen receptor T-cell therapy cycle. Front Immunol. (2023) 14:1303935. doi: 10.3389/fimmu.2023.1303935
106. Neelapu SS. CAR-T efficacy: is conditioning the key? Blood. (2019) 133:1799–800. doi: 10.1182/blood-2019-03-900928
107. Qin VM, Haynes NM, D'Souza C, Neeson PJ, Zhu JJ. CAR-T plus radiotherapy: A promising combination for immunosuppressive tumors. Front Immunol. (2021) 12:813832. doi: 10.3389/fimmu.2021.813832
108. Murty S, Haile ST, Beinat C, Aalipour A, Alam IS, Murty T, et al. Intravital imaging reveals synergistic effect of CAR T-cells and radiation therapy in a preclinical immunocompetent glioblastoma model. Oncoimmunology. (2020) 9:1757360. doi: 10.1080/2162402X.2020.1757360
109. DeSelm C, Palomba ML, Yahalom J, Hamieh M, Eyquem J, Rajasekhar VK, et al. Low-dose radiation conditioning enables CAR T cells to mitigate antigen escape. Mol Ther. (2018) 26:2542–52. doi: 10.1016/j.ymthe.2018.09.008
110. Mamola JA, Chen CY, Currier MA, Cassady K, Lee DA, Cripe TP. Opportunities and challenges of combining adoptive cellular therapy with oncolytic virotherapy. Mol Ther Oncolytics. (2023) 29:118–24. doi: 10.1016/j.omto.2023.04.008
111. Li Y, Xiao F, Zhang A, Zhang D, Nie W, Xu T, et al. Oncolytic adenovirus targeting TGF-β enhances anti-tumor responses of mesothelin-targeted chimeric antigen receptor T cell therapy against breast cancer. Cell Immunol. (2020) 348:104041. doi: 10.1016/j.cellimm.2020.104041
112. Chalise L, Kato A, Ohno M, Maeda S, Yamamichi A, Kuramitsu S, et al. Efficacy of cancer-specific anti-podoplanin CAR-T cells and oncolytic herpes virus G47Δ combination therapy against glioblastoma. Mol Ther Oncolytics. (2022) 26:265–74. doi: 10.1016/j.omto.2022.07.006
113. Tang X, Li Y, Ma J, Wang X, Zhao W, Hossain MA, et al. Adenovirus-mediated specific tumor tagging facilitates CAR-T therapy against antigen-mismatched solid tumors. Cancer Lett. (2020) 487:1–9. doi: 10.1016/j.canlet.2020.05.013
114. Crutchfield GG, Liu Y, Yan F, Li X, Lee H-H, Wang M, et al. Exploring biTE-integrated CAR T-cell therapy to overcome tumor antigen escape and reinforce CAR-T therapy in mantle cell lymphoma. Blood. (2023) 142:6820–. doi: 10.1182/blood-2023-190167
115. Choi BD, Yu X, Castano AP, Bouffard AA, Schmidts A, Larson RC, et al. CAR-T cells secreting BiTEs circumvent antigen escape without detectable toxicity. Nat Biotechnol. (2019) 37:1049–58. doi: 10.1038/s41587-019-0192-1
116. Torikai H, Reik A, Liu PQ, Zhou Y, Zhang L, Maiti S, et al. A foundation for universal T-cell based immunotherapy: T cells engineered to express a CD19-specific chimeric-antigen-receptor and eliminate expression of endogenous TCR. Blood. (2012) 119:5697–705. doi: 10.1182/blood-2012-01-405365
117. Poirot L, Philip B, Schiffer-Mannioui C, Le Clerre D, Chion-Sotinel I, Derniame S, et al. Multiplex genome-edited T-cell manufacturing platform for "Off-the-shelf" Adoptive T-cell immunotherapies. Cancer Res. (2015) 75:3853–64. doi: 10.1158/0008-5472.CAN-14-3321
118. Osborn MJ, Webber BR, Knipping F, Lonetree CL, Tennis N, DeFeo AP, et al. Evaluation of TCR gene editing achieved by TALENs, CRISPR/cas9, and megaTAL nucleases. Mol Ther. (2016) 24:570–81. doi: 10.1038/mt.2015.197
119. Melenhorst JJ, Leen AM, Bollard CM, Quigley MF, Price DA, Rooney CM, et al. Allogeneic virus-specific T cells with HLA alloreactivity do not produce GVHD in human subjects. Blood. (2010) 116:4700–2. doi: 10.1182/blood-2010-06-289991
120. Becker PS, Suck G, Nowakowska P, Ullrich E, Seifried E, Bader P, et al. Selection and expansion of natural killer cells for NK cell-based immunotherapy. Cancer Immunol Immunother. (2016) 65:477–84. doi: 10.1007/s00262-016-1792-y
121. Han J, Chu J, Keung Chan W, Zhang J, Wang Y, Cohen JB, et al. CAR-engineered NK cells targeting wild-type EGFR and EGFRvIII enhance killing of glioblastoma and patient-derived glioblastoma stem cells. Sci Rep. (2015) 5:11483. doi: 10.1038/srep11483
122. Capsomidis A, Benthall G, Van Acker HH, Fisher J, Kramer AM, Abeln Z, et al. Chimeric antigen receptor-engineered human gamma delta T cells: enhanced cytotoxicity with retention of cross presentation. Mol Ther. (2018) 26:354–65. doi: 10.1016/j.ymthe.2017.12.001
123. Vasic D, Lee JB, Leung Y, Khatri I, Na Y, Abate-Daga D, et al. Allogeneic double-negative CAR-T cells inhibit tumor growth without off-tumor toxicities. Sci Immunol. (2022) 7:eabl3642. doi: 10.1126/sciimmunol.abl3642
124. O'Neal J, Cooper ML, Ritchey JK, Gladney S, Niswonger J, González LS, et al. Anti-myeloma efficacy of CAR-iNKT is enhanced with a long-acting IL-7, rhIL-7-hyFc. Blood Adv. (2023) 7:6009–22. doi: 10.1182/bloodadvances.2023010032
125. Mo F, Watanabe N, McKenna MK, Hicks MJ, Srinivasan M, Gomes-Silva D, et al. Engineered off-the-shelf therapeutic T cells resist host immune rejection. Nat Biotechnol. (2021) 39:56–63. doi: 10.1038/s41587-020-0601-5
126. Wang D, Quan Y, Yan Q, Morales JE, Wetsel RA. Targeted disruption of the β2-microglobulin gene minimizes the immunogenicity of human embryonic stem cells. Stem Cells Transl Med. (2015) 4:1234–45. doi: 10.5966/sctm.2015-0049
127. Di Stasi A, Tey SK, Dotti G, Fujita Y, Kennedy-Nasser A, Martinez C, et al. Inducible apoptosis as a safety switch for adoptive cell therapy. N Engl J Med. (2011) 365:1673–83. doi: 10.1056/NEJMoa1106152
128. Bouquet L, Bôle-Richard E, Warda W, Neto Da Rocha M, Trad R, Nicod C, et al. RapaCaspase-9-based suicide gene applied to the safety of IL-1RAP CAR-T cells. Gene Ther. (2023) 30:706–13. doi: 10.1038/s41434-023-00404-2
129. Del Bufalo F, De Angelis B, Caruana I, Del Baldo G, De Ioris MA, Serra A, et al. GD2-CART01 for relapsed or refractory high-risk neuroblastoma. N Engl J Med. (2023) 388:1284–95. doi: 10.1056/NEJMoa2210859
130. Foster MC, Savoldo B, Lau W, Rubinos C, Grover N, Armistead P, et al. Utility of a safety switch to abrogate CD19.CAR T-cell–associated neurotoxicity. Blood. (2021) 137:3306–9. doi: 10.1182/blood.2021010784
131. Koneru M, O'Cearbhaill R, Pendharkar S, Spriggs DR, Brentjens RJ. A phase I clinical trial of adoptive T cell therapy using IL-12 secreting MUC-16(ecto) directed chimeric antigen receptors for recurrent ovarian cancer. J Transl Med. (2015) 13:102. doi: 10.1186/s12967-015-0460-x
132. Sahillioglu AC, Toebes M, Apriamashvili G, Gomez R, Schumacher TN. CRASH-IT switch enables reversible and dose-dependent control of TCR and CAR T-cell function. Cancer Immunol Res. (2021) 9:999–1007. doi: 10.1158/2326-6066.CIR-21-0095
133. Jan M, Scarfò I, Larson RC, Walker A, Schmidts A, Guirguis AA, et al. Reversible ON- and OFF-switch chimeric antigen receptors controlled by lenalidomide. Sci Transl Med. (2021) 13. doi: 10.1126/scitranslmed.abb6295
134. Salzer B, Schueller CM, Zajc CU, Peters T, Schoeber MA, Kovacic B, et al. Engineering AvidCARs for combinatorial antigen recognition and reversible control of CAR function. Nat Commun. (2020) 11:4166. doi: 10.1038/s41467-020-17970-3
135. Cho JH, Collins JJ, Wong WW. Universal chimeric antigen receptors for multiplexed and logical control of T cell responses. Cell. (2018) 173:1426–38.e11. doi: 10.1016/j.cell.2018.03.038
136. Cho JH, Okuma A, Sofjan K, Lee S, Collins JJ, Wong WW. Engineering advanced logic and distributed computing in human CAR immune cells. Nat Commun. (2021) 12:792. doi: 10.1038/s41467-021-21078-7
137. Kloss CC, Condomines M, Cartellieri M, Bachmann M, Sadelain M. Combinatorial antigen recognition with balanced signaling promotes selective tumor eradication by engineered T cells. Nat Biotechnol. (2013) 31:71–5. doi: 10.1038/nbt.2459
138. Fraessle SP, Tschulik C, Effenberger M, Cletiu V, Gerget M, Schober K, et al. Activation-inducible CAR expression enables precise control over engineered CAR T cell function. Commun Biol. (2023) 6:604. doi: 10.1038/s42003-023-04978-w
139. Smole A, Benton A, Poussin MA, Eiva MA, Mezzanotte C, Camisa B, et al. Expression of inducible factors reprograms CAR-T cells for enhanced function and safety. Cancer Cell. (2022) 40:1470–87.e7. doi: 10.1016/j.ccell.2022.11.006
140. Juillerat A, Tkach D, Busser BW, Temburni S, Valton J, Duclert A, et al. Modulation of chimeric antigen receptor surface expression by a small molecule switch. BMC Biotechnol. (2019) 19:44. doi: 10.1186/s12896-019-0537-3
141. Richman SA, Wang LC, Moon EK, Khire UR, Albelda SM, Milone MC. Ligand-induced degradation of a CAR permits reversible remote control of CAR T cell activity in vitro and in vivo. Mol Ther. (2020) 28:1600–13. doi: 10.1016/j.ymthe.2020.06.004
142. Wu Y, Liu Y, Huang Z, Wang X, Jin Z, Li J, et al. Control of the activity of CAR-T cells within tumours via focused ultrasound. Nat BioMed Eng. (2021) 5:1336–47. doi: 10.1038/s41551-021-00779-w
143. Kobayashi A, Nobili A, Neier SC, Sadiki A, Distel R, Zhou ZS, et al. Light-controllable binary switch activation of CAR T cells. ChemMedChem. (2022) 17:e202100722. doi: 10.1002/cmdc.202100722
144. Hiltbrunner S, Britschgi C, Schuberth P, Bankel L, Nguyen-Kim TDL, Gulati P, et al. Local delivery of CAR T cells targeting fibroblast activation protein is safe in patients with pleural mesothelioma: first report of FAPME, a phase I clinical trial. Ann Oncol. (2021) 32:120–1. doi: 10.1016/j.annonc.2020.10.474
145. Caimi PF, Pacheco Sanchez G, Sharma A, Otegbeye F, Ahmed N, Rojas P, et al. Prophylactic tocilizumab prior to anti-CD19 CAR-T cell therapy for non-Hodgkin lymphoma. Front Immunol. (2021) 12:745320. doi: 10.3389/fimmu.2021.745320
146. Strati P, Jallouk A, Deng Q, Li X, Feng L, Sun R, et al. A phase 1 study of prophylactic anakinra to mitigate ICANS in patients with large B-cell lymphoma. Blood Adv. (2023) 7:6785–9. doi: 10.1182/bloodadvances.2023010653
147. Park JH, Nath K, Devlin SM, Sauter CS, Palomba ML, Shah G, et al. CD19 CAR T-cell therapy and prophylactic anakinra in relapsed or refractory lymphoma: phase 2 trial interim results. Nat Med. (2023) 29:1710–7. doi: 10.1038/s41591-023-02404-6
148. Butt OH, Zhou AY, Ances BM, DiPersio JF, Ghobadi A. A systematic framework for predictive biomarkers in immune effector cell-associated neurotoxicity syndrome. Front Neurol. (2023) 14:1110647. doi: 10.3389/fneur.2023.1110647
149. Frigault M, Rotte A, Ansari A, Gliner B, Heery C, Shah B. Dose fractionation of CAR-T cells. A systematic review of clinical outcomes. J Exp Clin Cancer Res. (2023) 42:11. doi: 10.1186/s13046-022-02540-w
150. Sterner RM, Sakemura R, Cox MJ, Yang N, Khadka RH, Forsman CL, et al. GM-CSF inhibition reduces cytokine release syndrome and neuroinflammation but enhances CAR-T cell function in xenografts. Blood. (2019) 133:697–709. doi: 10.1182/blood-2018-10-881722
151. Kang L, Tang X, Zhang J, Li M, Xu N, Qi W, et al. Interleukin-6-knockdown of chimeric antigen receptor-modified T cells significantly reduces IL-6 release from monocytes. Exp Hematol Oncol. (2020) 9:11. doi: 10.1186/s40164-020-00166-2
152. Norelli M, Camisa B, Barbiera G, Falcone L, Purevdorj A, Genua M, et al. Monocyte-derived IL-1 and IL-6 are differentially required for cytokine-release syndrome and neurotoxicity due to CAR T cells. Nat Med. (2018) 24:739–48. doi: 10.1038/s41591-018-0036-4
153. Giavridis T, van der Stegen SJC, Eyquem J, Hamieh M, Piersigilli A, Sadelain M. CAR T cell-induced cytokine release syndrome is mediated by macrophages and abated by IL-1 blockade. Nat Med. (2018) 24:731–8. doi: 10.1038/s41591-018-0041-7
154. Francis N, Braun M, Neagle S, Peiffer S, Bohn A, Rosenthal A, et al. Development of an automated manufacturing process for large-scale production of autologous T cell therapies. Mol Ther Methods Clin Dev. (2023) 31:101114. doi: 10.1016/j.omtm.2023.101114
155. Jackson Z, Roe A, Sharma AA, Lopes F, Talla A, Kleinsorge-Block S, et al. Automated manufacture of autologous CD19 CAR-T cells for treatment of non-Hodgkin lymphoma. Front Immunol. (2020) 11:1941. doi: 10.3389/fimmu.2020.01941
156. Lock D, Mockel-Tenbrinck N, Drechsel K, Barth C, Mauer D, Schaser T, et al. Automated manufacturing of potent CD20-directed chimeric antigen receptor T cells for clinical use. Hum Gene Ther. (2017) 28:914–25. doi: 10.1089/hum.2017.111
157. Kedmi M, Shouval R, Fried S, Bomze D, Fein J, Cohen Z, et al. Point-of-care anti-CD19 CAR T-cells for treatment of relapsed and refractory aggressive B-cell lymphoma. Transplant Cell Ther. (2022) 28:251–7. doi: 10.1016/j.jtct.2022.02.017
158. Castella M, Caballero-Baños M, Ortiz-Maldonado V, González-Navarro EA, Suñé G, Antoñana-Vidósola A, et al. Point-of-care CAR T-cell production (ARI-0001) using a closed semi-automatic bioreactor: experience from an academic phase I clinical trial. Front Immunol. (2020) 11:482. doi: 10.3389/fimmu.2020.00482
159. Ghassemi S, Martinez-Becerra FJ, Master AM, Richman SA, Heo D, Leferovich J, et al. Enhancing chimeric antigen receptor T cell anti-tumor function through advanced media design. Mol Ther Methods Clin Dev. (2020) 18:595–606. doi: 10.1016/j.omtm.2020.07.008
160. Medvec AR, Ecker C, Kong H, Winters EA, Glover J, Varela-Rohena A, et al. Improved expansion and in vivo function of patient T cells by a serum-free medium. Mol Ther Methods Clin Dev. (2018) 8:65–74. doi: 10.1016/j.omtm.2017.11.001
161. Ganeeva I, Zmievskaya E, Valiullina A, Kudriaeva A, Miftakhova R, Rybalov A, et al. Recent advances in the development of bioreactors for manufacturing of adoptive cell immunotherapies. Bioengineering (Basel). (2022) 9. doi: 10.3390/bioengineering9120808
162. Hort S, Herbst L, Bäckel N, Erkens F, Niessing B, Frye M, et al. Toward rapid, widely available autologous CAR-T cell therapy - artificial intelligence and automation enabling the smart manufacturing hospital. Front Med (Lausanne). (2022) 9:913287. doi: 10.3389/fmed.2022.913287
163. Derraz B, Breda G, Kaempf C, Baenke F, Cotte F, Reiche K, et al. New regulatory thinking is needed for AI-based personalised drug and cell therapies in precision oncology. NPJ Precis Oncol. (2024) 8:23. doi: 10.1038/s41698-024-00517-w
164. Odeh-Couvertier VY, Dwarshuis NJ, Colonna MB, Levine BL, Edison AS, Kotanchek T, et al. Predicting T-cell quality during manufacturing through an artificial intelligence-based integrative multiomics analytical platform. Bioeng Transl Med. (2022) 7:e10282. doi: 10.1002/btm2.10282
165. Kirouac DC, Zmurchok C, Deyati A, Sicherman J, Bond C, Zandstra PW. Deconvolution of clinical variance in CAR-T cell pharmacology and response. Nat Biotechnol. (2023) 41:1606–17. doi: 10.1038/s41587-023-01687-x
166. Daniels KG, Wang S, Simic MS, Bhargava HK, Capponi S, Tonai Y, et al. Decoding CAR T cell phenotype using combinatorial signaling motif libraries and machine learning. Science. (2022) 378:1194–200. doi: 10.1126/science.abq0225
167. Heine R, Thielen FW, Koopmanschap M, Kersten MJ, Einsele H, Jaeger U, et al. Health economic aspects of chimeric antigen receptor T-cell therapies for hematological cancers: present and future. Hemasphere. (2021) 5:e524. doi: 10.1097/HS9.0000000000000524
168. Levine BL, Miskin J, Wonnacott K, Keir C. Global manufacturing of CAR T cell therapy. Mol Ther Methods Clin Dev. (2017) 4:92–101. doi: 10.1016/j.omtm.2016.12.006
169. Saleh S, Dabbous O, Sullivan SD, Ankleshwaria D, Trombini D, Toumi M, et al. A practical approach for adoption of a hub and spoke model for cell and gene therapies in low- and middle-income countries: framework and case studies. Gene Ther. (2024) 31:1–11. doi: 10.1038/s41434-023-00425-x
170. Geethakumari PR, Ramasamy DP, Dholaria B, Berdeja J, Kansagra A. Balancing quality, cost, and access during delivery of newer cellular and immunotherapy treatments. Curr Hematol Malig Rep. (2021) 16:345–56. doi: 10.1007/s11899-021-00635-3
171. Marks P. The FDA's regulatory framework for chimeric antigen receptor-T cell therapies. Clin Transl Sci. (2019) 12:428–30. doi: 10.1111/cts.12666
172. Locke FL, Davila ML. Regulatory challenges and considerations for the clinical application of CAR-T cell anti-cancer therapy. Expert Opin Biol Ther. (2017) 17:659–61. doi: 10.1080/14712598.2017.1322953
173. Mackensen A, Müller F, Mougiakakos D, Böltz S, Wilhelm A, Aigner M, et al. Anti-CD19 CAR T cell therapy for refractory systemic lupus erythematosus. Nat Med. (2022) 28:2124–32. doi: 10.1038/s41591-022-02017-5
174. Mullard A. CAR-T therapy for multiple sclerosis enters US trials for first time. Nature. (2024). doi: 10.1038/d41586-024-00470-5
175. Zhang L, Sosinowski T, Cox AR, Cepeda JR, Sekhar NS, Hartig SM, et al. Chimeric antigen receptor (CAR) T cells targeting a pathogenic MHC class II:peptide complex modulate the progression of autoimmune diabetes. J Autoimmun. (2019) 96:50–8. doi: 10.1016/j.jaut.2018.08.004
176. Amor C, Feucht J, Leibold J, Ho YJ, Zhu C, Alonso-Curbelo D, et al. Senolytic CAR T cells reverse senescence-associated pathologies. Nature. (2020) 583:127–32. doi: 10.1038/s41586-020-2403-9
177. Aghajanian H, Kimura T, Rurik JG, Hancock AS, Leibowitz MS, Li L, et al. Targeting cardiac fibrosis with engineered T cells. Nature. (2019) 573:430–3. doi: 10.1038/s41586-019-1546-z
Keywords: CAR T-cell therapy, immunotherapy, synthetic biology, gene editing, allogeneic CAR T cells, immunosuppressive microenvironment
Citation: Ali A and DiPersio JF (2024) ReCARving the future: bridging CAR T-cell therapy gaps with synthetic biology, engineering, and economic insights. Front. Immunol. 15:1432799. doi: 10.3389/fimmu.2024.1432799
Received: 14 May 2024; Accepted: 12 August 2024;
Published: 05 September 2024.
Edited by:
Xiuli Wang, City of Hope National Medical Center, United StatesReviewed by:
Caroline Arber, Centre Hospitalier Universitaire Vaudois (CHUV), SwitzerlandJiaqian Qi, The First Affiliated Hospital of Soochow University, China
Copyright © 2024 Ali and DiPersio. This is an open-access article distributed under the terms of the Creative Commons Attribution License (CC BY). The use, distribution or reproduction in other forums is permitted, provided the original author(s) and the copyright owner(s) are credited and that the original publication in this journal is cited, in accordance with accepted academic practice. No use, distribution or reproduction is permitted which does not comply with these terms.
*Correspondence: Alaa Ali, YWxhYS5hbGlAZ3VuZXQuZ2VvcmdldG93bi5lZHU=