- 1Department of Physical Medicine and Rehabilitation, The Affiliated Panyu Central Hospital, Guangzhou Medical University, Guangzhou, China
- 2Department of Rheumatology, Changde Hospital, Xiangya School of Medicine, Central South University, Changde, China
- 3Department of Acupuncture and Massage, The Affiliated Panyu Central Hospital, Guangzhou Medical University, Guangzhou, China
- 4Hainan Normal University, Haikou, China
Artemisinin and its derivatives are widely recognized as first-line treatments for malaria worldwide. Recent studies have demonstrated that artemisinin-based antimalarial drugs, such as artesunate, dihydroartemisinin, and artemether, not only possess excellent antimalarial properties but also exhibit antitumor, antifungal, and immunomodulatory effects. Researchers globally have synthesized artemisinin derivatives like SM735, SM905, and SM934, which offer advantages such as low toxicity, high bioavailability, and potential immunosuppressive properties. These compounds induce immunosuppression by inhibiting the activation of pathogenic T cells, suppressing B cell activation and antibody production, and enhancing the differentiation of regulatory T cells. This review summarized the mechanisms by which artemisinin and its analogs modulate excessive inflammation and immune responses in rheumatic and skeletal diseases, autoimmune inflammatory diseases, and autoimmune disorders, through pathways including TNF, Toll-like receptors, IL-6, RANKL, MAPK, PI3K/AKT/mTOR, JAK/STAT, and NRF2/GPX4. Notably, in the context of the NF-κB pathway, artemisinin not only inhibits NF-κB expression by disrupting upstream cascades and/or directly binding to NF-κB but also downregulates multiple downstream genes controlled by NF-κB, including inflammatory chemokines and their receptors. These downstream targets regulate various immune cell functions, apoptosis, proliferation, signal transduction, and antioxidant responses, ultimately intervening in systemic autoimmune diseases and autoimmune responses in organs such as the kidneys, nervous system, skin, liver, and biliary system by modulating immune dysregulation and inflammatory responses. Ongoing multicenter randomized clinical trials are investigating the effects of these compounds on rheumatic, inflammatory, and autoimmune diseases, with the aim of translating promising preclinical data into clinical applications.
1 Introduction
Autoimmune diseases are inflammatory conditions where the immune system mistakenly attacks normal cells, leading to impaired immune function, abnormal immune responses, and ultimately tissue damage and organ dysfunction (1). Although the exact mechanisms of autoimmune diseases remain unclear, they always exhibit several distinct characteristics (2, 3): (1) The causes are often unknown, with some cases linked to bacterial or viral infections or certain medications; (2) The incidence is higher in women than in men, with a female-to-male ratio of 10:1; (3) The disease course is typically chronic, with recurrent flares and progressive symptoms; (4) There is a noticeable familial predisposition; (5) A single patient may suffer from two or more autoimmune diseases simultaneously. Epidemiological studies indicate that approximately 10% of the global population is affected by various autoimmune diseases (4, 5). These conditions often involve the joints, muscles, bones, and other soft tissues throughout the body, and if left untreated, can lead to widespread organ or system damage. Autoimmune diseases are associated with high rates of disability, increased mortality, and a significant reduction in quality of life (6). As complex, multifactorial diseases influenced by genetic and environmental factors, they typically have a slow onset, with patients often testing positive for autoantibodies years before showing any clinical signs or symptoms (7–9). Clinically, corticosteroids and disease-modifying antirheumatic drugs (DMARDs) are commonly used to manage these conditions, but long-term or lifelong medication is often necessary. While these treatments can control disease activity, many patients continue to experience symptoms, and prolonged use of steroids and immunosuppressants can lead to metabolic disorders, immunodeficiency, secondary infections, and other adverse effects (10, 11). This highlights the need to explore alternative therapeutic biologics or novel traditional medicine formulations, particularly immunomodulatory or immunosuppressive DMARDs, to alleviate symptoms, reduce side effects, and improve quality of life (12, 13). The immune mechanisms underlying autoimmune diseases are thought to involve abnormal lymphocyte responses to target organs. Even with strict central and peripheral immune tolerance, a small number of potentially autoreactive lymphocytes can “escape” into the periphery, where they are repeatedly exposed to self-antigens and activated by costimulatory signals, initiating an immune response. Under the influence of various cytokines, naïve lymphocytes can become activated and differentiate into various types of inflammatory cells, ultimately leading to inflammation and soft tissue damage (14–16).
Artemisinin, an effective antimalarial compound, is extracted from the plant Artemisia annua L. using low-temperature ether extraction. Its structure, based on a sesquiterpene lactone core with a unique peroxide bridge, has led to the synthesis of various artemisinin derivatives, such as dihydroartemisinin (DHA) and artesunate (17). These derivatives have been improved in terms of water solubility, bioavailability, and pharmacological properties, making them some of the most widely used and effective antimalarial drugs today. DHA, the primary active metabolite of artemisinin, is produced by reducing artemisinin with sodium borohydride (18). With its unique peroxide bridge structure, DHA is a crucial natural drug from Artemisia annua L., offering advantages such as rapid absorption, fast metabolism and excretion, wide distribution, high efficacy, and low toxicity. Its oral bioavailability is more than ten times higher than that of artemisinin, and its antimalarial efficacy is 4 to 8 times greater (19). Additionally, studies have revealed that DHA possesses anticancer, anti-inflammatory, and antiparasitic properties (20). Notably, numerous studies have demonstrated that artemisinin and its derivatives have the capacity to regulate inflammation and autoimmune responses, marking significant progress in the treatment of various rheumatic and inflammatory diseases (21). This review provides an overview of the research on the mechanisms by which artemisinin-based drugs alleviate autoimmune diseases, aiming to serve as a reference for further in-depth studies.
2 Artemisinin and its main derivatives
With the deepening of research on artemisinin, the modification of artemisinin derivatives and analogs has become a hotspot of research. Artemisinin and its derivatives are all sesquiterpene lactone compounds. It has been demonstrated that deoxyartemisinin can inhibit the growth and reproduction of Plasmodium falciparum, which is related to the peroxide bridge in its structure (22). Therefore, the structural modifications of artemisinin are conducted based on the preservation of the peroxide bridge, and modifications at positions C-9 and C-10 are most common (23).
2.1 Artemisinin
Artemisinin was isolated in the 1950s by a research team at the China Academy of Chinese Medical Sciences from Artemisia annua L., becoming the second natural product used to treat malaria after quinine. Clinical studies have confirmed its exceptional antimalarial activity. In 1975, high-resolution mass spectrometry identified the compound as a sesquiterpene, and infrared spectroscopy, along with a quantitative reaction with triphenylphosphine, revealed the presence of a unique peroxide group. Subsequently, nuclear magnetic resonance and X-ray diffraction were used to determine the structure and relative configuration of artemisinin, while optical rotatory dispersion was employed to establish the absolute configuration of the lactone ring (24). The distinctive structure and outstanding antimalarial properties of artemisinin have since garnered ongoing scientific interest and research.
2.2 DHA
Reducing the C-10 carbonyl group in artemisinin’s structure with sodium borohydride produces DHA, the simplest compound in the semisynthetic process of artemisinin derivatives. DHA serves as a precursor for synthesizing other artemisinin-like compounds. Studies have shown that after artemisinin and its derivatives are absorbed by the human body, their pharmacological effects are primarily mediated by conversion to DHA, the active substance. DHA exhibits 4-8 times greater antimalarial efficacy than artemisinin and has significantly improved oral bioavailability, over 10 times higher (19). Additionally, it has a lower recurrence rate during treatment, lower toxicity, and better water solubility. However, DHA is less stable than artemisinin, and its water solubility remains suboptimal. Consequently, researchers are focused on developing new drug delivery materials and formulations to enhance DHA’s pharmaceutical properties and bioavailability, including sustained-release tablets (25), DHA nanoparticles (26–28), DHA liposomes (29), and magnetic DHA nanoliposomes (30).
2.3 Artesunate
Artesunate, also known as artemisinin succinate, is synthesized by esterifying DHA with succinic anhydride. Artesunate exhibits a range of pharmacological effects, including antimalarial, antiviral, anti-inflammatory, antitumor, and immunomodulatory properties. It is highly effective, fast-acting, and has low toxicity, making it less prone to drug resistance (31, 32). As a weakly acidic drug, artesunate primarily relies on simple diffusion for transport within the body, allowing it to easily pass through biological membranes. Its pKa values range from 3.5 to 5.5, indicating a low degree of ionization in acidic environments, though it is soluble in weak alkaline solutions. These characteristics enable artesunate to be formulated into various dosage forms, including injections, tablets, and suppositories, for intravenous, oral, or rectal administration (33).
2.4 Artemether and arteether
By replacing hydrogen atoms with alkyl groups at the hydroxyl positions on C-10 of DHA, artemisinin ether derivatives are produced. The most notable compounds in this category are artemether and arteether, both of which demonstrate higher activity than artemisinin. Although these ether derivatives have good lipid solubility, they suffer from poor water solubility, low bioavailability, and can cause irritation when administered by direct injection. To address these issues, some researchers have encapsulated artemether in aminopterin-modified targeted nanoliposomes. These liposomes have a uniform and stable structure. In vitro release studies have shown that this liposome system effectively sustains drug release in vivo, thereby enhancing the metabolism and bioavailability of artemether (34).
2.5 Other derivatives
Building on the unique peroxide bridge structure of artemisinin, researchers have synthesized various artemisinin analogs, polymers, and simplified structures, expanding the range of artemisinin-like compounds. Artemisinin dimers, which consist of two artemisinin monomers linked by a connector, are among these innovations. Common linkers include alkyl, ether, ester, and carbamate groups. Compared to artemisinin monomers, dimer compounds offer stronger pharmacological activity, fewer adverse reactions, and better physicochemical properties. Studies have demonstrated that artemisinin dimers exhibit excellent anticancer activity both in vitro and in vivo, with the type of linker significantly influencing their efficacy. Thus, optimizing the properties of artemisinin dimers can be achieved by modifying the linkers (35). Additionally, sodium artesunate, an alkaline salt derivative of artemisinin, offers good water solubility, rapid action, and high tolerance, making it suitable for intravenous or intramuscular injection (36).
3 In vivo and in vitro immunomodulatory and anti-inflammatory effects of artemisinin and its derivatives
3.1 Involvement in regulation of the innate immune system
Macrophages and dendritic cells (DCs) are key components of the human innate immune system. Macrophages can adopt different phenotypes depending on their microenvironment, while DCs are known for their strong antigen-presenting capabilities (37). Research in experimental colitis models has shown that artemisinin derivatives primarily target the innate immune system, modulating immune responses by acting on macrophages and DCs. When used alone or in combination with immunomodulators, these derivatives have demonstrated significant anti-inflammatory effects (37). Artesunate, for example, induces apoptosis in primary peritoneal macrophages and bone marrow-derived DCs in a time-dependent manner via the caspase-9 pathway. It also reduces the secretion of IL-12 by DCs and tumor necrosis factor (TNF)-α by macrophages, thereby mitigating inflammatory responses. A key mechanism behind these effects is the inhibition of the NF-κB signaling pathway, which decreases TNF-α release by macrophages—a classical pathway through which artemisinin and its derivatives exert their anti-inflammatory actions (38). In the RAW264.7 cell model, artesunate also inhibits macrophage autophagy activation through the TRAF6-Beclin1-PI3KC3 signaling pathway, reducing the release of TNF-α and IL-6 (39). Further research has revealed that the suppression of IL-1β, IL-6, IL-8, and other pro-inflammatory cytokines by artemisinin and its derivatives is closely linked to the NF-κB signaling pathway (40).
Additionally, artemisinin and its derivatives inhibit pro-inflammatory factors by mediating key signaling pathways, including mitogen-activated protein kinase (MAPK), phosphatidylinositol 3-kinase (PI3K)/AKT, Toll-like receptor (TLR), and nucleotide-binding oligomerization domain protein 2 (NOD2) pathways (41). Beyond inhibiting the production of pro-inflammatory mediators, artemisinin and its derivatives also promote the production of anti-inflammatory cytokines like IL-10, further contributing to their anti-inflammatory effects (42). As macrophages serve as a bridge between the innate and adaptive immune systems, their regulation often impacts adaptive immunity, including processes such as phagocytosis, internalization, and antigen presentation. For instance, evidence suggests that under the influence of IFN-γ secreted by T cells, macrophages and macrophage-like fibroblasts become activated, producing mediators like matrix metalloproteinases (MMPs) and nitric oxide (NO), which exacerbate tissue damage. These cells also form a positive feedback loop by secreting IL-12/IL-23, which enhances the Th1/Th17 response (43). Collectively, this evidence indicates that artemisinin and its derivatives can inhibit the activation of macrophages and DCs, regulate their quantity and functional levels, and participate in inflammation regulation, potentially influencing the adaptive immune system as well.
3.2 Involvement in regulation of the adaptive immune system
Current research indicates that artemisinin and its derivatives play a positive role in maintaining Th cells, regulatory T cells (Tregs), follicular helper T cells (Tfh), and follicular regulatory T cells (Tfr). First, artemisinin and its derivatives effectively inhibit T cell activation, suppressing Th1 and Th17 cells while enhancing Th2 function and differentiation. This helps maintain a balanced Th cell population and subtype ratio, influencing cytokine release and modulating immune function and inflammation levels (42, 44, 45). Second, artemisinin and its derivatives are involved in regulating Treg levels and function. Early studies found that artemether could effectively limit T cell proliferation and reduce IL-2 levels, thereby restricting Treg function (46). However, other derivatives, including SM934, artesunate, and dihydroartemisinin, have been shown to significantly increase Treg level and improve the Treg/Th17 balance (44, 47–52).
Third, artemisinin and its derivatives also regulate Tfh and Tfr levels, both of which play crucial roles in B cell function, germinal center (GC) formation, antibody affinity maturation, and memory B cell production. An imbalance, characterized by increased Tfh or decreased Tfr, is associated with excessive pathological autoantibody secretion and can promote disease progression (53, 54). Current research on the regulatory effects of artemisinin derivatives on Tfh and Tfr levels is inconsistent. In a systemic lupus erythematosus (SLE) model, artesunate was found to effectively reduce Tfh levels in the spleen of SLE mice, increase Tfr levels, maintain the Tfr/Tfh ratio, reduce SLE severity, and prolong survival (55). However, another study reported that artesunate regulated GC B cells without affecting Tfh levels (56). SM934, while not affecting the proportion of GC B cells, has been shown to exert immunosuppressive effects by inhibiting the TLR/MyD88/NF-κB signaling pathway, reducing pro-inflammatory mediator secretion by monocytes/macrophages, and thereby decreasing Tfh cell activation and Tfh/Th17 differentiation (57).
Artemisinin and its derivatives can inhibit the production of antibodies in various pathological conditions, providing important evidence for their involvement in humoral immune system regulation. To date, there is no research confirming the direct effects of artemisinin and its derivatives on B cells. Currently, more research suggests that the decreased antibody levels result from secondary changes mediated by T cell suppression. Artesunate, DHA, and SM934 have been shown to effectively reduce the levels of antinuclear antibodies (ANA) and double-stranded DNA (dsDNA), among other pathological antibodies, in SLE mice (44, 52, 55, 58). In rheumatoid arthritis (RA) models, artemisinin has been found to regulate B cell function by inhibiting GCB cell proliferation, limiting GC formation, and the production of autoantibodies, thereby preventing the occurrence and progression of RA (59). SM934 can directly inhibit splenic local B cell activation and plasma cell formation through the TLR/MyD88/NF-κB signaling pathway, reduce their proportions in B cells, and decrease inflammation levels (56).
4 Immunomodulatory and anti-inflammatory effects of artemisinin and its derivatives
4.1 Effects on inflammatory mediators
The process of inflammatory response is a protective mechanism that releases mediators to defend against damage and disease, resist foreign substances, and prevent infection (60, 61). Inhibiting inflammatory mediators is an effective strategy for treating acute or chronic inflammatory diseases (62). Cytokines, as mediators of intercellular communication, play important roles in the occurrence and maintenance of inflammatory diseases (63, 64). To date, most studies on the anti-inflammatory effects of DHA have focused on pro-inflammatory cytokines, including TNF-α and various interleukins (IL-1, IL-5, IL-6, IL-8, IL-17, IL-18, IL-22, IL-23) (65). Several studies have demonstrated the anti-inflammatory and anti-fibrotic effects of DHA. Dai et al. (66) found that DHA reduced TNF-α levels in serum and lung tissues in a radiation-induced lung injury model, thereby reducing inflammation and lung fibrosis. Similarly, Wang et al. (67) reported that DHA decreased TGF-β1 and TNF-α levels in a mouse model of carbon tetrachloride (CCl4)-induced liver fibrosis, highlighting its anti-fibrotic properties. In a nephritis model, Wu et al. (68) observed that DHA significantly lowered TNF-α and IL-6 levels in serum and improved kidney pathology. Yi et al. (69) showed that combining DHA with ibuprofen reduced IL-1β and TNF-α expression in rats with adjuvant-induced arthritis, compared to ibuprofen alone. Liu et al. (70) discovered that DHA inhibited the proliferation of HaCaT cells, elevated IL-4 and IL-10 levels, and reduced IFN-γ, IL-17, and IL-23 levels, suggesting modulation of the IL-23/IL-17 axis. In models of dextran sodium sulfate (DSS)-induced colitis, both Lei et al. (71) and Liu et al. (72) demonstrated that DHA reduced TNF-α, IL-1β, and IL-23 levels, improved intestinal symptoms, and restored epithelial integrity, indicating its potential in treating inflammatory bowel disease (IBD). Additionally, Li et al. (73) found that DHA reduced TNF-α, IL-1β, and IL-6 levels while increasing IL-10 in macrophages treated with ultra-high molecular weight polyethylene (UHMWPE), showing a dose-dependent anti-inflammatory effect. Macrophages are involved in the overproduction of inflammatory mediators [including nitric oxide (NO) and prostaglandin E2 (PGE2)] and play an important role in inflammation (74). NO, produced by inducible nitric oxide synthase (iNOS), is an important molecule for regulating the biological activity of blood vessels, nerves, and the immune system. However, excessive release of NO during the infection process can cause damage to target tissues (75). Yin et al. (76) found that DHA, at concentrations of 10, 20, and 50 μmol/L, reduced TNF-α and PGE2 expression in endothelial cells, and inhibited IL-6 and IL-1β upregulation induced by TNF-α or PGE2, highlighting its potential in treating infantile vascular diseases. Similarly, Yu et al. (77) reported that DHA inhibited the release of TNF-α, IL-6, and NO in LPS-induced macrophages, and suppressed iNOS protein expression, demonstrating its anti-inflammatory effects.
4.2 Effects of artemisinin and its main derivatives on signaling pathways
The effects of artemisinin and its main derivatives on signaling pathways are summarized in Figure 1.
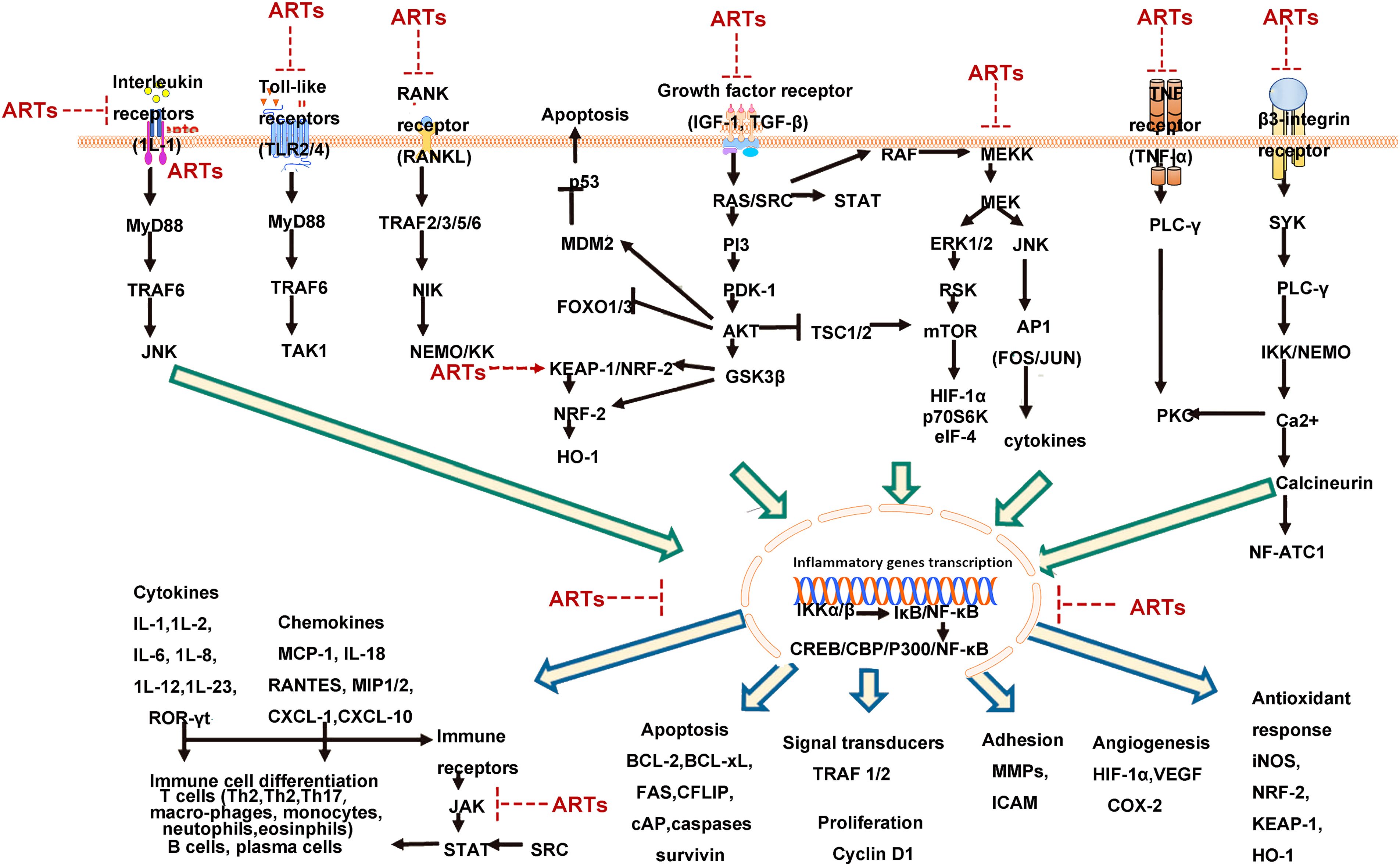
Figure 1. Hypothetical signaling network based on literature summary of immunosuppressive mechanisms of artemisinin and its derivatives [NF-κB plays a central role in the immunosuppressive activity of artemisinin and its derivatives (ARTs). The upstream mechanisms of NF-κB involve several receptor-driven signaling pathways, which are inhibited by artemisinin and its derivatives, subsequently suppressing numerous downstream mechanisms involved in inflammation and autoimmune diseases figures adapted from Efferth et al. 2021 (78), licensed under CC BY 4.0].
4.2.1 NF-κB signaling pathway
The NF-κB/Rel family members, as dimeric transcription factors, play important roles in regulating the expression of cytokines, growth factors, and anti-apoptotic genes. The NF-κB family consists of five related transcription factors, including Rel-C, NF-κB1 (p50/p105), NF-κB2 (p52/p100), Rel-A (p65), and Rel-B (79). NF-κB can be inhibited by NF-κB inhibitory protein (IκB) binding and suppression of the NF-κB/Rel proteins. The activation of the classical pathway of NF-κB affects various biological processes including immune response, inflammatory response, stress response, B cell development, and lymphoid organogenesis (80, 81), while the alternative pathway depends on the activation of IκB kinase (IKKα) (82). Sui et al. (83) established a radiation-induced lung injury model in Wistar rats and administered a single dose of 15 Gy radiation to both lungs of the treatment group rats, followed by oral administration of 60 mg/(kg·day) DHA. The results showed that DHA could inhibit the expression of TNF-α, IL-6, and NF-κB in lung tissues of rats, indicating the inhibitory effects of DHA on the expression of TNF-α and its anti-inflammatory effects in radiation-induced lung injury by regulating the NF-κB signaling pathway, thereby alleviating inflammation. Huang et al. (84) incorporated 80 mg/kg DHA into the basic diet of postpartum growth-retarded (IUGR) piglets. The study found that DHA activated nuclear factor erythroid 2-related factor 2 (Nrf2), which in turn inhibited the NF-κB pathway. This action suppressed the infiltration of inflammatory cells, enhanced myeloperoxidase activity, reduced oxidative stress, and decreased the production of IL-1β, TNF-α, and IL-6 induced by lipopolysaccharide (LPS), ultimately leading to a reduced inflammatory response. Yang et al. (85) divided rats into different groups: normal saline control group (NS group), bleomycin group, dexamethasone group, DHA-1, DHA-2, and DHA-3 groups. The bleomycin group received intratracheal instillation of bleomycin, the NS group received an equal amount of normal saline instead of bleomycin, and rats in the DHA-1, DHA-2, and DHA-3 groups received intraperitoneal injection of DHA (25, 50, and 100 mg/kg) in combination with intratracheal instillation of bleomycin. The results showed that compared with the bleomycin group, the number of neutrophils and macrophages in the lungs of rats in the DHA-1, DHA-2, and DHA-3 groups was significantly reduced, and the expression levels of TGF-β1, TNF-α, IL-1, alpha-smooth muscle actin (α-SMA), and NF-κB in lung tissues were significantly decreased, as well as the level ofcollagen synthesis. Liu et al. (86) pretreated male C57BL/6 mice with DHA (20 mg/kg) for 2 days and then induced sepsis-induced acute kidney injury (AKI) in mice by intraperitoneal injection of lipopolysaccharide (LPS) (10 mg/kg). The results showed that DHA deactivated the NF-κB signaling pathway in mice and inhibited the oxidative stress response elicited by LPS. You et al. (87) constructed a lupus model in MRL/lpr mice and studied the mechanism of DHA in treating lupus nephritis. The mice were divided into the normal control group, DHA group, prednisone group, and DHA+prednisone group, and were given 1 mL of normal saline, 60 mg/(kg·day) DHA, 9 mg/(kg·day) prednisone, or 60 mg/(kg·day) DHA + 9 mg/(kg·day) prednisone by gavage, respectively. The mRNA and protein expression levels of irregular chemokine fractalkine (FKN), NF-κB p65, and other markers in the renal cortex of mice were detected using reverse transcription-polymerase chain reaction and immunohistochemistry, respectively. The results showed that DHA reduced the expression levels of FKN, NF-κB p65 mRNA, and protein in the kidney cortex of mice after treatment, similar to the effects of prednisone treatment. Furthermore, the combination of DHA and prednisone exerted a more significant effect. The mechanism of action of DHA was through the regulation of the NF-κB signaling pathway to modulate FKN expression. Dong et al. (88) constructed a lupus nephritis model in BXSB mice and found that DHA treatment at low, medium, and high doses (5, 25, 125 mg/kg) reduced the activation of NF-κB and the expression of p65 protein in kidney tissues, thus effectively inhibiting the deposition of various immunoglobulins (such as IgG, IgA, IgM) and complement components (such as C3, C1q) in renal tissues. Du et al. (89) established rat models of adjuvant-induced arthritis (AIA) and collagen-induced arthritis (CIA) and treated them with DHA (30 mg/(kg·day)). In addition, RAW264.7 macrophages were cultured in vitro and treated with different concentrations (0.5, 1, 2, 4, 8 μmol/L) of DHA. The results showed that DHA reduced the ankle swelling index in AIA/CIA model rats, decreased the spleen index and thymus index in AIA model rats, and lowered the serum IL-6 level. Furthermore, it suppressed RAW264.7 cell viability and nuclear translocation of NF-κB p65, suggesting its anti-inflammatory mechanism may be related to the NF-κB signaling pathway.
Protein kinase B (Akt) regulates the activity of downstream signaling molecule NF-κB, and it has been found that the enhanced expression of NF-κB is achieved through the activation of Akt, which controls various transcription factors (90). Xu et al. (91) found that DHA induced apoptosis in RA synovial cells through the Akt/NF-κB signaling pathway, exerting an anti-inflammatory effect. NF-κB is one of the downstream signaling molecules in the TLR4 signaling pathway, and the activation of the TLR4/NF-κB signaling pathway controls the expression of various cytokines and plays an important role in various inflammatory diseases. Qin et al. (92) also found that DHA inhibited the expression of iNOS, IL-1β, IL-6, and TNF-α mRNA in activated BV-2 cells, reduced the expression of TLR4 protein and cytosolic IκBα, and inhibited the nuclear translocation of NF-κB. The mechanism may involve the regulation of the TLR4/NF-κB signaling pathway to suppress the release of pro-inflammatory cytokines, thereby exerting anti-inflammatory effects.
The MAPK pathway is involved in the signal cascade induced by various extracellular stimuli to regulate inflammatory responses, including the production of inflammatory mediators. The MAPK family consists of three major components: ERK1/2 or p42/p44, JNK/SAPK, and p38MAPK (93). Activated MAPKs can bind to and activate other kinase targets, translocate to the cell nucleus, and initiate the transcription of pro-inflammatory genes, with NF-κB serving as a key downstream component of the MAPK signaling pathway (93). Wei et al. (94) studied the effects and mechanisms of low and high doses of DHA (25, 50 mg/(kg·d)) on skin inflammation in a psoriasis-like model in mice. The results showed that DHA significantly inhibited erythema, scaling, and skin thickness in mice, and the inhibitory effects were enhanced with increasing doses. Furthermore, DHA significantly reduced nail psoriasis and infiltration of inflammatory cells. The study also found that DHA reduced the expression levels of IL-1β, IL-18, IL-6, and CXC chemokine ligand 1 (CXCL-1) in mouse skin lesions, inhibited the expression of NF-κB (p65) and phosphorylated p38 MAPK in a dose-dependent manner, and suggested that the mechanism of action may involve the MAPK/NF-κB signaling pathway, inhibiting excessive proliferation of keratinocytes and the secretion of cytokines.
4.2.2 AMPK/SIRT1 signaling pathway
The AMPK/SIRT1 signaling pathway is involved in various cellular metabolic processes. AMPK activation, indicated by phosphorylation, inhibits lipid synthesis, promotes fatty acid β-oxidation, and improves hepatic steatosis (95). SIRT1, a key enzyme involved in regulating glucose and lipid metabolism, plays a role in cellular differentiation and apoptosis (96). AMPK and SIRT1 can mutually regulate each other in physiological and pathological conditions and prevent the occurrence of inflammation (97). Zhao et al. (98) added 80 mg/kg DHA to the diet of IUGR piglets and compared them with piglets of normal body weight that did not receive DHA supplementation. The results showed that DHA increased the gene expression and activity of AMPK/SIRT1 signaling pathway in the liver of IUGR piglets, while the expression of inflammatory cytokine genes TNF-α, IL-1β, and IL-6 was suppressed. Zhao et al. (96) investigated the effects of adding 80 mg/kg DHA to the basal diet on hepatic inflammation and lipid metabolism in weanling piglets. The results showed that DHA treatment of LPS-induced hepatic inflammation model in piglets reduced the levels of triglycerides (TG), IL-1β, IL-6, TNF-α, and non-esterified fatty acids (NEFA) in the liver, inhibited the activity of fatty acid synthase (FAS), increased the mRNA expression of sterol regulatory element-binding protein 1c (SREBP-1c) and acetyl-CoA carboxylase β (ACCβ), and decreased the mRNA expression of carnitine palmitoyltransferase 1 (CPT1), SIRT1, AMP-activated AMPKα, and stearoyl-CoA desaturase (SCD), indicating the ability of DHA to alleviate hepatic inflammation and abnormal lipid metabolism through the AMPK/SIRT1 signaling pathway.
4.2.3 Mammalian target of mTOR/S6 kinase 1 pathway
The mTOR/S6K1 pathway regulates the activity of ribosomal S6 kinase (such as S6K1, S6K2) and eukaryotic initiation factor 4E (eIF4E). The mTOR/S6K1 pathway is an important cellular growth coordination pathway that plays a negative regulatory role in autophagy in mammals (99). Xi et al. (100) found that DHA treatment of IgA nephropathy mesangial cells significantly inhibited cell proliferation, downregulated the phosphorylation levels of mTOR/S6K1 signaling pathway, and increased the expression of autophagy-related protein LC3B, indicating that DHA has anti-inflammatory and autophagy-promoting effects in IgA nephropathy.
4.2.4 PI3K/Akt signaling pathway
The PI3K/Akt signaling pathway can be activated by various cellular stimuli or toxic injuries and regulates transcription, translation, proliferation, growth, and cell survival (100). Gao et al. (101) induced neuroinflammation in mice using LPS and found that treatment with DHA (40 mg/kg) improved the behavioral abnormalities in the LPS-induced neuroinflammatory mice and reduced the expression levels of glial fibrillary acidic protein (GFAP), IL-1β, IL-6, phosphorylated PI3K (p-PI3K)/PI3K, TNF, and phosphorylated protein kinase B (p-Akt)/Akt. The study demonstrated the inactivation of the PI3K/Akt signaling pathway in adult mice by DHA, protecting the hippocampus from neuroinflammatory damage.
4.2.5 MAPK signaling pathway
MAPKs are protein kinases that phosphorylate themselves on serine and threonine residues or are found phosphorylated on their substrates. They respond to various physiological signals such as hormones, cytokines, and growth factors (102). Artemisinin suppresses LPS-stimulated RAW264.7 macrophages from producing IL-12p40 by inhibiting JNK activity (103). L-A03, a DHA derivative, selectively inhibits the activation of JNK and promotes apoptosis in MCF-7 human breast cancer cells (104). DHA prevents dextran sulfate sodium-induced colitis by inhibiting NLRP3 inflammasome secretion and activation of the P38 MAPK signaling pathway (105). Artemisinin-based drugs have been used to treat concanavalin A (Con A)-induced autoimmunity in mice by suppressing the MAPK signaling pathway (106). Using single-cell transcriptome sequencing, Zhang et al. (107) constructed the first cellular transcriptome atlas of DHA-regulated immune cell subpopulations in the spleen. The study found that DHA significantly increased the proportion of Treg and cytotoxic CD8+IFN-γ+ T cells. It also revealed that Treg highly expressed inhibitory signaling molecules, such as CD73, CD274, and IL-10, while the expression of key effector molecules, including granzyme A and granzyme B, was downregulated in cytotoxic CD8+IFN-γ+ T cells. In terms of humoral immunity, DHA downregulated the expression of MHCII molecules in splenic follicular B cells and significantly decreased the expression levels of key molecules associated with B cell activation, such as CD79a, CD79b, and Ms4a1. These results suggest that DHA exerts different effects on humoral and cellular immunity. KEGG enrichment analysis and analysis of differential gene expression revealed that DHA significantly upregulated the expression of activating protein-1 (AP-1) transcription factors in various immune cells. The study confirmed the changes in AP-1 and its upstream kinase JNK expression levels regulated by DHA using flow cytometry and Western blot analysis. Superoxide dismutase 3 (SOD3), as a growth regulator, can drive the activation of AP-1. The study also used SOD3 knockout mice and confirmed that SOD3 knockout inhibited DHA-induced immune cell rearrangement and activation of the JNK-AP-1 signaling pathway. These findings indicate a close relationship between the immunomodulatory function of DHA and the activation of the SOD3-JNK-AP-1 signaling pathway, laying the groundwork for further understanding and revealing the molecular mechanisms underlying the immunomodulatory function of DHA.
In the context of the ERK-MAPK signaling pathway, the ERK cascade can be activated by various extracellular factors, such as growth factors, hormones, and cellular stress, leading to processes like cell proliferation and differentiation (108–110). Ouyi et al. treated BEAS-2B cells, which had been exposed to influenza A virus, with varying concentrations of DHA (12.5, 25, 50 μmol/L) and observed changes in TNF-α and IL-6 mRNA and protein levels, as well as p-ERK protein expression. The results showed that DHA inhibited the expression of TNF-α, IL-6 mRNA, TNF-α, IL-6, and p-ERK protein through the ERK signaling pathway, with the inhibition displaying a dose-dependent effect (111). Similarly, Wei et al. studied the regulatory effects of DHA on inflammatory mediators in an ovalbumin (OVA)-induced asthma mouse model, administering DHA orally at a dose of 30 mg/kg (112). Their findings revealed that DHA suppressed the activation of p38 MAPK and ERK, suggesting that DHA alleviates OVA-induced asthma by inhibiting the p38 MAPK and ERK pathways. These studies highlight the potential therapeutic role of DHA in managing allergic inflammation (112).
4.2.6 Nrf2 signaling pathway
Nuclear factor erythroid 2-related factor 2 (Nrf2) is a transcription factor negatively regulated by Kelch-like ECH-associated protein 1 (KEAP1), which controls the transcription of anti-inflammatory genes and inhibits the expression of antioxidant enzymes, thus suppressing inflammation (113, 114). The p62-mediated degradation of KEAP1 promotes Nrf2 nuclear translocation and transcription of its target genes. DHA treatment increased the expression of Nrf2 and its target gene heme oxygenase-1 (HO-1) in myeloid-derived suppressor cells (MDSCs) in systemic lupus erythematosus (SLE) mice and delayed the aging of MDSCs (52). Artemether-lumefantrine activated Nrf2 by increasing p62 expression in mouse bone marrow macrophages and upregulated the expression of HO-1 and/or NAD(P)H:quinone oxidoreductase 1 (NQO1) (115). Similarly, DC32 promoted Nrf2/HO-1 signaling and enhanced p62 transcription or KEAP1 degradation in DBA/1 mice and mouse embryonic cells (116). In conclusion, artemisinin derivatives promote the activation of the Nrf2 signaling pathway, leading to increased expression of anti-inflammatory genes and the alleviation of inflammation.
4.2.7 JAK/STAT signaling pathway
Janus kinases (JAKs) and signal transducers and activators of transcription (STAT) proteins control the signal transduction of many cytokines and growth factors involved in cell growth, survival, and differentiation (117). DHA reduced STAT1 phosphorylation in human umbilical vein endothelial cells (HUVECs) stimulated with IFN-α and inhibited the phosphorylation of JAK2 and STAT3 in the kidneys of MRL/lpr mice, thereby alleviating the symptoms of lupus nephritis (55, 118).
4.2.8 Others
Based on the farnesoid X receptor (FXR) as a potential target for the treatment of alcoholic liver disease (ALD), Xu et al. (119) investigated the effects of DHA on ALD and its underlying mechanism. They constructed a rat model of alcoholic liver injury and administered DHA at a dose of 7 mg/kg by intraperitoneal injection, in combination with ursodeoxycholic acid (10 mg/kg) and orphenadrine (30 mg/kg). The results showed that DHA inhibited the expression and activity of FXR in the liver of rats with ALD, reduced the expression of alanine aminotransferase (ALT), aspartate aminotransferase (AST), alkaline phosphatase (ALP), and lactate dehydrogenase (LD), improved liver injury, and suppressed the expression of inflammatory genes and infiltration of inflammatory cells. In conclusion, numerous studies have shown that artemisinin and its derivatives exert anti-inflammatory effects by modulating the levels of inflammatory cytokines through various signaling pathways, including the ERK, NF-κB, AMPK/SIRT1, ROS-JNK1/2, mTOR/S6K1, PI3K/Akt, Nrf2, JAK/STAT, and MAPK signaling pathways. See Figure 1.
5 Advances in the research on artemisinin and its derivatives in the treatment of autoimmune diseases
5.1 RA
RA is one of the most common immune-mediated diseases characterized by inflammatory arthritis, primarily affecting small joints of the hands and feet with symmetrical polyarthritis (120, 121). RA is a systemic disease with various co-existing diseases and extra-articular manifestations. The occurrence of synovial inflammation is a result of the interaction between genetic factors and specific environmental exposures (122). DHA is a derivative of artemisinin and one of the main active forms of artemisinin in the body (123). Du et al. (89) found that DHA had therapeutic effects on both AIA and CIA in rats, significantly reducing joint swelling and pathological scores, as well as the levels of the inflammatory cytokine IL-6 in RA rats. In vitro treatment of RAW264.7 macrophages with DHA for 24 hours resulted in a reduction in IL-6 in the culture supernatant and decreased nuclear expression of NF-κBp65, suggesting that the improvement of rheumatoid arthritis in rats may be related to the inhibition of the NF-κB signaling pathway by DHA. Another study found that DHA induced apoptosis in rheumatoid arthritis synovial cells by inhibiting the phosphorylation of Akt at serine 473 and the activation of NF-κB. These studies suggest the potential application of DHA in the treatment of rheumatoid arthritis (124). DC32, a derivative of DHA, has strong immune inhibitory properties. Studies have shown that DC32 can alleviate rheumatoid arthritis by activating the Nrf2/HO-1 antioxidant signaling pathway, increasing p62 protein transcription, and significantly reducing arthritis inflammation in CIA mice. In vitro intervention with DC32 promoted the degradation of Keap1 protein in NIH-3T3 embryonic fibroblasts and upregulated the expression of HO-1 and p62, indicating that DC32 could alleviate rheumatoid arthritis by activating the Nrf2/p62/Keap1 pathway (125). Another study demonstrated that DC32 inhibited Th17 cells, elevated the proportion of immunosuppressive Tregs, restored the Treg/Th17 balance, suppressed synovial inflammation, and reduced arthritis symptoms in CIA mice (50). Artemether-lumefantrine, a combination drug derived from artemisinin, reduced experimental systemic lupus erythematosus (SLE) symptoms by inhibiting Th17 and upregulating Treg cells in the CIA model (126). Studies have also shown that DHA combined with Torch Ginger Root has a therapeutic effect on AIA rats, possibly by inhibiting the expression of VEGF, MMP-1, and MIF and reducing the levels of inflammatory cytokines (127). DHA combined with hydroxychloroquine (an analog of artemisinin) has a better treatment effect than monotherapy. The therapeutic effect of DHA and hydroxychloroquine in the treatment of rheumatoid arthritis was achieved by blocking the NF-κB pathway (128). Regarding B cells, artemisinin was found to eliminate germinal center B cells and inhibit autoantibody-mediated autoimmune arthritis (59).
DHA modulates the proliferation, apoptosis, and autophagy of chondrocytes in the rheumatoid arthritis mouse model through the PI3K/AKT/mTOR signaling pathway (126). In vitro studies have shown that artemisinin can inhibit the differentiation of osteoclast precursors and bone resorption in RANKL-induced RAW264.7 cells and mouse bone marrow-derived macrophages (129, 130). Dimeric artesunate phospholipid-conjugated liposomes, a novel amphiphilic artemisinin compound with low cytotoxicity, significantly improved ankle joint swelling and inflammatory response in AIA mice. It exhibited higher inhibitory effects on pro-inflammatory factors and improved the condition of AIA mice compared to artemisinin (131). In summary, artemisinin and its derivatives can reduce collagen content, decrease fibroblast viability and proliferation, and increase cell apoptosis by inhibiting various inflammatory-related signaling pathways in rheumatic diseases. These research findings suggest great potential for the use of artemisinin derivatives in the treatment of rheumatoid arthritis, providing new insights and drug targets for its treatment.
5.2 SLE
SLE is an autoimmune disease characterized by multi-organ inflammation and widespread production of autoantibodies. Autoantibodies, especially anti-dsDNA and anti-Sm autoantibodies, have high specificity in SLE and are involved in immune complex formation and inflammatory damage to various end organs, including the kidneys, skin, and central nervous system (CNS) (132–134). In recent years, studies have revealed that artemisinin-based drugs can modulate the immune system and alleviate SLE (135). Myeloid-derived suppressor cells (MDSCs) as immunosuppressive cells are reduced in autoimmune disease. Zhang et al. (136) found that DHA could delay the senescence of MDSCs and improve lupus symptoms in mice by activating the antioxidant-related Nrf2/HO-1 signaling pathway. Another study found that both 125 mg/kg and 25 mg/kg DHA could suppress the production of anti-dsDNA antibodies and reduce the levels of TNF-α in the serum of BXSB mice (recombinant inbred strain of female C57BL mice and male SB mice), significantly improving lupus nephritis in mice (137). Liang et al. (138) demonstrated that the artemisinin analogs hydroxychloroquine and quinacrine can reduce autoimmune antibody production, B lymphocyte proportions, and alleviate the symptoms of lupus nephritis by inhibiting the KLF15/NF-κB signaling pathway. Studies have also shown that chloroquine and quinacrine, artemisinin analogs, can reduce the production of autoantibodies, such as anti-DNA antibodies, and the levels of pro-inflammatory cytokines TNF-α, IL-8, IL-6, and IFN-α in peripheral blood mononuclear cells (PBMCs) of SLE patients. In addition, in vitro interventions with chloroquine and quinacrine in HEK293 cells transfected with TLR3, TLR8, and TLR9 demonstrated a dose-dependent decrease in IL-6 in the culture supernatant and the expression levels of the three proteins, indicating that artemisinin analogs such as chloroquine and quinacrine can alleviate SLE symptoms by inhibiting TLR signaling pathways (139). Feng et al. (118) found that artemisinin can regulate macrophage migration inhibitory factor (MIF) and inhibit macrophage migration and phosphorylation of STAT1, thus ameliorating systemic lupus erythematosus-related atherosclerosis in patients. A study demonstrated that treatment with a TAT-modified cationic liposome co-delivering DHA and HMGB1 siRNA effectively prevented lupus nephritis in experimental SS mice (140). DHA has been shown to protect against the aging of bone marrow-derived suppressor cells (MDSCs) in NOD/Ltj mice and improve salivary gland secretion (52). The therapeutic effect of artesunate in lupus-susceptible MRL/lpr mice is dependent on T follicular helper cell differentiation and activation of the JAK2-STAT3 signalling pathway. It can regulate T cell subset ratios, reduce the generation of pro-inflammatory lymphocyte subsets Th1, Th17, and Tfh, and increase the level of immunosuppressive Tregs in NOD/Ltj model mice (55).
5.3 Sjögren’s syndrome
Primary Sjögren’s syndrome (pSS) is an autoimmune disease characterized by dry eyes and dry mouth caused by inflammation in the salivary and lacrimal glands. Currently, effective treatment methods for pSS patients are lacking (141–143). Artesunate may inhibit disease progression in experimental Sjögren’s syndrome and humanized Sjögren’s syndrome mice by suppressing Th17 responses (144). A recent network pharmacological study revealed that artemisinin corresponds to 412 targets, while pSS corresponds to 1495 genes. There are 40 overlapping genes between artemisinin and pSS. According to KEGG analysis, artemisinin’s therapeutic effect on pSS involves the IL-17 signaling pathway, HIF-1 signaling pathway, apoptosis signaling pathway, Th17 cell differentiation, PI3K-Akt signaling pathway, and MAPK signaling pathway (145). Molecular docking results further revealed that artemisinin molecules exhibited higher binding affinity to key nodes in the IL-17 signaling pathway. In in vivo experiments, artemisinin restored salivary gland secretory function in NOD/Ltj mice and improved glandular damage. It facilitated an increase in Treg levels and a decrease in IL-17 secretion in an NOD mouse model. In terms of B cell intervention, studies have found that artemisinin analogs such as artemisinin-lumefantrine suppress autoimmune responses resembling Sjögren’s syndrome by inhibiting TRAF6-mediated NF-κB signaling and excessive activation of B cells induced by BAP (B cell-activating factor) (146). Artemisinin-lumefantrine improves general conditions, increases salivary secretion, reduces fatigue, decreases the infiltration of lymphocytes in submandibular glands and lacrimal glands, inhibits the generation of pro-inflammatory lymphocyte subsets Th1, Th17, Tfh, and germinal center B cells, and increases the level of immunosuppressive Treg cells in NOD mice. Thus, it regulates the Th1/Th2 and Th17/Treg immunological balance. These actions may constitute specific mechanisms by which artemisinin-lumefantrine treats the dry symptoms of Sjögren’s syndrome in NOD mice. In terms of B cell intervention, artemisinin improves SS-like symptoms in mice and modulates the TRAF6-NF-κB signaling pathway, which determines the survival and proliferation of B cells (147). A recent study found that DHA promotes Treg proliferation and inhibits the expansion of germinal center B cells, resulting in reduced numbers of circulating plasma cells and serum immunoglobulin levels. Overall, these findings demonstrate that artemisinin effectively suppresses disease progression in ESS and humanized SS mice by inhibiting Th17 responses. Additionally, the study identified a novel metabolic regulation function of artemisinin, involving the promotion of IRF4 proteasomal degradation, which inhibits the glycolytic pathway in Th17 cells. In summary, these findings suggest that artemisinin may be a promising therapeutic candidate for the treatment of pSS (148).
5.4 Inflammatory neurological diseases
CNS autoimmune diseases involve the immune system—comprising autoimmune cells, autoantibodies, and other immune molecules—attacking the nervous system, including neurons, glial cells, and myelin (149). Such diseases include neuromyelitis optica spectrum disorder (NMOSD), myelin oligodendrocyte glycoprotein-IgG associated disorders (MOGAD), multiple sclerosis (MS), acute disseminated encephalomyelitis (ADEM), autoimmune encephalitis (AE), and CNS vasculitis. The complex pathogenesis of these conditions is driven by immune-inflammatory reactions targeting the nervous system (150–152).
Research indicates that artemisinin helps to normalize behavioral deficits and protect hippocampal tissue in neuroinflammatory conditions. Artemisinin-based drugs reduce the release of pro-inflammatory cytokines (TNF-α, IL-1β, IL-6, MCP-1) and downregulate inflammatory signaling pathways such as NF-κB, TLR-4, ERK, and MAPK in mice (153). Zhang et al. (154) demonstrated that a 100 mg/kg dose of an artemisinin derivative significantly reduced the severity of experimental autoimmune encephalomyelitis (EAE). This effect was attributed to the inhibition of the TLR-4/NF-κB pathway, regulation of pro-inflammatory cytokine expression, and maintenance of intestinal barrier integrity by downregulating MUC2 and claudin-1 in colonic mucosal layers (155).
Further studies on oral delivery of chitosan-coated artesunate (CPA), another artemisinin derivative, showed significant inhibition of the TLR-4/NF-κB pathway, reduced pro-inflammatory cytokine mRNA levels, and increased IL-10 in RAW264.7 cells. CPA also protected zonula occludens-1 (ZO-1) in Caco-2 cells and modulated macrophage polarization by reducing CD86 and increasing CD206 and p-STAT6 expression. These effects were dependent on STAT6, as they were not observed in STAT6-deficient cells (156). In a UC mouse model, CPA treatment inhibited TLR-4/NF-κB activation, M1 macrophage polarization, and mucosal barrier damage, while promoting STAT6 phosphorylation, an effect reversed by an HO-1 inhibitor. These findings suggest that CPA reduces Th1 and Th17 cell generation through AP1 inhibition, thereby alleviating EAE severity and showing potential as a therapeutic agent for multiple sclerosis (157).
Additionally, artemisinin administration significantly improved EAE symptoms by reducing IL-6, IL-17, and IL-1 expression in the spinal cord. Both artemisinin and tehranolid treatments stimulated the expression of anti-inflammatory genes such as TGF-β, IL-4, IL-10, FOXP3, GATA3, MBP, and AXL, while reducing T-bet expression. Neither treatment affected the expression of IFN-γ, RORγt, nestin, Gas6, Tyro3, or Mertk mRNA in the spinal cord. These results indicate that artemisinin and tehranolid effectively regulate genes responsible for inflammation and myelination, with tehranolid showing greater efficacy than antiretroviral treatment, suggesting its potential as an alternative therapeutic approach in MS management (158).
5.5 IBD
IBD, including ulcerative colitis (UC) and Crohn’s disease (CD), is a complex autoimmune disease with an unclear etiology (159, 160). Increasing evidence suggests that immune dysfunction, abnormal expression of inflammatory cytokines, and gut microbiome dysbiosis are major contributors to IBD pathogenesis (161, 162). NF-κB, a key transcription factor in M1 macrophages, induces the expression of various inflammatory factors, including TNF-α, IL-1β, IL-6, IL-10, IL-12, and COX-2. Dysregulation of NF-κB is closely linked to IBD development, making its inactivation crucial in IBD treatment (163, 164).
Artemisinin has been shown to inhibit the NF-κB signaling pathway, regulate inflammatory cytokine expression, and maintain intestinal barrier integrity by downregulating MUC2 and claudin-1 in the colonic mucosa, thereby suppressing DSS-induced colonic inflammation (165). CPA, another artemisinin derivative, significantly inhibited the TLR-4/NF-κB pathway and reduced pro-inflammatory cytokine mRNA levels while increasing IL-10 in RAW264.7 cells. CPA also protected ZO-1 in Caco-2 cells and modulated macrophage polarization by reducing CD86 and increasing CD206 and p-STAT6 expression, with STAT6 playing a critical role in these effects (166). Yang et al. (167) found that artemisinin reduced pro-inflammatory factors, M1 macrophages, and EMT-related proteins, while increasing M2 macrophages in colon tissues, improving disease activity index scores, and villus structure in colitis mice, indicating its efficacy in IBD treatment. DHA may also exert therapeutic effects on DSS-induced IBD by regulating inflammatory cytokines, cell junction-related genes, and gut microbiota (168). The Th/Treg imbalance plays a crucial role in IBD pathogenesis, with excessive CD4+ T cell activation being a potential mechanism (169). HO-1, a rate-limiting enzyme in heme metabolism, has anti-inflammatory and antioxidant effects and can induce apoptosis in activated CD4+ T cells through the Fas/CD95-FasL pathway (170, 171). Yan et al. (51) found that DHA treatment improved colitis symptoms, reduced lymphocyte infiltration and tissue fibrosis, decreased Th1, Th17, Th9, and Th22 cells, and increased Tregs in TNBS- and OXA-induced colitis models. DHA also inhibited CD4+ T lymphocyte activation and induced apoptosis, promoting HO-1 production, which was accompanied by CD4+ T cell apoptosis and restoration of the Th/Treg balance—effects blocked by the HO-1 inhibitor tin-protoporphyrin IX (SnPPIX). These findings suggest that DHA is a promising candidate for IBD treatment and Th/Treg immune modulation.
Network pharmacology, an emerging discipline studying drug-target interactions, revealed that artemisinin acts on multiple targets and signaling pathways involved in IBD. Lei et al. (172) showed that DHA treatment tended to restore and upregulate E-cadherin and significantly increased the abundance of Bacteroidales_S24-7_group in the gut microbiome, which was decreased in the DSS group. In conclusion, artemisinin exerts anti-inflammatory effects through multiple targets and signaling pathways, repairing the intestinal barrier and providing insights for its use in IBD treatment. Yu et al. (173) also found that DHA-bear bile acid conjugates are potential therapeutic agents for IBD, reducing disease activity index, colonic damage, and splenomegaly in DSS-induced colitis mice.
5.6 Myasthenia gravis
MG is an autoimmune disease characterized by muscle weakness, primarily affecting the motor function. It has been found that MG is not limited to motor symptoms such as weakness in the eye muscles, respiratory muscles, and overall muscle weakness, but also involves non-motor symptoms. These non-motor symptoms can affect various target organs, presenting in different forms, including sleep disorders, emotional disorders, pain, special sensory disorders, cognitive impairment, and autonomic dysfunction. These symptoms severely impact the quality of life of patients and can even shorten their lifespan (174, 175). The production of high-affinity pathogenic anti-acetylcholine receptor (AChR) antibodies in MG requires the involvement of CD4+ T helper cells and their cytokines. Th17 and Treg cells, as subsets of CD4+ T cells, play critical roles in the development and progression of various inflammatory diseases, including autoimmune disorders. Th17 cells and their cytokines promote inflammation, while Treg cells and their cytokines exert anti-inflammatory effects. These cytokines are also essential in generating and regulating autoimmune responses. Differentiated CD4+ T cells are classified into various subsets based on the cytokines they produce, such as Th1 cells, which secrete pro-inflammatory cytokines IL-12 and IFN-γ, Th17 cells, which produce IL-17, and Treg cells, which secrete transforming growth factor-β (TGF-β) and IL-10 (172, 176). Artemisinin improves the symptoms of experimental autoimmune myasthenia gravis by regulating the balance of Th1 cells, Th17 cells, and Treg cells (177). Artemisinin-lumefantrine has therapeutic potential in experimental autoimmune myasthenia gravis by upregulating Treg cells and regulating the Th1/Th2 cytokines (178).
5.7 Autoimmune liver diseases
AILD are a group of liver-damaging diseases caused by autoimmune abnormalities, including autoimmune hepatitis (AIH), primary biliary cholangitis (PBC), primary sclerosing cholangitis (PSC), and overlapping syndromes with simultaneous occurrence of two of these diseases (179). IL-17 is a pro-inflammatory cytokine belonging to the IL-17 family. IL-17A and IL-17F, mainly secreted by Th17 cells, can induce the secretion of IL-6 by hepatocytes, further activating Th17 cells in a positive feedback loop and promoting the release of other pro-inflammatory factors such as TNF-alpha (180, 181). A higher level of IL-17 has been found in the peripheral blood and liver tissues of AIH patients compared to healthy individuals, and it is positively correlated with disease activity and liver fibrosis (182). Studies have found that artemisinin may protect against Con A-induced autoimmune liver injury in mice by inhibiting the NF-κB signaling pathway (182). This study provides a scientific reference for the application of artemisinin in preventing autoimmune liver injury.It has been found that artemisinin effectively suppresses Con A-induced liver injury by inhibiting the activation of the TLR-4/NF-κB pathway and reducing the mRNA levels of pro-inflammatory cytokines while increasing the IL-10 level in RAW264.7 cells (106). Artemisinin treatment significantly improved the symptoms of Con A-induced experimental autoimmune hepatitis in mice. It reduced liver enzyme levels, inhibited the expression of inflammatory cytokines such as IFN-γ, TNF-α, IL-1β, IL-6, and IL-17, and increased the level of the anti-inflammatory cytokine IL-10. Additionally, artemisinin inhibited the phosphorylation of ERK, JNK, p38, IκBα, and NF-κB p65 in the liver, indicating its protective effect on autoimmune liver injury (183).
5.8 IgA nephropathy
IgA nephropathy is one of the most common forms of primary glomerulonephritis worldwide, characterized by the deposition of IgA immunoglobulin in the glomerular mesangium (184). Clinically, it often presents as microscopic hematuria and proteinuria, with 20% to 40% of patients progressing to end-stage kidney disease (ESKD) within 20 years of onset. The pathogenesis of IgA nephropathy, known as the “4 Hit” hypothesis, is closely linked to the immune system, involving B cells, T cells, TLRs, monocytes/macrophages, and the complement system (185–187). Activation of NF-κB-induced immune inflammation is a key factor in the disease’s progression (188).
Studies have shown that artemisinin combined with hydroxychloroquine significantly enhances the secretion of extracellular vesicles in renal tubular epithelial cells in rat models of IgA nephropathy. These vesicles, when absorbed by glomerular mesangial cells, inhibit NF-κB signaling and NLRP3 inflammasome activation, thereby reducing the expression of related inflammatory proteins and suppressing inflammation (189). CMap analysis revealed that artemisinin can reverse the expression of differentially expressed genes in IgA nephropathy, identifying 87 potential targets, with AKT1 and EGFR showing the highest binding affinity with artemisinin. In vivo, artemisinin improved kidney damage and fibrosis, while in vitro studies showed it weakened LPS-induced oxidative stress and fibrosis by promoting AKT phosphorylation and Nrf2 nuclear translocation (190).
Artemisinin has also been shown to significantly improve kidney function, reduce mesangial matrix expansion, and decrease immune complex deposition in the kidneys of IgA nephropathy rats. Mechanistic studies indicated that artemisinin modulates the differentiation of CD4+ T cell subgroups, reducing the high proportion of Th2 and Th17 cells and increasing Th1 and Treg cells, suggesting an immunosuppressive effect (191). Further research demonstrated that artemisinin facilitates the secretion of extracellular vesicles in kidney tissues, which in turn inhibits NF-κB/NLRP3 signaling and related protein expression. This effect was blocked by GW4869, indicating the crucial role of extracellular vesicles in this process (192).
In terms of autophagy, DHA was found to inhibit the proliferation of mesangial cells in IgA nephropathy through the mTOR signaling pathway (193). To further explore the therapeutic potential, Chen et al. designed a randomized, double-blind, placebo-controlled clinical trial involving 120 patients with IgA nephropathy. Participants will receive either 100 mg or 50 mg of artemisinin or a placebo for six months, with changes in proteinuria and kidney function measured post-intervention. The study will also assess levels of Gd-IgA1 and anti-Gd-IgA1 to explore potential immune mechanisms (194).
5.9 Autoimmune skin diseases
Autoimmune skin diseases involve the immune system attacking its own antigens, leading to an immune imbalance that causes skin damage, with potential involvement of other tissues and organs. Traditional immunosuppressive agents often come with a range of adverse effects (195). Research has explored the potential of artemisinin derivatives in treating conditions such as general skin rashes, rosacea, and psoriasis.
In a mouse model of rash, artemisinin was shown to reduce or eliminate symptoms, including decreased dermatitis scores, reduced ear, spleen, and lymph node weight, thinner skin epidermis, lower IgE levels, reduced inflammatory cell infiltration, decreased Th7 cells, increased Treg cells, downregulation of Th17-related factors (such as IL-6, IL-17, and IL-23), and upregulation of TGF-β and SOCS-3 (196). A clinical trial in patients with erythematotelangiectatic rosacea demonstrated that those treated with a combination of artemisinin drugs had significantly lower papule and pustule scores compared to those receiving standard metronidazole therapy over four weeks, with similar erythema scores (78).
Artemisinin has also been effective in alleviating psoriasis-like dermatitis in mice induced by imiquimod (IMQ) (197, 198). It significantly improved acute skin lesions and reduced relapse in low-dose IMQ-induced psoriasis by decreasing the frequency and number of CD8 central memory T cells (TCM) and CD8+ resident memory T cells (TRM). Artemisinin mitigated histopathological changes and T cell infiltration in the skin, inhibited the expression of inflammatory cytokines such as IL-15 and IL-17, and other pro-inflammatory factors. Artemisinin also downregulated the expression of EGF and BCL-6 in CD8 T cells and suppressed CD8CLA, CD8CD69, or CD8CD103+ TRM cells in the skin (199).
Moreover, artemisinin prevented psoriasis relapse when administered at the onset, unlike methotrexate, which only reduced CD8+ TCM cells but not CD8+ TRM cells. Recombinant IL-15 or CD8 (rather than CD4) TCM cell transfer led to complete relapse in previously artemisinin-treated psoriasis mice. Additionally, artemisinin alleviated psoriasis-like lesions in humanized NSG mice while reducing CD8+ TCM and CD103+ TRM cells (199).
In IL-17A-induced HaCaT cells, artemisinin significantly inhibited excessive proliferation, migration, and the expression of inflammatory factors. It also suppressed FGFR1 expression, which is highly expressed in these cells. The addition of an FGFR1 agonist reversed artemisinin’s inhibitory effects, suggesting that artemisinin targets FGFR1 to inhibit excessive proliferation and inflammation, presenting a new potential target for psoriasis treatment (200).
These findings highlight the potential applications and mechanisms of artemisinin in treating autoimmune disorders.
5.10 Autoimmune thyroiditis
AIT is a typical organ-specific autoimmune disease, characterized by immune imbalance leading to the production of specific antibodies against self-antigens, resulting in the onset of the disease under the influence of genetic and environmental factors (201). Several studies have confirmed the differentiation and functional imbalance of T cells in AIT patients, primarily characterized by an increase in Th1 and Th17 cells and a defect in Tregs (202, 203). Research has found that artemisinin treatment effectively decreases serum thyroglobulin antibody levels, improves thyroid lymphocyte infiltration and thyroid function, and reduces thyroid and spleen weights in AIT mice, indicating that artemisinin improves the condition of AIT mice by suppressing immune imbalance and lymphocyte infiltration (204).
5.11 Gout and gouty arthritis
Gout is a common and treatable disease caused by the deposition of monosodium urate (MSU) crystals in joints and non-articular structures. Hyperuricemia, an elevated level of uric acid in the blood, is the most important risk factor for gout (205). Gout is the most common inflammatory arthritis in adults. It is characterized by sterile inflammation caused by the deposition of monosodium urate (MSU) crystals in the joints and periarticular tissues. It presents with rapid onset and can lead to arthritis, tophi, renal stones, and urate nephropathy (206, 207). Studies have found that artemisinin exhibits anti-inflammatory effects in gout patients through its ability to inhibit the interaction between NEK7 and NLRP3, thereby suppressing the activation of the NLRP3 inflammasome. Artemisinin also reduces intra- and extracellular potassium efflux in macrophages stimulated by LPS and MSU crystals, thus alleviating joint inflammation in gouty arthritis mice. Artemisinin treatment effectively reduces foot and ankle swelling in mice with MSU crystal-induced arthritis. The study suggests that artemisinin inhibits NLRP3 inflammasome activation by suppressing the interaction between NEK7 and NLRP3 in uric acid-induced inflammation (208).
6 Prospects
In summary, artemisinin and its derivatives exhibit unique mechanisms of action, making them promising candidates for the treatment of autoimmune diseases. These compounds effectively intervene in the overactive inflammation and immune responses characteristic of rheumatic and autoimmune diseases by modulating various signaling pathways, including the TNF, Toll-like, IL-6, RANKL, MAPK, PI3K/AKT/mTOR, JAK/STAT, and NRF2/GPX4 pathways. Notably, within the NF-κB signaling pathway, both systemic and localized application of artemisinin has been shown to significantly improve a range of autoimmune conditions, including systemic autoimmune diseases, autoimmune kidney diseases, neurological disorders, skin diseases, and autoimmune liver and gallbladder conditions (4, 209–212).
The regulatory mechanisms of artemisinin primarily involve inhibiting abnormalities in both the innate and adaptive immune systems, such as the excessive proliferation and activation of immune cells, abnormal differentiation of functional subsets, and dysregulated cytokine expression. Additionally, artemisinin helps suppress the cascade reactions triggered by changes in the phenotype and function of pro-inflammatory immune cells at various stages of autoimmune disease progression, exerting targeted effects on organs impacted by these conditions (58, 118, 213, 214).
Artemisinin’s distinctive immunomodulatory effects focus on key nodes within pathological immune responses and cellular signaling cascades, promoting the restoration of immune homeostasis. This makes artemisinin and its derivatives particularly suitable for treating chronic rheumatic and autoimmune diseases with complex underlying mechanisms.
Clinically, several multicenter randomized controlled trials targeting rheumatic, inflammatory, and autoimmune diseases are currently underway, aiming to translate more preclinical findings into effective clinical treatments.
Author contributions
ZL: Conceptualization, Data curation, Formal analysis, Validation, Writing – original draft, Writing – review & editing. WaX: Conceptualization, Data curation, Formal analysis, Validation, Writing – original draft. WeX: Conceptualization, Data curation, Validation, Writing – original draft. YM: Conceptualization, Data curation, Formal analysis, Validation, Writing – original draft. QF: Conceptualization, Data curation, Formal analysis, Validation, Writing – original draft. BZ: Data curation, Formal analysis, Validation, Writing – original draft. LZ: Conceptualization, Data curation, Formal analysis, Validation, Writing – review & editing.
Funding
The author(s) declare that no financial support was received for the research, authorship, and/or publication of this article.
Conflict of interest
The authors declare that the research was conducted in the absence of any commercial or financial relationships that could be construed as a potential conflict of interest.
Publisher’s note
All claims expressed in this article are solely those of the authors and do not necessarily represent those of their affiliated organizations, or those of the publisher, the editors and the reviewers. Any product that may be evaluated in this article, or claim that may be made by its manufacturer, is not guaranteed or endorsed by the publisher.
References
1. Saferding V, Blüml S. Innate immunity as the trigger of systemic autoimmune diseases. J Autoimmun. (2020) 110:102382. doi: 10.1016/j.jaut.2019.102382
2. Chi X, Huang M, Tu H, Zhang B, Lin X, Xu H, et al. Innate and adaptive immune abnormalities underlying autoimmune diseases: the genetic connections. Sci China Life Sci. (2023) 66:1482–517. doi: 10.1007/s11427-021-2187-3
3. Long Z, Zeng L, He Q, Yang K, Xiang W, Ren X, et al. Research progress on the clinical application and mechanism of iguratimod in the treatment of autoimmune diseases and rheumatic diseases. Front Immunol. (2023) 14:1150661. doi: 10.3389/fimmu.2023.1150661
4. Altamimi E, Al Omari D, Obeidat H, Barham K. Retrospective, single-center analysis of autoimmune hepatitis in Jordanian children: clinical features, treatments, and outcomes. BMC Pediatr. (2024) 24:102. doi: 10.1186/s12887-024-04590-9
5. Conrad N, Misra S, Vernakel JY, Verbeke G, Molenberghs G, Taylor PN, et al. Incidence, prevalence, and co-occurrence of autoimmune disorders over time and by age, sex, and socioeconomic status: a population-based cohort study of 22 million individuals in the UK. Lancet. (2023). doi: 10.1016/S0140-6736(23)00457-9
6. Zeng L, He Q, Deng Y, Li Y, Chen J, Yang K, et al. Efficacy and safety of iguratimod in the treatment of rheumatic and autoimmune diseases: a meta-analysis and systematic review of 84 randomized controlled trials. Front Pharmacol. (2023) 14:1189142. doi: 10.3389/fphar.2023.1189142
7. Harroud A, Hafler DA. Common genetic factors among autoimmune diseases. Science. (2023) 380:485–90. doi: 10.1126/science.adg2992
8. Karagianni P, Tzioufas AG. Epigenetic perspectives on systemic autoimmune disease. J Autoimmun. (2019) 104:102315. doi: 10.1016/j.jaut.2019.102315
9. Fugger L, Jensen LT, Rossjohn J. Challenges, progress, and prospects of developing therapies to treat autoimmune diseases. Cell. (2020) 181:63–80. doi: 10.1016/j.cell.2020.03.007
10. Zeng L, Deng Y, Yang K, Chen J, He Q, Chen H. Safety and efficacy of fecal microbiota transplantation for autoimmune diseases and autoinflammatory diseases: A systematic review and meta-analysis. Front Immunol. (2022) 13:944387. doi: 10.3389/fimmu.2022.944387
11. Balderramo D, Quaresma AB, Olivera PA, Savio MC, Villamil MPG, Panaccione R, et al. Challenges in the diagnosis and treatment of inflammatory bowel disease in Latin America. Lancet Gastroenterol Hepatol. (2024) 9:263–72. doi: 10.1016/S2468-1253(23)00284-4
12. Zeng L, Yang T, Yang K, Yu G, Li J, Xiang W, et al. Curcumin and curcuma longa extract in the treatment of 10 types of autoimmune diseases: A systematic review and meta-analysis of 31 randomized controlled trials. Front Immunol. (2022) 13:896476. doi: 10.3389/fimmu.2022.896476
13. Zeng L, Yu G, Yang K, Xiang W, Li J, Chen H. Efficacy and safety of mesenchymal stem cell transplantation in the treatment of autoimmune diseases (Rheumatoid arthritis, systemic lupus erythematosus, inflammatory bowel disease, multiple sclerosis, and ankylosing spondylitis): A systematic review and meta-analysis of randomized controlled trial. Stem Cells Int. (2022) 2022:9463314. doi: 10.1155/2022/9463314
14. Tsokos GC, Lo MS, Costa Reis P, Sullivan KE. New insights into the immunopathogenesis of systemic lupus erythematosus. Nat Rev Rheumatol. (2016) 12:716–30. doi: 10.1038/nrrheum.2016.186
15. Anders HJ, Saxena R, Zhao MH, Parodis I, Salmon JE, Mohan C. Lupus nephritis. Nat Rev Dis Primers. (2020) 6:7. doi: 10.1038/s41572-019-0141-9
16. Yu C, Li P, Dang X, Zhang X, Mao Y, Chen X. Lupus nephritis: new progress in diagnosis and treatment. J Autoimmun. (2022) 132:102871. doi: 10.1016/j.jaut.2022.102871
17. Chan XS, White NJ, Hien TT. A temporizing solution to "Artemisinin resistance. N Engl J Med. (2019) 381:989–90. doi: 10.1056/NEJMc1909337
18. Yu R, Jin G, Fujimoto M. Dihydroartemisinin: A potential drug for the treatment of Malignancies and inflammatory diseases. Front Oncol. (2021) 11:722331. doi: 10.3389/fonc.2021.722331
19. Dolivo D, Weathers P, Dominko T. Artemisinin and artemisinin derivatives as anti-fibrotic therapeutics. Acta Pharm Sin B. (2021) 11:322–39. doi: 10.1016/j.apsb.2020.09.001
20. Dai X, Zhang X, Chen W, Chen Y, Zhang Q, Mo S, et al. Dihydroartemisinin: A potential natural anticancer drug. Int J Biol Sci. (2021) 17:603–22. doi: 10.7150/ijbs.50364
21. Gao X, Lin X, Wang Q, Chen J. Artemisinins: Promising drug candidates for the treatment of autoimmune diseases. Med Res Rev. (2024) 44:867–91. doi: 10.1002/med.22001
22. Liu CX. Discovery and development of artemisinin and related compounds. Chin Herbal Med. (2017) 9:101–14. doi: 10.1016/S1674-6384(17)60084-4
23. Luo W, Liu Y, Cong L, Sun L, Guo C. Progress in the study of artemisinin and its derivatives. Chin J Medicinal Chem. (2012) 22:155–66.
24. Ma N, Zhang Z, Liao F, Jiang T, Tu Y. The birth of artemisinin. Pharmacol Ther. (2020) 216:107658. doi: 10.1016/j.pharmthera.2020.107658
25. Guo F. Preparation and preliminary evaluation of dihydroartemisinin extended-release tablets. Beijing: China Academy of Traditional Chinese Medicine (2020).
26. Wang R. Preparation, pharmacokinetics and pharmacodynamics of self-assembled nanoparticles of four dihydroartemisinin precursors. Taiyuan: Shanxi Medical University (2019).
27. Ramazani A, Keramati M, Malvandi H, Danafar H, Kheiri Manjili H. Preparation and in vivo evaluation of anti-plasmodial properties of artemisinin-loaded PCL-PEG-PCL nanoparticles. Pharm Dev Technol. (2018) 23:911–20. doi: 10.1080/10837450.2017.1372781
28. Guan Y, Wang L, He B, Tan S. Progress of artemisinin and its derivatives nanoparticles in anti-tumour field. Chin Pharmacist. (2017) 20:1275–8.
29. Yu K, Hongjun X. Prescription preference and main drug content determination of OCT-modified liposomes of Zoerythromycin and dihydroartemisinin. Tibetan Med. (2019) 40:19–22.
30. Li H. Inhibition of human head and neck squamous carcinoma cell proliferation by magnetic dihydroartemisinin nanoliposomes. Shijiazhuang: Hebei Medical University (2017).
31. Ma L-L, Nong X-L. Progress on the anti-tumour mechanism of artesunate. Chin J Tumour Control Prev. (2020) 27:921–6.
32. Qian P, Chen JQ. Research progress on the mechanism of anti-hepatic fibrosis action of artesunate. Chongqing Med. (2017) 46:269–71.
33. Yanyan C, Zhao Y, Li P, Zeng X, Zhou H. Progress of pharmacokinetics and related pharmacological effects of artesunate. Chin J Traditional Chin Med. (2018) 43:3970–8.
34. Li XY, Li XT, Wang YL. Preparation of aminopterin-modified liposomes of artemether. Chin Herbal Med. (2019) 50:1569 1575.
35. Li TZ, Yang XT, Wang JP, Geng CA, Ma YB, Su LH, et al. Biomimetic synthesis of lavandiolides H, I, and K and artematrolide F via diels-alder reaction. Org Lett. (2021) 23:8380–4. doi: 10.1021/acs.orglett.1c03120
36. Adebayo JO, Tijjani H, Adegunloye AP, Ishola AA, Balogun EA, Malomo SO. Enhancing the antimalarial activity of artesunate. Parasitol Res. (2020) 119:2749–64. doi: 10.1007/s00436-020-06786-1
37. Sun W, Han X, Wu S, Wu J, Yang C, Li X. Unexpected mechanism of colitis amelioration by artesunate, a natural product from Artemisia annua L. Inflammopharmacology. (2020) 28:851–68. doi: 10.1007/s10787-019-00678-2
38. Li WD, Dong YJ, Tu YY, Lin ZB. Dihydroarteannuin ameliorates lupus symptom of BXSB mice by inhibiting production of TNF-alpha and blocking the signaling pathway NF-kappa B translocation. Int Immunopharmacol. (2006) 6:1243–50. doi: 10.1016/j.intimp.2006.03.004
39. Pang Y, Wu L, Tang C, Wang H, Wei Y. Autophagy-inflammation interplay during infection: balancing pathogen clearance and host inflammation. Front Pharmacol. (2022) 13:832750. doi: 10.3389/fphar.2022.832750
40. Xu H, He Y, Yang X, Liang L, Zhan Z, Ye Y, et al. Anti-malarial agent artesunate inhibits TNF-alpha-induced production of proinflammatory cytokines via inhibition of NF-kappaB and PI3 kinase/Akt signal pathway in human rheumatoid arthritis fibroblast-like synoviocytes. Rheumatol (Oxford). (2007) 46:920–6. doi: 10.1093/rheumatology/kem014
41. Ma JD, Jing J, Wang JW, Yan T, Li QH, Mo YQ, et al. A novel function of artesunate on inhibiting migration and invasion of fibroblast-like synoviocytes from rheumatoid arthritis patients. Arthritis Res Ther. (2019) 21:153. doi: 10.1186/s13075-019-1935-6
42. Hou LF, He SJ, Li X, Wan CP, Yang Y, Zhang XH, et al. SM934 treated lupus-prone NZB × NZW F1 mice by enhancing macrophage interleukin-10 production and suppressing pathogenic T cell development. PloS One. (2012) 7:e32424. doi: 10.1371/journal.pone.0032424
43. Roberts CA, Dickinson AK, Taams LS. The interplay between monocytes/macrophages and CD4(+) T cell subsets in rheumatoid arthritis. Front Immunol. (2015) 6:571. doi: 10.3389/fimmu.2015.00571
44. Hou LF, He SJ, Li X, Yang Y, He PL, Zhou Y, et al. Oral administration of artemisinin analog SM934 ameliorates lupus syndromes in MRL/lpr mice by inhibiting Th1 and Th17 cell responses. Arthritis Rheumatol. (2011) 63:2445–55. doi: 10.1002/art.30392
45. Wang JX, Tang W, Zhou R, Wan J, Shi LP, Zhang Y, et al. The new water-soluble artemisinin derivative SM905 ameliorates collagen-induced arthritis by suppression of inflammatory and Th17 responses. Br J Pharmacol. (2008) 153:1303–10. doi: 10.1038/bjp.2008.11
46. Wang JX, Tang W, Shi LP, Wan J, Zhou R, Ni J, et al. Investigation of the immunosuppressive activity of artemether on T-cell activation and proliferation. Br J Pharmacol. (2007) 150:652–61. doi: 10.1038/sj.bjp.0707137
47. Hou LF, He SJ, Wang JX, Yang Y, Zhu FH, Zhou Y, et al. SM934, a water-soluble derivative of arteminisin, exerts immunosuppressive functions in vitro and in vivo. Int Immunopharmacol. (2009)9:1509–17. doi: 10.1016/j.intimp.2009.09.003
48. Liu J, Hong X, Lin D, Luo X, Zhu M, Mo H. Artesunate influences Th17/Treg lymphocyte balance by modulating Treg apoptosis and Th17 proliferation in a murine model of rheumatoid arthritis. Exp Ther Med. (2017) 13:2267–73. doi: 10.3892/etm.2017.4232
49. Zhu MY, Lin D, Liu J, Mo HY. Artesunate interfere in modulation of Foxp3 expression in synovial cells in collagen-induced arthritis rats. Chin J Integr Med. (2016). doi: 10.1007/s11655-016-2611-1
50. Fan M, Li Y, Yao C, Liu X, Liu X, Liu J. Dihydroartemisinin derivative DC32 attenuates collagen-induced arthritis in mice by restoring the Treg/Th17 balance and inhibiting synovitis through down-regulation of IL-6. Int Immunopharmacol. (2018) 65:233–43. doi: 10.1016/j.intimp.2018.10.015
51. Yan SC, Wang YJ, Li YJ, Cai WY, Weng XG, Li Q, et al. Dihydroartemisinin Regulates the Th/Treg Balance by Inducing Activated CD4+ T cell Apoptosis via Heme Oxygenase-1 Induction in Mouse Models of Inflammatory Bowel Disease. Molecules. (2019) 24:2475. doi: 10.3390/molecules24132475
52. Li D, Qi J, Wang J, Pan Y, Li J, Xia X, et al. Protective effect of dihydroartemisinin in inhibiting senescence of myeloid-derived suppressor cells from lupus mice via Nrf2/HO-1 pathway. Free Radic Biol Med. (2019) 143:260–74. doi: 10.1016/j.freeradbiomed.2019.08.013
53. Krenács L, Krenács D, Borbényi Z, Tóth E, Nagy A, Piukovics K, et al. Comparison of follicular helper T-cell markers with the expression of the follicular homing marker CXCR5 in peripheral T-cell lymphomas-A reappraisal of follicular helper T-cell lymphomas. Int J Mol Sci. (2023) 25:428. doi: 10.3390/ijms25010428
54. Davies K, McLaren JE. Destabilisation of T cell-dependent humoral immunity in sepsis. Clin Sci (Lond). (2024) 138:65–85. doi: 10.1042/CS20230517
55. Dang WZ, Li H, Jiang B, Nandakumar KS, Liu KF, Liu LX, et al. Therapeutic effects of artesunate on lupus-prone MRL/lpr mice are dependent on T follicular helper cell differentiation and activation of JAK2-STAT3 signaling pathway. Phytomedicine. (2019) 62:152965. doi: 10.1016/j.phymed.2019.152965
56. Wu Y, He S, Bai B, Zhang L, Xue L, Lin Z, et al. Therapeutic effects of the artemisinin analog SM934 on lupus-prone MRL/lpr mice via inhibition of TLR-triggered B-cell activation and plasma cell formation. Cell Mol Immunol. (2016) 13:379–90. doi: 10.1038/cmi.2015.13
57. Lin ZM, Yang XQ, Zhu FH, He SJ, Tang W, Zuo JP. Artemisinin analogue SM934 attenuate collagen-induced arthritis by suppressing T follicular helper cells and T helper 17 cells. Sci Rep. (2016) 6:38115. doi: 10.1038/srep38115
58. Jin O, Zhang H, Gu Z, Zhao S, Xu T, Zhou K, et al. A pilot study of the therapeutic efficacy and mechanism of artesunate in the MRL/lpr murine model of systemic lupus erythematosus. Cell Mol Immunol. (2009) 6:461–7. doi: 10.1038/cmi.2009.58
59. Hou L, Block KE, Huang H. Artesunate abolishes germinal center B cells and inhibits autoimmune arthritis. PloS One. (2014) 9:e104762. doi: 10.1371/journal.pone.0104762
60. Briukhovetska D, Dörr J, Endres S, Libby P, Dinarello CA, Kobold S. Interleukins in cancer: from biology to therapy. Nat Rev Cancer. (2021) 21:481–99. doi: 10.1038/s41568-021-00363-z
61. Luo B, Xiang D, Ji X, Chen X, Li R, Zhang S, et al. The anti-inflammatory effects of exercise on autoimmune diseases: A twenty-year systematic review. J Sport Health Sci. (2024) S2095-2546:00019–X. doi: 10.1016/j.jshs.2024.02.002
62. Guzik TJ, Nosalski R, Maffia P, Drummond GR. Immune and inflammatory mechanisms in hypertension. Nat Rev Cardiol. (2024) 21:396–416. doi: 10.1038/s41569-023-00964-1
63. Saxton RA, Glassman CR, Garcia KC. Emerging principles of cytokine pharmacology and therapeutics. Nat Rev Drug Discovery. (2023) 22:21–37. doi: 10.1038/s41573-022-00557-6
64. Deckers J, Anbergen T, Hokke AM, de Dreu A, Schrijver DP, de Bruin K, et al. Engineering cytokine therapeutics. Nat Rev Bioeng. (2023) 1:286–303. doi: 10.1038/s44222-023-00030-y
65. Zhang C, Li Y, Yu Y, Li Z, Xu X, Talifu Z, et al. Impact of inflammation and Treg cell regulation on neuropathic pain in spinal cord injury: mechanisms and therapeutic prospects. Front Immunol. (2024) 15:1334828. doi: 10.3389/fimmu.2024.1334828
66. Dai X, Du X, Zhang X, Wang B, Bo J. Effect of dihydroartemisinin on TNF-α expression in rats with early radiation lung injury. Chin J Cancer Prev Control. (2010) 17:728–31. doi: CNKI:SUN:QLZL.0.2010-10-006
67. Wang Y, Wang J, Yang A, Yang L, Dong Y, Yun Q. Effects of dihydroartemisinin on TGF β1 and TNF-α in mice with liver fibrosis. Occupation Health. (2020) 36:1193 1196. doi: CNKI:SUN:ZYJK.0.2020-09-010
68. Wu P, Li Q, Xia Z, Zhang F, Xia Q. Preparation of a mouse nephritis model and the effect of dihydroartemisinin on the release of inflammatory factors. West China Med. (2011) 26:1028–31.
69. Yi J. Effect of ibuprofen and dihydroartemisinin on the expression of IL-1β and TNF-α in synovial membrane of rats with adjuvant arthritis. J Yichun Coll. (2009) 31:63–4.
70. Liu J, Jin L, Yu R, Wei Q, Li F, Jin Q, et al. Effects of dihydroartemisinin and oxidised artemisinin on the proliferation and cytokine expression of HaCaT cells. Yanbian Univ Med J. (2019) 42:95–8. doi: CNKI:SUN:YBYB.0.2019-02-005
71. Lei Z, Yang Y, Liu S, Lei Y, Yang L, Zhang X, et al. Dihydroartemisinin ameliorates dextran sulfate sodium induced inflammatory bowel diseases in mice. Bioorg Chem. (2020) 100:103915. doi: 10.1016/j.bioorg.2020.103915
72. Liu SM. Mechanistic study of EpCAM gene deletion inducing liver and intestinal diseases and dihydroartemisinin ameliorating inflammatory bowel disease. Guangzhou: Guangdong Pharmaceutical University (2019).
73. Li P, Cao X, Yang C, Guo Q, Yu L, Wu N, et al. Effect of dihydroartemisinin on macrophage-derived inflammatory factor release induced by ultrahigh molecular weight polyethylene particles. Adv Modern Biomedicine. (2014) 14:3601–5.
74. Chen J, Zhang Y, Yuan Y, Wu S, Ming J. Progress of immunomodulatory effects of plant polysaccharides on macrophages via NF-κB signalling pathway. Food Sci. (2015) 36:288–94.
75. Sibi G, Rabina S. Inhibition of pro-inflammatory mediators and cytokines by chlorella vulgaris extracts. Pharmacognosy Res. (2016) 8:118–22. doi: 10.4103/0974-8490.172660
76. Yin J, Xia W, Zhang Y, Ding G, Chen L, Yang G, et al. Role of dihydroartemisinin in regulating prostaglandin E2 synthesis cascade and inflammation in endothelial cells. Heart Vessels. (2018) 33:1411–22. doi: 10.1007/s00380-018-1190-9
77. Yu W, Kan W, Yu P, Li M, Song J, Zhao F. Anti-inflammatory effects and mechanisms of artemisinin and dihydroartemisinin. Chin J Traditional Chin Med. (2012) 37:2618–21.
78. Efferth T, Oesch F. The immunosuppressive activity of artemisinin-type drugs towards inflammatory and autoimmune diseases. Med Res Rev. (2021) 41:3023–61. doi: 10.1002/med.21842
79. Zhao N, Wang R, Zhou L, Zhu Y, Gong J, Zhuang SM. MicroRNA-26b suppresses the NF-κB signaling and enhances the chemosensitivity of hepatocellular carcinoma cells by targeting TAK1 and TAB3. Mol Cancer. (2014) 13:35. doi: 10.1186/1476-4598-13-35
80. Lv Y, Xu Q, Mao Y, Xu Y, Zhang R, Zhong H, et al. TRAF3 of blunt snout bream participates in host innate immune response to pathogenic bacteria via NF-κB signaling pathway. Fish Shellfish Immunol. (2020) 104:592–604. doi: 10.1016/j.fsi.2020.06.022
81. Zheng H, Liu Y, Deng Y, Li Y, Liu S, Yang Y, et al. Recent advances of NFATc1 in rheumatoid arthritis-related bone destruction: mechanisms and potential therapeutic targets. Mol Med. (2024) 30:20. doi: 10.1186/s10020-024-00788-w
82. Yi Y, Qi W-L, Zheng W-H. Advances in pro-injurious and protective signalling pathways in myocardial ischemia-reperfusion injury. Chem Life. (2020) 40:911–8.
83. Sui X, Zhu Q, Pan J, Wang Y, Sui Y. Therapeutic effects of dihydroartemisinin on radiological pneumonia in rats. J Weifang Med Coll. (2016) 38:338 341.
84. Huang XT, Liu W, Zhou Y, Hao CX, Zhou Y, Zhang CY, et al. Dihydroartemisinin attenuates lipopolysaccharide induced acute lung injury in mice by suppressing NF κB signaling in an Nrf2 dependent manner. Int J Mol Med. (2019) 44:2213–22. doi: 10.3892/ijmm.2019.4387
85. Yang D, Yuan W, Lv C, Li N, Liu T, Wang L, et al. Dihydroartemisinin supresses inflammation and fibrosis in bleomycine-induced pulmonary fibrosis in rats. Int J Clin Exp Pathol. (2015) 8:1270–81.
86. Liu X, Lu J, Liao Y, Liu S, Chen Y, He R, et al. Dihydroartemisinin attenuates lipopolysaccharide-induced acute kidney injury by inhibiting inflammation and oxidative stress. BioMed Pharmacother. (2019) 117:109070. doi: 10.1016/j.biopha.2019.109070
87. You Y, Liao P, Yang F, Lin X. Study on the regulatory effect of dihydroartemisinin on the expression of Fractalkine in renal cortex of MRL/lpr mice in lupus model. J Immunol. (2014) 30:617–22. doi: CNKI:SUN:MYXZ.0.2014-07-015
88. Dong Y, Li W, Tu Y, Zhang H, Zou W, Yang L, et al. Study on the effect and mechanism of dihydroartemisinin on wolf sore nephritis in BXSB mice. Chin J Pharmacol. (2003) 19:1125–8.
89. Du CC, Tan YQ, Shen JY, Yu R, Li F, Li H, et al. Effects and mechanisms of dihydroartemisinin on two models of rheumatoid arthritis. Chin J Exp Formulary. (2019) 25:48–56.
90. Wang HY, Qin HL, Zhang YF, et al. Effects of papaya triterpenes on the expression of Akt, NF-κB and pro-inflammatory factors in synovial tissues of rats with adjuvant arthritis. Chin J Exp Formulary. (2017) 23:141–6.
91. Xu C, Liu C, Zhao L. Dihydroartemisinin induces synovial cell apoptosis in rheumatoid arthritis through Akt signalling pathway. Chin Med J. (2009) 30:889–91.
92. Qin WX. Inhibitory effect of dihydroartemisinin on LPS-induced microglial cell inflammation and its mechanism. Chongqing: Chongqing Medical University (2018).
93. Gantke T, Sriskantharajah S, Sadowski M, Ley SC. IκB kinase regulation of the TPL-2/ERK MAPK pathway. Immunol Rev. (2012) 246:168–82. doi: 10.1111/j.1600-065X.2012.01104.x
94. Wei Q, Jin Q, Jin L, Yu R, Li F, Li H, et al. Dihydroartemisinin ameliorates psoriasis-like skin inflammation in mice by inhibiting proliferation of keratinocytes and production of pro-inflammatory factors. Chin J Immunol. (2020) 36:543–8.
95. Liu C, Guo X, Zhou Y, Wang H. AMPK signalling pathway: A potential strategy for the treatment of heart failure with chinese medicine. J Inflammation Res. (2023) 16:5451–64. doi: 10.2147/JIR.S441597
96. Zhao Y, Niu Y, He J, Ji S, Zhang L, Wang C, et al. Effects of dihydroartemisinin on lipopolysaccharide-induced hepatic inflammation and lipid metabolism in weanling piglets. Chin J Traditional Chin Med. (2020) 45:202–8.
97. Sharma A, Anand SK, Singh N, Dwivedi UN, Kakkar P. AMP-activated protein kinase: An energy sensor and survival mechanism in the reinstatement of metabolic homeostasis. Exp Cell Res. (2023) 428:113614. doi: 10.1016/j.yexcr.2023.113614
98. Zhao Y, Niu Y, He J, Gan Z, Ji S, Zhang L, et al. Effects of dietary dihydroartemisinin supplementation on growth performance, hepatic inflammation, and lipid metabolism in weaned piglets with intrauterine growth retardation. Anim Sci J. (2020) 91:e13363. doi: 10.1111/asj.13363
99. Morgos DT, Stefani C, Miricescu D, Greabu M, Stanciu S, Nica S, et al. Targeting PI3K/AKT/mTOR and MAPK signaling pathways in gastric cancer. Int J Mol Sci. (2024) 25:1848. doi: 10.3390/ijms25031848
100. Abu-Alghayth MH, Khan FR, Belali TM, Abalkhail A, Alshaghdali K, Nassar SA, et al. The emerging role of noncoding RNAs in the PI3K/AKT/mTOR signalling pathway in breast cancer. Pathol Res Pract. (2024) 255:155180. doi: 10.1016/j.prp.2024.155180
101. Gao Y, Cui M, Zhong S, Feng C, Nwobodo AK, Chen B, et al. Dihydroartemisinin ameliorates LPS-induced neuroinflammation by inhibiting the PI3K/AKT pathway. Metab Brain Dis. (2020) 35:661–72. doi: 10.1007/s11011-020-00533-2
102. Lee AS, Hur HJ, Sung MJ. The effect of artemisinin on inflammation-associated lymphangiogenesis in experimental acute colitis. Int J Mol Sci. (2020) 21:8068. doi: 10.3390/ijms21218068
103. Cho YC, Lee SH, Lee M, Kim HJ, Oak MH, Lee IS, et al. Enhanced IL-12p40 production in LPS-stimulated macrophages by inhibiting JNK activation by artemisinin. Arch Pharm Res. (2012) 35:1961–8. doi: 10.1007/s12272-012-1113-8
104. Yao GD, Ge MY, Li DQ, Chen L, Hayashi T, Tashiro SI, et al. L-A03, a dihydroartemisinin derivative, promotes apoptotic cell death of human breast cancer MCF-7 cells by targeting c-Jun N-terminal kinase. BioMed Pharmacother. (2018) 105:320–5. doi: 10.1016/j.biopha.2018.05.093
105. Liang R, Chen W, Fan H, Chen X, Zhang J, Zhu JS. Dihydroartemisinin prevents dextran sodium sulphate-induced colitisthrough inhibition of the activation of NLRP3 inflammasome and p38 MAPK signaling. Int Immunopharmacol. (2020) 88:106949. doi: 10.1016/j.intimp.2020.106949
106. Zhao X, Liu M, Li J, Yin S, Wu Y, Wang A. Antimalarial agent artesunate protects Concanavalin A-induced autoimmune hepatitis in mice by inhibiting inflammatory responses. Chem Biol Interact. (2017) 274:116–23. doi: 10.1016/j.cbi.2017.07.012
107. Zhang Y, Li Q, Jiang N, Su Z, Yuan Q, Lv L, et al. Dihydroartemisinin beneficially regulates splenic immune cell heterogeneity through the SOD3-JNK-AP-1 axis. Sci China Life Sci. (2022) 65:1636–54. doi: 10.1007/s11427-021-2061-7
108. Arkun Y, Yasemi M. Dynamics and control of the ERK signaling pathway: Sensitivity, bistability, and oscillations. PloS One. (2018) 13:e0195513. doi: 10.1371/journal.pone.0195513
109. Sugiura R, Satoh R, Takasaki T. ERK: a double-edged sword in cancer. ERK-dependent apoptosis as a potential therapeutic strategy for cancer. Cells. (2021) 10:2509. doi: 10.3390/cells10102509
110. Chen MJ, Ramesha S, Weinstock LD, Gao T, Ping L, Xiao H, et al. Extracellular signal-regulated kinase regulates microglial immune responses in Alzheimer’s disease. J Neurosci Res. (2021) 99:1704–21. doi: 10.1002/jnr.24829
111. Ou L, Qin K, Yang Z, Bie M. Effect of dihydroartemisinin on the expression of TNF-α and IL-6 in human bronchial epithelial cells induced by influenza A virus H1N1 and its mechanism. J Sichuan Univ (Medical Edition). (2020) 51:171–7.
112. Wei M, Xie X, Chu X, Yang X, Guan M, Wang D. Dihydroartemisinin suppresses ovalbumin-induced airway inflammation in a mouse allergic asthma model. Immunopharmacol Immunotoxicol. (2013) 35:382–9. doi: 10.3109/08923973.2013.785559
113. Yi M, Cruz Cisneros L, Cho EJ, Alexander M, Kimelman FA, Swentek L, et al. Nrf2 pathway and oxidative stress as a common target for treatment of diabetes and its comorbidities. Int J Mol Sci. (2024) 25:821. doi: 10.3390/ijms25020821
114. Glorieux C, Enríquez C, González C, Aguirre-Martínez G, Buc Calderon P. The multifaceted roles of NRF2 in cancer: friend or foe? Antioxidants (Basel). (2024) 13:70. doi: 10.3390/antiox13010070
115. Su X, Guo W, Yuan B, Wang D, Liu L, Wu X, et al. Artesunate attenuates bone erosion in rheumatoid arthritis by suppressing reactive oxygen species via activating p62/Nrf2 signaling. BioMed Pharmacother. (2021) 137:111382. doi: 10.1016/j.biopha.2021.111382
116. Fan M, Li Y, Yao C, Liu X, Liu J, Yu B. DC32, a dihydroartemisinin derivative, ameliorates collagen-induced arthritis through an nrf2-p62-keap1 feedback loop. Front Immunol. (2018) 9:2762. doi: 10.3389/fimmu.2018.02762
117. Goropevšek A, Holcar M, Pahor A, Avčin T. STAT signaling as a marker of SLE disease severity and implications for clinical therapy. Autoimmun Rev. (2019) 18:144–54. doi: 10.1016/j.autrev.2018.08.010
118. Feng X, Chen W, Xiao L, Gu F, Huang J, Tsao BP, et al. Artesunate inhibits type I interferon-induced production of macrophage migration inhibitory factor in patients with systemic lupus erythematosus. Lupus. (2017) 26:62–72. doi: 10.1177/0961203316651738
119. Xu W, Lu C, Yao L, Zhang F, Shao J, Zheng S. Dihydroartemisinin protects against alcoholic liver injury through alleviating hepatocyte steatosis in a farnesoid X receptor-dependent manner. Toxicol Appl Pharmacol. (2017) 315:23–34. doi: 10.1016/j.taap.2016.12.001
120. Gravallese EM, Firestein GS. Rheumatoid arthritis - common origins, divergent mechanisms. N Engl J Med. (2023) 388:529–42. doi: 10.1056/NEJMra2103726
121. Lewis MJ. Predicting best treatment in rheumatoid arthritis. Semin Arthritis Rheum. (2023) 152329. doi: 10.1016/j.semarthrit.2023.152329
122. Hanlon MM, McGarry T, Marzaioli V, Amaechi S, Song Q, Nagpal S, et al. Rheumatoid arthritis macrophages are primed for inflammation and display bioenergetic and functional alterations. Rheumatol (Oxford). (2023) 62:2611–20. doi: 10.1093/rheumatology/keac640
123. Luo Y, Zhang J, Jiao Y, Huang H, Ming L, Song Y, et al. Dihydroartemisinin abolishes cisplatin-induced nephrotoxicity in vivo. J Nat Med. (2024) 73:439–454. doi: 10.1007/s11418-024-01783-5
124. Xu C, Liu C, Wu N, Zhao L. Dihydroartemisinin induces synovial cell apoptosis in rheumatoid arthritis via Akt signalling pathway. Guangdong Med. (2009) 30:1043–5. doi: CNKI:SUN:GAYX.0.2009-07-010
125. Fan M, Li Y, Yao C, Liu X, Liu J, Yu B. DC32,a dihydroartemisinin derivative,Ameliorates collagen-induced arthritis through an nrf2-p62-keap1 feedback loop. Front Immunol. (2018) 9, 2762. doi: 10.3389/fimmu.2018.02762
126. Feng FB, Qiu HY. Effects of Artesunate on chondrocyte proliferation, apoptosis and autophagy through the PI3K/AKT/mTOR signaling pathway in rat models with rheumatoid arthritis. BioMed Pharmacother. (2018) 102:1209–20. doi: 10.1016/j.biopha.2018.03.142
127. He P, Tu R, Mei X, Zhang L, Huang C, Zhan M, et al. Protective effects of dihydroartemisinin and torch root in rats with adjuvant arthritis model. Proprietary Chin Med. (2022) 44:2290–4.
128. Lyu C, Zhang J, Chi H, Qiang Y, Zhang Q. Artemisinin combined with chloroquine treatment inhibits the inflammatory response in collagen-induced arthritis mice by blocking the NF-κB pathway. J Cell Mol Immunol. (2022) 38:632–9.
129. Li Z, Feng W, Wang C, Xiao F, Gao Y, Chen X. Dihydroartemisinin inhibition of nuclear factor κB receptor agonist ligand-induced differentiation of RAW264.7 cells into osteoblasts. Chin J Anat Clinics. (2017) 22:148–52. doi: 10.3760/cma.j.issn.2095-7041.2017.02.012
130. Li B, Zhang L, Wang J, Zheng J, Yu S, Luo P, Zhou H. Effect of dihydroartemisinin on cytokine release from CpG ODN-induced mouse RAW264.7 cells. Sichuan J Physiol Sci. (2005) 27:149–52. doi: 10.3969/j.issn.1671-3885.2005.04.003
131. Zhang Y, He W, Du Y, Du Y, Zhao C, Zhang Y, et al. Dimeric artesunate phospholipid-conjugated liposomes as promising anti-inflammatory therapy for rheumatoid arthritis. Int J Pharm. (2020) 579:119178. doi: 10.1016/j.ijpharm.2020.119178
132. Accapezzato D, Caccavale R, Paroli MP, Gioia C, Nguyen BL, Spadea L, et al. Advances in the pathogenesis and treatment of systemic lupus erythematosus. Int J Mol Sci. (2023) 24:6578. doi: 10.3390/ijms24076578
133. Zeng L, Yang K, Zhang T, Zhu X, Hao W, Chen H, et al. Research progress of single-cell transcriptome sequencing in autoimmune diseases and autoinflammatory disease: A review. J Autoimmun. (2022) 133:102919. doi: 10.1016/j.jaut.2022.102919
134. Crow MK. Pathogenesis of systemic lupus erythematosus: risks, mechanisms and therapeutic targets. Ann Rheum Dis. (2023) 82:999–1014. doi: 10.1136/ard-2022-223741
135. Zyad A, Tilaoui M, Jaafari A, Oukerrou MA, Mouse HA. More insights into the pharmacological effects of artemisinin. Phytother Res. (2018) 32:216–29. doi: 10.1002/ptr.5958
136. Zhang X. Study on the improvement of lupus symptoms by dihydroartemisinin through delaying the aging of MDSCs. Nanjing: Nanjing University (2018).
137. Dong Y, Li W, Tu Y. Effects of dihydroartemisinin on autoantibody production, TNFα secretion and pathological changes of lupus nephritis in BXSB lupus mice. Chin J Integr Med. (2003) 23:676–9. doi: 10.7661/CJIM.2003.9.676
138. Liang N, Zhong Y, Zhou J, Liu B, Lu R, Guan Y, et al. Immunosuppressive effects of hydroxychloroquine and artemisinin combination therapy via the nuclear factor-κB signaling pathway in lupus nephritis mice. Exp Ther Med. (2018) 15:2436–42. doi: 10.3892/etm.2018.5708
139. Kuznik A, Bencina M, Svajger U, Jeras M, Rozman B, Jerala R. Mechanism of endosomal TLR inhibition by antimalarial drugs and imidazoquinolines. J Immunol. (2011) 186:4794–804. doi: 10.4049/jimmunol.1000702
140. Diao L, Tao J, Wang Y, Hu Y, He W. Co-delivery of dihydroartemisinin and HMGB1 siRNA by TAT-modified cationic liposomes through the TLR4 signaling pathway for treatment of lupus nephritis. Int J Nanomedicine. (2019) 14:8627–45. doi: 10.2147/IJN.S220754
141. Longhino S, Chatzis LG, Dal Pozzolo R, Peretti S, Fulvio G, La Rocca G, et al. Sjögren's syndrome: one year in review 2023. Clin Exp Rheumatol. (2023) 41:2343–56. doi: 10.55563/clinexprheumatol/255qsx
142. Zabotti A, Giovannini I, Longhino S, Manfrè V, Rizzo MT, De Vita S, et al. Nuts and bolts of salivary gland pathology in primary Sjögren's syndrome. Clin Exp Rheumatol. (2023) 41:2525–37. doi: 10.55563/clinexprheumatol/fcjoqx
143. Wu T, Li S, Chen J, Liao J, Huang Z, Yang J, et al. A bibliometric analysis of primary Sjögren's syndrome-associated lymphoma from 1991 to 2022. Heliyon. (2023) 9:e21337. doi: 10.1016/j.heliyon.2023.e21337
144. Xiao F, Rui K, Han M, Zou L, Huang E, Tian J, et al. Artesunate suppresses Th17 response via inhibiting IRF4-mediated glycolysis and ameliorates Sjogüren's syndrome. Signal Transduct Target Ther. (2022) 7:274. doi: 10.1038/s41392-022-01103-x
145. Liao JH, He Q, Huang ZW, Yu XB, Yang JY, Zhang Y, et al. Network pharmacology-based strategy to investigate the mechanisms of artemisinin in treating primary Sjögren's syndrome. BMC Immunol. (2024) 25:16. doi: 10.1186/s12865-024-00605-3
146. Zhan T, Wang B, Fu J, Shao Y, Ye L, Shi H, et al. Artesunate inhibits Sjögren's syndrome-like autoimmune responses and BAFF-induced B cell hyperactivation via TRAF6-mediated NF-κB signaling. Phytomedicine. (2021) 80:153381. doi: 10.1016/j.phymed.2020.153381
147. Duan Y. Therapeutic effects and molecular mechanisms of artemisinin derivatives in mice with dry syndrome model. Beijing: Institute of Geriatrics, Ministry of Health (2022). doi: 10.27963/d.cnki.gbjln.2020.000002
148. Li Q, Jiang N, Zhang Y, Liu Y, Su Z, Yuan Q, et al. Dihydroartemisinin imposes positive and negative regulation on Treg and plasma cells via direct interaction and activation of c-Fos. Commun Biol. (2023) 6:52. doi: 10.1038/s42003-023-04454-5
149. Sarkar SK, Willson AML, Jordan MA. The plasticity of immune cell response complicates dissecting the underlying pathology of multiple sclerosis. J Immunol Res. (2024) 2024:5383099. doi: 10.1155/2024/5383099
150. Lei Z, Lin W. Mechanisms governing oligodendrocyte viability in multiple sclerosis and its animal models. Cells. (2024) 13:116. doi: 10.3390/cells13020116
151. Fornari Laurindo L, Aparecido Dias J, Cressoni Araújo A, Torres Pomini K, MaChado Galhardi C, Rucco Penteado Detregiachi C, et al. Immunological dimensions of neuroinflammation and microglial activation: exploring innovative immunomodulatory approaches to mitigate neuroinflammatory progression. Front Immunol. (2024) 14:1305933. doi: 10.3389/fimmu.2023.1305933
152. Amoriello R, Memo C, Ballerini L, Ballerini C. The brain cytokine orchestra in multiple sclerosis: from neuroinflammation to synaptopathology. Mol Brain. (2024) 17:4. doi: 10.1186/s13041-024-01077-7
153. Zhang Y, Lv J, Zhang S, Yang H, Shen J, Du C, et al. Synthesis and biological evaluation of artemisinin derivatives as potential MS agents. Bioorg Med Chem Lett. (2022) 64:128682. doi: 10.1016/j.bmcl.2022.128682
154. Khakzad MR, Ganji A, Ariabod V, Farahani I. Artemisinin therapeutic efficacy in the experimental model of multiple sclerosis. Immunopharmacol Immunotoxicol. (2017) 39:348–53. doi: 10.1080/08923973.2017.1379087
155. Lv J, Zhuang W, Zhang Y, Xie L, Xiang Z, Zhao Q, et al. 9,10-anhydrodehydroartemisinin attenuates experimental autoimmune encephalomyelitis by inhibiting th1 and th17 cell differentiation. Inflammation. (2021) 44:1793–802. doi: 10.1007/s10753-021-01456-5
156. Liu G, Jiang X, Han M, Lv J, Zhuang W, Xie L, et al. Artemisinin derivative TPN10466 suppresses immune cell migration and Th1/Th17 differentiation to ameliorate disease severity in experimental autoimmune encephalomyelitis. Cell Immunol. (2022) 373:104500. doi: 10.1016/j.cellimm.2022.104500
157. Xie L, Lv J, Saimaier K, Han S, Han M, Wang C, et al. The novel small molecule TPN10518 alleviates EAE pathogenesis by inhibiting AP1 to depress Th1/Th17 cell differentiation. Int Immunopharmacol. (2023) 123:110787. doi: 10.1016/j.intimp.2023.110787
158. Salehi N, Nourbakhsh M, Noori S, Rezaeizadeh H, Zarghi A. Tehranolid and artemisinin effects on ameliorating experimental autoimmune encephalomyelitis by modulating inflammation and remyelination. Mol Neurobiol. (2023) 60:5975–86. doi: 10.1007/s12035-023-03449-x
159. Ayoub M, Mattay S, Yarur AJ, Deepak P. Managing risks with newer oral small molecules in patients with inflammatory bowel diseases. Curr Gastroenterol Rep. (2024) 26:145–56. doi: 10.1007/s11894-024-00923-x
160. Xu C, Shao J. High-throughput omics technologies in inflammatory bowel disease. Clin Chim Acta. (2024) 555:117828. doi: 10.1016/j.cca.2024.117828
161. Kong L, Chen S, Huang S, Zheng A, Gao S, Ye J, et al. Challenges and opportunities in inflammatory bowel disease: from current therapeutic strategies to organoid-based models. Inflammation Res. (2024) 73:541–62. doi: 10.1007/s00011-024-01854-z
162. Qi J, Pan Z, Wang X, Zhang N, He G, Jiang X. Research advances of Zanthoxylum bungeanum Maxim. polyphenols inflammatory diseases. Front Immunol. (2024) 15:1305886. doi: 10.3389/fimmu.2024.1305886
163. Selinger CP, Rosiou K, Lenti MV. Biological therapy for inflammatory bowel disease: cyclical rather than lifelong treatment? BMJ Open Gastroenterol. (2024) 11:e001225. doi: 10.1136/bmjgast-2023-001225
164. Gandhi GR, Mohana T, Athesh K, Hillary VE, Vasconcelos ABS, Farias de Franca MN, et al. Anti-inflammatory natural products modulate interleukins and their related signaling markers in inflammatory bowel disease: A systematic review. J Pharm Anal. (2023) 13:1408–28. doi: 10.1016/j.jpha.2023.09.012
165. Yin S, Yang H, Tao Y, Wei S, Li L, Liu M, et al. Artesunate ameliorates DSS-induced ulcerative colitis by protecting intestinal barrier and inhibiting inflammatory response. Inflammation. (2020) 43:765–76. doi: 10.1007/s10753-019-01164-1
166. Tao Y, Xu L, Liu X, Wang P, Wei S, Huang Y, et al. Chitosan-coated artesunate protects against ulcerative colitis via STAT6-mediated macrophage M2 polarization and intestinal barrier protection. Int J Biol Macromol. (2024) 254. doi: 10.1016/j.ijbiomac.2023.127680
167. Yang Z, Ding J, Yang C, Gao Y, Li X, Chen X, et al. Immunomodulatory and anti-inflammatory properties of artesunate in experimental colitis. Curr Med Chem. (2012) 19:4541–51. doi: 10.2174/092986712803251575
168. Zhu X, Li T, Chen Z. Oral administration of artemisinin nanospheres reduces inflammation in mice with spontaneous ulcerative colitis. J Cell Mol Immunol. (2023) 39:787–92. doi: 10.13423/j.cnki.cjcmi.009634
169. Chen Y, Wang J, Li J, Zhu J, Wang R, Xi Q, et al. Astragalus polysaccharide prevents ferroptosis in a murine model of experimental colitis and human Caco-2 cells via inhibiting NRF2/HO-1 pathway. Eur J Pharmacol. (2021) 911:174518. doi: 10.1016/j.ejphar.2021.174518
170. Liang J, Yang C, Li P, Zhang M, Xie X, Xie X, et al. Astragaloside IV inhibits AOM/DSS-induced colitis-associated tumorigenesis via activation of PPARγ signaling in mice. Phytomedicine. (2023) 121:155116. doi: 10.1016/j.phymed.2023.155116
171. Ekhtiar M, Ghasemi-Dehnoo M, Mirzaei Y, Azadegan-Dehkordi F, Amini-Khoei H, Lorigooini Z, et al. The coumaric acid and syringic acid ameliorate acetic acid-induced ulcerative colitis in rats via modulator of Nrf2/HO-1 and pro-inflammatory cytokines. Int Immunopharmacol. (2023) 120:110309. doi: 10.1016/j.intimp.2023.110309
172. Golubovskaya V, Wu L. Different subsets of T cells, memory, effector functions, and CAR-T immunotherapy. Cancers (Basel). (2016) 8:36. doi: 10.3390/cancers8030036
173. Yu J, Sheng S, Zou X, Shen Z. Dihydroartemisinin-ursodeoxycholic acid conjugate is a potential treatment agent for inflammatory bowel disease. Int Immunopharmacol. (2023) 117:109918. doi: 10.1016/j.intimp.2023.109918
174. Willison AG, Pawlitzki M, Lunn MP, Willison HJ, Hartung HP, Meuth SG. SARS-coV-2 vaccination and neuroimmunological disease: A review. JAMA Neurol. (2024) 81:179–86. doi: 10.1001/jamaneurol.2023.5208
175. Crisafulli S, Boccanegra B, Carollo M, Bottani E, Mantuano P, Trifirò G, et al. Myasthenia gravis treatment: from old drugs to innovative therapies with a glimpse into the future. CNS Drugs. (2024) 38:15–32. doi: 10.1007/s40263-023-01059-8
176. Uzawa A, Kuwabara S, Suzuki S, Imai T, Murai H, Ozawa Y, et al. Roles of cytokines and T cells in the pathogenesis of myasthenia gravis. Clin Exp Immunol. (2021) 203:366–74. doi: 10.1111/cei.13546
177. Chen W, Li FF, Li C, Sui JK, Meng QF, Li XL, et al. Artemisinin ameliorates the symptoms of experimental autoimmune myasthenia gravis by regulating the balance of TH1 cells, TH17 cells and Treg cells. J Biol Regul Homeost Agents. (2018) 32:1217–23.
178. Meng QF, Zhang XX, Zhang Z, Chen W, Li XL, Wang YJ, et al. Therapeutic potential of artesunate in experimental autoimmune myasthenia gravis by upregulated T regulatory cells and regulation of Th1/Th2 cytokines. Pharmazie. (2018) 73:526–32. doi: 10.1691/ph.2018.8516
179. Trivedi PJ, Hirschfield GM, Adams DH, Vierling JM. Immunopathogenesis of primary biliary cholangitis, primary sclerosing cholangitis and autoimmune hepatitis: themes and concepts. Gastroenterology. (2024) 166:995–1019. doi: 10.1053/j.gastro.2024.01.049
180. Feng TT, Zou T, Wang X, Zhao WF, Qin AL. Clinical significance of changes in the Th17/Treg ratio in autoimmune liver disease. World J Gastroenterol. (2017) 23:3832–8. doi: 10.3748/wjg.v23.i21.3832
181. Pena Polanco NA, Levy C. Autoimmune hepatitis and pregnancy. Clin Liver Dis (Hoboken). (2024) 23:e0112. doi: 10.1097/CLD.0000000000000112
182. Cao J, Zhao X, Liu MJ, Zheng H, Li JG. Protective effects of artesunate against Con A-induced autoimmune liver injury in mice. Zhongguo Zhong Yao Za Zhi. (2018) 43:2123–8. doi: 10.19540/j.cnki.cjcmm.20180125.009
183. Wang C, Han M, Li X, Lv J, Zhuang W, Xie L, et al. TPN10475 alleviates concanavalin A-induced autoimmune hepatitis by limiting T cell development and function through inhibition of PI3K-AKT pathway. Int Immunopharmacol. (2023) 125:111110. doi: 10.1016/j.intimp.2023.111110
184. Selvaskandan H, Barratt J, Cheung CK. Novel treatment paradigms: primary igA nephropathy. Kidney Int Rep. (2023) 9:203–13. doi: 10.1016/j.ekir.2023.11.026
185. Parums DV. A review of igA vasculitis (Henoch-schönlein purpura) past, present, and future. Med Sci Monit. (2024) 30:e943912. doi: 10.12659/MSM.943912
186. Shimizu Y, Tomino Y, Suzuki Y. IgA nephropathy: beyond the half-century. Medicina (Kaunas). (2023) 60:54. doi: 10.3390/medicina60010054
187. Gentile M, Sanchez-Russo L, Riella LV, Verlato A, Manrique J, Granata S, et al. Immune abnormalities in IgA nephropathy. Clin Kidney J. (2023) 16:1059–70. doi: 10.1093/ckj/sfad025
188. Dybiec J, Frąk W, Kućmierz J, Tokarek J, Wojtasińska A, Młynarska E, et al. Liquid biopsy: A new avenue for the diagnosis of kidney disease: diabetic kidney disease, renal cancer, and igA nephropathy. Genes (Basel). (2024) 15:78. doi: 10.3390/genes15010078
189. Jin Q, Liu T, Chen D, Yang L, Mao H, Ma F, et al. Therapeutic potential of artemisinin and its derivatives in managing kidney diseases. Front Pharmacol. (2023) 14:1097206. doi: 10.3389/fphar.2023.1097206
190. Yang WG, Sun A, Zhu R, Liu N, He WJ, Liu LL. Exploration of artemisinin against igA nephropathy via AKT/nrf2 pathway by bioinformatics and experimental validation. Drug Des Devel Ther. (2023) 17:1679–97. doi: 10.2147/DDDT.S403422
191. Bai L, Li H, Li J, Song J, Zhou Y, Liu B, et al. Immunosuppressive effect of artemisinin and hydroxychloroquine combination therapy on IgA nephropathy via regulating the differentiation of CD4+ T cell subsets in rats. Int Immunopharmacol. (2019) 70:313–23. doi: 10.1016/j.intimp.2019.02.056
192. Bai L, Li J, Li H, Song J, Zhou Y, Lu R, et al. Renoprotective effects of artemisinin and hydroxychloroquine combination therapy on IgA nephropathy via suppressing NF-κB signaling and NLRP3 inflammasome activation by exosomes in rats. Biochem Pharmacol. (2019) 169:113619. doi: 10.1016/j.bcp.2019.08.021
193. Xia M, Liu D, Tang X, Liu Y, Liu H, Liu Y, et al. Dihydroartemisinin inhibits the proliferation of IgAN mesangial cells through the mTOR signaling pathway. Int Immunopharmacol. (2020) 80:106125. doi: 10.1016/j.intimp.2019.106125
194. Chen Q, Wang Z, Lv J, Liu L, Li H, Sun W, et al. Efficacy and safety of artesunate for patients with IgA nephropathy: a study protocol for a multicenter, double-blind, randomized, placebo-controlled trial. Trials. (2022) 23:444. doi: 10.1186/s13063-022-06336-3
195. Radu AM, Carsote M, Nistor C, Dumitrascu MC, Sandru F. Crossroads between skin and endocrine glands: the interplay of lichen planus with thyroid anomalies. Biomedicines. (2023) 12:77. doi: 10.3390/biomedicines12010077
196. Wang GJ, Gao XY, Wu Y, He HQ, Yu Y, Qin HH, et al. Evaluation of the efficacy and tolerance of artemether emulsion for the treatment of papulopustular rosacea: a randomized pilot study. J Dermatolog Treat. (2019) 30:809–12. doi: 10.1080/09546634.2019.1610549
197. Liu S, He M, Jiang J, Duan X, Chai B, Zhang J, et al. Triggers for the onset and recurrence of psoriasis: a review and update. Cell Commun Signal. (2024) 22:108. doi: 10.1186/s12964-023-01381-0
198. Huang ZZ, Xu Y, Xu M, Shi ZR, Mai SZ, Guo ZX, et al. Artesunate alleviates imiquimod-induced psoriasis-like dermatitis in BALB/c mice. Int Immunopharmacol. (2019) 75:105817. doi: 10.1016/j.intimp.2019.105817
199. Chen Y, Yan Y, Liu H, Qiu F, Liang CL, Zhang Q, et al. Dihydroartemisinin ameliorates psoriatic skin inflammation and its relapse by diminishing CD8+ T-cell memory in wild-type and humanized mice. Theranostics. (2020) 10:10466–82. doi: 10.7150/thno.45211
200. Chen B, Li C, Chang G, Wang H. Dihydroartemisinin targets fibroblast growth factor receptor 1 (FGFR1) to inhibit interleukin 17A (IL-17A)-induced hyperproliferation and inflammation of keratinocytes. Bioengineered. (2022) 13:1530–40. doi: 10.1080/21655979.2021.2021701
201. Chaker L, Cooper DS, Walsh JP, Peeters RP. Hyperthyroidism. Lancet. (2024) 403:768–80. doi: 10.1016/S0140-6736(23)02016-0
202. Vargas-Uricoechea H. Autoimmune thyroid disease and differentiated thyroid carcinoma: A review of the mechanisms that explain an intriguing and exciting relationship. World J Oncol. (2024) 15:14–27. doi: 10.14740/wjon1728
203. Tian Z, Li X, Yu X, Yan S, Sun J, Ma W, et al. The role of primary cilia in thyroid diseases. Front Endocrinol (Lausanne). (2024) 14:1306550. doi: 10.3389/fendo.2023.1306550
204. Liu H, Tian Q, Ai X, Qin Y, Cui Z, Li M, et al. Dihydroartemisinin attenuates autoimmune thyroiditis by inhibiting the CXCR3/PI3K/AKT/NF-κB signaling pathway. Oncotarget. (2017) 8:115028–40. doi: 10.18632/oncotarget.22854
205. Desai J, Steiger S, Anders HJ. Molecular pathophysiology of gout. Trends Mol Med. (2017) 23:756–68. doi: 10.1016/j.molmed.2017.06.005
206. Punzi L, Galozzi P, Luisetto R, Scanu A, Ramonda R, Oliviero F. Gout: one year in review 2023. Clin Exp Rheumatol. (2024) 42:1–9. doi: 10.55563/clinexprheumatol/uhyzcr
207. Jatuworapruk K, Louthrenoo W. Emerging therapeutic options for refractory gout. Nat Rev Rheumatol. (2023). doi: 10.1038/s41584-023-01066-5
208. Kim SK, Choe JY, Park KY. Anti-inflammatory effect of artemisinin on uric acid-induced NLRP3 inflammasome activation through blocking interaction between NLRP3 and NEK7. Biochem Biophys Res Commun. (2019) 517:338–45. doi: 10.1016/j.bbrc.2019.07.087
209. Tu Y. The discovery of artemisinin (qinghaosu) and gifts from Chinese medicine. Nat Med. (2011) 17:1217–20. doi: 10.1038/nm.2471
210. Efferth T. Beyond malaria: the inhibition of viruses by artemisinin-type compounds. Biotechnol Adv. (2018) 36:1730–7. doi: 10.1016/j.biotechadv.2018.01.001
211. Efferth T. From ancient herb to modern drug: artemisia annua and artemisinin for cancer therapy. Semin Cancer Biol. (2017) 46:65–83. doi: 10.1016/j.semcancer.2017.02.009
212. Hou L, Huang H. Immune suppressive properties of artemisinin family drugs. Pharmacol Ther. (2016) 166:123–7. doi: 10.1016/j.pharmthera.2016.07.002
213. Wu X, Zhang W, Shi X, An P, Sun W. Therapeutic effect of artemisinin on lupus nephritis mice and its mechanisms. Acta Biochim Biophys Sin. (2010) 42:916–23. doi: 10.1093/abbs/gmq101
Keywords: artemisinin, autoimmune diseases, autoimmune disorders, artememisinin derivatives, NF-κB pathway, immune modulation
Citation: Long Z, Xiang W, Xiao W, Min Y, Qu F, Zhang B and Zeng L (2024) Advances in the study of artemisinin and its derivatives for the treatment of rheumatic skeletal disorders, autoimmune inflammatory diseases, and autoimmune disorders: a comprehensive review. Front. Immunol. 15:1432625. doi: 10.3389/fimmu.2024.1432625
Received: 14 May 2024; Accepted: 16 September 2024;
Published: 25 October 2024.
Edited by:
Mohamed El-Shazly, Ain Shams University, EgyptReviewed by:
Leigh A. Frame, George Washington University, United StatesRafael Silva, Federal University of Rio de Janeiro, Brazil
Copyright © 2024 Long, Xiang, Xiao, Min, Qu, Zhang and Zeng. This is an open-access article distributed under the terms of the Creative Commons Attribution License (CC BY). The use, distribution or reproduction in other forums is permitted, provided the original author(s) and the copyright owner(s) are credited and that the original publication in this journal is cited, in accordance with accepted academic practice. No use, distribution or reproduction is permitted which does not comply with these terms.
*Correspondence: Zhiyong Long, MjIxMjQ3MTQzOEBxcS5jb20=; Wang Xiang, eGlhbmd3YW5nMTMyQDEyNi5jb20=; Wei Xiao, eGlhb3czMjEyQDEyNi5jb20=; Yu Min, bWluX3l1MTIzQDE2My5jb20=
†These authors have contributed equally to this work