- 1Ragon Institute of Massachusetts General Hospital, Massachusetts Institute of Technology, and Harvard University, Cambridge, MA, United States
- 2Seattle Children’s Research Institute, Center for Global Infectious Disease Research, Seattle, WA, United States
- 3Department of Pediatrics, University of Washington School of Medicine, Seattle, WA, United States
- 4Department of Global Health, University of Washington, Seattle, WA, United States
Tuberculosis (TB) is caused by infection with the bacterial pathogen Mycobacterium tuberculosis (M.tb) in the respiratory tract. There was an estimated 10.6 million people newly diagnosed with TB, and there were approximately 1.3 million deaths caused by TB in 2022. Although the global prevalence of TB has remained high for decades and is an annual leading cause of death attributed to infectious diseases, only one vaccine, Bacillus Calmette–Guérin (BCG), has been approved so far to prevent/attenuate TB disease. Correlates of protection or immunological mechanisms that are needed to control M.tb remain unknown. The protective role of antibodies after BCG vaccination has also remained largely unclear; however, recent studies have provided evidence for their involvement in protection against disease, as biomarkers for the state of infection, and as potential predictors of outcomes. Interestingly, the antibodies generated post-vaccination with BCG are linked to the activation of innate immune cascades, providing further evidence that antibody effector functions are critical for protection against respiratory pathogens such as M.tb. In this review, we aim to provide current knowledge of antibody application in TB diagnosis, prevention, and treatment. Particularly, this review will focus on 1) The role of antibodies in preventing M.tb infections through preventing Mtb adherence to epithelium, antibody-mediated phagocytosis, and antibody-mediated cellular cytotoxicity; 2) The M.tb-directed antibody response generated after vaccination and how humoral profiles with different glycosylation patterns of these antibodies are linked with protection against the disease state; and 3) How antibody-mediated immunity against M.tb can be further explored as early diagnosis biomarkers and different detection methods to combat the global M.tb burden. Broadening the paradigm of differentiated antibody profiling and antibody-based detection during TB disease progression offers new directions for diagnosis, treatment, and preventative strategies. This approach involves linking the aforementioned humoral responses with the disease state, progression, and clearance.
Introduction
Tuberculosis (TB), caused by Mycobacterium tuberculosis (M.tb), is an infectious disease of the respiratory tract. M.tb is a deadly and highly transmissible airborne pathogen that is passed from person to person through inhalation of respiratory secretions containing viable bacilli. Approximately 10.6 million patients were diagnosed as TB-positive and approximately 1.3 million deaths were attributed to TB in 2022 (1) The only licensed vaccine against TB, Bacillus Calmette–Guérin (BCG), which consists of attenuated Mycobacterium bovis, provides a protective effect in newborns and children against disseminated TB but offers less protection against pulmonary TB (2). In a systematic review including 14 reported studies and nearly 3900 children under 16, a 20% reduction in M.tb infection was attributed to BCG vaccination based on an interferon gamma (IFNγ) release assay (3). However, the protection rate may be influenced by infections that occurred prior to vaccination that were either undetectable, asymptomatic, and/or cleared. Due to the global health concern posed by TB and the lack of effective vaccines providing long-term protection against M.tb infection, novel methods for diagnosis, prevention, and therapy for TB are urgently needed.
M.tb infection occurs through the respiratory route, typically targeting the alveolar space of the lungs where alveolar macrophages recognize and engulf M.tb (4, 5). The infected alveolar macrophages provide a shelter for M.tb that shields them from extracellular antibodies and complement proteins, which could facilitate bacterial removal. Within 2-8 weeks of initial infection, if M.tb survives the formation of phagolysosomes, which is common in M.tb infection, it undergoes exponential replication and potentially infects other cells such as alveolar epithelium, endothelium, and other leukocytes present in the mucosa such as neutrophils and dendritic cells (5–8). Granulomas, aggregates of these immune cells, are the hallmark of TB and serve as a reservoir of M.tb infection. Mucosal antibodies can theoretically directly block M.tb binding to epithelial cells, while other functional antibody-mediated immune responses can occur in either serum or mucosal sites. These functional antibody responses, including antibody-dependent cellular phagocytosis (ADCP), neutrophil phagocytosis (ADNP), complement deposition (ADCD), and cellular cytotoxicity (ADCC), can play significant roles in identifying and killing infected cells, and help to clear extracellular M.tb, all of which provide additional host immune mechanisms contributing to killing the bacteria at the site of infection preventing dissemination (Figure 1).
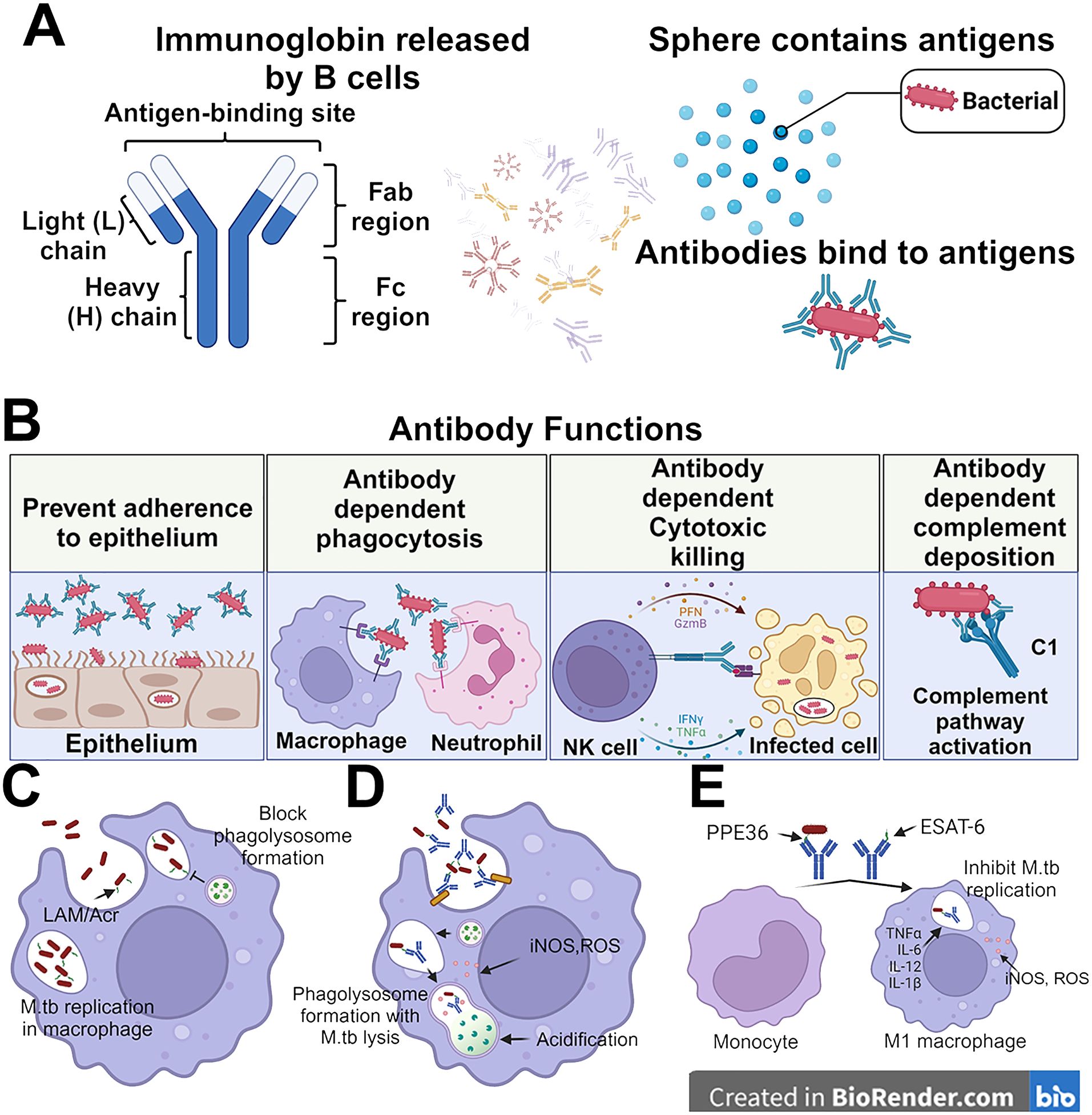
Figure 1. Protective roles for antibodies in M.tb control. (A) Antibodies consist of a Fab region which directly binds to target antigens and an Fc region which binds to cell-surfaced expressed receptors. The antibodies can bind to the surface of antigens and mediate different immunity functions. (B) Antibodies can leverage both their Fab and Fc domains to protect against M.tb. This can be done through direct binding of the bacteria to prevent adherence to the epithelium, opsinophagocytosis, cellular cytotoxicity activation, and complement deposition. (C) The M.tb not bound by the antibodies are able to replicate in the macrophages and inhibit the formation of phagolysosome. (D) while the antibody recognition helps the macrophage to lysis the M.tb via lysosome. (E) The monocytes also can be differentiated by the anti-PPE36 and anti-ESAT-6 antibodies into M1-polarized macrophages which release inflammatory cytokines to inhibit the self-replication of M.tb in the infected macrophage.
After decades of investigation, the immune responses and infection process of M.tb have been studied, yet the role of antibodies in preventing infection or disease development remains unclear. One of the challenges that has impeded progress in this area is the variable antibody profiling among TB cohorts, further complicated by the diversity of antibody profiles generated (9–13). We previously demonstrated a positive correlation between IgM, IgA, and IgG levels against LAM, Apa, and PstS1 in serum or bronchoalveolar lavage fluid (BALF) and a decreased M.tb infection rate in non-human primates (NHP) after BCG vaccination (14). Additionally, we found that FcγR binding was higher and ADCC was more activated in patients with latent TB infection (LTBI) compared to those with active TB (ATB). Here, IgG from LTBI patients limited more M.tb surviving in macrophages than IgG from ATB patients (13). These findings suggest that a boosted humoral antibody profile could prevent M.tb infection from its onset, and antibody-mediated functions were critical in controlling the LTBI to ATBI switch in people infected with M.tb.
Advancements in understanding the role of antibodies in preventing M.tb infections, TB development, and the diagnosis of M.tb infections are crucial for developing new clinical methods to prevent the spread of M.tb and the progression of TB disease. Antibodies that protect against M.tb infection could serve as potential standards for vaccine development, while antibody-mediated immunity triggered by M.tb infection could be leveraged for post-infection treatment. Furthermore, antibodies present in the early stages of infection could serve as biomarkers to (i) improve M.tb detection, (ii) classify the disease state, and (iii) predict responses to treatment. Several antibody responses correlated with M.tb infection or after M.tb vaccination, such as opsonophagocytic clearance (ADCP, ADNP), ADCC, and the functional diversity of antibodies against M.tb are discussed (15, 16). In this review, we expand on these concepts and provide a current overview of antibody profiling and its functions in preventing TB development as well as its potential as a diagnostic marker to limit the transmission of M.tb in TB-endemic regions.
Antibody maturation
Encounters with an antigen begin the process of antibody development and affinity maturation. A B cell receptor (BCR) can bind to an antigen and then signal for its presentation in the form of cleaved peptides to T cells through the major histocompatibility complex 2 (MHC II) on the cell surface. From there, the T cell stimulates B cell activation and proliferation through the interaction of CD40 ligand (CD40L) with CD40 on the B cell surface along with the secretion of various interleukins (ILs) such as IL-2 and IL-5. At this point, the B cell can begin the evolutionary marvel of somatic hypermutation (SHM) (17–19). The Fab domain of an antibody mediates its attachment to an antigen (Figure 1A), which is encoded within the heavy (IgH) and light (IgL) chains of the immunoglobulin genes. After stimulated expression of IgH and IgL, the B cell undergoes SHM to diversify the Fab’s binding pocket and generate immunoglobins targeting specific antigens. The B cells that produce low-affinity immunoglobin undergo apoptosis (20).
Once an antibody recognizes its targeted antigen, it can mediate a number of downstream processes. One of the most recognized functions is neutralization. Antibodies can directly bind to a region of a pathogen or toxin that is used for cellular entry (Figure 1B). While neutralizing antibodies typically target the receptor binding domain (RBD) of pathogen proteins and/or pathogen toxins, they can still exert neutralization at other sites. The primary mechanism of protection by neutralizing antibodies has long been proposed to be through direct steric hindrance of the RBD with its receptor. However, there are clearly other mechanisms by which neutralizing antibodies can inhibit cell entry of a toxin or whole organism/virus into a cell. Currently, no direct evidence proves that neutralizing antibodies exist in preventing M.tb infection.
Antibody-mediated functions and Mycobacterium tuberculosis infection
Prevention of adherence to lung epithelium
The abundance and repertoire of antibodies in the mucosa can be distinct from the serum profile within the same individual. The maturation of tissue-resident plasma cells to produce the specific antibody against M.tb antigens is in parallel with the progression of infection, which is common across most infectious diseases (21, 22). The specific mechanisms of antibody subclasses in preventing M.tb adherence to the mucosal surface may also depend on compartmentalization. Currently, although antibodies may play a role in defense against M.tb, there are no known mycobacteria-specific antibodies that can neutralize M.tb or inactivate M.tb extracellularly on their own which results in full sterilizing immunity. Immune responses to M.tb instead likely require many different cell types, representing both cellular and humoral immunity (for a review, see (23)). In BCG-immunized individuals, antibodies directed toward shared antigens should theoretically be able to inhibit or contribute to the prevention of infection. Antibodies specific for heparin-binding hemagglutinin (HBHA) as well as lipoarabinomannan (LAM), both of which are M.tb virulence factors on the surface of the bacilli, show some promise in host defense against M.tb infection. HBHA IgA antibodies at the mucosa, for example, can block M.tb infection of lung epithelial cells (9) which in turn can help prevent dissemination of M.tb from the lungs to other organs. Interestingly, anti-HBHA IgG antibodies were shown to have the opposite effect, instead facilitating M.tb infection (9). Considering the infection route and site of M.tb, mucosal antibodies would be more likely prominent players in preventing the infection state than antibodies circulating in serum. IgA is the most abundant immunoglobins at this interface, although this antibody can also be detected in serum as well (24–26). IgA, specific to several M.tb antigens, can be observed in the pleural fluid in pleural TB patients (27). Nasal-resident IgA also showed a protective effect in inhibiting the M.tb growth and infection with the necessary activation of FcαR (22). In addition to the effective role of IgA, relevant lung epithelial cells express Fc receptors that primarily interact with IgG, providing another potential layer of humoral immunity at this site of M.tb infection.
Opsonophagocytosis
Intracellular growth inhibition of M.tb following opsonophagocytosis, a process involving antibody-directed phagocytosis by macrophages and neutrophils (24, 26, 28–31), utilizing serum from BCG-immunized humans, has shown a potential protective role for arabinomannan-specific antibodies (32). Furthermore, the opsonophagocytosis process of killed M.tb in the presence of anti-LAM and anti-Acr (a major 16-kD a-crystallin membrane protein (33) donor-derived antibodies also results in phagosomal maturation of M.tb infected macrophages, leading to a cascade of host-mediated microbicidal responses such as the production of nitric oxide and acidification (34) (Figure 1B). Our group is currently studying the effects of adjuvants on M.tb vaccine-induced functional antibody responses against M.tb infection including opsonophagocytosis. Generating optimal antibody responses in the context of an immunotherapeutic M.tb vaccine, as an adjunct to drug treatment, could provide additional protection against persistent or recurrent M.tb (24, 26, 28–31).
For IgG subclasses, the Fc-domain can bind to FcγRs on the surface of phagocytic cells. Pro-phagocytic FcγRs include the high-affinity FcγRI (CD64) and the low-affinity FcγRIIA and FcγRIIIB (CD32a and CD16b, respectively). Phagocytosis can also occur through the recognition of complement deposited on an antigen through Fc-C1q. This deposition of complement on an antigen, or on the antibody itself bound to the antigen, can flag the complex for engulfment by phagocytes with complement receptors on their cell surface. Opsonophagocytic antibodies have been linked to protection against other intracellular bacteria as well as M.tb, including Staphylococcus aureus, Escherichia coli, Salmonella, and Shigella (35–37).
Regarding opsonophagocytosis, antibody-mediated phagocytosis is followed by phagolysosome fusion to digest antibody-bound complexes/microorganisms. This is enhanced through intracellular FcγR signaling activation (38). Interestingly, it is known that M.tb can inhibit the phagosome-lysosome fusion via a reduced Ca2+ pathway. Thus the bacteria can be engulfed by phagocytic cells such as macrophages, but are not killed through canonical opsonophagocytosis signaling (39). This phenomenon was shown to be antigen-specific, for example in the case of M.tb, anti-LAM mediated phagocytosis presented a higher rate of M.tb killing (39). Macrophage activation, especially M1 polarization, occurs during M.tb acute infection, initiates inflammatory cytokine release, and generates oxidative species to eliminate both the intracellular and local extracellular M.tb (40). The M.tb surface protein PPE36 inhibited the M1 polarization and reduced the inflammatory cytokine production from macrophages, which enhanced the survival of M.tb (41, 42). In TB patients, the levels of IgA against PPE36 were found to be significantly higher compared to healthy control, while less elevation was noticed in either IgG or IgM (43). As mentioned above, IgA is the most dominant antibody at the site of M.tb infection, and IgA against PPE36 could inactivate the function of PPE36 and activate the M1 polarization. Another surface protein, Rv1507A, promoted the polarization of M1 macrophages, enhanced the proinflammatory cytokine release of IFN-γ and tumor necrosis factor alpha (TNF-α), and upregulated the macrophage-driven phagocytosis (44). The secreted antigen ESAT-6 also can activate the M1 macrophage polarization, and anti-ESAT-6 immunoglobin helps to inhibit the proliferation of M.tb in the infected macrophages (45–47). Taken together, antibodies targeting either M.tb surface or secreted antigens may augment their immune-evading roles, and shift the humoral response to a more functional opsonophagocytic nature against M.tb. Antibody responses to specific antigens after administration of vaccines that developed against M.tb should be further evaluated to make sure specific categories of antibodies can mediate efficient phagolysosome formation and intracellular removal of M.tb.
In addition to macrophages, neutrophils are also recruited to the site of infection by the released cytokine and chemokines. These neutrophils produce hypochlorous acid, proteases, and cytokines, and can create neutrophil extracellular traps as well as mediate phagocytosis to eliminate extracellular M.tb (48). Our previous study reported that BCG-vaccinated NHP exhibited a higher level of antibody-dependent neutrophil phagocytosis facilitated by anti-LAM immunoglobulin (14). Another study showed that IgG from LTBI patients reduced M.tb burden in infected macrophages more effectively compared to the IgG from ATB patients. On the other hand, ADCP and ADNP were more triggered by antibodies from ATB patients compared to LTBI patients (13). M.tb can block the formation of the phagolysosome which makes the macrophages an ideal environment for M.tb replication and further infection. However, there is limited research on antibody-mediated neutrophil-driven M.tb removal. Understanding how specific antibodies engage neutrophils for phagocytosis and whether phagolysosome formation occurs within these innate cells in the alveoli remains largely unexplored.
Cellular cytotoxicity killing and natural killer cell activation
Natural killer cells (NK) are major innate lymphoid cells that play a similar role to CD8+ cytotoxic T cells. Unlike their CD8+T cell counterparts, however, NK cells do not express the T cell receptor (TCR) on their surface and can be thought of as more of a pan-cellular innate surveillance cell.
NK cells are activated by antibodies through binding of the FcγRIIIA (CD16a) on the surface (Figure 1B). Studies have shown that this binding is correlated with the afucosylation of the Fc-domain in IgG subclasses, predominantly IgG1 and IgG3 (49–52). Downstream activation signals funnel into the secretion of IFN-γ, cellular degranulation, and secretion of other inflammatory/recruiting cytokines such as TNF-α, macrophage inflammatory protein-1β (MIP-1β), and a host of interleukins (IL) such as IL-2, IL-12, and IL-18. The combined result of activation of these signaling networks results in ADCC by the NK cells.
ADCC has been shown to play an instrumental role in combating a wide range of infectious agents including viruses, intracellular bacteria, and protozoa (53–55). The coupled degranulation and recruitment of other inflammatory cells through cytokines allow for the rapid clearance of a localized infection. This same model can be translated to a growing mass of tumor cells whereby ADCC acts to wipe out a growing malignancy (56).
The role of NK cells in protecting against M.tb infection is yet to be fully investigated. Canonical mechanism(s) of elimination of M.tb by NK cells included releasing cytotoxic chemicals (perforin and granulysin) after binding to the bacterium and interacting with macrophages or neutrophils to remove the M.tb indirectly (57, 58). The surface receptors of NK cells, NKp46 and NKp44, can be activated by the M.tb surface antigens galactan and peptidoglycan, and initiate the direct killing of M.tb by NK cells (57, 59). Unlike the opsonized phagocytosis via macrophages and neutrophils, NK cells work differently when interacting with antibody-dependent mechanisms. Directly recognized M.tb antigen by the NKp44-Fc region activates the NK cells, and BCG vaccination enhances the surface expression of NKp44 on NK cells (59). NK cells also recognize and lyse the M.tb-infected macrophages via NKp46 (60).
In terms of antibody-mediated NK cell activation, Irvine et al. showed that the serum antibody targeting LAM in BCG-vaccinated NHP presented limited NK cell activation, while the antibody targeting LAM in lavage fluid showed activation of NK cells (14). The enrichment of activated NK cells in the mucosa can later recognize either the extracellular M.tb or M.tb infected monocytes via NKp44 or TLR‐2 to the peptidoglycan axis (59, 61). Abundance and maturation of NK cells within the pleural fluid are thought to be low, so many of these assays to quantify antibody-dependent effects are in artificial systems (14). That said, there is a growing body of literature implicating the role of NK cells in controlling and removing M.tb within the lower respiratory tract. Whereas some anti-M.tb immunoglobins are able to activate NK cells, including antibodies from the serum of humans immunized with ID93+GLA-SE (62),_further investigation is needed to understand the direct antibody-mediated mechanisms involved in the recognition and elimination of M.tb.
Antibodies and Mycobacterium tuberculosis vaccines
Antibody profiling and M.tb vaccine development
The role of antibodies in the control of M.tb has been historically controversial. The BCG vaccine has been licensed and deployed to protect against TB. While efficacy has been strong against severe TB in children and against pulmonary TB in low-endemicity regions, there is low protection against pulmonary TB in TB endemic countries. Moreover, BCG vaccination appears to offer low effectiveness in blunting onward transmission of M.tb (15, 63). The mechanism of protection against TB had been proposed to be mediated through cellular immunity, namely CD8+ and CD4+ T cells. This model was built up in large part due to the intracellular nature of M.tb and not in a cell-free state of the pathogen such as seen with respiratory viruses. However, as our appreciation for the non-neutralizing roles of antibodies has grown, we have had to revisit this long-standing dogma that cellular immunity is the sole means of controlling intracellular pathogens such as M.tb.
Several independent studies have shown that TB vaccines elicit antibody responses that correlate with protection. This includes the M72/AS01E vaccine that protects against disease progression in healthy IFN-γ release assays (IGRA)-positive M.tb exposed individuals (64, 65), pulmonary-delivered BCG in rhesus macaques (66), intravenous-delivered BCG (14), and arabinomannan-protein M.tb Ag85 conjugate vaccine (67). The ID93 vaccine candidate (made up of a fusion of 4 M.tb proteins: Rv2608, Rv3620, Rv1813, and Rv3619) administered with the TLR-4 agonist (GLA-SE), was shown to enhance vaccine-specific IgG1 and IgG3 antibody titers, NK activation, and opsonophagocytosis, in addition to an increased CD4+ T helper 1 (Th1) response (defined by IFN-γ, TNF-α, and IL-2 production from ID93-specific CD4+ T cells) (62). To that end, there is an emerging model in the field of M.tb vaccinations that cellular immunity is not solely responsible for protection against TB; similarly, antibody-mediated protection against TB is not functioning in a vacuum. Instead, both cellular and humoral immunity appear to be working in concert to limit the TB disease state (62).
The protective role of antibodies in TB disease progression has also been supported through the transfer of antibodies from an infected or vaccinated donor. In mice, high-dose IVIG attenuated bacterial growth in the lungs. This protection was lost in athymic mice, further supporting a model where antibodies and cellular immunity work in concert to limit pathogen spread and disease progression (68). Subsequent studies demonstrated that antibodies taken from individuals with high occupational exposure to M.tb could protect against aerosolized challenged mice. The antibodies were reactive towards surface-expressed M.tb antigens, and again, the protection offered by antibodies was dependent on the presence of T cells (69). Mechanistically, the glycosylation status of the Fc domain of the antibody appeared to be linked to bacterial killing. Moreover, the state of TB (active vs. latent) strongly influences the antibody response and activity (13). In ATB patients, higher levels of IgG1 to LAM and PPD, IgA1 against PPD, and IgG3 against groES were identified compared to LTBI patients (70).
Antibody glycosylation
Antibody glycosylation including fucosylation, galactosylation digalactosylation, and sialylation, has been used as biomarkers for differentiating between LTBI and ATB (13) (Figure 2). In one study, lower levels of fucose were observed in IgG from LTBI compared to ATB patients (71). Fucosylation/afucosulation status has been shown to be linked to FcγRIIIA binding and downstream signaling (49). Additionally, LTBI patients exhibited increased levels of di-galactosylation and sialylation, along with lower agalactosylation on IgG compared to ATB patients, indicating a heightened inflammatory state in ATB (13, 72–74). Specifically, glycosylation was predominantly observed in Fc regions rather than Fab regions and showed antigen specificity towards PPD and Ag85A (75). Furthermore, differentiated antibody glycosylation distinguishes not only between ATB and LTBI populations, but also identifies differences between ATB and treated ATB groups. Increased sialylation was found in treated ATB patients compared to untreated subjects, while no difference was observed regardless of the treatment (72). Despite extensive research into glycosylated antibodies in various M.tb infection cohorts, little work has been done to characterize the glycosylation status of antibodies following BCG vaccination, the only currently licensed vaccine (76). Notably, antibody glycosylation was only identified after M.tb infection in mice, indicating its potential as a marker of infection (76). Distinct glycosylated antibodies induced by M.tb infections could potentially aid in identifying infected macrophages and promoting phagocytosis or ADCC in cell-surfaced exposed antigens, whereas differentiated glycan removal may attenuate this process (13). RNA/DNA nucleic acid-derived M.tb vaccines expressing proteins that undergo glycosylation within the host cell may detrimentally provide ‘self-glycans’ on the protein masking the epitope needed for lymphocyte recognition and acquired immunity against M.tb (both humoral and cellular), whereas the mycobacterial-derived protein combined with an adjuvant may provide a more robust immune response (77).
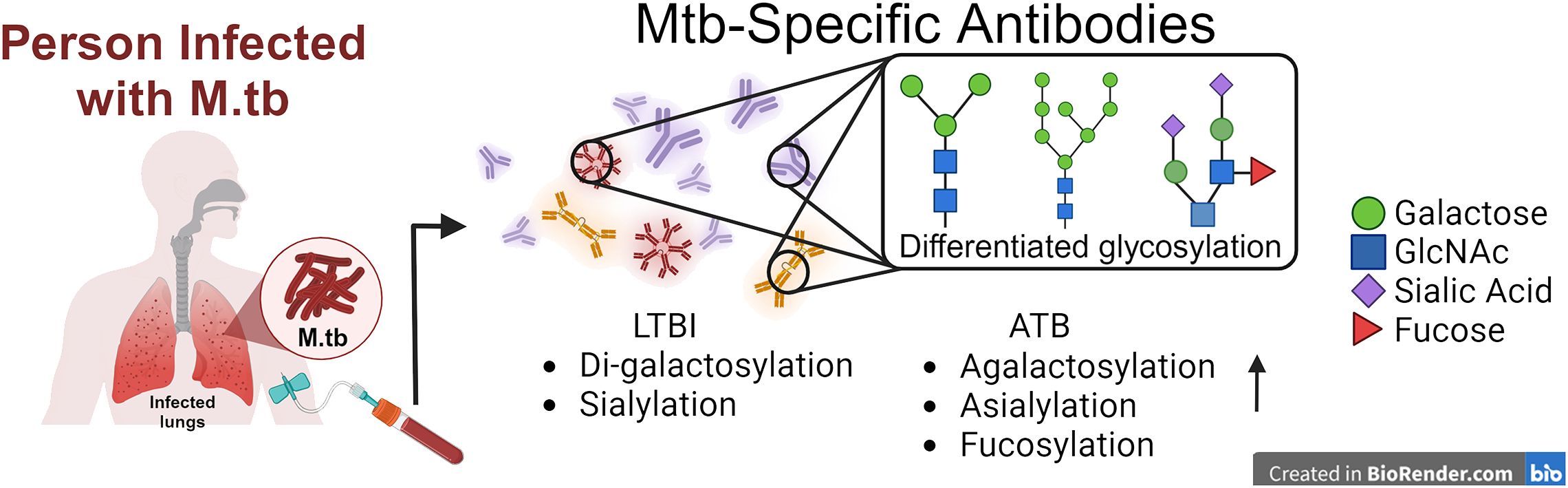
Figure 2. Translating antibody-based readouts novel vaccine platforms. Composite profiling of the total humoral profile can be investigated in the future with differentiated glycosylation signatures in favor of future vaccine designs against TB.
Antibodies as biomarkers for M.tb disease state
M.tb poses formidable challenges due to its ability to establish latent infection, leading to active disease in susceptible individuals (78). Timely and precise identification of TB plays a pivotal role in the efficient handling and containment of the disease. In recent years, there has been a growing interest in utilizing antibodies as biomarkers for assessing the disease state of TB, offering new avenues for improved diagnosis, treatment monitoring, and patient management (13, 72, 75).
Antibodies as biomarkers to M.tb infection
Upon infection with M.tb, the host immune system mounts a complex response involving various immune cells and immune signaling moieties, including antibodies. It is well established that B cells produce antibodies in response to specific antigens derived from M.tb (79, 80). As mentioned before, antibody treatment significantly decreased the bacterial burden in mice infected with M.tb (81), and IgM responses to BCG were strongly linked with suppressed M.tb survival in vivo (14). Studies have also highlighted distinctions in the antibody structure between ATB and LTBI in clinical serum samples (75). This distinction in antibody features in different disease states could be an important biomarker for TB.
Biomarkers are measurable indicators of biological processes, disease states, or responses to therapeutic interventions. In the context of TB, biomarkers represent a significant avenue for enhancing diagnostic precision, evaluating treatment effectiveness, and predicting disease outcomes. Antibodies produced in response to M.tb infection offer valuable insights as biomarkers owing to their specificity and fluctuating levels across the progression of the infection. However, the application of serological tests in TB diagnosis remains debatable due to the poor sensitivity and specificity compared to existing methods (82–84). Ongoing work seeking to standardize antibody signatures as biomarkers for TB will be highly beneficial.
To date, individual antibodies, or a clustered signature of antibodies against different antigens have both been studied with varied sensitivity and specificity in identifying ATB patients. Several studies have been done to investigate the use of antibodies as biomarkers for identifying TB infection status, as summarized in the tabular form (Table 1). The use of single antibody readouts and multivariate analyses have been reported with various sensitivity and specificity readouts as biomarkers. One study using anti-PPE17 IgG showed ~70% sensitivity in identifying an LTBI cohort from non-TB patients (85). IgG and IgA to different M.tb antigens consist of the majority of proposed biomarker candidates in TB diagnosis (80, 83, 87, 106, 107 (86, 87),. Antibody responses to M.tb antigens, specifically IgG against TBCM and CFP-10, and IgG and IgA against Ag85B, can effectively distinguish between active tuberculosis (ATB), latent tuberculosis infection (LTBI), and non-infected individuals, providing a potential biomarker for M.tb infection (86). IgG and IgA levels against the Rv2031 antigen significantly differ among patients with active TB, their household contacts, and non-infected controls (87). An interesting marker for ongoing inflammation and severity of TB disease is IgG4 in humans. In a study including healthy, LTBI, ATB, and treated ATB cohorts, PPD-specific IgG4 as well as HspX and GroEs-specific IgG4 levels were increased in ATB, and lower levels were associated with either LTBI or treated subjects (72). Despite being linked to M.tb control (14), there is limited study on IgM as a biomarker for M.tb infection. This could be due to the inherently low affinity of IgM compared to the affinity selected IgG and IgA (88). Considering the appearance duration of IgM in the humoral antibody profile throughout the infection, IgM may serve as biomarkers for an early, albeit lower-confidence, detection method of M.tb infection. Both IgG and IgA exist both in serum and mucosa and are more likely to play crucial roles in defending against M.tb re-infection or ATB development from LTBI (89). Additionally, it is well known that affinity-matured antibody responses to M.tb are stronger to select antigens such as those on the bacterial cell surface (4, 12, 13, 27, 64–66, 70, 80, 83, 90, 91). Therefore, a panel of IgA and IgG against multiple M.tb antigens could be considered as biomarker candidates to monitor the development of ATB from LTBI patients in TB endemic regions, which the IGRA test cannot identify (86). Figure 3 demonstrates how the immunoglobulins against antigens could be utilized not only for the detection of active TB but also for distinguishing between ATB and LTBI.
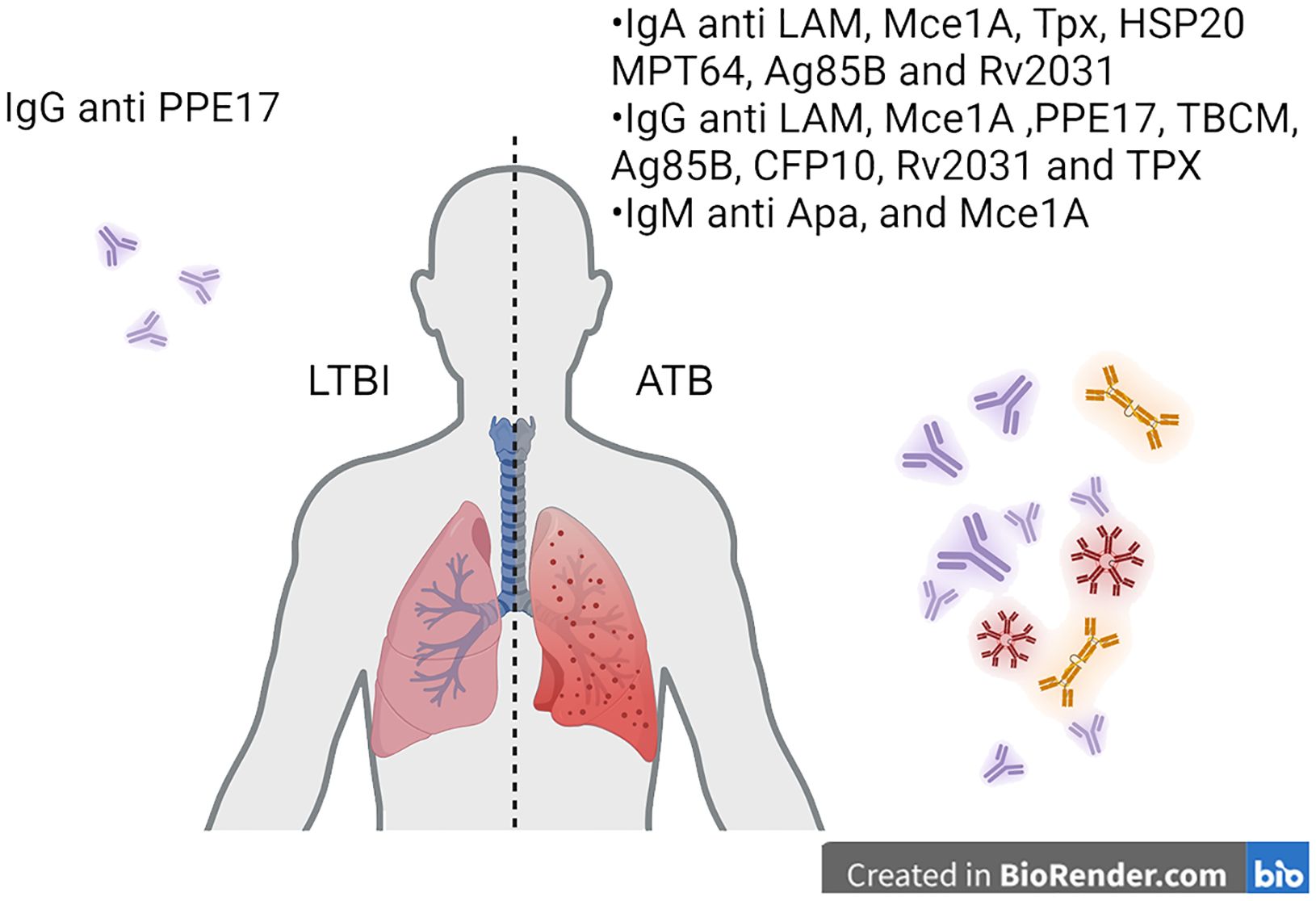
Figure 3. Translating antibody-based readouts to biomarkers of disease states. Antibodies specific to M.tb targets can be analyzed at the isotype and subclass level. Additionally, post-translational modifications can be identified on antigen-specific antibodies to identify signatures of disease states such as latency and active TB.
Detection methods
Several methods are available for detecting M.tb-specific antibodies in patient samples, each with its advantages and limitations. Enzyme-linked immunosorbent assay (ELISA) is a widely used technique that allows for the quantitative measurement of antibody levels in serum, plasma, or other bodily fluids (90, 92, 93). ELISA-based assays offer high sensitivity and specificity and are amenable to high-throughput screening (94). Other approaches, such as multiplex immunoassays (95) and lateral flow assays (96–98), provide rapid and cost-effective alternatives suitable for point-of-care settings where access to laboratory facilities may be limited. Since a combination of M.tb-specific antibodies as biomarkers to evaluate the M.tb infections showed higher sensitivity and specificity than applying individual antibody approaches (99), developing high-throughput methods with multiplexed measurements may not only be more accurate, but also more cost-effective as disease incidence and/or progression biomarkers due to the long experimental duration and high cost of ELISA in detecting individual antibody isotypes/subclasses to discreet antigens, and their body fluid concentration(s). Other than ELISA and Luminex-based measurement, antibody-antigen binding activity measured by surface plasmon resonance (SPR) and total internal reflectance fluorescence (TIRF) microscopy-based biosensors (100, 101) have also been studied. While these technologies are emerging and have unique strengths, they can be limited to the detection of a single antigen or a few antigens at a time. Super-resolution microscopy-based detection methods have also been employed for M.tb protein expression and detection (102). Such a tool, which can utilize antibodies as a detector, has been employed to identify M.tb responses to drug treatments (103).
Clinical utility
The use of antibodies as biomarkers for TB offers several advantages over traditional diagnostic methods. Antibody-based tests are non-invasive and can be performed on readily accessible samples, such as blood, saliva, or urine, making them suitable for use in diverse settings, including resource-limited areas (95, 96, 104). Moreover, antibody tests have the potential to detect TB infection at an earlier stage than conventional microbiological methods, enabling prompt initiation of treatment and reducing the risk of disease transmission.
Furthermore, longitudinal monitoring of antibody levels can provide valuable insights into treatment response and disease progression. Changes in antibody titers over time may serve as indicators of treatment efficacy, allowing clinicians to tailor therapy regimens and optimize patient care. Additionally, antibody-based biomarkers hold promise for predicting treatment outcomes and identifying individuals at increased risk of disease relapse, thereby informing clinical decision-making, and improving patient outcomes. Biomarkers and antibody signatures of disease/infection are an emerging field of study that warrants further research in the areas of vaccine design, antibody-based therapies, and disease states.
Challenges and future directions
Although studies have been conducted to understand the antibody differentiation during M.tb infection and TB development, limited vaccines have been developed to protect the adults who are exposed to M.tb, and there is still a knowledge gap in how antibody-mediated immunity controls M.tb infections. The discovery of dynamic antibody profiling at different stages of TB progression and M.tb infection is necessary to design new M.tb vaccines with better efficacy of M.tb removal after infection. Considering the principle of how NK cells participate in eliminating M.tb infected cells is not clear, further investigations are needed to understand the role of antibody-mediated NK cell activation in protecting against M.tb infection and TB development.
Despite their potential, antibody-based biomarkers for TB face several challenges that must be addressed to realize their full clinical utility. Studies show that recognition of M.tb antigens may vary among individuals, leading to the presence of different sets of antigen-specific antibody populations in samples (10), while cross-reactivity among different mycobacterial and nontuberculous mycobacterial species is also observed (105, 106). Variability in antibody responses among individuals, as well as cross-reactivity with other mycobacterial species or non-specific immune responses, may affect the accuracy of antibody-based assays. Standardization of assay protocols, validation in diverse populations, and integration into existing diagnostic algorithms are essential steps toward overcoming these challenges and ensuring the reliability and reproducibility of antibody-based tests for TB.
Furthermore, ongoing research efforts are focused on identifying novel antigen targets and developing innovative assay platforms capable of detecting multiple antibody specificities simultaneously (107). Additionally, the incorporation of antibody-based biomarkers into comprehensive diagnostic strategies, including imaging modalities and nucleic acid amplification tests, holds promise for enhancing the accuracy and efficiency of TB diagnosis and management.
Employment of therapeutic antibodies against TB is an emerging field, as is how to fine-tune humoral responses through vaccination platforms. It is known that vaccine platform and delivery route influence how antibodies leverage both Fab and Fc regions (14, 25, 88, 108–111). Given the emerging role of antibodies as mediators of protection against M.tb, further work characterizing precise mechanisms of protection at various stages within TB disease progress is needed. Such advances can reduce disease incidence and burden.
In conclusion, antibodies stand as hopeful treatments for disease and indicators for evaluating the progression of TB, presenting fresh avenues for enhanced diagnosis, treatment tracking, and patient care. Their unique specificity, ease of access, and fluctuating patterns over the infection timeline render them invaluable instruments for early TB detection, treatment progress monitoring, and prognosis forecasting (Figure 2). Through ongoing exploration and creativity, antibody-centric examinations hold the promise to transform TB diagnostics and bolster worldwide endeavors to reduce disease burden.
Concluding remarks
Antibody responses are not limited to pathogens that exist and/or circulate extracellularly. Moreover, antibody functions often bridge the adaptive and innate arms of the immune response. For example, an affinity-matured IgG or IgA isotype can bind to a target antigen and use its Fc domain to interact with FcRs on the surface of innate immune cells such as NK cells or neutrophils, and initiate inflammatory signaling cascades. This signaling can further recruit additional inflammatory cells to the site of the identified pathogen, helping to clear the infection.
Studies of antibody responses to M.tb have provided significant insight into how antibodies mobilize multiple aspects of the immune system to combat this pathogen. M.tb predominantly resides inside of cells which become constituents of granulomas in the lower respiratory tract. From there, the infection can spread to distant sites in the body including the spleen, kidneys, and brain (112). Antibody responses are not stagnant over time, much like disease states. Due to their adaptability, antibody signatures have been successfully used as biomarkers of disease. This approach has been successfully employed to distinguish between latent and active TB, further supporting the model of humoral fluidity.
Author contributions
QW: Writing – review & editing, Writing – original draft, Visualization. DN: Writing – review & editing, Writing – original draft, Visualization. SB: Writing – review & editing. RC: Writing – review & editing, Supervision, Resources, Funding acquisition, Conceptualization. RM: Writing – review & editing, Writing – original draft, Supervision, Resources, Funding acquisition, Conceptualization.
Funding
The author(s) declare that financial support was received for the research, authorship, and/or publication of this article. This project has been funded in whole or in part with Federal funds from the National Institute of Allergy and Infectious Diseases, National Institutes of Health, Department of Health and Human Services, under Contract No. 75N93021C00029, and additional funding from Seattle Tuberculosis Research Advancement Center under award P30 AI168034 (to RNC).
Conflict of interest
The authors declare that the research was conducted in the absence of any commercial or financial relationships that could be construed as a potential conflict of interest.
The author(s) declared that they were an editorial board member of Frontiers, at the time of submission. This had no impact on the peer review process and the final decision.
Publisher’s note
All claims expressed in this article are solely those of the authors and do not necessarily represent those of their affiliated organizations, or those of the publisher, the editors and the reviewers. Any product that may be evaluated in this article, or claim that may be made by its manufacturer, is not guaranteed or endorsed by the publisher.
References
2. Mangtani P, Abubakar I, Ariti C, Beynon R, Pimpin L, Fine PE, et al. Protection by BCG vaccine against tuberculosis: a systematic review of randomized controlled trials. Clin Infect Dis. (2014) 58(4):470–80. doi: 10.1093/cid/cit790
3. Roy A, Eisenhut M, Harris RJ, Rodrigues LC, Sridhar S, Habermann S, et al. Effect of BCG vaccination against Mycobacterium tuberculosis infection in children: systematic review and meta-analysis. BMJ. (2014) 349:g4643. doi: 10.1136/bmj.g4643
4. Delogu G, Sali M, Fadda G. The biology of mycobacterium tuberculosis infection. Mediterr J Hematol Infect Dis. (2013) 5(1):e2013070. doi: 10.4084/mjhid.2013.070
5. Haque MF, Boonhok R, Prammananan T, Chaiprasert A, Utaisincharoen P, Sattabongkot J, et al. Resistance to cellular autophagy by Mycobacterium tuberculosis Beijing strains. Innate Immun. (2015) 21:746–58. doi: 10.1177/1753425915594245
6. Lerner TR, Borel S, Greenwood DJ, Repnik U, Russell MR, Herbst S, et al. replicates within necrotic human macrophages. J Cell Biol. (2017) 216(3):583–94. doi: 10.1083/jcb.201603040
7. Huang L, Nazarova EV, Tan S, Liu Y, Russell DG. Growth of. J Exp Med. (2018) 215(4):1135–52. doi: 10.1084/jem.20172020
8. Toniolo C, Dhar N, McKinney JD. Uptake-independent killing of macrophages by extracellular Mycobacterium tuberculosis aggregates. EMBO J. (2023) 42(9):e113490. doi: 10.15252/embj.2023113490
9. Zimmermann N, Thormann V, Hu B, Köhler AB, Imai-Matsushima A, Locht C, et al. Human isotype-dependent inhibitory antibody responses against Mycobacterium tuberculosis. EMBO Mol Med. (2016) 8(11):1325–39. doi: 10.15252/emmm.201606330
10. Lyashchenko K, Colangeli R, Houde M, Al Jahdali H, Menzies D, Gennaro ML. Heterogeneous antibody responses in tuberculosis. Infect Immun. (1998) 66(8):3936–40. doi: 10.1128/IAI.66.8.3936-3940.1998
11. Mao L, LaCourse SM, Kim S, Liu C, Ning B, Bao D, et al. Evaluation of a serum-based antigen test for tuberculosis in HIV-exposed infants: a diagnostic accuracy study. BMC Med. (2021) 19(1):113. doi: 10.1186/s12916-021-01983-w
12. McIntyre S, Warner J, Rush C, Vanderven HA. Antibodies as clinical tools for tuberculosis. Front Immunol. (2023) 14:1278947. doi: 10.3389/fimmu.2023.1278947
13. Lu LL, Chung AW, Rosebrock TR, Ghebremichael M, Yu WH, Grace PS, et al. A functional role for antibodies in tuberculosis. Cell. (2016) 167(2):433–443.e14. doi: 10.1016/j.cell.2016.08.072
14. Irvine EB, O'Neil A, Darrah PA, Shin S, Choudhary A, Li W, et al. Robust IgM responses following intravenous vaccination with Bacille Calmette-Guérin associate with prevention of Mycobacterium tuberculosis infection in macaques. Nat Immunol. (2021) 22(12):1515–23. doi: 10.1038/s41590-021-01066-1
15. Kawahara JY, Irvine EB, Alter G. A case for antibodies as mechanistic correlates of immunity in tuberculosis. Front Immunol. (2019) 10:996. doi: 10.3389/fimmu.2019.00996
16. Carpenter SM, Lu LL. Leveraging antibody, B cell and fc receptor interactions to understand heterogeneous immune responses in tuberculosis. Front Immunol. (2022) 13:830482. doi: 10.3389/fimmu.2022.830482
17. Di Noia JM, Neuberger MS. Molecular mechanisms of antibody somatic hypermutation. Annu Rev Biochem. (2007) 76:1–22. doi: 10.1146/annurev.biochem.76.061705.090740
18. Young C, Brink R. The unique biology of germinal center B cells. Immunity. (2021) 54(8):1652–64. doi: 10.1016/j.immuni.2021.07.015
19. Chaudhary N, Wesemann DR. Analyzing immunoglobulin repertoires. Front Immunol. (2018) 9:462. doi: 10.3389/fimmu.2018.00462
20. Feng Y, Seija N, Di Noia JM, Martin A. AID in antibody diversification: there and back again. Trends Immunol. (2020) 41(7):586–600. doi: 10.1016/j.it.2020.04.009
21. Freund NT, Horwitz JA, Nogueira L, Sievers SA, Scharf L, Scheid JF, et al. A new glycan-dependent CD4-binding site neutralizing antibody exerts pressure on HIV-1 in vivo. PloS Pathog. (2015) 11(10):e1005238. doi: 10.1371/journal.ppat.1005238
22. Balu S, Reljic R, Lewis MJ, Pleass RJ, McIntosh R, van Kooten C, et al. A novel human IgA monoclonal antibody protects against tuberculosis. J Immunol. (2011) 186(5):3113–9. doi: 10.4049/jimmunol.1003189
23. Larsen SE, Williams BD, Rais M, Coler RN, Baldwin SL. It takes a village: the multifaceted immune response to. Front Immunol. (2022) 13:840225. doi: 10.3389/fimmu.2022.840225
24. Bartsch YC, Cizmeci D, Kang J, Zohar T, Periasamy S, Mehta N, et al. Antibody effector functions are associated with protection from respiratory syncytial virus. Cell. (2022) 185(26):4873–4886.e10. doi: 10.1016/j.cell.2022.11.012
25. Deng Y, Atyeo C, Yuan D, Chicz TM, Tibbitts T, Gorman M, et al. Beta-spike-containing boosters induce robust and functional antibody responses to SARS-CoV-2 in macaques primed with distinct vaccines. Cell Rep. (2023) 42(11):113292. doi: 10.1016/j.celrep.2023.113292
26. Horton RE, Vidarsson G. Antibodies and their receptors: different potential roles in mucosal defense. Front Immunol. (2013) 4:200. doi: 10.3389/fimmu.2013.00200
27. da Silva RJ, da Silva Corrêa R, Sardella IG, de Paulo Mulinari AC, Mafort TT, Santos AP, et al. IgA and IgG antibody detection of mycobacterial antigens in pleural fluid and serum from pleural tuberculous patients. BMC Immunol. (2019) 20(1):36. doi: 10.1186/s12865-019-0315-y
28. Zohar T, Hsiao JC, Mehta N, Das J, Devadhasan A, Karpinski W, et al. Upper and lower respiratory tract correlates of protection against respiratory syncytial virus following vaccination of nonhuman primates. Cell Host Microbe. (2022) 30(1):41–52.e5. doi: 10.1016/j.chom.2021.11.006
29. Hjelm F, Carlsson F, Getahun A, Heyman B. Antibody-mediated regulation of the immune response. Scand J Immunol. (2006) 64(3):177–84. doi: 10.1111/j.1365-3083.2006.01818.x
30. Vidarsson G, Dekkers G, Rispens T. IgG subclasses and allotypes: from structure to effector functions. Front Immunol. (2014) 5:520. doi: 10.3389/fimmu.2014.00520
31. Wang TT, Ravetch JV. Functional diversification of IgGs through Fc glycosylation. J Clin Invest. (2019) 129(9):3492–8. doi: 10.1172/JCI130029
32. Chen T, Blanc C, Eder AZ, Prados-Rosales R, Souza AC, Kim RS, et al. Association of human antibodies to arabinomannan with enhanced mycobacterial opsonophagocytosis and intracellular growth reduction. J Infect Dis. (2016) 214(2):300–10. doi: 10.1093/infdis/jiw141
33. Yuan Y, Crane DD, Simpson RM, Zhu YQ, Hickey MJ, Sherman DR, et al. The 16-kDa alpha-crystallin (Acr) protein of Mycobacterium tuberculosis is required for growth in macrophages. Proc Natl Acad Sci USA. (1998) 95(16):9578–83. doi: 10.1073/pnas.95.16.9578
34. Kumar SK, Singh P, Sinha S. Naturally produced opsonizing antibodies restrict the survival of Mycobacterium tuberculosis in human macrophages by augmenting phagosome maturation. Open Biol. (2015) 5(12):150171. doi: 10.1098/rsob.150171
35. Chen X, Schneewind O, Missiakas D. Engineered human antibodies for the opsonization and killing of Staphylococcus aureus. Proc Natl Acad Sci USA. (2022) 119(4). doi: 10.1073/pnas.2114478119
36. Vreede RW, Marcelis JH, Verhoef J. Antibodies raised against rough mutants of Escherichia coli and Salmonella strains are opsonic only in the presence of complement. Infect Immun. (1986) 52(3):892–6. doi: 10.1128/iai.52.3.892-896.1986
37. Mellouk N, Enninga J. Cytosolic access of intracellular bacterial pathogens: the shigella paradigm. Front Cell Infect Microbiol. (2016) 6:35. doi: 10.3389/fcimb.2016.00035
38. Joller N, Weber SS, Müller AJ, Spörri R, Selchow P, Sander P, et al. Antibodies protect against intracellular bacteria by Fc receptor-mediated lysosomal targeting. Proc Natl Acad Sci USA. (2010) 107(47):20441–6. doi: 10.1073/pnas.1013827107
39. Malik ZA, Denning GM, Kusner DJ. Inhibition of Ca(2+) signaling by Mycobacterium tuberculosis is associated with reduced phagosome-lysosome fusion and increased survival within human macrophages. J Exp Med. (2000) 191(2):287–302. doi: 10.1084/jem.191.2.287
40. Thiriot JD, Martinez-Martinez YB, Endsley JJ, Torres AG. Hacking the host: exploitation of macrophage polarization by intracellular bacterial pathogens. Pathog Dis. (2020) 78(1). doi: 10.1093/femspd/ftaa009
41. Gong Z, Yang W, Zhang H, Xiang X, Zeng J, Han S, et al. Mycobacterium tuberculosis Rv3717 enhances the survival of Mycolicibacterium smegmatis by inhibiting host innate immune and caspase-dependent apoptosis. Infect Genet Evol. (2020) 84:104412. doi: 10.1016/j.meegid.2020.104412
42. Peng Z, Yue Y, Xiong S. PPE36 modulates host inflammation by promoting E3 ligase smurf1-mediated myD88 degradation. Front Immunol. (2022) 13:690667. doi: 10.3389/fimmu.2022.690667
43. Le Moigne V, Le Moigne D, Mahana W. Antibody response to Mycobacterium tuberculosis p27-PPE36 antigen in sera of pulmonary tuberculosis patients. Tuberculosis (Edinb). (2013) 93(2):189–91. doi: 10.1016/j.tube.2012.10.006
44. Arora SK, Alam A, Naqvi N, Ahmad J, Sheikh JA, Rahman SA, et al. Immunodominant. Front Immunol. (2020) 11:1199. doi: 10.3389/fimmu.2020.01199
45. Pym AS, Brodin P, Majlessi L, Brosch R, Demangel C, Williams A, et al. Recombinant BCG exporting ESAT-6 confers enhanced protection against tuberculosis. Nat Med. (2003) 9(5):533–9. doi: 10.1038/nm859
46. Refai A, Gritli S, Barbouche MR, Essafi M. Virulent factor ESAT-6 drives macrophage differentiation toward the pro-inflammatory M1 phenotype and subsequently switches it to the anti-inflammatory M2 phenotype. Front Cell Infect Microbiol. (2018) 8:327. doi: 10.3389/fcimb.2018.00327
47. Bates TA, Trank-Greene M, Nguyenla X, Anastas A, Gurmessa SK, Merutka IR, et al. ESAT-6 undergoes self-association at phagosomal pH and an ESAT-6-specific nanobody restricts. Elife. (2024) 12. doi: 10.7554/eLife.91930
48. Parker HA, Forrester L, Kaldor CD, Dickerhof N, Hampton MB, et al. Antimicrobial activity of neutrophils against mycobacteria. Front Immunol. (2021) 12:782495. doi: 10.3389/fimmu.2021.782495
49. Tong X, McNamara RP, Avendaño MJ, Serrano EF, García-Salum T, Pardo-Roa C, et al. Waning and boosting of antibody Fc-effector functions upon SARS-CoV-2 vaccination. Nat Commun. (2023) 14(1):4174. doi: 10.1038/s41467-023-39189-8
50. Chakraborty S, Gonzalez J, Edwards K, Mallajosyula V, Buzzanco AS, Sherwood R, et al. Proinflammatory IgG Fc structures in patients with severe COVID-19. Nat Immunol. (2021) 22(1):67–73. doi: 10.1038/s41590-020-00828-7
51. Bryceson YT, Fauriat C, Nunes JM, Wood SM, Björkström NK, Long EO, et al. Functional analysis of human NK cells by flow cytometry. Methods Mol Biol. (2010) 612:335–52. doi: 10.1007/978-1-60761-362-6_23
52. Sakae Y, Satoh T, Yagi H, Yanaka S, Yamaguchi T, Isoda Y, et al. Conformational effects of N-glycan core fucosylation of immunoglobulin G Fc region on its interaction with Fcγ receptor IIIa. Sci Rep. (2017) 7(1):13780. doi: 10.1038/s41598-017-13845-8
53. Boudreau CM, Alter G. Extra-neutralizing fcR-mediated antibody functions for a universal influenza vaccine. Front Immunol. (2019) 10:440. doi: 10.3389/fimmu.2019.00440
54. Ackerman ME, Mikhailova A, Brown EP, Dowell KG, Walker BD, Bailey-Kellogg C, et al. Polyfunctional HIV-specific antibody responses are associated with spontaneous HIV control. PloS Pathog. (2016) 12(1):e1005315. doi: 10.1371/journal.ppat.1005315
55. Jegaskanda S, Weinfurter JT, Friedrich TC, Kent SJ. Antibody-dependent cellular cytotoxicity is associated with control of pandemic H1N1 influenza virus infection of macaques. J Virol. (2013) 87(10):5512–22. doi: 10.1128/JVI.03030-12
56. Lo Nigro C, Macagno M, Sangiolo D, Bertolaccini L, Aglietta M, Merlano MC. NK-mediated antibody-dependent cell-mediated cytotoxicity in solid tumors: biological evidence and clinical perspectives. Ann Transl Med. (2019) 7(5):105. doi: 10.21037/atm
57. Lu CC, Wu TS, Hsu YJ, Chang CJ, Lin CS, Chia JH, et al. NK cells kill mycobacteria directly by releasing perforin and granulysin. J Leukoc Biol. (2014) 96(6):1119–29. doi: 10.1189/jlb.4A0713-363RR
58. Allen M, Bailey C, Cahatol I, Dodge L, Yim J, Kassissa C, et al. Mechanisms of control of mycobacterium tuberculosis by NK cells: role of glutathione. Front Immunol. (2015) 6:508. doi: 10.3389/fimmu.2015.00508
59. Esin S, Batoni G, Counoupas C, Stringaro A, Brancatisano FL, Colone M, et al. Direct binding of human NK cell natural cytotoxicity receptor NKp44 to the surfaces of mycobacteria and other bacteria. Infect Immun. (2008) 76(4):1719–27. doi: 10.1128/IAI.00870-07
60. Vankayalapati R, Wizel B, Weis SE, Safi H, Lakey DL, Mandelboim O, et al. The NKp46 receptor contributes to NK cell lysis of mononuclear phagocytes infected with an intracellular bacterium. J Immunol. (2002) 168(7):3451–7. doi: 10.4049/jimmunol.168.7.3451
61. Marcenaro E, Ferranti B, Falco M, Moretta L, Moretta A. Human NK cells directly recognize Mycobacterium bovis via TLR2 and acquire the ability to kill monocyte-derived DC. Int Immunol. (2008) 20(9):1155–67. doi: 10.1093/intimm/dxn073
62. Coler RN, Day TA, Ellis R, Piazza FM, Beckmann AM, Vergara J, et al. The TLR-4 agonist adjuvant, GLA-SE, improves magnitude and quality of immune responses elicited by the ID93 tuberculosis vaccine: first-in-human trial. NPJ Vaccines. (2018) 3:34. doi: 10.1038/s41541-018-0057-5
63. Colditz GA, Berkey CS, Mosteller F, Brewer TF, Wilson ME, Burdick E, et al. The efficacy of bacillus Calmette-Guérin vaccination of newborns and infants in the prevention of tuberculosis: meta-analyses of the published literature. Pediatrics. (1995) 96(1 Pt 1):29–35. doi: 10.1542/peds.96.1.29
64. Van Der Meeren O, Hatherill M, Nduba V, Wilkinson RJ, Muyoyeta M, Van Brakel E, et al. Phase 2b controlled trial of M72/AS01. N Engl J Med. (2018) 379(17):1621–34. doi: 10.1056/NEJMoa1803484
65. Penn-Nicholson A, Geldenhuys H, Burny W, van der Most R, Day CL, Jongert E, et al. Safety and immunogenicity of candidate vaccine M72/AS01E in adolescents in a TB endemic setting. Vaccine. (2015) 33(32):4025–34. doi: 10.1016/j.vaccine.2015.05.088
66. Dijkman K, Sombroek CC, Vervenne RAW, Hofman SO, Boot C, Remarque EJ, et al. Prevention of tuberculosis infection and disease by local BCG in repeatedly exposed rhesus macaques. Nat Med. (2019) 25(2):255–62. doi: 10.1038/s41591-018-0319-9
67. Prados-Rosales R, Carreño L, Cheng T, Blanc C, Weinrick B, Malek A, et al. Enhanced control of Mycobacterium tuberculosis extrapulmonary dissemination in mice by an arabinomannan-protein conjugate vaccine. PloS Pathog. (2017) 13(3):e1006250. doi: 10.1371/journal.ppat.1006250
68. Roy E, Stavropoulos E, Brennan J, Coade S, Grigorieva E, Walker B, et al. Therapeutic efficacy of high-dose intravenous immunoglobulin in Mycobacterium tuberculosis infection in mice. Infect Immun. (2005) 73(9):6101–9. doi: 10.1128/IAI.73.9.6101-6109.2005
69. Li H, Wang XX, Wang B, Fu L, Liu G, Lu Y, et al. Latently and uninfected healthcare workers exposed to TB make protective antibodies against. Proc Natl Acad Sci USA. (2017) 114(19):5023–8. doi: 10.1073/pnas.1611776114
70. Nziza N, Cizmeci D, Davies L, Irvine EB, Jung W, Fenderson BA, et al. Defining discriminatory antibody fingerprints in active and latent tuberculosis. Front Immunol. (2022) 13(46):856906. doi: 10.3389/fimmu.2022.856906
71. Zou G, Ochiai H, Huang W, Yang Q, Li C, Wang LX. Chemoenzymatic synthesis and Fcγ receptor binding of homogeneous glycoforms of antibody Fc domain. Presence of a bisecting sugar moiety enhances the affinity of Fc to FcγIIIa receptor. J Am Chem Soc. (2011) 133:18975–91. doi: 10.1021/ja208390n
72. Grace PS, Dolatshahi S, Lu LL, Cain A, Palmieri F, Petrone L, et al. Antibody subclass and glycosylation shift following effective TB treatment. Front Immunol. (2021) 12:679973. doi: 10.3389/fimmu.2021.679973
73. Anthony RM, Kobayashi T, Wermeling F, Ravetch JV. Intravenous gammaglobulin suppresses inflammation through a novel T(H)2 pathway. Nature. (2011) 475(7354):110–3. doi: 10.1038/nature10134
74. Jefferis R. Glycosylation as a strategy to improve antibody-based therapeutics. Nat Rev Drug Discovery. (2009) 8(3):226–34. doi: 10.1038/nrd2804
75. Lu LL, Das J, Grace PS, Fortune SM, Restrepo BI, Alter G. Antibody fc glycosylation discriminates between latent and active tuberculosis. J Infect Dis. (2020) 222(12):2093–102. doi: 10.1093/infdis/jiz643
76. Kumagai T, Palacios A, Casadevall A, García MJ, Toro C, Tiemeyer M, et al. Serum igM glycosylation associated with tuberculosis infection in mice. mSphere. (2019) 4(2). doi: 10.1128/mSphere.00684-18
77. Ozdilek A, Paschall AV, Dookwah M, Tiemeyer M, Avci FY. Host protein glycosylation in nucleic acid vaccines as a potential hurdle in vaccine design for nonviral pathogens. Proc Natl Acad Sci USA. (2020) 117(3):1280–2. doi: 10.1073/pnas.1916131117
78. Kiazyk S, Ball TB. Latent tuberculosis infection: An overview. Can Commun Dis Rep. (2017) 43(3–4):62–6. doi: 10.14745/ccdr.v43i34a01
79. Glatman-Freedman A, Casadevall A. Serum therapy for tuberculosis revisited: reappraisal of the role of antibody-mediated immunity against Mycobacterium tuberculosis. Clin Microbiol Rev. (1998) 11(3):514–32. doi: 10.1128/CMR.11.3.514
80. Achkar JM, Chan J, Casadevall A. B cells and antibodies in the defense against Mycobacterium tuberculosis infection. Immunol Rev. (2015) 264(1):167–81. doi: 10.1111/imr.12276
81. Watson A, Li H, Ma B, Weiss R, Bendayan D, Abramovitz L, et al. Human antibodies targeting a Mycobacterium transporter protein mediate protection against tuberculosis. Nat Commun. (2021) 12(1):602. doi: 10.1038/s41467-021-20930-0
82. Steingart KR, Ramsay A, Dowdy DW, Pai M. Serological tests for the diagnosis of active tuberculosis: relevance for India. Indian J Med Res. (2012) 135(5):695–702.
83. Achkar JM, Ziegenbalg A. Antibody responses to mycobacterial antigens in children with tuberculosis: challenges and potential diagnostic value. Clin Vaccine Immunol. (2012) 19(12):1898–906. doi: 10.1128/CVI.00501-12
84. Steingart KR, Flores LL, Dendukuri N, Schiller I, Laal S, Ramsay A, et al. Commercial serological tests for the diagnosis of active pulmonary and extrapulmonary tuberculosis: an updated systematic review and meta-analysis. PloS Med. (2011) 8(8):e1001062. doi: 10.1371/journal.pmed.1001062
85. Abraham PR, Devalraju KP, Jha V, Valluri VL, Mukhopadhyay S. PPE17 (Rv1168c) protein of Mycobacterium tuberculosis detects individuals with latent TB infection. PloS One. (2018) 13(11):e0207787. doi: 10.1371/journal.pone.0207787
86. Lee JY, Kim BJ, Koo HK, Kim J, Kim JM, Kook YH, et al. Diagnostic potential of igG and igA responses to. Microorganisms. (2020) 8(7). doi: 10.3390/microorganisms8070979
87. Abebe F, Belay M, Legesse M, K LMCF, Ottenhoff THM. IgA and IgG against Mycobacterium tuberculosis Rv2031 discriminate between pulmonary tuberculosis patients, Mycobacterium tuberculosis-infected and non-infected individuals. PloS One. (2018) 13(1):e0190989. doi: 10.1371/journal.pone.0190989
88. Bowman KA, Kaplonek P, McNamara RP. Understanding Fc function for rational vaccine design against pathogens. mBio. (2023) 15(1):e0303623. doi: 10.1128/mbio.03036-23
89. Lund FE, Randall TD. Scent of a vaccine. Science. (2021) 373(6553):397–9. doi: 10.1126/science.abg9857
90. Takenami I, de Oliveira CC, Lima FR, Soares J, Machado A, Riley LW, et al. Immunoglobulin G response to mammalian cell entry 1A (Mce1A) protein as biomarker of active tuberculosis. Tuberculosis (Edinb). (2016) 100:82–8. doi: 10.1016/j.tube.2016.07.012
91. Khan IH, Ravindran R, Krishnan VV, Awan IN, Rizvi SK, Saqib MA, et al. Plasma antibody profiles as diagnostic biomarkers for tuberculosis. Clin Vaccine Immunol. (2011) 18(12):2148–53. doi: 10.1128/CVI.05304-11
92. Takenami I, de Oliveira CC, Petrilli JD, Machado A, Riley LW, Arruda S. Serum antiphospholipid antibody levels as biomarkers for diagnosis of pulmonary tuberculosis patients. Int J Tuberc Lung Dis. (2018) 22(9):1063–70. doi: 10.5588/ijtld.17.0874
93. Li LF, Lin MC, Chen NH, Hsieh MJ, Lee CH, Tsao TC. Serodiagnosis of tuberculosis by enzyme-linked immunosorbent assay for anti-A60 and anti-A38. Changgeng Yi Xue Za Zhi. (1998) 21(3):258–64.
94. Ben-selma W, Harizi H, Marzouk M, Ben Kahla I, Ben Lazreg F, Ferjeni A, et al. Evaluation of the diagnostic value of measuring IgG, IgM, and IgA antibodies to mycobacterial A60 antigen in active tuberculosis. Diagn Microbiol Infect Dis. (2010) 68(1):55–9. doi: 10.1016/j.diagmicrobio.2010.05.006
95. La Manna MP, Orlando V, Li Donni P, Sireci G, Di Carlo P, Cascio A, et al. Identification of plasma biomarkers for discrimination between tuberculosis infection/disease and pulmonary non tuberculosis disease. PloS One. (2018) 13(3):e0192664. doi: 10.1371/journal.pone.0192664
96. Gina P, Randall PJ, Muchinga TE, Pooran A, Meldau R, Peter JG, et al. Early morning urine collection to improve urinary lateral flow LAM assay sensitivity in hospitalised patients with HIV-TB co-infection. BMC Infect Dis. (2017) 17:339. doi: 10.1186/s12879-017-2313-0
97. Shah M, Ssengooba W, Armstrong D, Nakiyingi L, Holshouser M, Ellner JJ, et al. Comparative performance of urinary lipoarabinomannan assays and Xpert MTB/RIF in HIV-infected individuals. AIDS. (2014) 28(9):1307–14. doi: 10.1097/QAD.0000000000000264
98. Hanifa Y, Fielding KL, Chihota VN, Adonis L, Charalambous S, Karstaedt A, et al. Diagnostic accuracy of lateral flow urine LAM assay for TB screening of adults with advanced immunosuppression attending routine HIV care in South Africa. PloS One. (2016) 11(6):e0156866. doi: 10.1371/journal.pone.0156866
99. Awoniyi DO, Baumann R, Chegou NN, Kriel B, Jacobs R, Kidd M, et al. Detection of a combination of serum IgG and IgA antibodies against selected mycobacterial targets provides promising diagnostic signatures for active TB. Oncotarget. (2017) 8(23):37525–37. doi: 10.18632/oncotarget.v8i23
100. Lynch HE, Stewart SM, Kepler TB, Sempowski GD, Alam SM. Surface plasmon resonance measurements of plasma antibody avidity during primary and secondary responses to anthrax protective antigen. J Immunol Methods. (2014) 404(5):1–12. doi: 10.1016/j.jim.2013.11.026
101. Miyoshi T, Zhang Q, Miyake T, Watanabe S, Ohnishi H, Chen J, et al. Semi-automated single-molecule microscopy screening of fast-dissociating specific antibodies directly from hybridoma cultures. Cell Rep. (2021) 34(5):108708. doi: 10.1016/j.celrep.2021.108708
102. Möckl L, Moerner WE. Super-resolution microscopy with single molecules in biology and beyond-essentials, current trends, and future challenges. J Am Chem Soc. (2020) 142(42):17828–44. doi: 10.1021/jacs.0c08178
103. Botella H, Yang G, Ouerfelli O, Ehrt S, Nathan CF, Vaubourgeix J. Distinct spatiotemporal dynamics of peptidoglycan synthesis between. mBio. (2017) 8(5). doi: 10.1128/mBio.01183-17
104. Estévez O, Anibarro L, Garet E, Pallares Á, Pena A, Villaverde C, et al. Identification of candidate host serum and saliva biomarkers for a better diagnosis of active and latent tuberculosis infection. PloS One. (2020) 15(7):e0235859. doi: 10.1371/journal.pone.0235859
105. Reyes F, Tirado Y, Puig A, Borrero R, Reyes G, Fernández S, et al. Immunogenicity and cross-reactivity against Mycobacterium tuberculosis of proteoliposomes derived from Mycobacterium bovis BCG. BMC Immunol. (2013) 14(Suppl 1):S7. doi: 10.1186/1471-2172-14-S1-S7
106. Lin MY, Reddy TB, Arend SM, Friggen AH, Franken KL, van Meijgaarden KE, et al. Cross-reactive immunity to Mycobacterium tuberculosis DosR regulon-encoded antigens in individuals infected with environmental, nontuberculous mycobacteria. Infect Immun. (2009) 77(11):5071–9. doi: 10.1128/IAI.00457-09
107. Dai Z, Liu Z, Xiu B, Yang X, Zhao P, Zhang X, et al. A multiple-antigen detection assay for tuberculosis diagnosis based on broadly reactive polyclonal antibodies. Iran J Basic Med Sci. (2017) 20(4):360–7. doi: 10.22038/IJBMS.2017.8575
108. McMahan K, Wegmann F, Aid M, Sciacca M, Liu J, Hachmann NP, et al. Mucosal boosting enhances vaccine protection against SARS-CoV-2 in macaques. Nature. (2024) 626(7998):385–91. doi: 10.1038/s41586-023-06951-3
109. Tong X, Wang Q, Jung W, Chicz TM, Blanc R, Parker LJ, et al. Compartment-specific antibody correlates of protection to SARS-coV-2 omicron in macaques. iScience (2024) 27(9):110174. doi: 10.1016/j.isci.2024.110174
110. Jung W, Yuan D, Kellman B, Gonzalez IGDS, Clemens R, Milan EP, et al. Boosting with adjuvanted SCB-2019 elicits superior Fcγ-receptor engagement driven by IgG3 to SARS-CoV-2 spike. NPJ Vaccines. (2024) 9(1):7. doi: 10.1038/s41541-023-00791-y
111. Tong X, Deng Y, Cizmeci D, Fontana L, Carlock MA, Hanley HB, et al. Distinct functional humoral immune responses are induced after live attenuated and inactivated seasonal influenza vaccination. J Immunol. (2024) 212(1):24–34. doi: 10.4049/jimmunol.2200956
112. Manyelo CM, Solomons RS, Walzl G, Chegou NN. Tuberculous meningitis: pathogenesis, immune responses, diagnostic challenges, and the potential of biomarker-based approaches. J Clin Microbiol. (2021) 59(3). doi: 10.1128/JCM.01771-20
113. Lodes MJ, Dillon DC, Mohamath R, Day CH, Benson DR, Reynolds LD, et al. Serological expression cloning and immunological evaluation of MTB48, a novel Mycobacterium tuberculosis antigen. J Clin Microbiol. (2001) 39(7):2485–93. doi: 10.1128/JCM.39.7.2485-2493.2001
114. Shete PB, Ravindran R, Chang E, Worodria W, Chaisson LH, Andama A, et al. Evaluation of antibody responses to panels of M. tuberculosis antigens as a screening tool for active tuberculosis in Uganda. PloS One. (2017) 12(8):e0180122. doi: 10.1371/journal.pone.0180122
115. Khaliq A, Ravindran R, Hussainy SF, Krishnan VV, Ambreen A, Yusuf NW, et al. Field evaluation of a blood based test for active tuberculosis in endemic settings. PloS One. (2017) 12(4):e0173359. doi: 10.1371/journal.pone.0173359
116. Luo L, Zhu L, Yue J, Liu J, Liu G, Zhang X, et al. Antigens Rv0310c and Rv1255c are promising novel biomarkers for the diagnosis of Mycobacterium tuberculosis infection. Emerg Microbes Infect. (2017) 6(7):e64. doi: 10.1038/emi.2017.54
117. Burbelo PD, Keller J, Wagner J, Klimavicz JS, Bayat A, Rhodes CS, et al. Serological diagnosis of pulmonary Mycobacterium tuberculosis infection by LIPS using a multiple antigen mixture. BMC Microbiol. (2015) 15:205. doi: 10.1186/s12866-015-0545-y
118. Baumann R, Kaempfer S, Chegou NN, Oehlmann W, Loxton AG, Kaufmann SH, et al. Serologic diagnosis of tuberculosis by combining Ig classes against selected mycobacterial targets. J Infect. (2014) 69(6):581–9. doi: 10.1016/j.jinf.2014.05.014
119. Kumar G, Dagur PK, Singh PK, Shankar H, Yadav VS, Katoch VM, et al. Serodiagnostic efficacy of Mycobacterium tuberculosis 30/32-kDa mycolyl transferase complex, ESAT-6, and CFP-10 in patients with active tuberculosis. Arch Immunol Ther Exp (Warsz). (2010) 58(1):57–65. doi: 10.1007/s00005-009-0055-4
Keywords: tuberculosis, antibody, biomarkers, LTBI (latent TB infection), active tuberculosis (ATB), systems immunology, serology
Citation: Wang Q, Nag D, Baldwin SL, Coler RN and McNamara RP (2024) Antibodies as key mediators of protection against Mycobacterium tuberculosis. Front. Immunol. 15:1430955. doi: 10.3389/fimmu.2024.1430955
Received: 10 May 2024; Accepted: 13 August 2024;
Published: 02 September 2024.
Edited by:
Laurel L. Lenz, University of Colorado, United StatesReviewed by:
Rajko Reljic, St George’s, University of London, United KingdomStephen Cose, University of London, United Kingdom
Copyright © 2024 Wang, Nag, Baldwin, Coler and McNamara. This is an open-access article distributed under the terms of the Creative Commons Attribution License (CC BY). The use, distribution or reproduction in other forums is permitted, provided the original author(s) and the copyright owner(s) are credited and that the original publication in this journal is cited, in accordance with accepted academic practice. No use, distribution or reproduction is permitted which does not comply with these terms.
*Correspondence: Ryan P. McNamara, cnBtY25hbWFyYUBtZ2guaGFydmFyZC5lZHU=