- Department of Nuclear Medicine, The First Hospital of China Medical University, Shenyang, Liaoning, China
Currently, immunotherapy is being widely used for treating cancers. However, the significant heterogeneity in patient responses is a major challenge for its successful application. CD8-positive T cells (CD8+ T cells) play a critical role in immunotherapy. Both their infiltration and functional status in tumors contribute to treatment outcomes. Therefore, accurate monitoring of CD8+ T cells, a potential biomarker, may improve therapeutic strategy. Positron emission tomography (PET) is an optimal option which can provide molecular imaging with enhanced specificity. This review summarizes the mechanism of action of CD8+ T cells in immunotherapy, and highlights the recent advancements in PET-based tracers that can visualize CD8+ T cells and discusses their clinical applications to elucidate their potential role in cancer immunotherapy.
1 Introduction
Immunotherapy has advanced rapidly in the recent past (1–3). Key methods involving immune checkpoint inhibitors (ICIs) and adoptive cell therapy (ACT) have achieved notable success in the clinical management of various cancers (4, 5). However, durable and effective responses are only observed in a section of patients (6). Efforts have been made to further optimize immunotherapeutic strategies and several studies have attempted to identify potential biomarkers for improving the therapeutic efficacy (7–9).
CD8+ T lymphocytes, critical immune effector cells, play a vital role in cancer immunotherapy (10, 11). Studies have shown that improved outcomes correlate positively with CD8+ T cell infiltration in several types of tumors (12–14), including melanoma (15), non-small cell lung cancer (NSCLC) (16), breast cancer (17), and cervical cancer (18). In addition to the quantity of infiltration, the functional status of CD8+ T cells within the tumor microenvironment (TME), which is influenced by interactions with cells and may change with time, also significantly affect response to therapy (19, 20). Both infiltration and functional status greatly contribute to the heterogeneity in response to immunotherapy (6, 8, 9). Therefore, monitoring of CD8+ T cells in vivo is crucial for improving patient understanding and implementation of precision medicine.
Tumor biopsy, a conventional invasive method, is used in clinical practice to analyze CD8+ T cells. However, due to its inherent limitations, this invasive approach poses several challenges, including difficulty in re-assessment and the inability to provide spatial and dynamic information (21). In contrast, non-invasive methods using various imaging modalities and direct/indirect labeling of target cells or construction of radiolabeled agents play significant roles in monitoring the immune response in vivo (22, 23). Positron emission tomography (PET) is a promising molecular technique that can provide whole-body images with considerable specificity and sensitivity (24). The binding of targeted vectors to specific radionuclides forms the foundation of PET radiotracers, and PET enables non-invasive real-time monitoring of the target cells by detecting radionuclide decay emissions (25, 26). PET radiotracers have been extensively used to characterize CD8+ T cells, and thus effectively quantify early therapy-induced alterations in immune status (27).
Currently, existing PET radiotracers for visualizing CD8+ T cells can be generally divided into two categories: 1) those directly targeting CD8, a dimeric co-receptor, indicating the presence of CD8+ cells, and 2) those indirectly reflecting the functionality or status of CD8+ T cells by targeting potential biomarkers. This review offers an overview of the development of PET imaging of CD8+ T cells, briefly summarizes current information on relevant CD8+ T cell biology and innovative PET tracers and discusses the future potential applications of PET in the field of cancer immunotherapy.
2 Mechanisms of action of CD8+ T cells
2.1 CD8+ T cells in immunology
Common T cells originate from lymphoid progenitor cells in the red bone marrow. These immature precursor T cells then migrate to the thymus (28). CD8+ T cells gradually mature via several specific processes, including the development of the T cell receptor’s (TCR’s) affinity for major histocompatibility complex class-1 (MHC-1), positive selection, and negative selection (28, 29).
The direct interaction between CD8+ T cells and corresponding antigens is pivotal for CD8+ T cell activation. MHC-1, presented by malignant cells or antigen-presenting cells (APCs), is recognized by the TCR of CD8+ T cells (30). Following activation of the TCR signal, additional signals from co-receptors such as CD28 complexed with B7 molecules (CD80/86), along with the influence of cytokines or chemokines, further facilitate the activation of CD8+ T cells (31, 32). Consequently, CD8+ T cells can identify and target tumor sites. Upon reaching the site, CD8+ T cells begin to infiltrate and combat tumor cells.
2.2 CD8+ T cells in anti-tumor immunity
Several studies have established the critical function of CD8+ T cells in anti-tumor immunity (33, 34). The mechanisms via which CD8+ T cells contribute to tumor-killing activity are complex and involve multiple factors. A primary pathway involves the release of granules containing perforin and granzymes by CD8+ T cells, directly leading to the apoptosis of malignant cells (34). Perforin creates pores in tumor cell membranes, allowing granzymes to enter the TME and exert cytotoxic effect (35). The FAS ligand (FASL) pathway is another crucial pathway, which is cytotoxic for tumor cells (36). The interaction between FAS on malignant cells and FASL on CD8+ T cells triggers a signal that activates the FAS-associated death domain protein, resulting in caspase activation and subsequent apoptosis of tumor cells. Additionally, CD8+ T lymphocytes contribute to the destruction of tumor cells by secreting cytokines, including interferon-γ (IFN-γ) and tumor necrosis factor-alpha (37, 38). Various mechanisms collaborate to achieve tumor cell elimination, with several factors playing integral roles, including effector cytokines that impact the CD8+ T cells and the dynamic metabolic state of these cells (39, 40) (Figure 1).
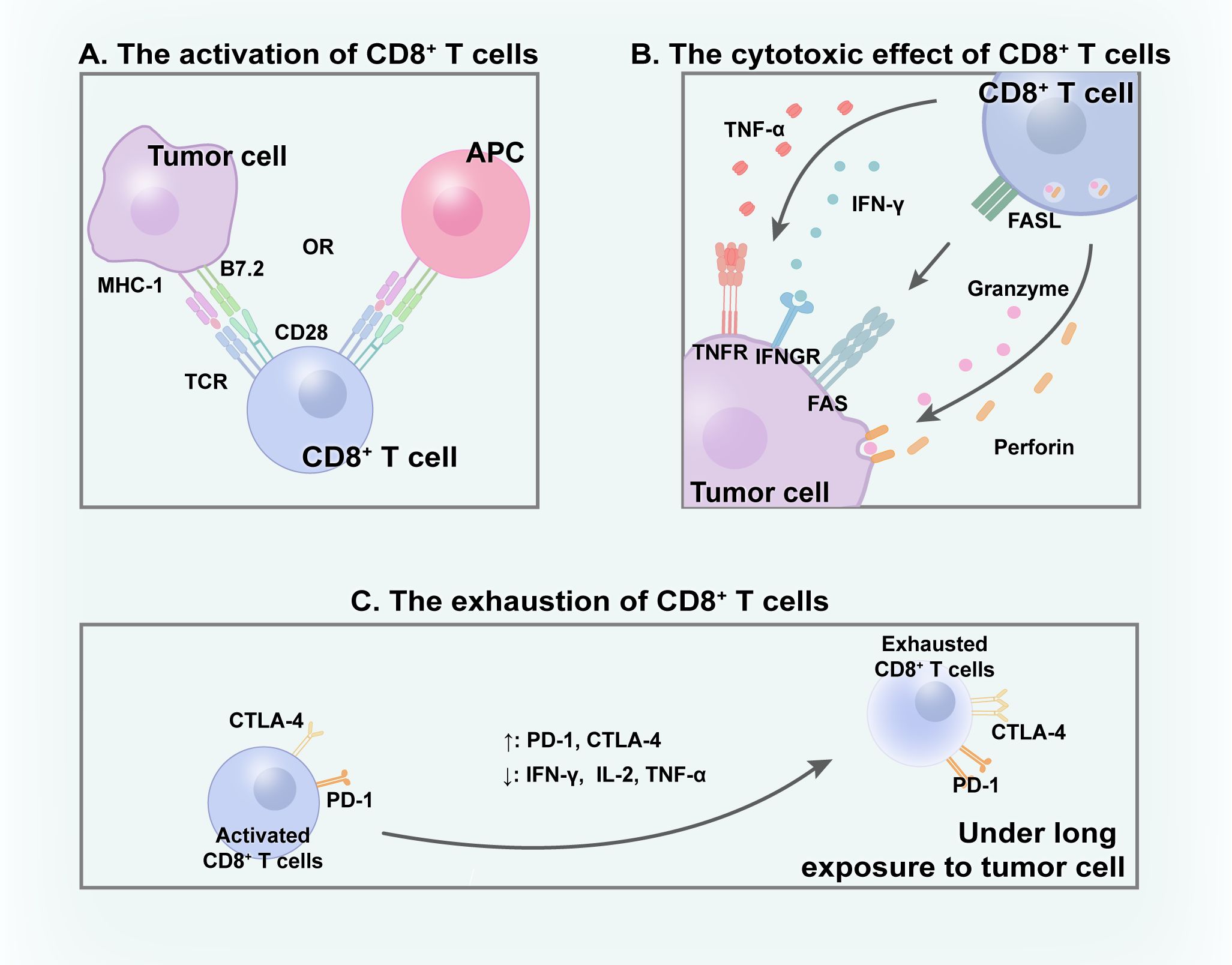
Figure 1 CD8+ T cells within TME. (A) With the interaction with APC/tumor cells, CD8+ T cells get activated and (B) subsequently excrete cytotoxic effect to eliminate tumor cells through various mechanisms. (C) Under long time exposure to tumor, activated CD8+ T cells gradually become exhausted.
2.3 CD8+ T cells in cancer immunotherapy
CD8+ T lymphocytes possess the potent ability to kill malignant cells. However, owing to prolonged exposure in the TME, many CD8+ T cells gradually exhibit characteristics of “exhaustion” (41, 42). In this state, the proliferation, effector cytokine production, and cytolytic activity of the CD8+ T cells tend to decrease, while cell surface expression of inhibitory receptors, including programmed death-1 receptor (PD-1) and cytotoxic T lymphocyte antigen-4 (CTLA-4), increase concurrently (43, 44). Tumor cells exploit this by overexpressing inhibitory immune checkpoints, thereby achieving immune escape and diminishing the effectiveness of the immune response against tumors (45).
Immunotherapy, which leverages natural immune function to eliminate tumor cells, can be generally categorized into ICIs, ACT, cancer vaccines, oncolytic virus therapies, and cytokine therapies (46). ICIs block immune checkpoint pathways, aiding in the reversal of the exhausted state of CD8+ T cells (47, 48). In recent years, ICIs have been shown to improve anti-tumor effects and exhibit excellent results (49, 50). A global study (KEYNOTE-042, NCT02220894) compared first-line monotherapy with pembrolizumab (a representative of ICIs) with platinum-based chemotherapy in patients with locally advanced/metastatic NSCLC without epidermal growth factor receptor/anaplastic lymphoma kinase alterations and programmed death-ligand 1 (PD-L1) tumor proportion score of ≥ 1%. Durable benefit was observed in pembrolizumab groups, in which higher 5-year overall survival (OS) rates were evident (51). Moreover, immunotherapy-based combinations demonstrated promise in further improving outcomes (52). The use of a combination of nivolumab, ipilimumab, and chemotherapy confirmed a significant improvement in OS compared with chemotherapy alone in a phase 3 trial (CheckMate 9LA, NCT03215706) involving patients with NSCLC (53). In addition to ICIs, ACT is a promising option for cancer therapy. Chimeric antigen receptor T cell therapy (CAR-T), based on gene editing in CD8+ T cells and reinfusion into the human body, enhances the effectiveness of immune cells (54). These engineered cells can target malignant cells better than other cells and exert cytotoxic effects (55). Moreover, oncolytic virus therapies selectively replicate in tumor cells while promoting the anti-tumor immunity of CD8+ T cells (56). Cancer vaccines activate APCs loaded with tumor antigens, inducing efficient CD8+ T cell responses (57). To some extent, various immunotherapy methods directly or indirectly boost the anti-tumor effect of CD8+ T lymphocytes, aiding in the eradication of tumor cells (58). Furthermore, novel combination therapies with radiotherapy aim to overcome immune resistance and increase CD8+ T cell infiltration, thereby enhancing efficacy (59). Consequently, CD8+ T lymphocytes play an indispensable part in immunotherapy (Figure 2).
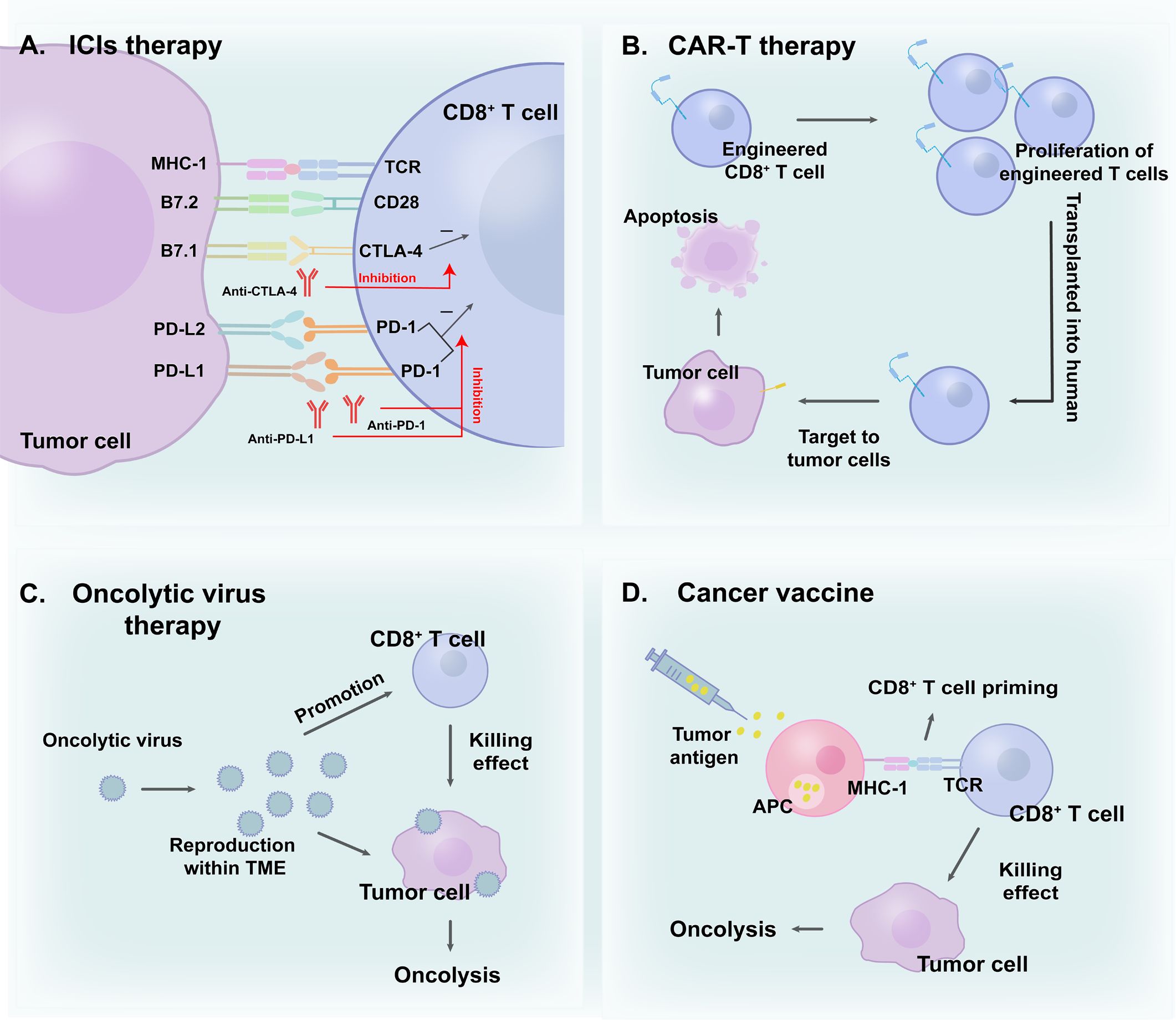
Figure 2 CD8+ T cells in (A) ICIs therapy; (B) CAR-T therapy; (C) Oncolytic virus therapy and (D) Cancer vaccine. CD8+ T cells play a vital role in cancer immunotherapies.
3 CD8+ T cell imaging tracers
PET is a powerful clinical technique (60) utilized for non-invasively and dynamically visualizing CD8+ T cells in cancer immunotherapy. Currently, the design and development of tracers for CD8+ T cells have improved considerably. Ideal radiotracers should possess high specificity, sensitivity, and a relatively low radiation burden. Both the choice of radionuclides and vectors are critical considerations in this process (27).
Previously, zirconium-89 (89Zr) was a popular choice. Owing to its relatively long half-life, this radionuclide pairs well with intact antibodies that also have long serum half-lives, potentially providing reliable information several hours or days after injection (61, 62). Copper-64 (64Cu) is another promising candidate for a long-lasting imaging agent that can be used in the human body (63). However, its high signal intensity in the liver and intestines may limit the clinical application of 64Cu-labeled probes (27). In contrast, gallium-68 (68Ga) and fluorine-18 (18F) are better options for obtaining sequential images in clinical settings due to their shorter half-lives (64).
Studies utilizing full-sized antibodies, which are relatively easy to produce as imaging agents, have been successfully used in immuno-PET (27). However, their use is challenging, because their size exceeds the renal filtration cutoff, which may impede diffusion and penetration (65). With the advancement of radiotracers, current choices for targeting vectors extend beyond full-length antibodies, with options such as minibodies, cys-diabodies, and nanobodies emerging. Smaller antibody fragments, particularly nanobodies, which consist solely of a heavy chain structure, are preferred in many studies for their rapid pharmacokinetics, better tissue penetration, and low immunogenicity (66, 67). When combined with radionuclides with short half-lives such as 68Ga and 18F, nanobodies can facilitate the creation of high-quality images with lower radiation doses, aiding their translation into clinical practice. The currently developed radiotracers used for visualizing CD8+ T cells are summarized in the Table 1.
4 Quantitative visualization of CD8+ T cells
The immune status within TME is dynamic, with CD8+ T cells, other immune cells, and effector molecules being constantly in flux (93, 94). Therefore, real-time monitoring of CD8+ T cell infiltration can provide accurate and significant information for personalized therapy. Due to its unique advantages, the PET tracer has emerged as an attractive tool, and many studies have investigated its potential clinical applications.
4.1 Feasibility of using CD8 PET tracers
4.1.1 Minibody
CD8-targeted tracers, primarily derived from antibodies, minibodies, cys-diabodies, and nanobodies, have shown promising results. The feasibility of using two 64Cu-labeled engineered minibodies, 64Cu-NOTA-2.43 and 64Cu-NOTA-YTS169, for monitoring CD8+ T cells has been confirmed in non-tumor murine models (68). The interaction between murine CD8α (one of the isoforms of CD8) and these tracers was verified without diminishing CD8+ T cell populations. Both tracers accumulated in the lymph nodes and spleen in antigen-positive mouse models, with notably decreased uptake in immunodeficient or antigen-depleted models (68). To delineate the TME more accurately in clinical patients, 64Cu-DOTA-IAB22M2C, a CD8-targeted minibody, has been effectively used in a xenograft model of orthotopic glioblastoma, demonstrating its ability to monitor both peripheral and intratumoral CD8+ T cells (79). Notably, tracer accumulation in the brain indicated that 64Cu-DOTA-IAB22M2C could potentially complement 18F-FDG, particularly in addressing its limitations in brain tumor imaging.
Building upon these animal studies, clinical trials using tracers with high affinity to human CD8 have been designed. Research on 89Zr-Df-IAB22M2C, a 89Zr-labeled anti-CD8 minibody, has progressed considerably (77, 95–97). Preclinical studies have demonstrated its uptake in targeted lesions with an ideal target-to-background ratio in mouse models. Subsequently, a phase I first-in-human study (NCT03107663) assessed the optimal mass doses (1.5 mg) and imaging timings (24 hours post-injection) among 15 patients in varying treatment states using 89Zr-Df-IAB22M2C (97). The optimal conditions for its clinical application are being investigated in an ongoing phase II clinical trial (NCT03802123).
4.1.2 Nanobody
Existing CD8-targeted minibody-based PET tracers are mainly labeled with 89Zr or 64Cu which has long half-live. Consequently, these tracers require a long time (usually several hours or days after injection) before the image acquisition and which may cause difficulties for clinical practice. In contrast, nanobodies have emerged as highly potent alternatives, offering rapid targeting, high signal-to-background ratios, and other superior characteristics (98).
Considering the advantages of 68Ga over 89Zr, a novel CD8-targeted nanobody, 68Ga-NOTA-SNA006a, was developed to monitor human CD8 antigen using PET (80). In vitro binding assays was conducted to assess the binding capacity of vectors to human CD8 protein and results demonstrated strong binding affinity with positive binding rate constant. In vivo studies assessing specificity and stability in humanized mouse models demonstrated its significant uptake in CD8-positive tumors and organs (lung, spleen and liver). An optimized variant with reduced kidney uptake, 68Ga-NODAGA-SNA006, was obtained by removing the His6 tag from 68Ga-NOTA-SNA006a (81). This nanobody’s ability to quantify CD8+ T lymphocytes has been shown not only in preclinical models but also in three lung cancer volunteers (NCT05126927). Notably, a patient who underwent immunotherapy displayed comparably high uptake in tumor lesions, suggesting the potential of this tracer to evaluate therapy-induced changes (81).
4.2 Patient stratification for immunotherapy
Immunotherapy has been successfully applied in various clinical settings, particularly in cancer treatment. However, its wider clinical application is hindered by the generally low patient response rate, with immune heterogeneity being a key deterrent. Therefore, visualization of CD8+ T cells may be a potent strategy for detecting immune heterogeneity and guiding patient stratification.
Kristensen developed 89Zr-DFO-CD8a, which was created from the F(ab’)2 fragments of a rat-anti-mouse CD8a antibody conjugated to the p-SCN-Bn-desferrioxamine chelator (72). This preclinical study investigated the correlation between baseline PET imaging of CD8 levels (tumor-to-heart ratios) and tumor suppression induced by a PD-1 checkpoint inhibitor. Groups with higher numbers of baseline CD8+ T lymphocytes exhibited significant tumor suppression, while the efficacy in groups with fewer CD8+ T lymphocytes was less pronounced. This finding suggests the potential for stratifying patients from responders to non-responders before starting immunotherapy (72). Notably, significant difference in the maximum 89Zr-DFO-CD8a tumor-to-heart ratio between responding and non-responding groups was not observed, highlighting the necessity of understanding the relationship between specific quantitative parameters and the patient population suitable for immunotherapy.
4.3 Evaluating the efficacy of immunotherapy
Owing to its unique mechanism, atypical patterns of response may emerge during immunotherapy (99, 100). Pseudoprogression, for example, is an atypical phenomenon that differs from actual progression, characterized by increasing tumor size due to therapy-induced immune cell infiltration (101). The existence of atypical patterns brings great challenges to the evaluation of efficacy in clinical practice (102). CD8 PET tracers can directly monitor the immune alterations caused by immunotherapy unaffected by atypical phenomenon, which may provide accurate evaluation at an early stage and thus favor follow-up decision making.
The use of 89Zr-malDFO-169cDb, an 89Zr-desferrioxamine-labeled anti-CD8 cys-diabody, enabled tracking of endogenous CD8+ T cells and assessment of changes induced by three different immunotherapies in murine models (70). The study compared tumor-to-blood ratios between responders and non-responders in each of the three models, noting that the difference in the anti-PD-L1 therapy group was less significant (70). In addition to monotherapy, Kristensen has evaluated the efficacy of combination therapy. The use of 64Cu-NOTA-CD8a for evaluating the response to combination therapy, external radiation therapy with anti-CTLA-4 therapy, has been validated demonstrating the tracer’s utility (74). This finding suggests a potential for using CD8-targeted probes for evaluating therapeutic efficacy and providing valuable information for clinical decision-making.
In clinical settings, a study involving patients with metastatic melanoma undergoing immunotherapy showed that CD8 PET tracer (89Zr-Df-IAB22M2C, performed 28 days after immunotherapy) was noticeably incorporated during metastases, indicating infiltration of CD8+ T cells. This positive indication was consistent with conventional computed tomography (CT) imaging (performed 3 months after immunotherapy) which suggested a complete response to therapy (Figure 3) (97). This study demonstrated the promising potential of CD8 PET in evaluating immunotherapeutic efficacy at rather early stage. Additionally, another clinical study suggested the feasibility of using 89Zr-Df-IAB22M2C PET/MRI in assessing CD8+ T cells infiltration for efficacy evaluation in a retrospective cohort of eight patients (78). However, future studies involving larger prospective cohorts are required to strengthen these findings.
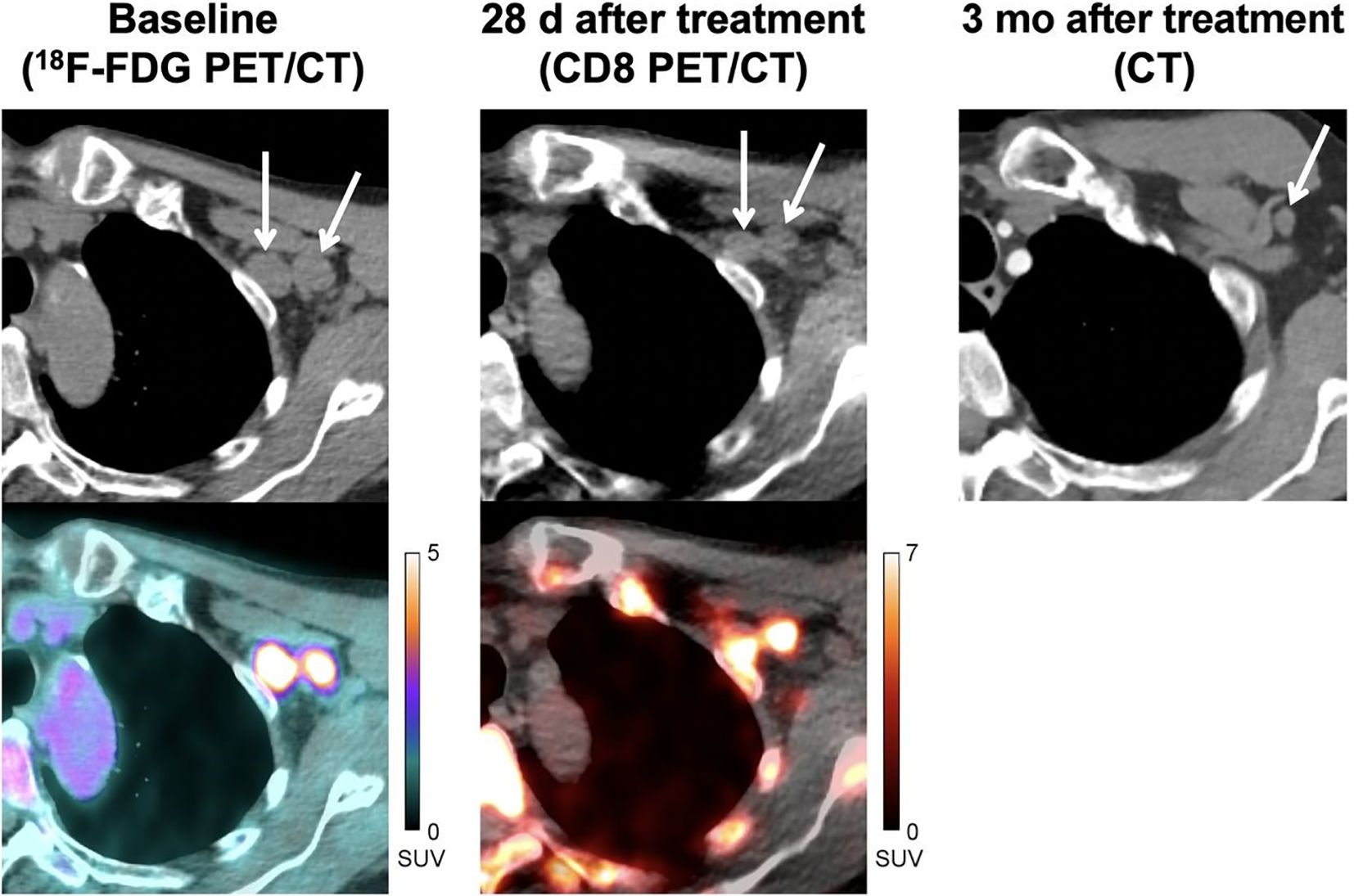
Figure 3 A 71 years old patient (locally advanced stage III melanoma) with immunotherapy (pembrolizumab). CD8 PET/CT images, which performed 28 days after pembrolizumab, show evident uptake in metastases indicating significant infiltration of CD8+ T cells. CT images performed 3 months after treatment confirmed the efficacy of immunotherapy. Reproduced from Farwell MD et al. (97).
4.4 Dynamic surveillance of ICI therapy
For more effective and precise immunotherapy, dynamic and non-invasive surveillance of the therapeutic process is urgently required. Rashidian used 89Zr-PEGylated camelid single-domain antibody fragments to successfully visualize the dynamic increase in CD8+ T lymphocytes within tumors during ICI therapy. They observed a significant trend of CD8+ T lymphocytes migrating from the periphery to the central position in tumor sites in murine models (73). This finding underscores the unique advantages of dynamic surveillance in this context.
Additionally, the occurrence of adverse events during immunotherapy, which are crucial factors in dynamic surveillance, should not be overlooked. Based on the results of a phase 1/2 trial (NCT04029181), the potential of ZED88082A in detecting inflammation in non-malignant areas has been investigated. The results suggest its ability to characterize CD8-related immune-related adverse events (irAEs) (76). This study investigated the potential applications of CD8-targeted PET tracers and provided practical directions for further clinical translation. However, irAEs may also be induced by other factors, such as B cells, indicating a potential limitation of CD8 PET tracers in obtaining comprehensive information on irAEs. More datasets in the future are required to confirm these findings.
4.5 Predicting the prognosis of ICI therapy
ZED88082A, based on a monoclonal antibody, was used to visualize CD8 infiltration in patients with solid tumors during ICI therapy (75, 76, 103). Thirty-nine patients (excluding one because of tracer extravasation) were used to assess tracer uptake both at baseline and during therapy (Figure 4) (76).
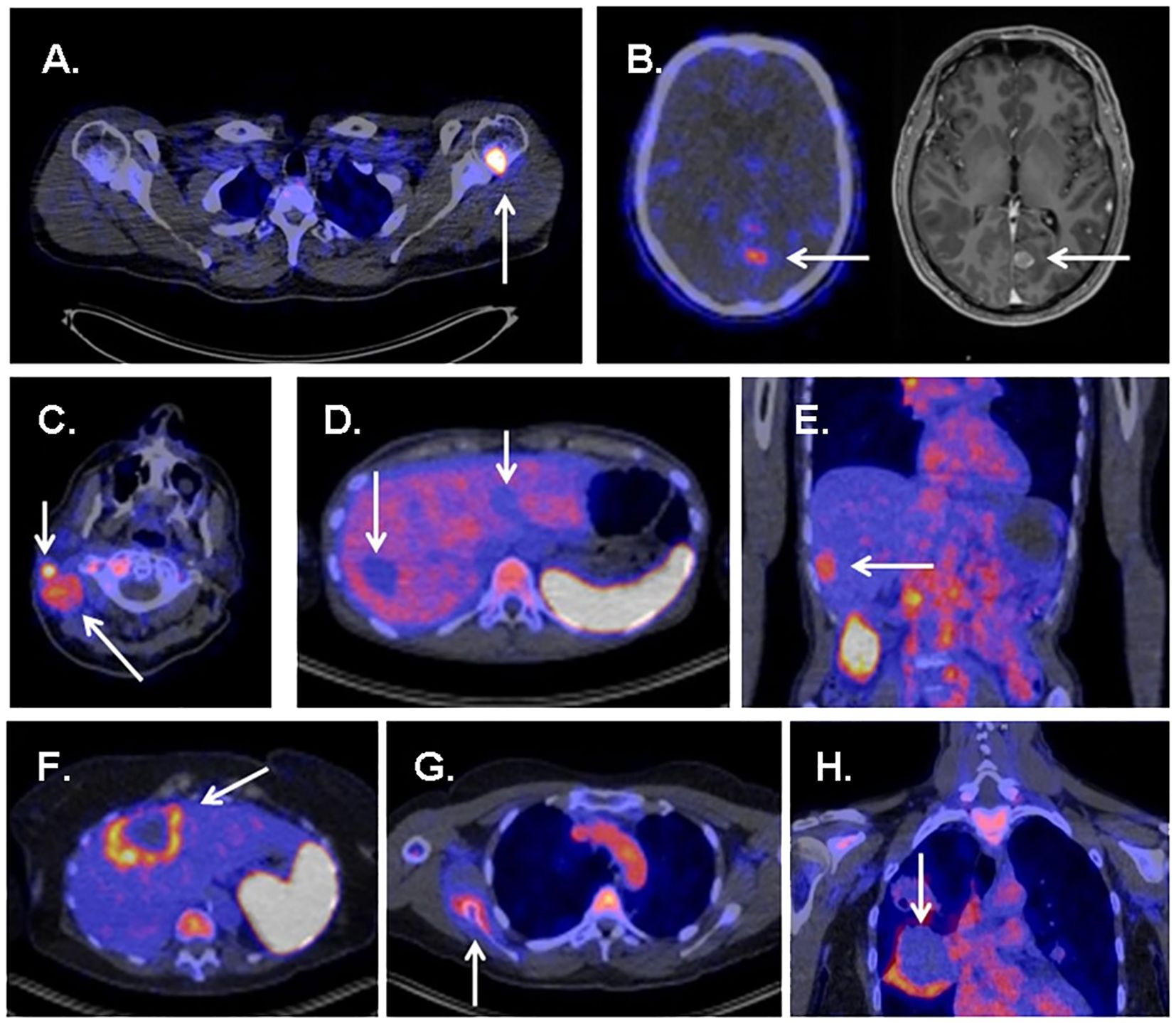
Figure 4 Several examples showing uptake of 89ZED88082A in tumor sites and metastases. (A) High uptake in bone metastasis of a patient with melanoma. (B) Uptake in a brain metastasis of a patient with melanoma. (C) Evident uptake in multiple cervical lymph node metastases in a patient with cutaneous squamous cell carcinoma. (D) Several liver metastases showing no uptake in a patient with ovarian clear cell carcinoma. (E) Uptake in a liver metastasis in a patient with squamous cell oesophageal cancer. (F) Liver metastases with rim uptake in a patient with colorectal cancer. (G) Uptake in bone lesion of a patient with squamous cell vulvar cancer. (H) Rim uptake in lung metastasis of a patient with cervical cancer. Reproduced from Kist de Ruijter L et al (76).
In an average follow-up of 5.6 months, baseline tracer uptake correlated positively with the best overall response per the Response Evaluation Criteria in Solid Tumors. The tracer accumulation in patients with no progressive disease, which included one showing complete response, eight showing partial responses, and four cases of stable disease, was 40% higher than that in patients with progressive disease. The study also found that patients with above-median baseline geometric mean maximum standardized uptake values (SUVmax) (> 5.2) tended to have better progression-free survival (PFS) and superior OS than others (76). This demonstrates the potential of ZED88082A in predicting prognosis for ICI therapy.
Studies focusing on CD8-targeted PET imaging have made significant advancements, and current developments demonstrate the feasibility of using CD8-targeted radiotracers in the field of immunotherapy. To a certain extent, these tracers have improved the efficacy of immunotherapies and may be used to assess the efficacy of newly developed treatments.
5 Functional visualization of CD8+ T cells
CD8-targeted PET tracers not only reveal the number of effector CD8+ T cells but also that of naïve and exhausted CD8+ T cells. However, the effector CD8+ T cells are the primary subtype contributing to anti-tumor immunity (104). Therefore, targeting of effector CD8+ T cells results in a functional representation of all CD8+ T cells, offering a comprehensive and accurate assessment of their status after combining both quantitative and functional visualization.
CD8 has been identified to be a reliable target for monitoring immunotherapy in numerous studies. Considering the variable functional states of CD8+ T lymphocytes (such as exhausted T cells), and the diverse impacts of various factors on these cells, several researchers have focused on the cytokines produced during the tumor-killing process and throughout T cell activation, which can indicate the activation of cytotoxic T lymphocytes (105).
5.1 Granzyme B
Granzyme B, released by activated effector CD8+ T lymphocytes, participates in the direct tumor-killing mechanism. As a potent representative of the anti-tumor immune response, granzyme B is considered a potential predictive biomarker for cancer therapy (106, 107). Larimer designed a novel peptide-based imaging probe, 68Ga-NOTA-GZP, which specifically represents granzyme B expression. They demonstrated a correlation between tracer uptake and therapeutic efficacy, verifying the granzyme B tracer’s potential as an immunotherapy predictive biomarker (85, 108). Another granzyme B-targeted tracer, 68Ga-grazytracer, showed comparably higher uptake at tumor sites than 68Ga-NOTA-GZP (87). This novel radiotracer, designed by Zhou, has demonstrated the ability to monitor granzyme B levels and the potential to evaluate the efficacy of ICIs and ACT therapy. Furthermore, it can assess intrinsically-induced immune responses, as shown by its ability to distinguish true progression from pseudoprogression in mouse models, potentially complementing the results obtained using 18F-FDG. This study also investigated the feasibility of clinical translation in five patients, obtaining positive results consistent with preclinical findings (Figure 5) (87).
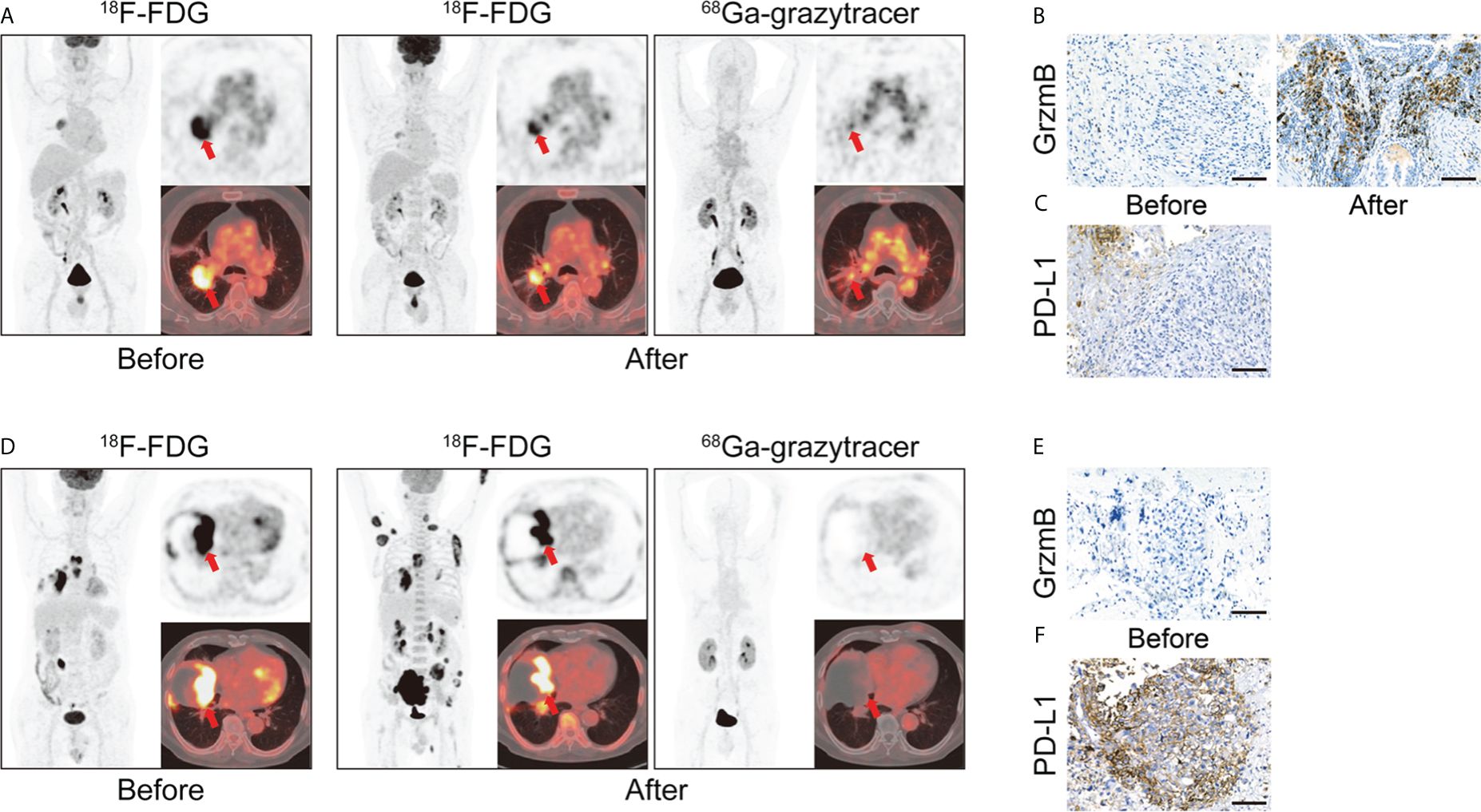
Figure 5 (A) A 66 years old patient (lung adenocarcinoma, cT2bN2M0 IIIa) with combination therapy (pemetrexed disodium + cisplatin + toripalimab). 68Ga-grazytracer PET/CT images, which performed 3 circles after treatment, demonstrate uptake in tumor lesions indicating the cytotoxic effect against tumor cells (SUVmax 4.1). Follow-up evaluation confirmed a positive prognosis for this patient. (B, C) Immunohistochemistry (IHC) staining of granzyme B and PD-L1 of corresponding patient. (D) A 70 years old patient (pulmonary sarcomatoid carcinoma, cT4N3M1c IVb) with immunotherapy (pembrolizumab). 68Ga-grazytracer PET/CT images, which performed 1 circle after treatment, reveal relatively fewer uptake (SUVmax 2.0) with follow-up evaluation suggesting a negative prognosis for this patient. (E, F) IHC staining of granzyme B and PD-L1 of corresponding patient. Reproduced from Zhou H et al. (87).
Evidence suggests that the granzyme B tracer is a valuable tool for characterizing the tumor-killing function in the context of immunotherapy. However, control of the imaging time point remains a hurdle to be overcome due to the variable time window between granzyme B secretion from cytotoxic CD8+ T cells and its arrival at malignant cells, which can be influenced by several factors (109).
5.2 IFN-γ
IFN-γ, which partially reflects the function of CD8+ T cells, may act as a promising biomarker for immuno-PET considering its significant role in the anti-tumor response. Studies have also investigated the feasibility of using IFN-γ as a therapeutic agent in immunotherapy (110, 111). 89Zr-anti-IFNγ, developed by Gibson, can be used to assess IFN-γ levels and may be potentially used as a probe for assessing active anti-tumor T cell activity and predicting treatment outcomes in animal models (88). Unlike CD8-targeted tracers, 89Zr-anti-IFNγ can directly represent effector function, addressing the limitation that CD8+ T cells might become dysfunctional despite the presence of tumor-infiltrating lymphocytes. However, CD8+ T cells are not the only subset capable of secreting this cytokine. Depending on the cell subset, IFN-γ might not only exert an anti-tumor effect but also potentially promote tumor progression (112). Therefore, the feasibility and practical value of targeting IFN-γ for immuno-PET has to be verified. Further investigation on IFN-γ-targeted tracers is required not only to evaluate the efficacy of immunotherapy but also to promote the development of IFN-γ-based therapeutic methods.
5.3 Arabinofuranosyl guanine
An 18F-labeled analog of AraG, which acts as a substrate for deoxyguanosine kinase (dGK), significantly influences T cell activation and functionality, particularly from a metabolic perspective, and can be used to elucidate the status of immune cells (113, 114). 18F-AraG can be used to detect the immune response to immunotherapy, as it shows pronounced accumulation in human immune cells (92, 115–116). In contrast, macrophages and dendritic cells negligibly affected the tracer uptake, while a significant increase in 18F-AraG uptake correlated with the activation of CD8+ T lymphocytes. Unlike 18F-FDG, which reflects tumor metabolism, 18F-AraG can directly illustrate the course of the immune response, overcoming a major limitation of 18F-FDG in immuno-PET applications (92). Levi and colleagues have demonstrated the significant value of 18F-AraG in evaluating anti-PD-1 therapy and chemotherapy. However, the variability in individual response kinetics may challenge its clinical translation. Further investigation is required to determine whether this tracer is sufficiently sensitive to detect authentic clinical changes and to optimize its use in the future.
5.4 Interleukin-2 receptor
IL-2 plays an active role in both differentiation and activation of T lymphocytes and induces cytotoxic effects by binding to their receptors on target cells (117, 118). IL-2 receptors can be categorized into monomeric, dimeric, and trimeric IL-2Rs. The high-affinity IL-2R consists of CD25, CD122, and CD132 subunits, all of which exist on activated T cells (119). A PET-based tracer, N-(4-18F-fluorobenzoyl) interleukin-2 (18F-FB-IL2), can be used to visualize activated T cells in animal models (90, 120). A clinical study (NCT02922283) involving 19 melanoma patients was conducted to investigate the biodistribution and kinetics in human subjects and assess its translational feasibility (91). Furthermore, 11 patients underwent 18F-FB-IL2 scans both at baseline and after receiving immunotherapy. Results indicated that the tracer could identify tumor lesions; however, uptake was generally low, and significant correlation was not observed between tracer uptake and therapy-induced changes (91). Therefore, related tracers have to be optimized further to determine whether IL-2R is a suitable target that can accurately and authentically reflect changes induced by ICIs. It is noteworthy that IL-2 has been approved by the Food and Drug Administration of USA for cancer immunotherapy; however, the subsequent results did not meet the initial expectations (121). IL-2R-based PET tracers may be used to develop innovative IL-2 therapeutic strategies.
6 Conclusion and future prospects
While immunotherapy is recognized as a groundbreaking advancement in oncology, questions regarding the varying immunogenic statuses of tumors, especially in terms of CD8+ T lymphocyte infiltration and their diverse responses to immunotherapy, remain. CD8+ T cells play a pivotal role in immune-mediated tumor killing, and hence, CD8-targeted PET radiotracers have become research hotspots. They can be used to track alterations in CD8+ T lymphocytes, contributing to the generation of a comprehensive immune profile. A series of preclinical and clinical studies have investigated the potential applications of such radiotracers, demonstrating their capacity to stratify patients, predict outcomes, and evaluate the efficacy of immunotherapies. Notably, baseline CD8 imaging has shown a correlation with better overall responses, indicating its potential as an early predictive biomarker. Furthermore, the emergence of PET tracers that depict the functional status of CD8+ T cells, distinguishing activated effector cells, offers the possibility of guiding individualized immunotherapy in a precise manner.
It is noteworthy that the patient numbers and tumor types in most clinical trials are relatively limited. For a more comprehensive investigation on the feasibility of clinical translation and standardized applications, future studies using larger cohorts, multidisciplinary integration, and long-term longitudinal design are required. The current tracers that visualize activated CD8+ T cells are limited, and additional biomarkers reflecting the function of CD8+ T cells remain underexplored. Some emerging PET imaging parameters require further validation in preclinical studies and multi-center collaborations. A large number of the existing PET tracers based on antibodies have yielded positive results in preclinical studies; however, their radiation burden and long serum half-lives will hinder their subsequent clinical application. Low molecular weight/peptide-based PET tracers coupled with short half-life radioisotopes such as 68Ga and 18F, which possess the advantages of low radiation burden and shorter image acquisition time, are required for clinical translation. In clinical strategies for tackling malignancies, the trend is shifting toward the use of combination therapy. The dynamic evaluation of such therapies using PET tracers still warrants further investigations.
In conclusion, in vivo, systematic, quantifiable, and visual molecular imaging technology may significantly aid clinicians in devising optimal regimens for immunotherapy in precision oncology.
Author contributions
JZ: Visualization, Writing – original draft, Writing – review & editing. BD: Funding acquisition, Writing – original draft, Writing – review & editing. YW: Writing – original draft, Writing – review & editing. YC: Investigation, Writing – review & editing. SW: Investigation, Writing – review & editing. YZ: Investigation, Writing – review & editing. YL: Funding acquisition, Supervision, Writing – review & editing. XL: Conceptualization, Investigation, Supervision, Writing – review & editing.
Funding
The author(s) declare financial support was received for the research, authorship, and/or publication of this article. This work was supported by National Natural Science Foundation of China (grant no. 81971652, 82171987, 82272043).
Conflict of interest
The authors declare that the research was conducted in the absence of any commercial or financial relationships that could be construed as a potential conflict of interest.
Publisher’s note
All claims expressed in this article are solely those of the authors and do not necessarily represent those of their affiliated organizations, or those of the publisher, the editors and the reviewers. Any product that may be evaluated in this article, or claim that may be made by its manufacturer, is not guaranteed or endorsed by the publisher.
References
1. Demaria O, Cornen S, Daëron M, Morel Y, Medzhitov R, Vivier E. Harnessing innate immunity in cancer therapy. Nature. (2019) 574:45–56. doi: 10.1038/s41586-019-1593-5
2. Peterson C, Denlinger N, Yang Y. Recent advances and challenges in cancer immunotherapy. Cancers. (2022) 14:3972. doi: 10.3390/cancers14163972
3. Yang Y. Cancer immunotherapy: harnessing the immune system to battle cancer. J Clin Invest. (2015) 125:3335–7. doi: 10.1172/JCI83871
4. Ribas A, Wolchok JD. Cancer immunotherapy using checkpoint blockade. Science. (2018) 359:1350–5. doi: 10.1126/science.aar4060
5. Singh AK, McGuirk JP. CAR T cells: continuation in a revolution of immunotherapy. Lancet Oncol. (2020) 21:e168–78. doi: 10.1016/S1470-2045(19)30823-X
6. Sharma P, Hu-Lieskovan S, Wargo JA, Ribas A. Primary, adaptive, and acquired resistance to cancer immunotherapy. Cell. (2017) 168:707–23. doi: 10.1016/j.cell.2017.01.017
7. Gibney GT, Weiner LM, Atkins MB. Predictive biomarkers for checkpoint inhibitor-based immunotherapy. Lancet Oncol. (2016) 17:e542–51. doi: 10.1016/S1470-2045(16)30406-5
8. Pan Y, Fu Y, Zeng Y, Liu X, Peng Y, Hu C, et al. The key to immunotherapy: how to choose better therapeutic biomarkers for patients with non-small cell lung cancer. biomark Res. (2022) 10:9. doi: 10.1186/s40364-022-00355-7
9. Zeng DQ, Yu YF, Ou QY, Li XY, Zhong RZ, Xie CM, et al. Prognostic and predictive value of tumor-infiltrating lymphocytes for clinical therapeutic research in patients with non-small cell lung cancer. Oncotarget. (2016) 7:13765–81. doi: 10.18632/oncotarget.v7i12
10. Hiam-Galvez KJ, Allen BM, Spitzer MH. Systemic immunity in cancer. Nat Rev Cancer. (2021) 21:345–59. doi: 10.1038/s41568-021-00347-z
11. Giles JR, Globig AM, Kaech SM, Wherry EJ. CD8+ T cells in the cancer-immunity cycle. Immunity. (2023) 56:2231–53. doi: 10.1016/j.immuni.2023.09.005
12. Duffy MJ, Crown J. Biomarkers for predicting response to immunotherapy with immune checkpoint inhibitors in cancer patients. Clin Chem. (2019) 65:1228–38. doi: 10.1373/clinchem.2019.303644
13. Dejardin D, Kraxner A, Blank A, Rieder N, Teichgräber V, Städler N, et al. A composite decision rule of CD8+ T-cell density in tumor biopsies predicts efficacy in early-stage, immunotherapy trials. Clin Cancer Res. (2024) 30:877–82. doi: 10.1158/1078-0432
14. Fridman WH, Zitvogel L, Sautès-Fridman C, Kroemer G. The immune contexture in cancer prognosis and treatment. Nat Rev Clin Oncol. (2017) 14:717–34. doi: 10.1038/nrclinonc.2017.101
15. Haywood S, Garioch J, Ramaiya A, Moncrieff M. Quantitative and spatial analysis of CD8+/PD-1 tumor-infiltrating lymphocytes as a predictive biomarker for clinical response of melanoma in-transit metastases to topical immunotherapy. Ann Surg Oncol. (2021) 28:1029–38. doi: 10.1245/s10434-020-08713-1
16. Yu Y, Zeng D, Ou Q, Liu S, Li A, Chen Y, et al. Association of survival and immune-related biomarkers with immunotherapy in patients with non-small cell lung cancer: A meta-analysis and individual patient-level analysis. JAMA Netw Open. (2019) 2:e196879. doi: 10.1001/jamanetworkopen.2019.6879
17. Semiglazov V, Tseluiko A, Kudaybergenova A, Artemyeva A, Krivorotko P, Donskih R. Immunology and immunotherapy in breast cancer. Cancer Biol Med. (2022) 19:609–18. doi: 10.20892/j.issn.2095-3941.2021.0597
18. Otter SJ, Chatterjee J, Stewart AJ, Michael A. The role of biomarkers for the prediction of response to checkpoint immunotherapy and the rationale for the use of checkpoint immunotherapy in cervical cancer. Clin Oncol (R Coll Radiol). (2019) 31:834–43. doi: 10.1016/j.clon.2019.07.003
19. Kurtulus S, Madi A, Escobar G, Klapholz M, Nyman J, Christian E, et al. Checkpoint blockade immunotherapy induces dynamic changes in PD-1-CD8+ Tumor-infiltrating T cells. Immunity. (2019) 50:181–194.e6. doi: 10.1016/j.immuni.2018.11.014
20. Wang C, Zeng Q, Gül ZM, Wang S, Pick R, Cheng P, et al. Circadian tumor infiltration and function of CD8+ T cells dictate immunotherapy efficacy. Cell. (2024) 187:2690–2702.e17. doi: 10.1016/j.cell.2024.04.015
21. Yamashita K, Iwatsuki M, Harada K, Koga Y, Kiyozumi Y, Eto K, et al. Can PD-L1 expression evaluated by biopsy sample accurately reflect its expression in the whole tumour in gastric cancer? Br J Cancer. (2019) 121:278–80. doi: 10.1038/s41416-019-0515-5
22. Liu Z, Li Z. Molecular imaging in tracking tumor-specific cytotoxic T lymphocytes (CTLs). Theranostics. (2014) 4:990–1001. doi: 10.7150/thno.9268
23. Wei W, Jiang D, Ehlerding EB, Luo Q, Cai W. Noninvasive PET imaging of T cells. Trends Cancer. (2018) 4:359–73. doi: 10.1016/j.trecan.2018.03.009
24. Phelps ME. PET: the merging of biology and imaging into molecular imaging. J Nucl Med. (2000) 41:661–81.
25. Juweid ME, Cheson BD. Positron-emission tomography and assessment of cancer therapy. N Engl J Med. (2006) 354:496–507. doi: 10.1056/NEJMra050276
26. Pantel AR, Mankoff DA. Molecular imaging to guide systemic cancer therapy: Illustrative examples of PET imaging cancer biomarkers. Cancer Lett. (2017) 387:25–31. doi: 10.1016/j.canlet.2016.05.008
27. Wei W, Rosenkrans ZT, Liu J, Huang G, Luo QY, Cai W. ImmunoPET: concept, design, and applications. Chem Rev. (2020) 120:3787–851. doi: 10.1021/acs.chemrev.9b00738
28. Kumar BV, Connors TJ, Farber DL. Human T cell development, localization, and function throughout life. Immunity. (2018) 48:202–13. doi: 10.1016/j.immuni.2018.01.007
29. Gascoigne NR, Rybakin V, Acuto O, Brzostek J. TCR signal strength and T cell development. Annu Rev Cell Dev Biol. (2016) 32:327–48. doi: 10.1146/annurev-cellbio-111315-125324
30. Guerder S, Flavell RA. T-cell activation. Two for T. Curr Biol. (1995) 5:866–8. doi: 10.1016/S0960-9822(95)00175-8
31. Zumerle S, Molon B, Viola A. Membrane rafts in T cell activation: A spotlight on CD28 costimulation. Front Immunol. (2017) 8:1467. doi: 10.3389/fimmu.2017.01467
32. Pennock ND, White JT, Cross EW, Cheney EE, Tamburini BA, Kedl RM. T cell responses: naive to memory and everything in between. Adv Physiol Educ. (2013) 37:273–83. doi: 10.1152/advan.00066.2013
33. Raskov H, Orhan A, Christensen JP, Gögenur I. Cytotoxic CD8+ T cells in cancer and cancer immunotherapy. Br J Cancer. (2021) 124:359–67. doi: 10.1038/s41416-020-01048-4
34. Farhood B, Najafi M, Mortezaee K. CD8+ cytotoxic T lymphocytes in cancer immunotherapy: A review. J Cell Physiol. (2019) 234:8509–21. doi: 10.1002/jcp.27782
35. Voskoboinik I, Whisstock JC, Trapani JA. Perforin and granzymes: function, dysfunction and human pathology. Nat Rev Immunol. (2015) 15:388–400. doi: 10.1038/nri3839
36. Fu Q, Fu TM, Cruz AC, Sengupta P, Thomas SK, Wang S, et al. Structural basis and functional role of intramembrane trimerization of the fas/CD95 death receptor. Mol Cell. (2016) 61:602–13. doi: 10.1016/j.molcel.2016.01.009
37. Bhat P, Leggatt G, Waterhouse N, Frazer IH. Interferon-γ derived from cytotoxic lymphocytes directly enhances their motility and cytotoxicity. Cell Death Dis. (2017) 8:e2836. doi: 10.1038/cddis.2017.67
38. van Horssen R, Ten Hagen TL, Eggermont AM. TNF-alpha in cancer treatment: molecular insights, antitumor effects, and clinical utility. Oncologist. (2006) 11:397–408. doi: 10.1634/theoncologist.11-4-397
39. Martínez-Lostao L, Anel A, Pardo J. How do cytotoxic lymphocytes kill cancer cells? Clin Cancer Res. (2015) 21:5047–56. doi: 10.1158/1078-0432.CCR-15-0685
40. Leone RD, Powell JD. Metabolism of immune cells in cancer. Nat Rev Cancer. (2020) 20:516–31. doi: 10.1038/s41568-020-0273-y
41. Philip M, Schietinger A. CD8+ T cell differentiation and dysfunction in cancer. Nat Rev Immunol. (2022) 22:209–23. doi: 10.1038/s41577-021-00574-3
42. Wang Q, Qin Y, Li B. CD8+ T cell exhaustion and cancer immunotherapy. Cancer Lett. (2023) 559:216043. doi: 10.1016/j.canlet.2022.216043
43. van der Leun AM, Thommen DS, Schumacher TN. CD8+ T cell states in human cancer: insights from single-cell analysis. Nat Rev Cancer. (2020) 20:218–32. doi: 10.1038/s41568-019-0235-4
44. Hashimoto M, Kamphorst AO, Im SJ, Kissick HT, Pillai RN, Ramalingam SS, et al. CD8 T cell exhaustion in chronic infection and cancer: opportunities for interventions. Annu Rev Med. (2018) 69:301–18. doi: 10.1146/annurev-med-012017-043208
45. Schreiber RD, Old LJ, Smyth MJ. Cancer immunoediting: integrating immunity's roles in cancer suppression and promotion. Science. (2011) 331:1565–70. doi: 10.1126/science.1203486
46. Zhang Y, Zhang Z. The history and advances in cancer immunotherapy: understanding the characteristics of tumor-infiltrating immune cells and their therapeutic implications. Cell Mol Immunol. (2020) 17:807–21. doi: 10.1038/s41423-020-0488-6
47. Pardoll DM. The blockade of immune checkpoints in cancer immunotherapy. Nat Rev Cancer. (2012) 12:252–64. doi: 10.1038/nrc3239
48. Sadreddini S, Baradaran B, Aghebati-Maleki A, Sadreddini S, Shanehbandi D, Fotouhi A, et al. Immune checkpoint blockade opens a new way to cancer immunotherapy. J Cell Physiol. (2019) 234:8541–9. doi: 10.1002/jcp.27816
49. Dermani FK, Samadi P, Rahmani G, Kohlan AK, Najafi R. PD-1/PD-L1 immune checkpoint: Potential target for cancer therapy. J Cell Physiol. (2019) 234:1313–25. doi: 10.1002/jcp.27172
50. Rowshanravan B, Halliday N, Sansom DM. CTLA-4: a moving target in immunotherapy. Blood. (2018) 131:58–67. doi: 10.1182/blood-2017-06-741033
51. de Castro G Jr, Kudaba I, Wu YL, Lopes G, Kowalski DM, Turna HZ, et al. Five-year outcomes with pembrolizumab versus chemotherapy as first-line therapy in patients with non-small-cell lung cancer and programmed death ligand-1 tumor proportion score ≥ 1% in the KEYNOTE-042 study. J Clin Oncol. (2023) 41:1986–91. doi: 10.1200/JCO.21.02885
52. Desai A, Peters S. Immunotherapy-based combinations in metastatic NSCLC. Cancer Treat Rev. (2023) 116:102545. doi: 10.1016/j.ctrv.2023.102545
53. Paz-Ares L, Ciuleanu TE, Cobo M, Schenker M, Zurawski B, Menezes J, et al. First-line nivolumab plus ipilimumab combined with two cycles of chemotherapy in patients with non-small-cell lung cancer (CheckMate 9LA): an international, randomised, open-label, phase 3 trial. Lancet Oncol. (2021) 22:198–211. doi: 10.1016/S1470-2045(20)30641-0
54. Hong M, Clubb JD, Chen YY. Engineering CAR-T cells for next-generation cancer therapy. Cancer Cell. (2020) 38:473–88. doi: 10.1016/j.ccell.2020.07.005
55. Ma S, Li X, Wang X, Cheng L, Li Z, Zhang C, et al. Current progress in CAR-T cell therapy for solid tumors. Int J Biol Sci. (2019) 15:2548–60. doi: 10.7150/ijbs.34213
56. Shalhout SZ, Miller DM, Emerick KS, Kaufman HL. Therapy with oncolytic viruses: progress and challenges. Nat Rev Clin Oncol. (2023) 20:160–77. doi: 10.1038/s41571-022-00719-w
57. Lin MJ, Svensson-Arvelund J, Lubitz GS, Marabelle A, Melero I, Brown BD, et al. Cancer vaccines: the next immunotherapy frontier. Nat Cancer. (2022) 3:911–26. doi: 10.1038/s43018-022-00418-6
58. Chen Y, Yu D, Qian H, Shi Y, Tao Z. CD8+ T cell-based cancer immunotherapy. J Transl Med. (2024) 22:394. doi: 10.1186/s12967-024-05134-6
59. Zboralski D, Osterkamp F, Christensen E, Bredenbeck A, Schumann A, Hoehne A, et al. Fibroblast activation protein targeted radiotherapy induces an immunogenic tumor microenvironment and enhances the efficacy of PD-1 immune checkpoint inhibition. Eur J Nucl Med Mol Imaging. (2023) 50:2621–35. doi: 10.1007/s00259-023-06211-6
60. Dercle L, Sun S, Seban RD, Mekki A, Sun R, Tselikas L, et al. Emerging and evolving concepts in cancer immunotherapy imaging. Radiology. (2023) 306:32–46. doi: 10.1148/radiol.210518
61. van Dongen GAMS, Beaino W, Windhorst AD, Zwezerijnen GJC, Oprea-Lager DE, Hendrikse NH, et al. The role of 89Zr-immuno-PET in navigating and derisking the development of biopharmaceuticals. J Nucl Med. (2021) 62:438–45. doi: 10.2967/jnumed.119.239558
62. De Feo MS, Pontico M, Frantellizzi V, Corica F, De Cristofaro F, De Vincentis G. 89Zr-PET imaging in humans: a systematic review. Clin Transl Imaging. (2022) 10:23–36. doi: 10.1007/s40336-021-00462-9
63. da Silva DA, De Luca A, Squitti R, Rongioletti M, Rossi L, MaChado CML, et al. Copper in tumors and the use of copper-based compounds in cancer treatment. J Inorg Biochem. (2022) 226:111634. doi: 10.1016/j.jinorgbio.2021.111634
64. Sanchez-Crespo A. Comparison of Gallium-68 and Fluorine-18 imaging characteristics in positron emission tomography. Appl Radiat Isot. (2013) 76:55–62. doi: 10.1016/j.apradiso.2012.06.034
65. Chomet M, van Dongen GAMS, Vugts DJ. State of the art in radiolabeling of antibodies with common and uncommon radiometals for preclinical and clinical immuno-PET. Bioconjug Chem. (2021) 32:1315–30. doi: 10.1021/acs.bioconjchem.1c00136
66. Rashidian M, Ploegh H. Nanobodies as non-invasive imaging tools. Immunooncol Technol. (2020) 7:2–14. doi: 10.1016/j.iotech.2020.07.001
67. Abousaway O, Rakhshandehroo T, Van den Abbeele AD, Kircher MF, Rashidian M. Noninvasive imaging of cancer immunotherapy. Nanotheranostics. (2021) 5:90–112. doi: 10.7150/ntno.50860
68. Tavaré R, McCracken MN, Zettlitz KA, Knowles SM, Salazar FB, Olafsen T, et al. Engineered antibody fragments for immuno-PET imaging of endogenous CD8+ T cells. vivo. Proc Natl Acad Sci U.S.A. (2014) 111:1108–13. doi: 10.1073/pnas.1316922111
69. Tavaré R, McCracken MN, Zettlitz KA, Salazar FB, Olafsen T, Witte ON, et al. Immuno-PET of murine T cell reconstitution postadoptive stem cell transplantation using anti-CD4 and anti-CD8 cys-diabodies. J Nucl Med. (2015) 56:1258–64. doi: 10.2967/jnumed.114.153338
70. Tavaré R, Escuin-Ordinas H, Mok S, McCracken MN, Zettlitz KA, Salazar FB, et al. An effective immuno-PET imaging method to monitor CD8-dependent responses to immunotherapy. Cancer Res. (2016) 76:73–82. doi: 10.1158/0008-5472.CAN-15-1707
71. Seo JW, Tavaré R, Mahakian LM, Silvestrini MT, Tam S, Ingham ES, et al. CD8+ T-cell density imaging with 64Cu-labeled cys-diabody informs immunotherapy protocols. Clin Cancer Res. (2018) 24:4976–87. doi: 10.1158/1078-0432.CCR-18-0261
72. Kristensen LK, Fröhlich C, Christensen C, Melander MC, Poulsen TT, Galler GR, et al. CD4+ and CD8a+ PET imaging predicts response to novel PD-1 checkpoint inhibitor: studies of Sym021 in syngeneic mouse cancer models. Theranostics. (2019) 9:8221–38. doi: 10.7150/thno.37513
73. Rashidian M, LaFleur MW, Verschoor VL, Dongre A, Zhang Y, Nguyen TH, et al. Immuno-PET identifies the myeloid compartment as a key contributor to the outcome of the antitumor response under PD-1 blockade. Proc Natl Acad Sci U.S.A. (2019) 116:16971–80. doi: 10.1073/pnas.1905005116
74. Kristensen LK, Christensen C, Alfsen MZ, Cold S, Nielsen CH, Kjaer A. Monitoring CD8a+ T cell responses to radiotherapy and CTLA-4 blockade using [64Cu]NOTA-CD8a PET imaging. Mol Imaging Biol. (2020) 22:1021–30. doi: 10.1007/s11307-020-01481-0
75. Gill H, Seipert R, Carroll VM, Gouasmat A, Yin J, Ogasawara A, et al. The production, quality control, and characterization of ZED8, a CD8-specific 89Zr-labeled immuno-PET clinical imaging agent. AAPS J. (2020) 22:22. doi: 10.1208/s12248-019-0392-0
76. Kist de Ruijter L, van de Donk PP, Hooiveld-Noeken JS, Giesen D, Elias SG, Lub-de Hooge MN, et al. Whole-body CD8+ T cell visualization before and during cancer immunotherapy: a phase 1/2 trial. Nat Med. (2022) 28:2601–10. doi: 10.1038/s41591-022-02084-8
77. Pandit-Taskar N, Postow MA, Hellmann MD, Harding JJ, Barker CA, O'Donoghue JA, et al. First-in-humans imaging with 89Zr-df-IAB22M2C anti-CD8 minibody in patients with solid Malignancies: preliminary pharmacokinetics, biodistribution, and lesion targeting. J Nucl Med. (2020) 61:512–9. doi: 10.2967/jnumed.119.229781
78. Schwenck J, Sonanini D, Seyfried D, Ehrlichmann W, Kienzle G, Reischl G, et al. In vivo imaging of CD8+ T cells in metastatic cancer patients: first clinical experience with simultaneous [89Zr]Zr-Df-IAB22M2C PET/MRI. Theranostics. (2023) 13:2408–23. doi: 10.7150/thno.79976
79. Nagle VL, Henry KE, Hertz CAJ, Graham MS, Campos C, Parada LF, et al. Imaging tumor-infiltrating lymphocytes in brain tumors with [64Cu]Cu-NOTA-anti-CD8 PET. Clin Cancer Res. (2021) 27:1958–66. doi: 10.1158/1078-0432.CCR-20-3243
80. Zhao H, Wang C, Yang Y, Sun Y, Wei W, Wang C, et al. ImmunoPET imaging of human CD8+ T cells with novel 68Ga-labeled nanobody companion diagnostic agents. J Nanobiotechnology. (2021) 19:42. doi: 10.1186/s12951-021-00785-9
81. Wang Y, Wang C, Huang M, Qin S, Zhao J, Sang S, et al. Pilot study of a novel nanobody 68 Ga-NODAGA-SNA006 for instant PET imaging of CD8+ T cells. Eur J Nucl Med Mol Imaging. (2022) 49:4394–405. doi: 10.1007/s00259-022-05903-9
82. Sriraman SK, Davies CW, Gill H, Kiefer JR, Yin J, Ogasawara A, et al. Development of an 18F-labeled anti-human CD8 VHH for same-day immunoPET imaging. Eur J Nucl Med Mol Imaging. (2023) 50:679–91. doi: 10.1007/s00259-022-05998-0
83. Tavaré R, Danton M, Giurleo JT, Makonnen S, Hickey C, Arnold TC, et al. Immuno-PET monitoring of lymphocytes using the CD8-specific antibody REGN5054. Cancer Immunol Res. (2022) 10:1190–209. doi: 10.1158/2326-6066.CIR-21-0405
84. Alsaid H, Cheng SH, Bi M, Xie F, Rambo M, Skedzielewski T, et al. Immuno-PET monitoring of CD8+ T cell infiltration post ICOS agonist antibody treatment alone and in combination with PD-1 blocking antibody using a 89Zr anti-CD8+ Mouse minibody in EMT6 syngeneic tumor mouse. Mol Imaging Biol. (2023) 25:528–40. doi: 10.1007/s11307-022-01781-7
85. Larimer BM, Wehrenberg-Klee E, Dubois F, Mehta A, Kalomeris T, Flaherty K, et al. Granzyme B PET imaging as a predictive biomarker of immunotherapy response. Cancer Res. (2017) 77:2318–27. doi: 10.1158/0008-5472.CAN-16-3346
86. Hartimath SV, Ramasamy B, Xuan TY, Rong TJ, Khanapur S, Cheng P, et al. Granzyme B PET imaging in response to in situ vaccine therapy combined with αPD1 in a murine colon cancer model. Pharmaceutics. (2022) 14:150. doi: 10.3390/pharmaceutics14010150
87. Zhou H, Wang Y, Xu H, Shen X, Zhang T, Zhou X, et al. Noninvasive interrogation of CD8+ T cell effector function for monitoring early tumor responses to immunotherapy. J Clin Invest. (2022) 132:e161065. doi: 10.1172/JCI161065
88. Gibson HM, McKnight BN, Malysa A, Dyson G, Wiesend WN, McCarthy CE, et al. IFNγ PET imaging as a predictive tool for monitoring response to tumor immunotherapy. Cancer Res. (2018) 78:5706–17. doi: 10.1158/0008-5472.CAN-18-0253
89. Rezazadeh F, Ramos N, Saliganan AD, Barr S, Peraino N, Schomburg F, et al. Evaluation and selection of a lead diabody for interferon-γ PET imaging. Nucl Med Biol. (2022) 114-115:162–7. doi: 10.1016/j.nucmedbio.2022.06.001
90. Di Gialleonardo V, Signore A, Glaudemans AW, Dierckx RA, De Vries EF. N-(4-18F-fluorobenzoyl)interleukin-2 for PET of human-activated T lymphocytes. J Nucl Med. (2012) 53:679–86. doi: 10.2967/jnumed.111.091306
91. van de Donk PP, Wind TT, Hooiveld-Noeken JS, van der Veen EL, Glaudemans AWJM, Diepstra A, et al. Interleukin-2 PET imaging in patients with metastatic melanoma before and during immune checkpoint inhibitor therapy. Eur J Nucl Med Mol Imaging. (2021) 48:4369–76. doi: 10.1007/s00259-021-05407-y
92. Levi J, Goth S, Huynh L, Lam T, Huynh TL, Schulte B, et al. 18F-araG PET for CD8 profiling of tumors and assessment of immunomodulation by chemotherapy. J Nucl Med. (2021) 62:802–7. doi: 10.2967/jnumed.120.249078
93. Gajewski TF, Schreiber H, Fu YX. Innate and adaptive immune cells in the tumor microenvironment. Nat Immunol. (2013) 14:1014–22. doi: 10.1038/ni.2703
94. Lei X, Lei Y, Li JK, Du WX, Li RG, Yang J, et al. Immune cells within the tumor microenvironment: Biological functions and roles in cancer immunotherapy. Cancer Lett. (2020) 470:126–33. doi: 10.1016/j.canlet.2019.11.009
95. Griessinger CM, Olafsen T, Mascioni A, Jiang ZK, Zamilpa C, Jia F, et al. The PET-tracer 89Zr-df-IAB22M2C enables monitoring of intratumoral CD8 T-cell infiltrates in tumor-bearing humanized mice after T-cell bispecific antibody treatment. Cancer Res. (2020) 80:2903–13. doi: 10.1158/0008-5472.CAN-19-3269
96. Maresca KP, Chen J, Mathur D, Giddabasappa A, Root A, Narula J, et al. Preclinical evaluation of 89Zr-df-IAB22M2C PET as an imaging biomarker for the development of the GUCY2C-CD3 bispecific PF-07062119 as a T cell engaging therapy. Mol Imaging Biol. (2021) 23:941–51. doi: 10.1007/s11307-021-01621-0
97. Farwell MD, Gamache RF, Babazada H, Hellmann MD, Harding JJ, Korn R, et al. CD8-targeted PET imaging of tumor-infiltrating T cells in patients with cancer: A phase I first-in-humans study of 89Zr-df-IAB22M2C, a radiolabeled anti-CD8 minibody. J Nucl Med. (2022) 63:720–6. doi: 10.2967/jnumed.121.262485
98. Yang E, Liu Q, Huang G, Liu J, Wei W. Engineering nanobodies for next-generation molecular imaging. Drug Discovery Today. (2022) 27:1622–38. doi: 10.1016/j.drudis.2022.03.013
99. Ramon-Patino JL, Schmid S, Lau S, Seymour L, Gaudreau PO, Li JJN, et al. iRECIST and atypical patterns of response to immuno-oncology drugs. J Immunother Cancer. (2022) 10:e004849. doi: 10.1136/jitc-2022-004849
100. Persigehl T, Lennartz S, Schwartz LH. iRECIST: how to do it. Cancer Imaging. (2020) 20:2. doi: 10.1186/s40644-019-0281-x
101. Chen MY, Zeng YC. Pseudoprogression in lung cancer patients treated with immunotherapy. Crit Rev Oncol Hematol. (2022) 169:103531. doi: 10.1016/j.critrevonc.2021.103531
102. Chai LF, Prince E, Pillarisetty VG, Katz SC. Challenges in assessing solid tumor responses to immunotherapy. Cancer Gene Ther. (2020) 27:528–38. doi: 10.1038/s41417-019-0155-1
103. Ogasawara A, Kiefer JR, Gill H, Chiang E, Sriraman S, Ferl GZ, et al. Preclinical development of ZED8, an 89Zr immuno-PET reagent for monitoring tumor CD8 status in patients undergoing cancer immunotherapy. Eur J Nucl Med Mol Imaging. (2023) 50:287–301. doi: 10.1007/s00259-022-05968-6
104. St Paul M, Ohashi PS. The roles of CD8+ T cell subsets in antitumor immunity. Trends Cell Biol. (2020) 30:695–704. doi: 10.1016/j.tcb.2020.06.003
105. Obst R. The timing of T cell priming and cycling. Front Immunol. (2015) 6:563. doi: 10.3389/fimmu.2015.00563
106. Trapani JA, Smyth MJ. Functional significance of the perforin/granzyme cell death pathway. Nat Rev Immunol. (2002) 2:735–47. doi: 10.1038/nri911
107. Rousalova I, Krepela E. Granzyme B-induced apoptosis in cancer cells and its regulation (review). Int J Oncol. (2010) 37:1361–78. doi: 10.3892/ijo_00000788
108. Larimer BM, Bloch E, Nesti S, Austin EE, Wehrenberg-Klee E, Boland G, et al. The effectiveness of checkpoint inhibitor combinations and administration timing can be measured by granzyme B PET imaging. Clin Cancer Res. (2019) 25:1196–205. doi: 10.1158/1078-0432.CCR-18-2407
109. Thiery J, Keefe D, Boulant S, Boucrot E, Walch M, Martinvalet D, et al. Perforin pores in the endosomal membrane trigger the release of endocytosed granzyme B into the cytosol of target cells. Nat Immunol. (2011) 12:770–7. doi: 10.1038/ni.2050
110. Dunn GP, Koebel CM, Schreiber RD. Interferons, immunity and cancer immunoediting. Nat Rev Immunol. (2006) 6:836–48. doi: 10.1038/nri1961
111. Ni L, Lu J. Interferon gamma in cancer immunotherapy. Cancer Med. (2018) 7:4509–16. doi: 10.1002/cam4.1700
112. Gocher AM, Workman CJ, Vignali DAA. Interferon-γ: teammate or opponent in the tumour microenvironment? Nat Rev Immunol. (2022) 22:158–72. doi: 10.1038/s41577-021-00566-3
113. Buck MD, O'Sullivan D, Klein Geltink RI, Curtis JD, Chang CH, Sanin DE, et al. Mitochondrial dynamics controls T cell fate through metabolic programming. Cell. (2016) 166:63–76. doi: 10.1016/j.cell.2016.05.035
114. Almeida L, Lochner M, Berod L, Sparwasser T. Metabolic pathways in T cell activation and lineage differentiation. Semin Immunol. (2016) 28:514–24. doi: 10.1016/j.smim.2016.10.009
115. Levi J, Lam T, Goth SR, Yaghoubi S, Bates J, Ren G, et al. Imaging of activated T cells as an early predictor of immune response to anti-PD-1 therapy. Cancer Res. (2019) 79:3455–65. doi: 10.1158/0008-5472.CAN-19-0267
116. Namavari M, Chang YF, Kusler B, Yaghoubi S, Mitchell BS, Gambhir SS. Synthesis of 2'-deoxy-2'-[18F]fluoro-9-β-D-arabinofuranosylguanine: a novel agent for imaging T-cell activation with PET. Mol Imaging Biol. (2011) 13:812–8. doi: 10.1007/s11307-010-0414-x
117. Sun Z, Ren Z, Yang K, Liu Z, Cao S, Deng S, et al. A next-generation tumor-targeting IL-2 preferentially promotes tumor-infiltrating CD8+ T-cell response and effective tumor control. Nat Commun. (2019) 10:3874. doi: 10.1038/s41467-019-11782-w
118. Damoiseaux J. The IL-2 - IL-2 receptor pathway in health and disease: The role of the soluble IL-2 receptor. Clin Immunol. (2020) 218:108515. doi: 10.1016/j.clim.2020.108515
119. Boyman O, Sprent J. The role of interleukin-2 during homeostasis and activation of the immune system. Nat Rev Immunol. (2012) 12:180–90. doi: 10.1038/nri3156
120. Di Gialleonardo V, Signore A, Willemsen AT, Sijbesma JW, Dierckx RA, de Vries EF. Pharmacokinetic modelling of N-(4-[(18)F]fluorobenzoyl)interleukin-2 binding to activated lymphocytes in an xenograft model of inflammation. Eur J Nucl Med Mol Imaging. (2012) 39:1551–60. doi: 10.1007/s00259-012-2176-y
Keywords: immunotherapy, CD8 + T cells, PET, clinical application, cancer
Citation: Zhang J, Du B, Wang Y, Cui Y, Wang S, Zhao Y, Li Y and Li X (2024) The role of CD8 PET imaging in guiding cancer immunotherapy. Front. Immunol. 15:1428541. doi: 10.3389/fimmu.2024.1428541
Received: 06 May 2024; Accepted: 27 June 2024;
Published: 12 July 2024.
Edited by:
Kelsey P. Kubelick, University of Virginia, United StatesReviewed by:
Xinpei Deng, Sun Yat-sen University Cancer Center (SYSUCC), ChinaAjay Kumar Sharma, Johns Hopkins University, United States
Copyright © 2024 Zhang, Du, Wang, Cui, Wang, Zhao, Li and Li. This is an open-access article distributed under the terms of the Creative Commons Attribution License (CC BY). The use, distribution or reproduction in other forums is permitted, provided the original author(s) and the copyright owner(s) are credited and that the original publication in this journal is cited, in accordance with accepted academic practice. No use, distribution or reproduction is permitted which does not comply with these terms.
*Correspondence: Yaming Li, eW1saTIwMDFAMTYzLmNvbQ==; Xuena Li, bGl4dWVuYWNtdW5tQDE2My5jb20=
†These authors have contributed equally to this work