- 1State Key Laboratory of Respiratory Disease, National Clinical Research Center for Respiratory Disease, National Center for Respiratory Medicine, Guangzhou Institute of Respiratory Health, The First Affiliated Hospital of Guangzhou Medical University, Guangzhou, China
- 2Guangzhou Laboratory, Bio-island, Guangzhou, China
Lactylation is a process where lactate, a cellular metabolism byproduct, is added to proteins, altering their functions. In the realm of macrophage activation, lactylation impacts inflammatory response and immune regulation. Understanding the effects of lactylation on macrophage activation is vital in lung diseases, as abnormal activation and function are pivotal in conditions like pneumonia, pulmonary fibrosis, COPD, and lung cancer. This review explores the concept of lactylation, its regulation of macrophage activation, and recent research progress in lung diseases. It offers new insights into lung disease pathogenesis and potential therapeutic targets.
1 Introduction
Lung diseases, a group of disorders that affect the respiratory system, are caused by a variety of factors, including genetic predisposition, environmental factors, infections, lifestyle, and occupational exposures (1). There is a wide range of lung diseases including, but not limited to, asthma, chronic obstructive pulmonary disease, pneumonia, tuberculosis, pulmonary fibrosis and lung cancer (1–4). The symptoms and severity of these diseases range from mild to severe, affecting respiratory function and life quality of the patients. The pathogenesis of lung diseases is complex, involving inflammatory response, cellular damage, tissue repair and fibrosis. Identification of pathogenic mechanisms and novel therapeutic approaches is particularly important to reduce the disease burden.
Epigenetic modifications are chemical alterations to DNA and proteins, rather than changes to the gene sequence itself. These changes mainly encompass DNA methylation, post-translational modifications (PTM) of histones, and non-coding RNAs. They have the potential to influence gene expression and the transmission of genetic information. Epigenetic modifications are indispensable in cell differentiation, disease progression, and environmental adaptation, playing a vital role in the development and survival of organisms. A strong correlation exists between cellular metabolism and epigenetic modifications (5). Metabolites produced during metabolic processes can impact epigenetic alterations in cells, which in turn regulate metabolic programming. The interaction between the two processes collaboratively modulates gene expression and cellular function (6).
Lactate in moderate amounts is not harmful to the body as it is a normal metabolic byproduct that can be efficiently eliminated under aerobic conditions. However, its excessive accumulation can disrupt the body’s homeostasis. Accumulated lactate triggers cell signaling pathways that regulate inflammatory progression and tumor immune tolerance. It is involved in various diseases and inhibits acute inflammation (7, 8). The tissue damage repair process is regulated by cytokines and growth factors. Lactate acts as an energy substrate to meet the high metabolic demands of repair (9, 10). Lactate and lactate-mediated activation play a significant role in various aspects of tumor progression, such as cell proliferation, invasion, angiogenesis, immune tolerance, and immune cell evasion (11, 12). Lactate was once seen as a byproduct of anaerobic metabolic processes, accumulating in the tissue microenvironment of inflammatory and neoplastic diseases, whereas some studies take lactate as a signaling molecule (6). Histone lactylation, a post-translational modification first described in 2019, involves glycolysis-derived lactate acting as a substrate for histone lactylation. This process directly influences the transcription of chromatin genes by modifying histone lysine residues (13). Further studies show that histone lactylation plays a role in regulating macrophage M1/2 polarization (14), cellular reprogramming (15), and tumorigenesis (16, 17). As research continues to deepen and expand, it has been found that lactate-driven lactylation occurs not only in histones, but also in other organelles and non-histone proteins (17, 18). This implies the important role of lactylation in various physiological and pathological processes. Lactate is now recognized beyond its metabolic function, as lactylation has emerged as a new area of research in protein post-translational modifications, particularly in the realms of tumor biology and immunity.
Macrophages, the firstline of immune response, uphold tissue homeostasis by recognizing and eliminating pathogens, killing target cells, presenting antigens, and regulating immunity. However, their excessive aggregation and activation can also cause tissue damage (1, 19, 20). Macrophages react to microorganisms and harmful factors by initiating inflammatory response to combat pathogenic threats; the shift from a pro-inflammatory to a reparative state is crucial for anti-inflammatory effects and restoring homeostasis. The molecular mechanisms governing this transition remain unclear. Lactylation regulates macrophage activation and function through diverse pathways. Further research on lactylation in macrophages could enhance our understanding of macrophage function and immune regulation, as well as offer new insights and targets for treating related diseases.
2 Lactate and lactylation
2.1 Sources of lactate
Lactate, an organic acid with the chemical formula C3H6O3, is a hydroxy acid and is also known as hydroxypropionic acid due to the hydroxyl group (-OH) in its chemical structure (21). Lactate is typically produced in three isomeric forms: D-lactate, L- lactate, and racemic mixture DL-lactate (22). L-lactate is the primary form in mammals, while D-lactate is an atypical mammalian metabolite and is primarily produced by glyoxalase to metabolize methylglyoxal (23). Lactate plays a crucial metabolic role in organisms via the family of transporter proteins monocarboxylic acid transporters (MCTs) (24) and its receptor G-protein-coupled receptor (GPR) 81 (25, 26). Lactate transfers inter- and intracellularly through MCTs and GPR81 to regulate physiological and pathological processes (Figure 1) (23). In mammalian cells, lactate is mainly produced from pyruvate, which is converted to lactate by lactate dehydrogenase A (LDHA). Intracellular pyruvate is derived from glycolysis and glutamine metabolism (27).
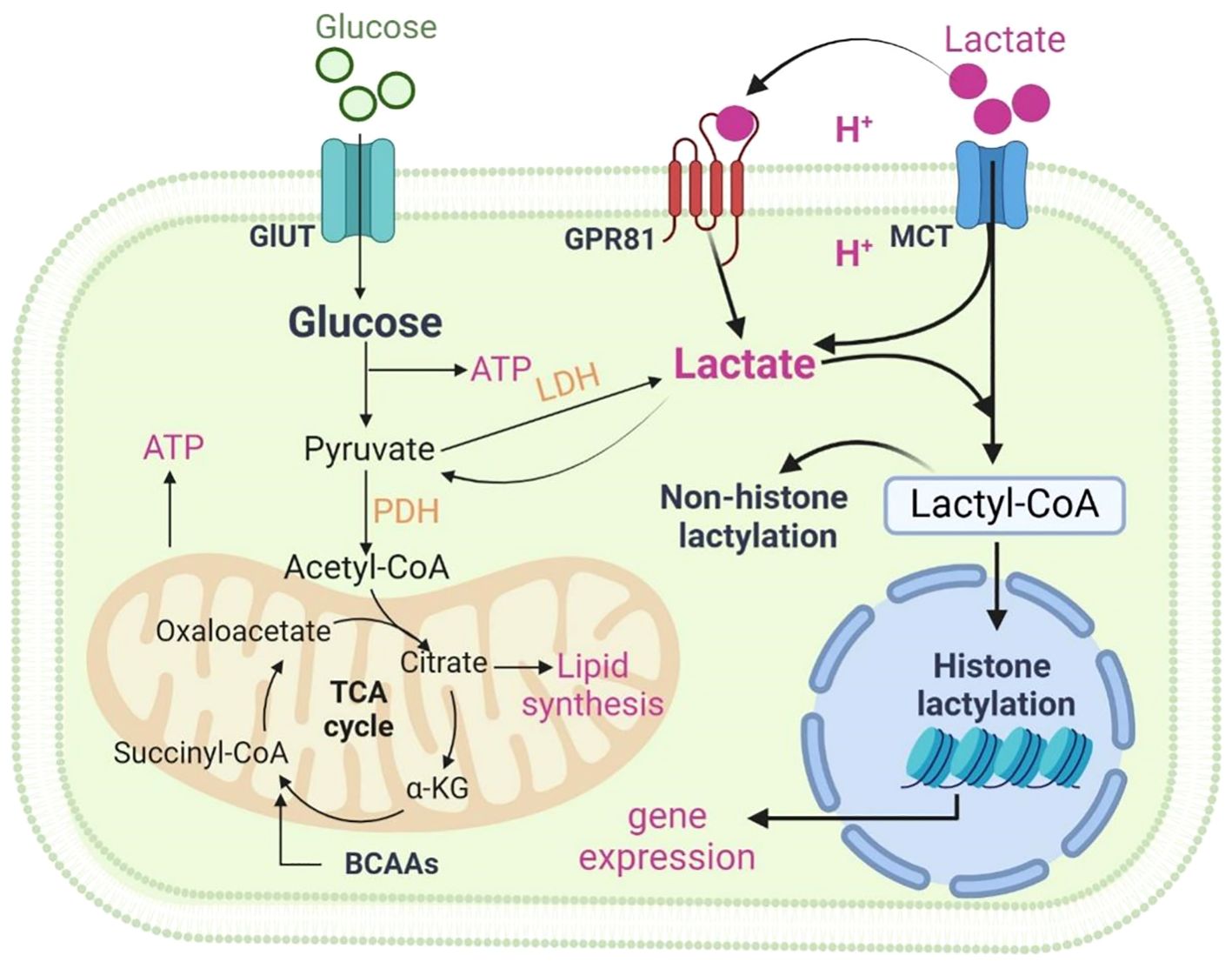
Figure 1 Metabolism of lactate. Two pathways of metabolic breakdown of lactic acid: lactate can be converted to pyruvate entering the tricarboxylic acid (TCA) cycle, or it can generate lactyl-coenzyme A (Lactyl-CoA), which is involved in lactylation of histones and non-histone proteins. Lactate transfers inter- and intracellularly through MCTs and GPR81. Figure was created with BioRender.com.
The metabolic breakdown of lactate involves two pathways: lactate can be converted to pyruvate to enter the tricarboxylic acid (TCA) cycle, or it can generate lactyl-coenzyme A (Lactyl-CoA), which is involved in lactylation of histones and non-histone proteins. Lactate metabolism involves the action of several key enzymes. Some of the major ones include: Lactate dehydrogenase (LDH), which converts pyruvate to lactate; Pyruvate kinase, facilitating the reaction between pyruvate and ADP to form pyruvate and ATP; Pyruvate dehydrogenase (PDH), converting pyruvate to Lactyl-CoA.
In addition to intracellular production, lactate can enter target cells through non-channel pathways or MCTs-mediated intercellular shuttling, influencing cellular physiological and pathological processes (24). Lactate shuttling involves the transportation and transformation of lactate within and between cells. This process allows rapid movement of lactate from its production site to other tissues or cells for further metabolism. Essential for maintaining lactate balance, shuttling regulates the concentration of lactate both inside and outside cells. MCT proteins primarily mediate lactate shuttling; MCT1 exhibits high lactate affinity, facilitating bidirectional transport across cell membranes, while MCT4, prevalent in highly glycolytic tissues, acts as a unidirectional transporter primarily for lactate export (28).
Factors influencing lactate levels include: 1. lactate production rate, determined by glycolysis and hypoxia levels. 2. lactate transport and metabolism, crucial for maintaining intracellular and extracellular lactate balance. 3. lactate clearance, involving liver and tissue processes that regulate its levels.
2.2 Lactylation
Lactylation, a histone modification introduced in 2019, has shown significant potential. Histone lysine lactylation has been observed to accumulate at gene promoters in cells under hypoxia, interferon (IFN)-γ, lipopolysaccharide (LPS), or bacterial attack, directly influencing gene expression for regulating bacterially-infected M1 macrophages (13, 16). As we mentioned earlier, lactate contains several forms and it has been found that L-lactate, not D-lactate, is used in the histone lactylation process (29). Apart from histones, other non-histone proteins can also undergo lactylation. Non-histone protein modifications are crucial in various cellular processes including gene transcription, DNA damage repair, cell division, signal transduction, protein folding, autophagy, and metabolism (30). Initial study on lactylation focused on macrophages, the cell type where histone lactylation was discovered, and their related diseases. To date, research on lactylation has focused on a diverse array of cell types (30). Remarkably, lactylation has been detected in a wide range of proteins found in the nucleus, cytoplasm, mitochondria, endoplasmic reticulum, and cell membrane (17, 18), suggesting that protein lactylation may be involved in regulating various biological activities. These findings emphasize the significant influence of lactylation as a posttranslational modification and suggest its potential role in a variety of cellular processes.
Histone lactylation by “writers” and “erasers”: In the process of histone modification, “writers” and “erasers” play significant roles in regulating gene expression dynamically and reversibly. In histone lactylation, the “writers” transfers lactate-generated lactyl-coenzyme A to the histone lysine tail for lactylation. The most studied lactylation writers are P300 (acetyltransferase), and two other histone acetyltransferase (HAT) family proteins, males absent on the first (MOF), and general control non-depressible 5 (GCN5) (13, 31, 32). The latest study reveals that lysine acetyltransferase 7 (HBO1) serves as a lactyltransferase to mediate a histone Kla (33). Other proteins such as CREBBP (CBP), peptidyl-lysine N-acetyltransferase YiaC, and lysine acetyltransferase 8 (KAT8) may also act as potential regulators of lactylation (30, 34). On the contrary, the “erasers” like histone deacetylase (HDAC)1–3 and Sirtuins (SIRT)1–3 are responsible for de-lactylation (29, 31, 32). Nevertheless, despite these discoveries, the specific enzymes and mechanisms that play a role in regulating lactylation in cells are still elusive, underscoring the necessity for additional research in this field.
2.3 The role of lactylation in pathogenic mechanisms
Lactylation is an important post-translational modification that can alter protein function and stability. It may influence organism function in various ways. Lactylation can modify gene expression and protein function, thus impacting their activity and stability within the cell (18, 32, 35, 36). This alteration can result in disruptions in intracellular signaling pathways, thereby affecting normal cell function. Additionally, lactylation might influence protein interactions with other proteins or ligands (37, 38), thereby affecting intracellular protein signaling and metabolic regulation. Furthermore, lactylation could disrupt progression of normal intracellular metabolic pathways (39–41), leading to irregularities in energy and material metabolism, consequently affecting cell function.
In essence, abnormal lactylation could detrimentally impact physiological cell functions, contributing to disease onset and progression. Hence, investigating the pathogenic mechanism of lactate modification is critical for understanding disease development at a molecular level and devising relevant therapeutic approaches.
3 Regulation of macrophage activation by lactylation
3.1 Subtypes of lung macrophages and their types of activation
Two types of macrophages exist in human lung tissue: tissue-resident macrophages (TRMs) and recruited monocyte-derived macrophages (Figure 2). Among TRMs, two main subtypes are identified: alveolar macrophages (AMs) and interstitial macrophages (IMs) (1). AMs are located within the alveoli, characterized by surface markers CD11blow CD11c++ CD169+, while IMs reside in the lung parenchyma with surface markers CD11b+ CD11clow CD169- (4, 42, 43).
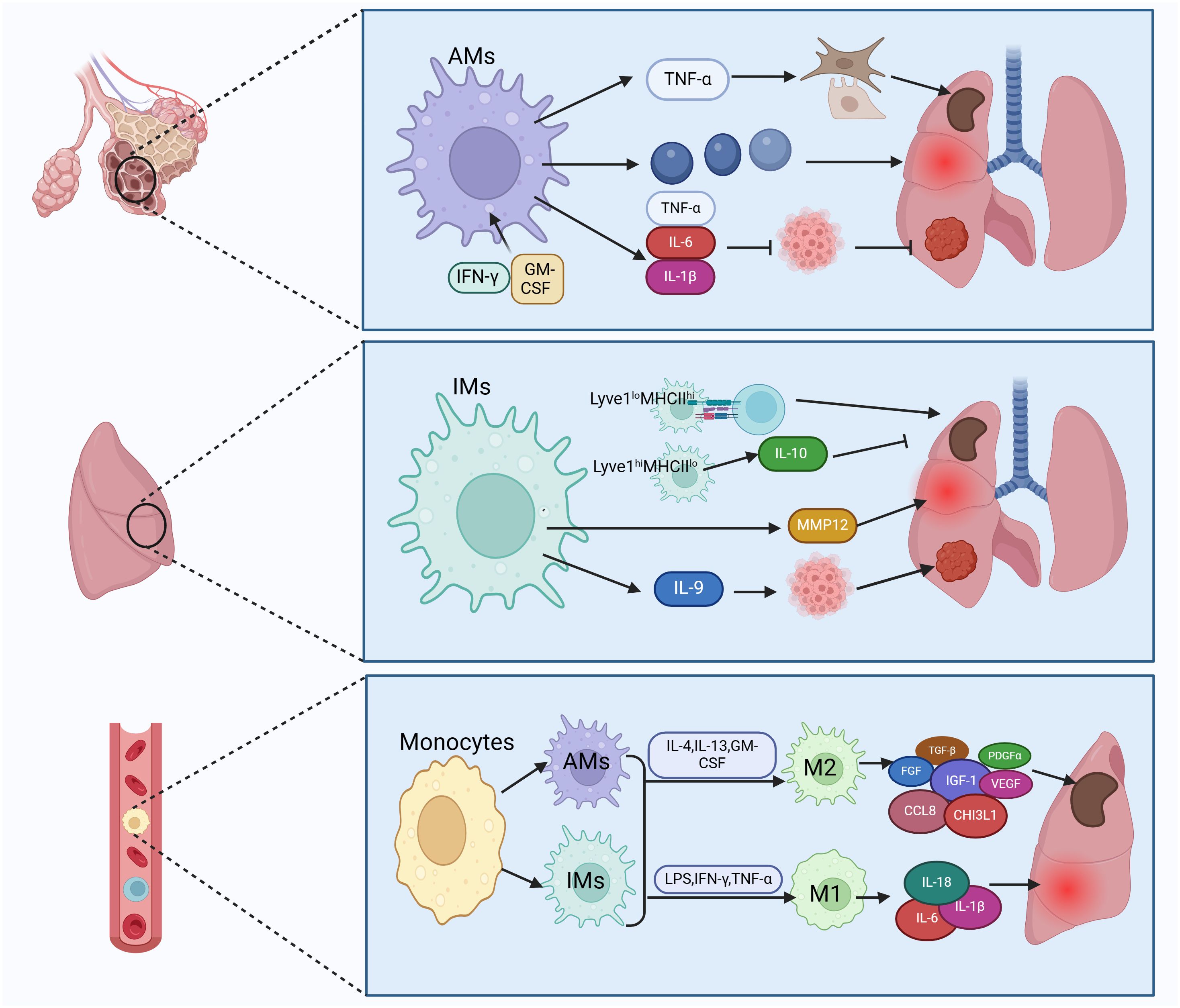
Figure 2 Subtypes of lung macrophages and their types of activation. Macrophages in lung tissue are categorized into tissue-resident macrophages and recruited mononuclear macrophages. Tissue-resident macrophages consist of alveolar macrophages (AMs) and interstitial macrophages (IMs). AMs can trigger fibroblasts and epithelium by secreting TNF-α, leading to fibrosis. They also release inflammatory factors causing lung injury and secrete IL-6 to inhibit lung cancer. IMs can enhance lung fibrosis, inflammation, and cancer by antigen presentation and secretion of IL-10, MMP12, and IL-9. Recruited monocytes transform into AMs and IMs, which can further differentiate into classically activated macrophages (M1) and alternatively activated macrophages (M2). M1 macrophages produce pro-inflammatory factors like IL-6 to boost lung inflammation, while M2 macrophages secrete TGF-β to induce pulmonary fibrosis. Figure was created with BioRender.com.
AMs are the predominant macrophages in the lungs and play a vital role in maintaining lung homeostasis, defending against pathogens, regulating lung inflammation, and possessing self-renewal capabilities (42, 44). AMs may promote fibrosis. Lineage tracing experiments in murine fibrosis models have shown an expansion of the AMs pool derived from monocytes expressing genes that promote fibrosis, and removing this subset of macrophages has been found to alleviate fibrosis (45, 46). During acute lung injury, AMs coordinate the initiation and resolution of inflammation to restore internal balance (47). In cases of chronic lung inflammation, AMs cause lung damage by producing various pro-inflammatory mediators such as chemokines, cytokines, proteases, reactive oxygen intermediates (ROI), and reactive nitrogen intermediates (RNI). Research suggests a link between matrix metalloproteinases (MMPs) and destruction or remodeling of lung tissue in chronic obstructive pulmonary disease (COPD) (2). Interestingly, TNF-α released by AMs promotes secretion of granulocyte-macrophage colony-stimulating factor (GM-CSF) from alveolar epithelial cells, supporting alveolar epithelial repair (48). The role of AMs in lung tumors is intricate (49). AMs obtained from lung cancer patients, when exposed to IFN-γ or GM-CSF, secrete TNF-α, IL-6, and IL-1β, enhancing anti-tumor activity (50). However, other studies indicate that many anti-tumor functions of AMs, such as phagocytosis and expression of cytokines that activate adaptive immunity, diminish as non-small cell lung cancer (NSCLC) progresses (51), suggesting a role of AMs in fostering an immunosuppressive microenvironment and immune dysfunction.
In contrast to the extensive research on AMs, there is relatively less study on IMs. IMs within tissue-resident macrophages can be further classified into Lyve1loMHCIIhi and Lyve1hiMHCIIlo subtypes, each exhibiting distinct functional characteristics. Lyve1loMHCIIhi IMs excel in antigen presentation, while Lyve1hiMHCIIlo IMs possess the ability to inhibit tissue fibrosis. Lyve1hiMHCIIlo IMs play an important role in suppressing collagen deposition, immune cell infiltration, and tissue inflammation, and their absence can exacerbate bleomycin-induced lung fibrosis (52). In COPD, IMs have been found to secrete matrix metalloproteinase 12 (MMP12), promoting formation of lung emphysema (53). In addition, IMs regulate virus-induced inflammation, serving as the primary source of the anti-inflammatory cytokine IL-10 in response to influenza A virus (IAV) infection and exposure to bacterial DNA (54). In the development of lung tumors, IMs act as the main tumor-associated macrophages (TAMs), with IL-9 signaling promoting the conversion of monocytes to IMs (19). Moreover, IMs represent the primary responders to IL-9 in lung cancer, and blocking IL-9 signaling on lung macrophages restricts the growth of murine lung tumors (55).
In the progression and advancement of pulmonary fibrosis, both AMs and IMs have the ability to polarize into classically activated macrophages (M1) and alternatively activated macrophages (M2), respectively (56, 57). Depending on the specific activating signals, M2 macrophages can be further classified into four subtypes: M2a, M2b, M2c, and M2d (20). In the early phases of lung damage, M1 macrophages get activated by LPS, IFN-γ, and TNF-α. These activated M1 macrophages contribute to pathogen elimination and escalate inflammation. With the progression of inflammation, the quantity of M2 macrophages rises as triggered by IL-4, IL-13, GM-CSF, and other factors, dominating the immune response. M2 macrophages, via the release of fibrosis-promoting molecules like TGF-β, fibroblast growth factor (FGF), platelet-derived growth factor alpha (PDGFα), insulin-like growth factor-1 (IGF-1), and vascular endothelial growth factor (VEGF), actively promote extracellular matrix (ECM) expansion and tissue restoration (58, 59). The prevalence of M2 macrophages tends to be elevated in idiopathic pulmonary fibrosis (IPF) (60). Macrophages stimulate fibroblasts by releasing fibrosis-promoting factors and remodeling ECM, thus advancing lung fibrosis (61–63). Studies have shown increased expression of the M2 macrophage-related chemokine CCL18 in bronchoalveolar lavage fluid (BALF) and serum of patients with IPF. The level of CCL18 is linked to the mortality rate of IPF patients (64, 65). CCL18 attracts T lymphocytes and maintains a positive feedback loop with lung fibroblasts to enhance collagen production (66). Another marker of M2 activation is chitinase 3-like protein 1 (CHI3L1), which is also elevated in the BALF of IPF patients and indicates a poor prognosis (4). Research indicates that inhibiting M2 macrophage polarization can reduce bleomycin-induced lung fibrosis in rats (67).
3.2 Regulation of macrophage M1/M2 by lactylation
Lactylation is a common post-translational modification that can influence protein stability, activity, and subcellular localization. Recent study indicates that lactylation can influence the M1/M2 polarization state of macrophages (14). As both AMs and IMs can undergo M1/M2 polarization, we focus on the regulatory mechanism of macrophage M1/M2 by lactylation. M1 macrophages typically trigger pro-inflammatory responses and cytotoxic activity, while M2 macrophages are linked to anti-inflammatory responses and reparative functions (56, 57). Macrophages polarize into different phenotypes in response to local microenvironmental cues. Activation of glycolytic pathways enhances production and release of lactate, leading to polarization of macrophages towards the M1 phenotype. In the early stages of inflammation, lactylation promotes M1 polarization of macrophages, with nitric oxide synthase 2 (NOS2) protein level peaking at 12 hours post-stimulation and decreasing thereafter (13). Furthermore, genes like arginase 1 (ARG1), modified by H3K18la, show significant upregulation primarily at 16–24 hours. The study demonstrates that the aerobic glycolysis observed during M1-type macrophage polarization sets off a “lactate clock” that drives M2-type gene expression during the later stages of M1 polarization by facilitating the lactylation of histone H3 lysine 18 (13). Mitochondria, the key metabolic organelles in cells, could adjust their energy production to support the transition of macrophages from a pro-inflammatory to a proresolving state by undergoing fission and fusion processes. Inflammatory M1 cells display elongated mitochondria, and blocking mitochondrial fusion enhances histone lactylation by affecting lactate levels, promoting M2 polarization in macrophages (68). Oncogenic M2-type macrophages isolated from melanoma and lung cancer tissues in mice exhibit a strong positive correlation with lactylation levels. This suggests that elevated lactate and histone lactylation in macrophages may play a role in regulating macrophage M2 polarization.
3.3 The role of lactylation in macrophage activation
Lactylation is a post-translational modification process that modifies proteins by adding lactate groups, altering their function and stability. Macrophages, vital in immune defense, primarily engage in phagocytosis and eliminating pathogens and waste. The interplay between lactylation and macrophage function is significant. Firstly, lactylation can modify macrophage activity and function by influencing protein structure and stability (18, 32, 35, 36), thus affecting cellular function. Lactate inhibits Toll-like receptor (TLR)-mediated monocyte-macrophage activation (26, 69), delays protein kinase B (Akt) phosphorylation and IκBα degradation. It also inhibits secretion of cytokines including tumor necrosis factor α (TNF-α), interleukin-23 (IL-23), and chemokines C-C motif chemokine ligand (CCL)2 and CCL7 (70). Lactylation plays a key role in regulating macrophage activity. Secondly, lactylation influences macrophage metabolic processes. The correlation between lactylation and macrophage metabolism is notable (71). The boost in VEGF production by polarized macrophages establishes a beneficial cycle that enhances angiogenesis and supports postischemic hemotransfusion and muscle regeneration (72). As a result of tissue hypoxia, lactate can also act as a marker for insufficient tissue perfusion. In clinical practice, monitoring serum lactate level is important for managing patients with ischemic injuries (73).
Macrophages require substantial energy and nutrients during phagocytosis and waste removal, with lactylation affecting their metabolic functions by controlling protein degradation and synthesis, energy metabolism, and other pathways (39–41). Lactylation can also influence metabolic functions of macrophages via altering their absorption and use of nutrients. Multiple connections between lactylation and other epigenetic changes influence macrophage activities and metabolic processes. Lactylation and methylation modifications frequently interact at the cellular level. A study verified the presence of H3K18la enrichment at the m6A reader YTH N6-methyladenosine RNA binding protein F2 (YTHDF2) promoter, demonstrating that H3K18la regulates YTHDF2 transcription (74). Hyper-H3K18la promotes the transcription of YTHDF1 in AECs of arsenite-related IPF (75), suggesting that lactylation adjustments can influence the methylation levels of DNA and histones, which in turn affect gene expression and transcriptional control. This interplay holds significant implications for the immune and inflammatory responses of macrophages. Lactylation and acetylation also collaborate within the nucleus. The levels of lactate and acetyl-CoA synergistically determine the cell fate and are manifested in histones as epigenetic marks (i.e., lactylation and acetylation) (76). In macrophages, the interplay between lactylation and acetylation can influence the inflammatory response and antigen presentation (17). Lactylation and ubiquitination also interact in the regulation of protein degradation and stability. Lactylation can control the protein degradation rate via altering the ubiquitination levels of proteins (3, 5, 77). This interaction plays an essential role in antigen processing and immunomodulation in macrophages. As previously stated, lactate-induced lactylation is integral to macrophage activation. However, the investigation into the crosstalk between lactylation and other epigenetic modifications, particularly in macrophages, is still in its nascent stages.
4 Lactylation modulates macrophage activation in lung disease
4.1 Lactylation regulates macrophage activation in inflammatory lung diseases
Recent research indicates that lactylation affects macrophage activation and progression of lung inflammation. Increased lactate was found in BALF from particulate matter (PM2.5)-exposed mice and lactylation was increased in macrophages (78). Lactylation has been shown to potentially stimulate M1-type macrophage activation, which intensifies inflammatory reactions. Activated macrophages release pro-inflammatory cytokines that worsen inflammation in the lungs. In sepsis patients, blood lactate levels correlate with high mobility group box 1 (HMGB1) levels, a type of damage-associated molecular pattern (DAMP), from activated macrophages. Macrophages in sepsis can induce HMGB1 lactylation by absorbing extracellular lactate through MCTs. Lactylated HMGB1 is then released by macrophages, disrupting endothelial integrity, increasing vascular permeability, and contributing to endothelial cell barrier dysfunction, thereby promoting sepsis progression (17). Pyroptosis is a programmed cell death associated with inflammatory diseases, occurring in macrophages mostly (79–81). In non-canonical pyroptosis, NEDD4 E3 ubiquitin protein ligase (NEDD4) acts as a negative regulator of Caspase-11, and lactylated NEDD4 leads to macrophage pyroptosis (82), suggesting that lactylation might enhance inflammation by influencing pyroptosis. Moreover, dexamethasone (DEX) treatment inhibits protein lactylation and pyroptosis in macrophages, thereby attenuating ovalbumin-induced aeroallergen (83). Interestingly, a study shows that lactate can inhibit M1 macrophage polarization and increase histone H3K18 lactylation in macrophages, thereby suppressing macrophage pyroptosis (84). Additionally, research indicates that lactylation can drive M2-type macrophage activation (14, 85), promoting anti-inflammatory responses and tissue repair. Drug delivery by nanoplatform-based system significantly decreased histone lactylation levels in LPS-induced macrophages and induced macrophage towards to M2 polarization, thereby attenuating LPS-induced acute lung injury in mice (86). Activated M2 macrophages release anti-inflammatory cytokines to dampen inflammation and support tissue healing. In spite of the controversy, these studies suggest that further investigation of the mechanisms by which lactylation regulates macrophage activation, as well as the development of drugs targeting lactylation, may be beneficial to control acute inflammation in lung tissues.
4.2 Effect of lactylation modulating macrophage activation on pulmonary fibrosis
Pulmonary fibrosis is a chronic progressive lung disease characterized by proliferation and deposition of fibrous tissue in the lung, leading to structural damage and impaired function (87). Abnormal activation and function of macrophages are closely tied to pulmonary fibrosis (55, 60, 88). When lung tissue is injured or inflamed, macrophages release inflammatory and growth factors, fueling the inflammatory response and fibrotic progression (55, 58). Moreover, macrophages stimulate fibrosis-related cell growth and collagen synthesis, contributing to lung scarring (89).
Upregulation of the TGF-β/Smad2 pathway is critical in fibrosis development and worsens fibrotic conditions. Lactylation of Snail1, a TGF-β transcription factor, enhances its nuclear localization and binding to the TGF-β promoter, leading to increased TGF-β production (90). Histone H3K18 lactylation interacts with m6A methylation to promote organic arsenic-induced pulmonary fibrosis (74). Also, lactate from the lung tissue can induce a pro-fibrotic phenotype in macrophages by promoting lactylation (85). This pro-fibrotic effect of lactylation may modulate macrophage metabolic phenotype and M2 polarization. During pulmonary fibrosis progression, glucose consumption increases, shifting macrophages’ metabolic status from lipid to glucose metabolism, particularly in the TCA cycle, and increasing glycolysis/gluconeogenesis in macrophages during the fibrotic phase (91, 92). Pyruvate kinase M2 (PKM2) plays a critical role in mediating metabolic adaptations in pro-inflammatory macrophages. Lactylation of PKM2 modulates the metabolic phenotypic shift of macrophages, contributing to polarization from pro-inflammatory M1 to pro-fibrotic M2 macrophages (39). In a mouse model of PM2.5-induced pulmonary fibrosis, macrophages with increased lactylation secreted more pro-fibrotic factors, which boosted EMT to exacerbate fibrosis through the TGF-β/Smad and VEGFA/ERK pathways. LDHA inhibitor treatment reduced macrophage lactylation and attenuated PM2.5-induced pulmonary fibrosis (78). In conclusion, studying lactylation would enhance our understanding of macrophage mechanisms in pulmonary fibrosis development and lay the groundwork for new immunomodulatory therapeutic strategies.
4.3 Lactylation modulates macrophage activation in lung cancer
Macrophage activation largely influences development and progression of lung cancer. Macrophages combat tumors by engulfing and destroying cancer cells, hindering tumor growth and spread (93). Additionally, they release cytokines like interleukins to boost immune cell activation and proliferation (94), bolstering the immune response against tumors. Macrophages also modulate the tumor microenvironment, impacting tumor cell growth, invasion, and metastasis, thereby shaping tumor advancement (95).
Lactate is the end product of glycolysis and a highly abundant metabolite in the tumor microenvironment, and enhanced glycolysis and lactate accumulation are common features of all types of cancer. Tumor cells often rely on glycolytic metabolism for energy production, favoring it over oxidative phosphorylation even in the presence of oxygen. This metabolic preference, known as “aerobic glycolysis” or the “Warburg effect” results in accumulation of large amounts of lactate (96). High levels of lactate in glycolysis-driven tumor cells are linked to increased aggressiveness and poor prognosis, highlighting the role of lactate in cancer progression (97). Indeed, lactate-driven lactacylation promotes lung cancer through a diversity of routes. Defective Numb/Parkin pathway leads to increased histone lactylation and promotes lung adenocarcinoma (LUAD) (98). In lung adenocarcinoma endothelial cells, H3K18la and H3K14la reduce the expression of solute carrier family 25 member 29 (SLC25A29), and low SLC25A29 expression is associated with LUAD progression (99). More importantly, inhibition of histone lactylation could suppress the malignant progression of LUAD (100). Nevertheless, whether and how lactate and lactylation regulate macrophage activation and lung carcinogenesis remain to be investigated.
In lung cancer, M1-type macrophages are primarily activated by cytokines like IL-12, IFN-γ, etc. (56). Activated M1-type macrophages induce tumor cell apoptosis and impede tumor growth, thereby suppressing lung cancer development (101). Conversely, activation of M2-type macrophages enhances tumor proliferation, invasion, and metastasis, thereby advancing lung cancer progression (95). Maintaining a balanced M1/M2 macrophage ratio is critical. An unbalanced M1/M2 ratio could alter the microenvironment, fostering tumor growth and metastasis. Twenty-eight lysine lactylation sites on core histones like H3, H4, H2A, and H2B were identified in human HeLa cells and mouse bone marrow-derived macrophages (BMDMs) (13). Studies on oncogenic M2-type macrophages from melanoma and lung cancer tissues in mice have shown a significant positive correlation between lactylation levels and these macrophages, suggesting a potential contribution of elevated lactate and histone lactylation in macrophages to tumor development and malignant advancement. In patients with lung squamous cell carcinoma, solute carrier family 2 member 1 (SLC2A1), encoding glucose transporter-1 (GLUT1), was highly expressed in SPP1+ macrophages. Upregulation of SLC2A1 was associated with high levels of protein lactylation and promoted macrophage polarization into the M2 phenotype, which was related to worse survival and poor pathological response to adjuvant immunochemotherapy (102).
5 Conclusion and outlook
In recent years, there has been a growing focus on the role of lactate as a signaling molecule and lactylation as a post-translational modification of proteins in various physiological and pathological conditions. Understanding how lactate influences macrophage activation is important for gaining insights into disease development. This review highlights key concepts in lactate metabolism, such as the shuttle, homeostasis, and microenvironment interactions of lactate. Recent research has shown that lactate plays a significant role in regulating gene expression related to inflammation, cytokine production, and metabolic pathways in macrophages, ultimately affecting their activation and function. Furthermore, the role of lactylation in modulating macrophage activation in lung diseases like inflammation, fibrosis, and cancer is discussed (Figure 3). Abnormal macrophage function is a key factor in the pathogenesis of lung diseases such as pneumonia, pulmonary fibrosis, COPD, and lung cancer, making the study of lactylation particularly relevant in these conditions. Understanding of lactylation’s impact on macrophage activation in lung diseases may offer new opportunities for therapeutic interventions.
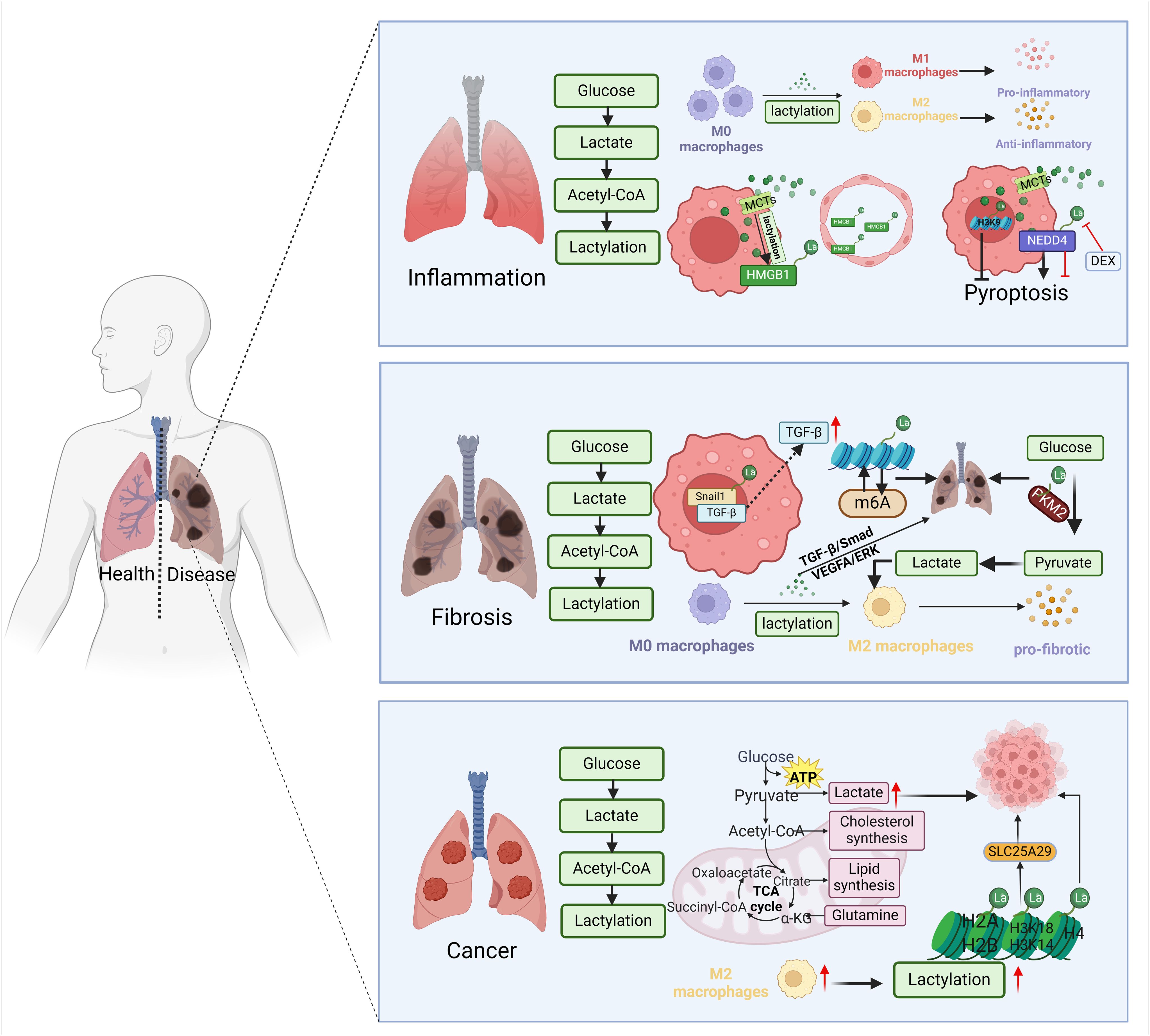
Figure 3 Lactylation modulates macrophage activation in lung disease. During the inflammatory phase, lactylation increases transformation of macrophages into M1 and M2 types. Cellular entry through MCTs boosts HMGB1 lactylation, leading to destruction of endothelial cells. Lactated NEDD4 induces macrophage pyroptosis, while lactated H3K9 inhibits it. Snail1 lactylation at the fibrosis stage stimulates TGF-β secretion, promoting M2 macrophage polarization. Histone lactylation and m6A methylation interaction contributes to lung fibrosis. PKM2 methylation enhances macrophage M2 polarization. The glycolysis/gluconeogenesis process in macrophages intensifies during the fibrotic phase. Higher lactate levels are observed in glycolysis-dominant tumor cells. Twenty-eight lysine lactation (Kla) sites were identified on core histones like H3, H4, H2A, and H2B. Oncogenic M2-type macrophages from melanoma and mouse lung cancer tissues exhibit a strong positive association with lactate levels. Figure was created with BioRender.com.
Nevertheless, the related research is still in its early stages. Could lactate potentially have similar applications in lung diseases in future? Additionally, could new medications be developed to target lactylation? What is the exact mechanism by which lactate influences macrophage activation, and how does it differ from other pathways of macrophage activation? In conclusion, a thorough exploration of lactylation and its specific role in regulating macrophage activation is essential for advancing our knowledge of lung disease mechanisms, as well as for providing innovative therapeutic approaches and identifying potential targets.
Author contributions
YW: Writing – original draft. HG: Writing – original draft. SC: Writing – original draft. XT: Writing – original draft, Conceptualization, Funding acquisition, Project administration, Resources, Supervision, Writing – review & editing.
Funding
The author(s) declare financial support was received for the research, authorship, and/or publication of this article. This study was supported by the National Natural Science Foundation of China (XT, 82270077), the National High-Level Talents Program (XT), R&D Program of Guangzhou National Laboratory, Local Innovative and Research Teams Project of Guangdong Pearl River Talents Program (2017BT01S155), Open Project of State Key Laboratory of Respiratory Disease (SKLRD-OP-202320), Guangzhou Institute of Respiratory Health Open Project (XT).
Conflict of interest
The authors declare that the research was conducted in the absence of any commercial or financial relationships that could be construed as a potential conflict of interest.
Publisher’s note
All claims expressed in this article are solely those of the authors and do not necessarily represent those of their affiliated organizations, or those of the publisher, the editors and the reviewers. Any product that may be evaluated in this article, or claim that may be made by its manufacturer, is not guaranteed or endorsed by the publisher.
References
1. Aegerter H, Lambrecht BN, Jakubzick CV. Biology of lung macrophages in health and disease. Immunity. (2022) 55:1564–80. doi: 10.1016/j.immuni.2022.08.010
2. Russell RE, Culpitt SV, DeMatos C, Donnelly L, Smith M, Wiggins J, et al. Release and activity of matrix metalloproteinase-9 and tissue inhibitor of metalloproteinase-1 by alveolar macrophages from patients with chronic obstructive pulmonary disease. Am J Respir Cell Mol Biol. (2002) 26:602–9. doi: 10.1165/ajrcmb.26.5.4685
3. Wang J, Wang Z, Wang Q, Li X, Guo Y. Ubiquitous protein lactylation in health and diseases. Cell Mol Biol Lett. (2024) 29:23. doi: 10.1186/s11658-024-00541-5
4. Bedoret D, Wallemacq H, Marichal T, Desmet C, Quesada Calvo F, Henry E, et al. Lung interstitial macrophages alter dendritic cell functions to prevent airway allergy in mice. J Clin Invest. (2009) 119:3723–38. doi: 10.1172/JCI39717
5. Wu H, Huang H, Zhao Y. Interplay between metabolic reprogramming and post-translational modifications: from glycolysis to lactylation. Front Immunol. (2023) 14:1211221. doi: 10.3389/fimmu.2023.1211221
6. Ye L, Jiang Y, Zhang M. Crosstalk between glucose metabolism, lactate production and immune response modulation. Cytokine Growth Factor Rev. (2022) 68:81–92. doi: 10.1016/j.cytogfr.2022.11.001
7. Zhang Y-T, Xing M-L, Fang H-H, Li W-D, Wu L, Chen Z-P. Effects of lactate on metabolism and differentiation of CD4+T cells. Mol Immunol. (2023) 154:96–107. doi: 10.1016/j.molimm.2022.12.015
8. Certo M, Tsai CH, Pucino V, Ho PC, Mauro C. Lactate modulation of immune responses in inflammatory versus tumour microenvironments. Nat Rev Immunol. (2021) 21:151–61. doi: 10.1038/s41577-020-0406-2
9. Linares JF, Cid-Diaz T, Duran A, Osrodek M, Martinez-Ordoñez A, Reina-Campos M, et al. The lactate-NAD(+) axis activates cancer-associated fibroblasts by downregulating p62. Cell Rep. (2022) 39:110792. doi: 10.1016/j.celrep.2022.110792
10. Kozlov AM, Lone A, Betts DH, Cumming RC. Lactate preconditioning promotes a HIF-1α-mediated metabolic shift from OXPHOS to glycolysis in normal human diploid fibroblasts. Sci Rep. (2020) 10:8388. doi: 10.1038/s41598-020-65193-9
11. Wang ZH, Peng WB, Zhang P, Yang XP, Zhou Q. Lactate in the tumour microenvironment: From immune modulation to therapy. EBioMedicine. (2021) 73:103627. doi: 10.1016/j.ebiom.2021.103627
12. Hayes C, Donohoe CL, Davern M, Donlon NE. The oncogenic and clinical implications of lactate induced immunosuppression in the tumour microenvironment. Cancer Lett. (2021) 500:75–86. doi: 10.1016/j.canlet.2020.12.021
13. Zhang D, Tang Z, Huang H, Zhou G, Cui C, Weng Y, et al. Metabolic regulation of gene expression by histone lactylation. Nature. (2019) 574:575–80. doi: 10.1038/s41586-019-1678-1
14. Irizarry-Caro RA, McDaniel MM, Overcast GR, Jain VG, Troutman TD, Pasare C. TLR signaling adapter BCAP regulates inflammatory to reparatory macrophage transition by promoting histone lactylation. Proc Natl Acad Sci U.S.A. (2020) 117:30628–38. doi: 10.1073/pnas.2009778117
15. Merkuri F, Rothstein M, Simoes-Costa M. Histone lactylation couples cellular metabolism with developmental gene regulatory networks. Nat Commun. (2024) 15(1):90. doi: 10.1038/s41467-023-44121-1
16. Yu J, Chai P, Xie M, Ge S, Ruan J, Fan X, et al. Histone lactylation drives oncogenesis by facilitating m(6)A reader protein YTHDF2 expression in ocular melanoma. Genome Biol. (2021) 22:85. doi: 10.1186/s13059-021-02308-z
17. Yang K, Fan M, Wang X, Xu J, Wang Y, Tu F, et al. Lactate promotes macrophage HMGB1 lactylation, acetylation, and exosomal release in polymicrobial sepsis. Cell Death Differ. (2022) 29:133–46. doi: 10.1038/s41418-021-00841-9
18. Xiong J, He J, Zhu J, Pan J, Liao W, Ye H, et al. Lactylation-driven METTL3-mediated RNA m(6)A modification promotes immunosuppression of tumor-infiltrating myeloid cells. Mol Cell. (2022) 82:1660–1677.e10. doi: 10.1016/j.molcel.2022.02.033
19. Rodríguez-Morales P, Franklin RA. Macrophage phenotypes and functions: resolving inflammation and restoring homeostasis. Trends Immunol. (2023) 44:986–98. doi: 10.1016/j.it.2023.10.004
20. Colin S, Chinetti-Gbaguidi G, Staels B. Macrophage phenotypes in atherosclerosis. Immunol Rev. (2014) 262:153–66. doi: 10.1111/imr.12218
21. Rabinowitz JD, Enerbäck S. Lactate: the ugly duckling of energy metabolism. Nat Metab. (2020) 2:566–71. doi: 10.1038/s42255-020-0243-4
22. Schütterle DM, Hegner R, Temovska M, Ortiz-Ardila AE, Angenent LT. Exclusive D-lactate-isomer production during a reactor-microbiome conversion of lactose-rich waste by controlling pH and temperature. Water Res. (2024) 250:121045. doi: 10.1016/j.watres.2023.121045
23. Xin Q, Wang H, Li Q, Liu S, Qu K, Liu C, et al. Lactylation: a passing fad or the future of posttranslational modification. Inflammation. (2022) 45:1419–29. doi: 10.1007/s10753-022-01637-w
24. Brooks GA. The science and translation of lactate shuttle theory. Cell Metab. (2018) 27:757–85. doi: 10.1016/j.cmet.2018.03.008
25. Brown TP, Ganapathy V. Lactate/GPR81 signaling and proton motive force in cancer: Role in angiogenesis, immune escape, nutrition, and Warburg phenomenon. Pharmacol Ther. (2020) 206:107451. doi: 10.1016/j.pharmthera.2019.107451
26. Hoque R, Farooq A, Ghani A, Gorelick F, Mehal WZ. Lactate reduces liver and pancreatic injury in Toll-like receptor- and inflammasome-mediated inflammation via GPR81-mediated suppression of innate immunity. Gastroenterology. (2014) 146:1763–74. doi: 10.1053/j.gastro.2014.03.014
27. Urbańska K, Orzechowski A. Unappreciated role of LDHA and LDHB to control apoptosis and autophagy in tumor cells. Int J Mol Sci. (2019) 20(9):2085. doi: 10.3390/ijms20092085
28. Benjamin D, Robay D, Hindupur SK, Pohlmann J, Colombi M, El-Shemerly MY, et al. Dual inhibition of the lactate transporters MCT1 and MCT4 is synthetic lethal with metformin due to NAD+ Depletion in cancer cells. Cell Rep. (2018) 25:3047–3058.e4. doi: 10.1016/j.celrep.2018.11.043
29. Moreno-Yruela C, Zhang D, Wei W, Bæk M, Liu W, Gao J, et al. Class I histone deacetylases (HDAC1-3) are histone lysine delactylases. Sci Adv. (2022) 8:eabi6696. doi: 10.1126/sciadv.abi6696
30. Hu Y, He Z, Li Z, Wang Y, Wu N, Sun H, et al. Lactylation: the novel histone modification influence on gene expression, protein function, and disease. Clin Epigenet. (2024) 16(1):72. doi: 10.1186/s13148-024-01682-2
31. Varner EL, Trefely S, Bartee D, von Krusenstiern E, Izzo L, Bekeova C, et al. Quantification of lactoyl-CoA (lactyl-CoA) by liquid chromatography mass spectrometry in mammalian cells and tissues. Open Biol. (2020) 10:200187. doi: 10.1098/rsob.200187
32. Wang N, Wang W, Wang X, Mang G, Chen J, Yan X, et al. Histone lactylation boosts reparative gene activation post-myocardial infarction. Circ Res. (2022) 131:893–908. doi: 10.1161/CIRCRESAHA.122.320488
33. Niu Z, Chen C, Wang S, Lu C, Wu Z, Wang A, et al. HBO1 catalyzes lysine lactylation and mediates histone H3K9la to regulate gene transcription. Nat Commun. (2024) 15(1):3561. doi: 10.1038/s41467-024-47900-6
34. Xie B, Zhang M, Li J, Cui J, Zhang P, Liu F, et al. KAT8-catalyzed lactylation promotes eEF1A2-mediated protein synthesis and colorectal carcinogenesis. Proc Natl Acad Sci U.S.A. (2024) 121:e2314128121. doi: 10.1073/pnas.2314128121
35. Meng Q, Sun H, Zhang Y, Yang X, Hao S, Liu B, et al. Lactylation stabilizes DCBLD1 activating the pentose phosphate pathway to promote cervical cancer progression. J Exp Clin Cancer Res. (2024) 43:36. doi: 10.1186/s13046-024-02943-x
36. Miao Z, Zhao X, Liu X. Hypoxia induced β-catenin lactylation promotes the cell proliferation and stemness of colorectal cancer through the wnt signaling pathway. Exp Cell Res. (2023) 422:113439. doi: 10.1016/j.yexcr.2022.113439
37. Gu J, Zhou J, Chen Q, Xu X, Gao J, Li X, et al. Tumor metabolite lactate promotes tumorigenesis by modulating MOESIN lactylation and enhancing TGF-β signaling in regulatory T cells. Cell Rep. (2022) 39:110986. doi: 10.1016/j.celrep.2022.110986
38. Zhang N, Zhang Y, Xu J, Wang P, Wu B, Lu S, et al. α-myosin heavy chain lactylation maintains sarcomeric structure and function and alleviates the development of heart failure. Cell Res. (2023) 33:679–98. doi: 10.1038/s41422-023-00844-w
39. Wang J, Yang P, Yu T, Gao M, Liu D, Zhang J, et al. Lactylation of PKM2 suppresses inflammatory metabolic adaptation in pro-inflammatory macrophages. Int J Biol Sci. (2022) 18:6210–25. doi: 10.7150/ijbs.75434
40. Yang Z, Yan C, Ma J, Peng P, Ren X, Cai S, et al. Lactylome analysis suggests lactylation-dependent mechanisms of metabolic adaptation in hepatocellular carcinoma. Nat Metab. (2023) 5:61–79. doi: 10.1038/s42255-022-00710-w
41. Chen AN, Luo Y, Yang YH, Fu JT, Geng XM, Shi JP, et al. Lactylation, a novel metabolic reprogramming code: current status and prospects. Front Immunol. (2021) 12:688910. doi: 10.3389/fimmu.2021.688910
42. Guilliams M, De Kleer I, Henri S, Post S, Vanhoutte L, De Prijck S, et al. Alveolar macrophages develop from fetal monocytes that differentiate into long-lived cells in the first week of life via GM-CSF. J Exp Med. (2013) 210:1977–92. doi: 10.1084/jem.20131199
43. Byrne AJ, Maher TM, Lloyd CM. Pulmonary macrophages: A new therapeutic pathway in fibrosing lung disease? Trends Mol Med. (2016) 22:303–16. doi: 10.1016/j.molmed.2016.02.004
44. Hashimoto D, Chow A, Noizat C, Teo P, Beasley MB, Leboeuf M, et al. Tissue-resident macrophages self-maintain locally throughout adult life with minimal contribution from circulating monocytes. Immunity. (2013) 38:792–804. doi: 10.1016/j.immuni.2013.04.004
45. Misharin AV, Morales-Nebreda L, Reyfman PA, Cuda CM, Walter JM, McQuattie-Pimentel AC, et al. Monocyte-derived alveolar macrophages drive lung fibrosis and persist in the lung over the life span. J Exp Med. (2017) 214:2387–404. doi: 10.1084/jem.20162152
46. McCubbrey AL, Barthel L, Mohning MP, Redente EF, Mould KJ, Thomas SM, et al. Deletion of c-FLIP from CD11b(hi) macrophages prevents development of bleomycin-induced lung fibrosis. Am J Respir Cell Mol Biol. (2018) 58:66–78. doi: 10.1165/rcmb.2017-0154OC
47. Malainou C, Abdin SM, Lachmann N, Matt U, Herold S. Alveolar macrophages in tissue homeostasis, inflammation, and infection: evolving concepts of therapeutic targeting. J Clin Invest. (2023) 133(19):e170501. doi: 10.1172/JCI170501
48. Cakarova L, Marsh LM, Wilhelm J, Mayer K, Grimminger F, Seeger W, et al. Macrophage tumor necrosis factor-alpha induces epithelial expression of granulocyte-macrophage colony-stimulating factor: impact on alveolar epithelial repair. Am J Respir Crit Care Med. (2009) 180:521–32. doi: 10.1164/rccm.200812-1837OC
49. Chang CY, Armstrong D, Corry DB, Kheradmand F. Alveolar macrophages in lung cancer: opportunities challenges. Front Immunol. (2023) 14:1268939. doi: 10.3389/fimmu.2023.1268939
50. Almatroodi SA, McDonald CF, Pouniotis DS. Alveolar macrophage polarisation in lung cancer. Lung Cancer Int. (2014) 2014:721087. doi: 10.1155/2014/721087
51. Pouniotis DS, Plebanski M, Apostolopoulos V, McDonald CF. Alveolar macrophage function is altered in patients with lung cancer. Clin Exp Immunol. (2006) 143:363–72. doi: 10.1111/j.1365-2249.2006.02998.x
52. Chakarov S, Lim HY, Tan L, Lim SY, See P, Lum J, et al. Two distinct interstitial macrophage populations coexist across tissues in specific subtissular niches. Science. (2019) 363(6432):eaau0964. doi: 10.1126/science.aau0964
53. Shibata S, Miyake K, Tateishi T, Yoshikawa S, Yamanishi Y, Miyazaki Y, et al. Basophils trigger emphysema development in a murine model of COPD through IL-4-mediated generation of MMP-12-producing macrophages. Proc Natl Acad Sci U.S.A. (2018) 115:13057–62. doi: 10.1073/pnas.1813927115
54. Ural BB, Yeung ST, Damani-Yokota P, Devlin JC, de Vries M, Vera-Licona P, et al. Identification of a nerve-associated, lung-resident interstitial macrophage subset with distinct localization and immunoregulatory properties. Sci Immunol. (2020) 5(45):eaax8756. doi: 10.1126/sciimmunol.aax8756
55. Fu Y, Pajulas A, Wang J, Zhou B, Cannon A, Cheung CCL, et al. Mouse pulmonary interstitial macrophages mediate the pro-tumorigenic effects of IL-9. Nat Commun. (2022) 13:3811. doi: 10.1038/s41467-022-31596-7
56. Zhang L, Wang Y, Wu G, Xiong W, Gu W, Wang CY. Macrophages: friend or foe in idiopathic pulmonary fibrosis? Respir Res. (2018) 19:170. doi: 10.1186/s12931-018-0864-2
57. Liu G, Zhai H, Zhang T, Li S, Li N, Chen J, et al. New therapeutic strategies for IPF: Based on the “phagocytosis-secretion-immunization” network regulation mechanism of pulmonary macrophages. BioMed Pharmacother. (2019) 118:109230. doi: 10.1016/j.biopha.2019.109230
58. Heukels P, Moor CC, von der Thüsen JH, Wijsenbeek MS, Kool M. Inflammation and immunity in IPF pathogenesis and treatment. Respir Med. (2019) 147:79–91. doi: 10.1016/j.rmed.2018.12.015
59. She YX, Yu QY, Tang XX. Role of interleukins in the pathogenesis of pulmonary fibrosis. Cell Death Discovery. (2021) 7:52. doi: 10.1038/s41420-021-00437-9
60. Wojtan P, Mierzejewski M, Osińska I, Domagała-Kulawik J. Macrophage polarization in interstitial lung diseases. Cent Eur J Immunol. (2016) 41:159–64. doi: 10.5114/ceji.2016.60990
61. Bitterman PB, Wewers MD, Rennard SI, Adelberg S, Crystal RG. Modulation of alveolar macrophage-driven fibroblast proliferation by alternative macrophage mediators. J Clin Invest. (1986) 77:700–8. doi: 10.1172/JCI112364
62. Madsen SJ, Gach HM, Hong SJ, Uzal FA, Peng Q, Hirschberg H. Increased nanoparticle-loaded exogenous macrophage migration into the brain following PDT-induced blood-brain barrier disruption. Lasers Surg Med. (2013) 45:524–32. doi: 10.1002/lsm.22172
63. Zhou Y, Peng H, Sun H, Peng X, Tang C, Gan Y, et al. Chitinase 3-like 1 suppresses injury and promotes fibroproliferative responses in Mammalian lung fibrosis. Sci Transl Med. (2014) 6:240ra76. doi: 10.1126/scitranslmed.3007096
64. Prasse A, Probst C, Bargagli E, Zissel G, Toews GB, Flaherty KR, et al. Serum CC-chemokine ligand 18 concentration predicts outcome in idiopathic pulmonary fibrosis. Am J Respir Crit Care Med. (2009) 179:717–23. doi: 10.1164/rccm.200808-1201OC
65. Cai M, Bonella F, He X, Sixt SU, Sarria R, Guzman J, et al. CCL18 in serum, BAL fluid and alveolar macrophage culture supernatant in interstitial lung diseases. Respir Med. (2013) 107:1444–52. doi: 10.1016/j.rmed.2013.06.004
66. Prasse A, Pechkovsky DV, Toews GB, Jungraithmayr W, Kollert F, Goldmann T, et al. A vicious circle of alveolar macrophages and fibroblasts perpetuates pulmonary fibrosis via CCL18. Am J Respir Crit Care Med. (2006) 173:781–92. doi: 10.1164/rccm.200509-1518OC
67. Guo Z, Li S, Zhang N, Kang Q, Zhai H. Schisandra inhibit bleomycin-induced idiopathic pulmonary fibrosis in rats via suppressing M2 macrophage polarization. BioMed Res Int. (2020) 2020:5137349. doi: 10.1155/2020/5137349
68. Susser LI, Nguyen M-A, Geoffrion M, Emerton C, Ouimet M, Khacho M, et al. Mitochondrial fragmentation promotes inflammation resolution responses in macrophages via histone lactylation. Mol Cell Biol. (2023) 43:531–46. doi: 10.1080/10985549.2023.2253131
69. Zhou HC, Yu WW, Yan XY, Liang XQ, Ma XF, Long JP, et al. Lactate-driven macrophage polarization in the inflammatory microenvironment alleviates intestinal inflammation. Front Immunol. (2022) 13:1013686. doi: 10.3389/fimmu.2022.1013686
70. Antoshina DV, Balandin SV, Bogdanov IV, Vershinina MA, Sheremeteva EV, Toropygin IY, et al. Antimicrobial activity and immunomodulatory properties of acidocin A, the pediocin-like bacteriocin with the non-canonical structure. Membranes (Basel). (2022) 12(12):1253. doi: 10.3390/membranes12121253
71. Pan RY, He L, Zhang J, Liu X, Liao Y, Gao J, et al. Positive feedback regulation of microglial glucose metabolism by histone H4 lysine 12 lactylation in Alzheimer’s disease. Cell Metab. (2022) 34:634–648.e6. doi: 10.1016/j.cmet.2022.02.013
72. Zhang J, Muri J, Fitzgerald G, Gorski T, Gianni-Barrera R, Masschelein E, et al. Endothelial lactate controls muscle regeneration from ischemia by inducing M2-like macrophage polarization. Cell Metab. (2020) 31:1136–1153.e7. doi: 10.1016/j.cmet.2020.05.004
73. Peoc’h K, Nuzzo A, Guedj K, Paugam C, Corcos O. Diagnosis biomarkers in acute intestinal ischemic injury: so close, yet so far. Clin Chem Lab Med. (2018) 56:373–85. doi: 10.1515/cclm-2017-0291
74. Wang P, Xie D, Xiao T, Cheng C, Wang D, Sun J, et al. H3K18 lactylation promotes the progression of arsenite-related idiopathic pulmonary fibrosis via YTHDF1/m6A/NREP. J Hazard Mater. (2024) 461:132582. doi: 10.1016/j.jhazmat.2023.132582
75. Zhao SS, Liu J, Wu QC, Zhou XL. Role of histone lactylation interference RNA m(6)A modification and immune microenvironment homeostasis in pulmonary arterial hypertension. Front Cell Dev Biol. (2023) 11:1268646. doi: 10.3389/fcell.2023.1268646
76. Dai X, Lv X, Thompson EW, Ostrikov KK. Histone lactylation: epigenetic mark of glycolytic switch. Trends Genet. (2022) 38:124–7. doi: 10.1016/j.tig.2021.09.009
77. Hong H, Chen X, Wang H, Gu X, Yuan Y, Zhang Z. Global profiling of protein lysine lactylation and potential target modified protein analysis in hepatocellular carcinoma. Proteomics. (2023) 23:e2200432. doi: 10.1002/pmic.202200432
78. Li J, Zeng G, Zhang Z, Wang Y, Shao M, Li C, et al. Urban airborne PM2.5 induces pulmonary fibrosis through triggering glycolysis and subsequent modification of histone lactylation in macrophages. Ecotoxicology Environ Saf. (2024) 273:116162. doi: 10.1016/j.ecoenv.2024.116162
79. Wei Y, You Y, Zhang J, Ban J, Min H, Li C, et al. Crystalline silica-induced macrophage pyroptosis interacting with mitophagy contributes to pulmonary fibrosis via modulating mitochondria homeostasis. J Hazard Mater. (2023) 454:131562. doi: 10.1016/j.jhazmat.2023.131562
80. Chen W, Chen S, Yan C, Zhang Y, Zhang R, Chen M, et al. Allergen protease-activated stress granule assembly and gasdermin D fragmentation control interleukin-33 secretion. Nat Immunol. (2022) 23:1021–30. doi: 10.1038/s41590-022-01255-6
81. Tao H, Zhao H, Mo A, Shao L, Ge D, Liu J, et al. VX-765 attenuates silica-induced lung inflammatory injury and fibrosis by modulating alveolar macrophages pyroptosis in mice. Ecotoxicology Environ Saf. (2023) 249:114359. doi: 10.1016/j.ecoenv.2022.114359
82. Li Q, Zhang F, Wang H, Tong Y, Fu Y, Wu K, et al. NEDD4 lactylation promotes APAP induced liver injury through Caspase11 dependent non-canonical pyroptosis. Int J Biol Sci. (2024) 20:1413–35. doi: 10.7150/ijbs.91284
83. Chen N, Xie Q-M, Song S-M, Guo S-N, Fang Y, Fei G-H, et al. Dexamethasone protects against asthma via regulating Hif-1α-glycolysis-lactate axis and protein lactylation. Int Immunopharmacol. (2024) 131:111791. doi: 10.1016/j.intimp.2024.111791
84. Sun S, Xu X, Liang L, Wang X, Bai X, Zhu L, et al. Lactic Acid-Producing Probiotic Saccharomyces cerevisiae Attenuates Ulcerative Colitis via Suppressing Macrophage Pyroptosis and Modulating Gut Microbiota. Front Immunol. (2021) 12:777665. doi: 10.3389/fimmu.2021.777665
85. Cui H, Xie N, Banerjee S, Ge J, Jiang D, Dey T, et al. Lung myofibroblasts promote macrophage profibrotic activity through lactate-induced histone lactylation. Am J Respir Cell Mol Biol. (2021) 64:115–25. doi: 10.1165/rcmb.2020-0360OC
86. Jin H, Luo R, Li J, Zhao H, Ouyang S, Yao Y, et al. Inhaled platelet vesicle-decoyed biomimetic nanoparticles attenuate inflammatory lung injury. Front Pharmacol. (2022) 13:1050224. doi: 10.3389/fphar.2022.1050224
87. Yu QY, Tang XX. Irreversibility of pulmonary fibrosis. Aging Dis. (2022) 13:73–86. doi: 10.14336/AD.2021.0730
88. She Y, Xu X, Yu Q, Yang X, He J, Tang XX. Elevated expression of macrophage MERTK exhibits profibrotic effects and results in defective regulation of efferocytosis function in pulmonary fibrosis. Respir Res. (2023) 24:118. doi: 10.1186/s12931-023-02424-3
89. Bhattacharyya A, Torre P, Yadav P, Boostanpour K, Chen TY, Tsukui T, et al. Macrophage cx43 is necessary for fibroblast cytosolic calcium and lung fibrosis after injury. Front Immunol. (2022) 13:880887. doi: 10.3389/fimmu.2022.880887
90. Fan M, Yang K, Wang X, Chen L, Gill PS, Ha T, et al. Lactate promotes endothelial-to-mesenchymal transition via Snail1 lactylation after myocardial infarction. Sci Adv. (2023) 9:eadc9465. doi: 10.1126/sciadv.adc9465
91. Lv J, Gao H, Ma J, Liu J, Tian Y, Yang C, et al. Dynamic atlas of immune cells reveals multiple functional features of macrophages associated with progression of pulmonary fibrosis. Front Immunol. (2023) 14:1230266. doi: 10.3389/fimmu.2023.1230266
92. Xie N, Tan Z, Banerjee S, Cui H, Ge J, Liu RM, et al. Glycolytic reprogramming in myofibroblast differentiation and lung fibrosis. Am J Respir Crit Care Med. (2015) 192:1462–74. doi: 10.1164/rccm.201504-0780OC
93. La Fleur L, Boura VF, Alexeyenko A, Berglund A, Pontén V, Mattsson JSM, et al. Expression of scavenger receptor MARCO defines a targetable tumor-associated macrophage subset in non-small cell lung cancer. Int J Cancer. (2018) 143:1741–52. doi: 10.1002/ijc.31545
94. La Fleur L, Botling J, He F, Pelicano C, Zhou C, He C, et al. Targeting MARCO and IL37R on immunosuppressive macrophages in lung cancer blocks regulatory T cells and supports cytotoxic lymphocyte function. Cancer Res. (2021) 81:956–67. doi: 10.1158/0008-5472.CAN-20-1885
95. Ren W, Hou J, Yang C, Wang H, Wu S, Wu Y, et al. Extracellular vesicles secreted by hypoxia pre-challenged mesenchymal stem cells promote non-small cell lung cancer cell growth and mobility as well as macrophage M2 polarization via miR-21-5p delivery. J Exp Clin Cancer Res. (2019) 38:62. doi: 10.1186/s13046-019-1027-0
96. Wang T, Ye Z, Li Z, Jing DS, Fan GX, Liu MQ, et al. Lactate-induced protein lactylation: A bridge between epigenetics and metabolic reprogramming in cancer. Cell Prolif. (2023) 56:e13478. doi: 10.1111/cpr.13478
97. Walenta S, Schroeder T, Mueller-Klieser W. Lactate in solid Malignant tumors: potential basis of a metabolic classification in clinical oncology. Curr Med Chem. (2004) 11:2195–204. doi: 10.2174/0929867043364711
98. He Y, Ji Z, Gong Y, Fan L, Xu P, Chen X, et al. Numb/Parkin-directed mitochondrial fitness governs cancer cell fate via metabolic regulation of histone lactylation. Cell Rep. (2023) 42(2):112033. doi: 10.1016/j.celrep.2023.112033
99. Zheng P, Mao Z, Luo M, Zhou L, Wang L, Liu H, et al. Comprehensive bioinformatics analysis of the solute carrier family and preliminary exploration of SLC25A29 in lung adenocarcinoma. Cancer Cell Int. (2023) 23(1):222. doi: 10.1186/s12935-023-03082-7
100. Wang M, He T, Meng D, Lv W, Ye J, Cheng L, et al. BZW2 modulates lung adenocarcinoma progression through glycolysis-mediated IDH3G lactylation modification. J Proteome Res. (2023) 22:3854–65. doi: 10.1021/acs.jproteome.3c00518
101. Garrido-Martin EM, Mellows TWP, Clarke J, Ganesan AP, Wood O, Cazaly A, et al. M1(hot) tumor-associated macrophages boost tissue-resident memory T cells infiltration and survival in human lung cancer. J Immunother Cancer. (2020) 8(2):e000778. doi: 10.1136/jitc-2020-000778
Keywords: macrophage, lactylation, immune regulation, metabolism, lung disease
Citation: Wei Y, Guo H, Chen S and Tang XX (2024) Regulation of macrophage activation by lactylation in lung disease. Front. Immunol. 15:1427739. doi: 10.3389/fimmu.2024.1427739
Received: 04 May 2024; Accepted: 24 June 2024;
Published: 04 July 2024.
Edited by:
Marco Orecchioni, Augusta University, United StatesReviewed by:
Felix Sebastian Nettersheim, University Hospital of Cologne, GermanyAdil Rasheed, University of Ottawa Heart Institute, Canada
Copyright © 2024 Wei, Guo, Chen and Tang. This is an open-access article distributed under the terms of the Creative Commons Attribution License (CC BY). The use, distribution or reproduction in other forums is permitted, provided the original author(s) and the copyright owner(s) are credited and that the original publication in this journal is cited, in accordance with accepted academic practice. No use, distribution or reproduction is permitted which does not comply with these terms.
*Correspondence: Xiao Xiao Tang, dGFuZ3hpYW94aWFvQGdpcmQuY24=