- 1NHC Key Laboratory of Drug Addiction Medicine, School of Forensic Medicine, Kunming Medical University, Kunming, China
- 2Department of Pathogen Biology and Immunology, School of Basic Medical Science, Kunming Medical University, Kunming, China
Combination antiretroviral therapy (cART) has dramatically reduced mortality in people with human immunodeficiency virus (HIV), but it does not completely eradicate the virus from the brain. Patients with long-term HIV-1 infection often show neurocognitive impairment, which severely affects the quality of life of those infected. Methamphetamine (METH) users are at a significantly higher risk of contracting HIV-1 through behaviors such as engaging in high-risk sex or sharing needles, which can lead to transmission of the virus. In addition, HIV-1-infected individuals who abuse METH exhibit higher viral loads and more severe cognitive dysfunction, suggesting that METH exacerbates the neurotoxicity associated with HIV-1. Therefore, this review focuses on various mechanisms underlying METH and HIV-1 infection co-induced neurotoxicity and existing interventions targeting the sigma 1 receptor, dopamine transporter protein, and other relevant targets are explored. The findings of this review are envisaged to systematically establish a theoretical framework for METH abuse and HIV-1 infection co-induced neurotoxicity, and to suggest novel clinical treatment targets.
1 Introduction
Acquired immunodeficiency syndrome (AIDS) is a highly infectious disease caused by infection with the human immunodeficiency virus (HIV). Most people with AIDS are infected with HIV-1, which is the most prevalent and more virulent subtype. It has been demonstrated that HIV-1 may trigger HIV-associated neurocognitive disorder (HAND) by inflicting damage to the neurovascular units (NVU), blood-brain barrier (BBB) dysfunction, and inflammatory response (1). It has been shown that the interplay between diverse HIV-1 viral proteins (Tat, Vpr, and gp120) and neural cells may be linked to the pathogenesis of HIV-1-induced neurotoxicity, which results in cellular injury and anomalous alterations in the CNS (2). However, the precise mechanism of action is not yet well defined. Despite a significant reduction in mortality rates among HIV-1 patients due to combination antiretroviral therapy (cART), chronic viral infections continue to persist in the brain (3). Moreover, it has been shown that long-term cART may disrupt mitochondrial function, affect metabolism, and even cause neurotoxicity and fatal complications (4, 5). Therefore, we need to explore safer and more effective ways for HIV treatment.
The global issue of substances misuse significantly affects the social welfare and public health. The World Drug Report 2024 by the United Nations Office on Drugs and Crime (UNODC) indicates that 13.9 million individuals engaged in substances injections in 2022, and about one in eight injecting substances abusers will be living with HIV-1 (6). Methamphetamine (METH), a highly addictive psychostimulant, is widely abused and can lead to severe health risks following withdrawal (7, 8). Prolonged METH use has also been reported to negatively impact memory, learning, and cognitive function, leading to symptoms such as paranoia, insomnia, irritability, hallucinations, and delusions (9). In severe cases, METH abuse may contribute to the development of neurodegenerative disorders such as Alzheimer’s disease (AD) and Parkinson’s disease (PD). And yet, there are currently no the US Food and Drug Administration (FDA) -approved drugs for the treatment of METH-induced neurotoxicity (10).
METH abuse is often associated with HIV-1 infection through needle sharing and high-risk sexual activity among users. HIV-1-infected individuals who abuse METH exhibit higher viral loads and more severe cognitive dysfunction (11). Concurrently, studies have shown that the pathways of neurotoxicity can be induced by METH and HIV are somewhat similar. For instance, they can cause mitochondrial dysfunction and altered metabolic pathways (12–14), which in turn can lead to programmed cell death and neuroinflammatory (15–17). However, the specific mechanisms underlying this synergistic neurotoxicity are not fully understood and effective treatments are lacking. Therefore, this paper reviews the mechanisms of synergistically induced neurotoxicity by METH and HIV-1 and proposes potential therapeutic targets.
2 Potential mechanisms of neurotoxicity co-induced by METH and HIV-1
2.1 Oxidative stress and endoplasmic reticulum stress
Oxidative stress, a harmful result of free oxygen radicals in the body, is commonly associated with the processes of ageing and disease (18). Endoplasmic reticulum stress (ERS) is characterized by an atypical accumulation of unfolded or misfolded proteins, resulting from an increased demand for properly folded proteins in response to external stimuli, ultimately leading to ER dysfunction (19). The interaction between ERS and oxidative stress was apparent. The disruption of redox homeostasis in the endoplasmic reticulum caused by oxidative stress has been shown to significantly affect endoplasmic reticulum function, leading to endoplasmic reticulum signaling activation, which could result in ERS development and could produce high levels of reactive oxygen species (ROS), thereby exacerbating oxidative stress (20).
METH has been demonstrated to damage dopaminergic neurons through oxidative stress processes. Upon entering the neuron, METH displaces dopamine (DA) from vesicles, leading to an increase in intracellular and synaptic gap DA levels, which results in elevated auto-oxidation and DA metabolism, leading to higher ROS production (21). The resulting damage to cellular proteins, lipids, and DNA leads to a loss of cellular function and subsequent neurotoxicity, which is further exacerbated when METH and HIV-1 act together (22). Within the human innate immune macrophage cell line system, METH induces significant cellular ROS production, thereby activating the expression of interleukine-1β (IL-1β) and tumor necrosis factor-alpha (TNF-α) in a dose-dependent manner. Additionally, METH-induced oxidative stress is further exacerbated by the presence of HIV-1 Tat protein (23). Combined exposure to METH and HIV-1 synergistically induces oxidative stress by activating transient receptor potential melastatin 2 (TRPM2) channels in endothelial cells, thereby resulting in ROS production, which further exacerbates the toxic effects on the nervous system by compromising the antioxidant defenses of catalase (CAT), glutathione peroxidase (GSH-Px), and superoxide dismutase (SOD) (1). TRPM2 channels can mediate oxidative stress by activating NLRP3 inflammasome and microglia or inducing TNF-α production, triggering autophagy in human cerebrovascular pericytes and damaging BBB (24).
It was also found that METH caused a significant increase in ERS-related proteins Bip, ATF-6, ATF-4, eIF2α, and CHOP in C57BL/6 mice, where memory loss and cognitive impairment were improved when treated with taurine, an ERS inhibitor, suggesting that METH-induced memory loss was associated with ERS (25).Abnormal mitochondrial function is a significant contributor to oxidative stress (26). Sirtuins (SIRTs) are NAD+-dependent deacetylases involved in mitochondrial biogenesis, protein responses, and intrinsic apoptosis. The activation of SIRTs has been reported to exert a neuroprotective effect in conditions such as AD and PD (27). In HIV-1 disease models, the miR-505/SIRT3 axis was implicated in mitochondrial oxidative stress, associated with the induction of the HIV-1 Tat protein-mediated microglia senescence phenotype, leading to elevated superoxide production within mitochondria (28). Consequently, HIV-1 Tat protein may induce oxidative stress and ERS by inhibiting SIRT1 and SIRT3, thereby diminishing their neuroprotective properties (28, 29). Mitochondria-associated endoplasmic reticulum membranes (MAMs) represent direct contact sites between the ER and mitochondria, functioning as critical platforms for the coordination of essential cellular processes, including mitochondrial dynamics and calcium homeostasis (30). Notably, the strategic targeting of MAMs to modulate astrocyte mitochondrial function holds potential as a promising approach to enhance the metabolic and antioxidant coupling between astrocytes and neurons, thereby promoting neuronal resilience against CNS pathologies (30). It has also been shown that METH and HIV-1-induced astrocyte excitatory amino acid transporter-2 dysfunction can be restored by maintaining calcium homeostasis (31).
Regulation of endoplasmic reticulum-related signaling pathways is critical for cellular homeostasis (19). Results of a study evaluating the effects of 7-day HIV-1 infection and chronic METH exposure on the endoplasmic reticulum and mitochondria showed that the expression level of ERS-related inositol-requiring protein 1α (IRE1α) was significantly upregulated (32). These results imply that ERS is implicated in the synergistic effect of HIV-1 and METH on the induction of neurocognitive impairment. Nonetheless, moderate ERS is beneficial for the body, but excessive ERS can cause neurocognitive dysfunction; thus, both oxidative stress and ERS are important factors in neurotoxicity caused by METH and HIV-1 (Figure 1).
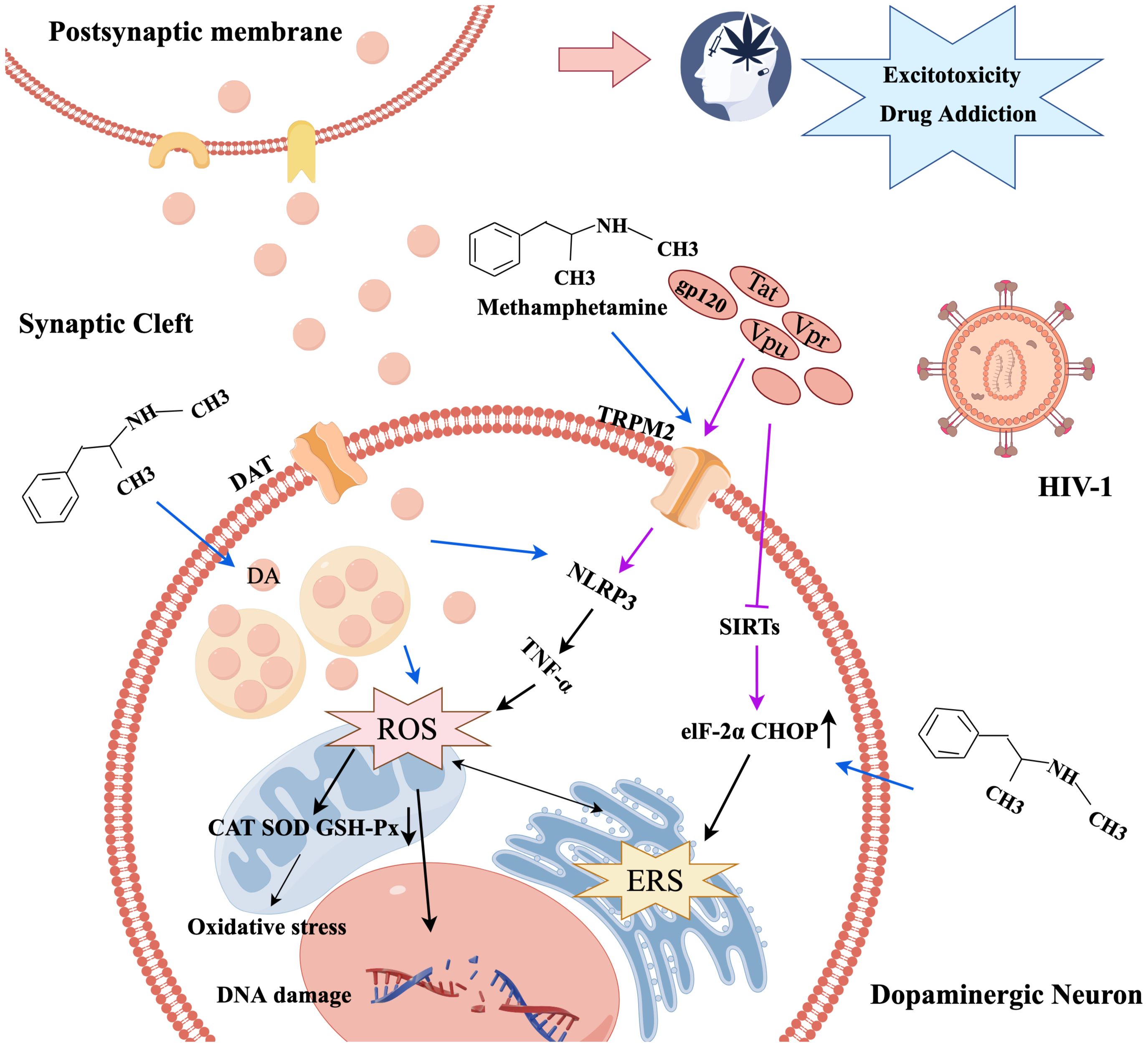
Figure 1. The mechanism diagram of oxidative stress and endoplasmic reticulum stress caused by METH and HIV-1.
2.2 Programmed cell death
Presently, the prevalent types of programmed cell death include apoptosis, pyroptosis, autophagy, necrosis, and ferroptosis (33). The molecular mechanisms underlying these forms of cell death are not self-contained, and recent findings indicate an intricate interplay between them, collectively promoting nerve cell death (Figure 2) (34).
2.2.1 Autophagy
Autophagy is a crucial physiological mechanism for preserving cellular homeostasis and energy conservation. This process is primarily initiated by the activation of ULK1, Atg13, and FIP200 complexes via the PI3K/Akt/mTOR pathway, which then triggers the commencement of autophagic program (35). The acceleration of cell death resulting from dysregulation of autophagy occurs when the body is overstimulated. Autophagy dysfunction has been identified as a contributing factor to mental disorders and synaptic damage in degenerative diseases (36). Meanwhile, autophagy is the primary pathway for the degradation of long-lived proteins and organelles and is closely associated with survival, death, and neuron transformation (33). The formation of double-membrane vesicles, known as autophagosomes, is facilitated by the copolymerization of a series of Atg complexes that can capture organelle fragments in the cytoplasm (37). The completion of autophagy depends on the correct formation, maturation, and fusion with lysosomes of autophagosomes.
The specific role of autophagy in the body is twofold. On the one hand, the potential for the accumulation of misfolded proteins following HIV-1 infection to induce ERS, autophagy can effectively remove these toxic proteins, thereby reducing ERS and inhibiting the progression of HAND. On the other hand, an autophagic imbalance may result in the build-up of harmful substances in neurons (38). Similarly, METH enhanced HIV-1 gp120-mediated autophagy via Beclin-1 and Atg5/Atg7, thereby exerting a protective effect on astrocytes (39). Recent research on the gut-brain axis has revealed that the METH-abused BALB/c mouse model with continuously increasing multiple doses can induce neurotoxicity and enteritis and alter the gut microbiota and fecal metabolites, which is closely associated with autophagy (40). Our previous study revealed that HIV-1 Tat protein can promote autophagy in nerve cells in a concentration-dependent manner, and the induction of autophagy by HIV-1 Tat protein was found to decrease cellular activity, signifying its detrimental impact on the nervous system (41).
METH abuse has been observed to result in elevated systemic inflammation and CNS impairment in HIV-1-infected individuals. METH was found to inhibit macrophage phagocytosis of aggregated amyloid-β, increase total ROS, and dysregulate the autophagic process in HIV-1-infected human macrophages (42). Furthermore, previous research demonstrated that the combined effects of HIV-1 Tat protein and METH can induce autophagy in primary tree shrew midbrain neuronal cells through mTOR signaling and the Atg5/Atg7 pathway (43). The combined impact of METH and HIV-1 Tat proteins results in a significant increase in LC3-II expression and a blockade of autophagic flow in primary human neuronal cells, resulting in increased mitochondrial DRP1-dependent breakdown or rupture and neuronal degenerative changes by inhibiting mitochondrial autophagy (44). These findings suggest that the combined action of METH and HIV-1 Tat proteins induces autophagy, thereby contributing to neurotoxic damage.
2.2.2 Ferroptosis
Ferroptosis is a recently discovered iron-dependent form of programmed cell death distinct from apoptosis, necrosis, and autophagy (45). Although Ferroptosis was initially proposed in 2012, prior research has identified irregularities in iron metabolism within the CNS (46). Ferroptosis is characterized by unique morphological and biochemical features, including depletion of intracellular GSH and inactivation of GSH-Px4, resulting in the accumulation of lipid peroxidation (34). Ferroptosis induction is closely associated with oxidative stress, a phenomenon that METH contributes to through various pathways, ultimately affecting neurocognitive function. Many studies have implied the importance of maintaining normal intracellular iron levels for proper development and functioning of the CNS. Consequently, Ferroptosis may be pivotal in METH-induced neurotoxicity.
Several studies have established a correlation between ferroptosis and various neurodegenerative disorders such as PD, AD, and HAND (47). Neurons and glial cells exhibit elevated susceptibility to ferroptosis and neurodegeneration, with microglia being the most iron-laden cells. Iron-activated microglia may participate in the aberrant elimination of neurons and synapses, thereby exacerbating ferroptosis-induced neurodegeneration (48). Researchers found, as early as the 1990s, that ferritin concentrations increased, and iron-mediated oxidative stress was evident as AIDS progressed (49). According to a recent clinical study, elevated concentrations of iron transport proteins in the cerebrospinal fluid are associated with the incidence of HAND (50). The underlying mechanism of HAND may be attributed to HIV-1-related proteins inducing ferroptosis, where HIV-1 Tat protein specifically triggers ferroptosis in microglia by suppressing miR-204 expression, which subsequently upregulates ACSL4 expression, leading to increased lipid peroxidation. This process ultimately results in microglial activation and secretion of proinflammatory cytokines, culminating in neuroinflammation and neurotoxicity (48).
SLC7A11 is a crucial cystine/glutamate transporter protein constituent that expedites GSH synthesis and impedes ferroptosis (51). Our research team discovered that METH and HIV-1 Tat proteins can collaboratively instigate ferroptosis in BV2 cells, which is antagonized by transcription factor NF-E2-related factor (Nrf2) by regulating SLC7A11, thus providing a theoretical basis for investigate the synergistic effects of METH and HIV-1 induced neurotoxicity (17). However, the precise mechanisms underlying ferroptosis are poorly understood and warrant further investigation.
2.2.3 Apoptosis
Apoptosis is among the earliest identified forms of programmed cell death mechanisms (52). Unlike necrosis, apoptosis is an activecell death process that does not involve rupture of the cell membrane. It is often characterized by cell shrinkage, nuclear breakage, budding of the cytoplasmic membrane, and formation of apoptotic vesicles (53). Apoptosis has been a research hotspot for METH and HIV-1 induced neurotoxicity.
Apoptosis can be divided into an initiation phase and an execution phase, where the former is generally mediated by caspases 8, 9, and 10 and the latter by caspases 3, 6, and 7 (54). Among them, caspases 8 and 10 are initiators of exogenous apoptosis, and caspase 9 is an initiator of endogenous apoptosis. The activation of caspase-3 heralds the beginning of the apoptotic process and is considered a key enzyme that leads to apoptosis. A variety of proteins play equally important roles in the development of apoptosis. Bcl-2 and Bcl-XL act as potent apoptosis inhibitors to prevent apoptosis. Factors such as Bak, Bad and Bax promote apoptosis. In addition, P53 and Cyt-c are also involved in apoptosis development (55).
As previously mentioned, autophagy may have deleterious effects, resulting in autophagic cell death, and multiple intermediates of programmed cell death engage in intricate interactions (56). In an in vitro astrocyte experiment, the combined effects of METH and gp120 were observed, where inhibition of autophagy resulted in significant astrocyte apoptosis, indicating that autophagy plays a protective role against apoptosis caused by METH and gp120 (39). Similarly, another study found that gp120 and METH-induced oxidative stress through the cytochrome (CYP) P450 and NADPH oxidase (NOX) pathways result in astrocyte apoptosis (57), implying that inhibiting these two pathways could significantly help suppress the apoptosis of astrocytes. These findings demonstrated that METH and gp120 can induce apoptosis in several ways. In addition to gp120, a significant increase in TUNEL-staining-positive cells was also found in the hCMEC/D3 model of human microvascular endothelial cells following the combined action of HIV-1 Tat protein and METH (24). This indicates that both METH and multiple viral proteins of HIV-1 can induce apoptosis and that efficacious interventions are currently unavailable.
2.2.4 Pyroptosis
Pyroptosis is a mode of inflammatory cell death activated through two main pathways: gasdermin D (GSDMD)-dependent activation regulated by caspase-1/4/5/11 and GSDME-dependent activation regulated by caspase 3 (58). Activated caspases cleave the hinge region between the N- and C-terminal domains of GSDMD or GSDME to recognize and bind to phospholipid molecules in the cell membrane, This results in the formation of pores or perforations within the cell membrane, leading to alterations in cellular osmolarity and ultimately cell membrane rupture and cell death (59).
Substances misuse has been shown to accelerate neurological symptoms in HIV-1-infected patients by facilitating HIV-1 entry into the brain and triggering an immune response, thereby mediating neuroinflammation and pyroptosis through the activation of microglia and the release of neurotoxins (60). Studies have disclosed that METH can induce GSDME-dependent cell death of hippocampal neurons through the ERS pathway (61). In addition, manifestations of cognitive impairment and changes in the NLRP1/Caspase1/GSDMD signaling pathway were found in an in vivo model of METH administration in rats (62). The use of aspirin and NLRP1 siRNA significantly attenuated METH-induced cognitive deficits while decreasing the activities of NLRP1 and cleaved caspase-1, IL-1β, and TNF-α, providing further evidence of complex programmed cell death mechanisms. Similarly, one study reported HIV-1 gp120-induced NLRP3-dependent pyroptosis and IL-1β production in microglia (63). Long-term administration of MCC950 (an NLRP3 inhibitor) to gp120 transgenic mice attenuated neuroinflammation and neuronal death, promoted neuronal regeneration, and restored impaired neurocognitive functions (64).
All the aforementioned findings suggest a link between METH and HIV-1 and pyroptosis. Indeed, a recent study showed that METH enhanced HIV-1 gp120-induced activation of microglia and NLRP3 inflammasome, while elevating GSDMD and GSDMD-N expression (65). Thus, activation of microglia-mediated neuroinflammation and pyroptosis might be one of the HAND etiological factors. Nevertheless, the investigation into METH and HIV-1 acting synergistically in inducing pyroptosis is still in its infancy. Further research is required to elucidate the interrelationships between pyroptosis and other mechanisms.
2.3 Neuroinflammation
Neuroinflammation is an important mechanism of neurotoxicity that has been closely associated with the activation of inflammasomes, glial cells, and cytokines (9, 66–68). The activation of microglia and astrocytes is a crucial component of the synergistic induction of neuroinflammation by METH and HIV-1. It has been shown that METH can activate inflammatory cells such as astrocytes and microglia, leading to the secretion of TNF-α and IL-1β inflammatory factors (23). Upon HIV-1 infection of human microglia, pro-IL-1β is secreted at 4 h, and mature IL-1β is secreted at 24 h, accompanied by caspase-1 activation, initiating the inflammatory process (69). Furthermore, HIV-1 infection induces a notable release of proinflammatory cytokines, namely IL-1β and IL-18. Exposure of monocytes to HIV-1 Tat has been observed to cause IL-1β release, which can lead to neuronal cell death and HAND through a direct or indirect proinflammatory mechanism (70). Similarly, the severity of this inflammatory response is elevated in HIV-1 patients with a history of METH abuse (71).
At present, five primary types of inflammasomes, namely NLRP3, NLRP1, NLRC4, IPAF, and AIM2, have been extensively studied and are known to play crucial roles in the onset and progression of various diseases (72). In a study involving METH abuse in rats, elevated NLRP1 activity was observed in the hippocampus, and NLRP1 inhibition significantly ameliorated METH-induced cognitive impairment (62). Moreover, inhibition of the NLRP3 inflammasome has the potential to mitigate motor impairments and cerebellar alterations induced by METH administration (68). Similarly, HIV-1 activates inflammasomes through diverse mechanisms, resulting in immune cell death and associated pathological changes (73). This shows a notable correlation between inflammasomes and neuroinflammation induced by METH and HIV-1.
The findings of our study indicated that microglia in the prefrontal cortex of METH abusers with concurrent HIV-1 infections exhibited significant activation (74). Additionally, METH and HIV-1 led to the activation of NLRP3 inflammasomes and caused neuroinflammation, as well as neuronal apoptosis in the prefrontal cortex and hippocampus (63). These results have suggested that neuroinflammation in HIV-1-infected individuals with METH abuse may contribute to neuronal apoptosis and consequent damage to the nervous system. Neuroinflammation involves a multifaceted interplay of mechanisms, including apoptosis and oxidative stress (75). Therefore, investigating the interplay between neuroinflammation and other mechanisms is a promising area for future research.
2.4 Blood-brain barrier damage
The BBB serves as a critical interface between the brain and the peripheral circulation and plays a vital role in maintaining homeostasis within the CNS (76). The neurovascular unit (NVU), which comprises endothelial cells, pericytes, astrocytes, microglia, neurons, and basement membranes, is the main functional unit of the blood-brain barrier (77). To access the CNS, both METH and HIV-1 must traverse the BBB. It has been established that the benzene ring and methyl group in the chemical structure of METH render it lipid-soluble and capable of traversing cell membranes and even the BBB, thereby exerting an effect on the central nervous system. HIV-1 may gain access to the central nervous system by infecting endothelial cells or mononuclear macrophages via a “Trojan horse” mechanism (78). Notably, HIV-1 infection and METH abuse synergistically impairs the BBB (79).
Disruption of BBB results in infiltration of various plasma components, and immune factors into the CNS, thereby inducing neuroinflammation and ultimately leading to toxic damage to the nervous system (80). The mechanisms of neuroinflammation and BBB damage caused by METH and HIV-1 are summarized in Figure 3. METH affects CNS homeostasis by impairing pericytes and astrocytes and disrupting tight junction proteins, such as occludin and claudins (81). Recent research has demonstrated that METH exposure induced neuroinflammation and BBB damage through neuronal-derived alpha-nucleoprotein transfer to astrocytes, as evidenced by the co-culturing of neurons with astrocytes (76). Upon entry into the BBB, HIV-1-infected cells secrete a range of HIV-1-associated viral proteins, including Tat, gp20, and Vpr. Notably, the HIV-1-associated viral proteins can exert disruptive effects on the BBB through various mechanisms (82), such as specifically enhancing vascular permeability, suppressing the expression of tight junction proteins, inducing a reduction in the expression of claudin-5 and laminin, and stimulating the expression of matrix metallopeptidase 9 (MMP9) and matrix metallopeptidase 2 (MMP2), ultimately resulting in the cleavage of tight junction proteins (83, 84). However, the mechanism of injury associated with HIV-1 Tat protein-induced BBB damage is multifaceted and not isolated. Despite extensive research, complete elucidation of the mechanism of injury remains elusive.
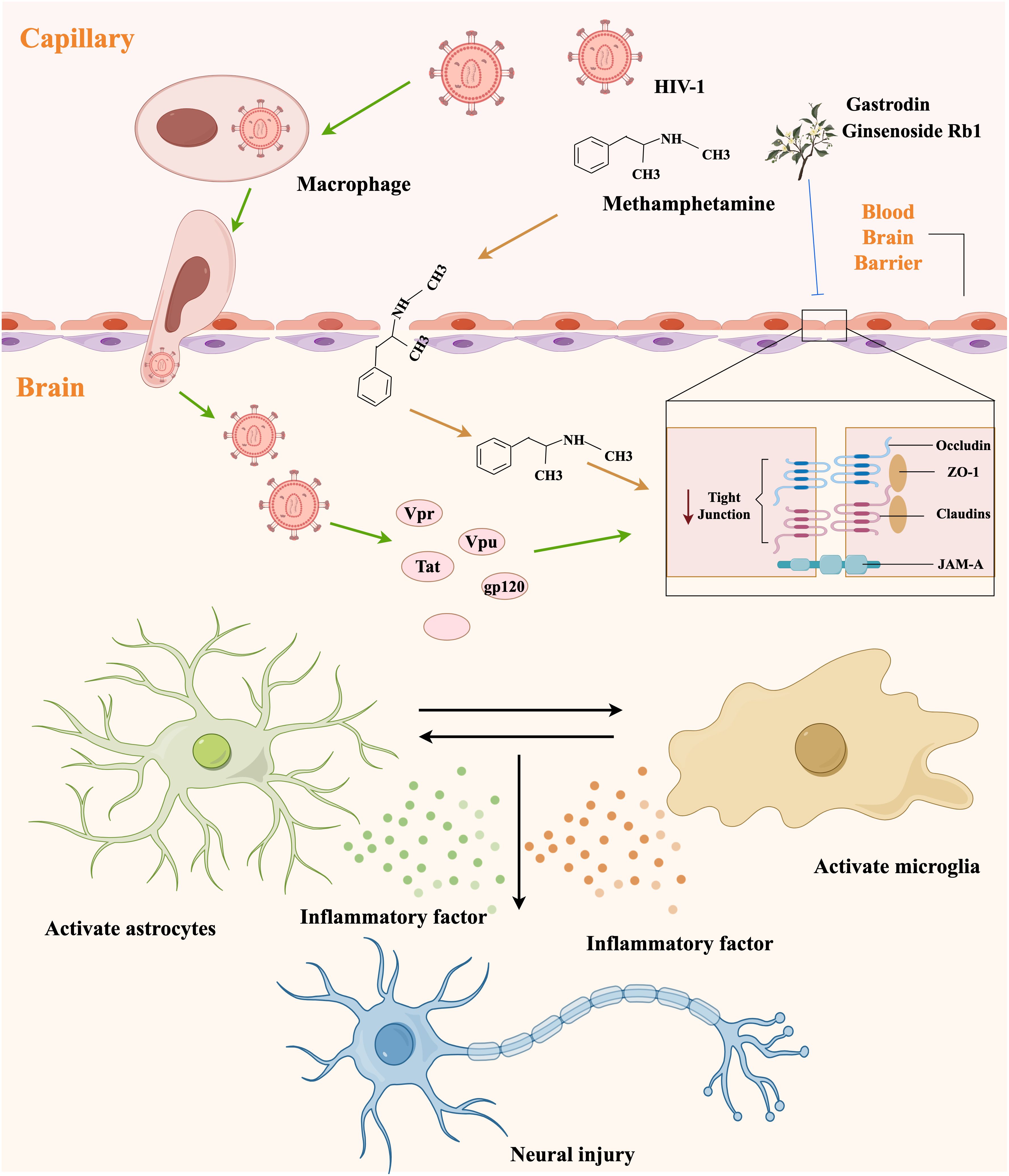
Figure 3. The mechanism diagram of neuroinflammation and blood-brain barrier damage caused by METH and HIV-1.
In our previous series of experiments, we found that ginsenoside Rb1 and gastrodin could alleviate the synergistically induced BBB damage by METH and HIV-1 Tat protein to a certain extent (85, 86). However, the neurotoxic effects caused by METH and HIV-1 are likely to arise from the interaction of various cellular and molecular mechanisms, and more in-depth studies, as well as additional clinical testing, may be required to achieve a therapeutic cure (22). Furthermore, the BBB presents significant challenges for future pharmacological interventions. Consequently, enhancing the ability of drugs across the BBB remains a prominent research focus and obstacle.
3 Potential therapeutic targets for neurotoxicity co-induced by METH and HIV-1
Despite the significant extension of life expectancy for individuals with HIV-1 through combination antiretroviral therapy (cART), chronic infections persist in the brain, and HIV-1-related neurocognitive impairment remains a formidable, incurable challenge (87). To date, the FDA has not approved any effective treatments for METH-induced neurotoxicity (88). Nevertheless, researchers continue to explore potential therapeutic agents for the neurotoxic effects induced by METH and HIV-1. The potential drugs that may treat METH and HIV-1-induced neurotoxicity are summarized in Table 1. For instance, ginsenoside Rb1 has shown promise in mitigating METH-induced neurotoxicity through the NR2B/ERK/CREB/BDNF pathway (89). Gastrodin, another agent, has demonstrated to attenuate METH-induced autophagy and apoptosis (41, 90). However, these agents have not yet provided a complete solution to the problem. Therefore, in this section, we offer a thorough overview of the targets for potential treatment of the neurotoxicity induced by METH and HIV-1 (Figure 4).
3.1 Sigma 1 receptor
Initially, Martin et al. proposed that the sigma 1 receptor (σ1R) was a new subtype of opioid receptor that facilitates specific effects induced by benzomorphans (109). Subsequent research has revealed that σ1R, an endoplasmic reticulum chaperone protein, is modulated by various ligands and serves as a potential therapeutic target for psychostimulant addiction (92). The σ1R has multiple functions within the cell, and its activation or inhibition may have different effects on the cell, depending on the specific biological context and pathological conditions (110).
A recent study suggests that supplementation with short-chain fatty acids can improve anxiety and depression-like behaviors induced by METH, through the activation of the σ1R/BDNF/TrkB pathway in the hippocampus of mice (13). Studies have found that HIV-1 gp120 can induce neuronal apoptosis and neuronal generation, while the 4-phenyl-1-(4-phenylbutyl) piperidine (PPBP), σ1R agonist, can weaken the neurotoxicity of gp120 (95). It suggests a protective role for σ1R. However, BD1047, a σ1R antagonist, has been found to mitigate METH-induced mortality and reduce seizures and convulsions in Swiss-Webster mice (93). Prior research also has demonstrated that cannabidiol can attenuate METH-induced conditioned place preference in rats through the σ1R/AKT/GSK-3β/CREB signaling pathway (94). The incidence of HIV-1 infection in quiescent CD4+ T cells is typically low; but even minor alterations in these cells can result in increased susceptibility to HIV-1 infection. Similarly, studies have indicated that the utilization of stimulants, such as cocaine, can enhance the likelihood of HIV-1 infection in quiescent CD4+ T cells through σ1R activation (111, 112). These findings suggest that σ1R may have damaging effects on the organism. In summary, σ1R plays a complex role in regulating homeostatic processes and may serve as a potential target for future therapeutic interventions.
3.2 Transcription factor NF-E2-related factor 2
Nrf2 is a significant transcriptional regulator with anti-inflammatory and antioxidant properties and a safeguarding effect on cellular survival (113). Under normal physiological conditions, Nrf2 is predominantly located in the cytoplasm and associates with Keap1 to form a complex. Upon exposure to stimuli such as ROS, Nrf2 disengages from Keap1 and translocates into the nucleus, where it interacts with antioxidant response elements and triggers the expression of antioxidant enzymes (114). When the extent of damage surpasses its regulatory capacity, the Keap1/Nrf2 pathway can have deleterious effects on the body, exacerbating oxidative stress.
The potential of targeting Nrf2 to treat METH and HIV-1-induced neurotoxicity has been extensively studied. Research has demonstrated that the endogenous antioxidant pathway, mediated by Nrf2, can mitigate METH-induced neurological dysfunction resulting from oxidative stress (96, 97). Resveratrol, a natural polyphenol, has been extensively studied for treating neurodegenerative diseases (115). Numerous studies have shown that multiple exposures to METH significantly impair cognitive function, leading to long-term memory deficits (116), whereas resveratrol may be effective in preventing memory impairment through interaction with Keap1, activation of the Keap1-Nrf2 pathway, and inhibition of DNA damage and apoptotic responses after METH exposure (98). Additionally, Nrf2 has also been reported to regulate iron homeostasis in vivo (117). It has been shown that Nrf2 can respectively prevent ferroptosis and autophagy induced by METH and HIV-1 Tat proteins by regulating the SLC7A11 and Nrf2/NQO1/HO-1 signalling pathways (17, 74). Consequently, further investigation of the functional role of Nrf2 provides great value for the treatment of METH and HIV-1-induced neurotoxicity.
3.3 Dopamine transporter protein
DA is crucial for regulating various physiological processes, such as reward, motivation, movement, working memory, and cognition (118). Dopaminergic neurons contain a transmembrane protein, DAT, which primarily promotes reuptake of DA released into the synaptic cleft (119).
Acute or single high dose METH abuse is indicated to cause a decrease in the amount of DAT and affect DA reuptake. This causes an increase in extracellular concentrations of DA, which in turn leads to the euphoria effect (120). However, the reduction in DAT can persist for up to 3 years after METH discontinuation. Prolonged or repeated METH administration can lead to a decrease in DA levels (121). The administration of traditional Chinese medicines, such as Chaihu-jia-Longgu-Muli decoction and levo-tetrahydropalmatine, has been demonstrated to regulate the levels of DA intracellular and extracellular, thereby mitigating the neurotoxicity associated with METH (99, 100). It has been posited that long-term HIV-1 viral protein exposure leads to a decreased dopaminergic state, which continues to persist despite the advent of cART (122).. Therefore, HIV-1 treatment should prioritize the restoration of DA function. The HIV-1 Tat protein plays a crucial role in the onset of HAND by binding to DAT, impeding DA transmission, and inducing neurotoxicity (123). Research has demonstrated that the HIV-1 Tat protein inhibits DA uptake by directly interacting with the human-dopamine transporter protein (hDAT) through mutation of crucial hDAT residues, namely D-H547, D-Y88, and D-D206, which are anticipated to participate in the binding process. This inhibition can be attenuated by disrupting the Tat-hDAT interaction via mutations in these residues (101). In the context of the combined influence of METH and HIV-1, the HIV-1 Tat protein has been observed to intensify the sensitizing impact of METH by altering the DA function (124). These findings indicate that extrasynaptic DA levels and DATs are crucial elements to focus on in managing neurotoxicity caused by METH and HIV-1, potentially paving the way for novel avenues in interventional research.
3.4 Peroxisome proliferator-activated receptor gamma
Peroxisome proliferator-activated receptor gamma (PPAR-γ) belongs to the nuclear hormone receptor superfamily of ligand-activated transcription factors that govern various functions, such as lipid metabolism and inflammation (125). Additionally, PPAR-γ plays a crucial anti-inflammatory role in brain injury and neurodegenerative diseases, is implicated in the pathogenesis of several diseases, including diabetes, cancer, and obesity, and is a promising therapeutic target for CNS disorders (126).
Activation of PPAR-γ results in its translocation to the nucleus, increasing anti-inflammatory gene expression and decreasing microglia/macrophage activation, ultimately downregulating proinflammatory factors (127). Behavioral sensitization, an experimental model of psychostimulant psychosis, is induced by repeated administrations of psychostimulants and has linked to brain inflammation. Notably, PPAR-γ activation attenuated METH-induced behavioral sensitization, potentially due to its anti-inflammatory properties (102). After the three-day METH injection, a gradual decrease in PPAR-γ expression was observed. However, subsequent treatments with aspirin and ibuprofen gradually reversed the DAT expression reduction, microglial activation, and PPAR-γ expression. These findings suggest a correlation between the reduction of PPAR-γ and METH-induced neurotoxicity, implying that the PPAR-γ agonistic effect of ibuprofen may underlie its ability to reverse neurotoxicity (103).
HIV-1 infection results in a range of neurological dysfunctions that entail activating glial cells and releasing inflammatory factors. These events lead to the occurrence of lethal toxic effects on nerve cells (104). Insulin treatment has been observed to upregulate PPAR-γ expression in HIV-1-infected primary microglia (106). Furthermore, HIV-1 primarily infects the CNS by disrupting the BBB. A reduction in PPAR-γ impedes MMP-9 and MMP-2 activity in human monocytes infected with HIV-1, leading to BBB impairment (105). Inflammation is a common factor in both METH and HIV-1 induced neurotoxicity, and the anti-inflammatory properties of PPAR-γ provide compelling evidence for synergistic therapeutic investigation of these conditions.
3.5 miRNA
The emergence of diverse genetic testing technologies, including transcriptome sequencing, has increased the interest in the in vivo function of non-coding RNAs (128), including rRNA, tRNA, snRNA, snoRNA, and microRNA. Recent extensive research has established that miRNAs can contribute to disease pathogenesis through various mechanisms and serve as viable targets for therapeutic drugs (129). This section provides a concise summary of the involvement of miRNAs in METH and HIV-1-induced neurotoxicity.
The pivotal function of miRNAs is contingent on their target genes, as established in the literature. Extensive studies have supported an important role for miRNAs in neurotoxicity induced by METH and HIV-1 (48, 130, 131). Specifically, METH induces the upregulation of miRNA-143 via σ1R, resulting in detrimental effects on human brain endothelial cells and disruption of tight junction proteins, ultimately compromising BBB integrity. Notably, silencing of miRNA-143 has been shown to confer protection to the BBB against METH-induced damage (107). Studies have demonstrated that chronic administration of METH leads to an increase in the expression of exosome-secreted miRNA-29a. However, ibudilast, an anti-inflammatory drug, can reduce the secretion of extracellular vesicles and subsequently downregulate miRNA-29a, mitigating synaptic damage (108). HIV-1 neurotoxicity is closely linked to its infectious capacity, and various stimuli can alter miRNA expression in vivo, thereby influencing HIV-1 replication. Activated CD4+ T cells can exhibit high levels of miRNA-132, the first miRNA discovered to enhance HIV-1 replication (132). Furthermore, the elevation of miR-34a by HIV-1 Tat protein impeded SIRT1, consequently triggering neuroinflammation (29). These results highlight the importance of miRNA-based gene therapy in addressing the neurotoxicity of both METH and HIV-1, as these miRNAs have been shown to be stably expressed in vivo.
4 Discussion
The excitotoxicity induced by METH leads to an elevated likelihood of engaging in risky sexual behavior and unhygienic injection practices among substance abusers, thereby increasing the risk of HIV-1 acquisition. This risk is further exacerbated in individuals with co-infection of HIV-1. Currently, there are no pharmacological interventions specifically targeting METH-induced neurotoxicity, with only symptomatic treatments available based on clinical presentation. Antiretroviral therapy can effectively manage HIV-1, but it does not completely remove the virus from the brain. In addition, a variety of complementary therapies can be employed, including psychotherapy, lifestyle changes, and social support. The above treatments primarily relieve clinical symptoms without preventing disease progression. Therefore, researchers are trying to find effective drugs that can treat the neurotoxicity they co-cause by exploring the common targets of METH and HIV-1. In this review, we have provided a comprehensive and up-to-date overview of the mechanisms and targets currently under investigation for METH and HIV-1-induced neurotoxicity, drawing on published literature and recent reports.
We have identified that σ1R, NRF2, DAT and PPAR-γ are all involved in METH and HIV-1 co-induced neurotoxicity (13, 17, 125, 133). Many preclinical studies have suggested that modulation of these targets may have significant therapeutic effects on METH and HIV-1 co-induced neurotoxicity. This provides new perspectives and potential breakthroughs for such future therapeutic strategies. However, future research should encompass more in-depth experiments and foster multidisciplinary communication to identify precise biomarkers. These biomarkers are instrumental for the early detection and classification of METH and HIV-1 co-induced neurotoxicity, thereby facilitating the development of personalized treatment plans. Meanwhile, we also found that METH and HIV-1 co-induced neurotoxicity has been significantly altered at the transcriptional level, and we briefly summarized some aberrant miRNAs, such as miRNA-143, miRNA-29a and miRNA-132 (108, 131, 134). The researchers have found that targeting these miRNAs could also ameliorate METH and HIV-1 co-induced neurotoxicity, providing a new strategy for treatment. However, the clinical application of miRNA therapy continues to face a lot of challenges, including issues of drug delivery, specificity, and safety, which need to be addressed through further research and clinical trials.
We believe that there are numerous unanswered questions for researchers to explore regarding the neurotoxicity associated with METH and HIV-1 co-exposure. Specific questions include: I. How METH affects HIV-1 latency and reactivation. II. The role of environmental factors in exacerbating or mitigating the neurotoxicity. III. The effects of simultaneous use of different drugs on the co-induced neurotoxicity of METH and HIV-1. IV. The specific roles of cellular populations and neural circuits in the neurotoxicity induced by the co-exposure to METH and HIV-1. Meanwhile, we should not overlook the impact of the social environment on patients. We must enhance public awareness of substance use disorder and HIV-1 infection through education and prevent such issues at their root. It is crucial to provide emotional support and practical assistance to individuals affected by HIV-1 and METH use, to promote societal understanding and acceptance of them, thereby reducing their psychological barriers to seeking help and improving their quality of life. In conclusion, we hope more researchers will focus on exploring treatments for METH and HIV-1-induced neurotoxicity.
5 Conclusion
The review provided an overview of the distinct mechanisms underlying neurotoxicity induced by METH and HIV-1, emphasizing their interplay. These mechanisms include oxidative stress, endoplasmic reticulum stress, neuroinflammation, programmed cell death, and blood-brain barrier damage. Additionally, potential targets for treating METH and HIV-1-induced neurotoxicity were discussed. The objective of this review was to establish a theoretical foundation for understanding METH and HIV-1-induced neurotoxicity and to suggest potential avenues for clinical research and treatment.
Author contributions
LM: Conceptualization, Writing – original draft. HW: Conceptualization, Writing – original draft. YL: Conceptualization, Writing – original draft. JH: Methodology, Supervision, Writing – review & editing. CW: Methodology, Supervision, Writing – review & editing. HT: Formal Analysis, Visualization, Writing – review & editing. LX: Formal Analysis, Visualization, Writing – review & editing. XY: Formal Analysis, Visualization, Writing – review & editing. YT: Formal Analysis, Visualization, Writing – review & editing. GY: Funding acquisition, Supervision, Writing – review & editing. JL: Funding acquisition, Supervision, Writing – review & editing. XZ: Funding acquisition, Supervision, Writing – review & editing.
Funding
The author(s) declare financial support was received for the research, authorship, and/or publication of this article. This work was supported by the National Natural Science Foundation of China (82160325, 82060382 and 82202081), the Yunnan Applied Basic Research Projects Joint Special Project (202201AY070001-020, 202301AY070001-234) and the Postgraduate Innovation Project of Kunming Medical University (2023S015, 2023S163).
Acknowledgments
We thank Home for Researchers editorial team (www.home-for-researchers.com) for language editing service. Figures were created by Figdraw (www.figdraw.com).
Conflict of interest
The authors declare that the research was conducted in the absence of any commercial or financial relationships that could be construed as a potential conflict of interest.
Publisher’s note
All claims expressed in this article are solely those of the authors and do not necessarily represent those of their affiliated organizations, or those of the publisher, the editors and the reviewers. Any product that may be evaluated in this article, or claim that may be made by its manufacturer, is not guaranteed or endorsed by the publisher.
References
1. Fattakhov N, Torices S, Stangis M, Park M, Toborek M. Synergistic impairment of the neurovascular unit by HIV-1 infection and methamphetamine use: implications for HIV-1-associated neurocognitive disorders. Viruses. (2021) 13:1883. doi: 10.3390/v13091883
2. Jadhav S, Nema V. HIV-associated neurotoxicity: the interplay of host and viral proteins. Mediators Inflammation. (2021) 2021:1267041. doi: 10.1155/2021/1267041
3. Mohammadzadeh N, Roda W, Branton WG, Clain J, Rabezanahary H, Zghidi-Abouzid O, et al. Lentiviral infections persist in brain despite effective antiretroviral therapy and neuroimmune activation. mBio. (2021) 12:e0278421. doi: 10.1128/mBio.02784-21
4. Lane T, Makarov V, Nelson JAE, Meeker RB, Sanna G, Riabova O, et al. N-Phenyl-1-(phenylsulfonyl)-1H-1,2,4-triazol-3-amine as a new class of HIV-1 non-nucleoside reverse transcriptase inhibitor. J Med Chem. (2023) 66:6193–217. doi: 10.1021/acs.jmedchem.2c02055
5. Oomen PGA, Hakkers CS, Arends JE, van der Berk GEL, Pas P, Hoepelman AIM, et al. Underlying neural mechanisms of cognitive improvement in Fronto-striatal response inhibition in people living with HIV switching off efavirenz: A randomized controlled BOLD fMRI trial. Infect Dis Ther. (2024) 13:1067–82. doi: 10.1007/s40121-024-00966-7
6. UNODC. World drug report 2024 (2024). Available online at: https://www.unodc.org/unodc/en/data-and-analysis/world-drug-report-2024.html (Accessed July 17, 2024).
7. Chomchai C, Chomchai S. Global patterns of methamphetamine use. Curr Opin Psychiatry. (2015) 28:269–74. doi: 10.1097/YCO.0000000000000168
8. Huang J, Yang G, Li Z, Leung CK, Wang W, Li Y, et al. Involvement of dopamine D3 receptor and dopamine transporter in methamphetamine-induced behavioral sensitization in tree shrews. Brain Behav. (2020) 10:e01533. doi: 10.1002/brb3.1533
9. Shaerzadeh F, Streit WJ, Heysieattalab S, Khoshbouei H. Methamphetamine neurotoxicity, microglia, and neuroinflammation. J Neuroinflamm. (2018) 15:341. doi: 10.1186/s12974-018-1385-0
10. Vearrier D, Greenberg MI, Miller SN, Okaneku JT, Haggerty DA. Methamphetamine: history, pathophysiology, adverse health effects, current trends, and hazards associated with the clandestine manufacture of methamphetamine. Dis Mon. (2012) 58:38–89. doi: 10.1016/j.disamonth.2011.09.004
11. Feldman MB, Thomas JA, Alexy ER, Irvine MK. Crystal methamphetamine use and HIV medical outcomes among HIV-infected men who have sex with men accessing support services in New York. Drug Alcohol Depend. (2015) 147:266–71. doi: 10.1016/j.drugalcdep.2014.09.780
12. Rawat P, Teodorof-Diedrich C, Spector SA. Human immunodeficiency virus Type-1 single-stranded RNA activates the NLRP3 inflammasome and impairs autophagic clearance of damaged mitochondria in human microglia. Glia. (2019) 67:802–24. doi: 10.1002/glia.23568
13. Zhang K, Chen L, Yang J, Liu J, Li J, Liu Y, et al. Gut microbiota-derived short-chain fatty acids ameliorate methamphetamine-induced depression- and anxiety-like behaviors in a Sigmar-1 receptor-dependent manner. Acta Pharm Sin B. (2023) 13:4801–22. doi: 10.1016/j.apsb.2023.09.010
14. Tran NKC, Jeong JH, Sharma N, Nguyen YND, Tran HP, Dang DK, et al. Ginsenoside Re blocks Bay k-8644-induced neurotoxicity via attenuating mitochondrial dysfunction and PKCδ activation in the hippocampus of mice: Involvement of antioxidant potential. Food Chem Toxicol. (2023) 178:113869. doi: 10.1016/j.fct.2023.113869
15. Yang J-Z, Zhang K-K, He J-T, Chen L-J, Ding J-F, Liu J-L, et al. Obeticholic acid protects against methamphetamine-induced anxiety-like behavior by ameliorating microbiota-mediated intestinal barrier impairment. Toxicology. (2023) 486:153447. doi: 10.1016/j.tox.2023.153447
16. Guo C, Chen L, Wang Y. Substance abuse and neurodegenerative diseases: focus on ferroptosis. Arch Toxicol. (2023) 97:1519–28. doi: 10.1007/s00204-023-03505-4
17. Lin S, Cheng H, Yang G, Wang C, Leung CK, Zhang S, et al. NRF2 antagonizes HIV-1 tat and methamphetamine-induced BV2 cell ferroptosis by regulating SLC7A11. Neurotox Res. (2023) 41:398–407. doi: 10.1007/s12640-023-00645-4
18. Zeng XF, Li Q, Li J, Wong N, Li Z, Huang J, et al. HIV-1 Tat and methamphetamine co-induced oxidative cellular injury is mitigated by N-acetylcysteine amide (NACA) through rectifying mTOR signaling. Toxicol Lett. (2018) 299:159–71. doi: 10.1016/j.toxlet.2018.09.009
19. Jayanthi S, Daiwile AP, Cadet JL. Neurotoxicity of methamphetamine: Main effects and mechanisms. Exp Neurol. (2021) 344:113795. doi: 10.1016/j.expneurol.2021.113795
20. Shrestha P, Katila N, Lee S, Seo JH, Jeong JH, Yook S. Methamphetamine induced neurotoxic diseases, molecular mechanism, and current treatment strategies. BioMed Pharmacother. (2022) 154:113591. doi: 10.1016/j.biopha.2022.113591
21. Turan C, Senormanci G, Neselioglu S, Budak Y, Erel O, Senormanci O. Oxidative stress and inflammatory biomarkers in people with methamphetamine use disorder. Clin Psychopharmacol Neurosci. (2023) 21:572–82. doi: 10.9758/cpn.22.1047
22. Banerjee A, Zhang X, Manda KR, Banks WA, Ercal N. HIV proteins (gp120 and Tat) and methamphetamine in oxidative stress-induced damage in the brain: potential role of the thiol antioxidant N-acetylcysteine amide. Free Radic Biol Med. (2010) 48:1388–98. doi: 10.1016/j.freeradbiomed.2010.02.023
23. Basova LV, Vien W, Bortell N, Najera JA, Marcondes MCG. Methamphetamine signals transcription of IL1β and TNFα in a reactive oxygen species-dependent manner and interacts with HIV-1 Tat to decrease antioxidant defense mechanisms. Front Cell Neurosci. (2022) 16:911060. doi: 10.3389/fncel.2022.911060
24. Huang J, Zhang R, Wang S, Zhang D, Leung C-K, Yang G, et al. Methamphetamine and HIV-Tat protein synergistically induce oxidative stress and blood-brain barrier damage via transient receptor potential melastatin 2 channel. Front Pharmacol. (2021) 12:619436. doi: 10.3389/fphar.2021.619436
25. Chen G, Wei X, Xu X, Yu G, Yong Z, Su R, et al. Methamphetamine inhibits long-term memory acquisition and synaptic plasticity by evoking endoplasmic reticulum stress. Front Neurosci. (2020) 14:630713. doi: 10.3389/fnins.2020.630713
26. Lenzi P, Biagioni F, Busceti CL, Lazzeri G, Polzella M, Frati A, et al. Alterations of mitochondrial structure in methamphetamine toxicity. Int J Mol Sci. (2022) 23:8926. doi: 10.3390/ijms23168926
27. Figarola-Centurión I, Escoto-Delgadillo M, González-Enríquez GV, Gutiérrez-Sevilla JE, Vázquez-Valls E, Torres-Mendoza BM. Sirtuins modulation: A promising strategy for HIV-associated neurocognitive impairmentsSirtuins. Int J Mol Sci. (2022) 23:643. doi: 10.3390/ijms23020643
28. Thangaraj A, Chivero ET, Tripathi A, Singh S, Niu F, Guo M-L, et al. HIV TAT-mediated microglial senescence: Role of SIRT3-dependent mitochondrial oxidative stress. Redox Biol. (2021) 40:101843. doi: 10.1016/j.redox.2020.101843
29. Zhang HS, Chen XY, Wu TC, Sang WW, Ruan Z. MiR-34a is involved in Tat-induced HIV-1 long terminal repeat (LTR) transactivation through the SIRT1/NFkappaB pathway. FEBS Lett. (2012) 586:4203–7. doi: 10.1016/j.febslet.2012.10.023
30. Proulx J, Park IW, Borgmann K. Cal'MAM'ity at the endoplasmic reticulum-mitochondrial interface: A potential therapeutic target for neurodegeneration and human immunodeficiency virus-associated neurocognitive disorders. Front Neurosci. (2021) 15:715945. doi: 10.3389/fnins.2021.715945
31. Cisneros IE, Ghorpade A, Borgmann K. Methamphetamine activates trace amine associated receptor 1 to regulate astrocyte excitatory amino acid transporter-2 via differential CREB phosphorylation during HIV-associated neurocognitive disorders. Front Neurol. (2020) 11:593146. doi: 10.3389/fneur.2020.593146
32. Proulx J, Stacy S, Park I-W, Borgmann K. A non-canonical role for IRE1α Links ER and mitochondria as key regulators of astrocyte dysfunction: implications in methamphetamine use and HIV-associated neurocognitive disorders. Front Neurosci. (2022) 16:906651. doi: 10.3389/fnins.2022.906651
33. Cui J, Zhao S, Li Y, Zhang D, Wang B, Xie J, et al. Regulated cell death: discovery, features and implications for neurodegenerative diseases. Cell Communication Signaling. (2021) 19:120. doi: 10.1186/s12964-021-00799-8
34. Guo D, Huang X, Xiong T, Wang X, Zhang J, Wang Y, et al. Molecular mechanisms of programmed cell death in methamphetamine-induced neuronal damage. Front Pharmacol. (2022) 13:980340. doi: 10.3389/fphar.2022.980340
35. Aki T, Funakoshi T, Unuma K, Uemura K. Impairment of autophagy: from hereditary disorder to drug intoxication. Toxicology. (2013) 311:205–15. doi: 10.1016/j.tox.2013.07.001
36. Limanaqi F, Busceti CL, Celli R, Biagioni F, Fornai F. Autophagy as a gateway for the effects of methamphetamine: From neurotransmitter release and synaptic plasticity to psychiatric and neurodegenerative disorders. Prog Neurobiol. (2021) 204:102112. doi: 10.1016/j.pneurobio.2021.102112
37. Abdullah CS, Remex NS, Aishwarya R, Nitu S, Kolluru GK, Traylor J, et al. Mitochondrial dysfunction and autophagy activation are associated with cardiomyopathy developed by extended methamphetamine self-administration in rats. Redox Biol. (2022) 58:102523. doi: 10.1016/j.redox.2022.102523
38. Chen X, Zhang T, Zhang Y. Endoplasmic reticulum stress and autophagy in HIV-1-associated neurocognitive disorders. J NeuroVirology. (2020) 26:824–33. doi: 10.1007/s13365-020-00906-4
39. Cao L, Fu M, Kumar S, Kumar A. Methamphetamine potentiates HIV-1 gp120-mediated autophagy via Beclin-1 and Atg5/7 as a pro-survival response in astrocytes. Cell Death Dis. (2016) 7:e2425. doi: 10.1038/cddis.2016.317
40. Chen L-J, Zhi X, Zhang K-K, Wang L-B, Li J-H, Liu J-L, et al. Escalating dose-multiple binge methamphetamine treatment elicits neurotoxicity, altering gut microbiota and fecal metabolites in mice. Food Chem Toxicol. (2021) 148:111946. doi: 10.1016/j.fct.2020.111946
41. Yang G, Zeng X, Li J, Leung CK, Zhang D, Hong S, et al. Protective effect of gastrodin against methamphetamine-induced autophagy in human dopaminergic neuroblastoma SH-SY5Y cells via the AKT/mTOR signaling pathway. Neurosci Lett. (2019) 707:134287. doi: 10.1016/j.neulet.2019.134287
42. Barbaro JM, Sidoli S, Cuervo AM, Berman JW. Methamphetamine dysregulates macrophage functions and autophagy to mediate HIV neuropathogenesis. Biomedicines. (2022) 10:1257. doi: 10.3390/biomedicines10061257
43. Li J, Wang W, Tong P, Leung C-K, Yang G, Li Z, et al. Autophagy Induction by HIV-Tat and Methamphetamine in Primary Midbrain Neuronal Cells of Tree Shrews via the mTOR Signaling and ATG5/ATG7 Pathway. Front Neurosci. (2018) 12:921. doi: 10.3389/fnins.2018.00921
44. Teodorof-Diedrich C, Spector SA. Human immunodeficiency virus type 1 and methamphetamine-mediated mitochondrial damage and neuronal degeneration in human neurons. J Virol. (2020) 94:e00924–20. doi: 10.1128/JVI.00924-20
45. Song Y, Wu Z, Xue H, Zhao P. Ferroptosis is involved in regulating perioperative neurocognitive disorders: emerging perspectives. J Neuroinflamm. (2022) 19:219. doi: 10.1186/s12974-022-02570-3
46. Dixon SJ, Lemberg KM, Lamprecht MR, Skouta R, Zaitsev EM, Gleason CE, et al. Ferroptosis: an iron-dependent form of nonapoptotic cell death. Cell. (2012) 149:1060–72. doi: 10.1016/j.cell.2012.03.042
47. Sfera A, Thomas KG, Andronescu CV, Jafri N, Sfera DO, Sasannia S, et al. Bromodomains in human-immunodeficiency virus-associated neurocognitive disorders: A model of ferroptosis-induced neurodegeneration. Front Neurosci. (2022) 16:904816. doi: 10.3389/fnins.2022.904816
48. Kannan M, Sil S, Oladapo A, Thangaraj A, Periyasamy P, Buch S. HIV-1 Tat-mediated microglial ferroptosis involves the miR-204-ACSL4 signaling axis. Redox Biol. (2023) 62:102689. doi: 10.1016/j.redox.2023.102689
49. Savarino A, Pescarmona GP, Boelaert JR. Iron metabolism and HIV infection: reciprocal interactions with potentially harmful consequences? Cell Biochem Funct. (1999) 17:279–87. doi: 10.1002/(SICI)1099-0844(199912)17:4<279::AID-CBF833>3.0.CO;2-J
50. Kallianpur AR, Gittleman H, Letendre S, Ellis R, Barnholtz-Sloan JS, Bush WS, et al. Cerebrospinal fluid ceruloplasmin, haptoglobin, and vascular endothelial growth factor are associated with neurocognitive impairment in adults with HIV infection. Mol Neurobiol. (2019) 56:3808–18. doi: 10.1007/s12035-018-1329-9
51. Ge C, Peng Y, Li J, Wang L, Zhu X, Wang N, et al. Hydroxysafflor yellow A alleviates acute myocardial ischemia/reperfusion injury in mice by inhibiting ferroptosis via the activation of the HIF-1alpha/SLC7A11/GPX4 signaling pathway. Nutrients. (2023) 15:3411. doi: 10.3390/nu15153411
52. Fleisher TA. Apoptosis. Ann Allergy Asthma Immunol. (1997) 78:245–9. doi: 10.1016/S1081-1206(10)63176-6
53. Xu X, Lai Y, Hua ZC. Apoptosis and apoptotic body: disease message and therapeutic target potentials. Biosci Rep. (2019) 39:BSR20180992. doi: 10.1042/BSR20180992
54. Ketelut-Carneiro N, Fitzgerald KA. Apoptosis, pyroptosis, and necroptosis-oh my! The many ways a cell can die. J Mol Biol. (2022) 434:167378. doi: 10.1016/j.jmb.2021.167378
55. Kowalski S, Karska J, Lapinska Z, Hetnal B, Saczko J, Kulbacka J. An overview of programmed cell death: Apoptosis and pyroptosis-Mechanisms, differences, and significance in organism physiology and pathophysiology. J Cell Biochem. (2023) 124:765–84. doi: 10.1002/jcb.30413
56. Cao L, Glazyrin A, Kumar S, Kumar A. Role of autophagy in HIV pathogenesis and drug abuse. Mol Neurobiol. (2017) 54:5855–67. doi: 10.1007/s12035-016-0118-6
57. Shah A, Kumar S, Simon SD, Singh DP, Kumar A. HIV gp120- and methamphetamine-mediated oxidative stress induces astrocyte apoptosis via cytochrome P450 2E1. Cell Death Dis. (2013) 4:e850. doi: 10.1038/cddis.2013.374
58. Hu L, Chen M, Chen X, Zhao C, Fang Z, Wang H, et al. Chemotherapy-induced pyroptosis is mediated by BAK/BAX-caspase-3-GSDME pathway and inhibited by 2-bromopalmitate. Cell Death Dis. (2020) 11:281. doi: 10.1038/s41419-020-2476-2
59. Kovacs SB, Miao EA. Gasdermins: effectors of pyroptosis. Trends Cell Biol. (2017) 27:673–84. doi: 10.1016/j.tcb.2017.05.005
60. Purohit V, Rapaka R, Shurtleff D. Drugs of abuse, dopamine, and HIV-associated neurocognitive disorders/HIV-associated dementia. Mol Neurobiol. (2011) 44:102–10. doi: 10.1007/s12035-011-8195-z
61. Liu Y, Wen D, Gao J, Xie B, Yu H, Shen Q, et al. Methamphetamine induces GSDME-dependent cell death in hippocampal neuronal cells through the endoplasmic reticulum stress pathway. Brain Res Bull. (2020) 162:73–83. doi: 10.1016/j.brainresbull.2020.06.005
62. Fan R, Shen Y, Li X, Luo H, Zhang P, Liu Y, et al. The effect of the NLRP1 inflammasome on methamphetamine-induced cognitive impairment in rats. Drug Alcohol Depend. (2022) 237:109537. doi: 10.1016/j.drugalcdep.2022.109537
63. He X, Yang W, Zeng Z, Wei Y, Gao J, Zhang B, et al. NLRP3-dependent pyroptosis is required for HIV-1 gp120-induced neuropathology. Cell Mol Immunol. (2020) 17:283–99. doi: 10.1038/s41423-019-0260-y
64. Adamczak SE, de Rivero Vaccari JP, Dale G, Brand FJ 3rd, Nonner D, Bullock MR, et al. Pyroptotic neuronal cell death mediated by the AIM2 inflammasome. J Cereb Blood Flow Metab. (2014) 34:621–9. doi: 10.1038/jcbfm.2013.236
65. Dutta D, Liu J, Xu E, Xiong H. Methamphetamine enhancement of HIV-1 gp120-mediated NLRP3 inflammasome activation and resultant proinflammatory responses in rat microglial cultures. Int J Mol Sci. (2024) 25:3588. doi: 10.3390/ijms25073588
66. Shi S, Chen T, Zhao M. The crosstalk between neurons and glia in methamphetamine-induced neuroinflammation. Neurochem Res. (2022) 47:872–84. doi: 10.1007/s11064-021-03513-9
67. Dang J, Tiwari SK, Agrawal K, Hui H, Qin Y, Rana TM. Glial cell diversity and methamphetamine-induced neuroinflammation in human cerebral organoids. Mol Psychiatry. (2021) 26:1194–207. doi: 10.1038/s41380-020-0676-x
68. Ding J, Shen L, Ye Y, Hu S, Ren Z, Liu T, et al. Inflammasome inhibition prevents motor deficit and cerebellar degeneration induced by chronic methamphetamine administration. Front Mol Neurosci. (2022) 15:861340. doi: 10.3389/fnmol.2022.861340
69. Walsh JG, Reinke SN, Mamik MK, McKenzie BA, Maingat F, Branton WG, et al. Rapid inflammasome activation in microglia contributes to brain disease in HIV/AIDS. Retrovirology. (2014) 11:35. doi: 10.1186/1742-4690-11-35
70. Borrajo A, Spuch C, Penedo MA, Olivares JM, Agis-Balboa RC. Important role of microglia in HIV-1 associated neurocognitive disorders and the molecular pathways implicated in its pathogenesis. Ann Med. (2021) 53:43–69. doi: 10.1080/07853890.2020.1814962
71. Walter TJ, Iudicello J, Cookson DR, Franklin D, Tang B, Young JW, et al. The relationships between HIV-1 infection, history of methamphetamine use disorder, and soluble biomarkers in blood and cerebrospinal fluid. Viruses. (2021) 13:1287. doi: 10.3390/v13071287
72. Barnett KC, Li S, Liang K, Ting JPY. A 360° view of the inflammasome: Mechanisms of activation, cell death, and diseases. Cell. (2023) 186:2288–312. doi: 10.1016/j.cell.2023.04.025
73. Chivero ET, Guo ML, Periyasamy P, Liao K, Callen SE, Buch S. HIV-1 tat primes and activates microglial NLRP3 inflammasome-mediated neuroinflammation. J Neurosci. (2017) 37:3599–609. doi: 10.1523/JNEUROSCI.3045-16.2017
74. Yang G, Li J, Leung CK, Shen B, Wang C, Xu Y, et al. Methamphetamine and HIV-1 Tat proteins synergistically induce microglial autophagy via activation of the Nrf2/NQO1/HO-1 signal pathway. Neuropharmacology. (2022) 220:109256. doi: 10.1016/j.neuropharm.2022.109256
75. Surnar B, Shah AS, Park M, Kalathil AA, Kamran MZ, Ramirez Jaime R, et al. Brain-accumulating nanoparticles for assisting astrocytes to reduce human immunodeficiency virus and drug abuse-induced neuroinflammation and oxidative stress. ACS Nano. (2021) 15:15741–53. doi: 10.1021/acsnano.0c09553
76. Huang J, Ding J, Wang X, Gu C, He Y, Li Y, et al. Transfer of neuron-derived alpha-synuclein to astrocytes induces neuroinflammation and blood-brain barrier damage after methamphetamine exposure: Involving the regulation of nuclear receptor-associated protein 1. Brain Behav Immun. (2022) 106:247–61. doi: 10.1016/j.bbi.2022.09.002
77. Maoz BM, Herland A, FitzGerald EA, Grevesse T, Vidoudez C, Pacheco AR, et al. A linked organ-on-chip model of the human neurovascular unit reveals the metabolic coupling of endothelial and neuronal cells. Nat Biotechnol. (2018) 36:865–74. doi: 10.1038/nbt.4226
78. Patel S, Leibrand CR, Palasuberniam P, Couraud PO, Weksler B, Jahr FM, et al. Effects of HIV-1 tat and methamphetamine on blood-brain barrier integrity and function in vitro. Antimicrob Agents Chemother. (2017) 61:e01307–17. doi: 10.1128/AAC.01307-17
79. Saloner R, Fields JA, Marcondes MCG, Iudicello JE, von Kanel S, Cherner M, et al. Methamphetamine and cannabis: A tale of two drugs and their effects on HIV, brain, and behavior. J Neuroimmune Pharmacol. (2020) 15:743–64. doi: 10.1007/s11481-020-09957-0
80. Sweeney MD, Sagare AP, Zlokovic BV. Blood-brain barrier breakdown in Alzheimer disease and other neurodegenerative disorders. Nat Rev Neurol. (2018) 14:133–50. doi: 10.1038/nrneurol.2017.188
81. Leitao RA, Fontes-Ribeiro CA, Silva AP. The effect of parthenolide on methamphetamine-induced blood-brain barrier and astrocyte alterations. Eur J Clin Invest. (2022) 52:e13694. doi: 10.1111/eci.13694
82. Bertrand L, Cho HJ, Toborek M. Blood-brain barrier pericytes as a target for HIV-1 infection. Brain. (2019) 142:502–11. doi: 10.1093/brain/awy339
83. Toborek M, Lee YW, Flora G, Pu H, Andras IE, Wylegala E, et al. Mechanisms of the blood-brain barrier disruption in HIV-1 infection. Cell Mol Neurobiol. (2005) 25:181–99. doi: 10.1007/s10571-004-1383-x
84. Mahajan SD, Aalinkeel R, Sykes DE, Reynolds JL, Bindukumar B, Fernandez SF, et al. Tight junction regulation by morphine and HIV-1 tat modulates blood-brain barrier permeability. J Clin Immunol. (2008) 28:528–41. doi: 10.1007/s10875-008-9208-1
85. Li J, Huang J, He Y, Wang W, Leung CK, Zhang D, et al. The protective effect of gastrodin against the synergistic effect of HIV-Tat protein and METH on the blood-brain barrier via glucose transporter 1 and glucose transporter 3. Toxicol Res (Camb). (2021) 10:91–101. doi: 10.1093/toxres/tfaa102
86. Li J, Zeng B, Hu X, Li Z, Zhang D, Yang G, et al. Protective effects of ginsenoside rb1 against blood-brain barrier damage induced by human immunodeficiency virus-1 tat protein and methamphetamine in sprague-dawley rats. Am J Chin Med. (2018) 46:551–66. doi: 10.1142/S0192415X18500283
87. Weber MT, Finkelstein A, Uddin MN, Reddy EA, Arduino RC, Wang L, et al. Longitudinal effects of combination antiretroviral therapy on cognition and neuroimaging biomarkers in treatment-naive people with HIV. Neurology. (2022) 99:e1045–e55. doi: 10.1212/WNL.0000000000200829
88. Petzold J, Szumlinski KK, London ED. Targeting mGlu(5) for methamphetamine use disorder. Pharmacol Ther. (2021) 224:107831. doi: 10.1016/j.pharmthera.2021.107831
89. Yang G, Li J, Peng Y, Shen B, Li Y, Liu L, et al. Ginsenoside Rb1 attenuates methamphetamine (METH)-induced neurotoxicity through the NR2B/ERK/CREB/BDNF signalings in vitro and in vivo models. J Ginseng Res. (2022) 46:426–34. doi: 10.1016/j.jgr.2021.07.005
90. Ma CL, Li L, Yang GM, Zhang ZB, Zhao YN, Zeng XF, et al. Neuroprotective effect of gastrodin in methamphetamine-induced apoptosis through regulating cAMP/PKA/CREB pathway in cortical neuron. Hum Exp Toxicol. (2020) 39:1118–29. doi: 10.1177/0960327120911438
91. Yang GM, Li L, Xue FL, Ma CL, Zeng XF, Zhao YN, et al. The potential role of PKA/CREB signaling pathway concerned with gastrodin administration on methamphetamine-induced conditioned place preference rats and SH-SY5Y cell line. Neurotox Res. (2020) 37:926–35. doi: 10.1007/s12640-019-00150-7
92. Ujike H, Kanzaki A, Okumura K, Akiyama K, Otsuki S. Sigma (sigma) antagonist BMY 14802 prevents methamphetamine-induced sensitization. Life Sci. (1992) 50:PL129–34. doi: 10.1016/0024-3205(92)90466-3
93. Ray A, Canal CE, Ehlen JC, Rice KC, Murnane KS. M100907 and BD 1047 attenuate the acute toxic effects of methamphetamine. Neurotoxicology. (2019) 74:91–9. doi: 10.1016/j.neuro.2019.05.011
94. Liu L, Li J, Wang C, Xu Y, Leung CK, Yang G, et al. Cannabidiol attenuates methamphetamine-induced conditioned place preference in male rats and viability in PC12 cells through the Sigma1R/AKT/GSK3beta/CREB signaling pathway. Am J Drug Alcohol Abuse. (2022) 48:548–61. doi: 10.1080/00952990.2022.2073450
95. Zhang Y, Shi Y, Qiao L, Sun Y, Ding W, Zhang H, et al. Sigma-1 receptor agonists provide neuroprotection against gp120 via a change in bcl-2 expression in mouse neuronal cultures. Brain Res. (2012) 1431:13–22. doi: 10.1016/j.brainres.2011.10.053
96. Zeng Q, Xiong Q, Lin K, Liang Z, Zhou M, Tian X, et al. Terminalia chebula extracts ameliorate methamphetamine-induced memory deficits via activating the ERK and Nrf2 pathway. Brain Res Bull. (2022) 184:76–87. doi: 10.1016/j.brainresbull.2022.04.002
97. Lee HS, Jeong GS. 6,7,4'-Trihydroxyflavanone mitigates methamphetamine-induced neurotoxicity in SH-SY5y cells via nrf2/heme oxyganase-1 and PI3K/Akt/mTOR signaling pathways. Molecules. (2021) 26:2442. doi: 10.3390/molecules26092442
98. Zeng Q, Xiong Q, Zhou M, Tian X, Yue K, Li Y, et al. Resveratrol attenuates methamphetamine-induced memory impairment via inhibition of oxidative stress and apoptosis in mice. J Food Biochem. (2021) 45:e13622. doi: 10.1111/jfbc.13622
99. Li Z, Qi Y, Liu K, Cao Y, Zhang H, Song C, et al. Effect of Chaihu-jia-Longgu-Muli decoction on withdrawal symptoms in rats with methamphetamine-induced conditioned place preference. Biosci Rep. (2021) 41:BSR20211376. doi: 10.1042/BSR20211376
100. Liu L, Liu M, Zhao W, Zhao YL, Wang Y. Levo-tetrahydropalmatine: A new potential medication for methamphetamine addiction and neurotoxicity. Exp Neurol. (2021) 344:113809. doi: 10.1016/j.expneurol.2021.113809
101. Quizon PM, Yuan Y, Zhu Y, Zhou Y, Strauss MJ, Sun WL, et al. Mutations of human dopamineTransporter at tyrosine88, aspartic acid206, and histidine547 influence basal and HIV-1 tat-inhibited dopamine transport. J Neuroimmune Pharmacol. (2021) 16:854–69. doi: 10.1007/s11481-021-09984-5
102. Maeda T, Kiguchi N, Fukazawa Y, Yamamoto A, Ozaki M, Kishioka S. Peroxisome proliferator-activated receptor gamma activation relieves expression of behavioral sensitization to methamphetamine in mice. Neuropsychopharmacology. (2007) 32:1133–40. doi: 10.1038/sj.npp.1301213
103. Tsuji T, Asanuma M, Miyazaki I, Miyoshi K, Ogawa N. Reduction of nuclear peroxisome proliferator-activated receptor gamma expression in methamphetamine-induced neurotoxicity and neuroprotective effects of ibuprofen. Neurochem Res. (2009) 34:764–74. doi: 10.1007/s11064-008-9863-x
104. Omeragic A, Hoque MT, Choi UY, Bendayan R. Peroxisome proliferator-activated receptor-gamma: potential molecular therapeutic target for HIV-1-associated brain inflammation. J Neuroinflamm. (2017) 14:183. doi: 10.1186/s12974-017-0957-8
105. Huang W, Andras IE, Rha GB, Hennig B, Toborek M. PPARalpha and PPARgamma protect against HIV-1-induced MMP-9 overexpression via caveolae-associated ERK and Akt signaling. FASEB J. (2011) 25:3979–88. doi: 10.1096/fj.11-188607
106. Mamik MK, Asahchop EL, Chan WF, Zhu Y, Branton WG, McKenzie BA, et al. Insulin treatment prevents neuroinflammation and neuronal injury with restored neurobehavioral function in models of HIV/AIDS neurodegeneration. J Neurosci. (2016) 36:10683–95. doi: 10.1523/JNEUROSCI.1287-16.2016
107. Bai Y, Zhang Y, Hua J, Yang X, Zhang X, Duan M, et al. Silencing microRNA-143 protects the integrity of the blood-brain barrier: implications for methamphetamine abuse. Sci Rep. (2016) 6:35642. doi: 10.1038/srep35642
108. Chand S, Gowen A, Savine M, Moore D, Clark A, Huynh W, et al. A comprehensive study to delineate the role of an extracellular vesicle-associated microRNA-29a in chronic methamphetamine use disorder. J Extracell Vesicles. (2021) 10:e12177. doi: 10.1002/jev2.12177
109. Martin WR, Eades CG, Thompson JA, Huppler RE, Gilbert PE. The effects of morphine- and nalorphine- like drugs in the nondependent and morphine-dependent chronic spinal dog. J Pharmacol Exp Ther. (1976) 197:517–32.
110. Sambo DO, Lebowitz JJ, Khoshbouei H. The sigma-1 receptor as a regulator of dopamine neurotransmission: A potential therapeutic target for methamphetamine addiction. Pharmacol Ther. (2018) 186:152–67. doi: 10.1016/j.pharmthera.2018.01.009
111. Prasad A, Kulkarni R, Shrivastava A, Jiang S, Lawson K, Groopman JE. Methamphetamine functions as a novel CD4(+) T-cell activator via the sigma-1 receptor to enhance HIV-1 infection. Sci Rep. (2019) 9:958. doi: 10.1038/s41598-018-35757-x
112. Kim SG, Jung JB, Dixit D, Rovner R Jr., Zack JA, Baldwin GC, et al. Cocaine exposure enhances permissiveness of quiescent T cells to HIV infection. J Leukoc Biol. (2013) 94:835–43. doi: 10.1189/jlb.1112566
113. Hybertson BM, Gao B, Bose SK, McCord JM. Oxidative stress in health and disease: the therapeutic potential of Nrf2 activation. Mol Aspects Med. (2011) 32:234–46. doi: 10.1016/j.mam.2011.10.006
114. Lal R, Dharavath RN, Chopra K. Nrf2 signaling pathway: a potential therapeutic target in combating oxidative stress and neurotoxicity in chemotherapy-induced cognitive impairment. Mol Neurobiol. (2024) 61:593–608. doi: 10.1007/s12035-023-03559-6
115. Zhang LX, Li CX, Kakar MU, Khan MS, Wu PF, Amir RM, et al. Resveratrol (RV): A pharmacological review and call for further research. BioMed Pharmacother. (2021) 143:112164. doi: 10.1016/j.biopha.2021.112164
116. Shukla M, Vincent B. Methamphetamine abuse disturbs the dopaminergic system to impair hippocampal-based learning and memory: An overview of animal and human investigations. Neurosci Biobehav Rev. (2021) 131:541–59. doi: 10.1016/j.neubiorev.2021.09.016
117. Kerins MJ, Ooi A. The roles of NRF2 in modulating cellular iron homeostasis. Antioxid Redox Signal. (2018) 29:1756–73. doi: 10.1089/ars.2017.7176
118. Volkow ND, Wise RA, Baler R. The dopamine motive system: implications for drug and food addiction. Nat Rev Neurosci. (2017) 18:741–52. doi: 10.1038/nrn.2017.130
119. Brucke T, Brucke C. Dopamine transporter (DAT) imaging in Parkinson's disease and related disorders. J Neural Transm (Vienna). (2022) 129:581–94. doi: 10.1007/s00702-021-02452-7
120. Kohno M, Dennis LE, McCready H, Hoffman WF. Dopamine dysfunction in stimulant use disorders: mechanistic comparisons and implications for treatment. Mol Psychiatry. (2022) 27:220–9. doi: 10.1038/s41380-021-01180-4
121. Prakash MD, Tangalakis K, Antonipillai J, Stojanovska L, Nurgali K, Apostolopoulos V. Methamphetamine: Effects on the brain, gut and immune system. Pharmacol Res. (2017) 120:60–7. doi: 10.1016/j.phrs.2017.03.009
122. McLaurin KA, Harris M, Madormo V, Harrod SB, Mactutus CF, Booze RM. HIV-associated apathy/depression and neurocognitive impairments reflect persistent dopamine deficits. Cells. (2021) 10:2158. doi: 10.3390/cells10082158
123. Zhu J, Ananthan S, Zhan CG. The role of human dopamine transporter in NeuroAIDS. Pharmacol Ther. (2018) 183:78–89. doi: 10.1016/j.pharmthera.2017.10.007
124. Kesby JP, Najera JA, Romoli B, Fang Y, Basova L, Birmingham A, et al. HIV-1 TAT protein enhances sensitization to methamphetamine by affecting dopaminergic function. Brain Behav Immun. (2017) 65:210–21. doi: 10.1016/j.bbi.2017.05.004
125. Wang Q, Imam MU, Yida Z, Wang F. Peroxisome proliferator-activated receptor gamma (PPARgamma) as a target for concurrent management of diabetes and obesity-related cancer. Curr Pharm Des. (2017) 23:3677–88. doi: 10.2174/1381612823666170704125104
126. Chen YC, Wu JS, Tsai HD, Huang CY, Chen JJ, Sun GY, et al. Peroxisome proliferator-activated receptor gamma (PPAR-gamma) and neurodegenerative disorders. Mol Neurobiol. (2012) 46:114–24. doi: 10.1007/s12035-012-8259-8
127. Bernardo A, Minghetti L. PPAR-gamma agonists as regulators of microglial activation and brain inflammation. Curr Pharm Des. (2006) 12:93–109. doi: 10.2174/138161206780574579
128. van Dijk EL, Jaszczyszyn Y, Naquin D, Thermes C. The third revolution in sequencing technology. Trends Genet. (2018) 34:666–81. doi: 10.1016/j.tig.2018.05.008
129. Diener C, Keller A, Meese E. Emerging concepts of miRNA therapeutics: from cells to clinic. Trends Genet. (2022) 38:613–26. doi: 10.1016/j.tig.2022.02.006
130. Klase Z, Houzet L, Jeang KT. MicroRNAs and HIV-1: complex interactions. J Biol Chem. (2012) 287:40884–90. doi: 10.1074/jbc.R112.415448
131. Deng B, Zhang Z, Zhou H, Zhang X, Niu S, Yan X, et al. MicroRNAs in methamphetamine-induced neurotoxicity and addiction. Front Pharmacol. (2022) 13:875666. doi: 10.3389/fphar.2022.875666
132. Chiang K, Liu H, Rice AP. miR-132 enhances HIV-1 replication. Virology. (2013) 438:1–4. doi: 10.1016/j.virol.2012.12.016
133. Wang S, Li M, Su L, Wang Y, Ma D, Wang H, et al. Knockout of dopamine D3 receptor gene blocked methamphetamine-induced distinct changes of dopaminergic and glutamatergic synapse in the nucleus accumbens shell of. Front Cell Neurosci. (2022) 16:893190. doi: 10.3389/fncel.2022.893190
Keywords: methamphetamine, psychostimulant, HIV-1, neurotoxicity, programmed cell death, blood-brain barrier, sigma 1 receptor
Citation: Miao L, Wang H, Li Y, Huang J, Wang C, Teng H, Xu L, Yang X, Tian Y, Yang G, Li J and Zeng X (2024) Mechanisms and treatments of methamphetamine and HIV-1 co-induced neurotoxicity: a systematic review. Front. Immunol. 15:1423263. doi: 10.3389/fimmu.2024.1423263
Received: 25 April 2024; Accepted: 02 August 2024;
Published: 19 August 2024.
Edited by:
Prasun K. Datta, Tulane University, United StatesReviewed by:
Santosh Kumar, University of Tennessee Health Science Center (UTHSC), United StatesJennifer Kelschenbach, Icahn School of Medicine at Mount Sinai, United States
Jerel Adam Fields, University of California, San Diego, United States
Copyright © 2024 Miao, Wang, Li, Huang, Wang, Teng, Xu, Yang, Tian, Yang, Li and Zeng. This is an open-access article distributed under the terms of the Creative Commons Attribution License (CC BY). The use, distribution or reproduction in other forums is permitted, provided the original author(s) and the copyright owner(s) are credited and that the original publication in this journal is cited, in accordance with accepted academic practice. No use, distribution or reproduction is permitted which does not comply with these terms.
*Correspondence: Genmeng Yang, MTIyMzk4NDg4NUBxcS5jb20=; Juan Li, MTIxMDkzMjU4QHFxLmNvbQ==; Xiaofeng Zeng, enhmMjAwNDAzM0AxNjMuY29t
†These authors have contributed equally to this work and share first authorship