- 1School of Laboratory Medicine, Medical Science, University of KwaZulu-Natal, Durban, South Africa
- 2Centre for the AIDS Programme of Research in South Africa (CAPRISA), Durban, South Africa
Variation within the non-coding genome may influence the regulation and expression of important genes involved in immune control such as the human leukocyte antigen (HLA) system. Class I and Class II HLA molecules are essential for peptide presentation which is required for T lymphocyte activation. Single nucleotide polymorphisms within non-coding regions of HLA Class I and Class II genes may influence the expression of these genes by affecting the binding of transcription factors and chromatin modeling molecules. Furthermore, an interplay between genetic and epigenetic factors may also influence HLA expression. Epigenetic factors such as DNA methylation and non-coding RNA, regulate gene expression without changing the DNA sequence. However, genetic variation may promote or allow genes to escape regulation by epigenetic factors, resulting in altered expression. The HLA system is central to most diseases, therefore, understanding the role of genetics and epigenetics on HLA regulation will tremendously impact healthcare. The knowledge gained from these studies may lead to novel and cost-effective diagnostic approaches and therapeutic interventions. This review discusses the role of non-coding variants on HLA regulation. Furthermore, we discuss the interplay between genetic and epigenetic factors on the regulation of HLA by evaluating literature based on polymorphisms within DNA methylation and miRNA regulatory sites within class I and Class II HLA genes. We also provide insight into the importance of the HLA non-coding genome on disease, discuss ethnic-specific differences across the HLA region and provide guidelines for future HLA studies.
Introduction
Most diseases found in humans are associated with a 4-megabase region known as the major histocompatibility complex (MHC). This region is central to the control of most diseases as it is rich in genes involved in inflammation and immune defense (1, 2). The human MHC, also known as the human leukocyte antigen (HLA) system is sorted into three classes. Class I HLA genes consist of classical (HLA-A, HLA-B, and HLA-C) and non-classical (HLA-E, HLA-F, and HLA-G) molecules (3). Class I molecules reside on the surface of all nucleated cells and are involved in presenting endogenous self and non-self peptides to cytotoxic CD8+ T lymphocytes (3). On the other hand, class II molecules (HLA-DP, HLA-DQ, and HLA-DR) are generally expressed on professional antigen-presenting cells and present extracellularly derived peptides to CD4+ T lymphocytes (3). Lastly, class III consists of more than 60 proteins that function in inflammation, activation of the complement cascade, and cellular stress (4, 5).
As the MHC locus is the most gene-dense region, it also contains the most genetic variation. More than 16,000 HLA alleles exist which encode for approximately 13,000 protein variants when considering only classical class I and II genes (6). The thousands of alleles are named according to the Human Genome Mapping Nomenclature Committee. Each unique allele name consists of the gene name followed by an asterisk and a number of up to four digits separated by a colon. The first two digits describe the serologic assignment. The third digit describes alleles that differ in at least one synonymous substitution in an exon, while the fourth digit describes alleles that differ by a single SNP in non-coding regions. Sometimes a suffix can be added to the unique allele to indicate the state of expression: “L” (Low), “S” (secreted), “N” (null), “C” (cytoplasm), “A” (Aberrant) and “Q” (questionable) (7).
The association of HLA variants and disease risk was first established nearly 50 years ago, and since then several hundreds of ailments including cancers, infectious diseases, autoimmune disorders, and neuropathologies have been linked to the presence of certain HLA alleles (8–11). The impact of the HLA region on disease is best seen in autoimmune conditions as the HLA region, mainly class II genes, accounts for 50% of genetic predisposition to autoimmune conditions (12). For instance, the DQA1*05:01-DQB1*02:01-DRB1*03:01 (DQ2-DR3) and DQA1*03:01-DQB1*03:02-DRB1*04 (DQ3-DR4) haplotypes confer the highest risk to type 1 diabetes mellitus (13). In contrast, HLA class I associations with autoimmune conditions are less common, however, they still have a strong association with conferring disease risk such as in the case of HLA-B*27 which the strongest genetic contributors to ankylosing spondylitis in Europeans (14, 15). Furthermore, sensitivity to certain drugs has also been linked to the presence of certain HLA alleles. For example, HLA-B*57-positive individuals are hypersensitive to the anti-retroviral known as Abacavir (16, 17).
Studies have largely focused on the variation within the coding region of HLA genes as it has a direct effect on antigen presentation. The frequency of single nucleotide polymorphisms (SNPs) in non-coding regions generally exceeds those found in the coding region. Zhao et al. (2003) found that intronic and untranslated regions had a SNP density of 8.21 and 7.51 SNPs per 10 kb respectively, while exonic regions only had a density of 5 SNPs per 10 kb (18). Due to genetic variation being an underlying factor contributing to disease risk, surely non-coding HLA variants should be closely examined.
The non-coding genome is dispersed across intergenic and intronic regions. It encompasses the UTRs, promoters, and distal regulatory elements. The 5’ and 3’ UTR flank the start and end of a gene and regulate protein levels by influencing translation, mRNA stability, and secondary structure (19, 20). The promoter regions were found ~0.5kb from the transcriptional start site. Theyact as a scaffold for transcriptional machinery such as transcription factors and RNA polymerases. Using enhancers and silencers found up to 1 megabase away from promoters, transcription factors can either initiate or suppress transcription. Non-coding SNPs typically regulate expression by influencing transcription factor binding, long-range chromosomal interactions, and mRNA stability (21). The induced changes in HLA expression can significantly impact antigen processing and presentation, thereby affecting the peptide repertoire presented to T cells and consequently altering T cell priming capacity. For instance, reduced HLA expression could result in decreased presentation of antigenic peptides to T cells and inadequate T cell activation. This diminishes the recognition and elimination of infected or cancerous cells by the immune system. Alternatively, higher HLA expression could enhance antigen presentation, broaden the peptide repertoire, and improve T cell priming capacity, leading to more effective immune responses (22). Differential expression of HLA genes and proteins has been associated with various diseases (23–26). Through its influence on gene expression, non-coding genetic variants may be a contributing factor to these diseases.
Genetic variation in non-coding regions may also influence gene expression through epigenetic mechanisms. Epigenetic mechanisms generally target non-coding regions and can influence gene expression without modifying DNA sequences. These factors include DNA methylation, histone modifications, and non-coding RNAs such as microRNAs and long non-coding RNAs (27). A few studies have identified epigenetic factors responsible for controlling HLA expression. For instance, Ramsuran et al. (2015) found that DNA methylation of HLA-A promoters is a major factor driving allele-specific expression of HLA-A (28). Kulkarni et al. (2013) also found that the 3’UTR of HLA-C contains binding sites for miR-148, resulting in lower HLA-C expression in individuals with Crohn’s disease (23). Polymorphisms found within these DNA methylation and non-coding RNA binding sites may alter the epigenetic regulation of these genes. It is therefore crucial to explore the interaction between genetic and epigenetic regulatory mechanisms.
In this review, we discuss variants found within the class I and class II HLA non-coding genome and the association it may have with disease outcomes. We further explore the link between genetic variation and its influence on epigenetic factors controlling HLA expression, the importance of the non-coding genome on disease, discuss ethnic specific differences across the HLA region, and provide guidelines for future HLA studies.
Genetic non-coding variants affecting HLA expression
Most studies have evaluated coding variations of HLA as they could lead to amino acid substitutions in the protein sequence, affecting protein structure, stability, antigen binding specificity, or interactions with other molecules involved in antigen presentation. The magnitude of change in HLA expression resulting from coding SNPs can also vary, depending on factors such as the location and nature of the amino acid substitution, and its impact on HLA expression and function (29). Non-coding variants have been shown to play a role in the regulation of both class I and II genes. Mutations in the non-coding genome can influence gene expression by affecting transcription factor binding, influencing long-range chromatin interactions, and modulating mRNA stability (21). It may also influence protein expression by altering post-transcriptional processes such as splicing, polyadenylation, cleavage, ribosome binding, and assembly. They also have greatly effect gene expression. for instance, rs2395471 and HLA-C 263 insertion/deletion polymorphisms account for 40% variation in HLA-C expression (23, 30).
A genome-wide association study (GWAS) conducted on Malaysian Chinese patients with nasopharyngeal carcinomas detected a significant association between certain SNPs found in the HLA-A gene and either susceptibility or resistance to nasopharyngeal carcinomas (31). Rs41545520 (G>T), which is found in the promoter region of HLA-A may affect HLA-A transcription as the G allele creates a binding site for activating transcription factor 3 (ATF3). Through its interaction with the cyclic adenosine monophosphate response element (CRE) at gene promoters with TGACATCA motifs, ATF3 represses transcription (32, 33). Using the Genevar Database, the authors found that rs41545520 (G>T) is in strong linkage disequilibrium with rs2860580 (A>G), an HLA-A intronic variant (31), which was previously associated with nasopharyngeal carcinomas (34, 35). Expression quantitative trait locus (eQTL) analysis using Genevar Database showed that the wild type G allele is associated with stronger binding with ATF3 and thus lower HLA-A expression. However, the mutant T allele is associated with higher HLA-A expression, due to its weaker ATF3 binding affinity (31). Furthermore, the T allele (rs41545520) marks a higher expression of the protective HLA-A*11:01 allele in nasopharyngeal carcinoma. The higher HLA-A expression allows for increased presentation of tumor-specific antigens to cytotoxic T cells and improved clearance of the tumor cells (31). Allelic polymorphisms have also been associated with higher expression of HLA-A*31 and HLA-A*33 alleles. Two polymorphisms, rs41272547 (C>A) found in the 5’UTR and rs1061235 (C>T) in the 3’UTR were only found in HLA-A*31 and HLA-A*33 alleles. The mutant alleles were associated with significantly higher HLA-A expression in heterozygous HLA-A*31 and HLA-A*33 individuals (36). Prediction analysis demonstrated that rs41272547 may disrupt a nuclear factor kappa B (NF-kB) and activating protein 2 (AP2) binding motifs and may be responsible for creating a site for glucocorticoid receptor binding. Transcriptional activity was assessed using luciferase reporter assay which found that the mutant A allele was associated with higher transcriptional activity compared to the reference allele (C) (36). While rs41272547 may affect transcriptional regulation, rs1061235 may affect post-transcriptional regulation of HLA-A. The consensus sequence (which contains a C allele for rs1061235) contains binding sites for miRNA binding (discussed later on) (36). Rs9260118 (T>C) and rs9260119 (T>A) were also found to reside in the 5’UTR. Wild type alleles were associated with significantly higher expression of HLA-A*01/A*11/A*03/A*30 alleles compared to the mutant alleles; however, these SNPs were not associated with altered transcription factor binding (36). These SNPs may be in linkage disequilibrium with additional functional SNPs. Although HLA-B is regarded as the most polymorphic gene region, consisting of approximately 3,000 SNPs, the non-coding region of HLA-B remains understudied as no polymorphisms within the non-coding region were shown to associate with HLA-B expression or disease. Furthermore, minor differences in the mRNA expression of HLA-B was reported across different HLA-B alleles suggesting that HLA-B non-coding SNPs may not play a significant role in HLA-B regulation (37, 38).
HLA-C has the lowest genetic diversity amongst the major class I genes. Like HLA-A, HLA-C is expressed in an allele-specific manner. HLA-C surface protein expression was imputed for 228 individuals from the 1000 Genomes study who were previously HLA-typed (30). Out of the 68,726 SNPs across the MHC region, rs2395471 (A>G) and, rs2249741 (A>G), were strongly associated with HLA-C imputed eQTL and surface expression. Rs2395471 is found 800 base pairs upstream of the core promoter. The association of rs2395471 and HLA-C surface expression was validated in two independent cohorts. Rs2395471 accounted for 36% variation in surface HLA-C expression in European Americans and a 28% variation in African Americans (30). A separate study supported the association of rs2395471 with HLA-C levels in peripheral blood mononuclear cells (PBMCs) from 273 European participants. Using the Alibaba prediction tool, Vince et al. (2016) found that rs2395471 may be located within a binding site for the Pit-Oct-Unc (POU) transcription factor family; however, rs2249741 did not seem to overlap with any transcription factor binding motifs (30). Electrophoretic mobility shift assays on the nuclear extracts obtained from HeLa and Jurkat cell lines as well PBMCs from healthy individuals found that rs2395471 only affects the binding of Oct1 and no other POU family members. This suggests that the G allele may account for lower HLA-C expression. The results were further validated by the use of luciferase reporter assays. High expressing HLA-C*01:02 and HLA-C*04:01 carry the A allele for rs2395471, while the G allele is present in low expressing HLA-C*03:04 and HLA-C*08:02. Substitution of A with G in high expressing alleles resulted in similar promoter activity of low expressing HLA-C alleles. However, the converse did not occur when the G was substituted for an A in low expressing HLA-C*03:04 and HLA-C*08:02; suggesting an additional regulatory factor may dominate over Oct1 transcriptional regulation in HLA-C*03:04 and HLA-C*08:02 (30). While the promoter activity of HLA-C*04:01 and HLA-C*08:02 differ drastically in HeLa and Jurkat cells; they are expressed at similar levels in CD3+ cells (39). Post-transcriptional regulatory mechanism may account for this discrepancy as HLA-C*04:01 contains a binding motif for miR-148a resulting in the degradation of HLA-C*04:01; however, HLA-C*08:02 contains a SNP which allows it to escape miR-148a binding (24). Not only does Oct1 stimulate gene transcription, it also interacts with other transcription factors such as NF-κB. Rs2395471 is located approximately 651 bp downstream of enhancer κB element in the HLA-C promoter. Rs2524094 (G>A) was found to disrupt enhancer sequence and led to cells being unresponsive to NF-κB stimulatory cytokines (TNF-α, IL-17A and IL-22). It would be interesting to note whether disruption of the Oct1 binding site may influence NF-κB binding at the enhancer κB element (40).
Unlike classical HLA genes, HLA-G functions through mediating immune tolerance rather than stimulation via antigen presentation. High levels of HLA-G can exert inhibitory functions against immune cells such as natural killer (NK) cells, T lymphocytes, and antigen-presenting cells allowing for successful maternal-fetal interactions and organ transplantations. Thus, polymorphisms causing low levels of HLA-G expression are more likely to result in spontaneous abortions, preeclampsia, and transplant rejection (41, 42). A 14bp insertion/deletion polymorphism found within the 3’UTR is the most well-studied HLA-G non-coding variant (42). The presence of the 14 bp insertion usually results in lower expression of circulating and membrane-bound HLA-G; while deletion of the sequence results in elevated HLA-G levels (43–46). The 14-bp insertion variant is related to the alternative splicing of the HLA-G primary transcript, which results in a more stable mRNA, but this higher stability does not compensate for the lower HLA-G expression. Although the presence of the 14bp sequence causes lower HLA-G expression, the spliced isoform that is formed is more stable than the isoforms where the 14bp sequence is removed (47). The presence of the insertion alleles has been associated with a higher risk of spontaneous abortions, preeclampsia, transplant rejections, and auto-immune conditions such as multiple sclerosis, and Crohn’s disease (43–46).
Non-coding variants also affect the expression of class II genes: HLA-DRB1, HLA-DQA1, HLA-DPA1, and HLA-DPB1 (48–51). SNPs within class II HLA genes strongly influence an individuals to risk of Hepatitis B virus (HBV) infection and viral clearance in Sichuan Han, non-Hispanic European, and Indonesian populations (49–55). Rs3077A (HLA-DPA1), rs9277535A (HLA-DPA1), and rs3135021A (HLA-DPB1) were observed at a higher frequency in healthy control than HBV carriers. The GA and AA genotypes of rs3077 were also associated with spontaneous clearance of HBV. The protective nature of rs3077A, rs9277535A, and rs3135021A could be due to changes in HLA expression. rs3077A and rs9277535A carriers have higher HLA-DPA1 expression. The increased expression of HLA-DPA1 may increase antigen presentation and T-cell priming allowing for protection against HBV. The mechanism behind the altered expression is unknown, however, rs3077A (HLA-DPA1), and rs9277535A are found within the 3′ UTR and may be regulated by miRNA (49–51).
Table 1 provides a list of non-coding variants affecting the expression of class I and class II genes and how they may be associated with certain disease outcomes. These studies demonstrate that disease phenotypes are not only a product of variation in the coding region but also changes in expression through non-coding genetic variants. While hundreds of non-coding variants exist, only a handful of studies have found a direct link between these variants and expression, Furthermore, even fewer studies have investigated the functional relevance of these polymorphisms.
Linking genetic variation with epigenetic regulation of HLA genes
Genetic variation has long been regarded as a major driver of disease-associated phenotypic variation. The contribution of epigenetic modifications to disease is becoming more recognized. Genetic and epigenetic changes are often studied independently, however, sometimes they may interact. SNPs can influence epigenetic mechanisms by affecting DNA methylation sites and altering non-coding RNA-target binding affinities. This in turn affects gene expression or results in the preferential expression of a specific allele (61). In the subsequent sections, we discuss the implications of genetic variation on DNA methylation and miRNA-mediated regulation of HLA expression.
Polymorphic methylation sites affecting HLA regulation
DNA methylation is the best characterized epigenetic modification. In mammals, methylation usually occurs on a cytosine base that is 5’ to a guanine base (CpG). This generally inhibits gene transcription by promoting a heterochromatin state and preventing transcription factor binding in the promoter regions of genes (62). Regulation of HLA genes is no exception to this phenomenon. Thus, methylation of HLA class I and II promoter regions are usually associated with reduced HLA mRNA expression (62–64). Promoter methylation of essential transcriptional HLA-regulating components such as the class II transactivator (CIITA) may also influence HLA expression (65, 66).
Genetic variants modulate quantitative changes in methylation levels at specific loci. This is known as methylation quantitative trait loci (meQTL) (67). meQTLs can affect a few CpG sites or influence the methylation of multiple CpG sites distributed across the extended genome and are often associated with changes to gene expression levels. SNPs usually underlie meQTLs (67, 68). SNPs can either introduce or abolish CpG sites leading to drastic changes in methylation at single CpG sites. For instance, a C-to-T transition on ‘C’ of CpG dinucleotides leads to a loss of a CpG site, resulting in a loss of methylation and an increase in gene expression (Figure 1) (69). Furthermore, these methylation-associated SNPs (meSNPs) may affect the methylation status of neighbouring CpG sites or influence expression through high linkage disequilibrium (69) (Table 2).
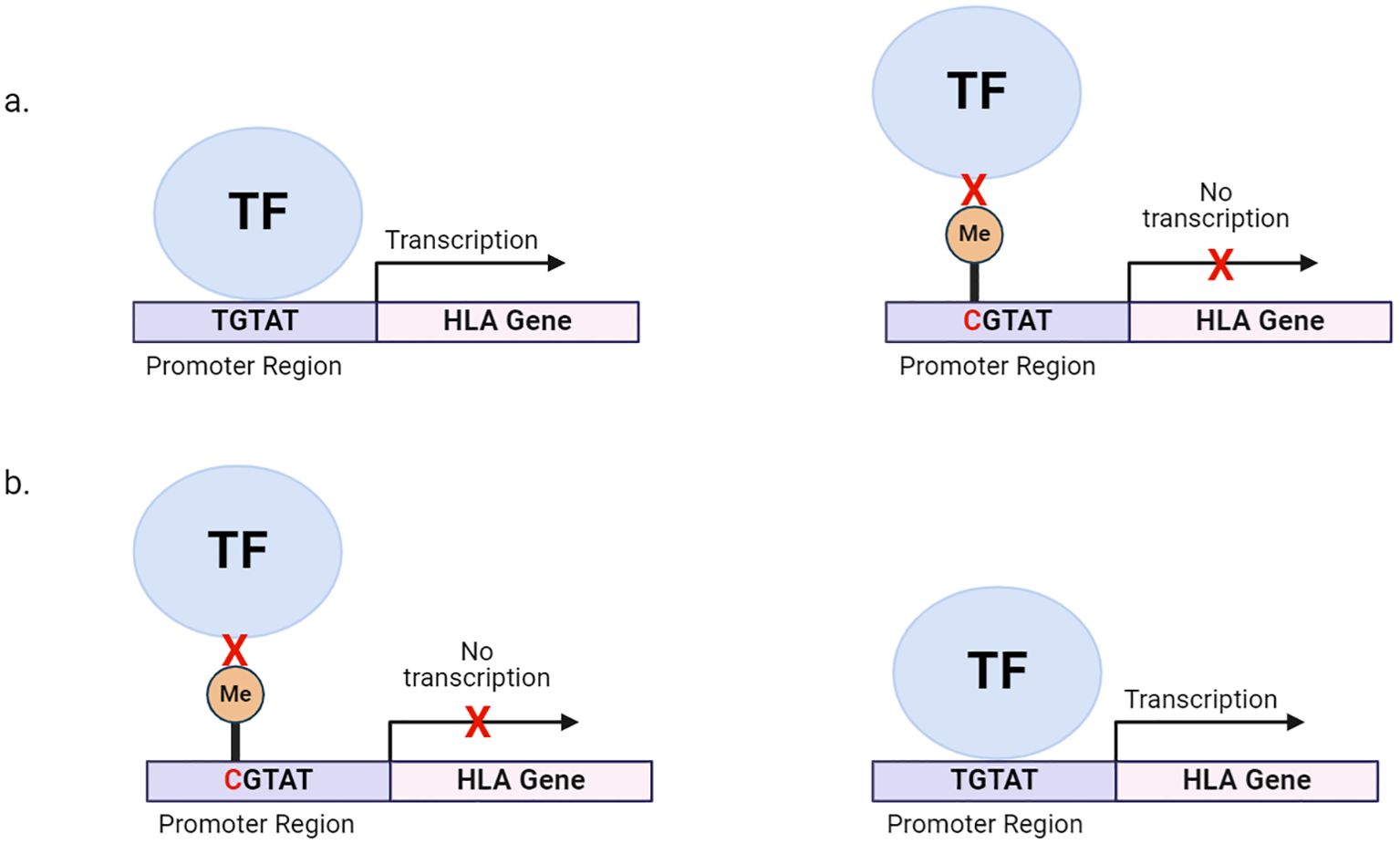
Figure 1. Genetic variation within HLA promoter regions can alter DNA methylation states, affecting HLA expression. (A) SNPs within the promoter can also create a CpG site. Methylation of CpG sites, preventing the binding of transcription factors and other chromatin factors and leading to reduced HLA transcription. (B) SNPs within the promoter can destroy CpG site, allowing for the binding of transcription factors and other chromatin factors and leading to HLA transcription. TF, transcription factor; Me, methyl group.
Individual genotypes at a given locus may result in different DNA methylation patterns and may influence the levels of expressed alleles. This is known as allele-specific methylation. Allele-specific methylation is common in genetic imprinting; where the inactive gene is significantly more methylated than the actively expressed gene (79). Ramsuran et al. (2015) observed allele-specific methylation in HLA-A (28). The group found several CpG sites including a CpG island within the promoter, and another spanning intron 1 to exon 3 of HLA-A. Deep-sequencing of a ∼300 bp DNA fragment upstream of the HLA-A transcription start site found that individuals homozygous for HLA-A*03 (a low expressing linage) had one or more individual CpG sites that were methylated within the promoter region. In contrast, individuals homozygous for HLA-A*24 (a high expressing lineage) had only one methylated CpG in the promoter region (28). Interestingly, a SNP within the HLA-A*24 lineage resulted in the loss of one CpG site reducing the number of potential methylation sites and potentially affecting HLA-A*24 expression. HLA-A allelic expression may also differ due to the methylation patterns within transcription factor binding sites. Ramsuran et al. (2015) observed two methylated CpG sites within the HLA class I regulatory complex (CRC) transcription factor binding site in the HLA-A*03 lineage; however, no methylation was observed in the CRC binding motif for HLA-A*24 (28). Methylation of the CRC motif probably prevented CRC from binding to the HLA-A promoter, hindering HLA-A*03 transcription while transcription of HLA-A*24 continued uninterrupted as methylation did not affect CRC binding (28). The HLA-A*24 promoter contains a polymorphism that disrupts a methylation site (CpG → TpG), while the CpG site remains in lower expressed HLA-A*03 lineage, arguing for methylation-mediated suppression of expression of the HLA-A locus (Ramsuran et al., 2015).
Zhao et al. (2023) evaluated the influence of DNA methylation patterns on women with endometriosis. Fifteen CpG sites were found to be differentially methylated between women with endometriosis and healthy controls. Five of these CpG sites were found in intron seven on HLA-C and four (cg03216697, cg01521131, cg09382842, and cg09556042) were found exclusively in HLA-C*07 allele (70). Higher methylation of intron 7 also led to higher expression of HLA-C*07 in women with endometriosis. It is possible that a silencer may be present on intron 7, and that the hypermethylation may inhibit the silencer resulting in increased expression of HLA-C*07.Furthermore, HLA-C*07 is a ligand for inhibitory KIR signaling of natural killer cells, which normally clear the endometrial tissue. It is possible that the higher expression of HLA-C*07 due to hypermethylation of intron seven, resulted in the silencing of natural killer cells, thus promoting the occurrence of endometriosis (70).
Allele-specific methylation has also been noted for HLA-G; however, the role non-coding variants may play has yet to be uncovered (80). Nevertheless, variation within the HLA-G promoter region has been shown to influence fetal loss (71). HLA-G plays an important role in embryo implantation, and fetal development as well as in protecting the fetus from the maternal immune system (81). Thus, it is not surprising that loss of HLA-G may result in adverse pregnancy outcomes such as preeclampsia, unsuccessful embryo implantation, and fetal loss (81, 82). Variation of the HLA-G promoter region was investigated within 42 Hutterites from South Dakota (71). Hutterites have a naturally high fertility rate despite the high level of consanguineous relationships within the population (83). Eighteen SNPs were identified in a 1,300bp region upstream of the HLA-G transcriptional start site. Only one (-725C>G) of these eighteen polymorphisms was associated with significant fetal loss. The presence of the -725G allele in both parents was associated with a significant risk of miscarriage (71). The G allele at -725 creates a CpG site at -726 and -275 which is located approximately 10bp from an IRF1 binding site. The presence of the G allele at -275 promoted the methylation of the C nucleotide found at -726. This may have disrupted IRF1 binding resulting in lower HLA-G expression. Low HLA-G expression leads to increased maternal immune responses against the fetus and impaired vascular remodeling of the placenta contributing to miscarriageand thus promoting fetal loss (71). However, in a study conducted on couples who had recurrent spontaneous abortions, no methylation was observed at -276C in the presence of the G allele at -275 and there was no association between the -275 SNP and recurrent spontaneous abortions (72). This difference in results may be attributed to the different study groups used. The first study used couples who suffered from miscarriages but also had pregnancies that were carried to full term, while the latter study focused on couples who had recurrent miscarriages.
Polymorphic methylation sites have also been noted in class II HLA genes. Several GWAS studies have identified SNP-CpG sites located in cis-regions of HLA-DQ and HLA-DR. These SNP-CpG sites were found to be associated with asthma, type 2 diabetes, food allergies, and congenital heart disease (74, 76, 77). A GWAS conducted on the airway epithelium of asthmatic individuals found that 59% of SNPs regulated cis-gene expression. Of that 59%, 89.9% of those SNPs mediated cis-gene expression via cis-methylation. rs6906021 (T>C), was one of the SNPs found to regulate HLA-DQA2 expression through methylation (74). Individuals homozygous for TT showed higher methylation for cg22933800 compared to individuals heterozygous for TC and homozygous for CC. The level of cg22933800 methylation directly correlated with HLA-DQA2 expression. Higher expression of HLA-DQA2 can increase asthma susceptibility by enhancing antigen presentation and promoting immune responses that drive allergic inflammation and hyperactivity of the immune cells within the airways (74).
The first GWAS of well-defined food allergies was conducted on 2,759 US participants and replicated in 2,197 participants of European ancestry (76). The study found that rs9275596 (C>T) an intergenic SNP found between (HLA-DQB1/HLA-DQA2) showed the most significant association with peanut allergies. The study showed that rs9275596 was a meQTL for the HLA-DRB1 and HLA-DQB1 genes, and that methylation was associated with risk of peanut allergies (76).
In addition to peanut allergies, variation in HLA-DR may also harbor a risk of congenital heart disease. rs9271573 (A>C), located near HLA-DRB6, was found to affect the methylation of cg08845336 located on HLA-DRB1 (77). Individuals with CC genotypes showed elevated methylation levels compared to individuals heterozygous for CA and homozygous for AA. Further, the SNP was found to colocalize with HLA-DRB1 expression (77).
Occasionally, CpG-SNPs can be in high linkage disequilibrium with other polymorphisms, making the altered methylation state a tag for other genetic polymorphisms (9). In human pancreatic islets, rs1063355 (C>A) was found to associate with HLA-DQB1 expression (75). Individuals who presented with CC genotypes had significantly higher levels of methylation at cg22984282 than individuals with CA or AA genotypes The study found that rs1063355 was found to be in linkage with rs9272346, (2KB upstream of HLA-DQA1) a variant which is associated with increased risk of type 1 diabetes (75). Similarly, Coit et al. (2019) set out to identify differentially methylated CpG sites in HLA-B*27-positive individuals with ankylosing spondylitis (78). The CpG site cg17616250 was the most hypomethylated in ankylosing spondylitis patients relative to the osteoarthritis control group (78). Furthermore, HLA-B*27 positive patients with ankylosing spondylitis had lower methylation at this CpG site compared to HLA-B*27 negative patients (78). However, cg17616250 is located in HLA Complex P5 RNA (HCP5). The methylation status of cg17616250 is determined by the SNP rs114212906 (C>T). Carriers of the CC genotype had higher methylation levels than CT and TT carriers as the minor T allele tends to disrupt the CpG site. Rs114212906 is in strong linage disequilibrium with rs4349859, a SNP often associated with HLA-B*27 positive ankylosing spondylitis patients (78). Table 2 summarizes studies investigating polymorphic methylation sites affecting HLA regulation.
Non-coding variants affecting miRNA regulation of class I and II HLA expression
Non-coding transcripts such as miRNAs play an essential role in regulating normal physiological processes and consequently human health and disease (84–86). MiRNAs belong to a class of small endogenous non-coding RNA molecules of approximately 20-24 nucleotides, with regulatory effects on cellular processes. Generally, a specific sequence within the 5’UTR of the miRNA, known as the “seed region” complementary base pairs with the 3’UTR of the miRNA-target transcript or mRNA. This interaction results in translational silencing and decay of the target transcript (87). The complementary relationship between miRNA-mRNA is evolutionarily conserved; however certain factors can disrupt miRNA-mRNA interactions. Genetic variation within the “seed region” of the miRNA or the 3’UTR of the targets may create, disrupt, or alter the affinity of miRNA-mRNA interactions, affecting the expression of the mRNA target and subsequently resulting in altered physiological processes or disease onset (87). Apart from affecting miRNA-mRNA interactions, SNPs within the miRNA gene may affect miRNA biogenesis or maturation, altering miRNA expression which may also affect the regulation of the target mRNA (Figure 2) (87, 88).
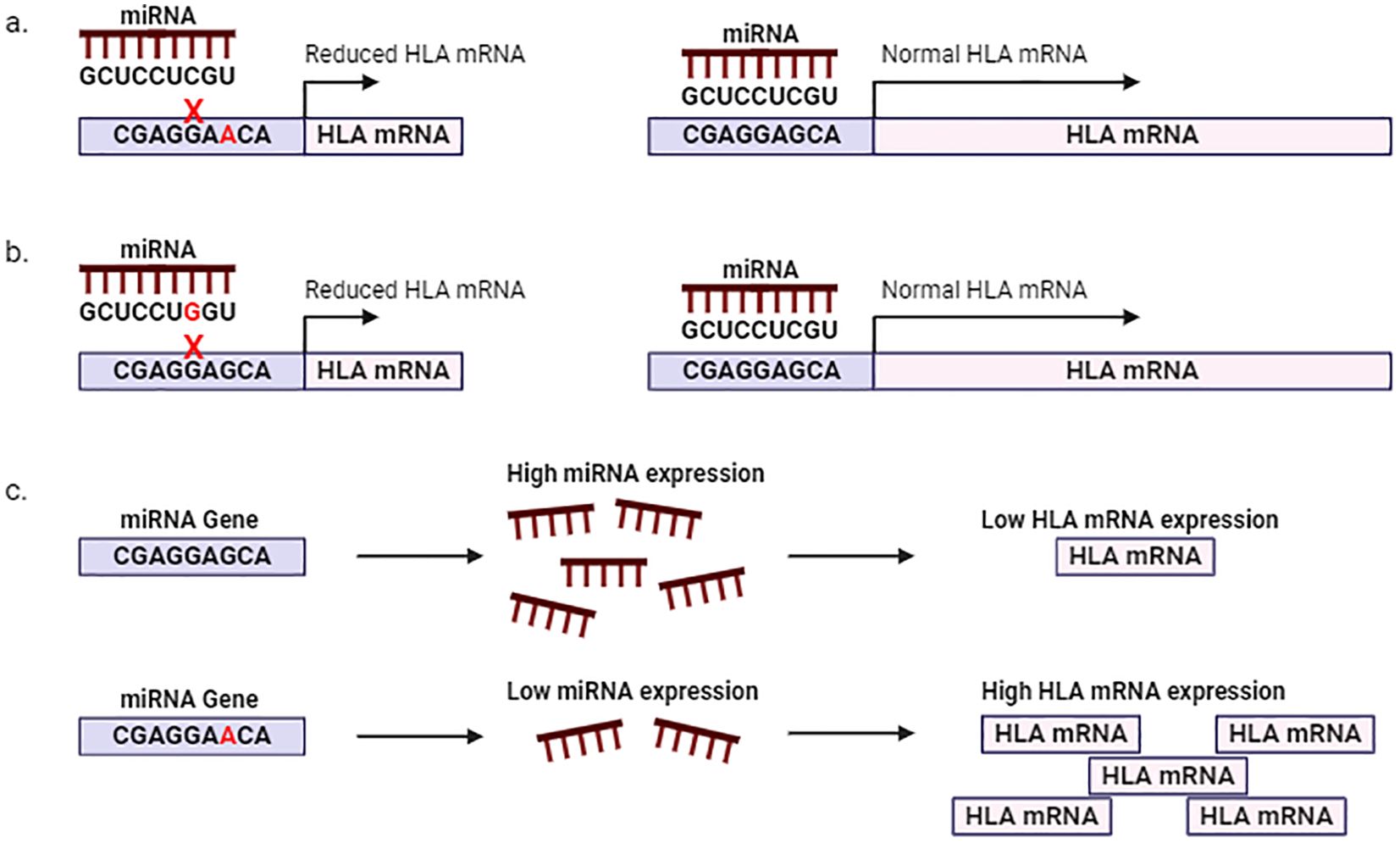
Figure 2. Polymorphisms affecting miRNA regulation of HLA mRNA. SNPs within the 3’UTR of HLA (A) or within the seed region of the miRNA (B) affect miRNA-HLA mRNA interactions. (C) SNPs within regulatory regions of the miRNA may affect miRNA expression and subsequently HLA expression.
A few studies have noted genetic variation affecting miRNA-HLA interactions (Table 3). We previously discussed a study by Rene et al. (2015) which found 5’UTR and 3’UTR SNPs involved in the allele-specific regulation of HLA-A (36). The presence of specific polymorphisms within the 3’UTR of certain HLA-A alleles may contribute to allele-specific regulation of HLA-A expression (36). Individuals with a single HLA-A*31 or HLA-A*33 allele tend to have high HLA-A levels. The polymorphism rs1061235 (A>T) is found in the 3’UTR of HLA-A (36). The A allele is the predicted target for the binding of miR-526b, -609, -1290, -342-5p, and -542-5p. While the T allele which is found in HLA-A*31 and HLA-A*33 disrupts the binding of miR-526b, -609, -1290, -342-5p, and -542-5p and instead creates predicted binding sites for miR-520f and miR-651. However, the miR-520f and miR-651 may not experimentally target HLA-A*31 and HLA-A*33 as the T allele is associated with higher HLA-A levels (36).
The miR-148/-152 family (comprising miR-148a, miR-148b, and miR-152) plays a versatile role in various diseases by modulating the expression of important immune genes [reviewed in (93)]. Polymorphisms within the miR-148 family as well as certain HLA genes affect miR-148 family regulation of HLA and disease states (89, 90). For instance, Kulkarni et al. (2011) performed a sequence analysis of the 3’UTR of common HLA-C alleles and found polymorphisms within the binding sites of miR-148a, miR-148b, and miR-657 (24). These three miRNAs were found to have the highest predicted binding scores of the 26 miRNAs that are predicted to bind to HLA-C (24). Variants within the miR-148a/-148b binding site included rs67384697 (G>T), located at position 263 downstream of the stop codon, as well as the linked variants +259C/T, +261T/C, and +266C/T. Low-expressing HLA-C alleles allotypes (Cw*0702) with the G allele were found to be more stable due to miRNA binding compared to high-expressing HLA-C alleles (Cw*0602) marked with the T allele (24). The T allele disrupts the miRNA-148 a/-148b binding site, thus restricting the control miR-148a/-148b would otherwise have on the regulation of HLA-C. HLA-C expression was highest amongst individuals homozygous for the T allele, while those homozygous for the G allele had the lowest expression. Experimentally, miR-148b and miR-657 binding sites were not shown to drive HLA-C expression; however, the interaction between miR-148a and rs67384697 could be the causal variant for differential HLA-C expression (85). Rs67384697 was found to be in strong LD with rs9264942 which was shown to associate with HLA-C expression and control of HIV disease (25, 94–96). It is possible that rs67384697 is the causal variant responsible for controlling HLA-C expression and controlling HIV disease. High levels of HLA-C are associated with better control of HIV through more efficient antigen presentation to CD8+ T lymphocytes. In a cohort of 2,527 HIV-infected European Americans, HIV controllers were found to have a higher frequency of the T allele (rs67384697) and potentially have higher HLA-C expression and thus lower viral loads; while the G allele was significantly frequent in non-controller (24).
Sequence variation within the miR-148 binding site may not be the only explanation for differential levels of HLA-C. Kulkarni et al. (2013) also identified sequence variation within the miR-148 gene which affected miR-148 and subsequently HLA-C expression (23). Twenty-six polymorphic sites were identified in a 7.7kb region of the miR-148a gene and its flanking regions within 219 European American individuals (23). rs735316 (G>A) and rs111299611 (ins/del), were found to be in perfect LD and associated most significantly with miR-148 expression. Individuals homozygous for the wild-type del variant for rs111299611 and GG for rs735316 had higher levels of miR-148 compared to individuals homozygous for the mutant ins (rs111299611) and AA (rs735316) alleles. Lower HLA-C expression is expected among individuals who carry the wild type rs111299611 and rs735316 alleles (higher miR-148 expression) and have an intact miR-148 binding site within HLA-C 3’UTR and carry the wild type rs111299611 and rs735316 alleles (23). In a longitudinal cohort of 2,918 HIV-infected individuals, rs753516 genotypes were associated with HIV viral load in individuals with an intact miR-148 binding site and had no effect in individuals with a disrupted binding site. Furthermore, Kulkarni et al. (2013) showed that HLA-C expression had opposing effects on HIV and Crohn’s disease (23). Low miRNA-148 levels and high HLA-C levels are associated with better control of HIV, but a higher risk of Crohn’s disease. While high HLA-C expression increases the presentation of viral antigens to cytotoxic T cells and viral clearance, it could also lead to inappropriate activation of T cells, contributing to chronic inflammation in the gastrointestinal tract leading to Crohn’s disease. Kulkarni et al. (2013) further tested whether rs735316 is associated with the risk of Crohn’s disease. A meta-analysis found that rs735316 (AA) was associated with an increased risk of Crohn’s disease by lowering miR-148 expression and subsequently HLA-C expression in individuals with intact miR-148 binding sites located in HLA-C (23). This study demonstrates the need to study non-coding variants in disease-specific cases as they influence different diseases differently.
Predictive and functional analysis by Tan et al. (2007) showed that the G allele of rs1063320 (G>C) has a high affinity for miRNA binding as the predicted minimum free energy between G and miR-148/-152 family was highly stable compared to the C allele and miR-148/-152 family (88). These results were experimentally validated. Luciferase assay found that the activity of the G allele in the presence of the miRNA-148/-152 family was significantly diminished compared to the presence of the C allele or deletion of the whole miRNA target site. Furthermore, HLA-G levels were significantly reduced by miR-148a in JEG3 cells, which naturally express high levels of HLA-G and are homozygous G at rs1063320 (91). Similar results were observed in a study evaluating the role of statin treatment on miR-148/-152 family in asthmatic patients, HLA-G expression negatively correlated with miR-148/-152 levels in individuals homozygous for GG. However, there was no correlation between HLA-G and miR-148/-152 in individuals carrying CC or CG. Furthermore, the authors did not directly test the interaction between rs1063320, miR-148/-152, and HLA-G (89). On the contrary, Manaster et al. (2012) showed that rs1063320 does not influence the binding efficacy of the miR-148/-152 family to HLA-G as luciferase activity was repressed in the presence of both G and C in the 3’UTR (97). It is possible that the results differed due to different cell lines being used. For instance, ATP is essential for luciferase assays since endogenous ATP levels differ among cell lines, which may impact the results. In silico analysis supported previous findings that the G allele has a high affinity for miRNA binding as the minimum free energy between HLA-G and miR-148/-152 family was similar to that estimated by Tan et al. (2007) (88, 91). In addition, the presence of the G allele is a predicted target of miR-19 and miR-218-2 as the minimum free energies observed were similar to the miR-148/-152 family (92).
Eight polymorphic sites (rs1704 ins>del, rs1063320 G>C, rs1707 T>C, rs1710 C>G, rs17179101 A>C, rs17179108 C>T, rs9380142 A>G and rs1610696 C>G) have been observed within the 3’UTR of HLA-G including rs1063320 which was previously discussed (98). Rs1704 represents a 14bp ins/del variant that is in strong LD with rs1063320 and significantly associated with HLA-G expression levels and mRNA stability. The presence of the insertion variant marks reduced HLA-G levels (92). A subset of these transcripts is further processed by the deletion of 92bp originating in exon 8 and extending into the 3’UTR. This region contained rs1707, rs1710, rs17179101, and rs17179108 which may affect the binding of specific miRNAs (miR-513a-5p, miR-518c*, miR-1262, miR 92a-1*, miR 92a-2*, and miR-661) according to in silico studies (92).
The HLA-DP polymorphism, rs9277534 (A>G) is strongly associated with HBV persistence due to the strong effect it has on HLA-DP mRNA and surface protein levels. Individuals in the GG group have approximately twofold higher expression than individuals in either the AG or AA groups (26). The association of the G allele with high HLA-DPB1 expression was also noted in acute graft versus host diseases and type 1 autoimmune hepatitis (99, 100). Computational assessment by Shieh et al. (2018) may explain the differences in expression. The A allele is present in low-expressing HLA-DPB1*04:01:01:01 (encoded by PGF B cell line); while high-expressing HLA-DPB1*03:01:01:01 (encoded by COX B cell line) transcripts contain the G allele. The 3’UTR of HLA-DPB1*04:01:01:01 was found to contain 27 predicted miRNA binding sites (101). Sixteen of these sites were also found in HLA-DPB1*03:01:01:01 and were targets of the same miRNAs. HLA-DPB1*03:01:01:01 had three additional miRNA binding sites and HLA-DPB1*04:01:01:01 contained one additional binding site that was not present in HLA-DPB1*03:01:01:01. Twenty and twenty-three miRNA binding sites were found in polymorphic regions of HLA-DPB1*03:01:01:01 and HLA-DPB1*04:01:01:01, respectively. The A allele of rs9277534 is associated with higher expression simply because it is targeted by a higher number of miRNAs (101). The authors further analyzed a publicly available data set of miRNA derived from 10 primary B cell samples (101, 102). Forty-four miRNAs were common in these 10 cell lines and the COX and PGF B cell lines. MiR-30e-3p was found to be the top candidate associated with rs9277534 as it is highly expressed and targets the A allele while disregarding the G allele (101, 102). However experimental validation and further functional analysis is necessary to identify if miR-30e-3p is indeed associated with rs9277534 or if one of the other predicted miRNAs is involved in rs9277534 mediated HLA-DPB1 expression.
The importance of the non-coding genome in HLA regulation and disease association
The HLA loci are strongly associated with a magnitude of diseases ranging from metabolic disorders to autoimmune conditions, cancers, and even infectious diseases. Due to HLA’s fundamental role in immune regulation, the HLA loci evolved to become the most genetically diverse gene region within humans (1, 2).
It is well known that polymorphisms within the coding regions of HLA genes influence antigen presentation. The function of HLA molecules is also impacted by their expression levels which also critically impacts normal immunological functioning. Differential HLA expression is shown to be associated with various diseases and transplantation (31, 44, 58). Non-coding regions of the genome predominately control the regulation of HLA expression. The binding of trans-regulatory factors to cis-regulatory regions such as promoters, enhancers, silencers influences gene expression and UTRs (103). Motifs within cis-regulatory sites allow for the binding transcriptional machinery that turns transcription “on” or “off”. The promoter region is located around the transcriptional start site of a gene and contains several elements that facilitate the binding of transcription factors and the assembly of transcriptional machinery (103). While promoters act on neighbouring genes, enhancers contain motifs that sequester transcription machinery to induce transcription of distant genes. Silencers act to down-regulate gene transcription, either through the binding of transcriptional repressor proteins or by passively preventing the binding of transcription factors. Thus, mutations within the cis-regulatory regions or trans-regulatory factors can interfere with the binding of trans-regulatory factors, altering gene transcription and thus expression (Figure 3) (103). While promoters, silencers, and enhancers regulate expression at the transcriptional level, the 5’ and 3’ UTR regulate expression at a post-transcriptional level by influencing mRNA stability, mRNA localization and transport, and protein expression. The 5’UTR contains elements that impact the binding of ribosomes to the mRNA and subsequently the initiation of translation. The 3’UTR contains AU-rich elements and miRNA binding sites that regulate mRNA stability. Some miRNAs bind to the 5’UTR. The binding of miRNAs leads to mRNA degradation or inhibition of translation affecting protein levels. Thus mutations within the UTR can effect miRNA binding and alter the efficiency of protein translation (104).
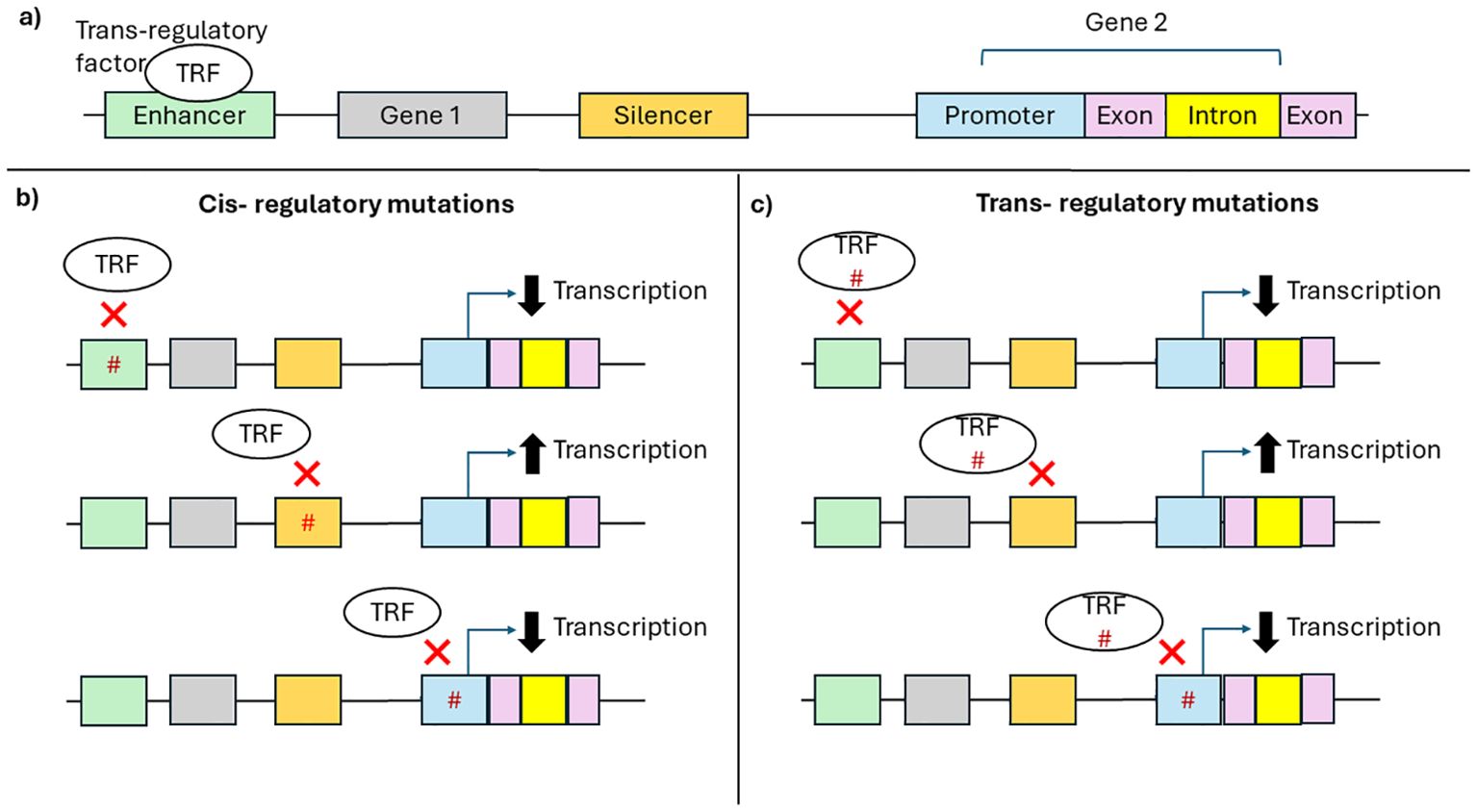
Figure 3. Effect of cis-regulatory mutations and trans-regulatory mutation of gene transcription. (A) Schematic representation of a gene region: cis-regulatory elements such as enhancer (green), silencer (orange), and promoter (blue); exons (pink), introns (yellow), and trans-regulatory factors such as transcription factors (white). (B) Mutations (hashtag) in cis-regulatory regions (C) or trans-regulatory factors can prevent the binding of trans-regulatory factors to cis-regulatory regions altering transcription.
We have discussed several studies in which non-coding variants lead to altered HLA expression and impact disease outcomes (23–25, 31, 40, 43, 44, 46, 48–55, 57–60, 70–78, 89–92). For instance, HLA-G plays a pivotal role in fetal-maternal immune tolerance and placental development. The -725 HLA-G variant is significantly associated with fetal loss. The G allele creates a CPG site near an IRF1 binding site. Methylation of the CpG site, prevents IRF1 biding resulting in lower HLA-G expression. Low HLA-G expression could lead to maternal immune activation against fetal antigens, leading to miscarriage (71). rs67384697G is present within low expressing HLA-C alleles as the G alleles allow for the binding of miR-148b, however, the presence of the T allele prevents miRNA-148b regulation of HLA-C and higher HLA-C expressions. rs67384697T has thus been associated with better control of HIV as the higher expression of HLA-C leads to more efficient presentation of HIV antigens to CD8+ T lymphocytes and viral clearance (24). Downregulation of HLA class I is a common escape mechanism for cancer cells from immunosurveillance and immunotherapy. rs41545520G creates a binding site for the transcriptional repressor, ATF3, leading to reduced HLA expression in nasopharyngeal carcinomas and consequently resulting in reduced presentation of tumour-associated antigens to CD8+ T lymphocytes and increased immune evasion (31). Somatic mutations add an extra layer to HLA diversity. In cancers, somatic mutations have been shown to contribute to changes in HLA expression and function supporting immune evasion (11, 105). Most studies investigating somatic mutations have been confined to coding regions, however, up to 96% of somatic mutations can occur within the non-coding genome and non-coding variants have been recognized as potential somatic catalysts and critic germline risk factors for cancer onset (106, 107). Thus, studying non-coding somatic mutations may also provide an improved understanding of the complexities of gene regulation and disease mechanisms.
Understanding mechanisms affecting the regulation of HLA expression is paramount for developing successful diagnostic and therapeutic approaches for different diseases (103). HLA-typing can be used to predict one’s susceptibility to disease and how an individual responds to the disease; however, it is an expensive and laborious process. In diseases where HLA expression is associated with severity or susceptibility to a disease, genotyping specific SNPs that play a role in regulating gene expression (such as those found in the non-coding genome) or determining the expression of certain HLA genes may be a more simple and cost-effective approach in predicting HLA-associated disease susceptibility and severity (108).
Altering HLA expression may also be important for certain therapeutic interventions. For instance, the onset of cancer is often associated with a decrease in class I HLA molecules. Several immunotherapeutic strategies have been designed to alter the tumor microenvironment. However, these strategies require normal HLA expression to be restored (109, 110). Targeting the non-coding genome either through genetic or epigenetic mechanisms will be essential for restoring HLA expression. According to ClinicalTrials.org, there are currently 509 and 332 clinical trials using miRNA and DNA methylation based therapy (111). This highlights the potential of epigenetic-based therapy that targets the most disease relevant region of the human genome.
Understanding the impact of the non-coding genome on HLA may further be used to optimize donor selection as well as broaden the donor pool for transplants. Hematopoietic stem cell transplantation is used in the treatment of hematological cancers and neoplastic disorders. It requires stringent matching of classical HLA class I and II alleles (HLA-A/-B/-C/-DRB1) between donor and recipient (112). Individuals requiring a transplant have a 30% chance of finding a first degree relative that has at least three matching HLA alleles (112). If a related donor cannot be found, an unrelated donor with a single mismatch can be used. This is however associated with a higher rate of mortality within a year (113). Furthermore, individuals from rare ethnic groups have a smaller chance of finding a suitable donor. One way of expanding the donor pool is by eliminating one of the HLA genes. Several studies have found HLA-A to be the most suitable candidate for elimination. Targeting of the non-coding genome using CRISPR-Cas9 and zinc finger nuclease was shown to effectively disrupt HLA-A (114, 115). This may improve the transplantation success involving unrelated individuals.
The importance of the non-coding genome in HLA regulation and pathophysiological states is extremely evident. More studies should be dedicated to exploring its potential for the design of diagnostic tools and therapeutic interventions.
Ethnic-specific differences across HLA genes
It is well known that the HLA region has the highest number of polymorphisms in the human genome with the identification of 13,412 unique HLA alleles in humans. Migration history, racial admixture, environmental pressure, and selection pressure due to pathogen exposure have been suggested to be major contributing factors to the high level of variation observed in the HLA region (116, 117). Historically, malaria has been suggested to be a major driver in the evolution of African populations (118), while tuberculosis infection was the major driver in Western European populations (119). The high selection pressure exerted by these pathogens was mainly on genes involved in immune regulation, such as the HLA region. This may account for the differences in HLA allele frequencies observed between European and African populations. Africa has the highest level of HLA diversity in the world (120). Furthermore, it has been observed that regions that are further away from Africa have lower HLA diversity than those regions closer to the African continent. The high degree of diversity is most probably due to Africa being the source of modern humans and the high burden of infectious diseases (116, 120). Although Africa has the highest number of classical HLA alleles, with 3,141 classical HLA class I alleles being present in North Africa alone; the African region has lower HLA class II diversity compared to Europe, North America, and South America (116). The higher class I diversity in Africa may stem from the high prevalence of infectious diseases in the region; while auto-immune conditions which are usually linked to HLA class II alleles are more common in European and American populations (116).
The main mechanism contributing to the generation of diverse HLA alleles is point mutations (Table 4). Point mutations in the form of nucleotide substitutions can result in changes in amino acid sequence (non-synonymous) or no change of amino acid sequence (synonymous). Insertion or deletion of a nucleotide are also forms of point mutations that can change the reading frame creating a premature stop in translation. These point mutations can create alternative splice sites diversifying mRNA transcripts generated (121). For instance, splicing of exon 3 of HLA-G gives rise to several HLA-G isoforms (122). The recombination events: gene conversion (donation of DNA segment from one chromosome to its homologs), and crossing-over (bidirectional exchange of DNA between to homologous chromosomes), also contribute to HLA diversity. Recombination allows for the intergenic or intragenic exchange of nucleotide sequences between two chromosomes. For example, the HLA-B*53:44 allele was generated by an intralocus gene conversion between exon 3 of HLA-B*38 or HLA-B*39 and exon 2, part of exon 3 and 4 of HLA-B*53:01:01 (123). The HLA-C*07:294 allele was created through the intergenic crossing over of nucleotide sequence between exon 3 HLA-C*07:27:02 and HLA-B alleles such as HLA-B*07:02:01(exon 3) (121).
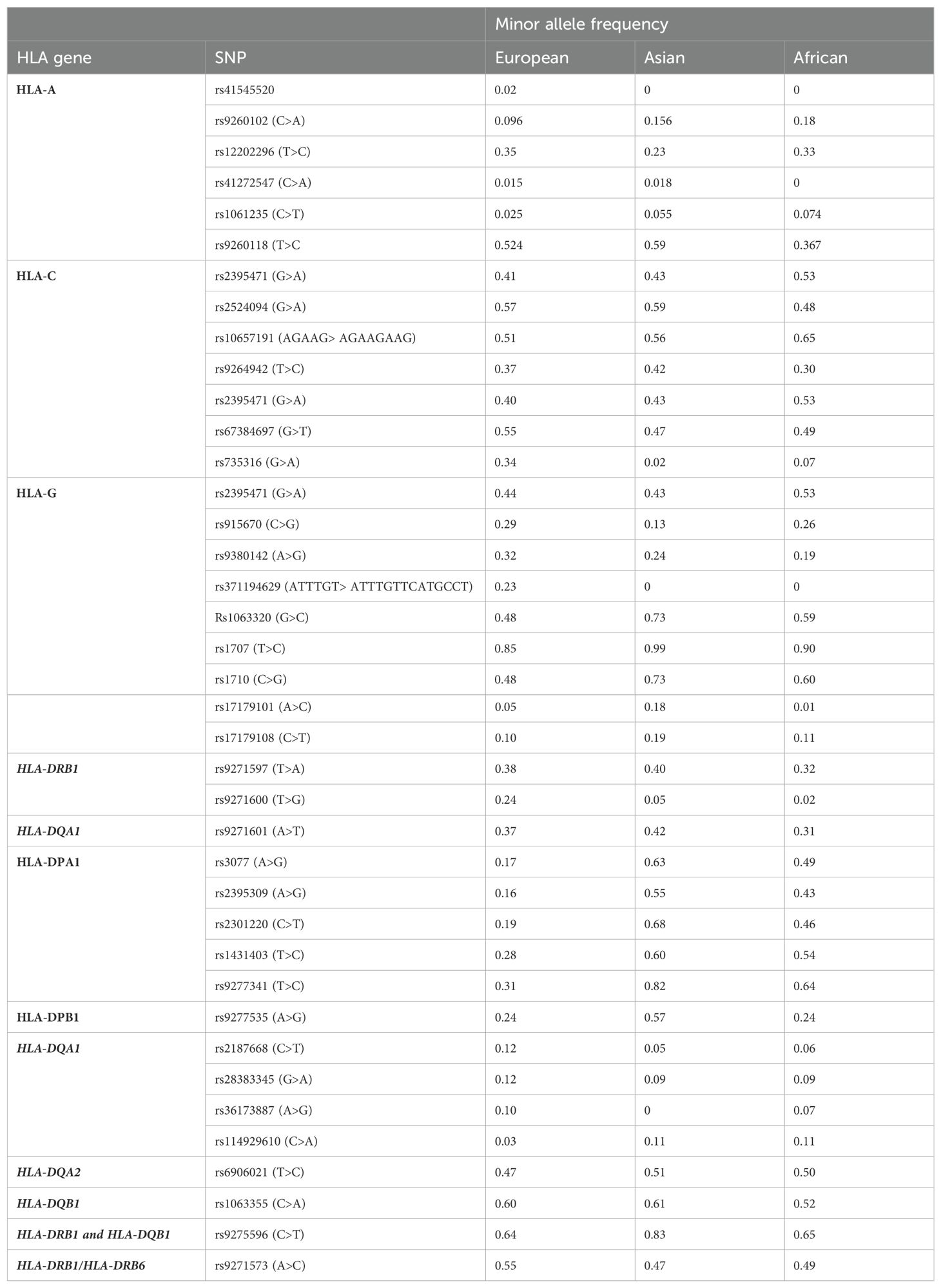
Table 4. Minor allele frequency of HLA non-coding variants in European, Asian and African populations.
HLA polymorphisms and alleles are observed at different frequencies in different populations. Certain HLA polymorphisms may be found in certain populations and may not even exist in other populations (117). For instance, within the HLA-A locus, the HLA-A*02 family is the most diverse allele family consisting of 31 known alleles. HLA-A*02 alleles are determined by a combination of specific SNPs found within the coding region of HLA-A. Unlike other HLA allele families, HLA-A*02 is frequent in all ethnic groups, however, the frequency of HLA-A*02 alleles is different in various populations (124). A USA-based study evaluated the frequencies of HLA-A*02 subtypes across different ethnic groups positive for HLA-A*02. HLA-A*02:011 was predominantly found in Caucasian (~95.7%) and Native American (94.3%) populations whereas HLA-A*02:011 was found in 59% of African Americans, and 50% of Chinese. Interestingly, certain HLA-A*02 alleles were not present in some ethnic groups. HLA-A*02:02 (25.8%) and HLA-A*02:05 (12.9%) were common in African Americans but no present in Caucasians, Pacific Islanders, and Chinese populations. HLA-A*02:03 and HLA-A*02:07 were found only in the Chinese population (124). Van Rensburg et al. (2021) showed that there were no common predominant HLA alleles between South African Black and Caucasian populations. HLA-A*30:01, HLA-B*58:02, HLA-C*06:02, and HLA-DRB1*13:01 were shown to be the predominant alleles in the black population, while HLA-A*02:01:01, HLA-B*07:02:01, HLA-C*07:01, and HLA-DRB1*03:01 was shown to be predominant in South African Caucasians. HLA-A*30:01:02, HLA-A*30:02:02, HLA-A*68:27, HLA-B*42:06, and HLA-B*45:07 were also shown to be unique to Black South Africans (125). Ethnic differences in the frequency of non-coding HLA SNPs can also occur. The National Center for Biotechnology Information created the single nucleotide polymorphism database (dbSNP) which contains allele frequency data for two thousand individuals across different populations. Using dbSNP, we identified the frequency of non-coding HLA SNPs (discussed in this review) within European, African, and Asian populations. For many of the SNPs, especially within class II genes, the frequency differed between ethnic groups. For instance, the minor allele of rs9277341 was found in 31% of Europeans, 82% of Asians and 64% of Africans. In some cases the SNP was absent in certain populations such as in the case of rs371194629 which is present in 23% of Europeans but absent in Asian and African populations.
The extensive differences in HLA allele diversity are associated with disease risk and progression in different ethnic groups (126, 127). For example, ethnic-specific differences regarding HLA were observed in Hepatitis C infection. Hepatitis C viral clearance correlated with HLA-DQB1*03, HLA-DRB1*11, and HLA-DRB3*02 in Caucasians, however, this was not observed in African individuals (126). Regarding ulcerative colitis, HLA-DRB1*15:03 is shown to be a risk allele for African American population, whereas HLA-DRB1*15:01, is a risk allele in Caucasian populations, where it is more frequent. Likewise, HLA-DRB1*09:01 is associated with ulcerative colitis in the Korean and Japanese populations (127). Ethnic-specific responses to disease are often associated with HLA coding regions as it induces changes in peptide binding; however, variation affecting HLA expression may also be associated with different responses to disease across different ethnicities.
Differences in HLA frequency across populations are also linked to adverse drug reactions experienced in certain populations (128). For instance, Abacavir is used in the treatment of HIV. Peptide fragments or metabolites of Abacavir complex with HLA-B*57, which activates T cells resulting in hypersensitivity to the drug (129). Abacavir hypersensitivity is more likely to affect Caucasians than Africans and Asians since the presence of HLA-B*57 is more frequent in Caucasians (129). Similarly, Carbamazepine is used in the treatment of neurological disorders such as epilepsy and bipolar. Carbamazepine hypersensitivity was shown to be more common in Asian populations compared to European populations (128). Asian populations with high frequency of HLA-B*15:02 such as Han Chinese, Malaysians, and Thai have been shown to associate with Carbamazepine hypersensitivity (128). HLA-B*15:11 and HLA-A*31:02 was shown to associate with Stevens–Johnson syndrome (SJS), and toxic epidermal necrolysis carbamazepine-induced hypersensitivity in Japanese and Koreans (128).
These marked differences in the distribution of HLA alleles across populations and ethnic groups make global treatment and vaccine strategies difficult to implement as certain groups may respond well, while others may not. This ethnic-specific bias requires ethnic-specific solutions to avoid disparities in global health care.
Guidelines for future HLA studies
Since its discovery, the HLA region has been one of the most well-studied gene regions in the human genome. However, there is still a lot to uncover about this region. In this section, we provide guidelines that should be considered in future studies involving the HLA region.
While variation within the coding region has been well studied as it affects peptide binding, the non-coding regions of HLA genes remain understudied (130, 131). The non-coding genome accounts for more genetic diversity than coding regions and SNPs within this region can affect the expression of specific HLA genes which may have disease consequences (18, 23–26). Yet from our literature search, only 23 studies evaluated the non-coding region of various HLA genes and their association with HLA expression and/or disease outcomes. To our surprise, no SNPs within the non-coding region of HLA-B were shown to associate with the regulation of HLA-B. This is surprising as HLA-B is the most polymorphic HLA gene, consisting of approximately 3000 different alleles (38). Furthermore, most studies discussed in this review had only investigated the effect non-coding variants had on HLA gene expression. Gene expression does not always correlate to protein levels, as approximately 40% of protein variance within mammalian cells correlates with mRNA abundance. Regarding, HLA expression, Aguair. V.R.C., et al. (2023) did find that HLA-C surface expression did correlate with mRNA expression (qPCR: r=0.59 and RNA-seq: r=0.67) (132). The discrepancy may be due to the multitude of regulatory events that occur between transcription and translation, such as alternative splicing or silencing by miRNAs (133). Furthermore, non-coding variants that occur within introns, 5’ and 3’ UTR may impact post-transcriptional processes such as splicing, polyadenylation, cleavage, ribosome binding, and assembly, thus impacting mRNA translation to protein. Therefore, it is important to evaluate whether these non-coding variants have an impact on HLA protein levels (134). We suggest that more research should be conducted on evaluating the variation within the non-coding genome and the functional consequences it may have on HLA gene and protein expression and disease outcomes.
Epigenetic studies provide valuable insight into the regulation of HLA genes across different diseases. Due to the limited number of studies evaluating epigenetic mechanisms regulating HLA, this review only discussed the epigenetic mechanisms DNA methylation and miRNAs. However, histone modifications and long non-coding RNAs (lncRNA) are also involved in HLA regulation. For example, the lncRNA, HOTAIR induces HLA-G expression by silencing the miR-152 in gastric cancer (135). Acetylation of lysine residues on H3 and H4 was also shown to induce HLA-G expression by promoting a permissive chromatin state (136). Unlike genetic factors, epigenetic factors are reversible and can be used to influence gene regulation without resulting in permanent changes (27). Epigenetic factors can increase the expression of low expressing HLA genes to improve pathogen clearance or decrease the expression of high expressing alleles to prevent hyperactive immune responses. Thus, understanding the epigenetic mechanisms involved in regulating HLA may have therapeutic advantages.
Although HLA diversity is the highest in African populations, only a limited number of African ethnic groups have been HLA-typed. African countries such as Angola, Lesotho, Malawi, Namibia, and Swaziland do not have any HLA data available (120). Where HLA data is available for African countries, this data is usually derived from disease association studies which is not a true reflection of the general population. Therefore, there is limited understanding of the HLA diversity observed in African populations and the associations it may have with diseases and vaccine development (120). Thus, to fully elucidate the extent of diversity within the HLA loci, more studies should evaluate the HLA diversity from the general population in the African region. Furthermore, resources similar to HLA-net which focuses on HLA diversity in Europeans and its application in population genetics, transplantation, and epidemiology may be used to improve donor selection, population studies, and disease association studies in Africa (120, 137, 138).
Lastly, to thoroughly evaluate HLA diversity, high resolution techniques such as next generation sequencing should be used. We previously discussed that HLA-A*02 is found in all ethnic groups but the frequency of HLA-A*02 subgroups differs amongst different ethnic groups. High resolution HLA typing will provide a more in-depth understanding of HLA diversity in specific ethnic groups (124, 139).
In summary, we suggest that future HLA-based studies should focus on: (I) the non-coding region of HLA, (II) epigenetic regulation of HLA, (III) understudied populations, and (IV) use high resolution typing. This will greatly improve our understanding of this complex loci.
Concluding remarks
With approximately 11,000 distinct protein variants, it is well known that the HLA loci are the most genetically diverse region. While genetic variants within the coding region have been long known as an important contributor to complex pathophysiological states, recent studies have identified functional variants within the non-coding regions of HLA. Furthermore, these genetic variants interact with epigenetic mechanisms further contributing to the complexity of HLA regulation. HLA association becomes even more complicated when we take into consideration the high level of diversity between different ethnic groups. Fully understanding the complexity of the HLA regions will result in improving the development of diagnostic approaches, therapeutic strategies, vaccine design, and transplantation procedures; improving overall global health. This review highlighted the importance of the non-coding genome concerning HLA regulation and different disease states. In this review, we: (i) discuss genetic non-coding variants that affect HLA expression, (ii) link genetic variation with epigenetic regulation of HLA genes, (iii) highlight the importance of the non-coding genome in HLA regulation and disease association, (iv) evaluate ethnic-specific differences across HLA genes and provide guidelines for future HLA studies.
Author contributions
TAr: Conceptualization, Funding acquisition, Writing – original draft, Writing – review & editing. TAd: Writing – original draft. AG: Writing – original draft. VR: Funding acquisition, Supervision, Writing – review & editing.
Funding
The author(s) declare financial support was received for the research, authorship, and/or publication of this article. VR was funded as a FLAIR Research Fellow (the Future Leader in African Independent Research (FLAIR) Fellowship Programme was a partnership between the African Academy of Sciences (AAS) and the Royal Society that was funded by the United Kingdom Government as part of the Global Challenge Research Fund (GCRF) (Grant No. FLAIR-FLR\R1\190204); supported by the South African Medical Research Council (SAMRC) with funds from the Department of Science and Technology (DST). Funding was also provided in part through the Sub-Saharan African Network for TB/HIV Research Excellence (SANTHE), a DELTAS Africa Initiative (Grant No. DEL-15-006) by the AAS. Support was also provided by the Grants, Innovation, and Product Development unit of the South African Medical Research Council with funds received from Novartis and GSK R&D (Grant No. GSKNVS2/202101/005). AG was funded by The Poliomyelitis Research Foundation (PRF) [Grant No. PRF22/77] and CHS Funding, College of Health Science, University of KwaZulu-Natal. TAr is funded by South African Medical Research Council Sir Grant and L’ORÉAL UNESCO Women in Science South African Young Talent fellow. The authors declare that this study received funding from Novartis and GSK R&D. TAd was funded by The Poliomyelitis Research Foundation (PRF). The funder was not involved in the study design, collection, analysis, interpretation of data, the writing of this article, or the decision to submit it for publication.
Acknowledgments
Biorender was used to generate the figures.
Conflict of interest
The authors declare that the research was conducted in the absence of any commercial or financial relationships that could be construed as a potential conflict of interest.
Publisher’s note
All claims expressed in this article are solely those of the authors and do not necessarily represent those of their affiliated organizations, or those of the publisher, the editors and the reviewers. Any product that may be evaluated in this article, or claim that may be made by its manufacturer, is not guaranteed or endorsed by the publisher.
References
1. Shiina T, Hosomichi K, Inoko H, Kulski JK. The HLA genomic loci map: expression, interaction, diversity and disease. J Hum Genet. (2009) 54:15–39. doi: 10.1038/jhg.2008.5
2. Matzaraki V, Kumar V, Wijmenga C, Zhernakova A. The MHC locus and genetic susceptibility to autoimmune and infectious diseases. Genome Biol. (2017) 18:76. doi: 10.1186/s13059-017-1207-1
3. Cruz-Tapias P, Castiblanco J, Anaya J-M. Major histocompatibility complex: antigen processing and presentation. In: Autoimmunity: From Bench to Bedside. Bogota, Colombia: El Rosario University Press (2013).
4. Xie T, Rowen L, Aguado B, Ahearn ME, Madan A, Qin S, et al. Analysis of the gene-dense major histocompatibility complex class III region and its comparison to mouse. Genome Res. (2003) 13:2621–36. doi: 10.1101/gr.1736803
5. Deakin JE, Papenfuss AT, Belov K, Cross JGR, Coggill P, Palmer S, et al. Evolution and comparative analysis of the MHC Class III inflammatory region. BMC Genomics. (2006) 7:281. doi: 10.1186/1471-2164-7-281
6. Lim J, Bae S-C, Kim K. Understanding HLA associations from SNP summary association statistics. Sci Rep. (2019) 9:1337–7. doi: 10.1038/s41598-018-37840-9
7. Hurley CK. Naming HLA diversity: A review of HLA nomenclature. Hum Immunol. (2021) 82:457–65. doi: 10.1016/j.humimm.2020.03.005
8. Walford RL, Finkelstein S, Neerhout R, Konrad P, Shanbrom E. Acute childhood leukaemia in relation to the HL–A human transplantation genes. Nature. (1970) 225:461–2. doi: 10.1038/225461a0
9. Amiel J, Curtoni E, Mattiuz P, Tosi M. Histocompatibility testing 1967. Munksgaard Copenhagen. (1967), 79–81.
10. Braun WE. HLA-disease Association in the Perspective of ABO Disease Associations, HLA and disease: a comprehensive review. 1st Edition. (2019) P27–28. doi: 10.1201/9781351073226
11. Shukla SA, Rooney MS, Rajasagi M, Tiao G, Dixon PM, Lawrence MS, et al. Comprehensive analysis of cancer-associated somatic mutations in class I HLA genes. Nat Biotechnol. (2015) 33:1152–8. doi: 10.1038/nbt.3344
12. Seldin MF. The genetics of human autoimmune disease: a perspective on progress in the field and future directions. J Autoimmun. (2015) 64:1–12. doi: 10.1016/j.jaut.2015.08.015
13. Simmonds M, Gough S. The HLA region and autoimmune disease: associations and mechanisms of action. Curr Genomics. (2007) 8:453–65. doi: 10.2174/138920207783591690
14. Brewerton DA, Hart FD, Nicholls A, Caffrey M, James DCO, Sturrock RD. Ankylosing spondylitis and HL-A 27. Lancet. (1973) 1:904–7. doi: 10.1016/S0140-6736(73)91360-3
15. Schlosstein L, Terasaki PI, Bluestone R, Pearson CM. High association of an HL-A antigen, W27, with ankylosing spondylitis. N Engl J Med. (1973) 288:704–6. doi: 10.1056/NEJM197304052881403
16. Hetherington S, Hughes AR, Mosteller M, Shortino D, Baker KL, Spreen W, et al. Genetic variations in HLA-B region and hypersensitivity reactions to abacavir. Lancet. (2002) 359:1121–2. doi: 10.1016/S0140-6736(02)08158-8
17. Mallal S, Nolan D, Witt C, Masel G, Martin A, Moore C, et al. Association between presence of HLA-B* 5701, HLA-DR7, and HLA-DQ3 and hypersensitivity to HIV-1 reverse-transcriptase inhibitor abacavir. Lancet. (2002) 359:727–32. doi: 10.1016/S0140-6736(02)07873-X
18. Zhao Z, Fu YX, Hewett-Emmett D, Boerwinkle E. Investigating single nucleotide polymorphism (SNP) density in the human genome and its implications for molecular evolution. Gene. (2003) 312:207–13. doi: 10.1016/S0378-1119(03)00670-X
19. Leppek K, Das R, Barna M. Functional 5′ UTR mRNA structures in eukaryotic translation regulation and how to find them. Nat Rev Mol Cell Biol. (2018) 19:158–74. doi: 10.1038/nrm.2017.103
20. Mayr C. Regulation by 3′-untranslated regions. Annu Rev Genet. (2017) 51:171–94. doi: 10.1146/annurev-genet-120116-024704
21. Consortium EP. An integrated encyclopedia of DNA elements in the human genome. Nature. (2012) 489:57. doi: 10.1038/nature11247
22. DhatChinamoorthy K, Colbert JD, Rock KL. Cancer immune evasion through loss of MHC class I antigen presentation. Front Immunol. (2021) 12. doi: 10.3389/fimmu.2021.636568
23. Kulkarni S, Qi Y, O'HUigin C, Pereyra F, Ramsuran V, McLaren P, et al. Genetic interplay between HLA-C and MIR148A in HIV control and Crohn disease. Proc Natl Acad Sci USA. (2013) 110:20705–10. doi: 10.1073/pnas.1312237110
24. Kulkarni S, Savan R, Qi Y, Gao X, Yuki Y, Bass SE, et al. Differential microRNA regulation of HLA-C expression and its association with HIV control. Nature. (2011) 472:495–8. doi: 10.1038/nature09914
25. Thomas R, Apps R, Qi Y, Gao X, Male V, O'HUigin C, et al. HLA-C cell surface expression and control of HIV/AIDS correlate with a variant upstream of HLA-C. Nat Genet. (2009) 41:1290–4. doi: 10.1038/ng.486
26. Thomas R, Thio CL, Apps R, Qi Y, Gao X, Marti D, et al. A novel variant marking HLA-DP expression levels predicts recovery from hepatitis B virus infection. J Virol. (2012) 86:6979–85. doi: 10.1128/JVI.00406-12
27. Gibney ER, Nolan CM. Epigenetics and gene expression. Heredity. (2010) 105:4–13. doi: 10.1038/hdy.2010.54
28. Ramsuran V, Kulkarni S, O'Huigin C, Yuki Y, Augusto DG, Gao X, et al. Epigenetic regulation of differential HLA-A allelic expression levels. Hum Mol Genet. (2015) 24:4268–75. doi: 10.1093/hmg/ddv158
29. de Bakker PIW, Raychaudhuri S. Interrogating the major histocompatibility complex with high-throughput genomics. Hum Mol Genet. (2012) 21:R29–36. doi: 10.1093/hmg/dds384
30. Vince N, Li H, Ramsuran V, Naranbhai V, Duh F-M, Fairfax BP, et al. HLA-C level is regulated by a polymorphic Oct1 binding site in the HLA-C promoter region. Am J Hum Genet. (2016) 99:1353–8. doi: 10.1016/j.ajhg.2016.09.023
31. Chin YM, Mushiroda T, Takahashi A, Kubo M, Krishnan G, Yap LF, et al. HLA-A SNPs and amino acid variants are associated with nasopharyngeal carcinoma in Malaysian Chinese. Int J Cancer. (2015) 136:678–87. doi: 10.1002/ijc.29035
32. Montminy MR, Bilezikjian LM. Binding of a nuclear protein to the cyclic-AMP response element of the somatostatin gene. Nature. (1987) 328:175–8. doi: 10.1038/328175a0
33. Deutsch PJ, Hoeffler J, Jameson JL, Lin J, Habener JF. Structural determinants for transcriptional activation by cAMP-responsive DNA elements. J Biol Chem. (1988) 263:18466–72. doi: 10.1016/S0021-9258(19)81381-9
34. Bei J-X, Li Y, Jia W-H, Feng B-J, Zhou G, Chen L-Z, et al. A genome-wide association study of nasopharyngeal carcinoma identifies three new susceptibility loci. Nat Genet. (2010) 42:599–603. doi: 10.1038/ng.601
35. Su WH, Hildesheim A, Chang YS. Human leukocyte antigens and epstein-barr virus-associated nasopharyngeal carcinoma: old associations offer new clues into the role of immunity in infection-associated cancers. Front Oncol. (2013) 3:299. doi: 10.3389/fonc.2013.00299
36. René C, Lozano C, Villalba M, Eliaou J-F. 5′ and 3′ untranslated regions contribute to the differential expression of specific HLA-A alleles. Eur J Immunol. (2015) 45:3454–63. doi: 10.1002/eji.201545927
37. Ramsuran V, Hernández-Sanchez PG, O'HUigin C, Sharma G, Spence N, Augusto DG, et al. Sequence and phylogenetic analysis of the untranslated promoter regions for HLA class I genes. J Immunol. (2017) 198:2320–9. doi: 10.4049/jimmunol.1601679
38. Robinson J, Halliwell JA, Hayhurst JD, Flicek P, Parham P, Marsh SG. The IPD and IMGT/HLA database: allele variant databases. Nucleic Acids Res. (2015) 43:D423–31. doi: 10.1093/nar/gku1161
39. Apps R, Qi Y, Carlson JM, Chen H, Gao X, Thomas R, et al. Influence of HLA-C expression level on HIV control. Science. (2013) 340:87–91. doi: 10.1126/science.1232685
40. Hundhausen C, Bertoni A, Mak RK, Botti E, Di Meglio P, Clop A, et al. Allele-specific cytokine responses at the HLA-C locus: implications for psoriasis. J Invest Dermatol. (2012) 132:635–41. doi: 10.1038/jid.2011.378
41. Donadi EA, Castelli EC, Arnaiz-Villena A, Roger M, Rey D, Moreau P. Implications of the polymorphism of HLA-G on its function, regulation, evolution and disease association. Cell Mol Life Sci CMLS. (2011) 68:369–95. doi: 10.1007/s00018-010-0580-7
42. de Almeida BS, Muniz YCN, Prompt AH, Castelli EC, Mendes-Junior CT, Donadi EA. Genetic association between HLA-G 14-bp polymorphism and diseases: A systematic review and meta-analysis. Hum Immunol. (2018) 79:724–35. doi: 10.1016/j.humimm.2018.08.003
43. Yie S-m, Li L-h, Xiao R, Librach CL. A single base-pair mutation in the 3′-untranslated region of HLA-G mRNA is associated with pre-eclampsia. Mol Hum Reprod. (2008) 14:649–53. doi: 10.1093/molehr/gan059
44. Twito T, Joseph J, Mociornita A, Rao V, Ross H, Delgado DH. The 14-bp deletion in the HLA-G gene indicates a low risk for acute cellular rejection in heart transplant recipients. J Heart Lung Transplant. (2011) 30:778–82. doi: 10.1016/j.healun.2011.01.726
45. Svendsen SG, Hantash BM, Zhao L, Faber C, Bzorek M, Nissen MH, et al. The expression and functional activity of membrane-bound human leukocyte antigen-G1 are influenced by the 3′-untranslated region. Hum Immunol. (2013) 74:818–27. doi: 10.1016/j.humimm.2013.03.003
46. Zidi I, Ben Yahia H, Bortolotti D, Mouelhi L, Laaribi AB, Ayadi S, et al. Association between sHLA-G and HLA-G 14-bp deletion/insertion polymorphism in Crohn’s disease. Int Immunol. (2015) 27:289–96. doi: 10.1093/intimm/dxv002
47. Rousseau P, Le Discorde M, Mouillot G, Marcou C, Carosella ED, Moreau P. The 14 bp Deletion-Insertion polymorphism in the 3′ UT region of the HLA-G gene influences HLA-G mRNA stability. Hum Immunol. (2003) 64:1005–10. doi: 10.1016/j.humimm.2003.08.347
48. Cavalli G, Hayashi M, Jin Y, Yorgov D, Santorico SA, Holcomb C, et al. MHC class II super-enhancer increases surface expression of HLA-DR and HLA-DQ and affects cytokine production in autoimmune vitiligo. Proc Natl Acad Sci. (2016) 113:1363–8. doi: 10.1073/pnas.1523482113
49. Ou G, Liu X, Yang L, Yu H, Ji X, Liu F, et al. Relationship between HLA-DPA1 mRNA expression and susceptibility to hepatitis B. J Viral Hepat. (2019) 26:155–61. doi: 10.1111/jvh.13012
50. O'Brien TR, Kohaar I, Pfeiffer RM, Maeder D, Yeager M, Schadt EE, et al. Risk alleles for chronic hepatitis B are associated with decreased mRNA expression of HLA-DPA1 and HLA-DPB1 in normal human liver. Genes Immun. (2011) 12:428–33. doi: 10.1038/gene.2011.11
51. Wasityastuti W, Yano Y, Ratnasari N, Triyono T, Triwikatmani C, Indrarti F, et al. Protective effects of HLA-DPA1/DPB1 variants against Hepatitis B virus infection in an Indonesian population. Infect Genet Evol. (2016) 41:177–84. doi: 10.1016/j.meegid.2016.03.034
52. Wang Z, Lu X, Yao X, Liu X, Zhao L, Chang S, et al. Relationship between HLA-DPA1 genetic polymorphism and anembryonic pregnancy. Mol Genet genomic Med. (2020) 8:e1046–6. doi: 10.1002/mgg3.1046
53. Morgan LZ, Rollins B, Sequeira A, Byerley W, DeLisi LE, Schatzberg AF, et al. Quantitative trait locus and brain expression of HLA-DPA1 offers evidence of shared immune alterations in psychiatric disorders. Microarrays (Basel Switzerland). (2016) 5:6. doi: 10.3390/microarrays5010006
54. Yang Z, Liu W, Yan T, Liu R. HLA-DPB1 rs9277535 polymorphism is associated with rheumatoid arthritis risk in a Chinese Han population. Aging (Albany NY). (2021) 13:11696–704. doi: 10.18632/aging.v13i8
55. Qin X-S, Liu J-H, Lyu G-T, Peng M-L, Yang F-N, Qin D-C, et al. Variants in the promoter region of HLA-DQA1 were associated with idiopathic membranous nephropathy in a Chinese Han population. Chin Med J. (2017) 130:1677–82. doi: 10.4103/0366-6999.209884
56. Abou El Hassan M, Huang K, Eswara MBK, Xu Z, Yu T, Aubry A, et al. Properties of STAT1 and IRF1 enhancers and the influence of SNPs. BMC Mol Biol. (2017) 18:6. doi: 10.1186/s12867-017-0084-1
57. Kawai Y, Hitomi Y, Ueta M, Khor S-S, Nakatani K, Sotozono C, et al. Mapping of susceptible variants for cold medicine-related Stevens–Johnson syndrome by whole-genome resequencing. NPJ Genomic Med. (2021) 6:9. doi: 10.1038/s41525-021-00171-2
58. Suzuki H, Joshita S, Hirayama A, Shinji A, Mukawa K, Sako M, et al. Polymorphism at rs9264942 is associated with HLA-C expression and inflammatory bowel disease in the Japanese. Sci Rep. (2020) 10:12424–4. doi: 10.1038/s41598-020-69370-8
59. Agrawal D, Prakash S, Misra MK, Phadke SR, Agrawal S. Implication of HLA-G 5′ upstream regulatory region polymorphisms in idiopathic recurrent spontaneous abortions. Reprod Biomed Online. (2015) 30:82–91. doi: 10.1016/j.rbmo.2014.09.015
60. Rizzo R, Bortolotti D, Fredj NB, Rotola A, Cura F, Castellazzi M, et al. Role of HLA-G 14bp deletion/insertion and +3142C>G polymorphisms in the production of sHLA-G molecules in relapsing-remitting multiple sclerosis. Hum Immunol. (2012) 73:1140–6. doi: 10.1016/j.humimm.2012.08.005
61. Vohra M, Sharma AR, Prabhu NB, Rai PS. SNPs in sites for DNA methylation, transcription factor binding, and miRNA targets leading to allele-specific gene expression and contributing to complex disease risk: A systematic review. Public Health Genomics. (2020) 23:155–70. doi: 10.1159/000510253
62. Moore LD, Le T, Fan G. DNA methylation and its basic function. Neuropsychopharmacology. (2013) 38:23–38. doi: 10.1038/npp.2012.112
63. Ye Q, Shen Y, Wang X, Yang J, Miao F, Shen C, et al. Hypermethylation of HLA class I gene is associated with HLA class I down-regulation in human gastric cancer. Tissue Antigens. (2010) 75:30–9. doi: 10.1111/j.1399-0039.2009.01390.x
64. Majumder P, Boss JM. DNA methylation dysregulates and silences the HLA-DQ locus by altering chromatin architecture. Genes Immun. (2011) 12:291–9. doi: 10.1038/gene.2010.77
65. Morris AC, Spangler WE, Boss JM. Methylation of class II trans-activator promoter IV: A novel mechanism of MHC class II gene control. J Immunol. (2000) 164:4143–9. doi: 10.4049/jimmunol.164.8.4143
66. Radosevich M, Jager M, Ono SJ. Inhibition of MHC class II gene expression in uveal melanoma cells is due to methylation of the CIITA gene or an upstream activator. Exp Mol Pathol. (2007) 82:68–76. doi: 10.1016/j.yexmp.2006.03.005
67. Smith AK, Kilaru V, Kocak M, Almli LM, Mercer KB, Ressler KJ, et al. Methylation quantitative trait loci (meQTLs) are consistently detected across ancestry, developmental stage, and tissue type. BMC Genomics. (2014) 15:145. doi: 10.1186/1471-2164-15-145
68. Zhi D, Aslibekyan S, Irvin MR, Claas SA, Borecki IB, Ordovas JM, et al. SNPs located at CpG sites modulate genome-epigenome interaction. Epigenetics. (2013) 8:802–6. doi: 10.4161/epi.25501
69. Zhou D, Li Z, Yu D, Wan L, Zhu Y, Lai M, et al. Polymorphisms involving gain or loss of CpG sites are significantly enriched in trait-associated SNPs. Oncotarget. (2015) 6:39995–40004. doi: 10.18632/oncotarget.v6i37
70. Zhao W, Lei L, Chen R, Zhang Y, Chang L, Cheng J. The association between deoxyribonucleic acid hypermethylation in intron VII and human leukocyte antigen-C∗07 expression in patients with endometriosis. Int J Clin Pract. (2023) 2023:2291156. doi: 10.1155/2023/2291156
71. Ober C, Aldrich CL, Chervoneva I, Billstrand C, Rahimov F, Gray HL, et al. Variation in the HLA-G promoter region influences miscarriage rates. Am J Hum Genet. (2003) 72:1425–35. doi: 10.1086/375501
72. Marik B, Nomani K, Agarwal N, Dadhwal V, Sharma A. Role of the HLA-G regulatory region polymorphisms in idiopathic recurrent spontaneous abortions (RSA). Am J Reprod Immunol. (2023) 90:e13740. doi: 10.1111/aji.13740
73. Kindt ASD, Fuerst RW, Knoop J, Laimighofer M, Telieps T, Hippich M, et al. Allele-specific methylation of type 1 diabetes susceptibility genes. J Autoimmun. (2018) 89:63–74. doi: 10.1016/j.jaut.2017.11.008
74. Kim S, Forno E, Yan Q, Jiang Y, Zhang R, Boutaoui N, et al. SNPs identified by GWAS affect asthma risk through DNA methylation and expression of cis-genes in airway epithelium. Eur Respir J. (2020) 55:1902079. doi: 10.1183/13993003.02079-2019
75. Olsson AH, Volkov P, Bacos K, Dayeh T, Hall E, Nilsson EA, et al. Genome-wide associations between genetic and epigenetic variation influence mRNA expression and insulin secretion in human pancreatic islets. PloS Genet. (2014) 10:e1004735. doi: 10.1371/journal.pgen.1004735
76. Hong X, Hao K, Ladd-Acosta C, Hansen KD, Tsai H-J, Liu X, et al. Genome-wide association study identifies peanut allergy-specific loci and evidence of epigenetic mediation in US children. Nat Commun. (2015) 6:6304–4. doi: 10.1038/ncomms7304
77. Li M, Lyu C, Huang M, Do C, Tycko B, Lupo PJ, et al. Mapping methylation quantitative trait loci in cardiac tissues nominates risk loci and biological pathways in congenital heart disease. BMC Genom Data. (2021) 22:20. doi: 10.1186/s12863-021-00975-2
78. Coit P, Kaushik P, Caplan L, Kerr GS, Walsh JA, Dubreuil M, et al. Genome-wide DNA methylation analysis in ankylosing spondylitis identifies HLA-B*27 dependent and independent DNA methylation changes in whole blood. J Autoimmun. (2019) 102:126–32. doi: 10.1016/j.jaut.2019.04.022
79. Shoemaker R, Deng J, Wang W, Zhang K. Allele-specific methylation is prevalent and is contributed by CpG-SNPs in the human genome. Genome Res. (2010) 20:883–9. doi: 10.1101/gr.104695.109
80. Verloes A, Spits C, Vercammen M, Geens M, LeMaoult J, Sermon K, et al. The role of methylation, DNA polymorphisms and microRNAs on HLA-G expression in human embryonic stem cells. Stem Cell Res. (2017) 19:118–27. doi: 10.1016/j.scr.2017.01.005
81. Xu X, Zhou Y, Wei H. Roles of HLA-G in the maternal-fetal immune microenvironment. Front Immunol. (2020) 11. doi: 10.3389/fimmu.2020.592010
82. Cecati M, Giannubilo SR, Emanuelli M, Tranquilli AL, Saccucci F. HLA-G and pregnancy adverse outcomes. Med Hypotheses. (2011) 76:782–4. doi: 10.1016/j.mehy.2011.02.017
83. Ober C, Hyslop T, Hauck WW. Inbreeding effects on fertility in humans: evidence for reproductive compensation. Am J Hum Genet. (1999) 64:225–31. doi: 10.1086/302198
84. Conrad R, Barrier M, Ford LP. Role of miRNA and miRNA processing factors in development and disease. Birth Defects Res Part C: Embryo Today: Rev. (2006) 78:107–17. doi: 10.1002/(ISSN)1542-9768
85. Tüfekci KU, Öner MG, Meuwissen RLJ, Genç Ş. The role of microRNAs in human diseases. In: miRNomics: MicroRNA biology and computational analysis. New York, USA: Springer (2014). p. 33–50.
86. Mendell JT, Olson EN. MicroRNAs in stress signaling and human disease. Cell. (2012) 148:1172–87. doi: 10.1016/j.cell.2012.02.005
87. Cammaerts S, Strazisar M, De Rijk P, Del Favero J. Genetic variants in microRNA genes: impact on microRNA expression, function, and disease. Front Genet. (2015) 6. doi: 10.3389/fgene.2015.00186
88. Landi D, Gemignani F, Landi S. Role of variations within microRNA-binding sites in cancer. Mutagenesis. (2012) 27:205–10. doi: 10.1093/mutage/ger055
89. Naidoo D, Wu AC, Brilliant MH, Denny J, Ingram C, Kitchner TE, et al. A polymorphism in HLA-G modifies statin benefit in asthma. pharmacogenomics J. (2015) 15:272–7. doi: 10.1038/tpj.2014.55
90. Ménard C, Rezende FA, Miloudi K, Wilson A, Tétreault N, Hardy P, et al. MicroRNA signatures in vitreous humour and plasma of patients with exudative AMD. Oncotarget. (2016) 7:19171–84. doi: 10.18632/oncotarget.v7i15
91. Tan Z, Randall G, Fan J, Camoretti-Mercado B, Brockman-Schneider R, Pan L, et al. Allele-specific targeting of microRNAs to HLA-G and risk of asthma. Am J Hum Genet. (2007) 81:829–34. doi: 10.1086/521200
92. Castelli EC, Moreau P, Chiromatzo AOe, Mendes-Junior CT, Veiga-Castelli LC, Yaghi L, et al. In silico analysis of microRNAS targeting the HLA-G 3′ untranslated region alleles and haplotypes. Hum Immunol. (2009) 70:1020–5. doi: 10.1016/j.humimm.2009.07.028
93. Friedrich M, Pracht K, Mashreghi M-F, Jäck H-M, Radbruch A, Seliger B. The role of the miR-148/-152 family in physiology and disease. Eur J Immunol. (2017) 47:2026–38. doi: 10.1002/eji.201747132
94. Fellay J, Shianna KV, Ge D, Colombo S, Ledergerber B, Weale M, et al. A whole-genome association study of major determinants for host control of HIV-1. science. (2007) 317:944–7. doi: 10.1126/science.1143767
95. Pereyra F, Jia X, McLaren PJ, Telenti A, de Bakker PI, Walker BD, et al. The major genetic determinants of HIV-1 control affect HLA class I peptide presentation. Science. (2010) 330:1551–7. doi: 10.1126/science.1195271
96. Stranger BE, Forrest MS, Clark AG, Minichiello MJ, Deutsch S, Lyle R, et al. Genome-wide associations of gene expression variation in humans. PloS Genet. (2005) 1:e78. doi: 10.1371/journal.pgen.0010078
97. Manaster I, Goldman-Wohl D, Greenfield C, Nachmani D, Tsukerman P, Hamani Y, et al. MiRNA-mediated control of HLA-G expression and function. PloS One. (2012) 7:e33395–5. doi: 10.1371/journal.pone.0033395
98. Castelli EC, Mendes-Junior CT, Deghaide NHS, de Albuquerque RS, Muniz YCN, Simões RT, et al. The genetic structure of 3′untranslated region of the HLA-G gene: polymorphisms and haplotypes. Genes Immun. (2010) 11:134–41. doi: 10.1038/gene.2009.74
99. Petersdorf EW, Malkki M, O'HUigin C, Carrington M, Gooley T, Haagenson MD, et al. High HLA-DP expression and graft-versus-host disease. N Engl J Med. (2015) 373:599–609. doi: 10.1056/NEJMoa1500140
100. Yamazaki T, Umemura T, Joshita S, Yoshizawa K, Tanaka E, Ota M. A cis-eQTL of HLA-DPB1 affects susceptibility to type 1 autoimmune hepatitis. Sci Rep. (2018) 8:11924–4. doi: 10.1038/s41598-018-30406-9
101. Shieh M, Chitnis N, Clark P, Johnson FB, Kamoun M, Monos D. Computational assessment of miRNA binding to low and high expression HLA-DPB1 allelic sequences. Hum Immunol. (2019) 80:53–61. doi: 10.1016/j.humimm.2018.09.002
102. Jima DD, Zhang J, Jacobs C, Richards KL, Dunphy CH, Choi WWL, et al. Deep sequencing of the small RNA transcriptome of normal and Malignant human B cells identifies hundreds of novel microRNAs. Blood. (2010) 116:e118–27. doi: 10.1182/blood-2010-05-285403
103. Riethoven JJ. Regulatory regions in DNA: promoters, enhancers, silencers, and insulators. Methods Mol Biol. (2010) 674:33–42. doi: 10.1007/978-1-60761-854-6_3
104. Barrett LW, Fletcher S, Wilton SD. Regulation of eukaryotic gene expression by the untranslated gene regions and other non-coding elements. Cell Mol Life Sci. (2012) 69:3613–34. doi: 10.1007/s00018-012-0990-9
105. Martínez-Jiménez F, Priestley P, Shale C, Baber J, Rozemuller E, Cuppen E. Genetic immune escape landscape in primary and metastatic cancer. Nat Genet. (2023) 55:820–31. doi: 10.1038/s41588-023-01367-1
106. Samur M, Szalat R, Aktas Samur A, Fulciniti M, Lopez M, Shammas M, et al. S119 THE ROLE OF RECURRENT SOMATIC ALTERATIONS IN THE NON-CODING GENOME WITH FUNCTIONAL IMPLICATIONS IN MM. HemaSphere. (2019) 3:11–2. doi: 10.1097/01.HS9.0000558696.90654.03
107. Pudjihartono M, Perry JK, Print C, O'Sullivan JM, Schierding W. Interpretation of the role of germline and somatic non-coding mutations in cancer: expression and chromatin conformation informed analysis. Clin Epigenet. (2022) 14:120. doi: 10.1186/s13148-022-01342-3
108. Johansson T, Partanen J, Saavalainen P. HLA allele-specific expression: Methods, disease associations, and relevance in hematopoietic stem cell transplantation. Front Immunol. (2022) 13. doi: 10.3389/fimmu.2022.1007425
109. Rodríguez JA. HLA−mediated tumor escape mechanisms that may impair immunotherapy clinical outcomes via T−cell activation (Review). Oncol Lett. (2017) 14:4415–27. doi: 10.3892/ol.2017.6784
110. del Campo AB, Carretero J, Aptsiauri N, Garrido F. Targeting HLA class I expression to increase tumor immunogenicity. Tissue Antigens. (2012) 79:147–54. doi: 10.1111/j.1399-0039.2011.01831.x
111. U.S. National Library of Medicine. (n.d.). Search of: microRNAs - List Results. ClinicalTrials.gov. Available online at: https://clinicaltrials.gov/ (accessed 2/06/2024).
112. Samur M, Szalat R, Aktas Samur A, Fulciniti M, Lopez M, Shammas M, et al. National marrow donor program HLA matching guidelines for unrelated adult donor hematopoietic cell transplants. Biol Blood Marrow Transplant. (2008) 14:45–53. doi: 10.1016/j.bbmt.2008.06.014
113. Lee SJ, Klein J, Haagenson M, Baxter-Lowe LA, Confer DL, Eapen M, et al. High-resolution donor-recipient HLA matching contributes to the success of unrelated donor marrow transplantation. Blood J Am Soc Hematol. (2007) 110:4576–83. doi: 10.1182/blood-2007-06-097386
114. Amiri F, Ranjbar M, Pirouzfar M, Nourigorji M, Dianatpour M. HLA-A gene knockout using CRISPR/Cas9 system toward overcoming transplantation concerns. Egyptian J Med Hum Genet. (2021) 22:37. doi: 10.1186/s43042-021-00155-y
115. Torikai H, Mi T, Gragert L, Maiers M, Najjar A, Ang S, et al. Genetic editing of HLA expression in hematopoietic stem cells to broaden their human application. Sci Rep. (2016) 6:21757. doi: 10.1038/srep21757
116. Prugnolle F, Manica A, Charpentier M, Guégan JF, Guernier V, Balloux F. Pathogen-driven selection and worldwide HLA class I diversity. Curr Biol. (2005) 15:1022–7. doi: 10.1016/j.cub.2005.04.050
117. Torikai H, Mi T, Gragert L, Maiers M, Najjar A, Ang S, et al. Tracking human migrations by the analysis of the distribution of HLA alleles, lineages and haplotypes in closed and open populations. Philos Trans R Soc Lond B Biol Sci. (2012) 367:820–9. doi: 10.1098/rstb.2011.0320
118. Miller LH. Impact of malaria on genetic polymorphism and genetic diseases in Africans and African Americans. Proc Natl Acad Sci. (1994) 91:2415–9. doi: 10.1073/pnas.91.7.2415
119. Cooke GS, Hill AV. Genetics of susceptibitlity to human infectious disease. Nat Rev Genet. (2001) 2:967–77. doi: 10.1038/35103577
120. Tshabalala M, Mellet J, Pepper MS. Human leukocyte antigen diversity: A southern african perspective. J Immunol Res. (2015) 2015:746151. doi: 10.1155/2015/746151
121. Adamek M, Klages C, Bauer M, Kudlek E, Drechsler A, Leuser B, et al. Seven novel HLA alleles reflect different mechanisms involved in the evolution of HLA diversity: description of the new alleles and review of the literature. Hum Immunol. (2015) 76:30–5. doi: 10.1016/j.humimm.2014.12.007
122. Paul P, Adrian Cabestre F, Ibrahim EC, Lefebvre S, Khalil-Daher I, Vazeux G, et al. Identification of HLA-G7 as a new splice variant of the HLA-G mRNA and expression of soluble HLA-G5, -G6, and -G7 transcripts in human transfected cells. Hum Immunol. (2000) 61:1138–49. doi: 10.1016/S0198-8859(00)00197-X
123. Fabreti-Oliveira RA, Lasmar MF, Oliveira CKF, Vale EMG, Nascimento E. Genetic mechanisms involved in the generation of HLA alleles in Brazilians: description and comparison of HLA alleles. Transplant Proc. (2018) 50:835–40. doi: 10.1016/j.transproceed.2018.02.011
124. Ellis JM, Henson V, Slack R, Ng J, Hartzman RJ, Katovich Hurley C. Frequencies of HLA-A2 alleles in five U.S. population groups: Predominance of A∗02011 and identification of HLA-A∗0231. Hum Immunol. (2000) 61:334–40. doi: 10.1016/S0198-8859(99)00155-X
125. van Rensburg WJJ, de Kock A, Bester C, Kloppers JF. HLA major allele group frequencies in a diverse population of the Free State Province, South Africa. Heliyon. (2021) 7(4):e06850. doi: 10.1016/j.heliyon.2021.e06850
126. Harris RA, Sugimoto K, Kaplan DE, Ikeda F, Kamoun M, Chang KM. Human leukocyte antigen class II associations with hepatitis C virus clearance and virus-specific CD4 T cell response among Caucasians and African Americans. Hepatology. (2008) 48:70–9. doi: 10.1002/hep.v48:1
127. Degenhardt F, Mayr G, Wendorff M, Boucher G, Ellinghaus E, Ellinghaus D, et al. Transethnic analysis of the human leukocyte antigen region for ulcerative colitis reveals not only shared but also ethnicity-specific disease associations. Hum Mol Genet. (2021) 30:356–69. doi: 10.1093/hmg/ddab017
128. Fan WL, Shiao MS, Hui RC, Su SC, Wang CW, Chang YC, et al. HLA association with drug-induced adverse reactions. J Immunol Res. (2017) 2017:3186328. doi: 10.1155/2017/3186328
129. Saag M, Balu R, Phillips E, Brachman P, Martorell C, Burman W, et al. High sensitivity of human leukocyte antigen-b* 5701 as a marker for immunologically confirmed abacavir hypersensitivity in white and black patients. Clin Infect Dis. (2008) 46:1111–8. doi: 10.1086/529382
130. Paul S, Weiskopf D, Angelo MA, Sidney J, Peters B, Sette A. HLA class I alleles are associated with peptide-binding repertoires of different size, affinity, and immunogenicity. J Immunol. (2013) 191:5831–9. doi: 10.4049/jimmunol.1302101
131. Ettinger RA, Papadopoulos GK, Moustakas AK, Nepom GT, Kwok WW. Allelic variation in key peptide-binding pockets discriminates between closely related diabeStes-protective and diabetes-susceptible HLA-DQB1* 06 alleles. J Immunol. (2006) 176:1988–98. doi: 10.4049/jimmunol.176.3.1988
132. Aguiar VRC, Castelli EC, Single RM, Bashirova A, Ramsuran V, Kulkarni S, et al. Comparison between qPCR and RNA-seq reveals challenges of quantifying HLA expression. Immunogenetics. (2023) 75:249–62. doi: 10.1007/s00251-023-01296-7
133. Vogel C, Marcotte EM. Insights into the regulation of protein abundance from proteomic and transcriptomic analyses. Nat Rev Genet. (2012) 13:227–32. doi: 10.1038/nrg3185
134. Peña-Martínez EG, Rodríguez-Martínez JA. Decoding non-coding variants: recent approaches to studying their role in gene regulation and human diseases. Front Biosci (Schol Ed). (2024) 16:4. doi: 10.31083/j.fbs1601004
135. Song B, Guan Z, Liu F, Sun D, Wang K, Qu H. Long non-coding RNA HOTAIR promotes HLA-G expression via inhibiting miR-152 in gastric cancer cells. Biochem Biophys Res Commun. (2015) 464:807–13. doi: 10.1016/j.bbrc.2015.07.040
136. Moreau P, Flajollet S, Carosella ED. Non-classical transcriptional regulation of HLA-G: an update. J Cell Mol Med. (2009) 13:2973–89. doi: 10.1111/j.1582-4934.2009.00800.x
137. Nunes JM, Buhler S, Roessli D, Sanchez‐Mazas A, Hn collaboration, Andreani M, et al. The HLA-net GENE [RATE] pipeline for effective HLA data analysis and its application to 145 population samples from Europe and neighbouring areas. Tissue Antigens. (2014) 83:307–23. doi: 10.1111/tan.12356
138. Sanchez-Mazas A, Vidan‐Jeras B, Nunes JM, Fischer G, Little AM, Bekmane U, et al. Strategies to work with HLA data in human populations for histocompatibility, clinical transplantation, epidemiology and population genetics: HLA-NET methodological recommendations. Int J immunogenetics. (2012) 39:459–76. doi: 10.1111/j.1744-313X.2012.01113.x
Keywords: human leukocyte antigen system (HLA), major histocompatibility complex (MHC), single nucleotide polymorphisms (SNP), epigenetics, DNA methylation, non-coding RNA, microRNA
Citation: Arumugam T, Adimulam T, Gokul A and Ramsuran V (2024) Variation within the non-coding genome influences genetic and epigenetic regulation of the human leukocyte antigen genes. Front. Immunol. 15:1422834. doi: 10.3389/fimmu.2024.1422834
Received: 24 April 2024; Accepted: 26 August 2024;
Published: 17 September 2024.
Edited by:
Sathi Babu Chodisetti, Thomas Jefferson University, United StatesReviewed by:
Margaret A. Jordan, James Cook University, AustraliaDolores Jaraquemada, Autonomous University of Barcelona, Spain
Alexandre Xavier, The University of Newcastle, Australia
Copyright © 2024 Arumugam, Adimulam, Gokul and Ramsuran. This is an open-access article distributed under the terms of the Creative Commons Attribution License (CC BY). The use, distribution or reproduction in other forums is permitted, provided the original author(s) and the copyright owner(s) are credited and that the original publication in this journal is cited, in accordance with accepted academic practice. No use, distribution or reproduction is permitted which does not comply with these terms.
*Correspondence: Thilona Arumugam, cyborglona@gmail.com