- Department of Emergency, The Second Affiliated Hospital, Jiangxi Medical College, Nanchang University, Jiangxi, China
Apolipoprotein A-I(ApoA-I) is a member of blood apolipoproteins, it is the main component of High density lipoprotein(HDL). ApoA-I undergoes a series of complex processes from its generation to its composition as spherical HDL. It not only has a cholesterol reversal transport function, but also has a function in modulating the inflammatory response. ApoA-I exerts its anti-inflammatory effects mainly by regulating the functions of immune cells, such as monocytes/macrophages, dendritic cells, neutrophils, and T lymphocytes. It also modulates the function of vascular endothelial cells and adipocytes. Additionally, ApoA-I directly exerts anti-inflammatory effects against pathogenic microorganisms or their products. Intensive research on ApoA-I will hopefully lead to better diagnosis and treatment of inflammatory diseases.
1 Introduction
ApoA-I is a member of blood apolipoproteins, which constitutes a major component of HDL (1). In addition to participating in cholesterol reversal, ApoA-I also has powerful anti-inflammatory functions (2). It is considered an anti-inflammatory protein, with levels reduced by at least 25% during acute inflammation (3). ApoA-I attenuates the inflammatory response through inhibit the production of TNF-a and IL-1 in rheumatoid arthritis, Crohn’s disease and other immune diseases (4). A negative correlation between ApoA-I levels and the severity of pancreatitis has also been found in pancreatitis (5–7). Furthermore, our previous study revealed a negative correlation between ApoA-I levels and disease severity in hypertriglyceridemic pancreatitis (8). In cases of sepsis, there is a negative correlation between ApoA-I and the severity of the condition (9). Additionally, the administration of ApoA-I mimetic peptide has been shown to improve survival rates in septic rats (10). The anti-inflammatory function of ApoA-I also plays an important role in the inhibition of atherosclerosis and anti-tumor growth (11). A negative correlation between reduced ApoA-I and disease severity was also found in COVID-19 infections (12, 13). However, the exact anti-inflammatory mechanism of ApoA-I is not well understood. In this article, we review the production, assembly and possible anti-inflammatory mechanism of ApoA-I.
2 Production and assembly
The human ApoA-I gene is located in the 11q23 region of human chromosome 11,and is thought to be of the same genetic origin as Apolipoprotein A-II, Apolipoprotein A-IV, Apolipoprotein C-I, Apolipoprotein C-III, and Apolipoprotein E (14). The liver and intestine are the main sites of ApoA-I production in human tissues, but small amounts of ApoA-mRNA expression have been demonstrated in other organs, such as the pancreas and heart (15). The regulation of human ApoA-I gene expression is very complex and is controlled at multiple levels. Hepatocyte Nuclear Factor 4, Liver Receptor Homologue 1 and ApoA-I Regulatory Protein 1 are thought to be the major regulators of ApoA-1 initiation and repression (16). Translated ApoA-I is pruned intracellularly and then secreted as a lipid-poor protein or lipid-free protein (Figure 1). ApoA-I gene mutations have been demonstrated to be associated with the development of numerous diseases, including those observed in diabetic patients. In particular, polymorphisms at the -75 bp locus of the ApoA-I gene have been linked to an increased risk of myocardial infarction in these patients (17). Individuals with the CC genotype of SNP rs5069 are more susceptible to oxidative imbalance and are at a higher risk of developing pancreatitis (18).
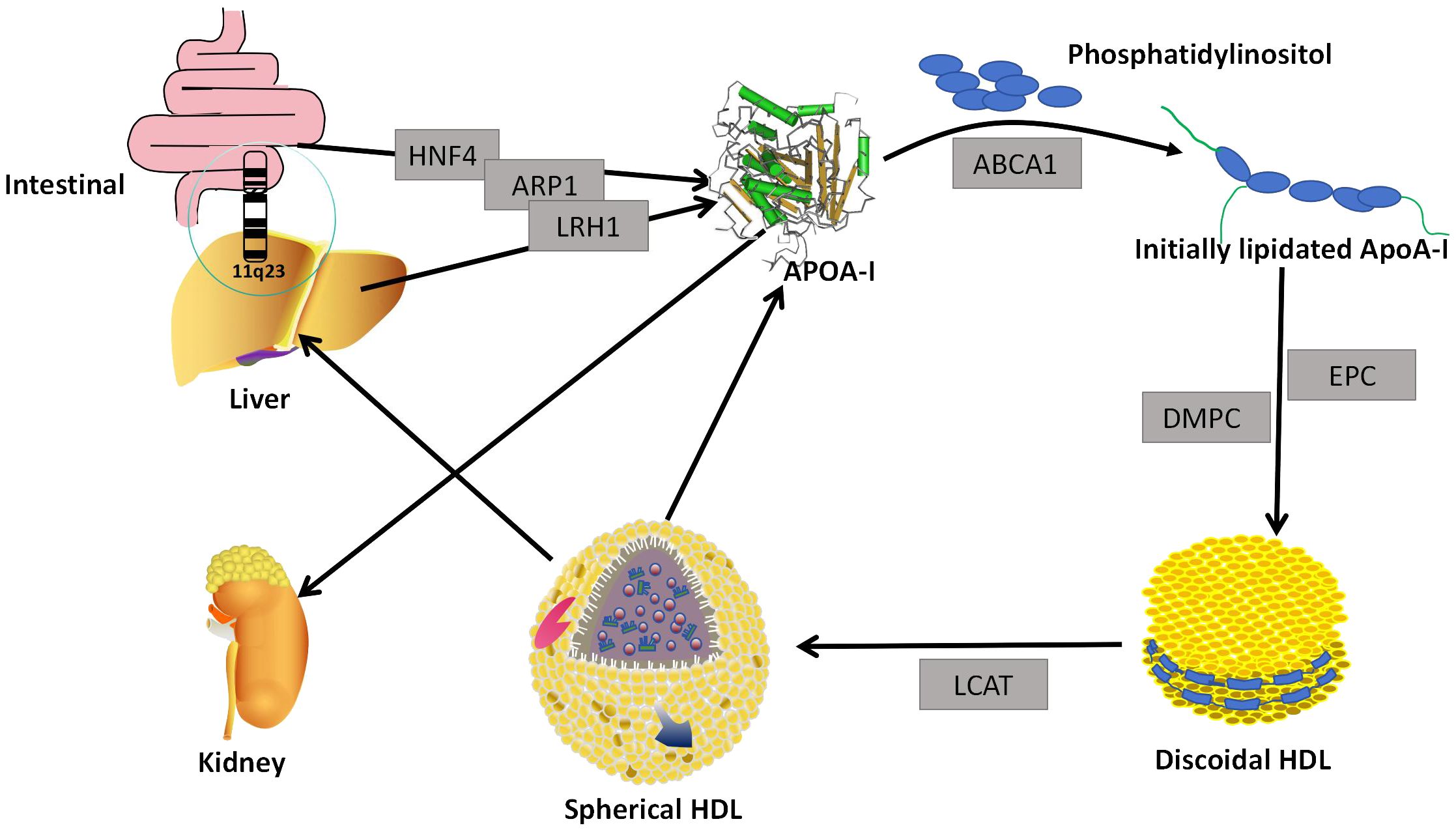
Figure 1 Generation and assembly. The ApoA-I gene located in the liver and small intestine secretes lipid-free ApoA-I under the regulation of HNF4, LRH1, and ARP1. Lipid-free ApoA-I binds to phosphatidylinositol to form Initially lipidated ApoA-I. Initially lipidated ApoA- I forms discoidal HDL in the presence of EPC and DMPC, and discoidal HDL forms globular HDL in the presence of LCAT. When globular HDL is reconstituted, lipid-free ApoA-I is formed. Most of the lipidated ApoA-I is metabolized in the liver, and the lipid-free ApoA-I is eliminated by the kidneys. HNF4, Hepatocyte Nuclear Factor4; ARP1, ApoA-I Regulatory Protein 1; LRH1, Liver Receptor Homologue 1; ABCA1, ATP-binding Cassette transport A1; DMPc, Dimyristoyl Phosphatidylcholine; EPC, egg phosphatidylcholine; LCAT, lecithin-cholesterol acyltransferase.
The primary structure of ApoA-I consists of 4 Tryptophan (Trp), 21 Lysine (Lys), 5Histidine (His), 16 Arginase (Arg), 16 Aspartic acid (Asp), 10 Threonine (Thr), 15 Serine (Ser), 27 Glutamic acid (Glu), 10 Proline(Pro), 10 Glycine (Gly), 19 Alanine (Ala), 13 Valine (Val), 3 Methionine (Met), 37 Leucine (Leu), 7 Tyrosine (Tyr), and 6 Phenylalanine (Phe), a total of 243 amino acid residues to form a single-chain peptide, which molecular weight is 28 KDa (19). This single-chain polypeptide may contain a plurality of 11 and 22 repeating amino acid residues, and these residues are typically separated by Pro residues. Among them, the 22 repeating amino acid residues can form the α-helix of ApoA-I, and the secondary structure of each ApoA-I contains about 8-9 tandem alpha helices (20). These α-helix play an important role in the biological function of ApoA-I (21–23). ApoA-I binds to the first extracellular domains(ECD1) of ATP-binding Cassette transport A1 (ABCA1), resulting in the transfer of cholesterol and cytosolic phospholipids from the cell membrane to ApoA-I, which then forms an initially lipidated ApoA-I (24). Initially lipidated ApoA-I can continue to form discoidal HDL in the presence of Dimyristoyl Phosphatidylcholine and egg phosphatidylcholine or palmitoyloleyl phosphatidylcholine (25). Studies of discoidal HDL mainly come from vitro models, as it is short-lived and difficult to isolate in plasma (26). Initially, it was believed that the binding of the α-helix to phospholipids in discoidal HDL was in the form of a spiked hedge fence. The α-helix consists of 22 repeating amino acids centered on a repeating proline residue crossing the edges of a bilayer parallel to the acyl chain (27). However, with research progressed, the ‘two-band’ model of discoidal HDL was considered the best possible model of α-helix binding to phospholipids. In this model, two cyclic ApoA-I molecules wrap a phospholipid bilayer in an antiparallel orientation. Discoidal HDL convers to spherical HDL by lecithin-cholesterol acyltransferase (LCAT) (28) (Figure 1). The globular HDLsubpopulation can be classified according to size and density into five major subpopulations: HDL3c, HDL3b, HDL3a, HDL2a, and HDL2b. Each subpopulation is distinguished by its unique molecular composition and biological function (29). ApoA-I is floating in the phospholipid molecules and serves to regulate HDL diameter in globular HDL (30).
ApoA-I constitutes the primary constituent of HDL, representing approximately 70%,ApoA-II accounts for 15-20%, while the remaining proteins are amphiphilic (1). ApoA-I not only determines the size and shape of HDL but is also a functional protein, such as determining the composition of HDL soluble lipids, transporting cholesterol from peripheral cells, activating LCAT activity to convert circulating cholesterol to cholesteryl esters, and delivering cholesteryl esters to the liver or to steroidogenic tissues via cell surface receptors (2). Most of ApoA-I is found in the blood associated with lipoproteins, with only about 5-10% present in a non-lipoprotein-associated state. This non-lipidated ApoA-I can be obtained from HDL remodeling or triglyceride-rich lipoproteins, or secreted directly by the liver or intestines (31). ApoA-I in plasma is about 100-150 mg/dl,and its half-life is about 4 days (32, 33). Most lipidated ApoA-I is metabolized in the liver (34), Non-lipidated ApoA-I can pass through glomerular filtration and be degraded, excreted in urine, or partially reabsorbed (35) (Figure 1).
3 Possible anti-inflammatory mechanism of ApoA-I
3.1 Regulation of immune cells
The inflammatory response is primarily determined on the function and activity of immune cells (36). Monocytes are an important component of the defense system of body, which play a crucial role in the immune system, and can induce a specific immune response in lymphocytes through antigen presentation (37). Macrophages are terminally differentiated monocyte, which have a variety of biological functions in the inflammatory response, including phagocytosis of microorganisms, mediation and promotion of the inflammatory response, antigen processing and presentation, modulation of the immune response, direct killing of target cells, adjuvant or inhibitory production of antibodies by B-lymphocytes, and production of cytokines (38). Immature dendritic cells have a strong migratory ability, and mature dendritic cells can effectively activate the initial T-cells to initiate the immune response (39). In the inflammatory response, neutrophils have multiple biological functions, such as chemotaxis, phagocytosis, apoptosis, degranulation, activation, production of reactive oxygen species and extra-neutral trapping networks (40). T cells play a key role in regulating the immune response, and which have responsible for mediating of the immune effector mechanisms (41).
ApoA-I exerts a modulatory effect on the immune functions of monocytes, macrophages, dendritic cells, neutrophils, and T lymphocytes. It also suppresses inflammatory responses through multiple pathways (42, 43). First, ApoA-I can exert anti-inflammatory effects by regulating the production of immune cells. In a mouse model of acute myocardial infarction, ApoA-I has been observed to inhibit the expansion of monocytes and macrophages in the blood, spleen, and myocardium of mice (44). Upon leaving the bloodstream and entering tissues, monocytes can differentiate into Dendritic Cells(DCs),ApoA-I upregulates Prostaglandin E2 (PGE2) and Interleukin-10 (IL-10) in monocytes, and inhibits their differentiation to dendritic cells (45). In neutrophil generation, ApoA-I can reduce neutrophil production by decreasing the production of granulocyte colony stimulating factor (G-CSF) (46). Furthermore, ApoA-I has been demonstrated to reduce neutrophil counts in patients with acute myocardial infarction, resulting in less myocardial inflammatory injury (47). ApoA-I also has an effect on lymphocyte production, it deficiency leads to increase of CD45RA+, CD16+, and CD56+ lymphocytes in the blood (48). Transplantation of bone marrow from ApoA-I knockout mice into LDL receptor knockout mice resulted in a significant increase in lymphocytes (49). Second, ApoA-I can exert anti-inflammatory effects by regulating the expression and production of related factors of immune cells. The expression and production of related factors of immune cells are closely related to anti-inflammatory functions, such as spreading, recognition and chemotaxis. ApoA-I can directly inhibit the spreading and antigen-presenting ability of monocytes by down-regulating the expression of monocyte CDC42, CD11c, CD86, CD14, and HLA-DR (50, 51). Expression of vascular cell adhesion molecule-1 (VCAM-1), monocyte chemotactic protein 1 (MCP-1), and macrophage inflammatory protein 1 (MIP-1) was also inhibited by ApoA-I, which also significantly reduced the release of sL-selectin and soluble Inter-cellular Adhesion molecule 1(sICAM-1), and decreased monocyte chemotaxis, adhesion and activation function (52, 53). ApoA-I inhibits the synthesis of IL-8 by activated neutrophils, limiting their chemotaxis to local sites of inflammation (53). It was also found that ApoA-I reduces the expression of CD11b in neutrophils, leading to the decreased of adhesion, migration and spreading capacity of neutrophils (54). In polymorphonuclear leukocytes, ApoA-I was also found to have the ability to inhibit the expression of CD11/CD18, resulting in the decreased of adhesion of polymorphonuclear leukocyte(PMN) (55). Third, ApoA-I can exert anti-inflammatory effects by regulating the interaction of immune cells. In the inflammatory response, immune cells can coordinate and influence each other. As previously discussed, ApoA-I can affect the differentiation between monocytes and dendritic cells. ApoA-I also inhibits T-cell proliferation via DCs, and inhibits the reciprocal response between DCs and NK cells, resulting in decreased of IFN-γ and IL-12p70 (56). Fourth, It can exert anti-inflammatory effects by altering the expression of ApoA-I in immune cells. It has been found that ApoA-I is also expressed in macrophages, and the effect of its expression also affects macrophage function (57, 58). In monocytes, the expression of CD11b, CD11c, and CD29 was negatively correlated with ApoA-I levels in all monocyte subpopulations, and ApoA-I levels directly affected the anti-inflammatory activity of monocytes (59) (Figure 2).
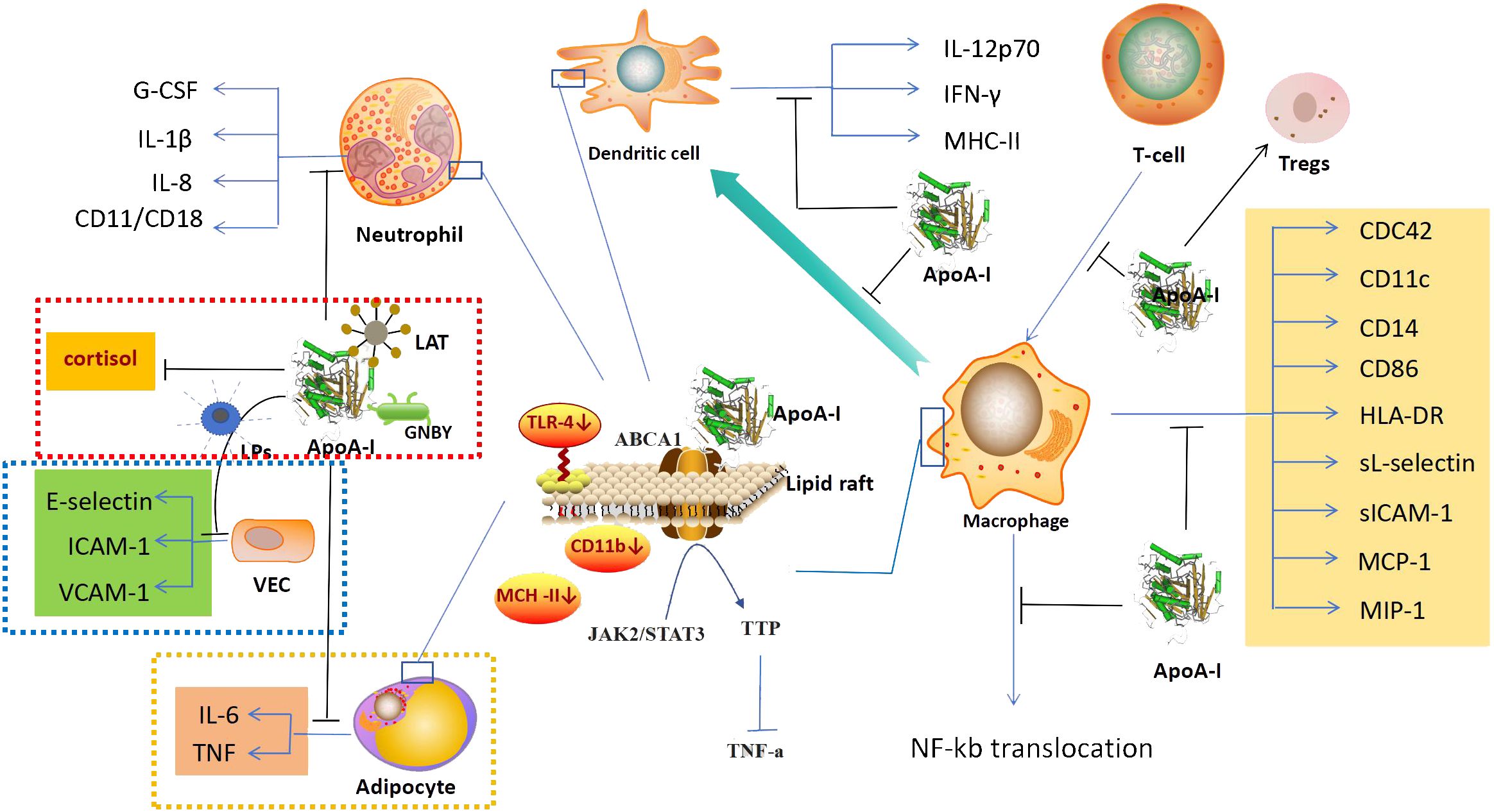
Figure 2 anti-inflammatory mechanism. In addition to its anti-inflammatory effects mainly through the regulation of immune cells,ApoA-I also exerts anti-inflammatory effects through the regulation of vascular endothelial cells (blue dashed area), the action on microorganisms and their products (red dashed area), and the regulation of adipocytes (yellow dashed area). In the regulation of immune cells, ApoA-I can inhibit the generation of immune cells, affect the expression of related factors, and inhibit the synergistic effect of immune cells. Its molecular mechanism is mainly to increase the efflux of cholesterol, regurate lipid raft-mediated signaling pathways and inhibit NF-kB nuclear translocation. ApoA-I, Apolipoprotein A-I; G-CSF, Granulocyte Colonystimulating Factor; IL-1β, interleukin 1β; IL-8, interleukin 8; LAT, Lipophosphatidic acid; LPs, lipopolysaccharide; GNBY, Gram-negative bacterium Yersinia; ICAM-1, Inter-cellular Adhesion molecule 1; VCAM-1, Vascular Cell Adhesion Molecule 1; VEC, Vascular endothelial cell; IL-6, interleukin 6; TNF, tumor necrosis factor; IL-12p70, interleukin 12p70; IFN-γ, Interferon γ; MHC-II, Major Histocompatibility Complex II; ABCA1, ATP-binding cassette transporter protein A1; TLR-4, Toll-like receptor; JAK2/STAT3, Janus kinase 2/Signal Transducer and Activator of Transcription 3; TTP, tristetraprolin; CDC42, Cell Division Cycle 42; HLA-DR, Human Leukoyte Antigen DR; sICAM-1, soluble Inter-cellular Adhesion molecule 1; MCP-1, Monocyte Chemotactic Protein 1; MIP-1, Macrophage Inflammatory Protein 1.
Based on the available research, the regulation of the anti-inflammatory function of immune cells by ApoA-I may be achieved through the following molecular mechanisms: First, ApoA-I causes cholesterol efflux from immune cells to affect the expression of related factors. ApoA-I has a powerful cholesterol transporter function, it can modulate cellular biological functions by altering the cholesterol in cells, as previously demonstrated, the regulation of cholesterol in arterial vascular endothelial cells (60), and the recently discovered of regulation in pancreatic islet cells (61). ApoA-I has the same cholesterol-transporting effect in immune cells (62). Studies have shown that the decrease of CD11b and TLR-4 expression in monocytes by ApoA-I is due to the exocytosis of cholesterol from monocyte lipid rafts as a result of ApoA-I binding to ABCA1 in monocyte lipid rafts (52, 63), and the inhibition of macrophage antigen presentation and activation of T cells is also due to ApoA-I causing a decrease in the cholesterol content in macrophage lipid rafts (64). Second, ApoA-I affects the expression of related factors through lipid raft-mediated signaling pathways. After treating macrophages with ApoA-I, Yin Kai et al. found that the binding of ApoA-I to ABCA1 activated the Janus kinase 2/Signal Transducer and Activator of Transcription 3 (JAK2/STAT3),and upregulated the expression the tristetraprolin(TTP),which in turn promoted the degradation of TNF-a mRNA through AU-rich elements (AREs) in the 3-untranslated regions (3-UTR), leading to a reduction in the production of TNF-a and a decrease of inflammatory response (65). Third, suppression of gene activation in immune cells by inhibiting NF-kB nuclear translocation. NF-kB translocation plays a crucial role in intracellular signaling pathways and regulates various biological processes (66). In immune cells, stimulation by various factors can cause the transfer of the NF-kB subunit to the nucleus, activating numerous NF-kB-specific target genes, this activation leads to the initiation or enhancement of the immune response (67). ApoA-I inhibits NF-kB nuclear translocation in THP-1 differentiated monocytes, and the expression of VCAM-1 was inhibited and the release of sL-selectin and sICAM-1 were decreased. This reduced the chemotaxis, adhesion and activation functions of monocytes and inhibited the anti-inflammatory effects of monocytes (52, 68) (Figure 2). However, whether the initiating link of ApoA-I for NF-kB translocation inhibition is also due to ApoA-I affecting monocyte cholesterol is not known, and more studies are expected to further explore this.
3.2 Regulation of vascular endothelial cells
Vascular endothelial cells are a single layer of flat epithelium that covers the inner surface of blood vessels. During inflammation, vascular endothelial cells express adhesion molecules and interact with leukocyte surface adhesion molecules in the bloodstream. They also regulate leukocyte crossing of the vessel wall through signaling (69). In a study of a mouse model of type 2 diabetes revascularization, ApoA-I was found to reduce the inflammatory response of endothelial cells in the reconstructed blood vessels and promote vascular repair (70). ApoA-I can directly bind to LPs and influence its stimulatory effect on vascular endothelial cells. This results in the inhibition of E-selectin and intercellular adhesion molecule 1 (ICAM-1) expression in vascular endothelial cells, which leads to reduced adhesion of neutrophils to endothelial cells (56). In vivo experiments were conducted on New Zealand white rabbits infused intravenously with lipid-free ApoA-I, the results showed a reduction in the expression of VCAM-1 and ICAM-1, as well as a decrease in endothelial neutrophil infiltration (71) (Figure 2). However, the specific molecular mechanisms by which ApoA-I regulates vascular endothelial cell function are not well understood.
3.3 Action on microorganisms and their products
ApoA-I binds to a wide range of Gram-positive and Gram-negative bacteria, as well as to lipopolysaccharides and lipophosphatidic acids. In addition, ApoA-I has in vitro antimicrobial activity against Gram-negative bacteria (72). The C-terminal structural domain of ApoA-I serves as an effector site that provides bactericidal activity and contributes to complement mediated killing of the Gram-negative bacterium Yersinia enterocolitica in the small intestine (73) (Figure 2). In addition, ApoA-I was also found to possess in vitro antimicrobial properties against Gram-positive and Gram-negative bacteria in studies with tilapia, and ApoA-I inhibited inflammation and apoptosis and increased the likelihood of survival to bacterial infection (74), and its antimicrobial effect is not affected by temperature, even when the a-helix of ApoA-I is damaged by high temperature, ApoA-I still has the activity of killing bacteria directly (75). Therefore, the structure of ApoA-I may not be closely related to its antimicrobial activity.
Lipoteichoic acid (LTA) is an amphiphilic cationic glycolipid and a major cell wall component of Gram-positive bacteria. It has a structure similar to that of LPs from Gram-negative bacteria. LTA has been implicated as one of the major immunostimulatory components that may trigger systemic inflammatory response syndromes (76). ApoA-I significantly reduces L-929 cell mortality induced by LTA activated macrophages in a dose-dependent manner (77). LPs is one of the most potent stimulators of innate immune activation, and have important effects on human monocyte and macrophage (78). ApoA-I can neutralize the inflammatory effects of LPs through direct binding to LPs and can also regulate cortisol hormone production to play an anti-inflammatory role. In the sepsis model with ApoA-I knockout mice, it was found that ApoA-I knockout mice had a decreased ability to neutralize LPs compared to wild-type mice, and their serum cortisol hormone production was impaired, and the sepsis protection of the mice was reduced (79) (Figure 2).
3.4 Others
In adipocytes, ApoA-I inhibits the expression of IL-6 and TNF induced by palmitate, and it translocate TLR-4 to lipid rafts, thereby inhibiting inflammatory responses in adipocytes (80) (Figure 2).
4 The anti-inflammatory of 4F and CSL
Based on the robustness of ApoA-I and findings in the clinic, ApoA-I supplementation is considered a viable approach to treatment disease. Therefore, ApoA-I which can be used as a medicine has become the focus of attention. Such as ApoA-I mimetic peptides and ApoA-I based infusions.
Anantharamaiah et al. synthesized the first apoA-I mimetic peptide 18A in, 1985,which comprises 18 amino acids (81). Subsequently, the amino acid and α-helix structures of ApoA-I mimetic peptides have been modified to enhance their functionality (82, 83). To date, ApoA-I mimetic peptides in the pilot study phase are 4F,6F,FX-5A,ATI-5261 and ETC-642 (84). Among the many mimetic peptides, 4F has the most prominent anti-inflammatory effects. In vitro,4 F reduces IL-6 secretion in SARS-CoV-2 infected Vero-E6 and Calu3 cells (85), and it has an inhibitory effect on IL-4-induced selective activation of macrophages (43), 4F also inhibits Reactive Oxygen Species(ROS) production and ameliorates oxidative damage in endothelial cells (86). It has been shown in animal studies that ApoA-I mimetic peptides have the inflammation inhibition (87). In mice,4F inhibits the expression of interleukin-6(IL-6), interleukin-1β(IL-1β) and tumor necrosis factor-α (TNF-α) (88), it reduce macrophage infiltration in the liver (89). It also upregulates vascular endothelial growth factor protein expression to improve endothelial cell function in animal studies (90). An in vivo study in humans also showed that oral administration of apoA-I mimetic peptides 6F and 4F reduced plasma and intestinal tissue cytokines (TNF-a, IL-6) and chemokines (CX3CL1), and reduced systemic and intestinal inflammation in chronic treatment of HIV (91).
ApoA-I based infusions include HDL-VHDL infusion, purified ApoA-I infusion, ApoA-IMilano infusion, CSL-111 and CSL-112 infusion,CER-001 infusion and modified ApoA-I (84). Of these,CSL-112 is considered the most viable infusion. CSL-112 consists of purified human ApoA-I and phosphatidylcholine, because it binds less phosphatidylcholine than CSL-111, it does not have the dose toxicity of CSL-111 (92). In previous studies, CSL-111 has been shown to have the effect of downregulating macrophages to reduce inflammation (93, 94). CSL112 also has shown anti-inflammatory effects ex vivo studies, including markers of monocyte chemotactic factor-1 and proinflammatory cytokines interleukin-1β (95).
5 Discussion
ApoA-I goes through a series of complex processes from generation to assembly (96). Thus, both the expression of the ApoA-I gene and the changes in its α-helix structure are susceptible to interference by external environmental factors, and these changes may be associated with the development of the disease (23, 97). ApoA-I is the primary component of HDL, and the biological functions of HDL, including cholesterol regulation (98), inflammatory response modulation (99), and tumor growth modulation are believed to be closely related to ApoA-I (100), which is an effective performer of HDL’s biological functions (101). As described above, ApoA-I plays a pivotal role in the inhibition of the inflammatory response, and this is achieved through the inhibition of immune cell production and activity, the reduction of the recognition of immune cells by vascular endothelial cells, and the direct destructive effect on microorganisms and their products. Specific molecular regulatory mechanisms have focused on the study of immune cells. According to the literature review, most of the regulatory effects of ApoA-I on immune cell function are related to its regulation of cholesterol, in which the effect on lipid rafts is an important target for ApoA-I to regulate immune cells, but a clearer and more systematic mechanism is still not well described, and more and more in-depth studies are expected to be conducted in the future. The decrease of ApoA-I has been detected in many diseases (102–105), especially in those related to inflammation (4–13). However, the underlying cause of the decrease is not clear. Whether the reduction is due to a decrease in ApoA-I production or an increase in ApoA-I depletions is necessary to explore.
As previously described, several studies have shown that ApoA-I mimetic peptides have some anti-inflammatory effects. However, even with studies showing that ApoA-I mimetic peptide does not play a role in suppressing inflammation. In the aortic constriction rabbit model, ApoA-I mimetic infusions did not significantly improve echocardiographic parameters nor molecular markers of cardiac inflammation, oxidative stress and fibrosis (106). This includes previous trial in patients with coronary artery disease or at high risk for cardiovascular disease that showed conflicting results on the effect of oral and parenteral administration of 4F on HDL inflammation indices (107). The contradictory test results may be attributed to the fact that the functions of the simulated components are distinct (108, 109) and that the final evaluation criteria differ for each test. ApoA-I mimetic peptide is a synthetic substance that is similar to ApoA-I. However, its molecular structure differs from ApoA-I, which means that it cannot exert the same effect when it enters the body. Furthermore, the pharmacokinetics and pharmacokinetics of ApoA-I mimetic peptides in vivo require further comprehensive and in-depth investigation. Research on the anti-inflammatory effects of ApoA-I mimetic peptides have been focused on in vitro and in vivo studies, with few clinical trials in humans. The main factors limiting the development of these may be related to the contradictory results of ApoA-I mimetic peptide tests and concerns about their safety. The development of ApoA-I mimetic peptides that are closer to the molecular structure of ApoA-I and safer may require more difficult work. However, from the theoretical standpoint, the structure of CSL-112 is more closely aligned with ApoA-I mimetic peptide, which should result in a more robust anti-inflammatory effect and enhanced safety profile. But the majority studies of CSL-111/112 on immune cells come from atheromatous plaque formation, lack of extensively studied as the ApoA-I mimetic peptides in inflammation. And concerns have been raised about the efficacy of CSL-112 due to the findings of recent clinical studies, which indicated that patients with acute myocardial infarction treated with CSL-112 did not experience a significant reduction in major adverse cardiovascular events (110). We are looking forward to deeper study and bigger breakthroughs in the anti-inflammatory treatment of CSL-112.
6 Conclusions
The production and assembly of ApoA-I is influenced by multiple factors, and ensuring the structural integrity of ApoA-I is a prerequisite for its anti-inflammatory effects. ApoA-I can exert its anti-inflammatory effects through regulate immune cells, vascular endothelial cells, and direct interaction with microorganisms and their products. Several experiments have demonstrated that ApoA-I mimetic peptides and ApoA-I based infusions inhibit inflammatory responses. It is anticipated that safer and more efficacious ApoA-I drugs will emerge in the near future as research progresses.
Author contributions
XT: Writing – original draft. TR: Writing – original draft. KW: Conceptualization, Writing – review & editing. LW: Writing – review & editing.
Funding
The author(s) declare that no financial support was received for the research, authorship, and/or publication of this article.
Conflict of interest
The authors declare that the research was conducted in the absence of any commercial or financial relationships that could be construed as a potential conflict of interest.
Publisher’s note
All claims expressed in this article are solely those of the authors and do not necessarily represent those of their affiliated organizations, or those of the publisher, the editors and the reviewers. Any product that may be evaluated in this article, or claim that may be made by its manufacturer, is not guaranteed or endorsed by the publisher.
References
1. Nazir S, Jankowski V, Bender G, Zewinger S, Rye K-A, van der Vorst EPC. Interaction between high-density lipoproteins and inflammation:Function matters more than concentration. Adv Drug Deliv Rev. (2020) 159:94–119. doi: 10.1016/j.addr.2020.10.006
2. Pownall HJ, Rosales C, Gillard BK, Gotto AM Jr. High-density lipoproteins, reverse cholesterol transport and atherogenesis. Nat Rev Cardiol. (2021) 18:712–. doi: 10.1038/s41569-021-00538-z
3. Gabay C, Kushner I. Acute-phase proteins and other systemicresponses to inflammation. N Engl J Med. (1999) 340:448 –54. doi: 10.1056/NEJM199902113400607
4. Georgila K, Vyrla D, Drako E. Apolipoprotein A-I (ApoA-I), immunity, inflammation and cancer. Cancers. (2019) 11:1097. doi: 10.3390/cancers11081097
5. Wu J, Wang Y, Li H, Tan W, Chen X, Ye. S. Serum apolipoprotein B-toapolipoprotein A1 ratio is independently associated with disease severity in patients with acute pancreatitis. Sci Rep. (2019) 9:7764. doi: 10.1038/s41598-019-44244-w
6. Yu Ge, Jiang W, Cheng Z, Wan R. Predictive value of serum apolipoprotein A-I in the organ failure of acute pancreatitis: a retrospective cohort study. Scand J Gastroenterol. (2023) 58:1049–55. doi: 10.1080/00365521.2023.2200500
7. Li Y, Zheng R, Gao F, Wang L, Feng S, Li J, et al. Association between high-density lipoprotein cholesterol and apolipoprotein A-I and severe acute pancreatitis: a case-control study. Eur J Gastroenterol Hepatol. (2021) 33:1517–23. doi: 10.1097/MEG.0000000000002095
8. Hu Y-q, Tao X, Wu H-b, Li W-g, Chen D-y, Liu Y-f, et al. Predicting severity in hypertriglyceridemiaInduced acute pancreatitis: the role of neutrophils, calcium, and apolipoproteins. Med Sci Monit. (2024) 30:942832. doi: 10.12659/MSM.942832
9. Wendel M, Paul R, Heller AR. Lipoproteins in inflammation and sepsis. II. Clinical aspects. Intensive Care Med. (2007) 33:25–35. doi: 10.1007/s00134-006-0433-x
10. Zhang Z, Datta G, Zhang Y, Miller AP, Mochon P, Chen Y-F, et al. Apolipoprotein A-I mimetic peptide treatment inhibits inflammatory responses and improves survival in septic rats. Am J Physiol Heart Circ Physiol. (2009) 297:H866–73. doi: 10.1152/ajpheart.01232.2008
11. Busnelli M, Manzini S, Chiara M. Aortic gene expression profiles show how apoA-I levels modulate inflammation, lysosomal activity, and sphingolipid metabolism in murine atherosclerosis. Arterioscler Thromb Vasc Biol. (2024) 41:651–67. doi: 10.1161/ATVBAHA.120.315669
12. Feingold KR. The bidirectional interaction of COVID-19 infections and lipoproteins. Best Pract Res Clin Endocrinol Metab. (2023) 37:101751. doi: 10.1016/j.beem.2023.101751
13. Hilser JR, Han Y, Biswas S, Gukasyan J, Cai Z, Zhu R, et al. Association of serum HDL-cholesterol and apolipoprotein A1 levels with risk of severe SARS-CoV-2 infection. J Lipid Res. (2021) 62:100061. doi: 10.1016/j.jlr.2021.100061
14. Arinami T, Hirano T, Kobayashi K, Yamanouchi Y, Hamaguchi H. Assignment of the apolipoprotein A-I gene to 11q23 based on RFLP in a case with a partial deletion of chromosome 11, del(11)(q23. 3—qter). Hum Genet. (1990) 85:39–40. doi: 10.1007/BF00276323
15. Zannis VI, Cole FS, Jackson CL, Kurnit DM, Karathanasis SK. Distribution of apolipoprotein A-I, C-II, C-III, and E mRNA in fetal human tissues. Time-dependent induction of apolipoprotein E mRNA by cultures of human monocyte-macrophages. Biochemistry. (1985) 24:4450–5. doi: 10.1021/bi00337a028
16. Kardassis D, Mosialou I, Kanaki M, Tiniakou I, Thymiakou E. Metabolism of HDL and its regulation. Curr Med Chem. (2014) 21:2864–80. doi: 10.2174/0929867321666140303153430
17. Casillas FA, Fernández DEM, Valle Y, Ramírez MA, Parra-Reyna B, Pulido SS, et al. APOA1 (-75 G>A and 83 C>T) and APOB (2488 C>T) polymorphisms and their association with myocardial infarction, lipids and apolipoproteins in patients with type 2 diabetes mellitus. Arch Med Sci. (2022) 18:1438–45. doi: 10.5114/aoms/108674
18. Sciskalska M, Milnerowicz H. Importance of Polymorphisms in the Gene of Paraoxonase-1 (SNP rs662) and Apolipoprotein A-I (SNP rs670 and rs5069) in Non-Smoking and Smoking Healthy Subjects and Patients with Acute Pancreatitis. GENES. (2022) 13:1968. doi: 10.3390/genes13111968
19. Brewer HB Jr, Fairwell T, LaRue A, Ronan R, Houser A, Bronzert TJ. The amino acid sequence of human ApoA-I,an apolipoprotein isolated from high density lipoproteins. Biochem Biophys Res Commun. (1978) 80:623–30. doi: 10.1016/0006-291X(78)91614-5
20. Segrest JP, Garber DW, Brouillette CG, Harvey SC, Anantharamaiah GM. The amphipathic a helix: A multifunctional structural motif in plasma apolipoproteins. Adv Protein Chem. (1994) 45:303–69. doi: 10.1016/S0065-3233(08)60643-9
21. Liu M, Mei X, Herscovitz H, Atkinson D. N-terminal mutation of apoA-I and interaction with ABCA1 reveal mechanisms of nascent HDL biogenesis. J Lipid Res. (2019) 60:44–57. doi: 10.1194/jlr.M084376
22. Gorshkova IN, Meyers NL, Herscovitz H, Mei X, Atkinson D. Human apoA-I[Lys107del] mutation affects lipid surface behavior of apoA-I and its ability to form large nascent HDL. J Lipid Res. (2023) 64:100319. doi: 10.1016/j.jlr.2022.100319
23. Battle S, Gogonea V, Willard B, Wang Z, Fu X, Huang Y, et al. The pattern of apolipoprotein A-I lysine carbamylation reflects its lipidation state and the chemical environment within human atherosclerotic aorta. J Biol Chem. (2022) 298:101832. doi: 10.1016/j.jbc.2022.101832
24. Kawanobe T, Shiranaga N, Kioka N, Kimura Y, Ueda K. Apolipoprotein A-I directly interacts with extracellular domain 1 of human ABCA1. Biosci Biotechnol Biochem. (2019) 83:490–7. doi: 10.1080/09168451.2018.1547106
25. Nichols AV, Gong EL, Blanche PJ, Forte TM. Characterization of discoidal complexes of phosphatidylcholine, apolipoprotein A-I and cholesterol by gradient gelelectrophore. Biochim Biophys Acta. (1983) 750:353–64. doi: 10.1016/0005-2760(83)90040-1
26. Brouillette CG, Anantharamaiah GM, Engle JA, Borhani DW. Structural models of human apolipoprotein A-I: a critical analysis and review. Biochim Biophys Acta. (2001) 1531:4–46. doi: 10.1016/S1388-1981(01)00081-6
27. Klon AE, Segrest JP, Harvey SC. Comparative models for human apolipoprotein A-I bound to lipid in discoidal high-density lipoprotein particles. Biochemistry. (2002) 41:10895–905. doi: 10.1021/bi020315m
28. Mishra VK, Palgunachari MN, Segrest JP, Anantharamaiah GMJ. Interactions of synthetic peptide analogs of the class A amphipathic helix with lipids. Evidence for the snorkel hypothesis. J Biol Chem. (1994) 269:7185–91. doi: 10.1016/S0021-9258(17)37266-6
29. Malajczuk CJ, Gandhi NS, Mancera RL. Structure and intermolecular interactions in spheroidal high-density lipoprotein subpopulations. J Struct Biol X. (2020) 10:5. doi: 10.1016/j.yjsbx.2020.100042
30. Melchior JT, Street SE, Vaisar T, Hart R, Jerome J, Kuklenyik Z, et al. Apolipoprotein A-I modulates HDL particle size in the absence of apolipoprotein A-II. J Lipid Res. (2021) 62:100099. doi: 10.1016/j.jlr.2021.100099
31. Frank PG, Marce YL. Apolipoprotein A-I: structure–function relationships. J Lipid Res. (2000) 41:853–72. doi: 10.1016/S0022-2275(20)32028-9
32. Gould R,G. Lipid metabolism and atherosclerosis. Am J Med. (1951) 11:209–27. doi: 10.1016/0002-9343(51)90107-6
33. Fidge N, Nestel P, Ishikawa T, Reardon M, Billington T. Turnover of apoproteins A-I and A-II of high density lipoprotein and the relationship to other lipoproteins in normal and hyperlipidemic individuals. Metabolism. (1980) 29:643–53. doi: 10.1016/0026-0495(80)90109-2
34. Christensen EI, Gburek J. Protein reabsorption in renal proximal tubule-function and dysfunction in kidney pathophysiology. Pediatr Nephrol. (2004) 19:714–21. doi: 10.1007/s00467-004-1494-0
35. Yang H, Fogo AB, Kon V. Kidneys: key modulators of high-density lipoprotein levels and function. Curr Opin Nephrol Hypertens. (2016) 25:174–9. doi: 10.1097/MNH.0000000000000217
36. Ward AC. Immune factors, immune cells and inflammatory diseases. Int J Mol Sci. (2024) 25:2417. doi: 10.3390/ijms25042417
37. Murray PJ. Immune regulation by monocytes. Semin Immunol. (2018) 35:12–8. doi: 10.1016/j.smim.2017.12.005
38. Verschoor CP, Puchta A, Bowdish DME. The macrophage. Methods Mol Biol. (2012) 844:139–56. doi: 10.1007/978-1-61779-527-5_10
39. Granucci F, Foti M, Ricciardi-Castagnoli P. Dendritic cell biology. Adv Immunol. (2005) 88:193–233. doi: 10.1016/S0065-2776(05)88006-X
40. Liew PX, Kubes P. The neutrophil’s role during health and disease. Physiol Rev. (2019) 99:1223–48. doi: 10.1152/physrev.00012.2018
41. Hickling JK. Measuring human T-lymphocyte function. Expert Rev Mol Med. (1998) 13:1–20. doi: 10.1017/S1462399498000313
42. Mu W, Sharma M, Heymans R, Ritou E, Rezek V, Hamid P, et al. Apolipoprotein A-I mimetics attenuate macrophage activation in chronic treated HIV. AIDS. (2021) 35:543–. doi: 10.1097/QAD.0000000000002785
43. Song X, Shi Y, You J, Wang Z, Xie L, Zhang C, et al. D-4F, an apolipoprotein A-I mimetic, suppresses IL-4 induced macrophage alternative activation and pro-fibrotic TGF-β1 expression. Pharm Biol. (2019) 57:470–6. doi: 10.1080/13880209.2019.1640747
44. Hamid T, Ismahil MA, Bansal SS, Patel B, Goel M, White CR, et al. The apolipoprotein A-I mimetic L-4F attenuates monocyte activation and adverse cardiac remodeling after myocardial infarction. Int J Mol Sci. (2020) 21:3519. doi: 10.3390/ijms21103519
45. Kim KD, Lim HY, Lee HG, Yoon D-Y, Choe Y-K, Choi I, et al. Apolipoprotein A-I induces IL-10 and PGE2 production in human monocytes and inhibits dendritic cell differentiation and maturation. Biochem Biophys Res Commun. (2005) 338:1126–36. doi: 10.1016/j.bbrc.2005.10.065
46. Dai C, Yao X, Keeran KJ, Zywicke GJ, Qu X, Yu Z-X, et al. Apolipoprotein A-I attenuates ovalbumin-induced neutrophilic airway inflammation via a granulocyte colony-stimulating factor dependent mechanism. Am J Respir Cell Mol Biol. (2012) 47:186–95. doi: 10.1165/rcmb.2011-0322OC
47. Kingwell BA, Duffy D, Clementi R, Velkoska E, Feaster J, Gibson CM. CSL112 (Apolipoprotein A-I [Human]) reduces the elevation in neutrophil-to-lymphocyte ratio induced by acute myocardial infarction. J Am Heart Assoc. (2024) 13:e033541. doi: 10.1161/JAHA.123.033541
48. Pashinskaya KO, Samodova AV, Dobrodeeva LK. The effect of the content of ApoA-I in peripheral blood on the state of immune homeostasis in people living in extreme climatic conditions of the Arctic. Klin Lab Diagn. (2021) 66:539–45. doi: 10.51620/0869-2084-2021-66-9-539-545
49. Ouweneel AB, Reiche ME, Snip OSC, Wever R, van der Wel EJ, Schaftenaar FH, et al. Apolipoprotein A1 deficiency in mice primes bone marrow stem cells for T cell lymphopoiesi. J Cell Sci. (2022) 135:jcs258901. doi: 10.1242/jcs.258901
50. Diederich W, Orso E, Drobnik W, Schmitz G. Apolipoprotein AI and HDL(3) inhibit spreading of primary human monocytes through a mechanism that involves cholesterol depletion and regulation of CDC42. Atherosclerosis. (2001) 159:313–24. doi: 10.1016/S0021-9150(01)00518-4
51. Richart AL, Reddy M, Khalaji M, Natoli AL, Heywood SE, Siebel AL, et al. ApoA-I nanoparticles delivered post myocardial infarction moderate inflammation. Circ Res. (2020) 127:1422–36. doi: 10.1161/CIRCRESAHA.120.316848
52. Wang Li, Chen W-Z, Wu M-P. Apolipoprotein A-I inhibits chemotaxis, adhesion, activation of THP-1 cells and improves the plasma HDL inflammatory index. Cytokine. (2010) 49:194–200. doi: 10.1016/j.cyto.2009.08.008
53. Smythies LE, White CR, Maheshwari A, Palgunachari MN, Anantharamaiah GM, Chaddha M, et al. Apolipoprotein A-I mimetic 4F alters the function of human monocyte-derived macrophages. Am J Physiol Cell Physiol. (2010) 298:C1538–48. doi: 10.1152/ajpcell.00467.2009
54. Cockerill GW, McDonald MC, Mota-Filipe H, Cuzzocrea S, Miller NE, Thiemermann C. High density lipoproteins reduce organ injury and organ dysfunction in a rat model of hemorrhagic shock. FASEB J. (2001) 15:1941–52. doi: 10.1096/fj.01-0075com
55. Murphy AJ, Woollard KJ, Suhartoyo A, Stirzaker RA, Shaw J, Sviridov D, et al. Neutrophil activation is attenuated by high-density lipoprotein and apolipoprotein A-I in in vitro and in vivo models of inflammation. Arterioscler Thromb Vasc Biol. (2011) 31:1333–41. doi: 10.1161/ATVBAHA.111.226258
56. Moundry R, Spycher M, Doran J. Reconstituted high density lipoprotein modulates adherence of polymorphonuclear leukocytes to human endothelial cells. Shock. (1997) 7:175–81. doi: 10.1097/00024382-199703000-00004
57. Chen W, Wu Y, Lu Q, Wang S, Xing D. Endogenous ApoA-I expression in macrophages: A potential target for protection against atherosclerosis. Clin Chim Acta. (2020) 505:55–9. doi: 10.1016/j.cca.2020.02.025
58. Nekrasova EV, Larionova EE, Danko K, Kuzmina DO, Shavva VS, Kudriavtsev IV, et al. Regulation of Apolipoprotein A-I Gene Expression in Human Macrophages by Oxidized Low-Density Lipoprotein. Biochemistry (Mosc). (2021) 86:1201–13. doi: 10.1134/S0006297921100047
59. Patel VK, Williams H, Li SCH, Fletcher JP, Medbury HJ. Monocyte subset recruitment marker profile is inversely associated with blood apoA1 level. Front Immunol. (2021) 12:616305. doi: 10.3389/fimmu.2021.616305
60. Borja MS, Zhao L, Hammerson B, Tang C, Yang R, Carson N, et al. HDL-apoA-I exchange: rapid detection and association with atherosclerosis. PloS One. (2013) 8:e71541. doi: 10.1371/journal.pone.0071541
61. Manandhar B, Pandzic E, Deshpande N, Chen S-Y, Wasinger VC, Kockx M, et al. ApoA-I protects pancreatic β-cells from cholesterol-induced mitochondrial damage and restores their ability to secrete insulin. Arterioscler Thromb Vasc Biol. (2024) 44:e20–38. doi: 10.1161/ATVBAHA.123.319378
62. Méndez-Lara KA, Farré Núria, Santos D. Human apoA-I overexpression enhances macrophage-specific reverse cholesterol transport but fails to prevent inherited diabesity in mice. Int J Mol Sci. (2019) 20:655. doi: 10.3390/ijms20030655
63. Ciesielska A, Matyjek M, Kwiatkowska K. TLR4 and CD14 trafficking and its influence on LPS-induced pro-inflammatory signaling. Cell Mol Life Sci. (2021) 78:1233–61. doi: 10.1007/s00018-020-03656-y
64. Wang S-h, Yuan S-g, Peng D-q, Zhao S-p. HDL and ApoA-I inhibit antigen presentation-mediated T cell activation by disrupting lipid rafts in antigen presenting cells. Atherosclerosis. (2012) 225:105–14. doi: 10.1016/j.atherosclerosis.2012.07.029
65. Yin K, Deng X, Mo Z-C, Zhao G-J, Jiang J, Cui L-B, et al. Tristetraprolin-dependent post-transcriptional regulation of inflammatory cytokine mRNA expression by apolipoprotein A-I. J Biol Chem. (2011) 286:13834 –13845. doi: 10.1074/jbc.M110.202275
66. Zinatizadeh MR, Schock B, Chalbatani GM. The Nuclear Factor Kappa B (NF-kB) signaling in cancer development and immune diseases. Genes Dis. (2020) 8:287–97. doi: 10.1016/j.gendis.2020.06.005
67. Mussbacher M, Derler M, Basílio José, Schmid JA. NF-κB in monocytes and macrophages - an inflammatory master regulator in multitalented immune cells. Front Immunol. (2023) 14:1134661. doi: 10.3389/fimmu.2023.1134661
68. Yan Y-j, Li Y, Lou B, Wu M-p. Beneficial effects of ApoA-I on LPS-induced acute lung injury and endotoxemia in mice. Life Sci. (2006) 79:210–5. doi: 10.1016/j.lfs.2006.02.011
69. Krüger-Genge A, Blocki A, Franke R-P, Jung F. Vascular endothelial cell biology: an update. Int J Mol Sci. (2019) 20:4411. doi: 10.3390/ijms20184411
70. Babu M, Devi D, Mäkinen P, Örd T, Aavik E, Kaikkonen M, et al. ApoA-I nanotherapy rescues postischemic vascular maladaptation by modulating endothelial cell and macrophage phenotypes in type 2 diabetic mice. Arterioscler Thromb Vasc Biol. (2023) 43:e46–61. doi: 10.1161/ATVBAHA.122.318196
71. Wu BJ, Chen K, Shrestha S, Ong KL, Barter PJ, Rye K-A. High-density lipoproteins inhibit vascular endothelial inflammation by increasing 3β-hydroxysteroid-Δ24 reductase expression and inducing heme oxygenase-1. Circ Res. (2013) 112:278–88. doi: 10.1161/CIRCRESAHA.111.300104
72. Wang W, Qu Q, Chen J. Identification,expression analysis, and antibacterial activity of Apolipoprotein A-I from amphioxus (Branchiostoma belcheri). Comp Biochem Physiol B Biochem Mol Biol. (2019) 8:110329. doi: 10.1016/j.cbpb.2019.110329
73. Biedzka-Sarek M, Metso J, Kateifides A. Apolipoprotein A-I Exerts Bactericidal Activity against Yersinia enterocolitica Serotype O:3. J Biol Chem. (2011) 286:38211–9. doi: 10.1074/jbc.M111.249482
74. Huang Y, Feng J, Li Q, Zhang Z, Jiang B, Amoah K, et al. Apolipoprotein A-I (ApoA-I) protects Nile tilapia (Oreochromis niloticus) against bacterial infection. Fish Shellfish Immunol. (2023) 139:108925. doi: 10.1016/j.fsi.2023.108925
75. Karan S, Mohapatra A, Ko P, et al. Structural-functional characterization of recombinant Apolipoprotein A-I fromLabeo rohitademonstrates heat-resistant antimicrobial activity. Microbiol Biotechnol. (2020) 104:145–59. doi: 10.1007/s00253-019-10204-
76. Jiao Y-l, Wu M-P. Apolipoprotein A-I diminishes acute lung injury and sepsis in mice induced by lipoteichoic acid. Cytokine. (2008) 43:83–7. doi: 10.1016/j.cyto.2008.04.002
77. Ahmad I, Xuan T, Wang Q, Zhang S, Wang L, Gu J, et al. Bacterial lipoteichoic acid induces capsular contracture by activating innate immune response. Plast Reconstr Surg. (2023) 8:342–56. doi: 10.1097/PRS.0000000000011054
78. Rossol M, Heine H, Meusch U, et al. LPS-induced cytokine production in human monocytes and macrophages. Crit Rev Immunol. (2011) 31:379–446. doi: 10.1615/CritRevImmunol.v31.i5
79. Yamada H, Umemoto T, Kawano M, et al. High-density lipoprotein inhibits human M1 macrophage polarization through redistribution of caveolin-1. Br J Pharmacol. (2016) 173:741–51. doi: 10.1111/bph.13319
80. Guo L, Ai J, Zheng Z, Howatt DA, Daugherty A, Huang B, et al. High density lipoprotein protects against polymicrobe-induced sepsis in mice. J Biol Chem. (2013) 288:17947–53. doi: 10.1074/jbc.M112.442699
81. Anantharamaiah GM, Jones JL, Brouillette CG, Schmidt CF, Chung BH, Hughes TA, et al. Studies of synthetic peptide analogs of the amphipathic helix. Structure of complexes with dimyristoyl phosphatidylcholine. J Biol Chem. (1985) 260:10248–55. doi: 10.1016/S0021-9258(17)39238-4
82. Venkatachalapathi YV, Phillips MC, Epand RM, Epand RF, Tytler EM, Segrest JP, et al. Effect of end group blockage on the properties of a class A amphipathic helical peptide. Proteins. (1993) 15:349–59. doi: 10.1002/prot.340150403
83. Wool GD, Reardon CA, Getz GS. Apolipoprotein A-I mimetic peptide helix number and helix linker influence potentially anti-atherogenic properties. J Lipid Res. (2008) 49:1268–83. doi: 10.1194/jlr.M700552-JLR200
84. Stoekenbroek RM, Stroes ES, Hovingh GK. ApoA-I mimetics. Handb Exp Pharmacol. (2015) 224:631–48. doi: 10.1007/978-3-319-09665-0_21
85. Kelesidis T, Madhav S, Petcherski A, Cristelle H, O'Connor E, Hultgren NW, et al. The ApoA-I mimetic peptide 4F attenuates in vitro replication of SARS-CoV-2, associated apoptosis, oxidative stress and inflammation in epithelial cells. Virulence. (2021) 12:2214–27. doi: 10.1080/21505594.2021.1964329
86. Guo Y, Li W, Qian M, Jiang T, Guo P, Du Q, et al. D-4F ameliorates contrast media-induced oxidative injuries in endothelial cells via the AMPK/PKC pathway. Front Pharmacol. (2021) 15:11. doi: 10.3389/fphar.2020.556074
87. Paul S, Gangwar A, Bharg K, Ahmad Y. D-4F prophylaxis enables redox and energy homeostasis while preventing inflammation during hypoxia exposure. BioMed Pharmacother. (2021) 133:111083. doi: 10.1016/j.biopha.2020.111083
88. Lu J, Gao J, Sun J, Wang H, Sun H, Huang Q, et al. Apolipoprotein A-I attenuates peritoneal fibrosis associated with peritoneal dialysis by inhibiting oxidative stress and inflammation. Front Pharmacol. (2023) 28:14. doi: 10.3389/fphar.2023.1106339
89. McGrath KC, Li X, Twigg SM, Heather AK. Apolipoprotein-AI mimetic peptides D-4F and L-5F decrease hepatic inflammation and increase insulin sensitivity in C57BL/6 mice. PloS One. (2020) 15:e0226931. doi: 10.1371/journal.pone.0226931
90. Moreira RS, Irigoyen MC, Capcha JMC, Sanches TR, Gutierrez PS, Garnica MR, et al. Synthetic apolipoprotein A-I mimetic peptide 4F protects hearts and kidneys after myocardial infarction. Am J Physiol Regul Integr Comp Physiol. (2020) 318:R529–44. doi: 10.1152/ajpregu.00185.2019
91. Daskou M, Mu W, Sharma M, Vasilopoulos H, Heymans R, Ritou E, et al. ApoA-I mimetics reduce systemic and gut inflammation in chronic treated HIV. PloS Pathog. (2022) 18:e1010160. doi: 10.1371/journal.ppat.1010160
92. Gille A, Easton R, D’Andrea D, Wright SD, Shear CL. CSL-112 enhances biomarkers of reverse cholesterol transport after single and multiple infusions in healthy subjects. Arterioscler Thromb Vasc Biol. (2014) 34:2106–14. doi: 10.1161/ATVBAHA.114.303720
93. Nasr H, Torsney E, Poston RN, Hayes L, Gaze DC, Basser R, et al. Investigating the efect of a single infusion of reconstituted highdensity lipoprotein in patients with symptomatic carotid plaques. Ann Vasc Surg. (2015) 29:1380–91. doi: 10.1016/j.avsg.2015.04.084
94. Richart AL, Reddy M, Khalaji M, Natoli AL, Heywood SE, Siebel AL, et al. ApoA-I nanoparticles delivered post myocardial infarction moderate infammation. Circ Res. (2020) 127:1422–36. doi: 10.1161/CIRCRESAHA.120.316848
95. Didichenko SA, Adam J, Navdaev AV, Wong M, Alhamdoosh M, Saxenhofer M, et al. Abstract 10491: CSL112 suppresses infammation in human monocyte-derived macrophages. Circulation. (2021) 144:A10491. doi: 10.1161/circ.144.suppl_1.10491
96. Mogilenko DA, Shavva VS, Dizhe EB, Orlov SV. Characterization of distal and proximal alternative promoters of the human apoA-I gene. Mol Biol (Mosk). (2019) 53:485–96. doi: 10.1134/S0026898419030121
97. Frankel R, Sparr E, Linse S. On the aggregation of apolipoprotein A-I. Int J Mol Sci. (2022) 23:8780. doi: 10.3390/ijms23158780
98. Nilsson O, Lindvall M, Obici L, Ekström S, Lagerstedt JO, Del Giudice R. Structure dynamics of ApoA-I amyloidogenic variants in small HDL increase their ability to mediate cholesterol efflux. J Lipid Res. (2021) 62:100004. doi: 10.1194/jlr.RA120000920
99. Su X, Zhang G, Cheng Ye, Wang B. New insights into the emerging effects of inflammatory response on HDL particles structure and function. Mol Biol Rep. (2021) 48:5723–33. doi: 10.1007/s11033-021-06553-0
100. Zamanian-Daryoush M, Lindner DJ, Buffa J, Gopalan B, Na J, Hazen SL, et al. Apolipoprotein A-I anti-tumor activity targets cancer cell metabolism. Oncotarget. (2020) 11:1777–96. doi: 10.18632/oncotarget.v11i19
101. Chroni A, Kardassis D. HDL dysfunction caused by mutations in apoA-I and other genes that are critical for HDL biogenesis and remodeling. Curr Med Chem. (2019) 26:1544–75. doi: 10.2174/0929867325666180313114950
102. King TW, Cochran BJ, Rye K-A. ApoA-I and diabetes. Arterioscler Thromb Vasc Biol. (2023) 43:1362–8. doi: 10.1161/ATVBAHA.123.318267
103. Lee M, Lim J-S, Kim Y, Park SH, Lee S-H, Kim C, et al. High apoB/apoA-I ratio predicts post-stroke cognitive impairment in acute ischemic stroke patients with large artery atherosclerosis. Nutrients. (2023) 15:4670. doi: 10.3390/nu15214670
104. Zuin M, Cervellati C, Trentini A, Passaro A, Rosta V, Zimetti F, et al. Association between serum concentrations of apolipoprotein A-I (ApoA-I) and alzheimer’s disease: systematic review and meta-analysis. Diagnostics (Basel). (2021) 11:984. doi: 10.3390/diagnostics11060984
105. Lin J, Si Z, Wang A. Predictive value of ApoB/ApoA-I for recurrence within 1 year after first incident stroke. Front Neurol. (2024) 11:14. doi: 10.3389/fneur.2023.1308442
106. Nachar W, Merlet N, Maafi F, Mihalache-Avram T, Mecteau M, Gélinas D, et al. ApoA-I mimetic does not improve left ventricular diastolic dysfunction in rabbits without aortic valve stenosis. Int J Cardiol. (2021) 15:331. doi: 10.1016/j.ijcard.2020.12.089
107. Bloedon LT, Dunbar R, Duffy D, Pinell-Salles P, Norris R, DeGroot BJ, et al. Safety, pharmacokinetics, and pharmacodynamics of oral apoA-I mimetic peptide D-4F in high-risk cardiovascular patients. J Lipid Res. (2008) 49:1344–52. doi: 10.1194/jlr.P800003-JLR200
108. Watson CE, Weissbach N, Kjems L, Ayalasomayajula S, Zhang Y, Chang I, et al. Treatment of patients with cardiovascular disease with L-4F, an apo-A1 mimetic, did not improve select biomarkers of HDL function. J Lipid Res. (2011) 52:361–73. doi: 10.1194/jlr.M011098
109. D’Souza W, Stonik JA, Murphy A, Demosky SJ, Sethi AA, Moore XL, et al. Structure/function relationships of apolipoprotein a-I mimetic peptides: implications for antiatherogenic activities of high-density lipoprotein. Circ Res. (2010) 107:217–27. doi: 10.1161/CIRCRESAHA.110.216507
Keywords: Apolipoprotein A-I, macrophage, dendritic cell, neutrophil, T lymphocyte, anti-inflammatory
Citation: Tao X, Tao R, Wang K and Wu L (2024) Anti-inflammatory mechanism of Apolipoprotein A-I. Front. Immunol. 15:1417270. doi: 10.3389/fimmu.2024.1417270
Received: 14 April 2024; Accepted: 19 June 2024;
Published: 08 July 2024.
Edited by:
Paola Patrignani, University of Studies G. d’Annunzio Chieti and Pescara, ItalyReviewed by:
Stefania Tacconelli, University of Studies G. d’Annunzio Chieti and Pescara, ItalyPietro Minuz, University of Verona, Italy
Copyright © 2024 Tao, Tao, Wang and Wu. This is an open-access article distributed under the terms of the Creative Commons Attribution License (CC BY). The use, distribution or reproduction in other forums is permitted, provided the original author(s) and the copyright owner(s) are credited and that the original publication in this journal is cited, in accordance with accepted academic practice. No use, distribution or reproduction is permitted which does not comply with these terms.
*Correspondence: Kaiyang Wang, a2FpeWFuZzM5MzdAMTI2LmNvbQ==
†These authors have contributed equally to this work and share first authorship