- 1Scientific Research Center, The Seventh Affiliated Hospital, Sun Yat-sen University, Shenzhen, Guangdong, China
- 2Department of Pharmaceutical Sciences, College of Pharmacy and Health Sciences, St. John’s University, New York, NY, United States
- 3Big Data Center, The Seventh Affiliated Hospital, Sun Yat-sen University, Shenzhen, Guangdong, China
- 4Department of Oncology, The Seventh Affiliated Hospital, Sun Yat-sen University, Shenzhen, Guangdong, China
The connections between cancer stem cells (CSCs) and epithelial-mesenchymal transition (EMT) is critical in cancer initiation, progression, metastasis, and therapy resistance, making it a focal point in cancer theragnosis. This review provides a panorama of associations and regulation pathways between CSCs and EMT, highlighting their significance in cancer. The molecular mechanisms underlined EMT are thoroughly explored, including the involvement of key transcription factors and signaling pathways. In addition, the roles of CSCs and EMT in tumor biology and therapy resistance, is further examined in this review. The clinical implications of CSCs-EMT interplay are explored, including identifying mesenchymal-state CSC subpopulations using advanced research methods and developing targeted therapies such as inhibitors and combination treatments. Overall, understanding the reciprocal relationship between EMT and CSCs holds excellent potential for informing the development of personalized therapies and ultimately improving patient outcomes.
1 Introduction
Cancer stem cells (CSCs) are a subpopulation with stem cell-like capabilities including self-renewal and differentiation capabilities. These characteristics allow CSCs to be tumorigenic and to shape tumor plasticity and heterogeneity (1). Therefore, CSCs are believed to be involved in tumor initiation, progression, recurrence, metastasis, and therapeutic resistance (2–4). Understanding the unique properties of CSCs has become a focus of cancer research, intending to develop targeted therapies that can effectively eliminate these cells. The CSC theory integrates genomic medicine with the broader context of the tumor microenvironment, emphasizing the interplay between genetic mutations and epigenetic modifications, cellular interactions, and extracellular signals. This holistic approach underscores the importance of considering the tumor as a complex ecosystem, where CSCs interact with other tumor cells and the surrounding stroma (5). Such interactions can influence the behavior of CSCs, including their ability to undergo epithelial-mesenchymal transition (EMT), which is a biological process that allows epithelial cells to acquire mesenchymal, stem cell-like properties, enhancing their migratory capacity, invasiveness, and resistance to apoptosis (6).
The correlation between CSCs and EMT is complex and multifaceted and has garnered substantial attention due to its implication in the orchestration of tumor heterogeneity and malignancy (Figure 1). For instance, in triple-negative breast cancer, tumor-associated macrophages have been found to promote EMT and enhance CSC properties via the activation of CCL2/AKT/β-catenin signaling (7). Similarly, Connexin46 expression was found to enhance CSC and EMT properties in human breast cancer MCF-7 cells (8). Furthermore, EMT signaling and CSCs present emerging biomarkers and opportunities for precision therapeutics, particularly in the context of prostate cancer (9, 10). It has become imperative to investigate the intricate relationship between EMT and CSCs in the context of cancer, as this understanding has emerged as a critical area of cancer research with significant implications for developing precision medicine. It is now recognized that EMT contributes to the acquisition of CSC traits in non-CSCs, promoting their self-renewal and differentiation abilities, and enhancing their resistance to therapy (11). Moreover, CSCs have been identified as critical drivers of metastasis, and EMT plays a pivotal role in disseminating these cells from the primary tumor to distant sites (12).
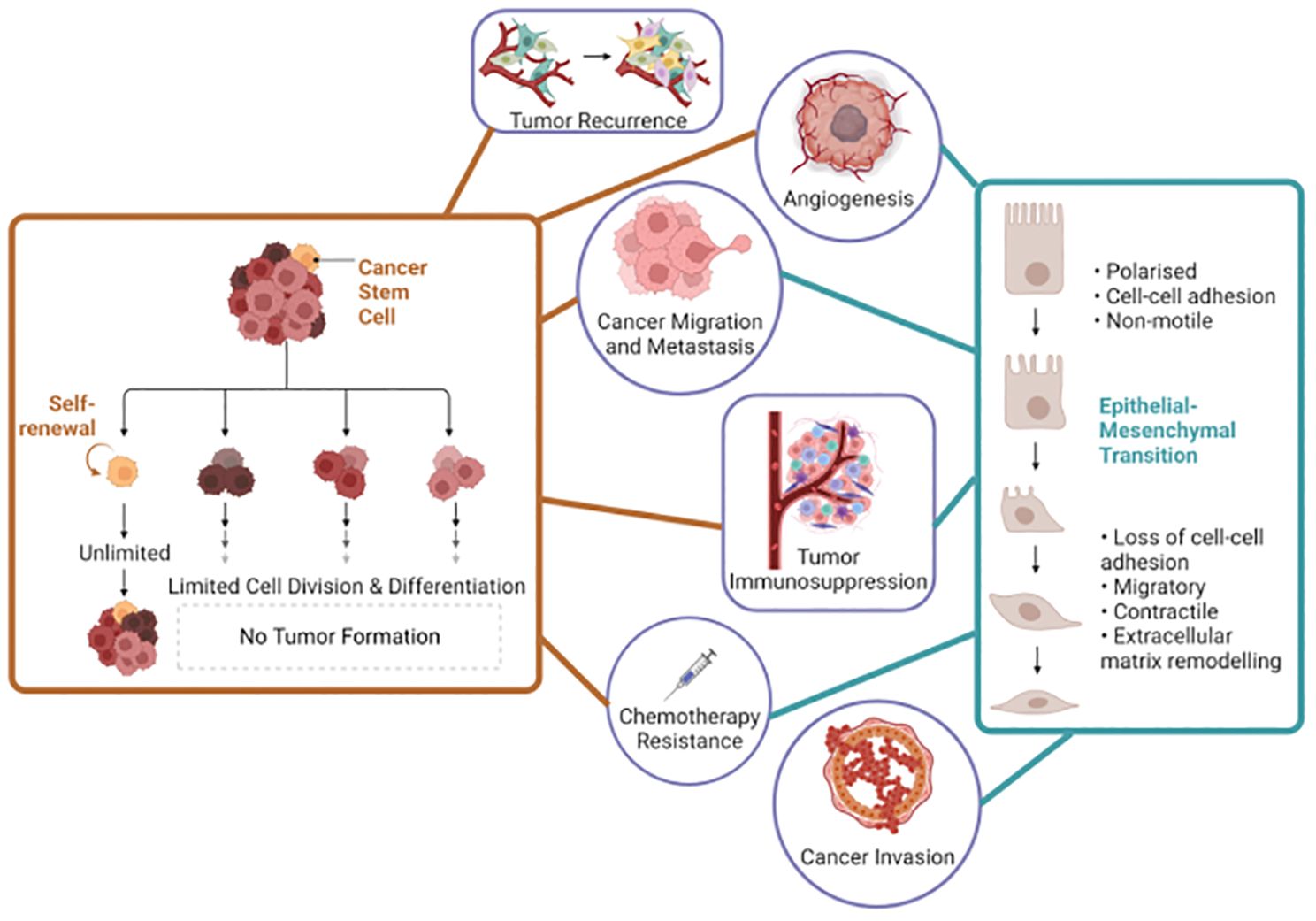
Figure 1. Interconnected roles of cancer stem cells and epithelial-mesenchymal transition in cancer biology. Left side the orange lines represent the key roles of cancer stem cells and the right side the blue lines represent the key roles of epithelial-mesenchymal transition in cancer progression and characteristics. This figure was created with Biorender.com.
Comprehending the dynamic interplay between EMT and CSCs is crucial for addressing the challenges of tumor heterogeneity, metastasis, and therapy resistance in clinical practice. This understanding forms the foundation for developing innovative, personalized therapeutic approaches that can improve patient outcomes and lead to more effective cancer management. EMT and CSCs are intricately linked, with EMT enhancing CSC properties and contributing to tumor heterogeneity and therapeutic resistance. Key signaling pathways, including TGF-β and Wnt/β-catenin, and transcription factors like Snail and SOX2, drive this interplay, complicating cancer treatment. Personalized medicine, leveraging insights into EMT-CSC dynamics, offers targeted strategies to overcome resistance and improve patient outcomes.
2 The interplay between EMT and CSCs
2.1 Characteristics of CSCs and EMT
CSCs are studied extensively for their role in tumor initiation, progression, and metastasis. Cancer-specific stem cell growth is regulated with the help of specific microenvironments, and interaction with these microenvironments plays a key role in the maintenance of CSC populations (13). This environment consists of a variety of factors such as hypoxic regions, stromal cells, immune cells, network of cytokines and growth factors, and the extracellular matrix (ECM), which helps in supporting the stemness of these CSCs by modifying the self-renewal pathways such as Wnt/β-catenin, Notch, NF-κB, JAK/STAT and Hedgehog or by hindering the transcriptional regulators such as NANOG, OCT-4, and SOX-2 (13–16). CSC-enriched populations can be distinguished by identifying specific normal stem cell markers and some distinct markers such as CD133, CD24, CD44, EpCAM (epithelial cell adhesion molecule), CD117, THY1, ALDH1, and CD200 when they are isolated from solid or hematological tumor tissues (16–21). Certain cancer cells have the flexibility to undergo a reversible dedifferentiation process, such as epithelial to mesenchymal transition(EMT), and convert from a differentiated state to a stem cell-like state depending on the distinct environmental stimulus (11). These cancer cells that have undergone EMT become more invasive and metastatic due to the presence of self-renewal EMT-mediating transcription factors such as Snail, Zebi, SOX2, KLF4, and many others which were discussed extensively before (13, 22). Numerous cellular processes, such as migration, metastasis, invasion, ECM alteration, and apoptosis, were controlled by EMT (12). EMT markers and stem cell markers (Table 1) are found to be expressed in circulating tumor cells in metastasized patients, which makes them potential targets for treating cancer in a targeted approach (52).
EMT is an intricate and highly dynamic cellular process that assumes a pivotal role in various physiological and pathological contexts (53), encompassing embryonic development (54), chronic inflammation (55), fibrotic diseases like renal fibrosis (56), as well as cancer progression and metastasis (57). Traditionally, epithelial cells were characterized as terminally differentiated with distinct apical-basal polarity (58)serving protective, supportive, and secretory roles (59, 60). However, recent findings show that epithelial cells can undergo a series of changes that entail the loss of cell polarity (61), disintegration of intercellular tight junctions and adherent junctions, acquisition of migratory capabilities (62, 63), and the adoption of mesenchymal cell morphology and attributes (64), culminating in what is defined as EMT. Therefore, EMT is mainly characterized by the loss of polarity of epithelial cells and the acquisition of mesenchymal properties (65), including fibroblast-like appearance (66) and the upregulation of genes like vimentin (67, 68), Snail (69), and osteopontin (70). Consequently, cells transition towards a fibroblast-like morphology or outright transformation into mesenchymal cells, accompanied by enhanced migration and metastatic potential (71). It is a complex dynamic process, mainly manifested as, on the one hand, it occurs between cells, leading to the relaxation of tight cell-cell junctions and ECM degradation through enzymatic hydrolysis (63); on the other hand, it occurs inside cells, involving profound cytoskeletal reorganization, diminished keratin expression, actin filament restructuring, and the induction of vimentin expression, ultimately morphing cells into spindle-shaped fibroblast-like entities (66, 68). These changes collectively amplify cellular migratory and invasive capacities, thus constituting a foundation for physiological and pathological phenomena.
2.2 Role of CSCs and EMT in tumor initiation, progression and metastasis
Previous literature has speculated that even one CSC can be enough to regenerate a tumor (6). However, in order to maintain their stemness, CSCs required to be in exposure of specialized microenvironments also known as stem cell niches (13, 72). Several niches, such as hypoxic conditions, vascular niche etc, were studied extensively to understand how these microenvironments were aiding in maintaining the CSCs and reprogramming normal cancer cells to CSCs thereby promoting more tumor formations. One such example of vascular niche involvement was a study that has demonstrated CSC populations expressing CD133+ marker in brain, liver, and pancreatic cancers produce increased levels of vascular endothelial growth factor (VEGF) and stromal-derived factor-1 (SDF-1), which stimulate angiogenesis thereby promoting metastasis (73–75). Accumulating evidence shows EMT is associated with many signaling pathways such as androgen receptor signaling, estrogen receptor signaling, TGF-β (transforming growth factor β) signaling, epidermal growth factor (EGF) signaling, Sonic hedgehog and WNT signaling pathways and its role in the related tissue development, wound healing and cancer (76, 77). Self-renewal pathways such as NF-κB and the Wnt/β-catenin pathway were shown to contribute to EMT activation, which is directly related to increased cancer invasiveness and aggressiveness, resulting in poor patient outcomes (20, 78). The interplay between CSC and EMT-involved pathways was regulated at a genetic level (79).
In particular scenarios like breast cancer, CSCs identified as BCSCs (Breast cancer stem cells) exist in specific development states, the first one being in a mesenchymal state expressing CD24-/CD44+ markers on their cell surface and the second being in an epithelial cell-like state expressing an enzyme known as Aldehyde dehydrogenase (ALDH) (80). A more significant tumor-initiating capacity was observed in BCSCs expressing both the marker and enzyme profiles (81).
2.3 Alteration of tumor heterogeneity, plasticity and tumor microenvironment alteration
Numerous literatures have shown that tumors contain explicit cell populations that vary in multiple factors like karyotype, phenotype, and chemoresistance (82–84). Sequencing studies have shown distinct epigenetic characteristics of multiple cell clones within the same tumor (85). This phenomenon is termed tumor heterogeneity and is primarily orchestrated by producing various phenotypically different subclones inside the tumor mass by CSCs (86–88). EMT also contributes to cell plasticity and promotes intra-tumor heterogeneity (89, 90).
Both EMT and CSC properties were related in tumors exhibiting resistance to cytotoxic T-lymphocytes (91). Tumor-associated macrophages (TAMs), a part of the stem niche in the tumor microenvironment, contributed to EMT characterization (92). Other studies have also indicated that TAMs can induce chemoresistance in myeloma cell lines by directly interacting with malignant cells and inhibiting the activation of caspase-dependent apoptotic signaling (19).
Overexpression of drug transporter proteins of the ABC family (mainly MDR1, ABCG2, and ABCB1) on the cell surface of various CSCs has been shown to contribute to chemo-drug resistance and promote disease relapse (93, 94). Self-renewal and cell proliferation signaling pathways like JNK were shown to be upregulated in CSCs and contribute towards cancer resistance by reducing the generation of intracellular ROS caused by 5-fluorouracil and gemcitabine (95, 96).
3 Molecular mechanisms and regulatory factors of EMT
3.1 Characteristics of EMT
The regulation of EMT involves a variety of molecular mechanisms and regulatory factors. Several key signaling pathways and transcription factors have been identified as central players in controlling EMT and its association with cancer stem cells (CSCs). For example, transforming growth factor-β (TGF-β) signaling is a potent inducer of EMT, promoting downregulation of E-cadherin and upregulation of N-cadherin and vimentin, thereby driving cells toward a mesenchymal phenotype development (97, 98). In addition, the Wnt/β-catenin pathway activates EMT-associated transcription factors such as Snail and Twist, suppressing epithelial markers and acquiring stem cell-like properties (99, 100). Furthermore, transcription factors such as Nanog, OCT4, SOX2, and KLF4, which are critical for maintaining stem cell pluripotency, have been found to interact with EMT regulators to enhance the stem-like characteristics of cancer cells (101–103).
Tumors are composed of heterogeneous cell populations. Under normal circumstances, maintaining the homeostasis of various tissues and organs in the body is based on self-renewal, multidirectional differentiation and proliferation of CSCs are all modulated by intricate regulatory mechanisms. However, the interplay of various stimulating factors in vivo and in vitro, in conjunction with the influence of CSCs, can trigger genetic mutations, promoting the development of tumors (5). In fact, CSCs tend to accumulate numerous gene mutations, which lead to the loss of normal regulation of cells, excessive proliferation, and even metastasis (2–4). Essential signaling pathways governing cellular division and differentiation, such as Notch, Wnt/β-Catenin, Ras/Raf/Mek/Erk, exert substantial influence within the context of CSCs (104). Altered or hyperactive signaling through any of these pathways can disrupt standard cellular regulatory mechanisms, leading to the occurrence of tumors (105). The complex network of molecular mechanisms and regulatory factors that control EMT and its association with CSCs highlights the complexity of this process and its importance in cancer biology.
3.2 Signaling pathways and transcription factors regulating EMT and CSCs
EMT is a complex process regulated by a network of signaling pathways and transcription factors intricately involved in controlling CSCs (Figure 2). Among these pathways, TGF-β stands out as a prominent inducer of EMT in various cancer types. TGF-β activates downstream Smad signaling, leading to upregulating transcription factors such as Snail, Slug, and Twist (106). These EMT-associated transcription factors actively downregulate E-cadherin while promoting the expression of mesenchymal markers, including N-cadherin and vimentin (107–109). Consequently, the epithelial cells undergo a metamorphosis toward a mesenchymal phenotype. TGF-β is paramount in EMT, often serving as a positive control in inducing EMT in experimental setups (109). In addition, TGF-β can enhance the self-renewal ability of CSCs, contributing to tumor progression and treatment resistance. As a pleiotropic cytokine, TGF-β exists in three subtypes: TGF-β1, TGF-β2, and TGF-β3 (110), with TGF-β1 being the extensively studied variant. Its presence is widespread in diverse tumors, exerting regulatory effects on diverse cellular processes such as immunosuppression, growth inhibition, EMT, and cell migration (111, 112). The role of TGF-β varies throughout tumorigenesis; in the early stages, it operates as a tumor suppressor, while in advanced tumors, it facilitates tumor growth (113, 114). TGF-β-induced EMT signaling pathways in tumor cells can be divided into two types according to whether Smad protein is involved, Smad-dependent classic TGF-β signaling pathway and Smad-independent TGF-β signaling pathway. In the TGF-β/Smad signaling pathway, TGF-β binds to specific receptors on the cell membrane, inducing phosphorylation of Smad2/Smad3, followed by heterotrimer formation with Smad4 (115). This complex translocate to the nucleus, upregulating transcription factors like Snail/Slug and Twist (106). Concurrently, E-cadherin expression is downregulated, effectively controlling EMT at the transcriptional level (97). For Smad-independent signaling pathways, on the other hand, alternate EMT-associated pathways like integrin, PI3K/AKT, and mitogen-activated protein kinase (MAPK) (116). TGF-β’s interaction with its transmembrane receptor activates PI3K, which generates the secondary messenger PIP3, subsequently phosphorylating AKT. This leads to AKT translocating to the nucleus, hindering GSK-3β-mediated degradation of β-catenin or activating nuclear factor-κB, thereby influencing EMT (117, 118). In addition to the PI3K/AKT signaling pathway, TGF-β’s involvement extends to the Ras-Raf-MAPK pathway, where downstream transcription factors, including extracellular signal-regulated kinases 1/2 (ERK1/2) and p38MAPK, facilitate EMT by modulating downstream target gene transcription (116).
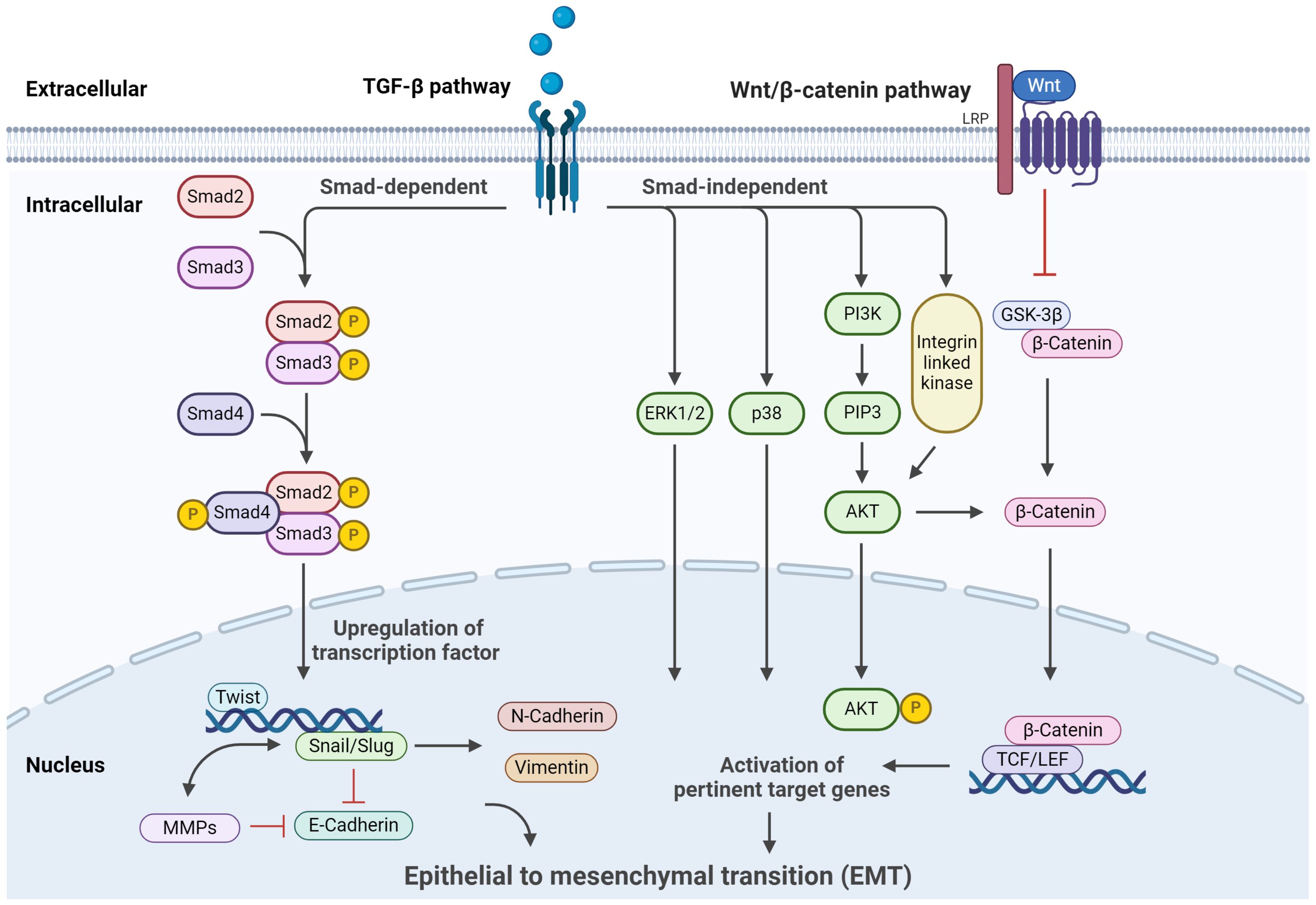
Figure 2. Signaling pathways regulating EMT. The primary signaling and crosstalk of the TGF-β/Smad signaling pathway, Wnt/β-catenin signaling pathway, Hedgehog signaling pathways, and their regulatory roles in cellular processes are illustrated. This figure was created with Biorender.com.
The Wnt/β-catenin pathway emerges as a pivotal regulator of EMT and CSC. In the Wnt/β-catenin signaling pathway, the Wnt protein binds to the corresponding transmembrane receptors, thereby inhibiting GSK-3β activity (118). This stymies the degradation of β-catenin, resulting in its intracellular accumulation. When β-catenin accumulates to a certain extent, it enters the nucleus, which collaborates with lymphoid enhancer factor/T cell factor (LEF/TCF) transcription factors to activate genes pertinent to EMT (119, 120). This sequence leads to the suppression of E-cadherin expression and facilitates EMT progression. In the process of EMT, an important molecular event is the downregulation of E-cadherin, and the transcription factor Snail can bind to the E-cadherin promoter region to inhibit the expression of E-cadherin (121). E-cadherin is a typical single transmembrane glycoprotein, which can regulate the adhesion between Ca2+-dependent cells and play an essential role in cell polarization and tissue formation (122). Downregulation or inhibition of E-cadherin expression will turn on EMT and lead to tumor invasion and metastasis. Batlle et al. determined that in many tumors, E-cadherin is considered to be the target gene directly acted by Snail, which directly binds to the E-box sequence of the promoter region of E-cadherin, which reduces the expression or defect of E-cadherin, thereby triggering EMT (123). In addition, Zhang et al. found that antisense Snail prevents the occurrence of EMT, and the process can be reversed by silencing the expression of Snail protein (124). Furthermore, the transcription factor Snail can indirectly upregulate the expression of matrix metalloproteinase (MMPS) family members MMP-1, MMP-2, and MMP-7, inhibit matrix synthesis, accelerate matrix component decomposition, and increase cell invasion ability (125). Therefore, Snail induces EMT and plays an essential role in tumor invasion and metastasis. Indeed, the significance of Snail’s involvement extends beyond EMT, encompassing various processes within tumor initiation and embryonic morphogenesis (126). Snail can initiate EMT from different pathways, and Snail plays a central role in this process, coordinating the induction of different signaling pathways. Experiments have observed that normal stem cell proliferation is disordered when the Wnt pathway is abnormally activated, and even tumor proliferation is formed. On the contrary, Fatima et al. shut down the Wnt signaling pathway of cells, reduced the content of β-catenin in liver cancer cells, and observed that the proliferation of liver cancer cells was weakened (127, 128). The Wnt signaling pathway plays a crucial role in colorectal carcinogenesis and has emerged as a target for stem cell therapy against colon cancer.
Moreover, the process of EMT is also affected by transcription factors that play an essential role in stem cell pluripotency. These factors, including Nanog, OCT4, SOX2, and KLF4, constitute pivotal determinants for maintaining the undifferentiated state of embryonic stem cells (101–103). Aberrant expression of these factors within the context of cancer cells can profoundly propel EMT processes and promote CSC properties. Specifically, Nanog, emerging as a standout influencer, has been shown to directly regulate EMT-related genes and promote the stemness of cancer cells (101, 102). The Notch pathway is highly conserved in the process of biological evolution, comprising Notch receptors (Notch1-4), Notch ligands (Jagged 1, Jagged 2, Delta-likeligand1-4) and CSL (DNA binding protein), dividing into CSL-dependent pathway and CSL-independent pathway (129). Its primary roles encompass the maintenance of stem cell existence and initiating postnatal embryonic or fetal cell differentiation. The Notch pathway affects cell differentiation, proliferation, and apoptosis, constituting a pivotal determinant in the genesis of malignant tumors (130). Likewise, OCT4 and SOX2 have been discerned to interact collaboratively with EMT transcription factors, thereby intensifying cancer cells’ plasticity and stem-like attributes. The hedgehog signaling pathway is essential in CSCs, mainly in basal cell tumors (131). This pathway is regulated by two receptors, namely Patched and Smoothened (SMO), positioned on the target cell membrane (132). Patched receptors inhibit the activation of the Hedgehog pathway, while SMO receptors promote the activation of the Hedgehog pathway. Activation of SMO receptors triggers downstream Hedgehog target gene activation, thereby delineating an intricate regulatory landscape governing cellular proliferation and survival (133, 134).
The intricate interplay among signaling pathways, such as TGF-β and Wnt/β-catenin, along with transcription factors including Snail, Nanog, OCT4, SOX2, and KLF4, controls the EMT process and the development of CSCs. Many factors regulate the occurrence of EMT in tumor cells. After undergoing EMT, tumor cells can migrate to adjacent organs or distant sites, concurrently conferring resistance against conventional chemotherapy and drug treatment. Understanding the convoluted regulatory networks that control EMT and CSCs can provide valuable insights into tumor progression, metastasis, and resistance to therapeutic interventions. However, studies have shown that EMT is not an irreversible process, and reversing or inhibiting EMT may be an effective way to inhibit tumor cell migration or distant metastasis (135). Targeting these pathways and factors holds great promise in developing novel and personalized cancer therapies. Further research in this area will help advance our knowledge and improve clinical outcomes for cancer patients.
4 Clinical implications of EMT and CSCs: biomarkers and targeted therapies
The research on EMT-related signaling pathways provides a direction for treating tumors and has also become the main target of clinical new drug development. Studies have found that integrin-linked kinase can activate AKT to lead to EMT, and down-regulating integrin-linked kinase can inhibit EMT and metastasis of tumor cells in endocrine cancer (136). Similarly, cysteine protease inhibitor C (CystC) modulates the TGF-β signaling pathway across both normal and tumor cells (137). The Ras pathway also suggests targets for the research of tumor therapeutic drugs. For example, farnesyltransferase inhibitors play an anti-tumor effect by inhibiting the binding of Ras to the cell membrane and have been applied to the treatment of various tumors (138). Src kinase inhibitors such as dasatinib can effectively inhibit the growth of cells undergoing EMT, thereby inhibiting tumor growth (139). Based on the close relationship between EMT and all aspects of tumor metastasis, designing targeted intervention strategies for the core regulatory mechanisms of EMT has become a research hotspot in metastasis treatment. The initial strategy to intervene in EMT is to directly block or reverse EMT to allow cells to regain their epithelial cell characteristics. This is exemplified by interventions like ADH-1 targeting N-cadherin (140), Fresolimumab targeting TGF-β (141), Catumaxomab targeting EpCAM (142), and GN-25 targeting Snail (143).
Furthermore, the landscape of tumor therapy has been redefined through the advent of RNA interference and (miRNA) silencing strategies. For example, the targeted silencing of Snail gene expression through short hairpin RNA has demonstrated the potential to reverse EMT and inhibit in vivo tumor growth (144). Similarly, the utilization of small interfering RNA targeting TGF-β has shown promise in attenuating tumor metastasis in vivo, substantiating the prospect of impeding EMT initiation via the inhibition of TGF-β secretion within the tumor cell matrix. Krutzfeldt et al. proposed that precise silencing of endogenous miRNAs and RNA antagonists could also silence specific miRNA expressions in vivo (145). Therefore, using miRNA as a therapeutic target to inhibit the occurrence of EMT is also a new strategy for tumor treatment.
Further research on EMT-related regulatory factors will help better understand the mechanism of tumor cell EMT and provide a new direction for understanding the mechanism of tumor metastasis and recurrence. Consequently, the multifaceted research imparts novel insights with the potential to reshape the approach to tumor treatment. Reducing the drug resistance of tumor cells by inhibiting EMT of tumor cells can be used as a supplement to conventional tumor therapy and become a new personalized tumor treatment method. Developing new anti-tumor drugs targeting EMT will be a new research direction.
Currently, the conventional cancer treatment modalities encompass surgical excision, chemotherapy, radiotherapy, ablation, and a combination of these methodologies. While these approaches have substantially enhanced the overall survival rate of cancer patients, the persisting challenge remains cancer recurrence. Although traditional approaches manage to eliminate the bulk of cancer cells, the enduring presence of cancer stem cells (CSCs), constituting a minute fraction of the total cellular population, invariably leads to cancer recurrence. The comprehensive eradication of these CSCs is a pivotal requisite for attaining curative outcomes. Consequently, CSCs are recognized as the origin of the occurrence and development of malignant tumors. By dissecting the attributes of CSCs, the potential emerges to elucidate the pertinent CSC surface markers, formulate tailored therapeutics targeting specific entities, and unearth novel avenues for malignancy management. In clinical spheres, the development of therapeutics and treatments singularly aimed at CSCs has gained traction, thereby conferring a degree of selectivity towards CSCs while preserving the homeostasis of normal stem cells. This selection arises from the observation that CSCs, equipped with ATP-binding cassette (ABC) transporters, can efflux drugs from their cytoplasm, thereby fortifying themselves against drug toxicity, endowing them with an inherent resistance to conventional chemotherapeutics and radiation therapy, along with a propensity for angiogenesis promotion.
Compared with paclitaxel, a commonly used chemotherapeutic drug for breast cancer, salinomycin demonstrated a significantly greater inhibitory effect on the growth of murine mammary tumors in breast cancer xenografted nude mice (146, 147). Salinomycin achieved this by targeting the EMT processes crucial for CSC maintenance and metastasis (148). Its efficacy is about 100 times greater than that of paclitaxel, due to its ability to inhibit EMT, thereby reducing the expression of key EMT markers such as Snail, Slug, and Twist (84, 149, 150). This process diminishes the CSC population, which is essential for tumor initiation, progression, and metastasis (151). Notably, the absence of breast cancer stem cell-associated gene expression, particularly the CD44+CD24- phenotype was observed with salinomycin treatment, further emphasizing its specific action against CSCs (152, 153). This heightened effect correlates with downregulating genes critical for CSC function, such as ALDH1 (154) and the ABC transporter genes (155, 156). Additionally, studies have shown that salinomycin disrupts the Wnt/β-catenin signaling pathway, which is often upregulated in CSCs, thereby enhancing its ability to target and eliminate these cells (157, 158). In contrast, paclitaxel primarily targets rapidly dividing cells but does not specifically affect the EMT or CSC pathways, which contributes to its reduced efficacy against CSCs (159, 160). Meanwhile, studies reported the capacity of pancreatic stem cells to undergo differentiation into functional pancreatic cells post-transplantation and play an essential role in the recovery of pancreatic endocrine function and the repair of pancreatic cell damage (161, 162). In addition, a small molecule Hedgehog signaling pathway inhibitor, cyclopamine, reduced acetaldehyde dehydrogenase by preventing aberrant pancreatic cancer Hedgehog signaling, which is often linked to CSC maintenance (163). This pharmacological approach presents promise in pancreatic cancer therapy by targeting pathways essential for CSC survival and EMT, similar to the mechanism of action of salinomycin in breast cancer.
Studies have shown that miRNA, combined with suitable carriers, can repair multiple disease-related abnormal pathways by restoring miRNA expression and targeting CSC (164), offering a novel approach to tumor therapy. In vitro assays and xenograft mice models demonstrated the effectiveness of inhibitors targeting signaling pathways like Wnt/β-catenin, Notch, and Hedgehog in effectively inhibiting CSC self-renewal (165, 166). The future focus of CSC research must be the development of precision drugs designed to selectively kill tumor cells to achieve the goal of eradicating tumors.
5 Summary
A growing body of research has illuminated the intricate and reciprocal relationship between EMT and CSCs, shedding light on their profound implications for cancer biology. EMT promotes CSC properties in non-CSCs, leading to tumor heterogeneity and enhanced metastatic potential (89, 90). Notably, EMT-induced phenotypic changes, including the loss of epithelial characteristics and the acquisition of mesenchymal traits, parallel the emergence of CSC traits (2–4). Moreover, the emergence of CSCs through EMT is closely linked to therapy resistance, as CSCs are known to be resilient to conventional treatments. EMT-driven CSCs often exhibit increased drug efflux mechanisms and the ability to evade the cytotoxic effects of therapeutic agents, presenting a formidable challenge in clinical oncology (93, 94).
Further investigations have unveiled the regulatory networks that govern the crosstalk between EMT and CSCs, highlighting key signaling pathways and transcription factors that serve as central orchestrators (97, 98). The TGF-β and Wnt/β-catenin pathways, among others, have been identified as critical inducers of EMT and are closely linked to CSC properties. Moreover, transcription factors such as Snail, Slug, Nanog, OCT4, SOX2, and KLF4 play pivotal roles in driving EMT-associated transformations and enhancing CSC properties (101–103). This intricate web of molecular mechanisms underscores the complexity of the EMT-CSC interplay. Disrupting this interplay is crucial for cancer therapy, potentially overcoming therapeutic resistance.
Additionally, the emergence of personalized medicine is intimately linked to understanding the EMT-CSC relationship. Through advanced research methods, it is now feasible to identify EMT-CSC subpopulations within individual tumors (167, 168). This provides a foundation for developing tailored treatments targeting a patient’s tumor’s specific molecular and cellular characteristics. Personalized therapies may involve a combination of EMT and CSC-targeted agents to maximize treatment efficacy while minimizing side effects.
In conclusion, the reciprocal relationship between EMT and CSCs has profound implications for developing targeted cancer therapies and personalized medicine. By disrupting this intricate interplay, we have the potential to transform the landscape of cancer treatment, offering new hope for patients and improving clinical outcomes.
Author contributions
JW: Conceptualization, Data curation, Formal Analysis, Funding acquisition, Investigation, Methodology, Project administration, Resources, Software, Supervision, Visualization, Writing – original draft, Writing – review & editing. Z-NL: Conceptualization, Data curation, Formal Analysis, Investigation, Software, Writing – original draft. Q-XT: Conceptualization, Data curation, Formal Analysis, Investigation, Resources, Software, Writing – original draft. JK: Conceptualization, Investigation, Software, Writing – original draft, Writing – review & editing. YRL: Conceptualization, Data curation, Investigation, Writing – review & editing. ZC: Conceptualization, Investigation, Writing – original draft, Writing – review & editing. LZ: Conceptualization, Data curation, Formal Analysis, Funding acquisition, Investigation, Writing – original draft. Z-XC: Conceptualization, Data curation, Investigation, Methodology, Project administration, Software, Supervision, Validation, Visualization, Writing – original draft, Writing – review & editing. SF: Conceptualization, Data curation, Formal Analysis, Funding acquisition, Investigation, Methodology, Resources, Software, Writing – original draft, Writing – review & editing. YCL: Conceptualization, Data curation, Formal Analysis, Funding acquisition, Investigation, Methodology, Project administration, Resources, Writing – original draft, Writing – review & editing. YP: Writing – original draft, Writing – review & editing.
Funding
The author(s) declare financial support was received for the research, authorship, and/or publication of this article. This work was supported by the National Key Research and Development Program (2018YFA0902801), National Natural Science Foundation of China (32270815), Guangdong Provincial Key Laboratory of Digestive Cancer Research (No. 2021B1212040006), the Postdoctoral Fellowship Program of CPSF under Grant Number (GZC20233233), the Guangdong basic and applied basic research foundation(2023A1515111044), the Shenzhen Science and Technology Program (Grant No. RCBS20231211090733052),and the Sanming Project of Medicine in Shenzhen (No. SZSM201911003).
Acknowledgments
We would like to acknowledge BioRender (https://biorender.com/) for the platform where the figures were generated.
Conflict of interest
The authors declare that the research was conducted in the absence of any commercial or financial relationships that could be construed as a potential conflict of interest.
The reviewer declared a shared parent affiliation with the authors JW, YL, ZC, LZ, SF, YL, YP to the handling editor at the time of review.
Publisher’s note
All claims expressed in this article are solely those of the authors and do not necessarily represent those of their affiliated organizations, or those of the publisher, the editors and the reviewers. Any product that may be evaluated in this article, or claim that may be made by its manufacturer, is not guaranteed or endorsed by the publisher.
Author disclaimer
The statements, opinions and data contained in all publications are solely those of the individual author(s) and contributor(s) and not of MDPI and/or the editor(s). MDPI and/or the editor(s) disclaim responsibility for any injury to people or property resulting from any ideas, methods, instructions or products referred to in the content.
References
1. Huang T, Song X, Xu D, Tiek D, Goenka A, Wu B, et al. Stem cell programs in cancer initiation, progression, and therapy resistance. Theranostics. (2020) 10(19):8721–43. doi: 10.7150/thno.41648
2. Zhou T, Wu L, Ma N, Tang F, Yu Z, Jiang Z, et al. SOX9-activated FARSA-AS1 predetermines cell growth, stemness, and metastasis in colorectal cancer through upregulating FARSA and SOX9. Cell Death Dis. (2020) 11(12):1071. doi: 10.1038/s41419-020-03273-4
3. Vainstein V, Kirnasovsky OU, Kogan Y, Agur Z. Strategies for cancer stem cell elimination: Insights from mathematical modeling. J Theor Biol. (2012) 298:32–41. doi: 10.1016/j.jtbi.2011.12.016
4. Nimmakayala RK, Leon F, Rachagani S, Rauth S, Nallasamy P, Marimuthu S, et al. Metabolic programming of distinct cancer stem cells promotes metastasis of pancreatic ductal adenocarcinoma. Oncogene. (2021) 40(1):215–31. doi: 10.1038/s41388-020-01518-2
5. Yoshida GJ. Emerging roles of Myc in stem cell biology and novel tumor therapies. J Exp Clin Cancer Res. (2018) 37(1):173. doi: 10.1186/s13046-018-0835-y
6. Hermann PC, Sainz B Jr.. Pancreatic cancer stem cells: A state or an entity? Semin Cancer Biol. (2018) 53:223–31. doi: 10.1016/j.semcancer.2018.08.007
7. Chen X, Yang M, Yin J, Li P, Zeng S, Zheng G, et al. Tumor-associated macrophages promote epithelial-mesenchymal transition and the cancer stem cell properties in triple-negative breast cancer through CCL2/AKT/β-catenin signaling. Cell communication Signaling Cell Commun Signal. (2022) 20:92. doi: 10.1186/s12964-022-00888-2
8. Acuña RA, Varas-Godoy M, Herrera-Sepulveda D, Retamal MA. Connexin46 expression enhances cancer stem cell and epithelial-to-Mesenchymal transition characteristics of human breast cancer MCF-7 cells. Int J Mol Sci. (2021) 22(22):12604. doi: 10.3390/ijms222212604
9. Chen L, Boleslaw Olszewski M, Kruithof-de Julio M, Snaar-Jagalska BE. Zebrafish microenvironment elevates EMT and CSC-like phenotype of engrafted prostate cancer cells. Cells. (2020) 9(4):797. doi: 10.3390/Cells9040797
10. Lee E, Wang J, Yumoto K, Jung Y, Cackowski FC, Decker AM, et al. DNMT1 regulates epithelial-mesenchymal transition and cancer stem cells, which promotes prostate cancer metastasis. Neoplasia. (2016) 18(9):553–66. doi: 10.1016/j.neo.2016.07.007
11. Cabrera MC, Hollingsworth RE, Hurt EM. Cancer stem cell plasticity and tumor hierarchy. World J Stem Cells. (2015) 7(1):27–36. doi: 10.4252/wjsc.v7.i1.27
12. Song J, Shi W. The concomitant apoptosis and EMT underlie the fundamental functions of TGF-β. Acta Biochim Biophys Sin (Shanghai). (2018) 50(1):91–7. doi: 10.1093/abbs/gmx117
13. Lau EY-T, Ho NP-Y, Lee TK-W. Cancer stem cells and their microenvironment: Biology and therapeutic implications. Stem Cells Int. (2017) 2017(0):3714190. doi: 10.1155/2017/3714190
14. Borah A, Raveendran S, Rochani A, Maekawa T, Kumar D. Targeting self-renewal pathways in cancer stem cells: Clinical implications for cancer therapy. Oncogenesis. (2015) 4(11):e177–e. doi: 10.1038/oncsis.2015.35
15. Kim J, Orkin SH. Embryonic stem cell-specific signatures in cancer: Insights into genomic regulatory networks and implications for medicine. Genome Med. (2011) 3(11):1–8. doi: 10.1186/gm291
16. Chen K, Huang Y-H, Chen J-I. Understanding and targeting cancer stem cells: Therapeutic implications and challenges. Acta Pharmacologica Sin. (2013) 34(6):732–40. doi: 10.1038/aps.2013.27
17. Dalerba P, Dylla SJ, Park I-K, Liu R, Wang X, Cho RW, et al. Phenotypic characterization of human colorectal cancer stem cells. Proc Natl Acad Sci. (2007) 104(24):10158–63. doi: 10.1073/pnas.0703478104
18. Yang ZF, Ho DW, Ng MN, Lau CK, Yu WC, Ngai P, et al. Significance of CD90+ cancer stem cells in human liver cancer. Cancer Cell. (2008) 13:153–66. doi: 10.1016/j.ccr.2008.01.013
19. Phi LTH, Sari IN, Yang Y-G, Lee S-H, Jun N, Kim KS, et al. Cancer stem cells (CSCs) in drug resistance and their therapeutic implications in cancer treatment. Stem Cells Int. (2018) 18:1–16. doi: 10.1155/2018/5416923
20. Kaltschmidt C, Banz-Jansen C, Benhidjeb T, Beshay M, Förster C, Greiner J, et al. A role for NF-κB in organ specific cancer and cancer stem cells. Cancers. (2019) 11(5):655. doi: 10.3390/cancers11050655
21. Walcher L, Kistenmacher A-K, Suo H, Kitte R, Dluczek S, Strauß A, et al. Cancer stem cells–origins and biomarkers: Perspectives for targeted personalized therapies. Front Immunol. (2020) 11:1280. doi: 10.3389/fimmu.2020.01280
22. Eun K, Ham SW, Kim H. Cancer stem cell heterogeneity: Origin and new perspectives on CSC targeting. BMB Rep. (2017) 50(3):117. doi: 10.5483/BMBRep.2017.50.3.222
23. Bao Z, Zeng W, Zhang D, Wang L, Deng X, Lai J, et al. SNAIL induces EMT and lung metastasis of tumours secreting CXCL2 to promote the invasion of M2-type immunosuppressed macrophages in colorectal cancer. Int J Biol Sci. (2022) 18(7):2867–81. doi: 10.7150/ijbs.66854
24. Liu A, Sun X, Xu J, Xuan Y, Zhao Y, Qiu T, et al. Relevance and prognostic ability of twist, slug and tumor spread through air spaces in lung adenocarcinoma. Cancer Med. (2020) 9(6):1986–98. doi: 10.1002/cam4.2858
25. Roudi R, Madjd Z, Ebrahimi M, Samani FS, Samadikuchaksaraei A. CD44 and CD24 cannot act as cancer stem cell markers in human lung adenocarcinoma cell line A549. Cell Mol Biol Lett. (2014) 19(1):23–36. doi: 10.2478/s11658-013-0112-1
26. Wang J, Shao F, Yang Y, Wang W, Yang X, Li R, et al. A non-metabolic function of hexokinase 2 in small cell lung cancer: Promotes cancer cell stemness by increasing USP11-mediated CD133 stability. Cancer Commun (Lond). (2022) 42(10):1008–27. doi: 10.1002/cac2.12351
27. Wang J, Yu H, Dong W, Zhang C, Hu M, Ma W, et al. N6-Methyladenosine-Mediated up-regulation of FZD10 regulates liver cancer stem cells' properties and lenvatinib resistance through WNT/β-catenin and hippo signaling pathways. Gastroenterology. (2023) 164(6):990–1005. doi: 10.1053/j.gastro.2023.01.041
28. Zhang C, Huang S, Zhuang H, Ruan S, Zhou Z, Huang K, et al. YTHDF2 promotes the liver cancer stem cell phenotype and cancer metastasis by regulating OCT4 expression Viam6A RNA methylation. Oncogene. (2020) 39(23):4507–18. doi: 10.1038/s41388-020-1303-7
29. Shi Y, Guo Q, Jing F, Shang X, Zhou C, Jing F. Ubenimex suppresses glycolysis mediated by CD13/Hedgehog signaling to enhance the effect of cisplatin in liver cancer. Transl Cancer Res. (2023) 12(10):2823–36. doi: 10.21037/tcr-23-435
30. Khosravi A, Jafari SM, Asadi J. Knockdown of TAZ decrease the cancer stem properties of ESCC cell line YM-1 by modulation of Nanog, OCT-4 and SOX2. Gene. (2021) 769:145207. doi: 10.1016/j.gene.2020.145207
31. Wang W, He S, Zhang R, Peng J, Guo D, Zhang J, et al. ALDH1A1 maintains the cancer stem-like cells properties of esophageal squamous cell carcinoma by activating the akt signal pathway and interacting with β-catenin. BioMed Pharmacother. (2020) 125:109940. doi: 10.1016/j.biopha.2020.109940
32. Jiang H, Zhou C, Zhang Z, Wang Q, Wei H, Shi W, et al. Jagged1-Notch1-Deployed tumor perivascular niche promotes breast cancer stem cell phenotype through Zeb1. Nat Commun. (2020) 11(1):5129. doi: 10.1038/s41467-020-18860-4
33. Liu C, Qiang J, Deng Q, Xia J, Deng L, Zhou L, et al. ALDH1A1 activity in tumor-initiating cells remodels myeloid-derived suppressor cells to promote breast cancer progression. Cancer Res. (2021) 81(23):5919–34. doi: 10.1158/0008-5472.Can-21-1337
34. Qiao X, Zhang Y, Sun L, Ma Q, Yang J, Ai L, et al. Association of human breast cancer CD44(-)/CD24(-) cells with delayed distant metastasis. Elife. (2021) 10:e65418. doi: 10.7554/eLife.65418
35. Cao G, Li P, He X, Jin M, Li M, Chen S, et al. FHL3 contributes to EMT and chemotherapy resistance through up-regulation of slug and activation of Tgfβ/Smad-independent pathways in gastric cancer. Front Oncol. (2021) 11:649029. doi: 10.3389/fonc.2021.649029
36. Wu C, Xie Y, Gao F, Wang Y, Guo Y, Tian H, et al. Lgr5 expression as stem cell marker in human gastric gland and its relatedness with other putative cancer stem cell markers. Gene. (2013) 525(1):18–25. doi: 10.1016/j.gene.2013.04.067
37. Hasegawa S, Nagano H, Konno M, Eguchi H, Tomokuni A, Tomimaru Y, et al. A crucial epithelial to mesenchymal transition regulator, Sox4/Ezh2 axis is closely related to the clinical outcome in pancreatic cancer patients. Int J Oncol. (2016) 48(1):145–52. doi: 10.3892/ijo.2015.3258
38. Wang R, Yao Y, Gao Y, Liu M, Yu Q, Song X, et al. CD133-targeted hybrid nanovesicles for Fluorescent/Ultrasonic imaging-guided HIFU pancreatic cancer therapy. Int J Nanomedicine. (2023) 18:2539–52. doi: 10.2147/ijn.S391382
39. Hutton C, Heider F, Blanco-Gomez A, Banyard A, Kononov A, Zhang X, et al. Single-cell analysis defines a pancreatic fibroblast lineage that supports anti-tumor immunity. Cancer Cell. (2021) 39(9):1227–44.e20. doi: 10.1016/j.cCell.2021.06.017
40. Wang J, Cai H, Liu Q, Xia Y, Xing L, Zuo Q, et al. Cinobufacini inhibits colon cancer invasion and metastasis Via suppressing WNT/β-catenin signaling pathway and EMT. Am J Chin Med. (2020) 48(3):703–18. doi: 10.1142/s0192415x20500354
41. de Sousa e Melo F, Kurtova AV, Harnoss JM, Kljavin N, Hoeck JD, Hung J, et al. A distinct role for Lgr5(+) stem cells in primary and metastatic colon cancer. Nature. (2017) 543(7647):676–80. doi: 10.1038/nature21713
42. Doberstein K, Bretz NP, Schirmer U, Fiegl H, Blaheta R, Breunig C, et al. miR-21-3p is a positive regulator of L1CAM in several human carcinomas. Cancer Lett. (2014) 354(2):455–66. doi: 10.1016/j.canlet.2014.08.020
43. Wang H, Wang X, Xu L, Zhang J, Cao H. A pan-cancer perspective analysis reveals the opposite prognostic significance of CD133 in lower grade glioma and papillary renal cell carcinoma. Sci Prog. (2021) 104(2):368504211010938. doi: 10.1177/00368504211010938
44. Matak D, Brodaczewska KK, Szczylik C, Koch I, Myszczyszyn A, Lipiec M, et al. Functional significance of CD105-positive cells in papillary renal cell carcinoma. BMC Cancer. (2017) 17(1):21. doi: 10.1186/s12885-016-2985-7
45. Gao S, Wang S, Zhao Z, Zhang C, Liu Z, Ye P, et al. TUBB4A interacts with MYH9 to protect the nucleus during cell migration and promotes prostate cancer Via GSK3β/β-catenin signalling. Nat Commun. (2022) 13(1):2792. doi: 10.1038/s41467-022-30409-1
46. Heidegger I, Fotakis G, Offermann A, Goveia J, Daum S, Salcher S, et al. Comprehensive characterization of the prostate tumor microenvironment identifies CXCR4/CXCL12 crosstalk as a novel antiangiogenic therapeutic target in prostate cancer. Mol Cancer. (2022) 21(1):132. doi: 10.1186/s12943-022-01597-7
47. Acikgoz E, Soner BC, Ozdil B, Guven M. CD133+/CD44+ prostate cancer stem cells exhibit embryo-like behavior patterns. Acta Histochem. (2021) 123(5):151743. doi: 10.1016/j.acthis.2021.151743
48. Ko H, Huh G, Jung SH, Kwon H, Jeon Y, Park YN, et al. Diospyros kaki leaves inhibit HGF/Met signaling-mediated EMT and stemness features in HepatoCellular carcinoma. Food Chem Toxicol. (2020) 142:111475. doi: 10.1016/j.fct.2020.111475
49. Erhart F, Blauensteiner B, Zirkovits G, Printz D, Soukup K, Klingenbrunner S, et al. Gliomasphere marker combinatorics: Multidimensional flow cytometry detects CD44+/CD133+/Itga6+/CD36+ signature. J Cell Mol Med. (2019) 23(1):281–92. doi: 10.1111/jcmm.13927
50. Zeng F, Miyazawa T, Kloepfer LA, Harris RC. Erbb4 deletion accelerates renal fibrosis following renal injury. Am J Physiol Renal Physiol. (2018) 314(5):F773–f87. doi: 10.1152/ajprenal.00260.2017
51. Heo SK, Noh EK, Ju LJ, Sung JY, Jeong YK, Cheon J, et al. CD45(dim)CD34(+)CD38(-)CD133(+) cells have the potential as leukemic stem cells in acute myeloid leukemia. BMC Cancer. (2020) 20(1):285. doi: 10.1186/s12885-020-06760-1
52. Oskarsson T, Batlle E, Massague J. Metastatic stem cells: sources, niches, and vital pathways. Cell Stem Cell. (2014) 14:306–21. doi: 10.1016/j.stem.2014.02.002
53. Debnath P, Huirem RS, Dutta P, Palchaudhuri S. Epithelial-mesenchymal transition and its transcription factors. Biosci Rep. (2022) 42:BSR20211754. doi: 10.1042/BSR20211754
54. Thomas R, Menon V, Mani R, Pruszak J. Glycan epitope and integrin expression dynamics characterize neural crest epithelial-to-Mesenchymal transition (EMT) in human pluripotent stem cell differentiation. Stem Cell Rev Rep. (2022) 18(8):2952–65. doi: 10.1007/s12015-022-10393-1
55. Sun Z, Ji N, Ma Q, Zhu R, Chen Z, Wang Z, et al. Epithelial-mesenchymal transition in asthma airway remodeling is regulated by the IL-33/CD146 axis. Front Immunol. (2020) 11:1598. doi: 10.3389/fimmu.2020.01598
56. Zhao X, Luo G, Fan Y, Ma X, Zhou J, Jiang H. ILEI is an important intermediate participating in the formation of TGF-β1-Induced renal tubular EMT. Cell Biochem Funct. (2018) 36(2):46–55. doi: 10.1002/cbf.3316
57. Pastushenko I, Mauri F, Song Y, de Cock F, Meeusen B, Swedlund B, et al. Fat1 deletion promotes hybrid EMT state, tumour stemness and metastasis. Nature. (2021) 589(7842):448–55. doi: 10.1038/s41586-020-03046-1
58. Cain S, Martinez G, Kokkinos MI, Turner K, Richardson RJ, Abud HE, et al. Differential requirement for beta-catenin in epithelial and fiber cells during lens development. Dev Bio. (2008) 321(2):420–33. doi: 10.1016/j.ydbio.2008.07.002
59. Cornick S, Tawiah A, Chadee K. Roles and regulation of the mucus barrier in the gut. Tissue Barriers. (2015) 3(1-2):e982426. doi: 10.4161/21688370.2014.982426
60. Zheng D, Liwinski T, Elinav E. Interaction between microbiota and immunity in health and disease. Cell Res. (2020) 30(6):492–506. doi: 10.1038/s41422-020-0332-7
61. Waghmare I, Kango-Singh M. Loss of cell adhesion increases tumorigenic potential of polarity deficient scribble mutant cells. PloS One. (2016) 11(6):e0158081. doi: 10.1371/journal.pone.0158081
62. Betanzos A, Zanatta D, Bañuelos C, Hernández-Nava E, Cuellar P, Orozco E. Epithelial cells expressing ehADH, an entamoeba histolytica adhesin, exhibit increased tight junction proteins. Front Cell Infection Microbiol. (2018) 8:340. doi: 10.3389/fcimb.2018.00340
63. Ogazon Del Toro A, Jimenez L, Hinojosa L, Martínez-Rendón J, Castillo A, Cereijido M, et al. Influence of endogenous cardiac glycosides, digoxin, and marinobufagenin in the physiology of epithelial cells. Cardiol Res Pract. (2019) 2019:8646787. doi: 10.1155/2019/8646787
64. Vijay GV, Zhao N, Den Hollander P, Toneff MJ, Joseph R, Pietila M, et al. GSK3β regulates epithelial-mesenchymal transition and cancer stem cell properties in triple-negative breast cancer. Breast Cancer Res. (2019) 21(1):37. doi: 10.1186/s13058-019-1125-0
65. Ozawa M, Kobayashi W. Reversibility of the snail-induced epithelial-mesenchymal transition revealed by the cre-loxp system. Biochem Biophys Res Commun. (2015) 458(3):608–13. doi: 10.1016/j.bbrc.2015.02.012
66. Takehara M, Sato Y, Kimura T, Noda K, Miyamoto H, Fujino Y, et al. Cancer-associated adipocytes promote pancreatic cancer progression through Saa1 expression. Cancer Sci. (2020) 111(8):2883–94. doi: 10.1111/cas.14527
67. Li Q, Deng MS, Wang RT, Luo H, Luo YY, Zhang DD, et al. PD-L1 upregulation promotes drug-induced pulmonary fibrosis by inhibiting vimentin degradation. Pharmacol Res. (2023) 187:106636. doi: 10.1016/j.phrs.2022.106636
68. Zhang N, Hua X, Tu H, Li J, Zhang Z, Max C. Isorhapontigenin (Iso) inhibits EMT through Foxo3a/Mettl14/Vimentin pathway in bladder cancer cells. Cancer Lett. (2021) 520:400–8. doi: 10.1016/j.canlet.2021.07.041
69. Lin X, Chai G, Wu Y, Li J, Chen F, Liu J, et al. RNA m(6)A methylation regulates the epithelial mesenchymal transition of cancer cells and translation of snail. Nat Commun. (2019) 10(1):2065. doi: 10.1038/s41467-019-09865-9
70. Ding T, Zhao T, Li Y, Liu Z, Ding J, Ji B, et al. Vitexin exerts protective effects against calcium oxalate crystal-induced kidney pyroptosis in vivo and in vitro. Phytomedicine. (2021) 86:153562. doi: 10.1016/j.phymed.2021.153562
71. Mittal V. Epithelial mesenchymal transition in tumor metastasis. Annu Rev Pathol. (2018) 13:395–412. doi: 10.1146/annurev-pathol-020117-043854
72. Li L, Xie T. Stem cell niche: Structure and function. Annu Rev Cell Dev Biol. (2005) 21:605–31. doi: 10.1146/annurev.Cellbio.21.012704.131525
73. Monzani E, Facchetti F, Galmozzi E, Corsini E, Benetti A, Cavazzin C, et al. Melanoma contains CD133 and ABCG2 positive cells with enhanced tumourigenic potential. Eur J Cancer. (2007) 43(5):935–46. doi: 10.1016/j.ejca.2007.01.017
74. Yang X-R, Xu Y, Yu B, Zhou J, Qiu S-J, Shi G-M, et al. High expression levels of putative hepatic Stem/Progenitor cell biomarkers related to tumour angiogenesis and poor prognosis of HepatoCellular carcinoma. Gut. (2010) 59(7):953–62. doi: 10.1136/gut.2008.176271
75. Bao S, Wu Q, Sathornsumetee S, Hao Y, Li Z, Hjelmeland AB, et al. Stem cell–like glioma cells promote tumor angiogenesis through vascular endothelial growth factor. Cancer Res. (2006) 66(16):7843–8. doi: 10.1158/0008-5472.CAN-06-1010
76. Zhang J, Tian X-J, Xing J. Signal transduction pathways of EMT induced by TGF-β, SHH, and WNT and their crosstalks. J Clin Med. (2016) 5(4):41. doi: 10.3390/jcm5040041
77. Tanabe S, Quader S, Cabral H, Ono R. Interplay of EMT and CSC in cancer and the potential therapeutic strategies. Front Pharmacol. (2020) 11:904. doi: 10.3389/fphar.2020.00904
78. Min C, Eddy SF, Sherr DH, Sonenshein GE. Nf-kappab and epithelial to mesenchymal transition of cancer. J Cell Biochem. (2008) 104(3):733–44. doi: 10.1002/jcb.21695
79. Loret N, Denys H, Tummers P, Berx G. The role of epithelial-to-Mesenchymal plasticity in ovarian cancer progression and therapy resistance. Cancers. (2019) 11(6):838. doi: 10.3390/cancers11060838
80. Hong D, Fritz AJ, Zaidi SK, Van Wijnen AJ, Nickerson JA, Imbalzano AN, et al. Epithelial-to-Mesenchymal transition and cancer stem cells contribute to breast cancer heterogeneity. J Cell Physiol. (2018) 233(12):9136–44. doi: 10.1002/jcp.26847
81. Liu S, Cong Y, Wang D, Sun Y, Deng L, Liu Y, et al. Breast cancer stem cells transition between epithelial and mesenchymal states reflective of their normal counterparts. Stem Cell Rep. (2014) 2(1):78–91. doi: 10.1016/j.stemcr.2013.11.009
82. Egeblad M, Nakasone ES, Werb Z. Tumors as organs: Complex tissues that interface with the entire organism. Dev Cell. (2010) 18(6):884–901. doi: 10.1016/j.devcel.2010.05.012
83. van den Bosch T, Derks S, Miedema DM. Chromosomal instability, selection and competition: Factors that shape the level of karyotype intra-tumor heterogeneity. Cancers (Basel). (2022) 14(20):4986. doi: 10.3390/cancers14204986
84. Diaz-Cano SJ. Tumor heterogeneity: Mechanisms and bases for a reliable application of molecular marker design. Int J Mol Sci. (2012) 13(2):1951–2011. doi: 10.3390/ijms13021951
85. Gerlinger M, Rowan AJ, Horswell S, Larkin J, Endesfelder D, Gronroos E, et al. Intratumor heterogeneity and branched evolution revealed by multiregion sequencing. New Engl J Med. (2012) 366(10):883–92. doi: 10.1056/NEJMoa1113205
86. Wang L, Zuo X, Xie K, Wei D. The role of CD44 and cancer stem cells. Cancer Stem Cells: Methods Protoc. (2018), 31–42. doi: 10.1007/978-1-4939-7401-6_3
87. Brooks MD, Burness ML, Wicha MS. Therapeutic implications of cellular heterogeneity and plasticity in breast cancer. Cell Stem Cell. (2015) 17(3):260–71. doi: 10.1016/j.stem.2015.08.014
88. Prasetyanti PR, Medema JP. Intra-tumor heterogeneity from a cancer stem cell perspective. Mol Cancer. (2017) 16(1):1–9. doi: 10.1186/s12943-017-0600-4
89. Wahl GM, Spike BT. Cell state plasticity, stem cells, EMT, and the generation of intra-tumoral heterogeneity. NPJ Breast Cancer. (2017) 3(1):14. doi: 10.1038/s41523-017-0012-z
90. Drápela S, Bouchal J, Jolly MK, Culig Z, Souček K1. ZEB1: A critical regulator of cell plasticity, DNA damage response, and therapy resistance. Front Mol Biosci. (2020) 7:36. doi: 10.3389/fmolb.2020.00036
91. Terry S, Chouaib S. EMT in immuno-resistance. Oncoscience. (2015) 2(10):841. doi: 10.18632/oncoscience.226
92. Li S, Xu F, Zhang J, Wang L, Zheng Y, Wu X, et al. Tumor-associated macrophages remodeling EMT and predicting survival in colorectal carcinoma. Oncoimmunology. (2018) 7(2):e1380765. doi: 10.1080/2162402X.2017.1380765
93. Li Y, Wang Z, Ajani JA, Song S. Drug resistance and cancer stem cells. Cell Communication Signaling. (2021) 19(1):1–11. doi: 10.1186/s12964-020-00627-5
94. Cho Y, Kim YK. Cancer stem cells as a potential target to overcome multidrug resistance. Front Oncol. (2020) 10:764. doi: 10.3389/fonc.2020.00764
95. Semba T, Sammons R, Wang X, Xie X, Dalby KN, Ueno NT. JNK signaling in stem cell self-renewal and differentiation. Int J Mol Sci. (2020) 21(7):2613. doi: 10.3390/ijms21072613
96. Stoica A-F, Chang C-H, Pauklin S. Molecular therapeutics of pancreatic ductal adenocarcinoma: Targeted pathways and the role of cancer stem cells. Trends Pharmacol Sci. (2020) 41(12):977–93. doi: 10.1016/j.tips.2020.09.008
97. Su J, Morgani SM, David CJ, Wang Q, Er EE, Huang YH, et al. TGF-β orchestrates fibrogenic and developmental EMTs Via the ras effector Rreb1. Nature. (2020) 577(7791):566–71. doi: 10.1038/s41586-019-1897-5
98. Li L, Liu J, Xue H, Li C, Liu Q, Zhou Y, et al. A TGF-β-Mta1-Sox4-Ezh2 signaling axis drives epithelial-mesenchymal transition in tumor metastasis. Oncogene. (2020) 39(10):2125–39. doi: 10.1038/s41388-019-1132-8
99. Kumari N, Reabroi S, North BJ. Unraveling the molecular nexus between GPCRs, ERS, and EMT. Mediators Inflammation. (2021) 2021:6655417. doi: 10.1155/2021/6655417
100. Yuan Z, Yu X, Ni B, Chen D, Yang Z, Huang J, et al. Overexpression of long non-coding RNA-CTD903 inhibits colorectal cancer invasion and migration by repressing WNT/β-catenin signaling and predicts favorable prognosis. Int J Oncol. (2016) 48(6):2675–85. doi: 10.3892/ijo.2016.3447
101. Yu AQ, Ding Y, Li CL, Yang Y, Yan SR, Li DS. Talen-induced disruption of Nanog expression results in reduced proliferation, invasiveness and migration, increased chemosensitivity and reversal of EMT in HepG2 cells. Oncol Rep. (2016) 35(3):1657–63. doi: 10.3892/or.2015.4483
102. Ma Y, Yu W, Shrivastava A, Srivastava RK, Shankar S. Inhibition of pancreatic cancer stem cell characteristics by α-Mangostin: Molecular mechanisms involving sonic hedgehog and Nanog. J Cell Mol Med. (2019) 23(4):2719–30. doi: 10.1111/jcmm.14178
103. Gheytanchi E, Naseri M, Karimi-Busheri F, Atyabi F, Mirsharif ES, Bozorgmehr M, et al. Morphological and molecular characteristics of spheroid formation in HT-29 and Caco-2 colorectal cancer cell lines. Cancer Cell Int. (2021) 21(1):204. doi: 10.1186/s12935-021-01898-9
104. McCubrey JA, Fitzgerald TL, Yang LV, Lertpiriyapong K, Steelman LS, Abrams SL, et al. Roles of gsk-3 and micrornas on epithelial mesenchymal transition and cancer stem cells. Oncotarget. (2017) 8(8):14221–50. doi: 10.18632/oncotarget.13991
105. Wang Q, Liang N, Yang T, Li Y, Li J, Huang Q, et al. DNMT1-mediated methylation of BEX1 regulates stemness and tumorigenicity in liver cancer. J Hepatol. (2021) 75(5):1142–53. doi: 10.1016/j.jhep.2021.06.025
106. Sun Z, Su Z, Zhou Z, Wang S, Wang Z, Tong X, et al. RNA demethylase ALKBH5 inhibits TGF-β-induced EMT by regulating TGF-β/SMAD signaling in non-small cell lung cancer. FASEB J. (2022) 36(5):e22283. doi: 10.1096/fj.202200005RR
107. Wei J, Wu L, Yang S, Zhang C, Feng L, Wang M, et al. E-cadherin to N-cadherin switching in the TGF-β1 mediated retinal pigment epithelial to mesenchymal transition. Exp Eye Res. (2022) 220:109085. doi: 10.1016/j.exer.2022.109085
108. Araki K, Shimura T, Suzuki H, Tsutsumi S, Wada W, Yajima T, et al. E/N-cadherin switch mediates cancer progression Via TGF-β-Induced epithelial-to-Mesenchymal transition in extrahepatic cholangiocarcinoma. Br J Cancer. (2011) 105(12):1885–93. doi: 10.1038/bjc.2011.452
109. Qiang L, Shah P, BarCellos-Hoff MH, He YY. TGF-β signaling links e-cadherin loss to suppression of nucleotide excision repair. Oncogene. (2016) 35(25):3293–302. doi: 10.1038/onc.2015.390
110. de Streel G, Bertrand C, Chalon N, Liénart S, Bricard O, Lecomte S, et al. Selective inhibition of TGF-β1 produced by garp-expressing tregs overcomes resistance to pd-1/PD-L1 blockade in cancer. Nat Commun. (2020) 11(1):4545. doi: 10.1038/s41467-020-17811-3
111. Liu Q, Hodge J, Wang J, Wang Y, Wang L, Singh U, et al. Emodin reduces breast cancer lung metastasis by suppressing macrophage-induced breast cancer cell epithelial-mesenchymal transition and cancer stem cell formation. Theranostics. (2020) 10(18):8365–81. doi: 10.7150/thno.45395
112. Wang G, Zhou X, Guo Z, Huang N, Li J, Lv Y, et al. The anti-fibrosis drug pirfenidone modifies the immunosuppressive tumor microenvironment and prevents the progression of renal cell carcinoma by inhibiting tumor autocrine TGF-β. Cancer Biol Ther. (2022) 23(1):150–62. doi: 10.1080/15384047.2022.2035629
113. Yang L, Pang Y, Moses HL. Tgf-beta and immune cells: An important regulatory axis in the tumor microenvironment and progression. Trends Immunol. (2010) 31(6):220–7. doi: 10.1016/j.it.2010.04.002
114. Peng P, Zhu H, Liu D, Chen Z, Zhang X, Guo Z, et al. TGFBI secreted by tumor-associated macrophages promotes glioblastoma stem cell-driven tumor growth Via integrin αvβ5-Src-Stat3 signaling. Theranostics. (2022) 12(9):4221–36. doi: 10.7150/thno.69605
115. Kim BG, Lee JH, Yasuda J, Ryoo HM, Cho JY. Phospho-Smad1 modulation by Nedd4 E3 ligase in Bmp/TGF-β signaling. J Bone Miner Res. (2011) 26(7):1411–24. doi: 10.1002/jbmr.348
116. Geng XQ, Ma A, He JZ, Wang L, Jia YL, Shao GY, et al. Ganoderic acid hinders renal fibrosis Via suppressing the TGF-β/Smad and mapk signaling pathways. Acta Pharmacol Sin. (2020) 41(5):670–7. doi: 10.1038/s41401-019-0324-7
117. Huang F, Kao CY, Wachi S, Thai P, Ryu J, Wu R. Requirement for both jAK-mediated PI3K signaling and ACT1/TRAF6/TAK1-dependent NF-kappaB activation by IL-17A in enhancing cytokine expression in human airway epithelial cells. J Immunol. (2007) 179(10):6504–13. doi: 10.4049/jimmunol.179.10.6504
118. Lv Q, Wang J, Xu C, Huang X, Ruan Z, Dai Y. Pirfenidone alleviates pulmonary fibrosis in vitro and in vivo through regulating Wnt/Gsk-3β/β-catenin and TGF-β1/Smad2/3 signaling pathways. Mol Med. (2020) 26(1):49. doi: 10.1186/s10020-020-00173-3
119. Kitagaki J, Iwamoto M, Liu JG, Tamamura Y, Pacifci M, Enomoto-Iwamoto M. Activation of beta-Catenin-Lef/Tcf signal pathway in chondrocytes stimulates ectopic endochondral ossification. Osteoarthritis Cartilage. (2003) 11(1):36–43. doi: 10.1053/joca.2002.0863
120. Yang X, Shao F, Guo D, Wang W, Wang J, Zhu R, et al. WNT/β-catenin-suppressed FTO expression increases m(6)A of c-Myc mRNA to promote tumor cell glycolysis and tumorigenesis. Cell Death Dis. (2021) 12(5):462. doi: 10.1038/s41419-021-03739-z
121. Wu F, Tian F, Qin C, Qin X, Zeng W, Liu X, et al. Peroxiredoxin2 regulates trophoblast proliferation and migration through spib-Hdac2 pathway. Exp Cell Res. (2023) 422(1):113428. doi: 10.1016/j.yexcr.2022.113428
122. Wendeler MW, Drenckhahn D, Gessner R, Baumgartner W. Intestinal li-cadherin acts as a Ca2+-dependent adhesion switch. J Mol Biol. (2007) 370(2):220–30. doi: 10.1016/j.jmb.2007.04.062
123. Batlle E, Sancho E, Francí C, Domínguez D, Monfar M, Baulida J, et al. The transcription factor snail is a repressor of e-cadherin gene expression in epithelial tumour cells. Nat Cell Biol. (2000) 2(2):84–9. doi: 10.1038/35000034
124. Zhang A, Chen G, Meng L, Wang Q, Hu W, Xi L, et al. Antisense-snail transfer inhibits tumor metastasis by inducing e-cadherin expression. Anticancer Res. (2008) 28(2a):621–8.
125. Chen Y, Yan H, Li G, Zhang Y. Higher TGF-β1, TGF-β2, mmp-2, and timp-1 levels in the aqueous humor of patients with acute primary angle closure. Ophthalmic Res. (2021) 64(1):62–7. doi: 10.1159/000507762
126. Richter A, Valdimarsdottir L, Hrafnkelsdottir HE, Runarsson JF, Omarsdottir AR, Ward-van Oostwaard D, et al. BMP4 promotes EMT and mesodermal commitment in human embryonic stem cells via SLUG and MSX2. Stem Cells. (2014) 32(3):636–48. doi: 10.1002/stem.1592
127. Fatima S, Lee NP, Tsang FH, Kolligs FT, Ng IO, Poon RT, et al. Dickkopf 4 (DKK4) acts on WNT/β-catenin pathway by influencing β-catenin in HepatoCellular carcinoma. Oncogene. (2012) 31(38):4233–44. doi: 10.1038/onc.2011.580
128. Fatima S, Shi X, Lin Z, Chen GQ, Pan XH, Wu JC, et al. 5-hydroxytryptamine promotes HepatoCellular carcinoma proliferation by influencing β-catenin. Mol Oncol. (2016) 10(2):195–212. doi: 10.1016/j.molonc.2015.09.008
129. Shi Y, Zhang D, Chen J, Jiang Q, Song S, Mi Y, et al. Interaction between BEND5 and RBPJ suppresses breast cancer growth and metastasis Via inhibiting notch signaling. Int J Biol Sci. (2022) 18(10):4233–44. doi: 10.7150/ijbs.70866
130. Del Bianco C, Vedenko A, Choi SH, Berger MF, Shokri L, Bulyk ML, et al. Notch and MAML-1 complexation do not detectably alter the DNA binding specificity of the transcription factor csl. PloS One. (2010) 5(11):e15034. doi: 10.1371/journal.pone.0015034
131. Luo W, Li S, Peng B, Ye Y, Deng X, Yao K. Embryonic stem cells markers SOX2, OCT4 and Nanog expression and their correlations with epithelial-mesenchymal transition in nasopharyngeal carcinoma. PloS One. (2013) 8(2):e56324. doi: 10.1371/journal.pone.0056324
132. Skoda AM, Simovic D, Karin V, Kardum V, Vranic S, Serman L. The role of the hedgehog signaling pathway in cancer: A comprehensive review. Bosnian J Basic Med Sci. (2018) 18(1):8–20. doi: 10.17305/bjbms.2018.2756
133. Wu X, Xiao S, Zhang M, Yang L, Zhong J, Li B, et al. A novel protein encoded by circular SMO RNA is essential for hedgehog signaling activation and glioblastoma tumorigenicity. Genome Biol. (2021) 22(1):33. doi: 10.1186/s13059-020-02250-6
134. Deng Q, Li P, Che M, Liu J, Biswas S, Ma G, et al. Activation of hedgehog signaling in mesenchymal stem cells induces cartilage and bone tumor formation Via WNT/β-catenin. Elife. (2019) 8:e50208. doi: 10.7554/eLife.50208
135. Ribatti D, Tamma R, Annese T. Epithelial-mesenchymal transition in cancer: A historical overview. Trans Oncol. (2020) 13(6):100773. doi: 10.1016/j.tranon.2020.100773
136. Ahmed N, Maines-Bandiera S, Quinn MA, Unger WG, Dedhar S, Auersperg N. Molecular pathways regulating egf-induced epithelio-mesenchymal transition in human ovarian surface epithelium. Am J Physiol Cell Physiol. (2006) 290(6):C1532–42. doi: 10.1152/ajpCell.00478.2005
137. Ghigo A, De Santi C, Hart M, Mitash N, Swiatecka-Urban A. Cell signaling and regulation of CFTR expression in cystic fibrosis cells in the era of high efficiency modulator therapy. J CysT Fibros. (2023) 22 Suppl 1:S12–s6. doi: 10.1016/j.jcf.2022.12.015
138. Klochkov SG, Neganova ME, Yarla NS, Parvathaneni M, Sharma B, Tarasov VV, et al. Implications of farnesyltransferase and its inhibitors as a promising strategy for cancer therapy. Semin Cancer Biol. (2019) 56:128–34. doi: 10.1016/j.semcancer.2017.10.010
139. Dosch AR, Dai X, Gaidarski Iii AA, Shi C, Castellanos JA, VanSaun MN, et al. Src kinase inhibition restores e-cadherin expression in dasatinib-sensitive pancreatic cancer cells. Oncotarget. (2019) 10(10):1056–69. doi: 10.18632/oncotarget.26621
140. Ye Q, Liu Z, Zhang S, Wang G, Wen G, Dong M. Development of (99m)Tc-Hynic-Adh-1 molecular probe specifically targeting N-cadherin and its preliminary experimental study in monitoring drug resistance of non-Small-Cell lung cancer. Cancers (Basel). (2023) 15(3):755. doi: 10.3390/cancers15030755
141. Rice LM, Padilla CM, McLaughlin SR, Mathes A, Ziemek J, Goummih S, et al. Fresolimumab treatment decreases biomarkers and improves clinical symptoms in systemic sclerosis patients. Jou Clin Invest. (2015) 125(7):2795–807. doi: 10.1172/jci77958
142. Kubo M, Umebayashi M, Kurata K, Mori H, Kai M, Onishi H, et al. Catumaxomab with activated t-cells efficiently lyses chemoresistant epcam-positive triple-negative breast cancer cell lines. Anticancer Res. (2018) 38(7):4273–9. doi: 10.21873/anticanres.12724
143. Azmi AS, Bollig-Fischer A, Bao B, Park BJ, Lee SH, Yong-Song G, et al. Systems analysis reveals a transcriptional reversal of the mesenchymal phenotype induced by SNAIL-inhibitor GN-25. BMC Syst Biol. (2013) 7:85. doi: 10.1186/1752-0509-7-85
144. Liu Y, Zhao R, Chi S, Zhang W, Xiao C, Zhou X, et al. Ube2c is upregulated by estrogen and promotes epithelial-mesenchymal transition via p53 in endometrial cancer. Mol Cancer Res. (2020) 18(2):204–15. doi: 10.1158/1541-7786.mcr-19-0561
145. Krützfeldt J, Rajewsky N, Braich R, Rajeev KG, Tuschl T, Manoharan M, et al. Silencing of micrornas vivo with ‘antagomirs’. Nature. (2005) 438(7068):685–9. doi: 10.1038/nature04303
146. Basu SM, Yadava SK, Singh R, Giri J. Lipid nanocapsules co-encapsulating paclitaxel and salinomycin for eradicating breast cancer and cancer stem cells. Colloids Surfaces B Biointerfaces. (2021) 204:111775. doi: 10.1016/j.colsurfb.2021.111775
147. Huang X, Borgström B, Kempengren S, Persson L, Hegardt C, Strand D, et al. Breast cancer stem cell selectivity of synthetic nanomolar-active salinomycin analogs. BMC Cancer. (2016) 16:145. doi: 10.1186/s12885-016-2142-3
148. Al Dhaheri Y, Attoub S, Arafat K, Abuqamar S, Eid A, Al Faresi N, et al. Salinomycin induces apoptosis and senescence in breast cancer: Upregulation of P21, downregulation of survivin and histone H3 and H4 hyperacetylation. Biochim Biophys Acta. (2013) 1830(4):3121–35. doi: 10.1016/j.bbagen.2013.01.010
149. Ou X, Tan Y, Xie J, Yuan J, Deng X, Shao R, et al. Methylation of GPRC5A promotes liver metastasis and docetaxel resistance through activating mtor signaling pathway in triple negative breast cancer. Drug Resist Update. (2024) 73:101063. doi: 10.1016/j.drup.2024.101063
150. Xie J, Deng X, Xie Y, Zhu H, Liu P, Deng W, et al. Multi-omics analysis of disulfidptosis regulators and therapeutic potential reveals glycogen synthase 1 as a disulfidptosis triggering target for triple-negative breast cancer. MedComm. (2020) 2024) 5(3):e502. doi: 10.1002/mco2.502
151. Ringel AE, Drijvers JM, Baker GJ, Catozzi A, García-Cañaveras JC, Gassaway BM, et al. Obesity shapes metabolism in the tumor microenvironment to suppress anti-tumor immunity. Cell. (2020) 183(7):1848–66.e26. doi: 10.1016/j.Cell.2020.11.009
152. Wang Y, Yang H, Jia A, Wang Y, Yang Q, Dong Y, et al. Dendritic cell Piezo1 directs the differentiation of T(H)1 and T(reg) cells in cancer. Elife. (2022) 11:e79957. doi: 10.7554/eLife.79957
153. Huang X, Borgström B, Månsson L, Persson L, Oredsson S, Hegardt C, et al. Semisynthesis of SY-1 for investigation of breast cancer stem cell selectivity of C-ring-modified salinomycin analogues. ACS Chem Biol. (2014) 9:1587–94. doi: 10.1021/cb5002153
154. Liu L, Wang Q, Mao J, Qin T, Sun Y, Yang J, et al. Salinomycin suppresses cancer cell stemness and attenuates TGF-β-Induced epithelial-mesenchymal transition of renal cell carcinoma cells. Chem Biol Interact. (2018) 296:145–53. doi: 10.1016/j.cbi.2018.09.018
155. Naemi AO, Dey H, Kiran N, Sandvik ST, Slettemeås JS, Nesse LL, et al. NarAB is an ABC-type transporter that confers resistance to the polyether ionophores narasin, salinomycin, and maduramicin, but not monensin. Front Microbiol. (2020) 11:104. doi: 10.3389/fmicb.2020.00104
156. Chen M, Yang Y, Ying Y, Huang J, Sun M, Hong M, et al. ABC transporters and CYP3A4 mediate drug interactions between enrofloxacin and salinomycin leading to increased risk of drug residues and resistance. Antibiotics (Basel). (2023) 12(2):403. doi: 10.3390/antibiotics12020403
157. Du H, Zhou B, Zhang H, Jin Y, Zhang D, Lin D. Salinomycin inhibits canine mammary carcinoma in vitro by targeting cancer stem cells. Oncol Lett. (2017) 14(1):427–32. doi: 10.3892/ol.2017.6164
158. Fu YZ, Yan YY, He M, Xiao QH, Yao WF, Zhao L, et al. Salinomycin induces selective cytotoxicity to MCF-7 mammosphere cells through targeting the hedgehog signaling pathway. Oncol Rep. (2016) 35(2):912–22. doi: 10.3892/or.2015.4434
159. Saha D, Mitra D, Alam N, Sen S, Mustafi SM, Majumder PK, et al. Lupeol and paclitaxel cooperate in hindering hypoxia induced vasculogenic mimicry Via suppression of HIF-1α-EphA2-Laminin-5γ2 network in human oral cancer. J Cell Commun Signal. (2023) 17(3):591–608. doi: 10.1007/s12079-022-00693-z
160. Navas T, Kinders RJ, Lawrence SM, Ferry-Galow KV, Borgel S, Hollingshead MG, et al. Clinical evolution of epithelial-mesenchymal transition in human carcinomas. Cancer Res. (2020) 80(2):304–18. doi: 10.1158/0008-5472.Can-18-3539
161. Wang RN, Klöppel G, Bouwens L. Duct- to islet-cell differentiation and islet growth in the pancreas of duct-ligated adult rats. Diabetologia. (1995) 38(12):1405–11. doi: 10.1007/bf00400600
162. Uday Chandrika K, Tripathi R, Kameshwari Y, Rangaraj N, Mahesh Kumar J, Singh S. Refunctionalization of DeCellularized organ scaffold of pancreas by ReCellularization: Whole organ regeneration into functional pancreas. Tissue Eng Regener Med. (2021) 18(1):99–112. doi: 10.1007/s13770-020-00296-y
163. Zhao J, Wang H, Hsiao CH, Chow DS, Koay EJ, Kang Y, et al. Simultaneous inhibition of hedgehog signaling and tumor proliferation remodels stroma and enhances pancreatic cancer therapy. Biomaterials. (2018) 159:215–28. doi: 10.1016/j.biomaterials.2018.01.014
164. Liu B, Liu J, Hu X, Xiang W, Hou W, Li C, et al. Recent advances in aptamer-based therapeutic strategies for targeting cancer stem cells. Mater Today Bio. (2023) 19:100605. doi: 10.1016/j.mtbio.2023.100605
165. Zhou JN, Zhang B, Wang HY, Wang DX, Zhang MM, Zhang M, et al. A functional screening identifies a new organic selenium compound targeting cancer stem cells: Role of c-Myc transcription activity inhibition in liver cancer. Adv Sci (Weinh). (2022) 9(22):e2201166. doi: 10.1002/advs.202201166
166. Laranjeira AB, Yang SX. Therapeutic target discovery and drug development in cancer stem cells for leukemia and lymphoma: From bench to the clinic. Expert Opin Drug Discovery. (2016) 11(11):1071–80. doi: 10.1080/17460441.2016.1236785
167. Amôr NG, Buzo RF, Ortiz RC, Lopes NM, Saito LM, Mackenzie IC, et al. In vitro and in vivo characterization of cancer stem cell subpopulations in oral squamous cell carcinoma. J Oral Pathol Med. (2021) 50(1):52–9. doi: 10.1111/jop.13101
168. Papadaki MA, Aggouraki D, Vetsika EK, Xenidis N, Kallergi G, Kotsakis A, et al. Epithelial-to-Mesenchymal transition heterogeneity of circulating tumor cells and their correlation with mdscs and tregs in Her2-negative metastatic breast cancer patients. Anticancer Res. (2021) 41(2):661–70. doi: 10.21873/anticanres.14817
Keywords: cancer stem cells, epithelial-mesenchymal transition, biomarkers, targeted therapy, molecular mechanism
Citation: Lei Z-N, Teng Q-X, Koya J, Liu Y, Chen Z, Zeng L, Chen Z-S, Fang S, Wang J, Liu Y and Pan Y (2024) The correlation between cancer stem cells and epithelial-mesenchymal transition: molecular mechanisms and significance in cancer theragnosis. Front. Immunol. 15:1417201. doi: 10.3389/fimmu.2024.1417201
Received: 14 April 2024; Accepted: 06 September 2024;
Published: 30 September 2024.
Edited by:
Xiaowei Qi, Army Medical University, ChinaReviewed by:
Peng Liu, Sun Yat-sen University, ChinaVinay Kumar Sharma, Eunice Kennedy Shriver National Institute of Child Health and Human Development (NIH), United States
Copyright © 2024 Lei, Teng, Koya, Liu, Chen, Zeng, Chen, Fang, Wang, Liu and Pan. This is an open-access article distributed under the terms of the Creative Commons Attribution License (CC BY). The use, distribution or reproduction in other forums is permitted, provided the original author(s) and the copyright owner(s) are credited and that the original publication in this journal is cited, in accordance with accepted academic practice. No use, distribution or reproduction is permitted which does not comply with these terms.
*Correspondence: Shuo Fang, fangsh9@mail.sysu.edu.cn; Jinxiang Wang, kingx_w@163.com; Yuchen Liu, liuych83@mail.sysu.edu.cn; Yihang Pan, panyih@mail.sysu.edu.cn
†These authors have contributed equally to this work