- 1Department of Hematology, The Second Affiliated Hospital of Xi’an Jiaotong University, Xi’an, Shaanxi, China
- 2Xi’an Key Laboratory of Hematological Diseases, Xi’an, Shaanxi, China
Chimeric antigen receptor T (CAR-T) cell therapy has revolutionized the treatment of hematological malignancies, demonstrably improving patient outcomes and prognosis. However, its application has introduced new challenges, such as safety concerns, off-target toxicities, and significant costs. Natural killer (NK) cells are crucial components of the innate immune system, capable of eliminating tumor cells without prior exposure to specific antigens or pre-activation. This inherent advantage complements the limitations of T cells, making CAR-NK cell therapy a promising avenue for hematological tumor immunotherapy. In recent years, preclinical and clinical studies have yielded preliminary evidence supporting the safety and efficacy of CAR-NK cell therapy in hematological malignancies, paving the way for future advancements in immunotherapy. This review aims to succinctly discuss the characteristics, significant therapeutic progress, and potential challenges associated with CAR-NK cell therapy.
1 Introduction
Chimeric antigen receptor T (CAR-T) cell therapy has emerged as a major breakthrough in cancer treatment in recent years. Notably, CARs targeting B-lineage-specific antigens, such as CD19 and CD20, have shown particular success (1). As of today, the Food and Drug Administration (FDA) has approved six CAR-T cell therapies: four CD19-directed T-cell products (tisagenlecleucel/KYMRIAH, axicabtagene ciloleucel/YESCARTA, brexucabtagene autoleucel/TECARTUS, and lisocabtagene maraleucel/BREYANZI) and two B-cell maturation antigen (BCMA)-targeted T-cell products (idecabtagene vicleucel/ABECMA and ciltacabtagene autoleucel/CARVYKTI). This approach has demonstrably improved outcomes for patients with recurrent/refractory hematological malignancies. However, significant challenges remain in the application of CAR-T cell therapy to solid tumors. These challenges include the scarcity of tumor-specific antigens and the limited infiltration of immune cells into solid tumors (2, 3). Despite its remarkable achievements, CAR-T cell therapy also faces inherent complexities and safety considerations associated with autologous T-cell therapy. These limitations, including the cytokine release syndrome (CRS) and the immune effector cell-associated neurotoxicity syndrome (ICANS), necessitate the exploration of alternative therapies (4, 5). These limitations have spurred interest in alternative therapies such as natural killer (NK) cell therapy.
2 Biological characteristics of NK cells
NK cells, which are derived from CD34+ lymphoid progenitor cells in the bone marrow, account for approximately 15% of the lymphocyte population and serve as important components of the innate immune defense system (6–9). Functionally similar to CD8+ cytotoxic T cells, NK cells lack antigen-specific receptors. This enables them to recognize and eliminate abnormal cells in an antigen-independent manner, distinguishing them from T cells within the lymphocyte lineage (10, 11). Circulating NK cells can be further categorized into two major subsets based on the surface expression density of CD56 (neural cell adhesion molecule) and CD16 (6): immature CD56brightCD16−/low NK cells and mature CD56dimCD16+ NK cells. The highly proliferative immature subset plays a critical role in immunoregulation through the production of cytokines, particularly interferon gamma (IFN-γ) (11). Upon cytokine stimulation, CD56brightCD16−/low NK cells can differentiate into cytotoxic CD56dimCD16+ NK cells (9). Notably, mature NK cells, constituting approximately 90% of peripheral blood NK cells, exhibit potent cytotoxic activity (12, 13).
NK cells distinguish abnormal cells from normal cells through a delicate interplay between the activating and inhibitory surface receptors. The functional state of an NK cell is ultimately determined by the balance of the signals received from these receptors (10, 14). The activating receptors encompass a diverse group, including partial cytokine-binding receptors (e.g., interleukin-2 receptor), natural cytotoxicity receptors (NCRs) such as NKp44, NKp30, and CD16, and others such as the SLAM family receptors and the activated forms of killer cell immunoglobulin-like receptors (KIRs) (11, 15). NKG2D is a typical activating receptor whose ligands are only generally expressed on the surface of abnormal cells such as tumor or stressed cells and can activate the ability of NK cells to kill abnormal cells upon binding to the NKG2D ligands (NKG2DL) (16). This property makes NKG2D an attractive target for cancer immunotherapy (17). Inhibitory receptors fall into two major categories: those recognizing the major histocompatibility complex class I (MHC-I) molecules and those that do not. MHC-I-specific inhibitory receptors include NKG2A, inhibitory KIRs (e.g., KIR2DL1/2/3 and KIR3DL1/2), and LLT1. PD-1 and 2B4 are examples of the non-MHC-I-specific inhibitory receptors (10, 11).
NK cells employ a multifaceted arsenal to eliminate target cells (Figure 1). Activated NK cells degranulate, releasing cytolytic granules containing perforin and granzymes. Perforin creates pores in the target cell membrane, allowing granzymes to enter and induce cell death (apoptosis) (18, 19). In addition, the activated NK cells upregulate death ligands, such as the Fas ligand (FasL) and the tumor necrosis factor (TNF)-related apoptosis-inducing ligand (TRAIL), on their surface. These ligands can bind to the death receptors on target cells, triggering apoptosis in a process known as the death receptor pathway (18, 20). Mature NK cells, characterized by a high CD16 expression, can also mediate antibody-dependent cellular cytotoxicity (ADCC). During this process, CD16 binds to the Fc portion of the antibodies already bound to the target cells, leading to target cell lysis (21). Finally, the activated NK cells secrete various cytokines that influence the immune response (22).
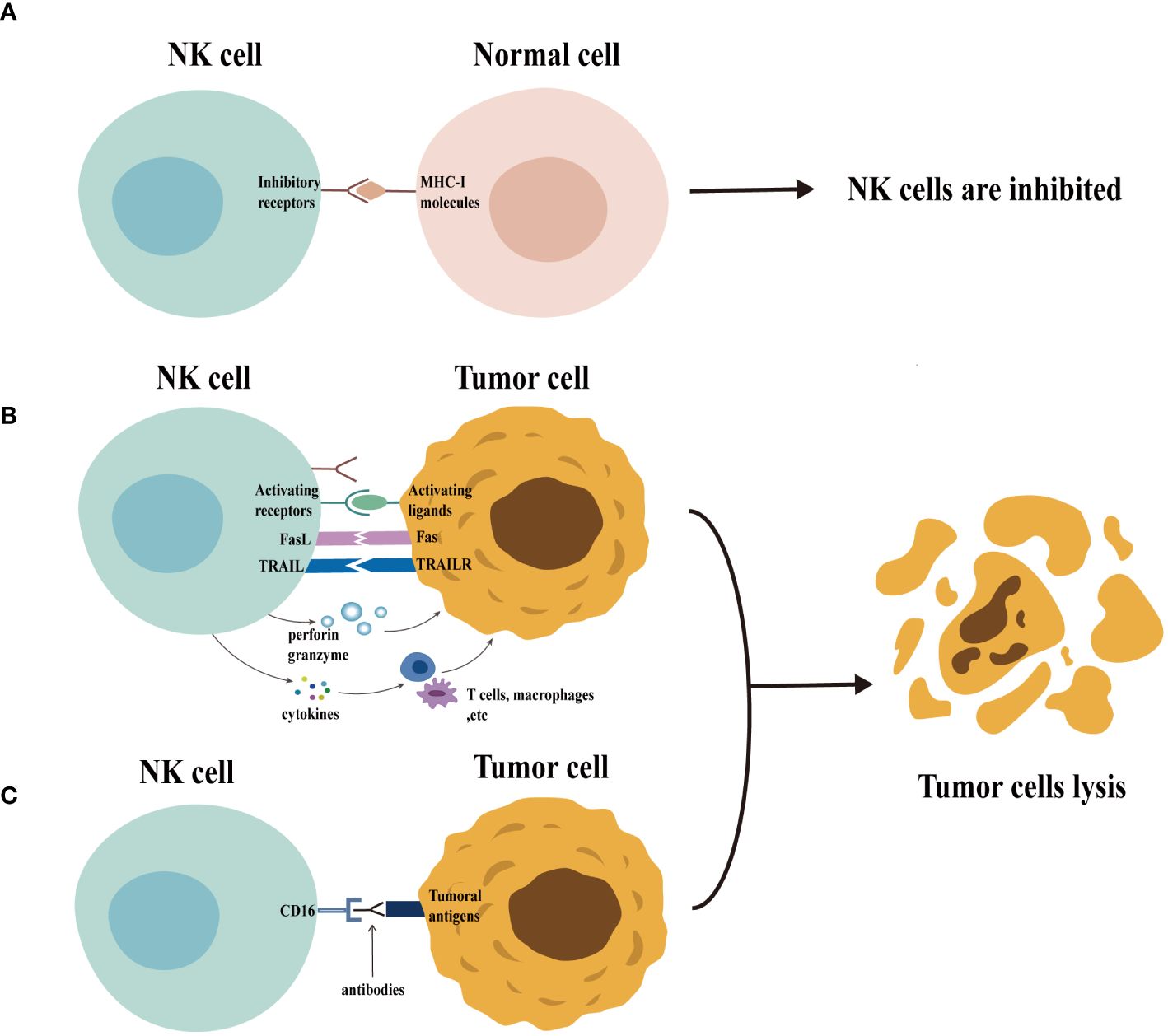
Figure 1 Function of natural killer (NK) cells. Normal cells express MHC-I molecules on their surface. When the inhibitory receptors on NK cells bind to these molecules, NK cells are inhibited and are unable to exert their cytotoxic potential (panel A) . In contrast (panel B), the expression of MHC-I molecules is usually downregulated in abnormal cells (e.g., tumor cells), accompanied by the expression of a variety of activating ligands, which leads to the activation of NK cells. The activated NK cells exert their cytotoxic potential by secreting granules containing perforin and granzyme and by the death receptor pathway. The activated NK cells can also secrete cytokines that regulate the function of other immune cells, including T cells and macrophages, thus enhancing the immune response. Furthermore, NK cells can be activated through CD16 recognition of target cell-binding antibodies, enabling them to exert their cytotoxic potential through ADCC effects (panel C).
3 Construction of CAR-NK
Chimeric antigen receptors (CARs) are engineered proteins composed of several key domains: extracellular antigen-binding domains for recognizing tumor-associated antigens (TAAs), flexible hinge regions, transmembrane domains anchoring the CAR to the cell membrane, and intracellular signaling domains that directly activate immune cells to eliminate the target cells (3, 23). The design of CARs has become increasingly sophisticated with advancements in research (Figure 2). The current exploration focuses on fifth-generation CARs, which may incorporate features such as inducible cytokine production through the inclusion of transcription factor binding sites. For instance, the integration of an interleukin-2 (IL-2) Rβ signaling domain, which activates STAT3, has been proposed to enhance T-cell activation and proliferation via both the CD3ζ/CD28 and JAK-STAT 3/5 pathways (24). Notably, most CAR-NK cell studies currently utilize first- or second-generation CAR structures adapted from CAR-T cell designs (23, 25). Research efforts are ongoing to improve the efficacy of CARs for targeted antitumor therapy. These efforts include optimizing the signaling domains, developing dual/multi-targeted CARs, and selecting less immunogenic extracellular fragments (26, 27). A recent study exploring a novel combination of intracellular signaling domains (CD3ζ-2B4) has demonstrated significant functional improvements in CAR-NK cells in vitro (28).
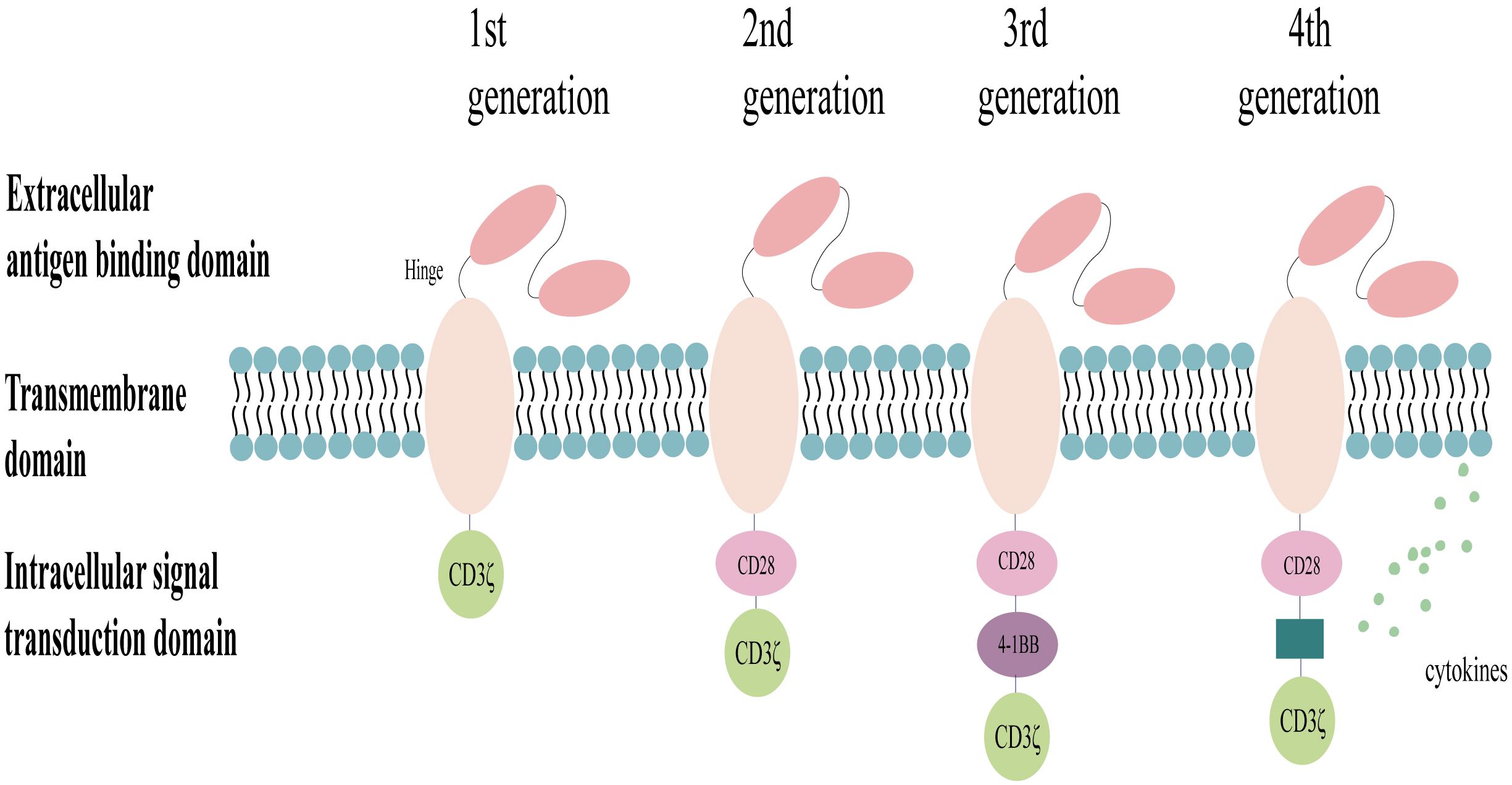
Figure 2 Structure of the chimeric antigen receptor (CAR). The design of CARs has evolved through generations, with the first generation incorporating a single CD3ζ signaling domain for transient activation, the second generation adding a co-stimulatory molecule (CD28 or 4–1BB) for enhanced signaling, the third generation incorporating two co-stimulatory molecules for further signal transduction efficiency, and the fourth generation co-expressing cytokines or suicide genes to improve targeting, efficacy, and the safety profiles.
NK cells can be derived from various sources (29), including peripheral blood (PB), umbilical cord blood (UCB), established NK cell lines (particularly the NK-92 cell line), and human pluripotent stem cells (hPSCs). This wider range of potential sources makes NK cells more readily available compared with T cells, with each source offering distinct characteristics, detailed in Table 1.
Currently, two main approaches are used to introduce genetic material into NK cells: viral transduction (including lentiviral and retroviral vectors) and non-viral transduction (encompassing mRNA electroporation and transposon systems). Of these, lentiviral vectors represent a classic and widely employed method. However, their lower efficiency in transducing NK cells can limit the application of CAR-NK therapy. Strategies to improve the lentiviral transduction efficiency, such as employing multiple transduction rounds or co-applying transduction enhancers, have been detailed in previous reviews (35, 42). A summary of the key characteristics of the different transduction systems is in Table 2.
4 Potential advantages of CAR-NK
At present, CAR-T cell therapy is expensive and time-consuming. The use of autologous T cells minimizes the risk of graft-versus-host disease (GvHD), a serious complication in allogeneic cell therapy (48, 49). However, this reliance on autologous T cells presents logistical challenges for large-scale clinical application due to limitations in T-cell collection and manipulation (50). NK cells, unlike CAR-T cells, pose a significantly lower risk of GvHD (51) and are abundantly available. This inherent accessibility presents a distinct advantage for NK-based therapies. In addition, the high costs associated with the currently approved CAR-T products, such as the Kymriah with a list price of $475,000, limit patient access (52). The study by Jagannath et al. (53) further highlighted the financial burden of CAR-T therapy, reporting pre- and peri-infusion healthcare costs exceeding US $15,000 for relapsed/refractory multiple myeloma (R/RMM) patients alone. Indeed, these high costs create a barrier for many patients and necessitate the exploration of alternative treatment options.
Despite promising response rates, CAR-T cell therapy carries a significant risk of CRS and ICANS, a concern that cannot be disregarded during clinical application (54). In contrast, preliminary clinical trials suggest a potentially safer profile for CAR-NK cell therapy (55). This difference may be attributed to the distinct cytokine profiles released by the activated CAR-T and CAR-NK cells (3). Upon activation, CAR-T cells release a surge of inflammatory cytokines, such as IL-2, IL-6, and tumor necrosis factor alpha (TNF-α), which are known contributors to CRS and ICANS (54). Conversely, the activated CAR-NK cells primarily produce IFN-γ and granulocyte–macrophage colony-stimulating factor (GM-CSF) (56), cytokines that lack a demonstrated role in the pathogenesis of CRS and ICANS (54). Supporting this notion, a clinical trial using cord blood-derived CAR-NK cells for B-cell malignancies observed minimal elevation of CRS-associated inflammatory factors such as IL-6 and TNF-α, suggesting a potentially safer therapeutic approach (55).
It is now understood that CAR-T cells recognize TAAs through their CARs. This recognition appears to require a higher TAA density compared with the activation of T cells via their T-cell receptors (TCRs) (50, 57, 58). Consequently, antigen escape, where tumor cells downregulate or lose TAA expression, is a frequent challenge in CAR-T cell therapy, limiting its in vivo efficacy. In contrast, CAR-NK cells exhibit a dual killing capacity: CAR-dependent and CAR-independent pathways. The latter pathway leverages the inherent cytotoxicity of NK cells, allowing them to eliminate tumor cells even with partial or complete TAA loss (59). This theoretical advantage suggests a potentially high antitumor potential for CAR-NK cells. However, the limited research available directly compares the antitumor efficacy of CAR-NK and CAR-T cells. A recent study by Egli et al. (60) compared autologous CD19-CAR-T cells with allogeneic CD19-CAR-NK cells in vitro and in vivo. The authors observed stronger antitumor activity with CAR-T cells. Conversely, a report by Marin et al. suggests that allogeneic CAR-NK cells may offer better clinical benefits for specific indolent diseases, such as low-grade non-Hodgkin’s lymphoma (NHL) and chronic lymphocytic leukemia (CLL) (61). These findings highlight the need for further clinical trials to definitively understand the differences in the antitumor efficacy between CAR-NK and CAR-T cells (Table 3).
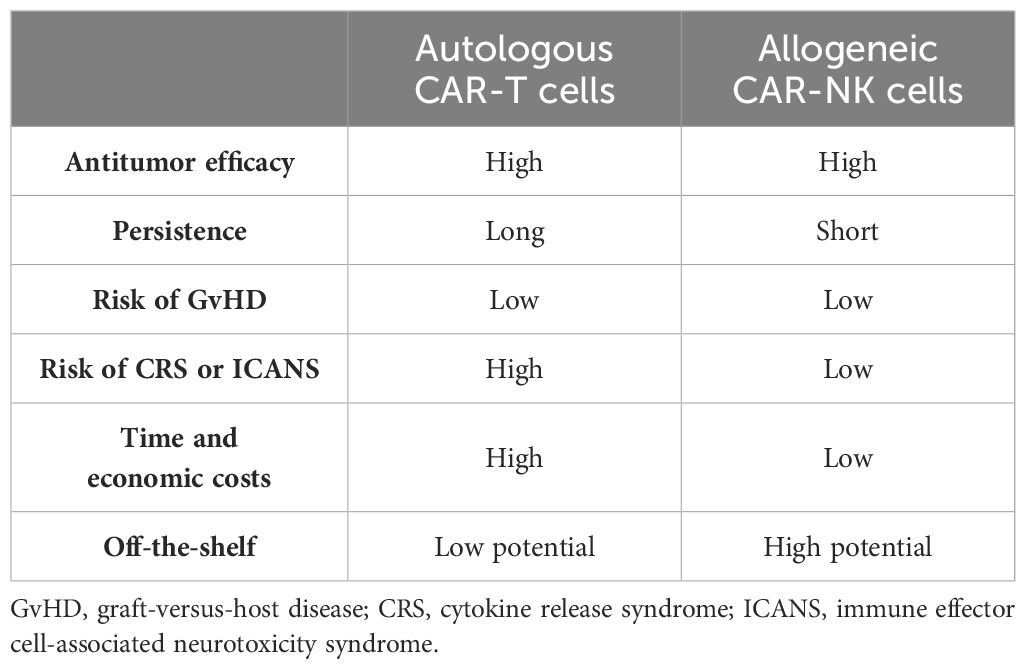
Table 3 Comparison of the characteristics of autologous chimeric antigen receptor T (CAR-T) and allogeneic chimeric antigen receptor natural killer (CAR-NK) cell therapies.
5 Preclinical research on CAR-NK cells
Preclinical studies have yielded promising preliminary data on the efficacy and safety of CAR-NK cell therapy, especially in the context of hematological malignancies. To this end, the following section provides a brief overview of the recent advancements in CAR-NK therapy for the treatment of common hematological tumors.
5.1 Leukemia
CD33 is a promising target antigen for CAR-NK cell therapy, being expressed on the surface of leukemia cells in over 90% of patients with acute myeloid leukemia (AML). In the study by Albinger et al. (62), lentiviral transduction was used to generate a CD33-CAR-NK cell model derived from PB-NK cells. These CAR-NK cells demonstrated potent in vitro and in vivo cytotoxicity against AML cells. Similarly, another study observed a significant reduction in tumor burden within a leukemia mouse model treated with CD33-CAR-PB-NK cells, with no apparent side effects (63). Building on these findings, Zhang et al. engineered CAR-NK cells co-expressing CD33-CAR and anti-CD16 antibodies. This approach achieved dual targeting of AML cells while also enhancing the activity of NK cells (64). Pediatric AML frequently exhibits high CD123 expression, making it another attractive target for CAR-NK therapy. Caruso et al. constructed second-generation CD123-CAR-NK cells utilizing 4–1BB co-stimulatory domains. These CAR-NK cells displayed a superior safety profile compared with CD123-CAR-T cells while maintaining remarkable anti-leukemia activity in both in vitro and in vivo models (65). The NPM1 mutation is common in AML and has also been targeted using CAR-NK cells. Initial studies suggest promising efficacy with NPM1–cytokine-induced memory-like (CIML) CAR-NK cells (66). CD38 represents another potential target for AML immunotherapy. A recent study has demonstrated that the combination of CD38-knockout CAR-NK cells expressing an affinity optimized CD38 with all-trans retinoic acid (ATRA) enhanced the targeting ability and the cytotoxicity of NK cells against CD38-positive mature myeloid cells (67). Research on CAR-NK therapy for acute lymphoblastic leukemia (ALL) is also progressing. Studies have explored CD5-CAR-NK cells (68) and CD7-CAR-NK cells (69) for T-cell acute lymphoblastic leukemia (T-ALL) treatment and FLT3-CAR-NK cells in B-cell acute lymphoblastic leukemia (B-ALL) (70). Notably, preclinical experiments using cryopreserved and thawed cord blood CD34+ cell-derived CAR-NK cells demonstrated potent and specific antitumor activity against ALL cells (71). The development of dual-targeted CAR-NK cells holds particular promise (72), as evidenced by recent reports of CD19/CD20 dual-targeted CAR-NK cells exhibiting enhanced cytotoxicity against ALL cells (73).
5.2 Multiple myeloma
BCMA is a highly expressed antigen on multiple myeloma (MM) cells and represents a promising target for CAR-NK cell therapy (74). CS1, a glycoprotein overexpressed on the MM cell membrane, also offers a potential therapeutic target. CAR-NK cells specifically designed to target CS1 have demonstrated significant antitumor efficacy in preclinical studies (75). GPRC5D, another tumor antigen on MM cells, holds promise for CAR-NK therapy. Yang et al. presented a GPRC5D-targeted CAR-NK product at a recent American Society of Hematology (ASH) meeting (76). This product exhibited potent cytotoxicity, with the efficacy maintained even after cryopreservation and long-distance transport. Dual-targeted CAR-NK cells offer potential advantages over single-targeted BCMA-CAR-NK cells. For instance, BCMA/GPRC5D-CAR-NK cells can effectively lyse BCMA-negative MM cells, leading to improved antitumor efficacy in in vivo and ex vivo models. This approach has been shown to prolong the survival of mice and to reduce the risk of tumor recurrence (77). FT555, a GPRC5D/CD38-CAR-NK cell product derived from induced pluripotent stem cells (iPSCs), incorporates several features: a GPRC5D-specific CAR structure; a high-affinity, non-cleavable CD16 (hnCD16) domain for enhanced ADCC activity; an IL-15/IL-15 receptor fusion protein (IL-15RF) for sustained cytokine signaling; and CD38 knockout. This product has demonstrated high and persistent killing efficiency, particularly when combined with daratumumab (78). Limited homing of NK cells to tumor sites can hinder the therapeutic efficacy. The co-expression of chemokine receptor 4 (CXCR4) in BCMA-CAR-NK cells has been proposed as a strategy to overcome this limitation, demonstrating promise in preclinical models (79).
5.3 Lymphomas
CAR-NK cell therapy has demonstrated continuous progress in the treatment of lymphomas. Gang et al. (80) developed CD19-CAR-CIML-NK cells. These CAR-NK cells exhibited significantly enhanced cytotoxicity against NK-resistant B-cell lymphoma (BCL) cells and improved the overall survival in mouse models. MHC class I chain (MIC)-related proteins act as ligands for NKG2D, effectively activating NK cells. Based on the NKG2D–NKG2DL interaction, Liu et al. designed a unique soluble CAR structure for NK cells named MS-Ig. This molecule comprises a MICA extracellular domain (MICA-ECD) for NKG2D binding, an anti-CD20 single-chain variable fragment (ScFv) for CD20 recognition on tumor cells, and a human IgG Fc fragment. During co-culture with CD20-positive lymphoma cells, the anti-CD20 ScFv binds to CD20 antigens, while MICA-ECD interacts with NKG2D on NK cells, directly triggering the cytotoxicity of NK cells (81). Studies have shown that the combination of anti-CD20 or anti-CD79 antibodies with NKTR-255 (a polymer-conjugated, IL-15Rα-dependent, recombinant human IL-15 agonist) can significantly enhance the in vitro cytotoxicity of CD19-CAR-NK cells against Burkitt’s lymphoma (BL) cells (82). CD70, which is predominantly expressed on lymphocytes, has emerged as a potential target for CAR-NK therapy (83). Given the high expression of CD70 on T-cell lymphomas (TCLs), Rafei et al. presented a study at ASH 2023 on a CD70-CAR-NK product for TCL treatment (84). Their findings suggest CD70 as a viable target for CAR-NK therapy against TCLs. Recent reports have further highlighted the excellent efficacy of CD70-CAR-NK cells in CD19-negative BCLs (85).
6 Clinical research on CAR-NK cells
Several clinical trials are underway to evaluate the efficacy and safety of CAR-NK cell therapy, with some demonstrating promising initial results. Liu et al. conducted a first-in-human clinical trial of CD19-CAR-UCB-NK cells for CD19-positive CLL or BCL in 11 patients. This trial demonstrated an encouraging overall response (OR) rate of 73% (8/11) and a complete response (CR) rate of 64% (7/11) with no severe side effects, suggesting favorable safety and efficacy (55). The updated follow-up data by Marin et al. (61) showed sustained positive outcomes, with OR rates of 100% for low-grade NHL, 67% for CLL without transformation, and 41% for diffuse large B-cell lymphoma (DLBCL). Notably, high CR rates were observed in indolent diseases (mainly low-grade NHL and CLL). This study also suggests that UCB-NK cells collected within 24 h and with low nucleated red blood cell content (≤8 × 107 cells) may have superior clinical value. Another ongoing clinical trial is evaluating FT596 (CD19-CAR-iPSC-NK cell therapy) for relapsed/refractory (R/R) BCL in 20 patients (86). Early results have indicated an OR rate exceeding 50% (9/17) after the first treatment cycle, with sustained clinical benefit observed in five patients who received a second cycle [four maintained CR and one achieved a deeper partial response (PR)]. The updated data presented at ASH 2021 included 13 patients treated with FT596 monotherapy and 19 treated with FT596 combined with rituximab. The trial also explored different cell doses (30 × 106, 90 × 106, 300 × 106, and 900 × 106) and demonstrated consistent clinical efficacy (NCT04245722). CNTY101, a CD19-CAR-iPSC-NK cell product, achieved its first human dose in February 2023 to assess its safety and efficacy in patients with R/R CD19-positive BCL. A case report presented at the 2023 ASH meeting indicated initial safety and efficacy (NCT05336409). A new PB-CAR-NK cell therapy targeting CD19, NKX019 (NCT05020678), recently reported results at the European Hematology Association (EHA) meeting in a trial involving 19 patients with R/R CD19-positive BCL. In the phase I dose-escalation study, 10 evaluable patients received doses of 1 billion or 1.5 billion CAR-NK cells. The final OR rate was 80% (8/10), with seven patients achieving CR. Importantly, no cases of GvHD or other serious adverse events were observed. While the trial included five patients with ALL/CLL, the therapeutic effect in these patients was less promising. This may be attributed to the limited sample size or the underlying disease mechanisms. Another product from the same company, NKX101 (NKG2D-CAR-NK, NCT04623944), is being evaluated in a clinical trial for AML. The phase I follow-up data presented at ASH 2023 showed a CR/CRi (complete remission with incomplete count recovery) rate as high as 67% (4/6). Additional ongoing clinical trials in AML are also yielding positive outcomes (87). Trials are also underway to assess CAR-NK cell therapy in MM. FT576, a BCMA-CAR-NK cell therapy derived from iPSCs, demonstrated excellent safety and tolerability in patients with R/RMM, both as a monotherapy and in combination with daratumumab. No cases of CRS, neurotoxicity, or GvHD were reported (88). The updated safety data, as of October 7, 2022, and presented at ASH 2022, confirmed no occurrence of serious adverse events (NCT05182073).
A search on ClinicalTrials.gov using the keyword “CAR-NK” identified 44 ongoing clinical trials evaluating CAR-NK cell therapy for hematological malignancies (Table 4). The majority of these trials are currently in phase I or II, suggesting that CAR-NK therapy remains in the early stages of clinical investigation.
7 Current challenges
Despite the promising preclinical and clinical data on the efficacy and safety of CAR-NK cell therapy for hematological malignancies, several limitations need to be addressed before its widespread clinical application. These limitations are summarized in Table 5.
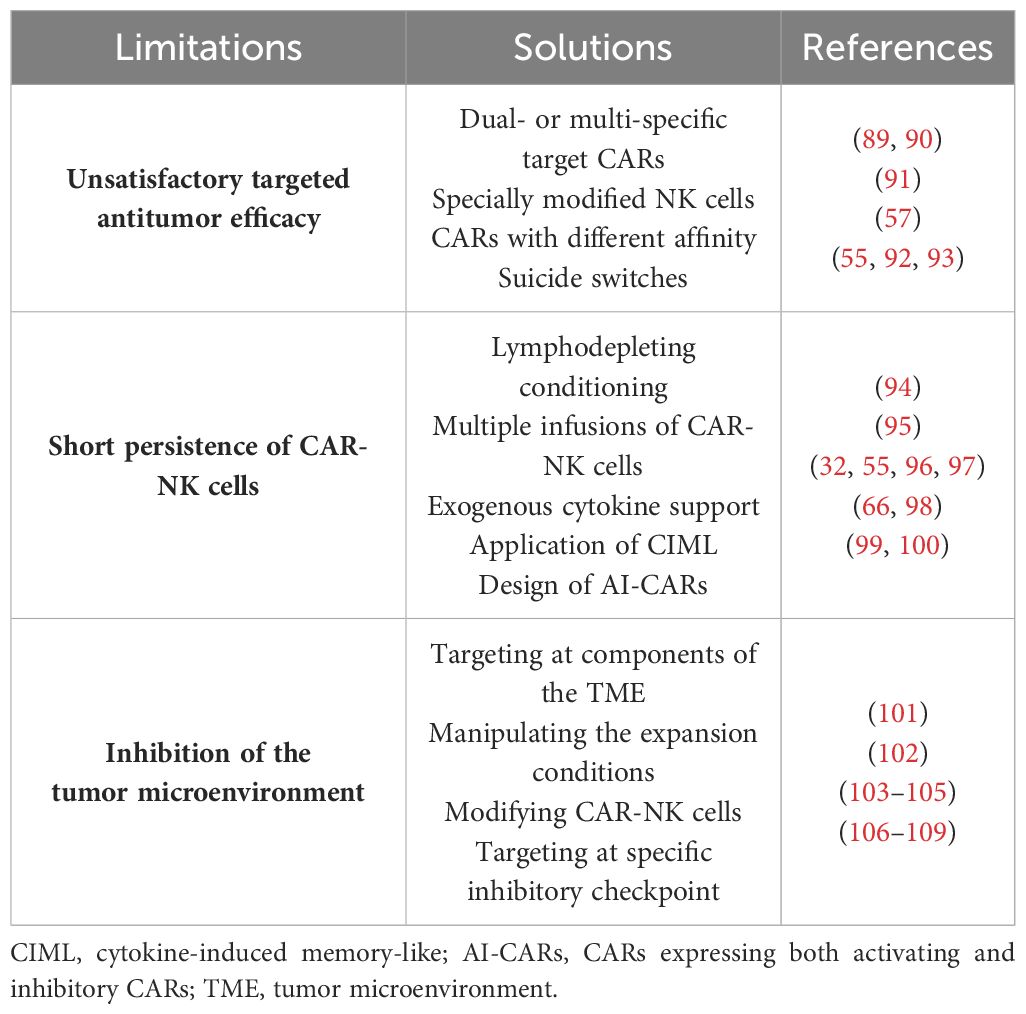
Table 5 Limitations of and potential solutions for chimeric antigen receptor natural killer (CAR-NK) cell therapy.
7.1 Improving the targeted antitumor efficacy
Ideally, the CAR targets should exhibit high specificity, a broad coverage of tumor cells, and stable expression on malignant cells. However, achieving all these characteristics simultaneously is challenging, and few targets in clinical application fully meet these criteria (110). To enhance the antitumor efficacy, the development of dual- or multi-specific CARs has emerged as a promising strategy (111). A clinical trial using CD19/CD22-CAR-T cells for refractory ALL has demonstrated the potential of dual-CARs. All patients achieved complete remission with no minimal residual disease (MRD), highlighting their clinical efficacy (89). Similarly, bispecific CAR-NK cells targeting both CD19 and BCMA for B-ALL and MM have shown potent cytotoxicity in vitro, warranting further investigation of their in vivo efficacy (90). Moreover, triple-modified NK cells incorporating a CD19-targeting CAR, hnCD16, and an IL-15/IL-15 receptor fusion protein (IL-15RF) have been designed, potentially mitigating tumor escape to a greater extent (91). Ensuring safety is paramount in CAR-NK cell therapy. The CAR design should not only accurately target tumors but also minimize the risk of “on-target, off-tumor” toxicity against normal tissues expressing the target antigen. Studies in various tumor models have demonstrated the feasibility of utilizing CARs with lower affinity for tumor tissues expressing high levels of TAA. This approach ensures that only those tumor cells with a sufficiently high antigen density can activate the CAR structure (57). Furthermore, incorporating suicide switches into CAR-NK cells offers an additional safety measure (92). The inducible caspase 9 (iCasp9) system has been successfully employed for this purpose, demonstrating a favorable safety profile (55, 93).
7.2 Extending the persistence of CAR-NK cells
Unlike T cells, which can differentiate into memory cells and maintain long-term antitumor efficacy (110), NK cells have a shorter life span in humans (approximately 2 weeks in blood) (112). This limited life span offers some safety advantages, but also hinders the efficacy of CAR-NK cell therapy (103). Various strategies have been implemented to prolong the survival of NK cells in vivo. Lymphodepleting conditioning regimens prior to adoptive cell transfer can mitigate the risk of rejection and significantly improve the efficacy of cell therapy through various mechanisms (94). Therefore, the combination of cyclophosphamide and fludarabine is often used as pretreatment before clinical treatment with allogeneic CAR-NK cells, whereas autologous NK cells do not necessitate pretreatment (113). Multiple infusions of CAR-NK cells have also been proposed to potentially safeguard the treatment outcomes (95). However, the inherent heterogeneity of the natural immune system requires close monitoring (114) and assessment of host immune rejection against allogeneic NK cells to prevent diminished efficacy (115, 116). Exogenous cytokine support, particularly IL-2 and IL-15, can maintain the proliferation and activity of NK cells in vivo (97, 117). However, systemic high-dose injections could induce additional safety risks, as exemplified by the association between high-dose IL-2 therapy and vascular leak syndrome (118). Liu et al. (32, 55) addressed these limitations by constructing CD19-CAR (iC9/CAR.19/IL-15)-CB-NK cells that ectopically secrete IL-15 and express suicide switches. These cells exhibited both longer persistence and stronger antitumor efficacy in vitro and in vivo, persisting at low levels for at least 12 months in patients. Building on this concept, Daher et al. (96) constructed CISH-knockout (KO)/IL-15 CAR-NK cells using CRISPR-Cas9 to knock down IL-15-associated negative immune checkpoint genes (CISH genes). These CISH-KO/IL-15 CAR-NK cells exhibited enhanced survival and antitumor activity compared with the control IL-15 CAR-NK cells. The induction of immune memory in NK cells represents another approach to improve their activity (119). NK cells can acquire memory-like properties upon stimulation with cytokines, particularly IL-12, IL-15, and IL-18. Therefore, the application of cytokines to induce the generation of memory-like NK cells might prolong their in vivo survival (66, 98). Interestingly, some studies have suggested that activating CAR (aCAR) NK cells can express and acquire trogocytic antigens (TROGs) through trogocytosis, leading to fratricide (killing of other CAR-NK cells). However, co-expressing an inhibitory CAR (iCAR) on these aCAR-NK cells can restrain their killing function, thereby reducing the risk of fratricide. This phenomenon suggests that CARs expressing both aCAR and iCAR (AI-CARs) could not only minimize NK cell depletion but also improve the durability of CAR-NK cell therapy in vivo (99, 100).
7.3 Overcoming the inhibitions of the tumor microenvironment
The tumor microenvironment (TME) presents a significant challenge for effective immune cell killing. Cancer-associated fibroblasts (CAFs), a major component of the tumor stroma, are well-established contributors to tumor progression (120). The targeting of CAFs represents a potential strategy to counteract the negative influence of the TME. Sakemura et al. (101) explored this concept by designing BCMA/CAF-CAR-T cells. Studies using human MM cells and mouse models demonstrated that this dual-targeted CAR structure could reverse CAR-T cell dysfunction and enhance the antitumor efficacy. In addition, manipulation of the expansion conditions of CAR-NK cells might mitigate the suppressive effects of the TME, as has been tentatively demonstrated with CAR-T cells (102). The TME harbors multiple immunosuppressive factors, including hypoxia, transforming growth factor beta (TGF-β), PGE-2, and extracellular metabolites such as lactate and adenosine, which can restrain immune cell activity (121). The modification of NK cells to overcome these suppressive factors presents a promising approach. Knockdown of the TGF-β receptor II (TGF-βRII) on NK cells has been shown to partially overcome the negative influence of the TME without compromising their anti-leukemia efficacy (103). Engineering CARs that express negative TGF-β receptors and combining treatment with TGF-β receptor inhibitors are also being explored (104, 105). Tumor cells can also upregulate the expression of ligands for immune cell inhibitory receptors, exploiting the negative feedback mechanisms of the immune system to evade immune clearance. Strategies to address this challenge include the knockdown of relevant receptors on NK cells (such as NKG2A) or combining therapy with specific inhibitory checkpoint antibodies to improve the antitumor effects (106–108). Furthermore, the design of CAR structures that target inhibitory checkpoints represents a promising approach (109), as initially demonstrated in solid tumors (122).
8 Conclusions
NK cells offer a novel avenue for cancer immunotherapy, and the development of CAR-NK cell therapy holds significant promise for the treatment of hematological malignancies. Preclinical studies suggest that CAR-NK cell therapy might address some of the limitations associated with CAR-T cell therapy while achieving comparable clinical efficacy. While NK cells and CAR-NK immunotherapy have inherent limitations, researchers are actively developing strategies to improve their treatment efficacy. Early studies have yielded promising results, paving the way for future clinical applications of CAR-NK therapy.
Author contributions
RY: Writing – original draft, Writing – review & editing. YY: Supervision, Writing – review & editing. RL: Writing – review & editing. YW: Writing – review & editing. RYY: Writing – review & editing. AH: Conceptualization, Supervision, Writing – review & editing.
Funding
The author(s) declare financial support was received for the research, authorship, and/or publication of this article. This work was supported by the Science and Technology Program of Shaanxi Province (Grant Number: 2023-ZDLSF-16).
Conflict of interest
The authors declare that the research was conducted in the absence of any commercial or financial relationships that could be construed as a potential conflict of interest.
Publisher’s note
All claims expressed in this article are solely those of the authors and do not necessarily represent those of their affiliated organizations, or those of the publisher, the editors and the reviewers. Any product that may be evaluated in this article, or claim that may be made by its manufacturer, is not guaranteed or endorsed by the publisher.
Abbreviations
ADCC, antibody-dependent cytotoxicity; ALL, acute lymphoblastic leukemia; AML, acute myeloid leukemia; ASH, American Society of Hematology; ATRA, all-trans retinoic acid; BCL, B-cell lymphoma; BCMA, B-cell maturation antigen; CAFs, cancer-associated fibroblasts; CAR, chimeric antigen receptor; CIML, cytokine-induced memory-like; CLL, chronic lymphoblastic leukemia; CR, complete response; CRi, CR with incomplete count recovery; CRS, cytokine release syndrome; CTL, CD8+ cytotoxic T cells; DLBCL, diffuse large B-cell lymphoma; FDA, Food and Drug Administration; GvHD, graft-versus-host disease; hnCD16, high-affinity and non-cleavable CD16; hPSC, human pluripotent stem cell; ICANS, immune effector cell-associated neurotoxicity syndrome; IFN-γ, interferon gamma; IL, interleukin; iPSC, induced pluripotent stem cell; MDS, myelodysplastic syndromes; MHC-I, major histocompatibility complex-I; MM, multiple myeloma; MRD, minimal residual disease; NCAM, nerve cell adhesion molecule; NCR, natural cytotoxicity receptors; NHL, non-Hodgkin’s lymphoma; NK cell, natural killer cell; OR, overall response; PB, peripheral blood; R/R, relapsed/refractory; TAA, tumor-associated antigens; TCL, T-cell lymphoma; TCR, T cell receptor; TME, tumor microenvironment; TNF-α, tumor necrosis factor-alpha; TROG, trogocytic antigen; UCB, umbilical cord blood.
References
1. Zhao J, Wu M, Li Z, Su S, Wen Y, Zhang L, et al. Chimeric antigen receptor therapy in hematological Malignancies: antigenic targets and their clinical research progress. Ann Hematol. (2020) 99:1681–99. doi: 10.1007/s00277-020-04020-7
2. Wagner DL, Fritsche E, Pulsipher MA, Ahmed N, Hamieh M, Hegde M, et al. Immunogenicity of CAR T cells in cancer therapy. Nat Rev Clin Oncol. (2021) 18:379–93. doi: 10.1038/s41571-021-00476-2
3. Pan K, Farrukh H, Chittepu V, Xu H, Pan CX, Zhu Z. Car race to cancer immunotherapy: from CAR T, CAR NK to car macrophage therapy. J Exp Clin Cancer Res CR. (2022) 41:119. doi: 10.1186/s13046-022-02327-z
4. June CH, Sadelain M. Chimeric antigen receptor therapy. New Engl J Med. (2018) 379:64–73. doi: 10.1056/NEJMra1706169
5. June CH, O'Connor RS, Kawalekar OU, Ghassemi S, Milone MC. CAR T cell immunotherapy for human cancer. Science. (2018) 359:1361–5. doi: 10.1126/science.aar6711
6. Caligiuri MA. Human natural killer cells. Blood. (2008) 112:461–9. doi: 10.1182/blood-2007-09-077438
7. Moretta A, Bottino C, Mingari MC, Biassoni R, Moretta L. What is a natural killer cell? Nat Immunol. (2002) 3:6–8. doi: 10.1038/ni0102-6
8. Crinier A, Narni-Mancinelli E, Ugolini S, Vivier E. Snapshot: natural killer cells. Cell. (2020) 180:1280–.e1. doi: 10.1016/j.cell.2020.02.029
9. Cooper MA, Fehniger TA, Caligiuri MA. The biology of human natural killer-cell subsets. Trends Immunol. (2001) 22:633–40. doi: 10.1016/S1471-4906(01)02060-9
10. Rezvani K. Adoptive cell therapy using engineered natural killer cells. Bone marrow Transplant. (2019) 54:785–8. doi: 10.1038/s41409-019-0601-6
11. Wu SY, Fu T, Jiang YZ, Shao ZM. Natural killer cells in cancer biology and therapy. Mol Cancer. (2020) 19:120. doi: 10.1186/s12943-020-01238-x
12. Angelo LS, Banerjee PP, Monaco-Shawver L, Rosen JB, Makedonas G, Forbes LR, et al. Practical nk cell phenotyping and variability in healthy adults. Immunologic Res. (2015) 62:341–56. doi: 10.1007/s12026-015-8664-y
13. Vivier E, Tomasello E, Baratin M, Walzer T, Ugolini S. Functions of natural killer cells. Nat Immunol. (2008) 9:503–10. doi: 10.1038/ni1582
14. Long EO, Kim HS, Liu D, Peterson ME, Rajagopalan S. Controlling natural killer cell responses: integration of signals for activation and inhibition. Annu Rev Immunol. (2013) 31:227–58. doi: 10.1146/annurev-immunol-020711-075005
15. Wolf NK, Kissiov DU, Raulet DH. Roles of natural killer cells in immunity to cancer, and applications to immunotherapy. Nat Rev Immunol. (2023) 23:90–105. doi: 10.1038/s41577-022-00732-1
16. Zhang J, Guo F, Li L, Zhang S, Wang Y. Immune evasion and therapeutic opportunities based on natural killer cells. Chin J Cancer Res = Chung-kuo yen cheng yen chiu. (2023) 35:283–98. doi: 10.21147/j.issn.1000-9604.2023.03.07
17. Lanier LL. NKG2D receptor and its ligands in host defense. Cancer Immunol Res. (2015) 3:575–82. doi: 10.1158/2326-6066.CIR-15-0098
18. Smyth MJ, Cretney E, Kelly JM, Westwood JA, Street SE, Yagita H, et al. Activation of NK cell cytotoxicity. Mol Immunol. (2005) 42:501–10. doi: 10.1016/j.molimm.2004.07.034
19. Prager I, Watzl C. Mechanisms of natural killer cell-mediated cellular cytotoxicity. J leukocyte Biol. (2019) 105:1319–29. doi: 10.1002/JLB.MR0718-269R
20. Wallin RP, Screpanti V, Michaëlsson J, Grandien A, Ljunggren HG. Regulation of perforin-independent NK cell-mediated cytotoxicity. Eur J Immunol. (2003) 33:2727–35. doi: 10.1002/eji.200324070
21. Farag SS, Caligiuri MA. Human natural killer cell development and biology. Blood Rev. (2006) 20:123–37. doi: 10.1016/j.blre.2005.10.001
22. Biron CA, Nguyen KB, Pien GC. Innate immune responses to Lcmv infections: natural killer cells and cytokines. Curr topics Microbiol Immunol. (2002) 263:7–27. doi: 10.1007/978-3-642-56055-2_2
23. Pang Z, Wang Z, Li F, Feng C, Mu X. Current progress of CAR-NK therapy in cancer treatment. Cancers. (2022) 14. doi: 10.3390/cancers14174318
24. Kagoya Y, Tanaka S, Guo T, Anczurowski M, Wang CH, Saso K, et al. A novel chimeric antigen receptor containing a JAK-STAT signaling domain mediates superior antitumor effects. Nat Med. (2018) 24:352–9. doi: 10.1038/nm.4478
25. Bashiri Dezfouli A, Yazdi M, Pockley AG, Khosravi M, Kobold S, Wagner E, et al. NK cells armed with chimeric antigen receptors (CAR): roadblocks to successful development. Cells. (2021) 10. doi: 10.3390/cells10123390
26. Włodarczyk M, Pyrzynska B. CAR-NK as a rapidly developed and efficient immunotherapeutic strategy against cancer. Cancers. (2022) 15. doi: 10.3390/cancers15010117
27. Ruppel KE, Fricke S, Köhl U, Schmiedel D. Taking lessons from CAR-T cells and going beyond: tailoring design and signaling for CAR-NK cells in cancer therapy. Front Immunol. (2022) 13:822298. doi: 10.3389/fimmu.2022.822298
28. de Azevedo JTTC, Silvestre RN, Covas D, Picanço-Castro V. 500 - immunotherapy: CD3Z-2B4 intracellular domain improves cytotoxicity function of CAR-NK cells against CD19+ Cell lines. Cytotherapy. (2022) 24:S108. doi: 10.1016/S1465-3249(22)00299-7
29. Xie G, Dong H, Liang Y, Ham JD, Rizwan R, Chen J. CAR-NK cells: A promising cellular immunotherapy for cancer. EBioMedicine. (2020) 59:102975. doi: 10.1016/j.ebiom.2020.102975
30. Sabbah M, Jondreville L, Lacan C, Norol F, Vieillard V, Roos-Weil D, et al. CAR-NK cells: A chimeric hope or a promising therapy? Cancers. (2022) 14. doi: 10.3390/cancers14153839
31. Zhu H, Kaufman DS. Engineered human pluripotent stem cell-derived natural killer cells: the next frontier for cancer immunotherapy. Blood Sci. (2019) 1:4–11. doi: 10.1097/BS9.0000000000000023
32. Liu E, Tong Y, Dotti G, Shaim H, Savoldo B, Mukherjee M, et al. Cord blood NK cells engineered to express il-15 and a CD19-targeted car show long-term persistence and potent antitumor activity. Leukemia. (2018) 32:520–31. doi: 10.1038/leu.2017.226
33. Heipertz EL, Zynda ER, Stav-Noraas TE, Hungler AD, Boucher SE, Kaur N, et al. Current perspectives on "Off-the-shelf" Allogeneic NK and CAR-NK cell therapies. Front Immunol. (2021) 12:732135. doi: 10.3389/fimmu.2021.732135
34. Li L, Chen H, Marin D, Xi Y, Miao Q, Lv J, et al. A novel immature natural killer cell subpopulation predicts relapse after cord blood transplantation. Blood Adv. (2019) 3:4117–30. doi: 10.1182/bloodadvances.2019000835
35. Zhang B, Yang M, Zhang W, Liu N, Wang D, Jing L, et al. Chimeric antigen receptor-based natural killer cell immunotherapy in cancer: from bench to bedside. Cell Death Dis. (2024) 15:50. doi: 10.1038/s41419-024-06438-7
36. Hermanson DL, Kaufman DS. Utilizing chimeric antigen receptors to direct natural killer cell activity. Front Immunol. (2015) 6:195. doi: 10.3389/fimmu.2015.00195
37. Daher M, Melo Garcia L, Li Y, Rezvani K. CAR-NK cells: the next wave of cellular therapy for cancer. Clin Trans Immunol. (2021) 10:e1274. doi: 10.1002/cti2.1274
38. Zhang C, Oberoi P, Oelsner S, Waldmann A, Lindner A, Tonn T, et al. Chimeric antigen receptor-engineered NK-92 cells: an off-the-shelf cellular therapeutic for targeted elimination of cancer cells and induction of protective antitumor immunity. Front Immunol. (2017) 8:533. doi: 10.3389/fimmu.2017.00533
39. Klingemann H, Boissel L, Toneguzzo F. Natural killer cells for immunotherapy - advantages of the NK-92 cell line over blood nk cells. Front Immunol. (2016) 7:91. doi: 10.3389/fimmu.2016.00091
40. Rezvani K, Rouce R, Liu E, Shpall E. Engineering natural killer cells for cancer immunotherapy. Mol Ther J Am Soc Gene Ther. (2017) 25:1769–81. doi: 10.1016/j.ymthe.2017.06.012
41. Moscarelli J, Zahavi D, Maynard R, Weiner LM. The next generation of cellular immunotherapy: chimeric antigen receptor-natural killer cells. Transplant Cell Ther. (2022) 28:650–6. doi: 10.1016/j.jtct.2022.06.025
42. Guo F, Zhang Y, Cui J. Manufacturing CAR-NK against tumors: who is the ideal supplier? Chin J Cancer Res = Chung-kuo yen cheng yen chiu. (2024) 36:1–16. doi: 10.21147/j.issn.1000-9604.2024.01.01
43. Zhang L, Meng Y, Feng X, Han Z. CAR-NK cells for cancer immunotherapy: from bench to bedside. biomark Res. (2022) 10:12. doi: 10.1186/s40364-022-00364-6
44. Vargas JE, Chicaybam L, Stein RT, Tanuri A, Delgado-Cañedo A, Bonamino MH. Retroviral vectors and transposons for stable gene therapy: advances, current challenges and perspectives. J Trans Med. (2016) 14:288. doi: 10.1186/s12967-016-1047-x
45. Kong JC, Sa'ad MA, Vijayan HM, Ravichandran M, Balakrishnan V, Tham SK, et al. Chimeric antigen receptor-natural killer cell therapy: current advancements and strategies to overcome challenges. Front Immunol. (2024) 15:1384039. doi: 10.3389/fimmu.2024.1384039
46. Gong Y, Klein Wolterink RGJ, Wang J, Bos GMJ, Germeraad WTV. Chimeric antigen receptor natural killer (CAR-NK) cell design and engineering for cancer therapy. J Hematol Oncol. (2021) 14:73. doi: 10.1186/s13045-021-01083-5
47. Schmidt P, Raftery MJ, Pecher G. Engineering NK cells for car therapy-recent advances in gene transfer methodology. Front Immunol. (2020) 11:611163. doi: 10.3389/fimmu.2020.611163
48. Smirnov S, Petukhov A, Levchuk K, Kulemzin S, Staliarova A, Lepik K, et al. Strategies to circumvent the side-effects of immunotherapy using allogeneic car-T cells and boost its efficacy: results of recent clinical trials. Front Immunol. (2021) 12:780145. doi: 10.3389/fimmu.2021.780145
49. Depil S, Duchateau P, Grupp SA, Mufti G, Poirot L. 'Off-the-shelf' Allogeneic car T cells: development and challenges. Nat Rev Drug Discovery. (2020) 19:185–99. doi: 10.1038/s41573-019-0051-2
50. Labanieh L, Mackall CL. Car immune cells: design principles, resistance and the next generation. Nature. (2023) 614:635–48. doi: 10.1038/s41586-023-05707-3
51. Ullrich E, Salzmann-Manrique E, Bakhtiar S, Bremm M, Gerstner S, Herrmann E, et al. Relation between acute Gvhd and NK cell subset reconstitution following allogeneic stem cell transplantation. Front Immunol. (2016) 7:595. doi: 10.3389/fimmu.2016.00595
52. Ran T, Eichmüller SB, Schmidt P, Schlander M. Cost of decentralized car T-cell production in an academic nonprofit setting. Int J Cancer. (2020) 147:3438–45. doi: 10.1002/ijc.33156
53. Jagannath S, Joseph N, Crivera C, Jackson CC, Valluri S, Cost P, et al. Total car-T cost of care beyond the price of car-T cell therapy in patients with multiple myeloma. Blood. (2021) 138:4964. doi: 10.1182/blood-2021-153438
54. Morris EC, Neelapu SS, Giavridis T, Sadelain M. Cytokine release syndrome and associated neurotoxicity in cancer immunotherapy. Nat Rev Immunol. (2022) 22:85–96. doi: 10.1038/s41577-021-00547-6
55. Liu E, Marin D, Banerjee P, Macapinlac HA, Thompson P, Basar R, et al. Use of car-transduced natural killer cells in CD19-positive lymphoid tumors. New Engl J Med. (2020) 382:545–53. doi: 10.1056/NEJMoa1910607
56. Marofi F, Al-Awad AS, Sulaiman Rahman H, Markov A, Abdelbasset WK, Ivanovna Enina Y, et al. CAR-NK cell: A new paradigm in tumor immunotherapy. Front Oncol. (2021) 11:673276. doi: 10.3389/fonc.2021.673276
57. Rafiq S, Hackett CS, Brentjens RJ. Engineering strategies to overcome the current roadblocks in car T cell therapy. Nat Rev Clin Oncol. (2020) 17:147–67. doi: 10.1038/s41571-019-0297-y
58. Sadelain M, Brentjens R, Rivière I. The promise and potential pitfalls of chimeric antigen receptors. Curr Opin Immunol. (2009) 21:215–23. doi: 10.1016/j.coi.2009.02.009
59. Zhang C, Hu Y, Xiao W, Tian Z. Chimeric antigen receptor- and natural killer cell receptor-engineered innate killer cells in cancer immunotherapy. Cell Mol Immunol. (2021) 18:2083–100. doi: 10.1038/s41423-021-00732-6
60. Egli L, Kaulfuss M, Mietz J, Picozzi A, Verhoeyen E, Münz C, et al. Car T cells outperform CAR NK cells in car-mediated effector functions in head-to-head comparison. Exp Hematol Oncol. (2024) 13:51. doi: 10.1186/s40164-024-00522-6
61. Marin D, Li Y, Basar R, Rafei H, Daher M, Dou J, et al. Safety, efficacy and determinants of response of allogeneic CD19-specific CAR-NK cells in CD19(+) B cell tumors: A phase 1/2 trial. Nat Med. (2024) 30:772–84. doi: 10.1038/s41591-023-02785-8
62. Albinger N, Pfeifer R, Nitsche M, Mertlitz S, Campe J, Stein K, et al. Primary CD33-targeting CAR-NK cells for the treatment of acute myeloid leukemia. Blood Cancer J. (2022) 12:61. doi: 10.1038/s41408-022-00660-2
63. Wang Y, Wang L, Shao M, He X, Yue Y, Zhou Y, et al. Off-the-shelf, multiplexed-engineered ipsc-derived CD33 CAR-NK cells for treatment of acute myeloid leukemia. Blood. (2022) 140:12685. doi: 10.1182/blood-2022-166420
64. Zhang R, Liu Q, Zhou S, He H, Zhao M, Ma W. Engineering CAR-NK cells targeting CD33 with concomitant extracellular secretion of anti-CD16 antibody revealed superior antitumor effects toward myeloid leukemia. Cancer Lett. (2023) 558:216103. doi: 10.1016/j.canlet.2023.216103
65. Caruso S, De Angelis B, Del Bufalo F, Ciccone R, Donsante S, Volpe G, et al. Safe and effective off-the-shelf immunotherapy based on car. CD123-NK cells for the treatment of acute myeloid leukaemia. J Hematol Oncol. (2022) 15:163. doi: 10.1186/s13045-022-01376-3
66. Dong H, Ham JD, Hu G, Xie G, Vergara J, Liang Y, et al. Memory-like NK cells armed with a neoepitope-specific car exhibit potent activity against npm1 mutated acute myeloid leukemia. Proc Natl Acad Sci United States America. (2022) 119:e2122379119. doi: 10.1073/pnas.2122379119
67. Gurney M, Stikvoort A, Nolan E, Kirkham-McCarthy L, Khoruzhenko S, Shivakumar R, et al. CD38 knockout natural killer cells expressing an affinity optimized CD38 chimeric antigen receptor successfully target acute myeloid leukemia with reduced effector cell fratricide. Haematologica. (2022) 107:437–45. doi: 10.3324/haematol.2020.271908
68. Chen KH, Wada M, Pinz KG, Liu H, Lin KW, Jares A, et al. Preclinical targeting of aggressive T-cell Malignancies using anti-Cd5 chimeric antigen receptor. Leukemia. (2017) 31:2151–60. doi: 10.1038/leu.2017.8
69. You F, Wang Y, Jiang L, Zhu X, Chen D, Yuan L, et al. A novel CD7 chimeric antigen receptor-modified NK-92MI cell line targeting T-cell acute lymphoblastic leukemia. Am J Cancer Res. (2019) 9:64–78.
70. Oelsner S, Waldmann A, Billmeier A, Röder J, Lindner A, Ullrich E, et al. Genetically engineered CAR NK cells display selective cytotoxicity against Flt3-positive B-all and inhibit in vivo leukemia growth. Int J Cancer. (2019) 145:1935–45. doi: 10.1002/ijc.32269
71. Stoltzman C, Chandrasekaran D, von Euw E, McKay C, Root C, Delaney C. Abstract 2 development of CAR-NK cell therapy for hematologic Malignancies. Stem Cells Trans Med. (2022) 11:S4–S. doi: 10.1093/stcltm/szac057.002
72. Davis Z, Cichocki F, Felices M, Wang H, Hinderlie P, Juckett M, et al. A novel dual-antigen targeting approach enables off-the-shelf CAR NK cells to effectively recognize and eliminate the heterogenous population associated with aml. Blood. (2022) 140:10288–9. doi: 10.1182/blood-2022-168981
73. Yang N, Zhang C, Zhang Y, Fan Y, Zhang J, Lin X, et al. CD19/CD20 dual-targeted chimeric antigen receptor-engineered natural killer cells exhibit improved cytotoxicity against acute lymphoblastic leukemia. J Trans Med. (2024) 22:274. doi: 10.1186/s12967-024-04990-6
74. Cho SF, Lin L, Xing L, Li Y, Yu T, Anderson KC, et al. Bcma-targeting therapy: driving a new era of immunotherapy in multiple myeloma. Cancers. (2020) 12. doi: 10.3390/cancers12061473
75. Chu J, Deng Y, Benson DM, He S, Hughes T, Zhang J, et al. CS1-specific chimeric antigen receptor (CAR)-engineered natural killer cells enhance in vitro and in vivo antitumor activity against human multiple myeloma. Leukemia. (2014) 28:917–27. doi: 10.1038/leu.2013.279
76. Fu J, Jiang L, Zhu Z, Yan Y, Wu G, Wei M, et al. Efficacy of human ipsc-derived CAR-NK cells targeting multiple myeloma cells. Blood. (2023) 142:4802. doi: 10.1182/blood-2023-181613
77. Cao Z, Yang C, Wang Y, Wang C, Wang Q, Ye G, et al. Allogeneic CAR-NK cell therapy targeting both Bcma and Gprc5d for the treatment of multiple myeloma. Blood. (2022) 140:7378. doi: 10.1182/blood-2022-159289
78. Reiser J, Chan SR, Mathavan K, Sillitti D, Mottershead C, Mattson B, et al. FT555: off-the-shelf CAR-NK cell therapy co-targeting Gprc5d and CD38 for the treatment of multiple myeloma. Blood. (2022) 140:4560–1. doi: 10.1182/blood-2022-170501
79. Ng YY, Du Z, Zhang X, Chng WJ, Wang S. CXCR4 and anti-bcma car co-modified natural killer cells suppress multiple myeloma progression in a xenograft mouse model. Cancer Gene Ther. (2022) 29:475–83. doi: 10.1038/s41417-021-00365-x
80. Gang M, Marin ND, Wong P, Neal CC, Marsala L, Foster M, et al. CAR-modified memory-like NK cells exhibit potent responses to NK-resistant lymphomas. Blood. (2020) 136:2308–18. doi: 10.1182/blood.2020006619
81. Liu R, Luo Q, Luo W, Wan L, Zhu Q, Yin X, et al. A soluble NK-CAR mediates the specific cytotoxicity of NK cells toward the target CD20(+) lymphoma cells. Aging Dis. (2022) 13:1576–88. doi: 10.14336/AD.2022.0415
82. Chu Y, Tian M, Marcondes MQ, Overwijk WW, Lee DA, Klein C, et al. 201 - optimizing chimeric antigen receptor (CAR) engineered NK cell-mediated cytotoxicity combined with anti-CD20 or anti-CD79 therapeutic antibodies and NKTR-255 in burkitt lymphoma (Bl). Transplant Cell Ther. (2022) 28:S164–S5. doi: 10.1016/S2666-6367(22)00362-1
83. Nilsson MB, Yang Y, Heeke S, Patel SA, Poteete A, Udagawa H, et al. CD70 is a therapeutic target upregulated in emt-associated EGFR tyrosine kinase inhibitor resistance. Cancer Cell. (2023) 41:340–55.e6. doi: 10.1016/j.ccell.2023.01.007
84. Rafei H, Basar R, Acharya S, Zhang P, Liu P, Moseley SM, et al. Targeting T-cell lymphoma using Cd70-directed cord blood-derived CAR-NK cells. Blood. (2023) 142:4811. doi: 10.1182/blood-2023-184864
85. Guo S, Lei W, Jin X, Liu H, Wang JQ, Deng W, et al. CD70-specific CAR-NK cells expressing il-15 for the treatment of CD19-negative B cell Malignancy. Blood Adv. (2024) 8:2635–45. doi: 10.1182/bloodadvances.2023012202
86. Bachanova V, Ghobadi A, Patel K, Park JH, Flinn IW, Shah P, et al. Safety and efficacy of FT596, a first-in-class, multi-antigen targeted, off-the-shelf, ipsc-derived CD19 CAR NK cell therapy in relapsed/refractory B-cell lymphoma. Blood. (2021) 138:823. doi: 10.1182/blood-2021-151185
87. Huang R, Wen Q, Wang X, Yan H, Ma Y, Mai-Hong W, et al. Off-the-shelf CD33 CAR-NK cell therapy for relapse/refractory aml: first-in-human, phase I trial. Blood. (2022) 140:7450–1. doi: 10.1182/blood-2022-170712
88. Dhakal B, Berdeja JG, Gregory T, Ly T, Bickers C, Zong X, et al. Interim phase I clinical data of FT576 as monotherapy and in combination with daratumumab in subjects with relapsed/refractory multiple myeloma. Blood. (2022) 140:4586–7. doi: 10.1182/blood-2022-166994
89. Dai H, Wu Z, Jia H, Tong C, Guo Y, Ti D, et al. Bispecific car-T cells targeting both CD19 and CD22 for therapy of adults with relapsed or refractory B cell acute lymphoblastic leukemia. J Hematol Oncol. (2020) 13:30. doi: 10.1186/s13045-020-00856-8
90. Roex G, Campillo-Davo D, Flumens D, Shaw PAG, Krekelbergh L, De Reu H, et al. Two for one: targeting Bcma and CD19 in B-cell Malignancies with off-the-shelf dual-car NK-92 cells. J Trans Med. (2022) 20:124. doi: 10.1186/s12967-022-03326-6
91. Rahnama R, Bonifant CL. Engineering builds multipotency for IPSC-NKS. Blood. (2022) 140:2414–6. doi: 10.1182/blood.2022017794
92. Glienke W, Esser R, Priesner C, Suerth JD, Schambach A, Wels WS, et al. Advantages and applications of car-expressing natural killer cells. Front Pharmacol. (2015) 6:21. doi: 10.3389/fphar.2015.00021
93. Stasi DA, Tey SK, Dotti G. Supplement to: inducible apoptosis as a safety switch for adoptive cell therapy. New Engl J Med. (2011) 365:1673–83. doi: 10.1056/NEJMoa1106152
94. Neelapu SS. CAR-T efficacy: is conditioning the key? Blood. (2019) 133:1799–800. doi: 10.1182/blood-2019-03-900928
95. Li L, Mohanty V, Dou J, Huang Y, Banerjee PP, Miao Q, et al. Loss of metabolic fitness drives tumor resistance after CAR-NK cell therapy and can be overcome by cytokine engineering. Sci Adv. (2023) 9:eadd6997. doi: 10.1126/sciadv.add6997
96. Daher M, Basar R, Gokdemir E, Baran N, Uprety N, Nunez Cortes AK, et al. Targeting a cytokine checkpoint enhances the fitness of armored cord blood CAR-NK cells. Blood. (2021) 137:624–36. doi: 10.1182/blood.2020007748
97. Sarkar S, Germeraad WT, Rouschop KM, Steeghs EM, van Gelder M, Bos GM, et al. Hypoxia induced impairment of NK cell cytotoxicity against multiple myeloma can be overcome by il-2 activation of the NK cells. PLoS One. (2013) 8:e64835. doi: 10.1371/journal.pone.0064835
98. Maia A, Tarannum M, Lérias JR, Piccinelli S, Borrego LM, Maeurer M, et al. Building a better defense: expanding and improving natural killer cells for adoptive cell therapy. Cells. (2024) 13. doi: 10.3390/cells13050451
99. Brunello L. Avoiding CAR-NK cell fratricide. Nat Cancer. (2022) 3:1445. doi: 10.1038/s43018-022-00476-w
100. Li Y, Basar R, Wang G, Liu E, Moyes JS, Li L, et al. Kir-based inhibitory cars overcome CAR-NK cell trogocytosis-mediated fratricide and tumor escape. Nat Med. (2022) 28:2133–44. doi: 10.1038/s41591-022-02003-x
101. Sakemura R, Hefazi M, Siegler EL, Cox MJ, Larson DP, Hansen MJ, et al. Targeting cancer-associated fibroblasts in the bone marrow prevents resistance to cart-cell therapy in multiple myeloma. Blood. (2022) 139:3708–21. doi: 10.1182/blood.2021012811
102. Kilgour MK, Bastin DJ, Lee SH, Ardolino M, McComb S, Visram A. Advancements in CAR-NK therapy: lessons to be learned from CAR-T therapy. Front Immunol. (2023) 14:1166038. doi: 10.3389/fimmu.2023.1166038
103. Daher M, Rezvani K. Outlook for new car-based therapies with a focus on CAR NK cells: what lies beyond car-engineered T cells in the race against cancer. Cancer Discovery. (2021) 11:45–58. doi: 10.1158/2159-8290.CD-20-0556
104. Rouce RH, Shaim H, Sekine T, Weber G, Ballard B, Ku S, et al. The TGF-Β/smad pathway is an important mechanism for NK cell immune evasion in childhood B-acute lymphoblastic leukemia. Leukemia. (2016) 30:800–11. doi: 10.1038/leu.2015.327
105. Otegbeye F, Ojo E, Moreton S, Mackowski N, Lee DA, de Lima M, et al. Inhibiting tgf-beta signaling preserves the function of highly activated, in vitro expanded natural killer cells in aml and colon cancer models. PLoS One. (2018) 13:e0191358. doi: 10.1371/journal.pone.0191358
106. Sun H, Sun C. The rise of NK cell checkpoints as promising therapeutic targets in cancer immunotherapy. Front Immunol. (2019) 10:2354. doi: 10.3389/fimmu.2019.02354
107. Kamiya T, Seow SV, Wong D, Robinson M, Campana D. Blocking expression of inhibitory receptor NKG2A overcomes tumor resistance to NK cells. J Clin Invest. (2019) 129:2094–106. doi: 10.1172/JCI123955
108. Ureña-Bailén G, Dobrowolski JM, Hou Y, Dirlam A, Roig-Merino A, Schleicher S, et al. Preclinical evaluation of crispr-edited CAR-NK-92 cells for off-the-shelf treatment of aml and B-all. Int J Mol Sci. (2022) 23. doi: 10.3390/ijms232112828
109. Yang K, Zhao Y, Sun G, Zhang X, Cao J, Shao M, et al. Clinical application and prospect of immune checkpoint inhibitors for CAR-NK cell in tumor immunotherapy. Front Immunol. (2022) 13:1081546. doi: 10.3389/fimmu.2022.1081546
110. Wei J, Han X, Bo J, Han W. Target selection for car-T therapy. J Hematol Oncol. (2019) 12:62. doi: 10.1186/s13045-019-0758-x
111. Ramakrishna S, Barsan V, Mackall C. Prospects and challenges for use of car T cell therapies in solid tumors. Expert Opin Biol Ther. (2020) 20:503–16. doi: 10.1080/14712598.2020.1738378
112. Zhang Y, Wallace DL, de Lara CM, Ghattas H, Asquith B, Worth A, et al. In vivo kinetics of human natural killer cells: the effects of ageing and acute and chronic viral infection. Immunology. (2007) 121:258–65. doi: 10.1111/j.1365-2567.2007.02573.x
113. Vivier E, Rebuffet L, Narni-Mancinelli E, Cornen S, Igarashi RY, Fantin VR. Natural killer cell therapies. Nature. (2024) 626:727–36. doi: 10.1038/s41586-023-06945-1
114. Ruggeri L, Capanni M, Urbani E, Perruccio K, Shlomchik WD, Tosti A, et al. Effectiveness of donor natural killer cell alloreactivity in mismatched hematopoietic transplants. Science. (2002) 295:2097–100. doi: 10.1126/science.1068440
115. Berrien-Elliott MM, Jacobs MT, Fehniger TA. Allogeneic natural killer cell therapy. Blood. (2023) 141:856–68. doi: 10.1182/blood.2022016200
116. Fabian KP, Hodge JW. The emerging role of off-the-shelf engineered natural killer cells in targeted cancer immunotherapy. Mol Ther oncolytics. (2021) 23:266–76. doi: 10.1016/j.omto.2021.10.001
117. Wu X, Matosevic S. Gene-edited and CAR-NK cells: opportunities and challenges with engineering of NK cells for immunotherapy. Mol Ther oncolytics. (2022) 27:224–38. doi: 10.1016/j.omto.2022.10.011
118. MacDonald A, Wu TC, Hung CF. Interleukin 2-based fusion proteins for the treatment of cancer. J Immunol Res. (2021) 2021:7855808. doi: 10.1155/2021/7855808
119. Lizana-Vasquez GD, Torres-Lugo M, Dixon RB, Powderly JD 2nd, Warin RF. The application of autologous cancer immunotherapies in the age of memory-NK cells. Front Immunol. (2023) 14:1167666. doi: 10.3389/fimmu.2023.1167666
120. Petrova V, Annicchiarico-Petruzzelli M, Melino G, Amelio I. The hypoxic tumour microenvironment. Oncogenesis. (2018) 7:10. doi: 10.1038/s41389-017-0011-9
121. Riggan L, Shah S, O'Sullivan TE. Arrested development: suppression of NK cell function in the tumor microenvironment. Clin Trans Immunol. (2021) 10:e1238. doi: 10.1002/cti2.1238
Keywords: chimeric antigen receptor, immunotherapy, NK cells, CAR-NK, hematological malignancies
Citation: Yang R, Yang Y, Liu R, Wang Y, Yang R and He A (2024) Advances in CAR-NK cell therapy for hematological malignancies. Front. Immunol. 15:1414264. doi: 10.3389/fimmu.2024.1414264
Received: 08 April 2024; Accepted: 10 June 2024;
Published: 28 June 2024.
Edited by:
Beatriz Martín-Antonio, University Hospital Fundación Jiménez Díaz, SpainReviewed by:
Francisco Borrego, Biobizkaia Health Research Institute, SpainJoyeeta Talukdar, All India Institute of Medical Sciences, India
Copyright © 2024 Yang, Yang, Liu, Wang, Yang and He. This is an open-access article distributed under the terms of the Creative Commons Attribution License (CC BY). The use, distribution or reproduction in other forums is permitted, provided the original author(s) and the copyright owner(s) are credited and that the original publication in this journal is cited, in accordance with accepted academic practice. No use, distribution or reproduction is permitted which does not comply with these terms.
*Correspondence: Aili He, aGVhaWxpQHhqdHUuZWR1LmNu