- 1Eco-environmental Protection Research Institute, Shanghai Academy of Agricultural Sciences, Shanghai, China
- 2Key Laboratory of Integrated Rice-Fish Farming Ecosystem, Ministry of Agriculture and Rural Affairs, Shanghai Academy of Agricultural Sciences, Shanghai, China
- 3Institute of Marine Sciences, University of Chittagong, Chattogram, Bangladesh
- 4State Key Laboratory of Freshwater Ecology and Biotechnology, Institute of Hydrobiology, Chinese Academy of Sciences, Hubei Hongshan Laboratory, Wuhan, China
Fish intestinal health under intensive aquaculture mode plays an important role in growth, development, and immune function. The present study was aimed to systematically investigate the differences of intestinal health between wild and cultured Monopterus albus by biochemical parameters, histomorphology, and molecular biology. A total of 15 healthy M. albus per group, with an average body weight of 45 g, were sampled to analyze intestinal health parameters. Compared with wild fish, the cultured M. albus in the foregut had lower trypsin, lipase, SOD, CAT, T-AOC, and GSH-Px activities (P < 0.05) and higher amylase activity and MDA content (P < 0.05). The villus circumference and goblet cells in the cultured group were significantly lower than those in the wild group (P < 0.05). In addition, the cultured fish showed lower relative expression levels of occludin, zo-1, zo-2, claudin-12, claudin-15, mucin5, mucin15, lysozyme, complement 3, il-10, tgf-β1, tgf-β2, and tgf-β3 (P < 0.05) and higher il-1β, il-6, il-8, tnf-a, and ifnγ mRNA expressions than those of wild fish (P < 0.05). In terms of gut microbiota, the cultured group at the phylum level displayed higher percentages of Chlamydiae and Spirochaetes and lower percentages of Firmicutes, Bacteroidetes, Actinobacteria, Cyanobacteria, and Verrucomicrobia compared to the wild group (P < 0.05). At the genus level, higher abundances of Pseudomonadaceae_Pseudomonas and Spironema and lower abundances of Lactococcus and Cetobacterium were observed in the cultured group than in the wild group (P < 0.05). To our knowledge, this is the first investigation of the intestinal health status between wild and cultured M. albus in terms of biochemistry, histology, and molecular biology levels. Overall, the present study showed significant differences in intestinal health between wild and cultured M. albus and the main manifestations that wild M. albus had higher intestinal digestion, antioxidant capacity, and intestinal barrier functions than cultured M. albus. These results would provide theoretical basis for the subsequent upgrading of healthy aquaculture technology and nutrient regulation of intestinal health of cultured M. albus.
1 Introduction
The global demand for fish has been accelerating owing to the increased population and raised awareness of the health benefits of fish consumption instead of meat products. The main sources of fish are natural resources (i.e., artisanal fisheries) and aquaculture (1). However, the increase in total fish consumption and unsustainable fishing operations have made it impossible to meet the growing demand with wild-caught fish alone (2), and the aquaculture industry has become a suitable means to satisfy the global demand for fish supply (3). In recent years, the wide acceptance of artificial compound feeds coupled with the continuous optimization of nutritional balancing has led to an increase in farmed fish production year by year (4). However, with the higher demand for unit production and the frequent occurrence of fish diseases, researchers are also conceding the vital relationship between growth performances and immune function in accord to intestinal health, a key indicator organ of fish health.
Fish intestinal health is a complex and comprehensive assessment system when considering the important role of the intestine in the organism, mainly involving the digestive and antioxidant enzymes, tissue morphology, various barrier functions, and microorganism composition (5), which covers a wide range of physiological functions. Factors that cause differences in intestinal health between wild and cultured fish include the living environment (water temperature, water quality, and water salinity), developmental stage, and dietary sources (6). Compared to cultured fish, wild fish live in environments with a high level of dissolved oxygen, low living density, and low ammonia–nitrogen level, but excess heavy metal contents in the wild environment may also be detrimental to intestinal health (7). Wild fish have a richer diet composition, and the food they consume may have specific nutrients that are easily digested to influence intestinal health, although a lower intake as well as excessive consumption can also lead to slower growth rates (8).
The Asian swamp eel Monopterus albus, known as rice field eel, an important traditional aquaculture species in China with a culture production of 334,000 tons in 2022 (9), is preferred due to its tasty flesh and high nutritional and medicinal value. However, currently, few studies have been reported on the intestinal health of wild and cultured fish of Paralichthys adspersus (10), Seriola lalandi (11), Huso dauricus (12), Oreochromis niloticus (13), and Genypterus chilensis (14), which mainly focused on the intestinal microorganism composition, while other aspects were poorly investigated. However, health status is a comprehensive characteristic system, which is difficult to be evaluated by only a few parameters. Therefore, this study aimed to analyze the relationship among the digestive enzymes, antioxidant enzymes, intestinal barrier function, and microbial composition of M. albus from biochemistry, histology, and molecular biology levels in order to systematically compare the intestinal health of farmed and wild M. albus. The results would provide a theoretical basis for the subsequent upgrading of healthy aquaculture technology and nutrient regulation of intestinal health of cultured M. albus.
2 Materials and methods
The Animal Ethics Committee of Shanghai Academy of Agricultural Sciences approved all animal procedures.
2.1 Sample collection
In this work, healthy wild (n = 15) and cultured (n = 15) M. albus were collected, with an average body weight of 45.3 ± 5.1 and 44.2 ± 3.8 g, and obtained from Jinshan District, Shanghai (30.78° N, 121.18° E) and Zhuanghang Comprehensive Experiment Station of Shanghai Academy of Agricultural Sciences (30.89°, 121.41° E), respectively. Among them, cultured M. albus with an initial body weight of 15.2 ± 0.5 g were fed commercial diet containing 43% crude protein and 7% crude lipid at 16:00 each day by hand for 10 weeks, and the daily feeding rate was 3% to 5% of the body weight. During the feeding trial, the water was continuously aerated, and one-third of the aeration water was replaced daily (dissolved O2≥ 5.8 mg/L, water temperature 28 ± 2°C, pH 7.3 ± 0.2, and NH4+–N < 0.5 mg/L).
Before sampling, the M. albus fish were fasted for at least 48 h to ensure that no chyme was observed in the intestines. The fish were dissected under aseptic conditions, and the foregut and hindgut were removed with a sterilized scalpel. A portion of the foregut was taken and stored frozen at -20°C for intestinal digestive enzyme and antioxidant parameter analysis, another portion of the foregut was placed in liquid nitrogen for intestinal gene expression determination, and the other portion of the foregut was collected and fixed in Bouin’s solution for observation of tissue morphology. The hindgut per fish was put into RNase-free tubes and stored in liquid nitrogen for intestinal microbiota analysis. Three fish were pooled as one sample, with a total of 15 fish per group (each with five replicates), to perform analyses of the digestive enzymes, antioxidant parameters, and intestinal microbiota as well as real-time quantitative PCR.
2.2 Sample analysis
2.2.1 Analysis of intestinal digestive enzymes and antioxidant parameters
The foregut samples were weighed and added four times the volume of pre-cooled saline. After homogenization, the samples were centrifuged at 4°C for 10 min (6,000 r/min), and the supernatant was extracted and stored at 4°C for the determination of intestinal digestive enzymes and antioxidant parameters within 24 h. The superoxide dismutase (SOD), glutathione peroxidase (GSH-Px), catalase (CAT), and total antioxidant capacity (TAOC) activities and malondialdehyde (MDA) content in the foregut were measured by using the corresponding kits produced by Nanjing Jiancheng Bioengineering Institute (Nanjing, China).
2.2.2 Intestinal tissue morphology
The foregut samples were fixed in Bouin’s solution for at least 48 h and then dehydrated with ethanol, transparent with xylene, embedded in paraffin, sectioned (8 μm), and stained with hematoxylin–eosin (H&E). The sections were photographed under an optical microscope to observe the intestinal morphology parameters (Nikon YS100 micrographic system). The goblet cell amounts were determined according to Shi et al. (15).
2.2.3 Real-time quantitative PCR analysis
Total RNA from intestine samples was extracted by using Trizol reagent following the manufacturer’s protocol, and the concentration and the purity of RNA were detected by using a UV spectrophotometer and agarose gel electrophoresis, respectively. Subsequently, cDNA synthesis was performed using the PrimeScript™ RT reagent kit (Takara, Dalian, China) and was then stored at –80°C until use. All real-time quantitative PCR analyses were performed using the SYBR® Premix Ex Taq (Perfect Real-Time) kit (TaKaRa) according to the manufacturer’s instructions. The total reaction volume was 20 μL, containing 10 μL SYBR® Premix Ex Taq™ (Tli RNaseH Plus), 0.5 μL upstream primer, 0.5 μL downstream primer, 1 μL cDNA template, and 8 μL ddH2O. The reaction program of real-time quantitative PCR was as follows: pre-denaturation at 95°C for 30 s, 35 cycles of denaturation at 95°C for 5 s, annealing at 58°C for 15 s, and extension at 72°C for 20 s; finally, the melting curve was performed to confirm the specificity. The PCR primers were obtained and designed based on M. albus sequences in the GenBank accession (Table 1), and RPL-17 was selected as a reference gene. The relative expression levels in intestine tissues were calculated by using the 2-ΔΔCt method.
2.2.4 Intestinal microbiota analysis
DNA extraction and PCR amplification were performed according to the instructions of the E.Z.N.A.® soil kit (Omega Bio-tek, Norcross, GA, USA), DNA concentration and purity were examined using NanoDrop2000, and the quality of the DNA extractions was examined using 1% agarose gel electrophoresis. The quality of the DNA extractions was determined using 338F (5′-ACTCCTACGGGGAGGCAGCAG-3′) and 806R (5′-GGACTACHVGGGTWT CTAAT-3′) primers for PCR amplification of the V3–V4 variable region. The PCR product was extracted from 2% agarose gel and purified using PCR Clean-Up Kit (YuHua, Shanghai, China) according to the manufacturer’s instructions and quantified using QuantiFluor™-ST (Promega, USA). Bioinformatic analysis was carried out using Illumina’s NovaSeq 6000 platform by Shanghai Personal Biotechnology Co., Ltd. The detailed information of intestinal microorganisms such as α-diversity, β-diversity, composition, and abundance were analyzed by the online platform of Personal Cloud Platform (www.genescloud.cn). The raw data are deposited in the National Center for Biotechnology Information (NCBI) sequence read archive (SRP504613).
2.3 Statistical analysis
The experimental results were expressed as mean with standard deviation. All data were subjected to equality of variances with Levene’s test with SPSS 22.0 software, and independent-sample t-tests were used to determine significant differences at P < 0.05.
3 Results
3.1 Intestinal digestive enzymes
As shown in Table 2, wild M. albus had higher activities of trypsin and lipase (P < 0.05) but lower activities of amylase than cultured M. albus (P < 0.05). Meanwhile, extremely significant differences were noted for lipase and amylase activities (P < 0.01).
3.2 Intestinal antioxidant parameters
As shown in Table 3, the intestinal antioxidant enzymes of the wild and cultured M. albus demonstrate very significant differences in this study (P < 0.01). Compared with the cultured group, increased SOD, CAT, GSH-Px, and T-AOC activities and a decreased MDA level were found in the wild M. albus group (P < 0.01).
3.3 Intestinal tissue morphology
The intestinal histology of the foregut is displayed in Table 4 and Figure 1. Significant changes were observed for villus circumference (VC) and goblet cell amount (GCA) (P < 0.05). The values of VC and GCA in wild M. albus presented to be higher than those in cultured M. albus (P < 0.01).
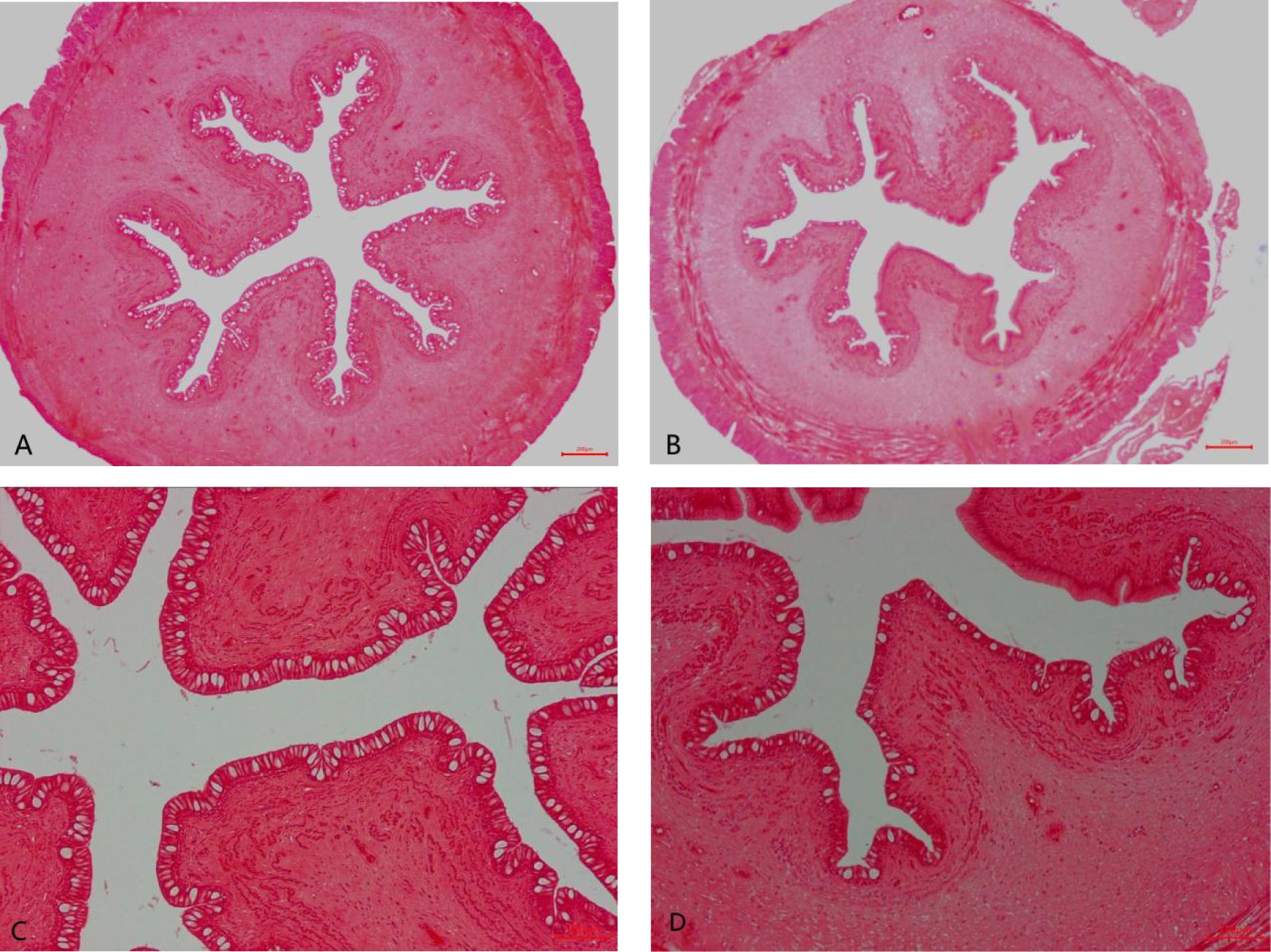
Figure 1 Foregut histological structure of the wild and cultured M. albus (H&E staining). (A) Wild M. albus (×40), (B) cultured M. albus (×40), (C) wild M. albus (×100), and (D) cultured M. albus (×100).
3.4 Intestinal chemical barrier
Compared with the wild fish, the relative expression levels of mucin5, mucin15, lysozyme, and complement 3 mRNA in the intestine were downregulated in cultured M. albus (Figure 2) (P < 0.05).
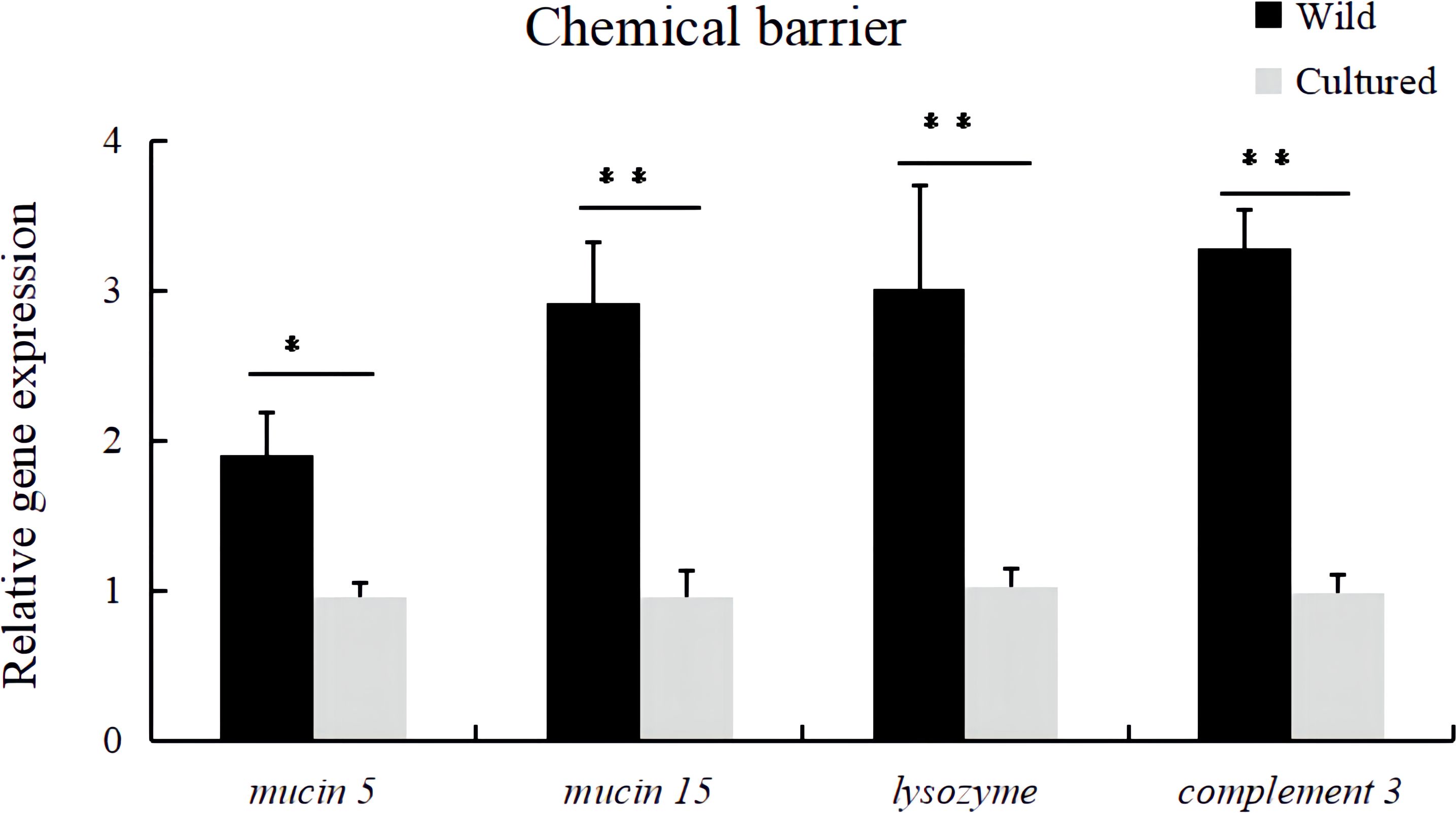
Figure 2 Relative expression levels of chemical barrier-related genes (mucin 5, mucin 15, lysozyme, and complement 3) in the intestine of the wild and cultured M. albus. Values marked with asterisks are significantly different (*P < 0.05 and **P < 0.01).
3.5 Intestinal physical barrier
In Figure 3, the occludin, zo-1, zo-2, claudin-12, and claudin-15 mRNA expression levels in the intestine of cultured M. albus were significantly lower than those of wild M. albus (P < 0.05).
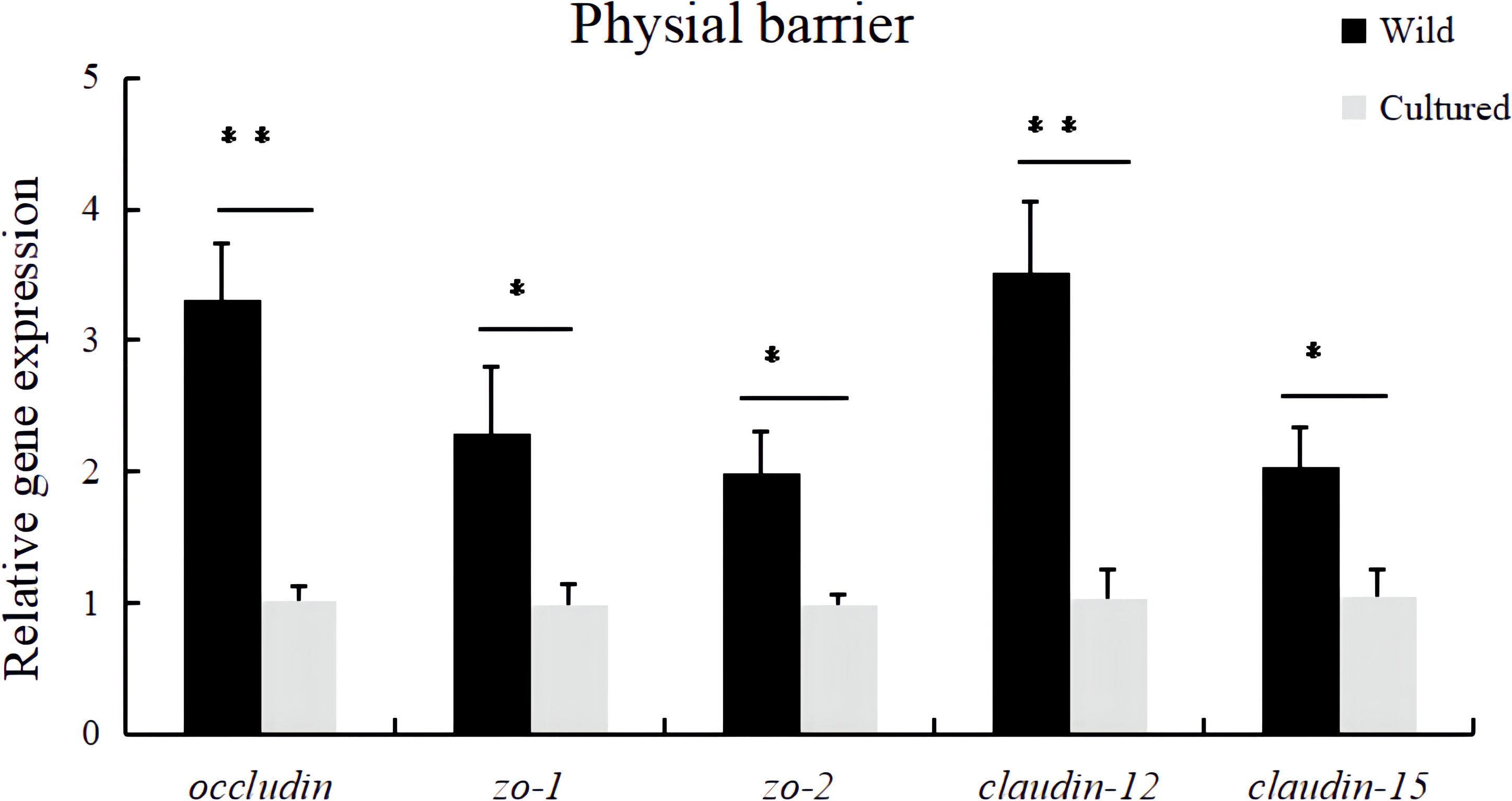
Figure 3 Relative expression levels of physical barrier-related gene (occludin, zo-1, zo-2, claudin-12, and claudin-15) in the intestine of the wild and cultured M. albus. Values marked with asterisks are significantly different (*P < 0.05 and **P < 0.01).
3.6 Intestinal immunological barrier
In terms of inflammation-related genes, the mRNA expressions of pro-inflammatory genes (il-1β, il-6, il-8, tnf-a, and ifnγ) and anti-inflammatory genes (il-10, tgf-β1, tgf-β2, and tgf-β3) were significantly affected (P < 0.05). The cultured group had significantly higher relative mRNA levels of il-1β, il-6, il-8, tnf-a, and ifnγ and lower relative mRNA levels of il-10, tgf-β1, tgf-β2, and tgf-β3 in the intestine of M. albus compared to the wild group (Figure 4).
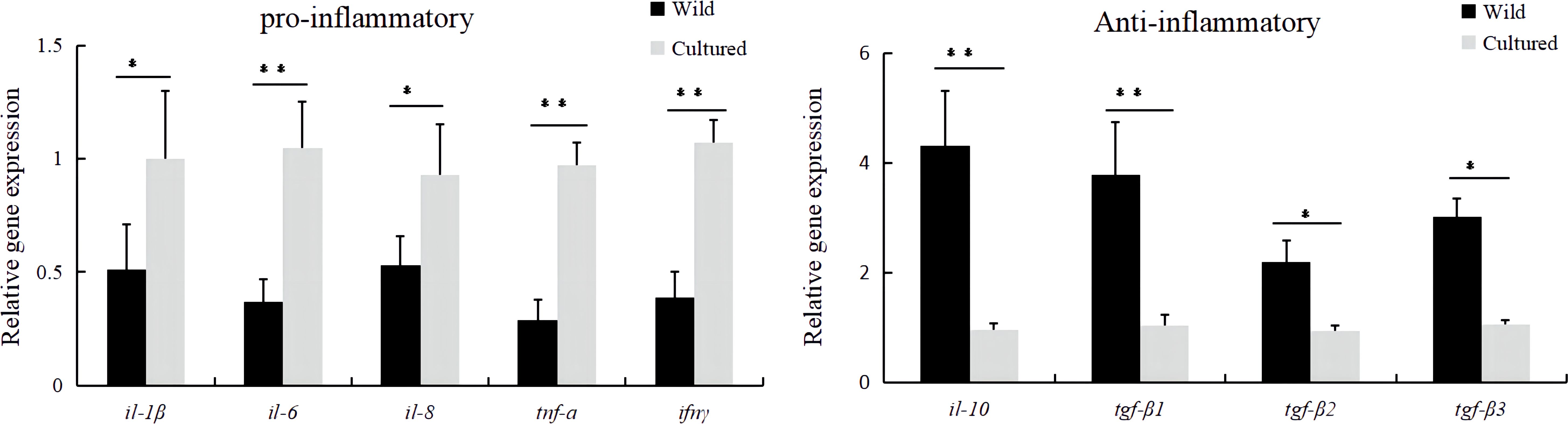
Figure 4 The mRNA expressions of inflammatory-related genes in the intestine of the wild and cultured M. albus. Values marked with asterisks are significantly different (*P < 0.05 and **P < 0.01).
3.7 Intestine microbiota analysis (microbiological barrier)
In order to have a more comprehensive assessment of the alpha diversity of the gut microbial communities of wild and cultured M. albus, this study characterized richness by Chao1 and observed species, diversity by Shannon and Simpson, and coverage by Goods coverage.
As can be seen from Table 5, a significant difference was observed in the α-diversity of the intestinal microbiota of cultured and wild groups. The coverage index is close to 1, which indicates that the bacterial community was adequately sampled and the data are representative of the population. Chao1, observed species, and Shannon and Simpson indices were found to be significantly higher in the wild than cultured M. albus, implying that wild M. albus had higher richness and diversity of intestinal flora.
The Venn diagram of operating taxonomic units (OTUs) is shown in Figure 5A, with 237 shared OTUs and 2,083 and 768 unique OTUs in the wild and cultured groups, respectively. Meanwhile, the β-diversity was displayed by principal coordinate analysis (PCoA) and non-metric multidimensional scaling analysis (NMDS). As shown in Figures 5B, C, there were no significant overlaps between the wild and cultured groups in PCoA (P = 0.025) and NMDS (P = 0.014).
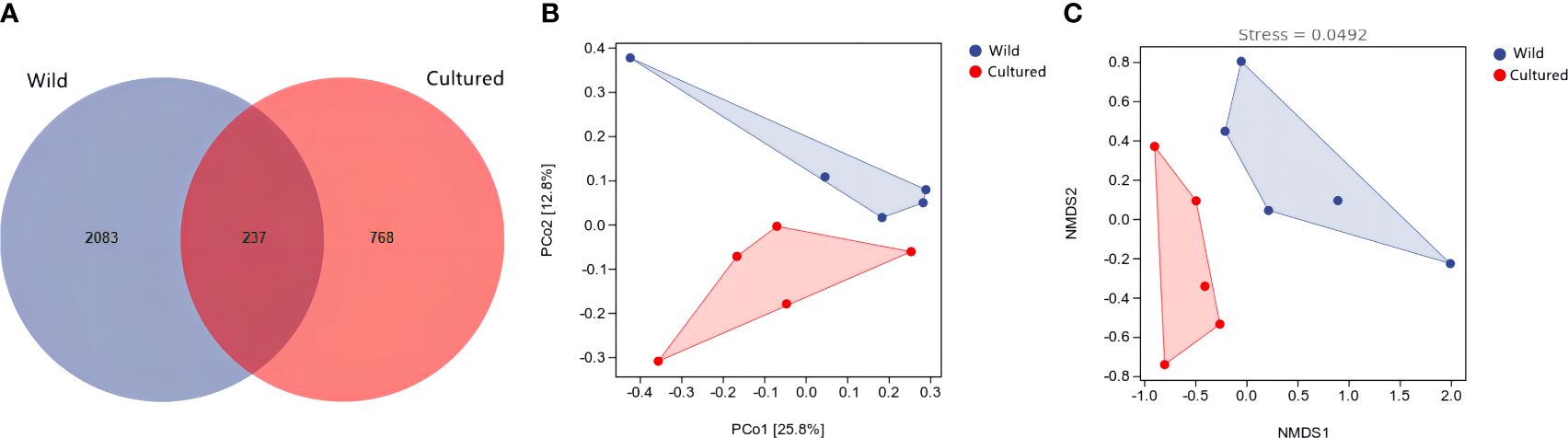
Figure 5 Venn diagram of operating taxonomic units (A) and β-diversity analysis (B, C) for the intestine microbiota of the wild and cultured M. albus.
As shown in Figure 6A, Table 6, the top 10 phyla according to the abundance of gut microbiota were observed as Proteobacteria, Chlamydiae, Firmicutes, Bacteroidetes, Actinobacteria, Spirochaetes, Cyanobacteria, Thermi, Fusobacteria, and Verrucomicrobia. The dominant phyla in the wild group were Proteobacteria (21.26%) and Firmicutes (20.50%) and in the cultured group were Proteobacteria (37.66%) and Chlamydiae (32.73%). Compared to the wild group, the cultured group had a higher abundance of Chlamydiae and Spirochaetes and a lower abundance of Firmicutes, Bacteroidetes, Actinobacteria, Cyanobacteria, and Verrucomicrobia.
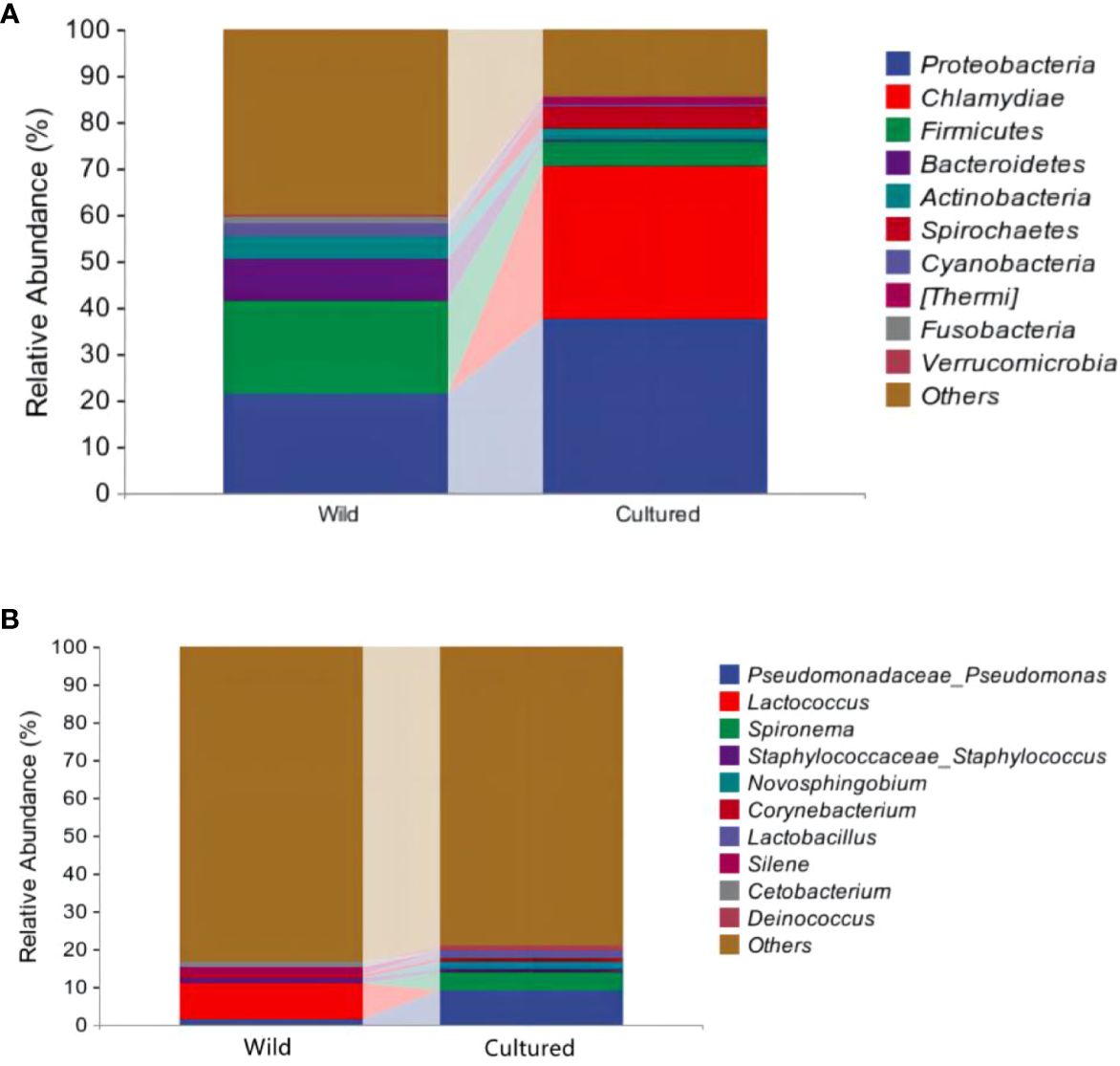
Figure 6 Community analysis at the phylum (A) and genus (B) levels for the intestinal microbiota of the wild and cultured M. albus.
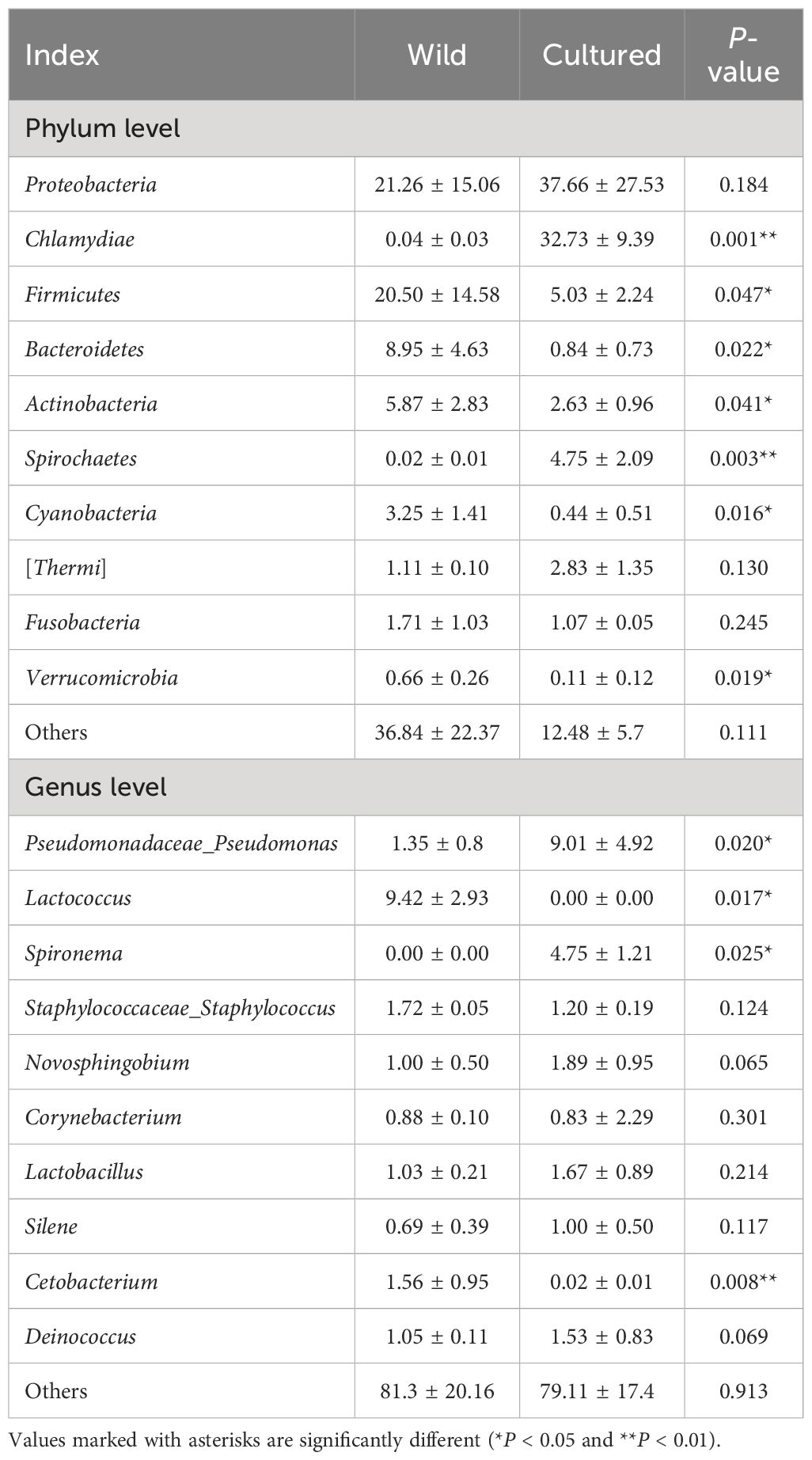
Table 6 Dominant bacteria and relative abundance (%) at phylum and genus levels for the intestinal microbiota of the wild and cultured M. albus.
At the genus level (Figure 6B; Table 6), the top 10 intestinal microorganisms were recorded as Pseudomonadaceae_Pseudomonas, Lactococcus, Spironema, Staphylococcaceae_Staphylococcus, Novosphingobium, Corynebacterium, Lactobacillus, Silene, Cetobacterium, and Deinococcus. Lactococcus (9.42%) and Pseudomonadaceae_Pseudomonas (9.01%) were found to be the dominant bacteria in the wild and cultured M. albus, respectively. Higher percentages of Pseudomonadaceae_Pseudomonas and Spironema and lower percentages of Lactococcus and Cetobacterium were observed in the cultured group than in the wild group.
4 Discussion
It is well known that the intestinal tract is an important site for the digestion and absorption of nutrients, while intestinal trypsin, lipase, and amylase are the main indicators of digestive function. The present study showed that wild M. albus had higher activities of trypsin and lipase and lower amylase than those of cultured ones, which may be due to the differences in ingestion capacities of varied diets in the living environments. The commercial diet may be rich in wide forms of carbohydrates, and the long-term dietary habits forced the cultured M. albus to adapt to high-starch diets, resulting in higher intestinal amylase activity. In addition, chronic satiation maybe also leads to weakened intestinal digestion (16). In wild environment, M. albus mainly ingest fish and shrimp, aquatic earthworms, aquatic insects, and a few plant roots and algae, which is more in line with M. albus’s dietary habits, coupled with greater exercise in the wild situation. Therefore, the secretion and the activity of intestinal protease and lipase are higher in the wild group, which can be inferred from the intestinal tissue morphology as related to digestive and absorptive functions (17). The increased villus circumference could enhance digestion by promoting the contact area with feed, thus facilitating digestion. Goblet cells are scattered among columnar cells and are capable of secreting digestive fluid. The villus circumference and goblet cells amount in the wild group were higher than those in the cultured group (Figure 1), which ascertained the robust intestinal digestive and absorptive functions of wild M. albus.
In addition to the function of nutrient digestion and absorption, the intestine is also an important barrier for animals to resist external environmental stimuli. In normal metabolism, the dynamic balance of redox is always maintained, and when fish are subjected to internal and external coercion, a large number of reactive oxygen molecules are produced, and the balance of the antioxidant system and the repair system in the body is impaired, which will cause the destruction of the organism’s tissues and functions, ultimately leading to the reduction of intestinal health, immunity, and growth performance (18). SOD, CAT, GSH-Px, T-AOC, and MDA are important indicators of antioxidant function. SOD and CAT are able to scavenge free radicals to reduce the damage caused by oxidative stress to the intestinal mucosa, which plays an important role in intestinal defense and repair (19); GSH-Px mirrors the body’s ability to scavenge free radicals, which protects the animal from damage caused by lipid peroxides (20); T-AOC is a comprehensive indicator of antioxidant function, reflecting the ability to compensate for external stimuli and the state of free radical metabolism (19); and MDA content represents the degree of lipid peroxidation and indirectly the degree of cellular damage (21). The present study showed that the SOD, CAT, GSH-Px, and T-AOC activities in wild M. albus were significantly higher, while the MDA level was significantly lower compared with the cultured ones, indicating that the antioxidant capacity of wild M. albus is stronger than that of cultured M. albus.
Fish and terrestrial animals have similar intestinal barrier compositions, which mainly include biological barrier, chemical barrier, physical barrier, and immunological barrier (22), while barrier function plays an important role in maintaining intestinal homeostasis. When the intestinal barrier function of fish is damaged, it firstly affects the intestinal health, and then it is susceptible to the attack of pathogenic bacteria, which ultimately leads to a decline in growth performance (23).
Chemical barriers are chemicals such as mucins, complement proteins, lysozyme, and intestinal antimicrobial peptides in the outer mucus layer of intestinal epithelial cells (24). Currently, there are no documented comparisons so far on exploring the intestinal chemical barrier function in wild and cultured fish. In this study, significantly higher mRNA expressions of chemical barrier-related genes (mucin 5, mucin 15, lysozyme, and complement 3) have been observed in wild M. albus compared with cultured M. albus, implying the superior intestinal chemical barrier functionality of wild M. albus.
The intestinal physical barrier mainly consists of intestinal epithelial cells, which are connected to each other by adhesive junctions and tight junctions and considered to be an important determinant of intestinal cell permeability (25). The physical barrier can be strengthened by regulating tight junction proteins, which are a major determinant of the magnitude of intercellular permeability. The tight junctions are mainly composed of several membrane proteins, namely, occludin, claudins and adhesion factors, and zonula occludens (ZO) (26). However, the differences of intestinal tight junction proteins in wild and cultured fish have not yet been investigated. In this study, the wild group had a higher mRNA relative expression of occludin, zo-1, zo-2, claudin-12, and claudin-15 in the gut than the cultured group, and these results provide a reference for future nutritional regulation in adjusting the integrity of the intestinal physical barrier in fish.
Inflammatory cytokines are important indicators of the intestinal immunological barrier in aquatic animals (27), which can usually be categorized into pro-inflammatory and anti-inflammatory cytokines based on the type of response effect (28). Pro-inflammatory cytokines play a role in inflammatory response by promoting inflammation; overexpression can exacerbate intestinal mucosal damage and can affect the distribution and expression of tight junction proteins, increasing intestinal permeability (29). Studies in fish have demonstrated that the downregulation of pro-inflammatory cytokine mRNA levels and upregulation of anti-inflammatory cytokine mRNA levels attenuate excessive inflammatory responses (30). Currently, no studies related to immunological barrier in wild and cultured M. albus have been reported. However, the present study revealed a reduced mRNA expression level of intestinal pro-inflammatory-related genes (il-1β, il-6, il-8, tnf-a, and ifnγ) and upregulated mRNA expression of anti-inflammatory genes (il-10, tgf-β1, tgf-β2, and tgf-β3) in wild M. albus, respectively.
The animal intestine contains a large number of complex microorganisms, which form a complex symbiotic relationship with the intestine, constituting a microbiological barrier, and changes in their levels are critical in maintaining homeostasis in the intestinal tract. In the present study, the results of alpha and beta diversity analyses showed significant differences in community richness, diversity, and flora structure of the gut microbiota between wild and cultured M. albus. Similar results have been reported in wild and cultured fine flounder Paralichthys adspersus (10), yellowtail kingfish Seriola lalandi (11), kaluga sturgeon Huso dauricus (12), Nile tilapia Oreochromis niloticus (13), and red cusk-eel Genypterus chilensis (14). Studies have shown that the intestinal flora alpha and beta diversity of fish is closely related to their living environment, variation in food habits, and nutritional intake (31, 32). Cao et al. (33) concluded by meta-analysis that, compared with the technical factors, host-associated and environmental factors influenced alpha and beta diversity to a larger extent, and the environmental factors led by diet impacted the alpha and beta diversity of gut bacteria among the host-associated and environmental factors.
In this experiment, at the phylum level, Proteobacteria, Firmicutes, Bacteroidetes, Actinobacteria, and Fusobacteria were found to be present in the wild and cultured groups, in line with the research on the types of gut microbial composition of healthy M. albus (34). The analysis revealed that the abundance of Firmicutes, Bacteroidetes, Actinobacteria, Cyanobacteria, and Verrucomicrobia in the wild group was significantly higher, and Chlamydiae and Spirochaetes were lower than those of cultured ones. Many members of Firmicutes are beneficial bacteria that produce acetate, butyrate, lactate, and antimicrobial substances that prevent pathogens from interfering with health and help maintain the integrity of the intestinal wall (35). The bacterial community under the classification of Bacteroidetes is closely associated with the conversion of organic matter such as proteins and lipids (14), and the increased abundance suggests a greater capacity for energy harvesting by their hosts, which may be due to the wild M. albus consuming less and irregular amounts of food in the wild, and they must digest and utilize their food more efficiently to maximize energy, a natural adaptation to their wild condition. Actinobacteria that were abundant in hindgut are widely known to produce antimicrobial secondary metabolites within the fish gut that protect the host fish from pathogens in vivo (36). Notwithstanding, Piazzon et al. (37) reported decreased gut Firmicutes and Bacteroidetes and promoted Proteobacteria compared with healthy fish after enteritis infection. Furthermore, the abundance analysis showed a shift in the level of Actinobacteria in healthy fish to Proteobacteria in infected ones (38). At present, the significantly lower abundance of Firmicutes, Bacteroidetes, and Actinobacteria was displayed in the cultured group, although the percentage of Proteobacteria in wild and cultured M. albus was not significantly different. Therefore, from the point of gut microorganisms, it was speculated that the gut health of wild M. albus was superior to that of cultured M. albus.
Cyanobacteria are mainly distributed in freshwater with a high content of organic matter. The wild environment has more organic matter, which may be one of the main reasons for the higher abundance of Cyanobacteria in the wild M. albus gut. Verrucomicrobia can break down polysaccharides such as mucopolysaccharides and cellulose, which provide energy and nutrients, and also produce short-chain fatty acids such as propionic acid and butyric acid, which are important for intestinal health and immune system regulation (39). The flora under Spirochaetes and Chlamydiae are mostly pathogenic bacteria that can cause a variety of fish diseases including enteritis and gill mucosal adhesion necrosis, which seriously jeopardize the health of cultured animals (40). Studies have shown that stress can lead to an increase in the abundance of Spirochaetes and Chlamydiae in the fish gut (41); therefore, it is hypothesized that the ammonia and nitrogen levels in aquaculture water may be higher than those in the wild environment, which, in turn, affects the harmful flora abundance.
At the genus level, higher abundances of Lactococcus and Cetobacterium and lower abundances of Pseudomonadaceae_Pseudomonas and Spironema were observed in the wild group than in the cultured group. Cetobacterium is commonly found in the intestinal tract of many fish species, and studies have shown a positive correlation with the content of digestive enzymes such as protease and lipase in carnivorous fish (42). Lactococcus maintains intestinal acid–base balance by producing lactic acid, which helps to improve digestion and absorption, promoting intestinal health, while as a probiotic it can balance the intestinal flora (43). Mougin and Joyce (44) reported that Pseudomonadaceae_Pseudomonas and Spironema are associated with intestinal barrier dysfunction and inflammatory bowel disease infections in fish. High abundance means that M. albus in cultured environments are more susceptible to infections than those in the wild, possibly due to excessive organic matter in the aquaculture environment, leading to susceptibility to pollution, which allows conditionally pathogenic bacteria to grow and multiply and produce toxins and other disease-causing factors (45). The environmental stresses experienced by fish reared at a high density in culture tanks differ from those experienced by fish living in natural water, with the former being subjected to significantly more stressful stimuli than the latter. It is the frequent exposure of cultured fish to a variety of stressors that tends to lead to a decline in the intestinal health and immunity of cultured fish, increasing their susceptibility to pathogens, and thus manifesting as an increase in the abundance of pathogenic bacteria (46). In addition, the large amount of feed consumed by cultured fish may place a greater burden for the fish gut, which may also contribute to the fact that the intestinal inflammation and intestinal barrier function of farmed M. albus are also worse than those of wild ones.
5 Conclusion
Overall, the present research showed significant differences in intestinal health between wild and cultured M. albus and the main manifestations that wild M. albus had higher intestinal digestion, antioxidant capacity, and intestinal barrier functions, including physical, chemical, immunological, and microbiological barriers, than cultured M. albus, providing theoretical references for nutrient regulation of the intestinal health of cultured M. albus.
Data availability statement
The original contributions presented in the study are included in the article/supplementary material. Further inquiries can be directed to the corresponding authors.
Ethics statement
The Animal Ethics Committee of Shanghai Academy of Agricultural Sciences approved all animal procedures. The study was conducted in accordance with the local legislation and institutional requirements.
Author contributions
HY: Formal analysis, Investigation, Methodology, Writing – original draft. QY: Conceptualization, Data curation, Writing – review & editing. MR: Conceptualization, Data curation, Writing – review & editing. WL: Investigation, Methodology, Writing – review & editing. WH: Resources, Software, Validation, Writing – review & editing. WWH: Visualization, Writing – review & editing. WZ: Funding acquisition, Project administration, Supervision, Writing – review & editing.
Funding
The author(s) declare financial support was received for the research, authorship, and/or publication of this article. This study was supported by China Agriculture Research System of MOF and MARA (CARS-46).
Conflict of interest
The authors declare that the research was conducted in the absence of any commercial or financial relationships that could be construed as a potential conflict of interest.
Publisher’s note
All claims expressed in this article are solely those of the authors and do not necessarily represent those of their affiliated organizations, or those of the publisher, the editors and the reviewers. Any product that may be evaluated in this article, or claim that may be made by its manufacturer, is not guaranteed or endorsed by the publisher.
References
1. Thong NT, Solgaard HS. Consumer's food motives and seafood consumption. Food Qual Prefer. (2017) 56:181–8. doi: 10.1016/j.foodqual.2016.10.008
2. Subasinghe R, Soto D, Jia J. Global aquaculture and its role in sustainable development. Rev Aquacult. (2009) 1:2–9. doi: 10.1111/j.1753-5131.2008.01002.x
3. Kobayashi M, Msangi S, Batka M, Vannuccini S, Dey MM, Anderson JL. Fish to 2030: the role and opportunity for aquaculture. Aquacult Econ Manag. (2015) 19:282–300. doi: 10.1080/13657305.2015.994240
4. Tacon AG, Metian M. Feed matters: satisfying the feed demand of aquaculture. Rev Fish Sci Aquac. (2015) 23:1–10. doi: 10.1080/23308249.2014.987209
5. Dawood MA. Nutritional immunity of fish intestines: Important insights for sustainable aquaculture. Rev Aquacult. (2021) 13:642–63. doi: 10.1111/raq.12492
6. Lorenzen K, Beveridge MC, Mangel M. Cultured fish: integrative biology and management of domestication and interactions with wild fish. Biol Rev. (2012) 87:639–60. doi: 10.1111/j.1469-185X.2011.00215.x
7. De Marco G, Cappello T, Maisano M. Histomorphological changes in fish gut in response to prebiotics and probiotics treatment to improve their health status: A Review. Animals. (2023) 13:2860. doi: 10.3390/ani13182860
8. Fuentes A, Fernández-Segovia I, Serra JA, Barat JM. Comparison of wild and cultured sea bass (Dicentrarchus labrax) quality. Food Chem. (2010) 119:1514–8. doi: 10.1016/j.foodchem.2009.09.036
9. China Fishery Statistical Yearbook. Ministry of Agriculture and Rural Affairs. Beijing, China: China Agriculture Press (2023). p. 25.
10. Salas-Leiva J, Opazo R, Remond C, Uribe E, Velez A, Romero J. Characterization of the intestinal microbiota of wild-caught and farmed fine flounder (Paralichthys adspersus). Lat Am J Aquat Res. (20172017) 45:370–8.
11. Ramírez C, Romero J. The microbiome of Seriola lalandi of wild and aquaculture origin reveals differences in composition and potential function. Front Microbiol. (2017) 8:1844. doi: 10.3389/fmicb.2017.01844
12. Lv S, Zhao W, Shi Z, Wang S, Wei J. Comparative study of the intestinal microbial community of wild and cultured kaluga sturgeon, Huso dauricus. Aquac Res. (2018) 49:2938–44. doi: 10.1111/are.2018.49.issue-9
13. Bereded NK, Abebe GB, Fanta SW, Curto M, Waidbacher H, Meimberg H, et al. The impact of sampling season and catching site (wild and aquaculture) on gut microbiota composition and diversity of Nile tilapia (Oreochromis niloticus). Biology. (20212021) 10:180. doi: 10.3390/biology10030180
14. Romero J, Díaz O, Miranda CD, Rojas R. Red cusk-eel (Genypterus Chilensis) gut microbiota description of wild and aquaculture specimens. Microorganisms. (2022) 10:105. doi: 10.3390/microorganisms10010105
15. Shi Y, Zhong L, Liu Y, Zhang J, Lv Z, Li Y, et al. Effects of dietary andrographolide levels on growth performance, antioxidant capacity, intestinal immune function and microbioma of rice field eel (Monopterus albus). Animals. (20202020) 10:1744. doi: 10.3390/ani10101744
16. Rønnestad I, Yufera M, Ueberschär B, Ribeiro L, Sæle Ø, Boglione C. Feeding behaviour and digestive physiology in larval fish: current knowledge, and gaps and bottlenecks in research. Rev Aquacult. (2013) 5:S59–98. doi: 10.1111/raq.12010
17. Yang H, Bian Y, Huang L, Lan Q, Ma L, Li X, et al. Effects of replacing fish meal with fermented soybean meal on the growth performance, intestinal microbiota, morphology and disease resistance of largemouth bass (Micropterus salmoides). Aquacult Rep. (2022) 22:100954. doi: 10.1016/j.aqrep.2021.100954
18. Kakade A, Salama ES, Pengya F, Liu P, Li X. Long-term exposure of high concentration heavy metals induced toxicity, fatality, and gut microbial dysbiosis in common carp, Cyprinus carpio. Environ pollut. (20202020) 266:115293. doi: 10.1016/j.envpol.2020.115293
19. Zheng Q, Cui L, Liao H, Junaid M, Li Z, Liu S, et al. Combined exposure to polystyrene nanoplastics and bisphenol A induces hepato-and intestinal-toxicity and disturbs gut microbiota in channel catfish (Ictalurus punctatus). Sci Total Environ. (2023) 891:164319. doi: 10.1016/j.scitotenv.2023.164319
20. Wang C, Yuan Z, Li J, Liu Y, Li R, Li S. Acute effects of antimony exposure on adult zebrafish (Danio rerio): from an oxidative stress and intestinal microbiota perspective. Fish Shellfish Immunol. (2022) 123:1–9. doi: 10.1016/j.fsi.2022.02.050
21. Chang X, Wang X, Feng J, Su X, Liang J, Li H, et al. Impact of chronic exposure to trichlorfon on intestinal barrier, oxidative stress, inflammatory response and intestinal microbiome in common carp (Cyprinus carpio L.). Environ pollut. (2020) 259:113846. doi: 10.1016/j.envpol.2019.113846
22. Nayak SK. Role of gastrointestinal microbiota in fish. Aquac Res. (2010) 41:1553–73. doi: 10.1111/are.2010.41.issue-11
23. Xiong JB, Nie L, Chen J. Current understanding on the roles of gut microbiota in fish disease and immunity. Zool Res. (2019) 40:70–6. doi: 10.24272/j.issn.2095-8137.2018.069
24. Rauta PR, Nayak B, Das S. Immune system and immune responses in fish and their role in comparative immunity study: a model for higher organisms. Immunol Lett. (2012) 148:23–33. doi: 10.1016/j.imlet.2012.08.003
25. Choi W, Yeruva S, Turner JR. Contributions of intestinal epithelial barriers to health and disease. Exp Cell Res. (2017) 358:71–7. doi: 10.1016/j.yexcr.2017.03.036
26. Chasiotis H, Kolosov D, Bui P, Kelly SP. Tight junctions, tight junction proteins and paracellular permeability across the gill epithelium of fishes: a review. Resp Physiol Neurobi. (2012) 184:269–81. doi: 10.1016/j.resp.2012.05.020
27. Standen BT, Peggs DT, Rawling MD, Foey A, Davies SJ, Santos GA, et al. Dietary administration of a commercial mixed-species probiotic improves growth performance and modulates the intestinal immunity of tilapia, Oreochromis niloticus. Fish Shellfish Immunol. (2016) 49:427–35. doi: 10.1016/j.fsi.2015.11.037
28. Baker OJ, Camden JM, Redman RS, Jones JE, Seye CI, Erb L, et al. Proinflammatory cytokines tumor necrosis factor-α and interferon-γ alter tight junction structure and function in the rat parotid gland Par-C10 cell line. Am J Physiol-Cell Ph. (2008) 295:C1191–201. doi: 10.1152/ajpcell.00144.2008
29. Secombes CJ, Wang T, Bird S. The interleukins of fish. Dev Comp Immunol. (2011) 35:1336–45. doi: 10.1016/j.dci.2011.05.001
30. Wang T, Secombes CJ. The cytokine networks of adaptive immunity in fish. Fish Shellfish Immunol. (20132013) 35:1703–18. doi: 10.1016/j.fsi.2013.08.030
31. Xia JH, Lin G, Fu GH, Wan ZY, Lee M, Wang L, et al. The intestinal microbiome of fish under starvation. BMC Genomics. (2014) 15:1–11. doi: 10.1186/1471-2164-15-266
32. Yukgehnaish K, Kumar P, Sivachandran P, Marimuthu K, Arshad A, Paray BA, et al. Gut microbiota metagenomics in aquaculture: factors influencing gut microbiome and its physiological role in fish. Rev Aquacult. (2020) 12:1903–27. doi: 10.1111/raq.12416
33. Cao S, Dicksved J, Lundh T, Vidakovic A, Norouzitallab P, Huyben D. A meta-analysis revealing the technical, environmental, and host-associated factors that shape the gut microbiota of Atlantic salmon and rainbow trout. Rev Aquacult. (2024), 1–18. doi: 10.1111/raq.12913
34. Wang Y. Studies on intestinal flora of Monopterus albus and application of probiotics. Nanchang: Jiangxi Agricultural University, Ph.D thesis (2021).
35. Wang AR, Ran C, Ringø E, Zhou ZG. Progress in fish gastrointestinal microbiota research. Rev Aquacult. (2018) 10:626–40. doi: 10.1111/raq.12191
36. Jami M, Ghanbari M, Kneifel W, Domig KJ. Phylogenetic diversity and biological activity of culturable Actinobacteria isolated from freshwater fish gut microbiota. Microbiol Res. (20152015) 175:6–15. doi: 10.1016/j.micres.2015.01.009
37. Piazzon MC, Calduch-Giner JA, Fouz B, Estensoro I, Simó-Mirabet P, Puyalto M, et al. Under control: how a dietary additive can restore the gut microbiome and proteomic profile, and improve disease resilience in a marine teleostean fish fed vegetable diets. Microbiome. (2017) 5:1–23. doi: 10.1186/s40168-017-0390-3
38. Bharathi RR, Tripathi G, Das BK, Jain R, Acharya A. Comparative analysis of gut microbiome in Pangasionodon hypopthalmus and Labeo catla during health and disease. Int Microbiol. (2024) 2024):1–15. doi: 10.1007/s10123-024-00494-x
39. Llewellyn MS, McGinnity P, Dionne M, Letourneau J, Thonier F, Carvalho GR, et al. The biogeography of the Atlantic salmon (Salmo salar) gut microbiome. ISME J. (2016) 10:1280–4. doi: 10.1038/ismej.2015.189
40. Sood N, Pradhan PK, Verma DK, Gupta S, Ravindra DAK, Yadav MK, et al. Epitheliocystis in rohu Labeo rohita (Hamilton, 1822) is caused by novel Chlamydiales. Aquaculture. (2019) 505:539–43. doi: 10.1016/j.aquaculture.2019.03.007
41. Wei MS, Zheng T, Lu SQ, Qiang J, Tao YF, Li Y, et al. Ammonia-N stress on tissue structure, enzyme activity and intestinal microbiota of Micropterus Salmoides. Acta Hydrobiol Sin. (2024) 48:10–22.
42. Xie M, Zhou W, Xie Y, Li Y, Zhang Z, Yang Y, et al. Effects of Cetobacterium somerae fermentation product on gut and liver health of common carp (Cyprinus carpio) fed diet supplemented with ultra-micro ground mixed plant proteins. Aquaculture. (2021) 543:736943. doi: 10.1016/j.aquaculture.2021.736943
43. Dawood MA, Koshio S, Ishikawa M, Yokoyama S, El Basuini MF, Hossain MS, et al. Effects of dietary supplementation of Lactobacillus rhamnosus or/and Lactococcus lactis on the growth, gut microbiota and immune responses of red sea bream, Pagrus major. Fish Shellfish Immunol. (2016) 49:275–85. doi: 10.1016/j.fsi.2015.12.047
44. Mougin J, Joyce A. Fish disease prevention via microbial dysbiosis-associated biomarkers in aquaculture. Rev Aquacult. (2023) 15:579–94. doi: 10.1111/raq.12745
45. Chen CZ, Li P, Liu L, Li ZH. Exploring the interactions between the gut microbiome and the shifting surrounding aquatic environment in fisheries and aquaculture: A review. Environ Res. (2022) 214:114202. doi: 10.1016/j.envres.2022.114202
Keywords: Monopterus albus, intestinal health, gut barrier, intestinal microbiota, intestinal tissue morphology
Citation: Yang H, Yuan Q, Rahman MM, Lv W, Huang W, Hu W and Zhou W (2024) Comparative studies on the intestinal health of wild and cultured ricefield eel (Monopterus albus). Front. Immunol. 15:1411544. doi: 10.3389/fimmu.2024.1411544
Received: 04 April 2024; Accepted: 21 May 2024;
Published: 10 June 2024.
Edited by:
Jiong Chen, Ningbo University, ChinaReviewed by:
Xu-Jie Zhang, Huazhong Agricultural University, ChinaGang Yang, Nanchang University, China
Gayatri Tripathi, Central Institute of Fisheries Education (ICAR), India
Copyright © 2024 Yang, Yuan, Rahman, Lv, Huang, Hu and Zhou. This is an open-access article distributed under the terms of the Creative Commons Attribution License (CC BY). The use, distribution or reproduction in other forums is permitted, provided the original author(s) and the copyright owner(s) are credited and that the original publication in this journal is cited, in accordance with accepted academic practice. No use, distribution or reproduction is permitted which does not comply with these terms.
*Correspondence: Wenzong Zhou, emhvdXd6MDAxQDE2My5jb20=
†These authors have contributed equally to this work