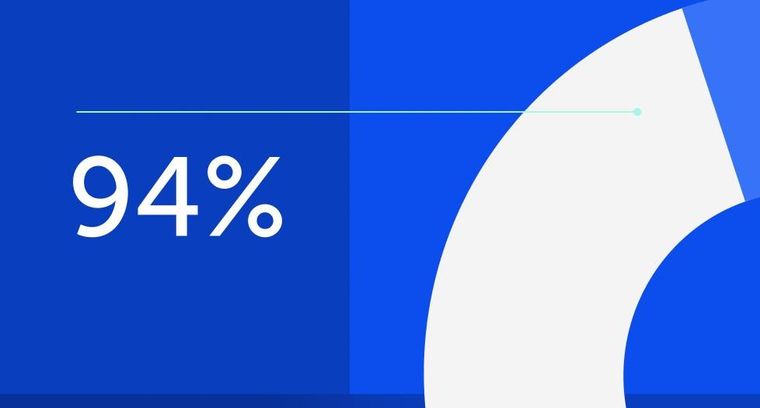
94% of researchers rate our articles as excellent or good
Learn more about the work of our research integrity team to safeguard the quality of each article we publish.
Find out more
ORIGINAL RESEARCH article
Front. Immunol., 01 May 2024
Sec. Parasite Immunology
Volume 15 - 2024 | https://doi.org/10.3389/fimmu.2024.1404297
This article is part of the Research TopicImmunology of Food-Borne Parasites: Recent Progress and Future AdvancesView all 5 articles
Introduction: Recently, the use of botanicals as an alternative to coccidiostats has been an appealing approach for controlling coccidiosis. Therefore, this study was conducted to evaluate the potential role of aqueous methanolic extract (200 mg/kg) of Krameria lappacea (roots) (KLRE) against infection induced by Eimeria papillata.
Methods: A total of 25 male C57BL/6 mice were divided into five groups (I, II, III, IV, and V). On 1st day of the experiment, all groups except groups I (control) and II (non-infected-treated group with KLRE), were inoculated orally with 103 sporulated E. papillata oocysts. On the day of infection, group IV was treated with KLRE. Group V served as an infected-treated group and was treated with amprolium (coccidiostat).
Results: Treatment with extract and coccidiostat was continued for five consecutive days. While not reaching the efficacy level of the reference drug (amprolium), KLRE exhibited notable anticoccidial activity as assessed by key criteria, including oocyst suppression rate, total parasitic stages, and maintenance of nutrient homeostasis. The presence of phenolic and flavonoid compounds in KLRE is thought to be responsible for its positive effects. The Eimeria infection increased the oxidative damage in the jejunum. KLRE treatment significantly increased the activity of catalase and superoxide dismutase. On the contrary, KLRE decreased the level of malondialdehyde and nitric oxide. Moreover, KLRE treatment decreased macrophage infiltration in the mice jejunal tissue, as well as the extent of CD4 T cells and NFkB. E. papillata caused a state of systemic inflammatory response as revealed by the upregulation of inducible nitric oxide synthase (iNOs)-mRNA. Upon treatment with KLRE, the activity of iNOs was reduced from 3.63 to 1.46 fold. Moreover, KLRE was able to downregulate the expression of pro-inflammatory cytokines interferon-γ, nuclear factor kappa B, and interleukin-10 -mRNA by 1.63, 1.64, and 1.38 fold, respectively. Moreover, KLRE showed a significant reduction in the expression of IL-10 protein level from 104.27 ± 8.41 pg/ml to 62.18 ± 3.63 pg/ml.
Conclusion: Collectively, K. lappacea is a promising herbal medicine that could ameliorate the oxidative stress and inflammation of jejunum, induced by E. papillata infection in mice.
The host immune response to the parasitic species is complex. Cell-mediated immunity driven by T lymphocytes, macrophages, and other effector cells plays a major role in host protection against coccidiosis (1). Upon activation by invading the coccidian Eimeria parasite, CD4 T cells can differentiate into various types of helper T cells that are responsible for regulating host immune responses by secreting cytokines and proinflammatory molecules (2). Eimeria species are highly species and site-specific within the host. Eimeria papillata is a significant cause of murine intestinal disease “coccidiosis” in mice jejunum. E. papillata is an intracellular obligate protozoan parasite having a complex life cycle of 5 days, comprising two developmental stages of exogenous (involving sporogony) and endogenous (involving schizogony and gametogony) within the host. Massive colonization of coccidia causes significant epithelial damage (3). The host may consequently suffer from diarrhea, malabsorption, inadequate weight gain, and overall poor performance (4). Also, coccidiosis leads to immune dysfunction and increases susceptibility to secondary bacterial infections, as it disrupts the balance of intestinal microflora (5).
Effective management of coccidiosis relies on vaccination and the use of chemoprophylaxis. However, the widespread and excessive use of synthetic coccidiostats has led to the development of drug resistance among various parasites (5–10). Consequently, alternative strategies for coccidiosis control have rapidly emerged and developed in response to these challenges. These strategies include the use of live anticoccidial vaccines, immunomodulators, prebiotics, and natural herbs (11). The utilization of various plants and their constituent parts for the control and treatment of E. papillata infection in mice has been documented. These plants have demonstrated therapeutic effects against coccidiosis, reducing mortality rate, oocyst numbers, and diarrhea, while also improving lesion scoring and production performance (12).
Krameria lappacea, commonly known as rhatany, belongs to the Krameriaceae family. It is renowned for its health-promoting characteristics attributed to its abundance in tannins, lignan derivatives, oligomeric proanthocyanidins, and benzofuran derivatives. Earlier investigators have confirmed their role as antioxidant (13), photoprotective (13), anti-inflammatory (14), antidiabetic (15), vasoprotective (16), anticancer (17, 18), and antimicrobial (19–22) activities. Moreover, its constituents are employed to alleviate diverse illnesses, among these, infections of the respiratory airways and gastrointestinal disorders (23).
Due to all the previously mentioned properties, this study was designed to investigate the anticoccidial and the antioxidant activity of Krameria lappacea roots extract (KLRE), as well as its role in the modulation of the expression of the inflammatory cytokines’ mRNA in the male C57BL/6 mice jejunum infected with the protozoan, Eimeria papillata.
The roots of Krameria lappacea, also known as rhatany, were obtained from a local market in Riyadh, Saudi Arabia A taxonomist from the Herbarium, College of Science (King Saud University), certified the plant identity with voucher number KSU-22958. The method outlined by Manikandan et al. (24) was employed to create a 70% methanolic extract from the roots of K. lappacea, referred to as KLRE. The crude extract was lyophilized and kept at -20°C.
The total phenolic content of KLRE was measured using the Folin-Ciocalteu procedure, as reported by Abdel Moneim (25). Also, the total flavonoid content of KLRE was estimated using the aluminum chloride colorimetric method published by Abdel Moneim (25). Absorbance was measured with the Spectra MAX 190 (SoftMax® Pro v.6.3.1). The values for phenolics and flavonoids are expressed as mg gallic acid/gram and mg quercetin/gram, respectively.
KLRE was found to be active in scavenging DPPH radicals. Initially, a fresh 0.08 mM DPPH radical solution was prepared in methanol. Then, 950 ml of the solution was combined with 50 ml of KLRE and incubated at 25°C for 5 minutes in the dark. The absorbance was measured at 515 nm using the Spectra MAX 190 (SoftMax® Pro v.6.3.1). Akillioglu and Karakaya (26) measure antioxidant activity as the percentage reduction of DPPH radicals.
E. papillata was used as a model coccidian murine parasite. To propagate oocysts, five laboratory mice (Mus musculus) were infected with 103 sporulated oocysts through oral gavage. Feces were collected at 5 days post-infection (p.i.), and oocysts were isolated using the floatation technique (27). Part of these oocysts could sporulate in 2.5% (w/v) potassium dichromate (K2Cr2O7) for three days before being washed in a phosphate buffer solution (Sigma Aldrich, Taufkirchen, Germany) and used in the in vivo study.
The animal facility of King Saud University (Riyadh, Saudi Arabia) provided twenty-five male C57BL/6 mice (10-12 weeks old, weighing 20-25 g). All mice were bred under pathogen-free conditions and allowed food and water ad libitum. Animals were housed in plastic cages under temperature-controlled conditions with a 12-hour light/dark cycle. Animals had been acclimated for one week before the start of the experiment. Mice were divided into five groups of five mice per group, as follows: G-I, Non-infected, non-treated (negative control), G-II, Non-infected, treated group with 200 mg/kg KLRE (28), G-III, Infected, non-treated (positive control), G-IV, Infected and treated group with 200 mg/kg KLRE (28), and G-V: Infected and treated group with 120 mg/kg Amprolium (29) for 5 days. All groups (except 1 and 2) were orally infected with 103 sporulated E. papillata oocysts in 100 µl physiological saline (30).
On day 5 p.i., fresh fecal pellets were collected from infected untreated and treated groups. The suppression of oocyst shedding was estimated using the formula of 100 - (oocysts output in the treated group/oocysts output in the infected group) × 100.
Mice were killed by CO2 asphyxiation on the 5th day p.i., and mice’s jejuna were isolated and cut into small pieces for the following: (a) Neutral buffered formalin was utilized for histological analysis. (b) In small tubes maintained at -80°C to investigate the oxidative status and protein expression (c) RNA later® (Invitrogen, Carlsbad, CA) was utilized for molecular analysis and kept at -80°C.
The jejuna were fixed in 10% neutral buffered formalin (NBF) for 24 hr, dehydrated, and embedded in paraffin wax, and then cut into 5 µm thick sections as per Adam and Caihak (31) method. Sections of jejuna were stained with hematoxylin-eosin (H&E) to detect parasite stages in both infected and infected-treated groups. The slides were examined and photographed using an Olympus B×61 microscope (Tokyo, Japan), and parasitic stages were counted on ten well-oriented villous-crypt units (VCU).
Other jejunal sections were stained using Hotchkiss (32) periodic acid-Schiff’s procedure for total carbohydrates and Mazia et al. (33) mercuric bromophenol blue method for total proteins. The slides were photographed using an Olympus B×61 microscope (Tokyo, Japan).
Sections (5µm thick) were picked onto Superfrost® glass slides (Thermo Scientific) and air-dried. Sections were deparaffinized using xylene and rehydrated through a serial grade of ethanol. Antigen retrieval was accomplished by steaming the slides in phosphate-buffered saline (PBS/pH=7.4) at various temperatures. To reduce non-specific background staining caused by endogenous peroxidase, H2O2 (3%) methanol solution was used. For immunostaining, the horseradish peroxidase amplified system, CD4 and NF-kB (nuclear factor kappa B) antibodies were used (Thermo Fisher Science, Waltham, MA, USA). Three components were used in this system: the primary antibody specific for the antigen to be localized, the secondary antibody capable of binding both primary antibodies and the horseradish peroxidase enzyme. Finally, the substrate/chromogen reagent diaminobenzidine (DAB) was used for reaction visualization. The number of immuno-histochemical-positive cells was presented as the mean number of brown cells per ten well-oriented villous-crypt units (VCU) and identified using an Olympus B×61 microscope (Tokyo, Japan).
To get 10% (w/v) jejunal homogenate, parts of jejunum were weighed, homogenized in an ice-cold medium with 50 mM Tris-HCl and 300 mM sucrose, and centrifuged for 10 min at 500×g at 4°C. The homogenate was then stored at -20°C until use (34). Oxidative stress markers were detected in the supernatant of jejunal homogenate. The appropriate chemical kits (Bio-Diagnostic kits, Bio-Diagnostic Co., Egypt) were used to determine Catalase (CAT), Nitric Oxide (NO), Malondialdehyde (MDA), and Superoxide Dismutase (SOD). Absorbance was measured with Spectra MAX 190 supported by software SoftMax® Pro v.6.3.1.
Total RNA was extracted from jejunal tissues using Trizol (Invitrogen, USA). RNA samples were processed with DNase (Applied Biosystems, Darmstadt, Germany) for at least 1 hour before being transformed into cDNA according to the manufacturer’s instructions using a reverse transcription kit (Qiagen, Hilden, Germany). qRT-PCR was carried out using an ABI Prism 7500HT sequence detection system (Applied Biosystems, Darmstadt, Germany) with Qiagen’s SYBR green PCR master mix (Hilden, Germany). The mRNA genes for interferon- γ (IFN- γ), inducible nitric oxide synthase (iNOs), interleukin-10 (IL-10), NF-kB, as well as beta-actin (β-actin) as a housekeeping control were studied using SYBR green (Hilden, Germany). All primer assays for qRT-PCR were obtained from Qiagen (Hildan, Germany). Real-time qPCR amplification and analysis were carried out using the Bio-Rad IMark Microplate Reader SW 1.04.02.E. The Ct method (2−ΔΔCT) described by Livak and Schmittgen (35) was used to analyze differences in gene expression.
Using a mouse IL-10 ELISA kit (IOK-05-P361, Creative Biolabs, USA) and following the protocol instructions, optical densities (OD) of outcomes from the jejunal samples were measured using the Bio-Rad IMark Microplate Reader SW 1.04.02.E. Based on a standard curve, OD values were converted to concentrations and presented as pg/ml.
Values were presented as mean ± standard deviation (SD). A one-way analysis of variance (ANOVA) with Duncan’s test was performed to compare the group means, with p-value ≤ 0.05 indicating statistical significance. SPSS version 18 for Windows (SPSS Inc., Chicago, Illinois, USA) was used for the analysis.
Using the Folin–Ciocalteu technique, KLRE had a total phenolic content of 214.30 ± 5.19 mg gallic acid/gram dry weight (Figure 1). Furthermore, the total flavonoid in KLRE measured using the aluminum chloride colorimetric method was 47.40 ± 3.20 mg quercetin/gram dry weight (Figure 1). KLRE’s antioxidant activity was measured using the 2,2-diphenyl-1-picrylhydrazyl (DPPH) method to measure free radical scavenging activity. Table 1 showed that KLRE had maximum DPPH (90.69%) at 250 µg/ml, while the lowest scavenging percentage (13.20%) occurred at 15.625 μg/ml.
Experimental E. papillata infection in infected and infected-treated mice groups was established as showed oocyst discharge in fecal pellets with a maximal level on the 5th day of infection. After treatment with KLRE, the output of Eimeria oocysts was inhibited by 75.71%, which was higher than the reference drug (65.65%) (Figure 2). Light microscopic examinations of hematoxylin/eosin stained sections indicated the presence of developmental Eimeria stages in the epithelial cells of the jejunal mouse tissue (Figure 3). KLRE treatment significantly reduced the total number of intracellular murine Eimeria stages, from 83.75 ± 19.36 stages/10 VCU in the infected group to 15.43 ± 4.07 stages/10 VCU in mice infected with E. papillata and treated with KLRE, and 19.73 ± 6.51 stages/10 VCU in mice treated with amprolium (Figure 4).
Figure 2 Suppression of E. papillata oocysts in infected and infected-treated mice. Significance At p ≤ 0.05 against the infected group (*).
Figure 3 Sections stained with hematoxylin and eosin (H&E) for the infected jejunum with Eimeria papillata on day 5 p.i. showing different developmental stages. (A, B) Infected jejunum with different Eimeria stages. (C) Macrogamonts. (D) Microgamonts. (E) Developing oocysts. Note: ME, meronts; MA, macrogamont; MI, macrogamont; DO, developing oocyst. Scale bar = 10 µm.
Figure 4 Treatment with 200 mg/kg KLRE and 120 mg/kg AMP induced changes in the total number of parasitic stages of Eimeria papillata in jejunum tissue per 10 VCU on day 5 p.i. All values are means ± SD. * significance (P ≤ 0.05) between the infected group and treated group.
Infection with E. papillata significantly altered the nutritional contents of the jejunal tissues. This was evidenced by the decrease in total carbohydrate content in the jejunal tissue for the infected group compared to the control one (Figure 5). KLRE treatment resulted in a significant change in carbohydrates compared to the infected group. Furthermore, E. papillata infection reduced jejunal protein content in contrast to the control group (Figure 6). KLRE treatment restored jejunal proteins to levels comparable to the infected group.
Figure 5 Carbohydrate content in jejunum sections stained with periodic acid Schiff’s (PAS) method. (A) control non-infected jejunum with normal content. (B) non-infected-treated group with 200 mg/kg KLRE. (C) E. papillata infected jejunum with depletion in their carbohydrate content. (D, E) infected treated mice (200 mg/kg KLRE and 120 mg/kg AMP, respectively) with improvement in their level. Scale bar = 100µm.
Figure 6 Protein content in jejunum sections stained with mercuric bromophenol blue method. (A) control non-infected jejunum with normal content. (B) non-infected-treated group with 200 mg/kg KLRE. (C) E. papillata infected jejunum with depletion in their protein content. (D, C) infected treated mice (200 mg/kg KLRE and 120 mg/kg AMP, respectively) with improvement in their level. Scale bar = 100µm.
CAT levels declined significantly from 8.40 ± 1.23 U/g in the non-infected group to 3.45 ± 0.78 U/g in the infected group. Mice treated with KLRE had higher levels of CAT (6.82 ± 0.66 U/g) compared to mice treated with the reference drug (6.44 ± 0.50 U/g) (Figure 7). Concerning NO production, E. papillata infection significantly increased jejunal NO, a free radical, to 26.99 ± 3.02 µmol/L compared to 17.13 ± 1.84 µmol/L in the control group. This marker contributes to the termination of lipid peroxidation reactions. Treatment with KLRE and amprolium resulted in considerable reductions in NO levels to 19.36 ± 2.29 and 19.59 ± 0.62 µmol/L, respectively (Figure 7). The infected group had a significantly higher level of malondialdehyde (MDA), a byproduct of polyunsaturated fatty acids peroxidation, compared to the non-infected group (269.71 ± 26.66 nmol/g vs. 485.62 ± 44.63 nmol/g). Mice treated with KLRE and amprolium significant decreases in MDA levels to 331.36 ± 36.93 and 323.03 ± 33.74 nmol/g, respectively (Figure 7). Furthermore, the activity of SOD reduced significantly from 7.05 ± 0.46 U/gm in the non-infected group to 4.03 ± 0.39 U/gm in the infected group. Mice treated with KLRE and amprolium showed significantly higher levels of SOD (5.91 ± 0.24 and 5.60 ± 0.56 U/gm, respectively) (Figure 7).
Figure 7 Effect of KLRE on the level of catalase, nitric oxide, malondialdehyde, and superoxide dismutase in mice infected with Eimeria papillata. * significant change concerning the control group, # significance change concerning the infected group.
Jejunal sections from experimental groups were stained for CD4 expression, which is thought to play an important role in the management of primary E. papillata infections (Figure 8). The Eimeria infection increased infiltration of positive immunohistochemical staining CD4 T cells into the infected mice jejunum, with the infected group having 233.33 ± 20.81 cells/10 VCU more positive cells than the control group, which had 113.33 ± 15.27 cells/10 VCU (Figure 9). CD4 T lymphocytes in murine coccidiosis may produce soluble cytokines such as IFN-γ and IL-10. Treatment resulted in a significantly lower CD4 expression (156.66 ± 5.77 cells/10 VCU) in the infected-treated KLRE group and (133.33 ± 15.27 cells/10 VCU) in the infected-treated amprolium group compared to the infected group (Figures 8, 9).
Figure 8 Immunohistochemical localization of CD4 in the jejuna of mice. (A) control non-infected jejunum. (B) non-infected-treated group with 200 mg/kg KLRE. (C) E. papillata infected jejunum with an increased number of CD4-positive cells. (D, E) infected treated mouse (200 mg/kg KLRE and 120 mg/kg AMP, respectively) with a decreased number of CD4-positive cells. Scale bar = 50µm.
Figure 9 Positive CD4 T cells number in mice infected with Eimeria papillata and for infected treated groups with 200 mg/kg KLRE and 120 mg/kg AMP. * significant change concerning the control group, # significance change concerning the infected group.
Moreover, jejunal sections were stained for NF-KB expression which play an important role in the inflammation and immune response caused by Eimeria infection (Figures 10, 11). It showed that the Eimeria infection induced an elevation of the NF-KB expression level with a number of positive cells reached 193.31 ± 15.02 in the infected group compared to the normal status in the control group 96.63 ± 15.20 (Figure 11). Upon treatment, the expression of NF-KB significantly changed in contrast to the infected group to be 136.54 ± 13.22 in the infected-treated KLRE group and 143.65 ± 11.17 in the infected-treated AMP group (Figures 10, 11).
Figure 10 Immunohistochemical localization of NF-KB in the jejuna of mice. (A) control non-infected jejunum. (B) non-infected-treated group with 200 mg/kg KLRE. (C) E. papillata infected jejunum with an increased number of NF-KB-positive cells. (D, E) infected treated mouse (200 mg/kg KLRE and 120 mg/kg AMP, respectively) with a decreased number of NF-KB positive cells. Scale bar = 50µm.
Figure 11 Positive NFkB cells number in mice infected with Eimeria papillata and for infected treated groups with 200 mg/kg KLRE and 120 mg/kg AMP. * significant change concerning the control group, # significance change concerning the infected group.
Eimeria infection increases mRNA expression of the IFN-γ gene in the jejunal tissue by around 3.81-fold compared to the control group (Figure 12). KLRE treatment significantly reduced IFN-γ gene expression by 1.63 fold, surpassing the reference drug’s 1.56 fold (Figure 12). Moreover, qRT-PCR demonstrated that E. papillata infection caused an increase in the mRNA expression level of the iNOs gene in the mice jejunum (Figure 13). Spontaneous enhanced nitric oxide (NO) production is linked to abnormal iNOs expression during Eimeria infection. After treatment with KLRE, iNOs gene expression was drastically reduced from 3.63 to 1.46 fold (Figure 13).
Figure 12 Effect of KLRE on the mRNA expression of IFN- γ in the jejunal samples from E. papillata-infected mice. The expression values obtained by RT-PCR analysis were normalized to the reference gene B-actin mRNA level and are shown as fold induction (in log 2 scale) relative to the mRNA level in the control. * significant change concerning the control group, # significance change concerning the infected group.
Figure 13 Effect of KLRE on the mRNA expression of iNOs in the jejunal samples from E. papillata-infected mice. The expression values obtained by RT-PCR analysis were normalized to the reference gene B-actin mRNA level and are shown as fold induction (in log 2 scale) relative to the mRNA level in the control. * significant change concerning the control group, # significance change concerning the infected group.
The upregulation in the mRNA expression of the NFkB gene was observed after the E. papillata infection. This elevation in mRNA expression of this gene was about 3.59 fold when compared with the control group (1.00 fold) (Figure 14). NFkB promotes T helper 1 cell differentiation by regulating toll-cell receptor (TCR) signaling as well as functioning in innate immune cells to mediate induction of cytokines. Upon treatment with KLRE, a significant downregulation to about 1.64 fold was observed for the expression of the NFkB gene which is quite similar to the reference drug (1.69 fold) (Figure 14).
Figure 14 Effect of KLRE on the mRNA expression of NF-kB in the jejunal samples from E. papillata-infected mice. The expression values obtained by RT-PCR analysis were normalized to the reference gene β-actin mRNA level and are shown as fold induction (in log 2 scale) relative to the mRNA level in the control. * significant change concerning the control group, # significance change concerning the infected group.
qRT-PCR was performed to assess changes in mRNA expression levels for inflammatory cytokines in the mice jejunum (Figure 15). E. papillata infection causes an increase in the mRNA expression of the IL-10 gene after the immune activation of lymphocytes and macrophages. This gene’s mRNA expression increased by approximately 3.46-fold when compared to the control group (Figure 15). IL-10 is a major anti-inflammatory mediator that protects mice against overactive reactions to E. papillata. Treatment with KLRE resulted in a significant downregulation of this gene’s expression by around 1.38 fold, which is similar to the reference drug (1.36 fold) (Figure 15). Moreover, analysis of IL-10 protein expression in the mice jejunum by ELISA revealed an increase in IL-10 production after infection with E. papillata reaching 104.27 ± 8.41 pg/ml, whereas KLRE treatment had significantly decreased IL-10 protein level (62.18 ± 3.63 pg/ml) at the 5th-day p.i. compared to the infected group (Figure 15).
Figure 15 The effect of KLRE on IL-10 levels in E. papillata-infected jejunum. Gene expression results are presented as mean ± SD from triplicate assays, normalized to GAPDH, while biochemical assay results are displayed as mean ± SD values (n = 5). Panels A, B showcase IL-10 expression and levels. * denotes significance against the control group at P < 0.05, # denotes significance compared to the sepsis group at P < 0.05.
Coccidiosis is typically treated with synthetic anticoccidial drugs; however, this strategy is under threat from the development of resistance in Eimeria strains (36). Different alternatives and techniques were successfully employed worldwide to treat and control diverse animal species (37). Among these options, numerous compounds derived from natural medicinal plants or natural health alternatives have been shown to have therapeutic effects that not only target the parasite but also preserve the host’s organs (38). This study sought to assess the anticoccidial and antioxidant properties as well as immunomodulation of K. lappacea roots against Eimeria infection. Previous studies reported the effective role of other root extracts like Cassia sieberiana (39), Beta vulgaris (40), Salvadora persica (41), and Glycyrrhiza glabra (42).
In addition to having a diminishing effect on the intracellular stages of the Eimeria parasite in the mice jejunum, the KLRE was an effective agent in mitigating infection, reducing oocyst shedding rate by approximately 75.71% on the 5th-day p.i. The majority of anticoccidial drugs have been shown to suppress Eimeria infection. K. lappacea derives its anticoccidial activity from many pharmacological compounds including antioxidants and phenolic compounds in its roots, which is consistent with Baumgartner et al. (14) Al-Oqail (18), and Alamari et al. (43). These compounds have a strong antimicrobial effect by disrupting the cell membrane of microbial pathogens leading to impaired membrane functions and leakage of cellular constituents and finally to cell death (19–22, 44). Most anticoccidial drugs have been shown to impede and inhibit the intracellular development of Eimeria stages in the intestinal tract, which is consistent with our findings.
The primary mechanism affected by Eimeria infection is host cell metabolism, and the parasite is very capable of manipulating host cells to its benefit by scavenging available host nutrients (45). Our data revealed that both carbohydrate and protein levels have been altered in the mice jejuna. Metwaly et al. (46) suggest that the lowered carbohydrate levels are due to the Eimeria stages’ excessive consumption of the stored carbohydrate content in the epithelial cells of mice jejuna. Regarding the bioactive components of KLRE, which disrupts the parasite’s ability to feed by lowering the enzymatic activity of glucose-6-phosphatase and thus plays an important role in the homeostatic regulation of tissue glycogen level, KLRE restored jejunal carbohydrate content. According to Al-Quraishy et al. (47), protein-losing enteropathy is characterized by the shedding of high amounts of proteins in the mice jejuna because of Eimeria infection. According to Bangoura and Daugschies (48), the amount of proteins in infected jejunal tissues is reduced, which is related to a higher rate of protein escaping into the intestinal lumen through the ruptured intestinal wall and being expelled via fecal pellets. The jejunal protein level improved after KLRE treatment due to a reduction in tissue protein catalytic processes.
The coccidian infection causes an imbalance in endogenous antioxidant defense and free radical production (49). Our findings showed that Eimeria infection induces oxidative damage to the mice’s jejunum, depleting antioxidant enzymes and lowering CAT and SOD levels. These oxidative indicators play an important role in protecting the animal body from free radical damage due to increased accumulation of reactive oxygen species (ROS) during Eimeria infection. Previous research has found that Eimeria infection disrupts the antioxidant defense system, resulting in detrimental cellular effects (10, 50–54). In this study, KLRE significantly prevented Eimeria infection-induced loss of these markers (CAT and SOD) and increased their activity, which is consistent with Carini et al. (55) for free radical scavenging properties that protect against oxidative damage and get rid of excess peroxides.
Furthermore, the elevated MDA and NO levels in infected mice are most likely caused by oxidative stress during Eimeria infection. Similar to the findings of Al-Otaibi et al. (45) Dominquez et al. (56)Al-Quraishy et al. (57), and Abdel-Gaber et al. (5) that Eimeria’s infective sporozoite stages, causing free radical overproduction and increased ROS production, resulting in lipid peroxidation. ROS causes pro-inflammatory cytokines and chemokines to be released, either directly or indirectly promoting inflammation due to Eimeria infection. In this study, E. papillata infection induced oxidative stress by upregulating the mRNA expression of iNOs, which is consistent with Metwaly et al. (58)Abdel-Latif et al. (59), and Abdel-Tawab et al. (30) reported that macrophages produce NO by oxidizing the guanidino nitrogen of L-arginine by an enzyme, nitric oxide synthase (NOS). This enzyme is inducible in macrophages by pathogen endotoxins and is termed inducible NOS (iNOS). The elevation of NO observed in this study is consistent with Allen and Fetterer (60) who stated that NO is involved in immunity and resistance against infectious diseases, as it exhibits toxicity towards pathogens. Administration of KLRE to the infected mice could significantly reduce the severity of the infection in the mice’s jejunum. Our findings showed that KLRE does not only target Eimeria stages within infected tissue but also exhibits anti-inflammatory activity protecting host tissues. The antioxidative, anti-inhibitory, and anti-inflammatory properties of KLRE may be attributed to the presence of phenolic and flavonoid components which mitigate the adverse effects of Eimeria infection on biological parameters of the affected animal. This is consistent with Awaad et al. (61) hypothesis that antioxidant compounds in different plants have played an important role in increasing the protection level against coccidiosis.
To understand the interaction between Eimeria infection and mice’s immune response, this study includes the determination of mRNA transcription levels for markers of T helper cell response. Lillehoj and Lillehoj (62) reported that T cell-mediated immunity by intestinal intraepithelial lymphocytes, including T helper (CD4) and T cytotoxic (CD8), confers the main component of protective immunity to Eimeria infections. This is consistent with the findings of this study that there is a significantly higher number of CD4 cells were detected in the jejunal tissue of infected mice compared to the control group, indicating macrophage involvement which migrates from circulation to the site of infection for the destruction of Eimeria stages. Previous studies by Dimier et al. (63) and Dalloul et al. (64) demonstrated that macrophages massively infiltrate into the chicken cecal lamina propria after E. tenella infection and secrete large amounts of cytokines. Generally, cytokines such as IL-1β, IL-12, IFN-γ, and TNF-α promote the development of cellular-mediated immunity against intracellular infections including coccidiosis (65). In addition, Mussbacher et al. (66) reported that NFkB may involve the expression of various pro-inflammatory genes, encoding cytokines and chemokines, and cell survival. This group of cytokines is associated with inflammatory responses, whilst cytokines such as IL-10 favor the development of humoral-mediated immunity and are implicated in anti-inflammatory responses (59, 67). This study showed that for mice inoculated with E. papillata oocysts, IL-10, NFkB, and IFN-γ during the 5th-day p.i., their gene expression levels were fold increased which in turn produces reactive oxygen (ROS) leading to the upregulated NO and iNOs levels, consistent with oocysts shedding compared with the control group. This is consistent with Lyons et al. (68) reported that a single infection with E. tenella evoked a host immune response which led to significant expression of cytokines such as IL-10 and IFN-γ in the ceca. Similar to the findings of Hong et al. (69) IL-10 and IFN-γ mRNA expression was robustly increased in CD4 cell populations following E. maxima-infected chickens. Our findings also indicated that the ELISA assay also detected massive IL-10 protein expression positively correlated with E. papillata replication and it is plausible E. papillata is inducing IL-10 as an immune evasion strategy. This indicated that T cells mediate their effects on the Eimeria parasite in primary infections through the secretion of cytokines. Similar to the findings of Bremner et al. (70) reported the increased IL-10 level in serum after E. maxima infection. Moreover, Chow et al. (71) and Dkhil et al. (72) reported that NFkB and IFN-γ is a key cytokine orchestrating the development of cellular-mediated immunity, and its expression is regulated by the induction of IL-10. It is believed that IFN-γ, which is known to activate intracellular cytotoxicity and produced by natural killer T cells, stimulates neutrophils and macrophages to migrate from circulation to the site of infection to destroy Eimeria sporozoites. This is consistent with previous studies demonstrated that a strong IFN-γ has been described to occur in the intestine upon infection with E. maxima (73), E. bovis and E. alabamensis (74). Our findings indicated that KLRE protects host tissues by acting as an immunomodulatory agent in addition to targeting intracellular Eimeria stages within the infected jejunal tissues associated with inhibiting fecal oocyst shedding as well as impairing the development and maturation of parasites. Following this view, our findings that KLRE attenuates the inflammatory response since it significantly downregulates the mRNA expression of IL-10, NFkB, and IFN-γ in the mouse jejunum infected with E. papillata. This reduction in iNOs, IL-10, NFkB, and IFN-γ in the intestinal tissue confirms the immunomodulatory and anti-inflammatory properties of flavonoid compounds in KLRE as well as their capacity to reduce the production of cytokines, which agreed with previous studies of Femández et al. (75), and Baumgartner et al. (14).
Our findings collectively demonstrate that the root extract of Krameria lappacea possesses anticoccidial properties, along with a notable enhancement in the nutritional status of jejunal tissue. Moreover, it exhibits antioxidant and anti-inflammatory activities, safeguarding host tissues from injuries induced by E. papillata. Further investigations are warranted to explore its potential protective role in other organs, as well as to conduct biochemical and molecular analyses to identify the genes regulated during infection.
The raw data supporting the conclusions of this article will be made available by the authors, without undue reservation.
This research was approved by the Research Ethics Committee (REC) at King Saud University (approval number KSU-SU-23-127). The study was conducted in accordance with the local legislation and institutional requirements.
RA-G: Conceptualization, Data curation, Formal analysis, Funding acquisition, Investigation, Methodology, Project administration, Resources, Software, Supervision, Validation, Visualization, Writing – original draft, Writing – review & editing. GA: Formal analysis, Investigation, Methodology, Visualization, Writing – original draft, Conceptualization, Data curation, Funding acquisition, Project administration, Resources, Software, Supervision, Validation, Writing – review & editing. MD: Formal analysis, Investigation, Methodology, Project administration, Software, Visualization, Writing – review & editing, Conceptualization, Data curation, Funding acquisition, Resources, Supervision, Validation, Writing – original draft. AM: Investigation, Validation, Visualization, Writing – review & editing, Conceptualization, Data curation, Formal analysis, Funding acquisition, Methodology, Project administration, Resources, Software, Supervision, Writing – original draft. EA-S: Data curation, Methodology, Resources, Validation, Visualization, Writing – review & editing, Conceptualization, Formal analysis, Funding acquisition, Investigation, Project administration, Software, Supervision, Writing – original draft. SA-Q: Investigation, Methodology, Project administration, Resources, Supervision, Validation, Visualization, Writing – review & editing, Conceptualization, Data curation, Formal analysis, Funding acquisition, Software, Writing – original draft.
The author(s) declare financial support was received for the research, authorship, and/or publication of this article. This study was supported by the Researchers Supporting Project (RSP2024R25), King Saud University, Riyadh, Saudi Arabia.
The authors declare that the research was conducted in the absence of any commercial or financial relationships that could be construed as a potential conflict of interest.
All claims expressed in this article are solely those of the authors and do not necessarily represent those of their affiliated organizations, or those of the publisher, the editors and the reviewers. Any product that may be evaluated in this article, or claim that may be made by its manufacturer, is not guaranteed or endorsed by the publisher.
1. Lillehoj HS, Trout JM. Avian gut-associated lymphoid tissues and intestinal immune responses to Eimeria parasites. Clin Microbiol Rev. (1996) 9:349–60. doi: 10.1128/CMR.9.3.349
2. Chen H, Pu J, Xiao J, Bai X, Zheng R, Gu X, et al. Evaluation of the immune protective effects of rEmMIC2 and rEmMIC3 from Eimeria magna in rabbits. Parasitol Res. (2023) 122:661–9. doi: 10.1007/s00436-022-07774-3
3. Dkhil MA, Abdel-Maksoud MA, Al-Quraishy S, Abdel-Baki AS, Wunderlich F. Gene expression in rabbit appendices infected with Eimeria coecicola. Veterinary Parasitol. (2012) 186:222–8. doi: 10.1016/j.vetpar.2011.11.031
4. Al-Shaebi EM, Al-Quraishy S, Maodaa SN, Abdel-Gaber R. In vitro studies for antiparasitic activities of Punica granatum extract. Microscopy Res Technique. (2023) 86:1655–66. doi: 10.1002/jemt.24401
5. Abdel-Gaber R, Hawsah MA, Al-Otaibi T, Alojayri G, Al-Shaebi EM, Mohammed OB, et al. Biosynthesized selenium nanoparticles to rescue coccidiosis mediated oxidative stress, apoptosis and inflammation in the jejunum of mice. Front Immunol. (2023) 14:1139899. doi: 10.3389/fimmu.2023.1139899
6. Rose ME, Hesketh P, Wakelin D. Immune control of murine coccidiosis: CD4+ and CD8+ T lymphocytes contribute differentially in resistance to primary and secondary infections. Parasitology. (1992) 105:349–54. doi: 10.1017/S0031182000074515
7. Innes EA, Vermeulen AN. Vaccination as a control strategy against the coccidial parasites Eimeria, Toxoplasma and Neospora. Parasitology. (2006) 133:S145–68. doi: 10.1017/S0031182006001855
8. Peek HW, Landman WJM. Coccidiosis in poultry: anticoccidial products, vaccines and other prevention strategies. Veterinary Q. (2011) 31:143–61. doi: 10.1080/01652176.2011.605247
9. Blake DP, Marugan-Hernandez V, Tomley FM. Spotlight on avian pathology: Eimeria and the disease coccidiosis. Avian Pathol. (2021) 50:209–13. doi: 10.1080/03079457.2021.1912288
10. Alajmi F, Al-Otaibi T, Al-Quraishy S, Al-Shaebi EM, Al-Hoshani N, Dkhil MA, et al. Persea americana extract protects intestinal tissue from Eimeria papillata-induced murine infection. BMC Veterinary Res. (2023) 19:248. doi: 10.1186/s12917-023-03810-1
11. Hamzić E, Buitenhuis B, Hérault F, Hawken R, Abrahamsen MS, Servin B, et al. Genome-wide association study and biological pathway analysis of the Eimeria maxima response in broilers. Genet Sel Evol. (2015) 47:91. doi: 10.1186/s12711-015-0170-0
12. Zaman MA, Iqbal Z, Abbas RZ, Khan MN. Anticoccidial activity of herbal complex in broiler chickens challenged with Eimeria tenella. Parasitology. (2012) 139:237–43. doi: 10.1017/S003118201100182X
13. Carini M, Aldini G, Orioli M, Facino RM. Antioxidant and photoprotective activity of a lipophilic extract containing neolignans from Krameria triandra roots. Planta Med. (2002) 68:193–7. doi: 10.1055/s-2002-23167
14. Baumgartner L, Schwaiger S, Stuppner H. Quantitative analysis of anti-inflammatory lignan derivatives in Ratanhiae radix and its tincture by HPLC-PDA and HPLC-MS. J Pharm Biomed Anal. (2011) 56:546–52. doi: 10.1016/j.jpba.2011.06.016
15. Heiss EH, Baumgartner L, Schwaiger S, Heredia RJ, Atanasov AG, Rollinger JM, et al. Ratanhiaphenol III from Ratanhiae radix is a PTP1B inhibitor. Planta Med. (2012) 78:678–81. doi: 10.1055/s-0031-1298242
16. Ladurner A, Atanasov AG, Heiss EH, Baumgartner L, Schwaiger S, Rollinger JM, et al. 2-(2,4-dihydroxyphenyl)-5-(E)-propenylbenzofuran promotes endothelial nitric oxide synthase activity in human endothelial cells. Biochem Pharmacol. (2012) 84:804–12. doi: 10.1016/j.bcp.2012.06.029
17. Calado A, Neves PM, Santos T, Ravasco P. The effect of flaxseed in breast cancer: A literature review. Front Nutr. (2018) 5:4. doi: 10.3389/fnut.2018.00004
18. Al-Oqail MM. Anticancer efficacies of Krameria lappacea extracts against human breast cancer cell line (MCF-7): Role of oxidative stress and ROS generation. Saudi Pharm J. (2021) 29:244–51. doi: 10.1016/j.jsps.2021.01.008
19. Bombardelli E, Morazzoni P, Cristoni A, Seghizzi R, Indena SA. Pharmaceutical and cosmetic formulations with antimicrobial activity. Canadian Patent 2268914 (2002).
20. Ortiz S, Lecsö-Bornet M, Bonnal C, Houze S, Michel S, Grougnet R, et al. Bioguided identification of triterpenoids and neolignans as bioactive compounds from anti-infectious medicinal plants of the Taira Atacama's community (Calama, Chile). J Ethnopharmacology. (2019) 231:217–29. doi: 10.1016/j.jep.2018.10.029
21. Genovese C, D’Angeli F, Bellia F, Distefano A, Spampinato M, Attanasio F, et al. In Vitro Antibacterial, Anti-Adhesive and Anti-Biofilm Activities of Krameria lappacea (Dombey) Burdet & B.B. Simpson Root Extract against Methicillin-Resistant Staphylococcus aureus Strains. Antibiotics. (2021) 10:428. doi: 10.3390/antibiotics10040428
22. Zabka M. Antifungal efficacy and convenience of krameria lappacea for the development of botanical fungicides and new alternatives of antifungal treatment. Agronomy. (2022) 12:2599. doi: 10.3390/agronomy12112599
23. Velasco-Lezama R, Aquilar-Carrillo MF, Tapia-Aguilar R, Velázquez-Vázquez MDL, Cerón-Ramírez R, Santana-Carrillo J. Determination of the antibacterial activity of Krameria pauciflora (Rose). J Drug Delivery Ther. (2023) 13:105–9. doi: 10.22270/jddt.v13i3.5996
24. Manikandan P, Letchoumy PV, Gopalakrishnan M, Nagini S. Evaluation of Azadirachta indica leaf fractions for in vitro antioxidant potential and in vivo modulation of biomarkers of chemoprevention in the hamster buccal pouch carcinogenesis model. Food Chem Toxicol. (2008) 46:2332–43. doi: 10.1016/j.fct.2008.03.013
25. Abdel Moneim A. The neuroprotective effects of purslane (Portulaca oleracea) on rotenone-induced biochemical changes and apoptosis in brain of rat. CNS Neurol Disord Drug Targets. (2013) 12:830–41. doi: 10.2174/18715273113129990081
26. Akillioglu HG, Karakaya S. Changes in total phenols, total flavonoids, and antioxidant activities of common beans and pinto beans after soaking, cooking, and in vitro digestion process. Food Sci Biotechnol. (2010) 19:633–9. doi: 10.1007/s10068-010-0089-8
27. Kumar S, Garg R, Moftah A, Clark EL, Macdonald SE, Chaudhry AS, et al. An optimised protocol for molecular identification of Eimeria from chickens. Vet Parasitol. (2014) 199:24–31. doi: 10.1016/j.vetpar.2013.09.026
28. Villareal-García L, Oranday-Cárdenas A, Garza-Ramos MA, Rivas-Morales C, Verde-Star M, Gómez-Treviño JA, et al. Neolignans from Krameria ramosissima (A. Gray) S. Watson with activity against Porphyromonas gingicalis, cytotoxical and mutagenic evaluations. Revista Mexicana de Ciencias Farmaceuticas. (2014) 45(2):69–76.
29. Abakar AD, Seri HI, Ismail AA, Musa HH. Comparative efficacy of selected anticoccidial drugs in ambarorow sheep naturally infected with enteric coccidia in South Darfur, Sudan. Sudan J Veterinary Res. (2005) 20:61–7.
30. Abdel-Tawab H, Abdel-Haleem HM, Abdel-Baki AAS, Al-Quraishy S, El-Mallah AM. Anticoccidial and antioxidant activities of Moringa oleifera leaf extract on murine intestinal eimeriosis. Acta Parasitologica. (2020) 65:823–30. doi: 10.2478/s11686-020-00219-w
31. Adam H, Caihak G. Grosses zoologisches parktikum tell. In: Arbeitsmethoden der makroskopischen und mikroskopischen anatomic Mit 283 Abbildungen Gustav. Stuttgart: Fischer Verlag (1964).
32. Hotchkiss RD. A microchemical reaction resulting in the staining of polysaccharide structures in fixed tissue preparations. Arch Biochem. (1948) 16:131–41.
33. Mazia D, Brewer PA, Alfert M. The cytochemical staining and measurement of protein with mercuric bromphenol blue. Biol Bull. (1953) 104:(1). doi: 10.2307/1538691
34. Tsakiris S, Schulpis KH, Marinou K, Behrakis P. Protective effect of l-cysteine and glutathione on the modulated suckling rat brain Plasmodium berghei ANKA after chronic exposure. Parasitol Res. (2004) 108:807–14. doi: 10.1515/znc-2000-3-421
35. Livak KJ, Schmittgen TD. Analysis of relative gene expression data using real-time quantitative PCR and the 2(-Delta Delta C(T)) Method. Methods. (2001) 25:402–8. doi: 10.1006/meth.2001.1262
36. Flores RA, Nguyen BT, Cammayo PL, Võ TC, Naw H, Kim S, et al. Epidemiological investigation and drug resistance of Eimeria species in Korean chicken farms. BMC Vet Res. (2022) 18:277. doi: 10.1186/s12917-022-03369-3
37. Lee Y, Lu M, Lillehoj HS. Coccidiosis: recent progress in host immunity and alternatives to antibiotic strategies. Vaccines. (2022) 10:215. doi: 10.3390/vaccines10020215
38. Ranasinghe S, Armson A, Lymbery AJ, Zahedi A, Ash A. Medicinal plants as a source of antiparasitics: an overview of experimental studies. Pathog Global Health. (2023) 117:535–53. doi: 10.1080/20477724.2023.2179454
39. Fall AD, Gbati OB, Diatta W, Lapo RA, Diatta-Badji K, Dieng M, et al. Anticoccidian activity of ethanol roots extract of Cassia sieberiana DC in chickens. Eur J Medicinal Plants. (2016) 11:1–7. doi: 10.9734/EJMP
40. Abbas A, Iqbal Z, Abbas RZ, Khan MK, Khan JA, Sindhu ZUD, et al. In vivo anticoccidial effects of Beta vulgaris (sugar beet) in broiler chickens. Microb Pathog. (2017) 111:139–44. doi: 10.1016/j.micpath.2017.07.052
41. Dkhil MA, Thagfan FA, Hassan AS, Al-Shaebi EM, AbdelGaber R, Al-Quraishy S. Anthelmintic, anticoccidial and antioxidant activity of Salvadora persica root extracts. Saudi J Biol Sci. (2019) 26:1223–6. doi: 10.1016/j.sjbs.2019.02.006
42. Hussain K, Iqbal Z, Abbas RZ, Khan MK, Saleem MK. Immunomodulatory activity of Glycyrrhiza glabra extract against mixed Eimeria infection in chickens. Int J Agric Biol. (2022) 19:928–32. doi: 10.17957/IJAB
43. Alamari G, Abdel-Gaber R, Al-Shaebi EM, Al-Quraishy S. Anticoccidial and jejunum-protective effects of Krameria lappacea roots extract on experimental Eimeria papillata infection. Microscopy Res Technique. (2024), 1–12. doi: 10.1002/jemt.24531
44. Neto C, Owens C, Langfield R, Comeau A, Onge J, Vaisberg A, et al. Antibacterial activity of some Peruvian medicinal plants from the Callejon de Huaylas. J Ethnopharmacology. (2002) 79:133–8. doi: 10.1016/S0378-8741(01)00398-1
45. Al-Otaibi T, Abu Hawsah M, Alojayri G, Al-Shaebi EM, Dkhil MA, Thagfan F, et al. Biological activities of Persea americana: in vitro and in vivo studies. Food Sci Technol. (2023) 43:e123722. doi: 10.1590/fst.123722
46. Metwaly MS, Dkhil MA, Gewik MM, Al-Ghamdy AO, Al-Quraishy S. Induced metabolic disturbance and growth depression in rabbits infected with Eimeria coecicola. Parasitol Res. (2013) 112:3109–14. doi: 10.1007/s00436-013-3485-1
47. Al-Quraishy S, Sherif NE, Metwaly MS, Dkhil MA. Berberine-induced amelioration of the pathological changes in nutrients’s homeostasis during murine intestinal Eimeria papillata infection. Pakistan J Zoology. (2014) 46:437–45.
48. Bangoura B, Daugschies A. Influence of experimental Eimeria zuernii infection in calves on electrolyte concentrations, acid base balance and blood gases. Parasitol Res. (2007) 101:1637–45. doi: 10.1007/s00436-007-0705-6
49. Benabdelhak AC, Derbak H, Titouah H, Aissanou S, Debbou-louknane N, Ayad A. Epidemiological survey on post mortem coccidiosis in broiler chicken in Bejaia province, Northern Algeria. Acta Parasitologica. (2024). doi: 10.1007/s11686-024-00806-1
50. Bosch SS, Kronenberger T, Meissner KA, Zimbres FM, Stegehake D, Izui NM, et al. Oxidative stress control by apicomplexan parasites. BioMed Res Int. (2015) 2015:10. doi: 10.1155/2015/351289
51. Dkhil MA, Metwaly MS, Al-Quraishy S, Sherif NE, Delic D, Al Omar SY, et al. Anti-Eimeria activity of berberine and identification of associated gene expression changes in the mouse jejunum infected with Eimeria papillata. Parasitol Res. (2015) 114:1581–93. doi: 10.1007/s00436-015-4344-z
52. Bozkurt M, Ege G, Aysul N, Akşit H, Tüzün AE, Küçükyılmaz K, et al. Effect of anticoccidial monensin with oregano essential oil on broilers experimentally challenged with mixed Eimeria spp. Poult Sci. (2016) 95:1858–68. doi: 10.3382/ps/pew077
53. Thagfan FA, Al-Megrin WA, Al-Shaebi EM, Al-Quraishy S, Dkhil MA. Protective role of Morus nigra leaf extracts against murine infection with Eimeria papillata. Combinatorial Chem High Throughput Screening. (2020) 24:1603–8. doi: 10.2174/1386207323666200903152811
54. Elmahallawy EK, Fehaid A, El-Shewehy DMM, Ramez AM, Alkhaldi AAM, Mady R, et al. S-Methylcysteine Ameliorates the Intestinal Damage Induced by Eimeria tenella Infection via Targeting Oxidative Stress and Inflammatory Modulators. Front Vet Sci. (2022) 8:754991. doi: 10.3389/fvets.2021.754991
55. Carini F, Mazzola M, Rappa F, Jurjus A, Geagea AG, Al Kattar S, et al. Colorectal carcinogenesis: role of oxidative stress and antioxidants. Anticancer Res. (2017) 37:4759–66. doi: 10.21873/anticanres
56. Dominquez PA, Pro-Martinez A, Narciso-Gaytán C, Hernández-Cázares A, Sosa-Montes E, Perez-Hernandez P, et al. Concurrent supplementation of arginine and antioxidant vitamin E and C reduces oxidative stress in broiler chicken after a challenge with Eimeria spp. Can J Anim Sci. (2015) 95:143–53. doi: 10.4141/cjas-2014-083
57. Al-Quraishy S, Qasem MAA, Al-Shaebi EM, Murshed M, Mares M, Dkhil MA. Rumex nervosus changed the oxidative status of chicken caecum infected with Eimeria tenella. Journal of King Saud University. Science. (2020) 32:2207–11. doi: 10.1016/j.jksus.2020.02.034
58. Metwaly MS, Dkhil MA, Al-Quraishy S. Anti-coccidial and anti-apoptotic activities of palm pollen grains on Eimeria papillata-induced infection in mice. Biologia. (2014) 69:254–9. doi: 10.2478/s11756-013-0297-9
59. Abdel-Latif M, Abdel-Haleem HM, Abdel-Baki AS. Anticoccidial activities of chitosan on Eimeria papillata- infected mice. Parasitol Res. (2016) 115:2845–52. doi: 10.1007/s00436-016-5035-0
60. Allen PC, Fetterer RH. Effect of Eimeria acervulina infections on plasma L-arginine. Poult Sci. (2000) 79:1414–7. doi: 10.1093/ps/79.10.1414
61. Awaad MHH, Afify MAA, Zoulfekar SA, Mohammed FF, Elmenawy MA, Hafez HM. Modulating effect of peppermint and eucalyptus essential oils on vVND infected chickens. Pak Vet J. (2016) 36:350–5.
62. Lillehoj HS, Lillehoj EP. Avian coccidiosis. A review of acquired intestinal immunity and vaccination strategies. Avian Dis. (2000) 44:408–25. doi: 10.2307/1592556
63. Dimier IH, Quéré P, Naciri M, Bout DT. Inhibition of Eimeria tenella development in vitro mediated by chicken macrophages and fibroblasts treated with chicken cell supernatants with IFN-γ activity. Avian Dis. (1998) 42:239–47. doi: 10.2307/1592473
64. Dalloul RA, Bliss TW, Hong YH, Ben-Chouikha I, Park DW, Keeler CL, et al. Unique responses of the avian macrophage to different species of Eimeria. Mol Immunol. (2007) 44:558–66. doi: 10.1016/j.molimm.2006.02.004
65. Dalloul RA, Lillehoj HS. Poultry coccidiosis: recent advancements in control measures and vaccine development. Expert Rev Vaccines. (2006) 5:143–63. doi: 10.1586/14760584.5.1.143
66. Mussbacher M, Derler M, Basílio J, Schmid JA. NF-kB in monocytes and macrophages – an inflammatory master regulator in multitalented immune cells. Front Immunol. (2023) 14:1134661. doi: 10.3389/fimmu.2023.1134661
67. Mantovani A, Sica A, Locati M. New vitas on macrophage differentiation and activation. Eur J Immunol. (2007) 37:14–6. doi: 10.1002/eji.200636910
68. Lyons RE, Anthony JP, Ferguson DJP, Byrne N, Alexander J, Roberts F, et al. Immunological studies of chronic ocular toxoplasmosis: up-regulation of major histocompatibility complex class I and transforming growth factor β and a protective role for interleukin-6. Infect Immun. (2001) 69:2589–95. doi: 10.1128/IAI.69.4.2589-2595.2001
69. Hong YH, Lillehoj HS, Lillehoj EP, Lee SH. Changes in immune-related gene expression and intestinal lymphocyte subpopulations following Eimeria maxima infection of chickens. Vet Immunol Immunopathol. (2006) 114:259–72. doi: 10.1016/j.vetimm.2006.08.006
70. Bremner A, Kim S, Morris KM, Nolan MJ, Borowska D, Wu Z, et al. Kinetics of the cellular and transcriptomic response to Eimeria maxima in relatively resistant and susceptible chicken lines. Front Immunol. (2021) 12:653085. doi: 10.3389/fimmu.2021.653085
71. Chow YP, Wan KL, Blake DP, Fiona T, Nathan S. Immunogenic Eimeria tenella glycosylphosphatidylinositol-anchored surface antigens (SAGs) induce inflammatory responses in Avian macrophages. PloS One. (2011) 6:e25233. doi: 10.1371/journal.pone.0025233
72. Dkhil MA, Abdel-Baki AS, Wunderlich F, Sies H, Al-Quraishy S. Anticoccidial and anti-inflammatory activity of garlic in immune Eimeria papillata infections. Veterinary Parasitol. (2011) 175:66–72. doi: 10.1016/j.vetpar.2010.09.009
73. Cornelissen JB, Swinkels WJ, Boersma WA, Rebel JM. Host response to simultaneous infections with Eimeria acervulina, maxima and tenella: a cumulation of single responses. Vet Parasitol. (2009) 162:58–66. doi: 10.1016/j.vetpar.2009.02.001
74. Alcala-Canto Y, Ibarra-Velarde F. Cytokine gene expression and NF-kappaB activation following infection of intestinal epithelial cells with Eimeria bovis or Eimeria alabamensis in vitro. Parasite Immunol. (2008) 30:175–9. doi: 10.1111/j.1365-3024.2007.01015.x
Keywords: Eimeria papillata, oxidative damage, Krameria lappacea, CD4 T cells, NFkB cells, IL-10
Citation: Abdel-Gaber R, Alamari G, Dkhil MA, Meryk A, Al-Shaebi EM and Al-Quraishy S (2024) Krameria lappacea root extract’s anticoccidial properties and coordinated control of CD4 T cells for IL-10 production and antioxidant monitoring. Front. Immunol. 15:1404297. doi: 10.3389/fimmu.2024.1404297
Received: 20 March 2024; Accepted: 18 April 2024;
Published: 01 May 2024.
Edited by:
Xochitl Hernandez-Velasco, National Autonomous University of Mexico, MexicoReviewed by:
Celio Geraldo Freire-de-Lima, Federal University of Rio de Janeiro, BrazilCopyright © 2024 Abdel-Gaber, Alamari, Dkhil, Meryk, Al-Shaebi and Al-Quraishy. This is an open-access article distributed under the terms of the Creative Commons Attribution License (CC BY). The use, distribution or reproduction in other forums is permitted, provided the original author(s) and the copyright owner(s) are credited and that the original publication in this journal is cited, in accordance with accepted academic practice. No use, distribution or reproduction is permitted which does not comply with these terms.
*Correspondence: Rewaida Abdel-Gaber, cmFiZGVsZ2FiZXJAa3N1LmVkdS5zYQ==
†ORCID: Rewaida Abdel-Gaber, orcid.org/0000-0002-0633-930X
Disclaimer: All claims expressed in this article are solely those of the authors and do not necessarily represent those of their affiliated organizations, or those of the publisher, the editors and the reviewers. Any product that may be evaluated in this article or claim that may be made by its manufacturer is not guaranteed or endorsed by the publisher.
Research integrity at Frontiers
Learn more about the work of our research integrity team to safeguard the quality of each article we publish.