- 1Department of Radiation Oncology, Hubei Cancer Hospital, TongJi Medical College, Huazhong University of Science and Technology, Wuhan, Hubei, China
- 2Department of Health Management, Hubei Cancer Hospital, TongJi Medical College, Huazhong University of Science and Technology, Wuhan, Hubei, China
- 3Department of Oncology, Yidu People’s Hospital, Yichang, Hubei, China
- 4Department of Urology, The First Affiliated Hospital of Zhengzhou University, Zhengzhou, Henan, China
Glioblastoma (GBM) is a highly malignant, invasive, and poorly prognosed brain tumor. Unfortunately, active comprehensive treatment does not significantly prolong patient survival. With the deepening of research, it has been found that gut microbiota plays a certain role in GBM, and can directly or indirectly affect the efficacy of immune checkpoint inhibitors (ICIs) in various ways. (1) The metabolites produced by gut microbiota directly affect the host’s immune homeostasis, and these metabolites can affect the function and distribution of immune cells, promote or inhibit inflammatory responses, affect the phenotype, angiogenesis, inflammatory response, and immune cell infiltration of GBM cells, thereby affecting the effectiveness of ICIs. (2) Some members of the gut microbiota may reverse T cell function inhibition, increase T cell anti-tumor activity, and ultimately improve the efficacy of ICIs by targeting specific immunosuppressive metabolites and cytokines. (3) Some members of the gut microbiota directly participate in the metabolic process of drugs, which can degrade, transform, or produce metabolites, affecting the effective concentration and bioavailability of drugs. Optimizing the structure of the gut microbiota may help improve the efficacy of ICIs. (4) The gut microbiota can also regulate immune cell function and inflammatory status in the brain through gut brain axis communication, indirectly affecting the progression of GBM and the therapeutic response to ICIs. (5) Given the importance of gut microbiota for ICI therapy, researchers have begun exploring the use of fecal microbiota transplantation (FMT) to transplant healthy or optimized gut microbiota to GBM patients, in order to improve their immune status and enhance their response to ICI therapy. Preliminary studies suggest that FMT may enhance the efficacy of ICI therapy in some patients. In summary, gut microbiota plays a crucial role in regulating ICIs in GBM, and with a deeper understanding of the relationship between gut microbiota and tumor immunity, it is expected to develop more precise and effective personalized ICI therapy strategies for GBM, in order to improve patient prognosis.
1 Introduction
Glioblastoma (GBM) is a highly malignant tumor of the nervous system that occurs mainly in the glial cells of the brain. It has one of the highest incidence rates and is the most complicated to treat among all brain tumors. It grows rapidly, with a short disease course and poor prognosis (1). For GBM, surgery combined with radiotherapy and chemotherapy is commonly used as the standard treatment. However, even with active treatment, the median survival period is only 15 months (2). Studies have shown that immune checkpoint inhibitors (ICIs) play a role in GBM (3), and are expected to become a new treatment strategy.
ICIs can relieve the inhibitory state of the patient’s immune system and activate T cells to attack tumors, thereby controlling the growth and spread of the tumor (4). However, ICI therapy has certain limitations. For example, not all patients benefit from ICI therapy; some patients may not respond to treatment or develop resistance, and ICI therapy may cause adverse reactions, such as immune-related adverse reactions, fatigue, and nausea (5). Researchers have found that gut microbiota plays a crucial role in regulating ICI therapy in GBM (6). The relationship between gut microbiota and ICI therapy for GBM is a complex topic of research. Currently, studies are underway on how the gut microbiota affects ICI therapy for GBM, and how to utilize it to enhance the effectiveness of ICI therapy. Notably, the composition and diversity of gut microbiota may affect the function of the immune system, thereby affecting the efficacy of ICIs in GBM treatment. For example, metabolites produced by the gut microbiota, such as short-chain fatty acids (SCFAs), tryptophan (Trp), and arginine, may affect immune cell activity, thereby affecting the effectiveness of ICI therapy (7). In addition, the gut microbiota may affect the immunogenicity of tumor cells, namely their ability to recognize and attack the immune system, which can also affect the effectiveness of ICI therapy (8). We are currently exploring methods to utilize the gut microbiota to enhance the efficacy of ICIs in GBM treatment. Some studies have shown that the activity and function of immune cells can be improved by regulating the composition and diversity of the gut microbiota, thereby enhancing the effectiveness of ICI therapy (9).
Taken together, the relationship between the gut microbiota and ICI therapy for GBM is an emerging research field. Although there is currently no clear conclusion, it has broad research prospects. This article reviews the role of gut microbiota in regulating ICIs in GBM to provide new ideas and methods for GBM.
2 Relationship between the gut microbiota and GBM
The gut microbiota includes a large number of microorganisms that colonize the human gut, affecting the body’s digestive function, defense ability, susceptibility to autoimmune diseases and tumors, and the body’s response to disease-treating drugs (10). Probiotics can promote intestinal peristalsis and food digestion, inhibit the growth of pathogenic bacteria, decompose harmful substances, and enhance immunity. Neutral bacteria have a dual function and are harmless to the human body under normal circumstances. When the bacterial community system is disrupted, bacteria can proliferate in large quantities and cause diseases; pathogenic bacteria, in particular, are harmful to the human body and benefit from microbial constraints. Once their quantity increases significantly, they can affect the immune system and cause diseases (11).
The gut microbiota plays a role in the growth and development of GBM (12), through various mechanisms. For example, the metabolites produced by intestinal bacteria promote the proliferation and migration of GBM cells and accelerate tumor growth and spread (13). Research has shown that in GBM, metabolites of gut microbiota such as 5-hydroxytryptamine, Norepinephrine, Glutamine, and lipopolysaccharide binding proteins (LBP) can promote the proliferation and migration of GBM cells, while dopamine, lipopolysaccharides (LPS) play the opposite role (14–16) (Figure 1). In addition, the composition and abundance of the gut microbiota can affect the activation and function of immune cells, thereby affecting the immune response to tumors. For example, dysbiosis of the gut microbiota can lead to immune system dysfunction, reduce the immune response to tumors, and promote tumor growth and progression (17) (Figure 2). Furthermore, the gut microbiota can influence GBM development by influencing the expression of host genes. Metabolites of the gut microbiota can interact with host cell genes, thereby affecting their expression and function. This can affect the proliferative, differentiative, and invasive abilities of GBM cells, affecting tumor development (18) (Figure 3). Understanding the relationship between gut microbiota and GBM will aid our understanding of the pathogenesis of GBM, which will help develop diagnostic and therapeutic strategies based on the gut microbiota to improve the therapeutic efficacy of GBM.
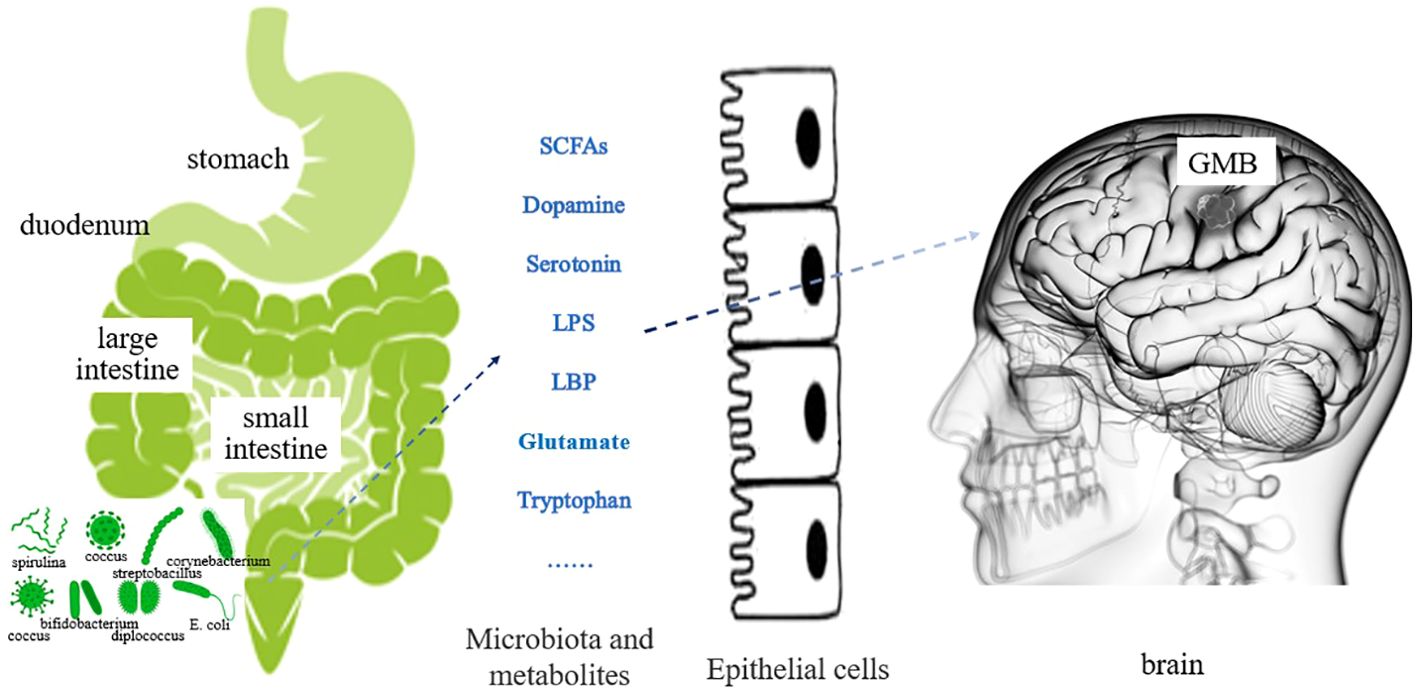
Figure 1 Effect of gut microbiota metabolites on GBM (The various metabolites of gut microbiota are secreted into the bloodstream through the intestinal mucosal epithelium. Regulating changes in GBM cells through blood circulation or affecting neurotransmitter function.).
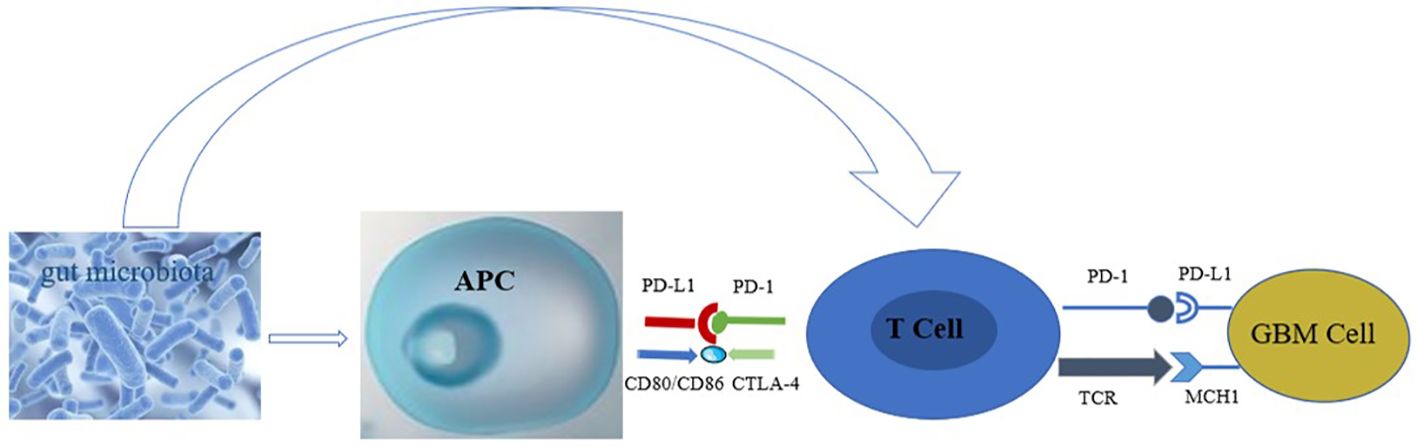
Figure 2 Effect of gut microbiota on immune cells in GBM (The gut microbiota stimulates the body to release antigens, which are taken up and processed by APC, presented to T cells, or directly acts on T cells, causing the PD-1/TCR receptors on the surface of T cells to bind to the corresponding ligands on the surface of GBM, inhibiting the proliferation of GBM cells).
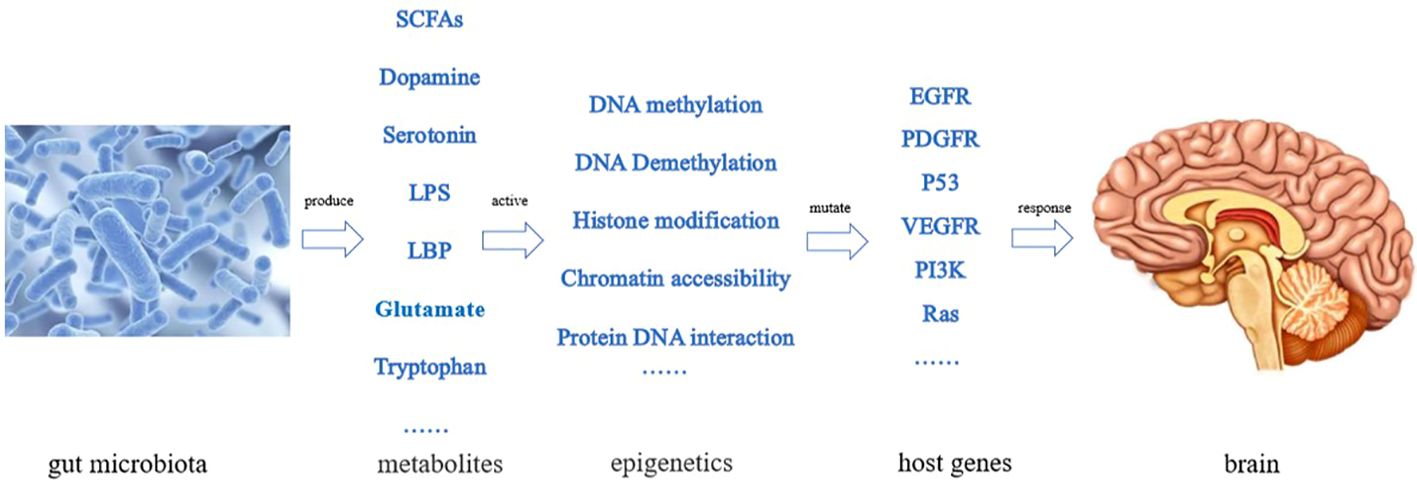
Figure 3 Effect of gut microbiota on GBM by affecting the expression of host genes (The gut microbiota produces various metabolic products, stimulating epigenetic changes in the body, leading to some gene mutations and causing proliferation and differentiation of GBM cells).
3 The mutual regulatory effect between gut microbiota and the tumor microenvironment of GBM
There is an interactive regulatory effect between gut microbiota and the tumor microenvironment (TME) of GBM, involving multiple levels such as immune regulation, metabolic regulation, neuroendocrine communication, and dietary intervention.
Immune system regulation: Some beneficial strains in the gut microbiota, such as lactobacilli and bifidobacteria (19), enhance the function of immune cells, especially the anti-tumor activity of T cells and macrophages, by producing SCFAs such as butyrate, propionate, and acetate (20). At the same time, these metabolites can promote the infiltration of immune cells in the TME, enhancing their recognition and attack ability against tumor cells (21). Imbalance of gut microbiota may lead to an increase in immunosuppressive cells, such as regulatory T cells (Tregs) and myeloid suppressive cells (MDSCs), as well as tumor associated macrophages (TAMs) leaning towards M2 type (immunosuppressive) (22). These immunosuppressive cells may weaken the anti-tumor immune response in the TME (23–25). Conversely, restoring gut microbiota balance may reduce the number or function of these immunosuppressive cells, thereby improving the TME (26).
Metabolites and signaling pathway regulation: Metabolites produced by gut microbiota can reach the brain through blood circulation, affecting the metabolism and signal transduction of GBM cells (27). For example, certain metabolites such as bile acids and indole compounds may activate specific signaling pathways within GBM cells, affecting tumor cell proliferation, migration, angiogenesis, and immune escape ability (28, 29). The chronic low-grade inflammatory state caused by imbalance of gut microbiota can lead to an increase in the levels of systemic inflammatory mediators (30), which may penetrate the brain through the blood-brain barrier (BBB), promote the sustained inflammatory response in the TME, and further exacerbate tumor progression and immune suppression (9).
The neuroendocrine communication regulation of the gut brain axis: gut microbiota can affect neurotransmitters (31). The synthesis and metabolism of aminobutyric acid, as well as the secretion of hormones such as cortisol and insulin, affect the function of neurons and glial cells in the brain through blood circulation, indirectly affecting the homeostasis of the GBM TME (16). Dysfunction of gut microbiota may affect the migration of immune cells to the brain by regulating neuroendocrine signals (32). For example, intestinal derived cytokines may stimulate the CXC ligand 12 / CXC receptor 4 (CXCL12/CXCR4) axis, promoting immune cell recruitment to the tumor site (33).
Dietary and therapeutic response: Dietary components directly affect the composition and activity of gut microbiota, thereby affecting the host’s nutritional metabolism (34). Specific dietary patterns, such as those rich in dietary fiber, antioxidants, or anti-inflammatory foods, may enhance the body’s response to chemotherapy drugs, radiation therapy, or ICI therapy by optimizing the gut microbiota, reducing therapeutic toxicity (35–37). The gut microbiota is involved in the biological transformation of drugs, including anti-tumor drugs (38). Some strains may alter the bioavailability, efficacy, or toxicity of drugs through enzymatic reactions, affecting the treatment efficacy and tolerance of patients (39).
Potential role of microbiome therapy: Fecal microbial transplantation (FMT) from healthy donors to GBM patients aims to restore gut microbiota balance, enhance anti-tumor immunity, and improve the TME (40, 41). Preliminary preclinical and clinical studies have explored its application in GBM treatment. By orally administering specific probiotics or prebiotics (such as dietary fiber, inulin, etc.) to regulate gut microbiota, promote beneficial bacterial growth, and inhibit harmful bacterial communities, it may indirectly improve the TME and overall therapeutic effect of cancer patients (42).
In depth study of these interaction mechanisms is expected to provide scientific basis for the development of new GBM treatment strategies, especially by combining existing treatment methods with gut microbiota regulation technologies.
4 Relationship between the gut microbiota, gut-brain axis, and GBM
The gut-brain axis comprises the bidirectional communication between the central nervous system and intestinal nervous system (43). Initially, researchers believed that the destruction of the gut microbiota would only produce pathological and physiological phenomena in the local gut, such as irritable bowel syndrome (44). However, recent studies have shown that dysbiosis of the gut microbiota not only causes pathological and physiological phenomena in the intestine but also leads to central nervous system lesions (45). Extensive preclinical studies using sterile and wild-type mice have confirmed that alterations in the gut microbiome can lead to abnormal brain signaling and behavior (46).
The gut microbiota gut-brain axis refers to the interaction and influence between the gut microbiota and the brain, which plays an important role in the occurrence and treatment of GBM (16). Metabolites of the gut microbiota can act on the autonomic nervous system and neuroendocrine axis, regulating brain function and behavior. This interaction can affect the growth and development of GBM and may effect the cognition and emotions of patients (47) (Figure 4). The effect of the gut microbiota gut-brain axis on GBM is multifaceted. First, gut microbiota can influence GBM progression by regulating the expression of neurotransmitters. For example, gut microbiota can regulate the expression of dopamine and serotonin. However, dopamine can activate the expression of epidermal growth factor receptor (EGFR) and promote the phosphorylation of mitogen-activated protein kinase by binding to the highly expressed dopamine receptor 2 in GBM cells, thereby promoting the progression of GBM. In addition, most serotonin in the body is metabolized by the gut microbiota, and excessive secretion of 5-hydroxytryptamine can promote the proliferation of gliomas by activating the protein phosphorylation signaling pathway (48). Second, GBM disrupts the blood-brain barrier during development, allowing circulating immune cells and inflammatory mediators, such as T cells, macrophages, B cells, IL-6, IL-8, and other inflammatory mediators, to enter the brain (49). However, immune cells are inhibited in an inhibitory environment. M2 macrophages secrete IL-10, EGF, and vascular endothelial growth factor (VEGF), which inhibit T cell proliferation and promote tumor growth and angiogenesis, leading to GBM progression (50). Inflammation is an important factor that promotes GBM progression. Munoz et al. confirmed that H-Ras isoforms upregulated the expression of IL-6 and IL-8 in GBM cells, promoting their survival, invasion, and proliferation (51). Third, metabolites related to the gut microbiota, such as SCFAs, affect the function of nuclear transcription factor-κB (NF-κB) in tumor cells and immune cells. A portion of circulating SCFAs can enter the central nervous system, especially when the blood-brain barrier is disrupted. Moreover, the abnormal activation of the B pathway activates survival genes, leading to the activation of signal transducer and activator of transcription 3 (STAT3) and increased invasiveness of GBM cells (52). In addition, SCFAs can downregulate STAT1 and histone deacetylase to inhibit INF-γ, whereas Indoleamine 2, 3-dioxygenase-1 (IDO-1) can induce tumor growth factor-β (TGF-β). Helper T cell-1 (Th1) and Th2 release triggers dysregulation, which is beneficial for the M2c phenotype of microglia and inhibits cytokine production, lymphocyte proliferation, and T cell differentiation, thereby promoting tumor growth (53).
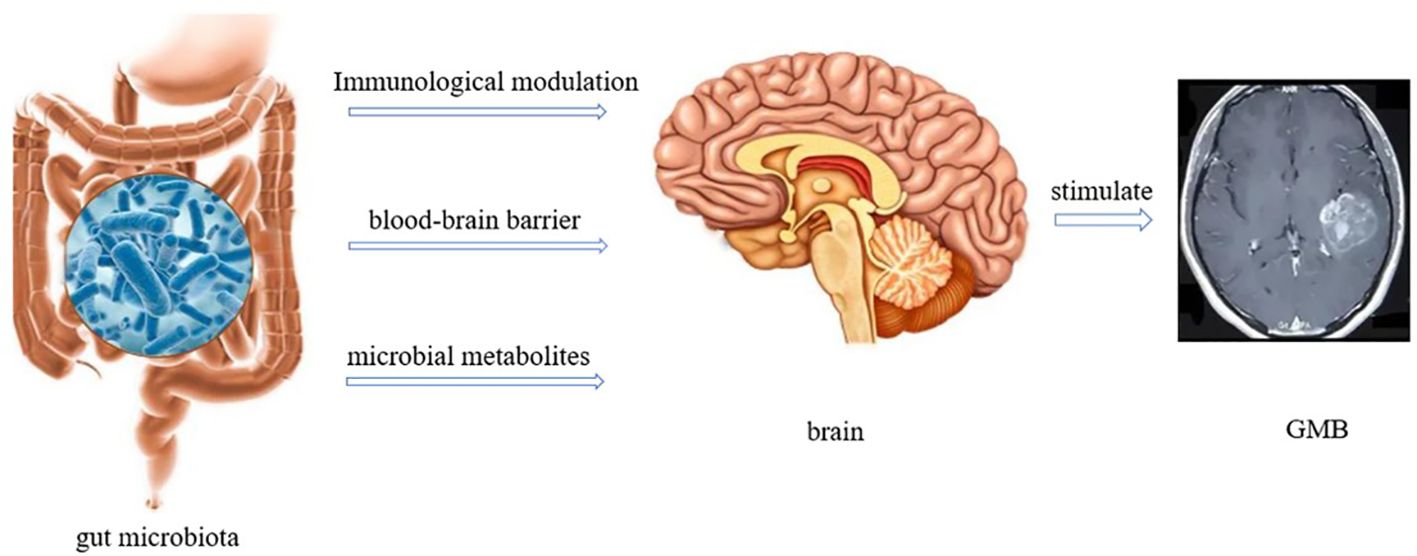
Figure 4 Interaction between gut microbiota and GBM (There are three ways in which gut microbiota interacts with the brain. 1) Immune regulation; 2) Directly acting through the blood-brain barrier; 3) Regulate through metabolites.).
5 Role of the gut microbiota and gut-brain axis in ICI therapy
The interplay between gut microbiota and ICIs is extremely complex. The gut microbiota not only directly regulates the activity and function of immune cells to affect the efficacy of ICIs but also regulates the immune system of the body through the gut-brain axis, affecting ICI efficacy against tumors (54). Routy et al. found that dysbiosis of the gut microbiota can lead to tumor resistance to ICI therapy (55). FMT from ICI-responsive tumor patients into sterile or antibiotic-treated mice can improve the antitumor effect of the programmed cell death protein 1 (PD-1) blockade, whereas FMT from unresponsive patients does not show any improvement in antitumor activity (56). Importantly, the FMT response overcame the resistance of patients with melanoma to PD-1 therapy (57). A close relationship has been observed between the gut-brain axis and ICIs of tumors; however, the specific underlying mechanisms are not yet clear.
Research has shown that the gut microbiota can downregulate granulocyte-macrophage colony-stimulating factor signaling through the gut-brain axis, leading to significant expression of reactive oxygen species in activated immature myeloid cells, thereby increasing the inhibitory activity of MDSCs on T cells and enhancing ICI therapy (58). The gut microbiota promotes the development of Foxp3 Tregs and improves intestinal barrier function through the gut-brain axis, thereby inhibiting the secretion of multiple pro-inflammatory Th17 cells. FOXP3+regulatory T cells are a subset of CD4+ Th cells that serve as checkpoints for immune activation and are crucial for peripheral autoimmune prevention (59). The SCFAs produced by the gut microbiota activate cellular receptors and affect cellular metabolism. They can also enter the central nervous system through the BBB and activate protein receptors such as free fatty acid receptor 3 (FFAR3) and FFA2/FFAR2, leading to the secretion of cytokines and chemokines and the regulation of cellular programs, supporting their role as immune response enhancers and anti-inflammatory agents (60). Antigen epitope tailband measurement protein 1 and proteasome subunits in Hirae Enterococcus β-Type 4 tumor antigens also exhibit high similarity, which can activate CD8+ T cells and improve the effectiveness of PD-1 blockade therapy (61).
6 Role of the gut microbiota and gut-brain axis in regulating ICI therapy for GBM
Over the years, researchers have found that the brain has immune functions and functional lymphatic vessels, challenging the view that the brain is an immune-privileged organ (62). It was always believed that GBM is in an immunosuppressive state with poor efficacy of ICI therapy. However, Chen et al. showed that ICI therapy (anti-cytotoxic T-lymphocyte antigen 4,CTLA-4) can generate antitumor immune responses in GBM, which can produce IFN-γ. CD4+ T cells and phagocytic microglia promote the phagocytic function of microglia and enhance their effectiveness in ICI therapy. This study provides new ideas for the application of ICI therapy in GBM (63).
Recent studies have shown that the gut microbiota plays an important role in ICI therapy for GBM and that appropriate microbial activity can reduce immune suppression in glioma models and improve the response to ICI therapy. Multiple studies have confirmed that specific gut microbiota induce the function of CD8+ T cells through the gut-brain axis to enhance the efficacy of ICIs. For example, melanoma patients with a higher relative abundance of gut microbiota show increased antigen presentation, improved CD4+ and CD8+ T cell function in the peripheral blood and TME, and enhanced antitumor effects of ICIs (64). Another study showed that Firmicutes and Actinobacteria are abundant in FMT and PD-1 blockade responses. The combination of FMT and PD-1 blockade stimulates mucosal-associated invariant T and CD56+CD8+ T cells in peripheral blood mononuclear cells and upregulates the expression of human leukocyte antigen class II genes CD74 and granzyme K on CD8+ T cells at the tumor site to improve the therapeutic effect of ICIs (65).
Inosine, a metabolic product of the intestinal microbiota, can significantly enhance the ability of tumor cells to present tumor antigens. Therefore, cytotoxic immune cells can easily recognize and kill tumor cells, thereby exerting antitumor effects (66). Further mechanistic studies have shown that in inosine-treated tumor cells, IFN-γ and TNF-α significantly increased the activation of signaling pathways. The cytotoxicity of tumor-specific T and NK cells can be activated by promoting the release of perforin and granzyme, and IFN-γ can enhance antigen presentation and promote the antitumor effect mediated by inosine. Inosine also enhances the inherent immunogenicity of tumors by directly binding and inhibiting the ubiquitin-activating enzyme, ubiquitin-like modifier activating enzyme 6, rendering tumor cells sensitive to T cell-mediated cytotoxicity. Additionally, inosine can enhance the efficacy of ICIs by acting on adenosine 2A receptors on T lymphocytes (67–69).
Furthermore, SCFAs can exert multiple effects through the gut-brain axis, including the enhancement of ICIs. The SCFAs propionic acid activates the cell cycle inhibitor p21 through G protein-coupled receptor 43 and downregulates apoptosis inhibitor protein, inhibiting cancer cell proliferation, inducing cell apoptosis, and enhancing the antitumor effect of ICIs (55). SCFAs can inhibit DNA binding 2-dependent IL-12 signaling; promote the antitumor cytotoxicity of CD8 T cells; provide energy to immune cells; regulate glycolysis, the tricarboxylic acid (TCA) cycle, and fatty acid oxidation of antitumor effector cells; and improve the efficiency of ICIs (70).
7 Dilemma of the gut microbiota in the treatment of GBM
Basic research has shown that gut microbiota plays a certain role in the treatment of GBM, but it also faces many dilemmas, including the complexity and individual differences of gut microbiota, unclear mechanisms of action, limitations of intervention methods, drug interactions, clinical translation difficulties, drug resistance and recurrence issues, and challenges of interdisciplinary collaboration and integration (71–73).
The complexity and individual differences of gut microbiota: Gut microbiota is a complex ecosystem composed of trillions of microorganisms, and its composition and function vary from individual to individual, influenced by various factors such as genetic background, dietary habits, living environment, and disease status (71). This highly individualized characteristic poses a challenge in designing GBM treatment strategies based on gut microbiota intervention, requiring precise identification of specific bacterial species or microbiota structures that have a critical impact on treatment outcomes, and considering how to achieve personalized adjustments among different patients (12).
The mechanism of action of gut microbiota is not yet fully understood: Although previous studies have shown that gut microbiota can indirectly affect the occurrence, development, and treatment response of GBM by regulating the immune system, affecting drug metabolism, and producing bioactive substances, the specific details, upstream and downstream relationships, and causal relationships of these mechanisms need to be further elucidated. Especially in tumor ICI therapy, the interaction mechanism between gut microbiota and ICIs is complex, and their mechanisms of action in reshaping the TME and activating anti-tumor immune responses still need further research (13).
Limitations of interventions for gut microbiota: Changing the state of gut microbiota to assist GBM treatment typically involves methods such as prebiotics, probiotics, synbiotics, and FMT. However, the evidence for the effectiveness and safety of these methods in GBM patients is not yet sufficient. The colonization effect and long-term stability of probiotics are uncertain. The selection and dosage of prebiotics require precise regulation. The effectiveness of synbiotics depends on the synergistic effect of multiple components and is difficult to standardize. FMT may face difficulties in donor screening, suboptimal post-transplant bacterial colonization, and potential risks of disease transmission. In addition, the dynamic changes in gut microbiota may lead to unstable intervention effects (41–43).
Interaction between gut microbiota and drugs: The gut microbiota can metabolize certain chemotherapy drugs and targeted drugs, changing their in vivo concentration, bioavailability, and toxicity (74, 75). The efficacy and toxicity of chemotherapy drugs such as temozolomide may be influenced by gut microbiota metabolism (76). However, predicting and regulating these interactions to optimize drug efficacy and reduce side effects remains challenging, especially in GBM where patients typically require multiple drug combinations, increasing the complexity of drug microbiota interactions (77).
Clinical transformation challenges of gut microbiota: Although basic research has revealed the potential value of gut microbiota in GBM, translating these findings into clinical practice still faces many challenges. This includes the need to design rigorous clinical trials to validate the effectiveness and safety of gut microbiota interventions, establish standardized methods for gut microbiota detection and analysis, and address ethical, regulatory, and patient acceptance issues.
Drug resistance and recurrence of GBM: GBM has the characteristics of high invasiveness, easy recurrence, and tolerance to traditional treatment methods. Although the gut microbiota may affect the response of tumors to treatment, it is currently unclear how to effectively overcome the therapeutic resistance of GBM or prevent and delay tumor recurrence by regulating the microbiota (78).
Multidisciplinary collaboration and integration: The interdisciplinary field of gut microbiota and GBM involves multiple disciplines such as oncology, immunology, microbiology, pharmacology, etc., requiring close collaboration among interdisciplin-ary experts to jointly promote research progress and clinical applications. However, in practical operation, there may be difficulties in disciplinary barriers, allocation of research resources, and standardization of research methods.
Based on the above difficulties, continuous scientific research, technological innovation, and in-depth interdisciplinary cooperation are needed to solve these problems, in order to truly bring survival benefits to patients with GBM through gut microbiota.
8 Summary and perspective
The gut microbiota has a significant effect on ICI therapy for GBM. The gut microbiota regulates the function, metabolism, and inflammatory response of the immune system through the gut-brain axis, affecting the efficacy and safety of ICIs for GBM. First, the gut microbiota can affect the function of the immune system, thereby affecting ICI therapy for GBM. The intestinal microbiota enhances the immune system by stimulating the activation and proliferation of immune cells, thereby improving the efficacy of ICI therapy. Second, the gut microbiota can affect the immune microenvironment of GBM by regulating the infiltration and activation of immune cells, thereby affecting the efficacy of ICIs. The immune microenvironment refers to the composition and state of immune cells, the matrix, and cytokines around tumors, which play an important regulatory role in tumor growth and spread. Third, gut microbiota can affect the nutritional and metabolic status of patients with GBM, thereby affecting the efficacy of ICI therapy. Intestinal microbiota participate in nutritional and metabolic processes in the human body, including vitamin synthesis and amino acid metabolism. The nutritional and metabolic statuses of patients can be improved by regulating the composition and activity of the gut microbiota, thereby enhancing the therapeutic effects of ICIs.
The regulation of the gut microbiota through the gut-brain axis in ICI therapy for GBM is a complex process that requires further research to gain a deeper understanding of its specific mechanisms and effects. Currently, relatively little research is available on the mechanism and efficacy of the gut microbiota in GBM, and further research is required to verify its specific effects and potential therapeutic value. Simultaneously, neural therapy targeting the gut microbiota must consider individual differences and safety issues.
Author contributions
HZ: Resources, Writing – original draft. YH: Resources, Writing – original draft. TW: Data curation, Writing – original draft. EB: Data curation, Resources, Writing – review & editing. SL: Supervision, Writing – review & editing. LH: Resources, Writing – original draft. TX: Data curation, Funding acquisition, Supervision, Writing – review & editing.
Funding
The author(s) declare financial support was received for the research, authorship, and/or publication of this article. This work was supported by Stone Medicine Tumor Research Fund-CSCO (No.sy2018-142).
Acknowledgments
Thank you for the funding from Stone Medicine Tumor Research Fund of CSCO.
Conflict of interest
The authors declare that the research was conducted in the absence of any commercial or financial relationships that could be construed as a potential conflict of interest.
Publisher’s note
All claims expressed in this article are solely those of the authors and do not necessarily represent those of their affiliated organizations, or those of the publisher, the editors and the reviewers. Any product that may be evaluated in this article, or claim that may be made by its manufacturer, is not guaranteed or endorsed by the publisher.
References
1. Liu H, Wei Z, Zhang Yu, Shi K, Li J. TGF-β based risk model to predict the prognosis and immune features in glioblastoma. Front Neurol. (2023) 14:1188383. doi: 10.3389/fneur.2023.1188383
2. Rončević A, Koruga N, Koruga AS, Rončević R, Rotim T, Šimundić T, et al. Personalized treatment of glioblastoma: current state and future perspective. Biomedicines. (2023) 11:1579. doi: 10.3390/biomedicines11061579
3. Pinheiro SLR, Lemos FFB, Marques HS, Luz MS, Silva LuísGdeO, Santos CFSM, et al. Immunotherapy in glioblastoma treatment: Current state and future prospects. World J Clin Oncol. (2023) 14:138–59. doi: 10.5306/wjco.v14.i4.138
4. Topalian SL, Forde PM, Emens LA, Yarchoan M, Smith KN, Pardoll DM. Neoadjuvant immune checkpoint blockade: A window of opportunity to advance cancer immunotherapy. Cancer Cell. (2023) 41:1551–66. doi: 10.1016/j.ccell.2023.07.011
5. Guo R, Li X, Hao M, Liang Y, Wang L, Yang Z. Advances in predictive biomarkers associated with immune checkpoint inhibitors for tumor therapy. Sheng Wu Gong Cheng Xue Bao. (2023) 39:1403–24. doi: 10.13345/j.cjb.220650
6. Naghavian R, Faigle W, Oldrati P, Wang J, Toussaint NC, Qiu Y, et al. Microbial peptides activate tumour-infiltrating lymphocytes in glioblastoma. Nature. (2023) 617:807–17. doi: 10.1038/s41586–023-06081-w
7. Dehhaghi M, Kazemi SPH, Heng B, Guillemin GJ. The gut microbiota, kynurenine pathway, and immune system interaction in the development of brain cancer. Front Cell Dev Biol. (2020) 8:562812. doi: 10.3389/fcell.2020.562812
8. Samami E, Aleebrahim-Dehkordi E, Mohebalizadeh M, Yaribash S, Saghazadeh A, Rezaei N. Inosine, gut microbiota, and cancer immunometabolism. Am J Physiol Endocrinol Metab. (2023) 324:E1–8. doi: 10.1152/ajpendo.00207.2022
9. Zhang X, Zhao L, Zhang He, Zhang Y, Ju H, Wang X, et al. The immunosuppressive microenvironment and immunotherapy in human glioblastoma. Front Immunol. (2022) 13:1003651. doi: 10.3389/fimmu.2022.1003651
10. Zuo WF, Pang Q, Yao LP, Zhang Y, Peng C, Huang W, et al. Gut microbiota: A magical multifunctional target regulated by medicine food homology species. J Adv Res. (2023) 52:151–70. doi: 10.1016/j.jare.2023.05.011
11. Laterza L, Putignani L, Settanni CR, Petito V, Varca S, Maio FDe, et al. Ecology and machine learning-based classification models of gut microbiota and inflammatory markers may evaluate the effects of probiotic supplementation in patients recently recovered from COVID-19. Int J Mol Sci. (2023) 24:6623. doi: 10.3390/ijms24076623
12. Zeng C, Zhang C, He C, Song H. Investigating the causal impact of gut microbiota on glioblastoma: a bidirectional Mendelian randomization study. BMC Genomics. (2023) 24:784. doi: 10.1186/s12864–023-09885–2
13. Lyu Y, Yang H, Chen L. Metabolic regulation on the Immune Environment of Glioma through Gut Microbiota. Semin Cancer Biol. (2021) 86:990–7. doi: 10.1016/j.semcancer.2021.05.005
14. Karmakar S, Lal G. Role of serotonergic system in regulating brain tumor-associated neuroin-flammatory responses. Methods Mol Biol. (2024) 2761:181–207. doi: 10.1007/978–1-0716–36626_14
15. Xie Li, Qiu S, Lu C, Gu C, Wang J, Lv J, et al. Gastric cancer-derived LBP promotes liver metastasis by driving intrahepatic fibrotic pre-metastatic niche formation. J Exp Clin Cancer Res. (2023) 42:258. doi: 10.1186/s13046–023-02833–8
16. D’Alessandro G, Lauro C, Quaglio D, Ghirga F, Botta B, Trettel F, et al. Neuro-signals from gut microbiota: perspectives for brain glioma. Cancers (Basel). (2021) 13:2810. doi: 10.3390/cancers13112810
17. Kim D, Sarvesh S, Valbak O, Backan O, Chen D, Morrow C, et al. The gut microbiota of brain tumor patients can impact immunotherapy efficacy in a preclinical model of glioma. Neuro-Oncology. (2023) 25:v299. doi: 10.1093/neuonc/noad179.1157
18. Montella L, Sarno F, Altucci L, Cioffi V, Sigona L, Colandrea SD, et al. A root in synapsis and the other one in the gut microbiome-brain axis: are the two poles of ketogenic diet enough to challenge glioblastoma? Front Nutr. (2021) 8:703392. doi: 10.3389/fnut.2021.703392
19. Cheng J, Hu H, Ju Y, Liu J, Wang M, Liu B, et al. Gut microbiota-derived short-chain fatty acids and depression: deep insight into biological mechanisms and potential applications. Gen Psychiatr. (2024) 37:e101374. doi: 10.1136/gpsych-2023–101374
20. Yao Y, Cai X, Fei W, Ye Y, Zhao M, Zheng C. The role of short-chain fatty acids in immunity, inflammation and metabolism. Crit Rev Food Sci Nutr. (2022) 62:1–12. doi: 10.1080/10408398.2020.1854675
21. González-Brito A, Uribe-Herranz M. The potential role of short chain fatty acids improving ex vivo T and CAR-T cell fitness and expansion for cancer immunotherapies. Front Immunol. (2023) 14:1083303. doi: 10.3389/fimmu.2023.1083303
22. Ma Y, Yang H, Pitt JM, Kroemer G, Zitvogel L. Therapy-induced microenvironmental changes in cancer. J Mol Med (Berl). (2016) 94:497–508. doi: 10.1007/s00109–016-1401–8
23. Tay C, Tanaka A, Sakaguchi S. Tumor-infiltrating regulatory T cells as targets of cancer immunotherapy. Cancer Cell. (2023) 41:450–65. doi: 10.1016/j.ccell.2023.02.014
24. Iglesias-Escudero M, Arias-González N, Martínez-Cáceres E. Regulatory cells and the effect of cancer immunotherapy. Mol Cancer. (2023) 22:26. doi: 10.1186/s12943–023-01714–0
25. Pan Y, Yu Y, Wang X, Zhang T. Tumor-associated macrophages in tumor immunity. Front Immunol. (2020) 11:583084. doi: 10.3389/fimmu.2020.583084
26. Schneider KM, Mohs A, Gui W, Galvez EJC, Candels LS, Hoenicke L, et al. Imbalanced gut microbiota fuels hepatocellular carcinoma development by shaping the hepatic inflammatory microenvironment. Nat Commun. (2022) 13:3964. doi: 10.1038/s41467–022-31312–5
27. Aljarrah D, Chalour N, Zorgani A, Nissan T, Pranjol MZI. Exploring the gut microbiota and its potential as a biomarker in gliomas. BioMed Pharmacother. (2024) 173:116420. doi: 10.1016/j.biopha.2024.116420
28. Sharpe MA, Baskin DS, Johnson RD, Baskin AM. Acquisition of immune privilege in GBM tumors: role of prostaglandins and bile salts. Int J Mol Sci. (2023) 24:3198. doi: 10.3390/ijms24043198
29. Fung S, Xu C, Hamel E, Wager-Miller JB, Woodruff G, Miller A. Novel indole-based compounds that differentiate alkylindole-sensitive receptors from cannabinoid receptors and microtubules: Characterization of their activity on glioma cell migration. Pharmacol Res. (2017) 115:233–41. doi: 10.1016/j.phrs.2016.10.025
30. Chen Y, Liu S, Leng SX. Chronic low-grade inflammatory phenotype (CLIP) and senescent immune dysregulation. Clin Ther. (2019) 41:400–9. doi: 10.1016/j.clinthera.2019.02.001
31. Chen Y, Xu J, Chen Y. Regulation of neurotransmitters by the gut microbiota and effects on cognition in neurological disorders. Nutrients. (2021) 13:2099. doi: 10.3390/nu13062099
32. Kasarello K, Cudnoch-Jedrzejewska A, Czarzasta K. Communication of gut microbiota and brainvia immune and neuroendocrine signaling. Front Microbiol. (2023) 14:1118529. doi: 10.3389/fmicb.2023.1118529
33. Hou JY, Xu H, Cao GZ, Tian LL, Wang LH, Zhu NQ, et al. Multi-omics reveals Dengzhan Shengmai formulation ameliorates cognitive impairments in D-galactose-induced aging mouse model by regulating CXCL12/CXCR4 and gut microbiota. Front Pharmacol. (2023) 10:14:1175970. doi: 10.3389/fphar.2023.1175970
34. Dasriya VL, Samtiya M, Ranveer S, Dhillon HS, Devi N, Sharma V, et al. Modulation of gut-microbiota through probiotics and dietary interventions to improve host health. J Sci Food Agric. (2024). doi: 10.1002/jsfa.13370
35. Sanati V, Sohouli MH, Dareini H, Esmailzadeh A, Sajadian AS, Raji LM, et al. The association between dietary patterns and chemotherapy side effects in patients with breast cancer (BrCa). Nutr Cancer. (2023) 75:948–59. doi: 10.1080/01635581.2023.2173552
36. Moraitis I, Guiu J, Rubert J. Gut microbiota controlling radiation-induced enteritis and intestinal regeneration. Trends Endocrinol Metab. (2023) 34:489–501. doi: 10.1016/j.tem.2023.05.006
37. Golonko A, Pienkowski T, Swislocka R, Orzechowska S, Marszalek K, Szczerbin- ski L, et al. Dietary factors and their influence on immunotherapy strategies in oncology: a comprehensive review. Cell Death Dis. (2024) 15:254. doi: 10.1038/s41419–024-06641–6
38. Klünemann M, Andrejev S, Blasche S, Mateus A, Phapale P, Devendran S, et al. Bioaccumulationof therapeutic drugs by human gut bacteria. Nature. (2021) 597:533–8. doi: 10.1038/s41586–021-03891–8
39. Weersma RK, Zhernakova A, Fu J. Interaction between drugs and the gut microbiome. Gut. (2020) 69:1510–9. doi: 10.1136/gutjnl-2019–320204
40. Fan Y, Su Q, Chen J, Wang Y, He S. Gut microbiome alterations affect glioma development and foxp3 expression in tumor microenvironment in mice. Front Oncol. (2022) 12:836953. doi: 10.3389/fonc.2022.836953
41. Kleber KT, Iranpur KR, Perry LM, Cruz SM, Razmara AM, Culp WTN, et al. Using the canine microbiome to bridge translation of cancer immunotherapy from pre-clinical murine models to human clinical trials. Front Immunol. (2022) 13:983344. doi: 10.3389/fimmu.2022.983344
42. Rau S, Gregg A, Yaceczko S, Limketkai B. Prebiotics and probiotics for gastrointestinalDisorders. Nutrients. (2024) 16:778. doi: 10.3390/nu16060778
43. Dono A, Nickles J, Rodriguez-Armendariz AG, McFarland BC, Ajami NJ, Ballester LY, et al. Glioma and the gut-brain axis: opportunities and future perspectives. Neurooncol Adv. (2022) 4:vdac054. doi: 10.1093/noajnl/vdac054
44. Mayer EA, Nance K, Chen S. The gut-brain axis. Annu Rev Med. (2022) 73:439–53. doi: 10.1146/annurev-med-042320–014032
45. Socała K, Doboszewska U, Szopa A, Serefko A, Włodarczyk M, Zielińska A, et al. The role of microbiota-gut-brain axis in neuropsychiatric and neurological disorders. Pharmacol Res. (2021) 172:105840. doi: 10.1016/j.phrs.2021.105840
46. Kim CH, Park J, Kim M. Gut microbiota-derived short-chain Fatty acids, T cells, and inflammation. Immune Netw. (2014) 14:277–88. doi: 10.4110/in.2014.14.6.277
47. Wang S, Yin F, Guo Z, Li R, Sun W, Wang Y, et al. Association between gut microbiota and glioblastoma: a Mendelian randomization study. Front Genet. (2024) 14:1308263. doi: 10.3389/fgene.2023.1308263
48. Caragher SP, Hall RR, Ahsan R, Ahmed. AU. Monoamines in glioblastoma: complex biology with therapeutic potential. Neuro Oncol. (2018) 20:1014–25. doi: 10.1093/neuonc/nox210
49. Elguindy M, Young JS, Mondal I, Lu RO, Ho WS. Glioma-immune cell crosstalk in tumor progression. Cancers (Basel). (2024) 16:308. doi: 10.3390/cancers16020308
50. Lisi L, Ciotti GMP, Braun D, Kalinin S, Currò D, Dello Russo C, et al. Expression of iNOS, CD163 and ARG-1 taken as M1 and M2 markers of microglial polarization in human glioblastoma and the surrounding normal parenchyma. Neurosci Lett. (2017) 645:106–12. doi: 10.1016/j.neulet.2017.02.076
51. Munoz L, Yeung YT, Grewal T. Oncogenic Ras modulates p38 MAPK-mediated inflammatory cytokine production in glioblastoma cells. Cancer Biol Ther. (2016) 17:355–63. doi: 10.1080/15384047.2016.1139249
52. Li W, Cai H, Ren L, Yang Y, Yang H, Liu J, et al. Sphingosine kinase 1 promotes growth of glioblastoma by increasing inflammation mediated by the NF-B/IL-6/STAT3 and JNK/PTX3 pathways. Acta Pharm Sin B. (2022) 12:4390–406. doi: 10.1016/j.apsb.2022.09.012
53. Roesch S, Rapp C, Dettling S, Herold-Mende C. When immune cells turn bad-tumor-associated microglia/macrophages in glioma. Int J Mol Sci. (2018) 19:436. doi: 10.3390/ijms19020436
54. Andrews MC, Vasanthakumar A. Gut microbiota a double edged sword in cancer immunotherapy. Trends Cancer. (2023) 9:3–5. doi: 10.1016/j.trecan.2022.08.003
55. Routy B, Chatelier ELe, Derosa L, Duong CPM, Alou MT, Daillère R, et al. Gut microbiome influences efficacy of PD-1-based immunotherapy against epithelial tumors. Science. (2018) 359:91–7. doi: 10.1126/science.Aan3706
56. Frankel AE, Coughlin LA, Kim J, Froehlich TW, Xie Y, Frenkel EP, et al. Metagenomic shotgun sequencing and unbiased metabolomic profiling identify specific human gut microbiota and metabolites associated with immune checkpoint therapy efficacy in melanoma patients. Neoplasia. (2017) 19:848–55. doi: 10.1016/j.neo.2017.08.004
57. Barbosa EC, Bucar EEC, Jubé GR, Silveira LB, Silva NCD, Faria PCC, et al. Fecal microbiota transplantation and its repercussions in patients with melanoma refractory to anti-PD-1 therapy: scope review. Rev Col Bras Cir. (2023) 50:e20233490. doi: 10.1590/0100–6991e-20233490-en
58. Li R, Hu Y, Hou S. An Exploration of Oral-Gut Pathogens Mediating Immune Escape of Pancreatic Cancer via miR-21/PTEN Axis. Front Microbiol. (2022) 13:928846. doi: 10.3389/fmicb.2022.928846
59. Choi J, Kim BR, Akuzum B, Chang L, Lee JY, Kwon HK. T(REG)king from gut to brain: the control of regulatory T cells along the gut-brain axis. Front Immunol. (2022) 13:916066. doi: 10.3389/fimmu.2022.916066
60. Abdelhalim KA. Short-chain fatty acids (SCFAs) from gastrointestinal disorders, metabolism, epigenetics, central nervous system to cancer - A mini-review. Chem Biol Interact. (2024) 388:110851. doi: 10.1016/j.cbi.2023.110851
61. Fluckiger Aurélie, Daillère R, Sassi M, Six BS, Liu P, Loos F, et al. Cross-reactivity between tumor MHC class I-restricted antigens and an enterococcal bacteriophage. Science. (2020) 369:936–42. doi: 10.1126/science.aax0701
62. Castellani G, Croese T, Peralta Ramos JM, Schwartz M. Transforming the understanding of brainimmunity. Science. (2023) 380:eabo7649. doi: 10.1126/science.abo7649
63. Chen D, Varanasi SK, Hara T, Traina K, Sun M, McDonald B, et al. CTLA-4 blockade induces a microglia-Th1 cell partnership that stimulates microglia phagocytosis and anti-tumor function in glioblastoma. Immunity. (2023) 56:2086–2104.e8. doi: 10.1016/j.immuni.2023.07.015
64. Pietrzak B, Tomela K, Olejnik-Schmidt A, Galus Ł, Mackiewicz J, Kaczmarek M, et al. A clinical outcome of the anti-PD-1 therapy of melanoma in polish patients is mediated by population-specific gut microbiome composition. Cancers (Basel). (2022) 14:5369. doi: 10.3390/cancers14215369
65. Routy B, Lenehan JG, Miller WH, Jamal R, Messaoudene M, Daisley BA, et al. Fecal microbiota transplantation plus anti-PD-1 immunotherapy in advancedmelanoma: a phase I trial. Nat Med. (2023) 29:2121–32. doi: 10.1038/s41591–023-02453-x
66. Bird L. Microbial metabolite boosts immunotherapy. Nat Rev Immunol. (2020) 20:648–9. doi: 10.1038/s41577–020-00465-z
67. Ulrike Harjes. Tumour-reactive T cells work remotely using IFNγ. Nat Rev Cancer. (2020) 20:261. doi: 10.1038/s41568–020-0255–0
68. Zhang L, Jiang Li, Yu L, Li Q, Tian X, He J, et al. Inhibition of UBA6 by inosine augments tumour immunogenicity and responses. Nat Commun. (2022) 13:5413. doi: 10.1038/s41467–022-33116-z
69. Mager LF, Burkhard R, Pett N, Cooke NCA, Brown K, Ramay H, et al. Microbiome-derived inosine modulates response to checkpoint inhibitor immunotherapy. Science. (2020) 369:1481–9. doi: 10.1126/science.abc3421
70. Luu M, Riester Z, Baldrich A, Reichardt N, Yuille S, Busetti A, et al. Microbial short-chain fatty acids modulate CD8+ T cell responses and improve adoptive immunotherapy for cancer. Nat Commun. (2021) 12:4077. doi: 10.1038/s41467–021-24331–1
71. Di Vincenzo F, Del Gaudio A, Petito V, Lopetuso LR, Scaldaferri F. Gut microbiota, intestinal permeability, and systemic inflammation: a narrative review. Intern Emerg Med. (2024) 19:275–93. doi: 10.1007/s11739–023-03374-w
72. Hofford RS, Kiraly DD. Clinical and preclinical evidence for gut microbiome mechanisms inSubstance use disorders. Biol Psychiatry. (2024) 95:329–38. doi: 10.1016/j.biopsych.2023.08.004
73. Gillette JS, Wang EJ, Dowd RS, Toms SA. Barriers to overcoming immunotherapy resistance in glioblastoma. Front Med (Lausanne). (2023) 10:1175507. doi: 10.3389/fmed.2023.1175507
74. Gori S, Inno A, Belluomini L, Bocus P, Bisoffi Z, Russo A, et al. Gut microbiota and cancer: How gut microbiota modulates activity, efficacy and toxicity of antitu-moral therapy. Crit Rev Oncol Hematol. (2019) 143:139–47. doi: 10.1016/j.critrevonc.2019.09.003
75. Zhu Y, Jian X, Chen S, An G, Jiang D, Yang Q, et al. Targeting gut microbial nitrogen recycling and cellular uptake of ammonium to improve bortezomib resistance in multiple myeloma. Cell Metab. (2024) 36:159–175.e8. doi: 10.1016/j.cmet.2023.11.019
76. Hou X, Du H, Deng Y, Wang H, Liu J, Qiao J, et al. Gut microbiota mediated the individualized efficacy of Temozolomide via immunomodulation in glioma. J Transl Med. (2023) 21:198. doi: 10.1186/s12967–023-04042–5
77. Rosito M, Maqbool J, Reccagni A, Giampaoli O, Sciubba F, Antonangeli F, et al. Antibiotics treatment promotes vasculogenesis in the brain of glioma-bearing mice. Cell Death Dis. (2024) 15:210. doi: 10.1038/s41419–024-06578-w
Keywords: glioblastoma, gut microbiota, immunotherapy, immune checkpoint inhibitor, gut-brain axis
Citation: Zhang H, Hong Y, Wu T, Ben E, Li S, Hu L and Xie T (2024) Role of gut microbiota in regulating immune checkpoint inhibitor therapy for glioblastoma. Front. Immunol. 15:1401967. doi: 10.3389/fimmu.2024.1401967
Received: 16 March 2024; Accepted: 28 May 2024;
Published: 10 June 2024.
Edited by:
Mingwei Zhang, First Affiliated Hospital of Fujian Medical University, ChinaReviewed by:
Xiang Hong, Southeast University, ChinaPing Zheng, Shanghai Pudong New Area People’s Hospital, China
Dingkang Xu, Chinese Academy of Medical Sciences and Peking Union Medical College, China
Copyright © 2024 Zhang, Hong, Wu, Ben, Li, Hu and Xie. This is an open-access article distributed under the terms of the Creative Commons Attribution License (CC BY). The use, distribution or reproduction in other forums is permitted, provided the original author(s) and the copyright owner(s) are credited and that the original publication in this journal is cited, in accordance with accepted academic practice. No use, distribution or reproduction is permitted which does not comply with these terms.
*Correspondence: Tao Xie, MjU0ODA5NTQ1QHFxLmNvbQ==; Eyi Ben, MzI5NDM5NTkyMUBxcS5jb20=; Shuai Li, enp1ZG9jbGlzaHVhaUAxNjMuY29t; Liu Hu, MzEzNjY0MDEzQHFxLmNvbQ==
†These authors have contributed equally to this work and share first authorship