- 1Urology Department, Hospital Universitario La Princesa, Madrid, Spain
- 2Immunology Department, Hospital Universitario La Princesa, Madrid, Spain
- 3Personalized Precision Medicine Chair, Universidad Autónoma de Madrid, Madrid, Spain
- 4Medical Oncology Department, Hospital Universitario Clínico San Carlos, Madrid, Spain
- 5Medical Oncology Department, Hospital Universitario 12 de Octubre, Madrid, Spain
- 6Medical Oncology Department, Hospital Universitario La Princesa, Madrid, Spain
- 7GU Translational Research Unit, Instituto de Investigación Sanitaria de la Princesa, Madrid, Spain
- 8Radiation Oncology Department, Hospital Universitario La Princesa, Madrid, Spain
- 9Medical Oncology Department, Clínica Universitaria de Navarra, Madrid, Spain
- 10Biocomputing Unit, Hospital Niño Jesús, Instituto de Investigación Sanitaria de la Princesa, Madrid, Spain
Tumor immune microenvironment (TIME) plays a key role to understand how tumors respond to prostate cancer (PC) therapies and potential mechanisms of resistance. Previous research has suggested that specific genomic aberrations, such as microsatellite instability (MSI) or CDK12 bi-allelic loss can allow PC patients more likely to respond to immune checkpoint inhibitors (ICI) or other immune therapies. However, responses to these treatments remain highly variable even in selected patients. Thus, it is essential to obtain more information about tumor immune cells that infiltrate these tumors, and on their plasticity and interactions, in order to better understand the underlying biology to allow development of new therapeutic strategies. This review analyzes: 1) How interactions among immune cell populations and other cells infiltrating the tumor stroma can modulate the progression of PC, 2) How the standard therapies to treat PC (such as androgen deprivation therapy, new androgen-directed hormone therapy or chemotherapy) may influence the dynamic changes of the immunome and 3) What are the limitations in characterizing the immune landscape of the host´s response to tumors.
1 Introduction
Prostate cancer (PC) is a heterogeneous malignancy and it is considered an immunologically “cold” tumor, unable of generating effective T-cell responses (1). It has been characterized by decreased number of cytotoxic cells and high density of regulatory T-cells (Tregs) that facilitate immune evasion. The number and proportions of immune cells with tumor immunity function, such as CD8+ T cells, NK or monocytes have been found significantly smaller in lymphatic metastases than in primary lesions of PC, implying immunosuppression in the tumor microenvironment (TME) of PC (2).
This immunosuppressive effect of PC cells may explain in part the poor results obtained by immunotherapy in advanced PC (3, 4). Peripheral blood myeloid expansion, indicated by a high neutrophil-to-lymphocyte ratio, has been associated with shorter survival (5). However, the identification of specific populations involved in immune response against the tumor, and their respective role and cell-cell interactions remain unclear. Below, we describe the immune microenvironment that characterizes PC (Figure 1, Table 1).
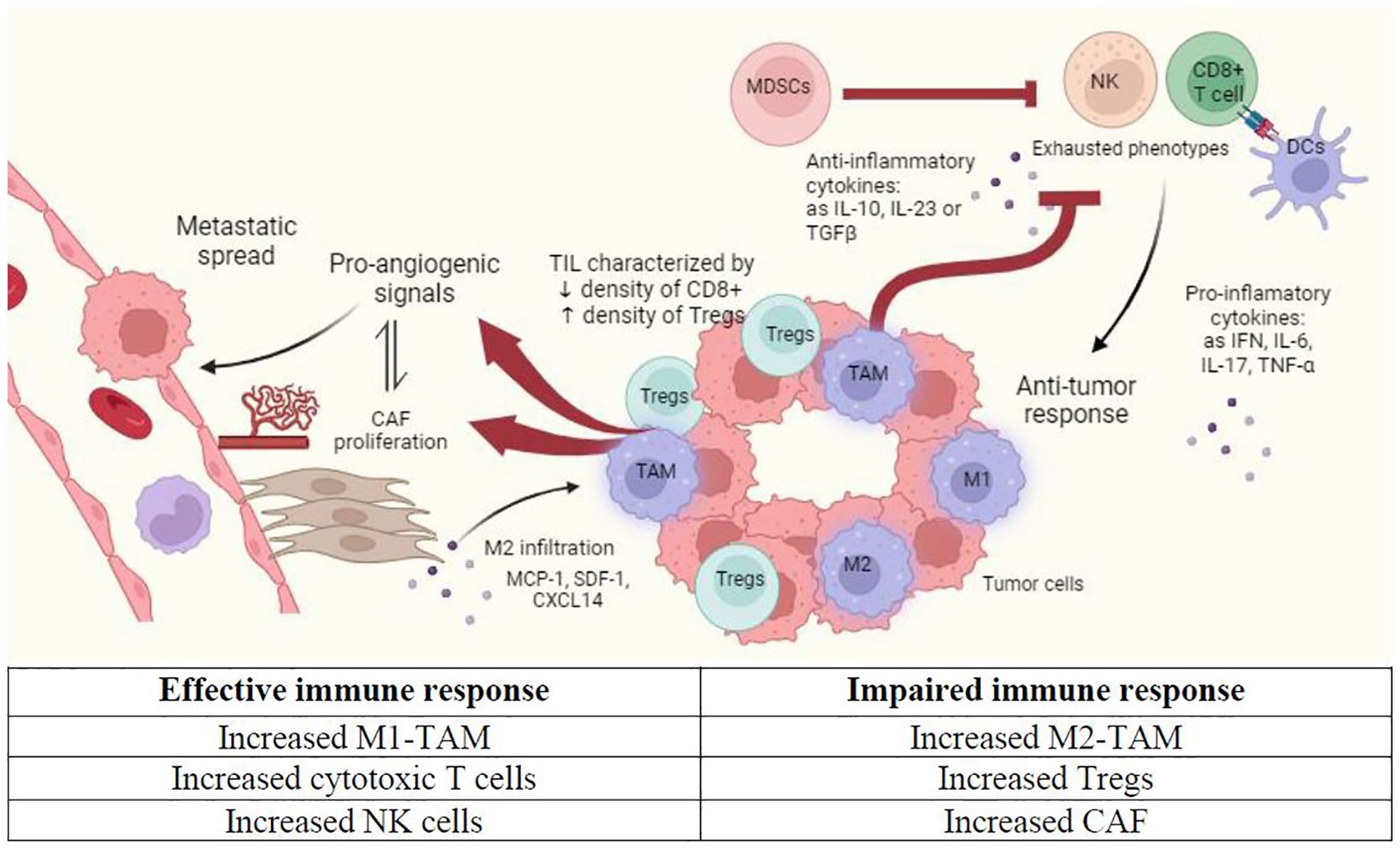
Figure 1. Immune microenvironment in prostate cancer (PC). This figure shows the main features that characterize tumor immune microenvironment in primary PC niche. CAF, Cancer-associated fibroblasts; DC, Dendritic cell; M1, Classically activated macrophages; M2, Alternatively activated macrophages; MDSC, Myeloid-derived suppressor cells; NK, Natural killer cell; TIL, Tumor infiltrating lymphocytes. Created with Biorender.com.
2 Immune phenotype in response to prostate cancer
An immune suppressive tumor microenvironment associated with suppressed myeloid populations and exhausted T-cell has been described (6). Despite detailed characterization of the epithelial and tumor cells, the immune phenotype in different clinical stages of PC is not well known.
2.1 Monocytes and tumor-associated macrophages
CD68+ tumor-associated macrophages (TAM) are the most abundant cell type in the TME of PC (7). Macrophages are a plastic immune population that can take on a wide range of phenotypes, ranging from anti-tumorigenic (M1, classically activated) to pro-tumorigenic (M2, alternatively activated). This fact makes identifying them correctly challenging. Recent studies based on RNA sequencing have revealed a third subpopulation annotated as monocyte-macrophage that suggests a transitional state from monocytes toward macrophages (6).
Classical M1 TAM carry out pathogen clearance by secreting pro-inflammatory factors such as IL-1β, IL-12, IFN-γ and TNF-α. They are characterized by higher levels of reactive oxygen species (ROS), inducible nitric oxide synthase 2 (iNOS), and major histocompatibility complex (MHC) class II. Conversely, M2 TAM are increased in the context of hypoxia, and high levels of IL-6 or IL-4, and these cells express autocrine factors such as TGF-β and IL-10 to promote their own maturation. Non-coding RNA and several transcription factors including interferon (IFN) regulatory factors and c-Myc, have been shown in M2 activation (8).
Macrophages depend on stimulation by different cytokines, hormones such as glucocorticoids, signaling extracellular vesicles, and extracellular matrix components (9). A prominent cytokine known to regulate macrophage differentiation and proliferation is the macrophage colony-stimulating factor (M-CSF or CSF1), which has been proposed as a therapeutic target (10). Other chemokines, like the granulocyte colony-stimulating factor (G-CSF) and chemokine ligand 2 (CCL-2), whether secreted from tumor or stromal host cells, are associated with TAM recruitment. Recently, macrophage receptor CXCR2 has been proposed as a major driver of TAM polarization, and a new therapeutic target in PC (7, 11, 12). In a feedback loop, macrophages also contribute to overproduction of chemokines (9).
M2 macrophages are thought to be related to cancer-associated fibroblasts (CAF). CAF are active recruiters of monocytes to tumor cells. They act through stromal-derived growth factor-1 secretion and promote trans-differentiation to M2. TAM and CAF cooperate in increasing tumor cell motility, to favor the escape of cancer cells from the primary site and their metastatic spread. In addition, TAM and CAF promote activation of endothelial cells and their bone marrow-derived precursors in de novo angiogenesis (13). CAF induce inflammation and stimulate macrophage infiltration via secretion of cytokines such as monocyte chemotactic protein-1 (MCP-1), stromal-derived growth factor-1 (SDF-1), CXCL14 and CCR-2. Clinical studies have shown the importance of CAF in biochemical recurrence and metastasis for patients undergoing radiotherapy or radical prostatectomy (14).
In peripheral blood, elevated monocyte counts have been correlated with aggressive tumor features and poor survival in patients with castration resistant prostate cancer (CRPC). However, studies in early stages have not found any association between monocyte counts and biochemical recurrence, CRPC or PC-specific mortality (15–17).
2.2 Tumor-infiltrating lymphocytes
Tumor-infiltrating lymphocytes (TIL) are key mediators of anti-tumor immune response given their ability to recognize peptides/MHC class I complexes through T-cell receptors (TCRs). The presence of cytotoxic and T helper cells within tumor margins has been associated with favorable prognosis across multiple cancer types (18).
However, in PC, an increase in T-cell infiltration has been frequently correlated with poor prognosis and shorter time to biochemical recurrence after radical prostatectomy (19, 20). This may be attributed to PC showing reduced lymphocyte infiltration, especially of the CD8+ cytotoxic subset, due to the hypoxic environment. In addition, CD8+ cells may have impaired cytotoxic responses despite tumor antigen stimulation (20). These so-called “exhausted” T-cells (Tex) are characterized by increased expression of coinhibitory receptors, the best known of which is PD-1, but also LAG3, TIM3, and TIGIT (21, 22).
Preclinical studies have shown that Tregs can suppress anti-tumor responses, which are directly related to an increased risk of cancer recurrence (23). Preliminary data suggest that the presence of other receptors such as CCR4 on Tregs may impact PC patient survival, although further research is required (24).
Recent studies based on RNA sequencing have described up to four CD4+ T-cell subpopulations (Th1, Th17, Tregs and Tnaïve) and three CD8+ T-cell subpopulations(CTL-1, CTL-2 and CD-8 effector) based on functional scores (6). These differences in the classification and the functional role of each TIL subtype are important to consider in future studies on immunome in PC.
2.3 Natural killer cells
Prostate tissue is generally poorly infiltrated by natural killer (NK) cells. Elevated NK cell expression in prostate tumors is associated with a lower risk of disease recurrence, as measured by biochemical recurrence after radical prostatectomy (25, 26).
NK cells infiltrating prostate tissues display unexpected immature features with decreased expression of activating receptors such as NKG2D and membrane proteins such as CD16, which are involved in antibody-dependent cellular cytotoxicity. The presence of this phenotype may be mediated by the action of TGFβ1 and PGE2, that would lead to the expression of immature NK cells with low cytotoxic potential and a hyposensitive functional status against target cells (27).
Four NK subpopulations have been described based on gene expression: NKT, CD56dim, CD56bright IL7R+ and CD56bright IL7R-. NKT cells are characterized by high expression of T-cell marker genes. CD56dim NK cells have high expression of HAVCR2. CD56bright NK cells are segregated in two subtypes depending on IL7R expression and the homing-receptor SELL (CD62L). Of the NK subpopulations, CD56dim cells score highest for exhaustion and are in higher abundance in PC compared to benign prostate samples (6).
In peripheral blood, detection of NK cells may play a role in early diagnosis. Barkin et al. observed in a small cohort (n=43) that patients with low levels of NK cells were more likely to have a positive prostate biopsy result (28). Recently, PC peripheral NK cells have been linked to enhanced CD9, CD49a, CXCR4, CXCL8, MMP-9 production, monocyte-recruiting and polarizing factors (29).
2.4 Myeloid-derived suppressor cells
Myeloid-derived suppressor cells (MDSC) are pathologically activated neutrophils and monocytes with potent immunosuppressive activity. They are classified as polymorphonuclear (PMN-MDSC: CD14+) or monocytic (M-MDSC: CD15+) and they can allow immune system evasion by inhibiting T-cell, B-cell and NK-cell mediated immune responses (30).
Higher levels of circulating MDSC have been linked to a worse overall survival in PC, and therefore the detection of this cell type has attracted a lot of attention (31). Previous studies have shown, using PC mouse models, that intratumor myeloid cells can drive paracrine oncogenic signaling, senescence evasion, and immunosuppression (7, 11, 32). High levels of PMN-MDSC in metastasis have been associated with higher levels of MDSC-recruiting chemokines in those areas, such as CXCL5/CXCR2 signaling (33). CXCR2 has been proposed as a new therapeutic target. A proof-of-concept trial with CXCR2 inhibitors has shown positive results with durable clinical benefits in terms of biochemical and radiological responses in patients with metastatic CRPC (12).
The inflammatory cytokine IL-23 produced by MDSC has been recently linked to CRPC development, since it induces the transcription of AR target genes through STAT3 transcription factor, leading to the proliferation of cancer cells and tumor survival (34). Thus, the IL-23 and STAT3 pathways have been proposed as new therapeutic targets in this setting (35, 36).
In bone metastasis, the chemokine CCL20 is overexpressed by myeloid cells, as is its CCR6 receptor on T cells. Disruption of CCL20-CCR6 axis in preclinical models restores T cell reactivity and improves survival, making it another attractive drug target linked to MDSC (37).
2.5 Dendritic cells
Dendritic cells (DC) are a heterogeneous group of antigen-presenting cells that can be classified into two basic subtypes: plasmacytoid DC, which accumulate in blood and lymphoid tissue, and classical DC, which infiltrate lymphoid and nonlymphoid tissues. However, plasmocytoid and classical DC markers can change in certain situations, such as inflammation or infection, making their identification more difficult (38).
DCs have a high capacity to induce and stimulate T-cell responses and improve the cytotoxic potential of NK cells, thus contributing to the elimination of tumor cells. For that reason, they have been considered a notable therapeutic target for the development of vaccines using autologous DC-based immunotherapy (39, 40). Sipuleucel-T, a DC based immunotherapy, improved overall survival in PC (39). Despite this initial success, there was controversy around a particularly unfavorable outcome of the control arm. Furthermore, subsequent DC vaccines have failed to produce significant benefit, and the utility of this strategy remains unproven.
2.6 Soluble immune-related factors in the tumor microenvironment
Multitude of soluble immune-related factors are secreted by immune cells within TME, as well as by the tumor itself, contributing to a complex landscape. Krueger et al. proposed that mesenchymal stem cells found in radical prostatectomy specimens may promote tumor progression by regulating the immunosuppressive microenvironment, suppressing T-cell proliferation and secreting soluble factors (TGFβ, IL-6, IL-10, IDO, among others) (41).
Nakashima et al. found that serum IL-6 correlated significantly with the clinical stage of PC (42). High expression of TGF-β and IL-6 in tissue has been also associated with poor prognosis (43). IL-10 has anti-inflammatory functions and supports tumor progression by limiting efficient antitumor response. It has been found to be related to Gleason score and considered a poor prognostic factor (44).
Different levels of TNF-α and IFN-γ have been associated with PC progression. High levels of TNF-α lead to tumor cell necrosis and apoptosis, contributing to immune cytotoxicity and release of other inflammatory cytokines. Paradoxically, a low dose paracrine TNF-α production in tumor areas may contribute to chronic inflammation and cancer progression. PC cells have been shown to be poorly sensitive to effects of IFN-γ. Furthermore, it has been suggested that IFN-γ could induce immunosuppressive effects in PC (45). Indoleamine 2,3-dioxygenase (IDO) is a tryptophan-depleting enzyme that has been extensively investigated due to its role in inhibiting the expansion of T-cells, suppressing adaptive immune responses. Evidence has shown that IDO and the tryptophan pathway are linked to immune tolerance (46). More recently, IDO has been shown to induce resistance to treatment with ICI, upon stimulation by pro-inflammatory cytokines, mainly IFN-γ (45).
3 Immunome response after prostate cancer therapy
The following section describes how the immune landscape evolves after the most common treatments for PC (Table 2).
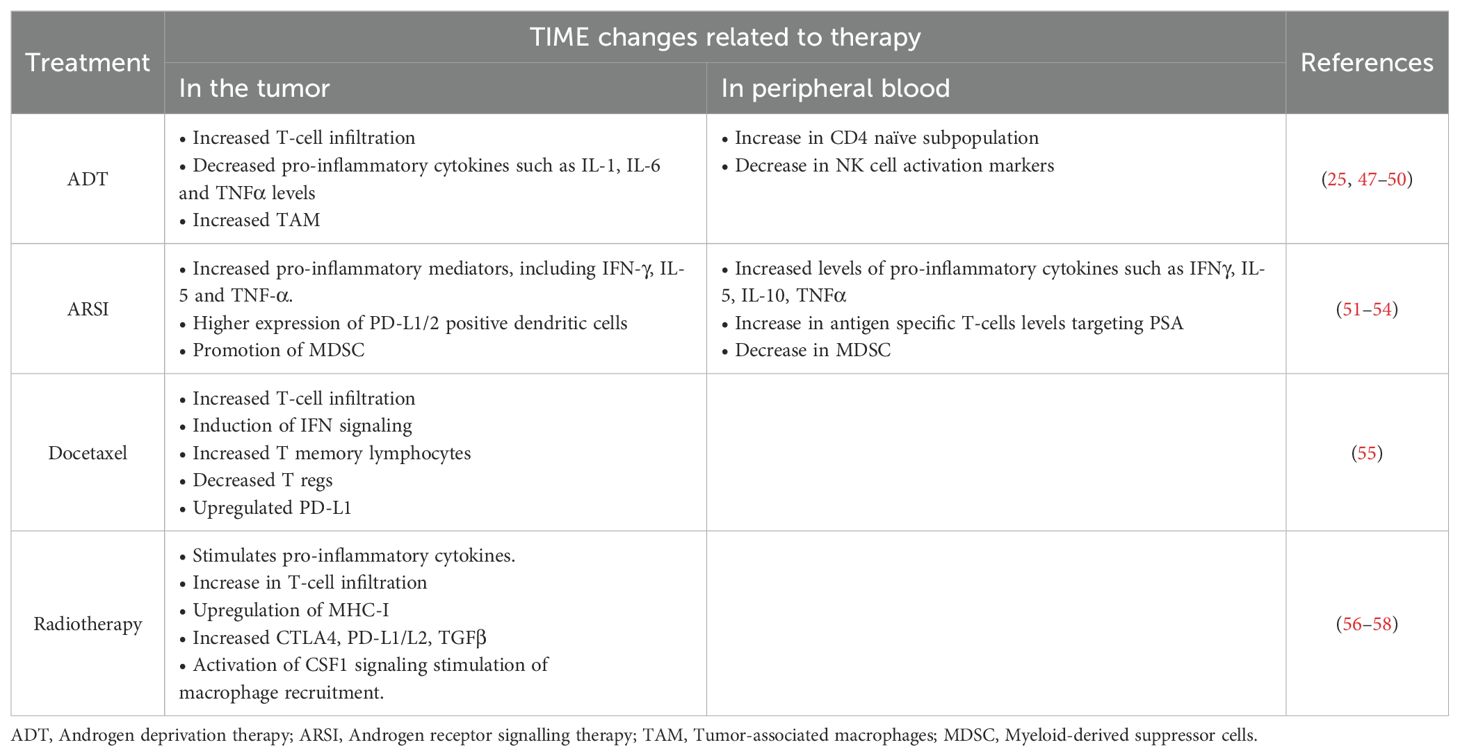
Table 2. Summary of evidence reporting changes in TIME with standard therapies used in advanced prostate cancer.
3.1 Androgen deprivation therapy
Androgen inhibition has been shown to result in thymic volume regeneration and enhanced T-cell lymphocyte production (59). In vivo studies have also linked ADT to a proliferative response to antigen-specific T-cell stimulation, faster lymphocyte recovery following chemotherapy, and T-cell infiltration into the prostate (60, 61). Under ADT, a prospective cohort of 20 patients showed an increase in the naïve CD4 subpopulation (CD45RO-/CCR7+) in peripheral blood after therapy. The other subpopulations (CD4+ and CD8+ effector, central memory, or effector memory) remained relatively stable over time. It suggests that ADT not only affects the frequency of T-cell subsets, but also their responsiveness to proliferation through co-stimulatory molecules (47). Other studies have also described how ADT induces CD4+ and CD8+ T-cell infiltration in PC compared to normal prostate tissue, which could be detected within the first month of treatment (48).
Although this may suggest a better response to immunotherapy in patients treated with ADT, it has been refuted by later studies. Furthermore, a decrease in inflammatory cytokines such as IL-1 IL-6, IL-8 and TNFα levels after initiation of ADT has been identified, with no effect on IFN levels (49, 50). These changes could potentially facilitate tumor growth by immune system suppression (62). An increase of CD68+ macrophages within the tumor has also been reported (26).
NK cell markers have been linked to prognosis in patients undergoing ADT (25). NK cells from metastatic PC patients with longer response to castration display phenotypic and functional patterns associated with high expression of activating receptors and molecules involved in NK cell maturation and degranulation.
In terms of progression to castration resistance, a key role for macrophages is emerging. El-Kenawi et al. demonstrated in a mouse PC model how macrophages took up cholesterol in the form of low-density lipoprotein and transported it to the cancer cells to support the synthesis of androgens. Macrophages also seemed to be able to stimulate AR translocation to the nucleus. Furthermore, they demonstrated that macrophage density correlated with ADT resistance and that macrophage depletion in mice (using a CSF1 antibody) reduced both tumor androgen levels and various surrogates of AR activation, as well as better outcome after ADT (63). This mechanism has been the basis for statin trials in PC patients under ADT.
3.2 Androgen receptor signalling inhibitors
Metastatic CRPC patients treated with abiraterone or enzalutamide have shown significantly lower levels of fibroblast growth factor, granulocyte-macrophage colony-stimulating factor (GM-CSF), IL-10 and IL-6 in plasma in ADT + ARSI sensitive compared to de novo resistant patients (51). Increased levels of IFNγ, IL-5, IL-10, TNFα and the chemokine macrophage inflammatory protein 1 alpha (MIP-1a)/CCL3 at week 8 after ARSI initiation suggest potential activation of T-cell-mediated immune responses to abiraterone/enzalutamide. The authors hypothesized that these immune markers could predict outcomes in patients treated with ARSIs.
Furthermore, in vivo studies showed how enzalutamide promotes MDSC-mediated immune suppression and tumor growth, leading to metabolism changes through the MPC-2 and MAPK pathways. This may be based on decreased mitochondrial respiration and increased glycolytic rate, showing the dynamic interactions between tumor, stroma, and immune cells. Therefore, it suggests a mechanism of ARSI resistance based on enhancement of myeloid tumor-promoting activity (52).
More recently, Madan et al. presented their results regarding the immunologic impact of enzalutamide in non-metastatic hormone sensitive PC patients. The study involved 38 patients treated with short-course enzalutamide, and found a rise in antigen specific T-cell levels targeting PSA and NK cells, plus a decrease in MDSC in blood (53). However, no significant association was found between clinical responses and immune changes, perhaps due to the small number of patients studied.
In metastatic CRPC treated by enzalutamide, a higher expression of PD-L1/2+ DC was identified compared with treatment-naïve or responder patients. This was suggested as a non-AR driven hyphen mechanism of resistance by the authors (54). In addition, PD-L1+ circulating tumor cells are more frequent in metastatic CRPC patients progressing to ARSI than those starting ARSI. This study also addressed heterogeneity in the frequency of immune related biomarkers such as PD-L1/L2, CTLA-4, and B7-H3, which could potentially account for the poor outcomes with ICI in metastatic unselected PC patients (64).
Macrophages have also been shown to be involved in resistance to ARSI. In metastatic CRPC patients, abiraterone- or enzalutamide-sensitive patients exhibited increased pro-inflammatory mediators, including IFN-γ, IL-5, and TNF-α, which were generally identified as M1 markers (56). Studies in PC models have shown how macrophages directly regulated AR nuclear translocation and resistance to enzalutamide (63). Inhibitors of CSF1 signaling have been tested in combination with abiraterone demonstrating that TAM blockade in this setting disrupted tumor promotion and maintained a more durable therapeutic response compared to abiraterone alone (10).
In peripheral whole blood, RNA deconvolution analysis from metastatic CRPC patients treated with enzalutamide (n=226) revealed that progression to this therapy was correlated with expansion of monocytes and contraction of CD8 lymphocytes (65).
3.3 Chemotherapy
Docetaxel regulates several immune-related pathways. T-cell (IFNγ and TNFα gene sets), B-cell, and NK cell mediated immunity are strengthened after docetaxel therapy, with an increase in CD8+, CD3+, and CD4+ T-cells, and a decrease in regulatory T-cells, mainly through the cGAS/STING-IFN pathway. Docetaxel-based chemohormonal therapy upregulated PD-L1 (55).
Baseline neutrophil-to-lymphocyte ratio and the systemic immune-inflammation index has proven to be related with worse prognosis in patients with mCRPC treated with docetaxel (15, 66, 67).
Preclinical studies have shown that chemoresistance to docetaxel is mediated by CCL5 cytokine secreted by CD4+, which can increase the aggressive potential and stem cell populations of PC. Also, this could potentially activate the PI3K/Akt and STAT3 pathways, which could be related to aggressiveness and cell migration (68). Baseline IL-6 levels have been inversely correlated with response, time-to-progression, and overall survival in docetaxel-treated patients (69). However, clinical attempts to demonstrate the efficacy of IL-6 antibodies in this setting have failed (70).
Preclinical studies have found that TAM promoted the survival of PC cells after docetaxel via the CSF1/CSF1R-CXCL12/CXCR4 axis in CRPC. The combination therapy of docetaxel and CSF1 inhibitors is currently under study (71). Regarding cabazitaxel, the CCL2-CCR2 axis has been found to be a key contributor to cabazitaxel resistance in CRPC (72).
Clinical studies have shown the emergence of polyaneuploid cancer cells, able to resist stress within the TME, as a survival strategy for the PC population. These cells correlate with poor response to docetaxel chemotherapy in the context of CRPC (73).
3.4 Radiotherapy
Radiotherapy (RT) has been shown to initiate a pro-inflammatory cascade and to increase systemic response rates to immunotherapy. Abscopal effect, although anecdotal, has been described in patients treated with RT and ICI (74). High-dose RT stimulates pro-inflammatory cytokines, such as TNFα, IL-1, IL-6, and IL-8, increasing the presence of activated T-cells; upregulation of MHC class I molecules to facilitate antigen presentation; and the presence of effector cells through the production of chemotactic chemokines and the upregulation of vascular adhesion molecules (75). Low-dose RT, additionally, can overcome immune desert tumors by increasing T-cell infiltration in TME (57).
In a series of 48 localized PC patients, several immune cell populations (Th1-cells, B-lymphocytes, CD8+ T-cells, Tregs, and TAM) were measured in tissue samples before and after treatment (with ADT or RT monotherapy or both). Overall, an increase in at least 3 over 5 of the immune populations were documented in all three arms. RT alone increased levels of Th1, B-cells or Tregs whereas RT in combination with neaoadjuvant ADT increased all subsets (76).
Keam et al. analyzed the effects of high dose brachytherapy on immune response mediators in localized PC (n=24). They found that many immune checkpoint molecules, such as CTLA-4, PD-L1 and PD-L2, as well as TGFβ levels, were increased in response to radiation, thus turning an immunologically “cold” tumor into a “hot” tumor, which may translate into better response to immunotherapy (58).
Clinical and preclinical studies have suggested that local irradiation induces ABL1-dependent CSF1 production, followed by activation of CSF1/CSF1R signaling leading to systemic macrophage recruitment (56).
3.5 Response to immune therapies
Only 10–30% of unselected patients respond to PD-1/PD-L1 blockade. However, it has been reported that those patients with MSI, around 3% of total PC, correlate with high tumor mutation burden, and subsequently, responses under ICI. This data led to an agnostic approval for MSI cancer patients. Interestingly, it has been suggested that in some of those patients, the MSI phenotype could be acquired by dynamic changes in TME and response to previous therapies (77). Delving into the underlying mechanisms could be crucial to improve current results of IT in PC.
There are no other predictive biomarkers for ICI in PC. The expression of PD-1 in lymphocytes is highly prevalent in PC compared with benign tissues and it has been associated with Gleason score. However, it has not been proven to be useful as a marker to select patients who would respond to ICI and its independent role in PC survival is yet to be validated (19, 78).
Combinations with anti-CTLA-4, chemotherapy, ARSIs, radionuclides and PARP inhibitors have been explored in phase I-III trials with negative results. Presence of androgen receptor splice variant 7 and alterations in DNA repair genes showed higher response rates, but this was not translated into better survival (62). Studies with (177)Lu-PSMA-617 and ICI are ongoing, based on immune-modulation of this drug and promising results of phase I trials (79). Loss of PTEN tumor suppressor is associated with poorly T cell-infiltrated tumors and resistance to anti-PD-1 immunotherapy, being present in 40-50% of primary and 70-90% of metastatic PC. Preclinical studies with intermittent PI3K inhibitors have shown promising results to overcome resistance to ICI (80).
Accumulated studies confirm that hypoxia potently induces HIF-1α-dependent PD-L1 expression on tumor cells, suggesting that PD-L1 expression can be upregulated in hypoxic tumor cells to promote immune escape from cytotoxic T cells. It has been suggested that co-blockade of PD-L1 and HIF-1α signaling might represent a promising approach to enhance the activity of cytotoxic T cells (81). However, combination of antiangiogenesis and ICI has been tested in phase III trials with differences in progression-free survival but no impact in OS (82).
Chimeric antigen receptor T cells (CAR-T) therapies targeting PSMA to re-direct the action of T cells have shown promising efficacy in preclinical studies, with Phase I-II trials ongoing (83). Bi-Specific T cell enhancers (BITE) bind simultaneously to immune cell markers (such as CD3 for T-cells or CD16 for NKs) and tumor-associated antigens to re-direct and potentiate the immune response. BITE immunotherapies targeting PSMA are currently being studied in early-stage clinical trials. Combination of ICI and BITE has shown preliminary efficacy in metastatic CRPC patients (84). Identifying the optimal moment to act on the immunome at each stage of the disease and in response to previous treatments may be crucial for the success of these new therapies.
4 Limitations to study TIME in prostate cancer
4.1 Main barriers
PC heterogeneity remains a challenge up to date, and currently we know that in addition to genetic factors, this diversity is derived also from the environmental landscape including fibroblast recruitment, immune cells migration, matrix remodeling, angiogenesis role and epigenetic influence (81). Moreover, TIME is dynamic with spatial and temporal changes in response to anticancer therapies. In fact, the continuing crosstalk between tumor cells and TME is fundamental for cancer progression, including therapeutic resistance. To capture this variability is one of the more important barriers when studying TIME in solid tumors. It may be improved if technical approaches to study immune response in peripheral blood are implemented, but validation and correlation with TME scenario in the primary tumor and metastasis is needed first. Moreover, to identify rare immune subsets is still challenging, and may lead to bias in data interpretation.
Recent studies have also provided a new perspective to understand antitumor immunity, which can be initiated directly at the tumor site or metastasis in spatially well-organized areas of infiltrative immune cell aggregates called tertiary lymphoid structures. These ectopic lymphoid structures have been correlated with prognosis in different tumors, with the potential of being modulated by different therapeutic strategies (85).
Beyond tumor heterogeneity, we have to consider the heterogeneity between individuals, since the immune response will depend on the characteristics of our body, such as age or the pre-existence of systemic inflammatory processes. Thus, studies published with matched-control normal biopsies that include a small number of controls may not be representative of the heterogeneity among healthy individuals (6, 86). Moreover, there is a lack of data about the immune environment in normal prostate tissue or pre-neoplastic lesions. All these factors should be included for PC TIME study design.
Another notable barrier to describe TIME is clonal heterogeneity, as PC is a multifocal disease and each tumor focus might have different phenotype. The impact of TIME on cancer cells is characterized by the regional heterogeneity in hypoxia, acidity and cytokines within the tumor environment. Intriguingly, many type of cells (such as CAF or TAM) possess tumor suppressive effects or tumor promoting effects depending on the dynamic interaction into the TME.
Different attempts to classify patients into different subtypes has been performed, but their utility in clinical practice remains controversial because they do not correlate precisely with response to standard therapy (87, 88). Recently, Weiner et al. published a molecular classification using gene expression, TIL data based on immunohistochemistry (IHC) and clinical characteristics, and they found 4 clusters: luminal differentiated, luminal proliferating, basal immune, and basal neuroendocrine. Tumors with the basal immune subtype represented 35.1% of the total sample and they were characterized by a significant IFN activity and increased TIL. However, no specific targeted therapy has been described for this subgroup (89).
4.2 Technical approaches
Beyond the simplicity of the Immunoscore test, additional data about the spatial location of the infiltrate and the interactions between different cell types and stroma are needed to understand the complexity of PC TIME. New approaches are being developed such as optimized multiplexing panels for immunofluorescence and the use of machine learning models. These advances allow for a precise definition of relevant patterns in terms of spatial clustering of the intratumoral immune infiltrate, and the association with clinical parameters such as survival or response to treatment. However, the algorithms developed must be carefully validated in independent cohorts.
Regarding expression analysis, first studies using arrays showed that immune response plays a key role in advanced PC (90). Soon after that, next-generation sequencing (NGS) obtained a complete immune landscape from high-throughput genomic data. NGS allows for bulk populations to be analyzed using deconvolution algorithms in order to infer the cell types present in the sample (such as xCell or EPIC). These signatures-based method have been developed from knowledge of thousands of pure cell types from various sources (14, 91). NGS, however, relies on the prior existence of known gene expression profiles, and does not provide information on the degree of heterogeneity of the population.
Classically, strategies have been used to analyze data obtained with different techniques separately and integrate them at a later stage, which usually implies that the data come from different cells within the sample. But the ideal integration system should aim at simultaneous acquisition of data derived from the same cell, to try to explain the fluidity and heterogeneity of the TIME in PC. For that reason, strategies based on integrated single-cell(sc) analysis are promising in this area (92).
RNA levels, however, do not always correspond directly with the presence of the protein, and can therefore provide misleading information about gene expression levels. Techniques such as CITE-Seq have been developed that allow for simultaneous detection of mRNA and protein levels in each cell, combining specific oligonucleotide-labeled antibodies against surface proteins with transcriptomic analysis.
At a transcriptional level, Han et al. have published the Tumor Immune Contexture Score (TICS), describing expression signatures related to immune microenvironment in patients with localized PC, that have shown a prognostic value of this RNA-based biomarker independent of TNM stage, PSA and Gleason score (93). A high TICS showed prolonged biochemical recurrence-free survival after radical prostatectomy. Previously, other authors had published RNA signatures in whole blood from patients with CRPC involving genes that suggested a possible dysregulation of the immune system. Immunogenic signatures have also been linked to identify the most lethal subtypes in PC (90, 94, 95).
Recently, an integrative analysis of TIME in metastatic CRPC has been published (n=100). Metastatic biopsies were analyzed using whole exome RNA sequencing, measuring tumor mutational burden and T-cell–inflamed gene expression profile score (Nanostring). IHC for PD-L1, ATM, PTEN, SOX2, and the presence of neuroendocrine features were also studied. The authors found that PD-L1, TcellinfGEP score, and SOX2 had prognostic value in this setting (96).
5 Discussion
The integration of the information obtained from different approaches (transcriptomic, genomic, epigenomic, proteomic, and topographic data) is of great relevance to obtain a complete picture of the landscape of the anti-tumor immunome (Figure 2) (97). Functional studies based on gene expression are currently indispensable to classify correctly different immune subpopulations and understand the dynamic changes in the TIME.
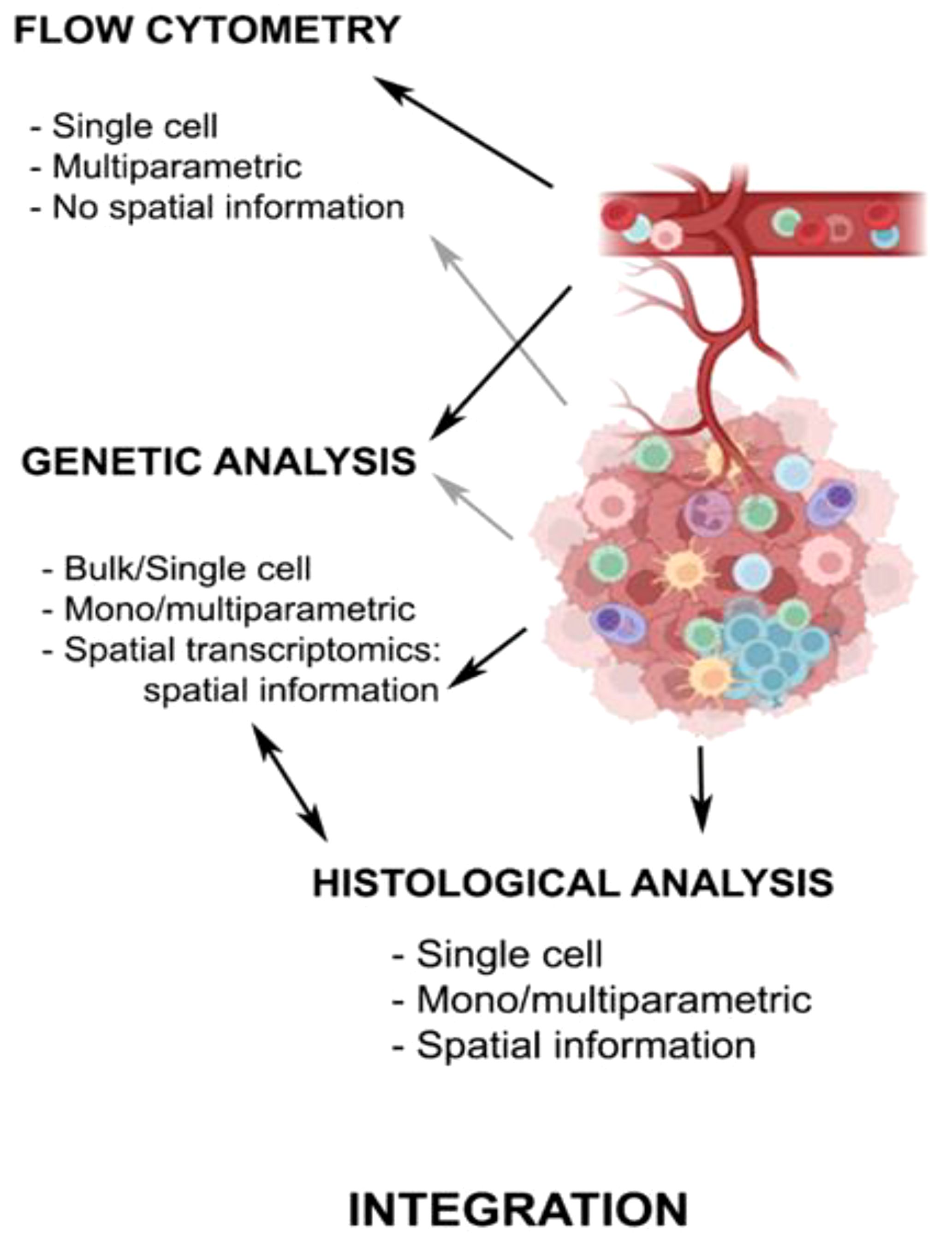
Figure 2. Technical approaches to analyze antitumor immune response. The chart summarizes the main characteristics of the techniques used in the study of the TIME in PC, with either peripheral blood or tumor samples. Created with Biorender.com.
Analysis of tumor samples from the selected small subgroup of PC patients who responded to anti PD-1/PD-L1 antibodies has revealed a specific phenotype with the presence of cytotoxic T-cell infiltrates, high PD-L1 receptor expression, and a higher mutational burden or MSI (98). However, the vast majority of PCs do not display these features. They show an immunosuppressive microenvironment mainly comprised of MDSC, TAM, and different soluble factors (TGF, IL-6/10/23) as main mediators of the downregulation of the innate immune response (41). This status frequently leads to immunological tolerance, a state of unresponsiveness of the immune system to the tumor. This could partly explain the lack of efficacy seen with ICI in molecularly unselected PC. Therefore, new strategies to overcome this dialed down turned-off immune system must be developed, with synthetic strategies based on BITE tested in clinical trials (99, 100).
In conclusion, the potential to discover new therapeutic approaches to improve PC outcomes relies in part on a better understanding of each step of the immune cycle, providing more accurate mechanistic insight (21). Many questions remain about how the immune system modulates, and how it can be modulated by the current standard therapies, thereby impacting PC progression. Therefore, further research integrating genomic, molecular, cellular, histologic and functional data is necessary to overcome these limitations.
Author contributions
LS-JM: Conceptualization, Writing – original draft, Writing – review & editing. AA: Conceptualization, Writing – original draft, Writing – review & editing. IM-P: Writing – original draft, Writing – review & editing. MR-V: Writing – original draft, Writing – review & editing. CV: Writing – original draft, Writing – review & editing. PT: Writing – original draft, Writing – review & editing. MP: Writing – original draft, Writing – review & editing. AZ: Writing – original draft, Writing – review & editing. DA: Writing – original draft, Writing – review & editing. GC: Writing – review & editing. EA: Writing – review & editing. M-DFM: Writing – review & editing. JG: Writing – review & editing. EC: Writing – review & editing. DO: Writing – review & editing. RC: Writing – review & editing. NR-L: Conceptualization, Formal analysis, Supervision, Validation, Writing – original draft, Writing – review & editing.
Funding
The author(s) declare financial support was received for the research, authorship, and/or publication of this article. NR-L team is supported by the CRIS Clinical Talent Programme 2022 from ‘Fundación CRIS contra el Cáncer’, Instituto Carlos III and Mutua Madrileña Foundation grants (PI21/01111, AP176822021).
Conflict of interest
The authors declare that the research was conducted in the absence of any commercial or financial relationships that could be construed as a potential conflict of interest.
Publisher’s note
All claims expressed in this article are solely those of the authors and do not necessarily represent those of their affiliated organizations, or those of the publisher, the editors and the reviewers. Any product that may be evaluated in this article, or claim that may be made by its manufacturer, is not guaranteed or endorsed by the publisher.
Abbreviations
ADT, androgen deprivation therapy; ARSI, androgen receptor signaling inhibitors; BITE, Bi-specific T cell enhancers; CAF, cancer-associated fibroblasts; CAR-T, Chimeric antigen receptor T cells; CCL-2, chemokine ligand 2; CRPC, castration resistance prostate cancer; DC, dendritic cells; G-CSF, granulocyte colony-stimulating factor; GM-CSF, granulocyte-macrophage colony-stimulating factor; ICI, immune checkpoint inhibitors; IDO, indoleamine 2,3-dioxygenase; IFN, interferon; IHC, immunohistochemistry; iNOS, inducible nitric oxide synthase; M1, classically activated macrophage (anti-tumorogenic); M2, alternatively activated macrophage (pro-tumorogenic); MCP-1, monocyte chemotactic protein-1; M-CSF or CSF1, macrophage colony-stimulating factor; MDSC, myeloid-derived suppressor cells; MHC, major histocompatibility complex; MIP-1a, macrophage inflammatory protein 1 alpha; M-MDSC, monocytic MDSC; MSI, microsatellite instability; NGS, next-generation sequencing; NK, natural killer; PC, prostate cancer; PMN-MDSC, polymorphonuclear MDSC; ROS, reactive oxygen species; RT, radiotherapy; SDF-1, stromal-derived growth factor-1; TAM, tumor-associated macrophages; TCR, T-cell receptor; Tex cell, “exhausted” T-cell; TICS, Tumor Immune Contexture Score; TIL, tumor-infiltrating lymphocytes; TIME, tumor immune microenvironment; TME, tumor microenvironment; Tregs, regulatory T-cells.
References
1. Melo CM, Vidotto T, Chaves LP, Lautert-Dutra W, Reis RBD, Squire JA. The role of somatic mutations on the immune response of the tumor microenvironment in prostate cancer. Int J Mol Sci. (2021) 22:9550. doi: 10.3390/ijms22179550
2. Xin S, Liu X, Li Z, Sun X, Wang R, Zhang Z, et al. ScRNA-seq revealed an immunosuppression state and tumor microenvironment heterogeneity related to lymph node metastasis in prostate cancer. Exp Hematol Oncol. (2023) 12:49. doi: 10.1186/s40164-023-00407-0
3. Beer TM, Kwon ED, Drake CG, Fizazi K, Logothetis C, Gravis G, et al. Randomized, double-blind, phase III trial of ipilimumab versus placebo in asymptomatic or minimally symptomatic patients with metastatic chemotherapy-naive castration-resistant prostate cancer. J Clin Oncol Off J Am Soc Clin Oncol. (2017) 35:40–7. doi: 10.1200/JCO.2016.69.1584
4. Antonarakis ES, Piulats JM, Gross-Goupil M, Goh J, Ojamaa K, Hoimes CJ, et al. Pembrolizumab for treatment-refractory metastatic castration-resistant prostate cancer: multicohort, open-label phase II KEYNOTE-199 study. J Clin Oncol Off J Am Soc Clin Oncol. (2020) 38:395–405. doi: 10.1200/JCO.19.01638
5. Templeton AJ, Pezaro C, Omlin A, McNamara MG, Leibowitz-Amit R, Vera-Badillo FE, et al. Simple prognostic score for metastatic castration-resistant prostate cancer with incorporation of neutrophil-to-lymphocyte ratio. Cancer. (2014) 120:3346–52. doi: 10.1002/cncr.v120.21
6. Hirz T, Mei S, Sarkar H, Kfoury Y, Wu S, Verhoeven BM, et al. Dissecting the immune suppressive human prostate tumor microenvironment via integrated single-cell and spatial transcriptomic analyses. Nat Commun. (2023) 14:663. doi: 10.1038/s41467-023-36325-2
7. Di Mitri D, Mirenda M, Vasilevska J, Calcinotto A, Delaleu N, Revandkar A, et al. Re-education of tumor-associated macrophages by CXCR2 blockade drives senescence and tumor inhibition in advanced prostate cancer. Cell Rep. (2019) 28:2156–2168.e5. doi: 10.1016/j.celrep.2019.07.068
8. Pello OM, De Pizzol M, Mirolo M, Soucek L, Zammataro L, Amabile A, et al. Role of c-MYC in alternative activation of human macrophages and tumor-associated macrophage biology. Blood. (2012) 119:411–21. doi: 10.1182/blood-2011-02-339911
9. Cassetta L, Fragkogianni S, Sims AH, Swierczak A, Forrester LM, Zhang H, et al. Human tumor-associated macrophage and monocyte transcriptional landscapes reveal cancer-specific reprogramming, biomarkers, and therapeutic targets. Cancer Cell. (2019) 35:588–602.e10. doi: 10.1016/j.ccell.2019.02.009
10. Escamilla J, Schokrpur S, Liu C, Priceman SJ, Moughon D, Jiang Z, et al. CSF1 receptor targeting in prostate cancer reverses macrophage-mediated resistance to androgen blockade therapy. Cancer Res. (2015) 75:950–62. doi: 10.1158/0008-5472.CAN-14-0992
11. Ruiz de Porras V, Wang XC, Palomero L, Marin-Aguilera M, Solé-Blanch C, Indacochea A, et al. Taxane-induced attenuation of the CXCR2/BCL-2 axis sensitizes prostate cancer to platinum-based treatment. Eur Urol. (2021) 79:722–33. doi: 10.1016/j.eururo.2020.10.001
12. Guo C, Sharp A, Gurel B, Crespo M, Figueiredo I, Jain S, et al. Targeting myeloid chemotaxis to reverse prostate cancer therapy resistance. Nature. (2023) 623:1053–61. doi: 10.1038/s41586-023-06696-z
13. Comito G, Giannoni E, Segura CP, Barcellos-de-Souza P, Raspollini MR, Baroni G, et al. Cancer-associated fibroblasts and M2-polarized macrophages synergize during prostate carcinoma progression. Oncogene. (2014) 33:2423–31. doi: 10.1038/onc.2013.191
14. Feng D, Xiong Q, Wei Q, Yang L. Cellular landscape of tumour microenvironment in prostate cancer. Immunology. (2023) 168:199–202. doi: 10.1111/imm.v168.2
15. Shigeta K, Kosaka T, Kitano S, Yasumizu Y, Miyazaki Y, Mizuno R, et al. High absolute monocyte count predicts poor clinical outcome in patients with castration-resistant prostate cancer treated with docetaxel chemotherapy. Ann Surg Oncol. (2016) 23:4115–22. doi: 10.1245/s10434-016-5354-5
16. Hayashi T, Fujita K, Tanigawa G, Kawashima A, Nagahara A, Ujike T, et al. Serum monocyte fraction of white blood cells is increased in patients with high Gleason score prostate cancer. Oncotarget. (2017) 8:35255–61. doi: 10.18632/oncotarget.13052
17. Yirga A, Oyekunle T, Howard LE, De Hoedt AM, Cooperberg MR, Kane CJ, et al. Monocyte counts and prostate cancer outcomes in white and black men: results from the SEARCH database. Cancer Causes Control CCC. (2021) 32:189–97. doi: 10.1007/s10552-020-01373-2
18. Fridman WH, Zitvogel L, Sautès-Fridman C, Kroemer G. The immune contexture in cancer prognosis and treatment. Nat Rev Clin Oncol. (2017) 14:717–34. doi: 10.1038/nrclinonc.2017.101
19. Petitprez F, Fossati N, Vano Y, Freschi M, Becht E, Lucianò R, et al. PD-L1 expression and CD8+ T-cell infiltrate are associated with clinical progression in patients with node-positive prostate cancer. Eur Urol. Focus. (2019) 5:192–6. doi: 10.1016/j.euf.2017.05.013
20. Ness N, Andersen S, Valkov A, Nordby Y, Donnem T, Al-Saad S, et al. Infiltration of CD8+ lymphocytes is an independent prognostic factor of biochemical failure-free survival in prostate cancer. Prostate. (2014) 74(14):1452–61. doi: 10.1002/pros.v74.14
21. Mellman I, Chen DS, Powles T, Turley SJ. The cancer-immunity cycle: Indication, genotype, and immunotype. Immunity. (2023) 56:2188–205. doi: 10.1016/j.immuni.2023.09.011
22. Wherry EJ, Kurachi M. Molecular and cellular insights into T cell exhaustion. Nat Rev Immunol. (2015) 15:486–99. doi: 10.1038/nri3862
23. Karpisheh V, Mousavi SM, Naghavi Sheykholeslami P, Fathi M, Mohammadpour Saray M, Aghebati-Maleki L, et al. The role of regulatory T cells in the pathogenesis and treatment of prostate cancer. Life Sci. (2021) 284:119132. doi: 10.1016/j.lfs.2021.119132
24. Watanabe M, Kanao K, Suzuki S, Muramatsu H, Morinaga S, Kajikawa K, et al. Increased infiltration of CCR4-positive regulatory T cells in prostate cancer tissue is associated with a poor prognosis. Prostate. (2019) 79:1658–65. doi: 10.1002/pros.v79.14
25. Pasero C, Gravis G, Granjeaud S, Guerin M, Thomassin-Piana J, Rocchi P, et al. Highly effective NK cells are associated with good prognosis in patients with metastatic prostate cancer. Oncotarget. (2015) 6:14360–73. doi: 10.18632/oncotarget.v6i16
26. Gannon PO, Poisson AO, Delvoye N, Lapointe R, Mes-Masson AM, Saad F. Characterization of the intra-prostatic immune cell infiltration in androgen-deprived prostate cancer patients. J Immunol Methods. (2009) 348:9–17. doi: 10.1016/j.jim.2009.06.004
27. Pasero C, Gravis G, Guerin M, Granjeaud S, Thomassin-Piana J, Rocchi P, et al. Inherent and tumor-driven immune tolerance in the prostate microenvironment impairs natural killer cell antitumor activity. Cancer Res. (2016) 76:2153–65. doi: 10.1158/0008-5472.CAN-15-1965
28. Barkin J, Rodriguez-Suarez R, Betito K. Association between natural killer cell activity and prostate cancer: a pilot study. Can J Urol. (2017) 24:8708–13.
29. Gallazzi M, Baci D, Mortara L, Bosi A, Buono G, Naselli A, et al. Prostate cancer peripheral blood NK cells show enhanced CD9, CD49a, CXCR4, CXCL8, MMP-9 production and secrete monocyte-recruiting and polarizing factors. Front Immunol. (2021) 11:586126. doi: 10.3389/fimmu.2020.586126
30. Veglia F, Sanseviero E, Gabrilovich DI. Myeloid-derived suppressor cells in the era of increasing myeloid cell diversity. Nat Rev Immunol. (2021) 21:485–98. doi: 10.1038/s41577-020-00490-y
31. Bronte G, Conteduca V, Landriscina M, Procopio AD. Circulating myeloid-derived suppressor cells and survival in prostate cancer patients: systematic review and meta-analysis. Prostate Cancer Prostatic Dis. (2023) 26:41–6. doi: 10.1038/s41391-022-00615-5
32. Garcia AJ, Ruscetti M, Arenzana TL, Tran LM, Bianci-Frias D, Sybert E, et al. Pten null prostate epithelium promotes localized myeloid-derived suppressor cell expansion and immune suppression during tumor initiation and progression. Mol Cell Biol. (2014) 34:2017–28. doi: 10.1128/MCB.00090-14
33. Wang G, Lu X, Dey P, Deng P, Wu CC, Jiang S, et al. Targeting YAP-dependent MDSC infiltration impairs tumor progression. Cancer Discovery. (2016) 6:80–95. doi: 10.1158/2159-8290.CD-15-0224
34. Calcinotto A, Spataro C, Zagato E, Di Mitri D, Gil V, Crespo M, et al. IL-23 secreted by myeloid cells drives castration-resistant prostate cancer. Nature. (2018) 559:363–9. doi: 10.1038/s41586-018-0266-0
35. Hossain DMS, Pal SK, Moreira D, Duttagupta P, Zhang Q, Won H, et al. TLR9-targeted STAT3 silencing abrogates immunosuppressive activity of myeloid-derived suppressor cells from prostate cancer patients. Clin Cancer Res Off J Am Assoc Cancer Res. (2015) 21:3771–82. doi: 10.1158/1078-0432.CCR-14-3145
36. Pal SK, Kortylewski M. Breaking bad habits: Targeting MDSCs to alleviate immunosuppression in prostate cancer. Oncoimmunology. (2015) 5(2):e1078060. doi: 10.1080/2162402X.2015.1078060
37. Kfoury Y, Baryawno N, Severe N, Mei S, Gustafsson K, Hirz T, et al. Human prostate cancer bone metastases have an actionable immunosuppressive microenvironment. Cancer Cell. (2021) 39:1464–1478.e8. doi: 10.1016/j.ccell.2021.09.005
38. Poltorak MP, Schraml BU. Fate mapping of dendritic cells. Front Immunol. (2015) 6:199. doi: 10.3389/fimmu.2015.00199
39. Kantoff PW, Higano CS, Shore ND, Berger ER, Small EJ, Penson DF, et al. Sipuleucel-T immunotherapy for castration-resistant prostate cancer. N Engl J Med. (2010) 363:411–22. doi: 10.1056/NEJMoa1001294
40. Jähnisch H, Füssel S, Kiessling A, Wehner R, Zastrow S, Bachmann M, et al. Dendritic cell-based immunotherapy for prostate cancer. Clin Dev Immunol. (2010) 2010:517493. doi: 10.1155/2010/517493
41. Krueger TE, Thorek DLJ, Meeker AK, Isaacs JT, Brennen WN. Tumor-infiltrating mesenchymal stem cells: Drivers of the immunosuppressive tumor microenvironment in prostate cancer? Prostate. (2019) 79:320–30. doi: 10.1002/pros.23738
42. Nakashima J, Tachibana M, Horiguchi Y, Oya M, Ohigashi T, Asakura H, et al. Serum interleukin 6 as a prognostic factor in patients with prostate cancer. Clin Cancer Res Off J Am Assoc Cancer Res. (2000) 6:2702–6.
43. dos Reis ST, Pontes-Júnior J, Antunes AA, Sousa-Canavez JM, Abe DK, Cruz JA, et al. Tgf-β1 expression as a biomarker of poor prognosis in prostate cancer. Clinics. (2011) 66:1143–7. doi: 10.1590/s1807-59322011000700004
44. Palano MT, Gallazzi M, Cucchiara M, Dehò F, Capogrosso P, Bruno A, et al. The tumor innate immune microenvironment in prostate cancer: an overview of soluble factors and cellular effectors. Explor Targeting Anti-Tumor Ther. (2022) 3:694–718. doi: 10.37349/etat
45. Banzola I, Mengus C, Wyler S, Hudolin T, Manzella G, Chiarugi A, et al. Expression of indoleamine 2,3-dioxygenase induced by IFN-γ and TNF-α as potential biomarker of prostate cancer progression. Front Immunol. (2018) 9:1051. doi: 10.3389/fimmu.2018.01051
46. Messerschmidt JL, Prendergast GC, Messerschmidt GL. How cancers escape immune destruction and mechanisms of action for the new significantly active immune therapies: helping nonimmunologists decipher recent advances. Oncologist. (2016) 21:233–43. doi: 10.1634/theoncologist.2015-0282
47. Morse MD, McNeel DG. Prostate cancer patients on androgen deprivation therapy develop persistent changes in adaptive immune responses. Hum Immunol. (2010) 71:496–504. doi: 10.1016/j.humimm.2010.02.007
48. Mercader M, Bodner BK, Moser MT, Kwon PS, Park ES, Manecke RG, et al. T cell infiltration of the prostate induced by androgen withdrawal in patients with prostate cancer. Proc Natl Acad Sci U. S. A. (2001) 98:14565–70. doi: 10.1073/pnas.251140998
49. Saylor PJ, Kozak KR, Smith MR, Ancukiewicz MA, Efstathiou JA, Zietman AL, et al. Changes in biomarkers of inflammation and angiogenesis during androgen deprivation therapy for prostate cancer. Oncologist. (2012) 17:212–9. doi: 10.1634/theoncologist.2011-0321
50. Singh J, Sohal SS, Ahuja K, Lim A, Duncan H, Thachil T, et al. Levels of plasma cytokine in patients undergoing neoadjuvant androgen deprivation therapy and external beam radiation therapy for adenocarcinoma of the prostate. Ann Transl Med. (2020) 8:636. doi: 10.21037/atm-19-1913
51. Pal SK, Moreira D, Won H, White SW, Duttagupta P, Lucia M, et al. Reduced T-cell numbers and elevated levels of immunomodulatory cytokines in metastatic prostate cancer patients. Novo Resistant to Abiraterone and/or Enzalutamide Ther Int J Mol Sci. (2019) 20(8):1831. doi: 10.3390/ijms20081831
52. Consiglio CR, Udartseva O, Ramsey KD, Bush C, Gollnick SO. Enzalutamide, an androgen receptor antagonist, enhances myeloid cell-mediated immune suppression and tumor progression. Cancer Immunol Res. (2020) 8:1215–27. doi: 10.1158/2326-6066.CIR-19-0371
53. Madan RA, Karzai F, Donahue RN, Al-Harthy M, Bilusic M, Rosner II, et al. Clinical and immunologic impact of short-course enzalutamide alone and with immunotherapy in non-metastatic castration sensitive prostate cancer. J Immunother. Cancer. (2021) 9:e001556. doi: 10.1136/jitc-2020-001556
54. Bishop JL, Sio A, Angeles A, Roberts ME, Azad AA, Chi KN, et al. PD-L1 is highly expressed in Enzalutamide resistant prostate cancer. Oncotarget. (2015) 6:234–42. doi: 10.18632/oncotarget.2703
55. Ma Z, Zhang W, Dong B, Xin Z, Ji Y, Su R, et al. Docetaxel remodels prostate cancer immune microenvironment and enhances checkpoint inhibitor-based immunotherapy. Theranostics. (2022) 12:4965–79. doi: 10.7150/thno.73152
56. Han C, Deng Y, Xu W, Liu Z, Wang T, Wang S, et al. The roles of tumor-associated macrophages in prostate cancer. J Oncol. (2022) 2022:8580043. doi: 10.1155/2022/8580043
57. Herrera FG, Ronet C, Ochoa de Olza M, Barras D, Crespo I, Andreatta M, et al. Low-dose radiotherapy reverses tumor immune desertification and resistance to immunotherapy. Cancer Discovery. (2022) 12:108–33. doi: 10.1158/2159-8290.CD-21-0003
58. Keam SP, Halse H, Nguyen T, Wang M, Van Kooten Losio N, Mitchell C, et al. High dose-rate brachytherapy of localized prostate cancer converts tumors from cold to hot. J Immunother. Cancer. (2020) 8:e000792. doi: 10.1136/jitc-2020-SITC2020.0580
59. Sutherland JS, Goldberg GL, Hammett MV, Uldrich AP, Berzins SP, Heng TS, et al. Activation of thymic regeneration in mice and humans following androgen blockade. J Immunol Baltim. Md 1950. (2005) 175:2741–53. doi: 10.4049/jimmunol.175.4.2741
60. Roden AC, Moser MT, Tri SD, Mercader M, Kuntz SM, Dong H, et al. Augmentation of T cell levels and responses induced by androgen deprivation. J Immunol Baltim. Md 1950. (2004) 173:6098–108. doi: 10.4049/jimmunol.173.10.6098
61. Tang S, Moore ML, Grayson JM, Dubey P. Increased CD8+ T-cell function following castration and immunization is countered by parallel expansion of regulatory T cells. Cancer Res. (2012) 72:1975–85. doi: 10.1158/0008-5472.CAN-11-2499
62. López-Campos F, Gajate P, Romero-Laorden N, Zafra-Martín J, Juan M, Hernando Polo S, et al. Immunotherapy in advanced prostate cancer: current knowledge and future directions. Biomedicines. (2022) 10:537. doi: 10.3390/biomedicines10030537
63. El-Kenawi A, Dominguez-Viqueira W, Liu M, Awasthi S, Abraham-Miranda J, Keske A, et al. Macrophage-derived cholesterol contributes to therapeutic resistance in prostate cancer. Cancer Res. (2021) 81:5477–90. doi: 10.1158/0008-5472.CAN-20-4028
64. Zhang T, Agarwal A, Almquist RG, Runyambo D, Park S, Bronson E, et al. Expression of immune checkpoints on circulating tumor cells in men with metastatic prostate cancer. biomark Res. (2021) 9:14. doi: 10.1186/s40364-021-00267-y
65. Navarro EP, Conteduca V, Alba del Mellado AG B, Cremaschi P, Calvo OF, et al. 589P Dynamics of peripheral blood immune profiling associated with tumour progression in metastatic castration resistant prostate cancer (mCRPC). Ann Oncol. (2021) 32:S637–8. doi: 10.1016/j.annonc.2021.08.1102
66. Buttigliero C, Pisano C, Tucci M, Vignani F, Bertaglia V, Iaconis D, et al. Prognostic impact of pretreatment neutrophil-to-lymphocyte ratio in castration-resistant prostate cancer patients treated with first-line docetaxel. Acta Oncol Stockh. Swed. (2017) 56:555–62. doi: 10.1080/0284186X.2016.1260772
67. Man Y-N, Chen Y-F. Systemic immune-inflammation index, serum albumin, and fibrinogen impact prognosis in castration-resistant prostate cancer patients treated with first-line docetaxel. Int Urol. Nephrol. (2019) 51:2189–99. doi: 10.1007/s11255-019-02265-4
68. Xiang P, Jin S, Yang Y, Sheng J, He Q, Song Y, et al. Infiltrating CD4+ T cells attenuate chemotherapy sensitivity in prostate cancer via CCL5 signaling. Prostate. (2019) 79:1018–31. doi: 10.1002/pros.23810
69. Visa L, Pineda E, Farrus B, Codony-Servat J, Filella X, Albiol S, et al. Correlation of serum interleukin-6 (IL-6) levels and clinical outcome in hormone-independent (HI) prostate cancer (PC) patients (PTS) treated with docetaxel. J Clin Oncol. (2009) 27:e16044–4. doi: 10.1200/jco.2009.27.15_suppl.e16044
70. Fizazi K, De Bono JS, Flechon A, Heidenreich A, Voog E, Davis NB, et al. Randomised phase II study of siltuximab (CNTO 328), an anti-IL-6 monoclonal antibody, in combination with mitoxantrone/prednisone versus mitoxantrone/prednisone alone in metastatic castration-resistant prostate cancer. Eur J Cancer Oxf. Engl 1990. (2012) 48:85–93. doi: 10.1016/j.ejca.2011.10.014
71. Guan W, Li F, Zhao Z, Zhang Z, Hu J, Zhang Y, et al. Tumor-Associated Macrophage Promotes the Survival of Cancer Cells upon Docetaxel Chemotherapy via the CSF1/CSF1R–CXCL12/CXCR4 Axis in Castration-Resistant Prostate Cancer. Genes. (2021) 12:773. doi: 10.3390/genes12050773
72. Izumi K, Mizokami A. Suppressive role of androgen/androgen receptor signaling via chemokines on prostate cancer cells. J Clin Med. (2019) 8:354. doi: 10.3390/jcm8030354
73. Pienta KJ, Hammarlund EU, Brown JS, Amend SR, Axelrod RM. Cancer recurrence and lethality are enabled by enhanced survival and reversible cell cycle arrest of polyaneuploid cells. Proc Natl Acad Sci U. S. A. (2021) 118:e2020838118. doi: 10.1073/pnas.2020838118
74. Rowell NP. The abscopal effect and its implications for radiotherapy-immunotherapy combinations. Transl Cancer Res. (2023) 12:8–12. doi: 10.21037/tcr-22-2354
75. Rudqvist N-P, Charpentier M, Lhuillier C, Wennerberg E, Spada S, Sheridan C, et al. Immunotherapy targeting different immune compartments in combination with radiation therapy induces regression of resistant tumors. Nat Commun. (2023) 14:5146. doi: 10.1038/s41467-023-40844-3
76. Erlandsson A, Lundholm M, Watz J, Bergh A, Petrova E, Alamdari F, et al. Infiltrating immune cells in prostate cancer tissue after androgen deprivation and radiotherapy. Int J Immunopathol Pharmacol. (2023) 37:3946320231158025. doi: 10.1177/03946320231158025
77. Abida W, Cheng ML, Armenia J, Middha S, Autio KA, Vargas HA, et al. Analysis of the prevalence of microsatellite instability in prostate cancer and response to immune checkpoint blockade. JAMA Oncol. (2019) 5:471–8. doi: 10.1001/jamaoncol.2018.5801
78. He J, Yi M, Tan L, Huang J, Huang L. The immune checkpoint regulator PD-L1 expression are associated with clinical progression in prostate cancer. World J Surg Oncol. (2021) 19:215. doi: 10.1186/s12957-021-02325-z
79. Aggarwal R, Starzinski S, de Kouchkovsky I, Koshkin V, Bose R, Chou J, et al. Single-dose 177Lu-PSMA-617 followed by maintenance pembrolizumab in patients with metastatic castration-resistant prostate cancer: an open-label, dose-expansion, phase 1 trial. Lancet Oncol. (2023) 24:1266–76. doi: 10.1016/S1470-2045(23)00451-5
80. Qi Z, Xu Z, Zhang L, Zou Y, Li J, Yan W, et al. Overcoming resistance to immune checkpoint therapy in PTEN-null prostate cancer by intermittent anti-PI3Kα/β/δ treatment. Nat Commun. (2022) 13:182. doi: 10.1038/s41467-021-27833-0
81. Ge R, Wang Z, Cheng L. Tumor microenvironment heterogeneity an important mediator of prostate cancer progression and therapeutic resistance. NPJ Precis. Oncol. (2022) 6:1–8. doi: 10.1038/s41698-022-00272-w
82. Agarwal N, Azad AA, Galceran JC, Matsubara N, Oudard S, Saad F, et al. LBA67 Cabozantinib (C) plus atezolizumab (A) versus 2nd novel hormonal therapy (NHT) in patients (Pts) with metastatic castration-resistant prostate cancer (mCRPC): Final overall survival (OS) results of the phase III, randomized, CONTACT-02 study. Ann Oncol. (2024) 35:S1256–7. doi: 10.1016/j.annonc.2024.08.2310
83. Zarrabi KK, Narayan V, Mille PJ, Zibelman MR, Miron B, Bashir B, et al. Bispecific PSMA antibodies and CAR-T in metastatic castration-resistant prostate cancer. Ther Adv Urol. (2023) 15:17562872231182219. doi: 10.1177/17562872231182219
84. Vaishampayan UN, Thakur A, Chen W, Deol A, Patel M, Dobson K, et al. Phase II trial of pembrolizumab and anti-CD3 x anti-HER2 bispecific antibody-armed activated T cells in metastatic castration-resistant prostate cancer. Clin Cancer Res Off J Am Assoc Cancer Res. (2023) 29:122–33. doi: 10.1158/1078-0432.CCR-22-1601
85. Esparcia-Pinedo L, Romero-Laorden N, Alfranca A. Tertiary lymphoid structures and B lymphocytes: a promising therapeutic strategy to fight cancer. Front Immunol. (2023) 14:1231315. doi: 10.3389/fimmu.2023.1231315
86. Bian X, Wang W, Abudurexiti M, Zhang X, Ma W, Shi G, et al. Integration analysis of single-cell multi-omics reveals prostate cancer heterogeneity. Adv Sci Weinh. Baden-Wurtt. Ger. (2024) 11:e2305724. doi: 10.1002/advs.202305724
87. Coleman IM, DeSarkar N, Morrissey C, Xin L, Roudier MP, Sayar E, et al. Therapeutic implications for intrinsic phenotype classification of metastatic castration-resistant prostate cancer. Clin Cancer Res. (2022) 28:3127–40. doi: 10.1158/1078-0432.CCR-21-4289
88. Cancer Genome Atlas Research Network. The molecular taxonomy of primary prostate cancer. Cell. (2015) 163:1011–25. doi: 10.1016/j.cell.2015.10.025
89. Weiner AB, Liu Y, Hakansson A, Zhao X, Proudfoot JA, Ho J, et al. A novel prostate cancer subtyping classifier based on luminal and basal phenotypes. Cancer. (2023) 129:2169–78. doi: 10.1002/cncr.v129.14
90. Olmos D, Brewer D, Clark J, Danila DC, Parker C, Attard G, et al. Prognostic value of blood mRNA expression signatures in castration-resistant prostate cancer: a prospective, two-stage study. Lancet Oncol. (2012) 13:1114–24. doi: 10.1016/S1470-2045(12)70372-8
91. Racle J, Gfeller D. EPIC: A tool to estimate the proportions of different cell types from bulk gene expression data. Methods Mol Biol Clifton NJ. (2020) 2120:233–48. doi: 10.1007/978-1-0716-0327-7_17
92. Feng D-C, Zhu WZ, Wang J, Li DX, Shi X, Xiong Q, et al. The implications of single-cell RNA-seq analysis in prostate cancer: unraveling tumor heterogeneity, therapeutic implications and pathways towards personalized therapy. Mil. Med Res. (2024) 11:21. doi: 10.1186/s40779-024-00526-7
93. Han S, Shi T, Liao Y, Chen D, Yang F, Wang M, et al. Tumor immune contexture predicts recurrence after prostatectomy and efficacy of androgen deprivation and immunotherapy in prostate cancer. J Transl Med. (2023) 21:194. doi: 10.1186/s12967-022-03827-4
94. Yamoah K, Awasthi S, Mahal BA, Zhao SG, Grass GD, Berglund A, et al. Novel transcriptomic interactions between immune content and genomic classifier predict lethal outcomes in high-grade prostate cancer. Eur Urol. (2022) 81:325–30. doi: 10.1016/j.eururo.2020.11.038
95. Ross RW, Galsky MD, Scher HI, Magidson J, Wassmann K, Lee GS, et al. A whole-blood RNA transcript-based prognostic model in men with castration-resistant prostate cancer: a prospective study. Lancet Oncol. (2012) 13:1105–13. doi: 10.1016/S1470-2045(12)70263-2
96. Fenor de la Maza MD, Chandran K, Rekowski J, Shui IM, Gurel B, Cross E, et al. Immune biomarkers in metastatic castration-resistant prostate cancer. Eur Urol. Oncol. (2022) 5:659–67. doi: 10.1016/j.euo.2022.04.004
97. Chaudagar K, Hieromnimon HM, Khurana R, Labadie B, Hirz T, Mei S, et al. Reversal of lactate and PD-1-mediated macrophage immunosuppression controls growth of PTEN/p53-deficient prostate cancer. Clin Cancer Res Off J Am Assoc Cancer Res. (2023) 29:1952–68. doi: 10.1158/1078-0432.CCR-22-3350
98. Rodrigues DN, Rescigno P, Liu D, Yuan W, Carreira S, Lambros MB, et al. Immunogenomic analyses associate immunological alterations with mismatch repair defects in prostate cancer. J Clin Invest. (2018) 128:4441. doi: 10.1172/JCI121924
99. Kamat NV, Yu EY, Lee JK. BiTE-ing into prostate cancer with bispecific T-cell engagers. Clin Cancer Res. (2021) 27:2675–7. doi: 10.1158/1078-0432.CCR-21-0355
Keywords: immunome, prostate cancer, biomarkers, immunophenotype, immunotherapy
Citation: San-Jose Manso L, Alfranca A, Moreno-Pérez I, Ruiz-Vico M, Velasco C, Toquero P, Pacheco M, Zapatero A, Aldave D, Celada G, Albers E, Fenor de la Maza M-D, García J, Castro E, Olmos D, Colomer R and Romero-Laorden N (2024) Immunome profiling in prostate cancer: a guide for clinicians. Front. Immunol. 15:1398109. doi: 10.3389/fimmu.2024.1398109
Received: 08 March 2024; Accepted: 28 October 2024;
Published: 20 November 2024.
Edited by:
Alvin Liu, University of Washington, United StatesReviewed by:
Alok Kumar Singh, Johns Hopkins Medicine, United StatesMireia Castillo-Martin, Champalimaud Foundation, Portugal
Copyright © 2024 San-Jose Manso, Alfranca, Moreno-Pérez, Ruiz-Vico, Velasco, Toquero, Pacheco, Zapatero, Aldave, Celada, Albers, Fenor de la Maza, García, Castro, Olmos, Colomer and Romero-Laorden. This is an open-access article distributed under the terms of the Creative Commons Attribution License (CC BY). The use, distribution or reproduction in other forums is permitted, provided the original author(s) and the copyright owner(s) are credited and that the original publication in this journal is cited, in accordance with accepted academic practice. No use, distribution or reproduction is permitted which does not comply with these terms.
*Correspondence: Nuria Romero-Laorden, bnVyaWFyb21lcm9sYW9yZGVuQGdtYWlsLmNvbQ==