- 1Department of Cellular and Molecular Medicine, Herbert Wertheim College of Medicine, Florida International University, Miami, FL, United States
- 2Department of Medical Biochemistry, Faculty of Medicine, South Valley University, Qena, Egypt
Vascular cell adhesion is a complex orchestration of events that commonly feature lectin–ligand interactions between circulating cells, such as immune, stem, and tumor cells, and endothelial cells (ECs) lining post-capillary venules. Characteristically, circulating cell adherence to the vasculature endothelium is initiated through interactions between surface sialo-fucosylated glycoprotein ligands and lectins, specifically platelet (P)- or endothelial (E)-selectin on ECs or between leukocyte (L)-selectin on circulating leukocytes and L-selectin ligands on ECs, culminating in circulating cell extravasation. This lectin–ligand interplay enables the migration of immune cells into specific tissue sites to help maintain effective immunosurveillance and inflammation control, the homing of stem cells to bone marrow or tissues in need of repair, and, unfortunately, in some cases, the dissemination of circulating tumor cells (CTCs) to distant metastatic sites. Interestingly, there is a growing body of evidence showing that the family of β-galactoside-binding lectins, known as galectins, can also play pivotal roles in the adhesion of circulating cells to the vascular endothelium. In this review, we present contemporary knowledge on the significant roles of host- and/or tumor-derived galectin (Gal)-3, -8, and -9 in facilitating the adhesion of circulating cells to the vascular endothelium either directly by acting as bridging molecules or indirectly by triggering signaling pathways to express adhesion molecules on ECs. We also explore strategies for interfering with galectin-mediated adhesion to attenuate inflammation or hinder the metastatic seeding of CTCs, which are often rich in galectins and/or their glycan ligands.
1 Introduction
It is well established that the rapid and tissue-specific homing of circulating immune or tumor cells relies on a series of interactions with the vascular endothelium, preceding transendothelial migration (also known as diapedesis or extravasation), chemotaxis, and adaptation to the tissue microenvironment (1, 2). The critical initial phase in this process is vascular cell adhesion, where the circulating cells adhere to the endothelial cells (ECs) lining blood vessels, primarily within distinct post-capillary venular segments (3, 4). Vascular cell adhesion plays a crucial role in various physiological processes, including embryonic development, immune response regulation, tissue repair, and inflammation control (5). Unsurprisingly, dysregulated vascular cell adhesion often directly contributes to diverse pathological conditions, such as psoriasis, rheumatoid arthritis, impaired wound healing, asthma, patient transplant rejection, and cancer metastasis (6).
The adhesion of circulating cells to the vascular endothelium involves several sequential steps, including cell tethering, rolling, and firm adhesion (7). These steps are orchestrated by surface molecules expressed on both circulating cells and ECs (8–10). Considering the fact that most cell surface and secreted molecules are glycosylated, it becomes evident that glycan–glycan binding protein interactions are pervasive in controlling, or at least tuning, human biological activities, and when dysregulated, can lead to cellular dysfunction and disease development (11). Glycan-binding proteins (also known as lectins) comprise a diverse group of proteins categorized into 25 distinct families, each of which binds discrete carbohydrate structures (12). C-type lectins (including selectins), S-type Lectins (galectins), and I-type lectins (sialic acid-binding immunoglobin-like lectins; siglecs) are three large families of mammalian lectins known to play crucial roles as mediators of vascular cell adhesion (13).
Galectin-mediated vascular cell adhesion is currently recognized as a significant contributing molecular mechanism (14). Galectins can facilitate both homotypic and heterotypic cell adhesion in a calcium-independent manner (11, 15–17). Galectin (Gal)-3, -8, and -9, in particular, have emerged as key players in mediating the adhesion between circulating cells and the vascular endothelium. Consequently, strategic inhibition of Gal-3, -8, and -9 expression or function has been documented to reduce vascular cell adhesion (18–24). In this review, we will explore the roles of Gal-3, -8, and -9 in vascular cell adhesion and their increasingly recognized importance in various aspects of autoimmunity, infectious diseases, and malignancy.
2 Canonical mechanisms of glycan-mediated cell adherence to vascular endothelium
Molecular mechanisms of circulating cell adherence to vascular ECs involve expression and presentation of lectins and cell surface glycosylation that serve as lectin ligands (25, 26). These glycosylated lectin ligands presented by circulating cells or ECs help initiate cell adhesion through binding interactions with lectins expressed on ECs or circulating cells, respectively, with a high degree of specificity (27).
Selectins are a group of calcium-dependent (C)-type lectins that are characteristically involved in vascular cell adhesion (28, 29). There are three members within the selectin family: Endothelial (E), Platelet (P), and Leukocyte (L)-selectin (30). Each selectin is comprised of an N-terminal carbohydrate recognition or lectin domain (CRD), epidermal growth factor (EGF)-like domain, a variable number of consensus repeats (CRs), a single transmembrane domain, and a short cytoplasmic tail (28, 31). Selectins play a crucial role in the initial stages of cell adhesion, namely in the “tethering and rolling” process, which is critical for the capture of circulating cells to the post-capillary venules that initiates diapedesis of circulating cells into tissues (2). Selectins extend from the cell surface, allowing for the CRD to recognize glycan ligands, notably sialyl Lewis X (sLeX) or A (sLeA), presented by surface glycoproteins and glycosphingolipids (2).
E-selectin (CD62E) expression is constitutive on dermal and bone marrow post-capillary venule ECs, though its levels on vascular ECs in other tissues are shown to be regulated by TNF-α, IL-1β, or bacterial lipopolysaccharides (2, 29, 32, 33).
P-selectin (CD62P) is stored in specialized secretory organelles in ECs, called Weibel-Palade bodies, and in α-granules of circulating platelets, and can be quickly translocated to the cell surface upon activation through mediators, such histamine, thrombin, or other components of the complement system (34, 35). Furthermore, other P-selectin ligands found on hematopoietic stem cells include CD24 and CD34 (36, 37).
L-selectin (CD62L) is variably expressed on the microvilli of circulating leukocytes depending on the state of differentiation and characteristically involved in lymphocyte homing to secondary lymphoid tissues and in facilitating leukocyte migration to sites of inflammation (38–40).
SLeX and sLeA are canonical glycan structures required for binding to selectins and are widely recognized as key molecular features in vascular cell adhesion due to their noted expression on circulating stem cells, leukocytes, CTCs, and ECs (41). SLeA is predominantly found on CTCs of epithelial origin (42), whereas sLeX expression is observed on a variety of circulating cells, including CTCs (43), granulocytes (44, 45), lymphocytes (46, 47), monocytes (46), dendritic cells (48), and hematopoietic stem cells (49). SLeX and sLeA can interact with E-selectin to mediate the initial first step of vascular cell adhesion, “tethering and rolling,” for circulating cells on the vascular endothelium that prompts firm adhesion and eventually transendothelial migration (2, 50–54). Similarly, SLeX-bearing surface glycoproteins, primarily P-selectin glycoprotein ligand-1 (PSGL-1) on leukocytes, for example, are crucial for vascular cell adhesion through their interaction with P-selectin, requiring tyrosine sulfation adjacent to these glycoproteins at the PSGL-1 terminus for effective binding (30, 55, 56). SLeX moieties, present on glycan ligands like Sgp200, GlyCAM-1, MAdCAM-1, endoglycan, endomucin, and podocalyxin-like protein (PCLP) on ECs lining high endothelial venules, bind L-selectin-bearing lymphocytes and are further modified by GlcNAc-6-O-sulfotransferases on CD34 O-glycans to generate 6-sulfo sLeX, enhancing L-selectin binding (11, 40, 57).. Furthermore, presentation of sLeX has been shown on a specialized glycoform of CD44, known as HCELL (hematopoietic stem cell E/L-selectin ligand) expressed by normal and malignant hematopoietic stem cells (41, 58, 59), CD34 as a ligand for E/P-selecting on hematopoietic cells (36), CD43 (60, 61) and PSGL-1, which is found on most leukocytes (62).
3 Galectin-mediated cell adherence to vascular endothelium
Galectins are a family of β-galactoside-binding lectins produced within the cytosol and extruded into the extracellular milieu through non-classical secretory routes due to lack of signal sequence (63–66). Galectins are categorized, based on their molecular structure, into three types: prototypical, chimeric, and tandem repeat galectins (Figure 1) (67). The prototypical galectins (Gal-1, -2, -5, -7, -10, -11, -13, -14, -15, and -16) have a single CRD, designed to directly interact with β-galactoside-bearing glycans and can assemble into homodimers (68). The chimeric galectin family contains only one member (Gal-3), which has a single CRD linked to an N-terminal domain (NTD) (67, 69). The tandem repeat galectins (Gal-4, -6, -8, -9, and -12) comprise two distinct CRDs with unique binding preferences connected together by a linker peptide crucial for their complete functionality (70).
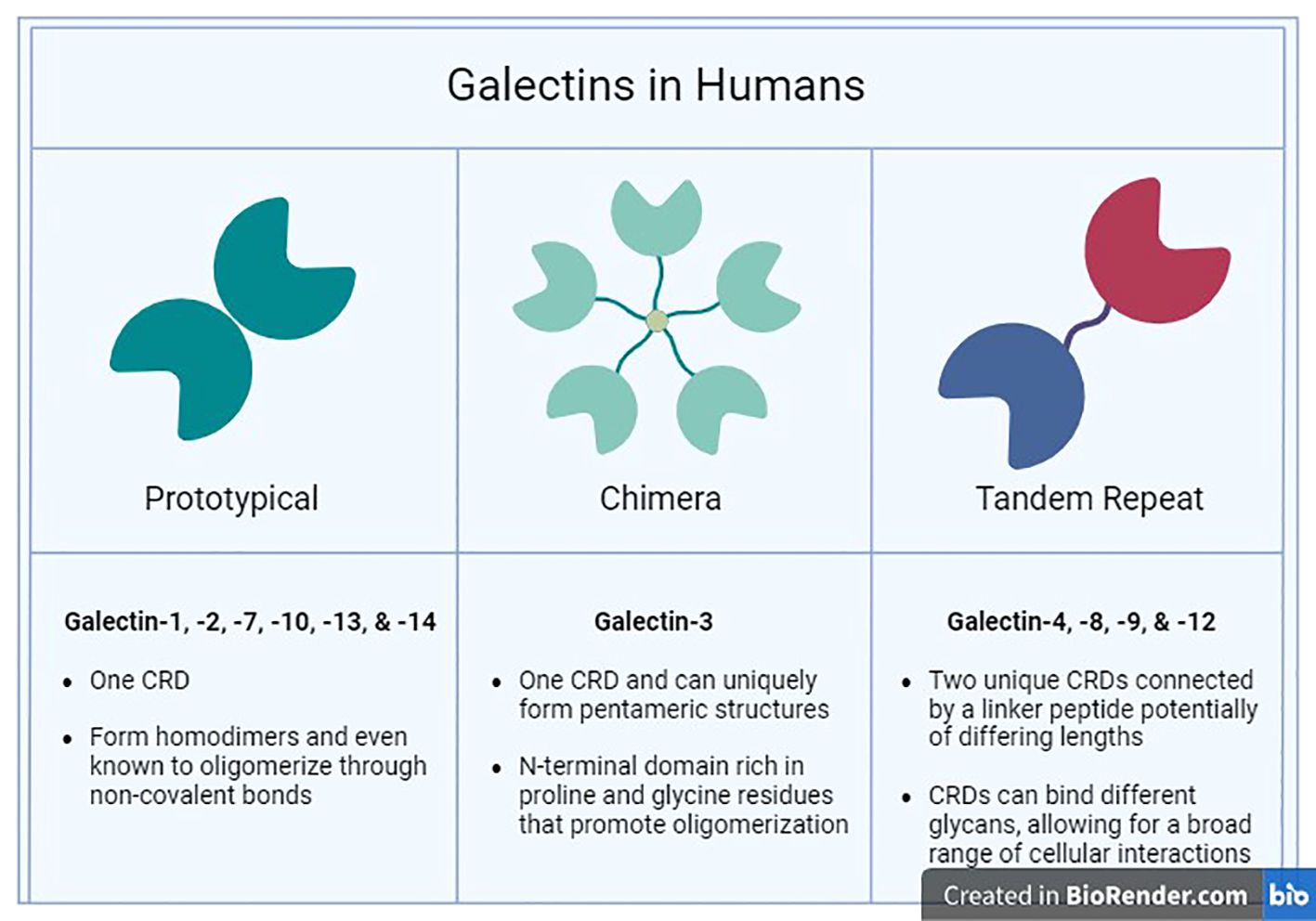
Figure 1 Human Galectins. The three major families (Prototypical, Chimera, and Tandem Repeat) of galectins found in humans are categorized by their structural characteristics and ligand selectivity properties.
Galectin functional expression varies enormously based on the tissue type and cellular/subcellular location, in which they function as intra- or extracellular modifiers, regulating a plethora of cellular activities such as cell differentiation, proliferation, apoptosis, and immune responses (11). Moreover, some galectins are involved in tumor progression through regulating intracellular oncogenic signaling, immune evasion, angiogenesis, and circulating tumor cell (CTC) survival, lodging, and proliferation in metastatic tissues (69, 71, 72). While adhesion molecules that mediate circulating cell interaction with vascular endothelium typically reside on the cell surface, some galectins have been suggested to function as soluble adhesion molecules that facilitate cross-linking of cell surface glycoconjugates (73). There is a large body of evidence emphasizing the importance of Gal-3, -8, and -9 in mediating circulating cells interactions with ECs, which we will delve into further in this section.
3.1 Gal-3
Gal-3 was first recognized in the early 1980s as a 35kDa β-galactoside-binding protein on murine peritoneal macrophages and referred to as Mac-2, IgE-binding protein, L-29, and L-31 (11, 27). Gal-3 is encoded by a single gene (LGALS3), located on chromosome 14, locus q21-q22, consisting of six exons and five introns (74). Gal-3 is synthesized by free cytosolic ribosomes and can translocate into the nucleus or be secreted into the extracellular space (75).
A hallmark glycan ligand of Gal-3 is a poly-N-acetyllactosamine (poly-LacNAc), which consists of repeating Galβ1,4GlcNAcβ1,3 disaccharides (76). Another ligand for Gal-3 is N,N′-diacetyllactosamine (GalNAcβ1,4GlcNAc or LacdiNAc) (77) found on a select group of N- and/or O-linked glycoproteins (76). Numerous studies have indicated that the interaction between Gal-3 and its ligands is influenced by the presence of terminal sialylation (78). In fact, α2,6 sialylation of terminal galactose residues on N-glycans via β-galactoside α2,6 sialyltransferase 1 (ST6GAL1) or of N-acetylgalactosamine on O-glycans by N-acetylgalactosamine α2,6 sialyltransferase 1 (ST6GALNAC1) significantly downregulates Gal-3 binding to glycan ligands (79, 80).
The characteristic structure of Gal-3 allows two forms of oligomerization: type N self-association mediated by the NTDs resulting in the formation of pentameric complexes, and type C self-association through the CRDs to form dimers or oligomers (81, 82). Extracellular Gal-3 oligomers are implicated in the adhesive and migratory activities of normal and cancer cells through binding interactions between glycosylated ligands present either on cell surfaces or in the ECM, including fibronectin, laminin, collagen IV, and elastin (83). In terms of vascular cell adhesion, Gal-3 facilitates the attachment of diverse cell types to ECs, initiating the homing of these cells to various tissues throughout the body, as elaborated below (84–86).
3.1.1 Gal-3-mediated immune cell adhesion to vascular endothelium
Mounting evidence supports Gal-3’s important role in coordinating the trafficking of leukocytes toward sites of injury or inflammation (84). Early in vivo studies utilizing a streptococcal pneumonia mouse model explore the role of Gal-3 as a modulator of neutrophil-mediated innate immunity (73). The authors report that Gal-3 is released extracellularly into alveolar spaces infected by Streptococcus pneumoniae at the onset of neutrophil extravasation (73). Immunohistochemistry analysis further showed an accumulation of Gal-3 in the vicinity of epithelial and EC layers within infected lungs, contrasting with its dispersed distribution in uninfected lungs (73). Moreover, data from in vitro experiments demonstrated that Gal-3 acts as an adhesion molecule facilitating direct cross-linking of neutrophils to ECs, a process dependent on Gal-3 oligomerization (73).
Considering data from intravital microscopy and flow chamber shear-based adhesion assays, Gittens et al. propose a dual role for Gal-3, both endogenous and exogenous, in promoting leukocyte migration to inflamed tissues (84). Their report shows that endogenous Gal-3 deficiency impairs leukocyte slow rolling and migration in response to IL-1β, likely due to reduced expression of cell adhesion molecules and changes in cell glycosylation profile (84). Additionally, exogenous administration of Gal-3 triggers the upregulated expression of proinflammatory cytokines and chemokines in the local microenvironment, thereby enhancing the recruitment of neutrophils and monocytes to inflamed sites (84).
Gal-3 also plays a key role in mediating eosinophil trafficking under flow conditions to sites of allergic inflammation (85). Data from studies conducted by Rao et al. show increased expression of Gal-3 in eosinophils obtained from allergic donors (85). They propose that eosinophil adhesion is initially enabled by the binding of eosinophil-expressed Gal-3 and α4β1 integrins to VCAM-1 expressed on ECs. EC-expressed Gal-3 further strengthens this interaction by relocating to the contact points and interacting with eosinophil-expressed Gal-3 and α4β1 integrins (85).
3.1.2 Gal-3-mediated cancer cell adhesion to vascular endothelium
Elevated serum Gal-3 levels in cancer patients have been reported in numerous studies across different types of cancer, including breast cancer (87, 88), colorectal cancer (87), prostate cancer (89), melanoma (90), bladder cancer (91), and lung cancer (92). In some studies, serum Gal-3 levels have been positively correlated with tumor progression, metastasis, and poor prognosis (69, 92, 93). These observations provide a foundation for theorizing that serum Gal-3 is involved in metastasis promotion, likely through supporting adhesion of CTCs to ECs and subsequent extravasation to metastatic sites (94).
Gal-3 is proposed to promote CTC adhesion to EC by binding to Thomsen-Friedenreich (TF) antigen carried by mucin protein 1 (MUC1) expressed on cancer cells, causing patchy redistribution of MUC1 on the cell surface (17, 95). This allows for the exposure of CTC surface adhesion molecules, such as CD44, as well as ligands to endothelial E-selectin, facilitating the interaction with their respective molecular counterparts on ECs (17, 95). The interaction between Gal-3 and cancer-associated MUC4 is also suggested to enhance the binding of tumor cells to ECs in a similar fashion (96). Nevertheless, the adherence of low MUC1-expressing cancer cells to ECs appears to be facilitated by both exogenous and cell-intrinsic Gal-3 through a β-catenin-dependent upregulation of CD44 and N-cadherin expression (97, 98).
Data from investigations led by Chen et al. demonstrate that high levels of Gal-3 enhance cancer cell adhesion to vascular endothelium by stimulating the secretion of various metastatic-promoting cytokines from ECs, such as IL-6 and granulocyte colony-stimulating factor (G-CSF) (99). These cytokines interact with the vascular ECs and cause increased expression of surface adhesion molecules, including αvβ1integrin, E-selectin, ICAM-1, and VCAM-1 (99). Further investigations carried out by the same research team reveal that Gal-3 binds to N-linked glycans on CD146/MCAM expressed on EC, inducing CD146 dimerization and subsequent activation of AKT signaling (100). Additionally, silencing of CD146 expression eliminates the Gal-3-induced secretion of metastatic-promoting cytokines from ECs, namely IL-6 and G-CSF (100).
Wang et al. have recently found that human EC-derived extracellular vesicles (H-EVs) mediate the adhesion of breast cancer cells to human umbilical vein endothelial cells (HUVECs), an effect that is enhanced by circulating Gal-3 (101). Mechanistically, their data show that Gal-3 induces ICAM-1 upregulation in HUVECs, which is transferred to cancer cells via H-Evs, promoting CTC-EC adhesion (101). Interestingly, Zhang et al. have identified Gal-3 as a potential mediator of integrin αvβ1 export into EVs derived from breast cancer cells, promoting their communication with other cell types, including ECs (102). The authors report that knockdown of Gal-3 results in reduced integrin levels on the EV surface and diminished metastatic potential of cancer cells in vivo (102).
Noteworthy, Krishnan et al. present evidence of significant constitutive expression of Gal-3 within the vascular ECs of the lungs, indicating that Gal-3 serves as a readily accessible anchoring point for CTCs bearing highly dense Gal-3-binding glycans to promote metastatic colonization of the lungs (86). Their results indicate that highly metastatic melanoma B16F10 cells exhibit upregulated expression of heavily glycosylated Gal-3 receptor ligand, lysosome-associated membrane protein-1 (LAMP-1), compared to the low metastatic melanoma B16F1 cells (86).
3.1.3 Gal-3-mediated stem cell adhesion to vascular endothelium
Mesenchymal stem cells (MSCs) have garnered significant attention over the past two decades for their clinical promise in regenerating damaged tissues and treating inflammatory diseases (103). Their versatility also spans the development of treatments for a wide range of conditions, including degenerative diseases and cancer (104, 105). In-depth comprehension of the dynamics between transplanted stem cells and the host vasculature is crucial for ensuring reliable delivery of stem cells to the targeted locations and maximizing their therapeutic effectiveness (106).
Data on the role of Gal-3 in facilitating stem cell adhesion to ECs is still emerging (107). Results from recent studies conducted by Sedlar et al. show that human adipose-derived stem cells (ADSCs) express an abundance of Gal-3, both inside the cells and on their surfaces, compared to HUVECs (107). Their data indicate that Gal-3-preadsorbed cultivation surfaces enhance the adhesion of both HUVECs and ADSCs, mediated, at least partially, by α5β1, αVβ3, and αVβ1 integrins (107). Interestingly, this adhesion is resistant to blocking with Gal-3 CRD ligand, LacdiNAc (GalNAcβ1,4GlcNAc), suggesting that this interaction is mediated by a mechanism independent of Gal-3 CRD canonical binding with glycoconjugates (107). These findings present a promising avenue for future research and are likely to gain significant attention in the field of vascular tissue engineering and regenerative medicine.
3.2 Gal-8
Gal-8 was first detected in rat livers as a 35kDa β-galactoside-binding protein (108). It was also called prostate carcinoma tumor antigen-1 (PCTA-1) due to its high expression on human prostate cancer cells, while absent on normal prostatic tissues and benign prostatic hyperplasia tissues (109). Later, it was revealed that Gal-8 is expressed by various normal cells throughout the body, with vascular and lymphatic ECs considered significant contributors to its production (110).
In humans, the gene encoding Gal-8, LGALS8, is located on chromosome 1 (1q42.11), spans 33kbp of genomic DNA, and contains 11 exons (111, 112). Human Gal-8 has at least six isoforms: three isoforms belong to the tandem repeat galectins, while the other three isoforms belong to the proto-type galectins (113). It has been suggested that galectins containing double CRD serve as hetero-bifunctional crosslinking molecules that can recognize distinct glycoconjugates, thus expanding their functional repertoire (98). In this regard, Gal-8 is conceptualized as comprising two distinct terminal domains with different affinities for oligosaccharides: a C-terminal domain (Gal-8C) that preferentially binds non-sialylated glycans such as blood group A and B antigens and poly-N-acetyllactosaminyl glycans, and an N-terminal domain (Gal-8N) which favors sulfated and sialylated glycans (114).
3.2.1 Gal-8-mediated immune cell adhesion to vascular endothelium
Gal-8 orchestrates the interaction between leukocytes, platelets, and vascular ECs, impacting many physiological and pathological processes such as hemostasis, infection, inflammation, and atherosclerosis (115). Data obtained from investigations led by Yamamoto et al. show that Gal-8 triggers marked increases in the adhesion of human peripheral blood leucocytes (T cells, B cells, neutrophils, eosinophils, and monocytes) to HUVECs (116).
Mechanistically, several studies propose that Gal-8 mediates leukocyte adhesion, either to ECs or ECM proteins, through direct interaction with certain members of the integrin family (116). For instance, integrin αM is implicated in modulating Gal-8-induced adhesion of neutrophils to plastic culture plates (117). For studies on human Jurkat T cell line, results obtained by Cárcamo et al. demonstrate that integrin α1β1, α3β1 and α5β1 participate in mediating Gal-8-induced adhesion to immobilized fibronectin (118), whereas investigations carried out by Yamamoto et al. present α4, α5, αL and β1 as the major Gal-8-binding proteins on Jurkat T cells (116).
Moreover, Gal-8 triggers EC activation and induces adhesion of normal platelets, likely through increased von Willebrand factor (vWF) exposure on ECs, which binds to platelet GPIb receptors (115). Gal-8 also stimulates the production of pro-inflammatory cytokines and chemokines within ECs, namely CCL2, CXCL3, IL-8, CXCL1, GM-CSF, IL-6, and CCL5, further exacerbating the inflammatory response (115).
3.2.2 Gal-8-mediated cancer cell adhesion to vascular endothelium
Various immunohistochemical studies have documented a wide variability of Gal-8 expression levels in malignant tissues compared to their normal counterparts, influenced by several factors including organ type, histologic type, and tumor stage (111, 113). However, ELISA-based studies have revealed markedly elevated levels of serum Gal-8 in cancer patients, including colorectal cancer (87), breast cancer (87), multiple myeloma (119), and melanoma (120). Some reports suggest a more pronounced elevation of Gal-8 in serum samples from patients who have developed metastases (87).
Experimental research indicates that elevated levels of circulating Gal-8 potentially facilitate the dissemination of tumor cells by promoting their adherence to vascular ECs (87, 119). Preincubation of colon cancer cells with exogenous Gal-8, at concentrations comparable to those found in sera of cancer patients, results in enhanced cancer cell adhesion to HUVECs monolayer, in a dose-dependent manner (87). This pro-adhesive effect is eliminated in the presence of lactose (87).
Interestingly, Friedel et al. have investigated the impact of two Gal-8 isoforms, Gal-8S and Gal-8L, on the adhesion of myeloma cells to vascular ECs (119). Results derived from this study indicate that both isoforms significantly increase myeloma cell adhesion to ECs under static and dynamic shear stress conditions, with Gal-8L showing a more pronounced effect compared to Gal-8S (119). Moreover, pre-stimulation of ECs with tumor necrosis factor (TNF) and subsequent treatment with Gal-8 leads to a 1.5–1.7-fold enhancement in myeloma cell adhesion compared to TNF-primed cells that are not treated (121). Unexpectedly, data from studies conducted by Gentilini et al. reveal that silencing of Gal-8 in prostate cancer cells impairs homotypic aggregation of cells, with no effect on their adherence to bovine aortic ECs (BAECs) (122). Taken together, these diverse findings suggest a complex role of Gal-8 in cancer cell adhesion to ECs, while also highlighting isoform-specific effects, which remain to be further investigated.
3.3 Gal-9
Gal-9, a 36kDa β-galactoside-binding protein belonging to the tandem-repeat type, was first identified in 1997 by three separate research groups (121, 123–125). In humans, the gene encoding Gal-9, LGALS9, is located on the long arm of chromosome 17 at locus 11.2 (17q11.2), consisting of 11 exons, in addition to two LGALS9-like genes (LGALS9B and LGALS9C), located on the short arm of the same chromosome at locus 11.2 (17p11.2) (126). Based on the length of the linker peptide, three main isoforms of Gal-9 are described: Gal-9L (large), Gal-9M (medium), and Gal-9S (small), each consisting of 355, 323, and 311 amino acids, respectively, giving Gal-9 further multivalent properties (127). Gal-9 prefers binding to internal LacNAc residues within poly-LacNAc chains and displays a specific affinity for i-linear N-glycans (128). The reactivity of the full-length Gal-9 is independent of sialylation status. However, desialylation results in an increased affinity of Gal-9 N-terminal domain to N-glycans (129, 130).
While Gal-9 is widely distributed in various tissues and variably expressed in the spleen, stomach, colon, lymph nodes, and appendix, studies elucidating the specific cell types within these organs that express Gal-9 remain lacking (131). Gal-9 is known for its immunomodulatory functions and has been studied in various pathological conditions associated with cancer, autoimmunity, graft rejection, inflammatory disease, and microbial infections (132). Importantly, Gal-9 is synthesized and secreted by activated ECs (133), with substantial evidence underscores its functions as an evolving player in EC biology (134). However, the exact role of Gal-9 in regulating circulating cell adhesion to vascular ECs remains to be elucidated (135, 136).
3.3.1 Gal-9-mediated immune cell adhesion to vascular endothelium
Gal-9 was initially described as a potent eosinophil chemoattractant agent in inflammatory processes (137). Although recent publications supporting this notion are deficient, emerging experimental studies have established the pro-adhesive function of Gal-9 on circulating leukocytes and further delved into investigating the underlying mechanisms (135). In line with observations regarding Gal-8, research conducted by Yamamoto et al. indicates that Gal-9 substantially boosts the adhesion of peripheral blood T-cells, B-cells, neutrophils, eosinophils, and monocytes to cultured HUVECs (116). Furthermore, data from studies done by Chakraborty et al. reveal that Gal-9, incidentally expressed at conspicuously high levels on human post-capillary venules, HEVs, and human B cells, facilitates the bridging of human circulating and naïve B cells to human vascular ECs, with concurrent deceleration of transendothelial migration (138). In neutrophils, both soluble and immobilized Gal-9 serve as an adhesion molecule enabling the interaction between neutrophils and ECs through CD44 and β2 integrin-dependent mechanisms (133). Results of a recent study by Mansour et al. point out that, under flow conditions, immobilized Gal-9 promotes the adhesion of CD4+ and CD8+ T cells through glycan and integrin-dependent mechanisms (135). Moreover, data from in vivo studies using Gal-9-deficient mice demonstrate impaired leukocyte trafficking and reduced local production of pro-inflammatory chemokines/cytokines (135).
More recently, Gal-9 has been shown to enhance the adhesion of leukocytes to ECs through interaction with blood group H antigen glycans present on EC surfaces (136). This investigation involved a model system utilizing galectin-positive Jurkat cells and EA.hy926 cells (immortalized human umbilical vein ECs expressing blood group H antigen) (136). Results indicate that Gal-9 binding to blood group H antigen facilitates adhesion, with loss of Gal-9 activity in defucosylated EA.hy926 cells, suggesting a potential mechanism for Gal-9-mediated leukocyte-EC interactions (136).
3.3.2 Gal-9-mediated cancer cell adhesion to vascular endothelium
Variation in tumor-intrinsic Gal-9 expression levels has been observed across studies. However, the prevailing trend suggests a low or almost absent Gal-9 in tumor cells compared to their normal counterparts (126). Moreover, Gal-9 expression levels in tumor tissues and cancer cell lines can inversely correlate with tumor progression in various types of cancer, such as breast cancer (139), cervical cancer (140), hepatocellular carcinoma (141), and melanoma (142), yet, high Gal-9 expression can associate with poor clinical outcomes in some other types of cancer (143–145). Conversely, elevated levels of Gal-9 have been reported in sera samples from patients with cancer, including chronic lymphocytic leukemia (146), pancreatic cancer (147), and ovarian cancer (148), with variable correlation with tumor stage and patient survival (146, 148). These observations have prompted speculation regarding a dual role for Gal-9 in mediating tumor metastasis (126). It has been suggested that decreased levels of Gal-9 in tumor tissues compromise cell-cell adhesion and confer anti-apoptotic features to cancer cells, leading to their detachment from the primary site (126). On the other hand, the observed heightened levels of circulating and EC-bound Gal-9 promote CTC-EC interaction, ultimately facilitating tumor cell extravasation and metastasis (126).
Using murine melanoma and colon cancer cell lines, Nobumoto et al. explore the role of Gal-9 in regulating cancer cell adhesion and metastasis (149). Intriguingly, daily i.v. administration of Gal-9 following i.v. inoculation of highly metastatic B16F10 melanoma cells into C57BL/6 mice results in a marked reduction of the number of metastatic nodules in the lung (149). The authors propose that this effect is due to the ability of Gal-9 to block the interaction between adhesion molecules on tumor cells, specifically CD44 and α4β1 integrin, with their respective ligands (hyaluronic acid and VCAM-1) on vascular ECs and ECM, thereby suppressing tumor cell adhesion and metastasis (149).
It is noteworthy that the three variants of Gal-9 modulate the expression levels of E-selectin on colon cancer cells differently, with Gal-9L downregulating E-selectin expression, whereas Gal-9S and Gal-9M upregulate its expression (150). Consequently, they exert varying effects on the adhesion of cancer cells to HUVECs, as evidenced by in vitro experiments conducted by Zhang et al. (150). However, future studies are needed to validate these findings in in vivo models.
4 Therapeutic potential of targeting circulating and EC-bound Gal-3, -8, and -9
There is a rise in research focusing on the development of therapeutic approaches that target galectin-glycan interactions aiming to treat various health conditions (2, 151–155), including inflammatory disorders (156), autoimmune diseases (157), and cancer (158–160). These experimental therapeutics encompass small molecule chemical inhibitors, natural polysaccharides, neutralizing antibodies, and synthetic peptides.
4.1 Small molecule inhibitors
The development of small molecule inhibitors against galectin-ligand interactions has concentrated on small β-galactoside derivatives (158). As carbohydrate molecules are rapidly cleared, degraded, and/or metabolized from circulation, identifying an efficacious, long-lasting inhibitor has been challenging (158). Attempts have been made to modify the C1 and C3 positions of β-galactose to improve the interactive profile within the CRD ligand-binding groove (161, 162). Efforts led by Zetterberg et al. to substitute C1 and C3 with chlorophenylthiols and fluoroaryl triazoles have resulted in the development of an α-thiogalactoside derivative for Gal-3, known as GB1107 (163). The orally active Gal-3 antagonist, GB1107, has demonstrated efficacy in attenuating lung cancer growth and metastasis in both human (A549) and mouse (LLC1) cell lines (22). Similarly, studies by Kim et al. have shown that GB1107 inhibits tumor growth in orthotopic mouse models of gastric cancer (164). Moreover, the next-generation orally active analog of GB1107, GB1211 (165), has shown promising results in combination with an anti-PD-L1 blocking antibody, leading to a significant reduction in the growth of LLC1 syngeneic lung cancer models (21). Currently, GB1211 is undergoing assessment in a phase I/II clinical trial (NCT05240131) in combination with the anti-PDL1 monoclonal antibody (mAb) atezolizumab in patients with non-small cell lung cancer (NSCLC) (22).
4.2 Natural polysaccharides
Studies conducted by Nangia-Makker et al. employ modified citrus pectin (MCP), a nondigestible, water-soluble polysaccharide fiber derived from citrus fruits, and demonstrate inhibitory effects on the adhesion of breast tumor cells to ECs (166). In other studies, MCP has been shown to blunt melanoma (167), thyroid (168), breast (169), and colon (166) tumor growth, and inhibit spontaneous metastasis of a rat prostate cancer model (170). Likewise, MCP variants, such as PectaSol MCP, PectaSol-C MCP, and GCS-100, have also shown the capacity to impede cancer cell proliferation in in vitro experiments (171–173). Another variant, belapectin has shown promise in clinical trials when administered in combination with pembrolizumab for patients with advanced melanoma or head and neck squamous cell carcinoma (NCT02117362 and NCT02575404) (174). However, the use of belapectin as an authentic Gal-3 inhibitor that can directly interact with and inhibit the CRD remains unclear (175).
4.3 Neutralizing antibodies
Neutralizing antibodies are emerging as important therapeutic tools for evaluating their inhibitory effect on galectins in disease (176). Neutralizing antibodies offer several advantages over small molecules, such as greater specificity for each galectin species, established pharmacokinetics/pharmacodynamics and bio-distribution knowledge, and exclusive targeting of extracellular galectins (176). Ortega-Ferreira et al. have developed a novel mAb targeting Gal-3, known as E07, for evaluation in a mouse model of systemic sclerosis (177). Data obtained from this study show that E07 significantly reduces the levels of Natural Killer and CD8+ T cells in the bronchoalveolar fluid, indicative of a protective function in damaged lungs (177). Two Gal-9 antibodies, Gal-Nab1 and Gal-Nab2, have been shown to limit the immunosuppressive activity of regulatory T cells (Tregs) and improve anti-tumor immunity (176). Moreover, Gal-Nab1 and Gal-Nab2 partially cross-react with murine Gal-9 and can be used in syngeneic murine tumor model experiments to assess their overall impact on host–tumor immunology (176). Further encouraging data shows that in vivo as well as in vitro treatment with LYT-200, a humanized anti-Gal-9 mAb developed by PureTech Health, are more effective in killing blood cancer cells compared to conventional treatments (178). The effect of Gal-8 antibodies has also been evaluated by Vicuna et al., revealing that anti-Gal-8 autoantibodies isolated from systemic lupus erythematosus patients can block the interaction of Gal-8 with lymphocyte function-associated antigen-1 (LFA-1), thereby negatively modulating LFA-1 function in the immune system (179).
4.4 Peptides
Several peptides targeting galectins have been reported. In studies using MDA-MB-435 breast cancer cells, the use of a T antigen peptide, specifically the Thomsen-Friedenreich antigen-specific peptide (P-30), significantly inhibits cancer cell adhesion to the vascular endothelium (50–74% reduction in a dose-dependent manner) (180). This inhibitory effect is crucial in impeding the early stages of cancer metastasis (180). Similarly, P-30 interferes with the homotypic aggregation of prostate cancer cells (180). Other Gal-3 peptides, G3–C12, have been shown to decrease metastasis formation in breast cancer in vivo (181). However, there is a need to explore the selectivity of peptide-based inhibitors towards different galectins and potential off-target effects.
Although galectin inhibitors show great promise as therapeutics, their specificity and selectivity within a particular pathological condition require further exploration. Systemic treatment may result in nonspecific effects on uninvolved organs or physiologies, potentially diminishing treatment efficacy (157). Therefore, employing a more targeted delivery approach to affected tissues and cells could enhance the potential for modulating the effects of galectins in vivo without impacting other tissues (157). A deeper understanding of galectin interactions with circulating cells and ECs, coupled with the ability to manipulate these interactions, could greatly enhance therapeutic strategies and improve patient treatment outcomes. Additionally, with their growing role in immunopathologies and cancer, there is a pressing need to develop more potent and selective inhibitors targeting Gal-8 and Gal-9. Nevertheless, targeted galectin therapy holds great promise for enhancing treatment efficacy in patients with inflammatory and autoimmune diseases, as well as cancer.
5 Conclusion
Extensive research on galectins has unfolded over the past few decades, with emphasis on their pivotal roles in diverse biological/pathobiological processes, including inflammation, immune response modulation, cancer progression, and tissue remodeling. Gal-3, -8, and -9 are emerging as key players in EC biology, contributing significantly to circulating cell entry into tissues via modulation of EC adhesion, vascular permeability, cytokine secretion/regulation, immune cell trafficking, neo/tumor angiogenesis, and tumor metastasis (Figure 2). Understanding the multifaceted functions of these galectins in regulating circulating cell adhesion to the vascular endothelium and identifying the underlying mechanisms holds promise for the development of targeted therapeutic interventions for inflammatory and autoimmune diseases and cancer.
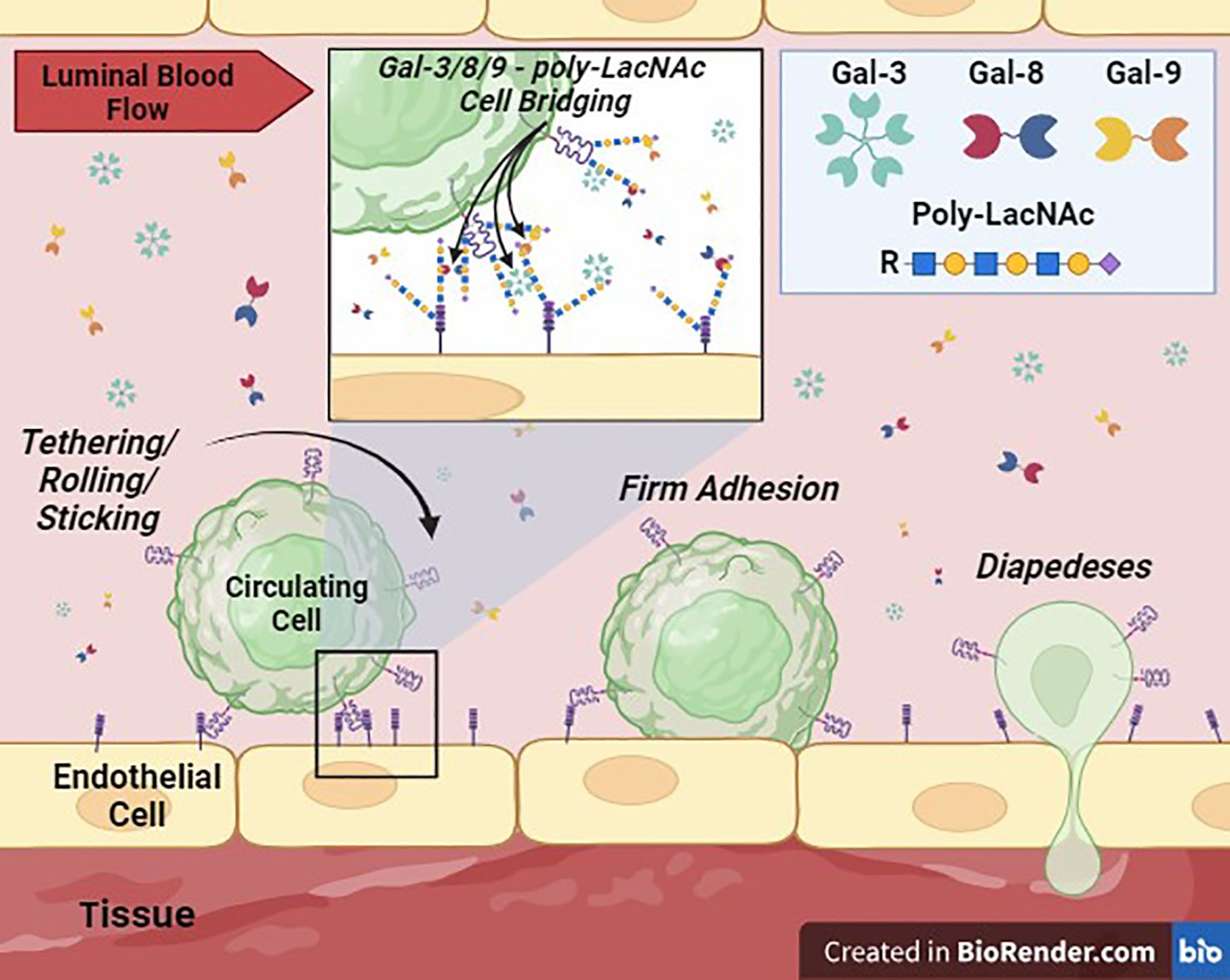
Figure 2 Galectin-Mediated Cell Adhesion to the Vascular Endothelium. This cartoon depicts the proposed critical role of Gal-3, -8, and -9, secreted into the extracellular space (lumen of a blood vessel) potentially by immune, stem, stromal, parenchymal and/or tumor cell sources, in supporting or ‘bridging’ circulating cell adhesion to vascular ECs. Membrane-bound galectins or galectins in solution can theoretically crosslink glycans, such poly-LacNAcs, displayed by surface glycoprotein ligands on cells to mediate intercellular adhesion. These interactions can then facilitate the transition to firm adhesion and diapedesis of cells into tissues.
Author contributions
JS: Writing – original draft, Writing – review & editing. NM: Writing – original draft, Writing – review & editing. LL: Writing – original draft, Writing – review & editing. CD: Writing – original draft, Writing – review & editing, Conceptualization, Data curation, Formal analysis, Funding acquisition, Investigation, Methodology, Project administration, Resources, Software, Supervision, Validation, Visualization.
Funding
The author(s) declare that no financial support was received for the research, authorship, and/or publication of this article.
Acknowledgments
This review was supported by the NIH/National Cancer Institute (NCI) Alliance of Glycobiologists for Cancer Research: Biological Tumor Glycomics Laboratory, U01 grant CA225644 (Dimitroff, CJ) and NIH/NCI R01 grant CA282520 (Dimitroff, CJ). The figures were created using Biorender illustrating tool (Biorender.com). The content is solely the responsibility of the authors and does not necessarily represent the official views of the NIH.
Conflict of interest
The authors declare that the research was conducted in the absence of any commercial or financial relationships that could be construed as a potential conflict of interest.
Publisher’s note
All claims expressed in this article are solely those of the authors and do not necessarily represent those of their affiliated organizations, or those of the publisher, the editors and the reviewers. Any product that may be evaluated in this article, or claim that may be made by its manufacturer, is not guaranteed or endorsed by the publisher.
References
1. Rutledge NS, Muller WA. Understanding molecules that mediate leukocyte extravasation. Curr Pathobiol. Rep. (2020) 8:25–35. doi: 10.1007/s40139-020-00207-9
2. Barthel SR, Gavino JD, Descheny L, Dimitroff CJ. Targeting selectins and selectin ligands in inflammation and cancer. Expert Opin Ther Targets. (2007) 11:1473–91. doi: 10.1517/14728222.11.11.1473
3. Fuhlbrigge RC, King SL, Dimitroff CJ, Kupper TS, Sackstein R. Direct real-time observation of E- and P-selectin-mediated rolling on cutaneous lymphocyte-associated antigen immobilized on Western blots. J Immunol. (2002) 168:5645–51. doi: 10.4049/jimmunol.168.11.5645
4. Konstantopoulos K, McIntire LV. Effects of fluid dynamic forces on vascular cell adhesion. J Clin Invest. (1996) 98:2661–5. doi: 10.1172/JCI119088
5. Alberts B. Molecular biology of the cell. Sixth edition Vol. 1. New York, NY: Garland Science, Taylor and Francis Group (2015). volume (various pagings) p.
6. Kong DH, Kim YK, Kim MR, Jang JH, Lee S. Emerging roles of vascular cell adhesion molecule-1 (VCAM-1) in immunological disorders and cancer. Int J Mol Sci. (2018) 19:1057. doi: 10.3390/ijms19041057
7. Golias C, Batistatou A, Bablekos G, Charalabopoulos A, Peschos D, Mitsopoulos P, et al. Physiology and pathophysiology of selectins, integrins, and IgSF cell adhesion molecules focusing on inflammation. A paradigm model on infectious endocarditis. Cell communication adhesion. (2011) 18:19–32. doi: 10.3109/15419061.2011.606381
8. Cerutti C, Ridley AJ. Endothelial cell-cell adhesion and signaling. Exp Cell Res. (2017) 358:31–8. doi: 10.1016/j.yexcr.2017.06.003
9. Benson BL, Li L, Myers JT, Dorand RD, Gurkan UA, Huang AY, et al. Biomimetic post-capillary venule expansions for leukocyte adhesion studies. Sci Rep. (2018) 8:9328. doi: 10.1038/s41598-018-27566-z
10. Mitroulis I, Alexaki VI, Kourtzelis I, Ziogas A, Hajishengallis G, Chavakis T. Leukocyte integrins: role in leukocyte recruitment and as therapeutic targets in inflammatory disease. Pharmacol Ther. (2015) 147:123–35. doi: 10.1016/j.pharmthera.2014.11.008
11. Varki A, Cummings RD, Esko JD, Stanley P, Hart GW, Aebi M, et al. Essentials of glycobiology. 4th ed. Cold Spring Harbor: Cold Spring Harbor Laboratory Press (2022). p. cm. p.
12. Naithani S, Komath SS, Nonomura A, Govindjee G. Plant lectins and their many roles: Carbohydrate-binding and beyond. J Plant Physiol. (2021) 266:153531. doi: 10.1016/j.jplph.2021.153531
13. Belardi B, Bertozzi CR. Chemical lectinology: Tools for probing the ligands and dynamics of mammalian lectins in vivo. Chem Biol. (2015) 22:983–93. doi: 10.1016/j.chembiol.2015.07.009
14. Inohara H, Raz A. Functional evidence that cell surface galectin-3 mediates homotypic cell adhesion. Cancer Res. (1995) 55:3267–71.
15. Fowlis D, Colnot C, Ripoche MA, Poirier F. Galectin-3 is expressed in the notochord, developing bones, and skin of the postimplantation mouse embryo. Dev Dyn. (1995) 203:241–51. doi: 10.1002/aja.1002030211
16. Zhao Q, Barclay M, Hilkens J, Guo X, Barrow H, Rhodes JM, et al. Interaction between circulating galectin-3 and cancer-associated MUC1 enhances tumour cell homotypic aggregation and prevents anoikis. Mol Cancer. (2010) 9:154. doi: 10.1186/1476-4598-9-154
17. Zhao Q, Guo X, Nash GB, Stone PC, Hilkens J, Rhodes JM, et al. Circulating galectin-3 promotes metastasis by modifying MUC1 localization on cancer cell surface. Cancer Res. (2009) 69:6799–806. doi: 10.1158/0008-5472.CAN-09-1096
18. Zou J, Glinsky VV, Landon LA, Matthews L, Deutscher SL. Peptides specific to the galectin-3 carbohydrate recognition domain inhibit metastasis-associated cancer cell adhesion. Carcinogenesis. (2005) 26:309–18. doi: 10.1093/carcin/bgh329
19. Hughes RC. Secretion of the galectin family of mammalian carbohydrate-binding proteins. Biochim Biophys Acta. (1999) 1473:172–85. doi: 10.1016/S0304-4165(99)00177-4
20. Ferragut F, Cagnoni AJ, Colombo LL, Sanchez Terrero C, Wolfenstein-Todel C, Troncoso MF, et al. Dual knockdown of Galectin-8 and its glycosylated ligand, the activated leukocyte cell adhesion molecule (ALCAM/CD166), synergistically delays in vivo breast cancer growth. Biochim Biophys Acta Mol Cell Res. (2019) 1866:1338–52. doi: 10.1016/j.bbamcr.2019.03.010
21. Mabbitt J, Holyer ID, Roper JA, Nilsson UJ, Zetterberg FR, Vuong L, et al. Resistance to anti-PD-1/anti-PD-L1: galectin-3 inhibition with GB1211 reverses galectin-3-induced blockade of pembrolizumab and atezolizumab binding to PD-1/PD-L1. Front Immunol. (2023) 14:1250559. doi: 10.3389/fimmu.2023.1250559
22. Vuong L, Kouverianou E, Rooney CM, McHugh BJ, Howie SEM, Gregory CD, et al. An orally active galectin-3 antagonist inhibits lung adenocarcinoma growth and augments response to PD-L1 blockade. Cancer Res. (2019) 79:1480–92. doi: 10.1158/0008-5472.CAN-18-2244
23. Zetterberg FR, Diehl C, Hakansson M, Kahl-Knutson B, Leffler H, Nilsson UJ, et al. Discovery of selective and orally available galectin-1 inhibitors. J medicinal Chem. (2023) 66:16980–90. doi: 10.1021/acs.jmedchem.3c01787
24. Aslanis V, Slack RJ, MacKinnon AC, McClinton C, Tantawi S, Gravelle L, et al. Safety and pharmacokinetics of GB1211, an oral galectin-3 inhibitor: a single- and multiple-dose first-in-human study in healthy participants. Cancer Chemother Pharmacol. (2023) 91:267–80. doi: 10.1007/s00280-023-04513-y
25. Dimitroff CJ, Descheny L, Trujillo N, Kim R, Nguyen V, Huang W, et al. Identification of leukocyte E-selectin ligands, P-selectin glycoprotein ligand-1 and E-selectin ligand-1, on human metastatic prostate tumor cells. Cancer Res. (2005) 65:5750–60. doi: 10.1158/0008-5472.CAN-04-4653
26. Rambaruth ND, Dwek MV. Cell surface glycan–lectin interactions in tumor metastasis. Acta histochemica. (2011) 113:591–600. doi: 10.1016/j.acthis.2011.03.001
27. Drickamer K, Taylor ME. Biology of animal lectins. Annu Rev Cell Biol. (1993) 9:237–64. doi: 10.1146/annurev.cb.09.110193.001321
29. Barthel SR, Hays DL, Yazawa EM, Opperman M, Walley KC, Nimrichter L, et al. Definition of molecular determinants of prostate cancer cell bone extravasation. Cancer Res. (2013) 73:942–52. doi: 10.1158/0008-5472.CAN-12-3264
30. Foxall C, Watson S, Dowbenko D, Fennie C, Lasky L, Kiso M, et al. The three members of the selectin receptor family recognize a common carbohydrate epitope, the sialyl Lewis(x) oligosaccharide. J Cell Biol. (1992) 117:895–902. doi: 10.1083/jcb.117.4.895
31. Kolbinger F, Patton JT, Geisenhoff G, Aenis A, Li X, Katopodis AG. The carbohydrate-recognition domain of E-selectin is sufficient for ligand binding under both static and flow conditions. Biochemistry. (1996) 35:6385–92. doi: 10.1021/bi9524528
32. Bevilacqua MP, Pober JS, Mendrick DL, Cotran RS, Gimbrone MA Jr. Identification of an inducible endothelial-leukocyte adhesion molecule. Proc Natl Acad Sci. (1987) 84:9238–42. doi: 10.1073/pnas.84.24.9238
33. Kang HW, Josephson L, Petrovsky A, Weissleder R, Bogdanov A. Magnetic resonance imaging of inducible E-selectin expression in human endothelial cell culture. Bioconjugate Chem. (2002) 13:122–7. doi: 10.1021/bc0155521
34. Wagner DD. The Weibel-Palade body: the storage granule for von Willebrand factor and P-selectin. Thromb haemostasis. (1993) 70:105–10.
35. Weibel ER, Palade GE. New cytoplasmic components in arterial endothelia. J Cell Biol. (1964) 23:101–12. doi: 10.1083/jcb.23.1.101
36. AbuSamra DB, Aleisa FA, Al-Amoodi AS, Jalal Ahmed HM, Chin CJ, Abuelela AF, et al. Not just a marker: CD34 on human hematopoietic stem/progenitor cells dominates vascular selectin binding along with CD44. Blood advances. (2017) 1:2799–816. doi: 10.1182/bloodadvances.2017004317
37. Aigner S, Sthoeger ZM, Fogel M, Weber E, Zarn, Ruppert M, et al. CD24, a mucin-type glycoprotein, is a ligand for P-selectin on human tumor cells. Blood J Am Soc Hematol. (1997) 89:3385–95. doi: 10.1182/blood.V89.9.3385
38. Foster GA, Gower RM, Stanhope KL, Havel PJ, Simon SI, Armstrong EJ. On-chip phenotypic analysis of inflammatory monocytes in atherogenesis and myocardial infarction. Proc Natl Acad Sci. (2013) 110:13944–9. doi: 10.1073/pnas.1300651110
39. Stein JV, Cheng G, Stockton BM, Fors BP, Butcher EC, von Andrian UH. L-selectin–mediated leukocyte adhesion in vivo: microvillous distribution determines tethering efficiency, but not rolling velocity. J Exp Med. (1999) 189:37–50. doi: 10.1084/jem.189.1.37
40. Ivetic A, Hoskins Green HL, Hart SJ. L-selectin: a major regulator of leukocyte adhesion, migration and signaling. Front Immunol. (2019) 10:1068. doi: 10.3389/fimmu.2019.01068
41. Ales E, Sackstein R. The biology of E-selectin ligands in leukemogenesis. Adv Cancer Res. (2023) 157:229–50. doi: 10.1016/bs.acr.2022.07.001
42. Ragupathi G, Damani P, Srivastava G, Srivastava O, Sucheck SJ, Ichikawa Y, et al. Synthesis of sialyl Lewis a (sLe a, CA19–9) and construction of an immunogenic sLe a vaccine. Cancer immunology Immunother. (2009) 58:1397–405. doi: 10.1007/s00262-008-0654-7
43. Lu Y, Lian S, Ye Y, Yu T, Liang H, Cheng Y, et al. S-Nitrosocaptopril prevents cancer metastasis in vivo by creating the hostile bloodstream microenvironment against circulating tumor cells. Pharmacol Res. (2019) 139:535–49. doi: 10.1016/j.phrs.2018.10.020
44. Chase S, Magnani J, Simon S. E-selectin ligands as mechanosensitive receptors on neutrophils in health and disease. Ann Biomed Eng. (2012) 40:849–59. doi: 10.1007/s10439-011-0507-y
45. Fukuda M, Hiraoka N, Yeh J-C. C-type lectins and sialyl Lewis X oligosaccharides: versatile roles in cell–cell interaction. J Cell Biol. (1999) 147:467–70. doi: 10.1083/jcb.147.3.467
46. Silva M, Fung RKF, Donnelly CB, Videira PA, Sackstein R. Cell-specific variation in E-selectin ligand expression among human peripheral blood mononuclear cells: implications for immunosurveillance and pathobiology. J Immunol. (2017) 198:3576–87. doi: 10.4049/jimmunol.1601636
47. Silva M, Martin KC, Mondal N, Sackstein R. Slex expression delineates distinct functional subsets of human blood central and effector memory T cells. J Immunol. (2020) 205:1920–32. doi: 10.4049/jimmunol.1900679
48. Silva Z, Tong Z, Cabral MG, Martins C, Castro R, Reis C, et al. Sialyl Lewisx-dependent binding of human monocyte-derived dendritic cells to selectins. Biochem Biophys Res Commun. (2011) 409:459–64. doi: 10.1016/j.bbrc.2011.05.026
49. Liesveld JL, Sharma N, Aljitawi OS. Stem cell homing: From physiology to therapeutics. Stem Cells. (2020) 38:1241–53. doi: 10.1002/stem.3242
50. Dimitroff CJ, Lechpammer M, Long-Woodward D, Kutok JL. Rolling of human bone-metastatic prostate tumor cells on human bone marrow endothelium under shear flow is mediated by E-selectin. Cancer Res. (2004) 64:5261–9. doi: 10.1158/0008-5472.CAN-04-0691
51. Cohen M. Notable aspects of glycan-protein interactions. Biomolecules. (2015) 5:2056–72. doi: 10.3390/biom5032056
52. Sundd P, Gutierrez E, Koltsova EK, Kuwano Y, Fukuda S, Pospieszalska MK, et al. ‘Slings’ enable neutrophil rolling at high shear. Nature. (2012) 488:399–403. doi: 10.1038/nature11248
53. Marki A, Gutierrez E, Mikulski Z, Groisman A, Ley K. Microfluidics-based side view flow chamber reveals tether-to-sling transition in rolling neutrophils. Sci Rep. (2016) 6:28870. doi: 10.1038/srep28870
54. Al Alwan B, AbuZineh K, Nozue S, Rakhmatulina A, Aldehaiman M, Al-Amoodi AS, et al. Single-molecule imaging and microfluidic platform reveal molecular mechanisms of leukemic cell rolling. Commun Biol. (2021) 4:868. doi: 10.1038/s42003-021-02398-2
55. Borges E, Tietz W, Steegmaier M, Moll T, Hallmann R, Hamann A, et al. P-selectin glycoprotein ligand-1 (PSGL-1) on T helper 1 but not on T helper 2 cells binds to P-selectin and supports migration into inflamed skin. J Exp Med. (1997) 185:573–8. doi: 10.1084/jem.185.3.573
56. Dimitroff CJ, Bernacki RJ, Sackstein R. Glycosylation-dependent inhibition of cutaneous lymphocyte–associated antigen expression: implications in modulating lymphocyte migration to skin. Blood. (2003) 101:602–10. doi: 10.1182/blood-2002-06-1736
57. Arata-Kawai H, Singer MS, Bistrup A, van Zante A, Wang Y-Q, Ito Y, et al. Functional contributions of N-and O-glycans to L-selectin ligands in murine and human lymphoid organs. Am J pathol. (2011) 178:423–33. doi: 10.1016/j.ajpath.2010.11.009
58. Dimitroff CJ, Lee JY, Schor KS, Sandmaier BM, Sackstein R. differential L-selectin binding activities of human hematopoietic cell L-selectin ligands, HCELL and PSGL-1. J Biol Chem. (2001) 276:47623–31. doi: 10.1074/jbc.M105997200
59. Dimitroff CJ, Lee JY, Rafii S, Fuhlbrigge RC, Sackstein R. CD44 is a major E-selectin ligand on human hematopoietic progenitor cells. J Cell Biol. (2001) 153:1277–86. doi: 10.1083/jcb.153.6.1277
60. Alcaide P, King SL, Dimitroff CJ, Lim YC, Fuhlbrigge RC, Luscinskas FW. The 130-kDa glycoform of CD43 functions as an E-selectin ligand for activated Th1 cells in vitro and in delayed-type hypersensitivity reactions in vivo. J Invest Dermatol. (2007) 127:1964–72. doi: 10.1038/sj.jid.5700805
61. Fuhlbrigge RC, King SL, Sackstein R, Kupper TS. CD43 is a ligand for E-selectin on CLA+ human T cells. Blood. (2006) 107:1421–6. doi: 10.1182/blood-2005-05-2112
62. Snapp KR, Ding H, Atkins K, Warnke R, Luscinskas FW, Kansas GS. A novel P-selectin glycoprotein ligand-1 monoclonal antibody recognizes an epitope within the tyrosine sulfate motif of human PSGL-1 and blocks recognition of both P-and L-selectin. Blood J Am Soc Hematol. (1998) 91:154–64. doi: 10.1182/blood.V91.1.154.154_154_164
63. Delacour D, Koch A, Jacob R. The role of galectins in protein trafficking. Traffic. (2009) 10:1405–13. doi: 10.1111/j.1600-0854.2009.00960.x
64. Barondes SH, Cooper DN, Gitt MA, Leffler H. Galectins. Structure and function of a large family of animal lectins. J Biol Chem. (1994) 269:20807–10. doi: 10.1016/S0021-9258(17)31891-4
65. Lahm H, Andre S, Hoeflich A, Kaltner H, Siebert HC, Sordat B, et al. Tumor galectinology: insights into the complex network of a family of endogenous lectins. Glycoconj J. (2004) 20:227–38. doi: 10.1023/B:GLYC.0000025817.24297.17
66. Cho M, Cummings RD. Galectin-1, a β-galactoside-binding lectin in Chinese hamster ovary cells: II. Localization and biosynthesis. J Biol Chem. (1995) 270:5207–12. doi: 10.1074/jbc.270.10.5207
67. Dumic J, Dabelic S, Flögel M. Galectin-3: an open-ended story. Biochim Biophys Acta (BBA)-General Subjects. (2006) 1760:616–35. doi: 10.1016/j.bbagen.2005.12.020
68. Kaminker JD, Timoshenko AV. Expression, regulation, and functions of the galectin-16 gene in human cells and tissues. Biomolecules. (2021) 11:1909. doi: 10.3390/biom11121909
69. Mohammed NB, Antonopoulos A, Dell A, Haslam SM, Dimitroff CJ. The pleiotropic role of galectin-3 in melanoma progression: Unraveling the enigma. In: Advances in Cancer Research, vol. 157. Cambridge, MA: Elsevier (2023). p. 157–93.
70. Earl LA, Bi S, Baum LG. Galectin multimerization and lattice formation are regulated by linker region structure. Glycobiology. (2011) 21:6–12. doi: 10.1093/glycob/cwq144
71. Girotti MR, Salatino M, Dalotto-Moreno T, Rabinovich GA. Sweetening the hallmarks of cancer: Galectins as multifunctional mediators of tumor progression. J Exp Med. (2020) 217. doi: 10.1084/jem.20182041
72. Rabinovich GA. Galectins: an evolutionarily conserved family of animal lectins with multifunctional properties; a trip from the gene to clinical therapy. Cell Death Differ. (1999) 6:711–21. doi: 10.1038/sj.cdd.4400535
73. Sato S, Ouellet N, Pelletier I, Simard M, Rancourt A, Bergeron MG. Role of galectin-3 as an adhesion molecule for neutrophil extravasation during streptococcal pneumonia. J Immunol. (2002) 168:1813–22. doi: 10.4049/jimmunol.168.4.1813
74. Zhong X, Qian X, Chen G, Song X. The role of galectin-3 in heart failure and cardiovascular disease. Clin Exp Pharmacol Physiol. (2019) 46:197–203. doi: 10.1111/1440-1681.13048
75. Hu S, Kuwabara R, Beukema M, Ferrari M, de Haan BJ, Walvoort MT, et al. Low methyl-esterified pectin protects pancreatic β-cells against diabetes-induced oxidative and inflammatory stress via galectin-3. Carbohydr polymers. (2020) 249:116863. doi: 10.1016/j.carbpol.2020.116863
76. Bumba L, Laaf D, Spiwok V, Elling L, Kren V, Bojarova P. Poly-N-acetyllactosamine neo-glycoproteins as nanomolar ligands of human galectin-3: binding kinetics and modeling. Int J Mol Sci. (2018) 19:372. doi: 10.3390/ijms19020372
77. Bocker S, Laaf D, Elling L. Galectin binding to neo-glycoproteins: lacDiNAc conjugated BSA as ligand for human galectin-3. Biomolecules. (2015) 5:1671–96. doi: 10.3390/biom5031671
78. Zhuo Y, Chammas R, Bellis SL. Sialylation of β1 integrins blocks cell adhesion to galectin-3 and protects cells against galectin-3-induced apoptosis. J Biol Chem. (2008) 283:22177–85. doi: 10.1074/jbc.M800015200
79. Nielsen MI, Stegmayr J, Grant OC, Yang Z, Nilsson UJ, Boos I, et al. Galectin binding to cells and glycoproteins with genetically modified glycosylation reveals galectin-glycan specificities in a natural context. J Biol Chem. (2018) 293:20249–62. doi: 10.1074/jbc.RA118.004636
80. Santos SN, Junqueira MS, Francisco G, Vilanova M, Magalhaes A, Dias Baruffi M, et al. O-glycan sialylation alters galectin-3 subcellular localization and decreases chemotherapy sensitivity in gastric cancer. Oncotarget. (2016) 7:83570–87. doi: 10.18632/oncotarget.v7i50
81. Halimi H, Rigato A, Byrne D, Ferracci G, Sebban-Kreuzer C, ElAntak L, et al. Glycan dependence of Galectin-3 self-association properties. PloS One. (2014) 9:e111836. doi: 10.1371/journal.pone.0111836
82. Lepur A, Salomonsson E, Nilsson UJ, Leffler H. Ligand induced galectin-3 protein self-association. J Biol Chem. (2012) 287:21751–6. doi: 10.1074/jbc.C112.358002
83. Fortuna-Costa A, Gomes AM, Kozlowski EO, Stelling MP, Pavao MS. Extracellular galectin-3 in tumor progression and metastasis. Front Oncol. (2014) 4:138. doi: 10.3389/fonc.2014.00138
84. Gittens BR, Bodkin JV, Nourshargh S, Perretti M, Cooper D. Galectin-3: A positive regulator of leukocyte recruitment in the inflamed microcirculation. J Immunol. (2017) 198:4458–69. doi: 10.4049/jimmunol.1600709
85. Rao SP, Wang Z, Zuberi RI, Sikora L, Bahaie NS, Zuraw BL, et al. Galectin-3 functions as an adhesion molecule to support eosinophil rolling and adhesion under conditions of flow. J Immunol. (2007) 179:7800–7. doi: 10.4049/jimmunol.179.11.7800
86. Krishnan V, Bane SM, Kawle PD, Naresh KN, Kalraiya RD. Altered melanoma cell surface glycosylation mediates organ specific adhesion and metastasis via lectin receptors on the lung vascular endothelium. Clin Exp Metastasis. (2005) 22:11–24. doi: 10.1007/s10585-005-2036-2
87. Barrow H, Guo X, Wandall HH, Pedersen JW, Fu B, Zhao Q, et al. Serum galectin-2, -4, and -8 are greatly increased in colon and breast cancer patients and promote cancer cell adhesion to blood vascular endothelium. Clin Cancer Res. (2011) 17:7035–46. doi: 10.1158/1078-0432.CCR-11-1462
88. Topcu TO, Kavgaci H, Gunaldi M, Kocoglu H, Akyol M, Mentese A, et al. The clinical importance of serum galectin-3 levels in breast cancer patients with and without metastasis. J Cancer Res Ther. (2018) 14:S583–S6. doi: 10.4103/0973-1482.176425
89. Nakajima K, Heilbrun LK, Hogan V, Smith D, Heath E, Raz A. Positive associations between galectin-3 and PSA levels in prostate cancer patients: a prospective clinical study-I. Oncotarget. (2016) 7:82266. doi: 10.18632/oncotarget.v7i50
90. Vereecken P, Zouaoui Boudjeltia K, Debray C, Awada A, Legssyer I, Sales F, et al. High serum galectin-3 in advanced melanoma: preliminary results. Clin Exp Dermatol: Exp Dermatol. (2006) 31:105–9. doi: 10.1111/j.1365-2230.2005.01992.x
91. Gendy HE, Madkour B, Abdelaty S, Essawy F, Khattab D, Hammam O, et al. Diagnostic and prognostic significance of serum and tissue galectin 3 expression in patients with carcinoma of the bladder. Curr urol. (2014) 7:185–90. doi: 10.1159/000365673
92. Jiang X-N, Dang Y-F, Gong F-L, Guo X-L. Role and regulation mechanism of Gal-3 in non-small cell lung cancer and its potential clinical therapeutic significance. Chemico-biological interactions. (2019) 309:108724. doi: 10.1016/j.cbi.2019.108724
93. Vereecken P, Awada A, Suciu S, Castro G, Morandini R, Litynska A, et al. Evaluation of the prognostic significance of serum galectin-3 in American Joint Committee on Cancer stage III and stage IV melanoma patients. Melanoma Res. (2009) 19:316–20. doi: 10.1097/CMR.0b013e32832ec001
94. Ahmed H, AlSadek DM. Galectin-3 as a potential target to prevent cancer metastasis. Clin Med Insights: Oncol. (2015) 9:CMO. S29462. doi: 10.4137/CMO.S29462
95. Yu LG, Andrews N, Zhao Q, McKean D, Williams JF, Connor LJ, et al. Galectin-3 interaction with Thomsen-Friedenreich disaccharide on cancer-associated MUC1 causes increased cancer cell endothelial adhesion. J Biol Chem. (2007) 282:773–81. doi: 10.1074/jbc.M606862200
96. Senapati S, Chaturvedi P, Chaney WG, Chakraborty S, Gnanapragassam VS, Sasson AR, et al. Novel INTeraction of MUC4 and galectin: potential pathobiological implications for metastasis in lethal pancreatic cancer. Clin Cancer Res. (2011) 17:267–74. doi: 10.1158/1078-0432.CCR-10-1937
97. Cao Z, Hao Z, Xin M, Yu L, Wang L, Zhang Y, et al. Endogenous and exogenous galectin-3 promote the adhesion of tumor cells with low expression of MUC1 to HUVECs through upregulation of N-cadherin and CD44. Lab Invest. (2018) 98:1642–56. doi: 10.1038/s41374-018-0119-3
98. Gopalkrishnan RV, Roberts T, Tuli S, Kang D-c, Christiansen KA, Fisher PB. Molecular characterization of prostate carcinoma tumor antigen-1, PCTA-1, a human galectin-8 related gene. Oncogene. (2000) 19:4405–16. doi: 10.1038/sj.onc.1203767
99. Chen C, Duckworth CA, Zhao Q, Pritchard DM, Rhodes JM, Yu L-G. Increased circulation of galectin-3 in cancer induces secretion of metastasis-promoting cytokines from blood vascular endothelium. Clin Cancer Res. (2013) 19:1693–704. doi: 10.1158/1078-0432.CCR-12-2940
100. Colomb F, Wang W, Simpson D, Zafar M, Beynon R, Rhodes JM, et al. Galectin-3 interacts with the cell-surface glycoprotein CD146 (MCAM, MUC18) and induces secretion of metastasis-promoting cytokines from vascular endothelial cells. J Biol Chem. (2017) 292:8381–9. doi: 10.1074/jbc.M117.783431
101. Wang L, Du D-D, Zheng Z-X, Shang P-F, Yang X-X, Sun C, et al. Circulating galectin-3 promotes tumor-endothelium-adhesion by upregulating ICAM-1 in endothelium-derived extracellular vesicles. Front Pharmacol. (2022) 13:979474. doi: 10.3389/fphar.2022.979474
102. Zhang DX, Dang XT, Vu LT, Lim CMH, Yeo EYM, Lam BWS, et al. αvβ1 integrin is enriched in extracellular vesicles of metastatic breast cancer cells: A mechanism mediated by galectin-3. J Extracell Vesicles. (2022) 11:e12234. doi: 10.1002/jev2.12234
103. Ma B, Wang T, Li J, Wang Q. Extracellular matrix derived from Wharton’s Jelly-derived mesenchymal stem cells promotes angiogenesis via integrin αVβ3/c-Myc/P300/VEGF. Stem Cell Res Ther. (2022) 13:327. doi: 10.1186/s13287-022-03009-5
104. Ahmadian-Moghadam H, Sadat-Shirazi M-S, Zarrindast M-R. Therapeutic potential of stem cells for treatment of neurodegenerative diseases. Biotechnol letters. (2020) 42:1073–101. doi: 10.1007/s10529-020-02886-1
105. Chu D-T, Nguyen TT, Tien NLB, Tran D-K, Jeong J-H, Anh PG, et al. Recent progress of stem cell therapy in cancer treatment: molecular mechanisms and potential applications. Cells. (2020) 9:563. doi: 10.3390/cells9030563
106. Schäfer R, Schwab M, Siegel G, von Ameln-Mayerhofer A, Buadze M, Lourhmati A, et al. Modulating endothelial adhesion and migration impacts stem cell therapies efficacy. EBioMedicine. (2020) 60:e102987. doi: 10.1016/j.ebiom.2020.102987
107. Sedlar A, Travnickova M, Bojarova P, Vlachova M, Slamova K, Kren V, et al. Interaction between galectin-3 and integrins mediates cell-matrix adhesion in endothelial cells and mesenchymal stem cells. Int J Mol Sci. (2021) 22:5144. doi: 10.3390/ijms22105144
108. Hadari YR, Paz K, Dekel R, Mestrovic T, Accili D, Zick Y. Galectin-8. A new rat lectin, related to galectin-4. J Biol Chem. (1995) 270:3447–53. doi: 10.1074/jbc.270.7.3447
109. Su ZZ, Lin J, Shen R, Fisher PE, Goldstein NI, Fisher PB. Surface-epitope masking and expression cloning identifies the human prostate carcinoma tumor antigen gene PCTA-1 a member of the galectin gene family. Proc Natl Acad Sci U S A. (1996) 93:7252–7. doi: 10.1073/pnas.93.14.7252
110. Cinkir U, Bir LS, Tekin S, Karagulmez AM, Cicek EA, Senol H. Investigation of anti-galectin-8 levels in patients with multiple sclerosis: A consort-clinical study. Medicine. (2023) 102:e32621. doi: 10.1097/MD.0000000000032621
111. Dong GW, Kim J, Park JH, Choi JY, il Cho S, Lim SC. Galectin-8 expression in laryngeal squamous cell carcinoma. Clin Exp otorhinolaryngology. (2009) 2:13–9. doi: 10.3342/ceo.2009.2.1.13
112. Zick Y, Eisenstein M, Goren RA, Hadari YR, Levy Y, Ronen D. Role of galectin-8 as a modulator of cell adhesion and cell growth. Glycoconjugate J. (2002) 19:517–26. doi: 10.1023/B:GLYC.0000014081.55445.af
113. Bidon-Wagner N, Le Pennec JP. Human galectin-8 isoforms and cancer. Glycoconj J. (2002) 19:557–63. doi: 10.1023/B:GLYC.0000014086.38343.98
114. Kumar S, Frank M, Schwartz-Albiez R. Understanding the specificity of human Galectin-8C domain interactions with its glycan ligands based on molecular dynamics simulations. PloS One. (2013) 8:e59761. doi: 10.1371/journal.pone.0059761
115. Cattaneo V, Tribulatti MV, Carabelli J, Carestia A, Schattner M, Campetella O. Galectin-8 elicits pro-inflammatory activities in the endothelium. Glycobiology. (2014) 24:966–73. doi: 10.1093/glycob/cwu060
116. Yamamoto H, Nishi N, Shoji H, Itoh A, Lu L-H, Hirashima M, et al. Induction of cell adhesion by galectin-8 and its target molecules in Jurkat T-cells. J Biochem. (2008) 143:311–24. doi: 10.1093/jb/mvm223
117. Nishi N, Shoji H, Seki M, Itoh A, Miyanaka H, Yuube K, et al. Galectin-8 modulates neutrophil function via interaction with integrin αM. Glycobiology. (2003) 13:755–63. doi: 10.1093/glycob/cwg102
118. Cárcamo C, Pardo E, Oyanadel C, Bravo-Zehnder M, Bull P, Cáceres M, et al. Galectin-8 binds specific β1 integrins and induces polarized spreading highlighted by asymmetric lamellipodia in Jurkat T cells. Exp Cell Res. (2006) 312:374–86. doi: 10.1016/j.yexcr.2005.10.025
119. Friedel M, Andre S, Goldschmidt H, Gabius HJ, Schwartz-Albiez R. Galectin-8 enhances adhesion of multiple myeloma cells to vascular endothelium and is an adverse prognostic factor. Glycobiology. (2016) 26:1048–58. doi: 10.1093/glycob/cww066
120. Chakraborty A, Perez M, Carroll JD, Antonopoulos A, Dell A, Ortega L, et al. Hypoxia controls the glycome signature and galectin-8–ligand axis to promote protumorigenic properties of metastatic melanoma. J Invest Dermatol. (2023) 143:456–69.e8. doi: 10.1016/j.jid.2022.07.033
121. Türeci Ö, Schmitt H, Fadle N, Pfreundschuh M, Sahin U. Molecular definition of a novel human galectin which is immunogenic in patients with Hodgkin's disease. J Biol Chem. (1997) 272:6416–22. doi: 10.1074/jbc.272.10.6416
122. Gentilini LD, Jaworski FM, Tiraboschi C, Perez IG, Kotler ML, Chauchereau A, et al. Stable and high expression of Galectin-8 tightly controls metastatic progression of prostate cancer. Oncotarget. (2017) 8:44654–68. doi: 10.18632/oncotarget.v8i27
123. Wada J, Ota K, Kumar A, Wallner EI, Kanwar YS. Developmental regulation, expression, and apoptotic potential of galectin-9, a beta-galactoside binding lectin. J Clin Invest. (1997) 99:2452–61. doi: 10.1172/JCI119429
124. Leal-Pinto E, Tao W, Rappaport J, Richardson M, Knorr BA, Abramson RG. Molecular cloning and functional reconstitution of a urate transporter/channel. J Biol Chem. (1997) 272:617–25. doi: 10.1074/jbc.272.1.617
125. Wada J, Kanwar YS. Identification and characterization of galectin-9, a novel beta-galactoside-binding mammalian lectin. J Biol Chem. (1997) 272:6078–86. doi: 10.1074/jbc.272.9.6078
126. Heusschen R, Griffioen AW, Thijssen VL. Galectin-9 in tumor biology: a jack of multiple trades. Biochim Biophys Acta. (2013) 1836:177–85. doi: 10.1016/j.bbcan.2013.04.006
127. John S, Mishra R. Galectin-9: From cell biology to complex disease dynamics. J Biosci. (2016) 41:507–34. doi: 10.1007/s12038-016-9616-y
128. Giovannone N, Liang J, Antonopoulos A, Geddes Sweeney J, King SL, Pochebit SM, et al. Galectin-9 suppresses B cell receptor signaling and is regulated by I-branching of N-glycans. Nat Commun. (2018) 9:3287. doi: 10.1038/s41467-018-05770-9
129. Fík Z, Valach J, Chovanec M, Mazánek J, Kodet R, Kodet O, et al. Loss of adhesion/growth-regulatory galectin-9 from squamous cell epithelium in head and neck carcinomas. J Oral Pathol Med. (2013) 42:166–73. doi: 10.1111/j.1600-0714.2012.01185.x
130. Solís D, Maté MJ, Lohr M, Ribeiro JP, López-Merino L, André S, et al. N-domain of human adhesion/growth-regulatory galectin-9: preference for distinct conformers and non-sialylated N-glycans and detection of ligand-induced structural changes in crystal and solution. Int J Biochem Cell Biol. (2010) 42:1019–29. doi: 10.1016/j.biocel.2010.03.007
131. Moar P, Tandon R. Galectin-9 as a biomarker of disease severity. Cell Immunol. (2021) 361:104287. doi: 10.1016/j.cellimm.2021.104287
132. Wiersma VR, de Bruyn M, Helfrich W, Bremer E. Therapeutic potential of Galectin-9 in human disease. Medicinal Res Rev. (2013) 33:E102–E26. doi: 10.1002/med.20249
133. Iqbal AJ, Krautter F, Blacksell IA, Wright RD, Austin-Williams SN, Voisin MB, et al. Galectin-9 mediates neutrophil capture and adhesion in a CD44 and β2 integrin-dependent manner. FASEB J. (2022) 36:e22065. doi: 10.1096/fj.202100832R
134. Aanhane E, Schulkens IA, Heusschen R, Castricum K, Leffler H, Griffioen AW, et al. Different angioregulatory activity of monovalent galectin-9 isoforms. Angiogenesis. (2018) 21:545–55. doi: 10.1007/s10456-018-9607-8
135. Mansour AA, Raucci F, Sevim M, Saviano A, Begum J, Zhi Z, et al. Galectin-9 supports primary T cell transendothelial migration in a glycan and integrin dependent manner. BioMed Pharmacother. (2022) 151:113171. doi: 10.1016/j.biopha.2022.113171
136. Rapoport EM, Ryzhov IM, Slivka EV, Korchagina EY, Popova IS, Khaidukov SV, et al. Galectin-9 as a potential modulator of lymphocyte adhesion to endothelium via binding to blood group H glycan. Biomolecules. (2023) 13:1166. doi: 10.3390/biom13081166
137. Matsushita N, Nishi N, Seki M, Matsumoto R, Kuwabara I, Liu F-T, et al. Requirement of divalent galactoside-binding activity of ecalectin/galectin-9 for eosinophil chemoattraction. J Biol Chem. (2000) 275:8355–60. doi: 10.1074/jbc.275.12.8355
138. Chakraborty A, Staudinger C, King SL, Erickson FC, Lau LS, Bernasconi A, et al. Galectin-9 bridges human B cells to vascular endothelium while programming regulatory pathways. J Autoimmun. (2021) 117:102575. doi: 10.1016/j.jaut.2020.102575
139. Irie A, Yamauchi A, Kontani K, Kihara M, Liu D, Shirato Y, et al. Galectin-9 as a prognostic factor with antimetastatic potential in breast cancer. Clin Cancer Res. (2005) 11:2962–8. doi: 10.1158/1078-0432.CCR-04-0861
140. Liang M, Ueno M, Oomizu S, Arikawa T, Shinonaga R, Zhang S, et al. Galectin-9 expression links to Malignant potential of cervical squamous cell carcinoma. J Cancer Res Clin Oncol. (2008) 134:899–907. doi: 10.1007/s00432-008-0352-z
141. Zhang Z-Y, Dong J-H, Chen Y-W, Wang X-Q, Li C-H, Wang J, et al. Galectin-9 acts as a prognostic factor with antimetastatic potential in hepatocellular carcinoma. Asian Pacific J Cancer Prev. (2012) 13:2503–9. doi: 10.7314/APJCP.2012.13.6.2503
142. Kageshita T, Kashio Y, Yamauchi A, Seki M, Abedin MJ, Nishi N, et al. Possible role of galectin-9 in cell aggregation and apoptosis of human melanoma cell lines and its clinical significance. Int J Cancer. (2002) 99:809–16. doi: 10.1002/ijc.10436
143. Jikuya R, Kishida T, Sakaguchi M, Yokose T, Yasui M, Hashizume A, et al. Galectin-9 expression as a poor prognostic factor in patients with renal cell carcinoma. Cancer Immunol Immunother. (2020) 69:2041–51. doi: 10.1007/s00262-020-02608-6
144. Fu H, Liu Y, Xu L, Liu W, Fu Q, Liu H, et al. Galectin-9 predicts postoperative recurrence and survival of patients with clear-cell renal cell carcinoma. Tumor Biol. (2015) 36:5791–9. doi: 10.1007/s13277-015-3248-y
145. Chen T-C, Chen C-H, Wang C-P, Lin P-H, Yang T-L, Lou P-J, et al. The immunologic advantage of recurrent nasopharyngeal carcinoma from the viewpoint of Galectin-9/Tim-3-related changes in the tumour microenvironment. Sci Rep. (2017) 7:10349. doi: 10.1038/s41598-017-10386-y
146. Wdowiak K, Gallego−Colon E, Francuz T, Czajka−Francuz P, Ruiz−Agamez N, Kubeczko M, et al. Increased serum levels of Galectin−9 in patients with chronic lymphocytic leukemia. Oncol letters. (2019) 17:1019–29. doi: 10.3892/ol.2018.9656
147. Seifert AM, Reiche C, Heiduk M, Tannert A, Meinecke A-C, Baier S, et al. Detection of pancreatic ductal adenocarcinoma with galectin-9 serum levels. Oncogene. (2020) 39:3102–13. doi: 10.1038/s41388-020-1186-7
148. Labrie M, De Araujo LOF, Communal L, Mes-Masson A-M, St-Pierre Y. Tissue and plasma levels of galectins in patients with high grade serous ovarian carcinoma as new predictive biomarkers. Sci Rep. (2017) 7:13244. doi: 10.1038/s41598-017-13802-5
149. Nobumoto A, Nagahara K, Oomizu S, Katoh S, Nishi N, Takeshita K, et al. Galectin-9 suppresses tumor metastasis by blocking adhesion to endothelium and extracellular matrices. Glycobiology. (2008) 18:735–44. doi: 10.1093/glycob/cwn062
150. Zhang F, Zheng M, Qu Y, Li J, Ji J, Feng B, et al. Different roles of galectin-9 isoforms in modulating E-selectin expression and adhesion function in LoVo colon carcinoma cells. Mol Biol Rep. (2009) 36:823–30. doi: 10.1007/s11033-008-9251-2
151. Lau LS, Mohammed NBB, Dimitroff CJ. Decoding strategies to evade immunoregulators galectin-1, -3, and -9 and their ligands as novel therapeutics in cancer immunotherapy. Int J Mol Sci. (2022) 23:15554. doi: 10.3390/ijms232415554
152. Cedeno-Laurent F, Dimitroff CJ. Galectin-1 research in T cell immunity: past, present and future. Clin Immunol. (2012) 142:107–16. doi: 10.1016/j.clim.2011.09.011
153. Cedeno-Laurent F, Opperman MJ, Barthel SR, Hays D, Schatton T, Zhan Q, et al. Metabolic inhibition of galectin-1-binding carbohydrates accentuates antitumor immunity. J Invest Dermatol. (2012) 132:410–20. doi: 10.1038/jid.2011.335
154. Dimitroff CJ. Leveraging fluorinated glucosamine action to boost antitumor immunity. Curr Opin Immunol. (2013) 25:206–13. doi: 10.1016/j.coi.2012.11.003
155. Mohammed NBB, Antonopoulos A, Dell A, Haslam SM, Dimitroff CJ. The pleiotropic role of galectin-3 in melanoma progression: Unraveling the enigma. Adv Cancer Res. (2023) 157:157–93. doi: 10.1016/bs.acr.2022.06.001
156. Chalasani N, Abdelmalek MF, Garcia-Tsao G, Vuppalanchi R, Alkhouri N, Rinella M, et al. Effects of belapectin, an inhibitor of galectin-3, in patients with nonalcoholic steatohepatitis with cirrhosis and portal hypertension. Gastroenterology. (2020) 158:1334–45.e5. doi: 10.1053/j.gastro.2019.11.296
157. Lightfoot A, McGettrick HM, Iqbal AJ. Vascular endothelial galectins in leukocyte trafficking. Front Immunol. (2021) 12:687711. doi: 10.3389/fimmu.2021.687711
158. Mariño KV, Cagnoni AJ, Croci DO, Rabinovich GA. Targeting galectin-driven regulatory circuits in cancer and fibrosis. Nat Rev Drug Discovery. (2023) 22:295–316. doi: 10.1038/s41573-023-00636-2
159. Goud NS, Soukya PSL, Ghouse M, Komal D, Alvala R, Alvala M. Human galectin-1 and its inhibitors: privileged target for cancer and HIV. Mini Rev Med Chem. (2019) 19:1369–78. doi: 10.2174/1389557519666190304120821
160. Tellez-Sanz R, Garcia-Fuentes L, Vargas-Berenguel A. Human galectin-3 selective and high affinity inhibitors. Present state Future Perspective Curr Med Chem. (2013) 20:2979–90. doi: 10.2174/09298673113209990163
161. Tejler J, Salameh B, Leffler H, Nilsson UJ. Fragment-based development of triazole-substituted O-galactosyl aldoximes with fragment-induced affinity and selectivity for galectin-3. Org Biomol Chem. (2009) 7:3982–90. doi: 10.1039/b909091f
162. Rajput VK, Leffler H, Nilsson UJ, Mukhopadhyay B. Synthesis and evaluation of iminocoumaryl and coumaryl derivatized glycosides as galectin antagonists. Bioorg Med Chem Lett. (2014) 24:3516–20. doi: 10.1016/j.bmcl.2014.05.063
163. Zetterberg FR, Peterson K, Johnsson RE, Brimert T, Håkansson M, Logan DT, et al. Monosaccharide derivatives with low-nanomolar lectin affinity and high selectivity based on combined fluorine-amide, phenyl-arginine, sulfur-π, and halogen bond interactions. ChemMedChem. (2018) 13:133–7. doi: 10.1002/cmdc.201700744
164. Kim SJ, Kang HG, Kim K, Kim H, Zetterberg F, Park YS, et al. Crosstalk between WNT and STAT3 is mediated by galectin-3 in tumor progression. Gastric Cancer. (2021) 24:1050–62. doi: 10.1007/s10120-021-01186-5
165. Zetterberg FR, MacKinnon A, Brimert T, Gravelle L, Johnsson RE, Kahl-Knutson B, et al. Discovery and optimization of the first highly effective and orally available galectin-3 inhibitors for treatment of fibrotic disease. J Med Chem. (2022) 65:12626–38. doi: 10.1021/acs.jmedchem.2c00660
166. Nangia-Makker P, Hogan V, Honjo Y, Baccarini S, Tait L, Bresalier R, et al. Inhibition of human cancer cell growth and metastasis in nude mice by oral intake of modified citrus pectin. J Natl Cancer Inst. (2002) 94:1854–62. doi: 10.1093/jnci/94.24.1854
167. Platt D, Raz A. Modulation of the lung colonization of B16-F1 melanoma cells by citrus pectin. J Natl Cancer Inst. (1992) 84:438–42. doi: 10.1093/jnci/84.6.438
168. Menachem A, Bodner O, Pastor J, Raz A, Kloog Y. Inhibition of Malignant thyroid carcinoma cell proliferation by Ras and galectin-3 inhibitors. Cell Death Discovery. (2015) 1:15047. doi: 10.1038/cddiscovery.2015.47
169. Wang L, Li Y-S, Yu L-G, Zhang X-K, Zhao L, Gong F-L, et al. Galectin-3 expression and secretion by tumor-associated macrophages in hypoxia promotes breast cancer progression. Biochem Pharmacol. (2020) 178:114113. doi: 10.1016/j.bcp.2020.114113
170. Pienta KJ, Naik H, Akhtar A, Yamazaki K, Replogle TS, Lehr J, et al. Inhibition of spontaneous metastasis in a rat prostate cancer model by oral administration of modified citrus pectin. J Natl Cancer Inst. (1995) 87:348–53. doi: 10.1093/jnci/87.5.348
171. Yan J, Katz A. PectaSol-C modified citrus pectin induces apoptosis and inhibition of proliferation in human and mouse androgen-dependent and- independent prostate cancer cells. Integr Cancer Ther. (2010) 9:197–203. doi: 10.1177/1534735410369672
172. Chauhan D, Li G, Podar K, Hideshima T, Neri P, He D, et al. A novel carbohydrate-based therapeutic GCS-100 overcomes bortezomib resistance and enhances dexamethasone-induced apoptosis in multiple myeloma cells. Cancer Res. (2005) 65:8350–8. doi: 10.1158/0008-5472.CAN-05-0163
173. Streetly MJ, Maharaj L, Joel S, Schey SA, Gribben JG, Cotter FE. GCS-100, a novel galectin-3 antagonist, modulates MCL-1, NOXA, and cell cycle to induce myeloma cell death. Blood. (2010) 115:3939–48. doi: 10.1182/blood-2009-10-251660
174. Curti BD, Koguchi Y, Leidner RS, Rolig AS, Sturgill ER, Sun Z, et al. Enhancing clinical and immunological effects of anti-PD-1 with belapectin, a galectin-3 inhibitor. J Immunother Cancer. (2021) 9:e002371. doi: 10.1136/jitc-2021-002371
175. Slack RJ, Mills R, Mackinnon AC. The therapeutic potential of galectin-3 inhibition in fibrotic disease. Int J Biochem Cell Biol. (2021) 130:105881. doi: 10.1016/j.biocel.2020.105881
176. Lhuillier C, Barjon C, Baloche V, Niki T, Gelin A, Mustapha R, et al. Characterization of neutralizing antibodies reacting with the 213–224 amino-acid segment of human galectin-9. PloS One. (2018) 13:e0202512. doi: 10.1371/journal.pone.0202512
177. Ortega-Ferreira C, Soret P, Robin G, Speca S, Hubert S, Le Gall M, et al. Antibody-mediated neutralization of galectin-3 as a strategy for the treatment of systemic sclerosis. Nat Commun. (2023) 14:5291. doi: 10.1038/s41467-023-41117-9
178. Henry CJ, Lee M, Filipovic A. Lyt-200, a humanized anti-galectin-9 antibody, exhibits Preclinical Efficacy in Models of Hematological Malignancies. Blood. (2022) 140:8837–. doi: 10.1182/blood-2022-169811
179. Vicuna L, Pardo E, Curkovic C, Doger R, Oyanadel C, Metz C, et al. Galectin-8 binds to LFA-1, blocks its interaction with ICAM-1 and is counteracted by anti-Gal-8 autoantibodies isolated from lupus patients. Biol Res. (2013) 46:275–80. doi: 10.4067/S0716-97602013000300008
180. Glinsky VV, Huflejt ME, Glinsky GV, Deutscher SL, Quinn TP. Effects of Thomsen-Friedenreich antigen-specific peptide P-30 on β-galactoside-mediated homotypic aggregation and adhesion to the endothelium of MDA-MB-435 human breast carcinoma cells. Cancer Res. (2000) 60:2584–8.
Keywords: galectin-3, galectin-8, galectin-9, endothelial cells, cell adhesion
Citation: Souchak J, Mohammed NBB, Lau LS and Dimitroff CJ (2024) The role of galectins in mediating the adhesion of circulating cells to vascular endothelium. Front. Immunol. 15:1395714. doi: 10.3389/fimmu.2024.1395714
Received: 04 March 2024; Accepted: 03 May 2024;
Published: 22 May 2024.
Edited by:
Charalampos Proestos, National and Kapodistrian University of Athens, GreeceReviewed by:
Pierre Busson, Centre National de la Recherche Scientifique (CNRS), FranceMaciej Ugorski, Wroclaw University of Environmental and Life Sciences, Poland
Jasmeen S Merzaban, King Abdullah University of Science and Technology, Saudi Arabia
Copyright © 2024 Souchak, Mohammed, Lau and Dimitroff. This is an open-access article distributed under the terms of the Creative Commons Attribution License (CC BY). The use, distribution or reproduction in other forums is permitted, provided the original author(s) and the copyright owner(s) are credited and that the original publication in this journal is cited, in accordance with accepted academic practice. No use, distribution or reproduction is permitted which does not comply with these terms.
*Correspondence: Charles J. Dimitroff, Y2RpbWl0cm9mZkBmaXUuZWR1