- 1College of Medical Technology, Chengdu University of Traditional Chinese Medicine, Chengdu, China
- 2Chongqing Key Laboratory of Sichuan-Chongqing Co-construction for Diagnosis and Treatment of Infectious Diseases Integrated Traditional Chinese and Western Medicine, Chongqing Traditional Chinese Medicine Hospital, Chongqing, China
In the recent history of the SARS-CoV-2 outbreak, vaccines have been a crucial public health tool, playing a significant role in effectively preventing infections. However, improving the efficacy while minimizing side effects remains a major challenge. In recent years, there has been growing interest in nanoparticle-based delivery systems aimed at improving antigen delivery efficiency and immunogenicity. Among these, self-assembled nanoparticles with varying sizes, shapes, and surface properties have garnered considerable attention. This paper reviews the latest advancements in the design and development of SARS-CoV-2 vaccines utilizing self-assembled materials, highlighting their advantages in delivering viral immunogens. In addition, we briefly discuss strategies for designing a broad-spectrum universal vaccine, which provides insights and ideas for dealing with possible future infectious sarbecoviruses.
1 Introduction
Since the emergence of SARS-CoV-2, the rapid expansion and mutation of the virus have led to a significant increase in morbidity and mortality worldwide. According to the World Health Organization, the COVID-19 pandemic has resulted in more than 776 million confirmed cases, including nearly 7 million deaths (https://www.who.int/). During this period, vaccines have played a crucial role as a powerful health tool for preventing viral infection. The approval of multiple COVID-19 vaccines and vaccination campaigns in more than 200 countries have increased herd immunity, reduced disease severity and mortality, and helped curb the spread of pandemics (1). Current COVID-19 vaccines based on traditional vaccine platforms include CoronaVac, BBIBP-CorV, NVX-CoV2373, and Ad26.COV2-S, etc. (2). Studies from various countries indicate that the efficacy of the inactivated vaccines CoronaVac and BBIBP-CorV ranges from 50.7% to 91% (3). The protein subunit vaccine NVX-CoV2373 has shown an efficacy of 95.6% against the SARS-CoV-2 wild-type (WT) (4). Additionally, one dose of the viral vector vaccine Ad26.COV2-S was 67% effective against moderate disease and 77% effective against severe disease (5). Despite their high efficacy, these vaccine platforms have the weakness of limited immunogenicity, insufficient cross-protection, and the induction of anti-vector immunity (6, 7). These challenges have prompted the development and exploration of novel vaccine platforms that offer higher efficacy and fewer side effects.
The ongoing development of nanotechnology presents new opportunities for creating highly effective vaccines. Nanoparticle vaccines can be broadly categorized into two main types based on their strategies for delivering antigens (8). The first category encapsulates the antigen within the carrier, such as liposomal nanoparticles and polymeric nanoparticles (8). Among the approved COVID-19 vaccines, mRNA vaccines are impressive and stand out, as they encapsulate nucleic acid molecules of the antigen in a vector that delivers them to human host cells (9). The efficacy of mRNA vaccines, represented by BNT162b2 and mRNA-1273, can reach as high as 96.7% and 94.1% against severe disease, respectively (10, 11). The rapid development of mRNA vaccines, driven by revolutionary vaccine technologies, has set historic milestones and significantly advanced the clinical translation of nanoparticle vaccines. However, the widespread use of these vaccines is limited by storage stability (12). The second category of nanoparticle vaccines delivers the antigen to the surface of the carrier, which includes protein nanoparticles, virus-like particles (VLPs), and micelles (8, 13). Proteins with self-assembly capabilities are popular in this field. The varying copy numbers and geometries of self-assembling proteins enable diverse vaccine delivery options. Not only can antigens be densely arranged on their surfaces, but multivalent antigen presentation can also be achieved (14, 15). Additionally, cell-penetrating peptides and antigen-presenting cell (APC)-targeting antibodies can be incorporated onto the surface to enhance APC targeting and boost the immune response (16, 17). Furthermore, the strong thermal and chemical stability of certain self-assembled proteins helps overcome the challenges associated with cold chain transportation in underserved areas. Given these advantages, self-assembled nanoparticle (SANP) vaccines appear promising and will be highlighted and summarized in this review.
2 SANPs
Generally, nanoparticles are tunable particles of nanoscale size that mimic the structural characteristics of viruses (8). When the attractive/repulsive forces within and between SANPs molecules are in equilibrium, the formation of various non-covalent interactions such as electrostatic, hydrophobic, hydrogen bonding, van der Waals, and π-π interactions allow the basic building blocks to assemble autonomously, a process that does not require human intervention (18, 19). Exogenous genes or antigenic proteins can be attached to specific positions in the basic building blocks of SANPs by gene fusion, tag coupling, etc., and antigens are presented to the surface of the particles as the basic building blocks of SANPs self-assemble (20) (Table 1) (Figure 1). Polysaccharides, ferritin, and other natural self-assembling molecules present in various organisms and the engineering self-assembling proteins have been widely used in the development of COVID-19 vaccines (Table 2).
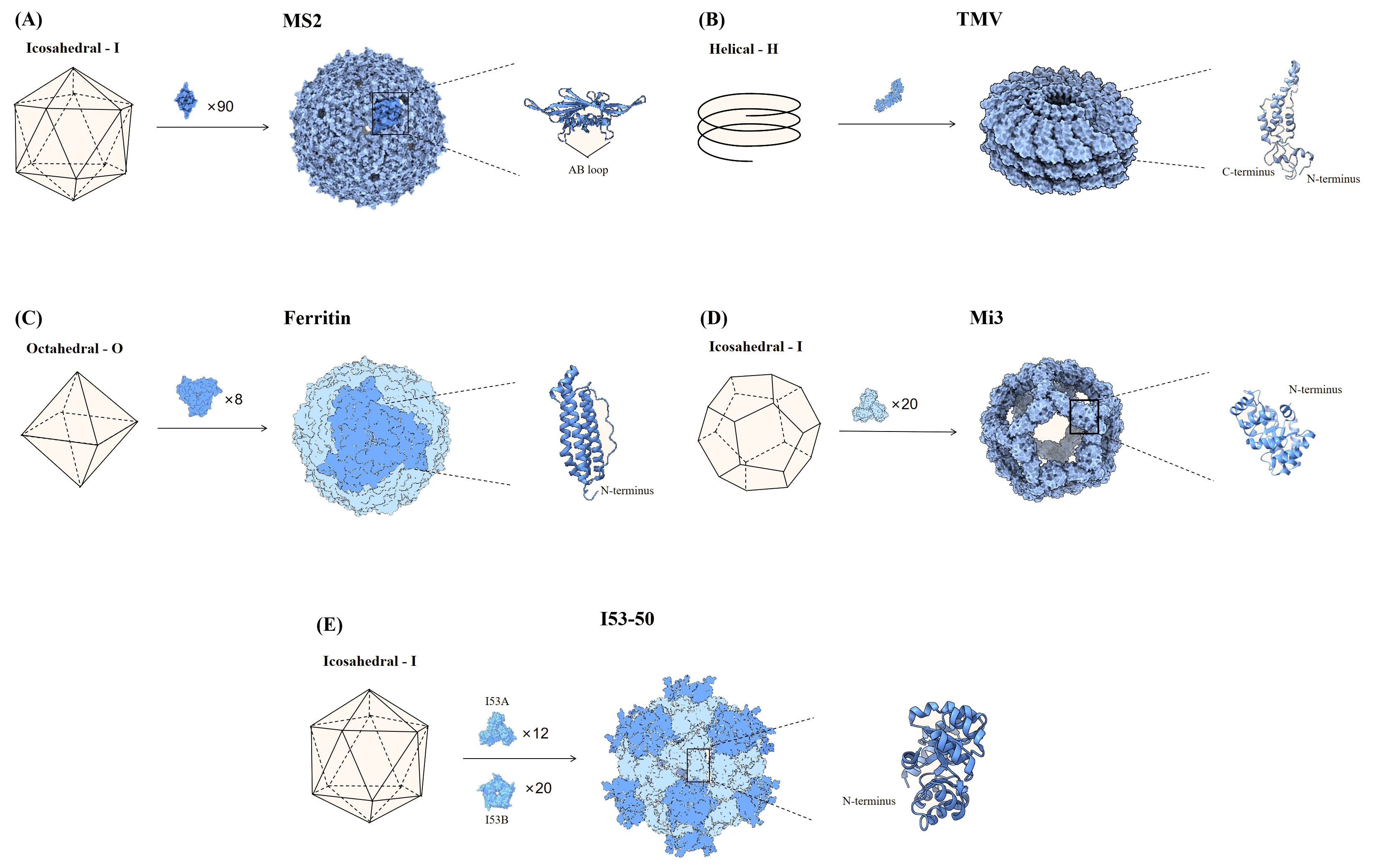
Figure 1. Partial nanoparticle structure and location of delivered antigens. (A) The MS2 phage consists of 90 dimers forming an icosahedron, and the AB loop of the homodimer is used for antigen presentation. (B) TMV is helical particle with the N- and C-terminus of the coat proteins exposed on the surface of the particle, which can be used to display antigens. (C) Ferritin consists of 8 trimers that form a sphere, and the N-terminus of each monomer is used to display antigenic. (D) Mi3 is shown as an icosahedron composed of 60 polymers, with the antigen attached to the N-terminus of each monomer. (E) I53-50 is an icosahedral nanosphere consisting of two parts, trimeric I53A and pentameric I53B. The N-terminus end of the monomeric I53A is connected to the antigen.
2.1 VLPs
VLPs are virus-like man-made nanostructures consisting of all or part of the proteins that make up the viral capsid, but lacking the viral genome (21). These structural proteins can self-assemble when produced in expression organisms such as bacteria, yeast, mammalian, and insect cells (22). However, not all pathogenic proteins can self-assemble into VLPs after overexpression, and 110 viral proteins in 35 virus families can self-assemble (23, 24).
2.1.1 Phage
MS2 phage is an icosahedral structure of 180 monomeric coat proteins self-assembling to form 90 homodimers (20) (Figure 1A). The AB loop in the homodimer is exposed on the surface and is a natural site for delivery of antigen (25). Genomic insertion of exogenous antigens into the AB loop tends to cause protein folding failure, a defect that can be ameliorated by constructing single-stranded homodimers, which greatly improves protein stability (25). Based on this research, AviTag was inserted into the single-stranded dimer and Spike (S) for biotinylation, then S was displayed on the surface of MS2 in the presence of high-affinity biotin-streptavidin. The vaccine provided protection against SARS-CoV-2 after a single injection in hamsters (26).
2.1.2 Plant viruses
Most plant viruses are envelope-free and their viral particles are formed by highly repetitive protein subunits assembled around the genome, which are basically divided into two symmetrical types of structure: helical and icosahedral (27). The most common helical plant virus is tobacco mosaic virus (TMV) (Figure 1B). Since WT TMV lacks exposed active lysine, Royal et al. mutated the N-terminal end of TMV with lysine (TMV NtK) to facilitate the chemical coupling of the SARS-CoV-2 receptor-binding domain (RBD)-Fc with it to construct a vaccine that triggered IgG antibody titers more than 10-fold higher than the RBD alone (28). The adjuvanted nanoparticle vaccine triggered more Th1-oriented cellular immunity, while the non-adjuvanted nanoparticle vaccine resulted in a more balanced Th1 and Th2 response (28). Free monomeric antigens stimulate mainly Th2 responses compared to nanoparticle vaccines (28). Papaya mosaic virus (PapMV) vaccine platforms are rod-like virus-like particles made of PapMV capsid protein self-assembled around single-stranded RNA (ssRNA) (29). PapMV nanoparticles are one of the few vaccine technologies capable of stimulating TLR7/8, other TLR7/8 agonists are not suitable for developing safe vaccines due to their toxicity (30). SRAS-CoV-2 S RBD coupled to PapMV by relying on sortase A, which can trigger the creation of covalent junctions between proteins (30). The sera produced after two immunizations of mice were 54, 111, and 5.2 times more efficient in neutralizing the ancestral SARS-CoV-2 strain, Delta and Omicron variant, respectively, than the uncoupled formulation (30). The RBD-PapMV vaccine can be stored stably at 4 ± 3°C for one month, which is an advantage over mRNA vaccines that require refrigeration (30). The generic T-cell epitopes derived from tetanus toxin was incorporated into the cucumber mosaic virus (CuMV) VLP platform (CuMVTT–VLP) for immune optimization to obtain T-cell help (31). The vaccine constructed by chemically coupling the RBD to CuMVTT-VLP prevents the binding of the RBD to the ACE2 receptor, and in addition, the antibodies induced in mice are effective in neutralizing SARS-CoV-2 (31). Delivery of oversized or positively charged proteins on the outer surface of plant viruses by genetic fusion can affect the yield of plant viruses (27). To improve yields, genetically fused smaller fragments of receptor-binding motif (RBM) was displayed into CuMVTT to construct the vaccine candidates (32). Sera from mice vaccinated with the CuMVTT-RBM vaccine candidate successfully recognized variants E484K, N501Y, K417N/E484K/N501Y and L452R/E484Q (32).
2.2 SANPs based on the ferritin system
Ferritin is one of the oldest molecule that is found in a variety of biological species such as fungi, archaea, bacteria, and viruses. Ferritin has excellent thermal and chemical stability and vaccines made upon it are useful in countries and regions with limited cold chain supply resources (23, 33). Ferritin is an octahedral nanoparticle consisting of eight identical trimeric subunits and therefore can display 24 copies of RBD or 8 copies of stable S-protein trimers on a single particle (8, 34) (Figure 1C). Simultaneously expression of SRAS-CoV-2 S RBD and heptad repeat 1(HR) on a single ferritin nanoparticle induced a 10-100-fold higher neutralizing potency than the monomeric antigens, as well as a safer and more effective Th1-based immune response (35). In mice injected with ferritin nanoparticle vaccines and monomeric antigens, respectively, and lymph nodes (LNs) were isolated for analysis of DCs and macrophages, the nanoparticle vaccine was found to be more susceptible to antigen capture and presentation by APCs, which in turn effectively activated CD8 T cells (35). Utilizing gene fusions, the prefusion-stabilized S has been expressed on the surface of ferritin to induce immune responses against SARS-CoV-2 (36). The vaccine triggered high levels of neutralizing antibodies in rhesus monkeys, an order of magnitude higher than human recovered serum, and induced Th1-biased CD4 T-cell helper responses (36). Serum from mice, immunized with Omicron RBD linked to ferritin by Fc interaction with protein A tags, showed strong neutralizing potency against BA.1 and BA.2 and Th1-biased cellular immune responses (37). Attached the WT strain S protein to ferritin by gene fusion and the nanoparticle vaccine demonstrated similar protection against WT, D614G, and Alpha in Syrian golden hamsters (38). A recombinant spike ferritin nanoparticle vaccine for SARS-CoV-2 has entered human clinical trials for the first time, demonstrating the ability to elicit neutralizing antibody titers exceeding 10,000 against the D614G variant in humans. This vaccine primarily causes self-limiting adverse reactions and shows no evidence of long-term toxicity (39). Additionally, conserved epitopes of pre-existing neutralizing antibodies (CePn) were delivered using Helicobacter pylori ferritin to generate nanoparticle vaccines that protect mice against the Delta, WIV04, and Omicron variants (40). Ferritin nanoparticle vaccines that present the RBD of SARS-CoV, MERS-CoV, and SARS-CoV-2 simultaneously elicited Th1-biased immune responses in mice, providing protection against all three types of β-coronaviruses (41).
2.3 Engineering self-assembling protein
The naturally occurring self-assembling proteins described above are generally chosen as the platform for antigen delivery, however, the number of naturally occurring self-assembling proteins is rather limited (8). With advances in bioengineering, it is currently possible to artificially modify the size of the nanoparticles, the spacing, and the number of antigens presented to further optimize the next generation of vaccines (8). Computationally constructed I53-50 and mi3 (the variant of I3-01) self-assembled nanoparticles have been successfully applied in SARS-CoV-2 vaccine design (42, 43). The mi3 is a dodecahedral one-component nanocage that can deliver 60 monomers on the surface (44) (Figure 1D). Construction of nanoparticle vaccine based on SpyTag/SpyCatcher technology for delivery of RBD on SpyCatcher003-mi3 platform induces stronger immune responses in mice and pigs compared to human recovered serum (45). Patel et al. developed an intranasal nanoparticle vaccine with modified the I3-01 protein into nanoparticles with a flexible SpyCatcher system to display the RBD. On day 5 after infection, the number of virus particles in golden Syrian hamsters vaccinated with the nanoparticle vaccine was 10 times lower than in those vaccinated with the monomer vaccine. Nanoparticle vaccine cleared viral particles from the respiratory tract much faster than the monomer vaccine (46). I53-50 is an icosahedral symmetric protein oligomer composed of 20 trimers (I53-50A) and 12 pentamers (I53-50B) (34, 43) (Figure 1E). The RBDs were linked to I53-50A by genetic fusion, and RBD-I53-50A and I53-50B were expressed independently and then mixed and assembled into nanoparticle vaccine (47). The vaccine induced a 10-fold higher neutralizing activity in mice at lower doses than the pre-fused stable S protein and a robust generating center (GC) B-cell response, favoring a durable humoral response (47). The ΔN1-SpyCatcher system was designed to express SARS-CoV-2 RBD on ferritin, mi3, and I53-50 NPs to construct nanoparticle vaccines, and the sera obtained after immunization of mice with the vaccines showed 8 to 120 times higher neutralizing activity against pseudoviruses and real viruses than sera from mice immunized with monomeric RBD (48). Three different sizes of nanoparticle vaccines were constructed by linking WT S1 to ferritin, mi3, and I50-53 via SpyTag/SpyCatcher system (49). Among them, the I50-53 nanoparticle vaccine induced the highest neutralizing antibody titer in mice, which was 39 times higher than that of the S1 monomeric vaccine, while the mi3 nanoparticle vaccine had the lowest neutralizing potency, which was only 8 times that of the S1 monomeric vaccine (49). The number of antigens on the surface of the particles does not necessarily correlate positively with the neutralizing antibody titer. Overall, all three vaccines produced high levels of neutralizing antibodies against multiple variants of SARS-CoV-2, including the Omicron variant, and protection against SARS-CoV and MERS-CoV (49).
2.4 Natural polysaccharides
Similar to the protein-based SANPs described above, natural polysaccharides can also be assembled through non-covalent interactions (50). A polysaccharide-protein conjugated nanoparticle vaccine against SARS-CoV-2 was constructed by coupling the SARS-CoV-2 recombinant RBD protein to capsular polysaccharide of streptococcus pneumoniae serotype 14 (PPS14) using a reductive amination method (51). This SANP triggers 3.7-fold higher neutralizing antibodies than the monomer RBDs vaccine and induces a Th1-biased immune response in mice while inducing a balanced Th1 and Th2 immune response in rhesus monkeys (51). A new natural polysaccharide was extracted from Bletilla striatal rhizomes (PRBS), which can be used for the development of a SARS-CoV-2 vaccine (52). Compared to the RBD monomeric vaccine, the PRBS-RBD vaccine triggered 5-fold higher neutralizing antibodies in mice and promoted phagocytosis of phagocytes and antigen presentation by B cells (52).
3 Nucleic acid-encoded nanoparticle vaccine platforms assembled in vivo
The production process of SANPs vaccines is mainly prepared in vitro, using protein expression systems and protein purification techniques, which can be costly. The incorporation of components like SpyTag/SpyCatcher necessitates even more expensive purification methods, such as size exclusion chromatography. In the event of an outbreak, these cumbersome preparation steps and high costs may hinder the rapid development and scaling of vaccines. Therefore, the in vivo assembly of self-assembled nanoparticle vaccines could represent a new paradigm in this field (53).
DNA and RNA vaccines are two approaches that enable in vivo self-assembly. Antigens encoded in DNA or RNA can enter host cells through methods like electroporation, jet delivery, or encapsulation in nanoparticles. Once inside, nanoparticle proteins are expressed and successfully assembled in vivo. For instance, Liu et al. designed a DNA plasmid encoding a Spike-HBsAg fusion protein, which was delivered to mice via intramuscular injection combined with electroporation. 2 µg DNA vaccine provided protection for over 7 months and exhibited higher neutralization activity compared to equal doses of soluble protein (54). Similarly, Konrath et al. discovered that DNA vaccines encoding nanoparticle proteins maintained high immunogenicity; a single dose of just 1 µg of a DNA-launched RBD g5.1 120-mer nanovaccine was sufficient to protect mice from SARS-CoV-2 challenges (55). Sun et al. prepared mRNA encoding RBD-ferritin nanoparticles, encapsulated in LNP, to induce a Th1-biased immune response in mice (56). These LNPs can simultaneously encapsulate three mRNAs corresponding to different mutant strains (WT, Alpha, Beta) of RBD, resulting in a trivalent vaccine that generates broad-spectrum neutralizing titers against a variety of pseudovirus (56).
4 Developing broad-spectrum vaccines with nanoparticle platforms
The RBD in S is a hot region of constant mutation and vaccines made using the WT S have shown varying degrees of reduced protection against multiple variants such as Alpha, Beta, Gamma, Delta, Lambda, and Omicron (38, 49). Thus, one of the biggest hurdles for a SARS-CoV-2 vaccine is the development of a universal vaccine that can overcome the viral immune escape and maintain neutralizing efficacy against multiple variants of concern.
Cocktail nanoparticle vaccines and mosaic nanoparticle vaccines are capable of inducing a wider range of neutralizing antibodies and are commonly used to construct universal vaccines against SARS-CoV-2. Cocktail nanoparticles are combinations of three or more independently assembled nanoparticles expressing only a single type of antigen (Figure 2). For instance, the mixing of three ferritin nanoparticle vaccines linked to D614G, Beta, and Delta RBD in a 1:1:1 ratio forms a cocktail of nanoparticle vaccines (57). There were no pathological changes in the lungs and the viral RNA was almost undetectable in mice injected with this trivalent vaccine after infection with the SARS-CoV-2 variant (57). Co-injection of D614G and Beta ferritin nanoparticle vaccines as booster shots into rhesus monkeys effectively induced antibodies to neutralize multiple variants of interest, including D614G, Alpha, Beta, Gamma, and Delta, and increased the neutralization potency by 21-110-fold (58). Three different RBD antigens derived from WT, Beta, and Epsilon were used to construct three ferritin nanoparticle vaccines utilizing genetic fusion, respectively (59). In mice immunized with monovalent and polyvalent vaccines, respectively, the number of memory cells in the LN was higher in the mice of the polyvalent vaccine group (59). In addition, mosaic nanoparticle vaccines can bind multiple antigens on the surface of a single nanoparticle, where the nanoparticles were mostly selected from I53-50 or mi3 (Figure 2). A quadrivalent mosaic nanoparticle vaccine was constructed by co-associating S of WT, Alpha, Beta, and Gamma with I50-53, which improved the breadth of neutralizing antibodies and showed 1.6-4 times higher neutralizing antibody titers against several variants of SARS-CoV-2 (Alpha, Beta, Gamma, Omicron, Lambda, Eta, and D614G) than the WT monovalent nanoparticle vaccine (60). Bivalent nanoparticle vaccines co-expressing WT and Beta S on I53-50 nanoparticles triggered 6.2, 4.7, 7.3, and 4.6-fold higher neutralizing antibody titers against Beta, Gamma, and Omicron BA.1 and BA.4/5, respectively, in rabbits than WT monovalent nanoparticle vaccines (61).
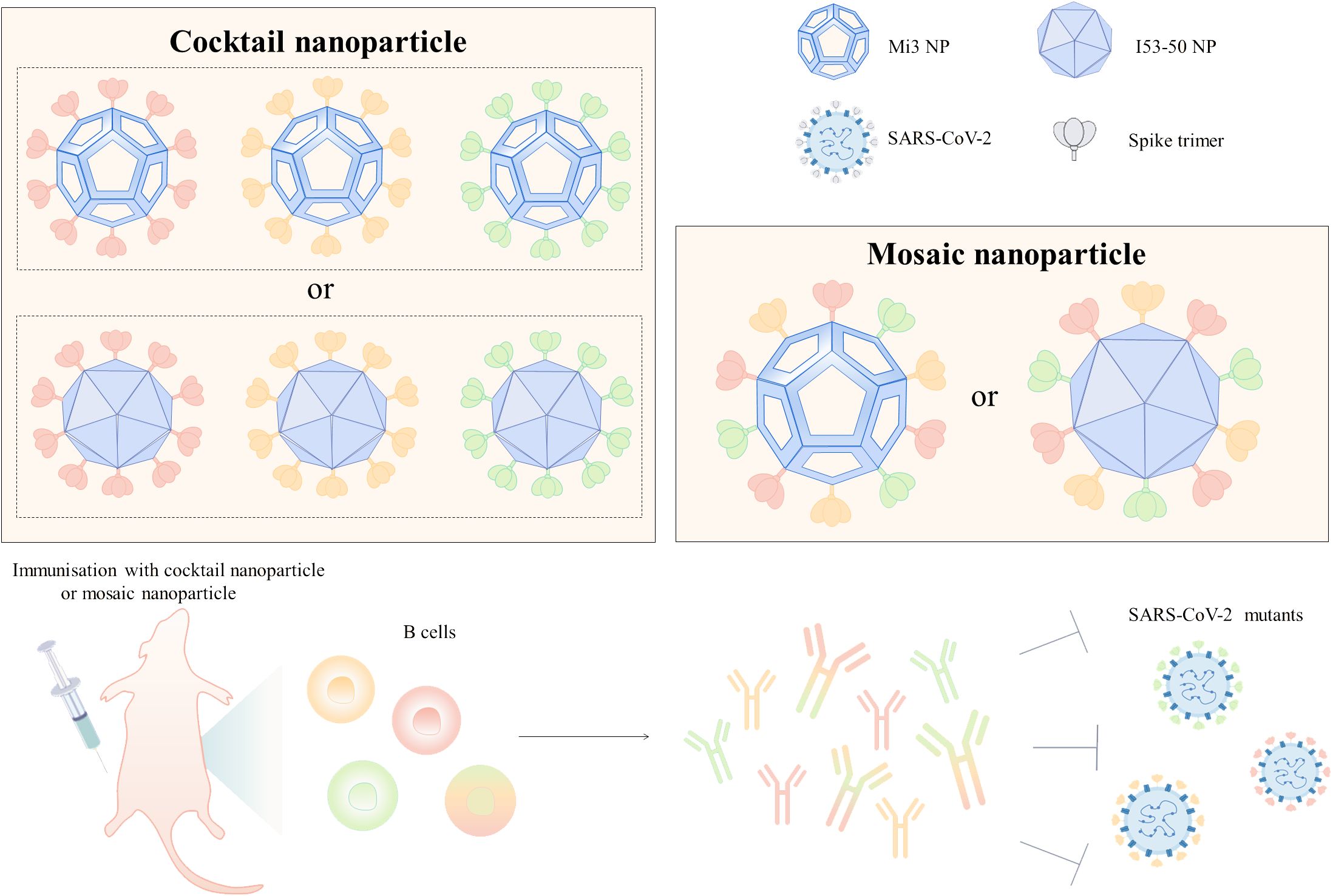
Figure 2. Design of broad-spectrum vaccines based on nanoparticle platforms. Cocktail or mosaic nanoparticle vaccines are commonly used strategies for broad-spectrum vaccine design, and Mi3 and I53-50 are mostly used for broad-spectrum vaccine design because of the high number of antigens that can be delivered on their surfaces. Mice injected with cocktail or mosaic nanoparticle vaccines developed resistance to infection with multiple SARS-CoV-2 mutant strains. In the figure, the different colored S trimers are from different strains.
The strategy of the mosaic nanoparticle vaccine is also applicable to the development of a universal vaccine against sarbecovirus. Bivalent mosaic nanoparticle vaccines co-expressing S constructs of SARS-CoV and SARS-CoV-2 on the surface of I53-50 nanoparticles produce potent and high-level neutralizing antibodies against SARS-CoV, SHC014, and WIV1 (61). An 8-valent mosaic mi3 nanoparticle vaccine expressing eight different zoonotic coronavirus RBDs simultaneously increased the breadth of antibody recognition of heterologous RBDs compared to homologous SARS-CoV-2 RBD nanoparticle vaccines (62). Similarly, an octavalent mosaic mi3 vaccine co-expressing SARS-CoV-2 Beta and seven animal sarbecoviruses RBDs increased targeting of conserved epitopes and induced antibodies binding mismatched viruses such as WA1, Delta, and Omicron RBDs in non-human primates, offering the possibility of preventing future sarbecoviruses -induced diseases (63). The mosaic-8 nanoparticles are currently undergoing clinical trials. However, the large-scale production of this type of vaccine is constrained by the need to produce nine components (eight different receptor-binding domains, or RBDs, along with SpyCatcher003-mi3). To address this challenge, Hills et al. have introduced an innovative solution by developing multiviral quartet nanocages. In this approach, four viral RBDs (SHC014, Rs4081, RaTG13, and SARS-CoV-2) are linked into a single peptide chain. This chain is then assembled into SpyCatcher003-mi3 using SpyTag, resulting in a nanoparticle vaccine with a branching morphology that elicits a broad immune response against sarbecoviruses (64). The development of broad-spectrum vaccines utilizing mosaic nanoparticles should prioritize the spatial arrangement and proportions of heterotypic antigens. Simply increasing the number of antigens without careful consideration can lead to a reduction in neutralization potency and may trigger excessive inflammatory responses (65).
5 Nanoparticle vaccine activates immune cells - APCs, B cells, and T cells
Vaccine antigens primarily activate the body’s adaptive immune system to fight off the invasion of foreign pathogens. The LNs are the primary site for the initiation of the adaptive immune response and therefore antigens first need to be transported from the injection site into the LN. Nanoparticles of different sizes enter the LN through different pathways after injection into the interstitium (66) (Figure 3A). Particles that are too small (<5nm) enter the capillaries because they diffuse faster than convection and vaccination is ineffective because of clearance effects (66, 67). Particles between 20 and 200 nm are effective in entering the lymphatic system (68), with medium-sized particles (20 to 50 nm) being the best size as they are more likely to be convectively governed to enter the lymphatic vessels (66). Larger particles above 50 nm slow down the rate of entry into the lymph vessels as they increase in size (66). Most protein-based SANPs and VLPs are concentrated in the 20-200 nm size range and are suitable for direct LN drainage. Particles over 200-500 nm become trapped in the interstitial space, increasing the chance of contact with APCs such as DCs in peripheral tissues and transport to lymph vessels via APCs (69).
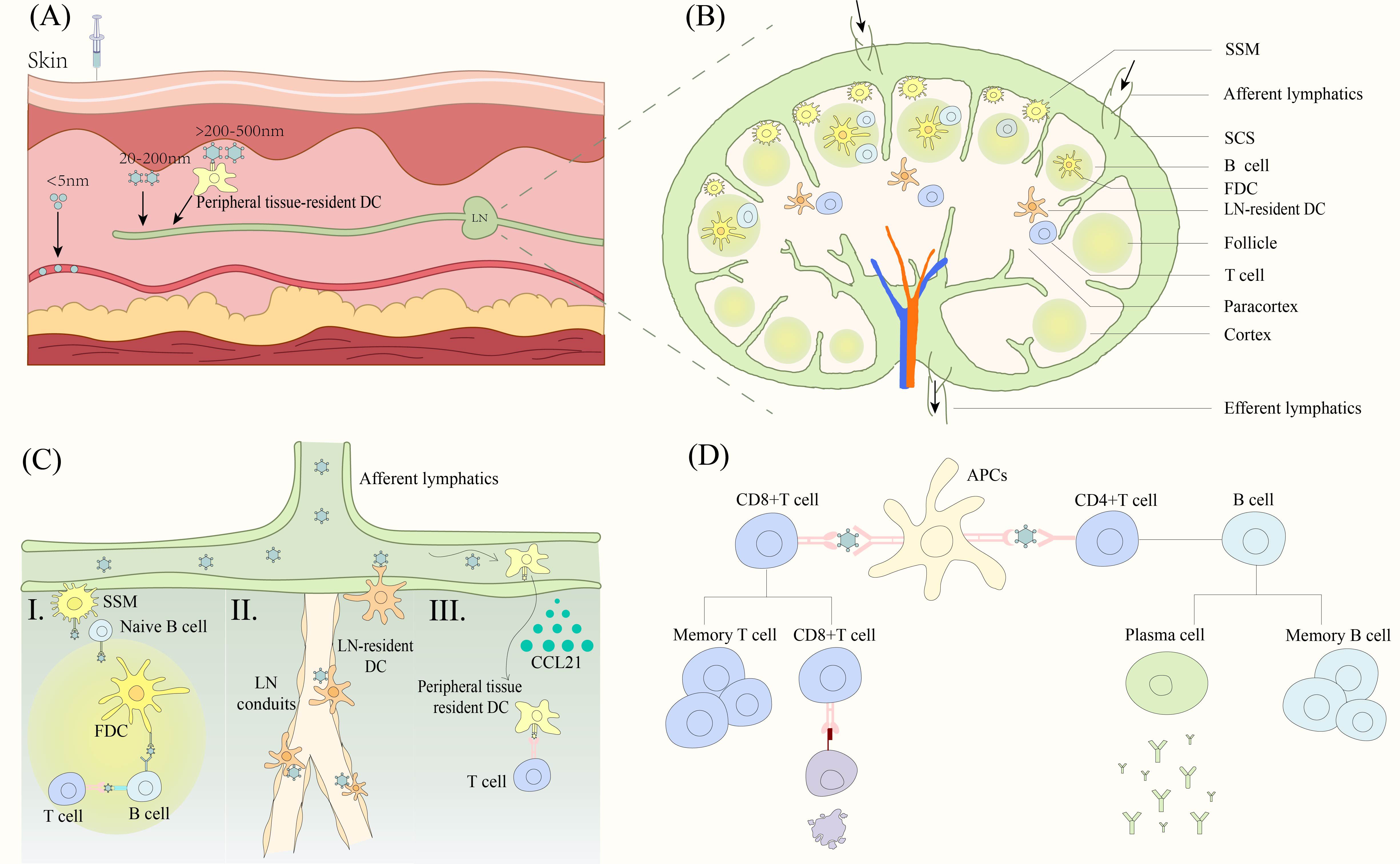
Figure 3. Nanoparticle vaccine triggers the immune response. (A) Nanoparticles of different sizes enter the LN. Particles smaller than 5 nm mainly enter capillaries. 20-200 nm particles drain directly into LNs. Particles larger than 200-500 nm enter the LNs by active transport of DC cells. (B) Structure of LN and cellular distribution. (C) Nanoparticles contacted with different APCs. (I) Antigen is presented to B cells via SSM, FDC to trigger an adaptive immune response. (II) LN resident DCs capture antigen at two sites. Antigens are captured by DCs near the lymphatic endothelium after entering the SSM or by DCs near the duct after entering the lymphatic conduit. (III) DCs that have captured antigen in peripheral tissues migrate to the paracortical region of LNs in response to low to high concentrations of CCL21, presenting the antigen to T cells to trigger an adaptive immune response. (D) APC presents antigens to T cells and B cells to trigger a cellular and humoral immune response respectively. DC, dendritic cells. LN, lymph nodes. SSM, subcapsular sinus macrophage. SCS, subcapsular sinus. FDC, follicular dendritic cells. APCs, antigen presenting cells.
The nanoparticles entering the lymphatic vessels reach the LN with the lymphatic fluid. The particles are distributed in the subcapsular sinus (SCS) and over time penetrate deeper into the lymphatic parenchyma such as the cortical and paracortical areas (69). The SCS is rich in macrophages and below it is the paracortical area with T cells and resident DCs as well as the cortical area with B cells (70, 71) (Figure 3B). The nanoparticles in turn trigger T and B cell responses upon contact with lymphatically resident APCs. However, the exact pathway of movement of nanoparticles deep into the LN parenchyma is not fully understood.
Deeper transfer of particles from the SCS and around the B-cell follicle to the interior of the B-cell follicle can be observed in the experiments of Manolova et al. (69). This process may be mediated by subcapsular sinus macrophages (SSM) (Figure 3C). SSM transports antigen to naive B cells, which transport it from the SCS to the follicle and the follicular dendritic cells (FDCs) in a complement receptor-dependent manner (72). FDCs endocytose antigen and present it to GC B cells, which are activated and differentiated into antibody-producing plasma cells or memory cells with the help of T cells (72). It is also possible that nanoparticles can penetrate from the lymphatic ducts deep into the T cell zone of the LN (Figure 3C). It is often thought that particles larger than 4-5 nm are excluded from the conduits (73), however, this is not absolute and cowpox and Zika particles have been shown to penetrate deeply into the parenchyma through the lymphatics (74). LN-resident DCs located near the LN conduits capture and present antigen to the T cells (74). In addition, there is a specialized population of DCs residing in the lymphatic endothelium, capable of capturing granular antigens from the lymphatic sinus (75) (Figure 3C). Finally, a few particles are possibly captured by peripheral tissue-resident DCs (69) (Figure 3C). Lymphatic endothelial cells secrete CCL21 chemokine, and CCRL1 can bind and remove CCL21 from the lumen of the lymphatic sinus, thus creating a gradient of high to low CCL21 concentrations from the LN parenchyma (inside the LN) to the subepithelial lymphatic sinus (LN margin), and peripheral tissue-resident DCs migrate to the paracortical layer by upregulating the chemokine receptor CCR7, which in turn presents antigen to surrounding T cells (76, 77).
Based on all these pathways, nanoparticle vaccines are exposed to various APCs and are phagocytosed into the endosome (66). CD8+T cells recognize the antigens presented by major histocompatibility complex (MHC) class I molecules through the T cell receptor (TCR), which are activated in the presence of co-stimulatory factors and cytokines, and subsequently activated CD8+T cells induce cytotoxicity to kill the virus-infected cells (78). CD4 helper T cells are activated by TCR recognition of peptides binding to MHC class II molecules on the surface of DCs (78). Activated CD4 helper T cells differentiate into memory T cells that play a role in secondary immunity and follicular T cells that secrete molecules such as IL-21 to aid B cell differentiation (79). B cells with the help of T cells differentiate into memory cells as well as plasma cells, which produce antibodies to bind to the virus (80) (Figure 3D).
6 Conclusion and perspective
Nanoparticle vaccines have great potential in vaccine development, where multiple vectors are applied, each with their own characteristics, to address the limitations of current vaccine technology. The diversity of VLP structures and their expression hosts, which are upwards of 170, make them attractive. The strong thermal and chemical stability of ferritin makes it ideal for carrying antigens. Certain modifications of proteins by computational design can further improve the stability or yield of nanoparticles. Engineering SANPs also favored the design of universal vaccines. Bacterial polysaccharides vaccines are well developed and the coupling of viral proteins to polysaccharides can prevent bacterial and viral co-infection. Nucleic acid-emitting nanovaccines offer a promising new approach that enables the in vivo synthesis of self-assembled proteins. This method helps to overcome the manufacturing and cost challenges typically associated with in vitro production. In future, the emergence of new viruses is unpredictable, and accelerating the development of nanoparticle vaccines could shorten the response time to unknown epidemics. Although there is no doubt that nanoparticle vaccines can improve immunogenicity, we should still work on continuous development and optimization of nanoparticle vaccines, such as improving the delivery efficiency of the carriers and optimizing the distribution and quantity of antigens. In addition, the deployment of viral vaccines still varies globally, and vaccine cost and storage are issues that need to be urgently addressed. Therefore, it is necessary to develop more stable and easier-to-produce nanoparticle vaccines. In conclusion, nanoparticle vaccines are an effective way to combat infectious viruses, and it is believed that they can be used to improve the health of all human beings in the near future.
Author contributions
KY: Writing – original draft. YZ: Writing – review & editing. XW: Writing – review & editing. JL: Writing – review & editing. JG: Writing – review & editing, Conceptualization.
Funding
The author(s) declare financial support was received for the research, authorship, and/or publication of this article. This work was supported by the COVID-19 Emergency Project of Sichuan Provincial Administration of TCM(2021XYCZ007).
Acknowledgments
JG conceived ideas and supervised the work. KY, YZ, wrote the initial draft and made figures. Other authors reviewed and edited the manuscript. All authors reviewed and approved the manuscript before submission.
Conflict of interest
The authors declare that the research was conducted in the absence of any commercial or financial relationships that could be construed as a potential conflict of interest.
Publisher’s note
All claims expressed in this article are solely those of the authors and do not necessarily represent those of their affiliated organizations, or those of the publisher, the editors and the reviewers. Any product that may be evaluated in this article, or claim that may be made by its manufacturer, is not guaranteed or endorsed by the publisher.
References
1. Chavda VP, Ghali E, Balar PC, Chauhan SC, Tiwari N, Shukla S, et al. Protein subunit vaccines: Promising frontiers against COVID-19. J Control Release. (2024) 366:761–82. doi: 10.1016/j.jconrel.2024.01.017
2. Li M, Wang H, Tian L, Pang Z, Yang Q, Huang T, et al. COVID-19 vaccine development: milestones, lessons and prospects. Signal Transduct Target Ther. (2022) 7:146. doi: 10.1038/s41392-022-00996-y
3. Kim JH, Marks F, Clemens JD. Looking beyond COVID-19 vaccine phase 3 trials. Nat Med. (2021) 27:205–11. doi: 10.1038/s41591-021-01230-y
4. Mahase E. Covid-19: Novavax vaccine efficacy is 86% against UK variant and 60% against South African variant. Bmj. (2021) 372:n296. doi: 10.1136/bmj.n296
5. Sadoff J, Gray G, Vandebosch A, Cárdenas V, Shukarev G, Grinsztejn B, et al. Safety and efficacy of single-dose ad26.COV2.S vaccine against covid-19. N Engl J Med. (2021) 384:2187–201. doi: 10.1056/NEJMoa2101544
6. García-Sastre A, Mena I. Novel vaccine strategies against emerging viruses. Curr Opin Virol. (2013) 3:210–6. doi: 10.1016/j.coviro.2013.02.001
7. Fausther-Bovendo H, Kobinger GP. Pre-existing immunity against Ad vectors: humoral, cellular, and innate response, what’s important? Hum Vaccin Immunother. (2014) 10:2875–84. doi: 10.4161/hv.29594
8. Vu MN, Kelly HG, Kent SJ, Wheatley AK. Current and future nanoparticle vaccines for COVID-19. EBioMedicine. (2021) 74:103699. doi: 10.1016/j.ebiom.2021.103699
9. Fang E, Liu X, Li M, Zhang Z, Song L, Zhu B, et al. Advances in COVID-19 mRNA vaccine development. Signal Transduct Target Ther. (2022) 7:94. doi: 10.1038/s41392-022-00950-y
10. Thomas SJ, Moreira ED Jr., Kitchin N, Absalon J, Gurtman A, Lockhart S, et al. Safety and Efficacy of the BNT162b2 mRNA Covid-19 Vaccine through 6 Months. N Engl J Med. (2021) 385:1761–73. doi: 10.1056/NEJMoa2110345
11. Baden LR, El Sahly HM, Essink B, Kotloff K, Frey S, Novak R, et al. Efficacy and safety of the mRNA-1273 SARS-coV-2 vaccine. N Engl J Med. (2021) 384:403–16. doi: 10.1056/NEJMoa2035389
12. Crommelin DJA, Anchordoquy TJ, Volkin DB, Jiskoot W, Mastrobattista E. Addressing the cold reality of mRNA vaccine stability. J Pharm Sci. (2021) 110:997–1001. doi: 10.1016/j.xphs.2020.12.006
13. Nguyen B, Tolia NH. Protein-based antigen presentation platforms for nanoparticle vaccines. NPJ Vaccines. (2021) 6:70. doi: 10.1038/s41541-021-00330-7
14. Machhi J, Shahjin F, Das S, Patel M, Abdelmoaty MM, Cohen JD, et al. Nanocarrier vaccines for SARS-coV-2. Adv Drug Delivery Rev. (2021) 171:215–39. doi: 10.1016/j.addr.2021.01.002
15. Irvine DJ, Read BJ. Shaping humoral immunity to vaccines through antigen-displaying nanoparticles. Curr Opin Immunol. (2020) 65:1–6. doi: 10.1016/j.coi.2020.01.007
16. Coolen AL, Lacroix C, Mercier-Gouy P, Delaune E, Monge C, Exposito JY, et al. Poly(lactic acid) nanoparticles and cell-penetrating peptide potentiate mRNA-based vaccine expression in dendritic cells triggering their activation. Biomaterials. (2019) 195:23–37. doi: 10.1016/j.biomaterials.2018.12.019
17. Vu MN, Pilkington EH, Lee WS, Tan HX, Davis TP, Truong NP, et al. Engineered ferritin nanoparticle vaccines enable rapid screening of antibody functionalization to boost immune responses. Adv Healthc Mater. (2023) 12:e2202595. doi: 10.1002/adhm.202202595
18. Whitesides GM, Grzybowski B. Self-assembly at all scales. Science. (2002) 295:2418–21. doi: 10.1126/science.1070821
19. Negahdaripour M, Golkar N, Hajighahramani N, Kianpour S, Nezafat N, Ghasemi Y. Harnessing self-assembled peptide nanoparticles in epitope vaccine design. Biotechnol Adv. (2017) 35:575–96. doi: 10.1016/j.biotechadv.2017.05.002
20. Sung HD, Kim N, Lee Y, Lee EJ. Protein-based nanoparticle vaccines for SARS-coV-2. Int J Mol Sci. (2021) 22:13445. doi: 10.3390/ijms222413445
21. Fuenmayor J, Gòdia F, Cervera L. Production of virus-like particles for vaccines. N Biotechnol. (2017) 39:174–80. doi: 10.1016/j.nbt.2017.07.010
22. Gupta R, Arora K, Roy SS, Joseph A, Rastogi R, Arora NM, et al. Platforms, advances, and technical challenges in virus-like particles-based vaccines. Front Immunol. (2023) 14:1123805. doi: 10.3389/fimmu.2023.1123805
23. Kim YI, Kim D, Yu KM, Seo HD, Lee SA, Casel MAB, et al. Development of spike receptor-binding domain nanoparticles as a vaccine candidate against SARS-coV-2 infection in ferrets. mBio. (2021) 12:e00230–21. doi: 10.1128/mBio.00230-21
24. Chroboczek J, Szurgot I, Szolajska E. Virus-like particles as vaccine. Acta Biochim Polonica. (2014) 61:531–9. doi: 10.18388/abp.2014_1875
25. Peabody DS, Manifold-Wheeler B, Medford A, Jordan SK, do Carmo Caldeira J, Chackerian B. Immunogenic display of diverse peptides on virus-like particles of RNA phage MS2. J Mol Biol. (2008) 380:252–63. doi: 10.1016/j.jmb.2008.04.049
26. Chiba S, Frey SJ, Halfmann PJ, Kuroda M, Maemura T, Yang JE, et al. Multivalent nanoparticle-based vaccines protect hamsters against SARS-CoV-2 after a single immunization. Commun Biol. (2021) 4:597. doi: 10.1038/s42003-021-02128-8
27. Steele JFC, Peyret H, Saunders K, Castells-Graells R, Marsian J, Meshcheriakova Y, et al. Synthetic plant virology for nanobiotechnology and nanomedicine. Wiley Interdiscip Rev Nanomed Nanobiotechnol. (2017) 9:e1447. doi: 10.1002/wnan.1447
28. Royal JM, Simpson CA, McCormick AA, Phillips A, Hume S, Morton J, et al. Development of a SARS-coV-2 vaccine candidate using plant-based manufacturing and a tobacco mosaic virus-like nano-particle. Vaccines (Basel). (2021) 9:1347. doi: 10.3390/vaccines9111347
29. Carignan D, Herblot S, Laliberté-Gagné M-È, Bolduc M, Duval M, Savard P, et al. Activation of innate immunity in primary human cells using a plant virus derived nanoparticle TLR7/8 agonist. Nanomedicine: Nanotechnology Biol Med. (2018) 14:2317–27. doi: 10.1016/j.nano.2017.10.015
30. Olivera-Ugarte SM, Bolduc M, Laliberté-Gagné M, Blanchette LJ, Garneau C, Fillion M, et al. A nanoparticle-based COVID-19 vaccine candidate elicits broad neutralizing antibodies and protects against SARS-CoV-2 infection. Nanomedicine. (2022) 44:102584. doi: 10.1016/j.nano.2022.102584
31. Zha L, Chang X, Zhao H, Mohsen MO, Hong L, Zhou Y, et al. Development of a vaccine against SARS-coV-2 based on the receptor-binding domain displayed on virus-like particles. Vaccines (Basel). (2021) 9:395. doi: 10.3390/vaccines9040395
32. Mohsen MO, Balke I, Zinkhan S, Zeltina V, Liu X, Chang X, et al. A scalable and highly immunogenic virus-like particle-based vaccine against SARS-CoV-2. Allergy. (2022) 77:243–57. doi: 10.1111/all.15080
33. He D, Marles-Wright J. Ferritin family proteins and their use in bionanotechnology. N Biotechnol. (2015) 32:651–7. doi: 10.1016/j.nbt.2014.12.006
34. Olshefsky A, Richardson C, Pun SH, King NP. Engineering self-assembling protein nanoparticles for therapeutic delivery. Bioconjug Chem. (2022) 33:2018–34. doi: 10.1021/acs.bioconjchem.2c00030
35. Ma X, Zou F, Yu F, Li R, Yuan Y, Zhang Y, et al. Nanoparticle vaccines based on the receptor binding domain (RBD) and heptad repeat (HR) of SARS-coV-2 elicit robust protective immune responses. Immunity. (2020) 53:1315–30.e9. doi: 10.1016/j.immuni.2020.11.015
36. Joyce MG, King HAD, Elakhal-Naouar I, Ahmed A, Peachman KK, Macedo Cincotta C, et al. A SARS-CoV-2 ferritin nanoparticle vaccine elicits protective immune responses in nonhuman primates. Sci Transl Med. (2022) 14:eabi5735. doi: 10.1126/scitranslmed.abi5735
37. Tai W, Chai B, Feng S, Zhuang X, Ma J, Pang M, et al. Development of a ferritin-based nanoparticle vaccine against the SARS-CoV-2 Omicron variant. Signal Transduct Target Ther. (2022) 7:173. doi: 10.1038/s41392-022-01041-8
38. Gu M, Torres JL, Li Y, Van Ry A, Greenhouse J, Wallace S, et al. One dose of COVID-19 nanoparticle vaccine REVC-128 protects against SARS-CoV-2 challenge at two weeks post-immunization. Emerg Microbes Infect. (2021) 10:2016–29. doi: 10.1080/22221751.2021.1994354
39. Ober Shepherd BL, Scott PT, Hutter JN, Lee C, McCauley MD, Guzman I, et al. SARS-CoV-2 recombinant spike ferritin nanoparticle vaccine adjuvanted with Army Liposome Formulation containing monophosphoryl lipid A and QS-21: a phase 1, randomised, double-blind, placebo-controlled, first-in-human clinical trial. Lancet Microbe. (2024) 5:e581–e93. doi: 10.1016/S2666-5247(23)00410-X
40. Wu X, Li W, Rong H, Pan J, Zhang X, Hu Q, et al. A nanoparticle vaccine displaying conserved epitopes of the preexisting neutralizing antibody confers broad protection against SARS-coV-2 variants. ACS Nano. (2024) 18:17749–63. doi: 10.1021/acsnano.4c03075
41. Zhang Y, Sun J, Zheng J, Li S, Rao H, Dai J, et al. Mosaic RBD nanoparticles elicit protective immunity against multiple human coronaviruses in animal models. Adv Sci (Weinh). (2024) 11:e2303366. doi: 10.1002/advs.202303366
42. Bruun TUJ, Andersson AC, Draper SJ, Howarth M. Engineering a rugged nanoscaffold to enhance plug-and-display vaccination. ACS Nano. (2018) 12:8855–66. doi: 10.1021/acsnano.8b02805
43. Bale JB, Gonen S, Liu Y, Sheffler W, Ellis D, Thomas C, et al. Accurate design of megadalton-scale two-component icosahedral protein complexes. Science. (2016) 353:389–94. doi: 10.1126/science.aaf8818
44. Hsia Y, Bale JB, Gonen S, Shi D, Sheffler W, Fong KK, et al. Design of a hyperstable 60-subunit protein dodecahedron. Nature. (2016) 535:136–9. doi: 10.1038/nature18010
45. Tan TK, Rijal P, Rahikainen R, Keeble AH, Schimanski L, Hussain S, et al. A COVID-19 vaccine candidate using SpyCatcher multimerization of the SARS-CoV-2 spike protein receptor-binding domain induces potent neutralising antibody responses. Nat Commun. (2021) 12:542. doi: 10.1038/s41467-020-20654-7
46. Patel DR, Minns AM, Sim DG, Field CJ, Kerr AE, Heinly TA, et al. Intranasal SARS-CoV-2 RBD decorated nanoparticle vaccine enhances viral clearance in the Syrian hamster model. Microbiol Spectr. (2024) 12:e0499822. doi: 10.1128/spectrum.04998-22
47. Walls AC, Fiala B, Schäfer A, Wrenn S, Pham MN, Murphy M, et al. Elicitation of potent neutralizing antibody responses by designed protein nanoparticle vaccines for SARS-coV-2. Cell. (2020) 183:1367–82.e17. doi: 10.1016/j.cell.2020.10.043
48. Kang YF, Sun C, Zhuang Z, Yuan RY, Zheng Q, Li JP, et al. Rapid development of SARS-coV-2 spike protein receptor-binding domain self-assembled nanoparticle vaccine candidates. ACS Nano. (2021) 15:2738–52. doi: 10.1021/acsnano.0c08379
49. Sun C, Yuan RY, Xie C, Sun JF, Fang XY, Hu YS, et al. Induction of broadly cross-reactive antibody responses to SARS-coV-2 variants by S1 nanoparticle vaccines. J Virol. (2022) 96:e0038322. doi: 10.1128/jvi.00383-22
50. Hu Y, Li Y, Xu FJ. Versatile functionalization of polysaccharides via polymer grafts: from design to biomedical applications. Acc Chem Res. (2017) 50:281–92. doi: 10.1021/acs.accounts.6b00477
51. Deng Y, Li J, Sun C, Chi H, Luo D, Wang R, et al. Rational development of a polysaccharide-protein-conjugated nanoparticle vaccine against SARS-coV-2 variants and streptococcus pneumoniae. Adv Mater. (2022) 34:e2200443. doi: 10.1002/adma.202200443
52. Chen S, Yang L, Ou X, Li JY, Zi CT, Wang H, et al. A new polysaccharide platform constructs self-adjuvant nanovaccines to enhance immune responses. J Nanobiotechnology. (2022) 20:320. doi: 10.1186/s12951-022-01533-3
53. Tursi NJ, Xu Z, Kulp DW, Weiner DB. Gene-encoded nanoparticle vaccine platforms for in vivo assembly of multimeric antigen to promote adaptive immunity. Wiley Interdiscip Rev Nanomed Nanobiotechnol. (2023) 15:e1880. doi: 10.1002/wnan.1880
54. Liu C, Wang L, Merriam JS, Shi W, Yang ES, Zhang Y, et al. Self-assembling SARS-CoV-2 spike-HBsAg nanoparticles elicit potent and durable neutralizing antibody responses via genetic delivery. NPJ Vaccines. (2023) 8:111. doi: 10.1038/s41541-023-00707-w
55. Konrath KM, Liaw K, Wu Y, Zhu X, Walker SN, Xu Z, et al. Nucleic acid delivery of immune-focused SARS-CoV-2 nanoparticles drives rapid and potent immunogenicity capable of single-dose protection. Cell Rep. (2022) 38:110318. doi: 10.1016/j.celrep.2022.110318
56. Sun W, He L, Zhang H, Tian X, Bai Z, Sun L, et al. The self-assembled nanoparticle-based trimeric RBD mRNA vaccine elicits robust and durable protective immunity against SARS-CoV-2 in mice. Signal Transduct Target Ther. (2021) 6:340. doi: 10.1038/s41392-021-00750-w
57. Chen R, Zhang X, Yuan Y, Deng X, Wu B, Xi Z, et al. Development of receptor binding domain (RBD)-conjugated nanoparticle vaccines with broad neutralization against SARS-coV-2 delta and other variants. Adv Sci (Weinh). (2022) 9:e2105378. doi: 10.1002/advs.202105378
58. Yuan Y, Zhang X, Chen R, Li Y, Wu B, Li R, et al. A bivalent nanoparticle vaccine exhibits potent cross-protection against the variants of SARS-CoV-2. Cell Rep. (2022) 38:110256. doi: 10.1016/j.celrep.2021.110256
59. Kim SA, Kim S, Kim GB, Goo J, Kim N, Lee Y, et al. A multivalent vaccine based on ferritin nanocage elicits potent protective immune responses against SARS-coV-2 mutations. Int J Mol Sci. (2022) 23:6123. doi: 10.3390/ijms23116123
60. Kang YF, Sun C, Sun J, Xie C, Zhuang Z, Xu HQ, et al. Quadrivalent mosaic HexaPro-bearing nanoparticle vaccine protects against infection of SARS-CoV-2 variants. Nat Commun. (2022) 13:2674. doi: 10.1038/s41467-022-30222-w
61. Brinkkemper M, Veth TS, Brouwer PJM, Turner H, Poniman M, Burger JA, et al. Co-display of diverse spike proteins on nanoparticles broadens sarbecovirus neutralizing antibody responses. iScience. (2022) 25:105649. doi: 10.1016/j.isci.2022.105649
62. Cohen AA, Gnanapragasam PNP, Lee YE, Hoffman PR, Ou S, Kakutani LM, et al. Mosaic nanoparticles elicit cross-reactive immune responses to zoonotic coronaviruses in mice. Science. (2021) 371:735–41. doi: 10.1101/2020.11.17.387092
63. Cohen AA, van Doremalen N, Greaney AJ, Andersen H, Sharma A, Starr TN, et al. Mosaic RBD nanoparticles protect against challenge by diverse sarbecoviruses in animal models. Science. (2022) 377:eabq0839. doi: 10.1126/science.abq0839
64. Hills RA, Tan TK, Cohen AA, Keeffe JR, Keeble AH, Gnanapragasam PNP, et al. Proactive vaccination using multiviral Quartet Nanocages to elicit broad anti-coronavirus responses. Nat Nanotechnol. (2024) 19:1216–23. doi: 10.1038/s41565-024-01655-9
65. Yang Y, Zhang J, Xu Y, Chen M, Wang S, Lin G, et al. Spatial engineering of heterotypic antigens on a DNA framework for the preparation of mosaic nanoparticle vaccines with enhanced immune activation against SARS-coV-2 variants. Angew Chem Int Ed Engl. (2024) 18:e202412294. doi: 10.1002/anie.202412294
66. Irvine DJ, Swartz MA, Szeto GL. Engineering synthetic vaccines using cues from natural immunity. Nat Mater. (2013) 12:978–90. doi: 10.1038/nmat3775
67. Ding Y, Li Z, Jaklenec A, Hu Q. Vaccine delivery systems toward lymph nodes. Adv Drug Delivery Rev. (2021) 179:113914. doi: 10.1016/j.addr.2021.113914
68. Bachmann MF, Jennings GT. Vaccine delivery: a matter of size, geometry, kinetics and molecular patterns. Nat Rev Immunol. (2010) 10:787–96. doi: 10.1038/nri2868
69. Manolova V, Flace A, Bauer M, Schwarz K, Saudan P, Bachmann MF. Nanoparticles target distinct dendritic cell populations according to their size. Eur J Immunol. (2008) 38:1404–13. doi: 10.1002/eji.200737984
70. Stein JV, FG S. Dynamic intravital imaging of cell-cell interactions in the lymph node. J Allergy Clin Immunol. (2017) 139:12–20. doi: 10.1016/j.jaci.2016.11.008
71. Cheng Z, Que H, Chen L, Sun Q, Wei X. Nanomaterial-based drug delivery system targeting lymph nodes. Pharmaceutics. (2022) 14:1372. doi: 10.3390/pharmaceutics14071372
72. Heesters BA, van der Poel CE, Das A, Carroll MC. Antigen presentation to B cells. Trends Immunol. (2016) 37:844–54. doi: 10.1016/j.it.2016.10.003
73. Roozendaal R, Mempel TR, Pitcher LA, Gonzalez SF, Verschoor A, Mebius RE, et al. Conduits mediate transport of low-molecular-weight antigen to lymph node follicles. Immunity. (2009) 30:264–76. doi: 10.1016/j.immuni.2008.12.014
74. Reynoso GV, Weisberg AS, Shannon JP, McManus DT, Shores L, Americo JL, et al. Lymph node conduits transport virions for rapid T cell activation. Nat Immunol. (2019) 20:602–12. doi: 10.1038/s41590-019-0342-0
75. Gerner MY, Torabi-Parizi P, Germain RN. Strategically localized dendritic cells promote rapid T cell responses to lymph-borne particulate antigens. Immunity. (2015) 42:172–85. doi: 10.1016/j.immuni.2014.12.024
76. Tal O, Lim HY, Gurevich I, Milo I, Shipony Z, Ng LG, et al. DC mobilization from the skin requires docking to immobilized CCL21 on lymphatic endothelium and intralymphatic crawling. J Exp Med. (2011) 208:2141–53. doi: 10.1084/jem.20102392
77. Ulvmar MH, Werth K, Braun A, Kelay P, Hub E, Eller K, et al. The atypical chemokine receptor CCRL1 shapes functional CCL21 gradients in lymph nodes. Nat Immunol. (2014) 15:623–30. doi: 10.1038/ni.2889
78. Zhou X, Jiang X, Qu M, Aninwene GE 2nd, Jucaud V, Moon JJ, et al. Engineering antiviral vaccines. ACS Nano. (2020) 14:12370–89. doi: 10.1021/acsnano.0c06109
79. Crotty S. Follicular helper CD4 T cells (TFH). Annu Rev Immunol. (2011) 29:621–63. doi: 10.1146/annurev-immunol-031210-101400
80. Morales-Hernández S, Ugidos-Damboriena N, López-Sagaseta J. Self-assembling protein nanoparticles in the design of vaccines: 2022 update. Vaccines (Basel). (2022) 10:1447. doi: 10.3390/vaccines10091447
81. McCormick AA, Palmer KE. Genetically engineered Tobacco mosaic virus as nanoparticle vaccines. Expert Rev Vaccines. (2008) 7:33–41. doi: 10.1586/14760584.7.1.33
Keywords: self-assembled nanoparticle, VLP, ferritin, SARS-CoV-2, vaccine
Citation: Yang K, Zeng Y, Wu X, Li J and Guo J (2024) Strategies for developing self-assembled nanoparticle vaccines against SARS-CoV-2 infection. Front. Immunol. 15:1392898. doi: 10.3389/fimmu.2024.1392898
Received: 28 February 2024; Accepted: 21 August 2024;
Published: 11 September 2024.
Edited by:
Susu M. Zughaier, Qatar University, QatarReviewed by:
Ebony Gary, Wistar Institute, United StatesSharon Vijayanand, Mercer University, United States
Copyright © 2024 Yang, Zeng, Wu, Li and Guo. This is an open-access article distributed under the terms of the Creative Commons Attribution License (CC BY). The use, distribution or reproduction in other forums is permitted, provided the original author(s) and the copyright owner(s) are credited and that the original publication in this journal is cited, in accordance with accepted academic practice. No use, distribution or reproduction is permitted which does not comply with these terms.
*Correspondence: Jinlin Guo, Z3VvNTk2QGNkdXRjbS5lZHUuY24=
†These authors share first authorship