- 1Programa de Becas Post-doctorales, Dirección General de Asuntos del Personal Académico, Universidad Nacional Autónoma de México, Mexico City, Mexico
- 2Departamento de Fisiología, Facultad de Medicina, Universidad Nacional Autónoma de México, Mexico City, Mexico
- 3Departamento de Anatomía, Facultad de Medicina, Universidad Nacional Autónoma de México, Mexico City, Mexico
- 4Unidad de Medicina Experimental “Ruy Pérez Tamayo”, Facultad de Medicina, Universidad Nacional Autónoma de México, Mexico City, Mexico
- 5Instituto de Fisiología Celular-Neurociencias, Universidad Nacional Autónoma de México, Mexico City, Mexico
Microglia are highly dynamic cells that have been mainly studied under pathological conditions. The present review discusses the possible implication of microglia as modulators of neuronal electrical responses in physiological conditions and hypothesizes how these cells might modulate hypothalamic circuits in health and during obesity. Microglial cells studied under physiological conditions are highly diverse, depending on the developmental stage and brain region. The evidence also suggests that neuronal electrical activity modulates microglial motility to control neuronal excitability. Additionally, we show that the expression of genes associated with neuron-microglia interaction is down-regulated in obese mice compared to control-fed mice, suggesting an alteration in the contact-dependent mechanisms that sustain hypothalamic arcuate-median eminence neuronal function. We also discuss the possible implication of microglial-derived signals for the excitability of hypothalamic neurons during homeostasis and obesity. This review emphasizes the importance of studying the physiological interplay between microglia and neurons to maintain proper neuronal circuit function. It aims to elucidate how disruptions in the normal activities of microglia can adversely affect neuronal health.
1 Introduction
Microglial cells, one of the resident macrophages of the central nervous system (CNS) in mammals, originate from mesodermal yolk sac myeloid progenitors during neurodevelopmental stages. These cells infiltrate the brain during embryogenesis and play a crucial role in neuronal differentiation and maturation (1). Microglia constitute approximately 10% of CNS cells and account for 5 to 20% of glial cells (2, 3).
Although there is no denying that microglia are the first responders against damage and infection, they are also crucial for maintaining brain homeostasis (4), as they can support neurons through several processes such as synaptic pruning, learning, memory, neurogenesis, and neuronal connectivity (2, 5). However, since their role in the brain was first discovered in pathological conditions, studies have paid particular attention to their pathologic role and relegated their physiological function to a dormant sentinel under physiological conditions.
Some studies suggest that microglial activities differ depending on brain region, age, and health status, suggesting that microglia function is heterogeneous, and not restricted to injury-related responses (6, 7). Microglia around various regions of the brain, including the arcuate nucleus (ARC) of the hypothalamus, play a crucial role in maintaining metabolic homeostasis and neuronal communication, so proper development and physiological functioning of microglial cells are essential for preventing metabolic disturbances linked to obesogenic diets. Disruptions in the normal activities of these resident immune cells can contribute significantly to the pathogenesis of obesity and related metabolic disorders (8, 9).
Understanding the mechanisms by which microglia in different brain regions influence metabolic processes is essential for developing targeted therapeutic strategies. This review aims to describe hypothalamic microglial function under physiological conditions, highlighting their critical role in maintaining proper neuronal activity and the physiological responses of ARC. Additionally, it explores the implications of ARC microglia in altered responses to dietary challenges, providing valuable insights into obesity-related neuroinflammatory conditions.
2 Brief scope on microglia
Microglia are the primary immunocompetent cells in the brain. As one of the main CNS resident macrophages, microglia play a critical role during physiological conditions. The induction of their immune program has been related to the development of diseases such as Alzheimer’s disease, ischemia, and even obesity (10–13).
Microglial cells present a highly dynamic resting non-immune state to surveil the brain parenchyma constantly; therefore, resting microglia does not mean “inactive” (14). Instead, they maintain baseline motility without inflammation, which consists of their processes’ extension, retraction, and movement. As a result, microglia can survey their environment, clear cellular debris, interact with neurons and other glial components, and remodel the extracellular matrix (15, 16). Furthermore, surveillance motility is highly correlated with morphological modifications such as the number, length, and ramification of their filipodia (17).
As CNS sentinels, quiescent microglia constantly survey the brain parenchyma, searching for damaging signals that may disrupt brain homeostasis (4). Damage-derived stimuli can be detected by microglia throughout four different types of pattern recognition receptors (PRRs): toll-like receptors (TLRs), nod-like receptors (NLR), rig-like receptors (RLR), and c-type lectin receptors (CLR) (11, 12, 16, 17). Activation of these receptors can initiate a multifaceted response in microglia, including phagocytosis, production of cytotoxic molecules, and promotion of signals that repair and restore brain tissue (17).
The microglial cytotoxic response is triggered by exposure to pathogen‐derived antigens like lipopolysaccharide (LPS), dying cells, or the accumulation of misfolded proteins in the extracellular matrix (4, 18). This function implies the production of pro-inflammatory cytokines such as tumor necrosis factor-α (TNFα), interleukin-1β (IL-1β), IL-6, IL-12, IL-23, nitric oxide (NO), reactive oxygen species (ROS), matrix metalloproteinases (MMPs), chemokines and redox molecules (e.g., NADPH oxidase or iNOS), among others. They also express other molecules like scavenger receptors (e.g., macrophage receptors with collagen structure), co-stimulatory proteins like the cluster of differentiation 40 (CD40), and the major histocompatibility complex II (MHC-II) (12, 18, 19). A similar pro-inflammatory response has been observed in the absence of infection, for example, during brain trauma, cell degeneration, or chemical exposure (19).
Microglia may also have a neuroprotective capacity characterized by the production of anti-inflammatory cytokines such as IL-4, IL-13, IL-10, transforming growth factor beta (TGF-β), and neurotrophic factors like vascular endothelial growth factor (VEGF), and epidermal growth factor (EGF) (12). After the initial pro-inflammatory immune activation, microglia gradually acquire a neuroprotective function to promote tissue repair, neuronal survival, and the reconstruction of the extracellular matrix (12, 20, 21).
Different stimuli, such as physical trauma, infection, systemic inflammation, tumor, ischemia, and neurodegeneration, may activate microglial immune functions. In the present review, we will not employ the terms M1 and M2 activated microglia since these profiles were coined based on studies exposing in vitro microglia to immune challenges such as LPS or combinations of pro-inflammatory cues, which are not replicated in vivo (21, 22).
Besides these widely studied immune functions, microglial cells might perform other tasks without any immune challenge. These tasks might be determined by different factors such as developmental stage, brain region, sexual dimorphism, and even animal species (22–27). The following sections will discuss microglia’s less-described physiological functions.
3 Microglia diversity in physiological conditions
Microglial cells are far more complex under physiological conditions. Despite the countless publications assessing the role of microglia under many pathological conditions, we still do not know the exact implications of microglial function under physiological conditions beyond the embryonic stages, such as synapse pruning, axon myelination, and trophic factor secretion for neurogenesis (28, 29).
In the yolk sac, early progenitor c-kit+ lineage cells give rise to microglia Cx3CR1+ colonizing brain tissue and accompanying neural precursors during neurodevelopmental stages (30). Afterward, during embryogenesis and early postnatal life, microglia respond to brain microenvironment changes (31). They can engulf presynaptic inputs and phagocyte apoptotic cells, pruning synapses, guiding neurogenesis, and refining synaptogenesis and myelin formation (32, 33). This microglial developmental role is thought to be associated with their immune function.
Microglia can trigger neuronal apoptosis by secreting TNFα, reactive oxygen species, and glutamate, among other factors (34), to initiate cell death programs in stressed or damaged cells to eventually phagocyte them through signaling pathways that include triggering the receptor expressed on myeloid cells 2 (TREM2), MER proto-oncogene, tyrosine kinase (MERTK), and milk fat globule EGF and factor V/VIII (MFG-E8) (35).
In addition, microglia maintain CNS homeostasis, and alterations in their function caused by deletions or mutations in TREM2 or the colony-stimulating factor 1 receptor (CSF1R) cause neurodegeneration or leukodystrophies, respectively (36–38).
During development, microglial cells contact synapses through CX3CR1 and the P2Y12 receptor (P2Y12R) by sensing and responding to neuronal activity. After the induction of long-term potentiation (LTP) in the hippocampus, ramified CX3CR1+ cells increase, thus establishing more contact with dendritic spines (39). These effects in LTP-induced microglial dynamic were absent after the administration of an NMDA antagonist. Importantly, microglia-spine contacts are rare and brief during basal synaptic hippocampal activity, suggesting that microglia sense high-frequency neuronal activity as indicated by the associations observed between dendrites, somas, and axons in the healthy brain (40, 41). Notwithstanding, it is unknown which signals may induce microglia-neuron associations, although glutamate has been a feasible candidate.
Embryonal and neonatal microglia express a highly characteristic transcriptomic profile, which differs from those encountered in adult microglia (6, 26), which might reflect their accelerated activity during these developmental stages. Hammond et al. identified transcriptionally distinct microglial subpopulations along distinct developmental ages, embryonic day 14.5 (E14.5), early postnatal day 4 or 5 (P4/5), late juvenile stage at postnatal day 30 (P30), adulthood at postnatal day 100 (P100), and old age at postnatal day 540 (P540), where the greatest microglial diversity was found at E14.5 and P4/5. Interestingly, the transcriptomic profile in these early stages completely differed from the microglia in old animals P540 and injured brains (6).
As previously mentioned, early postnatal brain microglia cells are involved in regulating axonal growth and fasciculation and in the refinement of synaptic circuits. These early-life microglial cells express high levels of genes like the insulin-like growth factor 1 (Igf1), which is an essential embryonic growth factor for myelinogenesis (42), the glycoprotein non-metastatic melanoma protein B (GpnmB), that is thought to provide neuroprotection (43), galectin-1 (Lgals1) and galectin-3 (Lgals3), well know immuno-modulators known to deactivate cytotoxic microglia (44) and the lysosomal markers; lysosomal-associated membrane protein 1 (Lamp1) (45) and (Cd68) (6, 46).
In the same study, eight microglial subpopulations were defined during early developmental stages by analyzing their specific transcriptional programs defined by the expression level of the following genes: arginase 1 (Arg1) (47), ribonucleotide reductase M2 (Rrm2) (48), ubiquitin-conjugating enzyme E2C (Ube2c) (49), centromere protein A (Cenpa) (50), fatty acid binding protein 5 (Fabp5) (51), osteopontin (Spp1) (52), heme oxygenase 1 (Hmox1) (53), and membrane-spanning 4-domains, subfamily A, member 7 (Ms4a7) (54), suggesting that each microglial subpopulation may perform specific functions (see Table 1).
Meanwhile, there is varying information about transcriptional profiles regarding juvenile and adult-derived microglial states. Some authors claim these are less diverse, identifying only three or two distinct subpopulations. These groups were sorted by their differential gene expression rather than specific genes (6, 70). In contrast, other studies using genome-wide chromatin and expression profiling, combined with single-cell transcriptomic analysis in the cortex, hippocampus, and spinal cord, describe a global gene expression pattern for adult microglia. It highlights Selectin P ligand (Selplg) (56), Prostate transmembrane protein androgen-induced 1 (Pmepa1) (63), cluster of differentiation 14 (Cd14) (63), Activator protein-1 family transcription factors Jun (Jun) and Fos (Fos) (61), Myocyte enhancer factor 2A (Mef2a) (60), and Maf family protein B (MafB) (59) as genes strongly correlated with adult microglia in physiological conditions (Table 1) (61, 71). Further studies have determined that markers like TMEM119, P2ry12, and P2ry13 are up-regulated in the mature brain (55, 57, 72).
Furthermore, the microglia derived from aged mice present transcriptional profiles with the up-regulation of genes involved in immune activation and the development of neurodegenerative diseases (64–69, 73, 74) (Table 1). All these data suggest that microglial function under physiological conditions varies according to the developmental stage.
Healthy microglia also present brain-region-associated transcriptional profiles. Masuda et al. also performed single-cell RNA sequencing (scRNA-seq) of microglia in homeostatic conditions of multiple anatomical regions of the CNS of embryonic, juvenile, and adult mice. Authors found two main clouds differentiating embryonic from juvenile and adult from postnatal microglia. Within the cloud derived from postnatal mice, t-SNE analysis showed six sub-clusters in postnatal microglia, while juvenile and adult microglia only presented four. Each sub-cluster had specific transcriptional patterns suggesting the existence of different microglial subclasses during both embryonic and juvenile/adult stages (27).
Similarly, the analysis of different regions within either embryonic, postnatal, and juvenile/adult mice revealed that each anatomical area of the CNS presented a regional distribution of transcript expression, denoted as microglial molecular signature, evidencing the existence of spatiotemporal microglial subclasses (27).
Later, Zheng et al. reported differences in the molecular signature between cortical and spinal microglia. This study characterized three distinct microglial clusters in the cortex and two in the spinal cord (7). Within cortical microglia, two sub-clusters exhibiting different expression levels of homeostatic genes were defined as homeostatic microglia 1 and 2 (HOM-M1 and HOM-M2), both differing in the expression of genes coding ribosomal proteins, molecular pathways involved in the establishment of homeostatic functions, among others (7). Furthermore, another subtype that expressed immune genes was identified as inflammatory microglia (IFLAM-M). This IFLAM-M was less represented in the cortex, while it constituted 45% of the spinal microglia in two-month-old mice; this percentage varied along the lifespan, suggesting that the immune function of these cells is age-related.
Interestingly, cortical microglia maintained a relatively low proportion of IFLAM-M, suggesting that the expression of inflammatory genes is more restrained in the cortex than in the spinal cord (7). Hammond et al. reported that the microglia of young mice are more heterogeneous and that the inflammatory pathways were mainly enriched in aged individuals (6). All these studies suggest that microglial molecular signatures differ according to age, brain region, and health status, indicating that these cells’ function is not homogeneous.
As dynamic cells, besides constantly surveilling the milieu, microglia are thought to detect neural electrical activity (40, 41, 75). Nimmerjahn et al., using in vivo two-photon microscopy, determined that microglial processes were significantly motile, where their filopodia experienced several extend-withdraw cycles under physiological conditions (14, 75). This motility process directs microglial podocytes to establish transitory contacts with dendritic spines in healthy mice’s somatosensory and visual cortexes in response to neuronal sensory stimulation (40). Conversely, sensory deprivation results in filopodial retraction, thus reducing the number of neuronal contacts in the visual cortex in vivo (40).
The exact signal microglia detect that redirects their processes toward activated neurons is unclear. Neuronal mitochondrial activity, induced by neuronal electrical activation, rapidly triggers the establishment of the microglia-neuron junction and is blocked by inhibition of P2Y12 receptors for adenosine 5’diphosphate (ADP), suggesting that the communication established through this receptor might allow the transitory junction observed between neurons and microglia (76).
Stimulation of hippocampal CA3 neurons that project to CA1 pyramidal cells through the Schaffer collateral pathway in mice results in an increase in microglial Ca2+ in early postnatal hippocampal CA1; this effect depends on neuronal action potentials since tetrodotoxin (TTX) administration significantly reduce microglial Ca2+ influx (77).
Microglial cells’ ability to detect and redirect their filopodia in response to neuronal electrical activity might be crucial to preserving a homeostatic neuronal firing rate (78–80). Blocking microglial capacity to redirect themselves toward firing neurons leads to the hyper-synchronicity of cortical circuits in response to sensory stimulation (79).
Although microglial transcriptional profiles vary depending on brain region, only a handful of studies approach region-specific microglial function in homeostatic conditions. Hypothalamic microglia have been approached to understand their implication in neuroinflammation resulting from consuming a high-fat diet (HFD), considering microglial cells as mere sentinels instead of active participants of the hypothalamic circuits’ physiology. In the following sections, we will explore the possible implications of hypothalamic microglia for its daily homeostatic function.
4 Brief scope of the medio-basal hypothalamic arcuate nucleus
Two primary problems in studying ARC microglial cells are that many of the models employed are not ARC-specific and that the studies do not focus on what this cell type does under physiological conditions.
Comparative studies in mammals suggest that species-specific developmental programs link anatomy, cellular differentiation, and gene expression to create hypothalamic ‘modules’ that can be gained or lost through evolution (81). Similar hypothalamic nuclei found in rodents and humans indicate possible homology at both anatomical and functional levels (82). In humans, the arcuate nucleus (ARC) is located in the medio-basal hypothalamus, adjacent to the third ventricle and attached to the median eminence (ME) (Figure 1). It is considered a circumventricular organ (83). This location allows cerebrospinal fluid (CSF) and blood-borne cues to enter the ARC parenchyma since the ME is highly vascularized with fenestrated capillaries originating from the hypophyseal portal system (84). Therefore, metabolic signals, like plasmatic glucose, triglycerides, leptin, insulin, and ghrelin, can freely reach and modulate the neuronal activity of the ARC (85–90).
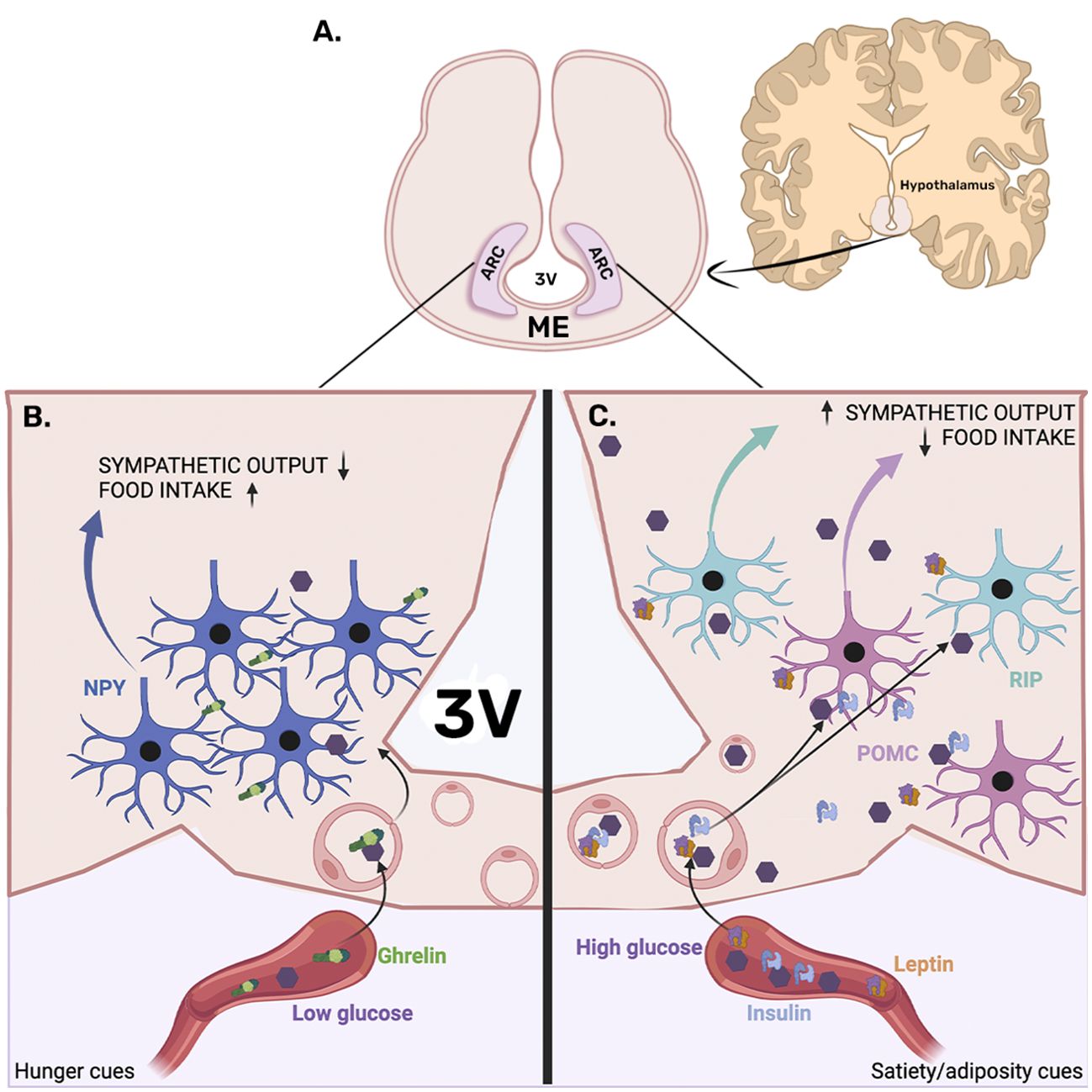
Figure 1. Arcuate nucleus location and neuronal organization. (A) The arcuate nucleus (ARC) is located in the medio-basal hypothalamus, adjacent to the third ventricle (3V), attached to the median eminence (ME). (B) The left panel presents ARC under fasting conditions, where hunger cues like low glucose levels and ghrelin secreted by the stomach can access the ARC parenchyma through the fenestrated vascularization of the median eminence, which eventually reaches the orexigenic neuropeptide Y (NPY) expressing neurons. NPY activation reduces sympathetic output, which consequently decreases energy expenditure. In addition, NPY neurons promote food intake. (C) The right panel presents the entrance of satiety/adiposity signals like insulin and leptin, which are known to activate pro-opiomelanocortin (POMC), promoting energy expenditure and inhibiting food intake. Furthermore, leptin is known to activate RIP neurons known to increase energy expenditure by promoting brown adipose tissue-induced thermogenesis.
In response to metabolic status, ARC neurons modulate both aspects of energy balance, food intake, and energy expenditure. In fasting conditions, plasmatic glucose levels decrease, and the hunger hormone ghrelin is secreted in response to gastric emptying. Both low glucose and ghrelin are known to activate ARC neuropeptide Y (NPY) and agouti-related peptide (AgRP) neurons to promote food intake and diminish energy expenditure (85). NPY and AgRP are also glucose-inhibited neurons since food intake-induced blood glucose elevations inhibit them from ceasing their orexigenic function and preventing overeating (91–94).
ARC-NPY afferences to secondary hypothalamic nuclei reduce the sympathetic output to the brown adipose tissue (BAT), a region that increases energy expenditure for heat production (95). In fasting conditions, NPY-mediated BAT thermogenic inhibition is observed (96). In addition, activation of the ARC-NPY neurons suppresses the sympathetic output of pre-autonomic pathways, thus decreasing blood pressure (97). Similarly, activation of the ARC-AgRP neurons promotes insulin resistance by inhibiting the sympathetic output that activates BAT glucose uptake (98). Furthermore, NPY projections to the paraventricular nucleus (PVN) are known to induce food intake (99, 100) (Figure 1).
Conversely, after food ingestion, satiety cues such as elevated glucose and insulin levels increase, and adipose-derived signals like leptin inhibit the NPY/AgRP neuronal activity and increase the firing rate of the pro-opiomelanocortin (POMC) and cocaine amphetamine-related transcript (CART) neurons, known to inhibit food intake and to increase energy expenditure (85, 101, 102, 103) (Figure 1). Additionally, ARC-GABAergic-RIP neurons modulate the sympathetic outflow, promoting energy expenditure by the noradrenergic stimulation of BAT-mediated thermogenesis (104) (Figure 1).
Although the neurons in the ARC are critical players in modulating the outputs that regulate several bodily functions involved in controlling metabolism, recent studies have demonstrated that ARC glial populations are also crucial for maintaining their function (105–107). Specifically, hypothalamic microglia maintain ARC neuronal function and are essential for developing metabolic diseases such as obesity (108, 109).
As previously mentioned, microglia are highly active cells under both physiological and pathological conditions; however, the ARC microglia have mainly been implicated in the hypothalamic inflammation resulting from obesity, although a few studies suggest that ARC microglia are constantly surveilling ARC neuronal function.
5 Microglia physiological functions in the ARC
The traditional view of microglia as mere phagocytic cells responsible for eliminating synapses, dead or apoptotic cells, and cellular debris is overly simplistic. Microglia play a crucial role in synaptic formation, reorganization, maturation, and neurogenesis. They achieve this through direct contact, the release of soluble factors, the engulfment of synaptic structures, and various microglia-neuronal signaling pathways during the remodeling of brain circuits. This dynamic process continues throughout life, allowing the brain to adapt to its ever-changing microenvironment. In addition, proper development and maintenance of hippocampal and hypothalamic neuronal circuits rely heavily on functional microglia (110). It has been suggested that the physiological implication of ARC microglia modulates feeding behavior and energy balance.
One piece of evidence is that the functional microglial marker, such as the cluster of differentiation 68 (CD68), a vesicle marker, changes according to the time of the day without immune stimulation (111). CD68, a member of the lysosome-associated membrane protein (LAMP) family, participates in vesicle mobilization, a process found in macrophages during phagocytosis, lysosome digestion, and solute secretion. The daily non-immune associated changes in CD68 expression in the ARC could be involved in any vesicle-forming process that repeats itself every 24 h (69).
Another ARC microglial action observed by Winkler et al. is the rearrangement of these cells in juxtaposition to NPY-activated neurons in response to a drop in plasmatic glucose levels elicited either by fasting or an insulin i.v. administration (112). This effect was inhibited by an intracerebroventricular (i.c.v.) minocycline microinjection, a microglial inhibitor (112). Furthermore, minocycline i.c.v. administration increased the counterregulatory glucose production in response to a hypoglycemic stimulus, indicating that ARC-NPY (glucose-inhibited) neuronal activity is sensed by microglial cells, thus modulating these cells’ response to hypoglycemia (Figure 2A).
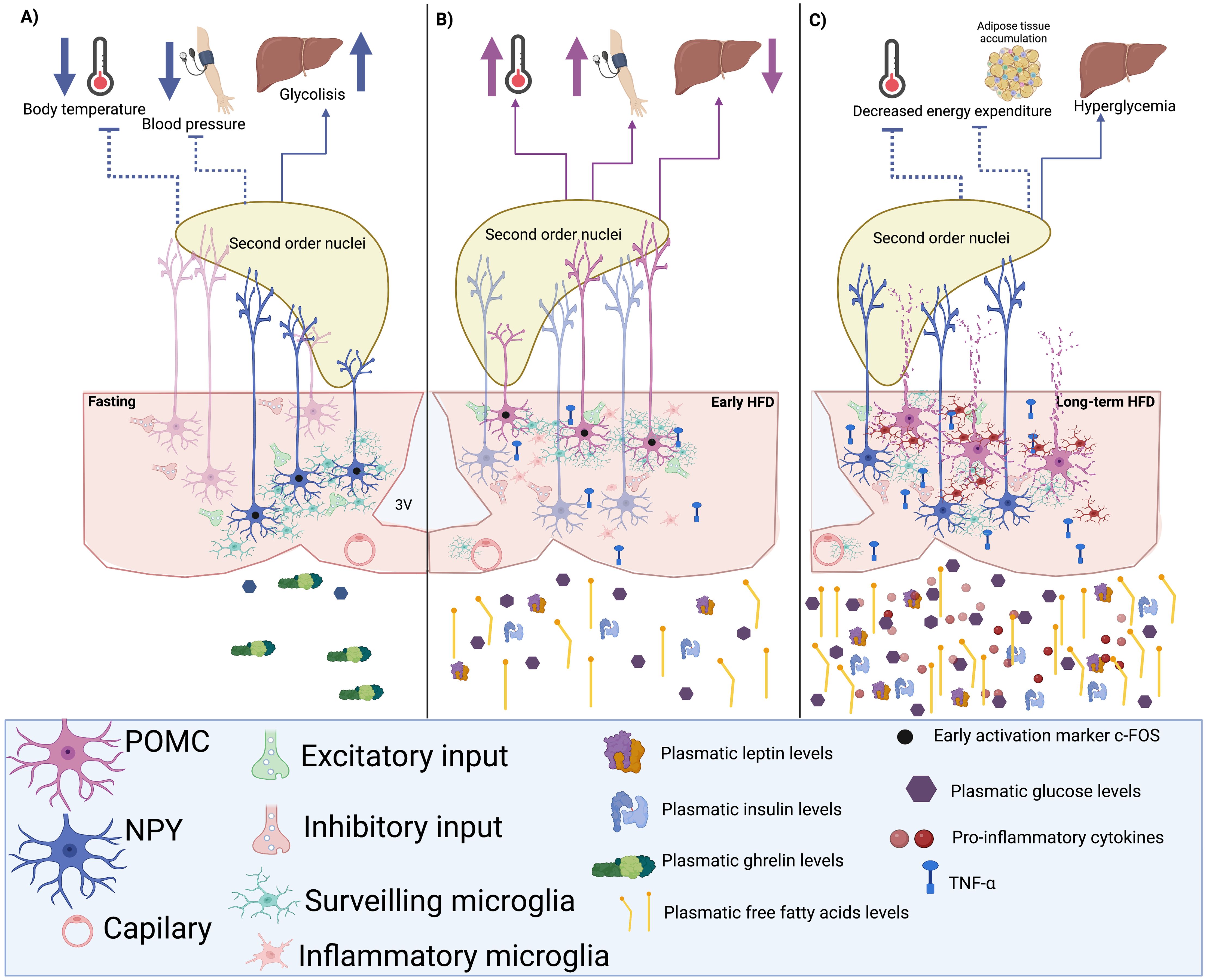
Figure 2. Hypothetic microglia-neuron circuit in the arcuate nucleus (ARC). (A) A hypoglycemic state induces the activation of neuropeptide Y (NPY) neurons and the rearrangement of microglial cells during fasting, increasing excitatory inputs to NPY neurons and their firing rate. Consequently, NPY output would inhibit the neuronal activity of second-order nuclei like the paraventricular nucleus (PVN), decreasing the sympathetic tone and reducing energy expenditure by inhibiting heat production and decreasing blood pressure. (B) The short-term consumption of a high-fat diet (HFD) could induce the rearrangement of microglia towards POMC neurons, raising their firing rate in response to glucose, insulin, and leptin to promote the activation of sympathetic outputs that improve energy expenditure and decrease hepatic glucose production. (C) When the consumption of an HFD is chronic, the inflammation of the adipose tissue induces the presence of pro-inflammatory circulating signals that reach and activate ARC microglia. The inflamed hypothalamus would cause neuronal damage, especially in POMC neurons and not in NPY cells, which may contribute to low metabolic rates and probably hyperglycemia.
In another study, Jin and collaborators demonstrated that stimulating the microglial TLR2 through an i.c.v. Pam3CSK4 administration rapidly triggers the rearrangement of these macrophages toward ARC-POMC neurons. This effect was associated with changes in the percentage of synaptic inputs contacting POMC neurons, increasing their excitatory inputs and raising their excitatory activity, ultimately resulting in anorexia and increased body temperature (113). In the same study, the minocycline-mediated microglial inhibition successfully prevented the observed anorexia and thermogenesis (113). Also, stimulation of microglial TLR4 promotes an excitatory response in POMC neurons, whereas inhibiting AgRP/NPY neurons (114). These data demonstrate that ARC microglia not only sense ARC neuronal activity but may also regulate neuronal excitability and their output, consequently modulating the biological effect of ARC neuronal populations.
In anorexic humans and rodent models, Iba1 brain expression and enrichment of microglia genes are increased (115, 116). Also, the administration of deoxynivalenol (DON), a compound known to induce microglial inflammatory function in circumventricular organs such as the ME, causes anorexia. Interestingly, PLX3397 microglial depletion enhanced DON sensitivity, causing food intake inhibition in response to non-anorectic DON doses and increased neuronal activation in the ARC and the PVN (117).
Furthermore, ARC microgliosis has been described in the early phases of pancreatic ductal adenocarcinoma, which has been associated with cachexia by altering the communication between POMC ARC neurons and the PVN (118). CSF1-R-mediated microglia depletion accelerates the cachexia onset and increases anorexia (118). These data suggest that microglia is a crucial modulator of ARC neuronal excitability, and its respective outputs control feeding behavior. Furthermore, microglial response to metabolic and immune challenges might contribute to preventing energy imbalance.
Other studies have also inhibited or depleted microglia and observed critical metabolic effects. Eight-week-old C57BL/6 mice subjected to whole-body irradiation received bone marrow transplants from green fluorescent protein (GFP)-transgenic C57BL/6 mice with a deletion of the BDNF gene, resulting in higher body weights. However, the establishing site of these cells is preferentially the PVN instead of the ARC (119). Likewise, conditional ablation of microglia in Cx3cr1-Dtr rats reduced food intake and energy expenditure (120). Interestingly, increasing brain CX3CL1 levels prevented diet-induced obesity in male mice (121), suggesting that the contact-dependent relationship established between microglia and neurons is crucial for maintaining energy homeostasis.
Campbell et al. performed a single-cell analysis of the ARC-ME of mice fed a normocaloric diet or an HFD, thus presenting a transcriptional census of these areas. They identified 50 distinct ARC-ME cell populations, such as tanycytes, leptin-sensing neurons, AgRP, and POMC subtypes, among others (122). Since ARC microglial cells respond to NPY and POMC neuronal activity, the mechanisms that connect these cellular populations might be deeply affected by an HFD.
The CD200-CD200R1 system is an in vivo “Off” signal that comprises the transmembrane glycoprotein ligand CD200, expressed by neurons and endothelial cells, and its receptor CD200R1, which is expressed in myeloid cells like microglia (123). Studies have demonstrated an up-regulation in CD200 in the neocortex, hippocampus, and striatum of the R6/1 transgenic mouse model of Huntington’s disease (HD), with unaltered expression in CD200R1 (124). This data indicates a counter-regulatory neuronal mechanism to maintain the neuronal–microglial communication to sustain neuronal function under a pro-inflammatory condition like HD or an HFD. The relationship established between microglia and the ARC-neuronal circuits has been widely studied during obesity; the following section will discuss how microglial non-physiological function in response to high-fat, high-carbohydrate diet consumption and the resulting low-grade inflammation during obesity may impair the ARC microglial-neuronal relationship therefore, de-regulating metabolic homeostasis.
6 Microglial response to obesity and its possible implications for ARC neuronal activity
Obesity is a pro-inflammatory state characterized by the hypertrophy and hyperplasia of the white adipose tissue (WAT), in which adipocytes secrete pro-inflammatory cytokines and chemokines, thus maintaining a mild inflammatory tone in the body for prolonged periods (125). Not only do cytokines and chemokines have inflammatory roles in obesity, but WAT can also react by producing and secreting biologically active substances as hormones or peptides, which are termed “adipokines” (126) that contribute to the obesity-derived chronic low-grade systemic pro-inflammatory condition, also known as “metainflammation” (127). The hypothalamus responds to the low-grade inflammation observed during obesity by further expressing pro-inflammatory cytokines (108, 128–131) that eventually impair hypothalamic insulin and leptin sensitivity (132, 133).
As previously mentioned, the ARC is a sensory region. Thus, its distinct cell populations can detect and respond to blood-borne circulating metabolic and inflammatory signals. The consumption of high-fat and high-carbohydrate diets increases plasmatic free fatty acids (134, 135), which, as they tend to accumulate in the white adipose depots, can cause inflammation and, eventually, neuroinflammation (136, 137), by initiating an innate immune response elicited in glial cells (138, 139).
Specifically, microglia are the first to respond to dietary saturated fatty acids, promoting lipid-induced neuronal stress, hypothalamic inflammation, leptin and insulin resistance, and hyperphagia in mice (140, 141). Furthermore, i.c.v. infusions of saturated and polyunsaturated fatty acids induce the expression of neuroinflammatory markers (131, 142–144), alter autophagic protection from cellular stress (145), and increase endoplasmic reticulum stress responses to unfolded proteins (130, 146).
In diet-resistant mice fed an HFD for only 1-2 weeks, which is not enough time to develop increased adiposity nor metabolic impairments, the number of inhibitory synapses directed towards the ARC-POMC neurons was elevated in the non-diet-resistant mice (control) that eventually became obese. Later, when HFD non-obesity resistant mice became obese, the number of inhibitory synapses associated with ARC-POMC neurons was significantly increased (147). As mentioned, POMC neurons inhibit food intake and promote energy expenditure by regulating the pathways controlling autonomic outputs. This suggests that microglial response during the first stages of HFD consumption highly regulates the synaptic inputs that modulate neuronal excitability.
Paradoxically, Douglass et al. recently showed that microglial inflammatory function during an HFD consumption enhances glucose physiological responses regardless of inducing adiposity (148) and preventing microglial IKKb signaling pathway in response to an HFD prevents obesity but impairs glucose tolerance (148). Furthermore, hypercaloric diets stimulate microglial TLR4, which responds to lipids (149, 131), thus inducing TNFα secretion, inhibiting NPY/AgRP neuronal activity (150) and increasing POMC neuronal excitability (114, 151).
Thaler et al.’s observations could explain this paradoxical effect of microglial pro-inflammatory response in glucose tolerance since a significant rise in hypothalamic pro-inflammatory gene expression was detected after only three days of consuming a hypercaloric diet. This gene profile was associated with increased ARC microglial markers, suggesting that the Iba1 increase within the first days of an obesogenic diet might reflect an increase in microglial function to counteract the excess in energy intake (108). This hypothesis is supported by the Douglass et al. report, where microglial activation promoted parasympathetic insulin secretion (148).
Furthermore, high-fat intake increases palmitate levels in cerebrospinal fluid and triggers a wave of microglial metabolic activation characterized by mitochondrial membrane activation, fission, and metabolic skewing towards aerobic glycolysis (152). Also, a hypercaloric diet increases microglial lipoprotein lipase (LPL) expression, an enzyme relevant for microglial lipid uptake. Mice lacking microglial-LPL are prone to become obese when fed both a control and an HFD (153), implying that ARC microglial immune activity might be part of the normal responses evoked by hypercaloric diets to prevent the metabolic impairments caused by the increased glucose disponibility before the development of obesity (Figures 2B, C). Further studies should assess if microglia can adapt or change their morphology, biomarkers, and cytokine secretion profile to the neuronal activity elicited by the consumption of HFD before developing a pro-inflammatory state.
Previous studies have suggested that defective regulation of POMC neurons precedes HFD inflammation and obesity development (154). RNA-seq studies of the POMC neurons of obese mice unveil an enrichment in apoptosis, chemokine signaling, and sphingolipid metabolism pathways, suggesting that an obesogenic diet causes POMC apoptotic neuronal loss (155). As previously mentioned, TNFα increases POMC neuronal excitability (151) and induces elevated blood pressure via a central mechanism involving sympathetic activation (156). This hypothesis is supported by the observation that during obesity, POMC neurons present a higher percentage of microglial contacts (151), suggesting that microglial TNFα constant release during obesity might affect POMC activity and even induce neurotoxicity since there is a significant decrease in the number of POMC neurons after chronic feeding with a high fat and carbohydrate diet (151). These observations indicate that TNFα secreting microglia may increase ARC-POMC neuronal activity, altering their autonomic output.
In contrast, postmortem studies in type II diabetic patients have shown an increase of NPY neurons in the ARC (157), implying that inflammatory signals’ effect on NPY neurons does not promote excitatory inputs, hence not hindering their survival. In fact, studies have demonstrated that long-term palmitate and TNFα exposure promotes NPY mRNA transcription (158); however, King et al. reported NPY neuronal inhibition after IL-1ß, IL-6, and TNFα administrations (159) (Figure 2C). Future studies of the exact effect of cytokines in NPY cell cycle programs or survival markers should be performed to understand how they survive an HFD while POMC neurons are significantly reduced.
Moreover, microglia trigger a complex hypothalamic immune response to dietary excess. After one week of a hypercaloric diet, mice presented a monocytic infiltration in the ARC; this infiltration was absent in control-fed mice (160). However, circulating monocyte recruitment is not the primary mechanism for microgliosis and its pro-inflammatory response during the development of obesity (161). Valdearcos et al. also defined two ARC microglial subpopulations: CX3CR1+/P2Y12+ and CX3CR1+/TMEM119+ microglial cells. After the HFD, GFP+CD68+ bone-marrow-derived cells were detected in the ARC; these cells were neither TMEM119+ nor P2Y12+, indicating their myeloid origin. These infiltrating cells arrive after the inflammatory response elicited by the hypothalamic parenchyma (162, 163), consequently recruiting further immune cells from the periphery, such as neutrophils, lymphocytes, and natural killer T cells, into the hypothalamus. Also, dendritic cell migration could be associated with the obesity-induced myeloid cell hypothalamic monocytic invasion contributing to hypothalamic inflammation (160).
Lee et al. demonstrated that perivascular macrophages secrete inducible nitric oxide synthase (iNOS) in mice fed an HFD, contributing to BBB leakage and increased vascular permeability in the hypothalamic parenchyma (164), probably facilitating peripheral immune cell infiltration. Likewise, hypothalamic infiltrated myeloid cells and perivascular macrophages secrete the VEGF (165), contributing to blood-borne metabolic signals’ increased permeability in the ARC (166). These data indicate that non-microglial macrophages are crucial for maintaining hypothalamic circuit homeostasis.
Since metainflammation has been correlated with a “low-grade” chronic microglial activation state, hypercaloric diets have been associated with hypothalamic dysfunction, including loss of synapses, lack of response to metabolic hormones, disturbed organelles function, and cell death (167). The sustained microglial immune response after the long-term consumption of a hypercaloric diet leads to hypothalamic injury and dysfunction, indicating that the relationship between ARC microglia and neurons is essential for preventing obesity. Taking into consideration the chemokines’ role in obesity-derived hypothalamic microglia activities, Dorfman et al. demonstrated that male mice fed an HFD for 18 weeks presented a reduction in the hypothalamic expression of the neuron-microglia binding protein CX3CL1 (fractalkine) and the mRNA levels of its receptor CX3CR1 (121).
CX3CL1 is a crucial axis for neuron-microglia communication (58, 62, 168). Dissociation of the contact established through CX3CL1 and its receptor promotes microglial pro-inflammatory response (169). I.c.v. CX3CL1 administration significantly suppressed food intake after 48 hours of fasting, while i.c.v. CX3CL1 and NPY co-administration prevented NPY-induced food intake (170). In contrast, maintaining CX3CL1-mediated microglial-neuronal interactions protects against diet-induced obesity (170), highlighting the importance of preserving the relationship between neurons and microglial cells to prevent obesity caused by dietary factors. Future studies should identify the molecular mechanisms involved in the hypothalamic neuron-microglial relationship between health and obesity. In addition, studies should focus on determining the exact moment this interaction is disrupted during a hypercaloric diet since identifying this specific moment might provide information regarding possible targets to restore this interaction and revert the metabolic impairments caused by obesity.
Additionally to the CX3CL1 role, other chemokines are also involved in hypothalamic neuroinflammation. CXCL12 is a chemokine that shows neuroactive effects by promoting the migration of dopaminergic neurons in the midbrain through the Akt-1/FOXO3a axis (171) and modulating electrical excitability in hypothalamic neurons through CXCR4, one of the CXCL12 receptors (172). HFD-fed rats increased expression of CXCL12 and its receptors CXCR4 and CXCR7, which correlated with cognitive decline and locomotor dysfunction (173). CCL2, also known as MCP-1, is produced by microglial cells after an inflammatory stimulus and has been associated with chemoattraction of monocytes in response to acute and chronic inflammatory responses through JAK2/STAT 3, MAPK, and PI3K Pathways (174). Peripheral myeloid cells can be recruited to ARC in hypercaloric diet conditions by crossing fenestrated blood vessels and the third ventricle, which has been related to hypothalamic microgliosis using the CCL2/CCR2 axis in obesogenic diet rodent models (175). CCL2 treatment attracted peripheral macrophage-like cells, and promoted microglial migration, and enhanced CCL2 and proinflammatory cytokine production (176).
As mentioned, cytokines and chemokines are not the only molecules involved in metainflammation and hypothalamic microglial responses. Adipokines have also activity over microglia function since it described that increased leptin, adiponectin, and resistin are correlated with metabolic dysfunction, decreasing food intake and increasing energy expenditure and insulin resistance [reviewed in Recinella et al. (177)]. The effects of the most relevant adipokines on the microglia function are described in Table 2.
Although the literature has explored the topic deeply, the mechanisms underlying microglial interactions in obesity remain unclear and require further studies.
7 Conclusion
Microglial function under physiological conditions is crucial for maintaining brain homeostasis. Even though not many studies describe the physiological role of microglia in the hypothalamus, it is clear that these cells respond to neuronal activity by rearranging themselves towards these activated neurons, suggesting that these cells play a role in maintaining adequate neuronal functioning in the hypothalamic area. The relationship between these two cell types becomes evident during a hypercaloric diet, where microglial cells surround ARC-POMC neurons and secrete pro-inflammatory molecules like TNFα. Future studies should describe 1) how ARC microglia sense neuronal activity, 2) the functional implication of the neuron-microglial associations, and 3) how these associations become dysregulated during metabolic impairments such as obesity.
Understanding the nature and physiological implication of the relationship between the ARC neuronal populations and microglial cells during health might contribute to identifying therapeutic targets aimed at maintaining this connection even under pathological conditions such as obesity.
Author contributions
MG-R: Conceptualization, Funding acquisition, Investigation, Resources, Visualization, Writing – original draft, Writing – review & editing. NGV: Writing – original draft, Writing – review & editing. JG-O: Writing – review & editing. BT-H: Writing – original draft, Writing – review & editing. MV-R: Writing – original draft, Writing – review & editing. RR-C: Writing – original draft, Writing – review & editing. RG-G: Writing – original draft, Writing – review & editing. AC: Funding acquisition, Supervision, Visualization, Writing – original draft, Writing – review & editing.
Funding
The author(s) declare financial support was received for the research, authorship, and/or publication of this article. The following grants supported this study: Consejo Nacional de Humanidades, Ciencias y Tecnologı́as (CONAHCYT) Ciencia de Frontera 2019-1783, CONAHCYT Ciencia Básica y de Frontera 2023-2024 CBF2023-2024-870, CONAHCYT FORDECYT-PRONACES/ 263957/2020, Dirección General de Asuntos del Personal Académico (DGAPA) PAPIIT (IN214821, IN22524, and IA205523).
Acknowledgments
MG-R received a postdoctoral fellowship from the Programa de Becas Post-doctorales, Dirección General de Asuntos del Personal Académico (DGAPA), Universidad Nacional Autónoma de México (UNAM). BT-H is part of the Programa de Apoyo y Fomento a la Investigación Estudiantil (AFINES), Facultad de Medicina, UNAM. MV-R is part of the Plan de Estudios Combinados en Medicina (PECEM), Facultad de Medicina, UNAM. RR-C is a doctoral student from Programa de Doctorado en Ciencias Biomédicas, UNAM, and received the fellowship (CVU 1020262) from the Consejo Nacional de Humanidades, Ciencias y Tecnologías (CONAHCYT).
Conflict of interest
The authors declare that the research was conducted in the absence of any commercial or financial relationships that could be construed as a potential conflict of interest.
The author(s) declared that they were an editorial board member of Frontiers, at the time of submission. This had no impact on the peer review process and the final decision.
Publisher’s note
All claims expressed in this article are solely those of the authors and do not necessarily represent those of their affiliated organizations, or those of the publisher, the editors and the reviewers. Any product that may be evaluated in this article, or claim that may be made by its manufacturer, is not guaranteed or endorsed by the publisher.
References
1. Ginhoux F, Greter M, Leboeuf M, Nandi S, See P, Gokhan S, et al. Fate mapping analysis reveals that adult microglia derive from primitive macrophages. Science. (2010) 330:841–5. doi: 10.1126/science.1194637
2. Zhang L, Zhang J, You Z. Switching of the microglial activation phenotype is a possible treatment for depression disorder. Front Cell Neurosci. (2018) 12:306. doi: 10.3389/fncel.2018.00306
3. Jurga AM, Paleczna M, Kuter KZ. Overview of general and discriminating markers of differential microglia phenotypes. Front Cell Neurosci. (2020) 14:198. doi: 10.3389/fncel.2020.00198
4. Li Q, Barres BA. Microglia and macrophages in brain homeostasis and disease. Nat Rev Immunol. (2018) 18:225–42. doi: 10.1038/nri.2017.125
5. Hammond TR, Robinton D, Stevens B. Microglia and the brain: complementary partners in development and disease. Annu Rev Cell Dev Biol. (2018) 34:523–44. doi: 10.1146/annurev-cellbio-100616-060509
6. Hammond TR, Dufort C, Dissing-Olesen L, Giera S, Young A, Wysoker A, et al. Single-cell RNA sequencing of microglia throughout the mouse lifespan and in the injured brain reveals complex cell-state changes. Immunity. (2019) 50:253–71 e6. doi: 10.1016/j.immuni.2018.11.004
7. Zheng J, Ru W, Adolacion JR, Spurgat MS, Liu X, Yuan S, et al. Single-cell RNA-seq analysis reveals compartment-specific heterogeneity and plasticity of microglia. iScience. (2021) 24:102186. doi: 10.1016/j.isci.2021.102186
8. Kim JD, Yoon NA, Jin S, Diano S. Microglial UCP2 mediates inflammation and obesity induced by high-fat feeding. Cell Metab. (2019) 30:952–62 e5. doi: 10.1016/j.cmet.2019.08.010
9. Banerjee J, Dorfman MD, Fasnacht R, Douglass JD, Wyse-Jackson AC, Barria A, et al. CX3CL1 action on microglia protects from diet-induced obesity by restoring POMC neuronal excitability and melanocortin system activity impaired by high-fat diet feeding. Int J Mol Sci. (2022) 23(12):6380. doi: 10.3390/ijms23126380
10. Huck JH, Freyer D, Bottcher C, Mladinov M, Muselmann-Genschow C, Thielke M, et al. De novo expression of dopamine D2 receptors on microglia after stroke. J Cereb Blood Flow Metab. (2015) 35:1804–11. doi: 10.1038/jcbfm.2015.128
11. Walker DG, Lue LF. Immune phenotypes of microglia in human neurodegenerative disease: challenges to detecting microglial polarization in human brains. Alzheimers Res Ther. (2015) 7:56. doi: 10.1186/s13195-015-0139-9
12. Tang Y, Le W. Differential roles of M1 and M2 microglia in neurodegenerative diseases. Mol Neurobiol. (2016) 53:1181–94. doi: 10.1007/s12035-014-9070-5
13. Milanova IV, Kalsbeek MJT, Wang XL, Korpel NL, Stenvers DJ, Wolff SEC, et al. Diet-induced obesity disturbs microglial immunometabolism in a time-of-day manner. Front Endocrinol (Lausanne). (2019) 10:424. doi: 10.3389/fendo.2019.00424
14. Nimmerjahn A, Kirchhoff F, Helmchen F. Resting microglial cells are highly dynamic surveillants of brain parenchyma in vivo. Science. (2005) 308:1314–8. doi: 10.1126/science.1110647
15. Graeber MB, Streit WJ. Microglia: immune network in the CNS. Brain Pathol. (1990) 1:2–5. doi: 10.1111/j.1750-3639.1990.tb00630.x
16. Garden GA, Moller T. Microglia biology in health and disease. J Neuroimmune Pharmacol. (2006) 1:127–37. doi: 10.1007/s11481-006-9015-5
17. Madry C, Attwell D. Receptors, ion channels, and signaling mechanisms underlying microglial dynamics. J Biol Chem. (2015) 290:12443–50. doi: 10.1074/jbc.R115.637157
18. Martinez FO, Gordon S. The M1 and M2 paradigm of macrophage activation: time for reassessment. F1000Prime Rep. (2014) 6:13. doi: 10.12703/P6-13
19. Chen GY, Nunez G. Sterile inflammation: sensing and reacting to damage. Nat Rev Immunol. (2010) 10:826–37. doi: 10.1038/nri2873
20. Prinz M, Priller J. Microglia and brain macrophages in the molecular age: from origin to neuropsychiatric disease. Nat Rev Neurosci. (2014) 15:300–12. doi: 10.1038/nrn3722
21. Orihuela R, McPherson CA, Harry GJ. Microglial M1/M2 polarization and metabolic states. Br J Pharmacol. (2016) 173:649–65. doi: 10.1111/bph.13139
22. Gosselin D, Skola D, Coufal NG, Holtman IR, Schlachetzki JCM, Sajti E, et al. An environment-dependent transcriptional network specifies human microglia identity. Science. (2017) 356(6344):eaal3222. doi: 10.1126/science.aal3222
23. Bennett ML, Bennett FC, Liddelow SA, Ajami B, Zamanian JL, Fernhoff NB, et al. New tools for studying microglia in the mouse and human CNS. Proc Natl Acad Sci U S A. (2016) 113:E1738–46. doi: 10.1073/pnas.1525528113
24. Keren-Shaul H, Spinrad A, Weiner A, Matcovitch-Natan O, Dvir-Szternfeld R, Ulland TK, et al. A unique microglia type associated with restricting development of alzheimer’s disease. Cell. (2017) 169:1276–90 e17. doi: 10.1016/j.cell.2017.05.018
25. Guneykaya D, Ivanov A, Hernandez DP, Haage V, Wojtas B, Meyer N, et al. Transcriptional and translational differences of microglia from male and female brains. Cell Rep. (2018) 24:2773–83 e6. doi: 10.1016/j.celrep.2018.08.001
26. Li Q, Cheng Z, Zhou L, Darmanis S, Neff NF, Okamoto J, et al. Developmental heterogeneity of microglia and brain myeloid cells revealed by deep single-cell RNA sequencing. Neuron. (2019) 101:207–23 e10. doi: 10.1016/j.neuron.2018.12.006
27. Masuda T, Sankowski R, Staszewski O, Bottcher C, Amann L, Sagar, et al. Spatial and temporal heterogeneity of mouse and human microglia at single-cell resolution. Nature. (2019) 566:388–92. doi: 10.1038/s41586-019-0924-x
28. Tong CK, Vidyadaran S. Role of microglia in embryonic neurogenesis. Exp Biol Med (Maywood). (2016) 241:1669–75. doi: 10.1177/1535370216664430
29. Cabirol MJ, Cardoit L, Courtand G, Mayeur ME, Simmers J, Pascual O, et al. Microglia shape the embryonic development of mammalian respiratory networks. Elife. (2022) 11:e80352. doi: 10.7554/eLife.80352
30. Kierdorf K, Katzmarski N, Haas CA, Prinz M. Bone marrow cell recruitment to the brain in the absence of irradiation or parabiosis bias. PloS One. (2013) 8:e58544. doi: 10.1371/journal.pone.0058544
31. Li YJ, Zhang X, Li YM. Antineuroinflammatory therapy: potential treatment for autism spectrum disorder by inhibiting glial activation and restoring synaptic function. CNS Spectr. (2020) 25:493–501. doi: 10.1017/S1092852919001603
32. Schafer DP, Stevens B. Microglia function in central nervous system development and plasticity. Cold Spring Harb Perspect Biol. (2015) 7:a020545. doi: 10.1101/cshperspect.a020545
33. Yu Q, Huang Q, Du X, Xu S, Li M, Ma S. Early activation of Egr-1 promotes neuroinflammation and dopaminergic neurodegeneration in an experimental model of Parkinson’s disease. Exp Neurol. (2018) 302:145–54. doi: 10.1016/j.expneurol.2018.01.009
34. Bessis A, BeChade C, Bernard D, Roumier A. Microglial control of neuronal death and synaptic properties. Glia. (2007) 55:233–8. doi: 10.1002/glia.20459
35. Brown GC, Neher JJ. Microglial phagocytosis of live neurons. Nat Rev Neurosci. (2014) 15:209–16. doi: 10.1038/nrn3710
36. Paloneva J, Kestila M, Wu J, Salminen A, Bohling T, Ruotsalainen V, et al. Loss-of-function mutations in TYROBP (DAP12) result in a presenile dementia with bone cysts. Nat Genet. (2000) 25:357–61. doi: 10.1038/77153
37. Rademakers R, Baker M, Nicholson AM, Rutherford NJ, Finch N, Soto-Ortolaza A, et al. Mutations in the colony stimulating factor 1 receptor (CSF1R) gene cause hereditary diffuse leukoencephalopathy with spheroids. Nat Genet. (2011) 44:200–5. doi: 10.1038/ng.1027
38. Hu B, Duan S, Wang Z, Li X, Zhou Y, Zhang X, et al. Insights into the role of CSF1R in the central nervous system and neurological disorders. Front Aging Neurosci. (2021) 13:789834. doi: 10.3389/fnagi.2021.789834
39. Pfeiffer T, Avignone E, Nagerl UV. Induction of hippocampal long-term potentiation increases the morphological dynamics of microglial processes and prolongs their contacts with dendritic spines. Sci Rep. (2016) 6:32422. doi: 10.1038/srep32422
40. Wake H, Moorhouse AJ, Jinno S, Kohsaka S, Nabekura J. Resting microglia directly monitor the functional state of synapses in vivo and determine the fate of ischemic terminals. J Neurosci. (2009) 29:3974–80. doi: 10.1523/JNEUROSCI.4363-08.2009
41. Tremblay ME, Lowery RL, Majewska AK. Microglial interactions with synapses are modulated by visual experience. PloS Biol. (2010) 8:e1000527. doi: 10.1371/journal.pbio.1000527
42. Wlodarczyk A, Holtman IR, Krueger M, Yogev N, Bruttger J, Khorooshi R, et al. A novel microglial subset plays a key role in myelinogenesis in developing brain. EMBO J. (2017) 36:3292–308. doi: 10.15252/embj.201696056
43. Satoh JI, Kino Y, Yanaizu M, Ishida T, Saito Y. Microglia express GPNMB in the brains of Alzheimer’s disease and Nasu-Hakola disease. Intractable Rare Dis Res. (2019) 8:120–8. doi: 10.5582/irdr.2019.01049
44. Starossom SC, Mascanfroni ID, Imitola J, Cao L, Raddassi K, Hernandez SF, et al. Galectin-1 deactivates classically activated microglia and protects from inflammation-induced neurodegeneration. Immunity. (2012) 37:249–63. doi: 10.1016/j.immuni.2012.05.023
45. BarraChina M, Maes T, Buesa C, Ferrer I. Lysosome-associated membrane protein 1 (LAMP-1) in Alzheimer’s disease. Neuropathol Appl Neurobiol. (2006) 32:505–16. doi: 10.1111/j.1365-2990.2006.00756.x
46. Kettenmann H, Hanisch UK, Noda M, Verkhratsky A. Physiology of microglia. Physiol Rev. (2011) 91:461–553. doi: 10.1152/physrev.00011.2010
47. Cherry JD, Olschowka JA, O’Banion MK. Neuroinflammation and M2 microglia: the good, the bad, and the inflamed. J Neuroinflamm. (2014) 11:98. doi: 10.1186/1742-2094-11-98
48. Zuo Z, Zhou Z, Chang Y, Liu Y, Shen Y, Li Q, et al. Ribonucleotide reductase M2 (RRM2): Regulation, function and targeting strategy in human cancer. Genes Dis. (2024) 11:218–33. doi: 10.1016/j.gendis.2022.11.022
49. Kumar D, Ambasta RK, Kumar P. Ubiquitin biology in neurodegenerative disorders: From impairment to therapeutic strategies. Ageing Res Rev. (2020) 61:101078. doi: 10.1016/j.arr.2020.101078
50. De Rop V, Padeganeh A, Maddox PS. CENP-A: the key player behind centromere identity, propagation, and kinetochore assembly. Chromosoma. (2012) 121:527–38. doi: 10.1007/s00412-012-0386-5
51. Low YL, Pan Y, Short JL, Nicolazzo JA. Profiling the expression of fatty acid-binding proteins and fatty acid transporters in mouse microglia and assessing their role in docosahexaenoic acid-d5 uptake. Prostaglandins Leukot Essent Fatty Acids. (2021) 171:102303. doi: 10.1016/j.plefa.2021.102303
52. Rosmus DD, Lange C, Ludwig F, Ajami B, Wieghofer P. The role of osteopontin in microglia biology: current concepts and future perspectives. Biomedicines. (2022) 10(4):840. doi: 10.3390/biomedicines10040840
53. Deininger MH, Meyermann R, Trautmann K, Duffner F, Grote EH, Wickboldt J, et al. Heme oxygenase (HO)-1 expressing macrophages/microglial cells accumulate during oligodendroglioma progression. Brain Res. (2000) 882:1–8. doi: 10.1016/s0006-8993(00)02594-4
54. Ni B, Huang G, Yang R, Wang Z, Song H, Li K, et al. The short isoform of MS4A7 is a novel player in glioblastoma microenvironment, M2 macrophage polarization, and tumor progression. J Neuroinflamm. (2023) 20:80. doi: 10.1186/s12974-023-02766-1
55. Ruan C, Elyaman W. A new understanding of TMEM119 as a marker of microglia. Front Cell Neurosci. (2022) 16:902372. doi: 10.3389/fncel.2022.902372
56. Rossi C, Cusimano M, Zambito M, Finardi A, Capotondo A, Garcia-Manteiga JM, et al. Interleukin 4 modulates microglia homeostasis and attenuates the early slowly progressive phase of amyotrophic lateral sclerosis. Cell Death Dis. (2018) 9:250. doi: 10.1038/s41419-018-0288-4
57. Kyrargyri V, Madry C, Rifat A, Arancibia-Carcamo IL, Jones SP, Chan VTT, et al. P2Y(13) receptors regulate microglial morphology, surveillance, and resting levels of interleukin 1beta release. Glia. (2020) 68:328–44. doi: 10.1002/glia.23719
58. Ho CY, Lin YT, Chen HH, Ho WY, Sun GC, Hsiao M, et al. CX3CR1-microglia mediates neuroinflammation and blood pressure regulation in the nucleus tractus solitarii of fructose-induced hypertensive rats. J Neuroinflamm. (2020) 17:185. doi: 10.1186/s12974-020-01857-7
59. Koshida R, Oishi H, Hamada M, Takahashi S. MafB antagonizes phenotypic alteration induced by GM-CSF in microglia. Biochem Biophys Res Commun. (2015) 463:109–15. doi: 10.1016/j.bbrc.2015.05.036
60. Cilenti F, Barbiera G, Caronni N, Iodice D, Montaldo E, Barresi S, et al. A PGE(2)-MEF2A axis enables context-dependent control of inflammatory gene expression. Immunity. (2021) 54:1665–82 e14. doi: 10.1016/j.immuni.2021.05.016
61. Holtman IR, Skola D, Glass CK. Transcriptional control of microglia phenotypes in health and disease. J Clin Invest. (2017) 127:3220–9. doi: 10.1172/jci90604
62. Fonseca MI, Chu SH, Hernandez MX, Fang MJ, Modarresi L, Selvan P, et al. Cell-specific deletion of C1qa identifies microglia as the dominant source of C1q in mouse brain. J Neuroinflamm. (2017) 14:48. doi: 10.1186/s12974-017-0814-9
63. Javanmehr N, Saleki K, Alijanizadeh P, Rezaei N. Microglia dynamics in aging-related neurobehavioral and neuroinflammatory diseases. J Neuroinflamm. (2022) 19:273. doi: 10.1186/s12974-022-02637-1
64. Daniels MJD, Lefevre L, Szymkowiak S, Drake A, McCulloch L, Tzioras M, et al. Cystatin F (Cst7) drives sex-dependent changes in microglia in an amyloid-driven model of Alzheimer’s disease. Elife. (2023) 12:e85279. doi: 10.7554/eLife.85279
65. Kremlev SG, Roberts RL, Palmer C. Differential expression of chemokines and chemokine receptors during microglial activation and inhibition. J Neuroimmunol. (2004) 149:1–9. doi: 10.1016/j.jneuroim.2003.11.012
66. Liu X, Quan N. Microglia and CNS interleukin-1: beyond immunological concepts. Front Neurol. (2018) 9:8. doi: 10.3389/fneur.2018.00008
67. Harmon E, Doan A, Bautista-Garrido J, Jung JE, Marrelli SP, Kim GS. Increased expression of interferon-induced transmembrane 3 (IFITM3) in stroke and other inflammatory conditions in the brain. Int J Mol Sci. (2022) 23(16):8885. doi: 10.3390/ijms23168885
68. He X, Ashbrook AW, Du Y, Wu J, Hoffmann HH, Zhang C, et al. RTP4 inhibits IFN-I response and enhances experimental cerebral malaria and neuropathology. Proc Natl Acad Sci U S A. (2020) 117:19465–74. doi: 10.1073/pnas.2006492117
69. Ghosh A, Shao L, Sampath P, Zhao B, Patel NV, Zhu J, et al. Oligoadenylate-Synthetase-Family Protein OASL Inhibits Activity of the DNA Sensor cGAS during DNA Virus Infection to Limit Interferon Production. Immunity. (2019) 50:51–63 e5. doi: 10.1016/j.immuni.2018.12.013
70. Masuda T, Sankowski R, Staszewski O, Prinz M. Microglia heterogeneity in the single-cell era. Cell Rep. (2020) 30:1271–81. doi: 10.1016/j.celrep.2020.01.010
71. Matcovitch-Natan O, Winter DR, Giladi A, Vargas Aguilar S, Spinrad A, Sarrazin S, et al. Microglia development follows a stepwise program to regulate brain homeostasis. Science. (2016) 353:aad8670. doi: 10.1126/science.aad8670
72. Ochocka N, Segit P, Walentynowicz KA, Wojnicki K, Cyranowski S, Swatler J, et al. Single-cell RNA sequencing reveals functional heterogeneity of glioma-associated brain macrophages. Nat Commun. (2021) 12:1151. doi: 10.1038/s41467-021-21407-w
73. Jin C, Shao Y, Zhang X, Xiang J, Zhang R, Sun Z, et al. A unique type of highly-activated microglia evoking brain inflammation via mif/cd74 signaling axis in aged mice. Aging Dis. (2021) 12:2125–39. doi: 10.14336/AD.2021.0520
74. Holland SD, Ramer MS. Microglial activating transcription factor 3 upregulation: An indirect target to attenuate inflammation in the nervous system. Front Mol Neurosci. (2023) 16:1150296. doi: 10.3389/fnmol.2023.1150296
75. Li Y, Du XF, Du JL. Resting microglia respond to and regulate neuronal activity in vivo. Commun Integr Biol. (2013) 6:e24493. doi: 10.4161/cib.24493
76. Cserep C, Posfai B, Lenart N, Fekete R, Laszlo ZI, Lele Z, et al. Microglia monitor and protect neuronal function through specialized somatic purinergic junctions. Science. (2020) 367:528–37. doi: 10.1126/science.aax6752
77. Logiacco F, Xia P, Georgiev SV, Franconi C, Chang YJ, Ugursu B, et al. Microglia sense neuronal activity via GABA in the early postnatal hippocampus. Cell Rep. (2021) 37:110128. doi: 10.1016/j.celrep.2021.110128
78. Badimon A, Strasburger HJ, Ayata P, Chen X, Nair A, Ikegami A, et al. Negative feedback control of neuronal activity by microglia. Nature. (2020) 586:417–23. doi: 10.1038/s41586-020-2777-8
79. Merlini M, Rafalski VA, Ma K, Kim KY, Bushong EA, Rios Coronado PE, et al. Microglial G(i)-dependent dynamics regulate brain network hyperexcitability. Nat Neurosci. (2021) 24:19–23. doi: 10.1038/s41593-020-00756-7
80. Umpierre AD, Wu LJ. How microglia sense and regulate neuronal activity. Glia. (2021) 69:1637–53. doi: 10.1002/glia.23961
81. Xie Y, Dorsky RI. Development of the hypothalamus: conservation, modification and innovation. Development. (2017) 144:1588–99. doi: 10.1242/dev.139055
82. Song J, Choi SY. Arcuate nucleus of the hypothalamus: anatomy, physiology, and diseases. Exp Neurobiol. (2023) 32:371–86. doi: 10.5607/en23040
83. Cone RD. Anatomy and regulation of the central melanocortin system. Nat Neurosci. (2005) 8:571–8. doi: 10.1038/nn1455
84. Kaur C, Ling EA. The circumventricular organs. Histol Histopathol. (2017) 32:879–92. doi: 10.14670/HH-11-881
85. Cone R, Cowley M, Butler A, Fan W, Marks D, Low M. The arcuate nucleus as a conduit for diverse signals relevant to energy homeostasis. Int J Obes. (2001) 25:S63–S7. doi: 10.1038/sj.ijo.0801913
86. Cowley MA, Smart JL, Rubinstein M, Cerdan MG, Diano S, Horvath TL, et al. Leptin activates anorexigenic POMC neurons through a neural network in the arcuate nucleus. Nature. (2001) 411:480–4. doi: 10.1038/35078085
87. Könner AC, Janoschek R, Plum L, Jordan SD, Rother E, Ma X, et al. Insulin action in agRP-expressing neurons is required for suppression of hepatic glucose production. Cell Metab. (2007) 5:438–49. doi: 10.1016/j.cmet.2007.05.004
88. Williams KW, Margatho LO, Lee CE, Choi M, Lee S, Scott MM, et al. Segregation of acute leptin and insulin effects in distinct populations of arcuate proopiomelanocortin neurons. J Neurosci. (2010) 30:2472–9. doi: 10.1523/JNEUROSCI.3118-09.2010
89. Zhang H, Zhang G, Gonzalez FJ, Park S-M, Cai D. Hypoxia-inducible factor directs POMC gene to mediate hypothalamic glucose sensing and energy balance regulation. PloS Biol. (2011) 9:e1001112. doi: 10.1371/journal.pbio.1001112
90. Sun J, Gao Y, Yao T, Huang Y, He Z, Kong X, et al. Adiponectin potentiates the acute effects of leptin in arcuate Pomc neurons. Mol Metab. (2016) 5:882–91. doi: 10.1016/j.molmet.2016.08.007
91. Belgardt BF, Okamura T, Bruning JC. Hormone and glucose signalling in POMC and AgRP neurons. J Physiol. (2009) 587:5305–14. doi: 10.1113/jphysiol.2009.179192
92. Marston OJ, Hurst P, Evans ML, Burdakov DI, Heisler LK. Neuropeptide Y cells represent a distinct glucose-sensing population in the lateral hypothalamus. Endocrinology. (2011) 152:4046–52. doi: 10.1210/en.2011-1307
93. Chalmers JA, Jang JJ, Belsham DD. Glucose sensing mechanisms in hypothalamic cell models: glucose inhibition of AgRP synthesis and secretion. Mol Cell Endocrinol. (2014) 382:262–70. doi: 10.1016/j.mce.2013.10.013
94. Alvarsson A, Stanley SA. Remote control of glucose-sensing neurons to analyze glucose metabolism. Am J Physiol Endocrinol Metab. (2018) 315:E327–E39. doi: 10.1152/ajpendo.00469.2017
95. Shi YC, Lau J, Lin Z, Zhang H, Zhai L, Sperk G, et al. Arcuate NPY controls sympathetic output and BAT function via a relay of tyrosine hydroxylase neurons in the PVN. Cell Metab. (2013) 17:236–48. doi: 10.1016/j.cmet.2013.01.006
96. Mano-Otagiri A, Ohata H, Iwasaki-Sekino A, Nemoto T, Shibasaki T. Ghrelin suppresses noradrenaline release in the brown adipose tissue of rats. J Endocrinol. (2009) 201:341–9. doi: 10.1677/JOE-08-0374
97. Cassaglia PA, Shi Z, Li B, Reis WL, Clute-Reinig NM, Stern JE, et al. Neuropeptide Y acts in the paraventricular nucleus to suppress sympathetic nerve activity and its baroreflex regulation. J Physiol. (2014) 592:1655–75. doi: 10.1113/jphysiol.2013.268763
98. Steculorum SM, Ruud J, Karakasilioti I, Backes H, Engstrom Ruud L, Timper K, et al. AgRP neurons control systemic insulin sensitivity via myostatin expression in brown adipose tissue. Cell. (2016) 165:125–38. doi: 10.1016/j.cell.2016.02.044
99. Kelley SP, Nannini MA, Bratt AM, Hodge CW. Neuropeptide-Y in the paraventricular nucleus increases ethanol self-administration. Peptides. (2001) 22:515–22. doi: 10.1016/s0196-9781(01)00361-8
100. Beck B. Neuropeptide Y in normal eating and in genetic and dietary-induced obesity. Philos Trans R Soc Lond B Biol Sci. (2006) 361:1159–85. doi: 10.1098/rstb.2006.1855
101. Schlenker EH. Muscimol microinjected in the arcuate nucleus affects metabolism, body temperature & ventilation. Respir Physiol Neurobiol. (2016) 227:34–40. doi: 10.1016/j.resp.2016.02.009
102. Timper K, Brüning JC. Hypothalamic circuits regulating appetite and energy homeostasis: pathways to obesity. Dis Models Mechanisms. (2017) 10:679–89. doi: 10.1242/dmm.026609
103. Sapru HN. Role of the hypothalamic arcuate nucleus in cardiovascular regulation. Autonomic Neurosci. (2013) 175:38–50. doi: 10.1016/j.autneu.2012.10.016
104. Kong D, Tong Q, Ye C, Koda S, Fuller PM, Krashes MJ, et al. GABAergic RIP-Cre neurons in the arcuate nucleus selectively regulate energy expenditure. Cell. (2012) 151:645–57. doi: 10.1016/j.cell.2012.09.020
105. Bolborea M, Langlet F. What is the physiological role of hypothalamic tanycytes in metabolism? Am J Physiol Regul Integr Comp Physiol. (2021) 320:R994–R1003. doi: 10.1152/ajpregu.00296.2020
106. García-Cáceres C, Balland E, Prevot V, Luquet S, Woods SC, Koch M, et al. Role of astrocytes, microglia, and tanycytes in brain control of systemic metabolism. Nat Neurosci. (2019) 22:7–14. doi: 10.1038/s41593-018-0286-y
107. Rodriguez-Cortes B, Hurtado-Alvarado G, Martinez-Gomez R, Leon-Mercado LA, Prager-Khoutorsky M, Buijs RM. Suprachiasmatic nucleus-mediated glucose entry into the arcuate nucleus determines the daily rhythm in blood glycemia. Curr Biol. (2022) 32:796–805 e4. doi: 10.1016/j.cub.2021.12.039
108. Thaler JP, Yi CX, Schur EA, Guyenet SJ, Hwang BH, Dietrich MO, et al. Obesity is associated with hypothalamic injury in rodents and humans. J Clin Invest. (2012) 122:153–62. doi: 10.1172/JCI59660
109. André C, Guzman-Quevedo O, Rey C, Rémus-Borel J, Clark S, Castellanos-Jankiewicz A, et al. Inhibiting microglia expansion prevents diet-induced hypothalamic and peripheral inflammation. Diabetes. (2017) 66:908–19. doi: 10.2337/db16-0586
110. Wang XL, Li L. Microglia regulate neuronal circuits in homeostatic and high-fat diet-induced inflammatory conditions. Front Cell Neurosci. (2021) 15:722028. doi: 10.3389/fncel.2021.722028
111. Wang XL, Kooijman S, Gao Y, Tzeplaeff L, Cosquer B, Milanova I, et al. Microglia-specific knock-down of Bmal1 improves memory and protects mice from high fat diet-induced obesity. Mol Psychiatry. (2021) 26(11):6336–49. doi: 10.1038/s41380-021-01169-z
112. Winkler Z, Kuti D, Polyák Á, Juhász B, Gulyás K, Lénárt N, et al. Hypoglycemia-activated hypothalamic microglia impairs glucose counterregulatory responses. Sci Rep. (2019) 9:6224. doi: 10.1038/s41598-019-42728-3
113. Jin S, Kim JG, Park JW, Koch M, Horvath TL, Lee BJ. Hypothalamic TLR2 triggers sickness behavior via a microglia-neuronal axis. Sci Rep. (2016) 6:29424. doi: 10.1038/srep29424
114. Reis WL, Yi CX, Gao Y, Tschop MH, Stern JE. Brain innate immunity regulates hypothalamic arcuate neuronal activity and feeding behavior. Endocrinology. (2015) 156:1303–15. doi: 10.1210/en.2014-1849
115. Nilsson I, Lindfors C, Fetissov SO, Hokfelt T, Johansen JE. Aberrant agouti-related protein system in the hypothalamus of the anx/anx mouse is associated with activation of microglia. J Comp Neurol. (2008) 507:1128–40. doi: 10.1002/cne.21599
116. Howard D, Negraes P, Voineskos AN, Kaplan AS, Muotri AR, Duvvuri V, et al. Molecular neuroanatomy of anorexia nervosa. Sci Rep. (2020) 10:11411. doi: 10.1038/s41598-020-67692-1
117. Gaige S, Barbouche R, Barbot M, Boularand S, Dallaporta M, Abysique A, et al. Constitutively active microglial populations limit anorexia induced by the food contaminant deoxynivalenol. J Neuroinflamm. (2022) 19:280. doi: 10.1186/s12974-022-02631-7
118. Burfeind KG, Zhu X, Norgard MA, Levasseur PR, Huisman C, Michaelis KA, et al. Microglia in the hypothalamus respond to tumor-derived factors and are protective against cachexia during pancreatic cancer. Glia. (2020) 68:1479–94. doi: 10.1002/glia.23796
119. Urabe H, Kojima H, Chan L, Terashima T, Ogawa N, Katagi M, et al. Haematopoietic cells produce BDNF and regulate appetite upon migration to the hypothalamus. Nat Commun. (2013) 4:1526. doi: 10.1038/ncomms2536
120. De Luca SN, Sominsky L, Soch A, Wang H, Ziko I, Rank MM, et al. Conditional microglial depletion in rats leads to reversible anorexia and weight loss by disrupting gustatory circuitry. Brain Behav Immun. (2019) 77:77–91. doi: 10.1016/j.bbi.2018.12.008
121. Dorfman MD, Krull JE, Douglass JD, Fasnacht R, Lara-Lince F, Meek TH, et al. Sex differences in microglial CX3CR1 signalling determine obesity susceptibility in mice. Nat Commun. (2017) 8:14556. doi: 10.1038/ncomms14556
122. Campbell JN, Macosko EZ, Fenselau H, Pers TH, Lyubetskaya A, Tenen D, et al. A molecular census of arcuate hypothalamus and median eminence cell types. Nat Neurosci. (2017) 20:484–96. doi: 10.1038/nn.4495
123. Shrivastava K, Gonzalez P, Acarin L. The immune inhibitory complex CD200/CD200R is developmentally regulated in the mouse brain. J Comp Neurol. (2012) 520:2657–75. doi: 10.1002/cne.23062
124. Comella Bolla A, Valente T, Miguez A, Brito V, Gines S, Sola C, et al. CD200 is up-regulated in R6/1 transgenic mouse model of Huntington’s disease. PloS One. (2019) 14:e0224901. doi: 10.1371/journal.pone.0224901
125. Pereira SS, Alvarez-Leite JI. Low-grade inflammation, obesity, and diabetes. Curr Obes Rep. (2014) 3:422–31. doi: 10.1007/s13679-014-0124-9
126. Lago F, Dieguez C, Gómez-Reino J, Gualillo O. The emerging role of adipokines as mediators of inflammation and immune responses. Cytokine Growth Factor Rev. (2007) 18:313–25. doi: 10.1016/j.cytogfr.2007.04.007
127. Hotamisligil GS. Inflammation, metaflammation and immunometabolic disorders. Nature. (2017) 542:177–85. doi: 10.1038/nature21363
128. De Souza CT, Araujo EP, Bordin S, Ashimine R, Zollner RL, Boschero AC, et al. Consumption of a fat-rich diet activates a proinflammatory response and induces insulin resistance in the hypothalamus. Endocrinology. (2005) 146:4192–9. doi: 10.1210/en.2004-1520
129. Kang YM, Zhang ZH, Xue B, Weiss RM, Felder RB. Inhibition of brain proinflammatory cytokine synthesis reduces hypothalamic excitation in rats with ischemia-induced heart failure. Am J Physiol Heart Circ Physiol. (2008) 295:H227–36. doi: 10.1152/ajpheart.01157.2007
130. Zhang X, Zhang G, Zhang H, Karin M, Bai H, Cai D. Hypothalamic IKKbeta/NF-kappaB and ER stress link overnutrition to energy imbalance and obesity. Cell. (2008) 135:61–73. doi: 10.1016/j.cell.2008.07.043
131. Milanski M, Degasperi G, Coope A, Morari J, Denis R, Cintra DE, et al. Saturated fatty acids produce an inflammatory response predominantly through the activation of TLR4 signaling in hypothalamus: implications for the pathogenesis of obesity. J Neurosci. (2009) 29:359–70. doi: 10.1523/JNEUROSCI.2760-08.2009
132. Chen W, Balland E, Cowley MA. Hypothalamic insulin resistance in obesity: effects on glucose homeostasis. Neuroendocrinology. (2017) 104:364–81. doi: 10.1159/000455865
133. El-Haschimi K, Pierroz DD, Hileman SM, Bjørbæk C, Flier JS. Two defects contribute to hypothalamic leptin resistance in mice with diet-induced obesity. J Clin Invest. (2000) 105:1827–32. doi: 10.1172/jci9842
134. Forsythe CE, Phinney SD, Fernandez ML, Quann EE, Wood RJ, Bibus DM, et al. Comparison of low fat and low carbohydrate diets on circulating fatty acid composition and markers of inflammation. Lipids. (2008) 43:65–77. doi: 10.1007/s11745-007-3132-7
135. Volk BM, Kunces LJ, Freidenreich DJ, Kupchak BR, Saenz C, Artistizabal JC, et al. Effects of step-wise increases in dietary carbohydrate on circulating saturated Fatty acids and palmitoleic Acid in adults with metabolic syndrome. PloS One. (2014) 9:e113605. doi: 10.1371/journal.pone.0113605
136. Parimisetty A, Dorsemans AC, Awada R, Ravanan P, Diotel N, Lefebvre d’Hellencourt C. Secret talk between adipose tissue and central nervous system via secreted factors-an emerging frontier in the neurodegenerative research. J Neuroinflamm. (2016) 13:67. doi: 10.1186/s12974-016-0530-x
137. Kawai T, Autieri MV, Scalia R. Adipose tissue inflammation and metabolic dysfunction in obesity. Am J Physiol Cell Physiol. (2021) 320:C375–C91. doi: 10.1152/ajpcell.00379.2020
138. Argente-Arizón P, Guerra-Cantera S, Garcia-Segura LM, Argente J, Chowen JA. Glial cells and energy balance. J Mol Endocrinol. (2017) 58:R59–71. doi: 10.1530/jme-16-0182
139. Robb JL, Morrissey NA, Weightman Potter PG, Smithers HE, Beall C, Ellacott KLJ. Immunometabolic changes in glia - A potential role in the pathophysiology of obesity and diabetes. Neuroscience. (2020) 447:167–81. doi: 10.1016/j.neuroscience.2019.10.021
140. Valdearcos M, Robblee MM, Benjamin DI, Nomura DK, Xu AW, Koliwad SK. Microglia dictate the impact of saturated fat consumption on hypothalamic inflammation and neuronal function. Cell Rep. (2014) 9:2124–38. doi: 10.1016/j.celrep.2014.11.018
141. Valdearcos M, Xu AW, Koliwad SK. Hypothalamic inflammation in the control of metabolic function. Annu Rev Physiol. (2015) 77:131–60. doi: 10.1146/annurev-physiol-021014-071656
142. Cheng L, Yu Y, Szabo A, Wu Y, Wang H, Camer D, et al. Palmitic acid induces central leptin resistance and impairs hepatic glucose and lipid metabolism in male mice. J Nutr Biochem. (2015) 26:541–8. doi: 10.1016/j.jnutbio.2014.12.011
143. Cheng L, Yu Y, Zhang Q, Szabo A, Wang H, Huang XF. Arachidonic acid impairs hypothalamic leptin signaling and hepatic energy homeostasis in mice. Mol Cell Endocrinol. (2015) 412:12–8. doi: 10.1016/j.mce.2015.04.025
144. Posey KA, Clegg DJ, Printz RL, Byun J, Morton GJ, Vivekanandan-Giri A, et al. Hypothalamic proinflammatory lipid accumulation, inflammation, and insulin resistance in rats fed a high-fat diet. Am J Physiol Endocrinol Metab. (2009) 296:E1003–12. doi: 10.1152/ajpendo.90377.2008
145. Portovedo M, Ignacio-Souza LM, Bombassaro B, Coope A, Reginato A, Razolli DS, et al. Saturated fatty acids modulate autophagy’s proteins in the hypothalamus. PloS One. (2015) 10:e0119850. doi: 10.1371/journal.pone.0119850
146. Ozcan L, Ergin AS, Lu A, Chung J, Sarkar S, Nie D, et al. Endoplasmic reticulum stress plays a central role in development of leptin resistance. Cell Metab. (2009) 9:35–51. doi: 10.1016/j.cmet.2008.12.004
147. Horvath TL, Sarman B, García-Cáceres C, Enriori PJ, Sotonyi P, Shanabrough M, et al. Synaptic input organization of the melanocortin system predicts diet-induced hypothalamic reactive gliosis and obesity. Proc Natl Acad Sci. (2010) 107:14875–80. doi: 10.1073/pnas.1004282107
148. Douglass JD, Ness KM, Valdearcos M, Wyse-Jackson A, Dorfman MD, Frey JM, et al. Obesity-associated microglial inflammatory activation paradoxically improves glucose tolerance. Cell Metab. (2023) 35(9):1613–29.e8. doi: 10.1016/j.cmet.2023.07.008
149. Maeshima N, Fernandez RC. Recognition of lipid A variants by the TLR4-MD-2 receptor complex. Front Cell Infect Microbiol. (2013) 3:3. doi: 10.3389/fcimb.2013.00003
150. Chaves FM, Mansano NS, Frazão R, Donato J Jr. Tumor necrosis factor alpha and interleukin-1β acutely inhibit AgRP neurons in the arcuate nucleus of the hypothalamus. Int J Mol Sci. (2020) 21(23):8928. doi: 10.3390/ijms21238928
151. Yi CX, Walter M, Gao Y, Pitra S, Legutko B, Kalin S, et al. TNFalpha drives mitochondrial stress in POMC neurons in obesity. Nat Commun. (2017) 8:15143. doi: 10.1038/ncomms15143
152. Drougard A, Ma EH, Wegert V, Sheldon R, Panzeri I, Vatsa N, et al. A rapid microglial metabolic response controls metabolism and improves memory. bioRxiv. (2023). doi: 10.1101/2023.04.03.535373
153. Gao Y, Vidal-Itriago A, Kalsbeek MJ, Layritz C, Garcia-Caceres C, Tom RZ, et al. Lipoprotein lipase maintains microglial innate immunity in obesity. Cell Rep. (2017) 20:3034–42. doi: 10.1016/j.celrep.2017.09.008
154. Souza GF, Solon C, Nascimento LF, De-Lima-Junior JC, Nogueira G, Moura R, et al. Defective regulation of POMC precedes hypothalamic inflammation in diet-induced obesity. Sci Rep. (2016) 6:29290. doi: 10.1038/srep29290
155. Lyu P, Huang Z, Feng Q, Su Y, Zheng M, Hong Y, et al. Unveiling the transcriptome alteration of POMC neuron in diet-induced obesity. Exp Cell Res. (2020) 389:111848. doi: 10.1016/j.yexcr.2020.111848
156. Rahmouni K, Davisson RL, Sigmund CD. Inflaming hypothalamic neurons raises blood pressure. Cell Metab. (2011) 14:3–4. doi: 10.1016/j.cmet.2011.06.006
157. Saderi N, Salgado-Delgado R, Avendaño-Pradel R, Basualdo MDC, Ferri G-L, Chávez-Macías L, et al. NPY and VGF immunoreactivity increased in the arcuate nucleus, but decreased in the nucleus of the tractus solitarius, of type-II diabetic patients. PloS One. (2012) 7:e40070. doi: 10.1371/journal.pone.0040070
158. Dalvi PS, Chalmers JA, Luo V, Han DY, Wellhauser L, Liu Y, et al. High fat induces acute and chronic inflammation in the hypothalamus: effect of high-fat diet, palmitate and TNF-α on appetite-regulating NPY neurons. Int J Obes. (2017) 41:149–58. doi: 10.1038/ijo.2016.183
159. King PJ, Widdowson PS, Doods H, Williams G. Effect of cytokines on hypothalamic neuropeptide Y release in vitro. Peptides. (2000) 21:143–6. doi: 10.1016/s0196-9781(99)00183-7
160. Valdearcos M, Douglass JD, Robblee MM, Dorfman MD, Stifler DR, Bennett ML, et al. Microglial inflammatory signaling orchestrates the hypothalamic immune response to dietary excess and mediates obesity susceptibility. Cell Metab. (2017) 26:185–97 e3. doi: 10.1016/j.cmet.2017.05.015
161. Lee CH, Shin SH, Kang GM, Kim S, Kim J, Yu R, et al. Cellular source of hypothalamic macrophage accumulation in diet-induced obesity. J Neuroinflamm. (2019) 16:221. doi: 10.1186/s12974-019-1607-0
162. Ataka K, Asakawa A, Nagaishi K, Kaimoto K, Sawada A, Hayakawa Y, et al. Bone marrow-derived microglia infiltrate into the paraventricular nucleus of chronic psychological stress-loaded mice. PloS One. (2013) 8:e81744. doi: 10.1371/journal.pone.0081744
163. Morari J, Anhe GF, Nascimento LF, de Moura RF, Razolli D, Solon C, et al. Fractalkine (CX3CL1) is involved in the early activation of hypothalamic inflammation in experimental obesity. Diabetes. (2014) 63:3770–84. doi: 10.2337/db13-1495
164. Lee CH, Kim HJ, Lee YS, Kang GM, Lim HS, Lee SH, et al. Hypothalamic macrophage inducible nitric oxide synthase mediates obesity-associated hypothalamic inflammation. Cell Rep. (2018) 25:934–46 e5. doi: 10.1016/j.celrep.2018.09.070
165. Jais A, Solas M, Backes H, Chaurasia B, Kleinridders A, Theurich S, et al. Myeloid-cell-derived VEGF maintains brain glucose uptake and limits cognitive impairment in obesity. Cell. (2016) 165:882–95. doi: 10.1016/j.cell.2016.03.033
166. Langlet F, Levin BE, Luquet S, Mazzone M, Messina A, Dunn-Meynell AA, et al. Tanycytic VEGF-A boosts blood-hypothalamus barrier plasticity and access of metabolic signals to the arcuate nucleus in response to fasting. Cell Metab. (2013) 17:607–17. doi: 10.1016/j.cmet.2013.03.004
167. Leon S, Nadjar A, Quarta C. Microglia-neuron crosstalk in obesity: melodious interaction or kiss of death? Int J Mol Sci. (2021) 22(10):5243. doi: 10.3390/ijms22105243
168. Bérangère Ré D, Przedborski S. Fractalkine: moving from chemotaxis to neuroprotection. Nat Neurosci. (2006) 9:859–61. doi: 10.1038/nn0706-859
169. Angelopoulou E, Paudel YN, Shaikh MF, Piperi C. Fractalkine (CX3CL1) signaling and neuroinflammation in Parkinson’s disease: Potential clinical and therapeutic implications. Pharmacol Res. (2020) 158:104930. doi: 10.1016/j.phrs.2020.104930
170. Kawamura N, Katsuura G, Yamada-Goto N, Nakama R, Kambe Y, Miyata A, et al. Brain fractalkine-CX3CR1 signalling is anti-obesity system as anorexigenic and anti-inflammatory actions in diet-induced obese mice. Sci Rep. (2022) 12:12604. doi: 10.1038/s41598-022-16944-3
171. Wu Y, Peng H, Cui M, Whitney NP, Huang Y, Zheng JC. CXCL12 increases human neural progenitor cell proliferation through Akt-1/FOXO3a signaling pathway. J Neurochem. (2009) 109:1157–67. doi: 10.1111/j.1471-4159.2009.06043.x
172. Callewaere C, Banisadr G, Desarmenien MG, Mechighel P, Kitabgi P, Rostene WH, et al. The chemokine SDF-1/CXCL12 modulates the firing pattern of vasopressin neurons and counteracts induced vasopressin release through CXCR4. Proc Natl Acad Sci U S A. (2006) 103:8221–6. doi: 10.1073/pnas.0602620103
173. Poon K, Barson JR, Ho HT, Leibowitz SF. Relationship of the chemokine, CXCL12, to effects of dietary fat on feeding-related behaviors and hypothalamic neuropeptide systems. Front Behav Neurosci. (2016) 10:51. doi: 10.3389/fnbeh.2016.00051
174. Dommel S, Blüher M. Does C-C motif chemokine ligand 2 (CCL2) link obesity to a pro-inflammatory state? Int J Mol Sci. (2021) 22(3):1500. doi: 10.3390/ijms22031500
175. Mendes NF, Kim YB, Velloso LA, Araujo EP. Hypothalamic microglial activation in obesity: A mini-review. Front Neurosci. (2018) 12:846. doi: 10.3389/fnins.2018.00846
176. Lee CH, Suk K, Yu R, Kim MS. Cellular contributors to hypothalamic inflammation in obesity. Mol Cells. (2020) 43:431–7. doi: 10.14348/molcells.2020.0055
177. Recinella L, Orlando G, Ferrante C, Chiavaroli A, Brunetti L, Leone S. Adipokines: new potential therapeutic target for obesity and metabolic, rheumatic, and cardiovascular diseases. Front Physiol. (2020) 11:578966. doi: 10.3389/fphys.2020.578966
178. Song J, Choi SM, Kim BC. Adiponectin regulates the polarization and function of microglia via PPAR-γ Signaling under amyloid β Toxicity. Front Cell Neurosci. (2017) 11:64. doi: 10.3389/fncel.2017.00064
179. Peng QM, Zhou JH, Xu ZW, Zhao QC, Li ZY, Zhao Q. Apelin−13 ameliorates LPS−induced BV−2 microglia inflammatory response through promoting autophagy and inhibiting H3K9ac enrichment of TNF−α and IL−6 promoter. Acta Neurobiol Exp. (2022) 82:65–76. doi: 10.55782/ane-2022-006
180. Ye L, Jia G, Li Y, Wang Y, Chen H, Yu L, et al. C1q/TNF-related protein 4 restores leptin sensitivity by downregulating NF-κB signaling and microglial activation. J Neuroinflamm. (2021) 18:159. doi: 10.1186/s12974-021-02167-2
181. Erfani S, Moghimi A, Aboutaleb N, Khaksari M. Nesfatin-1 improve spatial memory impairment following transient global cerebral ischemia/reperfusion via inhibiting microglial and caspase-3 activation. J Mol Neurosci. (2018) 65:377–84. doi: 10.1007/s12031-018-1105-3
182. Ji L, Zhang L, Liang Z, Zhong S, Liu X, Liu Z, et al. Role of omentin-1 in susceptibility to anxiety and depression like behaviors. Mol Cell Endocrinol. (2023) 574:111990. doi: 10.1016/j.mce.2023.111990
183. Jeon H, Kim JH, Kim JH, Lee WH, Lee MS, Suk K. Plasminogen activator inhibitor type 1 regulates microglial motility and phagocytic activity. J Neuroinflamm. (2012) 9:149. doi: 10.1186/1742-2094-9-149
184. Lloyd-Burton SM, York EM, Anwar MA, Vincent AJ, Roskams AJ. SPARC regulates microgliosis and functional recovery following cortical ischemia. J Neurosci. (2013) 33:4468–81. doi: 10.1523/JNEUROSCI.3585-12.2013
185. Yun H, Dumbell R, Hanna K, Bowen J, McLean SL, Kantamneni S, et al. The chemerin-CMKLR1 axis is functionally important for central regulation of energy homeostasis. Front Physiol. (2022) 13:897105. doi: 10.3389/fphys.2022.897105
186. Kang D, Kim HR, Kim KK, Kim DH, Jeong B, Jin S, et al. Brain-specific chemokine FAM19A5 induces hypothalamic inflammation. Biochem Biophys Res Commun. (2020) 523:829–34. doi: 10.1016/j.bbrc.2019.12.119
187. Xiao X, Zhang H, Ning W, Yang Z, Wang Y, Zhang T. Knockdown of FSTL1 inhibits microglia activation and alleviates depressive-like symptoms through modulating TLR4/MyD88/NF-κB pathway in CUMS mice. Exp Neurol. (2022) 353:114060. doi: 10.1016/j.expneurol.2022.114060
188. Jung BK, Ryu KY. Lipocalin-2: a therapeutic target to overcome neurodegenerative diseases by regulating reactive astrogliosis. Exp Mol Med. (2023) 55:2138–46. doi: 10.1038/s12276-023-01098-7
189. Fujita Y, Yamashita T. The effects of leptin on glial cells in neurological diseases. Front Neurosci. (2019) 13:828. doi: 10.3389/fnins.2019.00828
190. Pinteaux E, Inoue W, Schmidt L, Molina-Holgado F, Rothwell NJ, Luheshi GN. Leptin induces interleukin-1beta release from rat microglial cells through a caspase 1 independent mechanism. J Neurochem. (2007) 102:826–33. doi: 10.1111/j.1471-4159.2007.04559.x
191. Gao Y, Ottaway N, Schriever SC, Legutko B, García-Cáceres C, de la Fuente E, et al. Hormones and diet, but not body weight, control hypothalamic microglial activity. Glia. (2014) 62:17–25. doi: 10.1002/glia.22580
192. Xu Z, Bai S, Wu H, Fang M. Elevated retinal retinol-binding protein 4 levels in diabetic mice can induce retinal neurodegeneration through microglia. Microsc Res Tech. (2023) 86:223–31. doi: 10.1002/jemt.24258
Keywords: arcuate nucleus, hypothalamus, microglia, microglial-neuronal interaction, physiological function, obesity
Citation: Guzmán-Ruíz MA, Guerrero Vargas NN, Ramírez-Carreto RJ, González-Orozco JC, Torres-Hernández BA, Valle-Rodríguez M, Guevara-Guzmán R and Chavarría A (2024) Microglia in physiological conditions and the importance of understanding their homeostatic functions in the arcuate nucleus. Front. Immunol. 15:1392077. doi: 10.3389/fimmu.2024.1392077
Received: 26 February 2024; Accepted: 20 August 2024;
Published: 04 September 2024.
Edited by:
Francesca Granucci, University of Milano-Bicocca, ItalyReviewed by:
Magdalena Chadzińska, Jagiellonian University, PolandMariya Hristova, University College London, United Kingdom
Copyright © 2024 Guzmán-Ruíz, Guerrero Vargas, Ramírez-Carreto, González-Orozco, Torres-Hernández, Valle-Rodríguez, Guevara-Guzmán and Chavarría. This is an open-access article distributed under the terms of the Creative Commons Attribution License (CC BY). The use, distribution or reproduction in other forums is permitted, provided the original author(s) and the copyright owner(s) are credited and that the original publication in this journal is cited, in accordance with accepted academic practice. No use, distribution or reproduction is permitted which does not comply with these terms.
*Correspondence: Anahí Chavarría, YW5haGkuY2hhdmFycmlhQGZhY21lZC51bmFtLm14