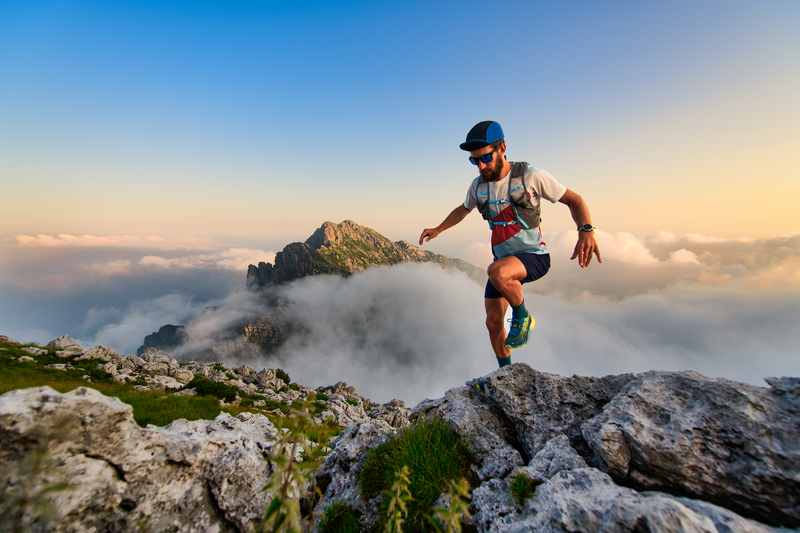
95% of researchers rate our articles as excellent or good
Learn more about the work of our research integrity team to safeguard the quality of each article we publish.
Find out more
REVIEW article
Front. Immunol. , 22 May 2024
Sec. Cancer Immunity and Immunotherapy
Volume 15 - 2024 | https://doi.org/10.3389/fimmu.2024.1389194
This article is part of the Research Topic Emerging Frontiers in Immunogenic Cell Death: Advancing Cancer Control and Immunotherapy View all 6 articles
Past research has identified that cancer cells sustain several cancer hallmarks by impairing function of the endolysosomal system (ES). Thus, maintaining the functional integrity of endolysosomes is crucial, which heavily relies on two key protein families: soluble hydrolases and endolysosomal membrane proteins. Particularly members of the TPC (two-pore channel) and TRPML (transient receptor potential mucolipins) families have emerged as essential regulators of ES function as a potential target in cancer therapy. Targeting TPCs and TRPMLs has demonstrated significant impact on multiple cancer hallmarks, including proliferation, growth, migration, and angiogenesis both in vitro and in vivo. Notably, endosomes and lysosomes also actively participate in various immune regulatory mechanisms, such as phagocytosis, antigen presentation, and the release of proinflammatory mediators. Yet, knowledge about the role of TPCs and TRPMLs in immunity is scarce. This prompts a discussion regarding the potential role of endolysosomal ion channels in aiding cancers to evade immune surveillance and destruction. Specifically, understanding the interplay between endolysosomal ion channels and cancer immunity becomes crucial. Our review aims to comprehensively explore the current knowledge surrounding the roles of TPCs and TRPMLs in immunity, whilst emphasizing the critical need to elucidate their specific contributions to cancer immunity by pointing out current research gaps that should be addressed.
For a long time after the discovery of lysosomes in 1955 (1) they were branded as the recycling compartment of the cell. However, research has shown that their major functions go beyond degradation of cellular compartments. As part of the ES, they are key regulators in endocytic uptake, intracellular trafficking, signaling and regulation of membrane proteins (2, 3). Also, lysosomes have emerged as regulators specifically for calcium signaling, as they hold high intravesicular calcium concentration estimated to be around 500μM (4–6). In the context of immunity it has become evident that the ES plays a significant role in orchestrating immune responses (7, 8).
In the innate immune system, the ES plays a pivotal role in recognizing pathogens through pattern recognition receptors (PRRs) expressed on immune cell surfaces. Activation of PRRs upon detection of pathogen-associated molecular patterns (PAMPs) triggers signaling cascades that activate immune cells and induce the production of proinflammatory cytokines and chemokines. Toll-like receptors (TLRs) located within endosomes and lysosomes, such as TLRs 3, 7, 8, and 9, specifically recognize pathogen-expressed nucleic acids and initiate kinase cascades to produce cytokines (9). The endolysosomal system’s ability to confine activation to foreign nucleic acids prevents recognition of self-DNA and RNA by degrading host nucleic acids using DNAse and RNAses (9, 10). Furthermore, the endolysosomal processing of TLRs is crucial for their activation, involving proteolytic cleavage by enzymes like AEP (asparagine endopeptidase) and cathepsins in various cell types (11).
In the adaptive immune system, endolysosomes are involved in the processing and presentation of antigens. The processing of antigens for presentation on MHC-II (major histocompatibility complex II) molecules involves proteases active in the endolysosomal pathway, facilitating their uptake from extracellular sources via icropinocytosis, phagocytosis or receptor-mediated endocytosis (12, 13). Within the endo-lysosomal compartments, the convergence of newly synthesized MHC-II molecules, proteolytic enzymes, and chaperones serves as a specialized environment for peptide loading, resembling a ‘reaction vessel’ for antigen processing (12, 13). While no specific endolysosomal protease is absolutely required in vivo (14, 15), efficient T cell activation can occur with lower antigen levels when captured by specific receptors like B cell membrane immunoglobulin (16). This mechanism involves early capture and stabilization of large antigen fragments, which can then be transferred to nearby MHC-II molecules. Moreover, the assembly of MHC-II/peptide complexes in late endocytic compartments prompts questions about their transfer to the cell surface, possibly achieved through fusion or vesicle transport (17). Notably, stimulation with TLR ligands or interaction with specific T cells induces tubulation of endolysosomes containing peptide/MHC complexes which offer an alternative mode of complex delivery (17, 18).
Interestingly, evidence suggests that the ES plays a significant role in the recycling and degradation of immune checkpoint molecules, with secretory lysosomes serving as temporary reservoirs for immune checkpoint proteins like cytotoxic T lymphocyte-associated antigen-4 (CTLA-4), programmed death-ligand 1 (PD-L1), TIM-3, CD70, CD200, and CD47 (19, 20). This degradation process might hinder the recognition of these immune checkpoint molecules by T cells, subsequently affecting the efficacy of T cell activation and immune checkpoint therapy.
Tumor immunotherapy represents an innovative approach leveraging the body’s immune system to identify and eradicate cancer cells, offering distinct advantages including high specificity, sustained efficacy, and minimal toxicity. This paradigm shift has emerged as a promising avenue in cancer treatment, evolving alongside conventional modalities like surgery, radiation therapy, and chemotherapy (21, 22). Notably, clinical immunotherapy methods encompass immune checkpoint inhibitors, adoptive cell therapy (ACT), and cancer vaccines, among others (23–25). Recent attention has turned to the pivotal role of lysosomes in cancer cell survival and the regulation of the tumor microenvironment (TME), positioning lysosomal targeting as a promising therapeutic avenue (26–28). For instance, Tang et al. introduced a pH-gated nano-adjuvant (PGN) designed to selectively modulate lysosomal pH and protease activity within tumor associated macrophages (TAMs), thereby promoting M1 polarization and enhancing antigen presentation (29). Additionally, lysosome degradation pathways have been explored for synergy with immune checkpoint inhibitors. Lysosome-targeting chimeric molecules (LYTACs) have demonstrated efficacy in degrading membrane proteins like epidermal growth factor receptor (EGFR) and PD-L1, bolstering therapeutic responses (26, 28). Also, lysosome trafficking modulation has been implicated in promoting the release of granular enzymes from tumor-infiltrating T cells (TILs) and activating innate immunity via toll-like receptors (TLRs), as demonstrated by enhanced immune responses observed with combination therapy involving cancer vaccines and TLR agonists (27, 30). Notably, the autophagy inhibitor chloroquine has shown promise in augmenting the efficacy of immune checkpoint inhibitors in clinical settings (31–33). CAR T cell therapy represents a form of cancer immunotherapy utilizing genetic manipulation to integrate chimeric antigen receptors (CARs) into the T cells of patients. While demonstrating significant efficacy in select cancer types (34), CAR T cell therapy encounters challenges concerning its persistence and functionality within the host organism, often resulting in tumor relapse or resistance (35). Lysosomes, specifically, exert a negative regulatory influence on CAR T cells by promoting the degradation of the CAR, thereby compromising CAR T cell activity and persistence. Recent research by Li et al. elucidated the underlying mechanisms, revealing that upon interaction with tumor antigens, the CAR undergoes ubiquitination and subsequent internalization, leading to lysosomal degradation and attenuation of CAR T cell function and persistence (36). To address this issue, a novel approach involving the development of a modified CAR, termed rCAR, was devised. This modified CAR features mutations in cytoplasmic lysine residues to reduce susceptibility to ubiquitination-induced downregulation. Notably, rCAR demonstrates the ability to resurface on the cell membrane following internalization, thereby maintaining functionality within intracellular compartments. In preclinical mouse tumor models, rCAR T cells exhibit elevated surface CAR expression, enhanced cytotoxicity, prolonged survival, and superior anti-tumor effects compared to conventional CAR T cells (36). In summary, lysosome-targeted cancer immunotherapy offers a multifaceted approach to disrupt tumor-immune interactions within the TME, potentially enhancing therapeutic outcomes. However, significant challenges including selective drug delivery, safety concerns, resistance mechanisms, and the need for elucidation of underlying mechanisms warrant further investigation.
This concise summary underscores the central role of the ES in both innate and adaptive immunity and in immunotherapy. Thus, proper function of endolysosomal compartments is crucial for proper regulation of immune response and surveillance. This connection is important to highlight, as impaired immune response and mechanisms that aid cancer cells to escape immune surveillance are prerequisites of cancer.
Impairment and manipulation of endolysosomal function have been shown to be one major mechanism of cells to sustain multiple cancer hallmarks (37). Therefore, one can say that proper lysosomal function is crucial for proper cell function. Lysosomal functionality is maintained by preserving low lysosomal pH and therefore securing enzymatic function through two main protein groups: the more than 60 hydrolytic enzymes located inside the lysosomes and lysosomal membrane proteins (2, 38, 39). The lysosomal membrane proteins and ion channels not only secure acidification of the lysosomal lumen, but also regulate protein imports from the cytosol, fusion with other organelles and transport of degradation products to the cytoplasm (40). The most prominent membrane proteins are the lysosome-associated membrane glycoproteins (LAMPs) and the vacuolar-type H+ ATPase (V-ATPase), an ATP-dependent proton pump. Past research has shown that V-ATPase does not only maintain the required low lysosomal pH (41), but it has become evident that it plays a significant role in cancer (42–45). Primarily, V-ATPase has been found to be overexpressed in cancer, which promotes ECM (extracellular matrix) degradation and consequently cancer cell invasion and migration (46). Additionally, inhibition of V-ATPase has been shown to reduce cancer cell proliferation, induce apoptosis and promote mitochondrial fission (43, 47). The unravelling of the role of V-ATPase and hence lysosomal ion homeostasis in cancer progression was followed by multiple clinical trials. However, V-ATPase inhibitors largely failed in clinical trials (48), which shifted the limelight to further lysosomal ion regulators, i.e. ion channels and their potential role in maintaining cancer hallmarks. The focus of this research field centers around two-pore channels (TPCs) and transient receptor potential cation channels (TRPMLs) both of which are cation channels abundantly co-expressed in the lysosomal membrane of non-carcinogenic and carcinogenic tissue (2, 49).
TPCs are ligand gated cation ion channels embedded in the membranes of endosomes, late endolysosomes, and lysosomes (50). In the human genome TPC1 and TPC2 are the two known paralogs, TPC1 is mainly found in endolysosomal organelles, whilst TPC2 is predominantly expressed in lysosomes [21]. Structurally, TPC2 is composed of two homologous building blocks, each consisting of six transmembrane domains with N- and C-termini reaching into the cytosol (51). Nicotinic acid adenine dinucleotide phosphate (NAADP)- and PI (3,5)P2 are both endogenous agonists of TPC2, which trigger the release of different cations in an agonist-depending manner (52–55). What has been recognized so far, is a well-established model of NAADP indirectly activating TPC2, resulting in a release of calcium from acidic stores (52, 55). Also, it has been proposed recently that TPC2 can function as sodium permeable ion channel upon direct activation by PI (3,5)P2 (54, 55). It has been shown that TPC2 plays a crucial role in tumorigenesis, as amplifications in the TPC2 gene and overexpression of the channel are associated with cancer hallmarks. Müller et al. demonstrated that TPC2 drives cancer cell proliferation and is crucial for sustaining cellular energy metabolism (56). Also, the genetic ablation of TPC2 resulted in a significant reduction of cancer cell proliferation in vitro (56–58) and prevented tumor growth in vivo (56). Besides driving proliferation, TPC2 is an activator of migration and invasion and therefore is involved in two critical steps in the development of metastases (57, 59). It has been indicated that silencing TPC2 leads to a minimization of lung metastases in vivo and reduced cell migration by impairment of the β1-integrin recycling pathway (57). Moreover, TPC2 is involved in promoting angiogenesis by being part of the VEGFR2/NAADP/TPC2/Ca2+ signaling pathway, which promotes VEGF-induced angiogenesis in vitro and in vivo (60, 61).
TRPML is a subfamily of the transient receptor potential (trp) family are non-selective cation channels, which are also ubiquitously expressed in late endosomes and lysosomes of mammalian cells (62). There are three known mammalian isoforms of TRPML: TRPML1, TRPML2 and TRPML3, which were established upon the identification of TRPML1 as the protein mutated in the lysosomal storage disease mucolipidosis type IV (MLIV) (63). PI (3,5)P2 and reactive oxygen species (ROS) act as endogenous activators and trigger lysosomal calcium release from TRPML1 in a pH-dependent manner, whereas PI (4, 5)P2 represents the endogenous inhibitor at membrane sites (64, 65). In addition to being considered the primary lysosomal calcium channel, TRPML1 also demonstrates the ability to transport heavy metals such as Fe2+ and Zn2+ from the lysosomal lumen into the cytosol (66). It has been suggested that the release of calcium through TRPML1 drives fission and fusion within the endocytic pathway and lysosomal membrane trafficking as many trafficking steps are calcium-dependent and altered in knockout cells (66–68). Likewise, the importance of TRPML1 in cell signal transduction, organelle homeostasis and endosomal maturation have been demonstrated (67, 69–71). In cancer, a study by Abrahamian et al. revealed elevated levels of TRPML1 in different cancer cell lines (72). Further research has revealed a significant impact of TRPML1 on sustaining multiple cancer hallmarks such as cell proliferation, survival, invasion and migration (73–77). Also, it has been recognized that TRPML2 plays an important role in cancer development and therapy. The expression of TRPML2 in cancer tissue has been connected to higher proliferation rates and impaired apoptosis, whilst overall survival of patients negatively correlates with TRPML2 expression levels (78–80).
Taken together, emerging research has highlighted the key role of two lysosomal membrane families TPCs and TRPMLs in cancer development and tumorigenesis by sustaining several cancer hallmarks, e.g. cell proliferation, evading apoptosis, chemoresistance and migration of invasive cancer cells (56, 57, 81, 82). The involvement of the ES and its ion channels in endocytosis, exocytosis, and trafficking processes in cancer cells, as well as the involvement of the ES in regulating major trafficking processes in immune cells prompts an intriguing query, which has not been addressed yet: Are TRPMLs and TPCs also regulating exocytosis processes in immune cells and therefore, responsible for cytokine release? As both ion channel families are expressed in different immune cells (Figure 1) they might also regulate immune cell function. Also, does impaired function of TRPMLs and TPCs in immune cells lead to altered expression of i.e.: MHC molecules? Are both ion channel families key regulators of the mechanisms we observe in cancer cells and immune cells, that aid to evade immune destruction and surveillance? In this review we want to summarize the current knowledge of both ion channel families in immune cells to then discuss their potential role in cancer immunity and to identify possible research gaps that should be addressed in the future.
Figure 1 Expression of TRPMLs and TPCs in immune cells. An overview of the expression of TRPMLs and TPCs among immune cells according to the literature used in our review and additional data from Human Protein Atlas: Blood Cell Type Expression (RNA) of TPCN1/TPCN2/MCOLN1/MCOLN2/MCOLN3 available at https://www.proteinatlas.org.
Mucolipins have been shown to play a pivotal role in the autophagic regulation, calcium homeostasis and cancer metastasis (81, 83, 84). Besides their role in cancer, recent research highlights their striving importance in immunity. In immune cells two of the three known isoforms, TRPML1 and TRPML2, are mainly expressed in monocytes and macrophages, whereas expression of TRPML3 is rather low (85). Thus, we will next focus on what is known about TRPML1 and TRPML2 in immune cell function (Figures 1, 2).
Figure 2 TRPML1 and TRPML2 in immune cells. Overview of the pivotal effects that cation channels of the TRPML family have in immune cells.
Macrophages play an important role within the innate immune system by secreting chemokines and cytokines after stimulation and thereby attracting more immune cells to the inflammation herd, as has been excellently reviewed elsewhere (86). The polarization state of macrophages is determined by the presence of different cytokines. On the one hand, classically activated macrophages (M1) are polarized through interferon-γ, TLR-ligands, or microbial substrates (e.g., LPS). The activation to M1 is linked to an increase in the production of pro-inflammatory cytokines such as TNF-α, IL-6, IL-1, and IL-23, consequently leading to the production of reactive oxygen species (ROS). On the other hand, M2 macrophages are generated in the presence of IL-4 and IL-13, and play a role in suppression of inflammation and tumor progression through the secretion of anti-inflammatory cytokines such as IL-10 and TGF-β (86, 87).
One of the main functions of macrophages is phagocytosis, a special form of endocytosis and a crucial step in the defense against pathogens and tumor cells (88). Recent work showed that TRPML1 plays different roles in the process of phagocytosis. Samie et al. were the first to provide information of the key role of TRPML1 in phagocytosis (89). They have claimed the involvement of TRPML1 in the process of engulfment of large particles by enlarging the cell surface/membrane through lysosomal exocytosis (89). They suggested that particle binding stimulated the endolysosomal kinase PIKfyve, which leads to a production of PI (3,5)P2 through phosphorylation of PI (3)P. PI (3,5)P2 is an endogenous activator of TRPML1 (90), thus the increased intracellular PI (3,5)P2 levels activate TPRML1, triggering a Ca2+ release which in return induced lysosomal exocytosis at the site of the phagocytic cup (89). In line with their observations, they demonstrated that a knockout of TRPML1 decreases the phagocytosis of large particles in bone marrow derived macrophages (BMDM), hinting that the TRPML1-mediated Ca2+ release is crucial for large particle phagocytosis (89). Moreover, Dayam et al. demonstrated that phagolysosomal biogenesis was impaired in TRPML1 silenced or PIKfyve inhibited cells and halted phagosome maturation (91). They indicate that TRPML1 regulates phagolysosomal fusion and phagosome maturation via PIKfyve-TRPML1-Ca2+ axis (91, 92), consequently leading to enhanced nuclear translocation of TFEB (transcription factor EB) (93). This observation suggests that nuclear translocation of lysosomal master regulator TFEB is dependent on phagosome maturation (93).
Besides phagocytosis, cytokine secretion by macrophages is a crucial step in the process of immune cell growth and activation. In this context, the treatment of macrophages with LPS triggers their activation followed by a strong upregulation of TRPML2 expression, while TRPML1 and TRPML3 expression remained unchanged (94). Plesch et al. followed up on this observation and showed that TRPML2 is localized on tubular compartments and early/recycling endosomes, where it promotes the release of major chemokine CCL2 (95). Subsequently, the release of CCL2 from macrophages results in a recruitment of more macrophages. In the TME release of cytokines like CCL2 and other growth factors from a network of different immune cells significantly contributes to tumor progression (96).
Moreover, alongside dendritic cells (DCs) and B-lymphocytes, macrophages are professional antigen presenting cells (APCs). Therefore, the presentation of exogenous antigens through MHC-I or MHC-II molecules counts as another major function of macrophages. Antigen presentation via MHC molecules builds a bridge between innate and adaptive immune system and is crucial for an effective immune response and surveillance. In retrospect TRPML1 was required for the efficient exit of MHC-II and MHC-I molecules that target the plasma membrane in macrophages (97).
DCs serve as crucial APCs in the adaptive immune response (98, 99). After capturing pathogenic antigens through macropinocytosis, immature DCs undergo maturation. Subsequently, they process and exhibit elevated levels of antigens, stimulatory molecules, and cytokines. Upon migration to lymph nodes, mature DCs present these antigens and activate T cell responses (100, 101). Bretou et al. have highlighted the importance of TRPML1 in DC chemotaxis and migration to lymph node by controlling the motor protein myosin II retrograde flow at the cell rear (102). Moreover, they demonstrated that TRPML1-mediated Ca2+ efflux promotes TFEB translocation in DCs. Translocation of TFEB results in an activation of the CLEAR network, which has been linked to a downregulation of MHC-I expression and an upregulation of MHC-II expression, IL-6, TNF-alpha and IL-1β production and most importantly DC migration (10, 102–105). Now, TRPML1-mediated TFEB translocation is responsible for reduced MHC-I expression on DCs. This prompts the question whether a TRPML1-dependent reduction is also observable on cancer cell surface and therefore accountable for a reduced detection by CD8+ T cells.
In addition, there is evidence that TRPML1 is a key regulator in the response of cells to ssRNA via TLR7 pathway (106). In this case, Li et al. have shown that by inhibiting PIKfyve/TRPML1 function the transport of ssRNA into the lysosomes is altered. An impairment of ssRNA transport, leads to an impaired TLR7 response, which is followed by reduced maturation/activation of dendritic cells and impeding their migration to the lymph nodes. Yet again, this highlights the important role of PIKfyve/TRPML1 axis in immunity (106).
The main functions of nature killer (NK) cells are to limit the spread of microbial infections and tumors and trigger “induced self-killing” via receptor binding (107). Conversely, in this class of immune cells TRPML1 plays a modulating role, regulating effector function and granzyme B content. A siRNA knockdown of TRPML1 or inhibition of upstream regulator PIKfyve led to an enlargement of lysosomes with increased granzyme B content (108). Therefore, Goodridge et al. suggested that by manipulating lysosomal calcium homeostasis, e.g.: targeting TRPML1 in NK cells, one can increase NK cell function and efficacy (108).
B lymphocytes constitute an integral component of the adaptive immune system, synergizing with other immune effectors such as T cells, macrophages, and DCs to eliminate foreign antigens. Their function involves the production and secretion of numerous antibody molecules, enabling them to recognize and respond to pathogens or foreign antigens (109, 110). Central to this process is the B cell receptor (BCR), an integral membrane protein characterized by its structural composition of two Ig light chains, two Ig heavy chains, and one heterodimer composed of Igα and Igβ. Notably, B cells harbor a specialized lysosomal compartment where antigens originating from endocytosed BCRs are loaded onto MHC-II molecules (109).
TRPML1 and TRPML2 have been shown to be expressed in B cells (111). Song et al. showed that TRPML1 deficient B cells do not show gross changes within the lysosomal compartments (112). This is in line with their observation that patients with mucolipidosis type IV (MLIV), who genetically lack TRPML1, do not show abnormalities in lymphocyte function or any immune function defects. Song et al. suggested compensatory lysosomal mechanisms by TRPML2 or other ion channels to be responsible for the maintenance of immune function upon TRPML1 ablation (111, 112).
Taken together there is growing evidence that TRPML1 and TRPML2 are essential for the rapid response of the innate immune system and are also key mediators between innate and adaptive immune response. There lays great potential in further elucidating their precise role in adaptive immunity in general, but also in elucidating their role in cancer immunity. The discussed data suggests that TRPML1 and TRPML2 are important regulators of phagocytosis, phagosome biogenesis, immune cell movement and are involved in mediating cytokine release. All named biological processes where both ion channels play key roles are also found in mechanisms that help cancer cells evade immune destruction. Thus, it would be relevant to know whether TRPML1 and TRPML2 play a role in the release of other cytokines important for tumor growth and securing a pro-tumorigenic TME. If TRPML1 affects MHC-I and II expression on macrophages, does it also regulate MHC-I expression in cancer cells and therefore cytotoxic T cell response? As TRPML1 affects cancer cell as well as DC migration and the recycling of adhesion molecules as well as actin remodeling, is leukocyte recruitment impacted by TRPML1? Could targeting TRPML1 ion channels in cancer present a dual role, by inhibiting cancer hallmarks on the one hand and fostering an anti-tumor response on the other?
Both isoforms of the TPC family, TPC1 and TPC2, have been reported to be involved in calcium homeostasis, cell regulation, cancer and the regulation of immune responses (38, 56) (Figure 3). TPC1 as well as TPC2 are expressed in all immune cells, yet they show higher expression levels in monocytes and neutrophils compared to other immune cells (113).
Figure 3 TPC1 and TPC2 in immune cells. Overview of the effects that cation channels of the TPC family have in immune cells.
As mentioned, phagocytosis is a crucial step in macrophages and part of the first line defense of the innate immune system against pathogens. A recent study by Davis et al. suggested that TPC1 or TPC2 may be a crucial player in phagosome scission from plasma membrane, a key step in the process of phagocytosis (114). They imply that Fcγ receptor binding triggers the release of local lysosomal Ca2+ via NAADP/TPC pathway, which in return activates the GTPase dynamin 2, an essential protein for phagosome scission from the plasma membrane (114). Moreover, Li et al. could show that a blockage of TPC2 in BMDMs resulted in reduced activation of the immune system, due to endolysosomal trapping. They have shown that TPC2 deficient cells are characterized by a reduced IL-1β levels and therefore diminished immune activation (115). Yet, further investigations in regard to the effect on cancer cells and the TME have not been made. Another question to be answered is whether TRPML or TPC channels are predominantly regulating macrophage function, or if both are mandatory for proper cell function.
Another mode of action has been described by Goretzko et al. emphasizing the role of TPC2 as a key element in controlling inflammatory leukocyte recruitment and adhesion via P-Selectin (116, 117). P-Selectin is a transmembrane protein that plays a crucial role in the initial recruitment of leukocytes to the site of inflammation. In the context of cancer immunity, P-Selectin has been linked to the development of cancer metastasis by complex forming with tumor cells and leukocytes, and thereby masking tumor cells from recognition by macrophages (118). Also, E-Selectin, which shows similar functional roles in leukocyte recruitment and cancer metastasis like P-Selectin, appears to be highly co-express with TPC2 in hepatocytes and in that way may promote an inflammatory TME (119, 120).
TPC1 has been linked to the exocytosis event of histamine secretion in mast cells (121). Histamine secretion is an inflammatory signal by mast cells and basophils and marks the main driver of allergic reaction and is dependent on intracellular calcium signals (122). Arlt et al. suggested TPC1-mediated Ca2+ release to be regulating histamine secretion in vivo and ex vivo in murine mast cells. The activation of Fcε receptors by IgE antibodies induces the activation of IP3 receptors, leading to the release of Ca2+ from the endoplasmic reticulum (ER) (123). The consequent elevation in cytosolic free Ca2+ concentration initiates the exocytosis of secretory granules. It was hypothesized that TPC1 predominantly resides at the interface between endolysosomes and the ER, facilitating the absorption of Ca2+ from the ER. It has been shown that TPCs play a role in the regulation of innate immune system and are invaluable during anaphylaxis (121). Thus, it may be important to shed more light on the presentation of chemokine signaling molecules and cytolytic factors in cytotoxic T cells, as ideally, a cytotoxic T cell response against tumor tissue is the aim of tumor immune therapy approaches.
Cytotoxic CD8+ T cells (CTLs) are indispensable in immune defense by targeting and eliminating infected cells, as well as malignant cells. They achieve their killing function by recognizing foreign or mutated peptides presented on the cell surface through MHC-I molecules. By secreting granzymes and perforin, they induce apoptosis and effectively eliminate their target cells. Indeed, the activation of TPCs in CTLs initiates the extracellular release of perforin and granzyme B from cytolytic granules. Notably, studies have identified the presence of both TPC1 and TPC2 within the immunological synapse, the interface between CTLs and target cells (124). However, the specific implications of TPC activation on anti-tumor responses have yet to be thoroughly investigated (125). In CD4+ T cells the role of TPCs is not fully understood and elucidated. Dammermann and Guse et al. have not seen a significant effect of NAADP mediated endolysosomal Ca2+ release in human Jurkat T cells (126). However, the importance of long lasting Ca2+ signaling for T cell activation is well elucidated (127–129). Thus, TPCs might be involved in the regulation of Ca2+ signals in CD4+ in the same manner as described in mast cells by Arlt et al. (121).
It becomes clear that research on the role of TPCs in immunity is emerging and it is of vast interest to address research gaps. TPCs are abundant for lysosomal trafficking in cancer cells as well as in immune cells, thus investigating their mechanisms and precise roles remains of great significance. Multiple queries remain elusive: Do TPCs regulate cytokine release in macrophages and cancer cells as observed for TPC1 in mediating histamine secretion in mast cells? Is the enhancement of CTL-mediated antitumor responses mediated by TPCs? Since there are no evidences for TPCs in the recycling of MHC molecules and consequently MHC-I and MHC-II antigen representation, we could wonder whether TPCs play a role in the process of antigen presentation.
The information presented here sheds light on the roles of TPCs and TRPMLs in cancer and immunity. Yet, a significant challenge persists in connecting these roles to elucidate their impact on cancer immunity, particularly in aiding cancer cells to evade immune destruction. To explore this, our discussion will be divided into two main sections: mechanisms contributing to the stabilization of the TME and mechanisms facilitating evasion of immune destruction (Figure 4).
Figure 4 Potential effects of TRPMLs and TPCs on cancer immunity. Effects that might be governed by TRPMLs or TPCs and therefore aid cancers to evade immune surveillance or destruction.
Tumor progression is very closely connected and dependent of the contribution of the environment surrounding the tumor. Thus, the concept of a TME has been studied extensively over the past century, elucidating that it is shaped and trained by cancer cells to assist the development of cancer hallmarks. The TME can be seen as a complex inflammatory system surrounding tumors, among others consisting of fibroblasts, endothelial cells, extracellular matrix, blood vessels, but also immune cells, signaling molecules and metabolites (130, 131). That immune cells are part of the TME has been known, since tumor-infiltrating lymphocytes (TILs) were identified (132). Ever since then, investigating and characterizing immune cells in the TME has been a high priority. Due to their altered characteristics, immune cells in the TME are regarded to serve as a potential target for cancer immunotherapy (133).
Recruiting immune cells to the TME and sustaining a pro-tumorigenic and inflammatory setting heavily relies on exocytosis processes such as cytokine secretion (131, 134). Specially TRPMLs have been linked to be key regulators of lysosomal exocytosis in healthy and malignant tissue (74, 89, 104, 135). In this context, the influence of TRPML1 on lysosomal exocytosis in healthy tissue was nicely described by Medina et al. They demonstrated that TFEB controls lysosomal exocytosis at the transcriptional level (136, 137) by promoting an increase in intracellular calcium levels via TRPML1 (138). Additionally, in Parkison Disease (PD) activation of TRPML1 rescued the impaired secretion of α-synuclein (α-syn) and prevented its accumulation within cells. These findings indicate that the levels of α-syn inside cells are influenced by lysosomal exocytosis in human dopaminergic neurons (139). This insight suggests that targeting TRPML1, and thereby lysosomal exocytosis, could offer a promising therapeutic strategy for PD and other disorders characterized by impaired lysosomal exocytosis. In macrophages, Plesch et al. demonstrated that TRPML2 governs release of chemokines, such as CCL2 (95). Further elucidation on this regard has not yet been made. However, the CCL2-CCR2 axis is one of the major chemokine signaling pathways (96), which is not only involved in tumor progression, but also helps to create an inflammatory TME. The TRPML2-mediated release of CCL2 leads to a M2-type polarization of macrophages in the TME. Furthermore, M2 macrophages in the TME or even TAMs, which are thought to have M2-like features, secrete CCL2 themselves to educate a larger number of macrophages to the TME, thus increasing their number of associates (111). In conclusion, the TRPML2/CCL2 axis might contribute to a pro-tumorigenic TME by recruiting and repolarization of immune cells and therefore in securing tumor growth (140).
To conclude, exocytosis is known to regulate TME adaptation, such as acidification and cytokine release, which shape vascularization and immune response (130, 131). TRPMLs have shown to be responsible for exocytosis processes and secretion of cytokines (95, 135). TPC1 is proposed to be involved in exocytosis behavior in mast cells (121), but no data in cancer is known so far. Still, there is a vast research gap on the effect of TPC and TRPML mediated lysosomal exocytosis on TME formation and maintenance. Thus, it would be beneficial to investigate, if and how, other lysosomal ion channels contribute to cytokine secretion and consequently the recruitment of immune cells to the TME? What cytokines/growth factors might be released through TPC or TRPML-mediated exocytosis and what effect does the release have on a pro-tumorigenic environment?
The lysosome plays a critical role in driving inflammatory responses that can facilitate proliferation, DNA damage, and angiogenesis (141). Lysosomal enzyme release can initiate inflammation in various contexts. For instance, cathepsin B-mediated cleavage of trypsinogen-1 in the pancreas can induce pancreatitis (142), a chronic inflammatory condition associated with heightened pancreatic cancer risk (143). Deficiencies in essential autophagy genes like Autophagy related 7 (ATG7) and Beclin 1 can also trigger chronic inflammation across multiple settings (144) and are implicated in the promotion of spontaneous cancers in organs such as the lung, liver, and lymphocytes through mechanisms that are not fully elucidated (145). Additionally, therapy-induced lysosomal membrane permeabilization may contribute to inflammation preceding cell death (146).
Heparin sulfate proteoglycans (HSPGs), a major component of the extracellular matrix, modulate the activity of cytokines and growth factors such as TGF-β (147). Additionally, HSPGs can induce inflammatory responses through TLR 4 signaling activation and leukocyte recruitment (148). Heparanase, the sole known mammalian endoglycosidase capable of degrading HSPGs (149), is synthesized as an inactive proenzyme and undergoes proteolytic activation in the lysosome. Inhibitors of heparanase, including heparin sulfate mimetics like PG545, PI-88, M402, and SST0001, are under investigation in clinical trials and have demonstrated promising efficacy in impeding progression and metastasis in ovarian cancer and hepatocellular carcinoma (150, 151).
Furthermore, the lysosome plays a crucial role in regulating glucocorticoid levels and anti-inflammatory responses in diseases like lupus erythematosus and rheumatoid arthritis (152). Cancer cells often display elevated expression of the glucocorticoid receptor (153–156), and inhibition of lysosomal function with compounds like chloroquine or the V-ATPase inhibitor bafilomycin A1 can mitigate inflammation. Besides lysosomal pump V-ATPase, do TPCs or TRPMLs affect the expression of glucocorticoid receptors? Conversely, knockdown of TFEB leads to increased glucocorticoid levels and suppression of proinflammatory cytokine transcription (152). In this context, TRPML1-mediated calcium efflux has been connected to mediate TFEB translocation to the nucleus (135, 157). Thus, the question arises, whether TRPML1 activation and/or overexpression in cancer cells, result in enhanced transcription of proinflammatory cytokines. Does targeting TRPML1 in cancer result in a reduced release of proinflammatory cytokines?
Inflammation mediated by heparanase, cathepsins and their substrates, or modulation of the glucocorticoid receptor may impact distinct tumor initiation pathways triggered by chronic inflammation. However, whether and how lysosomal ion channels are involved in tumor-promoting inflammation needs further investigation.
During cancer therapy, it has been noted that autophagy coupled to lysosomal degradation is a key mechanism of resistance to immunotherapy. In pancreatic cancer cells, it has been demonstrated that autophagic processes are linked to lysosomal degradation of MHC-I (33). Consequently, reducing the expression of MHC-I at the cancer cell surface and the recognition by CD8+ T cells, hampering the efficacy of immunotherapy. Interestingly, TRPML1 has been linked to regulate autophagic processes in cancer cells (64, 84, 158, 159). In the context of tumor cell survival within a complex environment, TRPML1 activation has been shown to promote autophagy in several cancer cell lines by disrupting the fusion process between autophagosomes and lysosomes. Rosato et al. demonstrated that TRPML1 activation leads to the formation of autophagic vacuoles (AVs) independently of TFEB, indicating TRPML1’s ability to influence autophagy through two distinct mechanisms: rapidly, by promoting both AV formation and fusion with lysosomes, and persistently, by facilitating TFEB nuclear translocation and subsequent transcription of genes essential for autophagy and lysosomal function (160), subsequently ensuring a sustained supply of autophagic substrates during prolonged autophagy stimulation. Furthermore, this process engages a feedback loop wherein TFEB positively regulates TRPML1 mRNA expression, thereby reinforcing long-term autophagic activity (84, 160). Moreover, it has been shown that the inhibition of autophagy induced by TRPML1 leads to disruptions in mitochondrial turnover and mitophagy. This disruption subsequently triggers an increase in ROS, causing significant DNA damage within cancer cells (64, 158) and activating ROS sensor TRPML1 (64). Therefore, it suggests that lysosomal degradation of MHC-I observed in pancreatic cancer is regulated by TRPML1-mediated autophagy.
Recently, immune checkpoint therapy has been proven as a breakthrough in cancer therapy (161). While many immune checkpoints have been identified in the past decades, only two are being directly targeted in cancer immunotherapy so far: CTLA-4 and PD-1 and its respective ligand PD-L1 (162, 163). CTLA-4 is an inhibitory checkpoint molecule, mainly located at the plasma membrane of T cells (164). Following T cell receptor (TCR) activation, CTLA-4 undergoes upregulation and binds to B7 with greater avidity than the T lymphocyte receptor CD28. This interaction leads to decreased T cell proliferation and reduced cytokine secretion (165–168). In the initial stages of tumorigenesis, CTLA-4 can reduce T cell activation by generating inhibitory signals, thereby attenuating the immune response against the tumor (169). Recently, research has shown that cancer cells express CTLA-4 in their favor. The binding of T cells to CTLA-4 expressed by cancer cells leads to a reduced T cell activation towards the cancer and subsequently reduced overall anti-tumor response (170–172). Lysosome have shown to play a central role in recycling and degrading CTLA-4. It is known that CTLA-4 can be internalized via endocytosis (173, 174) and subsequently degraded in the lysosome or bind to activator protein 1 (AP1) and AP2 to be degraded (175, 176). However, how recycling processes of CTLA-4 to the plasma membrane has not been highlighted yet.
Programmed cell death protein-1 (PD-1) serves as a prominent immunosuppressive checkpoint, primarily expressed in macrophages, B lymphocytes, dendritic cells (DCs), monocytes, tumor-specific activated T cells, myeloid cells, and natural killer (NK) cells during prolonged exposure to antigens (177, 178). One of the ligands for PD-1 is PD-L1, which is predominantly expressed in tumor cells, tumor-infiltrating cells, and APCs across various cancer types (177). In general, T cell activation predominantly hinges on dual signals. The initial signal involves the binding of MHC-presented antigens to the TCR. The second signal comprises co-stimulatory and co-inhibitory signals (179). The engagement between PD-1 on T cells and PD-L1 on tumor cells or APCs can actively impede T cell activation. This interaction is known to lead to T cell apoptosis, reduced cytokine production, T cell lysis, and the induction of antigen tolerance, enabling the tumor to evade immune surveillance (180, 181). PD-L1 is not solely found on the cell surface; it is also found intracellularly and its expression levels are dynamically regulated by lysosomal-related dynamic transport and degradation mechanisms (182–184). Moreover, patients treated with approved immune checkpoint inhibitors or nivolumab (mAb against PD-L1) (185) or ipilimumab (mAb against CTLA-4) (186), have been shown to develop resistance during therapy. Remarkably, poor outcomes of immunotherapy has been connected to lysosomal degradation of immune checkpoint PD-L1, yet specific mechanisms of resistance of immune therapy remains elusive (185, 187).
In summary, both immune checkpoints CTLA-4 and PD-L1 are expressed on cancer cells, contributing to immune evasion. Their expression on the cell membrane, as well as their recycling, has been shown to be regulated through the ES in which TRPML1 or TPC2 might be key players. Do TRPMLs or TPCs influence CTLA-4 or PD-L1 recycling and expression on cancer cells or immune cells? Are impaired TPCs or TRPMLs function responsible for the observed resistance mechanisms during immunotherapy?
Both ion channels have shown to regulate recycling of membranous proteins (E-cadherin and β1-integrin) in cancer cells favoring tumor progression (33, 57, 74). TPC2 is known to regulate recycling processes of β1-integrin in cancer cells, as ablation leads to attenuation in early endosomes (57). Very recent research has shown that TRPML1 mediates trafficking of E-cadherin to the plasma membrane by altering the expression of trafficking markers Rab11 or Rab5 in cancer (74). In addition, in macrophages TRPML1 has been shown to be required for the efficient transport of MHC-II complex molecules to the plasma membrane (97). Despite their undeniable influence on cellular trafficking and the expression of Rab proteins, the precise mechanisms through which TRPMLs and TPCs regulate the trafficking of receptors and membrane proteins have remained elusive so far. To shed light on these mechanisms, we propose investigating direct endolysosomal patch clamps of GTPases such as Rab5, Rab11, and Rab7, with a focus on protein-protein interactions.
In 2023, Davis et al. introduced a novel hypothesis suggesting that calcium nanodomains may be instrumental in ion channel-specific trafficking (188). They proposed a potential explanation for why TPC2 fails to induce lysosomal exocytosis during phagocytosis: the calcium it releases may not effectively reach the exocytotic machinery, which is presumed to be adjacent to TRPML1. They put forward a model wherein calcium released from lysosomal calcium channels does not create a continuous ‘halo’ of high calcium concentration around the lysosome, but rather forms a discontinuous ‘mosaic’ of calcium nanodomains associated with each channel point-source (188). While intriguing, their hypothesis requires validation in diverse cell lines and models. Consequently, we advocate for future research focusing on calcium nanodomains to elucidate precise molecular mechanisms for TRPMLs and TPCs.
In summary, this review highlights that despite significant progress in understanding the involvement of TRPMLs and TPCs in immunity, many unanswered questions persist regarding their role in cancer immunity. Specifically, TRPML1 exhibits numerous connections in maintaining the TME or aiding in immune evasion. However, a far-reaching study on its effect in cancer immunity is missing. Also, the precise role of TPC2 in this context remains an area that requires elucidation in future research. So far, there is a lack of knowledge on the role of both families of ion channels on the development of a pro-tumorigenic and pro-inflammatory TME and immune response, including the development of TAMs. What is the role of TPCs and TRPMLs in the regulation of cytokine secretion in macrophages and/or cancer cells? What are their roles in modulating MHC molecule and antigen presentation for proper T cell activation? Would the targeting of these ion channels present a dual role in treating cancer? Consequently, these channels may hold potential explanations or key contributions to the described mechanisms enabling cancer cells to evade immune destruction, uphold a resilient TME and reduce activation of T cells.
Here we summarize the current knowledge on the role of TRPMLs and TPCs in cancer development and in regulatory mechanisms in innate and adaptive immune response (Table 1). Both families of ion channels have shown to play key roles in the process of phagocytosis and immune cell signaling. Additionally, TRPMLs act as regulators between innate and adaptive immune system by secreting chemokines and regulating MHC-I expression. Due to rising resistance mechanisms in cancer immunotherapy, we emphasize the great potential that lays in elucidating research gaps in cancer immunity, especially mechanisms that aid evading immune destruction and surveillance.
LO: Writing – review & editing, Writing – original draft. KB: Conceptualization, Funding acquisition, Writing – review & editing.
The author(s) declare financial support was received for the research, authorship, and/or publication of this article. This work was supported by the German Research foundation: BA 7238/3-2.
The authors declare that the research was conducted in the absence of any commercial or financial relationships that could be construed as a potential conflict of interest.
All claims expressed in this article are solely those of the authors and do not necessarily represent those of their affiliated organizations, or those of the publisher, the editors and the reviewers. Any product that may be evaluated in this article, or claim that may be made by its manufacturer, is not guaranteed or endorsed by the publisher.
1. de Duve C, Pressman BC, Gianetto R, Wattiaux R, Appelmans F. Tissue fractionation studies. 6. Intracellular distribution patterns of enzymes in rat-liver tissue. Biochem J. (1955) 60:604–17. doi: 10.1042/bj0600604
2. Xu H, Ren D. Lysosomal physiology. Annu Rev Physiol. (2015) 77:57–80. doi: 10.1146/annurev-physiol-021014–071649
3. Ballabio A, Bonifacino JS. Lysosomes as dynamic regulators of cell and organismal homeostasis. Nat Rev Mol Cell Biol. (2020) 21:101–18. doi: 10.1038/s41580–019-0185–4
4. Brailoiu E, Hooper R, Cai X, Brailoiu GC, Keebler MV, Dun NJ, et al. An ancestral deuterostome family of two-pore channels mediates nicotinic acid adenine dinucleotide phosphate-dependent calcium release from acidic organelles. J Biol Chem. (2010) 285:2897–901. doi: 10.1074/jbc.C109.081943
5. Patel S, Docampo R. Acidic calcium stores open for business: expanding the potential for intracellular Ca2+ signaling. Trends Cell Biol. (2010) 20:277–86. doi: 10.1016/j.tcb.2010.02.003
6. Gerasimenko JV, Tepikin AV, Petersen OH, Gerasimenko OV. Calcium uptake via endocytosis with rapid release from acidifying endosomes. Curr Biol. (1998) 8:1335–8. doi: 10.1016/S0960–9822(07)00565–9
7. Watts C. Lysosomes and lysosome-related organelles in immune responses. FEBS Open Bio. (2022) 12:678–93. doi: 10.1002/2211–5463.13388
8. Watts C. The endosome-lysosome pathway and information generation in the immune system. Biochim Biophys Acta. (2012) 1824:14–21. doi: 10.1016/j.bbapap.2011.07.006
9. Miyake K, Shibata T, Ohto U, Shimizu T, Saitoh S-I, Fukui R, et al. Mechanisms controlling nucleic acid-sensing Toll-like receptors. Int Immunol. (2018) 30:43–51. doi: 10.1093/intimm/dxy016
10. Ewald SE, Lee BL, Lau L, Wickliffe KE, Shi G-P, Chapman HA, et al. The ectodomain of Toll-like receptor 9 is cleaved to generate a functional receptor. Nature. (2008) 456:658–62. doi: 10.1038/nature07405
11. Pelka K, Bertheloot D, Reimer E, Phulphagar K, Schmidt SV, Christ A, et al. The chaperone UNC93B1 regulates toll-like receptor stability independently of endosomal TLR transport. Immunity. (2018) 48:911–922.e7. doi: 10.1016/j.immuni.2018.04.011
12. Davidson HW, Reid PA, Lanzavecchia A, Watts C. Processed antigen binds to newly synthesized MHC class II molecules in antigen-specific B lymphocytes. Cell. (1991) 67:105–16. doi: 10.1016/0092-8674(91)90575-J
13. Neefjes JJ, Stollorz V, Peters PJ, Geuze HJ, Ploegh HL. The biosynthetic pathway of MHC class II but not class I molecules intersects the endocytic route. Cell. (1990) 61:171–83. doi: 10.1016/0092–8674(90)90224–3
14. Matthews SP, Werber I, Deussing J, Peters C, Reinheckel T, Watts C. Distinct protease requirements for antigen presentation in vitro and in vivo. J Immunol. (2010) 184:2423–31. doi: 10.4049/jimmunol.0901486
15. Manoury B, Hewitt EW, Morrice N, Dando PM, Barrett AJ, Watts C. An asparaginyl endopeptidase processes a microbial antigen for class II MHC presentation. Nature. (1998) 396:695–9. doi: 10.1038/25379
16. Lanzavecchia A. Receptor-mediated antigen uptake and its effect on antigen presentation to class II-restricted T lymphocytes. Annu Rev Immunol. (1990) 8:773–93. doi: 10.1146/annurev.iy.08.040190.004013
17. Kleijmeer M, Ramm G, Schuurhuis D, Griffith J, Rescigno M, Ricciardi-Castagnoli P, et al. Reorganization of multivesicular bodies regulates MHC class II antigen presentation by dendritic cells. J Cell Biol. (2001) 155:53–63. doi: 10.1083/jcb.200103071
18. Chow A, Toomre D, Garrett W, Mellman I. Dendritic cell maturation triggers retrograde MHC class II transport from lysosomes to the plasma membrane. Nature. (2002) 418:988–94. doi: 10.1038/nature01006
19. Xu J. Regulation of Cancer Immune Checkpoints: Molecular and Cellular Mechanisms and Therapy. Singapore: Springer Singapore Pte. Limited (2020). p. 657.
20. Qureshi OS, Zheng Y, Nakamura K, Attridge K, Manzotti C, Schmidt EM, et al. Trans-endocytosis of CD80 and CD86: a molecular basis for the cell-extrinsic function of CTLA-4. Science. (2011) 332:600–3. doi: 10.1126/science.1202947
21. Bagchi S, Yuan R, Engleman EG. Immune checkpoint inhibitors for the treatment of cancer: clinical impact and mechanisms of response and resistance. Annu Rev Pathol. (2021) 16:223–49. doi: 10.1146/annurev-pathol-042020–042741
22. Kirchhammer N, Trefny MP, der Maur PA, Läubli H, Zippelius A. Combination cancer immunotherapies: Emerging treatment strategies adapted to the tumor microenvironment. Sci Transl Med. (2022) 14:eabo3605. doi: 10.1126/scitranslmed.abo3605
23. He M, Yang T, Wang Y, Wang M, Chen X, Ding D, et al. Immune checkpoint inhibitor-based strategies for synergistic cancer therapy. Adv Healthc Mater. (2021) 10:e2002104. doi: 10.1002/adhm.202002104
24. Sahin U, Türeci Ö. Personalized vaccines for cancer immunotherapy. Science. (2018) 359:1355–60. doi: 10.1126/science.aar7112
25. Wang Z, Wu Z, Liu Y, Han W. New development in CAR-T cell therapy. J Hematol Oncol. (2017) 10:53. doi: 10.1186/s13045–017-0423–1
26. Ahn G, Riley NM, Kamber RA, Wisnovsky S, Moncayo von Hase S, Bassik MC, et al. Elucidating the cellular determinants of targeted membrane protein degradation by lysosome-targeting chimeras. Science. (2023) 382:eadf6249. doi: 10.1126/science.adf6249
27. Bonam SR, Wang F, Muller S. Lysosomes as a therapeutic target. Nat Rev Drug Discovery. (2019) 18:923–48. doi: 10.1038/s41573–019-0036–1
28. Pei J, Wang G, Feng L, Zhang J, Jiang T, Sun Q, et al. Targeting lysosomal degradation pathways: new strategies and techniques for drug discovery. J Med Chem. (2021) 64:3493–507. doi: 10.1021/acs.jmedchem.0c01689
29. Tang M, Chen B, Xia H, Pan M, Zhao R, Zhou J, et al. pH-gated nanoparticles selectively regulate lysosomal function of tumour-associated macrophages for cancer immunotherapy. Nat Commun. (2023) 14:1–16. doi: 10.1038/s41467–023-41592–0
30. Zhu S, Zhang T, Zheng L, Liu H, Song W, Liu D, et al. Combination strategies to maximize the benefits of cancer immunotherapy. J Hematol Oncol. (2021) 14:156. doi: 10.1186/s13045–021-01164–5
31. Ouyang Z, Gao Y, Shen S, Jia B, Yu H, Wang H, et al. A minimalist dendrimer nanodrug for autophagy inhibition-amplified tumor photothermo-immunotherapy. Nano Today. (2023) 51:101936. doi: 10.1016/j.nantod.2023.101936
32. Zhao X, Hu S, Zeng L, Liu X, Song Y, Zhang Y, et al. Irradiation combined with PD-L1-/- and autophagy inhibition enhances the antitumor effect of lung cancer via cGAS-STING-mediated T cell activation. iScience. (2022) 25:104690. doi: 10.1016/j.isci.2022.104690
33. Yamamoto K, Venida A, Yano J, Biancur DE, Kakiuchi M, Gupta S, et al. Autophagy promotes immune evasion of pancreatic cancer by degrading MHC-I. Nature. (2020) 581:100–5. doi: 10.1038/s41586–020-2229–5
34. Haslauer T, Greil R, Zaborsky N, Geisberger R. CAR T-cell therapy in hematological Malignancies. Int J Mol Sci. (2021) 22:8996–9008. doi: 10.3390/ijms22168996
35. Sterner RC, Sterner RM. CAR-T cell therapy: current limitations and potential strategies. Blood Cancer J. (2021) 11:69. doi: 10.1038/s41408–021-00459–7
36. Li W, Qiu S, Chen J, Jiang S, Chen W, Jiang J, et al. Chimeric antigen receptor designed to prevent ubiquitination and downregulation showed durable antitumor efficacy. Immunity. (2020) 53:456–470.e6. doi: 10.1016/j.immuni.2020.07.011
37. MaChado ER, Annunziata I, van de Vlekkert D, Grosveld GC, d’Azzo A. Lysosomes and cancer progression: A Malignant liaison. Front Cell Dev Biol. (2021) 9:642494. doi: 10.3389/fcell.2021.642494
38. Lawrence RE, Zoncu R. The lysosome as a cellular centre for signalling, metabolism and quality control. Nat Cell Biol. (2019) 21:133–42. doi: 10.1038/s41556–018-0244–7
39. Grimm C, Bartel K, Vollmar AM, Biel M. Endolysosomal cation channels and cancer-A link with great potential. Pharm (Basel). (2018) 11(1):4. doi: 10.3390/ph11010004
40. Eskelinen E-L, Tanaka Y, Saftig P. At the acidic edge: emerging functions for lysosomal membrane proteins. Trends Cell Biol. (2003) 13:137–45. doi: 10.1016/s0962–8924(03)00005–9
41. Mindell JA. Lysosomal acidification mechanisms. Annu Rev Physiol. (2012) 74:69–86. doi: 10.1146/annurev-physiol-012110–142317
42. Bartel K. Targeting V-ATPase influences cancer cell cholesterol metabolism and biophysical properties - a new option for HCC therapy. LMU Munich: lmu; Ludwig-Maximilians-Universität München (2017).
43. Bartel K, Winzi M, Ulrich M, Koeberle A, Menche D, Werz O, et al. V-ATPase inhibition increases cancer cell stiffness and blocks membrane related Ras signaling - a new option for HCC therapy. Oncotarget. (2017) 8:9476–87. doi: 10.18632/oncotarget.14339
44. Bartel K. Targeting V-ATPase influences cancer cell cholesterol metabolism and biophysical properties - a new option for HCC therapy. (2017). doi: 10.5282/edoc.20646
45. Bartel K, Müller R, von Schwarzenberg K. Differential regulation of AMP-activated protein kinase in healthy and cancer cells explains why V-ATPase inhibition selectively kills cancer cells. J Biol Chem. (2019) 294:17239–48. doi: 10.1074/jbc.RA119.010243
46. McGuire C, Cotter K, Stransky L, Forgac M. Regulation of V-ATPase assembly and function of V-ATPases in tumor cell invasiveness. Biochim Biophys Acta. (2016) 1857:1213–8. doi: 10.1016/j.bbabio.2016.02.010
47. von Schwarzenberg K, Wiedmann RM, Oak P, Schulz S, Zischka H, Wanner G, et al. Mode of cell death induction by pharmacological vacuolar H+-ATPase (V-ATPase) inhibition. J Biol Chem. (2013) 288:1385–96. doi: 10.1074/jbc.M112.412007
48. Eaton AF, Merkulova M, Brown D. The H+-ATPase (V-ATPase): from proton pump to signaling complex in health and disease. Am J Physiol Cell Physiol. (2021) 320:C392–414. doi: 10.1152/ajpcell.00442.2020
49. Yamaguchi S, Jha A, Li Q, Soyombo AA, Dickinson GD, Churamani D, et al. Transient receptor potential mucolipin 1 (TRPML1) and two-pore channels are functionally independent organellar ion channels. J Biol Chem. (2011) 286:22934–42. doi: 10.1074/jbc.M110.210930
50. Ruas M, Rietdorf K, Arredouani A, Davis LC, Lloyd-Evans E, Koegel H, et al. Purified TPC isoforms form NAADP receptors with distinct roles for Ca(2+) signaling and endolysosomal trafficking. Curr Biol. (2010) 20:703–9. doi: 10.1016/j.cub.2010.02.049
51. She J, Zeng W, Guo J, Chen Q, Bai X, Jiang Y. Cryo-EM structure of human TPC2 channel in the ligand-bound closed state. eLife. (2019). doi: 10.2210/pdb6nq2/pdb
52. Calcraft PJ, Ruas M, Pan Z, Cheng X, Arredouani A, Hao X, et al. NAADP mobilizes calcium from acidic organelles through two-pore channels. Nature. (2009) 459:596–600. doi: 10.1038/nature08030
53. Patel S, Yuan Y, Gunaratne GS, Rahman T, Marchant JS. Activation of endo-lysosomal two-pore channels by NAADP and PI(3,5)P2. Five things to know. Cell Calcium. (2022) 103:102543. doi: 10.1016/j.ceca.2022.102543
54. She J, Zeng W, Guo J, Chen Q, Bai X-C, Jiang Y. Structural mechanisms of phospholipid activation of the human TPC2 channel. Elife. (2019) 8:e45222. doi: 10.7554/eLife.45222
55. Gerndt S, Chen C-C, Chao Y-K, Yuan Y, Burgstaller S, Scotto Rosato A, et al. Agonist-mediated switching of ion selectivity in TPC2 differentially promotes lysosomal function. Elife. (2020) 9:e54712. doi: 10.7554/eLife.54712
56. Müller M, Gerndt S, Chao Y-K, Zisis T, Nguyen ON, Gerwien A, et al. Gene editing and synthetically accessible inhibitors reveal role for TPC2 in HCC cell proliferation and tumor growth. Cell Chem Biol. (2021) 28:1119–1131.e27. doi: 10.1016/j.chembiol.2021.01.023
57. Nguyen ON, Grimm C, Schneider LS, Chao Y-K, Atzberger C, Bartel K, et al. Two-pore channel function is crucial for the migration of invasive cancer cells. Cancer Res. (2017) 77:1427–38. doi: 10.1158/0008–5472.CAN-16–0852
58. Netcharoensirisuk P, Abrahamian C, Tang R, Chen C-C, Rosato AS, Beyers W, et al. Flavonoids increase melanin production and reduce proliferation, migration and invasion of melanoma cells by blocking endolysosomal/melanosomal TPC2. Sci Rep. (2021) 11:8515. doi: 10.1038/s41598–021-88196–6
59. Sun W, Yue J. TPC2 mediates autophagy progression and extracellular vesicle secretion in cancer cells. Exp Cell Res. (2018) 370:478–89. doi: 10.1016/j.yexcr.2018.07.013
60. Favia A, Pafumi I, Desideri M, Padula F, Montesano C, Passeri D, et al. NAADP-dependent ca(2+) signaling controls melanoma progression, metastatic dissemination and neoangiogenesis. Sci Rep. (2016) 6:18925. doi: 10.1038/srep18925
61. Favia A, Desideri M, Gambara G, D’Alessio A, Ruas M, Esposito B, et al. VEGF-induced neoangiogenesis is mediated by NAADP and two-pore channel-2-dependent Ca2+ signaling. Proc Natl Acad Sci U.S.A. (2014) 111:E4706–15. doi: 10.1073/pnas.1406029111
62. Pryor PR, Reimann F, Gribble FM, Luzio JP. Mucolipin-1 is a lysosomal membrane protein required for intracellular lactosylceramide traffic. Traffic. (2006) 7:1388–98. doi: 10.1111/j.1600-0854.2006.00475.x
63. Bargal R, Avidan N, Ben-Asher E, Olender Z, Zeigler M, Frumkin A, et al. Identification of the gene causing mucolipidosis type IV. Nat Genet. (2000) 26:118–23. doi: 10.1038/79095
64. Zhang X, Cheng X, Yu L, Yang J, Calvo R, Patnaik S, et al. MCOLN1 is a ROS sensor in lysosomes that regulates autophagy. Nat Commun. (2016) 7:12109. doi: 10.1038/ncomms12109
65. Li M, Zhang WK, Benvin NM, Zhou X, Su D, Li H, et al. Structural basis of dual Ca2+/pH regulation of the endolysosomal TRPML1 channel. Nat Struct Mol Biol. (2017) 24:205–13. doi: 10.1038/nsmb.3362
66. Wang W, Zhang X, Gao Q, Xu H. TRPML1: an ion channel in the lysosome. Handb Exp Pharmacol. (2014) 222:631–45. doi: 10.1007/978–3-642–54215-2_24
67. Shen D, Wang X, Li X, Zhang X, Yao Z, Dibble S, et al. Lipid storage disorders block lysosomal trafficking by inhibiting a TRP channel and lysosomal calcium release. Nat Commun. (2012) 3:731. doi: 10.1038/ncomms1735
68. Miedel MT, Rbaibi Y, Guerriero CJ, Colletti G, Weixel KM, Weisz OA, et al. Membrane traffic and turnover in TRP-ML1-deficient cells: a revised model for mucolipidosis type IV pathogenesis. J Exp Med. (2008) 205:1477–90. doi: 10.1084/jem.20072194
69. Grimm C, Butz E, Chen C-C, Wahl-Schott C, Biel M. From mucolipidosis type IV to Ebola: TRPML and two-pore channels at the crossroads of endo-lysosomal trafficking and disease. Cell Calcium. (2017) 67:148–55. doi: 10.1016/j.ceca.2017.04.003
70. Venkatachalam K, Wong C-O, Zhu MX. The role of TRPMLs in endolysosomal trafficking and function. Cell Calcium. (2015) 58:48–56. doi: 10.1016/j.ceca.2014.10.008
71. Venkatachalam K, Kiselyov K. TRPML1-dependent processes as therapeutic targets. TRP Channels as Ther Targets. (2015) 58:469–82. doi: 10.1016/B978–0-12–420024–1.00025–4
72. Abrahamian C, Grimm C. Endolysosomal cation channels and MITF in melanocytes and melanoma. Biomolecules. (2021) 11(7):1021. doi: 10.3390/biom11071021
73. Xu M, Almasi S, Yang Y, Yan C, Sterea AM, Rizvi Syeda AK, et al. The lysosomal TRPML1 channel regulates triple negative breast cancer development by promoting mTORC1 and purinergic signaling pathways. Cell Calcium. (2019) 79:80–8. doi: 10.1016/j.ceca.2019.02.010
74. Frey N, Ouologuem L, Blenninger J, Siow W-X, Thorn-Seshold J, Stöckl J, et al. Endolysosomal TRPML1 channel regulates cancer cell migration by altering intracellular trafficking of E-cadherin and β1-integrin. J Biol Chem. (2023) 0:105581. doi: 10.1016/j.jbc.2023.105581
75. Siow WX, Kabiri Y, Tang R, Chao Y-K, Plesch E, Eberhagen C, et al. Lysosomal TRPML1 regulates mitochondrial function in hepatocellular carcinoma cells. J Cell Sci. (2022) 135(6):jcs259455. doi: 10.1242/jcs.259455
76. Rühl P, Bracher F. Aza analogs of the TRPML1 inhibitor estradiol methyl ether (EDME). Molecules. (2023) 28:7428. doi: 10.3390/molecules28217428
77. Kasitinon SY, Eskiocak U, Martin M, Bezwada D, Khivansara V, Tasdogan A, et al. TRPML1 promotes protein homeostasis in melanoma cells by negatively regulating MAPK and mTORC1 signaling. Cell Rep. (2019) 28:2293–2305.e9. doi: 10.1016/j.celrep.2019.07.086
78. Santoni G, Santoni M, Maggi F, Marinelli O, Morelli MB. Emerging role of mucolipins TRPML channels in cancer. Front Oncol. (2020) 10:659. doi: 10.3389/fonc.2020.00659
79. Santoni G, Maggi F, Amantini C, Arcella A, Marinelli O, Nabissi M, et al. Coexpression of TRPML1 and TRPML2 mucolipin channels affects the survival of glioblastoma patients. Int J Mol Sci. (2022) 23:659. doi: 10.3390/ijms23147741
80. Morelli MB, Nabissi M, Amantini C, Tomassoni D, Rossi F, Cardinali C, et al. Overexpression of transient receptor potential mucolipin-2 ion channels in gliomas: role in tumor growth and progression. Oncotarget. (2016) 7:43654–68. doi: 10.18632/oncotarget.9661
81. Rühl P, Rosato AS, Urban N, Gerndt S, Tang R, Abrahamian C, et al. Estradiol analogs attenuate autophagy, cell migration and invasion by direct and selective inhibition of TRPML1, independent of estrogen receptors. Sci Rep. (2021) 11:8313. doi: 10.1038/s41598–021-87817–4
82. Geisslinger F, Müller M, Chao Y-K, Grimm C, Vollmar AM, Bartel K. Targeting TPC2 sensitizes acute lymphoblastic leukemia cells to chemotherapeutics by impairing lysosomal function. Cell Death Dis. (2022) 13:668. doi: 10.1038/s41419-022-05105-z
83. Di Paola S, Scotto-Rosato A, Medina DL. TRPML1: the ca(2+)retaker of the lysosome. Cell Calcium. (2018) 69:112–21. doi: 10.1016/j.ceca.2017.06.006
84. Medina DL, Di Paola S, Peluso I, Armani A, de SD, Venditti R, et al. Lysosomal calcium signalling regulates autophagy through calcineurin and TFEB. Nat Cell Biol. (2015) 17:288–99. doi: 10.1038/ncb3114
85. Karlsson M, Zhang C, Méar L, Zhong W, Digre A, Katona B, et al. A single-cell type transcriptomics map of human tissue. Sci. Adv. (2021) 7:eabh2169. doi: 10.1126/sciadv.abh2169
86. Gordon S. Alternative activation of macrophages. Nat Rev Immunol. (2003) 3:23–35. doi: 10.1038/nri978
87. Cavaillon JM. Cytokines and macrophages. Biomed Pharmacother. (1994) 48:445–53. doi: 10.1016/0753–3322(94)90005–1
88. Aderem A, Underhill DM. Mechanisms of phagocytosis in macrophages. Annu Rev Immunol. (1999) 17:593–623. doi: 10.1146/annurev.immunol.17.1.593
89. Samie M, Wang X, Zhang X, Goschka A, Li X, Cheng X, et al. A TRP channel in the lysosome regulates large particle phagocytosis via focal exocytosis. Dev Cell. (2013) 26:511–24. doi: 10.1016/j.devcel.2013.08.003
90. Dong X-P, Shen D, Wang X, Dawson T, Li X, Zhang Q, et al. PI(3,5)P(2) controls membrane trafficking by direct activation of mucolipin Ca(2+) release channels in the endolysosome. Nat Commun. (2010) 1:38. doi: 10.1038/ncomms1037
91. Dayam RM, Sun CX, Choy CH, Mancuso G, Glogauer M, Botelho RJ. The lipid kinase PIKfyve coordinates the neutrophil immune response through the activation of the rac GTPase. J Immunol. (2017) 199:2096–105. doi: 10.4049/jimmunol.1601466
92. Dayam RM, Saric A, Shilliday RE, Botelho RJ. The phosphoinositide-gated lysosomal ca(2+) channel, TRPML1, is required for phagosome maturation. Traffic. (2015) 16:1010–26. doi: 10.1111/tra.12303
93. Gray MA, Choy CH, Dayam RM, Ospina-Escobar E, Somerville A, Xiao X, et al. Phagocytosis enhances lysosomal and bactericidal properties by activating the transcription factor TFEB. Curr Biol. (2016) 26:1955–64. doi: 10.1016/j.cub.2016.05.070
94. Sun L, Hua Y, Vergarajauregui S, Diab HI, Puertollano R. Novel role of TRPML2 in the regulation of the innate immune response. J Immunol. (2015) 195:4922–32. doi: 10.4049/jimmunol.1500163
95. Plesch E, Chen C-C, Butz E, Scotto Rosato A, Krogsaeter EK, Yinan H, et al. Selective agonist of TRPML2 reveals direct role in chemokine release from innate immune cells. Elife. (2018) 7:e39720. doi: 10.7554/eLife.39720
96. Kadomoto S, Izumi K, Mizokami A. Roles of CCL2-CCR2 axis in the tumor microenvironment. Int J Mol Sci. (2021) 22(16):8530. doi: 10.3390/ijms22168530
97. Thompson EG, Schaheen L, Dang H, Fares H. Lysosomal trafficking functions of mucolipin-1 in murine macrophages. BMC Cell Biol. (2007) 8:54. doi: 10.1186/1471–2121-8–54
98. Lipscomb MF, Masten BJ. Dendritic cells: immune regulators in health and disease. Physiol Rev. (2002) 82:97–130. doi: 10.1152/physrev.00023.2001
99. Banchereau J, Steinman RM. Dendritic cells and the control of immunity. Nature. (1998) 392:245–52. doi: 10.1038/32588
100. MartIn-Fontecha A, Sebastiani S, Höpken UE, Uguccioni M, Lipp M, Lanzavecchia A, et al. Regulation of dendritic cell migration to the draining lymph node: impact on T lymphocyte traffic and priming. J Exp Med. (2003) 198:615–21. doi: 10.1084/jem.20030448
101. Mellman I. Dendritic cells: master regulators of the immune response. Cancer Immunol Res. (2013) 1:145–9. doi: 10.1158/2326–6066.CIR-13–0102
102. Bretou M, Sáez PJ, Sanséau D, Maurin M, Lankar D, Chabaud M, et al. Lysosome signaling controls the migration of dendritic cells. Sci Immunol. (2017) 2(16):eaak9573. doi: 10.1126/sciimmunol.aak9573
103. Park B, Brinkmann MM, Spooner E, Lee CC, Kim Y-M, Ploegh HL. Proteolytic cleavage in an endolysosomal compartment is required for activation of Toll-like receptor 9. Nat Immunol. (2008) 9:1407–14. doi: 10.1038/ni.1669
104. Inpanathan S, Botelho RJ. The lysosome signaling platform: adapting with the times. Front Cell Dev Biol. (2019) 7:113. doi: 10.3389/fcell.2019.00113
105. Vestre K, Persiconi I, Borg Distefano M, Mensali N, Guadagno NA, Bretou M, et al. Rab7b regulates dendritic cell migration by linking lysosomes to the actomyosin cytoskeleton. J Cell Sci. (2021) 134(18):jcs259221. doi: 10.1242/jcs.259221
106. Li X, Saitoh S-I, Shibata T, Tanimura N, Fukui R, Miyake K. Mucolipin 1 positively regulates TLR7 responses in dendritic cells by facilitating RNA transportation to lysosomes. Int Immunol. (2015) 27:83–94. doi: 10.1093/intimm/dxu086
107. Vivier E, Tomasello E, Baratin M, Walzer T, Ugolini S. Functions of natural killer cells. Nat Immunol. (2008) 9:503–10. doi: 10.1038/ni1582
108. Goodridge JP, Jacobs B, Saetersmoen ML, Clement D, Hammer Q, Clancy T, et al. Remodeling of secretory lysosomes during education tunes functional potential in NK cells. Nat Commun. (2019) 10:514. doi: 10.1038/s41467-019-08384-x
109. Ollila J, Vihinen M. B cells. Int J Biochem Cell Biol. (2005) 37:518–23. doi: 10.1016/j.biocel.2004.09.007
110. Pleiman CM, D’Ambrosio D, Cambier JC. The B-cell antigen receptor complex: structure and signal transduction. Immunol Today. (1994) 15:393–9. doi: 10.1016/0167–5699(94)90267–4
111. Chen Y, Song Y, Du W, Gong L, Chang H, Zou Z. Tumor-associated macrophages: an accomplice in solid tumor progression. J BioMed Sci. (2019) 26:78. doi: 10.1186/s12929-019-0568-z
112. Song Y, Dayalu R, Matthews SA, Scharenberg AM. TRPML cation channels regulate the specialized lysosomal compartment of vertebrate B-lymphocytes. Eur J Cell Biol. (2006) 85:1253–64. doi: 10.1016/j.ejcb.2006.08.004
114. Davis LC, Morgan AJ, Galione A. NAADP-regulated two-pore channels drive phagocytosis through endo-lysosomal Ca2+ nanodomains, calcineurin and dynamin. EMBO J. (2020) 39:e104058. doi: 10.15252/embj.2019104058
115. Li Y, Schön C, Chen C-C, Yang Z, Liegl R, Murenu E, et al. TPC2 promotes choroidal angiogenesis and inflammation in a mouse model of neovascular age-related macular degeneration. Life Sci Alliance. (2021) 4(8):e202101047. doi: 10.26508/lsa.202101047
116. Goretzko J, Heitzig N, Thomas K, Krogsaeter EK, Naß J, Linard Matos AL, et al. Leukocyte adhesion is governed by endolysosomal two pore channel 2 (TPC2). Preprint bioRxiv (2021). doi: 10.1101/2021.09.28.462104
117. Goretzko J, Pauels I, Heitzig N, Thomas K, Kardell M, Naß J, et al. P-selectin-dependent leukocyte adhesion is governed by endolysosomal two-pore channel 2. Cell Rep. (2023) 42:113501. doi: 10.1016/j.celrep.2023.113501
118. Borsig L, Wong R, Feramisco J, Nadeau DR, Varki NM, Varki A. Heparin and cancer revisited: mechanistic connections involving platelets, P-selectin, carcinoma mucins, and tumor metastasis. Proc Natl Acad Sci U.S.A. (2001) 98:3352–7. doi: 10.1073/pnas.061615598
119. Sawada R, Tsuboi S, Fukuda M. Differential E-selectin-dependent adhesion efficiency in sublines of a human colon cancer exhibiting distinct metastatic potentials. J Biol Chem. (1994) 269:1425–31. doi: 10.1016/S0021–9258(17)42275–7
120. Läubli H, Spanaus K-S, Borsig L. Selectin-mediated activation of endothelial cells induces expression of CCL5 and promotes metastasis through recruitment of monocytes. Blood. (2009) 114:4583–91. doi: 10.1182/blood-2008–10-186585
121. Arlt E, Fraticelli M, Tsvilovskyy V, Nadolni W, Breit A, O’Neill TJ, et al. TPC1 deficiency or blockade augments systemic anaphylaxis and mast cell activity. Proc Natl Acad Sci U.S.A. (2020) 117:18068–78. doi: 10.1073/pnas.1920122117
122. Plaut M, Pierce JH, Watson CJ, Hanley-Hyde J, Nordan RP, Paul WE. Mast cell lines produce lymphokines in response to cross-linkage of Fc epsilon RI or to calcium ionophores. Nature. (1989) 339:64–7. doi: 10.1038/339064a0
123. Kilpatrick BS, Eden ER, Hockey LN, Yates E, Futter CE, Patel S. An endosomal NAADP-sensitive two-pore ca2+ Channel regulates ER-endosome membrane contact sites to control growth factor signaling. Cell Rep. (2017) 18:1636–45. doi: 10.1016/j.celrep.2017.01.052
124. Davis LC, Morgan AJ, Chen J-L, Snead CM, Bloor-Young D, Shenderov E, et al. NAADP activates two-pore channels on T cell cytolytic granules to stimulate exocytosis and killing. Curr Biol. (2012) 22:2331–7. doi: 10.1016/j.cub.2012.10.035
125. Raskov H, Orhan A, Christensen JP, Gögenur I. Cytotoxic CD8+ T cells in cancer and cancer immunotherapy. Br J Cancer. (2021) 124:359–67. doi: 10.1038/s41416–020-01048–4
126. Dammermann W, Guse AH. Functional ryanodine receptor expression is required for NAADP-mediated local Ca2+ signaling in T-lymphocytes. J Biol Chem. (2005) 280:21394–9. doi: 10.1074/jbc.M413085200
127. Vaeth M, Feske S. Ion channelopathies of the immune system. Curr Opin Immunol. (2018) 52:39–50. doi: 10.1016/j.coi.2018.03.021
128. Kaufmann U, Shaw PJ, Kozhaya L, Subramanian R, Gaida K, Unutmaz D, et al. Selective ORAI1 inhibition ameliorates autoimmune central nervous system inflammation by suppressing effector but not regulatory T cell function. J Immunol. (2016) 196:573–85. doi: 10.4049/jimmunol.1501406
129. Gwack Y, Srikanth S, Oh-Hora M, Hogan PG, Lamperti ED, Yamashita M, et al. Hair loss and defective T- and B-cell function in mice lacking ORAI1. Mol Cell Biol. (2008) 28:5209–22. doi: 10.1128/MCB.00360–08
130. Binnewies M, Roberts EW, Kersten K, Chan V, Fearon DF, Merad M, et al. Understanding the tumor immune microenvironment (TIME) for effective therapy. Nat Med. (2018) 24:541–50. doi: 10.1038/s41591-018-0014-x
131. Denk D, Greten FR. Inflammation: the incubator of the tumor microenvironment. Trends Cancer. (2022) 8:901–14. doi: 10.1016/j.trecan.2022.07.002
132. Kalluri R, LeBleu VS. The biology, function, and biomedical applications of exosomes. Science. (2020) 367(6478):eaau6977. doi: 10.1126/science.aau6977
133. Thorsson V, Gibbs DL, Brown SD, Wolf D, Bortone DS, Ou Yang T-H, et al. The immune landscape of cancer. Immunity. (2018) 48:812–830.e14. doi: 10.1016/j.immuni.2018.03.023
134. Greten FR, Grivennikov SI. Inflammation and cancer: triggers, mechanisms, and consequences. Immunity. (2019) 51:27–41. doi: 10.1016/j.immuni.2019.06.025
135. Medina DL. TRPML1 and TFEB, an intimate affair. Handb Exp Pharmacol. (2023) 278:109–26. doi: 10.1007/164_2022_603
136. Settembre C, Di Malta C, Polito VA, Garcia Arencibia M, Vetrini F, Erdin S, et al. TFEB links autophagy to lysosomal biogenesis. Science. (2011) 332:1429–33. doi: 10.1126/science.1204592
137. Sardiello M, Palmieri M, Di Ronza A, Medina DL, Valenza M, Gennarino VA, et al. A gene network regulating lysosomal biogenesis and function. Science. (2009) 325:473–7. doi: 10.1126/science.1174447
138. Medina DL, Fraldi A, Bouche V, Annunziata F, Mansueto G, Spampanato C, et al. Transcriptional activation of lysosomal exocytosis promotes cellular clearance. Dev Cell. (2011) 21:421–30. doi: 10.1016/j.devcel.2011.07.016
139. Tsunemi T, Perez-Rosello T, Ishiguro Y, Yoroisaka A, Jeon S, Hamada K, et al. Increased lysosomal exocytosis induced by lysosomal ca2+ Channel agonists protects human dopaminergic neurons from α-synuclein toxicity. J Neurosci. (2019) 39:5760–72. doi: 10.1523/JNEUROSCI.3085–18.2019
140. Sierra-Filardi E, Nieto C, Domínguez-Soto A, Barroso R, Sánchez-Mateos P, Puig-Kroger A, et al. CCL2 shapes macrophage polarization by GM-CSF and M-CSF: identification of CCL2/CCR2-dependent gene expression profile. J Immunol. (2014) 192:3858–67. doi: 10.4049/jimmunol.1302821
141. Kobayashi T, Tanaka T, Toyama-Sorimachi N. How do cells optimize luminal environments of endosomes/lysosomes for efficient inflammatory responses? J Biochem. (2013) 154:491–9. doi: 10.1093/jb/mvt099
142. Lindkvist B, Fajardo I, Pejler G, Borgström A. Cathepsin B activates human trypsinogen 1 but not proelastase 2 or procarboxypeptidase B. Pancreatology. (2006) 6:224–31. doi: 10.1159/000091961
143. Wörmann SM, Algül H. Risk factors and therapeutic targets in pancreatic cancer. Front Oncol. (2013) 3:282. doi: 10.3389/fonc.2013.00282
144. Choi AJ, Ryter SW. Autophagy in inflammatory diseases. Int J Cell Biol. (2011) 2011:732798. doi: 10.1155/2011/732798
145. White E, Karp C, Strohecker AM, Guo Y, Mathew R. Role of autophagy in suppression of inflammation and cancer. Curr Opin Cell Biol. (2010) 22:212–7. doi: 10.1016/j.ceb.2009.12.008
146. Aits S, Jäättelä M. Lysosomal cell death at a glance. J Cell Sci. (2013) 126:1905–12. doi: 10.1242/jcs.091181
147. Lyon M, Rushton G, Gallagher JT. The interaction of the transforming growth factor-betas with heparin/heparan sulfate is isoform-specific. J Biol Chem. (1997) 272:18000–6. doi: 10.1074/jbc.272.29.18000
148. Akbarshahi H, Axelsson JB, Said K, Malmström A, Fischer H, Andersson R. TLR4 dependent heparan sulphate-induced pancreatic inflammatory response is IRF3-mediated. J Transl Med. (2011) 9:219. doi: 10.1186/1479–5876-9–219
149. Nakajima M, Irimura T, Di Ferrante N, Nicolson GL. Metastatic melanoma cell heparanase. Characterization of heparan sulfate degradation fragments produced by B16 melanoma endoglucuronidase. J Biol Chem. (1984) 259:2283–90. doi: 10.1016/S0021–9258(17)43350–3
150. Winterhoff B, Freyer L, Hammond E, Giri S, Mondal S, Roy D, et al. PG545 enhances anti-cancer activity of chemotherapy in ovarian models and increases surrogate biomarkers such as VEGF in preclinical and clinical plasma samples. Eur J Cancer. (2015) 51:879–92. doi: 10.1016/j.ejca.2015.02.007
151. Dredge K, Hammond E, Handley P, Gonda TJ, Smith MT, Vincent C, et al. PG545, a dual heparanase and angiogenesis inhibitor, induces potent anti-tumour and anti-metastatic efficacy in preclinical models. Br J Cancer. (2011) 104:635–42. doi: 10.1038/bjc.2011.11
152. He Y, Xu Y, Zhang C, Gao X, Dykema KJ, Martin KR, et al. Identification of a lysosomal pathway that modulates glucocorticoid signaling and the inflammatory response. Sci Signal. (2011) 4:ra44. doi: 10.1126/scisignal.2001450
153. Kach J, Conzen SD, Szmulewitz RZ. Targeting the glucocorticoid receptor in breast and prostate cancers. Sci Transl Med. (2015) 7:305ps19. doi: 10.1126/scitranslmed.aac7531
154. Abduljabbar R, Negm OH, Lai C-F, Jerjees DA, Al-Kaabi M, Hamed MR, et al. Clinical and biological significance of glucocorticoid receptor (GR) expression in breast cancer. Breast Cancer Res Treat. (2015) 150:335–46. doi: 10.1007/s10549–015-3335–1
155. Kassi E, Moutsatsou P. Glucocorticoid receptor signaling and prostate cancer. Cancer Lett. (2011) 302:1–10. doi: 10.1016/j.canlet.2010.10.020
156. Lu Y-S, Lien H-C, Yeh P-Y, Kuo S-H, Chang W-C, Kuo M-L, et al. Glucocorticoid receptor expression in advanced non-small cell lung cancer: clinicopathological correlation and in vitro effect of glucocorticoid on cell growth and chemosensitivity. Lung Cancer. (2006) 53:303–10. doi: 10.1016/j.lungcan.2006.05.005
157. Settembre C, Zoncu R, Medina DL, Vetrini F, Erdin S, Erdin S, et al. A lysosome-to-nucleus signalling mechanism senses and regulates the lysosome via mTOR and TFEB. EMBO J. (2012) 31:1095–108. doi: 10.1038/emboj.2012.32
158. Liu Y, Wang X, Zhu W, Sui Z, Wei X, Zhang Y, et al. TRPML1-induced autophagy inhibition triggers mitochondrial mediated apoptosis. Cancer Lett. (2022) 541:215752. doi: 10.1016/j.canlet.2022.215752
159. Qi X, Man SM, Malireddi RK, Karki R, Lupfer C, Gurung P, et al. Cathepsin B modulates lysosomal biogenesis and host defense against Francisella novicida infection. J Exp Med. (2016) 213:2081–97. doi: 10.1084/jem.20151938
160. Scotto Rosato A, Montefusco S, Soldati C, Di Paola S, Capuozzo A, Monfregola J, et al. TRPML1 links lysosomal calcium to autophagosome biogenesis through the activation of the CaMKKβ/VPS34 pathway. Nat Commun. (2019) 10:5630. doi: 10.1038/s41467-019-13572-w
161. Intlekofer AM, Thompson CB. At the bench: preclinical rationale for CTLA-4 and PD-1 blockade as cancer immunotherapy. J Leukoc Biol. (2013) 94:25–39. doi: 10.1189/jlb.1212621
162. de Sousa Linhares A, Leitner J, Grabmeier-Pfistershammer K, Steinberger P. Not all immune checkpoints are created equal. Front Immunol. (2018) 9:1909. doi: 10.3389/fimmu.2018.01909
163. Marin-Acevedo JA, Kimbrough EO, Lou Y. Next generation of immune checkpoint inhibitors and beyond. J Hematol Oncol. (2021) 14:45. doi: 10.1186/s13045–021-01056–8
164. Walker LS, Sansom DM. Confusing signals: recent progress in CTLA-4 biology. Trends Immunol. (2015) 36:63–70. doi: 10.1016/j.it.2014.12.001
165. Linsley PS, Brady W, Urnes M, Grosmaire LS, Damle NK, Ledbetter JA. CTLA-4 is a second receptor for the B cell activation antigen B7. J Exp Med. (1991) 174:561–9. doi: 10.1084/jem.174.3.561
166. Linsley PS, Greene JL, Tan P, Bradshaw J, Ledbetter JA, Anasetti C, et al. Coexpression and functional cooperation of CTLA-4 and CD28 on activated T lymphocytes. J Exp Med. (1992) 176:1595–604. doi: 10.1084/jem.176.6.1595
167. Chen L, Ashe S, Brady WA, Hellström I, Hellström KE, Ledbetter JA, et al. Costimulation of antitumor immunity by the B7 counterreceptor for the T lymphocyte molecules CD28 and CTLA-4. Cell. (1992) 71:1093–102. doi: 10.1016/s0092–8674(05)80059–5
168. Azuma M, Ito D, Yagita H, Okumura K, Phillips JH, Lanier LL, et al. B70 antigen is a second ligand for CTLA-4 and CD28. Nature. (1993) 366:76–9. doi: 10.1038/366076a0
169. Egen JG, Kuhns MS, Allison JP. CTLA-4: new insights into its biological function and use in tumor immunotherapy. Nat Immunol. (2002) 3:611–8. doi: 10.1038/ni0702–611
170. Chikuma S. CTLA-4, an essential immune-checkpoint for T-cell activation. Curr Top Microbiol Immunol. (2017) 410:99–126. doi: 10.1007/82_2017_61
171. Contardi E, Palmisano GL, Tazzari PL, Martelli AM, Falà F, Fabbi M, et al. CTLA-4 is constitutively expressed on tumor cells and can trigger apoptosis upon ligand interaction. Int J Cancer. (2005) 117:538–50. doi: 10.1002/ijc.21155
172. Leach DR, Krummel MF, Allison JP. Enhancement of antitumor immunity by CTLA-4 blockade. Science. (1996) 271:1734–6. doi: 10.1126/science.271.5256.1734
173. Chuang E, Alegre ML, Duckett CS, Noel PJ, Vander Heiden MG, Thompson CB. Interaction of CTLA-4 with the clathrin-associated protein AP50 results in ligand-independent endocytosis that limits cell surface expression. J Immunol. (1997) 159:144–51. doi: 10.4049/jimmunol.159.1.144
174. Bossi G, Griffiths GM. Degranulation plays an essential part in regulating cell surface expression of Fas ligand in T cells and natural killer cells. Nat Med. (1999) 5:90–6. doi: 10.1038/4779
175. Shiratori T, Miyatake S, Ohno H, Nakaseko C, Isono K, Bonifacino JS, et al. Tyrosine phosphorylation controls internalization of CTLA-4 by regulating its interaction with clathrin-associated adaptor complex AP-2. Immunity. (1997) 6:583–9. doi: 10.1016/S1074–7613(00)80346–5
176. Schneider H. Cytolytic T lymphocyte-associated antigen-4 and the TCRξ/CD3 complex, but not CD28, interact with clathrin adaptor complexes AP-1 and AP-2. J Immunol. (1999) 163(4):1868–79. doi: 10.4049/jimmunol.163.4.1868
177. Daud AI, Wolchok JD, Robert C, Hwu W-J, Weber JS, Ribas A, et al. Programmed death-ligand 1 expression and response to the anti-programmed death 1 antibody pembrolizumab in melanoma. J Clin Oncol. (2016) 34:4102–9. doi: 10.1200/JCO.2016.67.2477
178. Tang Q, Chen Y, Li X, Long S, Shi Y, Yu Y, et al. The role of PD-1/PD-L1 and application of immune-checkpoint inhibitors in human cancers. Front Immunol. (2022) 13:964442. doi: 10.3389/fimmu.2022.964442
179. O’Neill RE, Cao X. Co-stimulatory and co-inhibitory pathways in cancer immunotherapy. Adv Cancer Res. (2019) 143:145–94. doi: 10.1016/bs.acr.2019.03.003
180. Peña-Asensio J, Calvo H, Torralba M, Miquel J, Sanz-de-Villalobos E, Larrubia J-R. Anti-PD-1/PD-L1 based combination immunotherapy to boost antigen-specific CD8+ T cell response in hepatocellular carcinoma. Cancers. (2021) 13(8):1922. doi: 10.3390/cancers13081922
181. Banchereau R, Chitre AS, Scherl A, Wu TD, Patil NS, de AP, et al. Intratumoral CD103+ CD8+ T cells predict response to PD-L1 blockade. J immunother Cancer. (2021) 9(4):e002231. doi: 10.1136/jitc-2020–002231
182. Yao H, Lan J, Li C, Shi H, Brosseau J-P, Wang H, et al. Inhibiting PD-L1 palmitoylation enhances T-cell immune responses against tumours. Nat BioMed Eng. (2019) 3:306–17. doi: 10.1038/s41551–019-0375–6
183. Burr ML, Sparbier CE, Chan Y-C, Williamson JC, Woods K, Beavis PA, et al. CMTM6 maintains the expression of PD-L1 and regulates anti-tumour immunity. Nature. (2017) 549:101–5. doi: 10.1038/nature23643
184. Liang J, Li S, Li W, Rao W, Xu S, Meng H, et al. CMTM6, a potential immunotherapy target. J Cancer Res Clin Oncol. (2022) 148:47–56. doi: 10.1007/s00432–021-03835–9
185. Kashiwada T, Takano R, Ando F, Kuroda S, Miyabe Y, Asatsuma-Okumura T, et al. Lysosomal degradation of PD-L1 is associated with immune-related adverse events during anti-PD-L1 immunotherapy in NSCLC patients. Preprint medRvix (2024). doi: 10.1101/2024.01.21.24301536
186. Weber J. Ipilimumab: controversies in its development, utility and autoimmune adverse events. Cancer Immunol Immunother. (2009) 58:823–30. doi: 10.1007/s00262–008-0653–8
187. Wang Y, Deng S, Xu J. Proteasomal and lysosomal degradation for specific and durable suppression of immunotherapeutic targets. Cancer Biol Med. (2020) 17:583–98. doi: 10.20892/j.issn.2095–3941.2020.0066
188. Davis LC, Morgan AJ, Galione A. Optical profiling of autonomous Ca2+ nanodomains generated by lysosomal TPC2 and TRPML1. Cell Calcium. (2023) 116:102801. doi: 10.1016/j.ceca.2023.102801
Keywords: lysosome, ion channels, TRPML, TPC, cancer, cancer immunity, two-pore channels, mucolipins
Citation: Ouologuem L and Bartel K (2024) Endolysosomal transient receptor potential mucolipins and two-pore channels: implications for cancer immunity. Front. Immunol. 15:1389194. doi: 10.3389/fimmu.2024.1389194
Received: 21 February 2024; Accepted: 09 May 2024;
Published: 22 May 2024.
Edited by:
Jagadeesh Bayry, Indian Institute of Technology Palakkad, IndiaReviewed by:
Axel R. Concepcion, The University of Chicago, United StatesCopyright © 2024 Ouologuem and Bartel. This is an open-access article distributed under the terms of the Creative Commons Attribution License (CC BY). The use, distribution or reproduction in other forums is permitted, provided the original author(s) and the copyright owner(s) are credited and that the original publication in this journal is cited, in accordance with accepted academic practice. No use, distribution or reproduction is permitted which does not comply with these terms.
*Correspondence: Karin Bartel, a2FyaW4uYmFydGVsQGN1cC51bmktbXVlbmNoZW4uZGU=
Disclaimer: All claims expressed in this article are solely those of the authors and do not necessarily represent those of their affiliated organizations, or those of the publisher, the editors and the reviewers. Any product that may be evaluated in this article or claim that may be made by its manufacturer is not guaranteed or endorsed by the publisher.
Research integrity at Frontiers
Learn more about the work of our research integrity team to safeguard the quality of each article we publish.