- 1Laboratory of Therapeutic Vaccines, Mechnikov Research Institute for Vaccines and Sera, Moscow, Russia
- 2Laboratory of Glycoconjugate Chemistry, N. D. Zelinsky Institute of Organic Chemistry, Russian Academy of Science, Moscow, Russia
The disaccharide (β-D-glucopyranosyluronic acid)-(1→4)-β-D-glucopyranoside represents a repeating unit of the capsular polysaccharide of Streptococcus pneumoniae serotype 3. A conjugate of the disaccharide with BSA (di-BSA conjugate) adjuvanted with aluminum hydroxide induced — in contrast to the non-adjuvanted conjugate — IgG1 antibody production and protected mice against S. pneumoniae serotype 3 infection after intraperitoneal prime-boost immunization. Adjuvanted and non-adjuvanted conjugates induced production of Th1 (IFNγ, TNFα); Th2 (IL-5, IL-13); Th17 (IL-17A), Th1/Th17 (IL-22), and Th2/Th17 cytokines (IL-21) after immunization. The concentration of cytokines in mice sera was higher in response to the adjuvanted conjugate, with the highest level of IL-17A production after the prime and boost immunizations. In contrast, the non-adjuvanted conjugate elicited only weak production of IL-17A, which gradually decreased after the second immunization. After boost immunization of mice with the adjuvanted di-BSA conjugate, there was a significant increase in the number of CD45+/CD19+ B cells, TCR+ γδ T cell, CD5+ В1 cells, and activated cells with MHC II+ expression in the spleens of the mice. IL-17A, TCR+ γδ T cells, and CD5+ В1 cells play a crucial role in preventing pneumococcal infection, but can also contribute to autoimmune diseases. Immunization with the adjuvanted and non-adjuvanted di-BSA conjugate did not elicit autoantibodies against double-stranded DNA targeting cell nuclei in mice. Thus, the molecular and cellular markers associated with antibody production and protective activity in response to immunization with the di-BSA conjugate adjuvanted with aluminum hydroxide are IL-17A, TCR+ γδ T cells, and CD5+ В1 cells against the background of increasing MHC II+ expression.
1 Introduction
Streptococcus pneumoniae (pneumococcus) cause pneumonia, bacteremia, septic arthritis, meningitis, sinusitis, otitis media and some other diseases in humans (1, 2). The incidence of community-acquired pneumonia is one per one thousand adults. The mortality rate for pneumococcal pneumonia among hospitalized patients is 5–7% (3–7). Symptoms of pneumococcal infection depend on the localization of the infection. These may include fever, cough, chest pain, a stiff neck, chills, ear pain and others.
Pneumococcal polysaccharide and conjugate vaccines, which contain capsular polysaccharides (CPs) from clinically significant S. pneumoniae serotypes, are available (8). S. pneumoniae serotype 3 is predominant among other serotypes in various countries (9–12). Epidemiological data suggests a high incidence of disease caused by S. pneumoniae serotype 3 (13–15). However, the widespread use of pneumococcal vaccines should help to reduce the incidence of this disease (16–19). Improving the quality of S. pneumoniae type 3 in the composition of pneumococcal vaccines is essential.
Bacterial CPs contain a diverse mixture of oligosaccharides with varying chain lengths and frame shifts (20). Although their chemical preparation is practically possible (see, for example (21),), synthetic oligosaccharide derivatives represent more convenient antigenic components for the design of conjugate carbohydrate vaccines (22–25). Currently, a number of semisynthetic vaccines are under development, including those against Staphylococcus, Clostridium, Klebsiella, Shigella, and Enterococcus (25–33). The semi-synthetic glycoconjugate vaccine, Quimi-Hib, for the prevention of H. influenzae type b infection is licensed for use in Cuba (34). Optimization of the composition of pneumococcal vaccines using synthetic oligosaccharides conjugated with a protein carrier is a priority in contemporary vaccinology (25, 35–38).
Moreover, synthetic oligosaccharides with precisely defined chemical structures enable the study of the effect of bacterial antigens (39, 40), yielding a better understanding of the innate and cellular immunity, the antibody (Ab) response, and protective activity of CPs.
Immunization with glycoconjugate vaccines partially mimics the development of natural infection without actually causing the disease. In a mouse model, γδ T cells and natural killer T cells (NKT) have been shown to play a crucial role in anti-pneumococcal immunity by producing Th1 and/or Th17-related cytokines (41). The ability of semisynthetic glycoconjugates to stimulate cytokine production in vivo and their influence on the activation of cellular immunity remain unknown. Here, we report on the effect of a conjugate of the synthetic disaccharide, which represents a repeating unit of S. pneumoniae serotype 3 (42), on production of Th1/Th2/Th17 cytokines in mice, changes in expression of surface molecules on splenocytes, antibody response, and protection against S. pneumoniae infection. We also investigated the production of autoantibodies against double-stranded (ds) DNA.
2 Materials and methods
2.1 The synthetic disaccharide and its conjugate
The synthetic disaccharide (35, 43) was coupled to BSA (Sigma-Aldrich, St. Louis, MO, USA), as previously described (35, 44). The structure of the conjugate is illustrated in Figure 1. BSA is often used as a protein carrier in engineered immunogenic glycoconjugates and other biological systems (45). Previous studies using MALDI-TOF mass spectrometry have shown that the di-BSA conjugate contains, on average, 19 oligosaccharide ligands per protein molecule, which corresponds to a 9% carbohydrate content by weight (43, 44). The lyophilized di-BSA conjugate remains stable at +4°C, with no decrease in activity, for at least three years (i.e., observation period).
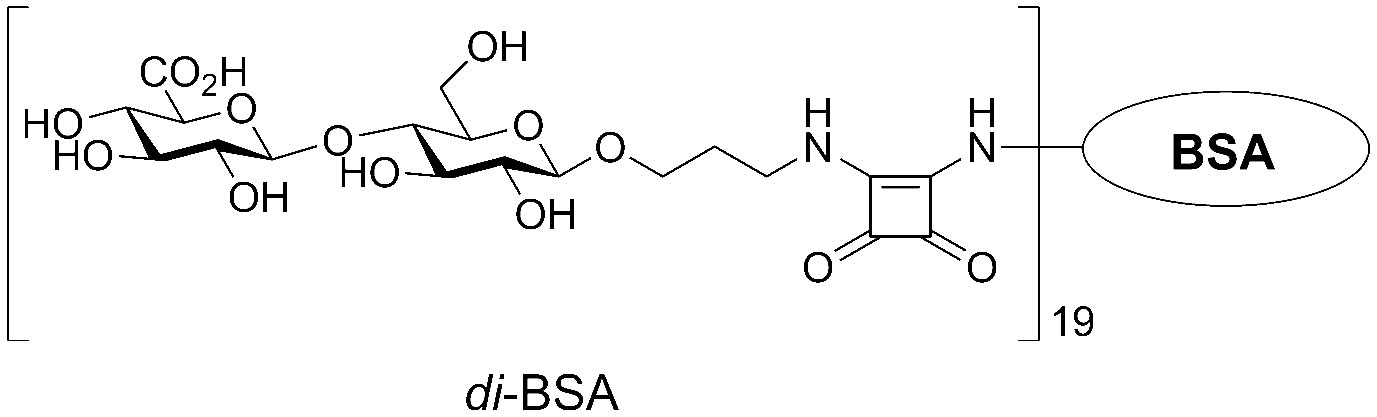
Figure 1 The structure of the BSA conjugate with the disaccharide that corresponds to a repeating unit of the CP from S. pneumoniae serotype 3.
2.2 Bacterial capsular polysaccharide
Bacterial CP was isolated from the S. pneumoniae type 3 laboratory strain, #10196, which was isolated on June 30, 2011, from the blood culture of a child suffering from bacteremia in the microbiology department of the “Scientific Center for Children’s Health” in Moscow, Russia. The strain had been grown in a semi-synthetic growth medium. The isolation process for CP has been previously described elsewhere (46). The presence of CP in the preparation was confirmed by NMR spectrometry.
2.3 Animals
BALB/c male mice, aged 6–8 weeks (n=162), were purchased from the Scientific and Production Centre for Biomedical Technologies in Moscow, Russia, and kept in the vivarium at the Mechnikov Institute for Vaccines and Sera. Housing, breeding, blood collection, and euthanasia conditions followed European Union guidelines for laboratory animal care and use. Experimental designs were reviewed and approved (Protocol No. 2, dated February 12th, 2019) by the Ethics Committee at the Institute.
2.4 Conjugated disaccharide-induced cytokine production
Quantitative determination of cytokines was performed as previously described (46). Male BALB/c mice (n=6) were sacrificed, and serum was collected and stored at –20°C until further quantification of cytokine levels. Using the Flow Cytomix Mouse Th1/Th2 10-plex test system, cytokine levels were measured by adding beads coated with monoclonal antibodies to IL-1α, IL-1β, IL-2, IL-4, IL-5, IL-6, IL-10, IL-12p70, IL-13, IL-17A, IL-21 and IL-22, as well as IFNγ and TNFα, following the manufacturer’s instructions (eBioscience, San Diego, USA) using a Cytomix FC-500 flow cytometer (Beckman Coulter, Brea, USA).
2.5 Immunization
Mice were intraperitoneally immunized with the di-BSA conjugate, either adjuvanted or not, with aluminum hydroxide (Sigma-Aldrich). The amount of carbohydrate in 0.5 mL of the experimental semisynthetic vaccine was 20 μg, BSA ~200 μg; aluminum hydroxide, as an adjuvant, standardized for aluminum, was added in an amount of 250 μg. The single immunizing dose per mice was 0.5 mL of the di-BSA conjugate. Animals were given the vaccine twice, on days 0 and 14 of the study.
Similar immunization schedules were used for the pneumococcal conjugate vaccine Prevnar 13 (Pfizer, New York, NY, USA), which contains aluminum phosphate as an adjuvant. A 0.5 mL dose contains 2.2 μg of polysaccharides from serotypes 1, 3, 4, 5, 6A, 7F, 9V, 14, 18C, 19A, 19F, and 23F, as well as 4.4 μg of the polysaccharide from serotype 6B. The vaccine also contains 32 μg of the carrier protein, CRM197, and 125 μg of aluminum as aluminum phosphate. Mice were immunized twice with a single dose of 1.1 μg of CP from S. pneumoniae type 3 per inoculation (equivalent to half of the recommended human dose). Control mice were injected with saline.
2.6 Content of bacterial endotoxins in glycoconjugates
Detection of bacterial endotoxin impurities in the di-BSA conjugate was performed using the Limulus amebocyte lysate ENDOCHROME ™ (Charles River Endosafe Div. of Charles River Laboratories, Inc., Charleston, US) test obtained from the Collective Usage Center of the Mechnikov Research Institute for Vaccine and Sera (Moscow, Russia), in accordance with the manufacturer’s instructions. The di-BSA conjugate contained 0.08–0.11 EU/mL of endotoxin (LAL-Center, Moscow, Russia).
2.7 Measurement of antibody response to the disaccharide conjugate
Antibody titers for CP in post-immunization sera were measured using ELISA. Briefly, plates coated with S. pneumoniae type 3 CP were incubated with antisera from 6 immunized mice (42). Wells were washed and secondary antibody was added, followed by incubation and washing. The results were then analyzed. Enzyme substrate aliquots (100 μL) were added, followed by incubation for 20 minutes at 22°C. The reactions were quenched with 1 M H2SO4. Optical densities (ODs) were determined using an iMark microplate absorbance reader (Bio-Rad, Osaka, Japan) at a wavelength of 450 nm. Antibody titers are expressed as the dilution of serum in which the antibody was detected.
2.8 Expression of surface molecules on splenic mononuclear cells
Splenocytes were isolated from mice that had been vaccinated with the glyconjugate either in the absence of or in the presence of aluminum hydroxide, one and seven days after primary and booster immunizations. Single-cell suspensions of splenocytes were prepared by manually mashing the spleens using the plunger from a disposable syringe. The ground spleen was then passed through a nylon mesh and the cells were suspended in PBS. Splenic single-cell suspensions were then stained with antibodies conjugated to phycoerythrin (PE) or fluorescein isothiocyanate (FITC) to detect specific proteins in the cells: CD3e-FITC (clone 145–2C11), CD4-FITC (clone GK1.5), CD8a-FITC (clone 53–6.7), CD19-FITC (eBio1D3), CD5-PE (clone 53–7.3), NK.1.1 (clone PK136), CD3/CD16/CD32 (NKT), CD25-PE (PC61.5), CD4/CD25/Foxp3 (Treg), γδT (clone γδ TCR-PE, eBioGL3), and MHCII-PE (I-EK) (clone 14–4-45). Treg cells were stained with CD4-FITC (clone GK1.5), together with CD25-PE (PC61.5), and after fixation with the fixation/permeabilization buffer, with Foxp3- APC (clone FJK-16s). Splenocytes were incubated with 50 µL of appropriate monoclonal antibodies (eBioscience, US) at 4°C for 30 minutes. Erythrocytes were then lysed using red blood cell lysis buffer (BioLegend, US). After washing with phosphate-buffered saline (PBS), the samples were fixed using a fixation solution (BioLegend, US) and analyzed by flow cytometry (Cytomix FC-500, Beckman Coulter, USA, with the CXP software). The cell population gate was determined based on forward and side scatter and cell size. 10,000 cells were recorded per gate.
2.9 Di-BSA-induced active protection in immunized mice
BALB/c mice were intraperitoneally immunized with the di-BSA conjugate adsorbed or non-adsorbed on aluminum hydroxide on days 0 and 14 (twenty animals per conjugate). The same animals were intraperitoneally challenged after 2 weeks with 105 colony-forming units of S. pneumoniae type 3/0.5 mL. Non-immunized control mice (twenty animals per conjugate) were also exposed to the bacteria. Mortality rates were determined at seven days post-infection.
2.10 Antibodies against double-stranded DNA
The analysis of antibodies against ds DNA in the immune sera of mice was conducted using ELISA. Salmon sperm DNA (Behringer GmbH, Germany), dissolved in a carbohydrate buffer solution at a concentration of 20 g/mL, was adsorbed onto the bottom of the wells. The plates were incubated for 2 hours at 37°C and then for additional 18 hours at 6°C. The serums were analyzed using dilutions of 1:10 to 1:1280. As secondary antibodies, secondary rabbit anti-mouse peroxidase conjugated IgG (Rockland Immunochemicals Inc., Pottstown, PA) was utilized (100 μL). After adding tetramethylbenzidine for 15 minutes, the reaction was terminated with 1 M sulfuric acid. Results were obtained utilizing a multi-channel automatic photometer (TiterTek Multiscan MC from Flow Laboratories, England), with excitation at 490nm. Serums from non-immunized mice, as well as mice immunized with either Prevnar-13 or BSA adjuvanted or non-adjuvanted with aluminum hydroxide, were used as controls.
2.11 Statistical analysis
Between-group comparisons were performed using Mann-Whitney rank sum tests for independent samples. Fisher exact tests were conducted to evaluate survival of mice after pneumococcal challenge. P values ≤0.05 were considered to indicate statistical significance. Statistical analyses were performed using the Statistical data analysis software system version 10 (StatSoft Inc., Tulsa, OK, USA).
3 Results
3.1 Antibodies induced by the di-BSA conjugate
Although the di-BSA conjugate adjuvanted with aluminum hydroxide was found to be less immunogenic than adjuvanted tri- and tetra-BSA conjugates, it was still able to induce the production of opsonizing antibodies and was sufficient for the development of serotype 3-protective immunity in mice (42).
In this study, we explored the ability of the di-BSA conjugate to induce antibodies capable of binding to the CP of S. pneumoniae serotype 3 in ELISA after primary and booster immunization with and without the adjuvant (Figure 2). The di-BSA conjugate without adjuvant did not induce Ab production after the prime and boost immunizations and no difference was observed relative to the control. The glycoconjugate adjuvanted with aluminum hydroxide induced no Ab production after prime immunization; however, after booster injection, the level of Abs increased in seven days (21 d) and was significantly elevated up to 28 d (14 d after boost). Prevnar 13 (1.1 µg/dose of carbohydrate of CP of S. pneumoniae serotype 3) induced IgG Ab production on day 14 after boost immunization (the time of the study) at a titer of 1:800 (data not shown).
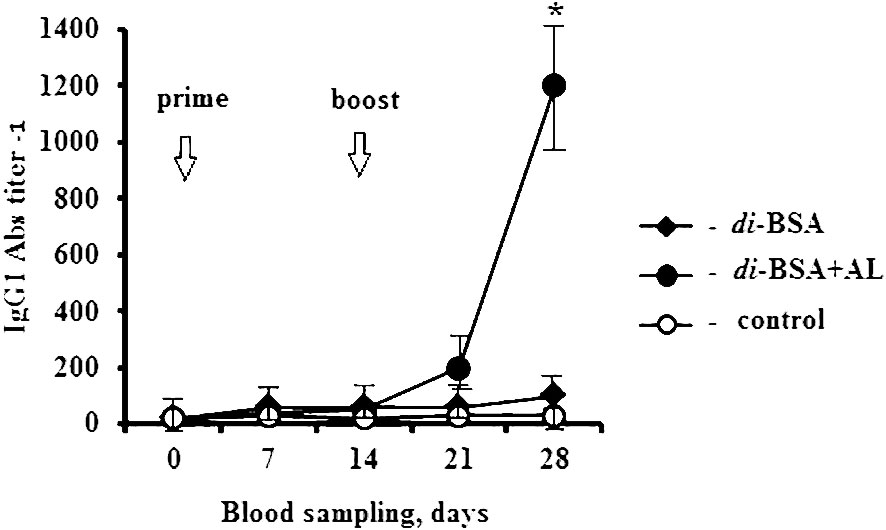
Figure 2 IgG1 antibody production induced by the adjuvanted and non-adjuvanted di-BSA conjugate. BALB/c mice (n = 6 per conjugate) were intraperitoneally injected with the di-BSA conjugate (20 µg/dose of carbohydrate) adjuvanted and non-adjuvanted with aluminum hydroxide, on days 0 and 14. The IgG1 Ab titer in the blood of mice was determined on days 0 (before prime immunization), days 7, 14, 21, and 28 (7 and 14 days after booster immunization, respectively), by ELISA, using CP of S. pneumoniae serotype 3 as the well-coating antigen. Mice (n=6) injected with saline at the same time served as a control group. AL - aluminum hydroxide. The data are presented as mean ± standard deviation (M ± SD). The Mann–Whitney rank sum test was used to determine significance, *P < 0.05.
3.2 Active protection upon challenge of mice immunized with the di-BSA conjugate
Mice immunized with the di-BSA conjugate and di-BSA conjugate adjuvanted with aluminum hydroxide were challenged with S. pneumoniae serotype 3 on day 28 (14 d after booster immunization). All control mice injected with saline and 18 out of 20 mice immunized with the non-adjuvanted di-BSA conjugate died on the second day after the challenge (Figure 3).
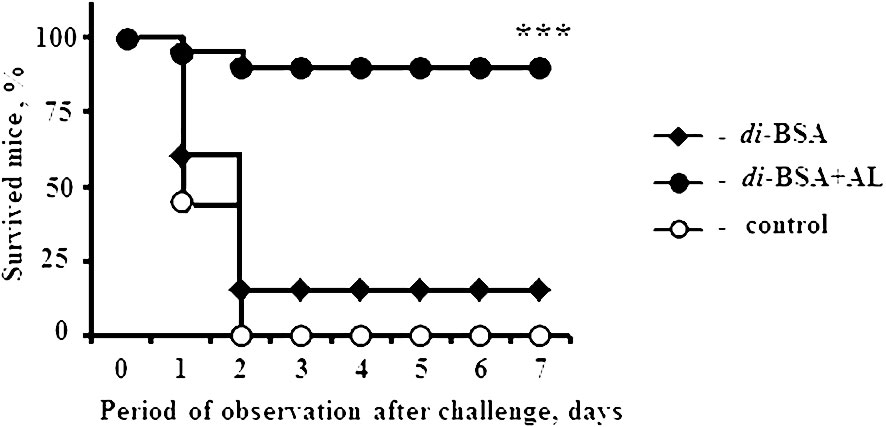
Figure 3 Protective activity of the adjuvanted and non-adjuvanted di-BSA conjugate. BALB/c mice (n = 20 per conjugate and control group) intraperitoneally injected with the di-BSA conjugate (20 µg/dose of carbohydrate) adjuvanted and non-adjuvanted with aluminum hydroxide on days 0 and 14 were challenged with 105 colony-forming units of S. pneumoniae serotype 3 on day 28. Mice injected with saline were used as a control. AL - aluminum hydroxide. The results of two experiments are summarized. The difference between mice immunized with the adjuvanted di-BSA conjugate and non-adjuvanted/non-immunized mice (control) is shown. Fisher exact test; ***P < 0.001.
The non-adjuvanted di-BSA conjugate that failed to induce Ab production also did not elicit any protection against challenge with S. pneumoniae serotype 3. However, the same conjugate administered to mice with aluminum hydroxide induced protection against S. pneumoniae serotype 3. Thus, aluminum hydroxide is indispensable for inducing protective immunity to the disaccharide conjugate. Prevnar 13 (1.1 µg/dose of carbohydrate of CP of S. pneumoniae serotype 3) protected all mice (n = 6) from the challenge (42).
3.3 Cytokine production in mice
To evaluate cytokine production, mice were intraperitoneally injected with the di-BSA conjugate adjuvanted or non-adjuvanted with aluminum hydroxide at a single dose of 20 µg (carbohydrate content). Serum cytokine levels were determined before injection of the glycoconjugate (d 0) and on days 1, 7, 15, and 21 (1 and 7 days after boost immunization, respectively) (Figure 4).
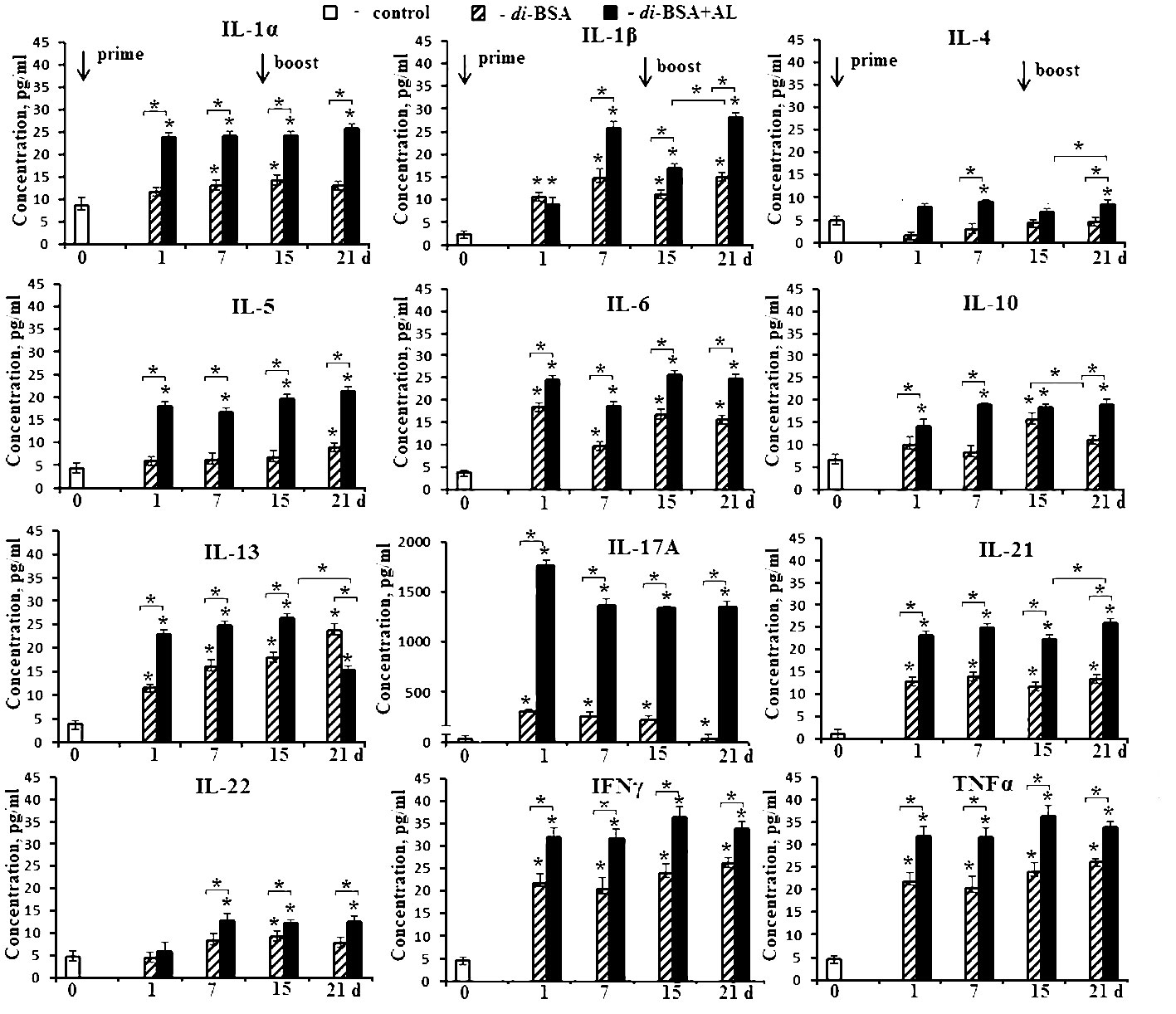
Figure 4 Cytokine production in mice induced by the adjuvanted and non-adjuvanted di-BSA conjugate. BALB/c mice were immunized with the di-BSA conjugate (20 μg of carbohydrate per mouse) adjuvanted or non-adjuvanted with aluminum hydroxide (n = 24 for each conjugate). Control mice (n = 6) were injected with saline 24 hours before the start of immunization (0 d). Serum was collected from mice (n=6 for each time point) after immunization. Cytokine levels were analyzed using flow cytometry. No increase in IL-2 or IL-12 p70 levels was observed in any of the time points (data not shown). The data is presented as the mean ± SD. Mann-Whitney rank sum tests were used to determine significant differences between control and other experimental groups; *P <0.05.
After prime immunization, the non-adjuvanted di-BSA conjugate induced an increase in the levels of IL-1α, IL-1β, IL-6, IL-13, IL-17A, IL-21, IFNγ, and TNFα compared with that in the control (0 d). After booster immunization with the conjugate, IL-5, IL-10, and IL-22 production was induced in addition to these cytokines. The concentration of IL-4 did not increase in any of the study periods.
After prime immunization, the di-BSA conjugate adjuvanted with aluminum hydroxide stimulated higher production of IL-1α, IL-1β, IL-4, IL-5, IL-6, IL-10, IL-13, IL-17A, IL-21, IL-22, IFNγ, and TNFα compared with the conjugate without the adjuvant. After booster immunization, all cytokines were found to be produced at high levels. When the conjugate was administered with the adjuvant, a very high level of IL-17A production was noted at all time points. In contrast, when mice were immunized with the conjugate without the adjuvant, the IL-17A level gradually decreased even after booster immunization. Regardless of the presence of the adjuvant, the levels of IL-2 and IL-12p70 did not increase during all follow-up periods. Free CP of S. pneumoniae serotype 3 (5 µg/mouse) elevated only the level of IFNγ (from 23.1 to 50.8 pg) after double immunization (data not shown). CP-CRM197 (Prevnar 13) is able to induce the production of IL-1, IL-2, IL-4, IL-5, IL-6, IL-10, IL-12, IL-17, IFNγ, and TNFα (47, 48). Free aluminum hydroxide did not elicit cytokine production when administered at the same time points (data not shown).
3.4 Expression of cell-surface molecules on splenic mononuclear cells
After first immunization with the di-BSA conjugate adjuvanted and non-adjuvanted with aluminum hydroxide, the number of CD45+/CD3+ T cells and CD45+/CD4+ T helper cells increased compared with that in the control. After booster immunization, regardless of the presence of adjuvant, there was no difference relative to the control (Figure 5).
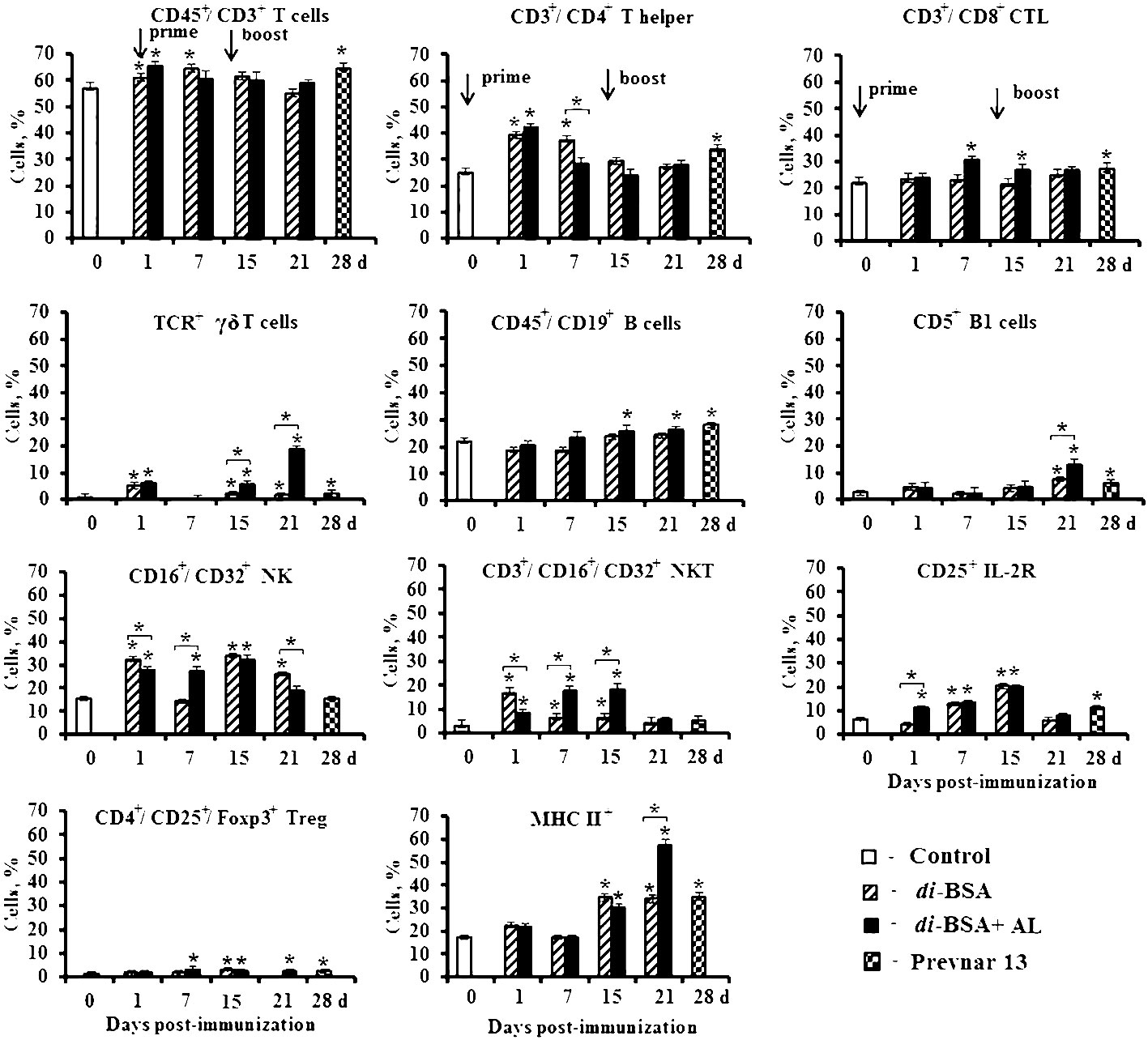
Figure 5 The number of splenocytes expressing membrane molecules in mice immunized with the di-BSA conjugate with and without adjuvant. BALB/c mice were immunized with the di-BSA conjugate (20 μg/dose of carbohydrate per mouse) adjuvanted or non-adjuvanted with aluminum hydroxide and with Prevnar 13 (1.1 μg/dose of carbohydrate of CP S. pneumoniae serotype 3 per mouse) adjuvanted with aluminum phosphate. Splenocytes were isolated from mice (n = 6 for each conjugate and each time point) on the indicated days after immunization. Control mice (n = 6) were injected with saline 24 hours before the start of immunization (0 d). Spleen cell suspensions were stained using antibodies against mouse CD3e-FITC (clone 145–2C11), CD4-FITC (clone GK1.5), CD8a-FITC (clone 53–6.7), γδT (clone γδ TCR-PE, eBioGL3), CD19-FITC (eBio1D3), CD5-PE (clone 53–7.3), NK.1.1 (clone PK136) CD25-PE (PC61.5), and MHCII-PE (I-EK) (clone 14–4-45). Treg: FITC anti-mouse CD4 (clone GK1.5). Staining with anti-Foxp3-APCconjugated Ab (clone FJK-16s) was performed according to the manufacturer’s protocol. The results were determined using flow cytometry. The data are shown as the mean ± SD. Mann-Whitney rank sum tests were used to calculate significant differences between control and other experimental groups; *P < 0.05.
After primary and booster immunization with the adjuvanted di-BSA conjugate, the number of CD45+/CD8+ cytotoxic T cells (CTLs) increased compared with that in the control. The non-adjuvanted conjugate did not induce any change in the number of CTLs during the entire observation period. An interesting result was revealed in relation to γδ T cells. One day after prime immunization of mice with the adjuvanted and non-adjuvanted di-BSA conjugate, the number of γδ T cells increased compared with that in the control and decreased to the initial levels on day 7. However, after booster immunization with the adjuvanted conjugate, the number of TCR+ γδ T cells increased on day 15 (1 d after boost), reaching high values on day 21 (7 d after boost). In contrast, in the absence of aluminum hydroxide, their values did not differ from the control level. After booster immunization with the di-BSA conjugate, the number of CD45+/CD19+ B cells increased only following booster immunization in the presence of aluminum hydroxide. After injection of the non-adjuvanted conjugate, the level of CD45+/CD19+ B cells did not differ from that in the control. The number of CD5+ B1 increased on day 1 after the first immunization with adjuvanted and non-adjuvanted conjugate compared with that in the control and then decreased on day 7. Booster immunization with the adjuvanted di-BSA conjugate led to an increase of number of CD5+ B1 cells on day 15 (1 d after boost) compared with that in the control, and on day 21 (7 d after boost) relative to the non-adjuvanted conjugate. The administration of the adjuvanted and non-adjuvanted conjugate increased the number of CD16+/CD32+ natural killer cells (NK) and CD3+/CD16+/CD32+ natural killer T cells (NKT) after primary and booster immunization. The adjuvanted and non-adjuvanted di-BSA conjugate led to increase in the number of cells expressing CD25+ and the IL-2 receptor and CD4+/CD25+/Foxp3+ T regulatory cells (Treg). The number of cells expressing MHC II+ increased only after booster immunization—to a greater extent on day 21 (7 d after boost)—and was higher than that in the case of conjugate administration without aluminum hydroxide.
Prevnar 13, containing a CRM197-CP of S. pneumoniae serotype 3 conjugate, induced similar changes on day 28 (14 d after booster immunization) in the number of cells expressing cell-surface molecules. Specifically, there was an increased number of (TCR+) γδ T cells, CD45+/CD8+ CTLs, CD5+ B1 cells, CD45+/CD19+ B cells, CD4+/CD25+/Foxp3+ Tregs, cells expressing CD25+, and cells expressing MHC II+. The number of NK- and NKT-cells did not differ from that in the control.
An elevation in Ab production and protection against S. pneumoniae serotype 3 was detected only after double immunization with the adjuvanted di-BSA conjugate. This finding suggests that the cells whose number showed a large increase after booster immunization (TCR+ γδT cells and CD5+ B1 cells), against the background of an increase in the number of activated cells expressing MHC II+, play a crucial role in the protective activity of the conjugate.
3.5 Antibodies against double-stranded DNA
No difference was observed in the level of Abs against ds DNA relative to the control at the dilution of 1:80 in sera of mice immunized with the di-BSA conjugate adsorbed and non-adsorbed on aluminum hydroxide, Prevnar 13, BSA, and free aluminum hydroxide (Figure 6).
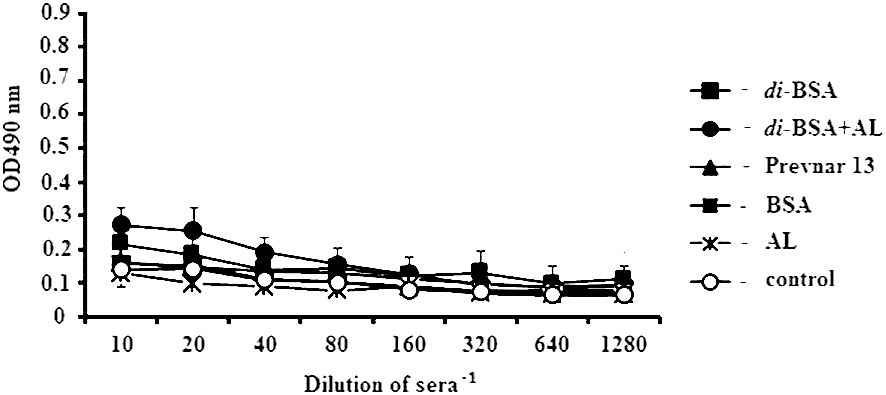
Figure 6 IgG antibodies to double-stranded DNA in immunized mice, analyzed by ELISA. ds DNA was used as the well-coating antigen. Sera to each conjugate, BSA, aluminum hydroxide, and control (non-immunized mice) (n = 6 for each antigen) was added to each well in dilutions from 1:10 to 1:1280. AL - aluminum hydroxide; control - mice injected with saline. After prime-boost immunization, autoantibodies to ds DNA, which target the cell nuclei, were not detected.
4 Discussion
In contrast to the conjugate without adjuvant, the di-BSA conjugate adjuvanted with aluminum hydroxide, induced production of IgG1 antibodies and protected mice against S. pneumoniae serotype 3 after prime-boost immunization. The role of adjuvants in enhancing the adaptive immune response to antigens, including semisynthetic glycoconjugates corresponds to the data of other authors (49–51).
The concentrations of IL-1α, IL-1β, IL-4, IL-5, IL-6, IL-10, IL-13, IL-17A, IL-21, IL-22, IFNγ, and TNFα in mice sera in response to the di-BSA conjugate adjuvanted with aluminum hydroxide were higher compared with those in response to the non-adjuvanted glycoconjugate. Free aluminum hydroxide is known to stimulate the production of IL-1β and IL-18, and, when administered with antigens, the spectrum of cytokines expands (52–58). IFNγ, IL-17A, and IL-22 (a member of the Th17 cytokine family) plays a role in the early stages of controlling S. pneumoniae infections (59–67). IL-17 has an important function in protecting against bacterial carriage and lung infection (59, 65, 68–71). The di-BSA conjugate adjuvanted with aluminum hydroxide induced a very high level of IL-17A after the prime and boost immunizations, while the conjugate without adjuvant caused a weak production of IL-17A that gradually decreased after the booster injection. A high level of Th2 cytokines (IL-4 and IL-5) was revealed in mice immunized with the adjuvanted di-BSA conjugate. Th2 cytokines promote switching from IgM to IgG, which is associated with high production of IgG1 antibodies (72). The conjugate without adjuvant did not elicit IL-4 production, only weakly stimulated the production of IL-5 even after boost immunization, and did not induce the antibody response. Prevnar 13 is known to induce the production of Th1/Th2 and Th17 cytokines (47, 48). In our previous studies, we have shown that Prevnar 13 induced anti-CP S. pneumoniae type 3 IgG1-antibodies and protected immunized mice from the challenge with S. pneumoniae type 3 (42).
The di-BSA conjugate and CPs, including that of S. pneumoniae serotype 3, are not Toll-like receptor (TLR) ligands (46). Purified CP from S. pneumoniae can bind to macrophages through the carbohydrate-recognition domains on the mannose receptor, leading to the production of proinflammatory cytokines such as IL-1, IL-6, and TNFα, as well as chemokines (73). Another receptor, the C-type lectin, also known as carbohydrate-binding protein, SIGN-R1, is expressed by macrophages, particularly in the marginal zone of the mouse spleen. This receptor is able to bind carbohydrates from several different serotypes of S. pneumoniae (73). Other carbohydrate-recognition receptors of macrophages remain to be identified (74). It is likely that macrophages play a significant role in the initial stage of the immune response to the di-BSA conjugate (36, 59, 74–77).
Regardless of the presence of the adjuvant, the number of CD4+ T helper cells involved in the adaptive immune response to the antigen increased only after the first immunization with the di-BSA conjugate. The number of CD4+ T cells after booster immunization with the BSA-conjugated synthetic hexasaccharide related to S. pneumoniae serotype 14 CP adsorbed on aluminum hydroxide did not differ from that in the control either (46). However, the number of CD4+ T helper cells increased on day 14 after booster immunization in mice immunized with CP of S. pneumoniae serotype 3 conjugated to CRM197 and adsorbed on aluminum phosphate (Prevnar 13). This result may be attributable to the multicomponent composition of the vaccine and the presence of a small amount of bacterial impurities remaining even after purification of CPs. The number of CD8+ cytotoxic cells (CTL) in response to the disaccharide conjugate and Prevnar 13 increased.
Both the adjuvanted di-BSA conjugate and Prevnar 13 significantly increased the number of (TCR+) γδ T cells among the splenocytes after booster immunization. γδ T cells play a crucial role in prevention of pneumococcal infection owing to their ability to recognize unprocessed non-peptide antigens (41). A large number of γδ T cell ligands remain unknown to date (78, 79). In mice, most γδ T cells are found in the body’s barrier tissues, with a small proportion in the blood and spleen (46, 80–83). The activation of γδ T cells through TCRs can be mediated by non-classical MHC molecules (e.g., T10/T22 and members of the CD1 family) and MHC-unrelated molecules (e.g., viral glycoproteins and butyrophilin 3A1) (79, 84–87). Putatively, γδ T cells bind the oligosaccharide portion of the glycoconjugate without processing in antigen-presenting cells (APCs) in combination with MHC-like molecules activate cytokine production. γδ T cells produce a large variety of cytokines and exhibit potent cytotoxic activity against pathogens through apoptosis-inducing receptors (FAS and TRAIL), as well as cytolytic proteins such as perforin and granzyme (88, 89). Furthermore, γδ T cells can function as professional APCs that require surface interactions with opsonized cells (90). The di-BSA conjugate has been shown to induce the formation of opsonizing antibodies (42). Certain subsets of γδ T cells express CD4. These cells have a Th1 or Th2 phenotype and produce IL-2, IL-4, IL-17A, IFNγ, and TNF (70). The di-BSA conjugate induced the production of IFNγ and TNFα (Th1 cytokines); IL-4, IL-5, and IL-13 (Th2 cytokines); IL-17A (Th17 cytokines); IL-21 (Th2 and Th17 subsets); and IL-22 (Th1 and Th17 subsets). γδ T cells play a crucial role in immune protection against extracellular respiratory bacteria (41, 91, 92). The potential role of γδ T cells in pneumococcal infection has only been investigated in animal models using S. pneumoniae serotype 3 (41). During infection, the number of γδ T cells can significantly increase, accounting for up to 50% of all peripheral lymphocytes (93, 94). In the mouse model, γδ T cells accumulate and become activated in the lungs during S. pneumoniae infection (95, 96). Mice with a lack of γδ T cells exhibit a higher bacterial load in their lungs and lower survival rates compared to control mice (66, 95, 97). The absence of γδ T cells is associated with impaired secretion of MIP-2, TNFα, and IL-17, as well as a poor recruitment of neutrophils (66, 95, 97). In addition, γδ T cells produce IFNγ during S. pneumoniae infections of serotypes 3 and 1. Along with their early role in defense against S. pneumoniae, γδ T cells participate in the resolution stage of pneumococcal pneumonia, eliminating inflammatory mononuclear phagocytes (98). Therefore, γδ T cells are essential for the host’s defense against S. pneumoniae (66, 95).
The di-BSA conjugate adjuvanted with aluminum hydroxide and Prevnar 13 adsorbed on aluminum phosphate induced a significant increase CD5+ B1 cells after booster immunization. CD5+ B1 cells are mainly located in the peritoneal and pleural cavities, but very small amounts were also found in the spleen (99, 100). CD5+ В1 cells are activated primarily by T-independent antigens (101, 102) and play an important role in protecting against pneumococcal infections (103) This role may be attributed to their production of natural antibodies as well as possible participation in the T-dependent immune response (102, 104–109). The B cell receptor (BCR) is involved in the phagocytosis of bacteria by B1 cells (110). CD5+ B1, isolated from the spleens of mice, primarily induce IL-17 production by T cells (111). B1 cells present antigen to antigen-specific T cells and induce more efficient proliferation than conventional CD19+ B cells (107, 108). After immunization with the di-BSA conjugate, the number of CD19+ B cells in the blood increased, regardless the presence of the adjuvant. The number of CD19+ B cells increased during all observation periods. Ovalbumin-presenting B1 cells were found to express a higher level of MHC class II compared to naïve B1 cells.
Immunization with either the adjuvanted or the non-adjuvanted di-BSA conjugate increases the number of natural killer (NK) cells and natural killer T (NKT) cells. NK cells, through the production of IFNγ, participate in the early immune response to pulmonary S. pneumoniae infection. NKT cells have a key role in protecting against pneumococcal infection. When mice lacking NKT cells were infected with S. pneumoniae serotype 3, they exhibited a higher mortality rate and bacterial load in their lungs compared to wild-type mice. It has been suggested that IFNγ derived from NKT cells has a critical function in protecting mice against pneumococcal pneumonia. Using S. pneumoniae serotype 1, it has also been found that NKT cells are an important innate immune effector in clearing pneumococci from the body. NKT cells can indirectly or directly assist B cells in mounting antibody responses and have a crucial role in the production of antibodies against pneumococcus and in the switch of classes in response to the administration of pneumococcal vaccines (112–114).
IL-17A, γδ T, and CD5+ В1 cells can also contribute to autoimmune diseases (115). In response to infection or immunization, autoreactive clones of B1 cells can be produced in the body’s own tissues (109, 116–118). The expansion of autoreactive clones of B cells is controlled by IL-10, leaving the BCR in a state of anergy. After booster immunization, there was an increase in the number of CD4+/CD25+/FoxP3+ T regulatory cells (Tregs) on the background of interleukin-10 (IL-10) production, which regulates the development of the immune response. After prime-boost immunization with the di-BSA conjugate or Prevnar 13, no formation of autoantibodies against ds DNA targeting cell nuclei was detected.
5 Conclusion
The key effectors of the immune response in mice following immunization with aluminum hydroxide adjuvanted di-BSA conjugate, associated with antibody response and protection from infection by S. pneumoniae serotype 3, were IL-17A, γδ T, and CD5+ B1 cells, with an increase in the number of MHC II-expressing cells after booster immunization. The roles of non-conventional γδ T cells, B1 cells, and production of IL-17A upon pneumococcal immunization with the semisynthetic glycoconjugate may provide an in-depth understanding of post-vaccination defense mechanisms, enabling the development of novel efficient therapies and improvement of existing vaccine formulations.
Data availability statement
The original contributions presented in the study are included in the article/supplementary material. Further inquiries can be directed to the corresponding author/s.
Ethics statement
The animal study was approved by Mechnikov Research Institute for Vaccines and Sera Ethics Committee. The study was conducted in accordance with the local legislation and institutional requirements.
Author contributions
EK: Conceptualization, Investigation, Writing – original draft. NA: Investigation, Methodology, Writing – review & editing. AZ: Investigation, Methodology, Writing – review & editing. EA: Investigation, Methodology, Writing – review & editing. NY: Investigation, Methodology, Writing – review & editing. ES: Investigation, Methodology, Writing – review & editing. DY: Investigation, Methodology, Writing – review & editing. YT: Investigation, Methodology, Writing – review & editing. NN: Conceptualization, Funding acquisition, Project administration, Writing – review & editing.
Funding
The author(s) declare financial support was received for the research, authorship, and/or publication of this article. This work was supported by the Russian Science Foundation (grant no. 19-73-30017-P).
Conflict of interest
The authors declare that the research was conducted in the absence of any commercial or financial relationships that could be construed as a potential conflict of interest.
Publisher’s note
All claims expressed in this article are solely those of the authors and do not necessarily represent those of their affiliated organizations, or those of the publisher, the editors and the reviewers. Any product that may be evaluated in this article, or claim that may be made by its manufacturer, is not guaranteed or endorsed by the publisher.
Abbreviations
Ab, antibody; BSA, bovine serum albumin; CP, capsular polysaccharide; CTL, cytotoxic T cells; EU, endotoxin unite; IL, interleukin; IFN γ, interferon gamma; IL-2 R, IL-2 receptor; MALDI-TOF, matrix assisted laser desorption ionization time-of-flight; MHC II, major histocompatibility complex class II; NK, natural killer cells; NKT, natural killer T cells; TCR, T cell receptor; TNF α, tumor necrosis factor alfa; Treg, T regulatory cells.
References
1. Rodgers GL, Arguedas A, Cohen R, Dagan R. Global serotype distribution among Streptococcus pneumoniae isolates causing otitis media in children: potential implications for pneumococcal conjugate vaccines. Vaccine. (2009) 27:3802–10. doi: 10.1016/j.vaccine.2009.04.021
2. O'Brien KL, Wolfson LJ, Watt JP, Henkle E, Deloria-Knoll M, McCall N, et al. Burden of disease caused by Streptococcus pneumoniae in children younger than 5 years: global estimates. Lancet. (2009) 374:893–902. doi: 10.1016/S0140–6736(09)61204–6
3. Martens P, Worm SW, Lundgren B, Konradsen HB. Benfield T Serotype-specific mortality from invasive Streptococcus pneumoniae disease revisited. BMC Infect Dis. (2004) 4:21. doi: 10.1186/1471-2334-4-21
4. Harboe ZB, Thomsen RW, Riis A, Valentiner-Branth P, Christensen JJ, Lambertsen L, et al. Pneumococcal serotypes and mortality following invasive pneumococcal disease: a population-based cohort study. PLoS Med. (2009) 6:e1000081. doi: 10.1371/journal.pmed.1000081
5. Weinberger DM, Harboe ZB, Sanders EA, Ndiritu M, Klugman KP, Rückinger S, et al. Association of serotype with risk of death due to pneumococcal pneumonia: a meta-analysis. Clin Infect Dis. (2010) 51:692–9. doi: 10.1086/655828
6. Grabenstein JD, Musey LK. Differences in serious clinical outcomes of infection caused by specific pneumococcal serotypes among adults. Vaccine. (2014) 32:2399–405. doi: 10.1016/j.vaccine.2014.02.096
7. Inverarity D, Lamb K, Diggle M, Robertson C, Greenhalgh D, Mitchell TJ, et al. Death or survival from invasive pneumococcal disease in Scotland: associations with serogroups and multilocus sequence types. J Med Microbiol. (2011) 60:793–802. doi: 10.1099/jmm.0.028803-0
8. PLoSker GL. 13-valent pneumococcal conjugate vaccine: a review of its use in infants, children, and adolescents. Pediatr Drugs. (2013) 15:403–23. doi: 10.1007/s40272-013-0047-z
9. Namkoong H, Ishii M, Funatsu Y, Kimizuka Y, Yagi K, Asami T, et al. Theory and strategy for pneumococcal vaccines in the elderly. Hum Vaccin Immunother. (2016) 12:336–43. doi: 10.1080/21645515.2015.1075678
10. Gransden WR, Eykyn SJ, Phillips I. Pneumococcal bacteraemia: 325 episodes diagnosed at St Thomas’s Hospital. Br Med J (Clin Res Ed). (1985) 290:505–8. doi: 10.1136/bmj.290.6467.505
11. Inostroza J, Vinet AM, Retamal G, Lorca P, Ossa G, Facklam RR, et al. Influence of patient age on Streptococcus pneumoniae serotypes causing invasive disease. Clin Diagn Lab Immunol. (2001) 8:556–9. doi: 10.1128/CDLI.8.3.556-559.2001
12. Scott JA, Hall AJ, Dagan R, Dixon JM, Eykyn SJ, Fenoll A, et al. Serogroup-specific epidemiology of Streptococcus pneumoniae: associations with age, sex, and geography in 7,000 episodes of invasive disease. Clin Infect Dis. (1996) 22:973–81. doi: 10.1093/clinids/22.6.973
13. España PP, Uranga A, Ruiz LA, Quintana JM, Bilbao A, Aramburu A, et al. Evolution of serotypes in bacteremic pneumococcal adult pneumonia in the period 2001–2014, after introduction of the pneumococcal conjugate vaccine in Bizkaia (Spain). Vaccine. (2019) 37:3840–8. doi: 10.1016/j.vaccine.2019.05.052
14. Lee S, Lee K, Kang Y, Bae S. Prevalence of serotype and multidrug-resistance of S. pneumoniae respiratory tract isolates in 265 adult and 36 children in Korea, 2002–2005. Microb Drug Resist. (2010) 16:135–42. doi: 10.1089/mdr.2009.0114
15. Jansen AG, Hak E, Veenhoven RH, Damoiseaux RA, Schilder AG, Sanders EA. Pneumococcal conjugate vaccines for preventing otitis media. Cochrane Database Syst Rev. (2009) 2:CD001480. doi: 10.1002/14651858.CD001480.pub3
16. Shiramoto M, Hanada R, Juergens C, Shoji Y, Yoshida M, Ballan B, et al. Immunogenicity and safety of the 13-valent pneumococcal conjugate vaccine compared to the 23-valent pneumococcal polysaccharide vaccine in elderly Japanese adults. Hum Vaccin Immunother. (2015) 11:2198–206. doi: 10.1080/21645515.2015.1030550
17. Andrews NJ, Waight PA, Burbidge P, Pearce E, Roalfe L, Zancolli M, et al. Serotype-specific effectiveness and correlates of protection for the 13-valent pneumococcal conjugate vaccine: a postlicensure indirect cohort study. Lancet Infect Dis. (2014) 14:839–46. doi: 10.1016/S1473-3099(14)70822-9
18. Schuerman L, Prymula R, Henckaerts I, Poolman J. ELISA IgG concentrations and opsonophagocytic activity following pneumococcal protein D conjugate vaccination and relationship to efficacy against acute otitis media. Vaccine. (2007) 25:1962–8. doi: 10.1016/j.vaccine.2006.12.008
19. Prymula R, Peeters P, Chrobok V, Kriz P, Novakova E, Kaliskova E, et al. Pneumococcal capsular polysaccharides conjugated to protein D provide protection against otitis media caused by both Streptococcus pneumoniae and non-typable Haemophilus influenzae: a randomized double blind efficacy study. Lancet. (2006) 367:740–8. doi: 10.1016/S0140-6736(06)68304-9
20. Yu X, Sun Y, Frasch C, Concepcion N, Nahm MH. Pneumococcal capsular polysaccharide preparations may contain non-C-polysaccharide contaminants that are immunogenic. Clin Diagn Lab Immunol. (1999) 6:519–24. doi: 10.1128/CDLI.6.4.519–524.1999
21. Kochetkov NK, Nifant'ev NE, Backinowsky LV. Synthesis of the capsular polysaccharide of Streptococcus pneumoniae type 14. Tetrahedron. (1987) 43:3109–21. doi: 10.1016/S0040–4020(01)86852–6
22. Sorieul C, Dolce M, Romano MR, Codée J, Adamo R. Glycoconjugate vaccines against antimicrobial resistant pathogens. Expert Rev Vaccines. (2023) 22:1055–78. doi: 10.1080/14760584.2023.2274955
23. del Bino L, Østerlid KE, Wu D-Y, Nonne F, Romano MR, Codée J, et al. Synthetic glycans to improve current glycoconjugate vaccines and fight antimicrobial resistance. Chem Rev. (2022) 122:15672–716. doi: 10.1021/acs.chemrev.2c00021
24. Krylov VB, Nifantiev NE. Synthetic carbohydrate based anti-fungal vaccines. Drug Discovery Today: Technol. (2020) 35–36:35–43. doi: 10.1016/j.ddtec.2020.11.002
25. Anish C, Schumann B, Pereira CL, Seeberger PH. Chemical biology approaches to designing defined carbohydrate vaccines. Chem Biol. (2014) 21:38–50. doi: 10.1016/j.chembiol.2014.01.002
26. Gening ML, Maira-Litran T, Kropec A, Skurnik D, Grout M, Tsvetkov YE, et al. Synthetic β(1→6)-linked N-acetylated and non-acetylated oligoglucosamines used to produce conjugate vaccines for bacterial pathogens. Infect Immun. (2010) 78:764–72. doi: 10.1128/IAI.01093–09
27. Parameswarappa SG, Reppe K, Geissner A, Ménová P, Govindan S, Calow ADJ, et al. A semi-synthetic oligosaccharide conjugate vaccine candidate confers protection against Streptococcus pneumoniae serotype 3 infection. Cell Chem Biol. (2016) 23:1407–16. doi: 10.1016/j.chembiol.2016.09.016
28. Seeberger PH, Pereira CL, Khan N, Xiao G, Diago-Navarro E, Reppe K, et al. A semi-synthetic glycoconjugate vaccine candidate for Carbapenem-resistant Klebsiella pneumoniae. Angew Chem Int Ed Eng. (2017) 56:13973–8. doi: 10.1002/anie.201700964
29. Broecker F, Hanske J, Martin CE, Baek JY, Wahlbrink A, Wojcik F, et al. Multivalent display of minimal Clostridium difficile glycan epitopes mimics antigenic properties of larger glycans. Nat Commun. (2016) 7:11224. doi: 10.1038/ncomms11224
30. Broecker F, Martin CE, Wegner E, Mattner J, Baek JY, Pereira CL, et al. Synthetic lipoteichoic acid glycans are potential vaccine candidates to protect from Clostridium difficile infections. Cell Chem Biol. (2016) 23:1014–22. doi: 10.1016/j.chembiol.2016.07.009
31. Solovev AS, Denisova EM, Kurbatova EA, Kutsevalova OY, Boronina LG, Ageevets VA, et al. Synthesis of methylphosphorylated oligomannosides structurally related to lipopolysaccharide O-antigens of Klebsiella pneumoniae serotype O3 and their application for detection of specific antibodies in rabbit and human sera. Org Biomol Chem. (2023) 21:8306–19. doi: 10.1039/D3OB01203D
32. van der Put RMF, Smitsman C, de Haan A, Hamzink M, Timmermans H, Uittenbogaard J, et al. The first-in-human synthetic glycan-based conjugate vaccine candidate against Shigella. ACS Cent Sci. (2022) 8:449–60. doi: 10.1021/acscentsci.1c01479
33. Laverde D, Romero-Saavedra F, Argunov DA, Enotarpi J, Krylov VB, Kalfopoulou E, et al. Synthetic Oligomers Mimicking Capsular Polysaccharide Diheteroglycan are Potential Vaccine Candidates against Encapsulated Enterococcal Infections. ACS Infect Dis. (2020) 6:1816–26. doi: 10.1021/acsinfecdis.0c00063
34. Aguilar-Betancourt A, González-Delgado CA, Cinza-Estévez Z, Martínez-Cabrera J, Véliz-Ríos G, Alemán-Zaldívar R, et al. Safety and immunogenicity of a combined hepatitis B virus-Haemophilus influenzae type B vaccine comprising a synthetic antigen in healthy adults. Hum Vaccin. (2008) 4:54–9. doi: 10.4161/hv.4.1.5257
35. Tsvetkov Y, Gening ML, Kurbatova EA, Akhmatova NK, Nifantiev NE. Oligosaccharide ligand tuning in design of third generation carbohydrate pneumococcal vaccines. Pure Appl Chem. (2017) 89:1403–1411. doi: 10.1515/pac-2016–1123
36. Gening ML, Kurbatova EA, Nifantiev NE. Synthetic analogs of Streptococcus pneumoniae capsular polysaccharides and immunogenic activities of glycoconjugates. Russ J Bioorganic Chem. (2021) 47:1–25. doi: 10.1134/S1068162021010076
37. Gening ML, Kurbatova EA, Tsvetkov YE, Nifantiev NE. Development of approaches to a conjugated carbohydrate vaccine of the third generation against Streptococcus pneumoniae: the search for optimal oligosaccharide ligands. Russ Chem Rev. (2015) 84:1100–13. doi: 10.1070/RCR4574
38. Micoli F, Romano MR, Carboni F, Adamo R, Berti F. Strengths and weaknesses of pneumococcal conjugate vaccines. Glycoconjugate J. (2023) 40:135–48. doi: 10.1007/s10719–023-10100–3
39. Jansen WT, Snippe H. Short-chain oligosaccharide protein conjugates as experimental pneumococcal vaccines. Indian J Med Res. (2004) 119:7–12.
40. Weishaupt MW, Matthies S, Hurevich M, Pereira CL, Hahm HS, Seeberger PH. Automated glycan assembly of a S. pneumoniae serotype 3 CPS antigen. Beilstein J Org Chem. (2016) 12:1440–6. doi: 10.3762/bjoc.12.139
41. Ivanov S, Paget C, Trottein F. Role of non-conventional T lymphocytes in respiratory infections: the case of the pneumococcus. PLoS Pathog. (2014) 10:e1004300. doi: 10.1371/journal.ppat.1004300
42. Kurbatova EA, Akhmatova NK, Zaytsev AE, Akhmatova EA, Egorova NB, Yastrebova NE, et al. and Nifantiev NE Higher cytokine and opsonizing antibody production induced by bovine serum albumin (BSA)-conjugated tetrasaccharide related to Streptococcus pneumoniae type 3 capsular polysaccharide. Front Immunol. (2020) 11:578019. doi: 10.3389/fimmu.2020.578019
43. Tsvetkov YE, Yashunsky DV, Sukhova EV, Nifantiev NE, Kurbatova EA. Synthesis of oligosaccharides structurally related to fragments of Streptococcus pneumoniae type 3 capsular polysaccharide. Russ Chem Bull. (2017) 66:111–22. doi: 10.1007/s11172-017-1708-9
44. Kurbatova EA, Akhmatov EA, Akhmatova NK, Egorova NB, Yastrebova NE, Romanenko EE, et al. The use of biotinylated oligosaccharides related to fragments of capsular polysaccharides from Streptococcus pneumoniae serotypes 3 and 14 as a tool for assessment of the level of vaccine-induced antibody response to neoglycoconjugates. Russ Chem Bull. (2017) 65:1608–16. doi: 10.1007/s11172–016-1488–7
45. Ananikov VP, Eremin DB, Yakukhnov SA, Dilman AD, Levin VV, Egorov MP, et al. Organic and hybrid systems: from science to practice. Mendeleev Commun. (2017) 27:425–38. doi: 10.1016/j.mencom.2017.09.001
46. Akhmatova NK, Kurbatova EA, Akhmatov EA, Egorova NB, Logunov DY, Gening ML, et al. The effect of a BSA conjugate of a synthetic hexasaccharide related to the fragment of capsular polysaccharide of Streptococcus pneumoniae type 14 on the activation of innate and adaptive immune responses. Front Immunol. (2016) 7:1–11. doi: 10.3389/fimmu.2016.00248
47. Lai Z, Schreiber JR. Outer membrane protein complex of meningococcus enhances the antipolysaccharide antibody response to pneumococcal polysaccharide-CRM197 conjugate vaccine. Clin Vaccine Immunol. (2011) 18:724–29. doi: 10.1128/CVI.00053–11
48. Karasartova D, Gazi U, Tosun O, Gureser AS, Sahiner IT, Dolapci M, et al. Anti-pneumococcal vaccine-induced cellular immune responses in post-traumatic splenectomized individuals. J Clin Immunol. (2017) 37:388–96. doi: 10.1007/s10875–017-0397–3
49. Lefeber DJ, Benaissa-Trouw B, Vliegenthart JF, Kamerling JP, Jansen WT, Kraaijeveld K, et al. Th1-directing adjuvants increase the immunogenicity of oligosaccharide-protein conjugate vaccines related to Streptococcus pneumoniae type 3. Infect Immun. (2003) 71:6915–20. doi: 10.1128/iai.71.12.6915–6920
50. Reppe K, Tschernig T, Luhrmann A, van Laak V, Grote K, Zemlin MV, et al. Immunostimulation with macrophage-activating lipopeptide-2 increased survival in murine pneumonia. Am J Respir Cell Mol Biol. (2009) 40:474–81. doi: 10.1165/rcmb.2008-0071OC
51. Witzenrath M, Pache F, Lorenz D, Koppe U, Gutbier B, Tabeling C, et al. The NLRP3 inflammasome is differentially activated by pneumolysin variants and contributes to host defense in pneumococcal pneumonia. J Immunol. (2011) 187:434–40. doi: 10.4049/jimmunol.1003143
52. He P, Zou Y, Hu Z. Advances in aluminum hydroxide-based adjuvant research and its mechanism. Hum Vaccin Immunother. (2015) 11:477–88. doi: 10.1080/21645515.2014.1004026
53. Gupta RK, Siber GR. Adjuvants for human vaccines—current status, problems and future prospects. Vaccine. (1995) 13:1263–76. doi: 10.1016/0264-410X(95)00011-O
54. Moingeon P, Haensler J, Lindeberg A. Towards the rational design of Th1 adjuvants. Vaccine. (2001) 19:4363–72. doi: 10.1016/S0264-410X(01)00193-1
55. Williams A, Flavell RA, Eisenbarth SC. The role of NOD-like Receptors in shaping adaptive immunity. Curr Opin Immunol. (2010) 22:34–40. doi: 10.1016/j.coi.2010.01.004
56. Franchi L, Núñez G. The NLRP3 inflammasome is critical for alum-mediated IL-1β secretion but dispensable for adjuvant activity. Eur J Immunol. (2008) 38:2085–9. doi: 10.1002/eji.200838549
57. Li H, Nookala S, Re F. Aluminum hydroxide adjuvants activate caspase-1 and induce IL-1beta and IL-18 release. J Immunol. (2007) 178:5271–6. doi: 10.4049/jimmunol.178.8.5271
58. Li H, Willingham SB, Ting JP-Y, Re F, Edge C. Inflammasome activation by Alum and Alum’s adjuvant effect are mediated by NLRP3. J Immunol. (2008) 181:17–21. doi: 10.4049/jimmunol.181.1.17
59. Zhang Z, Clarke TB, Weiser JN. Cellular effectors mediating Th17- dependent clearance of pneumococcal colonization in mice. J Clin Invest. (2009) 119:1899–909. doi: 10.1172/JCI36731
60. Yamamoto N, Kawakami K, Kinjo Y, Miyagi K, Kinjo T, Uezu K, et al. Essential role for the p40 subunit of interleukin-12 in neutrophil-mediated early host defense against pulmonary infection with Streptococcus pneumoniae: involvement of interferon-gamma. Microbes Infect. (2004) 6:1241–9. doi: 10.1016/j.micinf.2004.08.007
61. Sun K, Salmon SL, Lotz SA, Metzger DW. Interleukin-12 promotes gamma interferon-dependent neutrophil recruitment in the lung and improves protection against respiratory Streptococcus pneumoniae infection. Infect Immun. (2007) 75:1196–202. doi: 10.1128/IAI.01403-06
62. Nakamatsu M, Yamamoto N, Hatta M, Nakasone C, Kinjo T, Miyagi K, et al. Role of interferon-gamma in Valpha14+ natural killer T cell-mediated host defense against Streptococcus pneumoniae infection in murine lungs. Microbes Infect. (2007) 9:364–74. doi: 10.1016/j.micinf.2006.12.003
63. Yamada M, Gomez JC, Chugh PE, Lowell CA, Dinauer MC, Dittmer DP, et al. Interferon-gamma production by neutrophils during bacterial pneumonia in mice. Am J Respir Crit Care Med. (2011) 183:1391–401. doi: 10.1164/rccm.201004-0592OC
64. Weber SE, Tian H. Pirofski LA CD8+ cells enhance resistance to pulmonary serotype 3 Streptococcus pneumoniae infection in mice. J Immunol. (2011) 186:432–42. doi: 10.4049/jimmunol.1001963
65. Lu YJ, Gross J, Bogaert D, Finn A, Bagrade L, Zhang Q, et al. Interleukin-17A mediates acquired immunity to pneumococcal colonization. PLoS Pathog. (2008) 4:e1000159. doi: 10.1371/journal.ppat.1000159
66. Ma J, Wang J, Wan J, Charboneau R, Chang Y, Barke RA, et al. Morphine disrupts interleukin-23 (IL-23)/IL-17-mediated pulmonary mucosal host defense against Streptococcus pneumoniae infection. Infect Immun. (2010) 78:830–7. doi: 10.1128/IAI.00914-09
67. van Maele L, Carnoy C, Cayet D, Ivanov S, Porte R, Deruy E, et al. Activation of type 3 innate lymphoid cells and interleukin 22 secretion in the lungs during Streptococcus pneumoniae infection. J Infect Dis. (2014) 210:493–503. doi: 10.1093/infdis/jiu106
68. Kadioglu A, Coward W, Colston MJ, Hewitt CR. Andrew PW CD4-T lymphocyte interactions with pneumolysin and pneumococci suggest a crucial protective role in the host response to pneumococcal infection. Infect Immun. (2004) 72:2689–97. doi: 10.1128/IAI.72.5.2689-2697.2004
69. Malley R, Trzcinski K, Srivastava A, Thompson CM, Anderson PW, Lipsitch M, et al. CD4+ T cells mediate antibody-independent acquired immunity to pneumococcal colonization. Proc Natl Acad Sci USA. (2005) 102:4848–53. doi: 10.1073/pnas.0501254102
70. Trzciński K, Thompson CM, Srivastava A, Basset A, Malley R, Lipsitch M. Protection against nasopharyngeal colonization by Streptococcus pneumoniae is mediated by antigen-specific CD4+ T cells. Infect Immun. (2008) 76:2678–84. doi: 10.1128/IAI.00141–08
71. Wright AK, Bangert M, Gritzfeld JF, Ferreira DM, Jambo KC, Wright AD, et al. Experimental human pneumococcal carriage augments IL-17A-dependent T cell defence of the lung. PLoS Pathog. (2013) 9:e1003274. doi: 10.1371/journal.ppat.1003274
72. Coffman RL, Savelkoul HF, Lebman DA. Cytokine regulation of immunoglobulin isotype switching and expression. Semin Immunol. (1989) 1:55–63.
73. Zamz S, Martinez-Pomares L, Jones H, Taylor PR, Stillion RJ, Gordon S, et al. Recognition of bacterial capsular polysaccharides and lipopolysaccharides by the macrophage mannose receptor. J Biol Chem. (2002) 277:41613–23. doi: 10.1074/jbc.M207057200
74. Paterson GK, Mitchell TJ. Innate immunity and the pneumococcus. Microbiology. (2006) 152:285–93. doi: 10.1099/mic.0.28551–0
75. Kadioglu A, Weiser JN, Paton JC, Andrew PW. The role of Streptococcus pneumoniae virulence factors in host respiratory colonization and disease. Nat Rev Microbiol. (2008) 6:288–301. doi: 10.1038/nrmicro1871
76. van der Poll T, Opal SM. Pathogenesis, treatment, and prevention of pneumococcal pneumonia. Lancet. (2009) 374:1543–56. doi: 10.1016/S0140-6736(09)61114-4
77. Koppe U, Suttorp N, Opitz B. Recognition of Streptococcus pneumoniae by the innate immune system. Cell Microbiol. (2012) 14:460–66. doi: 10.1111/j.1462-5822.2011.01746.x
78. Ferreira LM. Gammadelta T cells: innately adaptive immune cells? Int Rev Immunol. (2013) 32:223–48. doi: 10.3109/08830185.2013.783831
79. Tanaka Y, Sano S, Nieves E, De Libero G, Rosa D, Modlin RL, et al. Nonpeptide ligands for human gamma delta T cells. ProcNatl Acad Sci USA. (1994) 91:8175–9. doi: 10.1073/pnas.91.17.8175
80. Sperling AI, Cron RQ, Decker DC, Stern DA, Bluestone JA. Peripheral T cell receptor γδ variable gene repertoire maps to the T cell receptor loci and is influenced by positive selection. J Immunol. (1992) 149:3200–207. doi: 10.4049/jimmunol.149.10.3200
81. Strominger JL. Developmental biology of T cell receptors. Science. (1989) 244:943–50. doi: 10.1126/science.2658058
82. Allison JP. Havran WL.The immunobiology of T cells with invariant gamma delta antigen receptors. Annu Rev Immunol. (1991) 9:679–705. doi: 10.1146/annurev.iy.09.040191.003335
83. Havran WL, Allison JP. Developmentally ordered appearance of thymocytes expressing different T cell antigen receptors. Nature. (1988) 335:443–5. doi: 10.1038/335443a0
84. Bonneville M, O’Brien RL, Born WK. Gammadelta T cell effector functions: a blend of innate programming and acquired plasticity. Nat Rev Immunol. (2010) 10:467–78. doi: 10.1038/nri2781
85. Kalyan S, Kabelitz D. Defining the nature of human gammadelta T cells: a biographical sketch of the highly empathetic. Cell Mol Immunol. (2013) 10:21–9. doi: 10.1038/cmi.2012.44
86. Vantourout P, Hayday A. Six-of-the-best: unique contributions of gammadelta T cells to immunology. Nat Rev Immunol. (2013) 13:88–100. doi: 10.1038/nri3384
87. Martin B, Hirota K, Cua DJ, Stockinger B, Veldhoen M. Interleukin-17- producing gammadelta T cells selectively expand in response to pathogen products and environmental signals. Immunity. (2009) 31:321–30. doi: 10.1016/j.immuni.2009.06.020
88. Dieli F, Troye-Blomberg M, Ivanyi J, Fournie JJ, Krensky AM, Bonneville M, et al. Granulysin-dependent killing of intracellular and extracellular Mycobacterium tuberculosis by Vgamma9/Vdelta2 T lymphocytes. J Infect Dis. (2001) 184:1082–5. doi: 10.1086/323600
89. Qin G, Mao H, Zheng J, Sia SF, Liu Y, Chan PL, et al. Phosphoantigen-expanded human gammadelta T cells display potent cytotoxicity against monocytederived macrophages infected with human and avian influenza viruses. J Infect Dis. (2009) 200:858–65. doi: 10.1086/605413
90. Himoudi N, Morgenstern DA, Yan M, Vernay B, Saraiva L, Wu Y, et al. Human gammadelta T lymphocytes are licensed for professional antigen presentation by interaction with opsonized target cells. J Immunol. (2012) 188:1708–16. doi: 10.4049/jimmunol.1102654
91. Zheng J, Liu Y, Lau YL, Tu W. gammadelta-T cells: an unpolished sword in human anti-infection immunity. Cell Mol Immunol. (2013) 10:50–7. doi: 10.1038/cmi.2012.43
92. Cheng P, Liu T, Zhou WY, Zhuang Y, Peng LS, Zhang JY, et al. Role of gammadelta T cells in host response against Staphylococcus aureus-induced pneumonia. BMC Immunol. (2012) 13:38. doi: 10.1186/1471-2172-13-38
93. Das H, Groh V, Kuijl C, Sugita M, Morita CT, Spies T, et al. MICA engagement by human Vgamma2Vdelta2 T cells enhances their antigen-dependent effector function. Immunity. (2001) 15:83–93. doi: 10.1016/S1074-7613(01)00168-6
94. Bertotto A, Gerli R, Spinozzi F, Muscat C, Scalise F, Castellucci G, et al. Lymphocytes bearing the gamma delta T cell receptor in acute Brucella melitensis infection. Eur J Immunol. (1993) 23:1177–80. doi: 10.1002/eji.1830230531
95. Nakasone C, Yamamoto N, Nakamatsu M, Kinjo T, Miyagi K, Uezu K, et al. Accumulation of gamma/delta T cells in the lungs and their roles in neutrophil-mediated host defense against pneumococcal infection. Microbes Infect. (2007) 9:251–8. doi: 10.1016/j.micinf.2006.11.015
96. Kirby AC, Newton DJ, Carding SR, Kaye PM. Evidence for the involvement of lung-specific gammadelta T cell subsets in local responses to Streptococcus pneumoniae infection. Eur J Immunol. (2007) 37:3404–13. doi: 10.1002/eji.200737216
97. Cao J, Wang D, Xu F, Gong Y, Wang H, Song Z, et al. Activation of IL-27 signalling promotes development of postinfluenza pneumococcal pneumonia. EMBO Mol Med. (2014) 6:120–40. doi: 10.1002/emmm.201302890
98. Kirby AC, Newton DJ, Carding SR, Kaye PM. Pulmonary dendritic cells and alveolar macrophages are regulated by gammadelta T cells during the resolution of S. pneumoniae-induced inflammation. J Pathol. (2007) 212:29–37. doi: 10.1002/path.2149
99. Deenen GJ, Kroese FG. Kinetics of peritoneal B-1a cells (CD5 B cells) in young adult mice. Eur J Immunol. (1993) 23:12–6. doi: 10.1002/eji.1830230104
100. Kroese FG, Ammerlaan WA, Deenen GJ. Location and function of B-cell lineages. Ann N Y Acad Sci. (1992) 651:44–58. doi: 10.1111/j.1749-6632.1992.tb24592.x
101. Martin F, Oliver AM, Kearney JF. Marginal zone and B1 B cells unite in the early response against T-independent blood-borne particulate antigens. Immunity. (2001) 14:617–29. doi: 10.1016/S1074–7613(01)00129–7
102. Margry B, Wieland WH, van Kooten PJ, van Eden W, Broere F. Peritoneal cavity B-1a cells promote peripheral CD4+ T-cell activation. Eur J Immunol. (2013) 43:2317–26. doi: 10.1002/eji.201343418
103. Sindhava VJ, Bondada S. Multiple regulatory mechanisms control B-1 B cell activation. Front Immunol. (2012) 3:372. doi: 10.3389/fimmu.2012.00372
104. Sato T, Ishikawa S, Akadegawa K, Ito T, Yurino H, Kitabatake M, et al. Aberrant B1 cell migration into the thymus results in activation of CD4 T cells through its potent antigen-presenting activity in the development of murine lupus. Eur J Immunol. (2004) 34:3346–58. doi: 10.1002/eji.200425373
105. Vigna AF, Godoy LC, Rogerio de Almeida S, Mariano M, Lopes JD. Characterization of B-1b cells as antigen presenting cells in the immune response to gp43 from Paracoccidioides brasiliensis in vitro. Immunol Lett. (2002) 83:61–6. doi: 10.1016/S0165–2478(02)00070–6
106. Wang Y, Rothstein TL. Induction of Th17 cell differentiation by B-1 cells. Front Immunol. (2012) 3:281. doi: 10.3389/fimmu.2012.00281
107. Zimecki M, Whiteley PJ, Pierce CW, Kapp JA. Presentation of antigen by B cells subsets. I. Lyb-5+ and Lyb-5- B cells differ in ability to stimulate antigen specific T cells. Arch Immunol Ther Exp (Warsz). (1994) 42:115–23.
108. Zimecki M, Kapp JA. Presentation of antigen by B cell subsets. II. The role of CD5 B cells in the presentation of antigen to antigen-specific T cells. Arch Immunol Ther Exp (Warsz). (1994) 42:349–53.
109. Berland R, Wortis HH. Origins and functions of B-1 cells with notes on the role of CD5. Annu Rev Immunol. (2002) 20:253–300. doi: 10.1146/annurev.immunol.20.100301.064833
110. Gao J, Ma X, Gu W, Fu M, An J, Xing Y, et al. Novel functions of murine B1 cells: active phagocytic and microbicidal abilities. Eur J Immunol. (2012) 42:982–92. doi: 10.1002/eji.201141519
111. Zhong X, Gao W, Degauque N, Bai C, Lu Y, Kenny J, et al. Reciprocal generation of Th1/Th17 and T(reg) cells by B1 and B2 B cells. Eur J Immunol. (2007) 37:2400–4. doi: 10.1002/eji.200737296
112. Kobrynski LJ, Sousa AO, Nahmias AJ, Lee FK. Cutting edge: antibody production to pneumococcal polysaccharides requires CD1 molecules and CD8+ T cells. J Immunol. (2005) 174:1787–90. doi: 10.4049/jimmunol.174.4.1787
113. Miyasaka T, Akahori Y, Toyama M, Miyamura N, Ishii K, Saijo S, et al. Dectin-2-dependent NKT cell activation and serotype-specific antibody production in mice immunized with pneumococcal polysaccharide vaccine. PLoS One. (2013) 8:e78611. doi: 10.1371/journal.pone.0078611
114. Miyasaka T, Aoyagi T, Uchiyama B, Oishi K, Nakayama T, Kinjo Y, et al. A possible relationship of natural killer T cells with humoral immune response to 23-valent pneumococcal polysaccharide vaccine in clinical settings. Vaccine. (2012) 30:3304–10. doi: 10.1016/j.vaccine.2012.03.007
115. Su D, Shen M, Li X, Sun L. Roles of γδ T cells in the pathogenesis of autoimmune diseases. Clin Dev Immunol. (2013) 2013:985753. doi: 10.1155/2013/985753
116. Choi YS, Baumgarth N. Dual role for B-1a cells in immunity to influenza virus infection. J Exp Med. (2008) 205:3053–64. doi: 10.1084/jem.20080979
117. Haas KM, Poe JC, Steeber DA, Tedder TF. B-1a and B-1b cells exhibit distinct developmental requirements and have unique functional roles in innate and adaptive immunity to S. pneumoniae. Immunity. (2005) 23:7–18. doi: 10.1016/j.immuni.2005.04.011
Keywords: Streptococcus pneumoniae serotype 3, synthetic disaccharide, cytokine, γδ T cells, B1 Cells, interleukin 17A, antibody, mice immunoprotection
Citation: Akhmatova NK, Kurbatova EA, Zaytsev AE, Akhmatova EA, Yastrebova NE, Sukhova EV, Yashunsky DV, Tsvetkov YE and Nifantiev NE (2024) Synthetic BSA-conjugated disaccharide related to the Streptococcus pneumoniae serotype 3 capsular polysaccharide increases IL-17A Levels, γδ T cells, and B1 cells in mice. Front. Immunol. 15:1388721. doi: 10.3389/fimmu.2024.1388721
Received: 20 February 2024; Accepted: 06 May 2024;
Published: 22 May 2024.
Edited by:
Helder Nakaya, University of São Paulo, BrazilReviewed by:
Sayan Das, University of Maryland, United StatesJutamas Shaughnessy, University of Massachusetts Medical School, United States
Copyright © 2024 Akhmatova, Kurbatova, Zaytsev, Akhmatova, Yastrebova, Sukhova, Yashunsky, Tsvetkov and Nifantiev. This is an open-access article distributed under the terms of the Creative Commons Attribution License (CC BY). The use, distribution or reproduction in other forums is permitted, provided the original author(s) and the copyright owner(s) are credited and that the original publication in this journal is cited, in accordance with accepted academic practice. No use, distribution or reproduction is permitted which does not comply with these terms.
*Correspondence: Ekaterina A. Kurbatova, a3VyYmF0b3ZhNjE2MkB5YW5kZXgucnU=; Nikolay E. Nifantiev, bmVuQGlvYy5hYy5ydQ==