- 1dsm-firmenich, Hørsholm, Denmark
- 2Immunology, Section for Preclinical Disease Biology, Department of Veterinary and Animal Sciences, University of Copenhagen, Frederiksberg, Denmark
Human milk oligosaccharides (HMOs) are present in high numbers in milk of lactating women. They are beneficial to gut health and the habitant microbiota, but less is known about their effect on cells from the immune system. In this study, we investigated the direct effect of three structurally different HMOs on human derived macrophages before challenge with Staphylococcus aureus (S. aureus). The study demonstrates that individual HMO structures potently affect the activation, differentiation and development of monocyte-derived macrophages in response to S. aureus. 6´-Sialyllactose (6’SL) had the most pronounced effect on the immune response against S. aureus, as illustrated by altered expression of macrophage surface markers, pointing towards an activated M1-like macrophage-phenotype. Similarly, 6’SL increased production of the pro-inflammatory cytokines TNF-α, IL-6, IL-8, IFN-γ and IL-1β, when exposing cells to 6’SL in combination with S. aureus compared with S. aureus alone. Interestingly, macrophages treated with 6’SL exhibited an altered proliferation profile and increased the production of the classic M1 transcription factor NF-κB. The HMOs also enhanced macrophage phagocytosis and uptake of S. aureus. Importantly, the different HMOs did not notably affect macrophage activation and differentiation without S. aureus exposure. Together, these findings show that HMOs can potently augment the immune response against S. aureus, without causing inflammatory activation in the absence of S. aureus, suggesting that HMOs assist the immune system in targeting important pathogens during early infancy.
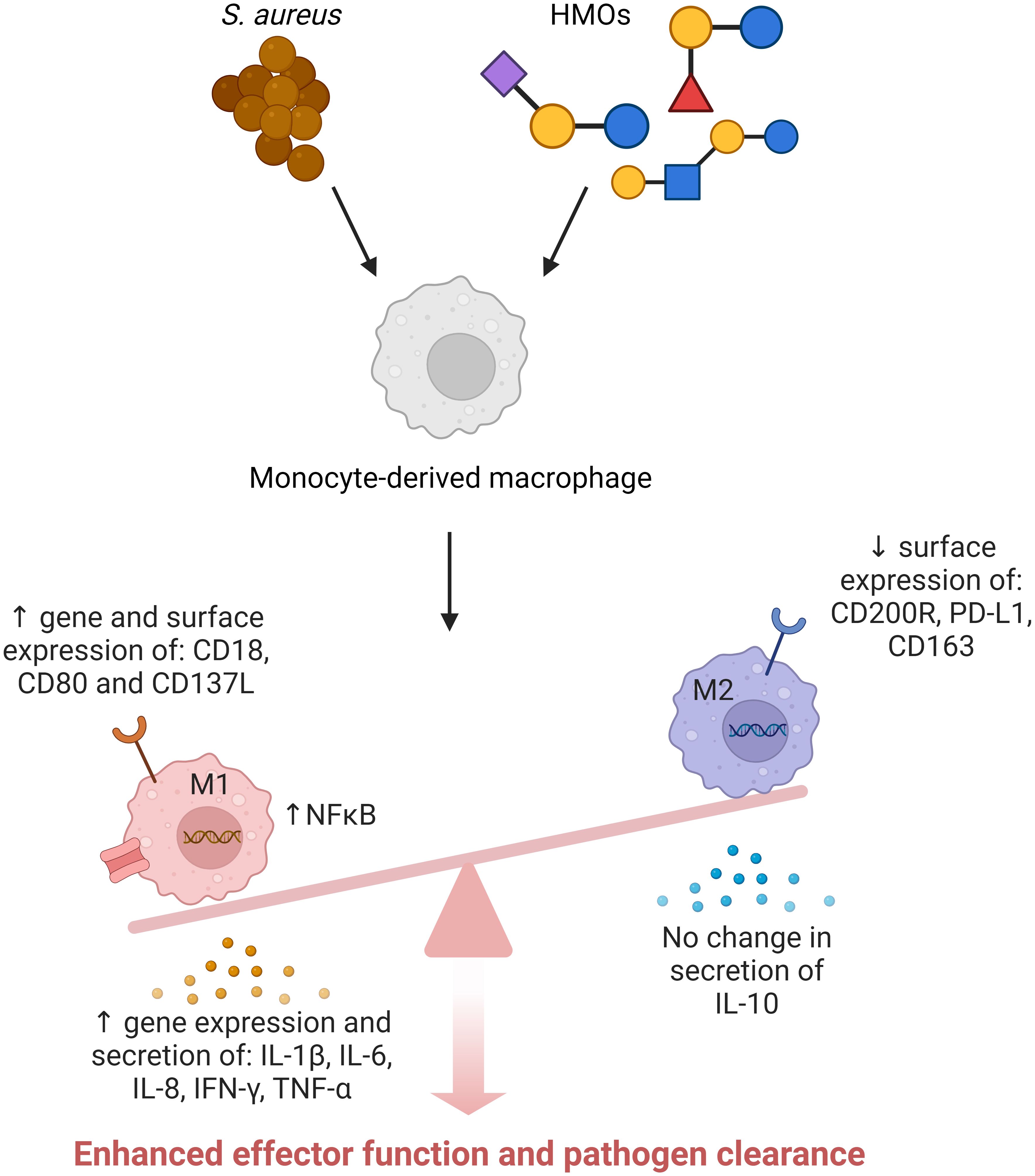
Graphical Abstract Created with BioRender.com.
Introduction
Human breast milk, the first and primary nutrition source for infants, is rich in nutrients and bioactive compounds that support development and growth (1, 2). Human milk oligosaccharides (HMOs) are complex sugar molecules, and the third most prevalent solid component in human breast milk. More than 200 different HMOs have been identified; however, only 10-15 structures make up the majority of the biomass (3). HMOs can be divided into three groups depending on their structural composition: 1) Non-fucosylated neutral core HMOs, made up of a neutral core backbone linked to one or more N-acetylglucosamine units; 2) Fucosylated neutral core HMOs with one or more fucose units; or 3) Sialylated HMOs with one or more sialylated units. HMOs are beneficial to microbiota and gut homeostasis (4–6), but have also shown to inhibit pathogen binding by functioning as decoy receptors (7, 8), and they can indirectly shape the intestinal immune system by producing immunomodulatory fermentation products, such as short chain fatty acids (9–14). HMOs have also been shown to affect the gut ecosystem and immune system in the absence of microbiota, as evaluated in germ-free mice (15). HMOs are able to regulate the human intestinal cell line HT-29 (16) and Zhang et al. revealed that a small fraction of HMOs with a high degree of polymerization increased the production of IL-1β, IL-6, IL-2, IL-10 and TNF-α in the murine macrophage cell line RAW264.7, and increased the expression of MAPKs and intracellular ROS (17). Likewise, Xiao et al. showed that a mixture of HMOs affected human monocyte derived dendritic cells, by increasing maturation, cytokine production, migration markers and anti-inflammatory properties in T cells (18). Recently, Boll et al. demonstrated that HMOs have structure-dependent functions and that HMOs belonging to the group of non-fucosylated neutral core and the group of fucosylated neutral core have beneficial effects on the epithelial cells, whereas HMOs belonging to the sialylated HMO-group have immunomodulatory functions in dendritic cells and macrophages (19). Lastly, the addition of HMOs to infant formula was demonstrated to reduce inflammatory cytokine production to resemble cytokine profiles of breastfed infants (20). Together, this strongly shows that HMOs can regulate cells of the immune system. Still, a thorough investigation of individual HMO structures and their effect on macrophage polarization is needed to fully understand the direct effect of individual HMOs.
Monocytes are blood-borne immune cells and when monocytes extravasate to tissues, they mature into macrophages. Monocytes and macrophages can differentiate in one of two directions: M1-like, which causes a potent immune response that is beneficial when combatting pathogens, and M2-like, that takes part in anti-inflammatory tissue remodeling (21). In the gut, the balance between the macrophage phenotypes is fine-tuned, and imbalances can cause persistent infection or chronic inflammation (22). The balance between M1 and M2-like states are dynamic, and macrophages are often in a state between the two extremes (23). Recently, changes in intracellular metabolism have been shown to be involved in this dynamic regulation of monocytes and macrophages (24). Several immune-relevant receptors found on macrophages are known for their glycan-binding properties, including C-type lectins, galectins and siglecs (25–27). Noll et al. described 2’FL binding to DC-SIGN (28), and have also previously described HMO binding to galectins (29). A similar finding was done by Hassan et al. demonstrating that 3′-Sialyllactose (3’SL) binds galectin-1 and galectin-3 (30). Finally, siglecs are sialic acid binding lectins, relevant in immune cell signaling and endocytosis (26, 31) and have been shown to be able to bind sialylated HMOs (32).
Staphylococcus aureus (S. aureus) is a commensal bacterium, colonizing 30-40% of the human population (33, 34). S. aureus is mainly present on the skin, in the nasopharyngeal tract and in the gut microbiota (35). The bacterium is often asymptomatic, but is characterized as an opportunistic pathogen and can cause damage ranging from mild and superficial infections to more severe disease such as bacteremia (36). Breastfed infants are at high risk of getting S. aureus from their mother’s skin, if the mothers are asymptomatic carriers (37, 38). S. aureus in the gut have been hypothesized to help develop the intestinal immune system (39, 40), but the intestines can also be an entry point for systemic dissemination. S. aureus can switch from being a harmless colonizer to an infectious pathogen, depending on the interplay between the gut, immune cells, microbiota, as well as gut integrity (41). S. aureus also have a potential to evade the immune system and develop antibiotic resistance (42, 43), and therefore relevant alternatives to antibiotics are of major interest in the treatment of S. aureus infections.
Monocytes and macrophages are highly relevant players in combatting S. aureus infections (35, 44). An abundance of M2-macrophages is associated with persistent infection and biofilm formation (45). Therefore, it is potentially relevant to enhance the M1-like macrophages to defeat infection (46, 47). Once the infection has been overcome, it is important that the homeostatic balance is reestablished to avoid excess damage.
The aim of this study was to investigate the direct effect of individual HMO structures on macrophage activation and polarization. S. aureus was chosen for macrophage activation in order to get a physiological relevant response, compared to artificial TLR ligand activation. The study shows that HMOs, especially 6´-Sialyllactose (6’SL), are able to significantly enhance effector functions of macrophages exposed to S. aureus. The results include increased expression of pro-inflammatory genes and surface molecules, enhanced cytokine responses, increased cell proliferation and nuclear factor kappa-light-chain-enhancer of activated B cells (NF-κB) induction and increased uptake of S. aureus. No noticeable macrophage activation was apparent in the absence of S. aureus challenge, demonstrating the safety of HMO-imposed immune regulation. This highlights the ability of HMOs to enhance specific effector functions in macrophages when encountering pathogens, which may be important for development of gut homeostasis and battle against infectious diseases.
Materials and methods
Cells and culture conditions
Primary cells, purification
Primary monocytes were isolated from buffy coats of healthy human volunteers obtained from the National Hospital (Copenhagen, Denmark). Monocytes were isolated using RosetteSep Human Monocyte Enrichment Cocktail (StemCell, 15068), followed by density centrifugation in Histopaque (Sigma, 10771) according to the manufacturer’s instructions. Red blood cells were removed by incubation in red blood cell-lysis buffer (eBioscience, 00-4333-57) for 10 minutes, and subsequently the cells were washed in PBS (Sigma, D8537) supplemented with 2% FBS (Sigma, F9665). Isolated monocytes were either set up for experiment or frozen in 90% FBS (Sigma, F9665) and 10% DMSO (Sigma, D2438), until further use.
Culture conditions
Primary monocytes and cell lines were cultivated in RPMI 1640 media (Sigma, R5886) supplemented with 10% FBS (Sigma, F9665), 2 mM L-glutamine (Sigma, G7513), and 2 mM penicillin/streptomycin (Sigma, P4333), henceforth referred to as standard 1640 RPMI media. The human monocytic (pre-macrophage) cell line U937 was purchased from Sigma (Sigma, 85011440) and the THP1–NF-kB–GFP reporter cells were kindly provided by Dr. Peter Steinberger (Institute of Immunology, Medical University of Vienna, Vienna, Austria) (48).
Human milk oligosaccharides
Chemically synthesized HMOs and HMOs produced by fermentation were prepared in house by Glycom A/S (Hørsholm, Denmark). Their structures were verified by 1H and 13C-NMR. Endotoxin measurements on HMOs produced by fermentation were performed with the Limulus Amebocyte Lysate Kinetic-QCL™ from Lonza (US License No. 1775, catalog No.:50-650U). HMO structures used in this study are depicted in Figure 1A.
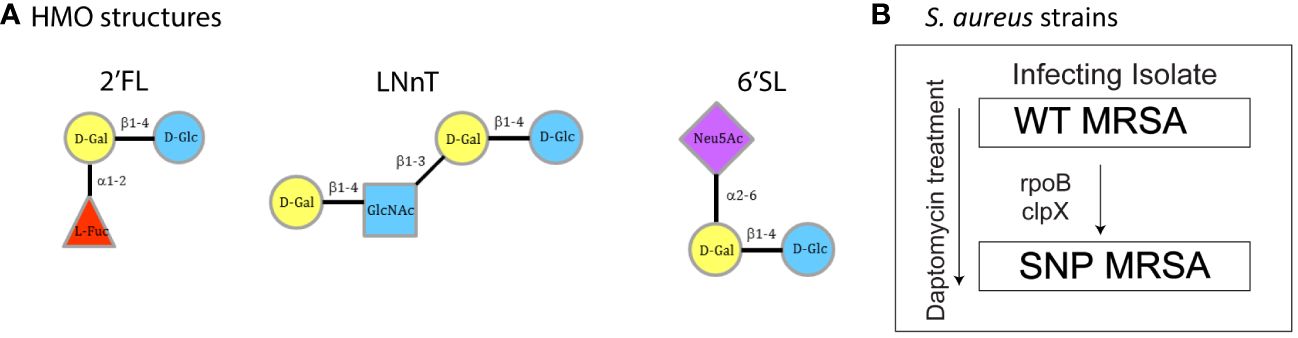
Figure 1 Overview of HMO structures and S. aureus strains. (A) Structural composition of the three individual HMO structures used in this experiment: 2’FL, LNnT and 6’SL. (B) Clinical S. aureus strains WT MRSA and SNP MRSA. The arrow indicates acquired SNPs and decreased daptomycin susceptibility. WT MRSA is the infecting isolate and SNP MRSA have decreased daptomycin susceptibility. Figure B modified from Mellergaard et al. (43, 49). Un, Untreated; 2’FL, 2′-Fucosyllactose; LNnT, Lacto-N-neotetraose; 6’SL, 6’-Sialyllactose; D-Gal, D-Galactose; D-Glc, D-Glucose; L-Fuc, L-Fucose; Glc-NAc, N-Acetylglucosamine; Neu5AC, N-Acetylneuraminic acid.
HMO-preparation for cell culture
Endotoxin-free HMOs were prepared in standard RPMI 1640 media at 37°C to a concentration of 20mM, passaged through a 0,2 µm sterile filter (Satorius, 16534 —GUK) and used immediately after preparation.
Preparation of UV-inactivated S. aureus strains
The clinically derived methicillin resistant S. aureus (MRSA) WT MRSA (SADR1/A9781) and SNP MRSA (SADR2/A9788) strains were previously described (original names in brackets) (49–51). Culture and UV-inactivation of S. aureus were performed as previously described (49). In brief, overnight S. aureus colonies were inoculated in trypsin soy broth to OD600 ~ 0.03 and grown in Erlenmeyer flasks to early stationary phase (OD600 = 5-6). Afterwards, the bacteria were washed and resuspended in PBS to OD600 = 1 (~109 S. aureus/ml). S. aureus solutions were transferred to petri dishes and subjected to pulsed UV-radiation of 10.000μJ/cm2 for 120 seconds (monochromatic wavelength of 254nm; CL-1000 crosslinker; UVP, Cambridge, United Kingdom) for UV-inactivation. Bacterial death was verified by plating and incubating on TSA plates at 37°C overnight (Supplementary Figure 1).
Primary macrophages: cell treatment and experimental set up
Primary monocytes were isolated from buffy coats, as described above. The isolated monocytes were seeded at 3 x 105 cells/ml and cultured in standard RPMI 1640 media, supplemented with 20 mM of HMO and 40 ng/mL M-CSF (Peprotech, 300-25). On day three, the cells received half new medium supplemented with the concentration of HMOs and M-CSF doubled. On day 6 all medium was discarded and replaced with fresh standard RPMI 1640 media containing 20 mM of HMO. The cells were then treated with 12,5 x 106 S. aureus/ml cell suspension and the monocyte-derived macrophages were further activated with S. aureus strains for two days. On day 8 the cells were taken for analysis.
Total RNA purification and transcriptomic profiling
Total RNA purification of primary monocytes was completed on day 8, after cultivation, as described above. Cell media was removed, the cells were lysed in RLT buffer (Qiagen, 79216) and stored at -80°C until purification. Total RNA purification was performed on a QIAcube Connect Instrument using the RNeasy Mini QIAcube Kit (Qiagen, 74116) with on-column DNase treatment, according to the manufacturer’s instructions. Purity and concentration were measured on a Denovix DS11 spectrophotometer. RNA quality was assessed on a bioanalyzer. An A260/A280 ratio above 1.95 and a RIN score above 7 was required for transcriptomic analysis. Hereafter RNA was stored at -20°C until further analysis. Transcriptomic profiling was done using Clariom™ D Assay (ThermoFischer Scientific, 902922) and performed by Center for Genomic Medicine, Copenhagen University Hospital. Data analysis was done using Transcriptome Analysis Console 4.0.2 (ThemoFischer Scientific). Mean probe signal intensity on each chip was used to normalize for loading differences between chips. Fold change values were reported by the program.
Flow cytometry
Counting
Adherent macrophages were counted by removing the supernatant and loosening the cells. Two different detachment methods were evaluated: A) Trypsination using trypsin/EDTA solution (Sigma, T4049) for 10 min at 37°C and resuspending the cells thoroughly: or B) Incubation of cells in citric saline for 15 min at 37°C followed by cell scraping using a cell lifter (Fischerbrand, 11577692). Afterwards, the cells were transferred to a 96-well plate and counted on a MacsQuant 16 Flow Cytometer.
Surface marker staining and analysis
Adherent cells were detached in citric saline followed by cell scraping using a cell lifter. For surface staining, cells were washed twice in cold FACS buffer containing PBS + 2% FBS, incubated with FcR Blocking Reagent (Miltenyi Biotec, 130-059-901) for 10 min on ice, and then washed and stained with fluorescent antibodies for 15 min on ice. Additionally, two washes were performed, and the cells were resuspended and analyzed in FACS buffer. Cells were analyzed with a MacsQuant 16 Flow Cytometer and data analysis was done using Flowlogic 8.6 Software and MACSQuantify™ 2.13.3 software. For every sample, technical duplicates were analyzed. Gating was carried out on viable cells (Supplementary Figure 2) and the results are presented as percent or mean fluorescence intensity average for technical duplicates (MFI). Isotype controls were included in the study and are presented in the supplementary section (Supplementary Figure 3). Specific antibodies (Supplementary Table 1) and isotypes controls (Supplementary Table 2) are listed in the supplementary section.
Cytokine measurements
Levels of secreted cytokines were measured in harvested cell culture supernatants. The analysis was performed using chemiluminescence-based assays from Meso Scale Discovery (MSD, Gaithersburg, MD, USA). The studies included two different assays from the V-PLEX platform: V-PLEX Human IL-8 Kit (K151RAD) singleplex and V-PLEX Viral Panel 2 Human Kit (K15346D). Assay set up, execution and analysis were done according to the manufacturer’s instructions. The analysis was performed using QuickPlex Q 120 instrument (MSD) and DISCOVRY WORKBENCH® 4.0 software.
NF-κB reporter assay
Investigation of the NF-κB-pathway was done using THP1-NF-κB-GFP reporter cells, kindly provided by Dr. Peter Steinberger (48). The reporter cells were seeded at a concentration of 3 x 105 cells/ml in standard RPMI media supplemented with HMO and incubated overnight at 37°C, 5% CO2. The next day S. aureus bacteria were added to the cells at a concentration of ~50 x 106 bacteria/ml cell suspension, and again incubated overnight at 37°C, 5% CO2. The GFP-signal was evaluated by flow cytometry the following day. The cells were harvested, transferred to a 96-well plate, and washed twice in PBS + 2% FBS, before analysis by flow cytometry.
Cell proliferation
To evaluate cell-proliferation, monocytes were stained with Carboxyfluorescein Succinimidyl Ester (CFSE, Molecular Probes, C34554) prior to seeding as previously described (49, 52). Shortly, this was done by dissolving cells in PBS + 5% FBS to a concentration of 5 x 106 cells/ml. The CFSE stock was diluted according to the manufacturer’s manual and added 1:1 to the cell suspension for final concentration of 5 μM. The cells were incubated dark for 5 min, while rotating at room temperature. Afterwards the cells were washed twice in PBS + 5% FBS and resuspended in standard 1640 RPMI media, supplemented with HMOs and M-CSF and set up as described above.
Brightfield microscopy
On day 8, prior to analysis, the cells were inspected in the microscope and photographed in the petri dish at 10x optics. Photos were taken using the ECHO Rebel Microscope.
Incucyte photos
Monocytic derived macrophages were cultured in the presence of 20 mM HMOs and 40 ng/mL M-CSF as earlier described and photographed on day 7. The photos of the cells were taken using Incucyte®, SX1 Live-Cell Analysis System.
Fluorescent labeling of UV-killed S. aureus
UV inactivated S. aureus strains were labelled with AF647 conjugated succinimidylester (SE-AF647; Molecular Probes, A-20006), as described in (49). Briefly, bacteria were resuspended in the original volume of sodium bicarbonate buffer (pH 8.5), SE-AF647 was added at a concentration of 10 ng/μL and bacteria were incubated at 4°C for 1 h with vigorous agitation. Stained bacteria were washed and resuspended to original volume in PBS and stored at -20°C until use. The staining was confirmed on the flow cytometer (Supplementary Figure 4). Data was acquired with an Accuri C6 instrument using Accuri C6 software and analyzed in Flowlogic 8.6 Software.
Uptake of fluorescent labelled and UV-killed S. aureus in U937 cells
U937 cells were seeded at a concentration of 3 x 105 cells/ml in standard 1640 RPMI media supplemented with 20 mM HMO and incubated overnight at 37°C, 5% CO2. The next day, UV-killed SE-AF647 labelled S. aureus bacteria were added to the cells at a concentration of ~50 x 106 bacteria/ml cell suspension and incubated overnight at 37°C, 5% CO2. The following day, the uptake of UV-killed SE-AF647-labelled S. aureus bacteria was evaluated. Cells were transferred to a 96-well plate, and washed twice in PBS + 2% FBS, before analyzing them by flow cytometry.
Phagocytosis of S. aureus bioparticles in primary macrophages
Primary monocytes were cultured as previously stated, supplemented with 20 mM HMOs and 40 ng/mL M-CSF (Peprotech, 300-25) for a total of 8 days. On day 8, 0,1μg S. aureus bioparticles (pHrodo® Red S. aureus Bioparticles® for Incucyte®, 4619) were added in 50µl/well (30.000 cells/well) in a 96-well plate. The plate was incubating for 15 minutes at room temperature to let the particles descend. The phagocytic uptake was analyzed by Incucyte®, SX1 Live-Cell Analysis System.
Figures
The graphical abstract was created in BioRender.com. Publication license, including confirmation of publication and licensing rights are available under agreement number is RM26AK4PC3.
Statistical analysis
Data preparation and statistical analysis was performed using GraphPad Prism, version 9.3.1 (GraphPad Software). Statistical analysis was performed as stated in the figure legends, and the level of statistical significance was determined by a p-value of less than 0.05 and is presented as follows: *p < 0.05, **p < 0.01, ***p < 0.001 and ****p < 0.0001.
Results
In this study, two previously described human infectious strains of S. aureus were used (49, 50). WT MRSA is the infecting isolate, whereas SNP MRSA is isolated from the same patient with bacteremia, after initiation of daptomycin treatment (Figure 1B). SNP MRSA has acquired two SNP mutations, in rpoB and clpP, known to be associated with antibiotic-resistance and immune evasion (49, 50). Here, we investigated the effect of individual HMO structures on macrophages exposed to an infecting S. aureus strain (WT MRSA). In addition to this primary focus, we explored the effect of individual HMO structures on the antibiotic-resistant SNP MRSA strain, since antibiotic resistance is an increasing problem affecting economy and global health (53). Results from challenge with the SNP MRSA strain are included throughout this article, but are specifically addressed at the end of the results section.
HMOs alter macrophage relevant genes towards an M1 phenotype in response to S. aureus
To investigate the effect of individual HMO structures on macrophages in response to S. aureus, transcriptomic profiling was performed on human blood monocyte-derived macrophages. Monocytes were isolated from healthy donors and treated with three different HMOs; 2’FL, LNnT or 6’SL (Figure 1A), as well as M-CSF, followed by treatment with S. aureus. On day 8, transcriptomic analysis was performed on total RNA, which showed that all three HMOs changed gene regulation of a high number of genes (Figure 2). The highest number of genes affected is seen in 6’SL-stimulated macrophages challenged with WT MRSA as depicted in Figures 2A, B. We observed an overlap in gene-regulation between the HMOs, but clearly the different structures also hold very specific regulatory potential (Figure 2A).
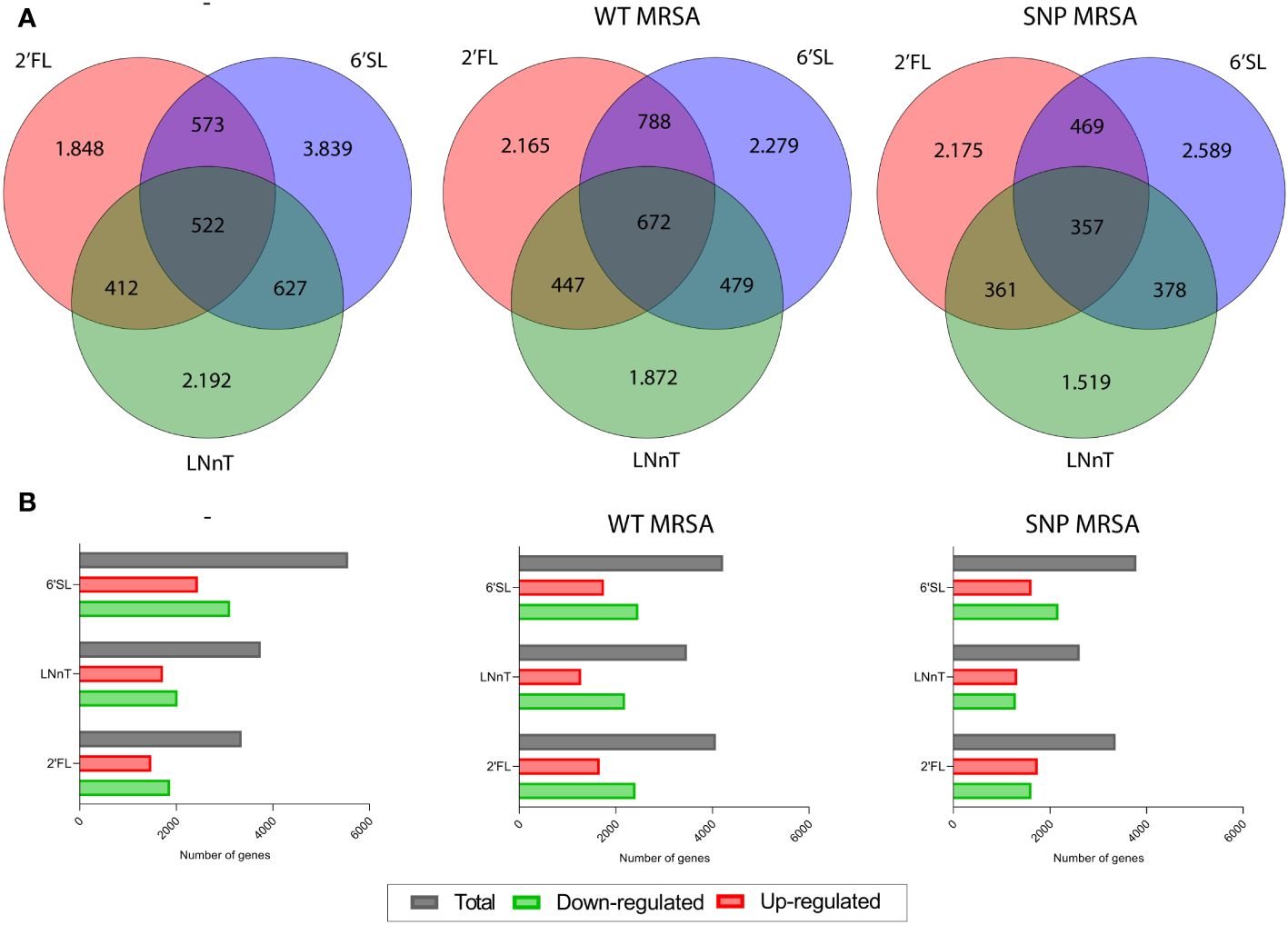
Figure 2 HMOs alter gene expression of several genes in monocyte-derived macrophages. Monocytes were treated with HMOs followed by treatment with WT MRSA or SNP MRSA. On day 8, total RNA was isolated, followed by transcriptomic profiling. Data is presented as (A) Venn diagrams and (B) bar graphs with data points showing number of differentially expressed genes in untreated macrophages and macrophages treated with WT MRSA and SNP MRSA from one experiment. Data is presented as number of genes with a fold change of < -2 or > 2 relative to untreated sample. 2’FL, 2′-Fucosyllactose; LNnT, Lacto-N-neotetraose; 6’SL, 6’-Sialyllactose.
HMOs change surface expression of macrophage-relevant proteins to mimic an M1 phenotype in response to S. aureus
To further explore the effect of HMOs on macrophages in response to S. aureus, the gene expression of several phenotypic macrophage-related surface molecules was investigated. From transcriptomic profiling, we found that all three HMO structures altered several macrophage-related surface proteins in response to S. aureus. Specifically, macrophages exposed to 6’SL had increased transcripts for the costimulatory activation marker CD137L (Supplementary Figure 5A), costimulatory molecule CD80 (Supplementary Figure 5B) and adhesion molecule CD18 (Supplementary Figure 5C). Concurrently, a decrease in the M2-markers CD200R and CD163 (Supplementary Figures 5D, F) was observed. The different responses are linked to the presence of S. aureus and are not notably affected by macrophage exposure to 6’SL alone. This indicates a boosted immune response against S. aureus.
To examine if the altered gene expression correlated with subsequent surface expression, human blood-derived monocytes were exposed to combinations of M-CSF, HMOs and S. aureus by flow cytometry. Data is gated on viable cells (Supplementary Figure 2). Isotype controls were included in all surface marker studies but showed no unspecific binding (Supplementary Figure 3). Moreover, cells were CD14 positive, indicating myeloid origin (Supplementary Figure 6). Surface molecule expression results confirmed that 6’SL is the most potent of the three HMO structures evaluated. Following S. aureus challenge 6’SL increased cell surface expression of the activating molecule CD137L (Figure 3A; Supplementary Figure 7A), the co-stimulatory molecule CD80 (Figure 3B; Supplementary Figure 7B) and adhesion molecule CD18 (Figure 3C; Supplementary Figure 7C), and at the same time decreased the anti-inflammatory and myeloid suppressive surface molecules CD200R, PD-L1 and CD163 in combination with S. aureus (Figures 3D–F; Supplementary Figures 7D–F). Interestingly, CD169 (Siglec-1) also decreased in the presence of HMOs and S. aureus (Figure 3G; Supplementary Figure 7G). Expression of additional classical myeloid cell surface molecules were analyzed but showed no difference (Supplementary Figure 8).
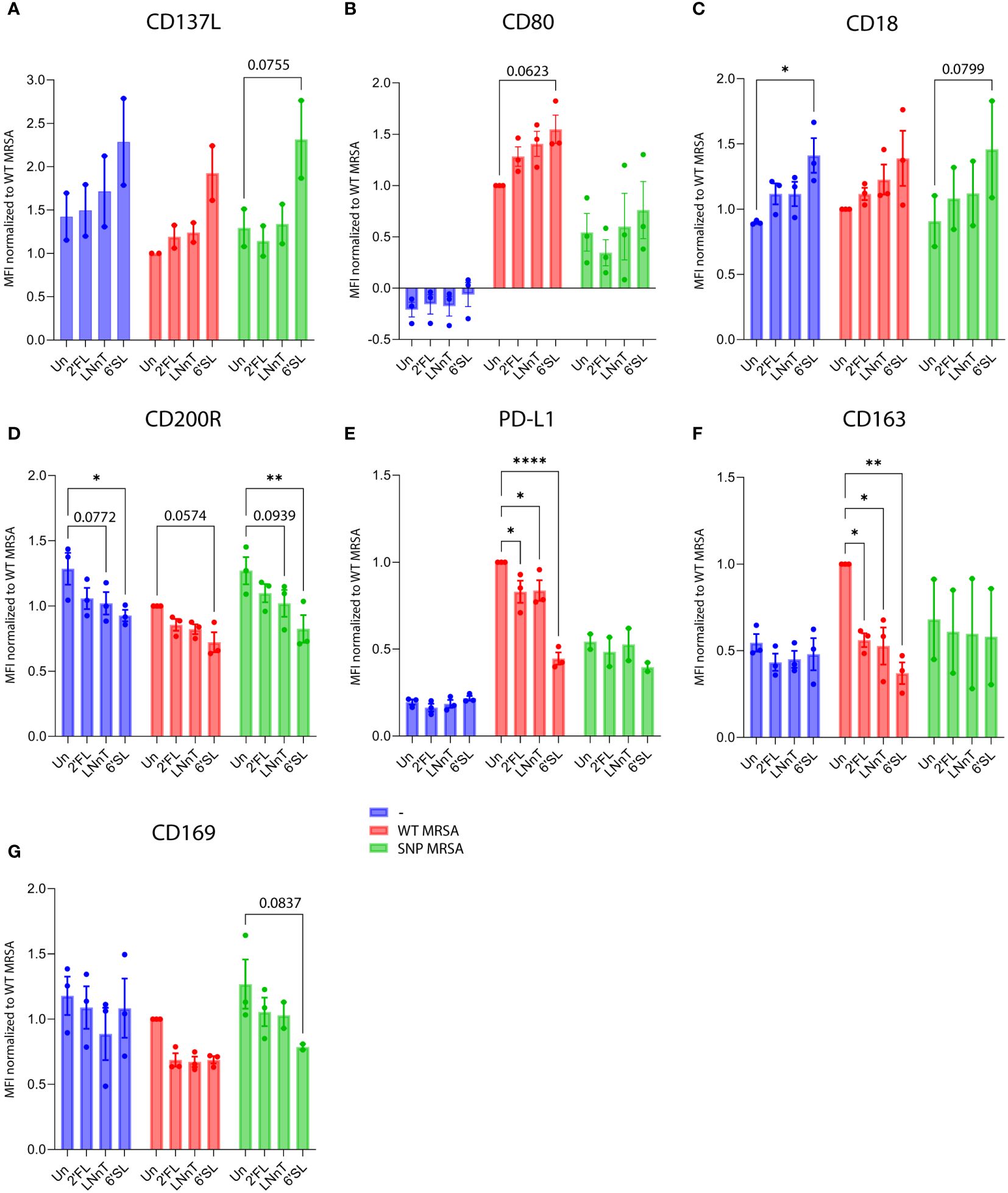
Figure 3 HMOs drive macrophage surface proteins towards a M1-characteristic phenotype in response to S. aureus. Monocytes were treated with HMOs followed by treatment with WT MRSA and SNP MRSA and analyzed by flow cytometry on day 8. Data is presented as bar graphs with data points showing cell surface expression of (A) CD137L, from two independent experiments and (B) CD80, (C) CD18, (D) CD200R, (E) PD-L1, (F) CD163 and (G) CD169 from three independent experiments. Data is presented as MFI values ± SEM, normalized to WT MRSA untreated. Statistical analysis was performed by 2-way ANOVA with Dunnett’s multiple comparison test and presented relative to untreated control. MFI, Mean Fluorescent Intensity; Un, Untreated; 2’FL, 2′-Fucosyllactose; LNnT, Lacto-N-neotetraose; 6’SL, 6’-Sialyllactose. *p < 0.05, **p < 0.01 and ****p < 0.0001.
These findings support the results from the gene array analysis and illustrates that most proteins regulated by HMOs on a transcriptional level are subsequently expressed on the cell surface. This is true for most of the displayed surface molecules. As an exception, S. aureus-induced gene expression of PD-L1 was potentiated by 6’SL and 2’FL, but did not translate into a higher surface expression. Contrarily, S. aureus-induced surface expression of PD-L1 was lower upon addition of HMOs (Figure 3E; Supplementary Figure 7E).
Treatment with HMOs increases secretion of pro-inflammatory cytokines from macrophages in response to S. aureus
Cytokine-genes were also potently regulated by HMOs (Supplementary Figure 9). Again, 6’SL was the most potent of the three tested HMOs, although 2´FL and LNnT also impacted cytokine gene transcription (Supplementary Figures 9A, B, D, E, G). The data show that macrophages treated with 6’SL before S. aureus challenge stimulate an increased production of genes encoding the pro-inflammatory cytokines: TNF-α, IL-6, IL-8, IFN-γ and IL-1β (Supplementary Figures 9A, D, E, F, G), and simultaneously decreased gene expression of the anti-inflammatory cytokine IL-10 (Supplementary Figure 9B). As with the expression of surface proteins, the HMO effect on the expression of pro-inflammatory cytokines is dependent on the presence of S. aureus bacteria for the majority of the tested cytokines.
In order to further investigate HMO-regulated secretion of cytokines, monocyte-derived macrophages were stimulated as described above to collect supernatants for cytokine measurements. The cytokine levels were measured by mesoscale multiplex analysis, and cell numbers were evaluated by flow cytometer-mediated counting. To ensure that the macrophage detachment method did not affect the read-out, two different methods were tested: Trypsination and citric saline (54) combined with cell scraping. As shown in Supplementary Figure 10, there was no difference between the methods, and the citric saline combined with cell scraping method was applied to enable subsequent flow cytometry analysis of the cells. In parallel with the observation from the transcriptomic profiling, HMO treatment (in particular 6’SL) followed by S. aureus challenge increased the pro-inflammatory cytokines TNF-α, IL-6, IL-8, IFN-γ and IL-1β (Figures 4A, D–G). No change in the regulation of the anti-inflammatory cytokine IL-10 was observed (Figure 4B), whereas IL-4 increased relative to the untreated control (Figure 4C). In summary, these observations are aligned with the results from the gene array and cell surface analysis, indicating that HMOs can boost the inflammatory response against the S. aureus pathogen without affecting unexposed macrophages.
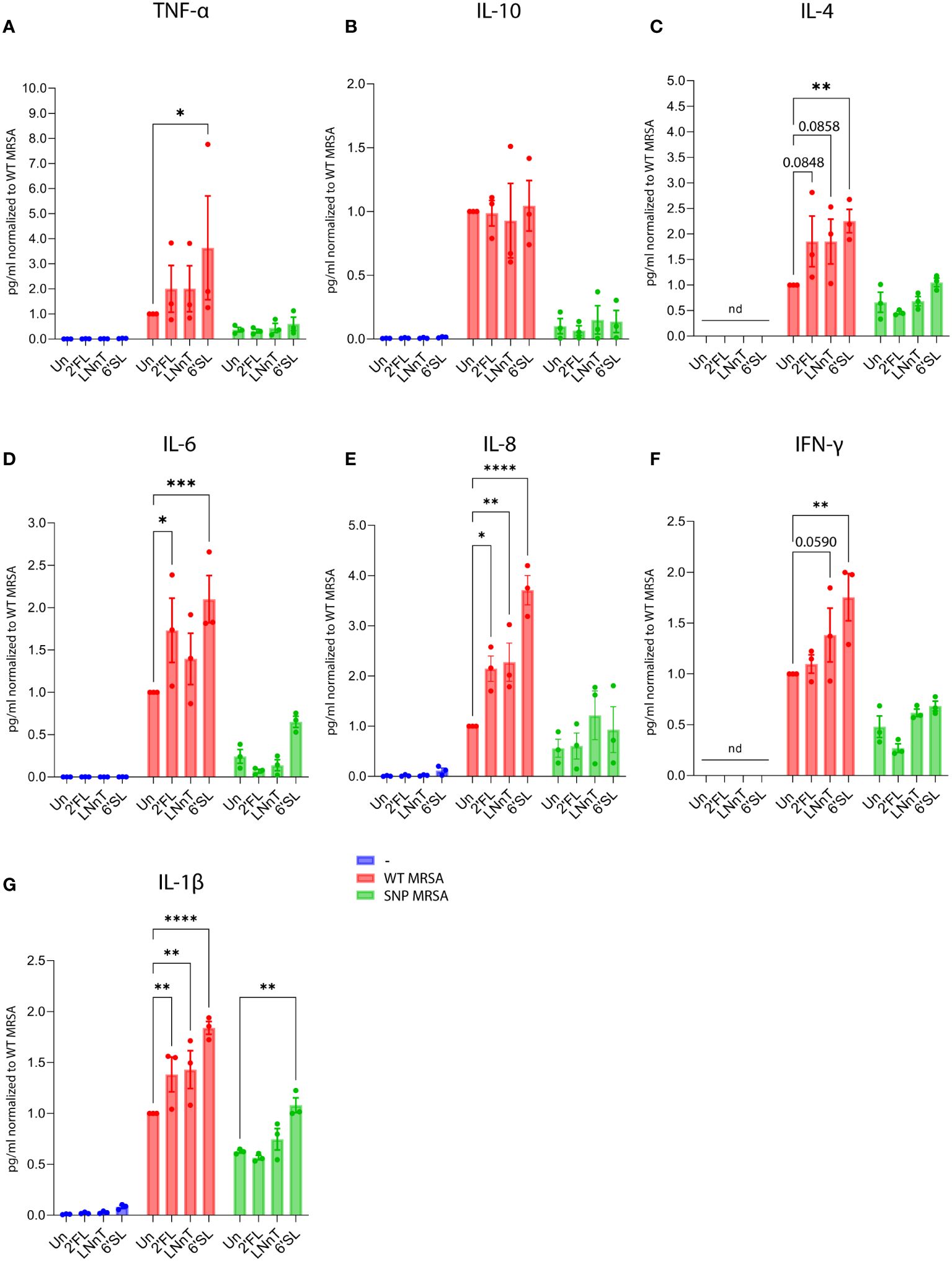
Figure 4 Treatment with HMOs increases secretion of pro-inflammatory cytokines from macrophages in response to S. aureus. Monocytes were treated with HMOs followed by treatment with WT MRSA and SNP MRSA. On day 8, cytokine levels were measured in culture supernatants by Mesoscale analysis. Bar graphs with data points show concentration of secreted cytokines normalized to WT MRSA untreated sample. Data represents three independent experiments, showing (A) TNF-α, (B) IL-10, (C) IL-4, (D) IL-6, (E) IL-8, (F) IFN-γ and (G) IL-1β. Concentration of cytokines were adjusted to the number of cells and presented as mean ± SEM, normalized to WT MRSA untreated. Statistical analysis was performed by 2-way ANOVA with Dunnett’s multiple comparison test. ND, Not detected; Un, Untreated; 2’FL, 2′-Fucosyllactose; LNnT, Lacto-N-neotetraose; 6’SL, 6’-Sialyllactose. *p < 0.05, **p < 0.01, ***p < 0.001 and ****p < 0.0001.
HMOs increase activation of the NF-κB-pathway in response to S. aureus in THP1 reporter cell line
Next, we investigated the potential of HMOs to activate the NF-κB-pathway. The NF-κB-pathway is a common pathway activated during infection and inflammation (55). We used a monocytic THP1-reporter cell line that expresses GFP in response to NF-κB activation, and it is therefore comprehensive to monitor myeloid cell activation by flow cytometry. As shown in Figure 5A, all tested HMOs increased the inflammatory NF-κB-response against S. aureus. Furthermore, the HMOs did not induce NF-κB-activity in macrophages without exposure to bacteria, highlighting that the effect is linked to the presence of pathogens (Figure 5A). Since HMO-mediated NF-κB activation was only observed together with S. aureus exposure, and as NF-κB can drive pro-inflammatory responses in macrophages (55), this could likely be a causative factor in the regulation of macrophages. Evaluation of data from microarray assay revealed that multiple genes relevant in the NF-κB-pathway were upregulated in macrophages treated with 6’SL and challenged with WT MRSA (Figure 5B). More specifically, there was an upregulation of the genes NFKBIE, NFKBIA, NFKB1, RELA, CHUK and IKBKG with 6’SL compared to untreated control. REL and IKBKB showed no difference in response to 6’SL. These upregulations were not seen in absence of the WT MRSA bacteria nor with the other HMOs (data not shown), pointing to a specific effect of 6’SL against pathogens.
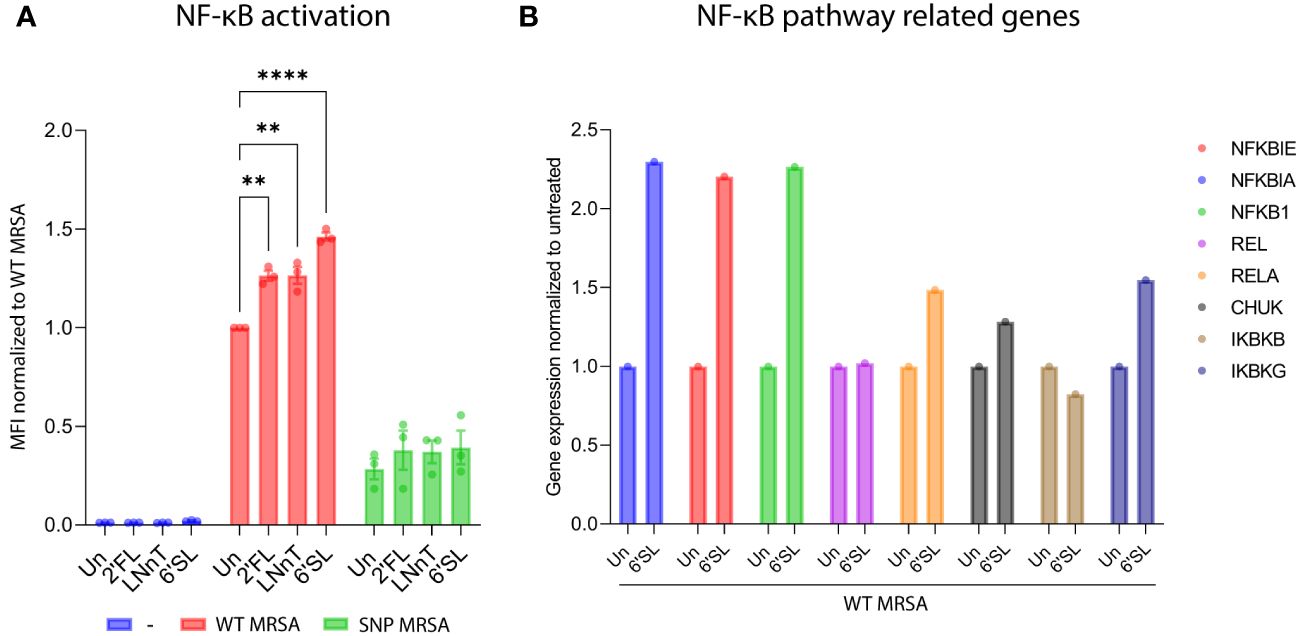
Figure 5 HMOs increases activation of the NF-κB-pathway as a response to S. aureus in a THP1 reporter cell line and NF-κB-related genes in macrophages. (A) NF-κB-THP1 reporter cell line was treated with HMOs followed by treatment with WT MRSA and SNP MRSA and analyzed by flow cytometry. Data is presented as bar graph with data points showing GFP-signal as a measure for NF-κB activation. Data represents three independent experiments and is presented as GFP-MFI values ± SEM normalized to untreated WT MRSA sample. The statistical analysis was performed by 2-way ANOVA with Dunnett’s multiple comparison test and presented relative to untreated control. (B) Monocytes were treated with 6’SL followed by treatment with WT MRSA. On day 8, total RNA was isolated, and genes relevant to the NF-κB-pathway were investigated by transcriptomic profiling. Data is presented as gene expression relative to untreated control and represents one experiment. MFI, Mean Fluorescent Intensity; NF-κB, Nuclear factor kappa-light-chain-enhancer of activated B cells; Un, Untreated; 2’FL, 2′-Fucosyllactose; LNnT, Lacto-N-neotetraose; 6’SL, 6’-Sialyllactose. **p < 0.01 and ****p < 0.0001.
Chemically synthesized and fermentation-produced HMOs show same myeloid activation profile
Most manufactured HMOs are produced by a fermentation process (56). To rule out that byproducts from the manufacturing process could impact or stimulate the immune cells, we did a comparison between HMOs produced by fermentation and HMOs produced chemically. Both fermented and chemically produced HMOs caused NF-κB activation, and there was no difference in activation between fermented and chemically produced HMOs (Figure 6). Moreover, an endotoxin analysis was performed on the fermented HMOs to rule out presence of endotoxins. The endotoxin test could not detect endotoxins above the assay threshold level (Table 1), clearly suggesting that the effect of HMOs is not caused by endotoxins. The studies in this article were carried out with the commercially available fermented HMOs.
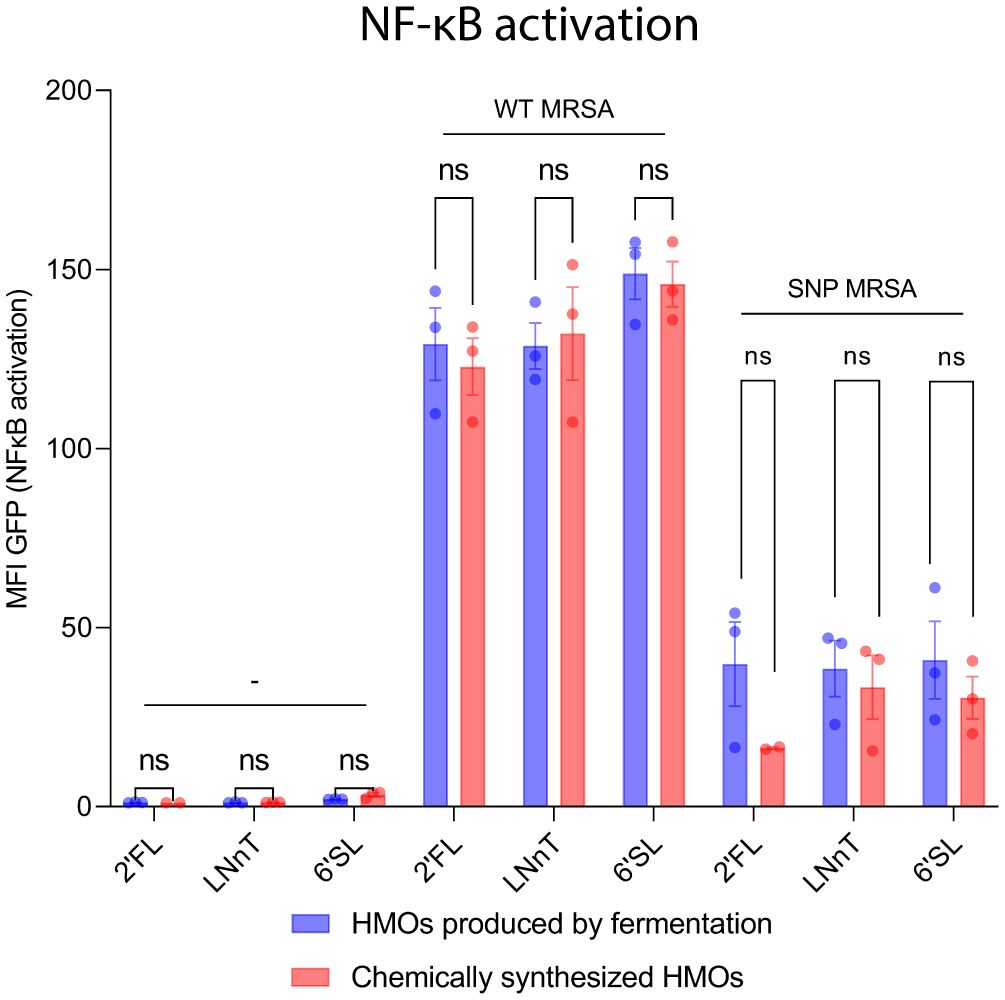
Figure 6 Chemically synthesized HMOs and HMOs produced by fermentation show same myeloid activation profile. NF-κB-THP1 reporter cells were treated with HMOs followed by treatment with WT MRSA and SNP MRSA and analyzed by flow cytometry. Data is presented as a bar graph with data points showing the GFP-signal ± SEM as a measure for NF-κB activation and represents three individual experiments. Statistical analysis was performed by 2-way ANOVA with Sidak´s multiple comparison test and presented as difference between fermented-produced- and chemically synthesized HMOs. Note that some of the data points included in this figure is identical to data in Figure 5. MFI, Mean Fluorescent Intensity; NF-κB, Nuclear factor kappa-light-chain-enhancer of activated B cells; Un, Untreated; HMOs, Human Milk Oligosaccharides; 2’FL, 2′-Fucosyllactose; LNnT, Lacto-N-neotetraose; 6’SL, 6’-Sialyllactose; ns, Not significant.
HMOs alter the proliferation and morphology of macrophages
Microscopic inspection showed that HMOs affected growth activation patterns. Therefore, proliferation of macrophages was evaluated by CFSE-labelling and culturing with combinations of M-CSF, HMOs and S. aureus. On day 8, the macrophages were analyzed by flow cytometry. As seen in Supplementary Figures 11A, B, cells receiving HMOs, most significant 6’SL, proliferated more compared to untreated controls, shown as a decrease in CFSE-MFI value, compared to untreated control (Supplementary Figure 11B). Microscopic evaluation further corroborated that 6’SL treatment affected macrophage differentiation and morphology by making them more adherent and elongated (Supplementary Figure 12). Together this indicates an altered activation profile for macrophages exposed to 6’SL compared with the untreated control.
HMOs increase S. aureus uptake by monocytes and phagocytosis in primary macrophages
Having established that the HMOs can push macrophages towards an M1-like phenotype, the ability of HMOs to affect bacterial uptake was evaluated in U937 monocytes, using fluorescent-labelled S. aureus (Supplementary Figure 4). Each HMO increased the uptake of S. aureus, suggesting that the phagocytic potential is augmented by HMOs (Supplementary Figures 13A, B). We further analyzed the phagocytic uptake by primary macrophages cultured with HMOs (Figures 7A, B; Supplementary Figure 13C). Here 6’SL significantly enhanced the phagocytosis of the S. aureus bioparticles compared to the 2’FL, LNnT and the untreated control. Together, this indicates that HMOs, and in particular 6’SL, have the potential to increase both the inflammatory and phagocytic response against a bacterial infection, in this case S. aureus.
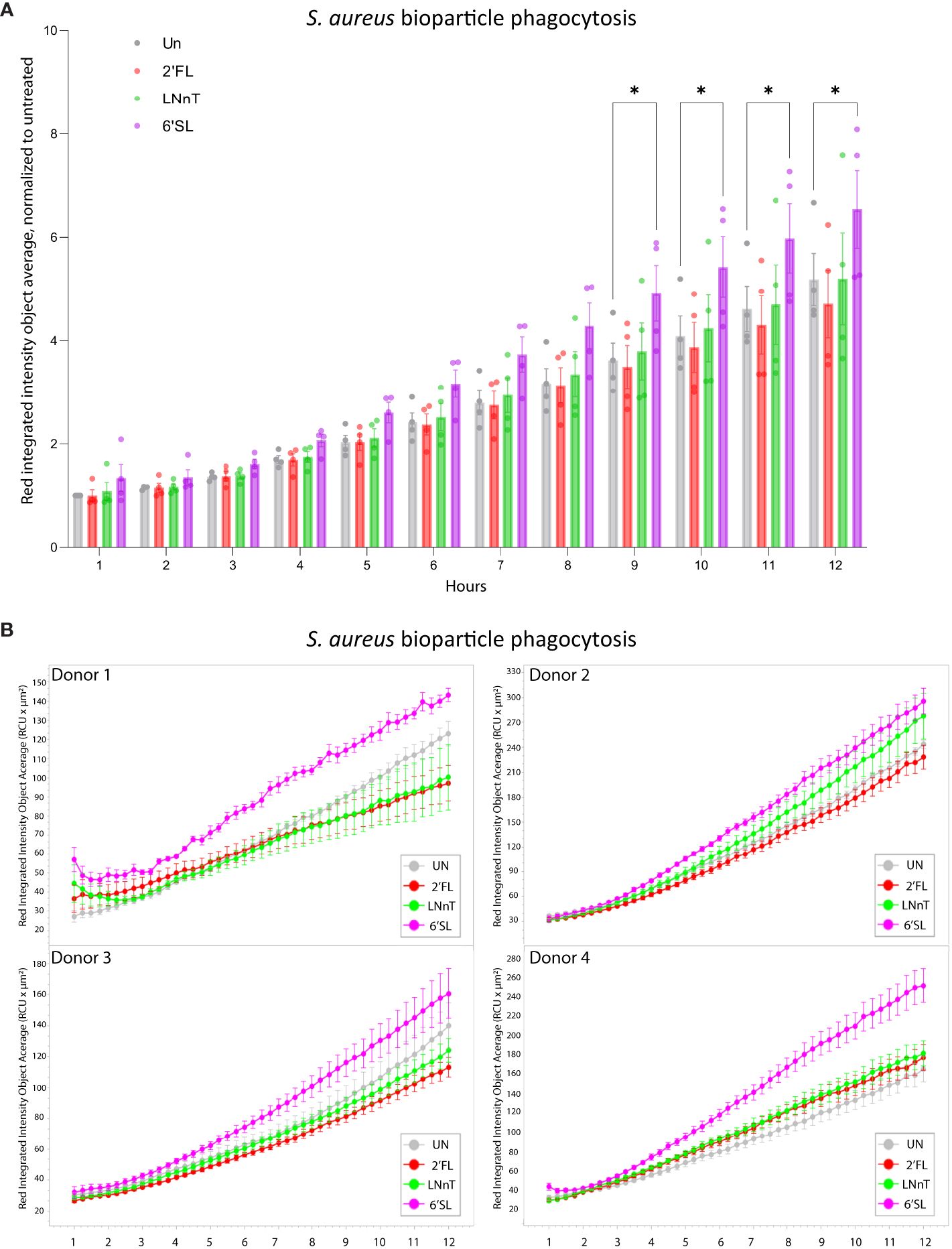
Figure 7 Treatment with HMOs increases phagocytosis of S. aureus bioparticles in primary macrophages. Primary macrophages were cultured for 8 days in the presence of 20 mM HMOs. On day 8 S. aureus bioparticles were added to the cells and the uptake was analyzed for 12 hours. Data is presented as (A) bar graph with data points showing red integrated intensity object average, normalized to untreated control at T-1h ± SEM from four individual experiments and (B) line graph representing the red integrated intensity object average over time for four donors (presented separately) ± SEM of three technical replicates. Statistical analysis was performed by 2-way ANOVA with Dunnett’s multiple comparison test and presented relative to untreated sample. Un, Untreated; 2’FL, 2′-Fucosyllactose; LNnT, Lacto-N-neotetraose; 6’SL, 6’-Sialyllactose. *p < 0.05.
HMOs enhance macrophage response upon exposure to S. aureus with mutations linked to antibiotic resistance
We further investigated the effect of HMOs on macrophages against a more antibiotic-resistant S. aureus strain. The SNP MRSA strain has clpP and rpoB SNP-mutations, related to increased antibiotic-resistance. The HMOs, especially 6’SL, increased the pro-inflammatory phenotype against the more antibiotic-resistant SNP MRSA, but to a lower extent than that observed for WT MRSA. This include increased gene expression of the adhesion molecule CD18 (Supplementary Figure 5C), and decreased gene expression of CD200R, CD163 and D169 (Supplementary Figures 5D, F, G). HMOs also increased gene expression of pro-inflammatory cytokine genes, including TNF, IL-6, IL-8 and IL-1β (Supplementary Figures 9A, D, E, G), and decreased the gene expression of IL-10 (Supplementary Figure 9B). When evaluating the effect of HMOs on surface expression of several proteins, 6’SL showed similar effect when co-cultured with SNP MRSA as when co-cultured with WT MRSA. This includes an increase in activating molecule CD137L and adhesion molecule CD18 (Figures 3A, C), and a decrease in the anti-inflammatory surface molecules CD200R and PD-L1 (Figures 3D, E), as well as CD169 (Figure 3G). Regulation of the co-stimulatory molecule CD80 and the M2-marker CD163 was not affected by SNP MRSA exposure, suggesting that the more resistant SNP MRSA has a potential to evade the immune system, as previously shown (43). However, HMOs can indeed activate macrophages against the resistant S. aureus strain. In agreement with this, the response of pro-inflammatory cytokines IL-6, IL-8 and IL-1β (Figures 4D, E, G) was enhanced by co-cultivating SNP MRSA with 6’SL. The macrophage proliferation was also higher in response to SNP MRSA, when co-culturing with HMOs (Supplementary Figure 11). In addition, a slight increase in SNP MRSA uptake was observed in U937 monocytes pretreated with 2’FL (Supplementary Figures 13A, B). However, the HMOs has no effect on the NF-κB pathway in SNP MRSA-exposed macrophages (Figure 5). These results indicate that HMOs, have the potential to enhance the immune response against S. aureus (WT MRSA), without causing aberrant activation of naïve cells. To a lower extent, a similar effect is observed for S. aureus with SNPs related to antibiotic resistance (SNP MRSA).
Discussion
The formation of the immune systems starts already in the embryonic phase within the uterus. However, the immune system of the newborn infant is still immature and relies heavily on external stimuli, e.g. stimuli from the microbiota, in order to fully develop (60). HMOs in breast milk have prebiotic functions and help shape and develop the gut eco system (61–63), and thereby the immune system, as the microbiota composition regulate specific immune responses. Vogel et al. showed that S. aureus enhanced a Th1 response, whereas Bifidobacterium, a classic infant-associated bacteria, had a mitigating effect on this immune response (64). HMOs are known to have a bifidogenic effect and can therefore indirectly support this microbiome-driven immune regulation. HMOs have also shown to affect immune cells, even without microbiota present, highlighting an effect that does not rely on microbial derivatives (15). Data have shown that HMOs have beneficial effects against various diseases such as rotavirus-induced diarrhea and necrotizing enterocolitis (65–67). It is also known that HMOs help shape the immune system in babies, as illustrated in a study by Goehring et al. where HMO-supplementation of infant formula decreased plasma levels of pro-inflammatory cytokines, bringing them closer to those of breastfed infants (20). Sialylated HMOs have been shown to increase the clearance of Pseudomonas aeruginosa in mice and in THP1 monocytes (68), and Xiao et al. demonstrated that a pool of HMOs have immunomodulatory functions on dendritic cells (18). HMOs have also been shown to be present in the amniotic fluid during pregnancy and could potentially already play a role in the development and modulation of the immune system in the embryonic state, and not only after birth (69).
While the effect of HMOs on the gut microbiota and their role in gut health is relatively well studied, the direct impact of HMOs on immune cells is still poorly understood. Here, we aimed to evaluate the direct effect of three individual HMOs with structures belonging to either the neutral, fucosylated or sialylated group. We found that HMOs can augment the response against S. aureus, illustrated by increased M1 surface markers, decreased expression of M2 markers (Figure 3), increased release of pro-inflammatory cytokines (Figure 4), enhanced NF-κB activation (Figure 5) and S. aureus phagocytosis (Figure 7; Supplementary Figure 13). We found that sialylated 6’SL had the highest immunomodulatory effect compared to the non-fucosylated neutral core HMO, LNnT and the fucosylated neutral core HMO, 2’FL. In parallel to our study, Boll et al. recently showed that M1 macrophages were strongly impacted by sialylated HMOs in response to LPS (19), through enhanced secretion of the inflammatory cytokines as IL-1β, IL-6 and TNF-α (19). This is similar to findings in our study, when challenging the cells with S. aureus, pointing to an immunomodulatory role for sialylated HMOs.
This study shows that HMOs can directly affect immune cells, though the molecular mode of action remains elusive. Hassan et al. demonstrated that the HMO 3’SL has several interactions with proteins found in murine macrophages, including well known glycan binding receptors as galectins (30). We hypothesize that HMOs work as surrogate TLR ligands that support a homeostatic immune activation, which is likely important for development of immune cells. In line with this, Kurakevich et al. observed that 3’SL mediated stimulatory effect on dendritic cells that was dependent on TLR4 (70). We did, however, not see changes in the surface expression of neither TLR2 nor TLR4 in this study. As previously mentioned, glycan-binding receptors, such as lectins and siglecs, are able to bind HMOs (28, 29, 32). In this study, a downregulation of CD169 (Siglec-1) was found, when macrophages were treated with HMOs and exposed to S. aureus, indicating a link between HMO-dependent macrophage activation and Siglec-1. We also found an increase in CD18 (Figure 3C), which is part of the important macrophage adhesion molecule Mac-1 (71). Surface expression ofCD11b (the other part of Mac-1) was however not changed (Supplementary Figure 8A). The HMO-mediated M1-like phenotype was highly linked to the presence of S. aureus. This indicates that the HMOs primarily work through co-stimulation, hypothetically through epigenetic regulation and dual-dependency between 6’SL and S. aureus, or through metabolic regulation of NF-κB, both interesting issues that needs further examination in further studies. Similarly, Ayechu-Muruzabal et al. demonstrated that 2′FL enhanced IFN-γ and IL-10 secretion in activated peripheral blood mononuclear cells co-cultured with the intestinal cell line HT-29, but only in the presence of CpG (mimicking bacterial trigger), whereas cytokine secretion was not affected by exposure to 2’FL alone (72).
In our study, we saw a minor change in the proliferation profile of macrophages by 6’SL, while this was consistently observed, we have to stress that the effect was small. The cell cycle phase is indeed important for macrophage plasticity and polarization (73). Further, IL-4 can lead to proliferation of macrophages (74), however this is likely not the causative effect of 6’SL, as the all HMOs examined in the current study induced similar levels of IL-4.
Human milk possesses many relevant immunomodulators including HMOs and antibodies (1). Current investigations also indicate that milk contains antigen fragments, believed to help develop and train the immune response in infants (75, 76). Previously, it was believed that the memory of the immune system was confined to the adaptive immune cells, and only developed as a response to infectious encounters. However, recent years, trained immunity of the innate immune cells has been highly investigated, challenging the assumption that memory is solely limited to cells of the adaptive immune system. It reveals that innate cells exposed to, and activated by, certain stimuli can enhance their response when encountering a non-specific pathogen at a later stage through epigenetic changes in the cell (77). Trained immunity can lead to enhanced effector functions such as improved phagocytosis and increased cytokine release upon pathogen encounter. Such booster effects of the infant immune system could be highly relevant in combatting infectious diseases. It has been shown that β-glucans can train the innate immune cells, such as monocytes and macrophages, to enhance the immune response at a later state (78, 79). Like β-glucans, HMOs possess the ability to bind pattern recognition receptors (28, 29). This, together with the immune-enhancing effects seen in this study, suggests that HMOs could have a role in developing and training innate cells of young infants, improving their ability to overcome infections. The impact of HMOs on trained immunity should be considered and investigated further.
Monocytes and macrophages are essential in pathogen clearing and are especially important in bacterial infections including clearance of S. aureus (44). Persistent activation of M2 macrophages is associated with persistent infections as well as biofilm formation (80). S. aureus has developed multiple mechanisms to manipulate and evade the host immune system (43), e.g.; S. aureus derived lactate-production can reprogram the immune response and maintain persistent infection (81). Therefore, it is highly relevant to identify methods to enhance M1-like effects against S. aureus. One way to augment the M1-response is by the use of metabolic reprogramming, as illustrated by Yamada et al. (47). In the current study, the immune-enhancing effect of HMOs were similar but lower for SNP MRSA compared to WT MRSA, indicating that the HMOs will have the same effect on different MRSA strains. As antibiotic-resistance and immune evasion are an increasing problem for S. aureus, alternatives to antibiotic treatments that influence activation of the immune response are of great interest and should be investigated further. Since HMOs have a well-regarded safety profile and are well-tolerated, this study supports a possible continuous use of HMOs to limit S. aureus infections, in infants, but also in elderly or people with immune defects.
In summary, this study characterized specific effects of a neutral, fucosylated and sialylated HMO structure on human monocyte derived macrophages in response to S. aureus, and we saw that HMOs have the potential to enhance the myeloid immune response against bacteria. Importantly, even though all HMOs were able to activate macrophages, the different HMO structures showed various degree of myeloid activation, which underlines the diversity of the different HMOs. This is further exemplified by a recent study by Boll et al. demonstrating particular biological properties depending on HMO structural class (19). Taken together, our study suggests that HMOs are able to enhance the immune response under infectious circumstances, without causing notable activation of naïve cells, highlighting a great potential of HMOs as a non-antibiotic alternative against infectious diseases.
Further studies are needed to assess the influence of HMOs on other immune cell populations. For example, HMOs were shown to dose-dependently reduce Mac-1 in neutrophils (82), which can have important implications for neutrophil-mediated pathogen clearance such as S. aureus-induced pneumonia (83). Moreover, the context of HMO exposure of macrophages and their precursors can have consequences for their clearance of infectious agents (84, 85).
Data availability statement
The original contributions presented in the study are included in the article/Supplementary Material, further inquiries can be directed to the corresponding author.
Ethics statement
Ethical approval was not required for the studies involving humans because Blood was purchased from National Hospital blood bank. The studies were conducted in accordance with the local legislation and institutional requirements. Written informed consent to participate in this study was not required from the participants in accordance with the national legislation and the institutional requirements.
Author contributions
SJ: Conceptualization, Data curation, Formal analysis, Funding acquisition, Investigation, Methodology, Project administration, Resources, Visualization, Writing – original draft, Writing – review & editing. AL: Data curation, Formal analysis, Investigation, Methodology, Project administration, Visualization, Writing – original draft, Writing – review & editing. MM: Formal analysis, Investigation, Methodology, Resources, Validation, Writing – original draft, Writing – review & editing. LA: Conceptualization, Formal analysis, Funding acquisition, Investigation, Methodology, Writing – original draft, Writing – review & editing. KC: Conceptualization, Funding acquisition, Investigation, Methodology, Project administration, Resources, Supervision, Writing – original draft, Writing – review & editing. SS: Conceptualization, Funding acquisition, Investigation, Methodology, Project administration, Resources, Supervision, Writing – original draft, Writing – review & editing.
Funding
The author(s) declare financial support was received for the research, authorship, and/or publication of this article. This work was supported by the Innovation Fund Denmark (9065-00193B). The authors declare that this study received funding from dsm-firmenich. The funder was not involved in the study design, collection, analysis, interpretation of data, the writing of this article, or the decision to submit it for publication.
Acknowledgments
We would like to thank Dr. Peter Steinberger (Institute of Immunology, Medical University of Vienna, Vienna, Austria) for providing the THP1-NF-κB-GFP reporter cells. We also acknowledge Anni Mehlsen (Department of Veterinary and Animal Sciences, University of Copenhagen, Copenhagen, Denmark) for great technical assistance and Maiken Mellergaard (Department of Clinical Biochemistry, Aalborg University Hospital, Aalborg, Denmark) for the invaluable help regarding S. aureus strains. We acknowledge Maryse Darch for thorough proofreading. Graphical abstract was created with BioRender.com.
Conflict of interest
SJ, MM and KC are employed by dsm-firmenich, a company producing human milk oligosaccharides. SS currently have research agreements with dsm-firmenich, Bioneer and Novo Nordisk. SS is a paid consultant for Novo Nordisk.
The remaining authors declare that the research was conducted in the absence of any commercial or financial relationships that could be construed as a potential conflict of interest.
The corresponding author declared that he was an editorial board member of Frontiers, at the time of submission. This had no impact on the peer review process and the final decision.
Publisher’s note
All claims expressed in this article are solely those of the authors and do not necessarily represent those of their affiliated organizations, or those of the publisher, the editors and the reviewers. Any product that may be evaluated in this article, or claim that may be made by its manufacturer, is not guaranteed or endorsed by the publisher.
Supplementary material
The Supplementary Material for this article can be found online at: https://www.frontiersin.org/articles/10.3389/fimmu.2024.1379042/full#supplementary-material
Abbreviations
CFSE, Carboxyfluorescein succinimidyl ester; FSC, Forward Scatter; HMO, Human Milk Oligosaccharide; LNnT, Lacto-N-neotetraose; MRSA, Methicillin-Resistant Staphylococcus aureus; NF-κB, Nuclear factor kappa-light-chain-enhancer of activated B cells; SE-AF647, AF647 conjugated succinimidylester; SSC, Side Scatter; S. aureus, Staphylococcus aureus; 2’FL, 2’-Fucosyllactose; 3’SL, 3′-Sialyllactose; 6’SL, 6′-Sialyllactose.
References
1. Hennet T, Borsig L. Breastfed at tiffany's. Trends Biochem Sci. (2016) 41:508–18. doi: 10.1016/j.tibs.2016.02.008
2. Järvinen KM, Martin H, Oyoshi MK. Immunomodulatory effects of breast milk on food allergy. Ann Allergy Asthma Immunol. (2019) 123:133–43. doi: 10.1016/j.anai.2019.04.022
3. Soyyılmaz B, Mikš MH, Röhrig CH, Matwiejuk M, Meszaros-Matwiejuk A, Vigsnæs LK. The mean of milk: A review of human milk oligosaccharide concentrations throughout lactation. Nutrients. (2021) 13(8):2737. doi: 10.3390/nu13082737
4. Bode L. Human milk oligosaccharides: Every baby needs a sugar mama. Glycobiology. (2012) 22:1147–62. doi: 10.1093/glycob/cws074
5. Iribarren C, Magnusson MK, Vigsnæs LK, Aziz I, Amundsen ID, Šuligoj T, et al. The effects of human milk oligosaccharides on gut microbiota, metabolite profiles and host mucosal response in patients with irritable bowel syndrome. Nutrients. (2021) 13:3836. doi: 10.3390/nu13113836
6. Elison E, Vigsnaes LK, Rindom Krogsgaard L, Rasmussen J, Sørensen N, McConnell B, et al. Oral supplementation of healthy adults with 2′-O-fucosyllactose and lacto-N-neotetraose is well tolerated and shifts the intestinal microbiota. Br J Nutr. (2016) 116:1356–68. doi: 10.1017/S0007114516003354
7. Hester SN, Chen X, Li M, Monaco MH, Comstock SS, Kuhlenschmidt TB, et al. Human milk oligosaccharides inhibit rotavirus infectivity in vitro and in acutely infected piglets. Br J Nutr. (2013) 110:1233–42. doi: 10.1017/S0007114513000391
8. Ruiz-Palacios GM, Cervantes LE, Ramos P, Chavez-Munguia B, Newburg DS. Campylobacter jejuni binds intestinal H(O) antigen (Fucα1, 2Galβ1, 4GlcNAc), and fucosyloligosaccharides of human milk inhibit its binding and infection. J Biol Chem. (2003) 278:14112–20. doi: 10.1074/jbc.M207744200
9. Mariño E, Richards JL, McLeod KH, Stanley D, Yap YA, Knight J, et al. Gut microbial metabolites limit the frequency of autoimmune T cells and protect against type 1 diabetes. Nat Immunol. (2017) 18:552–62. doi: 10.1038/ni.3713
10. Sun M, Wu W, Chen L, Yang W, Huang X, Ma C, et al. Microbiota-derived short-chain fatty acids promote Th1 cell IL-10 production to maintain intestinal homeostasis. Nat Commun. (2018) 9:3555. doi: 10.1038/s41467-018-05901-2
11. Høgh RI, Møller SH, Jepsen SD, Mellergaard M, Lund A, Pejtersen M, et al. Metabolism of short-chain fatty acid propionate induces surface expression of NKG2D ligands on cancer cells. FASEB J. (2020) 34:15531–46.
12. Bunesova V, Lacroix C, Schwab C. Mucin cross-feeding of infant bifidobacteria and eubacterium hallii. Microbial Ecol. (2018) 75:228–38. doi: 10.1007/s00248-017-1037-4
13. Schwab C, Ruscheweyh H-J, Bunesova V, Pham VT, Beerenwinkel N, Lacroix C. Trophic Interactions of Infant Bifidobacteria and Eubacterium hallii during L-Fucose and Fucosyllactose Degradation. Front Microbiol. (2017) 8:95–5. doi: 10.3389/fmicb.2017.00095
14. Pichler MJ, Yamada C, Shuoker B, Alvarez-Silva C, Gotoh A, Leth ML, et al. Butyrate producing colonic Clostridiales metabolise human milk oligosaccharides and cross feed on mucin via conserved pathways. Nat Commun. (2020) 11:3285. doi: 10.1038/s41467-020-17075-x
15. Rosa F, Sharma AK, Gurung M, Casero D, Matazel K, Bode L, et al. Human milk oligosaccharides impact cellular and inflammatory gene expression and immune response. Front Immunol. (2022) 13:907529. doi: 10.3389/fimmu.2022.907529
16. Lane JA, O'Callaghan J, Carrington SD, Hickey RM. Transcriptional response of HT-29 intestinal epithelial cells to human and bovine milk oligosaccharides. Br J Nutr. (2013) 110:2127–37. doi: 10.1017/S0007114513001591
17. Zhang W, Yan J, Wu L, Yu Y, Ye RD, Zhang Y, et al. In vitro immunomodulatory effects of human milk oligosaccharides on murine macrophage RAW264.7 cells. Carbohydr Polymers. (2019) 207:230–8. doi: 10.1016/j.carbpol.2018.11.039
18. Xiao L, van De Worp WRPH, Stassen R, van Maastrigt C, Kettelarij N, Stahl B, et al. Human milk oligosaccharides promote immune tolerance via direct interactions with human dendritic cells. Eur J Immunol. (2019) 49:1001–14. doi: 10.1002/eji.201847971
19. Boll EJ, Lopez DV, Terne M, Hessing S, Parschat K, Jensen SR. Human milk oligosaccharides differentially support gut barrier integrity and enhance Th1 and Th17 cell effector responses in vitro. Front Immunol. (2024) 15. doi: 10.3389/fimmu.2024.1359499
20. Goehring KC, Marriage BJ, Oliver JS, Wilder JA, Barrett EG, Buck RH. Similar to those who are breastfed, infants fed a formula containing 2′-fucosyllactose have lower inflammatory cytokines in a randomized controlled trial. J Nutr. (2016) 146:2559–66. doi: 10.3945/jn.116.236919
21. Atri C, Guerfali FZ, Laouini D. Role of human macrophage polarization in inflammation during infectious diseases. Int J Mol Sci. (2018) 19(6):1801. doi: 10.3390/ijms19061801
22. Jones G-R, Bain CC, Fenton TM, Kelly A, Brown SL, Ivens AC, et al. Dynamics of colon monocyte and macrophage activation during colitis. Front Immunol. (2018) 9. doi: 10.3389/fimmu.2018.02764
23. Liu PS, Wang H, Li X, Chao T, Teav T, Christen S, et al. α-ketoglutarate orchestrates macrophage activation through metabolic and epigenetic reprogramming. Nat Immunol. (2017) 18:985–94. doi: 10.1038/ni.3796
24. Liu P-S, Chen Y-T, Li X, Hsueh P-C, Tzeng S-F, Chen H, et al. CD40 signal rewires fatty acid and glutamine metabolism for stimulating macrophage anti-tumorigenic functions. Nat Immunol. (2023) 24:452–62. doi: 10.1038/s41590-023-01430-3
25. Bochner BS, Zimmermann N. Role of siglecs and related glycan-binding proteins in immune responses and immunoregulation. J Allergy Clin Immunol. (2015) 135:598–608. doi: 10.1016/j.jaci.2014.11.031
26. Crocker PR, Paulson JC, Varki A. Siglecs and their roles in the immune system. Nat Rev Immunol. (2007) 7:255–66. doi: 10.1038/nri2056
27. Rousseaux A, Brosseau C, Le Gall S, Piloquet H, Barbarot S, Bodinier M. Human milk oligosaccharides: their effects on the host and their potential as therapeutic agents. Front Immunol. (2021) 12. doi: 10.3389/fimmu.2021.680911
28. Noll AJ, Yu Y, Lasanajak Y, Duska-McEwen G, Buck RH, Smith DF, et al. Human DC-SIGN binds specific human milk glycans. Biochem J. (2016) 473:1343–53. doi: 10.1042/BCJ20160046
29. Noll AJ, Gourdine J-P, Yu Y, Lasanajak Y, Smith DF, Cummings RD. Galectins are human milk glycan receptors. Glycobiology. (2016) 26:655–69. doi: 10.1093/glycob/cww002
30. Hassan AA, Wozniak JM, Vilen Z, Li W, Jadhav A, Parker CG, et al. Chemoproteomic mapping of human milk oligosaccharide (HMO) interactions in cells. RSC Chem Biol. (2022) 3:1369–74. doi: 10.1039/D2CB00176D
31. Macauley MS, Crocker PR, Paulson JC. Siglec-mediated regulation of immune cell function in disease. Nat Rev Immunol. (2014) 14:653–66. doi: 10.1038/nri3737
32. Koliwer-Brandl H, Siegert N, Umnus K, Kelm A, Tolkach A, Kulozik U, et al. Lectin inhibition assays for the analysis of bioactive milk sialoglycoconjugates. Int Dairy J. (2011) 21:413–20. doi: 10.1016/j.idairyj.2011.01.005
33. Erikstrup LT, Dinh KM, Andersen PS, Skov RL, Kaspersen KA, Nielsen KR, et al. Cohort description: The Danish Blood Donor Staphylococcus aureus Carriage Study. Clin Epidemiol. (2019) 11:885–900. doi: 10.2147/CLEP
34. Turner NA, Sharma-Kuinkel BK, Maskarinec SA, Eichenberger EM, Shah PP, Carugati M, et al. Methicillin-resistant Staphylococcus aureus: an overview of basic and clinical research. Nat Rev Microbiol. (2019) 17:203–18. doi: 10.1038/s41579-018-0147-4
35. Howden BP, Giulieri SG, Wong Fok Lung T, Baines SL, Sharkey LK, Lee JYH, et al. Staphylococcus aureus host interactions and adaptation. Nat Rev Microbiol. (2023) 21:380–95. doi: 10.1038/s41579-023-00852-y
36. Tong SY, Davis JS, Eichenberger E, Holland TL, Fowler VG Jr. Staphylococcus aureus infections: epidemiology, pathophysiology, clinical manifestations, and management. Clin Microbiol Rev. (2015) 28:603–61. doi: 10.1128/CMR.00134-14
37. Leshem E, Maayan-Metzger A, Rahav G, Dolitzki M, Kuint J, Roytman Y, et al. Transmission of Staphylococcus aureus from mothers to newborns. Pediatr Infect Dis J. (2012) 31:360–3. doi: 10.1097/INF.0b013e318244020e
38. Benito D, Lozano C, Jiménez E, Albújar M, Gómez A, Rodríguez JM, et al. Characterization of Staphylococcus aureus strains isolated from faeces of healthy neonates and potential mother-to-infant microbial transmission through breastfeeding. FEMS Microbiol Ecol. (2015) 91(3):fiv007. doi: 10.1093/femsec/fiv007
39. Lundell A-C, Adlerberth I, Lindberg E, Karlsson H, Ekberg S, Åberg N, et al. Increased levels of circulating soluble CD14 but not CD83 in infants are associated with early intestinal colonization with Staphylococcus aureus. Clin Exp Allergy. (2007) 37:62–71. doi: 10.1111/j.1365-2222.2006.02625.x
40. Lundell A-C, Hesselmar B, Nordström I, Saalman R, Karlsson H, Lindberg E, et al. High circulating immunoglobulin A levels in infants are associated with intestinal toxigenic Staphylococcus aureus and a lower frequency of eczema. Clin Exp Allergy. (2009) 39:662–70. doi: 10.1111/j.1365-2222.2008.03176.x
41. Raineri EJM, Maaß S, Wang M, Brushett S, Palma Medina LM, Sampol Escandell N, et al. Staphylococcus aureus populations from the gut and the blood are not distinguished by virulence traits-a critical role of host barrier integrity. Microbiome. (2022) 10:239. doi: 10.1186/s40168-022-01419-4
42. Richardson JR, Armbruster NS, Günter M, Biljecki M, Klenk J, Heumos S, et al. PSM Peptides From Community-Associated Methicillin-Resistant Staphylococcus aureus Impair the Adaptive Immune Response via Modulation of Dendritic Cell Subsets in vivo. Front Immunol. (2019) 10. doi: 10.3389/fimmu.2019.00995
43. Mellergaard M, Skovbakke SL, Jepsen SD, Panagiotopoulou N, Hansen ABR, Tian W, et al. Clinical Staphylococcus aureus inhibits human T-cell activity through interaction with the PD-1 receptor. mBio. (2023) 0:e01349–23. doi: 10.1128/mbio.01349-23
44. Pidwill GR, Gibson JF, Cole J, Renshaw SA, Foster SJ. The role of macrophages in staphylococcus aureus infection. Front Immunol. (2020) 11:620339. doi: 10.3389/fimmu.2020.620339
45. Peng K-T, Hsieh C-C, Huang T-Y, Chen P-C, Shih H-N, Lee MS, et al. Staphylococcus aureus biofilm elicits the expansion, activation and polarization of myeloid-derived suppressor cells in vivo and in vitro. PloS One. (2017) 12:e0183271. doi: 10.1371/journal.pone.0183271
46. Hanke ML, Heim CE, Angle A, Sanderson SD, Kielian T. Targeting macrophage activation for the prevention and treatment of Staphylococcus aureus biofilm infections. J Immunol. (2013) 190:2159–68. doi: 10.4049/jimmunol.1202348
47. Yamada KJ, Heim CE, Xi X, Attri KS, Wang D, Zhang W, et al. Monocyte metabolic reprogramming promotes pro-inflammatory activity and Staphylococcus aureus biofilm clearance. PloS Pathog. (2020) 16:e1008354. doi: 10.1371/journal.ppat.1008354
48. Battin C, Hennig A, Mayrhofer P, Kunert R, Zlabinger GJ, Steinberger P, et al. A human monocytic NF-κB fluorescent reporter cell line for detection of microbial contaminants in biological samples. PloS One. (2017) 12:e0178220. doi: 10.1371/journal.pone.0178220
49. Mellergaard M, Thøgersen L, Mogenssen RG, Mellergaard M, Thomsen LE, Petersen A, et al. Staphylococcus aureus induces cell-surface expression of immune stimulatory NKG2D ligands on human monocytes. J Biol Chem. (2020) 295:11803–21. doi: 10.1074/jbc.RA120.012673
50. Bæk KT, Høgh RI, Lund A, Aldana BI, Guérillot R, Møller SH, et al. Stepwise decrease in daptomycin susceptibility in clinical Staphylococcus aureus isolates associated with an initial mutation in rpoB and a compensatory inactivation of the clpX gene. Antimicrob Agents Chemother. (2015) 59:6983–91. doi: 10.1128/AAC.01303-15
51. Peleg AY, Miyakis S, Ward DV, Earl AM, Rubio A, Cameron DR, et al. Whole genome characterization of the mechanisms of daptomycin resistance in clinical and laboratory derived isolates of staphylococcus aureus. PloS One. (2012) 7:e28316. doi: 10.1371/journal.pone.0028316
52. Quah BJC, Warren HS, Parish CR. Monitoring lymphocyte proliferation in vitro and in vivo with the intracellular fluorescent dye carboxyfluorescein diacetate succinimidyl ester. Nat Protoc. (2007) 2:2049–56. doi: 10.1038/nprot.2007.296
53. Wozniak TM, Dyda A, Merlo G, Hall L. Disease burden, associated mortality and economic impact of antimicrobial resistant infections in Australia. Lancet Reg Health West Pac. (2022) 27:100521. doi: 10.1016/j.lanwpc.2022.100521
54. Zhang B, Shan H, Li D, Li ZR, Zhu KS, Jiang ZB, et al. Different methods of detaching adherent cells significantly affect the detection of TRAIL receptors. Tumori. (2012) 98:800–3. doi: 10.1177/030089161209800619
55. Liu T, Zhang L, Joo D, Sun S-C. NF-κB signaling in inflammation. Signal Transduction Targeted Ther. (2017) 2:17023. doi: 10.1038/sigtrans.2017.23
56. Bych K, Mikš MH, Johanson T, Hederos MJ, Vigsnæs LK, Becker P. Production of HMOs using microbial hosts — from cell engineering to large scale production. Curr Opin Biotechnol. (2019) 56:130–7. doi: 10.1016/j.copbio.2018.11.003
57. Pérez Figueroa I, Horváth F, Dekany G, Ágoston K, Ágoston Á, Bajza I, et al. Production of 6'-O-sialyllactose and intermediates. (2011). Glycom A/S, patent no. WO 2011/100979 A1.
58. Dékany G, Bajza I, Boutet J, Pérez IF, Hederos M, Horvath F, et al. Synthesis of 2'-O-fucosyllactose. (2010). Glycom A/S, patent no. WO 2010/115935 A1.
59. Bajza I, Dekany G, Ágoston K, Pérez IF, Boutet J, Hederos M, et al. A method for preparation of the tetrasaccharide lacto-N-neotetraose (LNnT) containing N-acetyllactosamine. (2011). Glycom A/S, patent no. WO 2011/100980 A1.
60. Robertson RC, Manges AR, Finlay BB, Prendergast AJ. The human microbiome and child growth – first 1000 days and beyond. Trends Microbiol. (2019) 27:131–47. doi: 10.1016/j.tim.2018.09.008
61. Bajic D, Wiens F, Wintergerst E, Deyaert S, Baudot A, Van den Abbeele P. HMOs exert marked bifidogenic effects on children's gut microbiota ex vivo, due to age-related bifidobacterium species composition. Nutrients. (2023) 15(7):1701. doi: 10.3390/nu15071701
62. Iribarren C, Törnblom H, Aziz I, Magnusson MK, Sundin J, Vigsnæs LK, et al. Human milk oligosaccharide supplementation in irritable bowel syndrome patients: A parallel, randomized, double-blind, placebo-controlled study. Neurogastroenterol Motil. (2020) 32(10):e13920. doi: 10.1111/nmo.13920
63. Šuligoj T, Vigsnæs LK, Abbeele PVd, Apostolou A, Karalis K, Savva GM, et al. Effects of human milk oligosaccharides on the adult gut microbiota and barrier function. Nutrients. (2020) 12:2808. doi: 10.3390/nu12092808
64. Vogel K, Arra A, Lingel H, Bretschneider D, Prätsch F, Schanze D, et al. Bifidobacteria shape antimicrobial T-helper cell responses during infancy and adulthood. Nat Commun. (2023) 14:5943. doi: 10.1038/s41467-023-41630-x
65. Azagra-Boronat I, Massot-Cladera M, Knipping K, Van't Land B, Stahl B, Garssen J, et al. Supplementation with 2'-FL and scGOS/lcFOS ameliorates rotavirus-induced diarrhea in suckling rats. Front Cell infection Microbiol. (2018) 8:372–2. doi: 10.3389/fcimb.2018.00372
66. Azagra-Boronat I, Massot-Cladera M, Knipping K, Van't Land B, Tims S, Stahl B, et al. Oligosaccharides modulate rotavirus-associated dysbiosis and TLR gene expression in neonatal rats. Cells. (2019) 8(8):876. doi: 10.3390/cells8080876
67. Sodhi CP, Wipf P, Yamaguchi Y, Fulton WB, Kovler M, Niño DF, et al. The human milk oligosaccharides 2'-fucosyllactose and 6'-sialyllactose protect against the development of necrotizing enterocolitis by inhibiting toll-like receptor 4 signaling. Pediatr Res. (2020) 89:e00694-18.
68. Kim J, Kim Y-J, Kim JW. Bacterial clearance is enhanced by α2,3- and α2,6-sialyllactose via receptor-mediated endocytosis and phagocytosis. Infection Immun. (2019) 87:e00694–18. doi: 10.1128/IAI.00694-18
69. Jantscher-Krenn E, Schirnding L, Trötzmüller M, Köfeler H, Kurtovic U, Fluhr H, et al. Human milk oligosaccharides are present in amniotic fluid and show specific patterns dependent on gestational age. Nutrients. (2022) 14:2065. doi: 10.3390/nu14102065
70. Kurakevich E, Hennet T, Hausmann M, Rogler G, Borsig L. Milk oligosaccharide sialyl(α2,3)lactose activates intestinal CD11c+ cells through TLR4. Proc Natl Acad Sci. (2013) 110:17444–9. doi: 10.1073/pnas.1306322110
71. Erdei A, Lukácsi S, Mácsik-Valent B, Nagy-Baló Z, Kurucz I, Bajtay Z, et al. Non-identical twins: Different faces of CR3 and CR4 in myeloid and lymphoid cells of mice and men. Semin Cell Dev Biol. (2019) 85:110–21. doi: 10.1016/j.semcdb.2017.11.025
72. Ayechu-Muruzabal V, Overbeek SA, Kostadinova AI, Stahl B, Garssen J, Van't Land B, et al. Exposure of intestinal epithelial cells to 2'-fucosyllactose and cpG enhances galectin release and instructs dendritic cells to drive th1 and regulatory-type immune development. Biomolecules. (2020) 10(5):784. doi: 10.3390/biom10050784
73. Daniel B, Belk JA, Meier SL, Chen AY, Sandor K, Czimmerer Z, et al. Macrophage inflammatory and regenerative response periodicity is programmed by cell cycle and chromatin state. Mol Cell. (2023) 83:121–138.e7. doi: 10.1016/j.molcel.2022.11.017
74. Jenkins SJ, Ruckerl D, Thomas GD, Hewitson JP, Duncan S, Brombacher F, et al. IL-4 directly signals tissue-resident macrophages to proliferate beyond homeostatic levels controlled by CSF-1. J Exp Med. (2013) 210:2477–91. doi: 10.1084/jem.20121999
75. van den Elsen LWJ, Kollmann TR, Verhasselt V. Microbial antigen in human milk: a natural vaccine? Mucosal Immunol. (2022) 15:1058–9. doi: 10.1038/s41385-022-00561-4
76. Conti MG, Terreri S, Piano Mortari E, Albano C, Natale F, Boscarino G, et al. Immune response of neonates born to mothers infected with SARS-coV-2. JAMA Network Open. (2021) 4:e2132563–e2132563. doi: 10.1001/jamanetworkopen.2021.32563
77. Domínguez-Andrés J, Dos Santos JC, Bekkering S, Mulder WJM, van der Meer JWM, Riksen NP, et al. Trained immunity: adaptation within innate immune mechanisms. Physiol Rev. (2023) 103:313–46. doi: 10.1152/physrev.00031.2021
78. Keating ST, Groh L, van der Heijden CDCC, Rodriguez H, dos Santos JC, Fanucchi S, et al. The set7 lysine methyltransferase regulates plasticity in oxidative phosphorylation necessary for trained immunity induced by β-glucan. Cell Rep. (2020) 31:107548. doi: 10.1016/j.celrep.2020.107548
79. Bekkering S, Blok BA, Joosten LAB, Riksen NP, Crevel Rv, Netea MG. In vitro experimental model of trained innate immunity in human primary monocytes. Clin Vaccine Immunol. (2016) 23:926–33. doi: 10.1128/CVI.00349-16
80. Heim CE, Vidlak D, Kielian T. Interleukin-10 production by myeloid-derived suppressor cells contributes to bacterial persistence during Staphylococcus aureus orthopedic biofilm infection. J Leukocyte Biol. (2015) 98:1003–13. doi: 10.1189/jlb.4VMA0315-125RR
81. Heim CE, Bosch ME, Yamada KJ, Aldrich AL, Chaudhari SS, Klinkebiel D, et al. Lactate production by Staphylococcus aureus biofilm inhibits HDAC11 to reprogramme the host immune response during persistent infection. Nat Microbiol. (2020) 5:1271–84. doi: 10.1038/s41564-020-0756-3
82. Bode L, Rudloff S, Kunz C, Strobel S, Klein N. Human milk oligosaccharides reduce platelet-neutrophil complex formation leading to a decrease in neutrophil beta 2 integrin expression. J Leukoc Biol. (2004) 76:820–6. doi: 10.1189/jlb.0304198
83. Vignarajah M, Wood AJT, Nelmes E, Subburayalu J, Herre J, Nourshargh S, et al. Regulation of ICAM-1 in human neutrophils. J Leukoc Biol. (2024). doi: 10.1093/jleuko/qiae090
84. Kandalla PK, Sarrazin S, Molawi K, Berruyer C, Redelberger D, Favel A, et al. M-CSF improves protection against bacterial and fungal infections after hematopoietic stem/progenitor cell transplantation. J Exp Med. (2016) 213:2269–79. doi: 10.1084/jem.20151975
Keywords: human milk oligosaccharides, myeloid activation, immunology, 6′-sialyllactose, 2′-fucosyllactose, lacto-N-neotetraose, Staphylococcus aureus
Citation: Jepsen SD, Lund A, Matwiejuk M, Andresen L, Christensen KR and Skov S (2024) Human milk oligosaccharides regulate human macrophage polarization and activation in response to Staphylococcus aureus. Front. Immunol. 15:1379042. doi: 10.3389/fimmu.2024.1379042
Received: 30 January 2024; Accepted: 08 May 2024;
Published: 06 June 2024.
Edited by:
Javier E. Irazoqui, University of Massachusetts Medical School, United StatesReviewed by:
Qun Zhou, Sanofi Genzyme, United StatesJulien Subburayalu, Technical University Dresden, Germany
Copyright © 2024 Jepsen, Lund, Matwiejuk, Andresen, Christensen and Skov. This is an open-access article distributed under the terms of the Creative Commons Attribution License (CC BY). The use, distribution or reproduction in other forums is permitted, provided the original author(s) and the copyright owner(s) are credited and that the original publication in this journal is cited, in accordance with accepted academic practice. No use, distribution or reproduction is permitted which does not comply with these terms.
*Correspondence: Søren Skov, c29za0BzdW5kLmt1LmRr
†ORCID: Stine Dam Jepsen, orcid.org/0000-0001-5268-7383
Astrid Lund, orcid.org/0009-0009-7036-7311
Lars Andresen, orcid.org/0000-0002-6362-7973
Kristine Rothaus Christensen, orcid.org/0000-0003-2187-3858
Søren Skov, orcid.org/0000-0003-0732-0026