- 1Department of Urology, Urologic Surgery Center, Xinqiao Hospital, Third Military Medical University (Army Medical University), Chongqing, China
- 2Department of Medicinal Chemistry, College of Pharmacy, Third Military Medical University, Chongqing, China
- 3Department of Shigatse Branch, Xinqiao Hospital, Third Military Medical University, Shigatse, China
Tumors pose a significant global public health challenge, resulting in numerous fatalities annually. CD8+ T cells play a crucial role in combating tumors; however, their effectiveness is compromised by the tumor itself and the tumor microenvironment (TME), resulting in reduced efficacy of immunotherapy. In this dynamic interplay, extracellular vesicles (EVs) have emerged as pivotal mediators, facilitating direct and indirect communication between tumors and CD8+ T cells. In this article, we provide an overview of how tumor-derived EVs directly regulate CD8+ T cell function by carrying bioactive molecules they carry internally and on their surface. Simultaneously, these EVs modulate the TME, indirectly influencing the efficiency of CD8+ T cell responses. Furthermore, EVs derived from CD8+ T cells exhibit a dual role: they promote tumor immune evasion while also enhancing antitumor activity. Finally, we briefly discuss current prevailing approaches that utilize functionalized EVs based on tumor-targeted therapy and tumor immunotherapy. These approaches aim to present novel perspectives for EV-based tumor treatment strategies, demonstrating potential for advancements in the field.
1 Introduction
Cancer represents a significant global public health concern, with rapidly increasing incidence and mortality rates worldwide (1, 2). Currently, immunotherapy has emerged as a cutting-edge field in oncology, providing highly targeted and potent treatment options (3, 4). Immunotherapy harnesses the patient’ s immune system to combat tumors, presenting several advantages over conventional therapies, including rapid initiation, fewer side effects, and a broader range of treatment possibilities (5). CD8+ T lymphocytes play a critical role in immunotherapy (6, 7), serving as powerful effectors in antitumor immune responses and exhibiting the capacity to recognize and eliminate malignant cells (7–9). Once stimulated, antigen-specific CD8+ T cells undergo clonal expansion and differentiate into cytotoxic CD8+ T cells (CTLs), which then migrate throughout the body to identify and eradicate cancer cells (10, 11).
Although significant progress has been achieved in the field of immunotherapy, a substantial proportion of patients remain unresponsive to these treatment. Recent evidence from both human and murine cancer studies suggests that tumors and the tumor microenvironment (TME) play a crucial role in reshaping CD8+ T cells through various suppressive signals (12, 13). The TME refers to the ecosystem surrounding a tumor inside the body, comprising dendritic cells (DCs), tumor-associated macrophages (TAMs), myeloid-derived suppressor cells (MDSCs), regulatory T cells (Tregs), and other components that contribute to the immunosuppression of CD8+ T cells (14–17). However, the specific regulatory mechanisms through which tumors and the TME influence dysfunctional CD8+ T cells are still not fully understood.
Extracellular vesicles (EVs), which are released by most cells, are membranous vesicle-like structures comprising a lipid bilayer, and containing proteins, lipids, and nucleic acids (including DNA, mRNA, and noncoding RNA) (18–20). Based on their biogenesis and size, EVs are classified into three different categories (Table 1). Exosomes, with diameters ranging from 30 to 150 nm, originate and are released from endosomal compartments. Microvesicles (ectosomes or microparticles), sized between 100 and 1000 nm, bud directly from the plasma membrane. Apoptotic bodies, characterized by their larger sizes spanning from 50-5000 nm, are released from apoptotic cells (24, 43, 44). EVs play a crucial role in intercellular communication, facilitating the transport of various bioactive molecules and are considered as super vital in tumor progression and antitumor immune responses (45, 46). A growing body of research has been dedicated to investigating the involvement of EVs in tumors, primarily focusing on elucidating the direct impact of tumor-derived EVs (TDEs) on CD8+ T cells (47). Nevertheless, these studies often overlook the vital indirect regulatory role of the TME in this intricate process. The current review aims to address this gap by presenting recent study findings on EV-mediated direct and indirect bidirectional communication between CD8+ T cells and tumors. Specifically, we provide an overview of the latest research regarding the direct regulation (bioactive molecules inside or on the surface of EVs) of CD8+ T cells by TDEs. We also review the indirect regulatory effects of TDEs on immune cells within the TME (including DCs, TAMs, and MDSCs). Additionally, we summarize the bidirectional regulatory effects of CD8+ T cell-derived EVs on tumor cells. Furthermore, we explore the use of engineered EVs to activate CD8+ T cells (tumor-targeted therapy and tumor immunotherapy) and enhance their ability to kill tumors. The review offers a comprehensive insight into the interactions among EVs, CD8+ T cells, the TME, and tumors. Additionally, it provides a unique perspective on the therapeutic potentials of EVs in the treatment of tumors.
2 Effects of TDEs on CD8+ T cells
2.1 Direct effects
Tumors have the ability to evade the immune system’s antitumor response by specifically targeting CD8+ T cells that infiltrate the tumor. They employ various mechanisms to accomplish this evasion, such as inhibiting, rendering inactive, or deceiving CTLs, ultimately resulting in the evasion of cancer cells and, in some instances, triggering apoptosis in the T cells themselves (48). With advancements in TDE research, increasing attention is being directed toward comprehending the role of TDEs as communication messengers in inhibiting CD8+ T cells. In this context, we herein explore the mechanisms through which tumor cells directly impact CD8+ T cells via TDEs, with a focus on both the contents and membrane molecules involved (Figure 1).
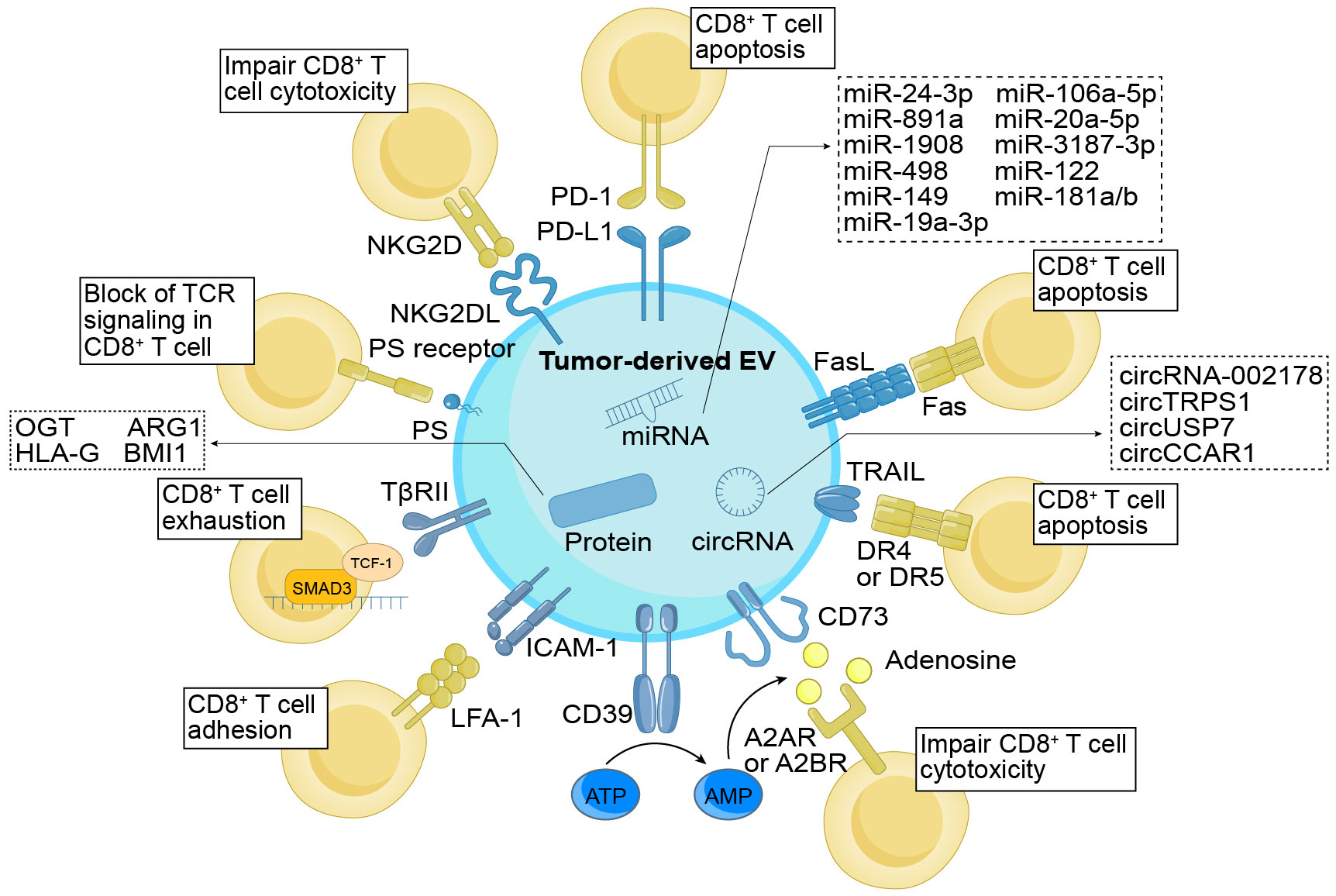
Figure 1 Direct immunomodulatory function of TDEs on CD8+ T cells. TDEs exert regulatory control over CD8+ T cells through their cargo and surface molecules. These surface molecules, including PD-L1, FasL, TRAIL, PS, and NKG2DL, directly interact with cognate ligands or receptors, impeding the activation and cytotoxicity of CD8+ T cells while promoting apoptosis. In addition, TDEs indirectly modulate CD8+ T cell function by influencing molecules like CD39 and CD73, which convert ATP into adenosine, thereby suppressing the cytotoxic abilities of CD8+ T cells. ICAM-1, through its interaction with LFA-1, promotes the adhesion of TDEs and CD8+ T cells. Additionally, TβRII induces CD8+ T cell exhaustion by activating the TGF-β signaling pathway. Simultaneously, the cargo enclosed within TDEs also holds significant importance in immune regulation. The TDEs contained miRNAs, circRNAs, and proteins function by suppressing the proliferation (e.g., miR-24-3p, ARG1, etc.), differentiation (e.g., miR-891a, miR-106a-5p, etc.), activation (e.g., miR-3187-3p, etc.), and cytotoxicity (e.g., HLA-G, etc.) of CD8+ T cells, while facilitating cellular exhaustion (e.g., circUSP7, OGT, etc.). As a result, they effectively undermine the anti-tumor effects of CD8+ T cells. TDEs, tumor-derived extracellular vesicles; PD-L1, programmed cell death ligand-1; FasL, Fas ligand; TRAIL, tumor necrosis factor-related apoptosis-inducing ligand; ICAM-1, intercellular adhesion molecule-1; TβRII, TGF-β type II receptor; PS, phosphatidylserine; NKG2DL, NKG2D ligand; PD-1, programmed cell death protein 1; LFA-1, lymphocyte function-associated antigen-1; TCR, T cell receptor; OGT, O-GlcNAc transferase; ARG1, arginase-1; HLA-G, human leukocyte antigen-G.
2.1.1 Components
Tumor cells employ EVs as carriers to transport a diverse range of cargo, including nucleic acids and proteins, to CD8+ T cells. Interestingly, TDEs containing micro RNAs (miRNAs) can actively participate in modulating of CD8+ T cell immune responses. Ye et al. identified five upregulated miRNAs—miR-24-3p, miR-891a, miR-106a-5p, miR-20a-5p, and miR-1908—from exosomes derived from nasopharyngeal carcinoma cells. They demonstrated that these miRNAs could downregulate the MARK1 signaling pathway, thereby affecting the proliferation and differentiation of CD8+ T cells (49). In EVs derived from melanoma, specific miRNAs, including miR-3187-3p, miR-498, miR-122, miR-149, and miR-181a/b, were found to downregulate T cell receptor (TCR) signaling and the secretion of tumor necrosis factor-α (TNF-α) in CD8+ T cells (50). Furthermore, miR-19a-3p was identified within EVs derived from leukemic cells and subsequently internalized by CD8+ T cells. This internalization induced immunosuppression through the SLC6A8-mediated creatine import (51). These studies provide evidence highlighting the significant roles that miRNAs play in the immunoregulation of CD8+ T cells.
Circular RNAs (circRNAs), an endogenous class of noncoding RNAs, play diverse roles and are integral components in various biological processes, particularly in tumor development and progression (52). Recently, circRNAs within TDEs have emerged as crucial signaling molecules involved in intercellular communication, notably influencing CD8+ T cell function modulation. In lung adenocarcinoma, it was observed that TDEs transported circRNA-002178 to CD8+ T cells, resulting in an upregulation of programmed cell death protein-1 (PD-1) expression and subsequently inducing CD8+ T cell exhaustion (53). Additionally, the presence of circTRPS1 in exosomes released by bladder cancer cells was implicated in inducing CD8+ T cell exhaustion by regulating intracellular reactive oxygen species (ROS) balance along the circTRPS1/miR141-3p/GLS1 pathway (54). In non-small cell lung cancer, TDEs containing circUSP7 were found to play crucial roles in promoting CD8+ T cell exhaustion through the miR-934/SHP2 axis, ultimately leading to immunosuppression (55). Following the absorption of exosomes derived from hepatocellular carcinoma cells containing circCCAR1, CD8+ T cells exhibited elevated exhaustion levels. This was primarily attributed to the inhibition of PD-1 ubiquitin degradation by these exosomes, consequently impairing CD8+ T cell function (56).
In addition to RNAs, TDEs are recognized for transferring proteins to tumor cells, thereby influencing CD8+ T cells. In cholangiocarcinoma, TDEs containing BMI1 impede chemokine recruitment by CD8+ T cells, accomplishing this inhibition through the enhancement of inhibitory H2A ubiquitination in tumor cells via autocrine or paracrine mechanisms (57). Proteins enclosed within TDEs have the capacity to modulate CD8+ T cell behaviors. O-GlcNAc transferase, for instance, enhances tumor growth and migration (58). According to Yuan et al., exosomal O-GlcNAc transferase from esophageal carcinoma stem cells is absorbed by CD8+ T cells, resulting in an upregulation of PD-1 in these cells and thereby protecting esophageal carcinoma stem cells (59). Furthermore, research has demonstrated that EVs containing arginase-1 directly impede CD8+ T cell proliferation by reducing L-arginine levels (60). Moreover, proteins can induce changes in CD8+ T cell phenotypes. Human leukocyte antigen-G (HLA-G) is a recently discovered IC inhibitor that binds to immunoglobulin-like transcript (ILT)-2 and ILT-4, which are inhibitory receptors found on immune cells that play an essential role in facilitating tumor growth (61). Recent evidence indicates that HLA-G EVs enhance the immunosuppressive characteristics of ILT-2-negative CD8+ T cells in breast cancer (BC). However, the specific impact of HLA-G on TDEs is yet to be explored (62).
Overall, gaining a comprehensive understanding of the precise mechanisms through which TDEs transport cargo to CD8+ T cells holds the potential to reveal novel avenues for cancer treatment.
2.1.2 Membrane molecules
The membrane molecules of TDEs play a pivotal role in the tumor immune response, encompassing functions such as immune evasion, modulation of immune cell polarization and function, and suppression of the initiation of antitumor immune responses. Recently, there has been an increasing interest in understanding the regulation of TDE membrane molecules in CD8+ T cells, with a particular focus on ICs as a the most noteworthy aspect. One of the most significant mechanisms in ICs is immunosuppression mediated by the PD-1/PD-L1 pathway. PD-L1 expression is widely observed on immune cells, particularly CD8+ T cells, while residing predominantly on the surface of various tumor cells (63). The interaction between PD-L1 and PD-1 can lead to the inhibition of immune function in CD8+ T cells. Recent research has demonstrated the presence of PD-L1 on the surface of TDEs from various malignancies, including head and neck squamous cell carcinoma, lung cancer, hypopharyngeal cancer, melanoma, and nasopharyngeal carcinoma (64–69). Notably, the expression of PD-L1 on the surface of TDEs is also regulated by various cytokines, such as interferon-γ (IFN-γ), transforming growth factor-β (TGF-β), and type I interferon (IFN-I) (70–74). Apart from PD-L1, other ICs have also been identified on the surface of TDEs. A previous study revealed that TDEs from prostate cancer carry Fas ligand (FasL), inducing the apoptosis of CD8+ T cells (75). Clayton et al. demonstrated that exosomes carry CD39 and CD73, resulting in the inhibition of CD8+ T cells by converting ATP into adenosine (76). Additionally, Azambuja et al. found that TDEs from glioblastoma express FasL, TRAIL, CD39, and CD73, along with a few immunostimulatory proteins, leading to the inhibition of TNF-α and INF-γ released from CD8+ T cells and the induction of apoptosis (77). Overall, these findings indicate that investigating the ICs present in EVs can potentially provide crucial insights into the complex mechanisms through which tumor cells evade immune responses mediated by CD8+ T cells.
In addition to ICs, other molecules present on the surface of TDEs actively participate in suppressing the immune function of CD8+ T cells. A crucial regulatory factor in inducing immune escape is the signal transduction of TGF-β. According to Xie et al., TDEs express TGF-β type II receptor (TβRII) on their surface in BC, and by delivering TβRII to CD8+ T cells, they stimulate TGF-β signal transduction, leading to the exhaustion of these T cells (78). The activating cytotoxicity receptor NKG2D is predominantly expressed on immune cells, including CD8+ T cells, whereas the soluble form of NKG2D ligand (NKG2DL) is utilized by tumors to evade immune responses (79, 80). Clayton et al. found that NKG2DL was presented on the surface of human TDEs and could downregulate the expression of NKG2D receptors on CD8+ T cells (81). Similarly, Lundholm et al. discovered that NKG2DL was also presented on the surface of TDEs derived from prostate cancer (82). Furthermore, adhesion molecules can facilitate interactions between TDEs and CD8+ T cells. Zhang et al. revealed that ICAM-1 was involved in mediating the adhesion between TDEs and CD8+ T cells through its interaction with LFA-1 on the surface of the CD8+ T cells, thus being indispensable for the functionality of exosomal PD-L1 in immunomodulation (83).
Apart from immunosuppressive proteins, the lipids present in EVs also exert inhibitory effects on the functionality of CD8+ T cells. The identification of phosphatidylserine on the surface of TDEs in ovarian tumors has been associated with the induction of a blockade in TCR signaling in CD8+ T cells. This discovery underscores the potential importance of targeting TDE-associated phosphatidylserine as a therapeutic approach for tumor treatment (84).
Based on the above findings from previous studies, TDEs not only carry transport cargoes within their lumen but can also express them on their surface, facilitating the efficient delivery of immunosuppressive cargoes to CD8+ T cells. Consequently, a more in-depth exploration of the specific molecules carried by TDEs is essential for a thorough understanding of the mechanisms underlying CD8+ T cell exhaustion.
2.2 Indirect effects through cells in the TME
The TME is a dynamic and intricate ecosystem during the process of tumorigenesis, comprising various immune cells (such as T and B lymphocytes, TAMs, DCs, natural killer cells, neutrophils, and MDSCs), stromal cells, the extracellular matrix, secreted molecules, as well as the blood and lymphatic vascular networks (85). The TME actively participates in the cancer-immune cycle and significantly influences tumor growth, advancement, and treatment outcomes (86). In the context of tumor therapy, the TME exerts immunomodulatory effects by targeting CD8+ T cells (87). TDEs also play a substantial role in immunomodulation within the TME (88), indirectly impacting CD8+ T cells through the components of the TME. In the subsequent discussion, we focus on the key immune cell populations within the TME and their role in mediating the indirect effects of TDEs on CD8+ T cells (Figure 2).
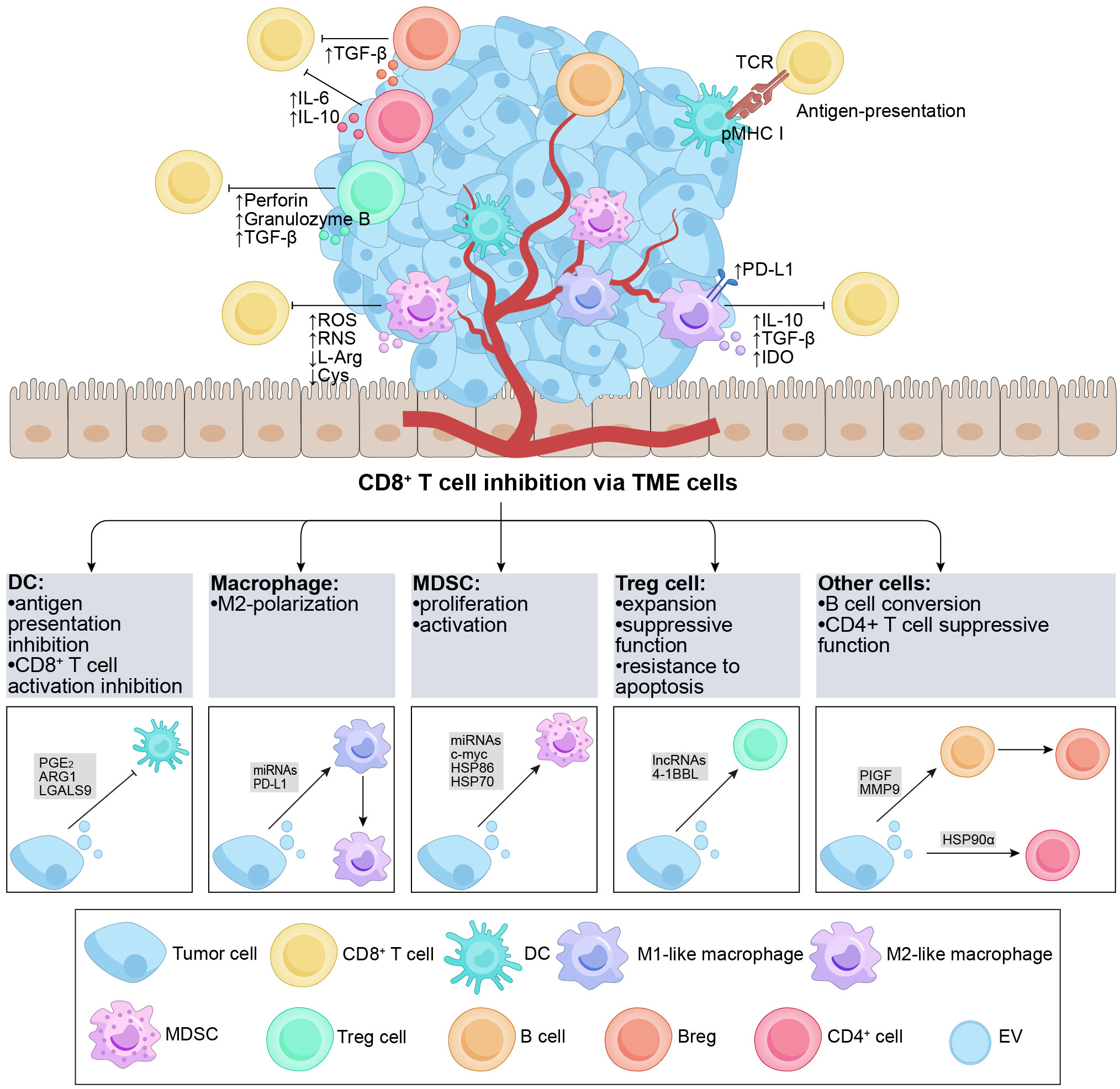
Figure 2 Overview of TDEs indirectly regulate CD8+ T cells via the TME cells. The TME contains various subgroups of immune cells, such as DCs, B cells, macrophages, Tregs, MDSCs, etc. The figure demonstrates the regulatory effects of different cell subgroups on CD8+ T cells. It is noteworthy that TDEs transport a diverse range of regulatory molecules that interact with immune cells in the TME through various pathways, resulting in inhibitory effects on CD8+ T cells. DCs possess the capability to effectively capture and present antigens. TDEs impede the activation of DCs and the presentation of antigens (e.g., PGE2, ARG1, etc.), thereby suppressing the activation of CD8+ T cells. Furthermore, TDEs can also hinder the functionality of CD8+ T cells by influencing cell polarization (e.g., PIGF, MMP9, miR-1231-5p, PD-L1, etc.) in M2-like macrophages and Bregs, ultimately promoting tumor growth. Additionally, TDEs proficiently inhibit anti-tumor immune responses by augmenting the activation, proliferation, and functionality of Tregs and MDSCs (e.g., 4-1BBL, HSP-86, HSP-70, miR-21, etc.). TDEs, tumor-derived extracellular vesicles; TME, tumor microenvironment; DC, dendritic cell; MDSC, myeloid-derived suppressor cell; Treg, regulatory T cell; PGE2, prostaglandin E+2; TCR, T cell receptor; pMHC I, peptide major histocompatibility complex class I; PD-L1, programmed cell death ligand-1; IL, interleukin; TGF-β, transforming growth factor-β; IDO, indoleamine 2,3-dioxygenase; ROS, reactive oxygen species; RNS, reactive nitrogen species; L-Arg, L-Arginine; ARG1, arginase-1; Cys, Cystine; PlGF, placenta growth factor; MMP9, matrix metalloprotease-9; HSP, heat shock protein.
2.2.1 DCs
DCs play a crucial role in initiating and controlling both innate and adaptive immune responses, serving as a distinct group of antigen-presenting cells (89). DCs possess the capability to efficiently capture and cross-present tumor antigens, activating CD8+ T cells and impeding tumor growth and progression (90, 91). Within the TME, TDEs indirectly suppress the immune response of CD8+ T cells by compromising the antigen-presenting function of DCs (88, 92). A study revealed that EVs derived from prostate cancer cells containing prostaglandin E+2 could enhance the expression of the CD73 molecule on the surface of DCs, inhibiting the cross-presentation of tumor-associated antigens by DCs and impairing the activation of CD8+ T cells (93). Similarly, EVs from ovarian cancer cells, containing arginase-1, could be internalized by DCs, impairing the activation of CD8+ T cells (60). Another study highlighted that exosomal LGALS9 from glioblastoma multiforme tumors could bind to the TIM3 receptor of DCs, inhibiting DC antigen presentation and suppressing CD8+ T cell activation (94). Furthermore, Lewis lung cancer cells (with EGFR E746-A750 deletion) secreted exosomes that transferred active E746-A750 deletion to the surface of DCs, resulting in suppressed DC function and enhanced tumor growth (95). Overall, tumor antigens carried by TDEs hinder the cross-presentation of DCs and the expression of maturation markers, ultimately impairing CD8+ T cell activation. This suggests that TDEs participate in immunomodulation within DCs as tumors progress, and the evaluation of the DC phenotype holds prognostic value for patients.
2.2.2 Macrophages
Macrophages are versatile cells that play essential roles in modulating immune responses and tumor development (96, 97). In tumor tissues, infiltrating macrophages, known as TAMs, can undergo polarization, resulting in the formation of M1-like macrophages that exhibit antitumor effects and M2-like macrophages that facilitate tumor growth by inhibiting CD8+ T cells (98, 99). M2 TAMs express PD-L1 and release inhibitory cytokines such as IL-10 and TGF-β, causing exhaustion and directly suppressing CD8+ T cell function. Moreover, M2 TAMs hinder CD8+ T cell proliferation and activity through the indoleamine-2,3-dioxygenase pathway (100). The reported findings suggest that TDEs have the potential to compromise the cytotoxic response of CD8+ T cells by inducing M2 polarization in macrophages. Specifically, exosomal miR-1231-5p and miR-92b-3p derived from bladder cancer cells activate the PTEN/AKT/STAT3/6 pathway, leading to the polarization of macrophages into the M2 phenotype. Consequently, this polarization inhibits the proliferation of CD8+ T cells (101). Meanwhile, one study reported that after the internalization of colorectal cancer-derived exosomes by macrophages, miR-21-5p and miR-200a could trigger the polarization of macrophages into an M2-like phenotype and upregulate the expression of PD-L1. This effect was achieved through the regulation of the PTEN/AKT and SCOS1/STAT1 pathways. Consequently, reduced activity of CD8+ T cells was observed, leading to enhancing tumor growth (102).
Notably, TDEs not only regulate the M2 differentiation of TAMs but also inhibit the function of CD8+ T cells by promoting the expression of PD-1/PD-L1 on the membrane of M2 TAMs. Exosomes derived from gastric cancer cells induce the differentiation of monocytes into PD-1+ TAMs. Upon interaction with PD-L1+ cells, PD-1+ TAMs produce IL-10 and impair the functions of CD8+ T cells (103). Another study reported that upon uptake of tumor cell-released autophagosomes (TRAPs), monocytes activate the MyD88-p38-STAT3 pathway via TLR4 to express an M2-like phenotype, leading to PD-L1 upregulation and IL-10 expression. This further inhibits the function of CD8+ T cells and enhances tumor progression (104).
Multiple factors contribute to the regulation of PD-1/PD-L1 expression in TAMs via TDEs, including endoplasmic reticulum (ER) stress, ROS, and proteins. When hepatocellular carcinoma cells are exposed to ER stress, it leads to the secretion of exosomal miR-23a-3p. This specific miRNA is transferred to M2 TAMs and enhances the expression of PD-L1 through the PTEN/AKT pathway, thereby exerting an inhibitory effect on CD8+ T cell-mediated immune responses (105). Additionally, ER stress-induced BC-derived exosomes containing miR-27a-3p can upregulate PD-L1 in M2 TAMs via the PTEN/AKT/PI3K pathway, ultimately promoting the inhibition of CD8+ T cells (106). In ovarian cancer, a high level of ROS can reduce the uptake of tumor-derived exosomal miR-155-5p by macrophages, resulting in the upregulation of PD-L1 and subsequent suppression of CD8+ T cells (107). Moreover, Golgi membrane protein 1 can inhibit the expression of Rab27b, promoting COP9 signalosome 5-mediated deubiquitination of PD-L1 and enhancing the transport of exosomal PD-L1 into M2 TAMs, ultimately causing increased suppression of CD8+ T cells (108).
Overall, TDEs have the ability to promote M2 polarization of macrophages and utilize immunosuppressive molecules to hinder CD8+ T cell activation.
2.2.3 MDSCs
MDSCs, (pathologically activated neutrophils) and monocytes, play a role in suppressing CD8+ T cell-mediated immune responses through various mechanisms, including the production of ROS and reactive nitrogen species as well as the depletion of L-arginine and cystine in the TME (109). MDSCs are essential components in tumor development, metastasis, and resistance to treatment. Within the TME, TDEs substantially contribute to promoting the proliferation and activation of MDSCs, thereby contributing to the suppression of CD8+ T cells (88). For example, in acute myeloid leukemia, MUC1 within TDEs promotes the expression of c-myc by inhibiting the expression of miR-34a, inducing the proliferation of MDSCs (110). Additionally, research has shown that hypoxia stimulates the release of glioma-derived exosomes, leading to the expression of miR-10a and miR-21 within these exosomes. Consequently, RAR-related orphan receptor alpha and PTEN are activated, resulting in the expansion and activation of MDSCs (111). Moreover, melanoma-derived EVs activate TLR4 on myeloid cells by inducing heat shock protein (HSP) 86 within the exosomes. This activation triggers the NF-κB pathway and upregulates PD-L1 expression, ultimately promoting the conversion of immature myeloid cells into MDSCs (112). In a separate laboratory study, exosomes derived from renal cancer were found to stimulate the proliferation and activation of MDSCs. This effect was attributed to the binding of HSP70 within these EVs to TLR2 receptors expressed on MDSCs. Consequently, this interaction hindered the cytotoxic impact of CD8+ T cells and facilitated the advancement of tumor growth (113). Moreover, a study revealed that miR-9 and miR-181a derived from exosomes in BC had the ability to activate the JAK/STAT signaling pathway. This activation occurred through the targeting of SOCS3 by miR-9 and PIAS3 by miR-181a. Consequently, these molecular interactions promoted early-stage MDSC expansion, inhibited CD8+ T cell proliferation, and induced CD8+ T cell apoptosis, ultimately facilitating tumor growth (114). Another study demonstrated that exosomes derived from BC cells could induce the differentiation of bone marrow cells into MDSCs. Notably, these exosomes significantly inhibited the proliferation of CD8+ T cells through the downregulation of CXCR4 expression and activation of the STAT3 signaling pathway (115). In summary, TDEs play a pivotal role in regulating the differentiation and phenotype of MDSCs by transporting specific cargo to these cells. This process leads to the amplification and activation of MDSCs, thereby providing further support for tumor progression.
2.2.4 Tregs
Tregs play a critical role in maintaining immune balance within the TME. They help prevent excessive immune reactions against normal body tissues (self-antigens) and hinder the immune system’s capacity to initiate a potent antitumor response (116, 117). Tregs primarily limit the activity of CD8+ T cells, employing various inhibitory mechanisms, including the direct killing of CD8+ T cells by activated Tregs through the release of substances like perforin or granzyme B. Additionally, Tregs can release TGF-β, which directly hinders the activation of CD8+ T cells (118).
Several studies have suggested that TDEs indirectly impede the functioning of CD8+ T lymphocytes by modulating Tregs. Within the TME, TDEs utilize receptor-ligand signaling interactions on their surface to facilitate the expansion of Tregs, enhance their suppressor function, and increase their resistance to apoptosis (119–121). In BC, γδ T cells were identified as the predominant lymphocyte population infiltrating the tumor, playing a critical role in immunosuppression (122). The study revealed that CD73+ γδT1 cells were the major subset of T lymphocytes with regulatory characteristics in BC. In another study, TDEs influenced the activation of the TGF-β1/SMAD5 pathway through the SNHG16/miR-16-5p/SMAD5 regulatory axis. This led to the upregulation of CD73 expression, which, in turn, inhibited immune function via adenosine signaling in γδ1 T cells. Consequently, CD73+γδT1 cells exhibited the ability to suppress the secretion of perforin and granzyme B by CD8+ T cells (123). TDEs possess the ability to induce the differentiation of Tregs and facilitate the acquisition of a suppressive phenotype and functional activity in these cells. Leukemic EVs containing 4-1BBL can enhance the suppressive activity and phenotype of Tregs through the regulation of mTOR and STAT5 signaling pathways, resulting in the inhibition of CD8+ T cell proliferation (124). In summary, these studies provide evidence that TDEs can modulate the immune system by influencing Tregs, ultimately leading to increased immunosuppression of CD8+ T cells. Moreover, targeting of Tregs by inhibiting TDEs is a promising approach for tumor treatment.
2.2.5 Other cells
In addition to the aforementioned cell populations, other cell types exert a significant influence on regulating CD8+ T cells. In recent years, EVs have emerged as key players in these processes.
B cells play a significant role in the TME, and recent studies have revealed intriguing findings regarding the impact of TDEs on CD8+ T cell function through their influence on these cells (125). Specifically, these studies have emphasized the role of exosomal placenta growth factor (PlGF), a member of the vascular endothelial growth factor family, released by glioma cells. PlGF has been observed to induce the generation of regulatory B cells (Bregs), subsequently suppressing the activities of CD8+ T cells. Naïve B cells uptake PlGF, promoting their differentiation into TGF-β+ Bregs. This process results in the suppression of CD8+ T cell proliferation and a reduction in the release of granzyme B and perforin (126, 127). Additionally, Li et al. revealed that microvesicles derived from esophageal cancer carry matrix metalloprotease-9 (MMP9), which converts latent transforming growth factor-β (LTGF-β) to its active form. Consequently, B cells transform into TGF-β+ Bregs, thereby suppressing CD8+ T cell activities. These mechanisms potentially contribute to tumor evasion of immune surveillance and facilitate tumor growth (128). Overall, TDEs have the ability to transfer cargo to B cells, inducing their conversion into Bregs, significantly impacting the inhibition of CD8+ T cell function.
CD4+ T cells, a subset of T lymphocytes characterized by the expression of the CD4 molecule on their cell surface, play a crucial role in antitumor immune responses by either enhancing or suppressing cytotoxic T cell activities (129). Although research in this area is limited, studies have reported that CD4+ T cells uptake TDEs, leading to disruptions in the functioning of CD8+ T cells. In malignancies involving fluid accumulation, HSP90α presented on the surface of TRAPs stimulates CD4+ T cells to produce IL-6 and IL-10 through a signaling cascade involving TLR2-MyD88-NF-κB. Importantly, CD4+ T cells activated by these TRAPs suppress the IFN-γ response of both CD4+ and CD8+ effector T cells through the action of IL-6 and IL-10. Consequently, these processes promote tumor growth and metastasis (130).
In summary, TDEs play a significant role in various mechanisms utilized by tumors to evade detection and immune attacks. Tumor antigens carried by TDEs hinder the cross-presentation of DCs and the expression of maturation markers, impair CD8+ T cell activation, and promote the development of tumors ultimately. TDEs that carry specific miRNA could trigger the polarization of macrophages into an M2-like phenotype and upregulate the expression of PD-L1, which reduce activity of CD8+ T cells and lead to enhancing tumor growth. TDEs with specific cargo could regulate the differentiation and phenotype of MDSCs and lead to the amplification and activation of MDSCs, which would inhibit CD8+ T cells and promote tumor growth. TDE carries specific biomolecules to promote Tregs, thereby inhibiting the immune function of T cells and achieving the purpose of promoting tumor development. These mechanisms suggest that TDE affects the growth of cells in the TME by carrying specific biological information molecules, thereby inhibiting the immune response of CD8+ T cells and thus promoting tumor growth. However, research on the impact of TDEs on cells within the TME lags behind studies focused on CD8+ T cells. It is crucial to investigate the functional molecules carried by TDEs to gain a comprehensive understanding of how tumor cells influence CD8+ T cells through interactions with TME cells. Furthermore, the functional molecules carried by TDEs have the potential to be targets for tumor immunotherapy, providing guidance for tumor treatment.
3 Effects of CD8+ T cell-derived EVs on tumors
In the cancer-immunity cycle, CD8+ T cells are widely perceived to directly engage and eliminate tumor cells through contact. Moreover, during the initiation of tumors, CD8+ T cells have the capability to release EVs in response to this event. CD8+ T cell-derived EVs exhibit a dual nature, simultaneously promoting tumor evasion while enhancing antitumor immune responses (Figure 3 and Table 2).
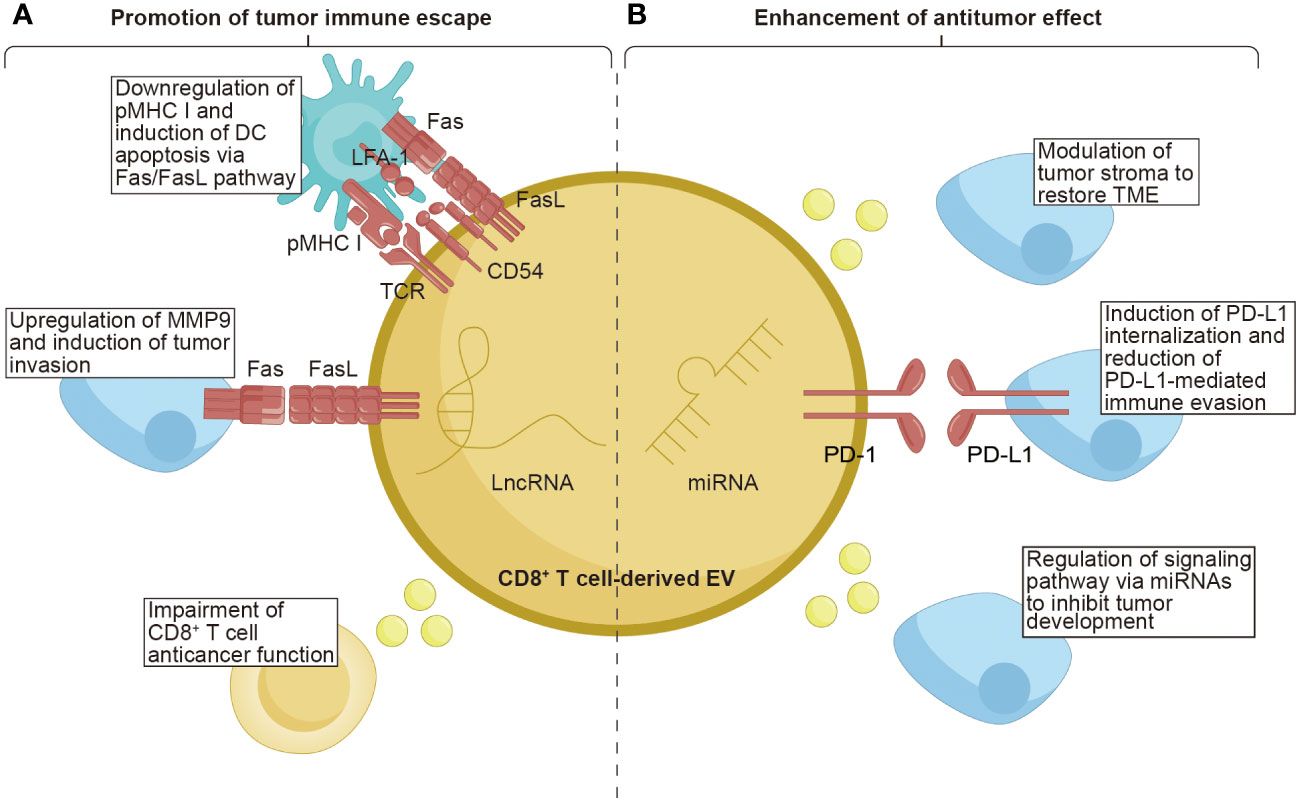
Figure 3 The function of CD8+ T cell-derived EVs on tumors. CD8+ T cell-derived EVs possess the unique properties of both promoting tumor immune escape and enhancing the anti-tumor effect within tumor tissues. (A) CD8+ T cell-derived EVs carrying TCR and FasL lead to the downregulation of pMHC I and induce DCs apoptosis via the Fas/FasL pathway. The FasL, as the ligand for Fas, can upregulate MMP9, promoting tumor invasion. Moreover, EVs from exhausted CD8+ T cells containing lncRNAs have been demonstrated to impair the anticancer function of CD8+ T cells. (B) CD8+ T cell-derived EVs contain miRNAs that exert a role in modulating the tumor stroma, restoring the TME, and regulating signaling pathways to inhibit tumor development. Additionally, the PD-1 molecules present on the surface of EVs derived from CD8+ T cells can induce internalization of PD-L1, thereby reducing PD-L1-mediated immune evasion. TCR, T cell receptor; pMHC I, peptide major histocompatibility complex class I; FasL, Fas ligand; LFA-1, lymphocyte function-associated antigen-1; PD-1, programmed cell death protein 1; PD-L1, programmed cell death ligand-1; MMP9, matrix metalloprotease-9; LncRNAs, long non-coding RNAs; TME, tumor microenvironment.
3.1 Contribution to immune evasion of tumors
Although still limited, some intriguing reports have indicated that CD8+ T cells can secrete EVs as one of the mechanisms through which tumors evade the immune system. Targeting the disruption of the Fas/FasL axis represents a focal point for EVs derived from CD8+ T cells. Xie et al. found that DCs uptook exosomes secreted by CD8+ T cells, expressing TCR and FasL on their surface. This uptake led to the interaction between pMHC I-TCR and CD54-LFA-1 on both DCs and the absorbed exosomes. Consequently, the expression of pMHC I in DCs was downregulated, and the DCs were susceptible to cell death through the Fas/FasL pathway. As a result, the antitumor CD8+ T cell response stimulated by DCs was inhibited (131). Another study showed that activated CD8+ T cells could generate exosomes that carried FasL. These exosomes could promote the invasion of melanoma and lung tumor cells by activating the Fas signaling pathway to upregulate MMP9 expression. Specifically, exosomes derived from activated T cells induced the accumulation of c-FLIP, an endogenous inhibitor of apoptotic death, causing the activation of the ERK and NF-κB pathways. This activation resulted in an increased expression of MMP9 (132).
Continuous antigenic stimulation of CD8+ T cells leads to their exhaustion, resulting in a decline in their functional capacity and proliferation ability (137). Additionally, exhausted CD8+ T cells can release EVs that have detrimental effects on normal T cells. Wang et al. revealed that normal CD8+ T cells are capable of internalizing exosomes released by exhausted CD8+ T cells, compromising their anticancer function. Furthermore, the exosomes derived from exhausted CD8+ T cells were found to harbor long noncoding RNAs implicated in modulating the behavior of normal CD8+ T cells. These findings contribute to our understanding of the varied states of CD8+ T cells and provide valuable insights into dysfunctional anticancer immunity (133). Overall, these studies confirm the immunosuppressive role of CD8+ T cell-derived EVs in tumor progression.
3.2 Enhancement of antitumor effects
Notably, CD8+ T cell-derived EVs exhibit dual effects, as they can both promote tumor metastasis and cancer evasion, as well as enhance antitumor immunity. The tumor stroma, a vital part of the TME, is composed of cells and noncellular connective tissue that provide structural support, exerting a crucial effect on the initiation, progression, and metastasis of tumors (138). Therefore, modulating the tumor stroma is considered an effective approach to restore the TME. Seo et al. revealed that activated CD8+ T cells isolated from healthy mice released cytotoxic EVs containing miR-298-5p, capable of triggering apoptotic depletion of mesenchymal tumor stromal cells. This mechanism effectively inhibited tumor invasion and metastasis (134). Interfering with the interaction between PD-1 and PD-L1 is a crucial factor in enhancing the cytotoxic potential of CD8+ T cell-derived EVs. Qiu et al. discovered that exosomal PD-1 derived from activated T cells played a role in attenuating PD-L1-induced immune evasion in triple-negative BC. This effect was achieved by inducing PD-L1 internalization through clathrin-mediated endocytosis suggesting that PD-1 molecules had a favorable influence on antitumor immune response (135). Manipulating signaling pathways through miRNA modulation serves as a powerful strategy employed by CD8+ T cells to inhibit tumor development. Zhou et al. found that the downregulation of miR-765 promoted cell proliferation, epithelial-mesenchymal transition, and invasion in uterine corpus endometrial cancer by activating the PLP2-Notch signaling pathway. Estrogen/ERβ, a known risk factor for uterine corpus endometrial cancer, regulated the miR-765/PLP2 axis to accelerate disease progression. However, it was observed that CD45RO-CD8+ T cell-derived EVs effectively ameliorated estrogen-driven endometrial cancer development by releasing significant amounts of miR-765 (136). The aforementioned studies indicate that EVs derived from CD8+ T cells can enhance their antitumor capabilities through various mechanisms. These findings highlight the unexplored avenues for CD8+ T cell-derived EVs in cancer therapy, further enriching their potential applications in the field.
It is worth mentioning that CTLs have the ability to secrete exosomes. Upon stimulation by IL-12, these exosomes can activate bystander CD8+ T cells, similar to observations made during infections (139). Additionally, CTL-derived exosomes can facilitate the activation of CTLs through low-affinity peptides in the presence of IL-12 (140). However, further investigation is warranted to explore these processes within the context of tumors.
4 Application of EVs to activate CD8+ T cell antitumor response
Exosomes are stable and have slow clearance with low toxicity and immunogenicity in circulation, moreover, they can spread in tumor tissues and pass the physical barriers like the blood-brain barrier (141). These characteristics of exosomes give them a natural advantage in tumor therapy. We classify exosome-mediated tumor therapy into two categories: tumor-targeted therapy and tumor immunotherapy.
4.1 Tumor-targeted therapy
In targeted tumor therapy, exosomes serve as carriers to deliver targeted therapeutic drugs to tumors. They can stably carry oncologic drugs to avoid enzymatic degradation of drugs, prolong the half-life of drugs during delivery, and target specificity with extremely high bioavailability (141, 142). It has been established that homotypic adhesion molecules on exosome membranes endow exosomes with strong preferential binding to the source cells (143). In addition, MSC-Exo was more preferred to be uptake by cancer cells in tumor tissue (144–146). The homing properties of exosomes make them effective vectors for tumor targeting. As an excellent carrier, exosomes can carry a variety of tumor treatment drugs (like chemotherapeutic drugs (147), radiosensitizing agents (148), photothermal agents (149), sonosensitizers (150), siRNA (151), miRNA (152), and CRISPR/Cas 9 (153)), providing more possibilities for tumor treatment (chemotherapy, radiotherapy, photodynamic therapy, photothermal therapy, sonodynamic therapy, and gene therapy). At present, in order to achieve more efficient tumor treatment, many multifunctional exosome therapy schemes have been designed. Wang et al. developed a multifunctional exosome tumor therapy platform (Exo-DOX-Fe3O4@PDA-MB) that combined chemotherapy, gene therapy, and photothermal therapy. Doxorubicin (DOX) was encapsulated into the isolated exosomes by electroporation, and the exosome membrane was coated with Fe3O4 which was modified with molecular beacon (MB) through polydopamine. The Exo-DOX- Fe3O4@PDA-MB were enriched at the tumor site by external magnetic field guidance. Then the application of near-infrared radiation (NIR) induced localized hyperthermia and triggered the release of DOX loaded inside the exosome, which led to about 91.04% of cancer cells being killed. The released MB could target the miR-21 for both imaging and gene silencing. However, there are still some disadvantages with the native exosomes. To improve the effect of tumor treatment and reduce adverse effects, more and more exosome modification methods have been developed to construct functional exosomes recently (154). Tao et al. engineered exosomes with HER2 ligand (CAR) by biological modification and then inserted transferrin receptor binding peptide modified with DSPE-PEG (DSPE-PEG-T7) into the exosome membrane through hydrophobic action. The obtained ExoCAR/T7 could not only accurately target HER2+ breast cancer cells but also increase the penetration rate of the blood-brain barrier (155). One more, Cho et al. utilized membrane-bound cytokine technology to genetically modify human primary CD8+ T cells, resulting in engineered EVs containing IL-2 and an anti-EGFR antibody on the surface. Each tether molecule of these engineered EVs enhanced tumor cytotoxicity and promoted cancer targeting (156).
4.2 Tumor immunotherapy
Tumor immunotherapy is a novel anti-cancer strategy that activates immune cells, unlike tumor-targeted therapy which primarily targets tumors themselves, these activated immune cells can spread tumor information to other unactivated immune cells throughout the body, and even if tumor cells try to spread to other sites, these primed immune cells can detect and clear them, providing a whole-body defense system for cancer (157, 158). CD8+ T cells play a crucial role in cancer immunotherapy as they are specifically targeted to destroy tumor cells. Among them, the most effective adoptive CD8+ T cell treatment for B cell lymphomas is anti-CD19 chimeric antigen receptor T cell therapy (159). EVs have emerged as a novel cancer immunotherapy in recent years owing to the rapid advancement of cancer research. The exosomes that mediate tumor immunotherapy include tumor cell-derived exosomes, immune cells-derived exosomes, and engineered exosomes. Here we focus on DC- and IC-mediated EV treatment which activate CD8+ T cells for tumor immunotherapy (Table 3).
4.2.1 DC-mediated EV treatment
DCs play a crucial role as mediators of immune responses by capturing, processing, and presenting antigens to CD8+ T cells. This interaction constitutes an essential function in the activation, proliferation, and differentiation of CD8+ T cells, ultimately leading to the elimination of tumor cells and the establishment of long-term immunity. Given the unique characteristics of DCs, DC-mediated EV therapy is emerging as a novel approach in cancer treatment. On one hand, EVs released by DCs directly activate CD8+ T cells, stimulating them to secrete cytokines and cytolytic molecules that contribute to tumor eradication. On the other hand, TDEs act indirectly through DCs to facilitate CD8+ T cells in killing tumor cells.
Currently, DC-derived exosomes (Dex) are being employed as an effective and promising antitumor strategy for CD8+ T cell-mediated immunotherapy. Dex vaccines, considered a novel form of immunotherapy, carry various molecules to exert their immune effects (175). Notably, proteins play a crucial role in Dex vaccines, significantly contributing to their ability to modulate the immune response. An example is the utilization of MUC1 glycopeptide-Dex conjugate vaccines, which enhance the antigen presentation capacity of DCs (Figure 4). These vaccines stimulate the generation of antigen-specific CTLs capable of effectively eliminating tumors and delaying tumor development (160). Ligands, which are proteins that bind to other biomolecules and have biological activities, serve as important regulators of Dex functions. Dex vaccines containing retinoic acid early inducible-1γ, a ligand of NKG2D, have demonstrated the capacity to effectively enhance the proliferation and effector functions of CD8+ T cells. In a mouse model of chronic myeloid leukemia, this vaccine not only exhibited potent therapeutic effects but also induced durable immune memory (161). Chimeric RNA (chiRNA), composed of exons from two or more different genes, can encode novel proteins, thereby altering cellular phenotypes (176). In recent years, chiRNA has been explored for use in Dex vaccines. For instance, the utilization of ASTN2-PAPPAantisense chiRNA-loaded Dex (DEXA-P) in cancer vaccination has shown promising results. This approach promotes antigen cross-presentation, thereby activating CD8+ T cells. In vitro experiments have indicated that DEXA-P vaccination prolongs the survival of mice with esophageal cancer (Figure 5), suggesting that transcription-induced chiRNAs could serve as a source of cancer-specific and mutation-independent neoantigens, demonstrating substantial potential for the treatment of malignancies with low mutational burden or lacking mutation-based antigens (162). Improving antigen presentation poses indeed a significant challenge in the development of Dex-based cancer vaccines. Identifying suitable cargo that effectively enhances antigen presentation and promotes robust immune responses, remains crucial. Determining the appropriate cargo for Dex vaccines is a vital direction for future research, contributing to the advancement of the field and enhancing the effectiveness of cancer immunotherapy approaches.
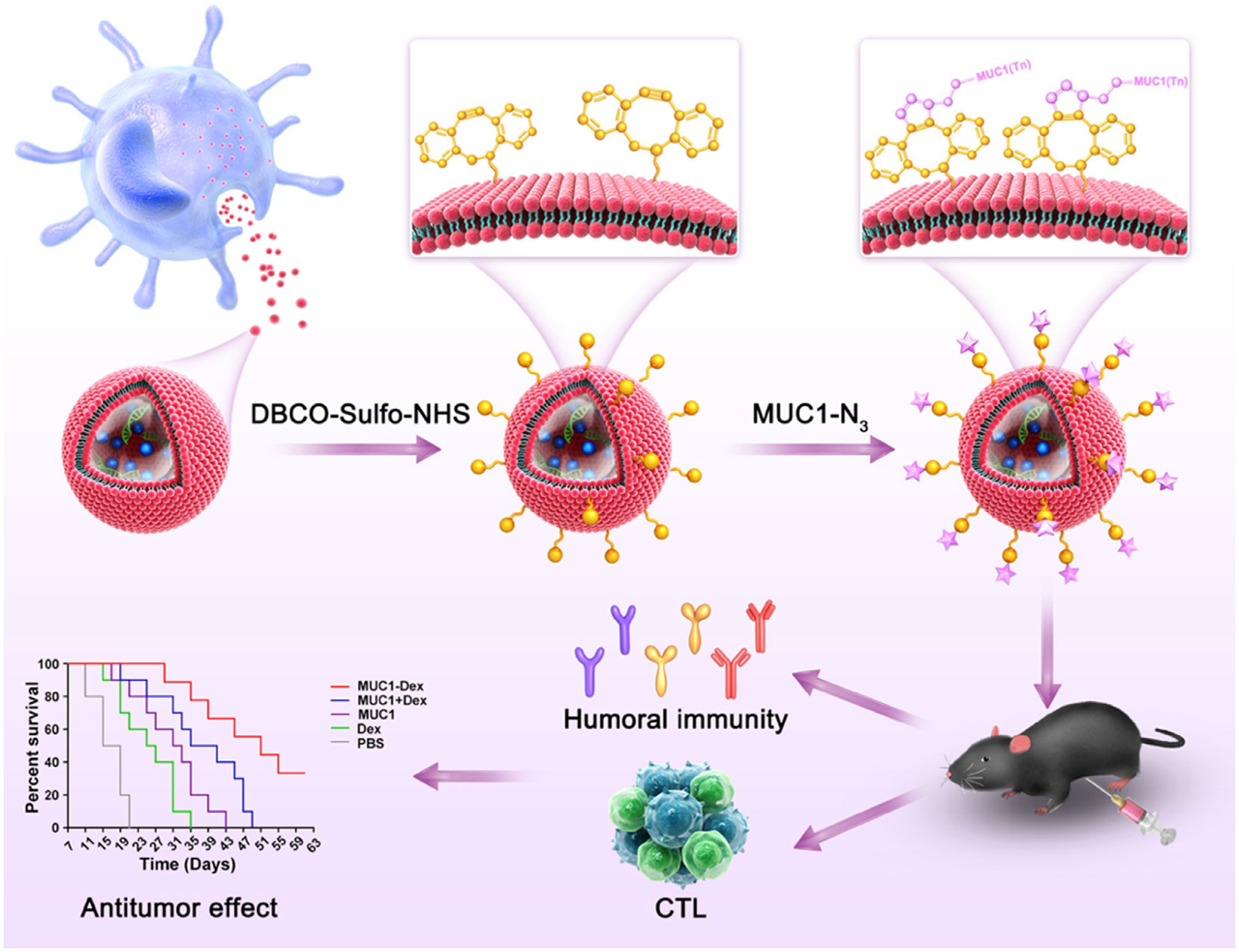
Figure 4 Schematic diagram of the preparation of MUC1 glycopeptide-Dex conjugate vaccines and the enhancement of CTL cytotoxicity (160). Copyright(2022), with permission from Acta Biomaterialia. CTL, cytotoxic CD8+ T cell.
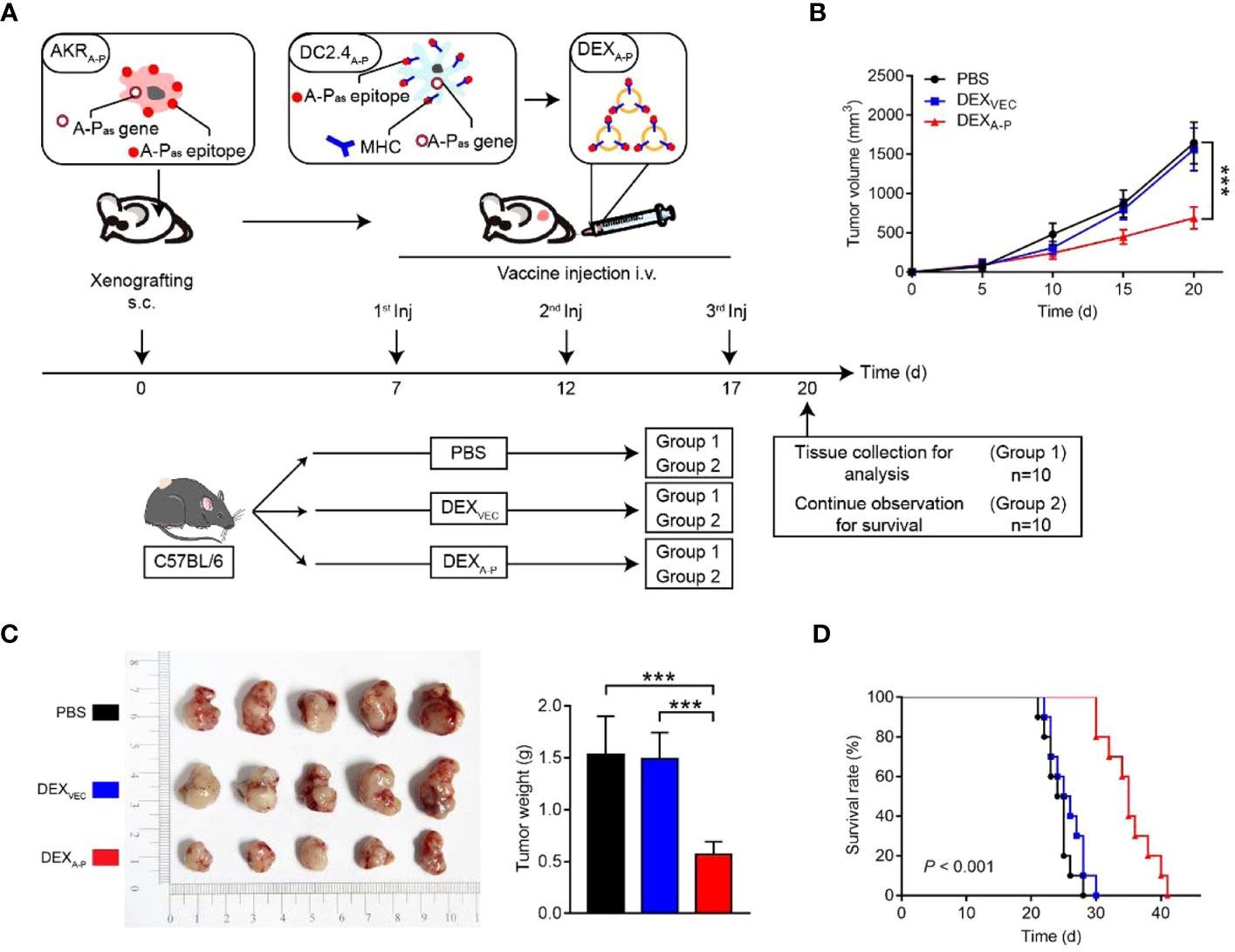
Figure 5 Therapeutic effect of DEXA-P vaccination in esophageal cancer model. (A) DEXA-P were i.v. injected in AKRA-P tumour-bearing mice. Treatment scheme indicates the timing of tumour inoculation and repeated vaccination of C57BL/6 mice. (B) Tumour growth curve after vaccination with DEXA-P, DEXVEC or PBS. (C) Representative images (left panel) and average weights (right panel) of tumours harvested on 20 d after AKRA-P cancer cell inoculation. (D) Kaplan-Meier survival curves of tumour-bearing mice vaccinated with DEXA-P, DEXVEC or PBS (162). Copyright(2022), with permission from Journal Of Extracellular Vesicles. *** p<0.001; DEXA-P, ASTN2-PAPPAantisense chiRNA-loaded dendritic cell-derived extracellular vesicle.
In addition to the previously mentioned strategies, an alternative approach with promising potential involves utilizing TDEs to stimulate CD8+ T cell-mediated immune responses by acting on DCs. Similarly, an effective method for enhancing antitumor immunity mediated by DCs through TDEs is to regulate the cargo carried by these vesicles. A potential strategy involves loading tumor-associated antigens onto TDEs. Microvesicles derived from tumors, carrying the MUC1 tumor glycoantigen, could be utilized to pulse DCs and activate an IFN-γ response mediated by MUC1-specific CD8+ T cells, ultimately promoting an antitumor response (163). Promising results have been observed in enhancing the antigen presentation of DCs by upregulating costimulatory molecules in TDEs. In the case of leukemia, exosomes derived from leukemia cells could be generated using lentiviral vectors encoding CD80 and CD86, two B7 costimulatory molecules. These exosomes exhibit high CD80 and CD86 expression levels. When administered to DCs, they increase the expression of CD80 and CD86, leading to the induction of CTL responses. Consequently, this approach enhances antileukemia immunity (164). Modifying TDEs using miRNAs holds potential for various applications. TDEs loaded with let7i and miR-142 have been shown to promote DC maturation and induce the release of a significant amount of granzyme-B from CD8+ T cells, proving to be effective in suppressing BC (165). The enrichment of miR-155 in TDEs has been observed to promote the differentiation, proliferation, and cytotoxicity of CTLs through DCs. This enhanced immune response effectively controls tumor growth and improves overall survival (166). Furthermore, TDEs can induce DCs to enhance the antitumor effectiveness of CTLs by silencing specific molecules. For instance, in the case of leukemia, exosomes derived from tumor cells with silenced TGF-β1 can effectively promote DC maturation by reducing TGF-β1 expression, resulting in a more effective induction of tumor-specific CTL responses (167). Additionally, exosomes derived from tumor cells with silenced PD-L1 could induce improved DC maturation, consequently leading to antigen-specific CTL responses (168). These studies highlight the feasibility of enhancing the antitumor immunity of DCs through the regulation of molecules on EVs. This approach offers a potential therapeutic strategy to facilitate tumor immunotherapy, aiming to improve treatment outcomes.
Furthermore, alternative approaches for designing EVs to fulfill a similar function are being explored. One such method involves combining engineering techniques with exosomes. Studies have demonstrated that using a lipid-anchoring technique to functionalize Dex membranes with an anti-CTLA-4 antibody could effectively enhance the CTL/Treg ratio (Figure 6), ultimately initiating antitumor T cell responses and inhibiting tumor growth (169). Loading specific cargo into engineered EVs is indeed a viable option. For example, Huang et al. loaded Hiltonol (a TLR3 agonist) and ELANE (human neutrophil elastase, an inducer of immunogenic cell death) into exosomes engineered with α-lactalbumin. The engineered exosomes displayed tremendous potential to enhance the activation of type one conventional DCs in their original location, subsequently leading to the cross-priming of tumor-reactive CD8+ T cell responses (Figure 7) and resulting in potent inhibition of tumor growth (170). It is well established that low molecular weight oligo hyaluronan can activate DCs. In this context, engineered EVs carrying PH20 hyaluronidase could facilitate DC maturation and migration. Importantly, these EVs have a particular affinity for CD103+ DCs, which playing an essential part in activating immune responses of tumor-specific CD8+ T cells. Consequently, this immune activation leads to the suppression of tumor growth (171). Collectively, these approaches offer valuable and innovative insights into the antitumor effects achieved through the utilization of DC-mediated engineered EVs. They effectively demonstrating the therapeutic potential of engineered EVs for treating tumors.
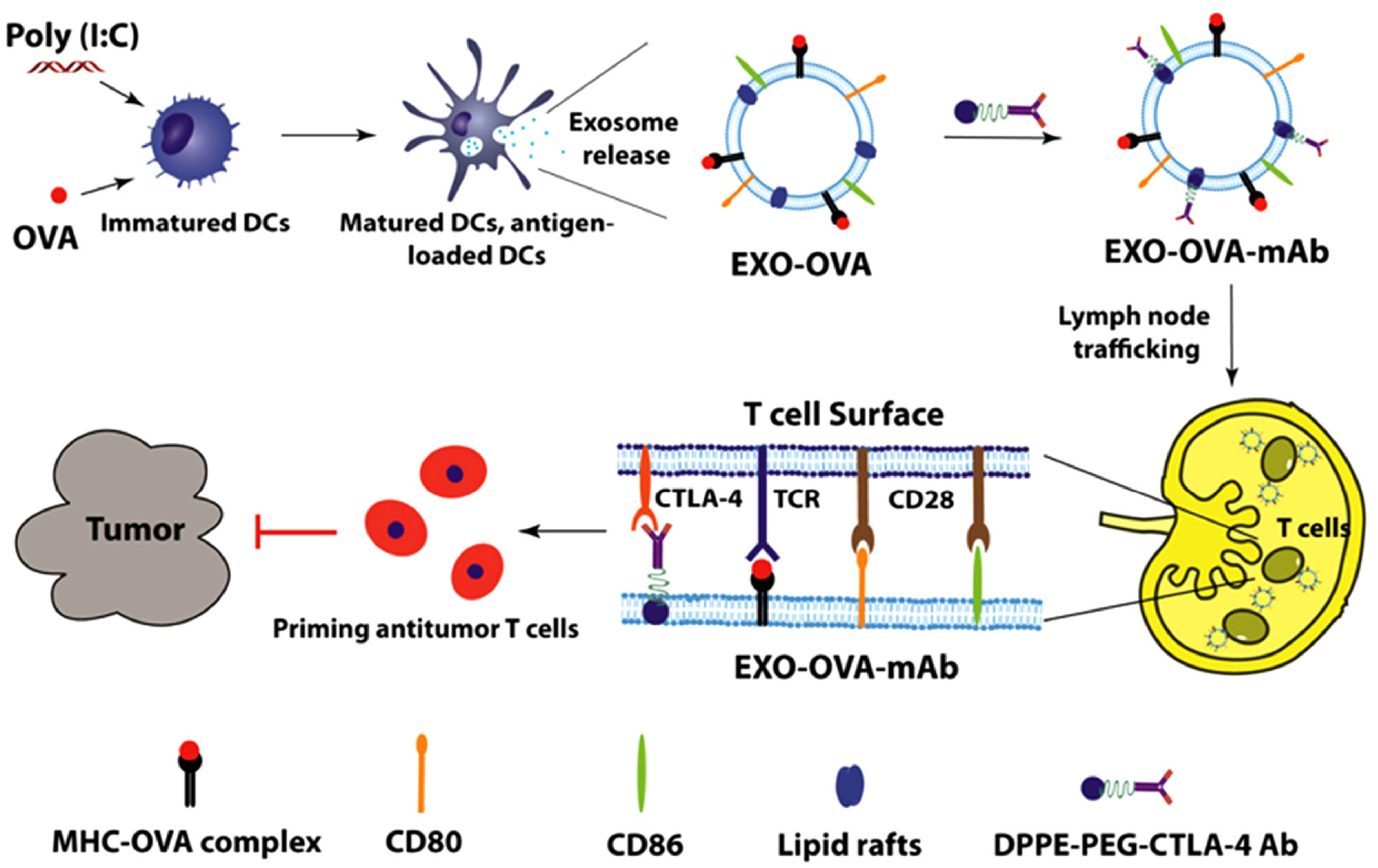
Figure 6 Schematic diagram of the preparation of anti-CTLA-4 antibody-functionalized Dex and the induction of tumor-specific T-cell responses (169). Copyright(2020), with permission from Acta Biomaterialia. Dex, DC-derived EVs.
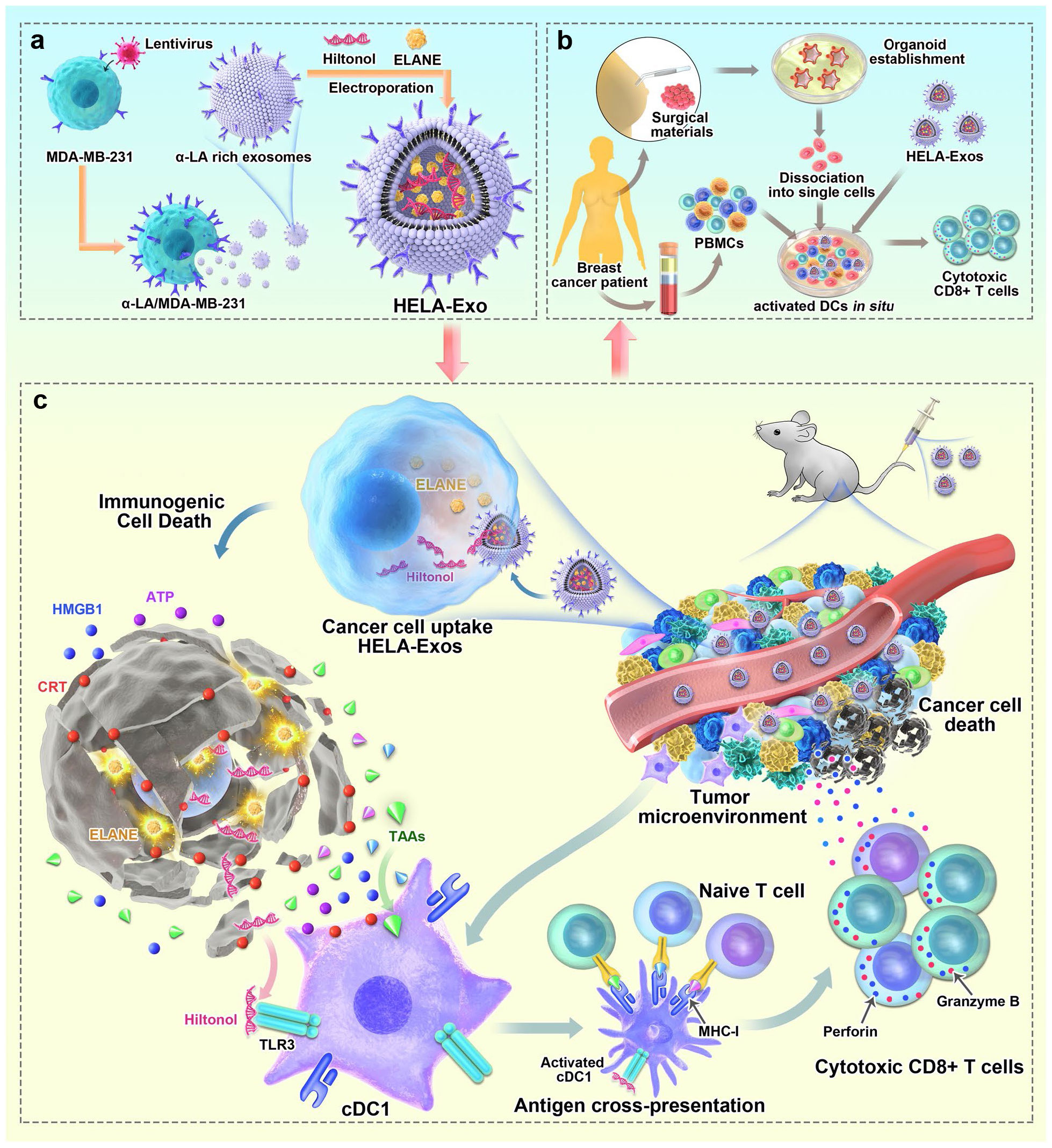
Figure 7 Schematic diagram of the preparation of HELA-Exos and their application as an in situ DC-primed vaccine for BC treatment. (A) The preparation of HELA-Exos. (B) The mechanism of HELA-Exos which activate DCs in situ in a TNBC mouse xenograft model. (C) The activation of DCs in situ by HELA-Exos in the PBMC-autologous tumor organoid coculture system of BC patients (170). Copyright(2022), with permission from Molecular Cancer. HELA-Exos, Hiltonol-ELANE-α-LA-engineered exosomes; BC, breast cancer; DC, dendritic cell; TNBC, triple-negative breast cancer; PBMC, peripheral blood mononuclear cells.
4.2.2 IC-based EV treatment
IC therapy (ICT) has revolutionized anticancer immunotherapy by disrupting the PD-1/PD-L1 interaction and rejuvenating exhausted CD8+ T cells, thereby increasing the antitumor immune response (7). ICT has significantly influenced the landscape of cancer treatment, with several IC-based drugs being approved for various cancers (177). However, it is essential to note that only a subset of patients with specific cancers respond positively to ICT (178, 179). The limited efficacy of ICT in certain cases has prompted researchers to investigate the underlying mechanisms. Interestingly, the immunosuppressive role of EVs has motivated researchers to explore their potential association with ICs.
Currently, there is significant research interest in exploring the correlation between EVs and PD-1/PD-L1. It has been reported that increased levels of PD-L1 on TDEs could inhibit the function of CD8+ T cells and promote cancer growth. This discovery served as the foundation for investigating the potential therapeutic targeting of exosomal PD-L1 (71). In the context of melanoma tumors, HRS phosphorylation restricted the migration of CD8+ T cells toward the tumor location by releasing inhibitory EVs containing PD-L1. This regulatory mechanism played a role in modulating anticancer immunity. Consequently, blocking HRS phosphorylation showed promise as a potentially novel solution for tumor immunotherapy (180).
To overcome limitations observed in ICT for tumors, researchers have made significant progress in identifying drugs that interfere with the PD-1/PD-L1 interaction. One such drug is macitentan, which is administered orally. Macitentan has been found to limit the secretion of PD-L1 by TDEs through its action on endothelin receptor A in BC cells. By attenuating the PD-1 interaction with TDE PD-L1, macitentan enhances the tumor-killing effects of CD8+ T cells. This discovery suggests that macitentan could potentially improve the limited response observed in PD-1/PD-L1 blockade therapy (172). Recent research findings indicate that temsirolimus could evidently limit the secretion of PD-L1 by EVs through the activation of autophagy, helping overcome the limited responsiveness observed in ICT (173). Furthermore, sulfisoxazole has also been identified to be capable of inhibiting the biogenesis of exosomes with PD-L1, thus improving the antitumor effect of anti-PD-1 monotherapy (Figure 8) (174). These findings provide potential avenues for enhancing the therapeutic efficacy of ICT by targeting EV-mediated PD-L1 signaling. However, the precise mechanisms by which these drugs affect EV PD-1/PD-L1 interactions and the role of the TME in this process require further investigation. It is also crucial to identify drugs targeting EV PD-1/PD-L1 that can be translated into clinical use. The exploration of such drugs has become a priority for researchers in this field. Additionally, it is important to investigate the immunosuppressive effects of IC molecules on EVs derived from other cell types and develop drugs to counteract these effects.
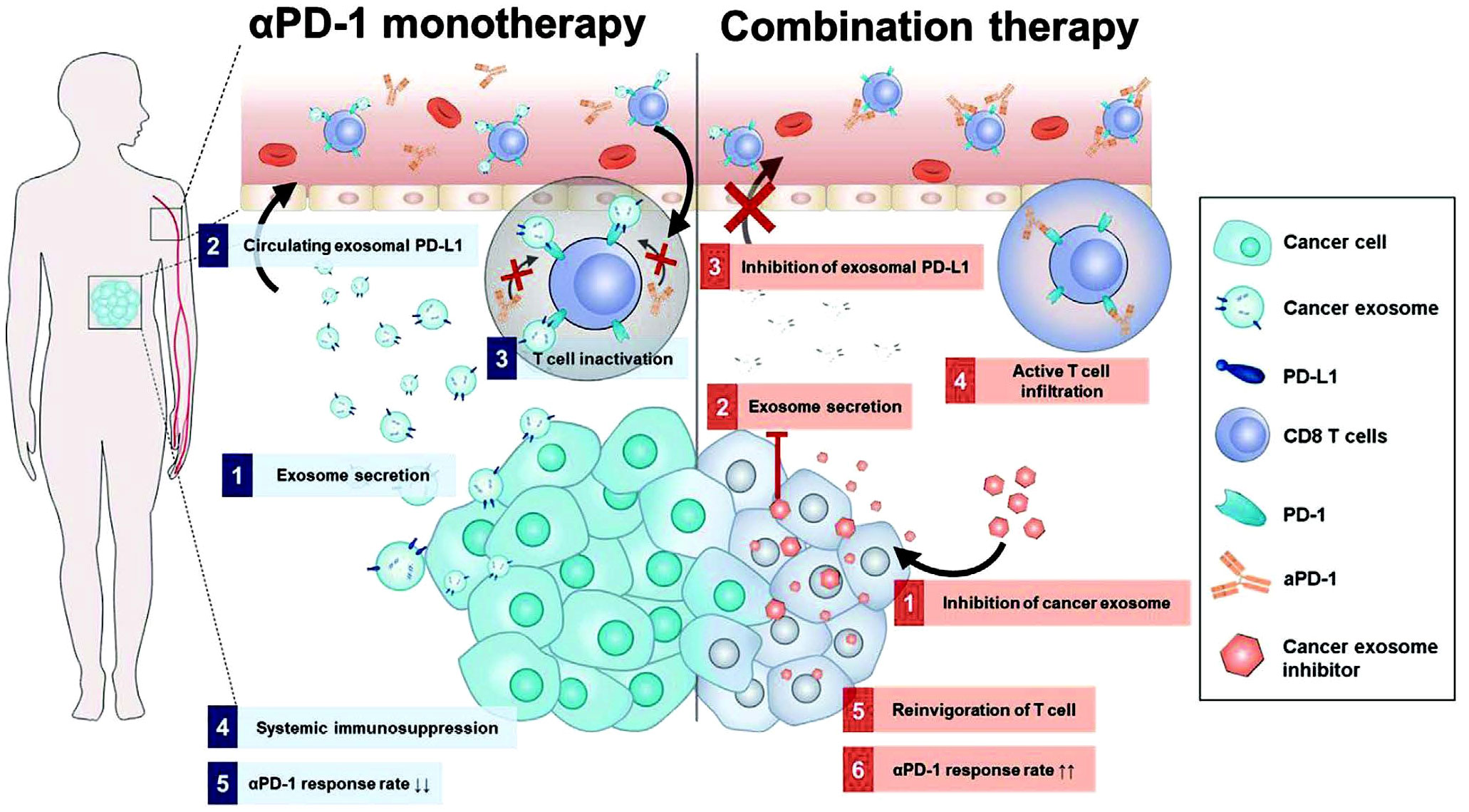
Figure 8 Mechanism diagram of combination treatment using sulfisoxazole and aPD-1. Sulfisoxazole suppresses tumor exosome biogenesis and cooperatively improves aPD-1 antitumor effect. 1) Tumors secrete exosomal PD-L1, which suppresses T cell activation to evade aPD-1 monotherapy immunity. 2) Sulfisoxazole suppresses the biogenesis of exosomes in tumors, thus improving aPD-1 antitumor efficacy (174). Copyright(2022), with permission from Advanced Science. Abbreviations: aPD-1, anti-PD-1.
5 Conclusions
The role of EVs in TME, as an important communication mediators between cells, has become a focus of research in recent years. Previous studies have primarily focused on elucidating the bidirectional communication between CD8+ T cells and tumor cells, as well as between various cells of the TME and tumor cells (47, 181, 182). However, we believe that EVs, serving as pivotal information carriers, exert influence not only on limited bidirectional communication but also on the intricate immunomodulatory network involving CD8+ T cells, TME, and tumor cells. Thus, this review provides insights into the functions performed by EVs in the dynamic interactions among tumors, CD8+ T cells, and the TME. It also highlights the utilization of functionalized EVs based on DCs and ICT in cancer immunotherapy. In particular, TDEs can directly or indirectly suppress the antitumor immune response mediated by CD8+ T cells. This occurs through the delivery of bioactive molecules, including proteins, miRNA, and circRNA, either on their surface or within their cargo. Consequently, CD8+ T cell activation, proliferation, differentiation, and function are inhibited, whereas promoting apoptosis and exhaustion are promoted. Furthermore, TDEs also indirectly hinder CD8+ T cell function by promoting the proliferation and activation of immunosuppressive cells such as MDSCs and Tregs, polarization of M2 TAMs and Bregs, and inhibition of antigen presentation by DCs. These mechanisms collectively facilitate tumor growth within the TME. Conversely, EVs derived from CD8+ T cells exhibit dual regulatory effects on tumors. On one hand, they enhance tumor growth by suppressing the antigen presentation functionality of DCs and promoting tumor cell invasion. On the other hand, through miRNAs and PD-1, these EVs modulate the tumor stroma, restore the TME, and promote PD-L1 internalization, ultimately inhibiting tumor growth. Due to the complexity of TME, numerous unsolved challenges still need to be addressed. The identification of EV origin remains challenging due to the presence of numerous subpopulations. Enhancing technological advancements for determining the source of EV represents a crucial future endeavor. Additionally, our understanding of EVs within the TME, particularly in the context immune cells, remains limited, necessitating further exploration of the intricate interactions between EVs and various immune cells in tumor settings. Targeting these EVs holds promising potential in enhancing the effectiveness of tumor immunotherapy. Conducting more detailed studies will help us better understand the intricate immunomodulatory networks associated with tumors.
Moreover, owing to their unique characteristics, such as traversing biological barriers, transporting molecules, low immunogenicity, and high safety, EVs already show promise as agents for tumor therapy. Based on this information, the review summarizes current common strategies involving functionalized EVs based on tumor-targeted therapy and tumor immunotherapy. We aim to provide new insights into EV-based therapeutic approaches for cancer. In recent years, extensive research on the role of EVs in tumor immunity has opened up diverse possibilities for cancer diagnosis and treatment. By delving into the crucial functions of EVs in regulating tumor immune evasion, activating immune responses, and combating tumor growth, we can achieve a deeper level of understanding and leverage the valuable information and signals carried by these minuscule vesicles. Although there are currently only a few relevant studies on CD8+ T cell-derived EVs in cancer, these EVs hold great potential as a novel approach for cancer treatment. However, several unresolved issues persist in EV preparation and study. Primarily, several methods are available for isolating and purifying EVs, making it challenging to establish standardized protocols for their isolation, purification, and characterization (183, 184). Furthermore, identifying the molecular cargo encapsulated within EVs could yield valuable biomarkers for tumor diagnosis and prognosis, presenting new avenues for early tumor detection (185, 186). Lastly, although the significance of EV PD-L1 in immune evasion is recognized, research on drugs that block EV PD-L1 to reactivate CD8+ T cells remains limited. Hence, the exploration and development of drugs targeting this specific pathway assume paramount importance in tumor immunotherapy. As of March 1, 2024, there are 191 clinical trials on exosome about tumor research searched on the website https://clinicaltrials.gov, and then only a few on tumor immunotherapy of exosomes. There are some controversial reports on whether tumor-derived exosomes and MSC-derived exosomes suppress or promote tumor growth. Besides, until exosome-based therapies enter clinical practice, there is currently a lack of standardized protocols to ensure consistent production of exosomes. At present, there is still a long way to go to realize the clinical application of exosome tumor therapy. Among them, exosome vaccine is the earliest clinical application of exosome in tumor immunotherapy. The researchers induced an immune response by inoculating patients with recurrent glioblastoma with exosomes released after therapeutic tumor cell death (NCT01550523), and the researchers used DC-based exosomes as a vaccine in combination with cyclophosphamide (mCTX) therapy to treat lung cancer (NCT01159288). Continued investigation on the role of EVs in tumor immunity holds the promise of yielding novel breakthroughs and innovations, ultimately expanding the prospects for improved health and increased survival rates among patients.
Author contributions
ZH: Writing – review & editing, Writing – original draft. XL: Writing – review & editing. QG: Writing – original draft. YZ: Writing – original draft. LS: Writing – original draft. QC: Writing – review & editing. ST: Writing – original draft. QO: Writing – review & editing. JZ: Writing – review & editing.
Funding
The author(s) declare that financial support was received for the research, authorship, and/or publication of this article. This work was supported by the Top Young and Middle-aged Medical Talent of Chongqing, Top Young and Middle-aged Medical Studio of Chongqing, Chongqing Science and Health Joint Fund for top young and Middle-aged Talent (2023GDRC007), the Key Project for Clinical Innovation of Army Medical University (CX2019LC107).
Conflict of interest
The authors declare that the research was conducted in the absence of any commercial or financial relationships that could be construed as a potential conflict of interest.
Publisher’s note
All claims expressed in this article are solely those of the authors and do not necessarily represent those of their affiliated organizations, or those of the publisher, the editors and the reviewers. Any product that may be evaluated in this article, or claim that may be made by its manufacturer, is not guaranteed or endorsed by the publisher.
References
1. Siegel RL, Miller KD, Fuchs HE, Jemal A. Cancer statistics, 2022. CA Cancer J Clin. (2022) 72:7–33. doi: 10.3322/caac.21708
2. Sung H, Ferlay J, Siegel RL, Laversanne M, Soerjomataram I, Jemal A, et al. Global cancer statistics 2020: GLOBOCAN estimates of incidence and mortality worldwide for 36 cancers in 185 countries. CA Cancer J Clin. (2021) 71:209–49. doi: 10.3322/caac.21660
3. Cerezo M, Robert C, Liu L, Shen S. The role of mRNA translational control in tumor immune escape and immunotherapy resistance. Cancer Res. (2021) 81:5596–604. doi: 10.1158/0008-5472.CAN-21-1466
4. Fu S, He K, Tian C, Sun H, Zhu C, Bai S, et al. Impaired lipid biosynthesis hinders anti-tumor efficacy of intratumoral iNKT cells. Nat Commun. (2020) 11:438. doi: 10.1038/s41467-020-14332-x
5. Szeto GL, Finley SD. Integrative approaches to cancer immunotherapy. Trends Cancer. (2019) 5:400–10. doi: 10.1016/j.trecan.2019.05.010
6. Farhood B, Najafi M, Mortezaee K. CD8(+) cytotoxic T lymphocytes in cancer immunotherapy: A review. J Cell Physiol. (2019) 234:8509–21. doi: 10.1002/jcp.27782
7. Raskov H, Orhan A, Christensen JP, Gogenur I. Cytotoxic CD8(+) T cells in cancer and cancer immunotherapy. Br J Cancer. (2021) 124:359–67. doi: 10.1038/s41416-020-01048-4
8. Durgeau A, Virk Y, Corgnac S, Mami-Chouaib F. Recent advances in targeting CD8 T-cell immunity for more effective cancer immunotherapy. Front Immunol. (2018) 9:14. doi: 10.3389/fimmu.2018.00014
9. Leclerc M, Voilin E, Gros G, Corgnac S, de Montpreville V, Validire P, et al. Regulation of antitumour CD8 T-cell immunity and checkpoint blockade immunotherapy by Neuropilin-1. Nat Commun. (2019) 10:3345. doi: 10.1038/s41467-019-11280-z
10. Menares E, Galvez-Cancino F, Caceres-Morgado P, Ghorani E, Lopez E, Diaz X, et al. Tissue-resident memory CD8(+) T cells amplify anti-tumor immunity by triggering antigen spreading through dendritic cells. Nat Commun. (2019) 10:4401. doi: 10.1038/s41467-019-12319-x
11. Tang S, Zhou L, Liu Z, Zou L, Xiao M, Huang C, et al. Ceria nanoparticles promoted the cytotoxic activity of CD8(+) T cells by activating NF-kappaB signaling. Biomater Sci. (2019) 7:2533–44. doi: 10.1039/C9BM00113A
12. Jiang Y, Li Y, Zhu B. T-cell exhaustion in the tumor microenvironment. Cell Death Dis. (2015) 6:e1792. doi: 10.1038/cddis.2015.162
13. Thommen DS, Schumacher TN. T cell dysfunction in cancer. Cancer Cell. (2018) 33:547–62. doi: 10.1016/j.ccell.2018.03.012
14. Dong L, Chen C, Zhang Y, Guo P, Wang Z, Li J, et al. The loss of RNA N(6)-adenosine methyltransferase Mettl14 in tumor-associated macrophages promotes CD8(+) T cell dysfunction and tumor growth. Cancer Cell. (2021) 39:945–57 e10. doi: 10.1016/j.ccell.2021.04.016
15. Fang W, Zhou T, Shi H, Yao M, Zhang D, Qian H, et al. Progranulin induces immune escape in breast cancer via up-regulating PD-L1 expression on tumor-associated macrophages (TAMs) and promoting CD8(+) T cell exclusion. J Exp Clin Cancer Res. (2021) 40:4. doi: 10.1186/s13046-020-01786-6
16. Zhao H, Teng D, Yang L, Xu X, Chen J, Jiang T, et al. Myeloid-derived itaconate suppresses cytotoxic CD8(+) T cells and promotes tumour growth. Nat Metab. (2022) 4:1660–73. doi: 10.1038/s42255-022-00676-9
17. Zhou X, Fang D, Liu H, Ou X, Zhang C, Zhao Z, et al. PMN-MDSCs accumulation induced by CXCL1 promotes CD8(+) T cells exhaustion in gastric cancer. Cancer Lett. (2022) 532:215598. doi: 10.1016/j.canlet.2022.215598
18. Li SR, Man QW, Gao X, Lin H, Wang J, Su FC, et al. Tissue-derived extracellular vesicles in cancers and non-cancer diseases: Present and future. J Extracell Vesicles. (2021) 10:e12175. doi: 10.1002/jev2.12175
19. Abels ER, Breakefield XO. Introduction to extracellular vesicles: biogenesis, RNA cargo selection, content, release, and uptake. Cell Mol Neurobiol. (2016) 36:301–12. doi: 10.1007/s10571-016-0366-z
20. Tkach M, Thery C. Communication by extracellular vesicles: where we are and where we need to go. Cell. (2016) 164:1226–32. doi: 10.1016/j.cell.2016.01.043
21. Zhang H, Freitas D, Kim HS, Fabijanic K, Li Z, Chen H, et al. Identification of distinct nanoparticles and subsets of extracellular vesicles by asymmetric flow field-flow fractionation. Nat Cell Biol. (2018) 20:332–43. doi: 10.1038/s41556-018-0040-4
22. Gyorgy B, Szabo TG, Pasztoi M, Pal Z, Misjak P, Aradi B, et al. Membrane vesicles, current state-of-the-art: emerging role of extracellular vesicles. Cell Mol Life Sci. (2011) 68:2667–88. doi: 10.1007/s00018-011-0689-3
23. Keerthikumar S, Gangoda L, Liem M, Fonseka P, Atukorala I, Ozcitti C, et al. Proteogenomic analysis reveals exosomes are more oncogenic than ectosomes. Oncotarget. (2015) 6:15375–96. doi: 10.18632/oncotarget.v6i17
24. Sanwlani R, Gangoda L. Role of extracellular vesicles in cell death and inflammation. Cells. (2021) 10(10):2663. doi: 10.3390/cells10102663
25. Beck S, Hochreiter B, Schmid JA. Extracellular vesicles linking inflammation, cancer and thrombotic risks. Front Cell Dev Biol. (2022) 10:859863. doi: 10.3389/fcell.2022.859863
26. Hoshino A, Costa-Silva B, Shen TL, Rodrigues G, Hashimoto A, Tesic Mark M, et al. Tumour exosome integrins determine organotropic metastasis. Nature. (2015) 527:329–35. doi: 10.1038/nature15756
27. Wang D, Zhao C, Xu F, Zhang A, Jin M, Zhang K, et al. Cisplatin-resistant NSCLC cells induced by hypoxia transmit resistance to sensitive cells through exosomal PKM2. Theranostics. (2021) 11:2860–75. doi: 10.7150/thno.51797
28. Leary N, Walser S, He Y, Cousin N, Pereira P, Gallo A, et al. Melanoma-derived extracellular vesicles mediate lymphatic remodelling and impair tumour immunity in draining lymph nodes. J Extracell Vesicles. (2022) 11:e12197. doi: 10.1002/jev2.12197
29. Li J, Li Z, Jiang P, Peng M, Zhang X, Chen K, et al. Circular RNA IARS (circ-IARS) secreted by pancreatic cancer cells and located within exosomes regulates endothelial monolayer permeability to promote tumor metastasis. J Exp Clin Cancer Res. (2018) 37:177. doi: 10.1186/s13046-018-0822-3
30. Thery C, Zitvogel L, Amigorena S. Exosomes: composition, biogenesis and function. Nat Rev Immunol. (2002) 2:569–79. doi: 10.1038/nri855
31. Menay F, Herschlik L, De Toro J, Cocozza F, Tsacalian R, Gravisaco MJ, et al. Exosomes isolated from ascites of T-cell lymphoma-bearing mice expressing surface CD24 and HSP-90 induce a tumor-specific immune response. Front Immunol. (2017) 8:286. doi: 10.3389/fimmu.2017.00286
32. Ma J, Wei K, Zhang H, Tang K, Li F, Zhang T, et al. Mechanisms by which dendritic cells present tumor microparticle antigens to CD8(+) T cells. Cancer Immunol Res. (2018) 6:1057–68. doi: 10.1158/2326-6066.CIR-17-0716
33. Yan W, Jiang S. Immune cell-derived exosomes in the cancer-immunity cycle. Trends Cancer. (2020) 6:506–17. doi: 10.1016/j.trecan.2020.02.013
34. Kugeratski FG, Kalluri R. Exosomes as mediators of immune regulation and immunotherapy in cancer. FEBS J. (2021) 288:10–35. doi: 10.1111/febs.15558
35. Baj-Krzyworzeka M, Szatanek R, Weglarczyk K, Baran J, Urbanowicz B, Brański P, et al. Tumour-derived microvesicles carry several surface determinants and mRNA of tumour cells and transfer some of these determinants to monocytes. Cancer Immunol Immunother. (2006) 55:808–18. doi: 10.1007/s00262-005-0075-9
36. Melzani G, Bugari G, Parrinello G, Mori G, Manganoni AM, de Panfilis G. Evaluation of soluble fas ligand as a serological marker for melanoma. Dermatology. (2002) 205:111 – 5. doi: 10.1159/000063894
37. Taraboletti G, D'Ascenzo S, Borsotti P, Giavazzi R, Pavan A, Dolo V. Shedding of the matrix metalloproteinases MMP-2, MMP-9, and MT1-MMP as membrane vesicle-associated components by endothelial cells. Am J Pathol. (2002) 160:673–80. doi: 10.1016/S0002-9440(10)64887-0
38. Grange C, Tapparo M, Collino F, Vitillo L, Damasco C, Deregibus MC, et al. Microvesicles released from human renal cancer stem cells stimulate angiogenesis and formation of lung premetastatic niche. Cancer Res. (2011) 71:5346–56. doi: 10.1158/0008-5472.CAN-11-0241
39. Gieseler F, Plattfaut C, Quecke T, Freund A, Ungefroren H, Ender F. Heterogeneity of microvesicles from cancer cell lines under inflammatory stimulation with TNF-alpha. Cell Biol Int. (2018) 42:1533–44. doi: 10.1002/cbin.11040
40. Szubert S, Szpurek D, Moszynski R, Nowicki M, Frankowski A, Sajdak S, et al. Extracellular matrix metalloproteinase inducer (EMMPRIN) expression correlates positively with active angiogenesis and negatively with basic fibroblast growth factor expression in epithelial ovarian cancer. J Cancer Res Clin Oncol. (2014) 140:361–9. doi: 10.1007/s00432-013-1569-z
41. Muralidharan-Chari V, Clancy JW, Sedgwick A, D'Souza-Schorey C. Microvesicles: mediators of extracellular communication during cancer progression. J Cell Sci. (2010) 123:1603–11. doi: 10.1242/jcs.064386
42. Holmgren L, Szeles A, Rajnavölgyi E, Folkman J, Klein G, Ernberg I, et al. Horizontal transfer of DNA by the uptake of apoptotic bodies. Blood. (1999) 93:3956–63. doi: 10.1182/blood.V93.11.3956
43. Thery C, Witwer KW, Aikawa E, Alcaraz MJ, Anderson JD, Andriantsitohaina R, et al. Minimal information for studies of extracellular vesicles 2018 (MISEV2018): a position statement of the International Society for Extracellular Vesicles and update of the MISEV2014 guidelines. J Extracell Vesicles. (2018) 7:1535750. doi: 10.1080/20013078.2018.1535750
44. Russell AE, Sneider A, Witwer KW, Bergese P, Bhattacharyya SN, Cocks A, et al. Biological membranes in EV biogenesis, stability, uptake, and cargo transfer: an ISEV position paper arising from the ISEV membranes and EVs workshop. J Extracell Vesicles. (2019) 8:1684862. doi: 10.1080/20013078.2019.1684862
45. Pegtel DM, Peferoen L, Amor S. Extracellular vesicles as modulators of cell-to-cell communication in the healthy and diseased brain. Philos Trans R Soc Lond B Biol Sci. (2014) 369(1652):20130516. doi: 10.1098/rstb.2013.0516
46. Becker A, Thakur BK, Weiss JM, Kim HS, Peinado H, Lyden D. Extracellular vesicles in cancer: cell-to-cell mediators of metastasis. Cancer Cell. (2016) 30:836–48. doi: 10.1016/j.ccell.2016.10.009
47. Del Vecchio F, Martinez-Rodriguez V, Schukking M, Cocks A, Broseghini E, Fabbri M. Professional killers: The role of extracellular vesicles in the reciprocal interactions between natural killer, CD8+ cytotoxic T-cells and tumour cells. J Extracell Vesicles. (2021) 10:e12075. doi: 10.1002/jev2.12075
48. Barsouk A, Rawla P, Hadjinicolaou AV, Aluru JS, Barsouk A. Targeted therapies and immunotherapies in the treatment of esophageal cancers. Med Sci (Basel). (2019) 7(10):100. doi: 10.3390/medsci7100100
49. Ye SB, Li ZL, Luo DH, Huang BJ, Chen YS, Zhang XS, et al. Tumor-derived exosomes promote tumor progression and T-cell dysfunction through the regulation of enriched exosomal microRNAs in human nasopharyngeal carcinoma. Oncotarget. (2014) 5:5439–52. doi: 10.18632/oncotarget.v5i14
50. Vignard V, Labbe M, Marec N, Andre-Gregoire G, Jouand N, Fonteneau JF, et al. MicroRNAs in tumor exosomes drive immune escape in melanoma. Cancer Immunol Res. (2020) 8:255–67. doi: 10.1158/2326-6066.CIR-19-0522
51. Peng M, Ren J, Jing Y, Jiang X, Xiao Q, Huang J, et al. Tumour-derived small extracellular vesicles suppress CD8+ T cell immune function by inhibiting SLC6A8-mediated creatine import in NPM1-mutated acute myeloid leukaemia. J Extracell Vesicles. (2021) 10:e12168. doi: 10.1002/jev2.12168
52. Kristensen LS, Jakobsen T, Hager H, Kjems J. The emerging roles of circRNAs in cancer and oncology. Nat Rev Clin Oncol. (2022) 19:188–206. doi: 10.1038/s41571-021-00585-y
53. Wang J, Zhao X, Wang Y, Ren F, Sun D, Yan Y, et al. circRNA-002178 act as a ceRNA to promote PDL1/PD1 expression in lung adenocarcinoma. Cell Death Dis. (2020) 11:32. doi: 10.1038/s41419-020-2230-9
54. Yang C, Wu S, Mou Z, Zhou Q, Dai X, Ou Y, et al. Exosome-derived circTRPS1 promotes Malignant phenotype and CD8+ T cell exhaustion in bladder cancer microenvironments. Mol Ther. (2022) 30:1054–70. doi: 10.1016/j.ymthe.2022.01.022
55. Chen SW, Zhu SQ, Pei X, Qiu BQ, Xiong D, Long X, et al. Cancer cell-derived exosomal circUSP7 induces CD8(+) T cell dysfunction and anti-PD1 resistance by regulating the miR-934/SHP2 axis in NSCLC. Mol Cancer. (2021) 20:144. doi: 10.1186/s12943-021-01448-x
56. Hu Z, Chen G, Zhao Y, Gao H, Li L, Yin Y, et al. Exosome-derived circCCAR1 promotes CD8 + T-cell dysfunction and anti-PD1 resistance in hepatocellular carcinoma. Mol Cancer. (2023) 22:55. doi: 10.1186/s12943-023-01759-1
57. Liu Z, Hu C, Zheng L, Liu J, Li K, Li X, et al. BMI1 promotes cholangiocarcinoma progression and correlates with antitumor immunity in an exosome-dependent manner. Cell Mol Life Sci. (2022) 79:469. doi: 10.1007/s00018-022-04500-1
58. Jiang M, Xu B, Li X, Shang Y, Chu Y, Wang W, et al. O-GlcNAcylation promotes colorectal cancer metastasis via the miR-101-O-GlcNAc/EZH2 regulatory feedback circuit. Oncogene. (2019) 38:301–16. doi: 10.1038/s41388-018-0435-5
59. Yuan Y, Wang L, Ge D, Tan L, Cao B, Fan H, et al. Exosomal O-GlcNAc transferase from esophageal carcinoma stem cell promotes cancer immunosuppression through up-regulation of PD-1 in CD8(+) T cells. Cancer Lett. (2021) 500:98–106. doi: 10.1016/j.canlet.2020.12.012
60. Czystowska-Kuzmicz M, Sosnowska A, Nowis D, Ramji K, Szajnik M, Chlebowska-Tuz J, et al. Small extracellular vesicles containing arginase-1 suppress T-cell responses and promote tumor growth in ovarian carcinoma. Nat Commun. (2019) 10:3000. doi: 10.1038/s41467-019-10979-3
61. Lin A, Yan WH. HLA-G/ILTs targeted solid cancer immunotherapy: opportunities and challenges. Front Immunol. (2021) 12:698677. doi: 10.3389/fimmu.2021.698677
62. Schwich E, Ho GT, LeMaoult J, Bade-Doding C, Carosella ED, Horn PA, et al. Soluble HLA-G and HLA-G bearing extracellular vesicles affect ILT-2 positive and ILT-2 negative CD8 T cells complementary. Front Immunol. (2020) 11:2046. doi: 10.3389/fimmu.2020.02046
63. Dermani FK, Samadi P, Rahmani G, Kohlan AK, Najafi R. PD-1/PD-L1 immune checkpoint: Potential target for cancer therapy. J Cell Physiol. (2019) 234:1313–25. doi: 10.1002/jcp.27172
64. Theodoraki MN, Yerneni SS, Hoffmann TK, Gooding WE, Whiteside TL. Clinical significance of PD-L1(+) exosomes in plasma of head and neck cancer patients. Clin Cancer Res. (2018) 24:896–905. doi: 10.1158/1078-0432.CCR-17-2664
65. Kim DH, Kim H, Choi YJ, Kim SY, Lee JE, Sung KJ, et al. Exosomal PD-L1 promotes tumor growth through immune escape in non-small cell lung cancer. Exp Mol Med. (2019) 51:1–13. doi: 10.1038/s12276-019-0295-2
66. Chen J, Song Y, Miao F, Chen G, Zhu Y, Wu N, et al. PDL1-positive exosomes suppress antitumor immunity by inducing tumor-specific CD8(+) T cell exhaustion during metastasis. Cancer Sci. (2021) 112:3437–54. doi: 10.1111/cas.15033
67. Gao Q, Liu HT, Xu YQ, Zhang L, Liu YR, Ren Q, et al. Serum-derived exosomes promote CD8+ T cells to overexpress PD-1, affecting the prognosis of hypopharyngeal carcinoma. Cancer Cell Int. (2021) 21:584. doi: 10.1186/s12935-021-02294-z
68. Dou X, Hua Y, Chen Z, Chao F, Li M. Extracellular vesicles containing PD-L1 contribute to CD8+ T-cell immune suppression and predict poor outcomes in small cell lung cancer. Clin Exp Immunol. (2022) 207:307–17. doi: 10.1093/cei/uxac006
69. Yang J, Chen J, Liang H, Yu Y. Nasopharyngeal cancer cell-derived exosomal PD-L1 inhibits CD8+ T-cell activity and promotes immune escape. Cancer Sci. (2022) 113:3044–54. doi: 10.1111/cas.15433
70. Castiello L, Sestili P, Schiavoni G, Dattilo R, Monque DM, Ciaffoni F, et al. Disruption of IFN-I signaling promotes HER2/neu tumor progression and breast cancer stem cells. Cancer Immunol Res. (2018) 6:658–70. doi: 10.1158/2326-6066.CIR-17-0675
71. Chen G, Huang AC, Zhang W, Zhang G, Wu M, Xu W, et al. Exosomal PD-L1 contributes to immunosuppression and is associated with anti-PD-1 response. Nature. (2018) 560:382–6. doi: 10.1038/s41586-018-0392-8
72. Chatterjee S, Chatterjee A, Jana S, Dey S, Roy H, Das MK, et al. Transforming growth factor beta orchestrates PD-L1 enrichment in tumor-derived exosomes and mediates CD8 T-cell dysfunction regulating early phosphorylation of TCR signalome in breast cancer. Carcinogenesis. (2021) 42:38–47. doi: 10.1093/carcin/bgaa092
73. Gong W, Donnelly CR, Heath BR, Bellile E, Donnelly LA, Taner HF, et al. Cancer-specific type-I interferon receptor signaling promotes cancer stemness and effector CD8+ T-cell exhaustion. Oncoimmunology. (2021) 10:1997385. doi: 10.1080/2162402X.2021.1997385
74. Fu Q, Liu X, Xia H, Li Y, Yu Z, Liu B, et al. Interferon-gamma induces immunosuppression in salivary adenoid cystic carcinoma by regulating programmed death ligand 1 secretion. Int J Oral Sci. (2022) 14:47. doi: 10.1038/s41368-022-00197-x
75. Abusamra AJ, Zhong Z, Zheng X, Li M, Ichim TE, Chin JL, et al. Tumor exosomes expressing Fas ligand mediate CD8+ T-cell apoptosis. Blood Cells Mol Dis. (2005) 35:169–73. doi: 10.1016/j.bcmd.2005.07.001
76. Clayton A, Al-Taei S, Webber J, Mason MD, Tabi Z. Cancer exosomes express CD39 and CD73, which suppress T cells through adenosine production. J Immunol. (2011) 187:676–83. doi: 10.4049/jimmunol.1003884
77. Azambuja JH, Ludwig N, Yerneni S, Rao A, Braganhol E, Whiteside TL. Molecular profiles and immunomodulatory activities of glioblastoma-derived exosomes. Neurooncol Adv. (2020) 2:vdaa056. doi: 10.1093/noajnl/vdaa056
78. Xie F, Zhou X, Su P, Li H, Tu Y, Du J, et al. Breast cancer cell-derived extracellular vesicles promote CD8(+) T cell exhaustion via TGF-beta type II receptor signaling. Nat Commun. (2022) 13:4461. doi: 10.1038/s41467-022-31250-2
79. Dhar P, Wu JD. NKG2D and its ligands in cancer. Curr Opin Immunol. (2018) 51:55–61. doi: 10.1016/j.coi.2018.02.004
80. Wensveen FM, Jelencic V, Polic B. NKG2D: A master regulator of immune cell responsiveness. Front Immunol. (2018) 9:441. doi: 10.3389/fimmu.2018.00441
81. Clayton A, Mitchell JP, Court J, Linnane S, Mason MD, Tabi Z. Human tumor-derived exosomes down-modulate NKG2D expression. J Immunol. (2008) 180:7249–58. doi: 10.4049/jimmunol.180.11.7249
82. Lundholm M, Schroder M, Nagaeva O, Baranov V, Widmark A, Mincheva-Nilsson L, et al. Prostate tumor-derived exosomes down-regulate NKG2D expression on natural killer cells and CD8+ T cells: mechanism of immune evasion. PloS One. (2014) 9:e108925. doi: 10.1371/journal.pone.0108925
83. Zhang W, Zhong W, Wang B, Yang J, Yang J, Yu Z, et al. ICAM-1-mediated adhesion is a prerequisite for exosome-induced T cell suppression. Dev Cell. (2022) 57:329–43 e7. doi: 10.1016/j.devcel.2022.01.002
84. Kelleher RJ Jr., Balu-Iyer S, Loyall J, Sacca AJ, Shenoy GN, Peng P, et al. Extracellular vesicles present in human ovarian tumor microenvironments induce a phosphatidylserine-dependent arrest in the T-cell signaling cascade. Cancer Immunol Res. (2015) 3:1269–78. doi: 10.1158/2326-6066.CIR-15-0086
85. Bejarano L, Jordāo MJC, Joyce JA. Therapeutic targeting of the tumor microenvironment. Cancer Discov. (2021) 11:933–59. doi: 10.1158/2159-8290.CD-20-1808
86. Kim SK, Cho SW. The evasion mechanisms of cancer immunity and drug intervention in the tumor microenvironment. Front Pharmacol. (2022) 13:868695. doi: 10.3389/fphar.2022.868695
87. Genova C, Dellepiane C, Carrega P, Sommariva S, Ferlazzo G, Pronzato P, et al. Therapeutic implications of tumor microenvironment in lung cancer: focus on immune checkpoint blockade. Front Immunol. (2021) 12:799455. doi: 10.3389/fimmu.2021.799455
88. Wang M, Zhang B. The immunomodulation potential of exosomes in tumor microenvironment. J Immunol Res. (2021) 2021:3710372. doi: 10.1155/2021/3710372
89. Wculek SK, Cueto FJ, Mujal AM, Melero I, Krummel MF, Sancho D. Dendritic cells in cancer immunology and immunotherapy. Nat Rev Immunol. (2020) 20:7–24. doi: 10.1038/s41577-019-0210-z
90. Fu C, Jiang A. Dendritic cells and CD8 T cell immunity in tumor microenvironment. Front Immunol. (2018) 9:3059. doi: 10.3389/fimmu.2018.03059
91. Jhunjhunwala S, Hammer C, Delamarre L. Antigen presentation in cancer: insights into tumour immunogenicity and immune evasion. Nat Rev Cancer. (2021) 21:298–312. doi: 10.1038/s41568-021-00339-z
92. Ma F, Vayalil J, Lee G, Wang Y, Peng G. Emerging role of tumor-derived extracellular vesicles in T cell suppression and dysfunction in the tumor microenvironment. J Immunother Cancer. (2021) 9(10):e003217. doi: 10.1136/jitc-2021-003217
93. Salimu J, Webber J, Gurney M, Al-Taei S, Clayton A, Tabi Z. Dominant immunosuppression of dendritic cell function by prostate-cancer-derived exosomes. J Extracell Vesicles. (2017) 6:1368823. doi: 10.1080/20013078.2017.1368823
94. Wang M, Cai Y, Peng Y, Xu B, Hui W, Jiang Y. Exosomal LGALS9 in the cerebrospinal fluid of glioblastoma patients suppressed dendritic cell antigen presentation and cytotoxic T-cell immunity. Cell Death Dis. (2020) 11:896. doi: 10.1038/s41419-020-03042-3
95. Yu S, Sha H, Qin X, Chen Y, Li X, Shi M, et al. EGFR E746-A750 deletion in lung cancer represses antitumor immunity through the exosome-mediated inhibition of dendritic cells. Oncogene. (2020) 39:2643–57. doi: 10.1038/s41388-020-1182-y
96. Chen D, Zhang X, Li Z, Zhu B. Metabolic regulatory crosstalk between tumor microenvironment and tumor-associated macrophages. Theranostics. (2021) 11:1016–30. doi: 10.7150/thno.51777
97. Yang D, Yang L, Cai J, Hu X, Li H, Zhang X, et al. A sweet spot for macrophages: Focusing on polarization. Pharmacol Res. (2021) 167:105576. doi: 10.1016/j.phrs.2021.105576
98. Pan Y, Yu Y, Wang X, Zhang T. Tumor-associated macrophages in tumor immunity. Front Immunol. (2020) 11:583084. doi: 10.3389/fimmu.2020.583084
99. Han S, Wang W, Wang S, Yang T, Zhang G, Wang D, et al. Tumor microenvironment remodeling and tumor therapy based on M2-like tumor associated macrophage-targeting nano-complexes. Theranostics. (2021) 11:2892–916. doi: 10.7150/thno.50928
100. Quaranta V, Schmid MC. Macrophage-mediated subversion of anti-tumour immunity. Cells. (2019) 8(7):747. doi: 10.3390/cells8070747
101. Jiang Z, Zhang Y, Zhang Y, Jia Z, Zhang Z, Yang J. Cancer derived exosomes induce macrophages immunosuppressive polarization to promote bladder cancer progression. Cell Commun Signal. (2021) 19:93. doi: 10.1186/s12964-021-00768-1
102. Yin Y, Liu B, Cao Y, Yao S, Liu Y, Jin G, et al. Colorectal cancer-derived small extracellular vesicles promote tumor immune evasion by upregulating PD-L1 expression in tumor-associated macrophages. Adv Sci (Weinh). (2022) 9:2102620. doi: 10.1002/advs.202102620
103. Wang F, Li B, Wei Y, Zhao Y, Wang L, Zhang P, et al. Tumor-derived exosomes induce PD1(+) macrophage population in human gastric cancer that promotes disease progression. Oncogenesis. (2018) 7:41. doi: 10.1038/s41389-018-0049-3
104. Wen ZF, Liu H, Gao R, Zhou M, Ma J, Zhang Y, et al. Tumor cell-released autophagosomes (TRAPs) promote immunosuppression through induction of M2-like macrophages with increased expression of PD-L1. J Immunother Cancer. (2018) 6:151. doi: 10.1186/s40425-018-0452-5
105. Liu J, Fan L, Yu H, Zhang J, He Y, Feng D, et al. Endoplasmic reticulum stress causes liver cancer cells to release exosomal miR-23a-3p and up-regulate programmed death ligand 1 expression in macrophages. Hepatology. (2019) 70:241–58. doi: 10.1002/hep.30607
106. Yao X, Tu Y, Xu Y, Guo Y, Yao F, Zhang X. Endoplasmic reticulum stress-induced exosomal miR-27a-3p promotes immune escape in breast cancer via regulating PD-L1 expression in macrophages. J Cell Mol Med. (2020) 24:9560–73. doi: 10.1111/jcmm.15367
107. Li X, Wang S, Mu W, Barry J, Han A, Carpenter RL, et al. Reactive oxygen species reprogram macrophages to suppress antitumor immune response through the exosomal miR-155-5p/PD-L1 pathway. J Exp Clin Cancer Res. (2022) 41:41. doi: 10.1186/s13046-022-02244-1
108. Chen J, Lin Z, Liu L, Zhang R, Geng Y, Fan M, et al. GOLM1 exacerbates CD8(+) T cell suppression in hepatocellular carcinoma by promoting exosomal PD-L1 transport into tumor-associated macrophages. Signal Transduct Target Ther. (2021) 6:397. doi: 10.1038/s41392-021-00784-0
109. Schouppe E, Van Overmeire E, Laoui D, Keirsse J, Van Ginderachter JA. Modulation of CD8(+) T-cell activation events by monocytic and granulocytic myeloid-derived suppressor cells. Immunobiology. (2013) 218:1385–91. doi: 10.1016/j.imbio.2013.07.003
110. Pyzer AR, Stroopinsky D, Rajabi H, Washington A, Tagde A, Coll M, et al. MUC1-mediated induction of myeloid-derived suppressor cells in patients with acute myeloid leukemia. Blood. (2017) 129:1791–801. doi: 10.1182/blood-2016-07-730614
111. Guo X, Qiu W, Liu Q, Qian M, Wang S, Zhang Z, et al. Immunosuppressive effects of hypoxia-induced glioma exosomes through myeloid-derived suppressor cells via the miR-10a/Rora and miR-21/Pten Pathways. Oncogene. (2018) 37:4239–59. doi: 10.1038/s41388-018-0261-9
112. Fleming V, Hu X, Weller C, Weber R, Groth C, Riester Z, et al. Melanoma extracellular vesicles generate immunosuppressive myeloid cells by upregulating PD-L1 via TLR4 signaling. Cancer Res. (2019) 79:4715–28. doi: 10.1158/0008-5472.CAN-19-0053
113. Gao Y, Xu H, Li N, Wang H, Ma L, Chen S, et al. Renal cancer-derived exosomes induce tumor immune tolerance by MDSCs-mediated antigen-specific immunosuppression. Cell Commun Signal. (2020) 18:106. doi: 10.1186/s12964-020-00611-z
114. Jiang M, Zhang W, Zhang R, Liu P, Ye Y, Yu W, et al. Cancer exosome-derived miR-9 and miR-181a promote the development of early-stage MDSCs via interfering with SOCS3 and PIAS3 respectively in breast cancer. Oncogene. (2020) 39:4681–94. doi: 10.1038/s41388-020-1322-4
115. Liu QW, Chen Y, Li JY, Xiao L, Zhang WJ, Zhao JL, et al. Bone marrow cells are differentiated into MDSCs by BCC-Ex through down-regulating the expression of CXCR4 and activating STAT3 signalling pathway. J Cell Mol Med. (2021) 25:5497–510. doi: 10.1111/jcmm.16559
116. Tanaka A, Sakaguchi S. Regulatory T cells in cancer immunotherapy. Cell Res. (2017) 27:109–18. doi: 10.1038/cr.2016.151
117. Munn DH, Sharma MD, Johnson TS. Treg destabilization and reprogramming: implications for cancer immunotherapy. Cancer Res. (2018) 78:5191–9. doi: 10.1158/0008-5472.CAN-18-1351
118. Zou W. Regulatory T cells, tumour immunity and immunotherapy. Nat Rev Immunol. (2006) 6:295–307. doi: 10.1038/nri1806
119. Wieckowski EU, Visus C, Szajnik M, Szczepanski MJ, Storkus WJ, Whiteside TL. Tumor-derived microvesicles promote regulatory T cell expansion and induce apoptosis in tumor-reactive activated CD8+ T lymphocytes. J Immunol. (2009) 183:3720–30. doi: 10.4049/jimmunol.0900970
120. Szajnik M, Czystowska M, Szczepanski MJ, Mandapathil M, Whiteside TL. Tumor-derived microvesicles induce, expand and up-regulate biological activities of human regulatory T cells (Treg). PloS One. (2010) 5:e11469. doi: 10.1371/journal.pone.0011469
121. Muller L, Simms P, Hong CS, Nishimura MI, Jackson EK, Watkins SC, et al. Human tumor-derived exosomes (TEX) regulate Treg functions via cell surface signaling rather than uptake mechanisms. Oncoimmunology. (2017) 6:e1261243. doi: 10.1080/2162402X.2016.1261243
122. Peng G, Wang HY, Peng W, Kiniwa Y, Seo KH, Wang RF. Tumor-infiltrating gammadelta T cells suppress T and dendritic cell function via mechanisms controlled by a unique toll-like receptor signaling pathway. Immunity. (2007) 27:334–48. doi: 10.1016/j.immuni.2007.05.020
123. Ni C, Fang QQ, Chen WZ, Jiang JX, Jiang Z, Ye J, et al. Breast cancer-derived exosomes transmit lncRNA SNHG16 to induce CD73+gammadelta1 Treg cells. Signal Transduct Target Ther. (2020) 5:41. doi: 10.1038/s41392-020-0129-7
124. Swatler J, Turos-Korgul L, Brewinska-Olchowik M, De Biasi S, Dudka W, Le BV, et al. 4-1BBL-containing leukemic extracellular vesicles promote immunosuppressive effector regulatory T cells. Blood Adv. (2022) 6:1879–94. doi: 10.1182/bloodadvances.2021006195
125. Fridman WH, Meylan M, Petitprez F, Sun CM, Italiano A, Sautes-Fridman C. B cells and tertiary lymphoid structures as determinants of tumour immune contexture and clinical outcome. Nat Rev Clin Oncol. (2022) 19:441–57. doi: 10.1038/s41571-022-00619-z
126. Ribatti D. The discovery of the placental growth factor and its role in angiogenesis: a historical review. Angiogenesis. (2008) 11:215–21. doi: 10.1007/s10456-008-9114-4
127. Han S, Feng S, Ren M, Ma E, Wang X, Xu L, et al. Glioma cell-derived placental growth factor induces regulatory B cells. Int J Biochem Cell Biol. (2014) 57:63–8. doi: 10.1016/j.biocel.2014.10.005
128. Li Y, An J, Huang S, He J, Zhang J. Esophageal cancer-derived microvesicles induce regulatory B cells. Cell Biochem Funct. (2015) 33:308–13. doi: 10.1002/cbf.3115
129. Ben Khelil M, Godet Y, Abdeljaoued S, Borg C, Adotevi O, Loyon R. Harnessing antitumor CD4(+) T cells for cancer immunotherapy. Cancers (Basel). (2022) 14(1):260. doi: 10.3390/cancers14010260
130. Chen YQ, Li PC, Pan N, Gao R, Wen ZF, Zhang TY, et al. Tumor-released autophagosomes induces CD4(+) T cell-mediated immunosuppression via a TLR2-IL-6 cascade. J Immunother Cancer. (2019) 7:178. doi: 10.1186/s40425-019-0646-5
131. Xie Y, Zhang H, Li W, Deng Y, Munegowda MA, Chibbar R, et al. Dendritic cells recruit T cell exosomes via exosomal LFA-1 leading to inhibition of CD8+ CTL responses through downregulation of peptide/MHC class I and Fas ligand-mediated cytotoxicity. J Immunol. (2010) 185:5268–78. doi: 10.4049/jimmunol.1000386
132. Cai Z, Yang F, Yu L, Yu Z, Jiang L, Wang Q, et al. Activated T cell exosomes promote tumor invasion via Fas signaling pathway. J Immunol. (2012) 188:5954–61. doi: 10.4049/jimmunol.1103466
133. Wang X, Shen H, He Q, Tian W, Xia A, Lu XJ. Exosomes derived from exhausted CD8+ T cells impaired the anticancer function of normal CD8+ T cells. J Med Genet. (2019) 56:29–31. doi: 10.1136/jmedgenet-2018-105439
134. Seo N, Shirakura Y, Tahara Y, Momose F, Harada N, Ikeda H, et al. Activated CD8(+) T cell extracellular vesicles prevent tumour progression by targeting of lesional mesenchymal cells. Nat Commun. (2018) 9:435. doi: 10.1038/s41467-018-02865-1
135. Qiu Y, Yang Y, Yang R, Liu C, Hsu JM, Jiang Z, et al. Activated T cell-derived exosomal PD-1 attenuates PD-L1-induced immune dysfunction in triple-negative breast cancer. Oncogene. (2021) 40:4992–5001. doi: 10.1038/s41388-021-01896-1
136. Zhou WJ, Zhang J, Xie F, Wu JN, Ye JF, Wang J, et al. CD45RO(-)CD8(+) T cell-derived exosomes restrict estrogen-driven endometrial cancer development via the ERbeta/miR-765/PLP2/Notch axis. Theranostics. (2021) 11:5330–45. doi: 10.7150/thno.58337
137. Hashimoto M, Kamphorst AO, Im SJ, Kissick HT, Pillai RN, Ramalingam SS, et al. CD8 T cell exhaustion in chronic infection and cancer: opportunities for interventions. Annu Rev Med. (2018) 69:301–18. doi: 10.1146/annurev-med-012017-043208
138. Valkenburg KC, de Groot AE, Pienta KJ. Targeting the tumour stroma to improve cancer therapy. Nat Rev Clin Oncol. (2018) 15:366–81. doi: 10.1038/s41571-018-0007-1
139. Li L, Jay SM, Wang Y, Wu SW, Xiao Z. IL-12 stimulates CTLs to secrete exosomes capable of activating bystander CD8(+) T cells. Sci Rep. (2017) 7:13365. doi: 10.1038/s41598-017-14000-z
140. Wu SW, Li L, Wang Y, Xiao Z. CTL-derived exosomes enhance the activation of CTLs stimulated by low-affinity peptides. Front Immunol. (2019) 10:1274. doi: 10.3389/fimmu.2019.01274
141. Kim MS, Haney MJ, Zhao Y, Yuan D, Deygen I, Klyachko NL, et al. Engineering macrophage-derived exosomes for targeted paclitaxel delivery to pulmonary metastases: in vitro and in vivo evaluations. Nanomedicine. (2018) 14:195–204. doi: 10.1016/j.nano.2017.09.011
142. Katakowski M, Chopp M. Exosomes as tools to suppress primary brain tumor. Cell Mol Neurobiol. (2016) 36:343–52. doi: 10.1007/s10571-015-0280-9
143. Li XQ, Liu JT, Fan LL, Liu Y, Cheng L, Wang F, et al. Exosomes derived from gefitinib-treated EGFR-mutant lung cancer cells alter cisplatin sensitivity via up-regulating autophagy. Oncotarget. (2016) 7:24585–95. doi: 10.18632/oncotarget.v7i17
144. Greco SJ, Rameshwar P. Mesenchymal stem cells in drug/gene delivery: implications for cell therapy. Ther Deliv. (2012) 3:997–1004. doi: 10.4155/tde.12.69
145. Wang J, Li G, Tu C, Chen X, Yang B, Huo Y, et al. High-throughput single-cell analysis of exosome mediated dual drug delivery, in vivo fate and synergistic tumor therapy. Nanoscale. (2020) 12:13742–56. doi: 10.1039/D0NR02344B
146. Kabashima-Niibe A, Higuchi H, Takaishi H, Masugi Y, Matsuzaki Y, Mabuchi Y, et al. Mesenchymal stem cells regulate epithelial-mesenchymal transition and tumor progression of pancreatic cancer cells. Cancer Sci. (2013) 104:157–64. doi: 0.1111/cas.12059
147. Kim MS, Haney MJ, Zhao Y, Mahajan V, Deygen I, Klyachko NL, et al. Development of exosome-encapsulated paclitaxel to overcome MDR in cancer cells. Nanomedicine. (2016) 12:655–64. doi: 10.1016/j.nano.2015.10.012
148. Zhu D, Liu Z, Li Y, Huang Q, Xia L, Li K. Delivery of manganese carbonyl to the tumor microenvironment using Tumor-Derived exosomes for cancer gas therapy and low dose radiotherapy. Biomaterials. (2021) 274:120894. doi: 10.1016/j.biomaterials.2021.120894
149. Wang J, Chen P, Dong Y, Xie H, Wang Y, Soto F, et al. Designer exosomes enabling tumor targeted efficient chemo/gene/photothermal therapy. Biomaterials. (2021) 276:121056. doi: 10.1016/j.biomaterials.2021.121056
150. Nguyen Cao TG, Truong Hoang Q, Kang JH, Kang SJ, Ravichandran V, Rhee WJ, et al. Bioreducible exosomes encapsulating glycolysis inhibitors potentiate mitochondria-targeted sonodynamic cancer therapy via cancer-targeted drug release and cellular energy depletion. Biomaterials. (2023) 301:122242. doi: 10.1016/j.biomaterials.2023.122242
151. Kamerkar S, LeBleu VS, Sugimoto H, Yang S, Ruivo CF, Melo SA, et al. Exosomes facilitate therapeutic targeting of oncogenic KRAS in pancreatic cancer. Nature. (2017) 546:498–503. doi: 10.1038/nature22341
152. O'Brien K, Lowry MC, Corcoran C, Martinez VG, Daly M, Rani S, et al. miR-134 in extracellular vesicles reduces triple-negative breast cancer aggression and increases drug sensitivity. Oncotarget. (2015) 6:32774–89. doi: 10.18632/oncotarget.v6i32
153. Kim SM, Yang Y, Oh SJ, Hong Y, Seo M, Jang M. Cancer-derived exosomes as a delivery platform of CRISPR/Cas9 confer cancer cell tropism-dependent targeting. J Control Release. (2017) 266:8–16. doi: 10.1016/j.jconrel.2017.09.013
154. Zhang M, Hu S, Liu L, Dang P, Liu Y, Sun Z, et al. Engineered exosomes from different sources for cancer-targeted therapy. Signal Transduct Target Ther. (2023) 8:124. doi: 10.1038/s41392-023-01382-y
155. Tao B, Du R, Zhang X, Jia B, Gao Y, Zhao Y, et al. Engineering CAR-NK cell derived exosome disguised nano-bombs for enhanced HER2 positive breast cancer brain metastasis therapy. J Control Release. (2023) 363:692–706. doi: 10.1016/j.jconrel.2023.10.007
156. Cho H, Jung I, Ju H, Baek MC, Yea K. Engineered CD8(+) T cell-derived extracellular vesicles induce enhanced anti-cancer efficacy and targeting to lung cancer cells. Cytokine. (2023) 169:156249. doi: 10.1016/j.cyto.2023.156249
157. Tang Q, Yang S, He G, Zheng H, Zhang S, Liu J, et al. Tumor-derived exosomes in the cancer immune microenvironment and cancer immunotherapy. Cancer Lett. (2022) 548:215823. doi: 10.1016/j.canlet.2022.215823
158. Li L, Wang C, Li Q, Guan Y, Zhang X, Kong F, et al. Exosomes as a modulator of immune resistance in human cancers. Cytokine Growth Factor Rev. (2023) 73:135–49. doi: 10.1016/j.cytogfr.2023.07.007
159. Jiang X, Xu J, Liu M, Xing H, Wang Z, Huang L, et al. Adoptive CD8(+) T cell therapy against cancer:Challenges and opportunities. Cancer Lett. (2019) 462:23–32. doi: 10.1016/j.canlet.2019.07.017
160. Zhu H, Wang K, Wang Z, Wang D, Yin X, Liu Y, et al. An efficient and safe MUC1-dendritic cell-derived exosome conjugate vaccine elicits potent cellular and humoral immunity and tumor inhibition in vivo. Acta Biomater. (2022) 138:491–504. doi: 10.1016/j.actbio.2021.10.041
161. Du Z, Huang Z, Chen X, Jiang G, Peng Y, Feng W, et al. Modified dendritic cell-derived exosomes activate both NK cells and T cells through the NKG2D/NKG2D-L pathway to kill CML cells with or without T315I mutation. Exp Hematol Oncol. (2022) 11:36. doi: 10.1186/s40164-022-00289-8
162. Xiong X, Ke X, Wang L, Lin Y, Wang S, Yao Z, et al. Neoantigen-based cancer vaccination using chimeric RNA-loaded dendritic cell-derived extracellular vesicles. J Extracell Vesicles. (2022) 11:e12243. doi: 10.1002/jev2.12243
163. Dionisi M, De Archangelis C, Battisti F, Rahimi Koshkaki H, Belleudi F, Zizzari IG, et al. Tumor-derived microvesicles enhance cross-processing ability of clinical grade dendritic cells. Front Immunol. (2018) 9:2481. doi: 10.3389/fimmu.2018.02481
164. Hu W, Huang F, Ning L, Hao J, Wan J, Hao S. Enhanced immunogenicity of leukemia-derived exosomes via transfection with lentiviral vectors encoding costimulatory molecules. Cell Oncol (Dordr). (2020) 43:889–900. doi: 10.1007/s13402-020-00535-3
165. Khani AT, Sharifzad F, Mardpour S, Hassan ZM, Ebrahimi M. Tumor extracellular vesicles loaded with exogenous Let-7i and miR-142 can modulate both immune response and tumor microenvironment to initiate a powerful anti-tumor response. Cancer Lett. (2021) 501:200–9. doi: 10.1016/j.canlet.2020.11.014
166. Asadirad A, Baghaei K, Hashemi SM, Dehnavi S, Ghanbarian H, Mortaz E, et al. Dendritic cell immunotherapy with miR-155 enriched tumor-derived exosome suppressed cancer growth and induced antitumor immune responses in murine model of colorectal cancer induced by CT26 cell line. Int Immunopharmacol. (2022) 104:108493. doi: 10.1016/j.intimp.2021.108493
167. Huang F, Wan J, Hao S, Deng X, Chen L, Ma L. TGF-beta1-silenced leukemia cell-derived exosomes target dendritic cells to induce potent anti-leukemic immunity in a mouse model. Cancer Immunol Immunother. (2017) 66:1321–31. doi: 10.1007/s00262-017-2028-5
168. Huang F, Li Z, Zhang W, Li J, Hao S. Enhancing the anti-leukemia immunity of acute lymphocytic leukemia-derived exosome-based vaccine by downregulation of PD-L1 expression. Cancer Immunol Immunother. (2022) 71:2197–212. doi: 10.1007/s00262-021-03138-5
169. Phung CD, Pham TT, Nguyen HT, Nguyen TT, Ou W, Jeong JH, et al. Anti-CTLA-4 antibody-functionalized dendritic cell-derived exosomes targeting tumor-draining lymph nodes for effective induction of antitumor T-cell responses. Acta Biomater. (2020) 115:371–82. doi: 10.1016/j.actbio.2020.08.008
170. Huang L, Rong Y, Tang X, Yi K, Qi P, Hou J, et al. Engineered exosomes as an in situ DC-primed vaccine to boost antitumor immunity in breast cancer. Mol Cancer. (2022) 21:45. doi: 10.1186/s12943-022-01515-x
171. Hong Y, Kim YK, Kim GB, Nam GH, Kim SA, Park Y, et al. Degradation of tumour stromal hyaluronan by small extracellular vesicle-PH20 stimulates CD103(+) dendritic cells and in combination with PD-L1 blockade boosts anti-tumour immunity. J Extracell Vesicles. (2019) 8:1670893. doi: 10.1080/20013078.2019.1670893
172. Lee CH, Bae JH, Choe EJ, Park JM, Park SS, Cho HJ, et al. Macitentan improves antitumor immune responses by inhibiting the secretion of tumor-derived extracellular vesicle PD-L1. Theranostics. (2022) 12:1971–87. doi: 10.7150/thno.68864
173. Park SS, Kim JI, Lee CH, Bae JH, Park JM, Choe EJ, et al. Temsirolimus enhances anti-cancer immunity by inducing autophagy-mediated degradation of the secretion of small extracellular vesicle PD-L1. Cancers (Basel). (2022) 14(17):4081. doi: 10.3390/cancers14174081
174. Shin JM, Lee CH, Son S, Kim CH, Lee JA, Ko H, et al. Sulfisoxazole elicits robust antitumour immune response along with immune checkpoint therapy by inhibiting exosomal PD-L1. Adv Sci (Weinh). (2022) 9:e2103245. doi: 10.1002/advs.202103245
175. Fu C, Zhou L, Mi QS, Jiang A. DC-based vaccines for cancer immunotherapy. Vaccines (Basel). (2020) 8(4):706. doi: 10.3390/vaccines8040706
176. Frenkel-Morgenstern M, Lacroix V, Ezkurdia I, Levin Y, Gabashvili A, Prilusky J, et al. Chimeras taking shape: potential functions of proteins encoded by chimeric RNA transcripts. Genome Res. (2012) 22:1231–42. doi: 10.1101/gr.130062.111
177. Bagchi S, Yuan R, Engleman EG. Immune checkpoint inhibitors for the treatment of cancer: clinical impact and mechanisms of response and resistance. Annu Rev Pathol. (2021) 16:223–49. doi: 10.1146/annurev-pathol-042020-042741
178. Marin-Acevedo JA, Kimbrough EO, Lou Y. Next generation of immune checkpoint inhibitors and beyond. J Hematol Oncol. (2021) 14:45. doi: 10.1186/s13045-021-01056-8
179. Sharma P, Siddiqui BA, Anandhan S, Yadav SS, Subudhi SK, Gao J, et al. The next decade of immune checkpoint therapy. Cancer Discov. (2021) 11:838–57. doi: 10.1158/2159-8290.CD-20-1680
180. Guan L, Wu B, Li T, Beer LA, Sharma G, Li M, et al. HRS phosphorylation drives immunosuppressive exosome secretion and restricts CD8(+) T-cell infiltration into tumors. Nat Commun. (2022) 13:4078. doi: 10.1038/s41467-022-31713-6
181. Fernandez-Delgado I, Calzada-Fraile D, Sanchez-Madrid F. Immune regulation by dendritic cell extracellular vesicles in cancer immunotherapy and vaccines. Cancers (Basel). (2020) 12(12):3558. doi: 10.3390/cancers12123558
182. Mortezaee K, Majidpoor J. Extracellular vesicle-based checkpoint regulation and immune state in cancer. Med Oncol (Northwood London England). (2022) 39:225. doi: 10.1007/s12032-022-01837-2
183. Xu R, Greening DW, Zhu HJ, Takahashi N, Simpson RJ. Extracellular vesicle isolation and characterization: toward clinical application. J Clin Invest. (2016) 126:1152–62. doi: 10.1172/JCI81129
184. Crescitelli R, Lasser C, Lotvall J. Isolation and characterization of extracellular vesicle subpopulations from tissues. Nat Protoc. (2021) 16:1548–80. doi: 10.1038/s41596-020-00466-1
185. Barile L, Vassalli G. Exosomes: Therapy delivery tools and biomarkers of diseases. Pharmacol Ther. (2017) 174:63–78. doi: 10.1016/j.pharmthera.2017.02.020
Keywords: CD8+ T cell, extracellular vesicles, tumor, tumor microenvironment, immunity
Citation: Huang Z, Liu X, Guo Q, Zhou Y, Shi L, Cai Q, Tang S, Ouyang Q and Zheng J (2024) Extracellular vesicle-mediated communication between CD8+ cytotoxic T cells and tumor cells. Front. Immunol. 15:1376962. doi: 10.3389/fimmu.2024.1376962
Received: 26 January 2024; Accepted: 05 March 2024;
Published: 18 March 2024.
Edited by:
Francisco Sanchez-Madrid, Autonomous University of Madrid, SpainReviewed by:
Arantzazu Alfranca, Hospital de la Princesa, SpainCarlos Cabañas, Spanish National Research Council (CSIC), Spain
Copyright © 2024 Huang, Liu, Guo, Zhou, Shi, Cai, Tang, Ouyang and Zheng. This is an open-access article distributed under the terms of the Creative Commons Attribution License (CC BY). The use, distribution or reproduction in other forums is permitted, provided the original author(s) and the copyright owner(s) are credited and that the original publication in this journal is cited, in accordance with accepted academic practice. No use, distribution or reproduction is permitted which does not comply with these terms.
*Correspondence: Ji Zheng, aml6aGVuZzAyM0BhbGl5dW4uY29t; Qin Ouyang, b3V5YW5ncUB0bW11LmVkdS5jbg==; Shupei Tang, c3BfdGFuZzIyQHlhaG9vLmNvbQ==
†These authors have contributed equally to this work