- 1Assisted Reproduction Unit, Department of Obstetrics and Gynecology, Sir Run Run Shaw Hospital, Zhejiang University School of Medicine, Hangzhou, China
- 2Department of Obstetrics and Gynecology, Key Laboratory of Reproductive Dysfunction, Management of Zhejiang Province, Hangzhou, China
- 3Department of Obstetrics and Gynecology, The Fourth Affiliated Hospital of Zhejiang University School of Medicine, Yiwu, China
Due to the physiological alteration during pregnancy, maternal gut microbiota changes following the metabolic processes. Recent studies have revealed that maternal gut microbiota is closely associated with the immune microenvironment in utero during pregnancy and plays a vital role in specific pregnancy complications, including preeclampsia, gestational diabetes, preterm birth and recurrent miscarriages. Some other evidence has also shown that aberrant maternal gut microbiota increases the risk of various diseases in the offspring, such as allergic and neurodevelopmental disorders, through the immune alignment between mother and fetus and the possible intrauterine microbiota. Probiotics and the high-fiber diet are effective inventions to prevent mothers and fetuses from diseases. In this review, we summarize the role of maternal gut microbiota in the development of pregnancy complications and the health condition of future generations from the perspective of immunology, which may provide new therapeutic strategies for the health management of mothers and offspring.
1 Introduction
During pregnancy, the maternal body experiences various hormonal, immunological, and metabolic changes (1, 2). To meet the fetus’s needs for growth and development, the maternal gut microbiota changes during pregnancy, predisposing mothers to metabolic syndrome (3). Gut microbiota has been considered closely associated with immune activation in the gut, systematic circulation, and other peripheral organs and tissues (4).
During this particular period, maternal gut microbiota can significantly impact the immune environment in utero. According to the available evidence, a wide range of diseases during pregnancy, including hypertensive disorders of pregnancy (HDP), gestational diabetes mellitus (GDM), preterm birth and recurrent miscarriages, are associated with gut dysbiosis owing to imbalances in the composition and diversity of gut microbiota (5–7). Furthermore, maternal gut microbiota profoundly influences the health status of the next generation (8–10), and disturbances in the maternal gut microbes can lead to diseases in the offspring’s later life (9, 11, 12). Therefore, it is crucial to develop effective inventions to restore the balance of maternal gut microbiota.
In this review, we concentrate on how maternal gut microbiota impacts the immune response in utero, leading to pregnancy complications and offspring disorders, which provides potent therapeutic strategies to restore microbiota balance.
2 The alteration of maternal gut microbiota during pregnancy
During a normal pregnancy, there are various changes in the maternal body compared to the non-pregnant women (1, 2). Production of estrogen, progesterone, prolactin, and human placental lactogen significantly increases, while hormones like gonadotropin decline sharply during pregnancy (1). Metabolic changes also occur during this period. For instance, in early pregnancy, body fat increases, followed by insulin sensitivity reduction later, which is beneficial for pregnancy as it helps mothers to support the fetal growth and provide the energy of breastfeeding (13). Interestingly, women may increase their food intake during pregnancy and sometimes uniquely prefer certain foods (14). As these changes in the maternal body are closely related to the gut microbiota, questions about whether gut microbiota is stable in normal pregnancy compared to non-pregnant populations and how the diversity and composition of bacterial community change have aroused great interest recently (3, 15–17).
Whether maternal gut microbiota changes during pregnancy is controversial. Previous studies have been conducted to sample the gut microbiota at longitudinal time points during pregnancy, and most of these studies reported that the diversity and composition of the gut microbiota remained stable primarily throughout pregnancy (17, 18). However, another study has shown the opposite. A study of 91 women with varying prepregnant BMI and gestational diabetes status by Koren et al. showed that during the first trimester (T1), gut microbiota was comparable to those of normal controls and similar to each other. However, the composition and structure shift dramatically throughout pregnancy. In the third trimester (T3), the β-diversity greatly expanded. In contrast, α-diversity is reduced, with a significant increase in Proteobacteria and Actinobacteria and pro-inflammatory cytokine concentrations in stool (3). Proteobacteria have been considered as pathogenic bacteria in the inflammation-associated dysbiosis (19). In the meantime, the abundance of health-related bacteria was depleted. For example, Faecalibacterium, one of the microbes producing butyrate, was at low average levels in the T3 (3). Of note, the expansion of β-diversity was not related to health status or diet, which strongly suggest that it was a widely shared phenomenon during pregnancy (3). However, whether other changes during pregnancy were common remained unknown. Similarly, another study found that maternal gut microbiota diversity changes as pregnancy progresses, and the alterations are closely correlated with gestational weight gain (16). Except for studies in human, Chen et al. found that in sows there were dramatic changes in the fecal microbiota from the early pregnancy to the late pregnancy, with significantly decreasing Firmicutes and increasing Bacteroidetes and Verrucomicrobia (20). Actinobacteria was also observed to increase in the late pregnancy (21, 22). In the genus level, Clostridiales, Desulfovibrio, Mogibacteriaceae and Prevotella in the gut of sows increased as the pregnancy progressed and decreased at weaning (23). The relative abundance of Streptococcus was found higher during the late pregnancy (24). However, these results in sows were not completely consistent with that in human.
The inconsistent data among different studies may result from multiple factors:
1. As the gut microbiota is correlated with clinical characteristics like race, age, diet, medication history, living environment, body weight, and health status (25–27), differential selection standards of the participants may lead to different concnlusions (3, 17). Sample size, sampling methods, time, and the study design are inevitable confounding factors. Because few studies have ruled out the interference of these factors, it remains unclear whether these results reflect physiological changes in normal pregnancy or dysbiosis caused by pathological processes.
2. Few studies have incorporated metagenomic measures, which provides more specific classifications of sequences when they were compared to 16S rRNA gene amplicon sequencing (28).
Dramatic shifts in the concentration of hormones during pregnancy, such as estrogen and progesterone, can be one of the contributors of the altered composition of gut microbiota. For instance, progesterone was found to promote Bifidobacterium growth during late pregnancy (15). Among overweight and obese women with dysregulated metabolic hormone levels, the gut microbiota profile was different. Insulin was positively correlated with the genus Collinsella (29). In turn, gut microbiota affects hormonal levels. For example, antibiotics contributed to lower estrogen levels in a clinical trial, which was associated with decreased abundance of β-glucuronidase-producing bacteria (30).
In brief, more extensive studies with multiple timepoint collections of fecal samples, improved sequencing methods, as well as adequate documentation and assessment of confounding factors are needed to investigate how and why maternal gut microbiota changes during pregnancy.
3 Maternal gut microbiota and the immune response during pregnancy
The gut microbiota and their metabolites greatly impact the immune system of the host and the fetus during pregnancy. Notably, hormonal changes are also highly associated with the host immune status (31). For example, progesterone during pregnancy is benefit for the balances of Th1/Th2 response and the regulation of pro-inflammatory cytokines (31). It seems that the interrelated hormonal and microbial factors jointly act on the host immune microenvironment at the maternal-fetal interface to main the stability. Here we mainly focused on the role of gut microbiota in dominating the immune response during pregnancy.
3.1 How to trigger the immune response
To trigger the immune response, maternal gut microbiota can adhere and translocate through the gut epithelial barrier to enter the systemic circulation for direct interaction between microorganisms and immune cells. In addition, the gut microbiota can release mediators, such as lipopolysaccharide (LPS), metabolites, or extracellular vesicles (EVs), to impact the immune system (Figure 1) (32).
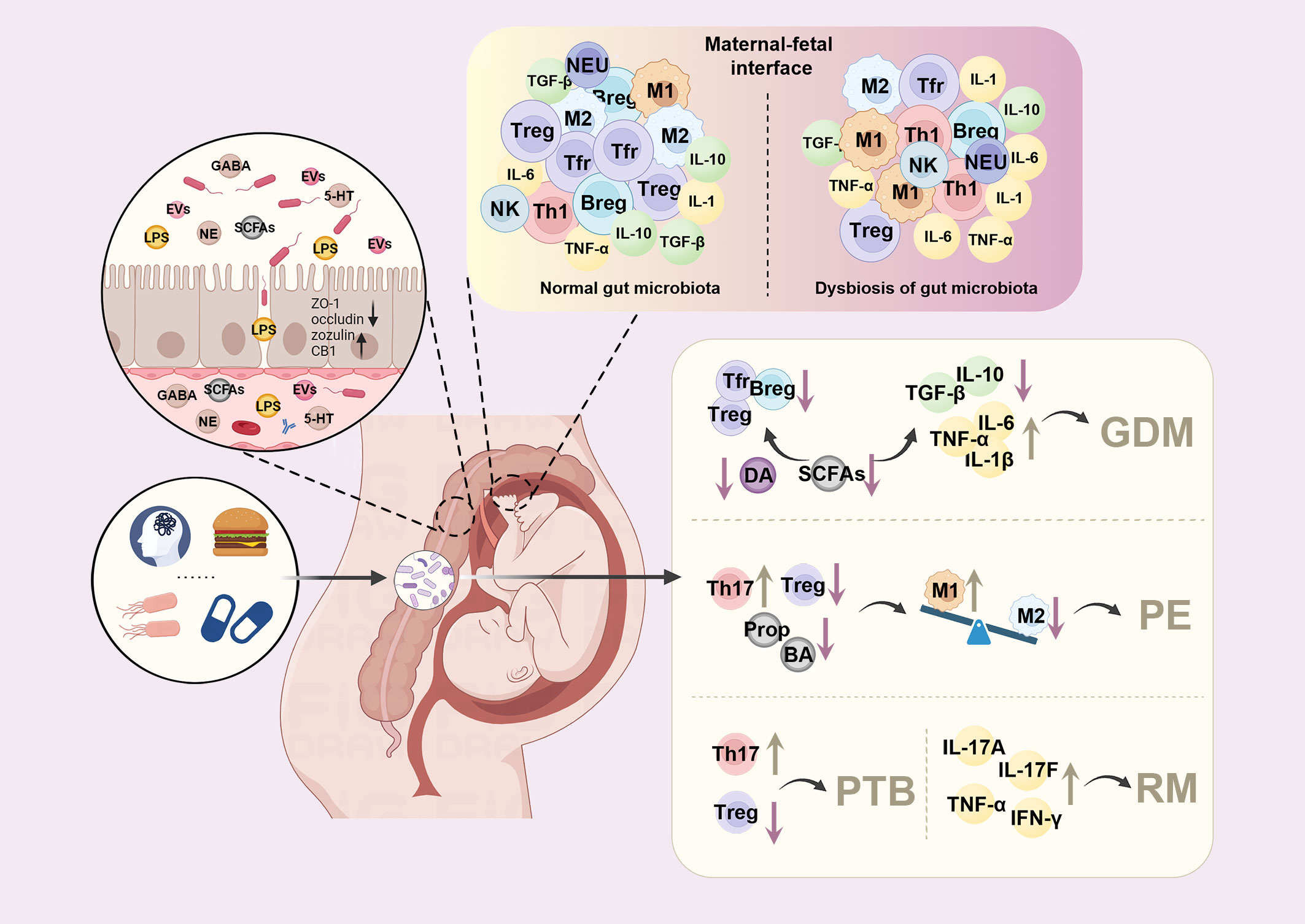
Figure 1 Dysbiosis of maternal gut microbiota alters the immune environment at the maternal-fetal interface and contributes to pregnancy complications. Factors such as diet, pressure, antibiotics, and infection can lead to dysbiosis of maternal gut microbiota during pregnancy. The altered maternal gut microbes can trigger the immune response by adhesion and translocation through the gut epithelial barrier or releasing mediators, such as lipopolysaccharide (LPS), short-chain fatty acids (SCFAs), or extracellular vesicles (EVs). At the maternal-fetal interface, the tolerogenic environment is disturbed, with increased pro-inflammatory macrophages (M1) phenotype and elevated levels of pro-inflammatory cytokines, including tumor necrosis factor-a (TNF-a), interleukin-6 (IL-6), and interleukin-1β (IL-1β). In contrast, decreased regulatory T cells (Tregs), regulatory B cells (Bregs), follicular regulatory T cells (Tfr), transforming growth factor-β (TGF-β) and interleukin-10 (IL-10) were observed during pregnancy, leading to various pregnancy complications. DA, dopamine; GDM, gestational diabetes mellitus; PE, preeclampsia; PTB, preterm birth; RM, recurrent miscarriages; Prop, propionate; BA, butyrate; GABA, gamma-aminobutyric acid; NE, norepinephrine; 5-HT, serotonin; NEU, neutrophils.
To enter the systemic circulation, maternal gut microbiota first crosses the epithelium of the gut by binding to receptors, such as toll-like receptors (TLR). Besides, the dendritic cells (DCs) can help to localize the pathobionts across the epithelium of the gut and into the systemic circulation by phagocytosis (32).
Gut microbiota triggered the immune response through its metabolites. Among all the metabolites, short-chain fatty acids (SCFAs) are the most extensively described. SCFAs, such as acetate, propionate, and butyrate, are produced by fermenting non-digestible fibers dominated by Bacteroidetes and Firmicutes (33, 34). It has been well established that SCFAs play a crucial role in host immunity (33). SCFAs and other microbial metabolites can cross the epithelium and stay in the host systemic circulation, where they are detected by immune cells (33).
Other substances can act as mediators for the maternal gut microbiota to influence the host immune response, among which EVs have aroused great interest. Gut microbiota is able to produce EVs, carrying products such as nucleic acids, proteins, and lipids (35). Growing studies have paid attention to the role of EVs in mediating the immune effects of gut bacteria (36–38). Recently, it has been reported that gut microbiota-derived EVs play a vital role in driving the prenatal immune system, which could be a potential area of research in the future (39).
3.2 Maternal gut microbiota: the role in the immune environment at the maternal-fetal interface
During pregnancy, the immune system of mothers tends to maintain tolerance to the fetal allograft while protecting from infections (40). The placenta and the decidua are crucial for pregnancy sustainment and preventing the fetus from maternal immune attacks (40). Maternal T helper 1 (Th1) activity was suppressed to maintain a successful pregnancy. At the same time, the immune profile switched to the Th2 dominant profile with the expansion of regulatory T cells (Tregs) in the placenta and decidua (41). Regulatory B cells (Bregs) were lowered in the systemic circulation and were considered to migrate to the maternal-fetal interface (42). More macrophages tend to be polarized into M2-like macrophages to maintain the immunosuppressive property (43).
Maternal gut microbiota has been reported to be closely associated with establishing a tolerogenic environment in utero through direct interaction, or their metabolites and components, such as LPS and SCFAs. Dysbiosis of gut microbiota is associated with a pro-inflammatory macrophage phenotype in the placenta with an elevated expression of tumor necrosis factor-α (TNF-α) and interleukin-6 (IL-6) (44). In recurrent miscarriages, metabolites like chenodeoxycholic acid sulfate, 1,4-Methylimidazoleacetic acid are positively associated with changes in levels of Th1 and Th17 cytokines, suggesting that they are involved in the pathogenesis of miscarriages (45). When fed with a diet enriched in prebiotics, the abundance of Tregs increased in the placenta, and Bregs increased in both the placenta and the uterus, followed by the alteration of gut microbiota (46). It has been proved recently that oral probiotics also increased Tregs, Bregs and follicular regulatory T cells (Tfr), as well as IL-10 and transforming growth factor-β (TGF-β), while decreased TNF-α and IL-6 to prevent inflammation in mice with GDM (Figure 1) (47). In a sow model, a higher abundance of Bacteroides fragilis was associated with higher levels of LPS, IL-1β and IL-6 as well as increased intestinal permeability (48).
LPS is a surface membrane component of Gram-negative bacteria. Elevation of LPS leads to metabolic endotoxemia, which can travel to the peripheral tissues, including the uterus and placenta, to trigger the immune response (49). LPS can increase the permeability of the gut epithelial layer by disrupting the mucosal layer and downregulating tight junction protein expression on gut epithelial cells, such as zonula occludens-1 (ZO-1) and occludin (50, 51). Meanwhile, cannabinoid receptor 1 (CB1) and zonulin were found significantly increased when gut microbiota was disturbed, leading to increased gut epithelial permeability (52, 53). LPS binds to TLR2/4 and recruits the adapter proteins myeloid differentiation factor 88 (MyD-88), IL-1 receptor-associated kinase (IRAK), TNF receptor-associated factor 6 (TRAF6), TGF-β-activated kinase1 (TAK1) and TAB1 (TAK1-binding protein 1), triggering the infiltration of macrophage and elevating pro-inflammatory cytokines, such as IL-1, IL-6, and TNF-α (54).
SCFAs could bind the G-protein coupled receptors (GPR), including GPR43, GPR41, GPR109A, and OLFR78/OR51E2 expressed on macrophages, neutrophils, eosinophils or DCs (55–57). Through inhibiting histone deacetylase (HDAC) activity (58, 59), SCFAs control gene transcription in various tissues and cells. Previous studies have shown that SCFAs promote Tregs via GPR-dependent and HDAC-dependent mechanisms (58, 60). Additionally, SCFAs can serve as energy substrates for immune cells and thus participate in cellular metabolism (61). Based on these mechanisms, SCFAs enhance the epithelial integrity and the anti-inflammatory effects in the regulation of cytokine production, suppression of macrophages, and expansion of Tregs in the gut and peripheral tissues (58, 62). SCFA supplements, such as sodium butyrate, decreased inflammatory cytokines (IL-1β, IL-6, and TGF-β) and reversed the symptoms of preeclampsia (63).
Gut microbiota was reported to produce or regulate neurotransmitters, such as glutamate, gamma-aminobutyric acid (GABA), and norepinephrine (NE), which are associated with emotional and cognitive disorders (64–66). The production of GABA, the inhibitory neurotransmitter, has been reported to be specially associated with Lactobacillus, Bifidobacterium and Bacteroides in the gut (64). In mice model, Lactobacillus rhamnosus JB-1 elevated the levels of GABA and the expression of GABA receptors in the brain, which led to the alleviation of anxiety and depression (67). Additionally, reduced levels of luminal NE in the gut have been found in germ-free mice, which could be reversed by colonization with Clostridium spp (66). It is likely that gut microbiota participated in regulating the nervous system of mothers during pregnancy and even played a role in the neurodevelopment of their offspring. However, up to now, evidence that linked gut microbiota-related neurotransmitters to the maternal-fetal diseases during pregnancy was still lacking.
Approximately 90% of serotonin is produced by enterochromaffin cells in the gut and its biosynthesis is largely regulated by commensal gut microbiota, such as spore-forming bacteria (68, 69). Several studies indicated that serotonin in maternal circulation, placenta, and cord blood apparently increased and its degradation is partly blocked in preeclampsia (70). Serotonin is key to maintain the function of DCs and modulate the activation and proliferation of T cells by decreasing the production of Th1/Th17 cytokines and enhancing Tregs (71, 72). Serotonin is also necessary for cytokine production, including IFN-γ, IL-1B, IL-8, IL-12, TNF-α, IL-17, and IL-6 (70).
Current findings that connect maternal gut microbiota with host immune profile at the maternal-fetal interface are limited. It is hard for researchers to identify the exact genus or species of gut microbiota or the metabolite that drives the immune alterations at the maternal-fetal interface of the host for the complexity of gut microbiota. Also, most studies have paid attention to the systemic change rather than the immune environment in the placenta or decidua. Therefore, improved evidence will be needed.
3.3 Gut microbiota-related immune alignment between mother and fetus
It has been widely recognized that most immune alterations driven by microbiota occur in the postnatal period (73–75). There are growing numbers of studies discussing how the maternal microbiota affects the offspring’s immune system. However, only some of these studies focus on the prenatal period instead of the vertical transmission in the postnatal period.
Maternal gut microbiota plays a vital role in developing the fetal immune system (76, 77). A recent study revealed that the Dialister, Escherichia, and Ruminococcus predominant cluster in maternal gut microbiota was closely associated with a lower proportion of granulocytes and higher proportions of central naïve CD4+ T cells and naïve Tregs in cord blood (76). A maternal fiber-rich diet, which profoundly affected the composition of maternal gut microbiota, was associated with lower proportions of RORγt-positive innate and adaptive immune cell subsets in the offspring (77).
Preparing the fetal immune system in utero for extrauterine conditions is crucial for survival. Humoral immunity, especially immunoglobulin G (IgG), has been well investigated (Figure 2) (78). Gomez et al. found that serum transfer from gestation-only colonized females to unexposed pregnant mice was adequate to shape intestinal group 3 innate lymphoid cells (ILC) populations in the neonates, but not when IgG was depleted, which may suggest that maternal IgG transfer of gut microbial components to the offspring during pregnancy is important for fetal innate immune development. Also, they showed that maternal antibodies increased the levels of microbial molecules in the fetus and the neonate. In other words, maternal antibodies have an effect on promoting microbial molecular transfer (8).
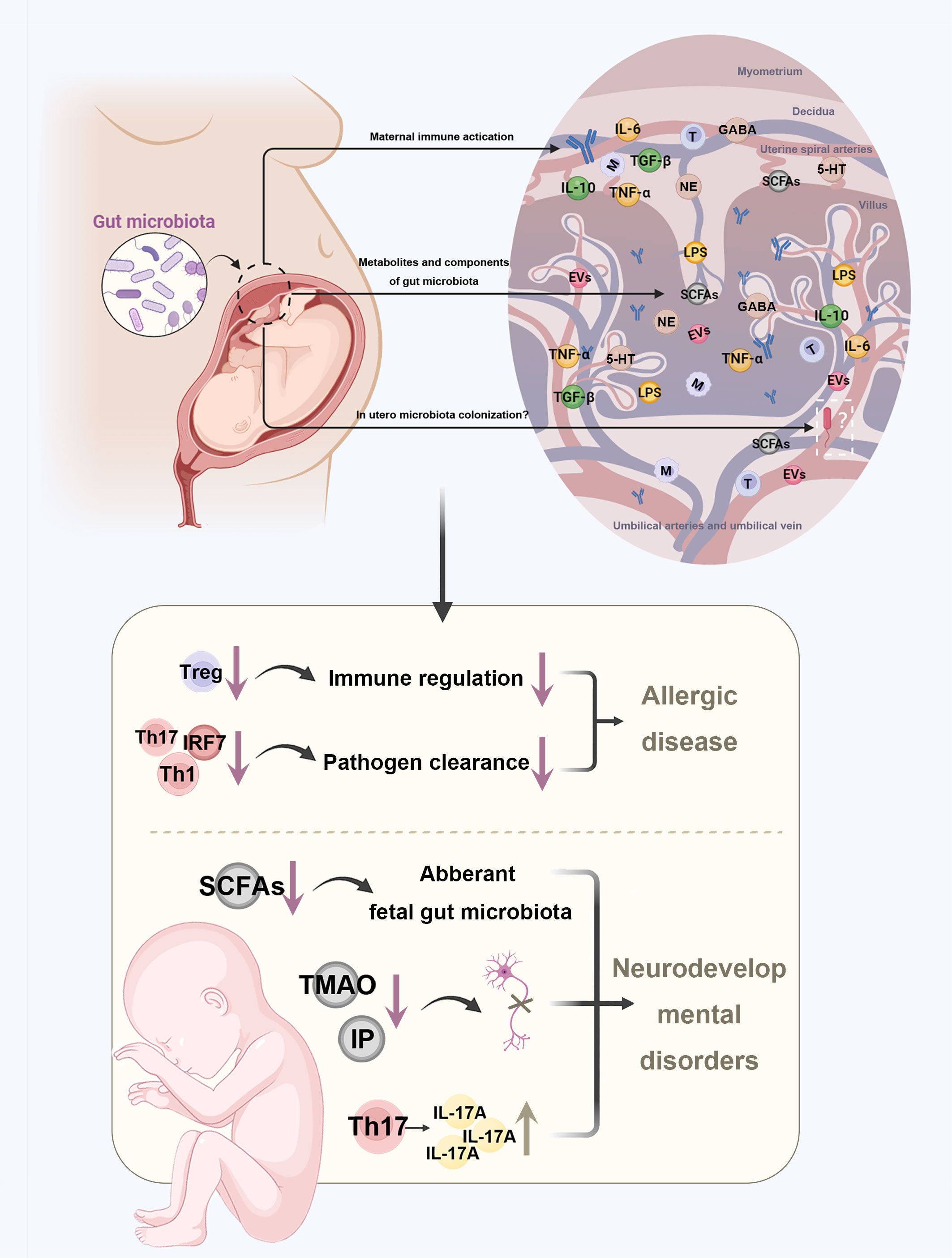
Figure 2 Disturbances in maternal gut microbiota increase the risk of diseases in the offspring. Maternal gut microbiota affects the health of the fetus by three potential pathways. First, after maternal immune activation, antibodies, immune molecules, and immune cells can cross the placental barrier to stay in fetal tissues. Next, the metabolites and components of maternal gut microbiota can be crucial substances that cross the placenta barrier. In addition, some microbes can colonize in utero as fetoplacental microbiota. Disturbances in maternal gut microbiota may further increase the risk of allergic diseases and neurodevelopmental disorders in the offspring through altered concentration of metabolites and imbalanced immune profile. TMAO, trimethylamine-N-oxide; IP, imidazole propionate; BA, butyrate; GABA, gamma-aminobutyric acid; NE, norepinephrine; 5-HT, serotonin.
Nevertheless, except for the antibody-dependent way, maternal microbiota-driven immune system development also depends on cellular immunity (Figure 2). The placenta provides a barrier preventing the immune cells traveling across the maternal-fetal interface, keeping the fetal and maternal immune cells separate (79). However, this concept has been challenged by a study providing strong evidence that a great number of maternal cells could cross the placental barrier to stay in fetal lymph nodes, before they induced the development of Tregs in fetus that suppress anti-maternal immunity (80). Also, fetal and maternal cells flow bi-directionally, with fetal cells being transferred to the mother (81). This early exchange shapes the offspring’s immune response and can, in part, explain why maternal gut microbiota can impact offspring’s immune condition.
Even though whether there are microbes in the placenta, amniotic fluid, or fetal tissues is questionable, the transport of immune molecules or microbiota-derived metabolites via the placental barrier is well established (Figure 2) (82–86). Recent evidence showed that a short and mild infection during prenatal development could lead to lasting alterations to gut epithelial stem cells of offspring mediated by IL-6, resulting in enhanced resistance to gut infection and increased susceptibility to mucosal inflammation (87). Metabolites of the maternal gut microbiota are also potential candidates for immune alignment. A study found that disturbances in the maternal microbiota that affect the generation of acetate may impair fetal Tregs development (88). Moreover, the serotonin dysregulation in placenta can disturb neurotransmitter signaling in the fetal brain (89).
3.4 The possible intrauterine microbiota and uterine immune tolerance in pregnancy
For about a century, the uterus was believed to be sterile, with microbes only colonizing the newborn during birth. However, this concept has been greatly challenged in recent studies. Several studies have detected microbes in human placenta and fetus (90–92), though others suggest that the presence of bacteria in pregnancy tissues may due to the DNA contamination (84, 85).
Current evidence revealed that placental microbiome in normal pregnancy is characterized by both gram-positive and gram-negative bacteria dominated by Lactobacillus, which plays a protective role in pregnancy (93). The dysbiosis of microbiota in placenta and the amniotic cavity has been reported to be associated with adverse maternal outcomes such as preterm birth, chorioamnionitis, intrauterine growth restriction, and postpartum hemorrhage (94, 95). Placental microbiota of women with GDM was characterized by lower relative abundance of the Pseudomonadales order and Acinetobacter genus, which was associated with increased O’Sullivan glucose and lower placental expression of anti-inflammatory cytokines IL-10 and TIMP3 (96). In placental microbiota of women with preeclampsia, pathogenic bacteria like Bacillus cereus, Listeria, Salmonella and Escherichia have been reported, which are often associated with infection (97).
Except for vaginal and oral microbiota, gut microbiota has been considered one of the origins of the intrauterine microbiota (98). It has been shown that in utero, bacterial colonization of the fetus can be affected by maternal oral uptake of microbiota. After pregnant mice were fed with a genetically labelled Enterococcus faecium strain, the bacteria could be detected in their offspring delivered by Caesarean section (99). Jeon et al. found that the maternal gut microbiota is the largest donor of the infant bacterial strains, whereas the maternal vaginal microbiota seems to be less important (100). One potential in utero bacterial colonization mechanism is translocation through the choriodecidual barriers (Figure 2) (91). After entering the maternal circulation, a small number of bacteria can be translocated to the placenta and go through the choriodecidual barriers due to higher intercellular junctional permeability and DC-mediated transport (91, 101). Therefore, it is possible for gut microbiota to impact maternal-fetal health via altering the intrauterine microbiota. However, evidence about how microbiota enters the fetoplacental compartment is still lacking.
The uterine immune environment can be affected by intrauterine microbiota. Nature killer (NK) cells are the most abundant immune cells (70–80%) in the decidua (102). As the core of the maternal-fetal immune environment, NK cells play a key role in decidual vascular transformation and IFN-γ production, which then stimulate macrophages, neutrophil cells, T cells and B cells to release antimicrobial substances (103, 104). Studies on uterine NK cells in response to local microbes during pregnancy are limited (105). Recently, a study indicated that intrauterine infection by Porphyromonas gingivalis was associated with increased uterine NK cell populations and decreased secretion of IL-18 in rat, accompanied by a reduction of TNF-α+ T cells (106). Another study showed that the depletion of NK cells, mainly uterine NK cells, played a protective role in Group B Streptococcus (GBS) fetal invasion in GDM mice (107). Crespo et al. show that human decidual NK cells, with higher expression of granulysin than peripheral NK cells, can defense Listeria monocytogenes infection and protect fetus by infusion of granulysin into placental trophoblast cells via nanotubes and removing the intracellular pathogen (108). In the gut, the mucosal NK cells are characterized by limited IFN-γ production and the absence of perforin as a result of the adaptation to the gut commensal microbiota (109, 110). However, whether this property exists when uterine NK cells adapt to the uterine microbiota remains to be clarified.
Small proportion of neutrophils are found at the maternal fetal interface during normal pregnancy. However, they increase dramatically and produce TNF-α and macrophage inflammatory protein-1β (MIP-1β) with intraamniotic bacterial infection, which often lead to preterm birth (111). Neutrophils can neutralize microorganisms and enhance the inflammatory response via forming neutrophil extracellular traps, producing reactive oxygen species and releasing antimicrobial enzymes (112). Additionally, specific placental microbiota and increased activation of neutrophils were found in women with preeclampsia (97, 113). However, to connect the intrauterine microbiota, neutrophils activation and the pathogenesis of preeclampsia, more evidence is still needed.
Antigen-presenting cells, such as macrophages and DCs, account for 10-20% of uterine leukocytes (114). As innate phagocytes, macrophages are likely to engage intrauterine microbiota. Evidence indicated that placental macrophages release macrophage extracellular traps (METs) in response to GBS infection via an oxidative burst-dependent mechanism (115). Other studies suggest that placental macrophages activated inflammatory pathways in response to GBS through Protein kinase D and eliminated Coxiella burnetii infection via an IFN-γ dependent way (116, 117). While intestinal macrophages have a diminished ability to express innate response receptors or to produce proinflammatory cytokines (118), it remains unclear in uterine macrophages.
T cells make up approximately 10–20% of uterine leukocytes in early pregnancy and become the major immune cells of the decidua in late pregnancy (114). Commensal gut microbiota promotes the generation and activation of mucosal Tregs (119), and disrupted gut microbiota leads to impaired differentiation of various T cell subsets (120). Similarly, intrauterine microbiota could be essential for programming the local immunity in the uterus. Specifically, Bacteroides fragilis, commonly present in the lower gut, is found in the uterus (121). Bacteroides fragilis have been reported to reverse systemic T cell deficiencies and the imbalance of Th1/Th2 via polysaccharide A (PSA) secretion (122). Another study found that PSA can promote the immunologic tolerance of Tregs through TLR2 at the intestinal mucosal surfaces in a mouse model (123). Therefore, it could be hypothesized that PSA derived from Bacteroides fragilis plays a role in uterine immune tolerance due to the immunomodulatory effect.
Microbiota has been found in fetal meconium and umbilical cord blood as well. Maternal gut microbiota is essential for the development of the fetal microbiota, which acts as an initiating stimulus for driving the fetal immune system (124). Beneficial bacteria, such as Bifidobacteria and Lactobacilli, present in the human placenta, are important for immune regulation. Their exposure to foreign antigens promotes fetal ILCs and prepares the offspring for colonization during delivery and postnatal life (8). Microbes in fetal tissues could also induce the activation of memory T cells in fetal mesenteric lymph nodes in vitro, suggesting the role of microbes in fetal immune priming (83).
Up to now, whether and how gut microbiota interact with intrauterine microbiota remains to be established. It remains to unclear whether different uterine microbial compositions are associated with altered uterine immune profiles. In addition, the uterine microbial composition may not be the cause but the consequence of the imbalanced immune state. Further studies are required to explore the interactions between intrauterine microbiota and the local immune cells.
4 Maternal gut microbiota in pregnancy complications
As gut microbiota plays an important role in physiological processes during pregnancy, recent studies have focused on the relationship between maternal gut microbiota and pregnancy complications. Recent studies showed that maternal gut microbiota was associated with GDM, HDP and preterm birth. Additionally, recent evidence showed that gut microbial dysbiosis impacted the levels of Th1/Th17 cytokines, thus leading to recurrent miscarriages (Figure 1) (45). Here, we review what kind of effects gut microbiota can bring about in pregnancy complications and the underlying mechanism by which it interacts with the host.
4.1 Maternal gut microbiota and GDM
GDM is a transient hyperglycemic condition during pregnancy. Insulin sensitivity is reduced, and increased maternal plasma glucose and free fatty acids (FFAs) are transported to provide sufficient energy for the development of the fetus through the placenta (125). However, women who are unable to compensate for insulin resistance suffer from hyperglycemia (125). In non-pregnant obese subjects, recent studies have stressed the role of gut microbiota in leading to metabolic disease (126, 127).
A strong association between gut microbiota during pregnancy and maternal metabolic milieu has been proposed in several studies (Table 1) (128–137, 146–170). Gomez-Arango et al. confirmed the positive correlation between circulating insulin at early gestation and the genus Collinsella, which is a strict anaerobic bacterium that produces lactic acid (168), suggesting that high Collinsella abundance may be responsible for the development of insulin resistance during pregnancy. However, as the study was conducted on overweight and obese women, another study investigated if the findings could be extended to non-obese pregnant women (169). Different from Gomez-Arango’s findings, the non-obese women with GDM presented a lower abundance of Collinsella at T1 and a higher abundance of Akkermansia in the second trimester (T2) than the normal controls (169). Several studies observed the increase of pathogenic bacteria and reduction of beneficial butyrate-producing microbes in GDM patients (131, 146–155). An alternative view is that women who developed GDM had significantly fewer changes in the classification and function of gut microbiota with advancing gestation than in gut microbiota during normal pregnancy (132, 165). Apart from bacteria, one study has observed alterations in the fungi in gut microbiota and found that glucose levels were negatively associated Ganoderma, a polysaccharide-producing genera (166).
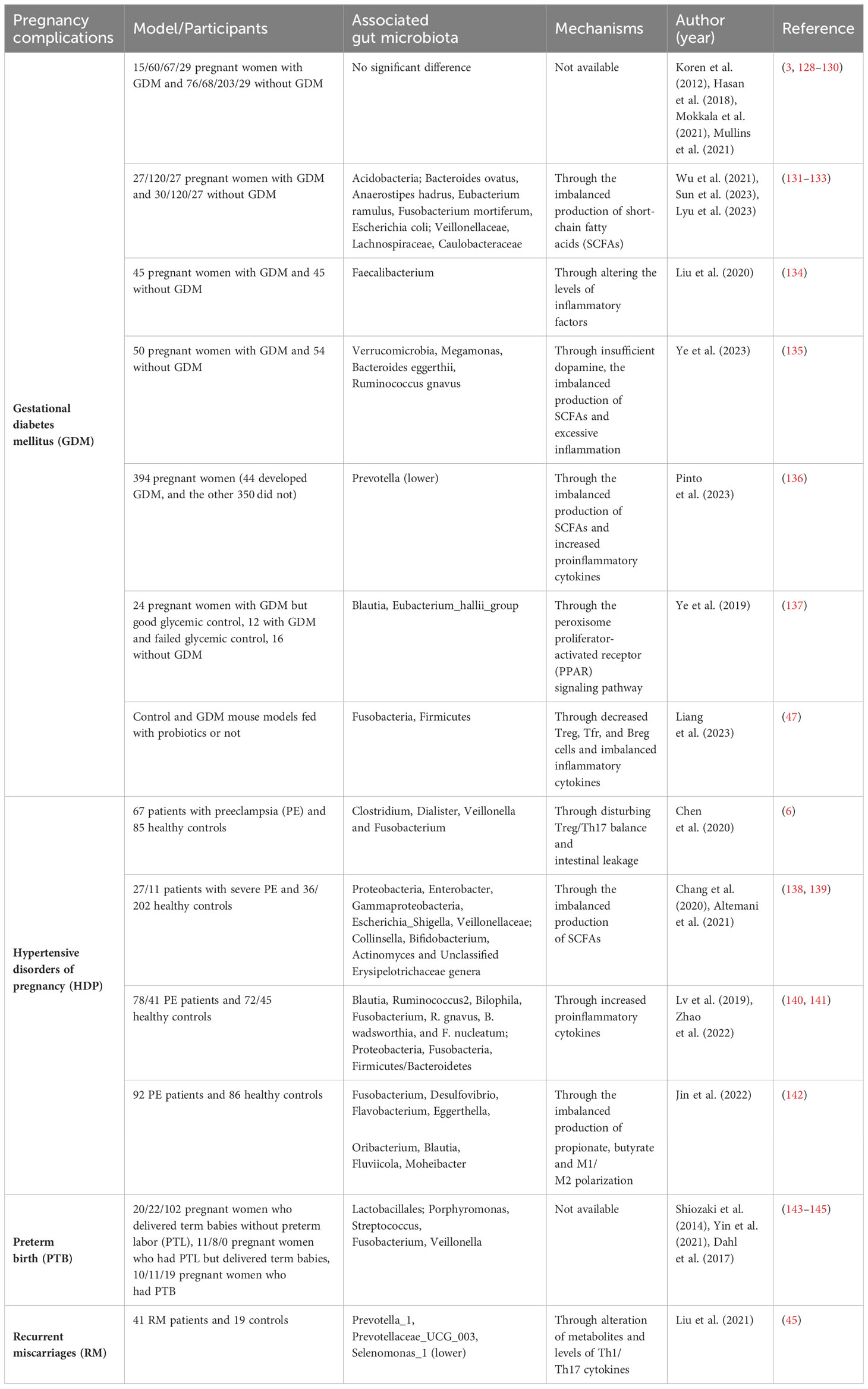
Table 1 Association between maternal gut microbiota and pregnancy complications in model/participants, associated gut microbiota and the underlying mechanisms.
Although there are many studies exploring the correlation between gut microbiota and GDM, few have confirmed a causal relationship between them. A prospective study with 75 overweight pregnant women with normal glucose tolerance found the relationship between the dysbiosis of gut microbiota in early pregnancy and the onset of GDM. After adjusting all potential confounders, there is still a significant association between the Ruminocococaceae family and glucose level (167). To clarify the causal relationship, Liu et al. transferred the fecal samples of GDM-positive women and non-GDM controls to germ-free mice. They found it led to different colonization patterns of gut microbiota, and microbiota from GDM patients induced hyperglycemia in mice (134).
There are also studies extending the time of sampling to the postnatal period (3, 128, 147, 170). Koren et al. reevaluated maternal gut microbiota one month after deliver and showed that gut microbiota dysbiosis and loss of bacteria richness persisted after delivery (3). Crusell et al. reported that the gut microbiota of women with previous GDM was still marked by an aberrant composition eight months after delivery, though the postpartum composition was different from that during pregnancy (147). However, five years after delivery, no significant differences in the composition of gut microbiota between GDM-positive women and non-GDM controls were observed (128).
Nonetheless, the link between gut microbiota and GDM is still controversial (3, 129, 130). Opposed to the above findings, a study by Koren et al. suggest there was no difference between the gut microbiotas of GDM-positive women and non-GDM controls (3). A study based on deep sequencing metagenomics pointed out that specific gut microbiota species did not influence GDM when adjusting all the confounders, except for the higher abundance of Ruminococcus obeum in late pregnancy in women with GDM (129).
Although growing evidence showed a correlation between the altered gut microbiota and GDM, few studies have investigated the mechanisms underlying the dysbiosis of the gut microbiota that leads to the development of GDM (Figure 1). Recent studies found that the insufficiency of circulating dopamine, imbalanced production of SCFAs, and excessive inflammation resulting from the dysbiosis of the gut microbiota led to the development of GDM (133, 135, 136). Meanwhile, restoring the balance of maternal gut microbiota limited the inflammatory response by increasing the production of Tregs, Tfrs, Bregs in the GDM mice model (47). Another study proposed that gut bacteria were responsible for shifting indoleamine 2,3-dioxygenase-dependent tryptophan anaerobic metabolism to kynurenine production, leading to intestinal inflammation and gestational insulin resistance in the mice model (171).
In summary, women with GDM often presented gut microbiota dysbiosis with increased pathogenic bacteria, while symbiotic butyrate-producing bacteria were depleted. The dysbiosis of the gut microbiota is closely related to immune imbalances and excessive inflammation. Given that the studies are not sufficient to confirm a causal relationship between gut microbiota and GDM, we need more prospective studies or try to transplant gut microbiota from GDM mice to germ-free mice to clarify the actual relationship and more detailed mechanisms between maternal gut microbiota and GDM.
4.2 Maternal gut microbiota and hypertensive disorders of pregnancy
Hypertensive disorders of pregnancy (HDP), characterized by increased blood pressure, include gestational hypertension, preeclampsia (PE), eclampsia and chronic hypertension with superimposed preeclampsia, seriously affecting the health of mothers and babies (172).
A growing number of studies have suggested a close relationship between the development of HDP and dysbiosis of the gut microbiota (Table 1) (6, 138–142, 173–182). Of the studies investigating these relationships, most have focused on PE. A study using shotgun metagenomic sequencing showed that some species that were classified in Blautia, Pauljensenia, Ruminococcus, and Collinsella were increased in the gut microbiota of PE donors (177). By transplanting feces from PE donors into antibiotic-treated mice, elevated pregestational blood pressure was observed in mice, suggesting that the gut microbiome has a direct impact on host blood pressure (6). The PE-transplanted group also demonstrated disturbed Treg/Th17 balance in the gut and spleen and more severe intestinal leakage than the controls (6). To clarify the causal effect of gut microbiota, a two-sample Mendelian randomization study was conducted to reveal that Bifidobacterium was causally associated with PE (175). Lv et al. found that the alterations of gut microbiota during pregnancy persisted 6 weeks postpartum (140).
Most studies have observed decreased SCFA-producing bacteria and SCFAs in the gut microbiota of individuals with PE (6, 138–142, 173–182). Recently, a study showed evidence that Akkermansia muciniphila, propionate, or butyrate significantly reversed the symptoms of PE rats by inducing autophagy and M2 phenotype of macrophages in the placenta (Figure 1, Table 1) (142). Tang et al. found that gut microbiota dysbiosis led to proliferation, invasion, and migration of trophoblast cells in preeclampsia (176).
To date, it is hard to conclude whether changes in the gut microbiome occur prior to HDP or as a result of it. We need to distinguish adaptive changes of gut microbiota from disease-promoting microbiota as well as eliminate environmental disturbances. There are still gaps in knowledge about the gut microbiome of HDP, including longitudinal analyses of the microbial profiles of the subjects during pregnancy and the underlying immune mechanisms linking microbes to the development of HDP. Moreover, we need more studies with fecal transplantation in animal models, which can clarify the cause-and-effect relationship between gut microbiota and HDP.
4.3 Maternal gut microbiota and preterm birth
Preterm birth has been a substantial problem in perinatal medicine worldwide in recent years. A great number of studies have investigated the relationship between vaginal microbiota and preterm delivery, while the research on gut microbiota is limited.
Only a few studies proposed the association between gut microbiota and preterm birth (Table 1). Shiozaki et al. first described that some clusters of Clostridium and Bacteroides depleted while the level of Lactobacillales increased in women delivering prematurely (143). Other studies revealed reduced α-diversity and lower species abundance in the Bifidobacterium, Streptococcus genera, and Clostridiales order in gut microbiota from individuals with preterm birth (7, 144). Recently, Yin et al. found that opportunistic pathogens, especially Porphyromonas, Streptococcus, Fusobacterium, and Veillonella, were increased. In contrast, Coprococcus and Gemmiger were markedly decreased in the gut microbiota of women with preterm birth. Interestingly, most of the enriched microbes were oral bacteria, implying the possibility of oral-to-gut migration (145).
The altered gut microbiota may impair the anti-inflammatory properties to increase the risk of preterm birth (Figure 1). Clostridia spp. is able to impact the number and function of Tregs in the colon, thereby attenuating inflammation (183). Bacteroides fragilis activates IL-10-secreting Tregs through PSA to reduce inflammatory response in the gut, which downregulates the TLR2 signaling pathway and suppresses Th17 responses (184). Multiple strains of Bifidobacterium have anti-inflammatory properties with the ability to inhibit LPS-induced NF-κB activation, IL-8, TNF-α, cyclooxygenase 2 (Cox-2), and intercellular adhesion molecule-1 (ICAM-1) in vitro (185). Thus, its decrease in abundance may increase the susceptibility of the host to inflammation-induced preterm delivery.
While these studies provide support for the potential role of gut microbiome in mediating preterm birth, more research is clearly needed in this area.
5 Maternal gut microbiota: the role in offspring health
Maternal gut microbiota is not only a candidate for the health of mothers but also an important factor that is beneficial or harmful to the health of the offspring. Maternal gut microbiota provides key metabolites and substrates essential for fetal immune and neural development (8–10). A range of mouse and human studies support that stable maternal gut microbiota can decrease the risk of offspring diseases. Conversely, disturbance in maternal gut microbiota, such as infection, malnutrition and exposure to antibiotics, may impair the health of offspring in a wide range (9, 11, 12).
5.1 Maternal microbiota and allergic diseases in offspring
Maternal gut microbiota plays a vital role in driving the early innate immune development of the fetus (76, 77). Allergic diseases, including atopic dermatitis, food allergy and asthma, are often linked to the immune system. A large number of studies have shown that elevated Th1 response, increased Tregs, and enhanced neutrophils are signs for the decreased risk of allergic disease (186–190). Dysbiosis of gut microbiota may impair the fetal immune system, making it susceptible to allergic diseases after birth.
Previous studies have explored the direct relationship between maternal gut microbiota and allergic disease in offspring in humans (Table 2). One study that collected stool samples from 60 pregnant women during T3 of their pregnancy showed that higher maternal total aerobes and enterococci were related to an increased risk of infant wheezing, which is associated with an increased risk of child asthma (205). Mothers with infants who had atopic dermatitis showed increased Candidatus_Stoquefichus and Pseudomonas during pregnancy (191). Instead, carriage of Holdemania and Prevotella copri in maternal gut microbiota during T3 protected infants from food allergies (192, 193). Another pilot study showed that the diversity of Proteobacteria and the relative abundance of Actinobacteria from maternal gut microbiota were negatively associated with dermatitis in early infancy (206).
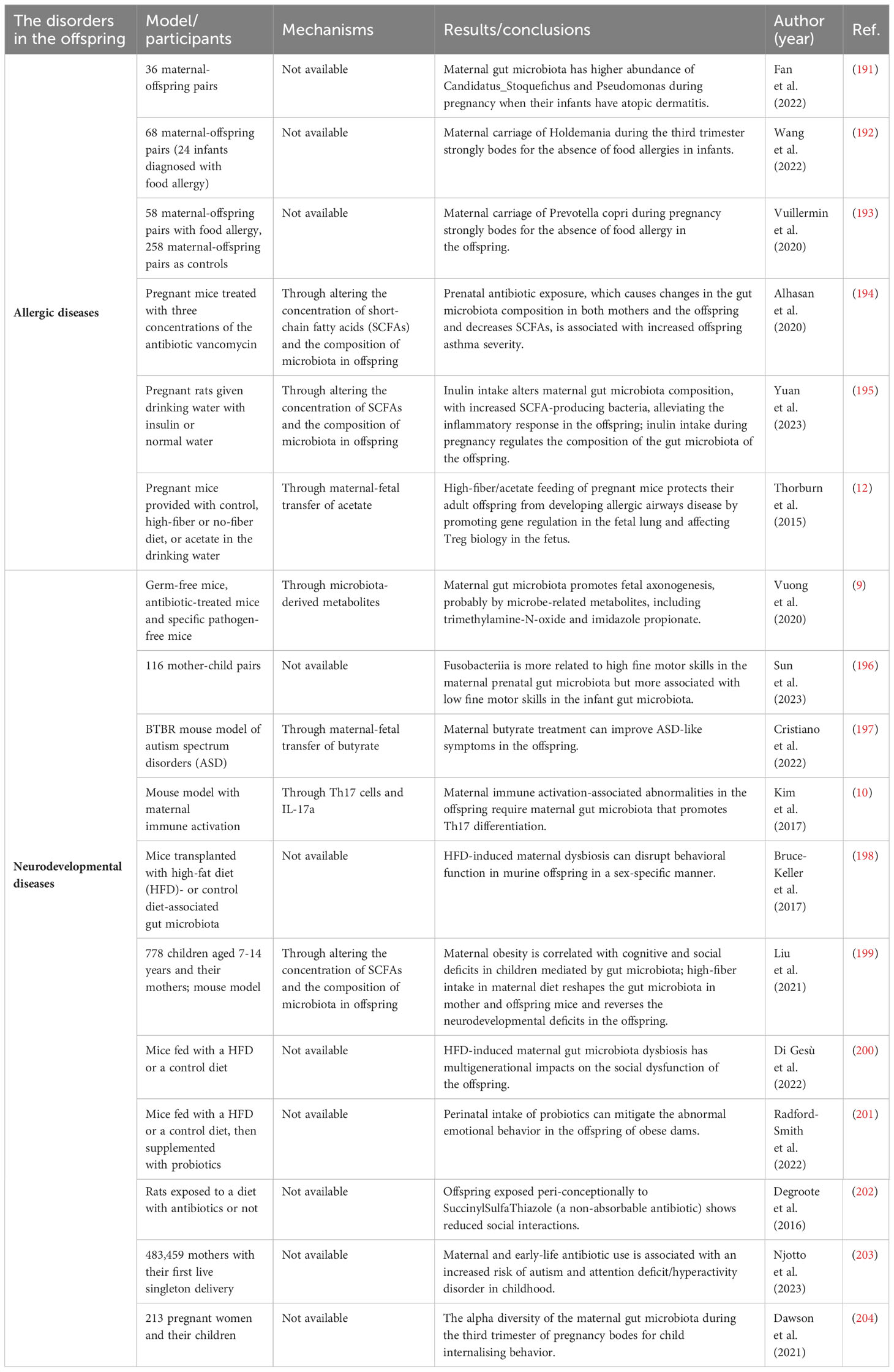
Table 2 Association between maternal gut microbiota and the disorders of the offspring in model/participants, mechanisms, and results/conclusions.
The association between maternal gut microbiota and allergic diseases in offspring may also depend on the maternal-fetal transfer of SCFAs, which often changes the composition of the gut microbiota of the offspring at the same time (12, 194, 195). SCFAs are known to have anti-inflammatory properties, and butyrate is able to protect against asthma by inducing FoxP3 on Tregs, suppressing inflammatory Th9 cells and inhibiting IL-13 and IL-5 production by ILC2s (207–209). Except for butyrate, propionate contributed to the alteration of DC biology to protect against allergic airway disease, for DCs had ability to promote Th2 responses (210),.
Additionally, maternal diet, nutrition, prenatal stress, supplementation of prebiotics and probiotics during pregnancy, as well as exposure to environmental pollutants, have all been proven to affect the allergic immune response across generations. However, it is unknown whether there is any direct involvement of maternal gut microbiota (211–215). Maternal treated with antibiotics during pregnancy was associated with increased asthma severity in the offspring in a dose-dependent way (194, 216–218).
5.2 Maternal gut microbiota and neurodevelopmental diseases in offspring
The prenatal and early postnatal periods are critical for the rapid development of the human nervous system. Disruptions in the development of the fetal gut microbiota in early life can affect neurodevelopment and may lead to poor mental health outcomes later in life (219). Recently, the role of maternal gut microbiota in the neurodevelopment of children has aroused heated discussion.
Maternal gut microbiota can have a great impact on the nervous system of the offspring (Table 2). In the formation of neural circuits, maternal gut microbiota produced metabolites, antibodies, and substrates during the prenatal period to provide important metabolic support (9). In human research, a large-scale study found that Fusobacteriia in maternal gut microbiota is more associated with the high fine motor skills of their children (196). In mice models, maternal butyrate treatment can reverse autism spectrum disorders in offspring (197). Embryos of antibiotic-treated and sterile mice showed lower expression of axonogenesis genes, defective thalamocortical axons and impaired thalamic axon growth, which suggest that dysbiosis of gut microbiota can have a permanent impact on fetal neurological development through altering microbially modulated metabolites which promote axonogenesis, including trimethylamine-N-oxide and imidazole propionate (9). Maternal immune activation (MIA) can lead to behavioral abnormalities and neurodevelopmental disorders in the offspring (220, 221), and IL-17a produced by Th17 cells plays a crucial role in inducing behavioral and cortical abnormalities (222). In 2017, Kim et al. proved that MIA-associated neurodevelopmental disorders in the offspring required maternal gut bacteria that promote Th17 cell differentiation. Mouse commensal segmented filamentous bacteria or human commensal bacteria that induced intestinal Th17 cells were more likely to increase the risk of MIA-associated abnormalities in the offspring (10). In addition to behavioral abnormalities, another study in 2022 proved that maternal gut microbiota could also drive inflammation in the gut of offspring with neurodevelopmental diseases by changing the chromatin landscape of naive CD4+ T cells (223).
Factors that impact the maternal gut microbiota, such as maternal diet, infections and prenatal stress, have all been linked to neurodevelopmental disorders in the offspring (198, 224). A maternal high-fat diet can induce long-term cognitive deficits in the next generation (225). However, maternal treatment with oral intestinal alkaline phosphatase, an enzyme that tightens the gut barrier and promotes the growth of commensal symbionts by detoxifying anti-inflammatory agents, mitigated high-fat diet-induced cognitive disorders in offspring mice (226). When there is antibiotic exposure during pregnancy, more severe anxiety and less social interactions were observed in rat offspring (202), and similar results were found in a human study (203). Maternal gut microbiota may work in both prenatal and postnatal phases to continuously influence the health of offspring.
Maternal gut microbiota dysbiosis participates in the development of neurological disorders in the offspring via microbially modulated metabolites and alterations in the composition of the microbiota of offspring (9, 199) or by mediating immune activation and IL-17a elevation (10). Given that most of the experiments were conducted with mice models, human research is needed for future exploration.
6 Potential therapeutic strategies
Given that maternal gut microbiota plays a crucial role in the pathogenesis of pregnancy complications and the health status of the offspring, it is particularly important to take effective interventions. Among the interventions, taking probiotics and improving the diet aimed at restoring gut microbiota balance are the most widely applied.
6.1 Probiotics
Probiotics are live microbes beneficial to the host when administered in adequate amounts (227). Bifidobacterium and Lactobacillus are the most common microbes used as medical interventions (228). Probiotics contribute to beneficial health outcomes by increasing the composition of symbiotic bacteria, reducing the adherence and penetration of pathogenic bacteria, strengthening the permeability of gut epithelium, and regulating immune response (Figure 3) (229, 230). The combination of Bifidobacterium, Lactobacillus, and Streptococcus increased the expression of tight junction proteins, including claudin-1 and occluding, and suppressed the expression of pro-inflammatory cytokines like IL-6 and IL-17 (231). Additionally, probiotics can induce the proliferation of Tregs and the secretion of anti-inflammatory cytokines like IL-10 (232). In general, probiotics are safe and play a protective role in mothers and infants (227, 233).
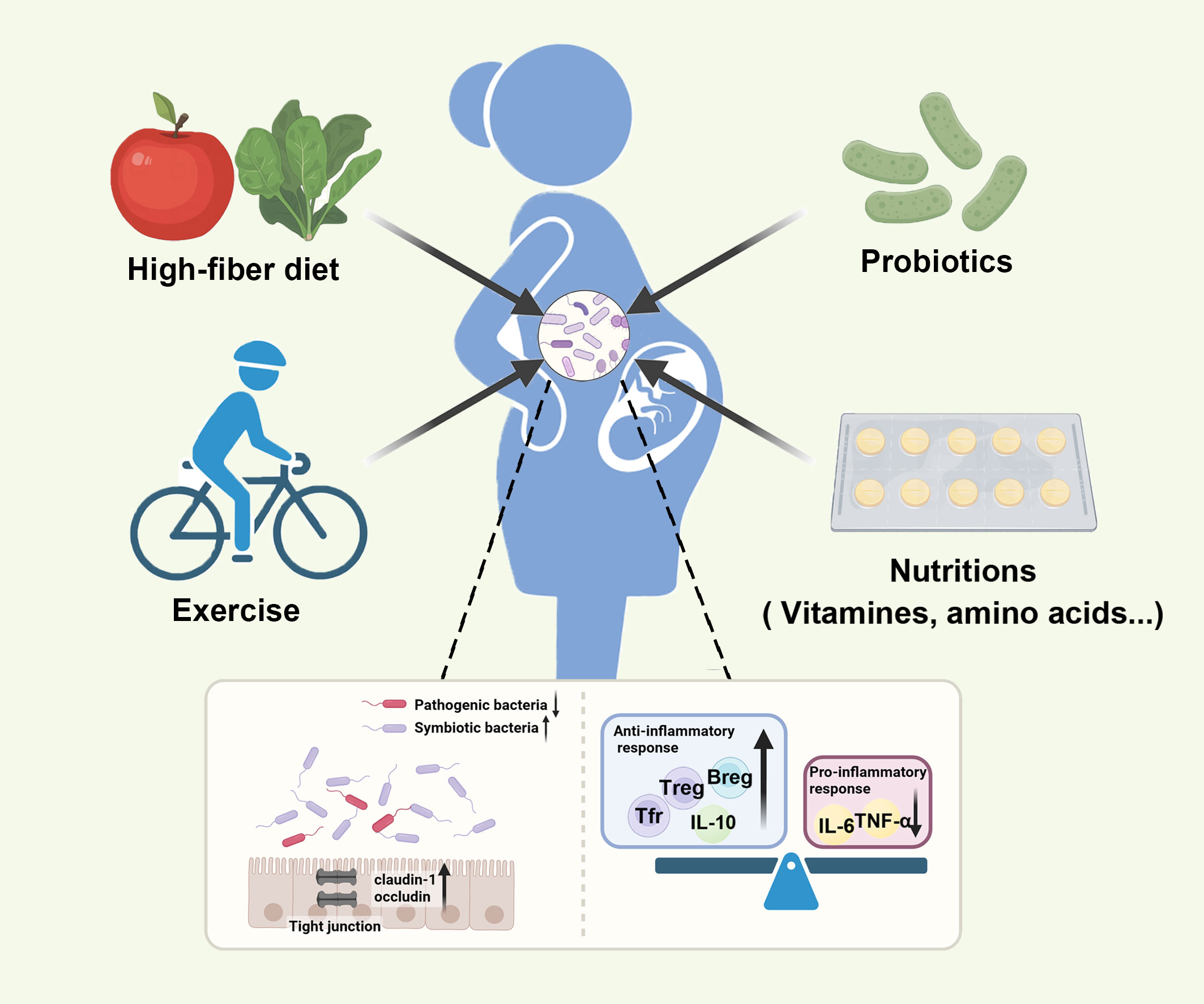
Figure 3 Various interventions can restore maternal gut microbiota balance and promote the health of mothers and infants. Various inventions, such as probiotics, the high-fiber diet, exercise, and nutrition supplementation, can restore the composition of gut microbiota by increasing symbiotic bacteria, reducing pathogenic bacteria, strengthening gut epithelial permeability, and regulating immune response.
Probiotics have a positive effect on preventing pregnancy complications. In GDM, a meta-analysis of 10 randomized control trials with 2921 pregnant women was published to assess the role of probiotics in GDM women (234). The results showed that probiotics reduced GDM incidence by 33%, and using multiple-strain probiotics had a greater effect on GDM. One study examined the therapeutic effects of probiotics on inflammatory factors. After the intervention, hypersensitive C-reactive protein (hs-CRP), TNF-α, and IL-6 levels were significantly reduced in women with GDM who were treated with probiotics compared to the placebo group (235). A recent study proved that oral probiotics increased Tregs, Tfrs and Bregs and decreased proinflammatory cytokines to prevent inflammation in mice with GDM (47), which showed the effect of probiotics on regulating the immune response. Additionally, probiotics can reverse the phenotype of GDM through the metabolism pathways of amino acids, bile acids, porphyrin, and chlorophyll (236). Limosilactobacillus reuteri could ameliorate PE in mice by improving endothelial dysfunction (237). However, a recent systematic review indicated that probiotics may slightly increase PE rates in pregnant women with comorbidities (238). There are also studies found that oral probiotics can prevent preterm birth in mice and human by reducing leucocyte infiltration and inflammation in reproductive tissue (239, 240). These findings emphasize the clinical value of regulating gut microbiota.
Probiotics taken by mothers can provide benefits for the offspring. A study in mice showed that maternal milk oligosaccharides and probiotics could promote immune organ development, splenocyte proliferation, and antibody production, as well as improve macrophage phagocytosis in the offspring (241). The systematic review and meta-analysis found that maternal probiotics supplementation prenatal reduced the risk of eczema in infants, but not on other allergic diseases (242, 243). However, another meta-analysis found that Lactobacillus rhamnosus GG supplementation did not reduce the risk of eczema (244). The heterogeneity between studies may due to different probiotic strains and protocols. In the prevention of neurodevelopmental diseases, a study showed that the intake of probiotics during pregnancy could alleviate the abnormal emotional behavior in the offspring of obese mice (201). Bifidobacterium feeding during pregnancy protected the offspring from depression-like behaviors (245).
The selection of an appropriate probiotic strain is important, which may impact the therapeutic effects of probiotics (234). Therefore, deeper investigation, including different strains of probiotics, was required in the future.
6.2 High-fiber diet
Dietary fiber, fermented by gut bacteria, is able to produce SCFAs and participated in the host’s physiologic process. The high-fiber diet has potential benefits for the health of mothers. Short-term diet management in GDM patients can bring a change in the ratio of Firmicutes/Bacteroidetes and even in some specific fungi, suggesting that the diet intervention could play a positive role during GDM pregnancy (131, 166). Recently, Huang et al. found that high dietary fiber, which increased abundances of Lachnospiraceae and butyrate, enhanced the gut barrier and inhibited the transfer of LPS, thus reducing placental inflammation and insulin resistance (246). Similar results were also found in pigs. A high-fiber diet reduced proinflammatory markers like IL-6 and increased the anti-inflammatory markers like IL-10 in sows and piglets through promoting beneficial bacteria and the production of SCFAs (247–249). However, studies that investigated the therapeutic effects of dietary fiber in HDP and preterm birth are still lacking.
While a high-fat diet may cause metabolic and neurological disadvantages in offspring, replacing it with a healthy diet may improve the disease status of offspring by improving infant gut microbiota diversity and reducing opportunistic pathogens, such as Enterococcaceae (250). An interesting finding showed that high-fiber/acetate feeding of pregnant mice, which yielded a distinctive gut microbiota, suppressed allergic airway disease responses in the offspring by suppressing the expression of certain genes in the fetal lung and promoting Treg function in the fetus (12). Given that a maternal high-fat diet can induce long-term cognitive disorders in the next generation (225), several studies found that maternal gut microbiota mediated maternal obesity-induced cognitive and social deficits in offspring through co-housing and fecal microbiota transplantation experiments, which could be reversed by a high-fiber diet in either pregnant mice or offspring (199, 200). Additionally, maternal high-fiber diet improved the testicular development in the offspring in sow model (251).
Dietary fiber provides benefits for the health of mothers and offspring via altering the composition of maternal gut microbiota, increasing SCFAs production, improving the antioxidant capacity, and reducing inflammation (248, 252). Li et al. proposed that dietary fiber promoted maternal serotonin synthesis in the gut and transported the serotonin to the placenta in sows, which improved placental function (253). A high-fiber diet was also found to lead to an increase in serum concentration of IL-10 and IgG, and a decrease in the serum concentration of IFN-γ and CRP (254, 255). Based on the solubility, dietary fiber has been classified into soluble fiber and insoluble fiber. Soluble fiber tends to have more extensive impact on gut microbiota and the expression of the gut barrier-related genes than insoluble fiber in sow model (256). Higher ratio of soluble fiber to insoluble fiber in pregnancy diets was associated with higher antioxidant capacity, higher total SCFAs concentrations and lower pro-inflammatory factors (252).
Except for probiotics and a healthy diet, other inventions, such as prebiotics, synbiotics, exercise, and nutrition supplementation (257–259), can also restore the composition of gut microbiota and even prevent detrimental outcomes, though current studies are still limited.
7 Conclusion and outlook
This review emphasizes the crucial role that maternal gut microbiota plays in the health of mothers and offspring from the perspective of immunology, linking maternal gut microbiota and its derivatives and metabolites to the immune response during pregnancy. The core findings lie in the abilities of maternal gut microbiota to alter the immune environment at the maternal-fetal interface and even affect the immune system of the offspring during pregnancy. From this point, the maternal-fetal health, is hopefully intervened through the reconstitution of the maternal gut microbiota. Lactobacillus and Bifidobacterium have been proved beneficial for patients with specific pregnancy-related diseases.
So far, the findings have been limited. The direct interaction between maternal gut microbiota and the immune response in utero has yet to be fully investigated. In current studies, the number of patients or animal models included is not always large enough to be statistically strong to draw clear and consistent conclusions. Likewise, there is great variability in inclusion criteria, methods, and doses and duration of medications between different studies, leading to contradictory findings.
Whether based on clinical cohorts or animal model, most of the studies proposed that gut microbiota dysbiosis is one of the etiological factors in the pregnancy-related diseases. However, due to the heterogeneity between human and animal model, the specific species of microbes that undergoes change is not always the same. While studies based on clinical patients are more suitable for exploring specific strains highly associated with the pathogenesis of GDM, animal models are more convenient for probing the underlying mechanisms and the causal relationship between gut microorganism and the diseases. Considering mouse model is more economical and convenient, we recommended to transplant specific constitution of microbes to germ-free mice for the in-depth study of this field.
More research should focus on identifying the exact species and strains of gut microbiota, or the specific molecules or metabolites that significantly improve or impair the maternal-fetal health, which may provide a potential therapeutic target. Researchers can pay more attention to the impact of gut microbiota on innate immune cells, like NK cells and neutrophils. The relationship between gut microbiota and intrauterine microbiota is also a research gap currently. Together, more valuable evidence is needed in the future research.
Author contributions
XL: Conceptualization, Writing – original draft, Writing – review & editing. ZS: Visualization, Writing – review & editing. LJ: Conceptualization, Visualization, Writing – review & editing. SZ: Funding acquisition, Supervision, Writing – review & editing.
Funding
The author(s) declare that financial support was received for the research, authorship, and/or publication of this article. This study was financially supported by Zhejiang Province “Leading Talents” research and development project (2023C03033), the National Natural Science Foundation of China (82271694), the Zhejiang Province Natural Science Foundation key project fund (LZ24H040002), the Zhejiang Medicine and Health Science and Technology Plan Project (2023KY800).
Acknowledgments
The authors would like to thank the participants for their contributions.
Conflict of interest
The authors declare that the research was conducted in the absence of any commercial or financial relationships that could be construed as a potential conflict of interest.
Publisher’s note
All claims expressed in this article are solely those of the authors and do not necessarily represent those of their affiliated organizations, or those of the publisher, the editors and the reviewers. Any product that may be evaluated in this article, or claim that may be made by its manufacturer, is not guaranteed or endorsed by the publisher.
References
1. Newbern D, Freemark M. Placental hormones and the control of maternal metabolism and fetal growth. Curr Opin endocrinology diabetes Obes. (2011) 18:409–16. doi: 10.1097/MED.0b013e32834c800d
2. Mor G, Cardenas I. The immune system in pregnancy: A unique complexity. Am J Reprod Immunol (New York NY 1989). (2010) 63:425–33. doi: 10.1111/j.1600-0897.2010.00836.x
3. Koren O, Goodrich JK, Cullender TC, Spor A, Laitinen K, Bäckhed HK, et al. Host remodeling of the gut microbiome and metabolic changes during pregnancy. Cell. (2012) 150:470–80. doi: 10.1016/j.cell.2012.07.008
4. Takiishi T, Fenero CIM, Câmara NOS. Intestinal barrier and gut microbiota: shaping our immune responses throughout life. Tissue barriers. (2017) 5:e1373208. doi: 10.1080/21688370.2017.1373208
5. Huang L, Thonusin C, Chattipakorn N, Chattipakorn SC. Impacts of gut microbiota on gestational diabetes mellitus: A comprehensive review. Eur J Nutr. (2021) 60:2343–60. doi: 10.1007/s00394-021-02483-6
6. Chen X, Li P, Liu M, Zheng H, He Y, Chen MX, et al. Gut dysbiosis induces the development of pre-eclampsia through bacterial translocation. Gut. (2020) 69:513–22. doi: 10.1136/gutjnl-2019-319101
7. Gershuni V, Li Y, Elovitz M, Li H, Wu GD, Compher CW. Maternal gut microbiota reflecting poor diet quality is associated with spontaneous preterm birth in a prospective cohort study. Am J Clin Nutr. (2021) 113:602–11. doi: 10.1093/ajcn/nqaa361
8. Gomez de Agüero M, Ganal-Vonarburg SC, Fuhrer T, Rupp S, Uchimura Y, Li H, et al. The maternal microbiota drives early postnatal innate immune development. Sci (New York NY). (2016) 351:1296–302. doi: 10.1126/science.aad2571
9. Vuong HE, Pronovost GN, Williams DW, Coley EJL, Siegler EL, Qiu A, et al. The maternal microbiome modulates fetal neurodevelopment in mice. Nature. (2020) 586:281–6. doi: 10.1038/s41586-020-2745-3
10. Kim S, Kim H, Yim YS, Ha S, Atarashi K, Tan TG, et al. Maternal gut bacteria promote neurodevelopmental abnormalities in mouse offspring. Nature. (2017) 549:528–32. doi: 10.1038/nature23910
11. Kimura I, Miyamoto J, Ohue-Kitano R, Watanabe K, Yamada T, Onuki M, et al. Maternal gut microbiota in pregnancy influences offspring metabolic phenotype in mice. Sci (New York NY). (2020) 367:eaaw8429. doi: 10.1126/science.aaw8429
12. Thorburn AN, McKenzie CI, Shen S, Stanley D, Macia L, Mason LJ, et al. Evidence that asthma is a developmental origin disease influenced by maternal diet and bacterial metabolites. Nat Commun. (2015) 6:7320. doi: 10.1038/ncomms8320
13. Nelson SM, Matthews P, Poston L. Maternal metabolism and obesity: modifiable determinants of pregnancy outcome. Hum Reprod Update. (2010) 16:255–75. doi: 10.1093/humupd/dmp050
14. Bowen DJ. Taste and food preference changes across the course of pregnancy. Appetite. (1992) 19:233–42. doi: 10.1016/0195-6663(92)90164-2
15. Nuriel-Ohayon M, Neuman H, Ziv O, Belogolovski A, Barsheshet Y, Bloch N, et al. Progesterone increases bifidobacterium relative abundance during late pregnancy. Cell Rep. (2019) 27:730–6.e3. doi: 10.1016/j.celrep.2019.03.075
16. Smid MC, Ricks NM, Panzer A, McCoy AN, Azcarate-Peril MA, Keku TO, et al. Maternal gut microbiome biodiversity in pregnancy. Am J perinatology. (2018) 35:24–30. doi: 10.1055/s-0037-1604412
17. DiGiulio DB, Callahan BJ, McMurdie PJ, Costello EK, Lyell DJ, Robaczewska A, et al. Temporal and spatial variation of the human microbiota during pregnancy. Proc Natl Acad Sci United States America. (2015) 112:11060–5. doi: 10.1073/pnas.1502875112
18. Dunlop AL, Knight AK, Satten GA, Cutler AJ, Wright ML, Mitchell RM, et al. Stability of the Vaginal, Oral, and Gut Microbiota across Pregnancy among African American Women: The Effect of Socioeconomic Status and Antibiotic Exposure. PeerJ. (2019) 7:e8004. doi: 10.7717/peerj.8004
19. Mukhopadhya I, Hansen R, El-Omar EM, Hold GL. Ibd-what role do proteobacteria play? Nat Rev Gastroenterol Hepatol. (2012) 9:219–30. doi: 10.1038/nrgastro.2012.14
20. Chen J, Li F, Yang W, Jiang S, Li Y. Comparison of gut microbiota and metabolic status of sows with different litter sizes during pregnancy. Front veterinary Sci. (2021) 8:793174. doi: 10.3389/fvets.2021.793174
21. Liu H, Hou C, Li N, Zhang X, Zhang G, Yang F, et al. Microbial and metabolic alterations in gut microbiota of sows during pregnancy and lactation. FASEB J Off Publ Fed Am Societies Exp Biol. (2019) 33:4490–501. doi: 10.1096/fj.201801221RR
22. Zhou P, Zhao Y, Zhang P, Li Y, Gui T, Wang J, et al. Microbial mechanistic insight into the role of inulin in improving maternal health in a pregnant sow model. Front Microbiol. (2017) 8:2242. doi: 10.3389/fmicb.2017.02242
23. Ji YJ, Li H, Xie PF, Li ZH, Li HW, Yin YL, et al. Stages of pregnancy and weaning influence the gut microbiota diversity and function in sows. J Appl Microbiol. (2019) 127:867–79. doi: 10.1111/jam.14344
24. Xie P, Hu C, Azad MAK, He Q, Zhu Q, Kong X. Dynamic alteration in the gut microbiota and metabolome of huanjiang mini-pigs during pregnancy. BMC veterinary Res. (2022) 18:385. doi: 10.1186/s12917-022-03477-0
25. David LA, Maurice CF, Carmody RN, Gootenberg DB, Button JE, Wolfe BE, et al. Diet rapidly and reproducibly alters the human gut microbiome. Nature. (2014) 505:559–63. doi: 10.1038/nature12820
26. Yatsunenko T, Rey FE, Manary MJ, Trehan I, Dominguez-Bello MG, Contreras M, et al. Human gut microbiome viewed across age and geography. Nature. (2012) 486:222–7. doi: 10.1038/nature11053
27. Spor A, Koren O, Ley R. Unravelling the effects of the environment and host genotype on the gut microbiome. Nat Rev Microbiol. (2011) 9:279–90. doi: 10.1038/nrmicro2540
28. Jovel J, Patterson J, Wang W, Hotte N, O'Keefe S, Mitchel T, et al. Characterization of the gut microbiome using 16s or shotgun metagenomics. Front Microbiol. (2016) 7:459. doi: 10.3389/fmicb.2016.00459
29. Gomez-Arango LF, Barrett HL, McIntyre HD, Callaway LK, Morrison M, Dekker Nitert M. Connections between the gut microbiome and metabolic hormones in early pregnancy in overweight and obese women. Diabetes. (2016) 65:2214–23. doi: 10.2337/db16-0278
30. Qi X, Yun C, Pang Y, Qiao J. The impact of the gut microbiota on the reproductive and metabolic endocrine system. Gut Microbes. (2021) 13:1–21. doi: 10.1080/19490976.2021.1894070
31. Robinson DP, Klein SL. Pregnancy and pregnancy-associated hormones alter immune responses and disease pathogenesis. Hormones Behav. (2012) 62:263–71. doi: 10.1016/j.yhbeh.2012.02.023
32. Amar J, Chabo C, Waget A, Klopp P, Vachoux C, Bermúdez-Humarán LG, et al. Intestinal mucosal adherence and translocation of commensal bacteria at the early onset of type 2 diabetes: molecular mechanisms and probiotic treatment. EMBO Mol Med. (2011) 3:559–72. doi: 10.1002/emmm.201100159
33. Koh A, De Vadder F, Kovatcheva-Datchary P, Bäckhed F. From dietary fiber to host physiology: short-chain fatty acids as key bacterial metabolites. Cell. (2016) 165:1332–45. doi: 10.1016/j.cell.2016.05.041
34. Flint HJ, Scott KP, Duncan SH, Louis P, Forano E. Microbial degradation of complex carbohydrates in the gut. Gut Microbes. (2012) 3:289–306. doi: 10.4161/gmic.19897
35. Macia L, Nanan R, Hosseini-Beheshti E, Grau GE. Host- and microbiota-derived extracellular vesicles, immune function, and disease development. Int J Mol Sci. (2019) 21:107. doi: 10.3390/ijms21010107
36. Kim MH, Choi SJ, Choi HI, Choi JP, Park HK, Kim EK, et al. Lactobacillus plantarum-derived extracellular vesicles protect atopic dermatitis induced by staphylococcus aureus-derived extracellular vesicles. Allergy Asthma Immunol Res. (2018) 10:516–32. doi: 10.4168/aair.2018.10.5.516
37. Kim JH, Jeun EJ, Hong CP, Kim SH, Jang MS, Lee EJ, et al. Extracellular vesicle-derived protein from bifidobacterium longum alleviates food allergy through mast cell suppression. J Allergy Clin Immunol. (2016) 137:507–16.e8. doi: 10.1016/j.jaci.2015.08.016
38. Díez-Sainz E, Milagro FI, Riezu-Boj JI, Lorente-Cebrián S. Effects of gut microbiota-derived extracellular vesicles on obesity and diabetes and their potential modulation through diet. J Physiol Biochem. (2022) 78:485–99. doi: 10.1007/s13105-021-00837-6
39. Kaisanlahti A, Turunen J, Byts N, Samoylenko A, Bart G, Virtanen N, et al. Maternal microbiota communicates with the fetus through microbiota-derived extracellular vesicles. Microbiome. (2023) 11:249. doi: 10.1186/s40168-023-01694-9
40. PrabhuDas M, Bonney E, Caron K, Dey S, Erlebacher A, Fazleabas A, et al. Immune mechanisms at the maternal-fetal interface: perspectives and challenges. Nat Immunol. (2015) 16:328–34. doi: 10.1038/ni.3131
41. Racicot K, Kwon JY, Aldo P, Silasi M, Mor G. Understanding the complexity of the immune system during pregnancy. Am J Reprod Immunol (New York NY 1989). (2014) 72:107–16. doi: 10.1111/aji.12289
42. Slawek A, Lorek D, Kedzierska AE, Chelmonska-Soyta A. Regulatory B cells with il-35 and il-10 expression in a normal and abortion-prone murine pregnancy model. Am J Reprod Immunol (New York NY 1989). (2020) 83:e13217. doi: 10.1111/aji.13217
43. Yao Y, Xu XH, Jin L. Macrophage polarization in physiological and pathological pregnancy. Front Immunol. (2019) 10:792. doi: 10.3389/fimmu.2019.00792
44. Wallace JG, Bellissimo CJ, Yeo E, Fei Xia Y, Petrik JJ, Surette MG, et al. Obesity during pregnancy results in maternal intestinal inflammation, placental hypoxia, and alters fetal glucose metabolism at mid-gestation. Sci Rep. (2019) 9:17621. doi: 10.1038/s41598-019-54098-x
45. Liu Y, Chen H, Feng L, Zhang J. Interactions between gut microbiota and metabolites modulate cytokine network imbalances in women with unexplained miscarriage. NPJ biofilms microbiomes. (2021) 7:24. doi: 10.1038/s41522-021-00199-3
46. Brosseau C, Selle A, Duval A, Misme-Aucouturier B, Chesneau M, Brouard S, et al. Prebiotic supplementation during pregnancy modifies the gut microbiota and increases metabolites in amniotic fluid, driving a tolerogenic environment in utero. Front Immunol. (2021) 12:712614. doi: 10.3389/fimmu.2021.712614
47. Liang W, Feng Y, Yang D, Qin J, Zhi X, Wu W, et al. Oral probiotics increased the proportion of treg, tfr, and breg cells to inhibit the inflammatory response and impede gestational diabetes mellitus. Mol Med (Cambridge Mass). (2023) 29:122. doi: 10.1186/s10020-023-00716-4
48. Chen Z, Yang H, Fu H, Wu L, Liu M, Jiang H, et al. Gut bacterial species in late trimester of pregnant sows influence the occurrence of stillborn piglet through pro-inflammation response. Front Immunol. (2022) 13:1101130. doi: 10.3389/fimmu.2022.1101130
49. Li ZH, Wang LL, Liu H, Muyayalo KP, Huang XB, Mor G, et al. Galectin-9 alleviates lps-induced preeclampsia-like impairment in rats via switching decidual macrophage polarization to M2 subtype. Front Immunol. (2018) 9:3142. doi: 10.3389/fimmu.2018.03142
50. Wright DP, Rosendale DI, Robertson AM. Prevotella enzymes involved in mucin oligosaccharide degradation and evidence for a small operon of genes expressed during growth on mucin. FEMS Microbiol Lett. (2000) 190:73–9. doi: 10.1111/fml.2000.190.issue-1
51. Cani PD, Bibiloni R, Knauf C, Waget A, Neyrinck AM, Delzenne NM, et al. Changes in gut microbiota control metabolic endotoxemia-induced inflammation in high-fat diet-induced obesity and diabetes in mice. Diabetes. (2008) 57:1470–81. doi: 10.2337/db07-1403
52. Muccioli GG, Naslain D, Bäckhed F, Reigstad CS, Lambert DM, Delzenne NM, et al. The endocannabinoid system links gut microbiota to adipogenesis. Mol Syst Biol. (2010) 6:392. doi: 10.1038/msb.2010.46
53. Demir E, Ozkan H, Seckin KD, Sahtiyancı B, Demir B, Tabak O, et al. Plasma zonulin levels as a non-invasive biomarker of intestinal permeability in women with gestational diabetes mellitus. Biomolecules. (2019) 9:24. doi: 10.3390/biom9010024
54. Akira S, Takeda K. Toll-like receptor signalling. Nat Rev Immunol. (2004) 4:499–511. doi: 10.1038/nri1391
55. Nilsson NE, Kotarsky K, Owman C, Olde B. Identification of a free fatty acid receptor, ffa2r, expressed on leukocytes and activated by short-chain fatty acids. Biochem Biophys Res Commun. (2003) 303:1047–52. doi: 10.1016/s0006-291x(03)00488-1
56. Le Poul E, Loison C, Struyf S, Springael JY, Lannoy V, Decobecq ME, et al. Functional characterization of human receptors for short chain fatty acids and their role in polymorphonuclear cell activation. J Biol Chem. (2003) 278:25481–9. doi: 10.1074/jbc.M301403200
57. Brown AJ, Goldsworthy SM, Barnes AA, Eilert MM, Tcheang L, Daniels D, et al. The orphan G protein-coupled receptors gpr41 and gpr43 are activated by propionate and other short chain carboxylic acids. J Biol Chem. (2003) 278:11312–9. doi: 10.1074/jbc.M211609200
58. Furusawa Y, Obata Y, Fukuda S, Endo TA, Nakato G, Takahashi D, et al. Commensal microbe-derived butyrate induces the differentiation of colonic regulatory T cells. Nature. (2013) 504:446–50. doi: 10.1038/nature12721
59. Arpaia N, Campbell C, Fan X, Dikiy S, van der Veeken J, deRoos P, et al. Metabolites produced by commensal bacteria promote peripheral regulatory T-cell generation. Nature. (2013) 504:451–5. doi: 10.1038/nature12726
60. Smith PM, Howitt MR, Panikov N, Michaud M, Gallini CA, Bohlooly YM, et al. The microbial metabolites, short-chain fatty acids, regulate colonic treg cell homeostasis. Sci (New York NY). (2013) 341:569–73. doi: 10.1126/science.1241165
61. Tan J, Ni D, Ribeiro RV, Pinget GV, Macia L. How changes in the nutritional landscape shape gut immunometabolism. Nutrients. (2021) 13:823. doi: 10.3390/nu13030823
62. Ohira H, Fujioka Y, Katagiri C, Mamoto R, Aoyama-Ishikawa M, Amako K, et al. Butyrate attenuates inflammation and lipolysis generated by the interaction of adipocytes and macrophages. J Atheroscl Thromb. (2013) 20:425–42. doi: 10.5551/jat.15065
63. Yong W, Zhao Y, Jiang X, Li P. Sodium butyrate alleviates pre-eclampsia in pregnant rats by improving the gut microbiota and short-chain fatty acid metabolites production. J Appl Microbiol. (2022) 132:1370–83. doi: 10.1111/jam.15279
64. Strandwitz P, Kim KH, Terekhova D, Liu JK, Sharma A, Levering J, et al. Gaba-modulating bacteria of the human gut microbiota. Nat Microbiol. (2019) 4:396–403. doi: 10.1038/s41564-018-0307-3
65. Kawase T, Nagasawa M, Ikeda H, Yasuo S, Koga Y, Furuse M. Gut microbiota of mice putatively modifies amino acid metabolism in the host brain. Br J Nutr. (2017) 117:775–83. doi: 10.1017/s0007114517000678
66. Asano Y, Hiramoto T, Nishino R, Aiba Y, Kimura T, Yoshihara K, et al. Critical role of gut microbiota in the production of biologically active, free catecholamines in the gut lumen of mice. Am J Physiol Gastrointestinal liver Physiol. (2012) 303:G1288–95. doi: 10.1152/ajpgi.00341.2012
67. Janik R, Thomason LAM, Stanisz AM, Forsythe P, Bienenstock J, Stanisz GJ. Magnetic resonance spectroscopy reveals oral lactobacillus promotion of increases in brain gaba, N-acetyl aspartate and glutamate. NeuroImage. (2016) 125:988–95. doi: 10.1016/j.neuroimage.2015.11.018
68. Yano JM, Yu K, Donaldson GP, Shastri GG, Ann P, Ma L, et al. Indigenous bacteria from the gut microbiota regulate host serotonin biosynthesis. Cell. (2015) 161:264–76. doi: 10.1016/j.cell.2015.02.047
69. Reigstad CS, Salmonson CE, Rainey JF 3rd, Szurszewski JH, Linden DR, Sonnenburg JL, et al. Gut microbes promote colonic serotonin production through an effect of short-chain fatty acids on enterochromaffin cells. FASEB J Off Publ Fed Am Societies Exp Biol. (2015) 29:1395–403. doi: 10.1096/fj.14-259598
70. Gumusoglu S, Scroggins S, Vignato J, Santillan D, Santillan M. The serotonin-immune axis in preeclampsia. Curr hypertension Rep. (2021) 23:37. doi: 10.1007/s11906-021-01155-4
71. Li N, Ghia JE, Wang H, McClemens J, Cote F, Suehiro Y, et al. Serotonin activates dendritic cell function in the context of gut inflammation. Am J Pathol. (2011) 178:662–71. doi: 10.1016/j.ajpath.2010.10.028
72. Sacramento PM, Monteiro C, Dias ASO, Kasahara TM, Ferreira TB, Hygino J, et al. Serotonin decreases the production of th1/th17 cytokines and elevates the frequency of regulatory cd4(+) T-cell subsets in multiple sclerosis patients. Eur J Immunol. (2018) 48:1376–88. doi: 10.1002/eji.201847525
73. Milani C, Duranti S, Bottacini F, Casey E, Turroni F, Mahony J, et al. The first microbial colonizers of the human gut: composition, activities, and health implications of the infant gut microbiota. Microbiol Mol Biol Rev MMBR. (2017) 81:e00036-17. doi: 10.1128/mmbr.00036-17
74. Olin A, Henckel E, Chen Y, Lakshmikanth T, Pou C, Mikes J, et al. Stereotypic immune system development in newborn children. Cell. (2018) 174:1277–92.e14. doi: 10.1016/j.cell.2018.06.045
75. Chung H, Pamp SJ, Hill JA, Surana NK, Edelman SM, Troy EB, et al. Gut immune maturation depends on colonization with a host-specific microbiota. Cell. (2012) 149:1578–93. doi: 10.1016/j.cell.2012.04.037
76. Gao Y, O'Hely M, Quinn TP, Ponsonby AL, Harrison LC, Frøkiær H, et al. Maternal gut microbiota during pregnancy and the composition of immune cells in infancy. Front Immunol. (2022) 13:986340. doi: 10.3389/fimmu.2022.986340
77. Grant ET, Boudaud M, Muller A, Macpherson AJ, Desai MS. Maternal diet and gut microbiome composition modulate early-life immune development. EMBO Mol Med. (2023) 15:e17241. doi: 10.15252/emmm.202217241
78. Patel DD, Bussel JB. Neonatal fc receptor in human immunity: function and role in therapeutic intervention. J Allergy Clin Immunol. (2020) 146:467–78. doi: 10.1016/j.jaci.2020.07.015
79. Tersigni C, Meli F, Neri C, Iacoangeli A, Franco R, Lanzone A, et al. Role of human leukocyte antigens at the feto-maternal interface in normal and pathological pregnancy: an update. Int J Mol Sci. (2020) 21:4756. doi: 10.3390/ijms21134756
80. Mold JE, Michaëlsson J, Burt TD, Muench MO, Beckerman KP, Busch MP, et al. Maternal alloantigens promote the development of tolerogenic fetal regulatory T cells in utero. Sci (New York NY). (2008) 322:1562–5. doi: 10.1126/science.1164511
81. Boddy AM, Fortunato A, Wilson Sayres M, Aktipis A. Fetal microchimerism and maternal health: A review and evolutionary analysis of cooperation and conflict beyond the womb. BioEssays News Rev molecular Cell Dev Biol. (2015) 37:1106–18. doi: 10.1002/bies.201500059
82. Robert-Gangneux F, Gangneux JP, Vu N, Jaillard S, Guiguen C, Amiot L. High level of soluble hla-G in amniotic fluid is correlated with congenital transmission of toxoplasma gondii. Clin Immunol (Orlando Fla). (2011) 138:129–34. doi: 10.1016/j.clim.2010.12.004
83. Mishra A, Lai GC, Yao LJ, Aung TT, Shental N, Rotter-Maskowitz A, et al. Microbial exposure during early human development primes fetal immune cells. Cell. (2021) 184:3394–409.e20. doi: 10.1016/j.cell.2021.04.039
84. Walter J, Hornef MW. A philosophical perspective on the prenatal in utero microbiome debate. Microbiome. (2021) 9:5. doi: 10.1186/s40168-020-00979-7
85. Theis KR, Romero R, Winters AD, Greenberg JM, Gomez-Lopez N, Alhousseini A, et al. Does the human placenta delivered at term have a microbiota? Results of cultivation, quantitative real-time pcr, 16s rrna gene sequencing, and metagenomics. Am J obstetrics gynecology. (2019) 220:267.e1–.e39. doi: 10.1016/j.ajog.2018.10.018
86. Kennedy KM, de Goffau MC, Perez-Muñoz ME, Arrieta MC, Bäckhed F, Bork P, et al. Questioning the fetal microbiome illustrates pitfalls of low-biomass microbial studies. Nature. (2023) 613:639–49. doi: 10.1038/s41586-022-05546-8
87. Lim AI, McFadden T, Link VM, Han SJ, Karlsson RM, Stacy A, et al. Prenatal maternal infection promotes tissue-specific immunity and inflammation in offspring. Sci (New York NY). (2021) 373:eabf3002. doi: 10.1126/science.abf3002
88. Hu M, Eviston D, Hsu P, Mariño E, Chidgey A, Santner-Nanan B, et al. Decreased maternal serum acetate and impaired fetal thymic and regulatory T cell development in preeclampsia. Nat Commun. (2019) 10:3031. doi: 10.1038/s41467-019-10703-1
89. Al-Haddad BJS, Oler E, Armistead B, Elsayed NA, Weinberger DR, Bernier R, et al. The fetal origins of mental illness. Am J obstetrics gynecology. (2019) 221:549–62. doi: 10.1016/j.ajog.2019.06.013
90. D'Argenio V. The prenatal microbiome: A new player for human health. High-throughput. (2018) 7:38. doi: 10.3390/ht7040038
91. Pelzer E, Gomez-Arango LF, Barrett HL, Nitert MD. Review: maternal health and the placental microbiome. Placenta. (2017) 54:30–7. doi: 10.1016/j.placenta.2016.12.003
92. Wassenaar TM, Panigrahi P. Is a foetus developing in a sterile environment? Lett Appl Microbiol. (2014) 59:572–9. doi: 10.1111/lam.12334
93. Olaniyi KS, Moodley J, Mahabeer Y, Mackraj I. Placental microbial colonization and its association with pre-eclampsia. Front Cell infection Microbiol. (2020) 10:413. doi: 10.3389/fcimb.2020.00413
94. Escobar MF, Hincapie MA, Barona JS. Immunological role of the maternal uterine microbiota in postpartum hemorrhage. Front Immunol. (2020) 11:504. doi: 10.3389/fimmu.2020.00504
95. DiGiulio DB, Romero R, Kusanovic JP, Gómez R, Kim CJ, Seok KS, et al. Prevalence and diversity of microbes in the amniotic fluid, the fetal inflammatory response, and pregnancy outcome in women with preterm pre-labor rupture of membranes. Am J Reprod Immunol (New York NY 1989). (2010) 64:38–57. doi: 10.1111/j.1600-0897.2010.00830.x
96. Bassols J, Serino M, Carreras-Badosa G, Burcelin R, Blasco-Baque V, Lopez-Bermejo A, et al. Gestational diabetes is associated with changes in placental microbiota and microbiome. Pediatr Res. (2016) 80:777–84. doi: 10.1038/pr.2016.155
97. Amarasekara R, Jayasekara RW, Senanayake H, Dissanayake VH. Microbiome of the placenta in pre-eclampsia supports the role of bacteria in the multifactorial cause of pre-eclampsia. J obstetrics gynaecology Res. (2015) 41:662–9. doi: 10.1111/jog.12619
98. Baker JM, Chase DM, Herbst-Kralovetz MM. Uterine microbiota: residents, tourists, or invaders? Front Immunol. (2018) 9:208. doi: 10.3389/fimmu.2018.00208
99. Jiménez E, Marín ML, Martín R, Odriozola JM, Olivares M, Xaus J, et al. Is meconium from healthy newborns actually sterile? Res Microbiol. (2008) 159:187–93. doi: 10.1016/j.resmic.2007.12.007
100. Jeon SJ, Cunha F, Vieira-Neto A, Bicalho RC, Lima S, Bicalho ML, et al. Blood as a route of transmission of uterine pathogens from the gut to the uterus in cows. Microbiome. (2017) 5:109. doi: 10.1186/s40168-017-0328-9
101. Prince AL, Chu DM, Seferovic MD, Antony KM, Ma J, Aagaard KM. The perinatal microbiome and pregnancy: moving beyond the vaginal microbiome. Cold Spring Harbor Perspect Med. (2015) 5:a023051. doi: 10.1101/cshperspect.a023051
102. Mei C, Yang W, Wei X, Wu K, Huang D. The unique microbiome and innate immunity during pregnancy. Front Immunol. (2019) 10:2886. doi: 10.3389/fimmu.2019.02886
103. Yeh CC, Chao KC, Huang SJ. Innate immunity, decidual cells, and preeclampsia. Reprod Sci (Thousand Oaks Calif). (2013) 20:339–53. doi: 10.1177/1933719112450330
104. Zhang J, Chen Z, Smith GN, Croy BA. Natural killer cell-triggered vascular transformation: maternal care before birth? Cell Mol Immunol. (2011) 8:1–11. doi: 10.1038/cmi.2010.38
105. Shmeleva EV, Colucci F. Maternal natural killer cells at the intersection between reproduction and mucosal immunity. Mucosal Immunol. (2021) 14:991–1005. doi: 10.1038/s41385-020-00374-3
106. Tavarna T, Wolfe B, Wu XJ, Reyes L. Porphyromonas gingivalis-mediated disruption in spiral artery remodeling is associated with altered uterine nk cell populations and dysregulated il-18 and htra1. Sci Rep. (2022) 12:14799. doi: 10.1038/s41598-022-19239-9
107. Mercado-Evans V, Mejia ME, Zulk JJ, Ottinger S, Hameed ZA, Serchejian C, et al. Gestational diabetes augments group B streptococcus infection by disrupting maternal immunity and the vaginal microbiota. Nat Commun. (2024) 15:1035. doi: 10.1038/s41467-024-45336-6
108. Crespo ÂC, Mulik S, Dotiwala F, Ansara JA, Sen Santara S, Ingersoll K, et al. Decidual nk cells transfer granulysin to selectively kill bacteria in trophoblasts. Cell. (2020) 182:1125–39.e18. doi: 10.1016/j.cell.2020.07.019
109. Satoh-Takayama N, Vosshenrich CA, Lesjean-Pottier S, Sawa S, Lochner M, Rattis F, et al. Microbial flora drives interleukin 22 production in intestinal nkp46+ Cells that provide innate mucosal immune defense. Immunity. (2008) 29:958–70. doi: 10.1016/j.immuni.2008.11.001
110. Sanos SL, Bui VL, Mortha A, Oberle K, Heners C, Johner C, et al. Rorgammat and commensal microflora are required for the differentiation of mucosal interleukin 22-producing nkp46+ Cells. Nat Immunol. (2009) 10:83–91. doi: 10.1038/ni.1684
111. Martinez-Varea A, Romero R, Xu Y, Miller D, Ahmed AI, Chaemsaithong P, et al. Clinical chorioamnionitis at term vii: the amniotic fluid cellular immune response. J perinatal Med. (2017) 45:523–38. doi: 10.1515/jpm-2016-0225
112. Cappelletti M, Presicce P, Kallapur SG. Immunobiology of acute chorioamnionitis. Front Immunol. (2020) 11:649. doi: 10.3389/fimmu.2020.00649
113. Hu Y, Li H, Yan R, Wang C, Wang Y, Zhang C, et al. Increased neutrophil activation and plasma DNA levels in patients with pre-eclampsia. Thromb haemostasis. (2018) 118:2064–73. doi: 10.1055/s-0038-1675788
114. Yang F, Zheng Q, Jin L. Dynamic function and composition changes of immune cells during normal and pathological pregnancy at the maternal-fetal interface. Front Immunol. (2019) 10:2317. doi: 10.3389/fimmu.2019.02317
115. Doster RS, Sutton JA, Rogers LM, Aronoff DM, Gaddy JA. Streptococcus agalactiae induces placental macrophages to release extracellular traps loaded with tissue remodeling enzymes via an oxidative burst-dependent mechanism. mBio. (2018) 9:e02084-18. doi: 10.1128/mBio.02084-18
116. Sutton JA, Rogers LM, Dixon B, Kirk L, Doster R, Algood HM, et al. Protein kinase D mediates inflammatory responses of human placental macrophages to group B streptococcus. Am J Reprod Immunol (New York NY 1989). (2019) 81:e13075. doi: 10.1111/aji.13075
117. Mezouar S, Benammar I, Boumaza A, Diallo AB, Chartier C, Buffat C, et al. Full-term human placental macrophages eliminate coxiella burnetii through an ifn-Γ Autocrine loop. Front Microbiol. (2019) 10:2434. doi: 10.3389/fmicb.2019.02434
118. Smythies LE, Sellers M, Clements RH, Mosteller-Barnum M, Meng G, Benjamin WH, et al. Human intestinal macrophages display profound inflammatory anergy despite avid phagocytic and bacteriocidal activity. J Clin Invest. (2005) 115:66–75. doi: 10.1172/jci19229
119. Geuking MB, Cahenzli J, Lawson MA, Ng DC, Slack E, Hapfelmeier S, et al. Intestinal bacterial colonization induces mutualistic regulatory T cell responses. Immunity. (2011) 34:794–806. doi: 10.1016/j.immuni.2011.03.021
120. Gaboriau-Routhiau V, Rakotobe S, Lécuyer E, Mulder I, Lan A, Bridonneau C, et al. The key role of segmented filamentous bacteria in the coordinated maturation of gut helper T cell responses. Immunity. (2009) 31:677–89. doi: 10.1016/j.immuni.2009.08.020
121. Verstraelen H, Vilchez-Vargas R, Desimpel F, Jauregui R, Vankeirsbilck N, Weyers S, et al. Characterisation of the human uterine microbiome in non-pregnant women through deep sequencing of the V1-2 region of the 16s rrna gene. PeerJ. (2016) 4:e1602. doi: 10.7717/peerj.1602
122. Mazmanian SK, Liu CH, Tzianabos AO, Kasper DL. An immunomodulatory molecule of symbiotic bacteria directs maturation of the host immune system. Cell. (2005) 122:107–18. doi: 10.1016/j.cell.2005.05.007
123. Round JL, Lee SM, Li J, Tran G, Jabri B, Chatila TA, et al. The toll-like receptor 2 pathway establishes colonization by a commensal of the human microbiota. Sci (New York NY). (2011) 332:974–7. doi: 10.1126/science.1206095
124. Romano-Keeler J, Weitkamp JH. Maternal influences on fetal microbial colonization and immune development. Pediatr Res. (2015) 77:189–95. doi: 10.1038/pr.2014.163
125. Plows JF, Stanley JL, Baker PN, Reynolds CM, Vickers MH. The pathophysiology of gestational diabetes mellitus. Int J Mol Sci. (2018) 19:3342. doi: 10.3390/ijms19113342
126. Vijay-Kumar M, Aitken JD, Carvalho FA, Cullender TC, Mwangi S, Srinivasan S, et al. Metabolic syndrome and altered gut microbiota in mice lacking toll-like receptor 5. Sci (New York NY). (2010) 328:228–31. doi: 10.1126/science.1179721
127. Cani PD, Amar J, Iglesias MA, Poggi M, Knauf C, Bastelica D, et al. Metabolic endotoxemia initiates obesity and insulin resistance. Diabetes. (2007) 56:1761–72. doi: 10.2337/db06-1491
128. Hasan S, Aho V, Pereira P, Paulin L, Koivusalo SB, Auvinen P, et al. Gut microbiome in gestational diabetes: A cross-sectional study of mothers and offspring 5 years postpartum. Acta obstetricia gynecologica Scandinavica. (2018) 97:38–46. doi: 10.1111/aogs.13252
129. Mokkala K, Paulin N, Houttu N, Koivuniemi E, Pellonperä O, Khan S, et al. Metagenomics analysis of gut microbiota in response to diet intervention and gestational diabetes in overweight and obese women: A randomised, double-blind, placebo-controlled clinical trial. Gut. (2021) 70:309–18. doi: 10.1136/gutjnl-2020-321643
130. Mullins TP, Tomsett KI, Gallo LA, Callaway LK, McIntyre HD, Dekker Nitert M, et al. Maternal gut microbiota displays minor changes in overweight and obese women with gdm. Nutrition metabolism Cardiovasc Dis NMCD. (2021) 31:2131–9. doi: 10.1016/j.numecd.2021.03.029
131. Wu N, Zhou J, Mo H, Mu Q, Su H, Li M, et al. The gut microbial signature of gestational diabetes mellitus and the association with diet intervention. Front Cell infection Microbiol. (2021) 11:800865. doi: 10.3389/fcimb.2021.800865
132. Sun Z, Pan XF, Li X, Jiang L, Hu P, Wang Y, et al. The gut microbiome dynamically associates with host glucose metabolism throughout pregnancy: longitudinal findings from a matched case-control study of gestational diabetes mellitus. Advanced Sci (Weinheim Baden-Wurttemberg Germany). (2023) 10:e2205289. doi: 10.1002/advs.202205289
133. Lyu X, Wang S, Zhong J, Cai L, Zheng Y, Zhou Y, et al. Gut microbiome interacts with pregnancy hormone metabolites in gestational diabetes mellitus. Front Microbiol. (2023) 14:1175065. doi: 10.3389/fmicb.2023.1175065
134. Liu Y, Qin S, Feng Y, Song Y, Lv N, Liu F, et al. Perturbations of gut microbiota in gestational diabetes mellitus patients induce hyperglycemia in germ-free mice. J Dev origins Health Dis. (2020) 11:580–8. doi: 10.1017/s2040174420000768
135. Ye D, Huang J, Wu J, Xie K, Gao X, Yan K, et al. Integrative metagenomic and metabolomic analyses reveal gut microbiota-derived multiple hits connected to development of gestational diabetes mellitus in humans. Gut Microbes. (2023) 15:2154552. doi: 10.1080/19490976.2022.2154552
136. Pinto Y, Frishman S, Turjeman S, Eshel A, Nuriel-Ohayon M, Shrossel O, et al. Gestational diabetes is driven by microbiota-induced inflammation months before diagnosis. Gut. (2023) 72:918–28. doi: 10.1136/gutjnl-2022-328406
137. Ye G, Zhang L, Wang M, Chen Y, Gu S, Wang K, et al. The gut microbiota in women suffering from gestational diabetes mellitus with the failure of glycemic control by lifestyle modification. J Diabetes Res. (2019) 2019:6081248. doi: 10.1155/2019/6081248
138. Chang Y, Chen Y, Zhou Q, Wang C, Chen L, Di W, et al. Short-chain fatty acids accompanying changes in the gut microbiome contribute to the development of hypertension in patients with preeclampsia. Clin Sci (London Engl 1979). (2020) 134:289–302. doi: 10.1042/cs20191253
139. Altemani F, Barrett HL, Gomez-Arango L, Josh P, David McIntyre H, Callaway LK, et al. Pregnant women who develop preeclampsia have lower abundance of the butyrate-producer coprococcus in their gut microbiota. Pregnancy hypertension. (2021) 23:211–9. doi: 10.1016/j.preghy.2021.01.002
140. Lv LJ, Li SH, Li SC, Zhong ZC, Duan HL, Tian C, et al. Early-onset preeclampsia is associated with gut microbial alterations in antepartum and postpartum women. Front Cell infection Microbiol. (2019) 9:224. doi: 10.3389/fcimb.2019.00224
141. Zhao Y, Wang B, Zhao X, Cui D, Hou S, Zhang H. The effect of gut microbiota dysbiosis on patients with preeclampsia. Front Cell infection Microbiol. (2022) 12:1022857. doi: 10.3389/fcimb.2022.1022857
142. Jin J, Gao L, Zou X, Zhang Y, Zheng Z, Zhang X, et al. Gut dysbiosis promotes preeclampsia by regulating macrophages and trophoblasts. Circ Res. (2022) 131:492–506. doi: 10.1161/circresaha.122.320771
143. Shiozaki A, Yoneda S, Yoneda N, Yonezawa R, Matsubayashi T, Seo G, et al. Intestinal microbiota is different in women with preterm birth: results from terminal restriction fragment length polymorphism analysis. PLoS One. (2014) 9:e111374. doi: 10.1371/journal.pone.0111374
144. Dahl C, Stanislawski M, Iszatt N, Mandal S, Lozupone C, Clemente JC, et al. Gut microbiome of mothers delivering prematurely shows reduced diversity and lower relative abundance of bifidobacterium and streptococcus. PLoS One. (2017) 12:e0184336. doi: 10.1371/journal.pone.0184336
145. Yin C, Chen J, Wu X, Liu Y, He Q, Cao Y, et al. Preterm birth is correlated with increased oral originated microbiome in the gut. Front Cell infection Microbiol. (2021) 11:579766. doi: 10.3389/fcimb.2021.579766
146. Kuang YS, Lu JH, Li SH, Li JH, Yuan MY, He JR, et al. Connections between the human gut microbiome and gestational diabetes mellitus. GigaScience. (2017) 6:1–12. doi: 10.1093/gigascience/gix058
147. Crusell MKW, Hansen TH, Nielsen T, Allin KH, Rühlemann MC, Damm P, et al. Gestational diabetes is associated with change in the gut microbiota composition in third trimester of pregnancy and postpartum. Microbiome. (2018) 6:89. doi: 10.1186/s40168-018-0472-x
148. Ferrocino I, Ponzo V, Gambino R, Zarovska A, Leone F, Monzeglio C, et al. Changes in the gut microbiota composition during pregnancy in patients with gestational diabetes mellitus (Gdm). Sci Rep. (2018) 8:12216. doi: 10.1038/s41598-018-30735-9
149. Chen F, Gan Y, Li Y, He W, Wu W, Wang K, et al. Association of gestational diabetes mellitus with changes in gut microbiota composition at the species level. BMC Microbiol. (2021) 21:147. doi: 10.1186/s12866-021-02207-0
150. Chen T, Zhang Y, Zhang Y, Shan C, Zhang Y, Fang K, et al. Relationships between gut microbiota, plasma glucose and gestational diabetes mellitus. J Diabetes Invest. (2021) 12:641–50. doi: 10.1111/jdi.13373
151. Li G, Yin P, Chu S, Gao W, Cui S, Guo S, et al. Correlation analysis between gdm and gut microbial composition in late pregnancy. J Diabetes Res. (2021) 2021:8892849. doi: 10.1155/2021/8892849
152. Su Y, Wang HK, Gan XP, Chen L, Cao YN, Cheng DC, et al. Alterations of gut microbiota in gestational diabetes patients during the second trimester of pregnancy in the shanghai han population. J Trans Med. (2021) 19:366. doi: 10.1186/s12967-021-03040-9
153. Hu P, Chen X, Chu X, Fan M, Ye Y, Wang Y, et al. Association of gut microbiota during early pregnancy with risk of incident gestational diabetes mellitus. J Clin Endocrinol Metab. (2021) 106:e4128–e41. doi: 10.1210/clinem/dgab346
154. Wei J, Qing Y, Zhou H, Liu J, Qi C, Gao J. 16s rrna gene amplicon sequencing of gut microbiota in gestational diabetes mellitus and their correlation with disease risk factors. J endocrinological Invest. (2022) 45:279–89. doi: 10.1007/s40618-021-01595-4
155. Wu Y, Bible PW, Long S, Ming WK, Ding W, Long Y, et al. Metagenomic analysis reveals gestational diabetes mellitus-related microbial regulators of glucose tolerance. Acta diabetologica. (2020) 57:569–81. doi: 10.1007/s00592-019-01434-2
156. Liu H, Pan LL, Lv S, Yang Q, Zhang H, Chen W, et al. Alterations of gut microbiota and blood lipidome in gestational diabetes mellitus with hyperlipidemia. Front Physiol. (2019) 10:1015. doi: 10.3389/fphys.2019.01015
157. Cortez RV, Taddei CR, Sparvoli LG, Ângelo AGS, Padilha M, Mattar R, et al. Microbiome and its relation to gestational diabetes. Endocrine. (2019) 64:254–64. doi: 10.1007/s12020-018-1813-z
158. Wang J, Zheng J, Shi W, Du N, Xu X, Zhang Y, et al. Dysbiosis of maternal and neonatal microbiota associated with gestational diabetes mellitus. Gut. (2018) 67:1614–25. doi: 10.1136/gutjnl-2018-315988
159. Sililas P, Huang L, Thonusin C, Luewan S, Chattipakorn N, Chattipakorn S, et al. Association between gut microbiota and development of gestational diabetes mellitus. Microorganisms. (2021) 9:1686. doi: 10.3390/microorganisms9081686
160. Wang X, Liu H, Li Y, Huang S, Zhang L, Cao C, et al. Altered gut bacterial and metabolic signatures and their interaction in gestational diabetes mellitus. Gut Microbes. (2020) 12:1–13. doi: 10.1080/19490976.2020.1840765
161. Dong L, Han L, Duan T, Lin S, Li J, Liu X. Integrated microbiome-metabolome analysis reveals novel associations between fecal microbiota and hyperglycemia-related changes of plasma metabolome in gestational diabetes mellitus. RSC Adv. (2020) 10:2027–36. doi: 10.1039/C9RA07799E
162. Ma S, You Y, Huang L, Long S, Zhang J, Guo C, et al. Alterations in gut microbiota of gestational diabetes patients during the first trimester of pregnancy. Front Cell infection Microbiol. (2020) 10:58. doi: 10.3389/fcimb.2020.00058
163. Xu Y, Zhang M, Zhang J, Sun Z, Ran L, Ban Y, et al. Differential intestinal and oral microbiota features associated with gestational diabetes and maternal inflammation. Am J Physiol Endocrinol Metab. (2020) 319:E247–e53. doi: 10.1152/ajpendo.00266.2019
164. Abdullah B, Daud S, Aazmi MS, Idorus MY, Mahamooth MIJ. Gut microbiota in pregnant Malaysian women: A comparison between trimesters, body mass index and gestational diabetes status. BMC pregnancy childbirth. (2022) 22:152. doi: 10.1186/s12884-022-04472-x
165. Zheng W, Xu Q, Huang W, Yan Q, Chen Y, Zhang L, et al. Gestational diabetes mellitus is associated with reduced dynamics of gut microbiota during the first half of pregnancy. mSystems. (2020) 5:e00109-20. doi: 10.1128/mSystems.00109-20
166. Wu N, Mo H, Mu Q, Liu P, Liu G, Yu W. The gut mycobiome characterization of gestational diabetes mellitus and its association with dietary intervention. Front Microbiol. (2022) 13:892859. doi: 10.3389/fmicb.2022.892859
167. Mokkala K, Houttu N, Vahlberg T, Munukka E, Rönnemaa T, Laitinen K. Gut microbiota aberrations precede diagnosis of gestational diabetes mellitus. Acta diabetologica. (2017) 54:1147–9. doi: 10.1007/s00592-017-1056-0
168. Gomez-Arango LF, Barrett HL, Wilkinson SA, Callaway LK, McIntyre HD, Morrison M, et al. Low dietary fiber intake increases collinsella abundance in the gut microbiota of overweight and obese pregnant women. Gut Microbes. (2018) 9:189–201. doi: 10.1080/19490976.2017.1406584
169. Tanaka K, Harata G, Miyazawa K, He F, Tanigaki S, Kobayashi Y. The gut microbiota of non-obese Japanese pregnant women with gestational diabetes mellitus. Bioscience microbiota Food Health. (2022) 41:4–11. doi: 10.12938/bmfh.2021-025
170. Fugmann M, Breier M, Rottenkolber M, Banning F, Ferrari U, Sacco V, et al. The stool microbiota of insulin resistant women with recent gestational diabetes, a high risk group for type 2 diabetes. Sci Rep. (2015) 5:13212. doi: 10.1038/srep13212
171. Priyadarshini M, Navarro G, Reiman DJ, Sharma A, Xu K, Lednovich K, et al. Gestational insulin resistance is mediated by the gut microbiome-indoleamine 2,3-dioxygenase axis. Gastroenterology. (2022) 162:1675–89.e11. doi: 10.1053/j.gastro.2022.01.008
172. Jabalie G, Ahmadi M, Koushaeian L, Eghbal-Fard S, Mehdizadeh A, Kamrani A, et al. Metabolic syndrome mediates proinflammatory responses of inflammatory cells in preeclampsia. Am J Reprod Immunol (New York NY 1989). (2019) 81:e13086. doi: 10.1111/aji.13086
173. Liu J, Yang H, Yin Z, Jiang X, Zhong H, Qiu D, et al. Remodeling of the gut microbiota and structural shifts in preeclampsia patients in south China. Eur J Clin Microbiol Infect Dis Off Publ Eur Soc Clin Microbiol. (2017) 36:713–9. doi: 10.1007/s10096-016-2853-z
174. Ishimwe JA, Akinleye A, Johnson AC, Garrett MR, Sasser JM. Gestational gut microbial remodeling is impaired in a rat model of preeclampsia superimposed on chronic hypertension. Physiol Genomics. (2021) 53:125–36. doi: 10.1152/physiolgenomics.00121.2020
175. Li P, Wang H, Guo L, Gou X, Chen G, Lin D, et al. Association between gut microbiota and preeclampsia-eclampsia: A two-sample mendelian randomization study. BMC Med. (2022) 20:443. doi: 10.1186/s12916-022-02657-x
176. Tang R, Xiao G, Jian Y, Yuan Q, Jiang C, Wang W. The gut microbiota dysbiosis in preeclampsia contributed to trophoblast cell proliferation, invasion, and migration via lncrna bc030099/nf-Κb pathway. Mediators Inflammation. (2022) 2022:6367264. doi: 10.1155/2022/6367264
177. Lv LJ, Li SH, Wen JY, Wang GY, Li H, He TW, et al. Deep metagenomic characterization of gut microbial community and function in preeclampsia. Front Cell infection Microbiol. (2022) 12:933523. doi: 10.3389/fcimb.2022.933523
178. Meijer S, Pasquinelli E, Renzi S, Lavasani S, Nouri M, Erlandsson L, et al. Gut micro- and mycobiota in preeclampsia: bacterial composition differences suggest role in pathophysiology. Biomolecules. (2023) 13:346. doi: 10.3390/biom13020346
179. Wu X, Li Q, Cai J, Huang H, Ma S, Tan H. Longitudinal change of gut microbiota in hypertensive disorders in pregnancy: A nested case-control and mendelian randomization study. Sci Rep. (2023) 13:16986. doi: 10.1038/s41598-023-43780-w
180. Xiong Z, Wang Q, Pei S, Zhu Z. The causal role of intestinal microbiome in development of pre-eclampsia. Funct Integr Genomics. (2023) 23:127. doi: 10.1007/s10142-023-01054-8
181. Wang J, Shi ZH, Yang J, Wei Y, Wang XY, Zhao YY. Gut microbiota dysbiosis in preeclampsia patients in the second and third trimesters. Chin Med J. (2020) 133:1057–65. doi: 10.1097/cm9.0000000000000734
182. Wang J, Gu X, Yang J, Wei Y, Zhao Y. Gut microbiota dysbiosis and increased plasma lps and tmao levels in patients with preeclampsia. Front Cell infection Microbiol. (2019) 9:409. doi: 10.3389/fcimb.2019.00409
183. Atarashi K, Tanoue T, Shima T, Imaoka A, Kuwahara T, Momose Y, et al. Induction of colonic regulatory T cells by indigenous clostridium species. Sci (New York NY). (2011) 331:337–41. doi: 10.1126/science.1198469
184. Round JL, Mazmanian SK. The gut microbiota shapes intestinal immune responses during health and disease. Nat Rev Immunol. (2009) 9:313–23. doi: 10.1038/nri2515
185. Riedel CU, Foata F, Philippe D, Adolfsson O, Eikmanns BJ, Blum S. Anti-inflammatory effects of bifidobacteria by inhibition of lps-induced nf-kappab activation. World J Gastroenterol. (2006) 12:3729–35. doi: 10.3748/wjg.v12.i23.3729
186. Tang ML, Kemp AS, Thorburn J, Hill DJ. Reduced interferon-gamma secretion in neonates and subsequent atopy. Lancet (London England). (1994) 344:983–5. doi: 10.1016/s0140-6736(94)91641-1
187. Prescott SL, King B, Strong TL, Holt PG. The value of perinatal immune responses in predicting allergic disease at 6 years of age. Allergy. (2003) 58:1187–94. doi: 10.1034/j.1398-9995.2003.00263.x
188. Ober C, Sperling AI, von Mutius E, Vercelli D. Immune development and environment: lessons from amish and hutterite children. Curr Opin Immunol. (2017) 48:51–60. doi: 10.1016/j.coi.2017.08.003
189. Hinz D, Bauer M, Röder S, Olek S, Huehn J, Sack U, et al. Cord blood tregs with stable foxp3 expression are influenced by prenatal environment and associated with atopic dermatitis at the age of one year. Allergy. (2012) 67:380–9. doi: 10.1111/j.1398-9995.2011.02767.x
190. Collier F, Ponsonby AL, O'Hely M, Tang MLK, Saffery R, Molloy J, et al. Naïve regulatory T cells in infancy: associations with perinatal factors and development of food allergy. Allergy. (2019) 74:1760–8. doi: 10.1111/all.13822
191. Fan X, Zang T, Dai J, Wu N, Hope C, Bai J, et al. The associations of maternal and children's gut microbiota with the development of atopic dermatitis for children aged 2 years. Front Immunol. (2022) 13:1038876. doi: 10.3389/fimmu.2022.1038876
192. Wang S, Zhang R, Li X, Gao Y, Dai N, Wei Y, et al. Relationship between maternal-infant gut microbiota and infant food allergy. Front Microbiol. (2022) 13:933152. doi: 10.3389/fmicb.2022.933152
193. Vuillermin PJ, O'Hely M, Collier F, Allen KJ, Tang MLK, Harrison LC, et al. Maternal carriage of prevotella during pregnancy associates with protection against food allergy in the offspring. Nat Commun. (2020) 11:1452. doi: 10.1038/s41467-020-14552-1
194. Alhasan MM, Cait AM, Heimesaat MM, Blaut M, Klopfleisch R, Wedel A, et al. Antibiotic use during pregnancy increases offspring asthma severity in a dose-dependent manner. Allergy. (2020) 75:1979–90. doi: 10.1111/all.14234
195. Yuan G, Wen S, Zhong X, Yang X, Xie L, Wu X, et al. Inulin alleviates offspring asthma by altering maternal intestinal microbiome composition to increase short-chain fatty acids. PLoS One. (2023) 18:e0283105. doi: 10.1371/journal.pone.0283105
196. Sun Z, Lee-Sarwar K, Kelly RS, Lasky-Su JA, Litonjua AA, Weiss ST, et al. Revealing the importance of prenatal gut microbiome in offspring neurodevelopment in humans. EBioMedicine. (2023) 90:104491. doi: 10.1016/j.ebiom.2023.104491
197. Cristiano C, Hoxha E, Lippiello P, Balbo I, Russo R, Tempia F, et al. Maternal treatment with sodium butyrate reduces the development of autism-like traits in mice offspring. Biomedicine pharmacotherapy = Biomedecine pharmacotherapie. (2022) 156:113870. doi: 10.1016/j.biopha.2022.113870
198. Bruce-Keller AJ, Fernandez-Kim SO, Townsend RL, Kruger C, Carmouche R, Newman S, et al. Maternal obese-type gut microbiota differentially impact cognition, anxiety and compulsive behavior in male and female offspring in mice. PLoS One. (2017) 12:e0175577. doi: 10.1371/journal.pone.0175577
199. Liu X, Li X, Xia B, Jin X, Zou Q, Zeng Z, et al. High-fiber diet mitigates maternal obesity-induced cognitive and social dysfunction in the offspring via gut-brain axis. Cell Metab. (2021) 33:923–38.e6. doi: 10.1016/j.cmet.2021.02.002
200. Di Gesù CM, Matz LM, Bolding IJ, Fultz R, Hoffman KL, Gammazza AM, et al. Maternal gut microbiota mediate intergenerational effects of high-fat diet on descendant social behavior. Cell Rep. (2022) 41:111461. doi: 10.1016/j.celrep.2022.111461
201. Radford-Smith DE, Probert F, Burnet PWJ, Anthony DC. Modifying the maternal microbiota alters the gut-brain metabolome and prevents emotional dysfunction in the adult offspring of obese dams. Proc Natl Acad Sci United States America. (2022) 119:e2108581119. doi: 10.1073/pnas.2108581119
202. Degroote S, Hunting DJ, Baccarelli AA, Takser L. Maternal gut and fetal brain connection: increased anxiety and reduced social interactions in wistar rat offspring following peri-conceptional antibiotic exposure. Prog Neuropsychopharmacol Biol Psychiatry. (2016) 71:76–82. doi: 10.1016/j.pnpbp.2016.06.010
203. Njotto LL, Simin J, Fornes R, Odsbu I, Mussche I, Callens S, et al. Maternal and early-life exposure to antibiotics and the risk of autism and attention-deficit hyperactivity disorder in childhood: A swedish population-based cohort study. Drug Saf. (2023) 46:467–78. doi: 10.1007/s40264-023-01297-1
204. Dawson SL, O'Hely M, Jacka FN, Ponsonby AL, Symeonides C, Loughman A, et al. Maternal prenatal gut microbiota composition predicts child behaviour. EBioMedicine. (2021) 68:103400. doi: 10.1016/j.ebiom.2021.103400
205. Lange NE, Celedón JC, Forno E, Ly NP, Onderdonk A, Bry L, et al. Maternal intestinal flora and wheeze in early childhood. Clin Exp Allergy J Br Soc Allergy Clin Immunol. (2012) 42:901–8. doi: 10.1111/j.1365-2222.2011.03950.x
206. Tanabe H, Sakurai K, Kato T, Kawasaki Y, Nakano T, Yamaide F, et al. Association of the maternal microbiome in Japanese pregnant women with the cumulative prevalence of dermatitis in early infancy: A pilot study from the chiba study of mother and child health birth cohort. World Allergy Organ J. (2019) 12:100065. doi: 10.1016/j.waojou.2019.100065
207. Thio CL, Chi PY, Lai AC, Chang YJ. Regulation of type 2 innate lymphoid cell-dependent airway hyperreactivity by butyrate. J Allergy Clin Immunol. (2018) 142:1867–83.e12. doi: 10.1016/j.jaci.2018.02.032
208. Vieira RS, Castoldi A, Basso PJ, Hiyane MI, Câmara NOS, Almeida RR. Butyrate attenuates lung inflammation by negatively modulating th9 cells. Front Immunol. (2019) 10:67. doi: 10.3389/fimmu.2019.00067
209. Tao R, de Zoeten EF, Ozkaynak E, Chen C, Wang L, Porrett PM, et al. Deacetylase inhibition promotes the generation and function of regulatory T cells. Nat Med. (2007) 13:1299–307. doi: 10.1038/nm1652
210. Trompette A, Gollwitzer ES, Yadava K, Sichelstiel AK, Sprenger N, Ngom-Bru C, et al. Gut microbiota metabolism of dietary fiber influences allergic airway disease and hematopoiesis. Nat Med. (2014) 20:159–66. doi: 10.1038/nm.3444
211. Buchenauer L, Junge KM, Haange SB, Simon JC, von Bergen M, Hoh AL, et al. Glyphosate differentially affects the allergic immune response across generations in mice. Sci total Environ. (2022) 850:157973. doi: 10.1016/j.scitotenv.2022.157973
212. Selle A, Brosseau C, Dijk W, Duval A, Bouchaud G, Rousseaux A, et al. Prebiotic supplementation during gestation induces a tolerogenic environment and a protective microbiota in offspring mitigating food allergy. Front Immunol. (2021) 12:745535. doi: 10.3389/fimmu.2021.745535
213. Bouchaud G, Castan L, Chesné J, Braza F, Aubert P, Neunlist M, et al. Maternal exposure to gos/inulin mixture prevents food allergies and promotes tolerance in offspring in mice. Allergy. (2016) 71:68–76. doi: 10.1111/all.12777
214. Kao HF, Wang YC, Tseng HY, Wu LS, Tsai HJ, Hsieh MH, et al. Goat milk consumption enhances innate and adaptive immunities and alleviates allergen-induced airway inflammation in offspring mice. Front Immunol. (2020) 11:184. doi: 10.3389/fimmu.2020.00184
215. Moustaki M, Tsabouri S, Priftis KN, Douros K. Prenatal stress enhances susceptibility to allergic diseases of offspring. Endocrine Metab Immune Disord Drug Targets. (2017) 17:255–63. doi: 10.2174/1871530317666170912160646
216. Loewen K, Monchka B, Mahmud SM, t Jong G, Azad MB. Prenatal antibiotic exposure and childhood asthma: A population-based study. Eur Respir J. (2018) 52:1702070. doi: 10.1183/13993003.02070-2017
217. Mulder B, Pouwels KB, Schuiling-Veninga CC, Bos HJ, de Vries TW, Jick SS, et al. Antibiotic use during pregnancy and asthma in preschool children: the influence of confounding. Clin Exp Allergy J Br Soc Allergy Clin Immunol. (2016) 46:1214–26. doi: 10.1111/cea.12756
218. Lee E, Park YM, Lee SY, Lee SH, Park MJ, Ahn K, et al. Associations of prenatal antibiotic exposure and delivery mode on childhood asthma inception. Ann allergy Asthma Immunol Off Publ Am Coll Allergy Asthma Immunol. (2023) 131:52–8.e1. doi: 10.1016/j.anai.2023.03.020
219. Borre YE, O'Keeffe GW, Clarke G, Stanton C, Dinan TG, Cryan JF. Microbiota and neurodevelopmental windows: implications for brain disorders. Trends Mol Med. (2014) 20:509–18. doi: 10.1016/j.molmed.2014.05.002
220. Malkova NV, Yu CZ, Hsiao EY, Moore MJ, Patterson PH. Maternal immune activation yields offspring displaying mouse versions of the three core symptoms of autism. Brain behavior Immun. (2012) 26:607–16. doi: 10.1016/j.bbi.2012.01.011
221. Bauman MD, Iosif AM, Smith SE, Bregere C, Amaral DG, Patterson PH. Activation of the maternal immune system during pregnancy alters behavioral development of rhesus monkey offspring. Biol Psychiatry. (2014) 75:332–41. doi: 10.1016/j.biopsych.2013.06.025
222. Choi GB, Yim YS, Wong H, Kim S, Kim H, Kim SV, et al. The maternal interleukin-17a pathway in mice promotes autism-like phenotypes in offspring. Sci (New York NY). (2016) 351:933–9. doi: 10.1126/science.aad0314
223. Kim E, Paik D, Ramirez RN, Biggs DG, Park Y, Kwon HK, et al. Maternal gut bacteria drive intestinal inflammation in offspring with neurodevelopmental disorders by altering the chromatin landscape of cd4(+) T cells. Immunity. (2022) 55:145–58.e7. doi: 10.1016/j.immuni.2021.11.005
224. Marques AH, O'Connor TG, Roth C, Susser E, Bjørke-Monsen AL. The influence of maternal prenatal and early childhood nutrition and maternal prenatal stress on offspring immune system development and neurodevelopmental disorders. Front Neurosci. (2013) 7:120. doi: 10.3389/fnins.2013.00120
225. Sarker G, Peleg-Raibstein D. Maternal overnutrition induces long-term cognitive deficits across several generations. Nutrients. (2018) 11:7. doi: 10.3390/nu11010007
226. Furlan Freguia C, Marriott A, Gill D, Kaleko M. Maternal treatment with oral intestinal alkaline phosphatase mitigates high fat diet-induced cognitive disorders in offspring mice. Behav Brain Res. (2020) 392:112701. doi: 10.1016/j.bbr.2020.112701
227. Sheyholislami H, Connor KL. Are probiotics and prebiotics safe for use during pregnancy and lactation? A systematic review and meta-analysis. Nutrients. (2021) 13:2382. doi: 10.3390/nu13072382
228. Syngai GG, Gopi R, Bharali R, Dey S, Lakshmanan GM, Ahmed G. Probiotics - the versatile functional food ingredients. J Food Sci Technol. (2016) 53:921–33. doi: 10.1007/s13197-015-2011-0
229. Mazziotta C, Tognon M, Martini F, Torreggiani E, Rotondo JC. Probiotics mechanism of action on immune cells and beneficial effects on human health. Cells. (2023) 12:184. doi: 10.3390/cells12010184
230. Li HY, Zhou DD, Gan RY, Huang SY, Zhao CN, Shang A, et al. Effects and mechanisms of probiotics, prebiotics, synbiotics, and postbiotics on metabolic diseases targeting gut microbiota: A narrative review. Nutrients. (2021) 13:3211. doi: 10.3390/nu13093211
231. Wang H, Gong J, Wang W, Long Y, Fu X, Fu Y, et al. Are there any different effects of bifidobacterium, lactobacillus and streptococcus on intestinal sensation, barrier function and intestinal immunity in pi-ibs mouse model? PLoS One. (2014) 9:e90153. doi: 10.1371/journal.pone.0090153
232. Curciarello R, Canziani KE, Salto I, Barbiera Romero E, Rocca A, Doldan I, et al. Probiotic lactobacilli isolated from kefir promote down-regulation of inflammatory lamina propria T cells from patients with active ibd. Front Pharmacol. (2021) 12:658026. doi: 10.3389/fphar.2021.658026
233. Gomez Arango LF, Barrett HL, Callaway LK, Nitert MD. Probiotics and pregnancy. Curr Diabetes Rep. (2015) 15:567. doi: 10.1007/s11892-014-0567-0
234. Pakmehr A, Ejtahed HS, Shirzad N, Hemmatabadi M, Farhat S, Larijani B. Preventive effect of probiotics supplementation on occurrence of gestational diabetes mellitus: A systematic review and meta-analysis of randomized controlled trials. Front Med. (2022) 9:1031915. doi: 10.3389/fmed.2022.1031915
235. Jafarnejad S, Saremi S, Jafarnejad F, Arab A. Effects of a multispecies probiotic mixture on glycemic control and inflammatory status in women with gestational diabetes: A randomized controlled clinical trial. J Nutr Metab. (2016) 2016:5190846. doi: 10.1155/2016/5190846
236. Zheng QX, Wang HW, Jiang XM, Ge L, Lai YT, Jiang XY, et al. Changes in the gut metabolic profile of gestational diabetes mellitus rats following probiotic supplementation. Front Microbiol. (2022) 13:779314. doi: 10.3389/fmicb.2022.779314
237. Li B, Shi Y, Qiu W, Lin Q, Zeng S, Hou Y, et al. Limosilactobacillus reuteri ameliorates preeclampsia in mice via improving gut dysbiosis and endothelial dysfunction. Biomedicine pharmacotherapy = Biomedecine pharmacotherapie. (2023) 161:114429. doi: 10.1016/j.biopha.2023.114429
238. Valiati N, Puel EM, Stefani CM, Lataro RM. Does probiotic ingestion reduce the risk of preeclampsia? A systematic review. Appl physiology nutrition Metab = Physiologie appliquee Nutr metabolisme. (2023) 49:135–147. doi: 10.1139/apnm-2023-0089
239. Kirihara N, Kamitomo M, Tabira T, Hashimoto T, Taniguchi H, Maeda T. Effect of probiotics on perinatal outcome in patients at high risk of preterm birth. J obstetrics gynaecology Res. (2018) 44:241–7. doi: 10.1111/jog.13497
240. Silvia Ventimiglia M, Jimena Valeff N, Pozo Albán M, Manuel Paturlanne J, Juriol L, Quadrana F, et al. Probiotic lactobacillus kefiri prevents endotoxin-induced preterm birth and stillbirth in mice. Reprod (Cambridge England). (2021) 161:657–67. doi: 10.1530/rep-20-0642
241. Xie Q, Cui D, Zhu Q, Qin X, Ren D, Xu X. Supplementing maternal diet with milk oligosaccharides and probiotics helps develop the immune system and intestinal flora of offsprings. Food Sci Nutr. (2023) 11:6868–77. doi: 10.1002/fsn3.3579
242. Cuello-Garcia CA, Brożek JL, Fiocchi A, Pawankar R, Yepes-Nuñez JJ, Terracciano L, et al. Probiotics for the prevention of allergy: A systematic review and meta-analysis of randomized controlled trials. J Allergy Clin Immunol. (2015) 136:952–61. doi: 10.1016/j.jaci.2015.04.031
243. Garcia-Larsen V, Ierodiakonou D, Jarrold K, Cunha S, Chivinge J, Robinson Z, et al. Diet during pregnancy and infancy and risk of allergic or autoimmune disease: A systematic review and meta-analysis. PLoS Med. (2018) 15:e1002507. doi: 10.1371/journal.pmed.1002507
244. Szajewska H, Horvath A. Lactobacillus rhamnosus gg in the primary prevention of eczema in children: A systematic review and meta-analysis. Nutrients. (2018) 10:1319. doi: 10.3390/nu10091319
245. Zhu H, Tian P, Qian X, Gu L, Zhao J, Wang G, et al. Perinatal transmission of a probiotic bifidobacterium strain protects against early life stress-induced mood and gastrointestinal motility disorders. Food Funct. (2022) 13:7520–8. doi: 10.1039/D2FO01164F
246. Huang S, Chen J, Cui Z, Ma K, Wu D, Luo J, et al. Lachnospiraceae-derived butyrate mediates protection of high fermentable fiber against placental inflammation in gestational diabetes mellitus. Sci Adv. (2023) 9:eadi7337. doi: 10.1126/sciadv.adi7337
247. Huang S, Wu D, Hao X, Nie J, Huang Z, Ma S, et al. Dietary fiber supplementation during the last 50 days of gestation improves the farrowing performance of gilts by modulating insulin sensitivity, gut microbiota, and placental function. J Anim Sci. (2023) 101:skad021. doi: 10.1093/jas/skad021
248. Liu B, Zhu X, Cui Y, Wang W, Liu H, Li Z, et al. Consumption of dietary fiber from different sources during pregnancy alters sow gut microbiota and improves performance and reduces inflammation in sows and piglets. mSystems. (2021) 6:e00591-20. doi: 10.1128/mSystems.00591-20
249. Sun C, Song R, Zhou J, Jia Y, Lu J. Fermented bamboo fiber improves productive performance by regulating gut microbiota and inhibiting chronic inflammation of sows and piglets during late gestation and lactation. Microbiol Spectr. (2023) 11:e0408422. doi: 10.1128/spectrum.04084-22
250. Sugino KY, Hernandez TL, Barbour LA, Kofonow JM, Frank DN, Friedman JE. A maternal higher-complex carbohydrate diet increases bifidobacteria and alters early life acquisition of the infant microbiome in women with gestational diabetes mellitus. Front Endocrinol. (2022) 13:921464. doi: 10.3389/fendo.2022.921464
251. Lin Y, Li L, Li Y, Wang K, Wei D, Xu S, et al. Interpretation of fiber supplementation on offspring testicular development in a pregnant sow model from a proteomics perspective. Int J Mol Sci. (2019) 20:4549. doi: 10.3390/ijms20184549
252. Li Y, Liu H, Zhang L, Yang Y, Lin Y, Zhuo Y, et al. Maternal dietary fiber composition during gestation induces changes in offspring antioxidative capacity, inflammatory response, and gut microbiota in a sow model. Int J Mol Sci. (2019) 21:31. doi: 10.3390/ijms21010031
253. Li Y, Yang M, Zhang L, Mao Z, Lin Y, Xu S, et al. Dietary fiber supplementation in gestating sow diet improved fetal growth and placental development and function through serotonin signaling pathway. Front veterinary Sci. (2022) 9:831703. doi: 10.3389/fvets.2022.831703
254. Wu J, Xiong Y, Zhong M, Li Y, Wan H, Wu D, et al. Effects of purified fibre-mixture supplementation of gestation diet on gut microbiota, immunity and reproductive performance of sows. J Anim Physiol Anim Nutr. (2020) 104:1144–54. doi: 10.1111/jpn.13287
255. Liu Y, Chen N, Li D, Li H, Fang Z, Lin Y, et al. Effects of dietary soluble or insoluble fiber intake in late gestation on litter performance, milk composition, immune function, and redox status of sows around parturition. J Anim Sci. (2020) 98:skaa303. doi: 10.1093/jas/skaa303
256. Chen T, Chen D, Tian G, Zheng P, Mao X, Yu J, et al. Soluble fiber and insoluble fiber regulate colonic microbiota and barrier function in a piglet model. BioMed Res Int. (2019) 2019:7809171. doi: 10.1155/2019/7809171
257. Zhang P. Influence of foods and nutrition on the gut microbiome and implications for intestinal health. Int J Mol Sci. (2022) 23:9588. doi: 10.3390/ijms23179588
258. Markowiak P, Śliżewska K. Effects of probiotics, prebiotics, and synbiotics on human health. Nutrients. (2017) 9:1021. doi: 10.3390/nu9091021
Keywords: gut microbiota, uterine microenvironment, immune cells, pregnancy complications, recurrent miscarriages, fetal development, probiotics
Citation: Lu X, Shi Z, Jiang L and Zhang S (2024) Maternal gut microbiota in the health of mothers and offspring: from the perspective of immunology. Front. Immunol. 15:1362784. doi: 10.3389/fimmu.2024.1362784
Received: 29 December 2023; Accepted: 28 February 2024;
Published: 13 March 2024.
Edited by:
Fuyan Wang, Qingdao Hiser Hospital Affiliated of Qingdao University, ChinaReviewed by:
Yang Li, Shandong Agricultural University, ChinaYonggang Zhou, University of Science and Technology of China, China
Copyright © 2024 Lu, Shi, Jiang and Zhang. This is an open-access article distributed under the terms of the Creative Commons Attribution License (CC BY). The use, distribution or reproduction in other forums is permitted, provided the original author(s) and the copyright owner(s) are credited and that the original publication in this journal is cited, in accordance with accepted academic practice. No use, distribution or reproduction is permitted which does not comply with these terms.
*Correspondence: Songying Zhang, emhhbmdzb25neWluZ0B6anUuZWR1LmNu; Lingling Jiang, bGluZ2xpbmdqaWFuZ0B6anUuZWR1LmNu
†These authors have contributed equally to this work