- 1Department of Pharmacy, Independent University Bangladesh, Dhaka, Bangladesh
- 2Department of Clinical Pharmacy and Pharmacology, Faculty of Pharmacy, University of Dhaka, Dhaka, Bangladesh
- 3Department of Pharmacy, University of Asia Pacific, Dhaka, Bangladesh
- 4Institute for Personalized Medicine, Department of Biochemistry and Molecular Biology, Pennsylvania State University College of Medicine, Hershey, PA, United States
- 5Department of Biochemistry and Molecular Biology, University of Dhaka, Dhaka, Bangladesh
Dengue, caused by the dengue virus (DENV), affects millions of people worldwide every year. This virus has two distinct life cycles, one in the human and another in the mosquito, and both cycles are crucial to be controlled. To control the vector of DENV, the mosquito Aedes aegypti, scientists employed many techniques, which were later proved ineffective and harmful in many ways. Consequently, the attention shifted to the development of a vaccine; researchers have targeted the E protein, a surface protein of the virus and the NS1 protein, an extracellular protein. There are several types of vaccines developed so far, such as live attenuated vaccines, recombinant subunit vaccines, inactivated virus vaccines, viral vectored vaccines, DNA vaccines, and mRNA vaccines. Along with these, scientists are exploring new strategies of developing improved version of the vaccine by employing recombinant DNA plasmid against NS1 and also aiming to prevent the infection by blocking the DENV life cycle inside the mosquitoes. Here, we discussed the aspects of research in the field of vaccines until now and identified some prospects for future vaccine developments.
1 History, epidemiology and etiology of dengue
The most widespread mosquito-borne disease in tropical as well as subtropical regions is dengue which affects 100 to 400 million people per year worldwide and poses a threat of dengue infection to nearly half the world’s population (1). Being the most frequent arboviral disease globally, it has already infected people across approximately 128 countries, a number that is about to increase even more in terms of incidence and geographic expansion, and is undoubtedly (2, 3) distressing international travelers (4, 5).
Southeast Asia, the Eastern Mediterranean, Africa, the Western Pacific, and the United States are among the native hotspots of DENV infections (6), however, this epidemic is emerging as an outbreak throughout the world. WHO has already declared dengue as the second most serious viral disease after COVID-19, since the number of infected cases surged in 2020 (1). The highest DENV infected cases were reported to take place in the Philippines, Vietnam, India, Colombia, and Brazil (7). Unplanned rapid urbanization along with improper vector control management are the prime causes of transmission of mosquito-borne diseases. Propagation is also accelerated via business or personal travel (8).
Originating from the Flaviviridae family, the DENV is the predominant cause of dengue fever (DF). Positive-sense RNA with a single strand makes up the DENV genome that encodes seven nonstructural proteins (NS) in addition to three structural proteins, which are the capsid protein (C), the pre-membrane protein (prM) and the envelop protein (E) (9). These are organized as 5′-UTR-C-prM-E-NS1-NS2A-NS2B-NS3-NS4A-NS4B-NS5-UTR-3′, where UTR means untranslated region. The mature virus particles are round with a diameter of 50 nm (10). The DENV E protein, which is found on the virion surface, contains the main epitopes needed to generate neutralizing antibodies. As a result, it is an ideal target for vaccine development. Additionally, the infected patient serum has high concentrations of a protein known as DENV NS1 released by affected cells of the body. As NS1 is an extracellular viral component, it could serve as another choice for a DENV infection vaccine (11, 12).
Aedes mosquitoes are the actual culprit for the transmission of four serotypes (DENV 1–4) in humans (9). The fifth and the latest addition to the existing serotypes of DENV is DENV-5 which was discovered in Sarawak, Malaysia in 2013 (13). In that case, DENV-4 was transmitted between non-human primates (NHP) and mosquitoes (A. nivalis) and was considered a sylvatic dengue infection (14). A thorough genetic verification process was done which led to a new DENV-5 serotype prevailing in Southeast Asian forests (13). Genetic conversion from sylvatic strains to human strains, higher mutation rates, and enormous deforestation are the underlying possible factors that lead to the upsurge in transmission. The inception of the DENV-5 serotype possessing a distinct phylogene (13) is an indication that novel DENV is evolving and will be continued by contact with the infected populations due to changes in ecosystem and climate (15).
In most of the cases, dengue infections are asymptomatic/subclinical (16). Primary symptomatic infection with any serotype is manifested as an acute febrile illness that cannot be distinguished from other febrile illnesses. Fever, headache, persistent pain in the eyelid, severe muscular and joint ache, abdominal pain, flushing, and anorexia are a few typical symptoms. Any of the dengue serotypes can induce DF following dengue hemorrhagic fever (DHF), and sometimes, dengue shock syndrome (DSS) (17). The most severe effects, DHF and DSS, are more frequently observed in infections of children and teenagers under the age of fifteen (17, 18).
DENV infection develops high neutralizing antibody titers comprising potential elements of the protective immune response (19, 20). It can provide long-term effectiveness in terms of homotypic protection, but efficacy lasts only for two years in the case of heterotypic protection (21). In addition, the cross-antibody titer concentration drops and the following heterotypic dengue infection becomes more severe than the initial infection (22). Non-neutralizing antibodies are the cause of the hazardous phenomena known as antibody-dependent enhancement (ADE). The ADE often occurs when non-neutralizing antibody causes enhanced entry of virus into the host cell leading to elevated infection. They combine with DENV particles to create complexes that facilitate infection of phagocytic cells through Fc receptors. This is particularly common when previous infection with a serotype leads to enhanced entry of other different serotypes. This enhanced infection results in DHF and DSS (17).
1.1 Life cycle of dengue between humans and mosquitoes
Before discussing the approaches to control dengue, we will delve into the lifecycle of dengue (Figure 1) to understand how the vector was aimed to be controlled. There are two different life cycles for DENV transmission among mosquitoes, primates, and humans - the sylvatic cycle and the urban cycle (23). Lower primates and humans are the only mammalian targets for dengue channeling. DENV-1 to DENV-4, all four serotypes cause infections in the urban cycle. When a mosquito bites a person who is infected with a virus, the mosquito’s midgut epithelial cells become infested with it. The virus rapidly spreads within the mosquito, moving from its hemocoel to its salivary glands and other tissues. Once this occurs, the mosquito becomes capable of transmitting the virus to uninfected humans through its bite (24). Because A. aegypti often breeds near human dwellings and is vulnerable to DENV, it is considered the main vector in tropical and subtropical regions. A. albopictus, deemed as the Asian tiger mosquito, can act as a secondary vector. For being able to survive in temperate regions, it has been rapidly spreading in all continents, and causing arboviral diseases, including DENV. A. albopictus plays a pivotal role in dengue transmission in Europe and China (8).
1.2 Approaches to dengue vector control
The development of a dengue vaccine and treatments for DHF and DSS took longer than anticipated. As a consequence, vector control continued to be essential for the prevention and management of dengue.
The most popular and easiest way to control dengue is mosquito repellents that are mixtures of synthetic or naturally occurring compounds. The main ingredient is N, N-diethyl-3-methylbenzamide (DEET) which is very effective against mosquitoes. But continuous exposure of it can cause toxic reactions to human body (25).
One of the earliest chemical control methods used to target the adult phases of the dengue vector was dichlorodiphenyltrichloroethane (DDT). Although substantial declines in vector populations were achieved, one of the main reasons for the resurgence of dengue from the dormant stage was the onset of DDT resistance in the 1960s and subsequent years (26). Second- and third-generation insecticides (such as malathion and pyrethroids) also became accessible during this time. In addition, there are various chemical-based techniques available for controlling adult mosquitoes and the larval and pupal aquatic stages of dengue vectors. Nevertheless, chemical-based control of dengue vectors has drawbacks, such as contamination of the environment, toxin-bioaccumulation, and the plausibility of human toxicity, which are particularly linked to the application of insecticides in potable water containers (27–29).
One of the proposed strategies for vector control is biological control. This includes the introduction of larvivorous predators, such as copepods, and insect larvae into water containers, the dissemination of transgenic vectors e.g. Wolbachia strain (30) (intended to lessen or supplant the wild-type vector population with populations that have a limited transmission and reproduction capability), and ecological management (such as limiting the potential larva development sites, identifying and extracting abandoned containers, masking functional water containers, reduction of water pollution and ensuring the availability of safe water, reducing human-vector contact by utilizing insecticide-treated nets, and screening doors and windows). However, these alternative vector control techniques have scant evidence of their efficacy (16, 31).
Therefore, the predominant strategy to counteract dengue outbreaks has been vector control. Simply put, by lowering insect concentrations below an entomological threshold, disease transmission can be slowed. However, research on vaccines to fight dengue is also ongoing as interest in preventative measures rises. Due to the growing public awareness and the invention of novel molecular techniques, dengue vaccine development efforts have substantially accelerated during the past ten years. Some prospective candidates are being assessed from preclinical to phase three for vaccine development, such as inactivated, live attenuated, DNA, recombinant subunit, and viral vectors (32).
2 Dengue vaccine research and development
In 1929, the earliest dengue vaccine evaluations took place (33, 34). Vaccine formulation has been, nevertheless, hampered by several issues. First, DENV, comprising of four antigenically distinct complicated serotypes, has made vaccine development a great challenge (35). Second, DHF and DSS resulting from a second heterotypic infection with unknown immune response and pathogenesis have hindered DENV vaccine development (36, 37). Third, the adapted immunological reaction to the virus is not fully understood and the precise trait of immune responses (protective or pathogenic) to the virus is perplexing the development of a vaccine. Also, we do not have a convenient animal model that is accessible, affordable, and that accurately mirrors the immune responses in humans following infection (32). NHPs can sustain viral replication and develop a robust immune response but are unable to develop overt disease. On the other hand, certain immunodeficient mouse models infected with mouse-adapted DENV strains show signs of severe disease, like the ‘vascular-leak’ syndrome seen in severe dengue in humans. Humanized mouse models can sustain DENV replication and show some signs of disease, but to validate the immune response, further development is necessary. In the case of immunocompetent mice being infected with DENV, they do not manifest the disease. Although the prevalence of DENV-containing immune complexes in serum suggests the possibility of an ADE-like phenomenon, the swine model for DENV still has some limitations of being asymptomatic (38, 39).
Despite these obstacles, vaccine development has evolved significantly in the last few years, and the current lineup for dengue vaccines in development and testing is advanced, varied, and encouraging. Currently, multiple candidates of dengue vaccine are undergoing phase III clinical studies, and others have been evaluated in human trials (40). Various strategies for developing a dengue vaccine are addressed herein.
2.1 Live attenuated vaccines
The first significant attempt to create a live attenuated vaccine was made by the University of Hawaii, employing the long-established strategy of virus passage in an NHP serially. Later, the project was moved to Mahidol University (Bangkok, Thailand) for additional virus passaging, vaccine formulation, and evaluation (41, 42). Even though the proposed vaccine was unsuccessful, the study acted as a spur for later advancements toward the development of a live attenuated tetravalent dengue vaccine (43).
The Walter Reed Army Institute of Research (WRAIR) had developed the second tissue-culture-passaged dengue vaccine. The original formulation of the tetravalent dengue vaccine manufactured by WRAIR similarly showed issues with uneven reactogenicity and immunogenicity (44). In a phase II investigation, changed formulations appeared as innocuous and immunogenic; nevertheless, additional research is required to fully assess their protective effects (45).
Using the mutagenesis technique, the US National Institutes of Health, launched a novel phase of dengue vaccine research. The genetic modification of dengue genomes was simple, resulting in attenuated strains that had been evaluated on adults in the US who had not previously been exposed to flaviviruses. Additionally, a molecularly attenuated, tetravalent dengue vaccine was developed by the US FDA and tested on primates from other species, except humans (46–48). In order to develop live attenuated tetravalent dengue vaccines, both methods offer an alternative strategy (48, 49).
A number of live attenuated dengue vaccines are being developed using recombinant DNA technology, including the tetra-live attenuated virus dengue vaccine (DENVax), the recombinant DENV-4 mutant vaccine carrying deletion of 30-nucleotide (rDEN4Δ30), and the tetravalent dengue vaccine with chimeric yellow fever 17D virus (CYD-TDV) (50, 51).
2.1.1 Live attenuated chimeric yellow fever–dengue vaccines
A tetravalent chimeric dengue vaccine was designed by the US Centers for Disease Control and Prevention (CDC) by incorporating the E genes and prM of DENV-1, DENV-3, and DENV-4 into the cDNA acquired from the effectively attenuated DENV-2 unit of the Mahidol University-Sanofi Pasteur live attenuated DENV vaccine (DEN-2, 16681 PDK-53) (49, 52). The infective cDNA component of the prominent yellow fever vaccine virus, strain 17D was modified to incorporate dengue structural genes. Beginnings for this can be found in the medical schools of Washington and St. Louis Universities. Sanofi Pasteur has been granted a manufacturing license by Acambis, Inc. to continue the commercial development of these yellow fever chimeras (53–55).
In consequence, Sanofi Pasteur (Lyon, France) developed the foremost authorized dengue vaccine named Dengvaxia. Using Vero cells as a substrate, this dengue vaccine is a tetravalent chimeric which has received marketing authorization for clinical studies in Mexico and the Philippines (56, 57). The four dengue serotypes’ matching sequences were substituted for the YF17D yellow fever virus prM/E RNAs to develop the respective vaccines (Figure 2) (58, 59). This putative vaccine was immune-modulating for all the DENV serotypes and may have provided protection against serotypes 1, 3, and 4 (60).
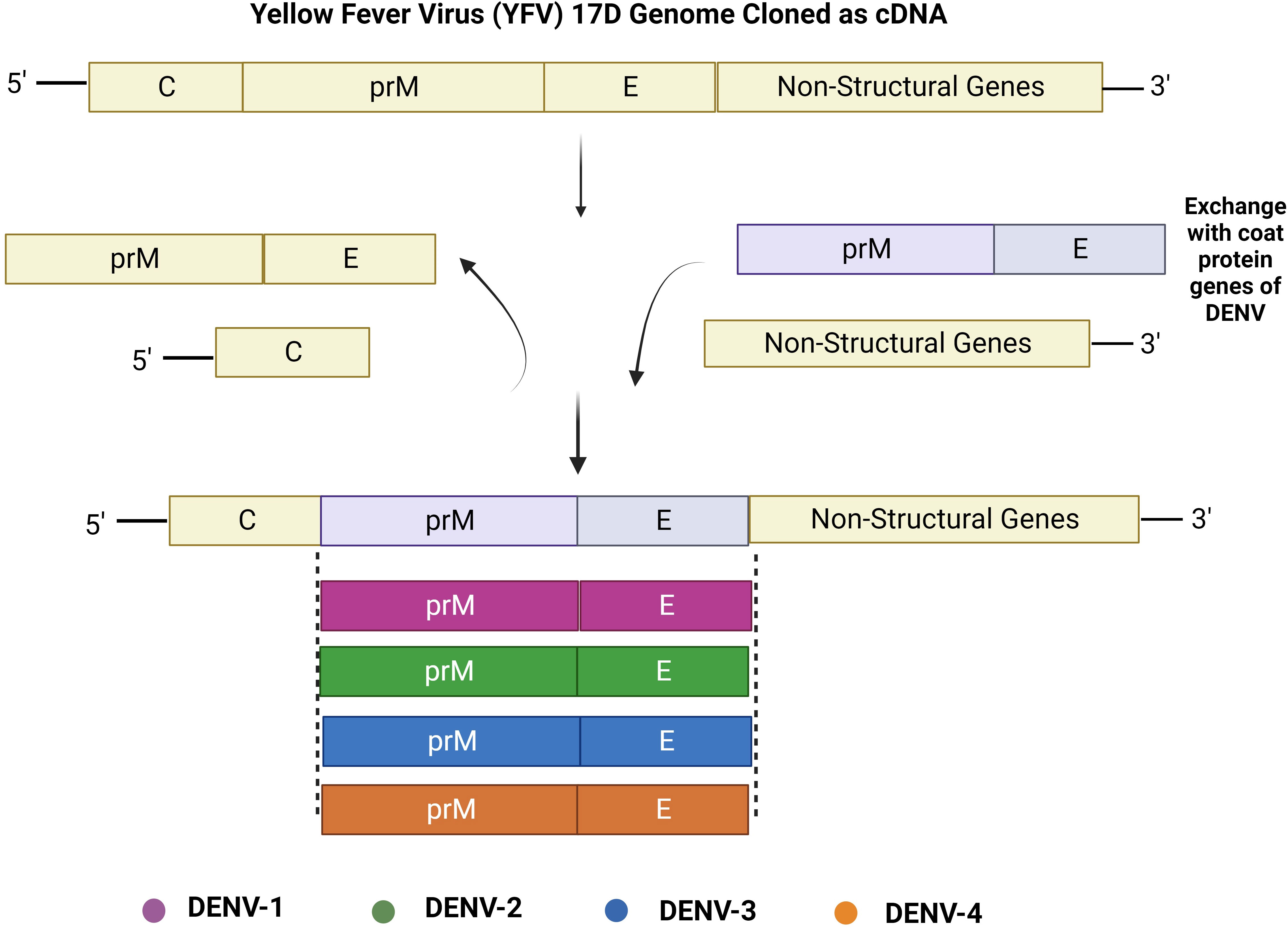
Figure 2 Construction of live attenuated chimeric yellow fever–dengue vaccines. Genes encoding for prM and E proteins from the cDNA backbone of yellow fever vaccine virus strain 17D are substituted with those of heterologous DENV serotypes: DENV-1, DENV-2, DENV-3, and DENV-4 to create these chimeric flavivirus vaccines.
In Thailand, a phase IIb investigation conducted on this particular vaccine reported high efficacy against DENV-3 and DENV-4 serotypes, moderate activity against DENV-1, and no potential to counter DENV-2 infection (55, 60, 61). Additionally, two phase III trials were carried out in the dengue-endemic Asia-Pacific region and five nations in Latin America. The results clearly demonstrated that the vaccines’ efficacy against DENV-1, DENV-2, DENV-3, and DENV-4 was 50.3%, 42.3%, 74.0%, and 77.7% in Latin America (62), and that its average efficacy against dengue-associated clinical indications and hospitalization was 56.5% in Asia-Pacific (63). These data demand further evaluation of the efficiency and protection by the Dengvaxia vaccine (64).
Immunity stimulated by the vaccine lasts up to four years, but the efficacy is affected by many factors such as virus serotype, age, and individual dengue sera status (65). It is reported that seropositive subjects aging over nine years gain conclusive protectivity by CYD-TDV. According to the recommendation of the WHO Strategic Advisory Panel, seronegative patients should not be vaccinated as the risk of severe dengue is increased via CYD-TDV vaccination (66). For the assessment of serum condition, the plaque reduction neutralization test, recognized as the gold standard, necessitates distinct technical and laboratory facilities.
Over conventional attenuation in cell culture, molecular clone-supported approaches for a tetravalent dengue vaccination provide significant benefits. These include a molecular explanation for attenuation and lessening the threat of inadvertent agents, both of which will lower the costs associated with product quality assurance.
2.1.2 Live attenuated rDENΔ30 vaccines
Remarkable studies based on the integration of the live attenuated tetravalent vaccine (LATV) with DENV monovalent vaccines have been conducted (67). Since the 3’-UTR of the genome of flavivirus is crucial for the RNA replication, it has been selected as the focal point (68, 69).
According to Durbin, on the 28th day after vaccination, a boost in serum neutralizing antibody titer has been increased to a seven fold or more (mean titer = 1:580), which was noted to have satisfactory tolerance (70). Obtaining rDEN-4Δ30 NS3-S158R through chemical mutagenesis from rDEN4Δ30 was a huge success (71–73).
Conversely, the TV003 vaccine, which combines four attenuated recombinant dengue vaccines (rDEN1Δ30, rDEN2/4Δ30, rDEN3Δ30/31, and rDEN4Δ30), exhibits higher resistance against DENV-2 compared to CYD-TDV (74). TV003 was reported to produce antibodies against each of the DENV serotypes among 91.7% of trial-subjects, and the potentiality to protect opposing DENV-2 was higher than CYD-TDV vaccination manifesting mild rash only as an adverse reaction (75). A comparative phase I clinical trial showed TV005 was combined with more DENV-2 attenuated virus components than TV003. A comparatively balanced immune response was produced in 90% of vaccines by a single vaccination of TV005, whereas TV003 vaccination produced an immune response in 76% of subjects only (76). TV003 and TV005 stimulate the proportionate production of neutralizing antibodies compared to other LATVs (TV001–TV005) since these vaccines have diverse virus particle structures, infectivity, and immunogenicity (77). Nonetheless, it can be challenging to generate a LATV that demonstrates an immunogenic response against every serotype and attenuates each monovalent component at the same time (46, 78, 79).
2.1.3 Live attenuated chimeric tetra-dengue vaccines
For producing the live attenuated chimeric tetra-dengue vaccines, at first the genetic code of the DENV-2, PDK-53 was altered and coding sequences of DENV-1, DENV- 3, and DENV-4 were included. Finally, by transferring the recombinant RNAs into Vero cells, the potential candidate DENVax was produced (80). Currently, these vaccines are undergoing clinical trials. However, research in AG129 mice indicates that the immunization effects on offspring, born by PDK53 immunized mothers, may be interfered due to maternal antibodies. Live attenuated vaccine contains definitive protectivity for the progeny over 9 years old. Due to high immunogenetic tolerance of DENVax, it is quite challenging to elicit systemic reactions from it (50).
2.2 Inactivated virus vaccines
Another way for the protection from live pathogens could be inactivated vaccines that comprise inactivated material derived from viruses or bacteria (Figure 3). S16803, which was produced utilizing formalin-inactivation and sucrose-centrifugal processes, is an illustration of a DENV-2-inactivated vaccine (81). Immunogenicity was assessed in Macaca mulatta using three different types of vaccines: a recombinant subunit protein vaccine (R80E), a live attenuated vaccine (DENV-2 PDK-50), and an inactivated vaccine (S16803). The result showed that stable titers of antibodies were only produced by DENV-2 PDK-50 (82). Initial vaccination of rhesus monkeys with tetravalent purified formalin-inactivated vaccine (TPIV) or tetravalent DNA vaccine (TVDV) followed by a tetravalent live attenuated vaccine (TLAV) revealed stimulated humoral immune responses against DENV in comparison to immunization with individual vaccine type (83, 84). The underlying mechanism is: initially TPIV helps reach all the titers of four serotypes of antibodies to a definite height and then immunization is enhanced by TLAV (84, 85).
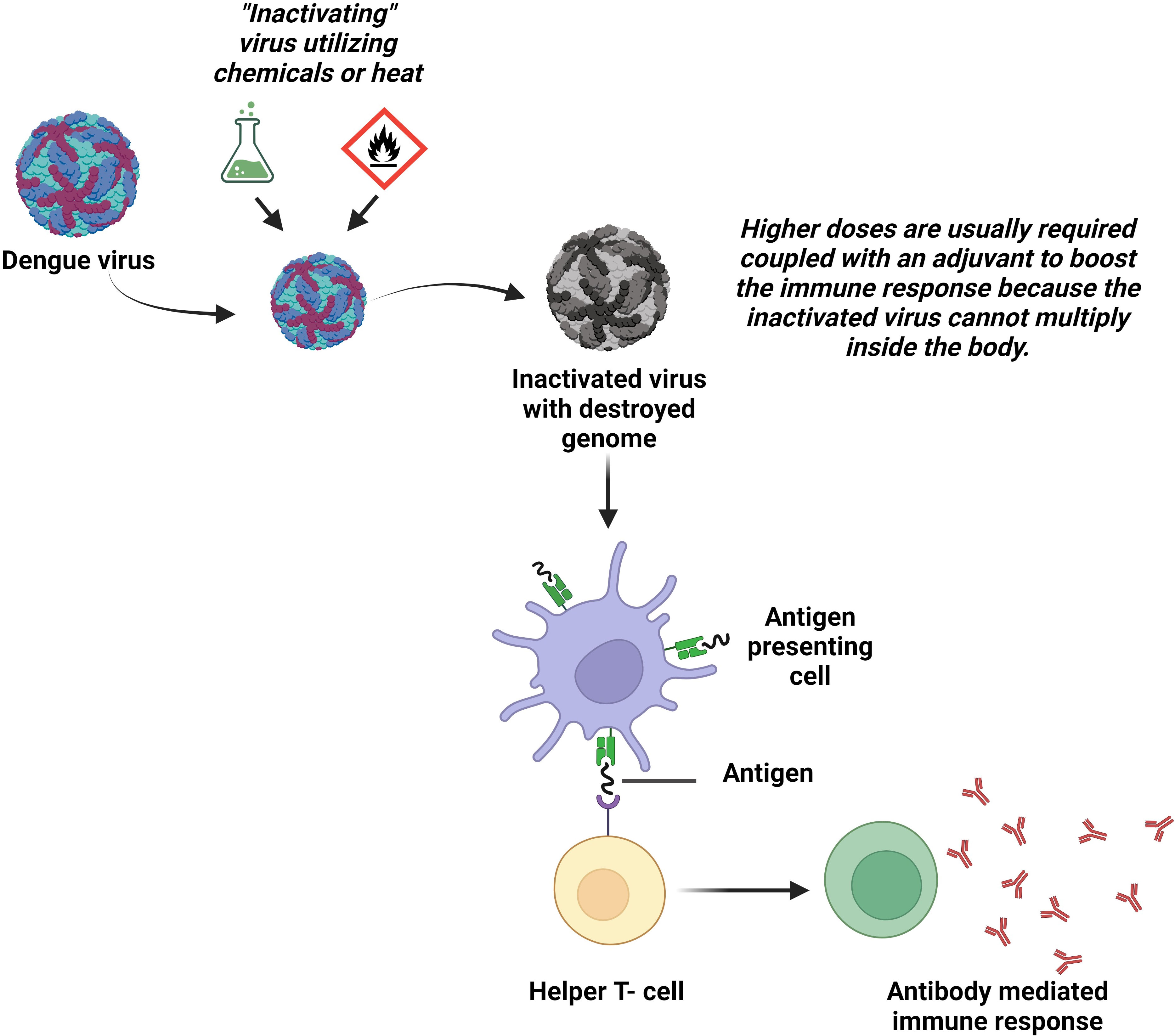
Figure 3 Inactivated virus vaccines. The wild DENV is rendered incapable of reproducing or causing diseases in the body by applying heat or chemicals in the laboratory. The body produces antibodies in response to the vaccinations made from these inactivated viruses.
Applying inactivated virus vaccines is quite challenging. This is because, these vaccines only express the part of the viral genome responsible for structural proteins, leaving them unable to stimulate immunity against the NS proteins. For gaining optimal immunogenicity in seronegative subjects, the addition of adjuvants may be an option, which in turn could increase the cost and reactogenicity of the vaccine. Another disadvantage is that, to ensure long-term immunity, multiple booster doses are required. In contrast, since they cannot change into a more pathogenic phenotype and are less likely to interact when combined, inactivated whole virus vaccines are advantageous. Additionally, it has been shown that inactivated flavivirus vaccines can induce both cell-mediated and humoral immune responses (86).
2.3 Recombinant subunit vaccines
A variety of expression techniques have been used to manufacture dengue antigens, especially E proteins, to create candidates for subunit vaccines (87). Eukaryotic or prokaryotic cells frequently express these antigenic proteins to elicit durable defensive or curative immune responses (Figure 4) (88).
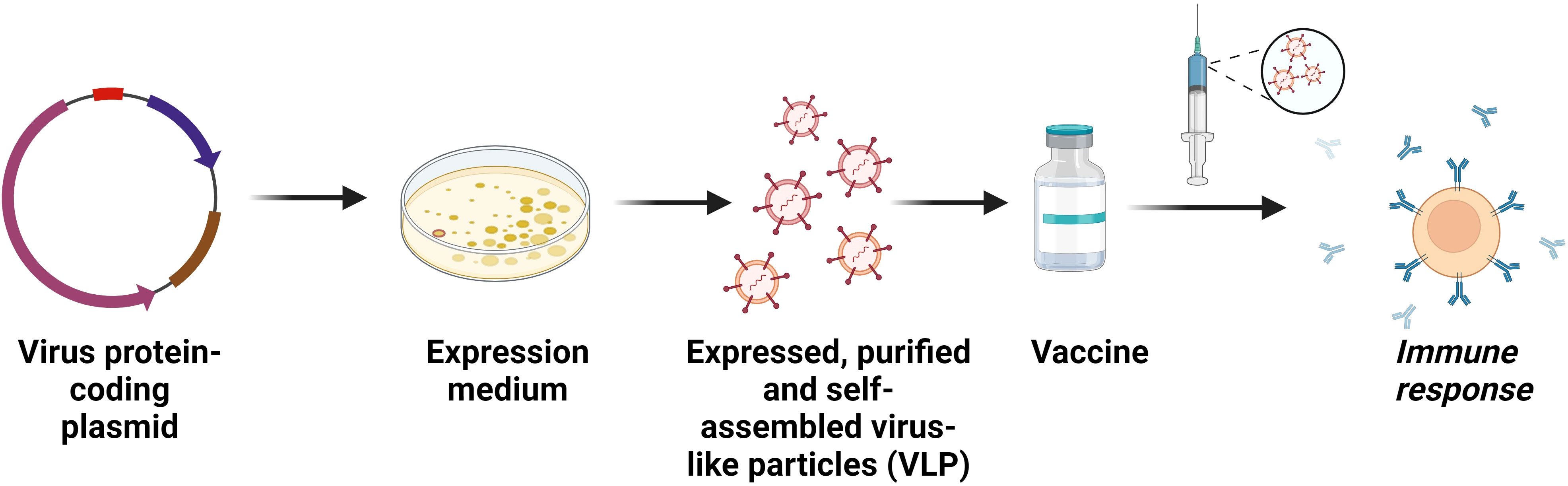
Figure 4 Recombinant subunit vaccines. Construction of subunit vaccines comprising purified viral proteins that act as antigens to induce immunity in host. A modified form of the subunit vaccine is the viral-like particles (VLP) vaccine. VLPs are entities made up of one or more proteins that resemble real viruses but do not have a viral genome. Effective immunological responses from T cells and B cells have been observed following the administration of these vaccines.
It was demonstrated that the recombinant envelope protein domain III (EDIII) from E. coli efficiently induces the production of antibodies against all the dengue serotypes in mice (89). Moreover, the protection of lactating mice from infection by these antibodies was also reported (90). A lipoprotein in combination with recombinant dengue proteins eliminated the use of adjuvants and immune responses against all the serotypes were provoked substantially. Here, lipoprotein is the main element of intact bacteria that activates monocytes through a CD14-dependent route in spirochaete and plays a significant role in determining the spirochaete’s pro-inflammatory potential (91–93).
One tetravalent dengue vaccine, called EDIII-P64K, contains adjuvant that expresses EDIII from various DENV serotypes and P64K from Neisseria meningitidis. Mice that received the vaccine three times, produced high levels of DENV1-3 and low levels of DENV-4 antibodies (94). Additionally, using E. coli to express DENV1–2 EDIII was combined with DENV3–4 EDIII via Gly-Ser linker, and mice receiving this combination of vaccinations successfully developed a defense against all serotypes of DENV (95, 96).
By using heat labile enterotoxin B subunit (LTB) derived from E. coli and synthetic consensus dengue envelope domain III (scEDIII), fusion protein LTB-scEDIII was produced. By immunizing with cell-free extracts (CFE) and recombinant yeast cells (rYC) via oral route, mucosal and systemic humoral immune responses have been observed. Concentration of neutralizing antibodies was higher in case of CFE rather than rYC (97).
V180 is considered another potential subunit vaccine made of insect cell-derived truncated protein DEN-80E. Vaccination of mice and rhesus monkeys with this showed remarkable immune protection against DENV with a low dose only (50, 98). On top of that, V180-immunization can protect rhesus monkeys from viremia (99).
A study showed that transgenic plants and ovarian cells of Chinese hamster were used to produce a protein containing polymeric immunoglobulin G scaffold and DENV consensus domain III (cEDIII). That protein induced large production of IgG antibodies in the mice, possessing DENV-2 neutralizing capacity (100).
The advantage of recombinant subunit vaccines is that they can induce balanced immunologic responses against all the DENV serotypes as well as reduce the incidence of ADE effect in comparison with live attenuated vaccines. A lack of post-translational protein processing, however, could initiate protein generation that are different from inherent responses of antibody and other proteins (101, 102).
2.4 Viral vector vaccines
Adenoviruses, alphaviruses, and the Vaccinia virus are some common vectors used for delivering dengue viral antigens in the formulation of vaccines (Figure 5) (103). Since the efficiency of DENV-4 proteins (prM, E, NS1, and NS2A) in the Cidofovir-resistant vaccinia (WR) strain was not up to the mark (103, 104), in order to increase protectivity, whole vaccinia virus or c-terminal truncated ones were recombined to generate the DENV E protein (50). MVA-DENV2-80%E and MVA-DENV4-80%E were created based on the innocuous, altered vaccinia Ankara (MVA) virus. Although the early viruses only mildly induced DENV-2 antibodies in rhesus monkeys, these viruses can produce large anti-E antibodies in mice models (105).
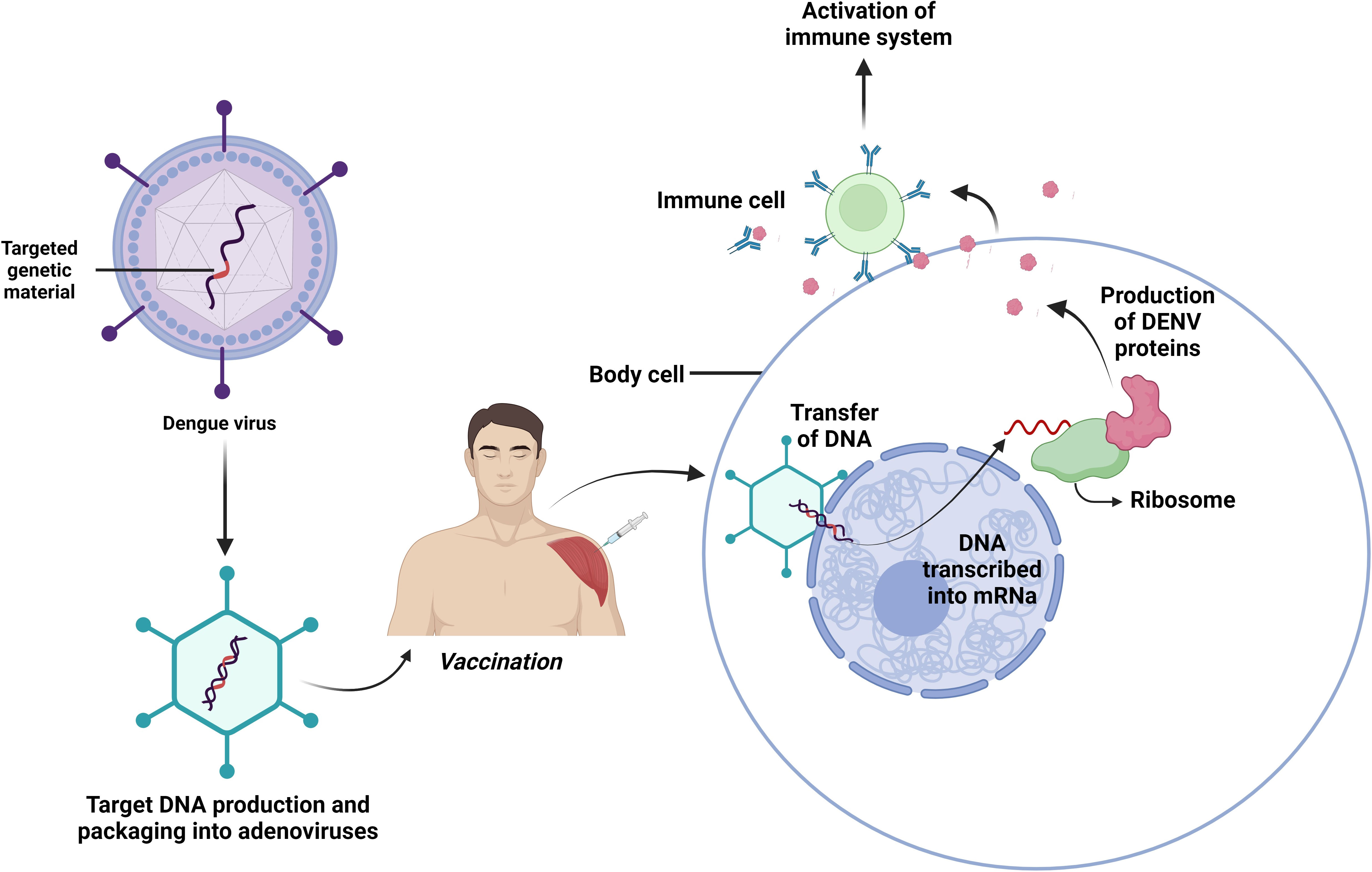
Figure 5 Viral vectored vaccines. Composed of a brief segment of a virus’s genetic material that contains the instructions for the target protein. Another virus that has undergone genetic modification so it cannot replicate itself in the human body delivers the gene safely to host cells to develop immunity against invaders.
In the development of recombinant replication-defective adenovirus (rAd), some factors such as- easy gene manipulation, high level of protein expression, and convenient identification of gene replication flaws were essential. The vaccine was administered to the mice intra-peritoneally and successful stimulation of DENV-2 antibodies and specified T-cell immunity via the E protein of DENV-2 had been witnessed (106, 107). Additionally, mice which were initially injected with rAd and subsequently given a boost with a DNA vaccine that expressed EDIII, displayed defense against DENV-2 and DENV-4 infection (108). Another potential agent is a divalent complex Adenovirus (cAd)-vectored vaccine e.g. cAdVaxD (1–2) and cAdVaxD (3–4). The rhesus monkeys developed antibodies against all serotypes of DENV after receiving prM and E of the DENVs, as well as a T-cell immunological response (109).
Venezuelan equine encephalitis virus (VEEV) replicon particles (VRP) are alphavirus-vectored vaccines against DENV that express large amounts of antigens in a single dose of vaccination (110, 111). The VRP which expresses DENV-1 proteins such as M and E, induced defensive antibodies in cynomolgus monkeys (112). On the other hand, DENV-2 VRP produced IgG and neutralized antibodies against DENV-2. Though both prM-E-VRP and E85-VRP were capable of producing antibodies against specific serotype in rhesus monkeys, against EDIII, the E85-VRP produced antibodies more rapidly and with higher concentration (113). Given that it gave monkeys a balanced immune response and made them safe to DENV1-4, the tetravalent E85-VRP dengue vaccine can be an important tool for overcoming serotype interference. Only after a single neonatal dose, the VRP vaccine developed robust immunity, though the magnitude was not even that of adult mice (114).
The viral vector vaccine is believed to have the greatest impact on cellular immunity and is likely to elicit greater humoral responses. In contrast to different viral vector vaccines, adenoviral vectors are better for simple genetic alterations, identifying abnormalities during replication, as well as elevated antigen expression.
2.5 DNA vaccines
DNA vaccines can be defined as a plasmid encoding specific antigens which, if injected into living organisms, express antigens and that is how trigger immune response (115). Neutralizing antibodies were successfully developed in mice and monkeys after receiving the TVDV. Injecting BALB/c mice with an intradermal DNA vaccine including structural prM and 92% of the DENV-2 E protein, resulted in the predicted antibodies (Figure 6) (115). The DNA vaccine that expressed prM and 100% of the E protein (ME100) induced antibodies more potently than one that expressed only 80% of the E protein (E80) (116). Safety and well-tolerance of D1ME100 had been proven in the first phase of vaccination with little discomfort or soreness at the injection site as side effects in human trials. Only 41.6% of vaccines in the high-dose group are reported to produce neutralizing antibodies with zero response in the low-dose group (117).
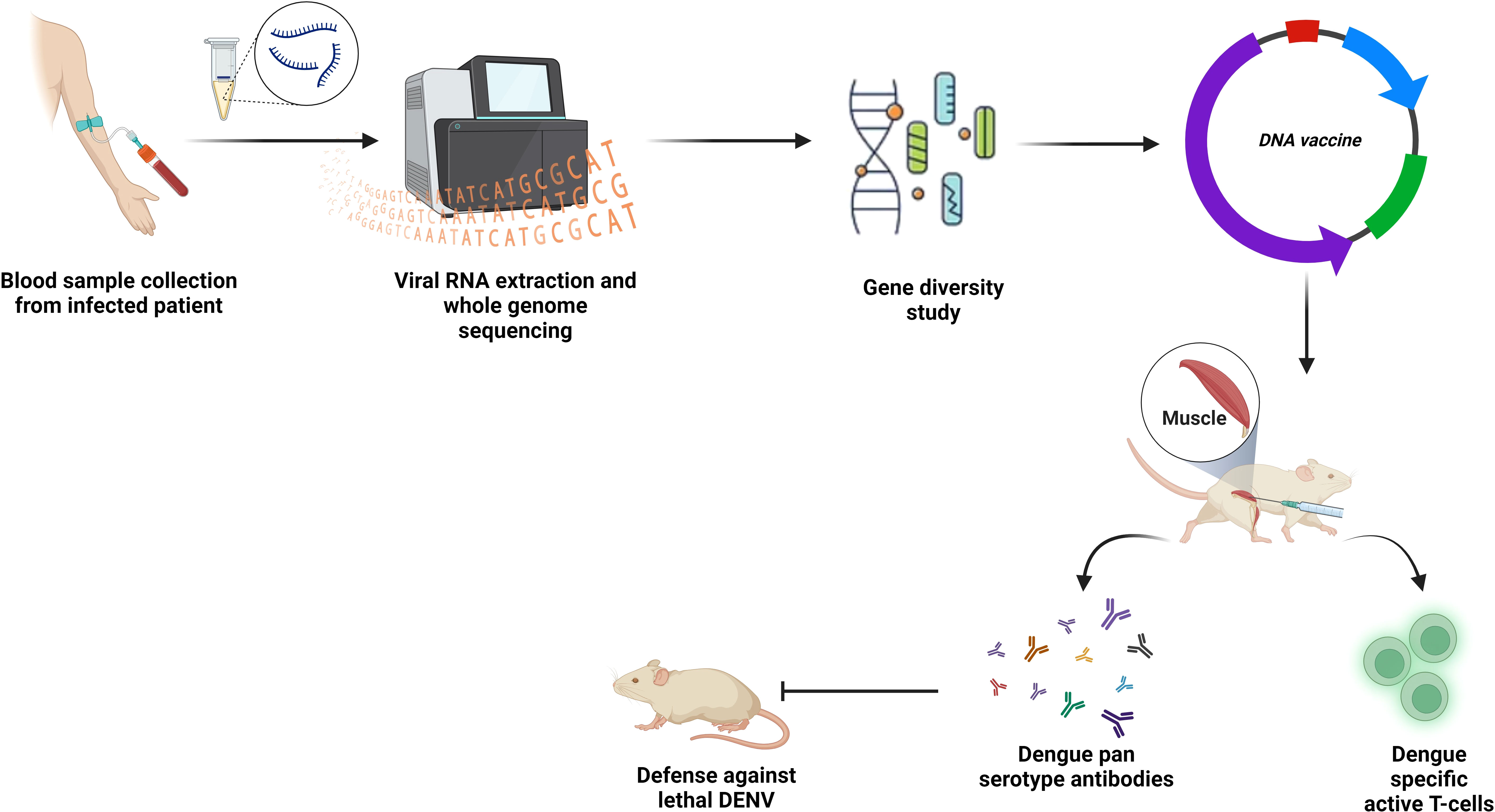
Figure 6 DNA vaccines. PCR, or synthetic generation, is used to obtain the desired gene sequence. To promote T cell and B cell mediated immunity in the host body, this sequence is introduced enzymatically into the multi-cloning region of a plasmid backbone, purified, and then administered at the inoculation site.
According to Porter et al, DENV-2 prM/E along with CpG motif is better in defense than DENV-2 prM/E alone (118).
It is also reported that a recombined DENV-2 EDIII and E. coli maltose binding protein (MBP), expressed by DENV-2 DNA vaccine was responsible for triggering the generation of neutralizing antibodies (119). A comparative study using DENV-2 prM/E DNA vaccine (D), DENV-2 EDIII and MBP recombinant fusion protein (R), and purified inactivated virus particles (P) on rhesus monkeys demonstrated the higher antibody level and higher neutralization, when administered as combined rather than alone. The upmost antibody titers were observed in DR/DR/DR, DP/DP/DP, and R/R/R vaccination, whereas D/D/D vaccination showed the least. However, animals vaccinated with only P showed substantial immune protection against viremia (120).
The use of myriads of guiding proteins that target the immune system, augments vaccine protection. One of the ways of developing the immunogenic response against DENV-2 prM/E DNA vaccine is ensuring increased synthesis of T-cells through incorporation of the antigen sequence into the lysosomal membrane protein, together with anti-CD4 antigens, which results in the enhanced production of MHC class II antigens (121). Another method for inducing robust immunity to a target antigen is through the fusion of the antigen with a single-chain Fv antibody (scFv) that is selective for the DC endocytic receptor (DEC205) (122).
To assess the efficacy of the TVDV, TPIV and TLAV immunization, Rhesus monkeys were used. Only TVDV/TVDV/TLAV treated monkeys were marginally protected, whereas monkeys inoculated with TRIV/TLAV were fully viremia free (123). It has been proved by a phase I clinical study that concurrent administration of TVDV with an adjuvant called Vaxfectin® (Vical, Boulder, CO, USA) induces anti-dengue T-cell IFN response safely (124).
While DNA vaccines offer advantages such as stability, easy preparation, cost-effectiveness, and apt for mass production, they require enhanced immunogenicity. Fortunately, the problem can be addressed by taking measures such as plasmid modification using alternative delivery strategies, promising promoters, multiple dosing systems, and co-immunization with adjuvants (125).
3 Newer strategies to immunize against dengue
Although numerous DENV vaccines have been approved or are currently undergoing clinical trials that target either the viral structural proteins or the DENV virions, there are a plethora of safety concerns that still need to be addressed. Conventional methods for developing an unerring dengue vaccine have been challenged by several issues relating to dengue pathogenesis, including the danger of ADE effects and pathogenicity entailed by cross-reactive T-cells throughout the disease progression. Having stated that, in addition to conventional approaches employed for dengue vaccine development, several unique aspects of DENV multiplication and life cycle may serve as the focal point for cutting-edge vaccine formulations.
In order to evade dengue disease, novel concepts like DENV NS1 based vaccines and mosquito based immunization approaches should be undertaken as prospective options (126). In addition to these, mRNA vaccines could be a promising choice, given the success of the mRNA COVID-19 vaccine during the pandemic.
3.1 Strategies based on NS1 proteins
The NS1 glycoprotein is composed of a hexamer alongside a dimer, which is the membrane-anchored type of glycosylphosphatidylinositol (127). The release of the NS1 protein in the sera of infected subjects usually ranges from 70 to 15,000 ng/mL, while in extraordinary circumstances, it reaches 50,000 ng/mL (11, 12). As an extracellular component, we can utilize NS1 as a potential vaccine candidate. According to Henchal, passive immunization against DENV in mice has been achieved via anti-NS1 antibodies (128). On the other hand, it is reported that, cranially challenged mice were partially protected by immunization with recombinant NS1 protein via the active intraperitoneal or subcutaneous route (129, 130). Two studies reported that NS1 completely prevented dengue-mediated vascular leakage while acting as a key factor in enhancing the permeability of blood vessels (37, 131). Furthermore, the mice which were deficient in interferon receptors, were protected from the fatal effects of DENV-2 (131). A promising alternative to NS1 immunization is to immunize using a protein combination consisting of protein E of DENV-2, N-terminal of NS1, along with protein A of Staphylococcus aureus, which helps protect the mice potentially by the generation of anti-DENV-2 antibodies (132).
NS1-based vaccines can be developed by using recombinant DNA plasmids. These DNA plasmids, by producing cytokines, DENV prM, NS1, E, and rat granulocyte-macrophage colony-stimulation factors, were reported to protect the mice from a fatal intracerebral DENV-2 challenge (133). Costa et al. also reported that, mice inoculated intracerebrally with DENV-2 NS1-consisting recombinant plasmid pcTRANS1 showed promising outcomes (134). Another study carried out in mice displayed NS1-specific humoral and cellular immunological reactions, resulting in retardation in the development of paralysis as well as a considerably extended survival after an intravenous challenge with fatal DENV-2 (135).
While recombinant vaccine viruses encoding DENV-2 or DENV-4 NS1 offer partial protection to BALB/c mice (136), several studies have suggested that NS1 antibodies may exacerbate dengue pathogenesis. This is due to their cross-reactivity with host proteins, leading to inhibited apoptosis of endothelial cells and triggering platelet aggregation (137). Further safety and effectiveness testing of the NS1 vaccination is required considering these issues.
3.2 Strategies based on blocking of transmission of infection
To address the spread of dengue, we must urgently implement all essential measures. For that, the number of infected vectors needs to be decreased by host specific vaccination. Vaccines must be designed such that they target the life cycles of pathogens in the environment to control the disease. By focusing on the mechanisms pathogens use to invade both vertebrate and invertebrate hosts, we can obstruct their pathways (126). For example, gametocytes of the Plasmodium species can be targeted to reduce the number of pathogens persisting in the mosquito gut, which in turn impairs the efficiency of microbial transmission efficiency (138–140). Immunizing against specific mosquito C-type lectins (mosGCTLs), which meet the DENV-2 virus and infect A. aegypti, it is possible to halt the life cycle of DENV. Research also indicates that a combination of antisera against several mosGCTLs can significantly decrease dengue infection post blood meal (141). Another study found that blocking of cysteine-rich venom protein (CRVP379) by either specific antisera or RNAi, retarded the DENV contamination in A. aegypti, which can be considered a promising prophylactic and curative action for controlling dengue disease (142). Combining immunization with DENV-related mosquito vulnerability variables may be a plausible concept for the development of a vaccine that prevents dengue transmission.
3.3 Advancements in dengue mRNA vaccine development
To develop an mRNA vaccine, scientists used to modify the natural structure of mRNA. The team of Wollner Clayton has pursued the same approach for the development of a dengue vaccine (Figure 7). They first transcribed DENV-1 membrane and envelop structural protein (prM/E) into a modified mRNA using pseudo uridine and then packed it into lipid nanoparticles (LNP). The mRNA-LNP complex was then injected intramuscularly and after the endocytosis by muscle cells, the LNP was degraded, leaving the mRNA for further translation of viral prM/E proteins. The viral polyproteins then exerted serotype-specific immune response in the study mice (143). Another group targeted prME, E80 and NS1 of DENV-2 using the same mRNA vaccine technology mentioned. In this case, they modified mRNA nucleoside to 1-methylpseudouridine-50-triphosphate (1 mψ). This candidate vaccine induces production of neutralizing antibodies against DENV-2 and triggers a T-cell immune response in mice (144).
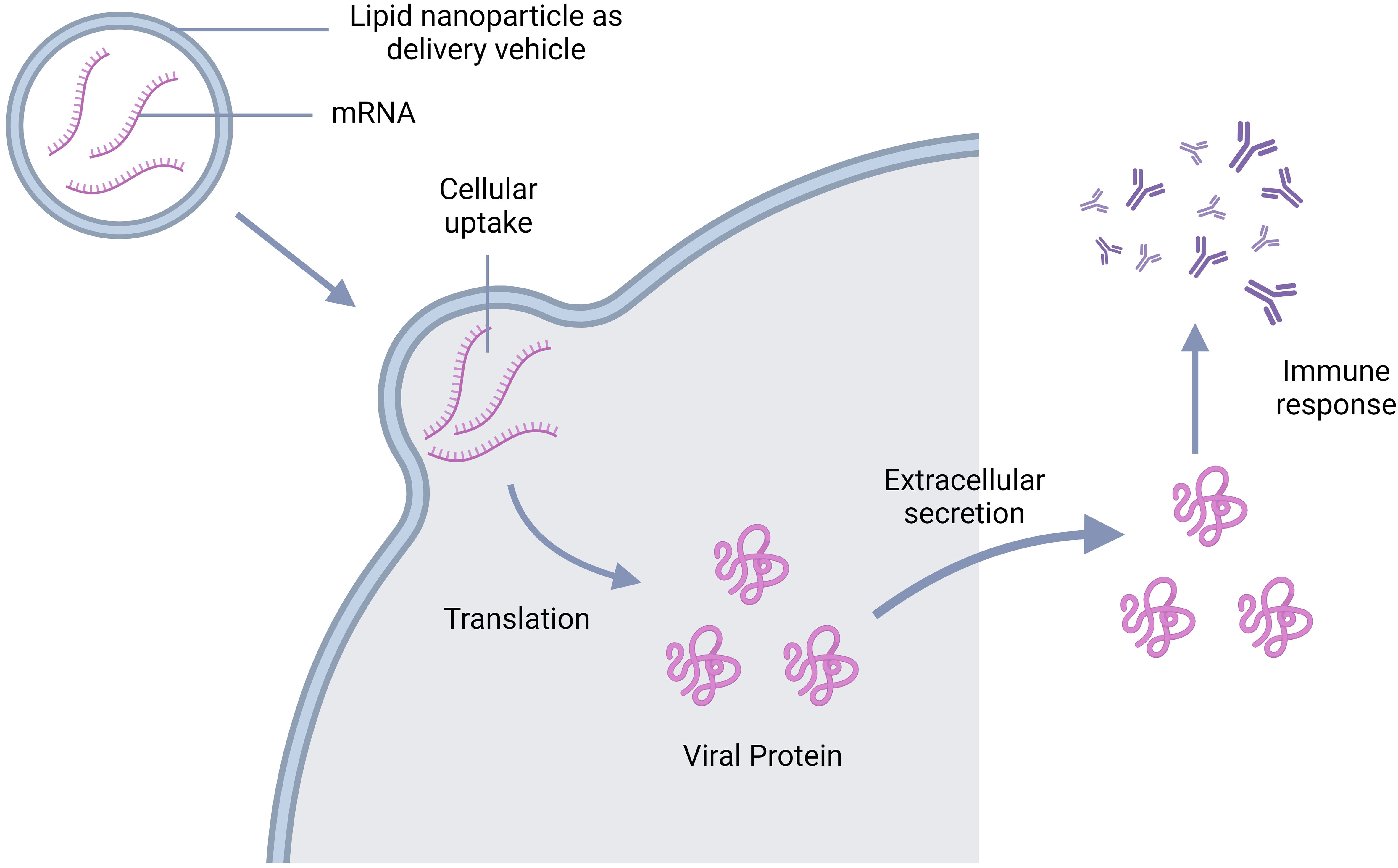
Figure 7 Schematic representation of the mechanism of action of mRNA vaccine; mRNA goes inside the cell leaving the vehicle outside and get translated into viral antigen. The antigens are then secreted extracellularly triggering the antibody production.
Both aforementioned vaccines are currently in initial stages, but hold promise for approval in upcoming clinical trials. Following table (Table 1) summarizes the stages of development of the dengue vaccines.
4 Summary and outlooks for the future
Currently, dengue stands as the most pressing arboviral disease impacting countries in the tropical and subtropical regions worldwide. The conventional vaccine approaches, such as the live attenuated vaccines, recombinant subunit vaccines, inactivated virus vaccines, viral vectored vaccines, DNA, and mRNA vaccines have been developed to prevent the transmission of all dengue serotypes in humans. These strategies primarily work by fostering defense against either the DENV virions or the envelope proteins exhibited on the surface of the causative virus. Tetravalent, live attenuated, chimeric dengue vaccines efficiently induce neutralizing-antibodies in human subjects, however, they lack the ability to neutralize the DENV-2 virus. In addition, female mice vaccinated with the live attenuated vaccine DENVax intervene with the vaccination outcome of their offspring. Despite triggering a well-balanced immune response against all the serotypes, recombinant subunit vaccines develop contamination of endotoxin with improper protein folding. Adenoviral vectored vaccines offer numerous benefits, such as the ease of genetic modifications, efficiency in detecting gene replication mistakes, and a high level of protein expression. Additionally, alphavirus-vectored dengue vaccines induce a robust immune response with only one dose of neonatal immunization in mice. On the other hand, DNA vaccines are extremely stable, economical, and conveniently producible; however, low immunogenicity limits their use. In terms of vaccination tactics, developing successful animal protection has involved priming and boosting immunizations using various vaccine combinations.
Computational advances, including advances in immunoinformatics (149), can enable improved dengue vaccine design strategies. Such approaches may include improvements in the identification of antigenic sites, structural modeling of the viral proteins, molecular docking experiments, and the examination of molecular biomimicry (150–152). By examining different serotypes and predicting how likely different parts of the dengue genome are to evolve over time, vaccines of improved efficacy, tailored for more dengue viral serotypes, can be achieved (153, 154).
Apart from NS1, Other NS proteins are NS2A, NS2B, NS3, NS4A, NS4B, and NS5. It is possible to develop inhibitors of NS3 and NS5 by evaluating their activity using biochemical assays, but there is no compound that reached clinical studies. On the other hand, NS2A, NS2B, NS3, NS4A, and NS4B do not retain any enzymatic activity. Also, challenges in crystallization and dynamic nature of these proteins hinder their structural studies (155).
Given the fragmentary effectiveness of current vaccines against dengue, we strongly advocate that future vaccine development incorporates cutting-edge vaccine concepts like mRNA, NS1-based, and mosquito-based immunization with conventional vaccines, for the improvement of their safety and efficiency against dengue infection in humans while minimizing mosquito dissemination and effectively reducing DENV presence in the environment.
Author contributions
RA: Methodology, Resources, Software, Writing – original draft. FT: Resources, Visualization, Writing – review & editing. SD: Visualization, Writing – review & editing. MAS: Validation, Writing – review & editing. IG-S: Validation, Writing – review & editing. RTJ: Validation, Visualization, Writing – review & editing, Software. SAS: Conceptualization, Project administration, Supervision, Validation, Writing – review & editing, Resources, Software, Writing – original draft.
Funding
The author(s) declare financial support was received for the research, authorship, and/or publication of this article. This project was supported by Huck Institutes of the Life Sciences at Penn State University through the Huck Innovative and Transformational Seed Grant (HITS). Content is the responsibility of the authors and does not represent the views of the Huck Institutes.
Acknowledgments
Authors created the Figures 2–7 using BioRender.com. Authors are grateful to the external reviewers for shaping the manuscript into its final form. The authors would also like to remember the people who suffered from Dengue over the recent years which made the authors in reviewing the topic.
Conflict of interest
The authors declare that the research was conducted in the absence of any commercial or financial relationships that could be construed as a potential conflict of interest.
Publisher’s note
All claims expressed in this article are solely those of the authors and do not necessarily represent those of their affiliated organizations, or those of the publisher, the editors and the reviewers. Any product that may be evaluated in this article, or claim that may be made by its manufacturer, is not guaranteed or endorsed by the publisher.
References
1. WHO. Dengue and severe dengue [Online] (2023). World Health Organization. Available online at: https://www.who.int/news-room/fact-sheets/detail/dengue-and-severe-dengue (Accessed 17 March 2023).
2. Jentes ES, Lash RR, Johansson MA, Sharp TM, Henry R, Brady OJ, et al. Evidence-based risk assessment and communication: a new global dengue-risk map for travellers and clinicians. J Travel Med. (2016) 23:taw062. doi: 10.1093/jtm/taw062.
3. Wilder-Smith A, Gubler DJ, Weaver SC, Monath TP, Heymann DL, Scott TW. Epidemic arboviral diseases: priorities for research and public health. Lancet Infect Dis. (2017) 17:e101–6. doi: 10.1016/S1473-3099(16)30518-7.
4. Riddell A, Babiker ZOE. Imported dengue fever in East London: a 6-year retrospective observational study. J Travel Med. (2017) 24:tax015. doi: 10.1093/jtm/tax015.
5. Masyeni S, Yohan B, Somia IKA, Myint KSA, Sasmono RT. Dengue infection in international travellers visiting Bali, Indonesia. J Travel Med. (2018) 25:tay061. doi: 10.1093/jtm/tay061.
6. Chaturvedi UC, Shrivastava R. Dengue haemorrhagic fever: a global challenge. Indian J Med Microbiol. (2004) 22:5–6. doi: 10.1016/S0255-0857(21)02943-1.
7. ECDC. Geographical distribution of dengue cases reported worldwid. European Centre for Disease Prevention and Control (2021). Available at: https://www.ecdc.europa.eu/en/publications-data/geographical-distribution-dengue-cases-reported-worldwide-2021 (Accessed April 20, 2023).
8. Kraemer MUG, Sinka ME, Duda KA, Mylne AQN, Shearer FM, Barker CM, et al. The global distribution of the arbovirus vectors Aedes aEgypti and Ae. albopictus. eLife. (2015) 4:e08347. doi: 10.7554/eLife.08347.
9. Clyde K, Kyle JL, Harris E. Recent advances in deciphering viral and host determinants of dengue virus replication and pathogenesis. J Virol. (2006) 80:11418–31. doi: 10.1128/JVI.01257-06.
10. WHO. Special programme for research and training in tropical diseases. Dengue: guidelines for diagnosis, treatment. Prev Control. (2009) 91–2.
11. Rigau-Pérez JG, Clark GG, Gubler DJ, Reiter P, Sanders EJ, Vance Vorndam A. Dengue and dengue haemorrhagic fever. Lancet. (1998) 352:971–7. doi: 10.1016/S0140-6736(97)12483-7.
12. Young Paul R, Hilditch Paige A, Bletchly C, Halloran W. An antigen capture enzyme-linked immunosorbent assay reveals high levels of the dengue virus protein NS1 in the sera of infected patients. J Clin Microbiol. (2000) 38:1053–7. doi: 10.1128/JCM.38.3.1053-1057.2000.
13. Mustafa MS, Rasotgi V, Jain S, Gupta V. Discovery of fifth serotype of dengue virus (DENV-5): A new public health dilemma in dengue control. Med J Armed Forces India. (2015) 71:67–70. doi: 10.1016/j.mjafi.2014.09.011.
14. Normile D. Surprising new dengue virus throws a spanner in disease control efforts. Science. (2013) 342:415–5. doi: 10.1126/science.342.6157.415.
15. Joob B, Wiwanikit V. Comment on: definitive tests for dengue fever: when and which should I use? Singapore Med J. (2018) 59:60–0. doi: 10.11622/smedj.2018008.
16. Bhatt S, Gething PW, Brady OJ, Messina JP, Farlow AW, Moyes CL, et al. The global distribution and burden of dengue. Nature. (2013) 496:504–7. doi: 10.1038/nature12060.
17. Murrell S, Wu S-C, Butler M. Review of dengue virus and the development of a vaccine. Biotechnol Adv. (2011) 29:239–47. doi: 10.1016/j.biotechadv.2010.11.008.
18. Hammon WM, Rundnick A, Sather GE. Viruses associated with epidemic hemorrhagic fevers of the Philippines and Thailand. Science. (1960) 131:1102–3. doi: 10.1126/science.131.3407.1102.
19. Rodrigo WWSI, Block OKT, Lane C, Sukupolvi-Petty S, Goncalvez AP, Johnson S, et al. Dengue virus neutralization is modulated by IgG antibody subclass and Fcγ receptor subtype. Virology. (2009) 394:175–82. doi: 10.1016/j.virol.2009.09.024.
20. Wu RSL, Chan KR, Tan HC, Chow A, Allen JC, Ooi EE. Neutralization of dengue virus in the presence of Fc receptor-mediated phagocytosis distinguishes serotype-specific from cross-neutralizing antibodies. Antiviral Res. (2012) 96:340–3. doi: 10.1016/j.antiviral.2012.09.018.
21. Montoya M, Gresh L, Mercado JC, Williams KL, Vargas MJ, Gutierrez G, et al. Symptomatic versus inapparent outcome in repeat dengue virus infections is influenced by the time interval between infections and study year. PloS Negl Trop Dis. (2013) 7:e2357. doi: 10.1371/journal.pntd.0002357.
22. Endy TP, Yoon I-K, Mammen MP. Prospective cohort studies of dengue viral transmission and severity of disease. In: Rothman AL, editor. Dengue virus. Springer Berlin Heidelberg, Berlin, Heidelberg (2010).
23. Carrington LB, Simmons CP. Human to mosquito transmission of dengue viruses. Front Immunol. (2014) 5:290. doi: 10.3389/fimmu.2014.00290.
24. Cheng G, Liu Y, Wang P, Xiao X. Mosquito defense strategies against viral infection. Trends Parasitol. (2016) 32:177–86. doi: 10.1016/j.pt.2015.09.009.
25. Azeem M, Zaman T, Tahir M, Haris A, Iqbal Z, Binyameen M, et al. Chemical composition and repellent activity of native plants essential oils against dengue mosquito, Aedes aEgypti. Ind Crops Products. (2019) 140:111609. doi: 10.1016/j.indcrop.2019.111609.
26. WHO. Dengue: guidelines for diagnosis, treatment, prevention and control. World Health Organization (2009). Available at: https://iris.who.int/bitstream/handle/10665/44188/9789241547871_eng.pdf?sequence=1.
27. Curtis CF, Lines JD. Should DDT be banned by international treaty? Parasitol Today. (2000) 16:119–21. doi: 10.1016/S0169-4758(99)01605-1.
28. Damalas CA, Eleftherohorinos IG. Pesticide exposure, safety issues, and risk assessment indicators. Int J Environ Res Public Health. (2011) 8:1402–19. doi: 10.3390/ijerph8051402.
29. Dalpadado R, Gunathilaka N, Amarasinghe D, Udayanaga L. A Challenge for a Unique Dengue Vector Control Programme: Assessment of the Spatial Variation of Insecticide Resistance Status amongst Aedes aEgypti and Aedes albopictus Populations in Gampaha District, Sri Lanka. BioMed Res Int. (2021) 2021:6619175. doi: 10.1155/2021/6619175.
30. Guruprasad NM, Jalali SK, Puttaraju HP. Wolbachia-a foe for mosquitoes. Asian Pacific J Trop Dis. (2014) 4:78–81. doi: 10.1016/S2222-1808(14)60319-4.
31. Brady OJ, Gething PW, Bhatt S, Messina JP, Brownstein JS, Hoen AG, et al. Refining the global spatial limits of dengue virus transmission by evidence-based consensus. PloS Negl Trop Dis. (2012) 6:e1760. doi: 10.1371/journal.pntd.0001760.
32. Yauch LE, Shresta S. Mouse models of dengue virus infection and disease. Antiviral Res. (2008) 80:87–93. doi: 10.1016/j.antiviral.2008.06.010.
34. Simmons JS, St John JH, Reynolds FHK. Experimental studies of dengue. Philippine J Sci. (1931) 44(1-2).
35. Halstead SB. Immunological parameters of togavirus disease syndromes. New York: Academic Press (1980). p. 107–73. doi: 10.1016/B978-0-12-625380-1.50010-2.
36. Halstead SB. Pathogenesis of dengue: challenges to molecular biology. Science. (1988) 239:476–81. doi: 10.1126/science.239.4839.476.
37. Modhiran N, Watterson D, Muller DA, Panetta AK, Sester DP, Liu L, et al. Dengue virus NS1 protein activates cells via Toll-like receptor 4 and disrupts endothelial cell monolayer integrity. Sci Trans Med. (2015) 7:304ra142–304ra142. doi: 10.1126/scitranslmed.aaa3863.
38. Zompi S, Harris E. Animal models of dengue virus infection. Viruses. (2012) 4(1):62-82. doi: 10.3390/v4010062.
39. Na W, Yeom M, Choi I-K, Yook H, Song D. Animal models for dengue vaccine development and testing. Clin Exp Vaccine Res. (2017) 6:104–10. doi: 10.7774/cevr.2017.6.2.104.
40. Torres-Flores JM, Reyes-Sandoval A, Salazar MI. Dengue vaccines: an update. BioDrugs. (2022) 36:325–36. doi: 10.1007/s40259-022-00531-z.
41. Bhamarapravati N, Sutee Y. Live attenuated tetravalent dengue vaccine. Vaccine. (2000) 18:44–7. doi: 10.1016/S0264-410X(00)00040-2.
42. Halstead SB, Marchette NJ. Biologic properties of dengue viruses following serial passage in primary dog kidney cells: studies at the University of Hawaii. Am J Trop Med hygiene. (2003) 69:5–11. doi: 10.4269/ajtmh.2003.69.6_suppl.0690005.
43. WHO. Dengue vaccine safety update. WHO weekly epidemiological report. (2018). Available at: https://www.who.int/groups/global-advisory-committee-on-vaccine-safety/topics/dengue-vaccines/safety-update#cms.
44. Sun W, Edelman R, Kanesa-Thasan N, Eckels KH, Putnak JR, King AD, et al. Vaccination of human volunteers with monovalent and tetravalent live-attenuated dengue vaccine candidates. Am J Trop Med hygiene. (2003) 69:24–31. doi: 10.4269/ajtmh.2003.69.6_suppl.0690024.
45. Thomas SJ, Eckels KH, Carletti I, de la Barrera R, Dessy F, Fernandez S, et al. A phase II, randomized, safety and immunogenicity study of a re-derived, live-attenuated dengue virus vaccine in healthy adults. Am J Trop Med Hyg. (2013) 88:73–88. doi: 10.4269/ajtmh.2012.12-0361.
46. Lai CJ, Zhao BT, Hori H, Bray M. Infectious RNA transcribed from stably cloned full-length cDNA of dengue type 4 virus. Proc Natl Acad Sci. (1991) 88:5139–43. doi: 10.1073/pnas.88.12.5139.
47. Polo S, Ketner G, Levis R, Falgout B. Infectious RNA transcripts from full-length dengue virus type 2 cDNA clones made in yeast. J Virol. (1997) 71:5366–74. doi: 10.1128/jvi.71.7.5366-5374.1997.
48. Halstead SB, Deen J. The future of dengue vaccines. Lancet. (2002) 360:1243–5. doi: 10.1016/S0140-6736(02)11276-1.
49. Thisyakorn U, Thisyakorn C. Latest developments and future directions in dengue vaccines. Ther Adv Vaccines. (2014) 2:3–9. doi: 10.1177/2051013613507862.
50. Deng S-Q, Yang X, Wei Y, Chen J-T, Wang X-J, Peng H-J. A review on dengue vaccine development. Vaccines. (2020) 8(1):63. doi: 10.3390/vaccines8010063.
51. Lee H-C, Butler M, Wu S-C. Using recombinant DNA technology for the development of live-attenuated dengue vaccines. Enzyme Microbial Technol. (2012) 51:67–72. doi: 10.1016/j.enzmictec.2012.05.005.
52. Osorio JE, Wallace D, Stinchcomb DT. A recombinant, chimeric tetravalent dengue vaccine candidate based on a dengue virus serotype 2 backbone. Expert Rev Vaccines. (2016) 15:497–508. doi: 10.1586/14760584.2016.1128328.
53. Rice CM, Grakoui A, Galler R, Chambers TJ. Transcription of infectious yellow fever RNA from full-length cDNA templates produced by in vitro ligation. New biologist. (1989) 1:285–96.
54. Guirakhoo F, Weltzin R, Chambers TJ, Zhang ZX, Soike K, Ratterree M, et al. Recombinant chimeric yellow fever-dengue type 2 virus is immunogenic and protective in nonhuman primates. J Virol. (2000) 74:5477–85. doi: 10.1128/JVI.74.12.5477-5485.2000.
55. Guirakhoo F, Pugachev K, Zhang Z, Myers G, Levenbook I, Draper K, et al. Safety and efficacy of chimeric yellow fever-dengue virus tetravalent vaccine formulations in nonhuman primates. J Virol. (2004) 78:4761–75. doi: 10.1128/JVI.78.9.4761-4775.2004.
56. Hadinegoro SR, Arredondo-García JL, Capeding MR, Deseda C, Chotpitayasunondh T, Dietze R, et al. Efficacy and long-term safety of a dengue vaccine in regions of endemic disease. New Engl J Med. (2015) 373:1195–206. doi: 10.1056/NEJMoa1506223.
57. Constenla D, Clark S. Financing dengue vaccine introduction in the Americas: challenges and opportunities. Expert Rev Vaccines. (2016) 15:547–59. doi: 10.1586/14760584.2016.1134329.
58. Vlaycheva Leonsia A, Chambers Thomas J. Neuroblastoma cell-adapted yellow fever 17D virus: characterization of a viral variant associated with persistent infection and decreased virus spread. J Virol. (2002) 76:6172–84. doi: 10.1128/JVI.76.12.6172-6184.2002.
59. Guy B, Barrere B, Malinowski C, Saville M, Teyssou R, Lang J. From research to phase III: Preclinical, industrial and clinical development of the Sanofi Pasteur tetravalent dengue vaccine. Vaccine. (2011) 29:7229–41. doi: 10.1016/j.vaccine.2011.06.094.
60. Sabchareon A, Wallace D, Sirivichayakul C, Limkittikul K, Chanthavanich P, Suvannadabba S, et al. Protective efficacy of the recombinant, live-attenuated, CYD tetravalent dengue vaccine in Thai schoolchildren: a randomised, controlled phase 2b trial. Lancet. (2012) 380:1559–67. doi: 10.1016/S0140-6736(12)61428-7.
61. Morrison D, Legg TJ, Billings CW, Forrat R, Yoksan S, Lang J. A novel tetravalent dengue vaccine is well tolerated and immunogenic against all 4 serotypes in flavivirus-naive adults. J Infect Dis. (2010) 201:370–7. doi: 10.1086/649916.
62. Villar L, Dayan GH, Arredondo-García JL, Rivera DM, Cunha R, Deseda C, et al. Efficacy of a tetravalent dengue vaccine in children in Latin America. N Engl J Med. (2014) 372(2):113–23. doi: 10.1056/NEJMoa1411037.
63. Capeding MR, Tran NH, Hadinegoro SR, Ismail HI, Chotpitayasunondh T, Chua MN, et al. Clinical efficacy and safety of a novel tetravalent dengue vaccine in healthy children in Asia: a phase 3, randomised, observer-masked, placebo-controlled trial. Lancet. (2014) 384(9951):1358–65. doi: 10.1016/S0140-6736(14)61060-6.
64. Halstead SB. Dengue vaccine development: a 75% solution? Lancet. (2012) 380:1535–6. doi: 10.1016/S0140-6736(12)61510-4
65. Guy B, Noriega F, Ochiai RL, L’azou M, Delore V, Skipetrova A, et al. A recombinant live attenuated tetravalent vaccine for the prevention of dengue. Expert Rev Vaccines. (2017) 16:671–84. doi: 10.1080/14760584.2017.1335201.
66. WHO. Dengue vaccine: WHO position paper, September 2018 – Recommendations. Vaccine. (2019) 37:4848–9. doi: 10.1016/j.vaccine.2018.09.063.
67. Durbin AP, Kirkpatrick BD, Pierce KK, Schmidt AC, Whitehead SS. Development and clinical evaluation of multiple investigational monovalent DENV vaccines to identify components for inclusion in a live attenuated tetravalent DENV vaccine. Vaccine. (2011) 29:7242–50. doi: 10.1016/j.vaccine.2011.07.023.
68. Men R, Bray M, Clark D, Chanock RM, Lai CJ. Dengue type 4 virus mutants containing deletions in the 3' noncoding region of the RNA genome: analysis of growth restriction in cell culture and altered viremia pattern and immunogenicity in rhesus monkeys. J Virol. (1996) 70:3930–7. doi: 10.1128/jvi.70.6.3930-3937.1996.
69. Ng WC, Soto-Acosta R, Bradrick SS, Garcia-Blanco MA, Ooi EE. The 5' and 3' Untranslated regions of the flaviviral genome. Viruses. (2017) 9(6):137. doi: 10.3390/v9060137.
70. Durbin AP, Karron RA, Sun W, Vaughn DW, Reynolds MJ, Perreault JR, et al. Attenuation and immunogenicity in humans of a live dengue virus type-4 vaccine candidate with a 30 nucleotide deletion in its 3'-untranslated region. Am J Trop Med hygiene. (2001) 65:405–13. doi: 10.4269/ajtmh.2001.65.405.
71. Blaney Joseph E, Johnson Daniel H, Firestone C-Y, Hanson Christopher T, Murphy Brian R, Whitehead Stephen S. Chemical mutagenesis of dengue virus type 4 yields mutant viruses which are temperature sensitive in vero cells or human liver cells and attenuated in mice. J Virol. (2001) 75:9731–40. doi: 10.1128/JVI.75.20.9731-9740.2001.
72. Hanley Kathryn A, Lee Jay J, Blaney Joseph E, Murphy Brian R, Whitehead Stephen S. Paired charge-to-alanine mutagenesis of dengue virus type 4 NS5 generates mutants with temperature-sensitive, host range, and mouse attenuation phenotypes. J Virol. (2002) 76:525–31. doi: 10.1128/JVI.76.2.525-531.2002.
73. Hanley KA, Manlucu LR, Manipon GG, Hanson CT, Whitehead SS, Murphy BR, et al. Introduction of mutations into the non-structural genes or 3′ untranslated region of an attenuated dengue virus type 4 vaccine candidate further decreases replication in rhesus monkeys while retaining protective immunity. Vaccine. (2004) 22:3440–8. doi: 10.1016/j.vaccine.2004.02.031.
74. Durbin AP, Kirkpatrick BD, Pierce KK, Elwood D, Larsson CJ, Lindow JC, et al. A single dose of any of four different live attenuated tetravalent dengue vaccines is safe and immunogenic in flavivirus-naive adults: A randomized, double-blind clinical trial. J Infect Dis. (2013) 207:957–65. doi: 10.1093/infdis/jis936.
75. Kirkpatrick BD, Whitehead SS, Pierce KK, Tibery CM, Grier PL, Hynes NA, et al. The live attenuated dengue vaccine TV003 elicits complete protection against dengue in a human challenge model. Sci Trans Med. (2016) 8:330ra36–330ra36. doi: 10.1126/scitranslmed.aaf1517.
76. Kirkpatrick BD, Durbin AP, Pierce KK, Carmolli MP, Tibery CM, Grier PL, et al. Robust and balanced immune responses to all 4 dengue virus serotypes following administration of a single dose of a live attenuated tetravalent dengue vaccine to healthy, flavivirus-naive adults. J Infect Dis. (2015) 212:702–10. doi: 10.1093/infdis/jiv082.
77. Whitehead SS. Development of TV003/TV005, a single dose, highly immunogenic live attenuated dengue vaccine; what makes this vaccine different from the Sanofi-Pasteur CYD™ vaccine? Expert Rev Vaccines. (2016) 15:509–17. doi: 10.1586/14760584.2016.1115727.
78. Sabchareon A, Lang J, Chanthavanich P, Yoksan S, Forrat R, Attanath P, et al. Safety and immunogenicity of a three dose regimen of two tetravalent live-attenuated dengue vaccines in five- to twelve-year-old Thai children. Pediatr Infect Dis J. (2004) 23(2):99-109. doi: 10.1097/01.inf.0000109289.55856.27.
79. Simasathien S, Thomas SJ, Watanaveeradej V, Nisalak A, Barberousse C, Innis BL, et al. Safety and immunogenicity of a tetravalent live-attenuated dengue vaccine in flavivirus naive children. Am J Trop Med hygiene. (2008) 78:426–33. doi: 10.4269/ajtmh.2008.78.426.
80. Brewoo JN, Kinney RM, Powell TD, Arguello JJ, Silengo SJ, Partidos CD, et al. Immunogenicity and efficacy of chimeric dengue vaccine (DENVax) formulations in interferon-deficient AG129 mice. Vaccine. (2012) 30:1513–20. doi: 10.1016/j.vaccine.2011.11.072.
81. Putnak R, Barvir DA, Burrous JM, Dubois DR, D'andrea VM, Hoke CH, et al. Development of a purified, inactivated, dengue-2 virus vaccine prototype in vero cells: immunogenicity and protection in mice and rhesus monkeys. J Infect Dis. (1996) 174:1176–84. doi: 10.1093/infdis/174.6.1176.
82. Robert Putnak J, Coller B-A, Voss G, Vaughn DW, Clements D, Peters I, et al. An evaluation of dengue type-2 inactivated, recombinant subunit, and live-attenuated vaccine candidates in the rhesus macaque model. Vaccine. (2005) 23:4442–52. doi: 10.1016/j.vaccine.2005.03.042.
83. Sun W, Nisalak A, Gettayacamin M, Eckels KH, Putnak JR, Vaughn DW, et al. Protection of Rhesus Monkeys against Dengue Virus Challenge after Tetravalent Live Attenuated Dengue Virus Vaccination. J Infect Dis. (2006) 193:1658–65. doi: 10.1086/503372.
84. Wilder-Smith A. Dengue vaccine development: status and future. Bundesgesundheitsblatt Gesundheitsforschung Gesundheitsschutz. (2020) 63:40–4. doi: 10.1007/s00103-019-03060-3.
85. Simmons M, Burgess T, Lynch J, Putnak R. Protection against dengue virus by non-replicating and live attenuated vaccines used together in a prime boost vaccination strategy. Virology. (2010) 396:280–8. doi: 10.1016/j.virol.2009.10.023.
86. Aihara H, Takasaki T, Toyosaki-Maeda T, Suzuki R, Okuno Y, Kurane I. T-cell activation and induction of antibodies and memory T cells by immunization with inactivated Japanese encephalitis vaccine. Viral Immunol. (2000) 13:179–86. doi: 10.1089/vim.2000.13.179.
87. Whitehead SS, Blaney JE, Durbin AP, Murphy BR. Prospects for a dengue virus vaccine. Nat Rev Microbiol. (2007) 5:518–28. doi: 10.1038/nrmicro1690.
88. Tripathi NK, Shrivastava A. Recent developments in recombinant protein–based dengue vaccines. Front Immunol. (2018) 9. doi: 10.3389/fimmu.2018.01919.
89. Babu JP, Pattnaik P, Gupta N, Shrivastava A, Khan M, Rao PVL. Immunogenicity of a recombinant envelope domain III protein of dengue virus type-4 with various adjuvants in mice. Vaccine. (2008) 26:4655–63. doi: 10.1016/j.vaccine.2008.07.006.
90. Yang J, Zhang J, Chen W, Hu Z, Zhu J, Fang X, et al. Eliciting cross-neutralizing antibodies in mice challenged with a dengue virus envelope domain III expressed in Escherichia coli. Can J Microbiol. (2012) 58:369–80. doi: 10.1139/w11-137.
91. Chiang C-Y, Hsieh C-H, Chen M-Y, Tsai J-P, Liu H-H, Liu S-J, et al. Recombinant lipidated dengue-4 envelope protein domain III elicits protective immunity. Vaccine. (2014) 32:1346–53. doi: 10.1016/j.vaccine.2014.01.041.
92. Chiang C-Y, Liu S-J, Hsieh C-H, Chen M-Y, Tsai J-P, Liu H-H, et al. Recombinant lipidated dengue-3 envelope protein domain III stimulates broad immune responses in mice. Vaccine. (2016) 34:1054–61. doi: 10.1016/j.vaccine.2016.01.009.
93. Christodoulides A, Boyadjian A, Kelesidis T. Spirochetal lipoproteins and immune evasion. Front Immunol. (2017) 8:364. doi: 10.3389/fimmu.2017.00364.
94. Lazo L, Izquierdo A, Suzarte E, Gil L, Valdés I, Marcos E, et al. Evaluation in mice of the immunogenicity and protective efficacy of a tetravalent subunit vaccine candidate against dengue virus. Microbiol Immunol. (2014) 58:219–26. doi: 10.1111/1348-0421.12140.
95. Zhao H, Jiang T, Zhou X-Z, Deng Y-Q, Li X-F, Chen S-P, et al. Induction of neutralizing antibodies against four serotypes of dengue viruses by mixBiEDIII, a tetravalent dengue vaccine. PloS One. (2014) 9:e86573. doi: 10.1371/journal.pone.0086573.
96. Wang WH, Urbina AN, Lin CY, Yang ZS, Assavalapsakul W, Thitithanyanont A, et al. Targets and strategies for vaccine development against dengue viruses. BioMed Pharmacother. (2021) 144:112304. doi: 10.1016/j.biopha.2021.112304.
97. Bal J, Luong NN, Park J, Song K-D, Jang Y-S, Kim D-H. Comparative immunogenicity of preparations of yeast-derived dengue oral vaccine candidate. Microbial Cell Factories. (2018) 17:1-14. doi: 10.1186/s12934-018-0876-0.
98. Clements DE, Coller B-AG, Lieberman MM, Ogata S, Wang G, Harada KE, et al. Development of a recombinant tetravalent dengue virus vaccine: Immunogenicity and efficacy studies in mice and monkeys. Vaccine. (2010) 28:2705–15. doi: 10.1016/j.vaccine.2010.01.022.
99. Govindarajan D, Meschino S, Guan L, Clements DE, Ter Meulen JH, Casimiro DR, et al. Preclinical development of a dengue tetravalent recombinant subunit vaccine: Immunogenicity and protective efficacy in nonhuman primates. Vaccine. (2015) 33:4105–16. doi: 10.1016/j.vaccine.2015.06.067.
100. Kim M-Y, Van Dolleweerd C, Copland A, Paul MJ, Hofmann S, Webster GR, et al. Molecular engineering and plant expression of an immunoglobulin heavy chain scaffold for delivery of a dengue vaccine candidate. Plant Biotechnol J. (2017) 15:1590–601. doi: 10.1111/pbi.12741.
101. Smucny JJ, Kelly EP, Macarthy PO, King AD. Murine immunoglobulin G subclass responses following immunization with live dengue virus or a recombinant dengue envelope protein. Am J Trop Med Hygiene. (1995) 53:432–7. doi: 10.4269/ajtmh.1995.53.432.
102. Kuriakose A, Chirmule N, Nair P. Immunogenicity of biotherapeutics: causes and association with posttranslational modifications. J Immunol Res. (2016) 2016:1298473. doi: 10.1155/2016/1298473.
103. Yauch LE, Shresta S. Dengue virus vaccine development. Adv Virus Res. (2014) 88:315–72. doi: 10.1016/B978-0-12-800098-4.00007-6.
104. Zhao BT, Prince G, Horswood R, Eckels K, Summers P, Chanock R, et al. Expression of dengue virus structural proteins and nonstructural protein NS1 by a recombinant vaccinia virus. J Virol. (1987) 61:4019–22. doi: 10.1128/jvi.61.12.4019-4022.1987.
105. Men R, Wyatt L, Tokimatsu I, Arakaki S, Shameem G, Elkins R, et al. Immunization of rhesus monkeys with a recombinant of modified vaccinia virus Ankara expressing a truncated envelope glycoprotein of dengue type 2 virus induced resistance to dengue type 2 virus challenge. Vaccine. (2000) 18:3113–22. doi: 10.1016/S0264-410X(00)00121-3.
106. Jaiswal S, Khanna N, Swaminathan S. Replication-defective adenoviral vaccine vector for the induction of immune responses to dengue virus type 2. J Virol. (2003) 77:12907–13. doi: 10.1128/JVI.77.23.12907-12913.2003.
107. Khanam S, Rajendra P, Khanna N, Swaminathan S. An adenovirus prime/plasmid boost strategy for induction of equipotent immune responses to two dengue virus serotypes. BMC Biotechnol. (2007) 7:10. doi: 10.1186/1472-6750-7-10.
108. Khanam S, Pilankatta R, Khanna N, Swaminathan S. An adenovirus type 5 (AdV5) vector encoding an envelope domain III-based tetravalent antigen elicits immune responses against all four dengue viruses in the presence of prior AdV5 immunity. Vaccine. (2009) 27:6011–21. doi: 10.1016/j.vaccine.2009.07.073.
109. Holman David H, Wang D, Raviprakash K, Raja Nicholas U, Luo M, Zhang J, et al. Two complex, adenovirus-based vaccines that together induce immune responses to all four dengue virus serotypes. Clin Vaccine Immunol. (2007) 14:182–9. doi: 10.1128/CVI.00330-06.
110. Macdonald Gene H, Johnston Robert E. Role of dendritic cell targeting in Venezuelan equine encephalitis virus pathogenesis. J Virol. (2000) 74:914–22. doi: 10.1128/JVI.74.2.914-922.2000.
111. Thompson JM, Whitmore AC, Konopka JL, Collier ML, Richmond EMB, Davis NL, et al. Mucosal and systemic adjuvant activity of alphavirus replicon particles. Proc Natl Acad Sci. (2006) 103:3722–7. doi: 10.1073/pnas.0600287103.
112. Chen L, Ewing D, Subramanian H, Block K, Rayner J, Alterson KD, et al. A heterologous DNA prime-Venezuelan equine encephalitis virus replicon particle boost dengue vaccine regimen affords complete protection from virus challenge in cynomolgus macaques. J Virol. (2007) 81:11634–9. doi: 10.1128/JVI.00996-07.
113. White Laura J, Sariol Carlos A, Mattocks Melissa D, Wahala MPBW, Yingsiwaphat V, Collier Martha L, et al. An alphavirus vector-based tetravalent dengue vaccine induces a rapid and protective immune response in macaques that differs qualitatively from immunity induced by live virus infection. J Virol. (2013) 87:3409–24. doi: 10.1128/JVI.02298-12.
114. Khalil SM, Tonkin DR, Mattocks MD, Snead AT, Johnston RE, White LJ. A tetravalent alphavirus-vector based dengue vaccine provides effective immunity in an early life mouse model. Vaccine. (2014) 32:4068–74. doi: 10.1016/j.vaccine.2014.05.053.
115. Kochel T, Wu S-J, Raviprakash K, Hobart P, Hoffman S, Porter K, et al. Inoculation of plasmids expressing the dengue-2 envelope gene elicit neutralizing antibodies in mice. Vaccine. (1997) 15:547–52. doi: 10.1016/S0264-410X(97)00215-6.
116. Raviprakash K, Kochel TJ, Ewing D, Simmons M, Phillips I, Hayes CG, et al. Immunogenicity of dengue virus type 1 DNA vaccines expressing truncated and full length envelope protein. Vaccine. (2000) 18:2426–34. doi: 10.1016/S0264-410X(99)00570-8.
117. Beckett CG, Tjaden J, Burgess T, Danko JR, Tamminga C, Simmons M, et al. Evaluation of a prototype dengue-1 DNA vaccine in a Phase 1 clinical trial. Vaccine. (2011) 29:960–8. doi: 10.1016/j.vaccine.2010.11.050.
118. Porter KR, Kochel TJ, Wu SJ, Raviprakash K, Phillips I, Hayes CG. Protective efficacy of a dengue 2 DNA vaccine in mice and the effect of CpG immuno-stimulatory motifs on antibody responses. Arch Virol. (1998) 143:997–1003. doi: 10.1007/s007050050348.
119. Simmons M, Murphy GS, Kochel T, Raviprakash K, Hayes CG. Characterization of antibody responses to combinations of a dengue-2 DNA and dengue-2 recombinant subunit vaccine. Am J Trop Med hygiene. (2001) 65:420–6. doi: 10.4269/ajtmh.2001.65.420.
120. Simmons M, Porter Kevin R, Hayes Curtis G, Vaughn David W, Putnak R. Characterization of antibody responses to combinations of a dengue virus type 2 DNA vaccine and two dengue virus type 2 protein vaccines in rhesus macaques. J Virol. (2006) 80:9577–85. doi: 10.1128/JVI.00284-06.
121. Raviprakash K, Marques E, Ewing D, Lu Y, Phillips I, Porter KR, et al. Synergistic neutralizing antibody response to a dengue virus type 2 DNA vaccine by incorporation of lysosome-associated membrane protein sequences and use of plasmid expressing GM-CSF. Virology. (2001) 290:74–82. doi: 10.1006/viro.2001.1136.
122. Zaneti AB, Yamamoto MM, Sulczewski FB, Almeida BDS, Souza HFS, Ferreira NS, et al. Dendritic cell targeting using a DNA vaccine induces specific antibodies and CD4+ T cells to the dengue virus envelope protein domain III. Front Immunol. (2019) 10. doi: 10.3389/fimmu.2019.00059.
123. Lazo L, Zulueta A, Hermida L, Blanco A, Sánchez J, Valdés I, et al. Dengue-4 envelope domain III fused twice within the meningococcal P64k protein carrier induces partial protection in mice. Biotechnol Appl Biochem. (2009) 52:265–71. doi: 10.1042/BA20080074.
124. Danko JR, Kochel T, Teneza-Mora N, Luke TC, Raviprakash K, Sun P, et al. Safety and immunogenicity of a tetravalent dengue DNA vaccine administered with a cationic lipid-based adjuvant in a phase 1 clinical trial. Am J Trop Med Hygiene. (2018) 98:849–56. doi: 10.4269/ajtmh.17-0416.
125. Danko JR, Beckett CG, Porter KR. Development of dengue DNA vaccines. Vaccine. (2011) 29:7261–6. doi: 10.1016/j.vaccine.2011.07.019.
126. Liu Y, Liu J, Cheng G. Vaccines and immunization strategies for dengue prevention. Emerging Microbes Infections. (2016) 5:1–6. doi: 10.1038/emi.2016.74.
127. Akey DL, Brown WC, Dutta S, Konwerski J, Jose J, Jurkiw TJ, et al. Flavivirus NS1 structures reveal surfaces for associations with membranes and the immune system. Science. (2014) 343:881–5. doi: 10.1126/science.1247749.
128. Henchal EA, Henchal LS, Schlesinger JJ. Synergistic interactions of anti-NS1 monoclonal antibodies protect passively immunized mice from lethal challenge with dengue 2 virus. J Gen Virol. (1988) 69:2101–7. doi: 10.1099/0022-1317-69-8-2101.
129. Schlesinger JJ, Brandriss MW, Walsh EE. Protection of mice against dengue 2 virus encephalitis by immunization with the dengue 2 virus non-structural glycoprotein NS1. J Gen Virol. (1987) 68:853–7. doi: 10.1099/0022-1317-68-3-853.
130. Amorim JH, Diniz MO, Cariri FAMO, Rodrigues JF, Bizerra RSP, Gonçalves AJS, et al. Protective immunity to DENV2 after immunization with a recombinant NS1 protein using a genetically detoxified heat-labile toxin as an adjuvant. Vaccine. (2012) 30:837–45. doi: 10.1016/j.vaccine.2011.12.034.
131. Beatty PR, Puerta-Guardo H, Killingbeck SS, Glasner DR, Hopkins K, Harris E. Dengue virus NS1 triggers endothelial permeability and vascular leak that is prevented by NS1 vaccination. Sci Trans Med. (2015) 7:304ra141–304ra141. doi: 10.1126/scitranslmed.aaa3787.
132. Srivastava AK, Putnak JR, Warren RL, Hoke CH. Mice immunized with a dengue type 2 virus E and NS1 fusion protein made in Escherichia coli are protected against lethal dengue virus infection. Vaccine. (1995) 13:1251–8. doi: 10.1016/0264-410X(94)00059-V.
133. Lu H, Xu X-F, Gao N, Fan D-Y, Wang J, An J. Preliminary evaluation of DNA vaccine candidates encoding dengue-2 prM/E and NS1: Their immunity and protective efficacy in mice. Mol Immunol. (2013) 54:109–14. doi: 10.1016/j.molimm.2012.11.007.
134. Costa SM, Freire MS, Alves AMB. DNA vaccine against the non-structural 1 protein (NS1) of dengue 2 virus. Vaccine. (2006) 24:4562–4. doi: 10.1016/j.vaccine.2005.08.022.
135. Wu S-F, Liao C-L, Lin Y-L, Yeh C-T, Chen L-K, Huang Y-F, et al. Evaluation of protective efficacy and immune mechanisms of using a non-structural protein NS1 in DNA vaccine against dengue 2 virus in mice. Vaccine. (2003) 21:3919–29. doi: 10.1016/S0264-410X(03)00310-4.
136. Falgout B, Bray M, Schlesinger JJ, Lai CJ. Immunization of mice with recombinant vaccinia virus expressing authentic dengue virus nonstructural protein NS1 protects against lethal dengue virus encephalitis. J Virol. (1990) 64:4356–63. doi: 10.1128/jvi.64.9.4356-4363.1990.
137. Wan S-W, Lin C-F, Yeh T-M, Liu C-C, Liu H-S, Wang S, et al. Autoimmunity in dengue pathogenesis. J Formosan Med Assoc. (2013) 112:3–11. doi: 10.1016/j.jfma.2012.11.006.
138. Miura K, Keister DB, Muratova OV, Sattabongkot J, Long CA, Saul A. Transmission-blocking activity induced by malaria vaccine candidates Pfs25/Pvs25 is a direct and predictable function of antibody titer. Malaria J. (2007) 6:107. doi: 10.1186/1475-2875-6-107.
139. Chowdhury DR, Angov E, Kariuki T, Kumar N. A potent malaria transmission blocking vaccine based on codon harmonized full length pfs48/45 expressed in escherichia coli. PloS One. (2009) 4:e6352. doi: 10.1371/journal.pone.0006352.
140. Theisen M, Jore MM, Sauerwein R. Towards clinical development of a Pfs48/45-based transmission blocking malaria vaccine. Expert Rev Vaccines. (2017) 16:329–36. doi: 10.1080/14760584.2017.1276833
141. Liu Y, Zhang F, Liu J, Xiao X, Zhang S, Qin C, et al. Transmission-blocking antibodies against mosquito C-type lectins for dengue prevention. PloS Pathog. (2014) 10:e1003931. doi: 10.1371/journal.ppat.1003931.
142. Londono-Renteria B, Troupin A, Conway MJ, Vesely D, Ledizet M, Roundy CM, et al. Dengue virus infection of aedes aEgypti requires a putative cysteine rich venom protein. PloS Pathog. (2015) 11:e1005202. doi: 10.1371/journal.ppat.1005202.
143. Wollner Clayton J, Richner M, Hassert Mariah A, Pinto Amelia K, Brien James D, Richner Justin M. A dengue virus serotype 1 mRNA-LNP vaccine elicits protective immune responses. J Virol. (2021) 95:10.1128/jvi.02482–20. doi: 10.1128/JVI.02482-20
144. Zhang M, Sun J, Li M, Jin X. Modified mRNA-LNP Vaccines Confer Protection against Experimental DENV-2 Infection in Mice. Mol Ther - Methods Clin Dev. (2020) 18:702–12. doi: 10.1016/j.omtm.2020.07.013.
145. Hou J, Ye W, Chen J. Current development and challenges of tetravalent live-attenuated dengue vaccines. Front Immunol. (2022) 13:840104. doi: 10.3389/fimmu.2022.840104.
146. Bauer K, Esquilin IO, Cornier AS, Thomas SJ, Quintero Del Rio AI, Bertran-Pasarell J, et al. A phase II, randomized, safety and immunogenicity trial of a re-derived, live-attenuated dengue virus vaccine in healthy children and adults living in Puerto Rico. Am J Trop Med Hyg. (2015) 93:441–53. doi: 10.4269/ajtmh.14-0625.
147. Diaz C, Lin L, Martinez LJ, Eckels KH, Campos M, Jarman RG, et al. Phase I randomized study of a tetravalent dengue purified inactivated vaccine in healthy adults from Puerto Rico. Am J Trop Med Hyg. (2018) 98:1435–43. doi: 10.4269/ajtmh.17-0627.
148. Pintado Silva J, Fernandez-Sesma A. Challenges on the development of a dengue vaccine: a comprehensive review of the state of the art. J Gen Virol. (2023) 104(3):001831. doi: 10.1099/jgv.0.001831.
149. Oli AN, Obialor WO, Ifeanyichukwu MO, Odimegwu DC, Okoyeh JN, Emechebe GO, et al. Immunoinformatics and vaccine development: an overview. Immunotargets Ther. (2020) 9:13–30. doi: 10.2147/ITT.
150. Meng XY, Zhang HX, Mezei M, Cui M. Molecular docking: a powerful approach for structure-based drug discovery. Curr Comput Aided Drug Des. (2011) 7:146–57. doi: 10.2174/157340911795677602.
151. Krishnan GS, Joshi A, Akhtar N, Kaushik V. Immunoinformatics designed T cell multi epitope dengue peptide vaccine derived from non structural proteome. Microb Pathog. (2021) 150:104728. doi: 10.1016/j.micpath.2020.104728.
152. Mukhtar M, Wajeeha AW, Zaidi N, Bibi N. Engineering Modified mRNA-Based Vaccine against Dengue Virus Using Computational and Reverse Vaccinology Approaches. Int J Mol Sci. (2022) 23(22):13911. doi: 10.3390/ijms232213911.
153. Ali M, Pandey RK, Khatoon N, Narula A, Mishra A, Prajapati VK. Exploring dengue genome to construct a multi-epitope based subunit vaccine by utilizing immunoinformatics approach to battle against dengue infection. Sci Rep. (2017) 7:9232. doi: 10.1038/s41598-017-09199-w.
154. Fadaka AO, Sibuyi NRS, Martin DR, Goboza M, Klein A, Madiehe AM, et al. Immunoinformatics design of a novel epitope-based vaccine candidate against dengue virus. Sci Rep. (2021) 11:19707. doi: 10.1038/s41598-021-99227-7.
Keywords: dengue, dengue vaccine, vector control, live attenuated vaccine, inactivated vaccine, recombinant vaccine, viral vector vaccine, DNA vaccine
Citation: Akter R, Tasneem F, Das S, Soma MA, Georgakopoulos-Soares I, Juthi RT and Sazed SA (2024) Approaches of dengue control: vaccine strategies and future aspects. Front. Immunol. 15:1362780. doi: 10.3389/fimmu.2024.1362780
Received: 29 December 2023; Accepted: 08 February 2024;
Published: 29 February 2024.
Edited by:
Henry Puerta-Guardo, Universidad Autónoma de Yucatán, MexicoReviewed by:
Srinivasa Reddy Bonam, University of Texas Medical Branch at Galveston, United StatesZheng Liu, Virginia Commonwealth University, United States
Copyright © 2024 Akter, Tasneem, Das, Soma, Georgakopoulos-Soares, Juthi and Sazed. This is an open-access article distributed under the terms of the Creative Commons Attribution License (CC BY). The use, distribution or reproduction in other forums is permitted, provided the original author(s) and the copyright owner(s) are credited and that the original publication in this journal is cited, in accordance with accepted academic practice. No use, distribution or reproduction is permitted which does not comply with these terms.
*Correspondence: Saiful Arefeen Sazed, c3pzNzMwMkBwc3UuZWR1