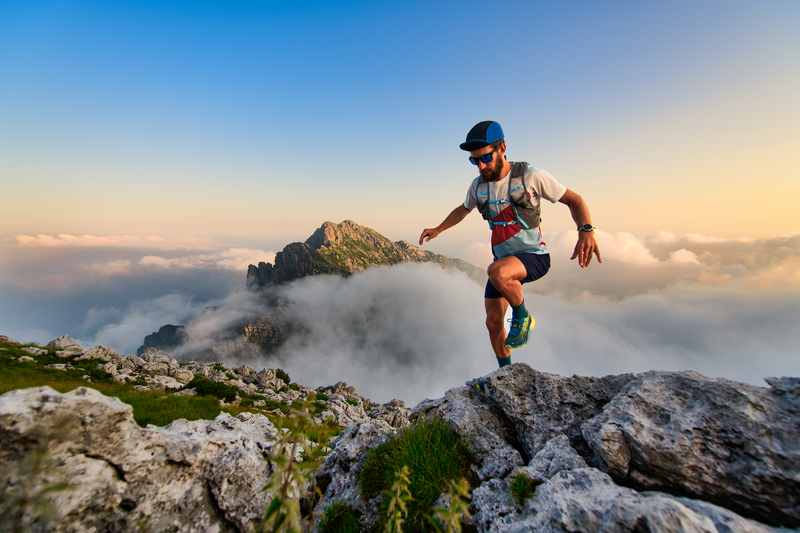
95% of researchers rate our articles as excellent or good
Learn more about the work of our research integrity team to safeguard the quality of each article we publish.
Find out more
REVIEW article
Front. Immunol. , 06 March 2024
Sec. Alloimmunity and Transplantation
Volume 15 - 2024 | https://doi.org/10.3389/fimmu.2024.1358153
This article is part of the Research Topic In-Vitro, In-Vivo, and Ex-Vivo Models of Ischemia-Reperfusion Injury in Lung Transplantation View all 8 articles
Primary graft dysfunction (PGD) is a common complication after lung transplantation. A plethora of contributing factors are known and assessment of donor lung function prior to organ retrieval is mandatory for determination of lung quality. Specialized centers increasingly perform ex vivo lung perfusion (EVLP) to further assess lung functionality and improve and extend lung preservation with the aim to increase lung utilization. EVLP can be performed following different protocols. The impact of the individual EVLP parameters on PGD development, organ function and postoperative outcome remains to be fully investigated. The variables relate to the engineering and function of the respective perfusion devices, such as the type of pump used, functional, like ventilation modes or physiological (e.g. perfusion solutions). This review reflects on the individual technical and fluid components relevant to EVLP and their respective impact on inflammatory response and outcome. We discuss key components of EVLP protocols and options for further improvement of EVLP in regard to PGD. This review offers an overview of available options for centers establishing an EVLP program and for researchers looking for ways to adapt existing protocols.
End-Stage Lung Disease (ESLD) often requires lung transplantation (LuTx) as a last resort for treatment. Despite the efforts to supply the demand, mortality on the waiting list remains a challenge (1). A possible countermeasure to this problem is the utilization of extended criteria donors (ECD). However, ECD lungs pose the risk of higher primary graft dysfunction (PGD). Extended criteria donor lungs have significantly higher early PGD 3 and lower intraoperative extracorporeal membrane oxygenation weaning rates in comparison to standard criteria donor lungs. However, survival and bronchiolitis obliterans syndrome rates do not differ (2, 3). To evaluate ECD lungs, pioneering centers established ex vivo lung perfusion (EVLP) as a means to assess organ quality. EVLP is a complex and multidimensional technology. The various individual specifications of the hardware, software and perfusate such as the type of pump, the ventilation modes or the composition of the perfusion solutions are evolving. While the core functions of EVLP have been well established, the evolution of this technology has only just started and a great many aspects remain to be advanced in order to eventually support stable multi-day long lung preservations suitable for organ regeneration and repair. Further to the immediate benefit for organ transplantation, EVLP may also serve as a research platform for primary or secondary lung cancer as described by Slama et al. (4) In order to create a meaningful platform for lung cancer research, EVLP might need to be prolonged to several days mimicking a physiological setting. First steps in this direction have been taken by Ali et al., who performed successful 3-day lung preservation utilizing a a cyclic normothermic ex vivo lung perfusion strategy. This strategy involved initial 6 hours of cold static storage at 4°C, followed by cold static storage at 10°C with two cycles of EVLP in-between (5). In liver perfusion models, extension of perfusion times allows for testing of new therapies ex vivo and thereby accelerate their development and eventual clinical use (6). This may add a valuable translational model system to the current armamentarium and help reduce animal testing.
At current, experimental, mostly porcine, setups report stable EVLP for up to 72 hours (5, 7). Clinical implementation of these prolonged protocols is still lacking. Previously described interventions for prolongation of stable EVLP include the optimization of the perfusate by adding nutritional factors and dextran, positioning maneuvers, negative pressure ventilation, perfusion pressure/volume or modifying the perfusion temperature and other (Figure 1) (8–12).
Figure 1 Overview of EVLP and PGD connections. EVLP, ex vivo lung perfusion, PGD, primary graft dysfunction.
Ischemia reperfusion injury (IRI) is a process induced by retrieval, storage and transplantation. In contrast to other solid organs, hypoxia is a reduced driving force amongst the mechanisms leading to injury since the lungs remain ventilated during the retrieval process and are cold stored in an inflated, but ischemic state. Hence tissue oxygenation is maintained to a certain extend. Nevertheless, the interruption of blood flow induces depolarizing mechanotransductional effects. This sparks production of reactive oxygen species (ROS) via the activation of NADPH oxidase and NO synthase eventually resulting in vasodilatation and angiogenesis (13). ROS can induce hypoxia-inducible factor 1α (HIF-1α) under normoxic conditions, which further promotes lung edema formation by inducing vascular endothelial growth factor (VEGF) (14–16). Mediated through this cascade, prolonged cold ischemia times leads to activation of necroptosis pathways and inflammatory cell infiltration (17). Nevertheless, literature is lacking data regarding anti-VEGF treatment during EVLP to reduce IRI.
Following reperfusion, oxidant production and complement activation is induced (18, 19). As a result, excess ROS production in the electron transport chain, as key factors in IRI, is prompted (20–22). With rising oxygen levels, also increased ROS production is triggered (18, 23). ROS cause oxidative stress and cell damage, death and inflammation through damage to nucleic acids, proteins and lipids. This damage activates apoptosis and necrosis and triggers an immune response through endogenous damage-associated molecular pattern molecules (23, 24). At the onset of apoptosis the redistribution of phosphatidylserine and loss of anticoagulant surface factors leads to increasing procoagulant tendencies in the endothelial cells (25). Hypoxia further causes a rise in plasminogen activator inhibitor-1 (PAI-1), which is associated with increased pulmonary fibrin deposition, intravascular fibrin and 125I-fibrinogen/fibrin. These mechanisms advance hypoxia-induced thrombosis and form a procoagulant and blood flow restricting milieu (26, 27).
Apoptosis can be induced via an extrinsic or an intrinsic pathway through caspase 8, or caspase 9. Extrinsic apoptosis is mediated through tumor necrosis factor alpha receptors and intrinsic apoptosis through intracellular mechanisms, such as oxidants or genotoxic effects (28). For intrinsic apoptosis Bcl-2 family proteins stabilize the outer membrane of mitochondria to keep cytochrome c in the cristae. Nevertheless, Bcl-2 proteins include proapoptotic proteins like Bax and Bac too. Bax and Bac lead to increased permeabilization of the outer membrane of the mitochondria. This starts a destabilizing cascade on the inner membrane of the mitochondria leading to a release of cytochrome c. If a specific threshold of cytochrome c is released, through damage to a large amount of mitochondria, apoptosis follows (29, 30). After binding to apoptotic protease-activating factor 1 apoptosomes are formed, activating procaspase 9 (31, 32). At the end of both induction pathways the executioner caspases 3 and 7 are activated (28). An overview of the extrinsic and intrinsic apoptosis pathway is shown in Figure 2.
Figure 2 Visualization of the extrinsic and intrinsic apoptosis pathway. APAF1, apoptotic peptidase activating factor 1; BAK, BCL2 antagonist/killer 1; BAX, BCL2 associated X; BCL-2, BCL2 apoptosis regulator; BCL-XL, B-cell lymphoma-extra large (encoded by the BCL2 like 1 gene); BH3, BCL2 homology region 3; BID, BH3 interacting domain death antagonist; FADD, Fas associated via death domain; FAS receptor, Fas cell surface death receptor; MCL 1, MCL1 apoptosis regulator; ROS, reactive oxygen species; SMAC, second mitochondria-derived activator of caspase; tBID, truncated BID; XIAP, X-linked inhibitor of apoptosis.
During hypoxia adenosine triphosphate (ATP) decreases and leads to accumulation of hypoxanthine (33). Fisher et al. report of a threshold of 7 mmHg or lower partial pressure of oxygen in the alveoli for this decrease in ATP to take place (34). Independently from ischemia, xanthine dehydrogenase is distributed in many tissues such as the lungs and liver. During ischemia xanthine dehydrogenase is increasingly converted to xanthine oxidase. When the partial pressure of oxygen rises again after reperfusion/reventilation, xanthine oxidase oxidizes the accumulated hypoxanthine and results in a burst of superoxide and hydrogen peroxide (35–37). Accompanying hypoxia seems to increase the activity of xanthine oxidase, while hyperoxia inversely influences activity levels (38). A rat model by Maia et al. proposes that xanthine dehydrogenase might be an even more efficient producer of superoxide when (hypo-)xanthine oxidation is induced (39). Additionally, interferon gamma, upregulated via interleukin-12 and interleukin 18, induces xanthine dehydrogenase and oxidase activity (40, 41).
A schematic outline of IRI is shown in Figure 3. PGD occurs as a result of IRI and negatively impacts the outcome. Even if PGD is treated successfully, patients are at a higher risk for developing Bronchiolitis obliterans syndrome (BOS) and face worse long-term lung function (42). Further factors influencing the development of IRI are described in the sections below.
Since its first clinical use in 2000 in Lund (Sweden), various commercially available EVLP systems have been developed (43). Most of the systems apply similar basic principles (Figure 4) and protocols described as the Lund, Toronto or the Organ Care System (OCS™) protocol. Currently commercially available systems include, but are not limited to, the OCS (Transmedics, Andover, MA, USA), XVIVO Perfusion System (XPS™,XVIVO Perfusion AB, Göteborg, Sweden) and TorEx Lung Perfusion System (Traferox,Technologies Inc., Mississauga, Canada).
Figure 5 Ferroptosis Signaling Pathway. Cys, cysteine; DMT1, divalent metal transporter 1; FE, iron; GCL, glutamate-cysteine ligase; Gln, glutamine; GLS, glutaminase; Glu, glutamate; Gly, glycine; GPX4, glutathione peroxidase 4; GSH, glutathione; GSS, glutathione synthetase; PUFA, polyunsaturated fatty acid; SLC1A5, solute carrier family 1 member 5; STEAP3, six-transmembrane epithelial antigen of prostate.
Systems vary in their ventilation and perfusion setting options and their respective recommended protocols. The XVIVO XPS and TorEx both use the Toronto protocol while for the OCS a separate protocol is recommended. The OCS system operates with an open circuit, meaning that no atrial cuff is used, and uses a pulsatile pump in comparison to a continuous one. An advantage of the XVIVO XPS represents its organ chamber design, which allows for easy radiographic imaging. Publicly available information for the TorEx Lung Perfusion System is scarce except that it is based on the Toronto protocol and is considered a plug-and-play system, which simplifies the setup.
These protocols vary in perfusate, perfusion parameters and ventilation. An overview can be seen in Table 1.
There are two types of perfusion solutions for EVLP: cellular and acellular. The Toronto protocol uses an acellular perfusate, while the Lund and OCS protocol utilize RBCs (47). The Lund and Toronto protocol both use STEEN Solution™ as a basis (XVIVO Perfusion, Goteborg, Sweden), while the Lund protocol employs red blood cell concentrates. The OCS is approved to be used with the OCS™ Lung Solution (Transmedics, Andover, MA, USA) with added red blood cell concentrates (44–46). The outcome of the respective studies comparing different perfusion solutions remains inconclusive (48–50).
Perfusion solutions may have a significant impact on ROS production making them a viable point for optimization. A promising option is to reduce cell-damage by introducing iron chelators with the perfusion solution, such as Custodiol-N or Custodiol-MP (Dr. F. Köhler Chemie, Bensheim, Germany). This has proven to significantly reduce ROS production and improve functional parameters (18, 51, 52). Ferroptosis leads to iron-dependent programmed cell death through lipid peroxidation (Figure 5). In ferroptosis the outer membrane of the mitochondria fragments while the cristae disappear. The pathway starts with iron entering the cell through transferrin receptors. After disintegration from endosomes iron is stored in the labile iron pool. An overload of the labile iron pool together with hydrogen peroxide leads to ROS production through the Fenton reaction. ROS is responsible for lipid peroxidation, because of reacting with polyunsaturated fatty acids of lipid membranes, which ultimately leads to ferroptosis (53, 54). An inhibitory pathway of ferroptosis is initiated with cystine entering the cell through cystine/glutamate reverse transporters. Afterwards it is reduced to cysteine. Glutamate-cysteine ligase and glutathione synthetase synthesize glutathione with the use of cysteine, glutamic acid and glycine. Glutathione then acts as a cofactor for glutathione peroxidase 4, which reduces lipid peroxidation and suppresses ferroptosis (54–56). These ferroptosis pathways can be targeted with iron chelators (e.g. deferoxamine) through a reduction of the iron load (53).
Figure 6 Visualization of atelectrauma through repeated recruitment and derecruitement of functional lung airway units.
RBC-based perfusates offer the advantage of assessing and perfusing the lungs under more physiological conditions. The addition of erythrocytes to the perfusate leads to better oxygen binding capacity and a more precise evaluation of the oxygenation capability. Acellular perfusion might lead to a flawed assessment of oxygenation capability using blood gas analysis as shunting during ex vivo perfusion might have reduced effects on the measured partial pressure of oxygen as a result of linear curve of oxygen content and partial pressure of oxygen (in comparison to the sigmoidal relationship for perfusates with hemoglobin) (57–59). This is of utmost importance, since blood gas analysis is a key determining factor during EVLP for predicting the outcome. Conversely, RBC based perfusates hold the risk of hemolysis during EVLP. Because of the mechanical stress towards RBCs through pumping of the perfusate, red blood cells may rupture and become dysfunctional or irreversibly stiffened. Thus the increased physical resistance may lead to consecutive mechanical lung injury (44, 60–62).
Further to functional aspects, RBC-based perfusion requires more personnel because of subsequently rising demand for blood concentrates. This also further aggravates costs related to EVLP and might deter from using EVLP with a RBC-based perfusate. Additionally, blood concentrates might be scarce or not available at all leading to a possible ethical dilemma for research protocols using cellular perfusates. For clinical settings alternative perfusion solutions should be in place to avoid unsuccessful EVLP due to constrained blood concentrate availability.
Recent data by Olbertz et al. suggest that using Custodiol-N with added glucose monohydrate, dextran 40 and albumin helps stabilizing lung function through a higher oxygen capacity and lower wet-to-dry ratio in comparison to STEEN Solution™. Moreover, Olbertz et al. report a lower peak airway pressures when using the above-mentioned perfusate (51). The same group also showed a significantly better oxygenation capacity, lower lactate dehydrogenase activity and lower lactate concentrations when using Custodiol-MP in a porcine EVLP model (52) (Table 2).
Table 2 Composition of modified Custodiol-N, Custodiol-MP base perfusion solution, Custodiol-MP final perfusion solution and Steen Solution™.
Huang et al. proposed a newly designed perfusate based on Dulbecco’s Modified Eagle Medium, containing essential and non-essential amino acids and specific vitamins, in combination with 5 g/L dextran 40 and 7% albumin. This perfusate (“D05D7A”) showed improved cell confluence, reduced apoptosis and better migration in a cell culture based study when compared to Steen Solution. D05D7A also lead to higher glutathione production, which is a key factor in inhibition of ferroptosis. Interestingly, the low apoptosis rate in the D05D7A group could be sustained for continuous 48h, which provides a possibility for further stabilizing prolonged EVLP protocols (64). A trial of this solution, outside of culture based protocols, is still missing.
The use of cytokine adsorption filters during EVLP might further mitigate pro-inflammatory effects of cytokines. Various studies have shown the positive effects of cytokine filtration on pulmonary edema development, microscopic lung injury, inflammatory response, peak airway pressure and decreased pro-inflammatory cytokines (65–68). Boffini et al. even report of reduced in-hospital mortality and 1-year death rate in a cytokine adsorption cohort when retrospectively comparing their EVLP patients. Yet it has to be noted, that the cohort without cytokine adsorption was in the beginning of their EVLP program and required cardiopulmonary bypass during transplantation more often (68).
In conclusion, the optimal perfusate remains unclear. Considering above-mentioned aspects, the use of acellular perfusates in prolonged EVLP might be advantageous. This might avoid detrimental effects of hemolysis and mechanical lung injury, which cumulate over time during EVLP. For shorter perfusion times and routine clinical use, RBC based perfusates may offer an advantage for evaluation of the donor lungs.
EVLP protocols differ with respect to flow rate and flow pattern. Favorable results have been shown for certain settings. Flow rates in clinical EVLP protocols range from fixed values (2 - 2.5 l/min) to 40 - 100% of cardiac output (47). Perfusion volume per minute is highest in the Lund protocol with a flow of 100% of cardiac output. The Toronto protocol applies 40% of cardiac output. Both protocols initiate EVLP with lower than targeted flow rates and gradually increase flow while temperature increases to 37°C (12, 69, 70). The OCS protocol predetermines the flow rate at 2 and 2.5 l/min and also gradually increases flow from start of EVLP until reaching the target temperature of 37°C (70–72).
Beller et al. suggest a low flow protocol with only 20% of predicted cardiac output. They have shown significantly higher pulmonary pO2, compliance, reduced lung wet-to-dry ratio and lower IL-1β (73). These low flow protocols were already successfully used in a rat and in a porcine model (73, 74). The improved results may be attributed to the reduced vascular stress and subsequent inflammation and fluid permeability (47, 73, 74).
The pulmonary arterial pressure (PAP) should not exceed 20 mm Hg perfusion pressure in both the Lund and OCS protocol, and 15 mmHg PAP in the Toronto protocol (11, 44, 47, 69, 75, 76). Upper limits for perfusion pressure are important for clinical evaluation of donor lungs since a higher PAP corresponds with impaired lung function and possible edema formation. Various study groups examined flow mechanics on EVLP and confirmed this finding (73, 77, 78).
Management of the left atrium further influences the vascular behavior and flow dynamics. The Lund and OCS protocol both use an open system, while the Toronto protocol attaches a cuff to the left atrium. With this cuff, the left atrial pressure is kept at about 3-5 mm Hg. Linacre et al. show that an open circuit in EVLP results in a lower success rate in prolonged EVLP because of worse oxygenation, decreased lung compliance, increased vascular resistance and peak inspiratory pressure. Consistent with these findings, the wet-to-dry ratio and lung edema score was worse in the open group. Linacre et al. hypothesize that this difference might be due to the missing venous afterload pressure with a subsequent change in lung perfusion zones and “a cyclical open-close phenomenon at the capillary level with ventilation exacerbated at low after-load pressures and leading to endothelial cell injury, vascular dysfunction, alteration of permeability and edema formation” (78). This may amplify the proinflammatory and procoagulant hypoxia-induced changes through translocation of phosphatidylserine, stimulating activated platelet adhesion (27). Phosphatidylserine offers a possibility for targeted therapy. Diannexin is a homodimer of Annexin V, which binds to phosphatidylserine and inhibits prothrombinase complexes and secretory phospholipase A2. Consequently, hydrolyzation of cells’ phospholipids is inhibited and reduces coagulation (79). Data from a murine transplant study showed lower wet-to-dry ratio, higher partial pressure of oxygen, lower alveolar fibrin deposition score and reduced caspase-cleaved cytokeratin 18, which acts as a marker for apoptosis (27). Clinical data of its usage as a prophylactic IRI therapy in EVLP is still lacking.
Furthermore, flow characteristics can be divided in continuous and pulsatile flow. Most EVLP devices use continuous flow, while the OCS Lung (Transmedics, Andover, MA, USA) applies pulsatile flow generated by a piston pump (47, 80). Even though effects of pulsatile flow on functional outcome parameters such as inflammation or pulmonary edema are still unclear, it might provide a more physiological setting for evaluation (80, 81).
During EVLP, donor lungs are mechanically ventilated to assess and preserve their function. Ventilator settings during EVLP are similar to conventional ventilation. However, mechanical ventilation (MVe) with positive pressure bares the risk of ventilator induced lung injury (VILI). VILI may be induced in the donor and prior to organ retrieval and and/or during EVLP MVe. The driving pressure during EVLP MVe is a result of various factors, which are dependent on the lungs and surrounding tissue, but also influenced by ventilator settings, including: flow at the tracheal opening, lung resistance (LE), lung elastance, and lung volume, which is relative to the functional residual capacity (82, 83). The most important and modifiable parameter is LE.
Volutrauma and atelectrauma are the two main stressors triggering LE and progression of VILI. Barotrauma does not seem to be relevant for the development of VILI. Data generated by Dreyfuss et al. suggest that the increased inflation volume through high pressures is responsible for tissue damage. Rat lungs ventilated with high tidal volume ventilation showed significantly increased edema development while high pressure ventilation did not (84). In-situ, damage through high driving pressure may be reduced as the thoracic cavity provides some natural resistance against massive distension of the lungs. With EVLP, this natural resistance is completely lost. Therefore, optimal settings of EVLP MVe need to focus on tidal volume and associated driving pressure.
Atelectrauma is the result of multiple opening and closing events of lung airway units, which are caused by recruitment of these units during inspiration and derecruitement during expiration. Emerging shear forces injure the epithelium and lead to epithelial necrosis, as is depicted in Figure 6.
To mitigate the risk of atelectrauma optimal positive end-expiratory pressure (PEEP) settings are warranted in order to reduce the number of recruitment cycles and keep lung units open (83, 85, 86). Therefore, all three clinical EVLP protocols utilize a volume-controlled ventilation mode with settings between 6 and 8 ml/kg donor weight. PEEP is kept at 5cmH2O in all protocols (47). With these settings, the dynamics of the driving pressure to achieve target volumes have merit as functional parameters for lung assessment.
Recent data suggest, that flow-controlled ventilation (FCV) may further reduce VILI. In flow-controlled ventilation, the expiration is linear, the inspiration/expiration-ratio equal and independent of recoil forces of the lung. Through this ventilation, the amount of dissipated energy during ventilation cycles is minimized and lung tissue damage prevented (87, 88). Moreover, FCV improves oxygenation and carbon dioxide elimination during MVe (89). Goebel et al. reported increased lung compliance, lower PEEP and lower wet-to-dry ratios in a porcine model (88). These results are reproducible in EVLP. Ordies et al. show that in a porcine EVLP model, FCV provides significantly better oxygenation and improved lung compliance in a larger area of ventilated lung tissue. Despite these superior functional results, total lung injury did not differ in comparison to volume controlled ventilation (90).
Another promising method is negative pressure ventilation (NPV) in which inspiration is modelled by placing the donor lungs in an air-tight chamber and applying negative pressure. This method does not rely on the force of positive pressure ventilation and might therefore reduce the risks of physical damage to the lung. Supporting evidence for this concept was generated in an EVLP model. Better compliance, reduced pulmonary vascular resistance and reduced weight gain during EVLP were found, while oxygenation remained unchanged (91). NPV is associated with reduced secretion of proinflammatory cytokines, such as tumor necrosis factor alpha, interleukin-6, and interleukin-8. Noteworthy, Aboelnazar et al. reported weight reduction and lower lung injury through NPV (92). Buchko et al. tested NPV in a clinical trial with 12 LuTx patients. They have established feasibility and demonstrated good outcomes with a PGD grade 0 rate at 72 hours of 83% (grade 1: 0%, grade 2: 17%, grade 3: 0%) (93).
The fraction of oxygen (FiO2) is set at 21% for the Toronto and OCS protocol and at 50% for the Lund protocol (47, 70). Various randomized trials are examining higher vs. lower FiO2 in ventilated patients on intensive care units displaying a trend towards an increase in mortality and serious adverse event, but the effects of higher FiO2 in EVLP remain unknown (94). To our knowledge, no studies addressing the influence of FiO2 in EVLP are available. In theory, higher FiO2 may lead to hyperoxemia and hyperoxia, which results in increased ROS (hydroxyl radicals and superoxide ions) production. This effect is evident in EVLP, since IRI is induced – albeit in a different form. Furthermore, higher FiO2 causes an alveolar nitrogen washout, which is subsequently responsible for atelectasis through the dislocation of oxygen from the alveoli to capillaries (23). Further studies are needed to assess the relevance of different FiO2 strategies in EVLP.
Respiratory rate is set at seven breaths per minute (bpm) in the Toronto protocol, 10 bpm in the OCS protocol and 10-15 bpm in the Lund protocol (47, 70). The standard MVe respiratory rate is 12-16 bpm and dynamically adjusted accordingly to achieve eucapnia. The respiratory rate may be increased to avoid hypercapnia or to compensate acidosis. Each increase of the respiratory rate, however, poses the risk of dynamic hyperinflation and volutrauma in donor lungs (95, 96). Specific data regarding the respiratory rate during EVLP are not available, but an extrapolation from in-vivo data may help. In an analysis of 102.632 cases, Santer et al. report that an increased respiratory rate (median 8 vs. 15 bpm) was associated with a significantly higher rate of postoperative respiratory complications and postoperative healthcare utilization (97). All three protocols use respiratory settings in or below the physiological range, but a direct comparison has not been performed. An overview of the influence of different ventilation modification on the lung during EVLP is visualized in Figure 7.
Figure 7 Aspects of the influence of ventilation during EVLP on the lungs. EVLP, ex vivo lung perfusion; FCV, flow-controlled ventilation; FiO2, fraction of inspired oxygen; ICU, intensive care unit; IL-6, interleukin 6; IL-8, interleukin 8; NPV, negative pressure ventilation; PEEP, positive end expiratory pressure; TNF-α, tumor necrosis factor-α; ROS, reactive oxygen species.
As primary graft dysfunction (PGD) is associated with elevated levels of interstitial fluid retention, gravitational forces should be considered. Conceptually, lungs could be treated as healthy lungs or rather as borderline intensive care unit patients. The typical EVLP setup places the donor lungs in a supine position. For treatment of acute respiratory distress syndrome (ARDS) and acute lung injury, prone positioning has shown to reduce mortality and positively impact oxygenation. Complete avoidance of edema formation during EVLP or ARDS seems difficult, but optimal management may help to reduce its negative impact. When edema is forming, the pressure of the superimposed lung parenchyma leads to a collapse of the surrounding parenchyma and impact organ function. Placing the lungs in a prone position helps to recover the dorsal regions through changing the direction of gravitational forces and respective hydrostatic pressure (98, 99). Ordies et al. demonstrated, that prone EVLP leads to a more homogenous distribution of pulmonary edema (100). The dorsal regions possess a higher volume of lung parenchyma, which may explain this observation.
Findings in EVLP confirmed this effect. Prone position EVLP improves partial pressure of oxygen/fraction of inspired oxygen ratio (P/F) and compliance. Interestingly, prone position reduces the amount of inflammatory cytokine expression (interleukin-1β, interleukin-8, tissue tumor necrosis factor alpha) in the lower lobes, while the interleukin-10, which has an anti-inflammatory effect through inhibition of macrophages or tissue tumor necrosis factor alpha among others, did not change significantly (100–102). A cytokine profile triggering increased granulocyte recruitment as a result of pro-inflammatory cytokines and reduced inhibition through interleukin-10, might be an important factor for PGD development. Neutrophils are capable of releasing ROS and neutrophil extracellular traps (NET). Through increased ROS production, NETosis increases and leads to further aggravation of inflammation and edema (103–105). The impact on lung injury and associated PGD was confirmed in a murine PGD model. In this study, NET formation and PGD development could be reduced with administration of acetylsalicylic acid and disrupted with direct intrabronchial administration of DNaseI (106).
Studies with human lungs demonstrate the benefit of prone position during EVLP. Niikawa et al. showed that three out of five human lungs, which were rejected for clinical use, could have been suitable for transplant after prone EVLP while zero out of five lungs placed in the supine position fulfilled the criteria for transplantation (102). Niikawa et al. also demonstrated the feasibility of prone EVLP in a case report, in which one of two human prone EVLP lungs, both with reduced lung edema after EVLP, was successfully transplanted (107).
During EVLP, donor lungs may be susceptible for colonization of microbial organisms. Contamination during organ retrieval is not uncommon and colonization during EVLP with massive germ transmission during transplantation represents a realistic threat. Heavy immunosuppression upon transplantation further aggravates this situation and makes is potentially life threatening. Aguilar-Guisado et al. reported an incidence of 72 episodes of post-transplant pneumonia per 100 LuTx. Bacterial infections were the most common cause (82.7%) (108). Colonization in the donor was responsible for 7.6% recipient infections (109). Such infections negatively affect short- and long-term outcome in LuTx patients (110). Andreasson et al. demonstrated that antimicrobial treatment with a single-dose of Meropenem (500mg) at the start of EVLP significantly reduces bacterial load after EVLP. Moreover, the yeast load increased during EVLP without anti-fungal treatment but decreased if Amphotericin B (50mg) was administered at the start of perfusion (110). Data by Nakajima et al. confirmed the importance of antimicrobial treatment during EVLP and the therapeutic possibilities. EVLP lungs, which were treated with Ciprofloxacin or Azithromycin, Vancomycin and Meropenem, showed significantly reduced bacterial counts in BAL, improved pulmonary oxygenation better compliance and reduced vascular resistance (111). These results also provide a cornerstone for EVLP as a therapeutic platform for reconditioning donor lungs with infections for transplantation, which would otherwise be rejected.
Because it is not possible to receive bacterial cultures for targeted anti-microbial therapy in time, treatment mostly remains empirical. Established treatment regimens show promising results, but further refinement of the anti-microbial drug therapy is warranted. Prolonged EVLP requires broad-spectrum anti-microbial treatment to prevent microbial infections and inflammation.
The lung microbiome is thought to play a role in PGD development, acute rejection, bronchiolitis obliterans syndrome and restrictive allograft syndrome (112, 113). Pseudomonas species, especially Pseudomonas aeruginosa (which is sensitive to Meropenem), are related to the development of BOS and inferior outcome (112, 114). Of note, the addition of Azithromycin to the perfusion solution improved survival in early stage BOS treatment and hence should be considered irrespective of its antimicrobial effect. This protective effect has been related to the effect on neutrophilic airway inflammation via decreased lipopolysaccharide stimulated release of Interleukin-8 and granulocyte macrophage colony stimulating factor (115, 116).
Apart from antibiotics application of high-dose (>160 ppm) nitric oxide (NO) may be used to treat infections caused by bacteria and viruses (117, 118). Although low-dose NO is already clinically used for treatment of ARDS its potential side effects such as hospital/ventilator-acquired pneumonia, acute kidney injury and increase in methemoglobin levels impairs prolonged and/or high-dose NO therapy (118–123). EVLP in combination with an acellular perfusate can overcome this obstacle and allows for continuous high-dose NO treatment. Michaelsen et al. showed the feasibility of high-dose inhaled NO for 12 hours in a porcine EVLP model and reported no differences in vascular resistance, static and dynamic compliance, graft oxygenation, peak airway pressure, levels of pro-inflammatory cytokines or edema formation. Methemoglobin stayed in a safe range during acellular EVLP (118). Although regimens with intermittent, in contrast to continuous, high-dose inhaled NO have been successfully clinically tested, they failed to achieve the same results as in-vitro studies (117).
EVLP is used for clinical and research purposes. It has become a routine procedure in specialized centers. EVLP plays an important role in the assessment of donor lungs and may ameliorate PGD. A plethora of mechanisms contribute to the effect of EVLP such as reduction of interstitial fluid retention and improvement of function.
Future studies assessing physiological lung assessment and determination of the susceptibility for PGD, might consider using pulsatile perfusion with a cellular perfusate at a flow rate similar to cardiac output and an NPV of 21% FiO2 as suitable settings. FiO2 should not be set to higher-than-normal in order to avoid a possible increase in ROS production, which in turn advances IRI (23). The commercially available perfusion systems and the established protocols have their distinct features while a head-to-head comparison is lacking.
Current trends aim at prolonging EVLP, minimizing PGD rates, and reconditioning of donor organs. For these goals to be achieved, a better understanding of edema formation during EVLP and PGD development after LuTx is key. An important goal in this context is the minimization of ROS and pro-inflammatory cytokine production. This can be achieved by altering the perfusate composition, e.g. through adding iron chelators, acetylsalicylic acid or DNaseI (18, 51, 52, 106). A low flow protocol may also contribute to reduce the wet-to-dry ratio and pro-inflammatory cytokine expression (73, 74). MVe settings impact PGD development, most likely through volu- and atelectrauma. FCV improved oxygenation, lung compliance and wet-to-dry ratio (88–90). NPV reduces secretion of pro-inflammatory cytokines and PGD rates in clinical studies (92, 93).
EVLP can be improved by placing the donor lungs in prone position. Prone positioning reduces pro-inflammatory cytokine expression, protects against PGD and improves P/F and compliance (100–106).
Nevertheless, most of these findings lack clinical implementation and testing, apart from porcine or murine models. As a result available protocols remained greatly unchanged over the last period.
No consensus on the EVLP setup exists and different indications might call for different settings. Although ex vivo perfusion of organs is a clinical reality, many questions remain unanswered. Further prolongation of perfusion times without diminishing organ quality is the immediate goal.
FP: Conceptualization, Data curation, Formal analysis, Investigation, Methodology, Project administration, Resources, Visualization, Writing – original draft, Writing – review & editing. JD: Investigation, Writing – original draft, Writing – review & editing. CK: Investigation, Writing – original draft, Writing – review & editing. AP: Investigation, Writing – original draft, Writing – review & editing. TH: Investigation, Writing – original draft, Writing – review & editing. DW: Investigation, Writing – original draft, Writing – review & editing. FA: Investigation, Writing – original draft, Writing – review & editing, Conceptualization, Methodology, Project administration, Supervision. SS: Conceptualization, Investigation, Methodology, Project administration, Supervision, Writing – original draft, Writing – review & editing, Resources.
The author(s) declare that no financial support was received for the research, authorship, and/or publication of this article.
Figure 1 created with BioRender.com. Figure 2 adapted from “Apoptosis Extrinsic and Intrinsic Pathways”, by BioRender.com (2023). Retrieved from https://app.biorender.com/biorender-templates Figure 4 created with BioRender.com. Figure 5 adapted from “Ferroptosis Signaling Pathway”, by BioRender.com (2023). Retrieved from https://app.biorender.com/biorender-templates. Figure 7 created with BioRender.com.
The authors declare that the research was conducted in the absence of any commercial or financial relationships that could be construed as a potential conflict of interest.
All claims expressed in this article are solely those of the authors and do not necessarily represent those of their affiliated organizations, or those of the publisher, the editors and the reviewers. Any product that may be evaluated in this article, or claim that may be made by its manufacturer, is not guaranteed or endorsed by the publisher.
1. Valapour M, Lehr CJ, Skeans MA, Smith JM, Miller E, Goff R, et al. OPTN/SRTR 2019 annual data report: lung. Am J Transplant. (2021) 21 Suppl 2:441–520. doi: 10.1111/ajt.16495
2. Somers J, Ruttens D, Verleden SE, Cox B, Stanzi A, Vandermeulen E, et al. A decade of extended-criteria lung donors in a single center: was it justified? Transpl Int. (2015) 28:170–9. doi: 10.1111/tri.2015.28.issue-2
3. Suh JW, Lee JG, Park MS, Kim SY, Jeong SJ, Paik HC. Impact of extended-criteria donor lungs according to preoperative recipient status and age in lung transplantation. Korean J Transplant. (2020) 34:185–92. doi: 10.4285/kjt.2020.34.3.185
4. Slama A, Raber C, Hedderich C, Stockhammer P, Hegedüs B, Koch A, et al. Implementation of an experimental isolated lung perfusion model on surgically resected human lobes. Sci Rep. (2019) 9:12193. doi: 10.1038/s41598-019-48719-8
5. Ali A, Nykanen AI, Beroncal E, Brambate E, Mariscal A, Michaelsen V, et al. Successful 3-day lung preservation using a cyclic normothermic ex vivo lung perfusion strategy. EBioMedicine. (2022) 83:104210. doi: 10.1016/j.ebiom.2022.104210
6. Cardini B, Oberhuber R, Fodor M, Hautz T, Margreiter C, Resch T, et al. Clinical implementation of prolonged liver preservation and monitoring through normothermic machine perfusion in liver transplantation. Transplantation. (2020) 104:1917–28. doi: 10.1097/TP.0000000000003296
7. Spratt JR, Mattison LM, Kerns NK, Huddleston SJ, Meyer L, Iles TL, et al. Prolonged extracorporeal preservation and evaluation of human lungs with portable normothermic ex vivo perfusion. Clin Transplant. (2020) 34:e13801. doi: 10.1111/ctr.13801
8. Takahashi M, Andrew Cheung HY, Watanabe T, Zamel R, Cypel M, Liu M, et al. Strategies to prolong homeostasis of ex vivo perfused lungs. J Thorac Cardiovasc Surg. (2021) 161:1963–73. doi: 10.1016/j.jtcvs.2020.07.104
9. Buchko MT, Stewart CJ, Hatami S, Himmat S, Freed DH, Nagendran J. Total parenteral nutrition in ex vivo lung perfusion: Addressing metabolism improves both inflammation and oxygenation. Am J Transplant. (2019) 19:3390–7. doi: 10.1111/ajt.15572
10. Sommer W, Salman J, Avsar M, Hoeffler K, Jansson K, Siemeni TN, et al. Prediction of transplant outcome after 24-hour ex vivo lung perfusion using the Organ Care System in a porcine lung transplantation model. Am J Transplant. (2019) 19:345–55. doi: 10.1111/ajt.15075
11. Loor G, Howard BT, Spratt JR, Mattison LM, Panoskaltsis-Mortari A, Brown RZ, et al. Prolonged EVLP using OCS lung: cellular and acellular perfusates. Transplantation. (2017) 101:2303–11. doi: 10.1097/TP.0000000000001616
12. Watanabe T, Cypel M, Keshavjee S. Ex vivo lung perfusion. J Thorac Dis. (2021) 13:6602–17. doi: 10.21037/jtd
13. Chatterjee S, Chapman KE, Fisher AB. Lung ischemia: a model for endothelial mechanotransduction. Cell Biochem Biophys. (2008) 52:125–38. doi: 10.1007/s12013-008-9030-7
14. Tao J-Q, Sorokina EM, Vazquez Medina JP, Mishra MK, Yamada Y, Satalin J, et al. Onset of inflammation with ischemia: implications for donor lung preservation and transplant survival. Am J Transplant. (2016) 16:2598–611. doi: 10.1111/ajt.13794
15. Paulus P, Holfeld J, Scheller B, Zacharowski K, Reissig C, Tybl E, et al. VEGF-A blockade reduces reperfusion edema but favors arterial thromboembolism in a rat model of orthotopic lung transplantation. Transplantation. (2014) 97:908–16. doi: 10.1097/TP.0000000000000056
16. Paulus P, Ockelmann P, Tacke S, Karnowski N, Ellinghaus P, Scheller B, et al. Deguelin attenuates reperfusion injury and improves outcome after orthotopic lung transplantation in the rat. PloS One. (2012) 7:e39265. doi: 10.1371/journal.pone.0039265
17. Wang X, O’Brien ME, Yu J, Xu C, Zhang Q, Lu S, et al. Prolonged cold ischemia induces necroptotic cell death in ischemia-reperfusion injury and contributes to primary graft dysfunction after lung transplantation. Am J Respir Cell Mol Biol. (2019) 61:244–56. doi: 10.1165/rcmb.2018-0207OC
18. Pak O, Sydykov A, Kosanovic D, Schermuly RT, Dietrich A, Schröder K, et al. Lung ischaemia-reperfusion injury: the role of reactive oxygen species. Adv Exp Med Biol. (2017) 967:195–225. doi: 10.1007/978-3-319-63245-2_12
19. Girn HRS, Ahilathirunayagam S, Mavor AID, Homer-Vanniasinkam S. Reperfusion syndrome: cellular mechanisms of microvascular dysfunction and potential therapeutic strategies. Vasc Endovascular Surg. (2007) 41:277–93. doi: 10.1177/1538574407304510
20. Rauen U, Groot H d. Mammalian cell injury induced by hypothermia- the emerging role for reactive oxygen species. Biol Chem. (2002) 383:477–88. doi: 10.1515/BC.2002.050
21. Ovechkin AV, Lominadze D, Sedoris KC, Robinson TW, Tyagi SC, Roberts AM. Lung ischemia-reperfusion injury: implications of oxidative stress and platelet-arteriolar wall interactions. Arch Physiol Biochem. (2007) 113:1–12. doi: 10.1080/13813450601118976
22. Weyker PD, Webb CAJ, Kiamanesh D, Flynn BC. Lung ischemia reperfusion injury: a bench-to-bedside review. Semin Cardiothorac Vasc Anesth. (2013) 17:28–43. doi: 10.1177/1089253212458329
23. Allardet-Servent J, Sicard G, Metz V, Chiche L. Benefits and risks of oxygen therapy during acute medical illness: Just a matter of dose! Rev Med Interne. (2019) 40:670–6. doi: 10.1016/j.revmed.2019.04.003
24. Helmerhorst HJF, Schultz MJ, van der Voort PHJ, Jonge E d, van Westerloo DJ. Bench-to-bedside review: the effects of hyperoxia during critical illness. Crit Care. (2015) 19:284. doi: 10.1186/s13054-015-0996-4
25. Bombeli T, Karsan A, Tait JF, Harlan JM. Apoptotic vascular endothelial cells become procoagulant. Blood. (1997) 89:2429–42. doi: 10.1182/blood.V89.7.2429
26. Pinsky DJ, Liao H, Lawson CA, Yan SF, Chen J, Carmeliet P, et al. Coordinated induction of plasminogen activator inhibitor-1 (PAI-1) and inhibition of plasminogen activator gene expression by hypoxia promotes pulmonary vascular fibrin deposition. J Clin Invest. (1998) 102:919–28. doi: 10.1172/JCI307
27. Hashimoto K, Kim H, Oishi H, Chen M, Iskender I, Sakamoto J, et al. Annexin V homodimer protects against ischemia reperfusion-induced acute lung injury in lung transplantation. J Thorac Cardiovasc Surg. (2016) 151:861–9. doi: 10.1016/j.jtcvs.2015.10.112
28. Henson PM, Tuder RM. Apoptosis in the lung: induction, clearance and detection. Am J Physiol Lung Cell Mol Physiol. (2008) 294:L601–11. doi: 10.1152/ajplung.00320.2007
29. Delivani P, Martin SJ. Mitochondrial membrane remodeling in apoptosis: an inside story. Cell Death Differ. (2006) 13:2007–10. doi: 10.1038/sj.cdd.4402049
30. Delivani P, Adrain C, Taylor RC, Duriez PJ, Martin SJ. Role for CED-9 and Egl-1 as regulators of mitochondrial fission and fusion dynamics. Mol Cell. (2006) 21:761–73. doi: 10.1016/j.molcel.2006.01.034
31. Chandra D, Bratton SB, Person MD, Tian Y, Martin AG, Ayres M, et al. Intracellular nucleotides act as critical prosurvival factors by binding to cytochrome C and inhibiting apoptosome. Cell. (2006) 125:1333–46. doi: 10.1016/j.cell.2006.05.026
32. Cain K, Bratton SB, Langlais C, Walker G, Brown DG, Sun XM, et al. Apaf-1 oligomerizes into biologically active approximately 700-kDa and inactive approximately 1.4-MDa apoptosome complexes. J Biol Chem. (2000) 275:6067–70. doi: 10.1074/jbc.275.9.6067
33. Perrot M d, Liu M, Waddell TK, Keshavjee S. Ischemia-reperfusion-induced lung injury. Am J Respir Crit Care Med. (2003) 167:490–511. doi: 10.1164/rccm.200207-670SO
34. Fisher AB, Dodia C. Lung as a model for evaluation of critical intracellular PO2 and PCO. Am J Physiol. (1981) 241:E47–50. doi: 10.1152/ajpendo.1981.241.1.E47
35. McCord JM. Oxygen-derived free radicals in postischemic tissue injury. N Engl J Med. (1985) 312:159–63. doi: 10.1056/NEJM198501173120305
36. Di Meo S, Venditti P. Evolution of the knowledge of free radicals and other oxidants. Oxid Med Cell Longev. (2020) 2020:9829176. doi: 10.1155/2020/9829176
37. Talaie T, DiChiacchio L, Prasad NK, Pasrija C, Julliard W, Kaczorowski DJ, et al. Ischemia-reperfusion injury in the transplanted lung: A literature review. Transplant Direct. (2021) 7:e652. doi: 10.1097/TXD.0000000000001104
38. Terada LS, Piermattei D, Shibao GN, McManaman JL, Wright RM. Hypoxia regulates xanthine dehydrogenase activity at pre- and posttranslational levels. Arch Biochem Biophys. (1997) 348:163–8. doi: 10.1006/abbi.1997.0367
39. Maia L, Duarte RO, Ponces-Freire A, Moura JJG, Mira L. NADH oxidase activity of rat and human liver xanthine oxidoreductase: potential role in superoxide production. J Biol Inorg Chem. (2007) 12:777–87. doi: 10.1007/s00775-007-0229-7
40. Dupont GP, Huecksteadt TP, Marshall BC, Ryan US, Michael JR, Hoidal JR. Regulation of xanthine dehydrogenase and xanthine oxidase activity and gene expression in cultured rat pulmonary endothelial cells. J Clin Invest. (1992) 89:197–202. doi: 10.1172/JCI115563
41. Mock JR, Tune MK, Dial CF, Torres-Castillo J, Hagan RS, Doerschuk CM. Effects of IFN-γ on immune cell kinetics during the resolution of acute lung injury. Physiol Rep. (2020) 8:e14368. doi: 10.14814/phy2.14368
42. Lee JC, Christie JD. Primary graft dysfunction. Proc Am Thorac Soc. (2009) 6:39–46. doi: 10.1513/pats.200808-082GO
43. Steen S, Sjöberg T, Pierre L, Liao Q, Eriksson L, Algotsson L. Transplantation of lungs from a non-heart-beating donor. Lancet. (2001) 357:825–9. doi: 10.1016/S0140-6736(00)04195-7
44. Cypel M, Yeung JC, Hirayama S, Rubacha M, Fischer S, Anraku M, et al. Technique for prolonged normothermic ex vivo lung perfusion. J Heart Lung Transplant. (2008) 27:1319–25. doi: 10.1016/j.healun.2008.09.003
45. Zeriouh M, Sabashnikov A, Mohite PN, Zych B, Patil NP, García-Sáez D, et al. Utilization of the organ care system for bilateral lung transplantation: preliminary results of a comparative study. Interact Cardiovasc Thorac Surg. (2016) 23:351–7. doi: 10.1093/icvts/ivw135
46. Ingemansson R, Eyjolfsson A, Mared L, Pierre L, Algotsson L, Ekmehag B, et al. Clinical transplantation of initially rejected donor lungs after reconditioning ex vivo. Ann Thorac Surg. (2009) 87:255–60. doi: 10.1016/j.athoracsur.2008.09.049
47. Andreasson ASI, Dark JH, Fisher AJ. Ex vivo lung perfusion in clinical lung transplantation–state of the art. Eur J Cardiothorac Surg. (2014) 46:779–88. doi: 10.1093/ejcts/ezu228
48. Prasad NK, Pasrija C, Talaie T, Krupnick AS, Zhao Y, Lau CL. Ex vivo lung perfusion: current achievements and future directions. Transplantation. (2021) 105:979–85. doi: 10.1097/TP.0000000000003483
49. Becker S, Steinmeyer J, Avsar M, Höffler K, Salman J, Haverich A, et al. Evaluating acellular versus cellular perfusate composition during prolonged ex vivo lung perfusion after initial cold ischaemia for 24 hours. Transpl Int. (2016) 29:88–97. doi: 10.1111/tri.2016.29.issue-1
50. Roman M, Gjorgjimajkoska O, Neil D, Nair S, Colah S, Parmar J, et al. Comparison between cellular and acellular perfusates for ex vivo lung perfusion in a porcine model. J Heart Lung Transplant. (2015) 34:978–87. doi: 10.1016/j.healun.2015.03.023
51. Olbertz C, Pizanis N, Bäumker H, Kalka K, Aigner C, Rauen U, et al. Use of modified Custodiol-N as perfusion solution in ex vivo lung perfusion. Am J Transl Res. (2020) 12:153–61.
52. Kalka K, Keldenich Z, Carstens H, Walter B, Rauen U, Ruhparwar A, et al. Custodiol-MP for ex vivo lung perfusion - A comparison in a porcine model of donation after circulatory determination of death. Int J Artif Organs. (2022) 45:162–73. doi: 10.1177/0391398821990663
53. Xie Y, Hou W, Song X, Yu Y, Huang J, Sun X, et al. Ferroptosis: process and function. Cell Death Differ. (2016) 23:369–79. doi: 10.1038/cdd.2015.158
54. Yu S, Jia J, Zheng J, Zhou Y, Jia D, Wang J. Recent progress of ferroptosis in lung diseases. Front Cell Dev Biol. (2021) 9:789517. doi: 10.3389/fcell.2021.789517
55. Hu Q, Zhang Y, Lou H, Ou Z, Liu J, Duan W, et al. GPX4 and vitamin E cooperatively protect hematopoietic stem and progenitor cells from lipid peroxidation and ferroptosis. Cell Death Dis. (2021) 12:706. doi: 10.1038/s41419-021-04008-9
56. Maiorino M, Conrad M, Ursini F. GPx4, lipid peroxidation, and cell death: discoveries, rediscoveries, and open issues. Antioxid Redox Signal. (2018) 29:61–74. doi: 10.1089/ars.2017.7115
57. Wallinder A, Ricksten S-E, Hansson C, Riise GC, Silverborn M, Liden H, et al. Transplantation of initially rejected donor lungs after ex vivo lung perfusion. J Thorac Cardiovasc Surg. (2012) 144:1222–8. doi: 10.1016/j.jtcvs.2012.08.011
58. Wallinder A, Ricksten S-E, Silverborn M, Hansson C, Riise GC, Liden H, et al. Early results in transplantation of initially rejected donor lungs after ex vivo lung perfusion: a case-control study. Eur J Cardiothorac Surg. (2014) 45:40–4. doi: 10.1093/ejcts/ezt250
59. Yeung JC, Cypel M, Machuca TN, Koike T, Cook DJ, Bonato R, et al. Physiologic assessment of the ex vivo donor lung for transplantation. J Heart Lung Transplant. (2012) 31:1120–6. doi: 10.1016/j.healun.2012.08.016
60. Roman MA, Nair S, Tsui S, Dunning J, Parmar JS. Ex vivo lung perfusion. Transplantation. (2013) 96:509–18. doi: 10.1097/TP.0b013e318295eeb7
61. Lee SS, Antaki JF, Kameneva MV, Dobbe JG, Hardeman MR, Ahn KH, et al. Strain hardening of red blood cells by accumulated cyclic supraphysiological stress. Artif Organs. (2007) 31:80–6. doi: 10.1111/j.1525-1594.2007.00344.x
62. Watanabe N, Sakota D, Ohuchi K, Takatani S. Deformability of red blood cells and its relation to blood trauma in rotary blood pumps. Artif Organs. (2007) 31:352–8. doi: 10.1111/j.1525-1594.2007.00392.x
63. Kniepeiss D, Houben P, Stiegler P, Berghold A, Riedl R, Kahn J, et al. A prospective, randomized, single-blind, multicentre, phase III study on organ preservation with Custodiol-N solution compared with Custodiol® solution in organ transplantation (kidney, liver and pancreas). Trials. (2020) 21:62. doi: 10.1186/s13063-019-3823-4
64. Huang L, Vellanki RN, Zhu Z, Wouters BG, Keshavjee S, Liu M. De novo design and development of a nutrient-rich perfusate for ex vivo lung perfusion with cell culture models. Int J Mol Sci. (2023) 24. doi: 10.3390/ijms241713117
65. Iskender I, Cosgun T, Arni S, Trinkwitz M, Fehlings S, Yamada Y, et al. Cytokine filtration modulates pulmonary metabolism and edema formation during ex vivo lung perfusion. J Heart Lung Transplant. (2017). doi: 10.1016/j.healun.2017.05.021
66. Iskender I, Arni S, Maeyashiki T, Citak N, Sauer M, Rodriguez JM, et al. Perfusate adsorption during ex vivo lung perfusion improves early post-transplant lung function. J Thorac Cardiovasc Surg. (2021) 161:e109–21. doi: 10.1016/j.jtcvs.2019.12.128
67. Ehrsam JP, Arni S, Weisskopf M, Nowack M, Inci I. Extracorporeal cytokine adsorption reduces systemic cytokine storm and improves graft function in lung transplantation. JTCVS Open. (2023) 15:497–507. doi: 10.1016/j.xjon.2023.06.011
68. Boffini M, Marro M, Simonato E, Scalini F, Costamagna A, Fanelli V, et al. Cytokines removal during ex-vivo lung perfusion: initial clinical experience. Transpl Int. (2023) 36:10777. doi: 10.3389/ti.2023.10777
69. Steen S, Liao Q, Wierup PN, Bolys R, Pierre L, Sjöberg T. Transplantation of lungs from non-heart-beating donors after functional assessment ex vivo. Ann Thorac Surg. (2003) 76:244–52. doi: 10.1016/S0003-4975(03)00191-7
70. Haam S. Ex vivo lung perfusion in lung transplantatio. J Chest Surg. (2022) 55:288–92. doi: 10.5090/jcs.22.056
71. Warnecke G, Moradiellos J, Tudorache I, Kühn C, Avsar M, Wiegmann B, et al. Normothermic perfusion of donor lungs for preservation and assessment with the Organ Care System Lung before bilateral transplantation: a pilot study of 12 patients. Lancet. (2012) 380:1851–8. doi: 10.1016/S0140-6736(12)61344-0
72. Warnecke G, van Raemdonck D, Smith MA, Massard G, Kukreja J, Rea F, et al. Normothermic ex-vivo preservation with the portable Organ Care System Lung device for bilateral lung transplantation (INSPIRE): a randomised, open-label, non-inferiority, phase 3 study. Lancet Respir Med. (2018) 6:357–67. doi: 10.1016/S2213-2600(18)30136-X
73. Beller JP, Byler MR, Money DT, Chancellor WZ, Zhang A, Zhao Y, et al. Reduced-flow ex vivo lung perfusion to rehabilitate lungs donated after circulatory death. J Heart Lung Transplant. (2020) 39:74–82. doi: 10.1016/j.healun.2019.09.009
74. Noda K, Shigemura N, Tanaka Y, Bhama JK, D’Cunha J, Luketich JD, et al. Successful prolonged ex vivo lung perfusion for graft preservation in rats. Eur J Cardiothorac Surg. (2014) 45:e54–60. doi: 10.1093/ejcts/ezt598
75. Cypel M, Yeung JC, Machuca T, Chen M, Singer LG, Yasufuku K, et al. Experience with the first 50 ex vivo lung perfusions in clinical transplantation. J Thorac Cardiovasc Surg. (2012) 144:1200–6. doi: 10.1016/j.jtcvs.2012.08.009
76. Lindstedt S, Eyjolfsson A, Koul B, Wierup P, Pierre L, Gustafsson R, et al. How to recondition ex vivo initially rejected donor lungs for clinical transplantation: clinical experience from lund university hospital. J Transplant. (2011) 2011:754383. doi: 10.1155/2011/754383
77. Peták F, Habre W, Hantos Z, Sly PD, Morel DR. Effects of pulmonary vascular pressures and flow on airway and parenchymal mechanics in isolated rat lungs. J Appl Physiol (1985). (2002) 92:169–78. doi: 10.1152/jappl.2002.92.1.169
78. Linacre V, Cypel M, Machuca T, Nakajima D, Hashimoto K, Zamel R, et al. Importance of left atrial pressure during ex vivo lung perfusion. J Heart Lung Transplant. (2016) 35:808–14. doi: 10.1016/j.healun.2016.02.008
79. Kuypers F, Larkin S, Emeis J, Allison A. Interaction of an annexin V homodimer (Diannexin) with phosphatidylserine on cell surfaces and consequent antithrombotic activity. Thromb Haemost. (2007) 97:478–86.
80. van Raemdonck D, Neyrinck A, Cypel M, Keshavjee S. Ex-vivo lung perfusion. Transpl Int. (2015) 28:643–56. doi: 10.1111/tri.12317
81. Brandes H, Albes JM, Conzelmann A, Wehrmann M, Ziemer G. Comparison of pulsatile and nonpulsatile perfusion of the lung in an extracorporeal large animal model. Eur Surg Res. (2002) 34:321–9. doi: 10.1159/000063067
82. Bates JHT. Lung mechanics: An inverse modeling approach. Cambridge: Cambridge University Press (2009). doi: 10.1017/CBO9780511627156
83. Bates JHT, Smith BJ. Ventilator-induced lung injury and lung mechanics. Ann Transl Med. (2018) 6:378. doi: 10.21037/atm
84. Dreyfuss D, Soler P, Basset G, Saumon G. High inflation pressure pulmonary edema. Respective effects of high airway pressure, high tidal volume, and positive end-expiratory pressure. Am Rev Respir Dis. (1988) 137:1159–64. doi: 10.1164/ajrccm/137.5.1159
85. Pintado M-C, Pablo RD, Trascasa M, Milicua J-M, Rogero S, Daguerre M, et al. Individualized PEEP setting in subjects with ARDS: a randomized controlled pilot study. Respir Care. (2013) 58:1416–23. doi: 10.4187/respcare.02068
86. Maggiore SM, Jonson B, Richard JC, Jaber S, Lemaire F, Brochard L. Alveolar derecruitment at decremental positive end-expiratory pressure levels in acute lung injury: comparison with the lower inflection point, oxygenation, and compliance. Am J Respir Crit Care Med. (2001) 164:795–801. doi: 10.1164/ajrccm.164.5.2006071
87. Barnes T, van Asseldonk D, Enk D. Minimisation of dissipated energy in the airways during mechanical ventilation by using constant inspiratory and expiratory flows - Flow-controlled ventilation (FCV). Med Hypotheses. (2018) 121:167–76. doi: 10.1016/j.mehy.2018.09.038
88. Goebel U, Haberstroh J, Foerster K, Dassow C, Priebe H-J, Guttmann J, et al. Flow-controlled expiration: a novel ventilation mode to attenuate experimental porcine lung injury. Br J Anaesth. (2014) 113:474–83. doi: 10.1093/bja/aeu058
89. Weber J, Schmidt J, Straka L, Wirth S, Schumann S. Flow-controlled ventilation improves gas exchange in lung-healthy patients- a randomized interventional cross-over study. Acta Anaesthesiol Scand. (2020) 64:481–8. doi: 10.1111/aas.13526
90. Ordies S, Orlitova M, Heigl T, Sacreas A, van Herck A, Kaes J, et al. Flow-controlled ventilation during EVLP improves oxygenation and preserves alveolar recruitment. Intensive Care Med Exp. (2020) 8:70. doi: 10.1186/s40635-020-00360-w
91. Bobba CM, Nelson K, Dumond C, Eren E, Black SM, Englert JA, et al. A novel negative pressure-flow waveform to ventilate lungs for normothermic ex vivo lung perfusion. ASAIO J. (2021) 67:96–103. doi: 10.1097/MAT.0000000000001168
92. Aboelnazar NS, Himmat S, Hatami S, White CW, Burhani MS, Dromparis P, et al. Negative pressure ventilation decreases inflammation and lung edema during normothermic ex-vivo lung perfusion. J Heart Lung Transplant. (2018) 37:520–30. doi: 10.1016/j.healun.2017.09.007
93. Buchko MT, Boroumand N, Cheng JC, Hirji A, Halloran K, Freed DH, et al. Clinical transplantation using negative pressure ventilation ex situ lung perfusion with extended criteria donor lungs. Nat Commun. (2020) 11:5765. doi: 10.1038/s41467-020-19581-4
94. Barbateskovic M, Schjørring OL, Russo Krauss S, Jakobsen JC, Meyhoff CS, Dahl RM, et al. Higher versus lower fraction of inspired oxygen or targets of arterial oxygenation for adults admitted to the intensive care unit. Cochrane Database Syst Rev. (2019) 2019. doi: 10.1002/14651858.CD012631.pub2
96. Mosier JM, Hypes C, Joshi R, Whitmore S, Parthasarathy S, Cairns CB. Ventilator strategies and rescue therapies for management of acute respiratory failure in the emergency department. Ann Emerg Med. (2015) 66:529–41. doi: 10.1016/j.annemergmed.2015.04.030
97. Santer P, Zheng S, Hammer M, Nabel S, Pannu A, Li Y, et al. Ventilatory frequency during intraoperative mechanical ventilation and postoperative pulmonary complications: a hospital registry study. Br J Anaesth. (2020) 125:e130–9. doi: 10.1016/j.bja.2020.02.018
98. Gattinoni L, Brusatori S, D’Albo R, Maj R, Velati M, Zinnato C, et al. Prone position: how understanding and clinical application of a technique progress with time. APS. (2023) 1. doi: 10.1007/s44254-022-00002-2
99. Abroug F, Ouanes-Besbes L, Dachraoui F, Ouanes I, Brochard L. An updated study-level meta-analysis of randomised controlled trials on proning in ARDS and acute lung injury. Crit Care. (2011) 15:R6. doi: 10.1186/cc9403
100. Ordies S, Frick AE, Claes S, Schols D, Verleden SE, van Raemdonck DE, et al. Prone positioning during ex vivo lung perfusion influences regional edema accumulation. J Surg Res. (2019) 239:300–8. doi: 10.1016/j.jss.2019.02.003
101. Niikawa H, Okamoto T, Ayyat KS, Itoda Y, Farver CF, McCurry KR. The protective effect of prone lung position on ischemia-reperfusion injury and lung function in an ex vivo porcine lung model. J Thorac Cardiovasc Surg. (2019) 157:425–33. doi: 10.1016/j.jtcvs.2018.08.101
102. Niikawa H, Okamoto T, Ayyat KS, Itoda Y, Sakanoue I, Farver CF, et al. Prone ex vivo lung perfusion protects human lungs from reperfusion injury. Artif Organs. (2022) 46:2226–33. doi: 10.1111/aor.14328
103. Scozzi D, Liao F, Krupnick AS, Kreisel D, Gelman AE. The role of neutrophil extracellular traps in acute lung injury. Front Immunol. (2022) 13. doi: 10.3389/fimmu.2022.953195
104. Yang H, Biermann MH, Brauner JM, Liu Y, Zhao Y, Herrmann M. New insights into neutrophil extracellular traps: mechanisms of formation and role in inflammation. Front Immunol. (2016) 7. doi: 10.3389/fimmu.2016.00302
105. Porto BN, Stein RT. Neutrophil extracellular traps in pulmonary diseases: too much of a good thing? Front Immunol. (2016) 7. doi: 10.3389/fimmu.2016.00311
106. Sayah DM, Mallavia B, Liu F, Ortiz-Muñoz G, Caudrillier A, DerHovanessian A, et al. Neutrophil extracellular traps are pathogenic in primary graft dysfunction after lung transplantation. Am J Respir Crit Care Med. (2015) 191:455–63. doi: 10.1164/rccm.201406-1086OC
107. Niikawa H, Okamoto T, Ayyat KS, Sakanoue I, Yun JJ, McCurry KR. Successful lung transplantation after acellular ex vivo lung perfusion with prone positioning. Ann Thorac Surg. (2020) 110:e285–7. doi: 10.1016/j.athoracsur.2020.02.045
108. Aguilar-Guisado M, Givaldá J, Ussetti P, Ramos A, Morales P, Blanes M, et al. Pneumonia after lung transplantation in the RESITRA Cohort: a multicenter prospective study. Am J Transplant. (2007) 7:1989–96. doi: 10.1111/j.1600-6143.2007.01882.x
109. Ruiz I, Gavaldà J, Monforte V, Len O, Román A, Bravo C, et al. Donor-to-host transmission of bacterial and fungal infections in lung transplantation. Am J Transplant. (2006) 6:178–82. doi: 10.1111/j.1600-6143.2005.01145.x
110. Andreasson A, Karamanou DM, Perry JD, Perry A, Özalp F, Butt T, et al. The effect of ex vivo lung perfusion on microbial load in human donor lungs. J Heart Lung Transplant. (2014) 33:910–6. doi: 10.1016/j.healun.2013.12.023
111. Nakajima D, Cypel M, Bonato R, Machuca TN, Iskender I, Hashimoto K, et al. Ex vivo perfusion treatment of infection in human donor lungs. Am J Transplant. (2016) 16:1229–37. doi: 10.1111/ajt.13562
112. Eskind CC, Shilts MH, Shaver CM, Das SR, Satyanarayana G. The respiratory microbiome after lung transplantation: Reflection or driver of respiratory disease? Am J Transplant. (2021) 21:2333–40. doi: 10.1111/ajt.16568
113. Becker J, Poroyko V, Bhorade S. The lung microbiome after lung transplantation. Expert Rev Respir Med. (2014) 8:221–31. doi: 10.1586/17476348.2014.890518
114. Dickson RP, Erb-Downward JR, Freeman CM, Walker N, Scales BS, Beck JM, et al. Changes in the lung microbiome following lung transplantation include the emergence of two distinct Pseudomonas species with distinct clinical associations. PloS One. (2014) 9:e97214. doi: 10.1371/journal.pone.0097214
115. Jain R, Hachem RR, Morrell MR, Trulock EP, Chakinala MM, Yusen RD, et al. Azithromycin is associated with increased survival in lung transplant recipients with bronchiolitis obliterans syndrome. J Heart Lung Transplant. (2010) 29:531–7. doi: 10.1016/j.healun.2009.12.003
116. Murphy DM, Forrest IA, Corris PA, Johnson GE, Small T, Jones D, et al. Azithromycin attenuates effects of lipopolysaccharide on lung allograft bronchial epithelial cells. J Heart Lung Transplant. (2008) 27:1210–6. doi: 10.1016/j.healun.2008.07.026
117. Del Sorbo L, Michaelsen VS, Ali A, Wang A, Ribeiro RVP, Cypel M. High doses of inhaled nitric oxide as an innovative antimicrobial strategy for lung infections. Biomedicines. (2022) 10. doi: 10.3390/biomedicines10071525
118. Michaelsen VS, Ribeiro RVP, Ali A, Wang A, Gazzalle A, Keshavjee S, et al. Safety of continuous 12-hour delivery of antimicrobial doses of inhaled nitric oxide during ex vivo lung perfusion. J Thorac Cardiovasc Surg. (2022) 163:841–849.e1. doi: 10.1016/j.jtcvs.2020.11.150
119. Al Sulaiman K, Korayem GB, Altebainawi AF, Al Harbi S, Alissa A, Alharthi A, et al. Evaluation of inhaled nitric oxide (iNO) treatment for moderate-to-severe ARDS in critically ill patients with COVID-19: a multicenter cohort study. Crit Care. (2022) 26:304. doi: 10.1186/s13054-022-04158-y
120. Gebistorf F, Karam O, Wetterslev J, Afshari A. Inhaled nitric oxide for acute respiratory distress syndrome (ARDS) in children and adults. Cochrane Database Syst Rev. (2016) 2016:CD002787. doi: 10.1002/14651858.CD002787.pub3
121. Rossaint R, Falke KJ, López F, Slama K, Pison U, Zapol WM. Inhaled nitric oxide for the adult respiratory distress syndrome. N Engl J Med. (1993) 328:399–405. doi: 10.1056/NEJM199302113280605
122. Taylor MB, Christian KG, Patel N, Churchwell KB. Methemoglobinemia: Toxicity of inhaled nitric oxide therapy. Pediatr Crit Care Med. (2001) 2:99–101. doi: 10.1097/00130478-200101000-00019
Keywords: EVLP, PGD, ex vivo, organ perfusion, transplantation, techniques
Citation: Ponholzer F, Dumfarth J, Krapf C, Pircher A, Hautz T, Wolf D, Augustin F and Schneeberger S (2024) The impact and relevance of techniques and fluids on lung injury in machine perfusion of lungs. Front. Immunol. 15:1358153. doi: 10.3389/fimmu.2024.1358153
Received: 19 December 2023; Accepted: 26 February 2024;
Published: 06 March 2024.
Edited by:
Ilker Iskender, University Hospital Zürich, SwitzerlandReviewed by:
Sandra Lindstedt, Lund University, SwedenCopyright © 2024 Ponholzer, Dumfarth, Krapf, Pircher, Hautz, Wolf, Augustin and Schneeberger. This is an open-access article distributed under the terms of the Creative Commons Attribution License (CC BY). The use, distribution or reproduction in other forums is permitted, provided the original author(s) and the copyright owner(s) are credited and that the original publication in this journal is cited, in accordance with accepted academic practice. No use, distribution or reproduction is permitted which does not comply with these terms.
*Correspondence: Florian Ponholzer, Zmxvcmlhbi5wb25ob2x6ZXJAaS1tZWQuYWMuYXQ=; Stefan Schneeberger, c3RlZmFuLnNjaG5lZWJlcmdlckBpLW1lZC5hYy5hdA==
Disclaimer: All claims expressed in this article are solely those of the authors and do not necessarily represent those of their affiliated organizations, or those of the publisher, the editors and the reviewers. Any product that may be evaluated in this article or claim that may be made by its manufacturer is not guaranteed or endorsed by the publisher.
Research integrity at Frontiers
Learn more about the work of our research integrity team to safeguard the quality of each article we publish.