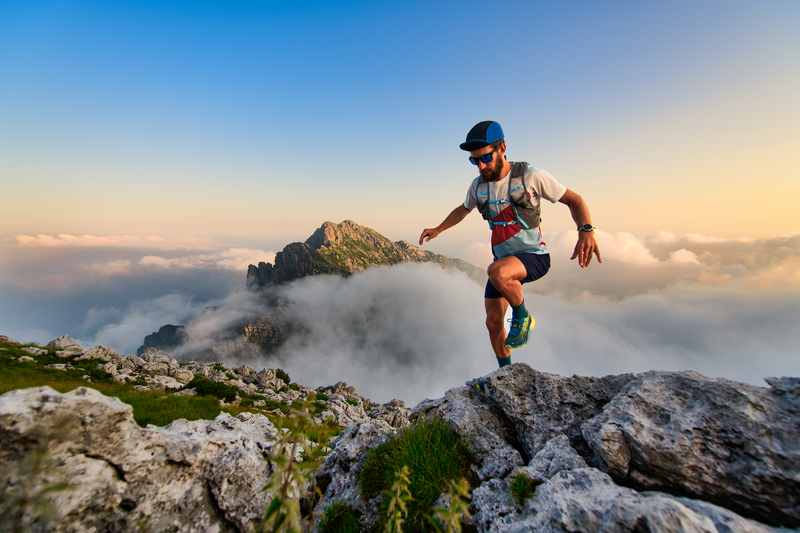
94% of researchers rate our articles as excellent or good
Learn more about the work of our research integrity team to safeguard the quality of each article we publish.
Find out more
REVIEW article
Front. Immunol. , 01 February 2024
Sec. Cancer Immunity and Immunotherapy
Volume 15 - 2024 | https://doi.org/10.3389/fimmu.2024.1356913
This article is part of the Research Topic Harnessing Tumor Microenvironment for Gynecologic Cancer Therapy View all 7 articles
The Mucin (MUC) family, a range of highly glycosylated macromolecules, is ubiquitously expressed in mammalian epithelial cells. Such molecules are pivotal in establishing protective mucosal barriers, serving as defenses against pathogenic assaults. Intriguingly, the aberrant expression of specific MUC proteins, notably Mucin 1 (MUC1) and Mucin 16 (MUC16), within tumor cells, is intimately associated with oncogenesis, proliferation, and metastasis. This association involves various mechanisms, including cellular proliferation, viability, apoptosis resistance, chemotherapeutic resilience, metabolic shifts, and immune surveillance evasion. Due to their distinctive biological roles and structural features in oncology, MUC proteins have attracted considerable attention as prospective targets and biomarkers in cancer therapy. The current review offers an exhaustive exploration of the roles of MUC1 and MUC16 in the context of cancer biomarkers, elucidating their critical contributions to the mechanisms of cellular signal transduction, regulation of immune responses, and the modulation of the tumor microenvironment. Additionally, the article evaluates the latest advances in therapeutic strategies targeting these mucins, focusing on innovations in immunotherapies and targeted drugs, aiming to enhance customization and accuracy in cancer treatments.
Mucins, categorized as high molecular weight glycoproteins, are divided into two subfamilies — secretory and transmembrane — based on molecular structures (1). Extensive glycosylation and growth factor-like domains in the C-terminal region, key attributes of mucins, are crucial for modulating cell surface microenvironments and enhancing interactions with other cellular receptors (2). Widely expressed on various epithelial cell surfaces, mucins undertake numerous physiological roles, including immune protection and regulation of signal transduction and transcription (3). A substantial body of research has demonstrated a strong association between the overexpression and abnormal glycosylation of mucins in various epithelial cancers with the processes of cancer cell proliferation, invasion, and metastasis (4–6). Dysregulated expression and glycosylation of MUC1 and MUC16 in cancer, notably affecting immune modulation and metastasis, impair dendritic cell function, resulting in heightened immunosuppression and advancing tumor progression (7). The comprehensive delineation of mucin dysregulation in cancer now leads to a focused analysis of MUC1 and MUC16, examining their intricate molecular interactions and pivotal influence in oncological processes.
Mucin 1 (MUC1) is a heterodimeric transmembrane glycoprotein, characterized by its polar distribution and function in providing a protective barrier (8). Its structure includes a highly glycosylated N-terminal subunit and the potentially oncogenic MUC1 C-terminal (MUC1-C), comprising a 58-amino acid extracellular domain, a 28-amino acid transmembrane region, and a 72-amino acid disordered cytoplasmic tail (9). Under normal physiological conditions, MUC1 serves as a protective barrier against external insults such as microbes, toxins, and mechanical stress, while also repairing damaged epithelium through mechanisms like epigenetic reprogramming and epithelial-mesenchymal transition (EMT), thus maintaining the stability of the epithelial layer (10). During tumorigenesis and progression, significant changes occur in the expression and function of MUC1, with over 90% of breast cancer cases exhibiting its abnormal overexpression (11, 12). In tumor cells, heightened MUC1 expression, combined with reduced glycosylation and altered polar distribution, modifies interactions with cell surface receptors (13). MUC1-C participates in several key biological processes in cancer cells, involving inflammation, proliferation, EMT, epigenetic reprogramming, and chromatin remodeling, thereby promoting cellular plasticity (14). Additionally, MUC1 enhances the stemness of cancer cells, primarily by activating pluripotency networks and also playing a role on the outer mitochondrial membrane, reducing drug-induced mitochondrial pro-apoptotic factor release and apoptosis (15, 16). Recent studies in pancreatic cancer treatment demonstrate that targeting tumor-associated MUC1 (tMUC1) with the monoclonal antibody TAB004 overcomes anoikis resistance by specifically binding to tMUC1, reducing cancer cell viability and promoting its degradation (17). Research has also indicated that MUC1 can induce chemoresistance, especially by promoting the accumulation of cancer stem cells in cervical cancer (18, 19). As an oncogenic driver, MUC1 increases the aggressiveness, metastatic potential, and drug resistance of cancer by promoting tumor cell proliferation (20, 21), EMT (22), and epigenetic changes (23, 24), and facilitates immune evasion through interactions with immune cells in the tumor microenvironment (25).
Mucin 16 (MUC16), known as the largest transmembrane mucin, has its secreted form, Cancer Antigen 125 (CA125), recognized as a serum biomarker for the diagnosis and poor prognosis of gynecological malignancies (26, 27). MUC16 is highly expressed in various epithelial cancers (28) and is considered to have a barrier function in certain organs (29, 30). Proteolytic cleavage of MUC16 releases CA-125 into the bloodstream, retaining the membrane-associated C-terminal on the cell surface; CA-125, a biomarker for ovarian cancer (31), is targeted by emerging therapies such as Chimeric Antigen Receptor (CAR) T cells (32) and antibody-drug conjugates (ADCs) (33). Approximately 80% of ovarian cancers exhibit MUC16 expression, which is present in all ovarian cancer subtypes (including serous, mucinous, endometrioid, and clear cell) though it varies in expression (34). Recent studies suggest that MUC16 may play a role in modulating immune responses in different cancers. Gubbels JA et al. reported that MUC16 can protect ovarian cancer cells from NK cell attacks by inhibiting the formation of immune synapses between NK cells and ovarian cancer cells (35). Belisle JA et al. found that MUC16 can bind to NK cells, B cells, and monocytes through Siglec-9, which, as an inhibitory receptor, can weaken the function of T cells and NK cells (36). Other research reveals that the proportion of circulating regulatory T (Treg) cells is related to the level of CA125 in the serum, and the C-terminal of MUC16 can activate the JAK2/STAT3 signaling pathway triggered by tumor-secreted IL-6, promoting the expression of Foxp3 and the accumulation of tumor-associated Tregs in pancreatic cancer (37). Additionally, studies show that inflammatory cytokines TNFα and IFNγ can stimulate MUC16 expression in breast cancer, endometrial cancer, and ovarian cancer cells via NFκB, and in these cancer tissues, increased MUC16 expression is associated with elevated cytokine levels (38). Inflammatory stimuli such as oxidative stress and treatment with cytokines IFNγ, IL-1α, TNFα have also been found to alter the glycosylation pattern of MUC16 in pancreatic cancer cells (39), suggesting that MUC16 may play a key role in promoting inflammatory signaling in cancer.
In summary, MUC1 and MUC16, key molecules within the mucin family, exhibit roles in cancer biology surpassing the conventional barrier formation in normal epithelial cells. These mucins contribute to various aspects of tumor development, affecting tumor cell proliferation, survival, migration, and interactions with the immune system. The roles of these mucins in tumor immune surveillance and as potential therapeutic targets present new opportunities for cancer treatment. This review examines the roles of MUC1 and MUC16 in cancer immune regulation and therapeutic effectiveness, exploring their potential in precision medicine. Challenges currently faced and future research directions are also discussed, offering novel insights and strategies for cancer therapy.
Extensive research has established MUC1 and MUC16 as key players in cancer development, with expression patterns significantly influencing tumor progression and serving as vital prognostic markers. Specifically, research by Ren et al. noted an increase in MUC1 expression across various tumors, enhanced by heregulin, which positions its C-terminal subunit in mitochondria, thereby inhibiting cisplatin-induced apoptotic signaling and reducing tumor cell sensitivity to chemotherapy drugs (21). Rajabi et al. showed that MUC1-C protein, by augmenting the activity of DNMT1 and DNMT3b, induces high levels of DNA methylation, leading to the silencing of tumor suppressor genes and thus exerting its carcinogenic effect (23). Moreover, MUC1-C’s role in cancer progression is further confirmed by enhancing the binding of BMI1 to the CDKN2A promoter and suppressing the expression of tumor suppressor genes like p16INK4a (24). In ovarian cancer research, Ma et al. identified MUC1 as a key regulatory factor in the disease’s development, primarily through upregulating EGFR expression and activating the AKT signaling pathway to promote tumor cell proliferation, while also highlighting Taxol’s significant therapeutic potential in targeting ovarian cancers with abnormally high MUC1 expression (40). Zong et al. indicated that circWHSC1, by binding with miR-145 and miR-1182, upregulates MUC1 expression in ovarian cancer, promoting cell proliferation and metastasis (41). MUC16 plays a significant role in ovarian cancer research, with its relationship to tumor growth, metastasis, and chemotherapy resistance extensively supported by research. Liu et al. found that the inflammatory microenvironment in ovarian cancer activates the MUC16 gene via the NF-κB pathway, leading to elevated CA125 levels, thereby exacerbating tumor progression and invasiveness (42). Furthermore, Wang et al. found that MUC16’s regulatory effect on GLUT1 expression might be a contributing factor to the proliferation and progression of ovarian epithelial cancer (43). In terms of tumor metastasis, Huo et al. noted that the CA125/MUC16 complex significantly enhances tumor cell migration and metastasis capabilities by downregulating DKK1 expression and activating the SGK3/FOXO3 pathway (44). Concurrently, Gubbels et al. demonstrated that MUC16’s high-affinity binding with mesothelin, mediated by N-glycan-dependent interactions, is crucial for peritoneal metastasis of ovarian tumors (45). Research by Matte et al. indicated that MUC16 can weaken TRAIL-induced apoptosis, a function related to its role in tumor formation and chemotherapy resistance (46).
The impact of MUC1 and MUC16 on the progression of breast cancer has been extensively studied and confirmed. Woo et al. showed that in breast cancer, abnormal overexpression of MUC1 promotes VEGF production by activating the AKT signaling pathway, a mechanism playing a vital role in tumor angiogenesis, growth, and metastasis (47). MUC1 also enhances the invasiveness of breast cancer cells by disrupting ATAD3A and activating Pink1-related mitochondrial autophagy, revealing the critical role of the MUC1/ATAD3A/Pink1 axis in breast cancer progression (48). Additionally, in HER2-positive breast cancer, Raina et al. revealed the potential efficacy of MUC1-C targeted therapy in reducing HER2 activity and reversing trastuzumab resistance, highlighting the therapeutic value of MUC1-C inhibitors (49). MUC1 glycosylation modifications also regulate the chemosensitivity of breast cancer cells, with Xi et al. confirming that knocking out MUC1 or removing specific glycosylation modifications enhances chemotherapy effects (50). In triple-negative breast cancer (TNBC) research, Yamashita et al. confirmed that MUC1-C promotes cancer progression by activating cytoplasmic nucleotide pattern recognition receptors (PRRs) such as RIG-I, MDA5, and cGAS, and the cGAS-STING pathway (51). Regarding MUC16, research by Chaudhary et al. found that its high expression in TNBC is associated with enhanced action of the RNA-binding protein ELAVL1/HuR, promoting TNBC metastasis to the lungs through regulation by the HuR/cMyc axis (52). Research by Lakshmanan et al. revealed that MUC16 overexpression in breast cancer alters interactions with JAK2, accelerating the G2/M phase transition of the cell cycle to promote cellular proliferation and apoptosis resistance, while its silencing induces cell cycle arrest and enhances TRAIL-mediated apoptosis (53). Additionally, the CDK4/6 inhibitor palbociclib, by downregulating MUC16 expression, exerts an inhibitory effect on the expression of key genes in the breast cancer cell cycle and suppresses ER(-) aggressive breast cancer cells (54). In cervical cancer research, Lv et al. found that the EGFR-TKI drug erlotinib, targeting MUC1-positive cervical cancer through the MUC1-EGFR-CREB/GRβ-IL-6 axis, significantly inhibits taxol-resistant tumor stem cells and reduces residual tumor stem cells after chemotherapy, offering the potential to improve patient prognosis (19).
The impact of MUC1 and MUC16 in lung cancer development has been further substantiated. Research by Raina et al. showed that MUC1-C activates the PI3K/Akt/mTOR pathway in non-small cell lung cancer (NSCLC) by interacting with PI3K p85, promoting tumor cell proliferation and significantly correlating with poor patient prognosis (55). Overexpression of MUC16 in lung cancer enhances tumor growth and migration, while also influencing development and metastasis through the JAK2/STAT3/GR axis, potentially contributing to chemotherapy resistance to agents like cisplatin and gemcitabine (56). Lei et al. disclosed that in lung cancer, ERO1L promotes IL6R secretion to activate the NF-κB pathway, leading to an upregulation of MUC16 gene expression, and the C-terminal of MUC16 further fosters the EMT phenotype and IL6 release, creating a positive feedback loop that exacerbates lung cancer progression (57). Research by Kanwal et al. also indicated that air pollution is associated with elevated MUC16 mRNA levels in lung cancer samples, and mutations in the MUC16 gene might enhance its expression, thereby increasing tumor resistance to chemotherapy and invasiveness (58).
MUC1 and MUC16 also play pivotal roles in gastrointestinal cancers. In colorectal cancer research, Morimoto et al. discovered that BRAF(V600E) mutant colorectal cancer exhibits overexpression of MUC1 protein, which, by activating the SHP2 phosphatase, further strengthens RAS/ERK signaling, leading to increased tumor cell proliferation and resistance to BRAF inhibitors; targeting MUC1-C could potentially enhance treatment sensitivity and inhibit tumor growth (59). In the progression from colitis to colorectal cancer, targeted inhibition of MUC1-C plays a crucial role in blocking LGR5+ intestinal stem cells (ISCs), Myc, and pluripotency factors signaling pathways, slowing down the worsening of colitis (10). Conversely, in liver cancer cell lines HepG2 and Huh7, Huang et al. found that MUC16 gene knockdown significantly enhances cell migration and invasion capabilities, with RNA-seq analysis confirming the molecular basis of these changes (60). Additionally, the role of MUC1 and MUC16 in pancreatic cancer progression has been extensively validated. Shukla et al. demonstrated that in pancreatic cancer cells, MUC1’s interaction with HIF-1α promotes glycolysis, leading to increased dCTP levels and decreased gemcitabine efficacy, while targeting HIF-1α markedly reduces tumor burden, underscoring MUC1’s essential role (61). Furthermore, Behrens et al. found that the cytoplasmic tail of MUC1 (MUC1.CT) regulates genes associated with invasion, angiogenesis, and metastasis, enhancing the tumor microenvironment’s carcinogenic properties (62). On the other hand, threonine deficiency and tRNA synthetase inhibition can reduce MUC1 levels in pancreatic cancer cells, thereby inhibiting tumor cell migration (63). Roy et al. further observed that in pancreatic cancer, MUC1 overexpression induces EMT, enhancing tumor cell invasion and metastasis through MUC1’s interaction with β-catenin, leading to its nuclear translocation and activation of genes associated with EMT and metastasis (22). Additionally, it has been discovered that in pancreatic ductal adenocarcinoma (PDAC), MUC1 overexpression induces non-canonical TGF-β signaling, where MUC1 overexpression activates the JNK pathway in response to TGF-β, thus altering its function from a tumor suppressor to a tumor promoter (64). In PDAC, MUC16’s role is also significant. Thomas et al. reported that in PDAC patients, high MUC16 protein expression correlates with faster tumor progression, more metastasis, aggressive subtypes like basal-like and squamous tumors, and shorter survival, with its abnormally glycosylated form activating AKT and GSK3β signaling pathways, thereby exacerbating tumor malignancy (65). Chirravuri-Venkata et al. showed that MUC16 overexpression in PDAC is closely associated with high tumor antigen load, polarization of heterogenous stromal fibroblasts (CAFs), and a significant increase in TP53 gene deletion risk, collectively indicating a poor prognosis (66). Marimuthu et al. revealed that MUC16 activates the JAK2/STAT1 signaling pathway, promoting liver metastasis in PDAC (67). Rajesh et al. revealed that in PDAC, truncated O-glycan forms of MUC16 interact with the α4β1 integrin complex, activating the ILK/FAK signaling pathway and enhancing tumor invasiveness (68). Muniyan et al. indicated that MUC16 significantly promotes PDAC proliferation and migration by interacting with extracellular matrix proteins like selectin-3 and endothelin and through FAK-mediated signaling pathways (69). Shimizu et al. confirmed that high expression of MUC16 in PDAC correlates with tumor invasiveness. MUC16 enhances pancreatic cancer cell invasion and migration by interacting with endothelin, thus playing a key role in the progression and worsening of PDAC (70). In gallbladder cancer research, Fan et al. established that the MUC16 carboxy-terminal peptide, by interacting with stathmin1, enhances the migration and invasion capabilities of gallbladder cancer cells (71). Deng et al.’s study revealed a positive correlation between GATA6 and EMT markers in cholangiocarcinoma, regulating β-catenin’s nuclear translocation through its downstream target MUC1, thereby promoting EMT and metastasis, indicating the critical role of the GATA6/MUC1/β-catenin signaling pathway in cholangiocarcinoma progression (72).
In prostate cancer, MUC1-C has been shown to activate the BAF complex in prostate cancer stem cells, enhancing the expression of NOTCH1 and NANOG, thus promoting self-renewal of tumor stem cells and accelerating the progression of neuroendocrine prostate cancer (15). Research by Shigeta et al. indicated that in urothelial cancer, increased activity of MUC1-C enhances resistance to cisplatin, while inhibition of MUC1 can restore drug sensitivity, a process involving regulation of the PI3K-AKT-mTOR pathway and ROS (73).
Figure 1 delineates the multifunctional roles of MUC1 and MUC16 in cancer development. Accompanying Tables 1 and 2 detail these mucins’ expression and functions across cancer types, underlining their importance in disease mechanisms and as potential therapeutic targets.
Figure 1 Oncogenic mechanisms of MUC1 and MUC16. The diagram delineates the complex functions of MUC1 and MUC16 in cancer mechanisms, illustrating their roles in promoting cell division, inhibiting apoptosis, facilitating metastasis, modifying DNA methylation patterns, conferring chemoresistance, and regulating immune interactions, which are pivotal in the progression of cancer and the response to therapies.
In tumor immune microenvironment research, the roles of MUC1 and MUC16 in regulating mechanisms by which tumor cells evade immune surveillance, and how these proteins influence immune escape and treatment response through different pathways, are currently focal points of investigation. Madsen et al. highlighted the role of MUC1 and MUC16 in breast and pancreatic cancer cells, showing that reducing their expression through COSMC gene knockdown enhances tumor cell sensitivity to natural killer cell-mediated antibody-dependent cellular cytotoxicity (ADCC) and cytotoxic T lymphocyte (CTL) killing. This suggests that high expression of MUC1 and MUC16 aids tumor cell immune evasion (74). Additionally, research by Menon et al. showed that MUC1 and MUC16 inhibit Toll-like receptor (TLR)-mediated innate immune responses, reducing expression of inflammatory factors IL-6, IL-8, and TNF-α, potentially linked to tumor immune escape (75). For MUC1, Beatson et al. revealed that tumor cell surface tumor-specific MUC1 glycoforms (MUC1-ST) bind to Siglec-9, activating myeloid cells to secrete key factors influencing tumor progression, induce macrophages to transform into tumor-associated macrophage (TAM) phenotypes, and increase PD-L1 expression (25). MUC1’s regulatory effect on the immune environment includes inhibiting myeloid-derived suppressor cells (MDSCs) proliferation and immunosuppressive functions, where its absence in mice leads to increased MDSCs numbers and activity, promoting tumor growth, and MUC1 deletion also heightens MDSCs’ release of factors such as iNOS, ARG1, and TGF-β, further inhibiting T cell activity (76). Chan et al. found that soluble MUC1 inhibits T cell proliferation and function, likely by causing T cell arrest at the G(0)/G(1) phase of the cell cycle, thus impeding T cell activation and playing a key role in tumor immune evasion (77). MUC16’s role in the tumor immune microenvironment is equally significant. Zhang et al.’s analysis of 10,195 solid tumor patients showed that MUC16 mutations correlate with a higher tumor mutational burden (TMB) and neoantigen load, increased CD8A and PD-L1 expression in the tumor immune microenvironment, and are linked to improved patient survival and clinical response rates, highlighting their significance as genomic markers in evaluating response to immune checkpoint inhibitors (ICIs) therapy (78). TNFα and IFNγ enhance MUC16 expression in breast, endometrial, and ovarian cancer cells through the NFκB pathway, with this upregulation linked to immune regulatory factor activity, indicating that MUC16 modulation may benefit treatment (38).
In ovarian cancer immunoregulation research, MUC16 plays a critical role, as evidenced by studies. Gubbels et al. confirmed that MUC16, as a highly glycosylated molecule expressed on the surface of ovarian cancer cells, promotes tumor immune evasion by blocking the establishment of immune synapses between NK cells and tumor cells, providing a selective survival mechanism for tumor metastasis (35). Similarly, Belisle et al. demonstrated that MUC16 binds with Siglec-9 on NK cells, B cells, and monocytes, inhibiting their functions and further facilitating immune escape, growth, and metastasis of ovarian cancer (36). Wu et al. also observed in ovarian cancer research that MUC16 activates neutrophils’ Siglec-9 receptors, inducing inflammatory and immunosuppressive characteristics in these cells and weakening NK cells’ killing ability, collectively promoting tumor immune evasion (79). Further, Belisle et al. found in ovarian cancer that MUC16 binding with a specific CD16(+) CD56(dim) NK cell subset induces their transition from an active to a passive or suppressed state, further aiding immune evasion in ovarian cancer (80). Felder et al. showed that in ovarian cancer, MUC16 is crucial for maintaining cytotoxicity of natural killer and macrophage cells, with its absence reducing these immune cells’ cytotoxic effects and increasing tumor cell sensitivity to ADCC, thereby prolonging survival in a mouse model (81). Long-term monitoring of ovarian cancer patients using digital cytometry enhanced with gold nanoparticles revealed that elevated MUC16 levels on peripheral blood mononuclear cells (PBMCs) surface predict tumor relapse and metastasis risk, with MUC16 levels in ovarian cancer patients’ PBMCs higher than in healthy controls (82). According to Zhai et al., overexpression of MUC16 in ovarian cancer activates the PI3K/AKT pathway, promoting tumor cell proliferation and invasion while also enhancing anti-tumor immune responses, primarily manifested in dendritic cell maturation and CD8+ T cell activation (83). Patankar et al. revealed that ovarian tumor marker CA125 (MUC16), through its specifically expressed oligosaccharides, inhibits NK cell cytotoxicity, significantly reducing their cytotoxicity and playing a key role in the immune evasion mechanism of ovarian tumors (84). Additionally, Winkler et al. discovered a significant negative correlation between iNKT+/CD3+/CD161+ lymphocytes in ovarian cancer patient tumor tissues and serum CA125 concentration, suggesting a potential role of CA125 in modulating immune cells in the tumor microenvironment (85). Innovations in ovarian cancer treatment targeting MUC16 have shown potential. Koneru et al. developed 4H11-28z CAR T cells targeting the MUC16ecto antigen, enhancing these CAR T cells’ immune response and tumor elimination capabilities against ovarian cancer by co-expressing IL-12 (86). Similarly, Li et al. employed PD1-antiMUC16 dual-target CAR-T cells to treat epithelial ovarian cancer, demonstrating in vivo that these dual-target CAR-T cells exhibited greater killing efficacy compared to their single-target counterparts and significantly prolonged survival in a mouse model, underscoring MUC16’s central role in immunotherapy (87). Additionally, Crawford et al. demonstrated that the dual-specific antibody REGN4018, targeting highly expressed MUC16 in ovarian cancer, effectively activates T cells and kills MUC16-positive tumor cells in vitro, with preclinical animal model studies revealing that its combination with anti-PD-1 antibodies significantly enhances anti-tumor effects (88). Boland et al. found that in epithelial ovarian cancer patients receiving immune checkpoint inhibitor therapy, increased serum MUC16 levels correlated with poor immunotherapy outcomes, suggesting its prognostic indicator value (89). Similarly, Baert et al. indicated that in high-grade serous ovarian cancer patients’ serum samples, an increase in MUC16 was positively correlated with the rise of immunosuppressive factors like IL-10 and negatively correlated with overall survival rates, suggesting MUC16 as an adverse prognostic marker (90). Kline et al. revealed that MUC16 inhibits ADCC by binding with antibodies and suppressing Fc-γ receptor activation, with patients having low MUC16 levels in recurrent platinum-sensitive ovarian cancer showing improved survival following farletuzumab treatment compared to a control group (91).
The role of MUC1 in breast cancer immunity research, particularly in modulating tumor immune escape mechanisms, has garnered significant attention. Maeda et al. showed that in TNBC, MUC1-C upregulation recruits MYC and NF-κB p65 to the PD-L1 promoter, enhancing PD-L1 transcription and leading to immune escape and reduced patient survival; targeted intervention against MUC1-C not only suppresses PD-L1 expression but also enhances infiltration and activity of CD8+ T cells in tumors (92). Beatson et al. further revealed that MUC1-ST binding to Siglec-9 promotes differentiation of monocytes into tumor-associated macrophages (TAMs), which recruit neutrophils, inhibit T cell function, and promote tumor cell invasion, closely associated with poor prognosis in breast cancer patients (93). Zhou et al. focused on MUC28z CAR T cells targeting tMUC1 in TNBC treatment, with these cells specifically recognizing tMUC1 in most TNBC subtypes and exhibiting potent targeted cytotoxicity by increasing Granzyme B and interferon-gamma (IFN-γ) production (94). Yamashita et al. further confirmed MUC1-C as a core regulatory factor of the TNBC transcriptome, playing a significant role in inducing the immunosuppressive IFN-γ pathway, with MUC1-C expression correlated with upregulation of immunosuppressive effector factors like IDO1 and COX2/PTGS2, and associated with CD8+ T cell exhaustion and dysfunction in the tumor immune microenvironment (TIME) (95). Another study revealed that high expression of MUC1-C in TNBC is closely associated with the absence of tumor-infiltrating lymphocytes (TILs), and its synergistic action with PBRM1 enhances STAT1 and IRF1 expression in the interferon pathway, affecting T and NK cell functions and promoting tumor DNA damage resistance and immune escape (96). Grosso et al. confirmed that expression of secretory mucin 1 (MUC1/sec) in breast tumor cells can initiate T cell-dependent immune rejection responses and promote the recruitment of immune cells by increasing chemokine CCL2 secretion, aiding in anti-tumor therapy (97). Lin et al.’s study on MUC1 mRNA nanoparticle vaccines for TNBC treatment demonstrated their ability to activate CTLs against MUC1-expressing tumor cells and, when used alongside CTLA-4 antibodies, reduce Tregs, thereby enhancing CTL cytotoxicity and effectively modulating the tumor microenvironment to strengthen the immune response (98). Similarly, Liu et al. demonstrated the effectiveness of using nanoparticles (NPs) to deliver MUC1 mRNA vaccines to lymph node dendritic cells (DCs) in TNBC treatment, a method that activates specific T cells and combines with anti-CTLA-4 monoclonal antibodies to enhance immune response (99). In other gynecological tumors, Hu et al. showed that MUC16 mutations improve patient prognosis by enhancing cytotoxic T lymphocyte infiltration and anti-tumor immunity in the endometrial cancer microenvironment (100). Wang et al. observed in cervical cancer that overexpression in MUC16 mutation samples is closely associated with enhanced immune cell activity in the tumor microenvironment and improved prognosis (101). In lung cancer research, Bouillez et al. found that targeting MUC1-C in NSCLC enhances CD8+ TILs’ cytotoxicity against tumor cells and plays a crucial role in promoting PD-L1 induction, aiding tumor cells in evading immune surveillance. Additionally, reducing PD-L1 expression and increasing IFN-γ levels through targeting MUC1-C bolsters the immune system’s ability to recognize and destroy tumor cells, effectively countering tumor progression (102). Concurrently, MUC1-C activates the NF-κB/ZEB1 pathway to promote PD-L1 (CD274) expression while suppressing immune effector genes like TLR9 and IFNG, thus enhancing PD-L1 expression and inhibiting immune responses, closely associated with decreased patient survival (103). Wang et al. demonstrated that dual-target Tan CAR-T cells, targeting both MUC1 and PSCA in NSCLC and used in combination with anti-PD-1 antibody therapy, exhibit superior anti-tumor effects compared to single-target CAR-T cells, with their efficacy significantly enhanced when combined with anti-PD-1 treatment (104). In NSCLC treatment, Jiang et al. discovered that evodiamine, by downregulating MUC1-C protein expression, modulates PD-L1 expression, thereby effectively inhibiting tumor growth and inducing apoptosis, while enhancing CD8+ T cells’ effector function, with its combination with anti-PD-1 monoclonal antibodies significantly bolstering tumor control (105). In MUC16-related research, Patel et al. revealed that in lung adenocarcinoma, a matrix metalloproteinase-resistant variant of MUC16 may lead to tumor cell evasion of the immune system, reducing tumor-specific peptide presentation through HLA-A and HLA-B molecules, thereby promoting tumor immune escape (106). In gastrointestinal tumor research, studies on MUC1 and MUC16 are crucial in unveiling tumor immune evasion mechanisms and progression. Saeland et al. demonstrated that abnormal glycosylation of MUC1 in colon cancer, particularly the exposure of Tn and TF antigens, may promote tumor immune evasion through binding with MGL expressed on DCs and macrophages; additionally, MUC1’s correlation with the adverse prognostic marker Helix pomatia agglutinin (HPA) indicates significant interactions between MUC1 and immune regulatory factors (107). Zhang et al. observed in the colon cancer tumor microenvironment that MUC1 presence leads to the accumulation of Tregs, MDSCs, and TAMs; blocking the PD1/PD-L1 pathway reduces immune suppressive cells in the tumor microenvironment, enhancing T cell cytotoxic responses and inhibiting tumor growth (108). In colitis-associated colorectal cancer (CAC), Sheng et al. found that abnormal overexpression of MUC1 significantly exacerbates tumor progression, while inhibiting MUC1 expression can reduce inflammation and tumor progression, marked by increased CD8+ T lymphocytes and reduced macrophages in tumors (109). Monti et al. revealed that in pancreatic cancer, MUC1 alters cytokine profiles of monocyte-derived DCs, turning them into regulatory cells with high IL-10 (an immunosuppressive cytokine) and low IL-12 (an immune-promoting cytokine), thereby inhibiting DCs’ ability to activate Th1 type immune responses and promoting tumor immune evasion (110). Beatty et al. emphasized that in pancreatic cancer precursor lesion intraductal papillary mucinous neoplasm (IPMN), abnormal expression of MUC1 promotes specific immune responses, including IgG production and T cell infiltration (111). Research on the C-terminal of MUC16 in pancreatic cancer has shown a positive correlation with Foxp3 expression, aligning with serum CA125 levels and the proportion of circulating Tregs, where MUC16c’s activation of the IL-6 JAK2/STAT3 pathway enhances Foxp3 expression, thereby promoting Treg accumulation in tumor tissue (37). In pancreatic ductal adenocarcinoma models with KrasG12D and Trp53R172H mutations, research by Lakshmanan et al. demonstrated that MUC16 deletion significantly impedes tumor progression and metastasis, leading to prolonged survival, and influences the tumor microenvironment, possibly by modulating the expression of genes like Actg2, Myh11, and Pdlim3 (112). The study by Chirravuri-Venkata et al. in PDAC indicated that MUC16, in conjunction with the TP53 family, regulates tumor-stromal heterogeneity. MUC16’s impact transcends tumor cells, prominently modulating the tumor environment by guiding the differentiation of CAFs (66). In cholangiocarcinoma research, Zhang et al. revealed that the interaction between MUC1 and EGFR activates the EGFR/PI3K/Akt pathway, leading to the accumulation of Foxp3+ Tregs, aiding tumor immune evasion and thus promoting cholangiocarcinoma development and metastasis (113).
In melanoma-related research, MUC1 and MUC16 have demonstrated distinct roles in tumor progression and immune responses. Regarding MUC1, a study by Wang et al. in a melanoma mouse model found that overexpression of TRAF6 significantly enhanced MUC1-specific Th1 and Tc1 responses while reducing the proportion of Tregs, thereby ameliorating the immunosuppressive state and inhibiting tumor growth (114). In melanoma treatment, immunotolerance induced by MUC1 vaccination was reversed by anti-PD-L1 antibodies, a process involving an increased CD80/PD-L1 ratio, promotion of dendritic cell maturation, activation of Th1 and Tc1 cells, and inhibition of Treg cells (115). Similarly, Zhang et al. demonstrated that the co-administration of a MUC1-MBP vaccine with αPD1 antibodies in the B16-MUC1 melanoma model markedly improved anti-tumor efficacy compared to the vaccine alone, mainly by elevating CD8+T cell, Th1, and Tc1 activities, and diminishing the proportion of MDSCs in the tumor microenvironment (116). In research on MUC16, Wang et al. found that individuals with MUC16/CA125 mutations exhibited higher TMB and were associated with an immune-activated microenvironment, elevated interferon gamma (IFNγ) and T cell inflammatory signatures, and enhanced cytotoxic activity. Notably, in male patients, this mutation correlated with better prognosis and higher immune therapy response rates (117). Further genomic data analysis indicated that MUC16 mutations are prevalent in melanoma patients, with such variations closely linked to increased TMB and improved prognosis, potentially by activating immune pathways and enhancing T cell memory functions (118). Additional studies confirmed that mutations in MUC16 in melanoma are closely associated with higher TMB, increased overall survival, and positive responses to anti-CTLA-4 and anti-PD-1 therapies (119).
In studies of urological cancers, MUC1 and MUC16 have been recognized for their significant roles in modulating the immune microenvironment, influencing chemotherapy resistance, and promoting immunosuppression. In clear cell renal carcinoma research, abnormally high expression of MUC1 activates the complement system, colocalizes with the immune marker PTX3, and leads to an increase in M2-type macrophages and a decrease in CD8+ T cells, thus fostering an immunosuppressive microenvironment (120). In castration-resistant prostate cancer (CRPC), MUC1-C protein activation promotes an immunosuppressive environment through the Type II IFN-γ pathway and affects chromatin remodeling, with MUC1-C regulating IDO1, WARS, and PTGES expression, thereby metabolically suppressing the tumor microenvironment and aiding tumor survival and progression, as observed by Hagiwara et al. (121). Yamashita et al. indicated that elevated CA125 expression in bladder cancer is linked to a gemcitabine/cisplatin-resistant tumor microenvironment and decreased survival, associated with regulatory T cells and M2-type macrophages’ infiltration, suggesting a pivotal role in chemotherapy resistance (122). In oral squamous cell carcinoma, Lan et al. found that high expression of MUC1 promotes immune escape, while Porphyromonas gingivalis, by reducing MUC1 and the immunosuppressive factor CXCL17 expression, improves the tumor microenvironment, enhancing the immune system’s ability to clear tumors (123).
Figure 2 delineates MUC1 and MUC16’s regulatory roles regarding immune interactions in oncogenesis, elucidating their contributions to modulating immune responses, from suppression and tolerance to activation, and highlighting their potential as targets for therapeutic intervention.
Figure 2 The immunological impact of MUC1 and MUC16. The diagram illustrates the multidimensional functions of MUC1 and MUC16 in immunoregulation, extending from suppression to enhancement of immune functions, highlighting these mucins’ significance as biomarkers for therapeutic interventions.
The roles and potential of MUC1 and MUC16 in tumor immunotherapy research have garnered widespread attention, especially their application as biomarkers in various cancer treatment strategies. This highlights their potential impact on tumor immune escape mechanisms and therapeutic value.
In Posey et al.’s study, CAR T cells targeting the MUC1 Tn glycoform demonstrated significant efficacy against adenocarcinoma, effectively controlling tumor growth and specifically targeting abnormal MUC1 glycosylation in multiple cancer types (124). Additionally, Heukamp et al. demonstrated that clonal CTLs targeting specific epitopes of MUC1 can effectively target tumor cells overexpressing MUC1 without harming normal tissues, highlighting their potential application in cancer immunotherapy (125). Moreover, the integration of anti-tMUC1-CAR T cells with inhibitors targeting IDO1, COX1/2, and Gal-9 has provided a significant strategy to enhance the cytotoxicity of CAR T cell therapy against PDAC cells, addressing the challenge of immunological resistance in pancreatic cancer treatment (126). Hong et al. showed that altering MUC1 epitope structures significantly increases the immunogenicity of pancreatic cancer, stimulating more cytotoxic T cells (CTLs), and indicating potential in enhancing pancreatic cancer immunotherapy (127). Li et al. further demonstrated that shRNA@Fe3O4 magnetic nanoparticles targeting the MUC1-C gene effectively inhibit TNBC progression (128). Parallel to MUC1, the modulation of MUC16 expression has emerged as a key factor in augmenting the response of cancer cells to therapeutic interventions. Morgado et al. found that reducing MUC16 expression through high-dose PPARγ agonists can increase the sensitivity of breast and ovarian cancer tumors to chemotherapy and immune responses (129). Wang et al. developed a dual-specificity T cell engager-modified oncolytic adenovirus (OAd-MUC16-BiTE) targeting MUC16, activating cytotoxic T cells to effectively overcome the immunosuppressive environment in ovarian cancer and enhance therapeutic efficacy (130). In another significant advancement, Mun et al. developed MUC16-specific CAR T cells (4H11) capable of dual attacks against WT1-expressing tumor cells, particularly showing enhanced therapeutic effects in tumors with lower MUC16 expression (131). Chekmasova’s team developed a range of CARs aimed at the extracellular domain of MUC16 (MUC-CD), resulting in T cells equipped with these CARs showing marked cytotoxicity against MUC-CD, thus effectively managing ovarian cancer (132). Further contributing to ovarian cancer therapy, Yue et al. found that an oncolytic adenovirus driven by the MUC16 promoter specifically targets CA-125 positive ovarian cancer cells, offering a new strategy for ovarian cancer treatment (133). Feely et al. developed immunomagnetic nanoparticles targeting MUC16, which efficiently extract circulating tumor cells (CTCs) in liquid biopsies, leveraging the widespread expression of MUC16 in high-grade serous ovarian cancer to provide an effective targeting technique for ovarian cancer detection (134).
Kondo et al.’s clinical study demonstrated that treatment of unresectable or recurrent pancreatic cancer with MUC1 peptide-pulsed dendritic cells (MUC1-DC) and activated cytotoxic T lymphocytes (MUC1-CTL) induced immune responses, achieving complete remission in one patient and stability in five, with an extended average survival time to 9.8 months, without observing severe toxic reactions. This underscores the efficacy and potential of MUC1-based immunotherapeutic strategies in pancreatic cancer treatment (135). Subsequently, Gonzalez et al. noted that peripheral blood mononuclear cells (PBMCs) in ovarian cancer patients exhibit significantly higher CA125 binding compared to healthy donors, providing key insights for the development of new diagnostic markers for ovarian cancer (136). In a Phase I clinical trial, Koneru et al. investigated the safety and initial effectiveness of IL-12-secreting CAR T cells that target MUC-16(ecto+) in patients with recurrent ovarian cancer. The results indicated a reduction in tumor size and symptom relief among patients, alongside enhanced cellular activity in the tumor microenvironment (32).
Fang et al. developed a dual-specific antibody (BsAb) targeting MUC1 and CD3, effectively enhancing T cell activation, cytokine release, and cytotoxicity, significantly inhibiting the growth of MUC1-positive tumors in xenograft mouse models (137). Subsequently, Panchamoorthy et al. developed the monoclonal antibody 3D1 targeting the MUC1-C subunit, which exhibits high affinity and specificity for MUC1-C expressing cancer cells, and its monomethyl auristatin E (MMAE) conjugate demonstrated antitumor activity in diverse human cancer models, supporting its clinical potential for treating tumors with MUC1-C overexpression (138). Additionally, Gong et al. developed a new anti-MUC1 antibody with desialylation, enhancing NK cell-mediated ADCC, thus exhibiting increased anti-tumor activity against tumors expressing MUC1-Tn/STn antigens (139). Heuser et al. showed that the C595scFv-Fc-IL2 fusion protein, by binding to MUC1, activates NK cells and enhances their ability to kill MUC1-positive tumor cells, indicating positive implications for clinical treatment of MUC1-positive tumors (140). Concurrently, regarding MUC16, Nicolaides et al. focused on the role of MUC16 (CA125) ADCs, discovering that a specific anti-MUC16 ADC (NAV-001) is significantly effective in eliminating tumor cells, highlighting MUC16’s potential as a therapeutic target (141). Moreover, Garg et al. showed that Meso-TR3, by specifically binding to MUC16-positive tumor cells, significantly promotes tumor cell death, underscoring its therapeutic potential (142).
MUC1 and MUC16, as key biomarkers in gynecological tumors, particularly ovarian and breast cancers, have attracted significant attention for their roles and potential in tumor immunotherapy. In this context, Mony et al. showed that ovarian cancer models expressing MUC1, after anti-PD-L1 antibody treatment, exhibited increased T cell infiltration in tumors and extended survival, indicating MUC1’s potential in ovarian cancer immunotherapy (143). In breast cancer treatment research, MUC1 has emerged as a significant therapeutic target. Kelly et al. discovered that humanized TAB004 (hTAB004), targeting transformed MUC1 (tMUC1), shows notable efficacy in treating TNBC by binding and internalizing tMUC1, effectively inhibiting tumor volume growth and improving patient survival (144). Similarly, Kim et al. isolated specific antibodies against MUC1-C using phage display technology, exhibiting significant inhibition of TNBC cells (145). Isla Larrain et al. indicated that the IgG response to MUC1 in breast cancer patients might contribute to improved prognosis, suggesting that enhancing this natural immune response could be a viable therapeutic strategy (146). Concerning MUC16’s impact, Yeku et al. confirmed the significant cytotoxicity of MUC16 ectodomain-specific bispecific single-chain variable fragments (BiTEDs) against ovarian cancer cells in vitro, effectively delaying tumor progression in vivo, especially when combined with anti-angiogenic treatment, showing potential for extending survival (147). Additionally, Babeker’s team’s investigation into the fully human monoclonal antibody M16Ab in ovarian cancer immune PET imaging revealed that the 89Zr-DFO-M16Ab conjugate specifically targets MUC16-positive tumor cells, underscoring the potential of fully human antibodies in diagnostic imaging and radiopharmaceutical development (148). Rao et al. identified the oncogenic potential of N-glycosylation sites on the extracellular C-terminal part of MUC16 in ovarian cancer, showing that monoclonal antibodies targeting these sites significantly inhibit ovarian cancer cell invasion and growth of xenograft tumors with MUC16, all driven by an MGAT5-dependent mechanism (149). Furthermore, Marcos-Silva et al. developed the monoclonal antibody 5E11 against a specific peptide segment FNTTER of ovarian cancer MUC16, which specifically recognizes MUC16, leading to the design of a MUC16-based vaccine to stimulate an immune response (150). Stasenko et al. discovered that the high-affinity antibody 14D11 targeting Gal3 inhibits tumor growth in high-grade serous ovarian cancers expressing MUC16, offering a new therapeutic strategy for MUC16-positive cancers (151).
Studies in pancreatic cancer have revealed that MUC1 in both cancer cells and exosomes presents specific dynamic epitopes identifiable by the anti-MUC1 antibody SN-131, which binds distinctively to core 1 type O-glycans on MUC1, as opposed to core 2 types in normal cells, demonstrating the therapeutic potential of targeting these unique O-glycosylation areas on MUC1 in pancreatic cancer cells (152). Schettini et al. demonstrated that coupling anti-MUC1 monoclonal antibodies with CpG ODN significantly enhances the ADCC effect of NK cells, augmenting the immune system’s ability to clear tumor cells, and reducing tumor burden in a mouse model of pancreatic cancer, underscoring the significant role of MUC1 in regulating NK cell functions (153). Additionally, in pancreatic cancer studies on MUC16, Thomas et al. showed that the monoclonal antibody AR9.6 significantly inhibits the AKT and GSK3β pathways activated by MUC16, effectively reducing tumor growth and metastasis and highlighting AR9.6’s potential as a novel immunotherapeutic approach for MUC16-mediated therapy in pancreatic cancer (65). Concurrently, Shah et al. demonstrated that the ch5E6 chimeric antibody against MUC16-Cter displayed anti-tumor effects in pancreatic cancer and non-small cell lung cancer by disrupting tumor growth and signaling, inhibiting tumor cell proliferation, and its interaction with MUC16 and N-cadherin underscored its potential in targeting tumor invasiveness (154).
Silk et al. discovered that patients with colorectal adenomas and cancers had higher anti-MUC1 antibody levels in their blood compared to healthy controls, suggesting that anti-MUC1 immune responses may contribute to tumor development inhibition (155). Advancing the insights into MUC16’s impact on cancer, Akita et al. found that differential expression of the sialyl-Tn antigen on MUC16 can distinguish between ovarian cancer and endometriosis, emphasizing MUC16’s potential importance in ovarian cancer diagnosis and treatment (156). Additionally, Liu et al.’s phase I study assessed the safety and pharmacokinetics of the antibody-drug conjugate DMUC5754A targeting MUC16 in patients with platinum-resistant ovarian cancer (OC) and unresectable pancreatic cancer (PC), observing significant tumor growth inhibition responses in ovarian cancer patients with high MUC16 expression (33).
Given the significance of MUC16 in ovarian cancer treatment, the study of immunotherapies and clinical trials demands special attention. In the 1990s, Wagner et al.’s clinical research already demonstrated the effectiveness of the ACA125 vaccine in inducing immune responses against CA125 in patients with advanced ovarian cancer. In this study, nine patients developed specific immune responses to ACA125, resulting in improved progression-free survival (157). In the MIMOSA trial, led by Battaglia et al., assessed ovarian cancer patients’ response to abagovomab, a MUC16-mimetic antibody. Results showed that patients with stronger immune functions, indicated by the elevated levels and counts of IFN-γ-producing CD8+ T cells, had better responses and improved relapse-free survival (RFS), highlighting the importance of robust immune system functionality in responding to MUC16-targeted treatment (158). Liu et al. conducted a Phase I trial on DMUC4064A, targeting high MUC16 expression in platinum-resistant ovarian cancer. The study demonstrated the drug’s tolerability and initial efficacy, with a notable proportion of patients showing partial response or disease stabilization, suggesting its potential in modulating tumor immunity (159). Similarly, Wang et al.’s Phase II clinical trial showed that in patients with advanced axillary melanoma, apatinib combined with carrelizumab presented positive anti-tumor effects, and mutations in the MUC16 gene were associated with better survival outcomes in patients (160). In a Phase II clinical trial by Nicolaides et al., the effect of the tumor marker CA125 on the immune efficacy of amatuximab (a monoclonal antibody against mesothelin) was explored. Results indicated that patients with baseline CA125 levels below 57 U/mL showed better treatment responses, suggesting that therapeutic strategies should consider patients’ baseline CA125 levels (161).
Advancements in vaccine development for MUC1 and MUC16 demonstrate promising outcomes in recent studies. Zhou et al.’s construction of a MUC1 vaccine, combined with aluminum adjuvant and TLR7 agonist, showed significant efficacy in enhancing antibody production and CD8+ T cell immune response against MUC1 (162). Additionally, Panasiuk et al. demonstrated that recombinant chimeric norovirus-like particles (VLPs) combined with MF59 adjuvant effectively induced high-titer IgG antibodies targeting MUC1 in mice, which specifically recognize and target MUC1 on tumor cell surfaces, thereby enhancing immune recognition and clearance of tumors (163). Moreover, Fang et al. revealed that combining MUC1-MBP with BCG stimulates a dual immune response, enhancing Th1-type immunity and the cytotoxic function of MUC1-specific CTLs, while also boosting NK cell activity, effectively inhibiting the growth of MUC1-positive tumor cells (164). Mehrab Mohseni et al. demonstrated that MUC1, a tumor-associated antigen in breast cancer, triggers a robust immune response, marked by the production of anti-glycoprotein serum IgG, IgA, and IFNɣ, effectively mobilizing the immune system against breast cancer proliferation and invasion (165). In Liu et al.’s study, a MUC1 vaccine combined with TLR7 agonist demonstrated significant immune activation and anti-tumor effects in a mouse model of breast cancer, enhancing immune response against MUC1-expressing tumor cells (166). Complementing this, Zhang et al. developed a vaccine containing MUC1 antigen and TLR7 agonist, significantly enhancing the immune response against MUC1-expressing tumors, increasing antibody titers and T cell activity, indicating its potential in breast cancer treatment (167). In immunotherapy research for digestive tract tumors, MUC1’s role is similarly pivotal. Yu et al. demonstrated that modified MUC1 peptides, by enhancing HLA-A0201 mediated T-cell activation, improved the recognition and attack of wild-type MUC1 gastric cancer cells (168). Equally, Guo et al. highlighted the central role of MUC1 in colorectal cancer stem cell vaccine immunotherapy, impacting tumor growth and metastasis, particularly notable in enhancing NK cell toxicity and promoting anti-MUC1 antibody generation (169). Furthermore, in urological tumors, Vang et al. demonstrated, using a MUC1 transgenic mouse model, the potential of specific immune responses to MUC1 for bladder cancer treatment; they found that vaccination with MUC1-specific peptides stimulated a Th1-type cytokine environment and elicited specific T-cell responses to MUC1 (170). Concerning MUC16’s impact on ovarian cancer, Lu et al. have developed an mRNA vaccine targeting neoantigens in MUC16 in breast and ovarian cancers, combined with CD40L and MHC-I targeting domains to enhance dendritic cell antigen presentation efficacy, with computational models predicting that this vaccine could activate IFN-γ and CD8+ T cells, playing a key role in tumor immunotherapy (171). Reinartz et al. demonstrated that fusing IL-6 to an anti-CA125 antibody targeting MUC16 significantly enhanced specific humoral immune responses, effectively inducing the immune system’s response to the CA125 marker (172).
Recent clinical trials have highlighted MUC1 and MUC16’s significant potential as immunotherapy targets in advanced cancer treatment, particularly in various solid tumors. As evidenced by the study by Scheid et al., Tn-MUC1 was established as an efficacious immunotherapeutic target in non-metastatic castration-resistant prostate cancer patients, with the Tn-MUC1 DC vaccine inducing T-cell responses in Phase I/II clinical trials and significantly extending PSADT, indicative of decelerated cancer progression and highlighting the therapeutic promise of Tn-MUC1 in immunotherapy (173). Complementing these findings, innovative vaccine strategies have shown initial success in eliciting specific T-cell responses, contributing to the inhibition of cancer progression and improvement in survival rates. A specific clinical example, such as Tan et al.’s Phase I clinical trial, utilized an adenoviral vector vaccine Ad-sig-hMUC1/ecdCD40L targeting the tumor-associated antigen MUC1 for treating advanced adenocarcinoma. Post-vaccination, patients exhibited improved immune network connectivity, particularly those with stable disease, characterized by a notable increase in CD8 T cells and B cells, highlighting the vaccine’s efficacy in activating multiple branches of the immune system and its significant impact on cancer treatment (174). Similarly, in Gatti-Mays et al.’s Phase I clinical trial, adenovirus 5 vector vaccines ETBX-011, ETBX-051, and ETBX-061, targeting CEA, MUC1, and brachyury, were administered safely to advanced cancer patients, eliciting CD4+ or CD8+ T cell responses to at least one vaccine-encoded antigen in all patients, with 83% demonstrating significant specificity to MUC1 (175). Further, in the same team’s Phase I dose-escalation trial, the BN-CV301 vaccine, incorporating MUC1 and CEA, was shown to be safe and to elicit specific T-cell responses against these tumor antigens in most patients, with significant disease stabilization observed particularly in patients with KRAS-mutated gastrointestinal tumors undergoing anti-PD-L1 therapy, underscoring its potential in immunotherapy (176). Concurrently, Musselli et al. conducted a study on breast cancer patients vaccinated with MUC1 peptide segments conjugated to KLH, revealing that while the immune response to KLH was enhanced, the MUC1-specific T-cell response showed limitations in consistency and intensity (177). In contrast, the phase II ABCSG 34 clinical trial with the tecemotide vaccine targeting MUC-1 failed to demonstrate increased efficacy in the neoadjuvant treatment of breast cancer (178). Meanwhile, Schoen et al. revealed that MUC1 peptide vaccines elicited significant immune responses in a subset of adenoma patients, with 25% showing at least a twofold increase in MUC1 IgG levels after 12 weeks, correlating with reduced adenoma recurrence rates and suggesting MUC1’s role in immune surveillance for intestinal adenoma prevention (179). In advanced NSCLC, the TG4010 vaccine, targeting MUC1 and interleukin-2, indicated enhanced progression-free survival (PFS) in the phase 2b/3 TIME trial, especially when used alongside first-line chemotherapy (180).
Tables 3 and 4 aggregate the contributions of MUC1 and MUC16 to immunological processes in oncogenesis, alongside their genetic and therapeutic implications. Figure 3, corresponding with Tables 3 and 4, systematically outlines a variety of immunotherapy methods targeting MUC1 and MUC16, including direct inhibitors, monoclonal antibodies, vaccination strategies, and cellular treatments, thus clarifying their application in cancer therapy. Clinical trials pertinent to these antigens, encompassing patient cohorts and therapeutic outcomes, are detailed in Table 5, offering a comprehensive view of current advancements in immunotherapy research.
Figure 3 Schematic of immunotherapies targeting MUC1/MUC16. The figure provides a systematic overview of treatments engaging MUC1 and MUC16, including inhibitors, monoclonal antibodies, vaccines, and adoptive therapies, detailing their role in cancer immunomodulation.
In the pursuit of furthering MUC1 and MUC16 research, the current understanding of their role in tumor biology and the tumor microenvironment presents a foundation for future exploration. The refinement of therapies such as vaccines, ADCs, and CAR-T cell treatments, alongside the elucidation of these proteins in therapeutic resistance, constitutes a critical research trajectory. The integration of precision medicine, leveraging genomic and proteomic technologies, is essential for tailoring patient-specific therapies. Furthermore, innovative clinical trial designs are imperative for the swift evaluation and enhancement of emerging treatments. As new strategies are introduced, their long-term safety and efficacy must be rigorously assessed, underscoring the need for a multidisciplinary approach in advancing cancer treatment.
XC: Formal analysis, Methodology, Writing – original draft, Writing – review & editing. IS: Data curation, Validation, Writing – review & editing. MY: Investigation, Resources, Writing – review & editing. JT: Investigation, Resources, Supervision, Writing – review & editing. XY: Conceptualization, Funding acquisition, Supervision, Writing – review & editing.
The author(s) declare financial support was received for the research, authorship, and/or publication of this article. This research received financial support from the State Key Program of the National Natural Science Foundation of China (Grant No. 82130092).
The authors declare that the research was conducted in the absence of any commercial or financial relationships that could be construed as a potential conflict of interest.
All claims expressed in this article are solely those of the authors and do not necessarily represent those of their affiliated organizations, or those of the publisher, the editors and the reviewers. Any product that may be evaluated in this article, or claim that may be made by its manufacturer, is not guaranteed or endorsed by the publisher.
MUC, Mucin; MUC1, Mucin 1; MUC16, Mucin 16; CA125, Cancer Antigen 125; MUC1-C, MUC1 C-terminal; CAR, Chimeric Antigen Receptor; ADCs, Antibody-Drug Conjugates; PRRs, Pattern Recognition Receptors; EMT, Epithelial-Mesenchymal Transition; ADCC, Antibody-Dependent Cellular Cytotoxicity; CTLs, Cytotoxic T Lymphocytes; DCs, Dendritic Cells; MDSCs, Myeloid-Derived Suppressor Cells; PBMCs, Peripheral Blood Mononuclear Cells; ICIs, Immune Checkpoint Inhibitors; TIME, Tumor Immune Microenvironment; TILs, Tumor-Infiltrating Lymphocytes; TMB, Tumor Mutational Burden; BsAb, Bispecific Antibody; BiTEDs, Bispecific Single-Chain Variable Fragments
1. Baldus SE, Engelmann K, Hanisch FG. MUC1 and the MUCs: a family of human mucins with impact in cancer biology. Crit Rev Clin Lab Sci (2004) 41(2):189–231. doi: 10.1080/10408360490452040
2. Malaker SA, Riley NM, Shon DJ, Pedram K, Krishnan V, Dorigo O, et al. Revealing the human mucinome. Nat Commun (2022) 13(1):3542. doi: 10.1038/s41467-022-31062-4
3. Hattrup CL, Gendler SJ. Structure and function of the cell surface (tethered) mucins. Annu Rev Physiol (2008) 70:431–57. doi: 10.1146/annurev.physiol.70.113006.100659
4. Kufe DW. Mucins in cancer: function, prognosis and therapy. Nat Rev Canc (2009) 9(12):874–85. doi: 10.1038/nrc2761
5. Bafna S, Kaur S, Batra SK. Membrane-bound mucins: the mechanistic basis for alterations in the growth and survival of cancer cells. Oncogene. (2010) 29(20):2893–904. doi: 10.1038/onc.2010.87
6. Remmers N, Anderson JM, Linde EM, DiMaio DJ, Lazenby AJ, Wandall HH, et al. Aberrant expression of mucin core proteins and o-linked glycans associated with progression of pancreatic cancer. Clin Cancer Res (2013) 19(8):1981–93. doi: 10.1158/1078-0432.CCR-12-2662
7. Bhatia R, Gautam SK, Cannon A, Thompson C, Hall BR, Aithal A, et al. Cancer-associated mucins: role in immune modulation and metastasis. Cancer Metastasis Rev (2019) 38(1-2):223–36. doi: 10.1007/s10555-018-09775-0
8. Singh R, Bandyopadhyay D. MUC1: a target molecule for cancer therapy. Cancer Biol Ther (2007) 6(4):481–6. doi: 10.4161/cbt.6.4.4201
9. Macao B, Johansson DG, Hansson GC, Hard T. Autoproteolysis coupled to protein folding in the SEA domain of the membrane-bound MUC1 mucin. Nat Struct Mol Biol (2006) 13(1):71–6. doi: 10.1038/nsmb1035
10. Li W, Zhang N, Jin C, Long MD, Rajabi H, Yasumizu Y, et al. MUC1-C drives stemness in progression of colitis to colorectal cancer. JCI Insight (2020) 5(12):e137112 . doi: 10.1172/jci.insight.137112
11. Rajabi H, Hiraki M, Kufe D. MUC1-C activates polycomb repressive complexes and downregulates tumor suppressor genes in human cancer cells. Oncogene. (2018) 37(16):2079–88. doi: 10.1038/s41388-017-0096-9
12. Siroy A, Abdul-Karim FW, Miedler J, Fong N, Fu P, Gilmore H, et al. MUC1 is expressed at high frequency in early-stage basal-like triple-negative breast cancer. Hum Pathol (2013) 44(10):2159–66. doi: 10.1016/j.humpath.2013.04.010
13. Chen W, Zhang Z, Zhang S, Zhu P, Ko JK, Yung KK. MUC1: structure, function, and clinic application in epithelial cancers. Int J Mol Sci (2021) 22(12):6567. doi: 10.3390/ijms22126567
14. Kufe DW. MUC1-C in chronic inflammation and carcinogenesis; emergence as a target for cancer treatment. Carcinogenesis. (2020) 41(9):1173–83. doi: 10.1093/carcin/bgaa082
15. Hagiwara M, Yasumizu Y, Yamashita N, Rajabi H, Fushimi A, Long MD, et al. MUC1-C activates the BAF (mSWI/SNF) complex in prostate cancer stem cells. Cancer Res (2021) 81(4):1111–22. doi: 10.1158/0008-5472.CAN-20-2588
16. Ren J, Bharti A, Raina D, Chen W, Ahmad R, Kufe D. MUC1 oncoprotein is targeted to mitochondria by heregulin-induced activation of c-Src and the molecular chaperone HSP90. Oncogene. (2006) 25(1):20–31. doi: 10.1038/sj.onc.1209012
17. Bose M, Sanders A, De C, Zhou R, Lala P, Shwartz S, et al. Targeting tumor-associated MUC1 overcomes anoikis-resistance in pancreatic cancer. Transl Res (2023) 253:41–56. doi: 10.1016/j.trsl.2022.08.010
18. Jin W, Liao X, Lv Y, Pang Z, Wang Y, Li Q, et al. MUC1 induces acquired chemoresistance by upregulating ABCB1 in EGFR-dependent manner. Cell Death Dis (2017) 8(8):e2980. doi: 10.1038/cddis.2017.378
19. Lv Y, Cang W, Li Q, Liao X, Zhan M, Deng H, et al. Erlotinib overcomes paclitaxel-resistant cancer stem cells by blocking the EGFR-CREB/GRbeta-IL-6 axis in MUC1-positive cervical cancer. Oncogenesis. (2019) 8(12):70. doi: 10.1038/s41389-019-0179-2
20. Kufe DW. MUC1-C oncoprotein as a target in breast cancer: activation of signaling pathways and therapeutic approaches. Oncogene. (2013) 32(9):1073–81. doi: 10.1038/onc.2012.158
21. Ren J, Agata N, Chen D, Li Y, Yu WH, Huang L, et al. Human MUC1 carcinoma-associated protein confers resistance to genotoxic anticancer agents. Cancer Cell (2004) 5(2):163–75. doi: 10.1016/S1535-6108(04)00020-0
22. Roy LD, Sahraei M, Subramani DB, Besmer D, Nath S, Tinder TL, et al. MUC1 enhances invasiveness of pancreatic cancer cells by inducing epithelial to mesenchymal transition. Oncogene. (2011) 30(12):1449–59. doi: 10.1038/onc.2010.526
23. Rajabi H, Tagde A, Alam M, Bouillez A, Pitroda S, Suzuki Y, et al. DNA methylation by DNMT1 and DNMT3b methyltransferases is driven by the MUC1-C oncoprotein in human carcinoma cells. Oncogene. (2016) 35(50):6439–45. doi: 10.1038/onc.2016.180
24. Hiraki M, Maeda T, Bouillez A, Alam M, Tagde A, Hinohara K, et al. MUC1-C activates BMI1 in human cancer cells. Oncogene. (2017) 36(20):2791–801. doi: 10.1038/onc.2016.439
25. Beatson R, Tajadura-Ortega V, Achkova D, Picco G, Tsourouktsoglou TD, Klausing S, et al. The mucin MUC1 modulates the tumor immunological microenvironment through engagement of the lectin Siglec-9. Nat Immunol (2016) 17(11):1273–81. doi: 10.1038/ni.3552
26. Vuento MH, Stenman UH, Pirhonen JP, Makinen JI, Laippala PJ, Salmi TA. Significance of a single CA 125 assay combined with ultrasound in the early detection of ovarian and endometrial cancer. Gynecol Oncol (1997) 64(1):141–6. doi: 10.1006/gyno.1996.4545
27. Chao A, Tang YH, Lai CH, Chang CJ, Chang SC, Wu TI, et al. Potential of an age-stratified CA125 cut-off value to improve the prognostic classification of patients with endometrial cancer. Gynecol Oncol (2013) 129(3):500–4. doi: 10.1016/j.ygyno.2013.02.032
28. Xiang X, Feng M, Felder M, Connor JP, Man YG, Patankar MS, et al. HN125: A novel immunoadhesin targeting MUC16 with potential for cancer therapy. J Canc (2011) 2:280–91. doi: 10.7150/jca.2.280
29. Davies JR, Kirkham S, Svitacheva N, Thornton DJ, Carlstedt I. MUC16 is produced in tracheal surface epithelium and submucosal glands and is present in secretions from normal human airway and cultured bronchial epithelial cells. Int J Biochem Cell Biol (2007) 39(10):1943–54. doi: 10.1016/j.biocel.2007.05.013
30. Gipson IK, Spurr-Michaud S, Tisdale A, Menon BB. Comparison of the transmembrane mucins MUC1 and MUC16 in epithelial barrier function. PloS One (2014) 9(6):e100393. doi: 10.1371/journal.pone.0100393
31. Mylonas I, Makovitzky J, Richter DU, Jeschke U, Briese V, Friese K. Immunohistochemical expression of the tumour marker CA-125 in normal, hyperplastic and Malignant endometrial tissue. Anticancer Res (2003) 23(2A):1075–80.
32. Koneru M, O'Cearbhaill R, Pendharkar S, Spriggs DR, Brentjens RJ. A phase I clinical trial of adoptive T cell therapy using IL-12 secreting MUC-16(ecto) directed chimeric antigen receptors for recurrent ovarian cancer. J Transl Med (2015) 13:102. doi: 10.1186/s12967-015-0460-x
33. Liu JF, Moore KN, Birrer MJ, Berlin S, Matulonis UA, Infante JR, et al. Phase I study of safety and pharmacokinetics of the anti-MUC16 antibody-drug conjugate DMUC5754A in patients with platinum-resistant ovarian cancer or unresectable pancreatic cancer. Ann Oncol (2016) 27(11):2124–30. doi: 10.1093/annonc/mdw401
34. Chauhan SC, Singh AP, Ruiz F, Johansson SL, Jain M, Smith LM, et al. Aberrant expression of MUC4 in ovarian carcinoma: diagnostic significance alone and in combination with MUC1 and MUC16 (CA125). Mod Pathol (2006) 19(10):1386–94. doi: 10.1038/modpathol.3800646
35. Gubbels JA, Felder M, Horibata S, Belisle JA, Kapur A, Holden H, et al. MUC16 provides immune protection by inhibiting synapse formation between NK and ovarian tumor cells. Mol Canc (2010) 9:11. doi: 10.1186/1476-4598-9-11
36. Belisle JA, Horibata S, Jennifer GA, Petrie S, Kapur A, Andre S, et al. Identification of Siglec-9 as the receptor for MUC16 on human NK cells, B cells, and monocytes. Mol Canc (2010) 9:118. doi: 10.1186/1476-4598-9-118
37. Fan K, Yang C, Fan Z, Huang Q, Zhang Y, Cheng H, et al. MUC16 C terminal-induced secretion of tumor-derived IL-6 contributes to tumor-associated Treg enrichment in pancreatic cancer. Cancer Lett (2018) 418:167–75. doi: 10.1016/j.canlet.2018.01.017
38. Morgado M, Sutton MN, Simmons M, Warren CR, Lu Z, Constantinou PE, et al. Tumor necrosis factor-alpha and interferon-gamma stimulate MUC16 (CA125) expression in breast, endometrial and ovarian cancers through NFkappaB. Oncotarget. (2016) 7(12):14871–84. doi: 10.18632/oncotarget.7652
39. Wu YM, Nowack DD, Omenn GS, Haab BB. Mucin glycosylation is altered by pro-inflammatory signaling in pancreatic-cancer cells. J Proteome Res (2009) 8(4):1876–86. doi: 10.1021/pr8008379
40. Ma Q, Song J, Wang S, He N. MUC1 regulates AKT signaling pathway by upregulating EGFR expression in ovarian cancer cells. Pathol Res Pract (2021) 224:153509. doi: 10.1016/j.prp.2021.153509
41. Zong ZH, Du YP, Guan X, Chen S, Zhao Y. CircWHSC1 promotes ovarian cancer progression by regulating MUC1 and hTERT through sponging miR-145 and miR-1182. J Exp Clin Cancer Res (2019) 38(1):437. doi: 10.1186/s13046-019-1437-z
42. Liu J, Li L, Luo N, Liu Q, Liu L, Chen D, et al. Inflammatory signals induce MUC16 expression in ovarian cancer cells via NF-kappaB activation. Exp Ther Med (2021) 21(2):163. doi: 10.3892/etm.2020.9594
43. Wang F, Zhang Q, Zhang H, Qiao X, Zhang X, Zhang K, et al. MUC16 promotes EOC proliferation by regulating GLUT1 expression. J Cell Mol Med (2021) 25(6):3031–40. doi: 10.1111/jcmm.16345
44. Huo Q, Xu C, Shao Y, Yu Q, Huang L, Liu Y, et al. Free CA125 promotes ovarian cancer cell migration and tumor metastasis by binding Mesothelin to reduce DKK1 expression and activate the SGK3/FOXO3 pathway. Int J Biol Sci (2021) 17(2):574–88. doi: 10.7150/ijbs.52097
45. Gubbels JA, Belisle J, Onda M, Rancourt C, Migneault M, Ho M, et al. Mesothelin-MUC16 binding is a high affinity, N-glycan dependent interaction that facilitates peritoneal metastasis of ovarian tumors. Mol Canc (2006) 5(1):50. doi: 10.1186/1476-4598-5-50
46. Matte I, Lane D, Boivin M, Rancourt C, Piche A. MUC16 mucin (CA125) attenuates TRAIL-induced apoptosis by decreasing TRAIL receptor R2 expression and increasing c-FLIP expression. BMC Canc (2014) 14:234. doi: 10.1186/1471-2407-14-234
47. Woo JK, Choi Y, Oh SH, Jeong JH, Choi DH, Seo HS, et al. Mucin 1 enhances the tumor angiogenic response by activation of the AKT signaling pathway. Oncogene. (2012) 31(17):2187–98. doi: 10.1038/onc.2011.410
48. Li Q, Chu Y, Li S, Yu L, Deng H, Liao C, et al. The oncoprotein MUC1 facilitates breast cancer progression by promoting Pink1-dependent mitophagy via ATAD3A destabilization. Cell Death Dis (2022) 13(10):899. doi: 10.1038/s41419-022-05345-z
49. Raina D, Uchida Y, Kharbanda A, Rajabi H, Panchamoorthy G, Jin C, et al. Targeting the MUC1-C oncoprotein downregulates HER2 activation and abrogates trastuzumab resistance in breast cancer cells. Oncogene. (2014) 33(26):3422–31. doi: 10.1038/onc.2013.308
50. Xi X, Wang J, Qin Y, Huang W, You Y, Zhan J. Glycosylated modification of MUC1 maybe a new target to promote drug sensitivity and efficacy for breast cancer chemotherapy. Cell Death Dis (2022) 13(8):708. doi: 10.1038/s41419-022-05110-2
51. Yamashita N, Fushimi A, Morimoto Y, Bhattacharya A, Hagiwara M, Yamamoto M, et al. Targeting MUC1-C suppresses chronic activation of cytosolic nucleotide receptors and STING in triple-negative breast cancer. Cancers (Basel) (2022) 14(11):2580. doi: 10.3390/cancers14112580
52. Chaudhary S, Appadurai MI, Maurya SK, Nallasamy P, Marimuthu S, Shah A, et al. MUC16 promotes triple-negative breast cancer lung metastasis by modulating RNA-binding protein ELAVL1/HUR. Breast Cancer Res (2023) 25(1):25. doi: 10.1186/s13058-023-01630-7
53. Lakshmanan I, Ponnusamy MP, Das S, Chakraborty S, Haridas D, Mukhopadhyay P, et al. MUC16 induced rapid G2/M transition via interactions with JAK2 for increased proliferation and anti-apoptosis in breast cancer cells. Oncogene. (2012) 31(7):805–17. doi: 10.1038/onc.2011.297
54. Kietzman WB, Graham GT, Ory V, Sharif GM, Kushner MH, Gallanis GT, et al. Short- and long-term effects of CDK4/6 inhibition on early-stage breast cancer. Mol Cancer Ther (2019) 18(12):2220–32. doi: 10.1158/1535-7163.MCT-19-0231
55. Raina D, Kosugi M, Ahmad R, Panchamoorthy G, Rajabi H, Alam M, et al. Dependence on the MUC1-C oncoprotein in non-small cell lung cancer cells. Mol Cancer Ther (2011) 10(5):806–16. doi: 10.1158/1535-7163.MCT-10-1050
56. Lakshmanan I, Salfity S, Seshacharyulu P, Rachagani S, Thomas A, Das S, et al. MUC16 regulates TSPYL5 for lung cancer cell growth and chemoresistance by suppressing p53. Clin Cancer Res (2017) 23(14):3906–17. doi: 10.1158/1078-0432.CCR-16-2530
57. Lei Y, Zang R, Lu Z, Zhang G, Huang J, Liu C, et al. ERO1L promotes IL6/sIL6R signaling and regulates MUC16 expression to promote CA125 secretion and the metastasis of lung cancer cells. Cell Death Dis (2020) 11(10):853. doi: 10.1038/s41419-020-03067-8
58. Kanwal M, Ding XJ, Song X, Zhou GB, Cao Y. MUC16 overexpression induced by gene mutations promotes lung cancer cell growth and invasion. Oncotarget. (2018) 9(15):12226–39. doi: 10.18632/oncotarget.24203
59. Morimoto Y, Yamashita N, Hirose H, Fushimi A, Haratake N, Daimon T, et al. MUC1-C is necessary for SHP2 activation and BRAF inhibitor resistance in BRAF(V600E) mutant colorectal cancer. Cancer Lett (2023) 559:216116. doi: 10.1016/j.canlet.2023.216116
60. Huang Y, Huang X, Zeng J, Lin J. Knockdown of MUC16 (CA125) enhances the migration and invasion of hepatocellular carcinoma cells. Front Oncol (2021) 11:667669. doi: 10.3389/fonc.2021.667669
61. Shukla SK, Purohit V, Mehla K, Gunda V, Chaika NV, Vernucci E, et al. MUC1 and HIF-1alpha signaling crosstalk induces anabolic glucose metabolism to impart gemcitabine resistance to pancreatic cancer. Cancer Cell (2017) 32(1):71–87.e7. doi: 10.1016/j.ccell.2017.06.004
62. Behrens ME, Grandgenett PM, Bailey JM, Singh PK, Yi CH, Yu F, et al. The reactive tumor microenvironment: MUC1 signaling directly reprograms transcription of CTGF. Oncogene. (2010) 29(42):5667–77. doi: 10.1038/onc.2010.327
63. Jeong SJ, Kim JH, Lim BJ, Yoon I, Song JA, Moon HS, et al. Inhibition of MUC1 biosynthesis via threonyl-tRNA synthetase suppresses pancreatic cancer cell migration. Exp Mol Med (2018) 50(1):e424. doi: 10.1038/emm.2017.231
64. Bose M, Grover P, Sanders AJ, Zhou R, Ahmad M, Shwartz S, et al. Overexpression of MUC1 induces non-canonical TGF-beta signaling in pancreatic ductal adenocarcinoma. Front Cell Dev Biol (2022) 10:821875. doi: 10.3389/fcell.2022.821875
65. Thomas D, Sagar S, Liu X, Lee HR, Grunkemeyer JA, Grandgenett PM, et al. Isoforms of MUC16 activate oncogenic signaling through EGF receptors to enhance the progression of pancreatic cancer. Mol Ther (2021) 29(4):1557–71. doi: 10.1016/j.ymthe.2020.12.029
66. Chirravuri-Venkata R, Dam V, Nimmakayala RK, Alsafwani ZW, Bhyravbhatla N, Lakshmanan I, et al. MUC16 and TP53 family co-regulate tumor-stromal heterogeneity in pancreatic adenocarcinoma. Front Oncol (2023) 13:1073820. doi: 10.3389/fonc.2023.1073820
67. Marimuthu S, Lakshmanan I, Muniyan S, Gautam SK, Nimmakayala RK, Rauth S, et al. MUC16 promotes liver metastasis of pancreatic ductal adenocarcinoma by upregulating NRP2-associated cell adhesion. Mol Cancer Res (2022) 20(8):1208–21. doi: 10.1158/1541-7786.MCR-21-0888
68. Rajesh C, Sagar S, Rathinavel AK, Chemparathy DT, Peng XL, Yeh JJ, et al. Truncated O-Glycan-Bearing MUC16 Enhances Pancreatic Cancer Cells Aggressiveness via alpha4beta1 Integrin Complexes and FAK Signaling. Int J Mol Sci (2022) 23(10):5459. doi: 10.3390/ijms23105459
69. Muniyan S, Haridas D, Chugh S, Rachagani S, Lakshmanan I, Gupta S, et al. MUC16 contributes to the metastasis of pancreatic ductal adenocarcinoma through focal adhesion mediated signaling mechanism. Genes Cancer (2016) 7(3-4):110–24. doi: 10.18632/genesandcancer.104
70. Shimizu A, Hirono S, Tani M, Kawai M, Okada K, Miyazawa M, et al. Coexpression of MUC16 and mesothelin is related to the invasion process in pancreatic ductal adenocarcinoma. Cancer Sci (2012) 103(4):739–46. doi: 10.1111/j.1349-7006.2012.02214.x
71. Fan K, Zhang D, Li M, Shen S, Wang J, Ni X, et al. Carboxyl-terminal polypeptide fragment of MUC16 combing stathmin1 promotes gallbladder cancer cell migration and invasion. Med Oncol (2020) 37(12):114. doi: 10.1007/s12032-020-01438-x
72. Deng X, Jiang P, Chen J, Li J, Li D, He Y, et al. GATA6 promotes epithelial-mesenchymal transition and metastasis through MUC1/beta-catenin pathway in cholangiocarcinoma. Cell Death Dis (2020) 11(10):860. doi: 10.1038/s41419-020-03070-z
73. Shigeta K, Hasegawa M, Kikuchi E, Yasumizu Y, Kosaka T, Mizuno R, et al. Role of the MUC1-C oncoprotein in the acquisition of cisplatin resistance by urothelial carcinoma. Cancer Sci (2020) 111(10):3639–52. doi: 10.1111/cas.14574
74. Madsen CB, Lavrsen K, Steentoft C, Vester-Christensen MB, Clausen H, Wandall HH, et al. Glycan elongation beyond the mucin associated Tn antigen protects tumor cells from immune-mediated killing. PloS One (2013) 8(9):e72413. doi: 10.1371/journal.pone.0072413
75. Menon BB, Kaiser-Marko C, Spurr-Michaud S, Tisdale AS, Gipson IK. Suppression of Toll-like receptor-mediated innate immune responses at the ocular surface by the membrane-associated mucins MUC1 and MUC16. Mucosal Immunol (2015) 8(5):1000–8. doi: 10.1038/mi.2014.127
76. Sahraei M, Bose M, Sanders JA, De C, DasRoy L, Nath S, et al. Repression of MUC1 promotes expansion and suppressive function of myeloid-derived suppressor cells in pancreatic and breast cancer murine models. Int J Mol Sci (2021) 22(11):5587. doi: 10.3390/ijms22115587
77. Chan AK, Lockhart DC, von Bernstorff W, Spanjaard RA, Joo HG, Eberlein TJ, et al. Soluble MUC1 secreted by human epithelial cancer cells mediates immune suppression by blocking T-cell activation. Int J Canc (1999) 82(5):721–6. doi: 10.1002/(SICI)1097-0215(19990827)82:5<721::AID-IJC16>3.0.CO;2-N
78. Zhang L, Han X, Shi Y. Association of MUC16 mutation with response to immune checkpoint inhibitors in solid tumors. JAMA Netw Open (2020) 3(8):e2013201.
79. Wu Y, Liu Q, Xie Y, Zhu J, Zhang S, Ge Y, et al. MUC16 stimulates neutrophils to an inflammatory and immunosuppressive phenotype in ovarian cancer. J Ovarian Res (2023) 16(1):181. doi: 10.1186/s13048-023-01207-0
80. Belisle JA, Gubbels JA, Raphael CA, Migneault M, Rancourt C, Connor JP, et al. Peritoneal natural killer cells from epithelial ovarian cancer patients show an altered phenotype and bind to the tumour marker MUC16 (CA125). Immunology. (2007) 122(3):418–29. doi: 10.1111/j.1365-2567.2007.02660.x
81. Felder M, Kapur A, Rakhmilevich AL, Qu X, Sondel PM, Gillies SD, et al. MUC16 suppresses human and murine innate immune responses. Gynecol Oncol (2019) 152(3):618–28. doi: 10.1016/j.ygyno.2018.12.023
82. Jeong S, Gonzalez G, Ho A, Nowell N, Austin LA, Hoballah J, et al. Plasmonic nanoparticle-based digital cytometry to quantify MUC16 binding on the surface of leukocytes in ovarian cancer. ACS Sens (2020) 5(9):2772–82. doi: 10.1021/acssensors.0c00567
83. Zhai Y, Lu Q, Lou T, Cao G, Wang S, Zhang Z. MUC16 affects the biological functions of ovarian cancer cells and induces an antitumor immune response by activating dendritic cells. Ann Transl Med (2020) 8(22):1494. doi: 10.21037/atm-20-6388
84. Patankar MS, Jing Y, Morrison JC, Belisle JA, Lattanzio FA, Deng Y, et al. Potent suppression of natural killer cell response mediated by the ovarian tumor marker CA125. Gynecol Oncol (2005) 99(3):704–13. doi: 10.1016/j.ygyno.2005.07.030
85. Winkler I, Wos J, Bojarska-Junak A, Semczuk A, Rechberger T, Baranowski W, et al. An association of iNKT+/CD3+/CD161+ lymphocytes in ovarian cancer tissue with CA125 serum concentration. Immunobiology. (2020) 225(6):152010. doi: 10.1016/j.imbio.2020.152010
86. Koneru M, Purdon TJ, Spriggs D, Koneru S, Brentjens RJ. IL-12 secreting tumor-targeted chimeric antigen receptor T cells eradicate ovarian tumors in vivo. Oncoimmunology (2015) 4(3):e994446. doi: 10.4161/2162402X.2014.994446
87. Li T, Wang J. Therapeutic effect of dual CAR-T targeting PDL1 and MUC16 antigens on ovarian cancer cells in mice. BMC Canc (2020) 20(1):678. doi: 10.1186/s12885-020-07180-x
88. Crawford A, Haber L, Kelly MP, Vazzana K, Canova L, Ram P, et al. A Mucin 16 bispecific T cell-engaging antibody for the treatment of ovarian cancer. Sci Transl Med (2019) 11(497). doi: 10.1126/scitranslmed.aau7534
89. Boland JL, Zhou Q, Iasonos AE, O'Cearbhaill RE, Konner J, Callahan M, et al. Utility of serum CA-125 monitoring in patients with ovarian cancer undergoing immune checkpoint inhibitor therapy. Gynecol Oncol (2020) 158(2):303–8. doi: 10.1016/j.ygyno.2020.04.710
90. Baert T, Van Camp J, Vanbrabant L, Busschaert P, Laenen A, Han S, et al. Influence of CA125, platelet count and neutrophil to lymphocyte ratio on the immune system of ovarian cancer patients. Gynecol Oncol (2018) 150(1):31–7. doi: 10.1016/j.ygyno.2018.05.004
91. Kline JB, Kennedy RP, Albone E, Chao Q, Fernando S, McDonough JM, et al. Tumor antigen CA125 suppresses antibody-dependent cellular cytotoxicity (ADCC) via direct antibody binding and suppressed Fc-gamma receptor engagement. Oncotarget. (2017) 8(32):52045–60. doi: 10.18632/oncotarget.19090
92. Maeda T, Hiraki M, Jin C, Rajabi H, Tagde A, Alam M, et al. MUC1-C induces PD-L1 and immune evasion in triple-negative breast cancer. Cancer Res (2018) 78(1):205–15. doi: 10.1158/0008-5472.CAN-17-1636
93. Beatson R, Graham R, Grundland Freile F, Cozzetto D, Kannambath S, Pfeifer E, et al. Cancer-associated hypersialylated MUC1 drives the differentiation of human monocytes into macrophages with a pathogenic phenotype. Commun Biol (2020) 3(1):644. doi: 10.1038/s42003-020-01359-5
94. Zhou R, Yazdanifar M, Roy LD, Whilding LM, Gavrill A, Maher J, et al. CAR T cells targeting the tumor MUC1 glycoprotein reduce triple-negative breast cancer growth. Front Immunol (2019) 10:1149. doi: 10.3389/fimmu.2019.01149
95. Yamashita N, Long M, Fushimi A, Yamamoto M, Hata T, Hagiwara M, et al. MUC1-C integrates activation of the IFN-gamma pathway with suppression of the tumor immune microenvironment in triple-negative breast cancer. J Immunother Cancer (2021) 9(1). doi: 10.1136/jitc-2020-002115
96. Yamashita N, Morimoto Y, Fushimi A, Ahmad R, Bhattacharya A, Daimon T, et al. MUC1-C dictates PBRM1-mediated chronic induction of interferon signaling, DNA damage resistance, and immunosuppression in triple-negative breast cancer. Mol Cancer Res (2023) 21(3):274–89. doi: 10.1158/1541-7786.MCR-22-0772
97. Grosso JF, Herbert LM, Owen JL, Lopez DM. MUC1/sec-expressing tumors are rejected in vivo by a T cell-dependent mechanism and secrete high levels of CCL2. J Immunol (2004) 173(3):1721–30. doi: 10.4049/jimmunol.173.3.1721
98. Lin X, Chen H, Xie Y, Zhou X, Wang Y, Zhou J, et al. Combination of CTLA-4 blockade with MUC1 mRNA nanovaccine induces enhanced anti-tumor CTL activity by modulating tumor microenvironment of triple negative breast cancer. Transl Oncol (2022) 15(1):101298. doi: 10.1016/j.tranon.2021.101298
99. Liu L, Wang Y, Miao L, Liu Q, Musetti S, Li J, et al. Combination immunotherapy of MUC1 mRNA nano-vaccine and CTLA-4 blockade effectively inhibits growth of triple negative breast cancer. Mol Ther (2018) 26(1):45–55. doi: 10.1016/j.ymthe.2017.10.020
100. Hu J, Sun J. MUC16 mutations improve patients' prognosis by enhancing the infiltration and antitumor immunity of cytotoxic T lymphocytes in the endometrial cancer microenvironment. Oncoimmunology. (2018) 7(10):e1487914. doi: 10.1080/2162402X.2018.1487914
101. Wang H, Yan C, Ye H. Overexpression of MUC16 predicts favourable prognosis in MUC16-mutant cervical cancer related to immune response. Exp Ther Med (2020) 20(2):1725–33. doi: 10.3892/etm.2020.8836
102. Bouillez A, Adeegbe D, Jin C, Hu X, Tagde A, Alam M, et al. MUC1-C promotes the suppressive immune microenvironment in non-small cell lung cancer. Oncoimmunology. (2017) 6(9):e1338998. doi: 10.1080/2162402X.2017.1338998
103. Bouillez A, Rajabi H, Jin C, Samur M, Tagde A, Alam M, et al. MUC1-C integrates PD-L1 induction with repression of immune effectors in non-small-cell lung cancer. Oncogene. (2017) 36(28):4037–46. doi: 10.1038/onc.2017.47
104. Wang A, Lv T, Song Y. Tandem CAR-T cells targeting MUC1 and PSCA combined with anti-PD-1 antibody exhibit potent preclinical activity against non-small cell lung cancer. Cell Immunol (2023) 391-392:104760. doi: 10.1016/j.cellimm.2023.104760
105. Jiang ZB, Huang JM, Xie YJ, Zhang YZ, Chang C, Lai HL, et al. Evodiamine suppresses non-small cell lung cancer by elevating CD8(+) T cells and downregulating the MUC1-C/PD-L1 axis. J Exp Clin Cancer Res (2020) 39(1):249. doi: 10.1186/s13046-020-01741-5
106. Patel JS, Callahan BM, Chobrutskiy BI, Blanck G. Matrix-metalloprotease resistant mucin-16 (MUC16) peptide mutants represent a worse lung adenocarcinoma outcome. Proteomics Clin Appl (2019) 13(4):e1800155. doi: 10.1002/prca.201800155
107. Saeland E, van Vliet SJ, Backstrom M, van den Berg VC, Geijtenbeek TB, Meijer GA, et al. The C-type lectin MGL expressed by dendritic cells detects glycan changes on MUC1 in colon carcinoma. Cancer Immunol Immunother (2007) 56(8):1225–36. doi: 10.1007/s00262-006-0274-z
108. Zhang Y, Dong X, Bai L, Shang X, Zeng Y. MUC1-induced immunosuppression in colon cancer can be reversed by blocking the PD1/PDL1 signaling pathway. Oncol Lett (2020) 20(6):317. doi: 10.3892/ol.2020.12180
109. Sheng YH, Davies JM, Wang R, Wong KY, Giri R, Yang Y, et al. MUC1-mediated macrophage activation promotes colitis-associated colorectal cancer via activating the interleukin-6/ signal transducer and activator of transcription 3 axis. Cell Mol Gastroenterol Hepatol (2022) 14(4):789–811. doi: 10.1016/j.jcmgh.2022.06.010
110. Monti P, Leone BE, Zerbi A, Balzano G, Cainarca S, Sordi V, et al. Tumor-derived MUC1 mucins interact with differentiating monocytes and induce IL-10highIL-12low regulatory dendritic cell. J Immunol (2004) 172(12):7341–9. doi: 10.4049/jimmunol.172.12.7341
111. Beatty PL, van der Geest R, Hashash JG, Kimura T, Gutkin D, Brand RE, et al. Immunobiology and immunosurveillance in patients with intraductal papillary mucinous neoplasms (IPMNs), premalignant precursors of pancreatic adenocarcinomas. Cancer Immunol Immunother (2016) 65(7):771–8. doi: 10.1007/s00262-016-1838-1
112. Lakshmanan I, Marimuthu S, Chaudhary S, Seshacharyulu P, Rachagani S, Muniyan S, et al. Muc16 depletion diminishes KRAS-induced tumorigenesis and metastasis by altering tumor microenvironment factors in pancreatic ductal adenocarcinoma. Oncogene. (2022) 41(48):5147–59. doi: 10.1038/s41388-022-02493-6
113. Zhang G, Zheng G, Zhang H, Qiu L. MUC1 induces the accumulation of Foxp3(+) Treg cells in the tumor microenvironment to promote the growth and metastasis of cholangiocarcinoma through the EGFR/PI3K/Akt signaling pathway. Int Immunopharmacol (2023) 118:110091. doi: 10.1016/j.intimp.2023.110091
114. Wang J, Liu Y, Ni W, Wu X, Zhou J, Zhang Z, et al. TRAF6-overexpressing dendritic cells loaded with MUC1 peptide enhance anti-tumor activity in B16-MUC1 melanoma-bearing mice. Int Immunopharmacol (2022) 107:108667. doi: 10.1016/j.intimp.2022.108667
115. Liu G, Zhang Z, Wu Y, Feng J, Lan Y, Dong D, et al. Anti-PD-L1 antibody reverses the immune tolerance induced by multiple MUC1-MBP vaccine immunizations by increasing the CD80/PD-L1 ratio, resulting in DC maturation, and decreasing Treg activity in B16-MUC1 melanoma-bearing mice. Int Immunopharmacol (2023) 121:110487. doi: 10.1016/j.intimp.2023.110487
116. Zhang Z, Zhou H, Liu Y, Ren J, Wang J, Sang Q, et al. Anti-PD1 antibody enhances the anti-tumor efficacy of MUC1-MBP fusion protein vaccine via increasing Th1, Tc1 activity and decreasing the proportion of MDSC in the B16-MUC1 melanoma mouse model. Int Immunopharmacol (2021) 101(Pt A):108173. doi: 10.1016/j.intimp.2021.108173
117. Wang Q, Yang Y, Yang M, Li X, Chen K. High mutation load, immune-activated microenvironment, favorable outcome, and better immunotherapeutic efficacy in melanoma patients harboring MUC16/CA125 mutations. Aging (Albany NY) (2020) 12(11):10827–43. doi: 10.18632/aging.103296
118. Wang Z, Hou H, Zhang H, Duan X, Li L, Meng L. Effect of MUC16 mutations on tumor mutation burden and its potential prognostic significance for cutaneous melanoma. Am J Transl Res (2022) 14(2):849–62.
119. Wang X, Yu X, Krauthammer M, Hugo W, Duan C, Kanetsky PA, et al. The association of MUC16 mutation with tumor mutation burden and its prognostic implications in cutaneous melanoma. Cancer Epidemiol Biomarkers Prev (2020) 29(9):1792–9. doi: 10.1158/1055-9965.EPI-20-0307
120. Lucarelli G, Netti GS, Rutigliano M, Lasorsa F, Loizzo D, Milella M, et al. MUC1 expression affects the immunoflogosis in renal cell carcinoma microenvironment through complement system activation and immune infiltrate modulation. Int J Mol Sci (2023) 24(5). doi: 10.3390/ijms24054814
121. Hagiwara M, Fushimi A, Bhattacharya A, Yamashita N, Morimoto Y, Oya M, et al. MUC1-C integrates type II interferon and chromatin remodeling pathways in immunosuppression of prostate cancer. Oncoimmunology. (2022) 11(1):2029298. doi: 10.1080/2162402X.2022.2029298
122. Yamashita T, Higashi M, Sugiyama H, Morozumi M, Momose S, Tamaru JI. Cancer antigen 125 expression enhances the gemcitabine/cisplatin-resistant tumor microenvironment in bladder cancer. Am J Pathol (2023) 193(3):350–61. doi: 10.1016/j.ajpath.2022.12.005
123. Lan Z, Zou KL, Cui H, Zhao YY, Yu GT. Porphyromonas gingivalis suppresses oral squamous cell carcinoma progression by inhibiting MUC1 expression and remodeling the tumor microenvironment. Mol Oncol (2023). doi: 10.1002/1878-0261.13517
124. Posey AD Jr., Schwab RD, Boesteanu AC, Steentoft C, Mandel U, Engels B, et al. Engineered CAR T cells targeting the cancer-associated tn-glycoform of the membrane mucin MUC1 control adenocarcinoma. Immunity. (2016) 44(6):1444–54. doi: 10.1016/j.immuni.2016.05.014
125. Heukamp LC, van Hall T, Ossendorp F, Burchell JM, Melief CJ, Taylor-Papadimitriou J, et al. Effective immunotherapy of cancer in MUC1-transgenic mice using clonal cytotoxic T lymphocytes directed against an immunodominant MUC1 epitope. J Immunother (2002) 25(1):46–56. doi: 10.1097/00002371-200201000-00005
126. Yazdanifar M, Zhou R, Grover P, Williams C, Bose M, Moore LJ, et al. Overcoming immunological resistance enhances the efficacy of A novel anti-tMUC1-CAR T cell treatment against pancreatic ductal adenocarcinoma. Cells (2019) 8(9). doi: 10.3390/cells8091070
127. Hong J, Guo G, Wu S, Lin S, Zhou Z, Chen S, et al. Altered MUC1 epitope-specific CTLs: A potential target for immunotherapy of pancreatic cancer. J Leukoc Biol (2022) 112(6):1577–90. doi: 10.1002/JLB.5MA0922-749R
128. Li Z, Guo T, Zhao S, Lin M. The therapeutic effects of MUC1-C shRNA@Fe(3)O(4) magnetic nanoparticles in alternating magnetic fields on triple-negative breast cancer. Int J Nanomed (2023) 18:5651–70. doi: 10.2147/IJN.S426849
129. Morgado M, Carson DD. PPARgamma modulation of cytokine-stimulated MUC16 (CA125) expression in breast and ovarian cancer-derived cells. J Cell Biochem (2017) 118(1):163–71. doi: 10.1002/jcb.25622
130. Wang Q, Ma X, Wu H, Zhao C, Chen J, Li R, et al. Oncolytic adenovirus with MUC16-BiTE shows enhanced antitumor immune response by reversing the tumor microenvironment in PDX model of ovarian cancer. Oncoimmunology. (2022) 11(1):2096362. doi: 10.1080/2162402X.2022.2096362
131. Mun SS, Meyerberg J, Peraro L, Korontsvit T, Gardner T, Malviya M, et al. Dual targeting ovarian cancer by Muc16 CAR T cells secreting a bispecific T cell engager antibody for an intracellular tumor antigen WT1. Cancer Immunol Immunother (2023) 72(11):3773–86. doi: 10.1007/s00262-023-03529-w
132. Chekmasova AA, Rao TD, Nikhamin Y, Park KJ, Levine DA, Spriggs DR, et al. Successful eradication of established peritoneal ovarian tumors in SCID-Beige mice following adoptive transfer of T cells genetically targeted to the MUC16 antigen. Clin Cancer Res (2010) 16(14):3594–606. doi: 10.1158/1078-0432.CCR-10-0192
133. Yue E, Yang G, Yao Y, Wang G, Mohanty A, Fan F, et al. Targeting CA-125 transcription by development of a conditionally replicative adenovirus for ovarian cancer treatment. Cancers (Basel) (2021) 13(17). doi: 10.3390/cancers13174265
134. Feely N, Wdowicz A, Chevalier A, Wang Y, Li P, Rollo F, et al. Targeting mucin protein enables rapid and efficient ovarian cancer cell capture: role of nanoparticle properties in efficient capture and culture. Small. (2023) 19(18):e2207154. doi: 10.1002/smll.202207154
135. Kondo H, Hazama S, Kawaoka T, Yoshino S, Yoshida S, Tokuno K, et al. Adoptive immunotherapy for pancreatic cancer using MUC1 peptide-pulsed dendritic cells and activated T lymphocytes. Anticancer Res (2008) 28(1B):379–87.
136. Gonzalez G, Lakatos K, Hoballah J, Fritz-Klaus R, Al-Johani L, Brooker J, et al. Characterization of cell-bound CA125 on immune cell subtypes of ovarian cancer patients using a novel imaging platform. Cancers (Basel) (2021) 13(9). doi: 10.3390/cancers13092072
137. Fang J, Lai S, Yu H, Ma L. Suppression of MUC1-overexpressing tumors by a novel MUC1/CD3 bispecific antibody. Antibodies (Basel) (2023) 12(3). doi: 10.3390/antib12030047
138. Panchamoorthy G, Jin C, Raina D, Bharti A, Yamamoto M, Adeebge D, et al. Targeting the human MUC1-C oncoprotein with an antibody-drug conjugate. JCI Insight (2018) 3(12). doi: 10.1172/jci.insight.99880
139. Gong Y, Klein Wolterink RGJ, Gulaia V, Cloosen S, Ehlers FAI, Wieten L, et al. Defucosylation of tumor-specific humanized anti-MUC1 monoclonal antibody enhances NK cell-mediated anti-tumor cell cytotoxicity. Cancers (Basel) (2021) 13(11). doi: 10.3390/cancers13112579
140. Heuser C, Ganser M, Hombach A, Brand H, Denton G, Hanisch FG, et al. An anti-MUC1-antibody-interleukin-2 fusion protein that activates resting NK cells to lysis of MUC1-positive tumour cells. Br J Canc (2003) 89(6):1130–9. doi: 10.1038/sj.bjc.6601267
141. Nicolaides NC, Kline JB, Grasso L. NAV-001, a high-efficacy antibody-drug conjugate targeting mesothelin with improved delivery of a potent payload by counteracting MUC16/CA125 inhibitory effects. PloS One (2023) 18(5):e0285161. doi: 10.1371/journal.pone.0285161
142. Garg G, Gibbs J, Belt B, Powell MA, Mutch DG, Goedegebuure P, et al. Novel treatment option for MUC16-positive Malignancies with the targeted TRAIL-based fusion protein Meso-TR3. BMC Canc (2014) 14:35. doi: 10.1186/1471-2407-14-35
143. Mony JT, Zhang L, Ma T, Grabosch S, Tirodkar TS, Brozick J, et al. Anti-PD-L1 prolongs survival and triggers T cell but not humoral anti-tumor immune responses in a human MUC1-expressing preclinical ovarian cancer model. Cancer Immunol Immunother (2015) 64(9):1095–108. doi: 10.1007/s00262-015-1712-6
144. Kelly VJ, Wu ST, Gottumukkala V, Coelho R, Palmer K, Nair S, et al. Preclinical evaluation of an (111)In/(225)Ac theranostic targeting transformed MUC1 for triple negative breast cancer. Theranostics. (2020) 10(15):6946–58. doi: 10.7150/thno.38236
145. Kim MJ, Choi JR, Tae N, Wi TM, Kim KM, Kim DH, et al. Novel antibodies targeting MUC1-C showed anti-metastasis and growth-inhibitory effects on human breast cancer cells. Int J Mol Sci (2020) 21(9). doi: 10.3390/ijms21093258
146. Isla Larrain MT, Colussi AG, Demichelis SO, Barbera A, Creton A, Segal-Eiras A, et al. Humoral immune response against tumoral mucin 1 (MUC1) in breast cancer patients. Int J Biol Markers (2013) 28(3):318–25. doi: 10.5301/JBM.5000036
147. Yeku OO, Rao TD, Laster I, Kononenko A, Purdon TJ, Wang P, et al. Bispecific T-cell engaging antibodies against MUC16 demonstrate efficacy against ovarian cancer in monotherapy and in combination with PD-1 and VEGF inhibition. Front Immunol (2021) 12:663379. doi: 10.3389/fimmu.2021.663379
148. Babeker H, Ketchemen JP, Annan Sudarsan A, Andrahennadi S, Tikum AF, Nambisan AK, et al. Engineering of a fully human anti-MUC-16 antibody and evaluation as a PET imaging agent. Pharmaceutics (2022) 14(12). doi: 10.3390/pharmaceutics14122824
149. Rao TD, Fernandez-Tejada A, Axelrod A, Rosales N, Yan X, Thapi S, et al. Antibodies against specific MUC16 glycosylation sites inhibit ovarian cancer growth. ACS Chem Biol (2017) 12(8):2085–96. doi: 10.1021/acschembio.7b00305
150. Marcos-Silva L, Ricardo S, Chen K, Blixt O, Arigi E, Pereira D, et al. A novel monoclonal antibody to a defined peptide epitope in MUC16. Glycobiology. (2015) 25(11):1172–82. doi: 10.1093/glycob/cwv056
151. Stasenko M, Smith E, Yeku O, Park KJ, Laster I, Lee K, et al. Targeting galectin-3 with a high-affinity antibody for inhibition of high-grade serous ovarian cancer and other MUC16/CA-125-expressing Malignancies. Sci Rep (2021) 11(1):3718. doi: 10.1038/s41598-021-82686-3
152. Wakui H, Yokoi Y, Horidome C, Ose T, Yao M, Tanaka Y, et al. Structural and molecular insight into antibody recognition of dynamic neoepitopes in membrane tethered MUC1 of pancreatic cancer cells and secreted exosomes. RSC Chem Biol (2023) 4(8):564–72. doi: 10.1039/D3CB00036B
153. Schettini J, Kidiyoor A, Besmer DM, Tinder TL, Roy LD, Lustgarten J, et al. Intratumoral delivery of CpG-conjugated anti-MUC1 antibody enhances NK cell anti-tumor activity. Cancer Immunol Immunother (2012) 61(11):2055–65. doi: 10.1007/s00262-012-1264-y
154. Shah A, Chaudhary S, Lakshmanan I, Aithal A, Kisling SG, Sorrell C, et al. Chimeric antibody targeting unique epitope on onco-mucin16 reduces tumor burden in pancreatic and lung Malignancies. NPJ Precis Oncol (2023) 7(1):74. doi: 10.1038/s41698-023-00423-7
155. Silk AW, Schoen RE, Potter DM, Finn OJ. Humoral immune response to abnormal MUC1 in subjects with colorectal adenoma and cancer. Mol Immunol (2009) 47(1):52–6. doi: 10.1016/j.molimm.2008.12.025
156. Akita K, Yoshida S, Ikehara Y, Shirakawa S, Toda M, Inoue M, et al. Different levels of sialyl-Tn antigen expressed on MUC16 in patients with endometriosis and ovarian cancer. Int J Gynecol Canc (2012) 22(4):531–8. doi: 10.1097/IGC.0b013e3182473292
157. Wagner U, Schlebusch H, Kohler S, Schmolling J, Grunn U, Krebs D. Immunological responses to the tumor-associated antigen CA125 in patients with advanced ovarian cancer induced by the murine monoclonal anti-idiotype vaccine ACA125. Hybridoma. (1997) 16(1):33–40. doi: 10.1089/hyb.1997.16.33
158. Battaglia A, Fossati M, Buzzonetti A, Scambia G, Fattorossi A. A robust immune system conditions the response to abagovomab (anti-idiotypic monoclonal antibody mimicking the CA125 protein) vaccination in ovarian cancer patients. Immunol Lett (2017) 191:35–9. doi: 10.1016/j.imlet.2017.09.006
159. Liu J, Burris H, Wang JS, Barroilhet L, Gutierrez M, Wang Y, et al. An open-label phase I dose-escalation study of the safety and pharmacokinetics of DMUC4064A in patients with platinum-resistant ovarian cancer. Gynecol Oncol (2021) 163(3):473–80. doi: 10.1016/j.ygyno.2021.09.023
160. Wang X, Wu X, Yang Y, Xu W, Tian H, Lian B, et al. Apatinib combined with camrelizumab in advanced acral melanoma patients: An open-label, single-arm phase 2 trial. Eur J Canc (2023) 182:57–65. doi: 10.1016/j.ejca.2022.12.027
161. Nicolaides NC, Schweizer C, Somers EB, Wang W, Fernando S, Ross EN, et al. CA125 suppresses amatuximab immune-effector function and elevated serum levels are associated with reduced clinical response in first line mesothelioma patients. Cancer Biol Ther (2018) 19(7):622–30. doi: 10.1080/15384047.2018.1449614
162. Zhou SH, Li YT, Zhang RY, Liu YL, You ZW, Bian MM, et al. Alum adjuvant and built-in TLR7 agonist synergistically enhance anti-MUC1 immune responses for cancer vaccine. Front Immunol (2022) 13:857779. doi: 10.3389/fimmu.2022.857779
163. Panasiuk M, Zimmer K, Czarnota A, Narajczyk M, Peszynska-Sularz G, Chraniuk M, et al. Chimeric virus-like particles presenting tumour-associated MUC1 epitope result in high titers of specific IgG antibodies in the presence of squalene oil-in-water adjuvant: towards safe cancer immunotherapy. J Nanobiotechnology (2022) 20(1):160. doi: 10.1186/s12951-022-01357-1
164. Fang F, Ma J, Ni W, Wang F, Sun X, Li Y, et al. MUC1 and maltose−binding protein recombinant fusion protein combined with Bacillus Calmette−Guerin induces MUC1−specific and nonspecific anti−tumor immunity in mice. Mol Med Rep (2014) 10(2):1056–64. doi: 10.3892/mmr.2014.2306
165. Mehrab Mohseni M, Amani J, Fasihi Ramandi M, Mahjoubi F, Jafaria M, Salmanian AH. Immunogenicity evaluation of recombinant edible vaccine candidate containing HER2-MUC1 against breast cancer. Iran J Allergy Asthma Immunol (2019) 18(5):511–22. doi: 10.18502/ijaai.v18i5.1921
166. Liu Y, Tang L, Gao N, Diao Y, Zhong J, Deng Y, et al. Synthetic MUC1 breast cancer vaccine containing a Toll-like receptor 7 agonist exerts antitumor effects. Oncol Lett (2020) 20(3):2369–77. doi: 10.3892/ol.2020.11762
167. Zhang S, Liu Y, Zhou J, Wang J, Jin G, Wang X. Breast cancer vaccine containing a novel toll-like receptor 7 agonist and an aluminum adjuvant exerts antitumor effects. Int J Mol Sci (2022) 23(23). doi: 10.3390/ijms232315130
168. Yu H, Ye C, Li J, Pan C, Lin W, Chen H, et al. An altered HLA-A0201-restricted MUC1 epitope that could induce more efficient anti-tumor effects against gastric cancer. Exp Cell Res (2020) 390(1):111953. doi: 10.1016/j.yexcr.2020.111953
169. Guo M, Luo B, Pan M, Li M, Zhao F, Dou J. MUC1 plays an essential role in tumor immunity of colorectal cancer stem cell vaccine. Int Immunopharmacol (2020) 85:106631. doi: 10.1016/j.intimp.2020.106631
170. Vang DP, Wurz GT, Griffey SM, Kao CJ, Gutierrez AM, Hanson GK, et al. Induction of invasive transitional cell bladder carcinoma in immune intact human MUC1 transgenic mice: a model for immunotherapy development. J Vis Exp (2013) 80:e50868. doi: 10.3791/50868
171. Lu L, Ma W, Johnson CH, Khan SA, Irwin ML, Pusztai L. In silico designed mRNA vaccines targeting CA-125 neoantigen in breast and ovarian cancer. Vaccine. (2023) 41(12):2073–83. doi: 10.1016/j.vaccine.2023.02.048
172. Reinartz S, Hombach A, Kohler S, Schlebusch H, Wallwiener D, Abken H, et al. Interleukin-6 fused to an anti-idiotype antibody in a vaccine increases the specific humoral immune response against CA125+ (MUC-16) ovarian cancer. Cancer Res (2003) 63(12):3234–40.
173. Scheid E, Major P, Bergeron A, Finn OJ, Salter RD, Eady R, et al. Tn-MUC1 DC vaccination of rhesus macaques and a phase I/II trial in patients with nonmetastatic castrate-resistant prostate cancer. Cancer Immunol Res (2016) 4(10):881–92. doi: 10.1158/2326-6066.CIR-15-0189
174. Tan TJ, Ang WXG, Wang WW, Chong HS, Tan SH, Cheong R, et al. A phase I study of an adenoviral vector delivering a MUC1/CD40-ligand fusion protein in patients with advanced adenocarcinoma. Nat Commun (2022) 13(1):6453. doi: 10.1038/s41467-022-33834-4
175. Gatti-Mays ME, Redman JM, Donahue RN, Palena C, Madan RA, Karzai F, et al. A phase I trial using a multitargeted recombinant adenovirus 5 (CEA/MUC1/brachyury)-based immunotherapy vaccine regimen in patients with advanced cancer. Oncologist. (2020) 25(6):479–e899. doi: 10.1634/theoncologist.2019-0608
176. Gatti-Mays ME, Strauss J, Donahue RN, Palena C, Del Rivero J, Redman JM, et al. A phase I dose-escalation trial of BN-CV301, a recombinant poxviral vaccine targeting MUC1 and CEA with costimulatory molecules. Clin Cancer Res (2019) 25(16):4933–44. doi: 10.1158/1078-0432.CCR-19-0183
177. Musselli C, Ragupathi G, Gilewski T, Panageas KS, Spinat Y, Livingston PO. Reevaluation of the cellular immune response in breast cancer patients vaccinated with MUC1. Int J Canc (2002) 97(5):660–7. doi: 10.1002/ijc.10081
178. Singer CF, Pfeiler G, Hubalek M, Bartsch R, Stoger H, Pichler A, et al. Efficacy and safety of the therapeutic cancer vaccine tecemotide (L-BLP25) in early breast cancer: Results from a prospective, randomised, neoadjuvant phase II study (ABCSG 34). Eur J Canc (2020) 132:43–52. doi: 10.1016/j.ejca.2020.03.018
179. Schoen RE, Boardman LA, Cruz-Correa M, Bansal A, Kastenberg D, Hur C, et al. Randomized, double-blind, placebo-controlled trial of MUC1 peptide vaccine for prevention of recurrent colorectal adenoma. Clin Cancer Res (2023) 29(9):1678–88. doi: 10.1158/1078-0432.CCR-22-3168
180. Quoix E, Lena H, Losonczy G, Forget F, Chouaid C, Papai Z, et al. TG4010 immunotherapy and first-line chemotherapy for advanced non-small-cell lung cancer (TIME): results from the phase 2b part of a randomised, double-blind, placebo-controlled, phase 2b/3 trial. Lancet Oncol (2016) 17(2):212–23. doi: 10.1016/S1470-2045(15)00483-0
Keywords: mucins, MUC1, MUC16, immunotherapy, tumor microenvironment, targeted drug therapy
Citation: Chen X, Sandrine IK, Yang M, Tu J and Yuan X (2024) MUC1 and MUC16: critical for immune modulation in cancer therapeutics. Front. Immunol. 15:1356913. doi: 10.3389/fimmu.2024.1356913
Received: 16 December 2023; Accepted: 18 January 2024;
Published: 01 February 2024.
Edited by:
Ming Yi, Zhejiang University, ChinaReviewed by:
Kasturi Banerjee, CSL, United StatesCopyright © 2024 Chen, Sandrine, Yang, Tu and Yuan. This is an open-access article distributed under the terms of the Creative Commons Attribution License (CC BY). The use, distribution or reproduction in other forums is permitted, provided the original author(s) and the copyright owner(s) are credited and that the original publication in this journal is cited, in accordance with accepted academic practice. No use, distribution or reproduction is permitted which does not comply with these terms.
*Correspondence: Xianglin Yuan, eXVhbnhpYW5nbGluQGh1c3QuZWR1LmNu; Jingyao Tu, dHVqaW5neWFvMDcwMkAxNjMuY29t
Disclaimer: All claims expressed in this article are solely those of the authors and do not necessarily represent those of their affiliated organizations, or those of the publisher, the editors and the reviewers. Any product that may be evaluated in this article or claim that may be made by its manufacturer is not guaranteed or endorsed by the publisher.
Research integrity at Frontiers
Learn more about the work of our research integrity team to safeguard the quality of each article we publish.