- 1Graduate Program in Immunology, University of California (UC) Davis, Davis, CA, United States
- 2Harvard Medical School, Brigham and Women’s Hospital, Boston, MA, United States
- 3Departments of Dermatology and Internal Medicine (Hematology/Oncology), University of California (UC) Davis School of Medicine, Sacramento, CA, United States
Immune responses to both SARS-CoV-2 infection and its associated vaccines have been highly variable within the general population. The increasing evidence of long-lasting symptoms after resolution of infection, called post-acute sequelae of COVID-19 (PASC) or “Long COVID,” suggests that immune-mediated mechanisms are at play. Closely related endemic common human coronaviruses (hCoV) can induce pre-existing and potentially cross-reactive immunity, which can then affect primary SARS-CoV-2 infection, as well as vaccination responses. The influence of pre-existing immunity from these hCoVs, as well as responses generated from original CoV2 strains or vaccines on the development of new high-affinity responses to CoV2 antigenic viral variants, needs to be better understood given the need for continuous vaccine adaptation and application in the population. Due in part to thymic involution, normal aging is associated with reduced naïve T cell compartments and impaired primary antigen responsiveness, resulting in a reliance on the pre-existing cross-reactive memory cell pool which may be of lower affinity, restricted in diversity, or of shorter duration. These effects can also be mediated by the presence of down-regulatory anti-idiotype responses which also increase in aging. Given the tremendous heterogeneity of clinical data, utilization of preclinical models offers the greatest ability to assess immune responses under a controlled setting. These models should now involve prior antigen/viral exposure combined with incorporation of modifying factors such as age on immune responses and effects. This will also allow for mechanistic dissection and understanding of the different immune pathways involved in both SARS-CoV-2 pathogen and potential vaccine responses over time and how pre-existing memory responses, including potential anti-idiotype responses, can affect efficacy as well as potential off-target effects in different tissues as well as modeling PASC.
Introduction: the diverse immunology underlying SARS-CoV-2 and vaccine responses
The ongoing SARS-CoV-2 (CoV2) pandemic, with its devastating health and economic effects, has generated an urgent need to gain more in-depth understanding of the complex and interdependent immune mechanisms at work in response to both the virus and to the vaccines that have been developed to combat it. This has been particularly important given the continual emergence of new viral variants which increase in their immune evasive properties, resulting in the need for further vaccine optimization and periodic application. This need has also been highlighted by the extreme diversity of immunological effects observed within the population after CoV2 infection (and reinfection) or vaccination. At one end of the spectrum, some infected patients can have over-reactive immune responses resulting in a life-threatening pro-inflammatory cytokine storm, necessitating the need for immune suppression (1). Aging and obesity appear be risk modifying factors on pathogenesis and outcome. Conversely, many younger individuals can present with relatively asymptomatic infections with rapid viral clearance and spontaneous resolution (1, 2). Similarly, immediate adverse immune reactions to the various vaccines in otherwise healthy adults, while rarer, are also diverse, with some developing rapid allergic reactions and some, even less frequently, with potentially serious off-target effects such as myocarditis and thrombotic events (3). It is still unclear as to what effects are due to vaccine application versus possible past or concurrent CoV2 exposure, especially given recent data indicating that both CoV2 and S protein can be detected in some patients long after viral infection resolution (4, 5). Finally, a growing body of data accrued from patients has shown that various symptoms can persist for many months after infection, called post-acute sequelae of COVID-19 (PASC) or, more colloquially, “Long COVID,” indicate that immune-mediated pathways are involved.
Regarding the potential of vaccine-mediated effects, some of these immune-mediated events may be intrinsic for the type and construct of vaccine applied (mRNA, adenovirus, inactivated virus, protein or protein fragment, use of carriers like polyethylene glycol, etc.), as well potential effects of the antigen targeted (i.e., the spike (S) protein in CoV2 which can have direct proinflammatory effects (6)). The immune responses induced to both the virus and vaccines likely play a driving role as well. The occurrence rate of these adverse effects are also notable and being increasingly appreciated as more data are generated. A recent report detailing that 70.79% of those that who were vaccinated and participated in a questionnaire had experienced side effects after the second dose of vaccine, while 46.76% of participants that had already experienced infection had adverse effects after the first injection (7). Another study observed that elderly patients experienced adverse events at a higher rate than other groups, with tachycardia, hypertension, and hypotension being commonly reported, though more serious events, like acute myocardial infarction and cardiac arrest, were also reported (8). Long-term effects of CoV2 infection have also been reported, with men being at an increased risk for cardiovascular complications after CoV2 infection (9, 10). Others have also reported similarly increased occurrence rates of adverse events, at elevated intensity, in the elderly population with repeated vaccination, with those having infection prior to vaccination typically having elevated rates of occurance (11). In comparison to adverse occurrence rates in the elderly with other common vaccinations (influenza, Td, Hepatitis B, etc.), adverse events have been reported to be lower than that observed with SARS-CoV-2 vaccination, though a direct comparison study has not yet been performed (12, 13). In addition, others have reported that mRNA SARS-CoV-2 vaccines have been associated with a higher occurrence of adverse events in comparison to adenoviral vector and inactivated virus vaccines (14, 15).
The diverse array of long-term and diverse effects defining PASC implicate multiple organ systems (cardiovascular, pulmonary, neurologic) being affected, similar to those reported with primary CoV2 infection itself (16). Data supporting the implication of the immune system mediating, at least in part, the development of PASC, is compelling, even considering the heterogeneity of immune responses reported. Primary infection has been demonstrated to cause apoptosis to hippocampal cells, as well as alteration of the neuronal landscape and cognitive impairment (17, 18), with preclinical studies also indicating neuronal inflammation even after clearance of the virus (19). Spike protein has been reported several months post-infection as well (5), with data demonstrating that the S protein has the ability to cross the blood brain barrier and cause inflammation through TLR triggering and inflammasome activation (20–22). While reports of possible long-term effects following vaccination have been shown (23), attributing these solely due to the vaccines is extremely problematic, given the reliance on clinical data and history. The presence of concurrent viral infections, such as asymptomatic CoV2, or common latent infections like Epstein-Barr virus (EBV) and cytomegalovirus (CMV), or continuous exposure to endemic hCoVs, can all be affecting immune responses and the symptoms reported. Additionally, the frequent emergence of CoV2 variants continuously alters immunologic epitopes targeted, which has further complicated the picture newer vaccine formulations are produced to combat them. Some of the initial data suggesting that pre-existing immune responses may affect CoV2 immunity revolved around the reported relatively rapid waning of protective antibody responses (within months), as well as reported reinfection rates (24, 25). More recent data has also demonstrated with both viral infection and vaccination that class switching of antibodies to IgG4 from IgG3 and IgG1 occurs, with IgG4 being a lower affinity subclass of IgG, compounding the issue of total antibody levels waning (26–28).
Given the tremendous heterogeneity of immune responses, pleiotropic pathologies reported, and the emergence of PASC, how can true mechanistic studies be performed to delineate not only causation but also treatment options? Unfortunately, most data regarding both CoV2 infection and vaccines have relied on imprecise measures of human immune function (primarily serum antibody levels or cytokines), variables known to be influenced by a number of clinical factors including underlying medical conditions, and to reflect in vivo immune function imperfectly as well as being highly variable and affected by numerous factors. It is only through the use of preclinical studies that controlled situations and experimental conditions can occur that delineate these different questions.
Preclinical insights gained through SARS-CoV and SARS-CoV-2 virus and vaccine modeling
Mus musculus (Mouse)
Preclinical modeling has already provided significant insights into the mechanisms of SARS-CoV-2 infection and vaccination. Understanding the advantages and limitations of the different animal models is critical for determining relevance to the human condition, especially given species differences in not only immune biology, but also CoV2 susceptibility.
The inbred laboratory mouse continues to be the bedrock for biomedical research, particularly immune-based studies, due to cost, reagent availability, and extensive immune and genetic characterization that already exists. Mice do, however, have significant immune differences between strains that need to be taken into consideration before extrapolating to humans (29, 30). Laboratory mice are also housed under specific pathogen free conditions which severely restricts pathogen exposure and results in, for the most part, a naïve immune repertoire even as they age (31). Mice are inherently resistant to CoV2 infection due to differences between mouse vs human angiotensin converting enzyme 2 (ACE2) (mACE2 and hACE2, respectively) (32, 33). This has led to the generation and use of transgenic hACE2 and Cre-Lox induced mouse models, as well as mouse-adapted strains of CoV2 which produce robust and severe infection pathologies (34).
While mice are resistant to CoV2, they can be infected with SARS-CoV (CoV) which, while similarly utilizing a S protein that also binds ACE2, has the capacity to bind mACE2 due to differences in binding domains (35). Earlier studies with CoV showed that wild type (WT) young mice, such as BALB/c and C57BL/6, have minimal pathology following infection. However, it has been demonstrated that aged BALB/c mice experience pathology similar to that observed in severe human infection, such as pneumonia, pulmonary fibrosis, and large inflammatory cytokine responses, indicating the importance of involving age in preclinical modeling (36, 37). Vaccine efficacy, using mRNA-based vaccines to the CoV S protein, also was demonstrated in mice (38–40), with protective anti-viral antibody responses being produced. However, the mouse strain used appeared to be critical, as BALB/c mice following vaccination and then CoV viral challenge exhibited eosinophilia, lung pathology, and elevated inflammatory cytokines, events not observed in C57BL/6 (B6) mice (39, 41, 42). Another recent report supports this, as a group demonstrated that CoV2 mRNA vaccination of BALB/c mice induced significant weight loss and elevated inflammatory cytokines 1-2 days post injection, with histopathology revealing that myocarditis, as evidenced by apoptosis and necrosis, was observable as far as two weeks after initial infection, with further boosting amplifying myocarditis pathology (43). BALB/c strain mice are skewed towards producing Th2-mediated immune responses which likely contributes to these allergic-type effects, while B6 mice are more skewed towards Th1-mediated responses (44). The vaccine adjuvant was also shown to play a role, as TLR-triggering adjuvants skewing to Th1-type cytokines could ameliorate these allergic effects in the vaccinated mice, while some Th2 promoting adjuvants have been shown to promote such effects (39). A mouse adapted CoV2 has also been recently demonstrated these effects in BALB/c mice mirroring the CoV studies (45). Given that allergic reactions have been reported after vaccine administration in some people, with some only experiencing severe adverse events after boosting (46), this suggests that it is important to use multiple mouse strains to develop a more complete immunologic picture of both vaccine and infection effects to be reflective of an outbred population.
Keratin 18 (K18) hACE2 transgenic mice have the hACE2 gene put on the cytokeratin 18 promoter, allowing expression of hACE2 (47). K18 mice were originally utilized to study CoV, being used to study severe pathology that wasn’t producible in young mice. K18 mice infected with CoV and CoV2 experience pathology similar to humans, such as elevated proinflammatory cytokines and cytokine storm with severe infection, innate immune infiltrates in the lungs, and lung and systemic tissue pathology (47–51). Neuropathology typical of CoV infection is also observable in K18 mice, with neuroinvasion via the nasal and central nervous system (CNS) occurring, followed by neuronal death and activation of immune cells such as microglia and T cells, consistent with human infection responses as well (52–55). While discussed at greater detail later, K18 mice have severe pathology that limits modeling of mild or asymptomatic infection.
Commonly used mRNA vaccines targeting the CoV2 S protein have been shown to be efficacious in K18 mice by generating robust antibody responses (56–60). Similarly, IgM (61–65), IgG (26–28, 66–69), and IgA (66, 70–72) mouse antibody kinetics mirroring those observed in humans have been demonstrated. While the vast majority of these vaccine studies have centered on antibody responses, T cell responses have also been characterized (72–74). While most preclinical studies have focused on vaccine efficacy, others have demonstrated adverse effects from mRNA and S1 protein vaccination. These adverse effects include weight loss, a proinflammatory response, acute lung injury, and presence of immune infiltrates (75, 76) suggesting these models may be appropriate in delineating immune-mediated effects due solely by the vaccine.
An issue in using transgenic K18 mice concerns the overexpression hACE2 due to use of the cytokeratin 18 promoter, which is present on all epithelial tissues, and can produce pathology not typical of humans (18, 77). Overexpression of hACE2 in the nasal passages and neuroepithelium has been shown to allow for aggressive SARS-CoV-2 neuroinvasion not observed with human infection (55), while neuropathology, such as neuronal death and immune infiltrates, has been shown by others to be more severe than typically seen in humans (52–54). This overexpression also can lead to more severe organ pathology in what would be considered non-critical SARS-CoV-2 targets, such as the spleen and liver (47–51). These limitations should also be thought of when interpreting the adverse events that have been demonstrated in K18 SARS-CoV-2 vaccine models. It should also be noted that K18 mice still maintain expression of mACE2, which could further alter viral and vaccine kinetics, as well as the immune response to these challenges. While K18 mice have shown a dose dependent response to SARS-CoV-2 (78), studies are still limited and capturing nuances of human infection may prove to be a challenge in the future due to the severity of infection. While Cre-Lox and adenovirus hACE2 mouse models, which can be induced to express hACE2 on target tissues like the lungs unlike K18 mice, may be able to overcome some of the disadvantages of the K18 mouse model, these also limit mirroring of the systemic effects of CoV2 infection on tissues outside the lung (79–81).
Mouse adapted SARS-CoV and SARS-CoV-2 strains have been generated through reverse genetic engineering (82) and serial passaging in BALB/c mice (82–86). These compatible strains, such as MA10, HRB26M, MASCp6, and others, allow for the infection of young mice, particularly those of a C57BL/6 background, and tend to generate a Th1 skewed immune response with pathology similar to that seen in humans, with acute respiratory distress, lung tissue damage, pneumonia, and lung infiltrates being reported (84, 86). Both non-severe and severe infections can be modeled in a dose dependent manner, while mouse age has been shown to exacerbate pathology. Vaccine efficacy, in the form of antibody responses and resistance to the mouse adapted strains, has also been shown, using different vaccine formulations (84, 87, 88). Due to the novelty of these mouse adapted viruses, limitations have not been as extensively characterized, though it has been observed that they have a different tropism than that observed in humans, and that serially passaging induced mutations could alter viral pathology and the resulting altered immune responses (84). Further studies need to performed to establish the exact pathogenesis and immunobiology of this mouse adapted viruses, with hACE2 mouse models still primarily being used for vaccine assessment efficacy.
Other small animal SARS-CoV-2 models – Mesocricetus auratus and Mustela putorius (Syrian hamsters and ferrets)
While most preclinical small animal CoV2 models use mice, others have had success using Syrian hamsters and ferrets due to inherent CoV2 susceptibility. Syrian hamsters offer several advantages over WT and transgenic hACE2 mouse models, with hamsters having a structurally similar ACE2 amino acid sequence and S1 protein binding site to humans (89), allowing for natural susceptibility to CoV and CoV2 infection (90–92). Because of these structural similarities, and because Syrian hamsters express ACE2 in the same tissues as humans, viral pathology is fairly similar, with the virus targeting the respiratory tract and lungs for replication, while still generating a systemic immune response (93). Viral pathology severity is dose dependent, allowing for both lethal and non-lethal SARS-CoV-2 infection, while other variables that correlate with disease severity in humans, such as age (91) and sex (94), have been observed to have similar effects on hamster outcomes following infection.
Reports on neuropathology have been conflicting, with some reporting neuronal invasion and pathology, while other have reported a lack of viral mRNA in the CNS, although neuronal immune activation and tissue damage have been consistently reported (19, 91, 95, 96). This neuropathology is more representative of human infection than what has been observed in K18 mice, and given similar viral clearance patterns to humans, hamsters have been used to model PASC (19, 96, 97). A recent report detailed structural and transcriptional changes to the lungs, kidneys, olfactory bulb, and olfactory epithelium following CoV2 infection in Syrian hamsters, with an elevated inflammatory transcriptional profile being observed in the hamsters’ brains 31 days after initial infection (19). This was accompanied by behavioral and cognitive changes. While not yet extensively performed, these models may be of particular use to also model PASC pathobiology.
While viral pathology in Syrian hamsters may more resemble humans, significant immune differences exist, although precise characterization of these differences has been hampered by limitations in validated reagents needed to delineate the complex immune responses occurring. The innate immune response is similar, with multiple groups reporting an increase in inflammatory cytokines such as TNF, IL-6, and IL-1B, as well as increase macrophage presence and activation in the lungs within the first 2-5 days of infection (91–93, 98). Adaptive immune characterization has been more difficult, however, due to limitations in hamster specific antibodies and reagents. While a robust virus specific T and B cell response has been observed, delineation and characterization of these adaptive immune responses beyond this has been limited (98). Infection also generates an antibody response similar to humans (98, 99). However, there has been acknowledgement on the limitations of hamsters in modeling human vaccine responses. Merkuleva, et al. demonstrated with a RBD-based vaccine that hamsters had a much smaller production of neutralizing antibodies in comparison to mice, rabbits, and ferrets when given the same vaccine formulation (100). Another group also demonstrated lower antibody titers in comparison to other animal models used (101), offering a potential explanation in Th2 skewing that could exist in hamsters, although this cannot be determined at this time due to the aforementioned lack of hamster specific antibodies for in-depth immune phenotyping.
Ferrets have also been extensively used in respiratory viral models, due to having a similar respiratory system to humans, as well as having similar clinical symptoms, such as coughing and sneezing (102). Ferret ACE2 is structurally similar to humans, and is also bound by the S1 protein, but actual disease severity has been shown to be mild, though severity has been shown to increase with age (not enough to be lethal however) (103–106). Neuropathology is also observable in ferrets, with viral RNA being detectable in the olfactory bulb and occipital lobe, though studies have been limited in regards to the neurological component of infection (107). PASC pathobiology has not been characterized in ferrets, and there are contrasting opinions on whether they are suitable for modeling due to the lack of disease severity (108, 109).
Studies on ferret immune responses to SARS-CoV-2 infection are limited, as, similarly to hamsters, immune-specific reagents that are ferret specific are limited. Current studies have shown the development of virus specific antibodies, with one study showing a similar response between mice and ferrets (91, 100, 110). Another group showed that with infection, a lung transcriptome profile indicating strong enrichment of genes related type 1 interferons, T cell activation, and M1 macrophage polarization, was observable, which was further elevated with age (91). Another group similarly demonstrated type 1 and type 2 interferon gene upregulation with infection, noting that this response was delayed in male ferrets (111).
Non-human primates
Non-human primate (NPH) large animal models offer significant advantages over mouse models, being closer to humans on a genetic, physiological, immune, and even behavioral level. NHPs have similar ACE2 to humans, only differing by a few amino acids, though the amino acids that do differ are those that would be used in S1 protein binding. The major disadvantages of NHP models, in general, is the expensiveness per animal, which severely limits the numbers of animals that can be used in studies. This can also make reproducibility of data and statistics difficult due to limited animal numbers.
NHPs have pathology resembling mild human infection when inoculated with SARS-CoV-2, with clinical scoring of pneumonia, weight loss, malaise, and fever being comparable but the reduced severity of infection is problematic (112, 113). While viral replication occurs, less efficient S1 protein binding limits infectivity and pathology. One study did observed coagulation abnormalities in Chlorocebus aethiops (African Green Monkeys) that would be associated with more severe human infection, but this did not seem to impact long-term overall health (114). Vaccine efficacy has also been demonstrated in NHPs through antibody, immune, and antiviral responses, although studies are limited and often of a short duration, and thus adverse events have not been well documented or even assessed (115–117).
Effects of cross-reactive secondary viral memory responses on CoV2 responses
Although the CoV2 pathogen itself may be new to our species, other human coronaviruses (hCoVs) are not and provide critical common immunological links. Seven hCoVs exist, all of which use a spike (S) protein for cellular entry. CoV2 has been linked with the original CoV due to similarities in virulence, origin, and a high molecular/genetic homology (76%) in their S-proteins (118–120). Four other hCoVs (hCoV-229E, hCoV-NL63, hCoV-HKU1, and hCoV-OC43) are endemic within the population and responsible for common seasonal minor respiratory tract infections worldwide, with the entire population generating immune responses from an early age (121–123). Significant similarities exist between CoV2 and these other hCoVs, suggesting potential cross-reactive immune responses. As with SARS-CoV and CoV2, NL63 also targets the ACE2 receptor via its S- protein. Furthermore, even stronger homologies of CoV2 exist with HKU1 and OC43, which are also beta-coronaviruses, with cross-reactive immune responses having been reported with both antibody and T cell responses (124–126). Others have already demonstrated that in addition to having similar sequence homologies, conservation of epitopes exists between SARS-CoV-2 and a few of these seasonal coronaviruses (127–129), while others have shown the potential of cross reactivity through antibody responses (124, 130, 131), which, taken together with other epidemiological evidence of cross-protective immunity (132), illustrates the potential of cross-reactive mechanisms. Immunological cross-reactivity exists not only among the hCoVs but even with coronaviruses from other species, as antibodies capable of neutralizing both human and mouse CoVs have demonstrated (133). These point to the tremendous potential of pre-existing cross-reactive secondary responses which can then affect not only resistance but also primary antigen-specific response capabilities (119). Furthermore, the endemic nature of these hCoVs indicates that continuous antigen exposure regularly occurs, further amplifying this reshaping of immune repertoire.
The tendency of the immune system to preferentially use immunologic memory from a previous infection when encountering a different version of the original stimulus has been referred to as the “Original Antigenic Sin.” This may represent a means to generate rapidly activated memory responses, even if not of high affinity, during acute infection, and provides a means to compensate in situations where optimal primary response induction is impaired. While not extensively characterized yet, the concept of original antigenic sin has been implicated in SARS-CoV-2 immunity, with recent publications supporting the impact this phenomenon could have on vaccine efficacy and disease outcome (134, 135). Other viruses, such as influenza, which have been published on more extensively in the context of antigenic sin, can potentially offer insight when extrapolated to CoV2. While cross-reactive antibody (xAb1) and T cell responses in CoV2 have been characterized, data on their overall role in efficacy of protection, either positive or negative, have been conflicting. However, many studies also illustrate the difficulty of relying on one readout especially with clinical data: use of antibody levels versus cell-mediated antigen- specific responses by T cells including an effector arm with CD8+ T cells and a helper arm with CD4+ T cells as surrogate predictors in ascertaining protection efficacy. The endemic nature of the hCoVs, with exposure occurring from a young age, combined with the presence of latent viral infections, such as CMV and EBV, add to T cell memory inflation over time, though the exact contribution of hCoVs to this memory inflation has not been well chracterized (136–139) (Figure 1). Preferential activation of these cross-reactive responses, even if of a lower affinity, is due to the tremendous speed advantage that memory responses have over generation of primary responses from naïve T cells. A critical question revolves around the effects of these cross-reactive pre-existing memory cells on overall efficacy towards the new pathogen, with the “net” effect highly contingent on host variables and degree of cross-reactivity. At one end, rapidly induced cross-reactive secondary immune responses, even though of lower affinity, may rapidly generate critically needed initial protection for the host and allow time for more specific primary responses to be generated, as has been suggested in CoV2 to be correlated with less severe disease (140), with a report that even prior responses to other vaccines such as to diphtheria, tetanus and pertussis (DPT), to be potential sources of protective cross-reactive responses to CoV2 (141). Another recent study demonstrated that CoV2 vaccination could induce long-lasting cross-reactive CD4+ T cell responses, suggesting overall better immune effects (142). Conversely, recent reports examining antibody response data from patients and mouse models demonstrated these lower affinity cross-reactive secondary responses to other hCoVs are not only less efficacious, but actually compete and inhibit primary response generation (143, 144), with suggestions that these pre-existing cross-reactive responses are deleterious in protection (145–148). These cross-reactive responses also could have a significant effect on the therapeutic application of convalescent plasma as a source of protective antibodies, which by themselves are immunogenic and also affected by anti-idiotype responses (131). How can one reconcile these potentially opposing effects being observed?
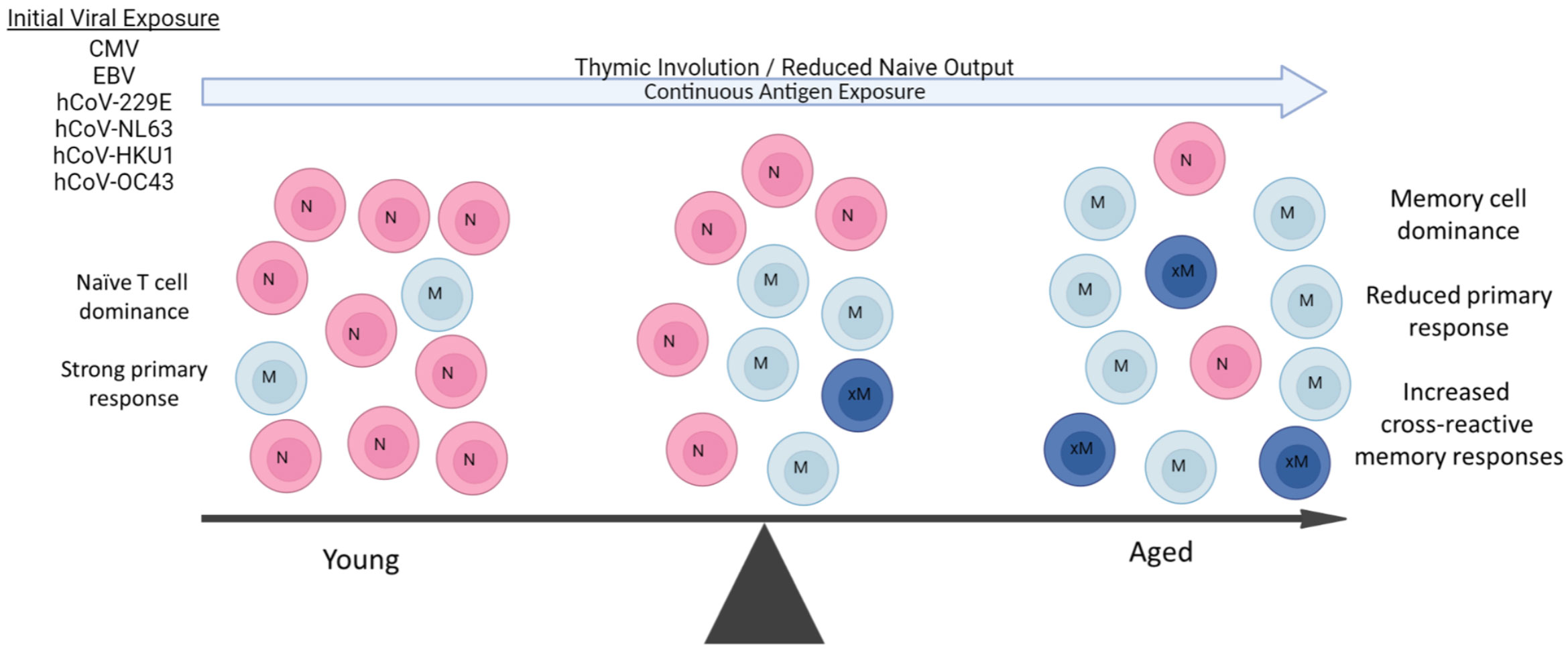
Figure 1 Aging Predisposes to Pre-existing Memory Responses. During aging, there is marked shift towards long-lived memory T cells due to both the massive reduction of naïve T cell output from thymic involution and constant antigenic exposure throughout life. Some viral pathogens are endemic and continuous (hCoVs), while others (CMV, EBV) are latent and a also continual source of antigen exposure. The resulting in contraction of the T cell repertoire due to naive T cell loss and predominance of memory T populations results in an increasingly impaired ability to mount primary immune responses with age. Some of these pre-existing memory cells can also be cross-reactive to new viral pathogens such as SARS-CoV-2 depending on extent of antigen similarities and affinity of original response., These cross-reactive responses can then be both of lower affinity and duration. A similar propensity for pre-existing memory B cell responses also occurs with aging, working in concert with long-lived memory CD4+ T cell help.
Aging and immune responses: the increasing role of memory and cross-reactive secondary responses to overall immunity
Normal aging has been well-documented to result in significantly impaired primary antigen-specific immune responses, in part due to massive changes in the naive T-cell pool caused by thymic involution (149, 150), as well as other alterations that contribute to immunosenescence (151). Aging and obesity are also associated with a persistent pro-inflammatory state that contributes to increased naïve to memory T cell conversion (152–154). Combined with continuous pathogen exposure throughout life, this results in a marked skewing towards long-lived tissue resident memory T-cell numbers from prior antigen responses (Figure 1). Accordingly, the overall TCR repertoire of the aging host markedly contracts in part due to continuous expansion of long-lived memory T cells directed towards common latent viral infections such as CMV and EBV, further impacting the ability of the immune system to respond to acute viral infections (154–156). These memory T cells markedly outcompete the ever-reduced numbers of naive T cells due to their ability to rapidly respond to antigen recall, even if of lower affinity. Aging therefore increases reliance on pre-existing memory T-cell responses by the host when encountering a new pathogen. This reduced ability to mount new primary antigen-specific CD4+ T-cell responses would then also impair the T-cell “help” needed for the generation of high-affinity B cell and antibody generation, also favoring pre-existing cross-reactive memory B-cell responses (157–159) (Figure 1). Thus, at both the B and T cell levels, activated cross-reactive memory responses can potentially suppress the primary CoV2 response in both specificity and duration while at the same time initially resulting in faster responses resulting in protective effects. However, even if activated, not all of these competitive cross-reactive responses may be efficacious, given a recent report of increased production of non-protective hCoV antibodies following CoV2 infection (160). Similarly, cross-reactive hCoV-specific T cells were observed in unexposed patients (125, 126), but it was observed that after infection, the CD4+ T cells were weaker in response and detectable for shorter time periods (161, 162), indicative of lower functional avidity. Several of the studies also correlated the presence of these cross-reactive antibody responses with increased disease severity, suggesting a net negative effect on protection (143, 144, 163).
While notable differences in antibody responses have been noted to occur in vaccinated vs naturally infected elderly patients, age as a factor has not been rigorously assessed in studies, although there was a report assessing T cell recall responses to OC43 and NL63, which showed an absence of T cell recall responses in elderly, but not younger individuals (164), with a caveat that one cannot rule out impaired function associated with aged T cell responses. Additionally, while reports on antibody responses and cross-reactive immune responses have centered on patients following CoV2 infection (165, 166), assessment of the potential effects of vaccines on cross-reactive activation have been lacking, although one study observed no effects of a vaccine on cross-reactive antibodies versus the virus itself (167). In contrast, activation of cross-reactive S-protein-specific CD4+ T-cell responses following vaccination was observed, which notably did not occur in the CD8+ T cell population (142), but the delineation of avidity and duration of these cells versus primed naïve T cell responses was assessed. More studies are needed to determine the effects of these, and potentially other, cross-reactive immune responses following different vaccination regimens. Outside of cross-reactive T memory or antibody responses directly competing with the induction of primary responses, these pre-existing immune responses can also potentially mediate effects through activation of cross-reactive anti-idiotype responses. Understanding immune system dynamics and regulation in the context of aging could be highly revealing, especially given the potential of therapeutics already being investigated for restoring immune function and attenuating dysregulated immune responses in the elderly (168).
Revisiting the network hypothesis: immunoregulatory effects of anti-idiotype and cross-reactive anti-idiotype responses and the role of aging
Both immunoglobulin and T-cell receptor (TCR) gene rearrangement results in the appearance of new antigenic determinants or idiotypes (Ab1 for antibody) to which the immune system has not been tolerized, which can then also induce immune responses. These anti-idiotype or Ab2 responses were postulated by Niels Jerne in the Network Theory as a means of immune-mediated regulation (169). This has been robustly demonstrated using inbred mouse models to defined antigens and monoclonal antibodies, with the bulk of research being performed in the 1980’s and 1990’s (170–173). The difficulty in demonstrating physiologically relevant Ab2 responses in humans partly stems from the tremendous heterogeneity of antigen responses in an outbred population and reliance on clinical data. Nonetheless, demonstration of anti-idiotype antibodies have been demonstrated validating the concept. The immunoregulatory effects of anti-idiotype response is due to the ability of Ab2 to bind Ab1 and neutralize it directly, or to act on the Ab1-producing B cells resulting in clearance or suppression (174–176). The cascade does not stop there however, as the Ab2 also induce down-regulatory “anti-anti-idiotype” or Ab3 responses (177). Some of the Ab3 are similar with the Ab1 idiotype response being also capable of binding the original antigen and possibly protective (178). Ab2 antibodies have even been explored to be surrogates to the original antigen as a vaccine approach. Furthermore, this Ab1>Ab2>Ab3 cascade would then allow for continuation of immune responses long after the antigen itself has disappeared which may also explain for long-lasting off-target effects of either infection or vaccination depending on the nature of the original antigen targeted.
Outside of directly affecting primary Ab1 efficacy, anti-idiotype (Ab2) responses could also potentially exert immune-mediated effects on the host which may account for longer-lasting pathologies following infection and possibly vaccination. A unique type of molecular mimicry attributed to Ab2 can mediate agonistic effects of the primary immunogen. It is important to note that Ab1 responses are polyclonal in nature, and not all Ab1 will induce the same Ab2 (which are also polyclonal), though some clones may predominate (Figure 2). The paratope or binding region of some, but not all, Ab2 can also represent a mirror image of the original antigenic epitope itself and as such, have the capability of binding to the cellular ligand targeted by the original antigen target. Diversity in the anti-idiotypic cascade may also partly explain the tremendous diversity of immune responses within the general population to both CoV2 infection and vaccines (179). Attempts to exploit this antigen-mimicry have included using Ab2 as surrogates for the antigen (180, 181). In the case of CoV2 or vaccines, the ACE2 receptor may be bound by Ab2 in a manner same as the CoV2 S protein (182). Detection of antibodies towards ACE2 following CoV2 infection in some patients supports this hypothesis (183). These Ab2 potentially could then mediate various off-target effects given the diverse expression of ACE2 in many tissues and cell-types, which, depending on the strength of Ab2 response, could possibly result in pathology (Figure 2) (182). Another receptor targeted by the CoV2 S protein, neuropilin-1 (NRP1), which is expressed in astrocytes and neurons, should also be considered in the context of Ab2 antibodies, especially given its roles in axon guidance and VEGF-A modulation. Studies have demonstrated that knockout of NRP1 can have detrimental effects, such as sympathetic nervous system dysregulated sinus bradycardia and neuronal abnormalities, such as poorly condensed ganglia and extended neurons (184). It has not yet been established if antibodies develop against NRP1 during CoV2 infection, however. Because of this, this review will focus on Ab2 responses in the context of ACE2, while noting that NRP1 should also be investigated as well.
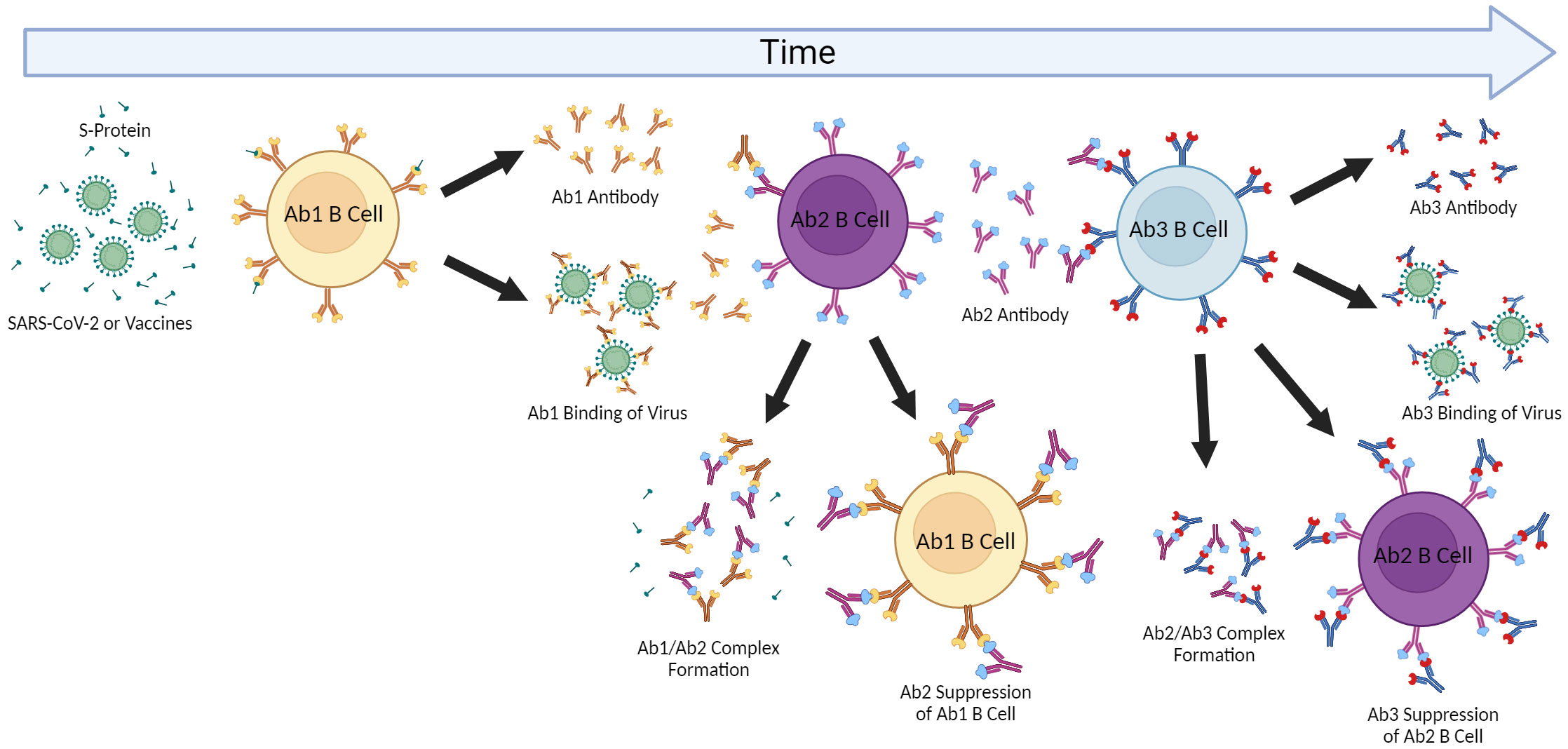
Figure 2 Anti-idiotype Response and the Network Cascade. The induction of antigen-specific antibody responses results in polyclonal antibodies (Ab1). The immunogenic nature of the paratope (antigen-binding region) of Ab1 then can result in polyclonal anti-idiotype (Ab2) antibodies capable of binding and inhibiting Ab1 by forming complexes resulting in Ab1 clearance. The paratope of some of these Ab2 antibodies may be a “mirror” to the original antigen and then induce anti-anti-idiotype antibody responses (Ab3) which can then regulate the down-regulatory Ab2 but also some of which may have similar binding as the Ab1 to the original antigen and be protective. This cascade and effects may be contingent on the extent of immunization or antigen exposure as repeated immunizations can result in greater Ab2 responses as well as effects on aging where cross-reactive Ab2 may also affect initial Ab1 responses.
The kinetics of Ab2 responses to both T-dependent and T-independent antigens have been extensively studied in mice, although rats and non-human primates also have been used. Initial responses to antigen demonstrated delayed Ab2 kinetics of lower magnitude (185). However, when boosting was applied, robust and rapid Ab2 responses were observed, even comparable to Ab1, while also resulting in lessening Ab1 (185). This network would then have a major effect on Ab1 efficacy and duration upon repeated stimulation. Importantly, higher Ab2 responses were also observed in older mice suggesting aging predisposes to Ab2 suppression (186). Similarly, T cells from aged mice were observed to play major role in the increased production of Ab2 at the expense of Ab1 (187). These data would suggest that cross-reactive secondary responses are induced after pathogen exposure. It is possible that cross-reactive secondary anti-idiotype (xAb2) responses may also be induced following CoV2 infection or vaccination, although this needs to be definitively shown.
Just as cross-reactive idiotype antibodies (xAb1) or T cells can potentially directly interfere with primary immune response generation and efficacy, triggered secondary xAb2 responses, along with induced specific Ab2, could augment this down-regulatory cascade causing reduction of Ab1 responses. This may also account for the rapid waning of protective CoV2 immunity following infection or vaccination. Furthermore, repeated antigenic challenge (either by re-infection, vaccine boosting, or even continuous exposure to the original hCoV) may further amplify these inhibitory pathways (182). Aging may exacerbate these as well due to impaired primary immune response capabilities. It was reported that elderly patients following CoV2 infection had no detectable Cov2 antibodies despite having antigen-specific memory B cells, pointing to potential effects of Ab2-mediated clearance being higher in this population (188). It is also worth considering that autoantibodies to ACE2 have been previously reported in patients with connective tissue diseases (189). Antigen mimicry effects by Ab2 could also be affected by cross-reactive memory xAb2 responses based on the recognition of shared epitopes. Pre-existing xAb2 from prior NL63 infections could then also bind the ACE2 receptor and contribute to Ab2 effects. Furthermore, the continuous exposure to endemic CoVs as well as repeated administration of CoV2 vaccines could further stimulate and preferentially expand cross-reactive memory (both xAb1 and xAb2) responses, particularly with aging (Figure 3), possibly resulting in long-term effects that may contribute to PASC symptoms given the perpetuating cascades (Ab1, Ab2, Ab3) involved in the Network Theory. Studies are needed to ascertain if similar anti-idiotype responses are also induced after vaccinations as well as induction of comparable xAb2, which could contribute to effects given the continuous antigenic exposure to these endemic hCoVs. Determining whether increased Ab2 capable of binding ACE2 are induced following vaccination given the restricted antigen exposure versus actual CoV2 infection are also needed.
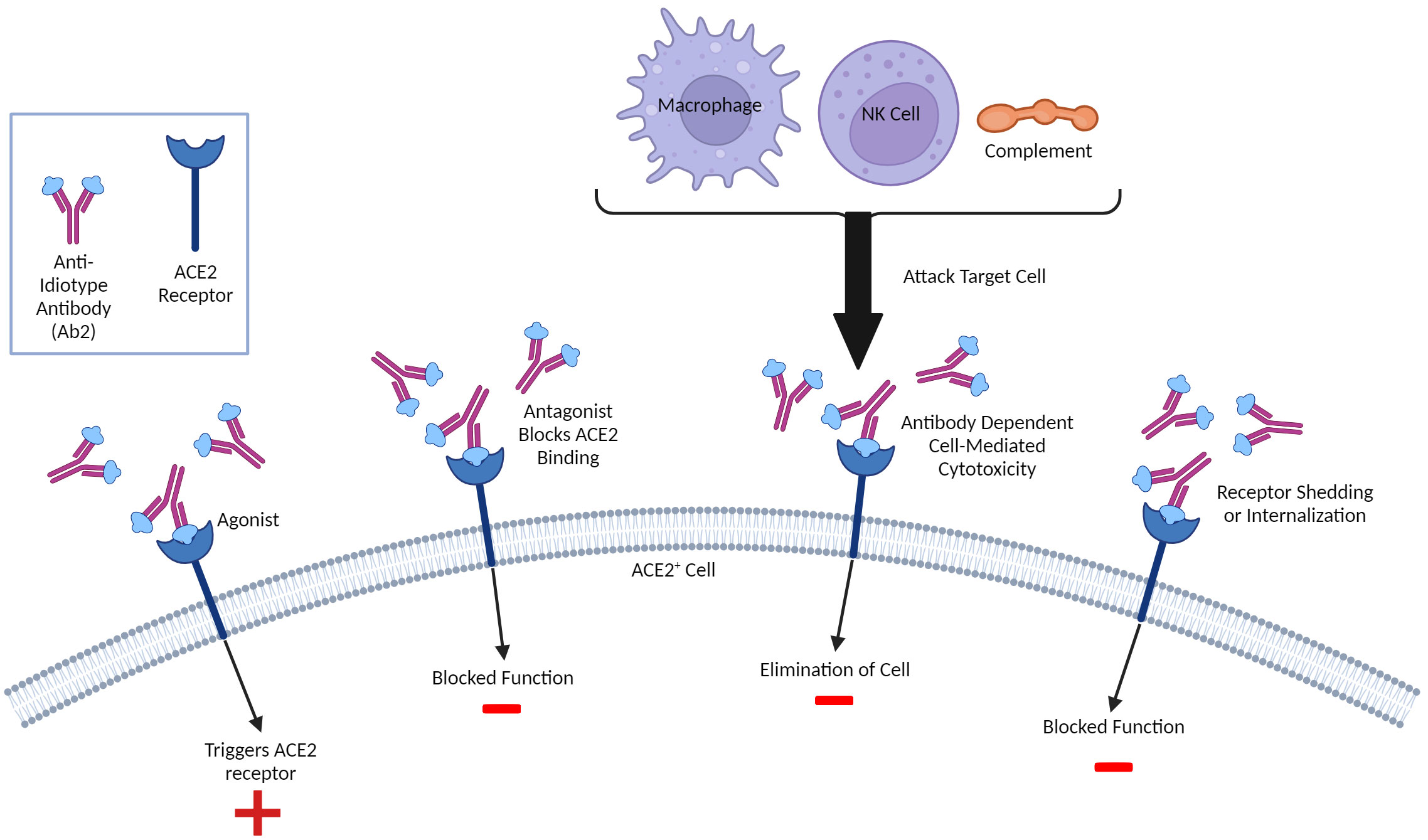
Figure 3 Molecular Mimicry and Potential Anti-Idiotype (Ab2)-Mediated Effects. Ab2 effects can be diverse and not limited to regulation of Ab1 responses. Ab2 are directed towards the Ab1 paratope or antigen- binding region and some can have their paratope be a mirror of the original antigen that is recognized. As such, these Ab2 can bind the same ligands the antigen which, in the case of SARS-CoV-2 infection or vaccination involving the Spike protein, can include the ACE2 receptor, resulting in multiple outcomes. If the Ab2 are antagonistic, they can competitively block ACE2 ligands from binding and inhibit function. The Ab2 upon binding can cause internalization thereby also being inhibitory. Some Ab2 could be agonistic and thereby stimulate ACE2 function. Finally, Ab2 binding ACE2+ cells can be targeted for attack by innate immune system due to ADCC (antibody-directed cell or complement-mediated cytotoxicity). A similar paradigm could exist with T cells, although this is much less characterized. Both inhibition and/or dysregulation of ACE2 function could result in potential pathology given critical role of ACE2 on multiple tissues/cells and in inflammation.
What are the implications of the anti-idiotype (either induced by Ab1 or cross-reactive) on viral protection and treatment? Other than contributing to loss of durability or down-regulation of the primary responses, they should be considered in immune-based therapies. As mentioned, therapeutic application of convalescent sera may be impacted. However, anti-idiotypic responses may have an even more profound down-regulatory effect when using monoclonal antibody-based therapeutics given the monoclonal nature of the therapeutic versus polyclonal Ab1 responses. Another issue centers on the vaccine formulation and schedule depending on the individual’s ability to mount primary immune responses. This also can be important when using vaccines targeting the original CoV2 S protein due to the continuous emergence of viral variants which result in increasing selection and loss of antigenic determinants. This extensive mutation rate of the CoV2 resulting in dominance of viral variants (Delta, Omicron, as well as the continuing emergence of new variants/subvariants), particularly within the S-protein, poses another challenge. The continuous loss of antigenic epitopes in the S-protein to which immune responses were initially generated and then expanded with current vaccine boosting could further limit efficacy due more reliance on the increasingly lower affinity cross-reactive responses. Continuous exposure to endemic hCoVs further complicates the picture by restimulating these memory responses. This has been viewed as a potential issue given the failure to generate a successful vaccine to the endemic hCoV viruses over time.
These effects may be even more pronounced in the aged population with an already impaired priming capability. One group that focused on PASC in the context of the elderly showed that an approximated 30% of patients over the age of 65 developed it (190), while another study showed that Long COVID symptoms were more severe in elderly patients (191), supporting data gathered by the US Census Bureau, though it should be noted that the elderly do not have the highest reported incidence rate of post-COVID symptoms following infection (192). Within the context of vaccination alone, the development of Long COVID has not been extensively addressed, though neuropathic symptoms have been reported, albeit at a lesser occurrence rate than primary infection without vaccination (193–195). While evidence suggests that those vaccinated before infection have protection against Long COVID (196), symptom development after vaccination alone is a phenomenon that is not understood and warrants preclinical investigation, particularly in the context of the elderly. Thus, understanding the immunology of aging in pathogen resistance is a critical parameter that needs to be incorporated into not only clinical studies but more importantly, preclinical studies, given the susceptibility of this population to not only CoV2, but other viral pathogens as well.
Understanding the potential roles of cross-reactive secondary responses and idiotypic regulation: the importance of preclinical modeling
Fortunately, many of the issues raised are very testable hypotheses which can be mechanistically addressed using appropriate preclinical modeling. Preclinical modeling is essential for delineating the complex immunological pathways that arise following infection or vaccination at a mechanistic level, as well as ascertaining potential off-target effects over time under controlled settings. Cross-reactive immune responses, including anti-idiotype responses, on Cov2 and vaccine immunity need to be incorporated into preclinical modeling (119). It is crucial that these studies incorporate important variables such as aging, sex, obesity, and pregnancy, common conditions which all result in significant immune alterations (197–201). Immunological data involving preclinical models on CoV2 have been minimal, especially regarding long-term assessment or dissection of immune pathways under those different conditions. It is important to also recognize that while these models will provide answers, they also have significant limitations since most of these mice are housed under specific-pathogen free conditions and lack significant pathogen exposure, resulting in immature immune phenotypes. In addition, insights on off-target immune-mediated effects can also be gleaned, but choosing the appropriate model is pivotal.Long-term studies as well as effects of repeated vaccination are needed, particularly in aged mice where anti-idiotype responses may be more dominant. It will also be important to use models in the context of prior immunization involving other hCoV viral antigens and repeated stimulation or with viral challenge to allow for accumulation of memory cells. These memory cells could potentially have cross-reactive responses, impacting the ability to later mount successful primary CoV2 antigen responses, which would require a monitoring of immunoregulatory anti-idiotype responses. As anti-idiotype responses are detected after CoV2 infection directed towards ACE2 and long-term effects associated with PASC can result, it is also important to understand the potential effects these antibodies can exert in such preclinical models. Publications have already shown that the spike protein itself can cross the blood brain barrier and cause acute pathology (202–204), particularly through activation of TLR-4 and NLRP3 inflammasome associated inflammatory pathways and mechanisms (21, 205). Direct administration of S protein to the hippocampus in mice has also been shown to induce cognitive defects, behavioral abnormalities, and neuronal death, acting primarily through glial activation and the upregulation of inflammatory cytokines like IL-1B (206). While publications investigating long term pathology in preclinical models have been limited, a recent preclinical model publication demonstrated that S protein administration intracranially produced long term synaptic damage and memory impairment, accompanied by upregulation of inflammatory cytokines, such as TNF and IL-6, complement proteins, most notably C1q, and increased microgliosis, which was also previously published on in relation to spike protein activation of the NLRP3 inflammasome (21, 22). Notably, this altered neurological landscape was associated with impaired cognitive and memory function, symptoms commonly associated with PASC (22). While elucidative, other potential immune mechanisms that could be related to PASC pathology must also be assessed preclinically.
ACE2 knock-out mice have been reported to develop cardiac dysfunction and pathology as they age, with predominantly male mice being affected (207), correlating to recent reports of increased susceptibility to cardiac disease in male patients recovering from CoV2 infection (10). Some tissues from ACE2 knock-out mice also can display increased inflammatory responses during certain stimuli (208) indicating that preclinical assessments should involve various immunostimulatory challenges in CoV2 models, although confounding issues may arise due to inappropriate expression of hACE2 in the tissues of these mice (209), as well as competition with mouse ACE2. Preclinical modeling using multiple approaches and conditions are needed, and it is crucial to take into consideration the limitations of each model before attempting to extrapolate results to the general population.
Preclinical studies on CoV2 responses are urgently needed to answer questions about potential effects of prior cross-reactive immunity on primary response generation and duration, tracking not only anti-idiotype responses but also other immunoregulatory pathways over time, as well as effects of heterologous and repeated vaccinations (Figure 4). It would also be pertinent to investigate these mechanisms in the context of viral infection as well, which is especially relevant given high reinfection rates reported with SARS-CoV-2. Such experiments would also allow for assessment of potential short- and long-term immune-mediated off-target effects on different tissues, a particularly pressing need given the complex biology and effects of ACE2 and other molecules by which the CoV2 S-protein can bind. The use of antibodies to ACE2 in mice as well as more complete characterization of the role of ACE2 on physiologic functions in various tissues may shed some light on issues surrounding PASC which is especially important given increased cardiac risks associated with infection after resolution.
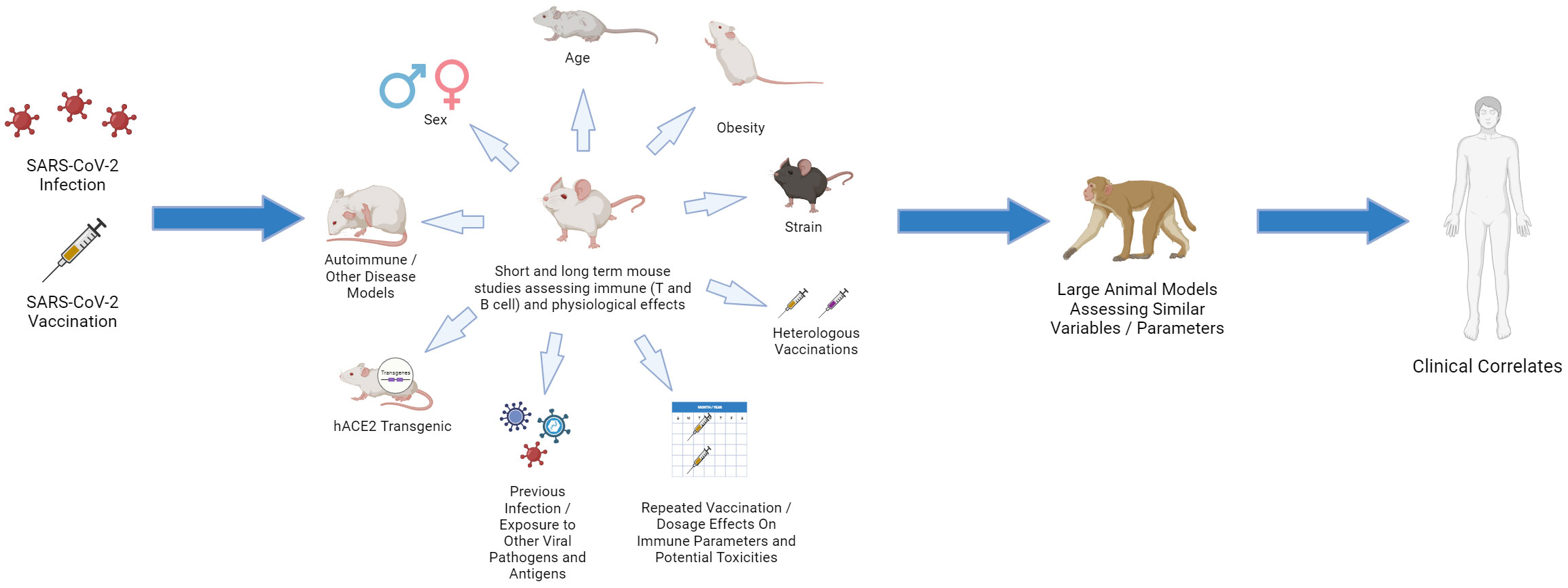
Figure 4 Choosing the Relevant Preclinical Models for CoV2: “Bench to Bedside Back to Bench”. Using young, inbred laboratory mice under SPF conditions fails to mirror the human landscape. Use of aged and obese mice, different strains, pregnant mice, mice that are prone to various autoimmune and disease states, and mice that have been exposed to various pathogens (including HCoV antigens) can all provide important insights. Studies in which repeated vaccinations, including heterologous, are applied and dissection of immune responses, including in different tissues, are assessed. These preclinical studies can then be linked with large animal models, such as non-human primate (NHP) using similar parameters as aging and obesity, which more faithfully represent human immune dynamics. The data can then be linked and validated with clinical results which then can drive questions using the various preclinical models on immune response efficacy and maintenance.
Therefore, increasing basic science investigation on the potential effects of pre-existing immune responses underlying prior viral infections and their potential impact on CoV2, as well as vaccine immune responses in the context of aging, are critical next steps in the continuing fight against the current, and possibly future, viral pandemics. Moving forward, given the significant gaps in knowledge on the effects of both the virus and associated vaccines on immune responses and effects, preclinical studies should emphasize human modifying variables such as obesity and aging combined with repeated stimulation on immune responses and possible off-target effects. It is only in preclinical models that control for exposure to the stimulus (virus or antigen) and incorporating these different variables can result in definitive data. The data observed with the original SARS-CoV vaccines and infection also point to potentially immunopathologic responses which were augmented in aged mice and in some cases, strain-dependent. Thus, it is perhaps not surprising that similar effects may occur with CoV2 and vaccines which need to be further delineated. The models should also be developed to better model PASC and the potential role of immune responses or the virus and associated antigens in perpetuating it these long-term effects. As the CoV2 virus continually changes, so do the vaccine formulations to combat it, and thus these variables also need to be incorporated in the preclinical models. Basic studies are still needed delineating the amount of protein being transcribed, by what cells and for how long as well as what variables affect it. The different components of the vaccine product need to also be more stringently studies regarding immune effects. In the case of the mRNA CoV2 vaccines, this involves understanding the immunogenicity of the NLP carrier, the mRNA itself, and finally the S protein made, both individually and as a composite on immune responses. Regarding anti-idiotype responses, studies in which transfer of anti-S antibodies to mice and assessing both longevity and generation of anti-idiotype responses will be revealing. This includes not only effects on adaptive (T and B cell) but also innate immune components. Finally, different dosing regimens need to be better assessed in determining boosting strategies also keeping in mind critical aspects such as age given the significant differences in immune status of young, adult, and advanced aged recipients towards any pathogen or antigenic challenge.
Author contributions
CC: Writing – original draft, Writing – review & editing. DL: Writing – original draft, Writing – review & editing. WM: Writing – original draft, Writing – review & editing.
Funding
The author(s) declare that no financial support was received for the research, authorship, and/or publication of this article.
Acknowledgments
Figures were made with BioRender.com.
Conflict of interest
The authors declare that the research was conducted in the absence of any commercial or financial relationships that could be construed as a potential conflict of interest.
Publisher’s note
All claims expressed in this article are solely those of the authors and do not necessarily represent those of their affiliated organizations, or those of the publisher, the editors and the reviewers. Any product that may be evaluated in this article, or claim that may be made by its manufacturer, is not guaranteed or endorsed by the publisher.
References
1. Harrison AG, Lin T, Wang P. Mechanisms of SARS-CoV-2 transmission and pathogenesis. Trends Immunol. (2020) 41:1100–15. doi: 10.1016/j.it.2020.10.004
2. Booth A, Reed AB, Ponzo S, Yassaee A, Aral M, Plans D, et al. Population risk factors for severe disease and mortality in COVID-19: A global systematic review and meta-analysis. PloS One. (2021) 16:e0247461. doi: 10.1371/journal.pone.0247461
3. Anand P, Stahel VP. Correction to: The safety of Covid-19 mRNA vaccines: a review. Patient Saf Surg. (2021) 15:22. doi: 10.1186/s13037-021-00296-4
4. Lai Y-C, Cheng Y-W, Chao C-H, Chang Y-Y, Chen C-D, Tsai W-J, et al. Antigenic cross-reactivity between SARS-CoV-2 S1-RBD and its receptor ACE2. Front Immunol. (2022) 13:868724. doi: 10.3389/fimmu.2022.868724
5. Swank Z, Senussi Y, Manickas-Hill Z, Yu XG, Li JZ, Alter G, et al. Persistent circulating severe acute respiratory syndrome coronavirus 2 spike is associated with post-acute coronavirus disease 2019 sequelae. Clin Infect Dis. (2023) 76:e487–90. doi: 10.1093/cid/ciac722
6. Haga S, Yamamoto N, Nakai-Murakami C, Osawa Y, Tokunaga K, Sata T, et al. Modulation of TNF-alpha-converting enzyme by the spike protein of SARS-CoV and ACE2 induces TNF-alpha production and facilitates viral entry. Proc Natl Acad Sci U.S.A. (2008) 105:7809–14. doi: 10.1073/pnas.0711241105
7. Ossato A, Tessari R, Trabucchi C, Zuppini T, Realdon N, Marchesini F. Comparison of medium-term adverse reactions induced by the first and second dose of mRNA BNT162b2 (Comirnaty, Pfizer-BioNTech) vaccine: a post-marketing Italian study conducted between 1 January and 28 February 2021. Eur J Hosp Pharm. (2023) 30:e15. doi: 10.1136/ejhpharm-2021-002933
8. Jeet Kaur R, Dutta S, Charan J, Bhardwaj P, Tandon A, Yadav D, et al. Cardiovascular adverse events reported from COVID-19 vaccines: A study based on WHO database. Int J Gen Med. (2021) 14:3909–27. doi: 10.2147/IJGM.S324349
9. Minhas AS, Shade JK, Cho S-M, Michos ED, Metkus T, Gilotra NA, et al. The role of sex and inflammation in cardiovascular outcomes and mortality in COVID-19. Int J Cardiol. (2021) 337:127–31. doi: 10.1016/j.ijcard.2021.05.011
10. Xie Y, Xu E, Bowe B, Al-Aly Z. Long-term cardiovascular outcomes of COVID-19. Nat Med. (2022) 28:583–90. doi: 10.1038/s41591-022-01689-3
11. Monami M, Gori D, Guaraldi F. COVID-19 vaccine hesitancy and early adverse events reported in a cohort of 7,881 italian physicians. ANNALI DI IGIENE MED PREVENTIVA E DI COMUNITÀ. (2021) 34(4):344–57. doi: 10.7416/ai.2021.2491
12. Govaert TM, Dinant GJ, Aretz K, Masurel N, Sprenger MJ, Knottnerus JA. Adverse reactions to influenza vaccine in elderly people: randomised double blind placebo controlled trial. BMJ. (1993) 307:988–90. doi: 10.1136/bmj.307.6910.988
13. Rodrigues D, de Cassia de Marchi Bacellos de Dalri R. Eventos adversos pós-vacinação contra influenza em idosos no Brasil/Adverse events following immunization against influenza the in elderly in Brazil. Rev Salúd Publica. (2019) 21:22–8. doi: 10.15446/rsap.v21n1.77308
14. Oudjedi A, Allali A, Bekli A, Lounis M, Ben Saad H, Boukoufa M. Reported COVID-19 vaccines side effects among Algerian athletes: a comparison between inactivated virus, adenoviral vector, and mRNA COVID-19 vaccines. Phys Sportsmed. (2023), 1–13. doi: 10.1080/00913847.2023.2186691
15. Kouhpayeh H, Ansari H. Adverse events following COVID-19 vaccination: A systematic review and meta-analysis. Int Immunopharmacol. (2022) 109:108906. doi: 10.1016/j.intimp.2022.108906
16. Nalbandian A, Sehgal K, Gupta A, Madhavan MV, McGroder C, Stevens JS, et al. Post-acute COVID-19 syndrome. Nat Med. (2021) 27:601–15. doi: 10.1038/s41591-021-01283-z
17. Borsini A, Merrick B, Edgeworth J, Mandal G, Srivastava DP, Vernon AC, et al. Neurogenesis is disrupted in human hippocampal progenitor cells upon exposure to serum samples from hospitalized COVID-19 patients with neurological symptoms. Mol Psychiatry. (2022) 27:5049–61. doi: 10.1038/s41380-022-01741-1
18. Yang J-H, Yang M-S, Kim D-M, Kim B, Tark D, Kang S-M, et al. Delta (B1.617.2) variant of SARS-CoV-2 induces severe neurotropic patterns in K18-hACE2 mice. Sci Rep. (2023) 13:3303. doi: 10.1038/s41598-023-29909-x
19. Frere JJ, Serafini RA, Pryce KD, Zazhytska M, Oishi K, Golynker I, et al. SARS-CoV-2 infection in hamsters and humans results in lasting and unique systemic perturbations after recovery. Sci Trans Med. (2022) 14:eabq3059. doi: 10.1126/scitranslmed.abq3059
20. Newell KL, Waickman AT. Inflammation, immunity, and antigen persistence in post-acute sequelae of SARS-CoV-2 infection. Curr Opin Immunol. (2022) 77:102228. doi: 10.1016/j.coi.2022.102228
21. Albornoz EA, Amarilla AA, Modhiran N, Parker S, Li XX, Wijesundara DK, et al. SARS-CoV-2 drives NLRP3 inflammasome activation in human microglia through spike protein. Mol Psychiatry. (2022) 28(7):2878–93. doi: 10.1038/s41380-022-01831-0
22. Fontes-Dantas FL, Fernandes GG, Gutman EG, De Lima EV, Antonio LS, Hammerle MB, et al. SARS-CoV-2 Spike protein induces TLR4-mediated long-term cognitive dysfunction recapitulating post-COVID-19 syndrome in mice. Cell Rep. (2023) 42:112189. doi: 10.1016/j.celrep.2023.112189
23. In rare cases, coronavirus vaccines may cause Long Covid–like symptoms . Available online at: https://www.science.org/content/article/rare-cases-coronavirus-vaccines-may-cause-long-covid-symptoms.
24. Choe PG, Kang CK, Suh HJ, Jung J, Song K-H, Bang JH, et al. Waning antibody responses in asymptomatic and symptomatic SARS-coV-2 infection. Emerg Infect Dis. (2021) 27:327–9. doi: 10.3201/eid2701.203515
25. Mao Y, Wang W, Ma J, Wu S, Sun F. Reinfection rates among patients previously infected by SARS-CoV-2: systematic review and meta-analysis. Chin Med J (Engl). (2022) 135:145–52. doi: 10.1097/CM9.0000000000001892
26. Buhre JS, Pongracz T, Künsting I, Lixenfeld AS, Wang W, Nouta J, et al. mRNA vaccines against SARS-CoV-2 induce comparably low long-term IgG Fc galactosylation and sialylation levels but increasing long-term IgG4 responses compared to an adenovirus-based vaccine. Front Immunol. (2022) 13:1020844. doi: 10.3389/fimmu.2022.1020844
27. Juno JA, Tan H-X, Lee WS, Reynaldi A, Kelly HG, Wragg K, et al. Humoral and circulating follicular helper T cell responses in recovered patients with COVID-19. Nat Med. (2020) 26:1428–34. doi: 10.1038/s41591-020-0995-0
28. Kiszel P, Sík P, Miklós J, Kajdácsi E, Sinkovits G, Cervenak L, et al. Class switch towards spike protein-specific IgG4 antibodies after SARS-CoV-2 mRNA vaccination depends on prior infection history. Sci Rep. (2023) 13:13166. doi: 10.1038/s41598-023-40103-x
29. Mestas J, Hughes CCW. Of mice and not men: differences between mouse and human immunology. J Immunol. (2004) 172:2731–8. doi: 10.4049/jimmunol.172.5.2731
30. Sellers RS, Clifford CB, Treuting PM, Brayton C. Immunological variation between inbred laboratory mouse strains: points to consider in phenotyping genetically immunomodified mice. Vet Pathol. (2012) 49:32–43. doi: 10.1177/0300985811429314
31. Hamilton SE, Badovinac VP, Beura LK, Pierson M, Jameson SC, Masopust D, et al. New insights into the immune system using dirty mice. J Immunol. (2020) 205:3–11. doi: 10.4049/jimmunol.2000171
32. Shang J, Ye G, Shi K, Wan Y, Luo C, Aihara H, et al. Structural basis of receptor recognition by SARS-CoV-2. Nature. (2020) 581:221–4. doi: 10.1038/s41586-020-2179-y
33. Li F, Li W, Farzan M, Harrison SC. Structure of SARS coronavirus spike receptor-binding domain complexed with receptor. Science. (2005) 309:1864–8. doi: 10.1126/science.1116480
34. Corleis B, Bastian M, Hoffmann D, Beer M, Dorhoi A. Animal models for COVID-19 and tuberculosis. Front Immunol. (2023) 14:1223260. doi: 10.3389/fimmu.2023.1223260
35. Saravanan UB, Namachivayam M, Jeewon R, Huang J-D, Durairajan SSK. Animal models for SARS-CoV-2 and SARS-CoV-1 pathogenesis, transmission and therapeutic evaluation. World J Virol. (2022) 11:40–56. doi: 10.5501/wjv.v11.i1.40
36. Roberts A, Paddock C, Vogel L, Butler E, Zaki S, Subbarao K. Aged BALB/c mice as a model for increased severity of severe acute respiratory syndrome in elderly humans. J Virol. (2005) 79:5833–8. doi: 10.1128/jvi.79.9.5833-5838.2005
37. Rockx B, Baas T, Zornetzer GA, Haagmans B, Sheahan T, Frieman M, et al. Early upregulation of acute respiratory distress syndrome-associated cytokines promotes lethal disease in an aged-mouse model of severe acute respiratory syndrome coronavirus infection. J Virol. (2009) 83:7062–74. doi: 10.1128/JVI.00127-09
38. Honda-Okubo Y, Barnard D, Ong CH, Peng B-H, Tseng C-TK, Petrovsky N. Severe acute respiratory syndrome-associated coronavirus vaccines formulated with delta inulin adjuvants provide enhanced protection while ameliorating lung eosinophilic immunopathology. J Virol. (2015) 89:2995–3007. doi: 10.1128/JVI.02980-14
39. Tseng C-T, Sbrana E, Iwata-Yoshikawa N, Newman PC, Garron T, Atmar RL, et al. Immunization with SARS coronavirus vaccines leads to pulmonary immunopathology on challenge with the SARS virus. PloS One. (2012) 7:e35421. doi: 10.1371/journal.pone.0035421
40. Iwata-Yoshikawa N, Nagata N, Takaki H, Matsumoto M, Suzuki T, Hasegawa H, et al. Prophylactic vaccine targeting TLR3 on dendritic cells ameliorates eosinophilic pneumonia in a mouse SARS-coV infection model. Immunohorizons. (2022) 6:275–82. doi: 10.4049/immunohorizons.2200020
41. Iwata-Yoshikawa N, Uda A, Suzuki T, Tsunetsugu-Yokota Y, Sato Y, Morikawa S, et al. Effects of toll-like receptor stimulation on eosinophilic infiltration in lungs of BALB/c mice immunized with UV-inactivated severe acute respiratory syndrome-related coronavirus vaccine. J Virol. (2014) 88:8597–614. doi: 10.1128/JVI.00983-14
42. Bolles M, Deming D, Long K, Agnihothram S, Whitmore A, Ferris M, et al. A double-inactivated severe acute respiratory syndrome coronavirus vaccine provides incomplete protection in mice and induces increased eosinophilic proinflammatory pulmonary response upon challenge. J Virol. (2011) 85:12201–15. doi: 10.1128/JVI.06048-11
43. Li C, Chen Y, Zhao Y, Lung DC, Ye Z, Song W, et al. Intravenous injection of coronavirus disease 2019 (COVID-19) mRNA vaccine can induce acute myopericarditis in mouse model. Clin Infect Dis. (2021) 74:1933–50. doi: 10.1093/cid/ciab707
44. Hsieh CS, Macatonia SE, O’Garra A, Murphy KM. T cell genetic background determines default T helper phenotype development in vitro. J Exp Med. (1995) 181:713–21. doi: 10.1084/jem.181.2.713
45. Iwata-Yoshikawa N, Shiwa N, Sekizuka T, Sano K, Ainai A, Hemmi T, et al. A lethal mouse model for evaluating vaccine-associated enhanced respiratory disease during SARS-CoV-2 infection. Sci Adv. (2022) 8:eabh3827. doi: 10.1126/sciadv.abh3827
46. Patone M, Mei XW, Handunnetthi L, Dixon S, Zaccardi F, Shankar-Hari M, et al. Risk of myocarditis after sequential doses of COVID-19 vaccine and SARS-coV-2 infection by age and sex. Circulation. (2022) 146:743–54. doi: 10.1161/CIRCULATIONAHA.122.059970
47. McCray PB, Pewe L, Wohlford-Lenane C, Hickey M, Manzel L, Shi L, et al. Lethal infection of K18-hACE2 mice infected with severe acute respiratory syndrome coronavirus. J Virol. (2007) 81:813–21. doi: 10.1128/jvi.02012-06
48. Moreau GB, Burgess SL, Sturek JM, Donlan AN, Petri WA, Mann BJ. Evaluation of K18-hACE2 mice as a model of SARS-coV-2 infection. Am J Trop Med Hyg. (2020) 103:1215–9. doi: 10.4269/ajtmh.20-0762
49. Oladunni FS, Park J-G, Pino PA, Gonzalez O, Akhter A, Allué-Guardia A, et al. Lethality of SARS-CoV-2 infection in K18 human angiotensin-converting enzyme 2 transgenic mice. Nat Commun. (2020) 11:6122. doi: 10.1038/s41467-020-19891-7
50. Winkler ES, Bailey AL, Kafai NM, Nair S, McCune BT, Yu J, et al. SARS-CoV-2 infection of human ACE2-transgenic mice causes severe lung inflammation and impaired function. Nat Immunol. (2020) 21:1327–35. doi: 10.1038/s41590-020-0778-2
51. Bao L, Deng W, Huang B, Gao H, Liu J, Ren L, et al. The pathogenicity of SARS-CoV-2 in hACE2 transgenic mice. Nature. (2020) 583:830–3. doi: 10.1038/s41586-020-2312-y
52. Seehusen F, Clark JJ, Sharma P, Bentley EG, Kirby A, Subramaniam K, et al. Neuroinvasion and neurotropism by SARS-coV-2 variants in the K18-hACE2 mouse. Viruses. (2022) 14:1020. doi: 10.3390/v14051020
53. Kumari P, Rothan HA, Natekar JP, Stone S, Pathak H, Strate PG, et al. Neuroinvasion and encephalitis following intranasal inoculation of SARS-coV-2 in K18-hACE2 mice. Viruses. (2021) 13:132. doi: 10.3390/v13010132
54. Fumagalli V, Ravà M, Marotta D, Di Lucia P, Laura C, Sala E, et al. Administration of aerosolized SARS-CoV-2 to K18-hACE2 mice uncouples respiratory infection from fatal neuroinvasion. Sci Immunol. (2021) 7:eabl9929. doi: 10.1126/sciimmunol.abl9929
55. Carossino M, Montanaro P, O’Connell A, Kenney D, Gertje H, Grosz KA, et al. Fatal neuroinvasion and SARS-CoV-2 tropism in K18-hACE2 mice is partially independent on hACE2 expression. Cold Spring Harbor Laboratory, 1 Bungtown Rd, Cold Spring Harbor, NY 11724: Preprint at bioRxiv (2021). doi: 10.1101/2021.01.13.425144
56. Ying B, Whitener B, VanBlargan LA, Hassan AO, Shrihari S, Liang C-Y, et al. Protective activity of mRNA vaccines against ancestral and variant SARS-CoV-2 strains. Sci Trans Med. (2021) 14:eabm3302. doi: 10.1126/scitranslmed.abm3302
57. van Oosten L, Yan K, Rawle DJ, Le TT, Altenburg JJ, Fougeroux C, et al. An S1-nanoparticle vaccine protects against SARS-coV-2 challenge in K18-hACE2 mice. J Virol. (2022) 96:e00844–22. doi: 10.1128/jvi.00844-22
58. Arieta CM, Xie YJ, Rothenberg DA, Diao H, Harjanto D, Meda S, et al. The T-cell-directed vaccine BNT162b4 encoding conserved non-spike antigens protects animals from severe SARS-CoV-2 infection. Cell. (2023) 186:2392–2409.e21. doi: 10.1016/j.cell.2023.04.007
59. Ying B, Scheaffer SM, Whitener B, Liang C-Y, Dmytrenko O, Mackin S, et al. Boosting with Omicron-matched or historical mRNA vaccines increases neutralizing antibody responses and protection against B.1.1.529 infection in mice. Cold Spring Harbor Laboratory, 1 Bungtown Rd, Cold Spring Harbor, NY 11724: Preprint at bioRxiv (2022). doi: 10.1101/2022.02.07.479419
60. Scheaffer SM, Lee D, Whitener B, Ying B, Wu K, Liang C-Y, et al. Bivalent SARS-CoV-2 mRNA vaccines increase breadth of neutralization and protect against the BA.5 Omicron variant in mice. Nat Med. (2023) 29:247–57. doi: 10.1038/s41591-022-02092-8
61. Röltgen K, Nielsen SCA, Arunachalam PS, Yang F, Hoh RA, Wirz OF, et al. mRNA vaccination compared to infection elicits an IgG-predominant response with greater SARS-CoV-2 specificity and similar decrease in variant spike recognition. medRxiv. (2021). doi: 10.1101/2021.04.05.21254952
62. Prabhu M, Yang YJ, Johnston CD, Murphy EA, Ketas TJ, Diaz-Tapia R, et al. Longitudinal antibody response kinetics following SARS-CoV-2 messenger RNA vaccination in pregnant and nonpregnant persons. Am J Obstet Gynecol MFM. (2023) 5:100796. doi: 10.1016/j.ajogmf.2022.100796
63. Garcia-Dominguez D, Henry C, Ma L, Jani H, Amato NJ, Manning T, et al. Altering the mRNA-1273 dosing interval impacts the kinetics, quality, and magnitude of immune responses in mice. Front Immunol. (2022) 13. doi: 10.3389/fimmu.2022.948335
64. Huang H, Zhang C, Yang S, Xiao W, Zheng Q, Song X. The investigation of mRNA vaccines formulated in liposomes administrated in multiple routes against SARS-CoV-2. J Controlled Release. (2021) 335:449–56. doi: 10.1016/j.jconrel.2021.05.024
65. Gebre MS, Rauch S, Roth N, Gergen J, Yu J, Liu X, et al. mRNA vaccines induce rapid antibody responses in mice. NPJ Vaccines. (2022) 7:1–7. doi: 10.1038/s41541-022-00511-y
66. Wisnewski AV, Luna JC, Redlich CA. Human IgG and IgA responses to COVID-19 mRNA vaccines. PloS One. (2021) 16:e0249499. doi: 10.1371/journal.pone.0249499
67. Gil-Manso S, Alonso R, Catalán P, Sánchez-Arcilla I, Marzola M, Correa-Rocha R, et al. IgG anti-RBD levels during 8-month follow-up post-vaccination with BNT162b2 and mRNA-1273 vaccines in healthcare workers: A one-center study. Front Cell Infect Microbiol. (2022) 12:1035155. doi: 10.3389/fcimb.2022.1035155
68. Huang Q, Ji K, Tian S, Wang F, Huang B, Tong Z, et al. A single-dose mRNA vaccine provides a long-term protection for hACE2 transgenic mice from SARS-CoV-2. Nat Commun. (2021) 12:776. doi: 10.1038/s41467-021-21037-2
69. Li J-Q, Zhang Z-R, Zhang H-Q, Zhang Y-N, Zeng X-Y, Zhang Q-Y, et al. Intranasal delivery of replicating mRNA encoding neutralizing antibody against SARS-CoV-2 infection in mice. Sig Transduct Target Ther. (2021) 6:1–8. doi: 10.1038/s41392-021-00783-1
70. Denis J, Garnier A, Cheutin L, Ferrier A, Timera H, Jarjaval F, et al. Long-term systemic and mucosal SARS-CoV-2 IgA response and its association with persistent smell and taste disorders. Front Immunol. (2023) 14:1140714. doi: 10.3389/fimmu.2023.1140714
71. Americo JL, Cotter CA, Earl PL, Liu R, Moss B. Intranasal inoculation of an MVA-based vaccine induces IgA and protects the respiratory tract of hACE2 mice from SARS-CoV-2 infection. Proc Natl Acad Sci U.S.A. (2022) 119:e2202069119. doi: 10.1073/pnas.2202069119
72. Lapuente D, Fuchs J, Willar J, Vieira Antão A, Eberlein V, Uhlig N, et al. Protective mucosal immunity against SARS-CoV-2 after heterologous systemic prime-mucosal boost immunization. Nat Commun. (2021) 12:6871. doi: 10.1038/s41467-021-27063-4
73. Joag V, Wijeyesinghe S, Stolley JM, Quarnstrom CF, Dileepan T, Soerens AG, et al. Cutting edge: mouse SARS-coV-2 epitope reveals infection and vaccine-elicited CD8 T cell responses. J Immunol. (2021) 206:931–5. doi: 10.4049/jimmunol.2001400
74. Zhuang Z, Lai X, Sun J, Chen Z, Zhang Z, Dai J, et al. Mapping and role of T cell response in SARS-CoV-2-infected mice. J Exp Med. (2021) 218:e20202187. doi: 10.1084/jem.20202187
75. Colunga Biancatelli RML, Solopov PA, Sharlow ER, Lazo JS, Marik PE, Catravas JD. The SARS-CoV-2 spike protein subunit S1 induces COVID-19-like acute lung injury in K18-hACE2 transgenic mice and barrier dysfunction in human endothelial cells. Am J Physiol Lung Cell Mol Physiol. (2021) 321:L477–84. doi: 10.1152/ajplung.00223.2021
76. Syenina A, Gan ES, Toh JZN, Alwis R, Lin LZ, Tham CYL, et al. Adverse effects following anti–COVID-19 vaccination with mRNA-based BNT162b2 are alleviated by altering the route of administration and correlate with baseline enrichment of T and NK cell genes. PloS Biol. (2022) 20:e3001643. doi: 10.1371/journal.pbio.3001643
77. Weerasinghe SVW, Ku N-O, Altshuler PJ, Kwan R, Omary MB. Mutation of caspase-digestion sites in keratin 18 interferes with filament reorganization, and predisposes to hepatocyte necrosis and loss of membrane integrity. J Cell Sci. (2014) 127:1464–75. doi: 10.1242/jcs.138479
78. Dong W, Mead H, Tian L, Park J-G, Garcia JI, Jaramillo S, et al. The K18-human ACE2 transgenic mouse model recapitulates non-severe and severe COVID-19 in response to an infectious dose of the SARS-coV-2 virus. J Virol. (2022) 96:e0096421. doi: 10.1128/JVI.00964-21
79. Winkler ES, Chen RE, Alam F, Yildiz S, Case JB, Uccellini MB, et al. SARS-coV-2 causes lung infection without severe disease in human ACE2 knock-in mice. J Virol. (2022) 96:e01511–21. doi: 10.1128/JVI.01511-21
80. Bruter AV, Korshunova DS, Kubekina MV, Sergiev PV, Kalinina AA, Ilchuk LA, et al. Novel transgenic mice with Cre-dependent co-expression of GFP and human ACE2: a safe tool for study of COVID-19 pathogenesis. Transgenic Res. (2021) 30:289–301. doi: 10.1007/s11248-021-00249-8
81. Rathnasinghe R, Strohmeier S, Amanat F, Gillespie VL, Krammer F, García-Sastre A, et al. Comparison of transgenic and adenovirus hACE2 mouse models for SARS-CoV-2 infection. Emerg Microbes Infect. (2020) 9:2433–45. doi: 10.1080/22221751.2020.1838955
82. Roberts A, Deming D, Paddock CD, Cheng A, Yount B, Vogel L, et al. A mouse-adapted SARS-coronavirus causes disease and mortality in BALB/c mice. PloS Pathog. (2007) 3:e5. doi: 10.1371/journal.ppat.0030005
83. Wang J, Shuai L, Wang C, Liu R, He X, Zhang X, et al. Mouse-adapted SARS-CoV-2 replicates efficiently in the upper and lower respiratory tract of BALB/c and C57BL/6J mice. Protein Cell. (2020) 11:776–82. doi: 10.1007/s13238-020-00767-x
84. Leist SR, Dinnon KH, Schäfer A, Tse LV, Okuda K, Hou YJ, et al. A mouse-adapted SARS-coV-2 induces acute lung injury and mortality in standard laboratory mice. Cell. (2020) 183:1070–1085.e12. doi: 10.1016/j.cell.2020.09.050
85. Dinnon KH, Leist SR, Schäfer A, Edwards CE, Martinez DR, Montgomery SA, et al. A mouse-adapted model of SARS-CoV-2 to test COVID-19 countermeasures. Nature. (2020) 586:560–6. doi: 10.1038/s41586-020-2708-8
86. Gu H, Chen Q, Yang G, He L, Fan H, Deng Y-Q, et al. Adaptation of SARS-CoV-2 in BALB/c mice for testing vaccine efficacy. Science. (2020) 369:1603–7. doi: 10.1126/science.abc4730
87. Zhang N-N, Li X-F, Deng Y-Q, Zhao H, Huang Y-J, Yang G, et al. A Thermostable mRNA Vaccine against COVID-19. Cell. (2020) 182:1271–1283.e16. doi: 10.1016/j.cell.2020.07.024
88. DiPiazza AT, Leist SR, Abiona OM, Moliva JI, Werner A, Minai M, et al. COVID-19 vaccine mRNA-1273 elicits a protective immune profile in mice that is not associated with vaccine-enhanced disease upon SARS-CoV-2 challenge. Immunity. (2021) 54:1869–1882.e6. doi: 10.1016/j.immuni.2021.06.018
89. Liu Y, Hu G, Wang Y, Ren W, Zhao X, Ji F, et al. Functional and genetic analysis of viral receptor ACE2 orthologs reveals a broad potential host range of SARS-CoV-2. Proc Natl Acad Sci U.S.A. (2021) 118:e2025373118. doi: 10.1073/pnas.2025373118
90. Zhang Y-N, Zhang Z-R, Zhang H-Q, Li N, Zhang Q-Y, Li X-D, et al. Different pathogenesis of SARS-CoV-2 Omicron variant in wild-type laboratory mice and hamsters. Sig Transduct Target Ther. (2022) 7:1–3. doi: 10.1038/s41392-022-00930-2
91. Osterrieder N, Bertzbach LD, Dietert K, Abdelgawad A, Vladimirova D, Kunec D, et al. Age-dependent progression of SARS-coV-2 infection in Syrian hamsters. Viruses. (2020) 12:779. doi: 10.3390/v12070779
92. Imai M, Iwatsuki-Horimoto K, Hatta M, Loeber S, Halfmann PJ, Nakajima N, et al. Syrian hamsters as a small animal model for SARS-CoV-2 infection and countermeasure development. Proc Natl Acad Sci U.S.A. (2020) 117:16587–95. doi: 10.1073/pnas.2009799117
93. Kreye J, Reincke SM, Kornau H-C, Sánchez-Sendin E, Corman VM, Liu H, et al. A therapeutic non-self-reactive SARS-coV-2 antibody protects from lung pathology in a COVID-19 hamster model. Cell. (2020) 183:1058–1069.e19. doi: 10.1016/j.cell.2020.09.049
94. Lieber CM, Cox RM, Sourimant J, Wolf JD, Juergens K, Phung Q, et al. SARS-CoV-2 VOC type and biological sex affect molnupiravir efficacy in severe COVID-19 dwarf hamster model. Nat Commun. (2022) 13:4416. doi: 10.1038/s41467-022-32045-1
95. Zhang AJ, Lee AC-Y, Chu H, Chan JF-W, Fan Z, Li C, et al. SARS-CoV-2 infects and damages the mature and immature olfactory sensory neurons of hamsters. Clin Infect Dis. (2020) 73(2):e503–12. doi: 10.1093/cid/ciaa995
96. Serafini RA, Frere JJ, Zimering J, Giosan IM, Pryce KD, Golynker I, et al. SARS-CoV-2 airway infection results in the development of somatosensory abnormalities in a hamster model. Sci Signaling. (2023) 16:eade4984. doi: 10.1126/scisignal.ade4984
97. Heydemann L, Ciurkiewicz M, Beythien G, Becker K, Schughart K, Stanelle-Bertram S, et al. Hamster model for post-COVID-19 alveolar regeneration offers an opportunity to understand post-acute sequelae of SARS-CoV-2. Nat Commun. (2023) 14:3267. doi: 10.1038/s41467-023-39049-5
98. Horiuchi S, Oishi K, Carrau L, Frere J, Møller R, Panis M, et al. Immune memory from SARS-CoV-2 infection in hamsters provides variant-independent protection but still allows virus transmission. Sci Immunol. (2021) 6:eabm3131. doi: 10.1126/sciimmunol.abm3131
99. Field CJ, Heinly TA, Patel DR, Sim DG, Luley E, Gupta SL, et al. Immune durability and protection against SARS-CoV-2 re-infection in Syrian hamsters. Emerg Microbes Infect. (2022) 11:1103–14. doi: 10.1080/22221751.2022.2058419
100. Merkuleva IA, Shcherbakov DN, Borgoyakova MB, Isaeva AA, Nesmeyanova VS, Volkova NV, et al. Are hamsters a suitable model for evaluating the immunogenicity of RBD-based anti-COVID-19 subunit vaccines? Viruses. (2022) 14:1060. doi: 10.3390/v14051060
101. Sui Y, Andersen H, Li J, Hoang T, Bekele Y, Kar S, et al. Protection from COVID-19 disease in hamsters vaccinated with subunit SARS-CoV-2 S1 mucosal vaccines adjuvanted with different adjuvants. Front Immunol. (2023) 14:1154496. doi: 10.3389/fimmu.2023.1154496
102. Belser JA, Katz JM, Tumpey TM. The ferret as a model organism to study influenza A virus infection. Dis Model Mech. (2011) 4:575–9. doi: 10.1242/dmm.007823
103. Sun H, Wang A, Wang L, Wang B, Tian G, Yang J, et al. Systematic tracing of susceptible animals to SARS-coV-2 by a bioinformatics framework. Front Microbiol. (2022) 13:781770. doi: 10.3389/fmicb.2022.781770
104. Richard M, Kok A, de Meulder D, Bestebroer TM, Lamers MM, Okba NMA, et al. SARS-CoV-2 is transmitted via contact and via the air between ferrets. Nat Commun. (2020) 11:3496. doi: 10.1038/s41467-020-17367-2
105. Ryan KA, Bewley KR, Fotheringham SA, Slack GS, Brown P, Hall Y, et al. Dose-dependent response to infection with SARS-CoV-2 in the ferret model and evidence of protective immunity. Nat Commun. (2021) 12:81. doi: 10.1038/s41467-020-20439-y
106. Zamoto A, Taguchi F, Fukushi S, Morikawa S, Yamada YK. Identification of ferret ACE2 and its receptor Function for Sars-Coronavirus. In: Perlman S, Holmes KV, editors. The Nidoviruses Advances in Experimental Medicine and Biology. One New York Plaza, Suite 4600, New York, NY 10004-1562, USA: Springer US (2006). p. 519–22. doi: 10.1007/978-0-387-33012-9_93
107. Au GG, Marsh GA, McAuley AJ, Lowther S, Trinidad L, Edwards S, et al. Characterisation and natural progression of SARS-CoV-2 infection in ferrets. Sci Rep. (2022) 12:5680. doi: 10.1038/s41598-022-08431-6
108. Jansen EB, Orvold SN, Swan CL, Yourkowski A, Thivierge BM, Francis ME, et al. After the virus has cleared-Can preclinical models be employed for Long COVID research? PloS Pathog. (2022) 18:e1010741. doi: 10.1371/journal.ppat.1010741
109. van de Ven K, van Dijken H, Wijsman L, Gomersbach A, Schouten T, Kool J, et al. Pathology and immunity after SARS-coV-2 infection in male ferrets is affected by age and inoculation route. Front Immunol. (2021) 12:750229. doi: 10.3389/fimmu.2021.750229
110. Shi J, Wen Z, Zhong G, Yang H, Wang C, Huang B, et al. Susceptibility of ferrets, cats, dogs, and other domesticated animals to SARS-coronavirus 2. Science. (2020) 368:1016–20. doi: 10.1126/science.abb7015
111. Francis ME, Richardson B, Goncin U, McNeil M, Rioux M, Foley MK, et al. Sex and age bias viral burden and interferon responses during SARS-CoV-2 infection in ferrets. Sci Rep. (2021) 11:14536. doi: 10.1038/s41598-021-93855-9
112. Lu S, Zhao Y, Yu W, Yang Y, Gao J, Wang J, et al. Comparison of nonhuman primates identified the suitable model for COVID-19. Sig Transduct Target Ther. (2020) 5:1–9. doi: 10.1038/s41392-020-00269-6
113. Munster VJ, Feldmann F, Williamson BN, van Doremalen N, Pérez-Pérez L, Schulz J, et al. Respiratory disease in rhesus macaques inoculated with SARS-CoV-2. Nature. (2020) 585:268–72. doi: 10.1038/s41586-020-2324-7
114. Woolsey C, Borisevich V, Prasad AN, Agans KN, Deer DJ, Dobias NS, et al. Establishment of an African green monkey model for COVID-19 and protection against re-infection. Nat Immunol. (2021) 22:86–98. doi: 10.1038/s41590-020-00835-8
115. Corbett KS, Nason MC, Flach B, Gagne M, O’Connell S, Johnston TS, et al. Immune correlates of protection by mRNA-1273 vaccine against SARS-CoV-2 in nonhuman primates. Science. (2021) 373:eabj0299. doi: 10.1126/science.abj0299
116. Corbett KS, Gagne M, Wagner DA, O’ Connell S, Narpala SR, Flebbe DR, et al. Protection against SARS-CoV-2 Beta variant in mRNA-1273 vaccine-boosted nonhuman primates. Science. (2021) 374:1343–53. doi: 10.1126/science.abl8912
117. Gagne M, Moliva JI, Foulds KE, Andrew SF, Flynn BJ, Werner AP, et al. mRNA-1273 or mRNA-Omicron boost in vaccinated macaques elicits similar B cell expansion, neutralizing responses, and protection from Omicron. Cell. (2022) 185:1556–1571.e18. doi: 10.1016/j.cell.2022.03.038
118. Kronvall G, Nordenfelt E. On the history of human coronaviruses. APMIS. (2021) 129(7):381–3. doi: 10.1111/apm.13109
119. Sealy RE, Hurwitz JL. Cross-reactive immune responses toward the common cold human coronaviruses and severe acute respiratory syndrome coronavirus 2 (SARS-coV-2): mini-review and a murine study. Microorganisms. (2021) 9:1643. doi: 10.3390/microorganisms9081643
120. Weiss SR. Forty years with coronaviruses. J Exp Med. (2020) 217:e20200537. doi: 10.1084/jem.20200537
121. Riski H, Hovi T. Coronavirus infections of man associated with diseases other than the common cold. J Med Virol. (1980) 6:259–65. doi: 10.1002/jmv.1890060309
122. Killerby ME, Biggs HM, Haynes A, Dahl RM, Mustaquim D, Gerber SI, et al. Human coronavirus circulation in the United States 2014-2017. J Clin Virol. (2018) 101:52–6. doi: 10.1016/j.jcv.2018.01.019
123. V’kovski P, Kratzel A, Steiner S, Stalder H, Thiel V. Coronavirus biology and replication: implications for SARS-CoV-2. Nat Rev Microbiol. (2021) 19:155–70. doi: 10.1038/s41579-020-00468-6
124. Ng KW, Faulkner N, Cornish GH, Rosa A, Harvey R, Hussain S, et al. Preexisting and de novo humoral immunity to SARS-CoV-2 in humans. Science. (2020) 370:1339–43. doi: 10.1126/science.abe1107
125. Mateus J, Grifoni A, Tarke A, Sidney J, Ramirez SI, Dan JM, et al. Selective and cross-reactive SARS-CoV-2 T cell epitopes in unexposed humans. Science. (2020) 370:89–94. doi: 10.1126/science.abd3871
126. Woldemeskel BA, Kwaa AK, Garliss CC, Laeyendecker O, Ray SC, Blankson JN. Healthy donor T cell responses to common cold coronaviruses and SARS-CoV-2. J Clin Invest. (2020) 130:6631–8. doi: 10.1172/JCI143120
127. Schulien I, Kemming J, Oberhardt V, Wild K, Seidel LM, Killmer S, et al. Characterization of pre-existing and induced SARS-CoV-2-specific CD8+ T cells. Nat Med. (2021) 27:78–85. doi: 10.1038/s41591-020-01143-2
128. Smit WL, van Tol S, Haas LEM, Limonard GJM, Bossink A, Reusken C, et al. Differential abundance of IgG antibodies against the spike protein of SARS-CoV-2 and seasonal coronaviruses in patients with fatal COVID-19. Virol J. (2023) 20:85. doi: 10.1186/s12985-023-02050-x
129. Simula ER, Manca MA, Jasemi S, Uzzau S, Rubino S, Manchia P, et al. HCoV-NL63 and SARS-coV-2 share recognized epitopes by the humoral response in sera of people collected pre- and during coV-2 pandemic. Microorganisms. (2020) 8:1993. doi: 10.3390/microorganisms8121993
130. Murray SM, Ansari AM, Frater J, Klenerman P, Dunachie S, Barnes E, et al. The impact of pre-existing cross-reactive immunity on SARS-CoV-2 infection and vaccine responses. Nat Rev Immunol. (2023) 23:304–16. doi: 10.1038/s41577-022-00809-x
131. Morgenlander WR, Henson SN, Monaco DR, Chen A, Littlefield K, Bloch EM, et al. Antibody responses to endemic coronaviruses modulate COVID-19 convalescent plasma functionality. J Clin Invest. (2021) 131:146927. doi: 10.1172/JCI146927
132. Nickbakhsh S, Ho A, Marques DFP, McMenamin J, Gunson RN, Murcia PR. Epidemiology of seasonal coronaviruses: establishing the context for the emergence of coronavirus disease 2019. J Infect Dis. (2020) 222:17–25. doi: 10.1093/infdis/jiaa185
133. Klompus S, Leviatan S, Vogl T, Mazor RD, Kalka IN, Stoler-Barak L, et al. Cross-reactive antibodies against human coronaviruses and the animal coronavirome suggest diagnostics for future zoonotic spillovers. Sci Immunol. (2021) 6:eabe9950. doi: 10.1126/sciimmunol.abe9950
134. Aguilar-Bretones M, Fouchier RA, Koopmans MP, van Nierop GP. Impact of antigenic evolution and original antigenic sin on SARS-CoV-2 immunity. J Clin Invest. (2023) 133:e162192. doi: 10.1172/JCI162192
135. Anderson EM, Li SH, Awofolaju M, Eilola T, Goodwin E, Bolton MJ, et al. SARS-CoV-2 infections elicit higher levels of original antigenic sin antibodies compared with SARS-CoV-2 mRNA vaccinations. Cell Rep. (2022) 41:111496. doi: 10.1016/j.celrep.2022.111496
136. Karrer U, Sierro S, Wagner M, Oxenius A, Hengel H, Koszinowski UH, et al. Memory inflation: continuous accumulation of antiviral CD8+ T cells over time. J Immunol. (2003) 170:2022–9. doi: 10.4049/jimmunol.170.4.2022
137. Shin HS, Kim Y, Kang J, Um J, Park JS, Park WB, et al. Longitudinal analysis of memory T-cell responses in survivors of middle east respiratory syndrome. Clin Infect Dis. (2022) 75:596–603. doi: 10.1093/cid/ciab1019
138. Taira N, Toguchi S, Miyagi M, Mori T, Tomori H, Oshiro K, et al. Altered pre-existing SARS-CoV-2-specific T cell responses in elderly individuals. Clin Immunol Commun. (2022) 2:6–11. doi: 10.1016/j.clicom.2021.12.001
139. Townsend JP, Hassler HB, Wang Z, Miura S, Singh J, Kumar S, et al. The durability of immunity against reinfection by SARS-CoV-2: a comparative evolutionary study. Lancet Microbe. (2021) 2:e666–75. doi: 10.1016/S2666-5247(21)00219-6
140. Sagar M, Reifler K, Rossi M, Miller NS, Sinha P, White LF, et al. Recent endemic coronavirus infection is associated with less-severe COVID-19. J Clin Invest. (2021) 131(1):e143380. doi: 10.1172/JCI143380
141. Reche PA. Potential cross-reactive immunity to SARS-coV-2 from common human pathogens and vaccines. Front Immunol. (2020) 11:586984. doi: 10.3389/fimmu.2020.586984
142. Mateus J, Dan JM, Zhang Z, Rydyznski Moderbacher C, Lammers M, Goodwin B, et al. Low-dose mRNA-1273 COVID-19 vaccine generates durable memory enhanced by cross-reactive T cells. Science. (2021) 374:eabj9853. doi: 10.1126/science.abj9853
143. Wratil PR, Schmacke NA, Karakoc B, Dulovic A, Junker D, Becker M, et al. Evidence for increased SARS-CoV-2 susceptibility and COVID-19 severity related to pre-existing immunity to seasonal coronaviruses. Cell Rep. (2021) 37:110169. doi: 10.1016/j.celrep.2021.110169
144. Lin C-Y, Wolf J, Brice DC, Sun Y, Locke M, Cherry S, et al. Pre-existing humoral immunity to human common cold coronaviruses negatively impacts the protective SARS-CoV-2 antibody response. Cell Host Microbe. (2022) 30:83–96.e4. doi: 10.1016/j.chom.2021.12.005
145. Beretta A, Cranage M, Zipeto D. Is cross-reactive immunity triggering COVID-19 immunopathogenesis? Front Immunol. (2020) 11:567710. doi: 10.3389/fimmu.2020.567710
146. Song G, He W-T, Callaghan S, Anzanello F, Huang D, Ricketts J, et al. Cross-reactive serum and memory B-cell responses to spike protein in SARS-CoV-2 and endemic coronavirus infection. Nat Commun. (2021) 12:2938. doi: 10.1038/s41467-021-23074-3
147. Chan KH, Cheng VCC, Woo PCY, Lau SKP, Poon LLM, Guan Y, et al. Serological responses in patients with severe acute respiratory syndrome coronavirus infection and cross-reactivity with human coronaviruses 229E, OC43, and NL63. Clin Diagn Lab Immunol. (2005) 12:1317–21. doi: 10.1128/CDLI.12.11.1317-1321.2005
148. de Assis RR, Jain A, Nakajima R, Jasinskas A, Felgner J, Obiero JM, et al. Analysis of SARS-CoV-2 antibodies in COVID-19 convalescent blood using a coronavirus antigen microarray. Nat Commun. (2021) 12:6. doi: 10.1038/s41467-020-20095-2
149. Aspinall R, Andrew D. Thymic involution in aging. J Clin Immunol. (2000) 20:250–6. doi: 10.1023/a:1006611518223
150. Liang Z, Dong X, Zhang Z, Zhang Q, Zhao Y. Age-related thymic involution: Mechanisms and functional impact. Aging Cell. (2022) 21:e13671. doi: 10.1111/acel.13671
151. Castelo-Branco C, Soveral I. The immune system and aging: a review. Gynecol Endocrinol. (2014) 30:16–22. doi: 10.3109/09513590.2013.852531
152. Wang Z, Aguilar EG, Luna JI, Dunai C, Khuat LT, Le CT, et al. Paradoxical effects of obesity on T cell function during tumor progression and PD-1 checkpoint blockade. Nat Med. (2019) 25:141–51. doi: 10.1038/s41591-018-0221-5
153. Jiang J, Fisher EM, Murasko DM. CD8 T cell responses to influenza virus infection in aged mice. Ageing Res Rev. (2011) 10:422–7. doi: 10.1016/j.arr.2011.02.001
154. Nikolich-Žugich J, Bradshaw CM, Uhrlaub JL, Watanabe M. Immunity to acute virus infections with advanced age. Curr Opin Virol. (2021) 46:45–58. doi: 10.1016/j.coviro.2020.09.007
155. Naylor K, Li G, Vallejo AN, Lee W-W, Koetz K, Bryl E, et al. The influence of age on T cell generation and TCR diversity. J Immunol. (2005) 174:7446–52. doi: 10.4049/jimmunol.174.11.7446
156. Yager EJ, Ahmed M, Lanzer K, Randall TD, Woodland DL, Blackman MA. Age-associated decline in T cell repertoire diversity leads to holes in the repertoire and impaired immunity to influenza virus. J Exp Med. (2008) 205:711–23. doi: 10.1084/jem.20071140
157. Eaton SM, Burns EM, Kusser K, Randall TD, Haynes L. Age-related defects in CD4 T cell cognate helper function lead to reductions in humoral responses. J Exp Med. (2004) 200:1613–22. doi: 10.1084/jem.20041395
158. Cancro MP, Hao Y, Scholz JL, Riley RL, Frasca D, Dunn-Walters DK, et al. B cells and aging: molecules and mechanisms. Trends Immunol. (2009) 30:313–8. doi: 10.1016/j.it.2009.04.005
159. Weksler ME, Russo C, Siskind GW. Peripheral T cells select the B-cell repertoire in old mice. Immunol Rev. (1989) 110:173–85. doi: 10.1111/j.1600-065x.1989.tb00033.x
160. Aguilar-Bretones M, Westerhuis BM, Raadsen MP, de Bruin E, Chandler FD, Okba NMA, et al. Seasonal coronavirus–specific B cells with limited SARS-CoV-2 cross-reactivity dominate the IgG response in severe COVID-19. J Clin Invest. (2021) 131(21):e150613. doi: 10.1172/JCI150613
161. Bacher P, Rosati E, Esser D, Martini GR, Saggau C, Schiminsky E, et al. Low-avidity CD4+ T cell responses to SARS-coV-2 in unexposed individuals and humans with severe COVID-19. Immunity. (2020) 53:1258–1271.e5. doi: 10.1016/j.immuni.2020.11.016
162. Dykema AG, Zhang B, Woldemeskel BA, Garliss CC, Cheung LS, Choudhury D, et al. Functional characterization of CD4+ T cell receptors crossreactive for SARS-CoV-2 and endemic coronaviruses. J Clin Invest. (2021) 131:146922. doi: 10.1172/JCI146922
163. Meyer-Arndt L, Schwarz T, Loyal L, Henze L, Kruse B, Dingeldey M, et al. Cutting Edge: Serum but Not Mucosal Antibody Responses Are Associated with Pre-Existing SARS-CoV-2 Spike Cross-Reactive CD4+ T Cells following BNT162b2 Vaccination in the Elderly. J Immunol. (2022) 208:1001–5. doi: 10.4049/jimmunol.2100990
164. Saletti G, Gerlach T, Jansen JM, Molle A, Elbahesh H, Ludlow M, et al. Older adults lack SARS CoV-2 cross-reactive T lymphocytes directed to human coronaviruses OC43 and NL63. Sci Rep. (2020) 10:21447. doi: 10.1038/s41598-020-78506-9
165. Müller L, Andrée M, Ostermann PN, Jazmati N, Flüh G, Fischer JC, et al. SARS-coV-2 infection in fully vaccinated individuals of old age strongly boosts the humoral immune response. Front Med. (2021) 8. doi: 10.3389/fmed.2021.746644
166. Bag Soytas R, Cengiz M, Islamoglu MS, Borku Uysal B, Yavuzer S, Yavuzer H. Antibody responses to COVID-19 vaccines in older adults. J Med Virol. (2022) 94:1650–4. doi: 10.1002/jmv.27531
167. Crowley AR, Natarajan H, Hederman AP, Bobak CA, Weiner JA, Wieland-Alter W, et al. Boosting of cross-reactive antibodies to endemic coronaviruses by SARS-CoV-2 infection but not vaccination with stabilized spike. eLife. (2022) 11:e75228. doi: 10.7554/eLife.75228
168. Aspinall R, Lang PO. Interventions to restore appropriate immune function in the elderly. Immun Ageing. (2018) 15:5. doi: 10.1186/s12979-017-0111-6
169. Jerne NK. Towards a network theory of the immune system. Ann Immunol (Paris). (1974) 125C:373–89.
170. Kearney JF, Barletta R, Quan ZS, Quintáns J. Monoclonal vs. heterogeneous anti-H-8 antibodies in the analysis of the anti-phosphorylcholine response in BALB/c mice. Eur J Immunol. (1981) 11:877–83. doi: 10.1002/eji.1830111106
171. Wittner MK, Bach MA, Köhler H. Immune response to phosphorylcholine. IX. Characterization of hybridoma anti-TEPC15 antibodies. J Immunol. (1982) 128:595–9.
172. Cosenza H. Detection of anti-idiotype reactive cells in the response to phosphorylcholine. Eur J Immunol. (1976) 6:114–6. doi: 10.1002/eji.1830060208
173. Cosenza H, Köhler H. Specific inhibition of plaque formation to phosphorylcholine by antibody against antibody. Science. (1972) 176:1027–9. doi: 10.1126/science.176.4038.1027
174. Urbain J, Wikler M, Franssen JD, Collignon C. Idiotypic regulation of the immune system by the induction of antibodies against anti-idiotypic antibodies. Proc Natl Acad Sci. (1977) 74:5126–30. doi: 10.1073/pnas.74.11.5126
175. Cazenave PA. Idiotypic-anti-idiotypic regulation of antibody synthesis in rabbits. Proc Natl Acad Sci. (1977) 74:5122–5. doi: 10.1073/pnas.74.11.5122
176. Geha RS. Idiotypic–anti-idiotypic interactions in man. Am J Dis Children. (1985) 139:417–20. doi: 10.1001/archpedi.1985.02140060099041
177. Jerne NK. The immune system: a web of V-domains. Harvey Lect 70 Series. Harvey Society of New York - New York City, New York (1974). pp. 93–110.
178. Stanova AK, Ryabkova VA, Utekhin SV, Shoenfeld VJ, Churilov LP, Shoenfeld Y. Anti-idiotypic agonistic antibodies: candidates for the role of universal remedy. Antibodies (Basel). (2020) 9:E19. doi: 10.3390/antib9020019
179. Murphy WJ, Longo DL. A possible role for anti-idiotype antibodies in SARS-coV-2 infection and vaccination. New Engl J Med. (2021) 386(4):394–6. doi: 10.1056/NEJMcibr2113694
180. Eichmann K, Rajewsky K. Induction of T and B cell immunity by anti-idiotypic antibody. Eur J Immunol. (1975) 5:661–6. doi: 10.1002/eji.1830051002
181. Jean-Francois MJ, Poskitt DC, MacDonald LM, Turnbull SJ, Yasmeen D. Production of monoclonal anti-idiotype antibodies which mimic an M-like protein of Streptococcus equi. Microbiol Immunol. (1993) 37:737–42. doi: 10.1111/j.1348-0421.1993.tb01699.x
182. Murphy WJ, Collins CP, Ashwood P. Potential role of anti-Idiotype responses on the neurological effects of post-acute sequelae of COVID-19 (PASC). Brain Behav Immun. (2023) 116:317–20. doi: 10.1016/j.bbi.2023.12.017
183. Arthur JM, Forrest JC, Boehme KW, Kennedy JL, Owens S, Herzog C, et al. Development of ACE2 autoantibodies after SARS-CoV-2 infection. PloS One. (2021) 16:e0257016. doi: 10.1371/journal.pone.0257016
184. Maden CH, Gomes J, Schwarz Q, Davidson K, Tinker A, Ruhrberg C. NRP1 and NRP2 cooperate to regulate gangliogenesis, axon guidance and target innervation in the sympathetic nervous system. Dev Biol. (2012) 369:277–85. doi: 10.1016/j.ydbio.2012.06.026
185. Schlueter AJ, Segre D, Kuhlenschmidt MS, Segre M. Behavior of the idiotypic network in conventional immune responses: I. Kinetics of idiotypic and anti-idiotypic antibodies following immunization with T-independent and T-dependent antigens. Cell Immunol. (1992) 144:311–23. doi: 10.1016/0008-8749(92)90247-M
186. Borghesi C, Nicoletti C. Increase of cross(auto)-reactive antibodies after immunization in aged mice: a cellular and molecular study. Int J Exp Pathol. (1994) 75:123–30.
187. Kim YT, Goidl EA, Samarut C, Weksler ME, Thorbecke GJ, Siskind GW. Bone marrow function. I. Peripheral T cells are responsible for the increased auto-antiidiotype response of older mice. J Exp Med. (1985) 161:1237–42. doi: 10.1084/jem.161.5.1237
188. Jeffery-Smith A, Burton AR, Lens S, Rees-Spear C, Davies J, Patel M, et al. SARS-CoV-2-specific memory B cells can persist in the elderly who have lost detectable neutralising antibodies. J Clin Invest. (2021) 132(2). doi: 10.1172/JCI152042
189. Takahashi Y, Haga S, Ishizaka Y, Mimori A. Autoantibodies to angiotensin-converting enzyme 2 in patients with connective tissue diseases. Arthritis Res Ther. (2010) 12:R85. doi: 10.1186/ar3012
190. Fung KW, Baye F, Baik SH, Zheng Z, McDonald CJ. Prevalence and characteristics of long COVID in elderly patients: An observational cohort study of over 2 million adults in the US. PloS Med. (2023) 20:e1004194. doi: 10.1371/journal.pmed.1004194
191. Sudre CH, Murray B, Varsavsky T, Graham MS, Penfold RS, Bowyer RC, et al. Attributes and predictors of long COVID. Nat Med. (2021) 27:626–31. doi: 10.1038/s41591-021-01292-y
192. Thaweethai T, Jolley SE, Karlson EW, Levitan EB, Levy B, McComsey GA, et al. Development of a definition of postacute sequelae of SARS-coV-2 infection. JAMA. (2023) 329:1934–46. doi: 10.1001/jama.2023.8823
193. Safavi F, Gustafson L, Walitt B, Lehky T, Dehbashi S, Wiebold A, et al. Neuropathic symptoms with SARS-CoV-2 vaccination. medRxiv. (2022). doi: 10.1101/2022.05.16.22274439
194. Finsterer J. Neurological adverse reactions to SARS-coV-2 vaccines. Clin Psychopharmacol Neurosci. (2023) 21:222–39. doi: 10.9758/cpn.2023.21.2.222
195. Yang Y, Huang L. Neurological disorders following COVID-19 vaccination. Vaccines (Basel). (2023) 11:1114. doi: 10.3390/vaccines11061114
196. Zisis SN, Durieux JC, Mouchati C, Perez JA, McComsey GA. The protective effect of coronavirus disease 2019 (COVID-19) vaccination on postacute sequelae of COVID-19: A multicenter study from a large national health research network. Open Forum Infect Dis. (2022) 9:ofac228. doi: 10.1093/ofid/ofac228
197. Effros RB, Walford RL. The immune response of aged mice to influenza: diminished T-cell proliferation, interleukin 2 production and cytotoxicity. Cell Immunol. (1983) 81:298–305. doi: 10.1016/0008-8749(83)90237-x
198. Klein SL, Flanagan KL. Sex differences in immune responses. Nat Rev Immunol. (2016) 16:626–38. doi: 10.1038/nri.2016.90
199. Frasca D, Reidy L, Cray C, Diaz A, Romero M, Kahl K, et al. Influence of obesity on serum levels of SARS-CoV-2-specific antibodies in COVID-19 patients. PloS One. (2021) 16:e0245424. doi: 10.1371/journal.pone.0245424
200. Bouchlaka MN, Sckisel GD, Chen M, Mirsoian A, Zamora AE, Maverakis E, et al. Aging predisposes to acute inflammatory induced pathology after tumor immunotherapy. J Exp Med. (2013) 210:2223–37. doi: 10.1084/jem.20131219
201. Murphy WJ. The urgent need for more basic research on SARS-Cov2 infection and vaccines in assessing potential psychoneurological effects using maternal immune activation (MIA) and other preclinical modeling. Brain Behav Immun. (2021) 97:1–3. doi: 10.1016/j.bbi.2021.06.009
202. Rhea EM, Logsdon AF, Hansen KM, Williams LM, Reed MJ, Baumann KK, et al. The S1 protein of SARS-CoV-2 crosses the blood–brain barrier in mice. Nat Neurosci. (2021) 24:368–78. doi: 10.1038/s41593-020-00771-8
203. Buzhdygan TP, DeOre BJ, Baldwin-Leclair A, Bullock TA, McGary HM, Khan JA, et al. The SARS-CoV-2 spike protein alters barrier function in 2D static and 3D microfluidic in-vitro models of the human blood-brain barrier. Neurobiol Dis. (2020) 146:105131. doi: 10.1016/j.nbd.2020.105131
204. Yang AC, Kern F, Losada PM, Agam MR, Maat CA, Schmartz GP, et al. Dysregulation of brain and choroid plexus cell types in severe COVID-19. Nature. (2021) 595:565–71. doi: 10.1038/s41586-021-03710-0
205. Zhao Y, Kuang M, Li J, Zhu L, Jia Z, Guo X, et al. SARS-CoV-2 spike protein interacts with and activates TLR41. Cell Res. (2021) 31:818–20. doi: 10.1038/s41422-021-00495-9
206. Oh J, Cho W-H, Barcelon E, Kim KH, Hong J, Lee SJ. SARS-CoV-2 spike protein induces cognitive deficit and anxiety-like behavior in mouse via non-cell autonomous hippocampal neuronal death. Sci Rep. (2022) 12:5496. doi: 10.1038/s41598-022-09410-7
207. Crackower MA, Sarao R, Oudit GY, Yagil C, Kozieradzki I, Scanga SE, et al. Angiotensin-converting enzyme 2 is an essential regulator of heart function. Nature. (2002) 417:822–8. doi: 10.1038/nature00786
208. Wang J, Kaplan N, Wysocki J, Yang W, Lu K, Peng H, et al. The ACE2-deficient mouse: A model for a cytokine storm-driven inflammation. FASEB J. (2020) 34:10505–15. doi: 10.1096/fj.202001020R
209. Netland J, Meyerholz DK, Moore S, Cassell M, Perlman S. Severe acute respiratory syndrome coronavirus infection causes neuronal death in the absence of encephalitis in mice transgenic for human ACE2. J Virol. (2008) 82:7264–75. doi: 10.1128/JVI.00737-08
Glossary
Keywords: immunology, vaccination, SARS-CoV-2, anti-idiotype antibodies, aging
Citation: Collins CP, Longo DL and Murphy WJ (2024) The immunobiology of SARS-CoV-2 infection and vaccine responses: potential influences of cross-reactive memory responses and aging on efficacy and off-target effects. Front. Immunol. 15:1345499. doi: 10.3389/fimmu.2024.1345499
Received: 27 November 2023; Accepted: 12 February 2024;
Published: 26 February 2024.
Edited by:
Huan Huu Nguyen, IGY Life Sciences, United StatesReviewed by:
Sivaram Gunisetty, Emory University, United StatesGüliz Tuba Barut, Institute of Virology and Immunology, Switzerland
Copyright © 2024 Collins, Longo and Murphy. This is an open-access article distributed under the terms of the Creative Commons Attribution License (CC BY). The use, distribution or reproduction in other forums is permitted, provided the original author(s) and the copyright owner(s) are credited and that the original publication in this journal is cited, in accordance with accepted academic practice. No use, distribution or reproduction is permitted which does not comply with these terms.
*Correspondence: William J. Murphy, d21qbXVycGh5QHVjZGF2aXMuZWR1