- 1The School of Medicine, Chongqing University, Chongqing, China
- 2Department of Pathogenic Biology, Army Medical University (Third Military Medical University), Chongqing, China
Attenuated sporozoites provide a valuable model for exploring protective immunity against the malarial liver stage, guiding the design of highly efficient vaccines to prevent malaria infection. Liver tissue-resident CD8+ T cells (CD8+ Trm cells) are considered the host front-line defense against malaria and are crucial to developing prime-trap/target strategies for pre-erythrocytic stage vaccine immunization. However, the spatiotemporal regulatory mechanism of the generation of liver CD8+ Trm cells and their responses to sporozoite challenge, as well as the protective antigens they recognize remain largely unknown. Here, we discuss the knowledge gap regarding liver CD8+ Trm cell formation and the potential strategies to identify predominant protective antigens expressed in the exoerythrocytic stage, which is essential for high-efficacy malaria subunit pre-erythrocytic vaccine designation.
1 Introduction
Malaria is one of the most devastating diseases worldwide. In 2022, 249 million cases and 608,000 deaths were recorded, most of which were children (1). Malarial infections are caused by the genus Plasmodium, including Plasmodium falciparum (P. falciparum), Plasmodium vivax (P. vivax), Plasmodium malariae, Plasmodium knowlesi, and Plasmodium ovale, with P. falciparum being the most common parasite responsible for malaria-related deaths.
Malarial infections are initiated by the bite of an infected Anopheles mosquito. The sporozoites are inoculated into the dermis of the host during a mosquito bite and subsequently enter the bloodstream and circulate into the liver sinusoids, where sporozoites pass through the sinusoidal cell layer and invade hepatocytes. Sporozoites in hepatocytes sequentially transform into trophozoites and schizonts, producing thousands of hepatic merozoites. One P. falciparum sporozoite produces ~40,000 merozoites (2). The pre-erythrocytic stage is approximately 2 days for rodent malaria and approximately 1 week for human malaria. The blood stage begins when the released hepatic merozoites invade red blood cells, leading to fever, weakness, headache, anemia, and even death, owing to malarial parasites replicating in the blood. In contrast to the blood stage, patients with malaria are clinically asymptomatic at the pre-erythrocytic stage (including sporozoite and liver stages). Moreover, the pre-erythrocytic stage is a key bottleneck in the life cycle of malarial parasites because mosquito bites deposit about tens to hundreds of sporozoites, and only a fraction reaches the liver (3). Vaccines targeting the pre-erythrocytic stage abrogate blood-stage infection, thus averting disease and disrupting transmission to the mosquito vector (4). Therefore, the pre-erythrocytic stage is an ideal target for malaria-preventative vaccines.
2 Whole sporozoite vaccine: a valuable model to dissect protective immunity against the pre-erythrocytic stage
Three approaches have been pursued to develop efficient pre-erythrocytic malaria vaccines: subunit vaccines, whole sporozoite vaccines (WSVs), and viral/bacterial vector-delivered vaccines (5). RTS, S/AS01E, the leading subunit vaccine, has been recently approved by the World Health Organization for use in moderate to high malaria endemic regions (6). However, the protective efficacy of RTS, S/AS01E is only approximately 30% for infants and 50% for children, and protection wanes after 18 months (7–9). R21/Matrix-M™ vaccine, which is regarded as the next-generation RTS, S-like vaccine, showed more than 70% protective efficacy in phase 2b trial (10), but its protective efficacy in phase III remains to be defined. In contrast, WSVs can induce high levels (> 90%) of sustained protection against malarial parasite infection (11–13). In whole sporozoite vaccination, subjects are immunized with sporozoites through mosquito bites or intradermal (i.d.)/intravenous (i.v.) injection, wherein sporozoite development in hepatocytes is arrested at the early or late stage due to attenuation, leading to persistent stimulation of the host immune system. To date, three WSVs, namely radiation-attenuated sporozoites (RAS) (14), genetically attenuated sporozoites (GAS) (15), and chemoprophylaxis vaccination (CVac) (16), have been developed using different attenuation approaches.
RAS were the first reported WSVs in which mosquitoes harboring infectious sporozoites were attenuated by X-ray irradiation. With a sufficient dose of irradiation for mosquito carrying sporozoites to prevent the completion of liver-stage infection but not over-irradiated to lose the immunogenicity required to induce immune-mediated protection (14). RAS development in the liver is arrested at an early stage and thus requires bites of more than 1,000 irradiated infected mosquitoes to induce sterile protection in human subjects (13). Unlike RAS production, the genetic attenuation of parasites is more precise and efficient since GAS are generated by deleting the specific gene responsible for parasite development in hepatocytes (15). Late liver stage-arresting GAS, generated by gene knockout, e.g., of the P. falciparum fabB/F gene, at the late liver stage, could develop into liver schizonts but fail to produce merozoites. The immunogenicity of late liver stage-arresting GAS is much higher than that of RAS or GAS arrested at the early stage because there are more antigens for the immune system to recognize (17). The safety issue for GAS is related to infection breakthrough. Clinical evaluation of p52/p36 GAP in humanized mouse models showed severe early liver-stage growth defects (18); nonetheless, infection breakthroughs have been achieved in human trials (19). After increasing the number of knockout genes, Pf GAP3KO (Pf p52/p36/SAP1) was demonstrated to be fully attenuated (20, 21), inducing relatively high protective immunity in controlled human malaria infection (CHMI) (22).
CVac involves vaccination with live sporozoites under anti-malarial drug prophylaxis, efficiently killing emerging blood-stage parasites but not liver-stage parasites (23). Under these circumstances, parasites can complete the entire liver-stage development, allowing a greater antigen repertoire to stimulate the host immune system. The negative effect of the blood stage on anti-liver-stage immune responses is also limited. Therefore, CVac immunogenicity is much higher than that of RAS, and 20-fold fewer infected mosquito bites are required for CVac to induce sterile protective immunity in controlling human malaria infection (16, 24). Initially, chloroquine was used to kill emerging blood-stage parasites after immunization with live sporozoites. However, the emergence and spread of chloroquine-resistant P. falciparum strains have raised safety concerns regarding chloroquine use in this vaccination approach; therefore, other anti-malarial drugs, such as mefloquine (24), artesunate (25), and pyrimethamine (12), have been used instead. Recently, primaquine and antibiotics (clindamycin and azithromycin) (26, 27), which arrest liver-stage parasite development, have been used as causal prophylaxis, eliciting high protective immunity.
Although attenuated sporozoites have proven to be the most efficient vaccines for preventing malaria infection, their wide application has been hampered by a requirement for mass production and safety limitations. Sporozoites must be aseptic, provided in large numbers, and transported via a cold chain (28). Sanaria (Rockville, MD, USA) established a facility for aseptic sporozoite production and has successfully produced infective P. falciparum sporozoites in vitro (29). Nevertheless, knowledge about the mechanism of protective immunity induced by attenuated sporozoites will help in designing high-efficacy next-generation subunit malaria vaccines.
3 Liver CD8+ Trm cells: correlation with WSV-induced protection
Determining the correlation between attenuated sporozoite-induced protection and immune effectors could guide the design of efficient subunit malaria vaccines. Immunization with RAS swiftly activates CD8+ T cells, which play a central role in the protective immunity induced by inoculating mice with RAS, as the sterile protection induced by RAS is abolished after CD8+ T cell depletion (30, 31). Adoptive transfer experiments have also shown that activated effector CD8+ T cells significantly resist sporozoite challenge (32). However, prolonged protection after vaccination is not dependent on these short-lived activated effector CD8+ T cells. Therefore, researchers have focused on memory CD8+ T cell subsets, which provide long-term protection when induced by vaccines. In fact, both effector memory CD8+ T (Tem) cells and central memory CD8+ T (Tcm) cells have been detected in protected mice immunized with RAS (33, 34); however, only a high frequency of CD8+ Tem cells can confer long-term protection induced after RAS immunization (35, 36).
CD8+ Tem cells patrol the blood and non-lymphoid tissues (NLTs) due to a lack of the expression of the secondary lymphoid organ (SLO)-homing receptors, such as L-selectin (CD62L), and exert effector functions during recall responses. Tcm cells are CD62L+ cells and are enriched in SLOs. They proliferate and differentiate into effector cells during recall responses (37, 38). Notably, apart from circulating memory CD8+ T cells (CD8+ Tem and Tcm cells), two pioneering studies discovered a new memory T cell subset—tissue-resident CD8+ memory T (Trm) cells—which enhances regional immunity in the host (39, 40). Like CD8+ Tem, CD8+ Trm cells do not express CD62L but highly express CD69, which contributes to their retention in tissues by forming a complex with sphingosine-1-phosphate receptor (S1PR1) and inhibiting S1PR1-induced tissue egress (41, 42). Liver CD8+ Trm cells were characterized by the upregulation of tissue retention molecules CD11a, CXCR3, and CXCR6 and the downregulation of tissue egress molecules CD62L and CCR7 (43). Although CD8+ Tem cells have also been implicated in protective immunity after RAS immunization (36), liver CD8+ Trm cells were found to patrol the hepatic sinuses and form the front-line defense against malarial liver-stage infection (43). The depletion of liver CD8+ Trm cells by anti-CXCR3 antibody abrogates RAS protection and demonstrates their essential roles in the protection induced by RAS (43).
As the liver CD8+ Trm cells cannot be detected in peripheral blood, the inability to obtain human liver samples greatly limited our knowledge about human liver CD8+ Trm against the malaria liver stage. The existence of human liver CD8+ Trm was demonstrated through a study of transplantation, in which T cells were detected in the donor liver transplanted for more than a decade (44). However, unlike liver CD8+ Trm cells in mice, 5–30% of human liver CD8+ Trm cells express CD103 (44–46). CD103+ liver Trm cells were specific for hepatotropic infections, but CD103- Trm cells were specific for both hepatotropic and non-hepatotropic infections (45). Human liver Trm cells have been associated with protective immunity against HBV infection (46). In malaria, intravenous RAS vaccination of non-human primates resulted in the generation of parasite-specific memory CD8+ T cells in the liver, but not in the blood. In contrast, parasite-specific memory CD8+T cells were not detected after subcutaneous RAS vaccination, which is markedly less protective (47). This indicated that liver CD8+ Trm cells are also essential for protection against liver-stage infection in non-human primates and humans.
Considering only 20% T cells in liver could be detected by flow cytometry (48), it is estimated that approximately 2.5 million liver CD8+ Trm cells are required to screen 99% of the whole liver for parasite infection during a 2-day window in mouse liver-stage malaria (43).This indicated that a large amount of liver CD8+ Trm cells are required to prevent progression to the blood stage, and the optimal generation of CD8+ Trm cells in the liver could guide the design of highly effective malaria vaccines.
4 Prospects for pre-erythrocytic stage vaccine designed to induce liver CD8+ Trm cells
Epithelial CD8+ Trm cells are thought to be derived from circulating effector CD8+ T cells wherein the Trm cell lineage is committed (49). Consistently, liver CD8+ Trm cells were also generated from circulating effector CD8+ T cells, as only the in vitro activated CD8+ T cells, but not naïve CD8+ T cells, intravenously adoptive transferred, were found to be seeded in sinusoids and transformed into liver CD8+ Trm cells (50). Therefore, the magnitude of CD8+ T cell responses during priming would affect the number of liver Trm cells that are finally generated.
Sporozoite injected intravenously could be detected in the spleen, lung, and liver, but only develop in the liver (51). Splenectomy prior to RAS immunization by i.v. greatly reduced the protection of the vaccinated mice, indicating the essential role of the spleen in the priming of parasite-specific CD8+ T cell responses (52). Further study showed that splenic CD11c+ DCs were responsible for the cross-priming of sporozoite circumsporozoite protein (CSP)-specific CD8+ T cells (53). During this process, CD4+ T cells are essential for activating and maintaining CSP-specific CD8+ T cells via the secretion of interleukin (IL)-4 (54, 55). However, parasite-infected hepatocytes are captured by monocyte-derived CD11c+ cells, and CD8+ T cells are primed in the liver-draining lymphoid nodes, after RAS successfully invade hepatocytes and develop into EEFs (56). Notwithstanding, there was much more pronounced CD8+T cell expansion in the spleen than in the liver draining lymphoid nodes following intravenous RAS vaccination (57). Moreover, γδ T cells are required to prime parasite-specific CD8+ T cells possibly through promoting CD8α+ DC influx into the liver (58). In contrast, malaria blood-stage infections significantly suppress protective CD8+ T cells against the liver stage by inhibiting splenic DC maturation (59). After priming, the activated CD8+ T cells either generated in the liver or circulated from the spleen, would convert into CD8+ Trm cells. The turnover of the circulating effector CD8+ T cells into liver CD8+ Trm cells was significantly affected by the local inflammatory status or antigen expression (50), which was consistent with the formation of resident memory CD8+ T cells in other tissues (60, 61) (Figure 1).
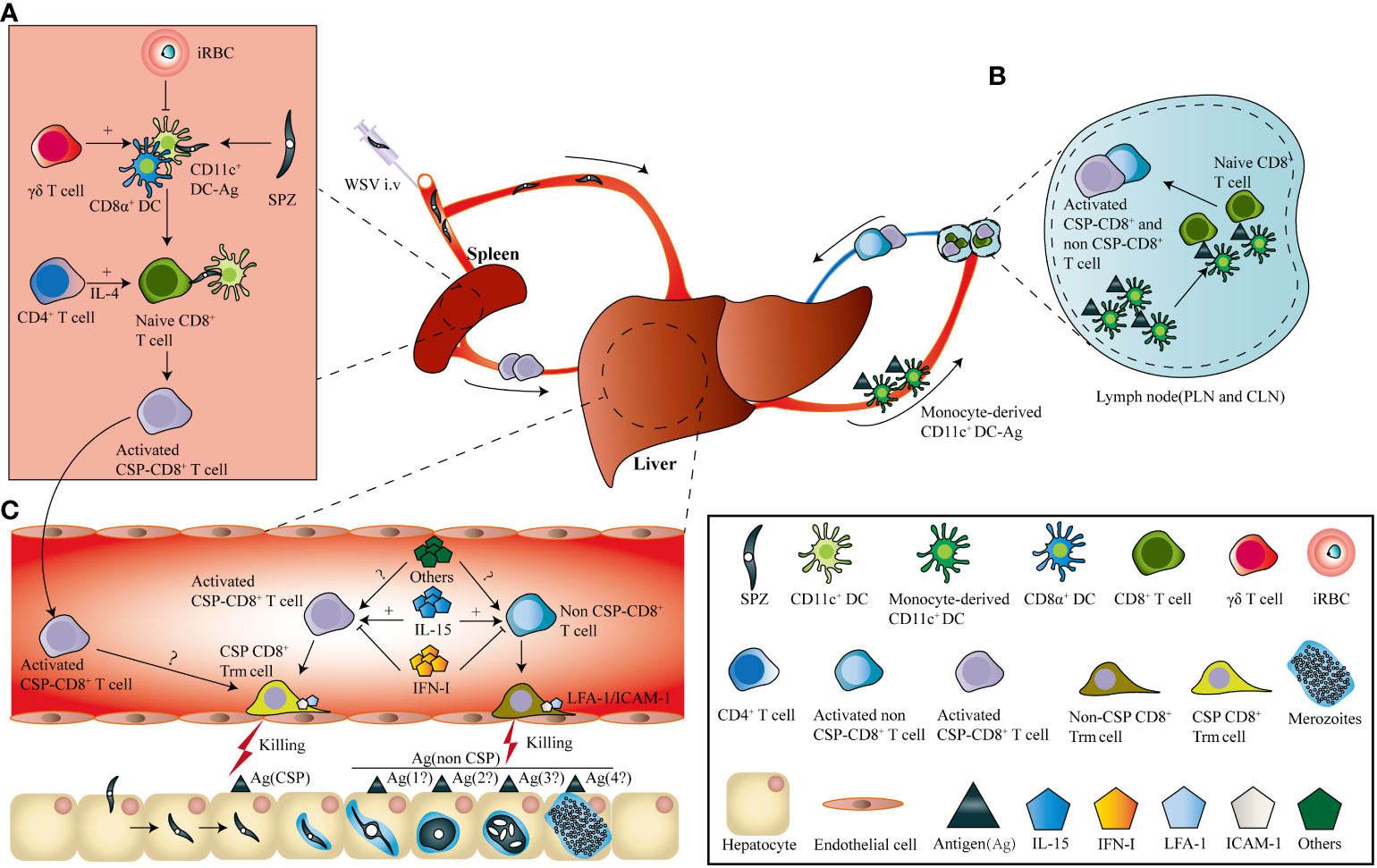
Figure 1 I.v immunization of WSV induces the generation of parasite-specific liver CD8+ Trm. (A). After i.v. immunization, sporozoites (SPZs) enter the spleen and are captured by conventional dendritic cells, and circumsporozoite protein (CSP)-specific CD8+ T cells are cross-primed. During this process, γδ T cells were required to prime the effector CD8+ T cells by inhibiting the influx of CD11c+ dendritic cells into the liver, and CD4+ T cells promote CD8+ T cell activation through interleukin (IL)-4 secretion. In contrast, malaria blood-stage infections suppress protective CD8+ T cells against the liver stage by inhibiting splenic DC maturation (B). Sporozoites invading the liver develop into exo-erythrocytic forms (EEFs), which are captured by monocyte-derived CD11c+ cells and prime both CSP- and non-CSP-specific CD8+ T cells in the liver-draining lymph nodes. (C). Both CSP-and non-CSP-specific CD8+ T cells primed in liver-draining lymph nodes, as well as CSP-specific CD8+ T cells activated in the spleen, circulate into the liver sinusoids and transform into CSP-and non-CSP-CD8+ Trm cells. The location of CD8+ Trm cells in liver sinusoids depends on the interaction between LFA-1 on CD8+ Trm cells and ICAM-1 on endothelial cells. The transformation of CD8+ Trm cells is positively and negatively regulated by IL-15 and type I interferon, as well as by other factors, respectively.
Based on the knowledge of liver Trm formation, a prime-trap/target strategy has been developed to generate high-frequency, parasite-specific CD8+ Trm cells in the liver. As the cross-priming by DGNR-1+ (CLEC9A+) DCs was essential for lung Trm precursor commitment (62), anti-Clec9A was fused to a malaria-specific epitope to increase the priming efficiency of Trm precursors (43, 62). After priming, parasite-specific Trm precursors would convert into Trm cells under the effect of the local inflammatory status or antigen expression in the liver. This goal was achieved by liver-targeting nanoparticles or intravenous infection with the recombinant adeno-associated virus vector or attenuated sporozoites (43, 63–65). Clinical trials have also shown that the delivery of recombinant chimpanzee adenovirus (ChAd) and modified vaccinia Ankara (MVA) viral vectors expressing protective liver-stage epitopes intramuscularly (i.m.) through a prime-boost strategy significantly induced circulating CD8+ T cell responses, but with low levels of protection in malaria-naïve humans. In contrast, the recombinant viral vector vaccine boosted with MVA i.v. generated higher protection through the induction of high frequency of liver CD8+ Trm cells (63) (Figure 2). It seems that the different protective immunity of RAS vaccinated by i.v. and i.d. might be closely associated with their ability to generate liver Trm. As compared to RAS immunized i.v, RAS injected by i.d. seldom enters into the liver and develops into EEFs in hepatocytes (66). Under this circumstance, fewer Trm precursors primed in the draining lymph nodes would convert into liver Trm, as i.d. injection of RAS does not lead to inflammatory response and parasite antigen expression in the liver.
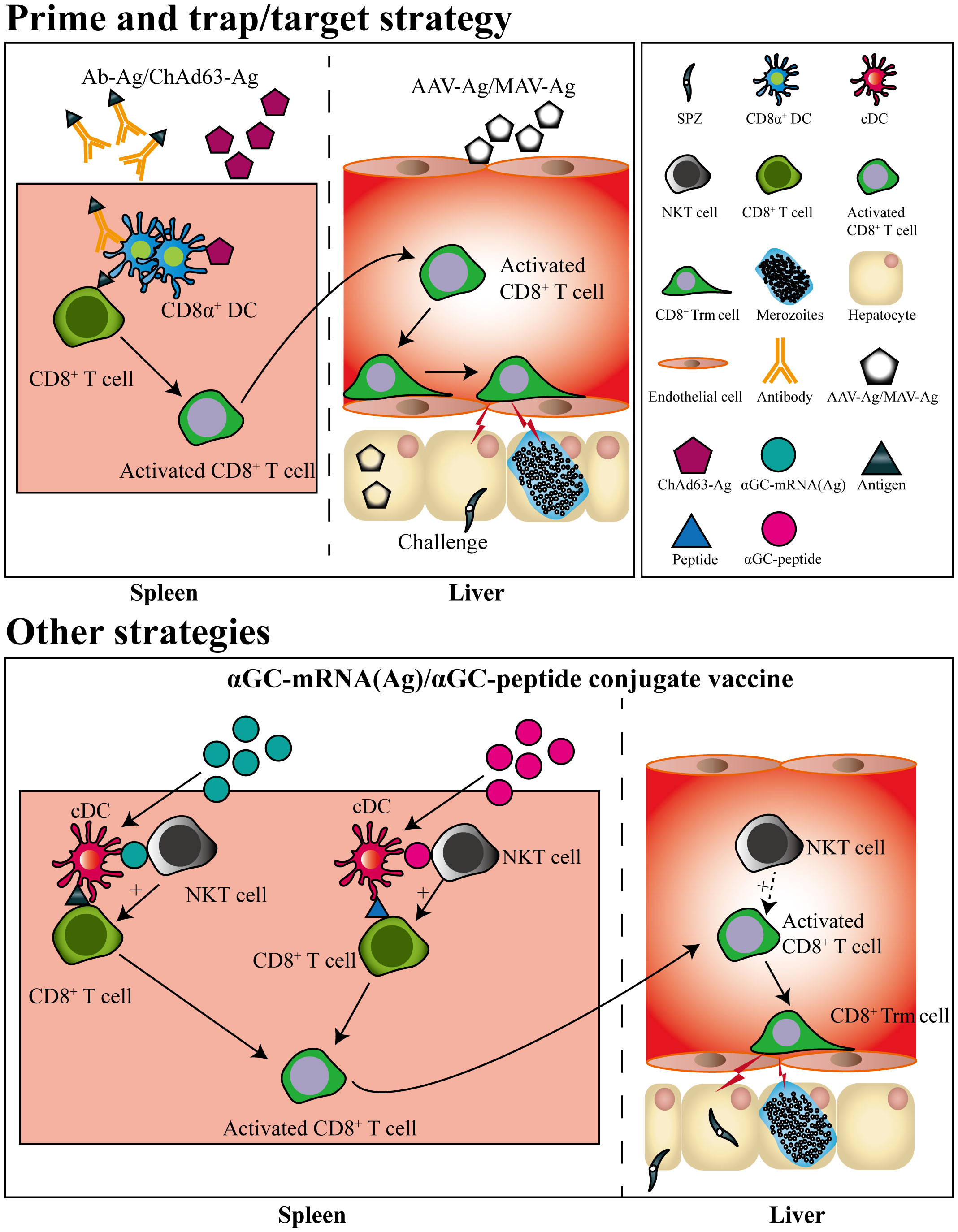
Figure 2 Pre-erythrocytic subunit vaccine designed by prime-and-trap/target strategies. CD8+ T cells are primed by antigens conjugated with a dendritic cell-targeted antibody or antigen-expressed chimpanzee Adenovirus (ChAd) 63 vector, after which the primed CD8+ T cells are trapped or targeted by delivering adeno-associated virus (AAV) or modified vaccinia Ankara (MVA) viral vector expressing the same antigen. The primed CD8+ T cells trapped or targeted in liver would convert into CD8+ Trm cells. Liver CD8+ Trm cells could also be generated by a single immunization of mRNA vaccine or self-adjuvating glycolipid-peptide conjugate vaccine both containing the natural killer T cell agonist αGC.
Strikingly, a single immunization with a self-adjuvating glycolipid-peptide conjugate vaccine, designed to simultaneously activate natural killer T cell (NKT) and DCs, has been reported to generate large numbers of liver CD8+ Trm cells and protect against malaria infection (67). As liposome nanoparticles (LNPs) have been suggested as the most promising platform for designing vaccines against a variety of infectious diseases (68), and mRNA in LNP delivered i.v efficiently targets and expresses in the liver (69). Thereby, a messenger RNA (mRNA)-based vaccine containing an NKT cell agonist has been designed and successfully induced sterile protection against sporozoite challenge, which was unaffected by previous exposure to blood-stage infection (70) (Figure 2). This indicated that the local inflammatory response induced by NKT cell agonist and targeted expression of malaria antigen in liver by i.v delivery of mRNA vaccine could efficiently promote the generation of liver CD8+ Trm.
5 Challenges with the research of liver CD8+ Trm cells against malaria liver stage
Great progress has been made in understanding the essential role of CD8+ Trm cells in the protection induced by RAS vaccination. Nonetheless, several knowledge gaps, including the mechanism of liver CD8+ Trm commitment, formation, and maintenance, and secondary responses to sporozoite challenge, as well as the protective antigens they recognized, warrant further investigation.
5.1 The regulatory mechanism of liver CD8+ Trm cells generation and maintenance
Two models have been proposed for the formation of CD8+ Trm cells. One is the “local divergent” model, in which the lineage of Trm was determined by the local tissue microenvironment. The other is the “systematic divergent” model, in which the lineage decision of Trm has been made during activation, and the local tissue micro-environment promotes the generation of CD8+ Trm. Most current studies focused on CD69+CD103+ Trm cells in epithelial tissues and supported the “systematic divergent” model for CD8+ Trm generation (49). In this model, epithelial CD8+ Trm precursors were poised in naïve CD8+ T cells (62, 71), and the activated CD8+ T cells were more prone to circulate into nonlymphoid tissues (NLTs), and differentiated into mature CD8+ Trm in the local tissue microenvironment (72, 73). Parasite-specific CD8+ T cells were cross-primed in different SLOs with the different immunization routes of RAS. For instance, CD8+ T cells were primed by DCs in skin-draining lymph nodes when RAS was i.d. or s.c. immunization, but sporozoite-specific CD8+ T cells and EEF-specific CD8+ T cells were primed in the spleen by CD8α+ DCs (57) and liver-draining lymph nodes by monocyte-derived CD11c+ cells (56, 66) after RAS vaccination i.v, respectively (Figure 1). It is well known that i.v. immunization of RAS is more prone to induce sterile protection than RAS vaccinated i.d. or s.c. in humans (47, 74). However, whether RAS immunized by different routes leads to their distinct abilities to commit liver CD8+ Trm cells is largely unknown. Although a vaccine designed to target DNGR-1+ DCs for cross-priming parasite-specific CD8+ T cells successfully induced the generation of liver CD8+ Trm (43), whether this approach well poised liver CD8+ Trm precursors also remains to be defined.
Liver and epithelial Trm cells share a common gene expression signature that is regulated by the transcription factors Hobit and Blimp1 (75); however, the regulatory mechanism underlying the formation of CD8+ Trm located in distinct tissues is different (76). For example, chemokines, such as CXCL9 and CXCL10, recruit - Trm precursors by acting on CXCR3 on their surface to the inflamed tissues, promoting Trm cell formation in the skin (73). In contrast, liver Trm cells also express CXCR3 (77), but this chemokine receptor is not necessary for the formation and maintenance of liver Trm (78). However, CXCR6, which is highly expressed by liver Trm cells, is required for their long-term maintenance (78). Transforming growth factor (TGF)-β signaling is essential for the maintaining of Trm cells in the intestine and salivary glands, but not for those in the fat, kidney, and liver (76). As compared to Trm in other tissues, an extreme difference was found between skin and liver CD8+ Trm (76, 79). Several inflammatory cytokines, such as IL-7, IL-15, IL-33, and TNF-α, have been reported to modulate epithelial CD8+ Trm formation (80, 81); only IL-15, but not TNF and IFN-γ, has a significant effect on the generation of liver Trm cells (50). Strikingly, type I IFN signaling, which is activated by EEFs in hepatocytes (82), even inhibits liver CD8+ Trm cell generation (83) (Figure 1). Recently, a system analysis of immune responses to the vaccination of the attenuated P. falciparum sporozoite showed that protection was associated with the inflammatory status of the human volunteers (84, 85). Although the formation of both hepatic and epithelial Trm did not always require antigen presentation (50, 61, 86, 87), local antigen presentation promoted liver CD8+ Trm formation (50). Liver Trm cells are located in the sinusoids, a part of the bloodstream, but epithelial Trm cells are found in the parenchyma of peripheral tissues. Integrin CD103, which is highly expressed in epithelial Trm cells of the skin and the gut, is required for T cell residence in the skin (39, 73, 88). However, differentiated liver CD8+ Trm cells do not express CD103 but upregulate the expression of the integrin LFA-1(CD11a/CD18). The interaction between LFA-1 and ICAM-1 allows liver CD8+ Trm cells to patrol and remain in the hepatic sinusoids (77) (Figure 1). Therefore, the regulation of liver CD8+ Trm cell generation and maintenance by tissue microenvironment is distinct from that of epithelial CD8+ Trm cell, and the regulatory mechanism of liver CD8+ Trm cell formation is required to be elucidated in the future.
5.2 Secondary responses of liver CD8+ Trm cells to sporozoite challenge
Upon reinfection, skin CD8+ Trm cells were found to expand locally, and the secondary Trm cells formed from pre-existing Trm cells, as well as from precursors recruited from the circulation (89). However, further study showed that the expansion of CD103+ Trm cells in situ was limited after secondary infection (90). Upon secondary challenge, Trm cells were mainly derived from CD103− Trm cells, with limited contribution from the circulating Tcm (90). As compared to skin CD8+ Trm cells, the adoptive transfer of liver CD8+ Trm cells exhibited a higher potential to trans-differentiate into circulating memory T cells and other tissue Trm cells in response to secondary challenge (79). In addition, skin CD8+ Trm cells could sense the invading pathogens (91) and activate both innate and adaptive immune responses upon secondary infection (92). Although liver CD8+ Trm cells expressed IFN-γ, TNF, granzyme B and CD107a (43), the protective mechanism of liver CD8+ Trm cells of the RAS-immunized mice against sporozoite challenge remains to be defined. Therefore, the dynamic response and protective mechanism of liver CD8+ Trm cells upon sporozoite challenge also needs to be clarified in the future researches.
5.3 Identification of protective antigens recognized by liver CD8+ Trm cells
CSP is the predominant protective antigen of RAS (93), but non-CSP antigens expressed by EEFs are also required for the full protection induced by attenuated sporozoites (93). This is confirmed by the finding that the immunogenicity of attenuated sporozoites arrested at an early stage was much lower than that of sporozoites arrested at a late stage (17). Thus, identifying antigens presented by MHC-I molecules in infected hepatocytes may uncover the unidentified antigens required for full protection of WSV.
In the pre-genomic era, a few protective antigens, such as CSP, thrombospondin-related anonymous protein (TRAP, also called SSP2), and liver-stage antigen-1, were primarily identified using immunized sera or oligonucleotide probe screening of sporozoites or P. falciparum genomic DNA expression libraries (94–96). In 2002, the genomes of P. falciparum and rodent malarial parasites were sequenced (97, 98), beginning the post-genomic era. With the availability of transcriptomic and proteomic data on the rodent malaria liver stage (99), two liver-stage antigens, ribosomal L3 protein and TRAP, were identified through using protective CD8+ T cells to screen H2b-restricted peptides predicted by genome-wide analysis (100, 101). Recently, ribosomal protein L6 (RPL6) of Plasmodium berghei, a novel protective liver-stage antigen, was identified by the approach of combinational peptide library scan and protein Blast within PlasmoDB (57, 65). Based on P. falciparum genomic and proteomic data and a combination of bioinformatics predictions and human leukocyte antigen analysis, 16 pre-erythrocytic antigenic proteins were identified in volunteers immunized with P. falciparum RAS (102).
With the development of T-cell receptor (TCR) repertoire sequencing techniques, a functional TCR-guided antigen discovery strategy, T-scan, has been developed (103). This strategy enabled genome-wide antigen library screening using a given T-cell clone with an orphan TCR of interest. Upon TCR-pMHC engagement, granzyme B (GzB) is delivered to target cells and cleaves the fluorescent protein (IFP)-based GzB reporter (IFPGZB) and activates the IFPGZB reporter. The target cells are then sorted by IFP, and the encoding antigen is identified by secondary generation sequencing. Similar strategies have been adopted to identify both MHC-I- and MHC-II-derived peptides of the malaria blood-stage (104, 105), but only the peptides in CSP recognized by the follicular helper T cell clones expanded in volunteers immunized with WSVs have been recently reported (106). Identifying peptides presented by MHC-I molecules against the malarial liver stage is greatly hampered by the difficulty of obtaining sufficient parasites to construct a cDNA library for screening. Since an extremely low rate of hepatocytes was often infected with rodent (< 5%) and human malaria parasites (< 2.5%) in vitro (107, 108), enough infected hepatocytes of sufficient purity could not be obtained for transcriptomic and proteomic analyses.
Protective antigens should not only be immunogenic to induce CD8+ T cell responses but also be presented to MHC-I on the surface of the infected cells for CD8+ T cells to recognize and kill the pathogens (109). For the malaria liver stage, protective antigens should be proteins with the ability to translocate from the parasitophorous vacuole into the cytosol of the infected hepatocyte and subsequently be presented to MHC-I molecules (110, 111). This was exemplified by the predominant protective antigen CSP, which can access hepatocyte cytoplasm and presented to MHC-I on hepatocytes (112–114). Therefore, the combination of in silico prediction of the candidate peptides of malaria liver-stage antigens presented by MHC-I molecules and TCR repertoire sequencing would be an alternative approach to identify the protective antigens recognized by CD8+ Trm after WSV immunization.
6 Concluding remarks
Recent scientific findings have demonstrated that liver CD8+ Trm cells are the predominant immune effectors of WSVs. With priming regulatory mechanisms and liver CD8+ Trm cell maintenance beginning to be elucidated, a prime-trap strategy has been developed for pre-erythrocytic vaccines to optimally generate liver CD8+ Trm cells. However, many knowledge gaps are still to be elucidated. Firstly, the environmental cues and cellular mechanisms promoting the optimal generation and maintenance of liver CD8+ Trm cells, as well as the dynamic secondary response to the sporozoite challenge, have not been completely defined. Secondly, non-CSP antigens are also important for the protective immunity induced by attenuated sporozoite vaccines, but only a few parasite antigens at the liver stage have been identified, limiting the designation of highly efficient subunit malaria vaccines. Finally, our understanding of the correlation between liver CD8+ Trm cells and the protection induced by WSVs stems mainly from studies in mouse models, and verification in human subjects is warranted for translational research.
Author contributions
CZ: Writing – original draft. SJ: Writing – original draft. WX: Writing – review & editing.
Funding
The author(s) declare financial support was received for the research, authorship, and/or publication of this article. This work was supported by the State Key Program of the National Natural Science Foundation of China (No. 81830067) and the National Natural Science Foundation of China (Nos. 82072299).
Acknowledgments
We would like to thank Editage (www.editage.cn) for English language editing.
Conflict of interest
The authors declare that the research was conducted in the absence of any commercial or financial relationships that could be construed as a potential conflict of interest.
Publisher’s note
All claims expressed in this article are solely those of the authors and do not necessarily represent those of their affiliated organizations, or those of the publisher, the editors and the reviewers. Any product that may be evaluated in this article, or claim that may be made by its manufacturer, is not guaranteed or endorsed by the publisher.
References
1. World Health Organization. World malaria report (2023). Available at: https://www.who.int/teams/global-malaria-programme/reports/world-malaria-report-2023 (Accessed December 26, 2023).
2. Miller LH, Ackerman HC, Su XZ, Wellems TE. Malaria biology and disease pathogenesis: insights for new treatments. Nat Med (2013) 19:156–67. doi: 10.1038/nm.3073
3. Amino R, Thiberge S, Martin B, Celli S, Shorte S, Frischknecht F, et al. Quantitative imaging of Plasmodium transmission from mosquito to mammal. Nat Med (2006) 12:220–4. doi: 10.1038/nm1350
4. Draper SJ, Sack BK, King CR, Nielsen CM, Rayner JC, Higgins MK, et al. Malaria vaccines: Recent advances and new horizons. Cell Host Microbe (2018) 24:43–56. doi: 10.1016/j.chom.2018.06.008
5. Duffy PE, Patrick Gorres J. Malaria vaccines since 2000: progress, priorities, products. NPJ Vaccines (2020) 5:48. doi: 10.1038/s41541-020-0196-3
6. World Health Organization. Approves Historic Rts, S Malaria Vaccine (2021). Available at: https://lenstapesmed.com/who-approves-historic-rtss-malaria-vaccine/ (Accessed August 15, 2023).
7. Agnandji ST, Lell B, Soulanoudjingar SS, Fernandes JF, Abossolo BP, Conzelmann C, et al. First results of phase 3 trial of RTS, S/AS01 malaria vaccine in African children. N Engl J Med (2011) 365:1863–75. doi: 10.1056/NEJMoa1102287
8. Agnandji ST, Lell B, Fernandes JF, Abossolo BP, Methogo BG, Kabwende AL, et al. A phase 3 trial of RTS, S/AS01 malaria vaccine in African infants. N Engl J Med (2012) 367:2284–95. doi: 10.1056/NEJMoa1208394
9. Rts SCTP. Efficacy and safety of RTS, S/AS01 malaria vaccine with or without a booster dose in infants and children in Africa: final results of a phase 3, individually randomised, controlled trial. Lancet (2015) 386:31–45. doi: 10.1016/S0140-6736(15)60721-8
10. Datoo MS, Natama MH, Somé A, Traoré O, Rouamba T, Bellamy D, et al. Efficacy of a low-dose candidate malaria vaccine, R21 in adjuvant Matrix-M, with seasonal administration to children in Burkina Faso: a randomised controlled trial. Lancet (2021) 397:1809–18. doi: 10.1016/S0140-6736(21)00943-0
11. Mordmuller B, Surat G, Lagler H, Chakravarty S, Ishizuka AS, Lalremruata A, et al. Sterile protection against human malaria by chemoattenuated PfSPZ vaccine. Nature (2017) 542:445–9. doi: 10.1038/nature21060
12. Mwakingwe-Omari A, Healy SA, Lane J, Cook DM, Kalhori S, Wyatt C, et al. Two chemoattenuated PfSPZ malaria vaccines induce sterile hepatic immunity. Nature (2021) 595:289–94. doi: 10.1038/s41586-021-03684-z
13. Hoffman SL, Goh LM, Luke TC, Schneider I, Le TP, Doolan DL, et al. Protection of humans against malaria by immunization with radiation-attenuated Plasmodium falciparum sporozoites. J Infect Dis (2002) 185:1155–64. doi: 10.1086/339409
14. Nussenzweig RS, Vanderberg J, Most H, Orton C. Protective immunity produced by the injection of x-irradiated sporozoites of Plasmodium berghei. Nature (1967) 216:160–2. doi: 10.1038/216160a0
15. Mueller AK, Labaied M, Kappe SHI, Matuschewski K. Genetically modified Plasmodium parasites as a protective experimental malaria vaccine. Nature (2005) 433:164–7. doi: 10.1038/nature03188
16. Roestenberg M, McCall M, Hopman J, Wiersma J, Luty AJF, Jan van Gemert G, et al. Protection against a malaria challenge by sporozoite inoculation. N Engl J Med (2009) 361:468–77. doi: 10.1056/NEJMoa0805832
17. Butler NS, Schmidt NW, Vaughan AM, Aly AS, Kappe SHI, Harty JT. Superior antimalarial immunity after vaccination with late liver stage-arresting genetically attenuated parasites. Cell Host Microbe (2011) 9:451–62. doi: 10.1016/j.chom.2011.05.008
18. VanBuskirk KM, O'Neill MT, de la Vega P, Maier AG, Krzych U, Williams J, et al. Preerythrocytic, live-attenuated Plasmodium falciparum vaccine candidates by design. Proc Natl Acad Sci U.S.A. (2009) 106:13004–9. doi: 10.1073/pnas.0906387106
19. Spring M, Murphy J, Nielsen R, Dowler M, Bennett JW, Zarling S, et al. First-in-human evaluation of genetically attenuated Plasmodium falciparum sporozoites administered by bite of Anopheles mosquitoes to adult volunteers. Vaccine (2013) 31:4975–83. doi: 10.1016/j.vaccine.2013.08.007
20. Kublin JG, Mikolajczak SA, Sack BK, Fishbaugher ME, Seilie A, Shelton L, et al. Complete attenuation of genetically engineered Plasmodium falciparum sporozoites in human subjects. Sci Transl Med (2017) 9:eaad9099. doi: 10.1126/scitranslmed.aad9099
21. Mikolajczak SA, Lakshmanan V, Fishbaugher M, Camargo N, Harupa A, Kaushansk A, et al. A next-generation genetically attenuated Plasmodium falciparum parasite created by triple gene deletion. Mol Ther (2014) 22:1707–15. doi: 10.1038/mt.2014.85
22. Murphy SC, Vaughan AM, Kublin JG, Fishbauger M, Seilie AM, Cruz KP, et al. A genetically engineered Plasmodium falciparum parasite vaccine provides protection from controlled human malaria infection. Sci Transl Med (2022) 14:eabn9709. doi: 10.1126/scitranslmed.abn9709
23. Belnoue E, Costa FTM, Frankenberg T, Vigário AM, Voza T, Leroy N, et al. Protective T cell immunity against malaria liver stage after vaccination with live sporozoites under chloroquine treatment. J Immunol (2004) 172:2487–95. doi: 10.4049/jimmunol.172.4.2487
24. Bijker EM, Schats R, Obiero JM, Behet MC, van Gemert G-J, van de Vegte-Bolmer M, et al. Sporozoite immunization of human volunteers under mefloquine prophylaxis is safe, immunogenic and protective: a double-blind randomized controlled clinical trial. PloS One (2014) 9:e112910. doi: 10.1371/journal.pone.0112910
25. Peng X, Keitany GJ, Vignali M, Chen L, Gibson C, Choi K, et al. Artesunate versus chloroquine infection-treatment-vaccination defines stage-specific immune responses associated with prolonged sterile protection against both pre-erythrocytic and erythrocytic Plasmodium yoelii infection. J Immunol (2014) 193:1268–77. doi: 10.4049/jimmunol.1400296
26. Friesen J, Silvie O, Putrianti ED, Hafalla JCR, Matuschewski K, Borrmann S. Natural immunization against malaria: causal prophylaxis with antibiotics. Sci Transl Med (2010) 2:40ra49. doi: 10.1126/scitranslmed.3001058
27. Putrianti ED, Silvie O, Kordes M, Borrmann S, Matuschewski K. Vaccine-like immunity against malaria by repeated causal-prophylactic treatment of liver-stage Plasmodium parasites. J Infect Dis (2009) 199:899–903. doi: 10.1086/597121
28. Luke TC, Hoffman SL. Rationale and plans for developing a non-replicating, metabolically active, radiation-attenuated Plasmodium falciparum sporozoite vaccine. J Exp Biol (2003) 206:3803–8. doi: 10.1242/jeb.00644
29. Eappen AG, Li T, Marquette M, Chakravarty S, Kc N, Zanghi G, et al. In vitro production of infectious Plasmodium falciparum sporozoites. Nature (2022) 612:534–9. doi: 10.1038/s41586-022-05466-7
30. Weiss WR, Sedegah M, Beaudoin RL, Miller LH, Good MF. CD8+ T cells (cytotoxic/suppressors) are required for protection in mice immunized with malaria sporozoites. Proc Natl Acad Sci U.S.A. (1988) 85:573–6. doi: 10.1073/pnas.85.2.573
31. Sano G, Hafalla JC, Morrot A, Abe R, Lafaille JJ, Zavala F. Swift development of protective effector functions in naive CD8(+) T cells against malaria liver stages. J Exp Med (2001) 194:173–80. doi: 10.1084/jem.194.2.173
32. Krzych U, Schwenk R, Guebre-Xabier M, Sun P, Palmer D, White K, et al. The role of intrahepatic lymphocytes in mediating protective immunity induced by attenuated Plasmodium berghei sporozoites. Immunol Rev (2000) 174:123–34. doi: 10.1034/j.1600-0528.2002.00013h.x
33. Guebre-Xabier M, Schwenk R, Krzych U. Memory phenotype CD8(+) T cells persist in livers of mice protected against malaria by immunization with attenuated Plasmodium berghei sporozoites. Eur J Immunol (1999) 29:3978–86. doi: 10.1002/(SICI)1521-4141(199912)29:12<3978::AID-IMMU3978>3.0.CO;2-0
34. Berenzon D, Schwenk RJ, Letellier L, Guebre-Xabier M, Williams J, Krzych U. Protracted protection to Plasmodium berghei malaria is linked to functionally and phenotypically heterogeneous liver memory CD8+ T cells. J Immunol (2003) 171:2024–34. doi: 10.4049/jimmunol.171.4.2024
35. Schmidt NW, Butler NS, Badovinac VP, Harty JT. Extreme CD8 T cell requirements for anti-malarial liver-stage immunity following immunization with radiation attenuated sporozoites. PloS Pathog (2010) 6:e1000998. doi: 10.1371/journal.ppat.1000998
36. Lefebvre MN, Surette FA, Anthony SM, Vijay R, Jensen IJ, Pewe LL, et al. Expeditious recruitment of circulating memory CD8 T cells to the liver facilitates control of malaria. Cell Rep (2021) 37:109956. doi: 10.1016/j.celrep.2021.109956
37. Sallusto F, Lenig D, Förster R, Lipp M, Lanzavecchia A. Two subsets of memory T lymphocytes with distinct homing potentials and effector functions. Nature (1999) 401:708–12. doi: 10.1038/44385
38. Masopust D, Vezys V, Marzo AL, Lefrançois L. Preferential localization of effector memory cells in nonlymphoid tissue. Science (2001) 291:2413–7. doi: 10.1126/science.1058867
39. Gebhardt T, Wakim LM, Eidsmo L, Reading PC, Heath WR, Carbone FR. Memory T cells in nonlymphoid tissue that provide enhanced local immunity during infection with herpes simplex virus. Nat Immunol (2009) 10:524–30. doi: 10.1038/ni.1718
40. Wakim LM, Waithman J, van Rooijen N, Heath WR, Carbone FR. Dendritic cell-induced memory T cell activation in nonlymphoid tissues. Science (2008) 319:198–202. doi: 10.1126/science.1151869
41. Walsh DA, Borges da Silva H, Beura LK, Peng C, Hamilton SE, Masopust D, et al. The functional requirement for CD69 in establishment of resident memory CD8(+) T cells varies with tissue location. J Immunol (2019) 203:946–55. doi: 10.4049/jimmunol.1900052
42. Mackay LK, Braun A, Macleod BL, Collins N, Tebartz C, Bedoui S, et al. Cutting edge: CD69 interference with sphingosine-1-phosphate receptor function regulates peripheral T cell retention. J Immunol (2015) 194:2059–63. doi: 10.4049/jimmunol.1402256
43. Fernandez-Ruiz D, Ng WY, Holz LE, Ma JZ, Zaid A, Wong YC, et al. Liver-resident memory CD8+ T cells form a front-line defense against malaria liver-stage infection. Immunity (2016) 45:889–902. doi: 10.1016/j.immuni.2016.08.011
44. Pallett LJ, Burton AR, Amin OE, Rodriguez-Tajes S, Patel AA, Zakeri N, et al. Longevity and replenishment of human liver-resident memory T cells and mononuclear phagocytes. J Exp Med (2020) 217:e20200050. doi: 10.1084/jem.20200050
45. Kim JH, Han JW, Choi YJ, Rha M-S, Koh JY, Kim KH, et al. Functions of human liver CD69+CD103-CD8+ T cells depend on HIF-2α activity in healthy and pathologic livers. J Hepatol (2020) 72:1170–81. doi: 10.1016/j.jhep.2020.01.010
46. Pallett LJ, Davies J, Colbeck EJ, Robertson F, Hansi N, Easom NJW, et al. IL-2high tissue-resident T cells in the human liver: Sentinels for hepatotropic infection. J Exp Med (2017) 214:1567–80. doi: 10.1084/jem.20162115
47. Epstein JE, Tewari K, Lyke KE, Sim BK, Billingsley PF, Laurens MB, et al. Live attenuated malaria vaccine designed to protect through hepatic CD8 T cell immunity. Science (2011) 334:475–80. doi: 10.1126/science.1211548
48. Steinert EM, Schenkel JM, Fraser KA, Beura LK, Manlove LS, Igyártó BZ, et al. Quantifying memory CD8 T cells reveals regionalization of immunosurveillance. Cell (2015) 161:737–49. doi: 10.1016/j.cell.2015.03.031
49. Kok L, Masopust D, Schumacher TN. The precursors of CD8(+) tissue resident memory T cells: from lymphoid organs to infected tissues. Nat Rev Immunol (2022) 22:283–93. doi: 10.1038/s41577-021-00590-3
50. Holz LE, Prier JE, Freestone D, Steiner TM, English K, Johnson DN, et al. CD8(+) T cell activation leads to constitutive formation of liver tissue-resident memory T cells that seed a large and flexible niche in the liver. Cell Rep (2018) 25:68–79 e4. doi: 10.1016/j.celrep.2018.08.094
51. Wen-yue X, Xing-xiang W, Jie Q, Jian-hua D, Fu-sheng H. Plasmodium yoelii: influence of immune modulators on the development of the liver stage. Exp Parasitol (2010) 126:254–8. doi: 10.1016/j.exppara.2010.05.005
52. Spitalny GL, Rivera-Ortiz CI, Nussenzweig RS. Plasmodium berghei: the spleen in sporozoite-induced immunity to mouse malaria. Exp Parasitol (1976) 40:179–88. doi: 10.1016/0014-4894(76)90080-1
53. Jung S, Unutmaz D, Wong P, Sano G, De los Santos K, Sparwasser T, et al. In vivo depletion of CD11c+ dendritic cells abrogates priming of CD8+ T cells by exogenous cell-associated antigens. Immunity (2002) 17:211–20. doi: 10.1016/S1074-7613(02)00365-5
54. Carvalho LH, Sano G, Hafalla JC, Morrot A, Curotto de Lafaille MA, Zavala F. IL-4-secreting CD4+ T cells are crucial to the development of CD8+ T-cell responses against malaria liver stages. Nat Med (2002) 8:166–70. doi: 10.1038/nm0202-166
55. Overstreet MG, Chen YC, Cockburn IA, Tse SW, Zavala F. CD4+ T cells modulate expansion and survival but not functional properties of effector and memory CD8+ T cells induced by malaria sporozoites. PloS One (2011) 6:e15948. doi: 10.1371/journal.pone.0015948
56. Kurup SP, Anthony SM, Hancox LS, Vijay R, Pewe LL, Moioffer SJ, et al. Monocyte-derived CD11c (+) cells acquire plasmodium from hepatocytes to prime CD8 T cell immunity to liver-stage malaria. Cell Host Microbe (2019) 25:565–77.e6. doi: 10.1016/j.chom.2019.02.014
57. Lau LS, Fernandez-Ruiz D, Mollard V, Sturm A, Neller MA, Cozijnsen A, et al. CD8+ T cells from a novel T cell receptor transgenic mouse induce liver-stage immunity that can be boosted by blood-stage infection in rodent malaria. PloS Pathog (2014) 10:e1004135. doi: 10.1371/journal.ppat.1004135
58. Zaidi I, Diallo H, Conteh S, Robbins Y, Kolasny J, Orr-Gonzalez S, et al. gammadelta T cells are required for the induction of sterile immunity during irradiated sporozoite vaccinations. J Immunol (2017) 199:3781–8. doi: 10.4049/jimmunol.1700314
59. Ocana-Morgner C, Mota MM, Rodriguez A. Malaria blood stage suppression of liver stage immunity by dendritic cells. J Exp Med (2003) 197:143–51. doi: 10.1084/jem.20021072
60. Linda M, Wakim AWD, Bevan MJ. Memory T cells persisting within the brain after local infection show functional adaptations to their tissue of residence. Proc Natl Acad Sci U.S.A. (2010) 107:17872–9. doi: 10.1073/pnas.1010201107
61. Mackay LK, Stock AT, Ma JZ, Jones CM, Kent SJ, Mueller SN, et al. Long-lived epithelial immunity by tissue-resident memory T (TRM) cells in the absence of persisting local antigen presentation. Proc Natl Acad Sci U.S.A. (2012) 109:7037–42. doi: 10.1073/pnas.1202288109
62. Iborra S, Martínez-López M, Khouili Sofía C, Enamorado M, Cueto Francisco J, Conde-Garrosa R, et al. Optimal generation of tissue-resident but not circulating memory T cells during viral infection requires crosspriming by DNGR-1+ dendritic cells. Immunity (2016) 45:847–60. doi: 10.1016/j.immuni.2016.08.019
63. Gola A, Silman D, Walters AA, Sridhar S, Uderhardt S, Salman AM, et al. Prime and target immunization protects against liver-stage malaria in mice. Sci Transl Med (2018) 10:eaap9128. doi: 10.1126/scitranslmed.aap9128
64. Olsen TM, Stone BC, Chuenchob V, Murphy SC. Prime-and-trap malaria vaccination to generate protective CD8(+) liver-resident memory T cells. J Immunol (2018) 201:1984–93. doi: 10.4049/jimmunol.1800740
65. Valencia-Hernandez AM, Ng WY, Ghazanfari N, Ghilas S, de Menezes MN, Holz LE, et al. A natural peptide antigen within the plasmodium ribosomal protein RPL6 confers liver TRM cell-mediated immunity against malaria in mice. Cell Host Microbe (2020) 27:950–62 e7. doi: 10.1016/j.chom.2020.04.010
66. Obeid M, Franetich JF, Lorthiois A, Gego A, Gruner AC, Tefit M, et al. Skin-draining lymph node priming is sufficient to induce sterile immunity against pre-erythrocytic malaria. EMBO Mol Med (2013) 5:250–63. doi: 10.1002/emmm.201201677
67. Holz LE, Chua YC, de Menezes MN, Anderson RJ, Draper SL, Compton BJ, et al. Glycolipid-peptide vaccination induces liver-resident memory CD8(+) T cells that protect against rodent malaria. Sci Immunol (2020) 5:eaaz8035. doi: 10.1126/sciimmunol.aaz8035
68. Chaudhary N, Weissman D, Whitehead KA. mRNA vaccines for infectious diseases: principles, delivery and clinical translation. Nat Rev Drug Discovery (2021) 20:817–38. doi: 10.1038/s41573-021-00283-5
69. Rizvi F, Everton E, Smith AR, Liu H, Osota E, Beattie M, et al. Murine liver repair via transient activation of regenerative pathways in hepatocytes using lipid nanoparticle-complexed nucleoside-modified mRNA. Nat Commun (2021) 12:613. doi: 10.1038/s41467-021-20903-3
70. Ganley M, Holz LE, Minnell JJ, de Menezes MN, Burn OK, Poa KCY, et al. mRNA vaccine against malaria tailored for liver-resident memory T cells. Nat Immunol (2023) 24:1487–98. doi: 10.1038/s41590-023-01562-6
71. Mani V, Bromley SK, Äijö T, Mora-Buch R, Carrizosa E, Warner RD, et al. Migratory DCs activate TGF-b to precondition naïve CD8+ T cells for tissue-resident memory fate. Science (2019) 366:eaav5728. doi: 10.1126/science.aav5728
72. Hirai T, Zenke Y, Yang Y, Bartholin L, Beura LK, Masopust D, et al. Keratinocyte-mediated activation of the cytokine TGF-β maintains skin recirculating memory CD8+ T cells. Immunity (2019) 50:1249–61.e5. doi: 10.1016/j.immuni.2019.03.002
73. Mackay LK, Rahimpour A, Ma JZ, Collins N, Stock AT, Hafon M-L, et al. The developmental pathway for CD103+CD8+ tissue-resident memory T cells of skin. Nat Immunol (2013) 14:1294–301. doi: 10.1038/ni.2744
74. Seder RA, Chang LJ, Enama ME, Zephir KL, Sarwar UN, Gordon IJ, et al. Protection against malaria by intravenous immunization with a nonreplicating sporozoite vaccine. Science (2013) 341:1359–65. doi: 10.1126/science.1241800
75. Mackay LK, Minnich M, Kragten NA, Liao Y, Nota B, Seillet C, et al. Hobit and Blimp1 instruct a universal transcriptional program of tissue residency in lymphocytes. Science (2016) 352:459–63. doi: 10.1126/science.aad2035
76. Crowl JT, Heeg M, Ferry A, Milner JJ, Omilusik KD, Toma C, et al. Tissue-resident memory CD8(+) T cells possess unique transcriptional, epigenetic and functional adaptations to different tissue environments. Nat Immunol (2022) 23:1121–31. doi: 10.1038/s41590-022-01229-8
77. McNamara HA, Cai Y, Wagle MV, Sontani Y, Roots CM, Miosge LA, et al. Up-regulation of LFA-1 allows liver-resident memory T cells to patrol and remain in the hepatic sinusoids. Sci Immunol (2017) 2:eaaj1996. doi: 10.1126/sciimmunol.aaj1996
78. Tse SW, Radtke AJ, Espinosa DA, Cockburn IA, Zavala F. The chemokine receptor CXCR6 is required for the maintenance of liver memory CD8(+) T cells specific for infectious pathogens. J Infect Dis (2014) 210:1508–16. doi: 10.1093/infdis/jiu281
79. Christo SN, Evrard M, Park SL, Gandolfo LC, Burn TN, Fonseca R, et al. Discrete tissue microenvironments instruct diversity in resident memory T cell function and plasticity. Nat Immunol (2021) 22:1140–51. doi: 10.1038/s41590-021-01004-1
80. Adachi T, Kobayashi T, Sugihara E, Yamada T, Ikuta K, Pittaluga S, et al. Hair follicle–derived IL-7 and IL-15 mediate skin-resident memory T cell homeostasis and lymphoma. Nat Med (2015) 21:1272–9. doi: 10.1038/nm.3962
81. Skon CN, Lee J-Y, Anderson KG, Masopust D, Hogquist KA, Jameson SC. Transcriptional downregulation of S1pr1 is required for the establishment of resident memory CD8+ T cells. Nat Immunol (2013) 14:1285–93. doi: 10.1038/ni.2745
82. Liehl P, Zuzarte-Luis V, Chan J, Zillinger T, Baptista F, Carapau D, et al. Host-cell sensors for Plasmodium activate innate immunity against liver-stage infection. Nat Med (2014) 20:47–53. doi: 10.1038/nm.3424
83. Minkah NK, Wilder BK, Sheikh AA, Martinson T, Wegmair L, Vaughan AM, et al. Innate immunity limits protective adaptive immune responses against pre-erythrocytic malaria parasites. Nat Commun (2019) 10:3950. doi: 10.1038/s41467-019-11819-0
84. Du Y, Hertoghs N, Duffy FJ, Carnes J, McDermott SM, Neal ML, et al. Systems analysis of immune responses to attenuated P. falciparum malaria sporozoite vaccination reveals excessive inflammatory signatures correlating with impaired immunity. PloS Pathog (2022) 18:e1010282. doi: 10.1371/journal.ppat.1010282
85. Oyong DA, Duffy FJ, Neal ML, Du Y, Carnes J, Schwedhelm KV, et al. Distinct immune responses associated with vaccination status and protection outcomes after malaria challenge. PloS Pathog (2023) 19:e1011051. doi: 10.1371/journal.ppat.1011051
86. Valencia-Hernandez AM, Zillinger T, Ge Z, Tan PS, Cozijnsen A, IM G, et al. Complexing CpG adjuvants with cationic liposomes enhances vaccine-induced formation of liver T(RM) cells. Vaccine (2023) 41:1094–107. doi: 10.1016/j.vaccine.2022.12.047
87. Casey KA, Fraser KA, Schenkel JM, Moran A, Abt MC, Beura LK, et al. Antigen-independent differentiation and maintenance of effector-like resident memory T cells in tissues. J Immunol (2012) 188:4866–75. doi: 10.4049/jimmunol.1200402
88. Masopust D, Choo D, Vezys V, Wherry EJ, Duraiswamy J, Akondy R, et al. Dynamic T cell migration program provides resident memory within intestinal epithelium. J Exp Med (2010) 207:553–64. doi: 10.1084/jem.20090858
89. Park SL, Zaid A, Hor JL, Christo SN, Prier JE, Davies B, et al. Local proliferation maintains a stable pool of tissue-resident memory T cells after antiviral recall responses. Nat Immunol (2018) 19:183–91. doi: 10.1038/s41590-017-0027-5
90. Fung HY, Teryek M, Lemenze AD, Bergsbaken T. CD103 fate mapping reveals that intestinal CD103–tissue-resident memory T cells are the primary responders to secondary infection. Sci Immunol (2022) 7:eabl9925. doi: 10.1126/sciimmunol.abl9925
91. Ariotti S, Hogenbirk MA, Dijkgraaf FE, Visser LL, Hoekstra ME, Song JY. T cell memory. Skin-resident memory CD8+ T cells trigger a state of tissue-wide pathogen alert. Science (2014) 346:101–5. doi: 10.1126/science.1254803
92. Schenkel JM, Fraser KA, Beura LK, Pauken KE, Vezys V, Masopust D. T cell memory. Resident memory CD8 T cells trigger protective innate and adaptive immune responses. Science (2014) 346:98–101. doi: 10.1126/science.1254536
93. Kumar KA, Sano G, Boscardin S, Nussenzweig RS, Nussenzweig MC, Zavala F, et al. The circumsporozoite protein is an immunodominant protective antigen in irradiated sporozoites. Nature (2006) 444:937–40. doi: 10.1038/nature05361
94. Guerin-Marchand C, Druilhe P, Galey B, Londono A, Patarapotikul J, Beaudoin RL, et al. A liver-stage-specific antigen of Plasmodium falciparum characterized by gene cloning. Nature (1987) 329:164–7. doi: 10.1038/329164a0
95. Yoshida N, Nussenzweig RS, Potocnjak P, Nussenzweig V, Aikawa M. Hybridoma produces protective antibodies directed against the sporozoite stage of malaria parasite. Science (1980) 207:71–3. doi: 10.1126/science.6985745
96. Rogers WO, Rogers MD, Hedstrom RC, Hoffman SL. Characterization of the gene encoding sporozoite surface protein 2, a protective Plasmodium yoelii sporozoite antigen. Mol Biochem Parasitol (1992) 53:45–51. doi: 10.1016/0166-6851(92)90005-5
97. Gardner MJ, Hall N, Fung E, White O, Berriman M, Hyman RW, et al. Genome sequence of the human malaria parasite Plasmodium falciparum. Nature (2002) 419:498–511. doi: 10.1038/nature01097
98. Carlton JM, Angiuoli SV, Suh BB, Kooij TW, Pertea M, Silva JC, et al. Genome sequence and comparative analysis of the model rodent malaria parasite Plasmodium yoelii yoelii. Nature (2002) 419:512–9. doi: 10.1038/nature01099
99. Mauduit M, Gruner AC, Tewari R, Depinay N, Kayibanda M, Chavatte JM, et al. A role for immune responses against non-CS components in the cross-species protection induced by immunization with irradiated malaria sporozoites. PloS One (2009) 4:e7717. doi: 10.1371/journal.pone.0007717
100. Hafalla JC, Bauza K, Friesen J, Gonzalez-Aseguinolaza G, Hill AV, Matuschewski K. Identification of targets of CD8(+) T cell responses to malaria liver stages by genome-wide epitope profiling. PloS Pathog (2013) 9:e1003303. doi: 10.1371/journal.ppat.1003303
101. Murphy SC, Kas A, Stone BC, Bevan MJ. A T-cell response to a liver-stage Plasmodium antigen is not boosted by repeated sporozoite immunizations. Proc Natl Acad Sci U.S.A. (2013) 110:6055–60. doi: 10.1073/pnas.1303834110
102. Doolan DL, Southwood S, Freilich DA, Sidney J, Graber NL, Shatney L, et al. Identification of Plasmodium falciparum antigens by antigenic analysis of genomic and proteomic data. Proc Natl Acad Sci U.S.A. (2003) 100:9952–7. doi: 10.1073/pnas.1633254100
103. Kula T, Dezfulian MH, Wang CI, Abdelfattah NS, Hartman ZC, Wucherpfennig KW, et al. T-Scan: A genome-wide method for the systematic discovery of T cell epitopes. Cell (2019) 178:1016–28 e13. doi: 10.1016/j.cell.2019.07.009
104. Draheim M, Wlodarczyk MF, Crozat K, Saliou JM, Alayi TD, Tomavo S, et al. Profiling MHC II immunopeptidome of blood-stage malaria reveals that cDC1 control the functionality of parasite-specific CD4 T cells. EMBO Mol Med (2017) 9:1605–21. doi: 10.15252/emmm.201708123
105. Howland SW, Poh CM, Gun SY, Claser C, Malleret B, Shastri N, et al. Brain microvessel cross-presentation is a hallmark of experimental cerebral malaria. EMBO Mol Med (2013) 5:984–99. doi: 10.1002/emmm.201202273
106. Wahl I, Obraztsova AS, Puchan J, Hundsdorfer R, Chakravarty S, Sim BKL, et al. Clonal evolution and TCR specificity of the human TFH cell response to Plasmodium falciparum CSP. Sci Immunol (2022) 7:eabm9644. doi: 10.1126/sciimmunol.abm9644
107. Sattabongkot J, Yimamnuaychoke N, Leelaudomlipi S, Rasameesoraj M, Jenwithisuk R, Coleman RE, et al. Establishment of a human hepatocyte line that supports in vitro development of the exo-erythrocytic stages of the malaria parasites Plasmodium falciparum and P. vivax. Am J Trop Med Hyg (2006) 74:708–15. doi: 10.4269/ajtmh.2006.74.708
108. Prudencio M, Rodrigues CD, Ataide R, Mota MM. Dissecting in vitro host cell infection by Plasmodium sporozoites using flow cytometry. Cell Microbiol (2008) 10:218–24. doi: 10.1111/j.1462-5822.2007.01032.x
109. Doll KL, Pewe LL, Kurup SP, Harty JT. Discriminating protective from nonprotective plasmodium-specific CD8+ T cell responses. J Immunol (2016) 196:4253–62. doi: 10.4049/jimmunol.1600155
110. Montagna GN, Beigier-Bompadre M, Becker M, Kroczek RA, Kaufmann SH, Matuschewski K. Antigen export during liver infection of the malaria parasite augments protective immunity. MBio (2014) 5(4):e01321–14. doi: 10.1128/mBio.01321-14
111. Muller K, Gibbins MP, Roberts M, Reyes-Sandoval A, Hill AVS, Draper SJ, et al. Low immunogenicity of malaria pre-erythrocytic stages can be overcome by vaccination. EMBO Mol Med (2021) 13:e13390. doi: 10.15252/emmm.202013390
112. Singh AP, Buscaglia CA, Wang Q, Levay A, Nussenzweig DR, Walker JR, et al. Plasmodium circumsporozoite protein promotes the development of the liver stages of the parasite. Cell (2007) 131:492–504. doi: 10.1016/j.cell.2007.09.013
113. Bongfen SE, Torgler R, Romero JF, Renia L, Corradin G. Plasmodium berghei-infected primary hepatocytes process and present the circumsporozoite protein to specific CD8+ T cells in vitro. J Immunol (2007) 178:7054–63. doi: 10.4049/jimmunol.178.11.7054
Keywords: malaria, attenuated sporozoite vaccine, liver tissue-resident CD8+ T cells, protective antigens, prime-trap/target
Citation: Zhu C, Jiao S and Xu W (2024) CD8+ Trms against malaria liver-stage: prospects and challenges. Front. Immunol. 15:1344941. doi: 10.3389/fimmu.2024.1344941
Received: 27 November 2023; Accepted: 08 January 2024;
Published: 22 January 2024.
Edited by:
Xiang Wu, Central South University, ChinaReviewed by:
Gaoqian Feng, Nanjing Medical University, ChinaDaniel Fernández Ruiz, University of New South Wales, Australia
Copyright © 2024 Zhu, Jiao and Xu. This is an open-access article distributed under the terms of the Creative Commons Attribution License (CC BY). The use, distribution or reproduction in other forums is permitted, provided the original author(s) and the copyright owner(s) are credited and that the original publication in this journal is cited, in accordance with accepted academic practice. No use, distribution or reproduction is permitted which does not comply with these terms.
*Correspondence: Wenyue Xu, eHV3ZW55dWVAdG1tdS5lZHUuY24=
†These authors have contributed equally to this work