- 1Department of Cell and Developmental Biology, The Faculty of Medical and Health Sciences, Tel Aviv University, Tel Aviv, Israel
- 2Department of Internal Medicine and Hematology and Research Group for Immunology and Hematology, Semmelweis University - Eötvös Loránd Research Network (Office for Supported Research Groups), Budapest, Hungary
Background: Mortalin/GRP75 is a ubiquitous mitochondrial chaperone related to the cytosolic heat shock protein 70. It protects cells from various types of damages and from senescence. Our goal was to determine whether COVID-19 patients have circulating mortalin in their blood and to assess its prognostic value in anticipating disease severity.
Methods: Mortalin was determined by ELISA in the sera of 83 COVID-19 patients enrolled in the study. Patients were categorized into 4 groups: critical patients who died (FATAL) or required intensive care and survived (ICU), patients of mild severity (hospitalized but not critical) who required nasal oxygen support (HOSP+O2), and patients who did not need oxygen therapy (HOSP).
Results: The mortalin concentration in the serum of all COVID-19 patients in the cohort was 194-2324 pg/mL. A comparison of the mortalin levels by peak severity among the various patient groups showed a highly significant difference between the HOSP and FATAL groups and a significant difference between the HOSP and the ICU groups. COVID-19 patients who eventually failed to survive had at hospitalization a markedly higher level of mortalin in their sera. Cox regression analysis revealed a high mortality hazard (HR=3.96, p<0.01) in patients with high mortalin circulating levels (above the median, ≥651 pg/mL). This was confirmed in survival curve analysis (Kaplan-Meier; p=0.0032, log-rank test). Mortalin remained an independent predictor of mortality even after adjusting for age and sex or various complement activation products. Complement activation data collected in an earlier study in the same cohort was compared regarding the mortalin levels. Patients with higher circulating mortalin levels also had higher levels of complement C3a but reduced levels of properdin.
Discussion: This is the first report on circulating mortalin in COVID-19 patients. Higher mortalin levels were associated with more severe illnesses and a higher risk of death. We claim that quantifying the blood levels of mortalin and activated complement proteins will provide important information on the prognosis of COVID-19 patients and will serve as a useful tool for guiding their clinical management and treatment.
Introduction
The COVID-19 pandemic started in December 2019 and rapidly spread throughout the world. As updated by the WHO in October 2023, the number of confirmed cases infected by the SARS-CoV-2 virus is higher than 770 million and the number of confirmed deaths is close to 7 million (1). Some of the infected patients develop a more severe illness, and experience an extensive systemic inflammatory response with a “cytokine storm”, severe lung damage, acute respiratory distress syndrome (ARDS), multiorgan failure, and a higher risk of death (2). This has been attributed to several risk factors, including age and medical conditions such as cardiovascular disease, diabetes, chronic respiratory disease, or cancer (3–5). Supportive life-saving treatments have been proposed and are being applied to severely sick COVID-19 patients with an understanding that patients who need them should receive them as early as possible (6). Hence, identifying early indicators of severe disease and mortality is essential.
To date, several markers have been proposed for early detection of severe COVID-19. SARS-CoV-2 virus activates the complement system of the patients, leading to the accumulation of cleaved fragments of complement C3 and C5, C3a, and C5a in the patients’ blood (7–9). Apparently, C3a and C5a play a significant role in triggering the cytokine storm and ARDS in the patients (8, 10–12). This has led to clinical trials targeting, for example, complement C5 with Ravulizumab and testing its effect on COVID-19-associated lung injuries and distress (7, 13). Moreover, complement activation as well as increased levels of C3a and C5a were found to be associated with advanced disease severity (8, 14). Soluble complement C5b-9 complex (sC5b-9) was also identified in the plasma of COVID-19 patients and was higher in patients with severe disease than in patients with moderate disease (15). It was proposed that sC5b-9 may be a more stable and reliable indicator of in-vivo complement activation than C5a (15).
Massive cellular damage occurs in the body after injury or during viral infection. This leads to the release of damage-associated molecular patterns (DAMPs), which are known to activate a systemic inflammatory response (16). Extensive cell damage may also affect the mitochondria, leading to the release of mitochondrial DAMPs, such as mitochondrial DNA (mtDNA) into the blood (17). Thus, higher levels of circulating mtDNA were measured in the blood of COVID-19 patients who eventually died or required ICU admission (18). High circulating mtDNA was apparently an indicator of poor COVID-19 outcomes.
Mortalin, also known as the mitochondrial stress protein 70 and GRP75 (gene: Hspa9), is an indispensable mitochondrial matrix protein. It is essential for the transport of proteins into mitochondria and has other important functions that support cell stress, survival, and aging (19). Once mortalin activity is blocked, cells fail to survive (20, 21). We previously reported that colorectal carcinoma patients have circulating mortalin in their blood plasma and that higher mortalin levels indicate a poor prognosis in terms of survival (22, 23). In addition, we have shown that mortalin can be released in extracellular vesicles from complement-activated cells (24). Identification of complement activation markers (increased levels of circulating C3a, C5a, and sC5b-9) as well as cellular mitochondrial damage indicators (circulating mtDNA) in COVID-19 patients, as described above, prompted us to look for circulating mortalin in the blood of COVID-19 patients. As reported here, variable levels of mortalin were found in COVID-19 patients’ blood. Interestingly, patients with higher mortalin levels experienced a more severe illness and had a shorter survival.
Methods
Patients, clinical categories, and serum samples
This study included a cohort of adult COVID-19 patients (83 individuals confirmed positive by PCR) who were admitted to two tertiary hospitals in Budapest between April 20 and July 2, 2020. Hence, this cohort represents the COVID-19 pathophysiology before the vaccine era, with wild-type SARS-CoV-2 infection. Additionally, 7 blood donors post-COVID-19 infection not requiring hospitalization served as the control group. All participants were part of a larger COVID-19 cohort described in an earlier report (14); however, the serum samples for the mortalin analysis were only available for the individuals described here. The severity of patients’ morbidity was categorized as described previously (14). In brief, critical patients were those who died (FATAL) or required intensive care and survived (ICU). Patients with milder severity (hospitalized but not critical) and that required nasal oxygen support constituted the HOSP+O2 group, whereas patients who did not need oxygen therapy constituted the HOSP group. Respiratory failure necessitating mechanical ventilation occurred in 60% and 78.3% of the ICU and FATAL groups, respectively. Macro-thromboembolic complications occurred in 46.7% and 4.3% of the ICU and FATAL groups, respectively. Acute kidney injury (KDIGO: 2-3) occurred in 8.3%, 6.7%, and 39.1% of the HOSP+O2, ICU, and FATAL groups, respectively (14).
For each enrolled patient, the sample taken at hospital admission was included in the study. Laboratory data (blood count, clinical chemistry, coagulation, and inflammatory parameters) were extracted from the hospital records. Blood samples were collected from the antecubital vein or from a central venous catheter and were immediately transferred to the processing laboratory for serum separation. The EDTA plasma and serum samples were immediately frozen and stored at -70°C or on dry ice until tested.
The study was conducted in accordance with the Declaration of Helsinki and its subsequent revisions and was approved by the Hungarian Scientific and Research Ethics Committee (ETT-TUKEB; No. IV/4403–2/2020/EKU). Written informed consent was obtained from the patients and the control subjects or from the closest relative available, if the patient was unable to give informed consent.
Mortalin ELISA
Mortalin levels in the serum samples were measured using the Mortalin ELISA kit from LSBio (Seattle, WA, USA), according to the manufacturer’s instructions. Briefly, test serum samples, diluted 1:3, in duplicate, were added to microtiter plate wells precoated with anti-mortalin antibodies (the capturing antibodies) for 1 h at 37°C. For the standard curve, wells were treated with serially diluted recombinant mortalin (5-40 pg/well) mixed with diluted (1:3) normal human serum. Wells treated with NHS (diluted 1:3) only served as negative controls and gave no signal above the Zero control provided in the kit. A biotin-conjugated anti-mortalin detection antibody was added for 1 h at room temperature. After a wash, the bound biotin was reacted with horseradish-peroxidase (HRP) conjugate, followed by washing and TMB substrate colorimetric detection at 450 nm using a microplate reader (Spectrafluor plus, Tecan, Austria). The Mortalin concentration in the test samples was calculated by using the standard curve.
Complement analyses
The complement classical, lectin, and alternative pathway activities as well as the concentrations of complement C3, C4, C1q, factors H, I, and B, and the activation markers C3a and sC5b-9 were determined as described earlier (14). The concentrations of properdin (HK334, Hycult Biotech), C1s/C1-INH complex (HK399, Hycult Biotech), MASP-1/C1-INH complex (HK3001, Hycult Biotech), C4a (A035, Quidel), C4d (A009, Quidel), and Bb (A027, Quidel) were determined in blood plasma-EDTA samples by using commercially available ELISA kits. All assays were performed according to the manufacturer’s instructions.
Statistical analyses
The distribution of most continuous variables was skewed; therefore, these data were presented as the median and interquartile range, and nonparametric tests were used: the Mann-Whitney test for comparing two independent groups, the Kruskal-Wallis test with Dunn’s post-test for comparing more than two independent groups, and Spearman’s rank correlation test for testing potential correlations between continuous variables. Cases with missing values were excluded pairwise. All applied tests were two-tailed, with the false discovery rate (alpha) of 0.05. The Benjamini-Hochberg procedure was used to correct the level of significance in order to maintain a false discovery rate of 5%. Uni- and multivariable Cox proportional hazard models were used to assess the effects of mortalin and various clinical and laboratory parameters on in-hospital mortality. Kaplan-Meier curves of selected subgroups were compared by the log-rank test in a pairwise manner. Statistical analyses were performed using Statistica 13.5 and GraphPad Prism 9 software.
Results
The concentration of soluble mortalin was quantified in the sera of 83 hospitalized COVID-19 patients and 7 controls. In the hospitalized patients, the mortalin levels ranged from 194-2324 pg/mL, with a median value of 651 pg/mL (Table 1). In the control group, the mortalin concentrations varied between 172 and 433 pg/mL. Comparison of the mortalin levels among the various patient groups (Figure 1A) showed a highly significant difference between the HOSP (627 (495-834) pg/mL) and the FATAL (1443 (651-2324) pg/mL) groups (p<0.0001) and a significant difference between the HOSP and the ICU (830 (328-1150) pg/mL) groups (p=0.0385). Comparison of the mortalin values by the Mann-Whitney test showed markedly higher levels of mortalin in sera from COVID-19 patients who eventually failed to survive (the difference between survived (510 (236-822) pg/mL) and deceased (1443 (651-2324) pg/mL) patients was highly significant; p<0.0001; Figure 1B). Survival analysis of the patients (Kaplan-Meier) divided into high mortalin (≥651 pg/mL median level) and low mortalin (<651 pg/mL) groups showed a significantly shorter survival (log-rank overall comparison of p=0.0032) of patients with high mortalin levels (Figure 2A).
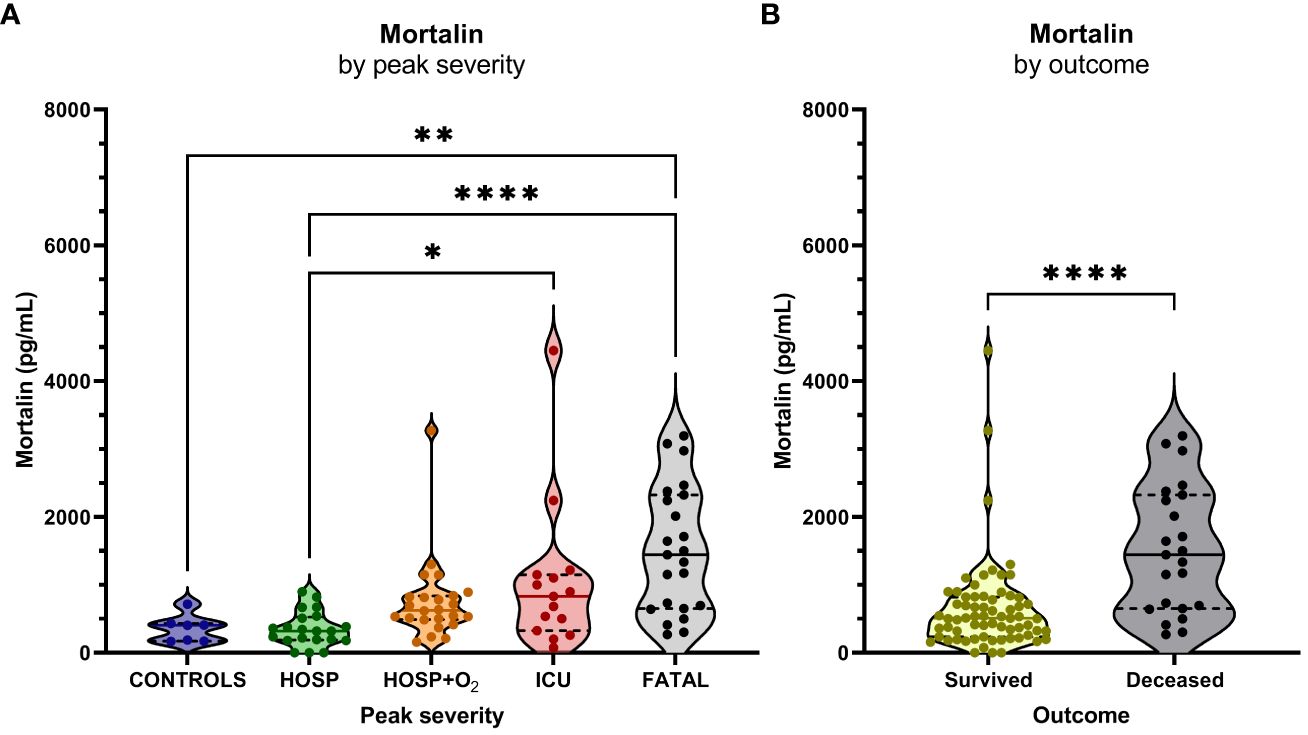
Figure 1 Mortalin levels and the COVID-19 disease outcome. (A) Mortalin levels by peak disease severity. Asterisks indicate the p-values obtained by the Kruskal-Wallis test with Dunn’s multiple comparison post hoc test (* p<0.05, ** p<0.01, and **** p<0.0001), non-significant differences are not indicated. (B) Relationship between mortalin levels and mortality. Asterisks indicate the p-value obtained by the Mann Whitney test (**** p<0.0001).
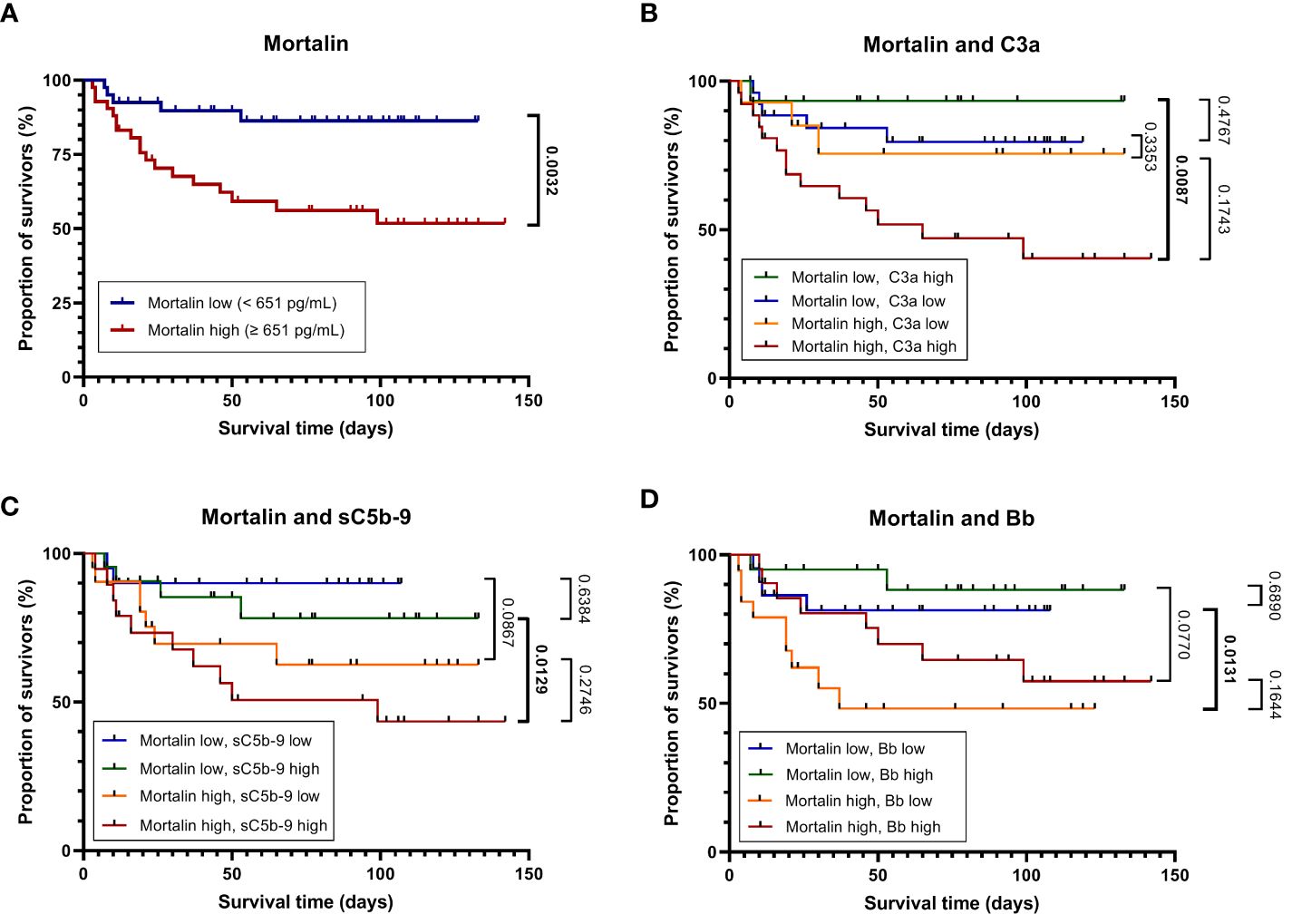
Figure 2 Mortality of patients with COVID-19 as stratified by high or low mortalin levels. Kaplan-Meier curves (in-hospital all-cause mortality plotted against the time from hospital admission to death or the last follow-up) for patients with mortalin levels above or below the median (651 pg/mL) are shown (A). The above groups were further divided based on the levels of the complement activation markers C3a, sC5b-9, and Bb above or below the median (251 and 301 ng/mL, and 1.723 μg/mL, respectively). The Kaplan-Meier curves of these subgroups are shown in (B–D), respectively. For pairwise comparisons of certain groups, the p-values of log-rank tests are shown; significant differences are denoted in bold.
More comorbidity and laboratory data of this cohort of patients is also shown in Table 1. Age of the patients and co-existence of a malignant disease significantly correlated with COVID-19 disease severity. Samples were taken at hospital admission, 9 (4-17) days after the appearance of the first symptoms. The time from disease onset until sampling did not differ in groups of hospitalized patients with different COVID-19 severity. Of the multiple laboratory tests performed, the parameters that were found to significantly reflect the severity of the disease were as follows: lymphocyte counts (Ly), RDW, INR, LDH, AST, ALT, troponin, protein, the neutrophil to lymphocyte ratio (Neu/Ly), CRP, PCT, IL-6, and ferritin. Consequently, those laboratory parameters were compared between patients who had higher mortalin levels (≥651 pg/mL) and patients with lower mortalin levels (Table 2). Interestingly, patients with higher mortalin levels in their blood also had higher Neu/Ly, RDW, INR, BUN, and PCT, and lower Ly levels in their blood. Their LDH, troponin, creatinine, CRP and ferritin levels also tended to be higher (with 0.013<p<0.1) in the high mortalin level patients (Table 2). Correlation analysis of the mortalin levels and the laboratory parameters confirmed that the levels of Neu/Ly, RDW, INR, LDH, troponin, BUN, Creatinine, CRP, PCT, and IL-6 indeed directly correlated with the mortalin levels (Figure 3).
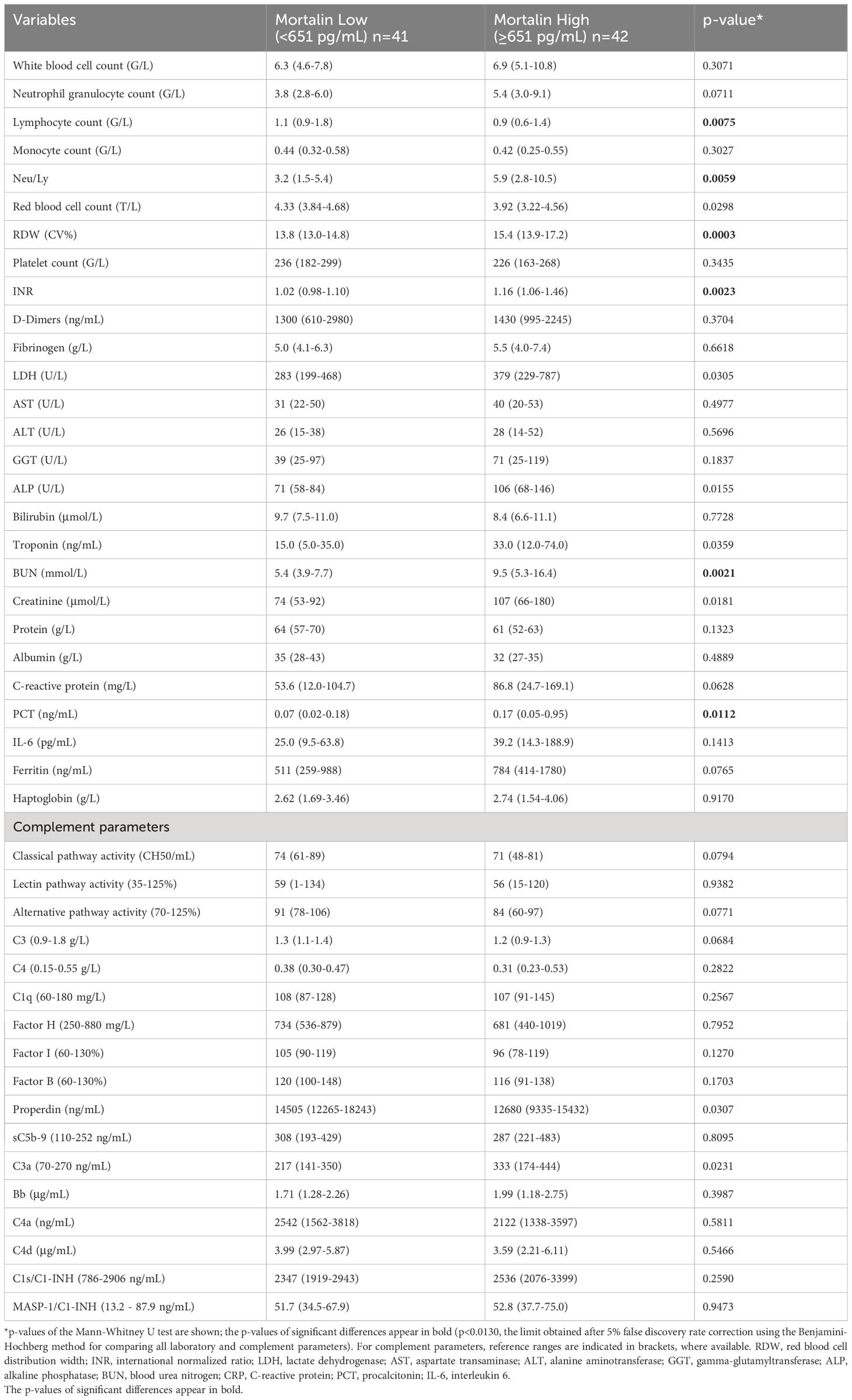
Table 2 Laboratory and complement data of cases with mortalin concentrations below or above the median.
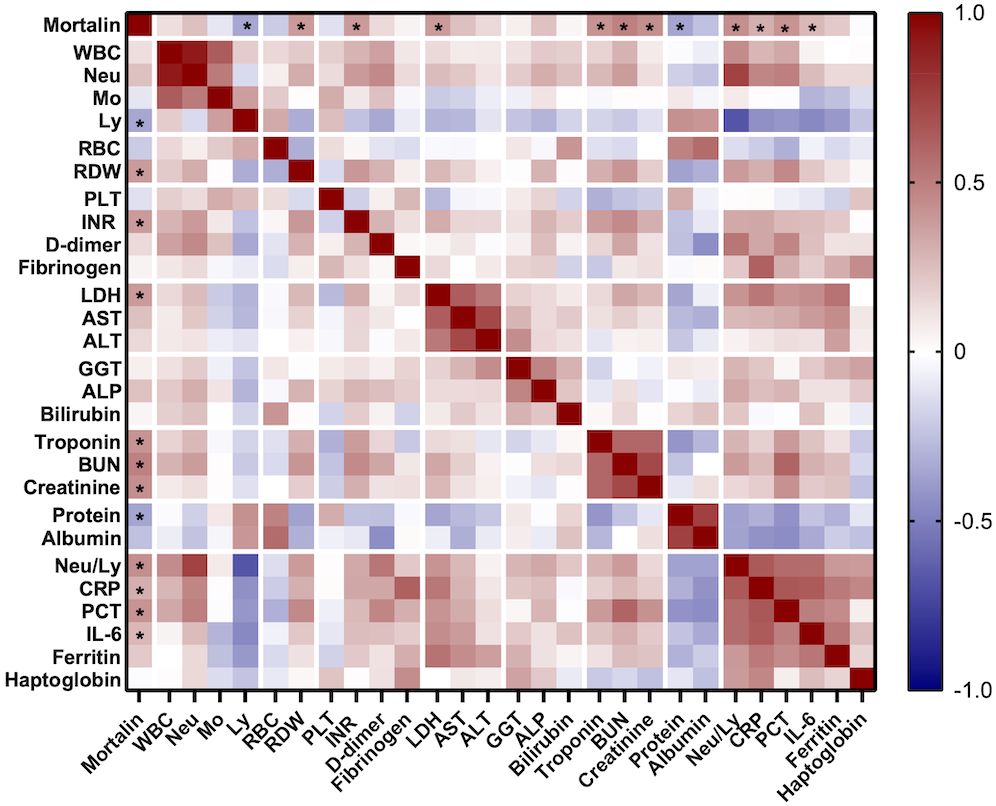
Figure 3 Correlation between mortalin levels and several routine laboratory parameters. Color-coding indicates the strength of each correlation (Spearman correlation coefficients); asterisks indicate significant correlations of mortalin levels (* p<0.018, the limit obtained after 5% false discovery rate correction using the Benjamini-Hochberg method for all correlations). WBC, white blood cell count; Neu, neutrophil granulocyte count; Mo, monocyte count; Ly, lymphocyte count; RBC, red blood cell count; RDW, red blood cell distribution width; PLT, platelet count; INR, international normalized ratio; LDH, lactate dehydrogenase; AST, aspartate transaminase; ALT, alanine aminotransferase; GGT, gamma-glutamyltransferase; ALP, alkaline phosphatase; BUN, blood urea nitrogen; CRP, C-reactive protein; PCT, procalcitonin; IL-6, interleukin 6.
Measurements of the complement pathway parameters were also available for these patients from an earlier study (14); they were also compared with patients having higher and lower mortalin levels. As shown in Table 2, of the various parameters tested, only reduced properdin levels and higher C3a levels (p<0.05) were associated with higher mortalin levels. Accordingly, correlation analysis revealed a significant correlation between mortalin and C3a levels (Figure 4). A positive correlation, yet statistically insignificant, perhaps due to the size of the cohort, was also seen between higher mortalin and higher sC5b-9 or Bb levels.
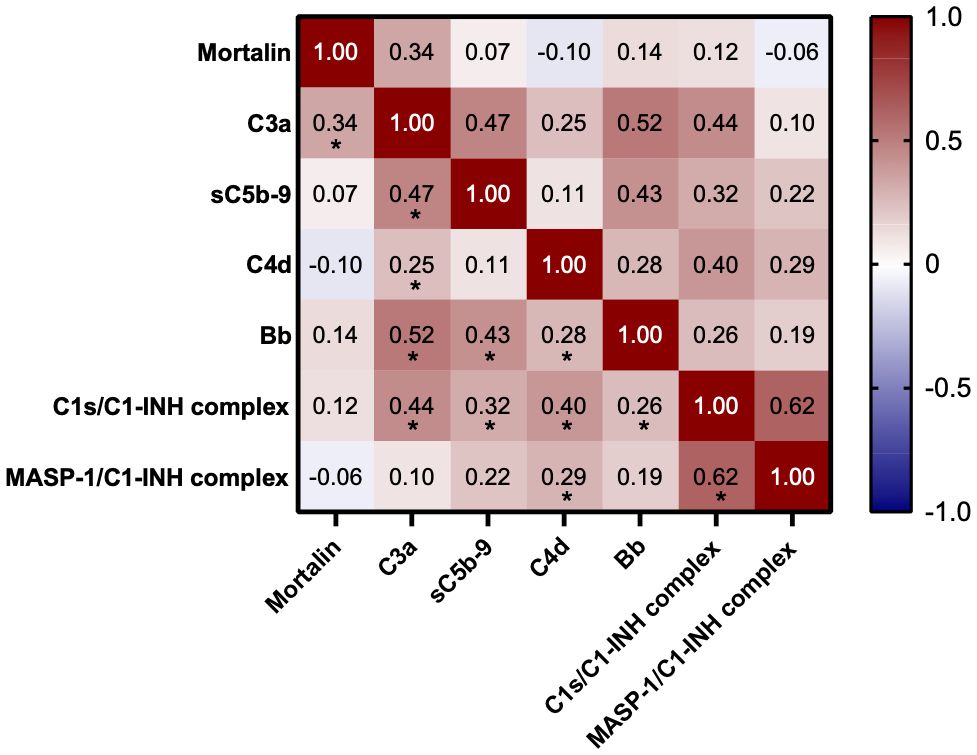
Figure 4 Correlation between mortalin levels and complement parameters. Color-coding indicates the strength of each correlation (Spearman correlation coefficients); asterisks indicate significance (* p<0.03, the limit obtained after 5% false discovery rate correction using the Benjamini-Hochberg method).
Univariate analysis of the risk of mortality by Cox regression of mortalin concentration is presented in Table 3. High mortalin levels (above the median) significantly (p=0.0026) increased the hazard ratio to 3.96 (95% CI 1.47-10.68), when compared to low mortalin levels (below the median). Multivariable analysis of the risk of mortality was also performed, where high/low mortalin levels were adjusted for age and sex. In the adjusted model, mortalin showed a significant age- and sex-independent association with mortality (HR 3.07, CI 1.13-8.33, Table 3). Multivariable analysis, combining mortalin levels with complement C3a, sC5b-9, or Bb levels, was also performed (Table 3). These analyses revealed that the HR of higher mortalin levels remained high (3.07, 3.58, and 4.02, correspondingly) even when combined with C3a, sC5b-9, or Bb levels, thus demonstrating their independence in predicting the disease severity.
To further examine the latter suggestion, we performed survival analyses of the patients by combining the mortalin and C3a levels, the mortalin and sC5b-9 levels, and the mortalin and Bb levels (Figures 2B–D). As shown in Figure 2B, patients who had high mortalin levels in addition to high (above the median) C3a levels had a significantly shorter survival (log-rank overall comparison of p=0.0087) relative to patients who had low mortalin and high C3a levels. Similarly, high mortalin, in addition to high sC5b-9 levels, predicted shorter patient survival (Figure 2C) compared with patients with equally high sC5b-9 but low mortalin levels. In contrast, high mortalin levels were associated with shorter survival in the group of low (and not high) Bb levels (Figure 2D). Interestingly, low Bb (rather than high) levels were associated with shorter survival (data not shown). Thus, we concluded that analysis of mortalin and markers of complement activation in COVID-19 patients’ sera has a high prognostic value and can help in identifying patients at low risk as well as high risk of mortality.
Discussion
Our results show, for the first time, that COVID-19 patients have elevated levels of circulating mortalin in their blood (the measured concentration levels varied between 194 and 2,324 pg/mL). Most importantly, patients with higher mortalin levels appear to be at significant risk of mortality. This in contrast to the insignificant levels of soluble mortalin found in the blood of healthy individuals (23) and the measured levels of circulating mortalin in the 7 blood donors post-COVID-19 infection, who did not require hospitalization (172-433 pg/mL). Comparing the survival curves of patients with high and low mortalin levels strongly supported the prognostic strength of the level of circulating mortalin in patients’ blood. Ninety percent of patients with below-median concentrations of mortalin survived after 120 days, but only 50% of the patients in the high mortalin group survived up to this time. Extracellular circulating mortalin has been shown to have prognostic value in identifying patients at high risk of shorter survival in patients with colorectal carcinoma (22, 23). In both cases, the source for the mortalin circulating in the blood remains to be determined.
As described in the Introduction, complement activation and variable levels of C3a (8, 14) and sC5b-9 (15) were identified in COVID-19 patients’ blood, showing a direct correlation with the disease severity. After dividing the patients into 2 groups according to their mortalin level (low vs. high, based on the median value), the data did not show a significant difference in complement activation patterns between the two groups. However, the observed data tendencies led us to hypothesize that having a larger number of patients in the two groups might have shown a significantly reduced classical and alternative pathway activity, reduced C3 and properdin levels, and elevated C3a levels in the high mortalin group (Table 2). A reduced C3 level may in part account for reduced classical and alternative pathway activities and a reduced properdin level may further account for reduced alternative pathway activity. Similar levels of sC5b-9 are seen in the 2 groups. The interactions between the mortalin levels and the levels of the complement activation products as predictors of mortality were tested by Cox proportional hazard models and by Kaplan Meier survival plotting. The fact that mortalin remains in the Cox proportional hazard model as an independent predictor of mortality even after adjusting for each of the tested complement activation products indicates that mortalin is also associated with mortality via complement-independent mechanisms. However, when analyzing the Kaplan-Meier curves of the different subgroups, we observed that high mortalin values are significantly associated with shorter survival times only in patients with high C3a or sC5b-9 or with low Bb values. This observation indicates that the association between high mortalin levels and a higher risk of mortality is partly caused by both complement-mediated mechanisms as well as complement-independent ones. The first two associations (interaction with C3a and sC5b-9) seem to be logical, since they indicate a complement activation process, but the association with low Bb is intriguing. However, taking into account that (a) the alternative complement pathway is more activated in high mortalin level patients, (b) the properdin level is reduced in high mortalin level patients, and (c) lower Bb is associated with a more severe disease, we hypothesize that COVID-19 patients have a more severe disease following activation of the alternative complement pathway, leading to a more stable fixation of the alternative pathway C3/C5 convertases C3bBbP and C3bBbC3bP on the affected tissue. Consequently, the C3a level in blood is elevated, whereas the properdin (P) level is reduced because it is attached to the affected tissue. Deposition of more stable convertases on the affected tissue will generate higher levels of C5a and deposited C5b-9, which will indirectly and directly cause more severe damage and mortality. These findings and the hypothesis should be tested further with a larger cohort of COVID-19 patients including patients from other medical centers.
The correlation between mortalin level and the level of the laboratory markers of inflammation and tissue damage (e.g., CRP, LDH) observed in COVID-19 patients suggests that mortalin is released from dying or damaged cells. This may parallel the observed spike in circulating mtDNA in COVID-19 patients’ blood (18). However, cumulative data supports a claim that mortalin protects cells against numerous insults (19, 25). We reported that in response to complement-mediated damage, cells use mortalin to limit their damage by relocating from the mitochondria to the plasma membrane and out of the cells while exporting the complement membrane attack complex C5b-9 (26). It is conceivable that some of the extracellular mortalin observed in COVID-19 patients’ blood reflects such an attempt of their affected tissues to reduce and avoid the damage. Here, the circulating mortalin should be regarded as a stress reporter rather than a tissue damage marker. Activation of the complement system has been described in COVID-19 patients and even implicated in the tissue damage evident in severely ill patients (7–10). It is possible that some of the mortalin that is released from complement-attacked cells in the patients’ body reflects the affected tissue’s attempt to repair the lytic damage and some is released from dying cells. As the complement activation increases, more extracellular mortalin is measured in the blood of COVID-19 patients. Whether mortalin released from stressed and dying cells has the same or a different molecular form remains to be determined.
Another important point to consider is whether mortalin plays a protective role against viral entry. It was reported that mortalin reduces the entry of porcine epidemic diarrhea virus into host cells by interfering with clathrin-mediated endocytosis of the virus (27). If this is also true for SARS-CoV-2 entry into host cells, it is possible that the release of mortalin in COVID-19 patients into their blood, accompanied by reduced intracellular mortalin levels, makes the patients’ cells more exposed to SARS-CoV-2 virus entry. However, this is still open to investigation.
In conclusion, our results suggest that quantification of circulating mortalin and activated complement proteins in the blood of COVID-19 patients will enable an early identification of patients at risk of developing a severe disease. Besides being a good prognostic marker, it is possible that mortalin plays an important role either in disease progression and/or in disease control. If mortalin plays a significant role in disease control, it should be further investigated and considered as a therapeutic target for COVID-19 disease.
Data availability statement
The original contributions presented in the study are included in the article/supplementary material. Further inquiries can be directed to the corresponding author.
Ethics statement
The studies involving humans were approved by Hungarian Scientific and Research Ethics Committee (ETT-TUKEB; No. IV/4403–2/2020/EKU). The studies were conducted in accordance with the local legislation and institutional requirements. The participants provided their written informed consent to participate in this study.
Author contributions
MA: Project administration, Writing – original draft, Data curation. GS: Data curation, Investigation, Methodology, Software, Writing – original draft, Writing – review & editing. LH: Investigation, Methodology, Software, Writing – review & editing. ZP: Conceptualization, Funding acquisition, Methodology, Resources, Supervision, Writing – review & editing. ZF: Conceptualization, Formal analysis, Funding acquisition, Methodology, Project administration, Resources, Supervision, Writing – original draft, Writing – review & editing.
Funding
The author(s) declare financial support was received for the research, authorship, and/or publication of this article. This research was supported by a grant from the Israel Science Foundation (to ZF) and by the Higher Education Institutional Excellence Programme of the Ministry of Human Capacities in Hungary, within the framework of the molecular biology thematic program of the Semmelweis University, “MOLORKIV” (TKP2021-EGA-24, to ZP), and by funds of the EU MSCA project CORVOS 860044 (to ZP and LH).
Conflict of interest
The authors declare that the research was conducted in the absence of any commercial or financial relationships that could be construed as a potential conflict of interest.
The author(s) declared that they were an editorial board member of Frontiers, at the time of submission. This had no impact on the peer review process and the final decision.
Publisher’s note
All claims expressed in this article are solely those of the authors and do not necessarily represent those of their affiliated organizations, or those of the publisher, the editors and the reviewers. Any product that may be evaluated in this article, or claim that may be made by its manufacturer, is not guaranteed or endorsed by the publisher.
References
1. WHO. Coronavirus disease (COVID-19) pandemic (2023). Available online at: https://www.who.int/emergencies/diseases/novel-coronavirus-2019.
2. Lipman M, Chambers RC, Singer M, Brown JS. SARS-CoV-2 pandemic: clinical picture of COVID-19 and implications for research. Thorax. (2020) 75:614–6. doi: 10.1136/thoraxjnl-2020-215024
3. Grasselli G, Greco M, Zanella A, Albano G, Antonelli M, Bellani G, et al. Risk factors associated with mortality among patients with COVID-19 in intensive care units in Lombardy, Italy. JAMA Intern Med. (2020) 180:1345–55. doi: 10.1001/jamainternmed.2020.3539
4. Gomes JJF, Ferreira A, Alves A, Sequeira BN. A risk scoring model of COVID-19 at hospital admission. PloS One. (2023) 18:e0288460. doi: 10.1371/journal.pone.0288460
5. Cruz JC, Martins CK, Katchborian Neto A, de Araujo CM, Dellalibera-Joviliano R. Age, gender, and 11 comorbidities as risk factors associated with COVID-19 mortality: A retrospective cohort including 1.8 million individuals. J Toxicol Environ Health A. (2023) 86:491–500. doi: 10.1080/15287394.2023.2223598
6. NIH. Coronavirus Disease 2019 (COVID-19). Treatment Guidelines (2023). Available online at: https://www.covid19treatmentguidelines.nih.gov/.
7. Zelek WM, Harrison RA. Complement and COVID-19: Three years on, what we know, what we don’t know, and what we ought to know. Immunobiology. (2023) 228:152393. doi: 10.1016/j.imbio.2023.152393
8. Prendecki M, Clarke C, Medjeral-Thomas N, McAdoo SP, Sandhu E, Peters JE, et al. Temporal changes in complement activation in haemodialysis patients with COVID-19 as a predictor of disease progression. Clin Kidney J. (2020) 13:889–96. doi: 10.1093/ckj/sfaa192
9. Noris M, Benigni A, Remuzzi G. The case of complement activation in COVID-19 multiorgan impact. Kidney Int. (2020) 98:314–22. doi: 10.1016/j.kint.2020.05.013
10. Gralinski LE, Sheahan TP, Morrison TE, Menachery VD, Jensen K, Leist SR, et al. Complement activation contributes to severe acute respiratory syndrome coronavirus pathogenesis. mBio. (2018) 9(5):e01753-18. doi: 10.1128/mBio.01753-18
11. Henry BM, Sinkovits G, Szergyuk I, de Oliveira MHS, Lippi G, Benoit JL, et al. Complement levels at admission reflecting progression to severe acute kidney injury (AKI) in coronavirus disease 2019 (COVID-19): A multicenter prospective cohort study. Front Med (Lausanne). (2022) 9:796109. doi: 10.3389/fmed.2022.796109
12. Carvelli J, Demaria O, Vely F, Batista L, Chouaki Benmansour N, Fares J, et al. Association of COVID-19 inflammation with activation of the C5a-C5aR1 axis. Nature. (2020) 588:146–50. doi: 10.1038/s41586-020-2600-6
13. Smith K, Pace A, Ortiz S, Kazani S, Rottinghaus S. A Phase 3 Open-label, Randomized, Controlled Study to Evaluate the Efficacy and Safety of Intravenously Administered Ravulizumab Compared with Best Supportive Care in Patients with COVID-19 Severe Pneumonia, Acute Lung Injury, or Acute Respiratory Distress Syndrome: A structured summary of a study protocol for a randomised controlled trial. Trials. (2020) 21:639. doi: 10.1186/s13063-020-04548-z
14. Sinkovits G, Mezo B, Reti M, Muller V, Ivanyi Z, Gal J, et al. Complement overactivation and consumption predicts in-hospital mortality in SARS-coV-2 infection. Front Immunol. (2021) 12:663187. doi: 10.3389/fimmu.2021.663187
15. Cugno M, Meroni PL, Gualtierotti R, Griffini S, Grovetti E, Torri A, et al. Complement activation in patients with COVID-19: A novel therapeutic target. J Allergy Clin Immunol. (2020) 146:215–7. doi: 10.1016/j.jaci.2020.05.006
16. Parthasarathy U, Martinelli R, Vollmann EH, Best K, Therien AG. The impact of DAMP-mediated inflammation in severe COVID-19 and related disorders. Biochem Pharmacol. (2022) 195:114847. doi: 10.1016/j.bcp.2021.114847
17. Zhang Q, Raoof M, Chen Y, Sumi Y, Sursal T, Junger W, et al. Circulating mitochondrial DAMPs cause inflammatory responses to injury. Nature. (2010) 464:104–7. doi: 10.1038/nature08780
18. Scozzi D, Cano M, Ma L, Zhou D, Zhu JH, O’Halloran JA, et al. Circulating mitochondrial DNA is an early indicator of severe illness and mortality from COVID-19. JCI Insight. (2021) 6(4):e143299. doi: 10.1172/jci.insight.143299
19. Esfahanian N, Knoblich CD, Bowman GA, Rezvani K. Mortalin: Protein partners, biological impacts, pathological roles, and therapeutic opportunities. Front Cell Dev Biol. (2023) 11:1028519. doi: 10.3389/fcell.2023.1028519
20. Jubran R, Saar-Ray M, Wawruszak A, Ziporen L, Donin N, Bairey O, et al. Mortalin peptides exert antitumor activities and act as adjuvants to antibody-mediated complement-dependent cytotoxicity. Int J Oncol. (2020) 57:1013–26. doi: 10.3892/ijo.2020.5101
21. Yoon AR, Wadhwa R, Kaul SC, Yun CO. Why is mortalin a potential therapeutic target for cancer? Front Cell Dev Biol. (2022) 10:914540. doi: 10.3389/fcell.2022.914540
22. Rozenberg P, Kocsis J, Saar M, Prohaszka Z, Fust G, Fishelson Z. Elevated levels of mitochondrial mortalin and cytosolic HSP70 in blood as risk factors in patients with colorectal cancer. Int J Cancer. (2013) 133:514–8. doi: 10.1002/ijc.28029
23. Jubran R, Kocsis J, Garam N, Malati E, Gombos T, Barabas L, et al. Circulating mitochondrial stress 70 protein/mortalin and cytosolic Hsp70 in blood: Risk indicators in colorectal cancer. Int J Cancer. (2017) 141:2329–35. doi: 10.1002/ijc.30918
24. Pilzer D, Fishelson Z. Mortalin/GRP75 promotes release of membrane vesicles from immune attacked cells and protection from complement-mediated lysis. Int Immunol. (2005) 17:1239–48. doi: 10.1093/intimm/dxh300
25. Pilzer D, Saar M, Koya K, Fishelson Z. Mortalin inhibitors sensitize K562 leukemia cells to complement-dependent cytotoxicity. Int J Cancer. (2010) 126:1428–35. doi: 10.1002/ijc.24888
26. Mazkereth N, Rocca F, Schubert JR, Geisler C, Hillman Y, Egner A, et al. Complement triggers relocation of Mortalin/GRP75 from mitochondria to the plasma membrane. Immunobiology. (2016) 221:1395–406. doi: 10.1016/j.imbio.2016.07.005
Keywords: COVID-19, mortalin, Grp75, complement, overall survival, risk factor
Citation: Avraham M, Sinkovits G, Hurler L, Prohászka Z and Fishelson Z (2024) Circulating mortalin in blood and activation of the alternative complement pathway as risk indicators in COVID-19 infection. Front. Immunol. 15:1337215. doi: 10.3389/fimmu.2024.1337215
Received: 12 November 2023; Accepted: 04 April 2024;
Published: 23 April 2024.
Edited by:
Heribert Stoiber, Innsbruck Medical University, AustriaReviewed by:
Paolo Macor, University of Trieste, ItalyElena Goicoechea De Jorge, Complutense University of Madrid, Spain
Copyright © 2024 Avraham, Sinkovits, Hurler, Prohászka and Fishelson. This is an open-access article distributed under the terms of the Creative Commons Attribution License (CC BY). The use, distribution or reproduction in other forums is permitted, provided the original author(s) and the copyright owner(s) are credited and that the original publication in this journal is cited, in accordance with accepted academic practice. No use, distribution or reproduction is permitted which does not comply with these terms.
*Correspondence: Zvi Fishelson, bGlmaXNoQHRhdWV4LnRhdS5hYy5pbA==
†These authors have contributed equally to this work