- Department of Microbiology and Immunology, Thomas Jefferson University, Philadelphia, PA, United States
The mRNA-LNP vaccine has received much attention during the COVID-19 pandemic since it served as the basis of the most widely used SARS-CoV-2 vaccines in Western countries. Based on early clinical trial data, these vaccines were deemed safe and effective for all demographics. However, the latest data raise serious concerns about the safety and effectiveness of these vaccines. Here, we review some of the safety and efficacy concerns identified to date. We also discuss the potential mechanism of observed adverse events related to the use of these vaccines and whether they can be mitigated by alterations of this vaccine mechanism approach.
The standard and non-standard components of the mRNA-LNP COVID-19 vaccines
Regulators approving the deployment of new therapeutic or prophylactic modalities insist on strict purity criteria for the product. However, the new mRNA vaccine platform offers some novel, untested, and previously unregulated aspects of impurities during manufacture. The mRNA vaccines consist of mRNA coding for the protein-of-interest complexed with and protected by a mixture of different lipids that form nanoparticles of around 100 nm in diameter (1). The mRNA is synthesized in vitro using an RNA-polymerase from an E. coli-derived DNA plasmid serving as a template. Ideally, the plasmid and other components are eliminated during the mRNA purification step (2). However, a recent preprint study using multiple sequencing assays reported levels of DNA contamination in both Moderna and Pfizer bivalent mRNA vaccines that exceeded the levels set by the European Medicines Agency (EMA) and The United States Food and Drug Administration (FDA) (3). Whether the contaminating plasmid DNA fragments can affect human health remains to be defined. Low levels of double-stranded RNAs (dsRNA) that can form during the production process and can activate innate immune sensors and induce inflammation (4), have also been reported for both Pfizer and Moderna mRNA vaccines (5, 6). The presence of physiologically relevant levels of the dsRNA in the Pfizer COVID-19 vaccine has also been confirmed experimentally, indicating that the adaptive immune responses induced by this vaccine in mice partially depend on MDA5 protein, a dsRNA-sensor (7).
The nucleoside modifications and removal of dsRNA using HPLC were initially introduced by Karikó et al., to circumvent the activation of innate immune sensors, a characteristic of unmodified mRNAs, and the production of inflammatory cytokines, such as type I interferon, that would limit protein translation from the mRNA (4, 8, 9). The long in vivo half-life was desirable because the mRNA technology was initially meant to replace or deliver a therapeutic protein (10). Nevertheless, the nucleoside modification was touted as a breakthrough discovery that allowed the human use of mRNA-based vaccines (11). The importance of the inflammatory components in the mRNA-LNP platform is highlighted by the fact that highly purified mRNA (no detectable dsRNA) combined with lipid nanoparticles (LNPs) that do not contain the inflammation-inducing ionizable lipid is unable to induce innate and adaptive immune responses in vivo, while the LNP containing the ionizable lipid mixed with protein or mRNA supported similar adaptive immune responses (12, 13). The contaminating dsRNA in the mRNA-LNP vaccines (7), in combination with the highly inflammatory nature of the LNPs (12, 14), might essentially obviate the need for nucleoside modification from the immune sensing perspective. Thus, the critical component that transformed the mRNA into an immune response-inducing vaccine is the inflammatory LNP – initially thought to be an inert carrier/delivery vehicle for mRNA (10) – and not the nucleoside modifications.
Different levels of contaminants between vaccine lots, besides storage, transportation, and clinical handling, might explain a recent finding from Denmark that different lots of the mRNA Pfizer vaccines induced distinct levels of adverse events. Some lots caused almost no side effects, while others were associated with a medium or very high incidence of adverse events (all suspected side effects, -serious and -related deaths) (15). It would be important to determine how the same vaccine lots behaved across different countries and demographics to define whether these findings can be generalized. While contaminants likely contribute to the inflammatory nature of this platform and induction of adverse events, the LNPs’ ionizable lipid component of the mRNA-LNP vaccine is highly inflammatory (12), and as we already discussed above, it is key for the reactogenicity and immunogenicity of this platform (12, 13). Thus, hypothetically, another potential explanation for the distinct lots triggering different levels of adverse events could be that the amounts of mRNA-LNP or the mRNA : LNP ratio differed between lots (16). To assess different possibilities, it would be essential to determine the level of adaptive immune responses triggered by the different vaccine lots and if any properly stored vaccine leftovers are still available to test them for impurities and the levels of therapeutic agents. In summary, these findings highlight the need for a strict assessment of purity criteria and allowable limits for this novel vaccine class.
Concerning assumptions made regarding the mRNA-LNP platform
Different experts and officials have made several assumption-based public statements regarding the mRNA-LNP vaccines. One of the most publicized ones was that the vaccine mRNA cannot be reverse transcribed into DNA; thus, there is no risk of insertion into the human genome (17–20). New DNA insertion into the human genome would be a serious concern if it happens on the level of stem cells of the reproductive system. In support of their statement, a modified version of Francis Crick’s central dogma of molecular biology (21) has often been cited that the information flow in eukaryotic cells is unidirectional, from DNA to RNA to protein. While the information flow in eukaryotic cells, in general, indeed is DNA to RNA to protein, in particular instances, RNA can be reverse transcribed into DNA. This process is mediated by reverse transcriptase, enzymes that are naturally associated with retroviruses. However, eukaryotic cells, including human cells, use reverse transcription-like processes to replicate telomeres and retrotransposons (22–25). With the Pfizer mRNA-LNP vaccine, it has been shown experimentally that the vaccine mRNA can be reverse-transcribed into DNA in an immortalized human hepatocyte cell line. Exposure to the mRNA-LNP vaccine also correlated with an increase in overall LINE-1 retrotransposon expression levels and localization to the nucleus (26). It has been proposed that the sequence features of the vaccines’ mRNA meet all known requirements for retroposition using LINE-1 (25). Whether these have any in vivo relevance remains to be determined (27). Spike protein localization to the nucleus was previously reported (28, 29). In support of studies revealing nuclear localization of the spike protein, a recent preprint study reported that the spike protein of the SARS-CoV-2, unlike other SARS viruses, contains a nuclear localization signal (NLS). The NLS allowed the transport of the spike protein to the nucleus, and it seems that the spike protein also shuttled the spike mRNA to the nucleus (30). A study also showed that SARS-CoV-2 RNA can be reverse-transcribed and integrated into the genome of cultured human cells, a process potentially mediated by LINE-1 elements, and can be expressed in patient-derived tissues. The authors propose that these findings could also possibly explain why some patients test PCR positive for SARS-CoV-2 even after clearance of the virus (31). These results, however, have been criticized as not reproducible (32, 33), infrequent and artefactual (34). While, to our knowledge, similar studies have not been performed with COVID-19 mRNA vaccines that code for full-length pre-fusion fixed form of SARS-CoV-2 spike protein, comparable transport of spike protein/mRNA to the nucleus could be expected. Because the mRNA can enter the nucleus, where it might be reverse-transcribed into DNA, this increases its potential to integrate into the genome. Furthermore, the mRNA-LNP diffuses throughout the body and can accumulate in both the testes and ovaries (5, 6) and is reported to alter the menstrual cycle in women (35, 36). Therefore, it could potentially be reaching the stem cells of the reproductive organs. These findings highlight the need to take these data and concerns seriously and conduct specific experiments to address them (25).
Another often touted feature of the vaccine mRNA is that it is degraded in vivo in hours or a few days, thus further limiting its potential to disrupt normal cell biology (17–20). This assumption likely arose since unmodified mRNAs have, in general, short in vivo half-life (37). However, human lymph node biopsies taken at different time points post-exposure to the mRNA-LNP revealed detectable levels of vaccine mRNA and spike proteins up to eight weeks (38). Circulating vaccine mRNA and spike protein have been detected in the plasma from a few weeks to several months post-vaccination (39–42). A recent post-mortem study also found vaccine mRNA in the lymph nodes of most subjects 30 days post-exposure and less frequently in their heart tissue but not in the liver and spleen (43). Thus, in light of these, we should admit to our limited understanding when it comes to how different modifications to the mRNA (5’ and 3’ modifications, the use of unique nucleotides, etc.) affect its in vivo half-life in the human body because no specific studies have been conducted to address this.
Modified ribonucleotides are commonly incorporated into the mRNAs of the COVID-19 vaccines to decrease their innate reactogenicity (8). However, unfortunately, their effects on mRNA translation fidelity were not defined until recently. Incorporation of N1-methylpseudouridine into mRNA resulted in +1 ribosomal frameshifting in vitro and cellular immunity in mice and humans to +1 frameshifted products from BNT162b2 vaccine mRNA translation occurred after vaccination (44). The presence of frameshifted products and associated adaptive immune responses were specific to this platform since they were not detectable with the adenovirus-based ChAdOx1 nCoV-19 vaccine (44). Whether the frameshifted products overlap with endogenous protein sequences and can contribute to developing autoimmune responses remains to be addressed.
Overall, this section highlights the danger when we assume and extrapolate in science and apply existing paradigms to new, untested platforms. Since the above issues concerning deploying this novel vaccine are particular to this platform, they must be better addressed for its future use in humans.
How safe and effective are the mRNA-LNP vaccines?
The mRNA-LNP COVID-19 vaccines, based on early analysis of the clinical trial data, were deemed safe and effective across demographics (45, 46). However, recent peer-reviewed research studies, a wide variety of continuously increasing case reports, and publicly available adverse events databases cast doubts on the safety and effectiveness of these products.
Safety
Careful analysis and re-analysis of the Pfizer and Moderna clinical trial data led by Dr. Doshi, an expert in clinical trials, revealed excess risk of severe adverse events (SAEs) of special interest with both the Pfizer and Moderna COVID-19 vaccines. Combined, there was a 16% higher risk of SAEs in mRNA vaccine recipients (47, 48). This concurs with a recent admission from the German Health Minister to ~1 in 10,000 severe/permanent damage events (49). The Countermeasures Injury Compensation Program(CICP) data show that out of a total of 13,406 CICP claims ever filed, 12,854 were COVID-19 countermeasure claims, out of which 9,682 allege injuries/deaths from COVID-19 vaccines (50). Peer-reviewed case reports, including but not limited to severe inflammatory/autoimmune events of bone marrow (51–55), liver (56–58), skin (59–64), cardiovascular- (65–77), musculoskeletal- (76, 78, 79), endocrine- (80–84), and nervous system (66, 84–87), etc. that sometimes had been fatal, have been steadily increasing. However, the incidence of SAEs is hard to judge based on the number of these reports alone (88). To supplement the case report data, we performed a limited analysis of the publicly available data from the Vaccine Adverse Event Reporting System (VAERS), co-managed by the Centers for Disease Control and Prevention (CDC) and FDA (89). According to VAERS, all death reports and selected serious events of interest are investigated (89). The analyses mirror the diverse adverse effects of these therapeutics reported in the literature and might provide some information on incidence (Figure 1). Compared to all other non-COVID-19 vaccines combined, the incidence of adverse events is far higher for the mRNA-LNP-based COVID-19 vaccines per million doses administered (Figure 1A). Deaths and SAEs also often occurred soon after injection (Figure 1B), making it more likely to be the consequence of the vaccine and not just a random event. Nevertheless, it is essential to emphasize that one cannot establish causation simply by looking at VAERS reports. The causative relationship between the mRNA-LNP vaccine and SAEs has only been officially recognized by the regulatory agencies for peri- and myocarditis affecting primarily young males (90). While most symptomatic patients might be young males, recent, in vivo physiological tests, such as heart glucose uptake, showed a 40% increase in asymptomatic vaccinated patients irrespective of gender and demographics (91), potentially suggesting a much broader impact. These findings are also supported by a postmortem study, in some of which an autopsy revealed heart inflammation and the presence of vaccine RNA (43). Whether the increase in heart attacks and death in young people (92, 93) might be linked to these vaccines remains to be determined.
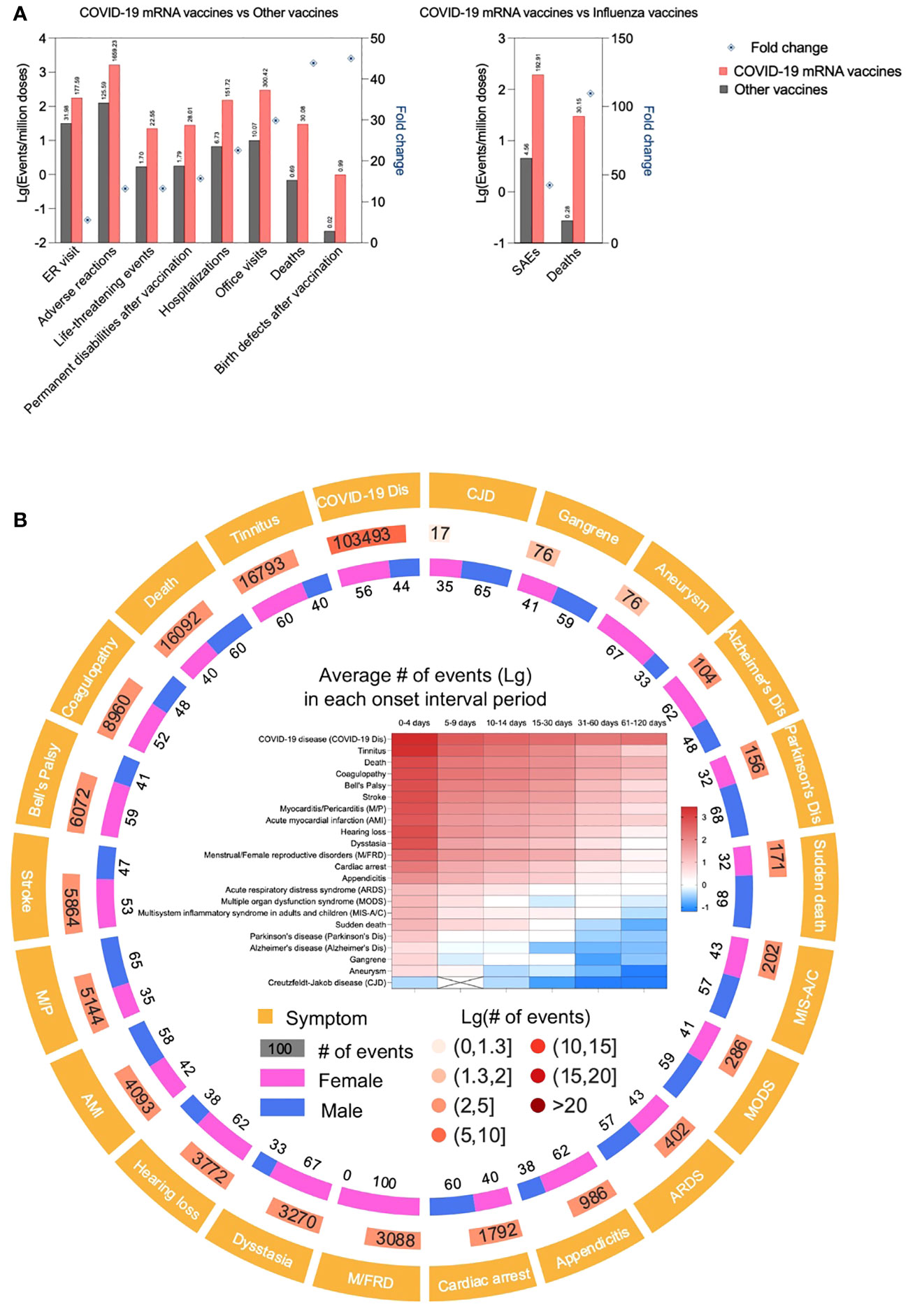
Figure 1 COVID-19 mRNA vaccines are associated with higher incidence of adverse events compared to other vaccines. (A). Data derived from CDC VAERS and National Vaccine Injury Compensation Program (NVICP) were depicted as Lg (events/million doses) in the bar chart. The data for COVID-19 mRNA vaccine and other vaccines were from December 2020 to September 2023 and January 2006 to December 2021, respectively. The fold change was calculated as the Lg (events/million doses) ratio of COVID-19 mRNA vaccine to other vaccines or influenza vaccines.(B). Adverse events of concern associated with COVID-19 mRNA vaccines. Data derived from CDC VAERS were analyzed, adverse events of concern (including AESI, adverse events of special interest, defined by CDC) were displayed as symptoms and their # of events, gender proportion and onset interval of each symptom.
Efficacy
The effectiveness of these therapeutics in preventing infections and limiting the spreading of the virus has been highly eroded from the early reports (94), and nowadays, their efficacy is mainly limited to potentially decreasing the disease severity and death in susceptible people (95). Excess inflammation caused by an overreacting immune system (cytokine storm) is one of the major pathological features in patients with severe COVID-19 (96). Thus, hypothetically, if exposure to the mRNA-LNP vaccine leads to a dampened systemic inflammatory response, that may explain why vaccination also reduced disease severity in the case of the delta and omicron variants, in which case the antibodies induced by the original vaccines were not (97), or minimally neutralizing (98). In support of this hypothesis, Dr. Netea’s group reported dampened transcriptional reactivity of the immune cells and decreased type I interferon responses in vaccinated individuals to secondary viral stimulation (97), while our group described inhibition of adaptive immune responses and alteration in innate immune fitness in mice with this platform (99). The immune-tolerant environment induced by these vaccines is further supported by recent studies that have discovered a correlation between an increased number of prior mRNA vaccine doses and a higher risk of catching COVID-19 (100–102). Thus, these data suggest that these vaccines’ efficacy in decreasing disease severity and death might lie with their previously undiscovered immune suppressive characteristics. These findings further highlight the need for rigorous pre-clinical studies to limit potential unexpected consequences for novel platforms.
mRNA-LNP-associated adverse events – both inflammatory and inhibitory
The SAEs reported with the mRNA-LNP platform are very diverse. The SAEs are likely caused by the combination of the mRNA-LNP vaccine components (103) and potentially by direct toxicity and biological action of the spike protein itself (104–108). Here, we will focus on SAEs likely mediated by the immune system. The SAEs can be divided into inflammatory and anergic/inhibitory categories from an immunological perspective. Inflammatory side effects include acute reactogenicities (fever, headache, fatigue, myalgia and arthralgia, chills, etc.) (45, 46), inflammatory/autoimmune, anti-PEG mediated CARPA (109), and other related events that involve activation of the innate and adaptive immune systems. Anergic side effects, i.e., immune suppression, are presented as virus reactivation where viruses like varicella-zoster virus (VZV) (110, 111) and hepatitis C virus (112) reoccur following COVID-19 mRNA vaccine injection. Furthermore, have also been reported to likely increase the risks in sensitivity to infections (100, 101, 113) and potentially disturbance of cancer immunosurveillance (114–117). How can a platform both activate and suppress immune responses?
Inflammatory responses
As discussed above, the inflammatory nature of this therapeutic is linked to the ionizable lipid component of the LNPs (12), which, in effect, might further be accentuated by potential pro-inflammatory contaminants, such as dsRNA (7). The acute reactogenicity responses observed with these therapeutics, such as fever, headache, fatigue, myalgia, arthralgia, chills, etc., are likely triggered by the release of a variety of high amounts of innate inflammatory cytokines, such as IL-1β, IL-6, GM-CSF and type I interferon (7, 12) upon exposure. This inflammatory environment induced by this therapeutic, and that the mRNA-LNP has additive effects with other inflammatory agents, such as LPS (118), could potentially support flareups with pre-existing autoimmune conditions and/or create conditions for novel autoimmune responses to develop in susceptible people. Since the mRNA-LNP diffuses throughout the body (6, 10, 41, 42), and LNPs can deliver mRNA into any cell type and enable its translation, it is reasonable to consider an off-target translation of protein-of-interest in non-APCs, which then could be under attack by the antigen-specific adaptive immune responses (103). Therefore, the destruction of spike protein- or frameshifted-protein-expressing cells by the immune system throughout the body might be responsible for some of the SAEs reported with this platform, such as COVID arm, peri/myocarditis, and inflammatory responses affecting the brain, liver, bone marrow, etc.
Tolerogenic responses
The observation that protection against omicron infection waned gradually by month after the booster and seventh month and thereafter, the incidence of infection was higher among people who had the booster compared to those with only the primary series (119), and COVID-19 incidence increases with the number of shots (100–102) and immunoreactivity decreases (97), and viruses can reactivate (110, 112), and cancer patients relapse or new cancer develop (114–117, 120), seems to indicate that exposure to the mRNA-LNP platform puts people or certain people into a semi-immunodeficient/immunosuppressed state. In line with this, mouse studies have shown that even one-time exposure to mRNA-LNP or LNP can inhibit adaptive immune responses and alter innate immune fitness in an inheritable fashion (99). While one-time exposure decreased susceptibility to subsequent influenza infection, the mice became significantly less resistant to systemic yeast infection. Whether the increase in sepsis- (121), nosocomial- (113, 122), and certain fungal infections (123–126) incidence are related to the vast exposure to the mRNA-LNP platform or COVID-19 and or other pandemic-related measures remains to be addressed.
The ionizable lipids of the LNPs are synthetic and are estimated to have a 20-30 day in vivo half-life (5). Thus, exposure to these vaccines may lead to an early high level of inflammation followed by a long-lasting low level of chronic inflammation. Chronic inflammation can lead to non-responsiveness and anergy of the immune system (127), thus potentially contributing to some of the viral reactivation and increased susceptibility to infections reported in association with this platform. Furthermore, as presented above, evidence suggests that contrary to expectations, spike mRNA can also remain intact for months following mRNA vaccination, allowing long-term spike protein expression. This correlates with the presence of germinal centers that last for months. Continuous stimulation with antigens can lead to aberrant T and B cell responses. Continuous antigen exposure might promote the isotype switch to IgG4 recently observed in roughly half of the individuals receiving three doses of an mRNA vaccine but not by adenovirus vaccines (128, 129). Prior exposure to natural SARS-CoV-2 infection prevented the mRNA vaccines from inducing IgG4 switching (130), indicating that the initial priming of the immune system imprints and determines the subsequent humoral immunity. IgG4 is generally considered anti-inflammatory and known to poorly facilitate opsonization, complement fixation, and antibody-dependent cellular toxicity. Still, it can also be pathogenic in the case of the autoimmune disease pemphigus vulgaris and some forms of myasthenia gravis (131). IgG4-related disease also appears to have a higher risk of overall cancer (132). The induction of IgG4-producing B cells relies on help from a subset of T follicular helper (Tfh) cells characterized by the production of high amounts of IL-10 (133). These IL-10-producing Tfh cells are generated in the presence of continuous antigen stimulation and were first identified in chronic viral infection (133), and were later shown to be present in IgG4-related human diseases (134). Whether the mRNA-LNP platform induces this unique type of Tfh cells and the in vivo relevance of the observed switching to IgG4 isotype with the mRNA-LNP platform remain to be determined (135).
Our laboratory has recently proposed another potential complementary mechanism to immune suppression (99). Activation and differentiation of T cells into effector cells are thought to rely on three signals received from antigen-presenting cells (APCs), such as dendritic cells (DCs). The first signal is in the form of peptide-MHC, the second signal is membrane-bound co-stimulation, and the third signal is in the form of soluble cytokines. If the T cells receive the third signal before the first one, it is called out-of-sequence stimulation, which can lead to the T cells’ death or render them anergic and non-responsive (136, 137). Exposure to mRNA-LNP or LNP leads to a rapid release of inflammatory cytokines (12), including type I interferons (7) that are known to induce out-of-sequence stimulation, which likely exposes the adaptive immune cells, including the T cells, to an inflammatory environment before the mRNA is translated into protein and presented on DCs. Thus, with a high likelihood, one of the potential flaws of the mRNA-LNP platform, unlike the old-school vaccines where the antigen presentation and inflammation coincide, is that it exposes the adaptive immune cells to an out-of-sequence stimulation, which might worsen upon further exposure. To bring experimental evidence that out-of-sequence stimulation might exist with the mRNA-LNP platform, we transferred naïve congenically marked Eα-specific CD4 T cells into WT B6 mice, and then we exposed the mice to mRNA-LNP or LNP or PBS. Two weeks later, some of the mice were left untreated while others were immunized with Eα antigen to trigger the proliferation of the transferred T cells. Four days later, the analysis of the skin-draining lymph nodes revealed a roughly tenfold decrease in the adoptively transferred T cell numbers in animals exposed to mRNA-LNP or LNP, irrespective of their immunization status (99). Thus, these data suggest that the inflammatory environment created by the LNPs is detrimental to naïve T cells. In another set of experiments, we compared the effect of mRNA-LNP and LNP to IFNα, or factors that induce inflammation or influenza infection, on the survival of adoptively transferred naïve CD4 T cells. Similar to mRNA-LNP and LNP, we found a decrease in T cell precursor numbers if we treated the mice with type I interferon (IFNα and poly (I:C) (triggers type I interferon secretion) (Figure 2). The high dose of LPS led to an intermediate phenotype, while sub-lethal influenza exposure had no significant effect on the adoptively transferred CD4 T cells (Figure 2). The effect was not limited to TEα cells since OT-II CD4 T cells responded similarly (data not shown). Whether endogenous T cells are similarly affected remains to be determined. Notably, the observed changes were likely systemic since the direct draining organs, the skin-draining lymph nodes, and the distant spleen showed similar trends. These data align with our previous findings that exposure to mRNA-LNP or LNP leads to systemic inhibition of adaptive immune responses (99). All the treatments used, except exposure to influenza, led to a significant decrease in the transferred naïve T cell numbers. The absence of an effect with influenza was not due to a lack of infection since mice exposed to the virus lost significant weight (data not shown). The LPS exposure, while at a lower degree, also reduced the naïve T cell numbers. Whether the effect in this case was mediated by other cytokines than type I interferon or by indirect induction of type I interferon, or the effect might be limited to the high dose of LPS that we used, remains to be determined. Overall, these data support the potential existence of a type I interferon-mediated out-of-sequence stimulation with the mRNA-LNP platform. However, further studies will be needed to identify the inhibition’s mechanistic details and determine whether these data are translatable to humans.
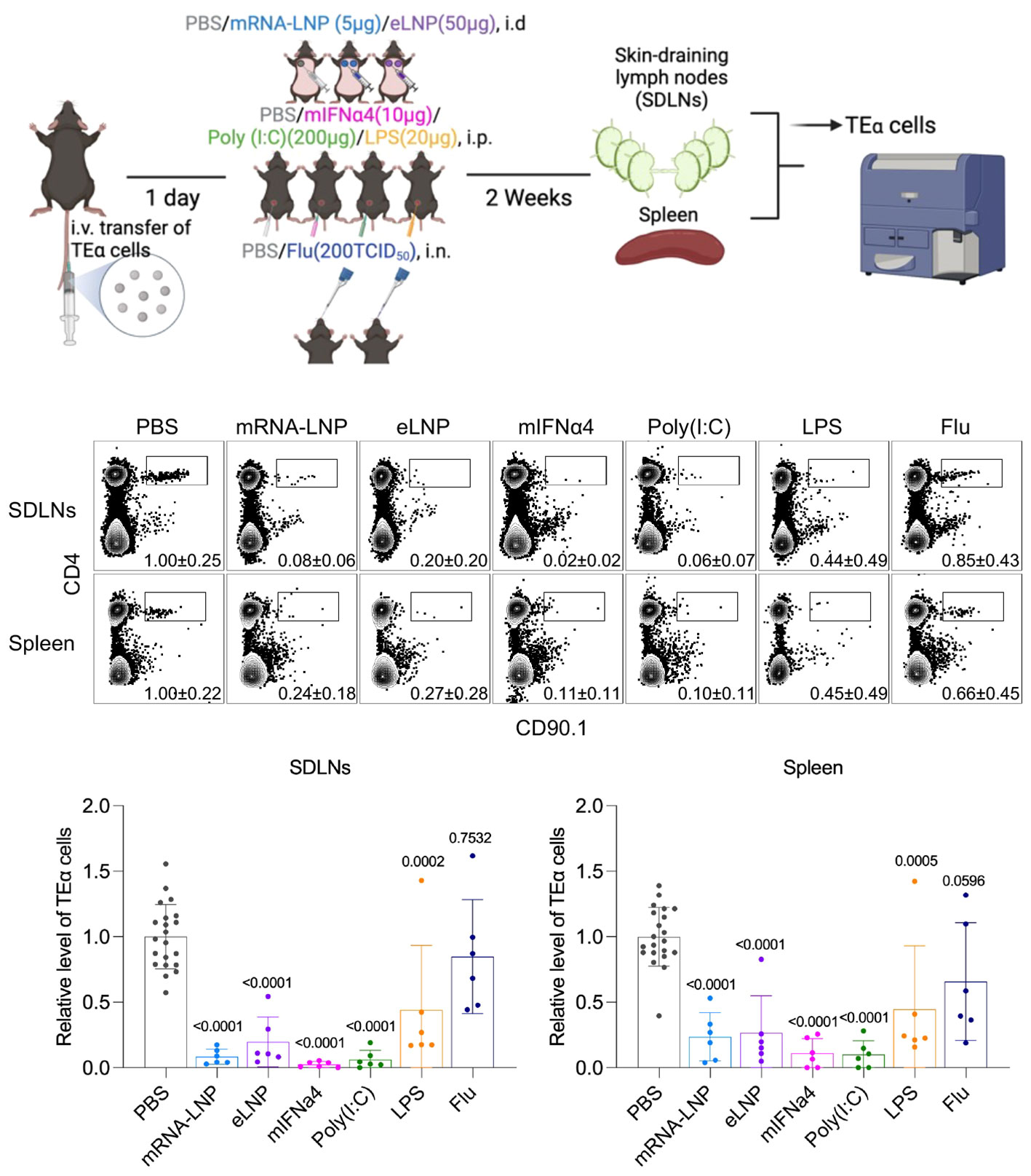
Figure 2 Exposure to mRNA-LNP and other type I IFN-producing reagents potentially induce out-of-sequence stimulation. Experimental design. Mice were adoptively transferred with TEα cells through tail vein one day before exposure to different types of inflammatory reagents at indicated routes. Two weeks later, draining and non-draining lymph nodes and spleens were harvested for detection of TEα cells by flow cytometry. Representative flow plots showing TEα cells (CD4+CD90.1+, gated from live CD3+ population) from different organs under each treatment condition, and corresponding summarized bar chart (bottom). Data were pooled from at least two independent experiments. Each dot stands for one mouse. The relative level of TEα cells was normalized to PBS group. Comparisons between PBS group and treatment groups were made by One-way ANOVA test.
Quo Vadis mRNA-LNP?
Several severe side effects have been reported with the mRNA-LNP platform, which, to preserve human health, should be carefully investigated and addressed before further use. We think that making the ionizable lipid biodegradable, thus decreasing its in vivo half-life, could potentially solve or limit the presence of chronic inflammation, which is detrimental to adaptive immune responses. Along the same line, the half-life of the mRNA should also be carefully adjusted to prevent chronic antigen stimulation of T and B cells. Whether the ionizable lipid component of the LNPs, similar to LPS (138, 139), can promote innate tolerance upon multiple exposures must be defined. When designing vaccines, especially booster shots targeting potential new variants, basic immunology on how antigen dose, pre-existing antibodies, and original antigenic sin (140) might affect the outcome should be considered. The out-of-sequence stimulation with the mRNA-LNP platform could be decreased using an “intelligent” design that would trigger inflammation only after the antigen has been translated from mRNA and it is ready to be presented on the surface of APCs. The present formulation of LNP makes them accumulate in specific organs more than in others, a characteristic that will persist regardless of the RNA cargo. Thus, to decrease the chance of inducing autoimmune responses and killing self-cells, the mRNA-LNP should be targeted to DCs to limit the expression of antigens to only APCs (103). The generation of frameshifted products due to the use of specific non-standard nucleotides should be remedied as proposed (44). To put people’s concerns to rest regarding the safety of the mRNA, studies should be conducted in NHPs and humans on the mRNAs’ in vivo half-life and whether there is any in vivo transcription into DNA and potential genomic insertion. Finally, since real-life data revealed potential severe gaps in quality control, stricter oversight of pharmaceutical companies from independent health officials is desirable and should be implemented without delay.
Brain bites
Several fundamental questions persist surrounding the pandemic measures and the adoption of this new vaccine platform. Rather than advocating for retraction and censorship (141), fostering open dialogue, considering all perspectives, and employing logic and reasoning for better future preparedness is crucial. While some, despite the concerns presented above or discussed elsewhere (142, 143), would likely argue that the measures were justifiable, citing lives saved, the robustness of supporting data raises important inquiries. Estimates relying on mathematical modeling (144) necessitate scrutiny, particularly concerning the quality of input data. For instance, reliance on official death toll statistics and not accounting for demographic variations in the virus infection fatality rate (IFR; the likelihood of death if you become infected) prompt questions about the accuracy of reported figures. Why did official statistics include both individuals who died from and with COVID-19? Why does the CDC estimate the flu-associated hospitalization and death for the adult population, arguing that it cannot be accurately determined (145) but can give us exact numbers for COVID-19? Does the IFR data (146, 147) support the official death toll numbers?
The unprecedented mass vaccination with a vaccine that minimally protects from getting infected and spreading the virus (94) during a pandemic prompts critical reflections: Was it a sound strategy? Was herd immunity a realistic expectation? Did this strategy inadvertently accelerate virus mutations, and could a more targeted focus on vulnerable populations have yielded better results? (148–156) The decision to opt for the mRNA-LNP platform over traditional methods requires scrutiny, considering both its advantages (speed of production, ease of updating) and limitations (patents, production constraints, affordability, unknown short- and long-term side effects). Why focus on a single virus protein with a high mutation rate? Was the vaccines’ ease of updating feature critical to fighting against variants? Why was basic immunology knowledge on how antigen dose, repeated boosters, pre-existing antibodies, antigenic sin, etc., affect the immune system mostly ignored during the pandemic?
Amidst a shared pool of scientific knowledge, divergent decisions by countries like Sweden, which chose not to recommend vaccinations for specific age groups (157), kept schools open and reported that no child with COVID-19 died (158, 159), underscore the need for a deeper understanding of varied perspectives. Why censor the “Great Barrington Declaration,” (160) which advocated a model similar to Sweden’s, emphasizing the protection of the vulnerable? (161) This ongoing list of inquiries highlights the nuanced complexity of these issues, urging a thorough examination.
Going forward
Numerous discussions have centered around the impact of influential scientists who advocate for drastic measures to address vaccine hesitancy and public trust erosion, potentially compromising academic freedom through censorship and intimidation. However, during the pandemic, actions like silencing dissenting voices, coupled with policy decisions often reliant on assumptions rather than robust experimental data, may have inadvertently undermined both science and public confidence. To rebuild trust, it is crucial to return to the fundamental principles of scientific inquiry. Scientists should embrace their training and be committed to questioning every assertion, regardless of the source. This approach guards against groupthink and herd mentality. Rigorous analysis of all available data using critical thinking and reasoned judgment, unaffected by conflict of interests, is essential to formulate a well-rounded perspective. Communicating transparently and honestly with society is equally vital. Acknowledging the inherent uncertainties in biology, our representatives should convey both what is known and what remains uncertain. Science is inherently dynamic, perpetually evolving as new knowledge emerges. It is imperative to emphasize that nothing in biology is absolute, and pursuing knowledge demands ongoing questioning and exploration. By adhering to these principles, we can foster a renewed trust in the scientific process and its capacity for growth and refinement.
Data availability statement
The original contributions presented in the study are included in the article/supplementary material. Further inquiries can be directed to the corresponding author.
Ethics statement
The animal study was approved by Institutional Care and Use Committee at Thomas Jefferson University. The study was conducted in accordance with the local legislation and institutional requirements.
Author contributions
BI: Conceptualization, Investigation, Supervision, Writing – original draft, Writing – review & editing. ZQ: Data curation, Formal Analysis, Investigation, Methodology, Visualization, Writing – review & editing.
Funding
The author(s) declare financial support was received for the research, authorship, and/or publication of this article. BI is supported by the National Institute of Allergy and Infectious Diseases (https://www.niaid.nih.gov) R01AI146420 and R01AI146101, and institutional start-up funds. The Flow Cytometry and Laboratory Animal Facilities at Sidney Kimmel Cancer Center at Thomas Jefferson University are supported by the National Cancer Institute (https://www.cancer.gov) P30CA056036.
Acknowledgments
We thank the Flow Cytometry and Laboratory Animal Facilities at Sidney Kimmel Cancer Center for their expert assistance in performing the presented experiments. We thank Dr. Adam G. Schrum at the University of Missouri, Dr. Tim Manser at Thomas Jefferson University, and other colleagues who chose to remain anonymous, for critically reading the manuscript.
Conflict of interest
The authors declare that the research was conducted in the absence of any commercial or financial relationships that could be construed as a potential conflict of interest.
The author(s) declared that they were an editorial board member of Frontiers, at the time of submission. This had no impact on the peer review process and the final decision
Publisher’s note
All claims expressed in this article are solely those of the authors and do not necessarily represent those of their affiliated organizations, or those of the publisher, the editors and the reviewers. Any product that may be evaluated in this article, or claim that may be made by its manufacturer, is not guaranteed or endorsed by the publisher.
References
1. Buschmann MD, Carrasco MJ, Alishetty S, Paige M, Alameh MG, Weissman D. Nanomaterial delivery systems for mrna vaccines. Vaccines (Basel) (2021) 9:1–30. doi: 10.3390/vaccines9010065
2. Pardi N, Hogan MJ, Porter FW, Weissman D. mRNA vaccines - a new era in vaccinology. Nat Rev Drug Discov (2018) 17:261–79. doi: 10.1038/nrd.2017.243
3. McKernan K, Helbert Y, Kane LT, McLaughlin S. Sequencing of bivalent Moderna and Pfizer mRNA vaccines reveals nanogram to microgram quantities of expression vector dsDNA per dose. OSFpreprint (2023). doi: 10.31219/osf.io/b9t7m
4. Karikó K, Muramatsu H, Ludwig J, Weissman D. Generating the optimal mRNA for therapy: HPLC purification eliminates immune activation and improves translation of nucleoside-modified, protein-encoding mRNA. Nucleic Acids Res (2011) 39:e142–2. doi: 10.1093/nar/gkr695
5. Comirnaty. Assessment report COVID-19 vaccine comirnaty. EMA/707383/2020 Corr1 (2021) 31:1–140. Available at: https://www.ema.europa.eu/en/documents/assessment-report/comirnaty-epar-public-assessment-report_en.pdf
6. Moderna. Assessment report COVID-19 Vaccine Moderna Common. EMA/15689/2021 Corr1*1 (2021) 31:1–169. Available at: https://www.ema.europa.eu/en/documents/assessment-report/spikevax-previously-covid-19-vaccine-moderna-epar-public-assessment-report_en.pdf.
7. Li C, Lee A, Grigoryan L, Arunachalam PS, Scott MKD, Trisal M, et al. Mechanisms of innate and adaptive immunity to the Pfizer-BioNTech BNT162b2 vaccine. Nat Immunol (2022) 23:543–55. doi: 10.1038/s41590-022-01163-9
8. Karikó K, Buckstein M, Ni H, Weissman D. Suppression of RNA recognition by toll-like receptors: the impact of nucleoside modification and the evolutionary origin of RNA. Immunity (2005) 23:165–75. doi: 10.1016/j.immuni.2005.06.008
9. Karikó K, Muramatsu H, Welsh FA, Ludwig J, Kato H, Akira S, et al. Incorporation of pseudouridine into mRNA yields superior nonimmunogenic vector with increased translational capacity and biological stability. Mol Ther (2008) 16:1833–1840. doi: 10.1038/mt.2008.200
10. Pardi N, Tuyishime S, Muramatsu H, Kariko K, Mui BL, Tam YK, et al. Expression kinetics of nucleoside-modified mRNA delivered in lipid nanoparticles to mice by various routes. J Control. Release (2015) 217:345–51. doi: 10.1016/j.jconrel.2015.08.007
11. Dolgin E, Ledford H. mRNA COVID vaccines saved lives and won a Nobel — what’s next for the technology? Nature (2023). doi: 10.1038/D41586-023-03119-X
12. Ndeupen S, Qin Z, Jacobsen S, Bouteau A, Estanbouli H, Igyártó BZ. The mRNA-LNP platform’s lipid nanoparticle component used in preclinical vaccine studies is highly inflammatory. iScience (2021) 24:103479. doi: 10.1016/j.isci.2021.103479
13. Alameh M-G, Tombácz I, Bettini E, Lederer K, Sittplangkoon C, Wilmore JR, et al. Lipid nanoparticles enhance the efficacy of mRNA and protein subunit vaccines by inducing robust T follicular helper cell and humoral responses. Immunity (2021) 54:2877–2892.e7. doi: 10.1016/j.immuni.2021.11.001
14. Tahtinen S, Tong A-J, Himmels P, Oh J, Paler-Martinez A, Kim L, et al. IL-1 and IL-1ra are key regulators of the inflammatory response to RNA vaccines. Nat Immunol (2022) 23:532–42. doi: 10.1038/s41590-022-01160-y
15. Schmeling M, Manniche V, Hansen PR. Batch-dependent safety of the BNT162b2 mRNA COVID-19 vaccine. Eur J Clin Invest (2023) 53:e13998. doi: 10.1111/eci.13998
16. Tinari S. The EMA covid-19 data leak, and what it tells us about mRNA instability. BMJ (2021) 372:n627. doi: 10.1136/bmj.n627
17. CDC. Understanding mRNA COVID-19 Vaccines. CDC. (2022). Available at: https://web.archive.org/web/20220722133644/https://www.cdc.gov/coronavirus/2019-ncov/vaccines/different-vaccines/mrna.html. (Accessed January 30, 2024)
18. Park JW, Lagniton PNP, Liu Y, Xu R-H. mRNA vaccines for COVID-19: what, why and how. Int J Biol Sci (2021) 2021:1446–60. doi: 10.7150/ijbs.59233
19. Nebraska Medicine. How long do mRNA and Spike proteins Last in the Body? Nebraska Medicine Omaha, NE: Nebraska Medicine. Available at: https://www.nebraskamed.com/COVID/where-mrna-vaccines-and-spike-proteins-go.
20. NIH. Understanding COVID-19 mRNA Vaccines . Available at: https://www.genome.gov/about-genomics/fact-sheets/Understanding-COVID-19-mRNA-Vaccines.
22. Chandramouly G, Zhao J, McDevitt S, Rusanov T, Hoang T, Borisonnik N, et al. Polθ reverse transcribes RNA and promotes RNA-templated DNA repair. Sci Adv (2021) 7:eabf1771. doi: 10.1126/sciadv.abf1771
23. Sciamanna I, De Luca C, Spadafora C. The reverse transcriptase encoded by LINE-1 retrotransposons in the genesis, progression, and therapy of cancer. Front Chem (2016) 4:6. doi: 10.3389/fchem.2016.00006
24. Nouvel P. The mammalian genome shaping activity of reverse transcriptase. Genetica (1994) 93:191–201. doi: 10.1007/BF01435251
25. Domazet-Lošo T. mRNA vaccines: why is the biology of retroposition ignored? Genes (Basel) (2022) 13:719. doi: 10.3390/genes13050719
26. Aldén M, Olofsson Falla F, Yang D, Barghouth M, Luan C, Rasmussen M, et al. Intracellular reverse transcription of pfizer bioNTech COVID-19 mRNA vaccine BNT162b2 in vitro human liver cell line. Curr Issues Mol Biol (2022) 44:1115–26. doi: 10.3390/cimb44030073
27. Merchant HA, Comment on Aldén, et al. Intracellular reverse transcription of pfizer bioNTech COVID-19 mRNA vaccine BNT162b2 in vitro in human liver cell line. Curr Issues Mol Biol (2022) 44:1115–26. doi: 10.3390/cimb44040113
28. Zhang J, Cruz-Cosme R, Zhuang M-W, Liu D, Liu Y, Teng S, et al. A systemic and molecular study of subcellular localization of SARS-CoV-2 proteins. Signal Transduction Targeting Ther (2020) 5:269. doi: 10.1038/s41392-020-00372-8
29. Kim ES, Jeon M-T, Kim K-S, Lee S, Kim S, Kim D-G. Spike proteins of sars-cov-2 induce pathological changes in molecular delivery and metabolic function in the brain endothelial cells. Viruses (2021) 13:2021. doi: 10.3390/v13102021
30. Sattar S, Kabat J, Jerome K, Feldmann F, Bailey K, Mehedi M. Nuclear translocation of spike mRNA and protein is a novel pathogenic feature of SARS-CoV-2. Front Microbiol (2023) 14:1073789. doi: 10.3389/fmicb.2023.1073789
31. Zhang L, Richards A, Barrasa MI, Hughes SH, Young RA, Jaenisch R. Reverse-transcribed SARS-CoV-2 RNA can integrate into the genome of cultured human cells and can be expressed in patient-derived tissues. Proc Natl Acad Sci USA (2021) 118:e2105968118. doi: 10.1073/pnas.2105968118
32. Parry R, Gifford RJ, Lytras S, Ray SC, Coin LJM. No evidence of SARS-CoV-2 reverse transcription and integration as the origin of chimeric transcripts in patient tissues. Proc Natl Acad Sci (2021) 118:e2109066118. doi: 10.1073/pnas.2109066118
33. Smits N, Rasmussen J, Bodea GO, Amarilla AA, Gerdes P, Sanchez-Luque FJ, et al. No evidence of human genome integration of SARS-CoV-2 found by long-read DNA sequencing. Cell Rep (2021) 36:109530. doi: 10.1016/j.celrep.2021.109530
34. Yan B, Chakravorty S, Mirabelli C, Wang L, Trujillo-Ochoa JL, Chauss D, et al. Host-virus chimeric events in SARS-coV-2-infected cells are infrequent and artifactual. J Virol (2021) 95:294–315. doi: 10.1128/JVI.00294-21
35. Lessans N, Rottenstreich A, Stern S, Gilan A, Saar TD, Porat S, et al. The effect of BNT162b2 SARS-CoV-2 mRNA vaccine on menstrual cycle symptoms in healthy women. Int J Gynaecol. Obstet. (2023) 160:313–8. doi: 10.1002/ijgo.14356
36. Edelman A, Boniface ER, Male V, Cameron ST, Benhar E, Han L, et al. Association between menstrual cycle length and covid-19 vaccination: global, retrospective cohort study of prospectively collected data. BMJ Med (2022) 1:e000297. doi: 10.1136/bmjmed-2022-000297
37. Yang E, van Nimwegen E, Zavolan M, Rajewsky N, Schroeder M, Magnasco M, et al. Decay rates of human mRNAs: correlation with functional characteristics and sequence attributes. Genome Res (2003) 13:1863–72. doi: 10.1101/gr.1272403
38. Röltgen K, Nielsen SCA, Silva O, Younes SF, Zaslavsky M, Costales C, et al. Immune imprinting, breadth of variant recognition, and germinal center response in human SARS-CoV-2 infection and vaccination. Cell (2022) 185:1025–1040.e14. doi: 10.1016/j.cell.2022.01.018
39. Fertig TE, Chitoiu L, Marta DS, Ionescu V-S, Cismasiu VB, Radu E, et al. Vaccine mRNA Can Be Detected in Blood at 15 Days Post-Vaccination. Biomedicines (2022) 10:1–11. doi: 10.3390/biomedicines10071538
40. Ogata AF, Cheng C-A, Desjardins M, Senussi Y, Sherman AC, Powell M, et al. Circulating severe acute respiratory syndrome coronavirus 2 (SARS-coV-2) vaccine antigen detected in the plasma of mRNA-1273 vaccine recipients. Clin Infect Dis (2022) 74:715–8. doi: 10.1093/cid/ciab465
41. Bansal S, Perincheri S, Fleming T, Poulson C, Tiffany B, Bremner RM, et al. Cutting Edge: Circulating Exosomes with COVID Spike Protein Are Induced by BNT162b2 (Pfizer–BioNTech) Vaccination prior to Development of Antibodies: A Novel Mechanism for Immune Activation by mRNA Vaccines. J Immunol (2021) 207:2405–10. doi: 10.4049/jimmunol.2100637
42. Maugeri M, Nawaz M, Papadimitriou A, Angerfors A, Camponeschi A, Na M, et al. Linkage between endosomal escape of LNP-mRNA and loading into EVs for transport to other cells. Nat Commun (2019) 10:4333. doi: 10.1038/s41467-019-12275-6
43. Krauson AJ, Casimero FVC, Siddiquee Z, Stone JR. Duration of SARS-CoV-2 mRNA vaccine persistence and factors associated with cardiac involvement in recently vaccinated patients. NPJ Vaccines (2023) 8:141. doi: 10.1038/s41541-023-00742-7
44. Mulroney TE, Pöyry T, Yam-Puc JC, Rust M, Harvey RF, Kalmar L, et al. N1-methylpseudouridylation of mRNA causes +1 ribosomal frameshifting. Nature (2023) 625:189–194. doi: 10.1038/s41586-023-06800-3
45. Baden LR, El Sahly HM, Essink B, Kotloff K, Frey S, Novak R, et al. Efficacy and safety of the mRNA-1273 SARS-coV-2 vaccine. N Engl J Med (2021) 384:403–16. doi: 10.1056/NEJMoa2035389
46. Polack FP, Thomas SJ, Kitchin N, Absalon J, Gurtman A, Lockhart S, et al. Safety and efficacy of the BNT162b2 mRNA covid-19 vaccine. N Engl J Med (2020) 383:2603–15. doi: 10.1056/NEJMoa2034577
47. Fraiman J, Erviti J, Jones M, Greenland S, Whelan P, Kaplan RM, et al. Letter to the editor. Vaccine (2023). doi: 10.1016/j.vaccine.2023.06.035
48. Fraiman J, Erviti J, Jones M, Greenland S, Whelan P, Kaplan RM, et al. Serious adverse events of special interest following mRNA COVID-19 vaccination in randomized trials in adults. Vaccine (2022) 40:5798–805. doi: 10.1016/j.vaccine.2022.08.036
49. Thomas F. Germany’s Health Minister Changes tune on Vaccine Injuries (2023). Available at: https://unherd.com/thepost/Germanys-health-minister-changes-tune-on-vaccine-injuries/.
50. HRSA. Countermeasures injury compensation program (CICP) data. HRSA (2024). Available at: https://www.hrsa.gov/cicp/cicp-data.
51. Sridhara S, Nair R, Stanek M. Severe aplastic anemia after receiving SARS-coV-2 moderna mRNA vaccination. J Hematol (2022) 11:34–9. doi: 10.14740/jh954
52. Mingot-Castellano ME, Butta N, Canaro M, Gómez Del Castillo Solano MDC, Sánchez-González B, Jiménez-Bárcenas R, et al. COVID-19 vaccines and autoimmune hematologic disorders. Vaccines (2022) 10:961. doi: 10.3390/vaccines10060961
53. Gadi SRV, Brunker PAR, Al-Samkari H, Sykes DB, Saff RR, Lo J, et al. Severe autoimmune hemolytic anemia following receipt of SARS-CoV-2 mRNA vaccine. Transfusion (2021) 61:3267–71. doi: 10.1111/trf.16672
54. Röth A, Bertram S, Schroeder T, Haverkamp T, Voigt S, Holtkamp C, et al. Acquired aplastic anemia following SARS-CoV -2 vaccination. Eur J Haematol (2022) 2:186–94. doi: 10.1111/ejh.13788
55. Tabata S, Hosoi H, Murata S, Takeda S, Mushino T, Sonoki T. Severe aplastic anemia after COVID-19 mRNA vaccination: Causality or coincidence? J Autoimmun (2022) 126:102782. doi: 10.1016/j.jaut.2021.102782
56. Schinas G, Polyzou E, Dimakopoulou V, Tsoupra S, Gogos C, Akinosoglou K. Immune-mediated liver injury following COVID-19 vaccination. World J Virol (2023) 12:100–8. doi: 10.5501/wjv.v12.i2.100
57. Zheng H, Zhang T, Xu Y, Lu X, Sang X. Autoimmune hepatitis after COVID-19 vaccination. Front Immunol (2022) 13:1035073. doi: 10.3389/fimmu.2022.1035073
58. Ozaka S, Kobayashi T, Mizukami K, Murakami K. COVID-19 vaccination and liver disease. World J Gastroenterol (2022) 28:6791–810. doi: 10.3748/wjg.v28.i48.6791
59. Pira A, Moltrasio C, Abeni D, Corrà A, Marzano AV, Caproni M, et al. Response: Commentary: Bullous pemphigoid associated with COVID-19 vaccines: An Italian multicenter study. Front Med (2023) 10:1160672. doi: 10.3389/fmed.2023.1160672
60. Petti S, Arduino PG. New-onset pemphigus vulgaris and pemphigus foliaceus following COVID-19 infection and vaccination, systematic review of case reports and a causal hypothesis. J Eur Acad Dermatol Venereol. (2023) 37:1–5. doi: 10.1111/jdv.19271
61. Fu P-A, Chen C-W, Hsu Y-T, Wei K-C, Lin P-C, Chen T-Y. A case of acquired hemophilia A and bullous pemphigoid following SARS-CoV-2 mRNA vaccination. J Formos. Med Assoc (2022) 121:1872–6. doi: 10.1016/j.jfma.2022.02.017
62. Blumenthal KG, Freeman EE, Saff RR, Robinson LB, Wolfson AR, Foreman RK, et al. Delayed Large Local Reactions to mRNA-1273 Vaccine against SARS-CoV-2. N Engl J Med (2021) 384:1273–7. doi: 10.1056/NEJMc2102131
63. Nakashima C, Kato M, Otsuka A. Cutaneous manifestations of COVID-19 and COVID-19 vaccination. J Dermatol (2023) 50:280–9. doi: 10.1111/1346-8138.16651
64. Agustin M, Trifitriana M, Danarti R. COVID arm as a common cutaneous manifestation after mRNA-1273 vaccination: a systematic review. BMC Infect Dis (2023) 23:1–11. doi: 10.1186/s12879-022-07973-4
65. Choi S, Lee S, Seo J-W, Kim M-J, Jeon YH, Park JH, et al. Myocarditis-induced Sudden Death after BNT162b2 mRNA COVID-19 Vaccination in Korea: Case Report Focusing on Histopathological Findings. J Korean Med Sci (2021) 36:1–7. doi: 10.3346/jkms.2021.36.e286
66. Mörz M. A Case Report: Multifocal Necrotizing Encephalitis and Myocarditis after BNT162b2 mRNA Vaccination against COVID-19. Vaccines (2022) 10:1651. doi: 10.3390/vaccines10101651
67. Akiyama Y, Inagaki T, Morioka S, Kusano E, Ohmagari N. Exacerbations of Idiopathic Systemic Capillary Leak Syndrome following BNT162b2 mRNA COVID-19 Vaccine (Pfizer-BioNTech). Intern Med (2023) 62:2013–7. doi: 10.2169/internalmedicine.1682-23
68. Devaux CA, Camoin-Jau L. Molecular mimicry of the viral spike in the SARS-coV-2 vaccine possibly triggers transient dysregulation of ACE2, leading to vascular and coagulation dysfunction similar to SARS-coV-2 infection. Viruses (2023) 15:1045. doi: 10.3390/v15051045
69. Gill JR, Tashjian R, Duncanson E. Autopsy histopathologic cardiac findings in 2 adolescents following the second COVID-19 vaccine dose. Arch Pathol Lab Med (2022) 146:925–9. doi: 10.5858/arpa.2021-0435-SA
70. Satomi H, Katano H, Kanno H, Kobayashi M, Ohkuma Y, Hashidume N, et al. An autopsy case of fulminant myocarditis after severe acute respiratory syndrome coronavirus 2 vaccine inoculation. Pathol Int (2022) 72:519–24. doi: 10.1111/pin.13267
71. Chow BT, Lai CK. Lymphohistiocytic Myocarditis Possibly Due to Moderna mRNA-1273 Vaccine A Case report. Am J Clin Pathol (2022) 158:167–72. doi: 10.1093/ajcp/aqac029
72. Barmada A, Klein J, Ramaswamy A, Brodsky NN, Jaycox JR, Sheikha H, et al. Cytokinopathy with aberrant cytotoxic lymphocytes and profibrotic myeloid response in SARS-CoV-2 mRNA vaccine–associated myocarditis. Sci Immunol (2023) 8:1–19. doi: 10.1126/sciimmunol.adh3455
73. Ohkubo Y, Ohmura SI, Ishihara R, Miyamoto T. Possible case of polyarteritis nodosa with epididymitis following COVID-19 vaccination: A case report and review of the literature. Mod Rheumatol Case Rep (2023) 7:172–6. doi: 10.1093/mrcr/rxac085
74. Fillon A. et al. De novo and relapsing necrotizing vasculitis after COVID-19 vaccination. Clin Kidney J (2022) 15:560–3. doi: 10.1093/ckj/sfab285
75. Wakabayashi H, Iwayanagi M, Sakai D, Sugiura Y, Hiruta N, Matsuzawa Y, et al. Development of giant cell arteritis after vaccination against SARS-CoV2: A case report and literature review. Med (United States) (2023) 102:E33948. doi: 10.1097/MD.0000000000033948
76. Sheka M, Coattrenec Y, Lorenzini KI, Nendaz M. A severe case of rhabdomyolysis after Moderna mRNA anti-COVID -19 vaccine with a literature review. Clin Case Rep (2023) 11:1–8. doi: 10.1002/ccr3.7184
77. Yasmin F, Najeeb H, Naeem U, Moeed A, Atif AR, Asghar MS, et al. Adverse events following COVID-19 mRNA vaccines: A systematic review of cardiovascular complication, thrombosis, and thrombocytopenia. Immunity Inflamm Dis (2023) 11:1–10. doi: 10.1002/iid3.807
78. Chen Y, Xu Z, Wang P, Li X, Shuai Z, Ye D, et al. New-onset autoimmune phenomena post-COVID-19 vaccination. Immunology (2022) 165:386–401. doi: 10.1111/imm.13443
79. Fong W, Woon TH, Chew L-C, Low A, Law A, Poh YJ, et al. Prevalence and factors associated with flares following COVID-19 mRNA vaccination in patients with rheumatoid arthritis, psoriatic arthritis and spondyloarthritis: a national cohort study. Adv Rheumatol (2023) 63. doi: 10.1186/s42358-023-00316-0
80. Pujol A, Gómez L-A, Gallegos C, Nicolau J, Sanchís P, González-Freire M, et al. Thyroid as a target of adjuvant autoimmunity/inflammatory syndrome due to mRNA-based SARS-CoV2 vaccination: from Graves’ disease to silent thyroiditis. J Endocrinol Invest. (2022) 45:875–82. doi: 10.1007/s40618-021-01707-0
81. Nakamura F, Awaya T, Ohira M, Enomoto Y, Moroi M, Nakamura M. Graves’ disease after mrna covid-19 vaccination, with the presence of autoimmune antibodies even one year later. Vaccines (2023) 11:934. doi: 10.3390/vaccines11050934
82. Patrizio A, Ferrari SM, Elia G, Ragusa F, Paparo SR, Mazzi V, et al. Graves’ Disease following SARS-coV-2 vaccination: A systematic review. Vaccines (2022) 10:1445. doi: 10.3390/vaccines10091445
83. Chee YJ, Liew H, Hoi WH, Lee Y, Lim B, Chin HX, et al. SARS-coV-2 mRNA vaccination and graves’ Disease: A report of 12 cases and review of the literature. J Clin Endocrinol Metab (2022) 107:E2324–30. doi: 10.1210/clinem/dgac119
84. Aliberti L, Gagliardi I, Rizzo R, Bortolotti D, Schiuma G, Franceschetti P, et al. Pituitary apoplexy and COVID-19 vaccination: a case report and literature review. Front Endocrinol (Lausanne). (2022) 13:1–7. doi: 10.3389/fendo.2022.1035482
85. Jarius S, Bieber N, Haas J, Wildemann B. MOG encephalomyelitis after vaccination against severe acute respiratory syndrome coronavirus type 2 (SARS-CoV-2): case report and comprehensive review of the literature. J Neurol (2022) 269:5198–212. doi: 10.1007/s00415-022-11194-9
86. Gao J-J, Tseng H-P, Lin C-L, Hsu R-F, Lee M-H, Liu C-H. Acute encephalitis after COVID-19 vaccination: A case report and literature review. Hum Vaccines Immunother. (2022) 18:2082206. doi: 10.1080/21645515.2022.2082206
87. Garg RK, Paliwal VK. Spectrum of neurological complications following COVID-19 vaccination Neurological Sci (2022) 43:3–40. doi: 10.1007/s10072-021-05662-9
88. Orient JM. Negative evidence: COVID-19 vaccines and cancer. J Am Physicians Surg (2022) 28:98–107.
89. Singleton JA, Lloyd JC, Mootrey GT, Salive ME, Chen RT. An overview of the vaccine adverse event reporting system (VAERS) as a surveillance system. Vaccine (1999) 17:2908–17. doi: 10.1016/S0264-410X(99)00132-2
90. Diaz GA, Parsons GT, Gering SK, Meier AR, Hutchinson IV, Robicsek A. Myocarditis and pericarditis after vaccination for COVID-19. JAMA (2021) 326:1210–1212. doi: 10.1001/jama.2021.13443
91. Nakahara T, Iwabuchi Y, Miyazawa R, Tonda K, Shiga T, Strauss HW, et al. Assessment of myocardial 18 F-FDG uptake at PET/CT in asymptomatic SARS-coV-2-vaccinated and nonvaccinated patients. Radiology (2023) 308. doi: 10.1148/radiol.230743
92. Yeo YH, Wang M, He X, Lv F, Zhang Y, Zu J, et al. Excess risk for acute myocardial infarction mortality during the COVID-19 pandemic. J Med Virol (2023) 95:e28187. doi: 10.1002/jmv.28187
93. Indiana Life Insurance CEO Says Deaths Up 40% From Pre-Pandemic Levels . Available at: https://va.org/news/Indiana-ceo-says-deaths-up-40.
94. Brown CM, Vostok J, Johnson H, Burns M, Gharpure R, Sami S, et al. Outbreak of SARS-coV-2 infections, including COVID-19 vaccine breakthrough infections, associated with large public gatherings — Barnstable county, massachusetts, july 2021. MMWR. Morb. Mortal. Wkly. Rep (2021) 70:1059–62. doi: 10.15585/mmwr.mm7031e2
95. Tenforde MW, Self WH, Adams K, Gaglani M, Ginde AA, McNeal T, et al. Association between mRNA vaccination and COVID-19 hospitalization and disease severity. JAMA - J Am Med Assoc (2021) 326:2043–54. doi: 10.1001/jama.2021.19499
96. Verma G, Dhawan M, Saied AA, Kaur G, Kumar R, Emran TB. Immunomodulatory approaches in managing lung inflammation in COVID-19: A double-edge sword. Immunity Inflamm Dis (2023) 11:e1020–e1020. doi: 10.1002/iid3.1020
97. Föhse K, Geckin B, Zoodsma M, Kilic G, Liu Z, Röring RJ, et al. The impact of BNT162b2 mRNA vaccine on adaptive and innate immune responses. Clin Immunol (2023) 255:109762. doi: 10.1016/j.clim.2023.109762
98. Edara V-V, Manning KE, Ellis M, Lai L, Moore KM, Foster SL, et al. mRNA-1273 and BNT162b2 mRNA vaccines have reduced neutralizing activity against the SARS-CoV-2 omicron variant. Cell Rep Med (2022) 3:100529. doi: 10.1016/j.xcrm.2022.100529
99. Qin Z, Bouteau A, Herbst C, Igyártó BZ. Pre-exposure to mRNA-LNP inhibits adaptive immune responses and alters innate immune fitness in an inheritable fashion. PloS Pathog (2022) 18:1–23. doi: 10.1371/journal.ppat.1010830
100. Shrestha NK, Burke PC, Nowacki AS, Simon JF, Hagen A, Gordon SM. Effectiveness of the coronavirus disease 2019 bivalent vaccine. Open Forum Infect Dis (2023) 10:1–8. doi: 10.1093/ofid/ofad209
101. Eythorsson E, Runolfsdottir HL, Ingvarsson RF, Sigurdsson MI, Palsson R. Rate of SARS-coV-2 reinfection during an omicron wave in Iceland. JAMA Netw Open (2022) 5:e2225320. doi: 10.1001/jamanetworkopen.2022.25320
102. Shrestha NK, Shrestha P, Burke PC, Nowacki AS, Terpeluk P, Gordon SM. Coronavirus disease 2019 vaccine boosting in previously infected or vaccinated individuals. Clin Infect Dis (2022) 75:2169–77. doi: 10.1093/cid/ciac327
103. Igyártó BZ, Jacobsen S, Ndeupen S. Future considerations for the mRNA-lipid nanoparticle vaccine platform. Curr Opin Virol (2021) 48:65–72. doi: 10.1016/j.coviro.2021.03.008
104. Lei Y, Zhang J, Schiavon CR, He M, Chen L, Shen H, et al. SARS-coV-2 spike protein impairs endothelial function via downregulation of ACE 2. Circ Res (2021) 128:1323–6. doi: 10.1161/CIRCRESAHA.121.318902
105. Panigrahi S, Goswami T, Ferrari B, Antonelli CJ, Bazdar DA, Gilmore H, et al. SARS-coV-2 spike protein destabilizes microvascular homeostasis. Microbiol Spectr. (2021) 9:15–20. doi: 10.1128/Spectrum.00735-21
106. Rhea EM, Logsdon AF, Hansen KM, Williams LM, Reed MJ, Baumann KK, et al. The S1 protein of SARS-CoV-2 crosses the blood–brain barrier in mice. Nat Neurosci (2021) 24:368–78. doi: 10.1038/s41593-020-00771-8
107. Nuovo GJ, Magro C, Shaffer T, Awad H, Suster D, Mikhail S, et al. Endothelial cell damage is the central part of COVID-19 and a mouse model induced by injection of the S1 subunit of the spike protein. Ann Diagn. Pathol (2021) 51:151682. doi: 10.1016/j.anndiagpath.2020.151682
108. Parry PI, Lefringhausen A, Turni C, Neil CJ, Cosford R, Hudson NJ, et al. ‘Spikeopathy’: COVID-19 spike protein is pathogenic, from both virus and vaccine mRNA. Biomedicines (2023) 11:2287. doi: 10.3390/biomedicines11082287
109. Szebeni J, Storm G, Ljubimova JY, Castells M, Phillips EJ, Turjeman K, et al. Applying lessons learned from nanomedicines to understand rare hypersensitivity reactions to mRNA-based SARS-CoV-2 vaccines. Nat Nanotechnol. (2022) 17:337–46. doi: 10.1038/s41565-022-01071-x
110. Katsikas Triantafyllidis K, Giannos P, Mian IT, Kyrtsonis G, Kechagias KS. Varicella zoster virus reactivation following COVID-19 vaccination: A systematic review of case reports. Vaccines (2021) 9:1013. doi: 10.3390/vaccines9091013
111. Daouk SK, Kamau E, Adachi K, Aldrovandi GM. Zoster meningitis in an immunocompetent child after COVID-19 vaccination, california, USA. Emerg Infect Dis (2022) 28:1523–4. doi: 10.3201/eid2807.220600
112. Lensen R, Netea MG, Rosendaal F. Hepatitis C virus reactivation following COVID-19 vaccination – A case report. Int Med Case Rep J Volume (2021) 14:573–6. doi: 10.2147/IMCRJ.S328482
113. Yamamoto K. Adverse effects of COVID-19 vaccines and measures to prevent them. Virol J (2022) 19:1–3. doi: 10.1186/s12985-022-01831-0
114. Goldman S, Bron D, Tousseyn T, Vierasu I, Dewispelaere L, Heimann P, et al. Rapid progression of angioimmunoblastic T cell lymphoma following BNT162b2 mRNA vaccine booster shot: A case report. Front Med (2021) 8:2409. doi: 10.3389/fmed.2021.798095
115. Sekizawa A, Hashimoto K, Kobayashi S, Kozono S, Kobayashi T, Kawamura Y, et al. Rapid progression of marginal zone B-cell lymphoma after COVID-19 vaccination (BNT162b2): A case report. Front Med (2022) 9:963393. doi: 10.3389/fmed.2022.963393
116. Cavanna L, Grassi SO, Ruffini L, Michieletti E, Carella E, Palli D, et al. Non-Hodgkin Lymphoma Developed Shortly after mRNA COVID-19 Vaccination: Report of a Case and Review of the Literature. Med (2023) 59:1–8. doi: 10.3390/medicina59010157
117. Eens S, Van Hecke M, Favere K, Tousseyn T, Guns P-J, Roskams T, et al. B-cell lymphoblastic lymphoma following intravenous BNT162b2 mRNA booster in a BALB/c mouse: A case report. Front Oncol (2023) 13:1158124. doi: 10.3389/fonc.2023.1158124
118. Parhiz H, Brenner JS, Patel PN, Papp TE, Shahnawaz H, Li Q, et al. Added to pre-existing inflammation, mRNA-lipid nanoparticles induce inflammation exacerbation (IE). J Control. Release (2022) 344:50–61. doi: 10.1016/j.jconrel.2021.12.027
119. Chemaitelly H, Ayoub HH, Tang P, Coyle P, Yassine HM, Al Thani AA, et al. Long-term COVID-19 booster effectiveness by infection history and clinical vulnerability and immune imprinting: a retrospective population-based cohort study. Lancet Infect Dis (2023) 23:816–27. doi: 10.1016/S1473-3099(23)00058-0
120. As an oncologist I am seeing People With Stable Cancer Rapidly Progress After Being Forced to Have a Booster – The Daily Sceptic . Available at: https://dailysceptic.org/2022/11/26/as-an-oncologist-i-am-seeing-people-with-stable-cancer-rapidly-progress-after-being-forced-to-have-a-booster/.
121. Reyes AE. While COVID Raged, Another Deadly Threat was on the Rise in Hospitals. Los Angeles Times (2023). Available at: https://www.latimes.com/california/story/2023-02-05/sepsis-california-hospitals-pandemic.
122. Lastinger LM, Alvarez CR, Kofman A, Konnor RY, Kuhar DT, Nkwata A, et al. Continued increases in the incidence of healthcare-associated infection (HAI) during the second year of the coronavirus disease 2019 (COVID-19) pandemic. Infect Control Hosp. Epidemiol. (2023) 44:997–1001. doi: 10.1017/ice.2022.116
123. Segrelles-Calvo G, De Saraújo GR, Frases S. Systemic mycoses: A potential alert for complications in COVID-19 patients. Future Microbiol (2020) 15:1405–13. doi: 10.2217/fmb-2020-0156
124. Aranjani JM, Manuel A, Abdul Razack HI, Mathew ST. COVID-19–associated mucormycosis: Evidence-based critical review of an emerging infection burden during the pandemic’s second wave in India. PloS Negl Trop Dis (2021) 15:e0009921. doi: 10.1371/journal.pntd.0009921
125. Costantini C, van de Veerdonk FL, Romani L. Covid-19-associated pulmonary aspergillosis: the other side of the coin. Vaccines (2020) 8:713. doi: 10.3390/vaccines8040713
126. Lyman M, Forsberg K, Sexton DJ, Chow NA, Lockhart SR, Jackson BR, et al. Worsening spread of candida auris in the United States, 2019 to 2021. Ann Intern Med (2023) 176:489–95. doi: 10.7326/m22-3469
127. Wherry EJ, Kurachi M. Molecular and cellular insights into T cell exhaustion. Nat Rev Immunol (2015) 15:486–99. doi: 10.1038/nri3862
128. Buhre JS, Pongracz T, Künsting I, Lixenfeld AS, Wang W, Nouta J, et al. mRNA vaccines against SARS-CoV-2 induce comparably low long-term IgG Fc galactosylation and sialylation levels but increasing long-term IgG4 responses compared to an adenovirus-based vaccine. Front Immunol (2023) 13:1020844. doi: 10.3389/fimmu.2022.1020844
129. Irrgang P, Gerling J, Kocher K, Lapuente D, Steininger P, Habenicht K, et al. Class switch toward noninflammatory, spike-specific IgG4 antibodies after repeated SARS-CoV-2 mRNA vaccination. Sci Immunol (2023) 8:eade2798–eade2798. doi: 10.1126/sciimmunol.ade2798
130. Kiszel P, Sík P, Miklós J, Kajdácsi E, Sinkovits G, Cervenak L, et al. Class switch towards spike protein-specific IgG4 antibodies after SARS-CoV-2 mRNA vaccination depends on prior infection history. Sci Rep (2023) 13:13166. doi: 10.1038/s41598-023-40103-x
131. Pillai S. Is it bad, is it good, or is IgG4 just misunderstood? Sci Immunol (2023) 8:1–4. doi: 10.1126/sciimmunol.adg7327
132. Yu T, Wu Y, Liu J, Zhuang Y, Jin X, Wang L. The risk of Malignancy in patients with IgG4-related disease: a systematic review and meta-analysis. Arthritis Res Ther (2022) 24:14. doi: 10.1186/s13075-021-02652-2
133. Xin G, Zander R, Schauder DM, Chen Y, Weinstein JS, Drobyski WR, et al. Single-cell RNA sequencing unveils an IL-10-producing helper subset that sustains humoral immunity during persistent infection. Nat Commun (2018) 9:5037. doi: 10.1038/s41467-018-07492-4
134. Munemura R, Maehara T, Murakami Y, Koga R, Aoyagi R, Kaneko N, et al. Distinct disease-specific Tfh cell populations in 2 different fibrotic diseases: IgG4-related disease and Kimura disease. J Allergy Clin Immunol (2022) 150:440–455.e17. doi: 10.1016/j.jaci.2022.03.034
135. Uversky VN, Redwan EM, Makis W, Rubio-Casillas A. IgG4 antibodies induced by repeated vaccination may generate immune tolerance to the SARS-coV-2 spike protein. Vaccines (2023) 11:991. doi: 10.3390/vaccines11050991
136. Crouse J, Kalinke U, Oxenius A. Regulation of antiviral T cell responses by type I interferons. Nat Rev Immunol (2015) 15:231–42. doi: 10.1038/nri3806
137. Urban SL, Welsh RM. Out-of-sequence signal 3 as a mechanism for virus-induced immune suppression of CD8 T cell responses. PloS Pathog (2014) 10:e1004357–e1004357. doi: 10.1371/journal.ppat.1004357
138. Beeson PB. Development of tolerance to typhoid bacterial pyrogen and its abolition by reticulo-endothelial blockade. Proc Soc Exp Biol Med (1946) 61:248–50. doi: 10.3181/00379727-61-15291P
139. Seeley JJ, Ghosh S. Molecular mechanisms of innate memory and tolerance to LPS J. Leukoc. Biol (2017) 101:107–19. doi: 10.1189/jlb.3mr0316-118rr
140. Schiepers A, van ’t Wout MFL, Greaney AJ, Zang T, Muramatsu H, Lin PJC, et al. Molecular fate-mapping of serum antibody responses to repeat immunization. Nature (2023) 615:482–9. doi: 10.1038/s41586-023-05715-3
141. Barrière J, Frank F, Besancon L, Samuel A, Saada V, Billy E, et al. Letter to Editor “Innate immune suppression by SARS-CoV-2 mRNA vaccinations: The role of G-quadruplexes, exosomes, and MicroRNAs”: Important concerns on the validity of this article. Food Chem Toxicol (2023) 178:113897. doi: 10.1016/j.fct.2023.113897
142. Seneff S, Nigh G, Kyriakopoulos AM, McCullough PA. Innate immune suppression by SARS-CoV-2 mRNA vaccinations: The role of G-quadruplexes, exosomes, and MicroRNAs. Food Chem Toxicol (2022) 164:113008. doi: 10.1016/j.fct.2022.113008
143. Igyártó BZ. “Don’t look up” Your science—Herd immunity or herd mentality? Microorganisms (2022) 10:1463. doi: 10.3390/microorganisms10071463
144. Watson OJ, Barnsley G, Toor J, Hogan AB, Winskill P, Ghani AC. Global impact of the first year of COVID-19 vaccination: a mathematical modelling study. Lancet Infect Dis (2022) 22:1293–302. doi: 10.1016/S1473-3099(22)00320-6
145. CDC. Why CDC Estimates the Burden of Season Influenza in the U.S. CDC (2018). Available at: https://www.cdc.gov/flu/about/burden/why-cdc-estimates.htm.
146. Ioannidis JPA. Infection fatality rate of COVID-19 inferred from seroprevalence data. Bull World Health Organ. (2021) 99:19–33F. doi: 10.2471/BLT.20.265892
147. O’Driscoll M, Ribeiro Dos Santos G, Wang L, Cummings DAT, Azman AS, Paireau J, et al. Age-specific mortality and immunity patterns of SARS-CoV-2. Nature (2021) 590:140–5. doi: 10.1038/s41586-020-2918-0
148. Read AF, Baigent SJ, Powers C, Kgosana LB, Blackwell L, Smith LP, et al. Imperfect vaccination can enhance the transmission of highly virulent pathogens. PloS Biol (2015) 13:e1002198–e1002198. doi: 10.1371/journal.pbio.1002198
149. Gaeta G. Mass vaccination in a roaring pandemic. Chaos Solitons Fractals (2022) 156:111786. doi: 10.1016/j.chaos.2021.111786
150. Miller IF, Metcalf CJE. Assessing the risk of vaccine-driven virulence evolution in SARS-CoV-2. R Soc Open Sci (2022) 9:211021. doi: 10.1098/rsos.211021
151. Bull JJ, Antia R. Which ‘imperfect vaccines’ encourage the evolution of higher virulence? Evol Med Public Heal (2022) 10:202–13. doi: 10.1093/emph/eoac015
152. Sasaki A, Lion S, Boots M. Antigenic escape selects for the evolution of higher pathogen transmission and virulence. Nat Ecol Evol (2022) 6:51–62. doi: 10.1038/s41559-021-01603-z
153. McLeod DV, Gandon S. Effects of epistasis and recombination between vaccine-escape and virulence alleles on the dynamics of pathogen adaptation. Nat Ecol Evol (2022) 6:786–93. doi: 10.1038/s41559-022-01709-y
154. Day T, Kennedy DA, Read AF, Gandon S. Pathogen evolution during vaccination campaigns. PloS Biol (2022) 20:e3001804. doi: 10.1371/journal.pbio.3001804
155. Rella SA, Kulikova YA, Dermitzakis ET, Kondrashov FA. Rates of SARS-CoV-2 transmission and vaccination impact the fate of vaccine-resistant strains. Sci Rep (2021) 11:15729. doi: 10.1038/s41598-021-95025-3
156. Mass Vaccination During Pandemic Historical Blunder: Nobel laureate-Telangana Today. Available at: https://telanganatoday.com/mass-vaccination-during-pandemic-historical-blunder-nobel-laureate.
157. Sweden Decides Against Recommending COVID Vaccines for kids aged 5-11. Available at: https://www.reuters.com/world/europe/Sweden-decides-against-recommending-covid-vaccines-kids-aged-5-12-2022-01-27/.
159. Ludvigsson JF, Engerström L, Nordenhäll C, Larsson E. Open Schools, Covid-19, and child and teacher morbidity in Sweden. N Engl J Med (2021) 384:669–71. doi: 10.1056/NEJMc2026670
160. NIH director Francis Collins wanted a ‘take-down’ to stifle Covid-19 debate . Available at: https://www.statnews.com/2021/12/23/at-a-time-when-the-u-s-needed-covid-19-dialogue-between-scientists-francis-collins-moved-to-shut-it-down/.
161. Kulldorff M, Gupta S, Bhattacharya J. Great Barrington Declaration. Great Barrington, United States: Great Barrington Declaration. (2020). Available at: https://gbdeclaration.org/(2020).
Keywords: mRNA vaccine, adverse event, side effect, Quo Vadis, suppression
Citation: Igyártó BZ and Qin Z (2024) The mRNA-LNP vaccines – the good, the bad and the ugly? Front. Immunol. 15:1336906. doi: 10.3389/fimmu.2024.1336906
Received: 11 November 2023; Accepted: 23 January 2024;
Published: 08 February 2024.
Edited by:
Cesar Lopez-Camacho, University of Oxford, United KingdomReviewed by:
Srinivasa Reddy Bonam, University of Texas Medical Branch at Galveston, United StatesCopyright © 2024 Igyártó and Qin. This is an open-access article distributed under the terms of the Creative Commons Attribution License (CC BY). The use, distribution or reproduction in other forums is permitted, provided the original author(s) and the copyright owner(s) are credited and that the original publication in this journal is cited, in accordance with accepted academic practice. No use, distribution or reproduction is permitted which does not comply with these terms.
*Correspondence: Botond Z. Igyártó, Ym90b25kLmlneWFydG9AamVmZmVyc29uLmVkdQ==