- Aix-Marseille Université, Centre National de la Recherche Scientifique (CNRS), Institut National de la Santé et de la Recherche Médicale (INSERM), CIML, Centre d’Immunologie de Marseille-Luminy, Marseille, France
The nervous and immune systems are the primary sensory interfaces of the body, allowing it to recognize, process, and respond to various stimuli from both the external and internal environment. These systems work in concert through various mechanisms of neuro-immune crosstalk to detect threats, provide defense against pathogens, and maintain or restore homeostasis, but can also contribute to the development of diseases. Among peripheral sensory neurons (PSNs), nociceptive PSNs are of particular interest. They possess a remarkable capability to detect noxious stimuli in the periphery and transmit this information to the brain, resulting in the perception of pain and the activation of adaptive responses. Pain is an early symptom of cancer, often leading to its diagnosis, but it is also a major source of distress for patients as the disease progresses. In this review, we aim to provide an overview of the mechanisms within tumors that are likely to induce cancer pain, exploring a range of factors from etiological elements to cellular and molecular mediators. In addition to transmitting sensory information to the central nervous system, PSNs are also capable, when activated, to produce and release neuropeptides (e.g., CGRP and SP) from their peripheral terminals. These neuropeptides have been shown to modulate immunity in cases of inflammation, infection, and cancer. PSNs, often found within solid tumors, are likely to play a significant role in the tumor microenvironment, potentially influencing both tumor growth and anti-tumor immune responses. In this review, we discuss the current state of knowledge about the degree of sensory innervation in tumors. We also seek to understand whether and how PSNs may influence the tumor growth and associated anti-tumor immunity in different mouse models of cancer. Finally, we discuss the extent to which the tumor is able to influence the development and functions of the PSNs that innervate it.
1 Introduction
A tumor is more than a mere collection of cells but a complex ecosystem known as the tumor microenvironment (TME), encompassing cells and soluble factors that play a pivotal role in tumor growth (1). Among these constituents, immune cells hold undeniable importance, as evidenced by the advent of immunotherapy (2). Nonetheless, despite unprecedented clinical benefit in various cancers, only a minority of patients respond to current immunotherapies. Therefore, it is crucial to explore the mechanisms within the TME impacting the development of an effective anti-tumor immune response either natural or therapeutically induced.
Nerve fibers in solid human tumors, often associated with a poor prognosis, have long been underappreciated for their potential influence on tumor progression and, more specifically, on the anti-tumor immune response (3). Among them, peripheral sensory neurons (PSNs) and, more specifically, nociceptive PSNs known as nociceptors innervate peripheral tissues and elicit painful sensations after sensitization of their terminals by noxious signals. They possess multiple receptors allowing the detection of pathogen-associated molecular patterns (PAMPs), damage-associated molecular patterns (DAMPs), and immune mediators during tissue injury or infection. Additionally, nociceptors release neuropeptides, including calcitonin gene-related peptide (CGRP) and substance P (SP), capable of modulating the functions of immune cells, which express the corresponding neuropeptide receptors (4). In the past decade, studies have revealed interactions between nociceptors and the immune system, impacting pain, inflammation, and host defense regulation. Specifically, nociceptors can modulate antimicrobial or inflammatory immune responses and contribute to chronic inflammatory disease pathogenesis (5–16). Furthermore, these studies have highlighted different capacities among nociceptor subsets, such as pro- or anti-inflammatory roles, in influencing the immune response (17).
While the influence of sympathetic and parasympathetic nerve fibers on tumor growth and anti-tumor immunity has been well-documented (18), PSNs have received relatively little attention in this context. Although a few studies have hinted at their potential role in tumor progression, apart from a few studies, their precise regulatory impact on the anti-tumor immune response remains poorly understood. We will present and thoroughly discuss these issues in this review. However, before delving into the details, considering our emphasis on nociceptors, we will first “set the stage” by addressing some basic questions about cancer pain.
Is cancer painful, and is pain a consistent feature across all cancer types? Although this question seems to be a simple one, the intricate nature of pain in various cancer types necessitates a clear definition of cancer pain and of the mechanisms underlying its triggering and perception. In this discussion, we will explore some pain-related mechanisms and with a special focus on the TME-induced signals that can activate nociceptors. We will also examine the extent of sensory innervation within tumors and its reciprocal relationship with carcinogenesis.
2 Cancer and pain
Given its heterogeneity, defining and treating cancer pain is a challenge, but a useful one. Indeed, pain is often the primary symptom prompting a cancer diagnosis but also a major source of patient distress during the disease’s progression.
2.1 What exactly is pain, and how is it generated?
PSNs form a family of neurons capable of detecting various stimuli in peripheral tissues. A significant proportion of these PSNs express the Nav1.8 sodium channel, including nociceptors/pruriceptors specialized in detecting and transmitting noxious stimuli that may induce pain. Historically, PSNs have been classified based on anatomical (e.g., fiber diameter, soma size, and localization), physiological (e.g., action potential velocities), and functional (e.g., neuropeptide production) properties. In addition, recent single-cell RNA sequencing studies have defined a comprehensive molecular and functional classification of PSN subsets in various species, including mice, macaques, and humans (19–23). Harmful stimuli provoking pain or itch are conveyed by different subsets of nociceptors/pruriceptors, which are further subdivided into peptidergic (PEP) or nonpeptidergic (NP), based on initial observation of their ability to produce canonical neuropeptides, SP and CGRP. Among PEPs, the PEP1 subgroup expresses Tac1, Calca, and Trpv1 (transcripts encoding SP, CGRP, and transient receptor potential vanilloid 1, respectively) and is therefore set to secrete CGRP and SP. Nociceptors are equipped with numerous receptors that enable them to detect perturbations in their environment. First, they express transient receptor potentials (TRPs), such as TRPV1, which are the main receptors involved in the detection and transduction of nociceptive stimuli. They also express receptors capable of recognizing various inflammatory or inflammation-associated mediators (microbial products, cytokines, neurotrophins, lipids and lipid-derived mediators, extracellular ATP, protons, etc.) produced by pathogens or cells. Finally, certain subtypes of nociceptors, including the C-low threshold mechanoreceptors (C-LTMRs) that express the Piezo2 receptor, can be excited by specific mechanical stimuli (24–26). The activation of these receptors by their ligands elicits their activation or increase in electrical activity, leading to hypersensitivity to external stimuli or amplification of pain perception and response.
What is the pain circuit? There are at least four control levels that filter information for cognitive pain perception (Figure 1). First, thanks to muliple specific recetors, PSNs detect noxious signals in the periphery and convert this information into action potentials (I, axon sensitization). These signals then reach sensory ganglia, including dorsal root ganglia (DRG), vagal ganglia (VG), and trigeminal ganglia (TG), which house PSN cell bodies. These ganglia are also composed of other cell types, notably glial and immune cells, that collectively respond to incoming signals (27–29) (II, sensory ganglia integration). PSNs from DRG afferent project nerve endings into the dorsal horn of the of the spinal cord, while PSNs from TG and NG project directly into the brainstem. In the spinal cord and brainstem, neuropeptides (mainly SP, CGRP, and somatostatin) and glutamate from primary afferent fibers, along with other neurotransmitters such as gamma-aminobutyric acid (GABA) and glycine produced by second-order nociceptive neurons and interneurons, exert their effects on spinal and supraspinal neurons (30, 31) (III, central sensitization). Finally, spinal and supraspinal projection neurons relay this information to higher brain regions including the brainstem, somatosensory, insular, cingulate and prefrontal cortices, and thalamus and subcortical areas, where their integration can lead to pain perception (32–34) (IV, cognitive perception). Thus, this sequence of transmission and integration of sensory signals ultimately leads to conscious perception and appropriate behavioral responses (31, 35).
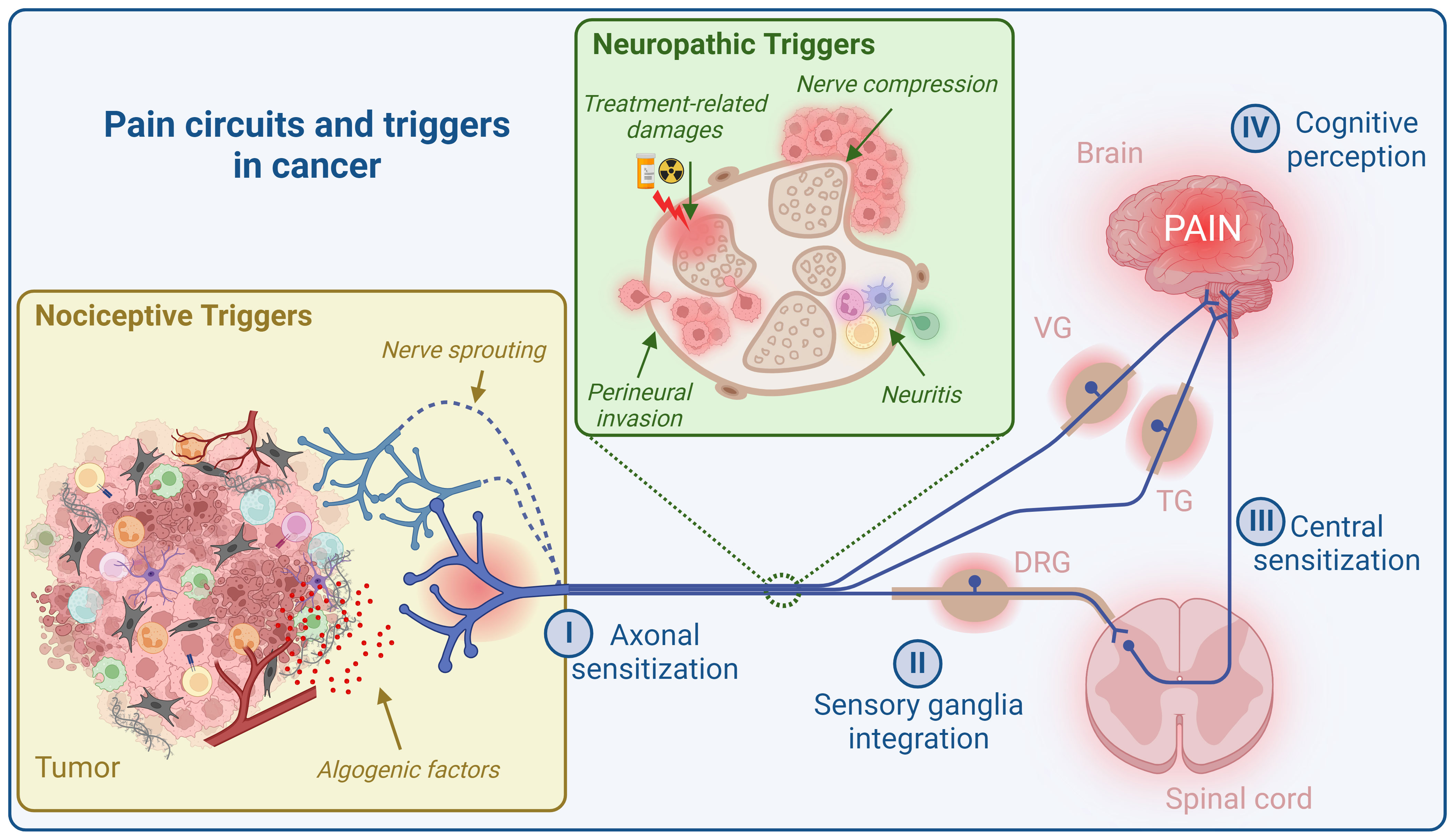
Figure 1 Pain circuit and its triggers in cancer. The cancer pain circuit comprises four levels of regulation. The initial level (I, axon sensitization) involves the sensitization of nerve endings of peripheral sensory neurons (PSNs) within the tumor microenvironment (TME), conveying neuronal information to sensory ganglia (DRG, VG, and TG) for integration (II, sensory ganglia integration). Next, PSNs from DRG afferent project nerve endings into the dorsal horn of the spinal cord, while PSNs from TG and VG project directly into the brainstem. In spinal cord and brainstem, neuropeptides (mainly SP, CGRP, and somatostatin) and glutamate from primary afferent fibers, along with other neurotransmitters such as gamma-aminobutyric acid (GABA) and glycine produced by second-order nociceptive neurons and interneurons, exert their effects on spinal and supraspinal neurons (III, central sensitization). Finally, spinal and supraspinal projection neurons relay this information to higher brain regions including the brainstem, somatosensory, insular, cingulate and prefrontal cortices, and thalamus and subcortical areas, where their integration can lead to pain perception (IV, cognitive perception). Cancer pain primarily arises from two main sources: “nociceptive triggers” and “neuropathic triggers”. Nociceptive stimuli encompass algogenic mediators released by the TME and nerve sprouting. Neuropathic triggers, on the other hand, are a consequence of nerve damage caused by tumor cell invasion (perineural invasion), immune cell invasion (neuritis), nerve compression, and the effects of radiotherapy and chemotherapy. DRG, dorsal root ganglia; VG, vagal ganglia; TG, trigeminal ganglia. Created with BioRender.com.
In the bidirectional communication between the brain and the body, a painful stimulus initially generates a danger signal that the brain perceives, subsequently triggering an adaptive response. The best illustration of this phenomenon is the withdrawal response, also known as the nociceptive flexion reflex, an automatic spinal cord reflex that plays a crucial role in protecting the organism against harmful stimuli perceived by nociceptors (36). In the case of acute inflammation, which is typically associated with pain, it can activate an anti-inflammatory reflex (37). However, a major problem arises when the stimulus becomes chronic, as in the case of chronic cancer pain. In such situations, the reflex that was suitable for an acute stimulus is unlikely to be suitable for one to a chronic, persistent one.
Are there different types of pain? There are indeed various pain types with different etiologies: nociceptive pain, inflammatory pain, neuropathic pain, and dysfunctional pain (38). Nociceptive pain may be viewed as a physiological pain due to the activation of high-threshold nociceptor neurons by noxious stimuli, not necessarily inflammatory ones. Inflammatory pain arises from inflammation in peripheral tissues, detected by nociceptors, leading to heightened sensitivity to pain. Neuropathic pain may result from somatosensory system damage or disease, altering nociceptive signal processing and leading to pain without stimuli or heightened responses to both innocuous and noxious stimuli. Dysfunctional pain occurs when there is no identifiable noxious stimulus, inflammation, or nervous system damage. More comprehensive and detailed information on these various types of pain, especially those involved in cancer, can be obtained from reviews specifically addressing these issues (31, 38–40).
2.2 Is a tumor always painful, and what is exactly cancer pain?
Is a tumor painful? Providing a concise response to this query is challenging due to numerous influencing factors. It hinges on the tumor’s characteristics, including its location, histological type, innervation level, immune cell infiltration, developpment stage/grade, and size. Additional factors, such as gender, psychological state, and genetic polymorphism, also play a role. As a result, pain tolerance varies from person to person.
However, it must be recalled that, often, cancer initially develops silently, without causing pain, making it difficult to diagnose. In addition, tumors are generally painless when small and only become painful or “unpleasant” when they grow, depending on their location. Therefore, it follows logically that cancer pain intensifies as the tumor grows and the disease advances. Thus, the prevalence of reported cancer pain varies primarily according to cancer stage, rather than cancer type (41). However, it is worth noting that the correlation between the level of pain and the tumor size is still debated, at least concerning oral cancers (42–44). Thus, in oral cancer, a report has been reported between tumor size and patient-reported pain and associated nociceptive behavior in a carcinogen mouse model (44), whereas such a correlation was not observed in other studies (42, 43). Moreover, although benign or precancerous lesions are generally painless, the transformation process may result in a sensation of pain. Thus, in a preclinical model of pancreatic cancer, it has been shown that neuroplastic changes occur in PSNs prior to transformation into cancer (45).
Certain types of cancer, notably oral, bone, and pancreatic cancer, are known to be highly painful (41, 46–49). In head and neck cancer (HNC), although the occurrence of pain is variable, almost all oral cancers are painful, and up to 85% of patients report pain at the time of diagnosis (46). In the case of bone, pain caused by bone metastases is the most frequent source of pain, and approximately 75% of patients with advanced cancer suffer from bone cancer pain (50). Moreover, systematic reviews have also indicated that the survival of advanced pancreatic cancer patients with cancer pain is notably shorter compared to those without (51). Because of their particularly painful nature, much of the knowledge about cancer pain discussed in this review comes from analysis of these cancer types.
What causes cancer pain? The etiology of cancer pain is multifaceted. Pain in cancer patients is believed to depend on factors such as the tumor’s mass, ulceration, inflammation, and infiltration. The underlying physiological mechanisms of cancer pain are usually viewed as a combination of inflammatory pain, arising from the release of numerous inflammatory factors within TME, and neuropathic pain, which results from damage to PSNs within the tumor, or during perineural invasion (PNI). However, a growing body of evidence suggests that cancer pain has unique characteristics that distinguish it from a simple combination of pain types already defined (39). This unique signature may explain the reduced efficacy of conventional analgesics (50).
Distinguishing between tumor-induced pain, treatment-related pain, and comorbid conditions is also important. Approximately 75% of pain is linked to the tumor itself, while 10%–20% results from treatments, especially chemotherapy and surgery, and the remaining 10% is attributed to comorbidities (52). For instance, cancer-related neuropathic pain can stem from treatment toxicity, vitamin deficiencies, tumor-induced nerve compression, or other problems such as diabetes and infection. Regardless of the cause, cancer pain becomes more prevalent as the disease advances, affecting approximately 64% of patients with advanced cancer (41, 49).
Why are certain cancers more painful than others? One obvious explanation is the level of tissue sensory innervation. For instance, the high degree of innervation of the oral cavity and bone tissue (53) may partly account for the heightened pain associated with cancers in these areas. However, this explanation does not hold entirely true, as melanoma, despite abundant skin innervation, is typically painless. The escalating frequency and intensity of pain as cancer progresses can be attributed to increased tissue innervation during tumor development. This occurs due to the remodeling of sensory and sympathetic nerve fibers through ectopic nerve sprouting (54–56). Additionally, differences in pain levels can be influenced by the histological nature and anatomical location of the cancer. Nevertheless, even within painful cancers, variations exist in pain phenotypes among histologically identical cancers at similar anatomical sites. These differences can be attributed to various factors, including etiology. For example, in the case of oropharyngeal squamous cell carcinoma, within the context of the same anatomical site, it has been shown that human papillomavirus (HPV)-negative tumors are more painful than HPV-positive ones (57). Regarding anatomical location, the pain associated with bone cancer has a specific feature. When tumor cells proliferate in the bone marrow, which is a space devoid of elasticity, this growth inevitably leads to physical constraints on other cell types within this microenvironment. This can result in various consequences, including anemia, bone fractures, and pain. Bone cancer is particularly significant because many metastatic cancers, regardless of the location of the primary tumor, tend to metastasize in the bone marrow for several reasons. One reason is the high vascularity of the bone marrow (58). Another one is the expression of CXCR4 by many metastatic tumor cells and by normal hematopoietic cells trapped in the bone marrow by this specific chemoreceptor (59).
Finally, TME is capable of generating factors that can sensitize nociceptors, called algogenic mediators, or, conversely, possess analgesic properties (29). The production of such mediators likely varies from one tumor type to another and, consequently, the balance of pro- or anti-nociceptive factors as well. For instance, in the early stages following a sunburn, keratinocytes can produce endogenous opioid peptide β-endorphin, which produces analgesia (60). It can be speculated that such a process could explain, for example, the relatively painless nature of melanoma. Thus, the TME of different cancers may contain different sets of algogenic and anti-nociceptive factors, able to either increase or dampen painful stimuli.
How to manage cancer pain? Cancer pain has a significant impact on the quality of life and mental health of patients. Consequently, it is crucial to tailor analgesic treatments based on the specific type of pain experienced by cancer patients. The primary strategy for managing cancer pain has traditionally followed the World Health Organization’s (WHO) guidelines for cancer pain relief. This strategy involves matching the potency of analgesia with the severity of pain, employing a range from basic analgesics to powerful opioids. Consequently, paracetamol and non-steroidal anti-inflammatory drugs (NSAIDs) are employed to address mild pain in patients, whereas mild and potent opioids such as codeine, tramadol, and morphine are utilized for the management of moderate to severe pain (61, 62). Accurately defining the type of cancer pain that a patient is experiencing is crucial, as it may necessitate the implementation of additional strategies like adjuvant analgesia (using antiepileptic or antidepressant drugs), corticosteroids, radiotherapy, and interventional procedures (63). For instance, neuropathic pain can be partially treated by combining opioids with antiepileptic or antidepressant drugs (64, 65). While these medications improve the quality of life for patients with cancer pain, their side effects are significant and require careful management during the treatment period (66).
2.3 Molecular and cellular mechanisms of pain in cancer
As mentioned earlier, the molecular and cellular mechanisms underlying cancer pain are numerous and occur at various levels of the pain circuit (Figure 1). In this review, our primary focus will be on the molecular and cellular mechanisms that take place within the tumor microenvironment (TME) or its vicinity. While we acknowledge the importance of neuropathic pain in cancer, we will not delve extensively into it due to space constraints. Nor will we discuss the mechanism of pain cancer regulation at the CNS level (termed central sensitization), which has been well documented elsewhere (31).
2.3.1 Algogenic mediators within the TME
One of the major mechanisms underlying cancer pain is the production and release of algogenic mediators within the TME. These mediators, either through their unique ligands or via other receptors and second messenger systems, can sensitize PSN, thereby playing a role in the development of hyperalgesia and allodynia within the TME (31, 46, 67) (Figure 2).
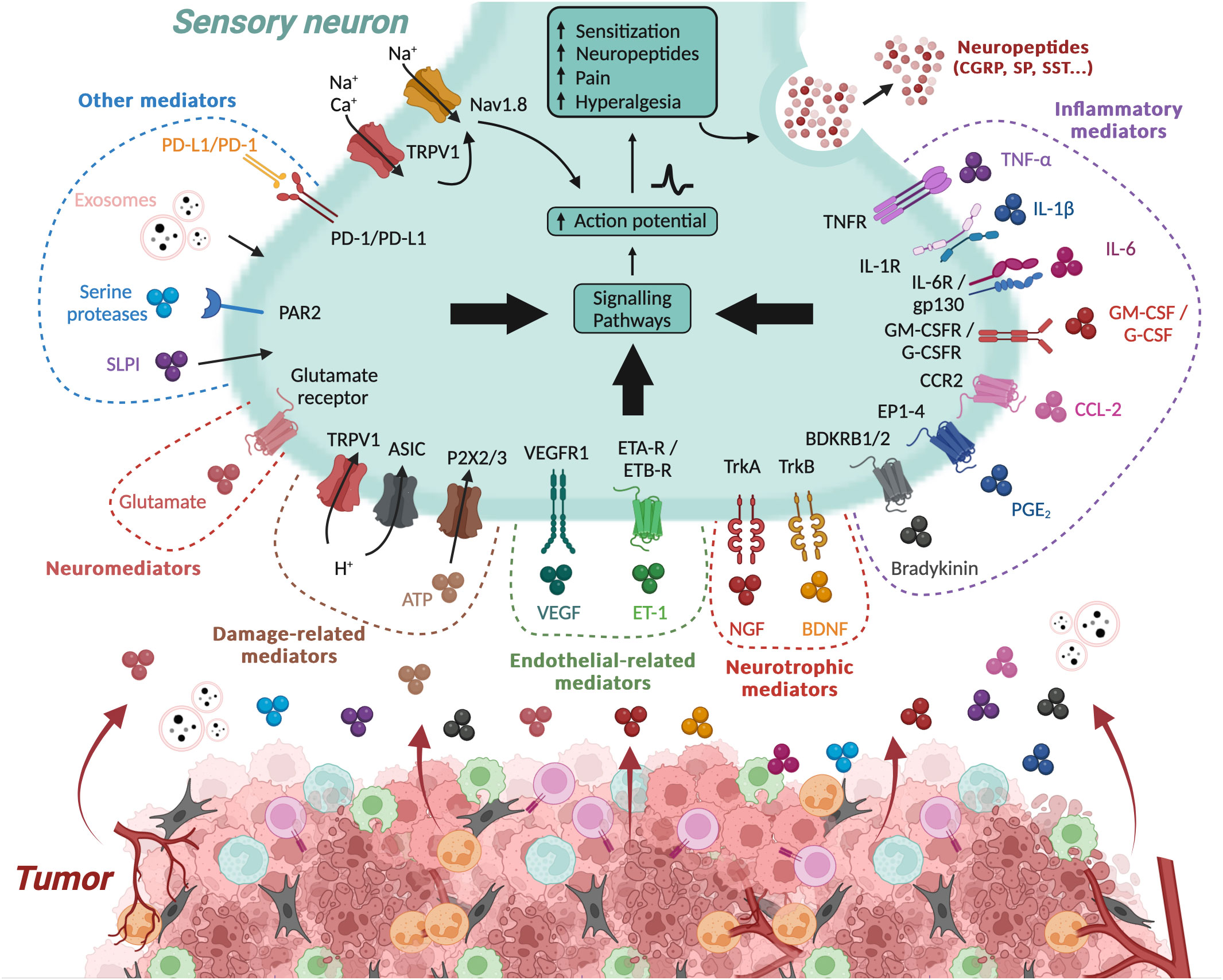
Figure 2 Algogenic factors in the tumor microenvironment. The tumor microenvironment (TME) generates numerous algogenic factors that are capable of sensitizing the peripheral sensory neurons (PSNs) innervating the tumor. This sensitization initiates a signaling cascade in the PSN, leading to sensations of pain or hyperalgesia, and also triggers the release of neuropeptides such as CGRP and SP. Some of these factors are associated to inflammation within the tumor, such as cytokines and chemokines, while other molecular factors are directly linked to tumor cells, such as exosomes. Inflammation and hypoxic conditions also promote tumor cell necrosis, leading to the production of damage-associated molecular patterns (DAMPs). Additionally, several other mediators, such as neurotrophic factors, neuromediators, and angiogenesis-related molecules, possess algogenic properties. On the contrary, certain molecule like PD-L1 can inhibit the activity of PSNs by limiting the activation of TRPV1. ASIC, acid-sensing ion channel; ATP, adenosine triphosphate; BDKRB1/2, bradykinin receptor B1/2; BDNF, brain-derived neurotrophic factor; cAMP, cyclic adenosine monophosphate; CGRP, calcitonin gene-related peptide; CCL-2, C–C motif chemokine 2; CCR2, C–C chemokine receptor type 2; EP1–4, prostaglandin E2 receptor 1-4; ETA-R, endothelin receptor type A; ETB-R, endothelin receptor type B; ET-1, endothelin 1; G-CSF, granulocyte colony-stimulating factor; G-SCFR, granulocyte colony-stimulating factor receptor; GM-CSF, granulocyte-macrophage colony-stimulating factor; GM-CSFR, granulocyte-macrophage colony-stimulating factor receptor; Gp130, glycoprotein 130; IL, interleukin; NGF, nerve growth factor; PAR2, proteinase-activated receptor 2; PD-1, programmed death 1; PD-L1, programmed death-ligand 1; PGE2, prostaglandin E2; P2X2/3, purinergic receptor P2X2/3; SLPI, secretory leukocyte protease inhibitor; SP, substance P; TNFα, tumor necrosis factor alpha; TNFR, tumor necrosis factor receptor; Trk, tropomyosin receptor kinase; TRPV1, transient receptor potential cation channel subfamily V member 1; VEGF, vascular endothelial growth factor; VEGFR1, vascular endothelial growth factor receptor 1. Created with BioRender.com.
The algogenic mediators can originate from a variety of sources within the TME, including tumor cells and immune cells, and encompass a diverse range of molecules such as inflammatory factors, cytokines, chemokines, colony-stimulating factors, and neurotrophic factors (Figure 2). Environmental changes (extracellular ATP and proton rises) can also lead to the generation of such mediators. These mediators have multiple mechanisms of action. Some interact directly with receptors or ion channels involved in the detection and signaling of noxious stimuli, such as protons by TRPV1 and acid-sensing ion channel (ASIC) receptors (68–72). Others induce sensitization of these receptors/channels. For example, they can induce the activation of various kinases, including protein kinase A, protein kinase C, and c-Src kinase, capable of phosphorylating TRPV1, leading to its sensitization (73–77). They can also increase the expression of these receptors/channels (78, 79). For example, by binding to its TrkA receptor, nerve growth factor (NGF) induces heightened expression of TRPV1 and ASIC3, and neurotransmitters such as SP, CGRP, brain-derived neurotrophic factor (BDNF), and various sodium and calcium channels that regulate nociceptor excitability (79). In addition, TRPV1 sensitization can result from functional interactions with other receptors, such as P2X3 or NMDA (80, 81). The list of algogenic mediators is too extensive to be exhaustively presented here. However, in Table 1, we have referenced a list of those known to play a role in cancer pain.
Regarding inflammatory mediators, PSNs express receptors for many of them, including cytokine receptors (TNFR, IL-1βR, IL-6R, and IL-17RA) and G-protein-coupled receptors for histamine, and prostanglandin E2 (PGE2) (Figure 2). The activation of these receptors is known to increase the excitability of nociceptive neurons and to enhance sensitization to subsequent stimuli, thus actively contributing to this process (120). The involvement of these mediators is well-documented in neuropathic pain, where the recruitment of pro-inflammatory cells like macrophages and T cells in the DRG and spinal cord has been evidenced (121–128). The importance of these immune cells and their pro-inflammatory cytokines in the maintenance of chronic pain is supported by experiments in which their depletion significantly reduced existing pain (123, 127, 128). Inflammatory mediators are not only produced by immune cells but also by glial cells like astrocytes and microglia. For instance, following peripheral nerve injury, neurons release chemokines such as CCL2 and CX3CL1 (129), along with other immune mediators like colony-stimulating factor 1 (CSF-1) and ATP (130, 131). These molecules can strongly activate spinal cord glia, leading to the release of pro-inflammatory cytokines (e.g., TNF-α, IL-1β, and IL-6), chemokines (e.g., CCL2), and nitric oxyde (NO) (132).
In the context of cancer pain, one must take into account components of TME, i.e., immune cells, which can produce substantial quantities of inflammatory algogenic mediators, and thus contribute to pain development and/or maintenance. The involvement of certain pro-inflammatory cytokines like TNF-α (82–86, 133), IL-1β (83, 86, 87, 133, 134), and IL-6 (78, 83, 86, 88) and other inflammatory mediators like PGE2 (133) in cancer pain has been reported in various cancers. Consequently, various NSAIDs are frequently used in clinical practice as adjuncts to stronger analgesics to potentially provide additional pain relief. For instance, in bone cancer where inflammatory mediators significantly stimulate afferent PSNs, NSAIDs are commonly used to alleviate cancer pain (135). However, clinical evidence supporting a substantial analgesic effect of classical NSAIDs like selective COX-1 and COX-2 inhibitors in cancer pain is generally lacking (136). Interestingly, drugs targeting pro-inflammatory molecules such as anti-TNF-α and anti-IL-1β are emerging as highly promising options for pain control and could potentially be employed in managing cancer pain (137).
In contrast to pro-nociceptive algogenic mediators, some factors present anti-nociceptive properties. One example is PD1/PD-L1 molecules (for programmed cell death protein 1/programmed death-ligand 1), when expressed on PSNs (138–141). The administration of PD-L1 to naive mice induces analgesia by activating PD-1 and downstream SHP-1 phosphorylation, leading to inhibition of the function of sodium channels and TRPV1, and enhancing the function of potassium channels (TREK2) in PSNs (138, 139, 142). In the context of cancer, the blockade of the PD-1/PD-L1 axis, either through anti-PD-1 administration or SHP-1 deletion in nociceptors, has been shown to aggravate cancer pain in mouse models of melanoma and bone cancer (138, 139). Moreover, in a recent study, Wanderley et al. demonstrated that combining anti-PD-L1 with chemotherapy can exacerbate chemotherapy-induced neuropathic pain (143). However, it is important to note that in a metastatic bone cancer model, despite a temporary increase in pain sensitivity after each treatment, anti-PD-1 immunotherapy leads to long-term advantages by preventing bone destruction and relieving bone cancer pain through the suppression of osteoclastogenesis (144). All these results have obviously many implications for the use of anti-PD1/anti-PD-L1 as immune checkpoint inhibitors and underscore their potential adverse effects on cancer pain. Other anti-nociceptive factors, such as endogenous opioids (enkephalins and endorphins), may also be secreted within the TME (145, 146). These molecules, typically released by immune cells during inflammation, interact with opioid receptors on the surface of nerve endings of sensory neurons to reduce pain sensitization (147). In a mouse model of oral cancer, tumor-infiltrating neutrophils have been shown to produce β-endorphins, thus alleviating tumor-associated pain (148). Paradoxically, in mammary tumor mice model, the presence of β-endorphins within the TME have a pro-nociceptive effect while promoting tumor development (149).
2.3.2 Ectopic nerve sprouting
In addition to nociceptor sensitization through algogenic mediators, cancer-related pain can also arise from heightened tissue innervation resulting from ectopic nerve sprouting (150)(Figure 1). Within the TME, various neurotrophic factors such as NGF, BDNF, glial cell–derived neurotrophic factor (GDNF), and vascular endothelial growth factor (VEGF) are released, leading to nerve sprouting (54–56, 97, 119, 151–155). Furthermore, it has been demonstrated that proteins involved in axonal guidance, such as molecules from the ephrin and netrin families, play a role in nerve sprouting within tumors. For instance, Netrin-1 induces the sprouting of sensory nerves and exacerbates pain sensitivity (156, 157). Additionally, Madeo et al. have shown that tumor cells can release the necessary molecules for axonogenesis, particularly ephrin family molecules, through exosome secretion (158). This disorganized sprouting results in a general increase in nerve fiber density, encompassing both sensory and sympathetic nerve fibers and the formation of neuroma-like structures. These changes contribute to episodes of severe acute pain and, in some cases, pain triggered by movement (54). In a healthy bone, sensory and sympathetic fibers are typically segregated. However, tumor-induced sprouting disrupts this separation, allowing sympathetic fibers to potentially activate nociceptive stimuli, thereby exciting adjacent sensory fibers (54).
2.3.3 Perineural invasion and neuritis
PNI is a process in which tumor cells infiltrate neighboring nerves (159). This process facilitates metastatic dissemination and nerve compression and promotes pain induction (Figure 1). Clinically, PNI is a recognized characteristic in cancer, often linked to a grim prognosis across various cancer types (160). PNI is prevalent in numerous malignant tumors, such as pancreatic cancer, oral cancer, gastric carcinoma, and biliary tract tumors. Interestingly, cancers that are notorious for causing pain tend to exhibit high rates of PNI. For instance, pancreatic cancer, known for its severe pain, frequently presents with PNI at rates ranging from 80% to 100% correlating with diminished survival and reduced quality of life (161, 162). PNI significantly contributes to the pain experienced by cancer patients, primarily due to the overlap between signaling molecules involved in PNI, such as neurotrophins and chemokines, and those involved in pain signaling (163). Furthermore, tumor cell invasion can lead to neuropathic pain by damaging the neural sheath, rendering nerve processes susceptible to detrimental extracellular matrix stimuli. Additionally, the communication between pancreatic cancer cells and nerves results in abnormal nerve growth and enlargement (48, 153).
In addition to tumor cell invasion, nearby nerves may also be infiltrated by immune cells, resulting in neuritis and neuropathic pain (164) (Figure 1). For example, in human pancreatic ductal adenocarcinoma (PDAC), pancreatic nerves were observed to be infiltrated by different types of leukocytes, and the presence of mast cells was strongly correlated with the sensation of abdominal pain in PDAC (165).
2.4 Cancer pain and psychological stress
In addition to pain, it is essential to consider that cancer also induces significant psychological stress. This stress primarily operates through stress hormones, namely, (nor-)adrenaline and glucocorticoids, which are released due to the activation of the sympathetic nervous system and the hypothalamic–pituitary–adrenal (HPA) axis (166). The influence of psychological stress and stress hormones on tumor development and progression has been extensively demonstrated and reviewed elsewhere (166). As a whole, the sympathetic nervous system is generally considered to promote tumorigenesis, notably by inhibiting CD8+ T cells priming and favoring their exhaustion (167, 168). Nevertheless, in the case of PDAC, it has been suggested that sympathetic neurons may exert an anti-tumor effect (152). Concerning glucocorticoids, recent studies have revealed that local glucocorticoid production can drive the dysfunctional or exhausted state of CD8+ T cells, undermining therapy-induced antitumor immunity and the response to immune checkpoint inhibitors (169, 170).
Surprisingly, there is a limited understanding of the connection between pain and stress, despite their frequent co-occurrence in cancer patients (Figure 3). However, it appears that the stress response, notably involving the sympathetic nervous system, plays a role in regulating cancer-related pain. For instance, the blockade of the sympathetic nervous system is a common approach to managing abdominal pain in cancer, although the precise underlying cellular and molecular mechanisms remain incompletely elucidated (171). In the context of oral cancer, specifically squamous cell carcinoma of the head and neck, recent research has demonstrated that the release of noradrenaline by sympathetic neurons stimulates the production of TNF-α by cancer cells. This TNF-α can then activate PSNs, leading to the experience of painful sensations (172). In addition to noradrenaline, sympathetic neurons secrete other molecules, including neuropeptide Y (NPY) and adenosine triphosphate (ATP), which can act on postganglionic sympathetic neurons expressing their specific receptors (173, 174). NPY has been implicated in both pro- and anti-nociceptive effects (173). Regarding ATP, it is stored and released along with noradrenaline in the synaptic vesicles of postganglionic sympathetic nerves (175). During stress-induced noradrenaline release, this ATP co-release could act on purinergic receptors expressed by PSNs and sensitized them. Additionally, considering that cancer induces neuropathic pain, it is plausible that post-ganglionic sympathetic neurons may sprout into DRG to activate PSNs and coordinate painful stimuli, as observed in nerve injury models (176). Finally, another compelling argument supporting the influence of stress on pain modulation is the fact that PSNs also express adrenergic receptors, which have the capacity to sensitize these neurons (172, 177–180).
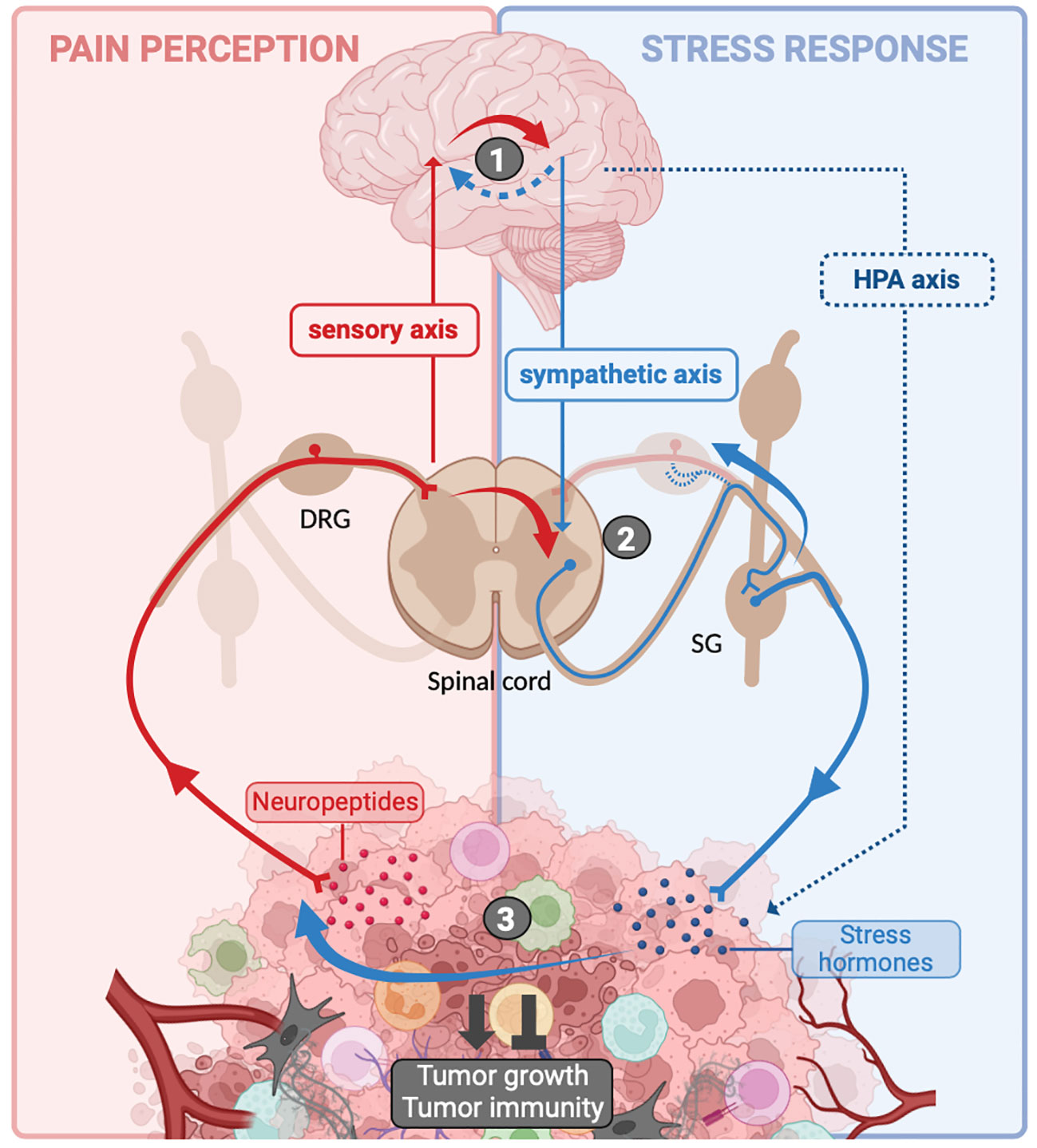
Figure 3 Crosstalk between pain and stress response in cancer. Sensitization of peripheral sensory neurons (PSNs) within the tumor microenvironment (TME) leads to the localized release of neuropeptides. Additionally, these neurons transmit an afferent nerve influx to the spinal cord, which relays this information to the brain. The cognitive processing of this information in the brain is responsible for pain perception (background). In the meantime, in response to the psychological stress caused by the disease, the brain activates the hypothalamic–pituitary–adrenal (HPA) axis and sympathetic neurons, resulting in the release of stress hormones [(nor-)adrenaline and glucocorticoids] into the TME (blue background). Interactions between the sensory axis and the sympathetic/HPA axis can take place at several levels: (1) at the brain level, afferent nerve impulses can trigger a stress response, resulting in activation of the HPA axis and the sympathetic system (solid red arrow). Similarly, it is possible that the stress induced by the disease may enhance pain perception (blue dotted arrow). (2) At the spinal cord and dorsal root ganglia (DRG) level, PSNs can transmit nerve impulses to interneurons that directly activate sympathetic neurons (solid red arrow). Additionally, due to nerve injury, sympathetic neurons from sympathetic ganglia (SG) may extend into DRG to activate PSNs. (3) Within the tumor itself, stress hormones may sensitize PSNs (solid blue arrow). All of these interactions result in the differential integration of pain and stress, and they influence tumor growth and the associated anti-tumor immune response. Created with BioRender.com.
Conversely, PSNs have been shown to promote efferent sympathetic nervous system feedback or HPA activation following stimulation of PSNs in a model of systemic inflammation (181) and atherosclerosis (182). The activity of the sympathetic neurons is regulated by descending supraspinal and primary afferent inputs. Indeed, some brain regions such as the rostral ventroLateral medulla (RVLM) and the paraventricular nucleus activate inhibitory or excitatory interneurons to modulate the activity of preganglionic sympathetic neurons (183). In addition to these descending inputs from the brain, afferent PSNs can also directly influence the activity of pre-ganglionic sympathetic neurons through the activation of propriospinal interneurons (Figure 3). This notably occurs after spinal nerve injury, where PSNs can overstimulate interneurons independently of supraspinal control. This results in the excessive activation of sympathetic neurons, leading to the dysfunction of multiple organs (184, 185). Finally, activation of PSNs can also attenuate sympathetic tone, as demonstrated in the bone marrow to regulate bone metabolism (186–188).
Collectively, there is therefore a body of evidence suggesting the existence of a potentially vicious cycle involving pain, stress, and tumor progression, warranting further investigation.
3 Cancer, sensory neurons and tumor immunity
3.1 Sensory innervation of the tumor
The peripheral nervous system plays a crucial role in maintaining the balance of healthy tissues by coordinating various molecular, cellular, and organic functions. Likewise, the homeostasis of tumors (like that of any organ) orchestrates these processes to optimize their functioning (189). Increasing evidence indicates that, just like in healthy tissues, tumors also modulate their innervation levels to optimize their growth and survival (190).
The observation that tumors can be highly innervated is not recent, and in a publication dating from 1897, Hugh H. Young already observed the presence of nerves in 50% of tumors (191). Despite this initial observation and the fact that as early as the mid-twentieth century, it had been shown that denervation could have an effect on tumor growth (192), the presence of nerve fibers in tumors and their influence on tumor growth remained controversial until the 2000s (193–195). Since then, numerous studies have clearly shown that human and murine tumors are extensively innervated by sympathetic and parasympathetic neurons. Although less well documented, sensory innervation of tumors has also been revealed in certain types of tumors (Table 2).
Several studies have indicated that tumor tissues from melanoma and ovarian cancer patients exhibit increased sensory innervation marked by TRPV1+ neurons when compared to healthy tissues (109, 203). As mentioned above, this could be the result of ectopic nerve sprouting. Additionally, various sensory neuronal markers in cancer patients have shown associations with different prognosis. The level of sensory innervation and the expression of sensory neuronal markers like TRPV1 and Nav1.8 have been linked to poor prognosis in breast cancer (196). In other cancer types, such as ovarian cancer, oral cancer, and melanoma, the relationship appears to be less clear, with some studies suggesting a favorable prognosis (200, 201, 205), while others indicate an unfavorable one (109, 198, 202, 206, 207). Furthermore, research has underscored the diagnostic significance of CGRP levels across various cancer types (208–212). In the case of SP, findings from patient samples data suggest that elevated SP levels are associated with poorly differentiated tumors in oral squamous cell carcinoma and gastric tumors (213, 214).
3.2 Influence of sensory neurons on cancer growth
While the impact of sympathetic and parasympathetic fibers on tumor growth has been extensively studied and well-documented (18), the role of sensory fibers, in particular, nociceptors, has only recently gained attention (Table 3, Figure 4).
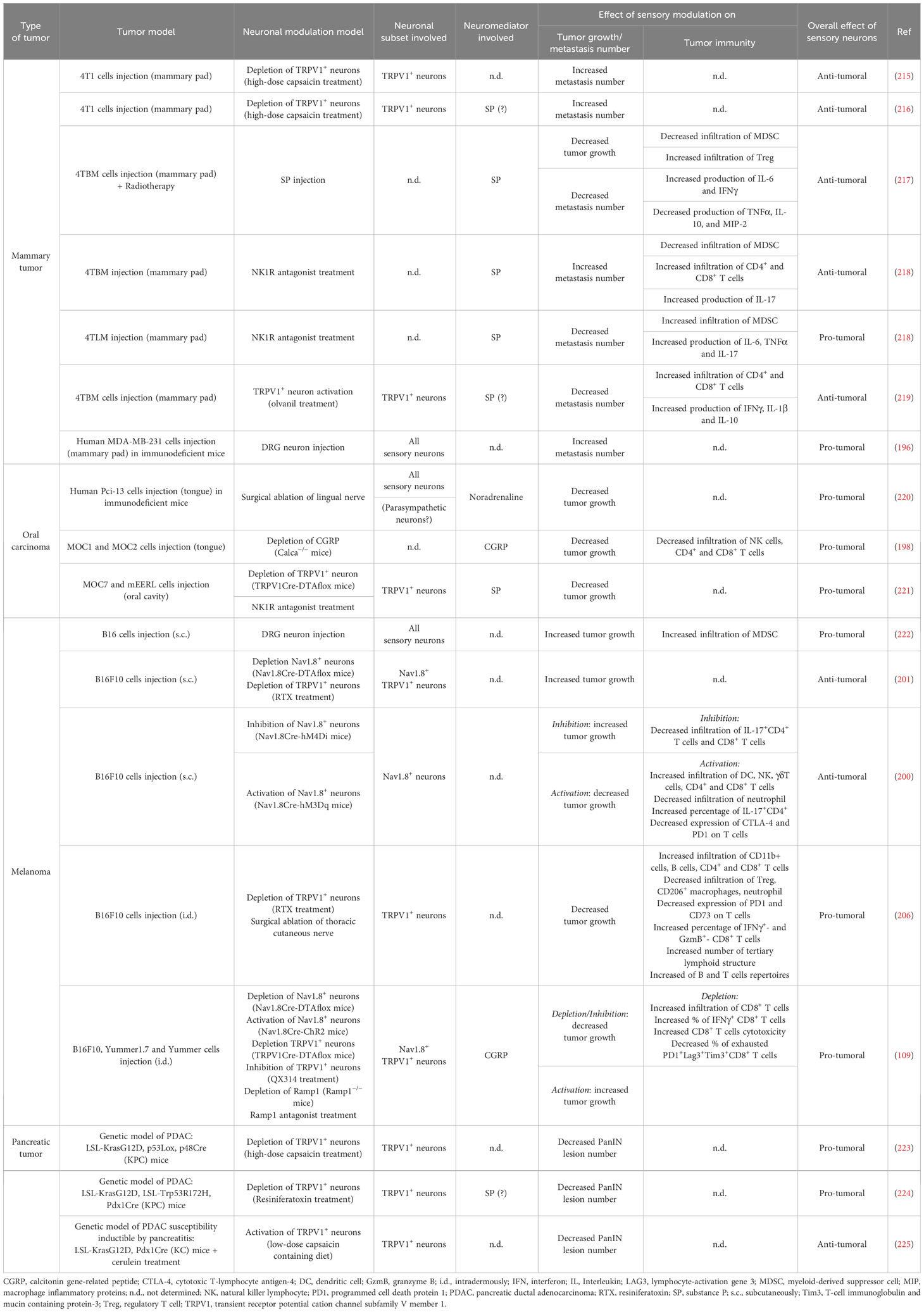
Table 3 Influence of peripheral sensory neurons on tumor growth and antitumor immune response in tumor mouse models.
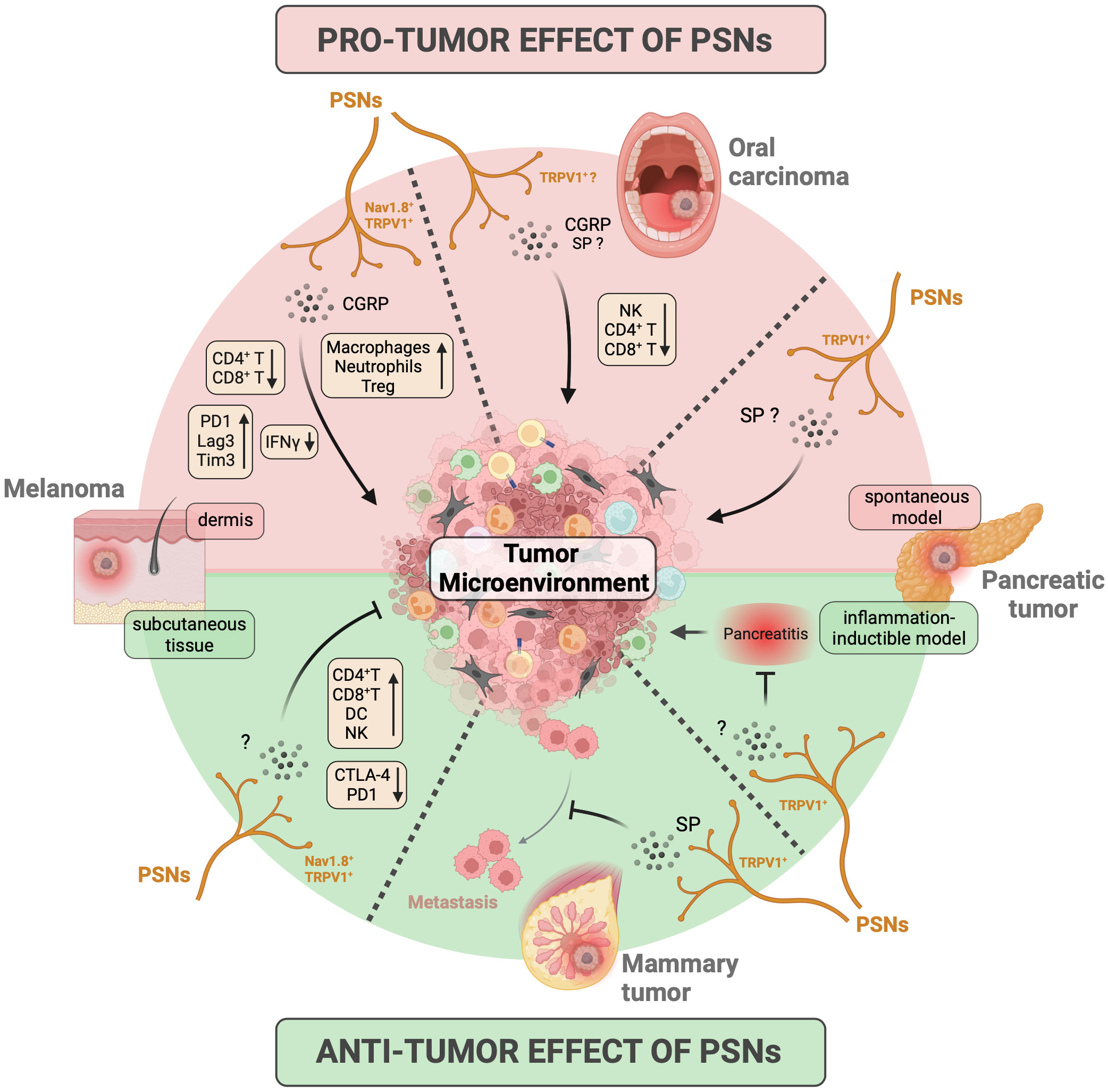
Figure 4 Influence of peripheral sensory neurons on tumor growth and antitumor immune response. The impact of peripheral sensory neurons (PSNs) on cancer development has primarily been investigated in murine models of melanoma, oral squamous carcinoma, pancreatic cancer, and breast cancer/metastasis. In these models, PSNs expressing Nav1.8 and TRPV1 ion channels release the neuropeptides CGRP and SP into the tumor microenvironment (TME). This leads to either pro-tumoral effects (indicated by the red background at the top) or anti-tumoral effects (indicated by the green background at the bottom) on primary tumors or metastases, depending on the specific cancer models studied. The results obtained for each tumor/model are represented in separate quadrants, delineated by dashed lines. Specifically, PSNs promote the growth of primary tumors in oral carcinoma models and inhibit the ability of mammary tumors to metastasize. Their influence on melanoma and pancreatic cancer is mixed and can depend on factors such as the location of melanoma tumor cell injection (pro-tumoral when injected in dermis and anti-tumoral when injected subcutaneously) and the genetic models of pancreatic cancer used (pro-tumoral in spontaneous development models and anti-tumoral in an inflammatory inducible model). Furthermore, PSNs also influence the anti-tumor immune response, affecting factors such as leukocyte recruitment, cytokine production, and the expression of exhaustion markers on T cells (as indicated in the yellow box). CGRP, calcitonin gene-related peptide; CTLA-4, cytotoxic T-lymphocyte antigen-4; DC, dendritic cell; IFN, interferon; NK, natural killer lymphocyte; PD1, programmed cell death protein 1; SP, substance P; Tim3, T-cell immunoglobulin and mucin containing protein-3; Treg, regulatory T cell; TRPV1, transient receptor potential cation channel subfamily V member 1. Created with BioRender.com.
The initial studies highlighting the impact of nociceptors on tumor development date back to the early 2000s, using mouse models of orthotopic 4T1 mammary tumors via chemical denervation experiments induced by administration of a high dose of capsaicin, a specific ligand of the TRPV1 receptor. Erin et al. first demonstrated that TRPV1+ PSNs do not affect the growth of primary mammary tumors but inhibit the formation of cardiac and pulmonary metastases, via a mechanism involving SP and its receptor neurokinin-1 receptor (NK-1R) (215, 216). However, they also showed that, conversely, NK1R antagonists could reduce the number of metastases when injecting a highly aggressive metastatic breast cancer cell line, suggesting a detrimental effect of SP in this case (218). Furthermore, in another model involving mammary tumor xenografts, it was demonstrated that co-administration of murine DRG neurons with human MDA-MB-231 cells in the orthotopic setting promoted pulmonary metastasis (196). In this case, in vitro experiments established a connection between the release of Semaphorin 5A by PSNs and tumor cell migration via Plexin B3 expression. Thus, in breast cancer, it appears that nociceptors primarily impact the metastatic process. Moreover, it is difficult to definitively determine the nature of their influence, whether it is beneficial or detrimental, as it seems to depend strongly on the model used and the degree of aggressiveness of the tumor cells.
In murine PDAC models, chemical denervation of TRPV1+ nociceptors leads to a decrease in the number of pancreatic intraepithelial neoplasms (PanIN) and PDAC lesions, resulting in improved mouse survival (223, 224). Co-culture experiments between DRG neurons and PDAC organoids have revealed that SP induces the secretion of trophic factors by neuroendocrine cells within neoplastic acini, thereby fostering tumor growth (224). Conversely, in a model stimulating PanIN lesion development through the induction of chronic pancreatitis, Bai et al. demonstrated that TRPV1+ nociceptor activation by diet supplemented with low-dose capsaicin exerts anti-tumor effects, leading to reduced PanIN damage and decreased expression of molecules associated with tumor cell proliferation (225). These discrepancies are likely due to differences in the models employed to induce PanIN/PDAC. Notably, in the pancreatitis-induced neoplasia model (225), the observed effects could be due to the control of inflammation by nociceptors rather than to its impact on carcinogenesis.
In oral cancer, tumor growth is diminished in mice lacking TRPV1+ PSNs (221) or CGRP (198) in orthotopic oral carcinoma mouse models. This effect might be attributed to the presence of receptors for CGRP (RAMP1 for receptor activity modifying protein 1) and SP (NK-1R) on tumor cells (198, 221) and/or immune cells (198). In another oral squamous cell carcinoma model induced by the carcinogen 4-nitroquinoline 1-oxyde (4NQO), Amit et al. have revealed that trigeminal PSNs undergo reprogramming into sympathetic-like neurons upon the integration of extracellular vesicles released by tumor cells (220). In this study, the release of noradrenaline by these reprogrammed PSNs fosters tumor growth, and the surgical removal of the lingual nerve leads to reduced tumor growth (220). Note, however, that the lingual nerve is not exclusively composed of PSNs but also contains parasympathetic fibers from the facial nerve (226), introducing a potential bias in the interpretation of these results. Hence, in the case of oral cancer, it appears that nociceptors may have a detrimental effect by promoting tumor growth.
Finally, studies using mouse models of melanoma have produced contradictory results. While some studies have indicated a pro-tumoral effect of Nav1.8+ and TRPV1+ PSNs (109, 206), others have reported the opposite (200, 201). They all used the B16 cell line as a melanoma model, but differ in the way the cells were injected. In experiments demonstrating pro-tumor effects, tumor cells are injected intradermally (109, 206), whereas those showing anti-tumor effects employ subcutaneous injection (200, 201). The different skin layers (epidermis, dermis, and subcutaneous tissue) exhibit distinct innervation and immune cell composition, the intradermic injection being the most immunogenic (227). This information is particularly significant, as Balood et al. have shown that the influence of nociceptors seems to depend on the degree of immunogenicity of the tumor cells used (109). Therefore, these anatomic and topological differences could account for these divergent outcomes. In addition, given that melanoma originates from malignant melanocytes located in the basal lamina of the epidermis, intradermal injection seems to correspond more to physiological conditions.
Due to the paucity of studies and to key experimental differences, one cannot definitively conclude that PSNs have a pro- or anti-tumor role. In addition to experimental differences, such as those observed in melanoma models, it seems that the impact of sensory fibers, harmful or beneficial, likely depends on the specific type of tumor and its anatomical location.
The observed impact of nociceptors on tumor growth might be a result of their influence on different cell types within the TME. For instance, one of the first known functions of neuropeptides is their capacity to regulate inflammation by directly acting on endothelial cells and smooth muscles, thereby controlling vascular permeability, extravasation, and blood flow (228). These vascular effects may impact tumor development by potentially influencing aspects such as the supply of nutrients and oxygen, or the infiltration of immune cells into the tumor. Other effects might also be attributed to the impact of nociceptors on tumor cells. Indeed, several studies have demonstrated that neuropeptide receptors are expressed on tumor cells, and their binding to neuropeptide ligands regulates various processes, including proliferation, survival, adhesion, and migration. This is particularly noteworthy, which have been shown to be involved in the progression of various cancer types, as shown in gastric, breast, and oral cancers, namely, through binding of SP to NK-1R expressed by tumor cells (213, 221, 229–242). Thus, sensory innervation could affect tumor growth not only by acting on tumor cells but also on tumor vascularization. This latter action could depend not only on neuropeptides but also on a structural factor, i.e., the fact that in most organs, the nervous and the vascular tree develop together. In addition, the effects of nociceptors on tumor growth may also result from their capacity to modulate tumor immunity, as discussed below.
3.3 Influence of sensory neurons on anti-tumor immunity
In addition to studying the effect of nociceptors on tumor growth, some studies have evaluated their influence on anti-tumor immunity (Table 3, Figure 4). As expected, these studies have observed a correlation between the influence of PSNs on tumor immunity and their effects on tumor growth.
In instances where PSNs exhibit a pro-tumor activity, they also tend to negatively affect the anti-tumor immune response (109, 198, 206). For example, in a mouse model of melanoma induced by intradermal injection of B16 cells, the depletion of nociceptors has been shown to increase the presence of immune cells with anti-tumoral properties, including CD4+ and CD8+ T cells, while reducing the abundance of pro-tumoral cells like regulatory T cells (Treg), tumor-associated macrophages (TAM), and neutrophils (109, 206). Moreover, in the absence of nociceptors, tumor-infiltrating CD8+ T lymphocytes (CD8+ TILs) display enhanced cytotoxicity; produce granzyme B, IFNγ, and TNF-α more effectively; and exhibit reduced exhaustion, as indicated by the lower expression of immune-checkpoint molecules PD-1, T-cell Immunoglobulin domain and mucin domain 3 (TIM-3), and lymphocyte activation gene 3 (LAG3). Balood et al. have shown that this is the result of a direct effect of CGRP on CD8+ TILs expressing RAMP1, the CGRP receptor, on their surface (109).. In this model, CGRP production by nociceptors is triggered by secretory leukocyte protease inhibitor (SLPI) released by B16 melanoma tumor cells. Vats et al. have also shown that PSNs prevent the formation of tertiary lymphoid structures (TLS), which play a role in the development of an effective anti-tumor melanoma response (206). Additionally, in an oral squamous cell carcinoma model, the depletion of CGRP leads to an increase in the infiltration of naturel killer (NK) and T cells (198). Thus, there is a whole set of studies in which nociceptors exert a pro-tumoral effect by inhibiting the anti-tumoral immune responses.
In contrast, in others studies, anti-tumor effects of nociceptors on tumor growth have been reported, mediated by an impact on anti-tumor immunity (200, 217, 219). In a melanoma mouse model induced by subcutaneous injection of B16 cells, activating nociceptors has been found to enhance the recruitment of NK, CD4+, and CD8+ T cells, which exhibit a less exhausted phenotype with reduced PD1 and cytotoxic T-lymphocyte-associated protein 4 (CTLA-4) expression (200). As for tumor growth, discrepancies in melanoma studies may be attributed to injection methods, either intradermal or subcutaneous. Additionally, in a model of metastatic breast cancer cell line injection, inhibiting the NK-1R receptor resulted in a decreased recruitment of CD4+ and CD8+ T cells and increased recruitment of myeloid-derived suppressor cells (MDSC) in metastasis (218). In the same model, stimulating nociceptors with TRPV1 agonist led to an enhanced recruitment of CD4+ and CD8+ T cells, along with improved cytokine production (243). However, it is worth noting that when a less aggressive metastatic breast cancer cell line was used, the authors observed the opposite outcome (218). Lastly, they also demonstrated that SP improved radiotherapy treatment efficacy by increasing IFN-γ and IL-6 production while reducing IL-10 production by restimulated leukocytes and by decreasing MDSC infiltration (217).
3.4 Putative effects of nociceptor mediators on tumor-infiltrating immune cells
In addition to these few studies, the putative effect of sensory neurons on the anti-tumor immune response can be apprehended with regard to the effects already described of nociceptor mediators, mainly CGRP and SP neuropeptides, on immune cells in a non-cancer context and/or within experiments carried out in vitro.
In most scenarios, SP boosts the immune response by increasing the release of inflammatory cytokines in the acute phase and enhancing the cytotoxicity of immune effectors. It improves mouse T-cell activation and IFN-γ production (244–246) and stimulates human T-cell proliferation by enhancing IL-2 production (247–249). SP may also promote the function of effector CD8+ T cells in autoimmune disorder, such has alopecia areata (250). It regulates neutrophil influx and enhances their phagocytic activity in inflamed tissues (251). SP potentiates the immunostimulatory role of skin-resident Langerhans cells, leading to the activation of T helper 1 (Th1)and IFN-γ-producing CD8+ T cells (252). However, in response to allergens in the skin, it acts also on dermal dendritic cells (DCs), promoting their migration to the draining lymph nodes (LNs), where they induce Th2 activation (253). SP may also elevate IL-12 production by DCs and macrophages (254–256). SP can additionally boost monocyte chemotaxis (257) and stimulate their release of pro-inflammatory cytokines, thus promoting Th17 cell generation (258). In a dose-dependent manner, SP increases NK cell migration and IFN-γ secretion (259, 260). SP also facilitates the generation of memory CD8+ T cells during the primary immune response by improving antigen-presenting cells (APCs) function (248). In specific conditions, it counteracts the immunosuppressive activity of Treg (261). Thus, SP may be viewed as a nerve-released, pleiotropic potentiator of the activation of pro-inflammatory immune cells.
Concerning the effects of CGRP on immune cells, these effects appear to be more contrasted than those of SP. On the one hand, a significant number of studies has pointed to its primarily immunosuppressive characteristics. For instance, CGRP suppresses the production of IFN-γ, TNF-α, and IL-2 by CD4+ T cells (262–265). Treatment of Langerhans cells with CGRP has also been shown to reduce their ability to migrate to draining LNs (266), to stimulate T-cell proliferation (267, 268), and to promote the production of IFN-γ, CXCL9, and CXCL10 by CD4+ T cells (269). Moreover, treatment of human monocytes or DCs with CGRP inhibits their functions and significantly decreases the proliferative response of allogeneic T cells (270–272). These effects may be attributed to CGRP downregulating the expression of class II major histocompatibility complex (MHC-II) and CD86 co-stimulatory molecules and inhibiting the release of IL-12, IL-1β, TNF-α, and CCL4 by APCs (268, 271–274). In the case of infection by Staphylococcus aureus in the lungs or Streptococcus pyogenes in the skin, CGRP also inhibits the recruitment of neutrophils to the affected tissues (10, 11). Lastly, CGRP plays a crucial role as an inhibitory factor for type 2 innate lymphoid cell (ILC2) responses, restraining acute airway inflammation (275, 276). In contrast, studies have shown that CGRP may have an immuno-activating effect. For instance, several studies across different models suggest that CGRP promotes type 17 immune response. First, it has been observed that CGRP exacerbates experimental autoimmune encephalomyelitis by promoting Th17 functions (277). In addition, in psoriasis and C. elegans infection models, CGRP stimulates DCs to produce IL-23 thereby facilitating the inappropriate activation of IL-17-producing γδT cells (5, 9). Finally, in a model of optogenetic activation to TRPV1+ nociceptors, induction of type 17 inflammation requires the vesicle release of CGRP (278). Recently, Hanc et al. conducted a detailed investigation into the effects of CGRP on DC biology, revealing that DC activation in the presence of nociceptors enhances their production of pro-inflammatory cytokines. Moreover, CGRP induces a heightened sentinel phenotype in DCs, characterized by increased pro-IL-1β in CGRP-treated DCs (279). To account for these seemingly contradictory results, we propose the following hypothesis: CGRP may interact with different types of target cells in the body, and these cells may exhibit different inflammatory responses. For example, CGRP may act on immune cells to decrease the production of proinflammatory cytokines, but it may also act on endothelial cells to promote vasodilation and increase inflammation under certain conditions. Moreover, the co-release of other peptides by PSNs producing CGRP, depending on the stimulus/challenge, could also account for some of theses contradictory results.
We have mainly focused on CGRP and SP here, but it is worth noting that PSNs release a whole range of other neuropeptides and factors that can influence immune cell responses. For example, vasoactive intestinal peptide (VIP) is involved in promoting allergic inflammation in asthma by acting on ILC2 and Th2 cells (6). In addition, PSN-derived TAFA-4 plays a role in facilitating tissue repair by modulating the inflammatory profile of macrophages (13). Finally, PSNs also have the ability to generate chemokines, including CCL2, which contribute to immune cell recruitment (122, 222, 279, 280).
All of these findings, mainly observed in non-cancer context or in vitro studies, emphasize the capacity of neuropeptides and, consequently, nociceptors to enhance or suppress cytotoxic responses. This could potentially impact the anti-tumor immune responses.
3.5 Transcriptional changes of PSNs induced by the tumor
As described above, PSNs can be sensitized by the tumor through algogenic mediators or neuronal lesions leading to cancer pain (Figure 2). The TME can also induce structural changes, such as growth and axon branching in PSNs, included in the ectopic neuronal sprouting evoked earlier (109, 197, 220, 222). Furthermore, tumor cells can induce a metabolic change in PSNs, leading to a reduced oxygen consumption and a deficit in neuronal electrical activity in a colon cancer model (281).
In addition, it is likely that tumors trigger profound transcriptional changes in the PSNs that innervate them. PNI, tumor compression causing neuronal damage, and chronic hypersensitivity of neurons innervating the tumor could be responsible for these transcriptomic changes. Such a phenomenon has been described after nerve injury, such as sciatic nerve ligation or transection (20). In this study, nerve injury induced transcriptomic changes leading to transient loss of identity of sensory neuron subsets in DRGs. This was associated with increased expression of nerve injury or activation markers such as the transcription factor Atf3 (activating transcription factor 3) and Sox11 (SRY-Box transcription factor 11). Interestingly, a similar induction of Atf3 is also observed in PSNs innervating the tumor. Thus, an increase in Atf3 expression has been shown in the PDAC model (45, 223) and in various bone cancer models (282–284). In the case of pancreatic cancer, this could be the consequence of its particular ability to induce PNI, causing neuronal damage and neuropathic pain (162). Regarding oral cancer, in a xenograft model, no Atf3 expression was observed (99), in contrast to a syngeneic model (285). However, even in the absence of Atf3 expression in PSNs, the tumor can induce a significant transcriptional change in the tumor-innervating PSNs, leading to increased transcription of multiple genes, including some involved in nociceptor sensitization (99, 281, 286). Furthermore, in an oral squamous cell carcinoma model, it was observed that trigeminal PSNs undergo reprogramming into sympathetic-like neurons upon the integration of extracellular vesicles released by tumor cells (220). Finally, in in vitro co-culture experiments, Ballod et al. have also shown that DRG neurons exposure to melanoma cells leads to changes in their transcriptome (109).
As done in nerve injury models (20), it is crucial to thoroughly document the transcriptomic changes induced by the tumor in PSNs and investigate its impact on tumor development and the associated immune response.
4 Key research questions
What we have discussed in this review paves the way for numerous questions and future research endeavors.
● Pain, resulting from the activation of nociceptors and the infiltration of the immune system both seem to carry prognostic significance in the context of cancer progression. Is there a connection between these two factors, and does a triple correlation exist between the pain level of a tumor, the extent of immune cell infiltration, and its progression?
● Considering that nociceptors may potentially exert a beneficial influence on the anti-tumoral immune response, it would be important to assess whether the use of analgesic treatments targeting these nociceptors (e.g., lidocaine) could lead to detrimental suppression of antitumor immunity.
● To date, most research into the effect of PSNs on the anti-tumor immune response has focused primarily on peptidergic nociceptors, specifically the Nav1.8+ TRPV1+ PSNs. Nevertheless, recent findings indicate that other types of PSNs, particularly non-peptidergic nociceptors, play a role in modulating inflammation and the immune response (13, 14). Could these PSNs also be involved in regulating the anti-tumor immune response?
● Current studies on the effects of nociceptors on tumor growth and immune response primarily evaluate their action through the local release of neuropeptides. However, one of their main features is to generate a signal that leads to an efferent response involving other types of neurons (e.g., sympathetic and parasympathetic) that can also influence tumor growth and immunity. This aspect of their influence, and their interactions with the different branches of the nervous system, is still largely unexplored and should be the focus of future investigations.
● The relationship between pain and psychological stress and how they influence each other is still relatively poorly documented. An intriguing question is whether the stress generated by the disease could promote cognitive sensations of pain. Given its prevalence and their significance for the well-being of patients, it is essential to gain a better understanding of the molecular and cellular mechanisms that underlie the relationship between pain and psychological stress.
5 Concluding remarks
Cancer pain is typically regarded as a byproduct of the disease, and it is managed as such. However, it also reflects the activation of PSNs, particularly nociceptors, which may have important consequences for cancer development, tumor immunity, and thus cancer treatment. Thus, in addition to the cognitive perception of pain, the recognition of noxious signals results in the activation of PSNs leading to the peripheral or central production of neuromediators, such as neuropeptides. These neuromediators have the capacity to directly impact not only tumor cells but also all cells within TME, including immune cells engaged in anti-tumor immune responses. They can modulate these cell functions, either positively or negatively. Conversely, the tumor itself exerts influence on PSNs via physical mechanisms (e.g., nerve compression or injury), PNI, or the release of algogenic mediators. This can result in hypersensitization or damage to these neurons, contributing to the generation of cancer-related pain, and potentially leading to significant deep transcriptomic alterations.
In summary, there exists a complex interplay between the tumor and the sensory nerve fibers within it. This interplay can take on either a detrimental or beneficial nature, depending on the specific characteristics of the cancer, and it undeniably has profound implications for tumor development and associated immunity. Beyond the fundamental interest in understanding the influence of PSNs on tumor growth and anti-tumor immunity, future research could lead to the discovery of new anti-cancer therapeutic targets, such as neuropeptides and their receptors, that could eventually be combined with other anti-cancer treatments, such as chemotherapy or immunotherapy, to improve their efficacy.
Author contributions
UM: Conceptualization, Writing – original draft, Writing – review & editing. NB: Conceptualization, Writing – original draft, Writing – review & editing. CD: Conceptualization, Writing – review & editing. VF: Conceptualization, Funding acquisition, Project administration, Supervision, Writing – original draft, Writing – review & editing.
Funding
The author(s) declare financial support was received for the research, authorship, and/or publication of this article. Fondation ARC pour la recherche sur le cancer (ARCPJA2021060003706), Fondation Française pour la Recherche contre le Myelome et les Gammapathies (00130253/EF-2022-25881).
Acknowledgments
We thank members of the laboratory for fruitful discussions. We also thank our colleagues in neurobiology, especially Aziz Moqrich, for his expertise and advice on pain neurobiology.
Conflict of interest
The authors declare that the research was conducted in the absence of any commercial or financial relationships that could be construed as a potential conflict of interest.
Publisher’s note
All claims expressed in this article are solely those of the authors and do not necessarily represent those of their affiliated organizations, or those of the publisher, the editors and the reviewers. Any product that may be evaluated in this article, or claim that may be made by its manufacturer, is not guaranteed or endorsed by the publisher.
References
1. Anderson NM, Simon MC. The tumor microenvironment. Curr Biol. (2020) 30:R921–R5. doi: 10.1016/j.cub.2020.06.081
2. Zhang Y, Zhang Z. The history and advances in cancer immunotherapy: understanding the characteristics of tumor-infiltrating immune cells and their therapeutic implications. Cell Mol Immunol. (2020) 17:807–21. doi: 10.1038/s41423-020-0488-6
3. Winkler F, Venkatesh HS, Amit M, Batchelor T, Demir IE, Deneen B, et al. Cancer neuroscience: state of the field, emerging directions. Cell. (2023) 186:1689–707. doi: 10.1016/j.cell.2023.02.002
4. Baral P, Udit S, Chiu IM. Pain and immunity: implications for host defence. Nat Rev Immunol. (2019) 19:433–47. doi: 10.1038/s41577-019-0147-2
5. Riol-Blanco L, Ordovas-Montanes J, Perro M, Naval E, Thiriot A, Alvarez D, et al. Nociceptive sensory neurons drive interleukin-23-mediated psoriasiform skin inflammation. Nature. (2014) 510(7503):157–61. doi: 10.1038/nature13199
6. Talbot S, Abdulnour RE, Burkett PR, Lee S, Cronin SJ, Pascal MA, et al. Silencing nociceptor neurons reduces allergic airway inflammation. Neuron. (2015) 87:341–54. doi: 10.1016/j.neuron.2015.06.007
7. Serhan N, Basso L, Sibilano R, Petitfils C, Meixiong J, Bonnart C, et al. House dust mites activate nociceptor-mast cell clusters to drive type 2 skin inflammation. Nat Immunol. (2019) 20(11):1435–43. doi: 10.1038/s41590-019-0493-z
8. Chiu IM, Heesters BA, Ghasemlou N, Von Hehn CA, Zhao F, Tran J, et al. Bacteria activate sensory neurons that modulate pain and inflammation. Nature. (2013) 501:52–7. doi: 10.1038/nature12479
9. Kashem SW, Riedl MS, Yao C, Honda CN, Vulchanova L, Kaplan DH. Nociceptive sensory fibers drive interleukin-23 production from cd301b+ Dermal dendritic cells and drive protective cutaneous immunity. Immunity. (2015) 43:515–26. doi: 10.1016/j.immuni.2015.08.016
10. Baral P, Umans BD, Li L, Wallrapp A, Bist M, Kirschbaum T, et al. Nociceptor sensory neurons suppress neutrophil and gammadelta T cell responses in bacterial lung infections and lethal pneumonia. Nat Med. (2018) 24:417–26. doi: 10.1038/nm.4501
11. Pinho-Ribeiro FA, Baddal B, Haarsma R, O'Seaghdha M, Yang NJ, Blake KJ, et al. Blocking neuronal signaling to immune cells treats streptococcal invasive infection. Cell. (2018) 173:1083–97 e22. doi: 10.1016/j.cell.2018.04.006
12. Filtjens J, Roger A, Quatrini L, Wieduwild E, Gouilly J, Hoeffel G, et al. Nociceptive sensory neurons promote cd8 T cell responses to hsv-1 infection. Nat Commun. (2021) 12:2936. doi: 10.1038/s41467-021-22841-6
13. Hoeffel G, Debroas G, Roger A, Rossignol R, Gouilly J, Laprie C, et al. Sensory neuron-derived tafa4 promotes macrophage tissue repair functions. Nature. (2021) 594:94–9. doi: 10.1038/s41586-021-03563-7
14. Zhang S, Edwards TN, Chaudhri VK, Wu J, Cohen JA, Hirai T, et al. Nonpeptidergic neurons suppress mast cells via glutamate to maintain skin homeostasis. Cell. (2021) 184:2151–66 e16. doi: 10.1016/j.cell.2021.03.002
15. Yang NJ, Neel DV, Deng L, Heyang M, Kennedy-Curran A, Tong VS, et al. Nociceptive sensory neurons mediate inflammation induced by bacillus anthracis edema toxin. Front Immunol. (2021) 12:642373. doi: 10.3389/fimmu.2021.642373
16. Lai NY, Musser MA, Pinho-Ribeiro FA, Baral P, Jacobson A, Ma P, et al. Gut-innervating nociceptor neurons regulate peyer's patch microfold cells and sfb levels to mediate salmonella host defense. Cell. (2019) 180(1):33–49. doi: 10.1016/j.cell.2019.11.014
17. Feuillet V, Ugolini S, Reynders A. Differential regulation of cutaneous immunity by sensory neuron subsets. Trends Neurosci. (2023) 46(8):640–53. doi: 10.1016/j.tins.2023.05.003
18. Kamiya A, Hiyama T, Fujimura A, Yoshikawa S. Sympathetic and parasympathetic innervation in cancer: therapeutic implications. Clin Auton Res. (2021) 31:165–78. doi: 10.1007/s10286-020-00724-y
19. Usoskin D, Furlan A, Islam S, Abdo H, Lonnerberg P, Lou D, et al. Unbiased classification of sensory neuron types by large-scale single-cell rna sequencing. Nat Neurosci. (2015) 18:145–53. doi: 10.1038/nn.3881
20. Renthal W, Tochitsky I, Yang L, Cheng YC, Li E, Kawaguchi R, et al. Transcriptional reprogramming of distinct peripheral sensory neuron subtypes after axonal injury. Neuron. (2020) 108:128–44 e9. doi: 10.1016/j.neuron.2020.07.026
21. Kupari J, Usoskin D, Parisien M, Lou D, Hu Y, Fatt M, et al. Single cell transcriptomics of primate sensory neurons identifies cell types associated with chronic pain. Nat Commun. (2021) 12:1510. doi: 10.1038/s41467-021-21725-z
22. Nguyen MQ, von Buchholtz LJ, Reker AN, Ryba NJ, Davidson S. Single-nucleus transcriptomic analysis of human dorsal root ganglion neurons. Elife. (2021) 10. doi: 10.7554/eLife.71752
23. Jung M, Dourado M, Maksymetz J, Jacobson A, Laufer BI, Baca M, et al. Cross-species transcriptomic atlas of dorsal root ganglia reveals species-specific programs for sensory function. Nat Commun. (2023) 14:366. doi: 10.1038/s41467-023-36014-0
24. Seal RP, Wang X, Guan Y, Raja SN, Woodbury CJ, Basbaum AI, et al. Injury-induced mechanical hypersensitivity requires C-low threshold mechanoreceptors. Nature. (2009) 462:651–5. doi: 10.1038/nature08505
25. Larsson M, Nagi SS. Role of C-tactile fibers in pain modulation: animal and human perspectives. Curr Opin Behav Sci. (2022) 43:138–44. doi: 10.1016/j.cobeha.2021.09.005
26. Murthy SE, Dubin AE, Patapoutian A. Piezos thrive under pressure: mechanically activated ion channels in health and disease. Nat Rev Mol Cell Biol. (2017) 18:771–83. doi: 10.1038/nrm.2017.92
27. Wang H, Xu C. A novel progress: glial cells and inflammatory pain. ACS Chem Neurosci. (2022) 13:288–95. doi: 10.1021/acschemneuro.1c00607
28. Domoto R, Sekiguchi F, Tsubota M, Kawabata A. Macrophage as a peripheral pain regulator. Cells. (2021) 10. doi: 10.3390/cells10081881
29. Ji RR, Chamessian A, Zhang YQ. Pain regulation by non-neuronal cells and inflammation. Science. (2016) 354:572–7. doi: 10.1126/science.aaf8924
30. Kuner R. Central mechanisms of pathological pain. Nat Med. (2010) 16:1258–66. doi: 10.1038/nm.2231
31. Basbaum AI, Bautista DM, Scherrer G, Julius D. Cellular and molecular mechanisms of pain. Cell. (2009) 139:267–84. doi: 10.1016/j.cell.2009.09.028
32. Ploner M, Sorg C, Gross J. Brain rhythms of pain. Trends Cognit Sci. (2017) 21:100–10. doi: 10.1016/j.tics.2016.12.001
33. Kuner R, Kuner T. Cellular circuits in the brain and their modulation in acute and chronic pain. Physiol Rev. (2021) 101:213–58. doi: 10.1152/physrev.00040.2019
34. Apkarian AV, Bushnell MC, Treede RD, Zubieta JK. Human brain mechanisms of pain perception and regulation in health and disease. Eur J Pain. (2005) 9:463–84. doi: 10.1016/j.ejpain.2004.11.001
35. Dubin AE, Patapoutian A. Nociceptors: the sensors of the pain pathway. J Clin Invest. (2010) 120:3760–72. doi: 10.1172/JCI42843
36. Skljarevski V, Ramadan NM. The nociceptive flexion reflex in humans – review article. Pain. (2002) 96:3–8. doi: 10.1016/s0304-3959(02)00018-0
37. Pavlov VA, Tracey KJ. Neural regulation of immunity: molecular mechanisms and clinical translation. Nat Neurosci. (2017) 20:156–66. doi: 10.1038/nn.4477
38. Costigan M, Scholz J, Woolf CJ. Neuropathic pain: A maladaptive response of the nervous system to damage. Annu Rev Neurosci. (2009) 32:1–32. doi: 10.1146/annurev.neuro.051508.135531
39. Schmidt BL. The neurobiology of cancer pain. Neuroscientist. (2014) 20:546–62. doi: 10.1177/1073858414525828
40. Caraceni A, Shkodra M. Cancer pain assessment and classification. Cancers (Basel). (2019) 11. doi: 10.3390/cancers11040510
41. Viderman D, Tapinova K, Aubakirova M, Abdildin YG. The prevalence of pain in chronic diseases: an umbrella review of systematic reviews. J Clin Med. (2023) 12. doi: 10.3390/jcm12237302
42. Connelly ST, Schmidt BL. Evaluation of pain in patients with oral squamous cell carcinoma. J Pain. (2004) 5:505–10. doi: 10.1016/j.jpain.2004.09.002
43. Ye Y, Dang D, Zhang J, Viet CT, Lam DK, Dolan JC, et al. Nerve growth factor links oral cancer progression, pain, and cachexia. Mol Cancer Ther. (2011) 10:1667–76. doi: 10.1158/1535-7163.MCT-11-0123
44. Naik K, Janal MN, Chen J, Bandary D, Brar B, Zhang S, et al. The histopathology of oral cancer pain in a mouse model and a human cohort. J Dent Res. (2021) 100:194–200. doi: 10.1177/0022034520961020
45. Stopczynski RE, Normolle DP, Hartman DJ, Ying H, DeBerry JJ, Bielefeldt K, et al. Neuroplastic changes occur early in the development of pancreatic ductal adenocarcinoma. Cancer Res. (2014) 74:1718–27. doi: 10.1158/0008-5472.CAN-13-2050
46. Epstein JB, Miaskowski C. Oral pain in the cancer patient. J Natl Cancer Inst Monogr. (2019) 2019. doi: 10.1093/jncimonographs/lgz003
47. Yang L, Liu B, Zheng S, Xu L, Yao M. Understanding the initiation, delivery and processing of bone cancer pain from the peripheral to the central nervous system. Neuropharmacology. (2023) 237:109641. doi: 10.1016/j.neuropharm.2023.109641
48. Ceyhan GO, Michalski CW, Demir IE, Muller MW, Friess H. Pancreatic pain. Best Pract Res Clin Gastroenterol. (2008) 22:31–44. doi: 10.1016/j.bpg.2007.10.016
49. van den Beuken-van Everdingen MH, Hochstenbach LM, Joosten EA, Tjan-Heijnen VC, Janssen DJ. Update on prevalence of pain in patients with cancer: systematic review and meta-analysis. J Pain Symptom Manage. (2016) 51:1070–90 e9. doi: 10.1016/j.jpainsymman.2015.12.340
50. Falk S, Dickenson AH. Pain and nociception: mechanisms of cancer-induced bone pain. J Clin Oncol. (2014) 32:1647–54. doi: 10.1200/JCO.2013.51.7219
51. Zylla D, Steele G, Gupta P. A systematic review of the impact of pain on overall survival in patients with cancer. Support Care Cancer. (2017) 25:1687–98. doi: 10.1007/s00520-017-3614-y
52. Bennett MI. Mechanism-based cancer-pain therapy. Pain. (2017) 158 Suppl 1:S74–S8. doi: 10.1097/j.pain.0000000000000825
53. Chartier SR, Mitchell SAT, Majuta LA, Mantyh PW. The changing sensory and sympathetic innervation of the young, adult and aging mouse femur. Neuroscience. (2018) 387:178–90. doi: 10.1016/j.neuroscience.2018.01.047
54. Mantyh WG, Jimenez-Andrade JM, Stake JI, Bloom AP, Kaczmarska MJ, Taylor RN, et al. Blockade of nerve sprouting and neuroma formation markedly attenuates the development of late stage cancer pain. Neuroscience. (2010) 171:588–98. doi: 10.1016/j.neuroscience.2010.08.056
55. Jimenez-Andrade JM, Bloom AP, Stake JI, Mantyh WG, Taylor RN, Freeman KT, et al. Pathological sprouting of adult nociceptors in chronic prostate cancer-induced bone pain. J Neurosci. (2010) 30:14649–56. doi: 10.1523/JNEUROSCI.3300-10.2010
56. Bloom AP, Jimenez-Andrade JM, Taylor RN, Castaneda-Corral G, Kaczmarska MJ, Freeman KT, et al. Breast cancer-induced bone remodeling, skeletal pain, and sprouting of sensory nerve fibers. J Pain. (2011) 12:698–711. doi: 10.1016/j.jpain.2010.12.016
57. McIlwain WR, Sood AJ, Nguyen SA, Day TA. Initial symptoms in patients with hpv-positive and hpv-negative oropharyngeal cancer. JAMA Otolaryngol Head Neck Surg. (2014) 140:441–7. doi: 10.1001/jamaoto.2014.141
58. Sivaraj KK, Adams RH. Blood vessel formation and function in bone. Development. (2016) 143:2706–15. doi: 10.1242/dev.136861
59. Shi J, Wei Y, Xia J, Wang S, Wu J, Chen F, et al. Cxcl12-cxcr4 contributes to the implication of bone marrow in cancer metastasis. Future Oncol. (2014) 10:749–59. doi: 10.2217/fon.13.193
60. Fell GL, Robinson KC, Mao J, Woolf CJ, Fisher DE. Skin beta-endorphin mediates addiction to uv light. Cell. (2014) 157:1527–34. doi: 10.1016/j.cell.2014.04.032
61. Fallon M, Giusti R, Aielli F, Hoskin P, Rolke R, Sharma M, et al. Management of cancer pain in adult patients: esmo clinical practice guidelines. Ann Oncol. (2018) 29:iv166–iv91. doi: 10.1093/annonc/mdy152
62. Paice JA, Bohlke K, Barton D, Craig DS, El-Jawahri A, Hershman DL, et al. Use of opioids for adults with pain from cancer or cancer treatment: asco guideline. J Clin Oncol. (2023) 41:914–30. doi: 10.1200/JCO.22.02198
63. Brozovic G, Lesar N, Janev D, Bosnjak T, Muhaxhiri B. Cancer pain and therapy. Acta Clin Croat. (2022) 61:103–8. doi: 10.20471/acc.2022.61.s2.13
64. Kane CM, Mulvey MR, Wright S, Craigs C, Wright JM, Bennett MI. Opioids combined with antidepressants or antiepileptic drugs for cancer pain: systematic review and meta-analysis. Palliat Med. (2018) 32:276–86. doi: 10.1177/0269216317711826
65. Yoon SY, Oh J. Neuropathic cancer pain: prevalence, pathophysiology, and management. Korean J Intern Med. (2018) 33:1058–69. doi: 10.3904/kjim.2018.162
66. Benyamin R, Trescot AM, Datta S, Buenaventura R, Adlaka R, Sehgal N, et al. Opioid complications and side effects. Pain Physician. (2008) 11:S105–20. doi: 10.36076/ppj.2008/11/S105
67. Schmidt BL, Hamamoto DT, Simone DA, Wilcox GL. Mechanism of cancer pain. Mol Interv. (2010) 10:164–78. doi: 10.1124/mi.10.3.7
68. Nagae M, Hiraga T, Yoneda T. Acidic microenvironment created by osteoclasts causes bone pain associated with tumor colonization. J Bone Miner Metab. (2007) 25:99–104. doi: 10.1007/s00774-006-0734-8
69. Hiasa M, Okui T, Allette YM, Ripsch MS, Sun-Wada GH, Wakabayashi H, et al. Bone pain induced by multiple myeloma is reduced by targeting V-atpase and asic3. Cancer Res. (2017) 77:1283–95. doi: 10.1158/0008-5472.CAN-15-3545
70. Wakabayashi H, Wakisaka S, Hiraga T, Hata K, Nishimura R, Tominaga M, et al. Decreased sensory nerve excitation and bone pain associated with mouse lewis lung cancer in trpv1-deficient mice. J Bone Miner Metab. (2018) 36:274–85. doi: 10.1007/s00774-017-0842-7
71. de Almeida AS, Bernardes LB, Trevisan G. Trp channels in cancer pain. Eur J Pharmacol. (2021) 904:174185. doi: 10.1016/j.ejphar.2021.174185
72. Duitama M, Moreno Y, Santander SP, Casas Z, Sutachan JJ, Torres YP, et al. Trp channels as molecular targets to relieve cancer pain. Biomolecules. (2021) 12. doi: 10.3390/biom12010001
73. Premkumar LS, Ahern GP. Induction of vanilloid receptor channel activity by protein kinase C. Nature. (2000) 408:985–90. doi: 10.1038/35050121
74. Vellani V, Mapplebeck S, Moriondo A, Davis JB, McNaughton PA. Protein kinase C activation potentiates gating of the vanilloid receptor vr1 by capsaicin, protons, heat and anandamide. J Physiol. (2001) 534:813–25. doi: 10.1111/j.1469-7793.2001.00813.x
75. Bhave G, Zhu W, Wang H, Brasier DJ, Oxford GS, Gereau R. Camp-dependent protein kinase regulates desensitization of the capsaicin receptor (Vr1) by direct phosphorylation. Neuron. (2002) 35:721–31. doi: 10.1016/s0896-6273(02)00802-4
76. Jin X, Morsy N, Winston J, Pasricha PJ, Garrett K, Akbarali HI. Modulation of trpv1 by nonreceptor tyrosine kinase, C-src kinase. Am J Physiol Cell Physiol. (2004) 287:C558–63. doi: 10.1152/ajpcell.00113.2004
77. Zhang X, Huang J, McNaughton PA. Ngf rapidly increases membrane expression of trpv1 heat-gated ion channels. EMBO J. (2005) 24:4211–23. doi: 10.1038/sj.emboj.7600893
78. Fang D, Kong LY, Cai J, Li S, Liu XD, Han JS, et al. Interleukin-6-mediated functional upregulation of trpv1 receptors in dorsal root ganglion neurons through the activation of jak/pi3k signaling pathway: roles in the development of bone cancer pain in a rat model. Pain. (2015) 156:1124–44. doi: 10.1097/j.pain.0000000000000158
79. Denk F, Bennett DL, McMahon SB. Nerve growth factor and pain mechanisms. Annu Rev Neurosci. (2017) 40:307–25. doi: 10.1146/annurev-neuro-072116-031121
80. Saloman JL, Chung MK, Ro JY. P2x(3) and trpv1 functionally interact and mediate sensitization of trigeminal sensory neurons. Neuroscience. (2013) 232:226–38. doi: 10.1016/j.neuroscience.2012.11.015
81. Lee J, Saloman JL, Weiland G, Auh QS, Chung MK, Ro JY. Functional interactions between nmda receptors and trpv1 in trigeminal sensory neurons mediate mechanical hyperalgesia in the rat masseter muscle. Pain. (2012) 153:1514–24. doi: 10.1016/j.pain.2012.04.015
82. Scheff NN, Ye Y, Bhattacharya A, MacRae J, Hickman DN, Sharma AK, et al. Tumor necrosis factor alpha secreted from oral squamous cell carcinoma contributes to cancer pain and associated inflammation. Pain. (2017) 158:2396–409. doi: 10.1097/j.pain.0000000000001044
83. Zhang J, Wang L, Wang H, Su Z, Pang X. Neuroinflammation and central pi3k/akt/mtor signal pathway contribute to bone cancer pain. Mol Pain. (2019) 15:1744806919830240. doi: 10.1177/1744806919830240
84. Zhao D, Han DF, Wang SS, Lv B, Wang X, Ma C. Roles of tumor necrosis factor-alpha and interleukin-6 in regulating bone cancer pain via trpa1 signal pathway and beneficial effects of inhibition of neuro-inflammation and trpa1. Mol Pain. (2019) 15:1744806919857981. doi: 10.1177/1744806919857981
85. Constantin CE, Mair N, Sailer CA, Andratsch M, Xu ZZ, Blumer MJ, et al. Endogenous tumor necrosis factor alpha (Tnfalpha) requires tnf receptor type 2 to generate heat hyperalgesia in a mouse cancer model. J Neurosci. (2008) 28:5072–81. doi: 10.1523/JNEUROSCI.4476-07.2008
86. Liu Y, Gao Y, Lin T. Expression of interleukin-1 (Il-1), il-6, and tumor necrosis factor-alpha (Tnf-alpha) in non-small cell lung cancer and its relationship with the occurrence and prognosis of cancer pain. Ann Palliat Med. (2021) 10:12759–66. doi: 10.21037/apm-21-3471
87. Baamonde A, Curto-Reyes V, Juarez L, Meana A, Hidalgo A, Menendez L. Antihyperalgesic effects induced by the il-1 receptor antagonist anakinra and increased il-1beta levels in inflamed and osteosarcoma-bearing mice. Life Sci. (2007) 81:673–82. doi: 10.1016/j.lfs.2007.07.003
88. Quarta S, Vogl C, Constantin CE, Uceyler N, Sommer C, Kress M. Genetic evidence for an essential role of neuronally expressed il-6 signal transducer gp130 in the induction and maintenance of experimentally induced mechanical hypersensitivity in vivo and in vitro. Mol Pain. (2011) 7:73. doi: 10.1186/1744-8069-7-73
89. Schweizerhof M, Stosser S, Kurejova M, Njoo C, Gangadharan V, Agarwal N, et al. Hematopoietic colony-stimulating factors mediate tumor-nerve interactions and bone cancer pain. Nat Med. (2009) 15:802–7. doi: 10.1038/nm.1976
90. Zhang F, Wang Y, Liu Y, Han H, Zhang D, Fan X, et al. Transcriptional regulation of voltage-gated sodium channels contributes to gm-csf-induced pain. J Neurosci. (2019) 39:5222–33. doi: 10.1523/JNEUROSCI.2204-18.2019
91. Khasabova IA, Stucky CL, Harding-Rose C, Eikmeier L, Beitz AJ, Coicou LG, et al. Chemical interactions between fibrosarcoma cancer cells and sensory neurons contribute to cancer pain. J Neurosci. (2007) 27:10289–98. doi: 10.1523/JNEUROSCI.2851-07.2007
92. Isono M, Suzuki T, Hosono K, Hayashi I, Sakagami H, Uematsu S, et al. Microsomal prostaglandin E synthase-1 enhances bone cancer growth and bone cancer-related pain behaviors in mice. Life Sci. (2011) 88:693–700. doi: 10.1016/j.lfs.2011.02.008
93. Sevcik MA, Ghilardi JR, Halvorson KG, Lindsay TH, Kubota K, Mantyh PW. Analgesic efficacy of bradykinin B1 antagonists in a murine bone cancer pain model. J Pain. (2005) 6:771–5. doi: 10.1016/j.jpain.2005.06.010
94. Fujita M, Andoh T, Ohashi K, Akira A, Saiki I, Kuraishi Y. Roles of kinin B1 and B2 receptors in skin cancer pain produced by orthotopic melanoma inoculation in mice. Eur J Pain. (2010) 14:588–94. doi: 10.1016/j.ejpain.2009.10.010
95. Halvorson KG, Kubota K, Sevcik MA, Lindsay TH, Sotillo JE, Ghilardi JR, et al. A blocking antibody to nerve growth factor attenuates skeletal pain induced by prostate tumor cells growing in bone. Cancer Res. (2005) 65:9426–35. doi: 10.1158/0008-5472.CAN-05-0826
96. Sevcik MA, Ghilardi JR, Peters CM, Lindsay TH, Halvorson KG, Jonas BM, et al. Anti-ngf therapy profoundly reduces bone cancer pain and the accompanying increase in markers of peripheral and central sensitization. Pain. (2005) 115:128–41. doi: 10.1016/j.pain.2005.02.022
97. Jimenez-Andrade JM, Ghilardi JR, Castaneda-Corral G, Kuskowski MA, Mantyh PW. Preventive or late administration of anti-ngf therapy attenuates tumor-induced nerve sprouting, neuroma formation, and cancer pain. Pain. (2011) 152:2564–74. doi: 10.1016/j.pain.2011.07.020
98. Sopata M, Katz N, Carey W, Smith MD, Keller D, Verburg KM, et al. Efficacy and safety of tanezumab in the treatment of pain from bone metastases. Pain. (2015) 156:1703–13. doi: 10.1097/j.pain.0000000000000211
99. Chodroff L, Bendele M, Valenzuela V, Henry M, Ruparel S. Express: bdnf signaling contributes to oral cancer pain in a preclinical orthotopic rodent model. Mol Pain. (2016) 12. doi: 10.1177/1744806916666841
100. Grayson M, Arris D, Wu P, Merlo J, Ibrahim T, Fang-Mei C, et al. Oral squamous cell carcinoma-released brain-derived neurotrophic factor contributes to oral cancer pain by peripheral tropomyosin receptor kinase B activation. Pain. (2022) 163:496–507. doi: 10.1097/j.pain.0000000000002382
101. Chichorro JG, Fiuza CR, Bressan E, Claudino RF, Leite DF, Rae GA. Endothelins as pronociceptive mediators of the rat trigeminal system: role of eta and etb receptors. Brain Res. (2010) 1345:73–83. doi: 10.1016/j.brainres.2010.04.075
102. Chichorro JG, Zampronio AR, Cabrini DA, Franco CR, Rae GA. Mechanisms operated by endothelin eta and etb receptors in the trigeminal ganglion contribute to orofacial thermal hyperalgesia induced by infraorbital nerve constriction in rats. Neuropeptides. (2009) 43:133–42. doi: 10.1016/j.npep.2008.12.001
103. Wacnik PW, Eikmeier LJ, Ruggles TR, Ramnaraine ML, Walcheck BK, Beitz AJ, et al. Functional interactions between tumor and peripheral nerve: morphology, algogen identification, and behavioral characterization of a new murine model of cancer pain. J Neurosci. (2001) 21:9355–66. doi: 10.1523/JNEUROSCI.21-23-09355.2001
104. Peters CM, Lindsay TH, Pomonis JD, Luger NM, Ghilardi JR, Sevcik MA, et al. Endothelin and the tumorigenic component of bone cancer pain. Neuroscience. (2004) 126:1043–52. doi: 10.1016/j.neuroscience.2004.04.027
105. Lam DK, Dang D, Zhang J, Dolan JC, Schmidt BL. Novel animal models of acute and chronic cancer pain: A pivotal role for par2. J Neurosci. (2012) 32:14178–83. doi: 10.1523/JNEUROSCI.2399-12.2012
106. Lam DK, Dang D, Flynn AN, Hardt M, Schmidt BL. Tmprss2, a novel membrane-anchored mediator in cancer pain. Pain. (2015) 156:923–30. doi: 10.1097/j.pain.0000000000000130
107. Scheff NN, Wall IM, Nicholson S, Williams H, Chen E, Tu NH, et al. Oral cancer induced trpv1 sensitization is mediated by par(2) signaling in primary afferent neurons innervating the cancer microenvironment. Sci Rep. (2022) 12:4121. doi: 10.1038/s41598-022-08005-6
108. Lam DK, Schmidt BL. Serine proteases and protease-activated receptor 2-dependent allodynia: A novel cancer pain pathway. Pain. (2010) 149:263–72. doi: 10.1016/j.pain.2010.02.010
109. Balood M, Ahmadi M, Eichwald T, Ahmadi A, Majdoubi A, Roversi K, et al. Nociceptor neurons affect cancer immunosurveillance. Nature. (2022) 611:405–12. doi: 10.1038/s41586-022-05374-w
110. Ye Y, Ono K, Bernabe DG, Viet CT, Pickering V, Dolan JC, et al. Adenosine triphosphate drives head and neck cancer pain through P2x2/3 heterotrimers. Acta Neuropathol Commun. (2014) 2:62. doi: 10.1186/2051-5960-2-62
111. Gilchrist LS, Cain DM, Harding-Rose C, Kov AN, Wendelschafer-Crabb G, Kennedy WR, et al. Re-organization of P2x3 receptor localization on epidermal nerve fibers in a murine model of cancer pain. Brain Res. (2005) 1044:197–205. doi: 10.1016/j.brainres.2005.02.081
112. Gonzalez-Rodriguez S, Pevida M, Roques BP, Fournie-Zaluski MC, Hidalgo A, Menendez L, et al. Involvement of enkephalins in the inhibition of osteosarcoma-induced thermal hyperalgesia evoked by the blockade of peripheral P2x3 receptors. Neurosci Lett. (2009) 465:285–9. doi: 10.1016/j.neulet.2009.09.015
113. Kaan TK, Yip PK, Patel S, Davies M, Marchand F, Cockayne DA, et al. Systemic blockade of P2x3 and P2x2/3 receptors attenuates bone cancer pain behaviour in rats. Brain. (2010) 133:2549–64. doi: 10.1093/brain/awq194
114. Hansen RR, Nasser A, Falk S, Baldvinsson SB, Ohlsson PH, Bahl JM, et al. Chronic administration of the selective P2x3, P2x2/3 receptor antagonist, a-317491, transiently attenuates cancer-induced bone pain in mice. Eur J Pharmacol. (2012) 688:27–34. doi: 10.1016/j.ejphar.2012.05.008
115. Wu JX, Xu MY, Miao XR, Lu ZJ, Yuan XM, Li XQ, et al. Functional up-regulation of P2x3 receptors in dorsal root ganglion in a rat model of bone cancer pain. Eur J Pain. (2012) 16:1378–88. doi: 10.1002/j.1532-2149.2012.00149.x
116. Liu M, Yang H, Fang D, Yang JJ, Cai J, Wan Y, et al. Upregulation of P2x3 receptors by neuronal calcium sensor protein vilip-1 in dorsal root ganglions contributes to the bone cancer pain in rats. Pain. (2013) 154:1551–68. doi: 10.1016/j.pain.2013.04.022
117. Bhattacharya A, Janal MN, Veeramachaneni R, Dolgalev I, Dubeykovskaya Z, Tu NH, et al. Oncogenes overexpressed in metastatic oral cancers from patients with pain: potential pain mediators released in exosomes. Sci Rep. (2020) 10:14724. doi: 10.1038/s41598-020-71298-y
118. Zhu YF, Linher-Melville K, Wu J, Fazzari J, Miladinovic T, Ungard R, et al. Bone cancer-induced pain is associated with glutamate signalling in peripheral sensory neurons. Mol Pain. (2020) 16:1744806920911536. doi: 10.1177/1744806920911536
119. Selvaraj D, Gangadharan V, Michalski CW, Kurejova M, Stosser S, Srivastava K, et al. A functional role for vegfr1 expressed in peripheral sensory neurons in cancer pain. Cancer Cell. (2015) 27:780–96. doi: 10.1016/j.ccell.2015.04.017
120. Vanderwall AG, Milligan ED. Cytokines in pain: harnessing endogenous anti-inflammatory signaling for improved pain management. Front Immunol. (2019) 10:3009. doi: 10.3389/fimmu.2019.03009
121. Hu P, Bembrick AL, Keay KA, McLachlan EM. Immune cell involvement in dorsal root ganglia and spinal cord after chronic constriction or transection of the rat sciatic nerve. Brain behavior Immun. (2007) 21:599–616. doi: 10.1016/j.bbi.2006.10.013
122. Kwon MJ, Shin HY, Cui Y, Kim H, Thi AH, Choi JY, et al. Ccl2 mediates neuron-macrophage interactions to drive proregenerative macrophage activation following preconditioning injury. J Neurosci. (2015) 35:15934–47. doi: 10.1523/JNEUROSCI.1924-15.2015
123. Zhang H, Li Y, de Carvalho-Barbosa M, Kavelaars A, Heijnen CJ, Albrecht PJ, et al. Dorsal root ganglion infiltration by macrophages contributes to paclitaxel chemotherapy-induced peripheral neuropathy. J Pain. (2016) 17:775–86. doi: 10.1016/j.jpain.2016.02.011
124. Cao L, DeLeo JA. Cns-infiltrating cd4+ T lymphocytes contribute to murine spinal nerve transection-induced neuropathic pain. Eur J Immunol. (2008) 38:448–58. doi: 10.1002/eji.200737485
125. Draleau K, Maddula S, Slaiby A, Nutile-McMenemy N, De Leo J, Cao L. Phenotypic identification of spinal cord-infiltrating cd4(+) T lymphocytes in a murine model of neuropathic pain. J Pain Relief. (2014) Suppl 3:3. doi: 10.4172/2167-0846.S3-003
126. Sun C, Zhang J, Chen L, Liu T, Xu G, Li C, et al. Il-17 contributed to the neuropathic pain following peripheral nerve injury by promoting astrocyte proliferation and secretion of proinflammatory cytokines. Mol Med Rep. (2017) 15:89–96. doi: 10.3892/mmr.2016.6018
127. Costigan M, Moss A, Latremoliere A, Johnston C, Verma-Gandhu M, Herbert TA, et al. T-cell infiltration and signaling in the adult dorsal spinal cord is a major contributor to neuropathic pain-like hypersensitivity. J. (2009) 29:14415–22. doi: 10.1523/JNEUROSCI.4569-09.2009
128. Kim CF, Moalem-Taylor G. Interleukin-17 contributes to neuroinflammation and neuropathic pain following peripheral nerve injury in mice. J Pain. (2011) 12:370–83. doi: 10.1016/j.jpain.2010.08.003
129. Zhang ZJ, Jiang BC, Gao YJ. Chemokines in neuron-glial cell interaction and pathogenesis of neuropathic pain. Cell Mol Life Sci. (2017) 74:3275–91. doi: 10.1007/s00018-017-2513-1
130. Tsuda M, Shigemoto-Mogami Y, Koizumi S, Mizokoshi A, Kohsaka S, Salter MW, et al. P2x4 receptors induced in spinal microglia gate tactile allodynia after nerve injury. Nature. (2003) 424:778–83. doi: 10.1038/nature01786
131. Guan Z, Kuhn JA, Wang X, Colquitt B, Solorzano C, Vaman S, et al. Injured sensory neuron-derived csf1 induces microglial proliferation and dap12-dependent pain. Nat Neurosci. (2016) 19:94–101. doi: 10.1038/nn.4189
132. Chen G, Zhang YQ, Qadri YJ, Serhan CN, Ji RR. Microglia in pain: detrimental and protective roles in pathogenesis and resolution of pain. Neuron. (2018) 100:1292–311. doi: 10.1016/j.neuron.2018.11.009
133. Mao M, Li Y, Wang L, Chen J, Ming X, Sun Y, et al. Aitongxiao improves pain symptoms of rats with cancer pain by reducing il-1, tnf-alpha, and pge2. Int J Clin Exp Pathol. (2021) 14:133–9.
134. Zhang RX, Liu B, Li A, Wang L, Ren K, Qiao JT, et al. Interleukin 1beta facilitates bone cancer pain in rats by enhancing nmda receptor nr-1 subunit phosphorylation. Neuroscience. (2008) 154:1533–8. doi: 10.1016/j.neuroscience.2008.04.072
135. Chapman EJ, Edwards Z, Boland JW, Maddocks M, Fettes L, Malia C, et al. Practice review: evidence-based and effective management of pain in patients with advanced cancer. Palliat Med. (2020) 34:444–53. doi: 10.1177/0269216319896955
136. Derry S, Wiffen PJ, Moore RA, McNicol ED, Bell RF, Carr DB, et al. Oral nonsteroidal anti-inflammatory drugs (Nsaids) for cancer pain in adults. Cochrane Database Syst Rev. (2017) 7:CD012638. doi: 10.1002/14651858.CD012638.pub2
137. Zhao J, Huh Y, Bortsov A, Diatchenko L, Ji RR. Immunotherapies in chronic pain through modulation of neuroimmune interactions. Pharmacol Ther. (2023) 248:108476. doi: 10.1016/j.pharmthera.2023.108476
138. Chen G, Kim YH, Li H, Luo H, Liu DL, Zhang ZJ, et al. Pd-L1 inhibits acute and chronic pain by suppressing nociceptive neuron activity via pd-1. Nat Neurosci. (2017) 20:917–26. doi: 10.1038/nn.4571
139. Liu BL, Cao QL, Zhao X, Liu HZ, Zhang YQ. Inhibition of trpv1 by shp-1 in nociceptive primary sensory neurons is critical in pd-L1 analgesia. JCI Insight. (2020) 5. doi: 10.1172/jci.insight.137386
140. Wang Z, Jiang C, He Q, Matsuda M, Han Q, Wang K, et al. Anti-pd-1 treatment impairs opioid antinociception in rodents and nonhuman primates. Sci Transl Med. (2020) 12. doi: 10.1126/scitranslmed.aaw6471
141. Meerschaert KA, Edwards BS, Epouhe AY, Jefferson B, Friedman R, Babyok OL, et al. Neuronally expressed pdl1, not pd1, suppresses acute nociception. Brain behavior Immun. (2022) 106:233–46. doi: 10.1016/j.bbi.2022.09.001
142. Xiao X, Zhao XT, Xu LC, Yue LP, Liu FY, Cai J, et al. Shp-1 dephosphorylates trpv1 in dorsal root ganglion neurons and alleviates cfa-induced inflammatory pain in rats. Pain. (2015) 156:597–608. doi: 10.1097/01.j.pain.0000460351.30707.c4
143. Wanderley CWS, Maganin AGM, Adjafre B, Mendes AS, Silva CEA, Quadros AU, et al. Pd-1/pd-L1 inhibition enhances chemotherapy-induced neuropathic pain by suppressing neuroimmune antinociceptive signaling. Cancer Immunol Res. (2022) 10:1299–308. doi: 10.1158/2326-6066.CIR-22-0003
144. Wang K, Gu Y, Liao Y, Bang S, Donnelly CR, Chen O, et al. Pd-1 blockade inhibits osteoclast formation and murine bone cancer pain. J Clin Invest. (2020) 130:3603–20. doi: 10.1172/JCI133334
145. Scheau C, Draghici C, Ilie MA, Lupu M, Solomon I, Tampa M, et al. Neuroendocrine factors in melanoma pathogenesis. Cancers (Basel). (2021) 13. doi: 10.3390/cancers13092277
146. Slominski RM, Raman C, Chen JY, Slominski AT. How cancer hijacks the body's homeostasis through the neuroendocrine system. Trends Neurosci. (2023) 46:263–75. doi: 10.1016/j.tins.2023.01.003
147. Corder G, Castro DC, Bruchas MR, Scherrer G. Endogenous and exogenous opioids in pain. Annu Rev Neurosci. (2018) 41:453–73. doi: 10.1146/annurev-neuro-080317-061522
148. Scheff NN, Alemu RG, Klares R 3rd, Wall IM, Yang SC, Dolan JC, et al. Granulocyte-colony stimulating factor-induced neutrophil recruitment provides opioid-mediated endogenous anti-nociception in female mice with oral squamous cell carcinoma. Front Mol Neurosci. (2019) 12:217. doi: 10.3389/fnmol.2019.00217
149. Argueta DA, Aich A, Lei J, Kiven S, Nguyen A, Wang Y, et al. Beta-endorphin at the intersection of pain and cancer progression: preclinical evidence. Neurosci Lett. (2021) 744:135601. doi: 10.1016/j.neulet.2020.135601
150. Silverman DA, Martinez VK, Dougherty PM, Myers JN, Calin GA, Amit M. Cancer-associated neurogenesis and nerve-cancer cross-talk. Cancer Res. (2021) 81:1431–40. doi: 10.1158/0008-5472.CAN-20-2793
151. Lindsay TH, Jonas BM, Sevcik MA, Kubota K, Halvorson KG, Ghilardi JR, et al. Pancreatic cancer pain and its correlation with changes in tumor vasculature, macrophage infiltration, neuronal innervation, body weight and disease progression. Pain. (2005) 119:233–46. doi: 10.1016/j.pain.2005.10.019
152. Guillot J, Dominici C, Lucchesi A, Nguyen HTT, Puget A, Hocine M, et al. Sympathetic axonal sprouting induces changes in macrophage populations and protects against pancreatic cancer. Nat Commun. (2022) 13:1985. doi: 10.1038/s41467-022-29659-w
153. Ceyhan GO, Demir IE, Rauch U, Bergmann F, Muller MW, Buchler MW, et al. Pancreatic neuropathy results in "Neural remodeling" and altered pancreatic innervation in chronic pancreatitis and pancreatic cancer. Am J Gastroenterol. (2009) 104:2555–65. doi: 10.1038/ajg.2009.380
154. Han H, Yang C, Zhang Y, Han C, Zhang G. Vascular endothelial growth factor mediates the sprouted axonogenesis of breast cancer in rat. Am J Pathol. (2021) 191:515–26. doi: 10.1016/j.ajpath.2020.12.006
155. Allen JK, Armaiz-Pena GN, Nagaraja AS, Sadaoui NC, Ortiz T, Dood R, et al. Sustained adrenergic signaling promotes intratumoral innervation through bdnf induction. Cancer Res. (2018) 78:3233–42. doi: 10.1158/0008-5472.CAN-16-1701
156. Diaz-delCastillo M, Palasca O, Nemler TT, Thygesen DM, Chavez-Saldana NA, Vazquez-Mora JA, et al. Metastatic infiltration of nervous tissue and periosteal nerve sprouting in multiple myeloma-induced bone pain in mice and human. J Neurosci. (2023) 43:5414–30. doi: 10.1523/JNEUROSCI.0404-23.2023
157. Ma Z, Wei Y, Liao T, Jie L, Yang N, Yu L, et al. Activation of vascular endothelial cells by synovial fibrosis promotes netrin-1-induced sensory nerve sprouting and exacerbates pain sensitivity. J Cell Mol Med. (2023) 27(23):3773–85. doi: 10.1111/jcmm.17950
158. Madeo M, Colbert PL, Vermeer DW, Lucido CT, Cain JT, Vichaya EG, et al. Cancer exosomes induce tumor innervation. Nat Commun. (2018) 9:4284. doi: 10.1038/s41467-018-06640-0
159. Liebig C, Ayala G, Wilks JA, Berger DH, Albo D. Perineural invasion in cancer: A review of the literature. Cancer. (2009) 115:3379–91. doi: 10.1002/cncr.24396
160. Schmitd LB, Beesley LJ, Russo N, Bellile EL, Inglehart RC, Liu M, et al. Redefining perineural invasion: integration of biology with clinical outcome. Neoplasia. (2018) 20:657–67. doi: 10.1016/j.neo.2018.04.005
161. Wang J, Chen Y, Li X, Zou X. Perineural invasion and associated pain transmission in pancreatic cancer. Cancers (Basel). (2021) 13. doi: 10.3390/cancers13184594
162. Bapat AA, Hostetter G, Von Hoff DD, Han H. Perineural invasion and associated pain in pancreatic cancer. Nat Rev Cancer. (2011) 11:695–707. doi: 10.1038/nrc3131
163. Chen Z, Fang Y, Jiang W. Important cells and factors from tumor microenvironment participated in perineural invasion. Cancers (Basel). (2023) 15. doi: 10.3390/cancers15051360
164. Demir IE, Friess H, Ceyhan GO. Neural plasticity in pancreatitis and pancreatic cancer. Nat Rev Gastroenterol Hepatol. (2015) 12:649–59. doi: 10.1038/nrgastro.2015.166
165. Demir IE, Schorn S, Schremmer-Danninger E, Wang K, Kehl T, Giese NA, et al. Perineural mast cells are specifically enriched in pancreatic neuritis and neuropathic pain in pancreatic cancer and chronic pancreatitis. PloS One. (2013) 8:e60529. doi: 10.1371/journal.pone.0060529
166. Eckerling A, Ricon-Becker I, Sorski L, Sandbank E, Ben-Eliyahu S. Stress and cancer: mechanisms, significance and future directions. Nat Rev Cancer. (2021) 21:767–85. doi: 10.1038/s41568-021-00395-5
167. Globig AM, Zhao S, Roginsky J, Maltez VI, Guiza J, Avina-Ochoa N, et al. The beta(1)-adrenergic receptor links sympathetic nerves to T cell exhaustion. Nature. (2023) 622(7982):383–92. doi: 10.1038/s41586-023-06568-6
168. Daher C, Vimeux L, Stoeva R, Peranzoni E, Bismuth G, Wieduwild E, et al. Blockade of beta-adrenergic receptors improves cd8(+) T-cell priming and cancer vaccine efficacy. Cancer Immunol Res. (2019) 7:1849–63. doi: 10.1158/2326-6066.CIR-18-0833
169. Yang H, Xia L, Chen J, Zhang S, Martin V, Li Q, et al. Stress-glucocorticoid-tsc22d3 axis compromises therapy-induced antitumor immunity. Nat Med. (2019) 25:1428–41. doi: 10.1038/s41591-019-0566-4
170. Acharya N, Madi A, Zhang H, Klapholz M, Escobar G, Dulberg S, et al. Endogenous glucocorticoid signaling regulates cd8(+) T cell differentiation and development of dysfunction in the tumor microenvironment. Immunity. (2020) 53:658–71.e6. doi: 10.1016/j.immuni.2020.08.005
171. Kurita GP, Sjogren P, Klepstad P, Mercadante S. Interventional techniques to management of cancer-related pain: clinical and critical aspects. Cancers (Basel). (2019) 11. doi: 10.3390/cancers11040443
172. Atherton M, Park S, Horan NL, Nicholson S, Dolan JC, Schmidt BL, et al. Sympathetic modulation of tumor necrosis factor alpha-induced nociception in the presence of oral squamous cell carcinoma. Pain. (2022) 164(1):27–42. doi: 10.1097/j.pain.0000000000002655
173. Diaz-delCastillo M, Woldbye DPD, Heegaard AM. Neuropeptide Y and its involvement in chronic pain. Neuroscience. (2018) 387:162–9. doi: 10.1016/j.neuroscience.2017.08.050
174. Inoue K, Tsuda M. Nociceptive signaling mediated by P2x3, P2x4 and P2x7 receptors. Biochem Pharmacol. (2021) 187:114309. doi: 10.1016/j.bcp.2020.114309
175. Burnstock G. Purinergic cotransmission. Exp Physiol. (2009) 94:20–4. doi: 10.1113/expphysiol.2008.043620
176. Zheng Q, Xie W, Luckemeyer DD, Lay M, Wang XW, Dong X, et al. Synchronized cluster firing, a distinct form of sensory neuron activation, drives spontaneous pain. Neuron. (2022) 110:209–20 e6. doi: 10.1016/j.neuron.2021.10.019
177. Yang X, Liu Z, Li Z. Effects of norepinephrine on galanin expression in dorsal root ganglion neurons in vitro. Curr Ther Res Clin Exp. (2009) 70:19–28. doi: 10.1016/j.curtheres.2009.02.002
178. Chen X, Levine JD. Epinephrine-induced excitation and sensitization of rat C-fiber nociceptors. J Pain. (2005) 6:439–46. doi: 10.1016/j.jpain.2005.02.004
179. Sato J, Yajima H, Banik RK, Kumazawa T, Mizumura K. Norepinephrine reduces heat responses of cutaneous C-fiber nociceptors in sprague-dawley rats in vitro. Neurosci Lett. (2005) 378:111–6. doi: 10.1016/j.neulet.2004.12.014
180. Sato J, Perl ER. Adrenergic excitation of cutaneous pain receptors induced by peripheral nerve injury. Science. (1991) 251:1608–10. doi: 10.1126/science.2011742
181. Liu S, Wang Z, Su Y, Qi L, Yang W, Fu M, et al. A neuroanatomical basis for electroacupuncture to drive the vagal-adrenal axis. Nature. (2021) 598:641–5. doi: 10.1038/s41586-021-04001-4
182. Mohanta SK, Peng L, Li Y, Lu S, Sun T, Carnevale L, et al. Neuroimmune cardiovascular interfaces control atherosclerosis. Nature. (2022) 605:152–9. doi: 10.1038/s41586-022-04673-6
183. Shouman K, Benarroch EE. Segmental spinal sympathetic machinery: implications for autonomic dysreflexia. Neurology. (2019) 93:339–45. doi: 10.1212/WNL.0000000000007973
184. Noble BT, Brennan FH, Wang Y, Guan Z, Mo X, Schwab JM, et al. Thoracic vglut2(+) spinal interneurons regulate structural and functional plasticity of sympathetic networks after high-level spinal cord injury. J Neurosci. (2022) 42:3659–75. doi: 10.1523/JNEUROSCI.2134-21.2022
185. Wulf MJ, Tom VJ. Consequences of spinal cord injury on the sympathetic nervous system. Front Cell Neurosci. (2023) 17:999253. doi: 10.3389/fncel.2023.999253
186. Chen H, Hu B, Lv X, Zhu S, Zhen G, Wan M, et al. Prostaglandin E2 mediates sensory nerve regulation of bone homeostasis. Nat Commun. (2019) 10:181. doi: 10.1038/s41467-018-08097-7
187. Picoli CC, Costa AC, Rocha BGS, Silva WN, Santos GSP, Prazeres P, et al. Sensory nerves in the spotlight of the stem cell niche. Stem Cells Transl Med. (2021) 10:346–56. doi: 10.1002/sctm.20-0284
188. Hu B, Lv X, Chen H, Xue P, Gao B, Wang X, et al. Sensory nerves regulate mesenchymal stromal cell lineage commitment by tuning sympathetic tones. J Clin Invest. (2020) 130:3483–98. doi: 10.1172/JCI131554
189. Hanahan D. Hallmarks of cancer: new dimensions. Cancer Discovery. (2022) 12:31–46. doi: 10.1158/2159-8290.CD-21-1059
190. Hanahan D, Monje M. Cancer hallmarks intersect with neuroscience in the tumor microenvironment. Cancer Cell. (2023) 41:573–80. doi: 10.1016/j.ccell.2023.02.012
191. Young HH. On the presence of nerves in tumors and of other structures in them as revealed by a modification of ehrlich's method of "Vital staining" with methylene blue. J Exp Med. (1897) 2:1–12. doi: 10.1084/jem.2.1.1
192. De Sousa Pereira A. A basis for sympathectomy for cancer of the cervix uteri. Arch Surg (1920). (1946) 52:260–85. doi: 10.1001/archsurg.1946.01230050265003
193. Seifert P, Spitznas M. Tumours may be innervated. Virchows Arch. (2001) 438:228–31. doi: 10.1007/s004280000306
194. Mitchell BS, Schumacher U, Kaiserling E. Are tumours innervated? Immunohistological investigations using antibodies against the neuronal marker protein gene product 9.5 (Pgp 9.5) in benign, Malignant and experimental tumours. Tumour Biol. (1994) 15:269–74. doi: 10.1159/000217901
195. Winkelmann RK. Cutaneous nerves in relation to epithelial tumors. J Invest Dermatol. (1956) 27:273–9. doi: 10.1038/jid.1956.102
196. Le TT, Payne SL, Buckwald MN, Hayes LA, Parker SR, Burge CB, et al. Sensory nerves enhance triple-negative breast cancer invasion and metastasis via the axon guidance molecule plexinb3. NPJ Breast Cancer. (2022) 8:116. doi: 10.1038/s41523-022-00485-z
197. Austin M, Elliott L, Nicolaou N, Grabowska A, Hulse RP. Breast cancer induced nociceptor aberrant growth and collateral sensory axonal branching. Oncotarget. (2017) 8:76606–21. doi: 10.18632/oncotarget.20609
198. McIlvried LA, Atherton MA, Horan NL, Goch TN, Scheff NN. Sensory neurotransmitter calcitonin gene-related peptide modulates tumor growth and lymphocyte infiltration in oral squamous cell carcinoma. Adv Biol (Weinh). (2022) 6:e2200019. doi: 10.1002/adbi.202200019
199. Rowe CW, Dill T, Griffin N, Jobling P, Faulkner S, Paul JW, et al. Innervation of papillary thyroid cancer and its association with extra-thyroidal invasion. Sci Rep. (2020) 10:1539. doi: 10.1038/s41598-020-58425-5
200. Costa PAC, Silva WN, Prazeres P, Picoli CC, Guardia GDA, Costa AC, et al. Chemogenetic modulation of sensory neurons reveals their regulating role in melanoma progression. Acta Neuropathol Commun. (2021) 9:183. doi: 10.1186/s40478-021-01273-9
201. Prazeres P, Leonel C, Silva WN, Rocha BGS, Santos GSP, Costa AC, et al. Ablation of sensory nerves favours melanoma progression. J Cell Mol Med. (2020) 24:9574–89. doi: 10.1111/jcmm.15381
202. Han GH, Chay DB, Nam S, Cho H, Chung JY, Kim JH. Prognostic significance of transient receptor potential vanilloid type 1 (Trpv1) and phosphatase and tension homolog (Pten) in epithelial ovarian cancer. Cancer Genomics Proteomics. (2020) 17:309–19. doi: 10.21873/cgp.20191
203. Barr JL, Kruse A, Restaino AC, Tulina N, Stuckelberger S, Vermeer SJ, et al. Intra-tumoral nerve-tracing in a novel syngeneic model of high-grade serous ovarian carcinoma. Cells. (2021) 10. doi: 10.3390/cells10123491
204. Lucido CT, Wynja E, Madeo M, Williamson CS, Schwartz LE, Imblum BA, et al. Innervation of cervical carcinoma is mediated by cancer-derived exosomes. Gynecol Oncol. (2019) 154:228–35. doi: 10.1016/j.ygyno.2019.04.651
205. Wang Z, Dong J, Tian W, Qiao S, Wang H. Role of trpv1 ion channel in cervical squamous cell carcinoma genesis. Front Mol Biosci. (2022) 9:980262. doi: 10.3389/fmolb.2022.980262
206. Vats K, Kruglov O, Sahoo B, Soman V, Zhang J, Shurin GV, et al. Sensory nerves impede the formation of tertiary lymphoid structures and development of protective antimelanoma immune responses. Cancer Immunol Res. (2022) 10:1141–54. doi: 10.1158/2326-6066.CIR-22-0110
207. Perez-Pacheco C, Schmitd LB, Furgal A, Bellile EL, Liu M, Fattah A, et al. Increased nerve density adversely affects outcome in oral cancer. Clin Cancer Res. (2023) 29:2501–12. doi: 10.1158/1078-0432.CCR-22-3496
208. Kelley MJ, Snider RH, Becker KL, Johnson BE. Small cell lung carcinoma cell lines express mrna for calcitonin and alpha- and beta-calcitonin gene related peptides. Cancer Lett. (1994) 81:19–25. doi: 10.1016/0304-3835(94)90159-7
209. Nagakawa O, Ogasawara M, Murata J, Fuse H, Saiki I. Effect of prostatic neuropeptides on migration of prostate cancer cell lines. Int J Urol. (2001) 8:65–70. doi: 10.1046/j.1442-2042.2001.00250.x
210. Takami H, Shikata J, Kakudo K, Ito K. Calcitonin gene-related peptide in patients with endocrine tumors. J Surg Oncol. (1990) 43:28–32. doi: 10.1002/jso.2930430108
211. Wimalawansa SJ. Cgrp radioreceptor assay: A new diagnostic tool for medullary thyroid carcinoma. J Bone Miner Res. (1993) 8:467–73. doi: 10.1002/jbmr.5650080411
212. Zhang Y, Lin C, Wang X, Ji T. Calcitonin gene-related peptide: A promising bridge between cancer development and cancer-associated pain in oral squamous cell carcinoma. Oncol Lett. (2020) 20:253. doi: 10.3892/ol.2020.12116
213. Feng F, Yang J, Tong L, Yuan S, Tian Y, Hong L, et al. Substance P immunoreactive nerve fibres are related to gastric cancer differentiation status and could promote proliferation and migration of gastric cancer cells. Cell Biol Int. (2011) 35:623–9. doi: 10.1042/CBI20100229
214. Mehboob R, Tanvir I, Warraich RA, Perveen S, Yasmeen S, Ahmad FJ. Role of neurotransmitter substance P in progression of oral squamous cell carcinoma. Pathol Res Pract. (2015) 211:203–7. doi: 10.1016/j.prp.2014.09.016
215. Erin N, Boyer PJ, Bonneau RH, Clawson GA, Welch DR. Capsaicin-mediated denervation of sensory neurons promotes mammary tumor metastasis to lung and heart. Anticancer Res. (2004) 24:1003–9.
216. Erin N, Zhao W, Bylander J, Chase G, Clawson G. Capsaicin-induced inactivation of sensory neurons promotes a more aggressive gene expression phenotype in breast cancer cells. Breast Cancer Res Treat. (2006) 99:351–64. doi: 10.1007/s10549-006-9219-7
217. Erin N, Korcum AF, Tanriover G, Kale S, Demir N, Koksoy S. Activation of neuroimmune pathways increases therapeutic effects of radiotherapy on poorly differentiated breast carcinoma. Brain behavior Immun. (2015) 48:174–85. doi: 10.1016/j.bbi.2015.02.024
218. Nizam E, Koksoy S, Erin N. Nk1r antagonist decreases inflammation and metastasis of breast carcinoma cells metastasized to liver but not to brain; phenotype-dependent therapeutic and toxic consequences. Cancer immunology immunotherapy CII. (2020) 69:1639–50. doi: 10.1007/s00262-020-02574-z
219. Erin N, Akman M, Aliyev E, Tanriover G, Korcum AF. Olvanil activates sensory nerve fibers, increases T cell response and decreases metastasis of breast carcinoma. Life Sci. (2022) 291:120305. doi: 10.1016/j.lfs.2022.120305
220. Amit M, Takahashi H, Dragomir MP, Lindemann A, Gleber-Netto FO, Pickering CR, et al. Loss of P53 drives neuron reprogramming in head and neck cancer. Nature. (2020) 578:449–54. doi: 10.1038/s41586-020-1996-3
221. Restaino AC, Walz A, Vermeer SJ, Barr J, Kovacs A, Fettig RR, et al. Functional neuronal circuits promote disease progression in cancer. Sci Adv. (2023) 9:eade4443. doi: 10.1126/sciadv.ade4443
222. Keskinov AA, Tapias V, Watkins SC, Ma Y, Shurin MR, Shurin GV. Impact of the sensory neurons on melanoma growth in vivo. PloS One. (2016) 11:e0156095. doi: 10.1371/journal.pone.0156095
223. Saloman JL, Albers KM, Li D, Hartman DJ, Crawford HC, Muha EA, et al. Ablation of sensory neurons in a genetic model of pancreatic ductal adenocarcinoma slows initiation and progression of cancer. Proc Natl Acad Sci United States America. (2016) 113:3078–83. doi: 10.1073/pnas.1512603113
224. Sinha S, Fu YY, Grimont A, Ketcham M, Lafaro K, Saglimbeni JA, et al. Panin neuroendocrine cells promote tumorigenesis via neuronal cross-talk. Cancer Res. (2017) 77:1868–79. doi: 10.1158/0008-5472.CAN-16-0899-T
225. Bai H, Li H, Zhang W, Matkowskyj KA, Liao J, Srivastava SK, et al. Inhibition of chronic pancreatitis and pancreatic intraepithelial neoplasia (Panin) by capsaicin in lsl-krasg12d/pdx1-cre mice. Carcinogenesis. (2011) 32:1689–96. doi: 10.1093/carcin/bgr191
226. Takezawa K, Kageyama I. Nerve fiber analysis on the morphology of the lingual nerve. Anat Sci Int. (2015) 90:298–302. doi: 10.1007/s12565-014-0267-5
227. Joncker NT, Bettini S, Boulet D, Guiraud M, Guerder S. The site of tumor development determines immunogenicity via temporal mobilization of antigen-laden dendritic cells in draining lymph nodes. Eur J Immunol. (2016) 46:609–18. doi: 10.1002/eji.201545797
228. Aalkjaer C, Nilsson H, De Mey JGR. Sympathetic and sensory-motor nerves in peripheral small arteries. Physiol Rev. (2021) 101:495–544. doi: 10.1152/physrev.00007.2020
229. Guo XD, Zhang N, Sha L. Mir-877-5p antagonizes the promoting effect of sp on the gastric cancer progression. Neoplasma. (2020) 67:1293–302. doi: 10.4149/neo_2020_200502N480
230. Davoodian M, Boroumand N, Mehrabi Bahar M, Jafarian AH, Asadi M, Hashemy SI. Evaluation of serum level of substance P and tissue distribution of nk-1 receptor in breast cancer. Mol Biol Rep. (2019) 46:1285–93. doi: 10.1007/s11033-019-04599-9
231. Al-Keilani MS, Elstaty R, Alqudah MA. The prognostic potential of neurokinin 1 receptor in breast cancer and its relationship with ki-67 index. Int J Breast Cancer. (2022) 2022:4987912. doi: 10.1155/2022/4987912
232. Ebrahimi S, Javid H, Alaei A, Hashemy SI. New insight into the role of substance P/neurokinin-1 receptor system in breast cancer progression and its crosstalk with micrornas. Clin Genet. (2020) 98:322–30. doi: 10.1111/cge.13750
233. Zhang L, Wang L, Dong D, Wang Z, Ji W, Yu M, et al. Mir-34b/C-5p and the neurokinin-1 receptor regulate breast cancer cell proliferation and apoptosis. Cell Prolif. (2019) 52:e12527. doi: 10.1111/cpr.12527
234. Wang L, Wang N, Zhang R, Dong D, Liu R, Zhang L, et al. Tgfbeta regulates nk1r-tr to affect the proliferation and apoptosis of breast cancer cells. Life Sci. (2020) 256:117674. doi: 10.1016/j.lfs.2020.117674
235. Yang C, Sun Y, Ouyang X, Li J, Zhu Z, Yu R, et al. Pain may promote tumor progression via substance P-dependent modulation of toll-like receptor-4. Pain Med. (2020) 21:3443–50. doi: 10.1093/pm/pnaa265
236. Wang JG, Yu J, Hu JL, Yang WL, Ren H, Ding D, et al. Neurokinin-1 activation affects egfr related signal transduction in triple negative breast cancer. Cell Signal. (2015) 27:1315–24. doi: 10.1016/j.cellsig.2015.03.015
237. Dong J, Feng F, Xu G, Zhang H, Hong L, Yang J. Elevated sp/nk-1r in esophageal carcinoma promotes esophageal carcinoma cell proliferation and migration. Gene. (2015) 560:205–10. doi: 10.1016/j.gene.2015.02.002
238. Wang F, Liu S, Liu J, Feng F, Guo Y, Zhang W, et al. Sp promotes cell proliferation in esophageal squamous cell carcinoma through the nk1r/hes1 axis. Biochem Biophys Res Commun. (2019) 514:1210–6. doi: 10.1016/j.bbrc.2019.05.092
239. Mohammadi F, Javid H, Afshari AR, Mashkani B, Hashemy SI. Substance P accelerates the progression of human esophageal squamous cell carcinoma via mmp-2, mmp-9, vegf-a, and vegfr1 overexpression. Mol Biol Rep. (2020) 47:4263–72. doi: 10.1007/s11033-020-05532-1
240. Zheng Y, Sang M, Liu F, Gu L, Li J, Wu Y, et al. Aprepitant inhibits the progression of esophageal squamous cancer by blocking the truncated neurokinin−1 receptor. Oncol Rep. (2023) 50(1):131. doi: 10.3892/or.2023.8568
241. Esteban F, Ramos-Garcia P, Munoz M, Gonzalez-Moles MA. Substance P and neurokinin 1 receptor in chronic inflammation and cancer of the head and neck: A review of the literature. Int J Environ Res Public Health. (2021) 19. doi: 10.3390/ijerph19010375
242. Munoz M, Rosso M, Covenas R. The nk-1 receptor antagonist L-732,138 induces apoptosis in human gastrointestinal cancer cell lines. Pharmacol Rep. (2017) 69:696–701. doi: 10.1016/j.pharep.2017.02.002
243. Erin N, Shurin GV, Baraldi JH, Shurin MR. Regulation of carcinogenesis by sensory neurons and neuromediators. Cancers (Basel). (2022) 14. doi: 10.3390/cancers14092333
244. Beinborn M, Blum A, Hang L, Setiawan T, Schroeder JC, Stoyanoff K, et al. Tgf-beta regulates T-cell neurokinin-1 receptor internalization and function. Proc Natl Acad Sci United States America. (2010) 107:4293–8. doi: 10.1073/pnas.0905877107
245. Blum AM, Metwali A, Elliott DE, Weinstock JV. T cell substance P receptor governs antigen-elicited ifn-gamma production. Am J Physiol Gastrointest Liver Physiol. (2003) 284:G197–204. doi: 10.1152/ajpgi.00271.2002
246. Morelli AE, Sumpter TL, Rojas-Canales DM, Bandyopadhyay M, Chen Z, Tkacheva O, et al. Neurokinin-1 receptor signaling is required for efficient ca(2+) flux in T-cell-receptor-activated T cells. Cell Rep. (2020) 30:3448–65 e8. doi: 10.1016/j.celrep.2020.02.054
247. Calvo CF, Chavanel G, Senik A. Substance P enhances il-2 expression in activated human T cells. J Immunol. (1992) 148:3498–504. doi: 10.4049/jimmunol.148.11.3498
248. Ikeda Y, Takei H, Matsumoto C, Mase A, Yamamoto M, Takeda S, et al. Administration of substance P during a primary immune response amplifies the secondary immune response via a long-lasting effect on cd8+ T lymphocytes. Arch Dermatol Res. (2007) 299:345–51. doi: 10.1007/s00403-007-0767-4
249. Rameshwar P, Gascon P, Ganea D. Stimulation of il-2 production in murine lymphocytes by substance P and related tachykinins. J Immunol. (1993) 151:2484–96. doi: 10.4049/jimmunol.151.5.2484
250. Siebenhaar F, Sharov AA, Peters EM, Sharova TY, Syska W, Mardaryev AN, et al. Substance P as an immunomodulatory neuropeptide in a mouse model for autoimmune hair loss (Alopecia areata). J Invest Dermatol. (2007) 127:1489–97. doi: 10.1038/sj.jid.5700704
251. Suvas S. Role of substance P neuropeptide in inflammation, wound healing, and tissue homeostasis. J Immunol. (2017) 199:1543–52. doi: 10.4049/jimmunol.1601751
252. Mathers AR, Tckacheva OA, Janelsins BM, Shufesky WJ, Morelli AE, Larregina AT. In vivo signaling through the neurokinin 1 receptor favors transgene expression by langerhans cells and promotes the generation of th1- and tc1-biased immune responses. J Immunol. (2007) 178:7006–17. doi: 10.4049/jimmunol.178.11.7006
253. Perner C, Flayer CH, Zhu X, Aderhold PA, Dewan ZNA, Voisin T, et al. Substance P release by sensory neurons triggers dendritic cell migration and initiates the type-2 immune response to allergens. Immunity. (2020) 53:1063–77.e7. doi: 10.1016/j.immuni.2020.10.001
254. Janelsins BM, Mathers AR, Tkacheva OA, Erdos G, Shufesky WJ, Morelli AE, et al. Proinflammatory tachykinins that signal through the neurokinin 1 receptor promote survival of dendritic cells and potent cellular immunity. Blood. (2009) 113:3017–26. doi: 10.1182/blood-2008-06-163121
255. Janelsins BM, Sumpter TL, Tkacheva OA, Rojas-Canales DM, Erdos G, Mathers AR, et al. Neurokinin-1 receptor agonists bias therapeutic dendritic cells to induce type 1 immunity by licensing host dendritic cells to produce il-12. Blood. (2013) 121:2923–33. doi: 10.1182/blood-2012-07-446054
256. Kincy-Cain T, Bost KL. Substance P-induced il-12 production by murine macrophages. J Immunol. (1997) 158:2334–9. doi: 10.4049/jimmunol.158.5.2334
257. Chernova I, Lai JP, Li H, Schwartz L, Tuluc F, Korchak HM, et al. (Sp) enhances ccl5-induced chemotaxis and intracellular signaling in human monocytes, which express the truncated neurokinin-1 receptor (Nk1r). J leukocyte Biol. (2009) 85:154–64. doi: 10.1189/jlb.0408260
258. Cunin P, Caillon A, Corvaisier M, Garo E, Scotet M, Blanchard S, et al. The tachykinins substance P and hemokinin-1 favor the generation of human memory th17 cells by monocytes. J Immunol. (2011) 186:4175–82. doi: 10.4049/jimmunol.1002535
259. Feistritzer C, Clausen J, Sturn DH, Djanani A, Gunsilius E, Wiedermann CJ, et al. Natural killer cell functions mediated by the neuropeptide substance P. Regul Pept. (2003) 116:119–26. doi: 10.1016/s0167-0115(03)00193-9
260. Lighvani S, Huang X, Trivedi PP, Swanborg RH, Hazlett LD. Substance P regulates natural killer cell interferon-gamma production and resistance to pseudomonas aeruginosa infection. Eur J Immunol. (2005) 35:1567–75. doi: 10.1002/eji.200425902
261. Paunicka KJ, Mellon J, Robertson D, Petroll M, Brown JR, Niederkorn JY. Severing corneal nerves in one eye induces sympathetic loss of immune privilege and promotes rejection of future corneal allografts placed in either eye. Am J Transplant. (2015) 15:1490–501. doi: 10.1111/ajt.13240
262. Inoue T, Ito Y, Nishizawa N, Eshima K, Kojo K, Otaka F, et al. Ramp1 in kupffer cells is a critical regulator in immune-mediated hepatitis. PloS One. (2018) 13:e0200432. doi: 10.1371/journal.pone.0200432
263. Kawamura N, Tamura H, Obana S, Wenner M, Ishikawa T, Nakata A, et al. Differential effects of neuropeptides on cytokine production by mouse helper T cell subsets. Neuroimmunomodulation. (1998) 5:9–15. doi: 10.1159/000026321
264. Tokoyoda K, Tsujikawa K, Matsushita H, Ono Y, Hayashi T, Harada Y, et al. Up-regulation of il-4 production by the activated camp/camp-dependent protein kinase (Protein kinase a) pathway in cd3/cd28-stimulated naive T cells. Int Immunol. (2004) 16:643–53. doi: 10.1093/intimm/dxh072
265. Wang F, Millet I, Bottomly K, Vignery A. Calcitonin gene-related peptide inhibits interleukin 2 production by murine T lymphocytes. J Biol Chem. (1992) 267:21052–7. doi: 10.1016/S0021-9258(19)36796-1
266. Mikami N, Matsushita H, Kato T, Kawasaki R, Sawazaki T, Kishimoto T, et al. Calcitonin gene-related peptide is an important regulator of cutaneous immunity: effect on dendritic cell and T cell functions. J Immunol. (2011) 186:6886–93. doi: 10.4049/jimmunol.1100028
267. Hosoi J, Murphy GF, Egan CL, Lerner EA, Grabbe S, Asahina A, et al. Regulation of langerhans cell function by nerves containing calcitonin gene-related peptide. Nature. (1993) 363:159–63. doi: 10.1038/363159a0
268. Asahina A, Moro O, Hosoi J, Lerner EA, Xu S, Takashima A, et al. Specific induction of camp in langerhans cells by calcitonin gene-related peptide: relevance to functional effects. Proc Natl Acad Sci United States America. (1995) 92:8323–7. doi: 10.1073/pnas.92.18.8323
269. Ding W, Stohl LL, Wagner JA, Granstein RD. Calcitonin gene-related peptide biases langerhans cells toward th2-type immunity. J Immunol. (2008) 181:6020–6. doi: 10.4049/jimmunol.181.9.6020
270. Rochlitzer S, Veres TZ, Kuhne K, Prenzler F, Pilzner C, Knothe S, et al. The neuropeptide calcitonin gene-related peptide affects allergic airway inflammation by modulating dendritic cell function. Clin Exp Allergy. (2011) 41:1609–21. doi: 10.1111/j.1365-2222.2011.03822.x
271. Carucci JA, Ignatius R, Wei Y, Cypess AM, Schaer DA, Pope M, et al. Calcitonin gene-related peptide decreases expression of hla-dr and cd86 by human dendritic cells and dampens dendritic cell-driven T cell-proliferative responses via the type I calcitonin gene-related peptide receptor. J Immunol. (2000) 164:3494–9. doi: 10.4049/jimmunol.164.7.3494
272. Fox FE, Kubin M, Cassin M, Niu Z, Hosoi J, Torii H, et al. Calcitonin gene-related peptide inhibits proliferation and antigen presentation by human peripheral blood mononuclear cells: effects on B7, interleukin 10, and interleukin 12. J Invest Dermatol. (1997) 108:43–8. doi: 10.1111/1523-1747.ep12285627
273. Harzenetter MD, Novotny AR, Gais P, Molina CA, Altmayr F, Holzmann B. Negative regulation of tlr responses by the neuropeptide cgrp is mediated by the transcriptional repressor icer. J Immunol. (2007) 179:607–15. doi: 10.4049/jimmunol.179.1.607
274. Torii H, Hosoi J, Beissert S, Xu S, Fox FE, Asahina A, et al. Regulation of cytokine expression in macrophages and the langerhans cell-like line xs52 by calcitonin gene-related peptide. J leukocyte Biol. (1997) 61:216–23. doi: 10.1002/jlb.61.2.216
275. Wallrapp A, Burkett PR, Riesenfeld SJ, Kim SJ, Christian E, Abdulnour RE, et al. Calcitonin gene-related peptide negatively regulates alarmin-driven type 2 innate lymphoid cell responses. Immunity. (2019) 51:709–23 e6. doi: 10.1016/j.immuni.2019.09.005
276. Nagashima H, Mahlakoiv T, Shih HY, Davis FP, Meylan F, Huang Y, et al. Neuropeptide cgrp limits group 2 innate lymphoid cell responses and constrains type 2 inflammation. Immunity. (2019) 51:682–95 e6. doi: 10.1016/j.immuni.2019.06.009
277. Mikami N, Watanabe K, Hashimoto N, Miyagi Y, Sueda K, Fukada S, et al. Calcitonin gene-related peptide enhances experimental autoimmune encephalomyelitis by promoting th17-cell functions. Int Immunol. (2012) 24:681–91. doi: 10.1093/intimm/dxs075
278. Cohen JA, Edwards TN, Liu AW, Hirai T, Jones MR, Wu J, et al. Cutaneous trpv1(+) neurons trigger protective innate type 17 anticipatory immunity. Cell. (2019) 178:919–32 e14. doi: 10.1016/j.cell.2019.06.022
279. Hanc P, Gonzalez RJ, Mazo IB, Wang Y, Lambert T, Ortiz G, et al. Multimodal control of dendritic cell functions by nociceptors. Science. (2023) 379:eabm5658. doi: 10.1126/science.abm5658
280. Chen O, Donnelly CR, Ji RR. Regulation of pain by neuro-immune interactions between macrophages and nociceptor sensory neurons. Curr Opin Neurobiol. (2020) 62:17–25. doi: 10.1016/j.conb.2019.11.006
281. Balogh M, Zhang J, Gaffney CM, Kalakuntla N, Nguyen NT, Trinh RT, et al. Sensory neuron dysfunction in orthotopic mouse models of colon cancer. J Neuroinflamm. (2022) 19:204. doi: 10.1186/s12974-022-02566-z
282. Ghilardi JR, Rohrich H, Lindsay TH, Sevcik MA, Schwei MJ, Kubota K, et al. Selective blockade of the capsaicin receptor trpv1 attenuates bone cancer pain. J Neurosci. (2005) 25:3126–31. doi: 10.1523/JNEUROSCI.3815-04.2005
283. Peters CM, Ghilardi JR, Keyser CP, Kubota K, Lindsay TH, Luger NM, et al. Tumor-induced injury of primary afferent sensory nerve fibers in bone cancer pain. Exp Neurol. (2005) 193:85–100. doi: 10.1016/j.expneurol.2004.11.028
284. Dore-Savard L, Otis V, Belleville K, Lemire M, Archambault M, Tremblay L, et al. Behavioral, medical imaging and histopathological features of a new rat model of bone cancer pain. PloS One. (2010) 5:e13774. doi: 10.1371/journal.pone.0013774
285. Horan NL, McIlvried LA, Atherton MA, Yuan MM, Dolan JC, Scheff NN. The impact of tumor immunogenicity on cancer pain phenotype using syngeneic oral cancer mouse models. Front Pain Res (Lausanne). (2022) 3:991725. doi: 10.3389/fpain.2022.991725
Keywords: peripheral sensory neurons, nociceptors, neuropeptides, pain, cancer, tumor microenvironment, immune cells, tumor immunity
Citation: Mardelle U, Bretaud N, Daher C and Feuillet V (2024) From pain to tumor immunity: influence of peripheral sensory neurons in cancer. Front. Immunol. 15:1335387. doi: 10.3389/fimmu.2024.1335387
Received: 08 November 2023; Accepted: 29 January 2024;
Published: 16 February 2024.
Edited by:
Abdelilah Soussi Gounni, University of Manitoba, CanadaReviewed by:
Huasheng Yu, University of Pennsylvania, United StatesNicole N Scheff, University of Pittsburgh, United States
Copyright © 2024 Mardelle, Bretaud, Daher and Feuillet. This is an open-access article distributed under the terms of the Creative Commons Attribution License (CC BY). The use, distribution or reproduction in other forums is permitted, provided the original author(s) and the copyright owner(s) are credited and that the original publication in this journal is cited, in accordance with accepted academic practice. No use, distribution or reproduction is permitted which does not comply with these terms.
*Correspondence: Vincent Feuillet, feuillet@ciml.univ-mrs.fr