- 1School of Rehabilitation, Capital Medical University, Beijing, China
- 2Department of Spinal and Neural Functional Reconstruction, China Rehabilitation Research Center, Beijing, China
- 3Institute of Rehabilitation medicine, China Rehabilitation Research Center, Beijing, China
- 4School of Population Medicine and Public Health, Chinese Academy of Medical Sciences/Peking Union Medical College, Beijing, China
- 5Cheeloo College of Medicine, Shandong University, Jinan, Shandong, China
Spinal cord injury is a severe neurological trauma that can frequently lead to neuropathic pain. During the initial stages following spinal cord injury, inflammation plays a critical role; however, excessive inflammation can exacerbate pain. Regulatory T cells (Treg cells) have a crucial function in regulating inflammation and alleviating neuropathic pain. Treg cells release suppressor cytokines and modulate the function of other immune cells to suppress the inflammatory response. Simultaneously, inflammation impedes Treg cell activity, further intensifying neuropathic pain. Therefore, suppressing the inflammatory response while enhancing Treg cell regulatory function may provide novel therapeutic avenues for treating neuropathic pain resulting from spinal cord injury. This review comprehensively describes the mechanisms underlying the inflammatory response and Treg cell regulation subsequent to spinal cord injury, with a specific focus on exploring the potential mechanisms through which Treg cells regulate neuropathic pain following spinal cord injury. The insights gained from this review aim to provide new concepts and a rationale for the therapeutic prospects and direction of cell therapy in spinal cord injury-related conditions.
1 Introduction
Spinal cord injury (SCI) occurs when there is injury or damage to the spinal cord due to an external force that may result in neurological dysfunction. This injury can affect, to varying degrees, the sensory, motor, and autonomic functions of the body (1). Such injuries result in severe deterioration in the quality of life of patients and increase disability and mortality rates for spinal cord injuries (2, 3). Trauma accounts for approximately 90% of spinal cord injuries (4). Neuropathic pain is a complex disorder caused by neurological lesion or disease and has become a major prognostic challenge for clinical patients due to its difficult-to-treat and often ineffective treatment options (5). A new definition of neuropathic pain was proposed by a panel of experts in 2008: ‘pain that occurs as a direct consequence of an injury or disease affecting the somatosensory system’ (6), which has since been endorsed by the International Association for the Study of Pain (IASP) (7). Peripheral or central neurological lesions can result in loss of sensation in the innervated areas of the damaged nerves or in areas of the body that correspond to areas of the spinal cord or brain that have been directly or indirectly damaged as a result of the lesion or disease. Therefore, sensory hypersensitivity in the affected area is often accompanied by sensory loss when most neuropathic pain occurs (8). Pain resulting from SCI can affect the patients’ quality of life and severely impact the prognosis, which can result in lifelong consequences. Neuropathic pain (NP), a complex and heterogeneous disorder, affects approximately 8% of the adult population and has significant implications for both patients and healthcare systems (9). The International Association for the Study of Pain (IASP) defines NP as pain that arises directly from a lesion or disease affecting the somatosensory system (10). The etiology of NP can be attributed to damage to either the peripheral nerves, resulting in peripheral neuropathic pain (PNP), or the central nerves, resulting in central neuropathic pain (CNP). PNP is commonly associated with conditions such as Complex Regional Pain Syndrome (CRPS) and Failed Back Surgery Syndrome (FBSS), including cancer and diabetes. CNP typically arises following a stroke, spinal cord injury, or multiple sclerosis (11). Around 8% of cases of central pain syndromes manifest in post-stroke patients (12), while spinal cord injury patients constitute approximately 30-50% of cases (13), and those suffering from multiple sclerosis comprise around 20-25% (14). NP is characterized by spontaneous pain (pain that occurs without provocation, such as burning sensations and tingling), allodynia (pain resulting from non-harmful stimuli), and hyperalgesia (an increased response to painful stimuli). Pain after SCI can manifest itself in a variety of ways, and as scar tissue recedes, chronic pain emerges, limiting the prognosis of SCI and affecting neuroplasticity (15, 16). The damage to nerve fibers and neurons following SCI can also cause chronic symptoms of neuropathic pain, which may be closely related to the neuroinflammatory response (17). Neuropathic pain is pain due to nerve fiber damage or chronic compression, which can manifest as a tingling, burning, or electric shock-like pain (8). It is an abnormal pain response due to damage to the nervous system, which may manifest as hypersensitivity or spreading of pain (18). Nerve fiber degeneration or sustained compression on nerve fibers can give rise to neuropathic pain, attributed to aberrant firing or heightened release of neurotransmitters. The propagation of nerve impulses becomes irregular, and these abnormal transmissions can lead to distortion or amplification of pain sensations (19). Subsequent to a spinal cord injury, certain neurons may exhibit augmented excitability, resulting in an excessive amplification of pain signaling. This abnormal excitability might involve neurons beyond the injury site, causing pain sensations to radiate into unaffected areas. The heightened neuronal activity engenders impaired nerve conduction, thereby disrupting the transmission of pain information through the central nervous system (20). A neuroinflammatory response occurs, characterized by tissue swelling and increased pressure in the vicinity of the nerves. Consequently, inflammatory mediators like tumor necrosis factor, prostaglandins, and cytokines are released, thereby leading to aberrant pain perception (8, 21, 22). Alterations and remodeling of neural circuits in the central nervous system manifest following spinal cord injury. This, in turn, can elicit a painful response to otherwise innocuous stimuli, consequently inducing neuropathic pain characterized by sensations of tingling, burning, and electric shock-like pain (8, 23).
Following SCI, the inflammatory response is complex and is driven by a variety of cellular and signaling molecules, including inflammatory factors and injury-associated molecules (e.g., high mobility group protein (HMGB1), heat shock protein (HSP), etc.). These inflammatory mediators subsequently recruit and activate immune cells to further exacerbate the inflammatory response (24). Intervention of the inflammatory response leads to inflammatory cell infiltration, neuronal degeneration, and abnormal neurotransmitter release, which in turn exacerbates the perception and development of neuropathic pain (25). Microenvironmental imbalance and parenchymal cell infiltration are key to secondary SCI (26, 27). The immune response is involved in post-injury microenvironmental regulation; its regulatory role is achieved through interactions with other immune cells, which can regulate the activation state of immune cells and control the intensity of the inflammatory and immune response through cell contact and cytokine secretion (28, 29).
Regulatory T (Treg) cells, a specialized subpopulation of immunosuppressive T cells, are essential for maintaining immune homeostasis (30). There is mounting evidence indicating the involvement of the adaptive T-cell immune response, within the immune system, in the development of neuropathic pain. Previous investigations have demonstrated the infiltration of T cells into the spinal cord (31, 32), the site of injury (33), and the dorsal root ganglion (DRG) subsequent to peripheral nerve injury (34). These studies highlight the indispensable role of T cell regulation in neuropathic pain. Although the precise contributions of distinct T cell subpopulations to neuropathic pain remain unclear, it has been observed that helper T (Th)1 cells are capable of augmenting pain sensitivity through the production of inflammatory cytokines, namely interferon gamma (33). Conversely, helper T (Th)2 cells have shown the ability to diminish pain sensitivity in animal models of nerve injury by generating anti-inflammatory cytokines, such as IL-10 (33). Simultaneous investigations have also suggested a potential link between microglia-mediated gender dimorphism in pain and Treg-mediated regulation of microglia activation and attenuation of pain hypersensitivity (35). Treg cells exert a localized immunosuppressive effect by targeting immune cells to reduce the inflammatory response and decrease self-attack (36). Regulation of Treg cell number and function is an important part of the pathogenesis of various immune diseases. Treg cells act as key negative regulators of inflammation in various pathological states, including autoimmunity, injury, and neurodegeneration (37–43). Regulatory T cells (Tregs) play a crucial role in maintaining self-tolerance and the dynamic equilibrium of the immune microenvironment (44). Given their involvement in immunoregulation during the inflammatory response to neuropathic pain, it is imperative to examine the functions of inflammation and Treg cell regulation in the context of neuropathic pain following spinal cord injury.
This review describes the relationship between the inflammatory response and Treg cell regulation following SCI (Figure 1), as well as the critical role of Treg cells in the development of neuropathic pain after SCI. The overarching objective of this review is to gain profound insights into the underlying mechanisms of neuropathic pain following SCI and to provide novel avenues for cellular therapeutic interventions.
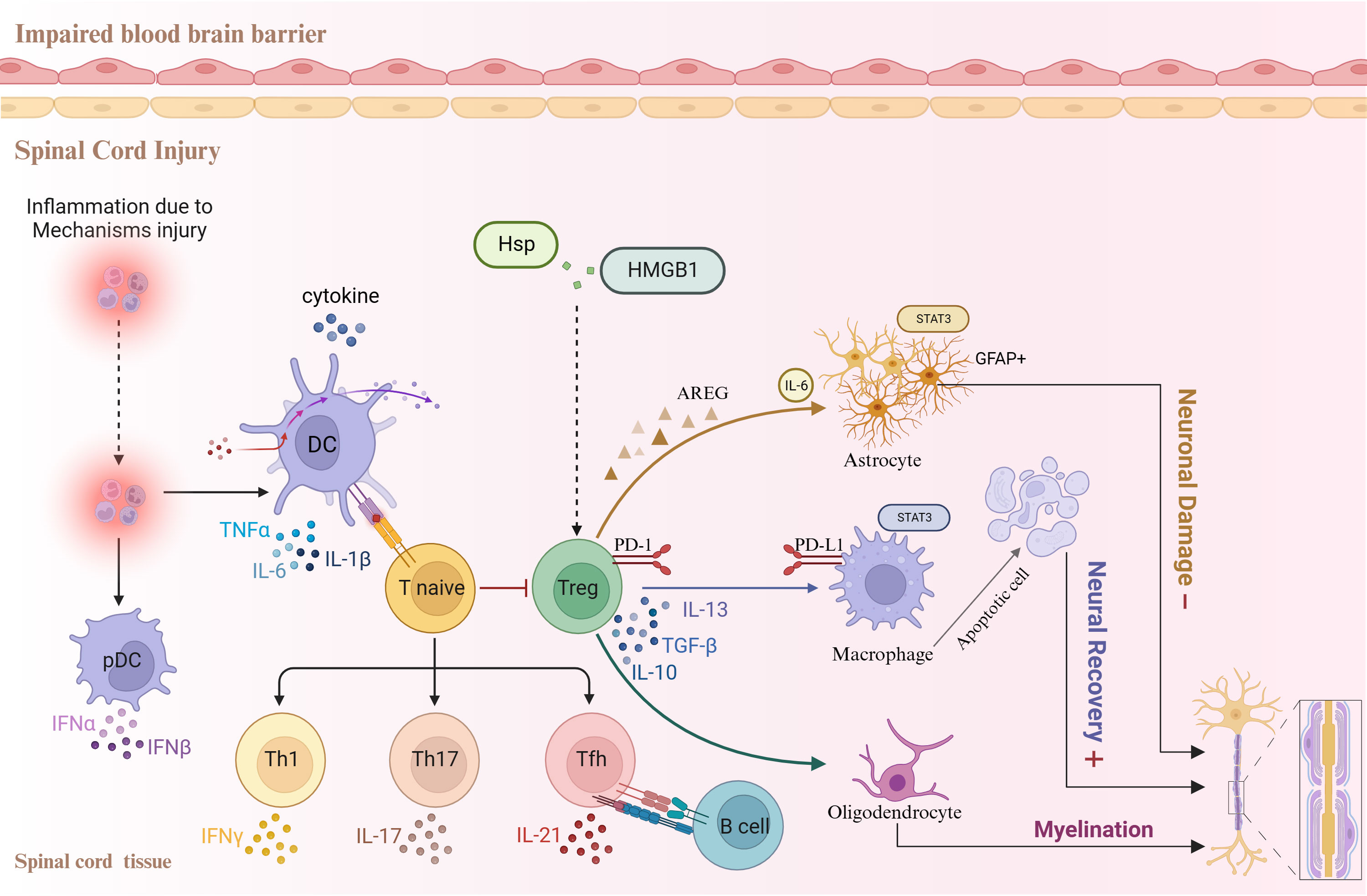
Figure 1 Relationships between the inflammatory response, Treg cells, and other cell types following spinal cord injury.
2 The inflammatory response is associated with neuropathic pain after SCI
2.1 Release of inflammatory mediators and inflammation-mediated pain transmission
The release of inflammatory mediators and inflammation-mediated pain transmission play an important role in the progression of disease after SCI (45). During the inflammatory response, immune cells and nerve cells interact to trigger the release of inflammatory mediators, including cytokines (tumor necrosis factor (TNF)-α, interleukin (IL)-1β) and chemokines (e.g., CXCL1, CXCL2). These inflammatory mediators are involved in the inflammatory response and regulate inflammation-related signaling pathways (46, 47).
In addition, the release of inflammatory mediators participates in the inflammation-mediated pain transduction process. Following SCI, inflammatory mediators stimulate sensory neurons and dorsal root ganglion cells, leading to increased neuronal excitability (48). This abnormal state of excitability induces pain perception, which is mediated through signaling pathways, during which inflammatory mediators act as signaling molecules that interact with their corresponding receptors and ion channels to regulate neuronal excitability (49, 50). Such receptors and channels include TRPV1 channels, ATP receptors, and acid-sensing ion channels. Inflammatory mediators alter neuronal excitatory thresholds and enhance neuropathic pain by modifying the activity and expression of these channels (51–54). Therefore, inflammatory mediators are involved in pain transmission following SCI.
2.2 Effect of the infiltration and activation of immune cells on pain after SCI
SCI elicits an immune response, resulting in the accumulation of inflammatory and immune cells. Immune cell infiltration occurs at the site of SCI where immune cells interact with neurons (55, 56). These infiltrating immune cells mainly include monocytes, macrophages, T cells, and B cells (57). Different cell types release different immune molecules at the site of injury after SCI, and the peak period of cellular infiltration varies (Figure 2). Immune cells are activated to release a series of cytokines and chemicals, including TNF-α, IL-1β, and IL-6, which an act directly on neurons to increase excitability and decrease inhibition (58). The interaction between immune cells and nerve cells leads to increased neuronal excitability, triggering or enhancing the nociceptive transmission of pain through direct stimulation of neurons or via effects on synaptic transmission (59). Related studies have shown that inhibiting the infiltration and activation of immune cells, or targeting and modulating cytokines and chemicals released by immune cells, can attenuate pain sensation following SCI (60–63). Therefore, modulation of the infiltration and activation of immune cells may be a novel approach to treat pain after SCI.
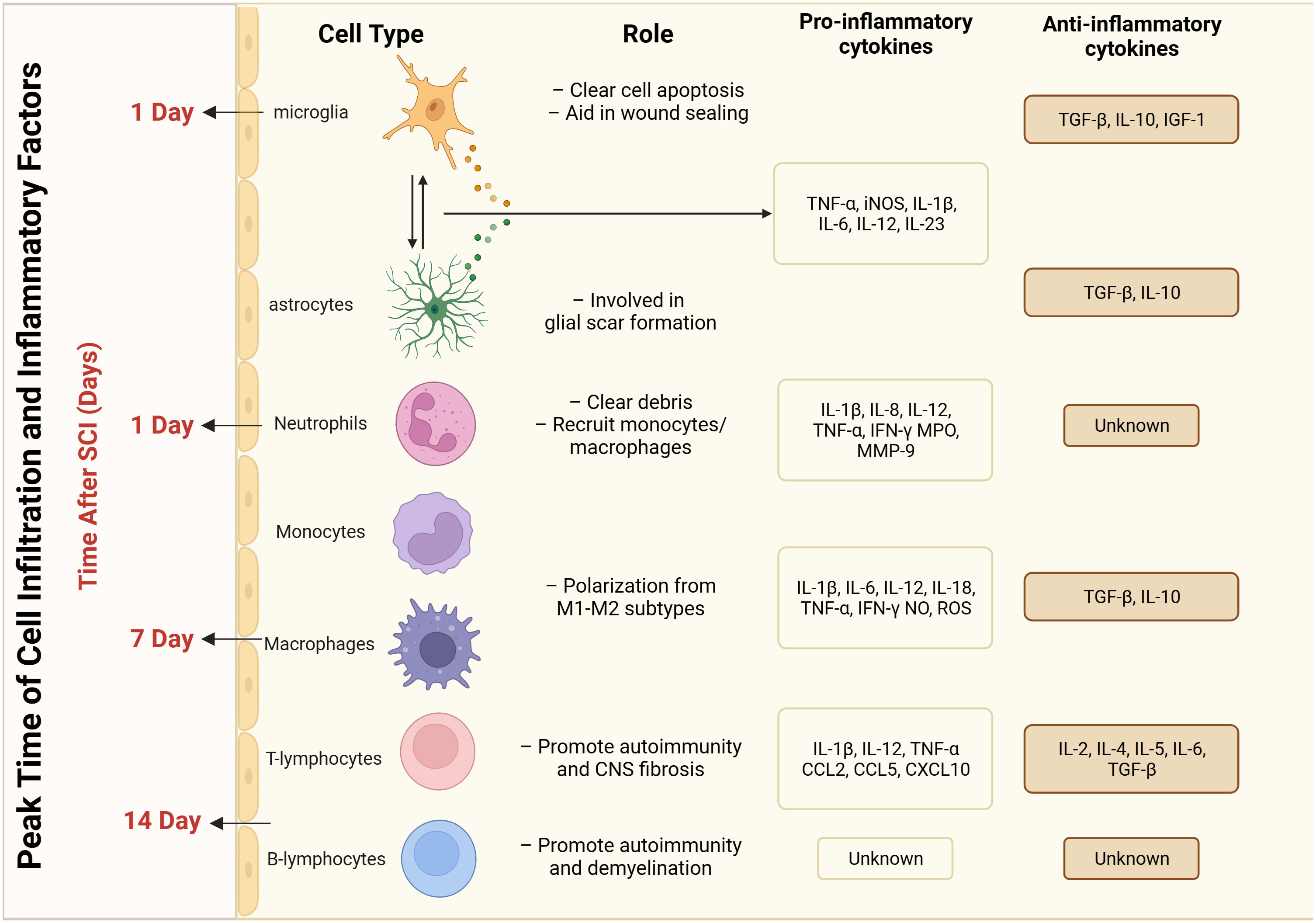
Figure 2 Peak time of infiltration and inflammatory factors released by different cell types after spinal cord injury.
2.3 The role of glial cells in the inflammatory response and the maintenance of neuropathic pain
Glial cells, including astrocytes and microglia, are involved in the inflammatory response following SCI (64–66). These glial cells become activated after SCI and release multiple inflammatory mediators (67, 68) (Figure 2). Primary injury is initiated by an initial insult to the spinal cord, leading to mechanical damage and subsequent opening of the blood-brain barrier (BBB). This process is characterized by oxidative damage, edema, ischemia, and heightened glutamate excitability (25, 69). Within the initial few hours, these mechanisms contribute to the onset of secondary damage, whereby immune cells infiltrate the damaged region via the vascular system, resulting in cell death and exacerbated injury. Various cell types are involved in this secondary phase, exerting distinct temporal influences on disease progression (25, 70). Spinal cord injury induces the activation and recruitment of multiple glial cells, leading to intricate downstream effects on neuronal function (71). The formation of the glial scar after SCI involves the participation of various cell types. Astrocyte activation begins on day 1 post-injury and reaches its peak at day 14 (72–75). Schwann cell recruitment starts 21 days after SCI (76, 77). Meningeal cells become involved 3 days after SCI and reach their peak at day 14 (78–80). Fibroblast activation initiates 3 days after SCI and peaks at day 7-14 (81–84). Finally, a limited degree of structural tissue regeneration and repair takes place in the weeks to months following spinal cord injury (25). Astrocytes, the most common glial cell type in the spinal cord, play an important role in maintaining normal neuronal function, regulating the blood–brain barrier, and removing intercellular metabolites (85, 86). The deleterious effects of astrocytes during SCI are produced through reactive astrocytes. Two types of reactive astrocytes have been identified: the A1 astrocyte and the A2 astrocyte (87, 88). The former plays a destructive role and promotes the inflammatory response, whereas the latter plays a restorative role in ischemia-induced inflammation and inhibits the inflammatory response (89). The imbalance between the A1 and A2 responses is an important mechanism in the development of neuropathic pain after SCI. Excessive A1 astrocyte responses and excessive release of inflammatory mediators may lead to neuronal activation and abnormal nociceptive transmission, resulting in the development of neuropathic pain (90). The A2 astrocyte response is a major contributor to the development of neuropathic pain. In addition, deficiencies in the A2 astrocyte response may affect tissue repair and anti-inflammatory mechanisms, perpetuating the inflammatory response and exacerbating the degree and duration of pain (91).
Microglia are mainly found in the gray matter regions of the central nervous system. Following SCI, microglia are also activated and participate in the inflammatory response, promoting neuronal excitability and inflammation-mediated nociceptive transmission (92). Microglia act as powerful neuromodulators to regulate salience transmission and pain transmission through multiple inflammatory mediators (e.g., pro-inflammatory and anti-inflammatory factors) acting on neurons and other glial cells (93) (Figure 2). Microglia have multiple cell surface receptors that dynamically and multifacetedly regulate the inflammatory response after SCI by interacting with neurons, astrocytes, immune cells, and others (94). Studies have reported that microglia are involved in inflammatory responses, pain signaling, and synaptic remodeling after SCI. Microglia maintain the inflammatory response and the enhancement of pain afferent signaling through the recruitment of immune cells after injury, forming synaptic structures with neurons. Vesicles released through these structures enhance neuronal excitability and strengthen pain signaling (95–98). Furthermore, it has been reported that the HMGB1–RAGE axis contributes to the major macrophage/microglia-mediated pro-inflammatory response, and that inhibition of this pathway exerts neuroprotective functions after SCI. This cascade modulation of the immune microenvironment has emerged as a prospective therapeutic approach for the treatment of SCI (99). In addition to the well-studied microglia and astrocytes, oligodendrocytes, as the main myelin-producing glial cells, are critical in maintaining myelin for fast and efficient conduction of electrical impulses along the axon and for maintaining axon integrity (100). Some studies have reported that Treg cells are involved in oligodendrocyte differentiation and myelination, which has a positive effect on SCI recovery (101).
3 Regulatory role of Treg cells in SCI
3.1 Function and characterization of Treg cells
Treg cells are an immunosuppressive subpopulation of CD4+ T helper cells, which have important immunomodulatory functions and unique characteristics that help to maintain immune homeostasis and inhibit overactivation of the immune response (102, 103). The functions of Treg cells are characterized by immunosuppression, immune tolerance, and immune homeostasis.
Recent advances in Treg cell biology have identified Treg cells residing in specific tissues for the maintenance of tissue homeostasis and repair (104), such as in the secondary prevention of ischemic stroke where they suppress immune responses by directly inhibiting the activation and function of other immune cells (105). Treg cells play an important role in the immune system as self-tolerance regulators, preventing damage to tissues from the immune response and reducing the occurrence of autoimmune diseases. Furthermore, Treg cells are involved in tumor development and progression by suppressing tumor immunity; Treg cells can be activated by chemokines (e.g., CCR4-CCL17/22, CCR8-CCL1, CCR10-CCL28, and CXCR3-CCL9/10/11), are chemotactically attracted to the tumor microenvironment, and participate in microenvironment regulation (106–108).
The dysregulation of the Th17 and Treg cell balance in neurological disorders can significantly impact disease progression (39). Excessive activation of Th17 cells and insufficient regulation by Treg cells can contribute to immune-mediated neuroinflammation and injury, thereby promoting disease progression (109, 110). Additionally, emerging evidence highlights the interconnectedness of the gut, spinal cord, and immune cells in spinal cord injury disorders, which establishes a “gut-spinal cord-immune” axis. Treg cell regulation in the intestinal environment, along with the promotion of IL-10 secretion, can modulate the dynamic equilibrium between Treg and IL-17γδ T cells, suppress inflammatory responses, and enhance motor function recovery in rats. Collectively, these studies underscore the crucial regulatory function of Treg cells in the pathogenesis of spinal cord injury (111).
CD25 is one of the hallmark features of Treg cells. Increased expression of CD25 by Treg cells is closely associated with immunosuppressive functions (112). In addition, Treg cells express the transcription factor FOXP3 in the nucleus, which is a major marker of Treg cell identity and a key molecule in the regulation of their function (113, 114). Transforming growth factor β (TGF-β) and IL-10 are examples of the multiple inflammatory suppressive cytokines produced by Treg cells; these factors inhibit the activation and immune response of other cells, leading to immunosuppression and immunomodulation (115, 116). Short-chain fatty acids (SCFAs) modulate Treg cells in the gut and affect the balance of Treg cells and IL-17+γδ T cells in the spinal cord, which has been reported to suppress inflammatory responses and promote locomotor function in SCI rats (111). Treg cells interact with amphiregulin (AREG) through the AREG/epidermal growth factor receptor (EGFR) signaling pathway to participate in immune regulation while controlling skeletal muscle function and regeneration (117). In summary, Treg cells play an important role in immune regulation by suppressing the activation and function of other immune cells, maintaining immune homeostasis, and preventing damage to host tissues from the immune response.
3.2 Treg cell regulation of the inflammatory response after SCI
A reduction in the number of Treg cells is closely associated with the inflammatory response following SCI. Treg cells regulate the degree of inflammation through a variety of mechanisms; they can inhibit the activity of other immune cells (e.g., Th1, Th2, and Th17 cells) and reduce the production and release of inflammatory mediators (118, 119). The number of Treg cells was reported to be significantly reduced in the spinal cord and its surrounding tissues, which resulted in a decrease in the immunomodulatory function of Treg cells, allowing an over-activated inflammatory response to develop at the site of injury (103). Treg cells reduce the extent of the inflammatory response by inhibiting the activation of other immune cells and the production of inflammatory factors. The inflammatory response in SCI is directly suppressed by inhibiting the activation signaling of other immune cells through the binding of the surface molecules cytotoxic T lymphocyte antigen 4 (CTLA4) and programmed cell death 1 (PD-1) to their ligands (120, 121). Treg cells act as regulatory antigen presenters through specific ligand–receptor interactions, inhibiting antigen activation and inflammatory responses by immune cells (120). Treg cells also interfere with inflammatory signaling pathways, and can reduce the production of inflammatory factors and inflammatory responses by inhibiting the activation of the nuclear transcription factor NF-κB (122–124). In addition, some studies have reported that by regulating the TUG1/miR-214/HSP27 signaling pathway, the proportion of Treg cells can be reduced, thereby alleviating acute SCI (125). Treg cells can also regulate neuroendocrine circuits by inhibiting cytokine secretion and release; therefore, regulating neuronal activity Janyga et al., 20231. Treg cells downregulate TNF-α and IL-6 expression in microglia by inhibiting STAT3 pathway activation, which ultimately improves the damaged spinal cord microenvironment and promotes the recovery of neurological function after SCI (126). MBP-Th2 cell transplantation after SCI changes the state dominated by Th1 and M1 cells to a state dominated by Th2, Treg, and M2 cells. This changes the local immune microenvironment by increasing the number of Th2 cells, thus producing beneficial effects on the spinal cord and promoting the repair of SCI.
3.3 Inhibition of neuropathic pain by Treg cells
Neuroimmune communication has emerged as a key neuropathic pain mechanism in previous studies, which reported that both the innate and adaptive immune systems are associated with neuroinflammatory changes in neuropathic pain (127). Local infiltration of macrophages, T cells, astrocytes, and activated microglia following SCI results in the release of multiple inflammatory mediators (pro-inflammatory cytokines, such as TNF-α, IL-1β, IL-17, and IFN-g) to maintain nociceptive hypersensitivity (128–131) (Figure 2). In mice, mechanical pain after nerve injury can be alleviated by intrathecal injection of Treg cells (132). Furthermore, CD28 agonists can alleviate mechanical pain hypersensitivity due to injury in rats with chronic compression injury of the sciatic nerve by modulating the number of T cells, macrophages, and other immune cells in the sciatic nerve and dorsal root ganglion (44, 133). The elimination of Treg cells using a CD25 antibody leads to prolonged mechanical abnormalities in sciatica mice (44). Depletion of FoxP31 Treg cells in transgenic DEREG mice leads to a transient increase in mechanical pain hypersensitivity (134).
Treg treatment also modulates the amount of reactive astrocytes of different phenotypes to reduce neurotoxicity by attenuating astrocyte GFAP expression. Interestingly this therapeutic effect of Treg cells manifests itself differently in female and male mice, with a reduction in the number of neurotoxic astrocytes in peripherally injured male mice, and conversely, in peripherally injured females, the number of protective astrocytes was increased with peripheral nerve injury (135). Brain Treg cells inhibit neurotoxic astrocyte proliferation and protect neurons from damage by producing the low-affinity EGFR ligand AREG (136). Male mouse meningeal Treg cell administration may induce an anti-inflammatory shift in microglia phenotype via Treg-associated effector cytokines IL-10 and TGF-β (137–139).
The inhibitory role of Treg cells in neuropathic pain has been confirmed by a series of animal studies, as described above. The results of clinical trials similarly suggest that the number of T helper cells producing IL-17 is reduced, while the number of Treg cells is increased in patients with chronic lower back pain. Correspondingly, mRNA expression levels of FOXP3 and TGF-β were elevated in peripheral blood mononuclear cells according to cytokine profiling assays (140, 141). This phenomenon has been speculated to reduce pain levels in patients through the suppression of inflammatory responses (142). In summary, Treg cells reduce inflammatory damage to neurons by modulating neuroimmune interactions, and reducing neuronal hyperexcitability and abnormal alterations in synaptic plasticity, which in turn attenuates the onset and progression of neuropathic pain.
4 Clinical application strategies for Treg cell enhancement and synergy
4.1 Therapeutic strategies for Treg cell increase and functional improvement
The number of Treg cells can be increased by exogenous donor acquisition or endogenous proliferation and expansion, for example, with the use of growth factors, immunomodulators, or cell therapy, among others (143–145). Targeting Treg cell ligand–receptor interactions using specific cytokines or drugs (e.g., IL-10, TGF-β, and IL-2, etc.) that enhance Treg cell immunosuppression, enhancing immunomodulation, improves the function of Treg cells (146, 147). Improving Treg cell migration and accurately localizing damaged sites by altering chemical gradients, enhancing cytokine adhesion, and modulating inflammatory factor expression is one of the important therapeutic strategies (148–150).
4.2 Synergistic effects of Treg cells with immunosuppressants and other treatments
Treg cells, as natural immunomodulatory cells, can inhibit immune cell activity, and can function synergistically with immunosuppressive agents (e.g., immunosuppressant drugs or cytokine inhibitors) to reduce inflammatory and autoimmune responses (151–153). Cell therapy for SCI is emerging as a new research hotspot (154). Currently common cell therapies, such as stem cell therapy or gene therapy, can repair and regenerate tissues. Treg cell combined with stem cell therapy is a potentially more desirable therapeutic tool, and the synergistic combination of drugs with Treg cells enhances the immune-suppressive effect of the drugs, reduces the side effects, and lowers the risk of immune tolerance (155). Treg deficiency affects the gut microbiota and bile acid metabolism, induces IL-6 expression, and triggers a lethal inflammatory response. Antibiotics can modulate the gut microbiota and bile acid metabolism by inhibiting IL-6 levels, thus preventing the lethal inflammation caused by Treg deficiency (156). In studies on immune-related diseases associated with tumors, Treg cells are implicated in tumor development and progression by suppressing anti-tumor immune responses. Therefore, there is a critical need in the field of cancer immunotherapy to deplete Treg cells and modulate their function to enhance anti-tumor immune responses (106). Research has demonstrated that dendrobine significantly reduces Foxp3 expression, increases serum IL-17 levels, and enhances Th17 cell function while suppressing Treg cell function. Additionally, in vivo, dendrobine and cisplatin may synergistically regulate the Treg/Th17 cell balance rather than induce apoptosis (157). All-trans retinoic acid is involved in regulating the differentiation of helper T cells (Th) and Treg cells. Furthermore, all-trans retinoic acid maintains the stability of thymus-derived Treg cells under inflammatory conditions (158). Severe asthma development is particularly associated with Th17 cell and neutrophil activation, and studies have shown that asthma patients can effectively suppress airway inflammation by increasing the Treg/Th17 cell ratio using statins in combination with corticosteroids (159, 160). Tregs are key target cells involved in asthma relief, and it has been suggested that glucocorticoid application reduces the number and activity of Tregs in various asthma mouse models, potentially through thymic T-cell production inhibition (161). Immunomodulators (e.g., anti-CD3 antibody, anti-CD25 antibody, etc.) can directly influence the number and activity of Treg cells, and targeted depletion of Treg cells can activate tumor-specific effector T cells and enhance the efficacy of tumor immunotherapy (162). Cancer immunotherapy primarily focuses on immune checkpoint molecules, and blocking CTLA-4 primarily activates T cells and suppresses Treg cells. PD-L1 plays a dominant role in Th1 and Th17 immunity, while PD-L2 primarily impacts Th2 immunity (163). The use of Treg cells as a cell-based therapeutic approach was initially demonstrated in mouse models; Treg cells were found to have a beneficial role in pathogenesis (36). However, the immune rejection faced by this therapeutic approach is considered to be one of the important challenges.
5 Prospects and challenges in the application of Treg cell therapy
From the current degree of clinical application, firstly, there still exists a certain technical difficulty in large-scale preparation of high-purity Treg cells. Second, further clinical research and validation are still needed to determine the therapeutic mechanism and safety of Treg cells. In addition, the survival time and functional stability of Treg cells also requires further improvement. Overall, although there are still some challenges and limitations in the clinical application of Treg cells, they have great potential in regulating inflammatory responses and treating immune-related diseases. Therefore, Treg cells are expected to be a potential target for regulating inflammatory responses and treating neuropathic pain after SCI, which will bring better therapeutic effects and treatment strategies for SCI patients.
6 Conclusions
Spinal cord injury (SCI) is a severe neurotraumatic condition that frequently results in the development of neuropathic pain. Neuropathic pain following spinal cord injury (SCI) is a complex spectrum of disorders characterized by a multitude of pathophysiologic mechanisms and associations with psychosocial factors, posing significant challenges in its management (164). While research in recent decades has shed light on the pathophysiology of neuropathic pain after SCI, therapeutic advancements have been limited. Given the high prevalence of chronic neuropathic pain, future research will prioritize the investigation of targeted therapies, identification of reliable biomarkers, and evaluation of combination therapies targeting multiple mechanisms to enhance treatment efficacy. Inflammation is known to play a critical role in the early stages following SCI, but excessive inflammation can exacerbate painful symptoms. Treg cells have a pivotal function in regulating inflammation and reducing neuropathic pain. Treg cells regulate inflammatory responses by influencing cytokine expression and other immune cell functions. However, inflammation also hinders the activity of Treg cells, thus exacerbating neuropathic pain. Therefore, besides suppression of the inflammatory response, enhancing the regulatory function of Treg cells may also offer new therapeutic avenues for the treatment of neuropathic pain caused by SCI. It is very valuable and meaningful to study the potential regulatory function of Treg cells in neuropathic pain after spinal cord injury or even central nervous system injury. Future research on neuropathic pain after spinal cord injury may focus on the development of new immunomodulatory drugs, assessment of the number and function of patients’ Tregs cells to form a personalized treatment plan, the development of vaccines to regulate the immune system, and novel cell therapies based on the in vitro expansion of Tregs technology and the transfer of Tregs cells into the patient’s body. However, it is important to note that the clinical application of Tregs for the treatment of neuropathic pain requires a careful consideration of human Treg cell purity, stability, and functional role in neuropathic pain disorders.
Author contributions
CZ: Data curation, Formal Analysis, Investigation, Methodology, Resources, Supervision, Validation, Writing – original draft. YL: Formal Analysis, Investigation, Writing – review & editing. YY: Formal Analysis, Investigation, Resources, Writing – review & editing. ZL: Software, Writing – review & editing. XX: Data curation, Formal Analysis, Writing – review & editing. ZT: Resources, Writing – review & editing. WL: Supervision, Visualization, Writing – review & editing. DY: Conceptualization, Writing – review & editing. FG: Project administration, Writing – review & editing. SW: Software, Writing – review & editing. LZ: Data curation, Writing – review & editing. HG: Methodology, Writing – review & editing. RP: Validation, Writing – review & editing. LD: Visualization, Writing – review & editing. LJ: Conceptualization, Data curation, Funding acquisition, Investigation, Methodology, Resources, Validation, Visualization, Writing – review & editing.
Funding
The author(s) declare financial support was received for the research, authorship, and/or publication of this article. This work was funded by the Fundamental Research Funds for Central Public Welfare Research Institutes (No. 2022CZ-3), the Fundamental Research Funds for Central Public Welfare Research Institutes (No. 2023CZ-3), and the Fundamental Research Funds for Central Public Welfare Research Institutes (No. 2019CZ-13).
Acknowledgments
The authors would like to thank Charlesworth Author Services (https://www.cwauthors.com.cn/) for its linguistic assistance during the preparation of this manuscript. We also thank https://biorender.com for the support of the diagram drawing.
Conflict of interest
The authors declare that the research was conducted in the absence of any commercial or financial relationships that could be construed as a potential conflict of interest.
Publisher’s note
All claims expressed in this article are solely those of the authors and do not necessarily represent those of their affiliated organizations, or those of the publisher, the editors and the reviewers. Any product that may be evaluated in this article, or claim that may be made by its manufacturer, is not guaranteed or endorsed by the publisher.
Footnotes
- ^ Janyga S, Kajdaniuk D, Czuba Z, Ogrodowczyk-Bobik M, Urbanek A, Kos-Kudła B, et al. Interleukin (IL)-23, IL-31, and IL-33 play a role in the course of autoimmune endocrine diseases. Endocr Metab Immune Disord Drug Targets. (Ahead of Print) (2023). doi: 10.2174/1871530323666230908143521
References
1. Zhang C, Talifu Z, Xu X, Liu W, Ke H, Pan Y, et al. MicroRNAs in spinal cord injury: A narrative review. Front Mol Neurosci (2023) 16:1099256. doi: 10.3389/fnmol.2023.1099256
2. Giger RJ, Hollis ER 2nd, Tuszynski MH. Guidance molecules in axon regeneration. Cold Spring Harb Perspect Biol (2010) 2:a001867. doi: 10.1101/cshperspect.a001867
3. Yan H, Hong P, Jiang M, Li H. MicroRNAs as potential therapeutics for treating spinal cord injury. Neural Regener Res (2012) 7:1352–9. doi: 10.3969/j.issn.1673-5374.2012.17.011
4. Alizadeh A, Dyck SM, Karimi-Abdolrezaee S. Traumatic spinal cord injury: an overview of pathophysiology, models and acute injury mechanisms. Front Neurol (2019) 10:282. doi: 10.3389/fneur.2019.00282
5. Colloca L, Ludman T, Bouhassira D, Baron R, Dickenson AH, Yarnitsky D, et al. Neuropathic pain. Nat Rev Dis Primers (2017) 3:17002. doi: 10.1038/nrdp.2017.2
6. Treede RD, Jensen TS, Campbell JN, Cruccu G, Dostrovsky JO, Griffin JW, et al. Neuropathic pain: redefinition and a grading system for clinical and research purposes. Neurology (2008) 70:1630–5. doi: 10.1212/01.wnl.0000282763.29778.59
7. Jensen TS, Baron R, Haanpää M, Kalso E, Loeser JD, Rice ASC, et al. A new definition of neuropathic pain. Pain (2011) 152:2204–5. doi: 10.1016/j.pain.2011.06.017
8. Finnerup NB, Kuner R, Jensen TS. Neuropathic pain: from mechanisms to treatment. Physiol Rev (2021) 101:259–301. doi: 10.1152/physrev.00045.2019
9. Vaegter HB, Andersen PG, Madsen MF, Handberg G, Enggaard TP. Prevalence of neuropathic pain according to the IASP grading system in patients with chronic non-malignant pain. Pain Med (2014) 15:120–7. doi: 10.1111/pme.12273
10. Treede RD, Rief W, Barke A, Aziz Q, Bennett MI, Benoliel R, et al. Chronic pain as a symptom or a disease: the IASP Classification of Chronic Pain for the International Classification of Diseases (ICD-11). Pain (2019) 160:19–27. doi: 10.1097/j.pain.0000000000001384
11. Schestatsky P, Nascimento OJ. What do general neurologists need to know about neuropathic pain? Arq Neuropsiquiatr (2009) 67:741–9. doi: 10.1590/S0004-282X2009000400039
12. Klit H, Finnerup NB, Jensen TS. Central post-stroke pain: clinical characteristics, pathophysiology, and management. Lancet Neurol (2009) 8:857–68. doi: 10.1016/S1474-4422(09)70176-0
13. Finnerup NB, Baastrup C. Spinal cord injury pain: mechanisms and management. Curr Pain Headache Rep (2012) 16:207–16. doi: 10.1007/s11916-012-0259-x
14. Foley PL, Vesterinen HM, Laird BJ, Sena ES, Colvin LA, Chandran S, et al. Prevalence and natural history of pain in adults with multiple sclerosis: systematic review and meta-analysis. Pain (2013) 154:632–42. doi: 10.1016/j.pain.2012.12.002
15. Hulsebosch CE, Hains BC, Crown ED, Carlton SM. Mechanisms of chronic central neuropathic pain after spinal cord injury. Brain Res Rev (2009) 60:202–13. doi: 10.1016/j.brainresrev.2008.12.010
16. Schomberg D, Ahmed M, Miranpuri G, Olson J, Resnick DK. Neuropathic pain: role of inflammation, immune response, and ion channel activity in central injury mechanisms. Ann Neurosci (2012) 19:125–32. doi: 10.5214/ans.0972.7531.190309
17. Chambel SS, Tavares I, Cruz CD. Chronic pain after spinal cord injury: is there a role for neuron-immune dysregulation? Front Physiol (2020) 11:748. doi: 10.3389/fphys.2020.00748
18. Cavalli E, Mammana S, Nicoletti F, Bramanti P, Mazzon E. The neuropathic pain: An overview of the current treatment and future therapeutic approaches. Int J Immunopathol Pharmacol (2019) 33:2058738419838383. doi: 10.1177/2058738419838383
19. Shiao R, Lee-Kubli CA. Neuropathic pain after spinal cord injury: challenges and research perspectives. Neurotherapeutics (2018) 15:635–53. doi: 10.1007/s13311-018-0633-4
20. Kang J, Cho SS, Kim HY, Lee BH, Cho HJ, Gwak YS. Regional hyperexcitability and chronic neuropathic pain following spinal cord injury. Cell Mol Neurobiol (2020) 40:861–78. doi: 10.1007/s10571-020-00785-7
21. Sawynok J, Liu XJ. Adenosine in the spinal cord and periphery: release and regulation of pain. Prog Neurobiol (2003) 69:313–40. doi: 10.1016/S0301-0082(03)00050-9
22. Alexander JK, Popovich PG. Neuroinflammation in spinal cord injury: therapeutic targets for neuroprotection and regeneration. Prog Brain Res (2009) 175:125–37. doi: 10.1016/S0079-6123(09)17508-8
23. Meacham K, Shepherd A, Mohapatra DP, Haroutounian S. Neuropathic pain: central vs. Peripheral mechanisms. Curr Pain Headache Rep (2017) 21:28. doi: 10.1007/s11916-017-0629-5
24. Freyermuth-Trujillo X, Segura-Uribe JJ, Salgado-Ceballos H, Orozco-Barrios CE, Coyoy-Salgado A. Inflammation: A target for treatment in spinal cord injury. Cells (2022) 11:2692. doi: 10.3390/cells11172692
25. Hellenbrand DJ, Quinn CM, Piper ZJ, Morehouse CN, Fixel JA, Hanna AS. Inflammation after spinal cord injury: a review of the critical timeline of signaling cues and cellular infiltration. J Neuroinflamm (2021) 18:284. doi: 10.1186/s12974-021-02337-2
26. Fan B, Wei Z, Yao X, Shi G, Cheng X, Zhou X, et al. Microenvironment imbalance of spinal cord injury. Cell Transplant (2018) 27:853–66. doi: 10.1177/0963689718755778
27. Fan B, Wei Z, Feng S. Progression in translational research on spinal cord injury based on microenvironment imbalance. Bone Res (2022) 10:35. doi: 10.1038/s41413-022-00199-9
28. Matsuda M, Huh Y, Ji RR. Roles of inflammation, neurogenic inflammation, and neuroinflammation in pain. J Anesth (2019) 33:131–9. doi: 10.1007/s00540-018-2579-4
29. Sterner RC, Sterner RM. Immune response following traumatic spinal cord injury: Pathophysiology and therapies. Front Immunol (2022) 13:1084101. doi: 10.3389/fimmu.2022.1084101
30. Dikiy S, Rudensky AY. Principles of regulatory T cell function. Immunity (2023) 56:240–55. doi: 10.1016/j.immuni.2023.01.004
31. Cao L, Deleo JA. CNS-infiltrating CD4+ T lymphocytes contribute to murine spinal nerve transection-induced neuropathic pain. Eur J Immunol (2008) 38:448–58. doi: 10.1002/eji.200737485
32. Costigan M, Moss A, Latremoliere A, Johnston C, Verma-Gandhu M, Herbert TA, et al. T-cell infiltration and signaling in the adult dorsal spinal cord is a major contributor to neuropathic pain-like hypersensitivity. J Neurosci (2009) 29:14415–22. doi: 10.1523/JNEUROSCI.4569-09.2009
33. Moalem G, Xu K, Yu L. T lymphocytes play a role in neuropathic pain following peripheral nerve injury in rats. Neuroscience (2004) 129:767–77. doi: 10.1016/j.neuroscience.2004.08.035
34. Hu P, Mclachlan EM. Macrophage and lymphocyte invasion of dorsal root ganglia after peripheral nerve lesions in the rat. Neuroscience (2002) 112:23–38. doi: 10.1016/S0306-4522(02)00065-9
35. Kuhn JA, Vainchtein ID, Braz J, Hamel K, Bernstein M, Craik V, et al. Regulatory T-cells inhibit microglia-induced pain hypersensitivity in female mice. Elife (2021) 10:e69056. doi: 10.7554/eLife.69056
36. Chen H, Peng H, Wang PC, Zou T, Feng XM, Wan BW. Role of regulatory T cells in spinal cord injury. Eur J Med Res (2023) 28:163. doi: 10.1186/s40001-023-01122-6
37. Solleiro-Villavicencio H, Rivas-Arancibia S. Effect of chronic oxidative stress on neuroinflammatory response mediated by CD4(+)T cells in neurodegenerative diseases. Front Cell Neurosci (2018) 12:114. doi: 10.3389/fncel.2018.00114
38. Wing JB, Tanaka A, Sakaguchi S. Human FOXP3(+) regulatory T cell heterogeneity and function in autoimmunity and cancer. Immunity (2019) 50:302–16. doi: 10.1016/j.immuni.2019.01.020
39. Yan JB, Luo MM, Chen ZY, He BH. The function and role of the Th17/Treg cell balance in inflammatory bowel disease. J Immunol Res (2020) 2020:8813558. doi: 10.1155/2020/8813558
40. Jiang Q, Yang G, Liu Q, Wang S, Cui D. Function and role of regulatory T cells in rheumatoid arthritis. Front Immunol (2021) 12:626193. doi: 10.3389/fimmu.2021.626193
41. Demaio A, Mehrotra S, Sambamurti K, Husain S. The role of the adaptive immune system and T cell dysfunction in neurodegenerative diseases. J Neuroinflamm (2022) 19:251. doi: 10.1186/s12974-022-02605-9
42. De Marchi F, Munitic I, Vidatic L, Papić E, Rački V, Nimac J, et al. Overlapping neuroimmune mechanisms and therapeutic targets in neurodegenerative disorders. Biomedicines (2023) 11:2793. doi: 10.3390/biomedicines11102793
43. Jafarzadeh A, Sheikhi A, Jafarzadeh Z, Nemati M. Differential roles of regulatory T cells in Alzheimer's disease. Cell Immunol (2023) 393-394:104778. doi: 10.1016/j.cellimm.2023.104778
44. Austin PJ, Kim CF, Perera CJ, Moalem-Taylor G. Regulatory T cells attenuate neuropathic pain following peripheral nerve injury and experimental autoimmune neuritis. Pain (2012) 153:1916–31. doi: 10.1016/j.pain.2012.06.005
45. Fakhri S, Abbaszadeh F, Jorjani M. On the therapeutic targets and pharmacological treatments for pain relief following spinal cord injury: A mechanistic review. BioMed Pharmacother (2021) 139:111563. doi: 10.1016/j.biopha.2021.111563
46. Liu X, Zhang Y, Wang Y, Qian T. Inflammatory response to spinal cord injury and its treatment. World Neurosurg (2021) 155:19–31. doi: 10.1016/j.wneu.2021.07.148
47. Subbarayan MS, Joly-Amado A, Bickford PC, Nash KR. CX3CL1/CX3CR1 signaling targets for the treatment of neurodegenerative diseases. Pharmacol Ther (2022) 231:107989. doi: 10.1016/j.pharmthera.2021.107989
48. Berger AA, Liu Y, Possoit H, Rogers AC, Moore W, Gress K, et al. Dorsal root ganglion (DRG) and chronic pain. Anesth Pain Med (2021) 11:e113020. doi: 10.5812/aapm.113020
49. Fujii T, Yamasaki R, Kira JI. Novel neuropathic pain mechanisms associated with allergic inflammation. Front Neurol (2019) 10:1337. doi: 10.3389/fneur.2019.01337
50. Huang Y, Chen SR, Pan HL. Calcineurin regulates synaptic plasticity and nociceptive transmission at the spinal cord level. Neuroscientist (2022) 28:628–38. doi: 10.1177/10738584211046888
51. De Logu F, Geppetti P. Ion channel pharmacology for pain modulation. Handb Exp Pharmacol (2019) 260:161–86. doi: 10.1007/164_2019_336
52. Dou B, Li Y, Ma J, Xu Z, Fan W, Tian L, et al. Role of neuroimmune crosstalk in mediating the anti-inflammatory and analgesic effects of acupuncture on inflammatory pain. Front Neurosci (2021) 15:695670. doi: 10.3389/fnins.2021.695670
53. Iftinca M, Defaye M, Altier C. TRPV1-targeted drugs in development for human pain conditions. Drugs (2021) 81:7–27. doi: 10.1007/s40265-020-01429-2
54. Hu SQ, Hu JL, Zou FL, Liu JP, Luo HL, Hu DX, et al. P2X7 receptor in inflammation and pain. Brain Res Bull (2022) 187:199–209. doi: 10.1016/j.brainresbull.2022.07.006
55. Allison DJ, Ditor DS. Immune dysfunction and chronic inflammation following spinal cord injury. Spinal Cord (2015) 53:14–8. doi: 10.1038/sc.2014.184
56. Zhou P, Guan J, Xu P, Zhao J, Zhang C, Zhang B, et al. Cell therapeutic strategies for spinal cord injury. Adv Wound Care (New Rochelle) (2019) 8:585–605. doi: 10.1089/wound.2019.1046
57. Hu X, Xu W, Ren Y, Wang Z, He X, Huang R, et al. Spinal cord injury: molecular mechanisms and therapeutic interventions. Signal Transduct Target Ther (2023) 8:245. doi: 10.1038/s41392-023-01477-6
58. Hirano T. IL-6 in inflammation, autoimmunity and cancer. Int Immunol (2021) 33:127–48. doi: 10.1093/intimm/dxaa078
59. Malcangio M. Role of the immune system in neuropathic pain. Scand J Pain (2019) 20:33–7. doi: 10.1515/sjpain-2019-0138
60. Ding W, You Z, Chen Q, Yang L, Doheny J, Zhou X, et al. Gut microbiota influences neuropathic pain through modulating proinflammatory and anti-inflammatory T cells. Anesth Analg (2021) 132:1146–55. doi: 10.1213/ANE.0000000000005155
61. Yao X, Sun C, Fan B, Zhao C, Zhang Y, Duan H, et al. Neurotropin exerts neuroprotective effects after spinal cord injury by inhibiting apoptosis and modulating cytokines. J Orthop Translat (2021) 26:74–83. doi: 10.1016/j.jot.2020.02.011
62. Ma P, Mo R, Liao H, Qiu C, Wu G, Yang C, et al. Gut microbiota depletion by antibiotics ameliorates somatic neuropathic pain induced by nerve injury, chemotherapy, and diabetes in mice. J Neuroinflamm (2022) 19:169. doi: 10.1186/s12974-022-02523-w
63. Wedel S, Mathoor P, Rauh O, Heymann T, Ciotu CI, Fuhrmann DC, et al. SAFit2 reduces neuroinflammation and ameliorates nerve injury-induced neuropathic pain. J Neuroinflamm (2022) 19:254. doi: 10.1186/s12974-022-02615-7
64. Tozaki-Saitoh H, Tsuda M. Microglia-neuron interactions in the models of neuropathic pain. Biochem Pharmacol (2019) 169:113614. doi: 10.1016/j.bcp.2019.08.016
65. Miranpuri GS, Bali P, Nguyen J, Kim JJ, Modgil S, Mehra P, et al. Role of microglia and astrocytes in spinal cord injury induced neuropathic pain. Ann Neurosci (2021) 28:219–28. doi: 10.1177/09727531211046367
66. Sideris-Lampretsas G, Malcangio M. Microglial heterogeneity in chronic pain. Brain Behav Immun (2021) 96:279–89. doi: 10.1016/j.bbi.2021.06.005
67. Zhang ZJ, Jiang BC, Gao YJ. Chemokines in neuron-glial cell interaction and pathogenesis of neuropathic pain. Cell Mol Life Sci (2017) 74:3275–91. doi: 10.1007/s00018-017-2513-1
68. Hu X, Du L, Liu S, Lan Z, Zang K, Feng J, et al. A TRPV4-dependent neuroimmune axis in the spinal cord promotes neuropathic pain. J Clin Invest (2023) 133:e161507. doi: 10.1172/JCI161507
69. Rust R, Kaiser J. Insights into the Dual Role of Inflammation after Spinal Cord Injury. J Neurosci (2017) 37:4658–60. doi: 10.1523/JNEUROSCI.0498-17.2017
70. Cofano F, Boido M, Monticelli M, Zenga F, Ducati A, Vercelli A, et al. Mesenchymal stem cells for spinal cord injury: current options, limitations, and future of cell therapy. Int J Mol Sci (2019) 20:2698. doi: 10.3390/ijms20112698
71. Orr MB, Gensel JC. Spinal cord injury scarring and inflammation: therapies targeting glial and inflammatory responses. Neurotherapeutics (2018) 15:541–53. doi: 10.1007/s13311-018-0631-6
72. Zamanian JL, Xu L, Foo LC, Nouri N, Zhou L, Giffard RG, et al. Genomic analysis of reactive astrogliosis. J Neurosci (2012) 32:6391–410. doi: 10.1523/JNEUROSCI.6221-11.2012
73. Burda JE, Sofroniew MV. Reactive gliosis and the multicellular response to CNS damage and disease. Neuron (2014) 81:229–48. doi: 10.1016/j.neuron.2013.12.034
74. Zhu Y, Soderblom C, Krishnan V, Ashbaugh J, Bethea JR, Lee JK. Hematogenous macrophage depletion reduces the fibrotic scar and increases axonal growth after spinal cord injury. Neurobiol Dis (2015) 74:114–25. doi: 10.1016/j.nbd.2014.10.024
75. Anderson MA, Burda JE, Ren Y, Ao Y, O'shea TM, Kawaguchi R, et al. Astrocyte scar formation aids central nervous system axon regeneration. Nature (2016) 532:195–200. doi: 10.1038/nature17623
76. David S, Aguayo AJ. Axonal elongation into peripheral nervous system "bridges" after central nervous system injury in adult rats. Science (1981) 214:931–3. doi: 10.1126/science.6171034
77. Beattie MS, Bresnahan JC, Komon J, Tovar CA, Van Meter M, Anderson DK, et al. Endogenous repair after spinal cord contusion injuries in the rat. Exp Neurol (1997) 148:453–63. doi: 10.1006/exnr.1997.6695
78. Abnet K, Fawcett JW, Dunnett SB. Interactions between meningeal cells and astrocytes in vivo and in vitro. Brain Res Dev Brain Res (1991) 59:187–96. doi: 10.1016/0165-3806(91)90099-5
79. Shearer MC, Fawcett JW. The astrocyte/meningeal cell interface–a barrier to successful nerve regeneration? Cell Tissue Res (2001) 305:267–73. doi: 10.1007/s004410100384
80. Bundesen LQ, Scheel TA, Bregman BS, Kromer LF. Ephrin-B2 and EphB2 regulation of astrocyte-meningeal fibroblast interactions in response to spinal cord lesions in adult rats. J Neurosci (2003) 23:7789–800. doi: 10.1523/JNEUROSCI.23-21-07789.2003
81. Kimura-Kuroda J, Teng X, Komuta Y, Yoshioka N, Sango K, Kawamura K, et al. An in vitro model of the inhibition of axon growth in the lesion scar formed after central nervous system injury. Mol Cell Neurosci (2010) 43:177–87. doi: 10.1016/j.mcn.2009.10.008
82. Göritz C, DIAS DO, TOMILIN N, BARBACID M, SHUPLIAKOV O, Frisén J. A pericyte origin of spinal cord scar tissue. Science (2011) 333:238–42. doi: 10.1126/science.1203165
83. Kawano H, Kimura-Kuroda J, Komuta Y, Yoshioka N, Li HP, Kawamura K, et al. Role of the lesion scar in the response to damage and repair of the central nervous system. Cell Tissue Res (2012) 349:169–80. doi: 10.1007/s00441-012-1336-5
84. Soderblom C, Luo X, Blumenthal E, Bray E, Lyapichev K, Ramos J, et al. Perivascular fibroblasts form the fibrotic scar after contusive spinal cord injury. J Neurosci (2013) 33:13882–7. doi: 10.1523/JNEUROSCI.2524-13.2013
85. Lukacova N, Kisucka A, Kiss Bimbova K, Bacova M, Ileninova M, Kuruc T, et al. Glial-neuronal interactions in pathogenesis and treatment of spinal cord injury. Int J Mol Sci (2021) 22:13577. doi: 10.3390/ijms222413577
86. Clifford T, Finkel Z, Rodriguez B, Joseph A, Cai L. Current advancements in spinal cord injury research-glial scar formation and neural regeneration. Cells (2023) 12:853. doi: 10.3390/cells12060853
87. Okada S, Hara M, Kobayakawa K, Matsumoto Y, Nakashima Y. Astrocyte reactivity and astrogliosis after spinal cord injury. Neurosci Res (2018) 126:39–43. doi: 10.1016/j.neures.2017.10.004
88. Zhou B, Zuo YX, Jiang RT. Astrocyte morphology: Diversity, plasticity, and role in neurological diseases. CNS Neurosci Ther (2019) 25:665–73. doi: 10.1111/cns.13123
89. Li X, Li M, Tian L, Chen J, Liu R, Ning B. Reactive astrogliosis: implications in spinal cord injury progression and therapy. Oxid Med Cell Longev (2020) 2020:9494352. doi: 10.1155/2020/9494352
90. Cho J, Huh Y. Astrocytic calcium dynamics along the pain pathway. Front Cell Neurosci (2020) 14:594216. doi: 10.3389/fncel.2020.594216
91. Pekny M, Wilhelmsson U, Tatlisumak T, Pekna M. Astrocyte activation and reactive gliosis-A new target in stroke? Neurosci Lett (2019) 689:45–55. doi: 10.1016/j.neulet.2018.07.021
92. Kohno K, Shirasaka R, Yoshihara K, Mikuriya S, Tanaka K, Takanami K, et al. A spinal microglia population involved in remitting and relapsing neuropathic pain. Science (2022) 376:86–90. doi: 10.1126/science.abf6805
93. Chen G, Zhang YQ, Qadri YJ, Serhan CN, Ji RR. Microglia in pain: detrimental and protective roles in pathogenesis and resolution of pain. Neuron (2018) 100:1292–311. doi: 10.1016/j.neuron.2018.11.009
94. Brockie S, Hong J, Fehlings MG. The role of microglia in modulating neuroinflammation after spinal cord injury. Int J Mol Sci (2021) 22:9706. doi: 10.3390/ijms22189706
95. Tsuda M. Microglia in the spinal cord and neuropathic pain. J Diabetes Investig (2016) 7:17–26. doi: 10.1111/jdi.12379
96. Bellver-Landete V, Bretheau F, Mailhot B, Vallières N, Lessard M, Janelle ME, et al. Microglia are an essential component of the neuroprotective scar that forms after spinal cord injury. Nat Commun (2019) 10:518. doi: 10.1038/s41467-019-08446-0
97. Li Y, He X, Kawaguchi R, Zhang Y, Wang Q, Monavarfeshani A, et al. Microglia-organized scar-free spinal cord repair in neonatal mice. Nature (2020) 587:613–8. doi: 10.1038/s41586-020-2795-6
98. Boakye PA, Tang SJ, Smith PA. Mediators of neuropathic pain; focus on spinal microglia, CSF-1, BDNF, CCL21, TNF-α, Wnt ligands, and interleukin 1β. Front Pain Res (Lausanne) (2021) 2:698157. doi: 10.3389/fpain.2021.698157
99. Fan H, Tang HB, Chen Z, Wang HQ, Zhang L, Jiang Y, et al. Inhibiting HMGB1-RAGE axis prevents pro-inflammatory macrophages/microglia polarization and affords neuroprotection after spinal cord injury. J Neuroinflamm (2020) 17:295. doi: 10.1186/s12974-020-01973-4
100. Kuhn S, Gritti L, Crooks D, Dombrowski Y. Oligodendrocytes in development, myelin generation and beyond. Cells (2019) 8:1424. doi: 10.3390/cells8111424
101. Al Mamun A, Wu Y, Monalisa I, Jia C, Zhou K, Munir F, et al. Role of pyroptosis in spinal cord injury and its therapeutic implications. J Adv Res (2021) 28:97–109. doi: 10.1016/j.jare.2020.08.004
102. Sakaguchi S, Mikami N, Wing JB, Tanaka A, Ichiyama K, Ohkura N. Regulatory T cells and human disease. Annu Rev Immunol (2020) 38:541–66. doi: 10.1146/annurev-immunol-042718-041717
103. Olson KE, Mosley RL, Gendelman HE. The potential for treg-enhancing therapies in nervous system pathologies. Clin Exp Immunol (2023) 211:108–21. doi: 10.1093/cei/uxac084
104. Lin S, Xu C, Lin J, Hu H, Zhang C, Mei X. Regulation of inflammatory cytokines for spinal cord injury recovery. Histol Histopathol (2021) 36:137–42. doi: 10.14670/HH-18-262
105. Wang HY, Ye JR, Cui LY, Chu SF, Chen NH. Regulatory T cells in ischemic stroke. Acta Pharmacol Sin (2022) 43:1–9. doi: 10.1038/s41401-021-00641-4
106. Ohue Y, Nishikawa H. Regulatory T (Treg) cells in cancer: Can Treg cells be a new therapeutic target? Cancer Sci (2019) 110:2080–9. doi: 10.1111/cas.14069
107. Tanaka A, Sakaguchi S. Targeting Treg cells in cancer immunotherapy. Eur J Immunol (2019) 49:1140–6. doi: 10.1002/eji.201847659
108. Wang P, Qi X, Xu G, Liu J, Guo J, Li X, et al. CCL28 promotes locomotor recovery after spinal cord injury via recruiting regulatory T cells. Aging (Albany NY) (2019) 11:7402–15. doi: 10.18632/aging.102239
109. Zhu L, Hua F, Ding W, Ding K, Zhang Y, Xu C. The correlation between the Th17/Treg cell balance and bone health. Immun Ageing (2020) 17:30. doi: 10.1186/s12979-020-00202-z
110. Zhang S, Gang X, Yang S, Cui M, Sun L, Li Z, et al. The alterations in and the role of the Th17/Treg balance in metabolic diseases. Front Immunol (2021) 12:678355. doi: 10.3389/fimmu.2021.678355
111. Liu P, Liu M, Xi D, Bai Y, Ma R, Mo Y, et al. Short-chain fatty acids ameliorate spinal cord injury recovery by regulating the balance of regulatory T cells and effector IL-17(+) γδ T cells. J Zhejiang Univ Sci B (2023) 24:312–25. doi: 10.1631/jzus.B2200417
112. De Candia P, Procaccini C, Russo C, Lepore MT, Matarese G. Regulatory T cells as metabolic sensors. Immunity (2022) 55:1981–92. doi: 10.1016/j.immuni.2022.10.006
113. Deng G, Song X, Fujimoto S, Piccirillo CA, Nagai Y, Greene MI. Foxp3 post-translational modifications and Treg suppressive activity. Front Immunol (2019) 10:2486. doi: 10.3389/fimmu.2019.02486
114. Ohkura N, Sakaguchi S. Transcriptional and epigenetic basis of Treg cell development and function: its genetic anomalies or variations in autoimmune diseases. Cell Res (2020) 30:465–74. doi: 10.1038/s41422-020-0324-7
115. Milling S, Edgar JM. How T'reg-ulate healing of the injured spinal cord? Immunology (2019) 158:253–4. doi: 10.1111/imm.13148
116. Lin X, Liu Y, Ma L, Ma X, Shen L, Ma X, et al. Constipation induced gut microbiota dysbiosis exacerbates experimental autoimmune encephalomyelitis in C57BL/6 mice. J Transl Med (2021) 19:317. doi: 10.1186/s12967-021-02995-z
117. Becker M, Joseph SS, Garcia-Carrizo F, Tom RZ, Opaleva D, Serr I, et al. Regulatory T cells require IL6 receptor alpha signaling to control skeletal muscle function and regeneration. Cell Metab (2023) 35:1736–51.e7. doi: 10.1016/j.cmet.2023.08.010
118. Hu JG, Shi LL, Chen YJ, Xie XM, Zhang N, Zhu AY, et al. Differential effects of myelin basic protein-activated Th1 and Th2 cells on the local immune microenvironment of injured spinal cord. Exp Neurol (2016) 277:190–201. doi: 10.1016/j.expneurol.2016.01.002
119. Wang C, Yang J, Xie L, Saimaier K, Zhuang W, Han M, et al. Methyl butyrate alleviates experimental autoimmune encephalomyelitis and regulates the balance of effector T cells and regulatory T cells. Inflammation (2022) 45:977–91. doi: 10.1007/s10753-021-01596-8
120. He X, Lin S, Yang L, Tan P, Ma P, Qiu P, et al. Programmed death protein 1 is essential for maintaining the anti-inflammatory function of infiltrating regulatory T cells in a murine spinal cord injury model. J Neuroimmunol (2021) 354:577546. doi: 10.1016/j.jneuroim.2021.577546
121. Tekguc M, WING JB, OSAKI M, LONG J, SAKAGUCHI S. Treg-expressed CTLA-4 depletes CD80/CD86 by trogocytosis, releasing free PD-L1 on antigen-presenting cells. Proc Natl Acad Sci U S A (2021) 118:e2023739118. doi: 10.1073/pnas.2023739118
122. Xie M, Wang J, Gong W, Xu H, Pan X, Chen Y, et al. NF-κB-driven miR-34a impairs Treg/Th17 balance via targeting Foxp3. J Autoimmun (2019) 102:96–113. doi: 10.1016/j.jaut.2019.04.018
123. Cui C, Zhang D, Sun K, Li H, Xu L, Lin G, et al. Propofol maintains Th17/Treg cell balance and reduces inflammation in rats with traumatic brain injury via the miR-145-3p/NFATc2/NF-κB axis. Int J Mol Med (2021) 48:135. doi: 10.3892/ijmm.2021.4968
124. Ke X, Chen Z, Wang X, Kang H, Hong S. Quercetin improves the imbalance of Th1/Th2 cells and Treg/Th17 cells to attenuate allergic rhinitis. Autoimmunity (2023) 56:2189133. doi: 10.1080/08916934.2023.2189133
125. He Y, Li M, Wujisiguleng LB, Huan Y, Liu B, Wang D, et al. Zhenbao Pill reduces Treg cell proportion in acute spinal cord injury rats by regulating TUG1/miR-214/HSP27 axis. Biosci Rep (2018) 38:BSR20180895. doi: 10.1042/BSR20180895
126. Liu R, Li Y, Wang Z, Chen P, Xie Y, Qu W, et al. Regulatory T cells promote functional recovery after spinal cord injury by alleviating microglia inflammation via STAT3 inhibition. CNS Neurosci Ther (2023) 29:2129–44. doi: 10.1111/cns.14161
127. Duffy SS, Keating BA, Perera CJ, Moalem-Taylor G. The role of regulatory T cells in nervous system pathologies. J Neurosci Res (2018) 96:951–68. doi: 10.1002/jnr.24073
128. Kim CF, Moalem-Taylor G. Interleukin-17 contributes to neuroinflammation and neuropathic pain following peripheral nerve injury in mice. J Pain (2011) 12:370–83. doi: 10.1016/j.jpain.2010.08.003
129. Gui WS, Wei X, Mai CL, Murugan M, Wu LJ, Xin WJ, et al. Interleukin-1β overproduction is a common cause for neuropathic pain, memory deficit, and depression following peripheral nerve injury in rodents. Mol Pain (2016) 12:1744806916646784. doi: 10.1177/1744806916646784
130. Sorkin LS, Doom CM. Epineurial application of TNF elicits an acute mechanical hyperalgesia in the awake rat. J Peripher Nerv Syst (2000) 5:96–100. doi: 10.1046/j.1529-8027.2000.00012.x
131. Tsuda M, Masuda T, Kitano J, Shimoyama H, Tozaki-Saitoh H, Inoue K. IFN-gamma receptor signaling mediates spinal microglia activation driving neuropathic pain. Proc Natl Acad Sci U S A (2009) 106:8032–7. doi: 10.1073/pnas.0810420106
132. Liu XJ, Zhang Y, Liu T, Xu ZZ, Park CK, Berta T, et al. Nociceptive neurons regulate innate and adaptive immunity and neuropathic pain through MyD88 adapter. Cell Res (2014) 24:1374–7. doi: 10.1038/cr.2014.106
133. Ahlström FHG, Mätlik K, Viisanen H, Blomqvist KJ, Liu X, Lilius TO, et al. Spared nerve injury causes sexually dimorphic mechanical Allodynia and differential gene expression in spinal cords and dorsal root ganglia in rats. Mol Neurobiol (2021) 58:5396–419. doi: 10.1007/s12035-021-02447-1
134. Lees JG, Duffy SS, Perera CJ, Moalem-Taylor G. Depletion of Foxp3+ regulatory T cells increases severity of mechanical allodynia and significantly alters systemic cytokine levels following peripheral nerve injury. Cytokine (2015) 71:207–14. doi: 10.1016/j.cyto.2014.10.028
135. Fiore NT, Keating BA, Chen Y, Williams SI, Moalem-Taylor G. Differential effects of regulatory T cells in the meninges and spinal cord of male and female mice with neuropathic pain. Cells (2023) 12:2317. doi: 10.3390/cells12182317
136. Ito M, Komai K, Mise-Omata S, Iizuka-Koga M, Noguchi Y, Kondo T, et al. Brain regulatory T cells suppress astrogliosis and potentiate neurological recovery. Nature (2019) 565:246–50. doi: 10.1038/s41586-018-0824-5
137. Zhao W, Beers DR, Liao B, Henkel JS, Appel SH. Regulatory T lymphocytes from ALS mice suppress microglia and effector T lymphocytes through different cytokine-mediated mechanisms. Neurobiol Dis (2012) 48:418–28. doi: 10.1016/j.nbd.2012.07.008
138. Chen NF, Huang SY, Chen WF, Chen CH, Lu CH, Chen CL, et al. TGF-β1 attenuates spinal neuroinflammation and the excitatory amino acid system in rats with neuropathic pain. J Pain (2013) 14:1671–85. doi: 10.1016/j.jpain.2013.08.010
139. Xie L, Choudhury GR, Winters A, Yang SH, Jin K. Cerebral regulatory T cells restrain microglia/macrophage-mediated inflammatory responses via IL-10. Eur J Immunol (2015) 45:180–91. doi: 10.1002/eji.201444823
140. Luchting B, Rachinger-Adam B, Zeitler J, Egenberger L, Möhnle P, Kreth S, et al. Disrupted TH17/Treg balance in patients with chronic low back pain. PloS One (2014) 9:e104883. doi: 10.1371/journal.pone.0104883
141. Luchting B, Rachinger-Adam B, Heyn J, Hinske LC, Kreth S, Azad SC. Anti-inflammatory T-cell shift in neuropathic pain. J Neuroinflamm (2015) 12:12. doi: 10.1186/s12974-014-0225-0
142. Heyn J, Luchting B, Hinske LC, Hübner M, Azad SC, Kreth S. miR-124a and miR-155 enhance differentiation of regulatory T cells in patients with neuropathic pain. J Neuroinflamm (2016) 13:248. doi: 10.1186/s12974-016-0712-6
143. Saravia J, Chapman NM, Chi H. Helper T cell differentiation. Cell Mol Immunol (2019) 16:634–43. doi: 10.1038/s41423-019-0220-6
144. Raffin C, Vo LT, Bluestone JA. T(reg) cell-based therapies: challenges and perspectives. Nat Rev Immunol (2020) 20:158–72. doi: 10.1038/s41577-019-0232-6
145. Moreau JM, Velegraki M, Bolyard C, Rosenblum MD, Li Z. Transforming growth factor-β1 in regulatory T cell biology. Sci Immunol (2022) 7:eabi4613. doi: 10.1126/sciimmunol.abi4613
146. Solomon I, Amann M, Goubier A, Arce Vargas F, Zervas D, Qing C, et al. CD25-T(reg)-depleting antibodies preserving IL-2 signaling on effector T cells enhance effector activation and antitumor immunity. Nat Cancer (2020) 1:1153–66. doi: 10.1038/s43018-020-00133-0
147. Shi L, Sun Z, Su W, Xu F, Xie D, Zhang Q, et al. Treg cell-derived osteopontin promotes microglia-mediated white matter repair after ischemic stroke. Immunity (2021) 54:1527–42.e8. doi: 10.1016/j.immuni.2021.04.022
148. Gentili M, Ronchetti S, Ricci E, Di Paola R, Gugliandolo E, Cuzzocrea S, et al. Selective CB2 inverse agonist JTE907 drives T cell differentiation towards a Treg cell phenotype and ameliorates inflammation in a mouse model of inflammatory bowel disease. Pharmacol Res (2019) 141:21–31. doi: 10.1016/j.phrs.2018.12.005
149. Cross AR, Lion J, Poussin K, Glotz D, Mooney N. Inflammation determines the capacity of allogenic endothelial cells to regulate human Treg expansion. Front Immunol (2021) 12:666531. doi: 10.3389/fimmu.2021.666531
150. Kotschenreuther K, Yan S, Kofler DM. Migration and homeostasis of regulatory T cells in rheumatoid arthritis. Front Immunol (2022) 13:947636. doi: 10.3389/fimmu.2022.947636
151. Ferreira LMR, Muller YD, Bluestone JA, Tang Q. Next-generation regulatory T cell therapy. Nat Rev Drug Discovery (2019) 18:749–69. doi: 10.1038/s41573-019-0041-4
152. Rana J, Biswas M. Regulatory T cell therapy: Current and future design perspectives. Cell Immunol (2020) 356:104193. doi: 10.1016/j.cellimm.2020.104193
153. Giganti G, Atif M, Mohseni Y, Mastronicola D, Grageda N, Povoleri GA, et al. Treg cell therapy: How cell heterogeneity can make the difference. Eur J Immunol (2021) 51:39–55. doi: 10.1002/eji.201948131
154. Ide C, Kanekiyo K. Points regarding cell transplantation for the treatment of spinal cord injury. Neural Regener Res (2016) 11:1046–9. doi: 10.4103/1673-5374.187021
155. Caplan HW, Prabhakara KS, Toledano Furman NE, Zorofchian S, Martin C, Xue H, et al. Human-derived Treg and MSC combination therapy may augment immunosuppressive potency in vitro, but did not improve blood brain barrier integrity in an experimental rat traumatic brain injury model. PLoS One (2021) 16:e0251601. doi: 10.1371/journal.pone.0251601
156. He B, Liu Y, Hoang TK, Tian X, Taylor CM, Luo M, et al. Antibiotic-modulated microbiome suppresses lethal inflammation and prolongs lifespan in Treg-deficient mice. Microbiome (2019) 7:145. doi: 10.1186/s40168-019-0751-1
157. Luo Y, Liu G, Hou P. Synergism effect of dendrobine on cisplatin in treatment of H1299 by modulating the balance of Treg/Th17. Anticancer Agents Med Chem (2023) 23:105–12. doi: 10.2174/1871520622666220520093837
158. Liu ZM, Wang KP, Ma J, Guo Zheng S. The role of all-trans retinoic acid in the biology of Foxp3+ regulatory T cells. Cell Mol Immunol (2015) 12:553–7. doi: 10.1038/cmi.2014.133
159. Maneechotesuwan K, Kasetsinsombat K, Wamanuttajinda V, Wongkajornsilp A, Barnes PJ. Statins enhance the effects of corticosteroids on the balance between regulatory T cells and Th17 cells. Clin Exp Allergy (2013) 43:212–22. doi: 10.1111/cea.12067
160. Ulivieri C, Baldari CT. Statins: from cholesterol-lowering drugs to novel immunomodulators for the treatment of Th17-mediated autoimmune diseases. Pharmacol Res (2014) 88:41–52. doi: 10.1016/j.phrs.2014.03.001
161. Olsen PC, Kitoko JZ, Ferreira TP, De-Azevedo CT, Arantes AC, Martins M. A. Glucocorticoids decrease Treg cell numbers in lungs of allergic mice. Eur J Pharmacol (2015) 747:52–8. doi: 10.1016/j.ejphar.2014.11.034
162. Chen BJ, Zhao JW, Zhang DH, Zheng AH, Wu GQ. Immunotherapy of cancer by targeting regulatory T cells. Int Immunopharmacol (2022) 104:108469. doi: 10.1016/j.intimp.2021.108469
163. Togashi Y, Shitara K, Nishikawa H. Regulatory T cells in cancer immunosuppression - implications for anticancer therapy. Nat Rev Clin Oncol (2019) 16:356–71. doi: 10.1038/s41571-019-0175-7
Keywords: spinal cord injury, neuropathic pain, Treg cells, inflammation, cell therapy
Citation: Zhang C, Li Y, Yu Y, Li Z, Xu X, Talifu Z, Liu W, Yang D, Gao F, Wei S, Zhang L, Gong H, Peng R, Du L and Li J (2024) Impact of inflammation and Treg cell regulation on neuropathic pain in spinal cord injury: mechanisms and therapeutic prospects. Front. Immunol. 15:1334828. doi: 10.3389/fimmu.2024.1334828
Received: 07 November 2023; Accepted: 03 January 2024;
Published: 29 January 2024.
Edited by:
Rajnikant Mishra, Banaras Hindu University, IndiaReviewed by:
Hermelinda Salgado-Ceballos, Mexican Social Security Institute (IMSS), MexicoFaezeh Sahebdel, University of Minnesota Twin Cities, United States
Copyright © 2024 Zhang, Li, Yu, Li, Xu, Talifu, Liu, Yang, Gao, Wei, Zhang, Gong, Peng, Du and Li. This is an open-access article distributed under the terms of the Creative Commons Attribution License (CC BY). The use, distribution or reproduction in other forums is permitted, provided the original author(s) and the copyright owner(s) are credited and that the original publication in this journal is cited, in accordance with accepted academic practice. No use, distribution or reproduction is permitted which does not comply with these terms.
*Correspondence: Jianjun Li, 13718331416@163.com