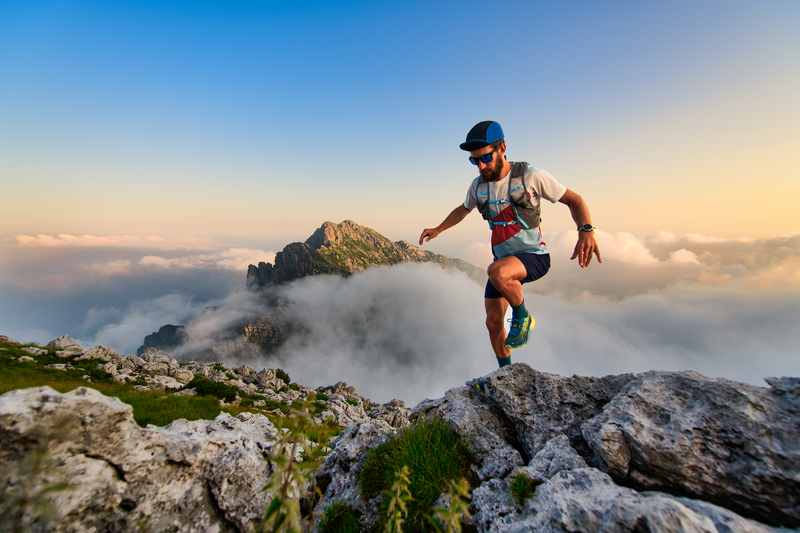
94% of researchers rate our articles as excellent or good
Learn more about the work of our research integrity team to safeguard the quality of each article we publish.
Find out more
ORIGINAL RESEARCH article
Front. Immunol. , 16 February 2024
Sec. Vaccines and Molecular Therapeutics
Volume 15 - 2024 | https://doi.org/10.3389/fimmu.2024.1330677
Introduction: Conventional foot-and-mouth disease (FMD) vaccines have been developed to enhance their effectiveness; however, several drawbacks remain, such as slow induction of antibody titers, short-lived immune response, and local side effects at the vaccination site. Therefore, we created a novel FMD vaccine that simultaneously induces cellular and humoral immune responses using the Dectin-2 agonist, D-galacto-D-mannan, as an adjuvant.
Methods: We evaluated the innate and adaptive (cellular and humoral) immune responses elicited by the novel FMD vaccine and elucidated the signaling pathway involved both in vitro and in vivo using mice and pigs, as well as immune cells derived from these animals.
Results: D-galacto-D-mannan elicited early, mid-, and long-term immunity via simultaneous induction of cellular and humoral immune responses by promoting the expression of immunoregulatory molecules. D-galacto-D-mannan also enhanced the immune response and coordinated vaccine-mediated immune response by suppressing genes associated with excessive inflammatory responses, such as nuclear factor kappa B, via Sirtuin 1 expression.
Conclusion: Our findings elucidated the immunological mechanisms induced by D-galacto-D-mannan, suggesting a background for the robust cellular and humoral immune responses induced by FMD vaccines containing D-galacto-D-mannan. Our study will help to facilitate the improvement of conventional FMD vaccines and the design of next-generation FMD vaccines.
Foot-and-mouth disease (FMD) is a highly contagious viral disease of livestock that causes significant economic losses. The FMD virus (FMDV) belongs to the Picornaviridae and Aphthovirus families and is classified into seven serotypes (1, 2). The inability of cross-protection among the seven FMDV serotypes complicates its prevention and control (3, 4). FMD is typically identified by symptoms, such as high fever, blisters in the mouth, and excessive secretion of sticky or foamy saliva (5). Additionally, adult animals can experience weight loss that they do not recover for several months, swelling in male testicles, and significant reduction in cattle milk production. Although several infected animals remain asymptomatic carriers, they can carry the virus and transmit it to other animals (6, 7).
Many countries have recommended vaccination to prevent FMD from acute spreading; however, available vaccines have several limitations, such as low antibody titers and local reactions at the injection site. Therefore, we investigated effective adjuvants to enhance the cellular and humoral immune responses of the vaccine and address safety concerns. Korea belongs to FMDV serotype pool 1 and is mainly exposed to FMDV serotypes O, A, and Asia 1 (8). Since 2000, FMD outbreaks in Korea have been primarily attributed to serotypes O and A. Indeed, recent outbreaks of FMD in Korea from 2017 to 2023 were caused by type O (ME-SA topotype) and type A (A/ASIA/Sea-97 topotype). Therefore, in this study, a test vaccine was prepared using FMD antigens O PA2 (ME-SA topotype) and A YC (A/ASIA/Sea-97 topotype).
Adjuvants enhance and prolong the immune response when used in combination with specific vaccine antigens (9); therefore, to develop a novel FMD vaccine, research on various adjuvants must be conducted.
Most FMD vaccines involve using inactivated viral antigens. Mineral oil-based adjuvants and aluminum hydroxide [Al(OH)3] with or without saponin have been used as traditional adjuvants for FMD vaccines to improve the stability and delivery of inactivated viral antigens (10–13). Several problems have been reported with FMD vaccines containing crude saponins, including hemolysis at the vaccination site and eliciting short-lived antibody responses. Therefore, Quil-A, which is safer than saponin and can induce a strong immune response, was used as an FMD vaccine adjuvant (14).
Despite improved FMD vaccines, repeated vaccination is recommended owing to low and short-lived antibody titers. Repeated vaccinations may cause local side effects at the injection site due to the mineral oil-based adjuvant contained in the FMD vaccine (11, 13, 15–17).
Thus, adjuvants, specifically immunostimulant combinations currently used in FMD vaccines, require improvement to enhance efficacy and safety. In a previous study, we confirmed that treatment of porcine peripheral blood mononuclear cells (PBMCs) with a dendritic cell (DC)-associated C-type lectin-2 (Dectin-2) agonist induced PBMC proliferation (18). Therefore, we hypothesized that Dectin-2 activation elicited a robust immune response in pigs.
Based on previous studies, we used D-galacto-D-mannan, a Dectin-2 agonist, as an adjuvant for the novel FMD vaccine in this study. Dectin-2 is a pattern recognition receptor (PRR) containing a C-type-like lectin domain with a mannose-binding glutamic acid-proline-asparagine motif that binds to high mannose-containing structures (19). Immunomodulatory responses through Dectin-2 have been reported in FMD-susceptible animals, including sheep and cattle (20, 21).
Dectin-2 is expressed in cells, including Langerhans cells, macrophages (MΦs), neutrophils, and several DC subsets (22, 23). Dectin-2 signaling is induced through the spleen tyrosine kinase (SYK), protein kinase C delta (PKCδ), and caspase-associated recruitment domain 9 (CARD9)-B-cell lymphoma 10 (BCL10)-mucosa-associated lymphoid tissue lymphoma translocation gene 1 (MALT1) downstream pathways, inducing several cytokines, such as interleukin (IL)-2, IL-10, IL-1β, IL-6, IL-12, and IL-23 (24, 25).
Dectin-2 signals also include phospholipase Cγ2 and mitogen-activated protein kinases, which selectively stimulate the c-Rel, nuclear factor kappa B (NF-κB) subunit through MALT1 to promote the secretion of T helper 17 (Th17) polar cytokines (IL-1β and IL-23) (26). Dectin-2 signaling also promotes the Th2 immune response by inducing cysteinyl leukotriene production (27). Considering these characteristics, Dectin-2 was selected among many PRRs and assessed as a target; D-galacto-D-mannan, a polysaccharide composed of a mannose backbone and galactose side group, was used as a Dectin-2 agonist. D-galacto-D-mannan, a hemicellulose derived from plant cell walls, has antioxidant activity against hydroxyl radical production (28). In this study, we assessed the innate and adaptive (cellular and humoral) immune responses induced by a novel FMD vaccine in mice and pigs, as well as immune cells derived from these animals (in vitro and in vivo), and elucidated the signaling pathway involved.
Purified antigens were prepared from BHK-21 (C-13) [baby hamster kidney; American Type Culture Collection (ATCC), VA, USA] cells infected with FMDV O PA2 (GenBank Accession No. AY593829.1) and FMDV A YC (GenBank Accession No. KY766148.1), as described previously (7, 29). For viral infection, O PA2 and A YC were inoculated into BHK-21 cells in serum-free culture medium—Dulbecco’s modified Eagle’s medium (DMEM; HyClone, UT, USA) and incubated at 37°C and 5% CO2 for 1 h. Extracellular viruses were removed; 16 h after infection, the virus was inactivated by treatment with 0.003 N binary ethylenimine (BEI) twice. The inactivated virus was precipitated with polyethylene glycol 6000 (Sigma-Aldrich, MO, USA) (30). Antigen (146S) was purified using a 15%–45% sucrose density gradient and ultracentrifuged. Approximate quantities of FMDV antigen were confirmed via optical density measurements using a lateral flow device (BioSign FMDV Ag; Princeton BioMeditech, NJ, USA). Prior to experimental use, the BEI-treated supernatant was confirmed to be free of live viruses with inactivation tests using ZZ-R 127 [fetal goat tongue epithelium; the Collection of Cell Lines in Veterinary Medicine (CCLV), Friedrich-Loeffler-Institut, Greifswald-Insel Riems, Germany)] and BHK-21 cells (7, 29).
BHK-21, LF-BK (fetal porcine kidney; Plum Island Animal Disease Center, NY, USA), and ZZ-R cells (2 × 104 cells/well) were treated and cultured for 48 h. Purified peritoneal exudate cells (PECs) and PBMCs (1 × 105 cells/well) were treated and stabilized for 1 h. D-galacto-D-mannan (0, 0.625, 1.25, 2.5, or 5 μg/mL; Sigma-Aldrich) was then added and cultured for 4 h. Cell viability was measured with an MTS (inner salt)-based colorimetric assay (Promega, WI, USA) following the manufacturer’s instruction. Data were obtained using a Hidex 300SL spectrophotometer (Hidex, Turku, Finland) at 490 nm.
Mice (experimental animals) and pigs (target animals) were managed as previously described (7, 29). Mice (C57BL/6, females, 6–7 weeks old) were purchased from KOSA BIO Inc. (Gyeonggi, Korea). Farm pigs (Landrace, 8–9 weeks old) that were negative for FMDV type O and A antibodies [using SP enzyme-linked immunosorbent assay (ELISA) in serum] were used. During the study, all animals were housed in a specific pathogen-free animal biosafety level 3 facility at the Animal and Plant Quarantine Agency (APQA) and were used for the experiment after at least 1 week of adaptation. The study and all experimental protocols were approved by the APQA Ethics Committee (Certification No.: IACUC-2022-670 and 2023-753).
For PEC isolation, naive mice (total n = 10) were anesthetized with CO2 and euthanized as previously described (7, 29). The abdominal cavity was flushed with 3 mL of Ca2+/Mg2+-free Dulbecco’s phosphate-buffered saline (DPBS; Gibco, MA, USA). The collected peritoneal lavage fluid was centrifuged, and the pelleted PECs were resuspended and counted using an automated cell counter (Bio-Rad TC20; Bio-Rad Laboratories, CA, USA). In particular, cryopreserved cells were not used in any experiments.
For PBMC isolation, whole blood of pigs (n = 5–6/group) was used as previously described (7, 29). Whole blood (10 mL/donor) was individually collected, and PBMCs were isolated using Lymphoprep™ (Stem Cell Technologies, BC, Canada). Red blood cells were lysed with ammonium–chloride–potassium (ACK) lysing buffer (Gibco). PBMCs were suspended in Ca2+/Mg2+-free Dulbecco’s PBS (Gibco) and analyzed using the automated cell counter (Bio-Rad). No cells used in the experiments were cryopreserved. Isolated PECs and PBMCs were incubated in RPMI1640 (Gibco) medium (7, 29).
D-galacto-D-mannan-mediated interferon γ (IFNγ) secretion with or without antigen (O PA2 or A YC) was assayed using commercial enzyme-linked immune absorbent spot (ELISpot) assay kits (R&D Systems, MN, USA) as per the manufacturer’s recommendations. Murine PECs or porcine PBMCs (5 × 105 cells/well) were cultured in 96-well polyvinylidene fluoride-backed microplates containing a monoclonal capture antibody specific for murine or porcine IFNγ and stimulated with 2 μg/mL (final concentration) of antigen mixed with 0.625, 1.25, 2.5, and 5 μg/mL of D-galacto-D-mannan sequentially, at 37°C and 5% CO2 for 18 h. Antigen and PBS were used as positive (PC) and negative controls (NC), respectively. Data were obtained using the ImmunoSpot ELISpot reader (AID iSpot Reader System; Autoimmune Diagnostika GmbH, Strassberg, Germany). The results were presented as spot-forming cells (SFC) per number of cells added to the well (7, 29).
Before evaluating the potential of D-galacto-D-mannan as an FMD vaccine adjuvant, the potential of D-galacto-D-mannan alone to elicit host protection against FMDV infection was evaluated.
The experimental (Exp) group mice received 100 μg of D-galacto-D-mannan/dose/mouse for a total volume of 100 μL, while the NC group received an equal volume of PBS. Mice (n = 5/group) were administered intramuscular (IM) injection [0 days post-injection (dpi)] and challenged with FMDV (100 LD50 of O/VET/2013 or 100 LD50 A/Malay/97) via intraperitoneal (IP) injection, 3 or 7 dpi. Survival rates and body weight were monitored for up to 7 days post-challenge (dpc).
The safety of the FMD vaccine containing D-galacto-D-mannan was evaluated in mice as previously described (29). The vaccine compositions for the usual vaccination in mice were as follows: purified antigens obtained via antigen purification from FMDV type O (O PA2) and type A (A YC) (0.375 μg + 0.375 μg/dose), ISA 206 (Seppic, Paris, France; 50% w/w), 10% aluminum hydroxide [Al(OH)3], and 15 μg/mouse Quil-A (InvivoGen, CA, USA), with the addition of 100 μg of D-galacto-D-mannan/dose/mouse in a total volume of 100 μL. Mice were administered with a vaccine equivalent to fivefold (500 μL) the volume of the usual vaccination dose (100 μL). All mice (n = 5/group) were vaccinated with IP injection into the peritoneum (0 dpi). To evaluate the safety of the vaccines, survival rates and body weight changes were evaluated up to 7 dpi.
The protective effect of FMD vaccine containing D-galacto-D-mannan was then verified in the early stages of viral infection in mice (n = 5/group). The vaccine formula for the PC group was as follows: purified antigens type O (O PA2) and type A (A YC) (0.375 μg + 0.375 μg/dose), 10% Al(OH)3, ISA 206 (Seppic; 50% w/w), and 15 μg/dose/mouse Quil-A (InvivoGen), in a total volume of 100 μL. The Exp group received vaccines with the same formula as the PC group, with the addition of 100 μg of D-galacto-D-mannan/dose/mouse as an adjuvant, via the same route. The NC group received an equal volume of PBS. Mice were vaccinated via IM injection [0 days post-vaccination (dpv)] and challenged with FMDV (100 LD50 of O/VET/2013 or 100 LD50 A/Malay/97) via IP injection 7 dpv. Survival rates and body weight were monitored for up to 7 dpc.
Early, mid-, and long-term immune responses were monitored in mice to evaluate the efficacy of the FMD vaccine containing D-galacto-D-mannan. The test vaccine formula was the same as described in section 2.7. Mice (n = 5/group) were immunized with the test vaccine and blood samples were collected 0, 7 (early), 28 (mid-term), 56, and 84 (long-term) dpv for serological analysis. Serum samples were stored at −80°C until analysis.
To assess the induction of early, mid-, and long-term immune responses of the FMD vaccine with D-galacto-D-mannan in target animals, a study was conducted as previously described (7, 29). Animals were randomly divided into three groups; NC, PC, and Exp group (n = 5–6/group). The vaccine formula for the PC group was as follows: purified antigens type O (O PA2) and type A (A YC) (15 + 15 μg/dose/mL), 10% Al(OH)3, ISA 206 (Seppic; 50% w/w), and 150 μg/dose/pig Quil-A (InvivoGen), in a total volume of 1 mL. The Exp group received test vaccines with the same formula as the PC group, with the addition of 1 mg of D-galacto-D-mannan/dose/pig as an adjuvant, via the same route. NC group pigs were administered an equal volume of PBS. After primary vaccination via IM injection, a booster shot was administered via the same route 28 dpv. Sera were collected from the vaccinated pigs 0, 7, 14 (early), 28, 42 (mid-term), 56, and 84 (long-term) dpv for serological assays. The local reaction was confirmed at the vaccination site (neck) by sacrificing pigs at 84 dpv. Under the observation of a veterinarian, both sides of the neck were cut into circular shapes with a diameter of 20 cm and a length of 40 cm and cut into 1.5-cm-thick pieces; the effect of suppressing abnormal meat formation was confirmed through visual inspection.
To detect SP antibodies in serum samples, VDPro® FMDV type O kit (Median Diagnostics, Gangwon-do, Korea) and PrioCheck™ FMDV type A kit (Prionics AG, Schlieren, Switzerland) were used as per the manufacturer’s instructions. Since the FMDV antigen subtypes coated on the VDPro® and PrioCheck™ kits were different, antibody titer was measured using both kits to prevent underestimation of the characteristics of the antigen coating the kit. Data were obtained using a spectrophotometer at 450 nm (7, 29), and the absorbance was converted to the percent inhibition (PI) value. When the PI value was ≥40% for the VDPro® FMDV kit or ≥50% for the PrioCheck™ FMDV kit, the animals were considered antibody-positive.
The VN test was conducted following the guidelines of the World Organization for Animal Health (31). Sera samples were heat-inactivated, diluted, and incubated with a 100 50% tissue culture infective dose (TCID50) in 50 μL of FMDV (O PA2 or A YC) media at 37°C for 1 h. Subsequently, 50 μL of LF-BK cells (106 cells/mL) was added to each well and incubated at 37°C and 5% CO2 for 3 days, and the wells were checked for cytopathic effects. VN titers were evaluated as the Log10 of the reciprocal antibody dilution required for neutralization of 100 TCID50 of viruses in 50% of the wells (32).
To detect antigen-specific antibodies [immunoglobulin (Ig) subtype], ELISAs were performed for porcine IgM, IgA, and IgG (Bethyl Laboratories Inc., TX, USA) on the serum samples, as per the manufacturer’s instructions. Data were obtained using a spectrophotometer at 450 nm (7, 29).
To investigate the immune response mechanism elicited by the FMD vaccine with D-galacto-D-mannan, an experiment was performed according to the previously described protocol (7, 29). Total RNA was extracted using TRIzol reagent (Invitrogen, CA, USA) and Rneasy Mini Kit (QIAGEN, CA, USA) as per the manufacturer’s instructions. Complementary DNA (cDNA) was prepared via reverse transcription using the GoScript Reverse Transcription System (Promega) as per the manufacturer’s recommendations. Synthesized cDNAs were amplified using quantitative real-time polymerase chain reaction (qRT-PCR) on a Bio-Rad iCycler using iQ SYBR Green Supermix (Bio-Rad). Gene expression levels were normalized to HPRT (reference gene) levels and presented as relative ratios compared with the control (29). The primers used in this study are listed in Supplementary Table 1.
To verify the effect of simultaneously inducing systemic immunity and mucosal immunity through a combined program of intramuscular vaccination of FMD vaccine and oral administration of D-galacto-D-mannan, an experiment was performed in mice (n = 5/group). The vaccine formula for the PC group was as follows: purified antigens type O (O PA2) (0.375 μg/dose), 10% Al(OH)3, ISA 206 (Seppic; 50% w/w), and 15 μg/dose/mouse Quil-A (InvivoGen), in a total volume of 100 μL. The Exp group received vaccines with the same formula as the PC group via the same route, and administered orally 100 μg of D-galacto-D-mannan/dose/mouse in 100 μL of PBS. The NC group received an equal volume of PBS. Mice were vaccinated via IM injection (0 dpv) and then received D-galacto-D-mannan or PBS orally daily until 28 dpv. Serological assays such as antibody titers by SP O ELISA and VN titers by VN test were performed as described in section 2.11.
Quantitative data are expressed as the mean ± standard error unless otherwise stated. Between-group statistical differences were assessed using a two-way or one-way analysis of variance, followed by Tukey’s or Dunnett’s post-hoc test. Statistical significance was denoted as *p < 0.05, **p < 0.01, ***p < 0.001, and ****p < 0.0001. Parametric tests were used to compare the different groups. Survival curves were constructed using the Kaplan–Meier method, and differences were analyzed using the log-rank sum test. Prism 10.0.2 (GraphPad, San Diego, CA, USA) was used for all statistical analyses.
Before conducting the study, we confirmed the cytotoxicity of D-galacto-D-mannan through in vitro experiments (Supplementary Figures 1A–E) and observed no cytotoxicity at the concentration of ≤5 μg/mL of D-galacto-D-mannan in tested cells. In an in vitro ELISpot assay performed using PECs isolated from mice, the Exp group containing D-galacto-D-mannan showed a more significant IFNγ secretion than the PC group administered with antigens (type O or A) or D-galacto-D-mannan alone (Figure 1A, Supplementary Figure 2A). In the in vitro ELISpot assay performed using PBMCs isolated from porcine whole blood, the Exp group containing D-galacto-D-mannan showed a significantly higher IFNγ secretion than the PC group administered with antigen or D-galacto-D-mannan alone (Figure 1B, Supplementary Figure 2B). These results demonstrated that D-galacto-D-mannan, used as an FMD vaccine adjuvant, enhances innate and cellular immune responses in mice and pigs.
Figure 1 D-galacto-D-mannan with FMDV type O (O PA2) or A (A YC) antigen induces potent innate and adaptive (cellular) immune responses in murine PECs and porcine PBMCs. IFNγ secretion mediated by O PA2 or A YC antigen, with or without D-galacto-D-mannan, was evaluated using ELISpot assay in murine PECs and porcine PBMCs. Data are presented as spot-forming cells per number of cells in the well and mean ± SEM of triplicate measurements (n = 3/group). (A, B) IFNγ-secreting cell spots in murine PECs (A) and porcine PBMCs (B). Statistical analyses were performed using one-way analysis of variance followed by Tukey’s post-hoc test. *p < 0.05; **p < 0.01; ***p < 0.001; ****p < 0.0001. FMDV, foot-and-mouth disease virus; PECs, peritoneal exudate cells; PBMCs, peripheral blood mononuclear cells; IFNγ, interferon γ.
Before evaluating the host protection in mice of the FMD vaccine containing D-galacto-D-mannan as an adjuvant, we investigated D-galacto-D-mannan-mediated host defense. However, D-galacto-D-mannan alone did not elicit host protection in mice (Supplementary Figures 3A–I). To investigate the safety of vaccine containing D-galacto-D-mannan, we administered a 500 μL (100 μL × 5-fold) intraperitoneal (I.P.) injection of an FMD vaccine containing D-galacto-D-mannan into the mouse peritoneal cavity and monitored it for up to 7 dpv. We found that all mice achieved a 100% survival rate, indicating that the FMD vaccine containing D-galacto-D-mannan as an adjuvant was safe (Supplementary Figures 4A, B).
To evaluate the initial host defense of the FMD vaccine containing D-galacto-D-mannan as an adjuvant against FMDV infection, experiments were conducted following the strategy presented in Figure 2A. A bivalent vaccine (with O PA2 + A YC antigen) containing D-galacto-D-mannan showed 100% survival rates against O/VET/2013 and A/Malay/97 (Figures 2B, C). Body weights of mice did not significantly change in the Exp group receiving the D-galacto-D-mannan-containing vaccine (Figures 2D, E). In the PC group not receiving D-galacto-D-mannan, the survival rate of FMDV type O and A infections were 60% and 40%, respectively, and the body weight decreased by more than 10% at 4 dpc. In the NC group, the mortality rate was 100% (survival rate 0%) 4 and 6 dpc for FMDV type O and A challenges, respectively. These results demonstrate that D-galacto-D-mannan plays a critical role in initial host defense in mice.
Figure 2 FMD vaccine containing D-galacto-D-mannan enhances vaccine efficacy and protective effects during the early stages of viral infection in mice. C57BL/6 mice (n = 5/group) were administered FMD vaccine containing inactivated FMDV antigens isolated from FMDV type O (O PA2) and A (A YC) (0.375 + 0.375 μg/dose/100 μL; 1/40 of the dose for pigs), 100 μg of D-galacto-D-mannan/dose/mouse, ISA 206 (oil-based emulsion, 50%, w/w), 10% aluminum hydroxide, and 15 μg of Quil-A. The PC group received vaccines of the same volume and formula as the Exp group but without D-galacto-D-mannan as an adjuvant. The NC group was injected with an equal volume of phosphate-buffered saline. Test vaccines were injected intramuscularly into mice later challenged with FMDV O (100 LD50 O/VET/2013) or FMDV A (100 LD50 A/Malay/97) 7 dpv via an intraperitoneal injection. Survival rates and body weights were monitored for 7 days post-challenge. (A–E) Experimental strategy (A); survival rates post-challenge with O/VET/2013 (B) and A/Malay/97 (C); body weight changes post-challenge with O/VET/2013 (D) and A/Malay/97 (E). Data are represented as mean ± SEM of triplicate measurements (n = 5/group). FMD, foot-and-mouth disease; FMDV, foot-and-mouth disease virus; PC, positive control; NC, negative control; dpv, days post-vaccination.
To evaluate the induction of humoral immune responses by the test vaccine using D-galacto-D-mannan as an adjuvant, an experiment was performed according to the design shown in Figure 3A.
Figure 3 FMD vaccine containing D-galacto-D-mannan elicits early, mid-, and long-term immune response in mice. C57BL/6 mice (n = 5/group) were administered FMD vaccine containing inactivated FMDV antigens isolated from FMDV type O (O PA2) and A (A YC) (0.375 + 0.375 μg/dose/100 μL; 1/40 of the dose for pigs, 100 μg of D-galacto-D-mannan/dose/mouse, ISA 206 (oil-based emulsion, 50%, w/w), 10% aluminum hydroxide, and 15 μg of Quil-A. The PC group received vaccines of the same volume and formula as the Exp group, but without D-galacto-D-mannan as an adjuvant. The NC group was injected with an equal volume of phosphate-buffered saline. Mice were vaccinated with test vaccine intramuscularly and blood was collected 0, 7, 28, 56, and 84 dpv for serological analysis using SP O and A ELISAs and VN titers for O/PKA/44/2008 (O PA2) and A/SKR/YC/2017 (A YC). (A–E) Experimental strategy (A); Antibody titers, as determined using SP O (B) and SP A (C) ELISAs; VN titers for O PA2 (D) or A YC (E), as determined using VN test. Data are presented as mean ± SEM of triplicate measurements (n = 5/group). Statistical analyses were performed using two-way analysis of variance followed by Tukey’s post-hoc test. *p < 0.05; **p < 0.01; ***p < 0.001; ****p < 0.0001. dpv, days post-vaccination; PC, positive control; NC, negative control; ELISA, enzyme-linked immunosorbent assay; VN, virus neutralization.
When the test vaccine containing D-galacto-D-mannan was administered to mice in the Exp group, the antibody titers at 7 dpv (p < 0.05, SP O ELISA using the VDPro® Kit; p < 0.01, SP A ELISA using the PrioCheck™ Kit), 28 dpv (p < 0.01, SP O ELISA; p < 0.001, SP A ELISA), 56 dpv (p < 0.001, both SP O and A ELISA), and 84 dpv (p < 0.0001, SP O ELISA; p < 0.001, SP A ELISA) were higher than those in the PC group vaccinated without D-galacto-D-mannan. Antibody titers in the NC group did not change (Figures 3B, C).
The VN titers were higher in the Exp group administered the vaccine containing D-galacto-D-mannan together with the O PA2 + A YC antigen, 7 dpv (p < 0.01, O PA2; p < 0.05, A YC), 28 dpv (p < 0.05, both O PA2 and A YC), 56 dpv (p < 0.001, O PA2; p < 0.01, A YC), and 84 dpv (p < 0.01, O PA2; p < 0.001, A YC), compared with the PC group. VN titers in the NC group did not change (Figures 3D, E).
The targeted animal experiments using FMD antibody-seronegative pigs to evaluate the induction of humoral immune response by test vaccine with D-galacto-D-mannan in pigs are shown in Figure 4A.
Figure 4 FMD vaccine containing D-galacto-D-mannan elicits early, mid-, and long-term immune responses in pigs. For the pig experiments, FMDV type O and type A antibody-seronegative animals (8–9 weeks old) were used. The pigs were divided into three groups (n = 5 or 6/group) and administered inactivated bivalent FMDV vaccine without (PC group) or with 1 mg/dose/pig D-galacto-D-mannan (Exp group). PC group received FMDV type O (O PA2) and type A (A YC) antigens (15 + 15 μg/dose/mL, one dose for cattle and pig use) with ISA 206 (oil-based emulsion, 50%, w/w), 10% aluminum hydroxide, and 150 μg of Quil-A. Vaccination was performed twice at 28-day intervals, with 1 mL of vaccine (one dose) injected via the deep intramuscular route into animal necks. The NC group was injected with an equal volume of phosphate-buffered saline. Blood samples were collected 0, 7, 14, 28, 42, 56, and 84 dpv for serological assays. (A–E) Experimental strategy (A); antibody titers, as determined using SP O (B) and SP A (C) ELISAs; VN titers for O PA2 (D) or A YC (E), as determined using VN test. Data are presented as mean ± SEM of triplicate measurements (n = 5 or 6/group). Statistical analyses were performed using two-way analysis of variance followed by Tukey’s post-hoc test. *p < 0.05; **p < 0.01; ***p < 0.001; ****p < 0.0001. FMD, foot-and-mouth disease; FMDV, foot-and-mouth disease virus; PC, positive control; NC, negative control; dpv, days post-vaccination; ELISA, enzyme linked immunosorbent assay; VN, virus neutralization.
The FMDV type O-specific antibody titers (SP O ELISA using the VDPro® kit) of the Exp group receiving the vaccine containing D-galacto-D-mannan were higher than that of the PC group at each sampling point. In particular, after boosting 28 dpv, the antibody titers of the Exp group were the highest at 42 dpv, and the elevated antibody titers were maintained until 84 dpv. The NC group showed no significant changes (Figure 4B).
When the antibody titers were measured using SP A ELISA using the PrioCheck™ kit, it was found to increase somewhat slowly compared with that determined using the SP O ELISA.
However, 7 dpv after the first vaccination, the Exp group antibody titers increased steadily and significantly up to 28 dpv compared with the PC group. After boosting, Exp group antibody titers were seropositive up to 84 dpv, whereas antibody titers in the PC group were seropositive up to 56 dpv. The NC group showed no significant changes (Figure 4C).
Similar to the antibody titers measured by SP ELISA, the VN titers were higher in all dpv in the Exp group compared with the PC group (Figures 4D, E).
The Ig isotype ELISAs performed using serum samples from vaccinated pigs at 56 dpv are shown in Figure 4A. The IgG and IgA concentrations were significantly higher in the Exp group than in the control group, whereas no significant difference was observed in the IgM concentration between the Exp and PC groups (Figures 5A–C).
Figure 5 FMD vaccine containing D-galacto-D-mannan elevates levels of immunoglobulin subtypes, such as IgG, IgA, and IgM in pigs. For the pig experiments, FMDV type O and type A antibody-seronegative animals (8–9 weeks old) were used. Pigs were divided into three groups (n = 5 or 6/group) and administered inactivated bivalent FMDV vaccine without (PC group) or with (Exp group) 1 mg/dose/pig D-galacto-D-mannan. The PC group received FMDV type O (O PA2) and A (A YC) antigens (15 + 15 μg/dose/mL, one dose for cattle and pig use) with ISA 206 (oil-based emulsion, 50%, w/w), 10% aluminum hydroxide, and 150 μg of Quil-A. Vaccination was performed twice at 28-day intervals with 1 mL of vaccine (one dose) injected via a deep intramuscular route into animal necks. The NC group was injected with an equal volume of phosphate-buffered saline. Blood samples were collected 0, 7, 14, 28, 42, 56, and 84 dpv for serological assays. Data are presented as mean ± SEM of triplicate measurements (n = 5 or 6/group). Statistical analyses were performed using two-way analysis of variance followed by Tukey’s post-hoc test. *p < 0.05; **p < 0.01; ***p < 0.001; and ****p < 0.0001. (A–C) IgG (A); IgA (B); and IgM (C) concentrations. FMD, foot-and-mouth disease; FMDV, foot-and-mouth disease virus; PC, positive control; NC, negative control; dpv, days post-vaccination.
Pigs vaccinated with a vaccine containing D-galacto-D-mannan showed no local side effects (granulomas or inflammation) at the injection site compared with the PC group (Supplementary Figures 5A–C).
These results demonstrated that the test vaccine containing D-galacto-D-mannan elicited superior early, mid-, and long-term immune responses.
To investigate the mechanisms underlying the innate and adaptive immune responses elicited by vaccines containing D-galacto-D-mannan, according to the strategy presented in Figure 4A, qRT-PCR was performed using porcine PBMCs at 14 and 56 dpv sampling points (Figures 6A–X).
Figure 6 FMD vaccine containing D-galacto-D-mannan induces gene expression of PRRs, transcription factors, cytokines, and costimulatory molecules in porcine PBMCs. Porcine PBMCs isolated from the whole blood of vaccinated pigs (n = 5 or 6/group), as described in Figure 4A, were used for quantitative real-time polymerase chain reaction. Gene expression levels were normalized to those of HPRT and are presented as relative ratios compared to control levels. (A–X) Gene expression levels of Dectin-2 (A); SYK (B); BCL10 (C); ICOS (D); CARD11 (E); AHNAK (F); MALT1 (G); SIRT1 (H); NF-κB (I); CARD9 (J); IFNα (K); IFNβ (L); IFNγ (M); IL-1β (N); IL-6 (O); IL-23p19 (P); IL-23R (Q); IL-17A (R); CD28 (S); CD21 (T); CD80 (U); CD86 (V); CD19 (W); and CD81 (X). Statistical analyses were performed using two-way analysis of variance followed by Tukey’s post-hoc test. *p < 0.05; **p < 0.01; ***p < 0.001; ****p < 0.001. FMD, foot-and-mouth disease; PRRs, pattern-recognition receptors; PBMCs, peripheral blood mononuclear cells.
The expression of PRR and transcription factors, such as Dectin-2 (Figure 6A), SYK (Figure 6B), BCL10 (Figure 6C), and ICOS (Figure 6D), was higher in the Exp group than the control groups (PC and NC) both at 14 and 56 dpv. The expression of CARD11 (Figure 6E) and AHNAK (Figure 6F) was higher in the Exp than the PC group at 56 dpv. Conversely, the expression of MALT1 (Figure 6G), SIRT1 (Figure 6H), and NF-κB (Figure 6I) was higher in the Exp than the PC group at 14 dpv, but lower at 56 dpv. Finally, the expression of CARD9 (Figure 6J) showed no significant difference compared with the PC and NC groups at both 14 and 56 dpv.
The cytokine expression levels in pigs vaccinated with D-galacto-D-mannan were generally higher than those in the PC group 14 dpv but tended to be lower at 56 dpv. IFNα (Figure 6K), IFNβ (Figure 6L), IFNγ (Figure 6M), IL-1β (Figure 6N), IL-6 (Figure 6O), IL-23p19 (Figure 6P), and IL-23R (Figure 6Q) expression in the Exp was higher than the PC group 14 dpv but lower at 56 dpv. IL-17A (Figure 6R) expression in the Exp was higher than the PC group at both 14 and 56 dpv.
The expression of co-stimulatory molecules, such as CD28 (Figure 6S) and CD21 (Figure 6T), was higher in the Exp group than the PC group at 14 and 56 dpv. Conversely, CD80 (Figure 6U), CD86 (Figure 6V), CD19 (Figure 6W), and CD81 (Figure 6X) expressions were higher in the Exp group than the PC group 14 dpv but lower or showed no difference at 56 dpv. Our findings elucidate the immunological mechanisms induced by D-galacto-D-mannan, suggesting a background for the strong cellular and humoral immune responses induced by FMD vaccines containing D-galacto-D-mannan.
To evaluate the effect of simultaneous induction of systemic immunity and mucosal immunity, and long-lasting immune response through a combined program of intramuscular vaccination of FMD vaccine and oral administration of D-galacto-D-mannan, an experiment was performed according to the strategy in Supplementary Figure 6A. When intramuscular single-dose vaccination of FMD vaccine was combined with oral administration of D-galacto-D-mannan for 28 days, the immune response using SP O ELISA and VN test lasted until 84 dpv (Supplementary Figure 6B, C).
To date, various approaches have been proposed to develop novel FMD vaccines; however, the shortcomings of commercialized vaccines, such as (1) slow antibody titer induction, (2) difficulty in initial host defense, (3) short antibody titer persistence, (4) periodic and repeated vaccinations, and (5) side effects at the vaccination site, have not been addressed (33, 34). Therefore, we developed a novel FMD vaccine containing D-galacto-D-mannan as an adjuvant (immunostimulant).
We have previously confirmed that furfurman, a Dectin-2 agonist, promoted porcine PBMC proliferation; when added as an adjuvant to a vaccine, it simultaneously induced cellular and humoral immunity in mice and pigs, the experimental and target animals, respectively (18). We also evaluated host defense against FMDV O and FMDV A challenge in pigs vaccinated with the FMD bivalent (O+A) vaccine containing furfurman as an adjuvant. The FMD vaccine containing furfurman completely protected the host against heterologous FMDV infection (data not shown).
Furfurman is a cell wall component derived from Malassezia furfur, an opportunistic skin fungal pathogen recognized by C-type lectin receptors, especially Dectin-2 (35). Its receptors play a pivotal role in antifungal innate immune response. Dectin-2 binds mannose carbohydrates, such as the mannose-coated lipoarabinomannan of mycobacteria (36) and α-mannan found in Candida albicans (37). Upon binding, Dectin-2 associates with the Fc receptor gamma chain (38) and signals via SYK and CARD9/BCL-10/MALT1 (CBM complex), triggering NF-κB activation and subsequent proinflammatory cytokine production (39). Despite the importance of furfurman in inducing immune responses, safety issues related to its origin in pigs remains a concern since the fungus could affect animals. Moreover, considering veterinary vaccine expenses, the cost of furfurman is high, complicating its application to FMD vaccines. Therefore, we established a strategy to use a furfurman replacement as an adjuvant with guaranteed safety and economic efficiency; hence, D-galacto-D-mannan extracted from the cell wall of Ceratonia siliqua was used in this study.
Prior to in vitro studies, D-galacto-D-mannan-mediated cytotoxicity was assessed. Since cytotoxicity was not observed at concentrations of 0–5 μg/mL (Supplementary Figure 1), subsequent experiments were performed assuming that treatment with D-galacto-D-mannan in this concentration range was safe. To evaluate D-galacto-D-mannan-mediated innate and adaptive (cellular) immune response, we quantified D-galacto-D-mannan-mediated IFNγ secretion using the ELISpot assay with murine PECs and porcine PBMCs, and confirmed that D-galacto-D-mannan exerted a promising adjuvant effect when co-treated with the FMD viral antigen (Figure 1). Both PECs and PBMCs contain immune cells closely related to innate and adaptive immune responses, including DCs, MΦs, T cells, B cells, and unconventional T cells [gamma delta T (γδ T), invariant natural killer T (iNKT), and mucosal-associated invariant T (MAIT) cells], making them suitable for evaluating cellular immune responses (18). IFNγ is mainly secreted by T, NK, NKT, antigen-presenting cells (MΦs and DCs), and B cells. In addition, IFNγ secreted by antigen presenting and NK cells is closely related to early host defense (40, 41). Oh et al. (42) reported that IFNγ secretion could be restimulated in vaccinated cows that displayed high VN titer levels on the day of challenge, indicating a direct correlation between FMD vaccine-induced protection and VN antibodies and IFNγ. Therefore, D-galacto-D-mannan has the potential to elicit strong host defense through an enhanced innate immune response and adaptive (cellular and humoral) immune response. The results in sections 3.2 (Supplementary Figure 2, Figure 2) and 3.3 (Figures 3, 4) demonstrated that D-galacto-D-mannan-mediated cellular immune responses promoted rapid increase in antibody and VN titers to host-defensible levels (3). To evaluate vaccine efficacy, long-term immunity is an important indicator to consider in addition to initial protection against viral infection. This study focused on the induction of not only early and mid-term immunity but also long-term immunity by inducing cellular and humoral immune responses of the FMD vaccine containing D-galacto-D-mannan.
The antibody and VN titers of the Exp group vaccinated with FMD vaccine containing D-galacto-D-mannan were superior to those of the control (PC and NC) groups in all aspects, including rate of increase, level, and maintenance. The Exp group showed significantly higher antibody and VN titers at 7, 28, 56, and 84 dpv compared with the control group. Even at 7 dpv, complete host protection was expected at 28, 56, and 84 dpv, provided that the challenged mice induced host protection against FMDV infection. These results demonstrate that the FMD vaccine containing D-galacto-D-mannan can induce early, mid-term, and long-term immunity after vaccination, leading to a rapid, long-lasting, and potent host defense.
Antibodies closely related to the humoral immune response are secreted by B cells. B cells produced in the bone marrow undergo random genetic recombination to produce antibodies that specifically bind to different antigens. Among others, VN antibodies are part of the adaptive (humoral) immune response that can fight and eliminate viral and microbial toxins. Unlike non-neutralizing antibodies, VN antibodies bind specifically to viral antigens and prevent viruses from infecting and destroying host cells (43, 44). Therefore, VN titers are an indirect indicator of host defense. VN titers >1.74 (Log10) have been reported to elicit host defense by the FMD vaccine (45). In addition, according to Korea’s FMD vaccine efficacy validation guidelines, host defense is induced when the VN titers are >1.65 (Log10) (7). Based on our previous study showing complete host protection against FMDV infection when VN titers > 1.65 (Log10), the Exp group in this study will also induce host protection against FMDV infection from 28 to 84 dpv (29). The VN titers of the group vaccinated with FMD vaccine with D-galacto-D-mannan was maintained above 2 (Log10) for a long duration following the second vaccination; hence, this novel FMD vaccine can induce long-term host protection.
Ig isotype ELISA confirmed the IgG, IgA, and IgM levels specifically induced by the bivalent (O PA2+A YC) FMD vaccine containing D-galacto-D-mannan, demonstrating that D-galacto-D-mannan significantly contributes to the host humoral immune response (Figure 5). Of the five isotypes (IgG, IgA, IgM, IgD, and IgE) found in mammals, IgG plays a critical role in humoral immunity, especially in the induction of neutralizing antibodies. IgG is classified into diverse subsets with their relative abundance, size, complement activation, immune complex formation, binding to Fc receptors, and effector functions (46, 47). IgA is detected in the serum and intestinal mucosa and mostly secreted by B-cell responses in the gut (48–50). IgA is also secreted by T-cell-independent and dependent pathways and based on the pathways, and has different functions (51). IgA produced by the T-cell-independent pathway targets non-invasive commensals, whereas IgA produced by the T-cell-dependent pathway coats penetrant commensals and invasive pathogens (52–55). Thus, the enhanced humoral immune response induced by FMD vaccine with D-galacto-D-mannan significantly increases IgG and IgA levels, enhancing systemic immunity and consequently contributing to long-term immunity.
In our previous study, similar to the qRT-PCR results of the Exp group including the Dectin-1 agonist, we assumed that the gene expression levels of downstream signals of the Dectin-2-mediated signaling pathway would be high for the Exp group including the Dectin-2 agonist (23, 29, 37). However, the results showed low expression levels of specific transcription factors (CARD9 and NF-κB) and several inflammatory cytokines (IL-1β and IL-6) in contrast to high expression levels of CARD11 and SIRT1. This maintains homeostasis within the host by suppressing excessive inflammatory immune responses.
Herein, D-galacto-D-mannan, used as a Dectin-2 agonist, is extracted from plant cell walls; Dectin-2 is essential for host protection against Candida albicans via Th17 cell response (24, 37). Previous studies have reported that D-galacto-D-mannan exhibits antioxidant activity (28), which, along with its anti-inflammatory activities, is associated with increased SIRT1 expression levels (56). In the Exp group, cytokines (IL-1β, IL-6, IL-23p19, and IL-17A) with higher expression at 14 than 56 dpv are essential for maturation and differentiation of cells involved in innate and humoral (cellular) immunity. IL-23 (IL-12p40/IL-23p19) contributes to the early immune response by stimulating Th17, γδ T, iNKT, and innate lymphoid cells (57–59). IL-23p19 also induces IFNγ secretion in Th17 cells, eliciting cellular and humoral immune responses (41, 60). IL-1β and IL-6 promote differentiation of Th17 cells. IL-1β enhances the metabolic fitness of rapidly dividing Th17 cells during an inflammatory response by inducing phosphorylation of the mammalian target of rapamycin (mTOR) in Th17 cells (61). IL-6 induces IL-17A and IL-23R by promoting Th17 cell-related gene expression (62, 63). Expression levels of genes involved in the adaptive immune response increased at 56 dpv compared with 14 dpv.
Increased CARD11, ICOS, AHNAK, CD28, and CD21 expression levels demonstrate enhanced adaptive immune response by promoting maturation and differentiation of T and B cells. First, as CARD11 is mainly activated by CD28, T-cell receptor co-stimulation, and B-cell receptor signaling to form the CBM complex, the increased CARD11 expression in long-term immunity demonstrates that T and B cells are significantly stimulated (64, 65). ICOS, a member of the B7 family that binds to CD28, can be derived from T cells during the immune response and exhibits high expression, especially on follicular T helper cells (66–68). Yamasaki et al. (36) reported that Dectin-2/SYK signaling induced IL-2 secretion upon nuclear factor of activated T cells (NFAT) and Ca2+ influx stimulation. AHNAK is also involved in Ca2+ influx/NFAT signaling and induces IL-2 and IFNγ secretion via CD4+ T and CD8+ T-cell activation (69, 70). CD28 and CD21 are involved in T-cell and B-cell activation, respectively, and are important costimulatory molecules in inducing adaptive immune responses (71). In the PC group, the expression of cytokines and costimulatory molecules 56 dpv after the second vaccination were enhanced compared with that observed at 14 dpv, whereas in the Exp group, the cytokine and costimulatory molecule expression was higher at 14 than 56 dpv after the first vaccination (Figure 6). These results provide evidence that the Exp group administered the FMD vaccine containing D-galacto-D-mannan induced a significantly faster and stronger cellular immune response than the control (PC, NC) groups, thereby effectively inducing humoral immune responses such as antibody titers and VN titers.
In addition to the well-known Dectin-2-related mechanism, we proposed novel findings in this study, including immunostimulatory mechanisms triggered by plant-derived Dectin-2 agonist, as well as immunoregulatory mechanisms of the host immune response through suppression of excessive inflammatory responses. However, repeated large-scale blood collection may cause side effects, such as hemorrhagic shock, and adversely impact normal growth and the formation of vaccine-mediated immune responses in animals. For Western blot, very few types of pig antibodies are commercially available, making it difficult to identify other potential mechanisms. Moreover, commercially available porcine primary antibodies for Western blot and cytokine ELISA kits are extremely limited, which hinders the complete assessment of the diverse immune-related mechanisms. Therefore, to better understand and elucidate the diverse and overall mechanism of the FMD vaccine containing D-galacto-D-mannan, we presented gene expression levels through qRT-PCR instead of quantifying protein expression levels related to immune response with Western blot or ELISA. Based on this study, we intend to identify various mechanisms caused by FMD vaccines containing D-galacto-D-mannan through a systemic approach, such as RNA sequencing, in future studies.
From the results in Figure 6, it can be observed that the expression of cytokine-related genes such as IL-1β, IL-23p19, and IL-17A, which play an important role in the induction mechanism of mucosal immunity, was significantly increased. Therefore, the effect of inducing an immune response by D-galacto-D-mannan was evaluated using vaccination routes that can induce mucosal immunity, such as oral administration in addition to intramuscular injection. A long-lasting immune response was demonstrated when D-galacto-D-mannan was administered orally daily for 28 days along with a single dose of control vaccine (Supplementary Figure 6). Based on these results, it was confirmed that D-galacto-D-mannan is effective in inducing not only systemic immunity but also mucosal immunity, and we plan to confirm the effect of inducing mucosal immune responses through various administration routes such as nasal administration in the future.
However, histological analysis was not performed to provide objective evidence regarding the D-galacto-D-mannan-mediated cellular response and subsequent viral clearance via challenge studies, which is a limitation of this study. Therefore, in the next study, we plan to conduct expanded animal research targeting more animals and present these results.
Our study provides novel perspectives for establishing vaccine formulas and vaccination strategies for FMD and other difficult-to-prevent and control viral diseases.
The original contributions presented in the study are included in the article/Supplementary Material. Further inquiries can be directed to the corresponding authors.
The animal study was approved by Animal and Plant Quarantine Agency (APQA) Ethics Committee (Certification No.: IACUC-2022-670 and 2023-753). The study was conducted in accordance with the local legislation and institutional requirements.
HWK: Formal Analysis, Investigation, Software, Validation, Visualization, Writing – original draft, Writing – review & editing. M-KK: Investigation, Writing – original draft. SHP: Investigation, Writing – original draft. SS: Investigation, Writing – original draft. GSK: Investigation, Writing – original draft. DYK: Investigation, Writing – original draft. J-HP: Resources, Writing – review & editing. S-MK: Resources, Writing – review & editing. J-SL: Supervision, Writing – review & editing. MJL: Conceptualization, Formal Analysis, Funding acquisition, Investigation, Methodology, Project administration, Resources, Software, Supervision, Validation, Visualization, Writing – original draft, Writing – review & editing.
The author(s) declare financial support was received for the research, authorship, and/or publication of this article. This work was supported by grants from the Animal and Plant Quarantine Agency (APQA) (B-1543386-2021-24) and the National Research Foundation of Korea (NRF) (Grant No. 2021R1A6A1A03045495).
We would like to thank the staff and researchers of the Center for Foot-and-Mouth Disease Vaccine Research at the APQA for help with this study.
The authors declare that the research was conducted in the absence of any commercial or financial relationships that could be construed as a potential conflict of interest.
All claims expressed in this article are solely those of the authors and do not necessarily represent those of their affiliated organizations, or those of the publisher, the editors and the reviewers. Any product that may be evaluated in this article, or claim that may be made by its manufacturer, is not guaranteed or endorsed by the publisher.
The Supplementary Material for this article can be found online at: https://www.frontiersin.org/articles/10.3389/fimmu.2024.1330677/full#supplementary-material
1. Raza S, Siddique K, Rabbani M, Yaqub T, Anjum AA, Ibrahim M, et al. In silico analysis of four structural proteins of aphthovirus serotypes revealed significant B and T cell epitopes. Microb Pathog (2019) 128:254–62. doi: 10.1016/j.micpath.2019.01.007.
2. Kitching P, Hammond J, Jeggo M, Charleston B, Paton D, Rodriguez L, et al. Global FMD control–is it an option? Vaccine (2007) 25:5660–4. doi: 10.1016/j.vaccine.2006.10.052.
3. Jamal SM, Belsham GJ. Foot-and-mouth disease: past, present and future. Vet Res (2013) 44:116. doi: 10.1186/1297-9716-44-116.
4. Robinson L, Knight-Jones TJ, Charleston B, Rodriguez LL, Gay CG, Sumption KJ, et al. Global foot-and-mouth disease research update and gap analysis: 7 - pathogenesis and molecular biology. Transbound Emerg Dis (2016) 63 Suppl 1:63–71. doi: 10.1111/tbed.2016.63.issue-S1.
5. Hwang JH, Lee G, Kim A, Park JH, Lee MJ, Kim B, et al. A vaccine strain of the A/ASIA/sea-97 lineage of foot-and-mouth disease virus with a single amino acid substitution in the P1 region that is adapted to suspension culture provides high immunogenicity. Vaccines (Basel) (2021) 9:308. doi: 10.3390/vaccines9040308.
6. Jo H, Kim BY, Park SH, Kim HM, Shin SH, Hwang SY, et al. The HSP70-fused foot-and-mouth disease epitope elicits cellular and humoral immunity and drives broad-spectrum protective efficacy. NPJ Vaccines (2021) 6:42. doi: 10.1038/s41541-021-00304-9.
7. Lee MJ, Kim HM, Shin S, Jo H, Park SH, Kim SM, et al. The C3d-fused foot-and-mouth disease vaccine platform overcomes maternally-derived antibody interference by inducing a potent adaptive immunity. NPJ Vaccines (2022) 7:70. doi: 10.1038/s41541-022-00496-8.
8. Sarry M, Romey A, Lefebvre D, Benfrid S, Dufour B, Durand B, et al. [Foot and mouth disease virus: transmission, pathogenesis, diagnosis and surveillance]. Virologie (Montrouge) (2022) 26:355–73. doi: 10.1684/vir.2022.0972
9. Wang ZB, Xu J. Better adjuvants for better vaccines: progress in adjuvant delivery systems, modifications, and adjuvant-antigen codelivery. Vaccines (Basel) (2020) 8:128. doi: 10.3390/vaccines8010128
10. Hunter P. Vaccination as a means of control of foot-and-mouth disease in sub-saharan Africa. Vaccine (1998) 16:261–4. doi: 10.1016/S0264-410X(97)00170-9.
11. Cloete M, Dungu B, Van Staden LI, Ismail-Cassim N, Vosloo W. Evaluation of different adjuvants for foot-and-mouth disease vaccine containing all the SAT serotypes. Onderstepoort J Vet Res (2008) 75:17–31. doi: 10.4102/ojvr.v75i1.84.
12. Park ME, Lee SY, Kim RH, Ko MK, Lee KN, Kim SM, et al. Enhanced immune responses of foot-and-mouth disease vaccine using new oil/gel adjuvant mixtures in pigs and goats. Vaccine (2014) 32:5221–7. doi: 10.1016/j.vaccine.2014.07.040.
13. Rathogwa NM, Scott KA, Opperman P, Theron J, Maree FF. Efficacy of SAT2 foot-and-mouth disease vaccines formulated with montanide ISA 206B and quil-A saponin adjuvants. Vaccines (Basel) (2021) 9:996. doi: 10.3390/vaccines9090996.
14. Cox JC, Coulter AR. Adjuvants–a classification and review of their modes of action. Vaccine (1997) 15:248–56. doi: 10.1016/S0264-410X(96)00183-1.
15. Barnett PV, Pullen L, Williams L, Doel TR. International bank for foot-and-mouth disease vaccine: assessment of Montanide ISA 25 and ISA 206, two commercially available oil adjuvants. Vaccine (1996) 14:1187–98. doi: 10.1016/S0264-410X(96)00055-2.
16. Patil PK, Bayry J, Ramakrishna C, Hugar B, Misra LD, Natarajan C. Immune responses of goats against foot-and-mouth disease quadrivalent vaccine: comparison of double oil emulsion and aluminium hydroxide gel vaccines in eliciting immunity. Vaccine (2002) 20:2781–9. doi: 10.1016/S0264-410X(02)00184-6.
17. Hunter P. The performance of southern African territories serotypes of foot and mouth disease antigen in oil-adjuvanted vaccines. Rev Sci Tech (1996) 15:913–22. doi: 10.20506/rst.issue.15.3.2492.
18. Lee MJ, Jo H, Shin SH, Kim SM, Kim B, Shim HS, et al. Mincle and STING-stimulating adjuvants elicit robust cellular immunity and drive long-lasting memory responses in a foot-and-mouth disease vaccine. Front Immunol (2019) 10:2509. doi: 10.3389/fimmu.2019.02509.
19. McGreal EP, Rosas M, Brown GD, Zamze S, Wong SY, Gordon S, et al. The carbohydrate-recognition domain of Dectin-2 is a C-type lectin with specificity for high mannose. Glycobiology (2006) 16:422–30. doi: 10.1093/glycob/cwj077.
20. Jin X, Zhang M, Cao GF, Yang YF. Saccharomyces cerevisiae mannan induces sheep beta-defensin-1 expression via Dectin-2-Syk-p38 pathways in ovine ruminal epithelial cells. Vet Res (2019) 50:8. doi: 10.1186/s13567-019-0624-4.
21. Bonkobara M, Hoshino M, Yagihara H, Tamura K, Isotani M, Tanaka Y, et al. Identification and gene expression of bovine C-type lectin dectin-2. Vet Immunol Immunopathol (2006) 110:179–86. doi: 10.1016/j.vetimm.2005.08.031.
22. McDonald JU, Rosas M, Brown GD, Jones SA, Taylor PR. Differential dependencies of monocytes and neutrophils on dectin-1, dectin-2 and complement for the recognition of fungal particles in inflammation. PloS One (2012) 7:e45781. doi: 10.1371/journal.pone.0045781.
23. Kerscher B, Willment JA, Brown GD. The Dectin-2 family of C-type lectin-like receptors: an update. Int Immunol (2013) 25:271–7. doi: 10.1093/intimm/dxt006.
24. Robinson MJ, Osorio F, Rosas M, Freitas RP, Schweighoffer E, Gross O, et al. Dectin-2 is a Syk-coupled pattern recognition receptor crucial for Th17 responses to fungal infection. J Exp Med (2009) 206:2037–51. doi: 10.1084/jem.20082818.
25. Strasser D, Neumann K, Bergmann H, Marakalala MJ, Guler R, Rojowska A, et al. Syk kinase-coupled C-type lectin receptors engage protein kinase C-δ to elicit Card9 adaptor-mediated innate immunity. Immunity (2012) 36:32–42. doi: 10.1016/j.immuni.2011.11.015.
26. Gringhuis SI, Wevers BA, Kaptein TM, van Capel TM, Theelen B, Boekhout T, et al. Selective C-Rel activation via Malt1 controls anti-fungal T(H)-17 immunity by dectin-1 and dectin-2. PloS Pathog (2011) 7:e1001259. doi: 10.1371/journal.ppat.1001259.
27. Barrett NA, Rahman OM, Fernandez JM, Parsons MW, Xing W, Austen KF, et al. Dectin-2 mediates Th2 immunity through the generation of cysteinyl leukotrienes. J Exp Med (2011) 208:593–604. doi: 10.1084/jem.20100793.
28. Pristov JB, Mitrović A, Spasojević I. A comparative study of antioxidative activities of cell-wall polysaccharides. Carbohydr Res (2011) 346:2255–9. doi: 10.1016/j.carres.2011.07.015.
29. Kim HW, Ko MK, Park SH, Hwang SY, Kim DH, Park SY, et al. Dectin-1 signaling coordinates innate and adaptive immunity for potent host defense against viral infection. Front Immunol (2023) 14:1194502. doi: 10.3389/fimmu.2023.1194502.
30. Bahnemann HG. Binary ethylenimine as an inactivant for foot-and-mouth disease virus and its application for vaccine production. Arch Virol (1975) 47:47–56. doi: 10.1007/BF01315592.
31. Fowler VL, Knowles NJ, Paton DJ, Barnett PV. Marker vaccine potential of a foot-and-mouth disease virus with a partial VP1 G-H loop deletion. Vaccine (2010) 28:3428–34. doi: 10.1016/j.vaccine.2010.02.074.
32. Fukai K, Morioka K, Yamada M, Nishi T, Yoshida K, Kitano R, et al. Comparative performance of fetal goat tongue cell line ZZ-R 127 and fetal porcine kidney cell line LFBK-αvβ6 for Foot-and-mouth disease virus isolation. J Vet Diagn Invest (2015) 27:516–21. doi: 10.1177/1040638715584156.
33. Lyons NA, Knight-Jones TJD, Bartels C, Paton DJ, Ferrari G, Vermillion MS, et al. Considerations for design and implementation of vaccine field trials for novel foot-and-mouth disease vaccines. Vaccine (2019) 37:1007–15. doi: 10.1016/j.vaccine.2018.12.064.
34. Mahapatra M, Parida S. Foot and mouth disease vaccine strain selection: current approaches and future perspectives. Expert Rev Vaccines (2018) 17:577–91. doi: 10.1080/14760584.2018.1492378.
35. Ishikawa T, Itoh F, Yoshida S, Saijo S, Matsuzawa T, Gonoi T, et al. Identification of distinct ligands for the C-type lectin receptors Mincle and Dectin-2 in the pathogenic fungus Malassezia. Cell Host Microbe (2013) 13:477–88. doi: 10.1016/j.chom.2013.03.008.
36. Yonekawa A, Saijo S, Hoshino Y, Miyake Y, Ishikawa E, Suzukawa M, et al. Dectin-2 is a direct receptor for mannose-capped lipoarabinomannan of mycobacteria. Immunity (2014) 41:402–13. doi: 10.1016/j.immuni.2014.08.005.
37. Saijo S, Ikeda S, Yamabe K, Kakuta S, Ishigame H, Akitsu A, et al. Dectin-2 recognition of alpha-mannans and induction of Th17 cell differentiation is essential for host defense against Candida albicans. Immunity (2010) 32:681–91. doi: 10.1016/j.immuni.2010.05.001.
38. Sato K, Yang XL, Yudate T, Chung JS, Wu J, Luby-Phelps K, et al. Dectin-2 is a pattern recognition receptor for fungi that couples with the Fc receptor gamma chain to induce innate immune responses. J Biol Chem (2006) 281:38854–66. doi: 10.1074/jbc.M606542200.
39. Sancho D, Reis e Sousa C. Signaling by myeloid C-type lectin receptors in immunity and homeostasis. Annu Rev Immunol (2012) 30:491–529. doi: 10.1146/annurev-immunol-031210-101352.
40. Frucht DM, Fukao T, Bogdan C, Schindler H, O’Shea JJ, Koyasu S. IFN-gamma production by antigen-presenting cells: mechanisms emerge. Trends Immunol (2001) 22:556–60. doi: 10.1016/S1471-4906(01)02005-1.
41. Kak G, Raza M, Tiwari BK. Interferon-gamma (IFN-γ): Exploring its implications in infectious diseases. Biomol Concepts (2018) 9:64–79. doi: 10.1515/bmc-2018-0007.
42. Oh Y, Fleming L, Statham B, Hamblin P, Barnett P, Paton DJ, et al. Interferon-γ induced by in vitro re-stimulation of CD4+ T-cells correlates with in vivo FMD vaccine induced protection of cattle against disease and persistent infection. PloS One (2012) 7:e44365. doi: 10.1371/journal.pone.0044365.
43. Klasse PJ. Neutralization of virus infectivity by antibodies: old problems in new perspectives. Adv Biol (2014) 2014:157895. doi: 10.1155/2014/157895.
44. Jung D, Alt FW. Unraveling V(D)J recombination; insights into gene regulation. Cell (2004) 116:299–311. doi: 10.1016/S0092-8674(04)00039-X.
45. Black L, Francis MJ, Rweyemamu MM, Umebara O, Boge A. The relationship between serum antibody titres and protection from foot and mouth disease in pigs after oil emulsion vaccination. J Biol Stand (1984) 12:379–89. doi: 10.1016/S0092-1157(84)80062-1.
46. Vidarsson G, Dekkers G, Rispens T. IgG subclasses and allotypes: from structure to effector functions. Front Immunol (2014) 5:520. doi: 10.3389/fimmu.2014.00520.
47. Paudyal B, Mwangi W, Rijal P, Schwartz JC, Noble A, Shaw A, et al. Fc-mediated functions of porcine igG subclasses. Front Immunol (2022) 13:903755. doi: 10.3389/fimmu.2022.903755.
48. Wilmore JR, Gaudette BT, Gomez Atria D, Hashemi T, Jones DD, Gardner CA, et al. Commensal microbes induce serum igA responses that protect against polymicrobial sepsis. Cell Host Microbe (2018) 23:302–11.e3. doi: 10.1016/j.chom.2018.01.005.
49. Gutzeit C, Magri G, Cerutti A. Intestinal IgA production and its role in host-microbe interaction. Immunol Rev (2014) 260:76–85. doi: 10.1111/imr.12189.
50. Kato LM, Kawamoto S, Maruya M, Fagarasan S. The role of the adaptive immune system in regulation of gut microbiota. Immunol Rev (2014) 260:67–75. doi: 10.1111/imr.12185.
51. Fagarasan S, Kawamoto S, Kanagawa O, Suzuki K. Adaptive immune regulation in the gut: T cell-dependent and T cell-independent IgA synthesis. Annu Rev Immunol (2010) 28:243–73. doi: 10.1146/annurev-immunol-030409-101314.
52. Palm NW, de Zoete MR, Cullen TW, Barry NA, Stefanowski J, Hao L, et al. Immunoglobulin A coating identifies colitogenic bacteria in inflammatory bowel disease. Cell (2014) 158:1000–10. doi: 10.1016/j.cell.2014.08.006.
53. Bunker JJ, Flynn TM, Koval JC, Shaw DG, Meisel M, McDonald BD, et al. Innate and adaptive humoral responses coat distinct commensal bacteria with immunoglobulin A. Immunity (2015) 43:541–53. doi: 10.1016/j.immuni.2015.08.007.
54. Bunker JJ, Erickson SA, Flynn TM, Henry C, Koval JC, Meisel M, et al. Natural polyreactive IgA antibodies coat the intestinal microbiota. Science (2017) 358:eaan6619. doi: 10.1126/science.aan6619.
55. Chen K, Magri G, Grasset EK, Cerutti A. Rethinking mucosal antibody responses: IgM, IgG and IgD join IgA. Nat Rev Immunol (2020) 20:427–41. doi: 10.1038/s41577-019-0261-1.
56. Singh V, Ubaid S. Role of silent information regulator 1 (SIRT1) in regulating oxidative stress and inflammation. Inflammation (2020) 43:1589–98. doi: 10.1007/s10753-020-01242-9.
57. Cua DJ, Tato CM. Innate IL-17-producing cells: the sentinels of the immune system. Nat Rev Immunol (2010) 10:479–89. doi: 10.1038/nri2800.
58. Marks BR, Nowyhed HN, Choi JY, Poholek AC, Odegard JM, Flavell RA, et al. Thymic self-reactivity selects natural interleukin 17-producing T cells that can regulate peripheral inflammation. Nat Immunol (2009) 10:1125–32. doi: 10.1038/ni.1783.
59. Gaffen SL, Jain R, Garg AV, Cua DJ. The IL-23-IL-17 immune axis: from mechanisms to therapeutic testing. Nat Rev Immunol (2014) 14:585–600. doi: 10.1038/nri3707.
60. Hirota K, Duarte JH, Veldhoen M, Hornsby E, Li Y, Cua DJ, et al. Fate mapping of IL-17-producing T cells in inflammatory responses. Nat Immunol (2011) 12:255–63. doi: 10.1038/ni.1993.
61. Gulen MF, Kang Z, Bulek K, Youzhong W, Kim TW, Chen Y, et al. The receptor SIGIRR suppresses Th17 cell proliferation via inhibition of the interleukin-1 receptor pathway and mTOR kinase activation. Immunity (2010) 32:54–66. doi: 10.1016/j.immuni.2009.12.003.
62. Yang XO, Panopoulos AD, Nurieva R, Chang SH, Wang D, Watowich SS, et al. STAT3 regulates cytokine-mediated generation of inflammatory helper T cells. J Biol Chem (2007) 282:9358–63. doi: 10.1074/jbc.C600321200.
63. Durant L, Watford WT, Ramos HL, Laurence A, Vahedi G, Wei L, et al. Diverse targets of the transcription factor STAT3 contribute to T cell pathogenicity and homeostasis. Immunity (2010) 32:605–15. doi: 10.1016/j.immuni.2010.05.003.
64. Brownlie RJ, Zamoyska R. T cell receptor signalling networks: branched, diversified and bounded. Nat Rev Immunol (2013) 13:257–69. doi: 10.1038/nri3403.
65. Ruland J, Hartjes L. CARD-BCL-10-MALT1 signalling in protective and pathological immunity. Nat Rev Immunol (2019) 19:118–34. doi: 10.1038/s41577-018-0087-2.
66. Hutloff A, Dittrich AM, Beier KC, Eljaschewitsch B, Kraft R, Anagnostopoulos I, et al. ICOS is an inducible T-cell co-stimulator structurally and functionally related to CD28. Nature (1999) 397:263–6. doi: 10.1038/16717.
67. Hu YL, Metz DP, Chung J, Siu G, Zhang M. B7RP-1 blockade ameliorates autoimmunity through regulation of follicular helper T cells. J Immunol (2009) 182:1421–8. doi: 10.4049/jimmunol.182.3.1421.
68. Hodgson R, Christiansen D, Ierino F, Sandrin M. Inducible Co-Stimulator (ICOS) in transplantation: A review. Transplant Rev (Orlando) (2022) 36:100713. doi: 10.1016/j.trre.2022.100713.
69. Matza D, Badou A, Kobayashi KS, Goldsmith-Pestana K, Masuda Y, Komuro A, et al. A scaffold protein, AHNAK1, is required for calcium signaling during T cell activation. Immunity (2008) 28:64–74. doi: 10.1016/j.immuni.2007.11.020.
70. Matza D, Badou A, Jha MK, Willinger T, Antov A, Sanjabi S, et al. Requirement for AHNAK1-mediated calcium signaling during T lymphocyte cytolysis. Proc Natl Acad Sci U.S.A (2009) 106:9785–90. doi: 10.1073/pnas.0902844106.
Keywords: foot-and-mouth disease, D-galacto-D-mannan, Dectin-2 agonist, vaccine adjuvant, cellular and humoral, immune response
Citation: Kim HW, Ko M-K, Park SH, Shin S, Kim GS, Kwak DY, Park J-H, Kim S-M, Lee J-S and Lee MJ (2024) D-galacto-D-mannan-mediated Dectin-2 activation orchestrates potent cellular and humoral immunity as a viral vaccine adjuvant. Front. Immunol. 15:1330677. doi: 10.3389/fimmu.2024.1330677
Received: 31 October 2023; Accepted: 30 January 2024;
Published: 16 February 2024.
Edited by:
Jai Rudra, Washington University in St. Louis, United StatesReviewed by:
Yashavanth Shaan Lakshmanappa, University of California, Davis, United StatesCopyright © 2024 Kim, Ko, Park, Shin, Kim, Kwak, Park, Kim, Lee and Lee. This is an open-access article distributed under the terms of the Creative Commons Attribution License (CC BY). The use, distribution or reproduction in other forums is permitted, provided the original author(s) and the copyright owner(s) are credited and that the original publication in this journal is cited, in accordance with accepted academic practice. No use, distribution or reproduction is permitted which does not comply with these terms.
*Correspondence: Jong-Soo Lee, am9uZ3Nvb2xAY251LmFjLmty; Min Ja Lee, aGVyYjEyQGtvcmVhLmty
Disclaimer: All claims expressed in this article are solely those of the authors and do not necessarily represent those of their affiliated organizations, or those of the publisher, the editors and the reviewers. Any product that may be evaluated in this article or claim that may be made by its manufacturer is not guaranteed or endorsed by the publisher.
Research integrity at Frontiers
Learn more about the work of our research integrity team to safeguard the quality of each article we publish.