- 1Department of Parasitology, Pasteur Institute of Iran, Tehran, Iran
- 2Department of Cardiology I-Coronary and Peripheral Vascular Disease, Heart Failure, University Hospital Münster, Westfälische Wilhelms-Universität, Münster, Germany
- 3Department of Medical Genetics, Third Faculty of Medicine, Charles University, Prague, Czechia
Understanding the immune response to Leishmania infection and identifying biomarkers that correlate with protection are crucial for developing effective vaccines. One intriguing aspect of Leishmania infection is the persistence of parasites, even after apparent lesion healing. Various host cells, including dendritic cells, fibroblasts, and Langerhans cells, may serve as safe sites for latent infection. Memory T cells, especially tissue-resident memory T cells (TRM), play a crucial role in concomitant immunity against cutaneous Leishmania infections. These TRM cells are long-lasting and can protect against reinfection in the absence of persistent parasites. CD4+ TRM cells, in particular, have been implicated in protection against Leishmania infections. These cells are characterized by their ability to reside in the skin and rapidly respond to secondary infections by producing cytokines such as IFN-γ, which activates macrophages to kill parasites. The induction of CD4+ TRM cells has shown promise in experimental immunization, leading to protection against Leishmania challenge infections. Identifying biomarkers of protection is a critical step in vaccine development and CD4+ TRM cells hold potential as biomarkers, as their presence and functions may correlate with protection. While recent studies have shown that Leishmania-specific memory CD4+ T-cell subsets are present in individuals with a history of cutaneous leishmaniasis, further studies are needed to characterize CD4+ TRM cell populations. Overall, this review highlights the importance of memory T cells, particularly skin-resident CD4+ TRM cells, as promising targets for developing effective vaccines against leishmaniasis and as biomarkers of immune protection to assess the efficacy of candidate vaccines against human leishmaniasis.
1 Introduction
Leishmaniasis, a disease caused by the protozoan parasites of the genus Leishmania, is a major worldwide health concern. The endemicity of the disease has been established in approximately 100 countries across the globe, with diverse clinical forms, including cutaneous (CL), mucocutaneous (MCL), visceral (VL), and post-kala-azar dermal (PKDL) leishmaniasis (1). CL typically manifests as a naturally healing skin lesion, yet there are exceptional cases where it does not follow the expected healing timeline and remains unresponsive to repeated antimonial treatments. Despite immense efforts to manage the disease through various approaches such as chemotherapy and vector/reservoir control, success rates remain limited in most endemic regions. It is important to note that, as of now, there is no vaccine accessible for any form of human leishmaniasis (2, 3).
While a wealth of information has been amassed regarding the factors influencing the pathogenesis of Leishmania infection and the immune responses mounted by the host, encompassing both animal models and human patients, there remains a notable absence of well-defined immune biomarkers that can reliably indicate recovery and protection in human leishmaniasis (4). It is well known that in a mouse model of leishmaniasis, the specific immune response elicited following infection with Leishmania parasite significantly influences the ultimate disease outcome, whether it leads to recovery and protection or exacerbation of the disease (5). Experimentally, resolution of the leishmanial lesion due to L. major infection in resistant mice is followed by the development of a lifelong immunity to reinfection, shown by cellular immune response and delayed-type hypersensitivity (DTH). It is widely acknowledged that individuals who develop CL through natural infection due to sand fly bites or through leishmanization experience robust protection against the development of additional lesions. This underscores the rationale for the development of a leishmaniasis vaccine (6, 7).
A safe vaccine with high efficacy that provides protection against both Old World and New World Leishmania species causing CL or VL would undoubtedly benefit the people in certain economically deprived areas of the world. During the last few years, a myriad of potential Leishmania vaccine candidates have been put forth, spanning various types such as live organisms, attenuated strains, genetically engineered parasites, inactivated forms, and subunit or fusion proteins. However, most of them have been evaluated in preclinical phases and shown immunogenicity in animal models [reviewed in (8, 9)] and only a limited number of candidates have a clear and timely path toward evaluation in clinical trials (Nateghi Rostami, et al., under review) (10). ChAd63-KH is a replication defective simian adenoviral vaccine expressing a synthetic gene (KH) and encoding two Leishmania proteins kinetoplastid membrane protein 11 (KMP-11) and hydrophilic acylated surface protein B (HASPB). It has progressed through a phase I human trial in healthy UK volunteers and a single intramuscular vaccination in Sudanese patients with persistent PKDL in phase IIa and phase IIb trials to evaluate therapeutic efficacy (11, 12). There are few other vaccine candidates that are in late-stage preclinical development. LmCen−/− cultures have been produced under GMP conditions, and phase I trials are being planned in the USA and India. The use of mRNA vaccine platforms for leishmaniasis has not been explored yet (13), but mRNA vaccines encoding Leishmania recombinant LEISH-F2 and LEISH-F3 antigens are in the late preclinical evaluation stage. This mRNA vaccine builds upon the promising outcomes observed in human efficacy trials of the LEISH-F1, F2, and F3 vaccines. LEISH-F vaccines consist of fusion recombinant proteins with adjuvants and were among the first second-generation vaccines to undergo human testing (14).
In addition to humans, various animal species including rodents and canids can serve as reservoirs or infected with Leishmania spp (15). as seen in the zoonotic cycle of CL due to L. major and of VL due to L. infantum. High infection rates in dogs, which usually live close to humans, facilitate the domestic transmission of L. infantum to humans, as indicated by several studies reporting a high incidence of canine VL in endemic regions of human VL (16, 17). Regarding canine VL, four vaccines have been licensed since 2000s, two in Brazil (Leishmune® and Leish-Tec®) and two in Europe (CaniLeish® and LetiFend®). The production and marketing license of Leishmune® vaccine was withdrawn due to lack of effectiveness. Some concerns regarding effectiveness and potential infectiousness remain for all of these vaccines (18).
Despite advances in experimental and preclinical vaccine research, one reason for the lack of an approved human leishmaniasis vaccine might stem from our incomplete comprehension of the factors that signify protective immunity (4). Additionally, animal models for leishmaniasis often do not precisely reproduce the human form of the disease, and the translation of findings from experimental animal models to humans is not always confident (Nateghi Rostami, et al., under review) (19).
2 T lymphocyte subsets in leishmaniasis
The effector T cells (TEFF) refer to a collection of cells comprising various T-cell types, including both CD4+ and CD8+ T cells, which actively respond to antigenic stimulations. Following the differentiation of an effector T cell in the lymphoid tissue, an effector T cell locates target cells presenting the MHC:peptide complex. CD8+ T cells recognize peptide fragments from cytosolic pathogens, bound to MHC class I molecules at the cell surface (20). Cytotoxic CD8+ T cells can limit intracellular infections by direct cytolysis via perforin/granzymes and Fas/FasL interactions or by activating immune responses through production of cytokines like IFN-γ. On the other hand, both CD4+ Th1 and CD4+ Th2 cells recognize antigen fragments from intracellular vesicles displayed on the cell surface by MHC class II molecules. In murine experimental L. major infection, CD4+ T cells differentiate into Th1 or Th2 subpopulations that determine infection outcomes. Resistant C57BL/6 inbred mice develop a strong Th1 response with high interferon (IFN)-γ production that promote healing of L. major lesions and parasite clearance, while susceptible BALB/c inbred mice develop a Th2 response with high IL-4 production that cause non-healing lesion and systemic dissemination of L. major infection and eventual death of the animal (21).
Besides the host’s genetic background, outcomes of the disease are influenced by the parasite strain, infection route, and parasite dose (22). For example, in contrast to L. major infection, L. braziliensis infection in BALB/c mice triggers an effective immune response resulting in lesion healing that coincides with parasite persistence in draining lymph nodes. Subcutaneous inoculation of a low dose of L. infantum induces a Th1 response accompanied by a low parasite burden, while high-dose infection results in a Th2 or mixed Th1/Th2 response and a high parasite burden in spleen and lymph nodes. Although Th1/Th2 polarized response is well established in the C57BL/6 and BALB/c mice strains, this paradigm sometimes falls short in explaining host immunity, especially in humans (23, 24) (Nateghi-Rostami, et al, under review).
Another T-cell population, Th17 cells, expressing skin and mucosal homing receptors such as CCR4 and CCR6, is involved in neutrophil and monocyte recruitment, producing cytokines (IL-17, IL-22, and IL-23) and providing antimicrobial immunity at epithelial and mucosal surfaces (25). The contributive role of Th17 cytokines in either protection against infection or exacerbation of the disease has been suggested in animal models of L. major and L. donovani infections (26–28). It is claimed that IL-17 and IL-22 may synergize with Th1 cytokines for protection against human VL due to L. donovani (29); in our study, patients with active VL due to L. infantum exhibit higher IL-22 production, decreasing after VL healing. No significant difference was found in IL-17 production in vitro between active and healed CL or VL patients (30, 31). It was suggested that Th17 cell population is suppressed by regulatory T (TREG) cells through TGF-β and IL-35 production during chronic VL (32).
CD4+CD25hiFOXP3+ TREG cells are known for their lymphocyte-suppressing mechanisms, including regulatory cytokine expression, such as IL-10, during inflammatory and infectious diseases. In both naive mice and humans, TREG cells make up as much as approximately 5%–10% of the peripheral CD4+ T-cell population. Their primary function is to suppress various potentially harmful immune responses in vivo, principally reactions targeting self-antigens.
In Table 1, a summary of human and mouse studies on the contribution of different T-cell subsets in the immunity against leishmaniasis is provided.
3 Persistent parasites and concomitant immunity
3.1 The host cells
Leishmania parasites survive within the host cells, primarily macrophages, and employ a range of immune evasion strategies to establish and maintain infection (79). The parasites transform into amastigotes within macrophages and replicate continuously, eventually leading to host cell death and rupture. Microbicidal mechanisms of macrophages, particularly reactive oxygen species (ROS) and nitric oxide (NO) productions, play a crucial role in eliminating intracellular parasites (80–82). Different signals such as IFN-γ, CCL2 [chemokine (CC-motif) ligand 2; also known as monocyte chemoattractant protein-1 or MCP-1], and MIP-1α (macrophage inflammatory protein-1 alpha or CCL3) mediate macrophage activation for NO release and subsequent parasite clearance (83). In the evasion mechanisms, some cell surface molecules of Leishmania, such as gp63, are involved (84, 85). Leishmania promastigotes are exposed to the complement system proteins in the blood and C3b is fixed to the surface of metacyclic parasites via LPG (lipophosphoglycan), but assembly of the C5b-9 attack complex is inhibited, as a result of phosphorylation activity that involves both classical and alternative pathways of the complement system. Leishmania promastigotes possess a cell surface protein kinase activity, and as they undergo transformation into metacyclic forms, there is an increase in both the activity of protein kinases and the phosphorylation of protein substrates in the parasite (86). Furthermore, Leishmania amastigotes inhibit several host cell phosphorylation signaling pathways (87, 88) and avoid superoxides during phagocytosis by interfering with NADPH oxidase assembly (89). For example, macrophages infected with L. donovani displayed impaired capacity to initiate phosphorylation in downstream molecules of Janus kinase (JAK)-signal transducer and activator of transcription (STAT) signaling pathways including STAT-1, JAK1, and JAK2 in response to IFN-γ (90). Downregulation of Toll-like receptors (TLRs) and JAK-STAT signaling pathway genes has also been observed in NK cells obtained from diffused CL (DCL) patients caused by L. mexicana, further highlighting the adaptability of Leishmania parasites (91). Recently, it was shown that Leishmania parasites use kinetoplast DNA to grow inside infected macrophages by taking advantage of the macrophage’s signaling pathway involving Cyclic GMP-AMP synthase (cGAS), stimulator of interferon genes (STING), and TANK-binding kinase 1 (TBK1) for their own benefit (92).
3.2 Parasite persistence in CL
Parasite persistence has been documented in both human and mouse models of Leishmania infection, but the specific host cells responsible for this prolonged persistence remain uncertain (93, 94). Various host cells, including dendritic cells (DCs), fibroblasts, and Langerhans cells (LCs), may serve as safe sites for latent infection (95–97). In mice resolved from L. major infection, viable parasites were found in both macrophages and DCs obtained from the draining lymph nodes (95), but only DCs have the ability to present endogenous parasite antigen to T cells. In vivo tracking analysis suggested that the infected DCs found in the lymph nodes were traced back to LCs that had previously migrated from the skin (95) and L. major-containing LCs were detected in infected mice skin (96). On the other hand, it was shown that fibroblasts play a substantial role as the host cell harboring a significant number of persisting parasites within the draining lymph nodes of mice following the resolution of cutaneous lesions caused by L. major infection (97). Interestingly, the infected fibroblasts failed to eradicate Leishmania, unlike infected macrophages. This suggests that fibroblasts might potentially serve as a secure host for the parasites during chronic infections. Leishmania amastigotes, residing within parasitophorous vacuoles (PVs) in host cells, rely on essential nutritional elements, including amino acids, purines, and lipids, to support their sustained growth and survival (98).
Belkaid’s group demonstrated that the persistence of L. major in the skin of resistant C57BL/6 mice, even after apparent healing, is regulated by an inherent population of CD4+CD25+ TREG cells (49). In L. major infection, CD4+CD25+ TREG cells recruit in dermis and, with or without IL-10 contribution, inhibit effector T cells from eliminating the parasite at the infection site (48). It was shown that following establishment of L. major infection in dermal regions, CCR5 plays a pivotal role in homing CD4+CD25+ TREG cells to the infected sites, thereby facilitating the parasite’s successful homing and enduring presence in the host (50). Even if the parasite is effectively eliminated in secondary infection sites, occurrence of Leishmania superinfection may reactivate the primary infection. This reactivation is orchestrated by TREG cells, which hinder the activation of effector memory responses (99). Both CD4+CD25+FoxP3+ and Foxp3− TREG cells were identified at the lesion site as the source of IL-10 production in the mouse model of Leishmania infection (99, 100).
Similarly in human leishmaniasis, TREG cells were characterized in skin lesions of patients with CL, PKDL, and MCL caused by different New World and Old World Leishmania species, suggesting a regulatory role of these cells through IL-10 production that contributes to the parasite persistence (53, 76, 101, 102).
3.3 Parasites persistence in VL
In the VL infection model, parasite persistence has been shown and the mechanisms of host immunity and immune response evasive mechanisms of the parasite have been discussed (103, 104). L. infantum parasites have been shown to persist in dogs in ulcerative skin lesions at primary sand fly bite sites and also at distal sites even after 6 months (105), and under experimental conditions, sand flies could efficiently obtain parasites upon feeding on lesions (105).
In a model of VL infection, it has been suggested that transition of the inflammatory milieu to a regulatory milieu, shown by the increased B-cell activity and IL-10 levels, is associated with the establishment of chronic infection and accumulation of Leishmania parasites in the skin (104). In human leishmaniasis, IL-10 plays a central role in the course of the immune response in VL, contributing to the chronicity of the disease through suppression of host immunity and effector T-cell functions (59, 106, 107). Indeed, it was shown that elevated levels of plasma IL-10 and IL-10 expression by keratinocytes indicate the likelihood of subsequent PKDL development in VL patients following treatment (108).
Both CD4+CD25+Foxp3+ and CD25−Foxp3− (Tr1) TREG cell populations were found as the source of IL-10 production in L. infantum infection in the mouse model (51, 52). Similarly, in dogs as the reservoirs of zoonotic VL, while both CD4+ and CD8+ T cells expressing CD25+FoxP3+ were found in the blood and bone marrow of infected animals, antileishmanial therapy caused an increase of CD4+CD25+FoxP3+ T cells in all tissues, ensuring the parasite’s survival and completion of the Leishmania life cycle (109).
Similar to mouse findings, controversial studies in L. donovani-infected patients introduced both CD4+CD25−Foxp3− T cells and CD4+CD25+FoxP3+ as the major source of IL-10 and TGFβ productions, contributing in the pathogenesis of human VL (59–61).
3.4 Concomitant immunity
Concomitant immunity, described as the immunological memory that is developed when a host is reinfected while presently hosting an initial infection caused by the same pathogen, plays a vital role in controlling Leishmania infections. Usually, the permanent survival of pathogens within a host result in the development of potent immunity against subsequent infections. In C57BL/6 mice, following resolution of a primary Leishmania infection, mice develop immunity against subsequent challenge infections while concurrently carrying a low level of persistent parasites, which continue to replicate within the host cell. The balance between parasite destruction and survival results in a relatively constant, low parasite count (110). It has been suggested that Leishmania parasite replication occurs continually in persistent infections, with most parasites found within activated APCs. Parasites inside iNOS+ APCs displayed typical morphology and intact genome compared to parasites within iNOS− cells. This implies that these parasites might possess an unexpected resistance to NO. Based on this study, a model of persistent parasite is proposed in which a population of rapidly replicating Leishmania acts as a permanent reservoir, whereas another population with slow or no replication rate is destroyed in APCs, leading to induction of immune response (110). Experimental investigations have revealed that following the resolution of leishmanial lesions, short-lived T-bet+ and Ly6C+ circulating TEFF offer protection against future challenges but cannot be sustained in the absence of persistent parasites (111). In a chronic L. major infection model in resistant C57BL/6 mice, it was shown that CD44+CD62L−T-bet+Ly6C+ effector T cells upon adoptive transfer were reactivated by secondary challenge, homed to the skin, and mediated concomitant immunity, shown by high IFN-γ production and inducing protection (111). It seems that the presence of persistent parasites is crucial for maintaining immunity against Leishmania, considering that TEFF cells need continuous stimulation to provide protection against reinfection. Supporting this hypothesis are the studies indicating that complete parasite elimination results in the loss of protection in infected mice (49, 112), probably due to depletion of the reservoir of circulating TEFF cells (113). Of note, in the absence of persistent parasite, it remains unclear whether a minor population of memory T cells persists, but their numbers are insufficient for providing protection, or if memory T cells indeed develop but are restricted due to the absence of IL-10 production (114, 115). In concomitant immunity, the time of effector Th1 response at the secondary challenge site on the skin is one of the most significant factors in eliminating parasites, which is linked to a change in how Leishmania influences the behavior of monocytic host cells. In experimental L. major infection, CXCR3-dependent recruitment of Ly6C+ Th1 effector cells and early interactions with phagocytes were shown to be essential for concomitant immunity and preventing the establishment of the pathogen (116).
Increasing the number of effector T cells to control Leishmania infection during the acute phase of VL typically results in a subsequent severe loss of effector cells accompanied by an increase in the memory T cells’ pool, poised for its efficient role upon reinfection. Nevertheless, some patients undergo immunosuppression and are susceptible to secondary infections due to a cellular immune response defect. Prolonged exposure to parasite antigens and chronic inflammation influence different functions of memory T cells, associated with immunopathology in VL. This phenomenon, identified as T-cell exhaustion, has variable effects on the disease outcome depending on the species of Leishmania involved (117). Recently, exhausted T-cell subsets have also been characterized in experimental CL caused by L. mexicana based on CXCR5 and TIM-3 (T-cell immunoglobulin and mucin domain-containing protein 3) expressions (118).
4 Memory T-cell subtypes and protection against the Leishmania infection
Effector T cells, once they migrate to the tissues, tend to have a short lifespan, but memory T cells are defined by their long-term presence following the resolution of an infection (119). The hallmark of T-cell memory is the ability to mount a robust and rapid response upon secondary challenges with a previously recognized pathogen (120–122). Memory T lymphocytes encompass heterogeneous populations, identified through surface markers and their specific functions, such as production of cytokines and their ability to proliferate (123–126) (Table 2). In general, three primary subsets of memory T cells have been identified, comprising circulating effector memory T cells (TEM) found in non-lymphoid tissues, circulating central memory T cells (TCM) located in secondary lymphoid organs, and non-circulating tissue-resident memory T cells (TRM) capable of persisting in non-lymphoid tissues.
Studies suggest that following an acute viral infection, memory CD8+ T cells do not need antigen for their survival, keep the capacity for homeostatic proliferation, and exhibit robust responsiveness to IL-7 and IL-15 cytokines. However, in the chronic infection, CD8+ T cells induced by viral infection are unable to acquire the feature of memory T cells to persist over the long term without requiring antigenic stimulation (127). Nevertheless, the development and maintenance of memory CD4+ T cells might be obviously different (128) and the nature of CD4+ memory responses is likely to vary depending on variations in antigenic exposure and persistence (129).
4.1 TEM/TCM subpopulations
The study of Lanzavecchia and colleagues demonstrated that memory T cells in human could be categorized into two subpopulations based on the expression of molecules governing T-cell migration (73). Some memory T cells express molecules required for migration to lymph nodes including CCR7 and CD62L, and are named TCM. CD62L, also known as L-selectin, is a C-type calcium-dependent lectin of the selectin subfamily expressed on most leukocytes. The most important function of CD62L is participation in lymphocyte homing to secondary lymphatic organs (130). Other memory T cells lack markers for lymph node homing and are able to migrate to various tissues. Notably, TCM cells do not produce effector cytokines like IFN-γ, whereas in peripheral tissues, TEM are capable of producing effector cytokines. Similarly, studies in mice have suggested the existence of heterogeneous populations of memory T cells with diverse migratory potentials and effector functions (119, 131).
There are several other surface, costimulation, and activation markers that might be used to distinguish the memory T-cell subpopulations (132) (Table 2); among them are CD27, belonging to the tumor necrosis factor/nerve growth factor receptor (TNF/NGF-R) family, and CD28, a member of the Ig superfamily required for both CD4+ and CD8+ memory T-cell expansion (133, 134).
4.1.1 TEM/TCM cells in leishmaniasis: mouse studies
Mice that have successfully recovered from an initial Leishmania infection display robust immunity to reinfection. To investigate the T cells responsible for this protection, CD4+ T cells from mice that recovered from L. major infection were purified and transferred to naive mice. Analysis revealed two distinct subpopulations of protective T cells in immune mice (135). One subset exhibited the characteristics similar to effector T cells, such as low expression of CD62L, capacity to induce DTH, production of IFN-γ, and lack of migration to the adjacent lymph nodes.
Another subset that exhibited characteristics of central memory T cells were CD62Lhi, with high IL-2 but no IFN-γ production. They migrated to the draining lymph node and proliferated upon challenge, which is notably accompanied by downregulation of CD62L expression, acquiring IFN-γ production capacity, and migration to infection sites (70). Adoptive transfer of sorted CD62Lhi and CD62Llow CD4+ T-cell subpopulations to naïve mice showed that both populations of T cells conferred protection upon a subsequent infection. The ability of TCM cells (CD62Lhi) to confer protection is aligned with the observation that upon a subsequent L. major challenge the lymph node-homing TCM cells can expand and become TEFF cells that proliferate and produce IFN-γ at the site of infection (70, 135).
Experiments with engineered thymidine auxotrophic L. major strain (dhfr-ts), which fails to survive long term in vivo (136), showed that following the eradication of the parasites, a group of Leishmania-specific CD4 T cells emerges, characterized by the TCM phenotype (CD62Lhi, CCR7hi) (136, 137). Remarkably, these cells can persist even when Leishmania parasites are no longer present. Consequently, existence of at least two distinct circulating subpopulations of Leishmania-specific CD4+ T cells are suggested in L. major-infected mice. One subset, referred to as TEFF cells, lacks the ability to survive without persistent parasites, while another population named TCM cells that emerges early post-infection displays the ability to survive once the parasites are eliminated. Some evidence suggests that the CD4+ TCM pool is heterogeneous with the potential to develop into either one of Th1 or Th2 cells (138, 139). Immunization of mice with a centrin gene-deleted strain of L. donovani (LdCen−/−) induced increased CD4+CD62Lhi, CCR7+ TCM cell (71) and CD8+CD62L+CD27+ TCM cell responses (72), which, upon restimulation with antigen in vitro, differentiated into TEFF cells.
4.1.2 TEM/TCM cells in leishmaniasis: human studies
Despite the fact that substantial knowledge exists regarding the development of primary effector T-cell responses and memory T-cell development in the murine model of Leishmania infection, the generation and maintenance of memory T cells in human leishmaniasis are less understood [14, 15]. Individuals with a history of cutaneous leishmaniasis (HCL) who have acquired protection against further infections provide a suitable population for investigating the potential role of memory T cells in protection against leishmaniasis. To this end, in tandem with experiments conducted in mouse model, we focused on HCL volunteers to study distinct subsets of CD4+ and CD8+ memory T-cell subpopulations and to understand the protective role of memory T cells in human CL. We have characterized different T cells from the blood of HCL and control volunteers and have shown that stimulation of sorted cells with Leishmania antigen in culture significantly increased CD4+CD45RA−CCR7− TEM cells from CD4+CD45RA+CCR7+ naïve T cells, and by carboxyfluorescein diacetate, succinimidyl ester (CFSE) labeling, CD4+CD45RA−CCR7+ TCM cells exhibited a greater capacity to proliferate compared to CD4+ TEM cells. Typically, both CD4+ and CD8+ T cells quickly engage in a proliferative response following a brief exposure to antigen stimulation. The phenotypic change of naïve cells to memory cells and commitment of TCM/TEM cells to high proliferation may guarantee the formation of a substantial memory T-cell pool, even when antigen levels decline in later stages of the immune response (140).
For the first time in human leishmaniasis, in individuals with a history of CL, a mixture of Leishmania-responsive CD4+ TEM cells capable of producing IFN-γ and Leishmania-responsive CD4+ TCM cells capable of producing IL-2 was detected. These cells may contribute to protective immune response against Leishmania infection (74). In a parallel study, the T-cell subset composition was assessed in peripheral blood CD8+ T cells obtained from individuals with HCL. When sorted CD8+ memory T cells from HCL volunteers were stimulated in vitro, there was a notably elevated levels of IFN-γ production in comparison to cells of healthy controls. A similar result was consistently observed in intracellular IFN-γ staining as well. The memory population was found to be accountable for the IFN-γ production triggered by Leishmania in culture. Among the subsets, Leishmania-responsive proliferating CD8+ TEM cells were the most prevalent, and they could potentially contribute to protection in the company of CD4+ T cells (44). Recently, the frequency of TCM and TEM cells during active CL and post-treatment has been analyzed in patients with L. braziliensis infection (75), and a higher frequency of CD4+ TEM, CD8+ TEM, and CD8+ TCM during CL development and following treatment has been observed (75).
The frequency and function of central and effector memory CD4+ and CD8+ T cells have also been investigated in New World CL patients due to L. braziliensis. In two separate studies, both CD4+ and CD8+ TEM cells were found to be higher than TCM or naive T cells in the skin lesions (76) and blood mononuclear cells following antigenic stimulation in active CL patients (34). Another recent study in Brazil assigns an even greater role to the CD8 compartment, showing that there is an increase in CD4+ TEM during CL, and CD8+ TEM and CD8+ TCM during CL and post-treatment (75), while it was previously suggested that CD4+ and CD8+ TEM compartments from healed CL individuals decreased gradually with time after clinical cure (141).
In an ex vivo study, a lower frequency of blood CD4+CD45RO+ memory cells without stimulation, and fewer CD4+ CD25hiFOXP3+ TREG cells after antigenic stimulation of mononuclear cells from symptomatic VL patients compared to recovered VL subjects were demonstrated, showing the immune impairment during symptomatic VL caused by L. infantum (77). However, the frequencies of the CD4+ and CD8+ TCM cells, responsible for producing IL-2, TNF-α, and IFN-γ, were notably elevated in the blood of VL patients following treatment (78).
4.2 TRM subpopulation
In Figure 1, the development of different T-cell subsets and their contribution in the immunity during experimental cutaneous Leishmania infection in mice are outlined (Figure 1). Following activation, a considerable fraction of T cells remains in circulation, while a distinct group of these activated T cells migrates into tissues, taking on a resident role as TRM cells. Among CD8+ T-cell compartments, the TRM cells are described as a subset that can be found in nearly all tissues of the body and are responsible for either defending against infections or occasionally triggering autoimmune responses (142, 143). There is growing support for the significant contribution of TRM cells in the relapse of chronic inflammatory diseases of the skin (144). On the other hand, compared to CD8+ T cells, knowledge regarding CD4+ TRM cells is limited, which have been identified in various tissues such as lungs, intestines, and skin (145).
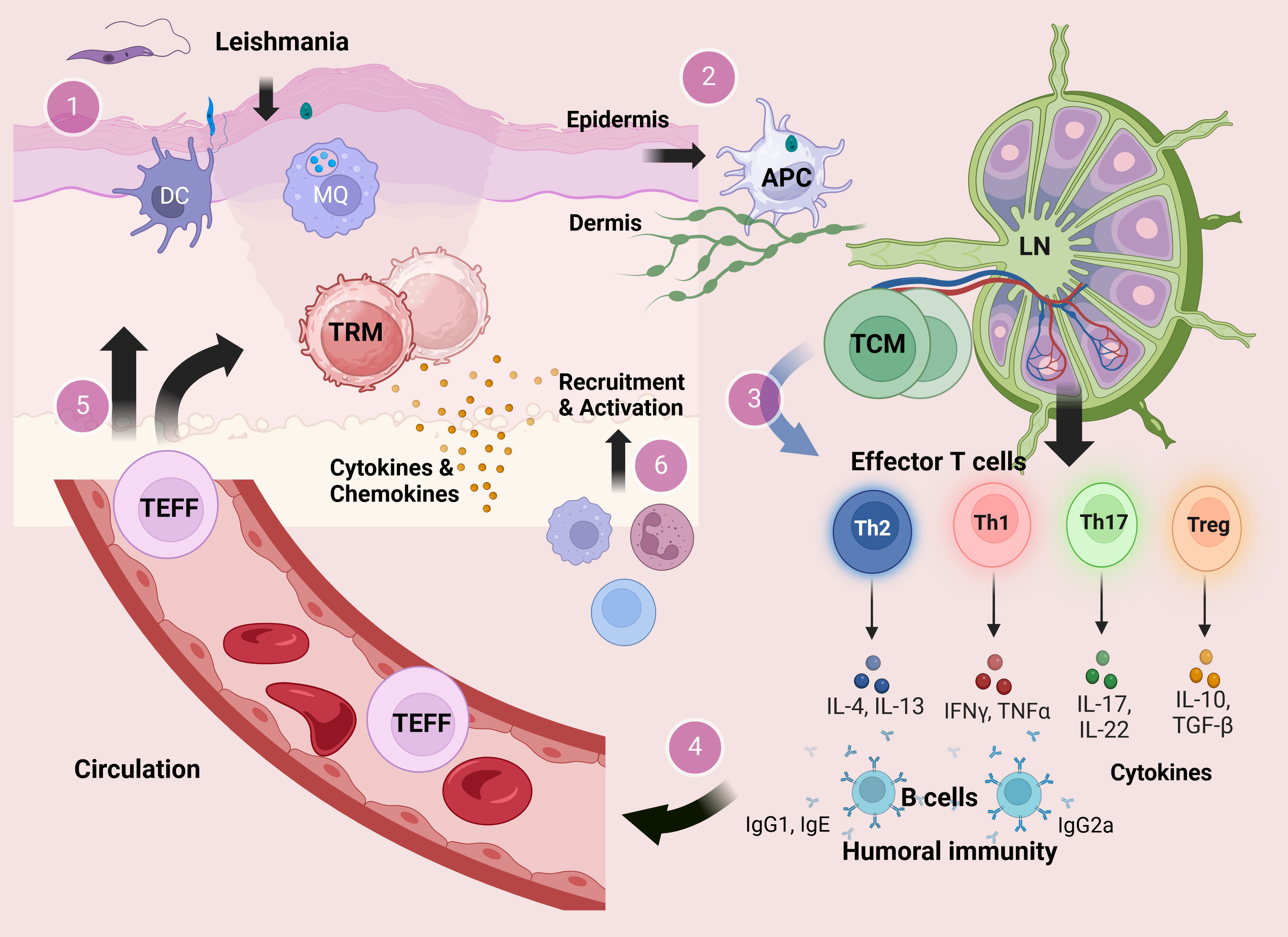
Figure 1 The development of T cells and their contribution in the immunity during experimental cutaneous Leishmania infection. At the site of sand fly bite, Leishmania metacyclic promastigotes infect cells of the monocyte–macrophage lineage including macrophages and DCs in the skin dermal layer. Within the host cells, promastigotes transform to the amastigote stage and replicate by binary fission (1). Infected antigen-presenting cells (APCs) carry antigens to lymphoid organs leading to activation of CD4+ T cells in the draining lymph nodes (2). Activated CD4+ T cells in the draining lymph nodes could develop into either effector T (TEFF) cells or central memory T (TCM) cells. In the course of secondary infection, the residing TCM cells could be reactivated and differentiated into different subsets of CD4+ TEFF cells (3) that provide a circulating pool of Leishmania-reactive T cells (4). Circulating CD4+ TEFF cells can be rapidly recruited into the site of infection, while some TEFF cells may migrate into skin distant from the lesion site and become resident memory T (TRM) cells (5). In response to challenge, TRM cells in the skin produce cytokines that mediate recruitment of inflammatory monocytes and effector T cells from the blood (6).
TRM cells are primarily characterized by high expression of canonical markers’ CD69 adhesion molecule and the αE integrin CD103 and downregulation of homing receptors CCR7 and CD62L. Various other markers, such as CXCR3 and CD49a, are also used to identify TRM cells in specific tissues (146). In Figure 2, a comparison between mouse and human tissue-resident memory T cells regarding the expression of surface markers and transcription molecules is illustrated (Figure 2) (147). The increased expression of four transcription factors plays a crucial role in the development and maintenance of TRM cells, including Runx3, Notch, Hobit, and Blimp1 (148–150).
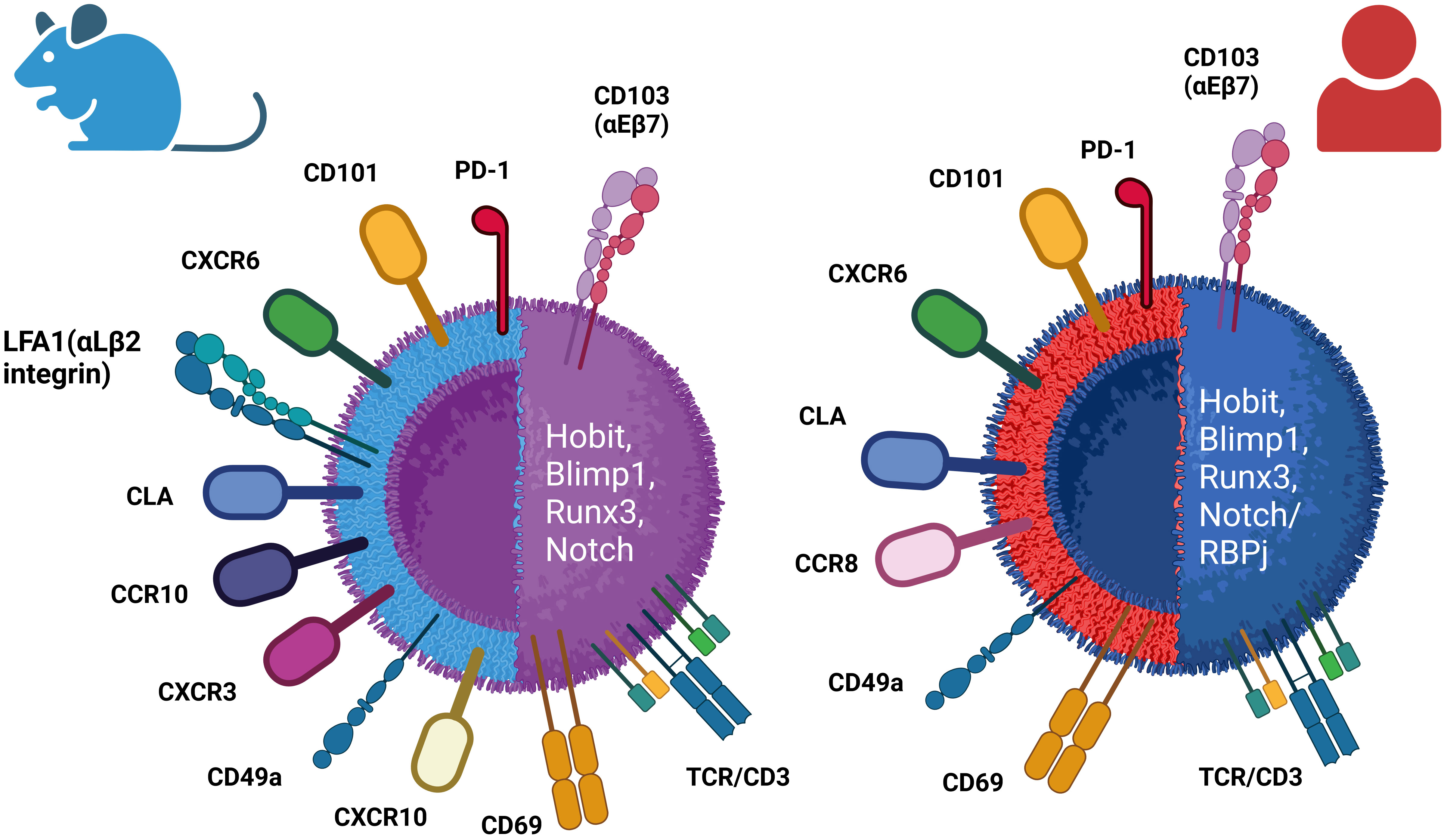
Figure 2 Differential phenotypes of mouse and human resident memory (TRM) cells. TRM cells are identified by the expression of canonical receptors CD103 and CD69, as well as several other surface markers depending on the specific resident tissues. TRM cells display upregulation of the expression of some transcriptional factors, including Hobit, Blimp1, Runx3, and Notch (/RBPj).
It was suggested that following an acute viral infection, CD8+ T cells are promptly recruited to the skin, a process facilitated by CD8+ T cells expressing E- and P-selectin ligands (151). Upon reaching the skin, additional factors facilitate the retention of T cells in the tissue. CD69 is a type II C-lectin receptor found on cell membranes of hematopoietic stem cells, T cells, and several other cells of the immune system. It is also implicated in T-cell differentiation as well as lymphocyte retention in lymphoid organs. CD69, as an early marker of T-cell activation, is detected in a substantial fraction of tissue-resident memory CD4+ and CD8+ T cells across both murine and human. The continuous expression of CD69 on TRM cells restricts tissue exit by antagonizing sphingosine-1-phosphate receptor 1 (S1PR1)-mediated extravasation. The presence of S1PR1, alongside the transcription factor Kruppel-like factor 2 (KFL-2), stimulates T-cell migration and is rather characteristic of circulating cells (152–154). It was demonstrated that in human, the transcriptional profile of the CD69+ subset of memory CD4+ and CD8+ T cells is distinct from that of CD69− memory cells in tissues and circulation (147, 149).
On the other hand, CD103, a heterodimeric transmembrane surface receptor, is an integrin that binds E-cadherin on epithelial cells, exhibited by some CD8+ memory T cells in mucosal tissues (155, 156). In both humans and mice, CD8+ TRM cells located in mucosal tissues including the skin, lungs, salivary glands, and small intestines are characterized by a notable enrichment of CD103 expression (157–160). The function of CD103 on CD4+ TRM cells is not fully understood, since a significant portion of CD4+ TRM cells in both mouse and human usually do not express the CD103 marker (161, 162), albeit, in specific tissue locations such as the skin in mice, populations of CD103+CD4+ TRM cells have been identified (163).
In mice, the skin harbors CD103+/−CD4+ TRM as the most frequent population (163), although significant quantities of CD103+CD8+ TRM could be found in epidermis post-infection (156). In healthy human skin, the majority of TRM cells located in the dermis express CD69, but not CD103 (CD4+CD69+CD103– cells), while in the epidermis layer, mixed populations of CD4+ and CD8+ TRM expressing CD103 are found (159), which show robust effector capabilities coupled with restricted proliferative potential in contrast to CD103− TRM cells. Elevated levels of cutaneous lymphocyte antigen (CLA) and distinct chemokine receptors like CCR4 have been observed in these TRM cells. Additionally, human skin contains TRM cells that express the chemokine receptor CCR8 upon infiltration into the skin (164, 165), and these CCR8-positive T cells exhibit all the predefined characteristics of resident memory T cells (Table 2; Figure 2).
Little is known about the transcriptional regulation and signaling pathways involved in the long-term maintenance of TRM cells in different tissues. IL-7 and IL-15 have an established role in controlling homeostatic proliferation and survival of memory T cells (166, 167). Increased levels of IL-7 and IL-15 receptors were demonstrated on TRM cells, similar to circulating memory T cells, implying that these cytokines might also play a role in survival of TRM cells (168). In the skin, the local mediators such as transforming growth factor (TGF)-β, IL-7, and IL-15 are required for regulation of long-lived CD8+ TRM, whereas IL-7 contributes to the persistence of CD4+ TRM (169). It seems that TRM in different tissues may rely on various signals to support their persistence and survival (147).
4.2.1 TRM cells in leishmaniasis: mouse studies
In experimental leishmaniasis, lesions harbor both CD4+ T cells that lack CD69 expression presumably in traveling through the tissues and a separate group of resident T cells expressing the CD69 marker (113). An experiment with L. major infection in the mouse model showed that residency-associated transcription factors Blimp-1 and Hobit (148) were more predominantly found in CD69+ T cells within leishmanial lesions as opposed to CD44+ T cells in the draining lymph nodes, whereas sorted CD4+CD69+ T cells from the lesion expressed a low level of both S1pr1 and Klf2 factors (113). This result suggested that CD4+CD69+ T cells are preferentially located in cutaneous Leishmania lesions as skin TRM cells. In leishmaniasis, the skin houses resident CD4+ T cells capable of producing IFN-γ upon restimulation (64). Following a primary infection with L. major, Leishmania-reactive Th1 effector T cells undergo proliferation within the draining lymph nodes and then migrate to the infection site, but they are not restricted to the infection site and can migrate to non-inflamed regions throughout the skin. A compartment of these cells transforms into CD4+ TRM cells, which in the secondary challenge infection produce IFN-γ to activate macrophages. The activated TRM cells also produce chemoattractant mediators like CCL2 and CCL7 [chemokine (C-C motif) ligand 7], which induce recruitment of inflammatory monocytes (65, 170). In secondary Leishmania infection, upon IFN-γ stimulation, this monocyte population promptly initiated the production of inducible nitric oxide synthase (iNOS), which activates parasite killing mechanisms (65, 171). Therefore, skin-resident memory CD4+ T cells protect against L. major challenge in mice and they are independent of persistent parasites for survival (65, 113).
In VL infection in animal models, resolution of Leishmania infection is dependent on the development of T cell-mediated immunity associated with the formation of granulomas in the liver. Development of immune memory subsets in the liver-resident T-cell population in response to L. infantum antigens has been suggested in experimental infection in BALB/c mice and treatment with meglumine antimoniate has shown to improve hepatic immune response by increasing the levels of effector and memory T cells (68).
4.2.2 TRM cells in leishmaniasis: human studies
Although the CD69 marker has been assessed as an activation marker in CD4+ and CD8+ T-cell compartments in blood and skin lesion of human leishmaniasis patients (76, 141), insufficient data are available regarding the involvement of TRM cells in human leishmaniasis.
Since the resolution of CL lesion and VL disease typically coincides with the development of a protective immune response and lifelong resistance to reinfection in human (30, 43, 172), further insight into the characterization of memory T-cell phenotypes and functions holds promise for using memory cells as potential biomarkers of protection and as a tool for assessing the effectiveness of candidate vaccines against leishmaniasis.
5 Biomarkers of immune protection in leishmaniasis
A key obstacle in the development of a leishmaniasis vaccine lies in the identification of reliable surrogate marker(s) for evaluating immune protection in human (6). The biomarkers might have different applications including evaluation of innovative diagnostic tools and monitoring the disease progression or the outcome of clinical interventions (173). In order to evaluate vaccine efficacy as a preventive intervention against human leishmaniasis, the researchers are required to clearly define measurable biomarkers as indicators of protection (4).
CD4+ Th1/Th2 population subtypes and their secreted cytokines in Leishmania infection has long been investigated and introduced as a putative biomarker of immunity against Leishmania infection (174). Several decades ago, Kellina observed differences in the susceptibility of various strains of inbred mice to L. major infection (175) and Mitchell showed that in the course of L. major infection in BALB/c nu/nu mice, the outcome may be either a progressive or a resolving lesion, contingent upon the quantity of T cells utilized for reconstitution (176). The role of CD4+ T cells in either resistance or susceptibility of different strains of mouse to infection with L. major has been established by several groups in the early 1980s (177). Mossman and Coffman defined two functionally distinct CD4+ T-cell subsets, Th1 and Th2, based on their functions and cytokine secretions (178, 179), and others explained differential function of Th1 and Th2 CD4+ subsets in the outcome of murine L. major infection: mice that effectively overcome the infection primarily demonstrate a Th1 type of immune response with high levels of IFN-γ production but little IL4, and mice with fatal, disseminating disease have a Th2 type of immune response, including a significant amount of IL4 and IL5 and lower levels of IFN-γ (177, 180–182). Although Th1/Th2 dichotomy is well established in the L. major infection model in the resistant C57BL/6 and susceptible BALB/c strains of inbred mice, this paradigm seems to be more complex in humans (183) and might be different in mice with other genetic backgrounds and in using other parasite strains (184–187). In addition, existence of non-cure phenotypes in spite of strong Th1 response suggested additional involving mechanisms (23, 188). Moreover, although some vaccines induce Th1-type cytokines, they have no or only a small effect on organ pathology (188) and protection could be achieved without activating a strong Th1 response (139).
The immune response to Leishmania infection in human is more complicated than what is usually retrieved from experiments in inbred animal models, and studies have yet to definitively demonstrate a clear-cut Th1/Th2 phenotype in CL (189). There are studies suggesting an association between Th1/Th2 dichotomy and the outcome of human leishmaniasis. It has been shown that in symptomatic VL and active DCL patients, a Th2 response was induced (190, 191), whereas a strong expression of IFN-γ and low IL-10 levels was observed in healing CL patients and after healing of skin lesions following treatment in DCL patients (30, 43, 172, 190, 192). Evidence shows that both antigen-specific IFN-γ-producing CD4+ and CD8+ T cells contribute to the immunity against human leishmaniasis, though the role of CD4+ T cells with two arms of Th1 and Th2 cells has more been highlighted in the outcome of disease (43, 193).
IL-10 produced by TREG cells has been identified as a factor that dampens both the intensity and effectiveness of the Th1 response (194), but despite this suppressive effect that leads to disease progression and parasite persistence, IL-10 is an indispensable immunoregulator that inhibits an exacerbated immunopathology and tissue damage caused by increased production of inflammatory cytokines, especially IFN-γ in CL (195).
It is believed that in human VL, while the majority of the infected individuals often experience a subclinical or asymptomatic infection accompanying robust cellular immune response, healing or protective responses are associated with the production of Th1-type cytokines, including IFN-γ, and conversion in the leishmanin skin test (LST) (196, 197). However, there are reports suggesting that VL might be exacerbated even when measurable amounts of Th1 cytokines are present, which are functionally neutralized by the function of other mediators, such as IL-10 (198, 199). Active VL is characterized by suppressed cell-mediated immunity, shown by a negative LST response, which is typically converted to positive in patients after cure or recovery from VL (200).
6 Memory T cells as putative biomarkers for protection and efficacy assessment of candidate vaccines
As mentioned above, the challenge in developing effective leishmaniasis vaccines lies in the identification of surrogate markers for protection. Active effector T cells, although vital in immune responses, are short-lived and require continuous stimulation, making them less favorable for vaccination strategies. However, recent advancements in understanding memory T cells, particularly parasite-independent subsets, raised new hope in this way.
Among these memory T-cell subsets, TCM and skin TRM have emerged as key players in Leishmania infection in mouse models. TRM cells, a population of CD4+ T cells resident in the skin, play a significant role in concomitant immunity against cutaneous Leishmania infection. They respond to challenge infection by rapid recruitment at infection site and producing IFN-γ upon restimulation, effectively reducing the parasite burden. A crucial aspect of Leishmania-reactive TRM cells is their potential to exist not only at the infection site but also in noninflamed skin regions distant from the lesions. This feature enables them to provide protection against challenge infections throughout the skin, even in the absence of Leishmania-specific TEFF cells. Therefore, since CD4+ TRM cells are long-lasting memory subsets independent of persistent parasites, successful vaccine strategies may include induction of CD4+ TRM cells as efficient immunogenicity outcome. In addition, assessing the induction of CD4+ TRM cells could be used as a biomarker of immune protection against leishmaniasis in evaluating the efficacy of candidate vaccines. While there are examples of successful leishmaniasis vaccines that have demonstrated strong immunogenicity in both humans and animal models, so far, there is no vaccine available for any type of human leishmaniasis (6). This challenge is not exclusive to leishmaniasis but extends to many infections reliant on sustaining a memory T-cell response (113). Recently, development of a series of live attenuated centrin gene-deleted Leishmania strains, including L. donovani, L. mexicana, L. braziliensis, and L. major, by using the CRISPR/Cas9 method, has shown promise in inducing protection against challenge infection (201). Mice immunized with a marker-less LmCen−/− L. major developed no evident lesions following challenge with the bite of L. major-infected sand flies (66). This protection is attributed to IFN-γ-secreting CD4+ TEFF cells, and immunization using LmCen−/− further triggered the development of CD4+ TRM cells in the skin, accompanied by the production of cytokines and chemokines required for their prolonged persistence, resembling the effects of leishmanization (67). Upon challenge with wild-type L. major, specific TRM cells were rapidly recruited and underwent proliferation at the infection site and triggered substantial production of IFN-γ and granzyme B in immunized mice compared to control mice (67). Similarly, immunization with the LmexCen−/− L. mexicana strain confers protection against challenge infection of wild-type L. mexicana parasites in both BALB/c and C57BL/6 mouse models (202). Interestingly, the same mutant strain (LmexCen−/−) also induced long-term protection against L. donovani VL infection in a hamster model (203). Mice immunized with LmexCen−/− exhibited a markedly elevated proportion of CD4+CD44+CL62L+ TCM cells in their lymph nodes compared to control mice injected with PBS, and this population of CD4+ memory T cells might contribute to long-term protection against L. mexicana infection (202).
7 Discussion
Over the last few years, a growing body of evidence has emerged, suggesting that TRM cells have both protective and pathogenic roles in different infectious diseases and immunological disorders (204). Our current knowledge implies that TRM cells serve as a first line of defense in peripheral tissues to protect human against different pathogens, including viruses, intracellular bacteria, and protozoan parasites [reviewed in (205)].
In Plasmodium infection, CD8+ TRM cells appear to play pivotal roles in protective immunity to liver-stage malaria, which is critical for vaccine development because parasites must be eliminated at the liver before development of blood-stage infection to prevent clinical manifestations of malaria (206). Induction of liver-resident memory T cells associated with protection has been shown in several experimental vaccine platforms including radiation-attenuated sporozoite (RAS) and the circumsporozoite protein (CSP)-based vaccines and a recent mRNA-containing lipid nanoparticle (mRNA-LNP) formulation (207–209). The protective role of TRM cells has also been investigated in Toxoplasma gondii, the intracellular protozoan parasite causing toxoplasmosis, which forms persistent cysts in various tissues in human and mammals. In a chronic infection model of T. gondii infection, the CD103+ CD8+ TRM phenotype accumulated within the brain and produced both IFN-γ and TNF-α cytokines, which are critical for parasite control within the central nervous system (CNS). It was suggested that a higher protective immunity was conferred by brain TRM against T. gondii infection in the CNS when contrasted with the protective capabilities of CD8+ TEM and TCM cells (210). Similarly, an experimental study on a mouse model of Trypanosoma (b.) brucei infection suggests that in the early stages of disease, most brain T cells exhibit a TEM phenotype, while during the late cerebral stage, T cells show a TRM phenotype and may have a potential role in causing sleep disorder during trypanosomiasis (211).
These accumulating lines of evidence on the contributing role of TRM cells in the pathogenesis of protozoan parasites suggest that exploring methods to generate and sustain TRM cells is essential for the advancement of vaccine development against parasitic infections.
In mice Leishmania infection, following resolution of leishmanial lesion, there are circulating TEFF cells that are capable of offering protection upon challenge, yet they fail to survive when parasites are no longer present. While vaccine formulation based on the permanent delivery of parasitic antigens to the immune system for induction of circulating TEFF cells that could provide protection to challenge is not a feasible strategy, vaccines could target antigen-independent memory CD4+ T cells subsets including long-lived skin-resident memory T cells that have the capacity for long-term maintenance in the absence of persistent parasites. Further research is required to characterize the specific T-cell populations and pathways activated by this type of vaccination, but in experiments with L. major infection in the murine model, the immunization led to the expansion of CD4+ T cells in the draining lymph node, which subsequently migrated to unaffected skin areas through circulation and differentiated into TRM cells. Experimental immunization has been successful in the induction of TRM cells that confer long-term protection against reinfection (67, 212). While a human vaccine for leishmaniasis remains unavailable, successful examples of experimental vaccines suggest that inducing protective, long-lived skin CD4+ TRM cells, independent of persistent parasites, might be a promising approach for future vaccine development and evaluating the protective efficacy induced by such vaccination (213).
The approval of a vaccine for human leishmaniasis still encounters significant challenges (214) and a more comprehensive understanding of the development and maintenance of protective memory T cells is necessary. Various parameters need to be taken into consideration to optimize vaccine formulations in leishmaniasis targeting skin tissue-resident memory responses (Figure 3). Defining outcome measures and finding biomarkers that correlate with TRM responses could provide a straightforward approach to assess skin-resident memory responses following vaccination in humans, as previously suggested (215).
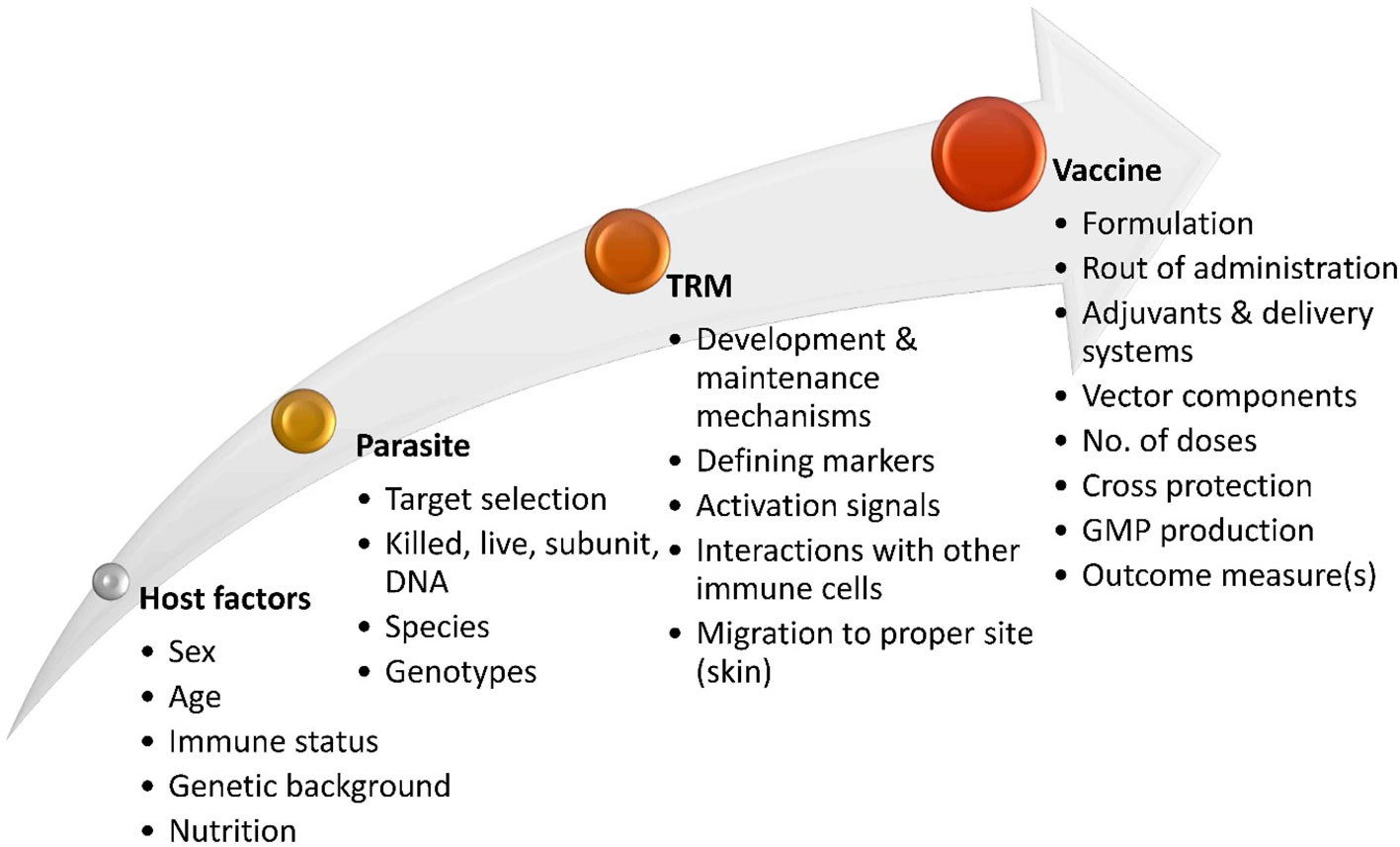
Figure 3 Factors involved in the development of Leishmaniasis vaccines aimed at inducing skin-resident memory T cell response. Effective vaccine strategies for inducing TRM cell responses in leishmaniasis will rely on a comprehensive understanding of the development and maintenance of protective TRM cells in Leishmania infection. Exploring the potential migration and localization of vaccine-induced TRM cells in specific sites in the skin and the signals needed to activate efficient TRM cells in an interactive microenvironment with other immune cells is also imperative. Various other factors of Leishmania parasite, host background, and vaccine design are involved in the effectiveness of candidate vaccines targeting the generation of protective TRM cells.
Given the encouraging and important result obtained concerning the role of TRM cells in the protection of immunized mice against Leishmania infection, they might be used as potential biomarkers of protection in the assessment of the efficacy of candidate vaccines in human leishmaniasis. However, before we could apply memory T cells as a biomarker, numerous critical questions remain to be answered, including the development of tissue-resident memory T-cell subsets, the possible differences between CD4+ and CD8+ memory T-cell subsets, and finally the role of persistent parasite in TRM survival in human leishmaniasis.
Author contributions
MN-R: Writing – original draft, Writing – review & editing, Conceptualization. YS: Writing – review & editing.
Funding
The author(s) declare that no financial support was received for the research, authorship, and/or publication of this article.
Conflict of interest
The authors declare that the research was conducted in the absence of any commercial or financial relationships that could be construed as a potential conflict of interest.
Publisher’s note
All claims expressed in this article are solely those of the authors and do not necessarily represent those of their affiliated organizations, or those of the publisher, the editors and the reviewers. Any product that may be evaluated in this article, or claim that may be made by its manufacturer, is not guaranteed or endorsed by the publisher.
References
1. Burza S, Croft SL, Boelaert M. Leishmaniasis. Lancet (London England) (2018) 392:951–70. doi: 10.1016/S0140-6736(18)31204-2
2. Ponte-Sucre A, Gamarro F, Dujardin JC, Barrett MP, López-Vélez R, García-Hernández R, et al. Drug resistance and treatment failure in leishmaniasis: A 21st century challenge. PloS Negl Trop Dis (2017) 11:e0006052. doi: 10.1371/journal.pntd.0006052
3. Kaye PM, Mohan S, Mantel C, Malhame M, Revill P, Le Rutte E, et al. Overcoming roadblocks in the development of vaccines for leishmaniasis. Expert Rev Vaccines (2021) 20:1419–30. doi: 10.1080/14760584.2021.1990043
4. Rostami MN, Khamesipour A. Potential biomarkers of immune protection in human leishmaniasis. Med Microbiol Immunol (2021) 210:81–100. doi: 10.1007/s00430-021-00703-8
5. Sacks D, Noben-Trauth N. The immunology of susceptibility and resistance to Leishmania major in mice. Nat Rev Immunol (2002) 2:845–58. doi: 10.1038/nri933
6. Khamesipour A. Therapeutic vaccines for leishmaniasis. Expert Opin Biol Ther (2014) 14:1641–9. doi: 10.1517/14712598.2014.945415
7. Iborra S, Solana JC, Requena JM, Soto M. Vaccine candidates against leishmania under current research. Expert Rev Vaccines (2018) 17:323–34. doi: 10.1080/14760584.2018.1459191
8. Dinc R. Leishmania vaccines: the current situation with its promising aspect for the future. Korean J Parasitol (2022) 60:379–91. doi: 10.3347/kjp.2022.60.6.379
9. Abdellahi L, Iraji F, Mahmoudabadi A, Hejazi SH. Vaccination in leishmaniasis: A review article. Iranian Biomed J (2022) 26:1–35. doi: 10.52547/ibj.26.1.35
10. Kaye PM, Matlashewski G, Mohan S, Le Rutte E, Mondal D, Khamesipour A, et al. Vaccine value profile for leishmaniasis. Vaccine (2023) 41 Suppl 2:S153–s75. doi: 10.1016/j.vaccine.2023.01.057
11. Younis BM, Osman M, Khalil EAG, Santoro F, Furini S, Wiggins R, et al. Safety and immunogenicity of ChAd63-KH vaccine in post-kala-azar dermal leishmaniasis patients in Sudan. Mol therapy: J Am Soc Gene Ther (2021) 29:2366–77. doi: 10.1016/j.ymthe.2021.03.020
12. Lacey C, Musa A, Khalil ET, Younis B, Osman M, Wiggins R, et al. LEISH2b - A phase 2b study to assess the safety, efficacy, and immunogenicity of the Leishmania vaccine ChAd63-KH in post-kala azar dermal leishmaniasis. Wellcome Open Res (2022) 7:200. doi: 10.12688/wellcomeopenres
13. Duthie MS, MaChado BAS, Badaró R, Kaye PM, Reed SG. Leishmaniasis vaccines: applications of RNA technology and targeted clinical trial designs. Pathog (Basel Switzerland) (2022) 11(11):259. doi: 10.3390/pathogens11111259
14. Coler RN, Duthie MS, Hofmeyer KA, Guderian J, Jayashankar L, Vergara J, et al. From mouse to man: safety, immunogenicity and efficacy of a candidate leishmaniasis vaccine LEISH-F3+GLA-SE. Clin Trans Immunol (2015) 4:e35. doi: 10.1038/cti.2015.6
15. Azami-Conesa I, Gómez-Muñoz MT, Martínez-díaz RA. A systematic review (1990-2021) of wild animals infected with zoonotic leishmania. Microorganisms (2021) 9(5):1101. doi: 10.3390/microorganisms9051101
16. Shokri A, Fakhar M, Teshnizi SH. Canine visceral leishmaniasis in Iran: A systematic review and meta-analysis. Acta Tropica (2017) 165:76–89. doi: 10.1016/j.actatropica.2016.08.020
17. Marcolino Silva D, Passarella Teixeira AI, Sierra Romero GA. Socioeconomic status of guardians as a risk factor for canine visceral leishmaniasis: A cohort study in an endemic area of the federal district, Brazil. Am J Trop Med Hygiene (2023) 108:328–34. doi: 10.4269/ajtmh.21-1170
18. Velez R, Gállego M. Commercially approved vaccines for canine leishmaniosis: a review of available data on their safety and efficacy. Trop Med Int Health: TM IH (2020) 25:540–57. doi: 10.1111/tmi.13382
19. Loría-Cervera EN, Andrade-Narváez FJ. Animal models for the study of leishmaniasis immunology. Rev do Instituto Medicina Trop Sao Paulo (2014) 56:1–11. doi: 10.1590/S0036-46652014000100001
20. Janeway CA Jr TP, Walport M, Shlomchik MJ. General properties of armed effector T cells. In: Immunobiology: The Immune System in Health and Disease. Garland Science, New York (2001).
21. Scott P, Novais FO. Cutaneous leishmaniasis: immune responses in protection and pathogenesis. Nat Rev Immunol (2016) 16:581–92. doi: 10.1038/nri.2016.72
22. Nateghi Rostami M, Keshavarz Valian H, Eskandari SE, Miramin Mohammadi A, Shahrestani ST, Sarraf-Nejad A, et al. Differential in vitro CD4+/CD8+ T-cell response to live vs. killed Leishmania major. Parasite Immunol (2010) 32:101–10. doi: 10.1111/pim.2010.32.issue-2
23. Sacks D, Anderson C. Re-examination of the immunosuppressive mechanisms mediating non-cure of Leishmania infection in mice. Immunol Rev (2004) 201:225–38. doi: 10.1111/j.0105-2896.2004.00185.x
24. Alexander J, Bryson K. T helper (h)1/Th2 and Leishmania: paradox rather than paradigm. Immunol Lett (2005) 99:17–23. doi: 10.1016/j.imlet.2005.01.009
25. Gonçalves-de-Albuquerque SDC, Pessoa ESR, Trajano-Silva LAM, de Goes TC, de Morais RCS, da COCN, et al. The equivocal role of th17 cells and neutrophils on immunopathogenesis of leishmaniasis. Front Immunol (2017) 8:1437. doi: 10.3389/fimmu.2017.01437
26. Khatonier R, Ahmed G, Sarmah P, Narain K, Khan AM. Immunomodulatory role of Th17 pathway in experimental visceral leishmaniasis. Immunobiology (2021) 226:152148. doi: 10.1016/j.imbio.2021.152148
27. Lopez Kostka S, Dinges S, Griewank K, Iwakura Y, Udey MC, von Stebut E. IL-17 promotes progression of cutaneous leishmaniasis in susceptible mice. J Immunol (Baltimore Md: 1950) (2009) 182:3039–46. doi: 10.4049/jimmunol.0713598
28. Gonzalez-Lombana C, Gimblet C, Bacellar O, Oliveira WW, Passos S, Carvalho LP, et al. IL-17 mediates immunopathology in the absence of IL-10 following Leishmania major infection. PloS Pathog (2013) 9:e1003243. doi: 10.1371/journal.ppat.1003243
29. Pitta MG, Romano A, Cabantous S, Henri S, Hammad A, Kouriba B, et al. IL-17 and IL-22 are associated with protection against human kala azar caused by Leishmania donovani. J Clin Invest (2009) 119:2379–87. doi: 10.1172/JCI38813
30. Nateghi Rostami M, Seyyedan Jasbi E, Khamesipour A, Mohammadi AM. Tumour Necrosis Factor-alpha (TNF-α) and its soluble receptor type 1 (sTNFR I) in human active and healed leishmaniases. Parasite Immunol (2016) 38:255–60. doi: 10.1111/pim.12305
31. Nateghi Rostami M, Seyyedan Jasbi E, Khamesipour A, Miramin Mohammadi A. Plasma levels of tumor necrosis factor-alpha (TNF-α), TNF-α soluble receptor type 1 (sTNFR I) and IL-22 in human leishmaniasis. Trop Biomed (2015) 32:478–84.
32. Asad M, Sabur A, Kamran M, Shadab M, Das S, Ali N. Effector functions of Th17 cells are regulated by IL-35 and TGF-β in visceral leishmaniasis. FASEB J (2021) 35:e21755. doi: 10.1096/fj.202002356RR
33. Kemp M, Hey AS, Kurtzhals JA, Christensen CB, Gaafar A, Mustafa MD, et al. Dichotomy of the human T cell response to Leishmania antigens. I. Th1-like response to Leishmania major promastigote antigens in individuals recovered from cutaneous leishmaniasis. Clin Exp Immunol (1994) 96:410–5. doi: 10.1111/j.1365-2249.1994.tb06043.x
34. Egui A, Ledesma D, Pérez-Antón E, Montoya A, Gómez I, Robledo SM, et al. Phenotypic and functional profiles of antigen-specific CD4(+) and CD8(+) T cells associated with infection control in patients with cutaneous leishmaniasis. Front Cell Infect Microbiol (2018) 8:393. doi: 10.3389/fcimb.2018.00393
35. Ajdary S, Riazi-Rad F, Alimohammadian MH, Pakzad SR. Immune response to Leishmania antigen in anthroponotic cutaneous leishmaniasis. J Infect (2009) 59:139–43. doi: 10.1016/j.jinf.2009.05.010
36. Ansari NA, Ramesh V, Salotra P. Interferon (IFN)-gamma, tumor necrosis factor-alpha, interleukin-6, and IFN-gamma receptor 1 are the major immunological determinants associated with post-kala azar dermal leishmaniasis. J Infect Dis (2006) 194:958–65. doi: 10.1086/506624
37. Bamorovat M, Sharifi I, Aflatoonian MR, Sadeghi B, Shafiian A, Oliaee RT, et al. Host’s immune response in unresponsive and responsive patients with anthroponotic cutaneous leishmaniasis treated by meglumine antimoniate: A case-control study of Th1 and Th2 pathways. Int Immunopharmacol (2019) 69:321–7. doi: 10.1016/j.intimp.2019.02.008
38. Babaloo Z, Kaye PM, Eslami MB. Interleukin-13 in Iranian patients with visceral leishmaniasis: relationship to other Th2 and Th1 cytokines. Trans R Soc Trop Med Hygiene (2001) 95:85–8. doi: 10.1016/S0035-9203(01)90344-X
39. Kemp M, Kurtzhals JA, Bendtzen K, Poulsen LK, Hansen MB, Koech DK, et al. Leishmania donovani-reactive Th1- and Th2-like T-cell clones from individuals who have recovered from visceral leishmaniasis. Infect Immun (1993) 61:1069–73. doi: 10.1128/iai.61.3.1069-1073.1993
40. Herath S, Kropf P, Müller I. Cross-talk between CD8(+) and CD4(+) T cells in experimental cutaneous leishmaniasis: CD8(+) T cells are required for optimal IFN-gamma production by CD4(+) T cells. Parasite Immunol (2003) 25:559–67. doi: 10.1111/j.0141-9838.2004.00668.x
41. Müller I, Kropf P, Etges RJ, Louis JA. Gamma interferon response in secondary Leishmania major infection: role of CD8+ T cells. Infect Immun (1993) 61:3730–8. doi: 10.1128/iai.61.9.3730-3738.1993
42. Uzonna JE, Joyce KL, Scott P. Low dose Leishmania major promotes a transient T helper cell type 2 response that is down-regulated by interferon gamma-producing CD8+ T cells. J Exp Med (2004) 199:1559–66. doi: 10.1084/jem.20040172
43. Nateghi Rostami M, Keshavarz H, Edalat R, Sarrafnejad A, Shahrestani T, Mahboudi F, et al. CD8+ T cells as a source of IFN-γ production in human cutaneous leishmaniasis. PloS Negl Trop Dis (2010) 4:e845. doi: 10.1371/journal.pntd.0000845
44. Khamesipour A, Nateghi Rostami M, Tasbihi M, Miramin Mohammadi A, Shahrestani T, Sarrafnejad A, et al. Phenotyping of circulating CD8+ T cell subsets in human cutaneous leishmaniasis. Microbes Infect (2012) 14:702–11. doi: 10.1016/j.micinf.2012.02.006
45. Faria DR, Souza PE, Durães FV, Carvalho EM, Gollob KJ, MaChado PR, et al. Recruitment of CD8(+) T cells expressing granzyme A is associated with lesion progression in human cutaneous leishmaniasis. Parasite Immunol (2009) 31:432–9. doi: 10.1111/j.1365-3024.2009.01125.x
46. Belkaid Y. The role of CD4(+)CD25(+) regulatory T cells in Leishmania infection. Expert Opin Biol Ther (2003) 3:875–85. doi: 10.1517/eobt.3.6.875.21257
47. Nylén S, Sacks D. Interleukin-10 and the pathogenesis of human visceral leishmaniasis. Trends Immunol (2007) 28:378–84. doi: 10.1016/j.it.2007.07.004
48. Belkaid Y, Hoffmann KF, Mendez S, Kamhawi S, Udey MC, Wynn TA, et al. The role of interleukin (IL)-10 in the persistence of Leishmania major in the skin after healing and the therapeutic potential of anti-IL-10 receptor antibody for sterile cure. J Exp Med (2001) 194:1497–506. doi: 10.1084/jem.194.10.1497
49. Belkaid Y, Piccirillo CA, Mendez S, Shevach EM, Sacks DL. CD4+CD25+ regulatory T cells control Leishmania major persistence and immunity. Nature (2002) 420:502–7. doi: 10.1038/nature01152
50. Yurchenko E, Tritt M, Hay V, Shevach EM, Belkaid Y, Piccirillo CA. CCR5-dependent homing of naturally occurring CD4+ regulatory T cells to sites of Leishmania major infection favors pathogen persistence. J Exp Med (2006) 203:2451–60. doi: 10.1084/jem.20060956
51. Rodrigues OR, Marques C, Soares-Clemente M, Ferronha MH, Santos-Gomes GM. Identification of regulatory T cells during experimental Leishmania infantum infection. Immunobiology (2009) 214:101–11. doi: 10.1016/j.imbio.2008.07.001
52. Eufrásio de Figueiredo WM, Heredia FF, Santos AS, da Rocha Braga R, Marciano Fonseca FR, Lúcia de Castro Rodrigues N, et al. CXCL10 treatment promotes reduction of IL-10(+) regulatory T (Foxp3(+) and Tr1) cells in the spleen of BALB/c mice infected by Leishmania infantum. Exp Parasitol (2019) 207:107789. doi: 10.1016/j.exppara.2019.107789
53. Barros N, Vasquez N, Woll F, Sanchez C, Valencia B, Llanos-Cuentas A, et al. Regulatory T-Cell Dynamics in Cutaneous and Mucocutaneous Leishmaniasis due to Leishmania Braziliensis. Am J Trop Med hygiene (2018) 98:753–8. doi: 10.4269/ajtmh.17-0624
54. Oliveira WN, Ribeiro LE, Schrieffer A, MaChado P, Carvalho EM, Bacellar O. The role of inflammatory and anti-inflammatory cytokines in the pathogenesis of human tegumentary leishmaniasis. Cytokine (2014) 66:127–32. doi: 10.1016/j.cyto.2013.12.016
55. Faria DR, Gollob KJ, Barbosa J Jr., Schriefer A, MaChado PR, Lessa H, et al. Decreased in situ expression of interleukin-10 receptor is correlated with the exacerbated inflammatory and cytotoxic responses observed in mucosal leishmaniasis. Infect Immun (2005) 73:7853–9. doi: 10.1128/IAI.73.12.7853-7859.2005
56. Campanelli AP, Roselino AM, Cavassani KA, Pereira MS, Mortara RA, Brodskyn CI, et al. CD4+CD25+ T cells in skin lesions of patients with cutaneous leishmaniasis exhibit phenotypic and functional characteristics of natural regulatory T cells. J Infect Dis (2006) 193:1313–22. doi: 10.1086/502980
57. Rodriguez-Pinto D, Navas A, Blanco VM, Ramírez L, Garcerant D, Cruz A, et al. Regulatory T cells in the pathogenesis and healing of chronic human dermal leishmaniasis caused by Leishmania (Viannia) species. PloS Negl Trop Dis (2012) 6:e1627. doi: 10.1371/journal.pntd.0001627
58. Bahrami F, Darabi H, Riazi-Rad F, Khaze V, Ajdary S, Alimohammadian MH. FOXP3 expression and frequency of regulatory T cells in healed individuals from Leishmania major infection and the asymptomatic cases. Hum Immunol (2014) 75:1026–33. doi: 10.1016/j.humimm.2014.08.204
59. Rai AK, Thakur CP, Singh A, Seth T, Srivastava SK, Singh P, et al. Regulatory T cells suppress T cell activation at the pathologic site of human visceral leishmaniasis. PloS One (2012) 7:e31551. doi: 10.1371/journal.pone.0031551
60. Nylén S, Maurya R, Eidsmo L, Manandhar KD, Sundar S, Sacks D. Splenic accumulation of IL-10 mRNA in T cells distinct from CD4+CD25+ (Foxp3) regulatory T cells in human visceral leishmaniasis. J Exp Med (2007) 204:805–17. doi: 10.1084/jem.20061141
61. Bhattacharya P, Ghosh S, Ejazi SA, Rahaman M, Pandey K, Ravi Das VN, et al. Induction of IL-10 and TGFβ from CD4+CD25+FoxP3+ T cells correlates with parasite load in Indian kala-azar patients infected with leishmania donovani. PloS Negl Trop Dis (2016) 10:e0004422. doi: 10.1371/journal.pntd.0004422
62. Bacellar O, Faria D, Nascimento M, Cardoso TM, Gollob KJ, Dutra WO, et al. Interleukin 17 production among patients with American cutaneous leishmaniasis. J Infect Dis (2009) 200:75–8. doi: 10.1086/599380
63. Boaventura VS, Santos CS, Cardoso CR, de Andrade J, Dos Santos WL, Clarêncio J, et al. Human mucosal leishmaniasis: neutrophils infiltrate areas of tissue damage that express high levels of Th17-related cytokines. Eur J Immunol (2010) 40:2830–6. doi: 10.1002/eji.200940115
64. Glennie ND, Yeramilli VA, Beiting DP, Volk SW, Weaver CT, Scott P. Skin-resident memory CD4+ T cells enhance protection against Leishmania major infection. J Exp Med (2015) 212:1405–14. doi: 10.1084/jem.20142101
65. Glennie ND, Volk SW, Scott P. Skin-resident CD4+ T cells protect against Leishmania major by recruiting and activating inflammatory monocytes. PloS Pathog (2017) 13:e1006349. doi: 10.1371/journal.ppat.1006349
66. Zhang WW, Karmakar S, Gannavaram S, Dey R, Lypaczewski P, Ismail N, et al. A second generation leishmanization vaccine with a markerless attenuated Leishmania major strain using CRISPR gene editing. Nat Commun (2020) 11:3461. doi: 10.1038/s41467-020-17154-z
67. Ismail N, Karmakar S, Bhattacharya P, Sepahpour T, Takeda K, Hamano S, et al. Leishmania major centrin gene-deleted parasites generate skin resident memory T-cell immune response analogous to leishmanization. Front Immunol (2022) 13:864031. doi: 10.3389/fimmu.2022.864031
68. Rodrigues A, Claro M, Alexandre-Pires G, Santos-Mateus D, Martins C, Valério-Bolas A, et al. Leishmania infantum antigens modulate memory cell subsets of liver resident T lymphocyte. Immunobiology (2017) 222:409–22. doi: 10.1016/j.imbio.2016.08.009
69. Colpitts SL, Scott P. The early generation of a heterogeneous CD4+ T cell response to Leishmania major. J Immunol (Baltimore Md: 1950) (2010) 185:2416–23. doi: 10.4049/jimmunol.1000483
70. Zaph C, Uzonna J, Beverley SM, Scott P. Central memory T cells mediate long-term immunity to Leishmania major in the absence of persistent parasites. Nat Med (2004) 10:1104–10. doi: 10.1038/nm1108
71. Ismail N, Kaul A, Bhattacharya P, Gannavaram S, Nakhasi HL. Immunization with live attenuated leishmania donovani centrin(-/-) parasites is efficacious in asymptomatic infection. Front Immunol (2017) 8:1788. doi: 10.3389/fimmu.2017.01788
72. Fiuza JA, Gannavaram S, Gaze ST, de Ornellas LG, Alves ÉA, Ismail N, et al. Deletion of MIF gene from live attenuated LdCen(-/-) parasites enhances protective CD4(+) T cell immunity. Sci Rep (2023) 13:7362. doi: 10.1038/s41598-023-34333-2
73. Sallusto F, Lenig D, Förster R, Lipp M, Lanzavecchia A. Two subsets of memory T lymphocytes with distinct homing potentials and effector functions. Nature (1999) 401:708–12. doi: 10.1038/44385
74. Keshavarz Valian H, Nateghi Rostami M, Tasbihi M, Miramin Mohammadi A, Eskandari SE, Sarrafnejad A, et al. CCR7+ central and CCR7- effector memory CD4+ T cells in human cutaneous leishmaniasis. J Clin Immunol (2013) 33:220–34. doi: 10.1007/s10875-012-9788-7
75. de Oliveira BC, da Silva AA, de Andrade Cavalcante MK, de Brito MEF, de Castro M, de Medeiros VLS, et al. Central and Effector Memory Human CD4+ and CD8+ T Cells during Cutaneous Leishmaniasis and after In Vitro Stimulation with Leishmania (Viannia) Braziliensis Epitopes. Vaccines (2023) 11(1):158. doi: 10.3390/vaccines11010158
76. de Oliveira Mendes-Aguiar C, Vieira-Gonçalves R, Guimarães LH, de Oliveira-Neto MP, Carvalho EM, Da-Cruz AM. Effector memory CD4(+) T cells differentially express activation associated molecules depending on the duration of American cutaneous leishmaniasis lesions. Clin Exp Immunol (2016) 185:202–9. doi: 10.1111/cei.12798
77. Rodrigues-Neto JF, Monteiro GR, Keesen TSL, Lacerda HG, Carvalho EM, Jeronimo SMB. CD45RO+ T Cells and T Cell Activation in the Long-Lasting Immunity after Leishmania infantum Infection. Am J Trop Med hygiene (2018) 98:875–82. doi: 10.4269/ajtmh.16-0747
78. Rodrigues LS, Barreto AS, Bomfim LGS, Gomes MC, Ferreira NLC, da Cruz GS, et al. Multifunctional, TNF-α and IFN-γ-secreting CD4 and CD8 T cells and CD8(High) T cells are associated with the cure of human visceral leishmaniasis. Front Immunol (2021) 12:773983. doi: 10.3389/fimmu.2021.773983
79. Olivier M, Gregory DJ, Forget G. Subversion mechanisms by which Leishmania parasites can escape the host immune response: a signaling point of view. Clin Microbiol Rev (2005) 18:293–305. doi: 10.1128/CMR.18.2.293-305.2005
80. Mukbel RM, Patten C Jr., Gibson K, Ghosh M, Petersen C, Jones DE. Macrophage killing of Leishmania amazonensis amastigotes requires both nitric oxide and superoxide. Am J Trop Med hygiene (2007) 76:669–75. doi: 10.4269/ajtmh.2007.76.669
81. Sadeghi S, Seyed N, Rafati S, Taheri T. Optimization of the timing of induction for the assessment of nitric oxide production in leishmania major infected macrophage cells. Iranian J Parasitol (2016) 11:325–31.
82. Van Assche T, Deschacht M, da Luz RA, Maes L, Cos P. Leishmania-macrophage interactions: insights into the redox biology. Free Radical Biol Med (2011) 51:337–51. doi: 10.1016/j.freeradbiomed.2011.05.011
83. Brandonisio O, Panaro MA, Fumarola I, Sisto M, Leogrande D, Acquafredda A, et al. Macrophage chemotactic protein-1 and macrophage inflammatory protein-1 alpha induce nitric oxide release and enhance parasite killing in Leishmania infantum-infected human macrophages. Clin Exp Med (2002) 2:125–9. doi: 10.1007/s102380200017
84. Gomez MA, Contreras I, Hallé M, Tremblay ML, McMaster RW, Olivier M. Leishmania GP63 alters host signaling through cleavage-activated protein tyrosine phosphatases. Sci Signal (2009) 2:ra58. doi: 10.1126/scisignal.2000213
85. Isnard A, Shio MT, Olivier M. Impact of Leishmania metalloprotease GP63 on macrophage signaling. Front Cell Infect Microbiol (2012) 2:72. doi: 10.3389/fcimb.2012.00072
86. Hermoso T, Fishelson Z, Becker SI, Hirschberg K, Jaffe CL. Leishmanial protein kinases phosphorylate components of the complement system. EMBO J (1991) 10:4061–7. doi: 10.1002/embj.1991.10.issue-13
87. Sen S, Roy K, Mukherjee S, Mukhopadhyay R, Roy S. Restoration of IFNγR subunit assembly, IFNγ signaling and parasite clearance in Leishmania donovani infected macrophages: role of membrane cholesterol. PloS Pathog (2011) 7:e1002229. doi: 10.1371/journal.ppat.1002229
88. Bhattacharyya S, Ghosh S, Jhonson PL, Bhattacharya SK, Majumdar S. Immunomodulatory role of interleukin-10 in visceral leishmaniasis: defective activation of protein kinase C-mediated signal transduction events. Infect Immun (2001) 69:1499–507. doi: 10.1128/IAI.69.3.1499-1507.2001
89. Lodge R, Descoteaux A. Phagocytosis of Leishmania donovani amastigotes is Rac1 dependent and occurs in the absence of NADPH oxidase activation. Eur J Immunol (2006) 36:2735–44. doi: 10.1002/eji.200636089
90. Blanchette J, Racette N, Faure R, Siminovitch KA, Olivier M. Leishmania-induced increases in activation of macrophage SHP-1 tyrosine phosphatase are associated with impaired IFN-gamma-triggered JAK2 activation. Eur J Immunol (1999) 29:3737–44. doi: 10.1002/(ISSN)1521-4141
91. Fernández-Figueroa EA, Imaz-Rosshandler I, Castillo-Fernández JE, Miranda-Ortíz H, Fernández-López JC, Becker I, et al. Down-regulation of TLR and JAK/STAT pathway genes is associated with diffuse cutaneous leishmaniasis: A gene expression analysis in NK cells from patients infected with leishmania mexicana. PloS Negl Trop Dis (2016) 10:e0004570. doi: 10.1371/journal.pntd.0004570
92. Yilmaz IC, Dunuroglu E, Ayanoglu IC, Ipekoglu EM, Yildirim M, Girginkardesler N, et al. Leishmania kinetoplast DNA contributes to parasite burden in infected macrophages: Critical role of the cGAS-STING-TBK1 signaling pathway in macrophage parasitemia. Front Immunol (2022) 13:1007070. doi: 10.3389/fimmu.2022.1007070
93. Martínez-Valencia AJ, Daza-Rivera CF, Rosales-Chilama M, Cossio A, Casadiego Rincón EJ, Desai MM, et al. Clinical and parasitological factors in parasite persistence after treatment and clinical cure of cutaneous leishmaniasis. PloS Negl Trop Dis (2017) 11:e0005713. doi: 10.1371/journal.pntd.0005713
94. Romero I, Téllez J, Suárez Y, Cardona M, Figueroa R, Zelazny A, et al. Viability and burden of Leishmania in extralesional sites during human dermal leishmaniasis. PloS Negl Trop Dis (2010) 4(9):e819. doi: 10.1371/journal.pntd.0000819
95. Moll H, Flohé S, Röllinghoff M. Dendritic cells in Leishmania major-immune mice harbor persistent parasites and mediate an antigen-specific T cell immune response. Eur J Immunol (1995) 25:693–9. doi: 10.1002/eji.1830250310
96. Blank C, Fuchs H, Rappersberger K, Röllinghoff M, Moll H. Parasitism of epidermal Langerhans cells in experimental cutaneous leishmaniasis with Leishmania major. J Infect Dis (1993) 167:418–25. doi: 10.1093/infdis/167.2.418
97. Bogdan C, Donhauser N, Döring R, Röllinghoff M, Diefenbach A, Rittig MG. Fibroblasts as host cells in latent leishmaniosis. J Exp Med (2000) 191:2121–30. doi: 10.1084/jem.191.12.2121
98. McConville MJ, Naderer T. Metabolic pathways required for the intracellular survival of Leishmania. Annu Rev Microbiol (2011) 65:543–61. doi: 10.1146/annurev-micro-090110-102913
99. Mendez S, Reckling SK, Piccirillo CA, Sacks D, Belkaid Y. Role for CD4(+) CD25(+) regulatory T cells in reactivation of persistent leishmaniasis and control of concomitant immunity. J Exp Med (2004) 200:201–10. doi: 10.1084/jem.20040298
100. Anderson CF, Oukka M, Kuchroo VJ, Sacks D. CD4(+)CD25(-)Foxp3(-) Th1 cells are the source of IL-10-mediated immune suppression in chronic cutaneous leishmaniasis. J Exp Med (2007) 204:285–97. doi: 10.1084/jem.20061886
101. Gonzalez K, Calzada JE, Tomokane TY, Pacheco CMS, Flores GVA, Castro Gomes CM, et al. In situ study of cellular immune response in human cutaneous lesions caused by Leishmania (Viannia) panamensis in Panama. Parasite Immunol (2021) 43:e12801. doi: 10.1111/pim.12801
102. Jafarzadeh A, Jafarzadeh S, Sharifi I, Aminizadeh N, Nozari P, Nemati M. The importance of T cell-derived cytokines in post-kala-azar dermal leishmaniasis. Cytokine (2021) 147:155321. doi: 10.1016/j.cyto.2020.155321
103. de Freitas EO, Leoratti FM, Freire-de-Lima CG, Morrot A, Feijó DF. The contribution of immune evasive mechanisms to parasite persistence in visceral leishmaniasis. Front Immunol (2016) 7:153. doi: 10.3389/fimmu.2016.00153
104. Arumugam S, Scorza BM, Petersen C. Visceral leishmaniasis and the skin: dermal parasite transmission to sand flies. Pathog (Basel Switzerland) (2022) 11(6):610. doi: 10.3390/pathogens11060610
105. Aslan H, Oliveira F, Meneses C, Castrovinci P, Gomes R, Teixeira C, et al. New Insights Into the Transmissibility of Leishmania infantum From Dogs to Sand Flies: Experimental Vector-Transmission Reveals Persistent Parasite Depots at Bite Sites. J Infect Dis (2016) 213:1752–61. doi: 10.1093/infdis/jiw022
106. Peixoto RF, Gois BM, Martins M, Palmeira PHS, Rocha JC, Gomes JAS, et al. Characterization of regulatory T cells in patients infected by leishmania infantum. Trop Med Infect Dis (2022) 8(1):18. doi: 10.3390/tropicalmed8010018
107. Kumar P, Misra P, Thakur CP, Saurabh A, Rishi N, Mitra DK. T cell suppression in the bone marrow of visceral leishmaniasis patients: impact of parasite load. Clin Exp Immunol (2018) 191:318–27. doi: 10.1111/cei.13074
108. Gasim S, Elhassan AM, Khalil EA, Ismail A, Kadaru AM, Kharazmi A, et al. High levels of plasma IL-10 and expression of IL-10 by keratinocytes during visceral leishmaniasis predict subsequent development of post-kala-azar dermal leishmaniasis. Clin Exp Immunol (1998) 111:64–9. doi: 10.1046/j.1365-2249.1998.00468.x
109. Santos MF, Alexandre-Pires G, Pereira MA, Gomes L, Rodrigues AV, Basso A, et al. Immunophenotyping of peripheral blood, lymph node, and bone marrow T lymphocytes during canine leishmaniosis and the impact of antileishmanial chemotherapy. Front Vet Sci (2020) 7:375. doi: 10.3389/fvets.2020.00375
110. Mandell MA, Beverley SM. Continual renewal and replication of persistent Leishmania major parasites in concomitantly immune hosts. Proc Natl Acad Sci USA (2017) 114:E801–e10. doi: 10.1073/pnas.1619265114
111. Peters NC, Pagán AJ, Lawyer PG, Hand TW, Henrique Roma E, Stamper LW, et al. Chronic parasitic infection maintains high frequencies of short-lived Ly6C+CD4+ effector T cells that are required for protection against re-infection. PloS Pathog (2014) 10:e1004538. doi: 10.1371/journal.ppat.1004538
112. Uzonna JE, Wei G, Yurkowski D, Bretscher P. Immune elimination of Leishmania major in mice: implications for immune memory, vaccination, and reactivation disease. J Immunol (Baltimore Md: 1950) (2001) 167:6967–74. doi: 10.4049/jimmunol.167.12.6967
113. Scott P. Long-lived skin-resident memory T cells contribute to concomitant immunity in cutaneous leishmaniasis. Cold Spring Harbor Perspect Biol (2020) 12(10):a038059. doi: 10.1101/cshperspect.a038059
114. Viana da Costa A, Huerre M, Delacre M, Auriault C, Correia Costa JM, Verwaerde C. IL-10 leads to a higher parasite persistence in a resistant mouse model of Leishmania major infection. Parasitol Int (2002) 51:367–79. doi: 10.1016/S1383-5769(02)00039-9
115. Okwor I, Uzonna J. Persistent parasites and immunologic memory in cutaneous leishmaniasis: implications for vaccine designs and vaccination strategies. Immunologic Res (2008) 41:123–36. doi: 10.1007/s12026-008-8016-2
116. Hohman LS, Mou Z, Carneiro MB, Ferland G, Kratofil RM, Kubes P, et al. Protective CD4+ Th1 cell-mediated immunity is reliant upon execution of effector function prior to the establishment of the pathogen niche. PloS Pathog (2021) 17:e1009944. doi: 10.1371/journal.ppat.1009944
117. Costa-Madeira JC, Trindade GB, Almeida PHP, Silva JS, Carregaro VT. Lymphocyte exhaustion during human and experimental visceral leishmaniasis. Front Immunol (2022) 13:835711. doi: 10.3389/fimmu.2022.835711
118. Diupotex M, Zamora-Chimal J, Gajón JA, Bonifaz LC, Becker I. CXCR5 and TIM-3 expressions define distinct exhausted T cell subsets in experimental cutaneous infection with Leishmania mexicana. Front Immunol (2023) 14:1231836. doi: 10.3389/fimmu.2023.1231836
119. Berard M, Tough DF. Qualitative differences between naïve and memory T cells. Immunology (2002) 106:127–38. doi: 10.1046/j.1365-2567.2002.01447.x
120. Stockinger B, Kassiotis G, Bourgeois C. CD4 T-cell memory. Semin Immunol (2004) 16:295–303. doi: 10.1016/j.smim.2004.08.010
121. Sallusto F, Geginat J, Lanzavecchia A. Central memory and effector memory T cell subsets: function, generation, and maintenance. Annu Rev Immunol (2004) 22:745–63. doi: 10.1146/annurev.immunol.22.012703.104702
122. Wu CY, Kirman JR, Rotte MJ, Davey DF, Perfetto SP, Rhee EG, et al. Distinct lineages of T(H)1 cells have differential capacities for memory cell generation in vivo. Nat Immunol (2002) 3:852–8. doi: 10.1038/ni832
123. Kaech SM, Ahmed R. Memory CD8+ T cell differentiation: initial antigen encounter triggers a developmental program in naïve cells. Nat Immunol (2001) 2:415–22. doi: 10.1038/87720
124. Reinhardt RL, Khoruts A, Merica R, Zell T, Jenkins MK. Visualizing the generation of memory CD4 T cells in the whole body. Nature (2001) 410:101–5. doi: 10.1038/35065111
125. Wherry EJ, Teichgräber V, Becker TC, Masopust D, Kaech SM, Antia R, et al. Lineage relationship and protective immunity of memory CD8 T cell subsets. Nat Immunol (2003) 4:225–34. doi: 10.1038/ni889
126. Okada R, Kondo T, Matsuki F, Takata H, Takiguchi M. Phenotypic classification of human CD4+ T cell subsets and their differentiation. Int Immunol (2008) 20:1189–99. doi: 10.1093/intimm/dxn075
127. Wherry EJ, Barber DL, Kaech SM, Blattman JN, Ahmed R. Antigen-independent memory CD8 T cells do not develop during chronic viral infection. Proc Natl Acad Sci USA (2004) 101:16004–9. doi: 10.1073/pnas.0407192101
128. Seder RA, Ahmed R. Similarities and differences in CD4+ and CD8+ effector and memory T cell generation. Nat Immunol (2003) 4:835–42. doi: 10.1038/ni969
129. Harari A, Vallelian F, Meylan PR, Pantaleo G. Functional heterogeneity of memory CD4 T cell responses in different conditions of antigen exposure and persistence. J Immunol (Baltimore Md: 1950) (2005) 174:1037–45. doi: 10.4049/jimmunol.174.2.1037
130. Imhof BA, Dunon D. Leukocyte migration and adhesion. Adv Immunol (1995) 58:345–416. doi: 10.1016/S0065-2776(08)60623-9
131. Künzli M, Masopust D. CD4(+) T cell memory. Nat Immunol (2023) 24:903–14. doi: 10.1038/s41590-023-01510-4
132. Todryk SM. T cell memory to vaccination. Vaccines (2018) 6(4):184. doi: 10.3390/vaccines6040084
133. Boesteanu AC, Katsikis PD. Memory T cells need CD28 costimulation to remember. Semin Immunol (2009) 21:69–77. doi: 10.1016/j.smim.2009.02.005
134. Schiött A, Lindstedt M, Johansson-Lindbom B, Roggen E, Borrebaeck CA. CD27- CD4+ memory T cells define a differentiated memory population at both the functional and transcriptional levels. Immunology (2004) 113:363–70. doi: 10.1111/j.1365-2567.2004.01974.x
135. Scott P, Artis D, Uzonna J, Zaph C. The development of effector and memory T cells in cutaneous leishmaniasis: the implications for vaccine development. Immunol Rev (2004) 201:318–38. doi: 10.1111/j.0105-2896.2004.00198.x
136. Titus RG, Gueiros-Filho FJ, de Freitas LA, Beverley SM. Development of a safe live Leishmania vaccine line by gene replacement. Proc Natl Acad Sci USA (1995) 92:10267–71. doi: 10.1073/pnas.92.22.10267
137. Scott P. Immunologic memory in cutaneous leishmaniasis. Cell Microbiol (2005) 7:1707–13. doi: 10.1111/j.1462-5822.2005.00626.x
138. Rivino L, Messi M, Jarrossay D, Lanzavecchia A, Sallusto F, Geginat J. Chemokine receptor expression identifies Pre-T helper (Th)1, Pre-Th2, and nonpolarized cells among human CD4+ central memory T cells. J Exp Med (2004) 200:725–35. doi: 10.1084/jem.20040774
139. Uzonna JE, Späth GF, Beverley SM, Scott P. Vaccination with phosphoglycan-deficient Leishmania major protects highly susceptible mice from virulent challenge without inducing a strong Th1 response. J Immunol (Baltimore Md: 1950) (2004) 172:3793–7. doi: 10.4049/jimmunol.172.6.3793
140. Kaech SM, Wherry EJ, Ahmed R. Effector and memory T-cell differentiation: implications for vaccine development. Nat Rev Immunol (2002) 2:251–62. doi: 10.1038/nri778
141. Pereira-Carvalho R, Mendes-Aguiar CO, Oliveira-Neto MP, Covas CJ, Bertho AL, Da-Cruz AM, et al. Leishmania Braziliensis-reactive T cells are down-regulated in long-term cured cutaneous Leishmaniasis, but the renewal capacity of T effector memory compartments is preserved. PloS One (2013) 8:e81529. doi: 10.1371/journal.pone.0081529
142. Willemsen M, Linkutė R, Luiten RM, Matos TR. Skin-resident memory T cells as a potential new therapeutic target in vitiligo and melanoma. Pigment Cell melanoma Res (2019) 32:612–22. doi: 10.1111/pcmr.12803
143. Wu H, Liao W, Li Q, Long H, Yin H, Zhao M, et al. Pathogenic role of tissue-resident memory T cells in autoimmune diseases. Autoimmun Rev (2018) 17:906–11. doi: 10.1016/j.autrev.2018.03.014
144. Chen L, Shen Z. Tissue-resident memory T cells and their biological characteristics in the recurrence of inflammatory skin disorders. Cell Mol Immunol (2020) 17:64–75. doi: 10.1038/s41423-019-0291-4
145. Clark RA. Resident memory T cells in human health and disease. Sci Trans Med (2015) 7:269rv1. doi: 10.1126/scitranslmed.3010641
146. Jameson SC, Masopust D. Understanding subset diversity in T cell memory. Immunity (2018) 48:214–26. doi: 10.1016/j.immuni.2018.02.010
147. Szabo PA, Miron M, Farber DL. Location, location, location: Tissue resident memory T cells in mice and humans. Sci Immunol (2019) 4(34):eaas9673. doi: 10.1126/sciimmunol.aas9673
148. Mackay LK, Minnich M, Kragten NA, Liao Y, Nota B, Seillet C, et al. Hobit and Blimp1 instruct a universal transcriptional program of tissue residency in lymphocytes. Sci (New York NY) (2016) 352:459–63. doi: 10.1126/science.aad2035
149. Kumar BV, Ma W, Miron M, Granot T, Guyer RS, Carpenter DJ, et al. Human tissue-resident memory T cells are defined by core transcriptional and functional signatures in lymphoid and mucosal sites. Cell Rep (2017) 20:2921–34. doi: 10.1016/j.celrep.2017.08.078
150. Milner JJ, Toma C, Yu B, Zhang K, Omilusik K, Phan AT, et al. Runx3 programs CD8(+) T cell residency in non-lymphoid tissues and tumours. Nature (2017) 552:253–7. doi: 10.1038/nature24993
151. Jiang X, Clark RA, Liu L, Wagers AJ, Fuhlbrigge RC, Kupper TS. Skin infection generates non-migratory memory CD8+ T(RM) cells providing global skin immunity. Nature (2012) 483:227–31. doi: 10.1038/nature10851
152. Carlson CM, Endrizzi BT, Wu J, Ding X, Weinreich MA, Walsh ER, et al. Kruppel-like factor 2 regulates thymocyte and T-cell migration. Nature (2006) 442:299–302. doi: 10.1038/nature04882
153. Shiow LR, Rosen DB, Brdicková N, Xu Y, An J, Lanier LL, et al. CD69 acts downstream of interferon-alpha/beta to inhibit S1P1 and lymphocyte egress from lymphoid organs. Nature (2006) 440:540–4. doi: 10.1038/nature04606
154. Mackay LK, Braun A, Macleod BL, Collins N, Tebartz C, Bedoui S, et al. Cutting edge: CD69 interference with sphingosine-1-phosphate receptor function regulates peripheral T cell retention. J Immunol (Baltimore Md: 1950) (2015) 194:2059–63. doi: 10.4049/jimmunol.1402256
155. Sathaliyawala T, Kubota M, Yudanin N, Turner D, Camp P, Thome JJ, et al. Distribution and compartmentalization of human circulating and tissue-resident memory T cell subsets. Immunity (2013) 38:187–97. doi: 10.1016/j.immuni.2012.09.020
156. Gebhardt T, Whitney PG, Zaid A, Mackay LK, Brooks AG, Heath WR, et al. Different patterns of peripheral migration by memory CD4+ and CD8+ T cells. Nature (2011) 477:216–9. doi: 10.1038/nature10339
157. Mackay LK, Rahimpour A, Ma JZ, Collins N, Stock AT, Hafon ML, et al. The developmental pathway for CD103(+)CD8+ tissue-resident memory T cells of skin. Nat Immunol (2013) 14:1294–301. doi: 10.1038/ni.2744
158. Thome JJ, Yudanin N, Ohmura Y, Kubota M, Grinshpun B, Sathaliyawala T, et al. Spatial map of human T cell compartmentalization and maintenance over decades of life. Cell (2014) 159:814–28. doi: 10.1016/j.cell.2014.10.026
159. Watanabe R, Gehad A, Yang C, Scott LL, Teague JE, Schlapbach C, et al. Human skin is protected by four functionally and phenotypically discrete populations of resident and recirculating memory T cells. Sci Trans Med (2015) 7:279ra39. doi: 10.1126/scitranslmed.3010302
160. Takamura S, Yagi H, Hakata Y, Motozono C, McMaster SR, Masumoto T, et al. Specific niches for lung-resident memory CD8+ T cells at the site of tissue regeneration enable CD69-independent maintenance. J Exp Med (2016) 213:3057–73. doi: 10.1084/jem.20160938
161. Iijima N, Iwasaki A. T cell memory. A local macrophage chemokine network sustains protective tissue-resident memory CD4 T cells. Sci (New York NY) (2014) 346:93–8. doi: 10.1126/science.1257530
162. Strutt TM, Dhume K, Finn CM, Hwang JH, Castonguay C, Swain SL, et al. IL-15 supports the generation of protective lung-resident memory CD4 T cells. Mucosal Immunol (2018) 11:668–80. doi: 10.1038/mi.2017.101
163. Collins N, Jiang X, Zaid A, Macleod BL, Li J, Park CO, et al. Skin CD4(+) memory T cells exhibit combined cluster-mediated retention and equilibration with the circulation. Nat Commun (2016) 7:11514. doi: 10.1038/ncomms11514
164. McCully ML, Ladell K, Andrews R, Jones RE, Miners KL, Roger L, et al. CCR8 expression defines tissue-resident memory T cells in human skin. J Immunol (Baltimore Md: 1950) (2018) 200:1639–50. doi: 10.4049/jimmunol.1701377
165. Clark RA, Chong B, Mirchandani N, Brinster NK, Yamanaka K, Dowgiert RK, et al. The vast majority of CLA+ T cells are resident in normal skin. J Immunol (Baltimore Md: 1950) (2006) 176:4431–9. doi: 10.4049/jimmunol.176.7.4431
166. Nolz JC, Richer MJ. Control of memory CD8(+) T cell longevity and effector functions by IL-15. Mol Immunol (2020) 117:180–8. doi: 10.1016/j.molimm.2019.11.011
167. Shin MS, Kim D, Yim K, Park HJ, You S, Dong X, et al. IL-7 receptor alpha defines heterogeneity and signature of human effector memory CD8(+) T cells in high dimensional analysis. Cell Immunol (2020) 355:104155. doi: 10.1016/j.cellimm.2020.104155
168. Mackay LK, Wynne-Jones E, Freestone D, Pellicci DG, Mielke LA, Newman DM, et al. T-box transcription factors combine with the cytokines TGF-β and IL-15 to control tissue-resident memory T cell fate. Immunity (2015) 43:1101–11. doi: 10.1016/j.immuni.2015.11.008
169. Adachi T, Kobayashi T, Sugihara E, Yamada T, Ikuta K, Pittaluga S, et al. Hair follicle-derived IL-7 and IL-15 mediate skin-resident memory T cell homeostasis and lymphoma. Nat Med (2015) 21:1272–9. doi: 10.1038/nm.3962
170. Goncalves R, Zhang X, Cohen H, Debrabant A, Mosser DM. Platelet activation attracts a subpopulation of effector monocytes to sites of Leishmania major infection. J Exp Med (2011) 208:1253–65. doi: 10.1084/jem.20101751
171. Romano A, Carneiro MBH, Doria NA, Roma EH, Ribeiro-Gomes FL, Inbar E, et al. Divergent roles for Ly6C+CCR2+CX3CR1+ inflammatory monocytes during primary or secondary infection of the skin with the intra-phagosomal pathogen Leishmania major. PloS Pathog (2017) 13:e1006479. doi: 10.1371/journal.ppat.1006479
172. Miramin-Mohammadi A, Javadi A, Eskandari SE, Nateghi-Rostami M, Khamesipour A. Immune Responses in Cutaneous Leishmaniasis: In vitro Thelper1/Thelper2 Cytokine Profiles Using Live Versus Killed Leishmania major. J Arthropod-borne Dis (2021) 15:126–35. doi: 10.18502/jad.v15i1.6491
173. Bahrami F, Harandi AM, Rafati S. Biomarkers of cutaneous leishmaniasis. Front Cell Infect Microbiol (2018) 8:222. doi: 10.3389/fcimb.2018.00222
174. Reiner SL, Locksley RM. Cytokines in the differentiation of Th1/Th2 CD4+ subsets in leishmaniasis. J Cell Biochem (1993) 53:323–8. doi: 10.1002/jcb.240530409
175. Kellina OI. [Differences in the sensitivity of inbred mice of different lines to Leishmania tropica major]. Meditsinskaia parazitologiia i parazitarnye bolezni (1973) 42:279–85.
176. Mitchell GF, Curtis JM, Scollay RG, Handman E. Resistance and abrogation of resistance to cutaneous leishmaniasis in reconstituted BALB/c nude mice. Aust J Exp Biol Med Sci (1981) 59:539–54. doi: 10.1038/icb.1981.47
177. Heinzel FP, Sadick MD, Holaday BJ, Coffman RL, Locksley RM. Reciprocal expression of interferon gamma or interleukin 4 during the resolution or progression of murine leishmaniasis. Evidence for expansion of distinct helper T cell subsets. J Exp Med (1989) 169:59–72. doi: 10.1084/jem.169.1.59
178. Mosmann TR, Cherwinski H, Bond MW, Giedlin MA, Coffman RL. Two types of murine helper T cell clone. I. Definition according to profiles of lymphokine activities and secreted proteins. J Immunol (Baltimore Md: 1950) (1986) 136:2348–57. doi: 10.4049/jimmunol.136.7.2348
179. Cher DJ, Mosmann TR. Two types of murine helper T cell clone. II. Delayed-type hypersensitivity is mediated by TH1 clones. J Immunol (Baltimore Md: 1950) (1987) 138:3688–94. doi: 10.4049/jimmunol.138.11.3688
180. Locksley RM, Heinzel FP, Holaday BJ, Mutha SS, Reiner SL, Sadick MD. Induction of Th1 and Th2 CD4+ subsets during murine Leishmania major infection. Res Immunol (1991) 142:28–32. doi: 10.1016/0923-2494(91)90007-6
181. Coffman RL, Chatelain R, Leal LM, Varkila K. Leishmania major infection in mice: a model system for the study of CD4+ T-cell subset differentiation. Res Immunol (1991) 142:36–40. doi: 10.1016/0923-2494(91)90009-8
182. Sadick MD, Locksley RM, Tubbs C, Raff HV. Murine cutaneous leishmaniasis: resistance correlates with the capacity to generate interferon-gamma in response to Leishmania antigens in vitro. J Immunol (Baltimore Md: 1950) (1986) 136:655–61. doi: 10.4049/jimmunol.136.2.655
183. Darzi F, Davoudian R, Nateghi Rostami M. Differential inflammatory responses associated with Leishmania major and L tropica in culture. Parasite Immunol (2021) 43:e12841. doi: 10.1111/pim.12841
184. Bodhale NP, Pal S, Kumar S, Chattopadhyay D, Saha B, Chattopadhyay N, et al. Inbred mouse strains differentially susceptible to Leishmania donovani infection differ in their immune cell metabolism. Cytokine (2018) 112:12–5. doi: 10.1016/j.cyto.2018.06.003
185. Childs GE, Lightner LK, McKinney L, Groves MG, Price EE, Hendricks LD. Inbred mice as model hosts for cutaneous leishmaniasis. I. Resistance and susceptibility to infection with Leishmania Braziliensis, L. mexicana and L. aethiopica. Ann Trop Med Parasitol (1984) 78:25–34. doi: 10.1080/00034983.1984.11811769
186. Nabors GS, Farrell JP. Site-specific immunity to Leishmania major in SWR mice: the site of infection influences susceptibility and expression of the antileishmanial immune response. Infect Immun (1994) 62:3655–62. doi: 10.1128/iai.62.9.3655-3662.1994
187. Baldwin TM, Elso C, Curtis J, Buckingham L, Handman E. The site of Leishmania major infection determines disease severity and immune responses. Infect Immun (2003) 71:6830–4. doi: 10.1128/IAI.71.12.6830-6834.2003
188. Fromm PD, Kling JC, Remke A, Bogdan C, Körner H. Fatal leishmaniasis in the absence of TNF despite a strong th1 response. Front Microbiol (2015) 6:1520. doi: 10.3389/fmicb.2015.01520
190. Bomfim G, Nascimento C, Costa J, Carvalho EM, Barral-Netto M, Barral A. Variation of cytokine patterns related to therapeutic response in diffuse cutaneous leishmaniasis. Exp Parasitol (1996) 84:188–94. doi: 10.1006/expr.1996.0104
191. Sundar S, Reed SG, Sharma S, Mehrotra A, Murray HW. Circulating T helper 1 (Th1) cell- and Th2 cell-associated cytokines in Indian patients with visceral leishmaniasis. Am J Trop Med hygiene (1997) 56:522–5. doi: 10.4269/ajtmh.1997.56.522
192. Castellano LR, Filho DC, Argiro L, Dessein H, Prata A, Dessein A, et al. Th1/Th2 immune responses are associated with active cutaneous leishmaniasis and clinical cure is associated with strong interferon-gamma production. Hum Immunol (2009) 70:383–90. doi: 10.1016/j.humimm.2009.01.007
193. da Silva Santos C, Brodskyn CI. The role of CD4 and CD8 T cells in human cutaneous leishmaniasis. Front Public Health (2014) 2:165. doi: 10.3389/fpubh.2014.00165
194. Darrah PA, Hegde ST, Patel DT, Lindsay RW, Chen L, Roederer M, et al. IL-10 production differentially influences the magnitude, quality, and protective capacity of Th1 responses depending on the vaccine platform. J Exp Med (2010) 207:1421–33. doi: 10.1084/jem.20092532
195. Gomes-Silva A, de Cássia Bittar R, Dos Santos Nogueira R, Amato VS, da Silva Mattos M, Oliveira-Neto MP, et al. Can interferon-gamma and interleukin-10 balance be associated with severity of human Leishmania (Viannia) Braziliensis infection? Clin Exp Immunol (2007) 149:440–4. doi: 10.1111/j.1365-2249.2007.03436.x
196. Khalil EA, Ayed NB, Musa AM, Ibrahim ME, Mukhtar MM, Zijlstra EE, et al. Dichotomy of protective cellular immune responses to human visceral leishmaniasis. Clin Exp Immunol (2005) 140:349–53. doi: 10.1111/j.1365-2249.2005.02768.x
197. Botana L, Matía B, San Martin JV, Romero-Maté A, Castro A, Molina L, et al. Cellular markers of active disease and cure in different forms of leishmania infantum-induced disease. Front Cell Infect Microbiol (2018) 8:381. doi: 10.3389/fcimb.2018.00381
198. Verma S, Kumar R, Katara GK, Singh LC, Negi NS, Ramesh V, et al. Quantification of parasite load in clinical samples of leishmaniasis patients: IL-10 level correlates with parasite load in visceral leishmaniasis. PloS One (2010) 5:e10107. doi: 10.1371/journal.pone.0010107
199. Mesquita I, Ferreira C, Barbosa AM, Ferreira CM, Moreira D, Carvalho A, et al. The impact of IL-10 dynamic modulation on host immune response against visceral leishmaniasis. Cytokine (2018) 112:16–20. doi: 10.1016/j.cyto.2018.07.001
200. Alimohammadian MH, Jones SL, Darabi H, Riazirad F, Ajdary S, Shabani A, et al. Assessment of interferon-γ levels and leishmanin skin test results in persons recovered for leishmaniasis. Am J Trop Med hygiene (2012) 87:70–5. doi: 10.4269/ajtmh.2012.11-0479
201. Volpedo G, Bhattacharya P, Gannavaram S, Pacheco-Fernandez T, Oljuskin T, Dey R, et al. The history of live attenuated centrin gene-deleted leishmania vaccine candidates. Pathog (Basel Switzerland) (2022) 11(4):431. doi: 10.3390/pathogens11040431
202. Volpedo G, Pacheco-Fernandez T, Holcomb EA, Zhang WW, Lypaczewski P, Cox B, et al. Centrin-deficient Leishmania mexicana confers protection against New World cutaneous leishmaniasis. NPJ Vaccines (2022) 7:32. doi: 10.1038/s41541-022-00449-1
203. Karmakar S, Volpedo G, Zhang WW, Lypaczewski P, Ismail N, Oliveira F, et al. Centrin-deficient Leishmania mexicana confers protection against Old World visceral leishmaniasis. NPJ Vaccines (2022) 7:157. doi: 10.1038/s41541-022-00574-x
204. Oja AE, van Lier RAW, Hombrink P. Two sides of the same coin: Protective versus pathogenic CD4(+) resident memory T cells. Sci Immunol (2022) 7:eabf9393. doi: 10.1126/sciimmunol.abf9393
205. Muruganandah V, Sathkumara HD, Navarro S, Kupz A. A systematic review: the role of resident memory T cells in infectious diseases and their relevance for vaccine development. Front Immunol (2018) 9:1574. doi: 10.3389/fimmu.2018.01574
206. Lefebvre MN, Harty JT. You shall not pass: memory CD8 T cells in liver-stage malaria. Trends Parasitol (2020) 36:147–57. doi: 10.1016/j.pt.2019.11.004
207. Nakamae S, Miyagawa S, Ogawa K, Kamiya M, Taniguchi M, Ono A, et al. Induction of liver-resident memory T cells and protection at liver-stage malaria by mRNA-containing lipid nanoparticles. Front Immunol (2023) 14:1116299. doi: 10.3389/fimmu.2023.1116299
208. Fernandez-Ruiz D, Ng WY, Holz LE, Ma JZ, Zaid A, Wong YC, et al. Liver-resident memory CD8(+) T cells form a front-line defense against malaria liver-stage infection. Immunity (2016) 45:889–902. doi: 10.1016/j.immuni.2016.08.011
209. Hoffman SL, Goh LM, Luke TC, Schneider I, Le TP, Doolan DL, et al. Protection of humans against malaria by immunization with radiation-attenuated Plasmodium falciparum sporozoites. J Infect Dis (2002) 185:1155–64. doi: 10.1086/339409
210. Landrith TA, Sureshchandra S, Rivera A, Jang JC, Rais M, Nair MG, et al. CD103(+) CD8 T cells in the toxoplasma-infected brain exhibit a tissue-resident memory transcriptional profile. Front Immunol (2017) 8:335. doi: 10.3389/fimmu.2017.00335
211. Olivera GC, Vetter L, Tesoriero C, Del Gallo F, Hedberg G, Basile J, et al. Role of T cells during the cerebral infection with Trypanosoma brucei. PloS Negl Trop Dis (2021) 15:e0009764. doi: 10.1371/journal.pntd.0009764
212. Louis L, Clark M, Wise MC, Glennie N, Wong A, Broderick K, et al. Intradermal Synthetic DNA Vaccination Generates Leishmania-Specific T Cells in the Skin and Protection against Leishmania major. Infect Immun (2019) 87(8):e00227–19. doi: 10.1128/IAI.00227-19
213. Gurunathan S, Prussin C, Sacks DL, Seder RA. Vaccine requirements for sustained cellular immunity to an intracellular parasitic infection. Nat Med (1998) 4:1409–15. doi: 10.1038/4000
214. Volpedo G, Huston RH, Holcomb EA, Pacheco-Fernandez T, Gannavaram S, Bhattacharya P, et al. From infection to vaccination: reviewing the global burden, history of vaccine development, and recurring challenges in global leishmaniasis protection. Expert Rev Vaccines (2021) 20:1431–46. doi: 10.1080/14760584.2021.1969231
Keywords: leishmaniasis, memory CD4+ T cells, vaccine, tissue resident memory, biomarkers
Citation: Nateghi-Rostami M and Sohrabi Y (2024) Memory T cells: promising biomarkers for evaluating protection and vaccine efficacy against leishmaniasis. Front. Immunol. 15:1304696. doi: 10.3389/fimmu.2024.1304696
Received: 29 September 2023; Accepted: 08 February 2024;
Published: 26 February 2024.
Edited by:
Soraya Gaze, Oswaldo Cruz Foundation (Fiocruz), BrazilReviewed by:
Vicente Larraga, Spanish National Research Council (CSIC), SpainDebabrata Mandal, National Institute of Pharmaceutical Education and Research, India
Greta Volpedo, University of Genoa, Italy
Copyright © 2024 Nateghi-Rostami and Sohrabi. This is an open-access article distributed under the terms of the Creative Commons Attribution License (CC BY). The use, distribution or reproduction in other forums is permitted, provided the original author(s) and the copyright owner(s) are credited and that the original publication in this journal is cited, in accordance with accepted academic practice. No use, distribution or reproduction is permitted which does not comply with these terms.
*Correspondence: Mahmoud Nateghi-Rostami, bV9uYW50ZWdoaUBwYXN0ZXVyLmFjLmly; Yahya Sohrabi, WWFoeWEuc29ocmFiaUB1a211ZW5zdGVyLmRl