- 1Columbia Center for Translational Immunology, Department of Medicine and Naomi Berrie Diabetes Center, Columbia University Irving Medical Center, New York, NY, United States
- 2Department of Pharmaceutical Chemistry, University of Kansas, Lawrence, KS, United States
- 3Department of Chemistry, University of Kansas, Lawrence, KS, United States
- 4Department of Pathology and Cell Biology, Columbia University Irving Medical Center and New York Presbyterian Hospital, New York, NY, United States
- 5Department of Pediatrics, Division of Allergy and Immunology, Columbia University Irving Medical Center, New York, NY, United States
- 6Department of Chemical and Petroleum Engineering, University of Kansas, Lawrence, KS, United States
Autoantigen-specific immunotherapy using peptides offers a more targeted approach to treat autoimmune diseases, but clinical implementation has been challenging. We previously showed that multivalent delivery of peptides as soluble antigen arrays (SAgAs) efficiently protects against spontaneous autoimmune diabetes in the non-obese diabetic (NOD) mouse model. Here, we compared the efficacy, safety, and mechanisms of action of SAgAs versus free peptides. SAgAs, but not their corresponding free peptides at equivalent doses, efficiently prevented the development of diabetes. SAgAs increased the frequency of regulatory T cells among peptide-specific T cells or induce their anergy/exhaustion or deletion, depending on the type of SAgA used (hydrolysable (hSAgA) and non-hydrolysable ‘click’ SAgA (cSAgA)) and duration of treatment, whereas their corresponding free peptides induced a more effector phenotype following delayed clonal expansion. Over time, the peptides induced an IgE-independent anaphylactic reaction, the incidence of which was significantly delayed when peptides were in SAgA form rather than in free form. Moreover, the N-terminal modification of peptides with aminooxy or alkyne linkers, which was needed for grafting onto hyaluronic acid to make hSAgA or cSAgA variants, respectively, influenced their stimulatory potency and safety, with alkyne-functionalized peptides being more potent and less anaphylactogenic than aminooxy-functionalized peptides. Immunologic anaphylaxis occurred in NOD mice in a dose-dependent manner but not in C57BL/6 or BALB/c mice; however, its incidence did not correlate with the level of anti-peptide antibodies. We provide evidence that SAgAs significantly improve the efficacy of peptides to induce tolerance and prevent autoimmune diabetes while at the same time reducing their anaphylactogenic potential.
Introduction
Type 1 diabetes (T1D) is an autoimmune disease mediated by lymphocytes reactive to insulin-producing pancreatic β-cell antigens that result in insulitis and loss of β-cells. T1D is affecting millions of Americans and has currently no cure. Its control requires managing blood glucose levels with regular insulin administrations, which is cumbersome, does not prevent the development of long-term complications in many patients, and does not tackle the root cause of the disease. There is also an unmet need for safe therapies that can be applied early enough so that β-cells can be preserved and life-long dependence on exogenous insulin averted. To that end, antigen-specific immunotherapy (ASIT) offers a more targeted and selective way of disabling disease-specific autoreactive lymphocytes to treat T1D without dampening the whole immune system.
The use of peptides as antigens for ASIT has several advantages over full proteins. Peptides are easier to manufacture, chemically modify, and customize for precision medicine (using a “mix & match” approach with peptides covering multiple antigens and restricted to specific human leukocyte antigen (HLA) haplotypes) (1–3). However, the use of peptides for clinical applications has been limited by their short half-life (due to enzymatic degradation), high dispersion resulting in low cellular uptake “per cell”, and poor stimulatory capacity in vivo (4). In some cases, repeated administration also leads to anaphylactic events (5–8). These limitations can be overcome by using an efficient nanodelivery platform and chemical modification of peptides. On one hand, a desirable delivery modality for peptides would increase their resistance to enzymatic degradation, facilitate their drainage to lymphoid tissues, and enhance their uptake, ideally resulting in more efficient and persistent antigen presentation in vivo (5, 9). Several delivery platforms including nanoparticles (10–13), nanofibers (14), cell penetrating peptides (9, 15), and soluble antigen arrays (SAgAs) (5, 16) have been shown to address some of the above limitations of peptides and to improve the efficacy and safety of ASIT. In some cases, anaphylaxis caused by peptides in free form could be averted by ensuring slow release (17) or non-free forms (18). On the other hand, peptide modifications such as PEGylation (19), side chain stapling (20), retro-inverso-D-amino acid peptides (21, 22), and lipidation (23, 24) can also improve the efficacy and safety of peptide-based ASIT. For instance, lipophilic modification of InsB9–23 or addition of RLGL to WE14 peptides at the N-terminus was shown to enhance antigen presentation and to induce antigen-specific immune tolerance in models of T1D (25, 26). Acidic residues can contribute to peptide-induced anaphylactic reactions, and amino acid additions or substitutions that neutralize these charges prevented these effects (6, 7). Likewise, modification of antibody contact residues within the peptide could prevent antibody recognition and overcome the risk of peptide-induced anaphylaxis (27). These examples support the feasibility of overcoming inherent limitations of peptide-based ASIT using suitable delivery modalities and chemical modifications.
We recently used SAgAs as a multivalent, versatile, and effective peptide delivery modality that features multiple copies of antigenic peptides bound to hyaluronic acid (HA). SAgAs have advantageous properties such as a small size (less than 10 nm radius) and improved solubility (relative to free peptides) that facilitate their efficient delivery to lymphoid tissues. SAgAs enhance peptide uptake and persistence of antigen presentation (5, 16, 28, 29). Thus, SAgAs address many of the aforementioned limitations of peptide-based ASIT. Peptides used to produce SAgAs require N-terminal aminooxy (ao) or homopropargyl (hp) modification for conjugation of multiple copies to HA using aminooxy chemistry (producing hydrolysable SAgAs (hSAgAs)) or using “click” chemistry (producing click SAgAs (cSAgAs)), respectively (5, 16, 30, 31). Following uptake of SAgAs by antigen-presenting cells (APCs), the peptides are released from the HA backbone (by hydrolysis at low pH for hSAgAs, or by unclear mechanisms, possibly involving degradation of HA itself for cSAgAs) and loaded onto MHC-II for presentation to CD4+ T cells. We previously showed that SAgAs carrying p79 mimotopes and 2.5 hybrid insulin peptides (2.5HIP) protect non-obese diabetic (NOD) mice from developing autoimmune diabetes in part by inducing regulatory CD4+ T cell populations (5, 30). The NOD mouse constitutes a polygenic and spontaneous model of autoimmune diabetes that shares many commonalities with human T1D (32, 33). Here, we compared the safety and efficacy of hSAgAs and cSAgAs to their corresponding free peptides at equivalent peptide doses and characterized the dynamics and phenotypes of responding T cells.
Materials and methods
Mice
All mice were used according to approved protocols by Columbia University Institutional Animal Care and Use Committee. Female NOD mice (Jax #001976) were obtained from The Jackson Laboratory at 7 weeks of age and were directly used for preclinical (treatment) and anaphylaxis incidence studies one week after their arrival in the animal barrier facility of the Columbia Center for Translational Immunology. For mechanistic studies, BDC2.5 T cell receptor (TCR) transgenic mice (Jax #004460) and NOD.CD45.2 congenic mice (Jax #014149) were originally procured from The Jackson Laboratory but bred together to produce BDC2.5 mice with the CD45.2 congenic marker and maintained in our animal barrier facility. They were used at 8–12 weeks of age as donors of antigen-specific T cells for in vivo tracking after adoptive transfer. Female NOD mice (8–12 weeks of age) were used for short mechanistic studies involving adoptive transfer (recipient mice) and MHC tetramer analysis. C57BL/6J (B6) mice (Jax #000664) were bred in our animal barrier facility, BALB/c mice (Jax #000651) were obtained from The Jackson Laboratory, and both strains were used for anaphylaxis studies as control mice.
Synthesis of hydrolysable hSAgA and non-hydrolysable (“click”) cSAgA
Alkyne-functionalized p79 bearing an N-terminal 4-pentynoic acid (homopropargyl) modification, alkyne-functionalized 2.5HIP bearing an N-terminal alkyne polyethylene glycol group modification, or aminooxy-functionalized p79 or 2.5HIP were purchased from PolyPeptide. SAgAs were synthesized by co-grafting approximately 10 hp-peptides to azide-functionalized HA to make cSAgAs using click chemistry or linking approximately 10 ao-peptides to HA to make hSAgAs using oxime conjugation chemistry and their physicochemical natures were characterized as previously reported (5, 16).
Preclinical studies
Starting at 8 weeks of age, NOD mice were treated subcutaneously (s.c.) at the neck fold with saline, SAgAmix, or peptidemix weekly at various peptide doses, ranging from 5 nmol to 125 nmol for each peptide from the mixture (p79 and 2.5HIP). Their blood glucose was monitored weekly (up to 30 weeks of age), and mice were diagnosed as diabetic after two consecutive blood glucose levels greater than 250 mg/dL two days apart.
Assessment of anaphylactic incidences and responses
Female NOD, B6 and BALB/c mice were treated weekly with hSAgAp79 (5 nmol), hSAgAmix (2.5–12.5 nmol each), ao-peptidemix (25–125 nmol each), cSAgAmix (2.5 nmol each), hp-peptidemix (25 nmol each), or saline for the period indicated in the figure legend. Incidence of anaphylaxis was recorded when mice developed fatal systemic anaphylaxis with typical type I hypersensitivity symptoms such as trouble breathing and loss of consciousness within 30 min. Blood samples were drawn every two weeks and were analyzed for complete blood count at the Department of Comparative Medicine using a Genesis instrument (Oxford Science Inc.). Serum samples were assessed for titers of different antibody isotypes using LegendPlex Mouse Immunoglobulin Isotyping Panel and IgE ELISA (BioLegend) according to manufacturer’s instructions. For antigen-specific indirect ELISA, the plate was coated with p79, ao-p79, hp-p79, ao-2.5HIP, or hp-2.5HIP (each at 10 µg/ml) for each immunoglobulin at 4°C overnight. After the plates were washed and blocked, four serially diluted serum samples from mice treated with saline, hSAgAp79, hSAgAmix, cSAgAmix, ao-peptidemix, or hp-peptidemix were added. The plates were washed and detection antibodies (biotinylated mouse anti-IgG1, anti-IgG2a or anti-IgE from BioLegend; anti-IgG2c from Mabtech) were added and incubated at room temperature for 1 hour. Finally, avidin-horseradish peroxidase solution was added to each well for a 30-min incubation at room temperature. The absorbance was measured at 450 nm and 570 nm on the same day. Anaphylaxis was confirmed by immunohistochemistry analysis of toluidine blue stained sections from formalin-fixed paraffin-embedded skin and small intestine samples collected after 5 doses of hSAgAmix (2.5 nmol). Intact and degranulated mast cells were identified as previously reported (34, 35). A total of 100 and 50 masts cells were counted per skin and intestine samples, respectively.
T cell response analysis
To evaluate BDC2.5 CD4+ T cell responses induced by unmodified free peptides vs modified in vitro, splenocytes from NOD.BDC2.5.CD45.2 mice were labeled with Violet Cell Proliferation Dye (eBioscience) and co-cultured (2x105 total cells/well) in the presence/absence of titrated p79, ao-p79, hp-p79, ao-2.5HIP, or hp-2.5HIP at 10-fold serial dilutions ranging from 10 pM to 1 µM concentrations. After 3 days of co-culture at 37°C and 5% CO2, the splenocytes were analyzed for activation markers (CD25) and proliferation by flow cytometry. For the mechanistic studies, female NOD mice were treated weekly by s.c. injection of saline or soluble peptidemix (25 nmol per peptide), or hSAgAmix (2.5 nmol each) or cSAgAmix (2.5 nmol each) for the period indicated in the legend. Spleen and various lymph nodes (LNs) including pancreatic LNs, pooled axillary and brachial LNs, or pooled LNs (pool of axillary, brachial, cervical, mesenteric and/or pancreatic LNs) were collected, and single cells suspensions were prepared. Analysis of polyclonal T cell responses by flow cytometry was performed at two time points: early time point (after two injections three days part) and late time point (following 23 weekly injections). Multiple panels were used to assess surface markers (CD4, CD25, CD44, CD73, FR4, Lag3, PD-1, KLRG1 and TIGIT), intracellular cytokines (IFN-γ, TNF-α, IL-2, and IL-10) and Foxp3 staining. Intracellular cytokine staining was performed after a 4-hour incubation with PMA (0.1 µg/mL), ionomycin (40 µg/mL), brefeldin A (1.5 µg/ml) and monensin (1 µM) using CytoFix/CytoPerm kit (BD Bioscience). Likewise, intracellular staining for transcription factor (Foxp3) was performed using True-Nuclear Factor kit (Biolegend) following manufacturer’s instructions. To identify endogenous antigen-specific T cells, allophycocyanin-conjugated I-Ag7/p79 (AAAAVRPLWVRMEAA) tetramer from the NIH Tetramer Core Facility was used. Fortessa (BD) was used for data acquisition by flow cytometry.
Cytokine analysis
NOD mice were untreated or treated with a single dose of soluble ao-peptidemix, hSAgAmix or cSAgAmix at 1 nmol or 5 nmol of peptide doses for each peptide (p79 or 2.5HIP) in the mix via s.c. route. At the same time, the mice received 5x105 purified and Violet Cell Proliferation Dye labeled BDC2.5 CD4+ CD25- T cells (with CD45.2 congenic marker) by intravenous injection. Three days after the treatment, the spleen was isolated and splenocytes (3x105 cells/well) were cultured in the presence of ao-p79 (5 nM), ao-2.5HIP (5 nM), or their mix (2.5 nM each) to assess cytokine recall responses ex vivo. After four days of ex vivo culture, thirteen cytokines, namely IFN-γ, TNF-α, IL-2, IL-4, IL-5, IL-6, IL-9, IL-10, IL-13, IL-17F, IL-17A, IL-21, and IL-22 were measured in the culture supernatants using the LEGENDPlex Mouse T Helper Cytokine Panel kit (BioLegend) following manufacturer’s instructions. Serum samples from treated mice were assessed with a Custom 9-plex LEGENDPlex kit (IFN-γ, TNF-α, IL-4, IL-5, IL-6, IL-10, IL-13, IL-21, and IL-22).
Statistical and data analysis
GraphPad Prism 4.0 was used to generate all graphs including the Kaplan-Meier curve and to perform statistical analyses. The log-rank (Mantel-Cox) test was used for the incidence of diabetes and anaphylaxis. Two-way analysis of variance (ANOVA) with Sidak’s post-hoc test correction or Tukey’s multiple comparisons, and/or unpaired T-tests were performed in other studies as indicated in legends. Flow cytometry data were analyzed with FCS Express 7. The threshold for statistical significance was set to p<0.05 (* p<0.05, ** p<0.01, *** p<0.001, **** p<0.0001, ns p>0.05).
Results
Peptide modifications and dosing influence the dynamic of anaphylactic responses
Our previous studies (5, 30) focused on comparing single SAgAs (carrying p79 or 2.5HIP) versus a mix thereof (SAgAmix) at different doses. We reported that both peptides (as SAgAmix at 2.5 nmol dose) were needed to achieve significant protection from T1D (5). However, some mice treated with hSAgAmix developed signs of anaphylaxis over time following repeated dosing, which precluded a full long-term assessment of efficacy. Thus, we set out to determine the conditions that influence the incidence of anaphylaxis. At 0.5 nmol, hSAgAmix treatment was safe, whereas it induced anaphylaxis after 8–11 weekly doses at 2.5 nmol (Figures 1A, B). The same dose given as free ao-peptidemix (25 nmol peptide ≈ 2.5 nmol SAgA as SAgA carry ~10 peptides in average) or higher dose of hSAgAmix (12.5 nmol) greatly accelerated the incidence of anaphylaxis (Figures 1A, B). We then compared hSAgAmix and cSAgAmix (2.5 nmol) against the corresponding peptides used to produce them (ao and hp; 25 nmol). While cSAgAmix was much safer than hSAgAmix (5), the hp-modified peptidemix was surprisingly also significantly less anaphylactogenic than its ao-modified counterpart (Figure 1C). Importantly, both SAgA forms were safer than their respective free peptides in delaying anaphylaxis (Figures 1A, C), underlining the safety advantage of SAgAs.
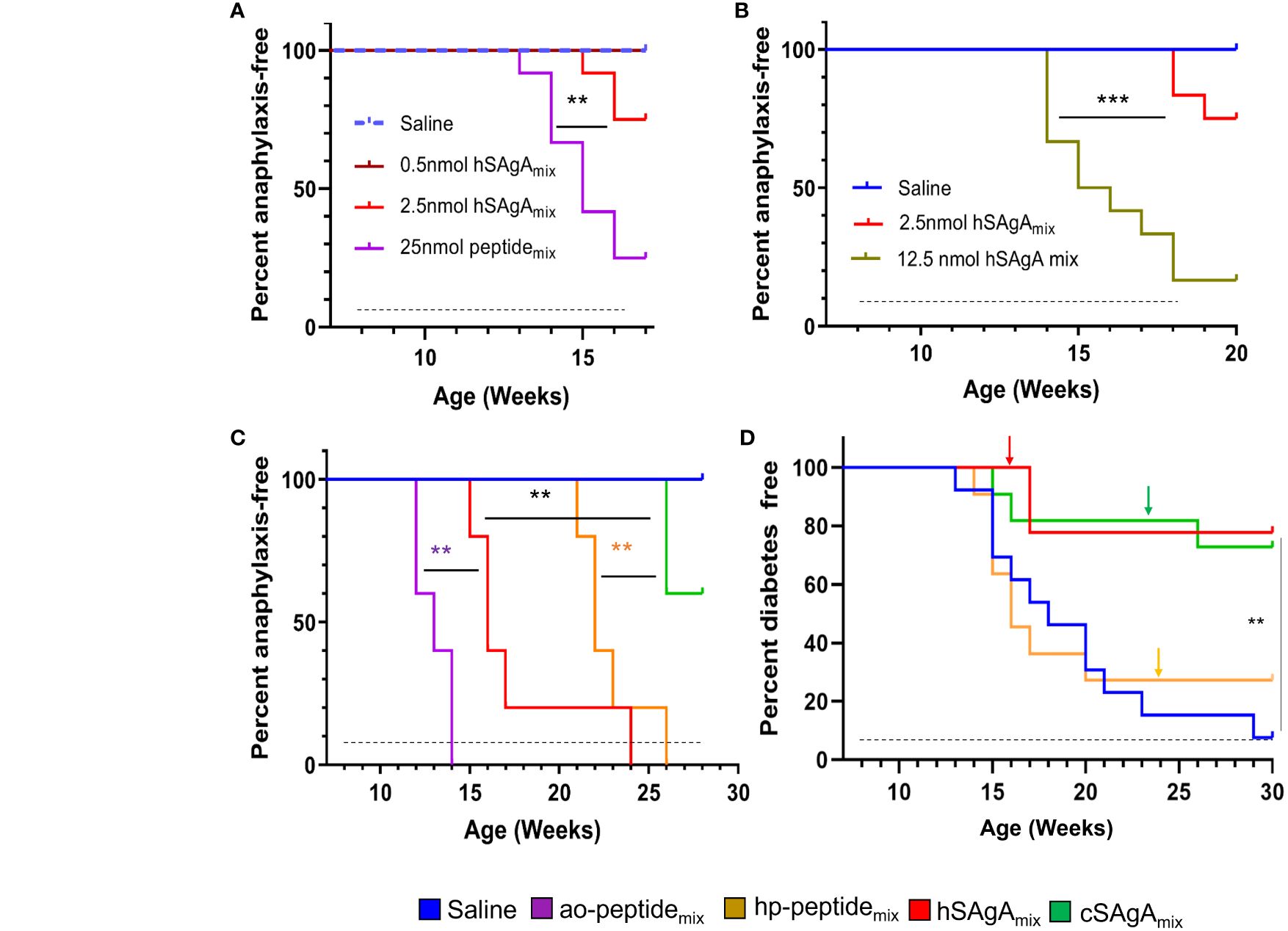
Figure 1 Incidence of anaphylaxis and diabetes in NOD mice. SAgAs mitigate anaphylaxis caused by free peptides in dose- and formulation-dependent manner (A–C). (A) Mice were treated with different doses of hSAgAmix (n=12), ao-peptidemix (n=12), or saline (n=12). (B) Incidence of anaphylaxis in mice treated with saline (n=12), 2.5 nmol (n=12), or 12.5 nmol (n=12) dose of hSAgAmix. (C) Comparison of incidence of anaphylaxis in mice treated with saline (n=5), hSAgAmix, and cSAgAmix (2.5 nmol, n=5 mice/group) and their respective peptidemix (ao and hp; 25 nmol, n=5 mice/group). (D) Incidence of diabetes in mice treated with saline (n=13), hp-peptidemix (25 nmol, n=13), hSAgAmix, or cSAgAmix (2.5 nmol, n=13). Arrows indicate tapering of the dose to 5 nmol each (peptidemix) or 0.5 nmol each (SAgAmix). In all panels, the dashed lines indicate the treatment period (weekly injections). Statistical analysis was performed using log-rank test for all panels. ** p<0.01, *** p<0.001.
SAgAs, but not free peptides, efficiently block the development of autoimmune diabetes
In our previous study (5), the efficacy of the free peptides was not evaluated, nor was a side-by-side comparison of hSAgAmix versus cSAgAmix conducted. Given that the non-anaphylactogenic 0.5 nmol dose of SAgA was not significantly protective when given from the beginning (5), we assessed the therapeutic efficacy of SAgAs and peptides in preventing diabetes in NOD mice using the 2.5 nmol dose but tapering to 0.5 nmol at the first sign of (or prior to) anaphylaxis (Figure 1D). The time of tapering (indicated by arrows) was consistent with the incidence of anaphylaxis assessed separately (Figure 1C), and we tapered cSAgAmix at the same time as its free peptide equivalent for better comparison, even though no sign of anaphylaxis was observed at that time. No anaphylaxis was observed in any of the treatment groups following dose tapering. Our results indicate that (1) these peptides (p79 and 2.5HIP) in free form do not confer any protection (already evident before dose tapering) but efficiently block the disease in SAgA form (2), hSAgA and cSAgA have a comparable efficacy if anaphylaxis is averted, and (3) dose tapering does not result in substantial loss of protection, but it efficiently overcame anaphylaxis. Of note, we excluded ao-modified peptides in this study due to their high anaphylactogenic nature. These data underline the therapeutic advantage of SAgAs as a peptide delivery modality over the free soluble form.
The nature and dynamics of antigen-specific T cell responses depend on the mode of delivery and dosing period
Using adoptive transfer of antigen-specific T cells, we previously showed that SAgAs were more stimulatory than their corresponding free peptides at the same dose in vivo, inducing higher expression of anergy markers and more IL-10 than IFN-γ production, which was mostly contributed by the response to the p79 mimotope (5). We sought to investigate additional aspects of the T cell response for further insights into the mechanism of action. Using the same model and following a single dose of cSAgAmix or hSAgAmix (0.5 nmol each) or free soluble ao-peptidemix (5 nmol each), the ex vivo recall response of transferred BDC2.5 CD4+ T cells was assessed 3 days after treatment by restimulating splenocytes with the ao-p79 or ao-2.5HIP peptides at 5 nM or their mix at 2.5 nM each. Analysis of culture supernatants 3 days later revealed the presence of substantial levels of Th2 cytokines (IL-4, IL-5, IL-10, IL-13), some Th1 cytokines (IFN-γ, TNF-α), as well as IL-6 and IL-22 induced by SAgAs (hSAgAmix most prominently), but not by the free peptides (Supplementary Figure S1). Cytokine production was consistently and significantly higher with hSAgAmix treatment than with cSAgAmix, except for IL-22, where cSAgAmix induced the same or higher levels (Supplementary Figure S1). When a five-times lower dose was used (0.1 nmol SAgAmix, 1 nmol free peptides) under the same conditions, no cytokine response was detected upon peptide recall ex vivo (data not shown).
T cell responses are expected to evolve over the course of repeated antigen administrations and continuous exposure to the delivered epitopes. When assessing the response of endogenous T cells with MHC tetramers, cSAgAmix induced a higher frequency of CD73+, CD73+ FR4+ (anergic cells), PD-1+, IL-10+, and IL-10+ IFN-γ + T cells among p79-reactive T cells than hSAgAmix (Supplementary Figure S2), while IL-2+ cells among p79-reactive T cells were comparable between cSAgA and hSAgA (Supplementary Figure S3), after two doses (three days apart), similar to responses seen after 3 and 6 weekly doses (5). In contrast, after 23 weeks of continuous weekly dosing, these endogenous T cell responses looked dramatically different. By that time (end of the experiment shown in Figure 1D), the frequency of p79-reactive CD4+ T cells in cSAgAmix-treated mice had returned to levels close to the control group, significantly lower than in hSAgAmix-treated mice (Figure 2A), possibly as a result of deletion or contraction. Surprisingly, these p79-reactive T cells in hp-peptidemix-treated mice reached their highest frequency (Figure 2A), indicating that T cells expand more slowly in response to free peptide than to SAgA. The high expression of CD73, FR4 and PD-1 seen at early time points was no longer observed, except to a limited extent in hSAgAmix-treated mice (Figures 2B–D). While p79-reactive T cells in cSAgAmix-treated mice were found at the lowest frequency out of all antigen-treated groups, these T cells retained the highest frequency of IL-10+ and IL-10+ IFN-γ+ (Figures 2E, F), Foxp3+ (Figure 2G and Supplementary Figure S4A), TIGIT+ and IL-2+ IL-10+ T cells (Supplementary Figures S4B, C). However, expression of IL-2+, TNF-α+ and IL-2+ TNF-α+ on p79-reactive T cells was lower in SAgAmix-treated groups than in hp-peptidemix-treated mice (Supplementary Figures S4D–F). IFN-γ+ (IL-10-) p79-reactive T cells did not change in frequency and were not significantly upregulated by the treatments at both early and late time points (Supplementary Figures S3D, S4G). Finally, expression of Lag3 and KLRG1 followed yet another pattern, with significant expression in mice treated with hp-peptidemix and its SAgA equivalent (cSAgAmix), but not with hSAgAmix (Supplementary Figures S4H, I).
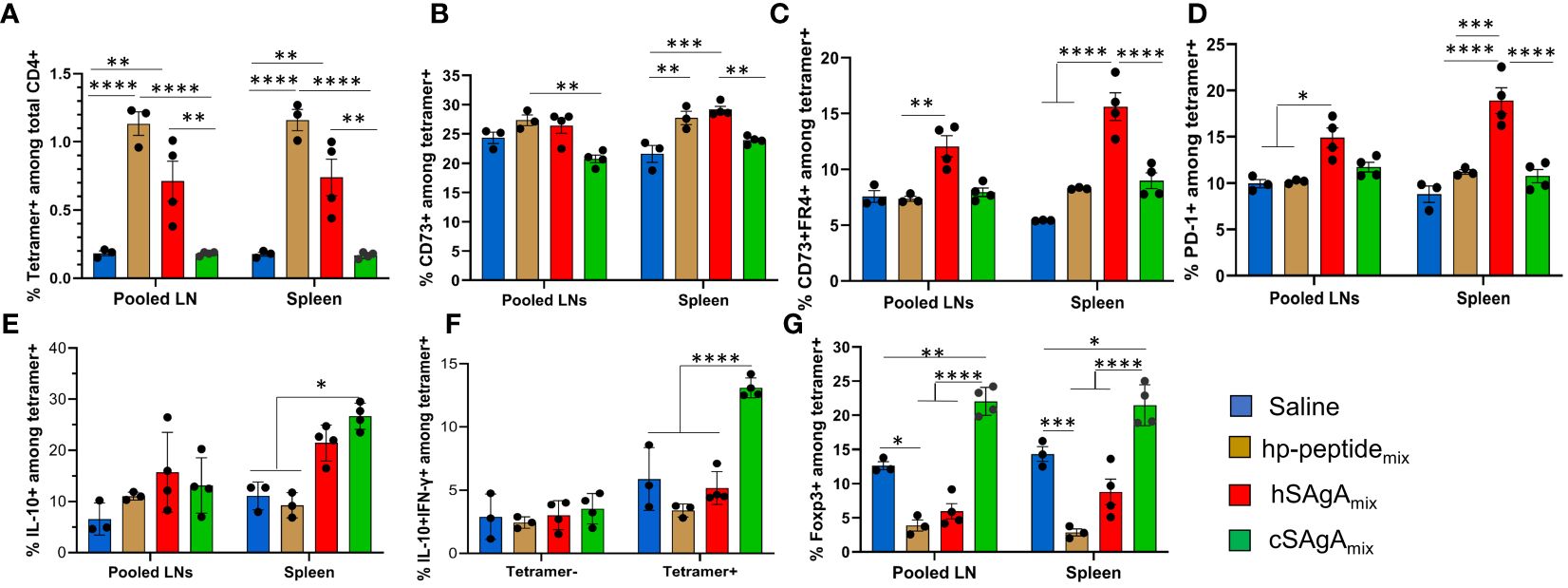
Figure 2 Comparison of polyclonal p79-reactive T cell responses between different modes of peptide delivery. T cell responses were measured in pooled lymph nodes (LNs) and spleen by flow cytometry after prolonged (23-week) weekly treatment with 2.5 nmol of hSAgAmix or cSAgAmix, or 25 nmol of hp-peptidemix (dose is for each peptide or SAgA (p79 and 2.5HIP) in the mix). Analysis was done on p79-tetramer+ CD4+ T cells and data are presented as: (A) % p79-tetramer+ cells among total CD4+ T cells, (B) % CD73+, (C) % CD73+/FR4+, (D) % PD-1+, (E) % IL-10+, (F) % IL-10+ IFN-γ+, and (G) % Foxp3+ among p79-tetramer+ CD4+ T cells. Data show the mean ± SEM from 3–4 mice per group. Statistical analysis was performed using two-way ANOVA/Tukey for all panels. * p<0.05, ** p<0.01, *** p<0.001, **** p<0.0001.
Targets and mechanisms of the anaphylactic reaction to peptides
We also investigated why the different peptide delivery modalities led to very different kinetics of anaphylactic reactions (Figure 1C) and the mechanisms underlying these responses. We initially encountered this adverse effect when treating with hSAgAmix. Thus, to assess the target and type of anaphylactic response in an amplified and accelerated manner, we treated NOD mice with 10 nmol hSAgAmix (a dose at which most mice developed anaphylaxis after only 5 weekly injections). Complete blood counts performed at the fifth dose indicate a dramatic elevation of all major leukocyte populations in the blood (Figure 3A). Serum collected at 0, 2 and 4 weeks’ time points was analyzed by multiplex assays to determine the level of different immunoglobulin isotypes. While levels of total IgG2a, IgG2b, IgG3 and IgM remained unchanged, IgG1 levels were significantly elevated after only two doses, and IgE levels significantly increased after four doses (Figure 3B). With another cohort of mice adding B6 and BALB/c mice as control, we demonstrated a significant increase in mast cell degranulation – a hallmark of anaphylaxis – in the skin and small intestine of NOD mice compared to the control strains following 5 weekly injections of 2.5 nmol hSAgAmix (Figures 3C, D). NOD mice showed signs of anaphylaxis after 4–5 injections, while the control strains did not. To assess the specificity of the antibodies elicited at the 10 nmol dose (Figure 3B), we performed ELISA using adsorbed ao-p79 or ao-2.5HIP peptides, followed by detection of antigen-specific IgG1 or IgE. Detection of anti-p79 IgG1 was evident at high serum dilutions, whereas that of anti-2.5HIP IgG1 required more concentrated serum (about 25x) (Figure 3E). However, low anti-2.5HIP IgG1 levels were highly variable and appear to preexist naturally as they were also detected in the untreated control group (Figure 3E), which is consistent with the fact that 2.5HIP is a naturally occurring epitope while p79 is an artificial mimotope. Interestingly, the later IgE response was directed at neither p79 nor 2.5HIP (Figure 3F). These data initially suggested that the anaphylactic response may be primarily driven by an anti-p79 IgG1 response, which may have been facilitated by an early anti-p79 Th2 response to hSAgAmix (Supplementary Figure S1). After subsequently observing that the free peptides led to even more severe and accelerated anaphylaxis, we repeated these studies to include all the treatment groups (Figure 1C) and using the same therapeutic dose as in preclinical studies (2.5 nmol SAgA or 25 nmol free peptide). Unexpectedly, both hSAgAmix and cSAgAmix led to significantly higher total IgG1 and IgE levels than the free peptides (ao- and hp-peptidemix) after dosing for 6 weeks (Figures 3G–J), whereas total IgG2a, IgG2b, IgG3 and IgM levels remained unchanged as before (data not shown). Moreover, both hSAgAmix and cSAgAmix induced higher levels of anti-p79 and anti-2.5HIP IgG1 than their corresponding free peptides (though not significantly, likely due to the lower (2.5 nmol) dose used) (Figures 3K, L). As before, no peptide-specific IgE was observed in all groups. Peptide-specific IgG2a levels were very low (expected, as IgG2c is the expressed isotype in NOD mice) while peptide-specific IgG2b, IgG3 and IgM were not measured. Fewer time points could be assessed for hSAgAmix and ao-peptidemix, due to the faster onset of anaphylaxis. These data indicate that the levels of anti-p79 and anti-2.5HIP IgG1, being greater with SAgA than with free peptide treatment, neither predict nor explain the occurrence of anaphylaxis in NOD mice.
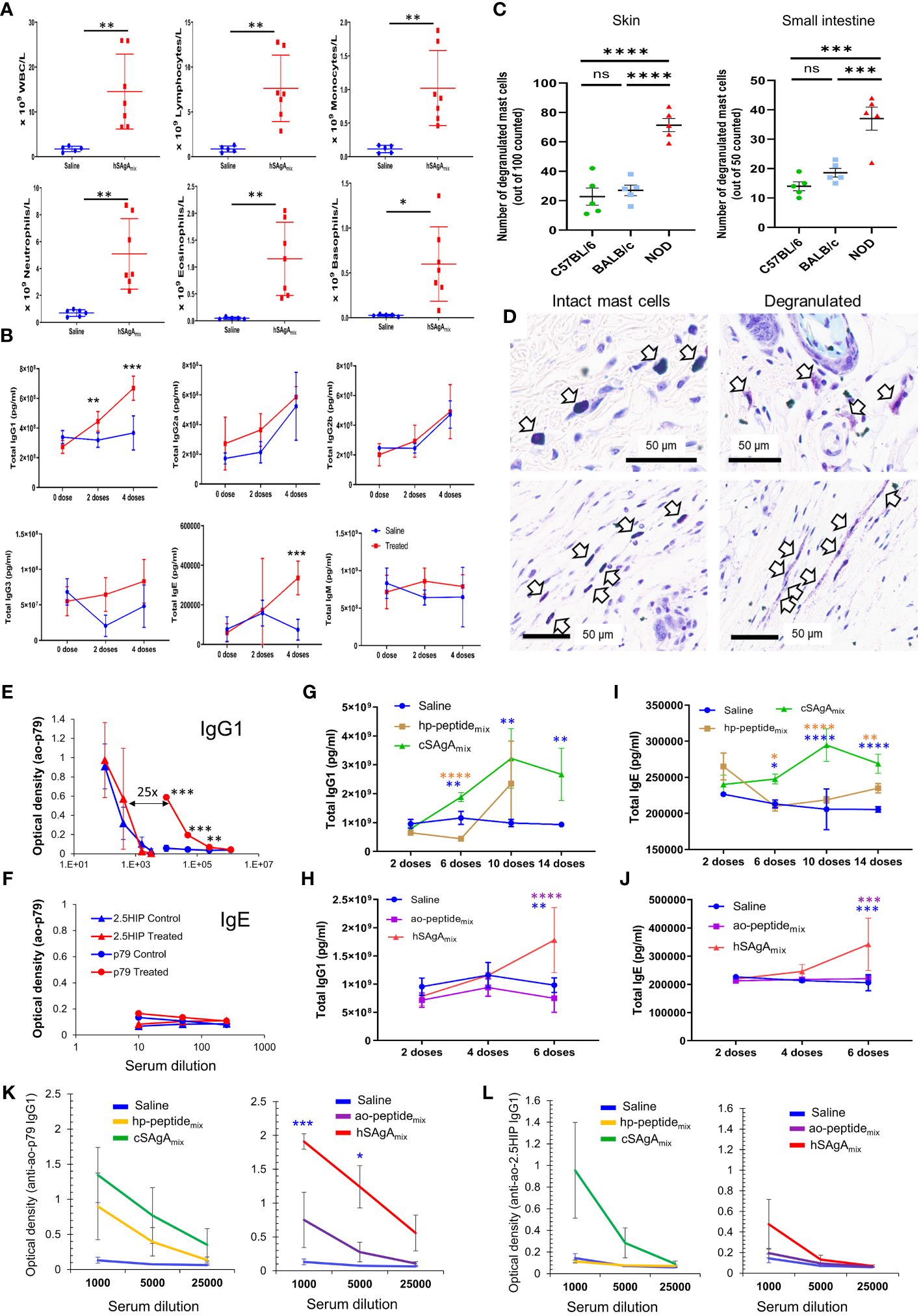
Figure 3 Characterization of the anaphylactic reaction to delivered peptides. (A) Changes in immune cell populations in the blood of mice developing anaphylaxis after 4 weekly doses of hSAgAmix at 10 nmol each. (B) Levels of total IgG1, IgG2a, IgG2b, IgG3, IgE and IgM at baseline and after 2 and 4 weekly doses of hSAgAmix (10 nmol each). No IgA was detected in all groups. Data in (A, B) show the mean ± SD from n=5 mice (control) and n=7 mice (treated). (C) Mast cell degranulation in the skin and small intestine of NOD, BALB/c and B6 mice treated with 5 doses of hSAgAmix at 2.5 nmol. Data show the mean ± SEM of degranulated mast cells per 100 (skin) or 50 (small intestine) mast cells (n=5 mice per group). (D) Representative toluidine blue histology of intact mast cells from B6 skin (left) and degranulated mast cells from NOD skin (right). (E, F) Relative levels of IgG1 (E) and IgE (F) specific for 2.5HIP or p79 peptides with or without treatment with hSAgAmix (4 weekly doses at 10 nmol each). Data show the mean ± SD from n=3 mice per group. (G–J) Comparison of total antibody isotype levels induced by both forms of SAgA and their corresponding peptides using the therapeutic dose of 2.5 nmol SAgA or 25 nmol free peptide as in preclinical studies and following the indicated number of doses. Total IgG1 (G, H) and IgE (I, J) were measured at 50,000- and 200-fold serum dilution, respectively. Other isotypes (IgG2a, IgG2b, IgG3 and IgM) were not changed at the former serum dilution. Data from cSAgAs/hp-peptides (G, I) and hSAgAs/ao-peptides (H, J) were separated for clarity and because of different kinetics of anaphylaxis development. Data show the mean ± SEM of 4–5 biological replicates. (K, L) Anti-p79 IgG1 (K) and anti-2.5HIP IgG1 (L) levels were measured at the indicated serum dilutions following treatment with saline, hp-peptidemix or cSAgAmix (10 doses) or ao-peptidemix or hSAgAmix (6 doses). Data from cSAgAs/hp-peptides and hSAgAs/ao-peptides were separated for clarity. Data show the mean ± SEM from 4–5 biological replicates. Statistical analysis was performed using non-parametric T-test for (A, E, F, K, L), multiple T-test for (B), one-way ANOVA with Tukey correction for (C), and two-way ANOVA/Tukey for (G–J). The color of the star for significance level indicates the group with which the point immediately below the star is compared to. * p<0.05, ** p<0.01, *** p<0.001, **** p<0.0001, ns not significant.
Intriguingly, unlike treatment with hSAgAmix at 2.5 nmol (each), treatment with a single hSAgA (p79 or 2.5HIP at 2.5 nmol) did not lead to anaphylaxis [Figures 4A, (5)]. In order to determine whether this difference was simply a dose issue, we tested the single hSAgAp79 at 5 nmol (a dose equal to both peptides of hSAgAmix combined) and we observed that these mice had an incidence of anaphylaxis comparable to hSAgAmix-treated mice (Figure 4B), as well as comparable levels of total IgG1 (Figure 4C), IgE (Figure 4D), and anti-p79 IgG1 (Figure 4E) after 6 weekly doses. Thus, we identified a threshold between 2.5 nmol and 5 nmol at which both anti-p79 responses and anaphylaxis were enabled, and in hSAgAmix, the 2.5HIP peptide helped p79 (only at 2.5 nmol) reach that threshold.
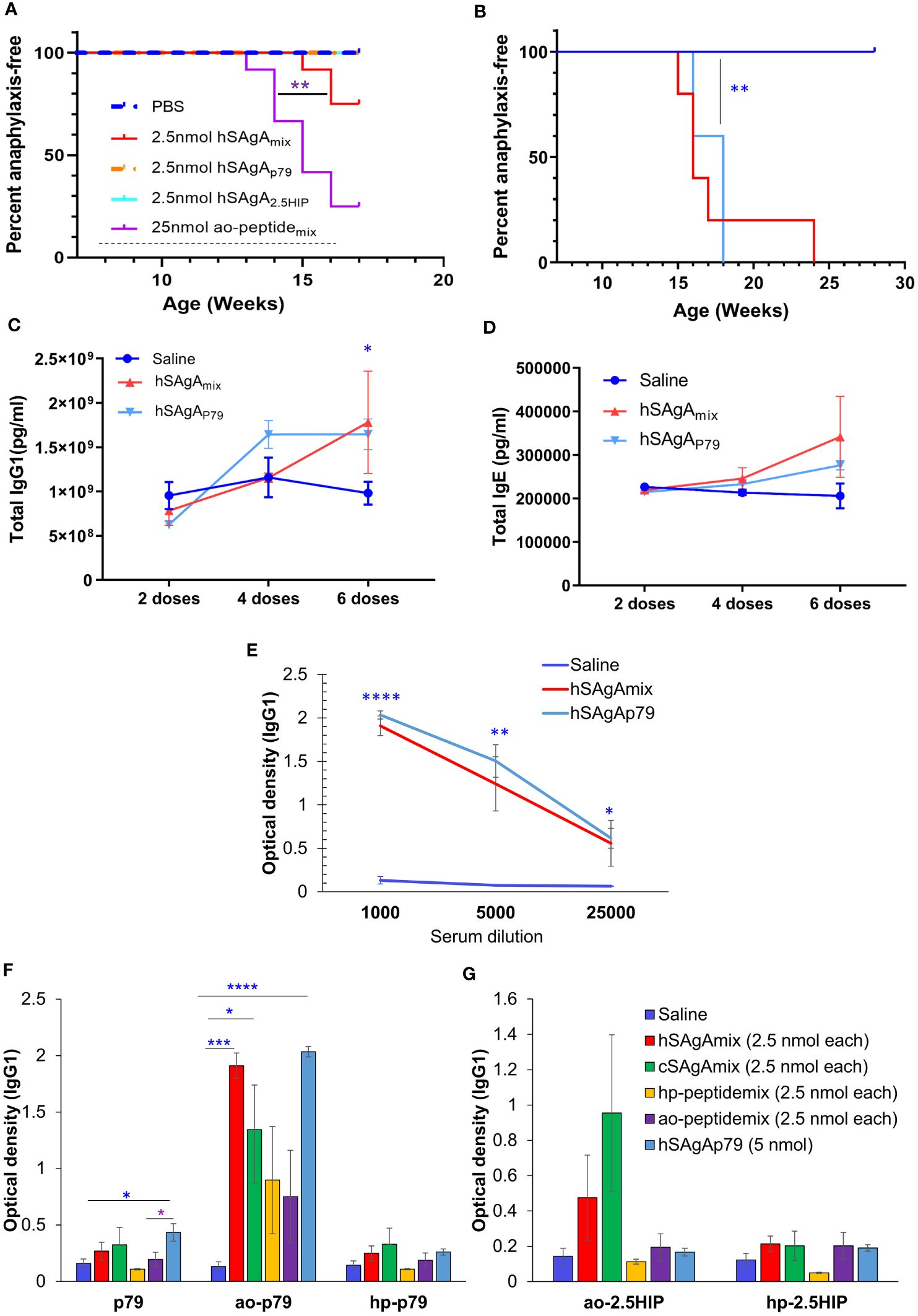
Figure 4 Comparison of anaphylaxis incidence and peptide-specific IgG1 levels between hSAgAp79 and hSAgAmix. (A) Incidence of anaphylaxis induced by a mix of the two hSAgAs (hSAgAmix) or single SAgA carrying p79 or 2.5HIP at the indicated dose (n=12 per group). The dashed line indicates the weekly treatment period. The saline group of this figure was the same as saline group of Figure 1A as both experiments were conducted concurrently. (B) Incidence of anaphylaxis induced by hSAgAmix at 2.5 nmol (each) versus single hSAgAp79 at 5 nmol (n=5 for each group). Mice were treated weekly for the duration of the experiment. (C–E) Total IgG1 (C), total IgE (D) and anti-ao-p79 IgG1 (E) induced by single hSAgAp79 at 5 nmol as compared to hSAgAmix at 2.5 nmol (each). (F, G) Peptide-specific IgG1 reactivity against ao-modified, hp-modified and unmodified p79 (F) and against ao-modified or hp-modified 2.5HIP (G) after 6 weekly doses for hSAgAmix, hSAgAp79, and ao-peptidemix and after 14 weekly doses for cSAgAmix and hp-peptidemix at 1:1000 serum dilution. Data in (C–G) show the mean ± SEM from 4–5 biological replicates. Statistical analysis was performed using log-rank test (A, B), two-way ANOVA/Tukey for (C, D) while non-parametric T-test was applied for (E–G). The blue stars indicate the level of significance when comparing single hSAgAp79 to saline control. * p<0.05, ** p<0.01, *** p<0.001, **** p<0.0001.
Because ao-peptidemix and its corresponding hSAgAmix were the most anaphylactogenic in our treatments, we considered the possibility of a response against the modified portion of the peptide. Thus, p79, ao-p79, hp-p79, ao-2.5HIP and hp-2.5HIP peptides were all assessed as targets in our ELISA assays to detect antigen-specific IgG1 and IgE levels induced by the different treatments. Surprisingly, both anti-p79 and anti-2.5HIP IgG1 levels were more pronounced against the adsorbed ao-modified peptides than the hp-modified peptides or unmodified peptides, regardless of the treatment (Figures 4F, G), while no peptide-specific IgE was detected for all conditions. Peptide modifications also accounted for differences in stimulatory activity: hp-modified p79 was 100x more stimulatory for BDC2.5 T cells than unmodified and ao-modified p79 in vitro [(30), Supplementary Figures S5A, B]. Likewise, hp-modified 2.5HIP was 10x more stimulatory than ao-modified 2.5HIP (Supplementary Figures S5C–F).
Strain-dependent differences in the anaphylactic responses to delivered peptides
Higher frequency of unwanted allergic responses has been associated with autoimmune diseases (36–38), thus we also evaluated our most anaphylactogenic mixes (hSAgAmix at 2.5 nmol and ao-peptidemix at 25 nmol) in the B6 mouse strain, which is not prone to develop autoimmune disease spontaneously. We continuously treated B6 mice with 19 weekly injections, past the time when anaphylaxis had occurred in all NOD mice, and none of them developed any sign of anaphylaxis (Figure 5A). For the last treatment, these mice received a higher dose of 10 nmol hSAgAmix or 100 nmol ao-peptidemix, and still did not show any sign of anaphylaxis. Separately, we tested whether discontinuing the treatment for a prolonged period before resuming may overcome the development of anaphylaxis in NOD mice. NOD mice (n=16) were treated with hSAgAmix at 2.5 nmol with 9 weekly doses until some of them started developing anaphylaxis (6/16). After 5 or 15 weeks of treatment interruption, the remaining NOD mice were rechallenged with a single injection of hSAgAmix at 2.5 nmol and all the mice that received the challenge (n=5 per interruption period) immediately developed fatal anaphylaxis (Figure 5B). Overall, treatment interruption did not appear to reset or delay the development of anaphylaxis, and once the remaining NOD mice were sensitized with 5–9 weekly treatments with hSAgAmix or free ao-peptidemix, they remained so for a prolonged period. In contrast, B6 mice were completely resistant to developing anaphylaxis during the same period of continuous treatment at the same dose, even with longer treatment. In another cohort of mice, we compared the anaphylaxis response to hSAgAmix in NOD, B6 and BALB/c mice. B6 mice had higher basal levels of total IgG1, IgG2a, IgG2b, IgG3, IgM and IgA than NOD or BALB/c mice, but the levels did not change during the course of 5 weekly injections with 2.5 nmol hSAgAmix (Supplementary Figure S6). Likewise, the levels of IgE did not change. BALB/c mice had high basal levels of IgE, which also did not change during treatment. In contrast, levels of total IgG1, IgG2a, IgG2b, IgG3 and IgM marginally increased during treatment (Supplementary Figure S6). In NOD mice, only total IgG1 levels increased during treatment (Supplementary Figure S6). For peptide-specific antibodies, we measured IgG1 and IgG2c isotypes. Anti-p79 and anti-2.5HIP IgG1 levels were significantly increased during treatment in NOD mice and not in the other two strains (Figures 5C, D). Serum samples were collected during the course of treatment and tested for IFN-γ, TNF-α, IL-4, IL-5, IL-6, IL-10, IL-13, IL-21, and IL-22. None of these cytokines were detected in NOD mice despite development of anaphylaxis after 4–5 doses (Supplementary Table 1). All BALB/c mice had detectable serum IL-5 after 4 doses. Most cytokines were detected in some of the B6 mice, even prior to treatment, consistent with the higher baseline of antibodies previously noted, suggesting that these mice may have been more active immunologically.
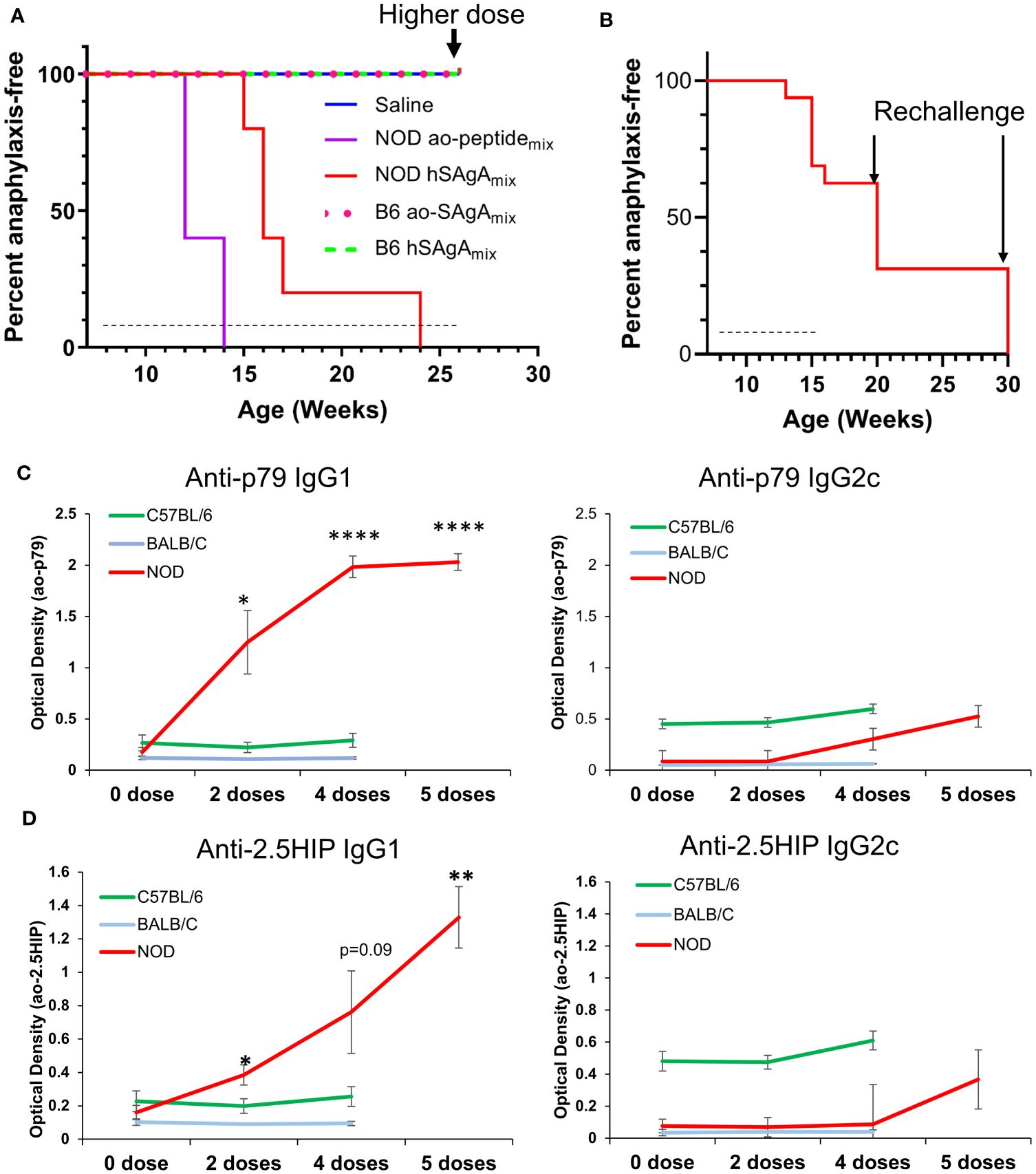
Figure 5 Incidence of anaphylactic reaction to ao-peptidemix and hSAgAmix is strain-dependent. (A) Incidence of anaphylaxis in NOD vs B6 mice (n=5 per strain) treated weekly with a 2.5 nmol dose of hSAgAmix or 25 nmol of the ao-peptidemix or saline. B6 mice also received an injection of a higher dose of 10 nmol hSAgAmix or 100 nmol ao-peptidemix as the final treatment. (B) Incidence of anaphylaxis in NOD mice in which treatment was resumed after a prolonged pause. Mice were initially treated with 2.5 nmol hSAgAmix weekly (for 8 weeks), and treatment was discontinued when some mice (n=6) succumbed to anaphylaxis. Among the remaining 10 mice, 5 were treated again after 5 weeks with a single injection of hSAgAmix at 2.5 nmol, and the other 5 were treated likewise after 15 weeks. In both cases, all mice developed fatal anaphylaxis. Dashed lines indicate the period of weekly treatments, arrows indicate a time point at which mice were rechallenged with a single injection of hSAgAmix at 10 nmol (A) or 2.5 nmol (B). (C, D) Relative levels of anti-p79 (C) and anti-2.5HIP (D) IgG1 and IgG2c in 1:100 diluted serum. The significance of increase was assessed between baseline level (0 dose) and various number of doses (one-way ANOVA). For each peptide, all measurements were done on the same plate, which could not accommodate the 5th dose of B6 and BALB/c mice * p<0.05, ** p<0.01, **** p<0.0001).
Discussion
SAgAs constitute a versatile peptide delivery modality that overcomes many inherent limitations of peptide-based immunotherapy to induce immune tolerance through different mechanisms involving T cells and B cells (5, 16, 28–31, 39, 40). In this study, we showed that the efficacy and safety of peptide-based immunotherapy may depend on the use of desirable peptide modifications and/or the type of delivery modality. While both SAgA variants efficiently protected NOD mice from developing T1D, their corresponding soluble peptides failed to confer any protection at equivalent doses, showing the critical role of this delivery modality and/or HA as a potential immunomodulator to achieve therapeutic efficacy. Once immune tolerance was established by an initial high dose (2.5 nmol) of SAgAs given for several weeks, a later dose tapering (0.5 nmol) was sufficient to maintain protection and abrogate the risk of anaphylaxis. As expected, the T cell dynamics following the treatment with the two SAgA variants and free soluble peptides were considerably different. Both SAgA variants induced more regulatory or anergic T cell phenotypes (CD73+ FR4+, PD-1+, IL-10+) and significantly less IL-2+ and TNF-α+ antigen-specific T cells than free peptides after prolonged treatment. In fact, the T cell responses induced by the latter, at a time of considerable clonal expansion, were more of an effector phenotype, with distinct upregulation of KLRG1, lack of anergy, exhaustion and regulatory markers upregulation, unlike what was seen with SAgAs when T cells were expanding (5). Overall, cSAgAs induced stronger initial immune responses than hSAgA variants or their free peptides, and its continued weekly dosing may have resulted in conversion of autoreactive T cells into regulatory T cells and/or their deletion, as evidenced by the low frequency of tetramer+ T cells, with a higher proportion of those now expressing Foxp3. hSAgAs induced weaker initial T cell responses than cSAgAs and their continued weekly dosing may have maintained anergic and/or IL-10+ T cells that protected the mice from T1D. In contrast, the free soluble peptides induced a weak response initially, but its continuous administration eventually resulted in increased frequency of antigen-specific T cells, which adopted a more classic effector phenotype and had no effect on disease progression in NOD mice. This weaker response to the free peptide in vivo was also evident from the cytokine analysis data with no detectable levels beyond the control at early time point following adoptive transfer of the transgenic BDC2.5 CD4+ T cells and ex vivo restimulation of splenocytes. On the contrary, recall response by splenocytes from mice treated with SAgAmix (particularly hSAgAmix) resulted in significantly higher production of Th2 cytokines as compared to the free ao-peptide or saline control, which may have also contributed to the protection. Interestingly, Lag3 expression on p79-reactive T cells was prominent in response to both hp-peptide and cSAgAs, suggesting that the greater stimulatory capacity conferred by the hp modification may influence Lag3 induction. Thus, the phenotype of antigen-specific T cells after a few administrations versus prolonged treatment differed substantially and was also dependent on the type of delivery modality used.
Modification of peptides was needed for SAgA formulations, and we unexpectedly found that the hp-modification improved both the potency and safety of the peptides. The hp-modified peptides were more stimulatory than their corresponding ao-modified peptides or unmodified p79 form at equivalent concentrations. In line with this, we previously showed that hp-p79 and ao-p79 differed in secondary structures, the former having a more hydrophobic modification (30). Thus, this secondary structure difference between the two modified peptides might alter the fitting and orientation of the peptides on the MHC groove for presentation to T cells. Whether this effect of hp modification on improving the peptide’s stimulatory potency could be generalizable to peptides restricted to other MHC II haplotypes/HLA alleles remains to be addressed. Moreover, hp-functionalized peptides and their SAgA form (cSAgAmix) significantly delayed the incidence of immunologic anaphylaxis as compared to the ao-functionalized peptides and hSAgAmix, pointing to a role of hp-peptide modification in minimizing the development of anaphylaxis. The lower levels of Th2 cytokines induced by cSAgAmix compared to hSAgAmix may provide one explanation for the lower anaphylactogenic potential of cSAgAmix. Indeed, a Th2 cytokine profile has been associated with a risk of developing anaphylactic reactivity to therapeutic peptides although shifting the antigen-specific immune response towards Th2 is one mechanism by which several ASITs were shown to suppress the progression of autoimmunity in mice (38, 41–43).
Systemic anaphylaxis is rapid in onset and features potentially life-threatening immune reactions mediated by immunologic or non-immunologic causes (7, 27, 44). The mechanism responsible for most cases of anaphylaxis in humans involves the classic pathway which is mediated by IgE engagement with high-affinity Fc receptor (FcϵRI) on mast cells and basophils, thereby inducing the release of inflammatory mediators (45). In humans, it may also be mediated by IgE and IgG1 combined (45, 46). For the alternative pathway, which is IgG-mediated and mainly reported in rodent models, platelet-activating factor, rather than histamine, is an important mediator in actively immunized mice and is released by basophils, monocytes/macrophages and neutrophils activated via their Fc gamma receptors (FcγRs) (46, 47). In this study, we report an antibody response that was consistently directed at p79 (an artificial mimotope), and in some cases at 2.5HIP (a natural β-cell neoepitope). Sudden exposure to large amounts of an artificial mimotope may explain stronger B cell reactivity, although anaphylactic reactions have also been reported in NOD mice injected with natural islet peptides (48, 49). Paradoxically, the two SAgA variants were associated with elevated levels of peptide-specific IgG1, total IgG1 and IgE, and Th2 cytokines relative to the free peptides, but were less anaphylactogenic. This was surprising given that whole allergen and multivalent antigens are more likely, for example, to crosslink IgE and induce mast cell and basophil degranulation and to provide 3D conformations that are optimal to function as B cell epitopes compared to soluble peptides (50, 51). SAgAs are more stimulatory than free peptides at equivalent doses, in part by increasing peptide load per cell and, in turn, the avidity of antigen recognition by T cells. We postulated that SAgAs may consequently induce more Th1-associated peptide-specific IgG2a (IgG2c in NOD and B6 mice [52)] that would outcompete peptide-specific IgG1, thereby reducing their anaphylactogenic potential. However, both SAgAs and peptides induced much lower levels of peptide-specific IgG2c than IgG1, making it difficult to reconcile the high anaphylaxis incidence of free peptides with their reduced or absent induction of immunoglobulins and cytokine responses. Alternatively, the higher frequency of antigen-specific IL-10+ regulatory T cells induced by SAgAs may contribute to a better control of anaphylactic responses in the long-term (53, 54). It should be noted that in humans, IgG4 and IgE are associated with Th2 responses, while IgG1 and IgG3 with Th1 and/or Th17 responses (55), and IgG2 may be T cell-independent (56).
Unlike NOD mice, we found B6 mice to be completely resistant to anaphylaxis induced by hSAgAmix or the ao-peptidemix even after an extended dosing period (19 weeks) and the use of 4 times higher dose for rechallenge. BALB/c mice also did not show signs of anaphylaxis during a 5-week treatment with hSAgAmix, though we cannot exclude a delayed incidence. The most plausible (and perhaps only) explanation for the resistance of the control strains is the lack of or poor binding of the studied peptides to the MHC class II molecules of these strains, minimizing T cell activation and B cell help. Interestingly, however, strains vary in their ability to develop anaphylaxis in response to the same antigen, and in some cases, NOD mice showed much greater susceptibility to developing anaphylaxis than common mouse strains, including B6 and BALB/c mice (43, 57), and even diabetes-resistant NOR mice that share the same MHC class II (57). Granted, these were responses to proteins, not peptides, which enable T cell responses to diverse epitopes in all strains; yet this reveals an interesting peculiarity of the NOD strain that could influence the direction of immune responses. Studies in the mouse model of multiple sclerosis indicated that anaphylactic reactions may be elicited with self-peptides that are not presented in the thymus, indicating that a lack of central tolerance, and possibly of thymic regulatory T cells, may create permissible conditions for anaphylaxis (38). Such lack of central tolerance is likely to apply to the p79 and 2.5HIP epitopes used in our studies. Independent from antigen presentation, chemically modified peptides may directly bind to and activate mast cells (58), which could provide an explanation for the greater anaphylactogenic potential of the free peptides, as their binding would be hindered in SAgA form. However, this does not seem to be the case, as these peptides had no effect in the control mice. Therefore, we propose that autoimmune strains/individuals may be more prone than others in developing anaphylactic reactions to peptides in ASIT, in part due to susceptible MHC alleles and impaired T cell regulation. As novel antigenic peptides are evaluated in ASIT, it is ineluctable that some may cause anaphylaxis, and thus, delivery via SAgA offers an additional safety advantage in mitigating this risk.
Data availability statement
The original contributions presented in the study are included in the article/Supplementary Material. Further inquiries can be directed to the corresponding authors.
Ethics statement
The animal study was approved by Columbia University Institutional Animal Care and Use Committee. The study was conducted in accordance with the local legislation and institutional requirements.
Author contributions
RF-F: Conceptualization, Formal analysis, Investigation, Methodology, Writing – original draft. SJ: Resources, Writing – review & editing. CBG: Formal analysis, Investigation, Writing – review & editing. ML: Resources, Writing – review & editing. AK: Formal analysis, Writing – review & editing. FOG: Formal analysis, Writing – review & editing. JM: Methodology, Writing – review & editing. JS: Funding acquisition, Resources, Writing – review & editing. CB: Conceptualization, Funding acquisition, Resources, Writing – review & editing. RC: Conceptualization, Funding acquisition, Methodology, Supervision, Writing – review & editing.
Funding
The author(s) declare financial support was received for the research, authorship, and/or publication of this article. RF-F was funded by postdoctoral fellowship 1-19-PMF-022 from the American Diabetes Association. SJ and ML were supported by the National Institutes of Health Graduate Training Program in Dynamic Aspects of Chemical Biology Grant (T32GM008545) from the National Institute of General Medical Sciences. CBG was supported by a Berrie Fellow in Diabetes Research award. These studies were originally funded by Juvenile Diabetes Research Foundation (JDRF) grant 2-SRA-2017-312-S-B to JS. RF-F and RC were also supported by grant R01DK127778. Research reported in this publication was performed using the Flow Cytometry Core of the Columbia Center for Translational Immunology, supported in part by awards S10RR027050, S10OD020056, P30CA013696 and P30DK063608, and the Molecular Pathology Shared Resource of the Herbert Irving Comprehensive Cancer Center, supported by award P30CA013696.
Acknowledgments
We thank the National Institutes of Health Tetramer Core Facility, supported by contract HHSN272201300006C from the National Institute of Allergy and Infectious Diseases, for the MHC tetramer used in these studies.
Conflict of interest
The authors declare that the research was conducted in the absence of any commercial or financial relationships that could be construed as a potential conflict of interest.
Publisher’s note
All claims expressed in this article are solely those of the authors and do not necessarily represent those of their affiliated organizations, or those of the publisher, the editors and the reviewers. Any product that may be evaluated in this article, or claim that may be made by its manufacturer, is not guaranteed or endorsed by the publisher.
Supplementary material
The Supplementary Material for this article can be found online at: https://www.frontiersin.org/articles/10.3389/fimmu.2024.1258369/full#supplementary-material
References
1. Skwarczynski M, Toth I. Peptide-based synthetic vaccines. Chem Sci. (2016) 7:842–54. doi: 10.1039/C5SC03892H
2. Crawford F, Stadinski B, Jin N, Michels A, Nakayama M, Pratt P, et al. Specificity and detection of insulin-reactive cd4+ T cells in type 1 diabetes in the nonobese diabetic (Nod) mouse. Proc Natl Acad Sci U.S.A. (2011) 108:16729–34. doi: 10.1073/pnas.1113954108
3. Firdessa-Fite R, Bechi Genzano C, Mallone R, Creusot RJ. Epitope-based precision immunotherapy of type 1 diabetes. Hum Vaccines Immunotherapeutics. (2023) 19(1):2154098. doi: 10.1080/21645515.2022.2154098
4. Di L. Strategic approaches to optimizing peptide adme properties. AAPS J. (2015) 17:134–43. doi: 10.1208/s12248–014-9687–3
5. Firdessa-Fite R, Johnson SN, Leon MA, Khosravi-Maharlooei M, Baker RL, Sestak JO, et al. Soluble antigen arrays efficiently deliver peptides and arrest spontaneous autoimmune diabetes. Diabetes. (2021) 70:1334–46. doi: 10.2337/db20–0845
6. Liu E, Moriyama H, Abiru N, Paronen J, Devendra D, Finkelman FD, et al. Preventing peptide-induced anaphylaxis: addition of C-terminal amino acids to produce a neutral isoelectric point. J Allergy Clin Immunol. (2004) 114:607–13. doi: 10.1016/j.jaci.2004.03.052
7. Smith CM, Bradding P, Neill DR, Baxendale H, Felici F, Andrew PW. Novel immunogenic peptides elicit systemic anaphylaxis in mice: implications for peptide vaccines. J Immunol. (2011) 187:1201–6. doi: 10.4049/jimmunol.1002152
8. Musio S, Pedotti P, Mantegazza R, Ohtsu H, Boon L, Steinman L, et al. Anaphylaxis to a self-peptide in the absence of mast cells or histamine. Lab Invest. (2009) 89:398–405. doi: 10.1038/labinvest.2009.4
9. Backlund CM, Holden RL, Moynihan KD, Garafola D, Farquhar C, Mehta NK, et al. Cell-penetrating peptides enhance peptide vaccine accumulation and persistence in lymph nodes to drive immunogenicity. Proc Natl Acad Sci U.S.A. (2022) 119:e2204078119. doi: 10.1073/pnas.2204078119
10. Jamison BL, DiLisio JE, Beard KS, Neef T, Bradley B, Goodman J, et al. Tolerogenic delivery of a hybrid insulin peptide markedly prolongs islet graft survival in the nod mouse. Diabetes. (2022) 71:483–96. doi: 10.2337/db20–1170
11. Bakay M, Pandey R, Grant SFA, Hakonarson H. The genetic contribution to type 1 diabetes. Curr Diabetes Rep. (2019) 19:116. doi: 10.1007/s11892–019-1235–1
12. Serra P, Santamaria P. Peptide-MHC-based nanomedicines for the treatment of autoimmunity: engineering, mechanisms, and diseases. Front Immunol. (2020) 11:621774. doi: 10.3389/fimmu.2020.621774
13. Singha S, Shao K, Yang Y, Clemente-Casares X, Sole P, Clemente A, et al. Peptide-MHC-based nanomedicines for autoimmunity function as T-cell receptor microclustering devices. Nat Nanotechnol. (2017) 12:701–10. doi: 10.1038/nnano.2017.56
14. Lau CYJ, Benne N, Lou B, Braake DT, Bosman E, van Kronenburg N, et al. Tuning surface charges of peptide nanofibers for induction of antigen-specific immune tolerance: an introductory study. J Pharm Sci. (2022). 111(4):1004–1011. doi: 10.1016/j.xphs.2022.01.030
15. Moynihan KD, Holden RL, Mehta NK, Wang C, Karver MR, Dinter J, et al. Enhancement of peptide vaccine immunogenicity by increasing lymphatic drainage and boosting serum stability. Cancer Immunol Res. (2018) 6:1025–38. doi: 10.1158/2326–6066.CIR-17–0607
16. Hartwell BL, Pickens CJ, Leon M, Northrup L, Christopher MA, Griffin JD, et al. Soluble antigen arrays disarm antigen-specific B cells to promote lasting immune tolerance in experimental autoimmune encephalomyelitis. J Autoimmun. (2018) 93:76–88. doi: 10.1016/j.jaut.2018.06.006
17. Quakkelaar ED, Fransen MF, van Maren WW, Vaneman J, Loof NM, van Heiningen SH, et al. Igg-mediated anaphylaxis to a synthetic long peptide vaccine containing a B cell epitope can be avoided by slow-release formulation. J Immunol. (2014) 192:5813–20. doi: 10.4049/jimmunol.1302337
18. Smith CE, Eagar TN, Strominger JL, Miller SD. Differential induction of ige-mediated anaphylaxis after soluble vs. Cell-bound tolerogenic peptide therapy of autoimmune encephalomyelitis. Proc Natl Acad Sci U.S.A. (2005) 102:9595–600. doi: 10.1073/pnas.0504131102
19. Roberts MJ, Bentley MD, Harris JM. Chemistry for peptide and protein pegylation. Adv Drug Delivery Rev. (2002) 54:459–76. doi: 10.1016/s0169–409x(02)00022–4
20. Bird GH, Madani N, Perry AF, Princiotto AM, Supko JG, He X, et al. Hydrocarbon double-stapling remedies the proteolytic instability of a lengthy peptide therapeutic. Proc Natl Acad Sci U.S.A. (2010) 107:14093–8. doi: 10.1073/pnas.1002713107
21. Garton M, Nim S, Stone TA, Wang KE, Deber CM, Kim PM. Method to generate highly stable D-amino acid analogs of bioactive helical peptides using a mirror image of the entire PDB. Proc Natl Acad Sci U.S.A. (2018) 115:1505–10. doi: 10.1073/pnas.1711837115
22. Lombardi A, Concepcion E, Hou H, Arib H, Mezei M, Osman R, et al. Retro-inverso D-peptides as a novel targeted immunotherapy for type 1 diabetes. J Autoimmun. (2020) 115:102543. doi: 10.1016/j.jaut.2020.102543
23. Menacho-Melgar R, Decker JS, Hennigan JN, Lynch MD. A review of lipidation in the development of advanced protein and peptide therapeutics. J Control Release. (2019) 295:1–12. doi: 10.1016/j.jconrel.2018.12.032
24. Kowalczyk R, Harris PWR, Williams GM, Yang SH, Brimble MA. Peptide lipidation - a synthetic strategy to afford peptide based therapeutics. Adv Exp Med Biol. (2017) 1030:185–227. doi: 10.1007/978–3-319–66095-0_9
25. Li M, Itoh A, Xi J, Yu C, Wu Y, Ridgway WM, et al. Enhancing antigen presentation and inducing antigen-specific immune tolerance with amphiphilic peptides. J Immunol. (2021) 207:2051–9. doi: 10.4049/jimmunol.1901301
26. Jin N, Wang Y, Crawford F, White J, Marrack P, Dai S, et al. N-terminal additions to the we14 peptide of chromogranin a create strong autoantigen agonists in type 1 diabetes. Proc Natl Acad Sci U.S.A. (2015) 112:13318–23. doi: 10.1073/pnas.1517862112
27. Leech MD, Chung CY, Culshaw A, Anderton SM. Peptide-based immunotherapy of experimental autoimmune encephalomyelitis without anaphylaxis. Eur J Immunol. (2007) 37:3576–81. doi: 10.1002/eji.200737148
28. Hartwell BL, Martinez-Becerra FJ, Chen J, Shinogle H, Sarnowski M, Moore DS, et al. Antigen-specific binding of multivalent soluble antigen arrays induces receptor clustering and impedes B cell receptor mediated signaling. Biomacromolecules. (2016) 17:710–22. doi: 10.1021/acs.biomac.5b01097
29. Griffin JD, Leon MA, Salash JR, Shao M, Hartwell BL, Pickens CJ, et al. Acute B-cell inhibition by soluble antigen arrays is valency-dependent and predicts immunomodulation in splenocytes. Biomacromolecules. (2019) 20:2115–22. doi: 10.1021/acs.biomac.9b00328
30. Leon MA, Firdessa-Fite R, Ruffalo JK, Pickens CJ, Sestak JO, Creusot RJ, et al. Soluble antigen arrays displaying mimotopes direct the response of diabetogenic T cells. ACS Chem Biol. (2019) 14:1436–48. doi: 10.1021/acschembio.9b00090
31. Hartwell BL, Pickens CJ, Leon M, Berkland C. Multivalent soluble antigen arrays exhibit high avidity binding and modulation of B cell receptor-mediated signaling to drive efficacy against experimental autoimmune encephalomyelitis. Biomacromolecules. (2017) 18:1893–907. doi: 10.1021/acs.biomac.7b00335
32. Anderson MS, Bluestone JA. The nod mouse: A model of immune dysregulation. Annu Rev Immunol. (2005) 23:447–85. doi: 10.1146/annurev.immunol.23.021704.115643
33. Aubin AM, Lombard-Vadnais F, Collin R, Aliesky HA, McLachlan SM, Lesage S. The nod mouse beyond autoimmune diabetes. Front Immunol. (2022) 13:874769. doi: 10.3389/fimmu.2022.874769
34. Joseph LB, Composto GM, Perez RM, Kim HD, Casillas RP, Heindel ND, et al. Sulfur mustard induced mast cell degranulation in mouse skin is inhibited by a novel anti-inflammatory and anticholinergic bifunctional prodrug. Toxicol Lett. (2018) 293:77–81. doi: 10.1016/j.toxlet.2017.11.005
35. Zhang S, Edwards TN, Chaudhri VK, Wu J, Cohen JA, Hirai T, et al. Nonpeptidergic neurons suppress mast cells via glutamate to maintain skin homeostasis. Cell. (2021) 184:2151–66 e16. doi: 10.1016/j.cell.2021.03.002
36. Krishna MT, Subramanian A, Adderley NJ, Zemedikun DT, Gkoutos GV, Nirantharakumar K. Allergic diseases and long-term risk of autoimmune disorders: longitudinal cohort study and cluster analysis. Eur Respir J. (2019) 54(5):1900476. doi: 10.1183/13993003.00476–2019
37. Watanabe Y, Yamaguchi Y. Drug allergy and autoimmune diseases. Allergol Int. (2022) 71:179–84. doi: 10.1016/j.alit.2022.02.001
38. Pedotti R, Mitchell D, Wedemeyer J, Karpuj M, Chabas D, Hattab EM, et al. An unexpected version of horror autotoxicus: anaphylactic shock to a self-peptide. Nat Immunol. (2001) 2:216–22. doi: 10.1038/85266
39. Kuehl C, Thati S, Sullivan B, Sestak J, Thompson M, Siahaan T, et al. Pulmonary administration of soluble antigen arrays is superior to antigen in treatment of experimental autoimmune encephalomyelitis. J Pharm Sci. (2017) 106:3293–302. doi: 10.1016/j.xphs.2017.06.008
40. Pickens CJ, Christopher MA, Leon MA, Pressnall MM, Johnson SN, Thati S, et al. Antigen-drug conjugates as a novel therapeutic class for the treatment of antigen-specific autoimmune disorders. Mol Pharm. (2019) 16:2452–61. doi: 10.1021/acs.molpharmaceut.9b00063
41. Rukma P. Glucagon for refractory anaphylaxis. Am J Ther. (2019) 26:e755–e6. doi: 10.1097/MJT.0000000000000910
42. Kow ASF, Chik A, Soo KM, Khoo LW, Abas F, Tham CL. Identification of soluble mediators in igg-mediated anaphylaxis via fcgamma receptor: A meta-analysis. Front Immunol. (2019) 10:190. doi: 10.3389/fimmu.2019.00190
43. Overbergh L, Decallonne B, Branisteanu DD, Valckx D, Kasran A, Bouillon R, et al. Acute shock induced by antigen vaccination in nod mice. Diabetes. (2003) 52:335–41. doi: 10.2337/diabetes.52.2.335
44. Shaker MS, Wallace DV, Golden DBK, Oppenheimer J, Bernstein JA, Campbell RL, et al. Anaphylaxis-a 2020 practice parameter update, systematic review, and grading of recommendations, assessment, development and evaluation (Grade) analysis. J Allergy Clin Immunol. (2020) 145:1082–123. doi: 10.1016/j.jaci.2020.01.017
45. Jonsson F, de Chaisemartin L, Granger V, Gouel-Cheron A, Gillis CM, Zhu Q, et al. An igG-induced neutrophil activation pathway contributes to human drug-induced anaphylaxis. Sci Transl Med. (2019) 11(500):eaat1479. doi: 10.1126/scitranslmed.aat1479
46. Finkelman FD, Khodoun MV, Strait R. Human ige-independent systemic anaphylaxis. J Allergy Clin Immunol. (2016) 137:1674–80. doi: 10.1016/j.jaci.2016.02.015
47. Khodoun MV, Strait R, Armstrong L, Yanase N, Finkelman FD. Identification of markers that distinguish IgE- from IgG-mediated anaphylaxis. Proc Natl Acad Sci U.S.A. (2011) 108:12413–8. doi: 10.1073/pnas.1105695108
48. Liu E, Moriyama H, Abiru N, Miao D, Yu L, Taylor RM, et al. Anti-peptide autoantibodies and fatal anaphylaxis in nod mice in response to insulin self-peptides B:9–23 and B:13–23. J Clin Invest. (2002) 110:1021–7. doi: 10.1172/JCI15488
49. Pedotti R, Sanna M, Tsai M, DeVoss J, Steinman L, McDevitt H, et al. Severe anaphylactic reactions to glutamic acid decarboxylase (Gad) self peptides in nod mice that spontaneously develop autoimmune type 1 diabetes mellitus. BMC Immunol. (2003) 4:2. doi: 10.1186/1471–2172-4–2
50. Richardson N, Wraith DC. Advancement of antigen-specific immunotherapy: knowledge transfer between allergy and autoimmunity. Immunother Adv. (2021) 1:ltab009. doi: 10.1093/immadv/ltab009
51. Oldfield WL, Kay AB, Larche M. Allergen-derived T cell peptide-induced late asthmatic reactions precede the induction of antigen-specific hyporesponsiveness in atopic allergic asthmatic subjects. J Immunol. (2001) 167:1734–9. doi: 10.4049/jimmunol.167.3.1734
52. Petrushina I, Tran M, Sadzikava N, Ghochikyan A, Vasilevko V, Agadjanyan MG, et al. Importance of IgG2c isotype in the immune response to beta-amyloid in amyloid precursor protein/transgenic mice. Neurosci Lett. (2003) 338:5–8. doi: 10.1016/s0304–3940(02)01357–5
53. Dawicki W, Li C, Town J, Zhang X, Gordon JR. Therapeutic reversal of food allergen sensitivity by mature retinoic acid-differentiated dendritic cell induction of LAG3(+)CD49b(-)Foxp3(-) regulatory T cells. J Allergy Clin Immunol. (2017) 139:1608–20 e3. doi: 10.1016/j.jaci.2016.07.042
54. O'Konek JJ, Landers JJ, Janczak KW, Goel RR, Mondrusov AM, Wong PT, et al. Nanoemulsion adjuvant-driven redirection of T(H)2 immunity inhibits allergic reactions in murine models of peanut allergy. J Allergy Clin Immunol. (2018) 141:2121–31. doi: 10.1016/j.jaci.2018.01.042
55. Kawasaki Y, Suzuki J, Sakai N, Isome M, Nozawa R, Tanji M, et al. Evaluation of T helper-1/-2 balance on the basis of igg subclasses and serum cytokines in children with glomerulonephritis. Am J Kidney Dis. (2004) 44:42–9. doi: 10.1053/j.ajkd.2004.03.029
56. Rijkers GT, Sanders EA, Breukels MA, Zegers BJ. Infant B cell responses to polysaccharide determinants. Vaccine. (1998) 16:1396–400. doi: 10.1016/S0264-410X(98)00098-X
57. Lu Y, Parker M, Pileggi A, Zhang B, Choi YK, Molano RD, et al. Human alpha 1-antitrypsin therapy induces fatal anaphylaxis in non-obese diabetic mice. Clin Exp Immunol. (2008) 154:15–21. doi: 10.1111/j.1365-2249.2008.03721.x
Keywords: soluble antigen array, peptide modification, peptide delivery, immunotherapy, autoimmune diabetes, anaphylaxis
Citation: Firdessa-Fite R, Johnson SN, Bechi Genzano C, Leon MA, Ku A, Ocampo Gonzalez FA, Milner JD, Sestak JO, Berkland C and Creusot RJ (2024) Soluble antigen arrays provide increased efficacy and safety over free peptides for tolerogenic immunotherapy. Front. Immunol. 15:1258369. doi: 10.3389/fimmu.2024.1258369
Received: 13 July 2023; Accepted: 21 May 2024;
Published: 12 June 2024.
Edited by:
Pere Santamaria, University of Calgary, CanadaReviewed by:
Joan Verdaguer Autonell, Universitat de Lleida, SpainNick Giannoukakis, Allegheny Health Network, United States
Copyright © 2024 Firdessa-Fite, Johnson, Bechi Genzano, Leon, Ku, Ocampo Gonzalez, Milner, Sestak, Berkland and Creusot. This is an open-access article distributed under the terms of the Creative Commons Attribution License (CC BY). The use, distribution or reproduction in other forums is permitted, provided the original author(s) and the copyright owner(s) are credited and that the original publication in this journal is cited, in accordance with accepted academic practice. No use, distribution or reproduction is permitted which does not comply with these terms.
*Correspondence: Rebuma Firdessa-Fite, cmVidW1hZmlyZGVzc2EuZml0ZUBlaW5zdGVpbm1lZC5lZHU=; Remi J. Creusot, cmpjMjE1MEBjb2x1bWJpYS5lZHU=
†Present address: Rebuma Firdessa-Fite, Norman Fleischer Institute of Diabetes and Metabolism, Departments of Medicine and Microbiology & Immunology, Albert Einstein College of Medicine, New York, NY, United States Cory Berkland, Department of Biomedical Engineering and Department of Chemistry, Washington University in St Louis, St Louis, MO, United States