- 1General Surgery Center, First Hospital of Jilin University, Changchun, Jilin, China
- 2School of Nursing, Jilin University, Changchun, China
Stroke poses a critical global health challenge, leading to substantial morbidity and mortality. Existing treatments often miss vital timeframes and encounter limitations due to adverse effects, prompting the pursuit of innovative approaches to restore compromised brain function. This review explores the potential of filamentous phages in enhancing stroke recovery. Initially antimicrobial-centric, bacteriophage therapy has evolved into a regenerative solution. We explore the diverse role of filamentous phages in post-stroke neurological restoration, emphasizing their ability to integrate peptides into phage coat proteins, thereby facilitating recovery. Experimental evidence supports their efficacy in alleviating post-stroke complications, immune modulation, and tissue regeneration. However, rigorous clinical validation is essential to address challenges like dosing and administration routes. Additionally, genetic modification enhances their potential as injectable biomaterials for complex brain tissue issues. This review emphasizes innovative strategies and the capacity of filamentous phages to contribute to enhanced stroke recovery, as opposed to serving as standalone treatment, particularly in addressing stroke-induced brain tissue damage.
Introduction
Stroke, an acute cerebrovascular condition that leads to cerebral tissue injury, ranks as the second leading cause of mortality worldwide and the third leading cause of disability. It accounts for over ten million cases annually, with ischemic strokes being predominant (1). The onset of a stroke is primarily attributed to cerebral ischemia or hemorrhage resulting from vascular blockage or rupture (2). Existing treatments rely on timely detection and intervention. Nevertheless, many cases fail to meet this critical timeframe. Moreover, conventional therapies are constrained by various limitations and adverse effects (3). To address these challenges, there is a growing momentum in the search for pioneering medical strategies aimed at restoring compromised brain function (4). Current interventions for acute stroke encompass hyperbaric oxygen therapy, rehabilitative training, and the administration of neurotrophic agents or anti-inflammatory compounds (5) (Table 1). However, these treatments are marred by complications such as oxygen toxicity, barotrauma, and decompression sickness, and their effectiveness in facilitating brain recovery is limited. In the context of post-stroke cerebral tissue rehabilitation, the establishment of a robust vascular network (angiogenesis) emerges as a pivotal factor. This process contributes to the restoration of both endogenous and transplanted neural stem cells (NSCs) and the regeneration of neuronal networks (neurogenesis) (6). However, achieving angiogenesis and neurogenesis in stroke potential therapy for brain regeneration remains a significant challenge. Although specific biomaterials like poly(lactic-co-glycolic acid) (PLGA) microparticles, hyaluronic acid hydrogels, and polymer scaffolds show potential in promoting angiogenesis or neurogenesis in stroke-affected areas, their overall effectiveness remains limited (7). For instance, an injectable dual-function angiogenic hydrogel, incorporating vascular endothelial growth factor but lacking neural stem cells (NSCs), has demonstrated the ability to induce angiogenesis and neuronal growth (8). However, this hydrogel’s potential in fostering extensive axonal growth within the cavity center was hampered due to challenges in cellular infiltration within the compromised brain tissue. Neuronal revival predominantly relied on the migration and differentiation of neuroblasts from the subventricular zone (SVZ). Nonetheless, temporal constraints on neuroblast proliferation within the SVZ and the spatial gap between the SVZ and the stroke lesion limited the effectiveness of this approach (9). Consequently, the demand for novel multifunctional materials becomes evident as a means to achieve more efficacious clinical treatments (10).
The formation of a cavity in brain tissue following a stroke stems from autophagy-induced degradation of the affected region, resulting in the absence of the usual extracellular matrix that supports cellular infiltration and tissue regeneration within the cavity (11). This distinctive cavity presents an ideal location for transplantation in stroke potential therapy (12). A nascent avenue, phage regenerative therapy harnesses phage attributes to facilitate tissue revitalization (13). Bacteriophages, nanostructured viruses capable of infecting and eradicating specific bacteria, have been employed to combat refractory bacterial infections (14) and offer a wide array of applications in the field of biological nanomaterials, presenting themselves as potential alternatives to antibiotics (15). The fusion of biology and materials science through phage display has been instrumental in propelling the advancement of biogenic nanomaterials-a pioneering concept first introduced by Nobel laureate George Smith in 1985 (16). This approach capitalizes on the diversity of phages, resulting in expansive libraries housing numerous clones with distinct peptides, facilitating the precise selection of receptors and enzymes (17). Recent insights extend beyond infection control, revealing bacteriophages’ impact on host immune and metabolic systems, contributing to the mitigation of pathological processes associated with chronic inflammation and metabolic disorders (18). For example, a specific peptide screened from a bacteriophage library, when conjugated to nano-complexes, exhibits optimal inhibition of activated hepatic stellate cell migration and achieves the highest uptake in rat livers, serving as an effective platform for alleviating liver fibrosis (19, 20). These revelations provide both theoretical underpinnings and empirical groundwork for the application of filamentous phage regenerative therapy. Its potential application in enhancing stroke recovery takes center stage, addressing a prevailing neurological disorder stemming from cerebral vascular blockages or ruptures, which induce ischemia and hypoxia. Stroke-triggered brain tissue experiences necrosis, apoptosis, autophagy, and inflammatory reactions that exacerbate damage (21). Filamentous phage regenerative therapy presents a promising pathway for enhancing stroke recovery, unveiling novel mechanisms for safeguarding and restoring post-stroke brain tissue. Bacteriophages showcase varied capabilities, spanning pathogen clearance, immune modulation, promotion of neurogenesis, and enhancement of vascular function (22–24). These combined effects culminate in neuroprotection, diminished edema, reduction in infarct size, and improved neural functionality. Despite its promise, the realm of filamentous phage regenerative therapy is still in its infancy, largely investigated in animal models, yet to undergo clinical trials. Aspects concerning safety, effectiveness, dosage, delivery, and timing demand additional investigation and validation. Challenges encompass selectivity, stability, tolerability, and resistance. Thus, the establishment of the potential therapy’s efficacy in stroke management necessitates meticulous scientific substantiation and technical reinforcement to create a dependable and efficacious treatment approach.
Recent investigations have illuminated the central role of regulatory T cells (Tregs) in orchestrating immune modulation and tissue restoration, infiltrating the aftermath of stroke and rousing microglia via osteopontin (OPN) secretion, thereby igniting the generation of oligodendrocyte progenitor cells (OPCs) and the rejuvenation of myelin sheaths (25). Experimental indications underscore that filamentous phages, operating via these intricate genetic modifications, strengthen neurological function and post-stroke prognosis. The modified filamentous bacteriophage promotes the generation of oligodendrocyte precursor cells, stimulates myelin regeneration, significantly enhances neural function and cognitive abilities, and facilitates neural repair. Additionally, it serves as a potential alternative to autologous nerve transplants for restoring extensive nerve damage (22, 26). These investigations not only underscore the promising potential of phage therapy in the context of enhancing stroke recovery but also emphasize the need for meticulous parameter optimization encompassing aspects like selection, dosing, administration routes, and temporal considerations to ensure heightened safety and efficacy. Furthermore, the attainment of comprehensive clinical trials remains imperative for the validation of its effects within human subjects.
In this review, we navigate through the multifaceted landscape of mechanisms employed in in situ tissue regeneration for stroke potential therapy. We commence by investigating the foundational principles of filamentous phage infective engineering and explore its applications in stroke therapy. Next, we dive into the biopanning techniques applied within the phage display framework, highlighting their significance in identifying therapeutic targets. Our journey continues with an examination of the pioneering utilization of filamentous phage-derived scaffolds for engineering cellular niches, shedding light on their role in fostering tissue regeneration. We delve deeper into the creation of engineered niche scaffolds, enriched with phage peptides, revealing their unique potential in enhancing post-stroke recovery. With this structured approach, we aim to provide a comprehensive insight into the potential of harnessing these mechanisms for enhancing stroke recovery, offering a detailed account of each element’s contribution to the field (Figure 1).
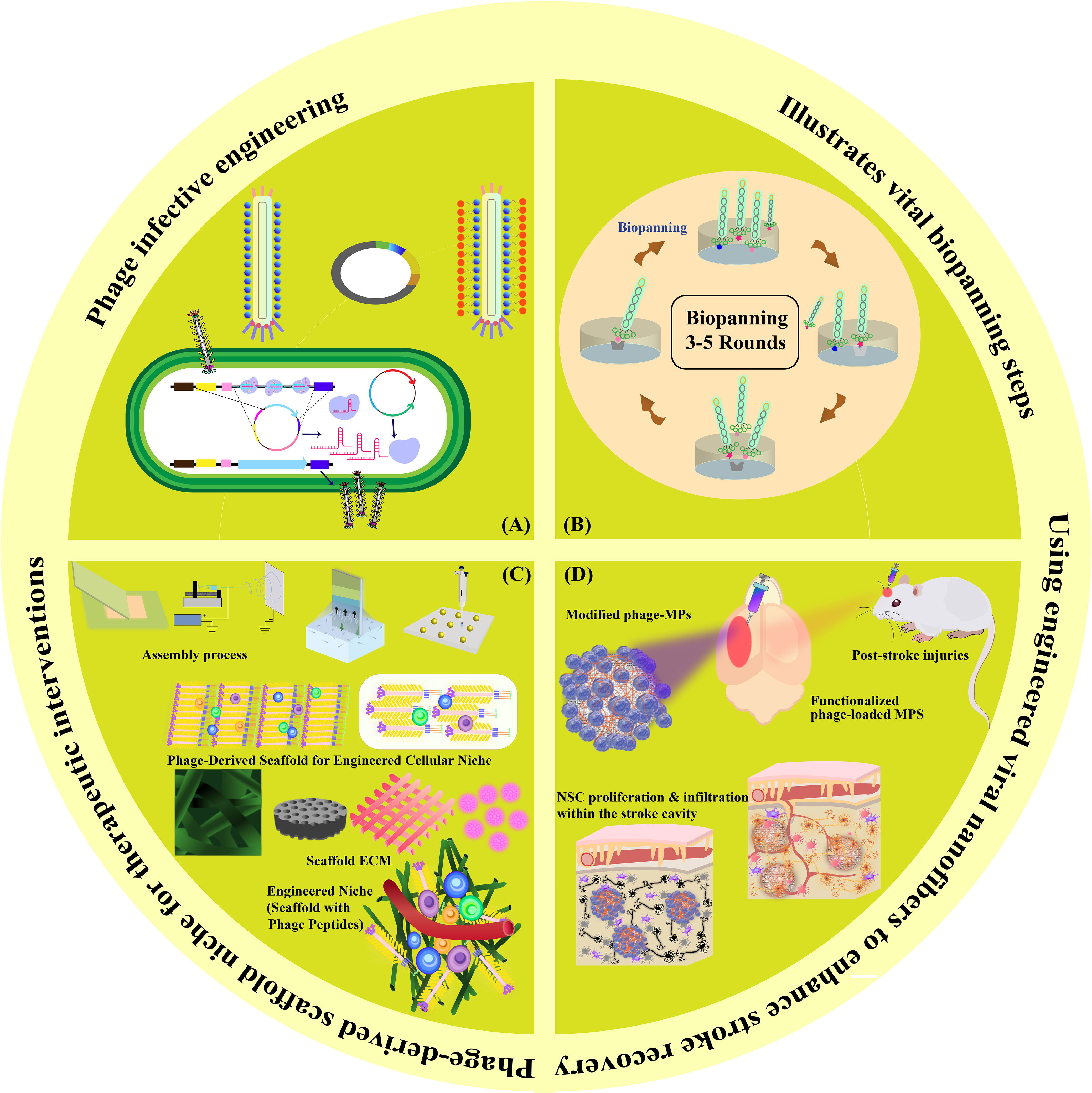
Figure 1 Graphical Abstract. This visual summary provides an overview of the multifaceted mechanisms explored in our review for in situ tissue regeneration in stroke potential therapy. The depiction encapsulates the sequential exploration of phage infective engineering, biopanning techniques in phage display, phage-derived scaffolds for cellular niches, engineered niche scaffolds with phage peptides, and functionalized phage-loaded MPS for NSC proliferation and infiltration within the stroke cavity.
Filamentous bacteriophage potential therapy for stroke recovery
The role of filamentous phages in therapeutics
Filamentous bacteriophages, such as fd, f1, and M13, belong to the Inovirus genus and possess a unique nanofiber-like structure. Among the various phage categories, filamentous phages, particularly M13 phages, emerge as widely adopted vectors for both display purposes and bionanomaterial manufacturing (27–31). M13 phages possess a semi-flexible, nanofiber-like structure, adept at self-assembling into diverse ordered forms responsive to their surroundings (17, 30, 32–35). Studies employing nuclear magnetic resonance spectroscopy have uncovered that both M13 and fd phages maintain highly conserved and resilient structures, capable of enduring severe conditions, with M13 phages displaying slightly augmented rigidity (36). Phages are generally categorized into various types, such as lytic phages and temperate phages. In contrast to the commonly observed lytic tailed phages of the Caudovirales family (e.g., T1, T4, T7) used in phage therapy, M13 phages exhibit lysogenic behavior, establishing persistent infections within their host without causing lytic destruction. Leveraging these characteristics, M13 phages have emerged as a preferred asset for various bionanomaterial applications (37–39). Its capability to host multiple peptides on a single phage, achieved through the insertion of foreign DNA fragments into filamentous phage gene III, offers a valuable characteristic for contemporary multifunctional nanomaterials, allowing precise protein modification and multi-peptide presentation (40, 41). Virion capsids, such as M13 phages, provide robust stability for drug delivery, enhancing precision and therapeutic potential. Abundant viral-like particles (VLPs) like M13 phages offer cost-effective reservoirs for tailored drug packaging and precise targeting in nanocarrier-based drug delivery systems, ensuring biocompatibility and uptake efficiency (30, 33, 34, 42).
Enhancing therapeutics through modification
Filamentous phages, notably M13 phages, present a promising trajectory in therapeutics owing to their distinctive nanofiber-like structure. Through genetic modifications, these phages enable a safe and stable multidimensional assembly. Presently, with the widespread application of phage therapy, the genetically engineered M13 phage, as a biological scaffold, facilitates easier purification and amplification compared to wild-type phages. Consequently, this chimeric phage-based therapy allows for the safe and effective identification and monitoring of bacteria, as well as the implementation of controlled antibacterial strategies through assembly with inorganic nanomaterials (43–46). Moreover, their genetic adaptability allows for precise and personalized medicine (47). The utilization of genetically engineered filamentous phages as bionanomaterials unlocks exciting opportunities for targeted cancer therapy, tissue regeneration, and drug delivery. These applications directly address the scarcity of tissue and organ donors, establishing these phages as creative resources in the field of nanomedicine (48). The filamentous bacteriophages stimulate the proliferation and differentiation of neural stem cells, fostering the regeneration of neurons and glial cells (49). In addition, they elevate cerebral blood flow, vascular function, neovascularization, and angiogenesis, collectively contributing to improved neurovascular outcomes (49). These diverse actions position bacteriophages as potential therapeutic agents to protect neurons, mitigate brain edema, minimize infarct size, and boost neurological function (Table 2). Phage display’s utility extends to developing peptides for drug transport across the blood-cerebrospinal fluid barrier (62). M13, fd, and f1 phages, with their versatile coat proteins, are prominent vectors for diverse applications (63). Figures 2A, B illustrates vital biopanning steps: rinsing to remove unbound virions, selectively collecting interacting virions, and propagating phages with strong ligand affinities via E. coli infection. The CRISPR/Cas system can be applied to engineer bacteriophages, enabling targeted alterations and creating novel phage variants (Figure 3) (47, 65). Utilizing the CRISPR-Cas system for genetic manipulation of filamentous or other bacteriophages has enabled the isolation of desired phage mutants, showcasing the significant utility of CRISPR-Cas in the genetic engineering of bacteriophages (47, 66). The development of genetic engineering techniques to modify phages such as M13, T5, and T7 involves diverse applications like single-base substitutions, deletions or insertions of base pairs, and more. This versatile technique is readily adaptable for use across a spectrum of bacterial and phage strains (47, 67).
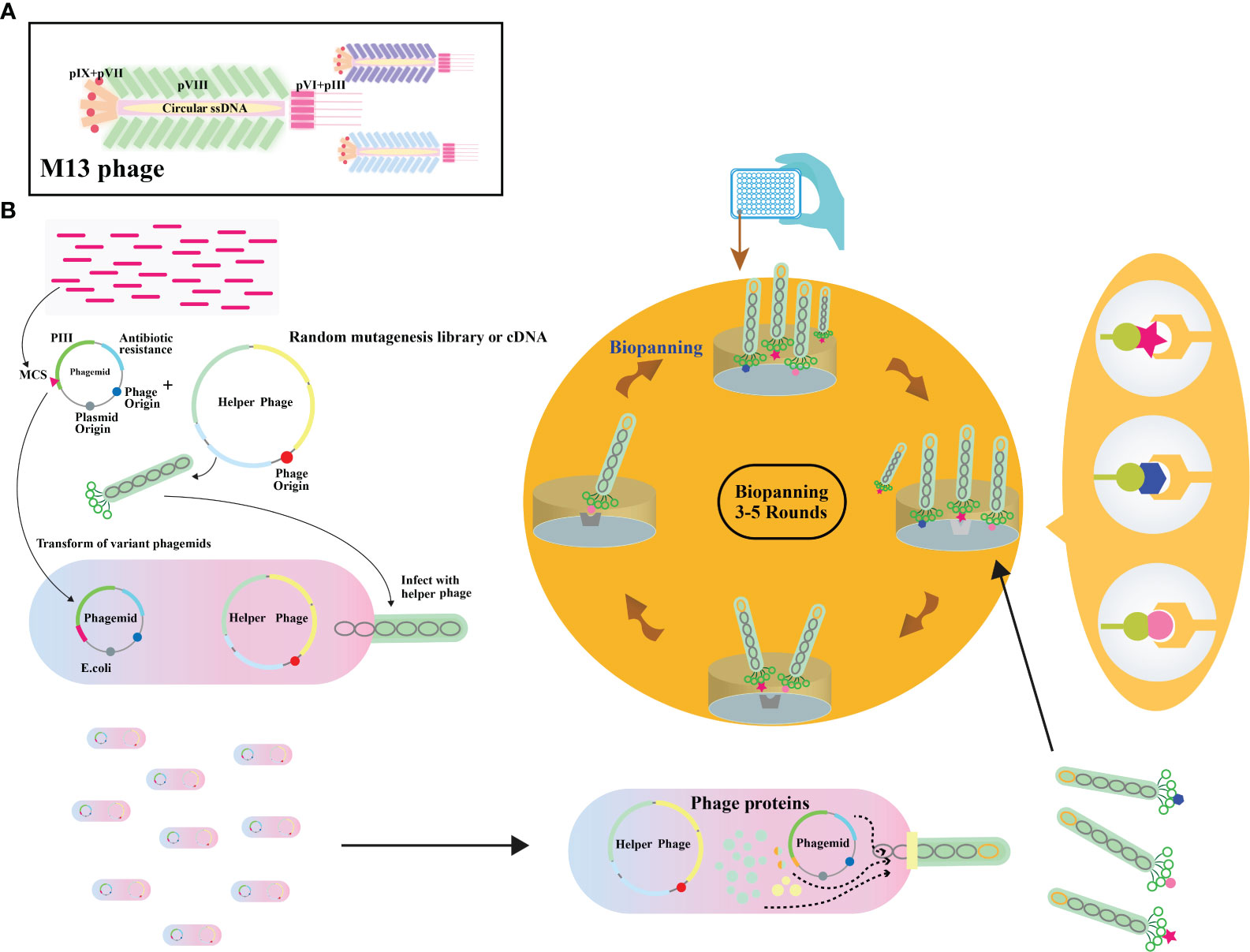
Figure 2 (A) Illustration depicting the overall architecture of the M13 phage. (B) Schematic representation of the phage display biopanning process. A DNA library, either natural or synthetic, is inserted between the signal peptide and pIII encoding gene on a phagemid vector. The phagemid pool is introduced into Escherichia coli cells already infected with a helper phage. A subset of these capsids carries the inserted peptides. The phage library then undergoes iterative rounds of biopanning. Adapted from (64).
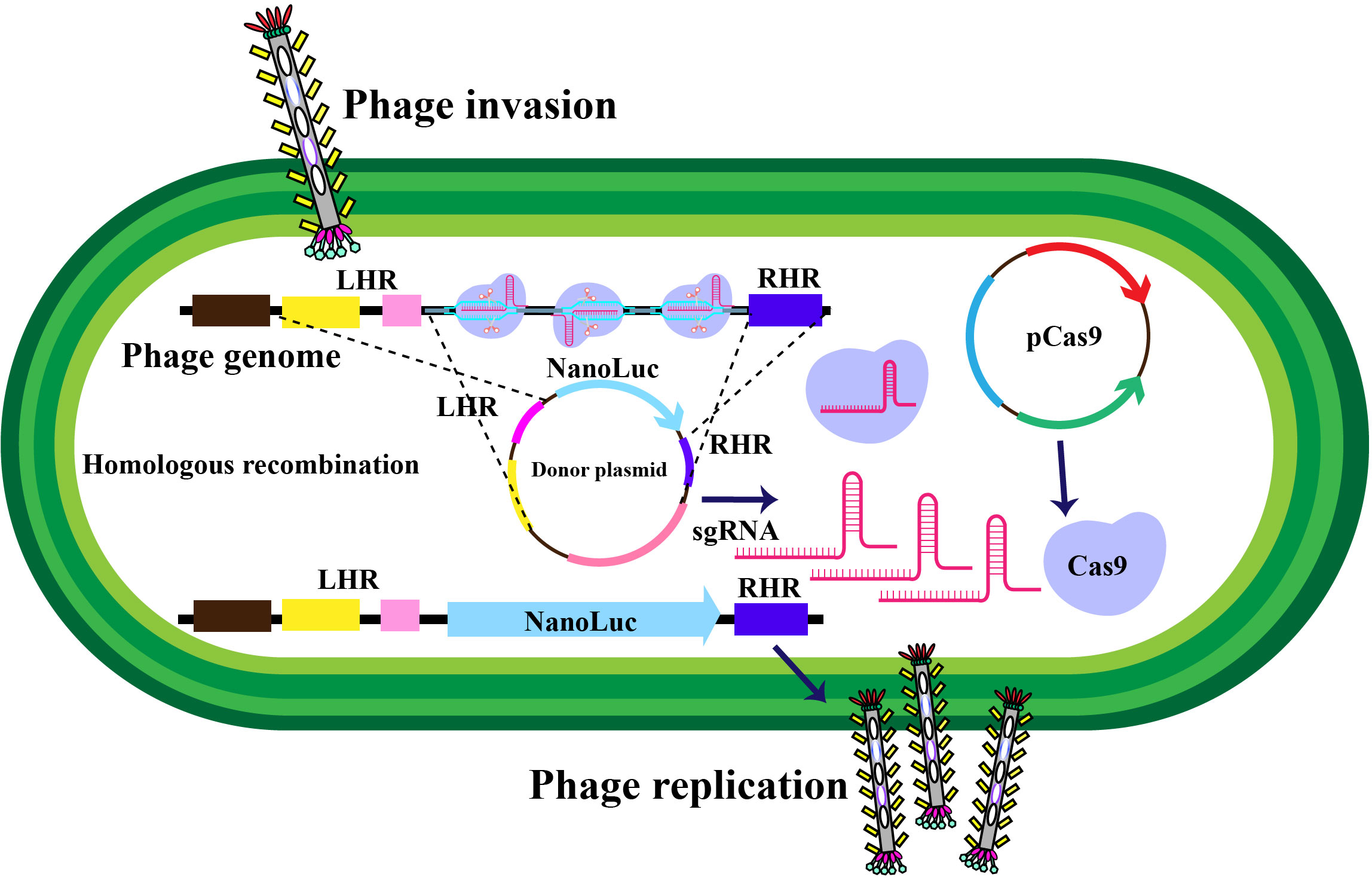
Figure 3 Schematic representation of in-vivo phage recombination facilitated by the CRISPR-Cas9 system. Host bacterial cells undergo transformation with a donor plasmid harboring the NanoLuc reporter gene flanked by left and right homology regions to the phage, alongside specifically chosen CRISPR RNA and genome-targeting sequences. Adapted from (65).
The surface proteins of bacteriophages offer a rich assortment of diverse amino acids, making them amenable to chemical modifications using various molecules (Figures 4A, B). Ongoing advancements in biotechnology now enable the in vitro synthesis of substantial DNA fragments, including entire bacteriophage genomes. This capability greatly facilitates the chemical alteration of bacteriophages, allowing for the creation of novel genomic sequences and streamlining genetic editing. It’s worth noting that there are some limitations when dealing with larger DNA molecules (70). Surface proteins, particularly in the case of the M13 bacteriophage, most notably pIII, serve as versatile platforms for the introduction of functional groups through chemical modifications (Figure 4C). Viral protein shells are chemically modified to enhance their functions or change their characteristics. Techniques include altering amino acid residues (71), natural chemical linkages (72), and incorporating non-natural amino acids (73). Eun-A. Kwak et al. showcased a method for site-specific chemical modification of fd bacteriophages using the formylglycine-generating enzyme (FGE). This enzyme selectively modifies the conserved peptide sequence CXPXR. By genetically modifying the fd bacteriophage, researchers can place the CXPXR motif at different locations, providing controlled sites for specific chemical attachment. Kwak et al. added the FGE recognition motif to the p3 protein shell, allowing site-specific enzyme-catalyzed modification to attach the bacteriophage to various surfaces with amino functional groups. This method introduces a new way of modifying viruses, affirming efficient viral engineering techniques by coupling them selectively to diverse chemical targets (74). This fine-tuning optimizes surface properties, supporting targeted therapeutic engineering in enhancing stroke recovery.
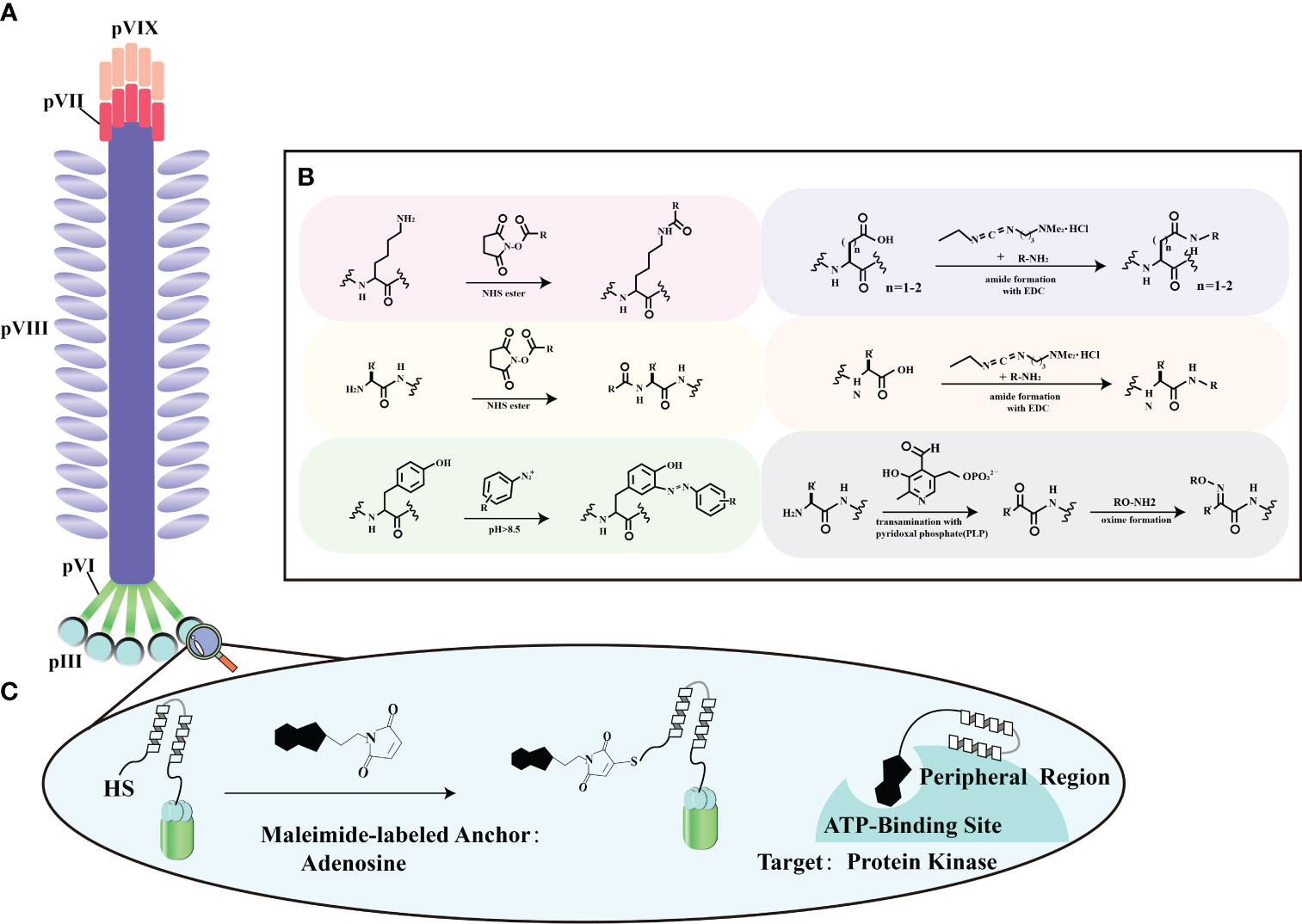
Figure 4 Chemical modification strategies for filamentous phage. (A) General locations of coat proteins. This panel illustrates the fundamental spatial distribution of coat proteins in filamentous phages, forming the basis for our chemical modification strategies. (B) Typical reactions for functional group modification. In this section, we outline the standard chemical reactions employed to selectively modify specific functional groups on these proteins, enhancing the adaptability of filamentous phage engineering. Adapted from (68) (C) Chemical modification of a phage-displayed peptide. Panel (C) depicts the chemical modification of a phage-displayed peptide using adenosine as an anchoring molecule. This modification is tailored to facilitate binding to the ATP binding site of protein kinases, demonstrating potential applications in advanced protein-protein interaction studies. Adapted from (69).
Harnessing mechanisms of in situ tissue regeneration for stroke recovery
Leveraging inherent regenerative capability within the human body, in conjunction with bio-nanomaterials, in a process termed in situ tissue regeneration, holds the promise of achieving tissue rejuvenation at its native location (75). Capitalizing on the versatile attributes of filamentous bacteriophages, encompassing neuronal protection, edema alleviation, infarct size reduction, and neurological improvement, opens avenues to integrate the mechanisms of in situ tissue regeneration into enhancing stroke recovery (26) (Figure 5). Currently, the therapeutic potential of extracellular matrix-mimicking scaffolds faces challenges associated with the incorporation of biomolecules. These challenges encompass the cost-effective production of bioactive agents and the intricate integration of these agents into scaffolds to maintain their bioactivity (76). Furthermore, the limitations in manipulating the fate of stem cells using chemically tethered peptides on biomaterial substrates could impede optimal outcomes (77). In contrast, the genetic incorporation of peptides onto M13 phage coat proteins offers an efficient and precise avenue, facilitating the seamless integration of biomolecules (78). Consequently, M13 phage emerges as a promising contender in the domain of tissue regenerative materials, leveraging its genetic control and modularity attributes to drive advancements in this field. These encompass its ability to precisely present functional peptides as signaling moieties on its surface (30, 33), its capacity to influence the behavior of macroscopic cells (79), its autonomous assembly into diverse structural configurations leading to varying topological effects (80), and its intrinsic biocompatibility and biodegradability (81). This amalgamation of attributes positions M13 phage as an exceptional choice for bionanomaterials, particularly in engineering artificial cellular “niches” (82) (Figure 6).
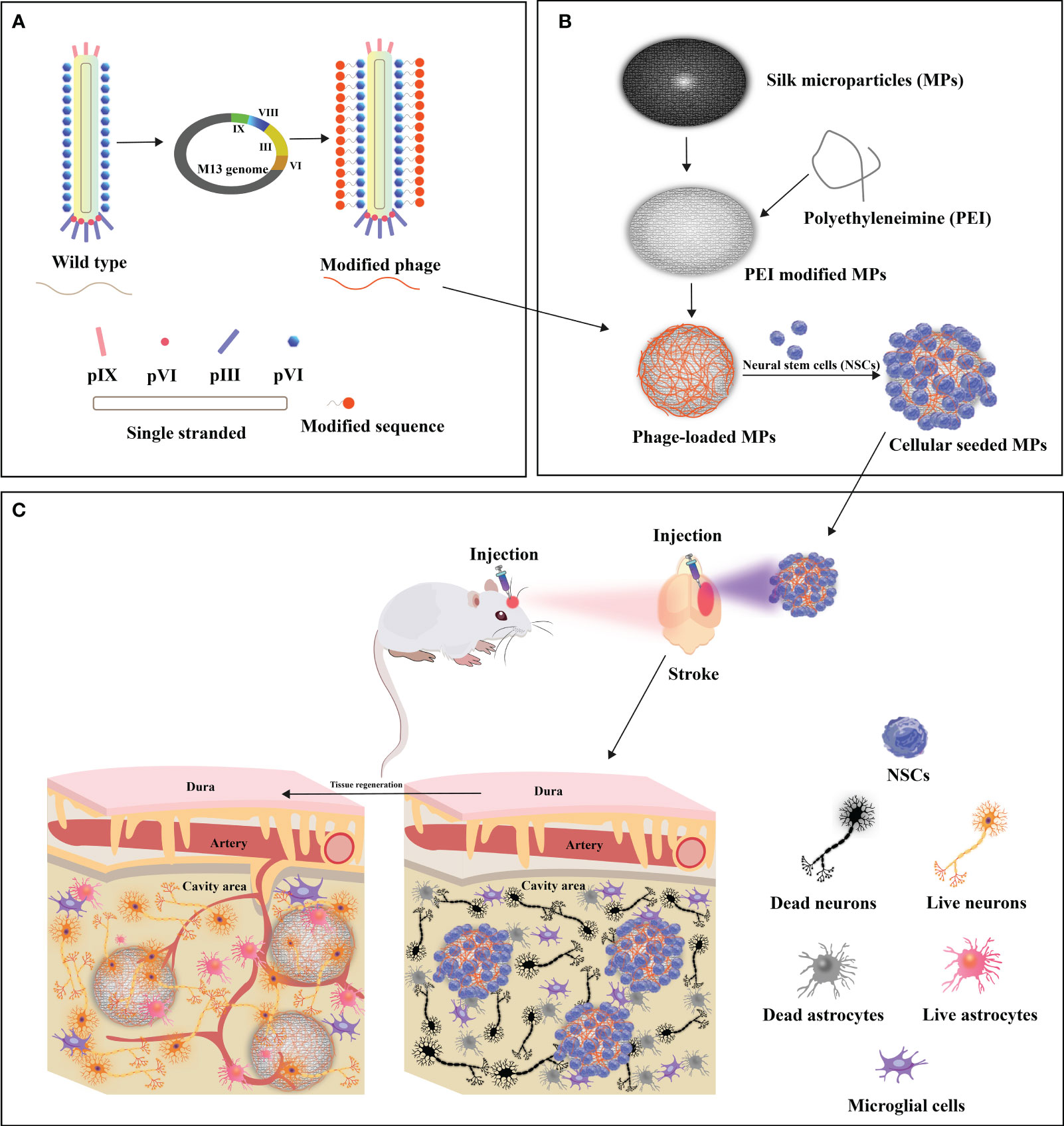
Figure 5 Design of functionalized filamentous phages-loaded microparticles(MPs) for post-stroke brain tissue repair. (A) Genetic linkage of modifying peptides to the N-terminus of the predominant capsid protein (pVIII) of WT-phage engenders the creation of modified phages. (B) Polyethyleneimine (PEI)-modified MPs undergo electrostatic adsorption with modified phages, thereby engendering phage-loaded MPs. Neural stem cells (NSCs) are subsequently implanted onto the surface of modified phage-MPs, and the cellular-seeded MPs are injected into the stroke-affected sites within rat brains. (C) The modified phage-MPs serve as physical scaffolds, fostering NSC proliferation and infiltration within the stroke cavity. The functionalized bacteriophage-loaded microparticles significantly amplify brain tissue repair in post-stroke injuries. Adapted from (26).
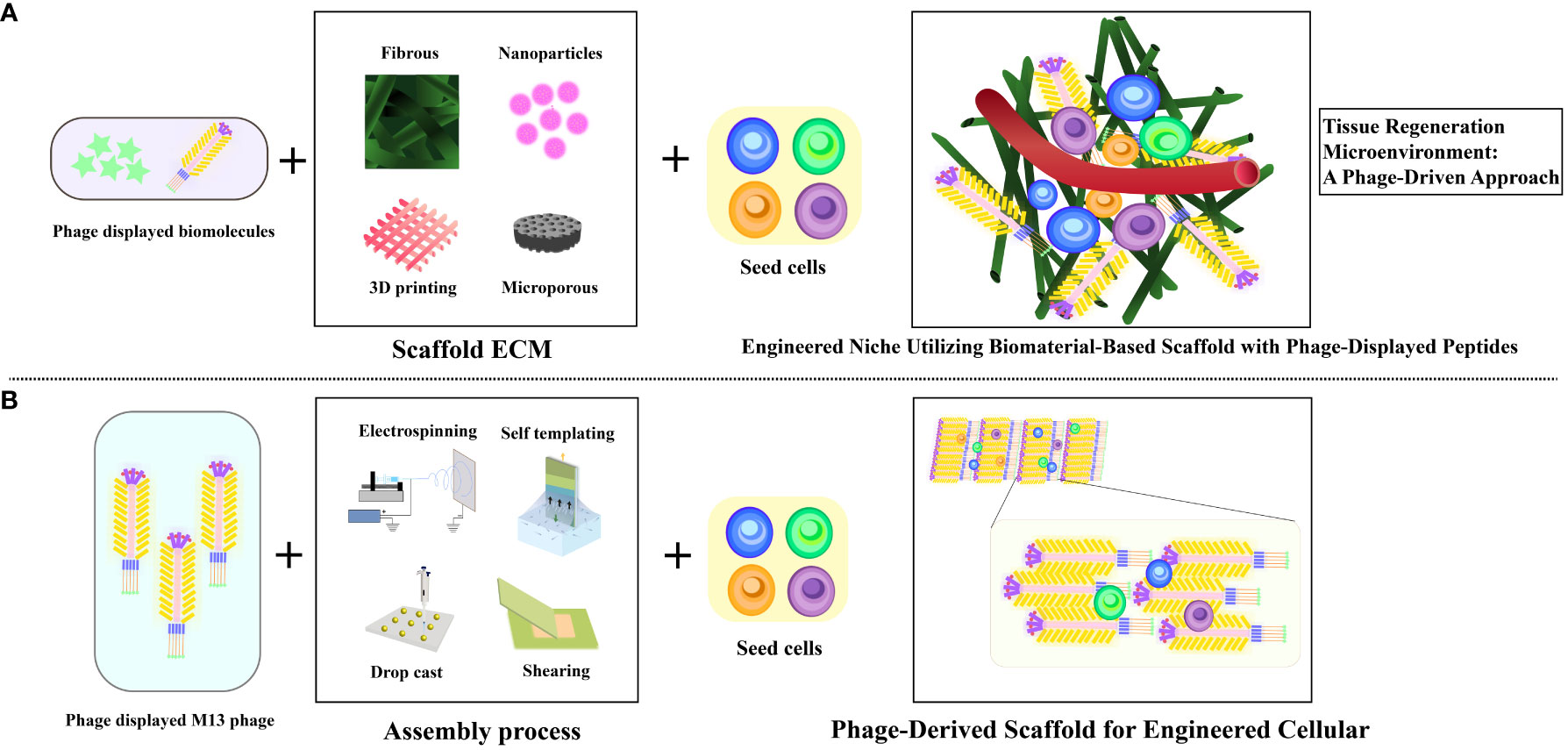
Figure 6 Illustrating diverse artificial cellular niches utilizing M13 phage as bionanomaterial. (A) Visualization of traditional biomaterial-infused scaffold featuring phage-displayed peptides and implanted stem cells to engineer a niche. (B) Depiction of biomimetic phage-infused scaffold encompassing implanted stem cells to engineer a niche. Adapted from (26).
Furthermore, through its integration with bioactive compounds, therapeutic cells, and polymer solutions during the electrospinning process, M13 phage transforms into electrospun nanofibers that replicate the characteristics of the extracellular matrix (ECM), facilitating the seamless incorporation of molecular constituents with heightened efficiency (83). Notably, it is worth mentioning that certain studies have employed M13 phage to display functional peptides and integrate them into complementary scaffolds, rather than using it as a primary element for constructing tissue scaffolds (84) (Figure 6). M13 phages leverage electrospinning to create bionanofibers, ideal for tissue engineering (85–89). In Shin et al.’s study, nanofiber matrices merged PLGA with RGD-displaying M13 phages, improving fibroblast attachment and proliferation. Electrospun nanofibers, as seen in research on rat hippocampus-derived neural stem/progenitor cells (rNSCs), finely modulate cell behavior. These fibers mimic the extracellular matrix, promoting wound healing processes and cellular differentiation. Additionally, nanofibrous architectures have been explored for astrocyte differentiation (90–94). Zhou et al. have unveiled an original nanoridge-in-microridge (NiM) structure through a unique dip-pulling technique, akin to window blinds. These microridges, formed by parallel phage bundles, induced rapid stem cell differentiation into neurons and astrocytes within 8 days. With versatile applications in tissue regeneration, materials templating, sensing, and electrodes, NiM structures, adorned with diverse peptides on various substrates, hold immense potential across technological domains (95, 96). This pioneering approach leverages NiM structures’ multifunctionality and balance of electrostatic forces for various applications (96). Vascular repair and regeneration.
The accumulation of metabolic byproducts within blood vessels often culminates in obstruction, hampering smooth blood circulation and culminating in vascular occlusion, thus fostering cerebral ischemia and hypoxia, a precursor to stroke incidence. Hence, the post-stroke recuperation is significantly contingent on the repair and regeneration of blood vessels. The formation of neovascularization in ischemic tissues stands pivotal in realizing satisfactory tissue restoration following ischemic insults (97). Employing genetically engineered M13 phage offers an avenue to target tissue repair with precision, unraveling the prospects of instigating vascular tissue regeneration, thereby aligning with the objectives of vascular tissue restoration. For instance, the engineered M13 phage with nanofiber arginine-glycine-aspartic acid (RGD) showcases both angiogenic and antioxidative attributes, surmounting pathological milieus and bolstering the implantation of cells and adjacent soft tissues at ischemic sites. Through elevating the survival rate of endothelial progenitor cells (EPCs) implanted in ischemic murine models, this construct efficaciously curtails myogenic degeneration and fibrosis, while amplifying angiogenesis by harnessing the polarization of M2 macrophages (23). Furthermore, an novel approach involves the utilization of M13 phage nanofibers featuring high-density RGD peptides for populating 3D-printed bio-ceramic scaffolds. By seeding mesenchymal stem cells (MSCs) onto these phage nanofibers and subsequently implanting them into bone defects, the cascade of events ensues whereby MSCs are prompted to endothelialize and differentiate into osteogenic lineages, thereby instigating osteogenesis and vascular genesis (98).
Neural repair and regeneration
Within the realm of Central Nervous System (CNS) Neural Regeneration, the restoration of axonal growth from emerging neural cells following traumatic brain injury is a paramount objective (99). Particularly in neurological conditions like cerebral stroke, the autophagy-mediated degradation of compromised tissue leads to the degradation of the Extracellular Matrix, thereby hindering the regenerative potential of brain tissue (100, 101). Leveraging delivery mechanisms such as M13 phages, therapeutic cells can be transported, and functional peptides carrying biochemical cues can be presented on the nanostructured virion, thereby creating an ECM-resembling scaffold or interfacing with compatible scaffolds effectively. Merzlyak and colleagues ingeniously engineered M13 phages to display neural cell-adhesive RGD or IKVAV peptides on the pVIII protein, orchestrating their assembly into intricate three-dimensional nanofiber matrices. This inventive approach concurrently incorporated neural progenitor cells (NPCs), fostering both cellular proliferation and differentiation, representing a promising strategy for neural tissue engineering (87). The results show that within seven days, adult rat neural progenitor cells (NPCs) exhibited a remarkable inclination to align along the M13 phage nanofibers, undergoing neural differentiation and extending neurites that remarkably mimicked the longitudinal axis of the phage fibers (87). This transformation orchestrated virion self-assembly into matrices with anisotropic topography, proficiently promoting neural progenitor cell (NPC) growth. The inclusion of biochemical and topographical cues within the RGD-phage films significantly increased NPC proliferation in comparison to the control group residing in wild-type-phage films. Most significantly, differentiating NPC neurites exhibited a strikingly accelerated growth rate, extending lengths four to seven times greater by the fourth day (23). Zhou et al. extended the paradigm to include not only neurons but also the crucial involvement of astrocytes in cerebral development. Astrocytes play a pivotal role in brain assembly and the growth of extracellular matrix constituents, including fibronectin and laminin (96). Their study explored the dual differentiation potential of human induced pluripotent stem cell (hiPSC)-derived neural progenitor cells (NPCs) into both neurons and astrocytes. They utilized a Nanofiber-mediated (NiM) construct ingeniously composed of M13 phage (96). The orchestrated manipulation of topographical influences, synergistically ameliorated by the inclusion of RGD peptides, has demonstrated a significant impact on NPCs. This effect is marked by the expression of neuron-specific markers and the accelerated generation of astrocytes within a remarkably short timeframe of just eight days, in contrast to the conventional approach of employing an astrocyte-inducing medium, which typically requires around 30 days to achieve similar outcomes (102).
The restoration of optimal functionality within compromised cerebral tissue is a complex process that relies on the establishment of intricate vascular networks. In the context of stroke potential therapy, Liu and colleagues conducted pioneering research on the potential of the M13 phage to promote dual neurogenesis and angiogenesis after cerebral infarction (26). Their approach involved genetically incorporating high-density RGD peptides onto the pVIII protein of the M13 phage, creating the customized “R-phage.” Simultaneously, silk fibroin microparticles (MPs) were crafted from silkworm cocoons, ensuring biocompatibility, and coated with polyethyleneimine (PEI) to enable the electrostatic attachment of R-phages onto the MPs. The strategic transplantation of NSC-seeded R-phage-MPs into ischemic regions within rat brains initiated a complex cascade of reparative events, ultimately enhancing brain tissue recovery post-stroke. This process resulted in the augmented differentiation of neural stem cells (NSCs), leading to the formation of densely interconnected, axon-rich neurons and an improvement in motor function. Moreover, this interplay stimulated a remarkable surge in revascularization and angiogenesis orchestrated through endothelial cell activation. This orchestrated response heighten nutrient and oxygen delivery to the growing neuronal environment, facilitating robust neurogenesis.
Progressing bacteriophage therapy for stroke recovery
In the realm of our investigation, significant and noteworthy advancements have emerged. For instance, the work by Xiangyu Liu and colleagues (26) has unveiled a groundbreaking development. Through genetic engineering, they have restructured filamentous phages to yield a novel biogenic material known as R-phage-MPs, specifically designed for regenerating cerebral tissue within the confines of stroke-induced cavities. This study underscores the material’s remarkable capacity to stimulate the regeneration of vascular and neural networks in stroke-affected regions, concomitantly mitigating the scar formation of astrocytes and the aberrant proliferation of neural progenitor cells. These findings present an effective therapeutic strategy for post-stroke neural recovery. Furthermore, this research has shed light on a novel approach to crafting biogenic materials for brain tissue regeneration, achieved through genetic means, allowing virtually any peptide to be displayed on the surface of bacteriophages. In a different line of pioneering investigations, exemplified by the work of Zhou, Li et al. (81), they introduced a novel concept, the Nanoridge-in-Microridge (NiM) architecture, derived from filamentous M13 bacteriophages. This revolutionary structural design holds the potential to address neurodegenerative diseases (NDD), offering fresh perspectives and therapeutic insights. Studies have indicated that NiM structures can be instrumental in the treatment of NDD. Furthermore, these structures enable the establishment of co-culture models involving neurons and astrocytes, facilitating the exploration of their intricate interactions. This, in turn, provides invaluable insights into potential NDD therapies. Additionally, Mao’s laboratory has explored the use of bacteriophages and peptides as biofunctional materials, as demonstrated in the work of (103). Using bacteriophage display techniques, they successfully fused gene sequences from two distinct peptides. One peptide segment binds to Bombyx mori silk fibroin membranes, while the other originates from bone growth factors. This novel chimeric peptide construct exhibits elevated osteogenic efficacy. Their pioneering work offers a universal strategy for combining different peptide segments to innovate approaches in the functionalization of biomaterials.
Furthermore, our research domain has witnessed the emergence of several significant review articles, providing comprehensive insights into the latest advancements. Notably, the work of Cheng Chang and colleagues (48) stands out. In their publication, they highlight the unique attributes of M13 bacteriophages as novel biogenic nanomaterials, rich with a diverse array of biological molecules. This seminal review delves into cutting-edge research and developments in the field of nanomedicine, with a particular focus on the utilization of M13 bacteriophages as therapeutic agents. In summary, bacteriophage therapy presents new opportunities for promoting stroke recovery. While there are currently no clinical cases using bacteriophage therapy for stroke treatment, we believe that with advancements in scientific technology and further research, this approach has the potential to become a therapeutic option in the field of stroke treatment (104), significantly improving patients’ health and quality of life. Nevertheless, continuous exploration and relentless efforts are still required.
Confronting dilemmas and challenges in stroke potential therapy
Stroke treatments, including pharmacological, interventional, and surgical methods, have shown limitations and side effects, often insufficient in fully restoring neural function. This drives the need to explore novel treatment modalities. Emerging biotechnological therapies such as stem cell, gene, and neurostimulation approaches aim to restore neural cells, facilitating cerebral tissue regeneration and functional recovery (105). These therapies offer advantages like precision and minimal invasiveness but are faced with challenges in safety and efficacy. Current stroke treatments, primarily reliant on acute measures, also possess limitations that impede the restoration of cerebral tissue.
Tissue regeneration
Stroke, an acute cerebrovascular disorder, results from the abrupt occlusion or rupture of cerebral blood vessels, leading to localized cerebral ischemia or hemorrhage and consequential focal brain injury (5). Post-stroke, the brain initiates a complex process of tissue repair and functional recovery. In the early stages, damaged neural cells release inflammatory cytokines, triggering a response that plays a crucial role in processes like cell apoptosis, necrosis, and the recruitment of immune cells, potentially worsening neural damage (106). Over the subsequent weeks post-stroke, tissue repair commences. Activated neural stem cells and progenitor cells migrate to the affected region, with their unique ability to proliferate and differentiate into various neural cell types, playing a pivotal role in the recovery process (107). Simultaneously, neovascularization takes place, restoring blood supply to the affected area, delivering vital oxygen and nutrients required for cerebral tissue rehabilitation (108). However, the journey of post-stroke tissue repair often faces challenges. Excessive inflammatory responses and reduced neural stem cell functionality can impede achieving optimal recovery outcomes. Consequently, there’s an active exploration of strategies to intervene and improve the post-stroke tissue regeneration process. Notably, challenges identified in previous studies demand a targeted research focus to overcome these issues. Specifically, studies aimed at investigating the modulation of inflammatory responses, enhancing neural stem cell functionality, and identifying novel approaches to facilitate post-stroke tissue regeneration are imperative. One significant area demanding further exploration is the thorough evaluation of bacteriophage-mediated mechanisms for promoting cerebral tissue repair after a stroke. This avenue holds substantial potential for advancing the field of post-stroke recovery.
Challenges in bacteriophage therapy for stroke recovery
Bacteriophage therapy for stroke has demonstrated promising outcomes in preclinical studies. Bacteriophages’ unique structure enables the fusion of exogenous peptides to their coat protein N-termini, achieving therapeutic objectives by presenting signaling peptides on the phage surface (109). Research indicates minimal adverse reactions and no observed side effects or detrimental brain tissue impairment upon introducing bacteriophages (110). Furthermore, phage display techniques allow the screening of target peptides and the synthesis of monoclonal antibodies with unique mechanisms of action. However, bacteriophage therapy faces significant limitations that demand further investigation. The potential demonstrated in preclinical studies necessitates comprehensive validation of its efficacy within the human body. Addressing this requires additional clinical trials that align therapeutic safety and efficacy findings from animal models with actual clinical settings. Overcoming the challenge of bypassing the blood-brain barrier in bacteriophage therapy remains crucial to ensure effective delivery to the damaged brain region. Additionally, the stability, persistence, and optimization of phage display technology warrant further exploration to optimize targeting efficiency (16). Advancements in molecular biology and genomics provide opportunities for furthering bacteriophage therapy. Tailoring bacteriophages for precise interventions using gene editing techniques is one such promising approach for stroke recovery. Reducing neuroinflammation is pivotal, necessitating research to optimize the therapy’s efficacy post-stroke (111). Moreover, combining bacteriophages with interventions like stem cell therapy and pharmacotherapy could lead to more potent treatment strategies. Innovation in monoclonal antibodies through phage display-driven screening could effectively address existing resistance concerns (112). As the field of molecular biology and genomics progresses, comprehensive research, thorough clinical trials, and technological advancements will propel phage therapy as a pioneering approach in enhancing stroke recovery (106).
Safety evaluation in bacteriophage therapy
The resurgence of bacteriophage therapy in disease treatment has highlighted its efficacy in recent years. However, due to its biological nature, bacteriophage formulations, production procedures, and treatment methods substantially differ from traditional pharmaceutical approaches. This distinction calls for a comprehensive safety evaluation. Existing safety assessment systems, primarily tailored for conventional antimicrobial drugs, lack sufficient specificity for bacteriophage formulations, especially those of genetically engineered variants. As a result, the establishment of a dedicated safety assessment protocol tailored to bacteriophage therapy is imperative (113). Safety evaluations should encompass a broad spectrum of assessments, including biological characteristics, toxicity, immunogenicity, and pathogenicity specific to genetically engineered bacteriophages (113, 114). Ethical considerations, patient autonomy, and compliance with legal regulations during production are crucial to ensuring patient safety and ethical standards (113, 115, 116). Conducting extensive safety assessments is indispensable before the clinical implementation of pioneering treatments, such as genetically engineered bacteriophages in stroke potential therapy (113). Essential components of patient care in this realm involve regular radiological examinations and an emphasis on psychological well-being (117–119). These practices are pivotal in ensuring comprehensive patient safety and holistic care in the context of bacteriophage therapy potential for enhancing stroke recovery.
Advancing stroke therapies to clinical application
To effectively transition the concept of novel stroke therapies to clinical practice, several crucial studies must be conducted. The current paradigm of stroke treatment faces limitations in fully restoring cerebral tissue and functional recovery. The pressing need for pioneering therapies, such as stem cell, gene, and neurostimulation approaches, to achieve more precise and minimally invasive interventions warrants rigorous examination. Clinical trials are imperative to assess the safety and efficacy of these emerging therapies in stroke treatment. Conducting comprehensive studies that move beyond preclinical findings to verify the feasibility of these therapies within the human body is essential. Kuriakose & Xiao (105) underscore the need for stringent clinical assessments to bridge the gap between laboratory research and real-world applicability. Long-term safety monitoring is critical to ascertain the enduring safety profile and effectiveness of these novel therapies over extended periods (105). Post-trial assessments are crucial to evaluate the sustained impact and side-effect profiles in real-world scenarios (120).
Comparative studies against existing acute measures in stroke treatment are vital to delineate the relative benefits and limitations of these pioneering approaches. Comparative analysis will provide a clearer understanding of the advantages and drawbacks of the emerging therapies compared to conventional treatment modalities (121). In-depth studies elucidating the underlying mechanisms of action of these novel therapies are essential to ensure targeted and effective interventions. Understanding the specific interactions and pathways involved in neural regeneration and tissue repair will guide the development of more precise and effective treatments (122). Evaluation of patient-centric outcomes and quality of life assessments after the application of these novel therapies is necessary. Analyzing the impact on patients’ quality of life, functional recovery, and long-term rehabilitation is vital to assess the true clinical significance of these pioneering approaches (105). Moving forward, these comprehensive studies are critical to establish the safety, efficacy, and practical applicability of the novel stroke therapies in real-world clinical settings, ensuring a transformative shift in stroke treatment modalities.
Conclusion
Stroke represents a neurological impairment resulting from acute focal injury within the central nervous system due to vascular causes, often leading to functional deficits in specific bodily regions (5). Conventional therapeutic approaches predominantly involve thrombolysis, surgical intervention, and postoperative physical rehabilitation. However, these methods are constrained by limitations and potential side effects, rendering them insufficient to substantially mitigate disability. Phage therapy introduces a novel avenue for enhancing stroke recovery (104, 123). Patients facing diseases lacking effective cures are increasingly exploring bacteriophage therapy, even driving medical tourism. Industrial development also continues to embrace bacteriophages (124). Reevaluating the applications of engineered bacteriophages reveals novel possibilities in enhancing stroke recovery and brain regenerative biomaterials (26). The nanofiber structure and genetic modifications of filamentous bacteriophages show promise as potential biomaterials for addressing complex brain tissue concerns. These nanofibers aid in enhancing neural stem cell activity and stimulating vascular generation at stroke sites, potentially enhancing stroke therapies. Genetic engineering techniques are rapidly advancing bacteriophage research (125). The distinctive architecture of bacteriophages enables integration of exogenous peptides onto their coat proteins, endowing these peptides with targeting attributes that bolster disease treatment (16). Employing phage display technology can uncover stroke-relevant targeting peptides, facilitating the development of monoclonal antibodies with unique mechanisms via targeted modifications. Furthermore, integrating peptides into the shell proteins of filamentous bacteriophages also contributes to facilitating the recovery process, reducing inflammatory responses and scar thickness of astrocytes, while fostering neuroblast reactions in the brain’s subventricular zone. Bacteriophage therapy faces limitations requiring more research. Preclinical studies’ potential needs validation in humans, requiring clinical trials aligning safety and efficacy outcomes. Overcoming challenges in blood-brain barrier penetration is crucial for effective brain delivery. Further exploration of bacteriophage display technology for stability and targeting is necessary. Establishing a safety assessment, covering biological traits, toxicity, and immunogenicity, is essential for genetically engineered bacteriophages.
Author contributions
YL: Conceptualization, Writing – original draft, Writing – review & editing. K-DY: Conceptualization, Investigation, Writing – original draft. D-CK: Investigation, Writing – review & editing. X–ML: Investigation, Writing – review & editing. HD: Investigation, Software, Writing – review & editing. J-FY: Investigation, Software, Writing – review & editing.
Funding
The author(s) declare financial support was received for the research, authorship, and/or publication of this article. This study was supported by Scientific Research Program of Jilin Provincial Department of Education (No.JJKH20231194KJ); Scientific Research Project of Jilin Provincial Department of Finance (No. JLSWSRCZX2020-0030; No.JLSWSRCZX2021-074); Research Project of Undergraduate Teaching Reform in Jilin University (No. 2021XZC087); Research Projects of Higher Education in Jilin Province (No. JGJX2021D53).
Conflict of interest
The authors declare that the research was conducted in the absence of any commercial or financial relationships that could be construed as a potential conflict of interest.
Publisher’s note
All claims expressed in this article are solely those of the authors and do not necessarily represent those of their affiliated organizations, or those of the publisher, the editors and the reviewers. Any product that may be evaluated in this article, or claim that may be made by its manufacturer, is not guaranteed or endorsed by the publisher.
References
1. Feigin VL, Stark BA, Johnson CO, Roth GA, Bisignano C, Abady GG, et al. Global, regional, and national burden of stroke and its risk factors, 1990-2019: a systematic analysis for the Global Burden of Disease Study 2019. Lancet Neurology (2021) 20(10):795–820. doi: 10.1016/s1474-4422(21)00252-0
2. Tsao CW, Aday AW, Almarzooq ZI, Alonso A, Beaton AZ, Bittencourt MS, et al. Heart disease and stroke statistics-2022 update: A report from the American heart association. Circulation (2022) 145(8):E153–639. doi: 10.1161/cir.0000000000001052
3. Saini V, Guada L, Yavagal DR. Global epidemiology of stroke and access to acute ischemic stroke interventions. Neurology (2021) 97(20S):S6–S16. doi: 10.1212/wnl.0000000000012781
4. Candelario-Jalil E, Dijkhuizen RM, Magnus T. Neuroinflammation, stroke, blood-brain barrier dysfunction, and imaging modalities. Stroke (2022) 53(5):1473–86. doi: 10.1161/strokeaha.122.036946
6. Xiong Y, Wakhloo AK, Fisher M. Advances in acute ischemic stroke therapy. Circ Res (2022) 130(8):1230–51. doi: 10.1161/circresaha.121.319948
7. Xin H, Li Y, Cui Y, Yang JJ, Zhang ZG, Chopp M. Systemic administration of exosomes released from mesenchymal stromal cells promote functional recovery and neurovascular plasticity after stroke in rats. J Cereb Blood Flow Metab (2013) 33(11):1711–5. doi: 10.1038/jcbfm.2013.152
8. Nih LR, Gojgini S, Carmichael ST, Segura T. Dual-function injectable angiogenic biomaterial for the repair of brain tissue following stroke. Nat Materials (2018) 17(7):642–+. doi: 10.1038/s41563-018-0083-8
9. Aimone JB, Li Y, Lee SW, Clemenson GD, Deng W, Gage FH. Regulation and function of adult neurogenesis: from genes to cognition [Review]. Physiol Rev (2014) 94(4):991–1026. doi: 10.1152/physrev.00004.2014
10. Phipps MS, Cronin CA. Management of acute ischemic stroke [Review]. Bmj-British Med J (2020) 368. doi: 10.1136/bmj.l6983
11. Li Z, Song Y, He T, Wen R, Li Y, Chen T, et al. M2 microglial small extracellular vesicles reduce glial scar formation via the miR-124/STAT3 pathway after ischemic stroke in mice. Theranostics 2021 (2021) 11(3):1232–48. doi: 10.7150/thno.48761
12. George PM, Steinberg GK. Novel stroke therapeutics: unraveling stroke pathophysiology and its impact on clinical treatments. Neuron (2015) 87(2):297–309. doi: 10.1016/j.neuron.2015.05.041
13. Kortright KE, Chan BK, Koff JL, Turner PE. Phage therapy: A renewed approach to combat antibiotic-resistant bacteria. Cell Host Microbe (2019) 25(2):219–32. doi: 10.1016/j.chom.2019.01.014
14. Strathdee SA, Hatfull GF, Mutalik VK, Schooley RT. Phage therapy: From biological mechanisms to future directions. Cell (2023) 186(1):17–31. doi: 10.1016/j.cell.2022.11.017
15. Salmond GPC, Fineran PC. A century of the phage: past, present and future. Nat Rev Microbiol (2015) 13(12):777–86. doi: 10.1038/nrmicro3564
16. Jaroszewicz W, Morcinek-Orlowska J, Pierzynowska K, Gaffke L, Wegrzyn G. Phage display and other peptide display technologies. FEMS Microbiol Rev (2022) 46(2). doi: 10.1093/femsre/fuab052
17. Dion MB, Oechslin F, Moineau S. Phage diversity, genomics and phylogeny. Nat Rev Microbiol (2020) 18(3):125–38. doi: 10.1038/s41579-019-0311-5
18. Roach DR, Leung CY, Henry M, Morello E, Singh D, Di Santo JP, et al. Synergy between the host immune system and bacteriophage is essential for successful phage therapy against an acute respiratory pathogen. Cell Host Microbe (2017) 22(1):38–+. doi: 10.1016/j.chom.2017.06.018
19. Chen Z, Jin W, Liu H, Zhao Z, Cheng K. Discovery of Peptide ligands for hepatic stellate cells using phage display. Mol Pharm (2015) 12(6):2180–8. doi: 10.1021/acs.molpharmaceut.5b00177
20. Zhao Z, Li Y, Jain A, Chen Z, Liu H, Jin W, et al. Development of a peptide-modified siRNA nanocomplex for hepatic stellate cells. Nanomedicine-Nanotechnology Biol Med (2018) 14(1):51–61. doi: 10.1016/j.nano.2017.08.017
21. Levard D, Buendia I, Lanquetin A, Glavan M, Vivien D, Rubio M. Filling the gaps on stroke research: Focus on inflammation and immunity. Brain Behav Immunity (2021) 91:649–67. doi: 10.1016/j.bbi.2020.09.025
22. Chen Y, Liu X, Yang M, Sun W, Mao C. Integration of genetically engineered virus nanofibers and fibrin to form injectable fibrous neuron-rich hydrogels and enable neural differentiation. J Materials Chem B (2023) 11(4):802–15. doi: 10.1039/d2tb01712a
23. Shrestha KR, Lee DH, Chung W, Lee S-W, Lee BY, Yoo SY. Biomimetic virus-based soft niche for ischemic diseases. Biomaterials (2022) 288:121747. doi: 10.1016/j.biomaterials.2022.121747
24. Sweere JM, Van Belleghem JD, Ishak H, Bach MS, Popescu M, Sunkari V, et al. Bacteriophage trigger antiviral immunity and prevent clearance of bacterial infection. Science (2019) 363(6434):1416–+. doi: 10.1126/science.aat9691
25. Wang M, Thomson AW, Yu F, Hazra R, Junagade A, Hu X. Regulatory T lymphocytes as a therapy for ischemic stroke. Semin Immunopathology (2023) 45(3):329–46. doi: 10.1007/s00281-022-00975-z
26. Liu X, Yang M, Lei F, Wang Y, Yang M, Mao C. Highly effective stroke therapy enabled by genetically engineered viral nanofibers. Adv Mater (2022) 34(20):e2201210. doi: 10.1002/adma.202201210
27. Chan BK, Abedon ST, Loc-Carrillo C. Phage cocktails and the future of phage therapy. Future Microbiol (2013) 8(6):769–83. doi: 10.2217/fmb.13.47
28. Hertveldt K, Beliën T, Volckaert G. General M13 phage display: M13 phage display in identification and characterization of protein-protein interactions. Methods Mol Biol (2009) 502:321–39. doi: 10.1007/978-1-60327-565-1_19
29. Ledsgaard L, Kilstrup M, Karatt-Vellatt A, McCafferty J, Laustsen AH. Basics of antibody phage display technology. Toxins (Basel) (2018) 10(6):236. doi: 10.3390/toxins10060236
30. Park JP, Do M, Jin H-E, Lee S-W, Lee H. M13 bacteriophage displaying DOPA on surfaces: fabrication of various nanostructured inorganic materials without time-consuming screening processes. ACS Appl Materials Interfaces. (2014) 6(21):18653–60. doi: 10.1021/am506873g
31. Szot-Karpińska K, Golec P, Leśniewski A, Pałys B, Marken F, Niedziółka-Jönsson J, et al. Modified filamentous bacteriophage as a scaffold for carbon nanofiber. Bioconjug Chem (2016) 27(12):2900–10. doi: 10.1021/acs.bioconjchem.6b00555
32. Herrera-Barrera M, Ryals RC, Gautam M, Jozic A, Landry M, Korzun T, et al. Peptide-guided lipid nanoparticles deliver mRNA to the neural retina of rodents and nonhuman primates. Sci Adv (2023) 9(2):eadd4623. doi: 10.1126/sciadv.add4623
33. Hou J, Qian X, Xu Y, Guo Z, Thierry B, Yang C-T, et al. Rapid and reliable ultrasensitive detection of pathogenic H9N2 viruses through virus-binding phage nanofibers decorated with gold nanoparticles. Biosensors Bioelectronics (2023) 237:115423. doi: 10.1016/j.bios.2023.115423
34. Karimi M, Mirshekari H, Basri SMM, Bahrami S, Moghoofei M, Hamblin MR. Bacteriophages and phage-inspired nanocarriers for targeted delivery of therapeutic cargos. Advanced Drug Delivery Rev (2016) 106:45–62. doi: 10.1016/j.addr.2016.03.003
35. Lee SW, Mao CB, Flynn CE, Belcher AM. Ordering of quantum dots using genetically engineered viruses. Science (2002) 296(5569):892–5. doi: 10.1126/science.1068054
36. Morag O, Abramov G, Goldbourt A. Similarities and differences within members of the ff family of filamentous bacteriophage viruses. J Phys Chem B (2011) 115(51):15370–9. doi: 10.1021/jp2079742
37. Jiang H, Li Y, Cosnier S, Yang M, Sun W, Mao C. Exploring phage engineering to advance nanobiotechnology. Materials Today Nano (2022) 19:100229. doi: 10.1016/j.mtnano.2022.100229
38. Kim H, Lee J-H, Lee JH, Lee BY, Lee BD, Okada K, et al. M13 virus triboelectricity and energy harvesting. Nano Letters. (2021) 21(16):6851–8. doi: 10.1021/acs.nanolett.1c01881
39. Merzlyak A, Indrakanti S, Lee S-W. Genetically engineered nanofiber-like viruses for tissue regenerating materials. Nano Letters. (2009) 9(2):846–52. doi: 10.1021/nl8036728
40. Pande J, Szewczyk MM, Grover AK. Phage display: Concept, innovations, applications and future. Biotechnol Advances (2010) 28(6):849–58. doi: 10.1016/j.bioteChadv.2010.07.004
41. Smith GP. Filamentous fusion phage: novel expression vectors that display cloned antigens on the virion surface [; Research Support, Non-U.S. Gov't; Research Support, U.S. Gov't, P.H.S.]. Sci (New York NY) (1985) 228(4705):1315–7. doi: 10.1126/science.4001944
42. Ma Y, Nolte RJM, Cornelissen JJLM. Virus-based nanocarriers for drug delivery. Advanced Drug Delivery Rev (2012) 64(9):811–25. doi: 10.1016/j.addr.2012.01.005
43. Peng H, Borg RE, Dow LP, Pruitt BL, Chen IA. Controlled phage therapy by photothermal ablation of specific bacterial species using gold nanorods targeted by chimeric phages. Proc Natl Acad Sci USA (2020) 117(4):1951–61. doi: 10.1073/pnas.1913234117
44. Peng H, Borg RE, Nguyen ABN, Chen IA. Chimeric phage nanoparticles for rapid characterization of bacterial pathogens: detection in complex biological samples and determination of antibiotic sensitivity. ACS Sensors (2020) 5(5):1491–9. doi: 10.1021/acssensors.0c00654
45. Peng H, Chen IA. Rapid colorimetric detection of bacterial species through the capture of gold nanoparticles by chimeric phages. ACS Nano (2019) 13(2):1244–52. doi: 10.1021/acsnano.8b06395
46. Peng H, Rossetto D, Mansy SS, Jordan MC, Roos KP, Chen IA. Treatment of wound infections in a mouse model using Zn2+-releasing phage bound to gold nanorods. ACS Nano (2022) 16(3):4756–74. doi: 10.1021/acsnano.2c00048
47. Fadaie M, Dianat-Moghadam H, Ghafouri E, Naderi S, Darvishali MH, Ghovvati M, et al. Unraveling the potential of M13 phages in biomedicine: Advancing drug nanodelivery and gene therapy. Environ Res (2023) 238(Pt 1):117132. doi: 10.1016/j.envres.2023.117132
48. Chang C, Guo W, Yu X, Guo C, Zhou N, Guo X, et al. Engineered M13 phage as a novel therapeutic bionanomaterial for clinical applications: From tissue regeneration to cancer therapy. Materials Today Bio (2023) 20:100612. doi: 10.1016/j.mtbio.2023.100612
49. Cao B, Li Y, Yang T, Bao Q, Yang M, Mao C. Bacteriophage-based biomaterials for tissue regeneration. Adv Drug Delivery Rev (2019) 145:73–95. doi: 10.1016/j.addr.2018.11.004
50. Caprini A, Silva D, Zanoni I, Cunha C, Volontè C, Vescovi A, et al. A novel bioactive peptide: assessing its activity over murine neural stem cells and its potential for neural tissue engineering. N Biotechnol (2013) 30(5):552–62. doi: 10.1016/j.nbt.2013.03.005
51. Cigognini D, Satta A, Colleoni B, Silva D, Donega M, Antonini S, et al. Evaluation of early and late effects into the acute spinal cord injury of an injectable functionalized self-assembling scaffold. PloS One (2011) 6(5):e19782. doi: 10.1371/journal.pone.0019782
52. Deng Q, Cai W, Li S, Su B. Identification of a NEP1-35 recognizing peptide that neutralizes CNS myelin inhibition using phage display library. Neurosci Lett (2013) 536:80–4. doi: 10.1016/j.neulet.2013.01.009
53. Deng Q, Cai W, Li S, Zhang Y, Su B. Small Nogo-66-binding peptide promotes neurite outgrowth through RhoA inhibition after spinal cord injury. Brain Res Bull (2013) 99:140–4. doi: 10.1016/j.brainresbull.2013.10.009
54. Gelain F, Cigognini D, Caprini A, Silva D, Colleoni B, Donegá M, et al. New bioactive motifs and their use in functionalized self-assembling peptides for NSC differentiation and neural tissue engineering. Nanoscale (2012) 4(9):2946–57. doi: 10.1039/c2nr30220a
55. Lee H-B, Kang J-I, Chung WJ, Lee DH, Lee BY, Lee S-W, et al. Engineered phage matrix stiffness-modulating osteogenic differentiation. ACS Appl Materials Interfaces. (2018) 10(5):4349–58. doi: 10.1021/acsami.7b17871
56. Safari Z, Sadeghizadeh M, Asgaritarghi G, Bardania H, Sadeghizadeh D, Soudi S. M13 phage coated surface elicits an anti-inflammatory response in BALB/c and C57BL/6 peritoneal macrophages. Int Immunopharmacol (2022) 107:108654. doi: 10.1016/j.intimp.2022.108654
57. Staquicini FI, Dias-Neto E, Li J, Snyder EY, Sidman RL, Pasqualini R, et al. Discovery of a functional protein complex of netrin-4, laminin gamma1 chain, and integrin alpha6beta1 in mouse neural stem cells. Proc Natl Acad Sci U S A. (2009) 106(8):2903–8. doi: 10.1073/pnas.0813286106
58. Sun Z, Dai X, Li Y, Jiang S, Lou G, Cao Q, et al. A novel Nogo-66 receptor antagonist peptide promotes neurite regeneration in vitro. Mol Cell Neurosci (2016) 71:80–91. doi: 10.1016/j.mcn.2015.12.011
59. Tang D-Y, Yu Y, Zhao X-J, Schachner M, Zhao W-J. Single chain fragment variable antibodies developed by using as target the 3rd fibronectin type III homologous repeat fragment of human neural cell adhesion molecule L1 promote cell migration and nemitogenesis. Exp Cell Res (2015) 330(2):336–45. doi: 10.1016/j.yexcr.10.021
60. Yang M, Zhang Z-C, Liu Y, Chen Y-R, Deng R-H, Zhang Z-N, et al. Function and mechanism of RGD in bone and cartilage tissue engineering. Front Bioengineering Biotechnol (2021) 9:773636. doi: 10.3389/fbioe.2021.773636
61. Yoo SY, Kobayashi M, Lee PP, Lee S-W. Early osteogenic differentiation of mouse preosteoblasts induced by collagen-derived DGEA-peptide on nanofibrous phage tissue matrices. Biomacromolecules (2011) 12(4):987–96. doi: 10.1021/bm1013475
62. Yang X, Li Y, Zhu Z, Huang X, Wang T, Yuan J, et al. Identification of a peptide that crosses the blood-cerebrospinal fluid barrier by phage display technology. Amino Acids (2021) 53(8):1181–6. doi: 10.1007/s00726-021-03016-5
63. Conners R, Leon-Quezada RI, McLaren M, Bennett NJ, Daum B, Rakonjac J, et al. Cryo-electron microscopy of the f1 filamentous phage reveals insights into viral infection and assembly. Nat Commun (2023) 14(1):2724. doi: 10.1038/s41467-023-37915-w
64. Hay ID, Lithgow T. Filamentous phages: masters of a microbial sharing economy. EMBO Rep (2019) 20(6):e47427. doi: 10.15252/embr.201847427
65. Alonzo LF, Jain P, Hinkley T, Clute-Reinig N, Garing S, Spencer E, et al. Rapid, sensitive, and low-cost detection of Escherichia coli bacteria in contaminated water samples using a phage-based assa. Sci Rep (2022) 12(1):7741. doi: 10.1038/s41598-022-11468-2
66. Meile S, Sarbach A, Du J, Schuppler M, Saez C, Loessner MJ, et al. Engineered reporter phages for rapid bioluminescence-based detection and differentiation of viable listeria cells. Appl Environ Microbiol (2020) 86(11):e00442-20. doi: 10.1128/aem.00442-20
67. Goren MG, Mahata T, Qimron U. An efficient, scarless, selection-free technology for phage engineering. RNA Biol (2023) 20(1):830–5. doi: 10.1080/15476286.2023.2270344
68. Bernard JM, Francis MB. Chemical strategies for the covalent modification of filamentous phage. Front Microbiol (2014) 5:734. doi: 10.3389/fmicb.2014.00734
69. Fujiwara D, Mihara K, Takayama R, Nakamura Y, Ueda M, Tsumuraya T, et al. Chemical modification of phage-displayed helix-loop-helix peptides to construct kinase-focused libraries. Chembiochem (2021) 22(24):3406–9. doi: 10.1002/cbic.202100450
70. Sun Q, Shen L, Zhang B-L, Yu J, Wei F, Sun Y, et al. Advance on engineering of bacteriophages by synthetic biology. Infection Drug Resistance. (2023) 16:1941–53. doi: 10.2147/idr.S402962
71. Li K, Chen Y, Li S, Huong Giang N, Niu Z, You S, et al. Chemical modification of M13 bacteriophage and its application in cancer cell imaging. Bioconjugate Chem (2010) 21(7):1369–77. doi: 10.1021/bc900405q
72. Dwyer MA, Lu W, Dwyer JJ, Kossiakoff AA. Biosynthetic phage display: a novel protein engineering tool combining chemical and genetic diversity. Chem Biol (2000) 7(4):263–74. doi: 10.1016/s1074-5521(00)00102-2
73. Carrico ZM, Romanini DW, Mehl RA, Francis MB. Oxidative coupling of peptides to a virus capsid containing unnatural amino acids. Chem Commun (2008) 10):1205–7. doi: 10.1039/b717826c
74. Kwak E-A, Jaworski J. Controlled surface immobilization of viruses via site-specific enzymatic modification. J Materials Chem B 2013 (2013) 1(28):3486–93. doi: 10.1039/c3tb20526f
75. Gaharwar AK, Singh I, Khademhosseini A. Engineered biomaterials for in situ tissue regeneration. Nat Rev Materials (2020) 5(9):686–705. doi: 10.1038/s41578-020-0209-x
76. Perez RA, Won J-E, Knowles JC, Kim H-W. Naturally and synthetic smart composite biomaterials for tissue regeneration. Advanced Drug Delivery Rev (2013) 65(4):471–96. doi: 10.1016/j.addr.2012.03.009
77. Yang K, Lee JS, Kim J, Lee YB, Shin H, Um SH, et al. Polydopamine-mediated surface modification of scaffold materials for human neural stem cell engineering [Article]. Biomaterials (2012) 33(29):6952–64. doi: 10.1016/j.biomaterials.2012.06.067
78. Chung W-J, Lee D-Y, Yoo SY. Chemical modulation of M13 bacteriophage and its functional opportunities for nanomedicine. Int J Nanomedicine. (2014) 9:5825–36. doi: 10.2147/ijn.S73883
79. Smeal SW, Schmitt MA, Pereira RR, Prasad A, Fisk JD. Simulation of the M13 life cycle II: Investigation of the control mechanisms of M13 infection and establishment of the carrier state. Virology (2017) 500:275–84. doi: 10.1016/j.virol.2016.08.015
80. He T, Abbineni G, Cao B, Mao C. Nanofibrous bio-inorganic hybrid structures formed through self-assembly and oriented mineralization of genetically engineered phage nanofibers. Small (2010) 6(20):2230–5. doi: 10.1002/smll.201001108
81. Peivandi A, Tian L, Mahabir R, Hosseinidoust Z. Hierarchically structured, self-healing, fluorescent, bioactive hydrogels with self-organizing bundles of phage nanofilaments. Chem Materials (2019) 31(15):5442–9. doi: 10.1021/acs.chemmater.9b00720
82. Wang J, Wang L, Li X, Mao C. Virus activated artificial ECM induces the osteoblastic differentiation of mesenchymal stem cells without osteogenic supplements. Sci Rep (2013) 3:1242. doi: 10.1038/srep01242
83. Potrc T, Baumgartner S, Roskar R, Planinsek O, Lavric Z, Kristl J, et al. Electrospun polycaprolactone nanofibers as a potential oromucosal delivery system for poorly water-soluble drugs. Eur J Pharm Sci (2015) 75:101–13. doi: 10.1016/j.ejps.2015.04.004
84. Wang J, Wang L, Yang M, Zhu Y, Tomsia A, Mao C. Untangling the effects of peptide sequences and nanotopographies in a biomimetic niche for directed differentiation of iPSCs by assemblies of genetically engineered viral nanofibers. Nano Lett (2014) 14(12):6850–6. doi: 10.1021/nl504358j
85. Christopherson GT, Song H, Mao H-Q. The influence of fiber diameter of electrospun substrates on neural stem cell differentiation and proliferation. Biomaterials (2009) 30(4):556–64. doi: 10.1016/j.biomaterials.2008.10.004
86. Hashi CK, Zhu Y, Yang G-Y, Young WL, Hsiao BS, Wang K, et al. Antithrombogenic property of bone marrow mesenchymal stem cells in nanofibrous vascular grafts. Proc Natl Acad Sci United States America. (2007) 104(29):11915–20. doi: 10.1073/pnas.0704581104
87. Shin YC, Lee JH, Jin L, Kim MJ, Oh J-W, Kim TW, et al. Cell-adhesive RGD peptide-displaying M13 bacteriophage/PLGA nanofiber matrices for growth of fibroblasts. Biomaterials Res (2014) 18:14. doi: 10.1186/2055-7124-18-14
88. Wang X-X, Yu G-F, Zhang J, Yu M, Ramakrishna S, Long Y-Z. Conductive polymer ultrafine fibers via electrospinning: Preparation, physical properties and applications. Prog Materials Sci (2021) 115:100704. doi: 10.1016/j.pmatsci.2020.100704
89. Yang F, Murugan R, Wang S, Ramakrishna S. Electrospinning of nano/micro scale poly(L-lactic acid) aligned fibers and their potential in neural tissue engineering. Biomaterials (2005) 26(15):2603–10. doi: 10.1016/j.biomaterials.2004.06.051
90. Khan FA, Almohazey D, Alomari M, Almofty SA. Impact of nanoparticles on neuron biology: current research trends. Int J Nanomedicine. (2018) 13::2767–2776. doi: 10.2147/ijn.S165675
91. Maysinger D, Behrendt M, Lalancette-Herbert M, Kriz J. Real-time imaging of astrocyte response to quantum dots:: In vivo screening model system for biocompatibility of nanoparticles. Nano Letters. (2007) 7(8):2513–20. doi: 10.1021/nl071611t
92. Moroni L, Licht R, de Boer J, de Wijn JR, van Blitterswijk CA. Fiber diameter and texture of electrospun PEOT/PBT scaffolds influence human mesenchymal stem cell proliferation and morphology, and the release of incorporated compounds. Biomaterials (2006) 27(28):4911–22. doi: 10.1016/j.biomaterials.2006.05.027
93. Tiwari SK, Agarwal S, Seth B, Yadav A, Nair S, Bhatnagar P, et al. Curcumin-loaded nanoparticles potently induce adult neurogenesis and reverse cognitive deficits in alzheimer's disease model via canonical Wnt/β-catenin pathway. ACS Nano (2014) 8(1):76–103. doi: 10.1021/nn405077y
94. Wen AM, Steinmetz NF. Design of virus-based nanomaterials for medicine, biotechnology, and energy. Chem Soc Rev (2016) 45(15):4074–126. doi: 10.1039/c5cs00287g
95. Yang T, Chen Y, Xu Y, Liu X, Yang M, Mao C. Viruses as biomaterials. Materials Sci Eng R-Reports (2023) 153:100715. doi: 10.1016/j.mser.2023.100715
96. Zhou N, Li Y, Loveland CH, Wilson MJ, Cao B, Qiu P, et al. Hierarchical ordered assembly of genetically modifiable viruses into nanoridge-in-microridge structures. Advanced Materials (2019) 31(52):e1905577. doi: 10.1002/adma.201905577
97. Zou T, Fan J, Fartash A, Liu H, Fan Y. Cell-based strategies for vascular regeneration. J Biomed Materials Res Part A. (2016) 104(5):1297–314. doi: 10.1002/jbm.a.35660
98. Wang J, Yang M, Zhu Y, Wang L, Tomsia AP, Mao C. Phage nanofibers induce vascularized osteogenesis in 3D printed bone scaffolds. Adv Mater (2014) 26(29):4961–6. doi: 10.1002/adma.201400154
99. Varadarajan SG, Hunyara JL, Hamilton NR, Kolodkin AL, Huberman AD. Central nervous system regeneration. Cell (2022) 185(1):77–94. doi: 10.1016/j.cell.2021.10.029
100. Lo EH, Dalkara T, Moskowitz MA. Mechanisms, challenges and opportunities in stroke. Nat Rev Neurosci (2003) 4(5):399–415. doi: 10.1038/nrn1106
101. Yong VW, Power C, Forsyth P, Edwards DR. Metalloproteinases in biology and pathology of the nervous system. Nat Rev Neurosci (2001) 2(7):502–11. doi: 10.1038/35081571
102. Ananthanarayanan B, Little L, Schaffer DV, Healy KE, Tirrell M. Neural stem cell adhesion and proliferation on phospholipid bilayers functionalized with RGD peptides. Biomaterials (2010) 31(33):8706–15. doi: 10.1016/j.biomaterials.2010.07.104
103. Lyu R, Chen Y, Shuai Y, Wang J, Lu L, Cheng Q, et al. Novel biomaterial-binding/osteogenic bi-functional peptide binds to silk fibroin membranes to effectively induce osteogenesis in vitro and in vivo. ACS Appl Materials Interfaces (2023) 15(6):7673–7685. doi: 10.1021/acsami.2c17554
104. Yeoh SG, Sum JS, Lai JY, Isa WYH, Lim TS. Potential of phage display antibody technology for cardiovascular disease immunotherapy. J Cardiovasc Trans Res (2022) 15(2):360–80. doi: 10.1007/s12265-021-10169-x
105. Kuriakose D, Xiao Z. Pathophysiology and treatment of stroke: present status and future perspectives. Int J Mol Sci (2020) 21(20):7609. doi: 10.3390/ijms21207609
106. Jayaraj RL, Azimullah S, Beiram R, Jalal FY, Rosenberg GA. Neuroinflammation: friend and foe for ischemic stroke. J Neuroinflamm (2019) 16(1):142. doi: 10.1186/s12974-019-1516-2
107. Jendelova P, Kubinova S, Sandvig I, Erceg S, Sandvig A, Sykova E. Current developments in cell- and biomaterial-based approaches for stroke repair. Expert Opin Biol Ther (2016) 16(1):43–56. doi: 10.1517/14712598.2016.1094457
108. Eelen G, Treps L, Li X, Carmeliet P. Basic and therapeutic aspects of angiogenesis updated. Circ Res (2020) 127(2):310–29. doi: 10.1161/circresaha.120.316851
109. Carmody CM, Goddard JM, Nugen SR. Bacteriophage capsid modification by genetic and chemical methods. Bioconjugate Chem (2021) 32(3):466–81. doi: 10.1021/acs.bioconjchem.1c00018
110. Malik DJ, Sokolov IJ, Vinner GK, Mancuso F, Cinquerrui S, Vladisavljevic GT, et al. Formulation, stabilisation and encapsulation of bacteriophage for phage therapy. Adv Colloid Interface Sci (2017) 249:100–33. doi: 10.1016/j.cis.2017.05.014
111. Okada T, Suzuki H. The role of tenascin-C in tissue injury and repair after stroke. Front Immunol (2021) 11:607587. doi: 10.3389/fimmu.2020.607587
112. Xu M, Lei G, Chen M, Wang K, Lv W, Zhang P, et al. Development of a novel, fully human, anti-PCSK9 antibody with potent hypolipidemic activity by utilizing phage display-based strategy. EBioMedicine (2021) 65:103250. doi: 10.1016/j.ebiom.2021.103250
113. Nale JY, Clokie MRJ. Preclinical data and safety assessment of phage therapy in humans. Curr Opin Biotechnol (2021) 68:310–7. doi: 10.1016/j.copbio.2021.03.002
114. Romero-Calle D, Benevides RG, Goes-Neto A, Billington C. Bacteriophages as alternatives to antibiotics in clinical care. Antibiotics-Basel (2019) 8(3):138. doi: 10.3390/antibiotics8030138
115. Miedzybrodzki R, Borysowski J, Weber-Dabrowska B, Fortuna W, Letkiewicz S, Szufnarowski K, et al. Clinical aspects of phage therapy. In: Lobocka M, Szybalski WT, editors. Advances in Virus Research, vol. 83 (2012). p. 73–121. doi: 10.1016/B978-0-12-394438-2.00003-7
116. Uyttebroek S, Chen B, Onsea J, Ruythooren F, Debaveye Y, Devolder D, et al. Safety and efficacy of phage therapy in difficult-to-treat infections: a systematic review. Lancet Infect Dis (2022) 22(8):E208–20. doi: 10.1016/s1473-3099(21)00612-5
117. Cushnaghan J, Coggon D, Reading I, Croft P, Byng P, Cox K, et al. Long-term outcome following total hip arthroplasty: A controlled longitudinal study. Arthritis Rheumatism-Arthritis Care Res (2007) 57(8):1375–80. doi: 10.1002/art.23101
118. Sahu P, Kashaw SK, Sau S, Kushwah V, Jain S, Iyer AK. Discovering pH triggered charge rebound surface modulated topical nanotherapy against aggressive skin papilloma. Materials Sci Eng C-Materials Biol Appl (2020) 107:110263. doi: 10.1016/j.msec.2019.110263
119. Xie W, Zhang G, Guo Z, Lu J, Ye J, Xu W, et al. Ultra-sensitive iron-doped palladium nanocrystals with enhanced hydroxyl radical generation for chemo-/chemodynamic nanotherapy. Advanced Funct Materials (2022) 32(12):2107518. doi: 10.1002/adfm.202107518
120. Guenl F, Mecate-Zambrano A, Rehlaender S, Hinse S, Ludwig S, Brunotte L. Shooting at a moving target-effectiveness and emerging challenges for SARS-CoV-2 vaccine development. Vaccines (2021) 9(10):1052. doi: 10.3390/vaccines9101052
121. Charmsaz S, Prencipe M, Kiely M, Pidgeon GP, Collins DM. Innovative technologies changing cancer treatment. Cancers (2018) 10(6):288. doi: 10.3390/cancers10060208
122. Orive G, Anitua E, Luis Pedraz J, Emerich DF. Biomaterials for promoting brain protection, repair and regeneration. Nat Rev Neurosci (2009) 10(9):682–U47. doi: 10.1038/nrn2685
123. Lu L, Bao Q, Miao Y, Cheng Q, Chen Y, Yang S, et al. Peptide-enabled thrombus-targeting nanoparticles for highly effective targeted CT imaging and eradication of thrombi. Advanced Funct Materials (2023) 33(41):2303331. doi: 10.1002/adfm.202303331
124. Gorski A, Miedzybrodzki R, Lobocka M, Glowacka-Rutkowska A, Bednarek A, Borysowski J, et al. Phage therapy: what have we learned? Viruses-Basel (2018) 10(6):288. doi: 10.3390/v10060288
Keywords: stroke, filamentous phages, bacteriophage therapy, neurological restoration, tissue regeneration
Citation: Li Y, Yang K-d, Kong D-c, Li X-m, Duan H-y and Ye J-f (2024) Harnessing filamentous phages for enhanced stroke recovery. Front. Immunol. 14:1343788. doi: 10.3389/fimmu.2023.1343788
Received: 24 November 2023; Accepted: 27 December 2023;
Published: 16 January 2024.
Edited by:
Muthuraman Muthuraman, University of Hospital Würzburg, GermanyReviewed by:
Zhaoting Li, University of Wisconsin-Madison, United StatesHuan Peng, University of California, Los Angeles, United States
Copyright © 2024 Li, Yang, Kong, Li, Duan and Ye. This is an open-access article distributed under the terms of the Creative Commons Attribution License (CC BY). The use, distribution or reproduction in other forums is permitted, provided the original author(s) and the copyright owner(s) are credited and that the original publication in this journal is cited, in accordance with accepted academic practice. No use, distribution or reproduction is permitted which does not comply with these terms.
*Correspondence: Jun-feng Ye, eWVqdW5mZW5nQGpsdS5lZHUuY24=