- 1State Key Laboratory of Biocatalysis and Enzyme Engineering, School of Life Sciences, Hubei University, Wuhan, China
- 2Infection and Innate Immunity Laboratory, Department of Biological Sciences, Institute for Biomedical Sciences, The George Washington University, Washington, DC, United States
- 3National Key Laboratory of Green Pesticide, Key Laboratory of Green Pesticide and Agricultural Bioengineering (Ministry of Education), Guizhou University, Guiyang, China
Insects constitute approximately 75% of the world’s recognized fauna, with the majority of species considered as pests. Entomopathogenic fungi (EPF) are parasitic microorganisms capable of efficiently infecting insects, rendering them potent biopesticides. In response to infections, insects have evolved diverse defense mechanisms, prompting EPF to develop a variety of strategies to overcome or circumvent host defenses. While the interaction mechanisms between EPF and insects is well established, recent findings underscore that their interplay is more intricate than previously thought, especially evident across different stages of EPF infection. This review primarily focuses on the interplay between EPF and the insect defense strategies, centered around three infection stages: (1) Early infection stage: involving the pre-contact detection and avoidance behavior of EPF in insects, along with the induction of behavioral responses upon contact with the host cuticle; (2) Penetration and intra-hemolymph growth stage: involving the initiation of intricate cellular and humoral immune functions in insects, while symbiotic microbes can further contribute to host resistance; (3) Host insect’s death stage: involving the ultimate confrontation between pathogens and insects. Infected insects strive to separate themselves from the healthy population, while pathogens rely on the infected insects to spread to new hosts. Also, we discuss a novel pest management strategy underlying the cooperation between EPF infection and disturbing the insect immune system. By enhancing our understanding of the intricate interplay between EPF and the insect, this review provides novel perspectives for EPF-mediated pest management and developing effective fungal insecticides.
1 Introduction
Insects constitute the most diverse and abundant clade within the animal kingdom with over a million known species inhabiting our planet, comprising approximately 75% of all described animal species (1, 2). However, nearly half of the known insect species are herbivorous pests as they directly consume crops and indirectly contribute to plant disease transmission (3–6). Currently, the most prevalent method for pest control involves the application of chemical pesticides (7). Nevertheless, concerns have arisen over the pesticide residues, prompting the need for more environmentally friendly and cost-effective pest control strategies (8, 9). Entomopathogenic fungi (EPF) comprise a specialized group of parasitic microorganisms with the remarkable ability to infect and ultimately kill insects and other arthropods. They stand as one of the most potent natural options for pest control, with over 60% of naturally occurring insect diseases attributed to EPF (10–12). These fungi possess several notable characteristics, including environmental adaptation, abundant strain resources, and limited resistance development, which position them as viable alternatives to chemical pesticides in numerous ecosystems (13–16). At present, around 1,000 species of EPF have been identified, and ongoing research continues to unveil new taxa within this group (15, 17, 18). The majority of EPF strains are found in the orders Onygenales, Entomophthorales, Neozygitales, and most prominently, Hypocreales (19, 20). Genera such as Beauveria, Metarhizium, Isaria, Lecanicilium, Nomuraea, Hirsutella, and Paecilomyces within the Hypocreales have been extensively studied and some have been developed into commercial EPF agents, particularly within the Beauveria and Metarhizium genera (21–25).
EPF employ direct penetration of the insect cuticle as their primary mode of infection, although recent studies have indicated that EPF can also utilize oral and respiratory routes to infect their hosts (26, 27). The invasive and developmental processes of EPF can be delineated into six key stages: attachment of conidia to the host, germination, appressorium formation and penetration, fungal growth within the hemolymph, conidia production on host, and ultimately, transmission and dispersal (28, 29). Recent studies have revealed that each step of EPF infection entails the intricate regulation of specific genes, or a combination of various genes, underscoring the existence of highly sophisticated mechanisms employed by EPF to eliminate the targeted insects (28, 30, 31). In the ongoing long-term coevolutionary arms race, insects have developed an array of defense mechanisms to resist potential pathogens. The primary line of defense is the cuticular integument, a robust protective barrier composed of diverse chemical components including n-alkane, fatty acids, chitin, and tanned proteins, which effectively safeguard the internal tissues from the external environment (32, 33). Should EPF breach this barrier, they encounter the second line of defense: the evolutionary conserved innate immune system, which can be categorized into cellular and humoral defenses (34–37).
The complex interaction between EPF and insects signifies a constant battle within the coevolutionary arms race. While previous reviews have detailed the interaction mechanisms, recent findings suggest a greater intricacy, particularly evident across different stages of EPF infection. In this review, we primarily emphasize the interplay between EPF and the insect immune system, focusing on three particular infection stages: (1) Early infection stage: EPF conidia adhere to the host surface and initiate pre-penetration. Insects exploit chemical components, behavioral response, and ectomicrobiomes to defend against EPF infection. (2) Penetration and intra-hemolymph growth phase: EPF penetrate the cuticle, and hyphal bodies grow and produce toxins in the hemolymph. This stage triggers a strong insect cellular and humoral immune response in insects. The involvement of symbiotic microorganisms further complicates the immune interactions. (3) Insect host death phase: this stage marks the ultimate confrontation between EPF and insects. Infected insects strive to isolate themselves from the population to shield it. Meanwhile, EPF secrete antimicrobial products to limit the growth of competitive microbes in the carcass. Finally, we propose a novel pest management strategy that centers around the host-EPF interaction. This involves modifying the EPF through genetic modification in combination with the use of chemicals, dsRNA, or microorganisms to defeat the insect immune system by enhancing EPF virulence.
2 During the early infection stage
2.1 The adhesion of EPF to the host cuticle and EPF appressorium differentiation
In the initial stage of EPF infection, the success rate and insect mortality are largely determined by the attachment of single-celled dispersive forms, such as conidia or blastospores, to the host cuticle (38, 39) (Figure 1A). Hydrophobins and adhesin proteins on the spores are thought to serve as successive stages in the attachment process: a non-specific (passive) adsorption step followed by a target-specific consolidation phase (40). Different types of fungal propagules exhibit varying adhesive properties: aerial conidia of B. bassiana can tightly adhere to hydrophobic surfaces. In contrast, their blastospores quickly bind to hydrophilic surfaces, and submerged conidia adhere to both hydrophobic and hydrophilic surfaces. All the three cell types are infectious, enabling B. bassiana to interact with a diverse range of substrates and bind to a broad spectrum of host targets (41, 42). B. bassiana possesses two hydrophobins, Hyd1 and Hyd2, localized on the surface of aerial conidia and submerged conidia, but not detected in blastospores. They are responsible for cell surface hydrophobicity, adhesion, and virulence (43, 44). Three adhesin genes (Adh1-3) of B. bassiana are functionally characterized and Adh2 plays a role in conidial adherence to insect cuticle (45). In Metarhizium anisopliae, two adhesin genes, Mad1 and Mad2 have been characterized, and Mad1 mediates spore adhesion to host surfaces (46). Moreover, several other cell wall proteins are involved in adhesion, for example, the non-hydrophobic cell-wall protein CWP10 of B. bassiana enhances conidial adhesion (47). A glycolytic enzyme, glyceraldehyde-3-phosphate dehydrogenase (GAPDH), in M. anisopliae, may also contribute to the adhesion of conidia to a host (48). In addition to proteins, the surface of fungal cells contains various carbohydrate moieties, playing diverse roles in adhesion, for example, the negatively charged sugars (sialic acids) on the surface of Aspergillus fumigatus are central to conidia adhere to basal lamina proteins (49). Carbohydrates moieties are also the main compounds commonly recognized by host for triggering immune signaling cascades (50, 51). However, carbohydrate analysis is complicated by the complexity of glycan structures and the challenges of separating and detecting carbohydrates experimentally, more investment is needed in this part (52).
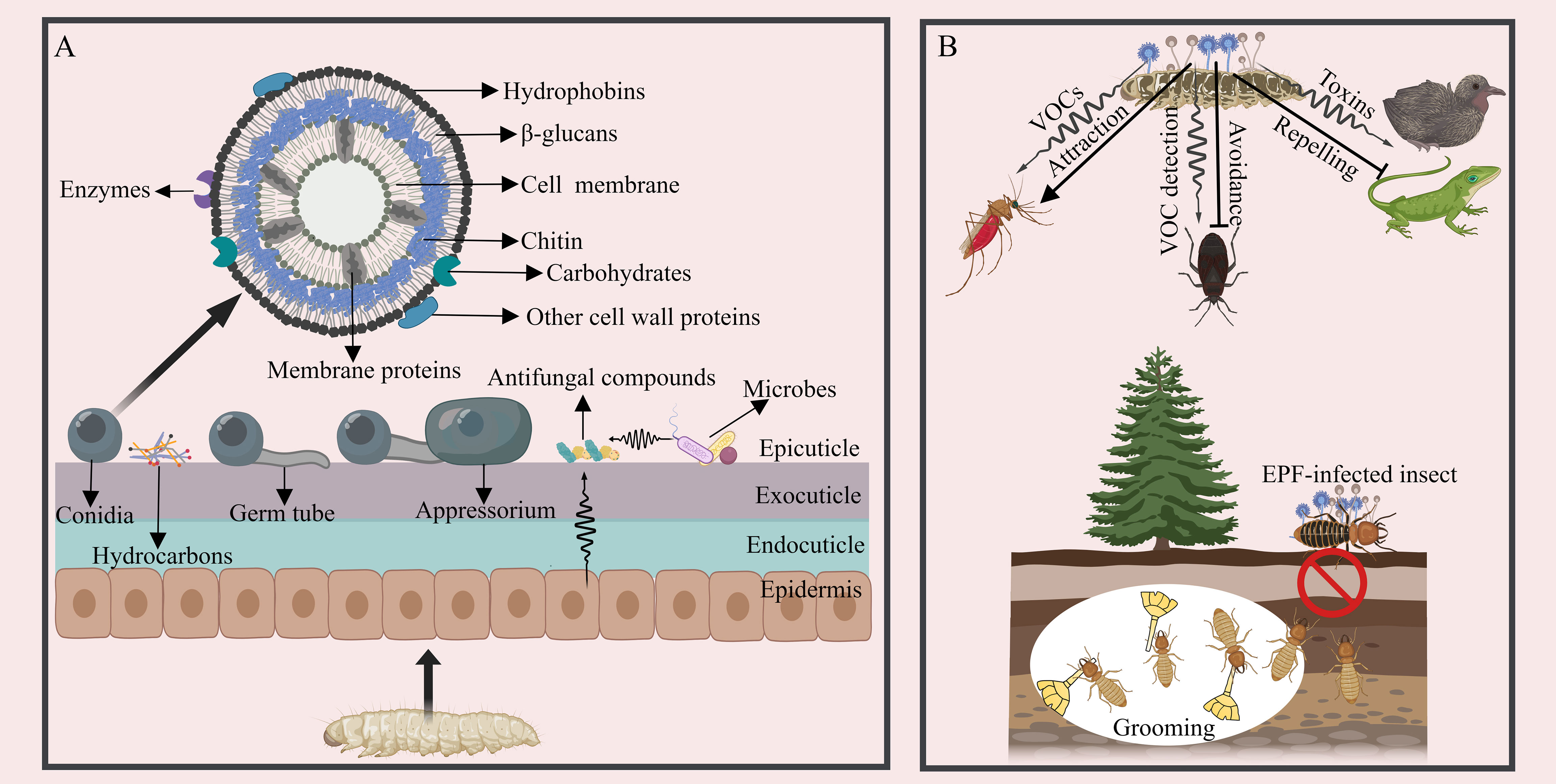
Figure 1 The interaction between EPF and insects during the early infection stage. (A) A schematic diagram of fungal adhesion, pre-penetration, and the cuticular defense. The surface of EPF conidia is equipped with hydrophobins and other components facilitating adhesion. The appressorium initiates growth. Antifungal compounds present in the insect cuticle, glandular secretion, and ectomicrobiomes act to inhibit EPF conidial growth. (B) Strategies employed by insects to prevent and counteract EPF infection prior to establishment. EPF can attract insects through VOCs, such as acetic acid, 1-octen-3-ol and Chokol K, but insects can detect VOCs from EPF and avoid infection. EPF can also generate toxins to deter other organisms from consuming the infected insect cadavers in the last stage of infection. Social insects exhibit collective behaviors, like grooming and self-removal as a means to circumvent EPF infection.
Following the attachment of EPF conidia to the host cuticle, appressoria may form at the tips of fungal germ tubes, displaying apical swelling structures adhering to the host cuticle with diverse morphologies (e.g., clavate, spherical, or cup-shaped structures) (53–55) (Figure 1A). However, certain EPF species, like Metarhizium rileyi, do not undergo appressorium formation, in which the germ tubes grow along the endocuticle and produce lateral branches (56). M. anisopliae can occasionally penetrate the cuticle directly without appressoria formation (57). Recent studies have also shown that B. bassiana may not always form appressoria during cuticle penetration (55, 58). To date, a series of key genes have been identified in appressoria differentiation like Protein kinase A (PKA) genes in M. anisopliae (MaPKA1) and B. bassiana (BbMPK1) (59, 60). In addition, a Rho3 homolog in Magnaporthe grisea (Mgrho3), G-protein coupled receptors (GPCR) genes and exopolymer galactosaminogalactan (GAG) biosynthetic genes in Metarhizium species, as well as the histone lysine methyltransferase ASH1 in Metarhizium robertsii are also essential for appressorium formulation, development and the formation of appressorium mucilage (61–64). Many other genes and pathways involved in regulating fungal sensing and infection of insects are also under investigation (55).
2.2 The strategies for insects to avoid
As EPF infect potential hosts, insects have developed strategies to detect and avoid them, both before coming into contact (pre-contact) or after contact (post-contact) (65) (Figure 1B). The odorant binding proteins of arthropods can respond actively to volatile organic compounds (VOCs) emitted by EPF, which further alters the insect immune response and induces the repellant effects on the pathogen and modulating host defense (66, 67). These abilities vary among insect species and across different developmental stages. For instance, Japanese beetle (Popillia japonica) larvae have been observed to avoid soil containing high concentrations of M. anisopliae (68). The generalist predator Anthocoris nemorum can detect the presence of B. bassiana and actively avoid it (69). Social insects, such as termites and ants, possess the ability to detect EPF and respond with avoidance behaviors. Reticulitermes flavipes termites can detect M. anisopliae-dusted termites and demonstrate alarm and aggregation reactions (70). The termite Macrotermes michaelseni presents similar ability (71, 72); Coptotermes lacteus displays an avoidance response by creating short tunnels into substrates containing M. anisopliae and sealing them off to prevent further contact with the fungus (73). It is intriguing that EPF can also release VOCs with attractant properties. For instance, female Anopheles stephensi mosquitoes are drawn to spores of B. bassiana and M. anisopliae, as well as other fungal-infected insects, however, the exact mechanisms involved in this behavior remain unclear (74); and the VOCs (such as acetic acid) of the genus Lecanicillium can attract female western flower thrips Frankliniella occidentalis (75), other VOCs from fungi such as 1-octen-3-ol and Chokol K are also insect attractive signals (76, 77). Additionally, certain plant root-associated EPF can alter host plants traits such as leaf reflectance, attracting herbivorous insects and promoting the EPF dispersal (78). Many phytopathogenic fungi-induced VOCs from plants that can attract insects have been identified (such as methyl salicylate, hexanal and 1-exanol) (79), but whether endophytic EPF can induce plants to produce insect attractive VOCs is less studied.
Moreover, grooming behavior serves as an efficient mechanism for removing conidia after the insect gets in contact with EPF. For example, over 90% of the M. anisopliae conidia on the body surface of Reticulitermes speratus can be groomed off by their nestmates (80). Mutual grooming behavior is highly effective in protecting Coptotermes formosanus termites from M. anisopliae infection (81). Similarly, when Solenopsis invicta and Lasius japonicus are exposed to fungus, both ant species can benefit through exhibiting grooming behavior (82, 83).
2.3 In defense against EPF pre-penetration
Upon adherence of EPF, the host cuticle employs various mechanisms to prevent spore adhesion and germination (Figure 1A). The inherent hydrophobicity of the insect cuticle generally makes it an ideal substrate for fungal spore adhesion. However, certain insects have developed mechanisms to counteract this trait in order to defend against the adhesion of EPF. For instance, the cuticular fatty amides found in booklice (Liposcelis bostrychophila) are able to deter the adhesion of dry EPF conidia by decreasing hydrophobicity and static charge (84). In addition to acting as a physical barrier, the insect cuticle plays a vital role in the molting process. Simple molting can serve as a means to evade infection. For example, rapid ecdysis observed in aphids and the diamondback moth (Plutella xylostella) contributes to limiting the EPF ability to infect the host (85, 86). Nevertheless, certain EPF have been observed to hinder the molting process of their hosts by means of oxidative inactivation of host ecdysteroid (87). The hydrocarbons found on the insect cuticle can serve as a carbon source for EPF adhesion and germination, including fatty acids and long chain hydrocarbons. Insects have the ability to alter the hydrocarbon content to evade EPF infection (88). For example, Metarhizium is unable to firmly attach to Aedes aegypti due to the absence of long-chain hydrocarbons in mosquito larvae, which are probably necessary for fungal development on the host cuticle (89). However, some insect cuticle components exhibit antimicrobial properties, including fatty acids, alkaloids, aldehydes, melanin and antimicrobial peptides (40, 90, 91). Additionally, certain insects release substances such as formic acid, quinones, terpenes, and glucanases, as well as proteinase and chitinase inhibitors, from their epidermal, salivary or poison glands, and these secretions serve to hinder spore adhesion, germination, or appressorium formation (92, 93). For instance, the invasive garden ants, Lasius neglectus, produce formic acid as a poison to cleanse the brood and body surfaces, inhibiting the growth of Metarhizium brunneum (94, 95). Tribolium castaneum produces cuticular secretions containing benzoquinone to inhibit the germination and growth of B. bassiana (96). Bedbugs (Cimex lectularius) utilize glandular aldehyde secretions, specifically (E)-2-hexenal and (E)-2-octenal, to coat their cuticle and inhibit the growth of M. anisopliae (97).
Besides, the ectomicrobiomes, which are resident antagonistic microbes residing on the surface of insects, confer colonization resistance to hosts by producing antimicrobial compounds to inhibit the germination and growth of fungal conidia (40, 58). Surfaces of Drosophila, for instance, were found to be densely populated with bacterial cells, which can deter fungal spore germination, and the controlled addition of isolated bacteria in a gnotobiotic setting significantly delays fungal infection of axenic flies (98). Out of 155 bacterial isolates from the cuticle of Dalbulus maidis and Delphacodes kuscheli, 91 were found to inhibit the growth of B. bassiana (99). Antagonistic interactions against fungi have also been observed in ectomicrobiomes residing on leaf-cutting ants (Acromyrmex subterraneus) (100), honeybees (Apis mellifera) (101), and the oriental fruit moth (Grapholita molesta) (102). Intriguingly, the beetle Lagria villosa possesses cuticular organs filled with bacterial symbionts, which protect larvae from EPF infection through the production of antifungal compounds (103). Several ant species have the ability to cultivate actinobacteria, which produce potent antimicrobial compounds, in order to safeguard conspecifics and brood from EPF pathogens (104, 105). However, recent research indicates that EPF have evolved strategies to defend against pathogens, for example, B. bassiana secrets the defensin-like peptide BbAMP1, which coats fungal spores in order to target and damage Gram-positive bacterial cells, thus suppressing the defensive microbiomes on the host surface (106). This highlights the dynamic and complex interactions between EPF and insect host defenses. Elucidating the critical chemical components, proteins, or ectomicrobiomes that play inhibitory or facilitating roles in EPF infection is essential for the development of highly effective EPF or synergistic agents.
3 Fungal penetration and growth in hemolymph and insect immune responses
3.1 EPF penetration of the insect cuticle and growth in hemolymph
The appressorium is responsible for generating a narrow penetration peg. This peg, under the pressure exerted by the turgor within the appressorium, can generate a downward force to breach the cuticle. Turgor is generated by the accumulation of solutes, cell compartmentalization and water uptake (107–109). Occasionally, the cuticle is slightly distorted at the penetration site, indicating substantial pressure during penetration (57). The penetration of the cuticle is not only achieved through mechanical pressure; it is also facilitated by enzymatic degradation of EPF. The cuticle mainly consists of a chitin framework, tanned proteins and lipids, and EPF are capable of producing a variety of extracellular hydrolytic enzymes that cooperate to degrade the cuticle (110, 111). The most commonly studied proteolytic enzymes are the subtilisin-like serine-protease Pr1 and trypsin-like protease Pr2 in B. bassiana and M. anisopliae (112). Chitinase genes chit1, chiti2, and chit3 in M. anisopliae and Bbchit1 in B. bassiana have also been well studied (113–115). It has been demonstrated that overexpressing the Pr‐like protease and chitinase in engineered B. bassiana strains significantly accelerates insect death (116). Moreover, EPF have evolved expansions of serine proteases and chitinases to facilitate cuticle degradation (117), and applied research onthese enzymes has been conducted (118). After successfully penetrating the cuticle, hyphae can invade the insect hemolymph (Figure 2). Within this environment, EPF generate hyphal bodies, using available nutrients for growth and reproduction through the budding process (119). These hyphal bodies exhibit a distinctive brush-like outermost structure and are believed to have modified carbohydrate epitopes, e.g. lower glucan contents compared to other propagules like submerged conidia and hyphae, which may contribute to their ability to evade host immune responses (31, 50, 120, 121). For example, hyphal bodies of M. rileyi induce weaker cellular immune responses in the body cavity of Helicoverpa armigera caterpillars compared to M. rileyi conidia (122). Additionally, EPF express a range of proteins to coat hyphal bodies, camouflaging their cell wall structures to further evade the host insect immunity (123, 124). An example of such proteins includes LysM domain-containing proteins in B. bassiana that bind to chitin in the cell wall to aid in evading insect immunity (125).
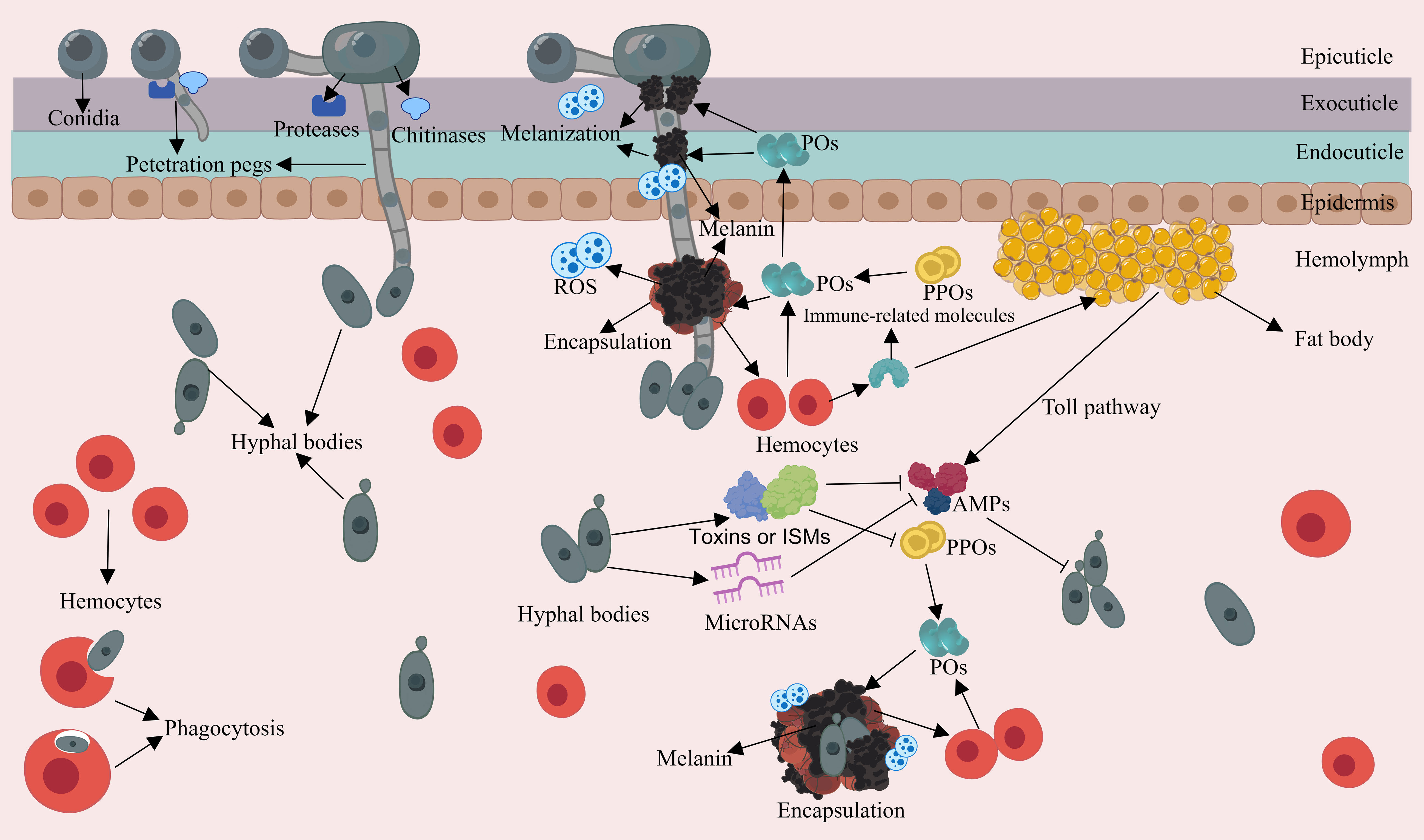
Figure 2 Schematic representation of the interaction between EPF and the insect immune system during the penetration and intra-hemolymph growth phase. Appressoria achieve turgidity and generate penetration pegs. EPF deploy extracellular hydrolytic enzymes to breach the cuticle. Hyphal bodies can produce insecticidal and immunosuppressive metabolites (ISMs), which can deactivate or degrade PPOs, and suppress AMP gene expression. Hyphal bodies can initiate cellular and humoral responses as well as activating the PPO cascade in the compromised insect.
3.2 Production of insecticidal and immunosuppressive metabolites by EPF
The primary source of nutrition for EPF growth is derived from the insect hemolymph, and the most abundant carbohydrate is the disaccharide trehalose. To access this vital resource, EPF secrete trehalase to break down trehalose and absorb the resulting glucose, or alternatively, they may transport trehalose into their cells for intracellular processing (126, 127). Furthermore, EPF are capable of secreting a range of insecticidal and immunosuppressive metabolites, which serve to hasten the death of the host or interfere with insect immune responses (128) (Figure 2). For example, B. bassiana produces certain compounds like beauvericin, beauveriolides, 2-pyridone tenellin, and benzoquinone oosporein, which possess insecticidal and cytotoxic properties, expediting fungal colonization (129–132). Cyclopeptide destruxins secreted by EPF such as M. anisopliae have been found to deactivate prophenoloxidases (PPOs) and suppress antimicrobial peptide (AMP) gene expression by targeting immunophilins (133, 134). The M35 family of metalloproteases in M. robertsii can degrade the PPOs of the host, and M. robertsii can directly degrade the inductive antifungal AMPs and protease inhibitors present in insects (135, 136). The extracellular laccase encoded by BbLac2 in the hyphal bodies of B. bassiana can oxidize and deactivate PPOs, as well as eliminate the reactive oxygen species (ROS) in the insect hemocoel (137). Furthermore, microRNA-like RNA is even utilized by EPF to silence the expression of the immune genes of insects, for example, B. bassiana exports a microRNA-like RNA (bba-milR1) that can silence expression of the mosquito Toll receptor ligand Spätzle 4 (Spz4), which finally attenuated mosquito immune responses (138). In summary, EPF appear to have evolved effector mechanisms akin to those found in phytopathogens, which serve to disrupt host immunity. Further research in this area is warranted to gain a deeper understanding of these interactions.
3.3 Insect immune responses during EPF infection
The insect cuticle serves crucial functions in wound healing and it can also produce immune responses (139). During the EPF penetration, the cuticle triggers a hemostatic response to minimize hemolymph leakage, while simultaneously initiating immune reactions, such as melanization (140) (Figure 2). Cuticular melanization is initiated by the PPO activation cascade and involves the conversion of phenolic substrates into black-brown pigments by the phenoloxidase (PO) enzyme (141, 142). In this reaction, ROS and toxic intermediates produced during melanin synthesis can encircle and neutralize pathogens, forming nodules through hemocyte aggregation (143, 144). Furthermore, end-product melanin pigments are important structural and protective elements of the cuticle. The accumulation of melanin in the insect cuticle aids in wound healing and acts as a barrier and toxin to retard cuticular penetration (145, 146). Moreover, hemocytes may migrate to the penetration sites to assist in wound repair and release AMPs by penetrating the basement membrane (33, 147).
When EPF successfully penetrate the cuticle and enter the hemocoel, the insect innate immune springs into action. Through a combination of humoral and cellular responses, along with the PPO cascade, the different layers of the immune system work together to combat the infection (Figure 2). This coordinated effort is initiated by the recognition of microbe-associated molecular patterns (MAMPs) by pathogen recognition receptors (PRRs) present in the hemocoel (148–150). As described before, the PPO the cascade is initiated through the interaction of MAMPs with hemocyte-bound PRRs in the hemocoel. Then PO converts phenolic substrates, facilitating the biosynthesis of microbicidal pigment and melanin with the cooperation of hemocytes (142, 151). Mutants with lower PPO activity in Drosophila and mosquitoes experience higher mortality when exposed to Metarhizium and Beauveria pathogens, underscoring the importance of melanization in defending EPF (152, 153).
Humoral immunity in insects engages the fat body in the biosynthesis of antimicrobial effectors such as AMPs and lysozyme (148, 154). These effectors are produced through the activation of Toll and Immune Deficiency (IMD) signaling pathways and are subsequently secreted into the body cavity to combat the invading microbes. Several antifungal peptides have been characterized, such as termicin from termites (155), drosomycin, metchnikowin, Baramicin A and thanatin from Drosophila (156–158), heliomycin from Heliothis virescens (159), gallerimycin from Galleria mellonella larvae (160), cecropins from Hyalophora cecropia (161). Mutations in certain genes of the Toll pathway lead to increased sensitivity to EPF infection (162, 163). Moreover, in Drosophila, Toll-dependent AMP responses require the Toll ligand Spätzle, indicating that the cellular immune response interacts with the humoral immunity (164).
Notably, the activation of Toll in the fat body and the overexpression of AMPs can lead to reduced glycogen and triglyceride storage in this tissue, negatively affecting the body growth. This suggests that insect immunity places an energy burden on the host (165, 166). However, EPF have evolved strategies to suppress PPO and AMP activities, as discussed above in 3.2 section. The outcome of pathogen-mediated suppression of the immune system’s excessive activation appears to allow insects to conserve energy for growth and development. However, the premise for this hypothesis is contingent on the host insect’s ability to successfully withstand pathogen invasion and proliferation through the restrained immune response.
3.4 The insect microbiome regulates the immune interactions between EPF and host
Microbes inhabit various parts of the insect body, including their cuticular surfaces, digestive tissues or cells (endosymbionts), and tract (gut microbiota). Many studies have reported that gut bacteria are essential for host physiology including growth and development, and the establishment and maintenance of the innate immune system (167–172). Interactions between the insect immune system and their EPF may have an impact on the structure of the microbiome, ultimately influencing whether infections are suppressed or promoted. For example, B. bassiana can interact with gut microbiota, resulting in EPF-infected mosquitoes containing gut microbiota dying significantly faster than those lacking microbiota, while also increasing the gut microbiota load (173). Similar results have also been observed in Dendroctonus valens (174). Conversely, the microbiota can suppress EPF infection. For example, the endosymbiotic bacterium Wolbachia in Drosophila confers a nonspecific resistance to insect pathogens including B. bassiana (175). Burkholderia bacterial species inhabiting the midgut crypts of the Southern chinch bug (Blissus insularis) can produce antifungal compounds to resist EPF (176). Gut microbiota in Delia antiqua assists in inhibiting B. bassiana infection, and the removal of the microbiota diminishes larval resistance to fungal infection (177). These effects are due to various EPF metabolites produced during growth in the hemolymph, which modify the expression of a wide range of host insect immune genes (67, 178, 179). The microbes on the cuticle can also shield the host from EPF infection, which has been discussed above in section 2.3.
Interestingly, as mentioned above, in different insects, the interaction between gut microbiota and EPF can have completely opposite effects. The majority of documented instances suggests that the surface microbiota on insects plays a protective role in shielding insects from EPF infections. This effect is likely due to the direct contact between surface microbiota and EPF, leading to more intense competition for nutrients and space between them. In contrast, gut bacteria and EPF have a greater spatial separation (as most EPF initiate infection from the surface) (54). However, it is important to note that these inferences require further investigation.
4 The battle between insects and EPF post infection
When the host’s nutrients are depleted and the insect is on the brink of death, the EPF must emerge from the insect to generate and disseminate its conidia. The fungi swiftly transition back to mycelial growth, initiating cuticular penetration once again. Only the hyphae that have successfully breached the insect cuticle are able to generate dispersal conidia, and the genes employed during the host invasion phase are subsequently repurposed for the process, enabling a swift and resource-efficient transformation (28, 31). An illustrative example is BbMPK1, known for its crucial role in virulence, as well as in the adhesion and differentiation of appressoria, and penetration of the insect cuticle (180). When mutant conidia lacking BbMPK1 are injected into the hemocoel, they can generate growing hyphae, but they are incapable of emerging from the surface of insect cadavers (180). Similar factors involved in both inward and outward cuticular penetration have also been explored in M. acridum (MaPls1 gene) (181). This genetic versatility enables a swift and efficient adaptation to new hosts. Conidia regenerated on the host surface are primarily dispersed by non-biological means, such as water and wind (182). The hydrophobic nature of EPF conidia serve not only for promoting the attachment to the insect cuticle, but also for facilitating dispersion via water (183). Additionally, some Metarhizium species can produce and accumulate the mycotoxin swainsonine, which protect the insect cadavers from being consumed by birds or other animals (184) (Figure 1B). Moreover, other EPF species can behaviorally manipulate infected hosts to ensure dispersal. This phenomenon is exemplified by the enticement of male houseflies (Musca domestica) by the cadavers of female flies that have previously been infected with Entomophthora mascae (185). Additionally, insect carcasses serve as a nutritional source for the insect or environmental microbiome, typically, only a few other microbes can proliferate from carcasses, and the fungal spores will be laden within the remains of the insect. This suggests that EPF can suppress the competitive proliferation of other microbes in insects or the environment, thus gaining an edge in assimilating host nutrients. For instance, oosporein released from B. bassiana have been suggested to participate in inhibiting the growth of bacteria originating from insect cadavers (131), and B. bassiana can also be induced to produce iron-chelating 2-pyridones to outcompete other antagonistic microbes (132). The isolation of other microbes with insecticidal activity from the insect carcasses may facilitate the development of synergistic mixtures.
Conversely, various strategies have evolved for infected insects to prevent the further spread of EPF, particularly among social group-living insects. For example, EPF infected individuals distance themselves from the nest to avoid disease transmission among nestmates. Ant workers of Myrmica rubra infected by M. brunneum are attracted to light, they display a decrease in their attraction towards nestmates or colony odor, and ultimately they withdraw from the nest, possibly because the EPF impair the olfactory system of the infected ants (186). Carpenter ants (Camponotus aethiops) infected by M. brunneum reduce social interactions and contact with brood, spending most of their time outside the nest until death (187). Sick honey bee workers (Apis mellifera) engage in altruistic self-removal, removing themselves from their colony to prevent disease transmission (188). Clearly, this disease-preventing strategy in social insects is detrimental to the dissemination of EPF, but it remains unclear how EPF responds to the defense strategies of social insects. The reasons behind this lack of understanding are uncertain, whether it is due to our limited information or because EPF have not yet evolved effective strategies remains unclear. Besides, behavioral changes in non-social insects to avoid further EPF infection will also be a subject of future investigation.
5 Synergy between EPF infection and disruption of insect defensive strategies
In light of our understanding of EPF infection mechanisms and insect anti-EPF defensive strategies, emerging practical evidence suggests that the EPF-mediated disruption of insect immune responses can amplify EPF virulence, offering a promising perspective for refining pest management tactics (Table 1). These synergistic approaches could be broadly categorized into four aspects corresponding to EPF infection: 1) Artificially enhancing EPF attachment or penetration capability through various methods. For example, an oil-in-glycerol formulation enhances the lethal infection of M. anisopliae conidia to the Red Palm Weevil (Rhynchophorus ferrugineus) by promoting adhesion (189); 2) Suppressing or evading host insect immunity through RNAi or specific chemicals. For example, ingestion of bacteria expressing immune-suppressive double-stranded RNAs (dsRNAs) leads to significantly increased mortality in the leaf beetle Plagiodera versicolora following B. bassiana infection (207). Similar results have been achieved by modifying either EPF or the insect host plant to successfully deliver dsRNA targeting crucial immune genes of the insect (200). 3) Disrupting the detection abilities of social insects to facilitate EPF dispersal. This can be accomplished by genetically modifying EPF to make the pathogens less recognizable to insects, thus avoiding social immunity. Alternatively, disrupting communication in social insects (e.g., using pesticides or dsRNA can increase EPF dissemination within populations) (208, 209). While applications in this area are currently limited, the hypothesis holds significant potential and warrants further exploration. 4) The development of mixed microbial formulations with synergistic effect alongside EPF, such as (Bacillus thuringiensis + Metarhizium species) pesticides, can offset the limitations of individual pesticides and enhance the control efficiency of EPF in field applications (210, 213). These combined agents present a more socially acceptable strategy compared to genetically modified strains, as they entail lower safety risks.
6 Concluding remarks
Overall, this review primarily delves into the interplay between EPF infection and insect defenses, emphasizing three pivotal infection stages. While our comprehension of the immunological interaction between insects and EPF is extensive, there are still some specific pathways that remain elusive. Moreover, the interaction between host-pathogen transcends mere immunological interplay, which encompasses pre-contact communication and the dispersal of EPF post-infection. Their interaction in these stages exhibits a wider array of forms, often involving more species and even producing important ecological or epizootic consequences. A comprehensive understanding of the intricate interplay not only provides molecular insights into fungus-insect interactions but also holds promise for the development of cost-effective and environmentally-friendly pest management strategies.
Author contributions
MM: Validation, Visualization, Writing – original draft, Writing – review & editing. JL: Writing – original draft, Writing – review & editing. CL: Writing – original draft. IE: Writing – review & editing. WZ: Conceptualization, Funding acquisition, Writing – original draft, Writing – review & editing. LX: Conceptualization, Funding acquisition, Supervision, Validation, Writing – original draft, Writing – review & editing. IE:.
Funding
The author(s) declare financial support was received for the research, authorship, and/or publication of this article. This research was supported by National Natural Science Foundation of China (31971663, 32370523, 32001961, 32160666), the Natural Science Foundation of Hubei Province (2022CFA061), Guizhou Province Science and Technology Support Project ([2022] General 239).
Conflict of interest
The authors declare that the research was conducted in the absence of any commercial or financial relationships that could be construed as a potential conflict of interest.
Publisher’s note
All claims expressed in this article are solely those of the authors and do not necessarily represent those of their affiliated organizations, or those of the publisher, the editors and the reviewers. Any product that may be evaluated in this article, or claim that may be made by its manufacturer, is not guaranteed or endorsed by the publisher.
References
1. Zhang ZQ, Hooper JNA, Soest RWMV, Pisera A, Richardson DJ. Animal biodiversity: An outline of higher-level classification and taxonomic richness. Zootaxa (2011) 3148(3148):7–237. doi: 10.11646/zootaxa.3703.1.1
2. Egambaram S. Insect biodiversity: the teeming millions. Bull Environment Pharmacol Life Sci (2017) 6:101–5. Available at: https://www.researchgate.net/publication/336924207
4. Popp J, Pető K, Nagy J. Pesticide productivity and food security. A review. Agron Sustain Dev (2012) 33(1):243–55. doi: 10.1007/s13593-012-0105-x
5. Jankielsohn A. The importance of insects in agricultural ecosystems. Adv Entomology (2018) 06(02):62–73. doi: 10.4236/ae.2018.62006
6. Kim KC. Biodiversity, conservation and inventory: why insects matter. Biodiversity Conserv (1993) 2(3):191–214. doi: 10.1007/BF00056668
7. Tudi M, Daniel Ruan H, Wang L, Lyu J, Sadler R, Connell D, et al. Agriculture development, pesticide application and its impact on the environment. Int J Environ Res Public Health (2021) 18(3):1112. doi: 10.3390/ijerph18031112
8. Bernardes MFF, Pazin M, Pereira LC, Dorta DJ. Impact of pesticides on environmental and human health. In: Andreazza AC, Scola G, editors. Toxicology Studies (Rijeka: IntechOpen) (2015) 8.
9. Zaim M, Guillet P. Alternative insecticides: an urgent need. Trends Parasitol (2002) 18(4):161–3. doi: 10.1016/s1471-4922(01)02220-6
10. Charnley AK, Collins SA. Entomopathogenic fungi and their role in pest control. Mycota: Environmental and microbial relationships. (2007) 4:159–87.
11. Lv C, Huang B, Qiao M, Wei J, Ding B. Entomopathogenic fungi on Hemiberlesia pitysophila. PLoS One (2011) 6(8):e23649. doi: 10.1371/journal.pone.0023649
12. Mantzoukas S, Kitsiou F, Natsiopoulos D, Eliopoulos PA. Entomopathogenic fungi: Interactions and applications. Encyclopedia (2022) 2(2):646–56. doi: 10.3390/encyclopedia2020044
13. Mann AJ, Davis TS. Entomopathogenic fungi to control bark beetles: a review of ecological recommendations. Pest Manag Sci (2021) 77(9):3841–6. doi: 10.1002/ps.6364
14. Litwin A, Nowak M, Różalska S. Entomopathogenic fungi: unconventional applications. Rev Environ Sci Bio/Technology (2020) 19(1):23–42. doi: 10.1007/s11157-020-09525-1
15. Inglis DG, Goettel MS, Butt TM, Strasser H. Use of hyphomycetous fungi for managing insect pests. Wallingford: CAB International (2001).
16. Gao T, Wang Z, Huang Y, Keyhani NO, Huang Z. Lack of resistance development in Bemisia tabaci to Isaria fumosorosea after multiple generations of selection. Sci Rep (2017) 7(1):42727. doi: 10.1038/srep42727
17. Shapiro-Ilan D, Hazir S, Glazer I. Chapter 6 - Basic and Applied Research: Entomopathogenic Nematodes. In: Lacey LA, editor. Microbial Control of Insect and Mite Pests. Academic Press, San Diego (2017) p. 91–105.
18. St. Leger RJ, Wang C. Genetic engineering of fungal biocontrol agents to achieve greater efficacy against insect pests. Appl Microbiol Biotechnol (2009) 85(4):901–7. doi: 10.1007/s00253-009-2306-z
19. Mora MAE, Castilho AMC, Fraga ME. Classification and infection mechanism of entomopathogenic fungi. Arquivos do Instituto Biológico (2018) 84(1-10):e0552015. doi: 10.1590/1808-1657000552015
20. Boomsma JJ, Jensen AB, Meyling NV, Eilenberg J. Evolutionary interaction networks of insect pathogenic fungi. Annu Rev Entomol (2014) 59:467–85. doi: 10.1146/annurev-ento-011613-162054
21. Kim JC, Lee MR, Kim S, Lee SJ, Park SE, Nai Y-S, et al. Tenebrio molitor-mediated entomopathogenic fungal library construction for pest management. J Asia-Pacif Entomol (2018) 21(1):196–204. doi: 10.1016/j.aspen.2017.11.018
22. Butt TM, Jackson C, Magan M. Introduction-fungal biological control agents: progress, problems and potential. In: Butt TM, Jackson C, Magan M, editors. Fungal biocontrol agents: progress, problems and potential. Wallingford: CAB International (2001). p. 1–8.
23. Lacey LA, Grzywacz D, Shapiro-Ilan DI, Frutos R, Brownbridge M, Goettel MS. Insect pathogens as biological control agents: Back to the future. J Invertebr Pathol (2015) 132:1–41. doi: 10.1016/j.jip.2015.07.009
24. Sullivan CF, Parker BL, Davari A, Lee MR, Kim JS, Skinner M. Evaluation of spray applications of Metarhizium anisopliae, Metarhizium brunneum and Beauveria bassiana against larval winter ticks, Dermacentor albipictus. Exp Appl Acarol (2020) 82(4):559–70. doi: 10.1007/s10493-020-00547-6
25. Faria MRD, Wraight SP. Mycoinsecticides and Mycoacaricides: A comprehensive list with worldwide coverage and international classification of formulation types. Biol Control (2007) 43(3):237–56. doi: 10.1016/j.biocontrol.2007.08.001
26. Mannino MC, Huarte-Bonnet C, Davyt-Colo B, Pedrini N. Is the insect cuticle the only entry gate for fungal fnfection? Insights into alternative modes of action of entomopathogenic fungi. J Fungi (2019) 5(2):33. doi: 10.3390/jof5020033
27. Rafaluk-Mohr C, Wagner S, Joop G. Cryptic changes in immune response and fitness in Tribolium castaneum as a consequence of coevolution with Beauveria bassiana. J Invertebr Pathol (2018) 152:1–7. doi: 10.1016/j.jip.2017.12.003
28. Shin TY, Lee MR, Park SE, Lee SJ, Kim WJ, Kim JS. Pathogenesis-related genes of entomopathogenic fungi. Arch Insect Biochem Physiol (2020) 105(4):e21747. doi: 10.1002/arch.21747
29. Mascarin GM, Jaronski ST. The production and uses of Beauveria bassiana as a microbial insecticide. World J Microbiol Biotechnol (2016) 32(11):177. doi: 10.1007/s11274-016-2131-3
30. Yang YT, Lee SJ, Nai YS, Kim S, Kim JS. Up-regulation of carbon metabolism-related glyoxylate cycle and toxin production in Beauveria bassiana JEF-007 during infection of bean bug, Riptortus pedestris (Hemiptera: Alydidae). Fungal Biol (2016) 120(10):1236–48. doi: 10.1016/j.funbio.2016.07.008
31. Valero-Jimenez CA, Wiegers H, Zwaan BJ, Koenraadt CJ, van Kan JA. Genes involved in virulence of the entomopathogenic fungus Beauveria bassiana. J Invertebr Pathol (2016) 133:41–9. doi: 10.1016/j.jip.2015.11.011
32. Ashida M, Brey PT. Role of the integument in insect defense: pro-phenol oxidase cascade in the cuticular matrix. Proc Natl Acad Sci (1995) 92(23):10698–702. doi: 10.1073/pnas.92.23.10698
33. Brey PT, Lee WJ, Yamakawa M, Koizumi Y, Perrot S, François M, et al. Role of the integument in insect immunity: epicuticular abrasion and induction of cecropin synthesis in cuticular epithelial cells. Proc Natl Acad Sci (1993) 90(13):6275–9. doi: 10.1073/pnas.90.13.6275
34. Strand MR. The insect cellular immune response. Insect Sci (2008) 15(1):1–14. doi: 10.1111/j.1744-7917.2008.00183.x
35. Irving P, Ubeda J-M, Doucet D, Troxler L, Lagueux M, Zachary D, et al. New insights into Drosophila larval haemocyte functions through genome-wide analysis. Cell Microbiol (2005) 7(3):335–50. doi: 10.1111/j.1462-5822.2004.00462.x
36. Hoffmann JA. The immune response of Drosophila. Nature (2003) 426(6962):33–8. doi: 10.1038/nature02021
37. Leulier F, Parquet C, Pili-Floury S, Ryu JH, Caroff M, Lee WJ, et al. The Drosophila immune system detects bacteria through specific peptidoglycan recognition. Nat Immunol (2003) 4(5):478–84. doi: 10.1038/ni922
38. Butt T, Goettel M. Bioassays of entomogenous fungi. Wallingford: CAB International (2000). pp. 141–95.
39. Clancy LM, Jones R, Cooper AL, Griffith GW, Santer RD. Dose-dependent behavioural fever responses in desert locusts challenged with the entomopathogenic fungus Metarhizium acridum. Sci Rep (2018) 8(1):14222. doi: 10.1038/s41598-018-32524-w
40. Ortiz-Urquiza A, Keyhani N. Action on the surface: Entomopathogenic fungi versus the insect cuticle. Insects (2013) 4(3):357–74. doi: 10.3390/insects4030357
41. Holder DJ, Keyhani NO. Adhesion of the entomopathogenic fungus Beauveria (Cordyceps) bassiana to substrata. Appl Environ Microbiol (2005) 71(9):5260–6. doi: 10.1128/aem.71.9.5260-5266.2005
42. Holder DJ, Kirkland BH, Lewis MW, Keyhani NO. Surface characteristics of the entomopathogenic fungus Beauveria (Cordyceps) bassiana. Microbiology (2007) 153(10):3448–57. doi: 10.1099/mic.0.2007/008524-0
43. Zhang S, Xia YX, Kim B, Keyhani NO. Two hydrophobins are involved in fungal spore coat rodlet layer assembly and each play distinct roles in surface interactions, development and pathogenesis in the entomopathogenic fungus, Beauveria bassiana. Mol Microbiol (2011) 80(3):811–26. doi: 10.1111/j.1365-2958.2011.07613.x
44. Cho E-M, Kirkland BH, Holder DJ, Keyhani NO. Phage display cDNA cloning and expression analysis of hydrophobins from the entomopathogenic fungus Beauveria (Cordyceps) bassiana. Microbiology (2007) 153(10):3438–47. doi: 10.1099/mic.0.2007/008532-0
45. Zhou Q, Yu L, Ying S-H, Feng M-G. Comparative roles of three adhesin genes (adh1–3) in insect-pathogenic lifecycle of Beauveria bassiana. Appl Microbiol Biotechnol (2021) 105(13):5491–502. doi: 10.1007/s00253-021-11420-w
46. Wang C, St Leger RJ. The MAD1 adhesin of Metarhizium anisopliae links adhesion with blastospore production and virulence to insects, and the MAD2 adhesin enables attachment to plants. Eukaryot Cell (2007) 6(5):808–16. doi: 10.1128/ec.00409-06
47. Li J, Ying S-H, Shan L-T, Feng M-G. A new non-hydrophobic cell wall protein (CWP10) of Metarhizium anisopliae enhances conidial hydrophobicity when expressed in Beauveria bassiana. Appl Microbiol Biotechnol (2009) 85(4):975–84. doi: 10.1007/s00253-009-2083-8
48. Broetto L, Da Silva WOB, Bailão AM, De Almeida Soares C, Vainstein MH, Schrank A. Glyceraldehyde-3-phosphate dehydrogenase of the entomopathogenic fungus Metarhizium anisopliae: cell-surface localization and role in host adhesion. FEMS Microbiol Lett (2010) 312(2):101–9. doi: 10.1111/j.1574-6968.2010.02103.x
49. Warwas ML, Watson JN, Bennet AJ, Moore MM. Structure and role of sialic acids on the surface of Aspergillus fumigatus conidiospores. Glycobiology (2007) 17(4):401–10. doi: 10.1093/glycob/cwl085
50. Wanchoo A, Lewis MW, Keyhani NO. Lectin mapping reveals stage-specific display of surface carbohydrates in in vitro and haemolymph-derived cells of the entomopathogenic fungus Beauveria bassiana. Microbiology (2009) 155(9):3121–33. doi: 10.1099/mic.0.029157-0
51. Brivio MF, Mastore M. When appearance misleads: The role of the entomopathogen surface in the relationship with its host. Insects (2020) 11(6):387. doi: 10.3390/insects11060387
52. Masuoka J. Surface glycans of Candida albicans and other pathogenic fungi: Physiological roles, clinical uses, and experimental challenges. Clin Microbiol Rev (2004) 17(2):281–310. doi: 10.1128/cmr.17.2.281-310.2004
53. Leger RJS, Butt TM, Goettel MS, Staples RC, Roberts DW. Production in vitro of appressoria by the entomopathogenic fungus Metarhizium anisopliae. Exp Mycology (1989) 13(3):274–88. doi: 10.1016/0147-5975(89)90049-2
54. Butt TM, Coates CJ, Dubovskiy IM, Ratcliffe NA. Entomopathogenic fungi. Adv Genet (2016) 94:307–64. doi: 10.1016/bs.adgen.2016.01.006
55. Hong S, Shang J, Sun Y, Tang G, Wang C. Fungal infection of insects: molecular insights and prospects. Trends Microbiol (2023) online ahead of print. doi: 10.1016/j.tim.2023.09.005
56. Srisukchayakul P, Wiwat C, Pantuwatana S. Studies on the pathogenesis of the local isolates of Nomuraea rileyi against Spodoptera litura. ScienceAsia (2005) 31(3), 273–6. doi: 10.2306/scienceasia1513-1874.2005.31.273
57. Butt TM, Ibrahim L, Clark SJ, Beckett A. The germination behaviour of Metarhizium anisopliae on the surface of aphid and flea beetle cuticles. Mycol Res (1995) 99(8):945–50. doi: 10.1016/S0953-7562(09)80754-5
58. Hong S, Sun Y, Chen H, Zhao P, Wang C. Fungus–insect interactions beyond bilateral regimes: the importance and strategy to outcompete host ectomicrobiomes by fungal parasites. Curr Opin Microbiol (2023) 74:102336. doi: 10.1016/j.mib.2023.102336
59. Fang W, Feng J, Fan Y, Zhang Y, Bidochka MJ, Leger RJS, et al. Expressing a fusion protein with protease and chitinase activities increases the virulence of the insect pathogen Beauveria bassiana. J Invertebr Pathol (2009) 102(2):155–9. doi: 10.1016/j.jip.2009.07.013
60. Fang W, Pava-ripoll M, Wang S, St. Leger R. Protein kinase A regulates production of virulence determinants by the entomopathogenic fungus, Metarhizium anisopliae. Fungal Genet Biol (2009) 46(3):277–85. doi: 10.1016/j.fgb.2008.12.001
61. Zheng W, Chen J, Liu W, Zheng S, Zhou J, Lu G, et al. A Rho3 homolog is essential for appressorium development and pathogenicity of Magnaporthe grisea. Eukaryot Cell (2007) 6(12):2240–50. doi: 10.1128/ec.00104-07
62. Shang J, Shang Y, Tang G, Wang C. Identification of a key G-protein coupled receptor in mediating appressorium formation and fungal virulence against insects. Sci China Life Sci (2020) 64(3):466–77. doi: 10.1007/s11427-020-1763-1
63. Wang L, Lai Y, Chen J, Cao X, Zheng W, Dong L, et al. The ASH1-PEX16 regulatory pathway controls peroxisome biogenesis for appressorium-mediated insect infection by a fungal pathogen. Proc Natl Acad Sci (2023) 120(4):e2217145120. doi: 10.1073/pnas.2217145120
64. Mei L, Wang X, Yin Y, Tang G, Wang C. Conservative production of galactosaminogalactan in Metarhizium is responsible for appressorium mucilage production and topical infection of insect hosts. PLoS Path (2021) 17(6):e1009656. doi: 10.1371/journal.ppat.1009656
65. Baverstock J, Roy HE, Pell JK. Entomopathogenic fungi and insect behaviour: from unsuspecting hosts to targeted vectors. BioControl (2009) 55(1):89–102. doi: 10.1007/s10526-009-9238-5
66. Zhang W, Xie M, Eleftherianos I, Mohamed A, Cao Y, Song B, et al. An odorant binding protein is involved in counteracting detection-avoidance and Toll-pathway innate immunity. J Adv Res (2023) 48, 1–16. doi: 10.1016/j.jare.2022.08.013
67. Zhang W, Jia C, Zang LS, Gu M, Zhang R, Eleftherianos I, et al. Entomopathogenic fungal-derived metabolites alter innate immunity and gut microbiota in the migratory locust. J Pest Sci (2023). doi: 10.1007/s10340-023-01685-7
68. Villani MG, Krueger SR, Schroeder PC, Frank C, Consolie NH, Preston-Wilsey LM, et al. Soil application effects of Metarhizium anisopliae on Japanese Beetle (Coleoptera: Scarabaeidae) behavior and survival in turfgrass microcosms. Environ Entomol (1994) 2):502–13. doi: 10.1093/ee/23.2.502
69. Meyling NV, Pell JK. Detection and avoidance of an entomopathogenic fungus by a generalist insect predator. Ecol Entomol (2006) 31(2):162–71. doi: 10.1111/j.0307-6946.2006.00781.x
70. Myles T. Alarm, aggregation, and defense by Reticulitermes flavipes in response to a naturally occurring isolate of Metarhizium anisopliae. Sociobiology (2002) 40:243–55. doi: 10.1006/pest.2001.2581
71. Mburu DM, Maniania NK, Hassanali A. Comparison of volatile blends and nucleotide sequences of two Beauveria Bassiana Isolates of different virulence and repellency towards the termite Macrotermes Michealseni. J Chem Ecol (2012) 39(1):101–8. doi: 10.1007/s10886-012-0207-6
72. Mburu DM, Ndung'u MW, Maniania NK, Hassanali A. Comparison of volatile blends and gene sequences of two isolates of Metarhizium anisopliae of different virulence and repellency toward the termite Macrotermes michaelseni. J Exp Biol (2011) 214(6):956–62. doi: 10.1242/jeb.050419
73. Staples JA, Milner RJ. A laboratory evaluation of the repellency of Metarhizium anisopliae conidia to Coptotermes lacteus (Isoptera: Rhinotermitidae). Sociobiology (2000) 36(1):133–48. Available at: https://www.researchgate.net/publication/279910098
74. George J, Jenkins NE, Blanford S, Thomas MB, Baker TC. Malaria mosquitoes attracted by fatal fungus. PLoS One (2013) 8(5):e62632. doi: 10.1371/journal.pone.0062632
75. Mitina GV, Stepanycheva EA, Choglokova AA, Cherepanova MA. Effect of volatile organic compounds of entomopathogenic fungi of the genus Lecanicillium and their component, acetic acid, on female behavior in the western flower thrips Frankliniella occidentalis (Pergande) (Thysanoptera, Thripidae). Entomol Rev (2023) 103(2):144–51. doi: 10.1134/S0013873823020033
76. Hung R, Lee S, Bennett JW. Fungal volatile organic compounds and their role in ecosystems. Appl Microbiol Biotechnol (2015) 99(8):3395–405. doi: 10.1007/s00253-015-6494-4
77. Davis TS, Crippen TL, Hofstetter RW, Tomberlin JK. Microbial volatile emissions as insect semiochemicals. J Chem Ecol (2013) 39(7):840–59. doi: 10.1007/s10886-013-0306-z
78. Cotes B, Thöming G, Amaya-Gómez CV, Novák O, Nansen C. Root-associated entomopathogenic fungi manipulate host plants to attract herbivorous insects. Sci Rep (2020) 10(1):22424. doi: 10.1038/s41598-020-80123-5
79. Raman A, Suryanarayanan TS. Fungus–plant interaction influences plant-feeding insects. Fungal Ecol (2017) 29:123–32. doi: 10.1016/j.funeco.2017.06.004
80. Shimizu S, Yamaji M. Effect of density of the termite, Reticulitermes speratus Kolbe (Isoptera: Rhinotermitidae), on the susceptibilities to Metarhizium anisopliae. Appl Entomology Zoology (2003) 38(1):125–30. doi: 10.1303/aez.2003.125
81. Yanagawa A, Shimizu S. Resistance of the termite, Coptotermes formosanus Shiraki to Metarhizium anisopliae due to grooming. BioControl (2006) 52(1):75–85. doi: 10.1007/s10526-006-9020-x
82. Qiu H-l, Lu L-h, Shi Q-x, He Y-r. Fungus exposed Solenopsis invicta ants benefit from grooming. J Insect Behav (2014) 27(5):678–91. doi: 10.1007/s10905-014-9459-z
83. Okuno M, Tsuji K, Sato H, Fujisaki K. Plasticity of grooming behavior against entomopathogenic fungus Metarhizium anisopliae in the ant Lasius japonicus. J Ethol (2011) 30(1):23–7. doi: 10.1007/s10164-011-0285-x
84. Lord JC, Howard RW. A proposed role for the cuticular fatty amides of Liposcelis bostrychophila (Psocoptera: Liposcelidae) in preventing adhesion of entomopathogenic fungi with dry-conidia. Mycopathologia (2004) 158(2):211–7. doi: 10.1023/B:MYCO.0000041837.29478.78
85. Vandenberg JD, Mark R, Altre JA. Dose-response and age- and temperature-related susceptibility of the diamondback moth (Lepidoptera: Plutellidae) to two isolates of Beauveria bassiana (Hyphomycetes: Moniliaceae). Environ Entomol (1998) 27(4):1017–21. doi: 10.1093/ee/27.4.1017
86. Kim JJ, Roberts DW. The relationship between conidial dose, moulting and insect developmental stage on the susceptibility of cotton aphid, Aphis gossypii, to conidia of Lecanicillium attenuatum, an entomopathogenic fungus. Biocontrol Sci Technol (2012) 22(3):319–31. doi: 10.1080/09583157.2012.656580
87. Kiuchi M, Yasui H, Hayasaka S, Kamimura M. Entomogenous fungus Nomuraea rileyi inhibits host insect molting by C22-oxidizing inactivation of hemolymph ecdysteroids. Arch Insect Biochem Physiol (2003) 52(1):35–44. doi: 10.1002/arch.10060
88. Pedrini N, Ortiz-Urquiza A, Huarte-Bonnet C, Zhang S, Keyhani NO. Targeting of insect epicuticular lipids by the entomopathogenic fungus Beauveria bassiana: hydrocarbon oxidation within the context of a host-pathogen interaction. Front Microbiol (2013) 4:24. doi: 10.3389/fmicb.2013.00024
89. Greenfield BPJ, Lord AM, Dudley E, Butt TM. Conidia of the insect pathogenic fungus, Metarhizium anisopliae, fail to adhere to mosquito larval cuticle. R Soc Open Sci (2014) 1(2):140193. doi: 10.1098/rsos.140193
90. Vilcinskas A. Coevolution between pathogen-derived proteinases and proteinase inhibitors of host insects. Virulence (2014) 1(3):206–14. doi: 10.4161/viru.1.3.12072
91. Sosa-Gomez DR, Boucias DG, Nation JL. Attachment of Metarhizium anisopliae to the southern green stink bug Nezara viridula cuticle and fungistatic effect of cuticular lipids and aldehydes. J Invertebr Pathol (1997) 69(1):31–9. doi: 10.1006/jipa.1996.4619
92. Bulmer MS, Bachelet I, Raman R, Rosengaus RB, Sasisekharan R. Targeting an antimicrobial effector function in insect immunity as a pest control strategy. Proc Natl Acad Sci U S A (2009) 106(31):12652–7. doi: 10.1073/pnas.0904063106
93. Bulmer MS. Duplication and diversifying selection among termite antifungal peptides. Mol Biol Evol (2004) 21(12):2256–64. doi: 10.1093/molbev/msh236
94. Tragust S, Mitteregger B, Barone V, Konrad M, Ugelvig LV, Cremer S. Ants disinfect fungus-exposed brood by oral uptake and spread of their poison. Curr Biol (2013) 23(1):76–82. doi: 10.1016/j.cub.2012.11.034
95. Ugelvig LV, Cremer S. Social prophylaxis: Group interaction promotes collective immunity in ant colonies. Curr Biol (2007) 17(22):1967–71. doi: 10.1016/j.cub.2007.10.029
96. Pedrini N, Ortiz-Urquiza A, Huarte-Bonnet C, Fan Y, Juárez MP, Keyhani NO. Tenebrionid secretions and a fungal benzoquinone oxidoreductase form competing components of an arms race between a host and pathogen. Proc Natl Acad Sci (2015) 112(28):E3651-60. doi: 10.1073/pnas.1504552112
97. Ulrich KR, Feldlaufer MF, Kramer M, St. Leger RJ. Inhibition of the entomopathogenic fungus Metarhizium anisopliae sensu lato in vitro by the bed bug defensive secretions (E)-2-hexenal and (E)-2-octenal. BioControl (2015) 60(4):517–26. doi: 10.1007/s10526-015-9667-2
98. Hong S, Sun Y, Sun D, Wang C. Microbiome assembly on Drosophila body surfaces benefits the flies to combat fungal infections. iScience (2022) 25(6):104408. doi: 10.1016/j.isci.2022.104408
99. Toledo AV, Alippi AM, de Remes Lenicov AMM. Growth Inhibition of Beauveria bassiana by bacteria isolated from the cuticular surface of the corn leafhopper, Dalbulus maidis and the planthopper, Delphacodes kuscheli, two important vectors of maize pathogens. J Insect Sci (2011) 11(29):1–13. doi: 10.1673/031.011.0129
100. Mattoso TC, Moreira DDO, Samuels RI. Symbiotic bacteria on the cuticle of the leaf-cutting ant Acromyrmex subterraneus subterraneus protect workers from attack by entomopathogenic fungi. Biol Lett (2011) 8(3):461–4. doi: 10.1098/rsbl.2011.0963
101. Miller DL, Smith EA, Newton ILG. A bacterial symbiont protects honey bees from fungal disease. MBio (2021) 12(3):e0050321. doi: 10.1128/mBio.00503-21
102. Wang X, Yang X, Zhou F, Tian ZQ, Cheng J, Michaud JP, et al. Symbiotic bacteria on the cuticle protect the oriental fruit moth Grapholita molesta from fungal infection. Biol Control: Theory Appl Pest Manage (2022) 169:104895. doi: 10.1016/j.biocontrol.2022.104895
103. Janke RS, Kaftan F, Niehs SP, Scherlach K, Rodrigues A, Svatoš A, et al. Bacterial ectosymbionts in cuticular organs chemically protect a beetle during molting stages. ISME J (2022) 16(12):2691–701. doi: 10.1038/s41396-022-01311-x
104. Little AEF, Murakami T, Mueller UG, Currie CR. Defending against parasites: fungus-growing ants combine specialized behaviours and microbial symbionts to protect their fungus gardens. Biol Lett (2006) 2(1):12–6. doi: 10.1098/rsbl.2005.0371
105. Folgarait P, Gorosito N, Poulsen M, Currie CR. Preliminary in vitro insights into the use of natural fungal pathogens of leaf-cutting ants as biocontrol agents. Curr Microbiol (2011) 63(3):250–8. doi: 10.1007/s00284-011-9944-y
106. Hong S, Sun Y, Chen H, Wang C. Suppression of the insect cuticular microbiomes by a fungal defensin to facilitate parasite infection. ISME J (2023) 17(1):1–11. doi: 10.1038/s41396-022-01323-7
107. Thines E, Weber RW, Talbot NJ. MAP kinase and protein kinase a-dependent mobilization of triacylglycerol and glycogen during appressorium turgor generation by Magnaporthe grisea. Plant Cell (2000) 12(9):1703–18. doi: 10.1105/tpc.12.9.1703
108. Wang C, St. Leger RJ. The Metarhizium anisopliae perilipin homolog MPL1 regulates lipid metabolism, appressorial turgor pressure, and virulence. J Biol Chem (2007) 282(29):21110–5. doi: 10.1074/jbc.M609592200
109. Hallsworth JE, Magan N. Culture age, temperature, and pH affect the polyol and trehalose contents of fungal propagules. Appl Environ Microbiol (1996) 62(7):2435–42. doi: 10.1128/aem.62.7.2435-2442.1996
110. Schrank A, Vainstein MH. Metarhizium anisopliae enzymes and toxins. Toxicon (2010) 56(7):1267–74. doi: 10.1016/j.toxicon.2010.03.008
111. Lee SJ, Lee MR, Kim S, Kim JC, Park SE, Shin TY, et al. Conidiogenesis-related DNA photolyase gene in Beauveria bassiana. J Invertebr Pathol (2018) 153:85–91. doi: 10.1016/j.jip.2018.02.013
112. Sánchez-Pérez L, Barranco-Florido JE, Rodríguez-Navarro S, Cervantes-Mayagoitia JF, Ramos-López MÁ. Enzymes of entomopathogenic fungi, advances and insights. Adv Enzyme Res (2014) 02(02):65–76. doi: 10.4236/aer.2014.22007
113. Staats CC, Kmetzsch L, Lubeck I, Junges A, Vainstein MH, Schrank A. Metarhizium anisopliae chitinase CHIT30 is involved in heat-shock stress and contributes to virulence against Dysdercus Peruvianus. Fungal Biol (2013) 117(2):137–44. doi: 10.1016/j.funbio.2012.12.006
114. Fang W, Leng B, Xiao Y, Jin K, Ma J, Fan Y, et al. Cloning of Beauveria bassiana chitinase gene Bbchit1 and its application to improve fungal strain virulence. Appl Environ Microbiol (2005) 71(1):363–70. doi: 10.1128/aem.71.1.363-370.2005
115. Liu Q, Ying S-H, Li J-G, Tian C-G, Feng M-G. Insight into the transcriptional regulation of Msn2 required for conidiation, multi-stress responses and virulence of two entomopathogenic fungi. Fungal Genet Biol (2013) 54:42–51. doi: 10.1016/j.fgb.2013.02.008
116. Lovett B, St. Leger RJ. Genetically engineering better fungal biopesticides. Pest Manage Sci (2017) 74(4):781–9. doi: 10.1002/ps.4734
117. Shang Y, Xiao G, Zheng P, Cen K, Zhan S, Wang C. Divergent and convergent evolution of fungal pathogenicity. Genome Biol Evol (2016) 8(5):1374–87. doi: 10.1093/gbe/evw082
118. Ferreira JM, Soares F. Entomopathogenic fungi hydrolytic enzymes: A new approach to biocontrol? J Natural Pesticide Res (2023) 3:100020. doi: 10.1016/j.napere.2023.100020
119. Lewis MW, Robalino IV, Keyhani NO. Uptake of the fluorescent probe FM4-64 by hyphae and haemolymph-derived in vivo hyphal bodies of the entomopathogenic fungus Beauveria bassiana. Microbiology (2009) 155(9):3110–20. doi: 10.1099/mic.0.029165-0
120. Andrianopoulos A, Zhang J, Jiang H, Du Y, Keyhani NO, Xia Y, et al. Members of chitin synthase family in Metarhizium acridum differentially affect fungal growth, stress tolerances, cell wall integrity and virulence. PLoS Path (2019) 15(8):e1007964. doi: 10.1371/journal.ppat.1007964
121. Rappleye CA, Wang H, Lu Z, Keyhani NO, Deng J, Zhao X, et al. Insect fungal pathogens secrete a cell wall-associated glucanase that acts to help avoid recognition by the host immune system. PLoS Path (2023) 19(8):e1011578. doi: 10.1371/journal.ppat.1011578
122. Li L, Zhong K, Wang JL, Liu XS. Mechanism of Metarhizium rileyi evading cellular immune responses in Helicoverpa armigera. Arch Insect Biochem Physiol (2021) 106(3):e21769. doi: 10.1002/arch.21769
123. Yang Z, Jiang H, Zhao X, Lu Z, Luo Z, Li X, et al. Correlation of cell surface proteins of distinct Beauveria bassiana cell types and adaption to varied environment and interaction with the host insect. Fungal Genet Biol (2017) 99:13–25. doi: 10.1016/j.fgb.2016.12.009
124. Wang C, St Leger RJ. A collagenous protective coat enables Metarhizium anisopliae to evade insect immune responses. Proc Natl Acad Sci U S A (2006) 103(17):6647–52. doi: 10.1073/pnas.0601951103
125. Cen K, Li B, Lu Y, Zhang S, Wang C. Divergent LysM effectors contribute to the virulence of Beauveria bassiana by evasion of insect immune defenses. PLoS Path (2017) 13(9):e1006604. doi: 10.1371/journal.ppat.1006604
126. Xia Y, Clarkson JM, Charnley AK. Trehalose-hydrolysing enzymes of Metarhizium anisopliae and their role in pathogenesis of the tobacco hornworm, Manduca sexta. J Invertebr Pathol (2002) 80(3):139–47. doi: 10.1016/S0022-2011(02)00105-2
127. Wang X-X, Ji X-P, Li J-X, Keyhani NO, Feng M-G, Ying S-H. A putative α-glucoside transporter gene BbAGT1 contributes to carbohydrate utilization, growth, conidiation and virulence of filamentous entomopathogenic fungus Beauveria bassiana. Res Microbiol (2013) 164(5):480–9. doi: 10.1016/j.resmic.2013.02.008
128. Zhang L, Fasoyin OE, Molnár I, Xu Y. Secondary metabolites from hypocrealean entomopathogenic fungi: novel bioactive compounds. Natural Product Rep (2020) 37(9):1181–206. doi: 10.1039/c9np00065h
129. Xu Y, Orozco R, Wijeratne EMK, Gunatilaka AAL, Stock SP, Molnár I. Biosynthesis of the cyclooligomer depsipeptide Beauvericin, a virulence factor of the entomopathogenic fungus Beauveria bassiana. Chem Biol (2008) 15(9):898–907. doi: 10.1016/j.chembiol.2008.07.011
130. Yin Y, Chen B, Song S, Li B, Yang X, Wang C. Production of diverse Beauveriolide analogs in closely related fungi: a rare case of fungal chemodiversity. MSphere (2020) 5(5):e00667-20. doi: 10.1128/mSphere.00667-20
131. Fan Y, Liu X, Keyhani NO, Tang G, Pei Y, Zhang W, et al. Regulatory cascade and biological activity of Beauveria bassiana oosporein that limits bacterial growth after host death. Proc Natl Acad Sci U S A (2017) 114(9):E1578–E86. doi: 10.1073/pnas.1616543114
132. Chen B, Sun Y, Li S, Yin Y, Wang C. Inductive production of the iron-chelating 2-pyridones benefits the producing fungus to compete for diverse niches. MBio (2021) 12(6):e0327921. doi: 10.1128/mbio.03279-21
133. Wang B, Kang Q, Lu Y, Bai L, Wang C. Unveiling the biosynthetic puzzle of destruxins in Metarhizium species. Proc Natl Acad Sci (2012) 109(4):1287–92. doi: 10.1073/pnas.1115983109
134. Han P, Gong Q, Fan J, Abbas M, Chen D, Zhang J. Destruxin A inhibits scavenger receptor B mediated melanization in Aphis citricola. Pest Manage Sci (2022) 78(5):1915–24. doi: 10.1002/ps.6809
135. Huang A, Lu M, Ling E, Li P, Wang C. A M35 family metalloprotease is required for fungal virulence against insects by inactivating host prophenoloxidases and beyond. Virulence (2020) 11(1):222–37. doi: 10.1080/21505594.2020.1731126
136. Mukherjee K, Vilcinskas A. The entomopathogenic fungus Metarhizium robertsii communicates with the insect host Galleria mellonella during infection. Virulence (2018) 9(1):402–13. doi: 10.1080/21505594.2017.1405190
137. Lu Z, Deng J, Wang H, Zhao X, Luo Z, Yu C, et al. Multifunctional role of a fungal pathogen-secreted laccase 2 in evasion of insect immune defense. Environ Microbiol (2021) 23(2):1256–74. doi: 10.1111/1462-2920.15378
138. Cui C, Wang Y, Liu J, Zhao J, Sun P, Wang S. A fungal pathogen deploys a small silencing RNA that attenuates mosquito immunity and facilitates infection. Nat Commun (2019) 10(1):4298. doi: 10.1038/s41467-019-12323-1
139. Dubovskiy IM, Whitten MMA, Kryukov VY, Yaroslavtseva ON, Grizanova EV, Greig C, et al. More than a colour change: insect melanism, disease resistance and fecundity. Proc Biol Sci (2013) 280(1763):20130584. doi: 10.1098/rspb.2013.0584
140. Theopold U, Li D, Fabbri M, Scherfer C, Schmidt O. The coagulation of insect hemolymph. Cell Mol Life Sci (2002) 59(2):363–72. doi: 10.1007/s00018-002-8428-4
141. Cerenius L, Lee BL, Soderhall K. The proPO-system: pros and cons for its role in invertebrate immunity. Trends Immunol (2008) 29(6):263–71. doi: 10.1016/j.it.2008.02.009
142. Whitten MMA, Coates CJ. Re-evaluation of insect melanogenesis research: Views from the dark side. Pigment Cell Melanoma Res (2017) 30(4):386–401. doi: 10.1111/pcmr.12590
143. Zhao P, Lu Z, Strand MR, Jiang H. Antiviral, anti-parasitic, and cytotoxic effects of 5,6-dihydroxyindole (DHI), a reactive compound generated by phenoloxidase during insect immune response. Insect Biochem Mol Biol (2011) 41(9):645–52. doi: 10.1016/j.ibmb.2011.04.006
144. Dubovskiy I, Kryukova N, Vv G, Ratcliffe N. Encapsulation and nodulation in insects. Invertebrate Survival J (2016) 13:229–46. doi: 10.25431/1824-307X/isj.v13i1.229-246
145. Du M-H, Yan Z-W, Hao Y-J, Yan Z-T, Si F-L, Chen B, et al. Suppression of Laccase 2 severely impairs cuticle tanning and pathogen resistance during the pupal metamorphosis of Anopheles sinensis (Diptera: Culicidae). Parasites Vectors (2017) 10(1):171. doi: 10.1186/s13071-017-2118-4
146. Qiao L, Du M, Liang X, Hao Y, He X, Si F, et al. Tyrosine Hydroxylase is crucial for maintaining pupal tanning and immunity in Anopheles sinensis. Sci Rep (2016) 6(1):29835. doi: 10.1038/srep29835
147. Gunnarsson S. Cellular immune reactions in the desert locust Schistocerca gregaria infected by the fungus Metarhizium anisopliae. J Insect Physiol (1987) 46(4):429–37. doi: 10.1016/S0022-1910(99)00128-6
148. Lemaitre B, Hoffmann J. The host defense of Drosophila melanogaster. Annu Rev Immunol (2007) 25:697–743. doi: 10.1146/annurev.immunol.25.022106.141615
149. Gay N, Nehme NT, Quintin J, Cho JH, Lee J, Lafarge M-C, et al. Relative roles of the cellular and humoral responses in the Drosophila host defense against three gram-positive bacterial infections. PLoS One (2011) 6(3):e14743. doi: 10.1371/journal.pone.0014743
150. Li S, Wang J, Tian X, Toufeeq S, Huang W. Immunometabolic regulation during the presence of microorganisms and parasitoids in insects. Front Immunol (2023) 14:905467. doi: 10.3389/fimmu.2023.905467
151. Nappi AJ, Christensen BM. Melanogenesis and associated cytotoxic reactions: applications to insect innate immunity. Insect Biochem Mol Biol (2005) 35(5):443–59. doi: 10.1016/j.ibmb.2005.01.014
152. Yassine H, Kamareddine L, Osta MA. The mosquito melanization response is implicated in defense against the entomopathogenic fungus Beauveria bassiana. PLoS Path (2012) 8(11):e1003029. doi: 10.1371/journal.ppat.1003029
153. Schneider DS, Binggeli O, Neyen C, Poidevin M, Lemaitre B. Prophenoloxidase activation is required for survival to microbial infections in Drosophila. PLoS Path (2014) 10(5):e1004067. doi: 10.1371/journal.ppat.1004067
154. Lehmann M. Endocrine and physiological regulation of neutral fat storage in Drosophila. Mol Cell Endocrinol (2018) 461:165–77. doi: 10.1016/j.mce.2017.09.008
155. Khaleel A, Nawaz M, Hindawi B. Sol–gel derived Cr(III) and Cu(II)/ γ -Al 2 O 3 doped solids: Effect of the dopant precursor nature on the structural, textural and morphological properties. Materials Res Bull (2013) 48(4):1709–15. doi: 10.1016/S1534-5807(02)00325-8
156. Da Silva P, Jouvensal L, Lamberty M, Bulet P, Caille A, Vovelle F. Solution structure of termicin, an antimicrobial peptide from the termite Pseudacanthotermes spiniger. Protein Sci (2009) 12(3):438–46. doi: 10.1110/ps.0228303
157. Levashina EA, Ohresser S, Bulet P, Reichhart JM, Hetru C, Hoffmann JA. Metchnikowin, a novel immune-inducible proline-rich peptide from Drosophila with antibacterial and antifungal properties. Eur J Biochem (1995) 233(2):694–700. doi: 10.1111/j.1432-1033.1995.694_2.x
158. Huang J, Lou Y, Liu J, Bulet P, Cai C, Ma K, et al. A Toll pathway effector protects Drosophila specifically from distinct toxins secreted by a fungus or a bacterium. Proc Natl Acad Sci U S A (2023) 120(12):e2205140120. doi: 10.1073/pnas.2205140120
159. Fehlbaum P, Bulet P, Michaut L, Lagueux M, Broekaert WF, Hetru C, et al. Insect immunity. Septic injury of Drosophila induces the synthesis of a potent antifungal peptide with sequence homology to plant antifungal peptides. J Biol Chem (1994) 269(52):33159–63. doi: 10.1016/S0021-9258(20)30111-3
160. Nappi AJ, Vass E. Cytotoxic Reactions Associated with Insect Immunity. In: Beck G, Sugumaran M, Cooper EL, editors. Phylogenetic Perspectives on the Vertebrate Immune System. Boston, MA: Springer US (2001), 329–48.
161. Faruck MO, Yusof F, Chowdhury S. An overview of antifungal peptides derived from insect. Peptides (2016) 80:80–8. doi: 10.1016/j.peptides.2015.06.001
162. Govind S. Innate immunity in Drosophila: Pathogens and pathways. Insect Sci (2008) 15(1):29–43. doi: 10.1111/j.1744-7917.2008.00185.x
163. Lu H-L, Wang JB, Brown MA, Euerle C, St. Leger RJ. Identification of Drosophila mutants affecting defense to an entomopathogenic fungus. Sci Rep (2015) 5(1):12350. doi: 10.1038/srep12350
164. Shia AK, Glittenberg M, Thompson G, Weber AN, Reichhart JM, Ligoxygakis P. Toll-dependent antimicrobial responses in Drosophila larval fat body require Spätzle secreted by haemocytes. J Cell Sci (2009) 122(Pt 24):4505–15. doi: 10.1242/jcs.049155
165. Clark RI, Tan SWS, Péan CB, Roostalu U, Vivancos V, Bronda K, et al. MEF2 is an in vivo immune-metabolic switch. Cell (2013) 155(2):435–47. doi: 10.1016/j.cell.2013.09.007
166. Boutros M, Agaisse H, Perrimon N. Sequential activation of signaling pathways during innate immune responses in Drosophila. Dev Cell (2002) 3(5):711–22. doi: 10.1016/S1534-5807(02)00325-8
167. Belkaid Y, Hand TW. Role of the microbiota in immunity and inflammation. Cell (2014) 157(1):121–41. doi: 10.1016/j.cell.2014.03.011
168. Nash MJ, Frank DN, Friedman JE. Early microbes modify immune system development and metabolic homeostasis-the "Restaurant" hypothesis revisited. Front Endocrinol (Lausanne) (2017) 8:349. doi: 10.3389/fendo.2017.00349
169. Sun H, Li H, Zhang X, Liu Y, Chen H, Zheng L, et al. The honeybee gut resistome and its role in antibiotic resistance dissemination. Integr Zoology (2023) 18(6):1014–26. doi: 10.1111/1749-4877.12714
170. Ma M, Tu C, Luo J, Lu M, Zhang S, Xu L. Metabolic and immunological effects of gut microbiota in leaf beetles at the local and systemic levels. Integr Zool (2021) 16(3):313–23. doi: 10.1111/1749-4877.12528
171. Xiong W. Intestinal microbiota in various animals. Integr Zoology (2022) 17(3):331–2. doi: 10.1111/1749-4877.12633
172. Zhu W, Shi X, Qi Y, Wang X, Chang L, Zhao C, et al. Commensal microbiota and host metabolic divergence are associated with the adaptation of Diploderma vela to spatially heterogeneous environments. Integr Zoology (2021) 17(3):346–65. doi: 10.1111/1749-4877.12590
173. Wei G, Lai Y, Wang G, Chen H, Li F, Wang S. Insect pathogenic fungus interacts with the gut microbiota to accelerate mosquito mortality. Proc Natl Acad Sci (2017) 114(23):5994–9. doi: 10.1073/pnas.1703546114
174. Xu L, Deng J, Zhou F, Cheng C, Zhang L, Zhang J, et al. Gut microbiota in an invasive bark beetle infected by a pathogenic fungus accelerates beetle mortality. J Pest Sci (2019) 92(1):343–51. doi: 10.1007/s10340-018-0999-4
175. Panteleev D, Goriacheva II, Andrianov BV, Reznik NL, Lazebnyĭ OE, Kulikov AM. The endosymbiotic bacterium Wolbachia enhances the nonspecific resistance to insect pathogens and alters behavior of Drosophila melanogaster. Genetika (2007) 43(9):1277–80. doi: 10.1134/S1022795407090153
176. Boucias DG, Garcia-Maruniak A, Cherry R, Lu H, Maruniak JE, Lietze V-U. Detection and characterization of bacterial symbionts in the Heteropteran, Blissus insularis. FEMS Microbiol Ecol (2012) 82(3):629–41. doi: 10.1111/j.1574-6941.2012.01433.x
177. Zhou F, Wu X, Xu L, Guo S, Chen G, Zhang X. Repressed Beauveria bassiana infections in Delia antiqua due to associated microbiota. Pest Manag Sci (2019) 75(1):170–9. doi: 10.1002/ps.5084
178. Lee K-A, Kim B, Bhin J, Kim DH, You H, Kim E-K, et al. Bacterial uracil modulates Drosophila DUOX-dependent gut immunity via Hedgehog-induced signaling endosomes. Cell Host Microbe (2015) 17(2):191–204. doi: 10.1016/j.chom.2014.12.012
179. Wang Z, Cheng Y, Wang Y, Yu X. Topical fungal infection induces shifts in the gut microbiota structure of brown planthopper, Nilaparvata lugens (Homoptera: Delphacidae). Insects (2022) 13(6):528. doi: 10.3390/insects13060528
180. Zhang Y, Zhang J, Jiang X, Wang G, Luo Z, Fan Y, et al. Requirement of a mitogen-activated protein kinase for appressorium formation and penetration of insect cuticle by the entomopathogenic fungus Beauveria bassiana. Appl Environ Microbiol (2010) 76(7):2262–70. doi: 10.1128/aem.02246-09
181. Luo S, He M, Cao Y, Xia Y. The tetraspanin gene MaPls1 contributes to virulence by affecting germination, appressorial function and enzymes for cuticle degradation in the entomopathogenic fungus, Metarhizium acridum. Environ Microbiol (2013) 15(11):2966–79. doi: 10.1111/1462-2920.12166
182. Zimmermann G. Review on safety of the entomopathogenic fungi Beauveria bassiana and Beauveria brongniartii. Biocontrol Sci Technol (2007) 17(6):553–96. doi: 10.1080/09583150701309006
183. Blango MG, Kniemeyer O, Brakhage AA. Conidial surface proteins at the interface of fungal infections. PloS Path (2019) 15(9):e1007939. doi: 10.1371/journal.ppat.1007939
184. Luo F, Hong S, Chen B, Yin Y, Tang G, Hu F, et al. Unveiling of swainsonine biosynthesis via a multibranched pathway in fungi. ACS Chem Biol (2020) 15(9):2476–84. doi: 10.1021/acschembio.0c00466
185. Naundrup A, Bohman B, Kwadha CA, Jensen AB, Becher PG, De Fine Licht HH. Pathogenic fungus uses volatiles to entice male flies into fatal matings with infected female cadavers. ISME J (2022) 16(10):2388–97. doi: 10.1038/s41396-022-01284-x
186. Leclerc J-B, Detrain C. Loss of attraction for social cues leads to fungal-infected Myrmica rubra ants withdrawing from the nest. Anim Behav (2017) 129:133–41. doi: 10.1016/j.anbehav.2017.05.002
187. Bos N, LefÈVre T, Jensen AB, D’Ettorre P. Sick ants become unsociable. J Evol Biol (2012) 25(2):342–51. doi: 10.1111/j.1420-9101.2011.02425.x
188. Rueppell O, Hayworth MK, Ross NP. Altruistic self-removal of health-compromised honey bee workers from their hive. J Evol Biol (2010) 23(7):1538–46. doi: 10.1111/j.1420-9101.2010.02022.x
189. Lei CJ, Ahmad RHIR, Halim NA, Asib N, Zakaria A, Azmi WA. Bioefficacy of an oil-emulsion formulation of entomopathogenic fungus, Metarhizium anisopliae against adult Red Palm Weevil, Rhynchophorus ferrugineus. Insects (2023) 14(5):482. doi: 10.3390/insects14050482
190. Birnbaum N, Reingold V, Matveev S, Kottakota C, Davidovitz M, Mani KA, et al. Not only a formulation: The effects of Pickering emulsion on the entomopathogenic action of Metarhizium brunneum. J Fungi (2021) 7(7):499. doi: 10.3390/jof7070499
191. Akbar W, Lord JC, Nechols JR, Howard RW. Diatomaceous earth increases the efficacy of Beauveria bassiana against Tribolium castaneum larvae and increases conidia attachment. J Econ Entomol (2004) 97(2):273–80. doi: 10.1603/0022-0493-97.2.273
192. Friuli M, Pellegrino R, Lamanna L, Nitti P, Madaghiele M, Demitri C. Materials engineering to help pest control: A narrative overview of biopolymer-based entomopathogenic fungi formulations. J Fungi (2023) 9(9):918. doi: 10.3390/jof9090918
193. Fan Y, Pei X, Guo S, Zhang Y, Luo Z, Liao X, et al. Increased virulence using engineered protease-chitin binding domain hybrid expressed in the entomopathogenic fungus Beauveria bassiana. Microb Pathog (2010) 49(6):376–80. doi: 10.1016/j.micpath.2010.06.013
194. Fan Y, Fang W, Guo S, Pei X, Zhang Y, Xiao Y, et al. Increased insect virulence in Beauveria bassiana strains overexpressing an engineered chitinase. Appl Environ Microbiol (2007) 73(1):295–302. doi: 10.1128/AEM.01974-06
195. Jin K, Peng GX, Liu YC, Xia YX. The acid trehalase, ATM1, contributes to the in vivo growth and virulence of the entomopathogenic fungus. Fungal Genet Biol (2015) 77:61–7. doi: 10.1016/j.fgb.2015.03.013
196. Wang S, Fang W, Wang C, St Leger RJ. Insertion of an esterase gene into a specific locust pathogen (Metarhizium acridum) enables it to infect caterpillars. PLoS Path (2011) 7(6):e1002097. doi: 10.1371/journal.ppat.1002097
197. Chen X, Li L, Hu Q, Zhang B, Wu W, Jin F, et al. Expression of dsRNA in recombinant Isaria fumosorosea strain targets the TLR7 gene in Bemisia tabaci. BMC Biotechnol (2015) 15(1):64. doi: 10.1186/s12896-015-0170-8
198. Hu Q, Wu W. Recombinant fungal entomopathogen RNAi target insect gene. Bioengineered (2016) 7(6):504–7. doi: 10.1080/21655979.2016.1146833
199. Wang Y, Xie X, Qin L, Yu D, Wang Z, Huang B. Integration of dsRNA against host immune response genes augments the virulence of transgenic Metarhizium robertsii strains in insect pest species. Microbial Biotechnol (2021) 14(4):1433–44. doi: 10.1111/1751-7915.13748
200. Han P, Chen D, Fan J, Zhang J, Jiang S, Zhu KY, et al. Genetically engineered Metarhizium anisopliae expressing dsRNA of Apolipophorin-D exhibits enhanced insecticidal virulence against Locusta migratoria. Entomol Gen (2023) 43:167–75. doi: 10.1127/entomologia/2023/1772
201. Moreira-Pinto CE, Coelho RR, Leite AGB, Silveira DA, de Souza DA, Lopes RB, et al. Increasing Anthonomus grandis susceptibility to Metarhizium anisopliae through RNAi-induced AgraRelish knockdown: a perspective to combine biocontrol and biotechnology. Pest Manage Sci (2021) 77(9):4054–63. doi: 10.1002/ps.6430
202. Grizanova EV, Coates CJ, Butt TM, Dubovskiy IM. RNAi-mediated suppression of insect metalloprotease inhibitor (IMPI) enhances Galleria mellonella susceptibility to fungal infection. Dev Comp Immunol (2021) 122:104126. doi: 10.1016/j.dci.2021.104126
203. Zhang J, Ye C, Wang Z-G, Ding B-Y, Smagghe G, Zhang Y, et al. DsRNAs spray enhanced the virulence of entomopathogenic fungi Beauveria bassiana in aphid control. J Pest Sci (2023) 96(1):241–51. doi: 10.1007/s10340-022-01508-1
204. Ye C, Wang Z-W, Sheng Y-L, Wang Z-G, Smagghe G, Christiaens O, et al. GNBP1 as a potential RNAi target to enhance the virulence of Beauveria bassiana for aphid control. J Pest Sci (2022) 95(1):87–100. doi: 10.1007/s10340-021-01388-x
205. Cui C, Wang Y, Li Y, Sun P, Jiang J, Zhou H, et al. Expression of mosquito miRNAs in entomopathogenic fungus induces pathogen-mediated host RNA interference and increases fungal efficacy. Cell Rep (2022) 41(4):111527. doi: 10.1016/j.celrep.2022.111527
206. Yu S-J, Pan Q, Luo R, Wang C-L, Cheng L-Y, Yang J-S, et al. Expression of exogenous dsRNA by Lecanicillium attenuatum enhances its virulence to Dialeurodes citri. Pest Manage Sci (2019) 75(4):1014–23. doi: 10.1002/ps.5210
207. Tu C, Zhang Y, Zhu P, Sun L, Xu P, Wang T, et al. Enhanced toxicity of entomopathogenic fungi Beauveria bassiana with bacteria expressing immune suppressive dsRNA in a leaf beetle. Pestic Biochem Physiol (2023) 193:105431. doi: 10.1016/j.pestbp.2023.105431
208. Fan Y, Pereira RM, Kilic E, Casella G, Keyhani NO. Pyrokinin β-neuropeptide affects necrophoretic behavior in fire ants (S. invicta), and expression of β-NP in a mycoinsecticide increases its virulence. PLoS One (2012) 7(1):e26924. doi: 10.1371/journal.pone.0026924
209. Hassan A, Mehmood N, Xu H, Muhammad Usman H, Wu J, Liu L, et al. Phosphofructokinase downregulation disturbed termite social behaviors and immunity against fungal infections. Entomol Gen (2022) 42(5):799–808. doi: 10.1127/entomologia/2022/1444
210. Yaroslavtseva ON, Dubovskiy IM, Khodyrev VP, Duisembekov BA, Kryukov VY, Glupov VV. Immunological mechanisms of synergy between fungus Metarhizium robertsii and bacteria Bacillus thuringiensis ssp. morrisoni on Colorado potato beetle larvae. J Insect Physiol (2017) 96:14–20. doi: 10.1016/j.jinsphys.2016.10.004
211. Mantzoukas S. The effect of Metarhizium robertsii and Bacillus thuringiensis against Helicoverpa armigera (Hübner) (Lepidoptera: Noctuidae). Sci Signpost Publishing (2019) 4(5):136–46. Available at: http://www.ss-pub.org/journals/aeer/vol-4/vol-4-issue-5-may-2019/
212. Sayed AMM, Behle RW. Evaluating a dual microbial agent biopesticide with Bacillus thuringiensis var. kurstaki and Beauveria bassiana blastospores. Biocontrol Sci Technol (2017) 27(4):461–74. doi: 10.1080/09583157.2017.1303662
213. Aynalem B, Muleta D, Jida M, Shemekite F, Aseffa F. Biocontrol competence of Beauveria bassiana, Metarhizium anisopliae and Bacillus thuringiensis against tomato leaf miner, Tuta absoluta Meyrick 1917 under greenhouse and field conditions. Heliyon (2022) 8(6):e09694. doi: 10.1016/j.heliyon.2022.e09694
214. Mantzoukas S, Milonas P, Kontodimas D, Angelopoulos K. Interaction between the entomopathogenic bacterium Bacillus thuringiensis subsp. kurstaki and two entomopathogenic fungi in bio-control of Sesamia nonagrioides (Lefebvre) (Lepidoptera: Noctuidae). Ann Microbiol (2012) 63(3):1083–91. doi: 10.1007/s13213-012-0565-x
Keywords: entomopathogenic fungi, infection stage, insect immunity, defense strategy, pest management
Citation: Ma M, Luo J, Li C, Eleftherianos I, Zhang W and Xu L (2024) A life-and-death struggle: interaction of insects with entomopathogenic fungi across various infection stages. Front. Immunol. 14:1329843. doi: 10.3389/fimmu.2023.1329843
Received: 30 October 2023; Accepted: 15 December 2023;
Published: 08 January 2024.
Edited by:
Qiuning Liu, Yancheng Teachers University, ChinaReviewed by:
Sheng-hua Ying, Zhejiang University, ChinaJorge Cime-Castillo, National Institute of Public Health, Mexico
Copyright © 2024 Ma, Luo, Li, Eleftherianos, Zhang and Xu. This is an open-access article distributed under the terms of the Creative Commons Attribution License (CC BY). The use, distribution or reproduction in other forums is permitted, provided the original author(s) and the copyright owner(s) are credited and that the original publication in this journal is cited, in accordance with accepted academic practice. No use, distribution or reproduction is permitted which does not comply with these terms.
*Correspondence: Wei Zhang, d3poYW5nOUBnenUuZWR1LmNu; Letian Xu, bGV0aWFuMDkyNkAxNjMuY29t
†These authors have contributed equally to this work