- 1Molecular Biology Division, National Institute of Nutrition, Indian Council of Medical Research, Hyderabad, India
- 2Department of Nutrition, Institute of Basic Medical Sciences, Faculty of Medicine, University of Oslo, Oslo, Norway
Bioactives significantly modify and maintain human health. Available data suggest that Bioactives might play a beneficial role in chronic inflammatory diseases. Although promised, defining their mechanisms and opting to weigh their benefits and limitations is imperative. Detailed mechanisms by which critical Bioactives, including probiotics and prebiotics such as dietary lipids (DHA, EPA, alpha LA), vitamin D, polysaccharides (fructooligosaccharide), polyphenols (curcumin, resveratrol, and capsaicin) potentially modulate inflammation and bone metabolism is limited. Certain dietary bioactive significantly impact the gut microbiota, immune system, and pain response via the gut-immune-bone axis. This narrative review highlights a recent update on mechanistic evidence that bioactive is demonstrated demonstrated to reduce osteoarthritis pathophysiology.
1 Introduction
Osteoarthritis (OA) is an age-related degenerative disease severely impacting bone health. The OA burden is prevalent globally (1) and also rapidly increasing in India in the past decade (2). The OA mainly contributes to activity limitations and burdens the Nation’s effective workforce and healthcare (3). Moreover, it has recently been reported to affect the young (4) due to several underlying risks, including obesity, dietary, lifestyle, environmental transitions, nutritional imbalances, gut dysbiosis, metaflammation, and chronic diseases. The risk of developing osteoarthritis can be reduced and prevented by managing these factors. However, the global emergence of the metabolic syndrome (5) led to an incremental burden that forced us to comprehend systemic inflammation and immunity before the symptoms influenced and directed toward osteoarthritis pathophysiology. Musculoskeletal disorders (osteoarthritis, rheumatoid arthritis, osteoporosis) are targets of unresolved inflammation associated with the metabolism of long bone tissues. The interaction between bone remodeling and inflammation is continuous and modulated by immune response. A thorough understanding of the disease associated with osteogenic changes, immunomodulation, inflammation, and pain could be a basis required for holistic therapeutic interventions.
Despite OA being a multifactorial disease, weight management remains a primary focus for its prevention. The prevalence of OA brought community awareness and frequent check-ups to detect the disease at its early stages. Treatment of OA includes dietary modifications, lifestyle changes, analgesics, intra-articular preparations, and surgical procedures (6). The non-steroidal anti-inflammatory drugs (NSAIDs) and corticosteroids are used with potentially serious side effects. Thus, alternate therapeutics delay and/or reduce the progression of chronic inflammatory diseases like osteoarthritis is warranted. Nutrition, in maintaining healthy bone and musculoskeletal health, in general, has led to the emergence of Bioactives, nutraceuticals, botanicals, or herbal formulations, helping delay the onset of the disease and sometimes treating conditions. Functional foods and supplements may help the immune system as their secondary metabolites can target molecular pathways, ameliorating the disease. The pathogenesis of bone disease is associated with disrupting the osteoclasts-osteoblasts balance that governs bone remodeling. With their bioactive properties and ability to intervene in signaling pathways, nutraceuticals promise to help restore this balance (7–9). Molecular mechanisms that may define the underlying benefits of dietary bioactive against inflammatory diseases are limited. Bioactivity can inhibit TLR4 (Toll-like Receptor 4) mediated inflammation by modulating the gut microbiome (10). Dietary bioactive takes on the intestinal flora diversity, and their release of metabolites, activates immunomodulation by receptor signaling, transmits a peripheral signal, modulates nociception, pain, and systemic inflammation, and alters blood parameters, tissue metabolism, and cytokine profile, as demonstrated by several studies (discussed later).
Although the functions of bioactive compounds influencing osteoarthritis pathophysiology are reported, their modulation of the intestinal microbiome via the gut-bone axis is not known. In addition, there is paramount evidence that gut microbiota modulates host immune responses in osteoarthritis; however, the exact mechanisms are still unknown (11). In that context, this narrative review highlights recent mechanistic evidence on using bioactive or functional foods to ameliorate osteoarthritis and inflammatory diseases. In this article, Bioactives and their roles in bone metabolism of osteoarthritis are proposed based on available data and evidence. A comprehensive literature review was conducted using PubMed, Scopus, and Science Direct databases. The tenure of the search ranged from 2009 to 2023. The main aim of this review is to describe the use of Bioactives in OA, highlighting their molecular mechanisms of action.
1.1 Osteoarthritis risk, pathophysiology, intervention, and scope of research
Obesity, mainly upper body adiposity, poses a significant risk of developing musculoskeletal disorders like osteoarthritis (12). The epidemiological data suggested that women suffering from obesity and people with a sedentary lifestyle had a higher prevalence of osteoarthritis (13). Obesity, diabetes, and osteoarthritis are interlinked, with the latter being a predominantly acquired risk factor due to excess adiposity, increased mechanical load, loss of muscle mass, and low-grade inflammation modulated by adipokines. Type 2 diabetes (T2D), the most common form of diabetes, is a significant consequence of physical inactivity and obesity (14). Glucose intolerance and resistance to insulin developed during obesity result in various metabolic disorders, including osteoarthritis. The most detrimental effect of obesity is the mechanical load on weight-bearing joints, leading to the progression of osteoarthritis (15). Studies indicate that adiposity leads to low-grade inflammation, stress on weight-bearing joints, and upregulates leptin, which can regulate chondrocyte apoptosis. Distinct differences are visible between normal bone and cartilage, and these features are influenced by osteoarthritis (16). Normal cartilage exists in an avascular state, which maintains the integrity of the tissue. However, cartilage no longer remains in the avascular, and neovascularization occurs in a diseased state. Chondrocyte clusters and osteophyte formation also occur (17). The cartilage matrix also degenerates owing to increased hydration and loss of proteoglycans, decreasing keratan sulfate and hyaluronic acid. The resultant mechanical stress leads to surface fissures in the articular cartilage (4, 18).
Both systemic and localized inflammation are associated with the progression of osteoarthritis (19, 20), where several pro-inflammatory cytokines and matrix metalloproteinase (MMPs) are involved at both early and late stages of osteoarthritis. Interleukin (IL)-1β, IL-6, TNF-α, matrix metalloproteinase (MMP)-1, MMP-3, and MMP-13 are secreted mainly by chondrocytes (21). Diseased chondrocytes and synoviocytes also express IL-1, type-1 IL-1 receptor, and type II collagen. The OA progression correlates with inflamed synovium, typically characterized by infiltrated neutrophils to the affected site, mediated by several small chemotactic proteins or chemokines, such as IL-1β, IL-6, IL-8, and others. Elevated IL-6 (22) and IL-8 (23) levels were reported in synovial fluid from OA patients compared to controls. These chemokines are known to induce chondrocyte hypertrophy and show an increased risk of cartilage loss. OA progression is also mediated by cross-talk between growth factors and chemokine productions. Connective tissue growth factor (CTGF or CCN2) is also involved in several events of skeletogenesis by acting as a molecular bridge for extracellular matrix (ECM) by modulating angiogenesis, chondrogenesis, and osteogenesis (24). CTGF acted as a pro-inflammatory mediator by producing IL-6 (25), a pro-angiogenic modulator by producing IL-8 (26), and other chemokines. CTGF is abundantly expressed in the OA (27), but its pathogenic role is unclear. OA synovium in obesity is enriched with excess T-cells and B-cells, where specific cartilage turnover markers are also elevated in obesity compared with healthy (28). Thus, a detailed understanding of mediators and pathways that impact initiation to the progression of osteoarthritis pathophysiology is required to design targeted therapies for its intervention.
Bone remodeling and bone loss are controlled by a balance between TNF-family protein, osteoprotegerin ligand (OPGL), and its decoy receptor osteoprotegerin (OPG), preventing RANKL (receptor activator of nuclear factor kappa β ligand) from binding to and activating RANK (receptor activator of nuclear factor kappa β). The OPGL receptor, RANK, is expressed on osteoclast precursors, chondrocytes, and mature osteoclasts. The binding of RANKL to RANK leads to osteoclastogenesis and inhibits osteoclast apoptosis (29). Thus, balancing activities of myeloid origin bone-resorbing cells, i.e., osteoclast with mesenchymal origin bone-forming cells, osteoblast is modulated by RANKL and OPG actions. A dysregulated RANK-RANKL-OPG system increases RANKL activity associated with osteoporosis and secondary inflammation of bone disease (30). It has been demonstrated that the RANKL/OPG ratio is consistently elevated in inflammatory diseases. The OPGL regulates lymphocyte development, organogenesis, and interactions between the immune system’s dendritic cells and T-cells. Studies suggest activated T-cells directly trigger osteoclastogenesis through OPGL and RANK (31). Thus, modulators of T-cell activators could influence bone metabolism by regulating OPGL production and bone loss.
The available treatment for osteoarthritis includes corticosteroid injections like methylprednisolone acetate and triamcinolone, hyaluronic acid injections, topical and oral non-steroidal anti-inflammatory drugs, opioids, and surgical procedures like knee arthroscopy (32). While corticosteroid injections and surgical procedures carry a significant risk of infection and possible hyperglycemia, NSAIDs and opioids can result in ulcers, gastrointestinal perforation, and iatrogenic addiction (33). Recent studies have shown the possible usage of regenerative medicine in treating osteoarthritis (34). These include platelet-rich plasma injections, proliferation therapy, and stem cell therapy. Mesenchymal stem cells aid cartilage tissue regeneration by upregulating growth factors, reducing the inflammatory response, and differentiating into chondrocytes (35). However, significant concerns loom about biosafety and disease risk over stem cell-based therapy for osteoarthritis. These include the possibility of tumor formation, immune rejection, formation of ectopic tissue, or differentiation into undesired cell types (36). Bioactive enriched nutraceuticals and functional foods demonstrated early evidence to relieve osteoarthritis (37), and promised NSAID-like effects in ameliorating pain and function (38). The ability of bioactive to function similarly to that of NSAIDs bereft of potential side effects and thus can be exploited to delay disease progression. However, current research is still in the early stages, and it is essential to understand the underlying mechanisms in the bioactive-mediated amelioration of osteoarthritis.
2 Molecular pathways involved in osteoarthritis pathophysiology
In a chronic inflammatory state, particularly in rheumatoid arthritis, persistent inflammation results in bone loss by volume and mass (39) with a concomitant increase in local RANKL expression. Excess pro-inflammatory cytokines, including IL-6, IL-1β, and TNF-α produced by osteoclasts and osteoblasts, triggered the bone microenvironment by boosting RANK expression and bone resorption phenotype (40). The interplay of bioactive mediators and target cells could shape the inflammatory and metabolic control of bone remodeling. Therefore, activator and resolution of inflammation can impact bone metabolism and disease.
Understanding molecular pathways associated with osteoarthritis ensures an understanding the underlying reasons for disease pathology. Various pathways play a key role in the onset and progression of osteoarthritis, including Wnt/β-catenin, transforming growth factor β (TGF-β), bone morphogenic protein (BMP), fibroblast growth factor (FGF) signaling, nuclear factor κB (NF-κB) pathway, transient receptor potential vanilloid 1 (TRPV1) pathway, and RANK/RANKL/OPG pathway. In addition, various transcription factors and regulators involved in osteoarthritis progression include RUNX2 (Runt-related transcription factor 2), ADAMTS (a disintegrin and metalloproteinase with thrombospondin motifs), mTOR (mammalian target of rapamycin) and MMPs (41).
2.1 RANK/RANKL/OPG pathway
The role of RANK/RANKL/OPG and Wnt/β-catenin signaling in osteoarthritis has been extensively studied (42). This diseased state results in the alterations of both Wnt/β-catenin and RANK/RANKL/OPG signaling, disrupting the homeostasis of bone remodeling and upregulating pro-inflammatory cytokines. RANKL activates RANK (43), while M-CSF (macrophage colony-stimulating factor) and RANK initiate osteoclast differentiation from hematopoietic stem cells (HSCs). The interaction between RANKL and RANK also activates NF-κβ and MAP kinases. On the other hand, OPG, an antagonist, inhibits the binding between RANK and RANKL, keeping the system in check. TNF-α, IL-6, and IL-10 are essential cytokines in regulating bone remodeling. While osteoclasts differentiate from HSCs, osteoblasts differentiate from mesenchymal stem cells via osterix and Runx2 (runt-related transcription factor-2). Runx2 is regulated by bone morphogenetic proteins (BMPs) and Wnt proteins. Several growth factors and hormones, including PGE2, bFGF, parathyroid hormone, glucocorticoids, TGF-β, estrogen, and vitamin D3, also modulate bone remodeling.
2.2 Wnt/β-catenin pathway
The Wnt (wingless-related integration site) signaling pathway plays a crucial role in the growth and proliferation of skeletal tissues and bone development. β-catenin is a transcriptional co-activator that regulates the transcription of Runx2 and other transcriptional factors. An inactive Wnt pathway results in the proteasomal degradation of β-catenin, hence inhibiting transcription of the Wnt target genes (44, 45). Inactive Wnt proteins are usually bound to sFRP3 (secreted frizzled-related protein 3). In the active state of Wnt signaling, the destruction complex degrades β-catenin, is inactivated, and cellular β-catenin levels rise. β-catenin then translocates and binds to the TCF (T-cell factor), activating the transcription of Wnt target genes. Canonical Wnt signaling is directly involved in osteoblastogenesis from MSCs. The signaling pathway maintains balance during bone remodeling by promoting bone formation via osteoblasts. The dysregulation of several Wnt signaling factors and their extended crosstalk with other signaling pathways could lead to a diseased state.
2.3 NLRP-3 inflammasome and Nrf2/HO-1 signaling
The relationship between NLRP-3 (NOD-, LRR- and pyrin domain-containing protein 3) inflammasome and Nrf2 (nuclear factor erythroid 2-related factor 2) signaling has been demonstrated in the osteoarthritis (46). In this state, human synovial tissues revealed a higher expression of Nrf2, HO-1 (heme oxygenase 1), NLRP-3 inflammasome, and ASC, suggesting increased oxidative stress. Nrf2 knock-down in rat chondrocytes showed an increased expression of NLRP-3 inflammasome, suggesting its role in NLRP-3 activation caused by increased oxidative stress during the diseased state (46). Hence, both Nrf2 and NLRP-3 could be therapeutic targets for the treatment of osteoarthritis. Several studies suggest the involvement of NLRP3 inflammasome in various bone ailments, activating pro-inflammatory cytokines. Osteoarthritis’s pathogenesis involves abnormal activating proinflammatory cytokines (47) (48), in which IL-1β primarily plays an important role. While stimulating RANKL-osteoclast activation, IL-1β inhibits osteoblast differentiation, resulting in increased bone resorption and reduced bone formation. During this process, IL-1β modulates NF-κB and MAPK (mitogen-activated protein kinases) pathways. NLRP3 inflammasome is also involved in gasdermin D-associated cell lysis and death & pyroptosis. Reactive oxygen species also upregulate NLRP3-mediated pyroptosis.
2.4 TRPV1 pathway
Transient receptor potential vanilloid subtype 1 (TRPV1) is majorly expressed in nerve fibers that involve the regulatory role of cardiovascular, respiratory, and digestive systems and play an essential role in specific diseases (49). Although capsaicin is a known agonist of TRPV1, various other mediators such as prostaglandin, nerve growth factor (NGF), substance P, physical and chemical features such as acidic pH, osmotic pressure, redox state, and noxious heat stimulation, all lead to activation of TRPV1 (50). Targeting the TRPV1 channel pathway to alleviate osteoarthritis pain is effective based on the current data. Several antagonists and agonists of TRPV1 are currently under various phases of preclinical and clinical trials (51). In both primary mouse chondrocytes and surgically induced-DMM (destabilization of medial meniscus) mice OA model, single cell RNA sequencing revealed that TRPV1 led to upregulation of selenoprotein glutathione peroxidase 4 (GPX4) and showed protective effective against ferroptosis of chondrocytes (52). Receptors of TRPV1 were detected in the synovium of osteoarthritic joints, following which an antagonist of TRPV1 was administered locally. Blockage of TRPV1 led to significant inhibition of joint pain and proved to be a promising therapeutic against osteoarthritis (53). Various agonists of TRPV1, such as capsaicin, resiniferatoxin, allicin, gingerol, and piperine, have been shown to interact and bind to TRPV1 (54, 55). Several clinical studies have shown the effect of topical or intra-articularly administered capsaicin in alleviating OA-related pain (56, 57). An analog of capsaicin, pellitorine extracted from Tetradium danielli is an antagonist of TRPV1 and can thus inhibit pain (58). Intra-articular treatment with resiniferatoxin, another agonist of TRPV1 led to reduced pain in the induced osteoarthritis model (59).
Available data suggest modulators of the TRPV1 channel pathway could be the target in ameliorating OA-related pain. Thus, the Bioactives that interact with the receptor are potential therapeutic targets.
3 Mechanisms of action of Bioactives on osteoarthritis: gut-immune-bone axis
Poor nutrition or nutritional deficiencies have a severe adverse impact on the health of bones. Diet plays a pivotal role in the pathogenesis of osteoarthritis, where obesity is a high-risk factor. Dietary changes influence the gut microbiome, affect body weight, and cause obesity (60). However, the interplay between diet, body weight, obesity, and gut microbiota in influencing gastrointestinal, metabolic effects, and immune modulation contributing to OA pathophysiology is unclear. Various studies show a bidirectional aspect where gut dysbiosis leads to obesity and vice versa, where obesity results in gut dysbiosis (61–63). The gut consists of a highly diverse composition of microorganisms that govern and modulate a wide range of functions like digestion, metabolism, absorption of nutrients, vitamin synthesis, signaling pathways, inhibiting the growth of pathogenic bacteria, immunity and interaction with sensory afferent fibers releasing neurotransmitters, forming a gut-brain axis. The various roles the intestinal microbiome plays in modulating the immune system and their modulation by bioactive is not understood yet.
The gut microbiome is majorly involved in directly interacting with the immune system based on diverse stimuli, generating an immune response, activation of specific immune cells, production of metabolites, acting as a barrier via tight junction proteins, maintaining homeostasis of the microbial composition, and recognizing commensal microbes of the intestine. Hence, the seemingly simple gut microbiome has vast involvement in the immune system at both innate and adaptive levels (64–66). Dietary habits constitute a major causative for maintaining gut homeostasis. The bioactive that are proven to have various health benefits modulate the microflora composition and affect the immune system in case of inflammatory disorders. With respect to osteoarthritis, the innate immune system plays a crucial role in inducing inflammatory responses during disease progression (67).
The most commonly found microbial phyla include Firmicutes, Actinobacteria, Proteobacteria, Bacteroidetes, Fusobacteriia, and Verrucomicrobia, among which Bacteroidetes and Firmicutes represent almost 90% of the microflora (68). However, variations in the gut microbial composition are found in different parts of the gastrointestinal tract, in addition to variations in age and environmental factors. Among individuals, these variations arise from BMI, diet, exercise, ethnicity, climate, and geographical location changes. The ratio of Firmicutes to Bacteroides increases during obesity and is a characteristic of gut dysbiosis (69, 70). Increased abundance of Firmicutes during obesity leads to increased production of short-chain fatty acids (SCFAs) and increased concentration in the samples that contribute to reduced energy expenditure (71), resulting in expanded body weight (72). Dysbiosis in the gut changes the metabolic pathways of certain microbes, leading to how lipids and carbohydrates are metabolized (73). High-fat diet-induced obesity also has a risk of developing low-grade inflammation (74, 75) that causes gut dysbiosis and disrupts the homeostasis of intestinal permeability by altering the expression of tight-junction proteins (76) leading to a leaky gut with increased plasma levels (77) of lipopolysaccharide (LPS).
The immune system influences the gut composition and develops tolerance towards non-pathogenic microbes from the early stages. Gut microbiota affects the development and maturation of various immune cells. Together, the immune system and the gut microbiota also act as a barrier at the intestinal lining against pathogenic microorganisms (78) (79) (64). Various luminal factors like microbial metabolites (SCFAs, tryptophan metabolites, succinate, vitamins, and lipid mediators), dietary ω-3 fatty acids, sphingolipid, and specific neuropeptides play an active role in the innate immune system of the intestine (80). In addition, the prevalence of the gut-immune-bone axis has also been demonstrated by several studies (81–84). TLRs have been a significant link between the gut microbiome and immune responses generated by the immune system. Specific microbial metabolites of C.sporogenes regulate intestinal permeability by directly interacting with xenobiotic receptors via TLR4 signaling (85). However, it has been reported that a high-fat diet leads to an increase in pro-inflammatory cytokines, causes changes in the gut microbial composition, increases the abundance of Enterobacteriaceae, which enhances endotoxin production, increases intestinal permeability, and induces TLR4 expression (86). This is because TLR4 is a critical protein that regulates gut permeability and is involved in acute and chronic inflammation (87, 88). Certain Prevotella species in the intestine (P. melaninogenica and P. copri) are involved in inducing the production of a subset of T helper cells (Th17) via their interaction with TLR2 (89). In addition to TLR-4 and TLR-2, TLR-1 is also actively involved in maintaining intestinal homeostasis and obstructs the translocation of microbes, which otherwise happens during either defective TLR-1 or an inflammatory state (90). Thus, abnormal TLR signaling negatively impacts the gut, compromising permeability and resulting in inflammation and dysbiosis. A schematic outline (Figure 1) suggests an emerging mechanism that Bioactives could play their roles in bone metabolism of osteoarthritis by gut-bone axis.
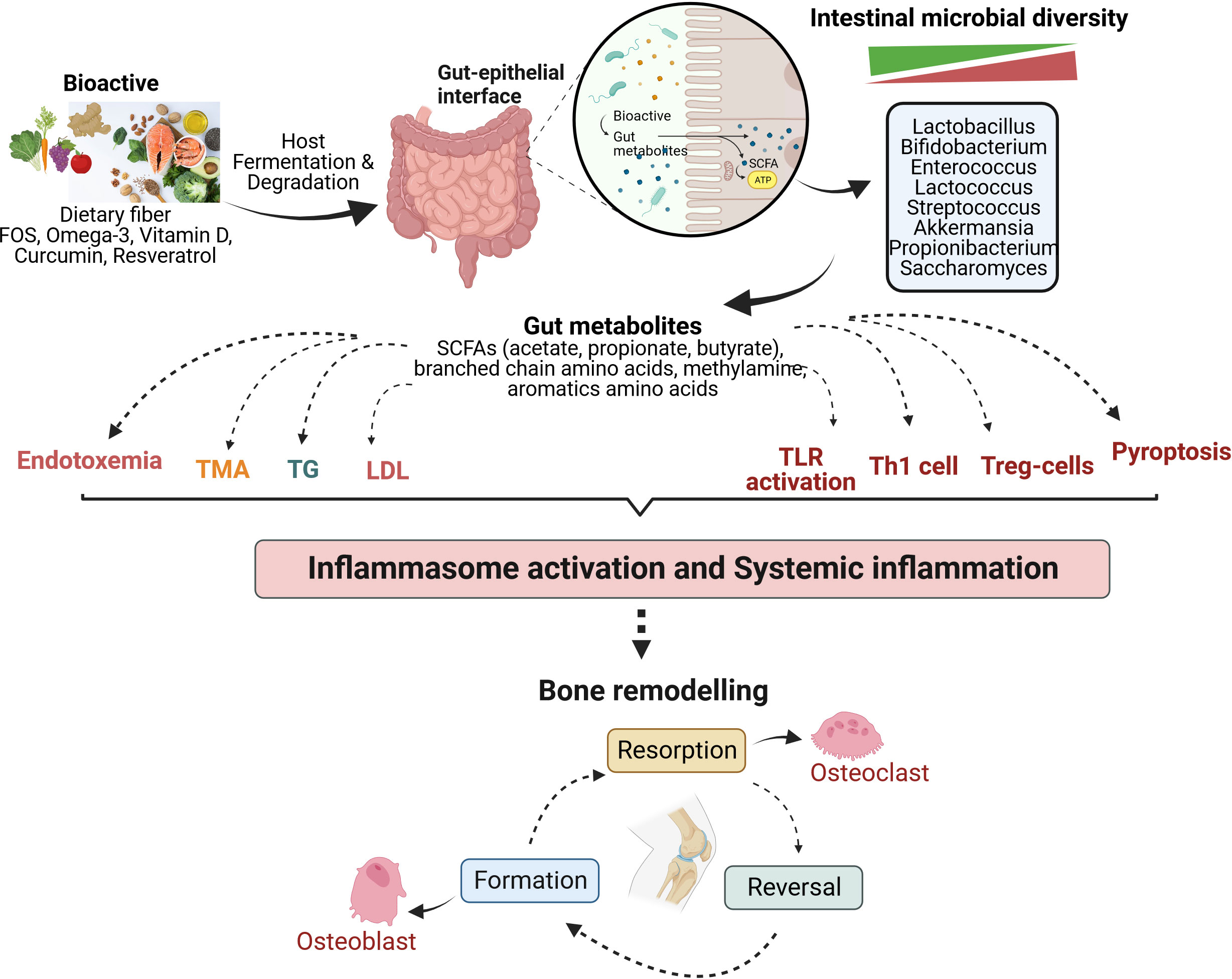
Figure 1 Mechanism of bioactives actions in modulating the bone metabolism of osteoarthritis by gut-immune-bone axis. Various bioactive compounds undergo fermentation in the host gut by intestinal microbiota, thereby regulating microbial diversity and generating metabolites that majorly modulate the immune system, among other significant functions. They are involved in lipid metabolism, influencing the levels of triglycerides and low-density lipoprotein. Gut metabolites also lead to differentiation and activation of immune cells to strengthen the intestinal barrier and maintain gut homeostasis. These mechanisms act at the system level, reducing inflammation and affecting bone remodelling to balance osteoclastogenesis (bone resorption) and osteoblastogenesis (bone formation). FOS, Fructooligosaccharide; SCFAs, Short chain fatty acids; TMA, Trimethylamine; TG, Triglyceride; LDL, Low density lipoprotein; TLR, Toll-like receptor; Th1, Helper T lymphocytes type 1; Treg, Regulatory T lymphocytes.
4 Bioactives and osteoarthritis: metabolic and anti-inflammatory functions
The Bioactives that include probiotics and prebiotics have recently been examined extensively for their efficacies and potential health benefits due to minimum side effects when included in the diet of healthy adults. The primary focus of the ongoing research is the gut microflora’s relationship to chronic diseases. In general, probiotics are food such as yoghurt, curd or supplements that contain live microbial strains such as Lactobacillus, which can help to maintain healthy microflora in the body. In contrast, prebiotics are foods rich in Bioactives, including polysaccharides, fructooligosaccharide, polyphenols, omega-3 fatty acids and other that typically serves foods for endogenous gut microflora.
4.1 Dietary habit, gut microbial diversity and osteoarthritis
It has been reported that gut dysbiosis worsens osteoarthritis (91). A causal relationship has been established among microbial taxa such as Methanobacteriaceae, Ruminiclostridium, Desulfovibionales and osteoarthritis (92). Knee osteoarthritis increases the abundance of microbes such as Peptococcus, Propionibacterium, Intestimonas, Parvimonas, and Shimwella (93). OA-related knee pain has been shown to correlate with the abundance of Streptococcus and Clostridium positively and reduced α- and β diversity (94) (95). A decrease in species of Bifidobacteria and Faecalibacteria is also observed. However, precise mechanisms that would increase the potential of gut microbiome being targeted to maintain immune homeostasis and prevent, delay, or treat inflammatory disorders like OA are required to investigate further.
The gut microbiome varies among populations from diverse geography, considering their subsistence and dietary patterns, ethnicity, and genetic factors (96). The urban Chinese population has comparatively less α and β-diversity and a decreased ratio of archaea-to-bacteria compared to the rural population (97). Methanobrevibacter smithii was abundant in rural populations, correlating with other SCFA-producing bacteria like Prevotella and Roseburia sp. Geography played a bigger role than ethnicity in influencing gut microbial composition in China (98). In India, across several regions and varied ethnic groups, the composition was dominated by Bacteroidetes, with Prevotella involved in carbohydrate metabolism being the most abundant (99, 100). Dietary patterns were also seen to bring in variations in the composition among different regions. Upon comparative analysis of the gut microbial composition of Indians with people from the United States, France, Germany, and Denmark, it was revealed that Prevotella, Bifidobacterium, and Lactobacillus were more abundant in Indians than people from these countries (100). Among the Spanish, Firmicutes and Bacteroidetes were the most dominant phyla, in which Faecalibacterium, Bacteroides, Alistipes, Oscillospiraceae, and Prevotella were prevalent at the genus level (101). Age, BMI, and diet-dependent associations with several human gut microbes were also noted. In Italy, variation in gut microbial composition was evident among samples from different geographical regions. Cyanobacteria and Nitrospirae were found in all samples of two individually separate regions (102). Still, they were absent in the other areas, proving the association between gut microbial composition and geographical location. Humanized gnotobiotic mice from various geographical locations (US, Fiji Islands, and Guatemala) had variations in their susceptibility to enteric infection caused by Citrobacterium rodentum (103). This establishes that geographic differences have a role in influencing health status by modulating gut variations. So far, studies have shown that Firmicutes and Bacteroidetes form the most dominant phyla, irrespective of geographical differences. However, a latitude-based correlation has been noted in affecting the Firmicutes-to-Bacteroidetes ratio. Europeans were abundant in Firmicutes, which decreased in the American, African, and Asian populations. On the contrary, Bacteroidetes were dominant in African and American and drastically reduced in European (104). Upon comparative analysis of the gut microbial composition among the obese population of France, French Polynesia, Amazonian French Guiana, and Saudi Arabia, it was revealed that the gut microbiome of obese Amazonians varied drastically compared to other populations because of different cultural and dietary habits (105). Consuming food rich in fiber, starch, and polysaccharides from plants led to increased microbial diversity in Polynesians and Amazonians compared to French and Saudi obese populations. Spirochaetes and specific Lactobacillus sp abundant in Amazonians were absent in other obese populations.
4.2 Polysaccharides, fructooligosaccharide and osteoarthritis
Since polysaccharides resist digestion, they are subjected to gut microbial fermentation. The beneficial effects of bioactive polysaccharides depend on their fermentability by gut microbiota, water-holding capacity, bile acid interaction, and other physicochemical properties. These bioactive slows down gastric emptying, acidosis, improving bowel function by modulating the gut microbiota structurally and functionally and thus protecting the immune system. Bioactive polysaccharides reshape intestinal microbial activity through bacterial fermentation. A healthy human gut microbiome abundantly expresses carbohydrate-active enzymes (CAZymes) essential in metabolizing specific complex polysaccharides like dietary fiber (106). The dietary shifts in infant from milk to solid food usually change the CAZymes (107). Varied dietary habits across geography and lifestyle contribute to variations in CAZymes. An Italian cohort compared the microflora of healthy and obese type 2 diabetes patients and found that healthy subjects who consumed a Mediterranean diet had more abundance of CAZymes-producing bacteria like F. prausntiznii and E. rectale. In contrast, the obese subjects suffering from T2D showed an abundance of R. bromii and S. variabile that would result in dysregulation of the gut (108). Adding dietary fiber or complex polysaccharides into the diet promotes the abundance of bacteria that can ferment it and use it as an energy source while generating various metabolites that prove beneficial for the hosts in terms of reducing inflammation via gut modulation. However, a refined diet can make these microbes redundant and alter the microbiome (109). Supplementation of dietary fiber in healthy adults increased the abundance of Bacteroidetes, thereby changing the ratio of Bacteroidetes: Firmicutes (110). A multicenter cohort suggests that intake of the recommended daily dietary fiber was associated with a reduced risk of knee pain over time (111). The prospective US cohorts over four and nine years showed that dietary fiber reduced the risk of developing symptomatic but not radiographic osteoarthritis (112). However, much work is required to emphasize how dietary fiber can reduce the risk of symptomatic osteoarthritis (113).
Supplementation with fructooligosaccharide (FOS) purportedly improves the concentration of Bifidobacteria and increases the function of dendritic cells (114). FOS supplementation in colitis-induced mice led to the manipulation of intestinal flora, reduced the abundance of Mucispirillum, downregulated expression of pro-inflammatory cytokines (TNF-α and IL-6) and upregulated IL-10 expression (115). FOS intake improved immune responses by ameliorating the hamster’s HFD-induced inflammation and lipid profile (116). FOS supplementation affected Treg and Th17 cells’ homeostasis using tryptophan metabolites and modulating the gut microbial composition (117). The in vitro fermentation of chicory (FOS) and native inulin utilizing pooled fecal inocula of infants exhibited reduced pro-inflammatory cytokines produced by immature dendritic cells via increased synthesis of succinate and lactate (118). A combined prebiotic and probiotic (Bacillus coagulans) supplementation in mice treated with cyclophosphamide showed increased expression of anti-inflammatory cytokines such as IFN-γ and IL-4 where the abundance of several beneficial microbes such as Enterococcus, Bacteroides, Ruminococcus, Oscillospira, and Anaerotruncus was found to increase (119). Prebiotic fiber like FOS showed an increased abundance of B. pseudolongum, a Bifidobacterium that is ablated during obesity, while reducing the number of microbes from Peptococcaceae family and other species associated with obesity, thereby reestablishing the microbiome associated with a lean gut (120). In addition, supplementation with oligofructose in obese mice with surgically-induced osteoarthritis led to reduced synovial inflammation, reduced mineralization of the meniscus, and downregulated chondrocyte hypertrophy (120).
Prebiotic supplementation in the form of short-chain galactooligosaccharides, long-chain FOS, and milk oligosaccharide 2’-fucosyllactose at early life in mice improved their immune response towards vaccine by generating specific antibodies, promoted the production of cytokines and variation in the relative abundance of various microbial phyla. Prebiotics led to an increase in the quantity of Actinobacteria and a decrease in Proteobacteria and Firmicutes (121). A specific prebiotic blend (mixture of anthocyanins, inulin, FOS, and galactooligosaccharides) was found to reduce pro-inflammatory cytokines and upregulated the expression of tight junction protein in vitro and in vivo models of inflammatory bowel disease (122). Prebiotic blend increased the relative abundance of specific genera such as Prevotella, Intestimonas, Butyricicoccus, Barnesiella, and Ochrobactrum. Supplementation of prebiotics (galactooligosaccharides and fructooligosaccharides) and postbiotics in healthy suckling rats resulted in specific changes in gut microflora composition and immune responses. Prebiotics led to variation in the composition of an increased abundance of Peptostreptococcaceae, Anaerotruncus, and Romboutsia, reduction in Akkermansia and Vibrionimona, altered the proportion of SCFAs and modulated the immunoglobulin profile (123). However, postbiotic supplementation alone and in combination with prebiotics increased the expression of Toll-like receptors such as TLR3, TLR4, TLR5, TLR7, and TLR9, indicating their role in generating an immune response.
The comprehensive systematic review suggests the benefit of antioxidant supplementation to reduce disease-related symptoms in knee osteoarthritis patients (124). Quercetin, an antioxidant used in the treatment of osteoarthritis, influenced rat metabolome and gut microbial composition by increasing Lactobacillus species and levels of SCFAs (125). The presence of Peptostreptococcaceae and Desulfovibrio, both of which hurt the intestine, and the absence of lactic acid-producing bacteria have been shown in rat models of osteoarthritis (126). Orally supplemented butyrate and Lactobacillus acidophillus (LA-1) ameliorated osteoarthritis in rats by downregulating inflammatory cytokines and improved autophagy by reducing necroptosis factors (126). In another study, LA-1 alleviated cartilage degradation and pain in the monoiodoacetate-induced osteoarthritis (127). A similar probiotic supplementation downregulated enzymes that degrade cartilage, inflammatory cytokines, and reduced expression of markers associated with pain in mice (128). The probiotic supplemented with Lactobacillus casei strain Shirota (LcS) showed improved WOMAC and VAS scores and significantly lowered C-reactive protein levels in knee osteoarthritis patients (129).
4.3 Polyphenols and osteoarthritis
Curcumin, one of the most researched nutraceuticals, has antioxidant and anti-inflammatory properties that modulate several pathways, including NF-κB, Wnt signaling, Nrf2 signaling, NOTCH, mTOR, and JAK/STAT (130). The role in alleviating osteoarthritis and protecting bone health due to its anti-inflammatory properties has been highlighted recently. The chondroprotective effects of curcumin alleviated apoptosis in chondrocytes upon IL-1β stimulation (131). Stimulating with IL-1β also caused increased p65 promoter activity of NF-κB, which curcumin reversed. In THP-1 cells treated with LPS and ATP, curcumin inhibited the expression of TNF-α and IL-1β in addition to downregulation of cleaved-caspase-1, thereby inhibiting NLRP3 activation (132). The increased stability and bioavailability of curcumin nanoformulation showed a protective effect on the articular cartilage in the OA model, which was visualized in the histological examination (133). The nanoformulation enhanced matrix staining and increased cellularity compared to native curcumin. Curcumin and nanocurcumin downregulate pro-inflammatory cytokines IL-1β, TNF-α. In the model, topically administered nanocurcumin slowed the progression of osteoarthritis, downregulated pro-inflammatory mediators, and showed chondroprotective effects and improved locomotor function (133). Oral curcumin was also effective in slowing the progression of osteoarthritis; however, it could not significantly reduce OA-related pain as compared to the nanoformulation (133). A clinical trial with curcumin nanomicelles significantly ameliorated osteoarthritis symptoms in knee patients over six weeks, which was analyzed through a questionnaire (134). Curcumin primed onto adipose-derived mesenchymal stem cells-derived small extracellular vesicle exhibited improved efficacy and chondro-protective effects against osteoarthritis by inhibiting oxidative stress and subsequent apoptosis of chondrocytes (135). In the anterior cruciate ligament transection surgery (ACLT) induced osteoarthritis mice, histological examination and oxidative biomarker assays confirmed reduced apoptosis of chondrocytes, thus showing protective effects on the cartilage when compared to only small extracellular vesicles and only curcumin in vivo.
The mechanism of bioactive curcumin in modulating osteoarthritis pathophysiology is elusive. Proinflammatory cytokines are involved in bone and joint-related diseases by inducing bone resorption and destroying cartilage (136). These cytokines are upregulated upon activation of NLRP3 inflammasome via PAMPs (pathogen-associated molecular patterns), DAMPs (damage-associated molecular patterns), and other signal molecules. Initially, NF-κB signaling is activated by toll-like receptors (TLR) upon recognition of PAMPs/DAMPs. This is called inflammasome priming and upregulates the expression of NLRP-3, pro-IL-1β, and pro-IL-18 (137). Post this, NLRP-3 forms NLRP-3 inflammasome, pro-caspase 1, and ASC. Pro-caspase 1 subsequently changes into caspase-1, which cleaves pro-IL-1β and pro-IL-18 into their active forms, such as IL-1β and IL-18. NLRP-3 inflammasome and NLRP-1 inflammasome induced pyroptosis in human primary fibroblast-like synoviocytes (FLS) collected from knee osteoarthritis patients under the stimulation of LPS/ATP (138). FLS cells, when stimulated with LPS and ATP, resulted in pyroptosis, which was, however, reversed in the presence of NLRP-1 and NLRP-3 siRNAs. The curcumin-ameliorating effects in patients with knee osteoarthritis symptoms could mediated by modulating NLRP3 inflammasome and its constituents that can effectively maintain the balance between osteoclastogenesis and osteoblastogenesis, reducing NLRP3-mediated inflammation oxidative stress and possibly improving osteoarthritis pathophysiology as shown in Figure 2.
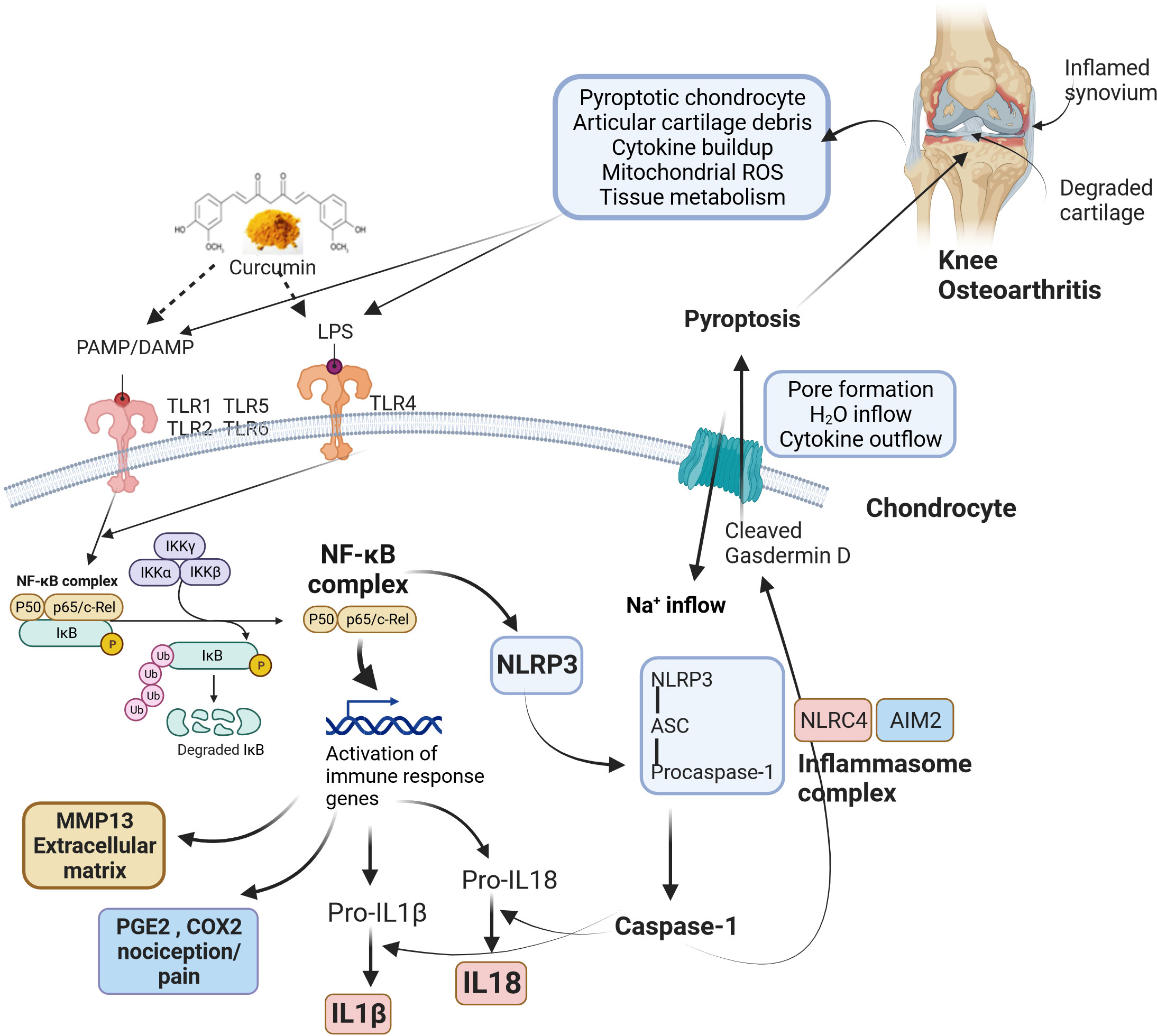
Figure 2 The roles of bioactive curcumin and modulation of osteoarthritis pathophysiology. Curcumin acts as a signalling molecule via PAMPs (pathogen-associated molecular patterns) and DAMPs (damage-associated molecular patterns) recognition that follows the activation of NLRP3 inflammasome, reduction of NLRP3-mediated inflammation, oxidative stress, possibly restoring the balance between osteoclastogenesis and osteoblastogenesis. Pathophysiological changes during knee osteoarthritis involve damaged cartilage tissue and inflamed synovium, which trigger various inflammatory pathways via PAMP/DAMP. The activated TLRs induce NF-κB signalling, leading to the upregulation of various genes related to immune responses. NF-κB complex also activates cytokines (IL-18 and IL-1β) and NLRP3. Activation of NLRP3 results in the formation of an inflammasome complex and cleaves pro-caspase 1 to caspase 1. Similarly, NLRC4 and AIM2 inflammasomes also form inflammasome complexes and activate caspase 1. Caspase 1 recognizes and cleaves Gasdermin D, producing transmembrane pore-forming channels that release pro-inflammatory cytokines. In addition, pore formation results in sodium and water inflow into cells. These factors cause programmed cell death (pyroptosis), further amplifying inflammation and aggravating knee osteoarthritis. ROS, Reactive oxygen species; LPS, Lipopolysaccharide; PAMP, Pathogen-associated molecular pattern; DAMP, Damage-associated molecular pattern; TLR, Toll-Like Receptor; NF-κB, Nuclear factor kappa B; IκB, I-kappa-B; IKK, Inhibitor of IκB kinase; MMP13, Matrix metalloproteinase 13; PGE2, Prostaglandin E2; COX-2, Cyclooxygenase 2; IL-1β, Interleukin-1β; IL-18, Interleukin 18; H2O, Water; Na+: Sodium; NLRP3, Nucleotide-binding domain, leucine-rich-containing (NLR) family, pyrin domain containing 3; ASC, Apoptosis-associated speck-like protein containing a caspase-recruitment domain; NLRC4, NLR family caspase activation and recruitment domain-containing protein 4; AIM2, Absent in melanoma 2.
Curcumin supplementation in mice showed neuroprotective effects by modulating the gut microbiota via the gut-brain axis (139). Curcumin was found to restore the profile of short-chain fatty acids and alleviated the expression of tight junction proteins of the intestine in comparison to diseased samples. Curcumin modulates the organization of tight junctions and downregulates LPS-mediated IL-1β expression, thus improving the intestinal barrier function (140). Supplementation with curcumin, Emblica, and Cassia extract in HFD fed-obese mice showed decreased intestinal inflammation and an improvement in the integrity of the gut epithelial barrier (141). Further, curcumin simultaneously reduced the plasma and mRNA levels of IL-1β and TNF-α downregulated the expression of MyD88, NF-κB, and TLR4 while upregulating major tight junction proteins such occludin and zonula occluden-1 in HFD-induced steatosis mice (142). Thus, there is strong evidence that dietary intervention with curcumin can improve the gut barrier function and potentially ameliorate inflammatory diseases.
Linking gut microbial composition, DHA-acylated curcumin diesters affected the abundance of microbes that metabolize trimethylamine and lipopolysaccharide. Thus, curcumin esters combined with DHA have significantly reduced renal tubal injury (143). Curcumin’s presence attenuated LPS-induced intestinal imbalance by reducing pro-inflammatory cytokine levels and impacting gut microbial diversity, leading to an increased abundance of Enterococcus and Butyricicoccus (144). Despite low absorption and low bioavailability, curcumin improves the function of the gut epithelial barrier via various mechanisms, including absorption into intestinal epithelial cells, binding to intercellular vitamin D receptor, direct interaction with IL-2, downregulation of sterol transporter NPC1L1, thus reducing intestinal cholesterol absorption (145). When combined with piperine, curcumin has also been shown to act on the mTORC inhibitor (TSC2), thereby downregulating mTORC signaling in human intestinal cells (146). Additionally, it has been shown that gut bacteria like Blautia sp. are involved in metabolizing curcuminoids into active metabolites such as demythylcurcumin, bisdemethylcurcumin, and demethyldemethoxycurcumin, all of which are promising synthetic analogs of curcumin that potentially show systemic and localized effects (147). Curcumin, thus, has the potential to modulate the epithelial barrier of the intestine (145). Table 1 represents data from various clinical trials conducted to test the efficacy of curcumin against osteoarthritis. In addition, a meta-analysis has revealed that both high- and low-dose curcuminoids significantly decrease pain relief (172) and promise an alternative or complementary agent for the management of osteoarthritis (173).
Inflammation due to dietary habits like HFD consumption is a significant causative for intestinal dysbiosis. The bioactive polyphenols have the potential to rescue gut dysbiosis and maintain intestinal homeostasis. Resveratrol has demonstrated protective effects on systemic and localized inflammation by modulating the gut in several HFD-induced metabolic studies. Intervention with resveratrol in healthy rats led to changes in the gut microbiota composition like enrichment of Parabacteroides, Bacteroides, Blautia, Lachnoclostridium, Ruminiclostridium, and Lachnospiraceae (174). Further, transplanting these microbiomes to mice fed with HFD increased insulin sensitivity, improved gut barrier function, reduced weight gain, decreased inflammation, and improved lipid metabolism (174). Resveratrol reduced systemic inflammation and fat accumulation in mice fed with HFD. A correlation was observed upon fecal microbiota transplantation in mice from HFD-fed mice who intervened with resveratrol, thus proving that resveratrol affects the gut microflora composition (175). An increased abundance of Ruminococcaceae, Akkermansia, and Lachnospiraceae and reduced Desulfovibriwere mitigated HFD-induced hepatic fat accumulation, inflammation, weight gain, and gut dysbiosis (176). A combination of resveratrol and quercetin in HFD-fed rats led to reduced ratio of Firmicutes to Bacteroidetes, enriched with Christensenellaceae, Ruminococcaceae, Bacteriodales, Akkermansia and a decreased abundance with Bilophila, Lachnoclostridium, Coriobacteriaceae, Acidaminococcaceae and Desulfovibrionaceae (177).
Similar to curcumin, metabolites of resveratrol such as lunularin and dihydroresveratrol are derived from the gut microbiota and distributed abundantly in the GI tract and tissues as compared to unmetabolized resveratrol and hence show better activity than native resveratrol (178). Resveratrol exhibited cytoprotective, antioxidant, and anti-inflammatory properties when exposed to the gut microbiota-derived uremic toxin by downregulating Nrf2 and upregulating NF-κB expression in RAW 264.7 cells (179). Trans-resveratrol has been shown to upregulate mRNA expression of an occluding gene that expresses a tight junction protein and other inflammatory markers like TLR2, TLR4, and IL-18 (180). An increased abundance of SCFA-producing bacteria - Dorea and Blautia sp. and a reduction in disease-causing Desulfovibrionaceae sp. has been observed upon resveratrol supplementation in rats fed with an HFD (181). Thus, resveratrol’s anti-oxidative and anti-inflammatory potential is modulated via gut microbiome, as evidenced by several studies.
Various evidences suggest the established use of another polyphenol, capsaicin (CAP), as a phytochemical analgesic that works by binding with TRPV1, a nociceptive fibre (182–185). The vanillyl moiety of capsaicin is majorly responsible for its bioactivity and is used widely for the treatment of chronic musculoskeletal and neuropathic pain, chronic migraines and in treating functional dyspepsia (186, 187). Capsaicin also shows an anti-inflammatory effect by down-regulating LPS-induced expression of COX-2 and NF-κB in RAW 264.7 macrophages (188). Capsaicin has similarly been found to suppress the LPS-induced expression of pro-inflammatory cytokines TNF-α, IL-1β, and IL-6 in THP-1 macrophages (189). Capsaicin significantly reduced TLR4 and ICAM-1 expression in primary Schwann cells (190). In muscle precursor myoblast cells, capsaicin could attenuate LPS-induced inflammation by downregulating the expression of TNF-α, Calpain-1, and Caspase-3 (191). A nanoemulsion of capsaicin with olive oil proved to be a more efficient anti-inflammatory agent and analgesic upon topical application in rats and rabbits (192).
Dietary capsaicin has also been shown to alleviate metabolic dysregulation in diabetic obese mice, thus exhibiting anti-obesity properties (193). Several mechanisms in this regard have been explored (194). Capsaicin has satiety-inducing properties and suppresses appetite (195). It also decreases the accumulation of lipids by enhancing their metabolism (196, 197). Capsaicin also exhibits properties of improving glucose homeostasis and insulin sensitivity. Administration of dietary capsaicin in db/db mice (diabetic) led to increased glycogen synthesis and reduced gluconeogenesis in addition to increasing the pool size of bile acid by suppressing FGF15 and upregulating expression of glucagon-like peptide (GLP-1) (198, 199).
In addition, gut microbial changes have also been associated with the anti-obesity effects of capsaicin. A disruption in glucose homeostasis is linked to alterations in the gut microbiome. Dietary capsaicin increased the ratio of Firmicutes/Bacteroides and an increased abundance of Roseburia in obese diabetic ob/ob mice (200). Capsaicin led to an increased abundance of Odoribacter, Coprococcus, Prevotella, Allobaculum, Muribaculaceae, Bacteroides, and Akkermansia, while reducing the abundance of Escherichia, Sutterella, Helicobacter, Proteobacteria and Desulfovibrio (201, 202). This increase in SCFA-producing species in HFD mice had an anti-obesity effect by increasing propionate and acetate concentrations. A combination of dietary fiber and capsaicin in HFD-rats increased gut-microbial diversity, reduced the abundance of Desulfovibrio, and increased Akkermansia and Allobaculum species (197). This evidence led us to believe that capsaicin might also function in a TRPV1-independent way by modulating the gut.
The ability of capsaicin to further increase gut microbial diversity has also been elucidated. Microbial community was maintained in vitro and supplemented with capsaicin for two weeks. Analysis of samples revealed a distinguished increase in microbial diversity and a modulation in the abundance of primary short-chain fatty acids (SCFAs) (203). Dietary intervention with capsaicin led to lowered plasma Ghrelin levels and increased levels of gastric inhibitory peptide (GIP) and GLP-1. It also led to an increased abundance of Faecalibacterium while increasing the ratio of Firmicutes-to-Bacteroide (204). Additionally, the administration of capsaicin also led to a dose-dependent increase in the production of SCFAs by regulating the abundance of Lactobacillus, Butryricimonas, Bifidobacterium, and Faecalibacterium (205). Faecalibacterium, Lactobacillus, and Roseburia are essential species of the gut microbial community, all of which have been shown to increase in abundance upon administration of dietary capsaicin, proving their role in positively modulating the gut microbiota (206).
Randomized controlled trials (RCT) studied the efficacy of dietary supplementation in improving bone and skeletal health. A recent systematic review of ten studies confirmed that turmeric or curcumin extract improved pain and function from baseline for individuals with knee osteoarthritis (38). Other Bioactives also demonstrated beneficial effects in improving bone disorders. For example, a combination of protein fortified with micronutrients was given as a nutraceutical, and markers related to bone turnover and bone metabolism were studied in premenopausal women. A decline in the expression of bone resorptive markers and increased vitamin B levels showed a promising outcome for the formulated supplement against skeletal health (207). The healthy post-menopausal women supplemented with pomegranate and grape seed juice showed downregulated expression of genes related to bone resorption and osteoclast differentiation and upregulated bone formation-related genes in ex-vivo microarray analysis (9). These data reiterate nutraceuticals’ ability to alter epigenetics through nutrient-gene interaction in bone and skeletal homeostasis. Table 2 represents clinical trials of various bioactive against osteoarthritis.
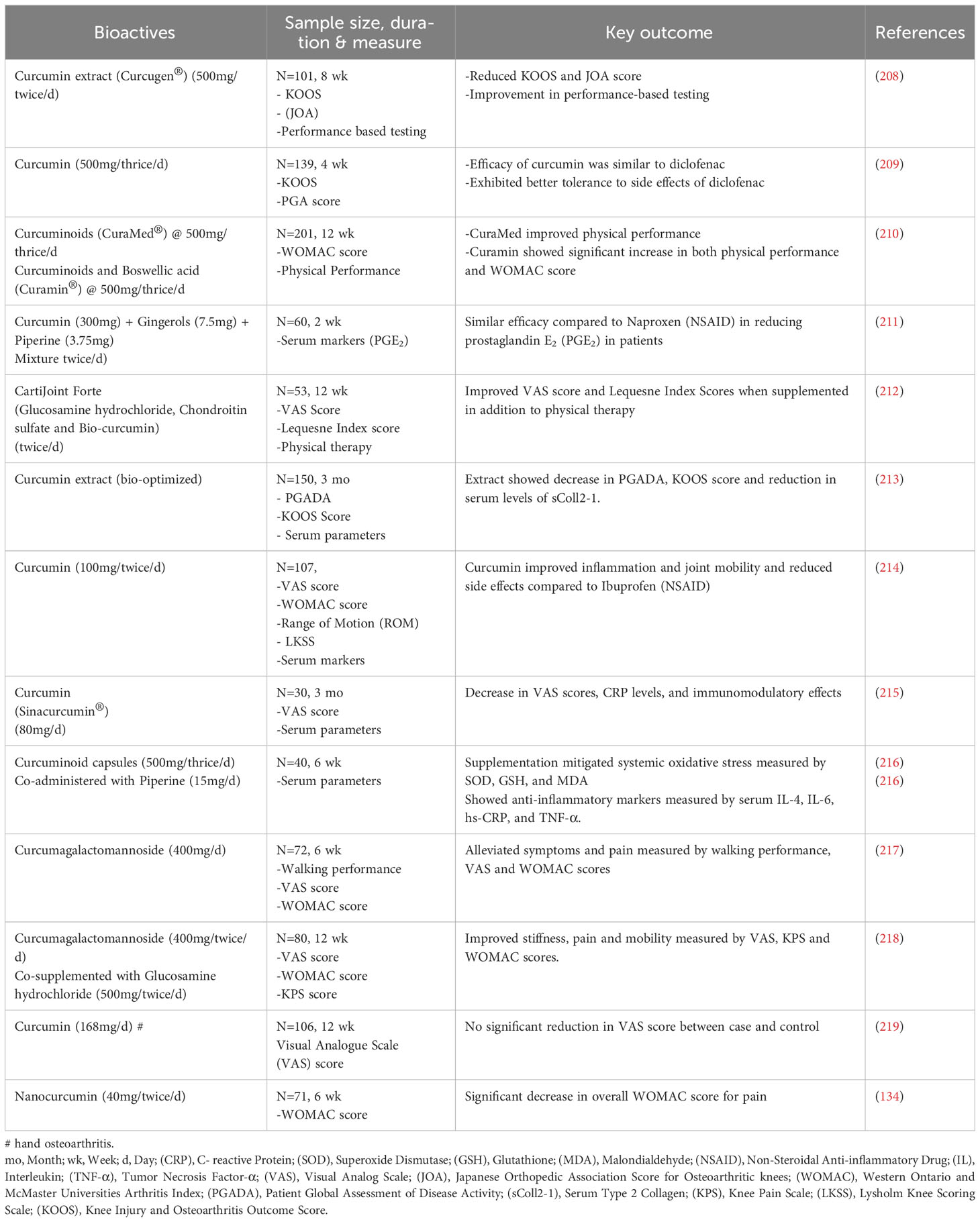
Table 2 Therapeutic effects of curcumin in managing knee osteoarthritis: a collection of clinical interventions.
4.4 Vitamin D and omega-3 fatty acids
The vitamin D is synthesized beneath the skin upon exposure to ultraviolet-B radiation from the sunlight. This biologically inactive form of vitamin D undergoes a series of reactions, including hydroxylation in the liver and kidney, to convert to an active form such as calcitriol (1,25-dihydroxy vitamin D). Calcitriol increases calcium reabsorption after being filtered by the kidneys. It mobilizes calcium in the bones and maintains calcium and phosphorous homeostasis in bones. Vitamin D deficiency leads to the disease rickets in children due to inadequate bone mineralization, while osteomalacia is reported in adults under similar conditions. Vitamin D deficiency has been associated with elevated inflammation, and long-term vitamin D insufficiency increases the risk of osteoporosis.
The bioactive vitamin D3 is the predominant form found in food. However, the roles of bioactive vitamin D on intestinal homeostasis and its impact on bone metabolism are unknown. Vitamin D and its receptor signaling play an essential role in maintaining intestinal homeostasis and the healthy gut microbiota by modulating effector T cells (Th1 and Th17) and regulatory T cells (220–222). Since vitamin D plays such a crucial role in maintaining gut homeostasis, it is evident that any deficiency will result in altered microbial composition. Several studies recently reported strong links between vitamin D synthesis, exposure to sunlight, and gut microbes, including mice, zebrafish, and humans (223, 224). Supplementation with vitamin D increased Firmicutes, Bacteroidetes, and Actinobacteria abundance at the phyla level and Faecalibacterium, Ruminococcaceae, Coprococcus, and Akkermansia at the genus level. Firmicutes are directly associated with vitamin D serum levels (225, 226). In rats, vitamin D deficiency impaired glucose tolerance and increased Desulfovibrio, Peptococcus, Roseburia, Lachnopriraceae, Lachnoclostridium, and Ruminiclostridium. Blautia linked to 2-picolinic acid was decreased, leading to a decrease in the metabolite that is crucial in generating an immunological response (227).
An association between serum vitamin D, ultraviolet rays (UV-B), and the gut microbiome was studied in the Canadian population. Exposure to narrow band (NB)-UVB among those who did not take vitamin D supplementation led to an aberrant increase in serum vitamin D levels in addition to increasing α- and β-diversity of the gut microbiome. This increase in diversity was similar to the microbial composition of those who took vitamin D supplements, among whom the NB-UVB exposure did not create a difference (228). The gut microbial composition in a semi-nomadic hunter-gatherer (Yanomami) showed similar to those with NB-UVB exposure in the previous study, thus establishing the role of sunlight and vitamin D levels in modulating the gut microbiota (229, 230). The association of dietary omega-3 fatty acids with vitamin D, as proven by several studies (231, 232), establishes the importance of omega-3 and other vital nutrients that directly or indirectly cause variation in the gut microbial composition and thereby affect the progression of inflammatory and musculoskeletal disorders. This aligns with the assumption that nutrition and diet are the significant causatives that trigger various diseases via intestinal dysbiosis.
The essential roles of long-chain fatty acids were evidenced first from the abnormal calcification due to essential fatty acid deficiency (233), suggesting the critical involvement of lipid mediators in bone metabolism. During acute inflammation, the immune cells are regulated by specialized lipid mediators such as lipoxins (derived from arachidonic acid, ARA), resolvins, maresins, and protectins (derived from eicosapentaenoic acid, EPA, and docosahexaenoic acid, DHA), those are collectively known as SPMs (specialized pro-resolving mediators), drive resolution of inflammation, remove inflammatory lesions and promotes apoptotic cell clearance through lymphatics (234). Resolvin E1 (RvE1), derived from EPA (omega-3), and other lipid mediators play a role in inflammation-associated models of arthritis (235) by modulating Cyclooxygenase 2 (COX-2) activity. Data showed that RvE1protectst inflammatory-induced bone damage by regulating bone cell gene expression in a model (236). Phospholipase-mediated releases of ARA, the substrate for prostaglandin E2 (PGE2) synthesis, are also involved in signaling bone turnover. COX2 converts ARA to PGE2 and modulates both osteoclastogenesis by stimulating the expression of RANK, RANKL, and osteoblastogenesis by promoting insulin-like growth factor (IGF-1) and Wnt signalling, respectively (237). PGE2 levels are a potent modulator of resorption and bone formation, while elevated PGE2 suppresses osteoblasts and promotes bone differentiation and resorption by osteoclasts, whereas low levels stimulate bone formation by osteoblasts (238). There is strong evidence that a diet rich in ω-3 fatty acid downregulates markers related to oxidative stress, cartilage degradation, and inflammation in chondrocytes while these markers are elevated with a higher ω-6 fatty acids (7, 239–243). Therefore, the quality of polyunsaturated fatty acids (PUFA) might have a distinct role in bone metabolism since metabolites derived from ω-6 and ω-3 fatty acids can act on precursor cells of osteoblast and adipocytes differentially (244). Bioactive rich in ω-6 fatty acids raises ω-6/ω-3 ratio and triggers adipogenesis lineage of the bone marrow by promoting adipogenic differentiation of mesenchymal cells, diverting their differentiation into osteoblasts and thus disrupting the homeostasis of the bone remodeling.
In contrast, bioactive rich with ω-3 fatty acids do not exert a strong adipogenesis induction and promote an inhibitory effect on osteoclastogenesis that helps maintain the bone mineral mass and thus allows osteoblastogenesis. Omega-3 fatty acids also regulate musculoskeletal growth (245) and bone homeostasis by causing a reduction in bone resorption when bone formation is consistent (238). Studies suggest that omega-3 fatty acids exert their beneficial effects on bone strength by improving microarchitecture with concomitant increases in trabecular network with decreased trabecular space, trabecular number, bone volume fraction and bone surface density (246, 247). Mice fed with fish oil after ovariectomy showed a reduced loss of bone mineral density (248), while femur bone mineral content positively correlated with ω-3 PUFAs in ovariectomized rats (249). DHA has been shown to accumulate in bone periosteum and bone marrow and correlates positively with bone mineral content (250). The ω-3 PUFAs contribute to the growth phase of the developing skeleton by accelerating growth plate, bone growth, chondrocyte proliferation and differentiation that collectively results in superior trabecular and cortical structure to stiffer bones and improved bone quality as evidenced in fat-1 mice (7). Clinical studies reiterated that ω-3 PUFAs may be linked with reduced structural damage in bones and improved function and pain in knee and hip OA patients (8, 251, 252).
A Rancho Bernardo study emphasized the roles of α-linoleic acid (ALA), EPA, and DHA in bone health to understand the relationship between ω-6/ω-3 ratio and bone mineral density (253). A higher percentage was associated with less bone mineral density (BMD), suggesting the crucial role of dietary ω-3 PUFA in preventing bone ailments. A similar study was conducted in older women and concluded dietary PUFA positively modulates BMD in the lumbar spine (L2-L4) as well as BMD of the total body (254). Studies have shown that ω-3 PUFAs and metabolites influence bone remodeling (255). The role of omega-3 fatty acids as a nutraceutical in managing osteoarthritis revealed that both oxidative stress and inflammation are involved (256). The ω-3 PUFAs and their metabolites have anti-inflammatory, antioxidant, and analgesic properties, which prevent bone loss by upregulating the Akt pathway and inhibiting the iNOS pathway (255), NF-κB pathway, MAPK signaling, JNK pathway, IL-1β, COX-2, and MMP-13. The pathogenesis of osteoarthritis has also been linked to adiposity. Adiposity is a potent risk factor for bone ailments with increased leptin levels. While excess ω-6 PUFAs are known to cause inflammation during adiposity, ω-3 PUFAs overturn this, reducing the risk and preventing bone ailments. The imbalance of the optimal ω-6/ω-3 ratio has also been linked to osteoarthritis. Hence, the use of ω-3 fatty acids, particularly EPA or DHA, as a nutraceutical supplementation resolves the skewed ω-6/ω-3 ratio, helps reduce inflammation, and results in the management of osteoarthritis and other bone-related conditions (257). High levels of ω-6 PUFA also cause synovial membrane inflammation due to their ability to produce pro-inflammatory eicosanoids (258). Available data suggest that bioactive rich with ω-3 PUFAs could have multiple benefits in modulating bone turnover, including its formation, re-absorption, and density of the bone cells and as an adjuvant with anti-inflammatory drugs to lower the systemic inflammation associated with OA.
Alterations in the ω-6/ω-3 ratio also affect the gut microbiome. Consumption of high ω-6 fatty acids led to low-grade inflammation at the systemic level, whereas ω-3 fatty acids increased the production of intestinal alkaline phosphatase that modulates gut homeostasis (259). Marine molluscs like Perna canaliculus contain high levels of omega-3 and various carbohydrates and proteins. Clinical studies have shown that mussel extract has anti-inflammatory effects and significantly affects the intestinal microbiome by reducing the abundance of Clostridia species (260). Fish oil rich in DHA, upon supplementation in ob/ob mice, results in significant production of SCFA, reducing the cholesteryl esters and triglycerides. DHA was found to upend intestinal dysbiosis and inhibit lipid droplet formation in the liver. The abundance of Akkkermansia and Muribaculaceae increased, whereas a reduction was observed in Lachnospiraceae, a pathogenic bacterium (261). Clinical interventions with various bioactive supplemented in knee osteoarthritis patient and its outcome are captured in Table 1.
5 Conclusions
Systemic inflammation is the root cause of most musculoskeletal disorders, where gut dysbiosis and pro-inflammatory triggers strongly modulate disease progression. The interplay between the gut, immune system, and local tissue metabolism drives osteoarthritis pathophysiology. Intestinal dysbiosis disrupts the abundance of essential commensals that protect the mucosal integrity of gut permeability, maintain immune tolerance, produce key metabolites or vitamin synthesis, and generate immune responses against pathogenic bacteria. Moreover, inflammatory states in osteoarthritis change microflora and accumulate bacterial LPS. TLR4 receptor activation is critical in regulating gut permeability and is associated with acute and chronic inflammation. The dysregulated TLR signaling negatively impacts the gut, compromising its permeability and resulting in inflammation and dysbiosis. Modulators of TLR signalling can play a crucial role in maintaining gut homeostasis. Despite the evidence, the specific sets of gut microbiotas in OA still needs to be understood in detail. Most of the evidence on the role of gut microbiota in OA, obtained from animal models, thus warrants control clinical trials. The association of dietary omega-3 fatty acids with vitamin D directly or indirectly causes variation in the gut microbial composition, affecting the progression of inflammatory and musculoskeletal disorders. The intestinal microbiome’s various roles in modulating the immune system and their impact by Bioactives still need to be understood. Further, the role of gut microbiome has yet to be elucidated in the various clinical trials, for example, bioactive curcumin used as an intervention against osteoarthritis (Table 2).
Curcumin promised to revert LPS-induced inflammation by improving synovial endotoxemia and releasing a specific set of gut microbiotas that potentially improve osteoarthritis functions. The absence of curcumin detected in the subjects’ serum after large intake indicates that anti-oxidant, anti-inflammatory, and other beneficial effects reported by several studies could mediate by signaling receptor activation. Curcumin might target NLRP3 inflammasome by reducing NLRP3-mediated inflammation and oxidative stress and possibly ameliorating osteoarthritis pathophysiology. Curcumin has the same vanilloid ring pharmacophore in its structure as capsaicin, which rationalizes curcumin’s affinity for the activation of TRPV1. Thus, the potential mechanisms involving nociception in pain-modulating pathways via TRV1 channel signaling are emerging with these Bioactives.
Bioactives could be potential alternative therapeutic in managing chronic inflammatory diseases like osteoarthritis. However, various confounders like geography, dietary habits, exposure to sunlight, serum vitamin D levels, history of medication, infection, endogenous gut microbial diversity, and other variability at inter-individual and intra-individual levels could influence the beneficial effects of Bioactives in managing osteoarthritis pathophysiology. The article highlighted the importance of bioactive compounds in preventing and treating OA. However, further work is required involving advanced technology to separate more bioactive compounds and explore the exact molecular mechanism and therapeutic targets of the bioactive compounds, which will be helpful for the treatment of OA in the early stage, preventing disability and enhancing the quality of patients’ lives.
Author contributions
SB: Conceptualization, Writing – original draft, Writing – review & editing. KS: Writing – original draft. AD: Conceptualization, Writing – original draft, Writing – review & editing.
Funding
The author(s) declare that no financial support was received for the research, authorship, and/or publication of this article.
Acknowledgments
KSNH was supported by the DST-Inspire Fellowship, Dep. of Science & Technology, Gov. of India.
Conflict of interest
The authors declare that the research was conducted in the absence of any commercial or financial relationships that could be construed as a potential conflict of interest.
Publisher’s note
All claims expressed in this article are solely those of the authors and do not necessarily represent those of their affiliated organizations, or those of the publisher, the editors and the reviewers. Any product that may be evaluated in this article, or claim that may be made by its manufacturer, is not guaranteed or endorsed by the publisher.
References
1. Steinmetz JD, Culbreth GT, Haile LM, Rafferty Q, Lo J, Fukutaki KG, et al. Global, regional, and national burden of osteoarthritis 1990–2020 and projections to 2050: a systematic analysis for the Global Burden of Disease Study 2021. Lancet Rheumatol (2023) 5:e508–22. doi: 10.1016/S2665-9913(23)00163-7
2. Singh A, Das S, Chopra A, Danda D, Paul BJ, March L, et al. Burden of osteoarthritis in India and its states 1990-2019: findings from the Global Burden of disease study 2019. Osteoarthritis Cartilage (2022) 30:1070–8. doi: 10.1016/j.joca.2022.05.004
3. Palazzo C, Ravaud JF, Papelard A, Ravaud P, Poiraudeau S. The burden of musculoskeletal conditions. PloS One (2014) 9:e90633. doi: 10.1371/journal.pone.0090633
4. Badley EM, Wilfong JM, Perruccio AV. Impact of osteoarthritis in younger adults. Osteoarthritis Cartilage (2021) 29:S278–9. doi: 10.1016/j.joca.2021.02.367
5. Noubiap JJ, Nansseu JR, Lontchi-Yimagou E, Nkeck JR, Nyaga UF, Ngouo AT, et al. Geographic distribution of metabolic syndrome and its components in the general adult population: A meta-analysis of global data from 28 million individuals. Diabetes Res Clin Pract (2022) 188:109924. doi: 10.1016/j.diabres.2022.109924
6. Khan M, Adili A, Winemaker M, Bhandari M. Management of osteoarthritis of the knee in younger patients. Cmaj (2018) 190:E72–e79. doi: 10.1503/cmaj.170696
7. Koren N, Simsa-Maziel S, Shahar R, Schwartz B, Monsonego-Ornan E. Exposure to omega-3 fatty acids at early age accelerate bone growth and improve bone quality. J Nutr Biochem (2014) 25:623–33. doi: 10.1016/j.jnutbio.2014.01.012
8. Hill CL, March LM, Aitken D, Lester SE, Battersby R, Hynes K, et al. Fish oil in knee osteoarthritis: a randomised clinical trial of low dose versus high dose. Ann Rheumatic Dis (2016) 75:23. doi: 10.1136/annrheumdis-2014-207169
9. Lin Y, Kazlova V, Ramakrishnan S, Murray MA, Fast D, Chandra A, et al. Bone health nutraceuticals alter microarray mRNA gene expression: A randomized, parallel, open-label clinical study. Phytomedicine (2016) 23:18–26. doi: 10.1016/j.phymed.2015.11.011
10. Coutinho-Wolino KS, Almeida PP, Mafra D, Stockler-Pinto MB. Bioactive compounds modulating Toll-like 4 receptor (TLR4)-mediated inflammation: pathways involved and future perspectives. Nutr Res (2022) 107:96–116. doi: 10.1016/j.nutres.2022.09.001
11. Sun C, Zhou X, Guo T, Meng J. The immune role of the intestinal microbiome in knee osteoarthritis: a review of the possible mechanisms and therapies. Front Immunol (2023) 14:1168818. doi: 10.3389/fimmu.2023.1168818
12. King LK, March L, Anandacoomarasamy A. Obesity & osteoarthritis. Indian J Med Res (2013) 138:185–93.
13. Zhang Y, Jordan JM. Epidemiology of osteoarthritis. Clin Geriatr Med (2010) 26:355–69. doi: 10.1016/j.cger.2010.03.001
15. Duclos M. Osteoarthritis, obesity and type 2 diabetes: The weight of waist circumference. Ann Phys Rehabil Med (2016) 59:157–60. doi: 10.1016/j.rehab.2016.04.002
16. Hamerman D. The biology of osteoarthritis. New Engl J Med (1989) 320:1322–30. doi: 10.1056/NEJM198905183202006
17. Caetano-Lopes J, Canhao H, Fonseca JE. Osteoblasts and bone formation. Acta Reumatol Port (2007) 32:103–10.
18. Boyce BF, Yao Z, Xing L. Osteoclasts have multiple roles in bone in addition to bone resorption. Crit Rev Eukaryot Gene Expr (2009) 19:171–80. doi: 10.1615/CritRevEukarGeneExpr.v19.i3.10
19. Robinson WH, Lepus CM, Wang Q, Raghu H, Mao R, Lindstrom TM, et al. Low-grade inflammation as a key mediator of the pathogenesis of osteoarthritis. Nat Rev Rheumatol (2016) 12:580–92. doi: 10.1038/nrrheum.2016.136
20. Scanzello CR. Role of low-grade inflammation in osteoarthritis. Curr Opin Rheumatol (2017) 29:79–85. doi: 10.1097/BOR.0000000000000353
21. Goldring MB, Otero M. Inflammation in osteoarthritis. Curr Opin Rheumatol (2011) 23:471–8. doi: 10.1097/BOR.0b013e328349c2b1
22. Livshits G, Zhai G, Hart DJ, Kato BS, Wang H, Williams FM, et al. Interleukin-6 is a significant predictor of radiographic knee osteoarthritis: The Chingford Study. Arthritis Rheum (2009) 60:2037–45. doi: 10.1002/art.24598
23. Takahashi A, De Andrés MC, Hashimoto K, Itoi E, Oreffo RO. Epigenetic regulation of interleukin-8, an inflammatory chemokine, in osteoarthritis. Osteoarthritis Cartilage (2015) 23:1946–54. doi: 10.1016/j.joca.2015.02.168
24. Omoto S, Nishida K, Yamaai Y, Shibahara M, Nishida T, Doi T, et al. Expression and localization of connective tissue growth factor (CTGF/Hcs24/CCN2) in osteoarthritic cartilage. Osteoarthritis Cartilage (2004) 12:771–8. doi: 10.1016/j.joca.2004.06.009
25. Liu SC, Hsu CJ, Chen HT, Tsou HK, Chuang SM, Tang CH. CTGF increases IL-6 expression in human synovial fibroblasts through integrin-dependent signaling pathway. PloS One (2012) 7:e51097. doi: 10.1371/journal.pone.0051097
26. Das MK, Basak S, Ahmed MS, Attramadal H, Duttaroy AK. Connective tissue growth factor induces tube formation and IL-8 production in first trimester human placental trophoblast cells. Eur J Obstet Gynecol Reprod Biol (2014) 181:183–8. doi: 10.1016/j.ejogrb.2014.07.045
27. Tu M, Yao Y, Qiao FH, Wang L. The pathogenic role of connective tissue growth factor in osteoarthritis. Biosci Rep (2019) 39. doi: 10.1042/BSR20191374
28. Nedunchezhiyan U, Varughese I, Sun AR, Wu X, Crawford R, Prasadam I. Obesity, inflammation, and immune system in osteoarthritis. Front Immunol (2022) 13:907750. doi: 10.3389/fimmu.2022.907750
29. Quinn JM, Gillespie MT. Modulation of osteoclast formation. Biochem Biophys Res Commun (2005) 328:739–45. doi: 10.1016/j.bbrc.2004.11.076
30. Poulsen RC, Moughan PJ, Kruger MC. Long-chain polyunsaturated fatty acids and the regulation of bone metabolism. Exp Biol Med (Maywood) (2007) 232:1275–88. doi: 10.3181/0704-MR-100
31. Kong YY, Feige U, Sarosi I, Bolon B, Tafuri A, Morony S, et al. Activated T cells regulate bone loss and joint destruction in adjuvant arthritis through osteoprotegerin ligand. Nature (1999) 402:304–9. doi: 10.1038/46303
32. Mora JC, Przkora R, Cruz-Almeida Y. Knee osteoarthritis: pathophysiology and current treatment modalities. J Pain Res (2018) 11:2189–96. doi: 10.2147/JPR.S154002
33. Charlesworth J, Fitzpatrick J, Perera NKP, Orchard J. Osteoarthritis- a systematic review of long-term safety implications for osteoarthritis of the knee. BMC Musculoskelet Disord (2019) 20:151. doi: 10.1186/s12891-019-2525-0
34. Im GI, Kim TK. Regenerative therapy for osteoarthritis: A perspective. Int J Stem Cells (2020) 13:177–81. doi: 10.15283/ijsc20069
35. Billesberger LM, Fisher KM, Qadri YJ, Boortz-Marx RL. Procedural treatments for knee osteoarthritis: A review of current injectable therapies. Pain Res Manage (2020) 2020:3873098. doi: 10.1155/2020/3873098
36. Thoene M, Bejer-Olenska E, Wojtkiewicz J. The current state of osteoarthritis treatment options using stem cells for regenerative therapy: A review. Int J Mol Sci (2023) 24:8925. doi: 10.3390/ijms24108925
37. Ameye LG, Chee WSS. Osteoarthritis and nutrition. From nutraceuticals to functional foods: a systematic review of the scientific evidence. Arthritis Res Ther (2006) 8:R127. doi: 10.1186/ar2016
38. Paultre K, Cade W, Hernandez D, Reynolds J, Greif D, Best TM. Therapeutic effects of turmeric or curcumin extract on pain and function for individuals with knee osteoarthritis: a systematic review. BMJ Open Sport Exerc Med (2021) 7:e000935. doi: 10.1136/bmjsem-2020-000935
39. Gough AK, Lilley J, Eyre S, Holder RL, Emery P. Generalised bone loss in patients with early rheumatoid arthritis. Lancet (1994) 344:23–7. doi: 10.1016/S0140-6736(94)91049-9
40. Kwon Y. Immuno-resolving ability of resolvins, protectins, and maresins derived from omega-3 fatty acids in metabolic syndrome. Mol Nutr Food Res (2020) 64:e1900824. doi: 10.1002/mnfr.201900824
41. Yao Q, Wu X, Tao C, Gong W, Chen M, Qu M, et al. Osteoarthritis: pathogenic signaling pathways and therapeutic targets. Signal Transduction Targeted Ther (2023) 8:56. doi: 10.1038/s41392-023-01330-w
42. Kovács B, Vajda E, Nagy EE. Regulatory effects and interactions of the wnt and OPG-RANKL-RANK signaling at the bone-cartilage interface in osteoarthritis. LID, Basel, Switzerland (2019). p. 4653. doi: 10.3390/ijms20184653
43. Pereira M, Petretto E, Gordon S, Bassett JHD, Williams GR, Behmoaras J. Common signalling pathways in macrophage and osteoclast multinucleation. J Cell Sci (2018) 131. doi: 10.1242/jcs.216267
44. Moon RT, Kohn AD, De Ferrari GV, Kaykas A. WNT and β-catenin signalling: diseases and therapies. Nat Rev Genet 2004 5:9 (2004) 5:691–701. doi: 10.1038/nrg1427
45. Amjadi-Moheb F, Akhavan-Niaki H. Wnt signaling pathway in osteoporosis: Epigenetic regulation, interaction with other signaling pathways, and therapeutic promises. J Cell Physiol (2019) 234:14641–50. doi: 10.1002/jcp.28207
46. Chen Z, Zhong H, Wei J, Lin S, Zong Z, Gong F, et al. Inhibition of Nrf2/HO-1 signaling leads to increased activation of the NLRP3 inflammasome in osteoarthritis. Arthritis Res Ther (2019) 21:300. doi: 10.1186/s13075-019-2085-6
47. Roškar S, Hafner-Bratkovič I. The role of inflammasomes in osteoarthritis and secondary joint degeneration diseases. Life (2022) 12:731. doi: 10.3390/life12050731
48. Jiang N, An J, Yang K, Liu J, Guan C, Ma C, et al. NLRP3 inflammasome: A new target for prevention and control of osteoporosis? Front Endocrinol (2021) 12. doi: 10.3389/fendo.2021.752546
49. Du Q, Liao Q, Chen C, Yang X, Xie R, Xu J. The role of transient receptor potential vanilloid 1 in common diseases of the digestive tract and the cardiovascular and respiratory system. Front Physiol (2019) 10:1064. doi: 10.3389/fphys.2019.01064
50. Alawi K, Keeble J. The paradoxical role of the transient receptor potential vanilloid 1 receptor in inflammation. Pharmacol Ther (2010) 125:181–95. doi: 10.1016/j.pharmthera.2009.10.005
51. Obeidat AM, Donner A, Miller RE. An update on targets for treating osteoarthritis pain: NGF and TRPV1. Curr Treatm Opt Rheumatol (2020) 6:129–45. doi: 10.1007/s40674-020-00146-x
52. Lv Z, Han J, Li J, Guo H, Fei Y, Sun Z, et al. Single cell RNA-seq analysis identifies ferroptotic chondrocyte cluster and reveals TRPV1 as an anti-ferroptotic target in osteoarthritis. EBioMedicine (2022) 84:104258. doi: 10.1016/j.ebiom.2022.104258
53. Kelly S, Chapman RJ, Woodhams S, Sagar DR, Turner J, Burston JJ, et al. Increased function of pronociceptive TRPV1 at the level of the joint in a rat model of osteoarthritis pain. Ann Rheum Dis (2015) 74:252–9. doi: 10.1136/annrheumdis-2013-203413
54. Vriens J, Nilius B, Vennekens R. Herbal compounds and toxins modulating TRP channels. Curr Neuropharmacol (2008) 6:79–96. doi: 10.2174/157015908783769644
55. Andrei C, Zanfirescu A, Nițulescu GM, Olaru OT, Negreș S. Natural active ingredients and TRPV1 modulation: focus on key chemical moieties involved in ligand-target interaction. Plants (Basel) (2023) 12:339. doi: 10.3390/plants12020339
56. Haywood AR, Hathway GJ, Chapman V. Differential contributions of peripheral and central mechanisms to pain in a rodent model of osteoarthritis. Sci Rep (2018) 8:7122. doi: 10.1038/s41598-018-25581-8
57. Persson MSM, Stocks J, Sarmanova A, Fernandes G, Walsh DA, Doherty M, et al. Individual responses to topical ibuprofen gel or capsaicin cream for painful knee osteoarthritis: a series of n-of-1 trials. Rheumatol (Oxford) (2021) 60:2231–7. doi: 10.1093/rheumatology/keaa561
58. Oláh Z, Rédei D, Pecze L, Vizler C, Jósvay K, Forgó P, et al. Pellitorine, an extract of Tetradium daniellii, is an antagonist of the ion channel TRPV1. Phytomedicine (2017) 34:44–9. doi: 10.1016/j.phymed.2017.06.006
59. Kim Y, Kim EH, Lee KS, Lee K, Park SH, Na SH, et al. The effects of intra-articular resiniferatoxin on monosodium iodoacetate-induced osteoarthritic pain in rats. Korean J Physiol Pharmacol (2016) 20:129–36. doi: 10.4196/kjpp.2016.20.1.129
60. Cardinelli CS, Sala PC, Alves CC, Torrinhas RS, Waitzberg DL. Influence of intestinal microbiota on body weight gain: a narrative review of the literature. Obes Surg (2015) 25:346–53. doi: 10.1007/s11695-014-1525-2
61. Gomes AC, Hoffmann C, Mota JF. The human gut microbiota: Metabolism and perspective in obesity. Gut Microbes (2018) 9:308–25. doi: 10.1080/19490976.2018.1465157
62. Amabebe E, Robert FO, Agbalalah T, Orubu ESF. Microbial dysbiosis-induced obesity: role of gut microbiota in homoeostasis of energy metabolism. Br J Nutr (2020) 123:1127–37. doi: 10.1017/S0007114520000380
63. Liu BN, Liu XT, Liang ZH, Wang JH. Gut microbiota in obesity. World J Gastroenterol (2021) 27:3837–50. doi: 10.3748/wjg.v27.i25.3837
64. Belkaid Y, Harrison OJ. Homeostatic immunity and the microbiota. Immunity (2017) 46:562–76. doi: 10.1016/j.immuni.2017.04.008
65. Yoo JY, Groer M, Dutra SVO, Sarkar A, Mcskimming DI. Gut microbiota and immune system interactions. Microorganisms (2020) 8:1587. doi: 10.3390/microorganisms8101587
66. Zheng D, Liwinski T, Elinav E. Interaction between microbiota and immunity in health and disease. Cell Res (2020) 30:492–506. doi: 10.1038/s41422-020-0332-7
67. Liu-Bryan R. Synovium and the innate inflammatory network in osteoarthritis progression. Curr Rheumatol Rep (2013) 15:323. doi: 10.1007/s11926-013-0323-5
68. Rinninella E, Raoul P, Cintoni M, Franceschi F, Miggiano GAD, Gasbarrini A, et al. What is the healthy gut microbiota composition? A changing ecosystem across age, environment, diet, and diseases. Microorganisms (2019) 7:14. doi: 10.3390/microorganisms7010014
69. Bell DS. Changes seen in gut bacteria content and distribution with obesity: causation or association? Postgrad Med (2015) 127:863–8. doi: 10.1080/00325481.2015.1098519
70. Magne F, Gotteland M, Gauthier L, Zazueta A, Pesoa S, Navarrete P, et al. The firmicutes/bacteroidetes ratio: A relevant marker of gut dysbiosis in obese patients? Nutrients (2020) 12:1474. doi: 10.3390/nu12051474
71. Turnbaugh PJ, Ley RE, Mahowald MA, Magrini V, Mardis ER, Gordon JI. An obesity-associated gut microbiome with increased capacity for energy harvest. Nature (2006) 444:1027–31. doi: 10.1038/nature05414
72. Ley RE, Bäckhed F, Turnbaugh P, Lozupone CA, Knight RD, Gordon JI. Obesity alters gut microbial ecology. Proc Natl Acad Sci U.S.A. (2005) 102:11070–5. doi: 10.1073/pnas.0504978102
73. Bäckhed F, Ding H, Wang T, Hooper LV, Koh GY, Nagy A, et al. The gut microbiota as an environmental factor that regulates fat storage. Proc Natl Acad Sci U.S.A. (2004) 101:15718–23. doi: 10.1073/pnas.0407076101
74. Ding S, Chi MM, Scull BP, Rigby R, Schwerbrock NM, Magness S, et al. High-fat diet: bacteria interactions promote intestinal inflammation which precedes and correlates with obesity and insulin resistance in mouse. PloS One (2010) 5:e12191. doi: 10.1371/journal.pone.0012191
75. Bleau C, Karelis AD, St-Pierre DH, Lamontagne L. Crosstalk between intestinal microbiota, adipose tissue and skeletal muscle as an early event in systemic low-grade inflammation and the development of obesity and diabetes. Diabetes Metab Res Rev (2015) 31:545–61. doi: 10.1002/dmrr.2617
76. Sanmiguel C, Gupta A, Mayer EA. Gut microbiome and obesity: A plausible explanation for obesity. Curr Obes Rep (2015) 4:250–61. doi: 10.1007/s13679-015-0152-0
77. De La Serre CB, Ellis CL, Lee J, Hartman AL, Rutledge JC, Raybould HE. Propensity to high-fat diet-induced obesity in rats is associated with changes in the gut microbiota and gut inflammation. Am J Physiol Gastrointest Liver Physiol (2010) 299:G440–448. doi: 10.1152/ajpgi.00098.2010
78. Thaiss CA, Zmora N, Levy M, Elinav E. The microbiome and innate immunity. Nature (2016) 535:65–74. doi: 10.1038/nature18847
79. Bauer H, Horowitz RE, Levenson SM, Popper H. The response of the lymphatic tissue to the microbial flora. Studies on germfree mice. Am J Pathol (1963) 42:471–83.
80. Higashiyama M, Miura S, Hokari R. Modulation by luminal factors on the functions and migration of intestinal innate immunity. Front Immunol (2023) 14. doi: 10.3389/fimmu.2023.1113467
81. Chisari E, Wouthuyzen-Bakker M, Friedrich AW, Parvizi J. The relation between the gut microbiome and osteoarthritis: A systematic review of literature. PloS One (2021) 16:e0261353. doi: 10.1371/journal.pone.0261353
82. Gleason B, Chisari E, Parvizi J. Osteoarthritis can also start in the gut: the gut-joint axis. Indian J Orthop (2022) 56:1150–5. doi: 10.1007/s43465-021-00473-8
83. Liu L, Tian F, Li GY, Xu W, Xia R. The effects and significance of gut microbiota and its metabolites on the regulation of osteoarthritis: Close coordination of gut-bone axis. Front Nutr (2022) 9:1012087. doi: 10.3389/fnut.2022.1012087
84. Zemanova N, Omelka R, Mondockova V, Kovacova V, Martiniakova M. Roles of gut microbiome in bone homeostasis and its relationship with bone-related diseases. Biol (Basel) (2022) 11:1402. doi: 10.3390/biology11101402
85. Venkatesh M, Mukherjee S, Wang H, Li H, Sun K, Benechet AP, et al. Symbiotic bacterial metabolites regulate gastrointestinal barrier function via the xenobiotic sensor PXR and Toll-like receptor 4. Immunity (2014) 41:296–310. doi: 10.1016/j.immuni.2014.06.014
86. Kim KA, Gu W, Lee IA, Joh EH, Kim DH. High fat diet-induced gut microbiota exacerbates inflammation and obesity in mice via the TLR4 signaling pathway. PloS One (2012) 7:e47713. doi: 10.1371/journal.pone.0047713
87. Velloso LA, Folli F, Saad MJ. TLR4 at the crossroads of nutrients, gut microbiota, and metabolic inflammation. Endocrine Rev (2015) 36:245–71. doi: 10.1210/er.2014-1100
88. Semin I, Ninnemann J, Bondareva M, Gimaev I, Kruglov AA. Interplay between microbiota, toll-like receptors and cytokines for the maintenance of epithelial barrier integrity. Front Med (2021) 8. doi: 10.3389/fmed.2021.644333
89. Huang Y, Tang J, Cai Z, Zhou K, Chang L, Bai Y, et al. Prevotella induces the production of th17 cells in the colon of mice. J Immunol Res (2020) 2020:9607328. doi: 10.1155/2020/9607328
90. Kamdar K, Johnson AMF, Chac D, Myers K, Kulur V, Truevillian K, et al. Innate recognition of the microbiota by TLR1 promotes epithelial homeostasis and prevents chronic inflammation. J Immunol (2018) 201:230–42. doi: 10.4049/jimmunol.1701216
91. Liu S, Li G, Xu H, Wang Q, Wei Y, Yang Q, et al. "Cross-talk" between gut microbiome dysbiosis and osteoarthritis progression: a systematic review. Front Immunol (2023) 14:1150572. doi: 10.3389/fimmu.2023.1150572
92. Yu XH, Yang YQ, Cao RR, Bo L, Lei SF. The causal role of gut microbiota in development of osteoarthritis. Osteoarthritis Cartilage (2021) 29:1741–50. doi: 10.1016/j.joca.2021.08.003
93. Ramasamy B, Magne F, Tripathy SK, Venugopal G, Mukherjee D, Balamurugan R. Association of gut microbiome and vitamin D deficiency in knee osteoarthritis patients: A pilot study. Nutrients (2021) 13:1272. doi: 10.3390/nu13041272
94. Chen J, Wang A, Wang Q. Dysbiosis of the gut microbiome is a risk factor for osteoarthritis in older female adults: a case control study. BMC Bioinf (2021) 22:299. doi: 10.1186/s12859-021-04199-0
95. Boer CG, Radjabzadeh D, Medina-Gomez C, Garmaeva S, Schiphof D, Arp P, et al. Intestinal microbiome composition and its relation to joint pain and inflammation. Nat Commun (2019) 10:4881. doi: 10.1038/s41467-019-12873-4
96. Gupta VK, Paul S, Dutta C. Geography, ethnicity or subsistence-specific variations in human microbiome composition and diversity. Front Microbiol (2017) 8:1162. doi: 10.3389/fmicb.2017.01162
97. Bai X, Sun Y, Li Y, Li M, Cao Z, Huang Z, et al. Landscape of the gut archaeome in association with geography, ethnicity, urbanization, and diet in the Chinese population. Microbiome (2022) 10:147. doi: 10.1186/s40168-022-01335-7
98. Lin D, Wang R, Luo J, Ren F, Gu Z, Zhao Y, et al. The core and distinction of the gut microbiota in chinese populations across geography and ethnicity. Microorganisms (2020) 8:1579. doi: 10.3390/microorganisms8101579
99. Tandon D, Haque M.M., R, S., Shaikh S., P, S., Dubey AK, Mande SS. A snapshot of gut microbiota of an adult urban population from Western region of India. PloS One (2018) 13:e0195643. doi: 10.1371/journal.pone.0195643
100. Kaur K, Khatri I, Akhtar A, Subramanian S, Ramya TNC. Metagenomics analysis reveals features unique to Indian distal gut microbiota. PloS One (2020) 15:e0231197. doi: 10.1371/journal.pone.0231197
101. Latorre-Pérez A, Hernández M, Iglesias JR, Morán J, Pascual J, Porcar M, et al. The Spanish gut microbiome reveals links between microorganisms and Mediterranean diet. Sci Rep (2021) 11:21602. doi: 10.1038/s41598-021-01002-1
102. Fontana A, Panebianco C, Picchianti-Diamanti A, Laganà B, Cavalieri D, Potenza A, et al. Gut microbiota profiles differ among individuals depending on their region of origin: an italian pilot study. Int J Environ Res Public Health (2019) 16:4065. doi: 10.3390/ijerph16214065
103. Porras AM, Shi Q, Zhou H, Callahan R, Montenegro-Bethancourt G, Solomons N, et al. Geographic differences in gut microbiota composition impact susceptibility to enteric infection. Cell Rep (2021) 36:109457. doi: 10.1016/j.celrep.2021.109457
104. Suzuki TA, Worobey M. Geographical variation of human gut microbial composition. Biol Lett (2014) 10:20131037. doi: 10.1098/rsbl.2013.1037
105. Angelakis E, Bachar D, Yasir M, Musso D, Djossou F, Melenotte C, et al. Comparison of the gut microbiota of obese individuals from different geographic origins. New Microbes New Infect (2019) 27:40–7. doi: 10.1016/j.nmni.2018.11.005
106. El Kaoutari A, Armougom F, Gordon JI, Raoult D, Henrissat B. The abundance and variety of carbohydrate-active enzymes in the human gut microbiota. Nat Rev Microbiol (2013) 11:497–504. doi: 10.1038/nrmicro3050
107. Ye L, Das P, Li P, Ji B, Nielsen J. Carbohydrate active enzymes are affected by diet transition from milk to solid food in infant gut microbiota. FEMS Microbiol Ecol (2019) 95. doi: 10.1093/femsec/fiz159
108. Soverini M, Turroni S, Biagi E, Quercia S, Brigidi P, Candela M, et al. Variation of carbohydrate-active enzyme patterns in the gut microbiota of italian healthy subjects and type 2 diabetes patients. Front Microbiol (2017) 8. doi: 10.3389/fmicb.2017.02079
109. Holscher HD. Dietary fiber and prebiotics and the gastrointestinal microbiota. Gut Microbes (2017) 8:172–84. doi: 10.1080/19490976.2017.1290756
110. Holscher HD, Caporaso JG, Hooda S, Brulc JM, Fahey GC Jr., Swanson KS. Fiber supplementation influences phylogenetic structure and functional capacity of the human intestinal microbiome: follow-up of a randomized controlled trial. Am J Clin Nutr (2015) 101:55–64. doi: 10.3945/ajcn.114.092064
111. Dai Z, Lu N, Niu J, Felson DT, Zhang Y. Dietary fiber intake in relation to knee pain trajectory. Arthritis Care Res (Hoboken) (2017) 69:1331–9. doi: 10.1002/acr.23158
112. Dai Z, Niu J, Zhang Y, Jacques P, Felson DT. Dietary intake of fibre and risk of knee osteoarthritis in two US prospective cohorts. Ann Rheum Dis (2017) 76:1411–9. doi: 10.1136/annrheumdis-2016-210810
113. Saidane O, Courties A, Sellam J. Dietary fibers in osteoarthritis: What are the evidences? Joint Bone Spine (2019) 86:411–4. doi: 10.1016/j.jbspin.2018.10.010
114. Lindsay JO, Whelan K, Stagg AJ, Gobin P, Al-Hassi HO, Rayment N, et al. Clinical, microbiological, and immunological effects of fructo-oligosaccharide in patients with Crohn's disease. Gut (2006) 55:348–55. doi: 10.1136/gut.2005.074971
115. Liao M, Zhang Y, Qiu Y, Wu Z, Zhong Z, Zeng X, et al. Fructooligosaccharide supplementation alleviated the pathological immune response and prevented the impairment of intestinal barrier in DSS-induced acute colitis mice. Food Funct (2021) 12:9844–54. doi: 10.1039/D1FO01147B
116. Mohammed S, Qadri SSY, Mir IA, Kondapalli NB, Basak S, Rajkumar H. Fructooligosaccharide ameliorates high-fat induced intrauterine inflammation and improves lipid profile in the hamster offspring. J Nutr Biochem (2022) 101:108925. doi: 10.1016/j.jnutbio.2021.108925
117. Yan X, Yan J, Xiang Q, Wang F, Dai H, Huang K, et al. Fructooligosaccharides protect against OVA-induced food allergy in mice by regulating the Th17/Treg cell balance using tryptophan metabolites. Food Funct (2021) 12:3191–205. doi: 10.1039/D0FO03371E
118. Logtenberg MJ, Akkerman R, An R, Hermes GDA, De Haan BJ, Faas MM, et al. Fermentation of chicory fructo-oligosaccharides and native inulin by infant fecal microbiota attenuates pro-inflammatory responses in immature dendritic cells in an infant-age-dependent and fructan-specific way. Mol Nutr Food Res (2020) 64:e2000068. doi: 10.1002/mnfr.202000068
119. Zhao S, Peng X, Zhou QY, Huang YY, Rao X, Tu JL, et al. Bacillus coagulans 13002 and fructo-oligosaccharides improve the immunity of mice with immunosuppression induced by cyclophosphamide through modulating intestinal-derived and fecal microbiota. Food Res Int (2021) 140:109793. doi: 10.1016/j.foodres.2020.109793
120. Schott EM, Farnsworth CW, Grier A, Lillis JA, Soniwala S, Dadourian GH, et al. Targeting the gut microbiome to treat the osteoarthritis of obesity. JCI Insight (2018) 3:e95997. doi: 10.1172/jci.insight.95997
121. Van Den Elsen LWJ, Tims S, Jones AM, Stewart A, Stahl B, Garssen J, et al. Prebiotic oligosaccharides in early life alter gut microbiome development in male mice while supporting influenza vaccination responses. Benef Microbes (2019) 10:279–91. doi: 10.3920/BM2018.0098
122. Chen Q, Ren Y, Lu J, Bartlett M, Chen L, Zhang Y, et al. A novel prebiotic blend product prevents irritable bowel syndrome in mice by improving gut microbiota and modulating immune response. Nutrients (2017) 9:1341. doi: 10.3390/nu9121341
123. Morales-Ferré C, Azagra-Boronat I, Massot-Cladera M, Tims S, Knipping K, Garssen J, et al. Effects of a postbiotic and prebiotic mixture on suckling rats' Microbiota and immunity. Nutrients (2021) 13:2975. doi: 10.3390/nu13092975
124. Nejadhosseinian M, Djalalinia S, Haerian H, Alikhani M, Mansour A, Mousavian AH, et al. The effects of antioxidants on knee osteoarthritis: A systematic review and meta-analysis. Front Nutr (2022) 9:1026450. doi: 10.3389/fnut.2022.1026450
125. Lan H, Hong W, Qian D, Peng F, Li H, Liang C, et al. Quercetin modulates the gut microbiota as well as the metabolome in a rat model of osteoarthritis. Bioengineered (2021) 12:6240–50. doi: 10.1080/21655979.2021.1969194
126. Cho KH, Na HS, Jhun J, Woo JS, Lee AR, Lee SY, et al. Lactobacillus (LA-1) and butyrate inhibit osteoarthritis by controlling autophagy and inflammatory cell death of chondrocytes. Front Immunol (2022) 13:930511. doi: 10.3389/fimmu.2022.930511
127. Lee SH, Kwon JY, Jhun J, Jung K, Park SH, Yang CW, et al. Lactobacillus acidophilus ameliorates pain and cartilage degradation in experimental osteoarthritis. Immunol Lett (2018) 203:6–14. doi: 10.1016/j.imlet.2018.07.003
128. O-Sullivan I, Natarajan Anbazhagan A, Singh G, Ma K, Green SJ, Singhal M, et al. Lactobacillus acidophilus mitigates osteoarthritis-associated pain, cartilage disintegration and gut microbiota dysbiosis in an experimental murine OA model. Biomedicines (2022) 10. doi: 10.3390/biomedicines10061298
129. Lei M, Guo C, Wang D, Zhang C, Hua L. The effect of probiotic Lactobacillus casei Shirota on knee osteoarthritis: a randomised double-blind, placebo-controlled clinical trial. Benef Microbes (2017) 8:697–703. doi: 10.3920/BM2016.0207
130. Sen S, Pathak Y. Nanotechnology in Nutraceuticals : Production to Cnsumption. Taylor & Francis, UK (2017).
131. Chen T, Zhou R, Chen Y, Fu W, Wei X, Ma G, et al. Curcumin ameliorates IL-1β-induced apoptosis by activating autophagy and inhibiting the NF-κB signaling pathway in rat primary articular chondrocytes. Cell Biol Int (2021) 45:976–88. doi: 10.1002/cbin.11541
132. Sun Y, Liu W, Zhang H, Li H, Liu J, Zhang F, et al. Curcumin prevents osteoarthritis by inhibiting the activation of inflammasome NLRP3. J Interferon Cytokine Res (2017) 37:449–55. doi: 10.1089/jir.2017.0069
133. Niazvand F, Khorsandi L, Abbaspour M, Orazizadeh M, Varaa N, Maghzi M, et al. Curcumin-loaded poly lactic-co-glycolic acid nanoparticles effects on mono-iodoacetate -induced osteoarthritis in rats. Veterinary Res Forum an Int Q J (2017) 8:155–61.
134. Hashemzadeh K, Davoudian N, Jaafari MR, Mirfeizi Z. The effect of nanocurcumin in improvement of knee osteoarthritis: A randomized clinical trial. Curr Rheumatol Rev (2020) 16:158–64. doi: 10.2174/1874471013666191223152658
135. Xu C, Zhai Z, Ying H, Lu L, Zhang J, Zeng Y. Curcumin primed ADMSCs derived small extracellular vesicle exert enhanced protective effects on osteoarthritis by inhibiting oxidative stress and chondrocyte apoptosis. J Nanobiotechnology (2022) 20:123. doi: 10.1186/s12951-022-01339-3
136. Murakami T, Nakaminami Y, Takahata Y, Hata K, Nishimura R. Activation and function of NLRP3 inflammasome in bone and joint-related diseases. Int J Mol Sci (2022) 23:5365. doi: 10.3390/ijms23105365
137. Mcallister MJ, Chemaly M, Eakin AJ, Gibson DS, Mcgilligan VE. NLRP3 as a potentially novel biomarker for the management of osteoarthritis. Osteoarthritis Cartilage (2018) 26:612–9. doi: 10.1016/j.joca.2018.02.901
138. Zhao LR, Xing RL, Wang PM, Zhang NS, Yin SJ, Li XC, et al. NLRP1 and NLRP3 inflammasomes mediate LPS/ATP−induced pyroptosis in knee osteoarthritis. Mol Med Rep (2018) 17:5463–9. doi: 10.3892/mmr.2018.8520
139. Cai B, Zhong L, Wang Q, Xu W, Li X, Chen T. Curcumin alleviates 1-methyl- 4-phenyl- 1,2,3,6-tetrahydropyridine- induced Parkinson's disease in mice via modulating gut microbiota and short-chain fatty acids. Front Pharmacol (2023) 14:1198335. doi: 10.3389/fphar.2023.1198335
140. Wang J, Ghosh SS, Ghosh S. Curcumin improves intestinal barrier function: modulation of intracellular signaling, and organization of tight junctions. Am J Physiol Cell Physiol (2017) 312:C438–c445. doi: 10.1152/ajpcell.00235.2016
141. D'antongiovanni V, Fornai M, Benvenuti L, Di Salvo C, Pellegrini C, Cappelli F, et al. Dietary supplement, containing the dry extract of curcumin, emblica and cassia, counteracts intestinal inflammation and enteric dysmotility associated with obesity. Metabolites (2023) 13:410. doi: 10.3390/metabo13030410
142. Feng D, Zou J, Su D, Mai H, Zhang S, Li P, et al. Curcumin prevents high-fat diet-induced hepatic steatosis in ApoE(-/-) mice by improving intestinal barrier function and reducing endotoxin and liver TLR4/NF-κB inflammation. Nutr Metab (Lond) (2019) 16:79. doi: 10.1186/s12986-019-0410-3
143. Shi HH, Chen LP, Wang CC, Zhao YC, Wang YM, Xue CH, et al. Docosahexaenoic acid-acylated curcumin diester alleviates cisplatin-induced acute kidney injury by regulating the effect of gut microbiota on the lipopolysaccharide- and trimethylamine-N-oxide-mediated PI3K/Akt/NF-κB signaling pathway in mice. Food Funct (2022) 13:6103–17. doi: 10.1039/D1FO04178A
144. Ruan D, Wu S, Fouad AM, Zhu Y, Huang W, Chen Z, et al. Curcumin alleviates LPS-induced intestinal homeostatic imbalance through reshaping gut microbiota structure and regulating group 3 innate lymphoid cells in chickens. Food Funct (2022) 13:11811–24. doi: 10.1039/D2FO02598A
145. Ghosh SS, He H, Wang J, Gehr TW, Ghosh S. Curcumin-mediated regulation of intestinal barrier function: The mechanism underlying its beneficial effects. Tissue Barriers (2018) 6:e1425085. doi: 10.1080/21688370.2018.1425085
146. Kaur H, He B, Zhang C, Rodriguez E, Hage DS, Moreau R. Piperine potentiates curcumin-mediated repression of mTORC1 signaling in human intestinal epithelial cells: implications for the inhibition of protein synthesis and TNFα signaling. J Nutr Biochem (2018) 57:276–86. doi: 10.1016/j.jnutbio.2018.04.010
147. Burapan S, Kim M, Han J. Curcuminoid demethylation as an alternative metabolism by human intestinal microbiota. J Agric Food Chem (2017) 65:3305–10. doi: 10.1021/acs.jafc.7b00943
148. Jin X, Jones G, Cicuttini F, Wluka A, Zhu Z, Han W, et al. Effect of vitamin D supplementation on tibial cartilage volume and knee pain among patients with symptomatic knee osteoarthritis: A randomized clinical trial. Jama (2016) 315:1005–13. doi: 10.1001/jama.2016.1961
149. Hussain SA, Marouf BH, Ali ZS, Ahmmad RS. Efficacy and safety of co-administration of resveratrol with meloxicam in patients with knee osteoarthritis: a pilot interventional study. Clin Interv Aging (2018) 13:1621–30. doi: 10.2147/CIA.S172758
150. Marouf BH, Hussain SA, Ali ZS. Correlation between serum pro inflammatory cytokines and clinical scores of knee osteoarthritic patients using resveratrol as a supplementary therapy with meloxicam. Indian J Pharmacol (2021) 53:270–7. doi: 10.4103/ijp.IJP_493_20
151. Nannoni G, Volterrani G, Mattarocci A, Minoretti P, Emanuele E. Comparative efficacy and safety of Verbascox(®) - a proprietary herbal extract capable of inhibiting human cyclooxygenase-2 - and celecoxib for knee osteoarthritis. Drug Discovery Ther (2020) 14:129–34. doi: 10.5582/ddt.2020.03034
152. Huseini HF, Mohtashami R, Sadeghzadeh E, Shadmanfar S, Hashem-Dabaghian F, Kianbakht S. Efficacy and safety of oral Nigella sativa oil for symptomatic treatment of knee osteoarthritis: A double-blind, randomized, placebo-controlled clinical trial. Complement Ther Clin Pract (2022) 49:101666. doi: 10.1016/j.ctcp.2022.101666
153. Mozaffari-Khosravi H, Naderi Z, Dehghan A, Nadjarzadeh A, Fallah Huseini H. Effect of ginger supplementation on proinflammatory cytokines in older patients with osteoarthritis: outcomes of a randomized controlled clinical trial. J Nutr Gerontol Geriatr (2016) 35:209–18. doi: 10.1080/21551197.2016.1206762
154. Hancke JL, Srivastav S, Cáceres DD, Burgos RA. A double-blind, randomized, placebo-controlled study to assess the efficacy of Andrographis paniculata standardized extract (ParActin®) on pain reduction in subjects with knee osteoarthritis. Phytother Res (2019) 33:1469–79. doi: 10.1002/ptr.6339
155. Maghsoumi-Norouzabad L, Alipoor B, Abed R, Eftekhar Sadat B, Mesgari-Abbasi M, Asghari Jafarabadi M. Effects of Arctium lappa L. (Burdock) root tea on inflammatory status and oxidative stress in patients with knee osteoarthritis. Int J Rheum Dis (2016) 19:255–61. doi: 10.1111/1756-185X.12477
156. Du C, Smith A, Avalos M, South S, Crabtree K, Wang W, et al. Blueberries improve pain, gait performance, and inflammation in individuals with symptomatic knee osteoarthritis. Nutrients (2019) 11:290. doi: 10.3390/nu11020290
157. Hashempur MH, Sadrneshin S, Mosavat SH, Ashraf A. Green tea (Camellia sinensis) for patients with knee osteoarthritis: A randomized open-label active-controlled clinical trial. Clin Nutr (2018) 37:85–90. doi: 10.1016/j.clnu.2016.12.004
158. Catanzaro R, Lorenzetti A, Solimene U, Zerbinati N, Milazzo M, Celep G, et al. Testing a novel bioactive marine nutraceutical on osteoarthritis patients. Acta BioMed (2013) 84:30–7.
159. Hunt S, Stebbings S, Mcnamara D. An open-label six-month extension study to investigate the safety and efficacy of an extract of Artemisia annua for managing pain, stiffness and functional limitation associated with osteoarthritis of the hip and knee. N Z Med J (2016) 129:97–102.
160. Stebbings S, Beattie E, Mcnamara D, Hunt S. A pilot randomized, placebo-controlled clinical trial to investigate the efficacy and safety of an extract of Artemisia annua administered over 12 weeks, for managing pain, stiffness, and functional limitation associated with osteoarthritis of the hip and knee. Clin Rheumatol (2016) 35:1829–36. doi: 10.1007/s10067-015-3110-z
161. Olsen NJ, Branch VK, Jonnala G, Seskar M, Cooper M. Phase 1, placebo-controlled, dose escalation trial of chicory root extract in patients with osteoarthritis of the hip or knee. BMC Musculoskelet Disord (2010) 11:156. doi: 10.1186/1471-2474-11-156
162. Ghoochani N, Karandish M, Mowla K, Haghighizadeh MH, Jalali MT. The effect of pomegranate juice on clinical signs, matrix metalloproteinases and antioxidant status in patients with knee osteoarthritis. J Sci Food Agric (2016) 96:4377–81. doi: 10.1002/jsfa.7647
163. Majeed M, Majeed S, Narayanan NK, Nagabhushanam K. A pilot, randomized, double-blind, placebo-controlled trial to assess the safety and efficacy of a novel Boswellia serrata extract in the management of osteoarthritis of the knee. Phytother Res (2019) 33:1457–68. doi: 10.1002/ptr.6338
164. Alazadeh M, Azadbakht M, Niksolat F, Asgarirad H, Moosazadeh M, Ahmadi A, et al. Effect of sweet fennel seed extract capsule on knee pain in women with knee osteoarthritis. Complement Ther Clin Pract (2020) 40:101219. doi: 10.1016/j.ctcp.2020.101219
165. Kasemsuk T, Saengpetch N, Sibmooh N, Unchern S. Improved WOMAC score following 16-week treatment with bromelain for knee osteoarthritis. Clin Rheumatol (2016) 35:2531–40. doi: 10.1007/s10067-016-3363-1
166. Farì G, Megna M, Scacco S, Ranieri M, Raele MV, Chiaia Noya E, et al. Hemp seed oil in association with β-caryophyllene, myrcene and ginger extract as a nutraceutical integration in knee osteoarthritis: A double-blind prospective case-control study. Medicina (Kaunas) (2023) 59:191. doi: 10.3390/medicina59020191
167. Jessberger S, Högger P, Genest F, Salter DM, Seefried L. Cellular pharmacodynamic effects of Pycnogenol® in patients with severe osteoarthritis: a randomized controlled pilot study. BMC Complement Altern Med (2017) 17:537. doi: 10.1186/s12906-017-2044-1
168. Salimzadeh A, Alipoor E, Dehghani S, Yaseri M, Hosseini M, Feinle-Bisset C, et al. The effect of 12-week garlic supplementation on symptom relief in overweight or obese women with knee osteoarthritis. Int J Clin Pract (2018) 72:e13208. doi: 10.1111/ijcp.13208
169. Dehghani S, Alipoor E, Salimzadeh A, Yaseri M, Hosseini M, Feinle-Bisset C, et al. The effect of a garlic supplement on the pro-inflammatory adipocytokines, resistin and tumor necrosis factor-alpha, and on pain severity, in overweight or obese women with knee osteoarthritis. Phytomedicine (2018) 48:70–5. doi: 10.1016/j.phymed.2018.04.060
170. Schell J, Scofield RH, Barrett JR, Kurien BT, Betts N, Lyons TJ, et al. Strawberries improve pain and inflammation in obese adults with radiographic evidence of knee osteoarthritis. Nutrients (2017) 9:949. doi: 10.3390/nu9090949
171. Basu A, Kurien BT, Tran H, Byrd B, Maher J, Schell J, et al. Strawberries decrease circulating levels of tumor necrosis factor and lipid peroxides in obese adults with knee osteoarthritis. Food Funct (2018) 9:6218–26. doi: 10.1039/C8FO01194J
172. Hsiao AF, Lien YC, Tzeng IS, Liu CT, Chou SH, Horng YS. The efficacy of high- and low-dose curcumin in knee osteoarthritis: A systematic review and meta-analysis. Complement Ther Med (2021) 63:102775. doi: 10.1016/j.ctim.2021.102775
173. Shokri-Mashhadi N, Bagherniya M, Askari G, Sathyapalan T, Sahebkar A. A systematic review of the clinical use of curcumin for the treatment of osteoarthritis. Adv Exp Med Biol (2021) 1291:265–82. doi: 10.1007/978-3-030-56153-6_16
174. Wang P, Li D, Ke W, Liang D, Hu X, Chen F. Resveratrol-induced gut microbiota reduces obesity in high-fat diet-fed mice. Int J Obes (Lond) (2020) 44:213–25. doi: 10.1038/s41366-019-0332-1
175. Wang P, Ma Y, Wang D, Zhao W, Hu X, Chen F, et al. Protective Effects of Dietary Resveratrol against Chronic Low-Grade Inflammation Mediated through the Gut Microbiota in High-Fat Diet Mice. Nutrients (2022) 14:1994. doi: 10.3390/nu14101994
176. Chen M, Hou P, Zhou M, Ren Q, Wang X, Huang L, et al. Resveratrol attenuates high-fat diet-induced non-alcoholic steatohepatitis by maintaining gut barrier integrity and inhibiting gut inflammation through regulation of the endocannabinoid system. Clin Nutr (2020) 39:1264–75. doi: 10.1016/j.clnu.2019.05.020
177. Zhao L, Zhang Q, Ma W, Tian F, Shen H, Zhou M. A combination of quercetin and resveratrol reduces obesity in high-fat diet-fed rats by modulation of gut microbiota. Food Funct (2017) 8:4644–56. doi: 10.1039/C7FO01383C
178. Li F, Han Y, Wu X, Cao X, Gao Z, Sun Y, et al. Gut microbiota-derived resveratrol metabolites, dihydroresveratrol and lunularin, significantly contribute to the biological activities of resveratrol. Front Nutr (2022) 9:912591. doi: 10.3389/fnut.2022.912591
179. Alvarenga L, Saldanha JF, Stockler-Pinto MB, Fouque D, Soulage CO, Mafra D. Effects of resveratrol on inflammation and oxidative stress induced by the uremic toxin indoxyl sulfate in Murine macrophage-like RAW 264.7. Biochimie (2023) 213:22–9. doi: 10.1016/j.biochi.2023.05.001
180. Chen K, Zhao H, Shu L, Xing H, Wang C, Lu C, et al. Effect of resveratrol on intestinal tight junction proteins and the gut microbiome in high-fat diet-fed insulin resistant mice. Int J Food Sci Nutr (2020) 71:965–78. doi: 10.1080/09637486.2020.1754351
181. Yang C, Deng Q, Xu J, Wang X, Hu C, Tang H, et al. Sinapic acid and resveratrol alleviate oxidative stress with modulation of gut microbiota in high-fat diet-fed rats. Food Res Int (2019) 116:1202–11. doi: 10.1016/j.foodres.2018.10.003
182. Caterina MJ, Schumacher MA, Tominaga M, Rosen TA, Levine JD, Julius D. The capsaicin receptor: a heat-activated ion channel in the pain pathway. Nature (1997) 389:816–24. doi: 10.1038/39807
183. Fattori V, Hohmann MS, Rossaneis AC, Pinho-Ribeiro FA, Verri WA. Capsaicin: current understanding of its mechanisms and therapy of pain and other pre-clinical and clinical uses. Molecules (2016) 21:844. doi: 10.3390/molecules21070844
184. Fernandes ES, Cerqueira AR, Soares AG, Costa SK. Capsaicin and its role in chronic diseases. Adv Exp Med Biol (2016) 929:91–125. doi: 10.1007/978-3-319-41342-6_5
185. Nisar A, Jagtap S, Vyavahare S, Deshpande M, Harsulkar A, Ranjekar P, et al. Phytochemicals in the treatment of inflammation-associated diseases: the journey from preclinical trials to clinical practice. Front Pharmacol (2023) 14:1177050. doi: 10.3389/fphar.2023.1177050
186. Basith S, Cui M, Hong S, Choi S. Harnessing the therapeutic potential of capsaicin and its analogues in pain and other diseases. Molecules (2016) 21:966. doi: 10.3390/molecules21080966
187. Liu T, Wan Y, Meng Y, Zhou Q, Li B, Chen Y, et al. Capsaicin: A novel approach to the treatment of functional dyspepsia. Mol Nutr Food Res (2023) 67:e2200793. doi: 10.1002/mnfr.202200793
188. Zheng Y, Chen J, Wu X, Zhang X, Hu C, Kang Y, et al. Enhanced anti-inflammatory effects of silibinin and capsaicin combination in lipopolysaccharide-induced RAW264.7 cells by inhibiting NF-κB and MAPK activation. Front Chem (2022) 10:934541. doi: 10.3389/fchem.2022.934541
189. Tang J, Luo K, Li Y, Chen Q, Tang D, Wang D, et al. Capsaicin attenuates LPS-induced inflammatory cytokine production by upregulation of LXRα. Int Immunopharmacol (2015) 28:264–9. doi: 10.1016/j.intimp.2015.06.007
190. Grüter T, Blusch A, Motte J, Sgodzai M, Bachir H, Klimas R, et al. Immunomodulatory and anti-oxidative effect of the direct TRPV1 receptor agonist capsaicin on Schwann cells. J Neuroinflamm (2020) 17:145. doi: 10.1186/s12974-020-01821-5
191. Shang K, Amna T, Amina M, Al-Musayeib NM, Al-Deyab SS, Hwang I. Influence of capsaicin on inflammatory cytokines induced by lipopolysaccharide in myoblast cells under in vitro environment. Pharmacogn Mag (2017) 13:S26–s32. doi: 10.4103/0973-1296.203984
192. Ghiasi Z, Esmaeli F, Aghajani M, Ghazi-Khansari M, Faramarzi MA, Amani A. Enhancing analgesic and anti-inflammatory effects of capsaicin when loaded into olive oil nanoemulsion: An in vivo study. Int J Pharm (2019) 559:341–7. doi: 10.1016/j.ijpharm.2019.01.043
193. Kang JH, Tsuyoshi G, Le Ngoc H, Kim HM, Tu TH, Noh HJ, et al. Dietary capsaicin attenuates metabolic dysregulation in genetically obese diabetic mice. J Med Food (2011) 14:310–5. doi: 10.1089/jmf.2010.1367
194. Narang N, Jiraungkoorskul W, Jamrus P. Current understanding of antiobesity property of capsaicin. Pharmacogn Rev (2017) 11:23–6. doi: 10.4103/phrev.phrev_48_16
195. Janssens PL, Hursel R, Westerterp-Plantenga MS. Capsaicin increases sensation of fullness in energy balance, and decreases desire to eat after dinner in negative energy balance. Appetite (2014) 77:44–9. doi: 10.1016/j.appet.2014.02.018
196. Lee MS, Kim CT, Kim IH, Kim Y. Effects of capsaicin on lipid catabolism in 3T3-L1 adipocytes. Phytother Res (2011) 25:935–9. doi: 10.1002/ptr.3339
197. Gong T, Zhou Y, Zhang L, Wang H, Zhang M, Liu X. Capsaicin combined with dietary fiber prevents high-fat diet associated aberrant lipid metabolism by improving the structure of intestinal flora. Food Sci Nutr (2023) 11:114–25. doi: 10.1002/fsn3.3043
198. Hui S, Liu Y, Chen M, Wang X, Lang H, Zhou M, et al. Capsaicin improves glucose tolerance and insulin sensitivity through modulation of the gut microbiota-bile acid-FXR axis in type 2 diabetic db/db mice. Mol Nutr Food Res (2019) 63:e1900608. doi: 10.1002/mnfr.201900608
199. Hui S, Huang L, Wang X, Zhu X, Zhou M, Chen M, et al. Capsaicin improves glucose homeostasis by enhancing glucagon-like peptide-1 secretion through the regulation of bile acid metabolism via the remodeling of the gut microbiota in male mice. FASEB J (2020) 34:8558–73. doi: 10.1096/fj.201902618RR
200. Song JX, Ren H, Gao YF, Lee CY, Li SF, Zhang F, et al. Dietary capsaicin improves glucose homeostasis and alters the gut microbiota in obese diabetic ob/ob mice. Front Physiol (2017) 8:602. doi: 10.3389/fphys.2017.00602
201. Shen W, Shen M, Zhao X, Zhu H, Yang Y, Lu S, et al. Anti-obesity effect of capsaicin in mice fed with high-fat diet is associated with an increase in population of the gut bacterium akkermansia muciniphila. Front Microbiol (2017) 8:272. doi: 10.3389/fmicb.2017.00272
202. Wang Y, Tang C, Tang Y, Yin H, Liu X. Capsaicin has an anti-obesity effect through alterations in gut microbiota populations and short-chain fatty acid concentrations. Food Nutr Res (2020) 64. doi: 10.29219/fnr.v64.3525
203. Mahalak KK, Bobokalonov J, Firrman J, Williams R, Evans B, Fanelli B, et al. Analysis of the ability of capsaicin to modulate the human gut microbiota in vitro. Nutrients (2022) 14:1283. doi: 10.3390/nu14061283
204. Kang C, Zhang Y, Zhu X, Liu K, Wang X, Chen M, et al. Healthy subjects differentially respond to dietary capsaicin correlating with specific gut enterotypes. J Clin Endocrinol Metab (2016) 101:4681–9. doi: 10.1210/jc.2016-2786
205. Xiang Q, Tang X, Cui S, Zhang Q, Liu X, Zhao J, et al. Capsaicin, the spicy ingredient of chili peppers: effects on gastrointestinal tract and composition of gut microbiota at various dosages. Foods (2022) 11:686. doi: 10.3390/foods11050686
206. Rosca AE, Iesanu MI, Zahiu CDM, Voiculescu SE, Paslaru AC, Zagrean AM. Capsaicin and gut microbiota in health and disease. Molecules (2020) 25:5681. doi: 10.3390/molecules25235681
207. Umarji PB, Verma P, Garg V, Schini M, Eastell R. Randomised controlled trial of nutritional supplement on bone turnover markers in Indian premenopausal women. Nutrients (2021) 13:364–79. doi: 10.3390/nu13020364
208. Lopresti AL, Smith SJ, Jackson-Michel S, Fairchild T. An investigation into the effects of a curcumin extract (Curcugen(®)) on osteoarthritis pain of the knee: A randomised, double-blind, placebo-controlled study. Nutrients (2021) 14:41. doi: 10.3390/nu14010041
209. Shep D, Khanwelkar C, Gade P, Karad S. Safety and efficacy of curcumin versus diclofenac in knee osteoarthritis: a randomized open-label parallel-arm study. Trials (2019) 20:214. doi: 10.1186/s13063-019-3327-2
210. Haroyan A, Mukuchyan V, Mkrtchyan N, Minasyan N, Gasparyan S, Sargsyan A, et al. Efficacy and safety of curcumin and its combination with boswellic acid in osteoarthritis: a comparative, randomized, double-blind, placebo-controlled study. BMC Complement Altern Med (2018) 18:7. doi: 10.1186/s12906-017-2062-z
211. Heidari-Beni M, Moravejolahkami AR, Gorgian P, Askari G, Tarrahi MJ, Bahreini-Esfahani N. Herbal formulation “turmeric extract, black pepper, and ginger” versus Naproxen for chronic knee osteoarthritis: A randomized, double-blind, controlled clinical trial. Phytother Res (2020) 34:2067–73. doi: 10.1002/ptr.6671
212. Sterzi S, Giordani L, Morrone M, Lena E, Magrone G, Scarpini C, et al. The efficacy and safety of a combination of glucosamine hydrochloride, chondroitin sulfate and bio-curcumin with exercise in the treatment of knee osteoarthritis: a randomized, double-blind, placebo-controlled study. Eur J Phys Rehabil Med (2016) 52:321–30.
213. Henrotin Y, Malaise M, Wittoek R, De Vlam K, Brasseur JP, Luyten FP, et al. Bio-optimized Curcuma longa extract is efficient on knee osteoarthritis pain: a double-blind multicenter randomized placebo controlled three-arm study. Arthritis Res Ther (2019) 21:179. doi: 10.1186/s13075-019-1960-5
214. Yuan T, Cai D, Hu B, Zhu Y, Qin J. Therapeutic effects of curcumin on osteoarthritis and its protection of chondrocytes through the wnt/B-catenin signaling pathway. Altern Ther Health Med (2022) 28:28–37.
215. Atabaki M, Shariati-Sarabi Z, Tavakkol-Afshari J, Mohammadi M. Significant immunomodulatory properties of curcumin in patients with osteoarthritis; a successful clinical trial in Iran. Int Immunopharmacol (2020) 85:106607. doi: 10.1016/j.intimp.2020.106607
216. Panahi Y, Alishiri GH, Parvin S, Sahebkar A. Mitigation of systemic oxidative stress by curcuminoids in osteoarthritis: results of a randomized controlled trial. J Diet Suppl (2016) 13:209–20. doi: 10.3109/19390211.2015.1008611
217. Thomas JV, Smina TP, Khanna A, Kunnumakkara AB, Maliakel B, Mohanan R, et al. Influence of a low-dose supplementation of curcumagalactomannoside complex (CurQfen) in knee osteoarthritis: A randomized, open-labeled, active-controlled clinical trial. Phytother Res (2021) 35:1443–55. doi: 10.1002/ptr.6907
218. Khanna A, Das SS, Smina TP, Thomas JV, Kunnumakkara AB, Maliakel B, et al. Curcumagalactomannoside/glucosamine combination improved joint health among osteoarthritic subjects as compared to chondroitin sulfate/glucosamine: double-blinded, randomized controlled study. J Altern Complement Med (2020) 26:945–55. doi: 10.1089/acm.2020.0128
219. Liu X, Robbins S, Eyles J, Fedorova T, Virk S, Deveza LA, et al. Efficacy and safety of a supplement combination on hand pain among people with symptomatic hand osteoarthritis an internet-based, randomised clinical trial the RADIANT study. Osteoarthritis Cartilage (2021) 29:667–77. doi: 10.1016/j.joca.2021.01.011
220. Clark A, Mach N. Role of vitamin D in the hygiene hypothesis: the interplay between vitamin D, vitamin D receptors, gut microbiota, and immune response. Front Immunol (2016) 7:627. doi: 10.3389/fimmu.2016.00627
221. Fakhoury HMA, Kvietys PR, Alkattan W, Anouti FA, Elahi MA, Karras SN, et al. Vitamin D and intestinal homeostasis: Barrier, microbiota, and immune modulation. J Steroid Biochem Mol Biol (2020) 200:105663. doi: 10.1016/j.jsbmb.2020.105663
222. Aggeletopoulou I, Tsounis EP, Mouzaki A, Triantos C. Exploring the role of vitamin D and the vitamin D receptor in the composition of the gut microbiota. Front Biosci (Landmark Ed) (2023) 28:116. doi: 10.31083/j.fbl2806116
223. Waterhouse M, Hope B, Krause L, Morrison M, Protani MM, Zakrzewski M, et al. Vitamin D and the gut microbiome: a systematic review of in vivo studies. Eur J Nutr (2019) 58:2895–910. doi: 10.1007/s00394-018-1842-7
224. Liao X, Lan Y, Wang W, Zhang J, Shao R, Yin Z, et al. Vitamin D influences gut microbiota and acetate production in zebrafish (Danio rerio) to promote intestinal immunity against invading pathogens. Gut Microbes (2023) 15:2187575. doi: 10.1080/19490976.2023.2187575
225. Bellerba F, Muzio V, Gnagnarella P, Facciotti F, Chiocca S, Bossi P, et al. The association between vitamin D and gut microbiota: A systematic review of human studies. Nutrients (2021) 13:3378. doi: 10.3390/nu13103378
226. Tangestani H, Boroujeni HK, Djafarian K, Emamat H, Shab-Bidar S. Vitamin D and the gut microbiota: a narrative literature review. Clin Nutr Res (2021) 10:181–91. doi: 10.7762/cnr.2021.10.3.181
227. Liu J, Liu J, Zhang J, Liu C, Qu C, Na L. Vitamin D deficiency in early life regulates gut microbiome composition and leads to impaired glucose tolerance in adult and offspring rats. Food Funct (2023) 14:5768–86. doi: 10.1039/D3FO00503H
228. Bosman ES, Albert AY, Lui H, Dutz JP, Vallance BA. Skin exposure to narrow band ultraviolet (UVB) light modulates the human intestinal microbiome. Front Microbiol (2019) 10:2410. doi: 10.3389/fmicb.2019.02410
229. Conteville LC, Oliveira-Ferreira J, Vicente ACP. Gut microbiome biomarkers and functional diversity within an amazonian semi-nomadic hunter-gatherer group. Front Microbiol (2019) 10:1743. doi: 10.3389/fmicb.2019.01743
230. Conteville LC, Vicente ACP. Skin exposure to sunlight: a factor modulating the human gut microbiome composition. Gut Microbes (2020) 11:1135–8. doi: 10.1080/19490976.2020.1745044
231. Laupheimer M. Vitamin D and omega-3 fatty acids in musculoskeletal medicine. Int Musculoskeletal Med (2014) 36. doi: 10.1179/1753614614Z.00000000063
232. Tessier AJ, Chevalier S. An update on protein, leucine, omega-3 fatty acids, and vitamin D in the prevention and treatment of sarcopenia and functional decline. Nutrients (2018) 10:1099. doi: 10.3390/nu10081099
233. Horrobin D. The role of dietary fat in bone and joint health. Prostaglandins Leukotrienes Essential Fatty Acids (2003) 68:359. doi: 10.1016/S0952-3278(03)00059-0
234. Bannenberg GL, Chiang N, Ariel A, Arita M, Tjonahen E, Gotlinger KH, et al. Molecular circuits of resolution: formation and actions of resolvins and protectins. J Immunol (2005) 174:4345–55. doi: 10.4049/jimmunol.174.7.4345
235. Norling LV, Perretti M. The role of omega-3 derived resolvins in arthritis. Curr Opin Pharmacol (2013) 13:476–81. doi: 10.1016/j.coph.2013.02.003
236. El Kholy K, Freire M, Chen T, Van Dyke TE. Resolvin E1 promotes bone preservation under inflammatory conditions. Front Immunol (2018) 9:1300. doi: 10.3389/fimmu.2018.01300
237. Yoshida K, Oida H, Kobayashi T, Maruyama T, Tanaka M, Katayama T, et al. Stimulation of bone formation and prevention of bone loss by prostaglandin E EP4 receptor activation. Proc Natl Acad Sci U.S.A. (2002) 99:4580–5. doi: 10.1073/pnas.062053399
238. Griel AE, Kris-Etherton PM, Hilpert KF, Zhao G, West SG, Corwin RL. An increase in dietary n-3 fatty acids decreases a marker of bone resorption in humans. Nutr J (2007) 6:2. doi: 10.1186/1475-2891-6-2
239. Bonnet N, Ferrari SL. Effects of long-term supplementation with omega-3 fatty acids on longitudinal changes in bone mass and microstructure in mice. J Nutr Biochem (2011) 22:665–72. doi: 10.1016/j.jnutbio.2010.05.006
240. Knott L, Avery NC, Hollander AP, Tarlton JF. Regulation of osteoarthritis by omega-3 (n-3) polyunsaturated fatty acids in a naturally occurring model of disease. Osteoarthritis Cartilage (2011) 19:1150–7. doi: 10.1016/j.joca.2011.06.005
241. Lukas R, Gigliotti JC, Smith BJ, Altman S, Tou JC. Consumption of different sources of omega-3 polyunsaturated fatty acids by growing female rats affects long bone mass and microarchitecture. Bone (2011) 49:455–62. doi: 10.1016/j.bone.2011.05.029
242. Weiler HA, Zhao J, Park L, Kohut JR, Burr LL, Fitzpatrick-Wong SC. Maternal supplementation with dietary arachidonic and docosahexaenoic acids during lactation elevates bone mass in weanling rat and Guinea pig offspring even if born small sized. Prostaglandins Leukotrienes Essential Fatty Acids (2012) 86:61–70. doi: 10.1016/j.plefa.2011.10.004
243. Huang M-J, Wang L, Jin D-D, Zhang Z-M, Chen T-Y, Jia C-H, et al. Enhancement of the synthesis of n-3 PUFAs in fat-1 transgenic mice inhibits mTORC1 signalling and delays surgically induced osteoarthritis in comparison with wild-type mice. Ann Rheumatic Dis (2014) 73:1719. doi: 10.1136/annrheumdis-2013-203231
244. Casado-Diaz A, Santiago-Mora R, Dorado G, Quesada-Gomez JM. The omega-6 arachidonic fatty acid, but not the omega-3 fatty acids, inhibits osteoblastogenesis and induces adipogenesis of human mesenchymal stem cells: potential implication in osteoporosis. Osteoporos Int (2013) 24:1647–61. doi: 10.1007/s00198-012-2138-z
245. Srinivas V, Molangiri A, Varma S, Mallepogu A, Kona SR, Ibrahim A, et al. Maternal omega-3 fatty acid deficiency affects fetal thermogenic development and postnatal musculoskeletal growth in mice. J Nutr Biochem (2023) 112:109218. doi: 10.1016/j.jnutbio.2022.109218
246. Hogstrom M, Nordstrom P, Nordstrom A. n-3 Fatty acids are positively associated with peak bone mineral density and bone accrual in healthy men: the NO2 Study. Am J Clin Nutr (2007) 85:803–7. doi: 10.1093/ajcn/85.3.803
247. Fallon EM, Nazarian A, Nehra D, Pan AH, O'loughlin AA, Nose V, et al. The effect of docosahexaenoic acid on bone microstructure in young mice and bone fracture in neonates. J Surg Res (2014) 191:148–55. doi: 10.1016/j.jss.2014.04.005
248. Sun L, Tamaki H, Ishimaru T, Teruya T, Ohta Y, Katsuyama N, et al. Inhibition of osteoporosis due to restricted food intake by the fish oils DHA and EPA and perilla oil in the rat. Biosci Biotechnol Biochem (2004) 68:2613–5. doi: 10.1271/bbb.68.2613
249. Watkins BA, Li Y, Seifert MF. Dietary ratio of n-6/n-3 PUFAs and docosahexaenoic acid: actions on bone mineral and serum biomarkers in ovariectomized rats. J Nutr Biochem (2006) 17:282–9. doi: 10.1016/j.jnutbio.2005.05.012
250. Maraldi T, Prata C, Caliceti C, Vieceli Dalla Sega F, Zambonin L, Fiorentini D, et al. VEGF-induced ROS generation from NAD(P)H oxidases protects human leukemic cells from apoptosis. Int J Oncol (2010) 36:1581–9. doi: 10.3892/ijo_00000645
251. Farina EK, Kiel DP, Roubenoff R, Schaefer EJ, Cupples LA, Tucker KL. Dietary intakes of arachidonic acid and alpha-linolenic acid are associated with reduced risk of hip fracture in older adults. J Nutr (2011) 141:1146–53. doi: 10.3945/jn.110.133728
252. Baker KR, Matthan NR, Lichtenstein AH, Niu J, Guermazi A, Roemer F, et al. Association of plasma n-6 and n-3 polyunsaturated fatty acids with synovitis in the knee: the MOST study. Osteoarthritis Cartilage (2012) 20:382–7. doi: 10.1016/j.joca.2012.01.021
253. Weiss LA, Barrett-Connor E, Von Mühlen D. Ratio of n–6 to n–3 fatty acids and bone mineral density in older adults: the Rancho Bernardo Study. Am J Clin Nutr (2005) 81:934–8. doi: 10.1093/ajcn/81.4.934
254. Järvinen R, Tuppurainen M, Erkkilä AT, Penttinen P, Kärkkäinen M, Salovaara K, et al. Associations of dietary polyunsaturated fatty acids with bone mineral density in elderly women. Eur J Clin Nutr (2011) 66:496–503. doi: 10.1038/ejcn.2011.188
255. Maggio M, Artoni A, Lauretani F, Ruggiero C, Cederholm T, Cherubini A, et al. The effect of polyunsaturated fatty acids on bone health. Rev Clin Gerontology (2011) 21:219–32. doi: 10.1017/S0959259810000456
256. Oppedisano F-O, Bulotta RM, Maiuolo J, Gliozzi M, Musolino V, Carresi C, et al. The role of nutraceuticals in osteoarthritis prevention and treatment. Focus n-3 PUFAs (2021) 2021. doi: 10.1155/2021/4878562
257. Thomas S, Browne H, Mobasheri A, Rayman MP. What is the evidence for a role for diet and nutrition in osteoarthritis? Rheumatology (2018) 57:iv61–74. doi: 10.1093/rheumatology/key011
258. Basak S, Vilasagaram S, Duttaroy AK. Maternal dietary deficiency of n-3 fatty acids affects metabolic and epigenetic phenotypes of the developing fetus. Prostaglandins Leukot Essent Fatty Acids (2020) 158:102109–20. doi: 10.1016/j.plefa.2020.102109
259. Kaliannan K, Wang B, Li XY, Kim KJ, Kang JX. A host-microbiome interaction mediates the opposing effects of omega-6 and omega-3 fatty acids on metabolic endotoxemia. Sci Rep (2015) 5:11276. doi: 10.1038/srep11276
260. Saltzman ET, Thomsen M, Hall S, Vitetta L. Perna canaliculus and the intestinal microbiome. Mar Drugs (2017) 15:207. doi: 10.3390/md15070207
Keywords: bioactive, bone remodeling, gut microbiota, inflammation, obesity, osteoarthritis, metabolism
Citation: Basak S, Hridayanka KSN and Duttaroy AK (2024) Bioactives and their roles in bone metabolism of osteoarthritis: evidence and mechanisms on gut-bone axis. Front. Immunol. 14:1323233. doi: 10.3389/fimmu.2023.1323233
Received: 17 October 2023; Accepted: 11 December 2023;
Published: 03 January 2024.
Edited by:
Wuquan Deng, Chongqing Emergency Medical Center, ChinaReviewed by:
Pei Shang, Mayo Clinic, United StatesNaheed Aryaeian, Iran University of Medical Sciences, Iran
Copyright © 2024 Basak, Hridayanka and Duttaroy. This is an open-access article distributed under the terms of the Creative Commons Attribution License (CC BY). The use, distribution or reproduction in other forums is permitted, provided the original author(s) and the copyright owner(s) are credited and that the original publication in this journal is cited, in accordance with accepted academic practice. No use, distribution or reproduction is permitted which does not comply with these terms.
*Correspondence: Asim K. Duttaroy, YS5rLmR1dHRhcm95QG1lZGlzaW4udWlvLm5v