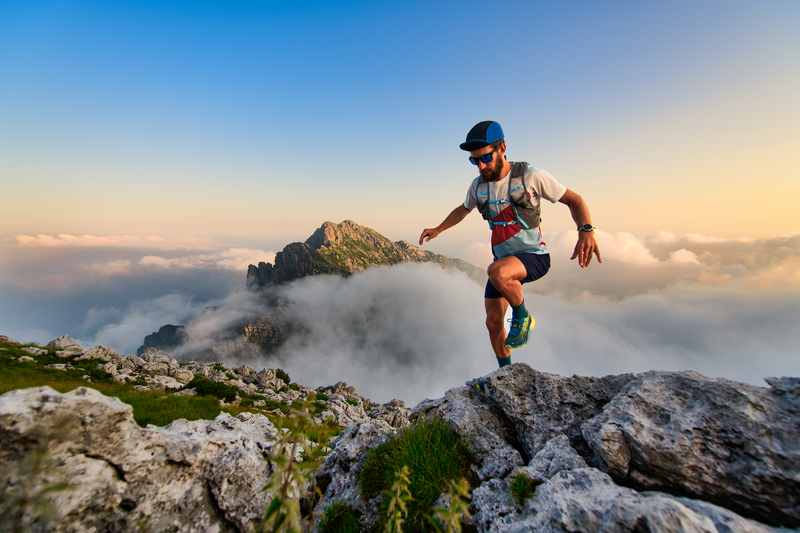
94% of researchers rate our articles as excellent or good
Learn more about the work of our research integrity team to safeguard the quality of each article we publish.
Find out more
REVIEW article
Front. Immunol. , 04 January 2024
Sec. Cytokines and Soluble Mediators in Immunity
Volume 14 - 2023 | https://doi.org/10.3389/fimmu.2023.1321051
Dendritic cells (DCs) are antigen presenting cells that link innate and adaptive immunity. DCs have been historically considered as the most effective and potent cell population to capture, process and present antigens to activate naïve T cells and originate favorable immune responses in many diseases, such as cancer. However, in the last decades, it has been observed that DCs not only promote beneficial responses, but also drive the initiation and progression of some pathologies, including inflammatory bowel disease (IBD). In line with those notions, different therapeutic approaches have been tested to enhance or impair the concentration and role of the different DC subsets. The blockade of inhibitory pathways to promote DCs or DC-based vaccines have been successfully assessed in cancer, whereas the targeting of DCs to inhibit their functionality has proved to be favorable in IBD. In this review, we (a) described the general role of DCs, (b) explained the DC subsets and their role in immunogenicity, (c) analyzed the role of DCs in cancer and therapeutic approaches to promote immunogenic DCs and (d) analyzed the role of DCs in IBD and therapeutic approaches to reduced DC-induced inflammation. Therefore, we aimed to highlight the “yin-yang” role of DCs to improve the understand of this type of cells in disease progression.
Dendritic cells (DCs), the bridge between innate and adaptive immune responses, are considered as the most potent antigen presenting cells (APCs) since they control both T cell immunity and tolerance (1). DCs represent a heterogeneous cell population which is differentiated from CD34+ hematopoietic precursors into other developed DC precursors. DCs comprise subsets in both lymphoid and nonlymphoid tissues, such as monocyte-derived DCs (moDCs) or inflammatory DC (infDC), plasmacytoid DCs (pDCs), and conventional DCs (cDC) 1 and cDC2s (also known as myeloid or classical DCs) (2). DCs mainly induce immune responses by capturing, processing and presenting unknown or self-antigens to adaptive immune cells. External antigens derive from diseases such as viral infections (3),or cancer (4), and self-antigens take part in autoimmune diseases (5), which comprise a set of disorders including (but not limited to) allergies, brain diseases, or inflammatory bowel disease (IBD) (6–8). However, in the last decades, it has been described that DCs have an interesting opposite behavior depending on their environment. That means, DCs have found to be widely downregulated in many diseases such as cancer where are called tolerogenic DCs (9), playing an important role in inducing peripheral tolerance via specific mechanisms, as activation of Treg cells (10), suppression of effector T cells, and negative modulation of Th1/Th2 immune responses. For this, DC has been successfully targeted by using inhibitory drugs or DC-based vaccines as immunotherapies (11, 12), whereas DC activation can play a contributing role in the pathogenesis of other disorders, including IBD (13) (see Figure 1). Of note, DCs have also demonstrated both beneficial and detrimental functions within the same disease (14).
Figure 1 Dual role of dendritic cells in disease progression. Dendritic cells are the most effective antigen-presenting cells to initiate immune responses, which lead to the activation of the cytotoxic machinery driven by T cells. This event is crucial to recognize tumor antigens and kill cancer cells. However, in the environment produced by gut inflammation, dendritic cells have an opposite behavior and promote a cascade of proinflammatory signals that ultimately induce inflammatory bowel disease.
The reasons why DCs have a ‘yin and yang’, dual role are not still completely elucidated and may have important implications in terms of therapeutic approaches. In this regard, it is known that the transcription factor β-catenin induces different characteristics over human moDCs in a dose-dependent manner, since its inhibition leads to a pro-inflammatory state by increasing IL-12p70 and reducing IL-10, whereas its activation enhances the nuclear β-catenin, which is associated with low levels of IL-12p70, higher IL-10 and the expression of inhibitory cell markers on DCs (15). C-type lectin receptors (CLRs) can also drive different behaviors in DCs. In fact, different nanovaccines have been designed to act on the CLRs from DCs and induce a regulatory response in allergic patients (16). Specifically, DC-specific ICAM-3–grabbing nonintegrin (DC-SIGN, also known as CD209) is a CLR that binds the nanovaccines changing the DC phenotype and improving Treg proliferation (17).
In turn, DC-SIGN also promotes the serine/threonine protein kinase RAF1 that may participate in the activation and proliferation of T cells since the inhibition of RAF kinases could impair DC activation in both human and mice, thus compromising T cell-mediated immune responses (18). Then, we aimed to review the dual behavior of DCs in two different microenvironments, cancer and IBD, putting the focus on their dysregulation and their pro-inflammatory function, respectively.
Immunogenicity is the ability of proteinic substances (e.g., foreign antigens) to promote immune responses. Currently, it is known that DCs activate their immunogenic machinery ex profeso to sample antigens by phagocytosis, receptor-mediated endocytosis, or micropinocytosis, and processing them for presentation to CD4+ T cells on major histocompatibility complex class II (MHC-II) molecules and CD8+ T cells on MHC-I (19). However, the discovery of the immunogenic capacity of DCs has been shown to be suitable for cancer therapy (20) and for other pathologies (21). In the last years, these immunogenic DCs have been used in personalized treatments in patients with HIV receiving antiretroviral treatment (22) or in ovarian cancer patients (23).
Another relevant aspect regarding immunogenicity is DC maturation, since mature DCs use different molecular mechanisms to tailor immune responses depending on the stimulus (24). In this sense, mature DCs require a set of receptors to have immunogenic properties including (but not limited to) CD31, CD40, CD80, CD83 and CD86 (25).
In addition, DCs have a tolerogenic ability in different immunological diseases (1). In this sense, DCs take part in the central and peripheral tolerance by controlling effector and regulatory mechanisms, especially self-reactivity associated to autoimmunity (26). However, another tolerogenic effect of DCs is their capacity to differentiate T cells into their regulatory phenotype (Tregs) (27). Those functions are carried out by immature or semi-mature DCs characterized by the expression of surface markers such as PD-L1 or CTLA-4 and the downregulation of MHC molecules, CD40, CD80 or CD86 (28, 29). Also, it has been found that the production of anti-inflammatory cytokines (e.g., IL-10 and TGF-β) by DCs induces tolerogenic effects in this cell population (30).
Different DC subsets have been found depending on their location in different tissues and lymphoid organs, including lymph nodes, spleen, thymus, gut, blood, or skin (31). In this regard, DC plasticity makes their categorization difficult, but a simplified classification based on the ontogeny divides this cell population into cDCs, moDCs or infDCs, pDCs, and Langerhans cells (LCs) (19), which could have immunogenic or tolerogenic effects.
In steady state, cDCs are in both non-lymphoid tissues and the spleen marginal zone and have a high capacity to migrate to T-lymphocyte zones (TLZs) of lymph nodes also during inflammation (32). cDC1s can be found within the lymph node paracortex and uptake cell-associated antigens (also dead cells) via receptors, such as DEC205 (also known as CD205) or T cell immunoglobulin and mucin domain-containing protein 3 (TIM3), preferentially by cross-presentation on MHC-I to CD8+ T cells, an essential pathway for both antiviral and antitumor immunity (33). cDC1s are also characterized by high expressions of CD103 and toll-like receptor (TLR) 3 (34), contribute to intracellular protection of T helper (Th) 1 cells by producing interleukin (IL)-12 (35), and promote Th17 responses in against influenza virus infection (36). cDC2s uptake antigens in the skin and migrate to TLZs by different pathways, including C-X-C Motif Chemokine Receptor 4 (CXCR4)- or CC-chemokine receptor 7 (CCR7)-dependent manners. In addition, cDC2s also uptake and cross-present tumor-associated antigens (TAAs) under certain conditions (33), and also express interferon regulatory factor 4 (IRF4), which makes cDC2s particularly efficient for antigen processing and presentation on MHC-II, thus inducing superior CD4+ T cell proliferation compared with cDC1s (33, 37) and supporting Th2 and Th17 polarization (38). Interestingly, the colony stimulating factor-1 (CSF-1), which is found in the airway and alveolar microenvironment, upregulates the expression of CCR7 on cDC2 (but not cDC1) in a IRF4-dependent manner in response to allergen stimuli, promoting Th2 responses (39). In turn, the deletion of IRF4 has been found both to promote and inhibit Th17 responses (40).
Similar to IRF4 in cDCs, moDCs have demonstrated dual roles by activating anti-tumoral CD8+ T cells (41) and suppressing respiratory CD8+ T cell memory in viral immunity (42). Moreover, moDCs or infDCs and cDC2s express CD11b during inflammation, making these cell populations phenotypically difficult to distinguish (19). Human pDCs are based on the coexpression of CD123 and CD303, whereas mouse pDCs are B220+ and CD11c+. pDCs have specific functions because they can recognize RNA and DNA viruses through TLR-7 and -9, leading to cell activation, and release high amounts of type I interferon (IFN-I) (43). In addition, pDCs not only have demonstrated to play a key role in the development of acute colitis and development of IBD, showing differences in the distribution, phenotype and function in patients with Crohn’s disease (CD) and ulcerative colitis (UC) (13), but also take part in viral infections together with cDC1s (44) by inducing IFN-I and recruiting natural killer (NK) lymphocytes (45). Additionally, LCs are in the epidermis but share common ontogeny with macrophages. LCs are needed for specific adaptive immune responses when antigens are highly found in the epidermis (46), and selectively promote the expansion and activation of skin-resident regulatory T cells (Tregs) to maintain the skin immune homeostasis (46, 47).
Cancer is one of the most common causes of death worldwide (48). Although the cancer death rate is continually falling every year, it was expected to have almost 2,000,000 new cancer cases and more than 600,000 cancer deaths in United States during the last year (49). As explained above, DCs acts as professional APCs to initiate immune responses. In cancer, the immunogenic capacity of DCs is called “cancer-immunity cycle”, a multistep and metabolic mechanism that explains how DCs capture, process and present TAAs to naïve T cells, which are consequently activated and infiltrated within the tumor microenvironment (TME) to kill cancer cells by specifically recognizing similar antigens, leading to the release of new TAAs and making the process starts over (50). However, inhibitory factors affect anti-tumor DC activity, including (but not limited to) tumor growth factor (TGF)-β (51), IL-10 (52), cytotoxic T-lymphocyte antigen (CTLA)-4 (53) and programmed cell death protein (PD)-1 expressions (51, 53), thus leading to cancer progression.
Of all existing types of DCs, pDCs and moDCs seem to have contradictory roles in cancer immunity. Evidence has demonstrated that pDCs infiltrate different types of tumors and are associated with poor outcomes (54), due to the expression of inhibitory markers including lymphocyte-activation gene (LAG)-3, PD-1, and CTLA-4 (55–57), the release of immunosuppressive cytokines (e.g., IL-10, TGF-β, and prostaglandin E2) (58) as well as the expansion and Treg accumulation (59). However, it has been suggested that pDCs may have a lytic ability against tumor cells (60, 61). MoDCs are phenotypically similar to antitumoral cDCs (62), and have demonstrated to mediate beneficial immune responses (63, 64), but are also involved in the maintenance of Th17 responses, which could induce a pro-tumoral state (65), and are related to monocytic myeloid-derived suppressor cells (MDSCs) (66), which have been correlated with tumor progression and poor outcomes in many oncological settings (67–69).
By contrast, cDCs have demonstrated a preferential capacity to promote antigen presentation to T cells in cancer (70). Specifically, cDC1s, which are characterized by the expression of integrin aE (also known as CD103) in mice or BDCA3 (CD141) in humans (31), have a superior ability to transport TAAs to the draining lymph node and cross-present antigens on MHC-I to activate cytotoxic T cells (71). The key role of cDC1s in cancer have been extensively supported by many studies in both humans and murine models. For example, the presence of cDC1s has been correlated with good outcomes in melanoma patients using anti-PD-1 (72). Mice lacking CD103+ cDC1s have driven an impaired CD40L-overexpressing chimeric antigen receptor (CAR) T cell antitumor response (73). Also, Batf3 DCs have shown to be necessary for effective antitumor responses driven by monoclonal antibodies and adoptive T cell therapy (74). On the contrary, cDC2s have a reduced capacity to cross-present antigens to CD8 T cells and are more efficient by priming CD4 T cells to induce antitumor immunity (37). Migratory CD301b+ cDC2s have demonstrated to be essential for an effective CD4 T cell priming (75). However, despite these well-established notions, it has been recently known that the deletion of MHC-II and CD40 in cDC1 also prevented early CD4 T cell priming and impaired tumor rejection in fibrosarcoma-bearing mice, thus suggesting that cDC1s are also required for CD4 T cell priming against TAAs (76).
Immunotherapies have demonstrated to improve outcomes in many different types of cancers (77, 78). Specifically, the infiltration of DCs into tumors has been positively correlated with prognosis and survival (79, 80), which has made the design of different therapeutic approaches possible to increase both concentration and functionality of DCs.
Inhibitory pathways and signals have been targeted since they maintain low concentrations of DCs within the TME and lead to tumor progression. Those mechanisms can be inhibited due to the immunosuppressive conditions found in the TME, MDSCs have the ability to decrease antitumor immunity (81). However, the PD-1/PD-L1 immune checkpoint also impairs the activation, proliferation, and cytotoxic function of T cells (82), which has been successfully reverted by using anti-PD-1 therapies, especially when combined with other treatments (83, 84). In addition, it has been observed that DCs could be necessary to boost anti-PD-1 efficacy due to the production of IFN-γ and IL-12 by this cell population (85). Another inhibitory signal, vascular endothelial growth factor (VEGF), has potent antiangiogenic properties and blocks DC maturation and proliferation (86, 87). Therefore, the inhibition of VEGF with targeted anti-VEGF therapies not only prevents angiogenesis, but also improves the capacity of DCs to carry out effective anti-tumor responses (88). IL-6 is another cytokine that promotes cancer progression by up-regulating different pathways that involve apoptosis, angiogenesis, invasiveness, metastasis, or tumor cell metabolism, among others. In fact, it has been reported that IL-6 inhibits anticancer immune responses generated by cytotoxic chemotherapy (89), and promotes breast cancer metastasis suppressing the anti-tumor immune response via IL-6/JAK/STAT3 signaling (90). In line with this notion, IL-6/JAK/STAT3 signaling has demonstrated to be a promising therapeutic target for hepatocellular carcinoma (91). On the contrary, although it has been shown that IL-10 levels are altered in cancer as well as IL-4 and IL-35 (92), there is evidence that IL-10 has dual functions in cancer (93). In this line, IL-10 suppression enhances T cell antitumor immunity and responses to checkpoint blockade in chronic lymphocytic leukemia (94).
Other alternatives to improve anti-tumor immunity are DC vaccines, that have been clinically evaluated and considered as safe therapies due to limited toxicities either alone or combined with other treatments (95–98). DCs are considered as the most effective APCs and promote immunological T cell response (33). Altogether, those characteristics made DCs as the most appropriate cell population for the development of cancer vaccines. Specifically, cDC1 vaccines have shown better anti-tumor efficacy compared with MoDC vaccines (99, 100), whereas another study reviewed that not only cDC but also pDC vaccines may be considered as more potent alternatives compared with MoDC vaccines (101). Another promising immunotherapeutic approach is the so-called in vivo vaccination, which consist of targeting DCs with DC receptor ligands, adjuvants, or other types of molecules that can accurately bind to DCs to exert better effects on anti-tumor T cell responses (102). In vivo vaccines target DC receptors such as TLRs (103), or adenosine receptors (104), and has concluded with promising results (105). Moreover, it has been demonstrated that DCs-pulsed with sulforaphane, a natural compound presents in cruciferous as broccoli induce T-cell activation through the modulation of regulatory molecules, JAK/STAT3- and microRNA-signaling in healthy conditions and in context of pancreatic cancer-derived antigens, proposing the possibility to use the sulforaphane in the co-treatment of cancer (106).
IBD is a disorder with high incidence (around 3.5 million people in the last decade only considering North America and Europe) and prevalence (currently exceeding 0.3%) (107). The etiology of IBD remains unclear, although it is known that involves the interaction between immune responses with genetic, environmental, and microbial factors, geographical location, or an inappropriate diet (108, 109). IBD is characterized by an altered epithelial barrier function due to exacerbated and continuing immune reactions toward the microbiota, including an improved chronic relapsing, and the inhibition of both adequate containments of luminal microorganisms and the ability to absorb nutrients (110). Specifically, UC involves aspect of the colon starting with mucosal inflammation in the rectum. Its main symptoms are bloody diarrhea, abdominal pain, fecal urgency, and tenesmus (111), whereas CD involves the whole gastrointestinal tract (although distal ileum is the most frequently affected part). CD presents periods of flares and remissions and causes transmural pleomorphic inflammation, leading to fistulas, abscesses, and granulomas (112).
Traditionally, it has been believed that gut inflammation has been only promoted by T helper cells (Th) 1, Th2, Th17 and Tregs, but now we known that inflammation is also induced by other immune cells, cytokines and processes, including macrophages, DCs, tumor necrosis factor (TNF), inflammasome activation, or autophagy (113–117). Particularly, autophagy deficiency decreases antigen sampling, increases DC maturation, and promotes pro-inflammatory DCs (118). Atg16l1 autophagy gene deficiency promotes the bacterial translocation of DSS-induced colitis in vivo and regulates autophagy and phagocytosis, which lead to an exacerbation of the intestinal inflammation (119). The immune microenvironment of UC inflamed colon is composed not only by follicular Th cells and IL17A+ Tregs, but also by memory cells (CD4+ T, IL17A+CD161+ T, and B cells), HLA-DR+CD56+ granulocytes, M1 macrophages, activated mast cells, neutrophils, and both resting and activated DCs (120, 121). In CD patients, peripheral blood mononuclear cells have high expression of IL-1B in the Treg, DC and monocyte fractions (121), whereas the inflamed mucosa of those patients is characterized by IL-1B in HLA-DR+CD38+ T cells, TNF+IFN-γ+ naïve B cells, and pDCs (121).
DCs promote IBD by expressing different markers in both human and mice (Figure 2). cDC subsets have been observed in gut mucosa from both human and mice in steady state (122), although the interaction with T cells to initiate immune responses seems conflicting (123), which could imply that some cell populations and cytokines play a dual role in the pathogenesis of IBD. In line with this notion, TGF-β not only has shown to play an unfavorable function by increasing collagen production and regulating fibrosis in CD patients with stricture (124) but also has been suggested to be necessary to inhibit inflammation in IBD (125). The role of TNF-α is essential in the immunological response of IBD (126). In fact, TNF-α regulates IL-22BP expression by colonic DCs and dampened IL-22-driven restitution of colonic epithelial functions in model of experimental colitis (127). Of note, DCs stimulated in vitro with TNF-α could not improve the activation and maturation of DCs compared with E. coli-stimulated DCs, which may suggest altered interactions between DCs and intestinal microflora in patients with UC and CD (116).
Figure 2 Types of dendritic cells with their most common pro-inflammatory surface markers in mice and humans with inflammatory bowel disease.
Intestinal SIRPα−CD103+ cDC1 constitute a small cell population in the human intestine and are molecularly similar to cross-presenting CD141+CLEC9A+CADM1+ cDC1, whereas SIRPα+CD103+ cDC2 are the main population in the small intestine in both mice and humans, and SIRPα+CD103− cDC2 predominate in the colon. CD103-expressing DCs have also been found in CD patients (128). CD103+ DC subsets with high expression of PD-L1/2 could be induced by the deficiency of the Smad7 protein, a negative regulator of TGF-β signaling (129). Also, CD103+ DCs have a colitogenic role and show impaired ability to produce Tregs (130) but generate Th1/Th2/Th17 responses through the induction of a huge variety of cytokines, including IFN-γ, IL-13, IL-6, IL-23, IL-12p35, IL-12p40, and TNF (131–133). The CD83 expression in DCs have also been found in IBD patients (134). Moreover, high expression of Receptor-interacting serine/threonine kinase 2 (RIPK2) signaling in DCs is a new therapeutic target in IBD (135, 136). In turn, the upregulation of other molecules, such as CXCL10 and CCL3 were positively correlated with activated DCs in CD and UC (128, 137), postulating them as effective diagnostic biomarkers in IBD. These molecules were found in UC patients compared with their CD counterparts following the exposure to cigarette smoke extract (CSE) in vitro, thus demonstrating that CSE modulates DC phenotypes and alters DC function, which results in Th1 polarization and increased levels of Foxp3+CD4+ T cells in UC (138). Of note, mutations (e.g., in NOD2fs gene) also promote a dysfunctional phenotype in DCs and increase CD susceptibility (139).
CD14+ cDCs have been found in the inflamed mucosa of IBD patients but are limited in mesenteric lymph nodes (MLNs), which have been less studied in relation to the DC phenotypes (122). By contrast, during the early stage of murine IBD, high levels of both mDCs and pDCs expressing CD11b and B220 have been found in colon (approximately, 50% of each subset) and MLNs (mainly mDCs) (140), but high levels of pDCs in MLNs has also been reported in other cases (141). Conversely, CD14-HLA-DRint pDCs have shown to be the most abundant subset in MLNs either expressing CD11c+ or not (122), especially in CD (141). High expression of CD11c have also been observed in CD103+ DCs (13) and CD123–HLA-DR+ DCs, which produce more IL-23 in CD patients than in their UC counterparts (142), demonstrating the importance of CD11c in the pathogenesis of IBD. In this sense, the chromofungin (CHR), a chromogranin-A derived peptide, CHR has demonstrated protective properties against intestinal inflammation by regulating CD11c of DCs (143). More CD14- DC subsets have been found in IBD by using transcriptomic analysis and high-dimensional phenotypic mapping, such as CD14−CD64dimCD11b+CD36+CD11c+ and CD14−CD64−CD163− DCs (141).
In addition, both CD103+ and CD11c- DCs have been associated with different levels of TLR expression (144). UC-derived DCs secrete pro-inflammatory cytokines and chemokines via activation of TLRs. TLRs induce infiltration of polymorphic neutrophils and activation of other innate immune cells, as well as the differentiation of naïve T cells to Th1 cells and the activation of DC to release IL-12 (140). In connection with this, regulatory or tolerogenic DCs increase the levels of colon-infiltrated Tregs and inhibited Th1 and Th17 cell-driven colon inflammation in a Galectin-3:TLR-4:Kynurenine-dependent manner, which demonstrated the importance of both TLR and galectin 3 in the immunosuppressive functions of Tregs (145). Similarly, another protein of the galectin family, galectin 1, has demonstrated to limit the immunogenic activity of DCs in a murine IBD, such as CD and UC (146).
DCs are emerging as central players since its levels tend to increase in the colonic mucosa and could have important functions in regulating response to gut microflora (13). There is a wide variety of cell-based therapies to improve outcomes in IBD (147), but we will focus on treatments that modulate or reduce DCs producing tolerogenic DCs (Table 1).
The first successful therapies for IBD consisted of targeting TNF-α, including infliximab or adalimumab (148). Antibodies against IL-12/IL-23 p40 (risankizumab) and IL-23 p19 (ustekinumab) have been tested to reduce the effects in IBD (133). Other antibodies target the α4 chain of integrin heterodimers on leukocytes (e.g., natalizumab), α4β7 integrin, which may reduce the inflammation by blocking the recruitment of pro-inflammatory monocytes and DCs to the intestine (e.g., vedolizumab) (148) or immunomodulatory agents such as thalidomide (149) and G-CSF (150).
Alternative therapies such as the use of glucocorticoids can inhibit cytokine secretion, as well as both T cell and DC activation by reducing the expression of MHC-II molecules in UC (151). Others such as thiopurine-based treatments have demonstrated to restore the migratory defects in autophagy-deficient DCs, thus improving DC-T cell interactions and the cytoskeletal regulation (154). Moreover, mesenchymal stem cell (MSC) administration reduced serum levels of inflammatory cytokines (e.g., IL-1β, IL-6, and IL-12) in mice with DSS-induced UC, thus improving the phenotype and function of colon infiltrating DCs (155). In CD, MSCs not only decreased the expression of CD80 and CD86 on mDCs and the production of IL-12 and TNF-α, but also improved the production of IL-10 via (165).
Antibiotics reduce the concentration of gut lumen bacteria such as Escherichia coli strains, Bacteroides spp, and Mycobacterium avium, that have been linked, together with DCs, to chronic inflammation in IBD (166). Specifically, betalactam antibiotics have demonstrated to alter DC maturation in allergic patients via MAPK and NF-kB signaling pathways (167). IBD is also promoted by DC migration and maturation, so the targeting of DCs with betalactam antibiotics may improve clinical outcomes in the disease (115, 116, 168). Probiotics have also been successfully proposed to modulate the gut microbiota in IBD (169). In this sense, Ghavami et al. (2020) studied the role of Lactobacillus salivarius, Bacillus coagulans, Bacillus subtilis and Bifidobacterium bifidum (Bb), concluding that the expression of CD80 and CD86 was enhanced by most of the probiotics in UC patients and only by Bb in CD patients. Also, DCs from UC patients increased the production of IL-10 and TGF-β and reduced the expression of TLR by using all probiotics except Bb, and DCs from CD patients increased the expression of integrin ß8 and reduced the expression of TLR-4, TLR-9, and IL-12p40 (14). Saccharomyces boulardii promoted epithelial restitution in IBD patients by improving IL-8 levels, inhibiting Th1 polarization induced by CD1c+CD11c+CD123- mDCs, and reducing TNF-α and IL-6 levels, as well as the expression of CD40, CD80, and CCR7 on mDCs (156, 157). Lactobacillus casei Shirota restored the stimulatory role of DCs in UC patients (170, 171) by improving gut DC ability to imprint homing molecules on T cells and promoting IL-22 production (158). Furthermore, Lactobacillus plantarum has reversed the function of altered gut DCs in UC patients (159).
Selective granulocyte/monocyte apheresis (SGMA) has been tested to remove DCs from IBD patients (172). Adacolumn apheresis (AA) could lead to a higher tolerogenic status since a significantly lower level of lymphocytes, pDCs and mDCs has been found in acute UC (160). In addition, AA increased IL-10 and reduced circulating TNF-α and CD16 expression on both mDC and pDCs in UC patients (173). Lymphocytapheresis has demonstrated to be clinically safe in those patients and contributed to downregulate CD83+ DCs, IL-6, and IL-8 (161).
Vitamin D also seems to have a role in the modulation of DCs. In fact, vitamin D metabolites are frequently used in protocols to develop therapeutic DC therapies for autoimmune diseases, such as IBD (174). Vitamin 1,25(OH)2 D has improved IBD outcomes, at least in part, by decreasing DC activity, inducing antimicrobial peptide secretion, and increasing the anti-/pro-inflammatory cytokine ratio (152). Vitamin D3 was positively associated with low disease activity in CD patients and had beneficial effects in vivo on the monocytic precursors of moDC (153).
In the same line, vitamin D deficiency has been suggested to contribute to the inflammatory process in CD based on data from in vitro experiments by stimulating mo-DC with lipopolysaccharides (LPS) (162). Conversely, LPS-activated DCs has been cultured with GLM, a luteolin derivative, downregulating pro-inflammatory cytokine production or antigen-presenting ability for MHC-II complex on DCs from UC (175). Other molecules with natural origin as fructo-oligosaccharides, have significantly increased the number of IL-10+, TLR2+, and TLR4+ DCs, and reduced IL-6+ DCs in CD patients, but the clinical benefit remains contradictory (163, 164).
Another molecule, sulforaphane, with anticancer properties (106), has preventive or therapeutic applications in some intestinal inflammatory diseases due to its activating effect of AMPK signaling pathway in mice (176), although more evidence would be necessary to confirm the role of this natural compound on DCs.
DCs have a crucial role in the establishing and maintaining immune homeostasis of the organism, because link innate and adaptive immunity since they initiate immune responses by taking up both antigens and pathogens, and migrating to secondary lymphoid organs, where DCs finally present molecules to naïve T cells, which are activated. This essential immune process is employed as therapeutic intervention tool to the cure or mitigate of many diseases such as cancer seeking to enhance the cytotoxic machinery of T cells to kill tumor cells. Conversely, under certain conditions, DCs have shown to play a key role in the induction and maintenance of chronic inflammation in other pathologies, including IBD, resulting in the so-called “yin-yang” role of DCs.
It is clear that not only DCs promote a pro-inflammatory state in the intestine, but also other recruited immune cells such as neutrophils, monocytes or macrophages. In this sense, monocytes and macrophages could be difficult to distinguish from some DC subsets because they express the same markers depending on their differentiation stage in the myeloid lineage. Even so, activated DCs have found to be accumulated at sites of intestinal inflammation expressing a wide variety of characteristics markers, including (but not limited to) CD80, CD86, CD103, CD83, IRF4 or TLRs, and producing cytokines such as IL-6, IL-8, IL-12, IL-23, TNF-α, which produce disruptions in the immune system and drive IBD progression.
Based on the existing evidence on the role of DCs in IBD, we strongly believe that this cell population can be considered as a good biomarker for the disease. In fact, most of in vivo and in vitro experiments have shown that DCs could be a valuable therapeutic target, since its depletion as well as the production of some cytokines (e.g., IL-10 or TGF-β) have been positively associated with good results, which could support the manipulation of DCs to generate DC-specific therapies. For that purpose, we would also need to fully understand the mechanisms that are promoted by DCs in the balance between immune cells, since the pro-inflammatory state in the intestine could be increased. In addition, further research is needed to better clarify the importance of some DCs markers in the disease (e.g., CD1c, CD11c, or CD123) since both their expression and lack on the cell surface have been associated with positive results in the disease. Moreover, the role of moDCs or infDCs and LCs has been less studied and may play a critical role in the pathogenesis of IBD, so those DCs subset would also need to be more investigated to reach innovative strategies to enhance their clinical efficacy in both IBD and cancer.
CJ-C: Writing – original draft, Writing – review & editing. FP: Writing – review & editing. GA: Writing – review & editing. CS-M: Writing – review & editing. LC-M: Writing – review & editing. VS-M: Conceptualization, Supervision, Writing – review & editing. SL-E: Conceptualization, Supervision, Writing – review & editing.
The author(s) declare that no financial support was received for the research, authorship, and/or publication of this article.
The authors declare that the research was conducted in the absence of any commercial or financial relationships that could be construed as a potential conflict of interest.
All claims expressed in this article are solely those of the authors and do not necessarily represent those of their affiliated organizations, or those of the publisher, the editors and the reviewers. Any product that may be evaluated in this article, or claim that may be made by its manufacturer, is not guaranteed or endorsed by the publisher.
1. Ness S, Lin S, Gordon JR. Regulatory dendritic cells, T cell tolerance, and dendritic cell therapy for immunologic disease. Front Immunol (2021) 12:633436. doi: 10.3389/fimmu.2021.633436
2. Patente TA, Pinho MP, Oliveira AA, Evangelista GCM, Bergami-Santos PC, Barbuto JAM. Human dendritic cells: their heterogeneity and clinical application potential in cancer immunotherapy. Front Immunol (2018) 9:3176. doi: 10.3389/fimmu.2018.03176
3. Martin-Gayo E, Yu XG. Role of dendritic cells in natural immune control of HIV-1 infection. Front Immunol (2019) 10:1306. doi: 10.3389/fimmu.2019.01306
4. Nin DS, Deng LW. Biology of cancer-testis antigens and their therapeutic implications in cancer. Cells (2023) 12(6):926. doi: 10.3390/cells12060926
5. Lopez de Padilla CM, Reed AM. Involvement of dendritic cells in autoimmune diseases in children. Pediatr Rheumatol Online J (2007) 5:16. doi: 10.1186/1546-0096-5-16
6. Brasal-Prieto M, Fernandez-Prades L, Dakhaoui H, Sobrino F, Lopez-Enriquez S, Palomares F. Update on in vitro diagnostic tools and treatments for food allergies, nutrients. Nutrients (2023) 15(17):3744. doi: 10.3390/nu15173744
7. Walker PR, Calzascia T, Tribolet N, Dietrich PY. T-cell immune responses in the brain and their relevance for cerebral Malignancies. Brain Res Brain Res Rev (2003) 42(2):97–122. doi: 10.1016/S0165-0173(03)00141-3
8. Peng V, Jaeger N, Colonna M. Innate lymphoid cells and inflammatory bowel disease. Adv Exp Med Biol (2022) 1365:97–112. doi: 10.1007/978-981-16-8387-9_7
9. Chrisikos TT, Zhou Y, Slone N, Babcock R, Watowich SS, Li HS. Molecular regulation of dendritic cell development and function in homeostasis, inflammation, and cancer. Mol Immunol (2019) 110:24–39. doi: 10.1016/j.molimm.2018.01.014
10. Jorgensen N, Persson G, Hviid TVF. The tolerogenic function of regulatory T cells in pregnancy and cancer. Front Immunol (2019) 10:911. doi: 10.3389/fimmu.2019.00911
11. Santos PM, Butterfield LH. Dendritic cell-based cancer vaccines. J Immunol (2018) 200(2):443–9. doi: 10.4049/jimmunol.1701024
12. Harari A, Graciotti M, Bassani-Sternberg M, Kandalaft LE. Antitumour dendritic cell vaccination in a priming and boosting approach. Nat Rev Drug Discov (2020) 19(9):635–52. doi: 10.1038/s41573-020-0074-8
13. Sun D, Li C, Chen S, Zhang X. Emerging role of dendritic cell intervention in the treatment of inflammatory bowel disease. BioMed Res Int (2022) 2022:7025634. doi: 10.1155/2022/7025634
14. Ghavami SB, Yadegar A, Aghdaei HA, Sorrentino D, Farmani M, Mir AS, et al. Immunomodulation and generation of tolerogenic dendritic cells by probiotic bacteria in patients with inflammatory bowel disease. Int J Mol Sci (2020) 21(17):6266. doi: 10.3390/ijms21176266
15. Azeem W, Bakke RM, Appel S, Oyan AM, Kalland KH. Dual pro- and anti-inflammatory features of monocyte-derived dendritic cells. Front Immunol (2020) 11:438. doi: 10.3389/fimmu.2020.00438
16. Nunez R, Rodriguez MJ, Lebron-Martin C, Martin-Astorga MDC, Palomares F, Ramos-Soriano J, et al. Methylation changes induced by a glycodendropeptide immunotherapy and associated to tolerance in mice. Front Immunol (2022) 13:1094172. doi: 10.3389/fimmu.2022.1094172
17. Palomares F, Gomez F, de la Fuente MC, Perez-Sanchez N, Torres MJ, Mayorga C, et al. Fucodendropeptides induce changes in cells of the immune system in food allergic patients via DC-SIGN receptor. Carbohydr Res (2022) 517:108580. doi: 10.1016/j.carres.2022.108580
18. Riegel K, Schloder J, Sobczak M, Jonuleit H, Thiede B, Schild H, et al. RAF kinases are stabilized and required for dendritic cell differentiation and function. Cell Death Differ (2020) 27(4):1300–15. doi: 10.1038/s41418-019-0416-4
19. Eisenbarth SC. Dendritic cell subsets in T cell programming: location dictates function. Nat Rev Immunol (2019) 19(2):89–103. doi: 10.1038/s41577-018-0088-1
20. Wculek SK, Cueto FJ, Mujal AM, Melero I, Krummel MF, Sancho D. Dendritic cells in cancer immunology and immunotherapy. Nat Rev Immunol (2020) 20(1):7–24. doi: 10.1038/s41577-019-0210-z
21. Yuan X, Qin X, Wang D, Zhang Z, Tang X, Gao X, et al. Mesenchymal stem cell therapy induces FLT3L and CD1c(+) dendritic cells in systemic lupus erythematosus patients. Nat Commun (2019) 10(1):2498. doi: 10.1038/s41467-019-10491-8
22. de Almeida Baptista MV, da Silva LT, Samer S, Oshiro TM, Shytaj IL, Giron LB, et al. Immunogenicity of personalized dendritic-cell therapy in HIV-1 infected individuals under suppressive antiretroviral treatment: interim analysis from a phase II clinical trial. AIDS Res Ther (2022) 19(1):2. doi: 10.1186/s12981-021-00426-z
23. Mastelic-Gavillet B, Balint K, Boudousquie C, Gannon PO, Kandalaft LE. Personalized dendritic cell vaccines-recent breakthroughs and encouraging clinical results. Front Immunol (2019) 10:766. doi: 10.3389/fimmu.2019.00766
24. Morante-Palacios O, Godoy-Tena G, Calafell-Segura J, Ciudad L, Martinez-Caceres EM, Sardina JL, et al. Vitamin C enhances NF-kappaB-driven epigenomic reprogramming and boosts the immunogenic properties of dendritic cells. Nucleic Acids Res (2022) 50(19):10981–94. doi: 10.1093/nar/gkac941
25. Tackett N, Bradley JH, Moore EK, Baker SH, Minter SL, DiGiacinto B, et al. Prolonged exposure to simulated microgravity diminishes dendritic cell immunogenicity. Sci Rep (2019) 9(1):13825. doi: 10.1038/s41598-019-50311-z
26. Morante-Palacios O, Fondelli F, Ballestar E, Martinez-Caceres EM. Tolerogenic dendritic cells in autoimmunity and inflammatory diseases. Trends Immunol (2021) 42(1):59–75. doi: 10.1016/j.it.2020.11.001
27. Bertolini TB, Biswas M, Terhorst C, Daniell H, Herzog RW, Pineros AR. Role of orally induced regulatory T cells in immunotherapy and tolerance. Cell Immunol (2021) 359:104251. doi: 10.1016/j.cellimm.2020.104251
28. Ritprajak P, Kaewraemruaen C, Hirankarn N. Current paradigms of tolerogenic dendritic cells and clinical implications for systemic lupus erythematosus. Cells (2019) 8(10):1291. doi: 10.3390/cells8101291
29. Palomares F, Gomez F, Bogas G, Campo P, Perkins JR, Diaz-Perales A, et al. Immunological changes induced in peach allergy patients with systemic reactions by pru p 3 sublingual immunotherapy. Mol Nutr Food Res (2018) 62(3). doi: 10.1002/mnfr.201700669
30. Guindi C, Cloutier A, Gaudreau S, Zerif E, McDonald PP, Tatsiy O, et al. Role of the p38 MAPK/C/EBPbeta pathway in the regulation of phenotype and IL-10 and IL-12 production by tolerogenic bone marrow-derived dendritic cells. Cells (2018) 7(12):256. doi: 10.3390/cells7120256
31. Balan S, Saxena M, Bhardwaj N. Dendritic cell subsets and locations. Int Rev Cell Mol Biol (2019) 348:1–68. doi: 10.1016/bs.ircmb.2019.07.004
32. Amon L, Lehmann CHK, Heger L, Heidkamp GF, Dudziak D. The ontogenetic path of human dendritic cells. Mol Immunol (2020) 120:122–9. doi: 10.1016/j.molimm.2020.02.010
33. Hilligan KL, Ronchese F. Antigen presentation by dendritic cells and their instruction of CD4+ T helper cell responses. Cell Mol Immunol (2020) 17(6):587–99. doi: 10.1038/s41423-020-0465-0
34. Roselli E, Araya P, Nunez NG, Gatti G, Graziano F, Sedlik C, et al. TLR3 activation of intratumoral CD103(+) dendritic cells modifies the tumor infiltrate conferring anti-tumor immunity. Front Immunol (2019) 10:503. doi: 10.3389/fimmu.2019.00503
35. Bottcher JP, Reis e Sousa C. The role of type 1 conventional dendritic cells in cancer immunity. Trends Cancer (2018) 4(11):784–92. doi: 10.1016/j.trecan.2018.09.001
36. Arabpour M, Lebrero-Fernandez C, Schon K, Stromberg A, Borjesson V, Lahl K, et al. ADP-ribosylating adjuvant reveals plasticity in cDC1 cells that drive mucosal Th17 cell development and protection against influenza virus infection. Mucosal Immunol (2022) 15(4):745–61. doi: 10.1038/s41385-022-00510-1
37. Binnewies M, Mujal AM, Pollack JL, Combes AJ, Hardison EA, Barry KC, et al. Unleashing type-2 dendritic cells to drive protective antitumor CD4(+) T cell immunity. Cell (2019) 177(3):556–571 e16. doi: 10.1016/j.cell.2019.02.005
38. Izumi G, Nakano H, Nakano K, Whitehead GS, Grimm SA, Fessler MB, et al. CD11b(+) lung dendritic cells at different stages of maturation induce Th17 or Th2 differentiation. Nat Commun (2021) 12(1):5029. doi: 10.1038/s41467-021-25307-x
39. Moon HG, Kim SJ, Jeong JJ, Han SS, Jarjour NN, Lee H, et al. Airway epithelial cell-derived colony stimulating factor-1 promotes allergen sensitization. Immunity (2018) 49(2):275–287 e5. doi: 10.1016/j.immuni.2018.06.009
40. Lee J, Zhang J, Chung YJ, Kim JH, Kook CM, Gonzalez-Navajas JM, et al. Inhibition of IRF4 in dendritic cells by PRR-independent and -dependent signals inhibit Th2 and promote Th17 responses. Elife (2020) 9:e49416. doi: 10.7554/eLife.49416
41. Backer RA, Probst HC, Clausen BE. Classical DC2 subsets and monocyte-derived DC: Delineating the developmental and functional relationship. Eur J Immunol (2023) 53(3):e2149548. doi: 10.1002/eji.202149548
42. Shane HL, Reagin KL, Klonowski KD. The respiratory environment diverts the development of antiviral memory CD8 T cells. J Immunol (2018) 200(11):3752–61. doi: 10.4049/jimmunol.1701268
43. Smith N, Rodero MP, Bekaddour N, Bondet V, Ruiz-Blanco YB, Harms M, et al. Control of TLR7-mediated type I IFN signaling in pDCs through CXCR4 engagement-A new target for lupus treatment. Sci Adv (2019) 5(7):eaav9019. doi: 10.1126/sciadv.aav9019
44. Cervantes-Barragan L, Vanderheiden A, Royer CJ, Davis-Gardner ME, Ralfs P, Chirkova T, et al. Plasmacytoid dendritic cells produce type I interferon and reduce viral replication in airway epithelial cells after SARS-CoV-2 infection. bioRxiv (2021). doi: 10.1101/2021.05.12.443948
45. Yang Y, Day J, Souza-Fonseca Guimaraes F, Wicks IP, Louis C. Natural killer cells in inflammatory autoimmune diseases. Clin Transl Immunol (2021) 10(2):e1250. doi: 10.1002/cti2.1250
46. Yan B, Liu N, Li J, Li J, Zhu W, Kuang Y, et al. The role of Langerhans cells in epidermal homeostasis and pathogenesis of psoriasis. J Cell Mol Med (2020) 24(20):11646–55. doi: 10.1111/jcmm.15834
47. Sconocchia T, Hochgerner M, Schwarzenberger E, Tam-Amersdorfer C, Borek I, Benezeder T, et al. Bone morphogenetic protein signaling regulates skin inflammation via modulating dendritic cell function. J Allergy Clin Immunol (2021) 147(5):1810–1822 e9. doi: 10.1016/j.jaci.2020.09.038
48. Pan H, Zhao Z, Deng Y, Zheng Z, Huang Y, Huang S, et al. The global, regional, and national early-onset colorectal cancer burden and trends from 1990 to 2019: results from the Global Burden of Disease Study 2019. BMC Public Health (2022) 22(1):1896. doi: 10.1186/s12889-022-14274-7
49. Siegel RL, Miller KD, Fuchs HE, Jemal A. Cancer statistics, 2021. CA Cancer J Clin (2021) 71(1):7–33. doi: 10.3322/caac.21654
50. Somarribas Patterson LF, Vardhana SA. Metabolic regulation of the cancer-immunity cycle. Trends Immunol (2021) 42(11):975–93. doi: 10.1016/j.it.2021.09.002
51. Yi M, Zhang J, Li A, Niu M, Yan Y, Jiao Y, et al. The construction, expression, and enhanced anti-tumor activity of YM101: a bispecific antibody simultaneously targeting TGF-beta and PD-L1. J Hematol Oncol (2021) 14(1):27. doi: 10.1186/s13045-021-01045-x
52. Batchu RB, Gruzdyn OV, Kolli BK, Dachepalli R, Umar PS, Rai SK, et al. IL-10 signaling in the tumor microenvironment of ovarian cancer. Adv Exp Med Biol (2021) 1290:51–65. doi: 10.1007/978-3-030-55617-4_3
53. Rotte A. Combination of CTLA-4 and PD-1 blockers for treatment of cancer. J Exp Clin Cancer Res (2019) 38(1):255. doi: 10.1186/s13046-019-1259-z
54. Plesca I, Benesova I, Beer C, Sommer U, Muller L, Wehner R, et al. Clinical significance of tumor-infiltrating conventional and plasmacytoid dendritic cells in pancreatic ductal adenocarcinoma. Cancers (Basel) (2022) 14(5):1216. doi: 10.3390/cancers14051216
55. Aggarwal V, Workman CJ, Vignali DAA. LAG-3 as the third checkpoint inhibitor. Nat Immunol (2023) 24(9):1415–22. doi: 10.1038/s41590-023-01569-z
56. Yin XK, Wang C, Feng LL, Bai SM, Feng WX, Ouyang NT, et al. Expression pattern and prognostic value of CTLA-4, CD86, and tumor-infiltrating lymphocytes in rectal cancer after neoadjuvant chemo(radio)therapy. Cancers (Basel) (2022) 14(22):5573. doi: 10.3390/cancers14225573
57. Han Y, Liu SM, Jin R, Meng W, Wu YL, Li H. A risk score combining co-expression modules related to myeloid cells and alternative splicing associates with response to PD-1/PD-L1 blockade in non-small cell lung cancer. Front Immunol (2023) 14:1178193. doi: 10.3389/fimmu.2023.1178193
58. Koucky V, Boucek J, Fialova A. Immunology of plasmacytoid dendritic cells in solid tumors: A brief review. Cancers (Basel) (2019) 11(4):470. doi: 10.3390/cancers11040470
59. Ding S, Qiao N, Zhu Q, Tong Y, Wang S, Chen X, et al. Single-cell atlas reveals a distinct immune profile fostered by T cell-B cell crosstalk in triple negative breast cancer. Cancer Commun (Lond) (2023) 43(6):661–84. doi: 10.1002/cac2.12429
60. Mitchell D, Chintala S, Dey M. Plasmacytoid dendritic cell in immunity and cancer. J Neuroimmunol (2018) 322:63–73. doi: 10.1016/j.jneuroim.2018.06.012
61. Poropatich K, Dominguez D, Chan WC, Andrade J, Zha Y, Wray B, et al. OX40+ plasmacytoid dendritic cells in the tumor microenvironment promote antitumor immunity. J Clin Invest (2020) 130(7):3528–42. doi: 10.1172/JCI131992
62. Tang-Huau TL, Segura E. Human in vivo-differentiated monocyte-derived dendritic cells. Semin Cell Dev Biol (2019) 86:44–9. doi: 10.1016/j.semcdb.2018.02.018
63. Lu C, Liu Y, Ali NM, Zhang B, Cui X. The role of innate immune cells in the tumor microenvironment and research progress in anti-tumor therapy. Front Immunol (2022) 13:1039260. doi: 10.3389/fimmu.2022.1039260
64. Wylie B, Macri C, Mintern JD, Waithman J. Dendritic cells and cancer: from biology to therapeutic intervention. Cancers (Basel) (2019) 11(4):521. doi: 10.3390/cancers11040521
65. Shahid A, Bharadwaj M. The connection between the Th17 cell related cytokines and cancer stem cells in cancer: Novel therapeutic targets. Immunol Lett (2019) 213:9–20. doi: 10.1016/j.imlet.2019.07.001
66. Bergenfelz C, Leandersson K. The generation and identity of human myeloid-derived suppressor cells. Front Oncol (2020) 10:109. doi: 10.3389/fonc.2020.00109
67. Palazon-Carrion N, Jimenez-Cortegana C, Sanchez-Leon ML, Henao-Carrasco F, Nogales-Fernandez E, Chiesa M, et al. Circulating immune biomarkers in peripheral blood correlate with clinical outcomes in advanced breast cancer. Sci Rep (2021) 11(1):17639. doi: 10.1038/s41598-021-93838-w
68. Lin S, Zhang X, Huang G, Cheng L, Lv J, Zheng D, et al. Myeloid-derived suppressor cells promote lung cancer metastasis by CCL11 to activate ERK and AKT signaling and induce epithelial-mesenchymal transition in tumor cells. Oncogene (2021) 40(8):1476–89. doi: 10.1038/s41388-020-01605-4
69. Jimenez-Cortegana C, Palazon-Carrion N, Martin Garcia-Sancho A, Nogales-Fernandez E, Carnicero-Gonzalez F, Rios-Herranz E, et al. Circulating myeloid-derived suppressor cells and regulatory T cells as immunological biomarkers in refractory/relapsed diffuse large B-cell lymphoma: translational results from the R2-GDP-GOTEL trial. J Immunother Cancer (2021) 9(6):e002323. doi: 10.1136/jitc-2020-002323
70. Lee KW, Yam JWP, Mao X. Dendritic cell vaccines: A shift from conventional approach to new generations. Cells (2023) 12(17):2147. doi: 10.3390/cells12172147
71. Noubade R, Majri-Morrison S, Tarbell KV. Beyond cDC1: emerging roles of DC crosstalk in cancer immunity. Front Immunol (2019) 10:1014. doi: 10.3389/fimmu.2019.01014
72. Wculek SK, Amores-Iniesta J, Conde-Garrosa R, Khouili SC, Melero I, Sancho D. Effective cancer immunotherapy by natural mouse conventional type-1 dendritic cells bearing dead tumor antigen. J Immunother Cancer (2019) 7(1):100. doi: 10.1186/s40425-019-0565-5
73. Kuhn NF, Lopez AV, Li X, Cai W, Daniyan AF, Brentjens RJ. CD103(+) cDC1 and endogenous CD8(+) T cells are necessary for improved CD40L-overexpressing CAR T cell antitumor function. Nat Commun (2020) 11(1):6171. doi: 10.1038/s41467-020-19833-3
74. Arnold IC, Zhang X, Artola-Boran M, Fallegger A, Sander P, Johansen P, et al. BATF3-dependent dendritic cells drive both effector and regulatory T-cell responses in bacterially infected tissues. PloS Pathog (2019) 15(6):e1007866. doi: 10.1371/journal.ppat.1007866
75. Tatsumi N, Codrington AL, El-Fenej J, Phondge V, Kumamoto Y. Effective CD4 T cell priming requires repertoire scanning by CD301b(+) migratory cDC2 cells upon lymph node entry. Sci Immunol (2021) 6(66):eabg0336. doi: 10.1126/sciimmunol.abg0336
76. Ferris ST, Durai V, Wu R, Theisen DJ, Ward JP, Bern MD, et al. cDC1 prime and are licensed by CD4(+) T cells to induce anti-tumour immunity. Nature (2020) 584(7822):624–9. doi: 10.1038/s41586-020-2611-3
77. Basu A, Ramamoorthi G, Jia Y, Faughn J, Wiener D, Awshah S, et al. Immunotherapy in breast cancer: Current status and future directions. Adv Cancer Res (2019) 143:295–349. doi: 10.1016/bs.acr.2019.03.006
78. Johdi NA, Sukor NF. Colorectal cancer immunotherapy: options and strategies. Front Immunol (2020) 11:1624. doi: 10.3389/fimmu.2020.01624
79. Melaiu O, Chierici M, Lucarini V, Jurman G, Conti LA, De Vito R, et al. Cellular and gene signatures of tumor-infiltrating dendritic cells and natural-killer cells predict prognosis of neuroblastoma. Nat Commun (2020) 11(1):5992. doi: 10.1038/s41467-020-19781-y
80. Szpor J, Streb J, Glajcar A, Fraczek P, Winiarska A, Tyrak KE, et al. Dendritic cells are associated with prognosis and survival in breast cancer. Diagnostics (Basel) (2021) 11(4):702. doi: 10.3390/diagnostics11040702
81. Sanchez-Leon ML, Jimenez-Cortegana C, Cabrera G, Vermeulen EM, de la Cruz-Merino L, Sanchez-Margalet V. The effects of dendritic cell-based vaccines in the tumor microenvironment: Impact on myeloid-derived suppressor cells. Front Immunol (2022) 13:1050484. doi: 10.3389/fimmu.2022.1050484
82. Han Y, Liu D, Li L. PD-1/PD-L1 pathway: current researches in cancer. Am J Cancer Res (2020) 10(3):727–42.
83. Hossain DMS, Javaid S, Cai M, Zhang C, Sawant A, Hinton M, et al. Dinaciclib induces immunogenic cell death and enhances anti-PD1-mediated tumor suppression. J Clin Invest (2018) 128(2):644–54. doi: 10.1172/JCI94586
84. Kato Y, Tabata K, Kimura T, Yachie-Kinoshita A, Ozawa Y, Yamada K, et al. Lenvatinib plus anti-PD-1 antibody combination treatment activates CD8+ T cells through reduction of tumor-associated macrophage and activation of the interferon pathway. PloS One (2019) 14(2):e0212513. doi: 10.1371/journal.pone.0212513
85. Garris CS, Arlauckas SP, Kohler RH, Trefny MP, Garren S, Piot C, et al. Successful anti-PD-1 cancer immunotherapy requires T cell-dendritic cell crosstalk involving the cytokines IFN-gamma and IL-12. Immunity (2018) 49(6):1148–1161 e7. doi: 10.1016/j.immuni.2018.09.024
86. Giovanelli P, Sandoval TA, Cubillos-Ruiz JR. Dendritic cell metabolism and function in tumors. Trends Immunol (2019) 40(8):699–718. doi: 10.1016/j.it.2019.06.004
87. Han Z, Dong Y, Lu J, Yang F, Zheng Y, Yang H. Role of hypoxia in inhibiting dendritic cells by VEGF signaling in tumor microenvironments: mechanism and application. Am J Cancer Res (2021) 11(8):3777–93.
88. Kudo M. Combination immunotherapy with anti-VEGF/TKI for hepatocellular carcinoma: present and future perspective. Hepatobil Surg Nutr (2021) 10(2):241–5. doi: 10.21037/hbsn-20-707
89. Bent EH, Millan-Barea LR, Zhuang I, Goulet DR, Frose J, Hemann MT. Microenvironmental IL-6 inhibits anti-cancer immune responses generated by cytotoxic chemotherapy. Nat Commun (2021) 12(1):6218. doi: 10.1038/s41467-021-26407-4
90. Manore SG, Doheny DL, Wong GL, Lo HW. IL-6/JAK/STAT3 signaling in breast cancer metastasis: biology and treatment. Front Oncol (2022) 12:866014. doi: 10.3389/fonc.2022.866014
91. Xu J, Lin H, Wu G, Zhu M, Li M. IL-6/STAT3 is a promising therapeutic target for hepatocellular carcinoma. Front Oncol (2021) 11:760971. doi: 10.3389/fonc.2021.760971
92. Mirlekar B. Tumor promoting roles of IL-10, TGF-beta, IL-4, and IL-35: Its implications in cancer immunotherapy. SAGE Open Med (2022) 10:20503121211069012. doi: 10.1177/20503121211069012
93. Ni G, Zhang L, Yang X, Li H, Ma B, Walton S, et al. Targeting interleukin-10 signalling for cancer immunotherapy, a promising and complicated task. Hum Vaccin Immunother (2020) 16(10):2328–32. doi: 10.1080/21645515.2020.1717185
94. Rivas JR, Liu Y, Alhakeem SS, Eckenrode JM, Marti F, Collard JP, et al. Interleukin-10 suppression enhances T-cell antitumor immunity and responses to checkpoint blockade in chronic lymphocytic leukemia. Leukemia (2021) 35(11):3188–200. doi: 10.1038/s41375-021-01217-1
95. Block MS, Dietz AB, Gustafson MP, Kalli KR, Erskine CL, Youssef B, et al. Th17-inducing autologous dendritic cell vaccination promotes antigen-specific cellular and humoral immunity in ovarian cancer patients. Nat Commun (2020) 11(1):5173. doi: 10.1038/s41467-020-18962-z
96. Liau LM, Ashkan K, Tran DD, Campian JL, Trusheim JE, Cobbs CS, et al. First results on survival from a large Phase 3 clinical trial of an autologous dendritic cell vaccine in newly diagnosed glioblastoma. J Transl Med (2018) 16(1):142. doi: 10.1186/s12967-018-1507-6
97. Esmaily M, Masjedi A, Hallaj S, Nabi Afjadi M, Malakotikhah F, Ghani S, et al. Blockade of CTLA-4 increases anti-tumor response inducing potential of dendritic cell vaccine. J Control Release (2020) 326:63–74. doi: 10.1016/j.jconrel.2020.06.017
98. Kodumudi KN, Ramamoorthi G, Snyder C, Basu A, Jia Y, Awshah S, et al. Sequential anti-PD1 therapy following dendritic cell vaccination improves survival in a HER2 mammary carcinoma model and identifies a critical role for CD4 T cells in mediating the response. Front Immunol (2019) 10:1939. doi: 10.3389/fimmu.2019.01939
99. Zhou Y, Slone N, Chrisikos TT, Kyrysyuk O, Babcock RL, Medik YB, et al. Vaccine efficacy against primary and metastatic cancer with in vitro-generated CD103(+) conventional dendritic cells. J Immunother Cancer (2020) 8(1):e000474. doi: 10.1136/jitc-2019-000474
100. Johnson P, Rosendahl N, Radford KJ. Conventional type 1 dendritic cells (cDC1) as cancer therapeutics: challenges and opportunities. Expert Opin Biol Ther (2022) 22(4):465–72. doi: 10.1080/14712598.2022.1994943
101. Bol KF, Schreibelt G, Rabold K, Wculek SK, Schwarze JK, Dzionek A, et al. The clinical application of cancer immunotherapy based on naturally circulating dendritic cells. J Immunother Cancer (2019) 7(1):109. doi: 10.1186/s40425-019-0580-6
102. Baldin AV, Savvateeva LV, Bazhin AV, Zamyatnin AA Jr. Dendritic cells in anticancer vaccination: rationale for ex vivo loading or in vivo targeting. Cancers (Basel) (2020) 12(3):590. doi: 10.3390/cancers12030590
103. Chiang CL, Kandalaft LE. In vivo cancer vaccination: Which dendritic cells to target and how? Cancer Treat Rev (2018) 71:88–101. doi: 10.1016/j.ctrv.2018.10.012
104. Arab S, Kheshtchin N, Ajami M, Ashurpoor M, Safvati A, Namdar A, et al. Increased efficacy of a dendritic cell-based therapeutic cancer vaccine with adenosine receptor antagonist and CD73 inhibitor. Tumour Biol (2017) 39(3):1010428317695021. doi: 10.1177/1010428317695021
105. Jang GY, Kim YS, Lee SE, Lee JW, Han HD, Kang TH, et al. Improvement of DC-based vaccines using adjuvant TLR4-binding 60S acidic ribosomal protein P2 and immune checkpoint inhibitors. Cancer Immunol Immunother (2021) 70(4):1075–88. doi: 10.1007/s00262-020-02759-6
106. Wang Y, Petrikova E, Gross W, Sticht C, Gretz N, Herr I, et al. Sulforaphane promotes dendritic cell stimulatory capacity through modulation of regulatory molecules, JAK/STAT3- and microRNA-signaling. Front Immunol (2020) 11:589818. doi: 10.3389/fimmu.2020.589818
107. Annese V, Annese M. Precision medicine in inflammatory bowel disease. Diagnostics (Basel) (2023) 13(17):2797. doi: 10.3390/diagnostics13172797
108. Petagna L, Antonelli A, Ganini C, Bellato V, Campanelli M, Divizia A, et al. Pathophysiology of Crohn’s disease inflammation and recurrence. Biol Direct (2020) 15(1):23. doi: 10.1186/s13062-020-00280-5
109. Seyedian SS, Nokhostin F, Malamir MD. A review of the diagnosis, prevention, and treatment methods of inflammatory bowel disease. J Med Life (2019) 12(2):113–22. doi: 10.25122/jml-2018-0075
110. Ardizzone A, Mannino D, Capra AP, Repici A, Filippone A, Esposito E, et al. New Insights into the Mechanism of Ulva pertusa on Colitis in Mice: Modulation of the Pain and Immune System. Mar Drugs (2023) 21(5):298. doi: 10.3390/md21050298
111. Feuerstein JD, Moss AC, Farraye FA. Ulcerative colitis. Mayo Clin Proc (2019) 94(7):1357–73. doi: 10.1016/j.mayocp.2019.01.018
112. Kelsen JR, Russo P, Sullivan KE. Early-onset inflammatory bowel disease. Immunol Allergy Clin North Am (2019) 39(1):63–79. doi: 10.1016/j.iac.2018.08.008
113. Sun Q, Lu Z, Ma L, Xue D, Liu C, Ye C, et al. Integrin beta6 deficiency protects mice from experimental colitis and colitis-associated carcinoma by altering macrophage polarization. Front Oncol (2023) 13:1190229. doi: 10.3389/fonc.2023.1190229
114. Na YR, Stakenborg M, Seok SH, Matteoli G. Macrophages in intestinal inflammation and resolution: a potential therapeutic target in IBD. Nat Rev Gastroenterol Hepatol (2019) 16(9):531–43. doi: 10.1038/s41575-019-0172-4
115. Smrekar N, Drobne D, Smid LM, Ferkolj I, Stabuc B, Ihan A, et al. Dendritic cell profiles in the inflamed colonic mucosa predict the responses to tumor necrosis factor alpha inhibitors in inflammatory bowel disease. Radiol Oncol (2018) 52(4):443–52. doi: 10.2478/raon-2018-0045
116. Radwan P, Radwan-Kwiatek K, Tabarkiewicz J, Radej S, Rolinski J. Enhanced phenotypic and functional maturation of monocyte-derived dendritic cells from patients with active Crohn’s disease and ulcerative colitis. J Physiol Pharmacol (2010) 61(6):695–703.
117. Huang B, Chen Z, Geng L, Wang J, Liang H, Cao Y, et al. Mucosal profiling of pediatric-onset colitis and IBD reveals common pathogenics and therapeutic pathways. Cell (2019) 179(5):1160–1176 e24. doi: 10.1016/j.cell.2019.10.027
118. Chen SL, Li CM, Li W, Liu QS, Hu SY, Zhao MY, et al. How autophagy, a potential therapeutic target, regulates intestinal inflammation. Front Immunol (2023) 14:1087677. doi: 10.3389/fimmu.2023.1087677
119. Zhang H, Wang D, Shihb DQ, Zhang XL. Atg16l1 in dendritic cells is required for antibacterial defense and autophagy in murine colitis. IUBMB Life (2020) 72(12):2686–95. doi: 10.1002/iub.2406
120. Zhang J, Shi G. Lymphocyte infiltration and key differentially expressed genes in the ulcerative colitis. Med (Baltimore) (2020) 99(35):e21997. doi: 10.1097/MD.0000000000021997
121. Mitsialis V, Wall S, Liu P, Ordovas-Montanes J, Parmet T, Vukovic M, et al. Single-cell analyses of colon and blood reveal distinct immune cell signatures of ulcerative colitis and Crohn’s disease. Gastroenterology (2020) 159(2):591–608 e10. doi: 10.1053/j.gastro.2020.04.074
122. Chapuy L, Sarfati M. Single-cell protein and RNA expression analysis of mononuclear phagocytes in intestinal mucosa and mesenteric lymph nodes of ulcerative colitis and Crohn’s disease patients. Cells (2020) 9(4):813. doi: 10.3390/cells9040813
123. Richter L, Landsverk OJB, Atlasy N, Bujko A, Yaqub S, Horneland R, et al. Transcriptional profiling reveals monocyte-related macrophages phenotypically resembling DC in human intestine. Mucosal Immunol (2018) 11(5):1512–23. doi: 10.1038/s41385-018-0060-1
124. Li C, Grider JR, Murthy KS, Bohl J, Rivet E, Wieghard N, et al. Endoplasmic reticulum stress in subepithelial myofibroblasts increases the TGF-beta1 activity that regulates fibrosis in Crohn’s disease. Inflamm Bowel Dis (2020) 26(6):809–19. doi: 10.1093/ibd/izaa015
125. Ghorbaninejad M, Abdollahpour-Alitappeh M, Shahrokh S, Fayazzadeh S, Asadzadeh-Aghdaei H, Meyfour A. TGF-beta receptor I inhibitor may restrict the induction of EMT in inflamed intestinal epithelial cells. Exp Biol Med (Maywood) (2023) 248(8):665–76. doi: 10.1177/15353702231151959
126. Vulliemoz M, Brand S, Juillerat P, Mottet C, Ben-Horin S, Michetti P. TNF-alpha blockers in inflammatory bowel diseases: practical recommendations and a user’s guide: an update. Digestion (2020) 101 Suppl 1:16–26. doi: 10.1159/000506898
127. Ninnemann J, Winsauer C, Bondareva M, Kuhl AA, Lozza L, Durek P, et al. TNF hampers intestinal tissue repair in colitis by restricting IL-22 bioavailability. Mucosal Immunol (2022) 15(4):698–716. doi: 10.1038/s41385-022-00506-x
128. Huang R, Wang W, Chen Z, Chai J, Qi Q, Zheng H, et al. Identifying immune cell infiltration and effective diagnostic biomarkers in Crohn’s disease by bioinformatics analysis. Front Immunol (2023) 14:1162473. doi: 10.3389/fimmu.2023.1162473
129. Garo LP, Ajay AK, Fujiwara M, Beynon V, Kuhn C, Gabriely G, et al. Smad7 controls immunoregulatory PDL2/1-PD1 signaling in intestinal inflammation and autoimmunity. Cell Rep (2019) 28(13):3353–3366 e5. doi: 10.1016/j.celrep.2019.07.065
130. Ferreira C, Abrantes P, Costa SS, Viveiros M, Couto I. Occurrence and Variability of the Efflux Pump Gene norA across the Staphylococcus Genus. Int J Mol Sci (2022) 23(23):15306. doi: 10.3390/ijms232315306
131. Dvornikova KA, Platonova ON, Bystrova EY. Inflammatory bowel disease: crosstalk between histamine, immunity, and disease. Int J Mol Sci (2023) 24(12):9937. doi: 10.3390/ijms24129937
132. Zheng K, Jia J, Yan S, Shen H, Zhu P, Yu J. Paeoniflorin ameliorates ulcerative colitis by modulating the dendritic cell-mediated T(H)17/T(reg) balance. Inflammopharmacology (2020) 28(6):1705–16. doi: 10.1007/s10787-020-00722-6
133. Neurath MF. IL-23 in inflammatory bowel diseases and colon cancer. Cytokine Growth Factor Rev (2019) 45:1–8. doi: 10.1016/j.cytogfr.2018.12.002
134. Despalatovic BR, Babic M, Bratanic A, Tonkic A, Vilovic K. Difference in presence and number of CD83(+) dendritic cells in patients with ulcerative colitis and Crohn’s disease. Sci Rep (2020) 10(1):10055. doi: 10.1038/s41598-020-67149-5
135. Honjo H, Watanabe T, Kamata K, Minaga K, Kudo M. RIPK2 as a new therapeutic target in inflammatory bowel diseases. Front Pharmacol (2021) 12:650403. doi: 10.3389/fphar.2021.650403
136. Lai Y, Wang X, Sun X, Wu S, Chen X, Yang C, et al. Discovery of a novel RIPK2 inhibitor for the treatment of inflammatory bowel disease. Biochem Pharmacol (2023) 214:115647. doi: 10.1016/j.bcp.2023.115647
137. Pan Z, Lin H, Fu Y, Zeng F, Gu F, Niu G, et al. Identification of gene signatures associated with ulcerative colitis and the association with immune infiltrates in colon cancer. Front Immunol (2023) 14:1086898. doi: 10.3389/fimmu.2023.1086898
138. Ueno A, Jijon H, Traves S, Chan R, Ford K, Beck PL, et al. Opposing effects of smoking in ulcerative colitis and Crohn’s disease may be explained by differential effects on dendritic cells. Inflamm Bowel Dis (2014) 20(5):800–10. doi: 10.1097/MIB.0000000000000018
139. Kramer M, Netea MG, de Jong DJ, Kullberg BJ, Adema GJ. Impaired dendritic cell function in Crohn’s disease patients with NOD2 3020insC mutation. J Leukoc Biol (2006) 79(4):860–6. doi: 10.1189/jlb.0805484
140. Yang ZJ, Wang BY, Wang TT, Wang FF, Guo YX, Hua RX, et al. Functions of dendritic cells and its association with intestinal diseases. Cells (2021) 10(3):583. doi: 10.3390/cells10030583
141. Chapuy L, Bsat M, Rubio M, Harvey F, Motta V, Schwenter F, et al. Transcriptomic analysis and high-dimensional phenotypic mapping of mononuclear phagocytes in mesenteric lymph nodes reveal differences between ulcerative colitis and Crohn’s disease. J Crohns Colitis (2020) 14(3):393–405. doi: 10.1093/ecco-jcc/jjz156
142. Korta A, Kula J, Gomulka K. The role of IL-23 in the pathogenesis and therapy of inflammatory bowel disease. Int J Mol Sci (2023) 24(12):10172. doi: 10.3390/ijms241210172
143. Kapoor K, Eissa N, Tshikudi D, Bernstein CN, Ghia JE. Impact of intrarectal chromofungin treatment on dendritic cells-related markers in different immune compartments in colonic inflammatory conditions. World J Gastroenterol (2021) 27(47):8138–55. doi: 10.3748/wjg.v27.i47.8138
144. Lu Y, Li X, Liu S, Zhang Y, Zhang D. Toll-like receptors and inflammatory bowel disease. Front Immunol (2018) 9:72. doi: 10.3389/fimmu.2018.00072
145. Volarevic V, Zdravkovic N, Harrell CR, Arsenijevic N, Fellabaum C, Djonov V, et al. Galectin-3 regulates indoleamine-2,3-dioxygenase-dependent cross-talk between colon-infiltrating dendritic cells and T regulatory cells and may represent a valuable biomarker for monitoring the progression of ulcerative colitis. Cells (2019) 8(7):709. doi: 10.3390/cells8070709
146. Cutine AM, Bach CA, Veigas F, Merlo JP, Laporte L, Manselle Cocco MN, et al. Tissue-specific control of galectin-1-driven circuits during inflammatory responses. Glycobiology (2021) 31(8):891–907. doi: 10.1093/glycob/cwab007
147. Cassinotti A, Passamonti F, Segato S. Cell therapy in inflammatory bowel disease. Pharmacol Res (2021) 163:105247. doi: 10.1016/j.phrs.2020.105247
148. Luzentales-Simpson M, Pang YCF, Zhang A, Sousa JA, Sly LM. Vedolizumab: potential mechanisms of action for reducing pathological inflammation in inflammatory bowel diseases. Front Cell Dev Biol (2021) 9:612830. doi: 10.3389/fcell.2021.612830
149. Deng L, Ding W, Granstein RD. Thalidomide inhibits tumor necrosis factor-alpha production and antigen presentation by Langerhans cells. J Invest Dermatol (2003) 121(5):1060–5. doi: 10.1046/j.1523-1747.2003.12565.x
150. Mannon PJ, Leon F, Fuss IJ, Walter BA, Begnami M, Quezado M, et al. Successful granulocyte-colony stimulating factor treatment of Crohn’s disease is associated with the appearance of circulating interleukin-10-producing T cells and increased lamina propria plasmacytoid dendritic cells. Clin Exp Immunol (2009) 155(3):447–56. doi: 10.1111/j.1365-2249.2008.03799.x
151. Yang X, Geng J, Meng H. Glucocorticoid receptor modulates dendritic cell function in ulcerative colitis. Histol Histopathol (2020) 35(12):1379–89. doi: 10.14670/HH-18-241
152. Nielsen OH, Rejnmark L, Moss AC. Role of vitamin D in the natural history of inflammatory bowel disease. J Crohns Colitis (2018) 12(6):742–52. doi: 10.1093/ecco-jcc/jjy025
153. Bartels LE, Bendix M, Hvas CL, Jorgensen SP, Agnholt J, Agger R, et al. Oral vitamin D3 supplementation reduces monocyte-derived dendritic cell maturation and cytokine production in Crohn’s disease patients. Inflammopharmacology (2014) 22(2):95–103. doi: 10.1007/s10787-013-0197-1
154. Prins MMC, Giugliano FP, van Roest M, van de Graaf SFJ, Koelink PJ, Wildenberg ME. Thiopurines correct the effects of autophagy impairment on intestinal healing - a potential role for ARHGAP18/RhoA. Dis Model Mech (2021) 14(4):dmm047233. doi: 10.1242/dmm.047233
155. Nikolic A, Simovic Markovic B, Gazdic M, Randall Harrell C, Fellabaum C, Jovicic N, et al. Intraperitoneal administration of mesenchymal stem cells ameliorates acute dextran sulfate sodium-induced colitis by suppressing dendritic cells. BioMed Pharmacother (2018) 100:426–32. doi: 10.1016/j.biopha.2018.02.060
156. Thomas S, Metzke D, Schmitz J, Dorffel Y, Baumgart DC. Anti-inflammatory effects of Saccharomyces boulardii mediated by myeloid dendritic cells from patients with Crohn’s disease and ulcerative colitis. Am J Physiol Gastrointest Liver Physiol (2011) 301(6):G1083–92. doi: 10.1152/ajpgi.00217.2011
157. Gao H, Li Y, Xu J, Zuo X, Yue T, Xu H, et al. Saccharomyces boulardii protects against murine experimental colitis by reshaping the gut microbiome and its metabolic profile. Front Microbiol (2023) 14:1204122. doi: 10.3389/fmicb.2023.1204122
158. Mann ER, Bernardo D, Ng SC, Rigby RJ, Al-Hassi HO, Landy J, et al. Human gut dendritic cells drive aberrant gut-specific t-cell responses in ulcerative colitis, characterized by increased IL-4 production and loss of IL-22 and IFNgamma. Inflamm Bowel Dis (2014) 20(12):2299–307. doi: 10.1097/MIB.0000000000000223
159. Al-Hassi HO, Mann ER, Sanchez B, English NR, Peake ST, Landy J, et al. Altered human gut dendritic cell properties in ulcerative colitis are reversed by Lactobacillus plantarum extracellular encrypted peptide STp. Mol Nutr Food Res (2014) 58(5):1132–43. doi: 10.1002/mnfr.201300596
160. Waitz G, Petermann S, Liebe S, Emmrich J, Ramlow W. Reduction of dendritic cells by granulocyte and monocyte adsorption apheresis in patients with ulcerative colitis. Dig Dis Sci (2008) 53(9):2507–15. doi: 10.1007/s10620-007-0168-8
161. Ikeda Y, Akbar SM, Matsui H, Murakami H, Onji M. Depletion and decreased function of antigen-presenting dendritic cells caused by lymphocytapheresis in ulcerative colitis. Dis Colon Rectum (2003) 46(4):521–8. doi: 10.1007/s10350-004-6593-2
162. Bartels LE, Jorgensen SP, Bendix M, Hvas CL, Agnholt J, Agger R, et al. 25-Hydroxy vitamin D3 modulates dendritic cell phenotype and function in Crohn’s disease. Inflammopharmacology (2013) 21(2):177–86. doi: 10.1007/s10787-012-0168-y
163. Lindsay JO, Whelan K, Stagg AJ, Gobin P, Al-Hassi HO, Rayment N, et al. Clinical, microbiological, and immunological effects of fructo-oligosaccharide in patients with Crohn’s disease. Gut (2006) 55(3):348–55. doi: 10.1136/gut.2005.074971
164. Benjamin JL, Hedin CR, Koutsoumpas A, Ng SC, McCarthy NE, Hart AL, et al. Randomised, double-blind, placebo-controlled trial of fructo-oligosaccharides in active Crohn’s disease. Gut (2011) 60(7):923–9. doi: 10.1136/gut.2010.232025
165. Amon L, Lehmann CHK, Baranska A, Schoen J, Heger L, Dudziak D. Transcriptional control of dendritic cell development and functions. Int Rev Cell Mol Biol (2019) 349:55–151. doi: 10.1016/bs.ircmb.2019.10.001
166. Fetter K, Weigel M, Ott B, Fritzenwanker M, Stricker S, de Laffolie J, et al. The microbiome landscape in pediatric Crohn’s disease and therapeutic implications. Gut Microbes (2023) 15(2):2247019. doi: 10.1080/19490976.2023.2247019
167. Lopez S, Gomez E, Torres MJ, Pozo D, Fernandez TD, Ariza A, et al. Betalactam antibiotics affect human dendritic cells maturation through MAPK/NF-kB systems. Role in allergic reactions to drugs. Toxicol Appl Pharmacol (2015) 288(3):289–99. doi: 10.1016/j.taap.2015.08.001
168. Stagg AJ, Hart AL, Knight SC, Kamm MA. The dendritic cell: its role in intestinal inflammation and relationship with gut bacteria. Gut (2003) 52(10):1522–9. doi: 10.1136/gut.52.10.1522
169. Alagon Fernandez Del Campo P, De Orta Pando A, Straface JI, Lopez Vega JR, Toledo Plata D, Niezen Lugo SF, et al. The use of probiotic therapy to modulate the gut microbiota and dendritic cell responses in inflammatory bowel diseases. Med Sci (Basel) (2019) 7(2):33. doi: 10.3390/medsci7020033
170. Mann ER, You J, Horneffer-van der Sluis V, Bernardo D, Omar Al-Hassi H, Landy J, et al. Dysregulated circulating dendritic cell function in ulcerative colitis is partially restored by probiotic strain Lactobacillus casei Shirota. Mediators Inflamm (2013) 2013:573576. doi: 10.1155/2013/573576
171. Qin D, Ma Y, Wang Y, Hou X, Yu L. Contribution of lactobacilli on intestinal mucosal barrier and diseases: perspectives and challenges of lactobacillus casei. Life (Basel) (2022) 12(11):1910. doi: 10.3390/life12111910
172. Leitner GC, Worel N, Vogelsang H. Selective granulocyte and monocyte apheresis as a non-pharmacological option for patients with inflammatory bowel disease. Transfus Med Hemother (2012) 39(4):246–52. doi: 10.1159/000341801
173. Sanchez-Garcia J, Serrano-Lopez J, Garcia-Sanchez V, Alvarez-Rivas MA, Jimenez-Moreno R, Perez-Seoane C, et al. Tumor necrosis factor-alpha-secreting CD16+ antigen presenting cells are effectively removed by granulocytapheresis in ulcerative colitis patients. J Gastroenterol Hepatol (2010) 25(12):1869–75. doi: 10.1111/j.1440-1746.2010.06377.x
174. Saul L, Mair I, Ivens A, Brown P, Samuel K, Campbell JDM, et al. 1,25-dihydroxyvitamin D(3) restrains CD4(+) T cell priming ability of CD11c(+) dendritic cells by upregulating expression of CD31. Front Immunol (2019) 10:600. doi: 10.3389/fimmu.2019.00600
175. Kim WS, Song HY, Han JM, Byun EB. GLM, a novel luteolin derivative, attenuates inflammatory responses in dendritic cells: Therapeutic potential against ulcerative colitis. Biochem Biophys Res Commun (2019) 518(1):87–93. doi: 10.1016/j.bbrc.2019.08.012
Keywords: dendritic cell, inflammation, immunity, cancer, inflammatory bowel disease
Citation: Jiménez-Cortegana C, Palomares F, Alba G, Santa-María C, de la Cruz-Merino L, Sánchez-Margalet V and López-Enríquez S (2024) Dendritic cells: the yin and yang in disease progression. Front. Immunol. 14:1321051. doi: 10.3389/fimmu.2023.1321051
Received: 16 October 2023; Accepted: 12 December 2023;
Published: 04 January 2024.
Edited by:
Helena Stabile, Sapienza University of Rome, ItalyReviewed by:
Zheng Yuan, China Academy of Chinese Medical Sciences, ChinaCopyright © 2024 Jiménez-Cortegana, Palomares, Alba, Santa-María, de la Cruz-Merino, Sánchez-Margalet and López-Enríquez. This is an open-access article distributed under the terms of the Creative Commons Attribution License (CC BY). The use, distribution or reproduction in other forums is permitted, provided the original author(s) and the copyright owner(s) are credited and that the original publication in this journal is cited, in accordance with accepted academic practice. No use, distribution or reproduction is permitted which does not comply with these terms.
*Correspondence: Carlos Jiménez-Cortegana, Y2pjb3J0ZWdhbmFAZ21haWwuY29t; Victor Sánchez-Margalet, bWFyZ2FsZXRAdXMuZXM=
†These authors share senior authorship
Disclaimer: All claims expressed in this article are solely those of the authors and do not necessarily represent those of their affiliated organizations, or those of the publisher, the editors and the reviewers. Any product that may be evaluated in this article or claim that may be made by its manufacturer is not guaranteed or endorsed by the publisher.
Research integrity at Frontiers
Learn more about the work of our research integrity team to safeguard the quality of each article we publish.