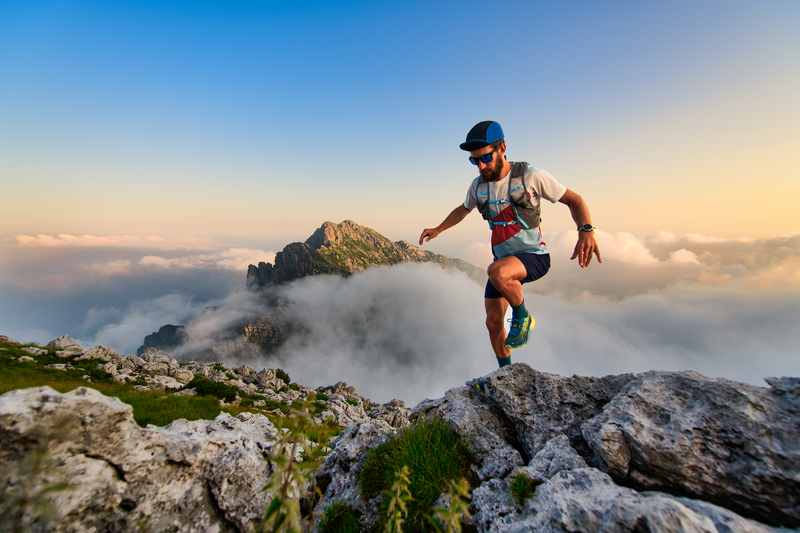
94% of researchers rate our articles as excellent or good
Learn more about the work of our research integrity team to safeguard the quality of each article we publish.
Find out more
MINI REVIEW article
Front. Immunol. , 02 November 2023
Sec. Cytokines and Soluble Mediators in Immunity
Volume 14 - 2023 | https://doi.org/10.3389/fimmu.2023.1303605
This article is part of the Research Topic Modulation of T cell or NK cell exhaustion by cytokines, soluble mediators or transcription factors in cancer immunity and immunotherapy View all articles
Natural killer (NK) cells kill mutant cells through death receptors and cytotoxic granules, playing an essential role in controlling cancer progression. However, in the tumor microenvironment (TME), NK cells frequently exhibit an exhausted status, which impairs their immunosurveillance function and contributes to tumor immune evasion. Emerging studies are ongoing to reveal the properties and mechanisms of NK cell exhaustion in the TME. In this review, we will briefly introduce the maturation, localization, homeostasis, and cytotoxicity of NK cells. We will then summarize the current understanding of the main mechanisms underlying NK cell exhaustion in the TME in four aspects: dysregulation of inhibitory and activating signaling, tumor cell-derived factors, immunosuppressive cells, and metabolism and exhaustion. We will also discuss the therapeutic approaches currently being developed to reverse NK cell exhaustion and enhance NK cell cytotoxicity in the TME.
Natural killer (NK) cells are an important component of the innate immune system and play a critical role in controlling malignancies and viral infections (1). Unlike T cells, NK cells kill tumor and virus-infected cells without antigen presentation, allowing NK cells to elicit a rapid immune response (2). NK cell-based therapies are currently being developed for the treatment of blood cancers as well as various solid tumors (3, 4). However, in the tumor microenvironment (TME), NK cells always exhibit an exhausted state, which facilitates the immune escape of tumor cells and reduces the efficacy of NK cell-based therapies (5). The main features of NK cell exhaustion include impaired cytotoxicity, decreased secretion of cytokines, upregulated expression of inhibitory receptors, downregulated expression of activating receptors, dysregulation of proliferation, and metabolic dysfunction (6, 7). In recent years, the mechanisms of NK cell exhaustion have been intensively studied, although the exact mechanisms have not been fully elucidated. In this review, we summarize the current understanding of the mechanisms of NK cell exhaustion in the TME.
NK cells arise from hematopoietic stem cells in the bone marrow. Mouse NK cells develop mainly in the bone marrow, whereas human NK cells can develop in the bone marrow as well as in other lymphoid tissues such as the thymus, spleen, lymph nodes, and liver (8, 9). Mouse NK cells are defined as CD3− NK1.1+ cells and can be classified into four types based on the presence of two markers, CD27 and CD11b, to indicate their maturation stages. CD27− CD11b− NK cells are immature NK cells with high differentiation potential. These NK cells differentiate into CD27+ CD11b− NK cells and then further develop into CD27+ CD11b+ NK cells that can migrate from bone marrow to peripheral tissues. CD27− CD11b+ NK cells are the mature NK cells with high cytotoxicity (10–15). Human NK cells are defined as CD3– CD56+ cells and can be further divided into two types: CD56bright CD16– and CD56dim CD16+ cells. CD56bright CD16– NK cells account for only 5-10% of NK cells and are less mature and less cytotoxic. They are thought to be the precursors of CD56dim CD16+ NK cells, i.e., mature NK cells. CD56dim CD16+ NK cells account for 90% of NK cells and have higher cytotoxicity (10, 12, 14).
NK cells are widely distributed in various tissues and organs of mammals. In recent decades, studies have shown that NK cells are mainly found in bone marrow, peripheral blood, liver, lung, spleen, and uterus. In addition, there are also significant numbers of NK cells in lymph nodes, thymus, mucosa-associated lymphoid tissues, skin, and kidney (16–20). In peripheral blood, 5-20% of lymphocytes are NK cells (9). In the liver, NK cells are enriched in the hepatic sinusoids. In mice, NK cells account for 5-10% of liver lymphocytes, whereas this proportion can reach 30-50% in humans (16). In the lung, NK cells can account for approximately 10-20% of total lymphocytes (21–23). Although NK cells account for only 2-4% of splenic lymphocytes in mice, their absolute number is quite high (21, 24). NK cells in utero are specialized NK cells that can make up 70% of lymphocytes at the maternal-fetal interface (25, 26).
Adequate numbers of NK cells in peripheral tissues are important for normal immune surveillance function. NK cells are continuously produced by development and differentiation from hematopoietic stem cells in the bone marrow and are replenished to peripheral tissues to replace dead NK cells (27, 28). Previously, mature NK cells were thought to have low proliferation ability. However, recent studies have shown that mature NK cells, similar to T cells, can undergo homeostatic proliferation to contribute to maintaining their numbers in peripheral tissues. This homeostatic proliferation depends on stimulation by several cytokines, including IL-2 and IL-15 (28–30). NK cells even have some memory properties similar to those of long-lived CD8+ T cells, and this immune memory can be maintained for several weeks to months. After re-stimulation by antigens, these long-lived memory NK cells rapidly proliferate and kill target cells (31–33).
Unlike CD8+ T cells, NK cell activation does not require antigen presentation but depends on the dynamic balance of activating and inhibitory signals from target cells, including cancer cells and infected cells (34). Cancer cells can escape the killing from cytotoxic T cells by downregulating the expression of MHC I molecules. However, this reduction of MHC I molecules on the surface of cancer cells triggers the activation of NK cells (35, 36). NK cells kill cancer cells mainly via four approaches. First, NK cells express FasL and TRAIL on the cell surface, which can bind to the corresponding receptors Fas and TRAILR on cancer cells, respectively. This binding triggers the activation of caspase-8 and, subsequently, caspase-3, leading to the apoptosis of target cells (37, 38). Second, NK cells release various cytotoxic granules such as perforin and granzyme B to induce lysis and apoptosis of tumor cells (37, 39–42). Third, the Fcγ receptor III or CD16 on NK cells can recognize the antibodies binding to cancer cells, which induces NK cells to produce more cytotoxic granules to eliminate cancer cells, an effect called antibody-dependent cell-mediated cytotoxicity (ADCC) (43–45). Fourth, NK cells can also secrete IFNγ to induce cancer cell death and regulate other cancer-fighting immune cells (46–48).
The control of NK cell activation is a balance between signals from inhibitory receptors (such as KIR, PD-1, TIGIT, TIM-3, LAG-3, NKG2A, CD96, IL-1R8, and KLRG1) and activating receptors (such as NKG2D, CD16, CD226, NKp30, NKp44, and NKp46) (49–53). In the TME, inhibitory receptors on NK cells and their ligands on tumor cells are always upregulated, whereas activating receptors and ligands are downregulated (Figure 1). For example, in ovarian cancer patients, the percentage of PD-1+ NK cells in peripheral blood was much higher than in healthy individuals (54). When PD-L1 expressed on the surface of tumor cells bound to PD-1 on NK cells, an exhausted phenotype and even apoptotic cell death were induced in NK cells (55–57). TIGIT is a co-inhibitory receptor expressed on NK cells, and the major ligand for TIGIT is CD155 (58). NK cells in intratumoral regions showed significantly higher expression of TIGIT than the NK cells in peritumoral regions. The binding of CD155, expressed on tumor cells, to TIGIT on the surface of NK cells reduced the expression levels of IFNγ and the death ligand TRAIL by NK cells (59). TIM3 is a maturation marker for NK cells, and upregulation of TIM-3 was found in several cancers, including lung, colorectal, and gastric cancer (60–62). At least four ligands for TIM-3 have been found, including galectin-9, CD66a, phosphatidylserine, and HMGB1 (63). The interaction between galectin-9 and TIM-3 was thought to suppress NK cell-mediated cytotoxicity in tumor tissues (64). However, some other studies suggested that galectin-9 might enhance NK cell activity (65, 66). NKG2D is a potent activating receptor expressed on the surface of NK cells. In hepatocellular carcinoma patients, a much higher DNA methylation frequency in the NKG2D promoter region was detected (67). Downregulation of NKG2D on NK cells was found in various cancers such as glioma, leukemia, head and neck cancer, and cervical cancer and contributed to the decreased activity of NK cells (68–71).
Figure 1 This figure summarizes the main mechanisms of NK cell exhaustion in the TME in four aspects: dysregulation of inhibitory and activating signaling, tumor cell-derived factors, immunosuppressive cells, and metabolic exhaustion.
Tumor cells can secrete various factors that inhibit NK cell activity (Figure 1). The binding of MICA/B on tumor cells and NKG2D on NK cells activates NK cells. However, several studies showed that tumor cells also produced and shed soluble MICA/B proteins that could bind to NKG2D and inhibit NK cell activation and cytotoxicity by mechanisms that are still not fully elucidated (72–74). Tumor cells secreted PGE2 to inhibit the killing effects of NK cells by decreasing the levels of NK receptors such as NKp30, NKp44, and NKG2D (75). In addition, PGE2 also reduced the killing effects of NK cells by suppressing IFNγ production (76, 77). TGF-β is an important immunosuppressive factor in balancing the immune response. Not surprisingly, tumor cells can secrete TGF-β to inhibit NK cell activation and cytotoxicity (78, 79). Some studies revealed that extracellular adenosine levels were increased in the TME, which could inhibit degranulation and cytokine production by NK cells (80–82). IL-10 is an important immunoregulatory cytokine with both protumoral and antitumoral effects. In its immunosuppressive role, IL-10 suppresses the expression of NKG2D ligands and increases the expression of inhibitory ligands on tumor cells to inhibit the activation and killing effects of NK cells (83, 84). Some studies showed that IL-10 could also enhance the activities of NK cells (85, 86). CD155 is a ligand for both activating and inhibitory receptors expressed on NK cells. The soluble form of CD155 secreted by melanoma cells was found to inhibit NK cell degranulation and cytotoxicity (87). Tumor cell-derived extracellular vesicles or exosomes can both activate and inhibit NK cell function, depending on the properties of the exosomes. Several studies have shown that tumor cells produce exosomes containing NKG2D ligands and TGF-β, which can impair NK cell proliferation and cytotoxicity (88–90). Tumor cells can also generate exosomes containing noncoding RNAs such as miR-92b, SNHG10, and circUHRF1 to inhibit NK cell activity in the TME (91, 92).
In the TME, there are a number of cells in addition to tumor cells and NK cells (Figure 1) (93). CD4+CD25+ regulatory T cells (Tregs), as immunosuppressive cells, impaired the proliferation and IFNγ production of NK cells through TGF-β signaling (94, 95). Myeloid-derived suppressor cells (MDSCs) in the TME could also inhibit NK cell activity through membrane-bound TGF-β, which decreased the expression levels of NKG2D and IFNγ in NK cells (96). MDSCs increased the level of arginase-1, which reduced IFNγ production but did not affect granzyme B release or NK cell viability (97). In clinical patients with hepatocellular carcinoma, MDSCs mediated the suppression of NK cells through NKp30 (98). Nitric oxide produced by MDSCs inhibited NK cell cytotoxicity by impairing the ADCC response (99). Tumor-associated macrophages (TAMs) play an important role in tumor immune evasion. Several studies showed that TAMs induced NK cell dysfunction through secretion of TGF-β (100, 101). TAMs also inhibited NK cell activity by expressing CD80 and CD86, which bound to CTLA-4 on NK cells. In addition, immunosuppressive chemokine ligands such as CCL5 and CCL22 secreted by TAMs could recruit Tregs to the TME, further suppressing the killing effects of NK cells (102). There are sufficient numbers of neutrophils in the TME to interfere with NK cell activity. For example, neutrophils reduced the expression of CCR1 on NK cells, which could reduce the infiltration of NK cells into tumor tissues. The PD-L1 molecules on neutrophils bound to PD-1 receptors on the surface of NK cells, reducing the amount of IFNγ expressed by NK cells (103). Neutrophils could also release ROS and cathepsin G to inhibit NK cell cytotoxicity (104, 105). In addition to immune cells, tumor stromal cells have been suggested to inhibit the proliferation of NK cells and suppress the expression of NKp44 and NKp46 on NK cells (106). While mesenchymal stromal cells (MSCs) initially promoted NK cell function by releasing type I interferon, they later induced NK cell dysfunction by secreting TGF-β and IL-6, ultimately leading to NK cell senescence (107).
Because of their unique metabolic properties, solid tumor cells generate various metabolic stresses such as nutrient depletion, low oxygen, low pH, and accumulation of waste products in the TME. These stresses not only affect the tumor cells but also severely impair the activity of NK cells in the TME (Figure 1) (108, 109). Adequate energy supply is important for proliferation, survival, and cytotoxicity of NK cells. Some studies suggested that steady-state NK cells preferred oxidative phosphorylation (OXPHOS) for ATP production, whereas activated NK cells relied more on glycolysis (109, 110). Disruption of either glycolysis or OXPHOS may impair NK cell function. Lung tumor cells secreted TGF-β in the TME, which upregulated the expression of fructose-1,6-bisphosphatase in NK cells, resulting in inhibition of glycolysis and decreased cytotoxicity and viability of NK cells (111). In melanoma, inhibition of Srebp activity led to a decrease in cytokine-enhanced glycolysis and OXPHOS in NK cells, which impaired the effector function of NK cells (112). Inhibition of glycolysis and OXPHOS could decrease the secretion of IFNγ by NK cells and attenuate their cytotoxicity to leukemia cells (113).
Hypoxia in the TME contributes to cancer cells escaping NK cells’ killing and developing into advanced tumors. Hypoxia inhibited the expression of heat shock protein 70 and MICA/B of tumor cells, which helped tumor cells escape recognition by NK cells (114). In multiple myeloma, hypoxia inhibited the expression of perforin and granzyme B by NK cells, impairing NK cell cytotoxicity (115). Hypoxia led to the upregulation of HIF-1ɑ and downregulation of NK cell activating receptors such as NKG2D and natural cytotoxicity receptors (116). In addition to NK cells, hypoxia-induced HIF-1ɑ could also increase the expression of PD-L1 on MDSCs, leading to the suppression of T cells (117). A liver cancer study showed that hypoxia induced activation of mTOR-Drp1 proteins in the tumor-infiltrating NK cells, leading to increased mitochondrial fragmentation in these NK cells. As a result, tumor-infiltrating NK cells exhibited lower cytotoxicity and were more prone to cell death compared with NK cells in tumor-adjacent tissues (118).
Most tumor cells rely on aerobic glycolysis for energy production, which is known as the “Warburg effect,” producing a large amount of lactate as a waste product (119). Several studies have found that lactate can lead to the acidification of the TME and impair NK cell activity. For example, melanoma cells highly expressed lactate dehydrogenase, an enzyme that catalyzes lactate synthesis from pyruvate, and the accumulation of lactate in melanomas decreased the number of NK cells and inhibited cytokine production by NK cells (120). To form liver metastases, colorectal cancer cells inhibited the function of liver-resident NK cells by secreting lactate, which lowered pH and damaged mitochondria inside NK cells (121).
Lipid metabolism is critical to the effector function of NK cells. A study showed that obesity-induced accumulation of lipids in NK cells decreased their anti-tumor activity by inhibiting cytotoxic machinery trafficking (122). In B-cell lymphomas, rich fatty acids in the TME impaired IFNγ secretion by NK cells (123). The formation of immunological synapses is critical for recognizing and killing cancer cells by NK cells. In liver cancer, inhibition of sphingomyelin biosynthesis in intratumoral NK cells severely dampened the membrane topology and synapse formation, which reduced NK cell cytotoxicity (124). Amino acid metabolism also regulates NK cell activity. Several works showed that tryptophan-derived kynurenine catalyzed by indoleamine 2,3-dioxygenase (IDO) inhibited NK cell proliferation and decreased activating receptors, including NKp46 and NKG2D, on the surface of NK cells (125, 126). IDO could also catalyze tryptophan to kynurenine in cancer cells, decreasing the expression of NKG2D ligands on the surface of cancer cells by ADAM10, and this reduced expression of NKG2D ligands inhibited degranulation and IFNγ release by NK cells (127).
Recombinant cytokine drugs are being developed to boost NK cell activation. IL-2 is a potent stimulator of NK cell and cytotoxic T cell survival and increases their killing activity. Several recombinant IL-2 drugs are currently under investigation or approved for the treatment of various cancers, such as lung, bladder, ovarian, and renal cell cancers (128–131). IL-12 is secreted mainly by antigen-presenting cells and enhances the proliferation, survival, and cytotoxicity of NK cells. Recombinant IL-12 is currently being tested in clinical trials for the treatment of head and neck cancer in combination with cetuximab, an EGFR inhibitor (132, 133). IL-15 has similar functions to IL-2 but does not have the activation-induced cell death effect (134). An IL-15 superagonist ALT-803 is being used in clinical trials to treat various types of cancers, including non-small-cell lung cancer, head and neck cancer, renal cell cancer, and melanoma (135, 136).
Immune checkpoint inhibitors have been used to block immunosuppressive signals on NK cells to enhance their anti-tumor activity. Blocking the PD-1/PD-L1 inhibitory axis has become a popular therapeutic strategy (137). PD-1/PD-L1 immune checkpoint inhibitors have been approved for the clinical treatment of various cancers (138, 139). Recently, a monoclonal antibody targeting LAG-3 has also been approved by the FDA for the treatment of melanoma (140). Dozens of monoclonal antibodies targeting other immune checkpoints, such as KIR, TIGIT, TIM-3, and NKG2A, are currently being developed and clinically tested (141–143). In addition to immune checkpoint inhibitors, agonistic antibodies are also being developed to activate costimulatory receptors on NK cells to enhance their anti-tumor activity. For example, monoclonal antibodies targeting 4-1BB are being tested in clinical trials for the treatment of lymphoma, melanoma, and non-small-cell lung cancer (144, 145).
Adoptive transfer of NK cells represents another strategy for cancer treatment. Therapeutic NK cells can be purified from peripheral blood or umbilical cord blood or derived from induced pluripotent stem cells. Some NK cell lines, such as NK-92, can also be used for transfusion (146, 147). Prior to transfusion, several cytokines such as IL-2, IL-12, IL-15, and IL-21 and feeder cells are used to promote NK cell activation and proliferation (148, 149). Genetically engineered NK cells such as chimeric antigen receptor (CAR)-NK cells and TCR-NK cells are generated to improve the killing ability and specificity of NK cells (150, 151). For example, anti-CD19 CAR-NK cells have been used in clinical trials to treat lymphoid tumors (152). Synthetic biology methods are also being investigated to help NK cells overcome immunosuppressive TME (153). The development of novel therapies based on NK cells and the combination of existing strategies will contribute greatly to cancer research and treatment.
HJ: Writing – original draft. HY: Writing – original draft. HX: Writing – original draft. KL: Writing – review & editing.
The author(s) declare financial support was received for the research, authorship, and/or publication of this article. This work was financially supported by the Multi-Year Research Grant of the University of Macau (File no. MYRG2020-00121-FHS and MYRG2022-00025-FHS), the Science and Technology Development Fund (FDCT) of Macao (File no. 0147/2020/A3, 044/2021/APD, and 0004/2021/AKP) and Ministry of Education Frontiers Science Centre for Precision Oncology (File no. SP2021-00001-FSCPO and SP2023-00001-FSCPO).
The authors declare that the research was conducted in the absence of any commercial or financial relationships that could be construed as a potential conflict of interest.
All claims expressed in this article are solely those of the authors and do not necessarily represent those of their affiliated organizations, or those of the publisher, the editors and the reviewers. Any product that may be evaluated in this article, or claim that may be made by its manufacturer, is not guaranteed or endorsed by the publisher.
1. Myers JA, Miller JS. Exploring the NK cell platform for cancer immunotherapy. Nat Rev Clin Oncol (2021) 18(2):85–100. doi: 10.1038/s41571-020-0426-7
2. Lanier LL. NK cell recognition. Annu Rev Immunol (2005) 23:225–74. doi: 10.1146/annurev.immunol.23.021704.115526
3. Mehta RS, Randolph B, Daher M, Rezvani K. NK cell therapy for hematologic Malignancies. Int J Hematol (2018) 107:262–70. doi: 10.1007/s12185-018-2407-5
4. Wrona E, Borowiec M, Potemski P. CAR-NK cells in the treatment of solid tumors. Int J Mol Sci (2021) 22(11):5899. doi: 10.3390/ijms22115899
5. Portale F, Di Mitri D. NK cells in cancer: mechanisms of dysfunction and therapeutic potential. Int J Mol Sci (2023) 24(11):9521. doi: 10.3390/ijms24119521
7. Roe K. NK-cell exhaustion, B-cell exhaustion and T-cell exhaustion—the differences and similarities. Immunology (2022) 166(2):155–68. doi: 10.1111/imm.13464
8. Vosshenrich CA, Samson-Villéger SI, Di Santo JP. Distinguishing features of developing natural killer cells. Curr Opin Immunol (2005) 17(2):151–8. doi: 10.1016/j.coi.2005.01.005
9. Abel AM, Yang C, Thakar MS, Malarkannan S. Natural killer cells: development, maturation, and clinical utilization. Front Immunol (2018) 9:1869. doi: 10.3389/fimmu.2018.01869
10. Béziat V, Duffy D, Quoc SN, Garff-Tavernier L, Decocq J, Combadière B, et al. CD56brightCD16+ NK cells: a functional intermediate stage of NK cell differentiation. J Immunol (2011) 186(12):6753–61. doi: 10.4049/jimmunol.1100330
11. Chiossone L, Chaix J, Fuseri N, Roth C, Vivier E, Walzer T. Maturation of mouse NK cells is a 4-stage developmental program. Blood (2009) 113(22):5488–96. doi: 10.1182/blood-2008-10-187179
12. Crinier A, Narni-Mancinelli E, Ugolini S, Vivier E. SnapShot: natural killer cells. Cell (2020) 180(6):1280–.e1. doi: 10.1016/j.cell.2020.02.029
13. Fu B, Wang F, Sun R, Ling B, Tian Z, Wei H. CD11b and CD27 reflect distinct population and functional specialization in human natural killer cells. Immunology (2011) 133(3):350–9. doi: 10.1111/j.1365-2567.2011.03446.x
14. Riggan L, Shah S, O’Sullivan TE. Arrested development: suppression of NK cell function in the tumor microenvironment. Clin Transl Immunol (2021) 10(1):e1238. doi: 10.1002/cti2.1238
15. Wang X, Sun R, Hao X, Lian Z-X, Wei H, Tian Z. IL-17 constrains natural killer cell activity by restraining IL-15–driven cell maturation via SOCS3. Proc Natl Acad Sci U.S.A. (2019) 116(35):17409–18. doi: 10.1073/pnas.1904125116
16. Shi F-D, Ljunggren H-G, La Cava A, Van Kaer L. Organ-specific features of natural killer cells. Nat Rev Immunol (2011) 11(10):658–71. doi: 10.1038/nri3065
17. Ebert LM, Meuter S, Moser B. Homing and function of human skin γδ T cells and NK cells: relevance for tumor surveillance. J Immunol (2006) 176(7):4331–6. doi: 10.4049/jimmunol.176.7.4331
18. Dogra P, Rancan C, Ma W, Toth M, Senda T, Carpenter DJ, et al. Tissue determinants of human NK cell development, function, and residence. Cell (2020) 180(4):749–63. doi: 10.1016/j.cell.2020.01.022
19. Ma K, Zheng Z-R, Meng Y. Natural killer cells, as the rising point in tissues, are forgotten in the kidney. Biomolecules (2023) 13(5):748. doi: 10.3390/biom13050748
20. Alizadeh D, Zhang L, Brown CE, Farrukh O, Jensen MC, Badie B. Induction of anti-glioma natural killer cell response following multiple low-dose intracerebral CpG therapy. Clin Cancer Res (2010) 16(13):3399–408. doi: 10.1158/1078-0432.CCR-09-3087
21. Grégoire C, Chasson L, Luci C, Tomasello E, Geissmann F, Vivier E, et al. The trafficking of natural killer cells. Immunol Rev (2007) 220(1):169–82. doi: 10.1111/j.1600-065X.2007.00563.x
22. Hervier B, Russick J, Cremer I, Vieillard V. NK cells in the human lungs. Front Immunol (2019) 10:1263. doi: 10.3389/fimmu.2019.01263
23. Cong J, Wei H. Natural killer cells in the lungs. Front Immunol (2019) 10:1416. doi: 10.3389/fimmu.2019.01416
24. Hensel JA, Khattar V, Ponnazhagan S. Characterization of immune cell subtypes in three commonly used mouse strains reveals gender and strain-specific variations. Lab Invest (2019) 99(1):93–106. doi: 10.1038/s41374-018-0137-1
25. Chen X, Mariee N, Jiang L, Liu Y, Wang CC, Li TC, et al. Measurement of uterine natural killer cell percentage in the periimplantation endometrium from fertile women and women with recurrent reproductive failure: establishment of a reference range. Am J Obstet Gynecol (2017) 217(6):680. e1–. e6. doi: 10.1016/j.ajog.2017.09.010
26. Sojka DK. Uterine natural killer cell heterogeneity: lessons from mouse models. Front Immunol (2020) 11:290. doi: 10.3389/fimmu.2020.00290
27. Knorr DA, Bachanova V, Verneris MR, Miller JS. Clinical utility of natural killer cells in cancer therapy and transplantation. Semin Immunol (2014) 26(2):161–72. doi: 10.1016/j.smim.2014.02.002
28. Zhang Y, Wallace DL, De Lara CM, Ghattas H, Asquith B, Worth A, et al. In vivo kinetics of human natural killer cells: the effects of ageing and acute and chronic viral infection. Immunology (2007) 121(2):258–65. doi: 10.1111/j.1365-2567.2007.02573.x
29. Lutz CT, Karapetyan A, Al-Attar A, Shelton BJ, Holt KJ, Tucker JH, et al. Human NK cells proliferate and die in vivo more rapidly than T cells in healthy young and elderly adults. J Immunol (2011) 186(8):4590–8. doi: 10.4049/jimmunol.1002732
30. Pfefferle A, Jacobs B, Haroun-Izquierdo A, Kveberg L, Sohlberg E, Malmberg K-J. Deciphering natural killer cell homeostasis. Front Immunol (2020) 11:812. doi: 10.3389/fimmu.2020.00812
31. Sun JC, Lanier LL. NK cell development, homeostasis and function: parallels with CD8+ T cells. Nat Rev Immunol (2011) 11(10):645–57. doi: 10.1038/nri3044
32. Sun JC, Beilke JN, Bezman NA, Lanier LL. Homeostatic proliferation generates long-lived natural killer cells that respond against viral infection. J Exp Med (2011) 208(2):357–68. doi: 10.1084/jem.20100479
33. Sun JC, Beilke JN, Lanier LL. Adaptive immune features of natural killer cells. Nature (2009) 457(7229):557–61. doi: 10.1038/nature07665
34. Vivier E, Tomasello E, Baratin M, Walzer T, Ugolini S. Functions of natural killer cells. Nat Immunol (2008) 9(5):503–10. doi: 10.1038/ni1582
35. Fruci D, Benevolo M, Cifaldi L, Lorenzi S, Monaco EL, Tremante E, et al. Major histocompatibility complex class I and tumour immuno-evasion: how to fool T cells and natural killer cells at one time. Curr Oncol (2012) 19(1):39–41. doi: 10.3747/co.19.945
36. Raulet DH. Missing self recognition and self tolerance of natural killer (NK) cells. Semin Immunol (2006) 18(3):145–50. doi: 10.1016/j.smim.2006.03.003
37. Prager I, Watzl C. Mechanisms of natural killer cell-mediated cellular cytotoxicity. J Leukoc Biol (2019) 105(6):1319–29. doi: 10.1002/JLB.MR0718-269R
38. Yang H, Jia H, Zhao Q, Luo KQ. Visualization of natural killer cell-mediated killing of cancer cells at single-cell resolution in live zebrafish. Biosens Bioelectron (2022) 216:114616. doi: 10.1016/j.bios.2022.114616
39. Masson D, Tschopp J. Isolation of a lytic, pore-forming protein (perforin) from cytolytic T-lymphocytes. J Biol Chem (1985) 260(16):9069–72. doi: 10.1016/S0021-9258(17)39328-6
40. Metkar SS, Wang B, Aguilar-Santelises M, Raja SM, Uhlin-Hansen L, Podack E, et al. Cytotoxic cell granule-mediated apoptosis: perforin delivers granzyme B-serglycin complexes into target cells without plasma membrane pore formation. Immunity (2002) 16(3):417–28. doi: 10.1016/s1074-7613(02)00286-8
41. Shi L, Mai S, Israels S, Browne K, Trapani JA, Greenberg AH. Granzyme B (GraB) autonomously crosses the cell membrane and perforin initiates apoptosis and GraB nuclear localization. J Exp Med (1997) 185(5):855–66. doi: 10.1084/jem.185.5.855
42. Trapani JA, Browne KA, Smyth MJ, Jans DA. Localization of granzyme B in the nucleus: a putative role in the mechanism of cytotoxic lymphocyte-mediated apoptosis. J Biol Chem (1996) 271(8):4127–33. doi: 10.1074/jbc.271.8.4127
43. Lanier LL, Ruitenberg JJ, Phillips J. Functional and biochemical analysis of CD16 antigen on natural killer cells and granulocytes. J Immunol (1988) 141(10):3478–85. doi: 10.4049/jimmunol.141.10.3478
44. Ravetch JV, Bolland S. IgG fc receptors. Annu Rev Immunol (2001) 19(1):275–90. doi: 10.1146/annurev.immunol.19.1.275
45. Wang W, Erbe AK, Hank JA, Morris ZS, Sondel PM. NK cell-mediated antibody-dependent cellular cytotoxicity in cancer immunotherapy. Front Immunol (2015) 6:368. doi: 10.3389/fimmu.2015.00368
46. Berthou C, Bourge J-F, Zhang Y, Soulié A, Geromin D, Denizot Y, et al. Interferon-γ–induced membrane PAF-receptor expression confers tumor cell susceptibility to NK perforin-dependent lysis. Blood (2000) 95(7):2329–36. doi: 10.1182/blood.V95.7.2329
47. Kaplan DH, Shankaran V, Dighe AS, Stockert E, Aguet M, Old LJ, et al. Demonstration of an interferon γ-dependent tumor surveillance system in immunocompetent mice. Proc Natl Acad Sci U.S.A. (1998) 95(13):7556–61. doi: 10.1073/pnas.95.13.7556
48. Cui F, Qu D, Sun R, Zhang M, Nan K. NK cell−produced IFN−γ regulates cell growth and apoptosis of colorectal cancer by regulating IL−15. Exp Ther Med (2020) 19(2):1400–6. doi: 10.3892/etm.2019.8343
49. Ayuso JM, Rehman S, Virumbrales-Munoz M, McMinn PH, Geiger P, Fitzgerald C, et al. Microfluidic tumor-on-a-chip model to evaluate the role of tumor environmental stress on NK cell exhaustion. Sci Adv (2021) 7(8):eabc2331. doi: 10.1126/sciadv.abc2331
50. Sun H, Sun C. The rise of NK cell checkpoints as promising therapeutic targets in cancer immunotherapy. Front Immunol (2019) 10:2354. doi: 10.3389/fimmu.2019.02354
51. Zhang C, Liu Y. Targeting NK cell checkpoint receptors or molecules for cancer immunotherapy. Front Immunol (2020) 11:1295. doi: 10.3389/fimmu.2020.01295
52. Chan CJ, Smyth MJ, Martinet L. Molecular mechanisms of natural killer cell activation in response to cellular stress. Cell Death Differ (2014) 21(1):5–14. doi: 10.1038/cdd.2013.26
53. Molgora M, Bonavita E, Ponzetta A, Riva F, Barbagallo M, Jaillon S, et al. IL-1R8 is a checkpoint in NK cells regulating anti-tumour and anti-viral activity. Nature (2017) 551(7678):110–4. doi: 10.1038/nature24293
54. Pesce S, Greppi M, Tabellini G, Rampinelli F, Parolini S, Olive D, et al. Identification of a subset of human natural killer cells expressing high levels of programmed death 1: A phenotypic and functional characterization. J Allergy Clin Immunol (2017) 139(1):335–46. doi: 10.1016/j.jaci.2016.04.025
55. Liu Y, Cheng Y, Xu Y, Wang Z, Du X, Li C, et al. Increased expression of programmed cell death protein 1 on NK cells inhibits NK-cell-mediated anti-tumor function and indicates poor prognosis in digestive cancers. Oncogene (2017) 36(44):6143–53. doi: 10.1038/onc.2017.209
56. Zitvogel L, Tesniere A, Kroemer G. Cancer despite immunosurveillance: immunoselection and immunosubversion. Nat Rev Immunol (2006) 6(10):715–27. doi: 10.1038/nri1936
57. Concha-Benavente F, Kansy B, Moskovitz J, Moy J, Chandran U, Ferris RL. PD-L1 mediates dysfunction in activated PD-1+ NK cells in head and neck cancer patients. Cancer Immunol Res (2018) 6(12):1548–60. doi: 10.1158/2326-6066.CIR-18-0062
58. Stanietsky N, Simic H, Arapovic J, Toporik A, Levy O, Novik A, et al. The interaction of TIGIT with PVR and PVRL2 inhibits human NK cell cytotoxicity. Proc Natl Acad Sci U.S.A. (2009) 106(42):17858–63. doi: 10.1073/pnas.0903474106
59. Zhang Q, Bi J, Zheng X, Chen Y, Wang H, Wu W, et al. Blockade of the checkpoint receptor TIGIT prevents NK cell exhaustion and elicits potent anti-tumor immunity. Nat Immunol (2018) 19(7):723–32. doi: 10.1038/s41590-018-0132-0
60. Datar I, Sanmamed MF, Wang J, Henick BS, Choi J, Badri T, et al. Expression analysis and significance of PD-1, LAG-3, and TIM-3 in human non–small cell lung cancer using spatially resolved and multiparametric single-cell analysis. Clin Cancer Res (2019) 25(15):4663–73. doi: 10.1158/1078-0432.CCR-18-4142
61. Li X, Lu H, Gu Y, Zhang X, Zhang G, Shi T, et al. Tim-3 suppresses the killing effect of Vγ9Vδ2 T cells on colon cancer cells by reducing perforin and granzyme B expression. Exp Cell Res (2020) 386(1):111719. doi: 10.1016/j.yexcr.2019.111719
62. Wang Z, Zhu J, Gu H, Yuan Y, Zhang B, Zhu D, et al. The clinical significance of abnormal Tim-3 expression on NK cells from patients with gastric cancer. Immunol Invest (2015) 44(6):578–89. doi: 10.3109/08820139.2015.1052145
63. Zhao L, Cheng S, Fan L, Zhang B, Xu S. TIM-3: An update on immunotherapy. Int Immunopharmacol (2021) 99:107933. doi: 10.1016/j.intimp.2021.107933
64. Komita H, Koido S, Hayashi K, Kan S, Ito M, Kamata Y, et al. Expression of immune checkpoint molecules of T cell immunoglobulin and mucin protein 3/galectin-9 for NK cell suppression in human gastrointestinal stromal tumors. Oncol Rep (2015) 34(4):2099–105. doi: 10.3892/or.2015.4149
65. Gleason MK, Lenvik TR, McCullar V, Felices M, O'Brien MS, Cooley SA, et al. Tim-3 is an inducible human natural killer cell receptor that enhances interferon gamma production in response to galectin-9. Blood (2012) 119(13):3064–72. doi: 10.1182/blood-2011-06-360321
66. Rahmati A, Bigam S, Elahi S. Galectin-9 promotes natural killer cells activity via interaction with CD44. Front Immunol (2023) 14:1131379. doi: 10.3389/fimmu.2023.1131379
67. Zhao N-H, Qian Y, Wu C-S, Wang J-W, Fang Y, Fan X-P, et al. Diagnostic value of NKG2D promoter methylation in hepatitis B virus-associated hepatocellular carcinoma. biomark Med (2019) 13(13):1093–105. doi: 10.2217/bmm-2019-0102
68. Crane CA, Han SJ, Barry JJ, Ahn BJ, Lanier LL, Parsa AT. TGF-β downregulates the activating receptor NKG2D on NK cells and CD8+ T cells in glioma patients. Neuro-Oncol (2010) 12(1):7–13. doi: 10.1093/neuonc/nop009
69. Dasgupta S, Bhattacharya-Chatterjee M, O’Malley BW, Chatterjee SK. Inhibition of NK cell activity through TGF-β1 by down-regulation of NKG2D in a murine model of head and neck cancer. J Immunol (2005) 175(8):5541–50. doi: 10.4049/jimmunol.175.8.5541
70. Garcia-Iglesias T, del Toro-Arreola A, Albarran-Somoza B, del Toro-Arreola S, Sanchez-Hernandez PE, Ramirez-Dueñas MG, et al. Low NKp30, NKp46 and NKG2D expression and reduced cytotoxic activity on NK cells in cervical cancer and precursor lesions. BMC Cancer (2009) 9:1–8. doi: 10.1186/1471-2407-9-186
71. Hilpert J, Grosse-Hovest L, Grünebach F, Buechele C, Nuebling T, Raum T, et al. Comprehensive analysis of NKG2D ligand expression and release in leukemia: implications for NKG2D-mediated NK cell responses. J Immunol (2012) 189(3):1360–71. doi: 10.4049/jimmunol.1200796
72. Baginska J, Viry E, Paggetti J, Medves S, Berchem G, Moussay E, et al. The critical role of the tumor microenvironment in shaping natural killer cell-mediated anti-tumor immunity. Front Immunol (2013) 4:490. doi: 10.3389/fimmu.2013.00490
73. Duan S, Guo W, Xu Z, He Y, Liang C, Mo Y, et al. Natural killer group 2D receptor and its ligands in cancer immune escape. Mol Cancer (2019) 18(1):1–14. doi: 10.1186/s12943-019-0956-8
74. Wang X, Lundgren AD, Singh P, Goodlett DR, Plymate SR, Wu JD. An six-amino acid motif in the α3 domain of MICA is the cancer therapeutic target to inhibit shedding. Biochem Biophys Res Commun (2009) 387(3):476–81. doi: 10.1016/j.bbrc.2009.07.062
75. Pietra G, Manzini C, Rivara S, Vitale M, Cantoni C, Petretto A, et al. Melanoma cells inhibit natural killer cell function by modulating the expression of activating receptors and cytolytic activity. Cancer Res (2012) 72(6):1407–15. doi: 10.1158/0008-5472.CAN-11-2544
76. Holt D, Ma X, Kundu N, Fulton A. Prostaglandin E 2 (PGE 2) suppresses natural killer cell function primarily through the PGE 2 receptor EP4. Cancer Immunol Immunother (2011) 60:1577–86. doi: 10.1007/s00262-011-1064-9
77. Knudsen NH, Manguso RT. Tumor-derived PGE2 gives NK cells a headache. Immunity (2020) 53(6):1131–2. doi: 10.1016/j.immuni.2020.11.018
78. Lee J-C, Lee K-M, Kim D-W, Heo DS. Elevated TGF-β1 secretion and down-modulation of NKG2D underlies impaired NK cytotoxicity in cancer patients. J Immunol (2004) 172(12):7335–40. doi: 10.4049/jimmunol.172.12.7335
79. Wilson EB, El-Jawhari JJ, Neilson AL, Hall GD, Melcher AA, Meade JL, et al. Human tumour immune evasion via TGF-β blocks NK cell activation but not survival allowing therapeutic restoration of anti-tumour activity. PloS One (2011) 6(9):e22842. doi: 10.1371/journal.pone.0022842
80. Hoskin DW, Mader JS, Furlong SJ, Conrad DM, Blay J. Inhibition of T cell and natural killer cell function by adenosine and its contribution to immune evasion by tumor cells. Int J Oncol (2008) 32(3):527–35. doi: 10.3892/ijo.32.3.527
81. Williams BA, Manzer A, Blay J, Hoskin DW. Adenosine acts through a novel extracellular receptor to inhibit granule exocytosis by natural killer cells. Biochem Biophys Res Commun (1997) 231(2):264–9. doi: 10.1006/bbrc.1997.6077
82. Lokshin A, Raskovalova T, Huang X, Zacharia LC, Jackson EK, Gorelik E. Adenosine-mediated inhibition of the cytotoxic activity and cytokine production by activated natural killer cells. Cancer Res (2006) 66(15):7758–65. doi: 10.1158/0008-5472.CAN-06-0478
83. Berti FCB, de Oliveira KB, Berti F, de Oliveira K. IL-10 in cancer: Just a classical immunosuppressive factor or also an immunostimulating one? AIMS Allergy Immunol (2018) 2(2):88–97. doi: 10.3934/Allergy.2018.2.88
84. Huang S, Ullrich SE, Bar-Eli M. Regulation of tumor growth and metastasis by interleukin-10: the melanoma experience. J Interferon Cytokine Res (1999) 19(7):697–703. doi: 10.1089/107999099313532
85. Mocellin S, Panelli M, Wang E, Rossi CR, Pilati P, Nitti D, et al. IL-10 stimulatory effects on human NK cells explored by gene profile analysis. Genes Immun (2004) 5(8):621–30. doi: 10.1038/sj.gene.6364135
86. Wang Z, Guan D, Huo J, Biswas SK, Huang Y, Yang Y, et al. IL-10 enhances human natural killer cell effector functions via metabolic reprogramming regulated by mTORC1 Signaling. Front Immunol (2021) 12:619195. doi: 10.3389/fimmu.2021.619195
87. Okumura G, Iguchi-Manaka A, Murata R, Yamashita-Kanemaru Y, Shibuya A, Shibuya K. Tumor-derived soluble CD155 inhibits DNAM-1–mediated antitumor activity of natural killer cells. J Exp Med (2020) 217(4):e20191290. doi: 10.1084/jem.20191290
88. Batista IA, Quintas ST, Melo SA. The interplay of exosomes and NK cells in cancer biology. Cancers (2021) 13(3):473. doi: 10.3390/cancers13030473
89. Hedlund M, Nagaeva O, Kargl D, Baranov V, Mincheva-Nilsson L. Thermal-and oxidative stress causes enhanced release of NKG2D ligand-bearing immunosuppressive exosomes in leukemia/lymphoma T and B cells. PloS One (2011) 6(2):e16899. doi: 10.1371/journal.pone.0016899
90. Hong C-S, Sharma P, Yerneni SS, Simms P, Jackson EK, Whiteside TL, et al. Circulating exosomes carrying an immunosuppressive cargo interfere with cellular immunotherapy in acute myeloid leukemia. Sci Rep (2017) 7(1):14684. doi: 10.1038/s41598-017-14661-w
91. Liu C-G, Chen J, Goh RMW-J, Liu Y-X, Wang L, Ma Z. The role of tumor-derived extracellular vesicles containing noncoding RNAs in mediating immune cell function and its implications from bench to bedside. Pharmacol Res (2023) (191):106756. doi: 10.1016/j.phrs.2023.106756
92. Tang Q, Yang S, He G, Zheng H, Zhang S, Liu J, et al. Tumor-derived exosomes in the cancer immune microenvironment and cancer immunotherapy. Cancer Lett (2022) (548):215823. doi: 10.1016/j.canlet.2022.215823
93. Azizi E, Carr AJ, Plitas G, Cornish AE, Konopacki C, Prabhakaran S, et al. Single-cell map of diverse immune phenotypes in the breast tumor microenvironment. Cell (2018) 174(5):1293–308. doi: 10.1016/j.cell.2018.05.060
94. Ghiringhelli F, Ménard C, Martin F, Zitvogel L. The role of regulatory T cells in the control of natural killer cells: relevance during tumor progression. Immunol Rev (2006) 214(1):229–38. doi: 10.1111/j.1600-065X.2006.00445.x
95. Ghiringhelli F, Ménard C, Terme M, Flament C, Taieb J, Chaput N, et al. CD4+ CD25+ regulatory T cells inhibit natural killer cell functions in a transforming growth factor–β–dependent manner. J Exp Med (2005) 202(8):1075–85. doi: 10.1084/jem.20051511
96. Li H, Han Y, Guo Q, Zhang M, Cao X. Cancer-expanded myeloid-derived suppressor cells induce anergy of NK cells through membrane-bound TGF-β1. J Immunol (2009) 182(1):240–9. doi: 10.4049/jimmunol.182.1.240
97. Goh CC, Roggerson KM, Lee H-C, Golden-Mason L, Rosen HR, Hahn YS. Hepatitis C virus–induced myeloid-derived suppressor cells suppress NK cell IFN-γ production by altering cellular metabolism via arginase-1. J Immunol (2016) 196(5):2283–92. doi: 10.4049/jimmunol.1501881
98. Hoechst B, Voigtlaender T, Ormandy L, Gamrekelashvili J, Zhao F, Wedemeyer H, et al. Myeloid derived suppressor cells inhibit natural killer cells in patients with hepatocellular carcinoma via the NKp30 receptor. Hepatology (2009) 50(3):799–807. doi: 10.1002/hep.23054
99. Stiff A, Trikha P, Mundy-Bosse B, McMichael E, Mace TA, Benner B, et al. Nitric oxide production by myeloid-derived suppressor cells plays a role in impairing Fc receptor–mediated natural killer cell function. Clin Cancer Res (2018) 24(8):1891–904. doi: 10.1158/1078-0432.CCR-17-0691
100. Nuñez SY, Ziblat A, Secchiari F, Torres NI, Sierra JM, Raffo Iraolagoitia XL, et al. Human M2 macrophages limit NK cell effector functions through secretion of TGF-β and engagement of CD85j. J Immunol (2018) 200(3):1008–15. doi: 10.4049/jimmunol.1700737
101. Krneta T, Gillgrass A, Poznanski S, Chew M, Lee AJ, Kolb M, et al. M2-polarized and tumor-associated macrophages alter NK cell phenotype and function in a contact-dependent manner. J Leukoc Biol (2017) 101(1):285–95. doi: 10.1189/jlb.3A1215-552R
102. Noy R, Pollard JW. Tumor-associated macrophages: from mechanisms to therapy. Immunity (2014) 41(1):49–61. doi: 10.1016/j.immuni.2014.06.010
103. Sun R, Xiong Y, Liu H, Gao C, Su L, Weng J, et al. Tumor-associated neutrophils suppress antitumor immunity of NK cells through the PD-L1/PD-1 axis. Transl Oncol (2020) 13(10):100825. doi: 10.1016/j.tranon.2020.100825
104. Romero AI, Thorén FB, Brune M, Hellstrand K. NKp46 and NKG2D receptor expression in NK cells with CD56dim and CD56bright phenotype: regulation by histamine and reactive oxygen species. Br J Haematol (2006) 132(1):91–8. doi: 10.1111/j.1365-2141.2005.05842.x
105. Valayer A, Brea D, Lajoie L, Avezard L, Combes-Soia L, Labas V, et al. Neutrophils can disarm NK cell response through cleavage of NKp46. J Leukoc Biol (2017) 101(1):253–9. doi: 10.1189/jlb.3AB0316-140RR
106. Johann P-D, Vaegler M, Gieseke F, Mang P, Armeanu-Ebinger S, Kluba T, et al. Tumour stromal cells derived from paediatric Malignancies display MSC-like properties and impair NK cell cytotoxicity. BMC Cancer (2010) 10:1–10. doi: 10.1186/1471-2407-10-501
107. Petri RM, Hackel A, Hahnel K, Dumitru CA, Bruderek K, Flohe SB, et al. Activated tissue-resident mesenchymal stromal cells regulate natural killer cell immune and tissue-regenerative function. Stem Cell Rep (2017) 9(3):985–98. doi: 10.1016/j.stemcr.2017.06.020
108. O’Brien KL, Finlay DK. Immunometabolism and natural killer cell responses. Nat Rev Immunol (2019) 19(5):282–90. doi: 10.1038/s41577-019-0139-2
109. Yang Y, Chen L, Zheng B, Zhou S. Metabolic hallmarks of natural killer cells in the tumor microenvironment and implications in cancer immunotherapy. Oncogene (2023) 42(1):1–10. doi: 10.1038/s41388-022-02562-w
110. Keating SE, Zaiatz-Bittencourt V, Loftus RM, Keane C, Brennan K, Finlay DK, et al. Metabolic reprogramming supports IFN-γ production by CD56bright NK cells. J Immunol (2016) 196(6):2552–60. doi: 10.4049/jimmunol.1501783
111. Cong J, Wang X, Zheng X, Wang D, Fu B, Sun R, et al. Dysfunction of natural killer cells by FBP1-induced inhibition of glycolysis during lung cancer progression. Cell Metab (2018) 28(2):243–55. doi: 10.1016/j.cmet.2018.06.021
112. Assmann N, O'Brien KL, Donnelly RP, Dyck L, Zaiatz-Bittencourt V, Loftus RM, et al. Srebp-controlled glucose metabolism is essential for NK cell functional responses. Nat Immunol (2017) 18(11):1197–206. doi: 10.1038/ni.3838
113. Wang Z, Guan D, Wang S, Chai LYA, Xu S, Lam K-P. Glycolysis and oxidative phosphorylation play critical roles in natural killer cell receptor-mediated natural killer cell functions. Front Immunol (2020) 11:202. doi: 10.3389/fimmu.2020.00202
114. Schilling D, Tetzlaff F, Konrad S, Li W, Multhoff G. A hypoxia-induced decrease of either MICA/B or Hsp70 on the membrane of tumor cells mediates immune escape from NK cells. Cell Stress Chaperones (2015) 20:139–47. doi: 10.1007/s12192-014-0532-5
115. Sarkar S, Germeraad WT, Rouschop KM, Steeghs EM, Mv G, GM B, et al. Hypoxia induced impairment of NK cell cytotoxicity against multiple myeloma can be overcome by IL-2 activation of the NK cells. PloS One (2013) 8(5):e64835. doi: 10.1371/journal.pone.0064835
116. Balsamo M, Manzini C, Pietra G, Raggi F, Blengio F, Mingari MC, et al. Hypoxia downregulates the expression of activating receptors involved in NK-cell-mediated target cell killing without affecting ADCC. Eur J Immunol (2013) 43(10):2756–64. doi: 10.1002/eji.201343448
117. Noman MZ, Desantis G, Janji B, Hasmim M, Karray S, Dessen P, et al. PD-L1 is a novel direct target of HIF-1α, and its blockade under hypoxia enhanced MDSC-mediated T cell activation. J Exp Med (2014) 211(5):781–90. doi: 10.1084/jem.20131916
118. Zheng X, Qian Y, Fu B, Jiao D, Jiang Y, Chen P, et al. Mitochondrial fragmentation limits NK cell-based tumor immunosurveillance. Nat Immunol (2019) 20(12):1656–67. doi: 10.1038/s41590-019-0511-1
119. Vander Heiden MG, Cantley LC, Thompson CB. Understanding the Warburg effect: the metabolic requirements of cell proliferation. Science (2009) 324:1029–33. doi: 10.1126/science.1160809
120. Brand A, Singer K, Koehl GE, Kolitzus M, Schoenhammer G, Thiel A, et al. LDHA-associated lactic acid production blunts tumor immunosurveillance by T and NK cells. Cell Metab (2016) 24(5):657–71. doi: 10.1016/j.cmet.2016.08.011
121. Harmon C, Robinson MW, Hand F, Almuaili D, Mentor K, Houlihan DD, et al. Lactate-mediated acidification of tumor microenvironment induces apoptosis of liver-resident NK cells in colorectal liver metastasis. Cancer Immunol Res (2019) 7(2):335–46. doi: 10.1158/2326-6066.CIR-18-0481
122. Michelet X, Dyck L, Hogan A, Loftus RM, Duquette D, Wei K, et al. Metabolic reprogramming of natural killer cells in obesity limits antitumor responses. Nat Immunol (2018) 19(12):1330–40. doi: 10.1038/s41590-018-0251-7
123. Kobayashi T, Lam PY, Jiang H, Bednarska K, Gloury R, Murigneux V, et al. Increased lipid metabolism impairs NK cell function and mediates adaptation to the lymphoma environment. Blood (2020) 136(26):3004–17. doi: 10.1182/blood.2020005602
124. Zheng X, Hou Z, Qian Y, Zhang Y, Cui Q, Wang X, et al. Tumors evade immune cytotoxicity by altering the surface topology of NK cells. Nat Immunol (2023) 24(5):802–13. doi: 10.1038/s41590-023-01462-9
125. Chiesa MD, Carlomagno S, Frumento G, Balsamo M, Cantoni C, Conte R, et al. The tryptophan catabolite L-kynurenine inhibits the surface expression of NKp46-and NKG2D-activating receptors and regulates NK-cell function. Blood (2006) 108(13):4118–25. doi: 10.1182/blood-2006-03-006700
126. Frumento G, Rotondo R, Tonetti M, Damonte G, Benatti U, Ferrara GB. Tryptophan-derived catabolites are responsible for inhibition of T and natural killer cell proliferation induced by indoleamine 2, 3-dioxygenase. J Exp Med (2002) 196(4):459–68. doi: 10.1084/jem.20020121
127. Fang X, Guo L, Xing Z, Shi L, Liang H, Li A, et al. IDO1 can impair NK cells function against non-small cell lung cancer by downregulation of NKG2D Ligand via ADAM10. Pharmacol Res (2022) 177:106132. doi: 10.1016/j.phrs.2022.106132
128. Gladkov O, Ramlau R, Serwatowski P, Milanowski J, Tomeczko J, Komarnitsky PB, et al. Cyclophosphamide and tucotuzumab (huKS-IL2) following first-line chemotherapy in responding patients with extensive-disease small-cell lung cancer. Anti-Cancer Drugs (2015) 26(10):1061–8. doi: 10.1097/CAD.0000000000000281
129. Gustavson L, Nadeau R, Oldfield N. Pharmacokinetics of teceleukin (recombinant human interleukin-2) after intravenous or subcutaneous administration to patients with cancer. J Biol Resp Modif (1989) 8(4):440–9.
130. Majidpoor J, Mortezaee K. Interleukin-2 therapy of cancer-clinical perspectives. Int Immunopharmacol (2021) 98:107836. doi: 10.1016/j.intimp.2021.107836
131. McDermott DF, Cheng S-C, Signoretti S, Margolin KA, Clark JI, Sosman JA, et al. The high-dose aldesleukin “select” trial: a trial to prospectively validate predictive models of response to treatment in patients with metastatic renal cell carcinoma. Clin Cancer Res (2015) 21(3):561–8. doi: 10.1158/1078-0432.CCR-14-1520
132. Luedke E, Jaime-Ramirez AC, Bhave N, Roda J, Choudhary MM, Kumar B, et al. Cetuximab therapy in head and neck cancer: Immune modulation with interleukin-12 and other natural killer cell–activating cytokines. Surgery (2012) 152(3):431–40. doi: 10.1016/j.surg.2012.05.035
133. McMichael EL, Benner B, Atwal LS, Courtney NB, Mo X, Davis ME, et al. A phase I/II trial of cetuximab in combination with interleukin-12 administered to patients with unresectable primary or recurrent head and neck squamous cell carcinoma. Clin Cancer Res (2019) 25(16):4955–65. doi: 10.1158/1078-0432.CCR-18-2108
134. Marks-Konczalik J, Dubois S, Losi JM, Sabzevari H, Yamada N, Feigenbaum L, et al. IL-2-induced activation-induced cell death is inhibited in IL-15 transgenic mice. Proc Natl Acad Sci U.S.A. (2000) 97(21):11445–50. doi: 10.1073/pnas.200363097
135. Margolin K, Morishima C, Velcheti V, Miller JS, Lee SM, Silk AW, et al. Phase I trial of ALT-803, a novel recombinant IL15 complex, in patients with advanced solid tumors. Clin Cancer Res (2018) 24(22):5552–61. doi: 10.1158/1078-0432.CCR-18-0945
136. Wrangle JM, Velcheti V, Patel MR, Garrett-Mayer E, Hill EG, Ravenel JG, et al. ALT-803, an IL-15 superagonist, in combination with nivolumab in patients with metastatic non-small cell lung cancer: a non-randomised, open-label, phase 1b trial. Lancet Oncol (2018) 19(5):694–704. doi: 10.1016/S1470-2045(18)30148-7
137. Hsu J, Hodgins JJ, Marathe M, Nicolai CJ, Bourgeois-Daigneault M-C, Trevino TN, et al. Contribution of NK cells to immunotherapy mediated by PD-1/PD-L1 blockade. J Clin Investig (2018) 128(10):4654–68. doi: 10.1172/JCI99317
138. Quatrini L, Mariotti FR, Munari E, Tumino N, Vacca P, Moretta L. The immune checkpoint PD-1 in natural killer cells: expression, function and targeting in tumour immunotherapy. Cancers (2020) 12(11):3285. doi: 10.3390/cancers12113285
139. Wang D, Bauersachs J, Berliner D. Immune checkpoint inhibitor associated myocarditis and cardiomyopathy: a translational review. Biology (2023) 12(3):472. doi: 10.3390/biology12030472
140. Albrecht LJ, Livingstone E, Zimmer L, SChadendorf D. The latest option: nivolumab and relatlimab in advanced melanoma. Curr Oncol Rep (2023) 25(6):647–57. doi: 10.1007/s11912-023-01406-4
141. Buckle I, Guillerey C. Inhibitory receptors and immune checkpoints regulating natural killer cell responses to cancer. Cancers (2021) 13(17):4263. doi: 10.3390/cancers13174263
142. Alturki NA. Review of the immune checkpoint inhibitors in the context of cancer treatment. J Clin Med (2023) 12(13):4301. doi: 10.3390/jcm12134301
143. Kohrt HE, Thielens A, Marabelle A, Sagiv-Barfi I, Sola C, Chanuc F, et al. Anti-KIR antibody enhancement of anti-lymphoma activity of natural killer cells as monotherapy and in combination with anti-CD20 antibodies. Blood (2014) 123(5):678–86. doi: 10.1182/blood-2013-08-519199
144. Hong DS, Gopal AK, Shoushtari AN, Patel SP, He AR, Ramalingam SS, et al. Utomilumab in patients with immune checkpoint inhibitor-refractory melanoma and non-small-cell lung cancer. Front Immunol (2022) 13:897991. doi: 10.3389/fimmu.2022.897991
145. Timmerman J, Herbaux C, Ribrag V, Zelenetz AD, Houot R, Neelapu SS, et al. Urelumab alone or in combination with rituximab in patients with relapsed or refractory B-cell lymphoma. Am J Hematol (2020) 95(5):510–20. doi: 10.1002/ajh.25757
146. Motallebnejad P, Kantardjieff A, Cichocki F, Azarin SM, Hu W-S. Process engineering of natural killer cell-based immunotherapy. Trends Biotechnol (2023) 41(10):1314–26. doi: 10.1016/j.tibtech.2023.03.018
147. Shin MH, Kim J, Lim SA, Kim J, Kim S-J, Lee K-M. NK cell-based immunotherapies in cancer. Immune Netw (2020) 20(2). doi: 10.4110/in.2020.20.e14
148. Guillerey C, Huntington ND, Smyth MJ. Targeting natural killer cells in cancer immunotherapy. Nat Immunol (2016) 17(9):1025–36. doi: 10.1038/ni.3518
149. Pérez-Martínez A, Fernández L, Valentín J, Martínez-Romera I, Corral MD, Ramírez M, et al. A phase I/II trial of interleukin-15–stimulated natural killer cell infusion after haplo-identical stem cell transplantation for pediatric refractory solid tumors. Cytotherapy (2015) 17(11):1594–603. doi: 10.1016/j.jcyt.2015.07.011
150. Karahan ZS, Aras M, Sütlü T. TCR-NK cells: a novel source for adoptive immunotherapy of cancer. Turk J Hematol (2023) 40(1):1. doi: 10.4274/tjh.galenos.2022.2022.0534
151. Poon AYC, Sugimura R. The prospect of genetically engineering natural killer cells for cancer immunotherapy. Biol Open (2022) 11(12):bio059396. doi: 10.1242/bio.059396
152. Liu E, Marin D, Banerjee P, Macapinlac HA, Thompson P, Basar R, et al. Use of CAR-transduced natural killer cells in CD19-positive lymphoid tumors. N Engl J Med (2020) 382(6):545–53. doi: 10.1056/NEJMoa1910607
Keywords: cancer, tumor microenvironment, NK cell, exhaustion, immunotherapy
Citation: Jia H, Yang H, Xiong H and Luo KQ (2023) NK cell exhaustion in the tumor microenvironment. Front. Immunol. 14:1303605. doi: 10.3389/fimmu.2023.1303605
Received: 28 September 2023; Accepted: 23 October 2023;
Published: 02 November 2023.
Edited by:
Bing Feng, Swiss Federal Institute of Technology Lausanne, SwitzerlandReviewed by:
Andrea Pelosi, Bambino Gesù Children’s Hospital (IRCCS), ItalyCopyright © 2023 Jia, Yang, Xiong and Luo. This is an open-access article distributed under the terms of the Creative Commons Attribution License (CC BY). The use, distribution or reproduction in other forums is permitted, provided the original author(s) and the copyright owner(s) are credited and that the original publication in this journal is cited, in accordance with accepted academic practice. No use, distribution or reproduction is permitted which does not comply with these terms.
*Correspondence: Kathy Qian Luo, a2x1b0B1bS5lZHUubW8=
†These authors have contributed equally to this work
Disclaimer: All claims expressed in this article are solely those of the authors and do not necessarily represent those of their affiliated organizations, or those of the publisher, the editors and the reviewers. Any product that may be evaluated in this article or claim that may be made by its manufacturer is not guaranteed or endorsed by the publisher.
Research integrity at Frontiers
Learn more about the work of our research integrity team to safeguard the quality of each article we publish.