- 1Clinical Research Center, The Second Affiliated Hospital of Guangdong Medical University, Zhanjiang, Guangdong, China
- 2Department of Hepatobiliary Surgery, The Second Affiliated Hospital of Guangdong Medical University, Zhanjiang, Guangdong, China
- 3Department of Hepatobiliary Surgery, Liaobu Hospital of Dongguan City, Dongguan, Guangdong, China
- 4Department of Medicine, Moores Cancer Center, and Sanford Stem Cell Institute, University of California, San Diego, La Jolla, CA, United States
Tumor-associated macrophages (TAMs) are integral to the tumor microenvironment (TME), influencing cancer progression significantly. Attracted by cancer cell signals, TAMs exhibit unparalleled adaptability, aligning with the dynamic tumor milieu. Their roles span from promoting tumor growth and angiogenesis to modulating metastasis. While substantial research has explored the fundamentals of TAMs, comprehending their adaptive behavior, and leveraging it for novel treatments remains challenging. This review delves into TAM polarization, metabolic shifts, and the complex orchestration of cytokines and chemokines determining their functions. We highlight the complexities of TAM-targeted research focusing on their adaptability and potential variability in therapeutic outcomes. Moreover, we discuss the synergy of integrating TAM-focused strategies with established cancer treatments, such as chemotherapy, and immunotherapy. Emphasis is laid on pioneering methods like TAM reprogramming for cancer immunotherapy and the adoption of single-cell technologies for precision intervention. This synthesis seeks to shed light on TAMs’ multifaceted roles in cancer, pinpointing prospective pathways for transformative research and enhancing therapeutic modalities in oncology.
1 Introduction
Tumor-associated macrophages (TAMs) are integral immune cells that occupy a pivotal position within the tumor microenvironment (TME). With their dual roles in both promoting and inhibiting tumor activities, they stand at the forefront of cancer progression research (1–5). Rather than mere bystanders, their active presence in the TME is influenced predominantly by signals from cancer cells, leading to their active recruitment (6, 7).
Numerous preclinical and clinical studies have highlighted the promising therapeutic implications of targeting TAMs. However, this journey presents its set of challenges, from understanding TAM subtypes to crafting precise therapies (8–11). The inherent adaptability of TAMs to diverse stimuli brings light to the unpredictable nature of therapeutic outcomes, further complicating the therapeutic landscape (12, 13).
This review has multifaceted objectives. We aim to deepen the understanding of both researchers and clinicians about TAMs, spotlighting their intricate roles in the complex landscape of cancer progression and potential interventions. We delve into aspects like TAM polarization, the influence of cytokines and chemokines, and related metabolic pathways. Furthermore, we investigate the potential of merging TAM-targeted strategies with conventional treatments, covering chemotherapy, radiation, and immunotherapy. This includes the innovative concept of utilizing reprogrammed TAMs for cancer immunotherapy (14) and employing single-cell-based technologies for personalized therapeutic interventions (15).
In summary, this review highlights the diverse roles of TAMs in oncology, offering a landscape teeming with both opportunities and complexities. We underscore the urgency to refine TAM-focused therapeutic strategies and shed light on ongoing advancements and challenges in cancer immunotherapy. Our intent is to pave a lucid path ahead, pinpointing areas warranting thorough exploration. In presenting this consolidated overview, we aim to equip our readers, whether researchers or medical professionals, with a comprehensive understanding of TAMs in the broader context of cancer progression and therapeutic intervention.
2 The multifaced role of TAMs in the TME
TAMs are essential components of the TME across various cancers. Originating from circulating monocytes, they migrate to the tumor site, these monocytes differentiate into macrophages (MΦ), which are TAMs under the influence of signals from both distressed tissues and neoplastic cells. As these macrophages immerse themselves within the tumor milieu, they further mature into the TAMs (6, 7). Due to their vast phenotypic diversity, TAMs possess the ability to transition between pro-tumor (M1) and anti-tumor (M2) roles, influenced by cues from the TME. Depending on their state and the TME’s signaling, TAMs might either bolster or hinder tumor progression (1, 16).
2.1 Phenotypic diversity and TAM interaction within the TME
TAMs are central players within the TME, characterized by a broad spectrum of phenotypic attributes. Their inherent phenotypic diversity equips them to assume varied roles, ranging from promoting to counteracting tumor activities (1, 4, 17, 18). This diversity becomes particularly pronounced when observing TAM behavior across different cancer types. For instance, in breast cancer, TAMs often support tumor growth by promoting angiogenesis (19) through releasing growth factors such as vascular endothelial growth factor (VEGF), transforming growth factor-β (TGF-β), and platelet-derived growth factor (PDGF) (20), while in melanoma, they predominantly exhibit immune-suppressive characteristics (21, 22).
Diving deeper into the specific factors released by TAMs, these cells secrete a variety of cytokines and growth factors that drive oncogenic activities (17, 23). Notably, the release of cytokines such as IL-6 and IL-10 promotes an immunosuppressive TME, facilitating tumor escape from immune surveillance (24, 25). On the angiogenic front, aside from the well-documented VEGF, TAMs release additional pro-angiogenic factors that augment tumor blood supply, enhancing nutrient availability for rapidly growing tumors (26–28). The interactions between these cytokines and growth factors not only fuel tumor growth and invasion but also shape the overall dynamics of the TME, reinforcing the pro-tumorigenic nature of TAMs (29, 30).
A deeper dive into the molecular mechanisms reveals that TAMs, while often manipulated by tumors to support their growth, can also be utilized in novel therapeutic strategies. There’s an ongoing shift in the therapeutic paradigm to not simply eliminate TAMs but to modulate their behavior. By targeting specific signaling pathways and employing checkpoint inhibitors, there’s potential to reprogram pro-tumorigenic M2-like TAMs into tumor-inhibiting M1-like counterparts (10, 31, 32). Such an approach aims to harness the inherent capabilities of TAMs in tissue repair and homeostasis, converting them from potential adversaries to allies in cancer therapy.
The differentiation into specific phenotypes is largely steered by the cytokines present in the TME, acting as molecular directives for TAMs. M1-type macrophages, which are pro-inflammatory, instigate immune responses against tumor cells and potentially restrict tumor expansion. Conversely, M2-type macrophages, which exhibit anti-inflammatory tendencies, generally facilitate tissue repair and boost angiogenesis. However, in the TME, they can inadvertently foster tumor progression and aid metastasis (33, 34).
TAMs’ presence in the TME isn’t solitary; they are in constant dialogue with other elements like cancer cells, stromal cells, and the extracellular matrix. Through these interactions, TAMs can support angiogenesis, helping form new blood vessels to feed the tumor, but also hinder immune responses, further aiding tumor cells in metastasizing to new locations (1, 31, 32).
2.2 Recruitment, maturation, and regulatory signaling pathways
The TME, serves as a hub of complex interactions, with its cellular and non-cellular components guiding the recruitment and maturation of TAMs. Molecular signals from tumor and stromal cells, notably the chemokine CCL2, actively attract circulating monocytes to the tumor vicinity. Upon arrival, monocytes are further influenced by diverse signals. Growth factors, prominently macrophage-colony stimulating factor (CSF-1) emanating from tumor cells, hypoxia-responsive elements like hypoxia-inducible factor 1-alpha (HIF-1α), and metabolic derivatives such as lactate play vital roles. The significance of contact-dependent signaling, exemplified by the CD200 and CD200R interaction, is also noteworthy. These myriad of cues steer monocytes to differentiate into macrophages, which further evolve into TAMs, reflecting the deep symbiosis between neoplastic cells and TAM adaptability. Such understanding hints at potential therapeutic interventions (6, 7).
In the realm of signaling pathways dictating TAM recruitment and maturation, the CSF1/CSF1R and CCL2/CCR2 axes stand out. The CSF1/CSF1R pathway is indispensable for macrophage survival and maturation, while the CCL2/CCR2 axis chiefly orchestrates monocyte trafficking to the TME (20, 33). Venturing beyond these, other pivotal pathways like STAT3, NF-κB, and PI3K are instrumental in shaping TAM activities, steering them toward either tumor promotion or suppression (35–37).
Given the central role of transcription factors, especially STAT3 and NF-κB, in TAM signaling, it’s pertinent to understand their modulators. Tyrosine kinase inhibitors (TKIs), such as Sunitinib and Sorafenib, have been identified to target the CSF1/CSF1R (38) and STAT3 (36, 39) pathways, respectively, implying potential avenues to redirect TAM functions. Additionally, small molecular inhibitors like BAY 11-7082, targeting the NF-κB pathway, offer promising leads. A deeper comprehension of these inhibitors can elucidate opportunities to alter TAM behavior favorably, rendering enhanced prognoses for patients.
Harnessing this intricate molecular knowledge paves the way for innovative therapeutic strategies, emphasizing the recalibration of TAM dynamics within the TME.
2.3 Modulation of TAM roles by tumor attributes
TAMs are pivotal constituents of the TME. Their behavior and roles are profoundly influenced by the broader tumor context. Key tumor attributes, including its stage and anatomical location, play pivotal roles in determining TAM behavior (40).
In the early stage of tumor development, the TME is characterized by a dominant immune response. This is primarily facilitated by M1-type TAMs known for their pro-inflammatory and anti-tumor properties. Their abundance in these early stages suggests the body’s proactive defense against tumor formation, which could be indicative of positive outcomes for patients (41).
As the tumor evolves, a significant shift occurs within the TME. M2-type TAMs, which assist tumor growth through angiogenesis, immune suppression, and tissue reconstruction, become more prevalent (4, 42). Their increased presence in the advanced stages reflects the tumor’s adeptness at modulating its surrounding environment. Such adaptability might enhance the tumor’s resilience against therapeutic strategies (31, 43–45).
Recognizing the dynamic roles of TAMs, influenced by tumor attributes, underscores the need for an in-depth understanding of the tumor’s condition. Grasping these changes can inform and refine therapeutic approaches, potentially enhancing treatment efficacy and overall patient prognosis.
3 Recognizing the limitations in TAM research
In the pursuit of understanding the role of TAMs in tumor biology, it’s imperative to address the inherent challenges and discrepancies that stand as obstacles to a thorough comprehension and its subsequent clinical application.
3.1 Challenges in TAM isolation techniques
TAMs, integral to the TME, play a pivotal role in influencing tumor dynamics and determining therapeutic responses (13, 46). Successfully isolating TAMs is essential for understanding their functions and devising effective therapeutic strategies (4, 47). However, each isolation technique presents distinct challenges.
For instance, while enzymatic digestion is adept at dismantling the extracellular matrix (ECM), its over-reliance on specific antigenic markers can lead to inaccurate TAM representation (47). Techniques like magnetic associated cell sorting (MACS) & fluorescence-activated cell sorting (FACS), though efficient, can be plagued by inconsistencies stemming from marker variability (48–51).
Adhesion-based techniques, tapping into TAMs’ natural propensity to adhere to certain surfaces, aren’t without their pitfalls. The adherence properties of TAMs, shaped by their originating tumor microenvironments, can vary. For example, TAMs from breast tumors may adhere differently than those from lung tumors, leading to disparities in isolation outcomes. Such variations underscore the influence of unique tumor microenvironments on TAM cellular behaviors, including adhesion (52, 53).
Other methods like density gradient centrifugation might produce heterogeneous cell populations, warranting further purification (54). While laser capture microdissection (LCM) offers precision, its stringent requirements could limit its widespread application (46, 55). Furthermore, tissue microarrays, despite their potential insight, might overlook specific TAM subsets depending on the selected markers (56).
3.2 Challenges in transitioning research to clinical settings
In various cancers, TAMs exhibit distinct interactions and behaviors shaped by the specific TME. For example, in breast cancer, TAMs often support tumor growth, and metastasis, and hinder anti-tumor immune responses (19, 20). In contrast, cervical cancer showcases a strong correlation between heightened TAM infiltration and advanced disease stages, with TAMs enhancing angiogenesis and suppressing immune reactions against cancer cells (57, 58). In melanoma, TAMs not only aid tumor progression but also sometimes foster resistance to standard treatments, although emerging therapies targeting TAMs offer hope (59, 60). Particularly in non-small cell lung cancer, a subtype of lung cancer, the presence of TAMs relates to tumor progression, metastasis, and even resistance to certain treatments, often indicating a reduced survival rate for affected patients (61, 62).
Transitioning these insights from laboratory studies to clinical applications is fraught with challenges. Central to this transition is the need to perfect TAM isolation methods suitable for clinical environments. Embracing and advocating for innovative, streamlined techniques will be critical, aiming to provide a consistent foundation for TAM research and thereby deepening our knowledge while fast-tracking TAM-centric therapeutic avenues.
4 TAM interactions and polarization dynamics
The intricate relationship between TAMs and the TME is a complex interweaving of factors, ranging from TAM activation dynamics, and polarization predispositions, to the distinctive features of the TME, governed by cancer type and its progression stage (17). Understanding this multifaceted interplay is essential to develop efficient TAM-targeted immunotherapies.
4.1 TAM regulatory mechanisms
Within the TME, the behavior and function of TAMs are influenced by multifarious factors including cytokines, metabolic cues, ECM components, and, crucially, hypoxia (63, 64). Hypoxia, characterized by reduced oxygen availability, is a hallmark in the TME and serves as a pivotal modulator of TAM polarization. In response to hypoxic conditions, TAMs are driven toward the M2 phenotype, which tends to support tumor growth and metastasis (65, 66). This hypoxia-induced M2 polarization activates specific transcriptional profiles that are critical for the cellular communication between TAMs and the tumor’s stromal components, including fibroblasts, endothelial cells, and other immune cells (67).
Additionally, TAMs display distinct metabolic signatures which further modulate their activities within the TME (68). Elevated glycolysis paired with decreased oxidative phosphorylation is often observed in TAMs, facilitating their survival in the nutrient depleted and hypoxic TME (68, 69). Increased fatty acid synthesis and uptake are also characteristic of TAMs and are correlated with their immunosuppressive functionalities (68, 70, 71). These unique metabolic patterns significantly contribute to angiogenesis, anti-tumor immunity suppression, and the facilitation of tumor cell invasion and metastasis.
Moreover, the molecular underpinnings governing TAM polarization are intricate (72, 73). When exposed to Th1 cytokines like IFN-γ, TAMs undergo a shift toward the M1 phenotype via the JAK-STAT1 pathway, augmenting their tumoricidal capabilities (74, 75). Lipopolysaccharide (LPS), an endotoxin predominantly derived from Gram-negative bacteria within the tumor microenvironment, serves as an influential modulator for TAMs. It interacts with TLR4 to strengthen the M1-type TAM response via the NF-κB and MAPK pathways (76–78). The source of this LPS can be multifaceted, stemming from tumor-associated bacteria, systemic sources, or even mimics from necrotic tumor cells within the TME (79, 80). Recent evidence suggests that certain tumors, especially in organs with a rich microbial environment, possess an associated microbiome. The gram-negative bacteria present within these tumors release LPS, which becomes a pivotal component of the TME, affecting the behavior of various immune cells, including TAMs. On the contrary, Th2 cytokines, notably IL-4 and IL-13, prompt a tilt toward the M2 phenotype through the activation of the JAK-STAT6 signaling pathway (81). This shift, in turn, promotes tissue repair, angiogenesis, and tumor progression (61). Other molecules such as TGFβ, chemokines, and PGE2 further emphasize the pro-tumorigenic M2 profile (44, 82–84).
Unraveling these multifaceted hypoxia-driven interactions, combined with the intricate molecular mechanisms governing TAM polarization, offers promising avenues for therapeutic interventions (85).
4.2 TAM-TME interaction dynamics
The hypoxic environment within the TME has profound implications for the behavior and functionality of TAM. Hypoxia not only drives TAM polarization but also modulates the complex interplay between TAMs and other cellular components of the TME, including immune cells, stromal cells, and cancer cells (29, 86). This low-oxygen condition intensifies the interactions between TAMs and other TME cells emphasizing the pivotal roles TAMs play, for instance, regulating T-cell functions (87, 88). Additionally, hypoxia augments TAM-associated processes like angiogenesis and communication with stromal cells, ultimately fostering tumor growth (89–91).
Within the TME, several cell types actively modify the microenvironment, each contributing distinct molecular mechanisms that collectively influence tumor dynamics (92). TAMs play a pivotal role by releasing various cytokines and growth factors, inducing angiogenesis via VEGF secretion, fostering tissue remodeling with MMPs, and suppressing immune responses through factors like IL-10 and TGF-β (4, 18, 32, 35). Complementing this, Cancer-Associated Fibroblasts (CAFs) actively remodel the extracellular matrix, heightening tumor stiffness that augments cancer cell migration (93). These fibroblasts secrete growth factors, notably TGF-β, propelling cancer cell proliferation and facilitating epithelial-mesenchymal transition (EMT) (94). CAFs also contribute to the immunosuppressive nature of the TME, recruiting regulatory T cells (Tregs) via CCL2 secretion (83). Similarly, Myeloid-Derived Suppressor Cells (MDSCs) are essential players in dampening the anti-tumor immune response, inhibiting T cell activation with reactive species, and depleting crucial amino acids, which further impede T cell functionality (95).
Macrophages are classically polarized into two primary phenotypes: M1, known for its pro-inflammatory attributes, and M2, recognized for its anti-inflammatory characteristics (74). The M1 macrophages predominantly defend against pathogens and participate in early wound healing, while M2 macrophages orchestrate tissue repair, immunoregulation, and inflammation resolution (96).
Diving deeper, M2 macrophages are further segmented into subtypes, namely M2a, M2b, M2c, and M2d, each distinguished by their specific roles, stimuli responsiveness, and cytokine production (97). Particularly, the M2b subtype, emerging as the focal point of numerous studies, exhibits hybrid pro- and anti-inflammatory activities, asserting their significance in varied physiological and pathological contexts (98).
Current research accentuates the diverse roles of M2b macrophages within both oncological and infectious contexts. M2b macrophages not only participate in cancer promotion, metastasis, and recurrence but also play crucial roles during infectious challenges. These cells, unique among macrophages, are characterized by their secretion of specific pro-inflammatory cytokines. Given their capability to accentuate infections while concomitantly suppressing certain immune responses, there is an increasing emphasis on understanding M2b polarization dynamics. This focus arises from the potential of targeting M2b macrophages for therapeutic innovations, both in cancer and infectious disease scenarios (99–101).
M2b macrophages can be identified based on their distinct cytokine secretion patterns. They are known to express high levels of the anti-inflammatory cytokine IL-10. At the same time, they exhibit reduced IL-12 levels. These cells also possess the capability to release significant quantities of pro-inflammatory agents, such as IL-1β, IL-6, and TNF-α. Interestingly, despite this pro-inflammatory profile, M2b macrophages maintain high IL-10 levels, demonstrating their multifaceted immunomodulatory roles (22). The following Table 1 elucidates the determinants guiding the polarization of macrophages into either M1 or M2 TAM phenotypes.
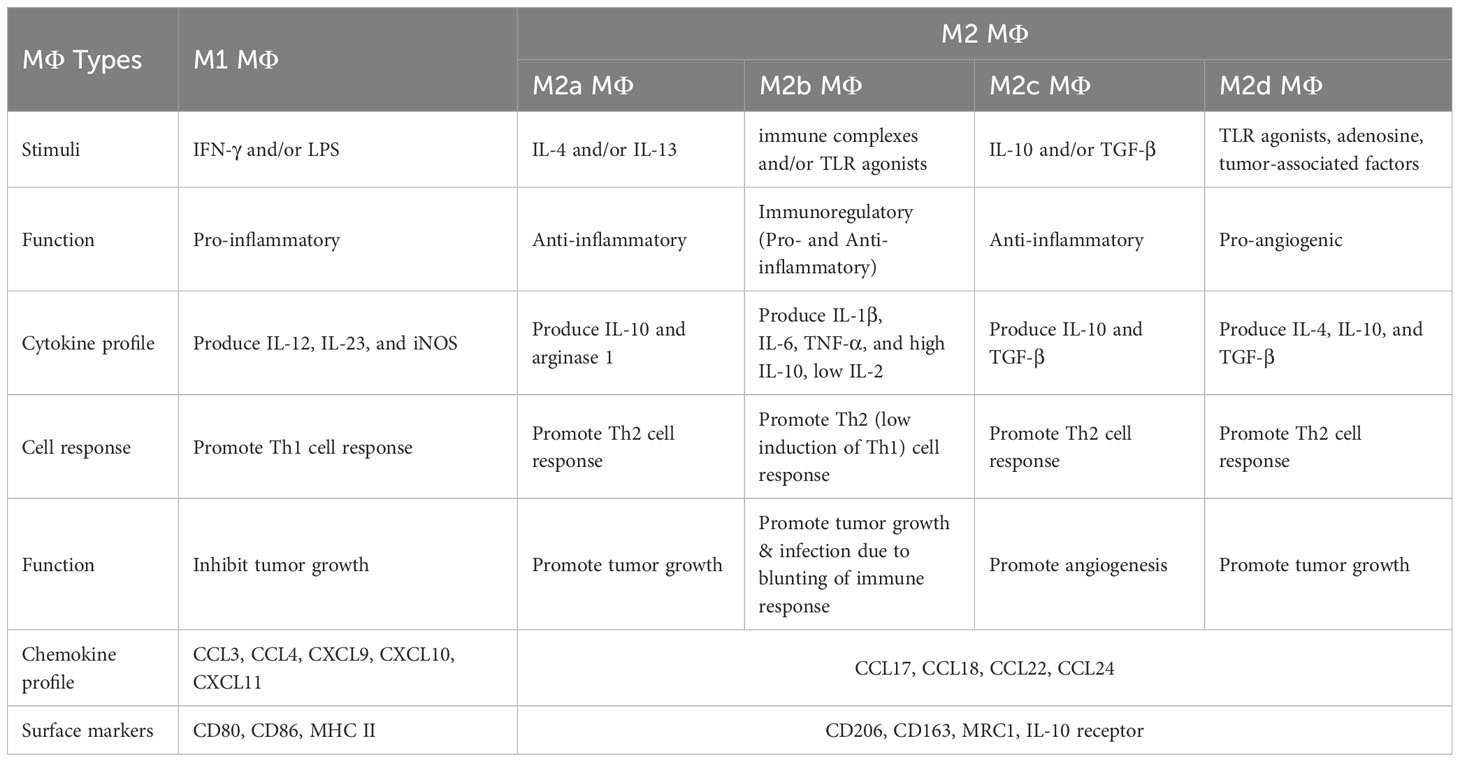
Table 1 Determinants of circulating macrophage polarization into M1 or M2 TAMs (22, 31, 32, 99–102).
4.3 TAM polarization in the TME
Hypoxia in the TME is a pivotal determinant in guiding TAM polarization. Under these low-oxygen conditions, CD8+ T cells lean toward M1-type polarization, whereas Tregs predominantly skew toward the M2-type (9, 27, 102, 103). The interplay between immune checkpoints, especially those involving PD-L1 and PD-1, further intricates TAM polarization within hypoxic TME. he implications of this interaction might have substantial consequences for tumor progression and its subsequent therapeutic targeting (104, 105).
Inflammatory cytokines, such as IL-6, TNF-α, and IL-1β, are instrumental in determining TAM behavior within the TME (106). These cytokines actively guide the polarization of macrophages toward an M2-like, tumor-promoting phenotype. For instance, IL-6, by activating the STAT3 signaling pathway, fosters M2 macrophage differentiation, which in turn is associated with activities that promote tumor growth and progression.
In addition to cytokines, ECM components present in the TME, notably hyaluronan and collagen, play significant roles in TAM functionality. Hyaluronan interacts with TAM receptors like CD44, triggering intracellular pathways that enhance the pro-tumorigenic functions. In contrast, collagen provides a structural scaffold that assists TAM. When collagen binds to integrin receptors on TAMs, it influences their differentiation and function within the TME (32, 107, 108).
A thorough comprehension of the interplay and dynamics of TAM in a hypoxic TME is essential for devising therapeutic strategies that amplify anti-tumor responses. Figure 1 illustrates the dynamics of TAM polarization within the TME, providing a visual representation of the factors that influence their differentiation and subsequent roles in tumor progression and immune response modulation.
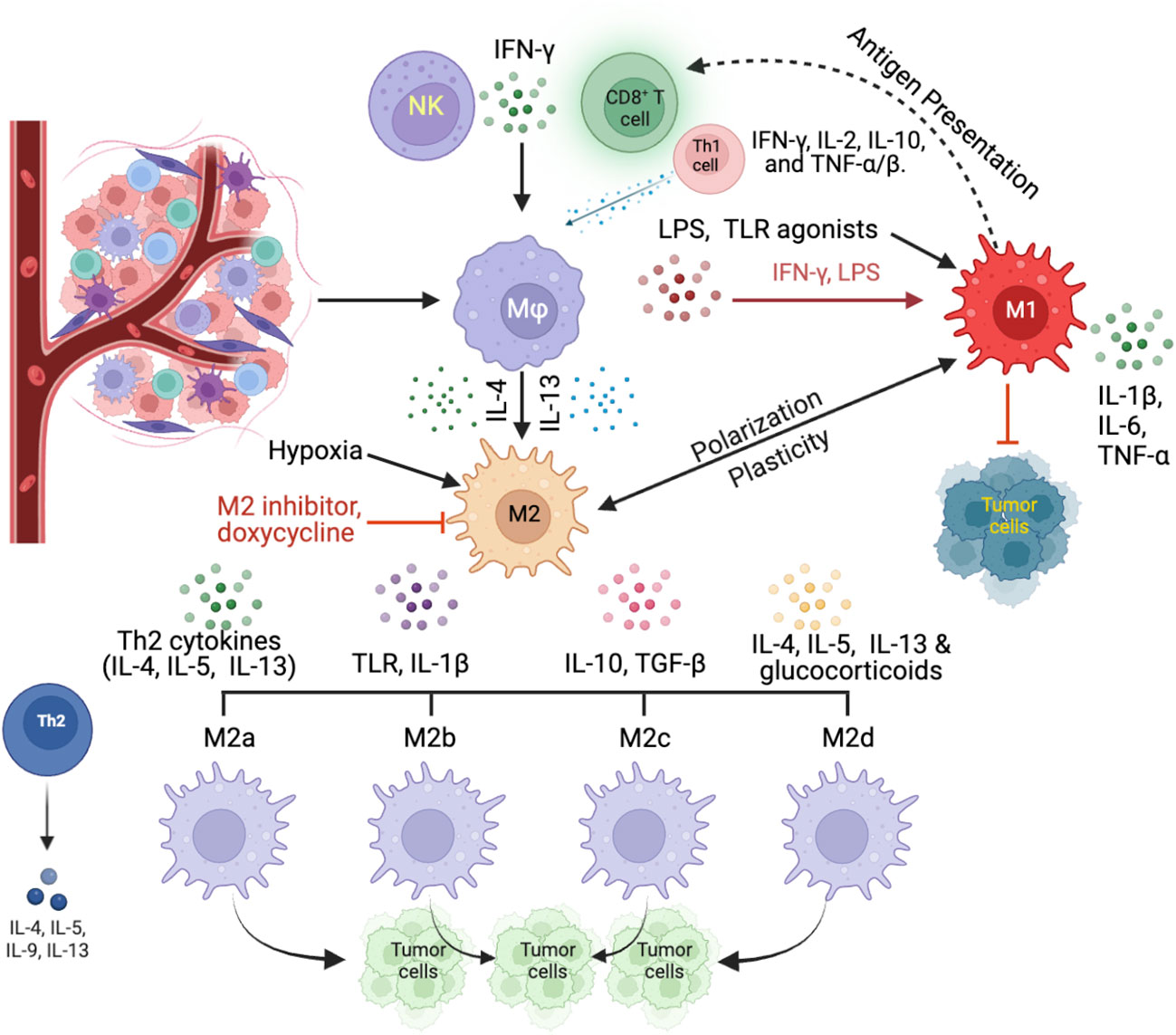
Figure 1 Dynamics of TAM polarization within the TME. TAMs, originating from circulating monocytes, differentiate into macrophages within the TME in response to specific signals. Key influencers include the cytokine IFN-γ, sourced from activated CD8+ T cells and NK cells, as well as a combination of IFN-γ, IL-2, IL-10, and TNF-α/β from Th1 cells (CD4+ T cells). External factors like LPS, predominantly derived from Gram-negative bacteria within the tumor microenvironment, and other TLR agonists, which are typically released from bacteria, and necrotic tumor cells, or systemic sources, favor the development of the M1 macrophage phenotype. In contrast, potent Th2 cytokines, such as IL-4 and IL-13, along with hypoxic conditions, steer macrophages toward the TAM2 direction. TAM1 macrophages, influenced dominantly by IFN-γ and LPS, assume a pro-inflammatory stance, producing cytokines like IL-1β, IL-6, and TNF-α that can promote tumor cell apoptosis. On the other hand, TAM2 macrophages, shaped by IL-4 and IL-13, exhibit an anti-inflammatory profile, secreting cytokines such as IL-10 and TGF-β, which not only suppress immune responses but also enhance angiogenesis processes.
5 Ongoing clinical trials
TAMs play a pivotal role in tumor progression and therapeutic outcomes. Given this, there is significant interest in the oncological domain regarding TAMs, with numerous clinical trials emphasizing TAM-centered therapies.
5.1 Clinical investigations centered around TAMs
TAMs have emerged as key players in the TME, guiding both tumor progression mechanisms and innovative therapeutic strategies. Several clinical trials are underway to harness the potential of TAMs, and we have sourced these from the extensive database www.clinicaltrials.gov. Table 2 provides a snapshot of these trials, both ongoing and completed.
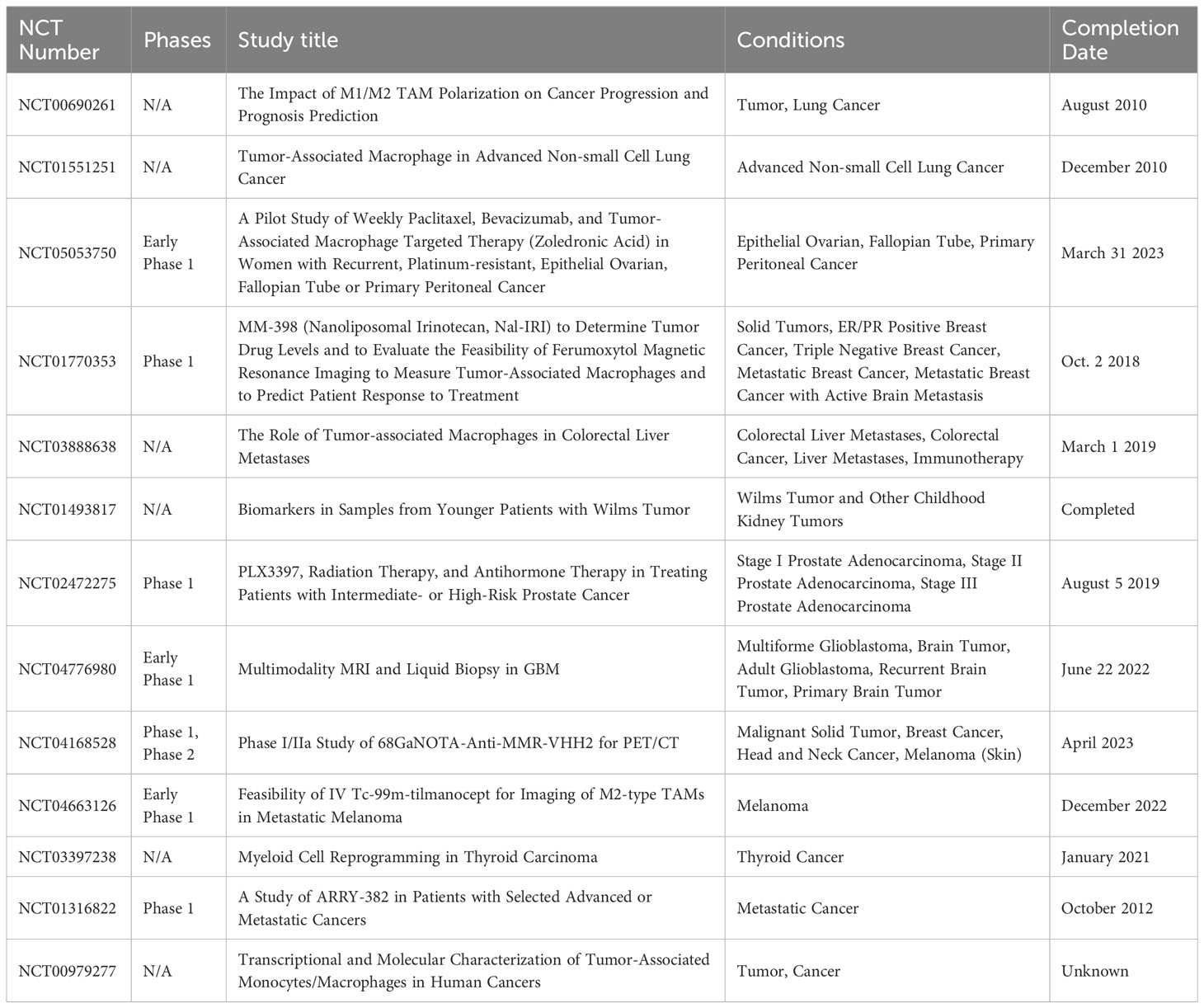
Table 2 Clinical trials involving TAMs in cancers (www.clinicaltrials.gov).
Among these trials of NCT04776980 and 687 NCT04168528, the former employs advanced imaging and biopsy methods to study TAMs within the glioblastoma multiforme (GBM) tumor environment. The latter focuses on a radiolabeled agent targeting the MMR (macrophage mannose receptor), predominantly found on M2-polarized TAMs, allowing researchers to visualize the TAM distribution in various malignancies. Another notable trial, NCT01316822, assesses ARRY-382, a targeted inhibitor against the CSF1/CSF1R pathway essential for TAM activity and differentiation. The emphasis on TAMs in these trials showcases their increasing importance in oncology, with potential applications in cancer treatment and patient care.
5.2 The potential of CSF1R as a therapeutic target
The Colony-stimulating factor 1 receptor (CSF1R) is fast gaining traction in the realm of cancer therapeutics. This receptor tyrosine kinase is a pivotal element found in TAMs. It plays an indispensable role in orchestrating the growth and survival of TAMs making it a compelling therapeutic target (109, 110).
The therapeutic potential of agents that inhibit CSF1R is a topic of considerable interest and is currently being scrutinized in several clinical trials. One standout Phase 2 clinical has been focusing on gauging the collective effectiveness of the CSF1R inhibitor, namely pexidartinib in tandem with pembrolizumab for treating patients with advanced melanoma cases (10, 111–113).
Currently, a commendable number of 20 clinical trials are actively in progress. These trials are delving into the potential implications of CSF1R in the field of Oncology. A detailed breakdown and specifics of these trials can be perused in Table 3.
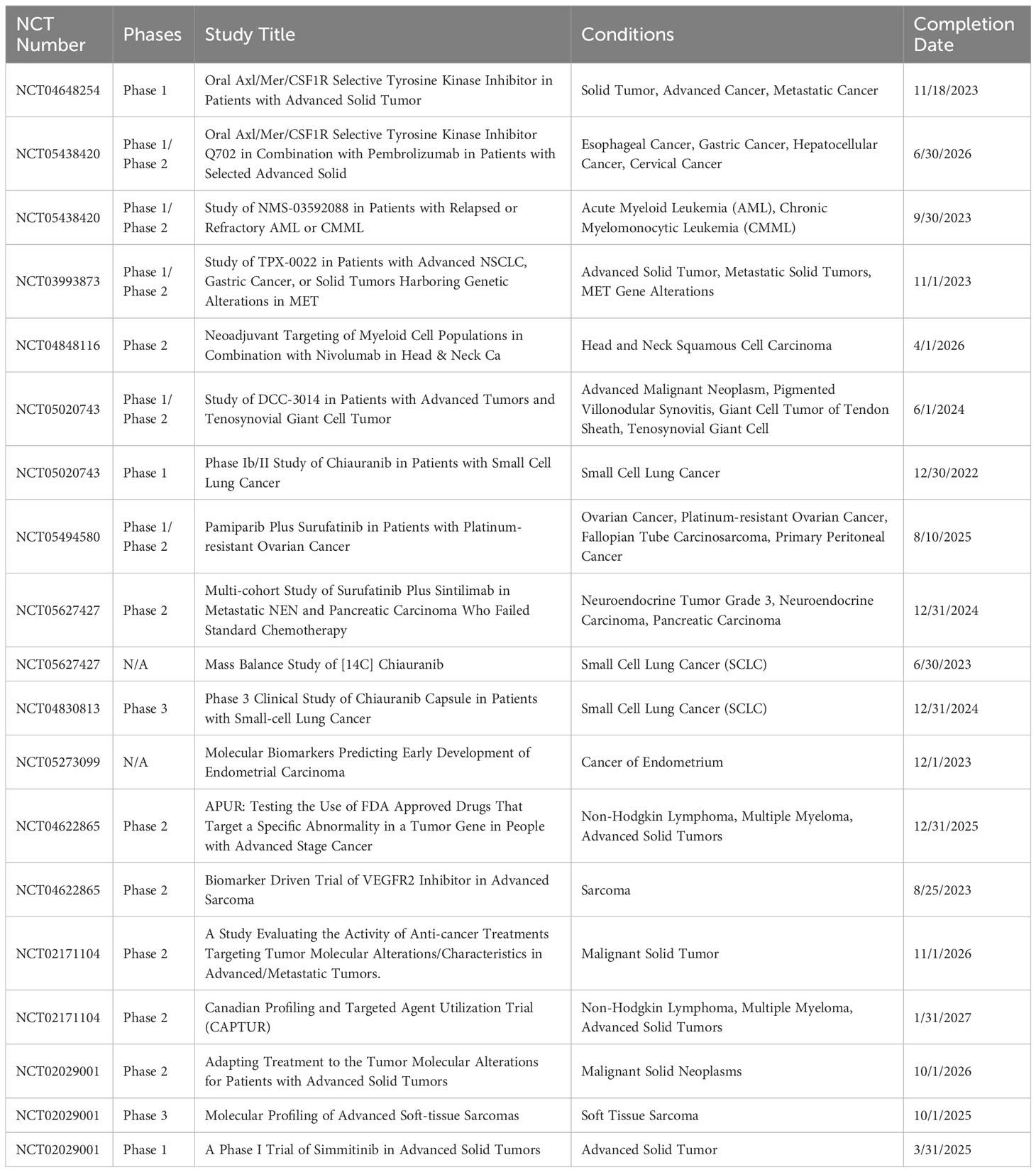
Table 3 Clinical trials involving CSF1R as a therapeutic target in cancers (www.clinicaltrials.gov).
6 Innovations in TAM-focused therapeutic strategies
Oncological advancements are revealing the significant role that TAMs play in the TME. This has inspired innovative therapeutic strategies that harness the complex biology of TAMs. These strategies, enriched by emerging technologies, are carving fresh and promising avenues in the treatment of cancer. Particularly notable are efforts to reprogram TAMs for cancer immunotherapy (14, 114) and the application of single-cell technologies for precision interventions (115–118).
6.1 Reprogramming TAMs for cancer immunotherapy
TAMs possess a dual nature in cancer development, particularly in aggressive cancers like gastric adenocarcinoma. They can transition between tumor-resistant M1 macrophages, which exert anti-tumor functions, and tumor-promoting M2 macrophages. Recent evidence suggests that M2-type macrophages, similar to TAMs, serve as prognostic markers for gastric cancer (119). Instead of eliminating TAMs altogether, current therapeutic strategies aim to modify their behavior by converting pro-tumorigenic M2-like TAMs into anti-tumor M1-like forms (14). This approach retains TAMs’ essential roles in tissue repair and homeostasis while harnessing their potential in the fight against cancer. M2 macrophages, which resemble TAMs, are increasingly recognized as independent prognostic factors in gastric cancer (119, 120). The utilization of TAMs as prognostic tools in clinical settings is on the rise, particularly in the context of therapeutic reprogramming. Instead of complete TAM removal, current strategies focus on behavior modification, transitioning pro-tumorigenic M2-like TAMs into anti-tumor M1-like counterparts (7, 14). This strategy maintains the advantageous properties of TAMs in tissue repair and homeostasis while engaging them in the battle against cancer.
6.2 Utilizing single-cell technologies for precision interventions
TAMs play critical roles in a range of cancers, notably endometrial and breast types. The impact of cancer on the transcriptional landscapes of monocytes and macrophages suggests consequential effects on patient outcomes. For instance, breast TAMs present a signature linked to aggressive cancer subtypes, correlating with decreased disease-specific survival (121, 122). Discovering the interactions between TAMs and cancer cells has led to the identification of potential therapeutic targets like SIGLEC1 and CCL8 (46, 121, 123, 124).
In stage II colon cancer, the varying effectiveness of post-surgery chemotherapy highlights the significance of reliable biomarkers. Notably, TAM ratios, such as CD206/CD68, are emerging as pivotal prognostic and predictive metrics for postoperative chemotherapy (125, 126). These insights not only deepen our grasp of TAMs’ function in cancer but also accentuate the imperative for precision interventions.
The evolving clarity of TAM populations within the TME underscores the necessity for sophisticated interventions. Advanced tools, like single-cell RNA sequencing (scRNA-seq) enable the identification of distinct TAM subsets, revealing their specific molecular imprints and operational states (12, 116, 127, 128). By combining lineage tracing with scRNA-seq, researchers like Casanova-Acebes et al. (117) have elucidated TAM dynamics in specific cancer models, advocating for bespoke therapeutic approaches that target distinct TAM groups. This refined comprehension, powered by cutting-edge technologies, foreshadows individualized treatments that reconfigure the TME to hinder tumor growth.
7 The future of TAM research
TAMs, prominent actors within TME, exhibit both facilitative and inhibitive roles in cancer progression, making them intriguing therapeutic targets. As TAM-centered clinical trials advance, their potential to redefine cancer immunotherapy becomes increasingly evident.
7.1 Precision in TAM-targeted therapeutics
Given the diverse roles of TAMs, precision in therapy is imperative. Recent studies advocate for restricting TAM influx into the TME to counter their tumor-promoting functions (13). Additionally, efforts to direct TAM polarization toward the anti-tumor M1 phenotype are gaining traction, which may enhance the TME’s immune responsiveness. Emphasis is also on curbing TAM-induced immune suppression (129). As we continue to gain insight, the targeting of distinct TAM subsets emerges as a crucial strategy, warranting an integrative understanding of the TME (130). Such holistic endeavors might propel a new epoch in cancer care.
7.2 Innovations in non-invasive TAM monitoring
Advancing TAM research necessitates the standardization of isolation techniques. In this context, cutting-edge tools, such as single-cell analysis (116, 118), have emerged as powerful assets. Single-cell analysis not only allows for the isolation and study of individual TAMs but also provides a window into the intricate nuances of TAM phenotypes. Furthermore, it sheds light on the dynamic interactions between TAMs and the TME (15). This innovative approach has the potential to revolutionize our understanding of TAMs by uncovering their heterogeneity and unveiling the intricacies of their crosstalk within the TME. These advancements in non-invasive TAM monitoring hold promise for more targeted and effective therapeutic strategies in the fight against cancer.
7.3 Navigating clinical trial & their implications
Optimizing TAM-oriented therapies requires rigorous clinical trials to validate both their efficacy and safety. Such endeavors also spotlight potential biomarkers to ascertain the most suitable patient groups for these interventions. By understanding the intricate interactions of TAM-TME engagements, opportunities for combinatorial treatments emerge, elevating the standards of cancer therapy.
7.4 Reflections and horizons
Within the complex world of oncology, TAMs are at the forefront. Unraveling their role in tumor dynamics will inspire novel therapeutic ventures. With this momentum, the domain of TAM research has the promise to revolutionize cancer immunotherapy, a sentiment gaining traction among researchers (131).
8 Conclusion
TAMs, central to cancer dynamics, both facilitate and counteract tumor progression. Their intricate roles pose significant therapeutic challenges. Yet, the advent of clinical trials reveals potential means to regulate TAMs, mitigating their immune-suppressive effects. Integrating these TAM-focused strategies with current treatments may revolutionize cancer immunotherapy.
A paramount shift in TAM research demands standardized methodologies for consistent and precise results. Breakthroughs such as single-cell analyses grant a profound understanding of TAM functions and their complex TME interplay. Concurrently, efforts to devise drugs targeting adverse TAM subsets are intensifying. Through careful assessment and biomarker application, patient selection for optimal benefits becomes feasible.
In summary, investigating TAMs isn’t merely scholarly; it bears the promise of transforming cancer treatment paradigms. Embracing the nuances and opportunities in TAM research brings us a step closer to improved outcomes for cancer patients.
Author contributions
SS: Conceptualization, Formal Analysis, Investigation, Methodology, Resources, Validation, Visualization, Writing – original draft, Writing – review & editing, Data curation. HM: Conceptualization, Data curation, Formal Analysis, Investigation, Methodology, Resources, Validation, Visualization, Writing – original draft, Writing – review & editing. WM: Conceptualization, Formal Analysis, Investigation, Methodology, Resources, Validation, Visualization, Writing – original draft, Writing – review & editing, Project administration, Software, Supervision.
Funding
The author(s) declare that no financial support was received for the research, authorship, and/or publication of this article.
Acknowledgments
This manuscript builds upon the authors’ interpretation of the latest relevant publications and existing literature in the field. We thank the clinical trials (https://www.clinicaltrials.gov) platform for making the data set publicly available.
Conflict of interest
The authors declare that the research was conducted in the absence of any commercial or financial relationships that could be construed as a potential conflict of interest.
The author(s) declared that they were an editorial board member of Frontiers, at the time of submission. This had no impact on the peer review process and the final decision.
Publisher’s note
All claims expressed in this article are solely those of the authors and do not necessarily represent those of their affiliated organizations, or those of the publisher, the editors and the reviewers. Any product that may be evaluated in this article, or claim that may be made by its manufacturer, is not guaranteed or endorsed by the publisher.
Abbreviations
CAFs, cancer-associated fibroblasts; CSF1R, colony-stimulating factor 1 receptor; ECM, extracellular matrix; EMT, epithelial to mesenchymal transition; FACS, fluorescence-activated cell sorting; GBM, glioblastoma multiforme; GM-CGF, granulocyte-monocyte colony-stimulating factor; HIF-1α, hypoxia-inducible factor 1-alpha; IL, interleukin; LCM, laser capture microdissection; LPS, lipopolysaccharide; MACS, magnetic associated cell sorting; PDGF, platelet-derived growth factor; MDSCs, myeloid-derived suppressor cells; MΦ, macrophages; PGE2, prostaglandin E2; scRNA-seq, single-cell RNA sequencing; TAMs, tumor-associated macrophages; TGF β, transforming growth factor β; TLR, toll-like receptor; TME, tumor microenvironment; VEGF, vascular endothelial growth factor.
References
1. Yang Q, Guo N, Zhou Y, Chen J, Wei Q, Han M. The role of tumor-associated macrophages (Tams) in tumor progression and relevant advance in targeted therapy. Acta Pharm Sin B (2020) 10(11):2156–70. doi: 10.1016/j.apsb.2020.04.004
2. Dallavalasa S, Beeraka NM, Basavaraju CG, Tulimilli SV, Sadhu SP, Rajesh K, et al. The role of tumor associated macrophages (Tams) in cancer progression, chemoresistance, angiogenesis and metastasis - current status. Curr Med Chem (2021) 28(39):8203–36. doi: 10.2174/0929867328666210720143721
3. Zhang J, Gao J, Cui J, Wang Y, Jin Y, Zhang D, et al. Tumor-associated macrophages in tumor progression and the role of traditional Chinese medicine in regulating tams to enhance antitumor effects. Front Immunol (2022) 13:1026898. doi: 10.3389/fimmu.2022.1026898
4. Pan Y, Yu Y, Wang X, Zhang T. Tumor-associated macrophages in tumor immunity. Front Immunol (2020) 11:583084. doi: 10.3389/fimmu.2020.583084
5. Schupp J, Krebs FK, Zimmer N, Trzeciak E, Schuppan D, Tuettenberg A. Targeting myeloid cells in the tumor sustaining microenvironment. Cell Immunol (2019) 343:103713. doi: 10.1016/j.cellimm.2017.10.013
6. Richards DM, Hettinger J, Feuerer M. Monocytes and macrophages in cancer: development and functions. Cancer Microenviron (2013) 6(2):179–91. doi: 10.1007/s12307-012-0123-x
7. Lin Y, Xu J, Lan H. Tumor-associated macrophages in tumor metastasis: biological roles and clinical therapeutic applications. J Hematol Oncol (2019) 12(1):76. doi: 10.1186/s13045-019-0760-3
8. Xiang X, Wang J, Lu D, Xu X. Targeting tumor-associated macrophages to synergize tumor immunotherapy. Signal Transduct Target Ther (2021) 6(1):75. doi: 10.1038/s41392-021-00484-9
9. Li M, He L, Zhu J, Zhang P, Liang S. Targeting tumor-associated macrophages for cancer treatment. Cell Biosci (2022) 12(1):85. doi: 10.1186/s13578-022-00823-5
10. Mantovani A, Allavena P, Marchesi F, Garlanda C. Macrophages as tools and targets in cancer therapy. Nat Rev Drug Discovery (2022) 21(11):799–820. doi: 10.1038/s41573-022-00520-5
11. Liu M, Yang J, Xu B, Zhang X. Tumor metastasis: mechanistic insights and therapeutic interventions. MedComm (2020) (2021) 2(4):587–617. doi: 10.1002/mco2.100
12. Wu K, Lin K, Li X, Yuan X, Xu P, Ni P, et al. Redefining tumor-associated macrophage subpopulations and functions in the tumor microenvironment. Front Immunol (2020) 11:1731. doi: 10.3389/fimmu.2020.01731
13. Tan Y, Wang M, Zhang Y, Ge S, Zhong F, Xia G, et al. Tumor-associated macrophages: A potential target for cancer therapy. Front Oncol (2021) 11:693517. doi: 10.3389/fonc.2021.693517
14. Khan SU, Khan MU, Azhar Ud Din M, Khan IM, Khan MI, Bungau S, et al. Reprogramming tumor-associated macrophages as a unique approach to target tumor immunotherapy. Front Immunol (2023) 14:1166487. doi: 10.3389/fimmu.2023.1166487
15. Khan F, Pang L, Dunterman M, Lesniak MS, Heimberger AB, Chen P. Macrophages and microglia in glioblastoma: heterogeneity, plasticity, and therapy. J Clin Invest (2023) 133(1):e163446. doi: 10.1172/JCI163446
16. Vitale I, Manic G, Coussens LM, Kroemer G, Galluzzi L. Macrophages and metabolism in the tumor microenvironment. Cell Metab (2019) 30(1):36–50. doi: 10.1016/j.cmet.2019.06.001
17. Feng Y, Ye Z, Song F, He Y, Liu J. The role of tams in tumor microenvironment and new research progress. Stem Cells Int (2022) 2022:5775696. doi: 10.1155/2022/5775696
18. Chen Y, Song Y, Du W, Gong L, Chang H, Zou Z. Tumor-associated macrophages: an accomplice in solid tumor progression. J BioMed Sci (2019) 26(1):78. doi: 10.1186/s12929-019-0568-z
19. Huang X, Cao J, Zu X. Tumor-associated macrophages: an important player in breast cancer progression. Thorac Cancer (2022) 13(3):269–76. doi: 10.1111/1759-7714.14268
20. Munir MT, Kay MK, Kang MH, Rahman MM, Al-Harrasi A, Choudhury M, et al. Tumor-associated macrophages as multifaceted regulators of breast tumor growth. Int J Mol Sci (2021) 22(12):6256. doi: 10.3390/ijms22126526
21. Bohn T, Rapp S, Luther N, Klein M, Bruehl TJ, Kojima N, et al. Tumor immunoevasion via acidosis-dependent induction of regulatory tumor-associated macrophages. Nat Immunol (2018) 19(12):1319–29. doi: 10.1038/s41590-018-0226-8
22. Gao J, Liang Y, Wang L. Shaping polarization of tumor-associated macrophages in cancer immunotherapy. Front Immunol (2022) 13:888713. doi: 10.3389/fimmu.2022.888713
23. Baghban R, Roshangar L, Jahanban-Esfahlan R, Seidi K, Ebrahimi-Kalan A, Jaymand M, et al. Tumor microenvironment complexity and therapeutic implications at a glance. Cell Commun Signal (2020) 18(1):59. doi: 10.1186/s12964-020-0530-4
24. Borowczak J, Szczerbowski K, Maniewski M, Kowalewski A, Janiczek-Polewska M, Szylberg A, et al. The role of inflammatory cytokines in the pathogenesis of colorectal carcinoma-recent findings and review. Biomedicines (2022) 10(7):1670. doi: 10.3390/biomedicines10071670
25. Verma NK, Wong BHS, Poh ZS, Udayakumar A, Verma R, Goh RKJ, et al. Obstacles for T-lymphocytes in the tumour microenvironment: therapeutic challenges, advances and opportunities beyond immune checkpoint. EBioMedicine (2022) 83:104216. doi: 10.1016/j.ebiom.2022.104216
26. Lugano R, Ramachandran M, Dimberg A. Tumor angiogenesis: causes, consequences, challenges and opportunities. Cell Mol Life Sci (2020) 77(9):1745–70. doi: 10.1007/s00018-019-03351-7
27. Larionova I, Kazakova E, Gerashchenko T, Kzhyshkowska J. New angiogenic regulators produced by tams: perspective for targeting tumor angiogenesis. Cancers (Basel) (2021) 13(13):3253. doi: 10.3390/cancers13133253
28. Liu ZL, Chen HH, Zheng LL, Sun LP, Shi L. Angiogenic signaling pathways and anti-angiogenic therapy for cancer. Signal Transduct Target Ther (2023) 8(1):198. doi: 10.1038/s41392-023-01460-1
29. Pernot S, Evrard S, Khatib AM. The give-and-take interaction between the tumor microenvironment and immune cells regulating tumor progression and repression. Front Immunol (2022) 13:850856. doi: 10.3389/fimmu.2022.850856
30. Bied M, Ho WW, Ginhoux F, Bleriot C. Roles of macrophages in tumor development: A spatiotemporal perspective. Cell Mol Immunol (2023) 20(9):983–92. doi: 10.1038/s41423-023-01061-6
31. Boutilier AJ, Elsawa SF. Macrophage polarization states in the tumor microenvironment. Int J Mol Sci (2021) 22(13):6995. doi: 10.3390/ijms22136995
32. Li C, Xu X, Wei S, Jiang P, Xue L, Wang J, et al. Tumor-associated macrophages: potential therapeutic strategies and future prospects in cancer. J Immunother Cancer (2021) 9(1):e001341. doi: 10.1136/jitc-2020-001341
33. Udeabor SE, Adisa AO, Orlowska A, Sader RA, Ghanaati S. Tumor-associated macrophages, angiogenesis, and tumor cell migration in oral squamous cell carcinoma. Ann Afr Med (2017) 16(4):181–5. doi: 10.4103/aam.aam_8_17
34. Italiani P, Boraschi D. From monocytes to M1/M2 macrophages: phenotypical vs. Functional differentiation. Front Immunol (2014) 5:514. doi: 10.3389/fimmu.2014.00514
35. Zhao H, Wu L, Yan G, Chen Y, Zhou M, Wu Y, et al. Inflammation and tumor progression: signaling pathways and targeted intervention. Signal Transduct Target Ther (2021) 6(1):263. doi: 10.1038/s41392-021-00658-5
36. Zou S, Tong Q, Liu B, Huang W, Tian Y, Fu X. Targeting stat3 in cancer immunotherapy. Mol Cancer (2020) 19(1):145. doi: 10.1186/s12943-020-01258-7
37. Anderson K, Ryan N, Alkhimovitch A, Siddiqui A, Oghumu S. Inhibition of pi3k isoform P110gamma increases both anti-tumor and immunosuppressive responses to aggressive murine head and neck squamous cell carcinoma with low immunogenicity. Cancers (Basel) (2021) 13(5):953. doi: 10.3390/cancers13050953
38. Moeller A, Kurzrock R, Botta GP, Adashek JJ, Patel H, Lee S, et al. Challenges and prospects of csf1r targeting for advanced Malignancies. Am J Cancer Res (2023) 13(7):3257–65.
39. Yang F, Van Meter TE, Buettner R, Hedvat M, Liang W, Kowolik CM, et al. Sorafenib inhibits signal transducer and activator of transcription 3 signaling associated with growth arrest and apoptosis of medulloblastomas. Mol Cancer Ther (2008) 7(11):3519–26. doi: 10.1158/1535-7163.MCT-08-0138
40. Zhang XM, Chen DG, Li SC, Zhu B, Li ZJ. Embryonic origin and subclonal evolution of tumor-associated macrophages imply preventive care for cancer. Cells (2021) 10(4):903. doi: 10.3390/cells10040903
41. Schweer D, McAtee A, Neupane K, Richards C, Ueland F, Kolesar J. Tumor-associated macrophages and ovarian cancer: implications for therapy. Cancers (Basel) (2022) 14(9):2220. doi: 10.3390/cancers14092220
42. Liu M, Liu L, Song Y, Li W, Xu L. Targeting macrophages: A novel treatment strategy in solid tumors. J Transl Med (2022) 20(1):586. doi: 10.1186/s12967-022-03813-w
43. Jayasingam SD, Citartan M, Thang TH, Mat Zin AA, Ang KC, Ch'ng ES. Evaluating the polarization of tumor-associated macrophages into M1 and M2 phenotypes in human cancer tissue: technicalities and challenges in routine clinical practice. Front Oncol (2019) 9:1512. doi: 10.3389/fonc.2019.01512
44. Malfitano AM, Pisanti S, Napolitano F, Di Somma S, Martinelli R, Portella G. Tumor-associated macrophage status in cancer treatment. Cancers (Basel) (2020) 12(7):1987. doi: 10.3390/cancers12071987
45. Larionova I, Tuguzbaeva G, Ponomaryova A, Stakheyeva M, Cherdyntseva N, Pavlov V, et al. Tumor-associated macrophages in human breast, colorectal, lung, ovarian and prostate cancers. Front Oncol (2020) 10:566511. doi: 10.3389/fonc.2020.566511
46. Ma RY, Black A, Qian BZ. Macrophage diversity in cancer revisited in the era of single-cell omics. Trends Immunol (2022) 43(7):546–63. doi: 10.1016/j.it.2022.04.008
47. Cassetta L, Noy R, Swierczak A, Sugano G, Smith H, Wiechmann L, et al. Isolation of mouse and human tumor-associated macrophages. Adv Exp Med Biol (2016) 899:211–29. doi: 10.1007/978-3-319-26666-4_12
48. George S, Georgouli M, Sanz-Moreno V. Protocol to drive human monocyte-to-macrophage polarization in vitro using tumor conditioned media. STAR Protoc (2022) 3(4):101666. doi: 10.1016/j.xpro.2022.101666
49. Madsen DH, Jurgensen HJ, Siersbaek MS, Kuczek DE, Grey Cloud L, Liu S, et al. Tumor-associated macrophages derived from circulating inflammatory monocytes degrade collagen through cellular uptake. Cell Rep (2017) 21(13):3662–71. doi: 10.1016/j.celrep.2017.12.011
50. Haque A, Moriyama M, Kubota K, Ishiguro N, Sakamoto M, Chinju A, et al. Cd206(+) tumor-associated macrophages promote proliferation and invasion in oral squamous cell carcinoma via egf production. Sci Rep (2019) 9(1):14611. doi: 10.1038/s41598-019-51149-1
51. Modak M, Mattes AK, Reiss D, Skronska-Wasek W, Langlois R, Sabarth N, et al. Cd206+ Tumor-associated macrophages cross-present tumor antigen and drive antitumor immunity. JCI Insight (2022) 7(11):e155022. doi: 10.1172/jci.insight.155022
52. Bennett S, Breit SN. Variables in the isolation and culture of human monocytes that are of particular relevance to studies of hiv. J Leukoc Biol (1994) 56(3):236–40. doi: 10.1002/jlb.56.3.236
53. Kubala MH, DeClerck YA. Conditional knockdown of gene expression in cancer cell lines to study the recruitment of monocytes/macrophages to the tumor microenvironment. J Vis Exp (2017) 129):56333. doi: 10.3791/56333
54. Zhang X, Zeng Y, Qu Q, Zhu J, Liu Z, Ning W, et al. Pd-L1 induced by ifn-gamma from tumor-associated macrophages via the jak/stat3 and pi3k/akt signaling pathways promoted progression of lung cancer. Int J Clin Oncol (2017) 22(6):1026–33. doi: 10.1007/s10147-017-1161-7
55. Massalha H, Bahar Halpern K, Abu-Gazala S, Jana T, Massasa EE, Moor AE, et al. A single cell atlas of the human liver tumor microenvironment. Mol Syst Biol (2020) 16(12):e9682. doi: 10.15252/msb.20209682
56. Zwager MC, Bense R, Waaijer S, Qiu SQ, Timmer-Bosscha H, de Vries EGE, et al. Assessing the role of tumour-associated macrophage subsets in breast cancer subtypes using digital image analysis. Breast Cancer Res Treat (2023) 198(1):11–22. doi: 10.1007/s10549-022-06859-y
57. Cheng Y, Song S, Wu P, Lyu B, Qin M, Sun Y, et al. Tumor associated macrophages and tams-based anti-tumor nanomedicines. Adv Healthc Mater (2021) 10(18):e2100590. doi: 10.1002/adhm.202100590
58. Guo F, Kong W, Zhao G, Cheng Z, Ai L, Lv J, et al. The correlation between tumor-associated macrophage infiltration and progression in cervical carcinoma. Biosci Rep (2021) 41(5):BSR20203145. doi: 10.1042/BSR20203145
59. Moeini P, Niedzwiedzka-Rystwej P. Tumor-associated macrophages: combination of therapies, the approach to improve cancer treatment. Int J Mol Sci (2021) 22(13):7239. doi: 10.3390/ijms22137239
60. Zhou Q, Fang T, Wei S, Chai S, Yang H, Tao M, et al. Macrophages in melanoma: A double−Edged sword and targeted therapy strategies (Review). Exp Ther Med (2022) 24(4):640. doi: 10.3892/etm.2022.11577
61. Sedighzadeh SS, Khoshbin AP, Razi S, Keshavarz-Fathi M, Rezaei N. A narrative review of tumor-associated macrophages in lung cancer: regulation of macrophage polarization and therapeutic implications. Transl Lung Cancer Res (2021) 10(4):1889–916. doi: 10.21037/tlcr-20-1241
62. Liang ZW, Ge XX, Xu MD, Qin H, Wu MY, Shen M, et al. Tumor-associated macrophages promote the metastasis and growth of non-small-cell lung cancer cells through nf-kappab/pp2ac-positive feedback loop. Cancer Sci (2021) 112(6):2140–57. doi: 10.1111/cas.14863
63. Sasidharan Nair V, Saleh R, Toor SM, Cyprian FS, Elkord E. Metabolic reprogramming of T regulatory cells in the hypoxic tumor microenvironment. Cancer Immunol Immunother (2021) 70(8):2103–21. doi: 10.1007/s00262-020-02842-y
64. Wei J, Hu M, Du H. Improving cancer immunotherapy: exploring and targeting metabolism in hypoxia microenvironment. Front Immunol (2022) 13:845923. doi: 10.3389/fimmu.2022.845923
65. Cunha PP, Minogue E, Krause LCM, Hess RM, Bargiela D, Wadsworth BJ, et al. Oxygen levels at the time of activation determine T cell persistence and immunotherapeutic efficacy. Elife (2023) 12:e84280. doi: 10.7554/eLife.84280
66. Park JH, Lee HK. Current understanding of hypoxia in glioblastoma multiforme and its response to immunotherapy. Cancers (Basel) (2022) 14(5):1176. doi: 10.3390/cancers14051176
67. Vasseur S, Guillaumond F. Lipids in cancer: A global view of the contribution of lipid pathways to metastatic formation and treatment resistance. Oncogenesis (2022) 11(1):46. doi: 10.1038/s41389-022-00420-8
68. Hasan MN, Capuk O, Patel SM, Sun D. The role of metabolic plasticity of tumor-associated macrophages in shaping the tumor microenvironment immunity. Cancers (Basel) (2022) 14(14):3331. doi: 10.3390/cancers14143331
69. He Z, Zhang S. Tumor-associated macrophages and their functional transformation in the hypoxic tumor microenvironment. Front Immunol (2021) 12:741305. doi: 10.3389/fimmu.2021.741305
70. Crezee T, Rabold K, de Jong L, Jaeger M, Netea-Maier RT. Metabolic programming of tumor associated macrophages in the context of cancer treatment. Ann Transl Med (2020) 8(16):1028. doi: 10.21037/atm-20-1114
71. Jin HR, Wang J, Wang ZJ, Xi MJ, Xia BH, Deng K, et al. Lipid metabolic reprogramming in tumor microenvironment: from mechanisms to therapeutics. J Hematol Oncol (2023) 16(1):103. doi: 10.1186/s13045-023-01498-2
72. Han J, Dong L, Wu M, Ma F. Dynamic polarization of tumor-associated macrophages and their interaction with intratumoral T cells in an inflamed tumor microenvironment: from mechanistic insights to therapeutic opportunities. Front Immunol (2023) 14:1160340. doi: 10.3389/fimmu.2023.1160340
73. Wang N, Liang H, Zen K. Molecular mechanisms that influence the macrophage M1-M2 polarization balance. Front Immunol (2014) 5:614. doi: 10.3389/fimmu.2014.00614
74. Chen S, Saeed A, Liu Q, Jiang Q, Xu H, Xiao GG, et al. Macrophages in immunoregulation and therapeutics. Signal Transduct Target Ther (2023) 8(1):207. doi: 10.1038/s41392-023-01452-1
75. Yadav S, Priya A, Borade DR, Agrawal-Rajput R. Macrophage subsets and their role: co-relation with colony-stimulating factor-1 receptor and clinical relevance. Immunol Res (2023) 71(2):130–52. doi: 10.1007/s12026-022-09330-8
76. Arora S, Dev K, Agarwal B, Das P, Syed MA. Macrophages: their role, activation and polarization in pulmonary diseases. Immunobiology (2018) 223(4-5):383–96. doi: 10.1016/j.imbio.2017.11.001
77. Ohadian Moghadam S, Nowroozi MR. Toll-like receptors: the role in bladder cancer development, progression and immunotherapy. Scand J Immunol (2019) 90(6):e12818. doi: 10.1111/sji.12818
78. Luiz JPM, Toller-Kawahisa JE, Viacava PR, Nascimento DC, Pereira PT, Saraiva AL, et al. Mek5/erk5 signaling mediates il-4-induced M2 macrophage differentiation through regulation of C-myc expression. J Leukoc Biol (2020) 108(4):1215–23. doi: 10.1002/JLB.1MA0520-016R
79. Ciernikova S, Sevcikova A, Stevurkova V, Mego M. Tumor microbiome - an integral part of the tumor microenvironment. Front Oncol (2022) 12:1063100. doi: 10.3389/fonc.2022.1063100
80. Huang X, Pan J, Xu F, Shao B, Wang Y, Guo X, et al. Bacteria-based cancer immunotherapy. Adv Sci (Weinh) (2021) 8(7):2003572. doi: 10.1002/advs.202003572
81. Ge Z, Ding S. The crosstalk between tumor-associated macrophages (Tams) and tumor cells and the corresponding targeted therapy. Front Oncol (2020) 10:590941. doi: 10.3389/fonc.2020.590941
82. Bai R, Li Y, Jian L, Yang Y, Zhao L, Wei M. The hypoxia-driven crosstalk between tumor and tumor-associated macrophages: mechanisms and clinical treatment strategies. Mol Cancer (2022) 21(1):177. doi: 10.1186/s12943-022-01645-2
83. Zhang C, Fei Y, Wang H, Hu S, Liu C, Hu R, et al. Cafs orchestrates tumor immune microenvironment-a new target in cancer therapy? Front Pharmacol (2023) 14:1113378. doi: 10.3389/fphar.2023.1113378
84. Sun R, Han R, McCornack C, Khan S, Tabor GT, Chen Y, et al. Trem2 inhibition triggers antitumor cell activity of myeloid cells in glioblastoma. Sci Adv (2023) 9(19):eade3559. doi: 10.1126/sciadv.ade3559
85. Kumari N, Choi SH. Tumor-associated macrophages in cancer: recent advancements in cancer nanoimmunotherapies. J Exp Clin Cancer Res (2022) 41(1):68. doi: 10.1186/s13046-022-02272-x
86. Mun JY, Leem SH, Lee JH, Kim HS. Dual relationship between stromal cells and immune cells in the tumor microenvironment. Front Immunol (2022) 13:864739. doi: 10.3389/fimmu.2022.864739
87. Zhang Z, Liu S, Zhang B, Qiao L, Zhang Y, Zhang Y. T cell dysfunction and exhaustion in cancer. Front Cell Dev Biol (2020) 8:17. doi: 10.3389/fcell.2020.00017
88. Fang L, Liu K, Liu C, Wang X, Ma W, Xu W, et al. Tumor accomplice: T cell exhaustion induced by chronic inflammation. Front Immunol (2022) 13:979116. doi: 10.3389/fimmu.2022.979116
89. Davis RJ, Van Waes C, Allen CT. Overcoming barriers to effective immunotherapy: mdscs, tams, and tregs as mediators of the immunosuppressive microenvironment in head and neck cancer. Oral Oncol (2016) 58:59–70. doi: 10.1016/j.oraloncology.2016.05.002
90. Li K, Shi H, Zhang B, Ou X, Ma Q, Chen Y, et al. Myeloid-derived suppressor cells as immunosuppressive regulators and therapeutic targets in cancer. Signal Transduct Target Ther (2021) 6(1):362. doi: 10.1038/s41392-021-00670-9
91. Tumino N, Fiore PF, Pelosi A, Moretta L, Vacca P. Myeloid derived suppressor cells in tumor microenvironment: interaction with innate lymphoid cells. Semin Immunol (2022) 61-64:101668. doi: 10.1016/j.smim.2022.101668
92. Elia I, Haigis MC. Metabolites and the tumour microenvironment: from cellular mechanisms to systemic metabolism. Nat Metab (2021) 3(1):21–32. doi: 10.1038/s42255-020-00317-z
93. Belhabib I, Zaghdoudi S, Lac C, Bousquet C, Jean C. Extracellular matrices and cancer-associated fibroblasts: targets for cancer diagnosis and therapy? Cancers (Basel) (2021) 13(14):3466. doi: 10.3390/cancers13143466
94. Wu F, Yang J, Liu J, Wang Y, Mu J, Zeng Q, et al. Signaling pathways in cancer-associated fibroblasts and targeted therapy for cancer. Signal Transduct Target Ther (2021) 6(1):218. doi: 10.1038/s41392-021-00641-0
95. Wang S, Zhao X, Wu S, Cui D, Xu Z. Myeloid-derived suppressor cells: key immunosuppressive regulators and therapeutic targets in hematological Malignancies. biomark Res (2023) 11(1):34. doi: 10.1186/s40364-023-00475-8
96. Hassanshahi A, Moradzad M, Ghalamkari S, Fadaei M, Cowin AJ, Hassanshahi M. Macrophage-mediated inflammation in skin wound healing. Cells (2022) 11(19):2953. doi: 10.3390/cells11192953
97. Xia T, Zhang M, Lei W, Yang R, Fu S, Fan Z, et al. Advances in the role of stat3 in macrophage polarization. Front Immunol (2023) 14:1160719. doi: 10.3389/fimmu.2023.1160719
98. Martin KE, Garcia AJ. Macrophage phenotypes in tissue repair and the foreign body response: implications for biomaterial-based regenerative medicine strategies. Acta Biomater (2021) 133:4–16. doi: 10.1016/j.actbio.2021.03.038
99. Abdelaziz MH, Abdelwahab SF, Wan J, Cai W, Huixuan W, Jianjun C, et al. Alternatively activated macrophages; a double-edged sword in allergic asthma. J Transl Med (2020) 18(1):58. doi: 10.1186/s12967-020-02251-w
100. Kang N, Liang X, Fan B, Zhao C, Shen B, Ji X, et al. Endothelial-specific molecule 1 inhibition lessens productive angiogenesis and tumor metastasis to overcome bevacizumab resistance. Cancers (Basel) (2022) 14(22):5681. doi: 10.3390/cancers14225681
101. Wang LX, Zhang SX, Wu HJ, Rong XL, Guo J. M2b macrophage polarization and its roles in diseases. J Leukoc Biol (2019) 106(2):345–58. doi: 10.1002/JLB.3RU1018-378RR
102. Liu J, Geng X, Hou J, Wu G. New insights into M1/M2 macrophages: key modulators in cancer progression. Cancer Cell Int (2021) 21(1):389. doi: 10.1186/s12935-021-02089-2
103. Zou Z, Lin H, Li M, Lin B. Tumor-associated macrophage polarization in the inflammatory tumor microenvironment. Front Oncol (2023) 13:1103149. doi: 10.3389/fonc.2023.1103149
104. Wicks EE, Semenza GL. Hypoxia-inducible factors: cancer progression and clinical translation. J Clin Invest (2022) 132(11):e159839. doi: 10.1172/JCI159839
105. Chen Z, Han F, Du Y, Shi H, Zhou W. Hypoxic microenvironment in cancer: molecular mechanisms and therapeutic interventions. Signal Transduct Target Ther (2023) 8(1):70. doi: 10.1038/s41392-023-01332-8
106. Habanjar O, Bingula R, Decombat C, Diab-Assaf M, Caldefie-Chezet F, Delort L. Crosstalk of inflammatory cytokines within the breast tumor microenvironment. Int J Mol Sci (2023) 24(4):4002. doi: 10.3390/ijms24044002
107. Reis-Sobreiro M, Teixeira da Mota A, Jardim C, Serre K. Bringing macrophages to the frontline against cancer: current immunotherapies targeting macrophages. Cells (2021) 10(9):2364. doi: 10.3390/cells10092364
108. Shi J, Song X, Traub B, Luxenhofer M, Kornmann M. Involvement of il-4, il-13 and their receptors in pancreatic cancer. Int J Mol Sci (2021) 22(6):2998. doi: 10.3390/ijms22062998
109. Barca C, Foray C, Hermann S, Herrlinger U, Remory I, Laoui D, et al. The colony stimulating factor-1 receptor (Csf-1r)-mediated regulation of microglia/macrophages as a target for neurological disorders (Glioma, stroke). Front Immunol (2021) 12:787307. doi: 10.3389/fimmu.2021.787307
110. Zhu M, Bai L, Liu X, Peng S, Xie Y, Bai H, et al. Silence of a dependence receptor csf1r in colorectal cancer cells activates tumor-associated macrophages. J Immunother Cancer (2022) 10(12):e005610. doi: 10.1136/jitc-2022-005610
111. Benner B, Good L, Quiroga D, Schultz TE, Kassem M, Carson WE, et al. Pexidartinib, a novel small molecule csf-1r inhibitor in use for tenosynovial giant cell tumor: A systematic review of pre-clinical and clinical development. Drug Des Devel Ther (2020) 14:1693–704. doi: 10.2147/DDDT.S253232
112. Johnson M, Dudek AZ, Sukari A, Call J, Kunk PR, Lewis K, et al. Arry-382 in combination with pembrolizumab in patients with advanced solid tumors: results from a phase 1b/2 study. Clin Cancer Res (2022) 28(12):2517–26. doi: 10.1158/1078-0432.CCR-21-3009
113. Belli C, Antonarelli G, Repetto M, Boscolo Bielo L, Crimini E, Curigliano G. Targeting cellular components of the tumor microenvironment in solid Malignancies. Cancers (Basel) (2022) 14(17):4278. doi: 10.3390/cancers14174278
114. Sarode P, Zheng X, Giotopoulou GA, Weigert A, Kuenne C, Gunther S, et al. Reprogramming of tumor-associated macrophages by targeting beta-catenin/fosl2/arid5a signaling: A potential treatment of lung cancer. Sci Adv (2020) 6(23):eaaz6105. doi: 10.1126/sciadv.aaz6105
115. Guruprasad P, Lee YG, Kim KH, Ruella M. The current landscape of single-cell transcriptomics for cancer immunotherapy. J Exp Med (2021) 218(1):e20201574. doi: 10.1084/jem.20201574
116. Yang Q, Zhang H, Wei T, Lin A, Sun Y, Luo P, et al. Single-cell rna sequencing reveals the heterogeneity of tumor-associated macrophage in non-small cell lung cancer and differences between sexes. Front Immunol (2021) 12:756722. doi: 10.3389/fimmu.2021.756722
117. Casanova-Acebes M, Dalla E, Leader AM, LeBerichel J, Nikolic J, Morales BM, et al. Tissue-resident macrophages provide a pro-tumorigenic niche to early nsclc cells. Nature (2021) 595(7868):578–84. doi: 10.1038/s41586-021-03651-8
118. Chen AX, Gartrell RD, Zhao J, Upadhyayula PS, Zhao W, Yuan J, et al. Single-cell characterization of macrophages in glioblastoma reveals marco as a mesenchymal pro-tumor marker. Genome Med (2021) 13(1):88. doi: 10.1186/s13073-021-00906-x
119. Raiha MR, Puolakkainen PA. Tumor-associated macrophages (Tams) as biomarkers for gastric cancer: A review. Chronic Dis Transl Med (2018) 4(3):156–63. doi: 10.1016/j.cdtm.2018.07.001
120. Hu J, Ma Y, Ma J, Yang Y, Ning Y, Zhu J, et al. M2 macrophage-based prognostic nomogram for gastric cancer after surgical resection. Front Oncol (2021) 11:690037. doi: 10.3389/fonc.2021.690037
121. Cassetta L, Fragkogianni S, Sims AH, Swierczak A, Forrester LM, Zhang H, et al. Human tumor-associated macrophage and monocyte transcriptional landscapes reveal cancer-specific reprogramming, biomarkers, and therapeutic targets. Cancer Cell (2019) 35(4):588–602.e10. doi: 10.1016/j.ccell.2019.02.009
122. Hey J, Halperin C, Hartmann M, Mayer S, Schonung M, Lipka DB, et al. DNA methylation landscape of tumor-associated macrophages reveals pathways, transcription factors and prognostic value relevant to triple-negative breast cancer patients. Int J Cancer (2023) 152(6):1226–42. doi: 10.1002/ijc.34364
123. Lou X, Zhao K, Xu J, Shuai L, Niu H, Cao Z, et al. Ccl8 as a promising prognostic factor in diffuse large B-cell lymphoma via M2 macrophage interactions: A bioinformatic analysis of the tumor microenvironment. Front Immunol (2022) 13:950213. doi: 10.3389/fimmu.2022.950213
124. Li Y, Wang R, Gao Q. The roles and targeting of tumor-associated macrophages. FBL (2023) 28(9):207. doi: 10.31083/j.fbl2809207
125. Feng Q, Chang W, Mao Y, He G, Zheng P, Tang W, et al. Tumor-associated macrophages as prognostic and predictive biomarkers for postoperative adjuvant chemotherapy in patients with stage ii colon cancer. Clin Cancer Res (2019) 25(13):3896–907. doi: 10.1158/1078-0432.CCR-18-2076
126. Wang H, Tian T, Zhang J. Tumor-associated macrophages (Tams) in colorectal cancer (Crc): from mechanism to therapy and prognosis. Int J Mol Sci (2021) 22(16):8470. doi: 10.3390/ijms22168470
127. Huang D, Ma N, Li X, Gou Y, Duan Y, Liu B, et al. Advances in single-cell rna sequencing and its applications in cancer research. J Hematol Oncol (2023) 16(1):98. doi: 10.1186/s13045-023-01494-6
128. Laviron M, Petit M, Weber-Delacroix E, Combes AJ, Arkal AR, Barthelemy S, et al. Tumor-associated macrophage heterogeneity is driven by tissue territories in breast cancer. Cell Rep (2022) 39(8):110865. doi: 10.1016/j.celrep.2022.110865
129. Zhou F, Liu Y, Liu C, Wang F, Peng J, Xie Y, et al. Knowledge landscape of tumor-associated macrophage research: A bibliometric and visual analysis. Front Immunol (2023) 14:1078705. doi: 10.3389/fimmu.2023.1078705
130. Falcomata C, Barthel S, Schneider G, Rad R, Schmidt-Supprian M, Saur D. Context-specific determinants of the immunosuppressive tumor microenvironment in pancreatic cancer. Cancer Discovery (2023) 13(2):278–97. doi: 10.1158/2159-8290.CD-22-0876
Keywords: tumor-associated macrophages (TAM), tumor microenvironment (TME), phenotypic diversity, regulatory signaling pathways, clinical trials, cancer immunotherapy
Citation: Shao S, Miao H and Ma W (2023) Unraveling the enigma of tumor-associated macrophages: challenges, innovations, and the path to therapeutic breakthroughs. Front. Immunol. 14:1295684. doi: 10.3389/fimmu.2023.1295684
Received: 17 September 2023; Accepted: 31 October 2023;
Published: 14 November 2023.
Edited by:
Sumit Kumar Hira, University of Burdwan, IndiaReviewed by:
Saptak Banerjee, Chittaranjan National Cancer Institute (CNCI), IndiaArmando Rojas, Catholic University of the Maule, Chile
Copyright © 2023 Shao, Miao and Ma. This is an open-access article distributed under the terms of the Creative Commons Attribution License (CC BY). The use, distribution or reproduction in other forums is permitted, provided the original author(s) and the copyright owner(s) are credited and that the original publication in this journal is cited, in accordance with accepted academic practice. No use, distribution or reproduction is permitted which does not comply with these terms.
*Correspondence: Wenxue Ma, d21hQGhlYWx0aC51Y3NkLmVkdQ==
†These authors have contributed equally to this work