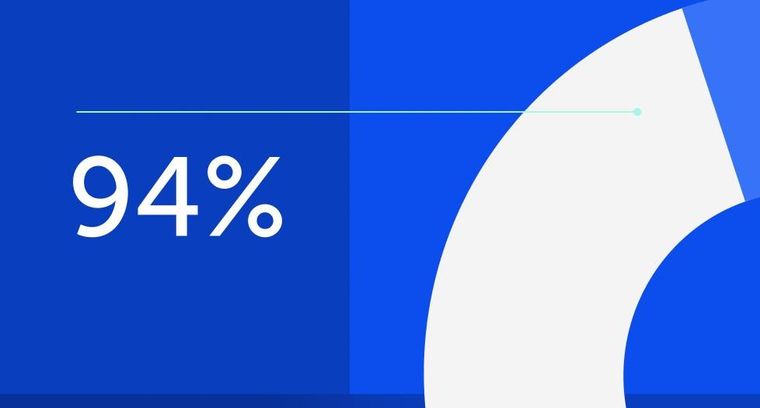
94% of researchers rate our articles as excellent or good
Learn more about the work of our research integrity team to safeguard the quality of each article we publish.
Find out more
REVIEW article
Front. Immunol., 26 January 2024
Sec. Microbial Immunology
Volume 14 - 2023 | https://doi.org/10.3389/fimmu.2023.1288256
The association between gut microbiota and central nervous system (CNS) development has garnered significant research attention in recent years. Evidence suggests bidirectional communication between the CNS and gut microbiota through the brain-gut axis. As a long and complex process, CNS development is highly susceptible to both endogenous and exogenous factors. The gut microbiota impacts the CNS by regulating neurogenesis, myelination, glial cell function, synaptic pruning, and blood-brain barrier permeability, with implication in various CNS disorders. This review outlines the relationship between gut microbiota and stages of CNS development (prenatal and postnatal), emphasizing the integral role of gut microbes. Furthermore, the review explores the implications of gut microbiota in neurodevelopmental disorders, such as autism spectrum disorder, Rett syndrome, and Angelman syndrome, offering insights into early detection, prompt intervention, and innovative treatments.
The mammalian central nervous system (CNS), consisting of the brain and spinal cord, exhibits unparalleled molecular, genetic, behavioral, developmental, and evolutionary complexity. A well-developed CNS is critical for survival, overseeing and directing behaviors through the transmission, storage, and processing of information. Recent research has emphasized the importance of interactions between gut microbes and the CNS, highlighting their ability to modulate host physiology, metabolism, immune function, brain function, and behavior (1). Early life represents a period of maximal bodily plasticity and heightened CNS sensitivity (2, 3). As the primary molecular interface, the intestine can influence the physiological environment during pregnancy, thus exposing the fetus to microbial signals before birth. Postnatally, the intestinal flora in infants undergoes rapid establishment and stabilization during the first two years of life, creating a persistent association between the host and commensal microbes (1, 3). The human gut microbiota exhibits remarkable genetic diversity, with over 22 million sequenced genes and an extensive library of unique enzymes capable of producing and modifying diverse chemical structures (4). Over evolutionary timeframes, microbial colonization has become integrated with CNS development programming, facilitated by a bidirectional connection between the gut and brain (4). In the prenatal phase, the fetus is exposed to microbial derivatives (e.g., metabolites, peptidoglycan) and maternal immune responses, both of which significantly influence CNS development (5, 6). Furthermore, gut microbial colonization during early postnatal life can impact CNS development and subsequent behavior (7, 8). Consequently, both prenatal and postnatal periods emerge as pivotal windows wherein gut microbes influence the CNS.
Neurodevelopmental disorders (NDDs) encompass a spectrum of chronic conditions impacting CNS functions during developmental stages, including motor skills, cognition, communication, and behavior (9, 10). Gastrointestinal (GI) comorbidities in NDDs, such as autism spectrum disorder (ASD), Rett syndrome (RTT), and Angelman syndrome (AS), exhibit a strong correlation with disease severity and pronounced symptoms of irritability, anxiety, and social withdrawal (11). These observations implicate the gut microbiome in the modulation of NDD severity and associated GI symptoms. While NDDs are usually studied from a genetic perspective, the above evidence has shifted attention toward the potential link between NDDs and gut microbiota.
In this review, the relationship between brain development and gut microbiome across various developmental stages is elaborated upon, with an emphasis on the role and mechanisms of microbes in related NDDs.
The mammalian body functions as an expansive “biochemical factory”. Beyond its native cells, it hosts trillions of microorganisms that create complex ecological niches both internally and externally (12). The gut, in particular, is a complex and dynamic ecosystem, inhabited by bacteria, archaea, viruses, and fungi (13, 14). This gut microbiota influences multiple aspects of host physiology, including nutritional balance, cellular metabolism, immune system, mucosal barrier permeability, and bidirectional communication between the gut and brain (15, 16).
The gut and brain communicate bidirectionally through integrated metabolic, endocrine, neurological, and immune pathways. Key components of this system include the vagus nerve, hypothalamic-pituitary-adrenal (HPA) axis, microbial metabolites, immune mediators such as cytokines, and enteroendocrine signaling (16, 17) (Figure 1). This interaction can be categorized into three primary pathways: Firstly, microbial metabolites can interface with the enteric nervous system (ENS), activating the vagus nerve and subsequently communicating with the CNS (18). Secondly, metabolites produced by gut microbes may traverse the intestinal barrier, entering the circulatory system and subsequently accessing the CNS via the blood-brain barrier (BBB) to modulate its functions (19, 20). Lastly, microbial-associated molecular patterns (MAMPs) such as lipopolysaccharide (LPS) and other microbiota-produced metabolites can elicit responses from the immune system, leading to cytokine release from immune cells, which directly influence the CNS (1). The gut-brain axis is not a linear system, but a circular feedback loop that communicates via multiple pathways. The bacterial-associated factors enter the circulation and modify peripheral immune cells via blood transport. Modified peripheral immune status promotes interactions with the BBB and neurovascular units (21). This allows microbiome-induced factors, cytokines, and immune substances to cross the BBB, affecting its integrity, transport rate, and triggering the release of neuroimmune substances from barrier cells (21). This involves simultaneously the nervous system, the immune system and the circulatory system. Multiple pathways are intertwined and together channel the bidirectional communication between the gut and the CNS. Notable metabolites in this context include short-chain fatty acids (SCFAs), bile acid metabolites, nervous system agonist transmitters such as γ-aminobutyric acid (GABA), tryptophan precursors, 5-hydroxytryptamine (5-HT), and catecholamines. These metabolites, by engaging in host signaling, can influence host metabolic processes and immune responses (22–25).
Figure 1 Microbiota-gut-brain axis. The bidirectional connection between the gut and the brain involves three major systems: the immune system, the circulatory system, and the nervous system. Multiple direct (e.g. vagus nerve) and indirect (e.g. short-chain fatty acids, cytokines, and key dietary amino acids, such as tryptophan) pathways exist to modulate the CNS with gut microbiota and to affect the gut microbial environment through the CNS. BMEC, brain microvascular endothelial cell; HPA, hypothalamic pituitary adrenal axis; SCFAs, short-chain fatty acids; 5-HT, 5-Hydroxytryptamine.
Within the regulatory framework of the microbiota-gut-brain axis, the gut engages in bidirectional communication with the brain, facilitating the influence of gut microbes on CNS development and related diseases. Investigating the relationship between the gut microbiota and CNS can offer insights into CNS pathologies and new therapeutic strategies. However, several unresolved questions remain: the exact mechanisms by which gut microbes influence CNS development and associated disorders; the repercussions of CNS-related diseases on the gut and its microbial community; and the efficacy of interventions involving intestinal probiotics and prebiotics for treating CNS diseases. These topics warrant further research.
CNS development is a long and complex process that continues from the fetal stages to early adulthood (Figure 2A). During embryonic development, the neural plate undergoes longitudinal folding to produce the neural tube, which forms the rudimentary CNS (26). Central neurodevelopment involves neurogenesis, neuronal migration, dendritic and axonal formation, synaptogenesis, and interneuronal connection formation (27, 28). Notably, processes such as dendritic expansion, dendritic spine and synapse formation, and synaptic pruning are the most time-consuming steps in human neuronal development (29–31). As the CNS matures, the developmental trajectories of neuronal and non-neuronal cells intertwine, including events such as myelinogenesis, angiogenesis, and blood-brain barrier formation (32). While most brain neurons are formed during the fetal stages, postnatal CNS development is dominated by an increase in glial cells and neural outgrowth (33).
Figure 2 Associations between different stages of CNS development and gut microbiota. (A). Critical processes in CNS development; (B). Influence of maternal gut microbial metabolites on fetal brain development; (C). Influence of bacterial LPS on fetal brain development; (D). Influence of maternal gut microbial mediation of immune system on fetal brain development; (E). Influence of establishment of gut microbes in early postnatal life on host CNS development. ABX, antibiotic-treated; TMAO, trimethylamine-N-oxide; TMAV, N, N, N-Trimethyl-5-aminovalerate; HIP, hippurate; 5-AV, 5-Aminovalerate; PG, peptidoglycan; PAFR, platelet activating factor receptor; BMEC, brain microvascular endothelial cell; MIA, maternal immune activation; SFB, segmented filamentous bacteria; FMT, fecal microbiota transplantation.
Given the prolonged and complex nature of CNS developmental processes, spanning both prenatal and postnatal periods, they are highly sensitive and vulnerable to internal and external environmental factors (34). Emerging evidence indicates that the gut microbiota plays a crucial role in CNS development. Germ-free (GF) mice serve as a valuable model for elucidating the effects of microbiota on CNS function and development (35, 36). Using this mouse model, key neurodevelopmental processes, including neurogenesis, cell migration, myelin formation, and microglia activation, have been linked to gut flora composition (37–40). Compared with conventional (specific pathogen-free; SPF) mice, GF mice display increased antidepressant-like behavior, risk-taking behavior, and hyperactivity, accompanied by deficits in learning and memory (22, 41, 42). Notably, these mice also show variations in the expression of 5-hydroxytryptamine receptors (5-HT 1A), synaptophysin, and neurotrophic factors (e.g., BDNF) in the hippocampus, as well as impaired blood-brain barrier functionality and increased myelin formation in the prefrontal cortex (42, 43). From a transcriptome sequencing perspective, the increase of certain immediate early response genes (e.g. Fos, Fosb, Egr2 or Nr4a1) in the GF mice amygdala is associated with increased CREB signaling, and suggests a link between the microbial establishment during early life and neurodevelopmental disorders (44). Studies have also highlighted the potential involvement of the microbiome in neurological disorders, such as depression, anxiety, schizophrenia, ASD, Alzheimer’s disease, Huntington’s disease, Parkinson’s disease, and multiple sclerosis (45, 46). Such findings underscore the pivotal contribution of the gut microbiota in CNS development and provide novel insights into the pathogenesis and therapeutic strategies for congenital CNS disorders.
The absence of fetal microbiota is commonly acknowledged. Nonetheless, the fetus is inevitably exposed to maternal-origin microbial-associated molecules, including metabolites, peptidoglycan (PG), and LPS (47–49) (Figures 2B–D). Various studies have established an association between maternal gut microbiota and abnormalities in offspring brain function and behavior. However, the precise mechanistic pathway by which this maternal impact shapes fetal brain development during the crucial prenatal period remains unknown.
During gestation, maternal gut microbial metabolites not only regulate maternal health but also exert effects on fetal brain development. While the molecular mechanisms underlying the effects of gut microbial metabolites on the brain remain elusive, certain metabolites, such as TMAO (Trimethylamine-N-oxide), TMAV (N, N, N-Trimethyl-5-aminovalerate), and HIP (Hippurate), have been implicated in neurological disorders and neurite growth (50) (Figure 2B).
Fetal brains from antibiotic-treated (ABX) dams exhibit decreased NTNG1 gene expression, which encodes the Netrin G1 protein essential for neuronal axonal growth, and fewer thalamocortical synapses relative to those from SPF dams (51–53). In vitro co-cultures pairing the thalamus with the striatum and hypothalamus demonstrate that thalamic neurons from ABX dams inadequately discern associated elements from the striatum and hypothalamus (53). Deficiencies in the maternal microbiome impede fetal thalamic reactions to tissue-related factors, leading to a reduction in thalamocortical synapses due to the lack of synaptic guidance signals (53). Specific microbial colonization, notably Clostridia, during pregnancy can ameliorate these alterations (53). Maternal serum and fetal cerebral concentrations of TMAO, TMAV, and HIP are diminished in ABX group relative to SPF group (53, 54). However, intraperitoneal delivery of TMAO, 5-AV (5-Aminovalerate), and HIP, or specialized gut microbe colonization in ABX dams, rescues these abnormalities, restoring fetal cerebral metabolite concentrations, thalamic synaptic densities, and tactile sensitivity in adults (53). These findings emphasize that the impact of maternal gut microbiota on fetal brain metabolic profiles and gene expression commences during the prenatal phase, underscoring the critical importance of gestation as a period during which maternal microbiota actively fosters fetal neurodevelopment.
Throughout pregnancy, maternal infections and inflammation can influence fetal health and development (55). Bacterial components produced during maternal infections can traverse the placental barrier, activating the fetal innate immune system and potentially impacting brain development and postnatal cognition (Figure 2C). These bacterial components are not only from maternal infection but may also be from the release of normal gut microbes (56, 57). Research has indicated that prenatal maternal exposure to PG is correlated with cognitive deficits in progeny. Notably, bacterial cell wall PG can cross the placenta, entering the developing fetal brain and inducing neuronal proliferation in the frontal cortex by increasing FOXG1 expression, a process dependent on Toll-like receptor 2 (TLR2) (58). FoxG1 is a transcription factor crucial for embryonic telencephalon development and patterning and a known spatiotemporal hub gene in the brain (59). Dysregulation of FoxG1 is associated with certain disorders, such as medulloblastoma and ASD (60, 61). These observations enhance our understanding of the link between maternal gut microbes and fetal brain and immune development, as well as the interactions between PAMPs and neuronal tissues and the discovery of novel cellular signaling pathways.
The maternal immune system is strongly associated with gut microbes and fetal development (Figure 2D). Activation of the maternal immune system during pregnancy can impact offspring physiology, neuropathology, behavior, and microbial composition. Epidemiological studies have shown that maternal prenatal infections and inflammation can significantly increase the risk of offspring developing schizophrenia and ASD (62). Researchers have developed primate and rodent maternal immune activation (MIA) models using synthetic double-stranded RNA (polyinosinic: polycytidylic acid (poly (I:C)) or TLR ligands (e.g., LPS). Offspring from these MIA models display behavioral anomalies indicative of NDDs, including diminished social behaviors, heightened repetitive actions, and communication irregularities (63–65). Changes in the gut microbiota of these offspring can significantly influence their serum metabolomic profiles (66, 67). While segmented filamentous bacteria in the prenatal gut of MIA dams can lead to atypical behaviors in offspring, intervention with Bacteroides fragilis can ameliorate some of these adverse effects (66, 67). The presence of gut microbes, combined with pro-inflammatory signals during pregnancy, induces TH17 cells, leading to elevated maternal plasma IL-17a concentrations (67). Certain gut microbes that promote TH17 cell proliferation may thereby increase NDD risks in MIA offspring. These findings indicate that MIA models can provide valuable insights into how the gut microbiome and immune response collaboratively impact the physiology, behavior, and neuropathology of offspring.
The commensal microbiota in organisms emerges through increasing interactions with the environment in early life, fostering enduring interactions between host and gut microbes. Influences on gut microbiota composition include host genetic susceptibility, environmental factors, lifestyle, diet, and antibiotic and non-antibiotic drug use (68) (Figure 2E). The early establishment of gut microbiota coincides with a crucial period of CNS development, during which antibiotic intervention and probiotic supplementation can have profound effects on brain development, structure, and function (69).
Research has linked antibiotic use in early life and adolescence with subsequent depression and behavioral challenges (70–72). Administering non-absorbable antibiotics to adult mice over a 7-day period is sufficient to reduce anxiety-like behavior, although this effect reverts to baseline upon cessation of antibiotic use and recovery of the gut microbiome within two weeks (65). Long-term antibiotic treatment from weaning to adulthood disrupts gut microbiota structure and affects brain development and behavior in mice (72). Similarly, GF animals exhibit distinctive behaviors and developmental phenotypes compared to SPF animals (22, 41, 42). Furthermore, imbalances in gut bacteria during early brain development may heighten neurodegeneration susceptibility in later life. Probiotic interventions can modulate or mitigate specific adverse conditions in both mice and humans by enhancing specific microbial populations, either temporarily or permanently (73). Current studies have also explored the potential reconstruction of healthy gut flora through fecal microbiota transplantation (FMT) (74, 75) (Figure 2E). These findings highlight the strong association between the gut microbiome in early postnatal life and CNS development and behavior. Approaches that utilize gut probiotics, prebiotics, and gut flora restoration are emerging as promising strategies for CNS disease treatment.
ASD represents a cluster of complex heterogeneous NDDs. The primary clinical manifestations of ASD include impaired social interactions, disordered language development, and restricted repetitive behaviors (76). Epidemiological studies indicate an increasing incidence of ASD in recent years. The World Health Organization (WHO) estimates a global ASD prevalence of about 1%, with a recent survey estimating a prevalence of 1%–5% in developed countries, potentially associated with changes in diagnostic criteria, improvements in screening and diagnostic tools, and increased public awareness (77, 78). The etiology and pathogenesis of ASD are complex, with contributions from genetic, epigenetic, and environmental factors. Environmental factors may increase the incidence of ASD by increasing genetic susceptibility (76). While the interval from prenatal and early postnatal life is considered critical for environmental impacts on ASD, the precise timing and mechanisms of action of these factors are yet to be fully elucidated.
Studies have shown that GI symptoms are strongly associated with autism severity, irritability, anxiety, and social withdrawal (79, 80). Interestingly, in addition to genetic differences, ASD patients also exhibit significant differences in gut bacterial species and abundance compared to typically developing (TD) individuals (81). Such findings have triggered further research on the link between ASD and gut microbiota as well as NDDs and gut dysbiosis. At the phylum level, the gut microbiota in ASD patients predominantly consists of Bacteroidetes, Firmicutes, and Actinobacteria, and Tenericutes (82), with a higher ratio of Bacteroidetes to Firmicutes in ASD than in TD (82, 83). At the genus level, ASD individuals exhibit higher levels of Bacteroides, Parabacteroides, Dorea, Phascolarctobacterium, Clostridium, Faecalibacterium, Ruminococcus, Lachnospiracea incertae sedis, Roseburia, and Lactobacillus, but lower relative abundances of Bifidobacterium, Coprococcus, Blautia, Veillonella, Dialister, Escherichia/Shigella, Prevotella, Clostridium XIVa, Streptococcus, Akkermansia, Sutterella, and Enterococcus (82–85) (Figure 3). The gut flora in ASD rodent models shows similar significant differences relative to normal rodents (81).
Figure 3 Venn diagram showing shared clinical and microbial features among ASD, RTT, and AS. NDDs with similar clinical features show some microbial compositional features that are enriched/reduced in the same taxa. The main overlap, both clinical and microbial. Among the three NDDs, ASD, RTT and AS, multiple studies have shown increased abundance of Bacteroides and decreased abundance of Streptococcus. Different colored circles represent different NDDs; pink, ASD; green, RTT; purple, AS. Overlapping regions have the same abundance trend. Red arrows, increasing relative abundance, blue arrows, decreasing relative abundance.
Dysbiosis in ASD-associated gut microbiota is characterized by an overabundance of pathogenic bacteria and a reduced presence of beneficial bacteria. Research indicates that GF mice receiving FMT from ASD mice display decreased communication and increased repetitive behaviors compared to GF mice receiving FMT from TD mice (81, 86). Treating ASD mice with Bacteroides fragilis has been shown to correct intestinal mucosal barrier defects and improve ASD symptoms, such as stereotyped behavior and anxiety (66, 87). Certain metabolites from the order Clostridiales are correlated with repetitive behaviors and GI issues in ASD, which can be reversed following antibiotic use (88). Elevated concentrations of propionic acid (PPA), primarily produced by the phylum Bacteroidetes, are recognized as significant neurotoxic SCFAs (89). SCFAs, when transported to the brain via the circulatory system, influence brain development by modulating serotonin and dopamine synthesis (90). Both animal models and clinical trials involving probiotics, such as Lactobacillus and Bifidobacterium, report improvements in mood, anxiety, sleep quality, and depression (91, 92). These findings further underscore the correlation between gut flora and neuropsychiatric and behavioral shifts in affected individuals.
Methyl-CpG binding protein 2 (MECP2) is an important gene in the pathogenesis of ASD and RTT and critical for regulating synaptic activity in early postnatal life (93). RTT is a progressive neurological disorder primarily caused by mutations in the MeCP2 gene on the X chromosome. GI dysfunction and constipation are commonly observed in RTT patients, suggesting a link between neurological abnormalities with intestinal function and gut microbiota (94). Research has documented structural alterations in the gut microbiome of human RTT subjects and MECP2-mutated animal models, characterized by diminished abundance and diversity (95, 96). At the phylum level, while Actinobacteria predominates in RTT and Firmicutes predominates in TD, RTT exhibits an increased relative abundance of Actinobacteria and Firmicutes, a reduced presence of Bacteroidetes, and a notably elevated Firmicutes/Bacteroidetes ratio, indicating gut ecological dysregulation associated with RTT (95, 96). At the genus level, Bacteroides, Bifidobacterium, Parabacteroides, Lachnospiracea incertae sedis, Blautia, Escherichia/Shigella, Actinomyces, Clostridium XIVa, Enterococcus, Clostridium, and Sutterella show an increase in relative abundance in RTT, but a decrease in Faecalibacterium, Streptococcus, Ruminococcus, Prevotella, Gemmiger, and Alistipes (94, 95, 97) (Figure 3) Functional disruptions in MeCP2 modify the gut microbial community structure, which, in turn, alters SCFA production and gastrointestinal pathophysiology in RTT, leading to constipation, inflammation, and host cytokine dysregulation (95). This impairment in MeCP2, mediated through the gut-brain axis, manifests as a dysregulated gut ecology in RTT patients.
RTT primarily affects females, with males harboring the MECP2 mutation tending to succumb shortly after birth. MECP2 is widely expressed in mammals, predominantly in the nervous system, with higher expression in neurons than in glial cells (93). Studies have shown that male ASD patients exhibit diminished MECP2 expression in brain astrocytes, concomitant with high methylation of the MECP2 promoter in the frontal cortex, alongside the genetic mutation observed in RTT patients (98, 99). Such evidence suggests that MECP2 DNA hypermethylation in astrocytes may underlie ASD pathogenesis in males. Several studies have shown that reintroducing MECP2 expression in astrocytes of MECP2-deficient mice can ameliorate certain behavioral and molecular abnormalities (100). In Mecp2-deficient mice, increased glutamine uptake by microglia can induce toxic effects due to glutamate overproduction in hippocampal neurons, leading to dendritic and synaptic damage (101, 102). Notably, glutamate, an excitatory neurotransmitter, is highly susceptible to gut microbes and can be metabolized by bacterial glutamate decarboxylase to produce gamma-aminobutyric acid (GABA). Subsequent research has shown that GABA-producing bacteria can reduce depression- and anxiety-like behaviors in mouse models (103, 104). Exploration into RTT immune dysfunction using targeted glial cell activation has also revealed that LPS stimulation can induce a dramatic increase in mixed astrocyte-microglia pro-inflammatory cytokine release in MECP2-deficient mice (105). These findings offer promising avenues for RTT treatment, potentially ameliorating physiological functions in patients or influencing disease progression through gut flora modulation.
Cholesterol is an important component of the brain, involved in membrane transport, signal transduction, myelin formation, dendritic remodeling, neuropeptide formation, and synaptogenesis (106). While evidence suggests that nascent neurons autonomously synthesize cholesterol, this capability diminishes with development, leading to a reliance on astrocytes for cholesterol production (107). This down-regulation is absent in RTT brains, leading to lipid accumulation and metabolic dysregulation (108). Intestinal microbes maintain cholesterol homeostasis in the body, playing a key role in bile acid (cholesterol derivative) metabolism and the conversion of cholesterol into distinct metabolites through dehydrogenase activity encoded by the IsmA gene (109). Previous studies have suggested that fecal metabolites are altered in Mecp2-mutated females and associated with lipid defects in the brain (108). Recent studies have applied RTT mouse models to investigate the role of the gut microbiome and metabolome perturbations in RTT disease progression.
Glial cell lesions and dysregulated brain lipid metabolism due to MECP2 dysfunction have been implicated as primary drivers of RTT and ASD pathogenesis (110, 111). Gut microbes play a critical role in RTT disease progression, which warrants further study. Changes in the intestinal microbiota of RTT patients may reflect clinical presentations and potentially impact disease evolution. A future research challenge is the restoration of intestinal flora or colonization with specific probiotics as a novel approach for RTT management.
AS is a rare genetic neurodevelopmental syndrome resulting from the gene expression deletion of maternally inherited ubiquitin ligase E3A (UBE3A) in brain neurons (112). While paternally inherited UBE3A is expressed in most peripheral organs, it is silenced in the CNS by long non-coding antisense transcripts (UBE3A-ATS) due to brain-specific imprinting. Thus, deletion of maternally inherited UBE3A can lead to complete loss of UBE3A expression in the brain (113). Clinical manifestations of AS include microcephaly, severe developmental delays, expressive communication deficits, distinct facial features, motor and coordination deficits, hypotonia, generalized epilepsy, and sleep disturbances (114). Beyond neurodevelopmental effects, GI issues are also frequently reported in AS patients (115). The gut microbiome is critical for the establishment of GI physiology and function, and alterations in microbial colonization are common in NDDs. At the phylum level, the gut microbiota of AS animal models shows an increased abundance of Bacteroidetes and Actinobacteria and a decreased abundance of Firmicutes compared to wild-type (WT) controls, leading to a lower Bacteroidetes to Firmicutes ratio in AS (116). At the genus level, AS animal models exhibit a decreased abundance of Lactobacillus, Bifidobacterium, Dubosiella, Streptococcus, and Akkermansia compared to WT controls, but increased levels of Bacteroides, Trichoderma, Lachnospiraceae, Desulfovibrio, Odoribacter, Faecalibacterium, Roseburia, Blautia, Ruminococcus, and Turicibacter (116) (Figure 3). Notably, Lactobacillus is associated with several diseases, including major depressive disorder and non-alcoholic fatty liver disease (117), while Desulfovibrio is associated with Parkinson’s disease, with its abundance in the gut directly related to disease severity (118). Furthermore, Odoribacter is associated with attention deficit and hyperactivity disorders, and can disrupt dopamine and serotonin levels in the gut (119). The changes observed in the AS gut microbiome are similar to those detected in the gut flora of other NDDs.
Most AS patients exhibit symptoms of impaired coordination, imbalance, and gait ataxia (120). PIEZO2, a mechanosensitive ion channel, is necessary for maintaining coordination and balance (121). Reduced PIEZO3 activity has been identified in sensory neurons from UBE3A-deficient mice and stem cell-derived neurons from AS patients. Linoleic acid (LA)-rich diets have been shown to increase PIEZO2 activity and mechanical excitability in male AS mice, ameliorating gait abnormalities. Furthermore, LA supplementation has been shown to increase PIEZO2 function in sensory neurons from UBE3A-deficient mice and in stem cell-derived neurons from AS patients (119). The gut microbiota has the capacity to metabolize lipids into bioactive metabolites (121). Recent studies have highlighted that LA-derived microbial metabolites can influence host lipid metabolism via the augmentation of peroxisomal β-oxidative metabolism (121). These metabolites also improve CNS self-immunity in multiple sclerosis mouse models, as evidenced by improved gut barrier function, attenuated inflammation, and increased in intestinal myeloid-derived suppressor-like cells (122). Strategic modulation of the gut microbiome may alleviate neurological and gastric symptoms in AS patients, thereby enhancing their overall quality of life.
Interestingly, NDDs with similar clinical features often exhibit microbial compositional similarities with enrichment or reduction in the same taxa (82–85, 94, 95, 97, 116). The main overlap, both clinical and microbial (Figure 3). The figure considers only the microbial changes reported in most studies (i.e., discarding inconsistent results). Bacteroides enrichment is frequently reported in the reviewed NDDs (82–85, 94, 95, 97, 116). This taxon, together with Clostridium, possesses remarkable proteolytic ability, leading to potentially toxic compounds that can disrupt gut homeostasis and permeability and affect the survival of other beneficial microorganisms, such as lactic bacteria (123, 124). Conversely, a decrease in the relative abundance of Streptococcus, including Streptococcus thermophilus, is common among NDD patients. While Streptococcus thermophilus is known to ferment lactose and sucrose (125), the overall relationship between Streptococcus and GI health remains largely unexplored. Remarkably, although Streptococcus species are generally non-pathogenic, some strains are associated with multiple metabolic disorders (126). Streptococcus thermophilus can increase the abundance of gut probiotics, including Bifidobacterium and Lactobacillus, via β-galactosidase (127). Predominant shifts in gut microbial communities can induce an increase of pro-inflammatory species and a decrease in probiotic species, which may promote alterations in gut permeability and barrier functionality.
Alterations in gut flora are associated with NDDs (e.g., ASD, RTT, AS) and GI disorders, highlighting the important role of gut microbes in gut-brain communication (11, 79, 80, 94, 115, 116). Whether these shifts in gut flora cause GI symptoms in NDDs and influence disease progression remains unclear. Based on current findings, evaluating gut flora alterations is necessary to study the effects of gut microbiota on GI physiology and motor behavior in NDD patients and related animal models.
In summary, the gut microbiota is closely linked to CNS development and related diseases. Gut microbes and their metabolites play a crucial role in brain-gut axis interactions. While exploration of the gut-brain axis remains at an early stage, certain basic circuits have begun to emerge and specific neurodevelopmental pathways may require a response from gut microbial signals. Mammalian CNS development is a long process that begins during pregnancy with the differentiation of neural progenitor cells and extends into late adolescence, potentially persisting across the whole life cycle. These developmental processes entail relevant gene expression and environmental inputs, with disruptions in any of these events fundamentally altering neural outcomes (128). Functioning as an environmental determinant, the gut microbiome is highly likely to exert an enduring influence on the structure and functionality of the CNS (129). Many studies have indicated that changes in the maternal gut microbiota can modulate offspring gut microbiota, neurodevelopment, and behavior (130). In humans and rodents, perinatal administration of antibiotics can affect the health and immune status of offspring (131). In mouse models, maternal exposure to antibiotics alters gut microbiota and reduces motility in dams and offspring, with anxiety-like behavior and motor deficits in the latter (132). As previously discusses, important processes (e.g., synaptic growth and neuronal proliferation) in fetal CNS development are affected by maternal microbial-associated molecules (e.g., metabolites, PG, and LPS), with maternal gut microbes contributing to fetal brain development through the maternal immune system. Furthermore, the establishment of gut microbiota in early postnatal life can influence host CNS development and behavior. These examples underscore the critical role of the gut microbiome in both prenatal and postnatal CNS development. Currently, the connection between gut microbes and the pathogenesis in CNS diseases is progressively gaining prominence as a frontier of scientific research. NDDs (e.g., ASD, RTT, AS) can disrupt gut microbial balance, potentially aggravating disease progression. By modulating gut microorganisms, it may be possible to attenuate CNS disease development and enhance host immunity, thereby ensuring human and animal well-being. Nonetheless, current clinical data regarding probiotic supplementation for disease treatment remain limited, with a lack of standardized probiotic protocols. Consequently, in-depth study of the regulatory mechanisms underpinning CNS diseases and gut microbiota holds profound implications not only for advancing gut health promotion but also for expanding the scope of CNS-related disease prevention.
YG: Conceptualization, Validation, Visualization, Writing – original draft, Writing – review & editing. YC: Investigation, Validation, Writing – review & editing. HZ: Validation, Writing – review & editing. ZL: Validation, Writing – review & editing. JG: Conceptualization, Funding acquisition, Supervision, Validation, Writing – review & editing. HW: Conceptualization, Funding acquisition, Investigation, Supervision, Validation, Writing – review & editing. WW: Conceptualization, Funding acquisition, Investigation, Supervision, Validation, Writing – original draft, Writing – review & editing.
The author(s) declare financial support was received for the research, authorship, and/or publication of this article. This study was supported by a grant from the National Natural Science Foundation of China (82160514, 82360395), Expert station of Yunnan province (Kunming) (YSZJGZZ-2020051), Eminent Doctors Program of Yunnan Province (YNWR-MY-2019-072), Fundamental Research Projects of Yunnan Province (202101AT070275, 202101AY070001-236, 202101AU070137) and the Foundation of First People’s Hospital of Yunnan Province (KHYJ-6-2020-001, KHBS-2022-028, 2022-KHRCBZ-C06).
The authors declare that the research was conducted in the absence of any commercial or financial relationships that could be construed as a potential conflict of interest.
All claims expressed in this article are solely those of the authors and do not necessarily represent those of their affiliated organizations, or those of the publisher, the editors and the reviewers. Any product that may be evaluated in this article, or claim that may be made by its manufacturer, is not guaranteed or endorsed by the publisher.
CNS, Central nervous system; 5-AV, 5-Aminovalerate; 5-HT, 5-Hydroxytryptamine; ABX, Antibiotic-treated; AS, Angelman syndrome; ASD, Autism spectrum disorder; BDNF, Brain-derived neurotrophic factor; NDDs, Neurodevelopmental disorders; GF, Germ-free; GI, Gastrointestinal; HIP, Hippurate; LA, Linoleic acid; LPS, Lipopolysaccharide; MIA, Maternal immune activation; RTT, Rett syndrome; PG, Peptidoglycan; SCFAs, Short-chain fatty acids; SPF, Specific pathogen-free; TD, Typically developing; TMAO, Trimethylamine-N-oxide; TMAV, N, N, N-Trimethyl-5-aminovalerate; WT, Wild-type.
1. Doroszkiewicz J, Groblewska M, Mroczko B. The role of gut microbiota and gut-brain interplay in selected diseases of the central nervous system. Int J Mol Sci (2021) 22(18). doi: 10.3390/ijms221810028
2. Stephenson J, Heslehurst N, Hall J, Schoenaker D, Hutchinson J, Cade JE, et al. Before the beginning: nutrition and lifestyle in the preconception period and its importance for future health. Lancet (2018) 391(10132):1830–41. doi: 10.1016/s0140-6736(18)30311-8
3. Grech A, Collins CE, Holmes A, Lal R, Duncanson K, Taylor R, et al. Maternal exposures and the infant gut microbiome: a systematic review with meta-analysis. Gut Microbes (2021) 13(1):1–30. doi: 10.1080/19490976.2021.1897210
4. Tierney BT, Yang Z, Luber JM, Beaudin M, Wibowo MC, Baek C, et al. The landscape of genetic content in the gut and oral human microbiome. Cell Host Microbe (2019) 26(2):283–295.e8. doi: 10.1016/j.chom.2019.07.008
5. Principi N, Esposito S. Gut microbiota and central nervous system development. J Infect (2016) 73(6):536–46. doi: 10.1016/j.jinf.2016.09.010
6. Knuesel I, Chicha L, Britschgi M, Schobel SA, Bodmer M, Hellings JA, et al. Maternal immune activation and abnormal brain development across CNS disorders. Nat Rev Neurol (2014) 10(11):643–60. doi: 10.1038/nrneurol.2014.187
7. Luk B, Veeraragavan S, Engevik M, Balderas M, Major A, Runge J, et al. Postnatal colonization with human "infant-type" Bifidobacterium species alters behavior of adult gnotobiotic mice. PloS One (2018) 13(5):e0196510. doi: 10.1371/journal.pone.0196510
8. Luck B, Engevik MA, Ganesh BP, Lackey EP, Lin T, Balderas M, et al. Bifidobacteria shape host neural circuits during postnatal development by promoting synapse formation and microglial function. Sci Rep (2020) 10(1):7737. doi: 10.1038/s41598-020-64173-3
9. Parenti I, Rabaneda LG, Schoen H, Novarino G. Neurodevelopmental disorders: from genetics to functional pathways. Trends Neurosci (2020) 43(8):608–21. doi: 10.1016/j.tins.2020.05.004
10. Tang X, Jaenisch R, Sur M. The role of GABAergic signalling in neurodevelopmental disorders. Nat Rev Neurosci (2021) 22(5):290–307. doi: 10.1038/s41583-021-00443-x
11. Iliodromiti Z, Triantafyllou AR, Tsaousi M, Pouliakis A, Petropoulou C, Sokou R, et al. Gut microbiome and neurodevelopmental disorders: A link yet to be disclosed. Microorganisms (2023) 11(2). doi: 10.3390/microorganisms11020487
12. Sender R, Fuchs S, Milo R. Revised estimates for the number of human and bacteria cells in the body. PloS Biol (2016) 14(8):e1002533. doi: 10.1371/journal.pbio.1002533
13. Puschhof J, Pleguezuelos-Manzano C, Clevers H. Organoids and organs-on-chips: Insights into human gut-microbe interactions. Cell Host Microbe (2021) 29(6):867–78. doi: 10.1016/j.chom.2021.04.002
14. Kho ZY, Lal SK. The human gut microbiome - A potential controller of wellness and disease. Front Microbiol (2018) 9:1835. doi: 10.3389/fmicb.2018.01835
15. Becattini S, Taur Y, Pamer EG. Antibiotic-induced changes in the intestinal microbiota and disease. Trends Mol Med (2016) 22(6):458–78. doi: 10.1016/j.molmed.2016.04.003
16. Kim YK, Shin C. The microbiota-gut-brain axis in neuropsychiatric disorders: pathophysiological mechanisms and novel treatments. Curr Neuropharmacol (2018) 16(5):559–73. doi: 10.2174/1570159x15666170915141036
17. Borkent J, Ioannou M, Laman JD, Haarman BCM, Sommer IEC. Role of the gut microbiome in three major psychiatric disorders. Psychol Med (2022) 52(7):1222–42. doi: 10.1017/s0033291722000897
18. Muller PA, Schneeberger M, Matheis F, Wang P, Kerner Z, Ilanges A, et al. Microbiota modulate sympathetic neurons via a gut-brain circuit. Nature (2020) 583(7816):441–6. doi: 10.1038/s41586-020-2474-7
19. Mayer EA, Nance K, Chen S. The gut-brain axis. Annu Rev Med (2022) 73:439–53. doi: 10.1146/annurev-med-042320-014032
20. Deng Y, Zhou M, Wang J, Yao J, Yu J, Liu W, et al. Involvement of the microbiota-gut-brain axis in chronic restraint stress: disturbances of the kynurenine metabolic pathway in both the gut and brain. Gut Microbes (2021) 13(1):1–16. doi: 10.1080/19490976.2020.1869501
21. Logsdon AF, Erickson MA, Rhea EM, Salameh TS, Banks WA. Gut reactions: How the blood-brain barrier connects the microbiome and the brain. Exp Biol Med (Maywood) (2018) 243(2):159–65. doi: 10.1177/1535370217743766
22. Clarke G, Grenham S, Scully P, Fitzgerald P, Moloney RD, Shanahan F, et al. The microbiome-gut-brain axis during early life regulates the hippocampal serotonergic system in a sex-dependent manner. Mol Psychiatry (2013) 18(6):666–73. doi: 10.1038/mp.2012.77
23. Wall R, Cryan JF, Ross RP, Fitzgerald GF, Dinan TG, Stanton C. Bacterial neuroactive compounds produced by psychobiotics. Adv Exp Med Biol (2014) 817:221–39. doi: 10.1007/978-1-4939-0897-4_10
24. Kiraly DD, Walker DM, Calipari ES, Labonte B, Issler O, Pena CJ, et al. Alterations of the host microbiome affect behavioral responses to cocaine. Sci Rep (2016) 6:35455. doi: 10.1038/srep35455
25. Jenkins TA, Nguyen JC, Polglaze KE, Bertrand PP. Influence of tryptophan and serotonin on mood and cognition with a possible role of the gut-brain axis. Nutrients (2016) 8(1). doi: 10.3390/nu8010056
26. Delhaye-Bouchaud N. [Development of the central nervous system in mammals]. Neurophysiol Clin (2001) 31(2):63–82. doi: 10.1016/s0987-7053(01)00249-0
27. Kriegstein AR, Noctor SC. Patterns of neuronal migration in the embryonic cortex. Trends Neurosci (2004) 27(7):392–9. doi: 10.1016/j.tins.2004.05.001
28. Geschwind DH, Rakic P. Cortical evolution: judge the brain by its cover. Neuron (2013) 80(3):633–47. doi: 10.1016/j.neuron.2013.10.045
29. Petanjek Z, Sedmak D, Džaja D, Hladnik A, Rašin MR, Jovanov-Milosevic N. The protracted maturation of associative layer IIIC pyramidal neurons in the human prefrontal cortex during childhood: A major role in cognitive development and selective alteration in autism. Front Psychiatry (2019) 10:122. doi: 10.3389/fpsyt.2019.00122
30. Bourgeois JP, Rakic P. Changes of synaptic density in the primary visual cortex of the macaque monkey from fetal to adult stage. J Neurosci (1993) 13(7):2801–20. doi: 10.1523/jneurosci.13-07-02801.1993
31. Petanjek Z, Judas M, Kostović I, Uylings HB. Lifespan alterations of basal dendritic trees of pyramidal neurons in the human prefrontal cortex: a layer-specific pattern. Cereb Cortex (2008) 18(4):915–29. doi: 10.1093/cercor/bhm124
32. Taverna E, Götz M, Huttner WB. The cell biology of neurogenesis: toward an understanding of the development and evolution of the neocortex. Annu Rev Cell Dev Biol (2014) 30:465–502. doi: 10.1146/annurev-cellbio-101011-155801
33. Jena A, Montoya CA, Mullaney JA, Dilger RN, Young W, McNabb WC, et al. Gut-brain axis in the early postnatal years of life: A developmental perspective. Front Integr Neurosci (2020) 14:44. doi: 10.3389/fnint.2020.00044
34. Rodier PM. Vulnerable periods and processes during central nervous system development. Environ Health Perspect (1994) 102 Suppl 2(Suppl 2):121–4. doi: 10.1289/ehp.94102121
35. Huang Y, Wu J, Zhang H, Li Y, Wen L, Tan X, et al. The gut microbiome modulates the transformation of microglial subtypes. Mol Psychiatry (2023) 28(4):1611–21. doi: 10.1038/s41380-023-02017-y
36. Lee J, Venna VR, Durgan DJ, Shi H, Hudobenko J, Putluri N, et al. Young versus aged microbiota transplants to germ-free mice: increased short-chain fatty acids and improved cognitive performance. Gut Microbes (2020) 12(1):1–14. doi: 10.1080/19490976.2020.1814107
37. Ogbonnaya ES, Clarke G, Shanahan F, Dinan TG, Cryan JF, O'Leary OF. Adult hippocampal neurogenesis is regulated by the microbiome. Biol Psychiatry (2015) 78(4):e7–9. doi: 10.1016/j.biopsych.2014.12.023
38. Erny D, Hrabě de Angelis AL, Jaitin D, Wieghofer P, Staszewski O, David E, et al. Host microbiota constantly control maturation and function of microglia in the CNS. Nat Neurosci (2015) 18(7):965–77. doi: 10.1038/nn.4030
39. Cryan JF, O'Riordan KJ, Sandhu K, Peterson V, Dinan TG. The gut microbiome in neurological disorders. Lancet Neurol (2020) 19(2):179–94. doi: 10.1016/s1474-4422(19)30356-4
40. Hoban AE, Stilling RM, Ryan FJ, Shanahan F, Dinan TG, Claesson MJ, et al. Regulation of prefrontal cortex myelination by the microbiota. Transl Psychiatry (2016) 6(4):e774. doi: 10.1038/tp.2016.42
41. Gareau MG, Wine E, Rodrigues DM, Cho JH, Whary MT, Philpott DJ, et al. Bacterial infection causes stress-induced memory dysfunction in mice. Gut (2011) 60(3):307–17. doi: 10.1136/gut.2009.202515
42. Diaz Heijtz R, Wang S, Anuar F, Qian Y, Bjorkholm B, Samuelsson A, et al. Normal gut microbiota modulates brain development and behavior. Proc Natl Acad Sci U.S.A. (2011) 108(7):3047–52. doi: 10.1073/pnas.1010529108
43. Ait-Belgnaoui A, Colom A, Braniste V, Ramalho L, Marrot A, Cartier C, et al. Probiotic gut effect prevents the chronic psychological stress-induced brain activity abnormality in mice. Neurogastroenterol Motil (2014) 26(4):510–20. doi: 10.1111/nmo.12295
44. Stilling RM, Ryan FJ, Hoban AE, Shanahan F, Clarke G, Claesson MJ, et al. Microbes & neurodevelopment–Absence of microbiota during early life increases activity-related transcriptional pathways in the amygdala. Brain Behav Immun (2015) 50:209–20. doi: 10.1016/j.bbi.2015.07.009
45. Foster JA, McVey Neufeld KA. Gut-brain axis: how the microbiome influences anxiety and depression. Trends Neurosci (2013) 36(5):305–12. doi: 10.1016/j.tins.2013.01.005
46. Needham BD, Kaddurah-Daouk R, Mazmanian SK. Gut microbial molecules in behavioural and neurodegenerative conditions. Nat Rev Neurosci (2020) 21(12):717–31. doi: 10.1038/s41583-020-00381-0
47. Vargas-Caraveo A, Sayd A, Maus SR, Caso JR, Madrigal JLM, García-Bueno B, et al. Lipopolysaccharide enters the rat brain by a lipoprotein-mediated transport mechanism in physiological conditions. Sci Rep (2017) 7(1):13113. doi: 10.1038/s41598-017-13302-6
48. Gomez de Agüero M, Ganal-Vonarburg SC, Fuhrer T, Rupp S, Uchimura Y, Li H, et al. The maternal microbiota drives early postnatal innate immune development. Science (2016) 351(6279):1296–302. doi: 10.1126/science.aad2571
49. Arentsen T, Qian Y, Gkotzis S, Femenia T, Wang T, Udekwu K, et al. The bacterial peptidoglycan-sensing molecule Pglyrp2 modulates brain development and behavior. Mol Psychiatry (2017) 22(2):257–66. doi: 10.1038/mp.2016.182
50. Ahmed H, Leyrolle Q, Koistinen V, Kärkkäinen O, Layé S, Delzenne N, et al. Microbiota-derived metabolites as drivers of gut-brain communication. Gut Microbes (2022) 14(1):2102878. doi: 10.1080/19490976.2022.2102878
51. Cline MM, Juarez B, Hunker A, Regiarto EG, Hariadi B, Soden ME, et al. Netrin-1 regulates the balance of synaptic glutamate signaling in the adult ventral tegmental area. Elife (2023) 12. doi: 10.7554/eLife.83760
52. Fujita Y, Nakanishi T, Ueno M, Itohara S, Yamashita T. Netrin-G1 regulates microglial accumulation along axons and supports the survival of layer V neurons in the postnatal mouse brain. Cell Rep (2020) 31(4):107580. doi: 10.1016/j.celrep.2020.107580
53. Vuong HE, Pronovost GN, Williams DW, Coley EJL, Siegler EL, Qiu A, et al. The maternal microbiome modulates fetal neurodevelopment in mice. Nature (2020) 586(7828):281–6. doi: 10.1038/s41586-020-2745-3
54. Pessa-Morikawa T, Husso A, Kärkkäinen O, Koistinen V, Hanhineva K, Iivanainen A, et al. Maternal microbiota-derived metabolic profile in fetal murine intestine, brain and placenta. BMC Microbiol (2022) 22(1):46. doi: 10.1186/s12866-022-02457-6
55. Lee YH, Cherkerzian S, Seidman LJ, Papandonatos GD, Savitz DA, Tsuang MT, et al. Maternal bacterial infection during pregnancy and offspring risk of psychotic disorders: variation by severity of infection and offspring sex. Am J Psychiatry (2020) 177(1):66–75. doi: 10.1176/appi.ajp.2019.18101206
56. Clarke TB, Davis KM, Lysenko ES, Zhou AY, Yu Y, Weiser JN. Recognition of peptidoglycan from the microbiota by Nod1 enhances systemic innate immunity. Nat Med (2010) 16(2):228–31. doi: 10.1038/nm.2087
57. Wolf AJ. Peptidoglycan-induced modulation of metabolic and inflammatory responses. Immunometabolism (Cobham) (2023) 5(2):e00024. doi: 10.1097/in9.0000000000000024
58. Humann J, Mann B, Gao G, Moresco P, Ramahi J, Loh LN, et al. Bacterial peptidoglycan traverses the placenta to induce fetal neuroproliferation and aberrant postnatal behavior. Cell Host Microbe (2016) 19(3):388–99. doi: 10.1016/j.chom.2016.02.009
59. Manuel M, Martynoga B, Yu T, West JD, Mason JO, Price DJ. The transcription factor Foxg1 regulates the competence of telencephalic cells to adopt subpallial fates in mice. Development (2010) 137(3):487–97. doi: 10.1242/dev.039800
60. Zhen J, Zhang H, Dong H, Tong X. miR-9-3p inhibits glioma cell proliferation and apoptosis by directly targeting FOXG1. Oncol Lett (2020) 20(2):2007–15. doi: 10.3892/ol.2020.11725
61. Mariani J, Coppola G, Zhang P, Abyzov A, Provini L, Tomasini L, et al. FOXG1-dependent dysregulation of GABA/glutamate neuron differentiation in autism spectrum disorders. Cell (2015) 162(2):375–90. doi: 10.1016/j.cell.2015.06.034
62. Al-Haddad BJS, Oler E, Armistead B, Elsayed NA, Weinberger DR, Bernier R, et al. The fetal origins of mental illness. Am J Obstet Gynecol (2019) 221(6):549–62. doi: 10.1016/j.ajog.2019.06.013
63. Murray KN, Edye ME, Manca M, Vernon AC, Oladipo JM, Fasolino V, et al. Evolution of a maternal immune activation (mIA) model in rats: Early developmental effects. Brain Behav Immun (2019) 75:48–59. doi: 10.1016/j.bbi.2018.09.005
64. Vlasova RM, Iosif AM, Ryan AM, Funk LH, Murai T, Chen S, et al. Maternal immune activation during pregnancy alters postnatal brain growth and cognitive development in nonhuman primate offspring. J Neurosci (2021) 41(48):9971–87. doi: 10.1523/jneurosci.0378-21.2021
65. Bauman MD, Iosif AM, Smith SE, Bregere C, Amaral DG, Patterson PH. Activation of the maternal immune system during pregnancy alters behavioral development of rhesus monkey offspring. Biol Psychiatry (2014) 75(4):332–41. doi: 10.1016/j.biopsych.2013.06.025
66. Hsiao EY, McBride SW, Hsien S, Sharon G, Hyde ER, McCue T, et al. Microbiota modulate behavioral and physiological abnormalities associated with neurodevelopmental disorders. Cell (2013) 155(7):1451–63. doi: 10.1016/j.cell.2013.11.024
67. Kim S, Kim H, Yim YS, Ha S, Atarashi K, Tan TG, et al. Maternal gut bacteria promote neurodevelopmental abnormalities in mouse offspring. Nature (2017) 549(7673):528–32. doi: 10.1038/nature23910
68. Sorboni SG, Moghaddam HS, Jafarzadeh-Esfehani R, Soleimanpour S. A comprehensive review on the role of the gut microbiome in human neurological disorders. Clin Microbiol Rev (2022) 35(1):e0033820. doi: 10.1128/cmr.00338-20
69. Ligezka AN, Sonmez AI, Corral-Frias MP, Golebiowski R, Lynch B, Croarkin PE, et al. A systematic review of microbiome changes and impact of probiotic supplementation in children and adolescents with neuropsychiatric disorders. Prog Neuropsychopharmacol Biol Psychiatry (2021) 108:110187. doi: 10.1016/j.pnpbp.2020.110187
70. Zeraati M, Enayati M, Kafami L, Shahidi SH, Salari AA. Gut microbiota depletion from early adolescence alters adult immunological and neurobehavioral responses in a mouse model of multiple sclerosis. Neuropharmacology (2019) 157:107685. doi: 10.1016/j.neuropharm.2019.107685
71. Tejkalová H, Jakob L, Kvasnová S, Klaschka J, Sechovcová H, Mrázek J, et al. The influence of antibiotic treatment on the behavior and gut microbiome of adult rats neonatally insulted with lipopolysaccharide. Heliyon (2023) 9(4):e15417. doi: 10.1016/j.heliyon.2023.e15417
72. Desbonnet L, Clarke G, Traplin A, O'Sullivan O, Crispie F, Moloney RD, et al. Gut microbiota depletion from early adolescence in mice: Implications for brain and behaviour. Brain Behav Immun (2015) 48:165–73. doi: 10.1016/j.bbi.2015.04.004
73. Snigdha S, Ha K, Tsai P, Dinan TG, Bartos JD, Shahid M. Probiotics: Potential novel therapeutics for microbiota-gut-brain axis dysfunction across gender and lifespan. Pharmacol Ther (2022) 231:107978. doi: 10.1016/j.pharmthera.2021.107978
74. Hourigan SK, Oliva-Hemker M. Fecal microbiota transplantation in children: a brief review. Pediatr Res (2016) 80(1):2–6. doi: 10.1038/pr.2016.48
75. Antushevich H. Fecal microbiota transplantation in disease therapy. Clin Chim Acta (2020) 503:90–8. doi: 10.1016/j.cca.2019.12.010
76. Hu T, Dong Y, He C, Zhao M, He Q. The gut microbiota and oxidative stress in autism spectrum disorders (ASD). Oxid Med Cell Longev (2020) 2020:8396708. doi: 10.1155/2020/8396708
77. Hirota T, King BH. Autism spectrum disorder: A review. Jama (2023) 329(2):157–68. doi: 10.1001/jama.2022.23661
78. Lord C, Elsabbagh M, Baird G, Veenstra-Vanderweele J. Autism spectrum disorder. Lancet (2018) 392(10146):508–20. doi: 10.1016/s0140-6736(18)31129-2
79. Chaidez V, Hansen RL, Hertz-Picciotto I. Gastrointestinal problems in children with autism, developmental delays or typical development. J Autism Dev Disord (2014) 44(5):1117–27. doi: 10.1007/s10803-013-1973-x
80. Restrepo B, Angkustsiri K, Taylor SL, Rogers SJ, Cabral J, Heath B, et al. Developmental-behavioral profiles in children with autism spectrum disorder and co-occurring gastrointestinal symptoms. Autism Res (2020) 13(10):1778–89. doi: 10.1002/aur.2354
81. Sharon G, Cruz NJ, Kang DW, Gandal MJ, Wang B, Kim YM, et al. Human gut microbiota from autism spectrum disorder promote behavioral symptoms in mice. Cell (2019) 177(6):1600–1618.e17. doi: 10.1016/j.cell.2019.05.004
82. Iglesias-Vázquez L, Van Ginkel Riba G, Arija V, Canals J. Composition of gut microbiota in children with autism spectrum disorder: A systematic review and meta-analysis. Nutrients (2020) 12(3). doi: 10.3390/nu12030792
83. Zou R, Xu F, Wang Y, Duan M, Guo M, Zhang Q, et al. Changes in the gut microbiota of children with autism spectrum disorder. Autism Res (2020) 13(9):1614–25. doi: 10.1002/aur.2358
84. Xu M, Xu X, Li J, Li F. Association between gut microbiota and autism spectrum disorder: A systematic review and meta-analysis. Front Psychiatry (2019) 10:473. doi: 10.3389/fpsyt.2019.00473
85. Andreo-Martínez P, García-Martínez N, Sánchez-Samper EP, Martínez-González AE. An approach to gut microbiota profile in children with autism spectrum disorder. Environ Microbiol Rep (2020) 12(2):115–35. doi: 10.1111/1758-2229.12810
86. Andreo-Martínez P, Rubio-Aparicio M, Sánchez-Meca J, Veas A, Martínez-González AE. A meta-analysis of gut microbiota in children with autism. J Autism Dev Disord (2022) 52(3):1374–87. doi: 10.1007/s10803-021-05002-y
87. Gilbert JA, Krajmalnik-Brown R, Porazinska DL, Weiss SJ, Knight R. Toward effective probiotics for autism and other neurodevelopmental disorders. Cell (2013) 155(7):1446–8. doi: 10.1016/j.cell.2013.11.035
88. Argou-Cardozo I, Zeidán-Chuliá F. Clostridium bacteria and autism spectrum conditions: A systematic review and hypothetical contribution of environmental glyphosate levels. Med Sci (Basel) (2018) 6(2). doi: 10.3390/medsci6020029
89. Abdelli LS, Samsam A, Naser SA. Propionic acid induces gliosis and neuro-inflammation through modulation of PTEN/AKT pathway in autism spectrum disorder. Sci Rep (2019) 9(1):8824. doi: 10.1038/s41598-019-45348-z
90. Frye RE, Nankova B, Bhattacharyya S, Rose S, Bennuri SC, MacFabe DF. Modulation of immunological pathways in autistic and neurotypical lymphoblastoid cell lines by the enteric microbiome metabolite propionic acid. Front Immunol (2017) 8:1670. doi: 10.3389/fimmu.2017.01670
91. Marotta A, Sarno E, Del Casale A, Pane M, Mogna L, Amoruso A, et al. Effects of probiotics on cognitive reactivity, mood, and sleep quality. Front Psychiatry (2019) 10:164. doi: 10.3389/fpsyt.2019.00164
92. Shaaban SY, El Gendy YG, Mehanna NS, El-Senousy WM, El-Feki HSA, Saad K, et al. The role of probiotics in children with autism spectrum disorder: A prospective, open-label study. Nutr Neurosci (2018) 21(9):676–81. doi: 10.1080/1028415x.2017.1347746
93. Kyle SM, Vashi N, Justice MJ. Rett syndrome: a neurological disorder with metabolic components. Open Biol (2018) 8(2). doi: 10.1098/rsob.170216
94. Gallucci A, Patterson KC, Weit AR, van der Pol WJ, Dubois LG, Percy AK, et al. Microbial community changes in a female rat model of Rett syndrome. Prog Neuropsychopharmacol Biol Psychiatry (2021) 109:110259. doi: 10.1016/j.pnpbp.2021.110259
95. Strati F, Cavalieri D, Albanese D, De Felice C, Donati C, Hayek J, et al. Altered gut microbiota in Rett syndrome. Microbiome (2016) 4(1):41. doi: 10.1186/s40168-016-0185-y
96. Neier K, Grant TE, Palmer RL, Chappell D, Hakam SM, Yasui KM, et al. Sex disparate gut microbiome and metabolome perturbations precede disease progression in a mouse model of Rett syndrome. Commun Biol (2021) 4(1):1408. doi: 10.1038/s42003-021-02915-3
97. Thapa S, Venkatachalam A, Khan N, Naqvi M, Balderas M, Runge JK, et al. Assessment of the gut bacterial microbiome and metabolome of girls and women with Rett Syndrome. PloS One (2021) 16(5):e0251231. doi: 10.1371/journal.pone.0251231
98. Nagarajan RP, Hogart AR, Gwye Y, Martin MR, LaSalle JM. Reduced MeCP2 expression is frequent in autism frontal cortex and correlates with aberrant MECP2 promoter methylation. Epigenetics (2006) 1(4):e1–11. doi: 10.4161/epi.1.4.3514
99. Liyanage VRB, Olson CO, Zachariah RM, Davie JR, Rastegar M. DNA methylation contributes to the differential expression levels of mecp2 in male mice neurons and astrocytes. Int J Mol Sci (2019) 20(8). doi: 10.3390/ijms20081845
100. Lioy DT, Garg SK, Monaghan CE, Raber J, Foust KD, Kaspar BK, et al. A role for glia in the progression of Rett's syndrome. Nature (2011) 475(7357):497–500. doi: 10.1038/nature10214
101. Khoury ES, Sharma A, Ramireddy RR, Thomas AG, Alt J, Fowler A, et al. Dendrimer-conjugated glutaminase inhibitor selectively targets microglial glutaminase in a mouse model of Rett syndrome. Theranostics (2020) 10(13):5736–48. doi: 10.7150/thno.41714
102. Maezawa I, Jin LW. Rett syndrome microglia damage dendrites and synapses by the elevated release of glutamate. J Neurosci (2010) 30(15):5346–56. doi: 10.1523/jneurosci.5966-09.2010
103. Zheng P, Zeng B, Liu M, Chen J, Pan J, Han Y, et al. The gut microbiome from patients with schizophrenia modulates the glutamate-glutamine-GABA cycle and schizophrenia-relevant behaviors in mice. Sci Adv (2019) 5(2):eaau8317. doi: 10.1126/sciadv.aau8317
104. Eicher TP, Mohajeri MH. Overlapping mechanisms of action of brain-active bacteria and bacterial metabolites in the pathogenesis of common brain diseases. Nutrients (2022) 14(13). doi: 10.3390/nu14132661
105. Nance E, Kambhampati SP, Smith ES, Zhang Z, Zhang F, Singh S, et al. Dendrimer-mediated delivery of N-acetyl cysteine to microglia in a mouse model of Rett syndrome. J Neuroinflamm (2017) 14(1):252. doi: 10.1186/s12974-017-1004-5
106. Pfrieger FW, Ungerer N. Cholesterol metabolism in neurons and astrocytes. Prog Lipid Res (2011) 50(4):357–71. doi: 10.1016/j.plipres.2011.06.002
107. Chen Z, Yuan Z, Yang S, Zhu Y, Xue M, Zhang J, et al. Brain energy metabolism: astrocytes in neurodegenerative diseases. CNS Neurosci Ther (2023) 29(1):24–36. doi: 10.1111/cns.13982
108. Buchovecky CM, Turley SD, Brown HM, Kyle SM, McDonald JG, Liu B, et al. A suppressor screen in Mecp2 mutant mice implicates cholesterol metabolism in Rett syndrome. Nat Genet (2013) 45(9):1013–20. doi: 10.1038/ng.2714
109. Kenny DJ, Plichta DR, Shungin D, Koppel N, Hall AB, Fu B, et al. Cholesterol metabolism by uncultured human gut bacteria influences host cholesterol level. Cell Host Microbe (2020) 28(2):245–257.e6. doi: 10.1016/j.chom.2020.05.013
110. Nagy G, Ackerman SL. Cholesterol metabolism and Rett syndrome pathogenesis. Nat Genet (2013) 45(9):965–7. doi: 10.1038/ng.2738
111. Derecki NC, Cronk JC, Lu Z, Xu E, Abbott SB, Guyenet PG, et al. Wild-type microglia arrest pathology in a mouse model of Rett syndrome. Nature (2012) 484(7392):105–9. doi: 10.1038/nature10907
112. Kishino T, Lalande M, Wagstaff J. UBE3A/E6-AP mutations cause Angelman syndrome. Nat Genet (1997) 15(1):70–3. doi: 10.1038/ng0197-70
113. Hsiao JS, Germain ND, Wilderman A, Stoddard C, Wojenski LA, Villafano GJ, et al. A bipartite boundary element restricts UBE3A imprinting to mature neurons. Proc Natl Acad Sci U.S.A. (2019) 116(6):2181–6. doi: 10.1073/pnas.1815279116
114. Buiting K, Williams C, Horsthemke B. Angelman syndrome - insights into a rare neurogenetic disorder. Nat Rev Neurol (2016) 12(10):584–93. doi: 10.1038/nrneurol.2016.133
115. Leader G, Whelan S, Chonaill NN, Coyne R, Tones M, Heussler H, et al. Association between early and current gastro-intestinal symptoms and co-morbidities in children and adolescents with Angelman syndrome. J Intellect Disabil Res (2022) 66(11):865–79. doi: 10.1111/jir.12975
116. Beitnere U, Vilanova-Cuevas B, Christian SG, Taylor C, Berg EL, Copping NA, et al. Unique features of the gut microbiome characterized in animal models of angelman syndrome. mSystems (2023) 8(1):e0060822. doi: 10.1128/msystems.00608-22
117. Vacca M, Celano G, Calabrese FM, Portincasa P, Gobbetti M, De Angelis M. The controversial role of human gut lachnospiraceae. Microorganisms (2020) 8(4). doi: 10.3390/microorganisms8040573
118. Murros KE, Huynh VA, Takala TM, Saris PEJ. Desulfovibrio bacteria are associated with parkinson's disease. Front Cell Infect Microbiol (2021) 11:652617. doi: 10.3389/fcimb.2021.652617
119. Wan L, Ge WR, Zhang S, Sun YL, Wang B, Yang G. Case-control study of the effects of gut microbiota composition on neurotransmitter metabolic pathways in children with attention deficit hyperactivity disorder. Front Neurosci (2020) 14:127. doi: 10.3389/fnins.2020.00127
120. Bruinsma CF, Schonewille M, Gao Z, Aronica EM, Judson MC, Philpot BD, et al. Dissociation of locomotor and cerebellar deficits in a murine Angelman syndrome model. J Clin Invest (2015) 125(11):4305–15. doi: 10.1172/jci83541
121. Chesler AT, Szczot M, Bharucha-Goebel D, Čeko M, Donkervoort S, Laubacher C, et al. The role of PIEZO2 in human mechanosensation. N Engl J Med (2016) 375(14):1355–64. doi: 10.1056/NEJMoa1602812
122. Fleck AK, Hucke S, Teipel F, Eschborn M, Janoschka C, Liebmann M, et al. Dietary conjugated linoleic acid links reduced intestinal inflammation to amelioration of CNS autoimmunity. Brain (2021) 144(4):1152–66. doi: 10.1093/brain/awab040
123. Mills RH, Dulai PS, Vázquez-Baeza Y, Sauceda C, Daniel N, Gerner RR, et al. Multi-omics analyses of the ulcerative colitis gut microbiome link Bacteroides vulgatus proteases with disease severity. Nat Microbiol (2022) 7(2):262–76. doi: 10.1038/s41564-021-01050-3
124. Nowak A, Libudzisz Z. Influence of phenol, p-cresol and indole on growth and survival of intestinal lactic acid bacteria. Anaerobe (2006) 12(2):80–4. doi: 10.1016/j.anaerobe.2005.10.003
125. Hutkins RW, Morris HA. Carbohydrate metabolism by streptococcus thermophilus : A review. J Food Prot (1987) 50(10):876–84. doi: 10.4315/0362-028x-50.10.876
126. Zeng H, Ishaq SL, Zhao FQ, Wright AG. Colonic inflammation accompanies an increase of β-catenin signaling and Lachnospiraceae/Streptococcaceae bacteria in the hind gut of high-fat diet-fed mice. J Nutr Biochem (2016) 35:30–6. doi: 10.1016/j.jnutbio.2016.05.015
127. Li Q, Hu W, Liu WX, Zhao LY, Huang D, Liu XD, et al. Streptococcus thermophilus inhibits colorectal tumorigenesis through secreting β-galactosidase. Gastroenterology (2021) 160(4):1179–1193.e14. doi: 10.1053/j.gastro.2020.09.003
128. Stiles J, Jernigan TL. The basics of brain development. Neuropsychol Rev (2010) 20(4):327–48. doi: 10.1007/s11065-010-9148-4
129. Nyangahu DD, Lennard KS, Brown BP, Darby MG, Wendoh JM, Havyarimana E, et al. Disruption of maternal gut microbiota during gestation alters offspring microbiota and immunity. Microbiome (2018) 6(1):124. doi: 10.1186/s40168-018-0511-7
130. Madany AM, Hughes HK, Ashwood P. Antibiotic treatment during pregnancy alters offspring gut microbiota in a sex-dependent manner. Biomedicines (2022) 10(5). doi: 10.3390/biomedicines10051042
131. Madany AM, Hughes HK, Ashwood P. Prenatal maternal antibiotics treatment alters the gut microbiota and immune function of post-weaned prepubescent offspring. Int J Mol Sci (2022) 23(21). doi: 10.3390/ijms232112879
Keywords: CNS development, gut microbes, autism spectrum disorder, Rett Syndrome, Angelman Syndrome
Citation: Gan Y, Chen Y, Zhong H, Liu Z, Geng J, Wang H and Wang W (2024) Gut microbes in central nervous system development and related disorders. Front. Immunol. 14:1288256. doi: 10.3389/fimmu.2023.1288256
Received: 04 September 2023; Accepted: 22 December 2023;
Published: 26 January 2024.
Edited by:
Karolina Skonieczna-Żydecka, Pomeranian Medical University, PolandReviewed by:
Quentin Leyrolle, Université Catholique de Louvain, BelgiumCopyright © 2024 Gan, Chen, Zhong, Liu, Geng, Wang and Wang. This is an open-access article distributed under the terms of the Creative Commons Attribution License (CC BY). The use, distribution or reproduction in other forums is permitted, provided the original author(s) and the copyright owner(s) are credited and that the original publication in this journal is cited, in accordance with accepted academic practice. No use, distribution or reproduction is permitted which does not comply with these terms.
*Correspondence: Wenxue Wang, V2VueHVlLldhbmdAa211c3QuZWR1LmNu, d2VueHVlX3dhbmdAMTYzLmNvbQ==; Huishan Wang, d2FuZ2h1aXNoYW5AbWFpbC5raXouYWMuY24=; Jiawei Geng, Smlhd2VpX0dlbmdAa211c3QuZWR1LmNu, amlhX3dlaV9nZW5nQDE2My5jb20=
Disclaimer: All claims expressed in this article are solely those of the authors and do not necessarily represent those of their affiliated organizations, or those of the publisher, the editors and the reviewers. Any product that may be evaluated in this article or claim that may be made by its manufacturer is not guaranteed or endorsed by the publisher.
Research integrity at Frontiers
Learn more about the work of our research integrity team to safeguard the quality of each article we publish.