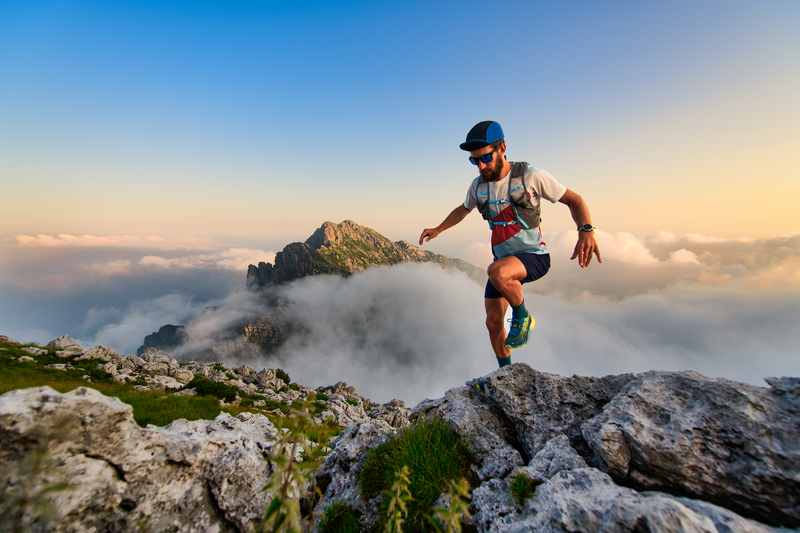
95% of researchers rate our articles as excellent or good
Learn more about the work of our research integrity team to safeguard the quality of each article we publish.
Find out more
REVIEW article
Front. Immunol. , 18 December 2023
Sec. Vaccines and Molecular Therapeutics
Volume 14 - 2023 | https://doi.org/10.3389/fimmu.2023.1281667
This article is part of the Research Topic Methods in Vaccines and Molecular Therapeutics: 2022 View all 17 articles
Arboviruses are a major threat to public health in tropical regions, encompassing over 534 distinct species, with 134 capable of causing diseases in humans. These viruses are transmitted through arthropod vectors that cause symptoms such as fever, headache, joint pains, and rash, in addition to more serious cases that can lead to death. Among the arboviruses, dengue virus stands out as the most prevalent, annually affecting approximately 16.2 million individuals solely in the Americas. Furthermore, the re-emergence of the Zika virus and the recurrent outbreaks of chikungunya in Africa, Asia, Europe, and the Americas, with one million cases reported annually, underscore the urgency of addressing this public health challenge. In this manuscript we discuss the epidemiology, viral structure, pathogenicity and integrated control strategies to combat arboviruses, and the most used tools, such as vaccines, monoclonal antibodies, treatment, etc., in addition to presenting future perspectives for the control of arboviruses. Currently, specific medications for treating arbovirus infections are lacking, and symptom management remains the primary approach. However, promising advancements have been made in certain treatments, such as Chloroquine, Niclosamide, and Isatin derivatives, which have demonstrated notable antiviral properties against these arboviruses in vitro and in vivo experiments. Additionally, various strategies within vector control approaches have shown significant promise in reducing arbovirus transmission rates. These encompass public education initiatives, targeted insecticide applications, and innovative approaches like manipulating mosquito bacterial symbionts, such as Wolbachia. In conclusion, combatting the global threat of arbovirus diseases needs a comprehensive approach integrating antiviral research, vaccination, and vector control. The continued efforts of research communities, alongside collaborative partnerships with public health authorities, are imperative to effectively address and mitigate the impact of these arboviral infections on public health worldwide.
The arbovirus (arthropod-borne viral) diseases have become serious public health problem with a wide geographic distribution, mainly in tropical regions (1), due to the presence of competent vectors (2). These infectious agents predominantly contain ribonucleic acid (RNA) as their genetic material, with an exception that is the Asfarviridae family, which have deoxyribonucleic acid (DNA) as their genetic material (3).
At the moment, there are more than 534 arboviruses identified, of which approximately 134 cause disease in humans (4). The families of arboviruses include Flaviviridae, Togaviridae, Asfarviridae, Orthomyxoviridae, Reoviridae, Rhabdoviridae and Bunyaviridae (5, 6). Most arboviruses are asymptomatic, but those of medical interest often cause symptoms (Figure 1) such as febrile illness, headache, muscle and joint pains. Rash may be present, as well as petechial rashes. In some cases, arboviruses can cause encephalitis, hemorrhagic fever, or polyarthralgia (6).
Figure 1 The arbovirus replication cycle and systematic infection. Arboviruses interact with multiple types of host attachment factors, including molecules that bind to the viral membrane or virion-associated N-linked carbohydrates. Virions are internalized by clathrin-dependent mechanisms that usurp host factors involved in the uptake of large macromolecules. Subsequently, viral RNA replication takes place, and infected cells migrate through skin into blood stream and lymphoid organs, such as lymph nodes, spleen, liver and brain. Host immune response, and each affected organ can trigger different sinptoms and pathologies.
Dengue virus (DENV) is the most prevalent arbovirus worldwide, found in more than 100 tropical and subtropical countries (7). According to the World Health Organization (WHO), it affects approximately 16.2 million people annually only in the Americas, with more than 500 million people at risk of contracting the disease in this region. As reported by the Pan American Health Organization (PAHO), in 2022, more than 2.8 million cases were registered in the Americas, with 4,607 of them being serious cases that caused 1,292 deaths. It is estimated that half of the world’s population is at risk of contracting the disease (8).
There are four genetically distinct serotypes of DENV that cause the dengue disease (9). However, in 2013, a fifth serotype was first detected in the blood of a patient in the state of Sarawak, Malaysia (10). In endemic countries, there can be co-circulation of more than one DENV serotype, as well as other arborviruses, all mainly transmitted by mosquitoes of the Aedes genus (11).
DENV-1 was first isolated in 1943 in Japan and later in 1945 in Hawaii. Since 1977, some countries in America have reported cases of DENV-1 (12–14). Over the years, new epidemics have emerged in several countries, leading to the reporting of other DENV serotypes. In 1944, DENV-2 was reported in Papua New Guinea and Indonesia, and in 1953 in Americas. In the 1970s, it started to spread to countries in Latin America, such as Puerto Rico and Brazil in 1984. DENV-3 and DENV-4 were first reported in 1953 in the Philippines and Thailand. The reports of DENV-3 in Brazil started around 2000s in Rio de Janeiro, and DENV-4 in 1981 (11, 14, 15).
The Zika virus (ZIKV) is another re-emerging flavivirus and human pathogen, that was first isolated in 1947 in the Zika forest in Uganda (16). Although the ZIKV has many similarity with other flavivirus, such as DENV, the ZIKV has an highly variable region present in its envelope, located close to an important glycosylation site that contains the amino acid asparagine at position 154 (Asn154) which is associated with its virulence (17). Before 2007, it was identified as a virus that cause a mild feverish disease in a small number of humans in Africa and parts of Asia. However, ZIKV has since spread to several countries, and in 2014, it was introduced in Brazil and other regions in the Americas (6). Genomic and phylogenetic analysis of virus isolates indicated the presence of two distinct lineages, African and Asian, both originating in East Africa (18). In addition to viral transmission through the bite of the Aedes mosquitoes, there are other forms, such as transmission through infected blood transfusion, sexual, and maternal-fetal transmission (19) which makes it even more difficult to control.
The chikungunya virus (CHIKV) was initially identified in patient serum during an epidemic in 1952 in Tanzania, and in 1953, the virus was isolated from Aedes and Culex spp. mosquitoes (20, 21). Phylogenetic studies show that the circulating strains of CHIKV have a common ancestor that originated 500 years ago (22). Currently, there are four identified CHIKV lineages, namely the Asian (AL), West African (WA), East/Central/South African (ECSA), and Indian Ocean (IOL) lineages. Among these, two main strains, responsible for several epidemics in Brazil, are the ECSA and AL (23, 24).
The ECSA genomic sequences isolated from patients of the outbreak in ‘La Reunion Island in the Indian Ocean’ showed a mutation in 90% of the viral sequences of the structural protein E1, where there was an exchange of the amino acid alanine for valine in region 226 (A226V) (25, 26). This mutation allowed the adaptation of a new vector, A. albopictus, which is present in temperate regions, favoring outbreaks in Italy and France (25, 26). A seroprevalence analysis between the years 2000-2019 found that the ECSA strain is the most frequent (27).
Since the first record of CHIKV in 1950s, several epidemics have emerged in Africa, Asia, Europe, and the Americas. Nowdays, CHIKV has been found in more than 100 countries (28). According to the WHO, more than one million cases are reported annually only in the Americas, with most cases occuring in Brazil (29). In 2016, in the Caribbean and the Americas, 185,000 cases of CHIKV were reported, with more than 90% of cases in the Americas being in Brazil (29).
The geographic distribution (Figure 2) of each arbovirus is related to ecological parameters that define the transmission cycle. Some factors include patterns of vegetation, temperature, and typical precipitation, which influence the distribution of the arthropod vector and the vertebrate host necessary for the maintenance of the virus. Social, demographic and climate change, deforestation, population migration, and urbanization in recent years have also had a strong impact on arbovirus infections (30).
Figure 2 The Arbovirus DENV, ZIKV, and CHIKV distribution around the world: The map shows the areas with the distribution of the risk of DENV throughout the world (A), the areas with the risk of ZIKV (B), and the areas of the risk of CHIKV (C). On (D), the incidence of DENV is shown in the Brazilian states, (E) ZIKV, and (F) CHIKV.
All these factors increase the possibility of human contact with vectors, contributing to the increasing transmission of viruses and the appearance of epidemics around the world. In recent decades, for example, the reemergence of arboviral infections has been observed in numerous countries (Figure 2). The reemergence of CHIKV in Asia, ZIKV outbreaks in the Americas, as well as West Nile virus (WNV) and Rift Valley fever virus (RVFV) outbreaks in Europe, are increasingly frequent (15, 31–33).
Therefore, control and prevention programs for arboviruses are essential, which must be based on the development of vaccines, treatment, vector control, and genomic surveillance programs based on tracking viruses using genomic sequence data as a cross-cutting activity (34). In this manuscript, we discuss the principal arboviruses, such as DENV, ZIKV and CHIKV, responsible for outbreaks in many countries. Furthermore, we address the perspectives of controlling these diseases.
Flaviviruses like DENV and ZIKV have a spherical and small composition of viral particles (~50nm in diameter). They contain a 10-11 kb genome and an open reading frame (ORFs) that encodes three structural proteins: capsid (C), premembrane/membrane (prM/M), and envelope (E), as well as seven non-structural proteins (NS1, NS2A, NS2B, NS3, NS4A, NS4B, and NS5), as seen in the Figure 3. The structural and non-structural proteins are important for correct virion assembly, cell receptor binding, and viral replication (Figure 1) (12, 35). The E protein is an essential structure responsible for viral attachment and membrane fusion. It is composed by three domains (E-DI, E-DII, and E-DIII) linked to the viral membrane by a helical region and two antiparallel transmembrane segments. The E-DI is responsible for the structural organization of the envelope, while E-DII allows the fusion of the virus with the host cell membrane (36, 37), and the E-DIII is highlighted as the most neutralizing antibody site and allows the virus to bind to the cell receptor (38, 39). Therefore, the E-DIII has been used as the best candidate for vaccine development, as well as for treatment (40).
Figure 3 Genomic Organization and Viral Structure of Flavivirus and Alphavirus. Flavivirus comprise a single Open Reading Frame with the genes for the Structural Proteins followed by the Non-Structural Proteins transcribed and translated and the resulting polyprotein undergoes proteolytic processing. Alphavirusus are comprised of the genes for the Non-Structural Proteins followed by the Structural Proteins, transcribed from two distinct ORFs, and each resulting polyprotein undergoes further proteolytical processing. Flavivirus structure have a mature virions with capsid (C) and membrane (M) covered by 90 dimers of (E) proteins. The E proteins exhibit three domain: E-DI (dark blue), E-DII (light blue) and E-DIII (green) anchored to the viral membrane (purple). The Alphavirus virion have the envelope glycoproteins in a shape of 240 dr trimer of E1(dark green), E2 (light green) ancored in lipid membrane (M) and the capsid (C) protein (blue). Showing heterodimers interacting with capsid protein.
The viral capsid (C) is a small helical protein with surfaces that bind to viral nucleic acids or host lipids and directs the incorporation of the viral genome into the host cells (17). The M protein originates from the cleavage of the precursor protein prM (41) and it is essential for the maturation of virions. For example, in the immature virions, prM prevents structural changes in the E glycoprotein, which are essential for the correct folding, maturation, and assembly of E protein during replication (Figure 1) (42).
As illustrated in Figure 3, the alphaviruses like CHIKV form spherical particles (~70nm in diameter) with icosahedral symmetry in their capsids. The virions consist of a host-derived lipid bilayer that is enriched in cholesterol. The lipid envelope contains 240 copies of E1 and E2 heterodimers glycoproteins arranged in a trimeric format (80 copies) (43, 44). The genome consists of two ORFs that encode four nonstructural proteins (nsP1-nsP4) and six structural proteins (Capsid, E3, E2, 6K, E1).
Some receptors involved are the Mxra8, αVβ3 integrins, C-type lectin receptors (CLR), TIM, TAM, AXL and phosphatidylserine receptor families (T cell immunoglobulin and mucin domain) (45). Most of the Arbovirus enter the cells by clathrin-mediated endocytosis (12, 46, 47). In the case of flaviviruses, the viral polyprotein (NS2-NS3) undergoes cleavage in the endoplasmic reticulum (ER) by host proteases to produce functional proteins. The viral genome is synthesized within a viral capsid (C) and surrounded by a lipid bilayer containing two transmembrane proteins, envelope (E) and membrane (M). Subsequently, the particles are released from the ER (Figure 1) (35).
In the case of alphaviruses, the E1 glycoprotein fuses the viral envelope in the endosome, while E2 interacts with the cell receptor, triggering endocytosis. The E2 glycoprotein conjugates with E3 glycoprotein (pE2) inside the cell. pE2 undergoes maturation in the Golgi complex and releases E2 and E3. Cleavage of E3 is crucial for particle fusion. E3 aids in spicule folding and prevents premature glycoprotein activation. 6K is involved in virion formation and growth (43, 48, 49). Nonstructural proteins play essential roles in genome replication and transcription, as well as in protecting the replication complex and genomic RNA from protease degradation (38). These proteins are translated into two forms: P123, which is more abundant, and P1234, generated by reading the opal stop codon at the junction of nsP3 and nsP4. Following cleavage, these proteins give rise to four distinct proteins: nsP1, nsP2, nsP3, and nsP4. The nonstructural proteins actively participate in the replication of the viral genome (50–55).
After attachment to the host cells, mainly innate immune cells, such as dendritic cells, monocytes, and macrophages, by receptor-mediated endocytosis, the viral RNA replicates. Once infected, dendritic cells migrate to lymphoid organs, allowing replication and dissemination to other organs (Figure 1) (56).
The arbovirus infects resident skin cells, such as fibroblasts, macrophages, and dendritic cells, through the bite of an infected female Aedes spp. mosquito (57). Once the virus is transferred to the tissues, it initiates the pre-acute phase of infection, characterized by an inflammatory response, including increased permeability and chemokine release from resident cells. After viral replication (Figure 1), it systemically migrates through lymph nodes, joints, spleen, liver, brain and muscles (58–61). During the initial days of infection, high titres of the virus can be detected in the blood (62–65).
The innate immunity plays an important role in controlling arboviruses in the early stages of infection. As is well known, the viral RNA is a pathogen-associated molecular patterns (PAMP) recognized by pattern recognition receptors (PRRs), mainly Toll-like receptors (TLRs 3, 7 and 8), RIG-I like receptors (RLRs) and Nod-like receptors (NLRs) (66, 67). As with other viral infections, type I interferon (IFN-α and IFN-β) acts as the first line of defense against arboviruses. These interferons stimulate the expression of many genes that interupt the virus replication and proliferation (68, 69). The IFNs activate the cells through a signal transducer such as Janus kinase, which induces the transcription factor JAK-STAT to produce interferon regulatory factors (IRFs 3, 5 and 7) and interferon-stimulated genes (ISG), that block viral replication by triggering an antiviral state in both infected and uninfected cells (70–72). Secondary infections, however, are known to cause serious diseases, specifically after a heterotypic infection (73). It is believed that the phenomenon of antibody-dependent enhancement (ADE) may cause increased virulence and pathogenicity (74).
The ADE phenomenon occurs when non-neutralizing antibodies from a previous heterotypic infection create a virus-antibody complex that is phagocytosed by cells that are generally not infected, via Fcγ receptors and complement receptors present in permissive cell for ADE, such as dendritic cells, monocytes and macrophages (75). ADE is primarily mediated by the IgG antibody, and in some instances, by IgM (76). It is expected to result in an increase in viral uptake and, therefore, contribute to the replication, leading to an exacerbated immune response to the infection, and cytokine storm, with an upregulation of IL-6, TNF-α and IL-10, whereas IL-12 and IFN-γ is downregulated (77).
It is worth mentioning Vo et al. (78) that conducted a study to investigate the phenomenon of ADE in the context of the four DENV serotypes by assessing plasma samples from patients with confirmed DENV-2 infections. Initially, during the early stages of infection, no significant differences in ADE activity were observed among the serotypes. However, intriguing distinctions emerged in later stages, specifically at 10 and 60 days post-infection confirmation. ADE activity was found to be notably higher against DENV-1 when compared to DENV-4 during these later time points. When analyzing the cumulative ADE activity by calculating the area under the curve (AUC), it was determined to be most pronounced against DENV-2, which happened to be the serotype responsible for the infection in the cohort under investigation (78).
Moreover, overall it is not new that DENV antibody avidity shifts from the previous infecting serotype to the current infecting serotype over time (79). Over time, there is a transition in the overall avidity of these antibodies, influenced by changing quantities and qualities. For example, the quantity of afucosylated Fc-IgG increases during convalescence compared to the acute phase of primary infections, potentially impacting the occurrence of ADE (80, 81). Additionally, antibody titers specific to DENV tend to decrease as time progresses, which may further contribute to the susceptibility to ADE (82, 83). This is of particular concern since antibodies targeting specific regions of viral proteins, such as the fusion loop of the envelope protein (E) and other surface proteins like (prM), have been identified as factors promoting ADE both in laboratory settings and animal models (84, 85). Structural aspects of the virus, including its maturation state, have also been found to influence ADE. Notably, severe cases of DENV infection can witness an increase in mortality rates of up to 20% (73).
Another important factor for pathogenicity is the NS1. This protein is found on the surface of infected cells and circulating viral particles in the host. The interaction of NS1 with cellular receptors and viral proteins contributes to viral replication, viable viral particles, viral persistence, and primarily the activation of immune system receptors, such as C3, C4, and C5 complement receptors, and even the membrane attack complex (86). TLR3, present in dendritic cells and macrophages, is activated via NS1, triggering the expression of antiviral factors. NS1 also activates TLR2 and TLR6 during DENV infection, increasing the production of proinflammatory cytokines like IL-6 and TNF-α (87). The recognition of NS1 by TLR4 causes the activation of peripheral blood mononuclear cells (PBMCs), leading to an increase in proinflammatory cytokines that induce endothelial tissue dysfunction (88). Studies using human endothelial cell cultures have shown that flavivirus NS1 can cause modulation and endothelial hyperpermeability in various tissues, favoring pathogenesis (89).
The most severe dengue clinical syndrome can manifest itself in the form of shock, including coagulation abnormalities, plasma leakage, and increased vascular fragility. Fluid loss due to increased capillary permeability leads to hypovolemic shock and multiorgan failure (90). Because the clinical diagnosis of these diseases is not specific (91), due to the wide spectrum of clinical manifestations and clinical overlap with other circulating arboviruses, molecular diagnostic techniques are necessary and are the most commonly used to confirm infection by some flavivirus at the beginning of symptoms (92, 93).
The presence of viral RNA in the amniotic fluid and placenta evidences the association of Congenital Zika Syndrome (SCZ) with neonatal complications, such as congenital microcephaly, optic neuropathy, congenital glaucoma, ventriculomegaly and lissencephaly (94, 95). Beyond neonatal complications, ZIKV infection in adults is related to Guillain Barré Syndrome (GBS), an autoimmune disease that attacks neural cells leading to gradual muscle weakness and even paralysis. Other complications such as arthralgia and cardiovascular problems have been reported; however, it is necessary to establish the exact connection (96, 97).
In the chronic phase, CHIKV propagation through the lymphatic, circulatory, and joint systems (98). The chronic-phase arthritis and the pro-inflammatory environment are associated, the persistence of viral RNA in macrophages, muscles and joints may favor persistent arthritis (99). Unlike other arboviruses, which show symptoms only in the acute infection phase, more than a third of CHIKV-caused infections are symptomatic and most patients experience joint pain years after the onset of the disease (100, 101).
It is necessary to understand in detail the Arbovirus’ biology, its relationship with the vectors, with the host and its history, so that is possible to develop integrated control strategies in an effective way, because multiple factors contribute to the emergence of arboviruses. For instance, population growth in areas with unplanned urbanization, climate changes, and viral genetic adaptation are significant contributors (30). Beyond their transmission between arthropods and humans, these viruses can infect a wide range of animal species, rendering complete eradication virtually impossible. Arbovirus infections exhibit diverse clinical presentations, ranging from mild to severe, and are categorized as either visceral or neurotropic, with some viruses displaying both characteristics (102, 103).
An effective vaccine against DENV must be able to induce a balanced response against all four DENV serotypes (104). However, its development is challenging due to the theoretical risk that an incomplete immunity generated by the vaccine could lead to increased pathogenesis upon subsequent natural infection, as discussed earlier with the ADE phenomenon.
Dengvaxia® (Sanofi Pasteur) is a live attenuated tetravalent vaccine containing chimeras of pre-structural membrane (prM) and envelope (E) genes from the four DENV types, combined with non-structural genes from the yellow fever vaccine strain 17D (dengue chimeric yellow fever – CYD) (105). It has been licensed in several American countries, including Mexico, Brazil, El Salvador, Costa Rica, Paraguay, Guatemala, and Peru (106). However, despite being licensed by ANVISA (The National Health Surveillance Agency) in 2015 (107), Dengvaxia® has raised numerous concerns in the scientific and health communities, particularly regarding its potential to predispose people with no prior DENV exposure to severe diseases. As a result, the vaccine is no longer considered a viable prevention tool, partly due to its high cost (106).
In the Philippines, immunizations with Dengvaxia® were suspended in 2017 after 14 vaccination-associated child deaths occurred (108). In Brazil, the vaccine was recommended for individuals aged 9 to 45 years old, with a documented history of prior laboratory-confirmed DENV infection (106). In the European Union and the United States, Dengvaxia® was also licensed for its use in people aged 9 or older living in DENV endemic areas, but limited to those who had experienced a previous DENV infection (109, 110). The Advisory Committee on Immunization Practices (ACIP) recommended in June 2021 Dengvaxia for routine use in children aged 9 to 16 years living in endemic areas with laboratory confirmation of previous DENV infection (111). Low acceptance of the vaccine is driven by concerns about an increased risk of severe dengue in vaccinated individuals without prior exposure to DENV.
Recently, the ANVISA approved the QDENGA® vaccine (TAK-003 – Takeda Pharma) to immunize individuals aged 4 - 60 years, without the need to confirm a previous infection, different from the Dengvaxia® vaccine. This vaccine, QDENGA®, is based on a live attenuated DENV-2 virus that serves as the genetic backbone for the four vaccine viruses, the chimeras (DENV-1, DENV-3, and DENV-4), which were generated by replacing the pre-membrane and envelope genes. The QDENGA® vaccine also received evaluation and a positive recommendation from the European Health Agency (EMA) under the “EU Medicines for all” program. Several clinical trials involving more than 20,000 subjects have demonstrated the vaccine’s safety and efficacy against DENV disease (112–114).
Despite the success of vaccines already licensed for certain flaviviruses such as Yellow Fever Virus (YFV), and Japanese Encephalitis Virus (JEV), combating epidemics caused by emerging flaviviruses poses significant challenges. The primary challenge stems from the extensive cross-reactivity observed in flavivirus-immune sera. While neutralization assays help to understanding antibody responses to both homologous and heterologous viruses in convalescent sera, the use of sera from acute infected individuals has been limited (115, 116).
The detection of flavivirus infection using molecular assays is limited due to the transient nature of viremia, making them sensitive only for relatively short periods. To develop vaccines against flaviviruses, new platforms must be explored, underscoring the necessity for further studies on the biology, structure, and heterogeneity of vaccine antigens (117).
The accessibility to vaccines also remains a significant challenge even after their development. This issue is particularly evident in regions like South America and Africa, where vaccine shortages have led to a continuous circulation of Yellow Fever Virus (YFV), prompting research on vaccine economics (118). Furthermore, the availability of effective vaccines does not always guarantee the expected impact on global health. An illustrative example was observed during the SARS-CoV-2 pandemic, where in many areas, the acceptance of vaccination was lower than anticipated, necessitating the implementation of public policies to ensure access and raise awareness about the importance of this preventive measure.
High safety, immunogenicity, and efficacy are always essential prerequisites for a vaccine to be embraced by both the public and the scientific community. However, a crucial question remains unanswered: Will these DENV vaccines offer superior protection to individuals with no prior exposure to DENV infections? Ensuring a vaccine’s effectiveness without the risk of sensitizing the population and exposing them to symptomatic or severe disease upon subsequent natural DENV infection is of utmost importance. Therefore, addressing these questions is imperative before we can consider using these vaccines as a significant tool in the prevention and control of dengue disease on a public scale.
Currently, no vaccines are available to prevent ZIKV and CHIKV infections. Nevertheless, several vaccine candidates are in the developmental pipeline (see Figure 4), with ongoing clinical trials. These vaccines utilize diverse technologies, encompassing live attenuated, inactivated virus, mRNA, DNA, recombinant, and VLP approaches.
Figure 4 Chord Diagram with candidate vaccine in clinical trials to DENV, ZIKV and CHIKV. The chord diagram employed in this study depicts a central circle representing the target virus of the vaccines, including DENV (dark green), ZIKV (red), and CHIKV (yellow). The diverse segments of the circle are assigned to distinct vaccine development methodologies: 3 trials on VLP (brown), 5 trials on inactivated virus (gray), 6 trials on recombinant methods (orange), 1 mRNA trial (yellow), 12 trials on live attenuated (blue), and 1 trials involving DNA (red). The phases of the clinical trials are indicated by symbols: dash (Phase 1), plus (Phase 2), and star (Phase 3). The vaccines mentioned in the chord diagram are registered in the ClinicalTrials.gov - National Library of Medicine (U.S.) database.
At the present, there are no specific therapy against arbovirus-causing infections, but some drugs are used to reduce symptoms (Table 1). Studies using “sofosbuvir” as an antiviral agent has been shown to inhibit ZIKV replication in hepatic and neural cell cultures, and also provided protection using animal model such as mice after being challenged with ZIKV (119). In another study, the antibiotic azithromycin was analyzed in vitro using glial cells, and it was capable to reduce viral proliferation (120). Even though these data are promising, it is known that the ideal drug against ZIKV should reduce viral load, symptoms, and prevent neurological complications in the fetus, what these experiments are far from achieving it (121, 122).
In the case of CHIKV, the treatment of arthralgia often involves the use of non-steroidal anti-inflammatory drugs, such as ribavirin and chloroquine. Additionally, the investigation of monoclonal antibodies as potential therapeutic agents has been explored (123–125). Passive transfer of immune serum protects against virus-induced lethality and studies in mice demonstrated a prophylactic efficacy when it is provided before or immediately after CHIKV challenge (124). Ribavirin has antiviral activity against several RNA viruses, and the authors (126, 127) suggest that it has a beneficial effect in relieving arthralgia and swelling associated with chronic arthralgia. Chloroquine is another treatment that has been shown to be effective as it inhibited CHIKV infection in cultured cells through endosomal acidification, thus interfering with the activation of Toll-like receptors (TLR), reducing inflammatory activity (128–130). Niclosamide, a medication used as an antiparasitic, was able to inhibit in vitro the entry of DENV, ZIKV, and CHIKV into the cells. This medication also interferes with endosomal acidification and inhibits membrane fusion (131, 132).
Isatin and its derivatives are heterocyclic organic compounds. They have broad applications in the pharmacological field, and several studies have pointed out their antiviral activities, such as spiropyrazolopyridone. Bin Zou and colleagues (133) used a viral infection model in mice with DENV and observed a reduction in viremia in the treated mice after oral administration of the drug. Another isatin derivative, 1-[(2-methylbenzimidazol-1-yl)methyl]-2-oxo-indolin-3-ylidene]-amino]thiourea (MBZM-N-IBT), demonstrated antiviral activity against CHIKV by inhibiting the nsP2 protease activity in vitro and in vivo experiments (134, 135).
Natural compounds belonging to the Flavonoid family, such as Delphinidin (D) and epigallocatechin gallate (EGCG), possess antioxidant activities, and according to recent studies, they also exhibit antiviral functions against some arboviruses, such as DENV, ZIKV, and CHIKV. Delphinidin likely acts during the interaction of viral proteins with receptors present in cells; this compound showed antiviral activity only during the early stages of viral infection tested in vitro (136). The EGCG was also able to inhibit CHIKV infection and attchament to cells, as shown by researchers (137) that transfected HEK cells with lentiviral vectors pseudotyped with CHIKV envelope proteins and subsequently added EGCG to assess antiviral effects.
Although there are several important studies in progress, the absence or limitation of effective therapies and vaccines makes the control of circulating mosquitoes vectors a commonly used strategy to combat arbovirus transmission. Vector control represents a viable approach to reduce the prevalence of these disease vectors (Table 1). Arboviruses are distributed worldwide, and their surveillance has become a crucial tool for detecting and controlling the circulation of viruses (138). Metatranscriptomics surveillance programs monitor and test mosquito populations for arboviruses, as demostrated by The Victorian Arbovirus Disease Control Program (VADCP) (139). Metatranscriptomics is a novel RNA sequencing approach used to analyze the RNA present in a sample. This technique aids in identifying viral mutations and even tracing the origin of cases. To achieve it, a complex bioinformatic analysis is required, as demonstrated in a study conducted in Australia (140). In this study, they analyzed the species of mosquitoes and the circulating virus to gain valuable insights into viral dynamics and transmission patterns.
Various methods targeting different stages of the mosquito life cycle can aid in controlling their population. Implementation of these strategies involves public education and raising awareness, encouraging measures like managing and preventing the deposition of eggs and larvae in water accumulations, tires, plant pots, and uncovered water tanks. Additionally, the application of insecticides to eliminate mosquito larvae and adults is crucial (141).
Furthermore, the incorporation of antiviral controls within mosquitoes has shown promise in preventing arbovirus transmission. Some recent studies have explored the use of genetically modified mosquitoes with antiviral genes that hinder viral replication (142).
Mosquitoes present a different defense system compared to mammals; they lack B and T lymphocytes, do not produce immunoglobulins, and do not develop specific antigen responses. However, they do exhibit defense mechanisms found in the innate immunity of mammals, such as Toll-like pathways, Janus kinase/signal transducer and activator of transcription (JAK/STAT), immune deficiency (IMD), and RNA interference (RNAi) pathways, which interfere with viral gene expression (143).
The Toll-like receptors (TLRs) bind to cytokine receptors like Spätzle, inducing signal transductions in the cytoplasm, where they interact with the myeloid differentiation primary response protein (MyD88)-Tube-Pelle complex, leading to the expression of B cell-dependent immune response-related NF-kB. The NF-kB expression can also be stimulated via IMD through the activation of peptidoglycan-recognition protein receptors PGPR-LC and PGPR-LG. Activation of the JAK/STAT pathway initiates the transcription of antiviral cytokines and growth factors.
Gene regulation is carried out by small non-coding RNAs, approximately 21-30 nucleotides long, classified as small interfering RNAs (siRNAs), microRNAs (miRNAs), and Piwi-associated interfering RNAs (piRNAs) (144, 145). siRNAs are double-stranded RNA molecules that target complementary messenger RNA (mRNA) sequences, subsequently degrading the gene and suppressing its function. miRNAs act in the post-transcriptional phase, repressing mRNA translation, while piRNAs regulate element transposition (144–147).
A better understanding of these insect modulation mechanisms aids in developing more efficient approaches for arbovirus control. This includes inhibiting specific genes to reduce virus transmission, enhancing insect resistance to the virus by using antimicrobial peptides, and even inhibiting viral replication in mosquitoes to prevent transmission.
Currently, a cutting-edge methodology for arbovirus control involves the manipulation of mosquito bacterial symbionts’ microbiota. The composition of the bacterial community can vary among different mosquito species (141). One notable example is Wolbachia pipiens, an α-proteobacterium found in a few arthropod species, which has been extensively researched for insect population control. Wolbachia is present in various mosquito tissues, including the Malpighian tubules, muscles, head, glands, and reproductive organs (148). The technique employed to introduce Wolbachia into insects is termed Insect Lineage Infection by Wolbachia. This method entails inoculating Wolbachia bacteria into insect eggs during the embryonic stage, resulting in the establishment of a persistent infection in the cells.Its effective spread is attributed to its ability to induce cytoplasmic incompatibility (CI), where mating between uninfected females and Wolbachia-infected males results in eggs that fail to develop, leading to the production of only Wolbachia-infected offspring. Additionally, Wolbachia can cause reduction of vectorial capacity by decreasing the mosquito’s lifespan (parthenogenesis) and inducing feminization. Moreover, Wolbachia inhibits virus replication within the mosquito and decreases saliva production, affecting feeding capacity during meals (149–151).
Research conducted in Brazil and Colombia has shown promising results in vector control, demonstrating that the release of modified Aedes aegypti mosquitoes containing Wolbachia led to the suppression of arbovirus transmission and replication. Consequently, Wolbachia has been proven capable of inhibiting the replication of DENV, ZIKV, and CHIKV (152–154). The use of Wolbachia as a vector control agent has been applied in several countries as part of programs aimed at eliminating arboviruses. One such example is the “Eliminate Dengue” project, an international program with the objective of controlling DENV circulation through Wolbachia infection in Aedes aegypti mosquitoes. This program has been implemented in countries such as Australia, Brazil, Indonesia, Vietnam, and Colombia, showing promising results in reducing DENV transmission rates. The initiative that combats the spread of arboviruses using Wolbachia is the “World Mosquito Program” (WMP). These prevention and control programs, which are based on the introduction of Wolbachia into insects, represent a promising and innovative approach (155).
The reemergence of arbovirus infections has been observed in several countries. Furthermore, the spread to new geographical areas is on the rise, underscoring the necessity for the implementation of effective control and prevention programs. These programs should be grounded in entomological surveillance activities and disease monitoring.
While specific pharmaceutical interventions for arbovirus infections remain elusive, we conclude that encouraging progress has been made with certain compounds, including Chloroquine, Niclosamide, and Isatin derivatives. These compounds have demonstrated substantial antiviral efficacy against these pathogens in both laboratory and animal studies.
It is crucial to implement strategic vector control measures to contain arbovirus transmission. These strategies encompass a range of interventions, as exemplified by the VADCP, along with educational campaigns to raise public awareness. Notably, advanced techniques like the manipulation of bacterial symbionts in mosquitoes, illustrated by the utilization of Wolbachia in Aedes aegypti mosquitoes, have shown remarkable success in suppressing the transmission of diseases like DENV across various nations, which holds potential such as pivotal component of integrated prevention strategy. By reducing vector competence, limiting viral replication, and influencing mosquito lifespan, Wolbachia contributes substantially to breaking the transmission cycle.
As we confront the complex challenges posed by arboviruses, it becomes evident that a holistic and collaborative approach is imperative. The integration of advancements in antiviral research, the development of effective vaccines, innovative vector control methodologies, and surveillance programs collectively fortify the global defense. This unified front not only prevents immediate outbreaks, but also lays the foundation for resilient and adaptative aproch to combat future arbovirus challegens.
NC: Conceptualization, Writing – original draft. AL: Conceptualization, Writing – original draft. WP: Writing – review & editing. JS: Writing – review & editing. LV: Writing – review & editing. WC: Writing – review & editing. AF: Writing – review & editing. OC: Writing – review & editing. GC: Writing – review & editing. RD: Writing – review & editing.
The author(s) declare financial support was received for the research, authorship, and/or publication of this article. The authors acknowledge the support provided by The São Paulo Research Foundation – FAPESP: GC-M: 2019/14526-0, 2020/04667-3; GC-M and NC: 2021/03508-1 (PhD Scholarship); GC-M and AL: 2021/03102-5 (PhD Scholarship); (FAPESP Young Investigator Program, 2021/03684-4, RD-C); (FAPESP grants: 2020/01688-0 and 2020/07069-0 to OC-M).
The authors declare that the research was conducted in the absence of any commercial or financial relationships that could be construed as a potential conflict of interest.
All claims expressed in this article are solely those of the authors and do not necessarily represent those of their affiliated organizations, or those of the publisher, the editors and the reviewers. Any product that may be evaluated in this article, or claim that may be made by its manufacturer, is not guaranteed or endorsed by the publisher.
1. Lopes N. Características gerais e epidemiologia dos arbovírus emergentes no Brasil. Rev Pan-Amaz Saude (2014) 5:55–64. doi: 10.5123/S2176-62232014000300007
2. Braack L, Gouveia de Almeida AP, Cornel AJ, Swanepoel R, de Jager C. Mosquito-borne arboviruses of African origin: review of key viruses and vectors. Parasit Vectors (2018) 11:29. doi: 10.1186/s13071-017-2559-9
3. Kleiboeker SB, Scoles GA. Pathogenesis of African swine fever virus in Ornithodoros ticks. Anim Health Res Rev (2001) 2:121–8. doi: 10.1079/AHRR200133
4. Karabatsos N. Supplement to International Catalogue of Arboviruses including certain other viruses of vertebrates. Am J Trop Med Hyg (1978) 27:372–440. doi: 10.4269/ajtmh.1978.27.372
5. Weaver SC, Charlier C, Vasilakis N, Lecuit M. Zika, Chikungunya, and other emerging vector-borne viral diseases. Annu Rev Med (2018) 69:395–408. doi: 10.1146/annurev-med-050715-105122
6. Young PR. Arboviruses: A family on the move. Adv Exp Med Biol (2018) 1062:1–10. doi: 10.1007/978-981-10-8727-1_1
7. Brady OJ, Gething PW, Bhatt S, Messina JP, Brownstein JS, Hoen AG, et al. Refining the global spatial limits of dengue virus transmission by evidence-based consensus. PLoS Negl Trop Dis (2012) 6:e1760. doi: 10.1371/journal.pntd.0001760
8. PAHO. Pan American Health Organization (PAHO) World Health Organization (WHO) Actualización epidemiológica semanal para dengue, chikunguña y zika en (2022). Available at: https://www3.paho.org/data/index.php/en/ (Accessed 10/05).
9. Thomas SJ, Endy TP, Rothman AL. Viral Infections Of Humans: Epidemiology and Control. Fifith edition. Kaslow RA, Stanberry LR, Duc JWL, editors. New York, NY: Springer (2007).
10. Mustafa MS, Rasotgi V, Jain S, Gupta V. Discovery of fifth serotype of dengue virus (DENV-5): A new public health dilemma in dengue control. Med J Armed Forces India (2015) 71:67–70. doi: 10.1016/j.mjafi.2014.09.011
11. Messina JP, Brady OJ, Scott TW, Zou C, Pigott DM, Duda KA, et al. Global spread of dengue virus types: mapping the 70 year history. Trends Microbiol (2014) 22:138–46. doi: 10.1016/j.tim.2013.12.011
12. Laureti M, Narayanan D, Rodriguez-Andres J, Fazakerley JK, Kedzierski L. Flavivirus receptors: diversity, identity, and cell entry. Front Immunol (2018) 9:2180. doi: 10.3389/fimmu.2018.02180
13. HOTTA S. Experimental studies on dengue. I. Isolation, identification and modification of the virus. J Infect Dis (1952) 90:1–9. doi: 10.1093/infdis/90.1.1
14. Figueiredo LTM. Human urban arboviruses can infect wild animals and jump to sylvatic maintenance cycles in South America. Front Cell Infect Microbiol (2019) 9:259. doi: 10.3389/fcimb.2019.00259
15. Figueiredo LT. Emergent arboviruses in Brazil. Rev Soc Bras Med Trop (2007) 40:224–9. doi: 10.1590/s0037-86822007000200016
16. DICK GW, KITCHEN SF, HADDOW AJ. Zika virus. I. Isolations and serological specificity. Trans R Soc Trop Med Hyg (1952) 46:509–20. doi: 10.1016/0035-9203(52)90042-4
17. Sirohi D, Kuhn RJ. Zika virus structure, maturation, and receptors. J Infect Dis (2017) 216:S935–44. doi: 10.1093/infdis/jix515
18. Gatherer D, Kohl A. Zika virus: a previously slow pandemic spreads rapidly through the Americas. J Gen Virol (2016) 97:269–73. doi: 10.1099/jgv.0.000381
19. Hills SL, Russell K, Hennessey M, Williams C, Oster AM, Fischer M, et al. Transmission of Zika virus through sexual contact with travelers to areas of ongoing transmission - continental United States, 2016. MMWR Morb Mortal Wkly Rep (2016) 65:215–6. doi: 10.15585/mmwr.mm6508e2
20. ROSS RW. The Newala epidemic. III. The virus: isolation, pathogenic properties and relationship to the epidemic. J Hyg (Lond) (1956) 54:177–91. doi: 10.1017/s0022172400044442
21. Chevillon C, Briant L, Renaud F, Devaux C. The Chikungunya threat: an ecological and evolutionary perspective. Trends Microbiol (2008) 16:80–8. doi: 10.1016/j.tim.2007.12.003
22. Volk SM, Chen R, Tsetsarkin KA, Adams AP, Garcia TI, Sall AA, et al. Genome-scale phylogenetic analyses of chikungunya virus reveal independent emergences of recent epidemics and various evolutionary rates. J Virol (2010) 84:6497–504. doi: 10.1128/JVI.01603-09
23. Burt FJ, Chen W, Miner JJ, Lenschow DJ, Merits A, Schnettler E, et al. Chikungunya virus: an update on the biology and pathogenesis of this emerging pathogen. Lancet Infect Dis (2017) 17:e107–17. doi: 10.1016/S1473-3099(16)30385-1
24. Nunes MR, Faria NR, de Vasconcelos JM, Golding N, Kraemer MU, de Oliveira LF, et al. Emergence and potential for spread of Chikungunya virus in Brazil. BMC Med (2015) 13:102. doi: 10.1186/s12916-015-0348-x
25. Schuffenecker I, Iteman I, Michault A, Murri S, Frangeul L, Vaney MC, et al. Genome microevolution of chikungunya viruses causing the Indian Ocean outbreak. PloS Med (2006) 3:e263. doi: 10.1371/journal.pmed.0030263
26. Rezza G, Nicoletti L, Angelini R, Romi R, Finarelli AC, Panning M, et al. Infection with chikungunya virus in Italy: an outbreak in a temperate region. Lancet (2007) 370:1840–6. doi: 10.1016/S0140-6736(07)61779-6
27. Li Z, Wang J, Cheng X, Hu H, Guo C, Huang J, et al. The worldwide seroprevalence of DENV, CHIKV and ZIKV infection: A systematic review and meta-analysis. PLoS Negl Trop Dis (2021) 15:e0009337. doi: 10.1371/journal.pntd.0009337
28. Vairo F, Haider N, Kock R, Ntoumi F, Ippolito G, Zumla A. Chikungunya: epidemiology, pathogenesis, clinical features, management, and prevention. Infect Dis Clin North Am (2019) 33:1003–25. doi: 10.1016/j.idc.2019.08.006
29. WHO. World Health Organization. A report about health. Chikungunya. Available at: https://www.who.int/news-room/fact-sheets/detail/chikungunya (Accessed 16/05).
30. Gubler DJ. Human arbovirus infections worldwide. Ann N Y Acad Sci (2001) 951:13–24. doi: 10.1111/j.1749-6632.2001.tb02681.x
31. Schatzmayr HG. [Emerging and reemerging viral diseases]. Cad Saude Publica (2001) 17(Suppl):209–13. doi: 10.1590/S0102-311X2001000700031
32. Vorou RM, Papavassiliou VG, Tsiodras S. Emerging zoonoses and vector-borne infections affecting humans in Europe. Epidemiol Infect (2007) 135:1231–47. doi: 10.1017/S0950268807008527
33. Powers AM, Logue CH. Changing patterns of chikungunya virus: re-emergence of a zoonotic arbovirus. J Gen Virol (2007) 88:2363–77. doi: 10.1099/vir.0.82858-0
34. Gubler; J,D, Kuno G, Markoff L. Flaviviruses; Field's Virology Vol. 1. . Philadelphia: Lippincott Williams & Wilkins (2007) p. 1153–252.
35. Chong HY, Leow CY, Abdul Majeed AB, Leow CH. Flavivirus infection-A review of immunopathogenesis, immunological response, and immunodiagnosis. Virus Res (2019) 274:197770. doi: 10.1016/j.virusres.2019.197770
36. Dai L, Song J, Lu X, Deng YQ, Musyoki AM, Cheng H, et al. Structures of the Zika virus envelope protein and its complex with a flavivirus broadly protective antibody. Cell Host Microbe (2016) 19:696–704. doi: 10.1016/j.chom.2016.04.013
37. Ye Q, Liu ZY, Han JF, Jiang T, Li XF, Qin CF. Genomic characterization and phylogenetic analysis of Zika virus circulating in the Americas. Infect Genet Evol (2016) 43:43–9. doi: 10.1016/j.meegid.2016.05.004
38. Zhang Y, Zhang W, Ogata S, Clements D, Strauss JH, Baker TS, et al. Conformational changes of the flavivirus E glycoprotein. Structure (2004) 12:1607–18. doi: 10.1016/j.str.2004.06.019
39. Kostyuchenko VA, Lim EX, Zhang S, Fibriansah G, Ng TS, Ooi JS, et al. Structure of the thermally stable Zika virus. Nature (2016) 533:425–8. doi: 10.1038/nature17994
40. Barba-Spaeth G, Dejnirattisai W, Rouvinski A, Vaney MC, Medits I, Sharma A, et al. Structural basis of potent Zika-dengue virus antibody cross-neutralization. Nature (2016) 536:48–53. doi: 10.1038/nature18938
41. Mukhopadhyay S, Kuhn RJ, Rossmann MG. A structural perspective of the flavivirus life cycle. Nat Rev Microbiol (2005) 3:13–22. doi: 10.1038/nrmicro1067
42. Stiasny K, Kiermayr S, Heinz FX. Entry functions and antigenic structure of flavivirus envelope proteins. Novartis Found Symp (2006) 277:57–65; discussion 65-73, 251-253. doi: 10.1002/0470058005.ch5
43. Holmes AC, Basore K, Fremont DH, Diamond MS. A molecular understanding of alphavirus entry. PLoS Pathog (2020) 16:e1008876. doi: 10.1371/journal.ppat.1008876
44. Brown RS, Wan JJ, Kielian M. The alphavirus exit pathway: what we know and what we wish we knew. Viruses (2018) 10(2):89. doi: 10.3390/v10020089
46. Cordero-Rivera CD, De Jesús-González LA, Osuna-Ramos JF, Palacios-Rápalo SN, Farfan-Morales CN, Reyes-Ruiz JM, et al. The importance of viral and cellular factors on flavivirus entry. Curr Opin Virol (2021) 49:164–75. doi: 10.1016/j.coviro.2021.05.001
47. Schnierle BS. Cellular attachment and entry factors for Chikungunya virus. Viruses (2019) 11(11):1078. doi: 10.3390/v11111078
48. Jose J, Snyder JE, Kuhn RJ. A structural and functional perspective of alphavirus replication and assembly. Future Microbiol (2009) 4:837–56. doi: 10.2217/fmb.09.59
49. Carey BD, Bakovic A, Callahan V, Narayanan A, Kehn-Hall K. New World alphavirus protein interactomes from a therapeutic perspective. Antiviral Res (2019) 163:125–39. doi: 10.1016/j.antiviral.2019.01.015
50. Rupp JC, Sokoloski KJ, Gebhart NN, Hardy RW. Alphavirus RNA synthesis and non-structural protein functions. J Gen Virol (2015) 96:2483–500. doi: 10.1099/jgv.0.000249
51. Ahola T, Kääriäinen L. Reaction in alphavirus mRNA capping: formation of a covalent complex of nonstructural protein nsP1 with 7-methyl-GMP. Proc Natl Acad Sci USA (1995) 92:507–11. doi: 10.1073/pnas.92.2.507
52. Peränen J, Rikkonen M, Liljeström P, Kääriäinen L. Nuclear localization of Semliki Forest virus-specific nonstructural protein nsP2. J Virol (1990) 64:1888–96. doi: 10.1128/JVI.64.5.1888-1896.1990
53. Rikkonen M, Peränen J, Kääriäinen L. ATPase and GTPase activities associated with Semliki Forest virus nonstructural protein nsP2. J Virol (1994) 68:5804–10. doi: 10.1128/JVI.68.9.5804-5810.1994
54. Wong KZ, Chu JJH. The interplay of viral and host factors in Chikungunya virus infection: targets for antiviral strategies. Viruses (2018) 10(6):294. doi: 10.3390/v10060294
55. Tomar S, Hardy RW, Smith JL, Kuhn RJ. Catalytic core of alphavirus nonstructural protein nsP4 possesses terminal adenylyltransferase activity. J Virol (2006) 80:9962–9. doi: 10.1128/JVI.01067-06
56. Briant L, Desprès P, Choumet V, Missé D. Role of skin immune cells on the host susceptibility to mosquito-borne viruses. Virology (2014) 464-465:26–32. doi: 10.1016/j.virol.2014.06.023
57. Suhrbier A, Jaffar-Bandjee MC, Gasque P. Arthritogenic alphaviruses–an overview. Nat Rev Rheumatol (2012) 8:420–9. doi: 10.1038/nrrheum.2012.64
58. Matusali G, Colavita F, Bordi L, Lalle E, Ippolito G, Capobianchi MR, et al. Tropism of the Chikungunya virus. Viruses (2019) 11(2):175. doi: 10.3390/v11020175
59. Schwartz O, Albert ML. Biology and pathogenesis of chikungunya virus. Nat Rev Microbiol (2010) 8:491–500. doi: 10.1038/nrmicro2368
60. Rohatgi A, Corbo JC, Monte K, Higgs S, Vanlandingham DL, Kardon G, et al. Infection of myofibers contributes to increased pathogenicity during infection with an epidemic strain of chikungunya virus. J Virol (2014) 88:2414–25. doi: 10.1128/JVI.02716-13
61. Ozden S, Huerre M, Riviere JP, Coffey LL, Afonso PV, Mouly V, et al. Human muscle satellite cells as targets of Chikungunya virus infection. PLoS One (2007) 2:e527. doi: 10.1371/journal.pone.0000527
62. Hiroki CH, Toller-Kawahisa JE, Fumagalli MJ, Colon DF, Figueiredo LTM, Fonseca BALD, et al. Neutrophil extracellular traps effectively control acute Chikungunya virus infection. Front Immunol (2019) 10:3108. doi: 10.3389/fimmu.2019.03108
63. Reddy V, Mani RS, Desai A, Ravi V. Correlation of plasma viral loads and presence of Chikungunya IgM antibodies with cytokine/chemokine levels during acute Chikungunya virus infection. J Med Virol (2014) 86:1393–401. doi: 10.1002/jmv.23875
64. Teng TS, Kam YW, Lee B, Hapuarachchi HC, Wimal A, Ng LC, et al. Systematic meta-analysis of immune signatures in patients with acute Chikungunya virus infection. J Infect Dis (2015) 211:1925–35. doi: 10.1093/infdis/jiv049
65. Silva LA, Dermody TS. Chikungunya virus: epidemiology, replication, disease mechanisms, and prospective intervention strategies. J Clin Invest (2017) 127:737–49. doi: 10.1172/JCI84417
66. Rudd PA, Wilson J, Gardner J, Larcher T, Babarit C, Le TT, et al. Interferon response factors 3 and 7 protect against Chikungunya virus hemorrhagic fever and shock. J Virol (2012) 86:9888–98. doi: 10.1128/JVI.00956-12
67. Ryman KD, Klimstra WB. Host responses to alphavirus infection. Immunol Rev (2008) 225:27–45. doi: 10.1111/j.1600-065X.2008.00670.x
68. Thurmond S, Wang B, Song J, Hai R. Suppression of type I interferon signaling by. Viruses (2018) 10(12):712. doi: 10.3390/v10120712
69. Makhluf H, Shresta S. Innate antiviral immunity against dengue virus. Crit Rev Immunol (2015) 35:253–60. doi: 10.1615/critrevimmunol.2015014251
70. Schoggins JW, Rice CM. Interferon-stimulated genes and their antiviral effector functions. Curr Opin Virol (2011) 1:519–25. doi: 10.1016/j.coviro.2011.10.008
71. Mamane Y, Heylbroeck C, Génin P, Algarté M, Servant MJ, LePage C, et al. Interferon regulatory factors: the next generation. Gene (1999) 237:1–14. doi: 10.1016/s0378-1119(99)00262-0
72. Fros JJ, Pijlman GP. Alphavirus infection: host cell shut-off and inhibition of antiviral responses. Viruses (2016) 8(6):166. doi: 10.3390/v8060166
73. Screaton G, Mongkolsapaya J, Yacoub S, Roberts C. New insights into the immunopathology and control of dengue virus infection. Nat Rev Immunol (2015) 15:745–59. doi: 10.1038/nri3916
74. Halstead SB. Observations related to pathogensis of dengue hemorrhagic fever. VI. Hypotheses and discussion. Yale J Biol Med (1970) 42:350–62.
75. Littaua R, Kurane I, Ennis FA. Human IgG Fc receptor II mediates antibody-dependent enhancement of dengue virus infection. J Immunol (1990) 144:3183–6. doi: 10.4049/jimmunol.144.8.3183
76. Cardosa MJ, Porterfield JS, Gordon S. Complement receptor mediates enhanced flavivirus replication in macrophages. J Exp Med (1983) 158:258–63. doi: 10.1084/jem.158.1.258
77. Chareonsirisuthigul T, Kalayanarooj S, Ubol S. Dengue virus (DENV) antibody-dependent enhancement of infection upregulates the production of anti-inflammatory cytokines, but suppresses anti-DENV free radical and pro-inflammatory cytokine production, in THP-1 cells. J Gen Virol (2007) 88:365–75. doi: 10.1099/vir.0.82537-0
78. Vo HTM, Upasani V, Auerswald H, Lay S, Sann S, Vanderlinden A, et al. Temporal patterns of functional anti-dengue antibodies in dengue infected individuals with different disease outcome or infection history. Sci Rep (2022) 12:17863. doi: 10.1038/s41598-022-21722-2
79. Puschnik A, Lau L, Cromwell EA, Balmaseda A, Zompi S, Harris E. Correlation between dengue-specific neutralizing antibodies and serum avidity in primary and secondary dengue virus 3 natural infections in humans. PLoS Negl Trop Dis (2013) 7:e2274. doi: 10.1371/journal.pntd.0002274
80. Bournazos S, Vo HTM, Duong V, Auerswald H, Ly S, Sakuntabhai A, et al. Antibody fucosylation predicts disease severity in secondary dengue infection. Science (2021) 372:1102–5. doi: 10.1126/science.abc7303
81. Thulin NK, Brewer RC, Sherwood R, Bournazos S, Edwards KG, Ramadoss NS, et al. Maternal anti-dengue igG fucosylation predicts susceptibility to dengue disease in infants. Cell Rep (2020) 31:107642. doi: 10.1016/j.celrep.2020.107642
82. Salje H, Cummings DAT, Rodriguez-Barraquer I, Katzelnick LC, Lessler J, Klungthong C, et al. Reconstruction of antibody dynamics and infection histories to evaluate dengue risk. Nature (2018) 557:719–23. doi: 10.1038/s41586-018-0157-4
83. Katzelnick LC, Gresh L, Halloran ME, Mercado JC, Kuan G, Gordon A, et al. Antibody-dependent enhancement of severe dengue disease in humans. Science (2017) 358:929–32. doi: 10.1126/science.aan6836
84. Dejnirattisai W, Jumnainsong A, Onsirisakul N, Fitton P, Vasanawathana S, Limpitikul W, et al. Cross-reacting antibodies enhance dengue virus infection in humans. Science (2010) 328:745–8. doi: 10.1126/science.1185181
85. Xu M, Zuest R, Velumani S, Tukijan F, Toh YX, Appanna R, et al. A potent neutralizing antibody with therapeutic potential against all four serotypes of dengue virus. NPJ Vaccines (2017) 2:2. doi: 10.1038/s41541-016-0003-3
86. Avirutnan P, Fuchs A, Hauhart RE, Somnuke P, Youn S, Diamond MS, et al. Antagonism of the complement component C4 by flavivirus nonstructural protein NS1. J Exp Med (2010) 207:793–806. doi: 10.1084/jem.20092545
87. Chen J, Ng MM, Chu JJ. Activation of TLR2 and TLR6 by dengue NS1 protein and its implications in the immunopathogenesis of dengue virus infection. PloS Pathog (2015) 11:e1005053. doi: 10.1371/journal.ppat.1005053
88. Modhiran N, Watterson D, Muller DA, Panetta AK, Sester DP, Liu L, et al. Dengue virus NS1 protein activates cells via Toll-like receptor 4 and disrupts endothelial cell monolayer integrity. Sci Transl Med (2015) 7:304ra142. doi: 10.1126/scitranslmed.aaa3863
89. Puerta-Guardo H, Glasner DR, Espinosa DA, Biering SB, Patana M, Ratnasiri K, et al. Flavivirus NS1 triggers tissue-specific vascular endothelial dysfunction reflecting disease tropism. Cell Rep (2019) 26:1598–1613.e1598. doi: 10.1016/j.celrep.2019.01.036
90. Pang X, Zhang R, Cheng G. Progress towards understanding the pathogenesis of dengue hemorrhagic fever. Virol Sin (2017) 32:16–22. doi: 10.1007/s12250-016-3855-9
91. Faye O, Dupressoir A, Weidmann M, Ndiaye M, Alpha Sall A. One-step RT-PCR for detection of Zika virus. J Clin Virol (2008) 43:96–101. doi: 10.1016/j.jcv.2008.05.005
92. Gourinat AC, O'Connor O, Calvez E, Goarant C, Dupont-Rouzeyrol M. Detection of Zika virus in urine. Emerg Infect Dis (2015) 21:84–6. doi: 10.3201/eid2101.140894
93. Plourde AR, Bloch EMA. Literature review of Zika virus. Emerg Infect Dis (2016) 22:1185–92. doi: 10.3201/eid2207.151990
94. de Araújo TVB, Rodrigues LC, de Alencar Ximenes RA, de Barros Miranda-Filho D, Montarroyos UR, de Melo APL, et al. Association between Zika virus infection and microcephaly in Brazil, January to May, 2016: preliminary report of a case-control study. Lancet Infect Dis (2016) 16:1356–63. doi: 10.1016/S1473-3099(16)30318-8
95. Baud D, Gubler DJ, Schaub B, Lanteri MC, Musso D. An update on Zika virus infection. Lancet (2017) 390:2099–109. doi: 10.1016/S0140-6736(17)31450-2
96. Edupuganti S, Natrajan MS, Rouphael N, Lai L, Xu Y, Feldhammer M, et al. Biphasic Zika illness with rash and joint pain. Open Forum Infect Dis (2017) 4:ofx133. doi: 10.1093/ofid/ofx133
97. Minhas AM, Nayab A, Iyer S, Narmeen M, Fatima K, Khan MS, et al. Association of Zika virus with myocarditis, heart failure, and arrhythmias: A literature review. Cureus (2017) 9:e1399. doi: 10.7759/cureus.1399
98. Baxter VK, Heise MT. Immunopathogenesis of alphaviruses. Adv Virus Res (2020) 107:315–82. doi: 10.1016/bs.aivir.2020.06.002
99. Paixão ES, Rodrigues LC, Costa MDCN, Itaparica M, Barreto F, Gérardin P, et al. Chikungunya chronic disease: a systematic review and meta-analysis. Trans R Soc Trop Med Hyg (2018) 112:301–16. doi: 10.1093/trstmh/try063
100. Erasmus JH, Rossi SL, Weaver SC. Development of vaccines for chikungunya fever. J Infect Dis (2016) 214:S488–96. doi: 10.1093/infdis/jiw271
101. Weaver SC, Lecuit M. Chikungunya virus and the global spread of a mosquito-borne disease. N Engl J Med (2015) 372:1231–9. doi: 10.1056/NEJMra1406035
102. Venter M. Assessing the zoonotic potential of arboviruses of African origin. Curr Opin Virol (2018) 28:74–84. doi: 10.1016/j.coviro.2017.11.004
103. Zhang W, Chen S, Mahalingam S, Wang M, Cheng A. An updated review of avian-origin Tembusu virus: a newly emerging avian Flavivirus. J Gen Virol (2017) 98:2413–20. doi: 10.1099/jgv.0.000908
104. Sabin AB, Schlesinger RW. Production of immunity to dengue with virus modified by propagation in mice. Science (1945) 101:640–2. doi: 10.1126/science.101.2634.640
105. Villar L, Dayan GH, Arredondo-García JL, Rivera DM, Cunha R, Deseda C, et al. Efficacy of a tetravalent dengue vaccine in children in Latin America. N Engl J Med (2015) 372:113–23. doi: 10.1056/NEJMoa1411037
106. World Health Organization. Dengue vaccine: WHO position paper – July 2016. Wkly Epidemiol Rec (2016) 91:349–64. doi: 10.1016/j.vaccine.2016.10.070
107. Diário Oficial da União, B., DF, Brazil. Available at: https://www.jusbrasil.com.br/diarios/106783857/dou-suplemento-secao-1-pg-4 (Accessed 28/05).
108. Reuters. Philippines says anti-dengue vaccine may be connected to three deaths. Available at: https://www.reuters.com/article/us-sanofidengue-Philippines/Philippines-says-anti-dengue-vaccine-may-beconnected-to-three-deaths-idUSKBN1FM0SP (Accessed 20/05).
109. European Medicines Agency. Dengvaxia vaccine approved for prevention of dengue in Europe. London, UK: European Medicine Agency (2018).
110. Administration, F.-U.F.a.D. First FDA-approved vaccine for the prevention of dengue disease in endemic regions. Swiftwater PA, USA: Sanofi Pasteur Inc. (2019).
111. Wong JM, Adams LE, Durbin AP, Muñoz-Jordán JL, Poehling KA, Sánchez-González LM, et al. Dengue: A growing problem with new interventions. Pediatrics (2022) 149(6). doi: 10.1542/peds.2021-055522
112. Biswal S, Reynales H, Saez-Llorens X, Lopez P, Borja-Tabora C, Kosalaraksa P, et al. Efficacy of a tetravalent dengue vaccine in healthy children and adolescents. N Engl J Med (2019) 381:2009–19. doi: 10.1056/NEJMoa1903869
113. Sáez-Llorens X, Tricou V, Yu D, Rivera L, Tuboi S, Garbes P, et al. Safety and immunogenicity of one versus two doses of Takeda's tetravalent dengue vaccine in children in Asia and Latin America: interim results from a phase 2, randomised, placebo-controlled study. Lancet Infect Dis (2017) 17:615–25. doi: 10.1016/S1473-3099(17)30166-4
114. Biswal S, Borja-Tabora C, Martinez Vargas L, Velásquez H, Theresa Alera M, Sierra V, et al. Efficacy of a tetravalent dengue vaccine in healthy children aged 4-16 years: a randomised, placebo-controlled, phase 3 trial. Lancet (2020) 395:1423–33. doi: 10.1016/S0140-6736(20)30414-1
115. Collins MH, McGowan E, Jadi R, Young E, Lopez CA, Baric RS, et al. Lack of durable cross-neutralizing antibodies against Zika virus from dengue virus infection. Emerg Infect Dis (2017) 23:773–81. doi: 10.3201/eid2305.161630
116. Montoya M, Collins M, Dejnirattisai W, Katzelnick LC, Puerta-Guardo H, Jadi R, et al. Longitudinal analysis of antibody cross-neutralization following Zika virus and dengue virus infection in asia and the Americas. J Infect Dis (2018) 218:536–45. doi: 10.1093/infdis/jiy164
117. Richner JM, Himansu S, Dowd KA, Butler SL, Salazar V, Fox JM, et al. Modified mRNA Vaccines Protect against Zika Virus Infection. Cell (2017) 169:176. doi: 10.1016/j.cell.2017.03.016
118. Casey RM, Harris JB, Ahuka-Mundeke S, Dixon MG, Kizito GM, Nsele PM, et al. Immunogenicity of fractional-dose vaccine during a yellow fever outbreak - final report. N Engl J Med (2019) 381:444–54. doi: 10.1056/NEJMoa1710430
119. Bullard-Feibelman KM, Govero J, Zhu Z, Salazar V, Veselinovic M, Diamond MS, et al. The FDA-approved drug sofosbuvir inhibits Zika virus infection. Antiviral Res (2017) 137:134–40. doi: 10.1016/j.antiviral.2016.11.023
120. Retallack H, Di Lullo E, Arias C, Knopp KA, Laurie MT, Sandoval-Espinosa C, et al. Zika virus cell tropism in the developing human brain and inhibition by azithromycin. Proc Natl Acad Sci USA (2016) 113:14408–13. doi: 10.1073/pnas.1618029113
121. Hamer DH, Wilson ME, Jean J, Chen LH. Epidemiology, prevention, and potential future treatments of sexually transmitted Zika virus infection. Curr Infect Dis Rep (2017) 19:16. doi: 10.1007/s11908-017-0571-z
122. McArthur MA. Zika virus: recent advances towards the development of vaccines and therapeutics. Viruses (2017) 9(6):143. doi: 10.3390/v9060143
123. Gardner J, Anraku I, Le TT, Larcher T, Major L, Roques P, et al. Chikungunya virus arthritis in adult wild-type mice. J Virol (2010) 84:8021–32. doi: 10.1128/JVI.02603-09
124. Couderc T, Khandoudi N, Grandadam M, Visse C, Gangneux N, Bagot S, et al. Prophylaxis and therapy for Chikungunya virus infection. J Infect Dis (2009) 200:516–23. doi: 10.1086/600381
125. Akahata W, Yang ZY, Andersen H, Sun S, Holdaway HA, Kong WP, et al. A virus-like particle vaccine for epidemic Chikungunya virus protects nonhuman primates against infection. Nat Med (2010) 16:334–8. doi: 10.1038/nm.2105
126. Smee DF, Alaghamandan HA, Kini GD, Robins RK. Antiviral activity and mode of action of ribavirin 5'-sulfamate against Semliki Forest virus. Antiviral Res (1988) 10:253–62. doi: 10.1016/0166-3542(88)90044-7
127. Sidwell RW, Smee DF. Viruses of the Bunya- and Togaviridae families: potential as bioterrorism agents and means of control. Antiviral Res (2003) 57:101–11. doi: 10.1016/s0166-3542(02)00203-6
128. Khan M, Santhosh SR, Tiwari M, Lakshmana Rao PV, Parida M. Assessment of in vitro prophylactic and therapeutic efficacy of chloroquine against Chikungunya virus in vero cells. J Med Virol (2010) 82:817–24. doi: 10.1002/jmv.21663
129. De Lamballerie X, Boisson V, Reynier JC, Enault S, Charrel RN, Flahault A, et al. On chikungunya acute infection and chloroquine treatment. Vector Borne Zoonotic Dis (2008) 8:837–9. doi: 10.1089/vbz.2008.0049
130. Ermann J, Bermas BL. The biology behind the new therapies for SLE. Int J Clin Pract (2007) 61:2113–9. doi: 10.1111/j.1742-1241.2007.01528.x
131. Wang YM, Lu JW, Lin CC, Chin YF, Wu TY, Lin LI, et al. Antiviral activities of niclosamide and nitazoxanide against chikungunya virus entry and transmission. Antiviral Res (2016) 135:81–90. doi: 10.1016/j.antiviral.2016.10.003
132. Kao JC, HuangFu WC, Tsai TT, Ho MR, Jhan MK, Shen TJ, et al. The antiparasitic drug niclosamide inhibits dengue virus infection by interfering with endosomal acidification independent of mTOR. PloS Negl Trop Dis (2018) 12:e0006715. doi: 10.1371/journal.pntd.0006715
133. Zou B, Chan WL, Ding M, Leong SY, Nilar S, Seah PG, et al. Lead optimization of spiropyrazolopyridones: a new and potent class of dengue virus inhibitors. ACS Med Chem Lett (2015) 6:344–8. doi: 10.1021/ml500521r
134. Mishra P, Kumar A, Mamidi P, Kumar S, Basantray I, Saswat T, et al. Inhibition of Chikungunya virus replication by 1-[(2-methylbenzimidazol-1-yl) methyl]-2-oxo-indolin-3-ylidene] amino] thiourea(MBZM-N-IBT). Sci Rep (2016) 6:20122. doi: 10.1038/srep20122
135. De S, Ghosh S, Keshry SS, Mahish C, Mohapatra C, Guru A, et al. MBZM-N-IBT, a novel small molecule, restricts Chikungunya virus infection by targeting nsP2 protease activity. Antimicrob Agents Chemother (2022) 66:e0046322. doi: 10.1128/aac.00463-22
136. Vázquez-Calvo Á, Jiménez de Oya N, Martín-Acebes MA, Garcia-Moruno E, Saiz JC. Antiviral properties of the natural polyphenols delphinidin and epigallocatechin gallate against the flaviviruses west nile virus, Zika virus, and dengue virus. Front Microbiol (2017) 8:1314. doi: 10.3389/fmicb.2017.01314
137. Weber C, Sliva K, von Rhein C, Kümmerer BM, Schnierle BS. The green tea catechin, epigallocatechin gallate inhibits chikungunya virus infection. Antiviral Res (2015) 113:1–3. doi: 10.1016/j.antiviral.2014.11.001
138. Kraemer MUG, Reiner RC, Brady OJ, Messina JP, Gilbert M, Pigott DM, et al. Past and future spread of the arbovirus vectors Aedes aEgypti and Aedes albopictus. Nat Microbiol (2019) 4:854–63. doi: 10.1038/s41564-019-0376-y
139. The Victorian Arbovirus Disease Control Program. Department of Natural Resources and Environment. Available at: https://www.betterhealth.vic.gov.au/mosquito-management-victoria (Accessed 07/24).
140. Batovska J, Mee PT, Sawbridge TI, Rodoni BC, Lynch SE. Enhanced arbovirus surveillance with high-throughput metatranscriptomic processing of field-collected mosquitoes. Viruses (2022) 14(12):2759. doi: 10.3390/v14122759
141. Schrieke H, Maignien L, Constancias F, Trigodet F, Chakloute S, Rakotoarivony I, et al. The mosquito microbiome includes habitat-specific but rare symbionts. Comput Struct Biotechnol J (2022) 20:410–20. doi: 10.1016/j.csbj.2021.12.019
142. Franz AW, Sanchez-Vargas I, Raban RR, Black WC, James AA, Olson KE. Fitness impact and stability of a transgene conferring resistance to dengue-2 virus following introgression into a genetically diverse Aedes aEgypti strain. PLoS Negl Trop Dis (2014) 8:e2833. doi: 10.1371/journal.pntd.0002833
143. Liu T, Xu Y, Wang X, Gu J, Yan G, Chen XG. Antiviral systems in vector mosquitoes. Dev Comp Immunol (2018) 83:34–43. doi: 10.1016/j.dci.2017.12.025
144. Tafesh-Edwards G, Kalukin A, Eleftherianos I. Zika virus induces sex-dependent metabolic changes in. Front Immunol (2022) 13:903860. doi: 10.3389/fimmu.2022.903860
145. Ding SW, Voinnet O. Antiviral immunity directed by small RNAs. Cell (2007) 130:413–26. doi: 10.1016/j.cell.2007.07.039
146. Zeng T, Jaffar S, Xu Y, Qi Y. The intestinal immune defense system in insects. Int J Mol Sci (2022) 23(23):15132. doi: 10.3390/ijms232315132
147. Sheehan G, Garvey A, Croke M, Kavanagh K. Innate humoral immune defences in mammals and insects: The same, with differences? Virulence (2018) 9:1625–39. doi: 10.1080/21505594.2018.1526531
148. Werren JH. Biology of wolbachia. Annu Rev Entomol (1997) 42:587–609. doi: 10.1146/annurev.ento.42.1.587
149. Frentiu FD, Robinson J, Young PR, McGraw EA, O'Neill SL. Wolbachia-mediated resistance to dengue virus infection and death at the cellular level. PLoS One (2010) 5:e13398. doi: 10.1371/journal.pone.0013398
150. Caragata EP, Dutra HL, Moreira LA. Inhibition of Zika virus by. Microb Cell (2016) 3:293–5. doi: 10.15698/mic2016.07.513
151. Wu M, Sun LV, Vamathevan J, Riegler M, Deboy R, Brownlie JC, et al. Phylogenomics of the reproductive parasite Wolbachia pipientis wMel: a streamlined genome overrun by mobile genetic elements. PLoS Biol (2004) 2:E69. doi: 10.1371/journal.pbio.0020069
152. Callaway E. Rio fights Zika with biggest release yet of bacteria-infected mosquitoes. Nature (2016) 539:17–8. doi: 10.1038/nature.2016.20878
153. Aliota MT, Peinado SA, Velez ID, Osorio JE. The wMel strain of Wolbachia Reduces Transmission of Zika virus by Aedes aEgypti. Sci Rep (2016) 6:28792. doi: 10.1038/srep28792
154. Dutra HL, Rocha MN, Dias FB, Mansur SB, Caragata EP, Moreira LA. Wolbachia blocks currently circulating zika virus isolates in Brazilian aedes aEgypti mosquitoes. Cell Host Microbe (2016) 19:771–4. doi: 10.1016/j.chom.2016.04.021
155. Monash Universit. World Mosquito Program. Available at: https://www.worldmosquitoprogram.org (Accessed 07/24).
Keywords: arboviruses, tropical regions, climate change, dengue virus, Zika virus, Chikungunya virus, strategies control, public health
Citation: Côrtes N, Lira A, Prates-Syed W, Dinis Silva J, Vuitika L, Cabral-Miranda W, Durães-Carvalho R, Balan A, Cabral-Marques O and Cabral-Miranda G (2023) Integrated control strategies for dengue, Zika, and Chikungunya virus infections. Front. Immunol. 14:1281667. doi: 10.3389/fimmu.2023.1281667
Received: 22 August 2023; Accepted: 24 November 2023;
Published: 18 December 2023.
Edited by:
P Bernard Fourie, University of Pretoria, South AfricaReviewed by:
Eva Gizella Rakasz, University of Wisconsin-Madison, United StatesCopyright © 2023 Côrtes, Lira, Prates-Syed, Dinis Silva, Vuitika, Cabral-Miranda, Durães-Carvalho, Balan, Cabral-Marques and Cabral-Miranda. This is an open-access article distributed under the terms of the Creative Commons Attribution License (CC BY). The use, distribution or reproduction in other forums is permitted, provided the original author(s) and the copyright owner(s) are credited and that the original publication in this journal is cited, in accordance with accepted academic practice. No use, distribution or reproduction is permitted which does not comply with these terms.
*Correspondence: Gustavo Cabral-Miranda, Z2NhYnJhbC5taXJhbmRhQHVzcC5icg==; Z2NhYnJhbC5taXJhbmRhQGdtYWlsLmNvbQ==
†These authors have contributed equally to this work and share first authorship
Disclaimer: All claims expressed in this article are solely those of the authors and do not necessarily represent those of their affiliated organizations, or those of the publisher, the editors and the reviewers. Any product that may be evaluated in this article or claim that may be made by its manufacturer is not guaranteed or endorsed by the publisher.
Research integrity at Frontiers
Learn more about the work of our research integrity team to safeguard the quality of each article we publish.