- 1Biomedical Sciences and Molecular Biology, College of Public Health, Medical and Veterinary Sciences, James Cook University, Douglas, QLD, Australia
- 2Australian Institute of Tropical Health and Medicine, James Cook University, Douglas, QLD, Australia
- 3Department of Microbiology and Immunology, Peter Doherty Institute for Infection and Immunity, University of Melbourne, Parkville, VIC, Australia
Tuberculosis (TB) is a leading cause of morbidity and mortality worldwide. Global research efforts to improve TB control are hindered by insufficient understanding of the role that antibodies play in protective immunity and pathogenesis. This impacts knowledge of rational and optimal vaccine design, appropriate diagnostic biomarkers, and development of therapeutics. Traditional approaches for the prevention and diagnosis of TB may be less efficacious in high prevalence, remote, and resource-poor settings. An improved understanding of the immune response to the causative agent of TB, Mycobacterium tuberculosis (Mtb), will be crucial for developing better vaccines, therapeutics, and diagnostics. While memory CD4+ T cells and cells and cytokine interferon gamma (IFN-g) have been the main identified correlates of protection in TB, mounting evidence suggests that other types of immunity may also have important roles. TB serology has identified antibodies and functional characteristics that may help diagnose Mtb infection and distinguish between different TB disease states. To date, no serological tests meet the World Health Organization (WHO) requirements for TB diagnosis, but multiplex assays show promise for improving the sensitivity and specificity of TB serodiagnosis. Monoclonal antibody (mAb) therapies and serum passive infusion studies in murine models of TB have also demonstrated some protective outcomes. However, animal models that better reflect the human immune response to Mtb are necessary to fully assess the clinical utility of antibody-based TB prophylactics and therapeutics. Candidate TB vaccines are not designed to elicit an Mtb-specific antibody response, but evidence suggests BCG and novel TB vaccines may induce protective Mtb antibodies. The potential of the humoral immune response in TB monitoring and control is being investigated and these studies provide important insight into the functional role of antibody-mediated immunity against TB. In this review, we describe the current state of development of antibody-based clinical tools for TB, with a focus on diagnostic, therapeutic, and vaccine-based applications.
1 Introduction
Tuberculosis (TB) remains a global health crisis, primarily affecting low- and middle-income countries (LMICs). With the rise of COVID-19 and the associated disruptions to healthcare services in LMICs, the TB crisis has only worsened (1). WHO estimates that 10.6 million people were infected with Mtb and 1.6 million died of TB in 2021 (2). Given the continued and significant global health burden of TB, the need for improved diagnosis, prevention, and treatment of Mtb infection is increasingly urgent, particularly in resource poor settings.
While a spectrum of TB presentations exists, it typically presents as a pulmonary disease (3). People with active pulmonary TB (PTB) produce airborne respiratory droplets through coughing and sneezing that contain Mtb bacteria. Inhalation of these infectious droplets can lead to Mtb infection and TB disease. Infectious droplets travel to the lower respiratory tract where Mtb encounters innate immune cells including alveolar macrophages (AMs), neutrophils, dendritic cells (DCs), and monocytes (4). The AMs phagocytose Mtb in the lower airways and alveolar spaces, allowing Mtb to preferentially infect and replicate within them. Uptake by AMs provides Mtb with an intracellular niche to grow and replicate that is largely protected from extracellular immune mediators (like antibody and complement) (4). However, active TB (ATB) is not always limited to the lungs and Mtb can disseminate to nearly any organ system including the lymph nodes, pleurae, gastrointestinal tract, skeleton, central nervous system, and the genitourinary tract (5, 6). This is termed extrapulmonary TB (EPTB), which accounts for ~15% of all Mtb infections (6). Although it can occur in immunocompetent individuals, there is a much higher incidence of EPTB in individuals with comorbidities like human immunodeficiency virus (HIV) infection (7). The pathogenesis of EPTB involves migration of Mtb into lymph nodes and eventually through the bloodstream to distal organs or tissues (8). As such, EPTB may present with no evidence of pulmonary Mtb infection (9).
The two primary outcomes that result from Mtb infection are early clearance of Mtb by the innate immune system, or latent persistence within lung granulomas (Figure 1). Innate immune clearance of Mtb occurs in a proportion of the human population, possibly due to a strong pro-inflammatory cytokine response and decreased recruitment of monocytes (21, 22). Individuals who cannot clear Mtb will go on to develop a latent TB infection (LTBI) (3). In LTBI, an adaptive immune response develops. The adaptive immune response to Mtb is characterised by T cell priming and the presence of Mtb-specific CD4+ T cells that secrete the macrophage-activating cytokine interferon gamma IFN-γ (Figure 1) (10). T cell priming occurs when infected DCs and macrophages present processed Mtb antigens to T cells in the lymph nodes (10). Both B and T lymphocytes, as well as innate immune cells, contribute to the formation of lung granulomas. These contain Mtb in a non-replicating latent phase for months to years (23). Development of ATB is estimated to occur in 5-15% of individuals infected with LTBI in the first few years (24).
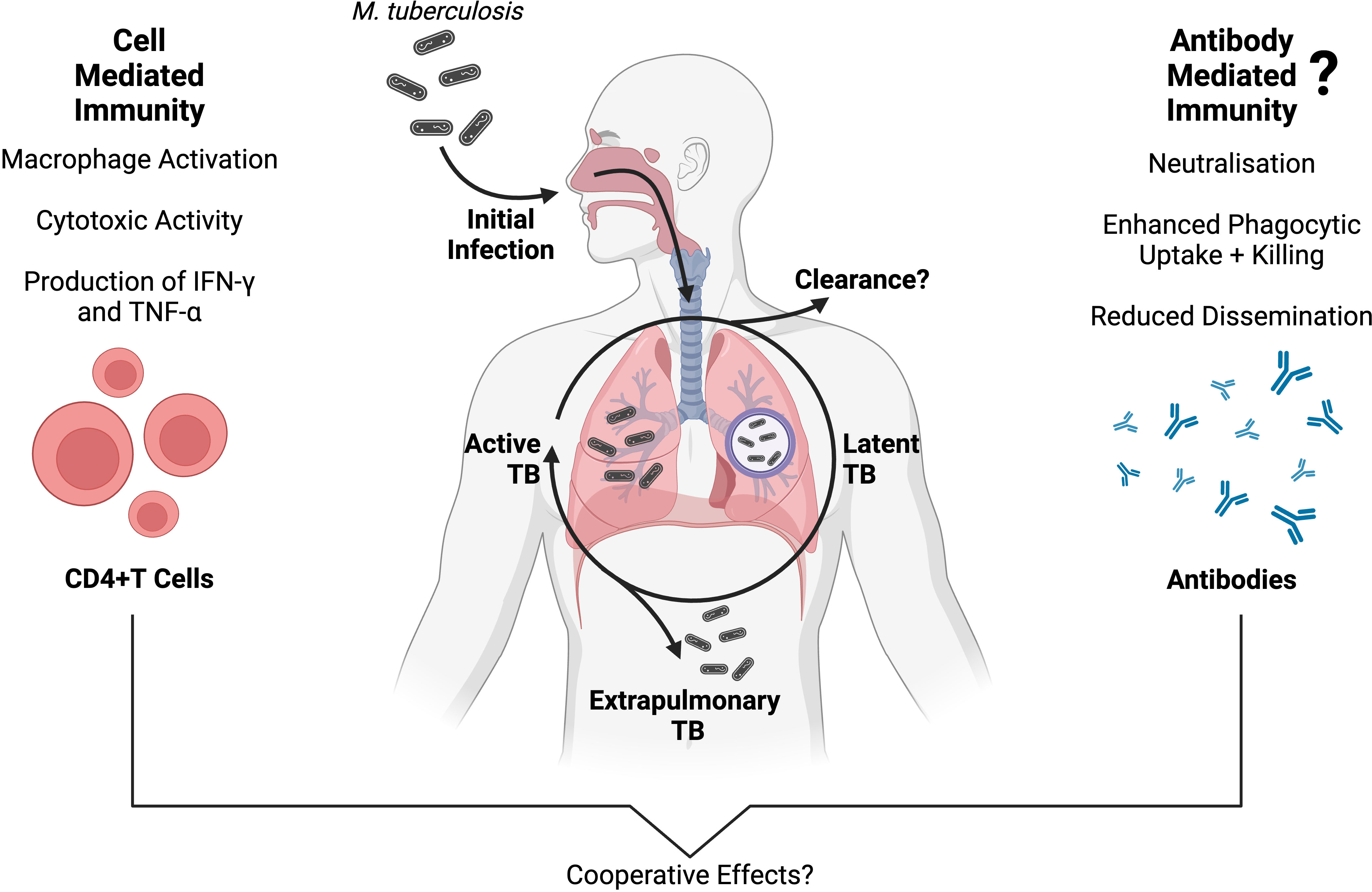
Figure 1 An overview of adaptive immunity to Mtb infection, and the progression of TB disease presentations. Protective cell-mediated immunity against Mtb is well established, and primarily involves CD4+ T cells producing inflammatory cytokines (such as IFN-γ and TNF-α) which activate the antimicrobial functions of Mtb-infected macrophages (10, 11). Conversely, antibody-mediated immunity has a less clear role in Mtb infection, although a growing body of evidence supports a protective association between Mtb-specific antibodies and TB disease outcomes (12–16). While the two branches of adaptive immunity have long been studied separately in TB, they are interconnected (17) and multiple independent studies suggest that cell- and antibody-mediated immunity are working cooperatively to protect against Mtb infection, inhibit Mtb growth and reduce disseminated TB disease (14, 18–20). Created with BioRender.com.
The interplay between Mtb and the host adaptive immune response is complex and may help determine the course of Mtb infection as well as the type of TB disease presentation (25, 26). It is becoming increasingly evident that a complete understanding of the immune response to Mtb requires the examination of both cell-mediated and humoral immunity (Figure 1) (17, 27, 28). In the last few decades, our understanding of TB immunity has advanced significantly, but the role of antibodies during Mtb infection is not yet fully characterised. Human infection with Mtb is known to induce a robust humoral immune response and the production of Mtb-specific antibodies against a variety of antigens (29). Overall, Mtb-specific antibody titres appear to increase with TB disease burden, likely because of increased antigen availability (12, 30–32). There is a growing interest in the antibody response to Mtb and there is increasing evidence that different TB disease states are associated with distinct antibody specificities and functions (12).
Antibody-mediated immunity (AMI) against Mtb occurs despite its largely intracellular lifecycle, which partially shields mycobacteria from humoral mediators. There are, however, multiple instances during its progression where Mtb and its antigens are extracellular, including during the initial infection, within the necrotic tissue of granulomas, and during the death of Mtb-infected cells (33, 34). Antibodies can also form immune complexes with soluble antigen or bind to infected cells and mediate effector functions, such as phagocytosis and targeted killing of infected cells, through engagement of Fc receptors on immune cells (Figure 2) (42).
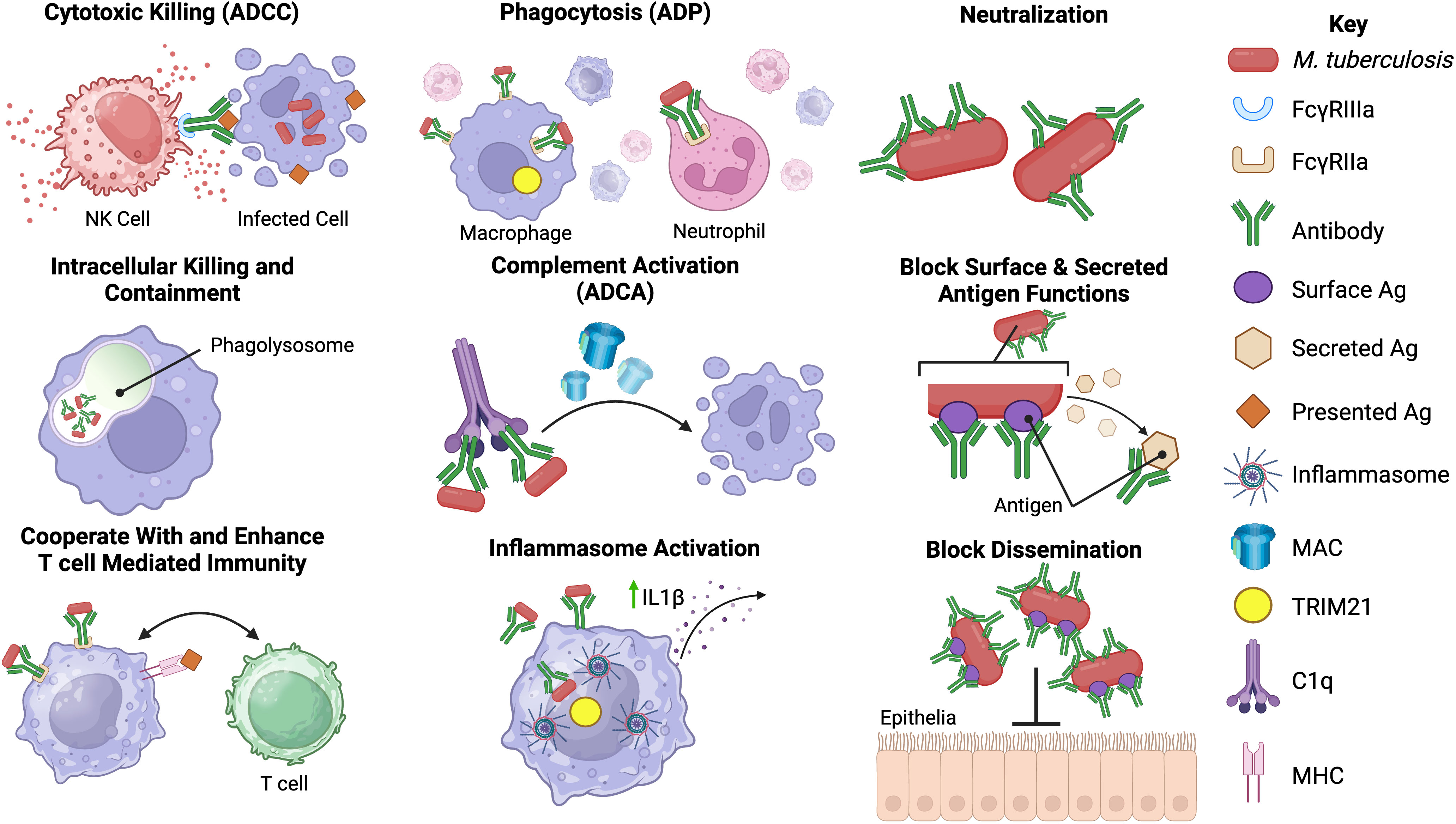
Figure 2 Potential functions of antibodies against Mtb. Antibodies may have an array of functions in the immune response against Mtb infection. The most well-known of these is neutralization of extracellular Mtb and secreted products via the antibody fragment antigen-binding (Fab) domain. Binding of antibody to Mtb may block entry of Mtb into host cells, aid in intracellular killing or control of replication, enhance phagocytosis by opsonizing Mtb, prevent the actions of secreted proteins and help prevent dissemination from the lungs (35–38). The fragment crystallizable (Fc) region of Mtb antibodies may also play a role in the immune response to Mtb. Fc-mediated antibody functions including antibody-dependent phagocytosis (ADP), antibody-dependent complement activation (ADCA), antibody-dependent cellular cytotoxicity (ADCC) and inflammasome activation may occur during Mtb infection (12, 13, 39, 40). Possible interactions with cell-mediated immunity, including enhancement of T-cell responses in the presence of specific antibodies, have been reported (14, 41). However, the precise mechanisms underlying some of the proposed Mtb antibody functions are not known or poorly defined in Mtb infection. Created with BioRender.com.
There is significant heterogeneity in the human antibody response to Mtb, and the proportion of Mtb-infected individuals that make antibodies against Mtb antigens varies widely between studies (13, 29, 43). As the antibody response to Mtb is so diverse, no consensus has been reached regarding the immunodominant antigens and target epitopes for humoral immunity. Consequently, studies measure responses to diverse panels of Mtb antigens and different isotypes/subclasses, making it challenging to compare results across studies (13). Associations between TB disease and antibody titre, isotype, or specificity are not well understood, but some progress has been made towards uncovering protective and pathological features of the antibody response to Mtb (44). Several immunodominant antigens targeted by Mtb antibodies have been identified including heat shock protein (HspX), lipoarabinomannan (LAM), arabinomannan (AM), heparin-binding hemagglutinin adhesin (HBHA), phosphate transporter subunit (PstS1), early secreted antigen (ESAT-6), and culture filtrate protein (CFP-10) (45, 46). These and many other target antigens play important roles in Mtb virulence, such as immunomodulation and adhesion to host cells (47–50). Mtb-specific antibodies could play a role in disrupting the functions of key virulence factors and may, therefore, influence TB disease progression.
Advancing our understanding of AMI during Mtb infection may provide new insights into the different TB disease states and may contribute to the development of clinical applications for Mtb-specific antibodies (Figure 3). This could include the discovery of novel antibody biomarkers to improve TB diagnosis or to aid in the identification individuals at risk of serious or progressive disease (51, 52). Antibodies with protective functions during Mtb infection could form the basis for new TB therapies and vaccines (53). Development of a more effective vaccine, shorter drug treatments, and more accessible diagnostics are all considered vital to the WHO End TB STRATEGY (52, 54). This review examines the clinical applications of Mtb antibodies including serodiagnostics, antibody-based therapeutics, and the humoral response to TB vaccines (Figure 3). Herein, we describe the state of development of serological tools to control and treat TB as well as the limitations that exist to their implementation, especially in TB endemic regions.
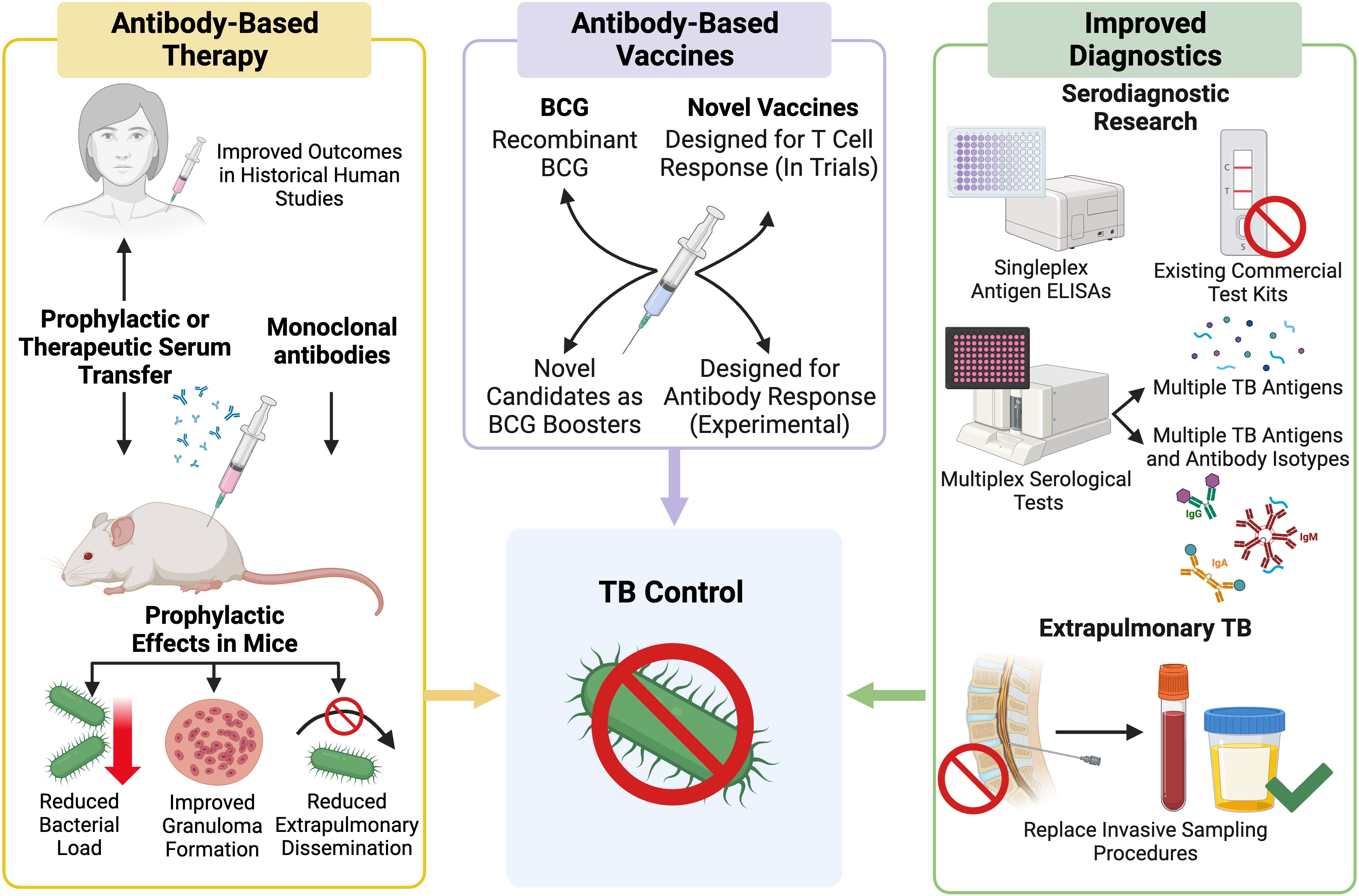
Figure 3 Outline of current research pathways and progress towards use of Mtb antibodies in a clinical setting. Created with BioRender.com.
2 Mtb antibodies as biomarkers in TB diagnosis
2.1 Existing TB diagnostics
Current methods for diagnosing TB in LMICs are limited in their sensitivity and cannot reliably distinguish different forms of TB (55). Given the high prevalence of TB, particularly LTBI and EPTB, in LMICs there is a need for rapid, sensitive, and accessible diagnostic tools. The ideal TB diagnostic test would be able to identify TB cases and differentiate between TB disease states (56, 57). Serological tests for Mtb may be advantageous due to their low cost and ease of use (58). Rapid serologic tests for COVID-19 have demonstrated the potential of antibody-based diagnostics for transmission control, although many COVID-19 tests were antigen based (59).
Reliance on acid fast bacilli staining (AFB) of sputum for TB diagnosis is common in LMICs due to relative affordability, rapid turnaround, and accessibility (58). Variable sensitivity (ranging from 20-80%) is a major drawback of AFB testing, which routinely misses Mtb-infected individuals with low or no lung bacillary load who may have subclinical PTB, LTBI, or EPTB (60, 61). The inability to reliably detect these Mtb-infected individuals contributes to higher TB transmission and mortality rates. Serological tests may circumvent these limitations by detecting Mtb-specific antibodies that correspond to different TB disease states. Alternative methods of diagnosing TB exist, including PCR-based Xpert MTB/RIF and bacterial culture. However, both PCR- and culture-based TB diagnostics have notable drawbacks (58). Culture-based diagnosis of Mtb infection is considered the gold-standard by the WHO, but bacterial culture has a long turnaround time and laboratory infrastructure requirements that limit its use in LMICs (62). The Xpert MTB/RIF assay is also WHO approved and used for fast molecular detection of Mtb. This PCR-based test has a similar specificity and sensitivity to Mtb culture, but its use in LMICs is limited by high cost and infrastructure requirements such as access to electricity (63–65).
The optimal biomarker test for TB would be simple, portable, rapid, and applicable to a broad range of Mtb-infected individuals, including those who are AFB negative (28). The WHO emphasises that new diagnostic tests for TB should have specificity and sensitivity similar to (or greater than) that of the Xpert MTB/RIF PCR assay for adults with ATB (both PTB and EPTB) and be able to distinguish between ATB, past TB and LTBI (28, 66). The only WHO-approved non-sputum-based point of care (POC) TB test measures Mtb LAM antigen in urine using a lateral-flow assay (LF-LAM). However, variable sensitivity (17.8-80.3%) and specificity (87.7-99%) have been reported for the LF-LAM assay (67–69), and it is only recommended for individuals with low CD4+ T cell counts (e.g. HIV infected) due to low sensitivity (< 20%) in immunocompetent individuals (70, 71).
2.2 Mtb antibodies as diagnostic biomarkers of ATB and LTBI
To date, the use of TB serodiagnostic tests has been contentious due to the poorer sensitivity and specificity of commercially available kits compared to conventional testing methods (72). As a result, the WHO has recommended against the use of current commercial serological tests for Mtb diagnosis (73–75). Despite this, the WHO has encouraged ongoing research efforts to develop new antibody-based TB diagnostics due to the advantages of serological testing formats in LMICs.
Current single antigen Mtb serodiagnostic tests have demonstrated low sensitivity and their development may be complicated by the heterogenous nature of the antibody response to human Mtb infection (46, 73, 76, 77). These diagnostic tests detect antibodies against various Mtb antigens including Mtb mammalian cell entry protein 1A (Mce1A), PstS1, proline-proline-glutamic acid protein 17 (PPE17), and A60 (Table 1). The detection of antibodies against multiple Mtb antigens and/or of multiple antibody isotypes may be more effective, and this approach may improve sensitivity (53). Multiple studies have aimed to identify which combinations of Mtb antibodies produce the optimal sensitivity and specificity for identifying TB across a broad range of Mtb-infected individuals. Serological assays would be particularly useful for TB groups that are more challenging to diagnose including smear (or MTB/RIF) negative patients with active PTB, individuals with LTBI, young children, and people with HIV co-infection. Various testing formats including ELISA, lateral flow and multiplex microbead assays have been utilised, although no study has directly compared different assay formats thus far.
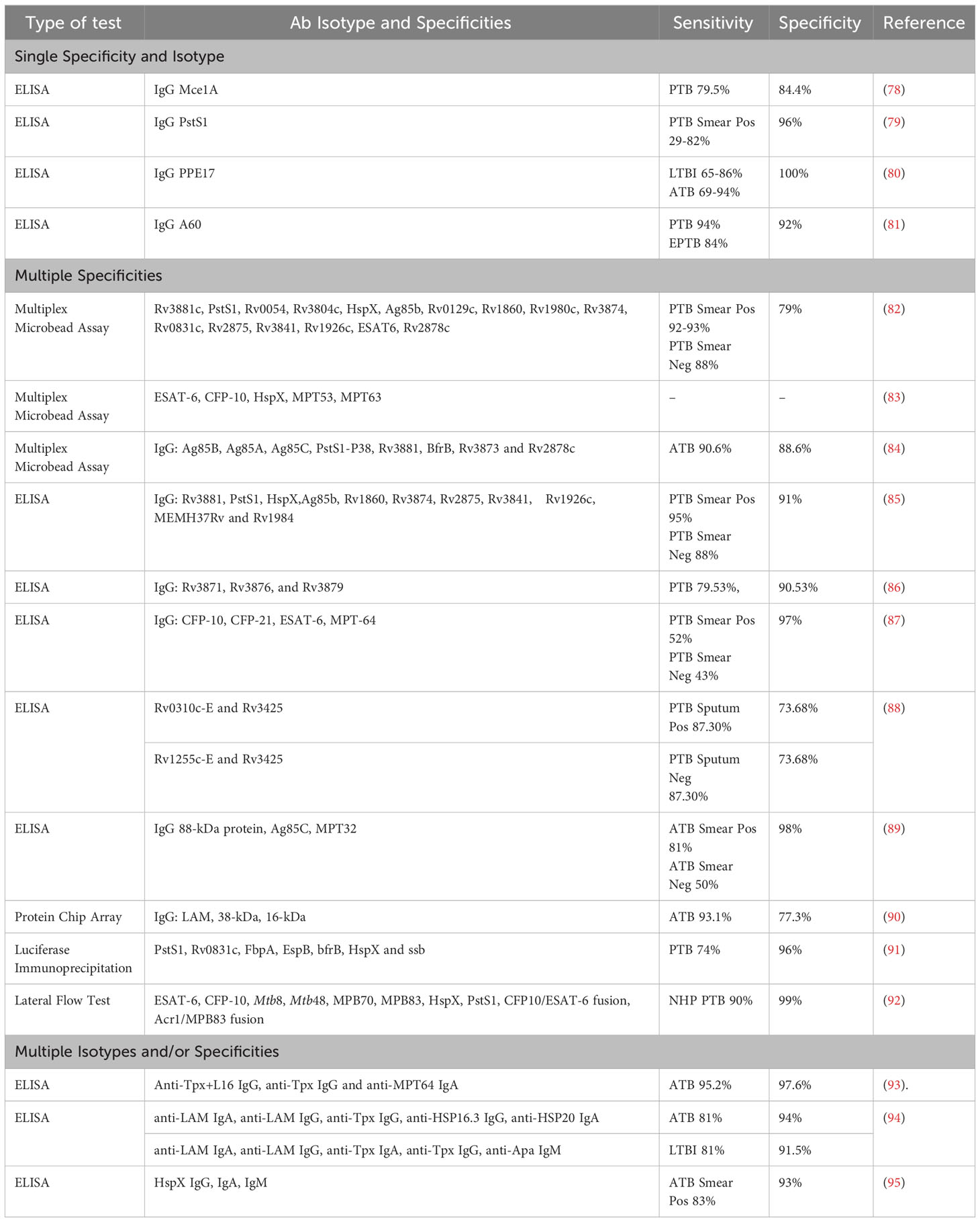
Table 1 Comparison of different serodiagnostic test formats and approaches for diagnosing Mtb from the last two decades.
A lateral-flow POC test that detects antibodies against multiple Mtb antigens, including ESAT-6, CFP-10, HspX, and PstS (Table 1), in Mtb-infected non-human primates (NHPs) showed high sensitivity and specificity (90% and 99%) (92). This POC test also demonstrated no cross reactivity with infections from non-tuberculous (NTB) mycobacterium species. Immunodominant Mtb antigens, such as ESAT-6, CFP-10, and HspX, have also been tested in a multiplex format to detect Mtb-specific antibodies in NHPs with both ATB and LTBI (83). Antibodies against ESAT-6 and CFP-10 were consistently detected in NHPs with LTBI, while NHPs with ATB had detectable antibodies against ESAT-6 and CFP-10 as well as HspX. These findings suggest that HspX antibodies may help differentiate ATB from LTBI in vivo. This antibody-based multiplex assay also showed no cross reactivity with Bacille Calmette-Guérin (BCG) in vaccinated NHPs, which is highly desirable in TB endemic LMICs. Antibodies targeting ESAT-6, CFP-10 and HspX appear to be detectable in NHP with ATB and LTBI, but it is unclear whether they could reliably distinguish between TB disease states in humans (83). Further testing of serological POC and multiplex assays in human cohorts is required to fully assess their diagnostic potential. Two multiplex assays that detect a combination of different Mtb-specific human immunoglobulin Gs (IgGs) (Table 1) had high sensitivity and specificity for diagnosing both smear-positive and negative ATB cases (88-95% sensitivity and 88.6-91% specificity) (84, 85). This indicates that these serological tests could be useful for diagnosing TB irrespective of lung bacillary load, circumventing the issue of negative smear results in individuals with TB. Other antibody-based TB tests have reported well-defined and consistent antibody profiles in people with ATB but had much poorer sensitivity and specificity relative to those described above (Table 1).
Multiplex assays using cytokines and other inflammatory biomarkers can also be used to diagnose ATB (96). However, these assays may have relatively low specificity as other respiratory diseases can induce similar inflammatory responses. Since antibody-based biomarker assays often lack sensitivity, an assay combining these two biomarker types could be highly effective. To date, multiple groups have developed TB diagnostic tests including both cytokines and antibodies in an attempt to raise test specificity and sensitivity (97). A microarray chip assay for diagnosis of ATB in serum samples found that a combined biomarker assay had similar or improved sensitivity and specificity compared to conventional TB tests (98). The criteria used for ATB diagnosis were positive expression of inflammatory markers (I-309, IL-8 and MIG) or the presence of IgG antibody specific for at least one of the selected Mtb antigens (Ag14-16kDa, Ag32kDa, Ag38kDa, and Ag85B). Individuals with ATB were compared to healthy controls and people with other respiratory diseases. Overall, this combined test demonstrated high sensitivity, specificity, and accuracy (91.03%, 92.59% and 91.82%) for diagnosing people with ATB (98). A marked increase in sensitivity and accuracy was observed when using a combination of biomarker types compared to using either biomarker alone. Conventional tests, including interferon-gamma release assay (IGRA) and TB culture, were also performed for this population, but the combined biomarker test was superior for ATB diagnosis (98). More recently, another combined biomarker assay demonstrated improved performance over single biomarker assays (97). Discriminant analysis was performed with antibody ELISA data and multiplex cytokine and inflammatory marker profiles in serum and plasma. Individuals with culture confirmed ATB or suspected TB were compared to a control population with other respiratory diseases. Two different combinations of biomarker profiles were found to have high sensitivity and specificity for detecting ATB. The most promising biomarker combination (including MTP64 IgA, Tpx IgA + NCAM-1, vitronectin, CFH, ferritin and A2M) had a sensitivity of 95% and a specificity of 88.5% for ATB diagnosis (97). Combined biomarker assays showed higher sensitivity and specificity than cytokine or antibody assays alone. The findings from these studies suggest that using multiple biomarker types may lead to the development of improved ATB diagnostic tests.
Control of LTBI is an important milestone in the WHO End TB Strategy as this population represents a significant global reservoir of TB (54). Some Mtb-specific antibodies have shown promise as biomarkers of LTBI, which can be difficult to diagnose in LMICs as chest X-ray and PCR-based diagnostics are often inaccessible (99). A recent study aimed to identify antibodies that could differentiate ATB from LTBI in a cohort of Mtb-infected people with or without HIV co-infection (100). This large-scale analysis examined 209 Mtb antigens, including well-characterised antigens like LAM and antigen 85 (Ag85) and novel antigens including Rv2435.C, Rv3583 and Rv1528. This study measured Mtb antibody levels and IgG affinity for a selection of different Fcγ receptors (FcγRIIAR, FcγRIIB, FcγRIIIAV, and FcγRIIIB). Antigen selection was based on predictions from in vivo and in vitro models that suggested enriched transcription in the hypoxic conditions of lung granulomas (101). While Mtb-specific antibody levels alone could not reliably discriminate between the TB disease states, Fcγ receptor binding profiles in combination with Mtb antibody levels could discriminate ATB from LTBI in the study cohort, irrespective of HIV status (100). The discriminatory parameters were enriched in people with ATB and included high levels of Rv2435.C IgG1 as well as increased binding of Rv3583, Rv1528 and LAM IgG1 to FcγRIIAR, FcγRIIB and FcγRIIIAV respectively. There may be an opportunity for future development of a rapid POC diagnostic test based on some of the identified parameters (100).
The robust IgG1 response mounted against the Mtb growth-associated ESAT-6 and latency-associated MDP1 antigens may represent potential biomarkers in individuals with recent LTBI (102). The Mtb antigens ESAT-6 and CFP-10 are already used in commercially available IGRA to measure the Mtb-specific memory T cell response. Associations between T- and B cell responses to ESAT-6 and CFP-10 have not been studied in TB endemic LMICs and this may provide further insight into the adaptive immune response to Mtb infection. Antibody against the PPE17 antigen may also be a useful marker for LTBI, as anti-PPE17 IgG titres are elevated in people with LTBI compared to Mtb unexposed individuals (80). Modulation of the host immune response by PPE17 leads to the release of the proinflammatory cytokine tumor necrosis factor-alpha (TNF-α), which is associated with granuloma formation in TB. The PPE17 antigen may, therefore, be involved in establishing and maintaining LTBI (103, 104). Interestingly, detection of anti-PPE17 antibody was able to identify individuals with LTBI who tested negative by QuantiFERON IGRA (QFT-IGRA) with a sensitivity of 65-86% and a specificity of 100% (80). Higher PPE17-specific antibody levels were detected in people with LTBI compared to the IGRA antigens (ESAT-6 and CFP-10), suggesting that anti-PPE17 antibody may be a useful adjunct marker for LTBI.
Furthermore, high levels of IgG against the Mce1A may also help differentiate LTBI from pulmonary ATB in both adults and children with a sensitivity of 79.5% and a specificity of 84.4% (78, 105). While this Mce1A IgG test did not meet WHO sensitivity cut-offs, it performed better than other serological TB tests in children (66, 106). Antibodies against the Mce1A antigen represent a promising biomarker that could be incorporated into improved serodiagnostic tests for TB to distinguish between disease states (28, 107). Serological assays that detect multiple antibody isotypes (IgG, IgA and IgM) against different Mtb antigens such as LAM, HspX, and MPT32 also show increased sensitivity and specificity for ATB and LTBI, with some tests producing values of ≥ 98% for both (Table 1) (93–95). These POC antibody tests are successfully used for other diseases like COVID-19 and may be helpful for improving TB diagnosis in LMICs (108).
A multiplex approach may be necessary for TB serodiagnosis, as tests must account for the variability of the antibody response both within and between individuals during the course of Mtb infection (29). Research in broader study populations is required to determine the clinical relevance of assays for diagnosing and distinguishing ATB from LTBI. The exact definitions of TB disease states are inconsistent and vary across studies, with different diagnostic tests and patient characteristics being used to define TB groups. A more standardised diagnostic definition of TB disease states is needed to produce comparable results between studies. Furthermore, a systematic approach, based on existing immunoproteomics data, should be used to guide the selection of Mtb antigens and antibody isotypes measured in future work.
3 Mtb antibody therapy and prophylaxis
3.1 Serum and IgG transfer prophylaxis for TB
Early studies suggest that TB prophylaxis, through passive infusion of Mtb antibodies prior to infection, may improve clinical outcome. Serum transfer trials from the late 1800s to early 1900s showed that patients with acute and localised TB had better outcomes following serum transfer than chronic cases (109). These positive outcomes included marked reduction in clinical symptoms and bacterial load. Antibody transfer studies for TB are ongoing in animal models, with a strong focus on prophylaxis. Chen et al. (2020) infused purified human anti-AM IgG from asymptomatic individuals with LTBI or PTB patients into mice one day before and one day after Mtb challenge (110). The LTBI IgG led to a greater reduction in lung colony forming units (CFU) than the PTB IgG in vivo (110). Intratracheal administration of total human IgG before Mtb challenge also reduced CFU in the lungs of treated mice compared to untreated controls (111). In this study, the observed protection was mediated by Mtb-specific antibodies as it was lost following absorption of Mtb-specific gamma globulin prior to inoculation (111). Polyclonal human IgG specific for mycobacterial surface proteins was protective in a murine TB model when administered five hours before lethal Mtb challenge (14). Additionally, IgG isolated from humans with LTBI and highly exposed but uninfected individuals significantly reduced lung CFU compared to IgG purified from humans with ATB. These results suggest that highly Mtb-exposed humans may generate surface-directed Mtb antibodies capable of preventing ATB and possibly LTBI, and that a lack of Mtb-specific IgG may contribute to ATB development. Identification of the key protective antigens and antibodies will be critical to unlocking the therapeutic potential of anti-Mtb IgG.
Antibody-based therapies and prophylactics are important avenues for further inquiry. While complete prevention of Mtb infection was not observed, these studies show that antibody-based prophylactics have the potential to prevent severe TB disease. This may be especially relevant for individuals who are at high risk of developing severe TB, including people living with HIV. No prophylactic antibody studies have focused on treating TB in HIV coinfection, likely due to the complexity and limitations of modelling HIV in mice (112). Current Mtb prophylaxis and vaccination regimens are not optimal for individuals with HIV coinfection due to compromised immune function and drug interactions (113). Several frontline TB antibiotics (including rifamycins) are contraindicated with common antiretroviral therapies due to reduced drug efficacy, increased toxicity, and TB immune reconstitution inflammatory syndrome (114). In LMICs, where access to specific HIV/TB drug combinations is limited, alternative treatment options such as antibody-based prophylactics or therapeutics may help remove this barrier to effective management of HIV and TB coinfection. However, the utility of antibody-based therapies in settings with poor access to healthcare may be limited, as passive infusion requires trained healthcare workers and specialist equipment that may not be present in remote, resource poor areas. There are, however, large populations with access to clinics who may benefit from antibody-based prophylaxis to help limit TB spread and reduce mortality.
3.2 Mtb-specific monoclonal antibodies
A variety of monoclonal antibodies (mAbs) against major Mtb virulence factors, including PstS1, HBHA, HspX, LAM, MPB83 and AM, are protective in murine models of TB (15, 35, 115–120). These Mtb-specific mAbs are associated with a range of outcomes in vivo including altered granulomatous pathology of the lungs, decreased dissemination of Mtb to the spleen and inhibition of intracellular bacterial growth (15, 35, 115–120).
Differential glycosylation of the antibody fragment crystallizable (Fc) domain affects structure and flexibility, altering Fc receptor binding and effector functions (121, 122). Early work showed that an aglycosylated mAb could not interact with FcγRs on cells, activate complement, induce antibody-dependent cellular cytotoxicity (ADCC) or efficiently clear immune complexes (123, 124). The Fc domain of IgG contains two N-glycosylation sites at asparagine 297 (N297), one on each heavy chain. The core Fc glycan on IgG is a biantennary heptasaccaride to which fucose, sialic acid and galactose can be added (125). Afucosylated IgG has a higher affinity for FcγRIIIA and enhances ADCC activity (126–128). The addition of sialic acid to the IgG Fc domain has been shown to have anti-inflammatory effects (129). Studies have reported reduced affinity for FcγRIIIA and lower ADCC with sialylated IgG (130, 131), whereas others showed that sialylation did not influence FcγR interactions (132–134). The role of galactose on FcγR binding and downstream functions is also controversial (135–139). Chung et al. demonstrated that increased galactosylation and total sialic acid induced robust antibody-dependent phagocytosis (ADP) (140). Anti-Mtb IgG from individuals with LTBI showed increased galatosylation and macrophage activation compared to those with ATB (12). Comparatively, the field of IgA glycosylation is in its early stages (141). Both IgA1 and IgA2 have multiple N-glycosylation sites and IgA1 has nine potential O-glycosylation sites in the hinge region (141). The IgA N-glycan can affect thermal stability, but may not impact binding to FcαRI (142). Lower sialylation of IgA2, but not IgA1, induced pro-inflammatory functions such as neutrophil activation and cytokine production when IgA was aggregated or immobilized to form immune complexes (143). The glycosylation of Mtb-specific mAbs can impact functionality and different glycoforms should be investigated in pre-clinical models to identify opportunities to improve mAb efficacy.
Memory B cells isolated from humans with ATB have been used to generate protective mAbs. In one study 85 mAbs from a single patient were analysed for activity against both Mtb and BCG (144). Of these, two mAbs (p4-36 and p4-163) targeting the PstS1 phosphate transporter protein displayed modest anti-Mtb activity. The PstS1 protein is an immunodominant virulence factor in Mtb, and both mAbs targeted different epitopes on this antigen (145, 146). In an ex vivo whole blood assay, only p4-36 or p4-163 mAbs could significantly inhibit the ability of Mtb and BCG to grow inside human blood cells as measured by a reduction in CFU (144). In THP-1 cells, these mAbs led to opsonisation and phagocytosis of Mtb in an Fc-dependent manner, as an aglycosylated Fc variant of the IgG1 mAbs did not exhibit the same activity as wild type IgG1. Furthermore, blocking of macrophage FcγRs (FcγRIIIA, FcγRIIA, and FcγRIIB in combination) abrogated the observed Mtb growth inhibition by p4-36 and p4-163. Depletion of T cells and MHC class II had no effect on mAb activity, suggesting that these mAbs act in a T cell independent manner unlike some previously described anti-Mtb mAbs (14, 144). Enhanced uptake of Mtb by macrophages (via mAb-FcγR interactions) was observed in these cell-based assays, but the mAbs did not increase bacterial load and appeared to restrict Mtb growth inside the macrophage through an undefined mechanism (144). Prophylactic administration of the p4-36 or p4-163 mAbs prior to aerosol infection with Mtb resulted in a 0.5 log reduction in lung bacterial CFU compared to untreated mice. The discovery of protective antibodies against an immunodominant virulence factor like PstS1 represents a significant step forward and could inform development of therapeutics as well as novel vaccines. Further characterisation of the mechanisms that underpin the in vivo efficacy of anti-PstS1 mAbs may provide key evidence for a protective role of antibodies during Mtb infection.
Two HspX-specific IgA mAbs (TBA61 and 2E9) were tested for prophylactic activity in mice, via either intranasal or intratracheal delivery (115, 147). The 2E9 human IgA1 mAb was given with IFN-γ, to FcαRI transgenic mice and the TBA61 murine IgA mAb was administered to non-transgenic mice (115). Both mAbs significantly reduced lung CFU and pathology in mice challenged with a lethal or sublethal dose of Mtb (115, 118, 120). Protection by TBA61 was at least partially isotype-specific, as an IgG1 mAb with the same specificity was less effective at reducing lung CFU (147). Since mice do not have a known homolog of human FcαRI, the observed TBA61-mediated protection was Fc-independent (42, 148). The HspX-specific IgA mAbs were not capable of clearing Mtb in the non-transgenic mouse model, but mice differ from humans in both Fc receptor expression and cellular distribution. Potential functions of the HspX-specific mAbs include fragment antigen-binding (Fab)-mediated neutralization, which may prevent critical HspX functions during Mtb infection such as intracellular survival and resilience to stressors (149–151). Murine models may not accurately reflect the Fc-mediated effector functions of human mAbs, limiting the translation of these results into therapeutic applications (30, 152). In addition to lacking a homolog of human FcαRI, the murine ortholog of human FcγRIIIa, FcγRIV, is not expressed on NK cells (42, 115, 148). These differences have important implications when using mice to model human IgA and IgG Fc-mediated effector functions like NK cell activation and antibody dependent cellular cytotoxicity (ADCC) (153). The use of Fc receptor transgenic or humanised mice for passive infusion studies represents a more suitable model system for future Mtb-specific mAb studies (115, 154). Further, most studies use the laboratory type-strain of Mtb (H37Rv) for in vivo challenges (17), but H37Rv does not elicit the same immune response and pathology as clinical Mtb isolates (155, 156). Improved animal models of TB that better represent human infection and humoral responses are necessary to advance the translation of mAb therapies.
A combined immunotherapy (CIT) strategy with IFN-γ or anti-IL-4 further enhanced TBA61 and 2E9 IgA protection in mice (157, 158). Use of CIT may also prevent post-chemotherapy Mtb infection relapse, and 2E9 IFN-γ CIT is effective against multidrug resistant-TB (MDR-TB) (115, 157, 159). These outcomes suggest that some mAbs could form part of an adjunct treatment regimen for MDR-TB, which is becoming increasingly challenging to treat. Effective and timely treatment of MDR-TB is vital for limiting spread in vulnerable communities. Prophylactic use of Mtb-specific mAbs alongside antibiotic treatment may help to discourage the spread of MDR-TB-causing bacteria by eliminating the resistant Mtb bacilli before they can cause post-treatment relapse (159, 160). While promising in animal models, these studies show Mtb-specific mAbs are clearly most effective when administered before or early after Mtb infection (15, 115, 147, 158). A study analysing temporal factors that affect mAb treatment found that prophylactic administration of the TBA61 mAb was required for protection in mice (158). Therefore, Mtb-specific mAbs may not have broad clinical applications as diagnosis of Mtb is difficult and slow in LMICs where MDR-TB is most widespread (161). Access to prophylactic mAbs is also limited in these areas due to their high cost, and the need for healthcare infrastructure to deliver infusions. Targeted administration to high-risk groups such as immunocompromised and treatment resistant individuals may provide the most benefit. Regimens including Mtb-specific IgA mAbs and CIT may be useful for improving TB outcomes in humans but require further examination in transgenic mice and NHPs that better model human immunity.
Anti-LAM and anti-AM mAbs have also been tested in murine models of TB (13, 117, 119). Pre-incubation of Mtb with an AM-specific IgG3 mAb (9d8) prolonged survival in healthy and immunocompromised mice infected intratracheally with a lethal dose of Mtb (119). Mice infected with Mtb that was pre-incubated with the 9d8 mAb had enhanced granuloma formation, which constitutes a protective effect, but there was no observed reduction in CFU in the murine lung, spleen, or liver. Prophylactic administration of 9d8 at 48 hrs, 24 hrs, and 4 hrs prior to Mtb challenge also did not impact survival (119). However, granuloma formation and Mtb burden outside of the lungs were not examined in the mice infused directly with 9d8 mAb, so evidence of prophylactic effects may have been missed. A LAM-specific IgG1 mAb (SMITB14) has also shown some efficacy in a murine TB model (117). In this study, the mice were either intravenously inoculated with SMITB14 mAb one hour prior to Mtb infection or given a SMITB14 mAb-Mtb mixture. The mice infused with SMITB14 mAb before Mtb infection had a significant reduction in bacterial CFUs in the lungs, livers and spleens and had lower CFUs compared to mice that received the SMITB14-Mtb mixture (117). The Fab domain of the SMITB14 mAb administered alone was prophylactic, suggesting that neutralization may be a primary mechanism of SMITB14 protection. An in vitro study also found human anti-LAM IgA mAbs decreased Mtb infection of human lung epithelial (HLE) and monocytic THP-1 cell lines (13). The observed reduction in Mtb infection was likely Fc-independent as IgA FcαR is not expressed on HLE cells and the use of FcαR-expressing THP-1 cells did not further decrease Mtb infection (13).
The testing of Mtb-specific mAbs in murine models, particularly in CIT, indicates that this modality may be a valuable tool to investigate mechanisms of humoral immunity in human TB. These Mtb-specific mAbs have the potential to treat patients with compromised cellular immunity, shorten treatment regimens, reduce relapse after chemotherapy and provide an alternative for drug-resistant TB cases (115, 162). The use of improved animal models of TB immunity and more clinically relevant Mtb strains for in vivo testing will help advance Mtb-specific mAbs as future prophylactic and therapeutic agents.
4 TB antibodies and vaccines
4.1 Antibody response to Bacille Calmette-Guérin
To date, BCG is the only approved vaccine for TB, but it has demonstrated limited protection against PTB and reactivation of LTBI in adults (163). Vaccination with BCG is able to prevent severe TB disease and Mtb dissemination in children, and in TB endemic areas BCG can halve mortality in the first 6-12 months of life (164). However, the lack of a protective vaccine against Mtb in the wider human population represents a significant hurdle to the control of TB globally (30). The BCG vaccine and the majority of candidate TB vaccines focus on enhancing cell-mediated immunity (CMI) against Mtb. Although novel TB vaccines are in development, some groups have instead focused on enhancing the protection provided by BCG. Various strategies to improve the efficacy of BCG have been investigated such as the addition of boosters that complement BCG, altering the route of BCG delivery and the creation of recombinant BCG vaccines (165).
In addition to increasing Mtb-specific CMI, the BCG vaccine also induces mycobacterium (Mb)-specific antibody responses similar to those elicited by Mtb infection (18). Standard intradermal BCG vaccination generates a strong Mb-specific IgG and IgM response in humans, including anti-LAM and anti-AM antibodies (18, 166–169). The subclass distribution of Mtb-specific IgG produced following BCG vaccination is skewed towards IgG1-3 (169). The IgG induced by BCG vaccination has been shown to increase phagocytic uptake, enhance phagolysosomal fusion, inhibit Mtb growth and increase Mb-specific CD4+ and CD8+ T cell proliferation and IFN-γ production in vitro (18, 168). Alternative routes of BCG administration have also been explored. In humans, mucosal administration of BCG significantly increased anti-LAM IgA in serum and nasal washes (18, 167). Mucosal BCG vaccination in rhesus macaques also boosted anti-purified protein derivative (anti-PPD) IgA in bronchoalveolar lavage (BAL) and serum, but the reduction in lung CFU and pathology after Mtb challenge did not correlate with IgA levels (170).
The antibodies elicited by BCG vaccination may be associated with TB protection in children but the lack of efficacy in adults suggests that BCG-induced antibodies do not provide sustained protection (36, 171, 172). Some trials aim to enhance BCG-mediated protection by adding novel subunit vaccines as boosters (like H4:IC31 and H56:IC31), although some of these subunit formulations are also being trialled as standalone vaccines (173–176). The H4:IC31 subunit vaccine consists of a fusion protein of two mycobacterial antigens Ag85B and TB10.4 (H4), while H56:IC31 is composed of a fusion protein of Ag85B, ESAT-6, and Rv2660c (H56). These novel subunit vaccines induced anti-H4 or -H56 antibody responses in humans, dominated by the IgG1 and IgG3 subclasses (173, 177). In NHP models, the inclusion of a H56:IC31 booster (post-vaccination with BCG) improved containment of Mtb infection, reduced lung pathology, and decreased Mtb dissemination from the lungs (176).
The efficacy of these TB booster vaccines in humans is not yet known, but they have been tested for safety and immunogenicity in phase I clinical trials (173, 175, 177). Future trials of TB subunit vaccines should include detailed serological analyses of Mtb-specific antibodies produced and their functions, as this may provide insight into the mechanisms of protection that underpin AMI to TB. Further trials in animal models may also be necessary to thoroughly assess the specific role of anti-H4 and -H56 antibodies on lung pathology, survival, and bacterial load. Administration of BCG with booster vaccines has the potential to provide greater and more sustained protection than BCG alone, and this may be attributed to the induction of both AMI and CMI.
4.2 Anti-Mtb antibody response to novel vaccines
There are a large number of TB vaccines in pre-clinical development and early clinical trials (178). The majority of TB vaccine studies focus on CMI, and none of the candidate TB vaccines that have progressed to clinical trials are specifically designed to elicit Mtb antibodies (53). However, numerous studies have shown an association between vaccine-induced Mtb antibodies and protection from severe TB (53). These findings suggest that some candidate TB vaccines may stimulate AMI capable of reducing TB disease severity in cooperation with cellular immunity. Most novel TB vaccines appear to induce detectable Mtb-specific antibodies (179). The antibody response to new TB vaccines is often reported in clinical trials, but analysis of Mtb antibodies is often limited to correlations with T helper cell type (Th1 vs Th2) and cytokine responses (180, 181). Few TB vaccine studies investigate the protective or pathological roles of Mtb antibodies in vitro or in animal models (16).
A phase IIb clinical trial for the viral vectored modified Vaccinia Ankara virus 85A (MVA85A) vaccine in BCG-vaccinated infants (aged 4-6 months) induced anti-Mtb antibodies that were associated with TB protection (182). While the original trial did not measure the antibody response, a retrospective case-controlled review found that increased Ag85A-specific IgG was associated with a decreased risk of TB disease (183). Interestingly, the MVA85A vaccine was designed to elicit a strong CD4+ T cell response but failed to be more efficacious than the current BCG vaccine. As such, there is a growing interest in developing TB vaccines that stimulate the humoral and cellular arms of adaptive immunity. A novel adenoviral vectored vaccine ChAdOx1 85A enhanced MVA85A-mediated protection and induced Ag85A-specific IgG in BCG-vaccinated adults (184, 185). The Mtb Ag85 complex is also the target of several DNA vaccines, which induced high levels of IgG1 and IgG2 against Ag85A or Ag85B (186–189). Recent advances in the development of viral-vectored vaccines for COVID-19 could be applicable to TB, alone or as a booster for BCG (190). A MVA vector expressing the HspX antigen was tested as a booster for BCG, and significantly reduced bacillary load in guinea pig models (191). Clinical trials for several viral vectored TB vaccines are ongoing, and the results from the MVA85A trial should encourage more detailed serological analyses to investigate whether humoral immunity is influencing vaccine efficacy (190).
The anti-LAM and -AM IgG induced by three separate TB subunit vaccines may confer some protection against Mtb infection (16, 36, 192, 193). One vaccine consisted of AM conjugated to Ag85b (AM-Ag85b) or B. anthracis protective antigen (AM-PA) (16). Mice immunised with AM-Ag85b had longer survival time, reduced lung pathology, and reduced CFU in the lung and spleen following lethal Mtb challenge compared to BCG (16). Survival time was increased by anti-AM antibodies as mice vaccinated with AM-Ag85b had longer survival times than mice that received Ag85b alone. However, both anti-AM and anti-Ag85b antibodies were required to achieve the full protective effect since AM-Ag85b vaccinated mice showed a greater reduction in lung CFU compared to AM-PA or Ag85b vaccinated groups. The protective effects of anti-Ag85 antibodies in AM-Ag85b vaccinated mice supports the observed association between high anti-Ag85A antibodies and a decreased risk of TB disease in MVA85A vaccinated children (16, 183). Induction of antibodies targeting the Mtb Ag85 complex appears to be a rational avenue for the development of protective TB vaccines. Future trials for the MVA85A vaccine should include assays to quantify anti-Ag85 antibodies as they represent a potential immune correlate of protection.
The live attenuated Mtb vaccine candidate MTBVAC is designed for newborns (194). This vaccine is composed of an Mtb isolate (Mt103) attenuated by the deletion of the phoP and fadD26 genes that encode important virulence factors (195). Mucosal vaccination with MTBVAC in rhesus macaques induced PPD-specific IgA, IgG, and IgM antibodies (196). The BAL IgG and IgM antibodies produced by mucosal MTBVAC vaccination showed increased binding to Mtb compared to BAL antibodies from subcutaneous vaccination (196). The BAL antibodies from mucosal vaccination with MTBVAC also increased ADP of Mtb by THP-1 cells in vitro (196). The specificity of these antibodies is currently unknown and should be elucidated to allow more focused analysis of their role, if any, in MTBVAC protection. Additional testing of MTBVAC-induced antibodies for Fc-mediated effector functions, such as NK cell activation, may further advance our understanding of their potential mechanisms of protection.
5 Conclusions
The WHO End TB Strategy outlines key pillars that are required to end TB by 2030, and one of these pillars is the development of new tools to diagnose, treat and manage TB (2, 54). The antibody response to Mtb infection and vaccination represents a potential avenue for developing these TB management strategies. While the functions of TB antibodies are not fully understood, it is clear that Mtb-specific antibodies have a role in the course of Mtb infection and disease. There is, however, a lack of available data to establish protective associations between the Mtb antibody profile and TB disease outcomes. Antibodies against Mtb may have useful applications in the diagnosis, treatment, and control of TB. Serology-based multiplex assays for TB have shown high sensitivity and specificity and could distinguish between different TB disease presentations. Identification of optimal Mtb antibody specificities and isotypes for serodiagnosis of TB is ongoing. Studies in animal models suggest that antibody-based prophylaxis may be useful for preventing severe TB in at-risk populations such as people living with HIV. New candidate TB vaccines can induce anti-Mtb antibodies that may work in conjunction with CMI to improve protection from Mtb infection in broader populations. Antibodies show some promise for the management and prevention of TB, but a greater understanding of TB antibodies is needed to progress antibody-based diagnostics and therapeutics beyond the research arena and into the clinic.
Author contributions
SM: Conceptualization, Writing – original draft. CR: Conceptualization, Supervision, Writing – review & editing. JW: Conceptualization, Writing – review & editing. HV: Supervision, Conceptualization, Funding acquisition, Writing – review & editing.
Funding
The author(s) declare financial support was received for the research, authorship, and/or publication of this article. The publication of this review was supported in part by a James Cook University Higher Degree by Research Enhancement Scheme grant.
Acknowledgments
All contributing authors and funding bodies are previously acknowledged.
Conflict of interest
The authors declare that the research was conducted in the absence of any commercial or financial relationships that could be construed as a potential conflict of interest.
Publisher’s note
All claims expressed in this article are solely those of the authors and do not necessarily represent those of their affiliated organizations, or those of the publisher, the editors and the reviewers. Any product that may be evaluated in this article, or claim that may be made by its manufacturer, is not guaranteed or endorsed by the publisher.
References
1. Sanduzzi Zamparelli S, Mormile M, Sanduzzi Zamparelli A, Guarino A, Parrella R, Bocchino M. Clinical impact of COVID-19 on tuberculosis. Infez Med (2022) 30(4):495–500. doi: 10.53854/liim-3004-3
2. World Health Organization. Global tuberculosis report 2022. 2 ed Vol. 2022. . Geneva: World Health Organization (2022).
3. Pai M, Behr MA, Dowdy D, Dheda K, Divangahi M, Boehme CC, et al. Tuberculosis. Nat Rev Dis Primers (2016) 2(1):16076. doi: 10.1038/nrdp.2016.76
4. Middleton AM, Chadwick MV, Nicholson AG, Dewar A, Groger RK, Brown EJ, et al. Interaction of mycobacterium tuberculosis with human respiratory mucosa. Tuberculosis (Edinb) (2002) 82(2):69–78. doi: 10.1054/tube.2002.0324
5. Gopalaswamy R, Dusthackeer VNA, Kannayan S, Subbian S. Extrapulmonary tuberculosis—an update on the diagnosis, treatment and drug resistance. J Respir (2021) 1(2):141–64. doi: 10.3390/jor1020015
6. Rodriguez-Takeuchi SY, Renjifo ME, Medina FJ. Extrapulmonary tuberculosis: pathophysiology and imaging findings. Radiographics (2019) 39(7):2023–37. doi: 10.1148/rg.2019190109
7. McLean MR, Lu LL, Kent SJ, Chung AW. An inflammatory story: antibodies in tuberculosis comorbidities. Front Immunol (2019) 10:2846. doi: 10.3389/fimmu.2019.02846
8. Baykan AH, Sayiner HS, Aydin E, Koc M, Inan I, Erturk SM. Extrapulmonary tuberculosıs: an old but resurgent problem. Insights Imaging (2022) 13(1):39. doi: 10.1186/s13244-022-01172-0
9. Purohit M, Mustafa T. Laboratory diagnosis of extra-pulmonary tuberculosis (Eptb) in resource-constrained setting: state of the art, challenges and the need. J Clin Diagn Res (2015) 9(4):Ee01–6. doi: 10.7860/jcdr/2015/12422.5792
10. Cooper AM. Cell-mediated immune responses in tuberculosis. Annu Rev Immunol (2009) 27(1):393–422. doi: 10.1146/annurev.immunol.021908.132703
11. Harari A, Rozot V, Enders FB, Perreau M, Stalder JM, Nicod LP, et al. Dominant tnf-A+ Mycobacterium tuberculosis–specific cd4+ T cell responses discriminate between latent infection and active disease. Nat Med (2011) 17(3):372–6. doi: 10.1038/nm.2299
12. Lu LL, Chung AW, Rosebrock TR, Ghebremichael M, Yu WH, Grace PS, et al. A functional role for antibodies in tuberculosis. Cell (2016) 167(2):433–43.e14. doi: 10.1016/j.cell.2016.08.072
13. Zimmermann N, Thormann V, Hu B, Köhler A-B, Imai-Matsushima A, Locht C, et al. Human isotype-dependent inhibitory antibody responses against mycobacterium tuberculosis. EMBO Mol Med (2016) 8(11):1325–39. doi: 10.15252/emmm.201606330
14. Li H, Wang X-x, Wang B, Fu L, Liu G, Lu Y, et al. Latently and uninfected healthcare workers exposed to tb make protective antibodies against mycobacterium tuberculosis. Proc Natl Acad Sci USA (2017) 114(19):5023–8. doi: 10.1073/pnas.1611776114
15. Buccheri S, Reljic R, Caccamo N, Meraviglia S, Ivanyi J, Salerno A, et al. Prevention of the post-chemotherapy relapse of tuberculous infection by combined immunotherapy. Tuberculosis (Edinb) (2009) 89(1):91–4. doi: 10.1016/j.tube.2008.09.001
16. Prados-Rosales R, Carreño L, Cheng T, Blanc C, Weinrick B, Malek A, et al. Enhanced control of mycobacterium tuberculosis extrapulmonary dissemination in mice by an arabinomannan-protein conjugate vaccine. PloS Pathog (2017) 13(3):e1006250. doi: 10.1371/journal.ppat.1006250
17. Li H, Javid B. Antibodies and tuberculosis: finally coming of age? Nat Rev Immunol (2018) 18(9):591–6. doi: 10.1038/s41577-018-0028-0
18. de Vallière S, Abate G, Blazevic A, Heuertz RM, Hoft DF. Enhancement of innate and cell-mediated immunity by antimycobacterial antibodies. Infect Immun (2005) 73(10):6711–20. doi: 10.1128/iai.73.10.6711-6720.2005
19. Masungi C, Temmerman S, Van Vooren JP, Drowart A, Pethe K, Menozzi FD, et al. Differential T and B cell responses against mycobacterium tuberculosis heparin-binding hemagglutinin adhesin in infected healthy individuals and patients with tuberculosis. J Infect Dis (2002) 185(4):513–20. doi: 10.1086/338833
20. Zanetti S, Bua A, Delogu G, Pusceddu C, Mura M, Saba F, et al. Patients with pulmonary tuberculosis develop a strong humoral response against methylated heparin-binding hemagglutinin. Clin Diagn Lab Immunol (2005) 12(9):1135–8. doi: 10.1128/cdli.12.9.1135-1138.2005
21. Verrall AJ, Schneider M, Alisjahbana B, Apriani L, van Laarhoven A, Koeken VACM, et al. Early clearance of mycobacterium tuberculosis is associated with increased innate immune responses. J Infect Dis (2019) 221(8):1342–50. doi: 10.1093/infdis/jiz147
22. Ringshausen FC, Nienhaus A, Schablon A, Schlösser S, Schultze-Werninghaus G, Rohde G. Predictors of persistently positive mycobacterium-tuberculosis-specific interferon-gamma responses in the serial testing of health care workers. BMC Infect Dis (2010) 10(1):220. doi: 10.1186/1471-2334-10-220
23. Russell DG. Who puts the tubercle in tuberculosis? Nat Rev Microbiol (2007) 5(1):39–47. doi: 10.1038/nrmicro1538
24. Vynnycky E, Fine PE. The natural history of tuberculosis: the implications of age-dependent risks of disease and the role of reinfection. Epidemiol Infect (1997) 119(2):183–201. doi: 10.1017/s0950268897007917
25. de Martino M, Lodi L, Galli L, Chiappini E. Immune response to mycobacterium tuberculosis: A narrative review. Front Pediatr (2019) 7. doi: 10.3389/fped.2019.00350
26. Liu CH, Liu H, Ge B. Innate immunity in tuberculosis: host defense vs pathogen evasion. Cell Mol Immunol (2017) 14(12):963–75. doi: 10.1038/cmi.2017.88
27. Kozakiewicz L, Phuah JY, Flynn JL, Chan J. The role of B cells and humoral immunity in mycobacterium tuberculosis infection. Adv Exp Med Biol (2013) 783:225–50. doi: 10.1007/978-1-4614-6111-1_12
28. World Health Organization. High priority target product profiles for new tuberculosis diagnostics: report of a consensus meeting. Geneva Switzerland (2014).
29. Lyashchenko KP, Colangeli R, Houde M, Jahdali HA, Menzies D, Gennaro ML. Heterogeneous antibody responses in tuberculosis. Infect Immun (1998) 66(8):3936–40. doi: 10.1128/iai.66.8.3936-3940.1998
30. Achkar JM, Casadevall A. Antibody-mediated immunity against tuberculosis: implications for vaccine development. Cell Host Microbe (2013) 13(3):250–62. doi: 10.1016/j.chom.2013.02.009
31. de Araujo LS, da Silva NBM, Leung JAM, Mello FCQ, Saad MHF. Igg subclasses' Response to a set of mycobacterial antigens in different stages of mycobacterium tuberculosis infection. Tuberculosis (Edinb) (2018) 108:70–6. doi: 10.1016/j.tube.2017.10.010
32. Lenzini L, Rottoli P, Rottoli L. The spectrum of human tuberculosis. Clin Exp Immunol (1977) 27(2):230–7.
33. Hoff DR, Ryan GJ, Driver ER, Ssemakulu CC, Groote MAD, Basaraba RJ, et al. Location of intra- and extracellular M. Tuberculosis populations in lungs of mice and Guinea pigs during disease progression and after drug treatment. PloS One (2011) 6(3):e17550. doi: 10.1371/journal.pone.0017550
34. Meena LS, Rajni N. Survival mechanisms of pathogenic mycobacterium tuberculosis H37rv. FEBS J (2010) 277(11):2416–27. doi: 10.1111/j.1742-4658.2010.07666.x
35. Pethe K, Sylvie A, Alonso S, Franck B, Biet F, Delogu G, et al. The heparin-binding haemagglutinin of M. Tuberculosis is required for extrapulmonary dissemination. Nature (2001) 412(6843):190–4. doi: 10.1038/35084083
36. Costello AM, Kumar A, Narayan V, Akbar MS, Ahmed S, Abou-Zeid C, et al. Does antibody to mycobacterial antigens, including lipoarabinomannan, limit dissemination in childhood tuberculosis? Trans R Soc Trop Med Hyg (1992) 86(6):686–92. doi: 10.1016/0035-9203(92)90192-f
37. McEwan WA, Tam JCH, Watkinson RE, Bidgood SR, Mallery DL, Leo CJ, et al. Intracellular antibody-bound pathogens stimulate immune signaling via the fc receptor trim21. Nat Immunol (2013) 14(4):327–36. doi: 10.1038/ni.2548
38. Armstrong JA, Hart PD. Phagosome-lysosome interactions in cultured macrophages infected with virulent tubercle bacilli. Reversal of the usual nonfusion pattern and observations on bacterial survival. J Exp Med (1975) 142(1):1–16. doi: 10.1084/jem.142.1.1
39. Cai Y, Yang Q, Tang Y, Zhang M, Liu H, Zhang G, et al. Increased complement C1q level marks active disease in human tuberculosis. PloS One (2014) 9(3):e92340. doi: 10.1371/journal.pone.0092340
40. Manivannan S, Rao NV, Ramanathan VD. Role of complement activation and antibody in the interaction between mycobacterium tuberculosis and human macrophages. Indian J Exp Biol (2012) 50(8):542–50.
41. Hao L, Hao L, Li H, Li H, Javid B. Antibodies and tuberculosis: finally coming of age? Nat Rev Immunol (2018) 18(9):591–6. doi: 10.1038/s41577-018-0028-0
42. Bruhns P, Jönsson F. Mouse and human fcr effector functions. Immunol Rev (2015) 268(1):25–51. doi: 10.1111/imr.12350
43. Mao L, LaCourse SM, Kim S, Liu C, Ning B, Bao D, et al. Evaluation of a serum-based antigen test for tuberculosis in hiv-exposed infants: A diagnostic accuracy study. BMC Med (2021) 19(1):113. doi: 10.1186/s12916-021-01983-w
44. Kunnath-Velayudhan S, Salamon H, Wang H-Y, Davidow AL, Molina DM, Huynh VT, et al. Dynamic antibody responses to the mycobacterium tuberculosis proteome. Proc Natl Acad Sci USA (2010) 107(33):14703–8. doi: 10.1073/pnas.1009080107
45. Matucci A, Maggi E, Vultaggio A. Cellular and humoral immune responses during tuberculosis infection: useful knowledge in the era of biological agents. J Rheumatol (2014) 91:17. doi: 10.3899/jrheum.140098
46. Steingart KR, Dendukuri N, Henry M, Schiller I, Nahid P, Hopewell PC, et al. Performance of purified antigens for serodiagnosis of pulmonary tuberculosis: A meta-analysis. Clin Vaccine Immunol (2009) 16(2):260–76. doi: 10.1128/cvi.00355-08
47. Esparza M, Palomares B, García T, Espinosa P, Zenteno E, Mancilla R. Psts-1, the 38-kda mycobacterium tuberculosis glycoprotein, is an adhesin, which binds the macrophage mannose receptor and promotes phagocytosis. Scand J Immunol (2015) 81(1):46–55. doi: 10.1111/sji.12249
48. Ragas A, Roussel L, Puzo G, Rivière M. The mycobacterium tuberculosis cell-surface glycoprotein apa as a potential adhesin to colonize target cells via the innate immune system pulmonary C-type lectin surfactant protein a*. J Biol Chem (2007) 282(8):5133–42. doi: 10.1074/jbc.M610183200
49. Geijtenbeek TBH, van Vliet SJ, Koppel EA, Sanchez-Hernandez M, Vandenbroucke-Grauls CMJE, Appelmelk B, et al. Mycobacteria target dc-sign to suppress dendritic cell function. J Exp Med (2003) 197(1):7–17. doi: 10.1084/jem.20021229
50. Mahon RN, Sande OJ, Rojas RE, Levine AD, Harding CV, Boom WH. Mycobacterium tuberculosis manlam inhibits T-cell-receptor signaling by interference with zap-70, lck and lat phosphorylation. Cell Immunol (2012) 275(1-2):98–105. doi: 10.1016/j.cellimm.2012.02.009
51. Gardiner JL, Karp CL. Transformative tools for tackling tuberculosis. J Exp Med (2015) 212(11):1759–69. doi: 10.1084/jem.20151468
52. Yong YK, Tan HY, Saeidi A, Wong WF, Vignesh R, Velu V, et al. Immune biomarkers for diagnosis and treatment monitoring of tuberculosis: current developments and future prospects. Front Microbiol (2019) 10:2789. doi: 10.3389/fmicb.2019.02789
53. Melkie ST, Arias L, Farroni C, Makek MJ, Goletti D, Vilaplana C. The role of antibodies in tuberculosis diagnosis, prophylaxis and therapy: A review from the esgmyc study group. Eur Respir Rev (2022) 31(163):210218. doi: 10.1183/16000617.0218-2021
55. Lange C, Mori T. Advances in the diagnosis of tuberculosis. Respirol (2010) 15(2):220–40. doi: 10.1111/j.1440-1843.2009.01692.x
56. Harries AD, Kumar AMV. Challenges and progress with diagnosing pulmonary tuberculosis in low- and middle-income countries. Diagnostics (2018) 8(4):78. doi: 10.3390/diagnostics8040078
57. Denkinger CM, Kik SV, Cirillo DM, Casenghi M, Shinnick T, Weyer K, et al. Defining the needs for next generation assays for tuberculosis. J Infect Dis (2015) 211 Suppl 2(Suppl 2):S29–38. doi: 10.1093/infdis/jiu821
58. Ireton GC, Greenwald R, Liang H, Esfandiari J, Lyashchenko KP, Reed SG. Identification of mycobacterium tuberculosis antigens of high serodiagnostic value. Clin Vaccine Immunol (2010) 17(10):1539–47. doi: 10.1128/CVI.00198-10
59. Dinnes J, Davenport C. COVID-19 rapid antigen testing strategies must be evaluated in intended use settings. Lancet Reg Health West Pac (2022) 25:100542. doi: 10.1016/j.lanwpc.2022.100542
60. Das P, Ganguly S, Mandal B. Sputum smear microscopy in tuberculosis: it is still relevant in the era of molecular diagnosis when seen from the public health perspective. BioMed Biotechnol Res J (2019) 3(2):77–9. doi: 10.4103/bbrj.bbrj_54_19
61. World Health Organization. Early detection of Tuberculosis: An Overview of Approaches, Guidelines and Tools. Geneva: World Health Organization (2011).
62. World Health Organization. Who Operational Handbook on Tuberculosis: Module 3: Diagnosis: Tests for Tuberculosis Infection Vol. 2022). . Geneva: World Health Organization (2022).
63. Boehme CC, Nabeta P, Hillemann D, Nicol MP, Shenai S, Krapp F, et al. Rapid molecular detection of tuberculosis and rifampin resistance. N Engl J Med (2010) 363(11):1005–15. doi: 10.1056/NEJMoa0907847
64. Brown S, Leavy JE, Jancey J. Implementation of genexpert for tb testing in low- and middle-income countries: A systematic review. Glob Health Sci Pract (2021) 9(3):698–710. doi: 10.9745/ghsp-d-21-00121
65. Maharjan B, Thapa J, Shah DK, Shrestha B, Avsar K, Suzuki Y, et al. Comparison of xpert mtb/rif to microscopy and culture for the diagnosis of tuberculosis in a referral laboratory in Nepal. J Infect Dis (2021) 74(6):517–21. doi: 10.7883/yoken.JJID.2020.921
66. Nonyane BAS, Nicol MP, Andreas NJ, Rimmele S, Schneiderhan-Marra N, Workman L, et al. Serologic responses in childhood pulmonary tuberculosis. Pediatr Infect Dis J (2017) 37(1):1–9. doi: 10.1097/inf.0000000000001683
67. Daley P, Michael JS, Hmar P, Latha A, Chordia P, Mathai D, et al. Blinded evaluation of commercial urinary lipoarabinomannan for active tuberculosis: A pilot study. Int J Tuberc Lung Dis (2009) 13(8):989–95.
68. Reither K, Saathoff E, Jung J, Minja LT, Kroidl I, Saad E, et al. Low sensitivity of a urine lam-elisa in the diagnosis of pulmonary tuberculosis. BMC Infect Dis (2009) 9(1):141. doi: 10.1186/1471-2334-9-141
69. Boehme C, Molokova E, Minja F, Geis S, Loscher T, Maboko L, et al. Detection of mycobacterial lipoarabinomannan with an antigen-capture elisa in unprocessed urine of Tanzanian patients with suspected tuberculosis. Trans R Soc Trop Med Hyg (2005) 99(12):893–900. doi: 10.1016/j.trstmh.2005.04.014
70. Shah M, Hanrahan C, Wang ZY, Dendukuri N, Lawn SD, Denkinger CM, et al. Lateral flow urine lipoarabinomannan assay for detecting active tuberculosis in hiv-positive adults. Cochrane Database Syst Rev (2016) 2016(5):Cd011420. doi: 10.1002/14651858.CD011420.pub2
71. Organization WH. The Use of Lateral Flow Urine Lipoarabinomannan Assay (Lf-Lam) for the Diagnosis and Screening of Active Tuberculosis in People Living with Hiv: Policy Guidance Vol. 2015). . Geneva: World Health Organization (2015).
72. Steingart KR, Ramsay A, Dowdy DW, Pai M. Serological Tests for the diagnosis of active tuberculosis: relevance for India. Indian J Med Res (2012) 135(5):695–702.
73. Steingart KR, Henry M, Laal S, Hopewell PC, Ramsay A, Menzies D, et al. A systematic review of commercial serological antibody detection tests for the diagnosis of extrapulmonary tuberculosis. Thorax (2007) 62(10):911–8. doi: 10.1136/thx.2006.075754
74. World Health Organization, Diseases Special Program for Research and Training in Tropical Diseases. Laboratory-Based Evaluation of 19 Commercially Available Rapid Diagnostic Tests for Tuberculosis Vol. 978. Geneva, Switzerland: World Health Organisation (2008). p. 92 4 159711 1.
75. Steingart KR, Flores LL, Dendukuri N, Schiller I, Laal S, Ramsay A, et al. Commercial serological tests for the diagnosis of active pulmonary and extrapulmonary tuberculosis: an updated systematic review and meta-analysis. PloS Med (2011) 8(8):e1001062. doi: 10.1371/journal.pmed.1001062
76. Abebe F, Holm-Hansen C, Wiker HG, Bjune G. Progress in serodiagnosis of mycobacterium tuberculosis infection. Scand J Immunol (2007) 66(2-3):176–91. doi: 10.1111/j.1365-3083.2007.01978.x
77. Demkow U, Filewska M, Michalowska-Mitczuk D, Kus J, Jagodzinski J, Zielonka T, et al. Heterogeneity of antibody response to myobacterial antigens in different clinical manifestations of pulmonary tuberculosis. J Physiol Pharmacol (2007) 58 Suppl 5(Pt 1):117–27.
78. Takenami I, de Oliveira CC, Lima FR, Soares J, MaChado A Jr., Riley LW, et al. Immunoglobulin G response to mammalian cell entry 1a (Mce1a) protein as biomarker of active tuberculosis. Tuberculosis (Edinb) (2016) 100:82–8. doi: 10.1016/j.tube.2016.07.012
79. Lodes MJ, Dillon DC, Mohamath R, Day CH, Benson DR, Reynolds LD, et al. Serological expression cloning and immunological evaluation of mtb48, a novel mycobacterium tuberculosis antigen. J Clin Microbiol (2001) 39(7):2485–93. doi: 10.1128/jcm.39.7.2485-2493.2001
80. Abraham PR, Devalraju KP, Jha V, Valluri VL, Mukhopadhyay S. Ppe17 (Rv1168c) protein of mycobacterium tuberculosis detects individuals with latent tb infection. PloS One (2018) 13(11):e0207787. doi: 10.1371/journal.pone.0207787
81. Ben-selma W, Harizi H, Marzouk M, Ben Kahla I, Ben Lazreg F, Ferjeni A, et al. Evaluation of the diagnostic value of measuring igg, igm, and iga antibodies to mycobacterial A60 antigen in active tuberculosis. Diagn Microbiol Infect Dis (2010) 68(1):55–9. doi: 10.1016/j.diagmicrobio.2010.05.006
82. Khan IH, Ravindran R, Krishnan VV, Awan IN, Rizvi SK, Saqib MA, et al. Plasma antibody profiles as diagnostic biomarkers for tuberculosis. Clin Vaccine Immunol (2011) 18(12):2148–53. doi: 10.1128/cvi.05304-11
83. Khan IH, Ravindran R, Yee J, Ziman M, Lewinsohn DM, Gennaro ML, et al. Profiling antibodies to mycobacterium tuberculosis by multiplex microbead suspension arrays for serodiagnosis of tuberculosis. Clin Vaccine Immunol (2008) 15(3):433–8. doi: 10.1128/cvi.00354-07
84. Shete PB, Ravindran R, Chang E, Worodria W, Chaisson LH, Andama A, et al. Evaluation of antibody responses to panels of M. Tuberculosis antigens as a screening tool for active tuberculosis in Uganda. PloS One (2017) 12(8):e0180122. doi: 10.1371/journal.pone.0180122
85. Khaliq A, Ravindran R, Hussainy SF, Krishnan VV, Ambreen A, Yusuf NW, et al. Field evaluation of a blood based test for active tuberculosis in endemic settings. PloS One (2017) 12(4):e0173359. doi: 10.1371/journal.pone.0173359
86. Meher AK, Bal NC, Chary KVR, Arora A. Mycobacterium tuberculosis H37rv esat-6–cfp-10 complex formation confers thermodynamic and biochemical stability. FEBS J (2006) 273(7):1445–62. doi: 10.1111/j.1742-4658.2006.05166.x
87. Kalra M, Khuller GK, Grover A, Behera D, Wanchu A, Verma I. Utility of a combination of rd1 and rd2 antigens as a diagnostic marker for tuberculosis. Diagn Microbiol Infect Dis (2010) 66(2):153–61. doi: 10.1016/j.diagmicrobio.2009.09.005
88. Luo L, Zhu L, Yue J, Liu J, Liu G, Zhang X, et al. Antigens rv0310c and rv1255c are promising novel biomarkers for the diagnosis of mycobacterium tuberculosis infection. Emerg Microbes Infect (2017) 6(1):1–8. doi: 10.1038/emi.2017.54
89. Samanich KM, Keen MA, Vissa VD, Harder JD, Spencer JS, Belisle JT, et al. Serodiagnostic potential of culture filtrate antigens of mycobacterium tuberculosis. Clin Diagn Lab Immunol (2000) 7(4):662–8. doi: 10.1128/cdli.7.4.662-668.2000
90. Deng S, Yuan T, Xia J, Huang H, Cheng X, Chen M. Clinical utility of a combination of lipoarabinomannan, 38-kda, and 16-kda antigens as a diagnosis tool for tuberculosis. Diagn Microbiol Infect Dis (2011) 71(1):46–50. doi: 10.1016/j.diagmicrobio.2011.04.015
91. Burbelo PD, Keller J, Wagner J, Klimavicz JS, Bayat A, Rhodes CS, et al. Serological diagnosis of pulmonary mycobacterium tuberculosis infection by lips using a multiple antigen mixture. BMC Microbiol (2015) 15:205. doi: 10.1186/s12866-015-0545-y
92. Lyashchenko KP, Greenwald R, Esfandiari J, Greenwald D, Nacy CA, Gibson S, et al. Primatb stat-pak assay, a novel, rapid lateral-flow test for tuberculosis in nonhuman primates. Clin Vaccine Immunol (2007) 14(9):1158–64. doi: 10.1128/cvi.00230-07
93. Awoniyi DO, Baumann R, Chegou NN, Kriel B, Jacobs R, Kidd M, et al. Detection of a combination of serum igg and iga antibodies against selected mycobacterial targets provides promising diagnostic signatures for active tb. Oncotarget (2017) 8(23):37525–37. doi: 10.18632/oncotarget.16401
94. Baumann R, Kaempfer S, Chegou NN, Oehlmann W, Loxton AG, Kaufmann SHE, et al. Serologic diagnosis of tuberculosis by combining ig classes against selected mycobacterial targets. J Infect (2014) 69(6):581–9. doi: 10.1016/j.jinf.2014.05.014
96. Jacobs R, Malherbe S, Loxton AG, Stanley K, van der Spuy G, Walzl G, et al. Identification of novel host biomarkers in plasma as candidates for the immunodiagnosis of tuberculosis disease and monitoring of tuberculosis treatment response. Oncotarget (2016) 7(36):57581–92. doi: 10.18632/oncotarget.11420
97. Jacobs R, Awoniyi DO, Baumann R, Stanley K, McAnda S, Kaempfer S, et al. Concurrent evaluation of cytokines improves the accuracy of antibodies against mycobacterium tuberculosis antigens in the diagnosis of active tuberculosis. Tuberculosis (Edinb) (2022) 133:102169. doi: 10.1016/j.tube.2022.102169
98. Chen T, Lin J, Wang W, Fleming J, Chen L, Wang Y, et al. Cytokine and antibody based diagnostic algorithms for sputum culture-positive pulmonary tuberculosis. PloS One (2015) 10(12):e0144705. doi: 10.1371/journal.pone.0144705
99. Pai M, O'Brien R. New diagnostics for latent and active tuberculosis: state of the art and future prospects. Semin Respir Crit Care Med (2008) 29(5):560–8. doi: 10.1055/s-0028-1085707
100. Nziza N, Cizmeci D, Davies L, Irvine EB, Jung W, Fenderson BA, et al. Defining discriminatory antibody fingerprints in active and latent tuberculosis. Front Immunol (2022) 13:856906. doi: 10.3389/fimmu.2022.856906
101. Coppola M, Ottenhoff THM. Genome wide approaches discover novel mycobacterium tuberculosis antigens as correlates of infection, disease, immunity and targets for vaccination. Semin Immunol (2018) 39:88–101. doi: 10.1016/j.smim.2018.07.001
102. Maekura R, Kitada S, Osada-Oka M, Tateishi Y, Ozeki Y, Fujicawa T, et al. Serum antibody profiles in individuals with latent mycobacterium tuberculosis infection. Microbiol Immunol (2019) 63(3-4):130–8. doi: 10.1111/1348-0421.12674
103. Mezouar S, Diarra I, Roudier J, Desnues B, Mege JL. Tumor necrosis factor-alpha antagonist interferes with the formation of granulomatous multinucleated giant cells: new insights into mycobacterium tuberculosis infection. Front Immunol (2019) 10:1947. doi: 10.3389/fimmu.2019.01947
104. Silva D, Silva MVD, Barros CCO, Alexandre PBD, Timóteo RP, Catarino JS, et al. Tnf-A Blockade impairs in vitro tuberculous granuloma formation and down modulate th1, th17 and treg cytokines. PloS One (2018) 13(3):e0194430. doi: 10.1371/journal.pone.0194430
105. Schmidt CM, Lovero KL, Carvalho FR, Dos Santos DCM, Barros A, Quintanilha AP, et al. Serum anti-mce1a immunoglobulin detection as a tool for differential diagnosis of tuberculosis and latent tuberculosis infection in children and adolescents. Tuberculosis (Edinb) (2020) 120:101893. doi: 10.1016/j.tube.2019.101893
106. Achkar JM, Ziegenbalg A. Antibody responses to mycobacterial antigens in children with tuberculosis: challenges and potential diagnostic value. Clin Vaccine Immunol (2012) 19(12):1898–906. doi: 10.1128/cvi.00501-12
107. Singh V, Tiwari S. Getting a better bacteriological diagnosis in children with tuberculosis. Pediatr Infect Dis (2012) 4(2):75–80. doi: 10.1016/S2212-8328(12)60027-7
108. Santoso MS, Masyeni S, Haryanto S, Yohan B, Hibberd ML, Sasmono RT. Assessment of dengue and COVID-19 antibody rapid diagnostic tests cross-reactivity in Indonesia. Virol J (2021) 18(1):54. doi: 10.1186/s12985-021-01522-2
109. Glatman-Freedman A, Casadevall A, Arturo C. Serum therapy for tuberculosis revisited: reappraisal of the role of antibody-mediated immunity against mycobacterium tuberculosis. Clin Microbiol Rev (1998) 11(3):514–32. doi: 10.1128/cmr.11.3.514
110. Chen T, Blanc C, Liu Y, Ishida E, Singer S, Xu J, et al. Capsular glycan recognition provides antibody-mediated immunity against tuberculosis. J Clin Invest (2020) 130(4):1808–22. doi: 10.1172/JCI128459
111. Olivares N, Puig A, Aguilar D, Moya A, Cádiz A, Otero O, et al. Prophylactic effect of administration of human gamma globulins in a mouse model of tuberculosis. Tuberculosis (Edinb) (2009) 89(3):218–20. doi: 10.1016/j.tube.2009.02.003
112. Hatziioannou T, Evans DT. Animal models for hiv/aids research. Nat Rev Microbiol (2012) 10(12):852–67. doi: 10.1038/nrmicro2911
113. Reljic R, Ivanyi J. A case for passive immunoprophylaxis against tuberculosis. Lancet Infect Dis (2006) 6(12):813–8. doi: 10.1016/S1473-3099(06)70658-2
114. Cerrone M, Bracchi M, Wasserman S, Pozniak A, Meintjes G, Cohen K, et al. Safety implications of combined antiretroviral and anti-tuberculosis drugs. Expert Opin Drug Saf (2020) 19(1):23–41. doi: 10.1080/14740338.2020.1694901
115. Balu S, Reljic R, Lewis MJ, Pleass RJ, McIntosh RS, McIntosh R, et al. A novel human iga monoclonal antibody protects against tuberculosis. J Immunol (2011) 186(5):3113–9. doi: 10.4049/jimmunol.1003189
116. Chambers MA, Gavier-Widén D, Hewinson RG. Antibody bound to the surface antigen mpb83 of mycobacterium bovis enhances survival against high dose and low dose challenge. FEMS Immunol Med Microbiol (2004) 41(2):93–100. doi: 10.1016/j.femsim.2004.01.004
117. Hamasur B, Haile M, Pawlowski A, Schröder U, Källenius G, Svenson SB, et al. A mycobacterial lipoarabinomannan specific monoclonal antibody and its F(Ab′)2 fragment prolong survival of mice infected with mycobacterium tuberculosis. Clin Exp Immunol (2004) 138(1):30–8. doi: 10.1111/j.1365-2249.2004.02593.x
118. López Y, Yero D, Falero-Diaz G, Olivares N, Sarmiento ME, Sifontes S, et al. Induction of a protective response with an iga monoclonal antibody against mycobacterium tuberculosis 16kda protein in a model of progressive pulmonary infection. Int J Med Microbiol (2009) 299(6):447–52. doi: 10.1016/j.ijmm.2008.10.007
119. Teitelbaum R, Glatman-Freedman A, Chen B, Robbins JB, Unanue ER, Casadevall A, et al. A mab recognizing a surface antigen of mycobacterium tuberculosis enhances host survival. Proc Natl Acad Sci U.S.A. (1998) 95(26):15688–93. doi: 10.1073/pnas.95.26.15688
120. Williams A, Reljic R, Naylor I, Clark SO, Falero-Diaz G, Singh M, et al. Passive protection with immunoglobulin a antibodies against tuberculous early infection of the lungs. Immunology (2004) 111(3):328–33. doi: 10.1111/j.1365-2567.2004.01809.x
121. Jennewein MF, Alter G. The immunoregulatory roles of antibody glycosylation. Trends Immunol (2017) 38(5):358–72. doi: 10.1016/j.it.2017.02.004
122. Krapp S, Mimura Y, Jefferis R, Huber R, Sondermann P. Structural analysis of human igg-fc glycoforms reveals a correlation between glycosylation and structural integrity. J Mol Biol (2003) 325(5):979–89. doi: 10.1016/s0022-2836(02)01250-0
123. Cobb BA. The history of igg glycosylation and where we are now. Glycobiology (2020) 30(4):202–13. doi: 10.1093/glycob/cwz065
124. Nose M, Wigzell H. Biological significance of carbohydrate chains on monoclonal antibodies. Proc Natl Acad Sci U.S.A. (1983) 80(21):6632–6. doi: 10.1073/pnas.80.21.6632
125. Wang TT. Igg fc glycosylation in human immunity. Curr Top Microbiol Immunol (2019) 423:63–75. doi: 10.1007/82_2019_152
126. Chung S, Quarmby V, Gao X, Ying Y, Lin L, Reed C, et al. Quantitative evaluation of fucose reducing effects in a humanized antibody on fcγ Receptor binding and antibody-dependent cell-mediated cytotoxicity activities. MAbs (2012) 4(3):326–40. doi: 10.4161/mabs.19941
127. Niwa R, Sakurada M, Kobayashi Y, Uehara A, Matsushima K, Ueda R, et al. Enhanced natural killer cell binding and activation by low-fucose igg1 antibody results in potent antibody-dependent cellular cytotoxicity induction at lower antigen density. Clin Cancer Res (2005) 11(6):2327–36. doi: 10.1158/1078-0432.Ccr-04-2263
128. Shields RL, Lai J, Keck R, O'Connell LY, Hong K, Meng YG, et al. Lack of fucose on human igg1 N-linked oligosaccharide improves binding to human fcgamma riii and antibody-dependent cellular toxicity. J Biol Chem (2002) 277(30):26733–40. doi: 10.1074/jbc.M202069200
129. Kaneko Y, Nimmerjahn F, Ravetch JV. Anti-inflammatory activity of immunoglobulin G resulting from fc sialylation. Science (2006) 313(5787):670–3. doi: 10.1126/science.1129594
130. Naso MF, Tam SH, Scallon BJ, Raju TS. Engineering host cell lines to reduce terminal sialylation of secreted antibodies. MAbs (2010) 2(5):519–27. doi: 10.4161/mabs.2.5.13078
131. Scallon BJ, Tam SH, McCarthy SG, Cai AN, Raju TS. Higher levels of sialylated fc glycans in immunoglobulin G molecules can adversely impact functionality. Mol Immunol (2007) 44(7):1524–34. doi: 10.1016/j.molimm.2006.09.005
132. Kapur R, Einarsdottir HK, Vidarsson G. Igg-effector functions: "The good, the bad and the ugly". Immunol Lett (2014) 160(2):139–44. doi: 10.1016/j.imlet.2014.01.015
133. Stadlmann J, Pabst M, Altmann F. Analytical and functional aspects of antibody sialylation. J Clin Immunol (2010) 30 Suppl 1(Suppl 1):S15–9. doi: 10.1007/s10875-010-9409-2
134. Thomann M, Schlothauer T, Dashivets T, Malik S, Avenal C, Bulau P, et al. In vitro glycoengineering of igg1 and its effect on fc receptor binding and adcc activity. PloS One (2015) 10(8):e0134949. doi: 10.1371/journal.pone.0134949
135. Boyd PN, Lines AC, Patel AK. The effect of the removal of sialic acid, galactose and total carbohydrate on the functional activity of campath-1h. Mol Immunol (1995) 32(17-18):1311–8. doi: 10.1016/0161-5890(95)00118-2
136. Hodoniczky J, Zheng YZ, James DC. Control of recombinant monoclonal antibody effector functions by fc N-glycan remodeling in vitro. Biotechnol Prog (2005) 21(6):1644–52. doi: 10.1021/bp050228w
137. Shinkawa T, Nakamura K, Yamane N, Shoji-Hosaka E, Kanda Y, Sakurada M, et al. The absence of fucose but not the presence of galactose or bisecting N-acetylglucosamine of human igg1 complex-type oligosaccharides shows the critical role of enhancing antibody-dependent cellular cytotoxicity. J Biol Chem (2003) 278(5):3466–73. doi: 10.1074/jbc.M210665200
138. Houde D, Peng Y, Berkowitz SA, Engen JR. Post-translational modifications differentially affect igg1 conformation and receptor binding. Mol Cell Proteomics (2010) 9(8):1716–28. doi: 10.1074/mcp.M900540-MCP200
139. Kumpel BM, Wang Y, Griffiths HL, Hadley AG, Rook GA. The biological activity of human monoclonal igg anti-D is reduced by beta-galactosidase treatment. Hum Antibodies Hybridomas (1995) 6(3):82–8. doi: 10.3233/HAB-1995-6301
140. Chung AW, Crispin M, Pritchard L, Robinson H, Gorny MK, Yu X, et al. Identification of antibody glycosylation structures that predict monoclonal antibody fc-effector function. AIDS (2014) 28(17):2523–30. doi: 10.1097/qad.0000000000000444
141. Ding L, Chen X, Cheng H, Zhang T, Li Z. Advances in iga glycosylation and its correlation with diseases. Front Chem (2022) 10:974854. doi: 10.3389/fchem.2022.974854
142. Gomes MM, Wall SB, Takahashi K, Novak J, Renfrow MB, Herr AB. Analysis of iga1 N-glycosylation and its contribution to fcalphari binding. Biochemistry (2008) 47(43):11285–99. doi: 10.1021/bi801185b
143. Steffen U, Koeleman CA, Sokolova MV, Bang H, Kleyer A, Rech J, et al. Iga subclasses have different effector functions associated with distinct glycosylation profiles. Nat Commun (2020) 11(1):120. doi: 10.1038/s41467-019-13992-8
144. Watson A, Li H, Ma B, Weiss R, Bendayan D, Abramovitz L, et al. Human antibodies targeting a mycobacterium transporter protein mediate protection against tuberculosis. Nat Commun (2021) 12(1):602. doi: 10.1038/s41467-021-20930-0
145. Peirs P, Lefèvre P, Boarbi S, Wang XM, Denis O, Braibant M, et al. Mycobacterium tuberculosis with disruption in genes encoding the phosphate binding proteins psts1 and psts2 is deficient in phosphate uptake and demonstrates reduced in vivo virulence. Infect Immun (2005) 73(3):1898–902. doi: 10.1128/iai.73.3.1898-1902.2005
146. Sharma S, Raj A, Singh N, Dahiya B, Sheoran A, Gupta KB, et al. Development of real-time immuno-pcr for the quantitative detection of mycobacterial psts1 in tuberculosis patients. J Microbiol Methods (2017) 132:134–8. doi: 10.1016/j.mimet.2016.12.006
147. López Y, Falero-Díaz G, Yero D, Solís RL, Sarmiento ME, Acosta A. Antibodies in the protection against mycobacterial infections: what have we learned? Proc Vaccinol (2010) 2(2):172–7. doi: 10.1016/j.provac.2010.07.011
148. Nimmerjahn F, Ravetch JV. Fcgamma receptors: old friends and new family members. Immunity (2006) 24(1):19–28. doi: 10.1016/j.immuni.2005.11.010
149. Rustad TR, Harrell MI, Liao R, Sherman DR. The enduring hypoxic response of mycobacterium tuberculosis. PloS One (2008) 3(1):e1502. doi: 10.1371/journal.pone.0001502
150. Saviola B. Mycobacterium tuberculosis adaptation to survival in a human host. London, United Kingdom: IntechOpen (2013).
151. Yuan Y, Crane DD, Simpson RM, Zhu Y, Hickey MJ, Sherman DR, et al. The 16-kda A-crystallin (Acr) protein of mycobacterium tuberculosis is required for growth in macrophages. Proc Natl Acad Sci U.S.A. (1998) 95(16):9578–83. doi: 10.1073/pnas.95.16.9578
152. Singh AK, Gupta UD. Animal models of tuberculosis: lesson learnt. Indian J Med Res (2018) 147(5):456–63. doi: 10.4103/ijmr.IJMR_554_18
153. de Taeye SW, Bentlage AEH, Mebius MM, Meesters JI, Lissenberg-Thunnissen S, Falck D, et al. Fcγr binding and adcc activity of human igg allotypes. Front Immunol (2020) 11. doi: 10.3389/fimmu.2020.00740
154. Calderon VE, Valbuena G, Goez Y, Judy BM, Huante MB, Sutjita P, et al. A humanized mouse model of tuberculosis. PloS One (2013) 8(5):e63331. doi: 10.1371/journal.pone.0063331
155. Mourik BC, de Steenwinkel JEM, de Knegt GJ, Huizinga R, Verbon A, Ottenhoff THM, et al. Mycobacterium tuberculosis clinical isolates of the beijing and east-african Indian lineage induce fundamentally different host responses in mice compared to H37rv. Sci Rep (2019) 9(1):19922. doi: 10.1038/s41598-019-56300-6
156. O'Toole RF, Gautam SS. Limitations of the mycobacterium tuberculosis reference genome H37rv in the detection of virulence-related loci. Genomics (2017) 109(5):471–4. doi: 10.1016/j.ygeno.2017.07.004
157. Reljic R, Clark SO, Williams A, Falero-Diaz G, Singh M, Challacombe S, et al. Intranasal ifngamma extends passive iga antibody protection of mice against mycobacterium tuberculosis lung infection. Clin Exp Immunol (2006) 143(3):467–73. doi: 10.1111/j.1365-2249.2006.03012.x
158. Buccheri S, Reljic R, Caccamo N, Ivanyi J, Singh M, Salerno A, et al. Il-4 depletion enhances host resistance and passive iga protection against tuberculosis infection in balb/C mice. Eur J Immunol (2007) 37(3):729–37. doi: 10.1002/eji.200636764
159. Tran AC, Diogo GR, Paul MJ, Copland A, Hart P, Mehta N, et al. Mucosal therapy of multi-drug resistant tuberculosis with iga and interferon-Γ. Front Immunol (2020) 11:582833. doi: 10.3389/fimmu.2020.582833
160. Rosini R, Nicchi S, Pizza M, Rappuoli R. Vaccines against antimicrobial resistance. Front Immunol (2020) 11:1048. doi: 10.3389/fimmu.2020.01048
161. Institute of Medicine Forum on Drug Discovery D, Science RAoM. A global perspective on drug-resistant tuberculosis Vol. 2011. . US: National Academies Press (2011).
162. Tran AC, Kim MY, Reljic R. Emerging themes for the role of antibodies in tuberculosis. Immune Netw (2019) 19(4):e24. doi: 10.4110/in.2019.19.e24
163. Martinez L, Cords O, Liu Q, Acuna-Villaorduna C, Bonnet M, Fox GJ, et al. Infant bcg vaccination and risk of pulmonary and extrapulmonary tuberculosis throughout the life course: A systematic review and individual participant data meta-analysis. Lancet Glob Health (2022) 10(9):e1307–e16. doi: 10.1016/S2214-109X(22)00283-2
164. Higgins JPT, Soares-Weiser K, López-López JA, Kakourou A, Chaplin K, Christensen H, et al. Association of bcg, dtp, and measles containing vaccines with childhood mortality: systematic review. BMJ (2016) 355:i5170. doi: 10.1136/bmj.i5170
165. Singh AK, Srikrishna G, Bivalacqua TJ, Bishai WR. Recombinant bcgs for tuberculosis and bladder cancer. Vaccine (2021) 39(50):7321–31. doi: 10.1016/j.vaccine.2021.09.040
166. Beyazova U, Rota S, Cevheroğlu C, Karsligil T. Humoral immune response in infants after bcg vaccination. Tuber Lung Dis (1995) 76(3):248–53. doi: 10.1016/S0962-8479(05)80013-9
167. Brown RM, Cruz O, Brennan MJ, Gennaro ML, Schlesinger LS, Skeiky YAW, et al. Lipoarabinomannan-reactive human secretory immunoglobulin a responses induced by mucosal bacille calmette-guérin vaccination. J Infect Dis (2003) 187(3):513–7. doi: 10.1086/368096
168. Chen T, Tingting C, Caroline B, Blanc C, Eder AZ, Prados-Rosales R, et al. Association of human antibodies to arabinomannan with enhanced mycobacterial opsonophagocytosis and intracellular growth reduction. J Infect Dis (2016) 214(2):300–10. doi: 10.1093/infdis/jiw141
169. Hoft DF, Kemp EB, Marinaro M, Cruz O, Kiyono H, McGhee JR, et al. A double-blind, placebo-controlled study of mycobacterium-specific human immune responses induced by intradermal bacille calmette-guérin vaccination. J Lab Clin Med (1999) 134(3):244–52. doi: 10.1016/s0022-2143(99)90204-4
170. Dijkman K, Sombroek CC, Vervenne RAW, Hofman SO, Boot C, Remarque EJ, et al. Prevention of tuberculosis infection and disease by local bcg in repeatedly exposed rhesus macaques. Nat Med (2019) 25(2):255–62. doi: 10.1038/s41591-018-0319-9
171. Fine PEM. Variation in protection by bcg: implications of and for heterologous immunity. Lancet (1995) 346(8986):1339–45. doi: 10.1016/S0140-6736(95)92348-9
172. Trunz BB, Fine PEM, Dye C. Effect of bcg vaccination on childhood tuberculous meningitis and miliary tuberculosis worldwide: A meta-analysis and assessment of cost-effectiveness. Lancet (2006) 367(9517):1173–80. doi: 10.1016/S0140-6736(06)68507-3
173. Luabeya AKK, Kagina BMN, Tameris MD, Geldenhuys H, Hoff ST, Shi Z, et al. First-in-human trial of the post-exposure tuberculosis vaccine H56:Ic31 in mycobacterium tuberculosis infected and non-infected healthy adults. Vaccine (2015) 33(33):4130–40. doi: 10.1016/j.vaccine.2015.06.051
174. Billeskov R, Elvang TT, Andersen PL, Dietrich J. The hyvac4 subunit vaccine efficiently boosts bcg-primed anti-mycobacterial protective immunity. PloS One (2012) 7(6):e39909. doi: 10.1371/journal.pone.0039909
175. Nemes E, Geldenhuys H, Rozot V, Rutkowski KT, Ratangee F, Bilek N, et al. Prevention of M. Tuberculosis infection with H4:Ic31 vaccine or bcg revaccination. N Engl J Med (2018) 379(2):138–49. doi: 10.1056/NEJMoa1714021
176. Lin PL, Dietrich J, Tan E, Abalos RM, Burgos J, Bigbee C, et al. The multistage vaccine H56 boosts the effects of bcg to protect cynomolgus macaques against active tuberculosis and reactivation of latent mycobacterium tuberculosis infection. J Clin Invest (2012) 122(1):303–14. doi: 10.1172/jci46252
177. Bekker L-G, Dintwe O, Fiore-Gartland A, Middelkoop K, Hutter J, Williams A, et al. A phase 1b randomized study of the safety and immunological responses to vaccination with H4:Ic31, H56:Ic31, and bcg revaccination in mycobacterium tuberculosis-uninfected adolescents in cape town, South Africa. EClinicalMedicine (2020) 21:100313. doi: 10.1016/j.eclinm.2020.100313
178. Scriba TJ, Netea MG, Ginsberg AM. Key recent advances in tb vaccine development and understanding of protective immune responses against mycobacterium tuberculosis. Semin Immunol (2020) 50:101431. doi: 10.1016/j.smim.2020.101431
179. Hansen SG, Zak DE, Xu G, Ford JC, Marshall EE, Malouli D, et al. Prevention of tuberculosis in rhesus macaques by a cytomegalovirus-based vaccine. Nat Med (2018) 24(2):130–43. doi: 10.1038/nm.4473
180. Bretscher P. On analyzing how the th1/th2 phenotype of an immune response is determined: classical observations must not be ignored. Front Immunol (2019) 10:1234. doi: 10.3389/fimmu.2019.01234
181. Kurono Y, Yamamoto M, Fujihashi K, Kodama S, Suzuki M, Mogi G, et al. Nasal immunization induces haemophilus influenzae—Specific th1 and th2 responses with mucosal iga and systemic igg antibodies for protective immunity. J Infect Dis (1999) 180(1):122–32. doi: 10.1086/314827
182. Tameris MD, Hatherill M, Landry BS, Scriba TJ, Snowden MA, Lockhart S, et al. Safety and efficacy of mva85a, a new tuberculosis vaccine, in infants previously vaccinated with bcg: A randomised, placebo-controlled phase 2b trial. Lancet (2013) 381(9871):1021–8. doi: 10.1016/S0140-6736(13)60177-4
183. Fletcher HA, Snowden MA, Landry B, Rida W, Satti I, Harris SA, et al. T-cell activation is an immune correlate of risk in bcg vaccinated infants. Nat Commun (2016) 7(1):11290. doi: 10.1038/ncomms11290
184. Stylianou E, Griffiths KL, Poyntz HC, Harrington-Kandt R, Dicks MD, Stockdale L, et al. Improvement of bcg protective efficacy with a novel chimpanzee adenovirus and a modified vaccinia ankara virus both expressing ag85a. Vaccine (2015) 33(48):6800–8. doi: 10.1016/j.vaccine.2015.10.017
185. Wilkie M, Satti I, Minhinnick A, Harris S, Riste M, Ramon RL, et al. A phase I trial evaluating the safety and immunogenicity of a candidate tuberculosis vaccination regimen, Chadox1 85a prime – mva85a boost in healthy uk adults. Vaccine (2020) 38(4):779–89. doi: 10.1016/j.vaccine.2019.10.102
186. Huygen K, Content J, Denis O, Montgomery DL, Yawman AM, Randall Deck R, et al. Immunogenicity and protective efficacy of a tuberculosis DNA vaccine. Nat Med (1996) 2(8):893–8. doi: 10.1038/nm0896-893
187. Teixeira FM, Teixeira HC, Ferreira AP, Rodrigues MF, Azevedo V, Macedo GC, et al. DNA vaccine using mycobacterium bovis ag85b antigen induces partial protection against experimental infection in balb/C mice. Clin Vaccine Immunol (2006) 13(8):930–5. doi: 10.1128/CVI.00151-06
188. Chang-hong S, Xiao-wu W, Hai Z, Ting-fen Z, Li-mei W, Zhi-kai X. Immune responses and protective efficacy of the gene vaccine expressing ag85b and esat6 fusion protein from mycobacterium tuberculosis. DNA Cell Biol (2007) 27(4):199–207. doi: 10.1089/dna.2007.0648
189. Palma C, Iona E, Giannoni F, Pardini M, Brunori L, Fattorini L, et al. The ltk63 adjuvant improves protection conferred by ag85b DNA-protein prime-boosting vaccination against mycobacterium tuberculosis infection by dampening ifn-Γ Response. Vaccine (2008) 26(33):4237–43. doi: 10.1016/j.vaccine.2008.05.050
190. Hu Z, Lu SH, Lowrie DB, Fan XY. Research advances for virus-vectored tuberculosis vaccines and latest findings on tuberculosis vaccine development. Front Immunol (2022) 13:895020. doi: 10.3389/fimmu.2022.895020
191. Nangpal P, Bahal RK, Tyagi AK. Boosting with recombinant mva expressing M. Tuberculosis A-crystallin antigen augments the protection imparted by bcg against tuberculosis in Guinea pigs. Sci Rep (2017) 7(1):17286. doi: 10.1038/s41598-017-17587-5
192. Glatman-Freedman A, Casadevall A, Dai Z, Jacobs WR Jr., Li A, Morris SL, et al. Antigenic evidence of prevalence and diversity of mycobacterium tuberculosis arabinomannan. J Clin Microbiol (2004) 42(7):3225–31. doi: 10.1128/jcm.42.7.3225-3231.2004
193. Hamasur B, Haile M, Pawlowski A, Schröder U, Williams A, Hatch GJ, et al. Mycobacterium tuberculosis arabinomannan-protein conjugates protect against tuberculosis. Vaccine (2003) 21(25):4081–93. doi: 10.1016/s0264-410x(03)00274-3
194. Gonzalo-Asensio J, Marinova D, Martin C, Aguilo N. Mtbvac: Attenuating the Human Pathogen of Tuberculosis (Tb) toward a Promising Vaccine against the Tb Epidemic. Front Immunol (2017) 8. doi: 10.3389/fimmu.2017.01803
195. Arbues A, Aguilo JI, Gonzalo-Asensio J, Marinova D, Uranga S, Puentes E, et al. Construction, characterization and preclinical evaluation of mtbvac, the first live-attenuated M. Tuberculosis-based vaccine to enter clinical trials. Vaccine (2013) 31(42):4867–73. doi: 10.1016/j.vaccine.2013.07.051
Keywords: tuberculosis, antibodies, immunotherapy, vaccines, mycobacteria, serodiagnostics, humoral immunity, monoclonal antibodies
Citation: McIntyre S, Warner J, Rush C and Vanderven HA (2023) Antibodies as clinical tools for tuberculosis. Front. Immunol. 14:1278947. doi: 10.3389/fimmu.2023.1278947
Received: 17 August 2023; Accepted: 27 November 2023;
Published: 14 December 2023.
Edited by:
Shoor Vir Singh, GLA University, IndiaReviewed by:
Taru S Dutt, Colorado State University, United StatesKavita Rawat, Washington University in St. Louis, United States
Copyright © 2023 McIntyre, Warner, Rush and Vanderven. This is an open-access article distributed under the terms of the Creative Commons Attribution License (CC BY). The use, distribution or reproduction in other forums is permitted, provided the original author(s) and the copyright owner(s) are credited and that the original publication in this journal is cited, in accordance with accepted academic practice. No use, distribution or reproduction is permitted which does not comply with these terms.
*Correspondence: Hillary A. Vanderven, aGlsbGFyeS52YW5kZXJ2ZW5AamN1LmVkdS5hdQ==